- Department of Obstetrics and Gynecology, Shengjing Hospital of China Medical University, Shenyang, China
Energy metabolism reprogramming is the characteristic feature of tumors. The tumorigenesis, metastasis, and drug resistance of ovarian cancer (OC) is dependent on energy metabolism. Even under adequate oxygen conditions, OC cells tend to convert glucose to lactate, and glycolysis can rapidly produce ATP to meet their metabolic energy needs. Non-coding RNAs (ncRNAs) interact directly with DNA, RNA, and proteins to function as an essential regulatory in gene expression and tumor pathology. Studies have shown that ncRNAs regulate the process of glycolysis by interacting with the predominant glycolysis enzyme and cellular signaling pathway, participating in tumorigenesis and progression. This review summarizes the mechanism of ncRNAs regulation in glycolysis in OC and investigates potential therapeutic targets.
1 Introduction
Ovarian cancer (OC) is currently the most deadly gynecologic malignancy with insidious and rapidly progressive onset. Most patients have advanced pelvic and abdominal metastases by the time of diagnosis, and the 5-years survival rate is only 20–30% worldwide (Vafadar et al., 2020; DiSilvestro et al., 2021; Vergote et al., 2021). OC account for 5% of all cancer deaths in women (Yang et al., 2021; Youssef et al., 2021) due to the low survival rates resulting from late diagnosis. The standard treatment for OC is tumor resection combined with platinum-based chemotherapy. However, the majority with advanced disease will replase or even develop drug resistance, leading to curative failure and ultimately mortality (Giudice et al., 2021; Xie H et al., 2021; Xu et al., 2021). Therefore, it is essential to investigate new treatment options to improve the outcome of OC.
Tumorigenesis is considered an energy metabolic disease. Compared with metabolism of healthy and neoplastic cells, researchers found the oxidative phosphorylation pathway is dominant to provide ATP in normal cells, while the glycolytic pathway is the primary energy supply in tumor cells (Nakagawa et al., 2020; Tyagi et al., 2021). Even in the presence of sufficient oxygen, the glycolytic pathway, an alteration known as the Warburg effect, or aerobic glycolysis, accounts for over 95% of energy supply (Sun et al., 2018; Harris and Fenton 2019; Lu 2019). The altered glycolytic pathway is a characteristic difference between neoplastic and healthy cells (Icard et al., 2018). Tumor cells can produce more nucleotides, fatty acids, proteins, and ATP through enhanced aerobic glycolysis as the material basis for rapid proliferation and invasiveness (Poff et al., 2019). Meanwhile, the Warburg effect reduces reactive oxygen species production, improves cellular antioxidant capacity, and reduces apoptosis (Yue et al., 2016; Shulman and Rothman 2017; Yue et al., 2019). In addition, aerobic glycolysis can produce large amounts of lactic acid, which creates an acidic microenvironment to facilitate invasion and metastasis of the tumor cells (Schwartz et al., 2017; Tekade and Sun 2017; Chen et al., 2018).
Noncoding RNAs (ncRNAs) primarily include microRNAs (miRNAs), long noncoding RNAs (lncRNAs), and circular RNAs (circRNAs) (Jusic et al., 2020; Deogharia and Gurha 2021; Rahimian et al., 2021). The ncRNAs bind to multiple molecular targets to form regulatory networks in various biological activities, including initiating specific cellular biological responses, regulating gene expression, intracellular signaling, and epigenetic modifications (Ding et al., 2021; Ducoli and Detmar 2021). NcRNAs are involved in a variety of life activities such as regulation of gene expression, intracellular signaling and epigenetic modifications. Apart from participation in tumorigenesis, ncRNAs also account paramount role in the glycolytic process of tumors (Li Q et al., 2021; Lu et al., 2021; Park et al., 2021; Razavi et al., 2021; Wang et al., 2021). This review summarizes the possible molecular mechanisms of ncRNAs in the process of glycolysis and potentially effective targeted therapies for OC.
2 Glucose Metabolism in Neoplastic Cells
Reprogramming of energy metabolism is the hallmark of cancer. Healthy cells generally undergo glycolysis to produce lactate only under anaerobic conditions with limited energy production, while the glycolysis of tumor cells in aerobic conditions (Chandel 2021; Reinfeld et al., 2021). Although glycolysis produces low levels of ATP compared to oxidative phosphorylation, cancer cells can rapidly uptake the available ATP and intermediates from glycolysis for the transduction of the biosynthetic pathway (Bacigalupa and Rathmell 2020; Cao et al., 2020). The reprogrammed metabolism contributes to tumor cell metastasis, preventing apoptosis and promoting other malignant features.
2.1 Warburg Effect
Warburg effect is mainly a compensatory activity of tumor to adapt to the external environment (Lu et al., 2015; Cassim et al., 2020) (Figure 1) Efficient aerobic glycolysis facilitates tumor cell proliferation allowing tumor cells to produce abundant ATP from extracellular nutrients. Although the total energy produced per glucose during the Warburg effect is less than that by oxidative phosphorylation, ATP production by aerobic glycolysis can exceed that of oxidative phosphorylation with glucose available (Linehan and Rouault 2013; Hitosugi and Chen 2014). On the other hand, the Warburg effect provides tumor cells with intermediates for biosynthetic pathways, including ribose for nucleotide synthesis, glycerol, citrate, and nonessential amino acids for lipid synthesis (Ward and Thompson 2012; Upadhyay et al., 2013). Glucose can also produce nicotinamide adenine dinucleotide phosphate via the pentose phosphate pathway. Therefore, the Warburg effect is vital for facilitating tumor cell bioenergetics and biosynthesis.
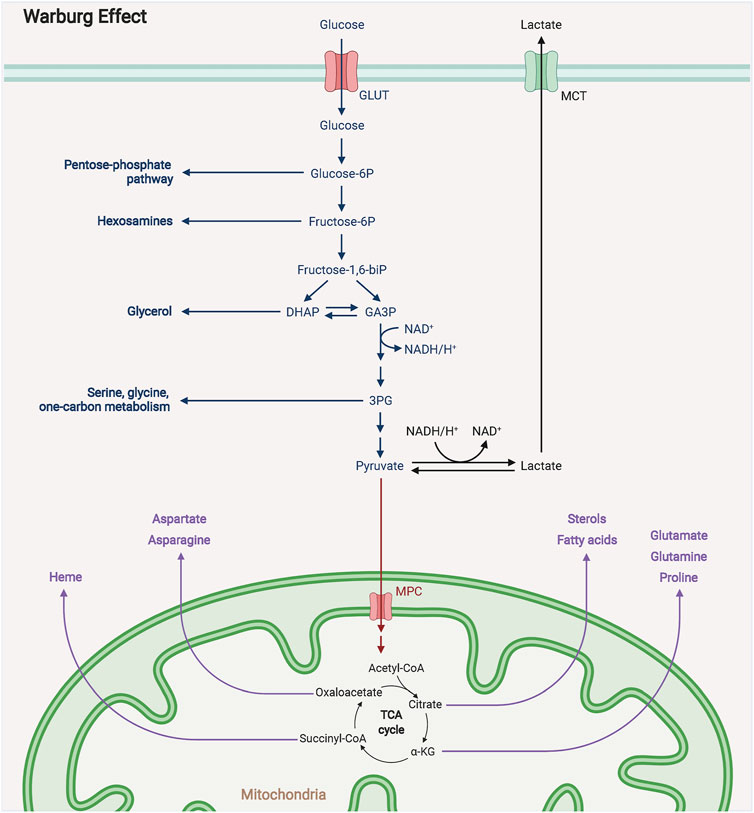
FIGURE 1. The mechanism diagram of Warburg effect. The Warburg effect states that in the presence of sufficient oxygen supply, tumor cells still prefer glycolysis for energy to the more efficient oxidative phosphorylation, a phenomenon known as the Warburg effect.
2.2 Factors Affecting Aerobic Glycolysis
2.2.1 GLUTs
Compared with healthy cells, tumor cells exhibit an efficient aerobic glycolysis rate, which requires increased glucose flux to improve the efficiency of glucose uptake (Yang et al., 2020). Therefore, the expression and activity of Glucose Transporters (GLUTs) and glycolytic rate-limiting enzymes, such as HK, PFK and PK were significantly upregulated in tumor cells to facilitate the inevitably increased glucose consumption (Foltynie 2019; Bommer et al., 2020; Faustman 2020). Oncogenes regulate GLUT1 to intervene the glucose intake and tumor cell metabolism. The c-myc induces GLUT1 overexpression leading to increased glucose uptake (Leen et al., 2013; Huang L et al., 2021; Su et al., 2021). P53 can inhibit GLUT1 expression in cells, resulting in decreased glucose uptake and thus inhibiting tumor development (Feng et al., 2018). GLUT3 is expressed in most cancer cells but rarely in normal cells, facilitating glucose consumption (Cazzato et al., 2021; Libby et al., 2021). Targeting GLUT can inhibit the degree of aerobic glycolysis, affecting tumorigenesis (Fu et al., 2021; Kim E et al., 2021).
2.2.2 HK Isoforms
Glycolysis is a complex process that starts with glucose catalyzation by various non-rate limiting and rate-limiting enzymes to form lactate (Ganapathy-Kanniappan 2018; Fan et al., 2019). The classical glycolysis involves three rate-limiting enzymes, HK, PFK, and PK, mediating different processes and playing essential roles in glucose metabolism (Shakespear et al., 2018; Yellen 2018), HK has four isoforms, HKI, HKII, HKIII, and HKIV, catalyzing glucose to glucose-6-phosphate (G6P) (Zuo et al., 2021). HKI and HKII present high affinity for mitochondria, and HK1 expression is present in most mammalian tissues (Zhong and Zhou 2017; Garcia et al., 2019). HKII is abundantly present in fat, heart, and skeletal muscle (Mathupala et al., 2009; Tan and Miyamoto 2015). with a higher glycolytic rate than HKI(Tan and Miyamoto 2015). HKIV, also known as glucokinase, is present in hepatocytes with the lowest affinity for glucose and no inhibition by G6P (Xu and Herschman 2019; Kasprzak 2021). HKII is essential for tumor metabolism. Increased expression of HKII promotes proliferation and is associated with poor prognosis in tumor patients (Roberts and Miyamoto 2015; Tan and Miyamoto 2015).
