- Biomedical Magnetic Resonance Research Group, Louvain Drug Research Institute, Université Catholique de Louvain (UCLouvain), Brussels, Belgium
Hypoxia is a common feature of solid tumors that contributes to angiogenesis, invasiveness, metastasis, altered metabolism and genomic instability. As hypoxia is a major actor in tumor progression and resistance to radiotherapy, chemotherapy and immunotherapy, multiple approaches have emerged to target tumor hypoxia. It includes among others pharmacological interventions designed to alleviate tumor hypoxia at the time of radiation therapy, prodrugs that are selectively activated in hypoxic cells or inhibitors of molecular targets involved in hypoxic cell survival (i.e., hypoxia inducible factors HIFs, PI3K/AKT/mTOR pathway, unfolded protein response). While numerous strategies were successful in pre-clinical models, their translation in the clinical practice has been disappointing so far. This therapeutic failure often results from the absence of appropriate stratification of patients that could benefit from targeted interventions. Companion diagnostics may help at different levels of the research and development, and in matching a patient to a specific intervention targeting hypoxia. In this review, we discuss the relative merits of the existing hypoxia biomarkers, their current status and the challenges for their future validation as companion diagnostics adapted to the nature of the intervention.
1 At-A-Glance View of Tumor Hypoxia: Causes and Consequences
The overall goal of this manuscript is to provide the rationale for the development of companion diagnostics that are crucially important when developing and evaluating emerging hypoxia-targeted therapies. A companion diagnostic is a test (in vitro or in vivo) used to help match a patient to a specific drug or therapy. Before describing how imaging modalities could be helpful to guide hypoxia-targeted therapies, it is important to first briefly introduce the factors contributing to the occurrence of hypoxic regions in solid tumors as well as the cellular consequences of acute or prolonged periods of hypoxia. This will give a sense for understanding approaches aimed at alleviating hypoxia, on the one hand, and/or at fighting against downstream cellular responses at the origin of malignant progression and resistance to therapies, on the other hand.
1.1 Pathogenesis of Tumor Hypoxia
The systematic detection of tumor hypoxia in the clinical setting has demonstrated that most solid tumors contain hypoxic regions that influence malignant progression and contribute to therapeutic resistance (Höckel and Vaupel, 2001; Vaupel et al., 2004; Vaupel et al., 2007; Vaupel and Mayer, 2007; Bayer et al., 2011; Busk and Horsman, 2013; Lee et al., 2014; Vaupel and Mayer, 2014; Horsman and Vaupel, 2016; Vaupel and Mayer, 2016; Hughes et al., 2019; Swartz et al., 2020; Sørensen and Horsman, 2020; Hompland et al., 2021; Vaup el et al., 2021). Mechanistically, tumor hypoxia is the result of an inadequate oxygen supply that cannot meet the oxygen demand by the cells present in the tumor microenvironment. Multiple factors contribute to the occurrence of tumor hypoxia (Figure 1) that exhibits a high degree of spatial and temporal heterogeneities (Dewhirst et al., 2008; Vaupel and Mayer, 2014; Vaupel and Mayer, 2016). While intrinsically related, the simplified view is to distinguish between chronic hypoxia and cycling hypoxia.
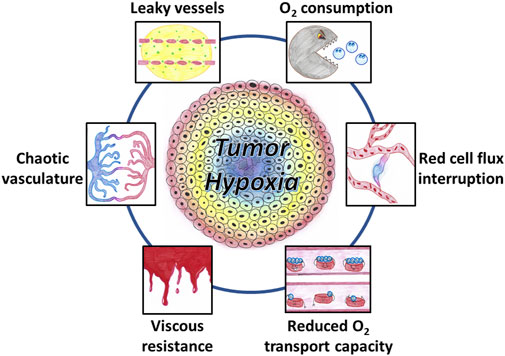
FIGURE 1. Factors contributing to the occurrence of tumor hypoxia. The tumor vasculature is chaotic, showing abnormal vascular density, contour irregularities, enlarged vessels, and vessels with blind ends. The increased leakiness of immature vessels results in an increased interstitial fluid pressure. Increased viscous resistance may contribute the vascular stasis. Tumor-associated anemia leads to a reduced oxygen transport capacity. Cycling hypoxia results from transient stasis in flow and transient interruption in red blood cell flux. Oxygen utilization by a large density of tumor cells with a high degree of metabolic activity and proliferation also contributes to tumor hypoxia.
