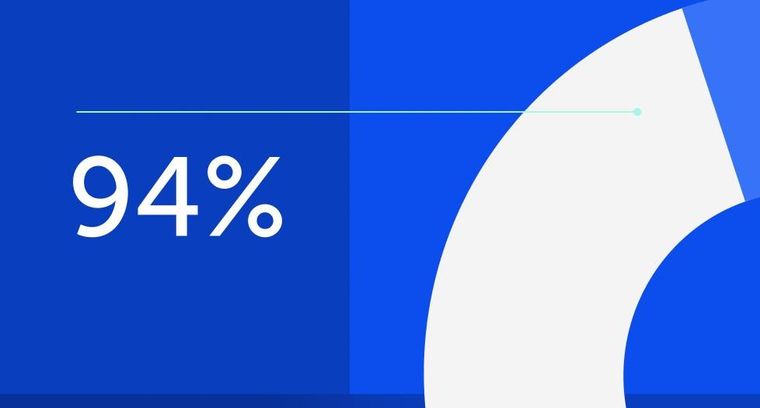
94% of researchers rate our articles as excellent or good
Learn more about the work of our research integrity team to safeguard the quality of each article we publish.
Find out more
REVIEW article
Front. Pharmacol., 02 June 2022
Sec. Gastrointestinal and Hepatic Pharmacology
Volume 13 - 2022 | https://doi.org/10.3389/fphar.2022.871100
This article is part of the Research TopicAdvances in Non-Alcoholic Fatty Liver Disease Therapeutics: Pathogenic Mechanisms and TargetsView all 15 articles
Thyroid hormone/thyroid hormone receptor (TH/TR) axis is characterized by TH with the assistance of plasma membrane transporters to combine with TR and mediate biological activities. Growing evidence suggests that TH/TR participates in plenty of hepatic metabolism. Thus, this review focuses on the role of the TH/TR axis in the liver diseases. To be specific, the TH/TR axis may improve metabolic-associated fatty liver disease, hepatitis, liver fibrosis, and liver injury while exacerbating the progression of acute liver failure and alcoholic liver disease. Also, the TH/TR axis has paradoxical roles in hepatocellular carcinoma. The TH/TR axis may be a prospecting target to cure hepatic diseases.
Thyroid hormones (THs), including thyroid hormones 3,5,3′,5′- tetraiodothyronine or thyroxine (T4) and 3,5,3′-triiodothyronine (T3), are secreted by the thyroid gland to mediate homeostasis of biological growth, development, and metabolism (Senese et al., 2019; Turan and Turksoy, 2021). Thyroid hormone receptor (TR), a member of the nuclear receptor superfamily, is a ligand-dependent transcriptional factor. TR isoforms include TRα1, TRα2, TRβ1, TRβ2, and v-erbA (Ventura-Holman et al., 2011; Knabl et al., 2020). TRα and TRβ are encoded by chromosome 17 and chromosome 3, respectively (Onigata and Szinnai, 2014). V-erbA, acting like a transcriptional suppressor, is a derivant after TRα1 is affected by the avian erythroblastosis virus (AEV) (Ciana et al., 1998). TRα1 is mainly expressed in most peripheral organs except the liver, while TRβ1 is highly expressed in the liver. Although the TRα1 and TRβ1 mRNA levels are similar in metabolically active fats and muscles, protein levels are quite different (TRβ: TRα = 1:10). Moreover, TRβ2 is highly expressed in the pituitary gland, and gender differences in the expression have been found (Minakhina et al., 2020). The active form of TH, T3, and its nuclear receptor assembles ligand-dependent TH/TR complexes, thus regulating gene expression and directing downstream transcriptional activities (Lin et al., 2020a). In addition, thyroid hormone-response element (TRE) is located on the promoters of T3 target genes and affects the activity of the TR transcription response (Cheng, 2005). In pituitary gland, thyroid-stimulating hormone (TSH) is responsible for synthesis and secretion of TH while the encoding genes of TSH are also regulated by TH in a negative way (Bargi-Souza et al., 2017).
Dysfunction of the TH/TR axis leads to numerous pathologies, especially including growth, skeletal development, heart diseases, cognitive dissonance, gastrointestinal function, obesity, dysmetabolism, and cancers (Brent, 2012; Ortiga-Carvalho et al., 2014; Ajdukovic et al., 2021; Moutzouri et al., 2021; Niedowicz et al., 2021; Salman et al., 2021). Therefore, the abnormity of the TH/TR axis elicits a series of diseases, the most common of which is metabolic disease (Malm, 2004). The correlation between the TH/TR axis and many metabolism-associated diseases has been well-elucidated. For example, the TH/TR axis plays a protective role in hyperlipidemia, obesity, and type 2 diabetes (Grover et al., 2007). Intriguingly, the TH/TR axis is intimately associated with the development of the brain and the cerebellar both in fetal and adults (Ishii et al., 2021). Also, the TH/TR axis acts as a promoter in arrhythmia, gastric tumors, and alcoholic-related liver injury (Puhr et al., 2020; Deng et al., 2021). Considering the aforementioned facts, the TH/TR axis may be an indispensable part in maintaining hepatic metabolism.
Indeed, accumulating evidence has demonstrated that the TH/TR axis plays an important role in liver diseases. For instance, TRβ1, a subtype of TH, is highly expressed in the liver, regulating the metabolism of cholesterol and carbohydrates (Dawson and Parini, 2018; Gautherot et al., 2018). Additionally, the TH/TR axis, a strong inducer of hepatic autophagy contributing to lipid droplet degradation, as well as maintaining mitochondrial biogenesis and turnover, causes the removal of damaged mitochondria and ROS, ultimately preventing hepatic injury (Chi et al., 2019). As the TH/TR axis is correlated with various hepatic physiological alterations, more emphasis should be placed on the mechanism of the TH/TR axis in liver diseases. This review summarizes the regulatory mechanism of the TH/TR axis in the liver and focuses on the role of the TH/TR axis in hepatic diseases.
TH has proven to be a hepatic mitogen, thus eliciting hepatocyte proliferating and liver repopulation. López-Fontal et al. (2010) also discovered that hypothyroidism and TR-deficient mice showed delayed recovery of liver mass. Interestingly, hypothyroidism can induce moderate non-alcoholic steatohepatitis, thereby promoting liver regeneration (Rodríguez-Castelán et al., 2017). A large number of studies reported that TRβ is involved in liver regeneration by the TH/TR axis (Sun et al., 2007). For instance, two TRβ agonists, TG68 and IS25, promote hepatocyte proliferation without TH/TR axis-dependent side effects (Perra et al., 2020). This aforementioned finding hints that the regulation of hepatocyte proliferation by the TH/TR axis is of great importance. Accordingly, studies have suggested the effect of the TH/TR axis on hepatocyte proliferation that TH promotes liver regeneration after 50% liver transplantation in mice via elevating histone 3 mRNA, proliferating cell nuclear antigen (PCNA), cyclin-dependent kinase 2 (cdk2), cyclin A, and cyclin D1 levels (Oren et al., 1999; Columbano et al., 2008; Taki-Eldin et al., 2011). The TH/TR axis activates β-catenin to induce hepatocyte proliferation through PKA and Wnt-dependent pathways (Fanti et al., 2014; Alvarado et al., 2016). Moreover, poly (ADP-ribose) polymerase (PARP), a nuclear enzyme involved in cell replication, is involved in the early steps of liver regeneration induced by TH after partial hepatectomy (PH) (Cesarone et al., 2000). The decrease of Dio3 elicits TH-dependent hepatocyte proliferation and liver regeneration (Kester et al., 2009). In addition, T3 bounds to nucleoprotein and then changes the interaction between nucleoprotein and TRE during liver regeneration (Hirose-Kumagai et al., 1995). These studies show that the regulation of hepatocyte proliferation by the TH/TR axis has been gradually demonstrated. Otherwise, the TH/TR axis is also involved in some specific regulation mechanisms of liver regeneration. For instance, Anan et al. (Abu Rmilah et al., 2020) summarized that TH mediates cell cycle regulators and apoptosis in liver regeneration. In addition, T3 improves liver regeneration by promoting the expression of VEGF and its receptor Flt-1 (Bockhorn et al., 2007). These studies suggest that the TH/TR axis may protect hepatocyte proliferation and liver regeneration (Figure 1).
FIGURE 1. Regulatory mechanism of the TH/TR axis in hepatic proliferation, liver regeneration, and MAFLD. The black lines depict the following: the effect of the TH/TR axis in hepatic proliferation and liver regeneration. TH promotes liver regeneration via elevating histone 3 mRNA, PCNA, cdk2, cyclin A, and cyclin D1. The axis activates β-catenin to induce hepatocyte proliferation through PKA and Wnt-dependent mechanisms. PARP participates in liver regeneration induced by TH. The decrease of Dio3 elicits TH-dependent hepatocyte proliferation and liver regeneration. T3 improves liver regeneration by promoting the VEGF and Flt-1 expression. The green lines represent the following: the TH/TR axis might improve MAFLD. Dio3 activates HIF-1a, thus inhibiting T3 signaling. Genes related to reverse cholesterol transport and lipase activity decrease with the downregulation of Dio2 in rats. TH is produced by Dio2 and then depletes H3K9me3. (For interpretation of the references to color in this figure legend, the reader is referred to the Web version of this article).
Hepatocyte proliferation, regeneration, and lipid homeostasis in the liver are involved in many hepatic diseases. Significantly, numerous studies have shown that the prevalence and development of hepatic diseases are related to TH/TR axis abnormity (Mishkin et al., 1981). The interplay between the TH/TR axis and liver diseases are summarized. These diseases mainly include metabolic-associated fatty liver disease (MAFLD), hepatocellular carcinoma (HCC), hepatitis of hepatitis B virus (HBV) and hepatitis C virus (HCV) infection, acute liver failure (ALF), liver fibrosis, alcoholic liver disease, and liver injury.
MAFLD, formerly named as non-alcoholic fatty liver disease, is a serious liver issue worldwide and will be the leading cause of liver transplantation in the forthcoming decades (Méndez-Sánchez and Díaz-Orozco, 2021). A large number of studies reported that TH regulates hepatic triglyceride and cholesterol metabolism (Zhao et al., 2020). Accordingly, TH increases the activity of hepatic lipase, thus enhancing lipid mobilization from fat droplets. Moreover, TR activation triggers free fatty acid transporting into the hepatocytes (Tanase et al., 2020). Considering the aforementioned facts, the TH/TR axis is likely to be intimately correlated with hepatic diseases such as MAFLD. Accumulating evidence has demonstrated that the TH/TR axis is involved in MAFLD. To be specific, MAFLD is positively related with hypothyroidism, elevated TSH, T3, and thyroid peroxidase antibody (TPOAb), and suppressed T4 (Gor et al., 2021; D'Ambrosio et al., 2021).
The fact that whether the TH/TR axis can be a risk factor in MAFLD is not clear. Martínez-Escudé A et al. (2021) reported that TSH, regarded as a risk factor of MAFLD, is involved in obesity, atherogenic dyslipidemia, metabolic syndrome (MetS), hypertransaminasemia, and altered cholesterol and triglycerides levels. Then, a recent research hint that TSH is a MAFLD risk factor but excludes the FT3 and FT4 levels (Tan et al., 2021). Intriguingly, the result in a middle-aged and elderly euthyroid subjects showed that high-normal FT3 and low-normal TSH independently predict the high incidence of MAFLD (Gu et al., 2021). In addition, Chao et al. thought that FT3 and FT4 are independent risk factors to MAFLD. Conversely, although the level of TSH in non-MAFLD and MAFLD subjects who are undergoing health examinations are significantly different, TSH is excluded as an independent risk factor of MAFLD (Zhang X. et al., 2020; Chao and Chen, 2021). As described earlier, there is still controversy to identify TSH and TH as independent risk factors of MAFLD.
Importantly, the TH/TR axis regulate hepatic lipid metabolism such as mitochondrial fatty acid β-oxidation, lipid autophagy, and expression of lipid-related genes (Sinha et al., 2012; Kagawa et al., 2018). Thus, selective TRβ agonists may improve hepatic lipid disorders and MAFLD (Kowalik et al., 2018; Senese et al., 2019; Ritter et al., 2020). These agonists include MGL-3196 (Taub et al., 2013; Raza et al., 2021; Kannt et al., 2021), MB07811 (Erion et al., 2007), KB-141 (Erion et al., 2007), sobetisome (GC-1) (Huang Y. Y. et al., 2013; Ferrara et al., 2018; Saponaro et al., 2020), KB2115 (Eprotirome) (Ladenson et al., 2010a; Senese et al., 2019), and DITPA (Ladenson et al., 2010b; Senese et al., 2019) (Table 1). The side effects of selective TRβ agonists mostly result from TRα-induced dose-dependent cardiac effects, muscle metabolism, and bone turnover (Erion et al., 2007; Kelly et al., 2014). In short, the TH/TR axis may act as a promising treatment method for MAFLD.
