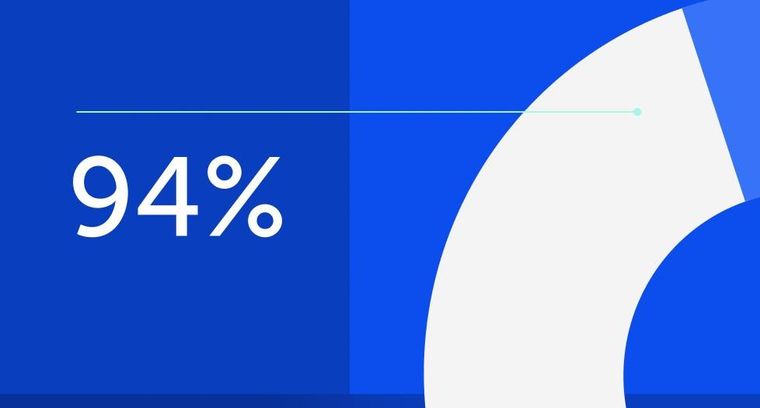
94% of researchers rate our articles as excellent or good
Learn more about the work of our research integrity team to safeguard the quality of each article we publish.
Find out more
ORIGINAL RESEARCH article
Front. Pharmacol., 17 May 2022
Sec. Predictive Toxicology
Volume 13 - 2022 | https://doi.org/10.3389/fphar.2022.862830
The complexity of chemical components of herbal medicines often causes great barriers to toxicity research. In our previous study, we have found the critical divergent hepatotoxic potential of a pair of stilbene isomers in a famous traditional Chinese herb, Polygonum multiflorum (Heshouwu in Chinese). However, the high-throughput in vitro evaluation for such stereoisomerism-dependent hepatotoxicity is a critical challenge. In this study, we used a hepatic organoids–based in vitro hepatotoxic evaluation system in conjunction with using high content imaging to differentiate in vivo organ hepatotoxicity of the 2,3,5,4′-tetrahydroxy-trans-stilbene-2-O-β-glucoside (trans-SG) and its cis-isomer (cis-SG). By using such an organoid platform, we successfully differentiated the two stereoisomers’ hepatotoxic potentials, which were in accordance with their differences in rodents and humans. The lesion mechanism of the toxic isomer (cis-SG) was further found as the mitochondrial injury by high-content imaging, and its hepatotoxicity could be dose-dependently inhibited by the mitochondrial protective agent. These results demonstrated the utility of the organoids-based high-content imaging approach in evaluating and predicting organ toxicity of natural products in a low-cost and high-throughput way. It also suggested the rationale to use long-term cultured organoids as an alternative toxicology platform to identify early and cautiously the hepatotoxic new drug candidates in the preclinical phase.
There is a safety problem in the clinical use of Chinese herbal medicine because of its constituent elements being complex. Especially, hepatotoxicity associated with herbal or botanical use is increasingly being recognized, as the use of these medicines has become widespread in many countries (Teschke et al., 2015; Liu et al., 2019; Björnsson and Björnsson 2022). Hundreds of Chinese medicines or their extracts have been reported to possess potential hepatotoxicity, which results in severe clinical adverse events, such as liver fibrosis, hepatitis, liver failure, and even death. Suspected cases of Chinese medicine–induced liver injury have been highlighted in many publications on case reports and case series (Hebels et al., 2014; Zhang et al., 2018).
Polygonum multiflorum (Heshouwu in Chinese), a widely used traditional Chinese medicine, has the functions of detoxification, carbuncle elimination, bowel relaxation, and malaria prevention and has also been recommended for the nourishment of the liver for many years. Recently, raw P. multiflorum has become popular in Europe and the United States as herbal and dietary supplements (Liu et al., 2018). Nevertheless, a series of reports of adverse hepatotoxic effects induced by raw P. multiflorum–containing drugs (Shou Wu Pian, Shen Min, etc.) have been occasionally reported in recent years, arousing great public concerns, and have also been summarized and recorded in the LiverTox® database (He et al., 2019). The hepatotoxicities induced by P. multiflorum are difficult to be defined due to its complex components and multiple functions (Li et al., 2017; Liu et al., 2018). Our previous work has shown that cotreatment with a nontoxic dose of lipopolysaccharide (LPS) and a therapeutic dose of P. multiflorum can induce liver injury in rats, whereas the solo treatment cannot induce observable injury (Dong et al., 2015; Li et al., 2017; Li et al., 2019; Zhang et al., 2020). The bioactive components of P. multiflorum include tetrahydroxystilbene glucoside, anthraquinone, phospholipids, and tannins. The 2,3,5,4′-tetrahydroxy-trans-stilbene-2-O-β-glucoside (trans-SG) as the major constituent has been concerning as the major hepatotoxic component in P. multiflorum (Wang et al., 2015). However, limited evidence exists to support this conclusion. The trans-isomer of stilbenes, such as trans-SG, can be transformed by ultraviolet (UV) light or sunlight into its cis-isomer (cis-SG). Stereoisomers account for quite a large percentage in medicines and have extremely similar structures but great differences in pharmacodynamics and pharmacokinetics, as well as in drug-induced toxicity. Interestingly, we collected P. multiflorum samples from patients who had experienced liver injury and found higher cis-SG content (Figure 1A). The opportunities for exposure to UV radiation in the course of sunlight drying, preparation, and preservation of the herb are known to be abundant. We still do not know the possible association of UV-induced cis-trans isomerization of trans-SG with the idiosyncratic hepatotoxicity of P. multiflorum.
FIGURE 1. Cis-SG in Polygonum multiflorum–induced DILI patients’ samples and its photoisomerization. (A) The content of cis-SG in normal Polygonum multiflorum samples and in samples from DILI patients. (B) The chemical structure of trans-SG and cis-SG. (C) The HPLC results of trans-SG and cis-SG without or with UV radiation. (D) The proportion of cis-SG to trans-SG without and with UV treatment. Values are expressed as mean ± SD. **p < 0.01.
