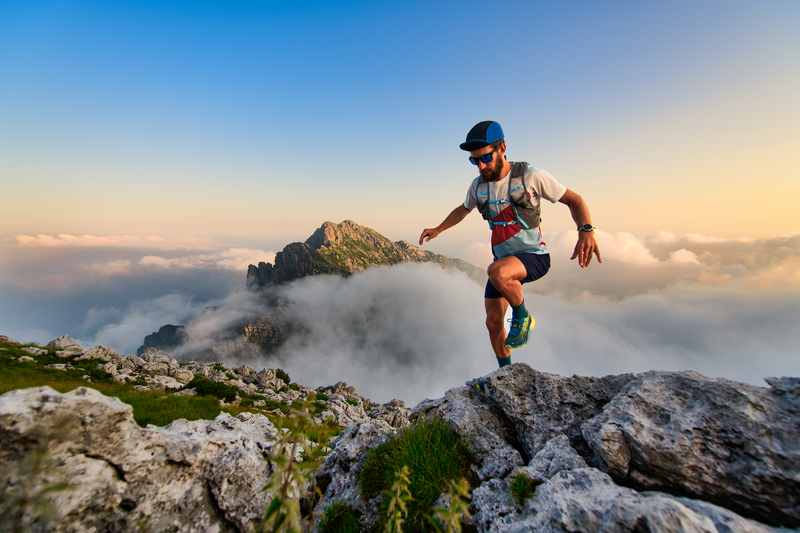
95% of researchers rate our articles as excellent or good
Learn more about the work of our research integrity team to safeguard the quality of each article we publish.
Find out more
SYSTEMATIC REVIEW article
Front. Pharmacol. , 16 June 2022
Sec. Ethnopharmacology
Volume 13 - 2022 | https://doi.org/10.3389/fphar.2022.857449
In recent years, neurological diseases including Alzheimer’s disease, Parkinson’s disease and stroke are one of the main causes of death in the world. At the same time, the incidence of psychiatric disorders including depression and anxiety has been increasing. Accumulating elderly and stressed people suffer from these brain disorders, which is undoubtedly a huge burden on the modern aging society. Neolignans, the main active ingredients in Magnolia officinalis cortex, were reported to have neuroprotective effects. In addition, the key bioactive ingredients of neolignans, magnolol (1) and honokiol (2), were proved to prevent and treat neurological diseases and psychiatric disorders by protecting nerve cells and brain microvascular endothelial cells (BMECs). Furthermore, neolignans played a role in protecting nerve cells via regulation of neuronal function, suppression of neurotoxicity, etc. This review summarizes the neuroprotective effect, primary mechanisms of the leading neolignans and provides new prospects for the treatment of brain disorders in the future.
Brain disorders including Alzheimer’s disease (AD), Parkinson’s disease (PD), stroke, anxiety, depression, are the diseases with increasing incidence in recent years. AD is one of the most common forms of dementia in the aging population, cause a growing death toll (Walsh and Selkoe, 2004; Oboudiyat et al., 2013; Silva et al., 2019; World Health Organization, 2020). From 1990 to 2015, the number of patients with PD approximately doubled due to the aging population (Dorsey et al., 2018). Cerebral vascular occlusion and rupture are the causes of stroke, followed by a series of nerve injuries. According to WHO, stroke alone accounted for 11% of the world’s total deaths in 2019 (World Health Organization, 2020). Anxiety and depression caused by great pressure are major psychiatric disorders, affecting 21% of the population in some developed countries annually (Alexeev et al., 2012; Cheng et al., 2018). The main therapeutic drugs for AD were cholinesterase inhibitors, memantine and so on (Taipale et al., 2016). Long term use of certain drugs in patients with AD might lead to drug interaction and toxicity (Cacabelos et al., 2008). Levodopa or other dopamine agonists were usually used as dopamine substitutes to treat PD, which often resulted in dopaminergic adverse reactions including involuntary movements, response fluctuations, etc. (Clarke 2004) When stroke occurred, it was usually treated by reperfusion and surgery, while there were few studies on chronic phase treatment (Kuriakose and Xiao, 2020; Szelenberger et al., 2020). Anti-anxiety drugs including benzodiazepines and anti-depressants including selective serotonin reuptake inhibitors were widely used in the pharmaceutical market (Nemeroff 2007; Ku et al., 2011). Sedation, cognitive and memory impairment, and other side effects often occurred after long-term medication (Lader 2008; Li L. F. et al., 2013; Millan et al., 2015). Therefore, the importance of constantly searching for new effective components for the treatment of brain disorders should be realized.
Neolignans are principal active ingredients of Magnolia officinalis cortex. Magnolol (MN) (1) and honokiol (HK) (2) are the most widely studied bioactive substances in neolignans of Magnolia officinalis. Current researches demonstrated that pharmacological activities of MN (1), HK (2)-based neolignans included gastrointestinal protection, anti-bacterial, anti-inflammatory, anti-oxidation, cardiovascular protection and anti-tumor (Luo et al., 2019; Rauf et al., 2021). Magnolia officinalis extract mainly including neolignans was used as an overall wellness nutritional supplement in United States and the recommended dosage was 200–800 mg/day per person for a variety of maladies including anxiety, depression, diabetes, inflammation, headache, muscle pain, weight loss, asthma, stroke, bacterial infection etc. (Poivre and Duez, 2017; Bui et al., 2020) In addition, neolignans were often available for daily life and added to mints, gums, mouthwash, insecticide, cosmetic products (Ye et al., 2015; Poivre and Duez, 2017; Bui et al., 2020; Ghorbani et al., 2021). Recently, accumulating studies showed that neolignans manifest neuroprotection on some brain disorders.
Neolignans have neuroprotective effects on brain disorders including AD, PD, stroke, anxiety, depression. AD is often accompanied by abnormal cholinergic nerve function, abnormal production and deposition of beta-amyloid (Aβ) and neuroinflammation. PD is characterized by the formation of Lewy bodies and dopaminergic neurological dysfunction (Xian et al., 2015). Stroke leads to neuroinflammation, oxidative stress, apoptosis and damages to BMECs, which can further damage the nerve (Xiaoyan 2016; Kou et al., 2017; Huang et al., 2018). Anxiety is mainly caused by abnormal GABAergic nerve function, and the improvement of serotonergic nerve function can play a therapeutic role in depression (Alexeev et al., 2012; Bai et al., 2018). It is concluded that the therapeutic effects of neolignans on these diseases can be divided into effects on nerve cells and brain microvascular endothelial cells (BMECs). The effects of neolignans on nerve cells include regulation of various neuronal function, reduction of neurotoxicity of Aβ and other protein deposition, suppression of apoptosis, neuroinflammation and nerve oxidation. While, the effects of neolignans on BMECs include normalization of blood glucose levels, promotion of vasodilation and reinforcement of tight junctions between BMECs. From the perspective of the mechanism of treating these diseases, this review mainly summarizes the neuroprotective effects of neolignans at the cellular level including nerve cells and BMECs.
The comprehensive information of this review came from electronic databases including the Web of Science (http://wokinfo.com/), ScienceDirect (www.sciencedirect.com), PubMed (http://ncbi.nlm.nih.gov/), CNKI (http://cnki.net/), Google Scholar (http://scholar.google.com.cn/) by using keywords “Magnolia officinalis,” “Neolignans,” “Houpo,” “Magnolol,” “Honokiol,” “Neurological disease,” “Psychiatric disorders,” “Alzheimer,” “Parkinson,” “Stroke,” “Anxiety,” “Depression” and their combinations.
Neolignans are polymerized by the side chain of one phenylpropylene and the benzene ring of another phenylpropylene. MN (1) (5,5′-Diallyl-2,2′-dihydroxybiphenyl) and HK (2) (5,3′-Diallyl-2,4′-dihydroxybiphenyl), two polyphenol compounds, are the leading bioactive ingredients of neolignans (Figure 1). Neolignans are a large class of natural compounds derived from the oxidative coupling of two C6–C3 units (Teponno et al., 2016). Their unique pharmacophore structure is two phenolic rings linked through a C–C bond, allowing the interaction with various biological targets (Hajduk et al., 2000). MN (1) and HK (2) were proved to have a series of pharmacological effects, including anti-cancer, anti-oxidant, anti-inflammatory, neuroprotective (Lin et al., 2007; Shen et al., 2010; Amorati et al., 2015; Ranaware et al., 2018; Cardullo et al., 2020). Moreover, MN (1) and HK (2) are isomers, whose only difference is the relative position of one hydroxyl group and allyl group. The 4′-hydroxy group and the 5-allyl group are the key to higher neurotrophic activity of HK (2) than that of MN (1) (Fukuyama et al., 2020). Furthermore, both HK (2) and MN (1) exerted neuroprotective effects, which might be related to penetrate the blood-brain barrier (BBB) (Kantham et al., 2017).
