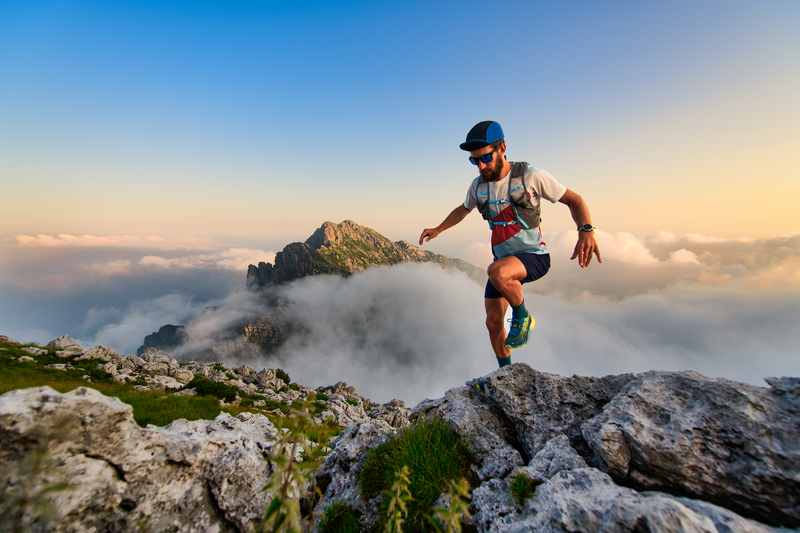
95% of researchers rate our articles as excellent or good
Learn more about the work of our research integrity team to safeguard the quality of each article we publish.
Find out more
REVIEW article
Front. Pharmacol. , 20 May 2022
Sec. Experimental Pharmacology and Drug Discovery
Volume 13 - 2022 | https://doi.org/10.3389/fphar.2022.875662
This article is part of the Research Topic MSPP 34th Scientific Meeting: Pharmacological Perspectives on Natural Products in Drug Discovery View all 10 articles
Retinal ganglion cells (RGCs) are neurons of the visual system that are responsible for transmitting signals from the retina to the brain via the optic nerve. Glaucoma is an optic neuropathy characterized by apoptotic loss of RGCs and degeneration of optic nerve fibers. Risk factors such as elevated intraocular pressure and vascular dysregulation trigger the injury that culminates in RGC apoptosis. In the event of injury, the survival of RGCs is facilitated by neurotrophic factors (NTFs), the most widely studied of which is brain-derived neurotrophic factor (BDNF). Its production is regulated locally in the retina, but transport of BDNF retrogradely from the brain to retina is also crucial. Not only that the interruption of this retrograde transport has been detected in the early stages of glaucoma, but significantly low levels of BDNF have also been detected in the sera and ocular fluids of glaucoma patients, supporting the notion that neurotrophic deprivation is a likely mechanism of glaucomatous optic neuropathy. Moreover, exogenous NTF including BDNF administration was shown reduce neuronal loss in animal models of various neurodegenerative diseases, indicating the possibility that exogenous BDNF may be a treatment option in glaucoma. Current literature provides an extensive insight not only into the sources, transport, and target sites of BDNF but also the intracellular signaling pathways, other pathways that influence BDNF signaling and a wide range of its functions. In this review, the authors discuss the neuroprotective role of BDNF in promoting the survival of RGCs and its possible application as a therapeutic tool to meet the challenges in glaucoma management. We also highlight the possibility of using BDNF as a biomarker in neurodegenerative disease such as glaucoma. Further we discuss the challenges and future strategies to explore the utility of BDNF in the management of glaucoma.
Retinal ganglion cells (RGCs) are essential to processing perceived images, and their loss can lead to irreversible blindness, such as that seen in glaucoma (Gupta et al., 2016). Optic neuropathies, such as glaucoma, the second leading cause of blindness globally, are associated with the loss of RGCs and gradual degeneration of the optic nerve head (ONH); hence producing a characteristic pattern of visual field loss (Weinreb et al., 2018; Smith et al., 2020).
Glaucoma is a group of ocular disorders with multiple clinical phenotypes, but regardless of the subtypes, increased intraocular pressure (IOP) remains a widely recognized risk factor for the development and progression of glaucoma. Hence, currently lowering IOP to a target level is the only treatment option for glaucoma (Weinreb et al., 2014). Its etiology is unclear and what constitutes a “major contributor” to the disease development remains ambiguous. Numerous studies have been conducted to understand the pathophysiology of glaucoma and to identify the cellular and molecular targets for therapeutic intervention. A comprehensive review by Agarwal et al. (2009) stated that IOP elevation and vascular dysregulation remain the primary pathophysiologic factors, while the excitotoxic and the oxidative damage of the neurons are the secondary factor contributing to glaucomatous RGC loss (Agarwal et al., 2009).
Numerous investigators have documented the potential cytotoxic stimuli that contribute to the RGC death in glaucoma, including neurotrophin deprivation, glutamate excitotoxicity, mitochondrial dysfunction, glial activation, inflammation, endoplasmic reticulum (ER) stress, ischemia, and oxidative stress (Almasieh et al., 2012; Almasieh and Levin, 2017). From a therapeutic standpoint, each of these mechanisms could be a potentially attractive strategy for the intervention to achieve neuroprotection. Thus, neuroprotectants that can block the downstream cascades evoked by various cytotoxic stimuli have extensively been studied in an attempt to eradicate or slow down the optic neurodegeneration. The favourable effects of any of the currently investigated neuroprotective candidates, although observed in animal glaucoma models have not been replicated in clinical trials (Claes et al., 2019) and, in fact, the proposed benefits of some of these potential agents have now been challenged (Gribkoff and Kaczmarek, 2017). Besides, the consensus on the neuroprotective properties of potential therapeutic intervention, mode of its delivery also remains a challenge (Cvenkel and Kolko, 2020). Of note, poor drug delivery has always been one of the main concerns in the treatment of glaucoma, hence there is ever growing need for novel drug delivery systems. This includes the applications of nanotechnology-based formulations such as nano-fibre (Omer and Zelkó, 2021; Rohde et al., 2022), hydrogels (Lynch et al., 2020; Balla et al., 2022), contact lenses (Fan et al., 2020; Dang et al., 2022), and implants (Adrianto et al., 2021; Boia et al., 2022). Discussion on this area is beyond the scope of this paper. Comprehensive reviews by Akhter et al. and others (Akhter et al., 2022; Peng et al., 2022).
The RGC loss in glaucoma is accomplished through apoptosis irrespective of the initiating pathological stimuli (Munemasa and Kitaoka, 2013). Although the precise factors that contribute to glaucoma are still being debated, the neurotrophin deprivation theory, having arisen from the observed failing of the axonal transport, currently presents as one of dominant contributors. Neurotrophins are used in neuroprotective therapies because of their effective role in maintaining and improving the survival of neuronal cells (Jeanneteau et al., 2020). The deprivation of essential neurotrophins leads to induction of the apoptosis. Studies have shown that the neurotrophin-dependent mechanisms of cell death inhibition include the regulation of Bcl-2 and Bad proteins (Miller and Kaplan, 2001). The repetitive neuronal activity increases the secretion and action of neurotrophins at the synapses and modulates the synaptic transmission and connectivity (Schinder and Poo, 2000). Brain-derived neurotrophic factor (BDNF), a potent trophic factor, is predominantly expressed in the central nervous system (CNS) and is crucial for synaptic and structural plasticity. Its enhanced expression offers protection after injury (Feng et al., 2017).
BDNF exerts neuroprotective effects directly via the Tropomyosin receptor kinase B (TrkB) expressed in RGCs (Vecino et al., 2002; Osborne et al., 2018) and/or indirectly via the TrkB expressed in glia (Dekeyster et al., 2015a). In addition to the RGCs, the amacrine cells in the retina also produce BDNF, which can be transported retrogradely, from the brain to retina via axons (Cohen-Cory et al., 1996; Vecino et al., 2002; Grishanin et al., 2008; Harada et al., 2015). There is evidence that both the local synthesis and retrograde transport of BDNF, get reduced subsequent to excitotoxic insult causing changes in the synaptic dynamics, which in turn leads to retinal neurodegeneration (Quigley et al., 2000). In this review, the authors discuss the role of BDNF deficiency in the glaucomatous RGC loss. Many published studies describe the link between the lack of BDNF support to the RGCs as a trigger for their apoptosis (Ko et al., 2001; Vecino et al., 2002; Johnson et al., 2009; Shoeb Ahmad et al., 2013). Therefore, it is likely that aberrant BDNF expression and the underlying signaling pathways in the visual system paly a key role in the pathophysiology of glaucoma. Indeed, previous studies revealed that BDNF preserves the RGCs after the optic nerve axotomy in chronically hypertensive rats (Peinado-Ramón et al., 1996; Ko et al., 2001; Dahlmann-Noor et al., 2010). Similarly, there is a consensus on the association of central and local alterations in the BDNF-TrkB signaling pathway with the retinal or the optic nerve damage, indicating the role of BDNF in preserving the inner retinal elements. (Pease et al., 2000; Gupta et al., 2014; Dekeyster et al., 2015a). However, the different roles of the BDNF/TrkB signaling pathway in RGC versus other retinal neurons and glia cells have yet to be elucidated.
In glaucomatous eyes, BDNF expression was observed to be significantly lower in aqueous humor, lacrimal fluid, and serum relative to the healthy subjects (Almasieh et al., 2012), suggesting a possible correlation between low BDNF levels and the early stages of glaucoma (Ghaffariyeh et al., 2011). Considering involvement of multiple interacting mechanisms, blocking a specific pathway at the point of onset may not be adequate to stop pathological progression (Almasieh et al., 2012). Therefore, a focus on altering pathological cascade close to the merging endpoints may prove to be more meaningful to stop the RGC death.
In this review, the authors discuss the role of BDNF as a potential biomarker for the early detection of glaucoma. We propose BDNF-based neuro-repair as a novel strategy to complement neuroprotection achieved by the current treatments, focusing primarily on cell death and conferring continuous neurotrophic support after the initial injury. It is noteworthy that, despite significant advances, no neuroprotective agent that protects the RGCs from damage has shown benefits in clinical trials. Therefore, investigations into molecular and cellular events leading to the RGC death in glaucoma are warranted. The authors highlight the exogenous application of BDNF in the experimental model of glaucoma and its limitations when translating research findings into clinical application. Indeed, future studies conducted to better understand the critical role of BDNF and its signaling in healthy versus glaucomatous retinas will provide new insights that may prove to be essential as neuroprotective strategies to preserve RGCs. This review was carried out using the key words, brain-derived neurotrophic factors; retinal ganglion cells; neurodegeneration; neuroprotection; retina; glaucoma on PubMed, SCOPUS, and Web of Science databases. English language papers published from the year 1951, 1982 to 2022 are included in this review.
Nerve growth factor (NGF) was the first growth factor identified in 1950s for its trophic (survival- and growth-promoting) effects on sensory and sympathetic neurons (Levi ‐ Montalcini and Hamburger, 1951). Later, in 1982, BDNF was discovered as the second member of the “neurotrophic” family of growth factors through isolation and purification from the pig brain. It was shown to promote survival of a subpopulation of neurons in dorsal root ganglion (Barde et al., 1982). Since the NGF and BDNF discovery, other members of the neurotrophin family have been described, such as neurotrophin-3 (NT-3), neurotrophin-4/5 (NT-4/5), ciliary neurotrophic factor (CNTF), and glial cell line-derived neurotrophic factor (GDNF) (Figure 1), each with a distinct profile of trophic effects on the subpopulations of neurons in the nervous system (Ip and Yancopoulos, 1996; Blum and Konnerth, 2005; Ibáñez and Andressoo, 2017). These molecules share several similarities, including their homologies in sequence, structure, and processing. They are synthesized as proneurotrophins, the immature precursors, and are converted to mature proteins after the proteolytic cleavage (Reichardt, 2006). These molecules bind to Tropomyosin receptor kinase (Trk) receptors and p75 neurotrophin receptor (p75NTR), and their affinity towards each of these receptors depends on their maturity (Lu et al., 2005). Mature Neurotrophic Factors (NTF) have a high affinity towards Trk receptors, which leads to cell survival and growth, while proneurotrophins have a high affinity towards p75NTR, which mainly elicits cell apoptosis. Each type of NTF binds selectively to specific Trk receptors: NGF binds specifically to TrkA; NT4 and BDNF activate TrkB; NT3 binds to TrkC, and all NF can bind to p75NTR (Reichardt, 2006). All the NTF-receptors bindings are not necessarily high affinity bindings. For example, the binding of BDNF to TrkB is of low affinity, but it can be changed when interacting with the Trk receptor and p75NTR (Chao, 2003). Upon activation, each receptor regulates several signaling pathways that are essential for neuronal development and function. Trk receptors regulate three major signaling pathways that mediate differentiation and survival, namely, mitogen-activated protein kinase (MAPK), phosphatidylinositol 3-kinase (PI3K), and phospholipase Cγ (PLC-γ).
FIGURE 1. The overview of human eye anatomy with focus on the retinal structure. The right side of the figure depicts the interactions of various NTFs with major intracellular signaling pathways activated through corresponding receptors. Adapted from “Structure of The Retina” by BioRender.com (2022). Retrieved from https://app.biorender.com/biorender-templates.
p75NTR with the intracellular death domain, similar to that in tumor necrosis factor receptors (TNFR), regulates survival and inflammation through nuclear factor kappa B (NF-κB), neuronal apoptosis through Jun-N terminal kinase (JNK), and reduced growth cone motility through RoA/ROCK signaling pathway (Huang and Reichardt, 2003). However, these two types of receptors could interact with each other or another type of receptor and transduce different binding affinities and signaling pathways that further contribute to additional functions of NTF and its receptors (Chao, 2003). Apart from forming complexes that produce high-affinity binding sites for NTF, activation of the Trk signaling pathway, such as NF-ΚB, by p75NTR has shown a synergistic contribution to neuronal survival (Huang and Reichardt, 2003). Although proapoptotic signaling of p75NTR is suppressed by Trk signaling, primarily through PI3K, this interaction is not always fully efficient, given that the crosstalk of p75NTR, and Trk signaling could also induce apoptosis in the presence of ceramide and regulate the number of mature cells (González-Hoyuela et al., 2001). The specificity of Trk receptors to each NTF is regulated by the presence or absence of an insert, a brief sequence of amino acids in the juxtamembrane region (Huang and Reichardt, 2003). For instance, TrkB without the insert can be activated by BDNF only, whereas the presence of the insert on TrkB makes it activable by NT3 and NT4 as well (Yacoubian and Lo, 2000). Hence, the receptors, either full length (TrkB-FL) or truncated (TrkB-Tc), would regulate distinct features of the NTF signaling. Compared to other NTFs, BDNF is highly expressed in the adult brain, mainly in the hippocampus, and is tightly regulated by neural activity. Apart from neuronal survival, BNDF is widely accepted to play a critical role in synaptic plasticity and memory (Sasi et al., 2017).