HCC is one of the most common malignant tumors. The TH/TR axis is involved in HCC. Some studies have demonstrated that mutations of TR genes are associated with human carcinoma (Anyetei-Anum et al., 2018; Piqué et al., 2020). A clinical study exhibited that hypothyroidism delays hepatocyte growth while hyperthyroidism promotes HCC (Mishkin et al., 1981). Additionally, the level of TR expression in adenomas (83%) and cancer (68%) is significantly lower than that in normal epithelium (96%) (Liu et al., 2019). Moreover, TH-related mitochondrial turnover protects hepatocytes from HBV hepatocarcinogenesis (Chi et al., 2017; Hossain et al., 2020). In addition, the TH/TR axis regulates proliferation, differentiation, metastasis, and drug resistance, autophagy in HCC (Jazdzewski et al., 2011; Rosen and Privalsky, 2011; Jerzak et al., 2015; Liu et al., 2019; Lin et al., 2020a).
Hepatitis of HBV and HCV infection are global issues, which have a risk to develop severe liver disease such as liver cirrhosis and HCC (Jing et al., 2020; Zhang et al., 2021). A study hinted that the FT3 level decreases in HBV patients, while the FT3 and FT4 levels increase in HCV patients (Orságová et al., 2014). More specifically is that along with the increasing inflammatory grade, the level of TT3 primary increased and then decreased, but only the increased level was significantly statistic (Hu et al., 2020). Interestingly, HBV/HCV coinfection elevates the probability of thyroid dysfunction (Ji et al., 2016). Meanwhile, one of the major problems with interferon therapy in hepatitis is the occurrence of aberrant TSH, T3, and T4 values, as well as autoantibodies and thyroid diseases (Ignatova et al., 1998; Orságová et al., 2014; Karwowska et al., 2018). Nevertheless, Huang MJ et al. (1999) indicated that seropositivity of thyroid autoantibodies should not be a contraindication to IFN therapy in HCV-infected patients. Similarly, a recent research reported that the antithyroid antibodies do not cause severe autoimmune disorders in children with chronic HBV infection and merely associated with subclinical hypothyroidism (Kansu et al., 2004).
Acute liver failure (ALF), characterized by elevated liver biochemistry, coagulopathy, and hepatic encephalopathy (HE) but with no underlying chronic liver disease (CLF), is a severe and complex clinical syndrome (Lopes and Samant, 2021). The TH/TR axis may be involved in ALF. On the one hand, type A HE is strongly related to low TSH in ALF patients with a concerning poor survival rate (Anastasiou et al., 2015; Wang et al., 2017). HINAT ACLF has proposed liver failure incorporating TSH into the standard (Feng and Shi, 2018; Wu et al., 2018). Interestingly, type C HE often happens to patients with cirrhosis and lower T3 and T4 levels (Wang et al., 2017). In addition, ALF induced by surgical liver devascularization in female pigs observed a decrease in serum-free T3 and T4 as well as TRα protein levels (Kostopanagiotou et al., 2009). Intriguingly, thioacetamide-induced ALF promotes hepatocyte proliferation in response to T3 in the rat (Malik et al., 2006). On the other hand, hypothyroidism prevents immune-mediated acute liver injury in mice, subsequently elevating TSH levels and survival rates and declining serum liver enzymes, blood ammonia, and prothrombin time. It has been reported that a patient with ALF results from non-controlled hyperthyroidism (Sousa Domínguez, 2015). Clinically, plasma exchange is an effective method to eliminate TH in acute liver failure with thyroid storm (Zeng et al., 2017). Mechanistically, low T3 and T4 levels in hypometabolism-associated hypothyroidism link to inflammation and oxidative stress (Bruck et al., 1998). In general, high TSH levels and low TH/TR functions manifest a protector in ALF.
Liver fibrosis is characterized by chronic inflammation and fibrous scar formation in the liver, finally resulting in hepatocyte deficiency and loss of hepatic function (Erhardtsen et al., 2021). Advanced fibrosis is associated with decreased serum FT3 levels (Du et al., 2021). As an independent risk factor, an elevated TSH level is significantly correlated with the risk of fibrosis (Martínez-Escudé et al., 2021). In a recent study, compared with 12.19% in chronic hepatitis C (CHC) patients without thyroid disease (TD), severe fibrosis is found at 92.85% among CHC patients with TD (Biciusca et al., 2020). However, studies have suggested that hypothyroidism was not highly associated with fibrosis (D'Ambrosio et al., 2021). Treating with TRβ agonist resmetirom in advanced NASH with fibrosis mice have lower α-smooth muscle actin, fibrogenesis-involved genes, and markers of fibrosis, especially including liver stiffness and N-terminal type III collagen pro-peptide (PRO-C3), which indicate that resmetirom can improve fibrosis (Harrison et al., 2021; Kannt et al., 2021). As a result, the TH/TR axis ameliorates liver fibrosis, although the deeper connection between hepatic fibrosis and the TH/TR axis needs more exploration.
Alcoholic liver disease is caused by long-term heavy drinking, initially manifesting as fatty liver, and hepatocyte necrosis, then developing into alcoholic hepatitis, liver fibrosis, cirrhosis, and liver failure (Wang and Mu, 2021). Papineni et al. (2017) indicated that TH-free T3 (fT3) decreases in alcoholic hepatitis and cirrhosis while fT3 and fT4 increase in chronic alcoholic liver disease patients after treatment. More specifically speaking, low fT3 not only probably reflects the severity of liver disease, the degree of liver damage but may also increase the withdrawal effects and craving for alcohol (Nomura et al., 1975; Israel et al., 1979; Burra et al., 1992). However, it has been reported that alcohol and TH also might cause a hypermetabolic state of the liver and liver cell damage. Accordingly, antithyroid drugs can cure alcoholic fatty liver via inhibiting ethanol metabolic rate (EMR) in chronic ethanol-consuming patients (Szilagyi et al., 1983). Overall, the TH/TR axis may aggravate alcoholic liver disease.
Liver injury is caused by multiple factors mainly including some drugs, poisons, or chronic liver, and extrahepatic diseases (Yamamoto, 1995). In CCl4-induced liver injury in rats, the serum T3 level is reduced due to the decreased release of T3 from liver cells rather than a decreased conversion of T4 to T3 (Ikeda et al., 1986). In general, the TH/TR axis protects against liver damage, and thyroid disorder aggravates the development of liver injury. From the protective aspect, T3 replenishment protects against liver injury via improving oxidative stress, cell ferroptosis, detoxification, and increasing drug transport proteins expression, inflammatory factors, autophagy, and lipid metabolism. DNA damage generated by reactive oxygen species upregulates 8-hydroxy-2-deoxyyguanosine (8-OHdG) levels. During diabetes, hypothyroidism, and hypothyroidism with diabetes, the use of TH downregulates the level of 8-OHdG, protein carbonyl content (PCO), protein oxidation, and advanced oxidation protein products (AOPPs) (Altan et al., 2010). In addition, TH synergizes with methylprednisolone (MP) to improve oxidative stress and liver damage and then realizing anti-inflammatory and antioxidant effects (D’Espessailles et al., 2013). Intriguingly, T3 scavenges lipid peroxyl free radicals and improves cell ferroptosis in the LPS/galactosamine-induced liver injury mouse model (Mishima et al., 2020). Moreover, the combined supplementation of T3 and n-3 polyunsaturated fatty acid (n-3 PUFA) in rat decreases ischemia-reperfusion (IR) liver injury and oxidative stress. From the aggravating aspect, hyperthyroidism promotes liver injury. Otherwise, thionamides, methimazole, and propylthiouracil are associated with drug-induced liver injury (LiverTox, 2012; Yan et al., 2017) (Table 2). In a word, the activation of the TH/TR axis can ameliorate liver injury.
TABLE 2. Regulatory mechanism of the TH/TR axis alleviates acute liver injury. The regulatory mechanisms are clarified into three categories, including the effects of protection or exacerbation, treating factors, and changed factors. TH downregulates 8-OHdG, PCO, and AOPP levels. TH can also synergize with MP to improve oxidative stress and liver damage and realize anti-inflammatory and antioxidant effects. T3 scavenges lipid peroxyl free radicals and improves cell. The combined supplementation of T3 and n-3 PUFA was given to rats to decrease IR liver injury and oxidative stress. T3 treatment recovers NF-κB activity, STAT3, TNF-α, and haptoglobin and increases liver GSH depletion and protein oxidation protection against IR. T3 upregulates the liver redox-sensitive nuclear transcription factor Nrf2 DNA, detoxification, and drug transport proteins expression, especially including protein levels of Eh1, NQO1, GST Ya, GST Yp, MRP-2, MRP-3, and MRP-4. The inactivation of Kupffer cell by GdCl3 can suppress T3-induced oxidative stress, thus ameliorating the development of liver injury. T3 induces liver PC against IR supported by triggering AMPK, ultimately accelerating the depletion of inflammatory factors such as hepatic NLRP3 and IL-1β. T3 induces hepatocyte proliferation in toxic liver injury. T3 injection protects liver IR damage by enhancing MEK/ERK/mTORC1 mediated autophagy. TH-induced MAO inhibitors inhibit the activity of MAO protecting against liver injury. The accumulation of TR mRNA may remove negative influences in fluoride-related liver injury via preventing disruption of lipid metabolism, oxidative damage, and apoptosis. Hyperthyroidism promotes liver injury. Thionamides, methimazole, and propylthiouracil are associated with drug-induced liver injury. The Yinning Tablet restores the expression of antiapoptotic Bcl-2 cytosol cytochrome c protein overexpression and downregulates the expression of l-thyroxine-induced overexpressed caspase-9, -8, -3, proapoptotic BAX and Dio1, thus ameliorating TH-induced liver injury in rats through regulating mitochondria-mediated apoptotic signals. (The arrows indicate factors are unregulated or downregulated.)
The regulatory mechanism of the TH/TR axis in liver diseases has been gradually elucidated. However, the understanding of the regulatory mechanism of the TH/TR axis in liver diseases is not entirely clear, which is still being explored. The study on the regulatory mechanism of the TH/TR axis in hepatic diseases is helpful to reveal the importance in liver diseases.
In MAFLD, the deiodinase family members, especially including types 1, 2, and 3 iodothyronine deiodinases (Dio1, 2, and 3) and responsible for the activation and inactivation of TH, can modulate the TH/TR axis. As hepatic enzymes, Dio1 and Dio2 convert T4 to T3 and increase T3/T4 levels. Conversely, Dio3 inactivates TH. To be specific, the activation of Dio3 activates hypoxia-inducible factor 1α (HIF-1α), thus inhibiting T3 signaling and the metabolic rate (Bianco and da Conceição, 2018; Luongo et al., 2019; Russo et al., 2021). Increased Dio1 level promotes β-oxidation of fatty acid and oxidative phosphorylation, then preventing hepatocyte steatosis. Moreover, the level of Dio1 mRNA depends on the dietary conditions. When fed a normal chow diet (NCD), Leprdb mice grows up with severe steatosis with only mild inflammation. The depletion of Dio1 in Leprdb mice upregulates hepatic Tnfa and Co1a1 mRNA levels, which are inflammation and fibrosis biomarkers, respectively (Bruinstroop et al., 2021). Genes related to reverse cholesterol transport and lipase activity decrease with the downregulation of Dio2 in rats (Russo et al., 2021). The sites of de novo DNA hypermethylation (H sites) disrupt long-distant chromatin interactions, looping enhancers, and promoters in hepatocytes. TH produced from Dio2 activation depletes H3K9me3 and interferes with the formation of more than a thousand H sites, subsequently maintaining the liver development and function (Fonseca et al., 2021). In general, the TH/TR axis modulated by the deiodinases may delay MAFLD progression. Nevertheless, the regulatory mechanism of the TH/TR axis in MAFLD remains to be further studied (Figure 1).
The TH/TR axis modulates cyclin-dependent kinase (CDK) and cyclins, MicroRNAs (miRNAs), long non-coding RNA (lncRNA), TGF-β signaling, hedgehog (Hh) (relying on the local deiodinase expression), and other tumor-related genes and -proteins to be involved in the growth, proliferation, and metastasis of HCC (Manka et al., 2018).