The major defining neuropathological features of AD are beta-amyloid (Aβ) accumulation in the cerebral cortex and hippocampus. In addition, accumulating researches showed that cholinergic injury and dysfunction of hippocampus neurons were the critical causes of weakened function of learning and memory (Mohapel et al., 2005; Gökçek-Saraç et al., 2012). Studies showed that neolignans could protect neurons of AD patients by reducing Aβ toxicity, regulating cholinergic nerve function, anti-inflammatory, anti-oxidant, etc. Lewy bodies involving the aggregation of α-synuclein (αS) into amyloid structures are a pathological hallmark of PD (Volpicelli-Daley et al., 2011; Luk et al., 2012; Iyer et al., 2014). Neolignans can regulate dopamine neurons and reduce αS toxicity to protect neurons. Neolignans treat stroke by protecting brain microvascular endothelial cells (BMECs) and inhibiting inflammation and oxidative stress caused by stroke. It is proved that neolignans can modulate HPA axis and regulate GABAergic nerves, treating anxiety and depression. In general, neolignans prevent and treat brain disorders mainly by protecting nerve cells and BMECs.
Neolignans protect nerve cells mainly through regulation of neuronal function, reduction of neurotoxicity, suppression of apoptosis, anti-inflammatory and anti-oxidation. Neolignans flexibly regulate acetylcholine and cholinesterase activity to protect cholinergic neurons; prevent striatal degeneration to protect dopamine neurons; enhance GABA by modulating the benzodiazepine site of GABA receptors and directly increasing GABA neurotransmission; improve serotonergic neurotransmission by increasing levels of 5-HT, 5-HT receptors, and brain-derived neurotrophic factor. Besides, neolignans decline Aβ toxicity through reducing APP, γ-secretase, BACE1 and increasing lysosomal degradation; reduce αS toxicity through stabilizing αS native conformations, leading to the reduction of neurotoxicity. In addition, neolignans reduce the levels of Bcl-2-associated X protein, caspase-3 and other apoptosis-related proteins, thereby inhibiting apoptosis. Moreover, neolignans achieve anti-inflammatory effect by down-regulating nuclear factor-κB and anti-oxidant by reducing reactive oxygen species.
The main pathway diagram is shown in Figure 2.
It has been established that the disadvantage in cholinergic system was likely the most general pathogenesis of AD (Walsh and Selkoe, 2004). But the cholinergic activity was even upregulated in the brain at the earliest stages of AD (Frölich 2002). In the passive avoidance and the Morris water maze tests, MN (1) maintained acetyl cholinesterase (AChE) positive nerve fiber density and AChE activity in hippocampus homogenate, thereby reversing memory impairment induced by scopolamine (Scop) in mice (Li Y. S. et al., 2013). It suggested that early therapeutic effect of MN (1) on AD was more suitable than AChE antagonist alone. On another stage, HK (2) could inhibit the activity of AChE and increased the level of acetylcholine (ACh) in the brain tissues of the Scop-treated mice (Xian et al., 2015). In another study, MN (1) or HK (2) were orally administered to SAMP8 mice once a day for 14 days, then researchers got consistent result. Besides, MN (1) and HK (2) compensated for the neurotrophic function of septal-hippocampal pathway and inhibited apoptosis by activating neurotrophin-mediated Akt pathway. (Matsui et al., 2009). These findings suggested that MN (1) and HK (2) maight be used cooperatively in the different states of AD.
The selective loss of nigral dopaminergic neurons resulted in a decrease of striatal dopamine, which could lead to PD (Lansbury and Brice, 2002). The plasma membrane dopamine transporter (DAT), tyrosine hydroxylase (TH) and the vesicular monoamine transporter (VMAT2) involved in the release, synthesis and removal of dopamine were essential for normal dopamine neurotransmission. Thus, the selective degeneration of DAT-, TH- and VMAT2-expressing in dopamine nerve terminals was thought to underlie PD (Miller et al., 1999; Chen et al., 2018). MN (1) was reported to attenuate the decrease in DAT and TH protein levels in the striatum, repressing MPTP-induced and 6-OHDA-lesioned neurodegeneration in mice and MPP+-induced cytotoxicity to human neuroblastoma SH-SY5Y cells (Chen et al., 2011; Muroyama et al., 2012). HK (2) ameliorated motor dysfunction in 6−OHDA-lesion hemiparkinsonian mice with higher DAT, TH and VMAT2 levels. Besides, HK (2) attenuated the increase of glial fibrillary acidic protein (GFAP) which is the marker of reactive astrocytes and nerve injury. In addition, these effects were found to impede by PPAR-γ antagonist (Chen et al., 2018). It suggested that MN (1) and HK (2) might partly and HK reverse dopaminergic nerve damage at least partly through PPAR-γ pathway.
It has been established that γ-aminobutyric acid (GABA), an important inhibitory neurotransmitter, could act on GABAA receptor to open Cl−-channel, thereby hyperpolarizing neurons and playing the role of sedation and anti-anxiety. Researchers found that MN (1) and HK (2) enhanced both phasic and tonic GABAergic neurotransmission in hippocampal dentate granule neurons of rats, which could be abolished by an antagonist at the benzodiazepine site of the GABAA receptor (Alexeev et al., 2012; Qu et al., 2012). Furthermore, a HK (2) derivative was reported to increased 36Cl− influx in mouse cerebral cortical synaptoneurosome (Maruyama et al., 2001). Interestingly, Ku et al. (2011) found that the anti-anxiety effect of HK (2) was similar to diazepam by the treatment of mice injected 7 days. Besides, the activity of hippocampal GABA synthesized enzymes of HK (2) treated mice was significantly increased than that of diazepam treated groups. In general, neolignans enhance GABAergic neurotransmission by modulating the benzodiazepine site of the GABAA receptor as well as directly increasing GABA.
Glial cells could produce brain-derived neurotrophic factor (BDNF), which had principle neurotrophic and supportive effects on neurons (Althaus and Richter-Landsberg, 2000). It was reported that in unpredictable chronic mild stress rats, MN (1) increased the level of glial fibrillary acidic protein (GFAP) that was an important marker and component of glial cells (Li L. F. et al., 2013). Accumulating studies have shown that the improvement of the serotonin (5-HT) system was regarded as one of the therapeutic targets for depression (Li L. F. et al., 2012). Serotonergic fibers densely dominated the hippocampus, which played an important role in emotional and cognitive processes (Xia et al., 2019). Abundant evidence suggested that the decrease of 5-HT neurotransmission or receptor expression could lead to depression (Dale et al., 2016). MN (1) and HK (2) were reported to effectively improve the levels of 5-HT. In a study, administration of MN (1) normalized the serotonergic system changes in the unpredictable chronic mild stress-treated rats. And in another research, HK (2) was found to have the similar effect. (Xu et al., 2008; Bai et al., 2018). 5-hydroxytryptamine receptor 1A (HTR1A) is a 5-HT receptor which has the highest affinity for 5-HT among all 5-HT receptor subtypes (Wang et al., 2016). Xia et al. (2019) found that a Chinese medicine containing MN (1) increased HTR1A protein and mRNA expression. cAMP-response element binding (CREB) is a key regulator of neuronal growth and related to the expression of BDNF. HTR1A could regulate cyclic adenosine monophosphate (cAMP)/protein kinase A (PKA)/CREB pathway in hippocampus. cAMP activated PKA and PKC subunits into the nucleus, phosphorylated and activated CREB transcription factor-specific serine residues to bind to the cAMP response element and then improved BDNF expression (Wang C. et al., 2017). On the other hand, BDNF had potent neurotrophic effects on serotonergic nerve and promoted the growth and differentiation of nerve synapses (Huang and Reichardt, 2001).
The main pathway diagram is shown in Figure 3.
Abnormal production and deposition of beta-amyloid (Aβ) induced neurotoxicity, leading to the irreversible degeneration and apoptosis of neurons (Tanokashira et al., 2017). Specifically, Aβ induced microglial and nuclear factor-κB (NF-κB) activation which contributed to the increase of inflammatory mediators including COX-2, ROS, iNOS, NO and prostaglandin E2 (PGE-2) (Maezawa et al., 2011; Cai et al., 2014). Besides, Aβ induced production of hydrogen peroxide from NADPH oxidase (Jekabsone et al., 2006). MN (1) has been proved to dose-dependently reduce Aβ deposition, toxicity and memory impairment caused by Aβ in transgenic C. elegans. In another experiment, HK (2) was found to have similar potency to MN (1) by protecting against Aβ-induced toxicity in transgenic C. elegans (Gökçek-Saraç et al., 2012; Kantham et al., 2017). Besides, ethanol extract of Magnolia officinalis including MN (1), HK (2) and other derivatives prevented memory dysfunction and amyloidogenesis in AD mouse model (Lee et al., 2013; Kubo 2015). Aβ was generated through successive cleavages of amyloid precursor protein (APP) by β-site APP cleaving enzyme 1 (BACE1) and γ-secretase (De Strooper et al., 2010). It has been reported that MN (1) and HK (2) might downregulate APP and BACE1 to block the generation of Aβ. (Lawrence 2009; Oboudiyat et al., 2013; Wang M. et al., 2017; Xie et al., 2020). Moreover, MN (1) inhibited the activities of β-secretase and γ-secretase and enhanced the Aβ-degrading enzymatic activities via adjusting the PI3K/Akt/GSK-3β pathway (Aggarwal et al., 2012; Xian et al., 2020). LXR, the target gene of PPAR-γ, was activated by MN (1) to upregulate other genes including ABCA1 and ApoE which mediated the lysosomal clearance of Aβ, reducing Aβ toxicity (Cramer et al., 2012; Oboudiyat et al., 2013; Bonet-Costa et al., 2016; Zhang et al., 2018; Xie et al., 2020). In general, MN (1) and HK (2) declined Aβ toxicity through reducing APP-, γ-secretase- and BACE1-mediated production of Aβ and increasing lysosomal-mediated degradation of Aβ.