The broad range of functions served by BDNF owe to the complexities of neurotrophin production, secretion, and receptor signaling in the nervous system. Once secreted, BDNF can be activated in two forms: prodomain of BDNF (cleaved precursor protein; pro-BDNF) and mature BDNF (mBDNF), which exert their functions primarily through p75NTR and sortilin signaling and TrkB and p75NTR/TrkB, respectively (Kutsarova et al., 2021). Although mBDNF has a high affinity of binding to TrkB, it binds to p75NTR when the expression level is aberrant, hence stimulating signaling cascades in the manner opposite to the TrkB receptor. Because of the opposing affinities, the intra-/extracellular cleavage of BDNF becomes another critical factor in regulating the downstream signaling effects of BDNF (Lee et al., 2001).
The Trk receptors dimerize in response to a ligand binding and autophosphorylate. There are several isoforms of TrkB, and the most abundantly expressed are the full-length (TrkB-FL) and the truncated (TrkB-Tc) forms. TrkB-Tc lacks an intracellular kinase domain (Eide et al., 1996), hence it functions as a dominant-negative receptor, forming heterodimers with full-length receptors and blocking neurotrophin signaling. In astrocytes and Schwann cells, the truncated form has been suggested to regulate the pool of neurotrophins and keep them from degrading or signaling until they are released into the extracellular space (Alderson et al., 2000). The pro-death receptor, p75NTR, comprises a cytosolic death domain that is highly expressed during development (Dechant and Barde, 2002). It acts canonically by mediating both pro-death and pro-survival signals, which depend entirely on associations with cytoplasmic proteins (Dechant and Barde, 2002). In contrast, several intracellular tyrosine residues of TrkB-FL can be phosphorylated (Huang and Reichardt, 2003). Because of this, the three signaling cascades (i.e., MAPK, PI3K, and PLC-γ pathways) promote and govern the activity-dependent and tissue-specific expression of BDNF (Chen et al., 2003). The promoters translocate to the nucleus, where transcription of mRNAs responsible for producing the heterogeneous population of BDNF occurs (Minichiello, 2009). The BDNF mRNA splice variant has been described in multiple species (Dekeyster et al., 2015a). Of importance, environmental experiences such as stress-induced epigenetic modifications can influence the BDNF gene activity and epigenetic marking of the BDNF gene (Roth and Sweatt, 2011).
Since these receptor-mediated actions are thought to act contradictory, the dynamics may help in balancing the growth and death of neurons. Of note, preferentially, pro-BDNF signaling through presynaptic p75NTR is essential for axonal retraction in growing neuromuscular synapses and results in antigrowth signaling (Lee et al., 2001). The modulator, pro-BDNF, would selectively promote N-methyl-D-aspartate (NMDA) activity, along with glutamate, through p75NTR. Unlike pro-BDNF, mBNDF via presynaptic TrkB leads to axonal stabilization and results in pro-growth signaling (Je et al., 2013). Mature BDNF-TrkB signaling mediates long-term potentiation (LTP) through pre- and post-synaptic mechanisms, such as by influencing local protein synthesis, spine remodeling, or gene transcription (Park and Poo, 2012). Since BDNF is predominantly secreted as pro-BDNF, proteins that cleave the prodomain may influence which receptors are triggered by the BDNF release, giving yet another regulatory mechanism for BDNF signaling (Chen et al., 2005). BDNF-TrkB signaling can act as both mediator and modulator for plasticity-inducing neuronal activity. Moreover, BDNF with neurotransmitter signaling released within a critical time window can act as the instructor for immediate synaptic plasticity. This is why BDNF has been of interest as a stimulant for protective and restorative treatments in both neurological and psychiatric disorders. What is also known about BDNF is that compared to other NTFs, the former is the superior factor for the RGC survival under glaucomatous conditions (Almasieh and Levin, 2017). This has been proven by the exogenous application of BDNF in the developing retinotectal system where the RGC axons showed arborization and growth, contrariwise to the depleted endogenous BDNF, which hampered presynaptic trafficking and axonal branch stabilization (Kutsarova et al., 2021).
Emerging evidence on the importance of BDNF as a neurotrophin in addition to NGF, was discovered by Barde et al. (1982). In this seminal study, BDNF was shown to exert trophic effects in the survival of a subpopulation of dorsal root ganglion neurons and the fiber growth in cultured embryonic chicks (Barde et al., 1982). The same effect was later identified in the adult human brain, where a sustained expression of BDNF was associated with increased number of receptors specific to dendrite growth indicating stimulation of neurogenesis and perhaps appearance of new neurons (Tyler et al., 2002). Importantly, BDNF is required for development and survival of dopaminergic, GABAergic, serotonergic, and cholinergic neurons. Indeed, an in-depth interpretation of the effects of BDNF on the development and survival of retinal neurons may provide more significant insights into role of BDNF/TrkB pathway in the pathogenesis and, ultimately, loss of RGCs.
Unlike other retinal neurons, the axons from RGCs project to various areas of brain via the optic nerve (Crair and Mason, 2016). Much of the information regarding projection of optic nerve fibres in brain has been gathered from studies involving animals like rodents, chicks, and tadpoles (Xenopus). However, the significant difference between these experimental species and humans is the distribution of RGC axon projections in brain. In higher mammals, such as macaque, the most RGC projections synapse in the lateral geniculate nucleus (LGN), with fewer axons extending to the superior colliculus (SC) (Perry et al., 1984). However, in other experimental species such as mice, 85%–95% of the RGCs project to the SC (Ito and Feldheim, 2018; Reinhard et al., 2019). The development of retinal axons and their projections undergoes changes over a broad time frame to regulate the structural morphology and connectivity. Most prominently 50% of RGCs undergo apoptosis during pre- and early postnatal period (Guerin et al., 2002). BDNF exhibits a spatiotemporal expression at this stage, which may play an essential role in maintaining the growth of the neuroretina as well as other structures of eye such as cornea, lens, trabecular meshwork, and ciliary body (Bennett et al., 1999).
As Frost et al. (2001) reported, while the BDNF protein expression in the hamster SC declines significantly to attain adult level by postnatal day 15, the same in retina increases significantly to attain adult level at the same time point. In SC, the BDNF protein level increases only during RGC branching but plateaus by the time arborization nears completion and then declines once the adult level is reached on the postnatal day 18 (Frost et al., 2001). It is clear that the relationship between the enhanced BDNF level and the neuronal activity of developing RGCs impacts their survival into adulthood. Likewise, when the RGC axons arborize in the SC, mature BDNF levels rise (Frost et al., 2001). Multiple events in the anatomical and functional maturation of the hamster retinal projection system are temporarily linked with developmental changes in retinal and SC BDNF protein concentrations. Moreover, BDNF expression is activity-dependent during the period of RGC death and synapse formation (Cohen-Cory and Lom, 2004). Both BDNF and TrkB mRNA and protein are expressed in the retina and SC at this time and are exceptionally high in RGC target sites (Perez and Caminos, 1995; Cohen-Cory et al., 1996; Frost et al., 2001; Cohen-Cory and Lom, 2004).
BDNF can be locally produced by RGCs and astrocytes in the retina and is transported to target areas via paracrine and autocrine actions (Cohen-Cory et al., 1996). However, it is still debatable whether BDNF/TrkB support of RGC survival throughout the development is due to retrograde or anterograde transport or the retinal BDNF sources persist into adulthood. What is undisputable is that BDNF promotes neuronal survival, axonal guidance and regulates the excitatory and inhibitory synaptic transmission in the visual system (Tyler et al., 2002). Indeed, BDNF was the major player of activity-dependent branching within the SC and remodeling of the RGC axons arborization (Marler et al., 2014). Owing to its highly regulated expression due to transcription, translation, and post-translational modifications (Ruiz et al., 2014), BDNF is believed to modulate critical protein synthesis in activity-dependent synaptic plasticity (Pollock et al., 2001). This complex regulation demonstrates the vitality and diversity of BDNF in supporting existing neurons after cellular insults in multiple neurodegenerative diseases (Pollock et al., 2001). For instance, reduced expression of BDNF and polymorphism -are closely associated with Alzheimer’s disease (AD) progression (Kunugi et al., 2001; Beeri and Sonnen, 2016). A meta-analysis reported that serum BDNF decrease in individuals affected by Parkinson Disease (PD), supporting the association of reduced BDNF level and PD (Jiang et al., 2019). The same was documented in the person with relapse-remitting multiple sclerosis, where BDNF concentration was significantly lower than in healthy individuals (Wens et al., 2016).
It is now well established from various experimental glaucoma studies that NTFs effectively promote the survival of neurons and prevent apoptotic ganglion cell death (Mallone et al., 2020). This was supported by the finding that the therapies that significantly preserved the RGCs in the rat model of glaucoma were associated with elevated BDNF expression as compared to the untreated controls (Martin et al., 2003). Further cementing the significance of BDNF as a neuroprotective agent, it has been observed that exogenously applied BDNF inhibits the RGC loss and optic nerve damage in various acute and chronic glaucoma models (Gao et al., 2002; Mohd Lazaldin et al., 2020; Fudalej et al., 2021). Similar evidence of reduced RGC loss was also observed in response to the topical application of NGF (Colafrancesco et al., 2011; Lambiase et al., 2011). Topically administered NGF rescued RGCs from degeneration and enhanced the visual function of individuals with advanced glaucoma (Lambiase et al., 2011). Interestingly, in a case-control study, serum BDNF and NGF levels were low in patients with early and moderate glaucoma, indicating that the NTFs have a potential to serve as diagnostic biomarkers for glaucoma (Oddone et al., 2017). The overexpressed CNTF has also been shown to exert a strong protective effect on RGCs in an experimental rat model (Pease et al., 2009). In an ocular hypertension-induced rat model of glaucoma, the administration of CNTF resulted in substantial reduction of the RGC loss, suggesting that CNTF promotes the survival of RGCs (Ji et al., 2004). CNTF has also been shown to promote regeneration in various retinal degeneration models (Li et al., 2010; Wen et al., 2012). Despite the evidence supporting the neuroprotective effects, the use of NTFs is challenging in clinical settings due to difficulties in their passage via anatomical barriers, such as, the blood-brain barrier, the blood-retinal barrier and the blood-aqueous barrier. Moreover, the challenges posed by their short half-lives and wide-ranging effects requires target-specific formulations (Mallone et al., 2020).
One of the hypotheses proposes that the hindered defense mechanism of RGCs stems from the compromised neurotrophin transport to the cell bodies (Munemasa and Kitaoka, 2013; Guo et al., 2020). Since neurotrophins, particularly BDNF, are transported to the retina primarily in a retrograde manner, the transport blockade prevents BNDF synthesized locally, in soma and dendrites of neurons, to bind to the Trk receptors at the axon terminals (Dekeyster et al., 2015a). The lack of trophic support to RGCs may trigger apoptotic signalling and resulting in RGC loss (Kimura et al., 2016). In theory, BDNF deprivation in RGCs exerts stress, which triggers the cellular apoptotic pathways via JNK-mediated signaling, resulting in activation of proapoptotic BCL-2 family of proteins and leading to mitochondrial dysfunction. As a response to disease or injury, RGCs are known to upregulate the BDNF gene expression to circumvent apoptosis signaling and support the survival of the remaining RGC population. The same trend can be seen in axonal growth rate; however, it occurs only within the axonal terminals (de Rezende Corrêa et al., 2015). Apart from the RGCs, the inner retinal cells and photoreceptors are responsive to BDNF, implying that the neurotrophins are locally synthesized in the inner nuclear layers (Perez and Caminos, 1995). TrkB is highly expressed in RGCs, amacrine, and Müller cells, suggesting that the cellular target of the trophic action of BDNF is in the inner retinal elements (Zhang et al., 2005; Weber et al., 2010).
Considering the role of neurotrophins in RGC survival, dampening the endogenous stimuli, especially during episodes of insult, leads to substantial RGC damage (Figure 2). Deficient BDNF-TrkB signaling has been shown to be associated with RGC loss in various studies (Pease et al., 2000; Quigley et al., 2000; Iwabe et al., 2007; Osborne et al., 2018). Quigley et al. (2000) demonstrated that acute IOP elevation substantially suppresses the retrograde BDNF delivery to the ONH from the SC in adult rats, contributing to neuronal loss due to BDNF deficits. This has been attributed to the aberrant distribution of the axoplasmic transport of the trophic factors from target neurons in the SC and dLGN (Pease et al., 2000; Tanaka et al., 2009). Similarly, Pease et al. (2000) reported that the obstructed retrograde transport of BDNF gives rise to abnormal TrkB axonal distribution, focal accumulation of TrkB and BDNF, increased levels of TrkB in GCL, and increased TrkB in glia (Pease et al., 2000).
FIGURE 2. BDNF deprivation theory in the adult human visual system. BDNF supply: in healthy conditions, BDNF is synthesized and secreted by cells in the brain and transported retrogradely via the optic nerve toward the somas (RGCs). BDNF deprivation: axonal transport is perturbed due to the elevated IOP and RGCs are deprived of target-derived (brain) BDNF, leading to the stressed RGCs undergoing apoptosis. IOP, intraocular pressure; ONH, optic nerve head. Created by Biorender.com (https://biorender.com/).
Multiple in vivo studies have suggested that the deficits of BDNF expression mark the RGC damage in glaucoma, and its interrupted axonal transport has been implicated in the progressive development of optic neuropathy in experimental models of glaucoma (Gupta et al., 2014; Feng et al., 2016; Osborne et al., 2018; Chitranshi et al., 2019; Wójcik-Gryciuk et al., 2020; Conti et al., 2021; Lazzara et al., 2021). The BDNF axonal transport in injured RGCs analysed via live-cell imaging was shown to exhibit reduced activity before the death of RGCs (Takihara et al., 2011). This finding is consistent with that in glaucomatous human eyes (Ghaffariyeh et al., 2009; Ghaffariyeh et al., 2011; Gupta et al., 2014; Shpak et al., 2018; Igarashi et al., 2021) as BDNF deficits were detected in serum (Ghaffariyeh et al., 2011; Shpak et al., 2018), aqueous humour (Shpak et al., 2018; Igarashi et al., 2021), and lacrimal fluid (tears) (Ghaffariyeh et al., 2009; Shpak et al., 2018) of patients with early glaucomatous changes. Although it stimulates the expression of BDNF and its receptors, excitotoxicity induced by NMDA may also alter the retrograde transport of BDNF in the optic nerve and deprive it of the neurotrophins (Lambuk et al., 2017). It is also noteworthy that upon acute insult, the expression of BDNF-TrkB in the mouse retina is enhanced above the normal levels with extended axon survival (Feng et al., 2017). It is also hypothesized that the neuronal compartments, including the soma, axon terminal, and dendrites, appear to start the orchestration of BDNF-TrkB signaling differently (Chowdary et al., 2012). Manipulating the RGC target regions in which the signal is initiated may be a way of preventing the RGC death and delaying the progression of glaucoma or.
