- 1Department of Cardiology, Nanjing First Hospital, Nanjing Medical University, Nanjing, China
- 2Department of Cardiology, Sir Run Run Hospital, Nanjing Medical University, Nanjing, China
ATP-sensitive potassium channels (KATP channels) play pivotal roles in excitable cells and link cellular metabolism with membrane excitability. The action potential converts electricity into dynamics by ion channel-mediated ion exchange to generate systole, involved in every heartbeat. Activation of the KATP channel repolarizes the membrane potential and decreases early afterdepolarization (EAD)-mediated arrhythmias. KATP channels in cardiomyocytes have less function under physiological conditions but they open during severe and prolonged anoxia due to a reduced ATP/ADP ratio, lessening cellular excitability and thus preventing action potential generation and cell contraction. Small active molecules activate and enhance the opening of the KATP channel, which induces the repolarization of the membrane and decreases the occurrence of malignant arrhythmia. Accumulated evidence indicates that mutation of KATP channels deteriorates the regulatory roles in mutation-related diseases. However, patients with mutations in KATP channels still have no efficient treatment. Hence, in this study, we describe the role of KATP channels and subunits in angiocardiopathy, summarize the mutations of the KATP channels and the functional regulation of small active molecules in KATP channels, elucidate the potential mechanisms of mutant KATP channels and provide insight into clinical therapeutic strategies.
Introduction
The aging of the population and improved survival after acute myocardial infarction have resulted in high morbidity and mortality, a poor clinical prognosis and high expenses due to heart failure (HF) (Savarese et al., 2022). The prevalence of HF is predicted to increase by 46% from 2012 to 2030. After several years of therapeutic exploration, the prognosis of HF remains poor, with a 5-years mortality of ≈40%–50%, and the projections suggest that the total costs for HF in 2030 will be close to $38.99 billion in the United States (Kaneko et al., 2021; Yu et al., 2021; Savarese et al., 2022). Sudden cardiac death (SCD) is the leading cause of death in HF, and malignant arrhythmia is regarded as the overriding risk within SCD (Akhtar et al., 2021; Grune et al., 2021; Mulder et al., 2021). HF involves numerous physiological and pathological processes, among which calcium (Ca2+) overload is a typical representative. Ca2+ overload destroys membranes, organelles and DNA, leading to structural and functional disruption of cells and tissues, eventually promoting cardiomyopathy, ventricular fibrillation and sudden death (Yang et al., 2021).
ATP-sensitive potassium channels (KATP channels) were first discovered in cardiac muscle in 1983 by Noma (Noma, 1983) and were successively found in skeletal muscle, the digestive system, urinary system, integumentary system, reproductive system, and central nervous system (Huang et al., 2019; Zhao et al., 2020) (Figure 1). Activating KATP channels shorten the action potential duration, reduce intracellular Ca2+ entry to suppress calcium overload, inhibit contractility, and prevent arrhythmias and cardiac insufficiency caused by calcium overload; however, completely opening KATP channels in the heart may result in complete cessation of cardiac electrical activity and contractile failure (Huang et al., 2019). Hence, KATP channels play an irreplaceable role in HF, whether from myocardial ischemia or arrhythmia.
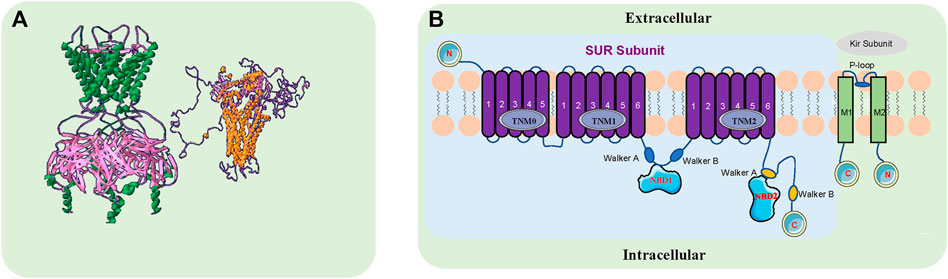
FIGURE 1. The Structure of the KATP Channel. (A). KATP channels are comprised of four sulfonylurea receptors (SURx) and four K+ inward rectifiers (Kir6. x) that assemble to form hetero-octameric protein complexes. The green and pink sections on the left represent the side view of the Kir6. x subunit, and the gold and purple sections on the right represent the side view of the SURx subunit. FASTA comes from NCBI, designed by www.swissmodel.com, Image designed by Swiss-Pdbviewer software. (B). The pore-forming subunit Kir6. x (Kir6.1 and Kir6.2) has intracellular N- and C-termini and two transmembrane segments M1 and M2, encoded by KCNJ8 and KCNJ11, respectively. The modulatory subunit SURX (SUR1, SUR2A, SUR2B) consists of three groups of transmembrane domains (TMD0, TMD1 and TMD2) and extracellular N- and intracellular C-termini, encoded by ABCC8 and ABCC9. There are two intracellular nucleotide binding folds (NBD1 and NBD2) within the SUR subunit. ABCC8 and KCNJ11 are adjacent to each other on chromosome 11p15.1, with ABCC9 and KCNJ8 on chromosome 12P12.1.
KATP channels are widely distributed in various organs, but the assembly of their subunits varies depending upon the tissue and they may confer different functional and pharmacological properties depending on which subunits are present (Li et al., 2021; Stewart and Turner, 2021) (Table 1). KATP channels are comprised of four sulfonylurea receptors (SURx) and four K+ inward rectifiers (Kir6. x) that assemble to form hetero-octameric protein complexes. The pore-forming subunit Kir6. x (Kir6.1 and Kir6.2) has intracellular N- and C-termini and two transmembrane segments M1 and M2, encoded by KCNJ8 and KCNJ11, respectively. The modulatory subunit SURx (SUR1, SUR2A, SUR2B) consists of three groups of transmembrane domains (TMD0, TMD1 and TMD2) and extracellular N- and intracellular C-termini, encoded by ABCC8 and ABCC9. There are two intracellular nucleotide binding folds (NBD1 and NBD2) within the SUR subunit. ABCC8 and KCNJ11 are adjacent to each other on chromosome 11p15.1, with ABCC9 and KCNJ8 on chromosome 12P12.1.
Cardiac KATP channels provide cardioprotection against ischemia/reperfusion injury; in contrast, overexpressed cardiac KATP channels have proarrhythmic effects, which associates them with profound value for clinical applications and exploration. There are three subtypes of KATP channels found within the cardiovascular system: two widely accepted channels, mitoKATP and sarcolemma KATP, and a controversial KATP channel, plasma membrane KATP (Iguchi et al., 2019; Pertiwi et al., 2019; Aziz et al., 2020; Jiang et al., 2021). Different cardiac KATP channels play different roles in the cardiovascular system, which will be explained here. Recent evidence has shown that several refractory diseases are closely related to mutations in KATP channel subunits. Disease-related clinical symptoms and high medical costs will burden the patient, the family, and society. Most encouraging, some KATP channel activators and antagonists have shown good results for treating KATP channel subunit mutation-related diseases, such as Cantú syndrome, congenital hyperinsulinism (CHI), neonatal diabetes mellitus (NDM), developmental delay epilepsy and neonatal diabetes (DEND) and ABCC9-related intellectual disability myopathy Syndrome (AIMS) (Demirbilek et al., 2019; Martin et al., 2020; McClenaghan et al., 2020).
In this review, we focus on the regulatory mechanism of KATP channels during angiocardiopathy and provide insights into how mutations in KATP channelopathies lead to some incurable diseases. Furthermore, we will explore the therapeutic strategy of targeting KATP channel drugs in clinical practice.
KATP Channels in Cardiovascular Diseases
Mitochondrial ATP-Sensitive Potassium Channels (mitoKATP Channels)
As an independent factor associated with high mortality, acute myocardial infarction is an irreversible process characterized by glycogen depletion, margination of nuclear chromatin, mitochondrial swelling and sarcolemmal breaks. Myocardial infarct size and the duration of ischemia are the main determinants of the prognosis (Heusch, 2020). Rapidly restoring blood flow is the key to successful salvage of ischemic myocardium; however, reperfusion not only salvages ischemic myocardium from infarction but also induces an increased risk of additional complications and further cardiomyocyte death, a process called myocardial ischemia reperfusion injury (MIRI) (Griffiths et al., 2021). In myocardial ischemia, the mitochondrial matrix is damaged and extensively broken, and it dissolves the mitochondrial crest, ruptures and vacuolates the mitochondrial membrane, significantly decreases glycogen granules, increases intracellular Ca2+, diminishes ATP production, and induces myocardial cell apoptosis (Paggio et al., 2019; Basalay et al., 2020; Wang et al., 2020; Bai et al., 2021; Wang et al., 2021). A novel autologous mitochondrial transplantation therapy, in which respiration-competent mitochondria are isolated from autologous nonischemic tissue and transplanted into ischemic myocardium, improves the contractile function and tissue viability of the injured myocardium, proving that mitochondrial injury is the main pathogenesis of MIRI (Shin et al., 2019).
mitoKATP channels are involved in a series of physiological and pathophysiological changes to mitigate cardiomyocyte injury and apoptosis. mitoKATP channels have been described as being located in the inner mitochondrial membrane and they have protective properties for ischemic myocardium; moreover, their existence has been the subject of heated debate (Bezerra Palácio et al., 2021). Recently, the molecular composition of mitoKATP was shown by Paggio et al., and they are comprised of pore-forming (MITOK, encoded by the CCDC51 gene (NCBI ID 79714)) and regulatory (MITOSUR, tissue expression correlates with ABCB8) subunits (Paggio et al., 2019) (Figure 2). The opening of mitoKATP channels promotes mitochondrial K+ inward flow into the deeply negative polarized matrix (mitochondrial membrane potential (Ψm)), decreases the transmembrane potential discrepancy, depolarizes the Ψm, reduces Ca2+ inward flow dynamics, inhibits Ca2+ inward flow, and prevents mitochondrial calcium overload, leading to mitochondrial relaxation, enhanced fatty acid oxidation, oxidative phosphorylation, respiratory function, and ATP production, thus improving myocardial cell survival (Sakamoto and Kurokawa, 2019; Jiang et al., 2021).