2.2.3 PFK and PK
Fructose 2, 6-bisphosphate (F26BP) can diminish the inhibition of ATP and increase glucose uptake by interacting with PFK1(Kalezic et al., 2021; Zuo et al., 2021). The substrate can abnormally inhibit PFK, and ATP has a dual effect on PFK (PK is an evolutionarily conserved metabolic enzyme that catalyzes pyruvate production from phosphoenolpyruvate) (Shen et al., 2020; Zhao et al., 2020). Almost all mammalian genomes, including humans, encode two PK genes, PKLR and PKM, which express four PK isoforms (L, R, M1, and M2) (Jyoti et al., 2020; Yang et al., 2021). PKL and PKR are encoded by the PKLR gene and are expressed in hepatocytes and erythrocytes, respectively (Park et al., 2020; Storkus et al., 2021). The PKM gene encodes PKM1 and PKM2 through selective splicing (Chen k et al., 2021; Itoyama et al., 2021). PKM1 is expressed in normal differentiated tissues (Zhong et al., 2021), while PKM2 is expressed in highly proliferative cells such as embryonic cells, stem cells and tumor cells (Wang et al., 2021). Physiologically, PKM1 exists as a tetramer, while PKM2 can exist as a tetramer or a dimer (Hu et al., 2020; Rai et al., 2020). Fructose 1,6-2 phosphate is a transactivator of PKM2 but has little effect on PKM1 (Xu et al., 2019; Angiari et al., 2020).
3 Tumor Aerobic Glycolytic Signaling Pathway
C-myc can regulate the transcriptional process of various glycolytic genes (Gu et al., 2017). C-myc can bind to the regulatory region of hexokinase 2 (HK2) and thus play an essential role in tumor aerobic glycolysis (Huang WL et al., 2021; Su et al., 2021). PK catalyzes the final step of glycolysis, PKM2, which is only found in self-renewable groups such as stem cells and tumors (Li et al., 2017; van Niekerk and Engelbrecht 2018). C-myc can directly activate the PKM2 promoter region and upregulate PKM2 expression, thus promoting tumor aerobic glycolysis (Li et al., 2017; Yin et al., 2019). In addition, c-myc can induce PKM2 splicing by indirectly regulating hnRNP protein, thus promoting aerobic glycolysis (Gu et al., 2017). Glucose-6-phosphate dehydrogenase is a key enzyme in the glucose metabolism pathway. C-myc binds to the promoter region of glucose-6-phosphate dehydrogenase to promote its expression and thus the pentose phosphate pathway (Tang et al., 2021).
Ras-mediated metabolic reprogramming provides vital functions in tumorigenesis (Lin et al., 2021). The Ras signaling pathway can promote aerobic glycolysis and provide lactate and α-ketoglutarate through various enzymes (Campbell and Philips 2021; Chen B et al., 2021). Ras can promote glucose uptake by upregulating the expression of GLUT1 on the cell membrane surface, which in turn increases aerobic glycolysis efficiency (Healy et al., 2021). In addition, PI3K-Akt-mTOR signaling is also a significant regulator of glucose uptake, promoting GLUT1 expression and protein translocation from the inner membrane to the cell surface (Krencz et al., 2021; Sanaei et al., 2021). P53 is the most critical oncogene, affecting the cell cycle by encoding transcription factors (Liu et al., 2019; Alvarado-Ortiz et al., 2020). P53 can inhibit aerobic glycolysis by regulating TP53-mediated glycolysis and apoptosis-inducing factor expression (Strycharz et al., 2017; Itahana and Itahana 2018; Smiles and Camera 2018), regulating mitochondrial respiratory function, pentose phosphate pathway, and glycolysis-related enzymes (Kruiswijk et al., 2015; Werner et al., 2016). PTEN proteins exert their tumor-suppressive effects through three predominant signaling pathways, PI3K/AKT, local adherens spot kinase and mitogen-activated protein kinase (Mendes et al., 2016). PTEN inhibits tumorigenesis by activating PI3K/AKT pathway (Ortega-Molina and Serrano 2013). Phosphoglycerate kinase 1 (PGK1) can function as a glycolytic enzyme or phosphorylated as a protein kinase (He et al., 2019; Zhang et al., 2019). PTEN directly interacts with PGK1 to control aerobic glycolysis in tumors, and PTEN encodes a protein with phosphatase activity that inhibits phosphorylated PGK1, which ultimately inhibits aerobic glycolysis and tumor cell proliferation (Nie et al., 2020; Chu et al., 2021).
4 The Regulatory Mechanism of ncRNAs in the Glycolysis of Ovarian Cancer
The ncRNAs can regulate the expression of criticalgenes or enzymes of glycolytic pathway through different cellular signaling pathways, which promote the malignant development by regulating glucose metabolism in OC. Here, we summarize the mechanisms of miRNAs, lncRNAs and circRNAs in the regulation of glycolysis in OC (Figure 2).
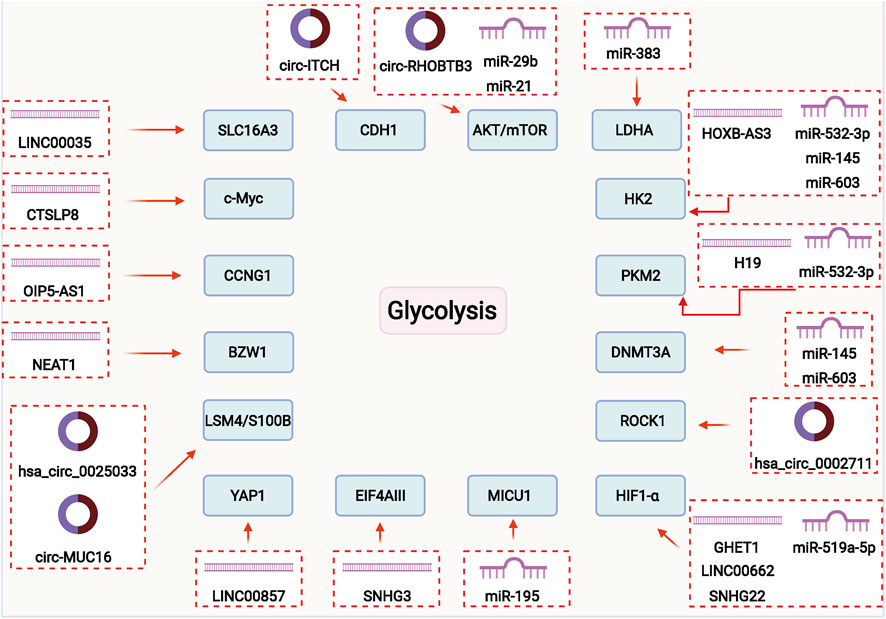
FIGURE 2. ncRNAs may play a vital role in regulating glycolysis of ovarian cancer through different signal pathways and mechanisms.
4.1 MicorRNAs in the Glycolysis of Ovarian Cancer
The miRNAs are a group of 18–24 nucleotide noncoding RNAs that bind to the 3-terminal noncoding region of the target mRNA, altering gene expression (Sakshi et al., 2021; Yang et al., 2021) (Figure 3). The aberrant expression of miRNA in tumor cells revealed that miRNAs play an essential role in tumor development by regulating the expression and function of their associated target genes and participating in a variety of physiological and pathological processes (Barrera-Rojas et al., 2021; Pidikova and Herichova 2021; Roy et al., 2021). Abundant miRNAs have been proved to regulate tumor metabolism and function as an essential role in the process of glycolysis in OC (Table 1).
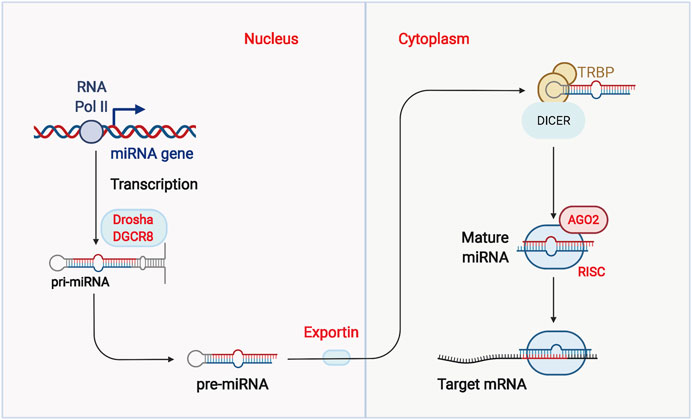
FIGURE 3. Biogenesis of micro RNAs (miRNAs). RNA polymerase II regulates the transcription of miRNAs. As pri-miRNAs are transcribed, pri-miRNAs are processed by several sequential cleavages to produce mature miRNAs. Finally, mature miRNAs are integrated into Argonaute to form the miRNA-induced silencing complex (RISC).
Studies have shown that miRNAs control the expression of several key enzymes of glycolysis to regulate the glycolytic process. As the critical rate-limiting enzymes of glycolysis, HK2 catalyzes the first irreversible step of glycolysis, which increases at significantly elevated levels in a variety of tumor cells. HK2 can significantly inhibit the function of mitochondria from regulating tumor growth, survival, and metastasis (Huang L et al., 2021; Yu et al., 2021). PKM2 becomes an essential component of tumorigenesis by providing a metabolic advantage that tumor cells can utilize the upstream lipids of glycolytic intermediates as precursors for lipid, amino acid, and nucleic acid synthesis (Xia et al., 2021; Yuan et al., 2021). Zhou et al., found that 20(S)-Rg3 significantly attenuated DNA methyltransferase 3 alpha (DNMT3A)-mediated methylation and promoted the inhibition of HK2 and PKM2 by miR-532–3p, thereby antagonizing the Warburg effect in OC cells (Zhou et al., 2018). Zhang et al., found that miR-145 could target DNMT3A to reduce methylation of the pre-miR-145 promoter region. The feedback loop between these two miRNA was a characteristic feature of the Warburg effect, promising a potential therapeutic target for OC(Mirzaei et al., 2016; Zhang et al., 2018). Lu et al., reported a similar regulatory machanism between miR-603 and DNMT3A, and the DNMT3A-miR-603-HK2 regulatory axis may be the critical molecular mechanism in the glycolytic pathway of OC(Lu et al., 2019; Pourhanifeh et al., 2020).
Lactate dehydrogenase A (LDHA) is an important metabolic enzyme belonging to the 2-hydroxy acid oxidoreductase family that plays a crucialrole in intracellular anaerobic sugar metabolism (Guan H et al., 2021; Huo et al., 2021). Hypoxic conditions induced the overexpression of LDHA, which shifts the metabolic pathway of ATP synthesis from oxidative phosphorylation to aerobic glycolysis. Therefore, the inhibition of LDHA is considered a promising strategy for tumor therapy (Jiang et al., 2021; Martinez-Ordonez et al., 2021). Han et al., demonstrated that miR-383 regulates LDHA expression in OC cells, impeding glycolysis, cell proliferation and invasion (Han et al., 2017). Tumor glycolytic activity is enhanced to adapt to ischemic and hypoxic environment by inducing an energy metabolic switch as the metabolic basis of its hypoxia tolerance (Wang et al., 2021). This process activateshypoxia-inducible factor-1 (HIF-1), a widely present dominant oxygen regulator in mammals, triggers various biological events, including glycolytic activation and tumorigenesis (Favier et al., 2015; Moldogazieva et al., 2020). Lu et al., reported that 20(S)-Rg3 upregulates miR-519a-5p expression by reducing DNMT3A-mediated DNA methylation of miR-519a-5p, thereby inhibiting HIF-1α and promoting the Warburg effect, leading to malignant progression of OC(Lu et al., 2020).