Predicting and studying the hepatotoxicity induced by drugs remain challenging for human health and pharmaceutical companies. As hepatotoxicity is one of the major reasons for developmental failures and withdrawal of new drugs, the development of evaluating models is needed to assess drugs and study the possible mechanisms of drug-induced liver injury (DILI) (Njoku 2014; Shehu et al., 2017). Nowadays, the development of innovative technologies brings opportunities for DILI forecasting, making it high throughput and highly sensitive and of low cost (Gomez-Lechon and Tolosa 2016; Funk and Roth 2017; Kuna et al., 2018). By using the techniques of molecular imaging, high-content screening, and cellular phenotype on suitable human hepatic cell models, most of the hepatotoxic-related molecular pathways can be determined, and they might be extended to the reactions at the tissue and organ levels to predict drug toxicity from molecular initiation to harmful outcome paths (Xu et al., 2008; Garside et al., 2014; Wink et al., 2018). In our previous work, we developed a simple but robust human-specific 3D HepaRG cell culture platform based on native liver extracellular matrix with multiparametric readouts to analyze the hepatotoxicity and possible mechanisms induced by drugs (Juan et al., 2018). The 3D cultured model, which has tight intracellular junctions, high-level expressions of metabolizing enzymes, and the ability of long-term culturing, has shown promising advantages in the evaluation of drug hepatotoxicity with simulation to in vivo organ functions. However, data about the utility of the 3D model in evaluating hepatotoxic differences between critically similar chemical structures have been sparse. Therefore, in this study, we tried to assess the possible hepatotoxic differences between a pair of stereoisomers, trans- and cis-SG, and to further elucidate the lesion mechanisms simultaneously.
2,3,5,4′-tetrahydroxy-trans-stilbene-2-O-β-glucoside (trans-SG) and its cis-isomer (cis-SG), as well as Schisandrin B (Sch B), were provided by the Chengdu Chroma Biotechnology Co., Ltd. The reference solutions (0.2 mg/ml) of cis-SG and trans-SG were prepared separately by methanol and stored in brown bottles. Lipopolysaccharide (LPS) was purchased from Sigma-Aldrich (Lot#113M4068V).
The P. multiflorum samples collected from patients were prepared through extraction for eight times using 50% ethanol-water solution via the ultrasound extraction method. Trans-SG was weighed precisely, dissolved in methanol to a concentration of 5 mg/ml, placed in a plain dish, and irradiated with UV light at 365 nm for 60 min. After volatilizing methanol in the fume cupboard, the samples were sealed and preserved in light-proof bags. The concentrations of cis-SG and trans-SG were determined by high-performance liquid chromatography.
Male Sprague Dawley (SD) rats weighing 200 ± 10 g were obtained from the Laboratory Animal Center of the Academy of Military Medical Sciences. They were housed in the Laboratory Animal Center of Military Medical Sciences and maintained under a 12-h light/dark cycle in a controlled temperature (25 ± 2°C) and humidity (50–60%) environment for a period of 1 week before evaluation. The animals were allowed access to food and water ad libitum.
The differential hepatotoxicity potential of these two stereoisomers was evaluated by a previously established rat model (Li et al., 2017), which used a nontoxic dosage of LPS pretreatment to induce minimal inflammatory stress. In a typical procedure, the animals were intragastrically administered with cis-SG or trans-SG or an equivalent volume of normal saline, separately, followed by a tail vein injection of LPS (2.8 mg/kg, Sigma-Aldrich) or normal saline 3 h later. At 7 h after the injection of LPS, the blood and liver samples were collected. The rat liver was fixed in 10% neutral buffered formalin for at least 72 h before being processed for histologic analysis. Paraffin-embedded sections were cut to 4-mm-thick pieces and stained with hematoxylin and eosin for microscopic examination. AST and ALT were also detected.
Human hepatoma cell lines and HepaRG cells were obtained from the Chinese Academy of Food and Drug Administration and cultured in Williams’ Media E (Gibco, United States) supplemented with 10% fetal bovine serum (FBS, Gibco, Canada), 2 mM GlutaMAX (Gibco, United States), 100 units/ml penicillin, and 100 μg/ml streptomycin. The medium was renewed every 2 days. The cells were maintained at 37°C with 5% CO2 in a cell incubator with a water reservoir.
To prepare the hepatic organoids, the HepaRG cells (400 cells per well) with liver extracellular matrix were seeded into round-bottomed 96-well plates with an ultra-low attachment surface (Costa, Corning) and were incubated at 37°C with 5% CO2. The culture medium was partially replaced by a fresh medium every 3 days.
Cell viability was detected by trans-SG and cis-SG. For the 2D cultured cell model, 100 μL of culture medium containing cells at a density of 8,000 cells/well was plated in 96-well plates, and after incubation of 24 h, the cells were treated with the drugs for 24 h. As for the 3D cultured HepaRG organoids model, the HepaRG cells were cultured under 3D culturing conditions for 14 days to form the organoids, and the organoids were then treated with testing drugs for 24 h once (as a single treatment) or for three repeated times of exposure at day 0, day 3, and day 5 and tested at day 7 (as repeated treatment). Cell viability was assessed using Alamar blue (Invitrogen) according to the manufacturer’s instructions. A microscope was used to observe the change of organoids.
The levels of lactic dehydrogenase (LDH), aspartate transaminase (AST), and alanine aminotransferase (ALT) in the supernatant gathered after treatment with drugs at different concentrations were tested using a reagent kit (Nanjing Jiancheng Bioengineering Institute, Nanjing, China) according to the manufacturer’s instructions.