While the interruption in the retrograde transport is present at the early phase of the damage, the BDNF protein is synthesized rapidly in RGCs as an endogenous neuroprotective response, corroborating the idea of locally produced vs. retrogradely transported BDNF (Vecino et al., 2002). In addition, BDNF and TrkB is abundantly expressed in RGCs after axotomy, indicating that the endogenous protective response may contribute to the short-term survival of the neurons (Hirsch et al., 2000). Similar to BDNF, TrkB may be transported and stored at the axon. The intense and consistent TrkB expression was detected in the nerve fiber layer (NFL) post optic nerve lesion in the adult rat retina (Cui et al., 2002). In short, TrkB receptors could be synthesized in the soma and transported anterogradely or isolated at the nerve terminals and retrogradely transported to the soma of RGCs. However, they are not likely to promote long-term survival of the cells due to the reduced expression of BDNF expression subsequent to initial upregulation in RGCs. The limited presence of BDNF could also be attributed to the metabolic changes in injured neurons (Hu et al., 2010).
It is also argued that interrupted retrograde BDNF delivery can not be considered as the only cause of RGC death in glaucoma. This argument is supported by the observation that the adult porcine RGCs in vitro continued to survive and maintain their regular interaction with neighbouring neurons despite the lack of exogenous BDNF and dissociation from target tissues and presynaptic inputs (García et al., 2003). BDNF is also anterogradely transported to the CNS, where it serves as a survival factor for postsynaptic neurons in the SC and dLGN (Caleo et al., 2000).
It is suggested that RGC axonal transport alterations are a critical pathological component concomitant with the early increase in the IOP. Anterograde axonal transport delivers proteins, lipids, and mitochondria to the distal synapse (Fahy et al., 2016). Since neuronal proteins and molecules are predominantly synthesized in the cell body, the long axon hinders soma-derived proteins from reaching their presynaptic destinations at the axonal terminals (Chowdary et al., 2012). However, anterograde transport is the vital means of transferring newly generated synaptic proteins, ion channels, lipids, and mitochondria to their axonal destinations (Chowdary et al., 2012). Conversely, retrograde axonal transport from the axon to the soma is involved in the transport of waste substances, for instance, degraded molecules and organelles for clearance (Guo et al., 2020). This axonal transport also serves as a channel for the intracellular transport of distal chemical and biological trophic signals back to the cell body. Alongside downregulated RGC-specific genes and metabolic changes, functional and mechanical impairment of the retrograde axonal transport can be an early indicator of glaucomatous damage (Vidal-Sanz et al., 2012). The failure could result from the distortion of the elements, including defects in the cytoskeletal filaments and motor protein, which is the key to the axonal traffic machinery (Perlson and Holzbaur, 2007). It may impact the delivery of factors essential for the cell survival and the retinal function (Lu et al., 2014; Fahy et al., 2016). Indeed, this idea corroborates the neurotrophin deprivation theory as one of the mechanisms of RGC loss (Fahy et al., 2016).
The anterograde axonal transport from RGCs may occur for both the endogenous and exogenously administered BDNF (Caleo et al., 2000; Caleo et al., 2003; Butowt and von Bartheld, 2005). A fraction of BDNF transported via the anterograde path is newly produced by RGCs or the neighbouring retinal cells (Butowt and von Bartheld, 2001). Several attempts have been made to show that the role of BDNF secreted and delivered from RGCs in an anterograde direction along axons is to promote survival factors for post-synaptic neurons after retinal injury (Caleo et al., 2003; Dengler-Crish et al., 2014). During the development of rodents, deficits of retinal BDNF-TrkB signaling retracted the RGC axons from the dLGN and affected the inner retinal neuronal circuit development, implying that the retrograde transport blockade in the retina does not affect the retinogeniculate connectivity (Menna et al., 2003; Grishanin et al., 2008). RGCs also continuously deliver BDNF to the SC in adulthood (Avwenagha et al., 2006). In adult rats RGCs express BDNF-TrkB post-axotomy, supporting the idea that the survival of damaged RGCs may depend on the sufficient BDNF levels (Vecino et al., 2002).
The overproduction of BDNF in RGCs ensures fine-tuning of proper target innervation in the visual cortex of the brain, including the dLGN, SC, the suprachiasmatic nucleus, and the pretectum (Leinonen and Tanila, 2018). Inadequate trajectory to these target areas could be due to the insufficient endogenous retinal BDNF or perturbed axonal transport of the neurotrophin (Johnson et al., 2009). BDNF is also synthesized in the SC, the primary target of optic projections, and can be delivered to the retina retrogradely via RGC axons (Spalding et al., 2004). These pieces of information highlight that BDNF is excessively produced after the onset of target innervation and at the early stages of RGC development. However, adult RGCs are supported by BDNF, which is primarily produced locally (Beros et al., 2021). The enhanced retrograde transport of BDNF is triggered when the local trophic support is progressively interrupted. In theory, although local intra-retinal BDNF supplies may be delivered anterogradely for long-term survival, RGCs are eventually considered to be dependent on the competition for limited amounts of retrograde BDNF support (Beros et al., 2021). Further studies are needed to explore this support of RGC survival by BDNF during the development and adulthood when experiencing injuries or stress.
The obstruction of BDNF delivery and accumulation of TrkB at the ONH plays a vital role in the pathogenesis of glaucoma (Pease et al., 2000). In animal models with elevated IOP, the retrograde transport of BDNF-TrkB was blocked at the ONH contributing to BDNF deficits eventually leading to gliosis and neuronal loss in the retina (Quigley et al., 2000; Gupta et al., 2014). Similar observations were made by Dekeyster et al. (2015a) in the mouse model of optic nerve crush due to blockage of the retrograde delivery of BDNF. Temporary upregulation of retinal BDNF-TrkB after injury suggests that it acts as a natural protection mechanism to overcome neurotrophin transport deficits (Gao et al., 1997; Johnson et al., 2009; Dekeyster et al., 2015a).
Interestingly, in mice, the absence of BDNF did not affect the number of RGCs in the mature retina (Chitranshi et al., 2019; Beros et al., 2021). Noteworthy, that for the target-dependent survival during the early retinal development, BDNF-TrkB signaling is not required, whereas in adult animals, it intensifies to reduce RGC degeneration in the presence of pathogenic stimuli. However, exogenous administration of BDNF to the optic tectum of developing Xenopus and chick improved the RGC dendritic arbor complexity (Lom and Cohen-Cory, 1999; Cohen-Cory and Lom, 2004). In fact, during the RGC development BDNF can be said to affect the RGC and optic tectum architecture differently depending on its source and transport. Despite the elegant series of studies, the interplay between retrograde and anterograde BDNF axonal transport in the human retina remains unclear. Most studies observed that BDNF differentially modulates the survival of RGCs in rodents, and there remains a need to investigate the same effects in humans.
Neuroprotection is an ideal therapeutic approach in glaucoma to keep RGCs alive (Almasieh and Levin, 2017). The goal of neuroprotection in glaucoma is to preserve the optic nerve independent of the IOP reduction and thus prevent or delay the RGC apoptosis and axonal degeneration (Tsai, 2020; Shalaby et al., 2021). Hence, any protective intervention that directly aims at promoting the health and survival of RGCs has the potential as an antiglaucoma agent. From this standpoint, BDNF seems an attractive option for further investigations.
As highlighted earlier, BDNF increases the number of receptor sites in neurons that lead to dendrite and axonal growth and stimulate neurogenesis (Miranda et al., 2019). It is required for both the development and survival of dopaminergic, GABAergic, serotonergic, and cholinergic neurons (Park and Poo, 2012). The cellular basis for learning and memory rests with the synapses within the hippocampus. The activation of the BDNF associated TrkB intracellular pathway was shown to improve cognition, which correlated with an increase in the synaptic density (Castello et al., 2014). Accordingly, the upregulation of both BDNF and TrkB was detected in the brain areas with neuronal plasticity. Because of this relationship, BDNF is considered a molecular mediator for regulating the synaptic plasticity, playing a pivotal role in memory formation and consolidation (Zeng et al., 2012). Disruption of the pathways that transport and produce BDNF can cause clinical symptoms of deteriorating memory and cognitive dysfunction (Leal et al., 2017). Clinical studies have shown a causal relationship between lower levels of BDNF and cognitive decline observed with aging, schizophrenia, and Rett syndrome (Zuccato et al., 2011; Autry and Monteggia, 2012; Soares et al., 2016).
In animal models of glaucoma, disrupted BDNF axonal transport was observed, and BDNF injection into the superior colliculus (SC) of neonatal hamsters resulted in a 13–15-fold reduction in RGC pyknosis, showing that the BDNF plays a significant role in promoting the RGC survival (Ma et al., 1998). In addition, clinical and experimental studies have shown that the BDNF/TrkB complex is downregulated in the inner retina and the optic nerve head of glaucomatous eyes (Pease et al., 2000; Gupta et al., 2014). TrkB also gets gradually downregulated in response to the neuronal damage, suggesting it may be less responsive to the BDNF levels (Shen et al., 1999). Compared to the control group, BDNF levels in the blood of primary open-angle glaucoma patients and in the tears of normal-tension glaucoma patients were significantly lower (Ghaffariyeh et al., 2011; Oddone et al., 2017). A study by Rudzinski et al. (2004) showed that changes in the retinal expression of neurotrophic factors significantly correlate with the RGC death. The same study observed a transient upregulation of both retinal NGF and TrkA receptors after 7 days of ocular hypertension (Rudzinski et al., 2004). The sustained upregulation of retinal BDNF after 28 days of ocular hypertension was also recorded. However, the expression of TrkB receptors as well as NT-3 levels remained unchanged; although, there was an early and sustained increase of TrkC receptors in Müller cells, but not in RGCs (Rudzinski et al., 2004). Thus, the asymmetric upregulation of neurotrophin and its receptor patterns may suggest that the dysregulated activity of neurotrophic factors plays a role in the RGC apoptosis.
Other studies also provide convincing evidence about the neuroprotective effects of BDNF in retina. Ma et al. (1998) showed a reduction in the RGC death after BDNF was injected into the SC (Ma et al., 1998). Studies by Pease et al. (2000) using an experimental model of glaucoma in monkeys and rats with acute IOP elevation showed that the BDNF transport in the optic nerve head was dysregulated (Pease et al., 2000). However, once BDNF was injected via an intravitreal injection, it reduced the RGC degeneration (Mansour-Robaey et al., 1994). Ko et al. (2001) also found that the injection of BDNF to rats with elevated IOP increased the survival rate of RGCs as compared to the untreated animals (Ko et al., 2001). Exogenous, topical, or intravitreal BDNF was found to be potent in activating the pro-survival signaling pathways in RGCs following induction of ocular hypertension in experimental animals (Ma et al., 1998; Ji et al., 2004). In another study, recombinant human BDNF eye drops caused recovery of the pattern electroretinogram (P-ERG) and the visual cortex evoked potential (VEP) damage (Domenici et al., 2014) in the presence of chronic intraocular hypertension. As measured by the Brn3 immunopositive cell density in the RGC layer using retinal immunocytochemistry, this was linked to an increase in the RGC survival (Domenici et al., 2014). In addition, three consecutive intraocular injections of BDNF at 1.0 g/L in moderately chronic hypertensive rat eyes resulted in a 2-week increase in the RGC survival with no cumulative effect (Ko et al., 2001). High-dose BDNF, when injected intravitreally in animal models of the optic nerve injury, induced a rapid and considerable downregulation of TrkB expression, reducing the BDNF efficiency (Chen and Weber, 2004). On the other hand, it was shown that cyclic AMP (cAMP) induced neuronal sensitivity and axonal regeneration in BDNF-treated culture. Enhanced survival was associated with the increased availability of TrkB (Park et al., 2004). This allowed more TrkB to bind to BDNF on the surface of RGCs, enhancing the cell survival. By combining the treatment with forskolin, a TrkB agonist, the cAMP level gets elevated and the responsiveness of RGCs to BDNF is enhanced (Hu et al., 2010). This may imply that injured RGCs are less active and more sensitive to BDNF, which may affect their overall activity more than that of their healthy counterparts. Furthermore, studies have shown that increased BDNF/TrkB expression might harm neuronal homeostasis by increasing the glutamate excitotoxicity (Maki et al., 2015). TrkB activation has been shown to accelerate the glutamate-induced mortality in rat neuroblastoma cells (Maki et al., 2015), and higher BDNF levels have been reported in muscles of amyotrophic lateral sclerosis (ALS) patients.
Use of high-dose subcutaneous or intrathecal rhBDNF in patients with ALS did not provide neuroprotective effect (Ochs et al., 2000). As a result, several efforts have been made to target TrkB specifically with low molecular weight substances. A flavonoid-based TrkB agonist, 7,8-dihydroxyflavone (7,8 DHF) has been tested for this purpose. In an animal model of Parkinson’s Disease and in an in vitro model of excitotoxic and oxidative stress-induced RGC apoptosis, this compound proved effective in activating TrkB downstream signaling and exerting neuroprotective effects (Jang et al., 2010; Gupta et al., 2013). Distinct TrkB agonist antibodies have enhanced the RGC survival in vitro and in vivo in acute and chronic glaucoma models (Bai et al., 2010; Hu et al., 2010). A cell-permeable phosphine–borane compound promoted the RGC protection in a rat model of optic nerve injury by stimulating the ERK1/2 pathway to directly activate the survival signaling downstream of TrkB (Almasieh et al., 2011). However, further research is needed to weigh the benefits and the drawbacks of activating BDNF/TrkB signaling pathway in the management of neurodegenerative diseases.
BDNF plays a role in a myriad of pathophysiologic pathways (TGF-β, MAP kinase, Rho kinase, JNK, PI-3/Akt, PTEN, Bcl-2, Caspase, Calcium-Calpain) and could serve as a promising candidate for devising therapies to enhance RGC survival in glaucoma (Chitranshi et al., 2018). Various studies targeting the BDNF-TrkB signaling pathways, primarily through topical or intravitreal applications, have shown BDNF to impede the RGC loss effectively in animal models. Indeed, NTFs have been a subject of interest for neuroprotection in the past two decades due to their pivotal roles in maintaining and enhancing neuronal survival (Poo, 2001; Mandolesi et al., 2005). Although, in the glaucomatous retina, BDNF and its receptor showed no distinct differences in the expression levels as compared to the normal retina, the treatment with topical drugs, such as prostaglandin analogs, caused an increase in the expression of BDNF and TrkB (Harper et al., 2020). The studies, however, have also demonstrated that the effect of the BDNF treatment on the RGC survival is short-lived, whereas the repeated or over-exposure decreased the responsiveness or even desensitized TrkB activation by BDNF (Klöcker et al., 1998; Dekeyster et al., 2015b).
