- 1Department of Pharmacology, Faculty of Medicine, Universiti Kebangsaan Malaysia, Cheras, Malaysia
- 2American River Nutrition, Hadley, MA, United States
Medication-related osteonecrosis of the jaw (ONJ) is a rare but significant adverse side effect of antiresorptive drugs. Bisphosphonate-related ONJ (BRONJ) is the most prevalent condition due to the extensive use of the drug in cancer and osteoporosis treatment. Nitrogen-containing bisphosphonates suppress osteoclastic resorption by inhibiting farnesyl pyrophosphate synthase in the mevalonate pathway, leading to deficiency of the substrate for GTPase prenylation. The bone remodelling process is uncoupled, subsequently impairing bone healing and causing ONJ. Targeted administration of geranylgeraniol (GGOH) represents a promising approach to mitigate BRONJ because GGOH is a substrate for GTPase prenylation. In the current review, the in vitro effects of GGOH on osteoclasts, osteoblasts and other related cells of the jaw are summarised. We also present and appraise the current in vivo evidence of GGOH in managing BRONJ in animal models. Lastly, several considerations of using GGOH in the clinical management of BRONJ are highlighted. As a conclusion, GGOH is a promising topical agent to manage BRONJ, pending more research on an effective delivery system and validation from a clinical trial.
Introduction
Osteonecrosis of the jaw (ONJ) is a rare adverse reaction towards medications that suppress bone resorption or angiogenesis. It is prominently associated with prolonged high-dose bisphosphonate administration, usually indicated for the treatment of cancer rather than osteoporosis. Other medications that have been associated with ONJ include denosumab (an antiresorptive), bevacizumab (an antiangiogenic) and tyrosine kinase inhibitors. Collectively, ONJ caused by these medications is called medication-related ONJ (MRONJ). ONJ is characterised by impaired bone healing of the craniofacial region (Chang et al., 2018; Kawahara et al., 2021). Clinically, this condition is diagnosed based on 1) exposed maxillofacial bone that is palpable through intraoral or extraoral fistula and does not heal within 8 weeks; 2) prior or current exposure to antiresorptive or antiangiogenic agents; 3) the absence of radiation therapy or apparent metastatic disease at the maxillofacial region (Ruggiero et al., 2014; Japanese Allied Committee on Osteonecrosis of the Jaw et al., 2017).
The unique anatomy and environment of the jaw predispose it to ONJ. The oral cavity serves as a habitat to a host of microorganisms, which can cause infection and inflammation directly to the jaw bone through the gap between the teeth and the oral epithelium or root canal, or indirectly through periodontal/periapical diseases and tooth decay, or any dental treatments that injure the oral mucosa (Japanese Allied Committee on Osteonecrosis of the Jaw et al., 2017). The pathogenesis of MRONJ remains elusive. Exposure to antiresorptive and antiangiogenic agents does not constitute an absolute cause because most patients do not develop MRONJ. The prevailing hypothesis is that MRONJ is a multifactorial disease (Kim et al., 2021). The combination of infection and inflammation caused by bone trauma (e.g. tooth extraction), suppressed bone remodelling by antiresorptive agents, and inhibition of new blood vessel formation by antiangiogenic agents contribute to the disease (Aghaloo et al., 2015). In addition, oral bisphosphonates can cause soft tissue toxicity which harms the mucosal layer (Psimma et al., 2021). However, the prevalence of MRONJ caused by denosumab, which lacks soft tissue toxicity, is as high as bisphosphonates (Stopeck et al., 2016; Srivastava et al., 2021), indicating it does not play a central role in disease development. In line with the pathogenesis, dental infection, poor oral hygiene, and bone-invasive dental treatment such as tooth extractions, dental implants and dentures are risk factors of MRONJ (Shibahara, 2019).
A meta-analysis of 12 observational studies involving patients with cancer receiving sequential bisphosphonate/bisphosphonate-denosumab therapy reported that the weighted pooled prevalence of MRONJ associated with sequential pamidronate-zoledronate therapy is 19% (95% confidence interval (CI) 10–27%), bisphosphonate-denosumab is 13% (95%CI 3–22%) and ibandronate-zoledronate is 10% (95% CI 3–22%). MRONJ prevalence for bisphosphonates per se was 5% (95% CI 0–9%) and denosumab per se is 4% (95% CI 3–5%) (Srivastava et al., 2021). However, studies included in this meta-analysis were highly heterogeneous with a wide range of MRONJ onset. A comparative study has highlighted that MRONJ occurs earlier in patients receiving sequential bisphosphonate-denosumab, especially during the first year after switching, compared to the bisphosphonate group (Loyson et al., 2018). The analysis of three large prospective trials of advanced breast cancer reported that MRONJ risk increases in patients receiving bevacizumab with bisphosphonate exposure (incidence: 0.9–2.4) compared to those without (incidence: 0–0.2%) (Guarneri et al., 2010).
Two major mitigation approaches of MRONJ currently are preventive premedication dental evaluation and drug holiday (Kim et al., 2021). A premedication dental evaluation involves baseline oral health assessment, scheduled follow up, oral care instructions, antiseptic rinses, preventive periodontal treatment, extraction of non-restorable teeth and denture adjustment. A meta-analysis of six studies reported that these measures reduced MRONJ incidence among cancer patients by 77.3% (95% CI 47.4–90.2%) (Karna et al., 2018). For patients at high risk for ONJ, withholding antiresorptive therapy before and following extensive oral surgery until the complete healing of the surgical site could be considered (Wan et al., 2020). However, for patients with serious diseases such as cancer and immunosuppression, cessation of treatment to reverse MRONJ is not recommended due to the lack of evidence on its benefits and concerns on disease relapse (Kawahara et al., 2021). The optimal treatment for MRONJ has not been established. In the early stages, surgical debridement and coverage, hyperbaric oxygen, and low-intensity laser therapy are effective. In severe cases, a segmental osteotomy is recommended (Rasmusson and Abtahi, 2014). The quest for an optimal strategy to manage MRONJ is ongoing, given the lack of effective options.
Since bisphosphonates pose a higher risk of ONJ compared to other medications and are used prevalently for osteoporosis and cancers, they are the focus of the current review. Nitrogen-containing bisphosphonates act by inhibiting farnesyl pyrophosphate synthase of the mevalonate pathway, thereby blocking the generation of substrate for protein prenylation in osteoclasts. This event will suppress differentiation, survival and function of the osteoclasts, and ultimately interferes with bone resorption rate (Hasan et al., 2018; Cremers et al., 2019). Nitrogen-containing bisphosphonates could also induce osteoclast apoptosis by inhibiting adenine nucleotide translocase due to the formation of ATP analogues as a result of mevalonate pathway suppression (Mönkkönen et al., 2006). Since bone remodelling is a coupled process, bone healing following trauma is also suppressed, especially in the presence of inflammation and infection (Loi et al., 2016). Thus, the revival of normal bone remodelling at the jawbone represents an avenue of intervention (Figure 1). Apart from that, bisphosphonates are toxic to gingival fibroblasts, blood vessel epithelial cells and osteoblasts important in mucosal and bone healing (Ziebart et al., 2011; Hagelauer et al., 2015).
