- Department of Pharmacology (The State-Province Key Laboratories of Biomedicine-Pharmaceutics of China, Key Laboratory of Cardiovascular Research, Ministry of Education), College of Pharmacy, Harbin Medical University, Harbin, China
In the past, different human disorders were described by scientists from the perspective of either environmental factors or just by genetically related mechanisms. The rise in epigenetic studies and its modifications, i.e., heritable alterations in gene expression without changes in DNA sequences, have now been confirmed in diseases. Modifications namely, DNA methylation, posttranslational histone modifications, and non-coding RNAs have led to a better understanding of the coaction between epigenetic alterations and human pathologies. Melatonin is a widely-produced indoleamine regulator molecule that influences numerous biological functions within many cell types. Concerning its broad spectrum of actions, melatonin should be investigated much more for its contribution to the upstream and downstream mechanistic regulation of epigenetic modifications in diseases. It is, therefore, necessary to fill the existing gaps concerning corresponding processes associated with melatonin with the physiological abnormalities brought by epigenetic modifications. This review outlines the findings on melatonin’s action on epigenetic regulation in human diseases including neurodegenerative diseases, diabetes, cancer, and cardiovascular diseases. It summarizes the ability of melatonin to act on molecules such as proteins and RNAs which affect the development and progression of diseases.
1 Introduction
Epigenetics can be redefined as any biological process that changes the genetic expression and activity minus alteration of the DNA sequence resulting in cellular modifications (Dupont et al., 2009). There are a few types of epigenetic modifications that have been uncovered: histone modifications, DNA methylation, and “RNA epigenetics.” Of the three, DNA methylation has been the most extensively studied modification in epigenetics and has been found to influence genetic stability, mutations, and gene expressions (Bird, 1986; Bell et al., 2011; Lind and Spagopoulou, 2018). Located strategically at position 5 of carbon on the CpG sites (cytosine and guanine separated by a phosphate) makes it available to convert cytosine to 5-methylcytosine. Due to heavy methylation in some regions than others, during cell division, genetic material transfer results in aberrantly programmed transcription inherited by the daughter cells aided by DNA methyltransferases (Edwards et al., 2017). Such modulations have been confirmed in many diseases making DNA methylation a very important agent in the study of the etiology of diseases (Wang et al., 2018a).
Histone modification is a post-translational process involving gene expression alterations through the small protein ‘tails’ from the nucleosomes. The covalent addition of these histone proteins including methylation, phosphorylation, sumoylation, acetylation, and ubiquitylation, have all been shown to be responsible for the epigenetic modifications (Paz et al., 2011). Aberrations in histone modifications can lead to the development and progression of diseases through disruption of gene regulations which could be transmitted from one generation to another (Renani et al., 2019).
The lately discovered epigenetic modification is the “RNA epigenetics” which includes the non-coding RNAs (miRNA, siRNA, lncRNA, lincRNA, and eRNA) and RNA methylation (N6-adenosine, 5-methylcytosine, pseudouridine, and N1-methyladenosine). The microRNA (miRNA) has been a notorious contributor to diseases when it binds to specific sites associated with the translation of mRNA, inhibiting the process. For instance, when binding occurs at the 3’ untranslated regions of mRNA, the actions of mRNA are suppressed (Sarshad et al., 2018). Collectively, epigenetic modifications play a key role in the biological processes and pathogenesis of diseases. Since its emergence, numerous studies on human diseases have been conducted in an attempt to design epigenetic therapies for the prevention and management of diseases.
Melatonin is a widely spread indoleamine derivative (chemically identified as N-acetyl-5-methoxytryptamine) hormone largely synthesized by the pineal gland and can also be found in small quantities in organs such as the retina, lens, gut, bone marrow, skin, and other solid organs with multifunctional facets (Acuña-Castroviejo et al., 2014). Because of its extensive distribution in diverse cells and well penetrability through bio-barriers, it is been reported to induce pathophysiological signals. For instance, in neurodegenerative disorders, supplementation of melatonin has been widely reported to alleviate cognition impairment through different mechanisms, including the normalization of brain insulin signaling (Xu et al., 2019), the elevation of antioxidant properties, restoration of antioxidants like glutathione (Paul et al., 2018), reduction of the amyloid-β (Aβ) and anti-inflammatory action (Lee et al., 2018a).
Favorable evidence has premised an antagonistic relationship between melatonin and insulin secretions observed in diabetic patients. Studies have demonstrated that the action of beta cells of the pancreas is suppressed by melatonin and vice versa (Li et al., 2018). That is, these two hormones tend to take turns to subdue each other, since the levels of melatonin are high at night in response to decreased insulin levels, and melatonin is low during the day in response to high levels of insulin. The presence of melatonin receptors MT1 and MT2 on the pancreases (Zibolka et al., 2015) provides a biochemical basis implicating the action of melatonin on insulin-dependent diabetic patients. Studies have revealed that melatonin improved aberrant glucose metabolism and decreased insulin secretion in diabetes (Li et al., 2018).
In the study of cancer, melatonin has exhibited positive results in the prevention and improvement of this disease. The vast knowledge of the hallmarks of cancer has expedited the understanding and development of different mechanisms involved in the treatment of cancer by melatonin. Mechanisms range from, targeting DNA repair, increasing the cytotoxicity of chemotherapy drugs, scavenging free radicals, and inhibiting tumor growth and metastasis (Chen et al., 2018). Studies performed on cardiovascular diseases have shown innumerable genes linked with the beneficial effects of melatonin receptors activation, suggesting its potential therapeutic action as it confers profound protective effects on the heart. Differentially expressed genes obtained via a networking analysis based on transcriptomes revealed transcriptional factors highly sensitive to melatonin treatment (Li et al., 2019a).
Based on the vast effects of melatonin on a variety of diseases, it is worth pondering the deep reason for the diverse functions and the common impression in these conditions. Regulation of epigenetic modification by melatonin seems to be the reasonable answer to the question. Yet, the study of the interaction between melatonin and epigenetics in human pathology is still in its infancy. There is no coherent and consistent picture to summarize the relativity of epigenetic modifications by melatonin currently. Therefore, we intend to narrow the gap by unraveling potential markers on the connections between previous and current work on the basic mechanisms involving epigenetic changes as a result of effects imparted by melatonin (both endogenous and exogenous) in pursuit of treatment of pathologies across the clinical spectrum. The information collated herein serves to account for the prospective formulation of ideas on melatonin’s function in alleviating various diseases. Furthermore, as a quick response guide, we have summarized in Table 1, the genes involved in the pathway and the mechanism of action by which melatonin alleviates these epigenetic-modulated diseases.
1.1 Neurodegenerative Disorders
For years, the aging brain has directly been linked with manifestations of neurodegenerative disorders such as Alzheimer’s disease (AD) and Parkinson's disease (PD). These were characterized by anomalies in the synthesis of proteins and a conglomeration of misfolded proteins as a result of altered signaling pathways by epigenetic manipulations (Shukla et al., 2019). These disorders otherwise associated with low levels of melatonin were significantly reversed when melatonin was administered and its receptors triggered (Bondy and Sharman, 2007).
1.1.1 Alzheimer’s Disease
Histone deacetylation (HDACs), one of the many epigenetic mechanisms now identified as playing a significant role in long-term potential and memory formation has been linked with memory and cognitive processes in the hippocampus and prefrontal cortex in Alzheimer’s disease. An experiment conducted on a Drosophila model showed how both knockdown and overexpression of HDACs resulted in long-term memory impairment in a specific way (Fitzsimons et al., 2013). By targeting specific classes of HDACs in these areas of the brain, the progression of the disease can therefore be seized and corrected. Recently, HDAC inhibitors have been established in patients with AD as potential targets due to their wide scope of effects, ranging from decreased deposition of Amyloid β-peptides, an immune response triggered by microglia, reduced β-secretase cleavage, regulated levels of SIRT1, BDNF, ABCA7, REST, LTP deficits and decreased phosphorylation and acetylation of Tau (Wang et al., 2019a; Rusek et al., 2019). Moreover, deacetylase classes of HDAC4 and HDAC2 levels were significantly upregulated in areas of the hippocampus, prefrontal cortex, and the basal forebrain region of neuronal cultured cells, in AD mouse models, (Chen et al., 2021). In the CK-p25 AD mouse model, increased binding of HDAC2 to promoter regions of genes with critical roles in learning and memory and synaptic plasticity was accompanied by reduced acetylation levels of H2BK5, H3K14, H4K5, andsq1 H4K12, reduced RNA polymerase II binding, and reduced gene expression (Giusti-Rodríguez et al., 2011). Thus, increased HDAC2/4 levels may lead to impaired neurological synaptic function and cognitive decline, a well-characterized pathological feature of AD.
De novo methylation and DNA methyltransferases (DNMTs) are critical in neurological function and behavior (Bayraktar and Kreutz, 2018). Consistent patterns of DNA methylation of particular interests have been reported in the replicated evidence of hypermethylation at CpG sites in neuropathology-associated epigenetic variations (Monti et al., 2020). Studies done on human postmortem brain samples revealed increased levels of mitochondrial 5-methylcytosine in CpG sites in the D-loop region of the entorhinal cortex, possibly leading to the formation of neurofibrillary tangles and accumulation of β-amyloid peptides (Blanch et al., 2016). Earlier on, it had been established that the D-loop region regulates mitochondrial transcription and regulation (Asin-Cayuela and Gustafsson, 2007), possibly explaining mitochondrial epigenetic mechanisms in AD. Corresponding immunoprecipitation followed by sequencing (ChIP-seq) analyses have revealed changes in the promoter (H3K4me3) or enhancer (H3K27ac) marks that correlate with gene expression alterations, while few alterations in heterochromatin or polycomb regions have been found (H3K9me3 and H3K27me3, respectively) (Akkers et al., 2009). H4K16ac is a histone mark localized to both enhancers and promoters generally associated with active gene expression, which is drastically lost in the AD cortex (Berson et al., 2018), pointing to an inability to upregulate H4K16ac in the aged AD brain.
Compelling evidence demonstrates melatonin’s neuroprotective activity in several models of brain injuries, impaired learning, and memory functions in AD (Shukla et al., 2019). Its antioxidant property has been shown to offer protective effects in AD through scavenging of the reactive oxygen and nitrogen species thereby decreasing Amyloid β-induced toxicity, and also solving sleep disturbance (Cardinali et al., 2010). Reduced formation of Aβ due to significant inhibition of inflammatory cytokines and interleukins triggered by microglial activation resulting in neuroinflammation, has been reported in transgenic AD mice when melatonin was administered (Olcese et al., 2009).
Lipopolysaccharide (LPS) has been widely used in studies including but not limited to, impaired learning and cognitive functions which are related to neurodegenerative diseases such as Parkinsonism and Alzheimer’s. Zhao et al. (2017), reported hyperactivity in the enzymatic action of the Histone acetyltransferases (HAT) coupled with an increase in histone acetylation H3 pattern in the fetal/immature brain of a maternal administered LPS. These effects were brought to control by maternally administered melatonin (Domínguez Rubio et al., 2017). LPS administered during pregnancy exhibited a decrease in histone acetylation activity of H4K8 and H3K9 in the hippocampus region, which exacerbated the impairment of learning and memory during the midlife and senectitude periods of the mice offspring (Li et al., 2016a). Previous studies have shown that impaired memory and learning are associated with the downregulation of histone acetylation, such as H3K9 (Singh et al., 2015) and H4K8 (Yan et al., 2015). This, therefore, increases the possibilities of melatonin as a potential drug in the treatment of LPS-induced neurodegenerative disorders through the mechanism process involving an increase of histone acetylation activity.
Abnormal regulation in phosphorylation of histone proteins modification by the histone enzymes, leading to accumulation of highly phosphorylated tau protein aggregates has been linked with cell growth and activation of gene transcription resulting in a dozen of neurodegenerative diseases including AD (Rossetto et al., 2012). The kinase activity of melatonin-induced phosphorylation and enhanced histone acetylation of proteins CBP (CREB-binding protein) and p300 was observed in Akt and MAPK/ERK1/2 signaling pathways on human neuroblastoma brain cells (Pan and Niles, 2015). Kinase enzymes of the MAPK/ERK1/2 signaling pathway have been implicated in the phosphorylation of histone proteins at serine -28 and -10 (H3S28 and H3S10) (He et al., 2005) resulting in the promotion of acetylation of histones at K9 (H3K9) (Zhong et al., 2003) and acetylation at K14 (H3K14) possibly causing tau phosphorylation. Xian li et al. demonstrated how melatonin enhanced the HAT activity of CPB/p300 histone proteins in the MAPK/ERK1/2 signaling pathway on neuronal stem cells correcting the abnormal phosphorylation through acetylation of histone proteins at lysine 14 (H3K14) (Li et al., 2017a), thus reducing the hyperphosphorylated effects on tau. A selective HDAC6 inhibitor effectively inhibited tau hyperphosphorylation leading to neuroprotection and promotion of neuronal neurite outgrowth (Wang et al., 2021). Furthermore, a synthesized hybrid molecule of melatonin and HDAC6 inhibitor showed a remarkable AD-modifying property having significantly higher efficacy compared to a positive control of HDAC6 inhibitor (He et al., 2021).
Additionally, phosphorylation of H3K14 and H3K9 have been demonstrated to cause activation of transcriptional regulation (Sawicka and Seiser, 2012), and enhanced chromatin structure relaxation (Wu et al., 2008), features seen in tau pathology of neurodegenerative diseases. Elsewhere, a comparison of tau extracted from the human brain diagnosed with AD with a normal brain shows upregulated levels of GSK-3β with elevated tau phosphorylation in the brain of the former, suggesting GSK-3β has a principal role in the pathophysiology of AD (Medina et al., 2011). Through MT2 receptor stimulation, melatonin causes a cascade of downstream activation of protein kinase C (PKC), phosphorylation, and inactivation of GSK-3β leading to the reduction of tau hyperphosphorylation (Shi et al., 2018). Studies have depicted downregulated levels of GSK-3β in neuronal cells exposed to melatonin, possibly due to inactivation of GSK-3β and consequently depletion of phosphorylation of tau at threonine/serine residues by melatonin (Majd et al., 2017).
The generation of the amyloid precursor protein initiates the development of the pathogenic peptides of Aβ, in the AD brain. Immunohistochemical analysis of the hippocampus and the frontal cortex revealed remarkable reductions in amyloid-beta deposition as a result of long-term treatment of melatonin through ERK phosphorylation and activation of transcription factors including CREB which in turn upregulates the ADAM 10 transactivation, causing a reduction in the generation of amyloid- β peptides (Shukla et al., 2015).
Collectively, these results show that further exploration of the role of melatonin enhanced transcriptional activation of these genes by their complementary proteins, together with the establishment of the connection between phosphorylated protein kinases and histone phosphorylation could give a deeper understanding and shade more light on the fate of the neuronal cells in the context of gene transcription and modulation of chromatin state and the pursuit of design and development of neurological therapeutics.
1.1.2 Parkinson's Disease
PD is a neurological disorder due to cytoplasmic aggregation of the α-synuclein protein within the neurons, jointly with the inadequate or loss of dopamine in the neurons in the regions of substantia nigra, resulting in motor and non-motor manifestations such as bradykinesia, tremor, rigidity, instability of the posture, random-eye movement, sleep behavioral disorder, cognitive impairments and autonomic dysfunction (Jankovic, 2008). Epigenetic mechanisms have been reported in PD as a vital factor in the etiology of this disease (Labbé et al., 2016). Alteration in the methylation process has been seen in CpG subset sites in PD patients. In one study, using 335 PD subjects, 82 CpG sites of DNA methylation were identified to be altered (Chuang et al., 2017). Another cohort study identified distinct patterns in methylation of CpG sites which was progressive as the disease advanced. The study also observed modulations in methylation when dopamine therapy was used (Henderson-Smith et al., 2019). Furthermore, the same study reported hypomethylation of α-synuclein which increased the expression of this protein possibly contributing to PD progression. The expression was however downregulated in levodopa exposure characterized by hypermethylation of α-synuclein, indicating that dopamine therapy may cause modulations in the methylation of this gene possibly controlling the progression of PD (Schmitt et al., 2015). Moreover, reduction in the action of sirtuin coupled with hyperacetylation of histone sites including H3K27, H3K9, and H2BK15 at the PD prefrontal cortex, have been profoundly dysregulated at genomic levels hence associated with the pathology of PD (Park et al., 2016). It was reported that disease-dependent elevation of histone acetylation seen in human PD could be due to degeneration of dopaminergic neurons and infiltrating activated microglia both contributing to the disease (Harrison et al., 2018) and that the use of HDACs inhibitors could offer a novel direction in the treatment approach of the PD (Sharma and Taliyan, 2015). Since their discovery, numerous miRNAs have been associated with the pathology of PD. As reviewed by SY Goh, miRNAs including miR-29, miR-485, let-7, miR-30, and miR-26 have recently been established as the miRNAs of interest in the pathogenesis of PD especially when their expression is dysregulation (Goh et al., 2019). For instance, miR-7 and miR-153 have been implicated in the pathology of PD where they have been identified to specifically bind to 3′-untranslated regions of α-synuclein, connotating that overexpression of these miRNAs can cause a significant decrease in the protein and mRNA levels of α-synuclein in patients with sporadic PD (Doxakis, 2010).
The biological effects of melatonin regarding PD are vastly widespread. Studies have demonstrated its neuroprotective effects in improving both motor and non-motor impairments experienced by PD patients (Medeiros et al., 2007). Melatonin alleviated oxidative stress involved in neurodegeneration possibly via increasing SOD, CAT, GPx, and through decreasing MDA and dopaminergic neuronal death in the SN of a rat model of PD (Ozsoy et al., 2015). Administration of melatonin was premised on the inhibition of autophagic-induced neuronal cell death and neurotoxicity in PD (Su et al., 2015). Similarly, neuroinflammation reported in PD caused by the cyclooxygenase type 2 pathway was decreased by melatonin (Ortiz et al., 2013). Pathologically, this supplement decreased the a-synuclein levels in the striatum in monkey models of PD and also protected against dopamine loss in the substantia nigra, and further replenished the striatal dopamine loss (Paul et al., 2018). Genetically, mutations of the leucine-rich repeat kinase 2 (LRRK2) gene associated with sleep disorders and PD were significantly decreased when melatonin was administered (Sun et al., 2016).