Although, some agents have shown promising results in preclinical studies, most are not ready for application in human trials. Some of these agents such as alpha 2-agonist brimonidine (BMD) (Lafuente et al., 2002), NMDA receptor antagonist (memantine) (Weinreb et al., 2018), ciliary neurotrophic factor (CNTF) (Kauper et al., 2012), rhNGF (Gala et al., 2021) or nicotinamide (vitamin B3) (Hui et al., 2020) have advanced to comprehensive randomized controlled trials (Osborne et al., 2018; Lee et al., 2012). However, the results of some of these trials are not favourable (Kolko, 2015). For example, BMD, commonly prescribed in clinics to reduce the IOP, was found to improve the BDNF production and preserve RGCs when administered systematically to mouse and rat models of IOP-independent glaucoma (Lee et al., 2012; Lee et al., 2010; Metoki et al., 2005). The neuroprotective effect of BMD was also shown in ocular hypertensive animals and those with optic nerve injury in terms of prevention of visual defects (Kitaoka et al., 2015; Semba et al., 2014). Clinically, monotherapy with 0.2% BMD tartrate causes significantly greater reduction in the progression of visual field defects compared to 0.5% timolol maleate eye drops in a 30-months Low-Pressure Glaucoma Study group trial (ClinicalTrials.gov identifier NCT00317577) (Krupin et al., 2011). However, the report raised concerns because the progression rate in the individuals treated with timolol was worse than that in the untreated group as observed in other trials, such as the Collaborative Normal Tension Glaucoma Study, suggesting that timolol enhances the disease progression rather than BMD reducing it, or it could be a combination of the two (Group, 1998). Besides, topical administration of BMD is associated with greater side effects, including hyperemia, discomfort, and hypersensitivity, as compared to other topical anti-glaucoma medications. A selectively higher dropout rate could have skewed the results in the BMD arm as compared to the timolol arm (Storgaard et al., 2021). Unfortunately, this observation is not limited to BMD only. None of the completed double-blind clinical trials for NTF administrations have met predefined endpoints for clinical efficacy (Shen et al., 2021). The overall progress of clinical trials demonstrated findings that could raise the uncertainty caused by ocular drug delivery challenges, thus highlighting the need to develop more relevant and appropriate clinical endpoints (Rusciano et al., 2017).
The rationale for the use of NTFs as therapeutic agents for glaucoma is their ability to promote RGC survival, regenerate axons, and increase the neuronal function and interconnectivity in such a way that their protection is not limited to preserving the remaining viable RGCs under the glaucomatous condition, but also to foster regeneration of the already lost nerve cells. For example, CNTF delivered by an encapsulated cell technology implant known as the NT-501 device is currently undergoing Phase II clinical trials against the geographic atrophy (age-related macular degeneration) and has been shown to slow down the progression of the vision loss (Zhang et al., 2011). Through this technology, the engineered retinal pigment epithelial cells with encapsulated CNTF were intravitreally implanted into the eyes to give a selective and sustained delivery of CNTF to the RGCs. More trials are conducted using the CNTF implants in POAG patients, although the outcomes are not yet published (Tian et al., 2015). Importantly, unlike CNTF, the human trials failed to show the benefit of the BDNF treatment for repairing the retinal damage. This could be because of the inability of the neurotrophic agents to cross blood ocular barriers. Hence, alternative methods of delivery to bypass the intrinsic biological barriers, should be sought.
It is reasonable to predict that BDNF will require a non-invasive delivery method in humans. Undoubtedly, the unfavourable pharmacokinetic properties (e.g., short half-life and low blood-brain/ocular barrier permeability) are the major hurdles to using BDNF-based therapies in clinical investigations (Houlton et al., 2019). Delivering BDNF to target site remains challenging due to its instability. Poor protein stability is detrimental to the therapeutic efficacy and may elicit potential immunogenic effects associated with the exposure of non-native peptide epitopes, which may act as the adjuvant for BDNF. Ultimately, the biological effects of BDNF will depend on the ability of the drug delivery system to provide a sustained and adequate drug release. With the right approach, it is possible to ameliorate functional axonal regeneration over a short period (Madduri and Gander, 2012). Furthermore, enormous challenges could arise for its human use due to a variety of genetic backgrounds, lifestyles, patterns of physical activity, and age of the patients with variable pathologies and additional medications, which may all affect the overall efficacy. Conclusively, successful NTF delivery requires dosage customization taking into account each of these factors and use of a delivery system optimal for clinical use.
Currently, there is an ongoing effort to achieve the selective activation of BDNF-TrkB via the administration of exogenous BDNF or its conjugation with other molecules with a high affinity to TrkB in order to achieve target-specific delivery (Ruiz et al., 2014). One of the approach is the exogenous administration of BDNF with nanoparticles as carriers (Schmidt et al., 2018). In cats with optic nerve damage, combined intravitreal injection of other molecules together with BDNF prolonged the RGC survival (Weber et al., 2010; Weber and Harman, 2013). It is likely that targeting BDNF-TrkB pathways via specific upstream or downstream molecules, such as inhibition of the Shp2 phosphatase and GSK-3β activity (Kimura et al., 2016) may prove to be beneficial. To achieve greater therapeutic efficacies, it may also be necessary to combine different compounds that target multiple mechanisms of RGC loss (Konstas et al., 2020). For instance, pharmacological approaches to reduce inflammation and oxidative stress in combination with gene therapy are currently being developed (Katsu-Jiménez et al., 2016; Yin et al., 2020). Although the use of combination therapies has been recommended, there are still no reports on their clinical efficacy.
Stem cell therapy is another approach with the potential to modulate BDNF signaling either by enhancing its production via activating multiple neuroprotective pathways or by acting as a nanocarrier. Stem cell-derived RGCs are an ideal treatment option to replace diseased or dead RGCs; however, the complexity of the retinal architecture makes the idea of the cell replacement difficult for functional repair. Alternatively, transplantation of stem cells, such as mesenchymal stem cells (MSCs), also holds a great prospect due to their capacity to secrete exosomes that can serve as extracellular vesicles encapsulating BDNF (Harrell et al., 2019). Interestingly, MSC-derived Exos (MSC-Exos) can survive in the vitreous humor for at least 4 weeks after the intravitreal injection and, because of their nanoscale dimensions, may rapidly reach RGCs to supply them with neurotrophins (Mathew et al., 2019). Accordingly, the intravitreal transplantation of MSCs that were engineered to produce and secrete BDNF at a constant and optimized level were found to preserve the functional and structural integrity of retina in a rat model of chronic ocular hypertension (Harper et al., 2011). Harrell et al. have extensively reviewed the therapeutic potential of the transplantation of MSCs-derived NTFs in glaucoma. The authors suggested that MSCs induced the production of neurotrophins and vasoactive and immunomodulatory factors, which triggered the expansion and regeneration of RGCs in animal models of glaucoma (Harrell et al., 2019). These findings suggest that the emerging role of stem-cell-based therapies as vectors for the delivery of BDNF may be beneficial for the glaucoma treatment. Exciting discoveries are underway by utilizing stem cell therapies, such as the engineered BDNF-producing cells that can be encapsulated (Deng et al., 2016; Pollock et al., 2016) or directly grafted with various moieties (Harper et al., 2011). BDNF gene delivery through recombinant adeno-associated viruses also seems to elicit a sustained increase in the BDNF concentration in the retina and support the survival of RGCs (Osborne et al., 2018). This method seems to hold a great potential for ensuing the BDNF delivery and release to the SC, which the RGCs target. Although viral vector-induced BDNF overexpression in the SC may improve the retrograde transport, it did not improve the BDNF levels in the retina nor did it protect RGCs in glaucomatous animals (Wójcik-Gryciuk et al., 2020).
The diagnostic criteria for glaucoma have been extensively debated and specific guidelines are now followed. Currently, diagnosis largely relies on the detection of abnormal changes in the optic disc and the visual field using various tools such as fundoscopy, optical coherence tomography (OCT) and the standard automated perimetry (SAP). However, it is suggested that most of the currently used methods can detect the disease only when 30%–50% of the RGCs have been irreparably lost (Quigley et al., 1992). Nonetheless, early detection of glaucomatous damage is ideal for preventing the progressive loss of RGCs (Aquino and Aquino, 2020). Hence, it is important to look for biomarkers that can predict the onset and/or progression of disease and can be objectively measured and evaluated as an indicator of biological processes in both normal and pathological conditions (Fiedorowicz et al., 2021). For example, an investigation into the relationship between the systemic levels of BDNF and the risk for the development and/or the rate of glaucoma progression may prove beneficial in predicting its possible utility as a biomarker (Oddone et al., 2017). It would also be interesting to explore the relationship of BDNF levels with treatment outcomes and prognosis. This proposition is based on the observations that BDNF levels are considerably lower in the sera, aqueous humors, and lacrimal fluids of patients with early stages of POAG (Shpak et al., 2018). A similar correlation of BDNF levels has been observed in patients with Alzheimer disease (Karege et al., 2005; Beeri and Sonnen, 2016; Eyileten et al., 2021). Since, BDNF is also generated by some non-neural cells, it remains debatable if the source of systemically detected BDNF is in fact the neuronal tissue. Studies have, however, shown that systemic BDNF levels corresponds to BDNF levels in the brain (Mojtabavi et al., 2020). The blood BDNF concentrations across species have been extensively reviewed by Klein et al. (2011). The authors suggest that the blood and plasma BDNF levels very closely reflect BDNF levels in brain tissues. These findings not only give insight into the pathophysiology of the disease but also indicate possible use of systemic BDNF levels as a biomarker for monitoring the onset and progression of neurodegenerative diseases such as glaucoma and Alzheimer disease.
To date, the bonafide intervention for glaucoma, whether it is a pharmaceutical or a surgical procedure, aims to slow down the progression of optic neuropathy and reduce visual field defects by lowering the IOP just enough to maintain good visual functions. Several published clinical trials have explicitly proven that the reduction of IOP could reduce the progression of the visual loss in both early and late stages of the disease. Yet, as it was reported in many cases, patients with excellent IOP readings have had worsening vision despite extensive therapy (Kim and Caprioli, 2018). Even with substantial improvements in therapeutic precision and knowledge on the disease progression, a subset of individuals with glaucoma is prone to aggressive progression, possibly owing to non-IOP-associated factors contributing to the RGC loss (Forchheimer et al., 2011). Besides that, there have been no substantial evidence of non-IOP lowering medications that could alter the glaucoma progression, and none of them could provide neuroprotection to recover the retinal and neural function in clinical trials (Lusthaus and Goldberg, 2019). An analytical review by Storgaard et al. suggests that despite several glaucoma related preclinical and clinical trials in the last 30 years, a successful translations to actual clinical use has not been achieved (Storgaard et al., 2021). The barriers to translate from preclinical into clinical practice may include heterogeneous nature of disease that is difficult to mimic fully in animal models leading to the variability in outcome measures, differences in ocular bioavailability, and the optimal timing of intervention. As opposed to therapeutic intervention after the diagnosis in human, similar interventions in animal studies are employed either before or during induction of disease process. Not to forget, human studies must account for disease variability induced by comorbidities and polypharmacy, which is generally not a component in preclinical studies. Furthermore, development of a formulation to circumvent anatomical and physiological barriers to drug permeation and allow a suitable route of administration, preferably topical, remains challenging.
RGC degeneration underlies a number of ocular disorders associated with terminal blindness, including glaucoma. Although various pathophysiological mechanisms have been described, deprivation of NTFs is a well-known factor contributing to RGC loss in glaucoma. Among the NTFs, BDNF has widely been investigated for its role in maintaining the integrity of retinal neuronal structure and function. It has a variety of roles extending from embryonic to adult life. The neuroprotective effects of BDNF have been observed by various researchers in preclinical studies; however, it application in clinical setting as monotherapy or adjuvant therapy remains to be explored. This review has highlighted various sources of BDNF, its transport mechanisms within neuronal cells and wide array of its functions. Considering the crucial role of BDNF in physiological functions, manipulations of its cellular pathways to specifically targeting the pathophysiological derangement would be the key to its successful therapeutic application. Additionally, its possible use as a biomarker requires further investigations. Additional medications that can operate concomitantly with the current IOP-lowering medications are desperately needed. However, various forms of glaucoma may require different additional therapies, necessitating an individualized therapeutic approach that considers the patients’ overall health and disease predispositions. Another critical matter is the need to create thoroughly reliable and precise screening procedures, which would enable an early detection of the neuronal injury in glaucoma. The development of clinical tools that are sensitive to retinal structure and changes in function on the scale of months instead of years will profoundly impact clinical trials by shortening their duration and fast-tracking the therapeutic development. This review suggests that BDNF is an exciting target as a biomarker. However, this vast subject still needs further investigation. Therefore, it is important that physicians remain updated on the most recent discoveries, particularly with respect to those highlighting therapeutic benefits of neuroprotective agents.
LL, MAML, and SA, performed literature search and drafted the manuscript. II, RA, VU, and RM supervised and revised the manuscript. All authors contributed to the manuscript and approved the submitted version.
This work was supported by grant from Ministry of Higher Education, Malaysia (Grant number: FRGS/1/2020/SKK06/USM/03/2).
Author VU is employed by TardigradeNano LLC, a biotech startup with no commercial or financial interest in the topic elaborated here.
The remaining authors declare that the research was conducted in the absence of any commercial or financial relationships that could be construed as a potential conflict of interest.
All claims expressed in this article are solely those of the authors and do not necessarily represent those of their affiliated organizations, or those of the publisher, the editors and the reviewers. Any product that may be evaluated in this article, or claim that may be made by its manufacturer, is not guaranteed or endorsed by the publisher.
Acknowledgement to “Ministry of Higher Education Malaysia” for Fundamental Research Grant Scheme with Project Code: FRGS/1/2020/SKK06/USM/03/2.
