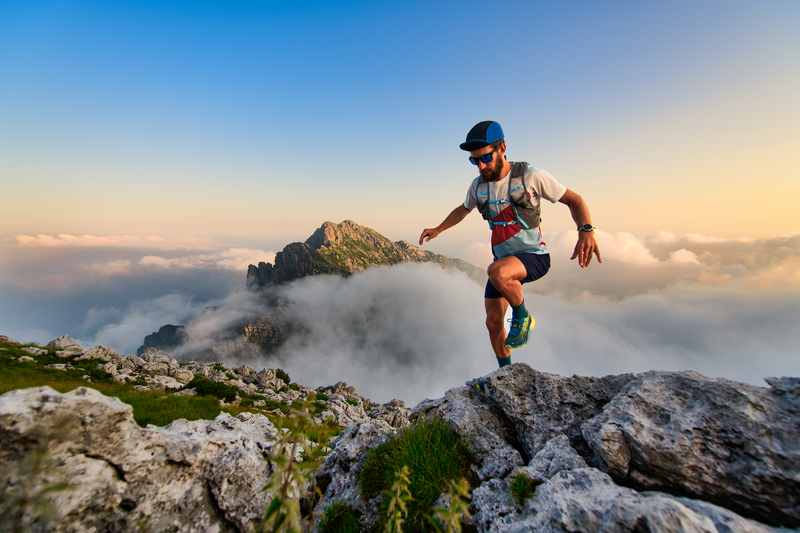
95% of researchers rate our articles as excellent or good
Learn more about the work of our research integrity team to safeguard the quality of each article we publish.
Find out more
ORIGINAL RESEARCH article
Front. Pharmacol. , 01 April 2022
Sec. Ethnopharmacology
Volume 13 - 2022 | https://doi.org/10.3389/fphar.2022.855393
This article is part of the Research Topic Bone and Cartilage Diseases – The Role and Potential of Natural Products View all 19 articles
A correction has been applied to this article in:
Corrigendum: Ginsenoside Compound K Enhances Fracture Healing via Promoting Osteogenesis and Angiogenesis
Fractures have an extraordinarily negative impact on an individual’s quality of life and functional status, particularly delayed or non-union fractures. Osteogenesis and angiogenesis are closely related to bone growth and regeneration, and bone modeling and remodeling. Recently Chinese medicine has been extensively studied to promote osteogenic differentiation in MSCs. Studies have found that Ginseng can be used as an alternative for tissue regeneration and engineering. Ginseng is a commonly used herbal medicine in clinical practice, and one of its components, Ginsenoside Compound K (CK), has received much attention. Evidence indicates that CK has health-promoting effects in inflammation, atherosclerosis, diabetics, aging, etc. But relatively little is known about its effect on bone regeneration and the underlying cellular and molecular mechanisms. In this study, CK was found to promote osteogenic differentiation of rat bone marrow mesenchymal stem cells (rBMSCs) by RT-PCR and Alizarin Red S staining in vitro. Mechanistically, we found CK could promote osteogenesis through activating Wnt/β-catenin signaling pathway by immunofluorescence staining and luciferase reporter assay. And we also showed that the tube formation capacity of human umbilical vein endothelial cells (HUVECs) was increased by CK. Furthermore, using the rat open femoral fracture model, we found that CK could improve fracture repair as demonstrated by Micro-CT, biomechanical and histology staining analysis. The formation of H type vessel in the fracture callus was also increased by CK. These findings provide a scientific basis for treating fractures with CK, which may expand its application in clinical practice.
Fractures have an extraordinarily negative influence on an individual’s quality of life and functional status. Bone is one of the organs that have the capacity to regenerate. Fracture disrupts bone circulation, leading to necrosis and hypoxia of adjacent bones (Glowacki 1998). Type H vessels, with high expression of Endomucin (Emcn) and CD31, have recently been identified and have the ability to induce bone formation (Peng et al., 2020). Fracture repair usually could restore the damaged bone to its pre-injury cellular composition, structure and biomechanical function, but approximately 10% of fractures do not heal properly (Einhorn and Gerstenfeld 2015). Some alternative therapies can promote fracture healing to prevent delayed healing or non-healing, such as herbal medicine.
Ginseng is a traditional Chinese herb that has been widely used in Asia for thousands of years to keep the physical vigor, improve immunity and resistance to aging, etc. Some ginsenosides have been found to prevent osteoporosis (Liu et al., 2020) and osteoarthritis (Chen et al., 2016), and also improve fracture healing (Gu et al., 2016). Ginsenoside compound K is a metabolite produced by ginsenosides Rb1, Rb2 and Rc through the metabolism of intestinal bacteria in vivo (Yang et al., 2015). The metabolic pathway of protopanaxadiol type ginsenosides by human intestinal bacteria is Rb1, Rb2 or Rc→Rd→F2→CK(Zhou et al., 2008), which has also been shown to be same in hydrolytic pathway (Zhou et al., 2018). Rb2 can reduce oxidative damage and bone resorption cytokines, reflecting the ability of anti-osteoporosis (Huang et al., 2014). Treatment of 3T3-L1 cells with CK inhibited adipocyte differentiation and expression of adipocyte-specific genes (Park and Yoon 2012). Meanwhile, a variety of studies have reported that ginsenosides could regulate angiogenesis. For example, ginsenoside-Rg1 has been shown to induce angiogenesis (Kwok et al., 2015). Ginsenoside Rg1 increased the expression of VEGF through PI3K/Akt/mTOR signaling pathway and promoted cerebral angiogenesis after ischemic stroke (Chen et al., 2019a). Ginsenoside F1-induced activation of the IGF-1/IGF1R pathway to promote angiogenesis is an effective approach to alleviate cerebral ischemia (Zhang et al., 2019).