Lewy bodies were the pathological marker of PD and the most abundant protein was α-Synuclein (αS) (Ganguly et al., 2021). Excessive αS disrupted the interactions of mitochondria and ER, leading to the decrease of mitochondrial ATP production and nerve cell injury (Paillusson et al., 2017). Ubiquitin-proteasome system (UPS), responsible for clearing mutated and misfolded proteins, was an important pathway involving protein degradation in vivo. It is reported that functional impairment of the UPS could lead to intracellular accumulation of α-synuclein probably by inhibiting its clearance in the proteasomal pathway (Ganguly et al., 2020). Over-expression of αS and functional impairment of the UPS in nerve cells reciprocally induced, then poisoned dopaminergic neurons, leading to PD (Bukhatwa et al., 2010; Egeler et al., 2011; Heo and Rutter, 2011; Taylor and Rutter, 2011). Rats were administered orally with Anchanling that was a Chinese medicine mainly containing MN (1), then Li Y. et al. (2012) found that UPS function was increased and αS expression was decreased. In vitro, HK (2) was reported to inhibit αS amyloidogenic aggregation by stabilizing αS native conformations (Das et al., 2018). The specific mechanism of MN (1) and HK (2) inhibiting over-expression of αS still need to be further studied.
Caspase family belonged to cysteine protease which played an important role in Aβ-induced apoptosis in vitro. MN (1) and HK (2) were reported to inhibit the elevation of caspase-3 activity, inhibiting apoptosis (Hoi et al., 2010). Furthermore, it has been shown that one of MN (1) derivative inhibited apoptosis via reducing the activity of caspase-3 in ischemic cerebral tissue (Li et al., 2015). In another study, a HK (2) derivative was found to have similar anti-apoptosis effect (Bu et al., 2014). In cerebral ischemic stroke rat model, the protein expression of Bcl-2-associated X protein (Bax), an apoptosis-related factor, were reduced by MN (1) (Liu et al., 2018). Bax activated Caspase-9, which initiated a cascade that ultimately activated caspase-3. The activated executor, Caspase-3, led to apoptosis by hydrolysis of caspase target proteins. Furthermore, the anti-apoptotic effect of MN (1) was related to Silent information regulator 1 (SIRT1) activation, a histone deacetylase, which was related to several cellular physiological regulatory pathways (Kou et al., 2017). The activation of SIRT1 led to the reduction in p53, resulting in a decrease of Bax (Yang et al., 2021). In addition, SH-SY5Y human neuroblastoma cells were preincubated with various concentrations of MN (1) (8, 16, and 32 μM) for 2 h, then researchers found that MN (1) increased the phosphorylation of Akt and FOXO1, resulting in repressing FOXO-mediated growth arrest and apoptosis in neuronal cells. Blockage of PI3K could block the activation of Akt by MN (1). It was also found that MN treatment suppressed ERK activation that was a pro-apoptosis signal mediator. These suggested that MN (1) protected models from apoptosis through activating the PI3K/Akt/FOXO1 pathway and inhibiting the MAPK/ERK pathway. Akt activated by PI3K phosphorylated and inhibited FOXO1, preventing FOXO1 activation from transactivating Bcl-2 family member Bim which produced apoptosis. Similarly, after treating SAMP8 mice with MN (1) or HK (2), phosphorylation of Akt in the forebrain was enhanced (Matsui et al., 2009; Dong et al., 2013). Moreover, transforming growth factor β1 (TGF-β1), an anti-apoptosis factor, was increased by MN (1) in the ischemic cortex (Wang et al., 2013). However, its specific mechanism need be further studied. The main pathway diagram is shown in Figure 4.
MN (1) and HK (2) were reported to reduce IL-1β, TNF-α, GRP78, CHOP, and other proinflammatory cytokine which played a significant role in the occurrence and development of hormonal and inflammatory cascade; elevated the level of anti-inflammatory cytokine IL-10 which suppressed the synthesis of many proinflammatory mediators. (Rubio-Perez and Morillas-Ruiz, 2012; Xian et al., 2015; Zhou et al., 2019). Moreover, HK (2) was found to inhibit expression of COX-2 mRNA and prostaglandin E2, reducing inflammatory response (Xian et al., 2015). NF-κB pathway which could be activated by pro-inflammatory factors is considered a prototypical proinflammatory signaling pathway (Lawrence 2009; Aggarwal et al., 2012; Zhang et al., 2013). NF-κB and inhibitor of NF-κB (IκB) usually existed in an inactivated state. After being activated, NF-κB separated from IκB and entered the nucleus, promoting transcription of various inflammatory mediators such as COX-2, IL-1 β, TNF- α, IL-6, etc (Lawrence 2009). HK (2) was reported to downregulate NF-κB by blocking TNF-α–induced NF-κB p65 nuclear translocation and degradation of the inhibitor of NF-κB α, thereby inhibiting production of inflammatory factors (Chen et al., 2016). In another study, intravenous administration of a HK (2) derivative to ischemia-reperfusion rats reduced the expression level of the nuclear NF-κB p65 subunit (Bu et al., 2014). Besides, MN (1) could inhibit NF-κB activation and IκB degradation in RAW264.7 cells stimulated with lipopolysaccharide (Fu et al., 2013). In addition, Male TgCRND8 mice were orally administered with MN (1) daily for 4 consecutive months, then researchers found that the regulation of NF-κB might be related to activation of PPAR-γ (Aggarwal et al., 2012; Oboudiyat et al., 2013; Xian et al., 2020; Xie et al., 2020). Specifically, the DNA-binding activity of NF-κB was inhibited by PPAR-γ activation. Meanwhile, PPAR-γ activation induced the degradation of NF-κB p65 (Hou et al., 2012; Zhao et al., 2015). Furthermore, HK (2) was reported to inhibit the expression of zinc finger transcription factor Krüppel-like factor 4 (KLF4) in microglia and astrocytes, which could also regulate proinflammatory cytokine and anti-inflammatory cytokine (Rickert et al., 2018). The main pathway diagram is shown in Figure 5.
Brain was rich in oxidizable lipid which oxidative damage caused the loss of nerve cells irreversibly, leading to nerve cells dysfunction (Loo et al., 1993). It has been reported that MN (1) and HK (2) inhibited reactive oxygen species (ROS) against oxidation by inhibiting NADPH oxidase activation and Glutathione (GSH) depletion. Scop-induced mouse model with MN (1) treatment was found that the activities of superoxide dismutase (SOD), total nitric oxide synthase (total NOS) returned to normal. (Hoi et al., 2010; Dong et al., 2013; Chen et al., 2018). In cerebral ischemia and stroke experiments, MN (1) has been proved to have anti-oxidation effect which was related to iNOS/p38/MAPK pathway (Chang et al., 2003; Chang et al., 2007; Chen et al., 2014; Huang et al., 2018). Concretely, MN (1) downregulated p38/MAPK pathway through inhibiting inducible NO synthase (iNOS) to prevent C/EBP homologous protein (CHOP) expression, thereby reduceing the production of ROS and oxidative stress (Chen et al., 2014). Mitochondria could produce ROS, which destroyed mitochondrial intima and ATP production. The oxidative disruption of mitochondria transition pore induced leakage of mitochondrial membrane, mitochondrial swelling, ATP deletion, thereby resulting in a vicious cycling in producing ROS burst (Corona and Duchen, 2015). The production of ATP is critical for the maintenance of the activity of Na+, K+-ATPase that is inhibited sensitively by increasing of ROS (Chaturvedi and Beal, 2008). The decrease of Na+, K+-ATPase activity in the cerebral ischemia was reversed by HK (2), which suggested that HK (2) inhibited ROS by improving synaptosomal mitochondrial metabolic function (Chen et al., 2007). The main pathway diagram is shown in Figure 6.
It has been shown that iNOS and neuronal NO synthase (nNOS) could produce a high level of NO, thereby inducing cell injury or death, but NO produced by endothelial NO synthase (eNOS) protected brain against stroke (Jiang et al., 2002; Li et al., 2014). HK (2) was reported to have effect on increasing cerebral blood supply in rats, and the mechanism might be associated with the vasodilation produced by eNOS activation and the protection of BMECs (Xiaoyan 2016). The activation of nNOS depended on translocation from cytoplasm to membrane and connection to N-methyl-D-aspartic acid receptor (NMDAR) via the scaffolding protein postsynaptic density 95 (PSD95), thereby stimulating NO production during ischemia (Fan et al., 2010; Zhou et al., 2010). HK (2) could block the PSD95 nNOS interaction and inhibit the translocation of nNOS from cytoplasm to membrane. In addition, HK (2) also inhibited NMDAR to partly (Hu et al., 2013). Adenosine 5′-monophosphate-activated protein kinase (AMPK), a serine/threonine kinase, was known to inhibit gluconeogenesis via suppression of phosphoenolpyruvate carboxy kinase (PEPCK) and other key enzymes in liver. AMPK was found to be increased by HK (2), resulting in decrease of blood glucose levels (Ronnett et al., 2009). Therefore, HK (2) might normalize blood glucose and decrease the extent of neuronal dysfunction by preventing post-ischemic glucose intolerance (Harada et al., 2012). Interestingly, in addition to protecting individual cells, neolignans also had effect on the structure of cells. Erythropoietin-producing hepatocellular A2 (EphA2) was one of transmembrane receptors tyrosine kinases and its phosphorylation led to the destruction of the tight junction between BMECs (Zhou et al., 2010). MN (1) was found to inhibit phosphorylation of EphA2 and enhance formation of the tight junction between BMEC, leading to improvement of BBB (Liu et al., 2017). The main pathway diagram is shown in Figure 7.