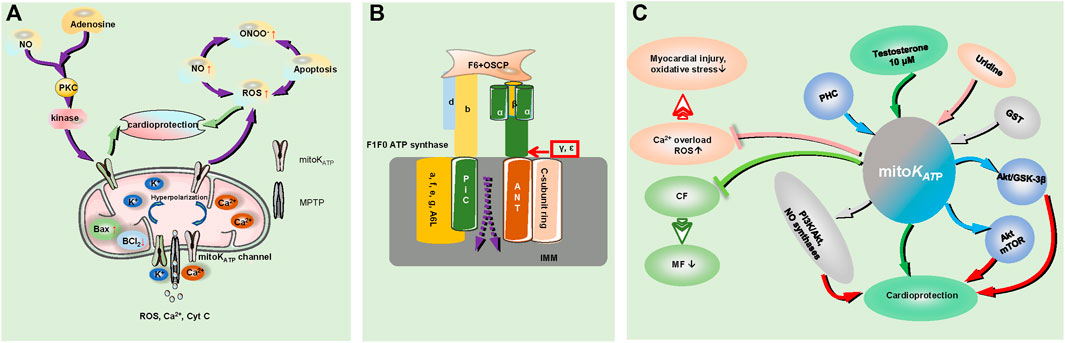
FIGURE 2. The role of mitoKATP in myocardial ischemia and the regulation of the signaling pathways involved. (A). Mitochondrial injury and mitoKATP play a role in cardiac protection during the process of MIRI; MIRI increased Bax expression and decreased BCL2 expression, triggering a cascade involving increased NO production and leading to the superficial formation of ONOO− with increased production of ROS. Activation of mitoKATP stimulates the anti-inflammatory and antioxidant effects of NO on ischemic myocardium by inhibiting the overproduction of ROS. Ischemic postadaptation activates protein kinase C and the reperfusion injury salvage kinase pathway through the intracellular concentration of adenosine and NO, and it acts on the mitoKATP channel to protect the myocardium; activation of the mitoKATP channel leads to cell hyperpolarization, resulting in reduced Ca2+ entry, and a reduced driving force of mitochondrial calcium uptake, prevent Ca2+ accumulation in the matrix and MPTP formation. The MPTP is the escape pore of ROS, Cyt C, Ca2+ and other signaling molecules. CF indicates cardiac fibroblasts; MF, myofibroblasts; MIRI, myocardial ischaemia reperfusion injury; and ONOO−, peroxynitrite. (B). The structure of MPTP; F1Fo ATP synthase: The Fo subunit consists of a, e, f, g and A6 L, the F1 component consists of α and ß subunits, labeled in bottle green and yellow, respectively, and the γ, ε subunits. The F1 peripheral stalk is comprised of subunits b, d, F6, and OSCP. The C cyclic subunit, ANT and PiC are overlaid by IMM; the dotted line represents the MPTP signal molecule outflow position. OSCP indicates oligomycin sensitivity conferring protein; IMM, inner mitochondrial membrane; ANT, adenine nucleotide translocase; and PiC, phosphate carrier. (C). mitoKATP channels prevent transdifferentiation of CF to MF to reduce MF maturation. Testosterone (10 μM) increases the opening probability of mitoKATP channels, PHC regulates the mitochondrial KATP channel, Akt/GSK-3β, and Akt/mTOR signaling pathways, GST depends on protein-kinase C and mitoKATP channel pathway activation by some typical pathways such as PI3K/Akt and NO synthases, and plays roles in cardioprotection. Uridine attenuates myocardial injury and oxidative stress by activating the mitoKATP channel to reduce excessive ROS production and prevent calcium overload. PHC indicates penehyclidine hydrochloride; GST, genistein.
During the process of MIRI, the activation of mitochondrial KATP channels depolarizes the mitochondrial membrane, reduces the driving force of mitochondrial calcium uptake, and prevents Ca2+ accumulation in the mitochondrial matrix, thus preventing the formation of the mitochondrial permeability transition pore (MPTP) (Testai et al., 2021). Currently, the main components of MPTP are as follows: adenine nucleotide translocator (ANT) and mitochondrial phosphate carrier (PiC) on the inner mitochondrial membrane (IMM), mammalian F1Fo(F)-ATP synthase (the Fo subunit consists of a, e, f, g and A6 L; the F1 subunit α, β, and the ε and γ subunits needed for ATP synthase dimer formation, the peripheral stem consists of b, d, and the F6 subunit and oligomycin sensitivity conferring protein (OSCP)) (Kent et al., 2021) (Figure 2). MPTP is the point at which reactive oxygen species (ROS), Ca2+, cytochrome C (Cyt C), and other small molecule modulators escape from the mitochondrial matrix, and its opening leads to the loss of oxidative phosphorylation capacity as well as the release of pro-death mitochondrial proteins (mitochondrial swelling and membrane rupture), increased Bax expression and decreased Bcl2 expression, ultimately activating the apoptotic cascade in the mitochondria in ischemia–reperfusion heart tissues (Jiang et al., 2021; Kent et al., 2021; Pereira and Kowaltowski, 2021). Mitochondrial ROS spreading from the electron transport chain damages the mitochondrial DNA, which can cause mitochondrial dysfunction and affect nuclear gene expression, ion handling, and mitochondrial metabolism, finally causing the activation of an inflammatory response, apoptotic signaling, and endoplasmic reticulum stress in the cardiovascular system (Bou-Teen et al., 2021). Indeed, MIRI triggers a cascade involving increased NO production (NO function; anti-inflammatory and antioxidant effects) and leads to the superfluous formation of peroxynitrite (ONOO−) with increased production of ROS, which mediates the pernicious impact of NO (Liu et al., 2021a). The activation of mitoKATP during ischemia plays a role in the cardioprotective function by inhibiting the overproduction of ROS and stimulating the NO effects on anti-inflammatory and antioxidant activities in the ischemic myocardium (Liu et al., 2021a; Rameshrad et al., 2021). Ischemic postconditioning activates protein kinase C and reperfusion injury salvage kinase pathways through modulating the intracellular concentrations of adenosine and NO, ultimately acting on the mitoKATP pathway to protect the myocardium (Li et al., 2020a).
mitoKATP channels also affect other cardiac components. Within rat cardiac fibroblasts (CFs), mitoKATP channels prevent the transdifferentiation of CFs to myofibroblasts (MFs) to reduce MF maturation and antagonize cardiac pathological remodeling following simulated ischemia-reperfusion injury (Stewart and Turner, 2021). ROMK (a kidney mRNA detectable in the thick ascending limb and the distal nephron) participates in K+ reabsorption and secretion. An experiment performed by Irina B. Krylova et al. implicated ROMK in Ca2+-induced MPTP opening but did not play a role in mitoKATP activity in the mouse heart (Papanicolaou et al., 2020). Electrophysiological analysis revealed that 10 μM testosterone increased the open probability of mitoKATP channels, which offered cytoprotection against MIRI (Sakamoto and Kurokawa, 2019).
The recently discovered signal-regulated pathways involved in mitoKATP channels are as follows. Penehyclidine hydrochloride (PHC) preconditioning plays a cardioprotective role by regulating the mitochondrial KATP channel and Akt/GSK-3β and Akt/mTOR signaling pathways (Zi et al., 2020). Uridine attenuates myocardial injury and oxidative stress in MIRI, which may be mediated by activation of the mitoKATP channel, achieved by reducing excessive ROS production and preventing the appearance of calcium overload (Krylova et al., 2021). GST (genistein, a phytoestrogen) provides a cardioprotective function that depends on protein kinase C and activates mitoKATP channels via a typical pathway, such as PI3K/Akt and NO synthases (Colareda et al., 2020).
Sarcolemma ATP-Sensitive Potassium Channels
Early evidence indicated that sarcolemma ATP-sensitive potassium (sarcKATP) channels play a crucial role in ischemic preconditioning and myocardial resistance to ischemia, which close during general conditions and open in response to increased [ADP]/[ATP], linking membrane excitability to the balance of ATP production and shortening action potential (AP) duration (APD) via the efflux of K+ (Garrott et al., 2017; Sudhir et al., 2020). SarcKATP channels improve adaptation to physical stress and profoundly alter membrane excitability and other membrane potential-related functions, such as Ca2+ overload, thus helping to maintain cellular homeostasis during cardiac challenge (i.v. adenosine) (Zhang et al., 2016). The partial opening of sarcKATP channels plays a crucial role in the regional depolarization of Ψm, which can transform cellular electrical excitability and increase the propensity for reentry arrhythmogenesis (Solhjoo and O'Rourke, 2015). An unstable or oscillating Ψm can expose cardiomyocytes to ROS or result in glutathione depletion, activate sarcKATP channels and abate the cellular ATP/ADP ratio, which has been deemed to be a dominant factor in arrhythmogenesis during MIRI (Solhjoo and O'Rourke, 2015).
Increased activation of the sarcKATP channel (a role in cardioprotection) does not participate in the protection provided by ordinary cardioprotective stimulation. sarcKATP opening actually occurs later during metabolic inhibition (after cardioprotection), cardioprotective stimuli prolong normal mitochondrial function during ischemia, and the delay in the opening of sarcKATP channels is a consequence of the continuation of ATP production, so sarcKATP channel opening is the last defense of cardiomyocytes to preserve ATP and limit the Ca2+ overload during ischemia (Brennan et al., 2015). The density of sarcKATP channels under physiological conditions plays a significant role in cardioprotection; however, certain pathophysiologic circumstances give rise to a declining density of sarcKATP channels, including hyperinsulinemia and cardiac ischemia (Yang et al., 2018). The lower basal expression level of sarcKATP channels in hESCs (human embryonic stem cells)-VCMs (ventricular cardiomyocytes) (∼1/8 of adults) means they were partially activated and sufficient to cause APD shortening and accelerate AP firing; when fully activated, sarcKATP channels silenced automaticity without compromising intrinsic cellular excitability (Keung et al., 2016).
Studies on the cardiac sarcKATP channel regulatory subunit SUR2A/SUR2B are ongoing. The activation of ß1-adrenoceptors upregulates SUR2B/Kir6.2, in which SUR2B physically associates with Kir6.2 to act as a regulatory subunit in sarcKATP channels to offer cardioprotection (Jovanovic et al., 2016). With an increasing number of sarcKATP channels, increased expression of SUR2A regulates cardiac physiology and improves the adaptation to physical stress by shortening the action potential and improving cardiac Ca2+ homeostasis (Zhang et al., 2016).
The recently discovered signal-regulated pathways and regulatory proteins involved in sarcKATP channels are as follows. Eps15 homology domain-containing protein (EHD)-2 affects the sarcKATP channel by stabilizing sarcKATP channel-containing caveolar structures to increase its surface density, which results in a reduced rate of endocytosis. Pathophysiologically, EHD-2 mutant-activated cardiomyocytes may be cardioprotective against ischemic damage (Yang et al., 2018). In rat cardiomyocytes, the sarcKATP channel exerts a cardioprotective effect against lipopolysaccharide (LPS)-induced apoptosis and it is mediated by mitochondrial Ca2+ (Zhang et al., 2016). The cardioprotective effect of BNP is related to sarcKATP channel opening. Additionally, the cardioprotective effects of ANP and cANP4-23 are mediated via sarcKATP channel opening (Krylatov et al., 2021). ANP (atrial natriuretic peptide) positively regulates the function of the sarcKATP channel in adult rabbit ventricular cardiomyocytes by activating NPR-A (natriuretic peptide receptor type A), an effect mediated by intracellular signaling mechanisms that cover PKG (cGMP-dependent protein kinase), ROS, ERK (extracellular signal-regulated protein kinase)1/2, CaMK II (calcium/calmodulin-dependent protein kinase II), and RyR (ryanodine receptor)-2; meanwhile, RyR2 (activation) is feasibly situated downstream of ROS/H2O2, which process enhances the opening frequency whereas it labilizes the long closures of the channel, thereby heightening channel activity (Zhang and Lin, 2020).
Mutation of KATP Channels
Kir 6.1
Endothelium-expressed Kir6.1 is located on human chromosome 12p, and via elevated endothelin-1 release it controls vascular tone. Smooth muscle Kir6.1 gain-of-function mutation causes overt hypertension and hypotension; notably, autosomal dominant hypertension is related to chromosome 12p recombination, and postural hypotension is related to chromosome 12 (Li et al., 2013) (Table 2). In gain-of-function mutation Kir6.1 [GD-QR] (point mutations in two C-terminal residues of Kir6.1; Gly343Asp and Gln53Arg), lymphatic smooth muscle and vascular dysfunction are present, and lymphatic smooth muscle-specific expression subunit mutations result in profound lymphatic contractile dysfunction and lymphatic smooth muscle hyperpolarization rather than lymphatic endothelial cells (Davis et al., 2020). In a CS animal model, the Kir6.1wt/VM mutation directly and/or indirectly affects the skeletal muscle through vascular dysfunction, resulting in reduced limb strength, skeletal muscle atrophy, autophagy, and myofiber connective tissue replacement (Scala et al., 2020). The S422 L mutation, a missense mutation in the KCNJ8 gene, leads to a gain-of-function Kir6.1 channel, which leads to shortened repolarization in ventricular tissue; nevertheless, it could shorten repolarization in the atrium to increase atrial fibrillation susceptibility (Delaney et al., 2012).