It has been reported that CK could significantly elevate the mRNA expression of genes regulating Wnt/β-catenin signaling, including Wnt10b, Wnt11, Lrp5 and β-catenin (Zhou et al., 2018). Many studies have shown that the Wnt/β-catenin signaling controls bone formation and osteoblast differentiation (Kobayashi et al., 2016; Yuan et al., 2016; Shen et al., 2020). Angiogenesis is an essentially biological process in bone regeneration and is also closely linked to the Wnt/β-catenin signaling pathway (Shi et al., 2020; Shen et al., 2021; Yu et al., 2021). However, it is unknown whether CK is effective on fracture repair, as well as the underlying mechanisms involved. In the present study, we investigated the effects of CK on rat fracture healing, including osteogenic differentiation and angiogenesis, and elucidated its potential regulation of Wnt/β-catenin signaling pathway.
CK was provided by Zhejiang Hongguan Bio-pharma Co., Ltd., and dissolved in DMSO, and diluted in PBS. Modified Eagle’s Medium of Alpha (α-MEM), Dulbecco’s Modified Eagle Medium/Nutrient Mixture F-12 (DMEM/F-12), fetal bovine serum (FBS), and penicillin/streptomycin were purchased from Gibco (United States). Beta-glycerolphosphate, dexamethasone, ascorbic acid phosphate, Safranine O, and Fast Green were purchased from Sigma (United States). Alizarin Red S was purchased from Solarbio (Beijing, China). Cell Counting Kit-8 (CCK-8) was purchased from Beyotime (Beijing, China). NucleoZOL reagent, Reverse Transcription Kit and SYBR-Green Master Mix were supplied by Takara (Japan). Hematoxylin-eosin (H&E) was purchased from biosharp (China). Primary antibodies against CD31, β-catenin, and DAPI were supplied by Santa Cruz Biotechnology (United States); Primary antibodies anti-CTSK, anti-ALP, anti-OPG, anti-RANKL, anti-Runx2, and anti-OPN were purchased from Bioss (China); Anti-GAPDH, and DAPI were obtained from Abcam (United States). Secondary antibodies HRP-conjugated Goat Anti-Rabbit IgG, Goat anti-Mouse IgG (H + L), Rabbit Anti-Rabbit IgM/Cy3 and Rabbit Anti-Mouse IgM/FITC were obtained from Bioss (China). Dual-Luciferase Reporter Assay System was supplied by Promega Company (United States). Matrigel was purchased from Becton Dickinson (United States).
After inducing differentiation for 3 days in 12-well plates with osteogenic induction medium, the total RNA was extracted using NucleoZOL, and cDNA was obtained from total RNA using a Reverse Transcription Kit. Next, qRT-PCR was performed using SYBR Green qPCR Master Mix. The relative gene expression was calculated by the 2–ΔCT method, and GAPDH was used as a reference for normalization. The primers were purchased from Invitrogen (United States) and primer sequences are shown in Table 1.
The method of rat bone marrow mesenchymal stem cells (BMSCs) isolation and cultivation has been described previously (Shen et al., 2018). BMSCs were isolated from Sprague-Dawley rats (male, 2 weeks old, 30–40 g) in a sterile environment. Rats were euthanized and the bone marrow of the bilateral femoral was flushed out with serum-free α-MEM to obtain a single-cell suspension. Flushed bone marrow cells was centrifuged at 1200 rpm for 6 min, the supernatant liquid was removed, and the cell pellets were resuspended in α-MEM supplemented with 10% fetal bovine serum, 1% penicillin and streptomycin. The medium was discarded after 72 h of primary culture and then changed once every 3 days. Upon 80—90% confluence, the adherent cells were further expanded with trypsin. The medium was changed several times to obtain pure BMSCs. Cells from passages 3 to 5 were used in the study.
The cell viability of CK on BMSCs was assessed using a Cell Counting Kit-8 (CCK-8) kit (C0037, Beyotime Biotechnology Co., Ltd., Shanghai, China). BMSCs or HUVECs were seeded in a 96-well plate with 5000 cells in each well and cultured without or with CK (0, 2.5,5, 10, 20, 30 and 40 μM) for 48 and 72 h. Subsequently, CCK-8 reagent was added to each well and the plates were incubated for 1 h at 37°C. The Optical density (OD) of the samples was measured at 450 nm with a spectrophotometric microplate reader (Xianke Instruments, Shanghai, China). The experiment was independently repeated 3 times.
8 week-old male Sprague Dawley (SD) rats (220 ± 10 g, n = 18) were purchased from the Guangzhou Medicine Laboratory Animal Center. The animals were housed in the First Affiliated Hospital of Guangzhou University of Chinese medicine animal center. All experimental methods were approved by the Animal Care and Use Committee of Guangzhou University of Traditional Chinese Medicine. All rats were fed with standard chow and free access to water with a 12 h light-dark cycle (24 ± 1°C). An open femoral fracture model with internal fixation was established. In brief, the procedure was performed under general anesthesia (pentobarbital sodium 100 mg/kg, intraperitoneally) and aseptic conditions. The right femur was exposed, and transverse osteotomy was performed with a hand saw to create a gap size of 2 mm. A K-wire (diameter: 1.2 mm, Stryker Ltd., United States) was inserted into the right femoral bone marrow cavity to fix the fracture. The rats were randomly assigned to the following two groups: fracture + PBS (n = 9, 100 μl/day), fracture + CK (n = 9, CK = 500 μM, 100 μl every other day). 5 days after the surgery, CK or PBS was locally injected at the fracture sites every other day for 4 weeks. Then the animals were sacrificed and the right femurs were collected for further analysis.