High-content imaging and analysis of organoids were performed using the Operetta High-Content Imaging System (PerkinElmer). The organoids were treated with trans-SG and cis-SG for 24 h and washed three times with PBS, and the corresponding probes were added to find the potential lesion mechanisms. All the fluorescence probes used in this study were purchased from Thermofisher. Live and dead cells were detected by calcein AM and EthD-1 probe (LIVE/DEAD™, L3224, 8 μM). Oxidative stress was detected by CellROX and mBcl probe (CellROX™ Deep Red Reagent, C10422, 5 Μm; Monochlorobimane, M1381MP, 100 μM). Mitochondria damage was detected by the MitoTracker probe (MitoTracker® Deep Red FM, M22426, 200 nM). Steatosis was detected by a Nile Red probe (Nile Red, N1142, 1 μM). Cholestasis was detected by the CMFDA probe (5-chloromethylfluorescein diacetate, C7025, 25 μM). All the probes were incubated for 30 min, the cells were washed three times with PBS, and pictures were taken and analyzed in 3D space.
The chemical compounds in traditional Chinese medicine and their targets were acquired from the TCM-SP and the TCM Database@Taiwan. We then used these keywords, namely, mitochondrial depolarization, depolarized mitochondria, damaged mitochondria, mitochondrial disorders, mitochondrial dysfunction, mitochondrial impairment, and mitochondrial damage, to retrieve the corresponding target information and establish the mitochondrial lesion target database for the subsequent analysis. Based on the Database of Interacting Proteins (DIP), the interacting network between drug compounds and mitochondrial lesion–related targets was established. The network characteristic parameters of the key node proteins, such as degree (D), betweenness centrality (BC), and closeness centrality (CC), were calculated. For the key node proteins with a D value greater than the median of the D value of all nodes, the key drug targets were screened out from the node proteins whose BC and CC values were greater than the median of the two parameters of all nodes. The screen parameters were set as CC ≥ 0.183, D ≥ 2, and BC ≥ 0.000906 and a total of 19 targets were selected according to the network analysis results. Based on the established compound–target database, the candidate mitochondria protective agents were predicted and selected by using a hypergeometric distribution model to enrich the compound–target interaction network involving the 19 targets (p < 0.01).
The DILI cases caused by PM which were then treated with preparations containing Sch B were collected from the Fifth Medical Centre, Chinese PLA General Hospital (inpatient records between 2007 and 2016).
Total RNA was isolated using the RNeasy Mini Kit (Qiagen), after which cDNA was synthesized with reverse transcriptase (ReverTra Ace® qPCR RT Master Mix, Toyobo) according to the manufacturer’s instructions. The qRT-PCR was performed with SYBR Green Master Mix (TOYOBO) on a Bio-Rad iQ5 real-time PCR detection system. Data were collected using the Bio-Rad CFX Manager software, and the expression of genes within a sample is normalized to the GAPDH expression by the 2−ΔΔCt method. The primers used in this study are listed in Table 1.
The 3D cultured organoids were isolated as a single cell separately by trypsinization, washed three times with PBS, and made into a cell suspension. The MitoProbe™ JC-1 Assay Kit (Invitrogen, M34152) was used to detect mitochondrial membrane potential. The cell suspension was incubated for 20 min with 10 ug/ml JC-1 at 37°C in the dark. The cells were incubated for 15 min with the FITC-Annexin V/Dead Cell Apoptosis Kit (ThermoFisher company, V13242) in the dark at room temperature for early apoptosis and immediately analyzed by flow cytometry according to the instructions. The TUNEL assay was used to detect late apoptosis, and the cells were incubated only with PI for the cell cycle analysis according to the instructions.
All statistical data are expressed as means and SDs. Unpaired two-tailed Student’s t test was used to compare differences between groups. Differences were considered statistically significant if the p-value was smaller than 0.05. Significance was indicated as *p < 0.05, **p < 0.01, and ***p < 0.001. Experiments were repeated at least three times.
We collected the rest P. multiflorum from the patients, who had been diagnosed as PM—induced liver injury, and tested the content of cis-SG in these samples and normal samples using high-performance liquid chromatography (HPLC). As shown in Figure 1A, there was a significant difference in the cis-SG content in the DILI patients’ samples which was much higher than in the normal samples. To our knowledge, the trans-SG to cis-SG conversion occurred under UV light conditions (Figure 1B). In HPLC fingerprinting, we observed that the intensity of cis-SG and trans-SG was changed after UV light radiation (Figure 1C). Most of the trans-SG converted to cis-SG with UV light treatment (Figure 1D), indicating that the illumination is important for the storage of P. multiflorum.
To determine which of the two isomers was associated with hepatotoxicity, we administered isolated trans-SG and cis-SG to LPS-treated rats to compare the hepatotoxic intensity of the two compounds. Results in Figure 2A show that the administration of cis-SG and LPS result in liver histologic damage and significantly increased plasma alanine aminotransferase (ALT) and aspartate aminotransferase (AST) (Figure 2B). By contrast, the administration of trans-SG and LPS was not associated with liver injury, suggesting that cis-SG is the major hepatotoxic component.
FIGURE 2. Hepatotoxicity evaluation of trans-SG and cis-SG in LPS-treated rat model. (A) The histological analysis of the liver sections with the treatment of trans-SG or cis-SG in the LPS-treated rat model (n = 9). (B) The serum ALT and AST levels. Scale bar = 100 μm. Values are expressed as mean ± SD. ***p < 0.001.
To further reveal the possible hepatotoxic mechanism induced by cis-SG, the monolayer cultured HepaRG cells were used to detect the hepatotoxicity of cis-SG and trans-SG. However, there was no difference between cis-SG and trans-SG–induced hepatotoxicities. The cell viability and related indicators, such as lactate dehydrogenase (LDH), ALT, and AST, did not show any statistical difference with the treatment of cis-SG and trans-SG (Figures 3A,B).