In addition, CDKs and regulatory subunits cyclins regulate the cell cycle in mammalian. P21, as a CDK inhibitor, halts G1/S and G2/M transitions of cell cycle progression by inhibiting CDK4,6/cyclin-D and CDK2/cyclin-E, respectively (Karimian et al., 2016). The inhibition of HCC cells growth and proliferation is dependent upon the activation of P21 by the TH/TR axis. A recent study reported that the activation of the TH/TR axis upregulates endoglin in HCC cells, thus restraining P21 polyubiquitination-induced cell proliferation (Lin et al., 2013a). In addition, the TH/TR axis inhibits hepatoma cell growth via repressing UHRF1 and relieves UHRF1-mediated P21 silence (Wu et al., 2015). Furthermore, TH induces the miR-214-3p expression, followed by interfering with the proto-oncogene serine/threonine-protein kinase (PIM-1) and activating P21, thus blocking cell proliferation (Huang et al., 2017). Other CDKs and cyclins also involve in the regulatory mechanism in HCC in a TH/TR axis-dependent fashion. Ezequiel et al. (Ridruejo et al., 2021) suggested that hexachlorobenzene (HCB) is an endocrine disruptor and a liver tumor promoter. In the HepG2 cell line, the depletion of HCB by TH leads to the downregulation of the TGF-β1/pSMAD-2/3 signaling pathway, thus increasing Dio1 levels and decreasing p21 and P27, ultimately suppressing cell proliferation. Beyond that, the silence of TGF-β mice promote the proliferation by increasing the expressions of CDK2, cyclin E, and cyclin A, as well as decreasing the expression of CDKn1a/p21 (Baek et al., 2010). It has been reported that depleting forkhead box M1 (FOXM1) by TH interferes with oncogenic expression of cyclin D1, cyclin E, and CDK2, thereby inhibits HCC cells proliferation (Barrera-Hernandez et al., 1999; Wu et al., 2020). These pathways all manifest that the TH/TR axis closely interacts with p21, CDKs, and its regulatory subunits cyclins to affect cell proliferation in HCC.
Moreover, miRNAs, a class of evolutionarily conserved non-protein-coding small RNA, are responsible for regulating gene expression at the translation level (Oura et al., 2020). The TH/TR axis regulates miRNAs, thereby producing various effects in HCC. For example, the downregulation of the TH/TR axis induces nodule regression and the increased expression of targeted microRNA, miR-27a, miR-181a, miR-204a, and miR-181a in the resistance-hepatocyte rat model (R-H model) and human cirrhotic peritumoral tissue (Frau et al., 2015). Beyond that, miR-214-3p, miR-130b, miR-17, miR-21, miR-424, and miR-503 also participate in the regulation of the TH/TR axis-mediated liver cancer (Huang Y. H. et al., 2013; Lin et al., 2013b; Ruiz-Llorente et al., 2014; Lin et al., 2015). Thus, miRNAs have the potential to be targets in TH/TR-involved HCC.
Furthermore, lncRNA, the human major transcriptional genome, is a length greater than 200 nucleotides, which is a non-coding protein (Dang et al., 2015). A recent study has reported that compared to the non-tumor samples, the expression of lncRNA related genes including MSC-AS1, POLR2J4, EIF3J-AS1, SERHL, RMST, and PVT1 are upregulated in tumor samples. Beyond that, lncRNA genes mostly cluster in the TGF-β signaling pathway, internal ribosome entry pathway, granzyme A mediated apoptosis, FAS signaling pathway, calcium signaling by HBx, and p38/MAPK signaling pathway (Gu et al., 2019). It has been reported that lncRNA CRNDE and lncRNA SNHG7 are independent risk factors of synchronous colorectal liver metastasis (SCLM), which also predict a high tumor recurrence rate (Zhang P. et al., 2020). Since lncRNA is associated with the occurrence of tumor, it is plausible that lncRNA may intimately be regulated by the TH/TR axis. Indeed, the TH/TR axis is related to lncRNA in HCC. For instance, brain cytoplasmic RNA 1 (BCYRN1 or BC200) is widely expressed in tumors. BC200 is also inhibited by T3/TR, then downregulating the expressions of CDK2, cyclin E1, and cyclin E2 and upregulating P21, thereby repressing cell growth and tumor sphere formation and preventing the evolvement of HCC (Lin et al., 2018). Otherwise, the downregulation of taurine upregulated gene 1 (TUG1) by the TH/TR axis also cause AFP mRNA, cyclin E, and H3K27me3 silence and cell growth inhibition (Lin et al., 2020b).
Finally, other TH/TR-related genes and -proteins are involved in HCC. Thyroid hormone receptor-interacting proteins (TRIP), the Zyxin family of LIM proteins, is responsible for regulating transcription of TR. Significantly, the transcriptional activation of the TH/TR axis in HCC may depend on TRIP. Specifically, when TRIP6 activates FOXC1, migration, invasion, and proliferation are strongly promoted. It is also found that TRIP6 induces cyclin D1 expression, decreases p21 and p27 activation, and HCC cell proliferation arrest (Lee et al., 1995; Zhao et al., 2017; Wang et al., 2020). Moreover, the downregulation of TRIP13 impairs the NHEJ repair process, increased apoptosis, and cell cycle arrest at the S-phase, ultimately inhibiting the proliferation, migration, and invasion of HCC cells (Ju et al., 2018). In addition, pituitary tumor transforming gene 1 (PTTG1) is silenced by Sp2, which is negatively mediated by T3/TR in Hep3B hepatoma cells (Chen et al., 2008). Ndrg2 is a Myc suppressor gene. The activation of V-erbA leads to the depletion of Ndrg2, thus exacerbating tumor invasion and metastasis (Ventura-Holman et al., 2011). As a tumor-associated protein, lipocalin 2 (Lcn2) can activate the Met/FAK pathway in a TH/TR axis-dependent manner, thus enhancing tumor cell migration and invasion (Chung et al., 2015). Intriguingly, T3/TR/MEK/ERK/NUPR1/PDGFA cascade may play a vital role in hepatocarcinogenesis. Consistently, T3/TR positively regulates nuclear protein 1(NUPR1) via binding to the NUPR1 promoter regions, therefore promoting vascular invasion (Chen et al., 2019). Recently, it has been found that increasing thyroid hormone responsive (THRSP) prevents the silence of the ERK/ZEB1 signaling pathway and inhibits the process of epithelial-to-mesenchymal transition, subsequently preventing hepatocellular carcinogenesis (Hu et al., 2021). A secreted protein named Dickkopf 4 (DKK 4) antagonizes the Wnt signal pathway and inhibits tumor metastasis, which is dependent upon the activation of the T3/TR axis (Chi et al., 2013).
In short, the TH/TR axis have paradoxical role in the growth, proliferation, invasion, metastasis, and migration of HCC. A relevant regulatory mechanism of the TH/TR axis in HCC remains to be further explored (Figures 2, 3).
FIGURE 2. TH/TR axis is involved in HCC growth, proliferation, invasion, and metastasis. The TH/TR axis upregulates endoglin, thus restraining P21 polyubiquitination-induced cell proliferation. The TH/TR axis inhibits hepatoma cell growth via repressing UHRF1 and relieves UHRF1-mediated P21 silence. TH induces the miR-214-3p expression, followed by interfering with PIM-1 and activating P21, thus blocking cell proliferation. The depletion of HCB by TH downregulates the TGF-β1/pSMAD-2/3 signaling pathway, thus increasing Dio1 levels and decreasing p21 and P27, ultimately suppressing cell proliferation. The silence of TGF-β mice promote the proliferation by increasing the expressions of CDK2, cyclin E, and cyclin A, as well as decreasing the expression of CDKn1a/p21. Depleting FOXM1 by TH interferes with cyclin D1, cyclin E, and CDK2, thereby inhibiting HCC cell proliferation.
FIGURE 3. The TH/TR axis inhibits nodule regression and decreases the expressions of miR-27a, miR-181a, and miR-204a. BC200 is inhibited by T3/TR and downregulates the expressions of CDK2, cyclin E1, and cyclin E2 and upregulates P21, thereby repressing cell growth. The downregulation of TUG1 by the TH/TR axis causes AFP mRNA, cyclin E, and H3K27me3 silence and cell growth inhibition. When TRIP is activated by FOXC1, migration, invasion, and proliferation are strongly promoted. TRIP6 induces the AKT signaling pathway, thereby preventing FOXO3a overexpression-induced cyclin D1 interference, p21 and p27 activation, and HCC cell proliferation arrest. The downregulation of PTTG1 is silenced by Sp2, which is negatively mediated by T3/TR. The activation of V-erbA leads to the depletion of Ndrg2, thus exacerbating tumor invasion and metastasis. Lcn2 can activate the Met/FAK pathway in a TH/TR axis-dependent manner, thus enhancing cell migration and invasion. T3/TR/MEK/ERK/NUPR1/PDGFA cascade may play a vital role in hepatocarcinogenesis. T3/TR upregulates NUPR1 via binding to the NUPR1 promoter regions, therefore promoting vascular invasion. THRSP prevents silence of the ERK/ZEB1 signaling pathway and inhibits the process of epithelial-to-mesenchymal transition. DKK 4 antagonizes the Wnt signal pathway and inhibits tumor metastasis, which depends upon the activation of the T3/TR axis.
In liver injury, the studies show that T3 treatment recovers NF-κB activity, signal transducer, and activator of transcription 3 (STAT3), TNF-α and haptoglobin and increases liver GSH depletion and protein oxidation protecting against IR (Fernández et al., 2007; Mardones et al., 2012). A study exhibited that T3 upregulates the liver redox-sensitive nuclear transcription factor erythroid 2-related factor 2 (Nrf2) DNA, detoxification, and drug transport proteins expression, mainly including protein levels of epoxide hydrolase 1 (Eh1), NADPH-quinone oxidoreductase 1 (NQO1), glutathione-S-transferases Ya (GST Ya), GST Yp, multidrug resistance-associated proteins 2 (MRP-2), mrp-3 ,and MRP-4 in male Sprague–Dawley rats, which may indicate the hepatocyte protective mechanism in liver injury attributed to ROS and chemical toxicity (Cornejo et al., 2013). In addition, the inactivation of Kupffer cell by gadolinium chloride (GdCl3) can suppress T3-induced oxidative stress, thus ameliorating the development of liver injury characterized by neutrophil infiltration and necrosis (Simon-Giavarotti et al., 2002). Accumulating evidence has demonstrated that T3 induces liver preconditioning (PC) against IR supported by triggering AMP-activated protein kinase (AMPK), ultimately accelerating the depletion of inflammatory factors such as hepatic NLRP3 and IL-1β (Fernández et al., 2009; Vargas and Videla, 2017). T3 induces hepatocyte proliferation in toxic liver injury (Malik et al., 2006). T3 injection protects liver IR damage by enhancing MEK/ERK/mTORC1-mediated autophagy in male C57BL/6 mice (Yang et al., 2015). In addition, TH-induced monoamine oxidase (MAO) inhibitors inhibit the activity of MAO protecting against liver injury in rats (Obata and Aomine, 2009). In addition to the TH/TR axis also prevents liver damage. It has been discovered that the accumulation of TR mRNA may remove negative influences in fluoride-related liver injury via preventing disruption of lipid metabolism, oxidative damage, and apoptosis (Bo et al., 2018). Particularly, traditional Chinese medicine is also involved in improving the TH/TR axis-induced liver injury. To be specific, the Yinning Tablet restores the expression of antiapoptotic Bcl-2 cytosol cytochrome c protein and downregulates the expression of l-thyroxine-induced overexpressed caspase-9, -8, -3, proapoptotic BAX and Dio1, thus ameliorating TH-induced liver injury in rats via regulating mitochondria-mediated apoptotic signals (Yang et al., 2020).
The TH/TR axis may be correlated with hepatitis. Some studies have demonstrated that ubiquitin-specific protease 18 (USP18), known as UBP43, participates in gene regulations of the TH signaling pathway. Li et al, (2017) discovered that USP18 regulates the signaling of antivirus by the TH signaling pathway, prolactin signaling pathway, insulin resistance and complement, and have crosstalk among them. Also, the thyroid hormone-uncoupling protein (TRUP) gene and thyroid hormone receptor-associated protein 150 alpha gene are associated to the integration of HBV DNA into liver cell DNA, which are the key regulators of cell proliferation and viability (Gozuacik et al., 2001; Paterlini-Bréchot et al., 2003). In recent years, the relationship between the TH/TR axis and hepatitis of HBV and HCV infection has been gradually elucidated. However, understanding of the antiviral mechanism of the TH/TR axis is not entirely clear, which is still being explored.