The femurs were harvested, fixed in 10% neutral formalin for 24 h, decalcified in 10% Ethylene Diamine Tetraacetic Acid (EDTA) for 21 days, dehydrated and then embedded in paraffin. After cutting into 5-μm-thick sections and dewaxing in xylene and rehydration in a decreasing alcohol gradient and distilled water, samples were processed for hematoxylin-eosin (H&E), and Safranine O-Fast Green (SO-FG) staining. For immunohistochemical staining, the sections were incubated in 0.3% hydrogen peroxide for 20 min and antigen retrieval in 0.01 M citrate buffer at 60°C for 30 min, and blocking with 5% g BSA in PBS for 1 h and then incubated overnight at 4°C with a primary antibody. The sections were then incubated with the secondary antibodies for 1 h at 37°C, counterstained with hematoxylin, and visualized using an HRP-streptavidin system. The primary antibodies used in this study included anti-OPG, anti-RANKL, anti-OPN and anti-ALP antibodies. For immunofluorescence staining, the slides were incubated in antigen retrieval in 0.01 M citrate buffer, and blocking with 5% g BSA in PBS for 1 h and then incubated overnight at 4°C with a primary antibody. The sections were then incubated with the fluorescent secondary antibodies for 1 h at 37°C. The primary antibodies used in this study included anti-Runx2, anti-Endomucin, anti-CD31 and anti-β-catenin antibodies. DAPI staining was carried out to stain the nuclei. Images were acquired with the fluorescence microscope (Olympus, IX73 L, United States).
Microcomputer tomography (micro-CT) examination was applied for the fractured femurs. Samples were scanned by Skyscan 1176 micro-CT scanner (Bruker micro-CT, Kontich, Belgium), with a source voltage of 80 kV, current of 114 μA, Al 0.5 mm filter and 10.5 μm isotropic resolution. The fractured callus sites were defined as the volume of interest. The bone volume/tissue volume (BV/TV), mean volumetric bone mineral density (BMD), and Callus Volume were measured. Three dimensional images were generated using CTvol software (Bruker micro-CT, Kontich, Belgium).
Tube formation assay was performed as previously reported (Lin et al., 2019). The wells of the 12-well plate were coated with Matrigel and incubated for 30 min hBMSCs (5000 cells/well) were treated with or without CK (10 μM), and co-cultured with HUVECs (105 cells/well). DMEM/F12 basal medium containing 2% FBS (Thermo Fisher Scientific) was used. Plates were incubated at 37°C, 5% CO2 for 8 h. Then the tube formation was observed using a microscope. Tubes were then assessed through an inverted fluorescent microscope at 10×(Olympus). Image J with the Angiogenesis Analyzer plugin were used to quantify the tube length and branch points of tube networks. Images taken at 5× magnification.
Experiments were performed as described previously (Ren et al., 2019). Briefly, 293FT cells were seeded on 24-well plates and allowed to grow to 80% confluence. Cells were then transfected with TOPflash (500 ng) and Renilla reporter plasmid pRL-CMV (100 ng) using Lipofectamine 8000. 24 h after transfection, cells were treated with CK (10 μM) for 24 h. The luciferase activity was measured using a GloMax™ 20/20 single-tube luminometer (Promega, Madison, WI, United States).
Three-point bending biomechanical testing was performed as previously reported (Lin et al., 2019). Fractured femurs were tested to failure with a constant displacement rate of 4 mm/min by a 3-point bending device (H25KS; Tinius Olsen, United Kingdom). The fractured femur was loaded in the front and back directions, and the span of the two support points was set as 10 mm. The force loading point was set at the fracture site. After testing, ultimate load to failure and energy absorbed to failure were recorded and analyzed by the QMAT software.
We applied GraphPad Prism 5 for comparison. Quantitative data were expressed as mean ± standard deviation (SD). Statistics were analyzed by t-test for two-group comparison, and One-way or two-way analysis of variance (ANOVA) for multi-comparison between groups. We used Tukey’s post hoc multiple comparisons test as the posttest method for ANOVA. P values <0.05 were considered statistically significant.
The chemical structure depiction of CK was shown in Figure 1A. BMSCs were cultured without or with CK (0, 2.5,5, 10, 20, 30 and 40 μM) for 48 and 72 h. The CCK-8 assay results showed that CK exhibited no obvious cytotoxicity to BMSCs even at the high concentration of 40µM, and CK at 10–40 µM increased the cell viability of BMSCs (Figure 1B). To investigate the effect of CK on osteogenic differentiation of BMSCs, BMSCs were cultured in the osteogenic induction medium (OIM) with or without CK at various concentrations (0, 1, and 10 μM) for 3 and 14 days. At the third day of osteogenic induction, the expression of osteogenesis-related genes was detected and it was found that the RNA levels of osteopontin (OPN), alkaline phosphatase (ALP), osteocalcin (OCN) and osterix (OSX) were significantly up-regulated by CK (Figures 1C–F). Furthermore, Alizarin Red S staining showed that CK significantly increased the formation of calcium deposits after 14 days of induction (Figures 1G,H). As 10 μM CK showed the best effect, it was used for the following in vitro experiments.