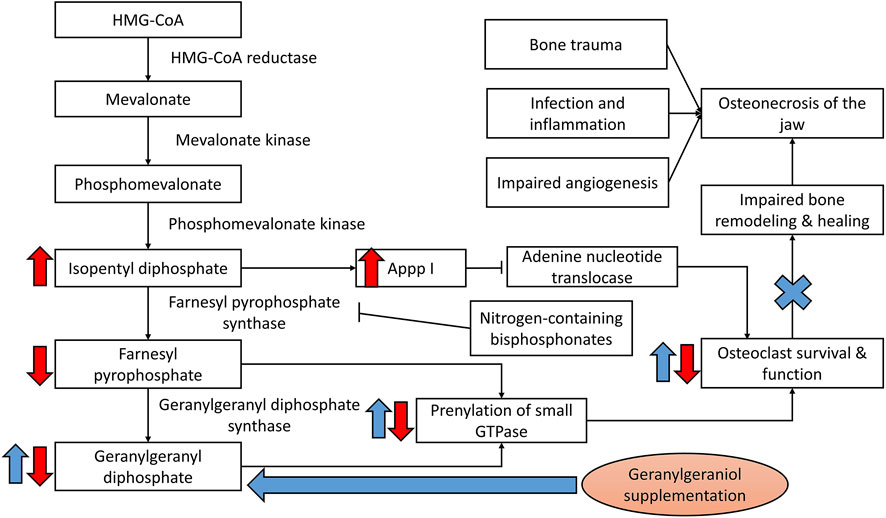
FIGURE 1. Bisphosphonates in the development of ONJ. Geranylgeraniol could replenish the substrate for prenylation of GTPase, thereby reactivating the bone remodelling cycle and healing process to mitigate osteonecrosis of the jaw induced by bisphosphonates. Notes: Red arrows indicate the effects of bisphosphonates; blue arrows indicate the potential effects of geranylgeraniol supplementation.
Many previous studies have utilised geranylgeraniol (GGOH), a diterpene alcohol or a polyprenol, to antagonise the effects of nitrogen-containing bisphosphonates. GGOH has been discovered as a wax ester from olives, annatto, sunflower, hemp and oil palm (Reiter and Lorbeer, 2001; Montserrat-de la Paz et al., 2014; Lucci et al., 2015; Silva et al., 2015). Being a central isoprenoid in the mevalonate pathway, GGOH is not only a substrate for the synthesis of menatetrenone/menaquinone-4 (MK-4) and a building block for CoQ10 (Shirakawa et al., 2018), but also acts as an important intermediate for the prenylation of proteins by GTPases (Shirakawa et al., 2018), providing the basis for its protective actions against bisphosphonate-related ONJ (BRONJ). Most recently, GGOH has been commercialized as a dietary supplement in the form of an extract from annatto, and is generally recognized as safe (GRAS) (Miyawaki et al., 2020), opening the doors to potential clinical trials with the endogenous nutrient.
This review aims to summarise the evidence of GGOH as an agent to prevent BRONJ. The biological effects of GGOH on osteoclasts, osteoblasts and other cells alone or in the presence of bisphosphonates are discussed. The in vivo evidence of GGOH in preventing BRONJ are also appraised and presented. We hope this review will provide the impetus for interested researchers to further explore the effects of GGOH as an interventional agent against BRONJ, and present a platform from which to launch human trials to illuminate the compound’s benefits clinically.
Cellular Effects of GGOH
To better understand the following content, the readers should know that osteoclasts originate from haematopoietic stem cells. The differentiation of these progenitors into functional, tartrate-resistant acid phosphate (TRAP)-positive multinucleated osteoclasts requires signals from osteoblasts from the mesenchymal lineage. In vitro experiments, osteoclasts are differentiated from murine spleen cells, murine or human macrophages cocultured with osteoblasts/stromal cells or osteoclastic differentiation stimulants. Osteoclasts initiate the bone remodelling cycle by degrading the bone matrix. Osteoblasts could be isolated from the long bones of animals or are available as commercial cell lines (e.g. MC3T3-E1 murine pre-osteoblasts) cultured with osteogenic medium (Jolly et al., 2018; Sieberath et al., 2020; Jolly et al., 2021). Osteoblasts rebuild bone by synthesising the bone matrix and mineralising it.
Effects of GGOH Alone on Osteoclasts
Earlier studies recognised that GGOH is the isoprenoid side chain of MK-4, one of the nine isoforms of vitamin K2 and might contribute to the biological activities of MK-4 (Ho et al., 2018). MK-4 displays the unique ability to suppress the formation of osteoclasts from bone marrow macrophages more effectively than other vitamin K isoforms. This property is not shared by vitamin K1 (Wu et al., 2015). Hara et al. reported that GGOH and MK-4 shares a similar ability to suppress TRAP-positive cell formation in primary murine spleen cultured with TMS stromal cells induced by 1,25-dihydroxyvitamin D3 (1,25(OH)D3), a stimulator of osteoclastogenesis. This effect is not shared by vitamin K1, phytol and other variations of similar molecules (geraniol, farnesol, generanylfarnesol, farnesylfarnesol and geranylgeranyl-farnesol) (Hara et al., 1995). Taira et al. demonstrated similar findings using human peripheral monocytes induced by RANKL and MCSF, and bone resorption activities of TRAP-positive cells in vitro was inhibited by MK-4 and GGOH (Taira et al., 2003). Hiruma et al. reported that 1,25(OH)D3 upregulates prostaglandin E2 and cyclooxygenase-2 expressions to stimulate osteoclast formation. In the same study, they found that GGOH is effective in suppressing TRAP-positive cells formation in co-culture of murine spleen cells and TMS bone marrow stromal cells induced by prostaglandin E2, while MK-4 is effective in suppressing a similar process induced by 1,25(OH)D3. These results indicate that GGOH act on pathways downstream of prostaglandin E2 signalling, while MK-4 acts on 1,25(OH)D3 signalling to inhibit osteoclast formation. As evidence, GGOH also reduces prostaglandin E2 production, cyclooxygenase-2 and RANKL expression in the co-culture (Hiruma et al., 2004).
Others have demonstrated the opposite results by showing that GGOH promotes osteoclast formation and bone resorption. Using murine foetal metatarsal explant culture, van Beek et al. reported that the effects of GGOH are dose-dependent, in which osteoclastic resorption is promoted at low doses (50–100 μM) but suppressed at high dose (500 μM) probably due to its cellular toxicity effects (van beek et al., 1999). Similar toxicity effects have been replicated by Fliefel et al., whereby low-dose GGOH (10–40 μM) promoted osteoclast viability but the inverse occurs at a high dose (80 μM) (Fliefel et al., 2019). Regarding the divergent actions of GGOH in different culture models, van Beek demonstrated that the effects of GGOH on osteoclasts are partially mediated by the retinoic acid receptor (van Beek et al., 2006), which is also the target of 1,25(OH)D3. In the foetal metatarsal explant model used by van Beek et al., which the authors claimed to mimic the natural bone environment better, GGOH promotes osteoclastic resorption (van Beek et al., 2006). In other in vitro osteoclast models, GGOH might compete with 1,25(OH)D3 to bind with retinoic acid receptor, a more effective osteoclastogenesis stimulant, leading to reduction of osteoclastic resorption (Table 1).