FIGURE 3. Organoid-based in vitro evaluation differentiated hepatotoxicity of cis-SG and trans-SG isomers. (A,B) Cell viability analysis (A) and biochemistry test (LDH, AST, and ALT) (B) on 2D cultured HepaRG cells showing no difference between the two isomers after 24 h of drug exposure. (C,D) Cell viability analysis of trans-SG and cis-SG on 3D cultured HepaRG organoids with single-dose (C) or repeated-dose (D) treatment. (E,F) The LDH, AST, and ALT levels of 3D cultured HepaRG organoids after being treated with trans-SG and cis-SG in single-dose (E) or repeated-dose (F). (G) The morphological images of HepaRG organoids after being treated with trans-SG and cis-SG for 1 or 7 days. (H) The fluorescent images of HepaRG organoids after being treated with trans-SG and cis-SG for 1 or 7 days with live (green)/dead (orange) staining. Scale bar = 50 μm. Values are expressed as mean ± SD. *p < 0.05; **p < 0.01; ***p < 0.001.
Therefore, the model which could reflect the hepatotoxic difference between cis-SG and trans-SG was required. Next, the 3D cultured HepaRG organoids were further prepared to assess cis-SG and trans-SG–induced hepatotoxicities. Fortunately, due to the enhanced hepatic functions, the results obtained from the 3D cultured HepaRG organoids were consistent with earlier overall animal outcomes. By using the established 3D cultured organoids model, the significantly different hepatotoxic potential between the cis-SG and trans-SG stereoisomers was observed via either single or repeated dose. The cis-SG showed higher toxicity with the lower half-maximal inhibitory concentration (IC50) than did trans-SG in single- or repeated-dose treatment on cells (Figures 3C,D). In addition, the cis-SG and trans-SG–induced cell death was in a concentration-dependent manner. The activity of LDH, ALT, and AST was significantly increased in cis-SG–treated cells (Figures 3E,F). Similarly, the recorded morphological images also confirmed that cis-SG caused more serious cell damage with visible loss of cells and impairment of spheroid structure, especially with the repeated-dose treatment (Figure 3G). Live/dead fluorescence staining was also used to qualitatively indicate the viability of the organoids with drug treatment. Figure 3H showed that more cells were alive (green) in trans-SG–treated organoids than in cis-SG–treated organoids. These aforementioned results suggest that long-term medication may increase the risk of liver injury. More importantly, the 3D HepaRG organoid culture system can be used to reveal the possible mechanism of hepatotoxicity induced by cis-SG.
To demonstrate the potential mechanisms of drug related to hepatotoxicity, multiparametric analysis of assessments in 3D cultured HepaRG organoids treated with trans-SG or cis-SG have been carried out. The cells were stained with selected fluorescence probes and monitored by high content imaging and analysis (HCA) after being treated with trans-SG or cis-SG for 24 h. HCA allows observation of drug-induced hepatotoxic alterations using hyper-multicolor cellular imaging. Different molecular probes were used to indicate the majority of reported changes in oxidative stress, mitochondria damage, steatosis, and cholestasis. For oxidative stress, the generation of reactive oxygen species (ROS) and depletion of intracellular glutathione (GSH) were detected by CellROX™ Deep Red Reagent and monochlorobimane (mBCl). As shown in Figure 4A, both trans-SG and cis-SG did not cause oxidative stress in cells. For mitochondrial damage, the change in mitochondrial membrane potential is the main reason for mitochondrial dysfunctions. MitoTracker® Deep Red FM was used to analyze the alteration of mitochondrial membrane potential. cis-SG could induce a significant decrease in fluorescence intensity, indicating the decrease of mitochondrial membrane potential (Figure 4B). For drug-induced steatosis, it indicates to the overaccumulation of lipids, mainly triglycerides, inside hepatocytes. Here, Nile red was used to stain and quantify the accumulation of neutral (e.g., triglycerides) and polar (e.g., phospholipids) lipids by its uptake and retention in cells. The images and quantified results showed that trans-SG and cis-SG did not cause steatosis. For cholestasis, the hepatic bile acid transporter substrate 5-chloromethylfluorescein diacetate (CMFDA) was used to stain the canalicular structures. Similar to the steatosis result, there was almost no alteration to the bile canaliculus structures of the 3D cultured HepaRG cells after being treated with trans-SG or cis-SG. These results demonstrated that the cis-SG–induced hepatotoxicity was mainly because of mitochondrial damages in the cells, and these results assist in gaining an understanding of the action mechanism of P. multiflorum–induced hepatotoxicity.
FIGURE 4. Multiparametric HCA of assessments on 3D cultured HepaRG organoids with trans-SG and cis-SG exposure for 24 h. (A) Representative confocal images shown corresponding to trans-SG and cis-SG. After incubation, 5 fluorescence-based endpoints were measured to assess chemically induced alterations in cellular function. (B) Image analysis readouts were derived to evaluate four kinds of different cellular alterations, including oxidative stress, mitochondria damage, steatosis, and cholestasis. Values are expressed as mean ± SD.
Mitochondrial protective compounds were screened by a computer-aided network pharmacology approach from the natural products database which are listed in Table 2. Among these, Schisandrin B (Sch B) was ranked first and was available to be procured as a pure compound (Figure 5A). The herb containing Sch B, named Schisandra chinensis, is a well-used traditional Chinese medicine to treat acute liver injury in clinical applications. Hence, Sch B was selected as the mitochondrial protective agent to further verify the mechanism of cis-SG–induced liver injury. Firstly, the cytotoxicity of Sch B was tested. There was no significant hepatotoxicity to HepaRG organoids after being treated with Sch B in the concentration range of 0.625–10 μM (Figure 5B). When co-exposed with Sch B, the cis-SG–induced decrease of cell viability was significantly and dose-dependently ameliorated (Figure 5C). And by mitochondrial imaging, it was shown that the mitochondrial protective agent, Sch B, reversed the mitochondrial membrane potential, suggesting that Sch B could decrease mitochondrial damage induced by cis-SG (Figure 5D). The mitochondrial membrane potential was further detected by the MitoProbe™ JC-1 Assay Kit, which in the healthy mitochondria accumulates as a red fluorescent dimer and following mitochondrial injury is released into the cytoplasm as a green fluorescent monomer. As shown in Figure 5E, the flow cytometric results showed that the co-treatment of cis-SG and Sch B effectively reduced the cis-SG–induced mitochondrial damage. In addition, the hepatoprotective effect of the preparations containing Sch B was demonstrated by clinical data with the decrease of ALT and AST levels in patients’ serum (Figure 5F and Table 3).