Furthermore, the TH/TR axis is associated with alcohol-related hepatic alterations. For example, TH has been proven to increase the level of Dio2, thereby elevating susceptibility to hepatic steatosis in a model of alcoholism (Fonseca et al., 2019; Hernandez, 2019). In addition, the mRNA level of TRIP12 is significantly different in alcohol-feed (AF) and control pair-feed (PF) mice (Zhang et al., 2018) (Figure 4).
FIGURE 4. TH/TR axis may be involved in hepatitis of hepatitis B virus and hepatitis C virus infection and accelerates alcoholic liver disease. USP18 regulates the signaling of antivirus by the thyroid hormone signaling pathway. TRUP gene and thyroid hormone receptor associated protein 150 alpha gene are associated to the integration of HBV DNA into the liver cell DNA, which are key regulators of cell proliferation and viability. TH has been proven to increase the level of Dio2, thereby elevating susceptibility to hepatic steatosis in a model of alcoholism. The mRNA level of TRIP12 is significantly different in alcohol liver disease.
Here, we amply reviewed the function of the TH/TR axis in hepatic diseases. The TH/TR axis may protect against metabolic-associated fatty liver disease, hepatitis B virus and hepatitis C virus infection, liver fibrosis, and drug-induced and extrahepatic liver injury but may accelerate the development of acute liver failure and alcoholic liver disease. Meanwhile, the axis has a dual role in hepatocellular carcinoma. As a result, targeting the TH/TR axis should be considered for treating liver disease, which may be a promising disease-reversing strategy for patients.
The total T3 in different stages of inflammation exhibits a trend of first increasing and then decreasing (Hu et al., 2020). Although there is no statistical significance of the total T3 decreases, it is still trustworthy, owing to the fact that the initial stage of TH also occurs booming in acute liver failure. Subsequently, the later declination may be due to the adjustment and balance of the body in different periods. In this retrospective study, the patient’s aggravation is not serious, and the samples are not large enough (n = 6), thus leading to an insignificant decrease.
TH protects liver cells from damage by mediating the HBV/HCV infectious signaling pathway and then improves hepatitis-related carcinogenic transformation. TSH and TT3 are promising aspects to be included in the evaluation criteria for inflammatory activities and served as biological markers to reduce the proportion of liver biopsy and the medical burden. Moreover, the treatment of interferon is not strongly related to autoimmune diseases except for thyroid. However, virus infection and antivirus endeavors lead to format a certain proportion of thyroid autoantibodies and related thyroid diseases such as hypothyroidism, and this cannot be ignored.
To date, it is reported that the mutation of the TRβ gene in thyroid hormone resistance patients leads to the impairment of TRβ signals in the hepatic steatosis. The mutation type of the THRβ gene is the substitution of glycine by arginine at position 243 (R243Q) of TRβ. Compared with patients with WT relatives, serum T3 and T4 of RTH β patients are higher than the upper limit of a reference range. However, there is no significant difference in TSH. They also found that the liver fat content, serum free fatty acids, and HDL cholesterol were higher (Chaves et al., 2021). At present, the fragments related to lipid metabolism are all located in the hinge region of TR. In the future, gene mutations may be used to discover the region that regulates TR lipid metabolism in the hinge region, as well as other regions and their functions, and this pathway exactly can become the therapy targets of NASH/NAFLD and HCC.
Post-translational modifications participate in the occurrence and development of liver disease. Both TRα and TRβ are regulated by the level of PTM, including SUMOylation (Liu and Brent, 2018). The small ubiquitin-like modifier (SUMO) family, existing widely in eukaryotes, is a highly conserved post-translational modification protein that regulate lipid metabolism, inflammatory response, bile acid homeostasis, autophagy, and other related biological functions in nuclear receptors (Zeng et al., 2020; Liu et al., 2021). The research team found that the sumo-3 protein (mainly expressed in the nucleus) and TR receptor have significantly upregulated in oleic acid (OA)-induced NAFLD in WRL68 cells and human liver tissue models (published in Chinese journals). TR can be SUMOylated (Anyetei-Anum et al., 2019). At the same time, nuclear autophagy can improve metabolic disorders (Fu et al., 2018). TR SUMOylation may associate with nuclear autophagy to improve metabolic disorders and prospectively become a therapeutic target in NAFLD.
In summary, the TH/TR axis provides effective insights into the treatment of hepatic diseases. The research and application prospects for the TH/TR axis in liver diseases are promising. TH/TR may provide new potential therapeutic targets
QT wrote the manuscript, and MZ searched for references. NF and LC were responsible for revising the manuscript.
This work was jointly supported by the National Natural Science Foundation of China (81973326), the Hunan Provincial Natural Science Foundation (2019JJ40255; 2021JJ30628), the Innovative Province Construction Special Project of Hunan Province (2021SK4031), the Clinical Medical Technology Innovation Guidance Project of Hunan Province (2020SK51904), the Postgraduate Scientific Research Innovation Project of Hunan Province (CX20210979), the Scientific Research Fund Project of Hunan Provincial Health Commission (20201901), the Scientific Research Project of University of South China [Documents of the University of South China (2019)02], and the Guiding Planning Project of Hengyang [Documents of the Hengyang science and Technology Bureau (2021)51-129].
The authors declare that the research was conducted in the absence of any commercial or financial relationships that could be construed as a potential conflict of interest.
All claims expressed in this article are solely those of the authors and do not necessarily represent those of their affiliated organizations, or those of the publisher, the editors, and the reviewers. Any product that may be evaluated in this article, or claim that may be made by its manufacturer, is not guaranteed or endorsed by the publisher.
Abu Rmilah, A. A., Zhou, W., and Nyberg, S. L. (2020). Hormonal Contribution to Liver Regeneration. Mayo Clin. Proc. Innov. Qual. Outcomes 4 (3), 315–338. doi:10.1016/j.mayocpiqo.2020.02.001
Ajdukovic, M., Vucic, T., and Cvijanovic, M. (2021). Effects of Thiourea on the Skull of Triturus Newts during Ontogeny. PeerJ 9, e11535. doi:10.7717/peerj.11535
Altan, N., Sepici-Dinçel, A., Sahin, D., Kocamanoğlu, N., Kosova, F., and Engin, A. (2010). Oxidative DNA Damage: the Thyroid Hormone-Mediated Effects of Insulin on Liver Tissue. Endocrine 38 (2), 214–220. doi:10.1007/s12020-010-9376-7
Alvarado, T. F., Puliga, E., Preziosi, M., Poddar, M., Singh, S., Columbano, A., et al. (2016). Thyroid Hormone Receptor β Agonist Induces β-Catenin-Dependent Hepatocyte Proliferation in Mice: Implications in Hepatic Regeneration. Gene Expr. 17 (1), 19–34. doi:10.3727/105221616x691631
Anastasiou, O., Sydor, S., Sowa, J. P., Manka, P., Katsounas, A., Syn, W. K., et al. (2015). Higher Thyroid-Stimulating Hormone, Triiodothyronine and Thyroxine Values Are Associated with Better Outcome in Acute Liver Failure. PLoS One 10 (7), e0132189. doi:10.1371/journal.pone.0132189
Anyetei-Anum, C. S., Evans, R. M., Back, A. M., Roggero, V. R., and Allison, L. A. (2019). Acetylation Modulates Thyroid Hormone Receptor Intracellular Localization and Intranuclear Mobility. Mol. Cel Endocrinol 495, 110509. doi:10.1016/j.mce.2019.110509
Anyetei-Anum, C. S., Roggero, V. R., and Allison, L. A. (2018). Thyroid Hormone Receptor Localization in Target Tissues. J. Endocrinol. 237 (1), R19–R34. doi:10.1530/JOE-17-0708
Baek, J. Y., Morris, S. M., Campbell, J., Fausto, N., Yeh, M. M., and Grady, W. M. (2010). TGF-beta Inactivation and TGF-Alpha Overexpression Cooperate in an In Vivo Mouse Model to Induce Hepatocellular Carcinoma that Recapitulates Molecular Features of Human Liver Cancer. Int. J. Cancer 127 (5), 1060–1071. doi:10.1002/ijc.25127
Bargi-Souza, P., Goulart-Silva, F., and Nunes, M. T. (2017). Novel Aspects of T3 Actions on GH and TSH Synthesis and Secretion: Physiological Implications. J. Mol. Endocrinol. 59 (4), R167–R178. doi:10.1530/JME-17-0068
Barrera-Hernandez, G., Park, K. S., Dace, A., Zhan, Q., and Cheng, S. Y. (1999). Thyroid Hormone-Induced Cell Proliferation in GC Cells Is Mediated by Changes in G1 Cyclin/cyclin-dependent Kinase Levels and Activity. Endocrinology 140 (11), 5267–5274. doi:10.1210/endo.140.11.7145
Bianco, A. C., and da Conceição, R. R. (2018). The Deiodinase Trio and Thyroid Hormone Signaling. Methods Mol. Biol. 1801, 67–83. doi:10.1007/978-1-4939-7902-8_8
Biciusca, V., Popescu, M., Petrescu, I. O., Stan, I. S., Durand, P., Petrescu, M., et al. (2020). Hepatic Pathological Features in Naive Patients with Chronic Hepatitis C Who Have Developed Thyroid Disorder. Rom. J. Morphol. Embryol. 61 (4), 1085–1097. doi:10.47162/RJME.61.4.11
Bo, X., Mu, D., Wu, M., Xiao, H., and Wang, H. (2018). The Morphological Changes and Molecular Biomarker Responses in the Liver of Fluoride-Exposed Bufo gargarizans Larvae. Ecotoxicol Environ. Saf. 151, 199–205. doi:10.1016/j.ecoenv.2018.01.027
Bockhorn, M., Frilling, A., Benko, T., Best, J., Sheu, S. Y., Trippler, M., et al. (2007). Tri-iodothyronine as a Stimulator of Liver Regeneration after Partial and Subtotal Hepatectomy. Eur. Surg. Res. 39 (1), 58–63. doi:10.1159/000098443
Brent, G. A. (2012). Mechanisms of Thyroid Hormone Action. J. Clin. Invest. 122 (9), 3035–3043. doi:10.1172/JCI60047
Bruck, R., Oren, R., Shirin, H., Aeed, H., Papa, M., Matas, Z., et al. (1998). Hypothyroidism Minimizes Liver Damage and Improves Survival in Rats with Thioacetamide Induced Fulminant Hepatic Failure. Hepatology 27 (4), 1013–1020. doi:10.1002/hep.510270417