Kir 6.2
Approximately 38.5% of mutations in the KCNJ11 gene, which encodes Kir 6.2 and consists of a single exon containing 390 amino acids, have been identified, which is associated with clinical diseases including but not limited to neonatal diabetes mellitus, maturity-onset diabetes of the young, type 2 diabetes mellitus, and even persistent hyperinsulinemic hypoglycemia of infancy (He et al., 2021). Patients with the E227K mutation in the KCNJ11 gene typically manifest with transient neonatal diabetes, which remits spontaneously, usually within 4–60 weeks of onset; however, more than half of these patients relapse into permanent diabetes in adolescence or early adulthood (Devaraja et al., 2020). rs5215 G/G (nucleotide change; G-A, amino acid change; Val337Ile) of the KCNJ11 gene, located at 11p15.1 and encoding the Kir6.2 subunit, causes valine-isoleucine substitution in exon 1,009 (ATC-GTC), and it is associated with a gain of function of the KATP channel, leading to vasodilation augmentation and shear stress reduction, which protects humans from lower coronary microvascular dysfunction, reducing the risk of ischemic heart disease in women (Severino et al., 2020). In a hypertension mouse model, the Kir6.2 mutation led to heart failure and death, involving knockout mutation-induced myocardial incommensurate remodeling (Liu et al., 2021b). In neurons, Kir6.2 has critical roles in glucose sensing and neuronal excitability in response to metabolic demands, and the KCNJ11 p. V59 M mutation was strongly associated with intellectual disability (Moriguchi et al., 2018; Svalastoga et al., 2020).
SUR1
SUR1 is mainly expressed in the pancreas, and its mutations may lead to neonatal diabetes by disrupting inhibitory binding/gating or enhancing nucleotide stimulation. Some SUR1 mutant models in mice did not recapitulate the human phenotype (Sachse et al., 2020; Usher et al., 2020). SUR1-mutant (a homozygous c.560T > A (V187D) mutation in exon four of the ABCC8 gene encoding the SUR1 protein) stem cell-derived islet-like clusters (SC islets) leads to increased beta-cell proliferation and mass, higher insulin secretion in hypoglycemia and makes KATP channels-acting pharmaceuticals ineffective (Lithovius et al., 2021). The homozygous p. H1401Tfs ABCC8 mutation could cause significant clinical heterogeneity congenital hyperinsulinemia, ranging from a late-onset and diazoxide-responsive mild form to an extremely early-onset severe form requiring multimodality treatment with a full-course assessment of neurodevelopment and glycometabolism (Takasawa et al., 2021). Some SUR1 mutations resulted in increased channel activity in MgATP/MgADP and drastically reduced KATP channel surface expression, which suggests that the overactive defects due to altered nucleotide sensitivities outweigh their biogenesis and surface expression defects and lead to an overall gain-of-channel-function effect and the neonatal diabetes mellitus disease phenotype (Balamurugan et al., 2019).
SUR2A
Due to the strong difficulties and inferior feasibility of single subunit mutation research, we mainly noted several common cases herein. In individuals with idiopathic dilated cardiomyopathy, two heterozygous mutations in exon 38 of ABCC9 encode at the C-terminal domain of SUR2A, Fs1524 (a frameshift at Leu1524, which introduces four anomalous terminal residues followed by a premature stop codon) and A1513T (a missense mutation (4537G→A) causing the amino acid substitution), substantially diminishing the maximal rate of the NBD2 ATPase reaction without altering the Michaelis-Menten constant of catalysis, resulting in abnormal hydrolytic dynamics of the regulatory channel subunits, disrupting catalysis-dependent gating and impairing metabolic decoding, resulting in severely dilated hearts with impaired systolic function and arrhythmia (Bienengraeber et al., 2004).
SUR2B
The SUR2B mutation R659C located in the secondary structure region in the L1 linker (it has the greatest α-helical propensity) most stably interacts with NBD1, which could cause heart disease and even lead to early repolarization syndrome, a life-threatening condition (Sooklal et al., 2018). During colonic inflammation, two specific mutations within SUR2B (C24S and C1455S) prevent the detrimental effects of sulfhydration and NaHS-induced tyrosine nitration from reducing the pore-forming subunit (Kir6.1) (Kang et al., 2015).
Multiple Subunits Mutations of KATP Channels
Cantú syndrome (CS) is an ultrarare autosomal dominant inherited disorder caused by dominant gain-of-function mutations in both the SUR2A and Kir6.1 subunits of the KATP channel, which is also characterized by multiple cardiovascular abnormalities, including edema, pericardial effusion, pulmonary hypertension, dilated and tortuous blood vessels with decreased systemic vascular resistance, cerebrovascular defects, patent ductus arteriosus, and marked cardiac hypertrophy (Chen et al., 2019; Chihara et al., 2020; McClenaghan et al., 2020; Zhang et al., 2021a) (Table 3). CHI is a rare genetically heterogeneous disorder caused by inactivating mutations in the SUR1 and Kir6.2 subunits of the KATP channel and it is characterized by persistent hypoglycemia in infants and children, which may increase the risk of permanent brain damage (Boodhansingh et al., 2019; Rosenfeld et al., 2019; Männistö et al., 2020; Rosenfeld et al., 2021). NDM is characterized by the development of hyperglycemia within the first 6 months of life, beta-cell destruction, pancreatic hypoplasia or aplasia, impaired beta-cell function or severe insulin resistance resulting from impaired insulin secretion caused by gain-of-function mutations in KCNJ11 and/or ABCC8 subunits of the KATP channel, which can be divided into two transient diabetes mellitus (TNDM) and perma-nent diabetes mellitus (PNDM) clinical subtypes, depending on the length of the disease course (Cao et al., 2020; Dahl and Kumar, 2020; Pipatpolkai et al., 2020; Horita et al., 2021). DEND syndrome is a severe pathological condition of neonatal diabetes with developmental delay, muscle weakness, and epilepsy caused by gain-of-function mutations in Kir 6.2 and SUR1 (Dahl and Kumar, 2020; Pipatpolkai et al., 2020; Gopi et al., 2021). AIMS is characterized by delayed psychomotor development with intellectual disability, anxiety, muscle weakness and fatigability and some shared dysmorphic features caused by loss-of-function mutations in ABCC9 (SUR2A and/or SUR2B) (Smeland et al., 2019).
Regulation of KATP Channels by Small Active Molecules
Hydrogen Sulfide
Hydrogen sulfide (H2S), as a gaseous signaling molecule, has a wide range of biological functions, including vasodilatation, anti-endoplasmic reticulum stress, anti-apoptotic and anti-inflammatory functions, and it contributes to ameliorating ventricular structural remodeling and cardiac function (Li et al., 2021). In cardiac tissue, the most important enzyme for the synthesis of H2S is cystathionine γ-lyase (CSE), which has reduced activity in atherosclerotic patients connected with angina and atrial fibrillation (Bibli et al., 2021) (Figure 3). H2S has many significant bioactivities, including cytoprotective, antioxidant, anti-inflammatory, anti-apoptotic, and smooth muscle relaxing effects, in part because it acts as a KATP channel opener (Fouad et al., 2020). H2S partially inhibits phosphodiesterase-5 through the activation of KATP channels and increases intracellular cGMP to evoke direct vasorelaxing responses (Citi et al., 2020). H2S activates the KATP channel and inhibits insulin secretion in INS-1E cells (a pancreatic ß-cell line), but the function of hyperpolarizing the plasma membrane and closing voltage-gated Ca2+ channels is not mediated by the KATP channel (Lu et al., 2019; Shoji et al., 2019). H2S modulates KATP channel activity, promotes protective effects against pulmonary hypertension and increases uterine blood flow by antagonizing vasoconstriction (Guerra and Hurt, 2019; Roubenne et al., 2021). H2S protects the embryonic heart from I/R injury by opening the KATP channel rather than increasing coronary artery flow, demonstrating that H2S treatment of the embryonic heart is independent of the mother and the underdeveloped placenta (Hess et al., 2020). NaHS, a rapid-releasing H2S donor, stimulates ANP secretion via the KATP channel under hypoxic conditions, resulting in decreased blood pressure, ECF volume and antiproliferation of vascular smooth muscle cells in the cardiovascular system (Yu et al., 2019). Briefly, the interaction between H2S and KATP plays an irreplaceable role in cardiovascular disease.
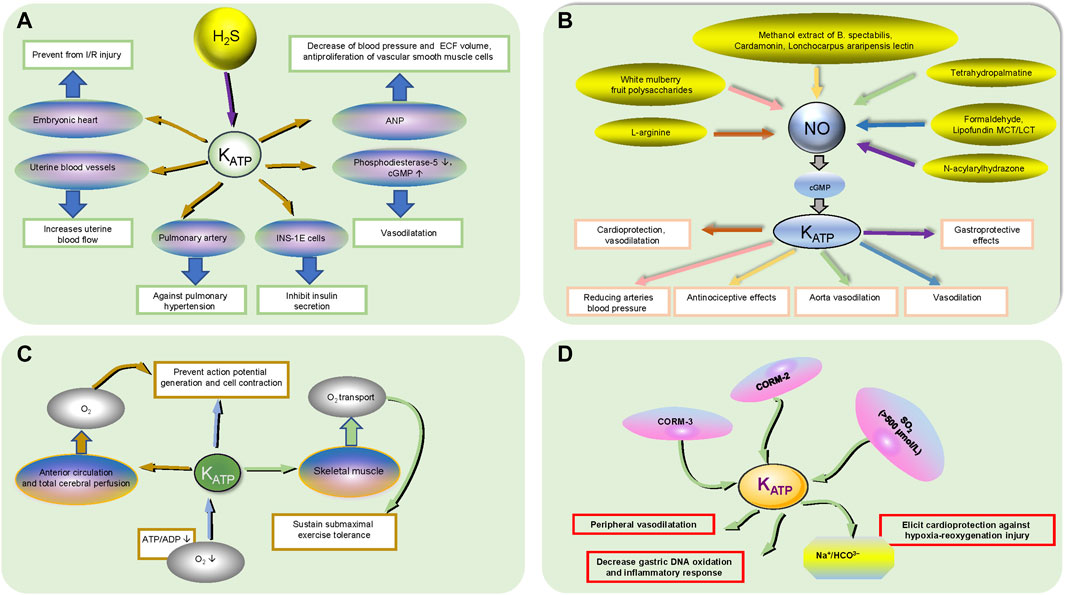
FIGURE 3. The functional regulation of active small molecules on KATP channels. (A) H2S participates in KATP channel regulation. H2S acts on the corresponding organ/tissues/cytochemical small molecules in the oval through KATP channels to produce physiological effects in a rectangular box. The black up and down arrows inside the ellipse represent increasing and decreasing, respectively. (B). NO participates in KATP channel regulation. The relative drugs in the ellipses produce physiological effects in the rectangular boxes through the KATP channels and NO/CGMP signaling pathways, which the reaction goes in the direction of the arrows in the same color. (C). O2 participates in KATP channel regulation. Hypoxia results in a decrease in ATP/ADP acting on KATP channels to prevent action potential generation and cell contraction; The KATP channel acts on skeletal muscle and affects O2 transport to sustain submaximal exercise tolerance; The KATP channel affects anterior cerebral circulation and total cerebral perfusion through O2 transport to prevent action potential generation and cell contraction. The black up and down arrows inside the ellipse represent increasing and decreasing, respectively. (D). Other small active molecules. CORM-3 can mimic the HO-1/CO pathway, activate mitoKATP channels and elicit cardioprotection against hypoxia-reoxygenation injury by inhibiting the Na+/HCO3− transporter; CORM-2 alleviates gastric lesions at the systemic level via KATP channels, reducing gastric DNA oxidation and inflammatory responses; SO2 plays a vasodilatory role through KATP channel activation in the peripheral cardiovascular system at high concentrations (>500 μmol/L).