Effects of GGOH on Osteoclasts in the Presence of Bisphosphonates
GGOH could antagonise the effects of alendronate in suppressing TRAP-positive cell formation and resorption area in murine bone marrow co-cultured with osteoblasts or primary leporine osteoclasts (Fisher et al., 1999). It can also prevent nuclear condensation and loss of actin rings in murine osteoclasts induced by risedronate or alendronate (Reszka et al., 1999; Hiruma et al., 2004). The suppression of Mst1 cleavage induced by risedronate or alendronate contributes to this event (Reszka et al., 1999). MK-4 did not share the property of GGOH in rescuing the actin ring of osteoclasts (Hiruma et al., 2004). However, GGOH cannot prevent apoptosis induced by non-nitrogen containing bisphosphonates, such as etidronate and clodronate (Reszka et al., 1999). Similarly, GGOH prevents suppression of osteoclast formation and bone resorption in murine foetal metatarsal explants induced by nitrogen-containing bisphosphonates, but not the non-nitrogen-containing bisphosphonates (van beek et al., 1999; Van Beek et al., 2002; Van Beek et al., 2003). These observations are expected because non-nitrogen containing bisphosphonates do not affect the cellular isoprenoid pool, which is the target of GGOH. However, GGOH only reverses pamidronate-mediated suppression of osteoclastic resorption partially, indicating that pamidronate may inhibit bone resorption through other mechanisms apart from the mevalonate pathway (Van Beek et al., 2003).
The reversal effects of GGOH are dependent on the dose of bisphosphonates. GGOH cannot reverse the effects of high-dose bisphosphonate resembling monthly or yearly administration (Fisher et al., 2013). Fisher et al. postulated that high-dose bisphosphonates deplete the cellular geranylgeranyl pyrophosphate pool beyond the level supplementation can reverse (Fisher et al., 2013). High-dose bisphosphonates also cause accumulation of proapoptotic ATP analogue, Appp I due to accumulation of isopentyl pyrophosphate and interaction with mitochondrial adenine nucleotide translocase (Mönkkönen et al., 2006). Since this step occurs before the action of farnesyl pyrophosphate synthase, it cannot be reversed by GGOH.
Other actions of GGOH include reversal of zoledronate-induced suppression of inward/outward chloride ion in the acidic environment (Ohgi et al., 2013), and reversal of zoledronate-induced suppression of NFATc1 and carbonyl anhydrase II expression (Nakagawa et al., 2015). In human peripheral mononuclear cells stimulated with RANKL and MCSF, GGOH downregulates tumour necrosis factor (TNF), chemokine ligand (CXCL) 9 and CXCL10, which are upregulated with zoledronic acid treatment (Zafar et al., 2020). TNF is a proinflammatory and antiangiogenic cytokine, which can upregulate CXCL9 and CXCL10 (Ohta et al., 2008), which are known to be antiangiogenic (Yates-Binder et al., 2012; Gao et al., 2017). In bone marrow macrophages stimulated with RANKL and MCSF, GGOH reactivates osteoclasts exposed to zoledronate by upregulating osteoclastic genes and proteins, such as TRAP, dendrocyte expressed seven transmembrane protein and osteoclast stimulatory transmembrane protein (Nagaoka et al., 2015). These events initiated by GGOH lead to increased protein expression of cathepsin K and calcitonin receptor, which are osteoclast differentiation markers (Nagaoka et al., 2015).
Overall, while the effects of GGOH alone on osteoclasts depends on the in vitro system used, it can negate the negative effects of nitrogen-containing bisphosphonates at a suitable dose range. Exceptions include high-dose bisphosphonates, which can activate non-mevalonate pathways that cause osteoclast apoptosis not restorable by GGOH (Table 2).
Effects of GGOH Alone on Osteoblasts
Evidence on the effects of GGOH on osteoblasts is limited compared to its effects on osteoclasts. Still et al. reported that GGOH alone can increase the colony-forming unit in rat bone marrow culture (Still et al., 2003). However, GGOH at a high dose (80 μM) is toxic to human mesenchymal stem cells which serve as osteoblast precursors (Fliefel et al., 2020). GGOH at low doses (10–40 μM) promoted human osteoblast viability but reduced it at a high dose (80 μM) (Fliefel et al., 2019). GGOH does not promote osteoblast migration (Hagelauer et al., 2015). Patntirapong et al. reported that GGOH alone does not alter the viability of MC3T3-E1 murine pre-osteoblasts but increases mineral deposition in culture (Patntirapong et al., 2021) (Table 3).
Effects of GGOH on Osteoblasts in the Presence of Bisphosphonates
Yoshida et al. demonstrated the ability of GGOH to prevent activation of caspase-3 and apoptosis by a geranylgeranyl transferase I inhibitor, probably by increasing the substrate pool for prenylation of GTPase (Yoshida et al., 2009). This response of GGOH could be extended to its reversal of the negative effects bisphosphonate have on osteoblasts or their precursors. Bisphosphonate treatment dramatically decreased viable mesenchymal stem cells by inhibiting geranylgeranylation. GGOH preserved these important differentiator cells by reversing apoptosis and cell cycle arrest through Rho-dependent yes-associated protein activation pathway (Singhatanadgit et al., 2021). Suppression of colony formation in rat bone marrow culture by alendronate and risedronate can be reversed by GGOH (Still et al., 2003). By extension, GGOH could reverse the negative effects of bisphosphonates on osteoblasts. In fact, multiple studies have reported that GGOH reverses bisphosphonate-mediated reductions in osteoblast viability, migration and mineralisation (Ziebart et al., 2011; Hagelauer et al., 2015; Patntirapong et al., 2021). Of note, zoledronate causes apoptotic features in human osteoblasts from the mandibular alveolar bone, such as chromatin condensation, organelle disintegration and irregular-shaped cellular membrane, which are reversed by GGOH (Zafar et al., 2016). GGOH also reverses destructive cellular morphology caused by alendronate, such as reduction in actin stress fibres, cell debris and condensation of nuclei in MC3T3-E1 cells (Patntirapong et al., 2021). Of note, actin cytoskeletal reorganisation is critical for osteoblast differentiation and functions (Suzuki et al., 2019). GGOH also relieves cell cycle arrest of MC3T3-E1 caused by alendronate (Patntirapong et al., 2021).
The rescue action of GGOH on osteoblast dysfunction could be attributed to the upregulation of Rap1A/B protein suppressed by zoledronic acid (Fliefel et al., 2019). This event eventually leads to the correction of the expression of genes coding for bone formation protein, such as fibroblast growth factor-2, vascular endothelial growth factor (VEGF), VEGF receptor 2 (VEGFR2), collagen 1 and osteopontin. Of note, VEGF and VEGFR2 are modulators of bone healing by promoting osteoblast differentiation and maturation, angiogenesis and recruitment of immune cells (Grosso et al., 2017; Hu and Olsen, 2017). Zafar et al. reported that GGOH suppressed zoledronate-initiated upregulation of 4 genes in primary human osteoblasts coding for regulators of angiogenesis, such as platelet-derived growth factor-beta polypeptide, chemokine (C-C motif) ligand 2, CD55 and thrombospondin 1 (Zafar et al., 2016). However, GGOH did not normalise the expression of osteogenic genes affected by zoledronate (Zafar et al., 2016). This finding indicates that the rescue effects of GGOH on osteoclasts could be more prominent than on osteoblasts.
Overall, GGOH alone promotes osteoblast viability at a low dose but may be cytotoxic to the cells at a high dose. At a low dose, it can prevent bisphosphonate-induced osteoblast damage (Table 3).