Epigenetic regulation of specific genes by HDACs through impairment of histone acetylation has been attributed to PD, accomplished through inhibitors of HDACs whose effects could lead to increased histone acetylation and transcription of genes that encode specific neurotrophic factors or via a reduction in the accumulation of neurotoxic proteins (such as α-synuclein) causing a neuroprotective effect (Shukla and Tekwani, 2020). A good example of this manifestation is depicted when HDAC inhibition by valproic acid demonstrated an upregulation of melatonin receptors and eventually reduced neurotoxic proteins thereby increasing the availability of melatonin hormone in the rat brain causing beneficial effects in neuronal related diseases (Bahna and Niles, 2017). Similarly, through inhibition of HDAC, valproic acid improved PD-like motor and non-motor symptoms in mice models (Kim et al., 2019) which eventually increased dopaminergic transmissions (Green et al., 2017). Although there are few studies on the epigenetic effects of melatonin on PD, melatonin functions on neuronal cells are undeniable great benefits, and further studies confirming its activities on HDACs via promotion of acetylation of histone proteins and prevention of dopamine loss in PD may prove vital.
DNA hypomethylation of the α-synuclein has been reported as a pathogenic substrate attributed to the development of Lewy bodies diseases such as PD. One such study, a cohort survey, showed how all PD subjects exhibited significant hypomethylation of the a-synuclein compared to the controls suggesting a possible nexus between a-synuclein, promoter hypomethylation, and the risk of PD (Pihlstrøm et al., 2015). Previously, melatonin has been used as a neuroprotective agent due to its anti-assembly effects against mutant α-synuclein-induced injury (Brito-Armas et al., 2013) and mitigation of neurotoxicity in the substantia nigra (Ono et al., 2012). Melatonin is well known for its powerful antioxidant effects by scavenging free radicals and also by preventing catecholamine auto-oxidation observed in PD via the O-methylation process, thereby preserving mitochondria and normal DNA methylation (Yang et al., 2019). Together, these findings suggest that melatonin’s beneficial effects in PD could occur through modulation of DNA methylation, and future research on this mechanism could prove vital.
2 Diabetes
2.1 Type 1 Diabetes
Type 1 diabetes (T1D) also known as insulin-dependent diabetes mellitus is an autoimmune condition characterized by inflammatory responses due to the influx of immune response cells in the pancreas, leading to the destruction of insulin-producing beta cells (Tai et al., 2016). Interaction between environmental and genetic factors has caused alteration of gene expression through epigenetic regulations in T1D (Cerna, 2019). Several studies point to the involvement of inflammatory inducers such as cytokines and lipopolysaccharides, which damage cellular and molecular components (Pestana et al., 2016) as among the causative agents of T1D. Cyclooxygenase-2 (COX-2) and inducible Nitric Oxide Synthase (iNOS) expressions have been vindicated in this inflammatory induced response (Chen et al., 2016b). These inflammatory inducible proteins have been reported as upstream factors of T1D via activation of transcription factors through binding of the kappa beta (NF-кB) promoter regions resulting in modulation of epigenetic chromatin remodeling (Hu et al., 2016a). Changes in DNA methylation were observed at the insulin region in the islets as a result of cross-talk between the β-cells affiliated with DNA methylation and the immune response which suggested that an increase in immune response such as cytokines, can result in an increase in DNA methylation and decrease in insulin levels, a phenomenon observed in T1D (Rui et al., 2016). Histone modification via hyperacetylation was implicated in T1D associated with COX-2 expression, whereby monocyte acetylated histone H4 levels were remarkably elevated in diabetic patients relative to non-diabetic patients (Chen et al., 2009). The same study furthermore highlighted how these hyperacetylations were restricted to patients who had no complications further suggesting the potential protective effect of histone hyperacetylation in T1D patients with complications such as endothelial and vascular dysfunctions (Chen et al., 2009).
Though inadequately studied, the association between miRNAs and immune cells has been established in T1D. Studies have found that dysregulation of expressed miRNAs which correlates with immune cells such as T cells and B lymphocytes was linked to the onset of T1D and that these miRNAs when manipulated to participate in the preservation of cells of the pancreas as reviewed by Mohammad Taheri et al. (2020).
The importance of melatonin in immune cells has been established through modulation of responses of pancreatic cells whereby melatonin exerted beneficial effects in type 1 diabetes through suppression of T cell proliferation in the islets (Lin et al., 2009). Wu Guo Deng et al. demonstrated melatonin’s anti-inflammatory mechanism via suppression of the expression of pro-inflammatory genes COX-2 and iNOS through epigenetic modulation (Deng et al., 2006). They showed how melatonin can reduce the p52 binding to the chromatin of COX-2 and iNOS promoter regions through inhibition of p300 HAT activity (Figure 1). The study further established that increase in p300 HAT activity consequently increased histone acetylation of p52 which increased the expression of the pro-inflammatory mediators. Several lines of studies have explained the relationship between the action of p300 HAT and that of iNOS and COX-2, that is, a decrease in p300 HAT activity leads to a decrease in transcriptional activities of the iNOS and COX-2 and vice versa (Deng et al., 2003). The exact mechanism by which melatonin inhibits p300 HAT activity is still elusive. However, overexpression of p300 protein interacts with the promoter regions, enhancing the transcriptional signal which results in the optimization of pro-inflammatory activation (Shi et al., 2012). Usually, these inflammatory mediators are bound by the p52 domain due to p300 HAT activity (and eventually increased histone acetylation of p52), which results in the opening up of the chromatin structures, making them available to trans-activators hence enabling their transcription (Deng et al., 2006), intensifying inflammation. However, this process was inhibited by melatonin, suggesting its regulatory role in pro-inflammatory genes by transcriptional mechanisms (Shi et al., 2012). Kellogg et al. (2008) postulated that the COX-2 pathway may provide a potential therapeutic alternative in diabetic peripheral neuropathy. In normal conditions, p52 is a transcriptional repressor protein generated from p100 under a tightly controlled process (Vallabhapurapu et al., 2015). The nexus of how transcription is activated through the p100 route via epigenetic mechanism is still a mystery and further investigations may provide a better therapeutic strategy in the development of target-specific drugs.
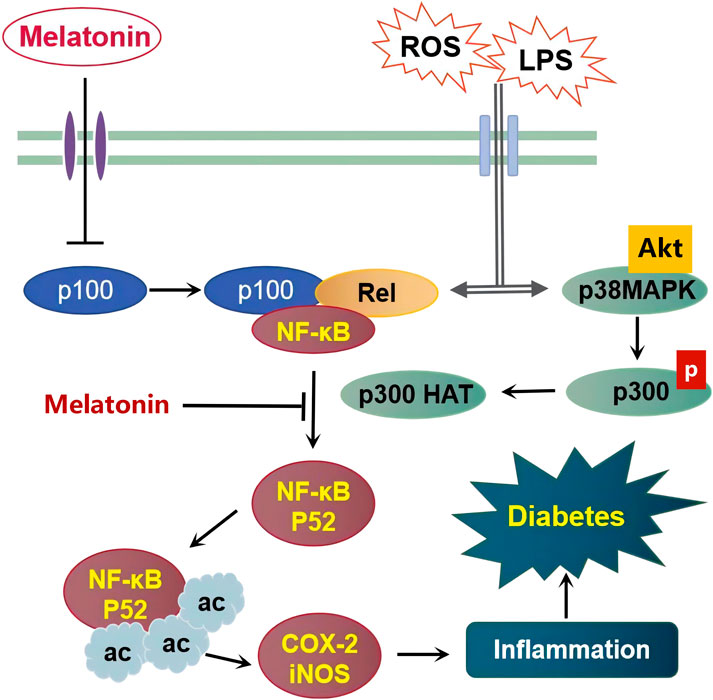
FIGURE 1. Schematic representation of the proposed mechanism underlying melatonin’s protective effects on diabetes caused by inflammatory inducers including Lipopolysaccharides (LPS) and Reactive Oxygen Species (ROS).
2.2 Type 2 Diabetes
P300 has further been investigated in Type 2 diabetes through different epigenetic modulated mechanisms to that of T1D. P300 protein has been implicated in insulin secretion patterns in the beta cells of the pancreas. Bompada et al. (2016) illustrated how p300 knockout led to misshapen secretion of insulin (a typical feature of T2D) and apoptosis of beta cells of pancreases, marked by a decrease in p300 acetylation in presence of high glucose, and an increase in acetylation when an HDAC inhibitor was introduced. Melatonin has been shown to promote beta cell survival by preventing glucotoxicity-induced beta cells loss via p38 MAPK inhibition. In the pancreases, it has been reported that phosphorylation of Akt and/or p38 MAPK plays an important role in beta-cell survival (Wijesekara et al., 2010). On the other hand, diabetes is characterized by dysfunction of pancreatic beta cells and the development of apoptosis due to raised levels of glucose above normal (Butler et al., 2003). Moreover, glucotoxicity and other inflammation-inducing agents have been implicated in the phosphorylation of p300 due to the activation of p38 MAPK in the pancreatic beta cells, leading to cell degradation and apoptosis (Ruiz et al., 2018). However, this process was reversed as the activation of pro-apoptotic factor p38 MAPK was blocked when melatonin was administered (Saldeen et al., 2001).
Overwhelming evidence shows activation of p38 MAPK in insulin-releasing cells causes a damaging effect on the beta cells. Natalia Makeeva et al. (2006) reported that upon activation of p38 MAPK due to the high levels of cytokines like iNOS, p38 MAPK was maintained at a hyper-phosphorylated state, the beta cells producing insulin was driven into apoptosis and eventually cell death. Melatonin signaling was shown to alleviate induced-cell apoptosis and improved the deleterious effects caused by insulin resistance (Figure 1) due to glucotoxicity and oxidative stress in human beta cells (Costes et al., 2015), through inhibition of the apoptotic factor p38 MAPK (Park et al., 2014) and reduction of p38 MAPK phosphorylation (Choi et al., 2018). In addition, Xiongfei Xu et al. (2018) demonstrated melatonin’s ability to attenuate LPS-induced inflammation in macrophages through Akt phosphorylation signaling. Akt phosphorylation has been reported severally as a critical modulator of GLUT 4 translocation (an effector of glucose uptake in tissues) thereby enhancing the effects of insulin on target tissues (Choi et al., 2018).
3 Cancer
Global statistics today place cancer as one of the leading causes of death next only to heart-related diseases in the order of incidence rate (Sung et al., 2021). Evidence shows studies and extensive reviews on different types of malignant neoplasia inhibited or controlled by the effects imparted by melatonin administration both in vivo and in vitro studies (Bondy and Campbell, 2018; Favero et al., 2018). Prospectively, several mechanistic approaches have been suggested in the treatment of cancer. Due to melatonin’s broad spectrum of action, epigenetic modification has been one of the fundamental tools used to unravel the possible mechanisms involved by this drug in numerous cancer types. Melatonin has also been widely implicated as a complementing drug in cancers where it enhances and synergizes the chemotherapeutic effects of other drugs (Gao et al., 2017).
3.1 Histone Modifications
COX-2 is an iso-enzyme responsible for the formation of prostanoids such as prostaglandins and thromboxanes, whose overexpression has been reported not only in inflammatory diseases but in the induction of numerous types of cancer as well (Roelofs et al., 2014). Melatonin on the other hand has been reported severally as an inhibitor of COX-2 in cancer cells (Yi et al., 2014; Shrestha et al., 2017). Several explanations have been explicated including the epigenetic approach which involves the regulation of trans-activator and co-activator genes. For instance, the Factor Nuclear kappa B (NF-ĸB) is a transcriptional factor located in the cell cytoplasm functioning to regulate gene expressions involved in cell survival, proliferation, inflammatory responses, and metastasis (Taniguchi and Karin, 2018). In normal cells, NF-ĸB is usually in a resting/inactive state in the cytosol and its activation is often regulated to maintain the cell homeostasis, and activation of immune responses thereby protecting the cell from environmental factors that would otherwise destroy it (Liu et al., 2017). In cancer cells, NF-ĸB is constitutively activated due to aberrant protein expression or alterations in normal regulation by the regulatory proteins leading to mutation (Park and Hong, 2016).
NF-ĸB activation has been reviewed in the inhibition of apoptosis, and induction of tumorigenic enhancing genes such as MMP-9, Bcl-xl, XIAP, and cyclin D1, leading to metastasis (Fuchs, 2013). Through suppression of NF-ĸB (p65) gene expression (Figure 2), melatonin can inhibit its activation and subsequent translocation to the nucleus (Qin et al., 2012), preventing metastasis in cancer cells (Gao et al., 2017). Huaiqiang Ju et al. attributed this to melatonin’s ability to decrease NF-ĸB protein activity via phosphorylation. The inhibitory protein, IкBα which usually binds to NF-кB, causes the latter to migrate into the nucleus when the IK kinase enzyme phosphorylates IкBα and is subsequently degraded by proteasomal enzyme 26S via ubiquitination, leading to NF-ĸB translocation into the nucleus. They concluded that melatonin administration to pancreatic ductal adenocarcinoma cells led to a decrease in phosphorylation of IкBα which helped to explain the low levels of p65 protein in the nucleus of melatonin treated groups (Ju et al., 2016). Further evidence on pancreatic cancer cells shows that upon activation (as a result of abnormal activation of p65 binding with p50 protein), a heterodimer is formed which enters the nucleus to begin the process of transcription and eventually tumor development and progression (Ling et al., 2012b). Zhen Wei Zou et al. (2018), reported that activation of NF- ĸB signaling evidenced by elevated levels of phosphorylated p65 and other gene products that enhance the invasion of cancer cells, was eradicated upon treatment with melatonin. Additionally, melatonin showed synergistic effects to 5-fluorouracil (5-FU) enhancing its antitumor activity by facilitating the migration of p50/p65 from the nucleus to the cytoplasm, reducing NF-кB dependent cancer development and progression (Gao et al., 2017). It is therefore evident that inhibition of the NF-ĸB pathway by targeting p65 protein could be a breakthrough in the discovery of drug therapy for various types of cancer. Melatonin offers a promising and noble target in this perspective, as far as drug efficacy and safety are concerned.
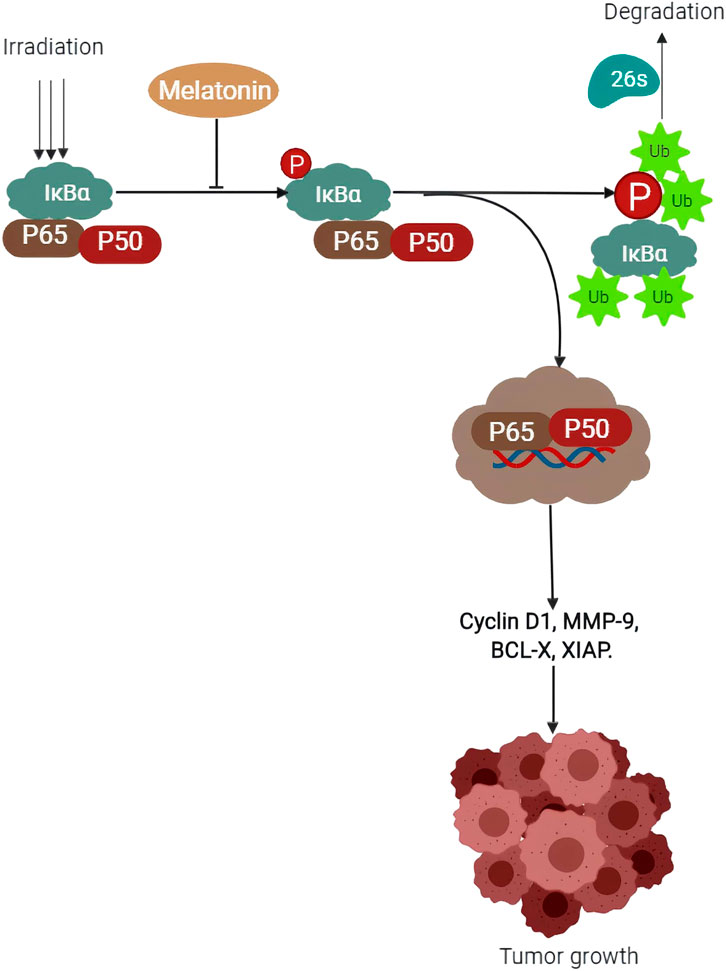
FIGURE 2. Schematic representation of the proposed mechanism on how melatonin prevents tumor growth by decreasing IK kinase enzyme (IkBα) phosphorylation.
3.2 Non-coding RNA
In the treatment of cancer, the actual mechanism underlying the association between miRNAs and melatonin remains elusory. In recent times, there has been a surge in the involvement of miRNAs in the advancement of tumors which has attracted attention in the field of research. These miRNAs have been demonstrated to regulate the expression of genes by inactivating messenger RNA by either preventing ribosomal binding or cutting the mRNA which is further destroyed by the cell leading to less or no translation of mRNA, a process resulting in gene silencing (Hammond et al., 2001). The study on miR-152 has been on the rise due to its tumor-suppressing activity. Constant overexpression of miR-152 in cancer cell lines caused a remarkable decline in cell proliferation and angiogenesis (Li et al., 2016b). Sun et al. (2017b) showed that miR-152-3p inhibited invasion and development of glioblastoma cells by down expressing DNA methyltransferase thereby hindering hypermethylation of neurofibromatosis 2 (NF2). Treatment with melatonin showed elevated levels of miR-152-3p in breast cancer cells when compared to control groups (Marques et al., 2018), suggesting melatonin’s role in cancer suppression.