Prolonged and repeated stress exposure caused hyperactivation of hypothalamic-pituitary-adrenal axis (HPA), which increased the level of corticosterone and affected the cognitive function, leading to depression and anxiety (Jangra et al., 2016). It has been shown that neolignans could restore the normal active level of HPA axis, which might be related to the decrease of glucocorticoid negative feedback and increase of BDNF. HK (2) was reported to increase the expression of glucocorticoid receptor α, reducing negative feedback, thereafter hyperactivity of HPA axis was regulated (Wang et al., 2018). In addition, it has been proved that the increase of BDNF had significance of maintaining the normal function of HPA axis (Xu et al., 2008). These results suggested that anti-depressant effect of neolignans was the result of the synergy of BDNF and HPA axis.
In a study, models were given 625, 1250, or 2500 mg/kg extract containing 94% MN (1) and 1.5% HK (2) orally for 14 days, and researchers did not find death or clinical toxicity (Li et al., 2007). Besides, Magnolia officinalis extract containing high content of MN (1) and HK (2) did not been found any mutagenicity and genotoxicity, even showed partly anti-mutagenic activity in vivo (Bai et al., 2003; Zhang et al., 2008; Fried and Arbiser, 2009; Lee et al., 2011). It has been reported that when administered orally or intraperitoneally, the most neolignans were excreted in feces and urine within 12 h (Hattori et al., 1986). Although neolignans could cause enterohepatic circulation, researchers have not found any special hepatotoxicity (Lee et al., 2011). It has been estimated that the safe dose of MN (1) available for teenage is up to 1.64 mg/kg per day (Zhang et al., 2019). Besides, to date, no serious side effects on human intake of prescriptions containing MN (1) or HK (2) have been reported. In general, therapeutic doses of neolignans could be considered safe.
Neolignans protect the nervous system from brain diseases including AD, PD, stroke, anxiety and depression mainly by protecting nerve cells and BMECs. It has the following effects on nerve cells: 1) regulating neuronal function mainly by normalizing neurotransmitters and their receptors 2) reducing neurotoxicity through reducing APP, γ-secretase, BACE1 and stabilizing αS native conformations 3) suppressing neuronal apoptosis through reducing the levels of Bax, caspase-3 and other apoptosis-related proteins 4) anti-inflammation in nerve by PPAR-γ/NF-κB pathway 5) anti-oxidation in nerve through MAPK pathway and PI3K/Akt pathway including p38/MAPK and MAPK/ERK. The combined action of these pathways allows neolignans to have pleiotropic neuroprotective effects in different links. For BMECs, neolignans can increase blood supply, normalize blood glucose and close the connection between BMEC. In addition, neolignans also regulate the humoral environment by modulating the function of HPA axis, keeping the nervous system away from stress.
However, there are few reports on the neuroprotective effect of neolignans except MN (1) and HK (2). The overall pharmacological effect and mechanism of neolignans can only be inferred from the existing researches of MN (1) and HK (2). Furthermore, the clinical applications of neolignans are limited due to low bioavailability and quick metabolism, which should be paid attention to and improved. In summary, neolignans can be considered as a promising neuroprotective resource for the treatment of brain diseases, and more clinical studies are needed.
The original contributions presented in the study are included in the article/Supplementary Material, further inquiries can be directed to the corresponding authors.
SZ wrote the article and designed the graphics. FL and SC came up with the idea of review and implemented the revision of the manuscripts. RZ and ZX participated in carried out the collection and analysis of references. QZ and LH revised the proof of manuscripts.
This work was supported by NSFC (No. 81603300), China Postdoctoral Science Foundation (No. 2020M673569XB), the Department of Science and Technology of Sichuan Province (No. 20YYJC0655) and Xinglin Scholar Research Promotion Project of Chengdu University of Traditional Chinese Medicine (No. QNXZ2018006). Sichuan Provincial Administration of Traditional Chinese Medicine (No. 2018QN33).
HSY was employed by Pharmaceutical Group Co., LTD.
The remaining authors declare that the research was conducted in the absence of any commercial or financial relationships that could be construed as a potential conflict of interest.
All claims expressed in this article are solely those of the authors and do not necessarily represent those of their affiliated organizations, or those of the publisher, the editors, and the reviewers. Any product that may be evaluated in this article, or claim that may be made by its manufacturer, is not guaranteed or endorsed by the publisher.
The Supplementary Material for this article can be found online at: https://www.frontiersin.org/articles/10.3389/fphar.2022.857449/full#supplementary-material
Aggarwal, B. B., Gupta, S. C., and Kim, J. H. (2012). Historical Perspectives on Tumor Necrosis Factor and its Superfamily: 25 Years Later, a Golden Journey. Blood 119 (3), 651–665. doi:10.1182/blood-2011-04-325225
Alexeev, M., Grosenbaugh, D. K., Mott, D. D., and Fisher, J. L. (2012). The Natural Products Magnolol and Honokiol Are Positive Allosteric Modulators of Both Synaptic and Extra-synaptic GABA(A) Receptors. Neuropharmacology 62 (8), 2507–2514. doi:10.1016/j.neuropharm.2012.03.002
Althaus, H. H., and Richter-Landsberg, C. (2000). Glial Cells as Targets and Producers of Neurotrophins. Int. Rev. Cytol. 197, 203–277. doi:10.1016/s0074-7696(00)97005-0
Amorati, R., Zotova, J., Baschieri, A., and Valgimigli, L. (2015). Antioxidant Activity of Magnolol and Honokiol: Kinetic and Mechanistic Investigations of Their Reaction with Peroxyl Radicals. J. Org. Chem. 80 (21), 10651–10659. doi:10.1021/acs.joc.5b01772
Bai, X., Cerimele, F., Ushio-Fukai, M., Waqas, M., Campbell, P. M., Govindarajan, B., et al. (2003). Honokiol, a Small Molecular Weight Natural Product, Inhibits Angiogenesis In Vitro and Tumor Growth In Vivo. J. Biol. Chem. 278 (37), 35501–35507. doi:10.1074/jbc.M302967200
Bai, Y., Song, L., Dai, G., Xu, M., Zhu, L., Zhang, W., et al. (2018). Antidepressant Effects of Magnolol in a Mouse Model of Depression Induced by Chronic Corticosterone Injection. Steroids 135, 73–78. doi:10.1016/j.steroids.2018.03.005
Bonet-Costa, V., Herranz-Pérez, V., Blanco-Gandía, M., Mas-Bargues, C., Inglés, M., Garcia-Tarraga, P., et al. (2016). Clearing Amyloid-β through PPARγ/ApoE Activation by Genistein Is a Treatment of Experimental Alzheimer's Disease. J. Alzheimers Dis. 51 (3), 701–711. doi:10.3233/JAD-151020
Bu, Q., Liu, X., Zhu, Y., Liu, Y., and Wang, Y. (2014). w007B Protects Brain against Ischemia-Reperfusion Injury in Rats through Inhibiting Inflammation, Apoptosis and Autophagy. Brain Res. 1558, 100–108. doi:10.1016/j.brainres.2014.02.034