Nitric Oxide (NO)
The NO-cyclic guanosine monophosphate (cGMP) signaling pathway is a potential therapeutic target for heart failure, and a reduction in NO bioavailability may result in the decreased production of cGMP, which could lead to decreased protection against myocardial injury, vascular and ventricular sclerosis, fibrosis, hypertrophy, and cardiorenal syndrome (Udelson et al., 2020). KATP channels can activate the L-arginine/NO/cGMP cascade pathway to induce membrane hyperpolarization, which results in shortening the action potential and restricting Ca2+ entry through Ca2+ channels, thus contributing to cardioprotection and vasodilatation (Wang et al., 2019a; Iguchi et al., 2019). Reductions in the arterial blood pressure effect of white mulberry fruit polysaccharides, the vascular relaxation effect of tetrahydropalmatine on rat aortae, and the vasodilatory effect of formaldehyde, either partially or completely, are all mediated by the NO/cGMP/KATP pathway (Wang et al., 2019a; Zhou et al., 2019a; Zhao et al., 2019). Lipofundin MCT/LCT is involved in attenuating KATP channel-induced vasodilation by inhibiting basally released endothelial NO and/or cGMP (Lee et al., 2020). The antinociceptive effects of methanol extracts of B. spectabilis, cardamonin and Lonchocarpus araripensis lectin ether are partially or completely mediated by the NO/cGMP/KATP pathways (Assreuy et al., 2020; Ferdous et al., 2020; Pui Ping et al., 2020). The KATP channel, a gastroprotective factor, is involved in the gastroprotective effects of N-acylarylhydrazone derivatives on ethanol-induced gastric lesions in mice via the NO/cGMP pathway (da Silva Monteiro et al., 2019). Therefore, the NO/cGMP/KATP pathway is involved in a variety of organoprotective and vasodilative pharmacological processes.
Oxygen(O2)
The heart operates exclusively under aerobic metabolism and three factors, heart rate, contractility, and ventricular wall tension, require myocardial mitochondria for maintaining sufficient O2 to sustain oxidative phosphorylation. Hypoxia causes the opening of the KATP channel due to a decline in the ATP:ADP ratio, which couples cellular metabolism to excitability to prevent action potential generation and cell contraction, ultimately leading to coronary artery smooth muscle cell hyperpolarization and the closure of voltage-dependent Ca2+ channels and relaxation (Yang et al., 2020a). Vascular KATP channels supporting skeletal muscle convective and diffusive O2 transport and oxidative phosphorylation sustain submaximal exercise tolerance; conversely, KATP channel inhibitors may exacerbate exercise intolerance in healthy rats (Colburn et al., 2020). Additionally, KATP channel activation modulates the anterior circulation and total cerebral perfusion, contributing to cerebral blood flow and oxygen delivery responses to hypoxia, maintaining a constant cerebral blood supply, avoiding disturbances in the precise regulation of cerebral perfusion and oxygen delivery, and preventing severe tissue damage and even death (Smith et al., 2020).
Regulated by Other Factors
CORM-3, a water-soluble CO-releasing molecule that can mimic the HO-1/CO (heme oxygenase-1/carbon monoxide) pathway by liberating CO under appropriate conditions in biological systems, activates mitoKATP channels and elicits cardioprotection against hypoxia-reoxygenation injury by inhibiting a bicarbonate transporter (most likely Na+/HCO3−) during reoxygenation (Portal et al., 2019). CORM-2 increases the gastric mucosal CO content and blood carboxyhemoglobin concentration, resulting in gastroprotection, alleviation of gastric lesions, decreased gastric DNA oxidation and the inflammatory response at the systemic level, which is partly mediated by KATP channels (Li et al., 2019). Sulfur dioxide (SO2), a major toxic gas and environmental pollutant, plays a vasodilatory role through KATP channel activation in the peripheral cardiovascular system at high concentrations (>500 μmol/L) (Magierowska et al., 2019). The search for signaling molecular regulatory pathways related to KATP channels is still in progress.
Highly specific HCN 2 (hyperpolarization-activated, cyclic-nucleotide gated channels 2) in ventricular myocytes, an integral part of ventricular electric remodeling, and the reduced expression of its mRNA, leads to the downregulation of the KATP channel current, which is one of the partial causes of arrhythmia in diabetic rats (Hadova et al., 2021; White et al., 2021). Exogenous cholesterol eliminated the increase in SUR2, suggesting that cholesterol may regulate KATP channel expression and explain why patients with hypercholesterolemia were also able to cope with ischemic events (Geiger et al., 2021). Low-density lipoprotein (LDL)5, the most negatively charged subfraction of circulating LDL, which has been considered a novel factor for predicting coronary vascular disease and stroke, prolongs the APD and increases the current density of KATP channels, and may induce arrhythmias (Ma et al., 2020). Under 5-Hz pacing conditions, the ATP-sensitive potassium current of ZFHX3 knockdown (zinc finger homeobox three gene) cells was increased compared with that under other conditions, confirming that ZFHX3 knockdown and tachypacing are related to increased stress (Lkhagva et al., 2021). Statins increased the ADP/ATP ratio and activated KATP channels to dedifferentiate myofibroblasts, while inhibition of KATP channels weakened the role of statin-induced myofibroblast dedifferentiation (Emelyanova et al., 2019). KATP channels participate in the cardiomyocyte-specific expression of photoinduced proton pump inhibitors, hyperpolarizing the intact heart to terminate ventricular arrhythmias (Funken et al., 2019). Resistin, secreted by PVAT (the fat reserve surrounding blood vessels consisting of fat cells, immune cells, fibroblasts, and endothelial cells), did not alter KATP channel-mediated relaxation in males, while KATP channel-mediated relaxation was significantly reduced in females (Small et al., 2019).
Clinical Therapy of Targeted KATP Channels
The functional regulation of small active molecules on KATP channels and the potential mechanisms of mutant KATP channels have been introduced in the previous content, and the relevant clinical effects and pharmacological mechanisms of some irre-placeable KATP channel openers and inhibitors will be introduced.
Nicorandil is a renowned cardioprotective drug that is characterized by opening KATP channels. It participates in the regulation of multiple signaling pathways and can be used to treat arrhythmias, chronic heart failure, stable angina, and acute coronary syndromes, including post-PCI (percutaneous coronary intervention). Nicorandil regulates coronary blood flow, protects cardiomyocytes from ischemia-reperfusion injury, alleviates endothelial dysfunction and reduces myocardial necrosis due to its KATP channel-opening effects, thereby relieving angina symptoms and limiting infarct size and subsequent severe ischemic insult (Jiang et al., 2021). A systematic review and meta-analysis demonstrated that nicorandil could effectively improve microvascular perfusion, alleviate microvascular spasms, reduce platelet aggregation, open KATP channels, reduce the excessive production of oxygen free radicals and myocardial ischemia, improve myocardial antioxidant capacity, and inhibit myocardial apoptosis and inflammatory reactions after ischemia to treat unstable angina pectoris and related microvascular complications (Zhang et al., 2021b).
Levosimendan is a calcium sensitization agent and KATP channel opener that is clinically used for the treatment of decompensated heart failure, which is characterized by inducing vasodilation of the pulmonary, coronary, and peripheral arteries and venous circulation, anti-inflammatory and antioxidant effects, and then it exerts a cardioprotective effect in various settings (Herpain et al., 2019; Efentakis et al., 2020). Levosimendan may be considered for the prevention of overt acute heart failure and cardiogenic shock due to its hemodynamic and anti-ischemia effects and its pharmacodynamic properties (Cosentino et al., 2020).
Sulfonamides, as an antibacterial drug, Marcel Janbon discovered its hypoglycemic side effects, and A. Loubatières proved that its hypoglycemic mechanism is to promote insulin secretion, so they were widely used in clinic as hypoglycemic drugs (Zhang et al., 2013). In pancreatic ß cells, when blood glucose concentration increases, intracellular ATP concentration increases with active glucose uptake and metabolism and inhibits KATP channels, leading to cell plasma membrane depolarization, activation of voltage-gated calcium channels, and calcium influx triggering insulin release (Yang et al., 2020b). Sulfonamides bind to KATP channel sulfonylurea receptors, inhibit the opening of KATP channel, promote the release of insulin, and reduce blood glucose and the risk of microvascular complications associated with diabetes (Wang et al., 2019b). Sulfonylureas might increase the risk of adverse cardiovascular events, due to KATP channel closure in the heart, in Neil Dhopeshwarkar et al. cohort included 268,094 glipizide users and 124,354 glimepiride users in Medicaid, they found that glimepiride (as opposed to glipizide) was associated with an elevated risk of sudden cardiac death/fatal ventricular arrhythmia, in Abdelmoneim et al. cohort included 7,441 gliclazide and 13 884 glyburide users, and they observed that statistically significant 14% higher risk of acute coronary syndrome was observed in patients taking glyburide compared with those taking gliclazide (Leroy et al., 2006; Rieg et al., 2020). In conclusion, the exploration of pancreatic specific KATP channel inhibitors can help control patients’ blood glucose, reduce microvascular complications.
At present, there is no specific pharmacotherapeutic treatment options are currently suitable for the diseases of KATP channels mutation (Rosenfeld et al., 2019). Glibenclamide inhibited KATP and slightly improved sensorimotor performance in DEND patients, but did not improve cognitive deficits caused by neuronal KATP gain-of-function expression (Jin et al., 2020). Glibenclamide directly act on SUR1, leading to the closure of KATP channel and the normal release of insulin, improving the growth imbalance, nervous system disorders and muscle strength of some PNDM children, but may cause hypoglycemia, temporary diarrhea, tooth staining, long Q-T syndrome and other adverse reactions (Cao et al., 2020). Chen et al. verified that Cantú Mutations C166S (Kir6.2) and S1020P (SUR2A) are inhibited by travoprost, betaxolol, and ritodrine, meanwhile, these compounds are not known to cause cardiac side effects or hypoglycemia (Chen et al., 2019). Scala et al. ‘s animal experiments demonstrated that glibenclamide treatment may help to reverse or avoid muscle weakness and atrophy in CS (Li et al., 2020b). In addition, in partially effective treatment regimens for patients with CHI, diazoxide opens the sarcKATP channel and inhibits insulin secretion, octreopeptide and long-acting somatostatin analogues act downstream of the KATP channel, inhibition of insulin secretion, subtotal pancreatectomy is used to reduce insulin production in focal and medically responsive non-focal cases (Rosenfeld et al., 2019). KATP openers may indeed prove beneficial in some AIMS patients (Bibli et al., 2021). KATP channel activator tifenazoxide, VU0071063 can be unlocked by opening the KATP channel, providing CHI patients with a new pharmacological option for CHI therapy to maintain normal blood glucose and reduce drug side effects and postoperative complications (Sim et al., 2002). Interestingly, CRISPR-based genome editing techniques were found to detect changes in ABCC8 and SUR1 expression levels in type 2 diabetes, suggesting that gene editing could be useful in diagnosing and treating KATP channel mutations in the future (Zhou et al., 2019b).
Conclusion
At present, no specific pharmacotherapeutic treatment options are currently suitable for CS, but glibenclamide can partially reverse the vascular symptoms of CS by inhibiting the overactivity of the KATP channel (McClenaghan et al., 2020; Laimon et al., 2021). Sulfonylureas can inhibit the effect of ABCC8 and KCNJ11 activation mutations that prevent the closure of KATP channels leading to insulin deficiency, reversing a condition that has historically been treated only with insulin (Laimon et al., 2021). The early ascertainment of a genetic diagnosis help us find the underlying cause which is the optimal treatment of the diseases of mutations in KATP channels. These facts support the hypothesis that the study of KATP channels may improve the prognosis, alleviate pain, and reduce the economic burden on patients. In the short run, Patients with cardiovascular disease and refractory KATP channel subunit mutations will have more treatment options, more promising outcomes, and acceptable medical costs.
Author Contributions
ZW contributed to literature search, and drafted the manuscript; WB, YY contributed to literature search; D-MZ contributed to review design, wrote and revised the manuscript. All authors reviewed the manuscript.
Funding
This work was supported by the National Natural Science Foundation of China (81370304, 81970342), Jiangsu Provincial Key Research and Development Program (BE2018611).
Conflict of Interest
The authors declare that the research was conducted in the absence of any commercial or financial relationships that could be construed as a potential conflict of interest.