Bruinstroop, E., Zhou, J., Tripathi, M., Yau, W. W., Boelen, A., Singh, B. K., et al. (2021). Early Induction of Hepatic Deiodinase Type 1 Inhibits Hepatosteatosis during NAFLD Progression. Mol. Metab. 53, 101266. doi:10.1016/j.molmet.2021.101266
Burra, P., Franklyn, J. A., Ramsden, D. B., Elias, E., and Sheppard, M. C. (1992). Severity of Alcoholic Liver Disease and Markers of Thyroid and Steroid Status. Postgrad. Med. J. 68 (804), 804–810. doi:10.1136/pgmj.68.804.804
Cesarone, C. F., Scarabelli, L., Demori, I., Balocco, S., and Fugassa, E. (2000). Poly(ADP-ribose) Polymerase Is Affected Early by Thyroid State during Liver Regeneration in Rats. Am. J. Physiol. Gastrointest. Liver Physiol. 279 (6), G1219–G1225. doi:10.1152/ajpgi.2000.279.6.G1219
Chao, G., and Chen, L. (2021). Study on the Independent Effect of Thyroid Hormone Based on Uric Acid Level on NAFLD. J. Health Popul. Nutr. 40 (1), 21. doi:10.1186/s41043-021-00247-w
Chaves, C., Bruinstroop, E., Refetoff, S., Yen, P. M., and Anselmo, J. (2021). Increased Hepatic Fat Content in Patients with Resistance to Thyroid Hormone Beta. Thyroid 31 (7), 1127–1134. doi:10.1089/thy.2020.0651
Chen, C. Y., Wu, S. M., Lin, Y. H., Chi, H. C., Lin, S. L., Yeh, C. T., et al. (2019). Induction of Nuclear Protein-1 by Thyroid Hormone Enhances Platelet-Derived Growth Factor A Mediated Angiogenesis in Liver Cancer. Theranostics 9 (8), 2361–2379. doi:10.7150/thno.29628
Chen, R. N., Huang, Y. H., Yeh, C. T., Liao, C. H., and Lin, K. H. (2008). Thyroid Hormone Receptors Suppress Pituitary Tumor Transforming Gene 1 Activity in Hepatoma. Cancer Res. 68 (6), 1697–1706. doi:10.1158/0008-5472.CAN-07-5492
Cheng, S. Y. (2005). Thyroid Hormone Receptor Mutations and Disease: beyond Thyroid Hormone Resistance. Trends Endocrinol. Metab. 16 (4), 176–182. doi:10.1016/j.tem.2005.03.008
Chi, H. C., Chen, S. L., Lin, S. L., Tsai, C. Y., Chuang, W. Y., Lin, Y. H., et al. (2017). Thyroid Hormone Protects Hepatocytes from HBx-Induced Carcinogenesis by Enhancing Mitochondrial Turnover. Oncogene 36 (37), 5274–5284. doi:10.1038/onc.2017.136
Chi, H. C., Liao, C. H., Huang, Y. H., Wu, S. M., Tsai, C. Y., Liao, C. J., et al. (2013). Thyroid Hormone Receptor Inhibits Hepatoma Cell Migration through Transcriptional Activation of Dickkopf 4. Biochem. Biophys. Res. Commun. 439 (1), 60–65. doi:10.1016/j.bbrc.2013.08.028
Chi, H. C., Tsai, C. Y., Tsai, M. M., Yeh, C. T., and Lin, K. H. (2019). Molecular Functions and Clinical Impact of Thyroid Hormone-Triggered Autophagy in Liver-Related Diseases. J. Biomed. Sci. 26 (1), 24. doi:10.1186/s12929-019-0517-x
Chung, I. H., Chen, C. Y., Lin, Y. H., Chi, H. C., Huang, Y. H., Tai, P. J., et al. (2015). Thyroid Hormone-Mediated Regulation of Lipocalin 2 through the Met/FAK Pathway in Liver Cancer. Oncotarget 6 (17), 15050–15064. doi:10.18632/oncotarget.3670
Ciana, P., Braliou, G. G., Demay, F. G., von Lindern, M., Barettino, D., Beug, H., et al. (1998). Leukemic Transformation by the V-ErbA Oncoprotein Entails Constitutive Binding to and Repression of an Erythroid Enhancer In Vivo. EMBO J. 17 (24), 7382–7394. doi:10.1093/emboj/17.24.7382
Columbano, A., Simbula, M., Pibiri, M., Perra, A., Deidda, M., Locker, J., et al. (2008). Triiodothyronine Stimulates Hepatocyte Proliferation in Two Models of Impaired Liver Regeneration. Cell Prolif 41 (3), 521–531. doi:10.1111/j.1365-2184.2008.00532.x
Cornejo, P., Vargas, R., and Videla, L. A. (2013). Nrf2-regulated Phase-II Detoxification Enzymes and Phase-III Transporters Are Induced by Thyroid Hormone in Rat Liver. Biofactors 39 (5), 514–521. doi:10.1002/biof.1094
D'Ambrosio, R., Campi, I., Maggioni, M., Perbellini, R., Giammona, E., Stucchi, R., et al. (2021). The Relationship between Liver Histology and Thyroid Function Tests in Patients with Non-alcoholic Fatty Liver Disease (NAFLD). PLoS One 16 (4), e0249614. doi:10.1371/journal.pone.0249614
D'Espessailles, A., Dossi, C., Intriago, G., Leiva, P., and Romanque, P. (2013). Hormonal Pretreatment Preserves Liver Regenerative Capacity and Minimizes Inflammation after Partial Hepatectomy. Ann. Hepatol. 12 (6), 881–891. doi:10.1016/s1665-2681(19)31293-1
Dang, Y., Wang, Y., Ouyang, X., Wang, L., and Huang, Q. (2015). High Expression of IncRNA-PCNA-AS1 in Human Gastric Cancer and its Clinical Significances. Clin. Lab. 61 (11), 1679–1685. doi:10.7754/clin.lab.2015.150312
Dawson, P. A., and Parini, P. (2018). Hepatic Thyroid Hormone Receptor β1 Agonism: Good for Lipids, Good for Bile? J. Lipid Res. 59 (9), 1551–1553. doi:10.1194/jlr.C088955
Deng, J., Guo, Y., Zhang, G., Zhang, L., Kem, D., Yu, X., et al. (2021). M2 Muscarinic Autoantibodies and Thyroid Hormone Promote Susceptibility to Atrial Fibrillation and Sinus Tachycardia in an Autoimmune Rabbit Model. Exp. Physiol. 106 (4), 882–890. doi:10.1113/EP089284
Du, J., Chai, S., Zhao, X., Sun, J., Zhang, X., and Huo, L. (2021). Association between Thyroid Hormone Levels and Advanced Liver Fibrosis in Patients with Type 2 Diabetes Mellitus and Non-alcoholic Fatty Liver Disease. Diabetes Metab. Syndr. Obes. 14, 2399–2406. doi:10.2147/DMSO.S313503
Erhardtsen, E., Rasmussen, D. G. K., Frederiksen, P., Leeming, D. J., Shevell, D., Gluud, L. L., et al. (2021). Determining a Healthy Reference Range and Factors Potentially Influencing PRO-C3 - A Biomarker of Liver Fibrosis. JHEP Rep. 3 (4), 100317. doi:10.1016/j.jhepr.2021.100317
Erion, M. D., Cable, E. E., Ito, B. R., Jiang, H., Fujitaki, J. M., Finn, P. D., et al. (2007). Targeting Thyroid Hormone Receptor-Beta Agonists to the Liver Reduces Cholesterol and Triglycerides and Improves the Therapeutic index. Proc. Natl. Acad. Sci. U S A. 104 (39), 15490–15495. doi:10.1073/pnas.0702759104
Fanti, M., Singh, S., Ledda-Columbano, G. M., Columbano, A., and Monga, S. P. (2014). Tri-iodothyronine Induces Hepatocyte Proliferation by Protein Kinase A-dependent β-catenin Activation in Rodents. Hepatology 59 (6), 2309–2320. doi:10.1002/hep.26775
Feng, X., and Shi, X. F. (2018). Research Progress in Prognostic Factors of Hepatitis B Virus-Associated End-Stage Liver Disease. Zhonghua Gan Zang Bing Za Zhi 26 (8), 626–629. doi:10.3760/cma.j.issn.1007-3418.2018.08.014
Fernández, V., Castillo, I., Tapia, G., Romanque, P., Uribe-Echevarría, S., Uribe, M., et al. (2007). Thyroid Hormone Preconditioning: protection against Ischemia-Reperfusion Liver Injury in the Rat. Hepatology 45 (1), 170–177. doi:10.1002/hep.21476
Fernández, V., Tapia, G., Varela, P., Cornejo, P., and Videla, L. A. (2009). Upregulation of Liver Inducible Nitric Oxide Synthase Following Thyroid Hormone Preconditioning: Suppression by N-Acetylcysteine. Biol. Res. 42 (4), 487–495. doi:10.4067/S0716-97602009000400010
Ferrara, S. J., Bourdette, D., and Scanlan, T. S. (2018). Hypothalamic-Pituitary-Thyroid Axis Perturbations in Male Mice by CNS-Penetrating Thyromimetics. Endocrinology 159 (7), 2733–2740. doi:10.1210/en.2018-00065
Fonseca, T. L., Fernandes, G. W., Bocco, B. M. L. C., Keshavarzian, A., Jakate, S., Donohue, T. M., et al. (2019). Hepatic Inactivation of the Type 2 Deiodinase Confers Resistance to Alcoholic Liver Steatosis. Alcohol. Clin. Exp. Res. 43 (7), 1376–1383. doi:10.1111/acer.14027
Fonseca, T. L., Garcia, T., Fernandes, G. W., Nair, T. M., and Bianco, A. C. (2021). Neonatal Thyroxine Activation Modifies Epigenetic Programming of the Liver. Nat. Commun. 12 (1), 4446. doi:10.1038/s41467-021-24748-8
Frau, C., Loi, R., Petrelli, A., Perra, A., Menegon, S., Kowalik, M. A., et al. (2015). Local Hypothyroidism Favors the Progression of Preneoplastic Lesions to Hepatocellular Carcinoma in Rats. Hepatology 61 (1), 249–259. doi:10.1002/hep.27399
Fu, N., Yang, X., and Chen, L. (2018). Nucleophagy Plays a Major Role in Human Diseases. Curr. Drug Targets 19 (15), 1767–1773. doi:10.2174/1389450119666180518112350
Gautherot, J., Claudel, T., Cuperus, F., Fuchs, C. D., Falguières, T., and Trauner, M. (2018). Thyroid Hormone Receptor β1 Stimulates ABCB4 to Increase Biliary Phosphatidylcholine Excretion in Mice. J. Lipid Res. 59 (9), 1610–1619. doi:10.1194/jlr.M084145
Gor, R., Siddiqui, N. A., Wijeratne Fernando, R., Sreekantan Nair, A., Illango, J., Malik, M., et al. (2021). Unraveling the Role of Hypothyroidism in Non-alcoholic Fatty Liver Disease Pathogenesis: Correlations, Conflicts, and the Current Stand. Cureus 13 (5), e14858. doi:10.7759/cureus.14858
Gozuacik, D., Murakami, Y., Saigo, K., Chami, M., Mugnier, C., Lagorce, D., et al. (2001). Identification of Human Cancer-Related Genes by Naturally Occurring Hepatitis B Virus DNA Tagging. Oncogene 20 (43), 6233–6240. doi:10.1038/sj.onc.1204835
Grover, G. J., Mellström, K., and Malm, J. (2007). Therapeutic Potential for Thyroid Hormone Receptor-Beta Selective Agonists for Treating Obesity, Hyperlipidemia and Diabetes. Curr. Vasc. Pharmacol. 5 (2), 141–154. doi:10.2174/157016107780368271
Gu, J. X., Zhang, X., Miao, R. C., Xiang, X. H., Fu, Y. N., Zhang, J. Y., et al. (2019). Six-long Non-coding RNA Signature Predicts Recurrence-free Survival in Hepatocellular Carcinoma. World J. Gastroenterol. 25 (2), 220–232. doi:10.3748/wjg.v25.i2.220
Gu, Y., Wu, X., Zhang, Q., Liu, L., Meng, G., Wu, H.., et al. (2021). High-normal Thyroid Function Predicts Incident Non-alcoholic Fatty Liver Disease Among Middle-Aged and Elderly Euthyroid Subjects. J. Gerontol. A. Biol. Sci. Med. Sci. 77 (1), 197–203. doi:10.1093/gerona/glab037
Harrison, S. A., Bashir, M., Moussa, S. E., McCarty, K., Pablo Frias, J., Taub, R., et al. (2021). Effects of Resmetirom on Noninvasive Endpoints in a 36-Week Phase 2 Active Treatment Extension Study in Patients with NASH. Hepatol. Commun. 5 (4), 573–588. doi:10.1002/hep4.1657
Hernandez, A. (2019). Thyroid Hormone and Alcoholic Fatty Liver: The Developmental Input. Alcohol. Clin. Exp. Res. 43 (9), 1834–1837. doi:10.1111/acer.14145
Hirose-Kumagai, A., Oda-Tamai, S., and Akamatsu, N. (1995). The Interaction between Nucleoproteins and Thyroid Response Element (TRE) during Regenerating Rat Liver. Biochem. Mol. Biol. Int. 35 (4), 881–888.