Bui, D., Li, L., Yin, T., Wang, X., Gao, S., You, M., et al. (2020). Pharmacokinetic and Metabolic Profiling of Key Active Components of Dietary Supplement Magnolia Officinalis Extract for Prevention against Oral Carcinoma. J. Agric. Food Chem. 68 (24), 6576–6587. doi:10.1021/acs.jafc.0c01475
Bukhatwa, S., Zeng, B. Y., Rose, S., and Jenner, P. (2010). A Comparison of Changes in Proteasomal Subunit Expression in the Substantia Nigra in Parkinson's Disease, Multiple System Atrophy and Progressive Supranuclear Palsy. Brain Res. 1326, 174–183. doi:10.1016/j.brainres.2010.02.045
Cacabelos, R., Meyyazhagan, A., Carril, J. C., Cacabelos, P., and Teijido, O. (2008). Pharmacogenetics of Vascular Risk Factors in Alzheimer's Disease. J. Pers. Med. 8 (1), 3. doi:10.3390/jpm8010003
Cai, Z., Hussain, M. D., and Yan, L. J. (2014). Microglia, Neuroinflammation, and Beta-Amyloid Protein in Alzheimer's Disease. Int. J. Neurosci. 124 (5), 307–321. doi:10.3109/00207454.2013.833510
Cardullo, N., Barresi, V., Muccilli, V., Spampinato, G., D'Amico, M., Condorelli, D. F., et al. (2020). Synthesis of Bisphenol Neolignans Inspired by Honokiol as Antiproliferative Agents. Molecules 25 (3), 733. doi:10.3390/molecules25030733
Chang, C. K., Chang, C. P., Liu, S. Y., and Lin, M. T. (2007). Oxidative Stress and Ischemic Injuries in Heat Stroke. Prog. Brain Res. 162, 525–546. doi:10.1016/s0079-6123(06)62025-6
Chang, C. P., Hsu, Y. C., and Lin, M. T. (2003). Magnolol Protects against Cerebral Ischaemic Injury of Rat Heatstroke. Clin. Exp. Pharmacol. Physiol. 30 (5-6), 387–392. doi:10.1046/j.1440-1681.2003.03847.x
Chaturvedi, R. K., and Beal, M. F. (2008). PPAR: a Therapeutic Target in Parkinson's Disease. J. Neurochem. 106 (2), 506–518. doi:10.1111/j.1471-4159.2008.05388.x
Chen, C. M., Liu, S. H., and Lin-Shiau, S. Y. (2007). Honokiol, a Neuroprotectant against Mouse Cerebral Ischaemia, Mediated by Preserving Na+, K+-ATPase Activity and Mitochondrial Functions. Basic Clin. Pharmacol. Toxicol. 101 (2), 108–116. doi:10.1111/j.1742-7843.2007.00082.x
Chen, H. H., Chang, P. C., Wey, S. P., Chen, P. M., Chen, C., and Chan, M. H. (2018). Therapeutic Effects of Honokiol on Motor Impairment in Hemiparkinsonian Mice Are Associated with Reversing Neurodegeneration and Targeting PPARγ Regulation. Biomed. Pharmacother. 108, 254–262. doi:10.1016/j.biopha.2018.07.095
Chen, H. H., Lin, S. C., and Chan, M. H. (2011). Protective and Restorative Effects of Magnolol on Neurotoxicity in Mice with 6-Hydroxydopamine-Induced Hemiparkinsonism. Neurodegener. Dis. 8 (5), 364–374. doi:10.1159/000323872
Chen, J. H., Kuo, H. C., Lee, K. F., and Tsai, T. H. (2014). Magnolol Protects Neurons against Ischemia Injury via the Downregulation of p38/MAPK, CHOP and Nitrotyrosine. Toxicol. Appl. Pharmacol. 279 (3), 294–302. doi:10.1016/j.taap.2014.07.005
Chen, P.-J., Wang, Y.-L., Kuo, L.-M., Lin, C.-F., Chen, C.-Y., Tsai, Y.-F., et al. (2016). Honokiol Suppresses TNF-α-Induced Neutrophil Adhesion on Cerebral Endothelial Cells by Disrupting Polyubiquitination and Degradation of IκBα. Sci. Rep. 6, 26554. doi:10.1038/srep26554
Cheng, J., Dong, S., Yi, L., Geng, D., and Liu, Q. (2018). Magnolol Abrogates Chronic Mild Stress-Induced Depressive-like Behaviors by Inhibiting Neuroinflammation and Oxidative Stress in the Prefrontal Cortex of Mice. Int. Immunopharmacol. 59, 61–67. doi:10.1016/j.intimp.2018.03.031
Clarke, C. E. (2004). Neuroprotection and Pharmacotherapy for Motor Symptoms in Parkinson's Disease. Lancet Neurol. 3 (8), 466–474. doi:10.1016/S1474-4422(04)00823-3
Corona, J. C., and Duchen, M. R. (2015). PPARγ and PGC-1α as Therapeutic Targets in Parkinson's. Neurochem. Res. 40 (2), 308–316. doi:10.1007/s11064-014-1377-0
Cramer, P. E., Cirrito, J. R., Wesson, D. W., Lee, C. Y., Karlo, J. C., Zinn, A. E., et al. (2012). ApoE-directed Therapeutics Rapidly Clear β-amyloid and Reverse Deficits in AD Mouse Models. Science 335 (6075), 1503–1506. doi:10.1126/science.1217697
Dale, E., Pehrson, A. L., Jeyarajah, T., Li, Y., Leiser, S. C., Smagin, G., et al. (2016). Effects of Serotonin in the hippocampus: How SSRIs and Multimodal Antidepressants Might Regulate Pyramidal Cell Function. CNS Spectr. 21 (2), 143–161. doi:10.1017/S1092852915000425
Das, S., Pukala, T. L., and Smid, S. D. (2018). Exploring the Structural Diversity in Inhibitors of α-Synuclein Amyloidogenic Folding, Aggregation, and Neurotoxicity. Front. Chem. 6, 181. doi:10.3389/fchem.2018.00181
De Strooper, B., Vassar, R., and Golde, T. (2010). The Secretases: Enzymes with Therapeutic Potential in Alzheimer Disease. Nat. Rev. Neurol. 6 (2), 99–107. doi:10.1038/nrneurol.2009.218
Dong, L., Zhou, S., Yang, X., Chen, Q., He, Y., and Huang, W. (2013). Magnolol Protects against Oxidative Stress-Mediated Neural Cell Damage by Modulating Mitochondrial Dysfunction and PI3K/Akt Signaling. J. Mol. Neurosci. 50 (3), 469–481. doi:10.1007/s12031-013-9964-0
Dorsey, E. R., Sherer, T., Okun, M. S., and Bloem, B. R. (2018). The Emerging Evidence of the Parkinson Pandemic. J. Park. Dis. 8 (s1), S3–S8. doi:10.3233/JPD-181474
Egeler, E. L., Urner, L. M., Rakhit, R., Liu, C. W., and Wandless, T. J. (2011). Ligand-switchable Substrates for a Ubiquitin-Proteasome System. J. Biol. Chem. 286 (36), 31328–31336. doi:10.1074/jbc.M111.264101
Fan, J., Vasuta, O. C., Zhang, L. Y., Wang, L., George, A., and Raymond, L. A. (2010). N-methyl-D-aspartate Receptor Subunit- and Neuronal-type Dependence of Excitotoxic Signaling through Post-synaptic Density 95. J. Neurochem. 115 (4), 1045–1056. doi:10.1111/j.1471-4159.2010.06994.x
Fried, L. E., and Arbiser, J. L. (2009). Honokiol, a Multifunctional Antiangiogenic and Antitumor Agent. Antioxid. Redox Signal 11 (5), 1139–1148. doi:10.1089/ars.2009.2440
Frölich, L. (2002). The Cholinergic Pathology in Alzheimer's Disease-Ddiscrepancies between Clinical Experience and Pathophysiological Findings. J. Neural Transm. (Vienna) 109 (7-8), 1003–1013. doi:10.1007/s007020200083
Fu, Y., Liu, B., Zhang, N., Liu, Z., Liang, D., Li, F., et al. (2013). Magnolol Inhibits Lipopolysaccharide-Induced Inflammatory Response by Interfering with TLR4 Mediated NF-Κb and MAPKs Signaling Pathways. J. Ethnopharmacol. 145 (1), 193–199. doi:10.1016/j.jep.2012.10.051
Fukuyama, Y., Kubo, M., and Harada, K. (2020). The Search for, and Chemistry and Mechanism of, Neurotrophic Natural Products. J. Nat. Med. 74 (4), 648–671. doi:10.1007/s11418-020-01431-8
Ganguly, U., Banerjee, A., Chakrabarti, S. S., Kaur, U., Sen, O., Cappai, R., et al. (2020). Interaction of α-synuclein and Parkin in Iron Toxicity on SH-Sy5y Cells: Implications in the Pathogenesis of Parkinson’s Disease. Biochem. J. 477 (6), 1109–1122. doi:10.1042/BCJ20190676
Ganguly, U., Singh, S., Pal, S., Prasad, S., Agrawal, B. K., Saini, R. V., et al. (2021). Alpha-Synuclein as a Biomarker of Parkinson's Disease: Good, but Not Good Enough. Front. Aging Neurosci. 13, 702639. doi:10.3389/fnagi.2021.702639
Ghorbani, F., Haghgoo, R., Aramjoo, H., Rakhshandeh, H., Jamehdar, S. A., and Zare-Bidaki, M. (2021). The Antibacterial Effect of Magnolia Mouthwash on the Levels of Salivary Streptococcus Mutans in Dental Plaque: a Randomized, Single-Blind, Placebo-Controlled Trial. Iran. J. Microbiol. 13 (1), 104–111. doi:10.18502/ijm.v13i1.5499