Publisher’s Note
All claims expressed in this article are solely those of the authors and do not necessarily represent those of their affiliated organizations, or those of the publisher, the editors and the reviewers. Any product that may be evaluated in this article, or claim that may be made by its manufacturer, is not guaranteed or endorsed by the publisher.
Acknowledgments
We thank all lab members for helpful discussion.
References
Akhtar, M. M., Lorenzini, M., Pavlou, M., Ochoa, J. P., O'Mahony, C., Restrepo-Cordoba, M. A., et al. (2021). Association of Left Ventricular Systolic Dysfunction Among Carriers of Truncating Variants in Filamin C with Frequent Ventricular Arrhythmia and End-Stage Heart Failure. JAMA Cardiol. 6 (8), 891–901. doi:10.1001/jamacardio.2021.1106
Assreuy, A. M. S., Amorim, R. M. F., Martins, S. L., de Queiroz Martins, M. G., Cajazeiras, J. B., da Silva, M. T. L., et al. (2020). Antinociceptive Effect of Lonchocarpus Araripensis Lectin: Activation of L-arginine/NO/cGMP/K+ATP Signaling Pathway. Inflammopharmacology 28 (6), 1623–1631. doi:10.1007/s10787-020-00729-z
Aziz, Q., Chen, J., Moyes, A. J., Li, Y., Anderson, N. A., Ang, R., et al. (2020). Vascular KATP Channels Protect from Cardiac Dysfunction and Preserve Cardiac Metabolism during Endotoxemia. J. Mol. Med. Berl. 98 (8), 1149–1160. doi:10.1007/s00109-020-01946-3
Bai, S., Wang, X., Wu, H., Chen, T., Li, X., Zhang, L., et al. (2021). Cardioprotective Effect of Anisodamine against Ischemia/reperfusion Injury through the Mitochondrial ATP-Sensitive Potassium Channel. Eur. J. Pharmacol. 901, 174095. doi:10.1016/j.ejphar.2021.174095
Balamurugan, K., Kavitha, B., Yang, Z., Mohan, V., Radha, V., and Shyng, S. L. (2019). Functional Characterization of Activating Mutations in the Sulfonylurea Receptor 1 (ABCC8) Causing Neonatal Diabetes Mellitus in Asian Indian Children. Pediatr. Diabetes 20 (4), 397–407. doi:10.1111/pedi.12843
Basalay, M. V., Yellon, D. M., and Davidson, S. M. (2020). Targeting Myocardial Ischaemic Injury in the Absence of Reperfusion. Basic Res. Cardiol. 115 (6), 63. doi:10.1007/s00395-020-00825-9
Bezerra Palácio, P., Brito Lucas, A. M., Varlla de Lacerda Alexandre, J., Oliveira Cunha, P. L., Ponte Viana, Y. I., Albuquerque, A. C., et al. (2021). Pharmacological and Molecular Docking Studies Reveal that Glibenclamide Competitively Inhibits Diazoxide-Induced Mitochondrial ATP-Sensitive Potassium Channel Activation and Pharmacological Preconditioning. Eur. J. Pharmacol. 908, 174379. doi:10.1016/j.ejphar.2021.174379
Bibli, S. I., Hu, J., Looso, M., Weigert, A., Ratiu, C., Wittig, J., et al. (2021). Mapping the Endothelial Cell S-Sulfhydrome Highlights the Crucial Role of Integrin Sulfhydration in Vascular Function. Circulation 143 (9), 935–948. doi:10.1161/CIRCULATIONAHA.120.051877
Bienengraeber, M., Olson, T. M., Selivanov, V. A., Kathmann, E. C., O'Cochlain, F., Gao, F., et al. (2004). ABCC9 Mutations Identified in Human Dilated Cardiomyopathy Disrupt Catalytic KATP Channel Gating. Nat. Genet. 36 (4), 382–387. doi:10.1038/ng1329
Boodhansingh, K. E., Kandasamy, B., Mitteer, L., Givler, S., De Leon, D. D., Shyng, S. L., et al. (2019). Novel Dominant KATP Channel Mutations in Infants with Congenital Hyperinsulinism: Validation by In Vitro Expression Studies and In Vivo Carrier Phenotyping. Am. J. Med. Genet. A 179 (11), 2214–2227. doi:10.1002/ajmg.a.61335
Bou-Teen, D., Kaludercic, N., Weissman, D., Turan, B., Maack, C., Di Lisa, F., et al. (2021). Mitochondrial ROS and Mitochondria-Targeted Antioxidants in the Aged Heart. Free Radic. Biol. Med. 167, 109–124. doi:10.1016/j.freeradbiomed.2021.02.043
Brennan, S., Jackson, R., Patel, M., Sims, M. W., Hudman, D., Norman, R. I., et al. (2015). Early Opening of Sarcolemmal ATP-Sensitive Potassium Channels Is Not a Key Step in PKC-Mediated Cardioprotection. J. Mol. Cell Cardiol. 79, 42–53. doi:10.1016/j.yjmcc.2014.10.016
Cao, L., He, Y., Huang, Q., Zhang, Y., Deng, P., Du, W., et al. (2020). Clinical Features and Partial Proportional Molecular Genetics in Neonatal Diabetes Mellitus: a Retrospective Analysis in Southwestern China. Endocrine 69 (1), 53–62. doi:10.1007/s12020-020-02279-4
Chen, X., Garon, A., Wieder, M., Houtman, M. J. C., Zangerl-Plessl, E. M., Langer, T., et al. (2019). Computational Identification of Novel Kir6 Channel Inhibitors. Front. Pharmacol. 10, 549. doi:10.3389/fphar.2019.00549
Chihara, M., Asahina, A., and Itoh, M. (2020). A Novel Mutation in the KCNJ8 Gene Encoding the Kir6.1 Subunit of an ATP-Sensitive Potassium Channel in a Japanese Patient with Cantú Syndrome. J. Eur. Acad. Dermatol Venereol. 34 (9), e476–e478. doi:10.1111/jdv.16384
Citi, V., Martelli, A., Bucci, M., Piragine, E., Testai, L., Vellecco, V., et al. (2020). Searching for Novel Hydrogen Sulfide Donors: The Vascular Effects of Two Thiourea Derivatives. Pharmacol. Res. 159, 105039. doi:10.1016/j.phrs.2020.105039
Colareda, G. A., Ragone, M. I., Bonazzola, P., and Consolini, A. E. (2020). The mKATP Channels and Protein-Kinase C Are Involved in the Cardioprotective Effects of Genistein on Estrogen-Deficient Rat Hearts Exposed to Ischemia/Reperfusion: Energetic Study. J. Cardiovasc Pharmacol. 75 (5), 460–474. doi:10.1097/FJC.0000000000000816
Colburn, T. D., Weber, R. E., Hageman, K. S., Caldwell, J. T., Schulze, K. M., Ade, C. J., et al. (2020). Vascular ATP-Sensitive K+ Channels Support Maximal Aerobic Capacity and Critical Speed via Convective and Diffusive O2 Transport. J. Physiol. 598 (21), 4843–4858. doi:10.1113/JP280232
Cosentino, N., Niccoli, G., Fracassi, F., Rebuzzi, A., Agostoni, P., and Marenzi, G. (2020). Rationale, Experimental Data, and Emerging Clinical Evidence on Early and Preventive Use of Levosimendan in Patients with Ventricular Dysfunction. Eur. Heart J. Cardiovasc Pharmacother. 6 (5), 310–316. doi:10.1093/ehjcvp/pvz065
da Silva Monteiro, C. E., Franco, Á. X., Sousa, J. A. O., Matos, V. E. A., de Souza, E. P., Fraga, C. A. M., et al. (2019). Gastroprotective Effects of N-Acylarylhydrazone Derivatives on Ethanol-Induced Gastric Lesions in Mice Are Dependent on the NO/cGMP/KATP Pathway. Biochem. Pharmacol. 169, 113629. doi:10.1016/j.bcp.2019.113629
Dahl, A., and Kumar, S. (2020). Recent Advances in Neonatal Diabetes. Diabetes Metab. Syndr. Obes. 13, 355–364. doi:10.2147/DMSO.S198932
Davis, M. J., Kim, H. J., Zawieja, S. D., Castorena-Gonzalez, J. A., Gui, P., Li, M., et al. (2020). Kir6.1-dependent KATP Channels in Lymphatic Smooth Muscle and Vessel Dysfunction in Mice with Kir6.1 Gain-Of-Function. J. Physiol. 598 (15), 3107–3127. doi:10.1113/JP279612
Delaney, J. T., Muhammad, R., Blair, M. A., Kor, K., Fish, F. A., Roden, D. M., et al. (2012). A KCNJ8 Mutation Associated with Early Repolarization and Atrial Fibrillation. Europace 14 (10), 1428–1432. doi:10.1093/europace/eus150
Demirbilek, H., Galcheva, S., Vuralli, D., Al-Khawaga, S., and Hussain, K. (2019). Ion Transporters, Channelopathies, and Glucose Disorders. Int. J. Mol. Sci. 20 (10), 2590. doi:10.3390/ijms20102590
Devaraja, J., Elder, C., and Scott, A. (2020). Non Classic Presentations of a Genetic Mutation Typically Associated with Transient Neonatal Diabetes. Endocrinol. Diabetes Metab. Case Rep. 2020, 2020. doi:10.1530/EDM-19-0125
Efentakis, P., Varela, A., Chavdoula, E., Sigala, F., Sanoudou, D., Tenta, R., et al. (2020). Levosimendan Prevents Doxorubicin-Induced Cardiotoxicity in Time- and Dose-dependent Manner: Implications for Inotropy. Cardiovasc Res. 116 (3), 576–591. doi:10.1093/cvr/cvz163
Emelyanova, L., Sra, A., Schmuck, E. G., Raval, A. N., Downey, F. X., Jahangir, A., et al. (2019). Impact of Statins on Cellular Respiration and De-differentiation of Myofibroblasts in Human Failing Hearts. Esc. Heart Fail 6 (5), 1027–1040. doi:10.1002/ehf2.12509
Ferdous, A., Janta, R. A., Arpa, R. N., Afroze, M., Khan, M., and Moniruzzaman, M. (2020). The Leaves of Bougainvillea Spectabilis Suppressed Inflammation and Nociception In Vivo through the Modulation of Glutamatergic, cGMP, and ATP-Sensitive K+ Channel Pathways. J. Ethnopharmacol. 261, 113148. doi:10.1016/j.jep.2020.113148
Fouad, A. A., Hafez, H. M., and Hamouda, A. (2020). Hydrogen Sulfide Modulates IL-6/STAT3 Pathway and Inhibits Oxidative Stress, Inflammation, and Apoptosis in Rat Model of Methotrexate Hepatotoxicity. Hum. Exp. Toxicol. 39 (1), 77–85. doi:10.1177/0960327119877437
Funken, M., Malan, D., Sasse, P., and Bruegmann, T. (2019). Optogenetic Hyperpolarization of Cardiomyocytes Terminates Ventricular Arrhythmia. Front. Physiol. 10, 498. doi:10.3389/fphys.2019.00498
Garrott, K., Kuzmiak-Glancy, S., Wengrowski, A., Zhang, H., Rogers, J., and Kay, M. W. (2017). KATP Channel Inhibition Blunts Electromechanical Decline during Hypoxia in Left Ventricular Working Rabbit Hearts. J. Physiol. 595 (12), 3799–3813. doi:10.1113/JP273873
Geiger, R., Fatima, N., Schooley, J. F., Smyth, J. T., Haigney, M. C., and Flagg, T. P. (2021). Novel Cholesterol-dependent Regulation of Cardiac KATP Subunit Expression Revealed Using Histone Deacetylase Inhibitors. Physiol. Rep. 8 (24), e14675. doi:10.14814/phy2.14675