TABLE 2. Network pharmacology predictions of mitochondria protective agents (Top 20) from natural products.
FIGURE 5. Protective effects of the computer-screened mitochondria protective agent and its preparations against liver injury on organoids and patients. (A) The Top 20 mitochondrial protective compounds screened by computer-aided network pharmacology approach from the natural products database. (B) The cell viability of HepaRG cells after being only treated with Sch B for 24 h. (C) The HepaRG cell viability recovered after being co-treated with Sch B and cis-SG. (D) The mitochondrial staining images of cells treated with different groups. (E) The flow cytometric results of HepaRG cells stained with the MitoProbe™ JC-1 Assay Kit, and the quantified ratio of JC-1 red fluorescence intensity to green fluorescence intensity, normalized to the control group. (F) Alleviation effect of the Chinese medicinal preparations containing Sch B on patients with Polygonum multiflorum–induced liver injury indicated by serum ALT and AST. Scale bar = 50 μm. Values are expressed as mean ± SD. **p < 0.01; ***p < 0.001.
Mitochondrial damage always induces cell apoptosis. cis-SG–induced apoptosis, indicated by either an increase of BAX and Caspase3 or a decrease of PCNA and Bcl2 gene expressions, was significantly reversed to control group levels (Figure 6A). Further analysis by flow cytometry showed that cis-SG induced 33.58% early apoptosis and 4.41% late apoptosis, while the combination of Sch B and cis-SG treatment showed a decreased proportion of either early or late apoptosis to 15.06% and 0.91%, respectively (Figure 6B). The inhibitory effect of Sch B on cis-SG–induced late apoptosis was also demonstrated by TUNEL analysis (Figure 6C). In addition, cell apoptosis was accompanied by DNA fragmentation. As shown in Figure 6D, the proportion of sub-G1 was increased after being treated with cis-SG, and Sch B could inhibit this effect.
FIGURE 6. Inhibiting apoptosis was involved in the blocking effect of mitochondria protective agents against cis-SG–induced hepatotoxicity on 3D cultured HepaRG organoids. (A) Sch B significantly reversed the cis-SG–induced abnormal expressions of mitochondrial dysfunction–related genes (BAX, Casp3, PCNA, and BCL2). (B) Flow cytometry assays of Annexin V/PI staining showed Sch B significantly decreased the proportions of early and late apoptosis induced by cis-SG. (C) TUNEL analysis showed Sch B significantly decreased cis-SG–induced late apoptosis. (D) Cell cycle–based apoptosis assay for HepaRG organoids showed that Sch B significantly reversed the cis-SG–induced DNA fragmentation. Values are expressed as the mean ± SD. *p < 0.05, **p < 0.01, and ***p < 0.001, cis-SG–treated group vs control group; #p < 0.05, ##p < 0.01, and ###p < 0.001, cis-SG and Sch B co-treated group vs cis-SG–treated group.
It is obviously important for an accurate in vitro evaluation model to reflect the in vivo organ circumstances as closely as possible and thus can predict drug hepatotoxicity and identify the possible mechanisms (Teschke et al., 2015). Because 3D cultured organoids can simulate the intercellular contacts of organs and provide a suitable microenvironment to maintain the organ-level molecular cell phenotypes (Pampaloni et al., 2007; Ravi et al., 2015), they are more competitive than monolayer cultured cell models in evaluating hepatotoxicity (Lauschke et al., 2016; Andersson 2017). In our previous work, we developed a hepatic cell organoids culturing system with the liver-specific extracellular matrix and HepaRG cells (Wang et al., 2011; Juan et al., 2018). Regarding the liver-specific microenvironment, the functional differences between the 2D cultured cells and organ-level hepatocytes are dramatically great. Considering many hepatotoxic drugs that injure liver cells via their metabolites (especially metabolizing enzyme-activated metabolites), the 3D cultured hepatic organoids model is more suitable to predict drug hepatotoxicity. Notably, most of the clinical drug-induced liver injuries occurred in a delayed pattern (usually days or months of repeated medication), and the organ concentration of drugs was relatively low. However, the current in vitro studies usually used significantly high concentrations and single or limited exposure time in evaluation, which have limited references to understanding the clinical hepatotoxicity. The hepatic organoids model is suitable to test the repeated or accumulative intoxication of drugs at low concentrations due to its long-term culturing nature. This is so important that the test concentration of drug compounds would be comparable to in vivo organ concentration, and therefore, this will represent more accordant results of hepatotoxic potential.