Another miRNA, miR-483 overexpression has been recently shown to play an oncogenic role in thyroid cancer (Zhang et al., 2019), breast cancer (Huang and Lyu, 2018), colorectal cancer (Liang et al., 2019), esophageal squamous cell carcinoma (Ma et al., 2016), ovarian cancer (Arrighetti et al., 2016), hepatocellular (Lupini et al., 2016), and pancreatic ductal adenocarcinoma (Wang et al., 2015). Earlier on, Clokie et al. (2012) had shown how the addition of miR-483-3p antagonist led to an increase in the synthesis of melatonin by the pineal gland and eventual attenuation of tumor invasion and progression. Thus, inhibition of miR-483-3p and subsequent elevation of melatonin present a promising tumor therapeutic target.
MiR-155 has been a gene of interest implicated in the development of cancer, just a while ago. Studies performed on hepatocellular carcinoma showed elevated levels of miR-155 which acted as an oncogene thereby promoting cell proliferation, progression of the cell cycle, and inhibition of apoptosis (Liao et al., 2018). Zhang et al. (2016) explained that this phenomenon was a result of the downregulation of ARID2, a tumor suppressor gene, which subsequently activated the Akt phosphorylation signaling pathway leading to cancer progression. Accruing evidence shows melatonin’s prowess in arresting cell cycle progression in hepatocellular carcinoma by downregulation of p-Akt dependent pathway (Long et al., 2017). Activation of the Akt pathway has been shown to cause oncogenic transcription factors to bind and inactivate phosphorylated Rb protein leading to the transcription factor E2 promoter-binding-protein-dimerization partner protein (E2F-DP) release, pushing the cell into the synthesis phase, a process that is reversed by melatonin (Long et al., 2017). On the same note, melatonin has been reported to inhibit miR-155 in glioma (Gu et al., 2017) and glioblastoma cells through suppression of Akt phosphorylation, inhibiting its epigenetic modified oncogenic transcriptional activity (Chen et al., 2016a).
Studies pointed towards miR-16-5p are the latest in trying to explain the epigenetic mechanism in cancer by the non-coding RNA molecules that regulate gene translation in various types of cancers. Studies reveal that aberrant expression of miR-16-5p resulted in malignancies including gastric (Zhang et al., 2015), (Krell et al., 2018), breast (Rinnerthaler et al., 2016), (Zhang et al., 2018a), bone (Sang et al., 2017), ovarian (Dwivedi et al., 2016), hepatic (Wu et al., 2015), prostate (Wang et al., 2019b), cervical (Wang et al., 2008), and lymphatic system (Calin et al., 2002). Treatment with melatonin suppressed gastric cancer (Figure 3) via upregulation of miR-16-5p expression and downregulation of SMAD3 (Zhu et al., 2018), indicating that the TGF-β/SMAD pathway might be a possible molecular mechanism by which melatonin precludes cancer progression. TGF-β is a protein known to control cell functions including cell growth, proliferation, and apoptosis (Lustri et al., 2017). Pathologically, the TGF-β/SMAD signaling pathway has been associated with migration, invasion, and metastasis (Ji et al., 2015). Here, the TGF-βI trans-phosphorylates type II (TGF-βII) induces phosphorylation of SMAD3, consequently activating the formation of a complex with SMAD4 which migrates and accumulates in the nucleus where they interact with transcription cofactors and promoters which in turn regulates transcription of target genes, eventually enhancing cell proliferation and metastasis (Ji et al., 2015). Melatonin exposure significantly attenuated gastric cancer by enhancing miR-16-5p expression which targeted SMAD3 and resulted in negative regulation of its abundance (Zhu et al., 2018). Elsewhere, melatonin was reported to alleviate liver fibrosis through TGF-β1/SMAD signaling pathway (Wang et al., 2018b). Hongliang Jiang et al. further demonstrated that miR-16-5p played a tumor suppressor role via downregulation of SMAD3 (Zhang et al., 2018a). How melatonin interacts with miR-16-5p to impart its cancer-suppressing activity still needs further clarification and future studies to confirm the molecular mechanism are vital. This approach puts melatonin and miR-16-5p/TGF-β/SMAD pathway as a possible candidate for therapeutic target and possibly understanding of the etiology of different types of cancer.
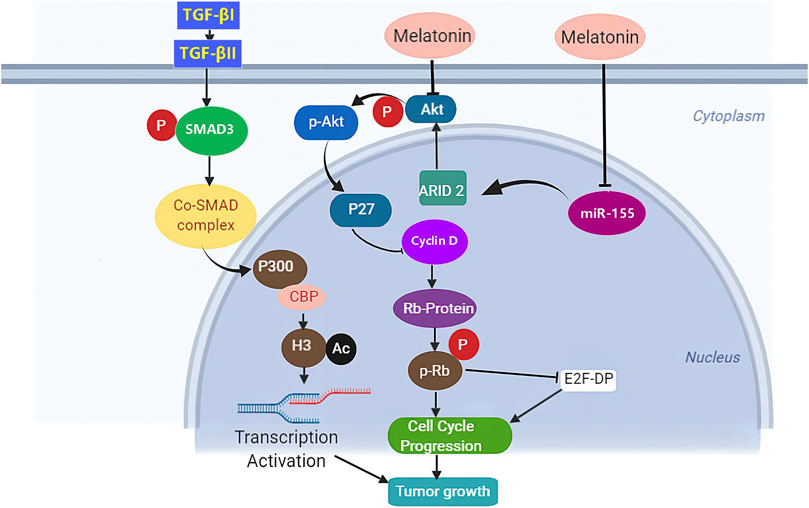
FIGURE. 3. The proposed mechanism underlying the anti-tumor effects of melatonin. Melatonin arrests cell cycle progression through downregulation of phosphorylated protein kinase B (p-Akt) dependent pathway.
3.3 Artificial Light and Histone Modification
Epidemiological studies show a strong association between artificial light and the prevalence of cancer (Garcia-Saenz et al., 2018). Modern artificial lights especially those with blue light emissions have been reported to cause a litany of health problems with cancer being among them (Kamat et al., 2016). The suprachiasmatic nucleus (SCN) functions to regulate the circadian cycle which is controlled by the external environment (day and night clock). Several reviews have been put forth trying to explain how environmental factors such as artificial light can cause circadian disruptions by causing desynchronization of the circadian pacemaker (SCN), resulting in decreased levels of melatonin secretions from the pineal gland (Benke and Benke, 2012). Concerns have been raised regarding the effects of the artificial light at night associated with hormonal imbalances (melatonin) and impaired circadian rhythms (Russart and Nelson, 2018), causing a myriad of diseases including cancer. Studies show that melatonin attenuates the effects of breast, prostate, liver, and skin cancers associated with ill-timed light exposures (early morning, late evening, night) (Dauchy et al., 2018). In the recent past, epigenetic modification affecting the circadian rhythm has been used to explain melatonin’s mechanism of action. Through the activation of the MT1 receptor, melatonin was able to prevent invasion and metastasis of breast cancer (Hill et al., 2015). Agbaria et al. (2019) demonstrated that, when artificial light at night (ALAN) with increased irradiances was administered in rats’ breast cancer, melatonin levels were significantly decreased, while the levels remained normal in the control group. This had been confirmed earlier by Sánchez et al. (2018) who implicated that melatonin resynchronized dysregulated circadian rhythm by preventing SIRT1 from binding to CLOCK/BMAL1 dimer which would otherwise cause deacetylation of BMAL1 resulting in less transcription and degradation of the period (PER) proteins. Earlier, SIRT1 had been implicated in the regulation of the circadian cycle through the deacetylation of PER2 protein (Asher et al., 2008). The CLOCK protein has been reported to exhibit histone acetylation activity necessary in the modulation of circadian rhythm in cancer animal models (Sun et al., 2017a).
It was also found that melatonin upregulated Casein Kinase 1 (CK1) protein levels, increasing the binding sites available for phosphorylation to the transcription factors (Sánchez et al., 2018). These findings together support melatonin’s effects on hepatocellular cells which might also have contributed to its protective activity through modulation of the circadian rhythm.
4 Cardiovascular Diseases
4.1 Cardiac Fibrosis
Cardiac fibrosis is a common manifestation of chronically stressed myocardial tissues characterized by abnormal thickening of the valves of the heart due to the proliferation of the fibroblasts (Kong et al., 2014). The functioning heart muscles are replaced by the thick dysfunctional fibrotic tissues resulting in heart failure and sometimes death. Currently, though there exists no convincing therapy that can prevent the onset and progression of this condition, understanding the pathophysiology of this process may prove crucial and beneficial.
Strides have been made in the interest of employing epigenetic therapies to arrest myocardial fibrotic degeneration through stimulation of human cardiac fibroblasts to suppress or express pro-fibrotic protein genes which have the effect of modulating DNA-methylating genes such as DNMT1 and DNMT3a/3b (Yu and Xu, 2015). Activated myocardial fibroblasts in the rat model resulted in an increase in DNMT3a which in turn led to overexpression of ERK1/2 coupled with suppression of RASAL1 (a Ras-GTPase-activating protein) genes, causing fibroblast activation and cardiac fibrosis (Grimaldi et al., 2017). A DNA methylation inhibitor 5-azacytidine has been reported in the sumoylation of interferon regulatory factor-1 (IRF-1) causing improvements in cardiac fibrosis (Jeong et al., 2015).
The protective effects of melatonin have been previously investigated, effectively alleviating cardiac fibroblast-induced cardiac fibrosis via inhibition of mitochondrial oxidative injury and regulation of SIRT3-mediated SOD2 deacetylation (Jiang et al., 2020). Melatonin also exerted anti‐fibrosis effects in all phases of fibrotic pathogenesis including inhibition of epithelial cell injury, blockading of inflammatory cytokine release, reduction in activation and proliferation of fibrogenic effector cells, and decrease in deposition of ECM (GAG and collagen I and III) (Hu et al., 2016b).
Big strides have been made in understanding melatonin-induced epigenetics on cardiac fibrosis and towards the identification of therapeutic targets. One such study conducted by Crespo et al. (2015) postulated that the development of fibrosis greatly depended on the pro-fibrogenic cytokine TGF- β, directly linked with hyperphosphorylation of SMAD3. TGF-β1/SMAD pathway has been associated with fibrosis in the kidney (Wang et al., 2016), heart (Bansal et al., 2017) liver (Lee et al., 2018b) skin (Qin et al., 2018), and lungs (Jia et al., 2019). They demonstrated that through downregulation of TGF-β and subsequent decrease in phosphorylation of SMAD3, melatonin abrogated the transcription of target genes induced by the TGF-β1/SMAD signaling pathway (Crespo et al., 2015). TGF-β is a well-known collagen synthesis enhancer (Qin et al., 2018). Additionally, melatonin significantly attenuated the fibrosis process by suppressing the activation of TGF-β1/SMAD signaling in heart (Yeung et al., 2015), which resulted in the reduction of collagen deposition in the heart tissues. Similarly, melatonin produced anti-fibrotic effects through activation of TGF-β1/SMAD signaling pathways as a result of down-regulation of lncRNA MALAT1 and knock-down of miR-141 (Che et al., 2020). Elsewhere, the elevated expression of histone deacetylases in cardiac fibrosis in juveniles was attributed to decreasing melatonin levels resulting in heart failure, a condition that was reversed when external melatonin was administered and playing an inhibitory role to the HDACs. Earlier on, Wu et al. (2018) had postulated that melatonin could prevent programmed hypertension by inhibiting HDACs (Wu et al., 2014). However, the underlying molecular mechanism behind this inhibition needs further elucidation.
4.2 Hypertension
The in-depth knowledge of the renal-angiotensin-aldosterone system (RAAS) alongside in vivo and in vitro studies have suggested possible epigenomic-related mechanisms underlying the etiology of hypertension. Unusual activation of RAAS by the associated genes has been previously reported to cause an increase in blood pressure (Crowley et al., 2006). Angiotensinogen (AGT) and angiotensin-converting enzyme (ACE) have been reported as the main genes which code to the angiotensinogen protein and angiotensin-converting enzyme respectively, regulating the RAAS pathway and eventually blood pressure (Pei et al., 2015). Moreover, activation of RAAS genes due to impaired functioning of the RAAS system has been associated with pathophysiological conditions including essential hypertension. One such gene 11-Betahydroxysteroid dehydrogenase type-2 (HSD11B2), performed in a rat model demonstrated a positive association between hypermethylation and hypertension (Baserga et al., 2010). Similar findings were reported in newborn babies whereby hypermethylation of the (HSD11B2) promoter gene was linked to a high risk of essential hypertension (Zhao et al., 2014). Elsewhere, hypomethylation of promoter gene coding AGT in rat adipose tissues (Wang et al., 2014) and hypomethylation of ACE during pregnancy (Goyal et al., 2010) both led to the occurrence of hypertension. Studies on non-coding RNA demonstrated repressed levels of AGT and elevated expression of ACE in hypertensive individuals (Litwin et al., 2013). MiR-27a/b and 21 have been linked with the expression of RAAS-regulated AGT and ACE genes respectively, both leading to hypertension (Zou et al., 2020).
Melatonin has been implicated in the epigenomic regulation of hypertension by decreasing the mRNA expression levels of agt1, ace, and agtr1 which promoted vasodilation and alleviating hypertension (Tain et al., 2017). Tain et al. (2016), demonstrated that melatonin could decrease renal levels of ace2, agt, and agtra1 genes. Additionally, melatonin attenuated hypertension through suppression of the renin-angiotensin system (Ohashi et al., 2019b). Recently, it has been reported and reviewed by several researchers, that aberrant methylation patterns of ACE and AGTR-1 promoter regions might be one of the underlying epigenetic mechanisms behind hypertension (Pei et al., 2015).
The non-coding RNAs could also play a role in the development and progression of hypertension through epigenetic modulations. Melatonin has been shown to regulate these non-coding RNAs thereby modulating the occurrence or progression of hypertension. Real-time PCR and western blotting results clearly illustrate how the overexpression of H19 and miR-675-3p coupled with downregulation of miR-200a in melatonin-treated rats (previously induced with hypertension) led to an increase in the expression of PDCD4 and a decrease in expression of IGFR1 proteins (Wang et al., 2018c). Changes in these two proteins eventually can result in apoptosis and suppression of smooth muscles of the pulmonary artery, a condition associated with the alleviation of hypertension (Liu et al., 2010). DNA-methylation induced by a methyl-donor diet resulted in programmed hypertension in women during the pregnancy period by altering renal transcriptomes, a condition that was reversed when maternal melatonin therapy was conducted. It was then reported that melatonin therapy could offer protective activity in adult male offspring against programmed hypertension mechanistically by regulating DNA-methylation (Tain et al., 2018) or playing a HDACs inhibitor role. To date, the actual nexus between melatonin and epigenetics in the pathophysiology of hypertension is still unknown. A deeper understanding of how the non-coding RNAs and DNA-methylation stimulates the RAAS, AGT, and ACE genes of the renal system leading to the development of hypertension, would be a step closer to better understanding the actual mechanism by which melatonin works to alleviate hypertension, making it easier to design and develop melatonin-based therapies.
4.3 Heart Failure
MiR-200 family is a target molecule in the pathology of heart failure. Liu et al. (2018) premised that upregulation of miR-200a due to increased secretion of protein CTRP3 (a paralog of adiponectin) in melatonin treated groups, led to activation of Nrf-2 and eventually abrogation of obesity-related heart failure. Contrarily, it was reported elsewhere that melatonin downregulated the expression of miR-200a which subsequently increased the apoptotic genes PDCD4 resulting in amelioration of pulmonary artery hypertension (Wang et al., 2018c). MiR-200 has also been implicated in arrhythmias (Daimi et al., 2015) and hypercholesterolemia associated with atherosclerosis (Martino et al., 2015).
Angiotensin II a well-known pro-inflammatory regulator has been implicated in cardiac dysfunction of a failing heart through its association with reactive oxygen species (ROS) (Zablocki and Sadoshima, 2013) and phosphorylation of p65/RelA when overly expressed as seen in heart failure (Kim et al., 2012). The result is an activated p65 which binds to the promoter site of NF-кB in the human antigen R (HuR) (Kong et al., 2017) eliciting a binding activation to the MMP2, leading to collagen degradation and eventual heart failure. An increase in hypomethylation of MMP2 has been previously reported in blood samples and cardiac tissues of heart failure patients (Glezeva et al., 2019). Additionally, the multifaceted sites of MMP2 available for phosphorylation contain NF-кB sites in the promoter domains which have been previously demonstrated to cause modulation in its activities (Sariahmetoglu et al., 2007; Jacob-Ferreira et al., 2013). Melatonin on the other hand has been shown to downregulate MMP2 protein expression (Figure 4) through inhibition of the NF-кB signaling pathway and via ROS scavenging (Kong et al., 2017). Although post-translational modulations such as phosphorylation can regulate MMP2 expression, melatonin involvement in this regulation is yet to be demystified.
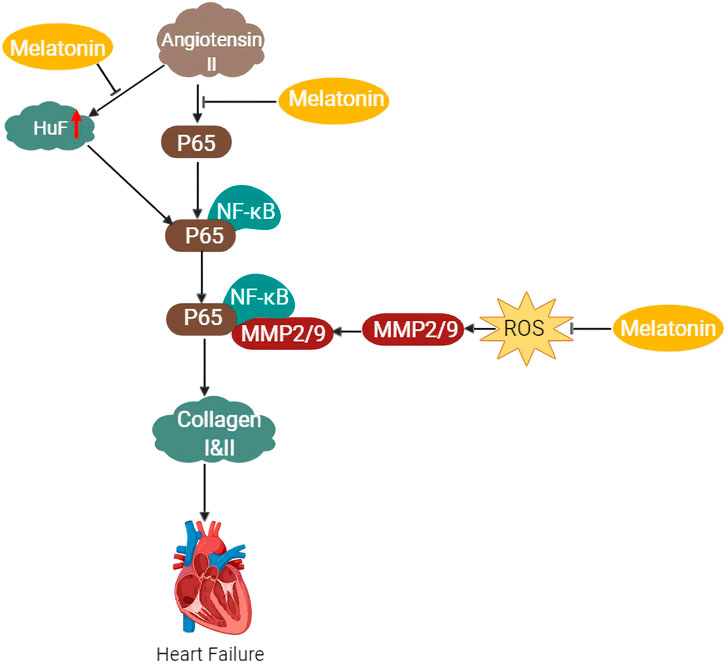
FIGURE 4. Schematic diagram of the proposed mechanism of the protective effects of melatonin against heart failure via inhibition of NF-kB and MMP2/9 pathways.