Gopi, S., Kavitha, B., Kanthimathi, S., Kannan, A., Kumar, R., Joshi, R., et al. (2021). Genotype-phenotype Correlation of KATP Channel Gene Defects Causing Permanent Neonatal Diabetes in Indian Patients. Pediatr. Diabetes 22 (1), 82–92. doi:10.1111/pedi.13109
Griffiths, K., Lee, J. J., Frenneaux, M. P., Feelisch, M., and Madhani, M. (2021). Nitrite and Myocardial Ischaemia Reperfusion Injury. Where Are We Now? Pharmacol. Ther. 223, 107819. doi:10.1016/j.pharmthera.2021.107819
Grune, J., Yamazoe, M., and Nahrendorf, M. (2021). Electroimmunology and Cardiac Arrhythmia. Nat. Rev. Cardiol. 18 (8), 547–564. doi:10.1038/s41569-021-00520-9
Guerra, D. D., and Hurt, K. J. (2019). Gasotransmitters in Pregnancy: from Conception to Uterine Involution. Biol. Reprod. 101 (1), 4–25. doi:10.1093/biolre/ioz038
Hadova, K., Kralova, E., Doka, G., Bies Pivackova, L., Kmecova, Z., Krenek, P., et al. (2021). Isolated Downregulation of HCN2 in Ventricles of Rats with Streptozotocin-Induced Diabetic Cardiomyopathy. BMC Cardiovasc Disord. 21 (1), 118. doi:10.1186/s12872-021-01929-3
He, B., Li, X., and Zhou, Z. (2021). Continuous Spectrum of Glucose Dysmetabolism Due to the KCNJ11 Gene Mutation-Case Reports and Review of the Literature. J. Diabetes 13 (1), 19–32. doi:10.1111/1753-0407.13114
Herpain, A., Bouchez, S., Girardis, M., Guarracino, F., Knotzer, J., Levy, B., et al. (2019). Use of Levosimendan in Intensive Care Unit Settings: An Opinion Paper. J. Cardiovasc Pharmacol. 73 (1), 3–14. doi:10.1097/FJC.0000000000000636
Hess, R. M., Niu, Y., Garrud, T. A. C., Botting, K. J., Ford, S. G., and Giussani, D. A. (2020). Embryonic Cardioprotection by Hydrogen Sulphide: Studies of Isolated Cardiac Function and Ischaemia-Reperfusion Injury in the Chicken Embryo. J. Physiol. 598 (19), 4197–4208. doi:10.1113/JP279978
Heusch, G. (2020). Myocardial Ischaemia-Reperfusion Injury and Cardioprotection in Perspective. Nat. Rev. Cardiol. 17 (12), 773–789. doi:10.1038/s41569-020-0403-y
Horita, S., Ono, T., Gonzalez-Resines, S., Ono, Y., Yamachi, M., Zhao, S., et al. (2021). Structure Based Analysis of KATP Channel with a DEND Syndrome Mutation in Murine Skeletal Muscle. Sci. Rep. 11 (1), 6668. doi:10.1038/s41598-021-86121-5
Huang, Y., Hu, D., Huang, C., and Nichols, C. G. (2019). Genetic Discovery of ATP-Sensitive K+ Channels in Cardiovascular Diseases. Circ. Arrhythm. Electrophysiol. 12 (5), e007322. doi:10.1161/CIRCEP.119.007322
Iguchi, K., Saotome, M., Yamashita, K., Hasan, P., Sasaki, M., Maekawa, Y., et al. (2019). Pinacidil, a KATP Channel Opener, Stimulates Cardiac Na+/Ca2+ Exchanger Function through the NO/cGMP/PKG Signaling Pathway in guinea Pig Cardiac Ventricular Myocytes. Schmiedeb. Arch. Pharmacol. 392 (8), 949–959. doi:10.1007/s00210-019-01642-1
Jiang, X., Wu, D., Jiang, Z., Ling, W., and Qian, G. (2021). Protective Effect of Nicorandil on Cardiac Microvascular Injury: Role of Mitochondrial Integrity. Oxid. Med. Cell Longev. 2021, 4665632. doi:10.1155/2021/4665632
Jin, X., Wu, Y., Cui, N., Jiang, C., and Li, S. S. (2020). Methylglyoxal-induced miR-223 Suppresses Rat Vascular KATP Channel Activity by Downregulating Kir6.1 mRNA in Carbonyl Stress. Vasc. Pharmacol. 128-129, 106666. doi:10.1016/j.vph.2020.106666
Jovanovic, S., Ballantyne, T., Du, Q., Blagojević, M., and Jovanović, A. (2016). Phenylephrine Preconditioning in Embryonic Heart H9c2 Cells Is Mediated by Up-Regulation of SUR2B/Kir6.2: A First Evidence for Functional Role of SUR2B in Sarcolemmal KATP Channels and Cardioprotection. Int. J. Biochem. Cell Biol. 70, 23–28. doi:10.1016/j.biocel.2015.10.029
Kaneko, H., Yano, Y., Itoh, H., Morita, K., Kiriyama, H., Kamon, T., et al. (2021). Association of Blood Pressure Classification Using the 2017 American College of Cardiology/American Heart Association Blood Pressure Guideline with Risk of Heart Failure and Atrial Fibrillation. Circulation 143 (23), 2244–2253. doi:10.1161/CIRCULATIONAHA.120.052624
Kang, M., Hashimoto, A., Gade, A., and Akbarali, H. I. (2015). Interaction between Hydrogen Sulfide-Induced Sulfhydration and Tyrosine Nitration in the KATP Channel Complex. Am. J. Physiol. Gastrointest. Liver Physiol. 308 (6), G532–G539. doi:10.1152/ajpgi.00281.2014
Kent, A. C., El Baradie, K. B. Y., and Hamrick, M. W. (2021). Targeting the Mitochondrial Permeability Transition Pore to Prevent Age-Associated Cell Damage and Neurodegeneration. Oxid. Med. Cell Longev. 2021, 6626484. doi:10.1155/2021/6626484
Keung, W., Ren, L., Sen Li, L., Wong, A. O., Chopra, A., Kong, C. W., et al. (2016). Non-cell Autonomous Cues for Enhanced Functionality of Human Embryonic Stem Cell-Derived Cardiomyocytes via Maturation of Sarcolemmal and Mitochondrial KATP Channels. Sci. Rep. 6, 34154. doi:10.1038/srep34154
Krylatov, A. V., Tsibulnikov, S. Y., Mukhomedzyanov, A. V., Boshchenko, A. A., Goldberg, V. E., Jaggi, A. S., et al. (2021). The Role of Natriuretic Peptides in the Regulation of Cardiac Tolerance to Ischemia/Reperfusion and Postinfarction Heart Remodeling. J. Cardiovasc Pharmacol. Ther. 26 (2), 131–148. doi:10.1177/1074248420952243
Krylova, I. B., Selina, E. N., Bulion, V. V., Rodionova, O. M., Evdokimova, N. R., Belosludtseva, N. V., et al. (2021). Uridine Treatment Prevents Myocardial Injury in Rat Models of Acute Ischemia and Ischemia/reperfusion by Activating the Mitochondrial ATP-dependent Potassium Channel. Sci. Rep. 11 (1), 16999. doi:10.1038/s41598-021-96562-7
Laimon, W., El-Ziny, M., El-Hawary, A., Elsharkawy, A., Salem, N. A., Aboelenin, H. M., et al. (2021). Genetic and Clinical Heterogeneity of Permanent Neonatal Diabetes Mellitus: a Single Tertiary Centre Experience. Acta Diabetol. 58 (12), 1689–1700. doi:10.1007/s00592-021-01788-6
Lee, S. H., Kang, D., Ok, S. H., Kim, J. Y., Bae, S. I., Hwang, Y., et al. (2020). Lipofundin MCT/LCT Inhibits Levcromakalim-Induced Vasodilation by Inhibiting Endothelial Nitric Oxide Release. Int. J. Mol. Sci. 21 (5), 1763. doi:10.3390/ijms21051763
Leroy, C., Privé, A., Bourret, J. C., Berthiaume, Y., Ferraro, P., and Brochiero, E. (2006). Regulation of ENaC and CFTR Expression with K+ Channel Modulators and Effect on Fluid Absorption across Alveolar Epithelial Cells. Am. J. Physiol. Lung Cell Mol. Physiol. 291 (6), L1207–L1219. doi:10.1152/ajplung.00376.2005
Li, A., Knutsen, R. H., Zhang, H., Osei-Owusu, P., Moreno-Dominguez, A., Harter, T. M., et al. (2013). Hypotension Due to Kir6.1 Gain-Of-Function in Vascular Smooth Muscle. J. Am. Heart Assoc. 2 (4), e000365. doi:10.1161/JAHA.113.000365
Li, B., Gao, M. X., Yang, W. L., Chai, C., Zhang, D. X., Cai, H. Y., et al. (2019). Inhibitory Effects of Sulfur Dioxide within the Nucleus Tractus Solitarii of Rats: Involvement of Calcium Ion Channels, Adenine Nucleoside Triphosphate-Sensitive Potassium Channels, and the Nitric Oxide/cyclic Guanine Trinucleotide Phosphate Pathway. Neuroreport 30 (13), 914–920. doi:10.1097/WNR.0000000000001304
Li, J., Zhou, W., Chen, W., Wang, H., Zhang, Y., and Yu, T. (2020). Mechanism of the Hypoxia Inducible Factor 1/hypoxic Response Element Pathway in Rat Myocardial Ischemia/diazoxide Post-conditioning. Mol. Med. Rep. 21 (3), 1527–1536. doi:10.3892/mmr.2020.10966
Li, Y., Aziz, Q., Anderson, N., Ojake, L., and Tinker, A. (2020). Endothelial ATP-Sensitive Potassium Channel Protects against the Development of Hypertension and Atherosclerosis. Hypertension 76 (3), 776–784. doi:10.1161/HYPERTENSIONAHA.120.15355
Li, Y., Feng, Y., Liu, L., Li, X., Li, X. Y., Sun, X., et al. (2021). The Baroreflex Afferent Pathway Plays a Critical Role in H2S-Mediated Autonomic Control of Blood Pressure Regulation under Physiological and Hypertensive Conditions. Acta Pharmacol. Sin. 42 (6), 898–908. doi:10.1038/s41401-020-00549-5
Lithovius, V., Saarimäki-Vire, J., Balboa, D., Ibrahim, H., Montaser, H., Barsby, T., et al. (2021). SUR1-mutant iPS Cell-Derived Islets Recapitulate the Pathophysiology of Congenital Hyperinsulinism. Diabetologia 64 (3), 630–640. doi:10.1007/s00125-020-05346-7
Liu, C., Lai, Y., Pei, J., Huang, H., Zhan, J., Ying, S., et al. (2021). Clinical and Genetic Analysis of KATP Variants with Heart Failure Risk in Patients with Decreased Serum ApoA-I Levels. J. Clin. Endocrinol. Metab. 106 (8), 2264–2278. doi:10.1210/clinem/dgab336
Liu, Y., Song, Y., Li, S., and Mo, L. (2021). Cardioprotective Effect of Quercetin against Ischemia/Reperfusion Injury Is Mediated through NO System and Mitochondrial K-ATP Channels. Cell J. 23 (2), 184–190. doi:10.22074/cellj.2021.7183
Lkhagva, B., Lin, Y. K., Chen, Y. C., Cheng, W. L., Higa, S., Kao, Y. H., et al. (2021). ZFHX3 Knockdown Dysregulates Mitochondrial Adaptations to Tachypacing in Atrial Myocytes through Enhanced Oxidative Stress and Calcium Overload. Acta Physiol. (Oxf) 231 (4), e13604. doi:10.1111/apha.13604
Lu, A., Chu, C., Mulvihill, E., Wang, R., and Liang, W. (2019). ATP-sensitive K+ Channels and Mitochondrial Permeability Transition Pore Mediate Effects of Hydrogen Sulfide on Cytosolic Ca2+ Homeostasis and Insulin Secretion in β-cells. Pflugers Arch. 471 (11-12), 1551–1564. doi:10.1007/s00424-019-02325-9
Ma, Y., Cheng, N., Sun, J., Lu, J. X., Abbasi, S., Wu, G., et al. (2020). Atherogenic L5 LDL Induces Cardiomyocyte Apoptosis and Inhibits KATP Channels through CaMKII Activation. Lipids Health Dis. 19 (1), 189. doi:10.1186/s12944-020-01368-7