Aberrant activation and inactivation of oncogenes regulate abnormal energy metabolism to adapt to tumor growth demands (Yeung et al., 2008; Meijer et al., 2012). Teng et al., demonstrated that inhibition of miR-29b promotes the expression of AKT2/3, pakt2/3, HK2, and PKM2 and regulates pyruvate and NAD+/NADH levels (Teng et al., 2015). The miR-29b regulates the Warburg effect in OC by modulating AKT2/AKT3, which is a potential therapeutic target for OC. Moreover, miR-21 could promote AKT phosphorylation and glycolysis enzymes expression in OC(Guo et al., 2017). The miR-1180 could activate the Wnt signaling pathway and regulate the glycolysis progression of OC(Gu et al., 2019). Rao et al., demonstrated that miR-195 significantly inhibited tumor growth, increased tumor proliferation time, and improved overall survival by targeting MICU1 to inhibite glycolysis and chemoresistance (Rao et al., 2020).
4.2 LncRNAs in the Glycolysis of Ovarian Cancer
LncRNAs are a category of noncoding RNAs with over 200 nucleotides in length, tissue specificity and low species conservation (Jalaiei et al., 2021; Zhao et al., 2021). LncRNAs bind to proteins through their unique secondary structure to form RNA-protein complexes (Dashti et al., 2021; Janaththani et al., 2021; Mardani et al., 2021) and interact with multiple RNAs to form complex gene expression regulatory networks (Sun and Feinberg 2021; Wu et al., 2021). LncRNAs also target miRNAs through their 3′UTR region to regulate the effective concentration and activity, which affects the repressive effect on the target mRNAs(Sun and Feinberg 2021; Wu et al., 2021). (Figure 4). Above all, lncRNAs are the critical regulators in the process of glycolysis in OC (Table 2).
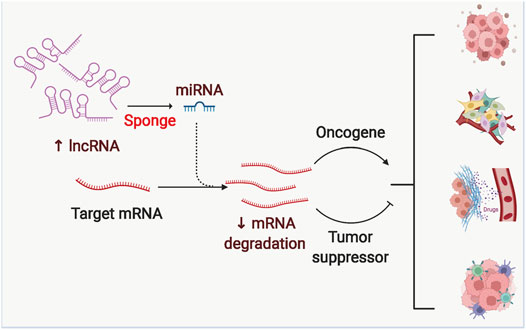
FIGURE 4. The competing endogenous RNA mechanism of Long noncoding RNAs (lncRNAs). LncRNAs can inhibit the degradation of downstream mRNAs by binding different miRNAs, which in turn regulates the expression of pro- or oncogenes, ultimately leading to malignant progression of tumors.
Small nucleolar RNA host gene 3 (SNHG3) promotes glycolysis and oxidative phosphorylation to induce OC drug resistance by binding to miR-186–5p and upregulating EIF4AIII expression (Li et al., 2018). H19 promotes glycolysis and malignant progression of OC by binding miR-324–5p to promote PKM2 expression (Zheng et al., 2018). LINC00857 acts as a pro-oncogene by binding miR-486–5p to promote Yes1 associated transcriptional regulator (YAP1) expression, promoting OC cell proliferation, migration, invasion, and glycolytic progression (Lin et al., 2020). Nuclear paraspeckle assembly transcript 1 (NEAT1) can play an essential role in OC malignant growth, metastasis and glycolysis by binding to miR-4500 and thus promoting basic leucine zipper and W2 domains 1 (BZW1) expression (Xu et al., 2020). HOXB-AS3 regulates both LDHA and ECAR expression by binding to miR-378a-3p in the glycolytic process of OC(Xu et al., 2021). OIP5-AS1 binds miR-128–3p to promote the expression of CCNG1, which leads to the malignant progression of OCthrough the glycolytic process (Liu et al., 2021). Moreover, LINC00504 is involved in the glycolytic process of OC by binding miR-1244. However, the specific downstream genes need more elaboration (Liu et al., 2020).
HIF is a nuclear transcription factor that facilitates cells to adapt to the hypoxic environment (Knutson et al., 2021; Cowman and Koh 2022). Liu et al., found that upregulation of gastric carcinoma proliferation enhancing transcript 1 (GHET1) positively correlated with tumor size, metastasis, proliferation, and colony formation in OC patients (Liu and Li 2019). Further studies confirmed that GHET1 interacted with von Hippel-Lindau (VHL) to prevent VHL-mediated hypoxia-inducible factor-1α (HIF-1α) degradation and increased HIF1α protein levels in OC cells. The up-regulated HIF-1α promoted glucose uptake and lactate production in OC cells. Tao et al., reported that LINC00662 was highly expressed in OC cells and was strongly associated with overall survival of OC patients (Tao et al., 2020). Mechanistic studies confirmed that LINC00662 act as a competitive RNA to regulate HIF-1α expression by directly binding to miR-375, which in turn regulates the proliferation and glycolysis of OC cells. Guan et al., found that SP1 and HIF1-α can promote SNHG22 expression and promote the glycolytic process and malignant progression of OC(Guan H et al., 2021).
In addition, there are lncRNAs that can directly regulate the expression of genes involved in the glycolytic process of OC. LINC00092 binds 6-phosphofructo-2-kinase/fructose-2,6-biphosphatase 2 (PFKFB2) and thus promotes malignant metastasis of OC by altering glycolysis and maintaining the local support function of cancer-associated fibroblasts (CAF) (Zhao et al., 2017; Hashemipour et al., 2021). Li et al., revealed that CTSLP8 expression increases in chemoresistant tumor tissues, which promotes c-Myc expression and thus upregulates glycolysis by facilitating the binding of PKM2 to the c-Myc promoter region (Li Q et al., 2021). Yang et al., demonstrated that LINC00035 promotes malignant progression of OC by regulating glycolysis and apoptosis through CEBPB-mediated SLC16A3 transcription (Yang et al., 2021).
4.3 circRNAs in the Glycolysis of Ovarian Cancer
Most circRNAs are expressed from known protein-coding genes and consist of exons forming a covalently closed loop structure by aberrant reverse splicing (Figure 5). CircRNA formation mechanisms included intron pairing-driven circularization, RNA-binding protein (RBP)-driven circularization, and lasso-driven circularization. The circRNAs play critical biological functions in eukaryotic organisms, which compete for miRNAs. By base-complementary pairing with the target mRNA 3-UTR, miRNAs can block the translation and stability of target RNA-binding Proteins (RBPs) can interact with circRNAs and regulate circRNA splicing, replication, folding, stabilization and localization (Huang and Zhu 2021; Zeng et al., 2021). In summary, the circRNAs act as miRNA sponges and interact with RBPs to perform transcriptional functions in organisms. The open reading frames in circRNAs enrich exosomes and can be translated into polypeptides for early diagnosis and prognosis (Kim H et al., 2021; Sinha et al., 2021; Wang et al., 2021). The circRNAs are critical in regulating the process of glycolysis in OC (Table 3).
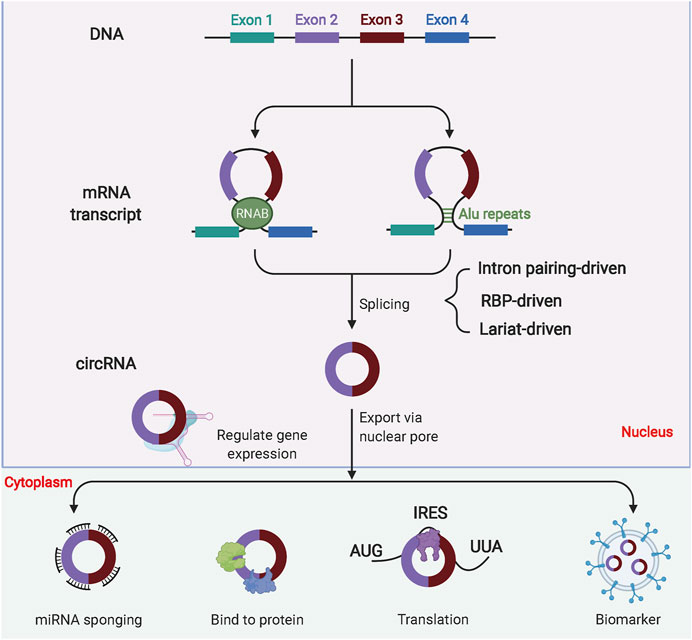
FIGURE 5. Biogenesis of circular RNAs (circRNAs). Most circRNAs are derived from pre-mRNA. Due to their composition, circRNAs are classified into several types, including exonic circRNAs, exon-intron circRNAs and intronic circRNAs. CircRNAs can perform biological functions by binding miRNAs, binding proteins or translating into polypeptides. In addition, circRNAs are also enriched in exosomes and are good markers for disease diagnosis.
Circ-ITCH was downregulated in OC and positively correlated with 5-years overall survival in OC patients (Lin et al., 2020) while the overexpression significantly inhibited proliferation, invasion, glycolysis and promoted apoptosis in OC cells. Sun et al., demonstrated the downregulation of circ-RHOBTB3 in OC tissues and cells, and overexpression significantly inhibited cell proliferation, metastasis, and glycolysis (Yalan et al., 2020). Circ-RHOBTB3 inhibited OC progression by inactivating the PI3K/AKT signaling pathway. The expression of hsa_circ_0025033 was found to be upregulated in OC, and downregulation of hsa_circ_0025033 significantly inhibited OC cell colony formation, migration/invasion and glycolytic metabolism (Hou and Zhang 2021). Hsa_circ_0025033 promotes LSM4 expression by binding miR-184. Xie et al., demonstrated that the hsa_circ_0002711/miR-1244/ROCK1 regulatory axis promotes malignant progression of OC in vivo by regulating Warburg effect and tumor growth (Xie W et al., 2021). Hsa_circ_MUC16 promotes OC cell proliferation, glycolytic metabolism, migration and invasion by targeting the miR-1182/S100B regulatory axis (Yang et al., 2021).
5 Future Perspectivesand Conclusion
The development and progression of OC is a complex physiological process. The invasion and metastasis of OC is a complicated process, which poses difficulties for early detection, intervention, and treatment (Tymon-Rosario et al., 2021; Wang et al., 2021). The Warburg effect is one of the recognized metabolic features of tumor cells (Abi Zamer et al., 2021; Nakagawa et al., 2021). Active glycolysis remains a common feature of cancer metabolism, and metabolic reprogramming increases the expression of critical enzymes and, ultimately, lactate secretion. Lactate in the tumor microenvironment can promote malignant progression and tumor immune escape (Hashemian et al., 2020; Mirzaei and Hamblin 2020; Holloway and Marignani 2021; Nakagawa et al., 2021). Various oncogenes and signaling pathways regulate the glycolytic enzymes to affect the rate of glycolysis (Almeida et al., 2021; Chandel 2021). Although the glycolytic process has drawn attention in the control of oncogenic features, the mechanisms of critical enzymes and complex interactions with signaling are not well studied in OC, considering the high heterogeneity of tumors.