FIGURE 1. CK enhanced cell viability, osteogenesis and angiogenesis in vitro. (A) The chemical structure depiction of CK. (B) Optical density (OD) values indicating the effects of CK treatment for 48 h and 72h on cell viability of BMSCs, *p < 0.05, n = 3, compared with 0 μM CK group. (C–F) Real-time PCR results of osteogenesis-related genes at 3 days treated with different concentrations of CK in OIM, *p < 0.05, n = 3, compared with OIM group. (G) Representative staining images and (H) Quantification of Alizarin Red S of BMSCs incubated with different concentrations of CK in OIM for 14 days, *p < 0.05, n = 3, compared with OIM group. (I) Optical density (OD) values indicating the effects of CK treatment for 48 h and 72h on cell viability of HUVECs, *p < 0.05, n = 3, compared with 0 μM CK group. (J) Representative images of tube formation assay and (K-L) Quantitative analysis of tube length and branch points in Matrigel MSCs and HUVEC co-culture system, Scale bars < 100 μm, *p < 0.05, compared with the Control group, n = 3.
HUVECs were cultured without or with CK (0, 2.5,5, 10, 20, 30 and 40 μM) for 48 and 72 h. The CCK-8 assay showed that CK exhibited no obvious cytotoxicity to HUVECs (Figure 1I). Some studies have reported MSCs can stimulate migration and angiogenesis of HUVECs (Chiang et al., 2018; Liu et al., 2019). To investigate the effect of CK on angiogenesis in the presence of MSCs, the HUVEC cells were co-cultured with BMSCs treated with or without CK (10 µM), and the tube length and branch points were evaluated (Figures 1J–L). We could observe that the tube formation capacity of HUVEC was increased by CK. The result demonstrated that CK enhanced angiogenesis in vitro compared with the control group.
It is well known that the Wnt/β-catenin signaling has been shown as an important regulatory pathway in the osteogenic differentiation of mesenchymal stem cells (Kim et al., 2013). To verify whether the Wnt/β-catenin signaling pathway is activated upon CK treatment in BMSCs, we performed immunofluorescence staining to detect the level of β-catenin and its co-location with Runx2 in rBMSCs. Immunofluorescence analysis revealed more nuclear translocation of β-catenin and an increased expression of Runx2 in the CK (10 μM) group, compared with the OIM group (Figures 2A,B). Additionally, the TOP flash assay was used to evaluate the effect of CK (10 μM) on the activation of the Wnt/β-catenin signaling pathway. After 24 h of stimulation, the luciferase activity was significantly increased by CK (Figure 2C).
FIGURE 2. CK increased β-catenin and Runx2 expression in BMSCs. (A) Representative images and (B) Quantification of immunofluorescence staining of β-catenin and Runx2 treated with 10 μM CK for 24 h. Scale bars = 50 μm, *p < 0.05, compared with the OIM group, n = 3 (C) The TOPflash luciferase activity was measured in BMSCs after treatment of CK (10 μM). *p < 0.05, compared with the Control group, n = 3.
We further conducted an open femoral fracture model to evaluate whether CK could accelerate fracture healing in rats. The time points of animal modeling and sample collection were shown in Figure 3A. The 3-dimensional images of the femurs obtained by micro-CT analysis showed that the fracture gap was almost filled by new bone in the CK-treated rats at 4w post-fracture, compared with that of the PBS group (Figure 3B). The BMD, BV/TV and callus volume at femoral callus sites of CK-treated rats were significantly higher than those in the PBS group (Figures 3C–E). In addition, results of biomechanical testing confirmed a much stronger biomechanical property in the femoral bones of CK- treated group than those of the PBS group (Figures 3F,G).
FIGURE 3. CK accelerated the progression of fracture healing. (A) Schematic illustration of time points of animal modeling and sample collection. (B) Representative 3-dimensional micro-CT images of femurs in each group. (C–E) Quantitative analysis of parameters, including CV, BMD, and BV/TV, *p < 0.05, compared with the PBS group, n = 3 (F–G) Biomechanical properties of the fractured bones by 3-point bending test, *p < 0.05, compared with the PBS group, n = 6.
Furthermore, H&E and SO-FG staining of the callus showed varying amounts of newly formed trabecular bone, cartilage tissue and fibrous-like tissue. Callus of CK-treated rats exhibited enhanced bone regeneration after 4 weeks, in comparison with the PBS group, which was evidenced by more neo-formed trabecular bone and less cartilaginous and fibrous-like tissue in the CK group (Figures 4A,B). Additionally, higher expression of OPG, OCN, ALP and lower expression of RANKL within the callus areas of the CK group was confirmed using immunohistochemical analysis (Figures 4C–F).
FIGURE 4. H&E, Safranin O-Fast Green, and immunohistochemical examination of fracture callus. (A) Representative images of H&E staining of fracture calluses in PBS and CK groups. Insets indicate the regions shown in the enlarged images (lower). Scale bar: 500 μm. (B) Representative images of Safranin O-Fast Green staining of fracture calluses in PBS and CK groups. Insets indicate the regions shown in the enlarged images (lower). (C–F) Representative images of immunohistochemical analysis of OPG, ALP, RANKL and OPN of fracture calluses in PBS and CK groups. Scale bar = 50 μm.
In addition, the H-type vessel was observed in vivo in sections of femur. The CD31 and Emcn double immunofluorescent staining revealed a greater population of CD31hiEmcnhi cells within the callus of the CK group, compared with the PBS group (Figures 5A,B).
FIGURE 5. CK regulated H-type vessel formation in fracture callus. (A) Representative immunofluorescence double staining and (B) Quantification of CD31 (green), EMCN (red) of fracture calluses in PBS and CK groups, Scale bars = 50 μm, *p < 0.05, compared with the PBS group, n = 3.
To detect the expression of β-catenin in fracture callus treated with CK, we performed immunofluorescent staining to detect the level of β-catenin and Runx2 in fracture callus. Immunofluorescence analysis revealed a higher expression of β-catenin and Runx2 within the callus of the CK group, compared with PBS group (Figures 6A,B), which is consistent with the in vitro experiment.