Gökçek-Saraç, Ç., Karakurt, S., Adalı, O., and Jakubowska-Doğru, E. (2012). Correlation between Hippocampal Levels of Neural, Epithelial and Inducible NOS and Spatial Learning Skills in Rats. Behav. Brain Res. 235 (2), 326–333. doi:10.1016/j.bbr.2012.08.005
Hajduk, P. J., Bures, M., Praestgaard, J., and Fesik, S. W. (2000). Privileged Molecules for Protein Binding Identified from NMR-Based Screening. J. Med. Chem. 43, 3443–3447. doi:10.1021/jm000164q
Harada, S., Kishimoto, M., Kobayashi, M., Nakamoto, K., Fujita-Hamabe, W., Chen, H. H., et al. (2012). Honokiol Suppresses the Development of Post-ischemic Glucose Intolerance and Neuronal Damage in Mice. J. Nat. Med. 66 (4), 591–599. doi:10.1007/s11418-011-0623-x
Hattori, M., Endo, Y., Takebe, S., Kobashi, K., Fukasaku, N., and Namba, T. (1986). Metabolism of Magnolol from Magnoliae Cortex. II. Absorption, Metabolism and Excretion of [ring-14C]magnolol in Rats. Chem. Pharm. Bull. (Tokyo) 34 (1), 158–167. doi:10.1248/cpb.34.158
Heo, J. M., and Rutter, J. (2011). Ubiquitin-dependent Mitochondrial Protein Degradation. Int. J. Biochem. Cell Biol. 43 (10), 1422–1426. doi:10.1016/j.biocel.2011.06.002
Hoi, C. P., Ho, Y. P., Baum, L., and Chow, A. H. (2010). Neuroprotective Effect of Honokiol and Magnolol, Compounds from Magnolia Officinalis, on Beta-Amyloid-Induced Toxicity in PC12 Cells. Phytother. Res. 24 (10), 1538–1542. doi:10.1002/ptr.3178
Hou, Y., Moreau, F., and Chadee, K. (2012). PPARγ Is an E3 Ligase that Induces the Degradation of NFκB/p65. Nat. Commun. 3 (1), 1300. doi:10.1038/ncomms2270
Hu, Z., Bian, X., Liu, X., Zhu, Y., Zhang, X., Chen, S., et al. (2013). Honokiol Protects Brain against Ischemia-Reperfusion Injury in Rats through Disrupting PSD95-nNOS Interaction. Brain Res. 1491, 204–212. doi:10.1016/j.brainres.2012.11.004
Huang, E. J., and Reichardt, L. F. (2001). Neurotrophins: Roles in Neuronal Development and Function. Annu. Rev. Neurosci. 24, 677–736. doi:10.1146/annurev.neuro.24.1.677
Huang, S. Y., Tai, S. H., Chang, C. C., Tu, Y. F., Chang, C. H., and Lee, E. J. (2018). Magnolol Protects against Ischemic-Reperfusion Brain Damage Following Oxygen-Glucose Deprivation and Transient Focal Cerebral Ischemia. Int. J. Mol. Med. 41 (4), 2252–2262. doi:10.3892/ijmm.2018.3387
Iyer, A., Petersen, N. O., Claessens, M. M., and Subramaniam, V. (2014). Amyloids of Alpha-Synuclein Affect the Structure and Dynamics of Supported Lipid Bilayers. Biophys. J. 106 (12), 2585–2594. doi:10.1016/j.bpj.2014.05.001
Jangra, A., Dwivedi, S., Sriram, C. S., Gurjar, S. S., Kwatra, M., Sulakhiya, K., et al. (2016). Honokiol Abrogates Chronic Restraint Stress-Induced Cognitive Impairment and Depressive-like Behaviour by Blocking Endoplasmic Reticulum Stress in the hippocampus of Mice. Eur. J. Pharmacol. 770, 25–32. doi:10.1016/j.ejphar.2015.11.047
Jekabsone, A., Mander, P. K., Tickler, A., Sharpe, M., and Brown, G. C. (2006). Fibrillar Beta-Amyloid Peptide Abeta1-40 Activates Microglial Proliferation via Stimulating TNF-Alpha Release and H2O2 Derived from NADPH Oxidase: a Cell Culture Study. J. Neuroinflammation 3, 24. doi:10.1186/1742-2094-3-24
Jiang, M. H., Kaku, T., Hada, J., and Hayashi, Y. (2002). Different Effects of eNOS and nNOS Inhibition on Transient Forebrain Ischemia. Brain Res. 946 (1), 139–147. doi:10.1016/s0006-8993(02)02870-6
Kantham, S., Chan, S., McColl, G., Miles, J. A., Veliyath, S. K., Deora, G. S., et al. (2017). Effect of the Biphenyl Neolignan Honokiol on Aβ42-Induced Toxicity in Caenorhabditis elegans, Aβ42 Fibrillation, Cholinesterase Activity, DPPH Radicals, and Iron(II) Chelation. ACS Chem. Neurosci. 8 (9), 1901–1912. doi:10.1021/acschemneuro.7b00071
Kou, D. Q., Jiang, Y. L., Qin, J. H., and Huang, Y. H. (2017). Magnolol Attenuates the Inflammation and Apoptosis through the Activation of SIRT1 in Experimental Stroke Rats. Pharmacol. Rep. 69 (4), 642–647. doi:10.1016/j.pharep.2016.12.012
Ku, T. H., Lee, Y. J., Wang, S. J., Fan, C. H., and Tien, L. T. (2011). Effect of Honokiol on Activity of GAD(65) and GAD(67) in the Cortex and hippocampus of Mice. Phytomedicine 18 (13), 1126–1129. doi:10.1016/j.phymed.2011.03.007
Kubo, M. (2015). Search of Neurotrophin-Mimic Natural Products for Prevention and Treatment of Neurodegenerative Disease. Yakugaku Zasshi 135 (10), 1147–1152. doi:10.1248/yakushi.15-00197
Kuriakose, D., and Xiao, C. (2020). Pathophysiology and Treatment of Stroke PresentStatus and Future Perspectives. Int. J. Mol. Sci. 21 (20), 7609–7632. doi:10.3390/ijms21207609
Lader, M. (2008). Effectiveness of Benzodiazepines: Do They Work or Not? Expert Rev. Neurother. 8 (8), 1189–1191. doi:10.1586/14737175.8.8.1189
Lansbury, P. T., and Brice, A. (2002). Genetics of Parkinson's Disease and Biochemical Studies of Implicated Gene Products. Curr. Opin. Genet. Dev. 12 (5), 299–306. doi:10.1016/s0959-437x(02)00302-7
Lawrence, T. (2009). The Nuclear Factor NF-kappaB Pathway in Inflammation. Cold Spring Harb. Perspect. Biol. 1 (6), a001651. doi:10.1101/cshperspect.a001651
Lee, Y. J., Choi, D. Y., Yun, Y. P., Han, S. B., Kim, H. M., Lee, K., et al. (2013). Ethanol Extract of Magnolia Officinalis Prevents Lipopolysaccharide-Induced Memory Deficiency via its Antineuroinflammatory and Antiamyloidogenic Effects. Phytother. Res. 27 (3), 438–447. doi:10.1002/ptr.4740
Lee, Y. J., Lee, Y. M., Lee, C. K., Jung, J. K., Han, S. B., and Hong, J. T. (2011). Therapeutic Applications of Compounds in the Magnolia Family. Pharmacol. Ther. 130 (2), 157–176. doi:10.1016/j.pharmthera.2011.01.010
Li, H., Liu, X., Zhu, Y., Liu, Y., and Wang, Y. (2015). Magnolol Derivative 002C-3 Protects Brain against Ischemia-Reperfusion Injury via Inhibiting Apoptosis and Autophagy. Neurosci. Lett. 588, 178–183. doi:10.1016/j.neulet.2015.01.007
Li, L. F., Lu, J., Li, X. M., Xu, C. L., Deng, J. M., Qu, R., et al. (2012). Antidepressant-like Effect of Magnolol on BDNF Up-Regulation and Serotonergic System Activity in Unpredictable Chronic Mild Stress Treated Rats. Phytother. Res. 26 (8), 1189–1194. doi:10.1002/ptr.3706
Li, L. F., Yang, J., Ma, S. P., and Qu, R. (2013). Magnolol Treatment Reversed the Glial Pathology in an Unpredictable Chronic Mild Stress-Induced Rat Model of Depression. Eur. J. Pharmacol. 711 (1-3), 42–49. doi:10.1016/j.ejphar.2013.04.008
Li, N., Song, Y., Zhang, W., Wang, W., Chen, J., Wong, A. W., et al. (2007). Evaluation of the In Vitro and In Vivo Genotoxicity of Magnolia Bark Extract. Regul. Toxicol. Pharmacol. 49 (3), 154–159. doi:10.1016/j.yrtph.2007.06.005
Li, S. T., Pan, J., Hua, X. M., Liu, H., Shen, S., Liu, J. F., et al. (2014). Endothelial Nitric Oxide Synthase Protects Neurons against Ischemic Injury through Regulation of Brain-Derived Neurotrophic Factor Expression. CNS Neurosci. Ther. 20 (2), 154–164. doi:10.1111/cns.12182
Li, Y., Wu, Z., Gao, X., Zhu, Q., Jin, Y., Wu, A., et al. (2012). Anchanling Reduces Pathology in a Lactacystin- Induced Parkinson's Disease Model. Neural Regen. Res. 7 (3), 165–170. doi:10.3969/j.issn.1673-5374.2012.03.001