Findings have confirmed the regulatory role of ncRNAs on the Warburg effect of tumor cells and highlight their significance in tumor biology research. The expression of specific ncRNAs in tumors predicts tumors’ biological properties and their possible outcomes and prognosis. On the other hand, ncRNAs may also become target sites for tumor treatment. However, there are still relatively few discoveries lacking systematic content and reliable clinical evidence. In summary, ncRNAs play an essential role in OC aerobic glycolysis, regulating the activity and content of specific enzymes and acting as transcriptional activators to regulate the expression of metabolism-related genes. In addition, these ncRNAs interact with other critical factors related to glucose metabolism and initiate various oncogenic processes. In the future, it is vital to confirm and elucidate the role of ncRNAs in OC aerobic glycolysis and their potential as molecular biomarkers. Investigating the correlation of ncRNA and aerobic glycolysis is promising for the interaction network of ncRNAs and the feedback regulation in tumorigenesis. Elucidating the mechanism of ncRNAs in the aerobic glycolysis of OC will provide new insights into OC research and provide new strategies for clinical treatment.
Author Contributions
Original draft preparation, allocation, supplementation and editing: CZ. Revision: NL and CZ, All authors have read and agreed to the published version of the manuscript.
Funding
This work was supported by the Shengjing Hospital of China Medical University (Shenyang) and China Medical University (Shenyang).
Conflict of Interest
The authors declare that the research was conducted in the absence of any commercial or financial relationships that could be construed as a potential conflict of interest.
Publisher’s Note
All claims expressed in this article are solely those of the authors and do not necessarily represent those of their affiliated organizations, or those of the publisher, the editors and the reviewers. Any product that may be evaluated in this article, or claim that may be made by its manufacturer, is not guaranteed or endorsed by the publisher.
Abbreviations
BZW1, basic leucine zipper and W2 domains 1; circRNAs, circular RNAs; CAF, cancer-associated fibroblasts; DNMT3A, DNA methyltransferase 3 alpha; F26BP, Fructose 2, 6-bisphosphate; GHET1, gastric carcinoma proliferation enhancing transcript 1; HK2, hexokinase 2; HIF-1, hypoxia-inducible factor-1; lncRNAs, long non-coding RNAs; LDHA, lactate dehydrogenase A; miRNAs, microRNAs; ncRNAs, Non-coding RNAs; NEAT1, nuclear paraspeckle assembly transcript 1; OC, Ovarian cancer; PKM, pyruvate kinase M1/2; PFKFB2, 6-phosphofructo-2-kinase/fructose-2,6-biphosphatase 2; RBP, RNA-binding protein; SNHG3, small nucleolar RNA host gene 3; VHL, von Hippel-Lindau; YAP1, Yes1 associated transcriptional regulator.
References
Abi Zamer, B., Abumustafa, W., Hamad, M., Maghazachi, A. A., and Muhammad, J. S. (2021). Genetic Mutations and Non-coding RNA-Based Epigenetic Alterations Mediating the Warburg Effect in Colorectal Carcinogenesis. Biology (Basel) 10, 849. doi:10.3390/biology10090847
Almeida, L., Dhillon-LaBrooy, A., Carriche, G., Berod, L., and Sparwasser, T. (2021). CD4+ T-Cell Differentiation and Function: Unifying Glycolysis, Fatty Acid Oxidation, Polyamines NAD Mitochondria. J. Allergy Clin. Immunol. 148, 16–32. doi:10.1016/j.jaci.2021.03.033
Alvarado-Ortiz, E., de la Cruz-López, K. G., Becerril-Rico, J., Sarabia-Sánchez, M. A., Ortiz-Sánchez, E., and García-Carrancá, A. (2020). Mutant P53 Gain-Of-Function: Role in Cancer Development, Progression, and Therapeutic Approaches. Front Cel Dev Biol 8, 607670. doi:10.3389/fcell.2020.607670
Angiari, S., Runtsch, M. C., Sutton, C. E., Palsson-McDermott, E. M., Kelly, B., Rana, N., et al. (2020). Pharmacological Activation of Pyruvate Kinase M2 Inhibits CD4+ T Cell Pathogenicity and Suppresses Autoimmunity. Cell Metab 31, 391–e8. doi:10.1016/j.cmet.2019.10.015
Bacigalupa, Z. A., and Rathmell, W. K. (2020). Beyond Glycolysis: Hypoxia Signaling as a Master Regulator of Alternative Metabolic Pathways and the Implications in clear Cell Renal Cell Carcinoma. Cancer Lett. 489, 19–28. doi:10.1016/j.canlet.2020.05.034
Barrera-Rojas, C. H., Otoni, W. C., and Nogueira, F. T. S. (2021). Shaping the Root System: the Interplay between miRNA Regulatory Hubs and Phytohormones. J. Exp. Bot. 72, 6822–6835. doi:10.1093/jxb/erab299
Bommer, G. T., Van Schaftingen, E., and Veiga-da-Cunha, M. (2020). Metabolite Repair Enzymes Control Metabolic Damage in Glycolysis. Trends Biochem. Sci. 45, 228–243. doi:10.1016/j.tibs.2019.07.004
Campbell, S. L., and Philips, M. R. (2021). Post-translational Modification of RAS Proteins. Curr. Opin. Struct. Biol. 71, 180–192. doi:10.1016/j.sbi.2021.06.015
Cao, L., Wu, J., Qu, X., Sheng, J., Cui, M., Liu, S., et al. (2020). Glycometabolic Rearrangements-Aaerobic Glycolysis in Pancreatic Cancer: Causes, Characteristics and Clinical Applications. J. Exp. Clin. Cancer Res. 39, 267. doi:10.1186/s13046-020-01765-x
Cassim, S., Vučetić, M., Ždralević, M., and Pouyssegur, J. (2020). Warburg and beyond: The Power of Mitochondrial Metabolism to Collaborate or Replace Fermentative Glycolysis in Cancer. Cancers (Basel) 12, 19. doi:10.3390/cancers12051119
Cazzato, G., Colagrande, A., Cimmino, A., Abbatepaolo, C., Bellitti, E., Romita, P., et al. (2021). GLUT1, GLUT3 Expression and 18FDG-PET/CT in Human Malignant Melanoma: What Relationship Exists? New Insights and Perspectives. Cells 10, 11.
Chandel, N. S. (2021). Glycolysis. Cold Spring Harb Perspect. Biol. 13, 5. doi:10.1101/cshperspect.a040535
Chen, B., Deng, Y. N., Wang, X., Xia, Z., He, Y., Zhang, P., et al. (2021). miR-26a Enhances Colorectal Cancer Cell Growth by Targeting RREB1 Deacetylation to Activate AKT-Mediated Glycolysis. Cancer Lett. 521, 1–13. doi:10.1016/j.canlet.2021.08.017
Chen, K., Zhang, Y., Qian, L., and Wang, P. (2021). Emerging Strategies to Target RAS Signaling in Human Cancer Therapy. J. Hematol. Oncol. 14, 116. doi:10.1186/s13045-021-01127-w
Chen, Z., Liu, M., Li, L., and Chen, L. (2018). Involvement of the Warburg Effect in Non-tumor Diseases Processes. J. Cel Physiol 233, 2839–2849. doi:10.1002/jcp.25998
Chu, Z., Huo, N., Zhu, X., Liu, H., Cong, R., Ma, L., et al. (2021). FOXO3A-induced LINC00926 Suppresses Breast Tumor Growth and Metastasis through Inhibition of PGK1-Mediated Warburg Effect. Mol. Ther. 29, 2737–2753. doi:10.1016/j.ymthe.2021.04.036
Cowman, S. J., and Koh, M. Y. (2022). Revisiting the HIF Switch in the Tumor and its Immune Microenvironment. Trends Cancer 8, 28–42. doi:10.1016/j.trecan.2021.10.004
Dashti, F., Mirazimi, S. M. A., Rabiei, N., Fathazam, R., Rabiei, N., Piroozmand, H., et al. (2021). The Role of Non-coding RNAs in Chemotherapy for Gastrointestinal Cancers. Mol. Ther. Nucleic Acids 26, 892–926. doi:10.1016/j.omtn.2021.10.004
Deogharia, M., and Gurha, P. (2021). The "guiding" Principles of Noncoding RNA Function. Wiley Interdiscip Rev RNA, e1704.