Effects of GGOH on Other Cells
Cozin et al. found that GGOH alone is inert to the primary gingival fibroblasts, but it reverses the suppression of proliferation, migration and adhesion defect due to loss of cell-substratum adhesion and F-actin bundles caused by zoledronate (Cozin et al., 2011). However, GGOH could not reverse the negative effects of pamidronate in the same study due to the more extensive damage marked by activation of caspase-3 and apoptosis (Cozin et al., 2011). Similar findings were obtained by Ziebart et al. and Hagelauer et al. (Ziebart et al., 2011; Hagelauer et al., 2015). Ziebart et al. also showed that GGOH could only rescue the viability of human gingival fibroblasts suppressed by zoledronate, but not ibandronate and pamidronate (Ziebart et al., 2011). However, GGOH restores migration suppressed by all nitrogen-containing bisphosphonates tested (Ziebart et al., 2011). Zafar et al. reported that GGOH alone reduces the viability of human gingival fibroblasts in contrast to the promoting effects of farnesol. GGOH reverses the degenerative changes, upregulation of bone morphogenetic protein-2 and downregulation of VEGF-A induced by zoledronic acid (Zafar et al., 2014).
The effects of GGOH on human umbilical vein endothelial cells (HUVECs) have also been examined. GGOH alone at high doses (50–100 µM) is toxic to HUVEC and does not affect cell migration (Hagelauer et al., 2015). On the other hand, GGOH antagonises the effects of zoledronate in suppressing HUVEC viability and migration (Hagelauer et al., 2015). Similar effects were observed with ibandronate but not with pamidronate (Ziebart et al., 2011).
Overall, GGOH prevents nitrogen-containing bisphosphonate-mediated damage in gingival fibroblasts and HUVECs, which can help oral mucosal healing and angiogenesis (Table 4).
Effects of GGOH on BRONJ in vivo
Only three animal studies investigating the effects of GGOH supplementation in animal models of BRONJ were identified in our literature search. Koneski et al. treated male Wistar rats with zoledronate (0.06 mg/kg) for 5 weeks and extracted their first mandibular molar. GGOH solution was administered at the tooth socket at 5 mM during the last 2 weeks of the experiment (Koneski et al., 2018). GGOH treatment attenuates soft tissue inflammation and jawbone defect in the rats (Koneski et al., 2018). As evidence, 80% of the untreated rats show signs of osteonecrosis while two-thirds of the treated rats show improved vascularity, tissue granulation, osteoblast lining and epithelial coverage, as well as reduced empty lacunae (Koneski et al., 2018).
Nagaoka et al. treated male C57BL/6J mice (6-week-old) with zoledronate (250 μg/kg, i. p.) and lipopolysaccharide (250 μg/kg, i. p.) twice a week (Nagaoka et al., 2015). The first maxillary molar was extracted after 2 weeks, and mice were treated with GGOH or geranylgeranyl pyrophosphate (GGPP) for 4 weeks after the tooth extraction. The researchers showed that GGOH and GGPP reverse the reduction of bone mineral density, bone volume and TRAP-positive cells at the tooth socket caused by zoledronate and lipopolysaccharide (Nagaoka et al., 2015).
In the study of Chen et al., male C57BL/6J mice (4-week-old) were given zoledronate (250 μg/kg, i. p.) twice a week and their first maxillary molar were extracted 1 week after zoledronate treatment. GGOH (250 μg/kg) was administered together with zoledronate after tooth extraction for 4 weeks (Chen et al., 2021). Zoledronate stimulates osteocyte and pre-osteoblast apoptosis in mice and suppressed efferocytosis of macrophages on apoptotic cells (Chen et al., 2021). GGOH reverse the negative effects of zoledronate on efferocytosis by restoring Rac1 homeostasis and shuttling Rac1 at the cellular membrane. Consequently, GGOH reduces osteocytic apoptosis at the alveolar crest and improves bone healing of the tooth sockets in mice (Chen et al., 2021). This is the only study that investigates the protective effects of GGOH on osteocytes under the influence of bisphosphonates. Considering the role of osteocytes as a master regulator of bone remodelling, more research should be invested to understand the effects of GGOH on osteocytes.
Overall, both topical and systemic administrations of GGOH are proven to prevent BRONJ in animal models (Table 5). Some limitations should be noted in all three animal studies, wherein small animals were used as a model. Significant intracortical bone remodelling important for cortical bone in humans is absent in rodents (Jilka, 2013). Therefore, larger animals such as minipigs, rabbits, dogs or sheep could be a better model of BRONJ because they have intracortical remodelling, barring their high cost (Allen, 2015). All studies also used male animals as a model, probably to minimise the influence of hormonal variation on bone in female animals. However, due to the prevalent use of bisphosphate among postmenopausal women to treat osteoporosis (Bor et al., 2015), women would be receiving the most benefits from GGOH intervention. Thus, the efficacy of GGOH in reversing BRONJ should be examined in animal models with oestrogen deficiency. However, sex difference in the occurrence of BRONJ is contentious, and women do not seem to be more susceptible than men (Kharazmi et al., 2014). Overall, more in vivo studies with longer-term applications are needed to show the efficacy of GGOH in managing BRONJ.
Perspective
Several important points should be considered in developing GGOH as an interventional agent for BRONJ. Several authors have proposed topical application of GGOH in contrast to systemic application, given the concerns that it might antagonise the therapeutic effects of bisphosphonates in the management of cancer and osteoporosis. This approach has been proven effective by Koneski et al. in a rat model of BRONJ using a GGOH solution at 5 mM, which can be translated to humans directly (Koneski et al., 2018). We further propose that GGOH can be incorporated into natural and synthetic dental implants or bone grafts to accelerate the healing process. This aspect would require further investigation at the preclinical stage. In a preprint article, GGOH (4 mM) has been incorporated in bone cement and shown the ability to reverse pamidronate-mediated suppression of osteoclast viability and resorption activity in vitro (Feldman et al., 2020).
In addition, dosing inconsistency and penetration of GGOH in deep tissue should be solved. Many in vitro studies highlighted that high-dose GGOH could be toxic to gingival fibroblasts, bone cells and HUVECs (Hagelauer et al., 2015; Fliefel et al., 2019). Exposure of human fibroblasts, osteoblasts and HUVECs to GGOH at concentrations below 50 μM appears to be safe (Hagelauer et al., 2015; Fliefel et al., 2019). Thus, delivery of GGOH at a precise dose through a slow-releasing strategy like nanomaterials should be adopted to achieve this. Besides, in vivo toxicity data of GGOH is scarce. An oral toxicity study reported that high doses of GGOH (725, 1,450, 2,900 mg/kg body weight) for 90 days induce significant pathological changes in the liver and forestomach in rats. The negative effects of the forestomach were considered to be local irritative effects attributed to oral gavage (Preece et al., 2021). However, the doses used are very high and might not be relevant if topical administration is considered. In the same study, the bacterial mutagenicity test and mammalian cell clastogenicity test of GGOH did not reveal any significant findings (Preece et al., 2021).
In a high-fat diet-induced bone loss study, Chung et al. showed that GGOH supplementation (800 mg/kg diet) for 14 weeks improved glucose metabolism and bone microarchitecture and strength in rats (Chung et al., 2021). GGOH also alters the gut microbiota diversity of the rats by increasing Butyricicoccus pullicaecorum (butyrate-producing bacteria) and reducing Dorea longicatena (glutamate-producing bacteria) in the caecum (Chung et al., 2021). This observation leads to the question of whether topical application of GGOH alters oral microflora and contributes to the prevention of BRONJ since microflora is one of the contributing factors to the disease. The currently limited evidence shows that GGOH possesses inhibitory action on Mycobacterium tuberculosis and Staphylococcus aureus in vitro. Its actions on other bacteria are largely unknown (Inoue et al., 2005; Vik et al., 2007).