Hossain, M. G., Akter, S., Ohsaki, E., and Ueda, K. (2020). Impact of the Interaction of Hepatitis B Virus with Mitochondria and Associated Proteins. Viruses 12 (2), 175. doi:10.3390/v12020175
Hu, J., Wang, Y., Jiang, G., Zheng, J., Chen, T., Chen, Z., et al. (2020). Predictors of Inflammatory Activity in Treatment-Naive Hepatitis B E-Antigen-Negative Patients with Chronic Hepatitis B Infection. J. Int. Med. Res. 48 (11), 300060520969582. doi:10.1177/0300060520969582
Hu, Q., Ma, X., Li, C., Zhou, C., Chen, J., and Gu, X. (2021). Downregulation of THRSP Promotes Hepatocellular Carcinoma Progression by Triggering ZEB1 Transcription in an ERK-dependent Manner. J. Cancer 12 (14), 4247–4256. doi:10.7150/jca.51657
Huang, M. J., Tsai, S. L., Huang, B. Y., Sheen, I. S., Yeh, C. T., and Liaw, Y. F. (1999). Prevalence and Significance of Thyroid Autoantibodies in Patients with Chronic Hepatitis C Virus Infection: a Prospective Controlled Study. Clin. Endocrinol. (Oxf) 50 (4), 503–509. doi:10.1046/j.1365-2265.1999.00686.x
Huang, P. S., Lin, Y. H., Chi, H. C., Chen, P. Y., Huang, Y. H., Yeh, C. T., et al. (2017). Thyroid Hormone Inhibits Growth of Hepatoma Cells through Induction of miR-214. Sci. Rep. 7 (1), 14868. doi:10.1038/s41598-017-14864-1
Huang, Y. H., Lin, Y. H., Chi, H. C., Liao, C. H., Liao, C. J., Wu, S. M., et al. (2013). Thyroid Hormone Regulation of miR-21 Enhances Migration and Invasion of Hepatoma. Cancer Res. 73 (8), 2505–2517. doi:10.1158/0008-5472.CAN-12-2218
Huang, Y. Y., Gusdon, A. M., and Qu, S. (2013). Cross-talk between the Thyroid and Liver: a New Target for Nonalcoholic Fatty Liver Disease Treatment. World J. Gastroenterol. 19 (45), 8238–8246. doi:10.3748/wjg.v19.i45.8238
Ignatova, T. M., Aprosina, Z. G., Serov, V. V., Mukhin, N. A., Krel', P. E., Semenkova, E. N., et al. (1998). Extrahepatic Manifestations of Chronic Hepatitis C. Ter Arkh 70 (11), 9–16.
Ikeda, T., Ito, Y., Murakami, I., Mokuda, O., Tominaga, M., and Mashiba, H. (1986). Conversion of T4 to T3 in Perfused Liver of Rats with Carbontetrachloride-Induced Liver Injury. Acta Endocrinol. (Copenh) 112 (1), 89–92. doi:10.1530/acta.0.1120089
Ishii, S., Amano, I., and Koibuchi, N. (2021). The Role of Thyroid Hormone in the Regulation of Cerebellar Development. Endocrinol. Metab. (Seoul) 36 (4), 703–716. doi:10.3803/EnM.2021.1150
Israel, Y., Walfish, P. G., Orrego, H., Blake, J., and Kalant, H. (1979). Thyroid Hormones in Alcoholic Liver Disease. Effect of Treatment with 6-N-Propylthiouracil. Gastroenterology 76 (1), 116–122. doi:10.1016/s0016-5085(79)80137-7
Jazdzewski, K., Boguslawska, J., Jendrzejewski, J., Liyanarachchi, S., Pachucki, J., Wardyn, K. A., et al. (2011). Thyroid Hormone Receptor Beta (THRB) Is a Major Target Gene for microRNAs Deregulated in Papillary Thyroid Carcinoma (PTC). J. Clin. Endocrinol. Metab. 96 (3), E546–E553. doi:10.1210/jc.2010-1594
Jerzak, K. J., Cockburn, J., Pond, G. R., Pritchard, K. I., Narod, S. A., Dhesy-Thind, S. K., et al. (2015). Thyroid Hormone Receptor α in Breast Cancer: Prognostic and Therapeutic Implications. Breast Cancer Res. Treat. 149 (1), 293–301. doi:10.1007/s10549-014-3235-9
Ji, S., Jin, C., Höxtermann, S., Fuchs, W., Xie, T., Lu, X., et al. (2016). Prevalence and Influencing Factors of Thyroid Dysfunction in HIV-Infected Patients. Biomed. Res. Int. 2016, 3874257. doi:10.1155/2016/3874257
Jing, W., Liu, J., and Liu, M. (2020). Eliminating Mother-To-Child Transmission of HBV: Progress and Challenges in China. Front. Med. 14 (1), 21–29. doi:10.1007/s11684-020-0744-2
Ju, L., Li, X., Shao, J., Lu, R., Wang, Y., and Bian, Z. (2018). Upregulation of Thyroid Hormone Receptor Interactor 13 Is Associated with Human Hepatocellular Carcinoma. Oncol. Rep. 40 (6), 3794–3802. doi:10.3892/or.2018.6767
Kagawa, T., Kozai, M., Masuda, M., Harada, N., Nakahashi, O., Tajiri, M., et al. (2018). Sterol Regulatory Element Binding Protein 1 Trans-activates 25-hydroxy Vitamin D3 24-hydroxylase Gene Expression in Renal Proximal Tubular Cells. Biochem. Biophys. Res. Commun. 500 (2), 275–282. doi:10.1016/j.bbrc.2018.04.058
Kannt, A., Wohlfart, P., Madsen, A. N., Veidal, S. S., Feigh, M., and Schmoll, D. (2021). Activation of Thyroid Hormone Receptor-β Improved Disease Activity and Metabolism Independent of Body Weight in a Mouse Model of Non-alcoholic Steatohepatitis and Fibrosis. Br. J. Pharmacol. 178 (12), 2412–2423. doi:10.1111/bph.15427
Kansu, A., Kuloğlu, Z., Demirçeken, F., and Girgin, N. (2004). Autoantibodies in Children with Chronic Hepatitis B Infection and the Influence of Interferon Alpha. Turk J. Gastroenterol. 15 (4), 213–218. https://pm.yuntsg.com/details.html?pmid=16249973&key=16249973
Karimian, A., Ahmadi, Y., and Yousefi, B. (2016). Multiple Functions of P21 in Cell Cycle, Apoptosis and Transcriptional Regulation after DNA Damage. DNA Repair (Amst) 42, 63–71. doi:10.1016/j.dnarep.2016.04.008
Karwowska, K., Wernik, J., and Abdulgater, A. (2018). New Possibilities for the Newborn's protection against Vertical HBV Trasmission - a Case Report. Pol. Merkur Lekarski 45 (269), 192–194. https://pm.yuntsg.com/details.html?pmid=30531668&key=30531668
Kelly, M. J., Pietranico-Cole, S., Larigan, J. D., Haynes, N. E., Reynolds, C. H., Scott, N., et al. (2014). Discovery of 2-[3,5-Dichloro-4-(5-Isopropyl-6-Oxo-1,6-Dihydropyridazin-3-Yloxy)phenyl]-3,5-Dioxo-2,3,4,5-Tetrahydro[1,2,4]triazine-6-Carbonitrile (MGL-3196), a Highly Selective Thyroid Hormone Receptor β Agonist in Clinical Trials for the Treatment of Dyslipidemia. J. Med. Chem. 57 (10), 3912–3923. doi:10.1021/jm4019299
Kester, M. H., Toussaint, M. J., Punt, C. A., Matondo, R., Aarnio, A. M., Darras, V. M., et al. (2009). Large Induction of Type III Deiodinase Expression after Partial Hepatectomy in the Regenerating Mouse and Rat Liver. Endocrinology 150 (1), 540–545. doi:10.1210/en.2008-0344
Knabl, J., de Maiziere, L., Hüttenbrenner, R., Hutter, S., Jückstock, J., Mahner, S., et al. (2020). Cell Type- and Sex-specific Dysregulation of Thyroid Hormone Receptors in Placentas in Gestational Diabetes Mellitus. Int. J. Mol. Sci. 21 (11), 4056. doi:10.3390/ijms21114056
Kostopanagiotou, G., Kalimeris, K., Mourouzis, I., Kostopanagiotou, K., Arkadopoulos, N., Panagopoulos, D., et al. (2009). Thyroid Hormones Alterations during Acute Liver Failure: Possible Underlying Mechanisms and Consequences. Endocrine 36 (2), 198–204. doi:10.1007/s12020-009-9210-2
Kowalik, M. A., Columbano, A., and Perra, A. (2018). Thyroid Hormones, Thyromimetics and Their Metabolites in the Treatment of Liver Disease. Front. Endocrinol. (Lausanne) 9, 382. doi:10.3389/fendo.2018.00382
Ladenson, P. W., Kristensen, J. D., Ridgway, E. C., Olsson, A. G., Carlsson, B., Klein, I., et al. (2010a). Use of the Thyroid Hormone Analogue Eprotirome in Statin-Treated Dyslipidemia. N. Engl. J. Med. 362 (10), 906–916. doi:10.1056/NEJMoa0905633
Ladenson, P. W., McCarren, M., Morkin, E., Edson, R. G., Shih, M. C., Warren, S. R., et al. (2010b). Effects of the Thyromimetic Agent Diiodothyropropionic Acid on Body Weight, Body Mass index, and Serum Lipoproteins: a Pilot Prospective, Randomized, Controlled Study. J. Clin. Endocrinol. Metab. 95 (3), 1349–1354. doi:10.1210/jc.2009-1209
Lee, J. W., Choi, H. S., Gyuris, J., Brent, R., and Moore, D. D. (1995). Two Classes of Proteins Dependent on Either the Presence or Absence of Thyroid Hormone for Interaction with the Thyroid Hormone Receptor. Mol. Endocrinol. 9 (2), 243–254. doi:10.1210/mend.9.2.7776974
Li, L., Lei, Q. S., Kong, L. N., Zhang, S. J., and Qin, B. (2017). Gene Expression Profile after Knockdown of USP18 in Hepg2.2.15 Cells. J. Med. Virol. 89 (11), 1920–1930. doi:10.1002/jmv.24819
Lin, Y. H., Huang, Y. H., Wu, M. H., Wu, S. M., Chi, H. C., Liao, C. J., et al. (2013a). Thyroid Hormone Suppresses Cell Proliferation through Endoglin-Mediated Promotion of P21 Stability. Oncogene 32 (33), 3904–3914. doi:10.1038/onc.2013.5
Lin, Y. H., Liao, C. J., Huang, Y. H., Wu, M. H., Chi, H. C., Wu, S. M., et al. (2013b). Thyroid Hormone Receptor Represses miR-17 Expression to Enhance Tumor Metastasis in Human Hepatoma Cells. Oncogene 32 (38), 4509–4518. doi:10.1038/onc.2013.309
Lin, Y. H., Lin, K. H., and Yeh, C. T. (2020a). Thyroid Hormone in Hepatocellular Carcinoma: Cancer Risk, Growth Regulation, and Anticancer Drug Resistance. Front. Med. (Lausanne) 7, 174. doi:10.3389/fmed.2020.00174
Lin, Y. H., Wu, M. H., Huang, Y. H., Yeh, C. T., Chi, H. C., Tsai, C. Y., et al. (2018). Thyroid Hormone Negatively Regulates Tumorigenesis through Suppression of BC200. Endocr. Relat. Cancer 25 (12), 967–979. doi:10.1530/ERC-18-0176
Lin, Y. H., Wu, M. H., Huang, Y. H., Yeh, C. T., and Lin, K. H. (2020b). TUG1 Is a Regulator of AFP and Serves as Prognostic Marker in Non-hepatitis B Non-hepatitis C Hepatocellular Carcinoma. Cells 9 (2), 262. doi:10.3390/cells9020262
Lin, Y. H., Wu, M. H., Liao, C. J., Huang, Y. H., Chi, H. C., Wu, S. M., et al. (2015). Repression of microRNA-130b by Thyroid Hormone Enhances Cell Motility. J. Hepatol. 62 (6), 1328–1340. doi:10.1016/j.jhep.2014.12.035
Liu, W., Zeng, M., and Fu, N. (2021). Functions of Nuclear Receptors SUMOylation. Clin. Chim. Acta 516, 27–33. doi:10.1016/j.cca.2021.01.007
Liu, Y. C., Yeh, C. T., and Lin, K. H. (2019). Molecular Functions of Thyroid Hormone Signaling in Regulation of Cancer Progression and Anti-apoptosis. Int. J. Mol. Sci. 20 (20), 4986. doi:10.3390/ijms20204986
Liu, Y. Y., and Brent, G. A. (2018). Posttranslational Modification of Thyroid Hormone Nuclear Receptor by Sumoylation. Methods Mol. Biol. 1801, 47–59. doi:10.1007/978-1-4939-7902-8_6
LiverTox (2012). “Antithyroid Agents,” in LiverTox: Clinical and Research Information on Drug-Induced Liver Injury (Bethesda (MD): National Institute of Diabetes and Digestive and Kidney Diseases).
Lopes, D., and Samant, H. (2021). “Hepatic Failure,” in StatPearls (Treasure Island (FL): StatPearls Publishing).