Adrianto, M. F., Annuryanti, F., Wilson, C. G., Sheshala, R., and Thakur, R. R. S. (2021). In Vitro dissolution Testing Models of Ocular Implants for Posterior Segment Drug Delivery. Drug Deliv. Transl. Res. 2021. doi:10.1007/s13346-021-01043-z
Agarwal, R., Gupta, S. K., Agarwal, P., Saxena, R., and Agrawal, S. S. (2009). Current Concepts in the Pathophysiology of Glaucoma. Indian J. Ophthalmol. 57 (4), 257–266. doi:10.4103/0301-4738.53049
Akhter, M. H., Ahmad, I., Alshahrani, M. Y., Al-Harbi, A. I., Khalilullah, H., Afzal, O., et al. (2022). Drug Delivery Challenges and Current Progress in Nanocarrier-Based Ocular Therapeutic System. Gels 8 (2), 82. doi:10.3390/gels8020082
Alderson, R. F., Curtis, R., Alterman, A. L., Lindsay, R. M., and DiStefano, P. S. (2000). Truncated TrkB Mediates the Endocytosis and Release of BDNF and Neurotrophin-4/5 by Rat Astrocytes and Schwann Cells In Vitro. Brain Res. 871 (2), 210–222. doi:10.1016/s0006-8993(00)02428-8
Almasieh, M., and Levin, L. A. (2017). Neuroprotection in Glaucoma: Animal Models and Clinical Trials. Annu. Rev. Vis. Sci. 3, 91–120. doi:10.1146/annurev-vision-102016-061422
Almasieh, M., Lieven, C. J., Levin, L. A., and Di Polo, A. (2011). A Cell-Permeable Phosphine-Borane Complex Delays Retinal Ganglion Cell Death after Axonal Injury through Activation of the Pro-survival Extracellular Signal-Regulated Kinases 1/2 Pathway. J. Neurochem. 118 (6), 1075–1086. doi:10.1111/j.1471-4159.2011.07382.x
Almasieh, M., Wilson, A. M., Morquette, B., Cueva Vargas, J. L., and Di Polo, A. (2012). The Molecular Basis of Retinal Ganglion Cell Death in Glaucoma. Prog. Retin Eye Res. 31 (2), 152–181. doi:10.1016/j.preteyeres.2011.11.002
Aquino, L. G., and Aquino, N. M. (2020). Evaluation of Macular Ganglion Cell Layer Thickness vs Peripapillary Retinal Nerve Fiber Layer Thickness for Glaucoma Detection Using Spectral-Domain Optical Coherence Tomography in a Tertiary Philippine Hospital. J. Curr. Glaucoma Pract. 14 (2), 50. doi:10.5005/jp-journals-10078-1278
Autry, A. E., and Monteggia, L. M. (2012). Brain-Derived Neurotrophic Factor and Neuropsychiatric Disorders. Pharmacol. Rev. 64 (2), 238–258. doi:10.1124/pr.111.005108
Avwenagha, O., Bird, M. M., Lieberman, A. R., Yan, Q., and Campbell, G. (2006). Patterns of Expression of Brain-Derived Neurotrophic Factor and Tyrosine Kinase B mRNAs and Distribution and Ultrastructural Localization of Their Proteins in the Visual Pathway of the Adult Rat. Neuroscience 140 (3), 913–928. doi:10.1016/j.neuroscience.2006.02.056
Bai, Y., Xu, J., Brahimi, F., Zhuo, Y., Sarunic, M. V., and Saragovi, H. U. (2010). An Agonistic TrkB mAb Causes Sustained TrkB Activation, Delays RGC Death, and Protects the Retinal Structure in Optic Nerve Axotomy and in Glaucoma. Invest. Ophthalmol. Vis. Sci. 51 (9), 4722–4731. doi:10.1167/iovs.09-5032
Balla, A., Ruponen, M., Valtari, A., Toropainen, E., Tuomainen, M., Alvarez-Lorenzo, C., et al. (2022). Understanding Dexamethasone Kinetics in the Rabbit Tear Fluid: Drug Release and Clearance from Solution, Suspension and Hydrogel Formulations. Eur. J. Pharm. Biopharm. 172, 53–60. doi:10.1016/j.ejpb.2022.01.005
Barde, Y. A., Edgar, D., and Thoenen, H. (1982). Purification of a New Neurotrophic Factor from Mammalian Brain. EMBO J. 1 (5), 549–553. doi:10.1002/j.1460-2075.1982.tb01207.x
Beeri, M. S., and Sonnen, J. (2016). Brain BDNF Expression as a Biomarker for Cognitive Reserve against Alzheimer Disease Progression. Neurology 86, 702-703. doi:10.1212/WNL.0000000000002389
Bennett, J. L., Zeiler, S. R., and Jones, K. R. (1999). Patterned Expression of BDNF and NT-3 in the Retina and Anterior Segment of the Developing Mammalian Eye. Invest. Ophthalmol. Vis. Sci. 40 (12), 2996–3005.
Beros, J., Rodger, J., and Harvey, A. R. (2021). Age Related Response of Neonatal Rat Retinal Ganglion Cells to Reduced TrkB Signaling In Vitro and In Vivo. Front. Cell Dev. Biol. 9, 1435. doi:10.3389/fcell.2021.671087
Blum, R., and Konnerth, A. (2005). Neurotrophin-mediated Rapid Signaling in the Central Nervous System: Mechanisms and Functions. Physiol. (Bethesda) 20 (1), 70–78. doi:10.1152/physiol.00042.2004
Boia, R., Dias, P. A. N., Galindo-Romero, C., Ferreira, H., Aires, I. D., Vidal-Sanz, M., et al. (2022). Intraocular Implants Loaded with A3R Agonist Rescue Retinal Ganglion Cells from Ischemic Damage. J. Control Release 343, 469–481. doi:10.1016/j.jconrel.2022.02.001
Butowt, R., and von Bartheld, C. S. (2005). Anterograde Axonal Transport of BDNF and NT-3 by Retinal Ganglion Cells: Roles of Neurotrophin Receptors. Mol. Cell Neurosci. 29 (1), 11–25. doi:10.1016/j.mcn.2005.02.004
Butowt, R., and von Bartheld, C. S. (2001). Sorting of Internalized Neurotrophins into an Endocytic Transcytosis Pathway via the Golgi System: Ultrastructural Analysis in Retinal Ganglion Cells. J. Neurosci. 21 (22), 8915–8930. doi:10.1523/jneurosci.21-22-08915.2001
Caleo, M., Medini, P., Von Bartheld, C. S., and Maffei, L. (2003). Provision of Brain-Derived Neurotrophic Factor via Anterograde Transport from the Eye Preserves the Physiological Responses of Axotomized Geniculate Neurons. J. Neurosci. 23 (1), 287–296. doi:10.1523/jneurosci.23-01-00287.2003
Caleo, M., Menna, E., Chierzi, S., Cenni, M. C., and Maffei, L. (2000). Brain-derived Neurotrophic Factor Is an Anterograde Survival Factor in the Rat Visual System. Curr. Biol. 10 (19), 1155–1161. doi:10.1016/s0960-9822(00)00713-2
Castello, N. A., Nguyen, M. H., Tran, J. D., Cheng, D., Green, K. N., and LaFerla, F. M. (2014). 7,8-Dihydroxyflavone, a Small Molecule TrkB Agonist, Improves Spatial Memory and Increases Thin Spine Density in a Mouse Model of Alzheimer Disease-like Neuronal Loss. PLoS One 9 (3), e91453. doi:10.1371/journal.pone.0091453
Chao, M. V. (2003). Neurotrophins and Their Receptors: A Convergence Point for Many Signalling Pathways. Nat. Rev. Neurosci. 4 (4), 299–309. doi:10.1038/nrn1078
Chen, H., and Weber, A. J. (2004). Brain-derived Neurotrophic Factor Reduces TrkB Protein and mRNA in the Normal Retina and Following Optic Nerve Crush in Adult Rats. Brain Res. 1011 (1), 99–106. doi:10.1016/j.brainres.2004.03.024
Chen, W. G., Chang, Q., Lin, Y., Meissner, A., West, A. E., Griffith, E. C., et al. (2003). Derepression of BDNF Transcription Involves Calcium-dependent Phosphorylation of MeCP2. Science 302 (5646), 885–889. doi:10.1126/science.1086446
Chen, Z. Y., Ieraci, A., Teng, H., Dall, H., Meng, C. X., Herrera, D. G., et al. (2005). Sortilin Controls Intracellular Sorting of Brain-Derived Neurotrophic Factor to the Regulated Secretory Pathway. J. Neurosci. 25 (26), 6156–6166. doi:10.1523/JNEUROSCI.1017-05.2005
Chitranshi, N., Dheer, Y., Abbasi, M., You, Y., Graham, S. L., and Gupta, V. (2018). Glaucoma Pathogenesis and Neurotrophins: Focus on the Molecular and Genetic Basis for Therapeutic Prospects. Curr. Neuropharmacol. 16 (7), 1018–1035. doi:10.2174/1570159X16666180419121247
Chitranshi, N., Dheer, Y., Mirzaei, M., Wu, Y., Salekdeh, G. H., Abbasi, M., et al. (2019). Loss of Shp2 Rescues BDNF/TrkB Signaling and Contributes to Improved Retinal Ganglion Cell Neuroprotection. Mol. Ther. 27 (2), 424–441. doi:10.1016/j.ymthe.2018.09.019
Chowdary, P. D., Che, D. L., and Cui, B. (2012). Neurotrophin Signaling via Long-Distance Axonal Transport. Annu. Rev. Phys. Chem. 63, 571–594. doi:10.1146/annurev-physchem-032511-143704
Claes, M., De Groef, L., and Moons, L. (2019). Target-derived Neurotrophic Factor Deprivation Puts Retinal Ganglion Cells on Death Row: Cold Hard Evidence and Caveats. Ijms 20 (17), 4314. doi:10.3390/ijms20174314
Cohen-Cory, S., Escandón, E., and Fraser, S. E. (1996). The Cellular Patterns of BDNF and trkB Expression Suggest Multiple Roles for BDNF during Xenopus Visual System Development. Dev. Biol. 179 (1), 102–115. doi:10.1006/dbio.1996.0244
Cohen-Cory, S., and Lom, B. (2004). Neurotrophic Regulation of Retinal Ganglion Cell Synaptic Connectivity: from Axons and Dendrites to Synapses. Int. J. Dev. Biol. 48 (8-9), 947–956. doi:10.1387/ijdb.041883sc
Colafrancesco, V., Parisi, V., Sposato, V., Rossi, S., Russo, M. A., Coassin, M., et al. (2011). Ocular Application of Nerve Growth Factor Protects Degenerating Retinal Ganglion Cells in a Rat Model of Glaucoma. J. Glaucoma 20 (2), 100–108. doi:10.1097/IJG.0b013e3181d787e5
Conti, F., Romano, G. L., Eandi, C. M., Toro, M. D., Rejdak, R., Di Benedetto, G., et al. (2021). Brimonidine Is Neuroprotective in Animal Paradigm of Retinal Ganglion Cell Damage. Front. Pharmacol. 2021, 1858. doi:10.3389/fphar.2021.705405
Crair, M. C., and Mason, C. A. (2016). Reconnecting Eye to Brain. J. Neurosci. 36 (42), 10707–10722. doi:10.1523/JNEUROSCI.1711-16.2016
Cui, Q., Tang, L. S., Hu, B., So, K. F., and Yip, H. K. (2002). Expression of trkA, trkB, and trkC in Injured and Regenerating Retinal Ganglion Cells of Adult Rats. Invest. Ophthalmol. Vis. Sci. 43 (6), 1954–1964.
Cvenkel, B., and Kolko, M. (2020). Current Medical Therapy and Future Trends in the Management of Glaucoma Treatment. J. Ophthalmol. 2020, 6138132. doi:10.1155/2020/6138132
Dahlmann-Noor, A., Vijay, S., Jayaram, H., Limb, A., and Khaw, P. T. (2010). Current Approaches and Future Prospects for Stem Cell Rescue and Regeneration of the Retina and Optic Nerve. Can. J. Ophthalmol. 45 (4), 333–341. doi:10.3129/i10-077
Dang, H., Dong, C., and Zhang, L. (2022). Sustained Latanoprost Release from PEGylated Solid Lipid Nanoparticle-Laden Soft Contact Lens to Treat Glaucoma. Pharm. Dev. Technol. 27, 127–133. doi:10.1080/10837450.2021.1999471
de Rezende Corrêa, G., Soares, V. H. P., de Araújo-Martins, L., Dos Santos, A. A., and Giestal-de-Araujo, E. (2015). Ouabain and BDNF Crosstalk on Ganglion Cell Survival in Mixed Retinal Cell Cultures. Cell. Mol. Neurobiol. 35 (5), 651–660.
Dechant, G., and Barde, Y. A. (2002). The Neurotrophin Receptor p75(NTR): Novel Functions and Implications for Diseases of the Nervous System. Nat. Neurosci. 5 (11), 1131–1136. doi:10.1038/nn1102-1131
Dekeyster, E., Geeraerts, E., Buyens, T., Van den Haute, C., Baekelandt, V., De Groef, L., et al. (2015). Tackling Glaucoma from within the Brain: an Unfortunate Interplay of BDNF and TrkB. PLoS One 10 (11), e0142067. doi:10.1371/journal.pone.0142067
Dekeyster, E., Geeraerts, E., Buyens, T., Van den Haute, C., Baekelandt, V., De Groef, L., et al. (2015). Tackling Glaucoma from within the Brain: An Unfortunate Interplay of BDNF and TrkB. PLoS One 10 (11), e0142067. doi:10.1371/journal.pone.0142067
Deng, P., Anderson, J. D., Yu, A. S., Annett, G., Fink, K. D., and Nolta, J. A. (2016). Engineered BDNF Producing Cells as a Potential Treatment for Neurologic Disease. Expert Opin. Biol. Ther. 16 (8), 1025–1033. doi:10.1080/14712598.2016.1183641
Dengler-Crish, C. M., Smith, M. A., Inman, D. M., Wilson, G. N., Young, J. W., and Crish, S. D. (2014). Anterograde Transport Blockade Precedes Deficits in Retrograde Transport in the Visual Projection of the DBA/2J Mouse Model of Glaucoma. Front. Neurosci. 8, 290. doi:10.3389/fnins.2014.00290
Domenici, L., Origlia, N., Falsini, B., Cerri, E., Barloscio, D., Fabiani, C., et al. (2014). Rescue of Retinal Function by BDNF in a Mouse Model of Glaucoma. PLoS One 9 (12), e115579. doi:10.1371/journal.pone.0115579
Eide, F. F., Vining, E. R., Eide, B. L., Zang, K., Wang, X. Y., and Reichardt, L. F. (1996). Naturally Occurring Truncated trkB Receptors Have Dominant Inhibitory Effects on Brain-Derived Neurotrophic Factor Signaling. J. Neurosci. 16 (10), 3123–3129. doi:10.1523/jneurosci.16-10-03123.1996
Eyileten, C., Sharif, L., Wicik, Z., Jakubik, D., Jarosz-Popek, J., Soplinska, A., et al. (2021). The Relation of the Brain-Derived Neurotrophic Factor with microRNAs in Neurodegenerative Diseases and Ischemic Stroke. Mol. Neurobiol. 58 (1), 329–347. doi:10.1007/s12035-020-02101-2
Fahy, E. T., Chrysostomou, V., and Crowston, J. G. (2016). Mini-review: Impaired Axonal Transport and Glaucoma. Curr. Eye Res. 41 (3), 273–283. doi:10.3109/02713683.2015.1037924
Fan, X., Torres-Luna, C., Azadi, M., Domszy, R., Hu, N., Yang, A., et al. (2020). Evaluation of Commercial Soft Contact Lenses for Ocular Drug Delivery: A Review. Acta Biomater. 115, 60–74. doi:10.1016/j.actbio.2020.08.025
Feng, L., Puyang, Z., Chen, H., Liang, P., Troy, J. B., and Liu, X. (2017). Overexpression of Brain-Derived Neurotrophic Factor Protects Large Retinal Ganglion Cells after Optic Nerve Crush in Mice. eNeuro 4 (1). doi:10.1523/ENEURO.0331-16.2016
Feng, L., Chen, H., Yi, J., Troy, J. B., Zhang, H. F., and Liu, X. (2016). Long-term Protection of Retinal Ganglion Cells and Visual Function by Brain-Derived Neurotrophic Factor in Mice with Ocular Hypertension. Invest. Ophthalmol. Vis. Sci. 57 (8), 3793–3802. doi:10.1167/iovs.16-19825
Fiedorowicz, E., Cieślińska, A., Kuklo, P., and Grzybowski, A. (2021). Protein Biomarkers in Glaucoma: A Review. J. Clin. Med. 10 (22), 5388. doi:10.3390/jcm10225388
Forchheimer, I., De Moraes, C., Teng, C., Folgar, F., Tello, C., Ritch, R., et al. (2011). Baseline Mean Deviation and Rates of Visual Field Change in Treated Glaucoma Patients. Eye 25 (5), 626–632. doi:10.1038/eye.2011.33
Frost, D. O., Ma, Y. T., Hsieh, T., Forbes, M. E., and Johnson, J. E. (2001). Developmental Changes in BDNF Protein Levels in the Hamster Retina and Superior Colliculus. J. Neurobiol. 49 (3), 173–187. doi:10.1002/neu.1073
Fudalej, E., Justyniarska, M., Kasarełło, K., Dziedziak, J., Szaflik, J. P., and Cudnoch-Jędrzejewska, A. (2021). Neuroprotective Factors of the Retina and Their Role in Promoting Survival of Retinal Ganglion Cells: A Review. Ophthalmic Res. 2021. doi:10.1159/000514441
Gala, B., Laurel, S., Sohail, H. M., Mariana, N., Lilia, P., Amy, D., et al. (2021). Phase 1b Randomized Controlled Study of Short Course Topical Recombinant Human Nerve Growth Factor (rhNGF) for Neuroenhancement in Glaucoma: Safety, Tolerability and Efficacy Measure Outcomes. Am. J. Ophthalmol. 2021.