Magierowska, K., Korbut, E., Hubalewska-Mazgaj, M., Surmiak, M., Chmura, A., Bakalarz, D., et al. (2019). Oxidative Gastric Mucosal Damage Induced by Ischemia/reperfusion and the Mechanisms of its Prevention by Carbon Monoxide-Releasing Tricarbonyldichlororuthenium (II) Dimer. Free Radic. Biol. Med. 145, 198–208. doi:10.1016/j.freeradbiomed.2019.09.032
Männistö, J. M. E., Maria, M., Raivo, J., Kuulasmaa, T., Otonkoski, T., Huopio, H., et al. (2020). Clinical and Genetic Characterization of 153 Patients with Persistent or Transient Congenital Hyperinsulinism. J. Clin. Endocrinol. Metab. 105 (4), dgz271. doi:10.1210/clinem/dgz271
Martin, G. M., Sung, M. W., and Shyng, S. L. (2020). Pharmacological Chaperones of ATP-Sensitive Potassium Channels: Mechanistic Insight from cryoEM Structures. Mol. Cell Endocrinol. 502, 110667. doi:10.1016/j.mce.2019.110667
McClenaghan, C., Huang, Y., Yan, Z., Harter, T. M., Halabi, C. M., Chalk, R., et al. (2020). Glibenclamide Reverses Cardiovascular Abnormalities of Cantu Syndrome Driven by KATP Channel Overactivity. J. Clin. Invest 130 (3), 1116–1121. doi:10.1172/JCI130571
Moriguchi, S., Ishizuka, T., Yabuki, Y., Shioda, N., Sasaki, Y., Tagashira, H., et al. (2018). Blockade of the KATP Channel Kir6.2 by Memantine Represents a Novel Mechanism Relevant to Alzheimer's Disease Therapy. Mol. Psychiatry 23 (2), 211–221. doi:10.1038/mp.2016.187
Mulder, B. A., van Veldhuisen, D. J., and Rienstra, M. (2021). Sudden Cardiac Death in Heart Failure: More Than Meets the Eye. Eur. J. Heart Fail 23 (8), 1361–1363. doi:10.1002/ejhf.2212
Noma, A. (1983). ATP-regulated K+ Channels in Cardiac Muscle. Nature 305 (5930), 147–148. doi:10.1038/305147a0
Paggio, A., Checchetto, V., Campo, A., Menabò, R., Di Marco, G., Di Lisa, F., et al. (2019). Identification of an ATP-Sensitive Potassium Channel in Mitochondria. Nature 572 (7771), 609–613. doi:10.1038/s41586-019-1498-3
Papanicolaou, K. N., Ashok, D., Liu, T., Bauer, T. M., Sun, J., Li, Z., et al. (2020). Global Knockout of ROMK Potassium Channel Worsens Cardiac Ischemia-Reperfusion Injury but Cardiomyocyte-specific Knockout Does Not: Implications for the Identity of mitoKATP. J. Mol. Cell Cardiol. 139, 176–189. doi:10.1016/j.yjmcc.2020.01.010
Pereira, O., and Kowaltowski, A. J. (2021). Mitochondrial K+ Transport: Modulation and Functional Consequences. Molecules 26 (10), 2935. doi:10.3390/molecules26102935
Pertiwi, K. R., Hillman, R. M., Scott, C. A., and Chilton, E. L. (2019). Ischemia Reperfusion Injury Produces, and Ischemic Preconditioning Prevents, Rat Cardiac Fibroblast Differentiation: Role of KATP Channels. J. Cardiovasc Dev. Dis. 6 (2), 22. doi:10.3390/jcdd6020022
Pipatpolkai, T., Usher, S., Stansfeld, P. J., and Ashcroft, F. M. (2020). New Insights into KATP Channel Gene Mutations and Neonatal Diabetes Mellitus. Nat. Rev. Endocrinol. 16 (7), 378–393. doi:10.1038/s41574-020-0351-y
Portal, L., Morin, D., Motterlini, R., Ghaleh, B., and Pons, S. (2019). The CO-releasing Molecule CORM-3 Protects Adult Cardiomyocytes against Hypoxia-Reoxygenation by Modulating pH Restoration. Eur. J. Pharmacol. 862, 172636. doi:10.1016/j.ejphar.2019.172636
Pui Ping, C., Akhtar, M. N., Israf, D. A., Perimal, E. K., and Sulaiman, M. R. (2020). Possible Participation of Ionotropic Glutamate Receptors and L-Arginine-Nitric Oxide-Cyclic Guanosine Monophosphate-ATP-Sensitive K+ Channel Pathway in the Antinociceptive Activity of Cardamonin in Acute Pain Animal Models. Molecules 25 (22), 5385. doi:10.3390/molecules25225385
Rameshrad, M., Omidkhoda, S. F., Razavi, B. M., and Hosseinzadeh, H. (2021). Evaluating the Possible Role of Mitochondrial ATP-Sensitive Potassium Channels in the Cardioprotective Effects of Morin in the Isolated Rat Heart. Life Sci. 264, 118659. doi:10.1016/j.lfs.2020.118659
Rieg, A. D., Suleiman, S., Bünting, N. A., Verjans, E., Spillner, J., Schnöring, H., et al. (2020). Levosimendan Reduces Segmental Pulmonary Vascular Resistance in Isolated Perfused Rat Lungs and Relaxes Human Pulmonary Vessels. PLoS One 15 (5), e0233176. doi:10.1371/journal.pone.0233176
Rosenfeld, E., Ganguly, A., and De Leon, D. D. (2019). Congenital Hyperinsulinism Disorders: Genetic and Clinical Characteristics. Am. J. Med. Genet. C Semin. Med. Genet. 181 (4), 682–692. doi:10.1002/ajmg.c.31737
Rosenfeld, E., Mitteer, L., Boodhansingh, K., Becker, S. A., McKnight, H., Boyajian, L., et al. (2021). Case Report: Two Distinct Focal Congenital Hyperinsulinism Lesions Resulting from Separate Genetic Events. Front. Pediatr. 9, 699129. doi:10.3389/fped.2021.699129
Roubenne, L., Marthan, R., Le Grand, B., and Guibert, C. (2021). Hydrogen Sulfide Metabolism and Pulmonary Hypertension. Cells 10 (6), 1477. doi:10.3390/cells10061477
Sachse, G., Haythorne, E., Proks, P., Stewart, M., Cater, H., Ellard, S., et al. (2020). Phenotype of a Transient Neonatal Diabetes Point Mutation (SUR1-R1183w) in Mice. Wellcome Open Res. 5, 15. doi:10.12688/wellcomeopenres.15529.2
Sakamoto, K., and Kurokawa, J. (2019). Involvement of Sex Hormonal Regulation of K+ Channels in Electrophysiological and Contractile Functions of Muscle Tissues. J. Pharmacol. Sci. 139 (4), 259–265. doi:10.1016/j.jphs.2019.02.009
Savarese, G., Stolfo, D., Sinagra, G., and Lund, L. H. (2022). Heart Failure with Mid-range or Mildly Reduced Ejection Fraction. Nat. Rev. Cardiol. 19 (2), 100–116. doi:10.1038/s41569-021-00605-5
Scala, R., Maqoud, F., Zizzo, N., Mele, A., Camerino, G. M., Zito, F. A., et al. (2020). Pathophysiological Consequences of KATP Channel Overactivity and Pharmacological Response to Glibenclamide in Skeletal Muscle of a Murine Model of Cantù Syndrome. Front. Pharmacol. 11, 604885. doi:10.3389/fphar.2020.604885
Severino, P., D'Amato, A., Netti, L., Pucci, M., Mariani, M. V., Cimino, S., et al. (2020). Susceptibility to Ischaemic Heart Disease: Focusing on Genetic Variants for ATP-Sensitive Potassium Channel beyond Traditional Risk Factors. Eur. J. Prev. Cardiol. 1, 1. doi:10.1177/2047487320926780
Shin, B., Saeed, M. Y., Esch, J. J., Guariento, A., Blitzer, D., Moskowitzova, K., et al. (2019). A Novel Biological Strategy for Myocardial Protection by Intracoronary Delivery of Mitochondria: Safety and Efficacy. JACC Basic Transl. Sci. 4 (8), 871–888. doi:10.1016/j.jacbts.2019.08.007
Shoji, T., Hayashi, M., Sumi, C., Kusunoki, M., Uba, T., Matsuo, Y., et al. (2019). Pharmacological Polysulfide Suppresses Glucose-Stimulated Insulin Secretion in an ATP-Sensitive Potassium Channel-dependent Manner. Sci. Rep. 9 (1), 19377. doi:10.1038/s41598-019-55848-7
Sim, J. H., Yang, D. K., Kim, Y. C., Park, S. J., Kang, T. M., So, I., et al. (2002). ATP-sensitive K(+) Channels Composed of Kir6.1 and SUR2B Subunits in guinea Pig Gastric Myocytes. Am. J. Physiol. Gastrointest. Liver Physiol. 282 (1), G137–G144. doi:10.1152/ajpgi.00057x.2002
Small, H. Y., McNeilly, S., Mary, S., Sheikh, A. M., and Delles, C. (2019). Resistin Mediates Sex-dependent Effects of Perivascular Adipose Tissue on Vascular Function in the Shrsp. Sci. Rep. 9 (1), 6897. doi:10.1038/s41598-019-43326-z
Smeland, M. F., McClenaghan, C., Roessler, H. I., Savelberg, S., Hansen, G. Å. M., Hjellnes, H., et al. (2019). ABCC9-related Intellectual Disability Myopathy Syndrome Is a KATP Channelopathy with Loss-Of-Function Mutations in ABCC9. Nat. Commun. 10 (1), 4457. doi:10.1038/s41467-019-12428-7
Smith, K. J., Neill, M., and Hoiland, R. L. (2020). Scratching the Surface of Hypoxic Cerebral Vascular Control: a Potentially Polarizing View of Mechanistic Research in Humans. J. Physiol. 598 (16), 3313–3315. doi:10.1113/JP280244
Solhjoo, S., and O'Rourke, B. (2015). Mitochondrial Instability during Regional Ischemia-Reperfusion Underlies Arrhythmias in Monolayers of Cardiomyocytes. J. Mol. Cell Cardiol. 78, 90–99. doi:10.1016/j.yjmcc.2014.09.024
Sooklal, C. R., López-Alonso, J. P., Papp, N., and Kanelis, V. (2018). Phosphorylation Alters the Residual Structure and Interactions of the Regulatory L1 Linker Connecting NBD1 to the Membrane-Bound Domain in SUR2B. Biochemistry 57 (44), 6278–6292. doi:10.1021/acs.biochem.8b00503
Stewart, L., and Turner, N. A. (2021). Channelling the Force to Reprogram the Matrix: Mechanosensitive Ion Channels in Cardiac Fibroblasts. Cells 10 (5), 990. doi:10.3390/cells10050990
Sudhir, R., Du, Q., Sukhodub, A., Jovanović, S., and Jovanović, A. (2020). Improved Adaptation to Physical Stress in Mice Overexpressing SUR2A Is Associated with Changes in the Pattern of Q-T Interval. Pflugers Arch. 472 (6), 683–691. doi:10.1007/s00424-020-02401-5
Svalastoga, P., Sulen, Å., Fehn, J. R., Aukland, S. M., Irgens, H., Sirnes, E., et al. (2020). Intellectual Disability in KATP Channel Neonatal Diabetes. Diabetes Care 43 (3), 526–533. doi:10.2337/dc19-1013
Takasawa, K., Miyakawa, Y., Saito, Y., Adachi, E., Shidei, T., Sutani, A., et al. (2021). Marked Clinical Heterogeneity in Congenital Hyperinsulinism Due to a Novel Homozygous ABCC8 Mutation. Clin. Endocrinol. (Oxf) 94 (6), 940–948. doi:10.1111/cen.14443