Ding, L., Wang, R., Shen, D., Cheng, S., Wang, H., Lu, Z., et al. (2021). Role of Noncoding RNA in Drug Resistance of Prostate Cancer. Cell Death Dis 12, 590. doi:10.1038/s41419-021-03854-x
DiSilvestro, P., Colombo, N., Harter, P., González-Martín, A., Ray-Coquard, I., and Coleman, R. L. (2021). Maintenance Treatment of Newly Diagnosed Advanced Ovarian Cancer: Time for a Paradigm Shift? Cancers (Basel) 13, 756. doi:10.3390/cancers13225756
Ducoli, L., and Detmar, M. (2021). Beyond PROX1: Transcriptional, Epigenetic, and Noncoding RNA Regulation of Lymphatic Identity and Function. Dev. Cel 56, 406–426. doi:10.1016/j.devcel.2021.01.018
Fan, T., Sun, G., Sun, X., Zhao, L., Zhong, R., and Peng, Y. (2019). Tumor Energy Metabolism and Potential of 3-Bromopyruvate as an Inhibitor of Aerobic Glycolysis: Implications in Tumor Treatment. Cancers (Basel) 11, 317. doi:10.3390/cancers11030317
Faustman, D. L. (2020). Benefits of BCG-Induced Metabolic Switch from Oxidative Phosphorylation to Aerobic Glycolysis in Autoimmune and Nervous System Diseases. J. Intern. Med. 288 6, 641–650. doi:10.1111/joim.13050
Favier, F. B., Britto, F. A., Freyssenet, D. G., Bigard, X. A., and Benoit, H. (2015). HIF-1-driven Skeletal Muscle Adaptations to Chronic Hypoxia: Molecular Insights into Muscle Physiology. Cell Mol Life Sci 72, 4681–4696. doi:10.1007/s00018-015-2025-9
Feng, Y., Wu, M., Li, S., He, X., Tang, J., Peng, W., et al. (2018). The Epigenetically Downregulated Factor CYGB Suppresses Breast Cancer through Inhibition of Glucose Metabolism. J. Exp. Clin. Cancer Res. 37, 313. doi:10.1186/s13046-018-0979-9
Foltynie, T. (2019). Glycolysis as a Therapeutic Target for Parkinson's Disease. Lancet Neurol. 18, 1072–1074. doi:10.1016/S1474-4422(19)30404-1
Fu, C., Fu, Z., Jiang, C., Xia, C., Zhang, Y., Gu, X., et al. (2021). CD205 + Polymorphonuclear Myeloid‐derived Suppressor Cells Suppress Antitumor Immunity by Overexpressing GLUT3. Cancer Sci. 112, 1011–1025. doi:10.1111/cas.14783
Ganapathy-Kanniappan, S. (2018). Molecular Intricacies of Aerobic Glycolysis in Cancer: Current Insights into the Classic Metabolic Phenotype. Crit. Rev. Biochem. Mol. Biol. 53, 667–682. doi:10.1080/10409238.2018.1556578
Garcia, S. N., Guedes, R. C., and Marques, M. M. (2019). Unlocking the Potential of HK2 in Cancer Metabolism and Therapeutics. Curr. Med. Chem. 26, 7285–7322. doi:10.2174/0929867326666181213092652
Giudice, E., Salutari, V., Ricci, C., Nero, C., Carbone, M. V., Ghizzoni, V., et al. (2021). Gut Microbiota and its Influence on Ovarian Cancer Carcinogenesis, Anticancer Therapy and Surgical Treatment: A Literature Review. Crit. Rev. Oncol. Hematol. 168, 103542. doi:10.1016/j.critrevonc.2021.103542
Gu, Z., Xia, J., Xu, H., Frech, I., Tricot, G., and Zhan, F. (2017). NEK2 Promotes Aerobic Glycolysis in Multiple Myeloma through Regulating Splicing of Pyruvate Kinase. J. Hematol. Oncol. 10, 17. doi:10.1186/s13045-017-0392-4
Gu, Z. W., He, Y. F., Wang, W. J., Tian, Q., and Di, W. (2019). MiR-1180 from Bone Marrow-Derived Mesenchymal Stem Cells Induces Glycolysis and Chemoresistance in Ovarian Cancer Cells by Upregulating the Wnt Signaling Pathway. J. Zhejiang Univ. Sci. B 20, 219–237. doi:10.1631/jzus.B1800190
Guan, H., Luo, W., Liu, Y., and Li, M. (2021). Novel Circular RNA circSLIT2 Facilitates the Aerobic Glycolysis of Pancreatic Ductal Adenocarcinoma via miR-510-5p/c-Myc/LDHA axis. Cel Death Dis 12, 645. doi:10.1038/s41419-021-03918-y
Guan, N., Zheng, H., Wu, X., Xie, L., and Tong, X. (2021). SP1-Regulated Non-coding RNA SNHG22 Promotes Ovarian Cancer Growth and Glycolysis. Cancer Manag. Res. 13, 7299–7309. doi:10.2147/CMAR.S318378
Guo, N. L., Zhang, J. X., Wu, J. P., and Xu, Y. H. (2017). Isoflurane Promotes Glucose Metabolism through Up-Regulation of miR-21 and Suppresses Mitochondrial Oxidative Phosphorylation in Ovarian Cancer Cells. Biosci. Rep. 37, 6. doi:10.1042/BSR20170818
Han, R. L., Wang, F. P., Zhang, P. A., Zhou, X. Y., and Li, Y. (2017). miR-383 Inhibits Ovarian Cancer Cell Proliferation, Invasion and Aerobic Glycolysis by Targeting LDHA. Neoplasma 64, 244–252. doi:10.4149/neo_2017_211
Harris, R. A., and Fenton, A. W. (20191871). A Critical Review of the Role of M2PYK in the Warburg Effect. Biochim. Biophys. Acta Rev. Cancer 1871, 225–239. doi:10.1016/j.bbcan.2019.01.004
Hashemian, S. M., Pourhanifeh, M. H., Fadaei, S., Velayati, A. A., Mirzaei, H., and Hamblin, M. R. (2020). Non-coding RNAs and Exosomes: Their Role in the Pathogenesis of Sepsis. Mol. Ther. Nucleic Acids 21, 51–74. doi:10.1016/j.omtn.2020.05.012
Hashemipour, M., Boroumand, H., Mollazadeh, S., Tajiknia, V., Nourollahzadeh, Z., Rohani Borj, M., et al. (2021). Exosomal microRNAs and Exosomal Long Non-coding RNAs in Gynecologic Cancers. Gynecol. Oncol. 161, 314–327. doi:10.1016/j.ygyno.2021.02.004
He, Y., Luo, Y., Zhang, D., Wang, X., Zhang, P., Li, H., et al. (2019). PGK1-mediated Cancer Progression and Drug Resistance. Am. J. Cancer Res. 9, 2280–2302.
Healy, F. M., Prior, I. A., and MacEwan, D. J. (2021). The Importance of Ras in Drug Resistance in Cancer. Br. J. Pharmacol. 15, 420. doi:10.1111/bph.15420
Hitosugi, T., and Chen, J. (2014). Post-translational Modifications and the Warburg Effect. Oncogene 33, 4279–4285. doi:10.1038/onc.2013.406
Holloway, R. W., and Marignani, P. A. (2021). Targeting mTOR and Glycolysis in HER2-Positive Breast Cancer. Cancers (Basel) 13, 122. doi:10.3390/cancers13122922
Hou, W., and Zhang, Y. (2021). Circ_0025033 Promotes the Progression of Ovarian Cancer by Activating the Expression of LSM4 via Targeting miR-184. Pathol. Res. Pract. 217, 153275. doi:10.1016/j.prp.2020.153275
Hu, X. K., Rao, S. S., Tan, Y. J., Yin, H., Luo, M. J., Wang, Z. X., et al. (2020). Fructose-coated Angstrom Silver Inhibits Osteosarcoma Growth and Metastasis via Promoting ROS-dependent Apoptosis through the Alteration of Glucose Metabolism by Inhibiting PDK. Theranostics 10, 7710–7729. doi:10.7150/thno.45858
Huang, L., He, C., Zheng, S., Wu, C., Ren, M., and Shan, Y. (2021). AKT1/HK2 Axis-mediated Glucose Metabolism: A Novel Therapeutic Target of Sulforaphane in Bladder Cancer. Weinheim, Germany: Mol Nutr Food Res, e2100738.
Huang, W. L., Abudureheman, T., Xia, J., Chu, L., Zhou, H., Zheng, W. W., et al. (2021). CDK9 Inhibitor Induces the Apoptosis of B-Cell Acute Lymphocytic Leukemia by Inhibiting C-Myc-Mediated Glycolytic Metabolism. Front. Cel Dev Biol 9, 641271. doi:10.3389/fcell.2021.641271
Huang, Y., and Zhu, Q. (2021). Mechanisms Regulating Abnormal Circular RNA Biogenesis in Cancer. Cancers (Basel) 13, 164185. doi:10.3390/cancers13164185
Huo, N., Cong, R., Sun, Z. J., Li, W. C., Zhu, X., Xue, C. Y., et al. (2021). STAT3/LINC00671 axis Regulates Papillary Thyroid Tumor Growth and Metastasis via LDHA-Mediated Glycolysis. Cel Death Dis 12, 799. doi:10.1038/s41419-021-04081-0
Icard, P., Shulman, S., Farhat, D., Steyaert, J. M., Alifano, M., and Lincet, H. (2018). How the Warburg Effect Supports Aggressiveness and Drug Resistance of Cancer Cells? Drug Resist. Updat 38, 1–11. doi:10.1016/j.drup.2018.03.001
Itahana, Y., and Itahana, K. (2018). Emerging Roles of P53 Family Members in Glucose Metabolism. Int. J. Mol. Sci. 19, 3. doi:10.3390/ijms19030776
Itoyama, R., Yasuda-Yoshihara, N., Kitamura, F., Yasuda, T., Bu, L., Yonemura, A., et al. (2021). Metabolic Shift to Serine Biosynthesis through 3-PG Accumulation and PHGDH Induction Promotes Tumor Growth in Pancreatic Cancer. Cancer Lett. 523, 29–42. doi:10.1016/j.canlet.2021.09.007
Jalaiei, A., Asadi, M. R., Sabaie, H., Dehghani, H., Gharesouran, J., Hussen, B. M., et al. (2021). Long Non-coding RNAs, Novel Offenders or Guardians in Multiple Sclerosis: A Scoping Review. Front. Immunol. 12, 774002. doi:10.3389/fimmu.2021.774002
Janaththani, P., Srinivasan, S. L., and Batra, J. (2021). Long Non-coding RNAs at the Chromosomal Risk Loci Identified by Prostate and Breast Cancer GWAS. Genes (Basel) 12, 122028. doi:10.3390/genes12122028
Jiang, Y., Li, F., Gao, B., Ma, M., Chen, M., Wu, Y., et al. (2021). KDM6B-mediated Histone Demethylation of LDHA Promotes Lung Metastasis of Osteosarcoma. Theranostics 11, 3868–3881. doi:10.7150/thno.53347
Jusic, A., Devaux, Y., and ActionU-CardioRNA Cost, E. (2020). Mitochondrial Noncoding RNA-Regulatory Network in Cardiovascular Disease. Basic Res. Cardiol. 115, 23. doi:10.1007/s00395-020-0783-5