Gao, H., Qiao, X., Cantor, L. B., and WuDunn, D. (2002). Up-regulation of Brain-Derived Neurotrophic Factor Expression by Brimonidine in Rat Retinal Ganglion Cells. Arch. Ophthalmol. 120 (6), 797–803. doi:10.1001/archopht.120.6.797
Gao, H., Qiao, X., Hefti, F., Hollyfield, J. G., and Knusel, B. (1997). Elevated mRNA Expression of Brain-Derived Neurotrophic Factor in Retinal Ganglion Cell Layer after Optic Nerve Injury. Invest. Ophthalmol. Vis. Sci. 38 (9), 1840–1847.
García, M., Forster, V., Hicks, D., and Vecino, E. (2003). In Vivo expression of Neurotrophins and Neurotrophin Receptors Is Conserved in Adult Porcine Retina In Vitro. Invest. Ophthalmol. Vis. Sci. 44 (10), 4532–4541. doi:10.1167/iovs.03-0419
Ghaffariyeh, A., Honarpisheh, N., Heidari, M. H., Puyan, S., and Abasov, F. (2011). Brain-derived Neurotrophic Factor as a Biomarker in Primary Open-Angle Glaucoma. Optom. Vis. Sci. 88 (1), 80–85. doi:10.1097/OPX.0b013e3181fc329f
Ghaffariyeh, A., Honarpisheh, N., Shakiba, Y., Puyan, S., Chamacham, T., Zahedi, F., et al. (2009). Brain-derived Neurotrophic Factor in Patients with Normal-Tension Glaucoma. Optometry 80 (11), 635–638. doi:10.1016/j.optm.2008.09.014
González-Hoyuela, M., Barbas, J. A., and Rodríguez-Tébar, A. (2001). The Autoregulation of Retinal Ganglion Cell Number. Development 128 (1), 117–124. doi:10.1242/dev.128.1.117
Gribkoff, V. K., and Kaczmarek, L. K. (2017). The Need for New Approaches in CNS Drug Discovery: Why Drugs Have Failed, and what Can Be Done to Improve Outcomes. Neuropharmacology 120, 11–19. doi:10.1016/j.neuropharm.2016.03.021
Grishanin, R. N., Yang, H., Liu, X., Donohue-Rolfe, K., Nune, G. C., Zang, K., et al. (2008). Retinal TrkB Receptors Regulate Neural Development in the Inner, but Not Outer, Retina. Mol. Cell Neurosci. 38 (3), 431–443. doi:10.1016/j.mcn.2008.04.004
Group, C. N-T. G. S. (1998). Comparison of Glaucomatous Progression between Untreated Patients with Normal-Tension Glaucoma and Patients with Therapeutically Reduced Intraocular Pressures. Am. J. Ophthalmol. 126 (4), 487–497. doi:10.1016/s0002-9394(98)00223-2
Guerin, M. B., Mckernan, D. P., O'Brien, C. J., and Cotter, T. G. (2002). Retinal Ganglion Cells: Dying to Survive. Int. J. Dev. Biol. 50 (8), 665–674. doi:10.1387/ijdb.062159mg
Guo, W., Dittlau, K. S., and Van Den Bosch, L. (2020). Axonal Transport Defects and Neurodegeneration: Molecular Mechanisms and Therapeutic Implications. Semin. Cell Dev. Biol. 99. 133-150. doi:10.1016/j.semcdb.2019.07.010
Gupta, M. P., Herzlich, A. A., Sauer, T., and Chan, C. C. (2016). Retinal Anatomy and Pathology. Dev. Ophthalmol. 55, 7–17. doi:10.1159/000431128
Gupta, V., You, Y., Li, J., Gupta, V., Golzan, M., Klistorner, A., et al. (2014). BDNF Impairment Is Associated with Age-Related Changes in the Inner Retina and Exacerbates Experimental Glaucoma. Biochim. Biophys. Acta 1842 (9), 1567–1578. doi:10.1016/j.bbadis.2014.05.026
Gupta, V. K., You, Y., Li, J. C., Klistorner, A., and Graham, S. L. (2013). Protective Effects of 7,8-dihydroxyflavone on Retinal Ganglion and RGC-5 Cells against Excitotoxic and Oxidative Stress. J. Mol. Neurosci. 49 (1), 96–104. doi:10.1007/s12031-012-9899-x
Harada, C., Azuchi, Y., Noro, T., Guo, X., Kimura, A., Namekata, K., et al. (2015). TrkB Signaling in Retinal Glia Stimulates Neuroprotection after Optic Nerve Injury. Am. J. Pathol. 185 (12), 3238–3247. doi:10.1016/j.ajpath.2015.08.005
Harper, M. M., Boese, E. A., Kardon, R. H., Ledolter, J., and Kuehn, M. H. (2020). High Correlation between Glaucoma Treatment with Topical Prostaglandin Analogs and BDNF Immunoreactivity in Human Retina. Curr. Eye Res. 46 (5), 1–7. doi:10.1080/02713683.2020.1822417
Harper, M. M., Grozdanic, S. D., Blits, B., Kuehn, M. H., Zamzow, D., Buss, J. E., et al. (2011). Transplantation of BDNF-Secreting Mesenchymal Stem Cells Provides Neuroprotection in Chronically Hypertensive Rat Eyes. Invest. Opthalmol Vis. Sci. 52 (7), 4506–4515. doi:10.1167/iovs.11-7346
Harrell, C. R., Fellabaum, C., Arsenijevic, A., Markovic, B. S., Djonov, V., and Volarevic, V. (2019). Therapeutic Potential of Mesenchymal Stem Cells and Their Secretome in the Treatment of Glaucoma. Stem cells Int. 2019. doi:10.1155/2019/7869130
Hirsch, S., Labes, M., and Bähr, M. (2000). Changes in BDNF and Neurotrophin Receptor Expression in Degenerating and Regenerating Rat Retinal Ganglion Cells. Restor. Neurol. Neurosci. 17 (2-3), 125–134.
Houlton, J., Abumaria, N., Hinkley, S. F., and Clarkson, A. N. (2019). Therapeutic Potential of Neurotrophins for Repair after Brain Injury: a Helping Hand from Biomaterials. Front. Neurosci. 13, 790. doi:10.3389/fnins.2019.00790
Hu, Y., Cho, S., and Goldberg, J. L. (2010). Neurotrophic Effect of a Novel TrkB Agonist on Retinal Ganglion Cells. Invest. Ophthalmol. Vis. Sci. 51 (3), 1747–1754. doi:10.1167/iovs.09-4450
Huang, E. J., and Reichardt, L. F. (2003). Trk Receptors: Roles in Neuronal Signal Transduction. Annu. Rev. Biochem. 72 (1), 609–642. doi:10.1146/annurev.biochem.72.121801.161629
Hui, F., Tang, J., Williams, P. A., McGuinness, M. B., Hadoux, X., Casson, R. J., et al. (2020). Improvement in Inner Retinal Function in Glaucoma with Nicotinamide (Vitamin B3) Supplementation: A Crossover Randomized Clinical Trial. Clin. Exp. Ophthalmol. 48 (7), 903–914. doi:10.1111/ceo.13818
Ibáñez, C. F., and Andressoo, J-O. (2017). Biology of GDNF and its Receptors—Relevance for Disorders of the Central Nervous System. Neurobiol. Dis. 97, 80–89. doi:10.1016/j.nbd.2016.01.021
Igarashi, T., Nakamoto, K., Kobayashi, M., Suzuki, H., Arima, T., Tobita, Y., et al. (2021). Brain-derived Neurotrophic Factor in the Aqueous Humor of Glaucoma Patients. J. Nippon. Med. Sch. 88 (2), 128–132. doi:10.1272/jnms.JNMS.2021_88-305
Ip, N. Y., and Yancopoulos, G. D. (1996). The Neurotrophins and CNTF: Two Families of Collaborative Neurotrophic Factors. Annu. Rev. Neurosci. 19 (1), 491–515. doi:10.1146/annurev.ne.19.030196.002423
Ito, S., and Feldheim, D. A. (2018). The Mouse Superior Colliculus: an Emerging Model for Studying Circuit Formation and Function. Front. Neural Circuits 12, 10. doi:10.3389/fncir.2018.00010
Iwabe, S., Moreno-Mendoza, N. A., Trigo-Tavera, F., Crowder, C., and García-Sánchez, G. A. (2007). Retrograde Axonal Transport Obstruction of Brain-Derived Neurotrophic Factor (BDNF) and its TrkB Receptor in the Retina and Optic Nerve of American Cocker Spaniel Dogs with Spontaneous Glaucoma. Vet. Ophthalmol. 10, 12–19. doi:10.1111/j.1463-5224.2007.00504.x
Jang, S. W., Liu, X., Yepes, M., Shepherd, K. R., Miller, G. W., Liu, Y., et al. (2010). A Selective TrkB Agonist with Potent Neurotrophic Activities by 7,8-dihydroxyflavone. Proc. Natl. Acad. Sci. U. S. A. 107 (6), 2687–2692. doi:10.1073/pnas.0913572107
Je, H. S., Yang, F., Ji, Y., Potluri, S., Fu, X. Q., Luo, Z. G., et al. (2013). ProBDNF and Mature BDNF as Punishment and Reward Signals for Synapse Elimination at Mouse Neuromuscular Junctions. J. Neurosci. 33 (24), 9957–9962. doi:10.1523/JNEUROSCI.0163-13.2013
Ji, J. Z., Elyaman, W., Yip, H. K., Lee, V. W., Yick, L. W., Hugon, J., et al. (2004). CNTF Promotes Survival of Retinal Ganglion Cells after Induction of Ocular Hypertension in Rats: the Possible Involvement of STAT3 Pathway. Eur. J. Neurosci. 19 (2), 265–272. doi:10.1111/j.0953-816x.2003.03107.x
Jiang, L., Zhang, H., Wang, C., Ming, F., Shi, X., and Yang, M. (2019). Serum Level of Brain-Derived Neurotrophic Factor in Parkinson's Disease: a Meta-Analysis. Prog. Neuropsychopharmacol. Biol. Psychiatry 88, 168–174. doi:10.1016/j.pnpbp.2018.07.010
Johnson, E. C., Guo, Y., Cepurna, W. O., and Morrison, J. C. (2009). Neurotrophin Roles in Retinal Ganglion Cell Survival: Lessons from Rat Glaucoma Models. Exp. Eye Res. 88 (4), 808–815. doi:10.1016/j.exer.2009.02.004
Karege, F., Bondolfi, G., Gervasoni, N., Schwald, M., Aubry, J-M., and Bertschy, G. (2005). Low Brain-Derived Neurotrophic Factor (BDNF) Levels in Serum of Depressed Patients Probably Results from Lowered Platelet BDNF Release Unrelated to Platelet Reactivity. Biol. psychiatry 57 (9), 1068–1072. doi:10.1016/j.biopsych.2005.01.008
Katsu-Jiménez, Y., Loría, F., Corona, J. C., and Díaz-Nido, J. (2016). Gene Transfer of Brain-Derived Neurotrophic Factor (BDNF) Prevents Neurodegeneration Triggered by FXN Deficiency. Mol. Ther. 24 (5), 877–889. doi:10.1038/mt.2016.32
Kauper, K., McGovern, C., Sherman, S., Heatherton, P., Rapoza, R., Stabila, P., et al. (2012). Two-year Intraocular Delivery of Ciliary Neurotrophic Factor by Encapsulated Cell Technology Implants in Patients with Chronic Retinal Degenerative Diseases. Invest. Ophthalmol. Vis. Sci. 53 (12), 7484–7491. doi:10.1167/iovs.12-9970
Kim, J. H., and Caprioli, J. (2018). Intraocular Pressure Fluctuation: Is it Important? J. ophthalmic & Vis. Res. 13 (2), 170. doi:10.4103/jovr.jovr_35_18
Kimura, A., Namekata, K., Guo, X., Harada, C., and Harada, T. (2016). Neuroprotection, Growth Factors and BDNF-TrkB Signalling in Retinal Degeneration. Int. J. Mol. Sci. 17 (9), 1584. doi:10.3390/ijms17091584
Kitaoka, Y., Kojima, K., Munemasa, Y., Sase, K., and Takagi, H. (2015). Axonal Protection by Brimonidine with Modulation of P62 Expression in TNF-Induced Optic Nerve Degeneration. Graefe's Archive Clin. Exp. Ophthalmol. 253 (8), 1291–1296. doi:10.1007/s00417-015-3005-3
Klein, A. B., Williamson, R., Santini, M. A., Clemmensen, C., Ettrup, A., Rios, M., et al. (2011). Blood BDNF Concentrations Reflect Brain-Tissue BDNF Levels across Species. Int. J. Neuropsychopharmacol. 14 (3), 347–353. doi:10.1017/S1461145710000738
Klöcker, N., Cellerino, A., and Bähr, M. (1998). Free Radical Scavenging and Inhibition of Nitric Oxide Synthase Potentiates the Neurotrophic Effects of Brain-Derived Neurotrophic Factor on Axotomized Retinal Ganglion CellsIn Vivo. J. Neurosci. 18 (3), 1038–1046.
Ko, M. L., Hu, D. N., Ritch, R., Sharma, S. C., and Chen, C. F. (2001). Patterns of Retinal Ganglion Cell Survival after Brain-Derived Neurotrophic Factor Administration in Hypertensive Eyes of Rats. Neurosci. Lett. 305 (2), 139–142. doi:10.1016/s0304-3940(01)01830-4
Kolko, M. (2015). Present and New Treatment Strategies in the Management of Glaucoma. Open Ophthalmol. J. 9, 89–100. doi:10.2174/1874364101509010089
Konstas, A. G., Schmetterer, L., Costa, V. P., Holló, G., Katsanos, A., Denis, P., et al. (2020). Current and Emerging Fixed Combination Therapies in Glaucoma: a Safety and Tolerability Review. Expert Opin. drug Saf. 19 (11), 1445–1460. doi:10.1080/14740338.2020.1826928
Krupin, T., Liebmann, J. M., Greenfield, D. S., Ritch, R., Gardiner, S., and Group, L-P. G. S. (2011). A Randomized Trial of Brimonidine versus Timolol in Preserving Visual Function: Results from the Low-Pressure Glaucoma Treatment Study. Am. J. Ophthalmol. 151 (4), 671–681. doi:10.1016/j.ajo.2010.09.026
Kunugi, H., Ueki, A., Otsuka, M., Isse, K., Hirasawa, H., Kato, N., et al. (2001). A Novel Polymorphism of the Brain-Derived Neurotrophic Factor (BDNF) Gene Associated with Late-Onset Alzheimer's Disease. Mol. Psychiatry 6 (1), 83–86. doi:10.1038/sj.mp.4000792
Kutsarova, E., Schohl, A., Munz, M., Wang, A., Zhang, Y. Y., Bilash, O. M., et al. (2021). BDNF Signaling in Hebbian and Stentian Structural Plasticity in the Developing Visual System. bioRxiv 2021.