Lastly, the effects of GGOH could only antagonise the side effects of nitrogen-containing bisphosphonates, not non-nitrogen-containing bisphosphonates, denosumab and antiangiogenic agents (Reszka et al., 1999; van beek et al., 1999). This limitation is due to its specificity in targeting the mevalonate pathway. GGOH intervention might not be effective when the dose of bisphosphonates is high, as in monthly or yearly regimens (Fisher et al., 2013). Recent studies have highlighted that bisphosphonates like zoledronate affect multiple cellular processes, and exert differential effects on cells. For example, stimulation of transient receptor potential cation channel subfamily V member 1 (TRPV1) by zoledronate at nanomolar concentrations increases osteoblast proliferation and mineralisation. This effect is absent in osteoclasts because they do not express TRPV1. However, at micromolar concentration, zoledronate inhibits farnesyl pyrophosphate synthase and geranylgeranyl pyrophosphate, leading to anti-proliferative effects on bone cells (Scala et al., 2019). Zoledronate also binds with KIR6.1/6.2 and the SUR2A/B subunits on ATP-sensitive potassium (KATP) channels of osteoblasts and muscle fibres, which potentially contribute to its musculoskeletal side effects (Maqoud et al., 2021). The effects of GGOH on these processes are not known and should be investigated.
These factors should be considered in applying GGOH in the management of MRONJ clinically in the future.
Conclusion
BRONJ is a multifactorial disease involving bone trauma, impaired bone healing due to suppressed bone remodelling and angiogenesis, soft tissue toxicity, microbial infection and inflammation of the injured site. The existing evidence shows that GGOH can antagonise the effects of nitrogen-containing bisphosphonates on bone remodelling, resolve soft tissue toxicity and promote angiogenetic factors locally. These properties make GGOH a potential adjunctive agent in the management of BRONJ. Further investigation on the method of delivery, precise dosing, and mechanisms of action should be undertaken both pre-clinically and clinically. With GGOH being generally recognized as safe and widely available as a dietary supplement, the nutrient can now be validated in well-planned clinical trials to show its efficacy in mitigating BRONJ in humans.
Author Contributions
K-YC wrote the initial draft of the manuscript. SOE and AT provided critical review for the manuscript. All authors agreed on the submission of the manuscript.
Funding
K-YC and SOE are funded by Universiti Kebangsaan Malaysia with Research University Grant (GUP-2020-021). SOE is a postdoctoral researcher fellow funded by FPR-1 Grant.
Conflict of Interest
Author AT was employed by American River Nutrition.
The remaining authors declare that the research was conducted in the absence of any commercial or financial relationships that could be construed as a potential conflict of interest.
Publisher’s Note
All claims expressed in this article are solely those of the authors and do not necessarily represent those of their affiliated organizations, or those of the publisher, the editors and the reviewers. Any product that may be evaluated in this article, or claim that may be made by its manufacturer, is not guaranteed or endorsed by the publisher.
Acknowledgments
The authors thank Universiti Kebangsaan Malaysia for supporting them via Research University Grant (GUP-2020-021).
References
Aghaloo, T., Hazboun, R., and Tetradis, S. (2015). Pathophysiology of Osteonecrosis of the Jaws. Oral Maxillofac. Surg. Clin. North Am. 27 (4), 489–496. doi:10.1016/j.coms.2015.06.001
Allen, M. R. (2015). “Animal Models of Medication-Related Osteonecrosis of the Jaw,” in Medication-Related Osteonecrosis of the Jaws: Bisphosphonates, Denosumab, and New Agents. Editor S. Otto (Berlin, Heidelberg: Springer Berlin Heidelberg), 155–167. doi:10.1007/978-3-662-43733-9_15
Bor, A., Matuz, M., Gyimesi, N., Biczók, Z., Soós, G., and Doró, P. (2015). Gender Inequalities in the Treatment of Osteoporosis. Maturitas 80 (2), 162–169. doi:10.1016/j.maturitas.2014.11.001
Chang, J., Hakam, A. E., and McCauley, L. K. (2018). Current Understanding of the Pathophysiology of Osteonecrosis of the Jaw. Curr. Osteoporos. Rep. 16 (5), 584–595. doi:10.1007/s11914-018-0474-4
Chen, X., Zhu, W., Xu, R., Shen, X., Fu, Y., and Cheng, J. (2021). Geranylgeraniol Restores Zoledronic Acid-Induced Efferocytosis Inhibition in Bisphosphonate-Related Osteonecrosis of the Jaw. Front. Cell. Dev. Biol. 9, 770899. doi:10.3389/fcell.2021.770899
Chung, E., Elmassry, M. M., Cao, J. J., Kaur, G., Dufour, J. M., Hamood, A. N., et al. (2021). Beneficial Effect of Dietary Geranylgeraniol on Glucose Homeostasis and Bone Microstructure in Obese Mice Is Associated with Suppression of Proinflammation and Modification of Gut Microbiome. Nutr. Res. 93, 27–37. doi:10.1016/j.nutres.2021.07.001
Cozin, M., Pinker, B. M., Solemani, K., Zuniga, J. M., Dadaian, S. C., Cremers, S., et al. (2011). Novel Therapy to Reverse the Cellular Effects of Bisphosphonates on Primary Human Oral Fibroblasts. J. Oral Maxillofac. Surg. 69 (10), 2564–2578. doi:10.1016/j.joms.2011.03.005
Cremers, S., Drake, M. T., Ebetino, F. H., Bilezikian, J. P., and Russell, R. G. G. (2019). Pharmacology of Bisphosphonates. Br. J. Clin. Pharmacol. 85 (6), 1052–1062. doi:10.1111/bcp.13867
Feldman, G. J., Yagnik, A., Taub, D., and Diecidue, R. (2020). Geranylgeraniol in Bone Cement Rescues Osteoclasts from the Toxic Effects of Pamidronate and Restores Function. Pre-print. doi:10.21203/rs.3.rs-29946/v1
Fisher, J. E., Rogers, M. J., Halasy, J. M., Luckman, S. P., Hughes, D. E., Masarachia, P. J., et al. (1999). Alendronate Mechanism of Action: Geranylgeraniol, an Intermediate in the Mevalonate Pathway, Prevents Inhibition of Osteoclast Formation, Bone Resorption, and Kinase Activation In Vitro. Proc. Natl. Acad. Sci. U. S. A. 96 (1), 133–138. doi:10.1073/pnas.96.1.133