For drugs, although they have the same functional groups but only a stereoscopic difference in structure, there might be divergent activity or toxicity of stereoisomers (Srinivas et al., 2001; Shim et al., 2016). The stereoscopic structural differences of drug candidates are sometimes ignored in drug research and development; for instance, there are 25% of marketed drugs of mixed isomers but not the single isomer (Hutt, 2002). However, the stereoisomers are difficult to differentiate regarding hepatotoxicity. Currently, there is not much concern about the toxicity of stilbene derivatives and their stereoisomers. The trans-form stilbenes, such as trans-resveratrol and trans-SG, are generally considered to be of good safety as potent drug candidates, as well as for their cis-isomers (Wang and Chatterjee, 2017). However, we have discovered that the cis-SG, other than the trans-form, contributed as the major hepatotoxic component in clinical cases and animal models (Li et al., 2017). The hepatotoxic potential of cis-stilbenes has not been addressed before, despite the isomerization of trans-stilbenes to their cis-forms occurring spontaneously when being exposed to ultraviolet light or sunlight (Koppen et al., 2012; Sharma et al., 2017; Xiao et al., 2018). Thus, there is a great need to elucidate the divergent hepatotoxicity and lesion mechanisms of structurally similar drug candidates. By using the established hepatic organoids model, we demonstrated the differential hepatotoxicity of the cis- and trans-isomers in vitro for the first time. By contrast, there was no difference in cytotoxicity between the two isomers in the common 2D cultured hepatocyte model. Interestingly, there was much more difference in hepatotoxicity when the culture time was prolonged for the two isomers. This suggested that there might be delayed or accumulated hepatotoxicity of the toxic isomer, which was in accordance with the clinical onset characteristics of DILI (Zhu et al., 2016). Combined with HCA, we further discovered the lesion mechanism of the hepatotoxic cis-SG, focusing on impairment of the mitochondria instead of the other common hepatotoxic mechanisms, such as oxidative stress, cholestasis, and steatosis. In order to further confirm the mitochondrial lesion mechanism, we screened and used the mitochondrial protective reagent to co-administer with cis-SG. The results showed that if we protected the mitochondria, the hepatotoxicity of cis-SG was partly blocked. This result demonstrated that the hepatotoxicity of cis-SG is mitochondria-dependent and illustrated the reliability of HCA results. Nevertheless, the more specific toxicity target of cis-SG–induced mitochondrial lesion needs to be further studied. These results suggested a necessary concern on the toxicity of cis-form stilbenes rather than their normally occurring trans-stereoisomers.
This study illustrated the utility of the organoids-based high-content imaging approach to differentiate and predict organ hepatotoxicity of a pair of cis- or trans-stereoisomers. The results also highlighted the remarkable influence of stereoisomerism on the toxicity of lead compounds in new drug development. Considering the liver as a complicated organ consisting of either parenchymal cells or non-parenchymal cells, it is interesting to test if there is an additional influence of non-parenchymal cells on the pathogenesis of drug hepatotoxicity in the organoids model. More specially, the resident and recruited immune cells in this organ might play pivotal roles in DILI pathogenesis (Kim et al., 2017), but this organ-level multiple cell typic interactions on hepatotoxicity in organoids are nearly unknown. A most recent study demonstrated that the transwell co-culture of hepatocytes with macrophages had the additional release of pro-inflammatory cytokines after drug exposure (Wewering et al., 2017). Thus, it is of great interest to establish the hepatocytes and immune cells co-cultured organoids and test drug hepatotoxicity in the future. Although there was no positive result of cis-SG causing injuries to cholangiocyte-like cells, the established organoids model has the ability to evaluate the potential of drugs to cause cholestasis due to the ability of HepaRG cells to differentiate into both hepatocytes and cholangiocytes in 3D cultured organoids. In summary, using the in vitro organoids model to simulate in vivo organ-level circumstances is important to evaluate and predict drug toxicity and thus decreases the possibility of drug research and developmental failure and withdrawal caused by unpredicted severe adverse drug reactions.
The original contributions presented in the study are included in the article; further inquiries can be directed to the corresponding authors.
The animal study was reviewed and approved by the Laboratory Animal Center of the Academy of Military Medical Sciences.
JL, TL, JaW, and YW conceived the project, designed the experiments, and wrote the manuscript; JL, TL, RL, JeW, PL, MN, LZ, and CL conceived of and performed the experiments, interpreted the data, and drafted the manuscript; XX edited the manuscript; All authors contributed to writing and providing feedback.
This work was supported by the National Natural Science Foundations of China (Nos 32000970, 81730052, 82074112, and U21A20412), the Natural Science Foundation of Beijing (7214306), the Beijing Talent Scholar Project (JQ21026), the Tsinghua University Spring Breeze Fund (2021Z99CFZ008), the Capital Health Research and Development of Special (2021-1G-2241), the Beijing Hospitals Authority Ascent Plan (DFL20190901), and the Beijing Hospitals Authority Youth Programme (QMS20200903).
The authors declare that the research was conducted in the absence of any commercial or financial relationships that could be construed as a potential conflict of interest.
All claims expressed in this article are solely those of the authors and do not necessarily represent those of their affiliated organizations, or those of the publisher, the editors, and the reviewers. Any product that may be evaluated in this article, or claim that may be made by its manufacturer, is not guaranteed or endorsed by the publisher.