FIGURE 6. CK up-regulated β-catenin and Runx2 in fracture callus. (A) Immunofluorescence staining images of β-catenin and Runx2 in PBS and CK groups and (B) Quantification of the immunofluorescence density of β-catenin and Runx2 of fracture calluses in PBS and CK groups, Scale bars = 50 μm, *p < 0.05, compared with the PBS group, n = 3.
Osteogenic differentiation of MSCs is the principal mechanism of bone regeneration and fracture repair. It has been reported that the expressions of alkaline phosphatase (ALP), type I collagen (COL-I) and mineralization were significantly increased after CK treatment in H2O2-stimulated MC3T3-E1 cells (Kang et al., 2016). Studies have shown that CK improved the biocompatibility and morphology of microsphere scaffolds without affecting the biocompatibility, and CK impregnated porous microsphere scaffold system may be applicable as a promising microsphere scaffold for bone regeneration (Thangavelu et al., 2020). In this study, we found that CK promoted the osteogenic differentiation of BMSCs. Our results demonstrated that CK enhanced the mineralization and mRNA expression of osteogenic markers in rat BMSCs, including ALP, Runx2, OPN and OCN. Fracture healing is closely related to the number and activity of BMSCs near the fracture site (Gu et al., 2016). What’s more, human umbilical vein endothelial cells (HUVECs) play an important role as a model system for studying the regulation of endothelial cell function and angiogenesis. It is well known that both osteogenesis and angiogenesis are integrated parts of bone regeneration (Huang et al., 2015). Interestingly, our results showed CK increased tube formation when HUVEC and BMSCs were co-cultured. Therefore, the in vitro experiments indicated that CK might up-regulate osteogenesis coupled with angiogenesis in bone regeneration.
CK is an initial bacterial metabolite of ginsenoside Rb1, which has many advantages of pharmacological properties, such as anti-cancer, anti-inflammatory, anti-aging, anti-allergenic, anti-diabetic, and anti-diabetic (Yoon et al., 2007; Yang et al., 2015; Wang et al., 2017; Chen et al., 2019b; Yin et al., 2021). In open femoral fracture rats, micro-CT examination showed that callus growth in rats treated with CK was substantially faster than that in control rats after fracture, and BMD, BV/TV, and callus volume were significantly increased in the CK-treated group. In addition, biomechanical testing confirmed a much stronger biomechanical property in the femoral bones of CK-treated rats than those of PBS-treated rats. The results of H&E, Safranin-O/Fast Green and IHC staining revealed that, compared with the PBS group, fracture callus in the CK treatment group had a significantly higher proportion of trabecular bone and better fracture healing but a much lower proportion of fibers and cartilage components inside the callus.
Recent studies have revealed that ginsenoside CK regulates multiple signaling pathways, such as PI3K/mTOR/p70S6K1, HIF-1α/NF-κB, Nrf2/Keap1, RhoA/ROCKs/YAP, and PI3K-Akt signaling pathway (Li et al., 2018; Yang et al., 2019; Chen et al., 2020; Tian et al., 2021; Zhang et al., 2021). Wnt/β-catenin signaling pathway is important not only in the growth and development of mineralized tissues, but also in regulating the skeletal response to load and unloading and the vitality and health of adult and aging bones (Duan and Bonewald 2016). Wnt/β-catenin signaling pathway has been widely reported in the regulation of osteogenesis and angiogenesis (Jiang et al., 2015; Shen et al., 2020). Runx2 is a master transcription factor governing osteogenesis (Vimalraj et al., 2015). In the present study, we found that the expression of Runx2 and β-catenin was significantly elevated in vivo by immunofluorescent staining, which is consistent with the in vitro result. Additionally, the luciferase activity further verified that CK activated β-catenin expression.
The vascular system is a major source of oxygen, nutrients, hormones, neurotransmitters and growth factors to bone cells and is essential for bone development, regeneration and remodeling (Filipowska et al., 2017). Bone regeneration is closely related to angiogenesis and impaired angiogenesis often leads to failure of fracture healing. H-type vessels, highly positive for CD31 and Endomucin, could mediate local growth of the vascular system, combine angiogenesis with osteogenesis by mediating the selective location of Osterix positive cells around blood vessels and the differentiation of these bone progenitor cells (Kusumbe et al., 2014; Ramasamy et al., 2014). In this study, we proved that CK could stimulate type H vessel formation, and promote bone formation in fracture rats.
Taken together, our study suggested that Wnt/β-catenin signaling contributed to the enhancement in the coupling of osteogenesis and angiogenesis induced by CK treatment during fracture healing. CK may serve as an effective component of Ginseng in bone tissue regeneration. However, many more potential mechanisms remain undiscovered. Further experiments or clinical trials are needed to be conducted to expand its clinical application.
The original contributions presented in the study are included in the article/Supplementary Material, further inquiries can be directed to the corresponding authors.
The animal study was reviewed and approved by Animal Care and Use Committee of Guangzhou University of Traditional Chinese Medicine. Written informed consent was obtained from the owners for the participation of their animals in this study.
LX, GZ and HZ: conceptualization, methodology, and supervision. LD and SG: manuscript writing—original draft preparation, review and editing. BZ, MW, YZ and SW: investigation, data curation and formal analysis. LX and ZG: project administration and funding acquisition. All authors have read and agreed to the published version of the manuscript.
This work is supported by the grants from National Natural Science Foundation of China (81871778), and Key Project of the Sports Research Foundation of Guangdong Province (No. GDSS2020M006).
The authors declare that the research was conducted in the absence of any commercial or financial relationships that could be construed as a potential conflict of interest.