Fisher, J. E., Rosenberg, E., Santora, A. C., and Reszka, A. A. (2013). In Vitro and In Vivo Responses to High and Low Doses of Nitrogen-Containing Bisphosphonates Suggest Engagement of Different Mechanisms for Inhibition of Osteoclastic Bone Resorption. Calcif. Tissue Int. 92 (6), 531–538. doi:10.1007/s00223-013-9711-0
Fliefel, R., El Ashwah, A., Entekhabi, S., Kumbrink, J., Ehrenfeld, M., and Otto, S. (2020). Bifunctional Effect of Zoledronic Acid (ZA) on Human Mesenchymal Stem Cells (hMSCs) Based on the Concentration Level. J. Stomatol. Oral Maxillofac. Surg. 121 (6), 634–641. doi:10.1016/j.jormas.2020.03.004
Fliefel, R. M., Entekhabi, S. A., Ehrenfeld, M., and Otto, S. (2019). Geranylgeraniol (GGOH) as a Mevalonate Pathway Activator in the Rescue of Bone Cells Treated with Zoledronic Acid: An In Vitro Study. Stem Cells Int. 2019, 4351327. doi:10.1155/2019/4351327
Gao, N., Liu, X., Wu, J., Li, J., Dong, C., Wu, X., et al. (2017). CXCL10 Suppression of Hem- and Lymph-Angiogenesis in Inflamed Corneas through MMP13. Angiogenesis 20 (4), 505–518. doi:10.1007/s10456-017-9561-x
Grosso, A., Burger, M. G., Lunger, A., Schaefer, D. J., Banfi, A., and Di Maggio, N. (2017). It Takes Two to Tango: Coupling of Angiogenesis and Osteogenesis for Bone Regeneration. Front. Bioeng. Biotechnol. 5, 68. doi:10.3389/fbioe.2017.00068
Guarneri, V., Miles, D., Robert, N., Dieras, V., Glaspy, J., Smith, I., et al. (2010). Bevacizumab and Osteonecrosis of the Jaw: Incidence and Association with Bisphosphonate Therapy in Three Large Prospective Trials in Advanced Breast Cancer. Breast Cancer Res. Treat. 122 (1), 181–188. doi:10.1007/s10549-010-0866-3
Hagelauer, N., Ziebart, T., Pabst, A. M., and Walter, C. (2015). Bisphosphonates Inhibit Cell Functions of HUVECs, Fibroblasts and Osteogenic Cells via Inhibition of Protein Geranylgeranylation. Clin. Oral Investig. 19 (5), 1079–1091. doi:10.1007/s00784-014-1320-4
Hara, K., Akiyama, Y., Nakamura, T., Murota, S., and Morita, I. (1995). The Inhibitory Effect of Vitamin K2 (Menatetrenone) on Bone Resorption May Be Related to its Side Chain. Bone 16 (2), 179–184. doi:10.1016/8756-3282(94)00027-w
Hasan, W. N. W., Chin, K. Y., Jolly, J. J., Ghafar, N. A., and Soelaiman, I. N. (2018). Identifying Potential Therapeutics for Osteoporosis by Exploiting the Relationship between Mevalonate Pathway and Bone Metabolism. Endocr. Metab. Immune Disord. Drug Targets 18 (5), 450–457. doi:10.2174/1871530318666180423122409
Hiruma, Y., Nakahama, K., Fujita, H., and Morita, I. (2004). Vitamin K2 and Geranylgeraniol, its Side Chain Component, Inhibited Osteoclast Formation in a Different Manner. Biochem. Biophys. Res. Commun. 314 (1), 24–30. doi:10.1016/j.bbrc.2003.12.051
Ho, H. J., Shirakawa, H., Giriwono, P. E., Ito, A., and Komai, M. (2018). A Novel Function of Geranylgeraniol in Regulating Testosterone Production. Biosci. Biotechnol. Biochem. 82 (6), 956–962. doi:10.1080/09168451.2017.1415129
Hu, K., and Olsen, B. R. (2017). Vascular Endothelial Growth Factor Control Mechanisms in Skeletal Growth and Repair. Dev. Dyn. 246 (4), 227–234. doi:10.1002/dvdy.24463
Inoue, Y., Hada, T., Shiraishi, A., Hirose, K., Hamashima, H., and Kobayashi, S. (2005). Biphasic Effects of Geranylgeraniol, Teprenone, and Phytol on the Growth of Staphylococcus aureus. Antimicrob. Agents Chemother. 49 (5), 1770–1774. doi:10.1128/aac.49.5.1770-1774.2005
Jilka, R. L. (2013). The Relevance of Mouse Models for Investigating Age-Related Bone Loss in Humans. J. Gerontol. A Biol. Sci. Med. Sci. 68 (10), 1209–1217. doi:10.1093/gerona/glt046
Jolly, J. J., Chin, K. Y., Farhana, M. F. N., Alias, E., Chua, K. H., Hasan, W. N. W., et al. (2018). Optimization of the Static Human Osteoblast/Osteoclast Co-culture System. Iran. J. Med. Sci. 43 (2), 208–213.
Jolly, J., Mohd Fozi, N. F., Chin, K.-Y., Wong, S., Chua, K., Alias, E., et al. (2021). Skeletal Microenvironment System Utilising Bovine Bone Scaffold Co-cultured with Human Osteoblasts and Osteoclast-like Cells. Exp. Ther. Med. 22 (1), 680. doi:10.3892/etm.2021.10112
Karna, H., Gonzalez, J., Radia, H. S., Sedghizadeh, P. P., and Enciso, R. (2018). Risk-reductive Dental Strategies for Medication Related Osteonecrosis of the Jaw Among Cancer Patients: A Systematic Review with Meta-Analyses. Oral Oncol. 85, 15–23. doi:10.1016/j.oraloncology.2018.08.003
Kawahara, M., Kuroshima, S., and Sawase, T. (2021). Clinical Considerations for Medication-Related Osteonecrosis of the Jaw: a Comprehensive Literature Review. Int. J. Implant Dent. 7 (1), 47. doi:10.1186/s40729-021-00323-0
Kharazmi, M., Hallberg, P., and Michaelsson, K. (2014). Gender Related Difference in the Risk of Bisphosphonate Associated Atypical Femoral Fracture and Osteonecrosis of the Jaw. Ann. Rheum. Dis. 73 (8), 1594. doi:10.1136/annrheumdis-2013-205080
Kim, J. W., Kwak, M. K., Han, J. J., Lee, S. T., Kim, H. Y., Kim, S. H., et al. (2021). Medication Related Osteonecrosis of the Jaw: 2021 Position Statement of the Korean Society for Bone and Mineral Research and the Korean Association of Oral and Maxillofacial Surgeons. J. Bone Metab. 28 (4), 279–296. doi:10.11005/jbm.2021.28.4.279
Koneski, F., Popovic-Monevska, D., Gjorgoski, I., Krajoska, J., Popovska, M., Muratovska, I., et al. (2018). In Vivo effects of Geranylgeraniol on the Development of Bisphosphonate-Related Osteonecrosis of the Jaws. J. Craniomaxillofac Surg. 46 (2), 230–236. doi:10.1016/j.jcms.2017.11.007
Loi, F., Córdova, L. A., Pajarinen, J., Lin, T.-h., Yao, Z., and Goodman, S. B. (2016). Inflammation, Fracture and Bone Repair. Bone 86, 119–130. doi:10.1016/j.bone.2016.02.020
Loyson, T., Van Cann, T., Schoffski, P., Clement, P. M., Bechter, O., Spriet, I., et al. (2018). Incidence of Osteonecrosis of the Jaw in Patients with Bone Metastases Treated Sequentially with Bisphosphonates and Denosumab. Acta Clin. Belg 73 (2), 100–109. doi:10.1080/17843286.2017.1348001