Jyoti, P., Shree, M., Joshi, C., Prakash, T., Ray, S. K., Satapathy, S. S., et al. (2020). The Entner-Doudoroff and Nonoxidative Pentose Phosphate Pathways Bypass Glycolysis and the Oxidative Pentose Phosphate Pathway in Ralstonia Solanacearum. mSystems 5, 20. doi:10.1128/mSystems.00091-20
Kalezic, A., Udicki, M., Srdic Galic, B., Aleksic, M., Korac, A., Jankovic, A., et al. (2021). Tissue-Specific Warburg Effect in Breast Cancer and Cancer-Associated Adipose Tissue-Relationship between AMPK and Glycolysis. Cancers (Basel) 13, 112731. doi:10.3390/cancers13112731
Kasprzak, A. (2021). Insulin-Like Growth Factor 1 (IGF-1) Signaling in Glucose Metabolism in Colorectal Cancer. Int. J. Mol. Sci. 22, 126434. doi:10.3390/ijms22126434
Kim, E., Kim, Y. K., and Lee, S. V. (2021). Emerging Functions of Circular RNA in Aging. Trends Genet. 4, 14. doi:10.1016/j.tig.2021.04.014
Kim, H., Son, S., Ko, Y., and Shin, I. (2021). CTGF Regulates Cell Proliferation, Migration, and Glucose Metabolism through Activation of FAK Signaling in Triple-Negative Breast Cancer. Oncogene 40, 2667–2681. doi:10.1038/s41388-021-01731-7
Knutson, A. K., Williams, A. L., Boisvert, W. A., and Shohet, R. V. (2021). HIF in the Heart: Development, Metabolism, Ischemia, and Atherosclerosis. J. Clin. Invest. 131, 17. doi:10.1172/JCI137557
Krencz, I., Sztankovics, D., Danko, T., Sebestyen, A., and Khoor, A. (2021). Progression and Metastasis of Small Cell Lung Carcinoma: the Role of the PI3K/Akt/mTOR Pathway and Metabolic Alterations. Cancer Metastasis Rev. 21, 12. doi:10.1007/s10555-021-10012-4
Kruiswijk, F., Labuschagne, C. F., and Vousden, K. H. (2015). p53 in Survival, Death and Metabolic Health: a Lifeguard with a Licence to Kill. Nat. Rev. Mol. Cel Biol 16, 393–405. doi:10.1038/nrm4007
Leen, W. G., Wevers, R. A., Kamsteeg, E. J., Scheffer, H., Verbeek, M. M., and Willemsen, M. A. (2013). Cerebrospinal Fluid Analysis in the Workup of GLUT1 Deficiency Syndrome: a Systematic Review. JAMA Neurol. 70, 1440–1444. doi:10.1001/jamaneurol.2013.3090
Li, K., Cheng, J., Lu, H., Yang, W., Zhou, J., and Cen, K. (2017). Transcriptome-based Analysis on Carbon Metabolism of Haematococcus pluvialis Mutant under 15% CO2. Bioresour. Technol. 233, 313–321. doi:10.1016/j.biortech.2017.02.121
Li, N., Zhan, X., and Zhan, X. (2018). The lncRNA SNHG3 Regulates Energy Metabolism of Ovarian Cancer by an Analysis of Mitochondrial Proteomes. Gynecol. Oncol. 150, 343–354. doi:10.1016/j.ygyno.2018.06.013
Li, Q., Sun, H., Luo, D., Gan, L., Mo, S., Dai, W., et al. (2021). Lnc-RP11-536 K7.3/SOX2/HIF-1α Signaling axis Regulates Oxaliplatin Resistance in Patient-Derived Colorectal Cancer Organoids. J. Exp. Clin. Cancer Res. 40, 348. doi:10.1186/s13046-021-02143-x
Li, X., Zhang, Y., Wang, X., Lin, F., Cheng, X., Wang, Z., et al. (2021). Long Non-coding RNA CTSLP8 Mediates Ovarian Cancer Progression and Chemotherapy Resistance by Modulating Cellular Glycolysis and Regulating C-Myc Expression through PKM2. Cell Biol Toxicol 21, 9. doi:10.1007/s10565-021-09650-9
Libby, C. J., Gc, S., Benavides, G. A., Fisher, J. L., Williford, S. E., Zhang, S., et al. (2021). A Role for GLUT3 in Glioblastoma Cell Invasion that Is Not Recapitulated by GLUT1. Cell Adhes. Migration 15, 101–115. doi:10.1080/19336918.2021.1903684
Lin, C., Xu, X., Yang, Q., Liang, L., and Qiao, S. (2020). Circular RNA ITCH Suppresses Proliferation, Invasion, and Glycolysis of Ovarian Cancer Cells by Up-Regulating CDH1 via Sponging miR-106a. Cancer Cel Int 20, 336. doi:10.1186/s12935-020-01420-7
Lin, L., Miao, L., Lin, H., Cheng, J., Li, M., Zhuo, Z., et al. (2021). Targeting RAS in Neuroblastoma: Is it Possible? Pharmacol. Ther. 236, 108054. doi:10.1016/j.pharmthera.2021.108054
Lin, X., Feng, D., Li, P., and Lv, Y. (2020). LncRNA LINC00857 Regulates the Progression and Glycolysis in Ovarian Cancer by Modulating the Hippo Signaling Pathway. Cancer Med. 9, 8122–8132. doi:10.1002/cam4.3322
Linehan, W. M., and Rouault, T. A. (2013). Molecular Pathways: Fumarate Hydratase-Deficient Kidney Cancer-Ttargeting the Warburg Effect in Cancer. Clin. Cancer Res. 19, 3345–3352. doi:10.1158/1078-0432.CCR-13-0304
Liu, D., and Li, H. (2019). Long Non-coding RNA GEHT1 Promoted the Proliferation of Ovarian Cancer Cells via Modulating the Protein Stability of HIF1α. Biosci. Rep. 39, 5. doi:10.1042/BSR20181650
Liu, J., Zhang, C., Hu, W., and Feng, Z. (2019). Tumor Suppressor P53 and Metabolism. J. Mol. Cel Biol 11, 284–292. doi:10.1093/jmcb/mjy070
Liu, Y., He, X., Chen, Y., and Cao, D. (2020). Long Non-coding RNA LINC00504 Regulates the Warburg Effect in Ovarian Cancer through Inhibition of miR-1244. Mol. Cel Biochem 464 (1-2), 39–50. doi:10.1007/s11010-019-03647-z
Liu, Y., Fu, X., Wang, X., Liu, Y., and Song, X. (2021). Long Noncoding RNA OIP5AS1 Facilitates the Progression of Ovarian Cancer via the miR1283p/CCNG1 axis. Mol. Med. Rep. 23, 5. doi:10.3892/mmr.2021.12027
Lu, J., Chen, H., He, F., You, Y., Feng, Z., Chen, W., et al. (2020). Ginsenoside 20(S)-Rg3 Upregulates HIF-1α-Targeting miR-519a-5p to Inhibit the Warburg Effect in Ovarian Cancer Cells. Clin. Exp. Pharmacol. Physiol. 47, 1455–1463. doi:10.1111/1440-1681.13321
Lu, J., Tan, M., and Cai, Q. (2015). The Warburg Effect in Tumor Progression: Mitochondrial Oxidative Metabolism as an Anti-metastasis Mechanism. Cancer Lett. 356, 156–164. doi:10.1016/j.canlet.2014.04.001
Lu, J. (2019). The Warburg Metabolism Fuels Tumor Metastasis. Cancer Metastasis Rev. 38 (1-2), 157–164. doi:10.1007/s10555-019-09794-5
Lu, J., Wang, L., Chen, W., Wang, Y., Zhen, S., Chen, H., et al. (2019). miR-603 Targeted Hexokinase-2 to Inhibit the Malignancy of Ovarian Cancer Cells. Arch. Biochem. Biophys. 661, 1–9. doi:10.1016/j.abb.2018.10.014
Lu, S., Han, L., Hu, X., Sun, T., Xu, D., Li, Y., et al. (2021). N6-methyladenosine Reader IMP2 Stabilizes the ZFAS1/OLA1 axis and Activates the Warburg Effect: Implication in Colorectal Cancer. J. Hematol. Oncol. 14, 188. doi:10.1186/s13045-021-01204-0
Mardani, M., Rashedi, S., Keykhaei, M., Farrokhpour, H., Azadnajafabad, S., Tavolinejad, H., et al. (2022). Long Non-coding RNAs (lncRNAs) as Prognostic and Diagnostic Biomarkers in Multiple Myeloma: A Systematic Review and Meta-Analysis. Pathol. Res. Pract. 229, 153726. doi:10.1016/j.prp.2021.153726
Martínez-Ordoñez, A., Seoane, S., Avila, L., Eiro, N., Macía, M., Arias, E., et al. (2021). POU1F1 Transcription Factor Induces Metabolic Reprogramming and Breast Cancer Progression via LDHA Regulation. Oncogene 40, 2725–2740. doi:10.1038/s41388-021-01740-6
Mathupala, S. P., Ko, Y. H., and Pedersen, P. L. (2009). Hexokinase-2 Bound to Mitochondria: Cancer's Stygian Link to the "Warburg Effect" and a Pivotal Target for Effective Therapy. Semin. Cancer Biol. 19, 17–24. doi:10.1016/j.semcancer.2008.11.006
Meijer, T. W., Kaanders, J. H., Span, P. N., and Bussink, J. (2012). Targeting Hypoxia, HIF-1, and Tumor Glucose Metabolism to Improve Radiotherapy Efficacy. Clin. Cancer Res. 18, 5585–5594. doi:10.1158/1078-0432.CCR-12-0858
Mendes, R. D., Canté-Barrett, K., Pieters, R., and Meijerink, J. P. (2016). The Relevance of PTEN-AKT in Relation to NOTCH1-Directed Treatment Strategies in T-Cell Acute Lymphoblastic Leukemia. Haematologica 101, 1010–1017. doi:10.3324/haematol.2016.146381
Mirzaei, H., and Hamblin, M. R. (2020). Regulation of Glycolysis by Non-coding RNAs in Cancer: Switching on the Warburg Effect. Mol. Ther. Oncolytics 19, 218–239. doi:10.1016/j.omto.2020.10.003
Mirzaei, H., Yazdi, F., Salehi, R., and Mirzaei, H. R. (2016). SiRNA and Epigenetic Aberrations in Ovarian Cancer. J. Cancer Res. Ther. 12, 498–508. doi:10.4103/0973-1482.153661
Moldogazieva, N. T., Mokhosoev, I. M., and Terentiev, A. A. (2020). Metabolic Heterogeneity of Cancer Cells: An Interplay between HIF-1, GLUTs, and AMPK. Cancers (Basel) 12, 40862. doi:10.3390/cancers12040862
Nakagawa, T., Lanaspa, M. A., Millan, I. S., Fini, M., Rivard, C. J., Sanchez-Lozada, L. G., et al. (2020). Fructose Contributes to the Warburg Effect for Cancer Growth. Cancer Metab. 8, 16. doi:10.1186/s40170-020-00222-9
Nakagawa, T., Sanchez-Lozada, L. G., Andres-Hernando, A., Kojima, H., Kasahara, M., Rodriguez-Iturbe, B., et al. (2021). Endogenous Fructose Metabolism Could Explain the Warburg Effect and the Protection of SGLT2 Inhibitors in Chronic Kidney Disease. Front. Immunol. 12, 694457. doi:10.3389/fimmu.2021.694457
Nie, H., Ju, H., Fan, J., Shi, X., Cheng, Y., Cang, X., et al. (2020). O-GlcNAcylation of PGK1 Coordinates Glycolysis and TCA Cycle to Promote Tumor Growth. Nat. Commun. 11, 36. doi:10.1038/s41467-019-13601-8