4.4 Atherosclerosis
Atherosclerosis is a condition characterized by the buildup of plaques from cholesterol, deposited on the inner walls of the endothelium, causing stenosis and heart attack, and sometimes death (Insull, 2009). Endothelium dysfunction due to degeneration causes the formation of these plaques which upon rupture have been reported to cause sudden coronary death, myocardial infarction, and heart attack (Hsiao et al., 2015). Therefore, plaque stabilization is an important approach in the pharmacotherapy of heart diseases stemming from atherosclerotic plaque. Changing the morphology and content of the atherosclerotic plaque could help alter the function of the plaque in the endothelium by either preventing or reducing its stringent effects when it ruptures (Libby and Aikawa, 2003).
Increasing the fibrous content through collagen synthesis is the basic idea of stabilizing atherosclerotic plaque. Recently, Li et al. (2019b) demonstrated this phenomenon by using the smooth muscle cells from ApoE−/− mice. They found out that at high doses, melatonin was able to cause significant stabilization of atherosclerotic plaque. They further explained this occurrence to be caused by melatonin-induced Akt phosphorylation of the specificity protein 1 (Sp1), which cleaved to the promoter site and elevated the expression of the PH4alpha-1 gene. The PH4alpha-1 gene has been associated with the formation of a major component of the extracellular matrix known as 4- hydroxyproline in collagens (Abrams and Andrew, 2002). Sp1 is a ubiquitously expressed transcription factor illustrated to be activated through Akt phosphorylation (Chuang et al., 2013). Earlier on, Sp1 was shown to cause transactivation of the VEGF promoter region via Akt phosphorylation (Pore et al., 2004). Additionally, the pineal hormone has been implicated in the downregulation of MMPs and interstitial increase in collagen, leading to stabilization of the atherosclerotic plaque, as a result of elevated levels of fibrous tissues (Aikawa et al., 1998). An increase in MMPs has been widely reported to degrade and suppress collagen synthesis (Bertelsen et al., 2018). This reduction of collagen due to degradation can lead to the weakening of the plaque, inevitably causing its rupture (Braganza and Bennett, 2001). Computational chemistry studies showed that melatonin could inhibit the enzymatic activity of MMP-9 by docking on its active site preventing further action of the enzyme (Rudra et al., 2013). This pineal hormone has also been premised to cause an upswing in collagen synthesis in the experimental model (Drobnik et al., 2008), which could unwind the process of plaque rupture.
Different experimental reports have deduced molecular pathways involving inflammatory processes leading to atherosclerosis (Moriya, 2019). The role of miR-223 in the regulation of atherosclerosis has been studied in the recent past with a growing body of evidence reporting exciting findings on the association of miR-223 with inflammation-induced atherosclerosis (You et al., 2022). Tang et al. (2015) explained that through suppression of TLR4 and its subsequent downstream gene NF-кB, they managed to cease inflammation-induced atherosclerotic plaque. Lately, miR-223 has been linked to the inhibition of inflammatory processes related to endothelium dysfunction in atherosclerosis (Cavallari et al., 2019). The expression of tissue factor (TF) also known as thromboplastin was reportedly suppressed by miR-223, suggesting a possible anti-thrombogenesis mechanism in the formation of atherosclerotic plaque (Li et al., 2014). We have previously reported the progression of atherosclerosis through the pyroptotic process via lncRNA-MEG3/miR-223/NLRP3 pathway. Any attempts of knocking down miR-223 led to the blocking of melatonin’s inhibitory effects on pyroptosis, suggesting that there is a nexus between miR-223 and melatonin in the alleviation of atherosclerotic plaque (Zhang et al., 2018b). Although it is not yet defined how melatonin directly collaborates with miR-223 to impact anti-atherosclerosis action, the recent research findings on melatonin seem to corroborate its putative actions against atherosclerotic plaque.
4.5 Cardiac Senescence
The concept of aging and growing old seems not to go down well with many people; some people believe once they grow old, they might lose their worth, good looks, or importance in society. For a long period, several factors majorly environmental stimuli had been attributed to aging. In recent decades, scientists have been able to demonstrate that aging is hugely dependent and associated with genetic and epigenetic pathways which influence lifespan. Senescence involves a prolonged condition requiring the adaptation of the cell to a stressful microenvironment initiated by a deep epigenetic reconditioning response resulting in irreparable DNA damage. For instance, when mitochondrial DNA methylation was examined during the senescence period, a study concluded that hypomethylation of the mitochondrial CpG region was directly linked to cellular senescence as a result of oxidative stress (Yu et al., 2017). Likewise, the histone deacetylase inhibitor enzymes were intensely involved in the promotion of cellular senescence as a result of the upregulation of p16 and p21 gene expression via potent stimulation of cardiac fibroblasts (Tao et al., 2016). MiRNAs have also been involved in the physiological process of cellular senescence and aging. Through the TGF-β signaling pathway, miR-29 negatively regulates H4K20me3, an epigenetic target that leads to the progression of senescence (Lyu et al., 2018). These epigenetic alterations could be identified as targets for epigenetic therapies, from suppression of senescence-inducing triggers to positive activation of signaling pathways associated with anti-senescence.
Melatonin has been used substantially in many vital life processes, and its secretion and circulation decline gradually over time. For instance, Lahiri postulated that the levels of melatonin in the serum decreased significantly with age, in body tissues including the heart (Lahiri et al., 2004), and that chronic treatment with melatonin exhibited anti-senescence effects and also reduced deteriorative and oxidative changes associated with cardiac aging (Guo et al., 2017). Although a relatively new area of research, the role played by miRNAs in aging has gained interest among researchers in an attempt to prolong human life. Studies on these miRNAs associated with cardiac senescence and aging have shed much light on prospects, as to how they can be regulated to slow down cardiac senescence processes. LncRNA H19 has recently been implicated in the promotion of cardiomyocytes senescence by regulating the aging markers p53 and p21 (Zhuang et al., 2021). The lncRNA H19 silenced the MALAT1 and MIAT in fibrosis-related cardiac hypertrophy resulting in increasing in senescence protein markers p53 and p21 (Su et al., 2021). Melatonin on the hand actively produced antifibrotic action in cardiac fibrosis through inhibition of MALAT1 (Che et al., 2020). Additionally, H19-derived miR-675 has been implicated as a regulator of cellular senescence in aging-induced vascular dysfunction (Han et al., 2019). MiR-675 overexpression exhibited anti-senescence activity in cardiac progenitor cells in presence of melatonin through regulation of transcriptional factors p53 and p21 (Cai et al., 2016). Further, accumulation of p53 and p21 proteins have been reported in cardiovascular studies as promulgators of senescence through p53/p21 DNA-induced damage and cell cycle arrest rendering the cells senescence (Li et al., 2019c), a function that was reversed by exposure to melatonin (Cai et al., 2016). Altogether, these findings provide basic evidence of melatonin’s cardio-protective action, especially in an aging state.
5 Discussion
Melatonin has a broad spectrum of functions that are not well understood due to the vast effects mediated by its receptors MT1 and MT2. The expression of these receptors in different peripheral body cells insinuates that melatonin may associate and affect cellular functions ubiquitously in a fashion related to circadian rhythm. The characteristic rhythm of secretion of melatonin by the pineal gland is known to decline in an aged and/or diseased state (Hardeland, 2012). Although the actual mechanism by which melatonin and its receptors are aberrantly expressed is unknown, increasing evidence suggests that epigenetic modifications influence the development and progression of several diseases, such as neurodegenerative diseases, cancers, dysfunctional metabolic conditions such as diabetes, and cardiovascular diseases. For instance, melatonin receptors and eventually their signaling was significantly upregulated by the HDAC inhibitors via histone acetylation (Bahna and Niles, 2018), presenting a novel therapeutic strategy in counteracting negative gene transcription that might lead to age-related and/or disease condition as a result of impaired melatonin receptors. Despite this, the strategy is still inadequate since it is unclear which of the melatonin receptors is associated with the histone process. This, therefore, calls for strict discrimination and selection of specific receptor subtypes as targets and matching them with individual epigenetic isoforms of the histone process in an attempt to formulate personalized therapies. Furthermore, the susceptibility and possibility of pathological occurrence are linked with the interaction between genetic polymorphism and melatonin expression. The melatonin receptor B1 (MTNR1B) gene, a genetic polymorph, was implicated in T2D as an insulin controller, by regulating its secretion by beta cells of the pancreas (Prokopenko et al., 2009). This, therefore, suggests, that further information collected on gene polymorphism and the variants associated with controlled hyperglycemia in presence of melatonin could help develop therapies for patients with glucose disturbance.
Despite the well-collated information on the benefits of melatonin, there are inadequacies due to poor investigations on the subject. The nexus between melatonin and melatonin receptors in specific organs is not clearly explained. This is to say, the signaling routes by which melatonin regulates the epigenetic process, require further investigation. Secondly, we could not decipher how the action of melatonin affects different cells. For instance, in cancer, melatonin is seen doing the exact opposite of what is known of non-cancer cells (Li et al., 2017b). It is well illustrated that melatonin significantly declines in epigenetic regulated diseases (Bahna and Niles, 2018), and attempting to assess whether, this reduction has etiological significance or whether it is simply a basal epiphenomenon elementary to different disorders, is still a mystery. Hence, the need for more investigations in these areas to avoid unnecessary controversies.
Clinical trials on the safety and efficacy of melatonin in the field of medicine demonstrate low toxicity with a wide range of doses (Sánchez-Barceló et al., 2010). Doses up to 300 mg/d have been administered over a long period with effective results and no manifestations of adverse effects (Weishaupt et al., 2006), demonstrating its high safety profile. Available in pure pharmacological form, melatonin has a lipid-soluble phase that enables it to be effectively absorbed from all biological membranes (Hardeland, 2005). Additionally, melatonin as an adjuvant of tumor therapy demonstrated tremendous remission of cancer by inducing self-destruction of cells indicating its synergistic potential and also reducing the collateral side effects of chemotherapy drugs (Wang et al., 2012).
The regulatory interaction of melatonin and epigenetics has so far been studied under the conditions of early development. The study of miRNAs interaction with melatonin, though in its infancy, has elicited huge interest in the research field. The signaling pathway of melatonin can be affected by miRNAs, as numerous diseases display alterations in levels and the general composition of miRNAs indicating their importance in the pathology of diseases. Pointing out, the beneficial role of melatonin has been reported in atherosclerosis in elevated levels of miR-29b (Zhu et al., 2014), neoplasm involving miR-374 (Chuffa et al., 2020), memory and cognitive disorders associated with miR-124 (Wang et al., 2013), and so on. However, the direct information available on the action of melatonin to date is missing and does not give a coherent picture as investigations touching on this subject are scattered all over other topics of melatonin. Nevertheless, this field promises to be important as the findings already obtained show miRNAs modulating circadian rhythms (Hardeland, 2014).
6 Conclusion
There are but a handful of studies demonstrating the mechanism by which melatonin influences epigenetic processes. Herein, we have highlighted the effects of melatonin on histone modification, DNA methylation, and non-coding RNAs and summarized its significance in alleviating aberrant regulations often leading to diseases. Several other proteins which directly regulate epigenetic modifications causing mutation or functionality loss have resulted in a series of human disorders. This has enabled a precedential focus on the transcription factors and miRNAs in the quest to unlock the mystery behind their association with diseases and melatonin action on them. This, therefore, provides exciting prospects about melatonin as an oscillator of the epigenetic gene in human diseases, and great potential in the development of “epigenetic therapies” as an adjuvant and/or alternative treatment to the currently available therapies.
Author Contributions
SM (who wrote the manuscript) and XL (Corresponding author) has revised and approved the final version of the manuscript.
Funding
This study was funded by the National Natural Science Foundation of China (81903610) and General projects of China Postdoctoral Science Foundation (2018M641880).
Conflict of Interests
The authors declare that the research was conducted in the absence of any commercial or financial relationships that could be construed as a potential conflict of interest.
Publisher’s Note
All claims expressed in this article are solely those of the authors and do not necessarily represent those of their affiliated organizations, or those of the publisher, the editors and the reviewers. Any product that may be evaluated in this article, or claim that may be made by its manufacturer, is not guaranteed or endorsed by the publisher.