Andersson, T. B. (2017). Evolution of Novel 3d Culture Systems for Studies of Human Liver Function and Assessments of the Hepatotoxicity of Drugs and Drug Candidates. Basic Clin. Pharmacol. Toxicol. 121, 234–238. doi:10.1111/bcpt.12804
Björnsson, H. K., and Björnsson, E. S. (2022). Drug-Induced Liver Injury: Pathogenesis, Epidemiology, Clinical Features, and Practical Management. Eur. J. Intern. Med. 97, 26–31. doi:10.1016/j.ejim.2021.10.035
Dong, Q., Li, N., Li, Q., Zhang, C. E., Feng, W. W., Li, G. Q., et al. (2015). Screening for Biomarkers of Liver Injury Induced by Polygonum Multiflorum: A Targeted Metabolomic Study. Front. Pharmacol. 6, 217. doi:10.3389/fphar.2015.00217
Funk, C., and Roth, A. (2017). Current Limitations and Future Opportunities for Prediction of Dili from In Vitro. Arch. Toxicol. 91, 131–142. doi:10.1007/s00204-016-1874-9
Garside, H., Marcoe, K. F., Chesnut-Speelman, J., Foster, A. J., Muthas, D., Kenna, J. G., et al. (2014). Evaluation of the Use of Imaging Parameters for the Detection of Compound-Induced Hepatotoxicity in 384-well Cultures of Hepg2 Cells and Cryopreserved Primary Human Hepatocytes. Toxicol. Vitro 28, 171–181. doi:10.1016/j.tiv.2013.10.015
Gómez-Lechón, M. J., and Tolosa, L. (2016). Human Hepatocytes Derived from Pluripotent Stem Cells: A Promising Cell Model for Drug Hepatotoxicity Screening. Arch. Toxicol. 90, 2049–2061. doi:10.1007/s00204-016-1756-1
He, S., Zhang, X., Lu, S., Zhu, T., Sun, G., and Sun, X. (2019). A Computational Toxicology Approach to Screen the Hepatotoxic Ingredients in Traditional Chinese Medicines: Polygonum Multiflorum Thunb as a Case Study. Biomolecules 9, 577. doi:10.3390/biom9100577
Hebels, D. G., Jetten, M. J., Aerts, H. J., Herwig, R., Theunissen, D. H., Gaj, S., et al. (2014). Evaluation of Database-Derived Pathway Development for Enabling Biomarker Discovery for Hepatotoxicity. Biomarkers Med. 8, 185–200. doi:10.2217/bmm.13.154
Hutt, A. J. (2002). The Development of Single-Isomer Molecules: Why and How. CNS Spectr. 7, 14–22. doi:10.1017/s1092852900028558
Juan, L., Ruihong, L., Rui, X., Tingting, L., Ling, L., Yi, W., et al. (2018). Liver Extracellular Matrices Bioactivated Hepatic Spheroids as a Model System for Drug Hepatotoxicity Evaluations. Adv. Biosyst. 2, 1800110. doi:10.1002/adbi.201800110
Kim, D. E., Jang, M. J., Kim, Y. R., Lee, J. Y., Cho, E. B., Kim, E., et al. (2017). Prediction of Drug-Induced Immune-Mediated Hepatotoxicity Using Hepatocyte-like Cells Derived from Human Embryonic Stem Cells. Toxicology 387, 1–9. doi:10.1016/j.tox.2017.06.005
Köppen, R., Riedel, J., Proske, M., Drzymala, S., Rasenko, T., Durmaz, V., et al. (2012). Photochemical Trans-/cis-isomerization and Quantitation of Zearalenone in Edible Oils. J. Agric. Food Chem. 60, 11733–11740. doi:10.1021/jf3037775
Kuna, L., Bozic, I., Kizivat, T., Bojanic, K., Mrso, M., Kralj, E., et al. (2018). Models of Drug Induced Liver Injury (Dili) - Current Issues and Future Perspectives. Curr. Drug Metab. 19, 830–838. doi:10.2174/1389200219666180523095355
Lauschke, V. M., Hendriks, D. F. G., Bell, C. C., Andersson, T. B., and Ingelman-Sundberg, M. (2016). Novel 3D Culture Systems for Studies of Human Liver Function and Assessments of the Hepatotoxicity of Drugs and Drug Candidates. Chem. Res. Toxicol. 29, 1936–1955. doi:10.1021/acs.chemrestox.6b00150
Li, C. Y., He, Q., Gao, D., Li, R. Y., Zhu, Y., Li, H. F., et al. (2017a). Idiosyncratic Drug-Induced Liver Injury Linked to Polygonum Multiflorum: A Case Study by Pharmacognosy. Chin. J. Integr. Med. 23, 625–630. doi:10.1007/s11655-017-2543-9
Li, C. Y., Niu, M., Bai, Z., Zhang, C., Zhao, Y., Li, R., et al. (2017b). Screening for Main Components Associated with the Idiosyncratic Hepatotoxicity of a Tonic Herb, Polygonum Multiflorum. Front. Med. 11, 253–265. doi:10.1007/s11684-017-0508-9
Li, C., Rao, T., Chen, X., Zou, Z., Wei, A., Tang, J., et al. (2019). Hla-b*35:01 Allele Is a Potential Biomarker for Predicting Polygonum Multiflorum-Induced Liver Injury in Humans. Hepatology 70, 346–357. doi:10.1002/hep.30660
Liu, Y., Wang, Q., Yang, J., Guo, X., Liu, W., Ma, S., et al. (2018). Polygonum Multiflorum thunb.: A Review on Chemical Analysis, Processing Mechanism, Quality Evaluation, and Hepatotoxicity. Front. Pharmacol. 9, 364. doi:10.3389/fphar.2018.00364
Liu, Z., He, X., Wang, L., Zhang, Y., Hai, Y., and Gao, R. (2019). Chinese Herbal Medicine Hepatotoxicity: The Evaluation and Recognization Based on Large-Scale Evidence Database. Curr. Drug Metab. 20, 138–146. doi:10.2174/1389200219666180813144114
Njoku, D. B. (2014). Drug-Induced Hepatotoxicity: Metabolic, Genetic and Immunological Basis. Int. J. Mol. Sci. 15, 6990–7003. doi:10.3390/ijms15046990
Pampaloni, F., Reynaud, E. G., and Stelzer, E. H. K. (2007). The Third Dimension Bridges the Gap between Cell Culture and Live Tissue. Nat. Rev. Mol. Cel. Biol. 8, 839–845. doi:10.1038/nrm2236
Ravi, M., Paramesh, V., Kaviya, S. R., Anuradha, E., and Solomon, F. D. (2015). 3d Cell Culture Systems: Advantages and Applications. J. Cel. Physiol. 230, 16–26. doi:10.1002/jcp.24683