All claims expressed in this article are solely those of the authors and do not necessarily represent those of their affiliated organizations, or those of the publisher, the editors and the reviewers. Any product that may be evaluated in this article, or claim that may be made by its manufacturer, is not guaranteed or endorsed by the publisher.
Chen, J., Si, M., Wang, Y., Liu, L., Zhang, Y., Zhou, A., et al. (2019a). Ginsenoside Metabolite Compound K Exerts Anti-inflammatory and Analgesic Effects via Downregulating COX2. Inflammopharmacology 27 (1), 157–166. doi:10.1007/s10787-018-0504-y
Chen, J., Zhang, X., Liu, X., Zhang, C., Shang, W., Xue, J., et al. (2019b). Ginsenoside Rg1 Promotes Cerebral Angiogenesis via the PI3K/Akt/mTOR Signaling Pathway in Ischemic Mice. Eur. J. Pharmacol. 856, 172418. doi:10.1016/j.ejphar.2019.172418
Chen, K., Jiao, J., Xue, J., Chen, T., Hou, Y., Jiang, Y., et al. (2020). Ginsenoside CK Induces Apoptosis and Suppresses Proliferation and Invasion of Human Osteosarcoma Cells through the PI3K/mTOR/p70S6K1 Pathway. Oncol. Rep. 43 (3), 886–896. doi:10.3892/or.2020.7460
Chen, Y., Lin, S., Sun, Y., Pan, X., Xiao, L., Zou, L., et al. (2016). Translational Potential of Ginsenoside Rb1 in Managing Progression of Osteoarthritis. J. Orthop. Translat 6, 27–33. doi:10.1016/j.jot.2016.03.001
Chiang, Y. H., Lin, C. C., Chen, Y. C., and Lee, O. K. (2018). Treatment of Arsenite Intoxication-Induced Peripheral Vasculopathy with Mesenchymal Stem Cells. Int. J. Mol. Sci. 19 (4), 1026. doi:10.3390/ijms19041026
Duan, P., and Bonewald, L. F. (2016). The Role of the Wnt/β-Catenin Signaling Pathway in Formation and Maintenance of Bone and Teeth. Int. J. Biochem. Cel Biol 77 (Pt A), 23–29. doi:10.1016/j.biocel.2016.05.015
Einhorn, T. A., and Gerstenfeld, L. C. (2015). Fracture Healing: Mechanisms and Interventions. Nat. Rev. Rheumatol. 11 (1), 45–54. doi:10.1038/nrrheum.2014.164
Filipowska, J., Tomaszewski, K. A., Niedźwiedzki, Ł., Walocha, J. A., and Niedźwiedzki, T. (2017). The Role of Vasculature in Bone Development, Regeneration and Proper Systemic Functioning. Angiogenesis 20 (3), 291–302. doi:10.1007/s10456-017-9541-1
Glowacki, J. (1998). Angiogenesis in Fracture Repair. Clin. Orthop. Relat. Res. 355 (Suppl. l), S82–S89. doi:10.1097/00003086-199810001-00010
Gu, Y., Zhou, J., Wang, Q., Fan, W., and Yin, G. (2016). Ginsenoside Rg1 Promotes Osteogenic Differentiation of rBMSCs and Healing of Rat Tibial Fractures through Regulation of GR-dependent BMP-2/SMAD Signaling. Sci. Rep. 6, 25282. doi:10.1038/srep25282
Huang, C., Ness, V. P., Yang, X., Chen, H., Luo, J., Brown, E. B., et al. (2015). Spatiotemporal Analyses of Osteogenesis and Angiogenesis via Intravital Imaging in Cranial Bone Defect Repair. J. Bone Miner Res. 30 (7), 1217–1230. doi:10.1002/jbmr.2460
Huang, Q., Gao, B., Jie, Q., Wei, B. Y., Fan, J., Zhang, H. Y., et al. (2014). Ginsenoside-Rb2 Displays Anti-osteoporosis Effects through Reducing Oxidative Damage and Bone-Resorbing Cytokines during Osteogenesis. Bone 66, 306–314. doi:10.1016/j.bone.2014.06.010
Jiang, L., Yin, M., Wei, X., Liu, J., Wang, X., Niu, C., et al. (2015). Bach1 Represses Wnt/β-Catenin Signaling and Angiogenesis. Circ. Res. 117 (4), 364–375. doi:10.1161/circresaha.115.306829
Kang, S., Siddiqi, M. H., Yoon, S. J., Ahn, S., Noh, H. Y., Kumar, N. S., et al. (2016). Therapeutic Potential of Compound K as an IKK Inhibitor with Implications for Osteoarthritis Prevention: an In Silico and In Vitro Study. In Vitro Cel Dev Biol Anim 52 (9), 895–905. doi:10.1007/s11626-016-0062-9
Kim, J. H., Liu, X., Wang, J., Chen, X., Zhang, H., Kim, S. H., et al. (2013). Wnt Signaling in Bone Formation and its Therapeutic Potential for Bone Diseases. Ther. Adv. Musculoskelet. Dis. 5 (1), 13–31. doi:10.1177/1759720x12466608
Kobayashi, Y., Uehara, S., Udagawa, N., and Takahashi, N. (2016). Regulation of Bone Metabolism by Wnt Signals. J. Biochem. 159 (4), 387–392. doi:10.1093/jb/mvv124
Kusumbe, A. P., Ramasamy, S. K., and Adams, R. H. (2014). Coupling of Angiogenesis and Osteogenesis by a Specific Vessel Subtype in Bone. Nature 507 (7492), 323–328. doi:10.1038/nature13145