López-Fontal, R., Zeini, M., Través, P. G., Gómez-Ferrería, M., Aranda, A., Sáez, G. T., et al. (2010). Mice Lacking Thyroid Hormone Receptor Beta Show Enhanced Apoptosis and Delayed Liver Commitment for Proliferation after Partial Hepatectomy. PLoS One 5 (1), e8710. doi:10.1371/journal.pone.0008710
Luongo, C., Dentice, M., and Salvatore, D. (2019). Deiodinases and Their Intricate Role in Thyroid Hormone Homeostasis. Nat. Rev. Endocrinol. 15 (8), 479–488. doi:10.1038/s41574-019-0218-2
Malik, R., Saich, R., Rahman, T., and Hodgson, H. (2006). During Thioacetamide-Induced Acute Liver Failure, the Proliferative Response of Hepatocytes to Thyroid Hormone Is Maintained, Indicating a Potential Therapeutic Approach to Toxin-Induced Liver Disease. Dig. Dis. Sci. 51 (12), 2235–2241. doi:10.1007/s10620-006-9275-1
Malm, J. (2004). Thyroid Hormone Ligands and Metabolic Diseases. Curr. Pharm. Des. 10 (28), 3525–3532. doi:10.2174/1381612043382873
Manka, P., Coombes, J. D., Boosman, R., Gauthier, K., Papa, S., and Syn, W. K. (2018). Thyroid Hormone in the Regulation of Hepatocellular Carcinoma and its Microenvironment. Cancer Lett. 419, 175–186. doi:10.1016/j.canlet.2018.01.055
Mardones, M., Valenzuela, R., Romanque, P., Covarrubias, N., Anghileri, F., Fernández, V., et al. (2012). Prevention of Liver Ischemia Reperfusion Injury by a Combined Thyroid Hormone and Fish Oil Protocol. J. Nutr. Biochem. 23 (9), 1113–1120. doi:10.1016/j.jnutbio.2011.06.004
Martínez-Escudé, A., Pera, G., Costa-Garrido, A., Rodríguez, L., Arteaga, I., Expósito-Martínez, C., et al. (2021). TSH Levels as an Independent Risk Factor for NAFLD and Liver Fibrosis in the General Population. J. Clin. Med. 10 (13), 2907. doi:10.3390/jcm10132907
Méndez-Sánchez, N., and Díaz-Orozco, L. E. (2021). Editorial: International Consensus Recommendations to Replace the Terminology of Non-alcoholic Fatty Liver Disease (NAFLD) with Metabolic-Associated Fatty Liver Disease (MAFLD). Med. Sci. Monit. 27, e933860. doi:10.12659/MSM.933860
Minakhina, S., Bansal, S., Zhang, A., Brotherton, M., Janodia, R., De Oliveira, V., et al. (2020). A Direct Comparison of Thyroid Hormone Receptor Protein Levels in Mice Provides Unexpected Insights into Thyroid Hormone Action. Thyroid 30 (8), 1193–1204. doi:10.1089/thy.2019.0763
Mishima, E., Sato, E., Ito, J., Yamada, K. I., Suzuki, C., Oikawa, Y., et al. (2020). Drugs Repurposed as Antiferroptosis Agents Suppress Organ Damage, Including AKI, by Functioning as Lipid Peroxyl Radical Scavengers. J. Am. Soc. Nephrol. 31 (2), 280–296. doi:10.1681/ASN.2019060570
Mishkin, S. Y., Pollack, R., Yalovsky, M. A., Morris, H. P., and Mishkin, S. (1981). Inhibition of Local and Metastatic Hepatoma Growth and Prolongation of Survival after Induction of Hypothyroidism. Cancer Res. 41 (8), 3040–3045.
Moutzouri, E., Lyko, C., Feller, M., Blum, M. R., Adam, L., Blum, S., et al. (2021). Subclinical Thyroid Function and Cardiovascular Events in Patients with Atrial Fibrillation. Eur. J. Endocrinol. 185 (3), 375–385. doi:10.1530/eje-20-1442
Niedowicz, D. M., Wang, W.-X., Price, D. A., and Nelson, P. T. (2021). Modulating Thyroid Hormone Levels in Adult Mice: Impact on Behavior and Compensatory Brain Changes. J. Thyroid Res. 2021, 1–13. doi:10.1155/2021/9960188
Nomura, S., Pittman, C. S., Chambers, J. B., Buck, M. W., and Shimizu, T. (1975). Reduced Peripheral Conversion of Thyroxine to Triiodothyronine in Patients with Hepatic Cirrhosis. J. Clin. Invest. 56 (3), 643–652. doi:10.1172/JCI108134
Obata, T., and Aomine, M. (2009). Changes in Monoamine Oxidase Activity in Hepatic Injury: a Review. Res. Commun. Mol. Pathol. Pharmacol. 122-123, 51–63.
Onigata, K., and Szinnai, G. (2014). Resistance to Thyroid Hormone. Endocr. Dev. 26, 118–129. doi:10.1159/000363159
Oren, R., Dabeva, M. D., Karnezis, A. N., Petkov, P. M., Rosencrantz, R., Sandhu, J. P., et al. (1999). Role of Thyroid Hormone in Stimulating Liver Repopulation in the Rat by Transplanted Hepatocytes. Hepatology 30 (4), 903–913. doi:10.1002/hep.510300418
Orságová, I., RoŽnovský, L., Petroušová, L., Konečná, M., Kabieszová, L., Šafarčík, K., et al. (2014). Thyroid Dysfunction during Interferon Alpha Therapy for Chronic Hepatitis B and C - Twenty Years of Experience. Klin Mikrobiol Infekc Lek 20 (3), 92–97. https://pm.yuntsg.com/details.html?pmid=25702290&key=25702290
Ortiga-Carvalho, T. M., Sidhaye, A. R., and Wondisford, F. E. (2014). Thyroid Hormone Receptors and Resistance to Thyroid Hormone Disorders. Nat. Rev. Endocrinol. 10 (10), 582–591. doi:10.1038/nrendo.2014.143
Oura, K., Morishita, A., and Masaki, T. (2020). Molecular and Functional Roles of MicroRNAs in the Progression of Hepatocellular Carcinoma-A Review. Int. J. Mol. Sci. 21 (21), 8362. doi:10.3390/ijms21218362
Papineni, J. K., Pinnelli, V. B. K., and Davanum, R. (2017). Thyroid Hormone Levels in Chronic Alcoholic Liver Disease Patients before and after Treatment. J. Clin. Diagn. Res. 11 (7), BC13–BC16. doi:10.7860/JCDR/2017/24552.10276
Paterlini-Bréchot, P., Saigo, K., Murakami, Y., Chami, M., Gozuacik, D., Mugnier, C., et al. (2003). Hepatitis B Virus-Related Insertional Mutagenesis Occurs Frequently in Human Liver Cancers and Recurrently Targets Human Telomerase Gene. Oncogene 22 (25), 3911–3916. doi:10.1038/sj.onc.1206492
Perra, A., Kowalik, M. A., Cabras, L., Runfola, M., Sestito, S., Migliore, C., et al. (2020). Potential Role of Two Novel Agonists of Thyroid Hormone Receptor-β on Liver Regeneration. Cel Prolif 53 (5), e12808. doi:10.1111/cpr.12808
Piqué, D. G., Greally, J. M., and Mar, J. C. (2020). Identification of a Novel Subgroup of Endometrial Cancer Patients with Loss of Thyroid Hormone Receptor Beta Expression and Improved Survival. BMC Cancer 20 (1), 857. doi:10.1186/s12885-020-07325-y
Puhr, H. C., Wolf, P., Berghoff, A. S., Schoppmann, S. F., Preusser, M., and Ilhan-Mutlu, A. (2020). Elevated Free Thyroxine Levels Are Associated with Poorer Overall Survival in Patients with Gastroesophageal Cancer: A Retrospective Single Center Analysis. Horm. Cancer 11 (1), 42–51. doi:10.1007/s12672-019-00374-1
Raza, S., Rajak, S., Upadhyay, A., Tewari, A., and Anthony Sinha, R. (2021). Current Treatment Paradigms and Emerging Therapies for NAFLD/NASH. Front. Biosci. (Landmark Ed. 26, 206–237. doi:10.2741/4892
Ridruejo, E., Romero Caimi, G., Miret, N., Obregón, M. J., Randi, A., Deza, Z., et al. (2021). TGF-β1 Mediates Cell Proliferation and Development of Hepatocarcinogenesis by Downregulating Deiodinase 1 Expression. Medicina (B Aires) 81 (3), 346–358.
Ritter, M. J., Amano, I., and Hollenberg, A. N. (2020). Thyroid Hormone Signaling and the Liver. Hepatology 72 (2), 742–752. doi:10.1002/hep.31296
Rodríguez-Castelán, J., Corona-Pérez, A., Nicolás-Toledo, L., Martínez-Gómez, M., Castelán, F., and Cuevas-Romero, E. (2017). Hypothyroidism Induces a Moderate Steatohepatitis Accompanied by Liver Regeneration, Mast Cells Infiltration, and Changes in the Expression of the Farnesoid X Receptor. Exp. Clin. Endocrinol. Diabetes 125 (3), 183–190. doi:10.1055/s-0042-112367
Rosen, M. D., and Privalsky, M. L. (2011). Thyroid Hormone Receptor Mutations in Cancer and Resistance to Thyroid Hormone: Perspective and Prognosis. J. Thyroid Res. 2011, 361304. doi:10.4061/2011/361304
Ruiz-Llorente, L., Ardila-González, S., Fanjul, L. F., Martínez-Iglesias, O., and Aranda, A. (2014). microRNAs 424 and 503 Are Mediators of the Anti-proliferative and Anti-invasive Action of the Thyroid Hormone Receptor Beta. Oncotarget 5 (10), 2918–2933. doi:10.18632/oncotarget.1577
Russo, S. C., Salas-Lucia, F., and Bianco, A. C. (2021). Deiodinases and the Metabolic Code for Thyroid Hormone Action. Endocrinology 162 (8), bqab059. doi:10.1210/endocr/bqab059
Salman, M. A., Rabiee, A., Salman, A., Qassem, M. G., Ameen, M. A., Hassan, A. M., et al. (2021). Laparoscopic Sleeve Gastrectomy Has A Positive Impact on Subclinical Hypothyroidism Among Obese Patients: A Prospective Study. World J. Surg. 45 (10), 3130–3137. doi:10.1007/s00268-021-06201-5
Saponaro, F., Sestito, S., Runfola, M., Rapposelli, S., and Chiellini, G. (2020). Selective Thyroid Hormone Receptor-Beta (TRβ) Agonists: New Perspectives for the Treatment of Metabolic and Neurodegenerative Disorders. Front. Med. (Lausanne) 7, 331. doi:10.3389/fmed.2020.00331
Senese, R., Cioffi, F., Petito, G., Goglia, F., and Lanni, A. (2019). Thyroid Hormone Metabolites and Analogues. Endocrine 66 (1), 105–114. doi:10.1007/s12020-019-02025-5
Simon-Giavarotti, K. A., Giavarotti, L., Gomes, L. F., Lima, A. F., Veridiano, A. M., Garcia, E. A., et al. (2002). Enhancement of Lindane-Induced Liver Oxidative Stress and Hepatotoxicity by Thyroid Hormone Is Reduced by Gadolinium Chloride. Free Radic. Res. 36 (10), 1033–1039. doi:10.1080/1071576021000028280
Sinha, R. A., You, S. H., Zhou, J., Siddique, M. M., Bay, B. H., Zhu, X., et al. (2012). Thyroid Hormone Stimulates Hepatic Lipid Catabolism via Activation of Autophagy. J. Clin. Invest. 122 (7), 2428–2438. doi:10.1172/JCI60580
Sousa Domínguez, A. (2015). Severe Acute Liver Failure and Thyrotoxicosis: an Unusual Association. Rev. Esp Enferm Dig. 107 (9), 575–576. doi:10.17235/reed.2015.3607/2014
Sun, Y., Deng, X., Li, W., Yan, Y., Wei, H., Jiang, Y., et al. (2007). Liver Proteome Analysis of Adaptive Response in Rat Immediately after Partial Hepatectomy. Proteomics 7 (23), 4398–4407. doi:10.1002/pmic.200600913
Szilagyi, A., Lerman, S., and Resnick, R. H. (1983). Ethanol, Thyroid Hormones and Acute Liver Injury: Is There a Relationship? Hepatology 3 (4), 593–600. doi:10.1002/hep.1840030420
Taki-Eldin, A., Zhou, L., Xie, H. Y., Chen, K. J., Zhou, W. H., Zhang, W., et al. (2011). Tri-iodothyronine Enhances Liver Regeneration after Living Donor Liver Transplantation in Rats. J. Hepatobiliary Pancreat. Sci. 18 (6), 806–814. doi:10.1007/s00534-011-0397-2