References
Abrams, E. W., and Andrew, D. J. (2002). Prolyl 4-hydroxylase Alpha-Related Proteins in Drosophila melanogaster: Tissue-specific Embryonic Expression of the 99F8-9 Cluster. Mech. Dev. 112 (1-2), 165–171. doi:10.1016/s0925-4773(01)00636-0
Acuña-Castroviejo, D., Escames, G., Venegas, C., Díaz-Casado, M. E., Lima-Cabello, E., López, L. C., et al. (2014). Extrapineal Melatonin: Sources, Regulation, and Potential Functions. Cell Mol Life Sci 71 (16), 2997–3025. doi:10.1007/s00018-014-1579-2
Agbaria, S., Haim, A., Fares, F., and Zubidat, A. E. (2019). Epigenetic Modification in 4T1 Mouse Breast Cancer Model by Artificial Light at Night and Melatonin - the Role of DNA-Methyltransferase. Chronobiol Int. 36, 629–643. doi:10.1080/07420528.2019.1574265
Aikawa, M., Rabkin, E., Okada, Y., Voglic, S. J., Clinton, S. K., Brinckerhoff, C. E., et al. (1998). Lipid Lowering by Diet Reduces Matrix Metalloproteinase Activity and Increases Collagen Content of Rabbit Atheroma: a Potential Mechanism of Lesion Stabilization. Circulation 97 (24), 2433–2444. doi:10.1161/01.cir.97.24.2433
Akkers, R. C., van Heeringen, S. J., Jacobi, U. G., Janssen-Megens, E. M., Françoijs, K. J., Stunnenberg, H. G., et al. (2009). A Hierarchy of H3K4me3 and H3K27me3 Acquisition in Spatial Gene Regulation in Xenopus Embryos. Dev. Cel 17 (3), 425–434. doi:10.1016/j.devcel.2009.08.005
Arrighetti, N., Cossa, G., De Cecco, L., Stucchi, S., Carenini, N., Corna, E., et al. (2016). PKC-alpha Modulation by miR-483-3p in Platinum-Resistant Ovarian Carcinoma Cells. Toxicol. Appl. Pharmacol. 310, 9–19. doi:10.1016/j.taap.2016.08.005
Asher, G., Gatfield, D., Stratmann, M., Reinke, H., Dibner, C., Kreppel, F., et al. (2008). SIRT1 Regulates Circadian Clock Gene Expression through PER2 Deacetylation. Cell 134 (2), 317–328. doi:10.1016/j.cell.2008.06.050
Asin-Cayuela, J., and Gustafsson, C. M. (2007). Mitochondrial Transcription and its Regulation in Mammalian Cells. Trends Biochem. Sci. 32 (3), 111–117. doi:10.1016/j.tibs.2007.01.003
Bahna, S. G., and Niles, L. P. (2017). Epigenetic Induction of Melatonin MT1 Receptors by Valproate: Neurotherapeutic Implications. Eur. Neuropsychopharmacol. 27 (8), 828–832. doi:10.1016/j.euroneuro.2017.06.002
Bahna, S. G., and Niles, L. P. (2018). Epigenetic Regulation of Melatonin Receptors in Neuropsychiatric Disorders. Br. J. Pharmacol. 175 (16), 3209–3219. doi:10.1111/bph.14058
Bansal, T., Chatterjee, E., Singh, J., Ray, A., Kundu, B., Thankamani, V., et al. (2017). Arjunolic Acid, a Peroxisome Proliferator-Activated Receptor α Agonist, Regresses Cardiac Fibrosis by Inhibiting Non-canonical TGF-β Signaling. J. Biol. Chem. 292 (40), 16440–16462. doi:10.1074/jbc.M117.788299
Baserga, M., Kaur, R., Hale, M. A., Bares, A., Yu, X., Callaway, C. W., et al. (2010). Fetal Growth Restriction Alters Transcription Factor Binding and Epigenetic Mechanisms of Renal 11beta-Hydroxysteroid Dehydrogenase Type 2 in a Sex-specific Manner. Am. J. Physiol. Regul. Integr. Comp. Physiol. 299 (1), R334–R342. doi:10.1152/ajpregu.00122.2010
Bayraktar, G., and Kreutz, M. R. (2018). Neuronal DNA Methyltransferases: Epigenetic Mediators between Synaptic Activity and Gene Expression? Neuroscientist 24 (2), 171–185. doi:10.1177/1073858417707457
Bell, J. T., Pai, A. A., Pickrell, J. K., Gaffney, D. J., Pique-Regi, R., Degner, J. F., et al. (2011). DNA Methylation Patterns Associate with Genetic and Gene Expression Variation in HapMap Cell Lines. Genome Biol. 12 (1), R10. doi:10.1186/gb-2011-12-1-r10
Benke, K. K., and Benke, K. E. (2012). Uncertainty in Health Risks from Artificial Lighting Due to Disruption of Circadian Rhythm and Melatonin Secretion: A Review. Hum. Ecol. Risk Assess. Int. J. 19 (4), 916–929. doi:10.1080/10807039.2012.702608
Berson, A., Nativio, R., Berger, S. L., and Bonini, N. M. (2018). Epigenetic Regulation in Neurodegenerative Diseases. Trends Neurosci. 41 (9), 587–598. doi:10.1016/j.tins.2018.05.005
Bertelsen, D. M., Neergaard, J. S., Bager, C. L., Nielsen, S. H., Secher, N. H., Svendsen, J. H., et al. (2018). Matrix Metalloproteinase Mediated Type I Collagen Degradation Is an Independent Predictor of Increased Risk of Acute Myocardial Infarction in Postmenopausal Women. Sci. Rep. 8 (1), 5371. doi:10.1038/s41598-018-23458-4
Bird, A. P. (1986). CpG-rich Islands and the Function of DNA Methylation. Nature 321 (6067), 209–213. doi:10.1038/321209a0
Blanch, M., Mosquera, J. L., Ansoleaga, B., Ferrer, I., and Barrachina, M. (2016). Altered Mitochondrial DNA Methylation Pattern in Alzheimer Disease-Related Pathology and in Parkinson Disease. Am. J. Pathol. 186 (2), 385–397. doi:10.1016/j.ajpath.2015.10.004
Bompada, P., Atac, D., Luan, C., Andersson, R., Omella, J. D., Laakso, E. O., et al. (2016). Histone Acetylation of Glucose-Induced Thioredoxin-Interacting Protein Gene Expression in Pancreatic Islets. Int. J. Biochem. Cel Biol 81, 82–91. doi:10.1016/j.biocel.2016.10.022
Bondy, S. C., and Campbell, A. (2018). Mechanisms Underlying Tumor Suppressive Properties of Melatonin. Int. J. Mol. Sci. 19 (8). doi:10.3390/ijms19082205
Bondy, S. C., and Sharman, E. H. (2007). Melatonin and the Aging Brain. Neurochem. Int. 50 (4), 571–580. doi:10.1016/j.neuint.2006.12.014
Braganza, D. M., and Bennett, M. R. (2001). New Insights into Atherosclerotic Plaque Rupture. Postgrad. Med. J. 77 (904), 94–98. doi:10.1136/pmj.77.904.94
Brito-Armas, J. M., Baekelandt, V., Castro-Hernández, J. R., González-Hernández, T., Rodríguez, M., and Castro, R. (2013). Melatonin Prevents Dopaminergic Cell Loss Induced by Lentiviral Vectors Expressing A30P Mutant Alpha-Synuclein. Histol. Histopathol 28 (8), 999–1006. doi:10.14670/HH-28.999
Butler, A. E., Janson, J., Bonner-Weir, S., Ritzel, R., Rizza, R. A., and Butler, P. C. (2003). Beta-cell Deficit and Increased Beta-Cell Apoptosis in Humans with Type 2 Diabetes. Diabetes 52 (1), 102–110. doi:10.2337/diabetes.52.1.102
Cai, B., Ma, W., Bi, C., Yang, F., Zhang, L., Han, Z., et al. (2016). Long Noncoding RNA H19 Mediates Melatonin Inhibition of Premature Senescence of C-Kit(+) Cardiac Progenitor Cells by Promoting miR-675. J. Pineal Res. 61 (1), 82–95. doi:10.1111/jpi.12331
Calin, G. A., Dumitru, C. D., Shimizu, M., Bichi, R., Zupo, S., Noch, E., et al. (2002). Frequent Deletions and Down-Regulation of Micro- RNA Genes miR15 and miR16 at 13q14 in Chronic Lymphocytic Leukemia. Proc. Natl. Acad. Sci. U S A. 99 (24), 15524–15529. doi:10.1073/pnas.242606799
Cardinali, D. P., Furio, A. M., and Brusco, L. I. (2010). Clinical Aspects of Melatonin Intervention in Alzheimer's Disease Progression. Curr. Neuropharmacol 8 (3), 218–227. doi:10.2174/157015910792246209
Cavallari, C., Dellepiane, S., Fonsato, V., Medica, D., Marengo, M., Migliori, M., et al. (2019). Online Hemodiafiltration Inhibits Inflammation-Related Endothelial Dysfunction and Vascular Calcification of Uremic Patients Modulating miR-223 Expression in Plasma Extracellular Vesicles. J. Immunol. 202 (8), 2372–2383. doi:10.4049/jimmunol.1800747
Cerna, M. (2019). Epigenetic Regulation in Etiology of Type 1 Diabetes Mellitus. Int. J. Mol. Sci. 21 (1). doi:10.3390/ijms21010036
Che, H., Wang, Y., Li, H., Li, Y., Sahil, A., Lv, J., et al. (2020). Melatonin Alleviates Cardiac Fibrosis via Inhibiting lncRNA MALAT1/miR-141-Mediated NLRP3 Inflammasome and TGF-β1/Smads Signaling in Diabetic Cardiomyopathy. FASEB J. 34 (4), 5282–5298. doi:10.1096/fj.201902692R
Chen, C. C., Chen, C. Y., Wang, S. H., Yeh, C. T., Su, S. C., Ueng, S. H., et al. (2018). Melatonin Sensitizes Hepatocellular Carcinoma Cells to Chemotherapy through Long Non-coding RNA RAD51-AS1-Mediated Suppression of DNA Repair. Cancers (Basel) 10 (9). doi:10.3390/cancers10090320
Chen, L., Teng, H., Fang, T., and Xiao, J. (2016). Agrimonolide from Agrimonia Pilosa Suppresses Inflammatory Responses through Down-Regulation of COX-2/iNOS and Inactivation of NF-Κb in Lipopolysaccharide-Stimulated Macrophages. Phytomedicine 23 (8), 846–855. doi:10.1016/j.phymed.2016.03.016
Chen, S. S., Jenkins, A. J., and Majewski, H. (2009). Elevated Plasma Prostaglandins and Acetylated Histone in Monocytes in Type 1 Diabetes Patients. Diabet Med. 26 (2), 182–186. doi:10.1111/j.1464-5491.2008.02658.x
Chen, X., Hao, A., Li, X., Du, Z., Li, H., Wang, H., et al. (2016). Melatonin Inhibits Tumorigenicity of Glioblastoma Stem-like Cells via the AKT-EZH2-STAT3 Signaling axis. J. Pineal Res. 61 (2), 208–217. doi:10.1111/jpi.12341
Chen, Y. A., Lu, C. H., Ke, C. C., Chiu, S. J., Chang, C. W., Yang, B. H., et al. (2021). Evaluation of Class IIa Histone Deacetylases Expression and In Vivo Epigenetic Imaging in a Transgenic Mouse Model of Alzheimer's Disease. Int. J. Mol. Sci. 22 (16). doi:10.3390/ijms22168633
Choi, J., Kim, K. J., Koh, E. J., and Lee, B. Y. (2018). Gelidium Elegans Extract Ameliorates Type 2 Diabetes via Regulation of MAPK and PI3K/Akt Signaling. Nutrients 10 (1). doi:10.3390/nu10010051
Chuang, C. W., Pan, M. R., Hou, M. F., and Hung, W. C. (2013). Cyclooxygenase-2 Up-Regulates CCR7 Expression via AKT-Mediated Phosphorylation and Activation of Sp1 in Breast Cancer Cells. J. Cel Physiol 228 (2), 341–348. doi:10.1002/jcp.24136
Chuang, Y. H., Paul, K. C., Bronstein, J. M., Bordelon, Y., Horvath, S., and Ritz, B. (2017). Parkinson's Disease Is Associated with DNA Methylation Levels in Human Blood and Saliva. Genome Med. 9 (1), 76. doi:10.1186/s13073-017-0466-5
Chuffa, L. G. A., Carvalho, R. F., Justulin, L. A., Cury, S. S., Seiva, F. R. F., Jardim-Perassi, B. V., et al. (2020). A Meta-Analysis of microRNA Networks Regulated by Melatonin in Cancer: Portrait of Potential Candidates for Breast Cancer Treatment. J. Pineal Res. 69 (4), e12693. doi:10.1111/jpi.12693
Clokie, S. J. H., Lau, P., Kim, H. H., Coon, S. L., and Klein, D. C. (2012). MicroRNAs in the Pineal Gland. J. Biol. Chem. 287 (30), 25312–25324. doi:10.1074/jbc.m112.356733
Costes, S., Boss, M., Thomas, A. P., and Matveyenko, A. V. (2015). Activation of Melatonin Signaling Promotes β-Cell Survival and Function. Mol. Endocrinol. 29 (5), 682–692. doi:10.1210/me.2014-1293
Crespo, I., San-Miguel, B., Fernández, A., Ortiz de Urbina, J., González-Gallego, J., and Tuñón, M. J. (2015). Melatonin Limits the Expression of Profibrogenic Genes and Ameliorates the Progression of Hepatic Fibrosis in Mice. Transl Res. 165 (2), 346–357. doi:10.1016/j.trsl.2014.10.003
Crowley, S. D., Gurley, S. B., Herrera, M. J., Ruiz, P., Griffiths, R., Kumar, A. P., et al. (2006). Angiotensin II Causes Hypertension and Cardiac Hypertrophy through its Receptors in the Kidney. Proc. Natl. Acad. Sci. U S A. 103 (47), 17985–17990. doi:10.1073/pnas.0605545103
Daimi, H., Lozano-Velasco, E., Haj Khelil, A., Chibani, J. B., Barana, A., Amorós, I., et al. (2015). Regulation of SCN5A by microRNAs: miR-219 Modulates SCN5A Transcript Expression and the Effects of Flecainide Intoxication in Mice. Heart Rhythm 12 (6), 1333–1342. doi:10.1016/j.hrthm.2015.02.018
Dauchy, R. T., Wren-Dail, M. A., Dupepe, L. M., Hill, S. M., Xiang, S., Anbalagan, M., et al. (2018). Effect of Daytime Blue-Enriched LED Light on the Nighttime Circadian Melatonin Inhibition of Hepatoma 7288CTC Warburg Effect and Progression. Comp. Med. 68 (4), 269–279. doi:10.30802/AALAS-CM-17-000107
Deng, W. G., Tang, S. T., Tseng, H. P., and Wu, K. K. (2006). Melatonin Suppresses Macrophage Cyclooxygenase-2 and Inducible Nitric Oxide Synthase Expression by Inhibiting P52 Acetylation and Binding. Blood 108 (2), 518–524. doi:10.1182/blood-2005-09-3691
Deng, W. G., Zhu, Y., and Wu, K. K. (2003). Up-regulation of P300 Binding and P50 Acetylation in Tumor Necrosis Factor-Alpha-Induced Cyclooxygenase-2 Promoter Activation. J. Biol. Chem. 278 (7), 4770–4777. doi:10.1074/jbc.M209286200
Domínguez Rubio, A. P., Correa, F., Aisemberg, J., Dorfman, D., Bariani, M. V., Rosenstein, R. E., et al. (2017). Maternal Administration of Melatonin Exerts Short- and Long-Term Neuroprotective Effects on the Offspring from Lipopolysaccharide-Treated Mice. J. Pineal Res. 63 (4). doi:10.1111/jpi.12439
Doxakis, E. (2010). Post-transcriptional Regulation of Alpha-Synuclein Expression by Mir-7 and Mir-153. J. Biol. Chem. 285 (17), 12726–12734. doi:10.1074/jbc.M109.086827
Drobnik, J., Karbownik-Lewińska, M., Szczepanowska, A., Słotwińska, D., Olczak, S., Jakubowski, L., et al. (2008). Regulatory Influence of Melatonin on Collagen Accumulation in the Infarcted Heart Scar. J. Pineal Res. 45 (3), 285–290. doi:10.1111/j.1600-079X.2008.00588.x
Dupont, C., Armant, D. R., and Brenner, C. A. (2009). Epigenetics: Definition, Mechanisms and Clinical Perspective. Semin. Reprod. Med. 27 (5), 351–357. doi:10.1055/s-0029-1237423
Dwivedi, S. K., Mustafi, S. B., Mangala, L. S., Jiang, D., Pradeep, S., Rodriguez-Aguayo, C., et al. (2016). Therapeutic Evaluation of microRNA-15a and microRNA-16 in Ovarian Cancer. Oncotarget 7 (12), 15093–15104. doi:10.18632/oncotarget.7618
Edwards, J. R., Yarychkivska, O., Boulard, M., and Bestor, T. H. (2017). DNA Methylation and DNA Methyltransferases. Epigenetics Chromatin 10, 23. doi:10.1186/s13072-017-0130-8
Favero, G., Moretti, E., Bonomini, F., Reiter, R. J., Rodella, L. F., and Rezzani, R. (2018). Promising Antineoplastic Actions of Melatonin. Front. Pharmacol. 9, 1086. doi:10.3389/fphar.2018.01086
Fitzsimons, H. L., Schwartz, S., Given, F. M., and Scott, M. J. (2013). The Histone Deacetylase HDAC4 Regulates Long-Term Memory in Drosophila. PLoS One 8 (12), e83903. doi:10.1371/journal.pone.0083903
Fuchs, O. (2013). Targeting of NF-kappaB Signaling Pathway, Other Signaling Pathways and Epigenetics in Therapy of Multiple Myeloma. Cardiovasc. Hematol. Disord. Drug Targets 13 (1), 16–34. doi:10.2174/1871529x11313010003
Gao, Y., Xiao, X., Zhang, C., Yu, W., Guo, W., Zhang, Z., et al. (2017). Melatonin Synergizes the Chemotherapeutic Effect of 5-fluorouracil in colon Cancer by Suppressing PI3K/AKT and NF-κB/iNOS Signaling Pathways. J. Pineal Res. 62 (2). doi:10.1111/jpi.12380
Garcia-Saenz, A., Sánchez de Miguel, A., Espinosa, A., Valentin, A., Aragonés, N., Llorca, J., et al. (2018). Evaluating the Association between Artificial Light-At-Night Exposure and Breast and Prostate Cancer Risk in Spain (MCC-Spain Study). Environ. Health Perspect. 126 (4), 047011. doi:10.1289/EHP1837
Giusti-Rodríguez, P., Gao, J., Gräff, J., Rei, D., Soda, T., and Tsai, L. H. (2011). Synaptic Deficits Are Rescued in the p25/Cdk5 Model of Neurodegeneration by the Reduction of β-secretase (BACE1). J. Neurosci. 31 (44), 15751–15756. doi:10.1523/JNEUROSCI.3588-11.2011
Glezeva, N., Moran, B., Collier, P., Moravec, C. S., Phelan, D., Donnellan, E., et al. (2019). Targeted DNA Methylation Profiling of Human Cardiac Tissue Reveals Novel Epigenetic Traits and Gene Deregulation across Different Heart Failure Patient Subtypes. Circ. Heart Fail. 12 (3), e005765. doi:10.1161/CIRCHEARTFAILURE.118.005765
Goh, S. Y., Chao, Y. X., Dheen, S. T., Tan, E. K., and Tay, S. S. (2019). Role of MicroRNAs in Parkinson's Disease. Int. J. Mol. Sci. 20 (22). doi:10.3390/ijms20225649
Goyal, R., Goyal, D., Leitzke, A., Gheorghe, C. P., and Longo, L. D. (2010). Brain Renin-Angiotensin System: Fetal Epigenetic Programming by Maternal Protein Restriction during Pregnancy. Reprod. Sci. 17 (3), 227–238. doi:10.1177/1933719109351935
Green, A. L., Zhan, L., Eid, A., Zarbl, H., Guo, G. L., and Richardson, J. R. (2017). Valproate Increases Dopamine Transporter Expression through Histone Acetylation and Enhanced Promoter Binding of Nurr1. Neuropharmacology 125, 189–196. doi:10.1016/j.neuropharm.2017.07.020
Grimaldi, V., De Pascale, M. R., Zullo, A., Soricelli, A., Infante, T., Mancini, F. P., et al. (2017). Evidence of Epigenetic Tags in Cardiac Fibrosis. J. Cardiol. 69 (2), 401–408. doi:10.1016/j.jjcc.2016.10.004
Gu, J., Lu, Z., Ji, C., Chen, Y., Liu, Y., Lei, Z., et al. (2017). Melatonin Inhibits Proliferation and Invasion via Repression of miRNA-155 in Glioma Cells. Biomed. Pharmacother. 93, 969–975. doi:10.1016/j.biopha.2017.07.010
Guo, X. H., Li, Y. H., Zhao, Y. S., Zhai, Y. Z., and Zhang, L. C. (2017). Anti-aging E-ffects of M-elatonin on the M-yocardial M-itochondria of R-ats and A-ssociated M-echanisms. Mol. Med. Rep. 15 (1), 403–410. doi:10.3892/mmr.2016.6002
Hammond, S. M., Caudy, A. A., and Hannon, G. J. (2001). Post-transcriptional Gene Silencing by Double-Stranded RNA. Nat. Rev. Genet. 2 (2), 110–119. doi:10.1038/35052556
Han, C., Zhou, J., Liu, B., Liang, C., Pan, X., Zhang, Y., et al. (2019). Delivery of miR-675 by Stem Cell-Derived Exosomes Encapsulated in Silk Fibroin Hydrogel Prevents Aging-Induced Vascular Dysfunction in Mouse Hindlimb. Mater. Sci. Eng. C Mater. Biol. Appl. 99, 322–332. doi:10.1016/j.msec.2019.01.122
Hardeland, R. (2005). Antioxidative protection by Melatonin: Multiplicity of Mechanisms from Radical Detoxification to Radical Avoidance. Endocrine 27 (2), 119–130. doi:10.1385/endo:27:2:119
Hardeland, R. (2012). Melatonin in Aging and Disease -multiple Consequences of Reduced Secretion, Options and Limits of Treatment. Aging Dis. 3 (2), 194–225.