Kwok, H. H., Chan, L. S., Poon, P. Y., Yue, P. Y., and Wong, R. N. (2015). Ginsenoside-Rg1 Induces Angiogenesis by the Inverse Regulation of MET Tyrosine Kinase Receptor Expression through miR-23a. Toxicol. Appl. Pharmacol. 287 (3), 276–283. doi:10.1016/j.taap.2015.06.014
Li, X., Huang, Q., Wang, M., Yan, X., Song, X., Ma, R., et al. (2018). Compound K Inhibits Autophagy-Mediated Apoptosis through Activation of the PI3K-Akt Signaling Pathway Thus Protecting against Ischemia/Reperfusion Injury. Cell Physiol Biochem 47 (6), 2589–2601. doi:10.1159/000491655
Lin, W., Xu, L., Pan, Q., Lin, S., Feng, L., Wang, B., et al. (2019). Lgr5-overexpressing Mesenchymal Stem Cells Augment Fracture Healing through Regulation of Wnt/ERK Signaling Pathways and Mitochondrial Dynamics. FASEB J. 33 (7), 8565–8577. doi:10.1096/fj.201900082RR
Liu, J., Chuah, Y. J., Fu, J., Zhu, W., and Wang, D. A. (2019). Co-culture of Human Umbilical Vein Endothelial Cells and Human Bone Marrow Stromal Cells into a Micro-cavitary Gelatin-Methacrylate Hydrogel System to Enhance Angiogenesis. Mater. Sci. Eng. C Mater. Biol. Appl. 102, 906–916. doi:10.1016/j.msec.2019.04.089
Liu, Q., Zhou, J., Yang, Z., Xie, C., Huang, Y., Ling, L., et al. (2020). The Ginsenoside Exhibits Antiosteoporosis Effects in Ketogenic-Diet-Induced Osteoporosis via Rebalancing Bone Turnover. Front. Pharmacol. 11, 593820. doi:10.3389/fphar.2020.593820
Park, D., and Yoon, M. (2012). Compound K, a Novel Ginsenoside Metabolite, Inhibits Adipocyte Differentiation in 3T3-L1 Cells: Involvement of Angiogenesis and MMPs. Biochem. Biophys. Res. Commun. 422 (2), 263–267. doi:10.1016/j.bbrc.2012.04.142
Peng, Y., Wu, S., Li, Y., and Crane, J. L. (2020). Type H Blood Vessels in Bone Modeling and Remodeling. Theranostics 10 (1), 426–436. doi:10.7150/thno.34126
Ramasamy, S. K., Kusumbe, A. P., Wang, L., and Adams, R. H. (2014). Endothelial Notch Activity Promotes Angiogenesis and Osteogenesis in Bone. Nature 507 (7492), 376–380. doi:10.1038/nature13146
Ren, L., Chen, H., Song, J., Chen, X., Lin, C., Zhang, X., et al. (2019). MiR-454-3p-Mediated Wnt/β-Catenin Signaling Antagonists Suppression Promotes Breast Cancer Metastasis. Theranostics 9 (2), 449–465. doi:10.7150/thno.29055
Shen, G., Ren, H., Shang, Q., Zhao, W., Zhang, Z., Yu, X., et al. (2020). Foxf1 Knockdown Promotes BMSC Osteogenesis in Part by Activating the Wnt/β-Catenin Signalling Pathway and Prevents Ovariectomy-Induced Bone Loss. EBioMedicine 52, 102626. doi:10.1016/j.ebiom.2020.102626
Shen, J., Sun, Y., Liu, X., Zhu, Y., Bao, B., Gao, T., et al. (2021). EGFL6 Regulates Angiogenesis and Osteogenesis in Distraction Osteogenesis via Wnt/β-Catenin Signaling. Stem Cel Res Ther 12 (1), 415. doi:10.1186/s13287-021-02487-3
Shen, W., Luo, H., Xu, L., Wu, Z., Chen, H., Liu, Y., et al. (2018). Wnt5a Mediates the Effects of Bushen Huoxue Decoction on the Migration of Bone Marrow Mesenchymal Stem Cells In Vitro. Chin. Med. 13, 45. doi:10.1186/s13020-018-0200-2
Shi, L., Feng, L., Zhu, M. L., Yang, Z. M., Wu, T. Y., Xu, J., et al. (2020). Vasoactive Intestinal Peptide Stimulates Bone Marrow-Mesenchymal Stem Cells Osteogenesis Differentiation by Activating Wnt/β-Catenin Signaling Pathway and Promotes Rat Skull Defect Repair. Stem Cell Dev 29 (10), 655–666. doi:10.1089/scd.2019.0148
Thangavelu, M., Adithan, A., John Peter, J. S., Hossain, M. A., Kim, N. S., Hwang, K. C., et al. (2020). Ginseng Compound K Incorporated Porous Chitosan/biphasic Calcium Phosphate Composite Microsphere for Bone Regeneration. Int. J. Biol. Macromol 146, 1024–1029. doi:10.1016/j.ijbiomac.2019.09.228
Tian, F., Wang, X., Ni, H., Feng, X., Yuan, X., and Huang, Q. (2021). The Ginsenoside Metabolite Compound K Stimulates Glucagon-like Peptide-1 Secretion in NCI-H716 Cells by Regulating the RhoA/ROCKs/YAP Signaling Pathway and Cytoskeleton Formation. J. Pharmacol. Sci. 145 (1), 88–96. doi:10.1016/j.jphs.2020.11.005
Vimalraj, S., Arumugam, B., Miranda, P. J., and Selvamurugan, N. (2015). Runx2: Structure, Function, and Phosphorylation in Osteoblast Differentiation. Int. J. Biol. Macromol 78, 202–208. doi:10.1016/j.ijbiomac.2015.04.008
Wang, L., Zhang, F., Cao, Z., Xiao, Y., Li, S., Yu, B., et al. (2017). Ginsenoside F2 Induces the Release of Mediators Associated with Anaphylactoid Reactions. Fitoterapia 121, 223–228. doi:10.1016/j.fitote.2017.07.010