Ortega-Molina, A., and Serrano, M. (2013). PTEN in Cancer, Metabolism, and Aging. Trends Endocrinol. Metab. 24, 184–189. doi:10.1016/j.tem.2012.11.002
Park, J. S., Burckhardt, C. J., Lazcano, R., Solis, L. M., Isogai, T., Li, L., et al. (2020). Mechanical Regulation of Glycolysis via Cytoskeleton Architecture. Nature 578, 621–626. doi:10.1038/s41586-020-1998-1
Park, M. K., Zhang, L., Min, K.-W., Cho, J.-H., Yeh, C.-C., Moon, H., et al. (2021). NEAT1 Is Essential for Metabolic Changes that Promote Breast Cancer Growth and Metastasis. Cel Metab. 33, 2380–2397. doi:10.1016/j.cmet.2021.11.011
Pidíková, P., and Herichová, I. (2021). miRNA Clusters with Up-Regulated Expression in Colorectal Cancer. Cancers (Basel) 13, 122979. doi:10.3390/cancers13122979
Poff, A., Koutnik, A. P., Egan, K. M., Sahebjam, S., D'Agostino, D., and Kumar, N. B. (2019). Targeting the Warburg Effect for Cancer Treatment: Ketogenic Diets for Management of Glioma. Semin. Cancer Biol. 56, 135–148. doi:10.1016/j.semcancer.2017.12.011
Pourhanifeh, M. H., Darvish, M., Tabatabaeian, J., Fard, M. R., Mottaghi, R., Azadchehr, M. J., et al. (2020). Therapeutic Role of Curcumin and its Novel Formulations in Gynecological Cancers. J. Ovarian Res. 13, 130. doi:10.1186/s13048-020-00731-7
Rahimian, N., Razavi, Z. S., Aslanbeigi, F., Mirkhabbaz, A. M., Piroozmand, H., Shahrzad, M. K., et al. (2021). Non-coding RNAs Related to Angiogenesis in Gynecological Cancer. Gynecol. Oncol. 161, 896–912. doi:10.1016/j.ygyno.2021.03.020
Rai, G., Urban, D. J., Mott, B. T., Hu, X., Yang, S. M., Benavides, G. A., et al. (2020). Pyrazole-Based Lactate Dehydrogenase Inhibitors with Optimized Cell Activity and Pharmacokinetic Properties. J. Med. Chem. 63, 10984–11011. doi:10.1021/acs.jmedchem.0c00916
Rao, G., Dwivedi, S. K. D., Zhang, Y., Dey, A., Shameer, K., Karthik, R., et al. (2020). MicroRNA-195 Controls MICU1 Expression and Tumor Growth in Ovarian Cancer. EMBO Rep. 21, e48483. doi:10.15252/embr.201948483
Razavi, Z. S., Tajiknia, V., Majidi, S., Ghandali, M., Mirzaei, H. R., Rahimian, N., et al. (2021). Gynecologic Cancers and Non-coding RNAs: Epigenetic Regulators with Emerging Roles. Crit. Rev. Oncol. Hematol. 157, 103192. doi:10.1016/j.critrevonc.2020.103192
Reinfeld, B. I., Rathmell, W. K., Kim, T. K., and Rathmell, J. C. (2021). The Therapeutic Implications of Immunosuppressive Tumor Aerobic Glycolysis. Cell Mol Immunol 21, 727. doi:10.1038/s41423-021-00727-3
Roberts, D. J., and Miyamoto, S. (2015). Hexokinase II Integrates Energy Metabolism and Cellular protection: Akting on Mitochondria and TORCing to Autophagy. Cell Death Differ 22, 364–257. doi:10.1038/cdd.2014.208
Roy, B., Ghose, S., and Biswas, S. (2021). Therapeutic Strategies for miRNA Delivery to Reduce Hepatocellular Carcinoma. Semin. Cel Dev Biol 4, 6. doi:10.1016/j.semcdb.2021.04.006
Sakshi, S., Jayasuriya, R., Ganesan, K., Xu, B., and Ramkumar, K. M. (2021). Role of circRNA-miRNA-mRNA Interaction Network in Diabetes and its Associated Complications. Mol. Ther. Nucleic Acids 26, 1291–1302. doi:10.1016/j.omtn.2021.11.007
Sanaei, M. J., Baghery Saghchy Khorasani, A., Pourbagheri-Sigaroodi, A., Shahrokh, S., Zali, M. R., and Bashash, D. (2021). The PI3K/Akt/mTOR axis in Colorectal Cancer: Oncogenic Alterations, Non-coding RNAs, Therapeutic Opportunities, and the Emerging Role of Nanoparticles. J. Cel Physiol. [Online ahead of print] doi:10.1002/jcp.30655
Schwartz, L., Seyfried, T., Alfarouk, K. O., Da Veiga Moreira, J., and Fais, S. (2017). Out of Warburg Effect: An Effective Cancer Treatment Targeting the Tumor Specific Metabolism and Dysregulated pH. Semin. Cancer Biol. 43, 134–138. doi:10.1016/j.semcancer.2017.01.005
Shakespear, M. R., Iyer, A., Cheng, C. Y., Das Gupta, K., Singhal, A., Fairlie, D. P., et al. (2018). Lysine Deacetylases and Regulated Glycolysis in Macrophages. Trends Immunol. 39, 473–488. doi:10.1016/j.it.2018.02.009
Shen, J., Jin, Z., Lv, H., Jin, K., Jonas, K., Zhu, C., et al. (2020). PFKP Is Highly Expressed in Lung Cancer and Regulates Glucose Metabolism. Cel Oncol (Dordr) 43, 617–629. doi:10.1007/s13402-020-00508-6
Shulman, R. G., and Rothman, D. L. (2017). The Glycogen Shunt Maintains Glycolytic Homeostasis and the Warburg Effect in Cancer. Trends Cancer 3, 761–767. doi:10.1016/j.trecan.2017.09.007
Sinha, T., Panigrahi, C., Das, D., and Chandra, Panda. A. (2021). Circular RNA Translation, a Path to Hidden Proteome. Wiley Interdiscip Rev RNA, e1685.
Smiles, W. J., and Camera, D. M. (2018). The Guardian of the Genome P53 Regulates Exercise-Induced Mitochondrial Plasticity beyond Organelle Biogenesis. Acta Physiol. (Oxf) 222, 3. doi:10.1111/apha.13004
Storkus, W. J., Maurer, D., Lin, Y., Ding, F., Bose, A., Lowe, D., et al. (2021). Dendritic Cell Vaccines Targeting Tumor Blood Vessel Antigens in Combination with Dasatinib Induce Therapeutic Immune Responses in Patients with Checkpoint-Refractory Advanced Melanoma. J. Immunother. Cancer 9, 11. doi:10.1136/jitc-2021-003675
Strycharz, J., Drzewoski, J., Szemraj, J., and Sliwinska, A. (2017). Erratum to "Is P53 Involved in Tissue-specific Insulin Resistance Formation?". Oxid Med. Cel Longev 2017, 8036902. doi:10.1155/2017/8036902
Su, H., Huang, J., Weng, S., Zhang, B., Zhang, T., and Xu, Y. (2021). Glutathione Synthesis Primes Monocytes Metabolic and Epigenetic Pathway for β-glucan-trained Immunity. Redox Biol. 48, 102206. doi:10.1016/j.redox.2021.102206
Sun, L., Suo, C., Li, S. T., Zhang, H., and Gao, P. (2018). Metabolic Reprogramming for Cancer Cells and Their Microenvironment: Beyond the Warburg Effect. Biochim. Biophys. Acta Rev. Cancer 1870, 51–66. doi:10.1016/j.bbcan.2018.06.005
Sun, X., and Feinberg, M. W. (2021). Vascular Endothelial Senescence: Pathobiological Insights, Emerging Long Noncoding RNA Targets, Challenges and Therapeutic Opportunities. Front. Physiol. 12, 693067. doi:10.3389/fphys.2021.693067
Tan, V. P., and Miyamoto, S. (2015). HK2/hexokinase-II Integrates Glycolysis and Autophagy to Confer Cellular protection. Autophagy 11, 963–964. doi:10.1080/15548627.2015.1042195
Tang, Y.-C., Hsiao, J.-R., Jiang, S.-S., Chang, J.-Y., Chu, P.-Y., Liu, K.-J., et al. (2021). c-MYC-directed NRF2 Drives Malignant Progression of Head and Neck Cancer via Glucose-6-Phosphate Dehydrogenase and Transketolase Activation. Theranostics 11, 5232–5247. doi:10.7150/thno.53417
Tao, L. M., Gong, Y. F., Yang, H. M., Pei, J. H., Zhao, X. J., and Liu, S. S. (2020). LINC00662 Promotes Glycolysis and Cell Survival by Regulating miR- 375/HIF-1α axis in Ovarian Cancer. J. Biol. Regul. Homeost Agents 34 (3), 467–477. doi:10.23812/19-300-A-18
Tekade, R. K., and Sun, X. (2017). The Warburg Effect and Glucose-Derived Cancer Theranostics. Drug Discov. Today 22, 1637–1653. doi:10.1016/j.drudis.2017.08.003
Teng, Y., Zhang, Y., Qu, K., Yang, X., Fu, J., Chen, W., et al. (2015). MicroRNA-29B (Mir-29b) Regulates the Warburg Effect in Ovarian Cancer by Targeting AKT2 and AKT3. Oncotarget 6, 40799–40814. doi:10.18632/oncotarget.5695
Tyagi, K., Mandal, S., and Roy, A. (2021). Recent Advancements in Therapeutic Targeting of the Warburg Effect in Refractory Ovarian Cancer: A Promise towards Disease Remission. Biochim. Biophys. Acta Rev. Cancer 1876, 188563. doi:10.1016/j.bbcan.2021.188563
Tymon-Rosario, J., Adjei, N. N., Roque, D. M., and Santin, A. D. (2021). Microtubule-Interfering Drugs: Current and Future Roles in Epithelial Ovarian Cancer Treatment. Cancers (Basel) 13, 24. doi:10.3390/cancers13246239
Upadhyay, M., Samal, J., Kandpal, M., Singh, O. V., and Vivekanandan, P. (2013). The Warburg Effect: Insights from the Past Decade. Pharmacol. Ther. 137, 318–330. doi:10.1016/j.pharmthera.2012.11.003
Vafadar, A., Shabaninejad, Z., Movahedpour, A., Fallahi, F., Taghavipour, M., Ghasemi, Y., et al. (2020). Quercetin and Cancer: New Insights into its Therapeutic Effects on Ovarian Cancer Cells. Cell Biosci 10, 32. doi:10.1186/s13578-020-00397-0
van Niekerk, G., and Engelbrecht, A. M. (2018). Role of PKM2 in Directing the Metabolic Fate of Glucose in Cancer: a Potential Therapeutic Target. Cel Oncol (Dordr) 41, 343–351. doi:10.1007/s13402-018-0383-7
Vergote, I., Gonzalez-Martin, A., Ray-Coquard, I., Harter, P., Colombo, N., Pujol, P., et al. (2021). European Experts Consensus: BRCA/homologous Recombination Deficiency Testing in First-Line Ovarian Cancer. Ann. Oncol. 33 (3), 276–287. doi:10.1016/j.annonc.2021.11.013