Lafuente, M. P., Villegas-Pérez, M. P., Mayor, S., Aguilera, M. E., Miralles de Imperial, J., and Vidal-Sanz, M. (2002). Neuroprotective Effects of Brimonidine against Transient Ischemia-Induced Retinal Ganglion Cell Death: a Dose Response In Vivo Study. Exp. Eye Res. 74 (2), 181–189. doi:10.1006/exer.2001.1122
Lambiase, A., Mantelli, F., Sacchetti, M., Rossi, S., Aloe, L., and Bonini, S. (2011). Clinical Applications of NGF in Ocular Diseases. Arch. Ital. Biol. 149 (2), 283–292. doi:10.4449/aib.v149i2.1363
Lambuk, L., Jafri, A. J., Arfuzir, N. N., Iezhitsa, I., Agarwal, R., Rozali, K. N., et al. (2017). Neuroprotective Effect of Magnesium Acetyltaurate against NMDA-Induced Excitotoxicity in Rat Retina. Neurotox. Res. 31 (1), 31–45. doi:10.1007/s12640-016-9658-9
Lazzara, F., Amato, R., Platania, C. B. M., Conti, F., Chou, T. H., Porciatti, V., et al. (2021). 1α,25-dihydroxyvitamin D3 Protects Retinal Ganglion Cells in Glaucomatous Mice. J. Neuroinflammation 18 (1), 206–219. doi:10.1186/s12974-021-02263-3
Leal, G., Bramham, C. R., and Duarte, C. B. (2017). BDNF and Hippocampal Synaptic Plasticity. Vitamins Hormones 104, 153–195. doi:10.1016/bs.vh.2016.10.004
Lee, D., Kim, K-Y., Noh, Y. H., Chai, S., Lindsey, J. D., Ellisman, M. H., et al. (2012). Brimonidine Blocks Glutamate Excitotoxicity-Induced Oxidative Stress and Preserves Mitochondrial Transcription Factor a in Ischemic Retinal Injury. PLoS ONE 7 (10), e47098.
Lee, K. Y. C., Nakayama, M., Aihara, M., Chen, Y-N., and Araie, M. (2010). Brimonidine Is Neuroprotective against Glutamate-Induced Neurotoxicity, Oxidative Stress, and Hypoxia in Purified Rat Retinal Ganglion Cells. Mol. Vis. 16, 246.
Lee, R., Kermani, P., Teng, K. K., and Hempstead, B. L. (2001). Regulation of Cell Survival by Secreted Proneurotrophins. Science 294 (5548), 1945–1948. doi:10.1126/science.1065057
Leinonen, H., and Tanila, H. (2018). Vision in Laboratory Rodents-Tools to Measure it and Implications for Behavioral Research. Behav. Brain Res. 352, 172–182. doi:10.1016/j.bbr.2017.07.040
Levi‐Montalcini, R., and Hamburger, V. (1951). Selective Growth Stimulating Effects of Mouse Sarcoma on the Sensory and Sympathetic Nervous System of the Chick Embryo. J. Exp. Zoology 116 (2), 321–361.
Li, Y., Tao, W., Luo, L., Huang, D., Kauper, K., Stabila, P., et al. (2010). CNTF Induces Regeneration of Cone Outer Segments in a Rat Model of Retinal Degeneration. PLoS One 5 (3), e9495. doi:10.1371/journal.pone.0009495
Lom, B., and Cohen-Cory, S. (1999). Brain-derived Neurotrophic Factor Differentially Regulates Retinal Ganglion Cell Dendritic and Axonal Arborization In Vivo. J. Neurosci. 19 (22), 9928–9938. doi:10.1523/jneurosci.19-22-09928.1999
Lu, B., Nagappan, G., and Lu, Y. (2014). BDNF and Synaptic Plasticity, Cognitive Function, and Dysfunction. Handb. Exp. Pharmacol. 220, 223–250. doi:10.1007/978-3-642-45106-5_9
Lu, B., Pang, P. T., and Woo, N. H. (2005). The Yin and Yang of Neurotrophin Action. Nat. Rev. Neurosci. 6 (8), 603–614. doi:10.1038/nrn1726
Lusthaus, J., and Goldberg, I. (2019). Current Management of Glaucoma. Med. J. Aust. 210 (4), 180–187. doi:10.5694/mja2.50020
Lynch, C. R., Kondiah, P. P. D., Choonara, Y. E., du Toit, L. C., Ally, N., and Pillay, V. (2020). Hydrogel Biomaterials for Application in Ocular Drug Delivery. Front. Bioeng. Biotechnol. 8, 228. doi:10.3389/fbioe.2020.00228
Ma, Y. T., Hsieh, T., Forbes, M. E., Johnson, J. E., and Frost, D. O. (1998). BDNF Injected into the Superior Colliculus Reduces Developmental Retinal Ganglion Cell Death. J. Neurosci. 18 (6), 2097–2107. doi:10.1523/jneurosci.18-06-02097.1998
Madduri, S., and Gander, B. (2012). Growth Factor Delivery Systems and Repair Strategies for Damaged Peripheral Nerves. J. Control. Release 161 (2), 274–282. doi:10.1016/j.jconrel.2011.11.036
Maki, T., Arishima, K., Yamamoto, M., and Sakaue, M. (2015). TrkB Is Involved in the Mechanism by Which BDNF Accelerates the Glutamate-Induced Death of Rat Neuroblastoma B35 Cells. Neurol. Res. 37 (1), 30–34. doi:10.1179/1743132814Y.0000000403
Mallone, F., Sacchetti, M., Bruscolini, A., Scuderi, L., Marenco, M., and Lambiase, A. (2020). Neurotrophic Factors in Glaucoma and Innovative Delivery Systems. Appl. Sci. 10 (24), 9015. doi:10.3390/app10249015
Mandolesi, G., Menna, E., Harauzov, A., Von Bartheld, C. S., Caleo, M., and Maffei, L. (2005). A Role for Retinal Brain-Derived Neurotrophic Factor in Ocular Dominance Plasticity. Curr. Biol. 15 (23), 2119–2124. doi:10.1016/j.cub.2005.10.045
Mansour-Robaey, S., Clarke, D. B., Wang, Y. C., Bray, G. M., and Aguayo, A. J. (1994). Effects of Ocular Injury and Administration of Brain-Derived Neurotrophic Factor on Survival and Regrowth of Axotomized Retinal Ganglion Cells. Proc. Natl. Acad. Sci. U. S. A. 91 (5), 1632–1636. doi:10.1073/pnas.91.5.1632
Marler, K. J., Suetterlin, P., Dopplapudi, A., Rubikaite, A., Adnan, J., Maiorano, N. A., et al. (2014). BDNF Promotes Axon Branching of Retinal Ganglion Cells via miRNA-132 and p250GAP. J. Neurosci. 34 (3), 969–979. doi:10.1523/JNEUROSCI.1910-13.2014
Martin, K. R., Quigley, H. A., Zack, D. J., Levkovitch-Verbin, H., Kielczewski, J., Valenta, D., et al. (2003). Gene Therapy with Brain-Derived Neurotrophic Factor as a Protection: Retinal Ganglion Cells in a Rat Glaucoma Model. Invest. Ophthalmol. Vis. Sci. 44 (10), 4357–4365. doi:10.1167/iovs.02-1332
Mathew, B., Ravindran, S., Liu, X., Torres, L., Chennakesavalu, M., Huang, C-C., et al. (2019). Mesenchymal Stem Cell-Derived Extracellular Vesicles and Retinal Ischemia-Reperfusion. Biomaterials 197, 146–160. doi:10.1016/j.biomaterials.2019.01.016
Menna, E., Cenni, M. C., Naska, S., and Maffei, L. (2003). The Anterogradely Transported BDNF Promotes Retinal Axon Remodeling during Eye Specific Segregation within the LGN. Mol. Cell Neurosci. 24 (4), 972–983. doi:10.1016/s1044-7431(03)00258-6
Metoki, T., Ohguro, H., Ohguro, I., Mamiya, K., Ito, T., and Nakazawa, M. (2005). Study of Effects of Antiglaucoma Eye Drops on N-Methyl-D-Aspartate-Induced Retinal Damage. Jpn. J. Ophthalmol. 49 (6), 453–461. doi:10.1007/s10384-005-0253-5
Miller, F. D., and Kaplan, D. R. (2001). Neurotrophin Signalling Pathways Regulating Neuronal Apoptosis. Cell Mol. Life Sci. 58 (8), 1045–1053. doi:10.1007/PL00000919
Minichiello, L. (2009). TrkB Signalling Pathways in LTP and Learning. Nat. Rev. Neurosci. 10 (12), 850–860. doi:10.1038/nrn2738
Miranda, M., Morici, J. F., Zanoni, M. B., and Bekinschtein, P. (2019). Brain-Derived Neurotrophic Factor: A Key Molecule for Memory in the Healthy and the Pathological Brain. Front. Cell Neurosci. 13 (363), 363. doi:10.3389/fncel.2019.00363
Mohd Lazaldin, M. A., Iezhitsa, I., Agarwal, R., Bakar, N. S., Agarwal, P., and Mohd Ismail, N. (2020). Neuroprotective Effects of Brain-Derived Neurotrophic Factor against Amyloid Beta 1-40-induced Retinal and Optic Nerve Damage. Eur. J. Neurosci. 51 (12), 2394–2411. doi:10.1111/ejn.14662
Mojtabavi, H., Saghazadeh, A., van den Heuvel, L., Bucker, J., and Rezaei, N. (2020). Peripheral Blood Levels of Brain-Derived Neurotrophic Factor in Patients with Post-traumatic Stress Disorder (PTSD): A Systematic Review and Meta-Analysis. PLoS One 15 (11), e0241928. doi:10.1371/journal.pone.0241928
Munemasa, Y., and Kitaoka, Y. (2013). Molecular Mechanisms of Retinal Ganglion Cell Degeneration in Glaucoma and Future Prospects for Cell Body and Axonal Protection. Front. Cell Neurosci. 6, 60. doi:10.3389/fncel.2012.00060
Ochs, G., Penn, R. D., York, M., Giess, R., Beck, M., Tonn, J., et al. (2000). A Phase I/II Trial of Recombinant Methionyl Human Brain Derived Neurotrophic Factor Administered by Intrathecal Infusion to Patients with Amyotrophic Lateral Sclerosis. Amyotroph. Lateral Scler. Other Mot. Neuron Disord. 1 (3), 201–206. doi:10.1080/14660820050515197
Oddone, F., Roberti, G., Micera, A., Busanello, A., Bonini, S., Quaranta, L., et al. (2017). Exploring Serum Levels of Brain Derived Neurotrophic Factor and Nerve Growth Factor across Glaucoma Stages. PLoS One 12 (1), e0168565. doi:10.1371/journal.pone.0168565
Omer, S., and Zelkó, R. (2021). A Systematic Review of Drug-Loaded Electrospun Nanofiber-Based Ophthalmic Inserts. Pharmaceutics 13 (10), 1637. doi:10.3390/pharmaceutics13101637
Osborne, A., Khatib, T. Z., Songra, L., Barber, A. C., Hall, K., Kong, G. Y. X., et al. (2018). Neuroprotection of Retinal Ganglion Cells by a Novel Gene Therapy Construct that Achieves Sustained Enhancement of Brain-Derived Neurotrophic Factor/tropomyosin-Related Kinase Receptor-B Signaling. Cell Death Dis. 9 (10), 1007–1018. doi:10.1038/s41419-018-1041-8
Park, H., and Poo, M. M. (2012). Neurotrophin Regulation of Neural Circuit Development and Function. Nat. Rev. Neurosci. 14 (1), 7–23. doi:10.1038/nrn3379
Park, K., Luo, J. M., Hisheh, S., Harvey, A. R., and Cui, Q. (2004). Cellular Mechanisms Associated with Spontaneous and Ciliary Neurotrophic Factor-cAMP-Induced Survival and Axonal Regeneration of Adult Retinal Ganglion Cells. J. Neurosci. 24 (48), 10806–10815. doi:10.1523/JNEUROSCI.3532-04.2004
Pease, M., Zack, D., Berlinicke, C., Bloom, K., Cone, F., Wang, Y., et al. (2009). CNTF Over-expression Leads to Increased Retinal Ganglion Cell Survival in Experimental Glaucoma. Invest. Opthalmol Vis. Sci. 50 (13), 4052. doi:10.1167/iovs.08-3013
Pease, M. E., McKinnon, S. J., Quigley, H. A., Kerrigan-Baumrind, L. A., Baumrind, L. A., and Zack, D. J. (2000). Obstructed Axonal Transport of BDNF and its Receptor TrkB in Experimental Glaucoma. Invest. Ophthalmol. Vis. Sci. 41 (3), 764–774.
Peinado-Ramón, P., Salvador, M., Villegas-Pérez, M. P., and Vidal-Sanz, M. (1996). Effects of Axotomy and Intraocular Administration of NT-4, NT-3, and Brain-Derived Neurotrophic Factor on the Survival of Adult Rat Retinal Ganglion Cells. A Quantitative In Vivo Study. Invest. Ophthalmol. Vis. Sci. 37 (4), 489–500.