Hardeland, R. (2014). Melatonin, Noncoding RNAs, Messenger RNA Stability and Epigenetics-Eevidence, Hints, Gaps and Perspectives. Int. J. Mol. Sci. 15 (10), 18221–18252. doi:10.3390/ijms151018221
Harrison, I. F., Smith, A. D., and Dexter, D. T. (2018). Pathological Histone Acetylation in Parkinson's Disease: Neuroprotection and Inhibition of Microglial Activation through SIRT 2 Inhibition. Neurosci. Lett. 666, 48–57. doi:10.1016/j.neulet.2017.12.037
He, F., Chou, C. J., Scheiner, M., Poeta, E., Yuan Chen, N., Gunesch, S., et al. (2021). Melatonin- and Ferulic Acid-Based HDAC6 Selective Inhibitors Exhibit Pronounced Immunomodulatory Effects In Vitro and Neuroprotective Effects in a Pharmacological Alzheimer's Disease Mouse Model. J. Med. Chem. 64 (7), 3794–3812. doi:10.1021/acs.jmedchem.0c01940
He, Z., Cho, Y. Y., Ma, W. Y., Choi, H. S., Bode, A. M., and Dong, Z. (2005). Regulation of Ultraviolet B-Induced Phosphorylation of Histone H3 at Serine 10 by Fyn Kinase. J. Biol. Chem. 280 (4), 2446–2454. doi:10.1074/jbc.M402053200
Henderson-Smith, A., Fisch, K. M., Hua, J., Liu, G., Ricciardelli, E., Jepsen, K., et al. (2019). DNA Methylation Changes Associated with Parkinson's Disease Progression: Outcomes from the First Longitudinal Genome-wide Methylation Analysis in Blood. Epigenetics 14 (4), 365–382. doi:10.1080/15592294.2019.1588682
Hill, S. M., Belancio, V. P., Dauchy, R. T., Xiang, S., Brimer, S., Mao, L., et al. (2015). Melatonin: an Inhibitor of Breast Cancer. Endocr. Relat. Cancer 22 (3), R183–R204. doi:10.1530/ERC-15-0030
Hsiao, H. M., Wu, Y. Y., Tsai, B. C., Chen, Y. C., and Cheng, Y. H. (2015). Investigation of Fibrous Cap Stresses on Vulnerable Plaques Leading to Heart Attacks. Technol. Health Care 24 (Suppl. 1), S155–S161. doi:10.3233/THC-151064
Hu, G., Gong, A. Y., Wang, Y., Ma, S., Chen, X., Chen, J., et al. (2016). LincRNA-Cox2 Promotes Late Inflammatory Gene Transcription in Macrophages through Modulating SWI/SNF-Mediated Chromatin Remodeling. J. Immunol. 196 (6), 2799–2808. doi:10.4049/jimmunol.1502146
Hu, W., Ma, Z., Jiang, S., Fan, C., Deng, C., Yan, X., et al. (2016). Melatonin: the Dawning of a Treatment for Fibrosis? J. Pineal Res. 60 (2), 121–131. doi:10.1111/jpi.12302
Huang, X., and Lyu, J. (2018). Tumor Suppressor Function of miR-483-3p on Breast Cancer via Targeting of the Cyclin E1 Gene. Exp. Ther. Med. 16 (3), 2615–2620. doi:10.3892/etm.2018.6504
Insull, W. (2009). The Pathology of Atherosclerosis: Plaque Development and Plaque Responses to Medical Treatment. Am. J. Med. 122 (1 Suppl. l), S3–S14. doi:10.1016/j.amjmed.2008.10.013
Jacob-Ferreira, A. L., Kondo, M. Y., Baral, P. K., James, M. N., Holt, A., Fan, X., et al. (2013). Phosphorylation Status of 72 kDa MMP-2 Determines its Structure and Activity in Response to Peroxynitrite. PLoS One 8 (8), e71794. doi:10.1371/journal.pone.0071794
Jankovic, J. (2008). Parkinson's Disease: Clinical Features and Diagnosis. J. Neurol. Neurosurg. Psychiatry 79 (4), 368–376. doi:10.1136/jnnp.2007.131045
Jeong, H. Y., Kang, W. S., Hong, M. H., Jeong, H. C., Shin, M. G., Jeong, M. H., et al. (2015). 5-Azacytidine Modulates Interferon Regulatory Factor 1 in Macrophages to Exert a Cardioprotective Effect. Sci. Rep. 5, 15768. doi:10.1038/srep15768
Ji, Q., Liu, X., Han, Z., Zhou, L., Sui, H., Yan, L., et al. (2015). Resveratrol Suppresses Epithelial-To-Mesenchymal Transition in Colorectal Cancer through TGF-β1/Smads Signaling Pathway Mediated Snail/E-Cadherin Expression. BMC Cancer 15, 97. doi:10.1186/s12885-015-1119-y
Jia, L., Sun, P., Gao, H., Shen, J., Gao, Y., Meng, C., et al. (2019). Mangiferin Attenuates Bleomycin-Induced Pulmonary Fibrosis in Mice through Inhibiting TLR4/p65 and TGF-beta1/Smad2/3 Pathway. J. Pharm. Pharmacol. 71, 1017–1028. doi:10.1111/jphp.13077
Jiang, J., Liang, S., Zhang, J., Du, Z., Xu, Q., Duan, J., et al. (2020). Melatonin Ameliorates PM2.5 -induced Cardiac Perivascular Fibrosis through Regulating Mitochondrial Redox Homeostasis. J. Pineal Res., e12686. doi:10.1111/jpi.12686
Ju, H. Q., Li, H., Tian, T., Lu, Y. X., Bai, L., Chen, L. Z., et al. (2016). Melatonin Overcomes Gemcitabine Resistance in Pancreatic Ductal Adenocarcinoma by Abrogating Nuclear Factor-Κb Activation. J. Pineal Res. 60 (1), 27–38. doi:10.1111/jpi.12285
Kamat, A. M., Cookson, M., Witjes, J. A., Stenzl, A., and Grossman, H. B. (2016). The Impact of Blue Light Cystoscopy with Hexaminolevulinate (HAL) on Progression of Bladder Cancer - A New Analysis. Bladder Cancer 2 (2), 273–278. doi:10.3233/BLC-160048
Kellogg, A. P., Cheng, H. T., and Pop-Busui, R. (2008). Cyclooxygenase-2 Pathway as a Potential Therapeutic Target in Diabetic Peripheral Neuropathy. Curr. Drug Targets 9 (1), 68–76. doi:10.2174/138945008783431691
Kim, J. M., Heo, H. S., Ha, Y. M., Ye, B. H., Lee, E. K., Choi, Y. J., et al. (2012). Mechanism of Ang II Involvement in Activation of NF-Κb through Phosphorylation of P65 during Aging. Age (Dordr) 34 (1), 11–25. doi:10.1007/s11357-011-9207-7
Kim, T., Song, S., Park, Y., Kang, S., and Seo, H. (2019). HDAC Inhibition by Valproic Acid Induces Neuroprotection and Improvement of PD-like Behaviors in LRRK2 R1441G Transgenic Mice. Exp. Neurobiol. 28 (4), 504–515. doi:10.5607/en.2019.28.4.504
Kong, J., Zhang, Y., Liu, S., Li, H., Liu, S., Wang, J., et al. (2017). Melatonin Attenuates Angiotensin II-Induced Abdominal Aortic Aneurysm through the Down-Regulation of Matrix Metalloproteinases. Oncotarget 8 (9), 14283–14293. doi:10.18632/oncotarget.15093
Kong, P., Christia, P., and Frangogiannis, N. G. (2014). The Pathogenesis of Cardiac Fibrosis. Cel Mol Life Sci 71 (4), 549–574. doi:10.1007/s00018-013-1349-6
Krell, A., Wolter, M., Stojcheva, N., Hertler, C., Liesenberg, F., Zapatka, M., et al. (2018). MiR-16-5p Is Frequently Down-Regulated in Astrocytic Gliomas and Modulates Glioma Cell Proliferation, Apoptosis and Response to Cytotoxic Therapy. Neuropathol. Appl. Neurobiol. 45, 441–458. doi:10.1111/nan.12532
Labbé, C., Lorenzo-Betancor, O., and Ross, O. A. (2016). Epigenetic Regulation in Parkinson's Disease. Acta Neuropathol. 132 (4), 515–530. doi:10.1007/s00401-016-1590-9
Lahiri, D. K., Ge, Y. W., Sharman, E. H., and Bondy, S. C. (2004). Age-related Changes in Serum Melatonin in Mice: Higher Levels of Combined Melatonin and 6-hydroxymelatonin Sulfate in the Cerebral Cortex Than Serum, Heart, Liver and Kidney Tissues. J. Pineal Res. 36 (4), 217–223. doi:10.1111/j.1600-079X.2004.00120.x
Lee, B., Shim, I., Lee, H., and Hahm, D. H. (2018). Melatonin Ameliorates Cognitive Memory by Regulation of cAMP-Response Element-Binding Protein Expression and the Anti-inflammatory Response in a Rat Model of post-traumatic Stress Disorder. BMC Neurosci. 19 (1), 38. doi:10.1186/s12868-018-0439-7
Lee, I. C., Ko, J. W., Park, S. H., Shin, N. R., Shin, I. S., Moon, C., et al. (2018). Copper Nanoparticles Induce Early Fibrotic Changes in the Liver via TGF-β/Smad Signaling and Cause Immunosuppressive Effects in Rats. Nanotoxicology 12 (6), 637–651. doi:10.1080/17435390.2018.1472313
Li, B., Xie, Z., and Li, B. (2016). miR-152 Functions as a Tumor Suppressor in Colorectal Cancer by Targeting PIK3R3. Tumour Biol. 37 (8), 10075–10084. doi:10.1007/s13277-016-4888-2
Li, H., Li, J., Jiang, X., Liu, S., Liu, Y., Chen, W., et al. (2019). Melatonin Enhances Atherosclerotic Plaque Stability by Inducing Prolyl-4-Hydroxylase α1 Expression. J. Hypertens. 37 (5), 964–971. doi:10.1097/HJH.0000000000001979
Li, K., Hu, F., Xiong, W., Wei, Q., and Liu, F. F. (2019). Network-based Transcriptomic Analysis Reveals Novel Melatonin-Sensitive Genes in Cardiovascular System. Endocrine 64 (2), 414–419. doi:10.1007/s12020-019-01925-w
Li, S., Chen, H., Ren, J., Geng, Q., Song, J., Lee, C., et al. (2014). MicroRNA-223 Inhibits Tissue Factor Expression in Vascular Endothelial Cells. Atherosclerosis 237 (2), 514–520. doi:10.1016/j.atherosclerosis.2014.09.033
Li, T., Ni, L., Zhao, Z., Liu, X., Lai, Z., Di, X., et al. (2018). Melatonin Attenuates Smoking-Induced Hyperglycemia via Preserving Insulin Secretion and Hepatic Glycogen Synthesis in Rats. J. Pineal Res. 64 (4), e12475. doi:10.1111/jpi.12475
Li, W. Q., Tan, S. L., Li, X. H., Sun, T. L., Li, D., Du, J., et al. (2019). Calcitonin Gene-Related Peptide Inhibits the Cardiac Fibroblasts Senescence in Cardiac Fibrosis via Up-Regulating Klotho Expression. Eur. J. Pharmacol. 843, 96–103. doi:10.1016/j.ejphar.2018.10.023
Li, X., Chen, X., Zhou, W., Ji, S., Li, X., Li, G., et al. (2017). Effect of Melatonin on Neuronal Differentiation Requires CBP/p300-mediated Acetylation of Histone H3 Lysine 14. Neuroscience 364, 45–59. doi:10.1016/j.neuroscience.2017.07.064
Li, X. W., Cao, L., Wang, F., Yang, Q. G., Tong, J. J., Li, X. Y., et al. (2016). Maternal Inflammation Linearly Exacerbates Offspring Age-Related Changes of Spatial Learning and Memory, and Neurobiology until Senectitude. Behav. Brain Res. 306, 178–196. doi:10.1016/j.bbr.2016.03.011
Li, Y., Li, S., Zhou, Y., Meng, X., Zhang, J. J., Xu, D. P., et al. (2017). Melatonin for the Prevention and Treatment of Cancer. Oncotarget 8 (24), 39896–39921. doi:10.18632/oncotarget.16379
Liang, H., Xu, Y., Zhang, Q., Yang, Y., Mou, Y., Gao, Y., et al. (2019). MiR-483-3p Regulates Oxaliplatin Resistance by Targeting FAM171B in Human Colorectal Cancer Cells. Artif. Cell Nanomed Biotechnol 47 (1), 725–736. doi:10.1080/21691401.2019.1569530
Liao, W. W., Zhang, C., Liu, F. R., and Wang, W. J. (2018). Effects of miR-155 on Proliferation and Apoptosis by Regulating FoxO3a/BIM in Liver Cancer Cell Line HCCLM3. Eur. Rev. Med. Pharmacol. Sci. 22 (5), 1277–1285. doi:10.26355/eurrev_201803_14468
Libby, P., and Aikawa, M. (2003). Mechanisms of Plaque Stabilization with Statins. Am. J. Cardiol. 91 (4A), 4B–8B. doi:10.1016/s0002-9149(02)03267-8
Lin, G. J., Huang, S. H., Chen, Y. W., Hueng, D. Y., Chien, M. W., Chia, W. T., et al. (2009). Melatonin Prolongs Islet Graft Survival in Diabetic NOD Mice. J. Pineal Res. 47 (3), 284–292. doi:10.1111/j.1600-079X.2009.00712.x
Lind, M. I., and Spagopoulou, F. (2018). Evolutionary Consequences of Epigenetic Inheritance. Heredity (Edinb) 121 (3), 205–209. doi:10.1038/s41437-018-0113-y
Ling, J., Kang, Y., Zhao, R., Xia, Q., Lee, D. F., Chang, Z., et al. (2012). KrasG12D-induced IKK2/β/NF-Κb Activation by IL-1α and P62 Feedforward Loops Is Required for Development of Pancreatic Ductal Adenocarcinoma. Cancer Cell 21 (1), 105–120. doi:10.1016/j.ccr.2011.12.006
Ling, J., Kang, Y. a., Zhao, R., Xia, Q., Lee, D.-F., Chang, Z., et al. (2012). KrasG12D-Induced IKK2/β/NF-Κb Activation by IL-1α and P62 Feedforward Loops Is Required for Development of Pancreatic Ductal Adenocarcinoma. Cancer Cell 21 (1), 105–120. doi:10.1016/j.ccr.2011.12.006
Litwin, M., Michałkiewicz, J., Trojanek, J., Niemirska, A., Wierzbicka, A., and Szalecki, M. (2013). Altered Genes Profile of Renin-Angiotensin System, Immune System, and Adipokines Receptors in Leukocytes of Children with Primary Hypertension. Hypertension 61 (2), 431–436. doi:10.1161/HYPERTENSIONAHA.111.00181
Liu, T., Zhang, L., Joo, D., and Sun, S. C. (2017). NF-κB Signaling in Inflammation. Signal. Transduct Target. Ther. 2. doi:10.1038/sigtrans.2017.23
Liu, X., Cheng, Y., Yang, J., Krall, T. J., Huo, Y., and Zhang, C. (2010). An Essential Role of PDCD4 in Vascular Smooth Muscle Cell Apoptosis and Proliferation: Implications for Vascular Disease. Am. J. Physiol. Cel Physiol 298 (6), C1481–C1488. doi:10.1152/ajpcell.00413.2009
Liu, Y., Li, L. N., Guo, S., Zhao, X. Y., Liu, Y. Z., Liang, C., et al. (2018). Melatonin Improves Cardiac Function in a Mouse Model of Heart Failure with Preserved Ejection Fraction. Redox Biol. 18, 211–221. doi:10.1016/j.redox.2018.07.007
Long, F., Dong, C., Jiang, K., Xu, Y., Chi, X., Sun, D., et al. (2017). Melatonin Enhances the Anti-tumor Effect of Sorafenib via AKT/p27-mediated Cell Cycle Arrest in Hepatocarcinoma Cell Lines. RSC Adv. 7 (34), 21342–21351. doi:10.1039/c7ra02113e
Lupini, L., Pepe, F., Ferracin, M., Braconi, C., Callegari, E., Pagotto, S., et al. (2016). Over-expression of the miR-483-3p Overcomes the miR-145/TP53 Pro-apoptotic Loop in Hepatocellular Carcinoma. Oncotarget 7 (21), 31361–31371. doi:10.18632/oncotarget.8913
Lustri, A. M., Di Matteo, S., Fraveto, A., Costantini, D., Cantafora, A., Napoletano, C., et al. (2017). TGF-β Signaling Is an Effective Target to Impair Survival and Induce Apoptosis of Human Cholangiocarcinoma Cells: A Study on Human Primary Cell Cultures. PLoS One 12 (9), e0183932. doi:10.1371/journal.pone.0183932
Lyu, G., Guan, Y., Zhang, C., Zong, L., Sun, L., Huang, X., et al. (2018). TGF-β Signaling Alters H4K20me3 Status via miR-29 and Contributes to Cellular Senescence and Cardiac Aging. Nat. Commun. 9 (1), 2560. doi:10.1038/s41467-018-04994-z
Ma, J., Hong, L., Xu, G., Hao, J., Wang, R., Guo, H., et al. (2016). miR-483-3p Plays an Oncogenic Role in Esophageal Squamous Cell Carcinoma by Targeting Tumor Suppressor EI24. Cell Biol Int 40 (4), 448–455. doi:10.1002/cbin.10585
Majd, S., Power, J. H. T., Koblar, S. A., and Grantham, H. J. M. (2017). The Impact of Tau Hyperphosphorylation at Ser262 on Memory and Learning after Global Brain Ischaemia in a Rat Model of Reversible Cardiac Arrest. IBRO Rep. 2, 1–13. doi:10.1016/j.ibror.2016.12.002
Makeeva, N., Myers, J. W., and Welsh, N. (2006). Role of MKK3 and P38 MAPK in Cytokine-Induced Death of Insulin-Producing Cells. Biochem. J. 393 (Pt 1), 129–139. doi:10.1042/BJ20050814