Wang, D., Li, C., Zhu, Y., Song, Y., Lu, S., Sun, H., et al. (2021). TEPP-46-Based AIE Fluorescent Probe for Detection and Bioimaging of PKM2 in Living Cells. Anal. Chem. 93, 12682–12689. doi:10.1021/acs.analchem.1c02529
Ward, P. S., and Thompson, C. B. (2012). Metabolic Reprogramming: a Cancer Hallmark Even Warburg Did Not Anticipate. Cancer Cell 21 (3), 297–308. doi:10.1016/j.ccr.2012.02.014
Werner, H., Sarfstein, R., LeRoith, D., and Bruchim, I. (2016). Insulin-like Growth Factor 1 Signaling Axis Meets P53 Genome Protection Pathways. Front. Oncol. 6, 159. doi:10.3389/fonc.2016.00159
Wu, M., Yang, L.-Z., and Chen, L.-L. (2021). Long Noncoding RNA and Protein Abundance in lncRNPs. RNA 27, 1427–1440. doi:10.1261/rna.078971.121
Xia, Q., Jia, J., Hu, C., Lu, J., Li, J., Xu, H., et al. (2021). Tumor-associated Macrophages Promote PD-L1 Expression in Tumor Cells by Regulating PKM2 Nuclear Translocation in Pancreatic Ductal Adenocarcinoma. Oncogene. doi:10.1038/s41388-021-02133-5
Xie, H., Wang, W., Qi, W., Jin, W., and Xia, B. (2021). Targeting DNA Repair Response Promotes Immunotherapy in Ovarian Cancer: Rationale and Clinical Application. Front. Immunol. 12, 661115. doi:10.3389/fimmu.2021.661115
Xie W, W., Liu, L. U., He, C., Zhao, M., Ni, R., Zhang, Z., et al. (2021). Circ_0002711 Knockdown Suppresses Cell Growth and Aerobic Glycolysis by Modulating miR-1244/ROCK1 axis in Ovarian Cancer. J. Biosci. 46, 136. doi:10.1007/s12038-020-00136-0
Xu, D., Liang, J., Lin, J., and Yu, C. (2019). PKM2: A Potential Regulator of Rheumatoid Arthritis via Glycolytic and Non-glycolytic Pathways. Front. Immunol. 10, 2919. doi:10.3389/fimmu.2019.02919
Xu, H., Sun, X., Huang, Y., Si, Q., and Li, M. (2020). Long Non-coding RNA NEAT1 M-odifies C-ell P-roliferation, colony F-ormation, A-poptosis, M-igration and I-nvasion via the miR-4500/BZW1 axis in O-varian C-ancer. Mol. Med. Rep. 22, 3347–3357. doi:10.3892/mmr.2020.11408
Xu, S., and Herschman, H. R. (2019). A Tumor Agnostic Therapeutic Strategy for Hexokinase 1-Null/Hexokinase 2-Positive Cancers. Cancer Res. 79, 5907–5914. doi:10.1158/0008-5472.CAN-19-1789
Xu, S., Jia, G., Zhang, H., Wang, L., Cong, Y., Lv, M., et al. (2021). LncRNA HOXB-AS3 Promotes Growth, Invasion and Migration of Epithelial Ovarian Cancer by Altering Glycolysis. Life Sci. 264, 118636. doi:10.1016/j.lfs.2020.118636
Xu, X. L., Deng, S. L., Lian, Z. X., and Yu, K. (2021). Resveratrol Targets a Variety of Oncogenic and Oncosuppressive Signaling for Ovarian Cancer Prevention and Treatment. Antioxidants (Basel) 10, 1718. doi:10.3390/antiox10111718
Yalan, S., Yanfang, L., He, C., and Yujie, T. (2020). Circular RNA circRHOBTB3 Inhibits Ovarian Cancer Progression through PI3K/AKT Signaling Pathway. Panminerva Med. doi:10.23736/s0031-0808.20.03957-9
Yang, C., Wang, R., and Hardy, P. (2021). Potential of miRNA-Based Nanotherapeutics for Uveal Melanoma. Cancers (Basel) 13, 5192. doi:10.3390/cancers13205192
Yang, G. J., Wu, J., Leung, C. H., Ma, D. L., and Chen, J. (2021). A Review on the Emerging Roles of Pyruvate Kinase M2 in Anti-leukemia Therapy. Int. J. Biol. Macromol 193, 1499–1506. doi:10.1016/j.ijbiomac.2021.10.213
Yang, J., Ren, B., Yang, G., Wang, H., Chen, G., You, L., et al. (2020). The Enhancement of Glycolysis Regulates Pancreatic Cancer Metastasis. Cel Mol Life Sci 77, 305–321. doi:10.1007/s00018-019-03278-z
Yellen, G. (2018). Fueling Thought: Management of Glycolysis and Oxidative Phosphorylation in Neuronal Metabolism. J. Cel Biol 217, 2235–2246. doi:10.1083/jcb.201803152
Yeung, S. J., Pan, J., and Lee, M. H. (2008). Roles of P53, MYC and HIF-1 in Regulating Glycolysis - the Seventh Hallmark of Cancer. Cel Mol Life Sci 65, 3981–3999. doi:10.1007/s00018-008-8224-x
Yin, X., Choudhury, M., Kang, J. H., Schaefbauer, K. J., Jung, M. Y., Andrianifahanana, M., et al. (2019). Hexokinase 2 Couples Glycolysis with the Profibrotic Actions of TGF-β. Sci. Signal. 12, 612. doi:10.1126/scisignal.aax4067
Youssef, A., Haskali, M. B., and Gorringe, K. L. (2021). The Protein Landscape of Mucinous Ovarian Cancer: Towards a Theranostic. Cancers (Basel) 13, 596. doi:10.3390/cancers13225596
Yu, H., Yang, W., Huang, J., Miao, X., Wang, B., Ren, X., et al. (2021). GPR120 Induces Regulatory Dendritic Cells by Inhibiting HK2-dependent Glycolysis to Alleviate Fulminant Hepatic Failure. Cel Death Dis 13, 1. doi:10.1038/s41419-021-04394-0
Yuan, Q., Zhang, J., Liu, Y., Chen, H., Liu, H., Wang, J., et al. (2021). MyD88 in Myofibroblasts Regulates Aerobic Glycolysis-Driven Hepatocarcinogenesis via ERK-dependent PKM2 Nuclear Relocalization and Activation. J. Pathol. 256 (4), 414–426. doi:10.1002/path.5856
Yue, J., Jin, S., Gu, S., Sun, R., and Liang, Q. (2019). High Concentration Magnesium Inhibits Extracellular Matrix Calcification and Protects Articular Cartilage via Erk/autophagy Pathway. J. Cel Physiol 234, 23190–23201. doi:10.1002/jcp.28885
Yue, J., Jin, S., Li, Y., Zhang, L., Jiang, W., Yang, C., et al. (2016). Magnesium Inhibits the Calcification of the Extracellular Matrix in Tendon-Derived Stem Cells via the ATP-P2r and Mitochondrial Pathways. Biochem. Biophys. Res. Commun. 478, 314–322. doi:10.1016/j.bbrc.2016.06.108
Zeng, X., Yuan, X., Cai, Q., Tang, C., and Gao, J. (2021). Circular RNA as an Epigenetic Regulator in Chronic Liver Diseases. Cells 339, 1945. doi:10.3390/cells10081945
Zhang, J., Zhang, J., Wei, Y., Li, Q., and Wang, Q. (2019). ACTL6A Regulates Follicle-Stimulating Hormone-Driven Glycolysis in Ovarian Cancer Cells via PGK1. Cel Death Dis 10, 811. doi:10.1038/s41419-019-2050-y
Zhang, S., Pei, M., Li, Z., Li, H., Liu, Y., and Li, J. (2018). Double-negative Feedback Interaction between DNA Methyltransferase 3A and microRNA-145 in the Warburg Effect of Ovarian Cancer Cells. Cancer Sci. 109, 2734–2745. doi:10.1111/cas.13734
Zhao, L., Ji, G., Le, X., Wang, C., Xu, L., Feng, M., et al. (2017). Long Noncoding RNA LINC00092 Acts in Cancer-Associated Fibroblasts to Drive Glycolysis and Progression of Ovarian Cancer. Cancer Res. 77, 1369–1382. doi:10.1158/0008-5472.CAN-16-1615
Zhao, Q., Li, J., Wu, B., Shang, Y., Huang, X., Dong, H., et al. (2020). Smart Biomimetic Nanocomposites Mediate Mitochondrial Outcome through Aerobic Glycolysis Reprogramming: A Promising Treatment for Lymphoma. ACS Appl. Mater. Inter. 12, 22687–22701. doi:10.1021/acsami.0c05763
Zhao, S., Heng, N., Weldegebriall Sahlu, B., Wang, H., and Zhu, H. (2021). Long Noncoding RNAs: Recent Insights into Their Role in Male Infertility and Their Potential as Biomarkers and Therapeutic Targets. Int. J. Mol. Sci. 22, 24. doi:10.3390/ijms222413579
Zheng, X., Zhou, Y., Chen, W., Chen, L., Lu, J., He, F., et al. (2018). Ginsenoside 20(S)-Rg3 Prevents PKM2-Targeting miR-324-5p from H19 Sponging to Antagonize the Warburg Effect in Ovarian Cancer Cells. Cell Physiol Biochem 51, 1340–1353. doi:10.1159/000495552
Zhong, J., Kang, Q., Cao, Y., He, B., Zhao, P., Gou, Y., et al. (2021). BMP4 Augments the Survival of Hepatocellular Carcinoma (HCC) Cells under Hypoxia and Hypoglycemia Conditions by Promoting the Glycolysis Pathway. Am. J. Cancer Res. 11, 793–811.
Zhong, J. T., and Zhou, S. H. (2017). Warburg Effect, Hexokinase-II, and Radioresistance of Laryngeal Carcinoma. Oncotarget 8, 14133–14146. doi:10.18632/oncotarget.13044
Zhou, Y., Zheng, X., Lu, J., Chen, W., Li, X., and Zhao, L. (2018). Ginsenoside 20(S)-Rg3 Inhibits the Warburg Effect via Modulating DNMT3A/ MiR-532-3p/HK2 Pathway in Ovarian Cancer Cells. Cel Physiol Biochem 45, 2548–2559. doi:10.1159/000488273
Keywords: circular RNAs, long non-coding RNAs, microRNAs, glycolysis, ovarian cancer
Citation: Zhang C and Liu N (2022) Noncoding RNAs in the Glycolysis of Ovarian Cancer. Front. Pharmacol. 13:855488. doi: 10.3389/fphar.2022.855488
Received: 15 January 2022; Accepted: 15 March 2022;
Published: 30 March 2022.
Edited by:
Na Li, University of California, San Diego, United StatesReviewed by:
Patricia Zancan, Federal University of Rio de Janeiro, BrazilShiv Verma, Case Western Reserve University, United States
Copyright © 2022 Zhang and Liu. This is an open-access article distributed under the terms of the Creative Commons Attribution License (CC BY). The use, distribution or reproduction in other forums is permitted, provided the original author(s) and the copyright owner(s) are credited and that the original publication in this journal is cited, in accordance with accepted academic practice. No use, distribution or reproduction is permitted which does not comply with these terms.
*Correspondence: Ning Liu, bmluZ2xpdUBjbXUuZWR1LmNu