Lucci, P., Pacetti, D., Frega, N. G., and Mozzon, M. (2015). Phytonutrient Concentration and Unsaturation of Glycerides Predict Optimal Harvest Time for Elaeis Oleifera × E. Guineensis Palm Oil Hybrids. Eur. J. Lipid Sci. 117 (7), 1027–1036. doi:10.1002/ejlt.201400599
Maqoud, F., Scala, R., Tragni, V., Pierri, C. L., Perrone, M. G., Scilimati, A., et al. (2021). Zoledronic Acid as a Novel Dual Blocker of KIR6.1/2-SUR2 Subunits of ATP-Sensitive K+ Channels: Role in the Adverse Drug Reactions. Pharmaceutics 13 (9), 1350. doi:10.3390/pharmaceutics13091350
Miyawaki, A., Rojasawasthien, T., Hitomi, S., Aoki, Y., Urata, M., Inoue, A., et al. (2020). Oral Administration of Geranylgeraniol Rescues Denervation-Induced Muscle Atrophy via Suppression of Atrogin-1. vivo (Athens, Greece) 34 (5), 2345–2351. doi:10.21873/invivo.12047
Mönkkönen, H., Auriola, S., Lehenkari, P., Kellinsalmi, M., Hassinen, I. E., Vepsäläinen, J., et al. (2006). A New Endogenous ATP Analog (ApppI) Inhibits the Mitochondrial Adenine Nucleotide Translocase (ANT) and Is Responsible for the Apoptosis Induced by Nitrogen-Containing Bisphosphonates. Br. J. Pharmacol. 147 (4), 437–445. doi:10.1038/sj.bjp.0706628
Montserrat-de la Paz, S., Marín-Aguilar, F., García-Giménez, M. D., and Fernández-Arche, M. A. (2014). Hemp (Cannabis Sativa L.) Seed Oil: Analytical and Phytochemical Characterization of the Unsaponifiable Fraction. J. Agric. Food Chem. 62 (5), 1105–1110. doi:10.1021/jf404278q
Nagaoka, Y., Kajiya, H., Ozeki, S., Ikebe, T., and Okabe, K. (2015). Mevalonates Restore Zoledronic Acid-Induced Osteoclastogenesis Inhibition. J. Dent. Res. 94 (4), 594–601. doi:10.1177/0022034514564187
Nakagawa, T., Ohta, K., Kubozono, K., Ishida, Y., Naruse, T., Takechi, M., et al. (2015). Zoledronate Inhibits Receptor Activator of Nuclear Factor Kappa-B Ligand-Induced Osteoclast Differentiation via Suppression of Expression of Nuclear Factor of Activated T-Cell C1 and Carbonic Anhydrase 2. Arch. Oral Biol. 60 (4), 557–565. doi:10.1016/j.archoralbio.2014.09.012
Ohgi, K., Kajiya, H., Okamoto, F., Nagaoka, Y., Onitsuka, T., Nagai, A., et al. (2013). A Novel Inhibitory Mechanism of Nitrogen-Containing Bisphosphonate on the Activity of Cl- Extrusion in Osteoclasts. Naunyn Schmiedeb. Arch. Pharmacol. 386 (7), 589–598. doi:10.1007/s00210-013-0857-0
Ohta, K., Shigeishi, H., Taki, M., Nishi, H., Higashikawa, K., Takechi, M., et al. (2008). Regulation of CXCL9/10/11 in Oral Keratinocytes and Fibroblasts. J. Dent. Res. 87 (12), 1160–1165. doi:10.1177/154405910808701211
Patntirapong, S., Korjai, N., Matchimapiro, M., Sungkaruk, P., and Suthamporn, Y. (2021). Geranylgeraniol Reverses Alendronate-Induced MC3T3 Cell Cytotoxicity and Alteration of Osteoblast Function via Cell Cytoskeletal Maintenance. J. Oral Pathol. Med. 50 (2), 191–199. doi:10.1111/jop.13120
Preece, K., Glavits, R., Foster, J. R., Murbach, T., Endres, J. R., Hirka, G., et al. (2021). A Toxicological Evaluation of Geranylgeraniol. Regul. Toxicol. Pharmacol. 124, 104975. doi:10.1016/j.yrtph.2021.104975
Psimma, C., Psimma, Z., Willems, H. C., Klüter, W. J., and van der Maarel-Wierink, C. D. (2021). Oral Bisphosphonates: Adverse Effects on the Oral Mucosa Not Related to the Jaw Bones. A Scoping Review. Gerodontology, 12590. doi:10.1111/ger.12590
Rasmusson, L., and Abtahi, J. (2014). Bisphosphonate Associated Osteonecrosis of the Jaw: an Update on Pathophysiology, Risk Factors, and Treatment. Int. J. Dent. 2014, 471035. doi:10.1155/2014/471035
Reiter, B., and Lorbeer, E. (2001). Analysis of the Wax Ester Fraction of Olive Oil and Sunflower Oil by Gas Chromatography and Gas Chromatography-Mass Spectrometry. J. Amer Oil Chem. Soc. 78 (9), 881–888. doi:10.1007/s11746-001-0359-z
Reszka, A. A., Halasy-Nagy, J. M., Masarachia, P. J., and Rodan, G. A. (1999). Bisphosphonates Act Directly on the Osteoclast to Induce Caspase Cleavage of Mst1 Kinase during Apoptosis. A Link between Inhibition of the Mevalonate Pathway and Regulation of an Apoptosis-Promoting Kinase. J. Biol. Chem. 274 (49), 34967–34973. doi:10.1074/jbc.274.49.34967
Ruggiero, S. L., Dodson, T. B., Fantasia, J., Goodday, R., Aghaloo, T., Mehrotra, B., et al. (2014). American Association of Oral and Maxillofacial Surgeons Position Paper on Medication-Related Osteonecrosis of the Jaw--2014 Update. J. Oral Maxillofac. Surg. 72 (10), 1938–1956. doi:10.1016/j.joms.2014.04.031
Scala, R., Maqoud, F., Angelelli, M., Latorre, R., Perrone, M. G., Scilimati, A., et al. (2019). Zoledronic Acid Modulation of TRPV1 Channel Currents in Osteoblast Cell Line and Native Rat and Mouse Bone Marrow-Derived Osteoblasts: Cell Proliferation and Mineralization Effect. Cancers 11 (2), 206. doi:10.3390/cancers11020206
Shibahara, T. (2019). Antiresorptive Agent-Related Osteonecrosis of the Jaw (ARONJ): A Twist of Fate in the Bone. Tohoku J. Exp. Med. 247 (2), 75–86. doi:10.1620/tjem.247.75
Shirakawa, H., Ho, H.-J., and Komai, M. (2018). The Function of Geranylgeraniol. Oleoscience 18 (3), 99–106. doi:10.5650/oleoscience.18.99
Sieberath, A., Della Bella, E., Ferreira, A. M., Gentile, P., Eglin, D., and Dalgarno, K. (2020). A Comparison of Osteoblast and Osteoclast In Vitro Co-culture Models and Their Translation for Preclinical Drug Testing Applications. Int. J. Mol. Sci. 21 (3). doi:10.3390/ijms21030912
Silva, E. K., Zabot, G. L., and Meireles, M. A (2015). Ultrasound-assisted Encapsulation of Annatto Seed Oil: Retention and Release of a Bioactive Compound with Functional Activities. Food Res. Int. 78, 159–168. doi:10.1016/j.foodres.2015.10.022
Singhatanadgit, W., Hankamolsiri, W., and Janvikul, W. (2021). Geranylgeraniol Prevents Zoledronic Acid-Mediated Reduction of Viable Mesenchymal Stem Cells via Induction of Rho-dependent YAP Activation. R. Soc. Open Sci. 8(6), 202066. doi:10.1098/rsos.202066