Marques, J. H. M., Mota, A. L., Oliveira, J. G., Lacerda, J. Z., Stefani, J. P., Ferreira, L. C., et al. (2018). Melatonin Restrains Angiogenic Factors in Triple-Negative Breast Cancer by Targeting miR-152-3p: In Vivo and In Vitro Studies. Life Sci. 208, 131–138. doi:10.1016/j.lfs.2018.07.012
Martino, F., Carlomosti, F., Avitabile, D., Persico, L., Picozza, M., Barillà, F., et al. (2015). Circulating miR-33a and miR-33b Are Up-Regulated in Familial Hypercholesterolaemia in Paediatric Age. Clin. Sci. (Lond) 129 (11), 963–972. doi:10.1042/CS20150235
Medeiros, C. A., Carvalhedo de Bruin, P. F., Lopes, L. A., Magalhães, M. C., de Lourdes Seabra, M., and de Bruin, V. M. (2007). Effect of Exogenous Melatonin on Sleep and Motor Dysfunction in Parkinson's Disease. A Randomized, Double Blind, Placebo-Controlled Study. J. Neurol. 254 (4), 459–464. doi:10.1007/s00415-006-0390-x
Medina, M., Garrido, J. J., and Wandosell, F. G. (2011). Modulation of GSK-3 as a Therapeutic Strategy on Tau Pathologies. Front. Mol. Neurosci. 4, 24. doi:10.3389/fnmol.2011.00024
Monti, N., Cavallaro, R. A., Stoccoro, A., Nicolia, V., Scarpa, S., Kovacs, G. G., et al. (2020). CpG and Non-CpG Presenilin1 Methylation Pattern in Course of Neurodevelopment and Neurodegeneration Is Associated with Gene Expression in Human and Murine Brain. Epigenetics 15 (8), 781–799. doi:10.1080/15592294.2020.1722917
Moriya, J. (2019). Critical Roles of Inflammation in Atherosclerosis. J. Cardiol. 73 (1), 22–27. doi:10.1016/j.jjcc.2018.05.010
Ohashi, N., Ishigaki, S., and Isobe, S. (2019). The Pivotal Role of Melatonin in Ameliorating Chronic Kidney Disease by Suppression of the Renin-Angiotensin System in the Kidney. Hypertens. Res. 42 (6), 761–768. doi:10.1038/s41440-018-0186-2
Ohashi, N., Ishigaki, S., and Isobe, S. (2019). The Pivotal Role of Melatonin in Ameliorating Chronic Kidney Disease by Suppression of the Renin-Angiotensin System in the Kidney. Hypertens. Res. doi:10.1038/s41440-018-0186-2
Olcese, J. M., Cao, C., Mori, T., Mamcarz, M. B., Maxwell, A., Runfeldt, M. J., et al. (2009). Protection against Cognitive Deficits and Markers of Neurodegeneration by Long-Term Oral Administration of Melatonin in a Transgenic Model of Alzheimer Disease. J. Pineal Res. 47 (1), 82–96. doi:10.1111/j.1600-079X.2009.00692.x
Ono, K., Mochizuki, H., Ikeda, T., Nihira, T., Takasaki, J., Teplow, D. B., et al. (2012). Effect of Melatonin on α-synuclein Self-Assembly and Cytotoxicity. Neurobiol. Aging 33 (9), 2172–2185. doi:10.1016/j.neurobiolaging.2011.10.015
Ortiz, G. G., Pacheco-Moisés, F. P., Gómez-Rodríguez, V. M., González-Renovato, E. D., Torres-Sánchez, E. D., and Ramírez-Anguiano, A. C. (2013). Fish Oil, Melatonin and Vitamin E Attenuates Midbrain Cyclooxygenase-2 Activity and Oxidative Stress after the Administration of 1-Methyl-4-Phenyl-1,2,3,6- Tetrahydropyridine. Metab. Brain Dis. 28 (4), 705–709. doi:10.1007/s11011-013-9416-0
Ozsoy, O., Yildirim, F. B., Ogut, E., Kaya, Y., Tanriover, G., Parlak, H., et al. (2015). Melatonin Is Protective against 6-Hydroxydopamine-Induced Oxidative Stress in a Hemiparkinsonian Rat Model. Free Radic. Res. 49 (8), 1004–1014. doi:10.3109/10715762.2015.1027198
Pan, Y., and Niles, L. P. (2015). Epigenetic Mechanisms of Melatonin Action in Human SH-Sy5y Neuroblastoma Cells. Mol. Cel Endocrinol 402, 57–63. doi:10.1016/j.mce.2015.01.003
Park, G., Tan, J., Garcia, G., Kang, Y., Salvesen, G., and Zhang, Z. (2016). Regulation of Histone Acetylation by Autophagy in Parkinson Disease. J. Biol. Chem. 291 (7), 3531–3540. doi:10.1074/jbc.M115.675488
Park, J. H., Shim, H. M., Na, A. Y., Bae, K. C., Bae, J. H., Im, S. S., et al. (2014). Melatonin Prevents Pancreatic β-cell Loss Due to Glucotoxicity: the Relationship between Oxidative Stress and Endoplasmic Reticulum Stress. J. Pineal Res. 56 (2), 143–153. doi:10.1111/jpi.12106
Park, M. H., and Hong, J. T. (2016). Roles of NF-Κb in Cancer and Inflammatory Diseases and Their Therapeutic Approaches. Cells 5 (2). doi:10.3390/cells5020015
Paul, R., Phukan, B. C., Justin Thenmozhi, A., Manivasagam, T., Bhattacharya, P., and Borah, A. (2018). Melatonin Protects against Behavioral Deficits, Dopamine Loss and Oxidative Stress in Homocysteine Model of Parkinson's Disease. Life Sci. 192, 238–245. doi:10.1016/j.lfs.2017.11.016
Paz, S., Vilasco, M., Werden, S. J., Arguello, M., Joseph-Pillai, D., Zhao, T., et al. (2011). A Functional C-Terminal TRAF3-Binding Site in MAVS Participates in Positive and Negative Regulation of the IFN Antiviral Response. Cell Res 21 (6), 895–910. doi:10.1038/cr.2011.2
Pei, F., Wang, X., Yue, R., Chen, C., Huang, J., Huang, J., et al. (2015). Differential Expression and DNA Methylation of Angiotensin Type 1A Receptors in Vascular Tissues during Genetic Hypertension Development. Mol. Cel Biochem 402 (1-2), 1–8. doi:10.1007/s11010-014-2295-9
Pestana, R. M., Domingueti, C. P., Duarte, R. C., Fóscolo, R. B., Reis, J. S., Rodrigues, A. M., et al. (2016). Cytokines Profile and its Correlation with Endothelial Damage and Oxidative Stress in Patients with Type 1 Diabetes Mellitus and Nephropathy. Immunol. Res. 64 (4), 951–960. doi:10.1007/s12026-016-8806-x
Pihlstrøm, L., Berge, V., Rengmark, A., and Toft, M. (2015). Parkinson's Disease Correlates with Promoter Methylation in the α-synuclein Gene. Mov Disord. 30 (4), 577–580. doi:10.1002/mds.26073
Pore, N., Liu, S., Shu, H. K., Li, B., Haas-Kogan, D., Stokoe, D., et al. (2004). Sp1 Is Involved in Akt-Mediated Induction of VEGF Expression through an HIF-1-independent Mechanism. Mol. Biol. Cel 15 (11), 4841–4853. doi:10.1091/mbc.e04-05-0374
Prokopenko, I., Langenberg, C., Florez, J. C., Saxena, R., Soranzo, N., Thorleifsson, G., et al. (2009). Variants in MTNR1B Influence Fasting Glucose Levels. Nat. Genet. 41 (1), 77–81. doi:10.1038/ng.290
Qin, W., Lu, W., Li, H., Yuan, X., Li, B., Zhang, Q., et al. (2012). Melatonin Inhibits IL1β-induced MMP9 Expression and Activity in Human Umbilical Vein Endothelial Cells by Suppressing NF-Κb Activation. J. Endocrinol. 214 (2), 145–153. doi:10.1530/JOE-12-0147
Qin, Z., Fisher, G. J., Voorhees, J. J., and Quan, T. (2018). Actin Cytoskeleton Assembly Regulates Collagen Production via TGF-β Type II Receptor in Human Skin Fibroblasts. J. Cel Mol Med 22 (9), 4085–4096. doi:10.1111/jcmm.13685
Renani, P. G., Taheri, F., Rostami, D., Farahani, N., Abdolkarimi, H., Abdollahi, E., et al. (2019). Involvement of Aberrant Regulation of Epigenetic Mechanisms in the Pathogenesis of Parkinson's Disease and Epigenetic-Based Therapies. J. Cel Physiol 234 (11), 19307–19319. doi:10.1002/jcp.28622
Rinnerthaler, G., Hackl, H., Gampenrieder, S. P., Hamacher, F., Hufnagl, C., Hauser-Kronberger, C., et al. (2016). miR-16-5p Is a Stably-Expressed Housekeeping MicroRNA in Breast Cancer Tissues from Primary Tumors and from Metastatic Sites. Int. J. Mol. Sci. 17 (2). doi:10.3390/ijms17020156
Roelofs, H. M., Te Morsche, R. H., van Heumen, B. W., Nagengast, F. M., and Peters, W. H. (2014). Over-expression of COX-2 mRNA in Colorectal Cancer. BMC Gastroenterol. 14, 1. doi:10.1186/1471-230X-14-1
Rossetto, D., Avvakumov, N., and Côté, J. (2012). Histone Phosphorylation: a Chromatin Modification Involved in Diverse Nuclear Events. Epigenetics 7 (10), 1098–1108. doi:10.4161/epi.21975
Rudra, D. S., Pal, U., Maiti, N. C., Reiter, R. J., and Swarnakar, S. (2013). Melatonin Inhibits Matrix Metalloproteinase-9 Activity by Binding to its Active Site. J. Pineal Res. 54 (4), 398–405. doi:10.1111/jpi.12034
Rui, J., Deng, S., Lebastchi, J., Clark, P. L., Usmani-Brown, S., and Herold, K. C. (2016). Methylation of Insulin DNA in Response to Proinflammatory Cytokines during the Progression of Autoimmune Diabetes in NOD Mice. Diabetologia 59 (5), 1021–1029. doi:10.1007/s00125-016-3897-4
Ruiz, L., Gurlo, T., Ravier, M. A., Wojtusciszyn, A., Mathieu, J., Brown, M. R., et al. (2018). Proteasomal Degradation of the Histone Acetyl Transferase P300 Contributes to Beta-Cell Injury in a Diabetes Environment. Cell Death Dis 9 (6), 600. doi:10.1038/s41419-018-0603-0
Rusek, M., Pluta, R., Ułamek-Kozioł, M., and Czuczwar, S. J. (2019). Ketogenic Diet in Alzheimer's Disease. Int. J. Mol. Sci. 20 (16). doi:10.3390/ijms20163892
Russart, K. L. G., and Nelson, R. J. (2018). Light at Night as an Environmental Endocrine Disruptor. Physiol. Behav. 190, 82–89. doi:10.1016/j.physbeh.2017.08.029
Saldeen, J., Lee, J. C., and Welsh, N. (2001). Role of P38 Mitogen-Activated Protein Kinase (P38 MAPK) in Cytokine-Induced Rat Islet Cell Apoptosis. Biochem. Pharmacol. 61 (12), 1561–1569. doi:10.1016/s0006-2952(01)00605-0
Sánchez, D. I., González-Fernández, B., Crespo, I., San-Miguel, B., Álvarez, M., González-Gallego, J., et al. (2018). Melatonin Modulates Dysregulated Circadian Clocks in Mice with Diethylnitrosamine-Induced Hepatocellular Carcinoma. J. Pineal Res. 65 (3), e12506. doi:10.1111/jpi.12506
Sánchez-Barceló, E. J., Mediavilla, M. D., Tan, D. X., and Reiter, R. J. (2010). Clinical Uses of Melatonin: Evaluation of Human Trials. Curr. Med. Chem. 17 (19), 2070–2095. doi:10.2174/092986710791233689
Sang, S., Zhang, Z., Qin, S., Li, C., and Dong, Y. (2017). MicroRNA-16-5p Inhibits Osteoclastogenesis in Giant Cell Tumor of Bone. Biomed. Res. Int. 2017, 3173547. doi:10.1155/2017/3173547
Sariahmetoglu, M., Crawford, B. D., Leon, H., Sawicka, J., Li, L., Ballermann, B. J., et al. (2007). Regulation of Matrix Metalloproteinase-2 (MMP-2) Activity by Phosphorylation. FASEB J. 21 (10), 2486–2495. doi:10.1096/fj.06-7938com
Sarshad, A. A., Juan, A. H., Muler, A. I. C., Anastasakis, D. G., Wang, X., Genzor, P., et al. (2018). Argonaute-miRNA Complexes Silence Target mRNAs in the Nucleus of Mammalian Stem Cells. Mol. Cel 71 (6), 1040–e8. doi:10.1016/j.molcel.2018.07.020
Sawicka, A., and Seiser, C. (2012). Histone H3 Phosphorylation - a Versatile Chromatin Modification for Different Occasions. Biochimie 94 (11), 2193–2201. doi:10.1016/j.biochi.2012.04.018
Schmitt, I., Kaut, O., Khazneh, H., deBoni, L., Ahmad, A., Berg, D., et al. (2015). L-Dopa Increases α-synuclein DNA Methylation in Parkinson's Disease Patients In Vivo and In Vitro. Mov Disord. 30 (13), 1794–1801. doi:10.1002/mds.26319
Sharma, S., and Taliyan, R. (2015). Targeting Histone Deacetylases: a Novel Approach in Parkinson's Disease. Parkinsons Dis. 2015, 303294. doi:10.1155/2015/303294
Shi, C., Zeng, J., Li, Z., Chen, Q., Hang, W., Xia, L., et al. (2018). Melatonin Mitigates Kainic Acid-Induced Neuronal Tau Hyperphosphorylation and Memory Deficits through Alleviating ER Stress. Front. Mol. Neurosci. 11, 5. doi:10.3389/fnmol.2018.00005
Shi, D., Xiao, X., Wang, J., Liu, L., Chen, W., Fu, L., et al. (2012). Melatonin Suppresses Proinflammatory Mediators in Lipopolysaccharide-Stimulated CRL1999 Cells via Targeting MAPK, NF-Κb, c/EBPβ, and P300 Signaling. J. Pineal Res. 53 (2), 154–165. doi:10.1111/j.1600-079X.2012.00982.x
Shrestha, S., Zhu, J., Wang, Q., Du, X., Liu, F., Jiang, J., et al. (2017). Melatonin Potentiates the Antitumor Effect of Curcumin by Inhibiting IKKβ/NF-Κb/cox-2 Signaling Pathway. Int. J. Oncol. 51 (4), 1249–1260. doi:10.3892/ijo.2017.4097
Shukla, M., Chinchalongporn, V., Govitrapong, P., and Reiter, R. J. (2019). The Role of Melatonin in Targeting Cell Signaling Pathways in Neurodegeneration. Ann. N. Y Acad. Sci. 1443 (1), 75–96. doi:10.1111/nyas.14005
Shukla, M., Htoo, H. H., Wintachai, P., Hernandez, J. F., Dubois, C., Postina, R., et al. (2015). Melatonin Stimulates the Nonamyloidogenic Processing of βAPP through the Positive Transcriptional Regulation of ADAM10 and ADAM17. J. Pineal Res. 58 (2), 151–165. doi:10.1111/jpi.12200
Shukla, S., and Tekwani, B. L. (2020). Histone Deacetylases Inhibitors in Neurodegenerative Diseases, Neuroprotection and Neuronal Differentiation. Front. Pharmacol. 11, 537. doi:10.3389/fphar.2020.00537
Singh, P., Konar, A., Kumar, A., Srivas, S., and Thakur, M. K. (2015). Hippocampal Chromatin-Modifying Enzymes Are Pivotal for Scopolamine-Induced Synaptic Plasticity Gene Expression Changes and Memory Impairment. J. Neurochem. 134 (4), 642–651. doi:10.1111/jnc.13171
Su, L. Y., Li, H., Lv, L., Feng, Y. M., Li, G. D., Luo, R., et al. (2015). Melatonin Attenuates MPTP-Induced Neurotoxicity via Preventing CDK5-Mediated Autophagy and SNCA/α-synuclein Aggregation. Autophagy 11 (10), 1745–1759. doi:10.1080/15548627.2015.1082020
Su, W., Huo, Q., Wu, H., Wang, L., Ding, X., Liang, L., et al. (2021). The Function of LncRNA-H19 in Cardiac Hypertrophy. Cell Biosci 11 (1), 153. doi:10.1186/s13578-021-00668-4
Sun, J., Tian, X., Zhang, J., Huang, Y., Lin, X., Chen, L., et al. (2017). Regulation of Human Glioma Cell Apoptosis and Invasion by miR-152-3p through Targeting DNMT1 and Regulating NF2 : MiR-152-3p Regulate Glioma Cell Apoptosis and Invasion. J. Exp. Clin. Cancer Res. 36 (1), 100. doi:10.1186/s13046-017-0567-4
Sun, X., Ran, D., Zhao, X., Huang, Y., Long, S., Liang, F., et al. (2016). Melatonin Attenuates hLRRK2-Induced Sleep Disturbances and Synaptic Dysfunction in a Drosophila Model of Parkinson's Disease. Mol. Med. Rep. 13 (5), 3936–3944. doi:10.3892/mmr.2016.4991
Sun, Y., Jin, L., Sui, Y. X., Han, L. L., and Liu, J. H. (2017). Circadian Gene CLOCK Affects Drug-Resistant Gene Expression and Cell Proliferation in Ovarian Cancer SKOV3/DDP Cell Lines through Autophagy. Cancer Biother. Radiopharm. 32 (4), 139–146. doi:10.1089/cbr.2016.2153
Sung, H., Ferlay, J., Siegel, R. L., Laversanne, M., Soerjomataram, I., Jemal, A., et al. (2021). Global Cancer Statistics 2020: GLOBOCAN Estimates of Incidence and Mortality Worldwide for 36 Cancers in 185 Countries. CA Cancer J. Clin. 71 (3), 209–249. doi:10.3322/caac.21660