Tan, Y., Tang, X., Mu, P., Yang, Y., Li, M., Nie, Y., et al. (2021). High-Normal Serum Thyrotropin Levels Increased the Risk of Non-alcoholic Fatty Liver Disease in Euthyroid Subjects with Type 2 Diabetes. Diabetes Metab. Syndr. Obes. 14, 2841–2849. doi:10.2147/DMSO.S313224
Tanase, D. M., Gosav, E. M., Neculae, E., Costea, C. F., Ciocoiu, M., Hurjui, L. L., et al. (2020). Hypothyroidism-Induced Nonalcoholic Fatty Liver Disease (HIN): Mechanisms and Emerging Therapeutic Options. Int. J. Mol. Sci. 21 (16), 5927. doi:10.3390/ijms21165927
Taub, R., Chiang, E., Chabot-Blanchet, M., Kelly, M. J., Reeves, R. A., Guertin, M. C., et al. (2013). Lipid Lowering in Healthy Volunteers Treated with Multiple Doses of MGL-3196, a Liver-Targeted Thyroid Hormone Receptor-β Agonist. Atherosclerosis 230 (2), 373–380. doi:10.1016/j.atherosclerosis.2013.07.056
Turan, E., and Turksoy, V. A. (2021). Selenium, Zinc, and Copper Status in Euthyroid Nodular Goiter: A Cross-Sectional Study. Int. J. Prev. Med. 12, 46. doi:10.4103/ijpvm.IJPVM_337_19
Vargas, R., and Videla, L. A. (2017). Thyroid Hormone Suppresses Ischemia-Reperfusion-Induced Liver NLRP3 Inflammasome Activation: Role of AMP-Activated Protein Kinase. Immunol. Lett. 184, 92–97. doi:10.1016/j.imlet.2017.01.007
Ventura-Holman, T., Mamoon, A., Subauste, M. C., and Subauste, J. S. (2011). The Effect of Oncoprotein V-erbA on Thyroid Hormone-Regulated Genes in Hepatocytes and Their Potential Role in Hepatocellular Carcinoma. Mol. Biol. Rep. 38 (2), 1137–1144. doi:10.1007/s11033-010-0211-2
Wang, F., Zhang, B., Xu, X., Zhu, L., and Zhu, X. (2020). TRIP6 Promotes Tumorigenic Capability through Regulating FOXC1 in Hepatocellular Carcinoma. Pathol. Res. Pract. 216 (4), 152850. doi:10.1016/j.prp.2020.152850
Wang, L., Yu, W., Cao, W., and Lu, W. (2017). The Abnormality of Thyroid Hormones in Patients with Type A Hepatic Encephalopathy. Oncotarget 8 (40), 67821–67828. doi:10.18632/oncotarget.18869
Wang, R., and Mu, J. (2021). Arbutin Attenuates Ethanol-Induced Acute Hepatic Injury by the Modulation of Oxidative Stress and Nrf-2/HO-1 Signaling Pathway. J. Biochem. Mol. Toxicol. 35, e22872. doi:10.1002/jbt.22872
Wu, C. H., Yeh, C. T., and Lin, K. H. (2020). Thyroid Hormones Suppress FOXM1 Expression to Reduce Liver Cancer Progression. Oncol. Rep. 44 (4), 1686–1698. doi:10.3892/or.2020.7716
Wu, D., Sun, Z., Liu, X., Rao, Q., Chen, W., Wang, J., et al. (2018). HINT: a Novel Prognostic Model for Patients with Hepatitis B Virus-Related Acute-On-Chronic Liver Failure. Aliment. Pharmacol. Ther. 48 (7), 750–760. doi:10.1111/apt.14927
Wu, S. M., Cheng, W. L., Liao, C. J., Chi, H. C., Lin, Y. H., Tseng, Y. H., et al. (2015). Negative Modulation of the Epigenetic Regulator, UHRF1, by Thyroid Hormone Receptors Suppresses Liver Cancer Cell Growth. Int. J. Cancer 137 (1), 37–49. doi:10.1002/ijc.29368
Yan, L. D., Thomas, D., Schwartz, M., Reich, J., and Steenkamp, D. (2017). Rescue of Graves Thyrotoxicosis-Induced Cholestatic Liver Disease without Antithyroid Drugs: A Case Report. J. Endocr. Soc. 1 (3), 231–236. doi:10.1210/js.2016-1065
Yang, J., Wang, Y., Sui, M., Liu, F., Fu, Z., and Wang, Q. X. (2015). Tri-iodothyronine Preconditioning Protects against Liver Ischemia Reperfusion Injury through the Regulation of Autophagy by the MEK/ERK/mTORC1 axis. Biochem. Biophys. Res. Commun. 467 (4), 704–710. doi:10.1016/j.bbrc.2015.10.080
Yang, Q., Liu, W., Sun, D., Wang, C., Li, Y., Bi, X., et al. (2020). Yinning Tablet, a Hospitalized Preparation of Chinese Herbal Formula for Hyperthyroidism, Ameliorates Thyroid Hormone-Induced Liver Injury in Rats: Regulation of Mitochondria-Mediated Apoptotic Signals. J. Ethnopharmacol 252, 112602. doi:10.1016/j.jep.2020.112602
Zeng, F., Takaya, T., Yoshida, N., Ito, T., Suto, M., Hatani, Y., et al. (2017). A Case of Fatal Heart and Liver Failure Accompanied by Thyroid Storm Treated with Prompt Plasma Exchange. J. Cardiol. Cases 15 (3), 100–103. doi:10.1016/j.jccase.2016.11.001
Zeng, M., Liu, W., Hu, Y., and Fu, N. (2020). Sumoylation in Liver Disease. Clin. Chim. Acta 510, 347–353. doi:10.1016/j.cca.2020.07.044
Zhang, L., Zeyu, W., Liu, B., Jang, S., Zhang, Z., and Jiang, Y. (2021). Pyroptosis in Liver Disease. Rev. Esp Enferm Dig. 113 (4), 280–285. doi:10.17235/reed.2020.7034/2020
Zhang, P., Shi, L., Song, L., Long, Y., Yuan, K., Ding, W., et al. (2020). LncRNA CRNDE and lncRNA SNHG7 Are Promising Biomarkers for Prognosis in Synchronous Colorectal Liver Metastasis Following Hepatectomy. Cancer Manag. Res. 12, 1681–1692. doi:10.2147/CMAR.S233147
Zhang, X., Li, R., Chen, Y., Dai, Y., Chen, L., Qin, L., et al. (2020). The Role of Thyroid Hormones and Autoantibodies in Metabolic Dysfunction Associated Fatty Liver Disease: TgAb May Be a Potential Protective Factor. Front. Endocrinol. (Lausanne) 11, 598836. doi:10.3389/fendo.2020.598836
Zhang, Y., Zhan, C., Chen, G., and Sun, J. (2018). Label-free Quantitative Proteomics and Bioinformatics Analyses of Alcoholic Liver Disease in a Chronic and Binge Mouse Model. Mol. Med. Rep. 18 (2), 2079–2087. doi:10.3892/mmr.2018.9225
Zhao, L. F., Iwasaki, Y., Han, B. L., Wang, J., Zhang, Y., Han, J., et al. (2020). Triiodothyronine Activates Glycerol-3-Phosphate Acyltransferase 3 via Aggtca-Like-Direct-Repeat-4 Type Thyroid Hormone Response Element. Acta Endocrinol. (Buchar) 16 (2), 129–135. doi:10.4183/aeb.2020.129
Zhao, W., Dai, Y., Dai, T., Xie, T., Su, X., Li, J., et al. (2017). TRIP6 Promotes Cell Proliferation in Hepatocellular Carcinoma via Suppression of FOXO3a. Biochem. Biophys. Res. Commun. 494 (3-4), 594–601. doi:10.1016/j.bbrc.2017.10.117
TH/TR axis thyroid hormone/thyroid hormone receptor axis
T4 thyroid hormones 3,5,3′,5′- tetraiodothyronine or thyroxine
T3 3,5,3′-triiodothyronine
AEV avian erythroblastosis virus
TRE thyroid hormone-response element
TSH thyroid-stimulating hormone
PCNA proliferating cell nuclear antigen
cdk2 cyclin-dependent kinase 2
PARP Poly (ADP-ribose) polymerase
PH partial hepatectomy
MAFLD metabolic-associated fatty liver disease
HCC hepatocellular carcinoma
HBV hepatitis B virus
HCV hepatitis C virus
ALF acute liver failure
GC-1 sobetisome
Eprotirome KB2115
MetS metabolic syndrome
TPOAb thyroid peroxidase antibody
SBP systolic blood pressure
DBP diastolic blood pressure
FBG fasting blood glucose
TG triglyceride
HDLC high-density lipoprotein
ALT alanine aminotransferase
AST aspartate aminotransferase
BUN urea nitrogen
CR creatinine
Dio iodothyronine deiodinases
HIF-1α hypoxia-inducible factor 1α
NCD normal chow diet
H sites sites of de novo DNA hypermethylation
CDK cyclin-dependent kinase
miRNAs microRNAs
lncRNA long non-coding RNA
Hh Hedgehog
PIM-1 the proto-oncogene serine/threonine-protein kinase-1
HCB hexachlorobenzene
FOXM1 forkhead box M1
R-H model resistance-hepatocyte rat model
SCLM synchronous colorectal liver metastasis
BC200 brain cytoplasmic RNA 1
TUG1 taurine upregulated gene 1
TRIP Thyroid hormone receptor-interacting proteins
PTTG1 pituitary tumor-transforming gene 1
Lcn2 lipocalin 2
NUPR1 nuclear protein 1
THRSP thyroid hormone responsive
DKK 4 dickkopf 4
USP18 ubiquitin-specific protease 18
TRUP thyroid hormone uncoupling protein
ALF acute liver failure
HE hepatic encephalopathy
CLF chronic liver disease
CHC chronic hepatitis C
TD thyroid disease
PRO-C3 N-terminal type III collagen pro-peptide
PBC primary biliary cirrhosis
fT3 TH-free T3
EMR ethanol metabolic rate
AF alcohol-feed
PF pair-feed
8-OHdG 8-hydroxy-2-deoxyyguanosine
PCO protein carbonyl content
AOPPs advanced oxidation protein products
MP methylprednisolone
n-3 PUFA n-3 polyunsaturated fatty acid
IR ischemia-reperfusion
STAT3 signal transducer and activator of transcription 3
Nrf2 nuclear transcription factor erythroid 2-related factor 2
Eh1 epoxide hydrolase 1
NQO1 NADPH-quinone oxidoreductase 1
GST glutathione-S-transferases
MRP multidrug resistance-associated proteins
GdCl3 gadolinium chloride
PC preconditioning
AMPK AMP-activated protein kinase
MAO monoamine oxidase
R243Q substitution of glycine by arginine at position 243
SUMO small ubiquitin-like modifier
OA oleic acid
Keywords: TH/TR axis, thyroid hormone receptor, thyroid hormone, metabolic-associated fatty liver disease, hepatocellular carcinoma
Citation: Tang Q, Zeng M, Chen L and Fu N (2022) Targeting Thyroid Hormone/Thyroid Hormone Receptor Axis: An Attractive Therapy Strategy in Liver Diseases. Front. Pharmacol. 13:871100. doi: 10.3389/fphar.2022.871100
Received: 07 February 2022; Accepted: 19 April 2022;
Published: 02 June 2022.
Reviewed by:
Simone Wajner, Federal University of Rio Grande do Sul, BrazilEdited by:
Francisco Javier Cubero, Complutense University of Madrid, SpainCopyright © 2022 Tang, Zeng, Chen and Fu. This is an open-access article distributed under the terms of the Creative Commons Attribution License (CC BY). The use, distribution or reproduction in other forums is permitted, provided the original author(s) and the copyright owner(s) are credited and that the original publication in this journal is cited, in accordance with accepted academic practice. No use, distribution or reproduction is permitted which does not comply with these terms.
*Correspondence: Linxi Chen, MTk5NTAwMTc2NUB1c2MuZWR1LmNu; Nian Fu, MjAwMmZ1bmlhbkAxNjMuY29t
†These authors have contributed equally to this work
Disclaimer: All claims expressed in this article are solely those of the authors and do not necessarily represent those of their affiliated organizations, or those of the publisher, the editors and the reviewers. Any product that may be evaluated in this article or claim that may be made by its manufacturer is not guaranteed or endorsed by the publisher.
Research integrity at Frontiers
Learn more about the work of our research integrity team to safeguard the quality of each article we publish.