Srivastava, A., Nogueras Gonzalez, G. M., Geng, Y., Won, A. M., Cabanillas, M. E., Naing, A., et al. (2021). Prevalence of Medication Related Osteonecrosis of the Jaw in Patients Treated with Sequential Antiresorptive Drugs: Systematic Review and Meta-Analysis. Support Care Cancer 29 (5), 2305–2317. doi:10.1007/s00520-020-05882-3
Still, K., Phipps, R. J., and Scutt, A. (2003). Effects of Risedronate, Alendronate, and Etidronate on the Viability and Activity of Rat Bone Marrow Stromal Cells In Vitro. Calcif. Tissue Int. 72 (2), 143–150. doi:10.1007/s00223-001-2066-y
Stopeck, A. T., Fizazi, K., Body, J. J., Brown, J. E., Carducci, M., Diel, I., et al. (2016). Safety of Long-Term Denosumab Therapy: Results from the Open Label Extension Phase of Two Phase 3 Studies in Patients with Metastatic Breast and Prostate Cancer. Support Care Cancer 24 (1), 447–455. doi:10.1007/s00520-015-2904-5
Suzuki, H., Tatei, K., Ohshima, N., Sato, S., and Izumi, T. (2019). Regulation of MC3T3-E1 Differentiation by Actin Cytoskeleton through Lipid Mediators Reflecting the Cell Differentiation Stage. Biochem. Biophys. Res. Commun. 514 (2), 393–400. doi:10.1016/j.bbrc.2019.04.093
Taira, H., Fujikawa, Y., Kudo, O., Itonaga, I., and Torisu, T. (2003). Menatetrenone (Vitamin K2) Acts Directly on Circulating Human Osteoclast Precursors. Calcif. Tissue Int. 73 (1), 78–85. doi:10.1007/s00223-002-2061-y
van Beek, E., Löwik, C., Karperien, M., and Papapoulos, S. (2006). Independent Pathways in the Modulation of Osteoclastic Resorption by Intermediates of the Mevalonate Biosynthetic Pathway: the Role of the Retinoic Acid Receptor. Bone 38 (2), 167–171. doi:10.1016/j.bone.2005.08.011
van beek, E., Löwik, C., van der Pluijm, G., and Papapoulos, S. (1999). The Role of Geranylgeranylation in Bone Resorption and its Suppression by Bisphosphonates in Fetal Bone Explants In Vitro: A Clue to the Mechanism of Action of Nitrogen-Containing Bisphosphonates. J. Bone Min. Res. 14 (5), 722–729. doi:10.1359/jbmr.1999.14.5.722
Van Beek, E. R., Cohen, L. H., Leroy, I. M., Ebetino, F. H., Löwik, C. W. G. M., and Papapoulos, S. E. (2003). Differentiating the Mechanisms of Antiresorptive Action of Nitrogen Containing Bisphosphonates. Bone 33 (5), 805–811. doi:10.1016/j.bone.2003.07.007
Van Beek, E. R., Lowik, C., and Papapoulos, S. E. (2002). Bisphosphonates Suppress Bone Resorption by a Direct Effect on Early Osteoclast Precursors without Affecting the Osteoclastogenic Capacity of Osteogenic Cells: The Role of Protein Geranylgeranylation in the Action of Nitrogen-Containing Bisphosphonates on Osteoclast Precursors. BONE 30 (1), 64–70. doi:10.1016/S8756-3282(01)00655-X
Vik, A., James, A., and Gundersen, L. L. (2007). Screening of Terpenes and Derivatives for Antimycobacterial Activity; Identification of Geranylgeraniol and Geranylgeranyl Acetate as Potent Inhibitors of Mycobacterium tuberculosis In Vitro. Planta Med. 73 (13), 1410–1412. doi:10.1055/s-2007-990238
Wan, J. T., Sheeley, D. M., Somerman, M. J., and Lee, J. S. (2020). Mitigating Osteonecrosis of the Jaw (ONJ) through Preventive Dental Care and Understanding of Risk Factors. Bone Res. 8, 14. doi:10.1038/s41413-020-0088-1
Wu, W. J., Kim, M. S., and Ahn, B. Y. (2015). The Inhibitory Effect of Vitamin K on RANKL-Induced Osteoclast Differentiation and Bone Resorption. Food Funct. 6 (10), 3351–3358. doi:10.1039/c5fo00544b
Yates-Binder, C. C., Rodgers, M., Jaynes, J., Wells, A., Bodnar, R. J., and Turner, T. (2012). An IP-10 (CXCL10)-Derived Peptide Inhibits Angiogenesis. PLoS One 7 (7), e40812. doi:10.1371/journal.pone.0040812
Japanese Allied Committee on Osteonecrosis of the Jaw, Yoneda, T., Hagino, H., Sugimoto, T., Ohta, H., Takahashi, S., Soen, S., et al. (2017). Antiresorptive Agent-Related Osteonecrosis of the Jaw: Position Paper 2017 of the Japanese Allied Committee on Osteonecrosis of the Jaw. J. Bone Min. Metab. 35 (1), 6–19. doi:10.1007/s00774-016-0810-710.1007/s00774-017-0816-9
Yoshida, T., Clark, M. F., and Stern, P. H. (2009). The Small GTPase RhoA Is Crucial for MC3T3-E1 Osteoblastic Cell Survival. J. Cell. Biochem. 106 (5), 896–902. doi:10.1002/jcb.22059
Zafar, S., Coates, D. E., Cullinan, M. P., Drummond, B. K., Milne, T., and Seymour, G. J. (2016). Effects of Zoledronic Acid and Geranylgeraniol on the Cellular Behaviour and Gene Expression of Primary Human Alveolar Osteoblasts. Clin. Oral Investig. 20 (8), 2023–2035. doi:10.1007/s00784-015-1706-y
Zafar, S., Coates, D. E., Cullinan, M. P., Drummond, B. K., Milne, T., and Seymour, G. J. (2014). Zoledronic Acid and Geranylgeraniol Regulate Cellular Behaviour and Angiogenic Gene Expression in Human Gingival Fibroblasts. J. Oral Pathol. Med. 43 (9), 711–721. doi:10.1111/jop.12181
Zafar, S., Cullinan, M, P., Drummond, B, K., Seymour, G, J., and Coates, D, E. (2020). Effects of Zoledronic Acid and Geranylgeraniol on Angiogenic Gene Expression in Primary Human Osteoclasts. J. Oral Sci. 62 (1), 79–83. doi:10.2334/josnusd.19-0130
Keywords: angiogenesis, bone, gingival fibroblast, osteoblasts, osteoclasts
Citation: Chin K-Y, Ekeuku SO and Trias A (2022) The Role of Geranylgeraniol in Managing Bisphosphonate-Related Osteonecrosis of the Jaw. Front. Pharmacol. 13:878556. doi: 10.3389/fphar.2022.878556
Received: 21 February 2022; Accepted: 20 April 2022;
Published: 04 May 2022.
Edited by:
Domenico Tricarico, University of Bari Aldo Moro, ItalyReviewed by:
Harika Atmaca, Celal Bayar University, TurkeyEric D. Jensen, University of Minnesota Twin Cities, United States
Copyright © 2022 Chin, Ekeuku and Trias. This is an open-access article distributed under the terms of the Creative Commons Attribution License (CC BY). The use, distribution or reproduction in other forums is permitted, provided the original author(s) and the copyright owner(s) are credited and that the original publication in this journal is cited, in accordance with accepted academic practice. No use, distribution or reproduction is permitted which does not comply with these terms.
*Correspondence: Kok-Yong Chin, Y2hpbmtva3lvbmdAcHB1a20udWttLmVkdS5teQ==