Sharma, A., Bányiová, K., Vrana, B., Justan, I., and Čupr, P. (2017). Investigation of Cis-Trans Isomer Dependent Dermatotoxicokinetics of Uv Filter Ethylhexyl Methoxycinnamate through Stratum Corneum In Vivo. Environ. Sci. Pollut. Res. Int. 24, 25061–25070. doi:10.1007/s11356-017-0172-2
Shehu, A. I., Ma, X., and Venkataramanan, R. (2017). Mechanisms of Drug-Induced Hepatotoxicity. Clin. Liver Dis. 21, 35–54. doi:10.1016/j.cld.2016.08.002
Shim, J. S., Li, R. J., Bumpus, N. N., Head, S. A., Kumar Pasunooti, K., Yang, E. J., et al. (2016). Divergence of Antiangiogenic Activity and Hepatotoxicity of Different Stereoisomers of Itraconazole. Clin. Cancer Res. 22, 2709–2720. doi:10.1158/1078-0432.Ccr-15-1888
Srinivas, N. R., Barbhaiya, R. H., and Midha, K. K. (2001). Enantiomeric Drug Development: Issues, Considerations, and Regulatory Requirements. J. Pharm. Sci. 90, 1205–1215. doi:10.1002/jps.1074
Teschke, R., Zhang, L., Long, H., Schwarzenboeck, A., Schmidt-Taenzer, W., Genthner, A., et al. (2015). Traditional Chinese Medicine and Herbal Hepatotoxicity: A Tabular Compilation of Reported Cases. Ann. Hepatol. 14, 7–19. doi:10.1016/s1665-2681(19)30796-3
Wang, F., and Chatterjee, S. (2017). Dominant Carbons in Trans- and Cis-Resveratrol Isomerization. J. Phys. Chem. B 121, 4745–4755. doi:10.1021/acs.jpcb.7b02115
Wang, Y., Cui, C. B., Yamauchi, M., Miguez, P., Roach, M., Malavarca, R., et al. (2011). Lineage Restriction of Human Hepatic Stem Cells to Mature Fates Is Made Efficient by Tissue-specific Biomatrix Scaffolds. Hepatology 53, 293–305. doi:10.1002/hep.24012
Wang, J., Ma, Z., Niu, M., Zhu, Y., Liang, Q., Zhao, Y., et al. (2015). Evidence Chain-Based Causality Identification in Herb-Induced Liver Injury: Exemplification of a Well-Known Liver-Restorative Herb Polygonum Multiflorum. Front. Med. 9, 457–467. doi:10.1007/s11684-015-0417-8
Wewering, F., Jouy, F., Wissenbach, D. K., Gebauer, S., Blüher, M., Gebhardt, R., et al. (2017). Characterization of Chemical-Induced Sterile Inflammation In Vitro: Application of the Model Compound Ketoconazole in a Human Hepatic Co-culture System. Arch. Toxicol. 91, 799–810. doi:10.1007/s00204-016-1686-y
Wink, S., Hiemstra, S. W., Huppelschoten, S., Klip, J. E., and van de Water, B. (2018). Dynamic Imaging of Adaptive Stress Response Pathway Activation for Prediction of Drug Induced Liver Injury. Arch. Toxicol. 92, 1797–1814. doi:10.1007/s00204-018-2178-z
Xiao, Y. D., Huang, W. Y., Li, D. J., Song, J. F., Liu, C. Q., Wei, Q. Y., et al. (2018). Thermal Degradation Kinetics of All-Trans and Cis-Carotenoids in a Light-Induced Model System. Food Chem. 239, 360–368. doi:10.1016/j.foodchem.2017.06.107
Xu, J. J., Henstock, P. V., Dunn, M. C., Smith, A. R., Chabot, J. R., and de Graaf, D. (2008). Cellular Imaging Predictions of Clinical Drug-Induced Liver Injury. Toxicol. Sci. 105, 97–105. doi:10.1093/toxsci/kfn109
Zhang, H. Y., Wang, H. L., Zhong, G. Y., and Zhu, J. X. (2018). Molecular Mechanism and Research Progress on Pharmacology of Traditional Chinese Medicine in Liver Injury. Pharm. Biol. 56, 594–611. doi:10.1080/13880209.2018.1517185
Zhang, L., Niu, M., Wei, A. W., Tang, J. F., Tu, C., Bai, Z. F., et al. (2020). Risk Profiling Using Metabolomic Characteristics for Susceptible Individuals of Drug-Induced Liver Injury Caused by Polygonum Multiflorum. Arch. Toxicol. 94, 245–256. doi:10.1007/s00204-019-02595-3
Keywords: hepatotoxicity, organoids, high-content imaging, stereoisomer, Polygonum multiflorum
Citation: Liu J, Li T, Li R, Wang J, Li P, Niu M, Zhang L, Li C, Wang T, Xiao X, Wang J-b and Wang Y (2022) Hepatic Organoid-Based High-Content Imaging Boosts Evaluation of Stereoisomerism-Dependent Hepatotoxicity of Stilbenes in Herbal Medicines. Front. Pharmacol. 13:862830. doi: 10.3389/fphar.2022.862830
Received: 26 January 2022; Accepted: 28 March 2022;
Published: 17 May 2022.
Edited by:
Petr Pavek, Charles University, CzechiaReviewed by:
Yun Zhang, Biology Institute of Shandong Academy of Sciences, ChinaCopyright © 2022 Liu, Li, Li, Wang, Li, Niu, Zhang, Li, Wang, Xiao, Wang and Wang. This is an open-access article distributed under the terms of the Creative Commons Attribution License (CC BY). The use, distribution or reproduction in other forums is permitted, provided the original author(s) and the copyright owner(s) are credited and that the original publication in this journal is cited, in accordance with accepted academic practice. No use, distribution or reproduction is permitted which does not comply with these terms.
*Correspondence: Jia-bo Wang, cGhhcm1fc2NpQDEyNi5jb20=; Yunfang Wang, d3lmYTAyNzE3QGJ0Y2guZWR1LmNu, d2FuZ3lmMTk3MkBnbWFpbC5jb20=
†These authors have contributed equally to this work
Disclaimer: All claims expressed in this article are solely those of the authors and do not necessarily represent those of their affiliated organizations, or those of the publisher, the editors and the reviewers. Any product that may be evaluated in this article or claim that may be made by its manufacturer is not guaranteed or endorsed by the publisher.
Research integrity at Frontiers
Learn more about the work of our research integrity team to safeguard the quality of each article we publish.