Testai, L., Sestito, S., Martelli, A., Gorica, E., Flori, L., Calderone, V., et al. (2021). Synthesis and Pharmacological Characterization of Mitochondrial KATP Channel Openers with Enhanced Mitochondriotropic Effects. Bioorg Chem. 107, 104572. doi:10.1016/j.bioorg.2020.104572
Udelson, J. E., Lewis, G. D., Shah, S. J., Zile, M. R., Redfield, M. M., Burnett, J., et al. (2020). Effect of Praliciguat on Peak Rate of Oxygen Consumption in Patients with Heart Failure with Preserved Ejection Fraction: The CAPACITY HFpEF Randomized Clinical Trial. JAMA 324 (15), 1522–1531. doi:10.1001/jama.2020.16641
Usher, S. G., Ashcroft, F. M., and Puljung, M. C. (2020). Nucleotide Inhibition of the Pancreatic ATP-Sensitive K+ Channel Explored with Patch-Clamp Fluorometry. Elife 9, e52775. doi:10.7554/eLife.52775
Wang, C., Cheng, W., Bai, S., Ye, L., Du, J., Zhong, M., et al. (2019). White Mulberry Fruit Polysaccharides Enhance Endothelial Nitric Oxide Production to Relax Arteries In Vitro and Reduce Blood Pressure In Vivo. Biomed. Pharmacother. 116, 109022. doi:10.1016/j.biopha.2019.109022
Wang, C., Liu, L., Wang, Y., and Xu, D. (2021). Advances in the Mechanism and Treatment of Mitochondrial Quality Control Involved in Myocardial Infarction. J. Cell Mol. Med. 25 (15), 7110–7121. doi:10.1111/jcmm.16744
Wang, S., Guo, X., Long, C. L., Li, C., Zhang, Y. F., Wang, J., et al. (2019). SUR2B/Kir6.1 Channel Openers Correct Endothelial Dysfunction in Chronic Heart Failure via the miR-1-3p/ET-1 Pathway. Biomed. Pharmacother. 110, 431–439. doi:10.1016/j.biopha.2018.11.135
Wang, W. L., Ge, T. Y., Chen, X., Mao, Y., and Zhu, Y. Z. (2020). Advances in the Protective Mechanism of NO, H2S, and H2 in Myocardial Ischemic Injury. Front. Cardiovasc Med. 7, 588206. doi:10.3389/fcvm.2020.588206
White, D. S., Chowdhury, S., Idikuda, V., Zhang, R., Retterer, S. T., Goldsmith, R. H., et al. (2021). cAMP Binding to Closed Pacemaker Ion Channels Is Non-cooperative. Nature 595 (7868), 606–610. doi:10.1038/s41586-021-03686-x
Yang, H. Q., Jana, K., Rindler, M. J., and Coetzee, W. A. (2018). The Trafficking Protein, EHD2, Positively Regulates Cardiac Sarcolemmal KATP Channel Surface Expression: Role in Cardioprotection. FASEB J. 32 (3), 1613–1625. doi:10.1096/fj.201700027R
Yang, H. Q., Martinez-Ortiz, W., Hwang, J., Fan, X., Cardozo, T. J., and Coetzee, W. A. (2020). Palmitoylation of the KATP Channel Kir6.2 Subunit Promotes Channel Opening by Regulating PIP2 Sensitivity. Proc. Natl. Acad. Sci. U. S. A. 117 (19), 10593–10602. doi:10.1073/pnas.1918088117
Yang, L., Li, R. C., Xiang, B., Li, Y. C., Wang, L. P., Guo, Y. B., et al. (2021). Transcriptional Regulation of Intermolecular Ca2+ Signaling in Hibernating Ground Squirrel Cardiomyocytes: The Myocardin-Junctophilin axis. Proc. Natl. Acad. Sci. U. S. A. 118 (14), e2025333118. doi:10.1073/pnas.2025333118
Yang, M., Dart, C., Kamishima, T., and Quayle, J. M. (2020). Hypoxia and Metabolic Inhibitors Alter the Intracellular ATP:ADP Ratio and Membrane Potential in Human Coronary Artery Smooth Muscle Cells. PeerJ 8, e10344. doi:10.7717/peerj.10344
Yu, H., Zhang, F., Yan, P., Zhang, S., Lou, Y., Geng, Z., et al. (2021). LARP7 Protects against Heart Failure by Enhancing Mitochondrial Biogenesis. Circulation 143 (20), 2007–2022. doi:10.1161/CIRCULATIONAHA.120.050812
Yu, L., Li, W., Park, B. M., Lee, G.-J., and Kim, S. H. (2019). Hypoxia Augments NaHS-Induced ANP Secretion via KATP Channel, HIF-1α and PPAR-γ Pathway. Peptides 121, 170123. doi:10.1016/j.peptides.2019.170123
Zhang, D. M., and Lin, Y. F. (2020). Functional Modulation of Sarcolemmal KATP Channels by Atrial Natriuretic Peptide-Elicited Intracellular Signaling in Adult Rabbit Ventricular Cardiomyocytes. Am. J. Physiol. Cell Physiol. 319 (1), C194–C207. doi:10.1152/ajpcell.00409.2019
Zhang, H., Hanson, A., de Almeida, T. S., Emfinger, C., McClenaghan, C., Harter, T., et al. (2021). Complex Consequences of Cantu Syndrome SUR2 Variant R1154Q in Genetically Modified Mice. JCI Insight 6 (5), e145934. doi:10.1172/jci.insight.145934
Zhang, H. X., Silva, J. R., Lin, Y. W., Verbsky, J. W., Lee, U. S., Kanter, E. M., et al. (2013). Heterogeneity and Function of K(ATP) Channels in Canine Hearts. Heart rhythm. 10 (10), 1576–1583. doi:10.1016/j.hrthm.2013.07.020
Zhang, X., Zhang, X., Xiong, Y., Xu, C., Liu, X., Lin, J., et al. (2016). Sarcolemmal ATP-Sensitive Potassium Channel Protects Cardiac Myocytes against Lipopolysaccharide-Induced Apoptosis. Int. J. Mol. Med. 38 (3), 758–766. doi:10.3892/ijmm.2016.2664
Zhang, Y., Wang, X., Liu, R., Li, Q., Tian, W., Lei, H., et al. (2021). The Effectiveness and Safety of Nicorandil in the Treatment of Patients with Microvascular Angina: A Protocol for Systematic Review and Meta-Analysis. Med. Baltim. 100 (2), e23888. doi:10.1097/MD.0000000000023888
Zhao, G., Kaplan, A., Greiser, M., and Lederer, W. J. (2020). The Surprising Complexity of KATP Channel Biology and of Genetic Diseases. J. Clin. Invest 130 (3), 1112–1115. doi:10.1172/JCI135759
Zhao, Y., Ge, J., Li, X., Guo, Q., Zhu, Y., Song, J., et al. (2019). Vasodilatory Effect of Formaldehyde via the NO/cGMP Pathway and the Regulation of Expression of KATP, BKCa and L-type Ca2+ Channels. Toxicol. Lett. 312, 55–64. doi:10.1016/j.toxlet.2019.04.006
Zhou, M., Yoshikawa, K., Akashi, H., Miura, M., Suzuki, R., Li, T. S., et al. (2019). Localization of ATP-Sensitive K+ Channel Subunits in Rat Liver. World J. Exp. Med. 9 (2), 14–31. doi:10.5493/wjem.v9.i2.14
Zhou, Z. Y., Zhao, W. R., Shi, W. T., Xiao, Y., Ma, Z. L., Xue, J. G., et al. (2019). Endothelial-Dependent and Independent Vascular Relaxation Effect of Tetrahydropalmatine on Rat Aorta. Front. Pharmacol. 10, 336. doi:10.3389/fphar.2019.00336
Zi, C., Zhang, C., Yang, Y., and Ma, J. (2020). Penehyclidine Hydrochloride Protects against Anoxia/reoxygenation Injury in Cardiomyocytes through ATP-Sensitive Potassium Channels, and the Akt/GSK-3β and Akt/mTOR Signaling Pathways. Cell Biol. Int. 44 (6), 1353–1362. doi:10.1002/cbin.11329
Glossary
ADP adenosine diphosphate
AIMS ABCC9-related intellectual disability myopathy Syndrome
ANP atrial natriuretic peptide
ANT adenine nucleotide translocator
AP action potential
APD action potential duration
ATP adenosine triphosphate
BCL2 B-cell lymphoma-2
CaMK calcium/calmodulin-dependent protein kinase
CF cardiac fibroblasts
cGMP cyclic guanosine monophosphate
CHI congenital hyperinsulinism
CO carbon monoxide
CORM-3 CO-releasing molecule-3
CS cantú syndrome
CSE cystathionine γ-lyase
Cyt C cytochrome C
DEND syndrome developmental delay epilepsy and neonatal diabetes syndrome
EAD early afterdepolarization
EHD-2 Eps15 homology domain-containing protein-2
ERK extracellular signal-regulated protein kinase
GST genistein
H2S hydrogen sulfide
HCN hyperpolarization-activated cyclic-nucleotide gated channels
hESC human embryonic stem cell
HF heart failure
HO-1/CO heme oxygenase-1/carbon monoxide
IMM inner mitochondrial membrane
INS-1E cells a pancreatic ß cell line
K+ potassium
KATP channels ATP-sensitive potassium channels;
Kir K+ inward rectifiers
LDL low-density lipoprotein
LPS lipopolysaccharide
MF myofibroblasts
MIRI myocardial ischaemia reperfusion injury
mitoKATP mitochondrial ATP-sensitive potassium
MPTP permeability transition pore
NBF nucleotide binding folds
NDM neonatal diabetes mellitus
NO nitric oxide
NPR-A natriuretic peptide receptor type A
O2 oxygen
ONOO- peroxynitrite
PCI percutaneous coronary intervention
PHC penehyclidine hydrochloride
PNDM permanent neonatal diabetes mellitus
PiC phosphate carrier
PKG cGMP-dependent protein kinase
PLN phospholamban
ROS reactive oxygen species
RyR ryanodine receptor
sarcKATP sarcolemma ATP-sensitive potassium
SCD sudden cardiac death
SO2 sulfur dioxide
SUR sulfonylurea receptors
TMD transmembrane domains
TNDM transient neonatal diabetes mellitus
VCMs ventricular cardiomyocytes
ZFHX3 zinc finger homeobox three gene
Keywords: KATP channels, mitoKATP channels, myocardial ischemia, channelopathy, small active molecules, mutation
Citation: Wang Z, Bian W, Yan Y and Zhang D-M (2022) Functional Regulation of KATP Channels and Mutant Insight Into Clinical Therapeutic Strategies in Cardiovascular Diseases. Front. Pharmacol. 13:868401. doi: 10.3389/fphar.2022.868401
Received: 02 February 2022; Accepted: 03 June 2022;
Published: 28 June 2022.
Edited by:
Ismail Laher, University of British Columbia, CanadaReviewed by:
Khaled S. Abd-Elrahman, University of Ottawa, CanadaMasayo Koide, University of Vermont, United States
Michael Christian Puljung, Trinity College, United States
Pei-Chun Chen, National Cheng Kung University, Taiwan
Copyright © 2022 Wang, Bian, Yan and Zhang. This is an open-access article distributed under the terms of the Creative Commons Attribution License (CC BY). The use, distribution or reproduction in other forums is permitted, provided the original author(s) and the copyright owner(s) are credited and that the original publication in this journal is cited, in accordance with accepted academic practice. No use, distribution or reproduction is permitted which does not comply with these terms.
*Correspondence: Dai-Min Zhang, ZGFpbWluemhAMTI2LmNvbQ==
†These authors have contributed equally to this work