Peng, C., Kuang, L., Zhao, J., Ross, A. E., Wang, Z., and Ciolino, J. B. (2022). Bibliometric and Visualized Analysis of Ocular Drug Delivery from 2001 to 2020. J. Control Release 345, 625–645. doi:10.1016/j.jconrel.2022.03.031
Perez, M. T., and Caminos, E. (1995). Expression of Brain-Derived Neurotrophic Factor and of its Functional Receptor in Neonatal and Adult Rat Retina. Neurosci. Lett. 183 (1-2), 96–99. doi:10.1016/0304-3940(94)11123-z
Perlson, E., and Holzbaur, E. L. F. (2007). The Role of Molecular Motors in Axonal Transport. Protein Trafficking in Neurons 2007, 29–43. doi:10.1016/b978-012369437-9/50004-9
Perry, V. H., Oehler, R., and Cowey, A. (1984). Retinal Ganglion Cells that Project to the Dorsal Lateral Geniculate Nucleus in the Macaque Monkey. Neuroscience 12 (4), 1101–1123. doi:10.1016/0306-4522(84)90006-x
Pollock, G. S., Vernon, E., Forbes, M. E., Yan, Q., Ma, Y. T., Hsieh, T., et al. (2001). Effects of Early Visual Experience and Diurnal Rhythms on BDNF mRNA and Protein Levels in the Visual System, hippocampus, and Cerebellum. J. Neurosci. 21 (11), 3923–3931. doi:10.1523/jneurosci.21-11-03923.2001
Pollock, K., Dahlenburg, H., Nelson, H., Fink, K. D., Cary, W., Hendrix, K., et al. (2016). Human Mesenchymal Stem Cells Genetically Engineered to Overexpress Brain-Derived Neurotrophic Factor Improve Outcomes in Huntington's Disease Mouse Models. Mol. Ther. 24 (5), 965–977. doi:10.1038/mt.2016.12
Poo, M. M. (2001). Neurotrophins as Synaptic Modulators. Nat. Rev. Neurosci. 2 (1), 24–32. doi:10.1038/35049004
Quigley, H. A., Katz, J., Derick, R. J., Gilbert, D., and Sommer, A. (1992). An Evaluation of Optic Disc and Nerve Fiber Layer Examinations in Monitoring Progression of Early Glaucoma Damage. Ophthalmology 99 (1), 19–28. doi:10.1016/s0161-6420(92)32018-4
Quigley, H. A., McKinnon, S. J., Zack, D. J., Pease, M. E., Kerrigan-Baumrind, L. A., Baumrind, L. A., et al. (2000). Retrograde Axonal Transport of BDNF in Retinal Ganglion Cells Is Blocked by Acute IOP Elevation in Rats. Invest. Ophthalmol. Vis. Sci. 41 (11), 3460–3466.
Reichardt, L. F. (2006). Neurotrophin-regulated Signalling Pathways. Philos. Trans. R. Soc. Lond B Biol. Sci. 361 (1473), 1545–1564. doi:10.1098/rstb.2006.1894
Reinhard, K., Li, C., Do, Q., Burke, E. G., Heynderickx, S., and Farrow, K. (2019). A Projection Specific Logic to Sampling Visual Inputs in Mouse Superior Colliculus. Elife 8, e50697. doi:10.7554/eLife.50697
Rohde, F., Walther, M., Wächter, J., Knetzger, N., Lotz, C., and Windbergs, M. (2022). In-situ Tear Fluid Dissolving Nanofibers Enable Prolonged Viscosity-Enhanced Dual Drug Delivery to the Eye. Int. J. Pharm. 616, 121513. doi:10.1016/j.ijpharm.2022.121513
Roth, T. L., and Sweatt, J. D. (2011). Epigenetic Marking of the BDNF Gene by Early-Life Adverse Experiences. Horm. Behav. 59 (3), 315–320. doi:10.1016/j.yhbeh.2010.05.005
Rudzinski, M., Wong, T. P., and Saragovi, H. U. (2004). Changes in Retinal Expression of Neurotrophins and Neurotrophin Receptors Induced by Ocular Hypertension. J. Neurobiol. 58 (3), 341–354. doi:10.1002/neu.10293
Ruiz, C. R., Shi, J., and Meffert, M. K. (2014). Transcript Specificity in BDNF-Regulated Protein Synthesis. Neuropharmacology 76, 657–663. doi:10.1016/j.neuropharm.2013.05.004
Rusciano, D., Pezzino, S., Mutolo, M. G., Giannotti, R., Librando, A., and Pescosolido, N. (2017). Neuroprotection in Glaucoma: Old and New Promising Treatments. Adv. Pharmacol. Sci. 2017. doi:10.1155/2017/4320408
Sasi, M., Vignoli, B., Canossa, M., and Blum, R. (2017). Neurobiology of Local and Intercellular BDNF Signaling. Pflugers Arch. 469 (5-6), 593–610. doi:10.1007/s00424-017-1964-4
Schinder, A. F., and Poo, M. (2000). The Neurotrophin Hypothesis for Synaptic Plasticity. Trends Neurosci. 23 (12), 639–645. doi:10.1016/s0166-2236(00)01672-6
Schmidt, N., Schulze, J., Warwas, D. P., Ehlert, N., Lenarz, T., Warnecke, A., et al. (2018). Long-term Delivery of Brain-Derived Neurotrophic Factor (BDNF) from Nanoporous Silica Nanoparticles Improves the Survival of Spiral Ganglion Neurons In Vitro. PLoS One 13 (3), e0194778. doi:10.1371/journal.pone.0194778
Semba, K., Namekata, K., Kimura, A., Harada, C., Mitamura, Y., and Harada, T. (2014). Brimonidine Prevents Neurodegeneration in a Mouse Model of Normal Tension Glaucoma. Cell death Dis. 5 (7), e1341. doi:10.1038/cddis.2014.306
Shalaby, W. S., Ahmed, O. M., Waisbourd, M., and Katz, L. J. (2021). A Review of Potential Novel Glaucoma Therapeutic Options Independent of Intraocular Pressure. Surv Ophthal 2021, 3. doi:10.1016/j.survophthal.2021.12.003
Shen, J., Wang, Y., and Yao, K. (2021). Protection of Retinal Ganglion Cells in Glaucoma: Current Status and Future. Exp. Eye Res. 2021, 108506. doi:10.1016/j.exer.2021.108506
Shen, S., Wiemelt, A. P., McMorris, F. A., and Barres, B. A. (1999). Retinal Ganglion Cells Lose Trophic Responsiveness after Axotomy. Neuron 23 (2), 285–295. doi:10.1016/s0896-6273(00)80780-1
Shoeb Ahmad, S., Abdul Ghani, S., and Hemalata Rajagopal, T. (2013). Current Concepts in the Biochemical Mechanisms of Glaucomatous Neurodegeneration. J. Curr. Glaucoma Pract. 7 (2), 49–53. doi:10.5005/jp-journals-10008-1137
Shpak, A. A., Guekht, A. B., Druzhkova, T. A., Kozlova, K. I., and Gulyaeva, N. V. (2018). Brain-derived Neurotrophic Factor in Patients with Primary Open-Angle Glaucoma and Age-Related Cataract. Curr. Eye Res. 43 (2), 224–231. doi:10.1080/02713683.2017.1396617
Smith, C. A., West, M. E., Sharpe, G. P., Hutchison, D. M., Shuba, L. M., Rafuse, P. E., et al. (2020). Asymmetry Analysis of Macular Optical Coherence Tomography Angiography in Patients with Glaucoma and Healthy Subjects. Br. J. Ophthalmol. 104 (12), 1724–1729. doi:10.1136/bjophthalmol-2019-315592
Soares, A. T., Andreazza, A. C., Rej, S., Rajji, T. K., Gildengers, A. G., Lafer, B., et al. (2016). Decreased Brain-Derived Neurotrophic Factor in Older Adults with Bipolar Disorder. Am. J. Geriatr. Psychiatry 24 (8), 596–601. doi:10.1016/j.jagp.2016.02.052
Spalding, K. L., Rush, R. A., and Harvey, A. R. (2004). Target-derived and Locally Derived Neurotrophins Support Retinal Ganglion Cell Survival in the Neonatal Rat Retina. J. Neurobiol. 60 (3), 319–327. doi:10.1002/neu.20028
Storgaard, L., Tran, T. L., Freiberg, J. C., Hauser, A. S., and Kolko, M. (2021). Glaucoma Clinical Research: Trends in Treatment Strategies and Drug Development. Front. Med. 2021, 1492. doi:10.3389/fmed.2021.733080
Takihara, Y., Inatani, M., Hayashi, H., Adachi, N., Iwao, K., Inoue, T., et al. (2011). Dynamic Imaging of Axonal Transport in Living Retinal Ganglion Cells In Vitro. Invest. Ophthalmol. Vis. Sci. 52 (6), 3039–3045. doi:10.1167/iovs.10-6435
Tanaka, H., Ito, Y., Nakamura, S., Shimazawa, M., and Hara, H. (2009). Involvement of Brain-Derived Neurotrophic Factor in Time-dependent Neurodegeneration in the Murine Superior Colliculus after Intravitreal Injection of N-Methyl-D-Aspartate. Mol. Vis. 15, 662–669.
Tian, K., Shibata-Germanos, S., Pahlitzsch, M., and Cordeiro, M. F. (2015). Current Perspective of Neuroprotection and Glaucoma. Clin. Ophthalmol. Auckl. NZ) 9, 2109. doi:10.2147/OPTH.S80445
Tsai, J. C. (2020). Innovative IOP-independent Neuroprotection and Neuroregeneration Strategies in the Pipeline for Glaucoma. J. Ophthalmol. 2020, 9329310. doi:10.1155/2020/9329310
Tyler, W. J., Perrett, S. P., and Pozzo-Miller, L. D. (2002). The Role of Neurotrophins in Neurotransmitter Release. Neuroscientist 8 (6), 524–531. doi:10.1177/1073858402238511
Vecino, E., García-Crespo, D., García-Grespo, D., García, M., Martinez-Millán, L., Sharma, S. C., et al. (2002). Rat Retinal Ganglion Cells Co-express Brain Derived Neurotrophic Factor (BDNF) and its Receptor TrkB. Vis. Res. 42 (2), 151–157. doi:10.1016/s0042-6989(01)00251-6
Vidal-Sanz, M., Salinas-Navarro, M., Nadal-Nicolás, F. M., Alarcón-Martínez, L., Valiente-Soriano, F. J., de Imperial, J. M., et al. (2012). Understanding Glaucomatous Damage: Anatomical and Functional Data from Ocular Hypertensive Rodent Retinas. Prog. Retin Eye Res. 31 (1), 1–27. doi:10.1016/j.preteyeres.2011.08.001
Weber, A. J., and Harman, C. D. (2013). BDNF Treatment and Extended Recovery from Optic Nerve Trauma in the Cat. Invest. Opthalmol Vis. Sci. 54 (10), 6594–6604. doi:10.1167/iovs.13-12683
Weber, A. J., Viswanáthan, S., Ramanathan, C., and Harman, C. D. (2010). Combined Application of BDNF to the Eye and Brain Enhances Ganglion Cell Survival and Function in the Cat after Optic Nerve Injury. Invest. Ophthalmol. Vis. Sci. 51 (1), 327–334. doi:10.1167/iovs.09-3740
Weinreb, R. N., Aung, T., and Medeiros, F. A. (2014). The Pathophysiology and Treatment of Glaucoma: a Review. Jama 311 (18), 1901–1911. doi:10.1001/jama.2014.3192
Weinreb, R. N., Liebmann, J. M., Cioffi, G. A., Goldberg, I., Brandt, J. D., Johnson, C. A., et al. (2018). Oral Memantine for the Treatment of Glaucoma: Design and Results of 2 Randomized, Placebo-Controlled, Phase 3 Studies. Ophthalmology 125 (12), 1874–1885. doi:10.1016/j.ophtha.2018.06.017
Wen, R., Tao, W., Luo, L., Huang, D., Kauper, K., Stabila, P., et al. (2012). Regeneration of Cone Outer Segments Induced by CNTF. Retinal Degenerative Diseases. Springer, 93–99. doi:10.1007/978-1-4614-0631-0_13
Wens, I., Keytsman, C., Deckx, N., Cools, N., Dalgas, U., and Eijnde, B. O. (2016). Brain Derived Neurotrophic Factor in Multiple Sclerosis: Effect of 24 Weeks Endurance and Resistance Training. Eur. J. Neurol. 23 (6), 1028–1035. doi:10.1111/ene.12976
Wójcik-Gryciuk, A., Gajewska-Woźniak, O., Kordecka, K., Boguszewski, P. M., Waleszczyk, W., and Skup, M. (2020). Neuroprotection of Retinal Ganglion Cells with AAV2-BDNF Pretreatment Restoring Normal TrkB Receptor Protein Levels in Glaucoma. Int. J. Mol. Sci. 21 (17), 6262.
Yacoubian, T. A., and Lo, D. C. (2000). Truncated and Full-Length TrkB Receptors Regulate Distinct Modes of Dendritic Growth. Nat. Neurosci. 3 (4), 342–349. doi:10.1038/73911
Yin, X., He, T., Chen, R., Cui, H., and Li, G. (2020). Impact of Neurotrophic Factors Combination Therapy on Retinitis Pigmentosa. J. Int. Med. Res. 48 (11), 0300060520967833. doi:10.1177/0300060520967833
Zeng, Y., Liu, Y., Wu, M., Liu, J., and Hu, Q. (2012). Activation of TrkB by 7,8-Dihydroxyflavone Prevents Fear Memory Defects and Facilitates Amygdalar Synaptic Plasticity in Aging. J. Alzheimers Dis. 31, 765–778. doi:10.3233/JAD-2012-120886
Zhang, C. W., Lu, Q., You, S. W., Zhi, Y., Yip, H. K., Wu, W., et al. (2005). CNTF and BDNF Have Similar Effects on Retinal Ganglion Cell Survival but Differential Effects on Nitric Oxide Synthase Expression Soon after Optic Nerve Injury. Invest. Ophthalmol. Vis. Sci. 46 (4), 1497–1503. doi:10.1167/iovs.04-0664
Zhang, K., Hopkins, J. J., Heier, J. S., Birch, D. G., Halperin, L. S., Albini, T. A., et al. (2011). Ciliary Neurotrophic Factor Delivered by Encapsulated Cell Intraocular Implants for Treatment of Geographic Atrophy in Age-Related Macular Degeneration. Proc. Natl. Acad. Sci. 108 (15), 6241–6245. doi:10.1073/pnas.1018987108
Keywords: brain-derived neurotrophic factor, glaucoma, neurodegeneration, neuroprotection, retina, retinal ganglion cell
Citation: Lambuk L, Mohd Lazaldin MA, Ahmad S, Iezhitsa I, Agarwal R, Uskoković V and Mohamud R (2022) Brain-Derived Neurotrophic Factor-Mediated Neuroprotection in Glaucoma: A Review of Current State of the Art. Front. Pharmacol. 13:875662. doi: 10.3389/fphar.2022.875662
Received: 14 February 2022; Accepted: 28 April 2022;
Published: 20 May 2022.
Edited by:
Mohd Farooq Shaikh, Monash University, MalaysiaReviewed by:
Ana Raquel Santiago, University of Coimbra, PortugalCopyright © 2022 Lambuk, Mohd Lazaldin, Ahmad, Iezhitsa, Agarwal, Uskoković and Mohamud. This is an open-access article distributed under the terms of the Creative Commons Attribution License (CC BY). The use, distribution or reproduction in other forums is permitted, provided the original author(s) and the copyright owner(s) are credited and that the original publication in this journal is cited, in accordance with accepted academic practice. No use, distribution or reproduction is permitted which does not comply with these terms.
*Correspondence: Rohimah Mohamud, cm9oaW1haG1AdXNtLm15
Disclaimer: All claims expressed in this article are solely those of the authors and do not necessarily represent those of their affiliated organizations, or those of the publisher, the editors and the reviewers. Any product that may be evaluated in this article or claim that may be made by its manufacturer is not guaranteed or endorsed by the publisher.
Research integrity at Frontiers
Learn more about the work of our research integrity team to safeguard the quality of each article we publish.