Li, Y. S., Hong, Y. F., He, J., Lin, J. X., Shan, Y. L., Fu, D. Y., et al. (2013). Effects of Magnolol on Impairment of Learning and Memory Abilities Induced by Scopolamine in Mice. Biol. Pharm. Bull. 36 (5), 764–771. doi:10.1248/bpb.b12-00880
Lin, Y. R., Chen, H. H., Ko, C. H., and Chan, M. H. (2007). Effects of Honokiol and Magnolol on Acute and Inflammatory Pain Models in Mice. Life Sci. 81 (13), 1071–1078. doi:10.1016/j.lfs.2007.08.014
Liu, X., Chen, X., Zhu, Y., Wang, K., and Wang, Y. (2017). Effect of Magnolol on Cerebral Injury and Blood Brain Barrier Dysfunction Induced by Ischemia-Reperfusion In Vivo and In Vitro. Metab. Brain Dis. 32 (4), 1109–1118. doi:10.1007/s11011-017-0004-6
Liu, Z., Xie, J., Lin, K., and Qi, L. (2018). Influencing Mechanism of Magnolol on Expression of BDNF and Bax in Rats with Cerebral Ischemic Stroke. Exp. Ther. Med. 16 (6), 4423–4428. doi:10.3892/etm.2018.6807
Loo, D. T., Copani, A., Pike, C. J., Whittemore, E. R., Walencewicz, A. J., and Cotman, C. W. (1993). Apoptosis Is Induced by Beta-Amyloid in Cultured Central Nervous System Neurons. Proc. Natl. Acad. Sci. U. S. A. 90 (17), 7951–7955. doi:10.1073/pnas.90.17.7951
Luk, K. C., Kehm, V., Carroll, J., Zhang, B., O'Brien, P., Trojanowski, J. Q., et al. (2012). Pathological α-synuclein Transmission Initiates Parkinson-like Neurodegeneration in Nontransgenic Mice. Science 338 (6109), 949–953. doi:10.1126/science.1227157
Luo, H., Wu, H., Yu, X., Zhang, X., Lu, Y., Fan, J., et al. (2019). A Review of the Phytochemistry and Pharmacological Activities of Magnoliae Officinalis Cortex. J. Ethnopharmacol. 236, 412–442. doi:10.1016/j.jep.2019.02.041
Maezawa, I., Zimin, P. I., Wulff, H., and Jin, L. W. (2011). Amyloid-beta Protein Oligomer at Low Nanomolar Concentrations Activates Microglia and Induces Microglial Neurotoxicity. J. Biol. Chem. 286 (5), 3693–3706. doi:10.1074/jbc.M110.135244
Maruyama, Y., Kuribara, H., Kishi, E., Weintraub, S. T., and Ito, Y. (2001). Confirmation of the Anxiolytic-like Effect of Dihydrohonokiol Following Behavioural and Biochemical Assessments. J. Pharm. Pharmacol. 53 (5), 721–725. doi:10.1211/0022357011775848
Matsui, N., Takahashi, K., Takeichi, M., Kuroshita, T., Noguchi, K., Yamazaki, K., et al. (2009). Magnolol and Honokiol Prevent Learning and Memory Impairment and Cholinergic Deficit in SAMP8 Mice. Brain Res. 1305, 108–117. doi:10.1016/j.brainres.2009.09.107
Millan, M. J., Goodwin, G. M., Meyer-Lindenberg, A., and Ove Ögren, S. (2015). Learning from the Past and Looking to the Future: Emerging Perspectives for Improving the Treatment of Psychiatric Disorders. Eur. Neuropsychopharmacol. 25 (5), 599–656. doi:10.1016/j.euroneuro.2015.01.016
Miller, G. W., Gainetdinov, R. R., Levey, A. I., and Caron, M. G. (1999). Dopamine Transporters and Neuronal Injury. Trends Pharmacol. Sci. 20 (10), 424–429. doi:10.1016/s0165-6147(99)01379-6
Mohapel, P., Leanza, G., Kokaia, M., and Lindvall, O. (2005). Forebrain Acetylcholine Regulates Adult Hippocampal Neurogenesis and Learning. Neurobiol. Aging 26 (6), 939–946. doi:10.1016/j.neurobiolaging.2004.07.015
Muroyama, A., Fujita, A., Lv, C., Kobayashi, S., Fukuyama, Y., and Mitsumoto, Y. (2012). Magnolol Protects against MPTP/MPP(+)-Induced Toxicity via Inhibition of Oxidative Stress in In Vivo and In Vitro Models of Parkinson's Disease. Park. Dis. 2012, 985157. doi:10.1155/2012/985157
Nemeroff, C. B. (2007). The Burden of Severe Depression: a Review of Diagnostic Challenges and Treatment Alternatives. J. Psychiatr. Res. 41 (3-4), 189–206. doi:10.1016/j.jpsychires.2006.05.008
Oboudiyat, C., Glazer, H., Seifan, A., Greer, C., and Isaacson, R. S. (2013). Alzheimer's Disease. Semin. Neurol. 33 (4), 313–329. doi:10.1055/s-0033-1359319
Paillusson, S., Gomez-Suaga, P., Stoica, R., Little, D., Gissen, P., Devine, M. J., et al. (2017). α-Synuclein Binds to the ER-Mitochondria Tethering Protein VAPB to Disrupt Ca2+ Homeostasis and Mitochondrial ATP Production. Acta Neuropathol. 134 (1), 129–149. doi:10.1007/s00401-017-1704-z
Poivre, M., and Duez, P. (2017). Biological Activity and Toxicity of the Chinese Herb Magnolia Officinalis Rehder & E. Wilson (Houpo) and its Constituents. J. Zhejiang Univ. Sci. B 18 (3), 194–214. doi:10.1631/jzus.B1600299
Qu, W. M., Yue, X. F., Sun, Y., Fan, K., Chen, C. R., Hou, Y. P., et al. (2012). Honokiol Promotes Non-rapid Eye Movement Sleep via the Benzodiazepine Site of the GABA(A) Receptor in Mice. Br. J. Pharmacol. 167 (3), 587–598. doi:10.1111/j.1476-5381.2012.02010.x
Ranaware, A. M., Banik, K., Deshpande, V., Padmavathi, G., Roy, N. K., Sethi, G., et al. (2018). Magnolol: A Neolignan from the Magnolia Family for the Prevention and Treatment of Cancer. Int. J. Mol. Sci. 19 (8), 2362. doi:10.3390/ijms19082362
Rauf, A., Olatunde, A., Imran, M., Alhumaydhi, F. A., Aljohani, A. S. M., Khan, S. A., et al. (2021). Honokiol: A Review of its Pharmacological Potential and Therapeutic Insights. Phytomedicine 90, 153647. doi:10.1016/j.phymed.2021.153647
Rickert, U., Cossais, F., Heimke, M., Arnold, P., Preuße-Prange, A., Wilms, H., et al. (2018). Anti-inflammatory Properties of Honokiol in Activated Primary Microglia and Astrocytes. J. Neuroimmunol. 323, 78–86. doi:10.1016/j.jneuroim.2018.07.013
Ronnett, G. V., Ramamurthy, S., Kleman, A. M., Landree, L. E., and Aja, S. (2009). AMPK in the Brain: its Roles in Energy Balance and Neuroprotection. J. Neurochem. 109 (Suppl. 1), 17–23. doi:10.1111/j.1471-4159.2009.05916.x
Rubio-Perez, J. M., and Morillas-Ruiz, J. M. (2012). A Review: Inflammatory Process in Alzheimer's Disease, Role of Cytokines. ScientificWorldJournal 2012, 756357. doi:10.1100/2012/756357
Shen, J. L., Man, K. M., Huang, P. H., Chen, W. C., Chen, D. C., Cheng, Y. W., et al. (2010). Honokiol and Magnolol as Multifunctional Antioxidative Molecules for Dermatologic Disorders. Molecules 15 (9), 6452–6465. doi:10.3390/molecules15096452
Silva, M. V. F., Loures, C. M. G., Alves, L. C. V., de Souza, L. C., Borges, K. B. G., and Carvalho, M. D. G. (2019). Alzheimer's Disease: Risk Factors and Potentially Protective Measures. J. Biomed. Sci. 26 (1), 33. doi:10.1186/s12929-019-0524-y
Szelenberger, R., Kostka, J., Saluk-Bijak, J., and Miller, E. (2020). Pharmacological Interventions and Rehabilitation Approach for Enhancing Brain Self-Repair and Stroke Recovery. Curr. Neuropharmacol. 18 (1), 51–64. doi:10.2174/1570159X17666190726104139
Taipale, H., Koponen, M., Tanskanen, A., Tolppanen, A. M., Tiihonen, J., and Hartikainen, S. (2016). Drug Use in Persons with and without Alzheimer's Disease Aged 90 Years or More. Age Ageing 45 (6), 900–904. doi:10.1093/ageing/afw141
Tanokashira, D., Mamada, N., Yamamoto, F., Taniguchi, K., Tamaoka, A., Lakshmana, M. K., et al. (2017). The Neurotoxicity of Amyloid β-protein Oligomers Is Reversible in a Primary Neuron Model. Mol. Brain 10 (1), 4. doi:10.1186/s13041-016-0284-5
Taylor, E. B., and Rutter, J. (2011). Mitochondrial Quality Control by the Ubiquitin-Proteasome System. Biochem. Soc. Trans. 39 (5), 1509–1513. doi:10.1042/BST0391509
Teponno, R. B., Kusari, S., and Spiteller, M. (2016). Recent Advances in Research on Lignans and Neolignans. Nat. Prod. Rep. 33 (9), 1044–1092. doi:10.1039/c6np00021e