Taheri, M., Eghtedarian, R., Dinger, M. E., and Ghafouri-Fard, S. (2020). Emerging Roles of Non-coding RNAs in the Pathogenesis of Type 1 Diabetes Mellitus. Biomed. Pharmacother. 129, 110509. doi:10.1016/j.biopha.2020.110509
Tai, N., Wong, F. S., and Wen, L. (2016). The Role of the Innate Immune System in Destruction of Pancreatic Beta Cells in NOD Mice and Humans with Type I Diabetes. J. Autoimmun. 71, 26–34. doi:10.1016/j.jaut.2016.03.006
Tain, Y. L., Chan, J. Y. H., Lee, C. T., and Hsu, C. N. (2018). Maternal Melatonin Therapy Attenuates Methyl-Donor Diet-Induced Programmed Hypertension in Male Adult Rat Offspring. Nutrients 10 (10). doi:10.3390/nu10101407
Tain, Y. L., Lee, C. T., Chan, J. Y., and Hsu, C. N. (2016). Maternal Melatonin or N-Acetylcysteine Therapy Regulates hydrogen Sulfide-Generating Pathway And renal transcriptome to Prevent Prenatal NG-Nitro-L-arginine-methyl Ester (L-NAME)-induced Fetal Programming of Hypertension in Adult Male Offspring. Am. J. Obstet. Gynecol. 215 (5), 636–e72. doi:10.1016/j.ajog.2016.07.036
Tain, Y. L., Lin, Y. J., Chan, J. Y. H., Lee, C. T., and Hsu, C. N. (2017). Maternal Melatonin or Agomelatine Therapy Prevents Programmed Hypertension in Male Offspring of Mother Exposed to Continuous Light. Biol. Reprod. 97 (4), 636–643. doi:10.1093/biolre/iox115
Tang, Y. L., Jiang, J. H., Wang, S., Liu, Z., Tang, X. Q., Peng, J., et al. (2015). TLR4/NF-κB Signaling Contributes to Chronic Unpredictable Mild Stress-Induced Atherosclerosis in ApoE-/- Mice. PLoS One 10 (4), e0123685. doi:10.1371/journal.pone.0123685
Taniguchi, K., and Karin, M. (2018). NF-κB, Inflammation, Immunity and Cancer: Coming of Age. Nat. Rev. Immunol. 18 (5), 309–324. doi:10.1038/nri.2017.142
Tao, H., Yang, J. J., Shi, K. H., and Li, J. (2016). Epigenetic Factors MeCP2 and HDAC6 Control α-tubulin Acetylation in Cardiac Fibroblast Proliferation and Fibrosis. Inflamm. Res. 65 (5), 415–426. doi:10.1007/s00011-016-0925-2
Vallabhapurapu, S. D., Noothi, S. K., Pullum, D. A., Lawrie, C. H., Pallapati, R., Potluri, V., et al. (2015). Transcriptional Repression by the HDAC4-RelB-P52 Complex Regulates Multiple Myeloma Survival and Growth. Nat. Commun. 6, 8428. doi:10.1038/ncomms9428
Wang, C., Sun, Y., Wu, H., Yu, S., Zhang, L., Meng, Y., et al. (2015). Elevated miR-483-3p Expression Is an Early Event and Indicates Poor Prognosis in Pancreatic Ductal Adenocarcinoma. Tumour Biol. 36 (12), 9447–9456. doi:10.1007/s13277-015-3690-x
Wang, D. B., Kinoshita, C., Kinoshita, Y., Sopher, B. L., Uo, T., Lee, R. J., et al. (2019). Neuronal Susceptibility to Beta-Amyloid Toxicity and Ischemic Injury Involves Histone Deacetylase-2 Regulation of Endophilin-B1. Brain Pathol. 29 (2), 164–175. doi:10.1111/bpa.12647
Wang, F., Demura, M., Cheng, Y., Zhu, A., Karashima, S., Yoneda, T., et al. (2014). Dynamic CCAAT/enhancer Binding Protein-Associated Changes of DNA Methylation in the Angiotensinogen Gene. Hypertension 63 (2), 281–288. doi:10.1161/HYPERTENSIONAHA.113.02303
Wang, F., Mao, A., Tang, J., Zhang, Q., Yan, J., Wang, Y., et al. (2019). microRNA-16-5p Enhances Radiosensitivity through Modulating Cyclin D1/E1-pRb-E2f1 Pathway in Prostate Cancer Cells. J. Cel Physiol 234 (Suppl. ment_1), 13182–13190. doi:10.1002/jcp.27989
Wang, H., Lou, D., and Wang, Z. (2018). Crosstalk of Genetic Variants, Allele-specific DNA Methylation, and Environmental Factors for Complex Disease Risk. Front. Genet. 9, 695. doi:10.3389/fgene.2018.00695
Wang, H. W., Shi, L., Xu, Y. P., Qin, X. Y., and Wang, Q. Z. (2016). Oxymatrine Inhibits Renal Fibrosis of Obstructive Nephropathy by Downregulating the TGF-Β1-Smad3 Pathway. Ren. Fail. 38 (6), 945–951. doi:10.3109/0886022X.2016.1164185
Wang, R., Zhou, S., Wu, P., Li, M., Ding, X., Sun, L., et al. (2018). Identifying Involvement of H19-miR-675-3p-Igf1r and H19-miR-200a-PDCD4 in Treating Pulmonary Hypertension with Melatonin. Mol. Ther. Nucleic Acids 13, 44–54. doi:10.1016/j.omtn.2018.08.015
Wang, X., Tang, S., Le, S. Y., Lu, R., Rader, J. S., Meyers, C., et al. (2008). Aberrant Expression of Oncogenic and Tumor-Suppressive microRNAs in Cervical Cancer Is Required for Cancer Cell Growth. PLoS One 3 (7), e2557. doi:10.1371/journal.pone.0002557
Wang, X., Wang, Z. H., Wu, Y. Y., Tang, H., Tan, L., Wang, X., et al. (2013). Melatonin Attenuates Scopolamine-Induced Memory/synaptic Disorder by Rescuing EPACs/miR-124/Egr1 Pathway. Mol. Neurobiol. 47 (1), 373–381. doi:10.1007/s12035-012-8355-9
Wang, X. X., Xie, F., Jia, C. C., Yan, N., Zeng, Y. L., Wu, J. D., et al. (2021). Synthesis and Biological Evaluation of Selective Histone Deacetylase 6 Inhibitors as Multifunctional Agents against Alzheimer's Disease. Eur. J. Med. Chem. 225, 113821. doi:10.1016/j.ejmech.2021.113821
Wang, Y. M., Jin, B. Z., Ai, F., Duan, C. H., Lu, Y. Z., Dong, T. F., et al. (2012). The Efficacy and Safety of Melatonin in Concurrent Chemotherapy or Radiotherapy for Solid Tumors: a Meta-Analysis of Randomized Controlled Trials. Cancer Chemother. Pharmacol. 69 (5), 1213–1220. doi:10.1007/s00280-012-1828-8
Wang, Y. R., Hong, R. T., Xie, Y. Y., and Xu, J. M. (2018). Melatonin Ameliorates Liver Fibrosis Induced by Carbon Tetrachloride in Rats via Inhibiting TGF-β1/Smad Signaling Pathway. Curr. Med. Sci. 38 (2), 236–244. doi:10.1007/s11596-018-1871-8
Weishaupt, J. H., Bartels, C., Pölking, E., Dietrich, J., Rohde, G., Poeggeler, B., et al. (2006). Reduced Oxidative Damage in ALS by High-Dose Enteral Melatonin Treatment. J. Pineal Res. 41 (4), 313–323. doi:10.1111/j.1600-079X.2006.00377.x
Wijesekara, N., Krishnamurthy, M., Bhattacharjee, A., Suhail, A., Sweeney, G., and Wheeler, M. B. (2010). Adiponectin-induced ERK and Akt Phosphorylation Protects against Pancreatic Beta Cell Apoptosis and Increases Insulin Gene Expression and Secretion. J. Biol. Chem. 285 (44), 33623–33631. doi:10.1074/jbc.M109.085084
Wu, T. H., Kuo, H. C., Lin, I. C., Chien, S. J., Huang, L. T., and Tain, Y. L. (2014). Melatonin Prevents Neonatal Dexamethasone Induced Programmed Hypertension: Histone Deacetylase Inhibition. J. Steroid Biochem. Mol. Biol. 144, 253–259. doi:10.1016/j.jsbmb.2014.07.008
Wu, W. L., Wang, W. Y., Yao, W. Q., and Li, G. D. (2015). Suppressive Effects of microRNA-16 on the Proliferation, Invasion and Metastasis of Hepatocellular Carcinoma Cells. Int. J. Mol. Med. 36 (6), 1713–1719. doi:10.3892/ijmm.2015.2379
Wu, X., Chen, P. S., Dallas, S., Wilson, B., Block, M. L., Wang, C. C., et al. (2008). Histone Deacetylase Inhibitors Up-Regulate Astrocyte GDNF and BDNF Gene Transcription and Protect Dopaminergic Neurons. Int. J. Neuropsychopharmacol. 11 (8), 1123–1134. doi:10.1017/S1461145708009024
Wu, Y., Si, F., Luo, L., Jing, F., Jiang, K., Zhou, J., et al. (2018). The Effect of Melatonin on Cardio Fibrosis in Juvenile Rats with Pressure Overload and Deregulation of HDACs. Korean J. Physiol. Pharmacol. 22 (6), 607–616. doi:10.4196/kjpp.2018.22.6.607
Xu, J., Gao, H., Zhang, L., Rong, S., Yang, W., Ma, C., et al. (2019). Melatonin Alleviates Cognition Impairment by Antagonizing Brain Insulin Resistance in Aged Rats Fed a High-Fat Diet. J. Pineal Res. 67, e12584. doi:10.1111/jpi.12584
Xu, X., Wang, G., Ai, L., Shi, J., Zhang, J., and Chen, Y. X. (2018). Melatonin Suppresses TLR9-Triggered Proinflammatory Cytokine Production in Macrophages by Inhibiting ERK1/2 and AKT Activation. Sci. Rep. 8 (1), 15579. doi:10.1038/s41598-018-34011-8
Yan, W. W., Chen, G. H., Wang, F., Tong, J. J., and Tao, F. (2015). Long-term Acarbose Administration Alleviating the Impairment of Spatial Learning and Memory in the SAMP8 Mice Was Associated with Alleviated Reduction of Insulin System and Acetylated H4K8. Brain Res. 1603, 22–31. doi:10.1016/j.brainres.2015.01.042
Yang, M., Tao, J., Wu, H., Guan, S., Liu, L., Zhang, L., et al. (2019). Aanat Knockdown and Melatonin Supplementation in Embryo Development: Involvement of Mitochondrial Function and DNA Methylation. Antioxid. Redox Signal. 30 (18), 2050–2065. doi:10.1089/ars.2018.7555
Yeung, H. M., Hung, M. W., Lau, C. F., and Fung, M. L. (2015). Cardioprotective Effects of Melatonin against Myocardial Injuries Induced by Chronic Intermittent Hypoxia in Rats. J. Pineal Res. 58 (1), 12–25. doi:10.1111/jpi.12190
Yi, C., Zhang, Y., Yu, Z., Xiao, Y., Wang, J., Qiu, H., et al. (2014). Melatonin Enhances the Anti-tumor Effect of Fisetin by Inhibiting COX-2/iNOS and NF-κB/p300 Signaling Pathways. PLoS One 9 (7), e99943. doi:10.1371/journal.pone.0099943
You, D., Qiao, Q., Ono, K., Wei, M., Tan, W., Wang, C., et al. (2022). miR-223-3p Inhibits the Progression of Atherosclerosis via Down-Regulating the Activation of MEK1/ERK1/2 in Macrophages. Aging (Albany NY) 14 (4), 1865–1878. doi:10.18632/aging.203908
Yu, D., Du, Z., Pian, L., Li, T., Wen, X., Li, W., et al. (2017). Mitochondrial DNA Hypomethylation Is a Biomarker Associated with Induced Senescence in Human Fetal Heart Mesenchymal Stem Cells. Stem Cell Int 2017, 1764549. doi:10.1155/2017/1764549
Yu, L. M., and Xu, Y. (2015). Epigenetic Regulation in Cardiac Fibrosis. World J. Cardiol. 7 (11), 784–791. doi:10.4330/wjc.v7.i11.784
Zablocki, D., and Sadoshima, J. (2013). Angiotensin II and Oxidative Stress in the Failing Heart. Antioxid. Redox Signal. 19 (10), 1095–1109. doi:10.1089/ars.2012.4588
Zhang, H., Yang, K., Ren, T., Huang, Y., Tang, X., and Guo, W. (2018). miR-16-5p Inhibits Chordoma Cell Proliferation, Invasion and Metastasis by Targeting Smad3. Cel Death Dis 9 (6), 680. doi:10.1038/s41419-018-0738-z
Zhang, J., Song, Y., Zhang, C., Zhi, X., Fu, H., Ma, Y., et al. (2015). Circulating MiR-16-5p and MiR-19b-3p as Two Novel Potential Biomarkers to Indicate Progression of Gastric Cancer. Theranostics 5 (7), 733–745. doi:10.7150/thno.10305
Zhang, L., Wang, W., Li, X., He, S., Yao, J., Wang, X., et al. (2016). MicroRNA-155 Promotes Tumor Growth of Human Hepatocellular Carcinoma by Targeting ARID2. Int. J. Oncol. 48 (6), 2425–2434. doi:10.3892/ijo.2016.3465
Zhang, X., Liu, L., Deng, X., Li, D., Cai, H., Ma, Y., et al. (2019). MicroRNA 483-3p Targets Pard3 to Potentiate TGF-Β1-Induced Cell Migration, Invasion, and Epithelial-Mesenchymal Transition in Anaplastic Thyroid Cancer Cells. Oncogene 38 (5), 699–715. doi:10.1038/s41388-018-0447-1
Zhang, Y., Liu, X., Bai, X., Lin, Y., Li, Z., Fu, J., et al. (2018). Melatonin Prevents Endothelial Cell Pyroptosis via Regulation of Long Noncoding RNA MEG3/miR-223/NLRP3 axis. J. Pineal Res. 64 (2). doi:10.1111/jpi.12449
Zhao, Y., Gong, X., Chen, L., Li, L., Liang, Y., Chen, S., et al. (2014). Site-specific Methylation of Placental HSD11B2 Gene Promoter Is Related to Intrauterine Growth Restriction. Eur. J. Hum. Genet. 22 (6), 734–740. doi:10.1038/ejhg.2013.226
Zhao, Y., Jaber, V., and Lukiw, W. J. (2017). Secretory Products of the Human GI Tract Microbiome and Their Potential Impact on Alzheimer's Disease (AD): Detection of Lipopolysaccharide (LPS) in AD Hippocampus. Front Cel Infect Microbiol 7, 318. doi:10.3389/fcimb.2017.00318
Zhong, S., Goto, H., Inagaki, M., and Dong, Z. (2003). Phosphorylation at Serine 28 and Acetylation at Lysine 9 of Histone H3 Induced by Trichostatin A. Oncogene 22 (34), 5291–5297. doi:10.1038/sj.onc.1206507
Zhu, C., Huang, Q., and Zhu, H. (2018). Melatonin Inhibits the Proliferation of Gastric Cancer Cells through Regulating the miR-16-5p-Smad3 Pathway. DNA Cel Biol 37 (3), 244–252. doi:10.1089/dna.2017.4040
Zhu, H. Q., li, Q., Dong, L. Y., Zhou, Q., Wang, H., and Wang, Y. (2014). MicroRNA-29b Promotes High-Fat Diet-Stimulated Endothelial Permeability and Apoptosis in apoE Knock-Out Mice by Down-Regulating MT1 Expression. Int. J. Cardiol. 176 (3), 764–770. doi:10.1016/j.ijcard.2014.07.095
Zhuang, Y., Li, T., Xiao, H., Wu, J., Su, S., Dong, X., et al. (2021). LncRNA-H19 Drives Cardiomyocyte Senescence by Targeting miR-19a/socs1/p53 Axis. Front. Pharmacol. 12, 631835. doi:10.3389/fphar.2021.631835
Zibolka, J., Mühlbauer, E., and Peschke, E. (2015). Melatonin Influences Somatostatin Secretion from Human Pancreatic δ-cells via MT1 and MT2 Receptors. J. Pineal Res. 58 (2), 198–209. doi:10.1111/jpi.12206
Zou, X., Wang, J., Chen, C., Tan, X., Huang, Y., Jose, P. A., et al. (2020). Secreted Monocyte miR-27a, via Mesenteric Arterial Mas Receptor-eNOS Pathway, Causes Hypertension. Am. J. Hypertens. 33 (1), 31–42. doi:10.1093/ajh/hpz112
Keywords: epigenetic modification, DNA methylation, histone modification, non-coding RNAs, melatonin
Citation: Monayo SM and Liu X (2022) The Prospective Application of Melatonin in Treating Epigenetic Dysfunctional Diseases. Front. Pharmacol. 13:867500. doi: 10.3389/fphar.2022.867500
Received: 01 February 2022; Accepted: 14 April 2022;
Published: 20 May 2022.
Edited by:
Gauthaman Kalamegam, Saveetha Dental College And Hospitals, IndiaReviewed by:
Nurul ’Izzah Ibrahim, National University of Malaysia, MalaysiaDafang Wang, Delta State University, United States
Copyright © 2022 Monayo and Liu. This is an open-access article distributed under the terms of the Creative Commons Attribution License (CC BY). The use, distribution or reproduction in other forums is permitted, provided the original author(s) and the copyright owner(s) are credited and that the original publication in this journal is cited, in accordance with accepted academic practice. No use, distribution or reproduction is permitted which does not comply with these terms.
*Correspondence: Xin Liu, ZnJleWFsaXV4aW5AMTYzLmNvbQ==