Yang, Q., Lin, J., Zhang, H., Liu, Y., Kan, M., Xiu, Z., et al. (2019). Ginsenoside Compound K Regulates Amyloid β via the Nrf2/Keap1 Signaling Pathway in Mice with Scopolamine Hydrobromide-Induced Memory Impairments. J. Mol. Neurosci. 67 (1), 62–71. doi:10.1007/s12031-018-1210-3
Yang, X. D., Yang, Y. Y., Ouyang, D. S., and Yang, G. P. (2015). A Review of Biotransformation and Pharmacology of Ginsenoside Compound K. Fitoterapia 100, 208–220. doi:10.1016/j.fitote.2014.11.019
Yin, Q., Chen, H., Ma, R. H., Zhang, Y. Y., Liu, M. M., Thakur, K., et al. (2021). Ginsenoside CK Induces Apoptosis of Human Cervical Cancer HeLa Cells by Regulating Autophagy and Endoplasmic Reticulum Stress. Food Funct. 12 (12), 5301–5316. doi:10.1039/d1fo00348h
Yoon, S. H., Han, E. J., Sung, J. H., and Chung, S. H. (2007). Anti-diabetic Effects of Compound K versus Metformin versus Compound K-Metformin Combination Therapy in Diabetic Db/db Mice. Biol. Pharm. Bull. 30 (11), 2196–2200. doi:10.1248/bpb.30.2196
Yu, X., Rong, P. Z., Song, M. S., Shi, Z. W., Feng, G., Chen, X. J., et al. (2021). lncRNA SNHG1 Induced by SP1 Regulates Bone Remodeling and Angiogenesis via Sponging miR-181c-5p and Modulating SFRP1/Wnt Signaling Pathway. Mol. Med. 27 (1), 141. doi:10.1186/s10020-021-00392-2
Yuan, Z., Li, Q., Luo, S., Liu, Z., Luo, D., Zhang, B., et al. (2016). PPARγ and Wnt Signaling in Adipogenic and Osteogenic Differentiation of Mesenchymal Stem Cells. Curr. Stem Cel Res Ther 11 (3), 216–225. doi:10.2174/1574888x10666150519093429
Zhang, J., Liu, M., Huang, M., Chen, M., Zhang, D., Luo, L., et al. (2019). Ginsenoside F1 Promotes Angiogenesis by Activating the IGF-1/IGF1R Pathway. Pharmacol. Res. 144, 292–305. doi:10.1016/j.phrs.2019.04.021
Zhang, J., Ma, X., and Fan, D. (2021). Ginsenoside CK Inhibits Hypoxia-Induced Epithelial-Mesenchymal Transformation through the HIF-1α/nf-Κb Feedback Pathway in Hepatocellular Carcinoma. Foods 10 (6), 1195. doi:10.3390/foods10061195
Zhou, W., Huang, H., Zhu, H., Zhou, P., and Shi, X. (2018). New Metabolites from the Biotransformation of Ginsenoside Rb1 by Paecilomyces Bainier sp.229 and Activities in Inducing Osteogenic Differentiation by Wnt/β-Catenin Signaling Activation. J. Ginseng Res. 42 (2), 199–207. doi:10.1016/j.jgr.2017.03.004
Zhou, W., Li, J., Li, X., Yan, Q., and Zhou, P. (2008). Development and Validation of a Reversed-phase HPLC Method for Quantitative Determination of Ginsenosides Rb1, Rd, F2, and Compound K during the Process of Biotransformation of Ginsenoside Rb1. J. Sep. Sci. 31 (6-7), 921–925. doi:10.1002/jssc.200700406
Keywords: CK, fracture healing, Wnt/β-catenin, osteogenesis, angiogenesis
Citation: Ding L, Gu S, Zhou B, Wang M, Zhang Y, Wu S, Zou H, Zhao G, Gao Z and Xu L (2022) Ginsenoside Compound K Enhances Fracture Healing via Promoting Osteogenesis and Angiogenesis. Front. Pharmacol. 13:855393. doi: 10.3389/fphar.2022.855393
Received: 15 January 2022; Accepted: 07 March 2022;
Published: 01 April 2022.
Edited by:
Longhuo Wu, Gannan Medical University, ChinaReviewed by:
Xiaowei Wei, Affiliated Zhongshan Hospital of Dalian University, ChinaCopyright © 2022 Ding, Gu, Zhou, Wang, Zhang, Wu, Zou, Zhao, Gao and Xu. This is an open-access article distributed under the terms of the Creative Commons Attribution License (CC BY). The use, distribution or reproduction in other forums is permitted, provided the original author(s) and the copyright owner(s) are credited and that the original publication in this journal is cited, in accordance with accepted academic practice. No use, distribution or reproduction is permitted which does not comply with these terms.
*Correspondence: Guoping Zhao, Z3B6aGFvQHNpYnMuYWMuY24=; Zhao Gao, cWlxaW4xOTkwQDE2My5jb20=; Liangliang Xu, eHVsbC0yMDE2QGd6dWNtLmVkdS5jbg==
Disclaimer: All claims expressed in this article are solely those of the authors and do not necessarily represent those of their affiliated organizations, or those of the publisher, the editors and the reviewers. Any product that may be evaluated in this article or claim that may be made by its manufacturer is not guaranteed or endorsed by the publisher.
Research integrity at Frontiers
Learn more about the work of our research integrity team to safeguard the quality of each article we publish.