Volpicelli-Daley, L. A., Luk, K. C., Patel, T. P., Tanik, S. A., Riddle, D. M., Stieber, A., et al. (2011). Exogenous α-synuclein Fibrils Induce Lewy Body Pathology Leading to Synaptic Dysfunction and Neuron Death. Neuron 72 (1), 57–71. doi:10.1016/j.neuron.2011.08.033
Walsh, D. M., and Selkoe, D. J. (2004). Deciphering the Molecular Basis of Memory Failure in Alzheimer's Disease. Neuron 44 (1), 181–193. doi:10.1016/j.neuron.2004.09.010
Wang, C., Gan, D., Wu, J., Liao, M., Liao, X., and Ai, W. (2018). Honokiol Exerts Antidepressant Effects in Rats Exposed to Chronic Unpredictable Mild Stress by Regulating Brain Derived Neurotrophic Factor Level and Hypothalamus-Pituitary-Adrenal Axis Activity. Neurochem. Res. 43 (8), 1519–1528. doi:10.1007/s11064-018-2566-z
Wang, C., Guo, J., and Guo, R. (2017). Effect of XingPiJieYu Decoction on Spatial Learning and Memory and cAMP-PKA-CREB-BDNF Pathway in Rat Model of Depression through Chronic Unpredictable Stress. BMC Complement. Altern. Med. 17 (1), 73. doi:10.1186/s12906-016-1543-9
Wang, C. C., Lin, K. C., Lin, B. S., Chio, C. C., and Kuo, J. R. (2013). Resuscitation from Experimental Traumatic Brain Injury by Magnolol Therapy. J. Surg. Res. 184 (2), 1045–1052. doi:10.1016/j.jss.2013.04.059
Wang, L., Zhou, C., Zhu, D., Wang, X., Fang, L., Zhong, J., et al. (2016). Serotonin-1A Receptor Alterations in Depression: a Meta-Analysis of Molecular Imaging Studies. BMC Psychiatry 16 (1), 319. doi:10.1186/s12888-016-1025-0
Wang, M., Li, Y., Ni, C., and Song, G. (2017). Honokiol Attenuates Oligomeric Amyloid β1-42-Induced Alzheimer's Disease in Mice through Attenuating Mitochondrial Apoptosis and Inhibiting the Nuclear Factor Kappa-B Signaling Pathway. Cell Physiol. Biochem. 43 (1), 69–81. doi:10.1159/000480320
World Health Organization (2020). Top 10 Causes of Death. Available at: https://www.who.int/zh/news-room/fact-sheets/detail/the-top-10-causes-of-death (Accessed November 12, 2021).
Xia, Z., Zhang, C., Du, Y., Huang, W., Xing, Z., Cao, H., et al. (2019). The Effect of Traditional Chinese Medicine Zhike-Houpu Herbal Pair on Depressive Behaviors and Hippocampal Serotonin 1A Receptors in Rats after Chronic Unpredictable Mild Stress. Psychosom. Med. 81 (1), 100–109. doi:10.1097/PSY.0000000000000639
Xian, Y. F., Ip, S. P., Mao, Q. Q., Su, Z. R., Chen, J. N., Lai, X. P., et al. (2015). Honokiol Improves Learning and Memory Impairments Induced by Scopolamine in Mice. Eur. J. Pharmacol. 760, 88–95. doi:10.1016/j.ejphar.2015.04.013
Xian, Y. F., Qu, C., Liu, Y., Ip, S. P., Yuan, Q. J., Yang, W., et al. (2020). Magnolol Ameliorates Behavioral Impairments and Neuropathology in a Transgenic Mouse Model of Alzheimer's Disease. Oxid. Med. Cell Longev. 2020, 5920476. doi:10.1155/2020/5920476
Xiaoyan, L. (2016). Protective Effects of Orally Administered Honokiol on Cerebral Ischemia Reperfusion in Rats and on Stroke in SHRsp. J. Chin. Pharm. Sci. 25 (12), 882–891. doi:10.5246/jcps.2016.12.099
Xie, Z., Zhao, J., Wang, H., Jiang, Y., Yang, Q., Fu, Y., et al. (2020). Magnolol Alleviates Alzheimer’s Disease-like Pathology in Transgenic C. elegans by Promoting Microglia Phagocytosis and the Degradation of Beta-Amyloid through Activation of PPAR-Gamma. Biomed. Pharmacother. 124, 109886. doi:10.1016/j.biopha.2020.109886
Xu, Q., Yi, L. T., Pan, Y., Wang, X., Li, Y. C., Li, J. M., et al. (2008). Antidepressant-like Effects of the Mixture of Honokiol and Magnolol from the Barks of Magnolia Officinalis in Stressed Rodents. Prog. Neuropsychopharmacol. Biol. Psychiatry 32 (3), 715–725. doi:10.1016/j.pnpbp.2007.11.020
Yang, A. J. T., Bagit, A., and MacPherson, R. E. K. (2021). Resveratrol, Metabolic Dysregulation, and Alzheimer's Disease: Considerations for Neurogenerative Disease. Int. J. Mol. Sci. 22 (9), 4628. doi:10.3390/ijms22094628
Ye, Y. H., Li, C., Yang, J., Ma, L., Xiao, Y., Hu, J., et al. (2015). Construction of an Immobilised Acetylcholinesterase Column and its Application in Screening Insecticidal Constituents from Magnolia Officinalis. Pest Manag. Sci. 71 (4), 607–615. doi:10.1002/ps.3908
Zhang, B., Maniatis, T., Song, Y., Zhang, W., Zhang, X., Li, N., et al. (2008). Evaluation of Magnolia Bark Extract in Chromosomal Aberration Assays. Mutat. Res. 654 (2), 133–137. doi:10.1016/j.mrgentox.2008.05.009
Zhang, J., Chen, Z., Huang, X., Shi, W., Zhang, R., Chen, M., et al. (2019). Insights on the Multifunctional Activities of Magnolol. Biomed. Res. Int. 2019, 1847130. doi:10.1155/2019/1847130
Zhang, M., Qian, C., Zheng, Z. G., Qian, F., Wang, Y., Thu, P. M., et al. (2018). Jujuboside A Promotes Aβ Clearance and Ameliorates Cognitive Deficiency in Alzheimer's Disease through Activating Axl/HSP90/PPARγ Pathway. Theranostics 8 (15), 4262–4278. doi:10.7150/thno.26164
Zhang, P., Liu, X., Zhu, Y., Chen, S., Zhou, D., and Wang, Y. (2013). Honokiol Inhibits the Inflammatory Reaction during Cerebral Ischemia Reperfusion by Suppressing NF-Κb Activation and Cytokine Production of Glial Cells. Neurosci. Lett. 534, 123–127. doi:10.1016/j.neulet.2012.11.052
Zhao, X. R., Gonzales, N., and Aronowski, J. (2015). Pleiotropic Role of PPARγ in Intracerebral Hemorrhage: an Intricate System Involving Nrf2, RXR, and NF-Κb. CNS Neurosci. Ther. 21 (4), 357–366. doi:10.1111/cns.12350
Zhou, F., Jiang, Z., Yang, B., and Hu, Z. (2019). Magnolol Exhibits Anti-inflammatory and Neuroprotective Effects in a Rat Model of Intracerebral Haemorrhage. Brain Behav. Immun. 77, 161–167. doi:10.1016/j.bbi.2018.12.018
Keywords: neolignans, Magnolia officinalis, neuroprotective effect, multiple pathways, brain disorders
Citation: Zhu S, Liu F, Zhang R, Xiong Z, Zhang Q, Hao L and Chen S (2022) Neuroprotective Potency of Neolignans in Magnolia officinalis Cortex Against Brain Disorders. Front. Pharmacol. 13:857449. doi: 10.3389/fphar.2022.857449
Received: 18 January 2022; Accepted: 20 May 2022;
Published: 16 June 2022.
Edited by:
Paul Chazot, Durham University, United KingdomReviewed by:
Shupeng Li, Peking University, ChinaCopyright © 2022 Zhu, Liu, Zhang, Xiong, Zhang, Hao and Chen. This is an open-access article distributed under the terms of the Creative Commons Attribution License (CC BY). The use, distribution or reproduction in other forums is permitted, provided the original author(s) and the copyright owner(s) are credited and that the original publication in this journal is cited, in accordance with accepted academic practice. No use, distribution or reproduction is permitted which does not comply with these terms.
*Correspondence: Fang Liu, Mjk3OTMzOTk1QHFxLmNvbQ==; Shiyin Chen, NzY4MTc2MDBAcXEuY29t
Disclaimer: All claims expressed in this article are solely those of the authors and do not necessarily represent those of their affiliated organizations, or those of the publisher, the editors and the reviewers. Any product that may be evaluated in this article or claim that may be made by its manufacturer is not guaranteed or endorsed by the publisher.
Research integrity at Frontiers
Learn more about the work of our research integrity team to safeguard the quality of each article we publish.