Chronic hypoxia (or diffusion-limited hypoxia) occurs with low-frequency variations (timeframe of hours, days or weeks) (Vaupel and Mayer, 2014; Vaupel and Mayer, 2016; Dewhirst et al., 2008). Chronic hypoxia is mainly caused by limitations in the diffusion of oxygen from the blood vessels to reach distant cells. Two types of oxygen gradient are existing in tumors as demonstrated by studies in window chamber tumors: a radial gradient due to enlarged diffusion distances from the perivascular space to distant cells, and a longitudinal gradient corresponding to a decline in vascular pO2 along the afferent path of blood flow (Dewhirst et al., 1994; Dewhirst et al., 1999). Several factors contribute to the occurrence of chronic hypoxia (Figure 1). Compared to the well-organized blood supply of normal tissues, the vascular system in tumors is chaotic. The tumor vascular supply shows abnormal vascular density, contour irregularities, enlarged vessels, and vessels with blind ends (Konerding et al., 1999; Horsman and Vaupel, 2016; Vaupel and Mayer, 2016; Sørensen and Horsman, 2020). In addition, the vessels originating from angiogenesis are often immature and highly permeable allowing significant plasma leakage. The increased leakiness and the absence of functional lymphatic drainage results in an increased interstitial fluid pressure leading to a decrease in pressure differences between arterial and venous ends causing blood flow stasis (Horsman and Vaupel, 2016). Moreover, increased viscous resistance may also contribute the vascular stasis. The lower pH resulting from metabolic adaptation to hypoxia and/or high glycolysis rate increases the rigidity of red blood cells and increases the blood viscosity (Jain, 1988; Sevick and Jain, 1989). Tumor-associated or therapy-induced anemia leads to a reduced oxygen transport capacity and can also contribute to the development of hypoxia (known as “anemic hypoxia” or “hypoxemic hypoxia”) (Vaupel and Mayer, 2007; Vaupel and Mayer, 2016).
Cycling hypoxia (or equivalently acute hypoxia or perfusion-limited hypoxia or fluctuating hypoxia or transient hypoxia) is the second major type of tumor hypoxia. Cycling hypoxia is characterized by episodes of hypoxia varying over shorter periods of time than chronic hypoxia (Brown, 1979; Chaplin et al., 1987; Dewhirst et al., 2008; Vaupel and Mayer, 2014). Experimental evidences demonstrated the occurrence of rapid cycles of fluctuating hypoxia (timeframe of a few seconds or less) and slow cycles (minutes to hours) (Braun et al., 1999; Braun et al., 2001; Baudelet et al., 2004; Baudelet et al., 2006; Cárdenas-Navia et al., 2008; Magat et al., 2010; Matsumoto et al., 2010; Yasui et al., 2010). Rapid cycles of hypoxia mainly result from transient stasis in flow or transient interruption in red blood cell flux (Dewhirst et al., 1996; Kimura et al., 1996) while it is speculated that slow cycles of hypoxia could be more related to vascular remodeling and presence of vascular smooth muscles (Baudelet et al., 2006; Bayer and Vaupel, 2012; Vaupel and Mayer, 2014; Bader et al., 2020).
While most factors described previously are related to the delivery of oxygen through the perfusion of the tumor, the oxygen utilization by cells present in the tumor microenvironment should not be neglected. First, solid tumors are composed of a large density of tumor cells with a high degree of metabolic activity and proliferation. Many tumor cells exhibit a glycolytic phenotype that provides a rapid production of ATP (much faster than mitochondria) and sustains cell proliferation through the pentose phosphate pathway. However, contrarily to the historical dogma, mitochondria remain functional in cancer cells that may exhibit a high respiratory capacity, and other substrates may fuel the electron transport chain (Zhdanov et al., 2014; De Preter et al., 2016a; Corbet and Feron, 2017; Marchetti et al., 2020; Vaupel and Multhoff, 2021a; Vaupel and Multhoff, 2021b). In addition, it has been shown that other cells present in the tumor microenvironment such as tumor associated macrophages (TAMs) present high oxidative phosphorylation with high basal and maximal oxygen consumption rate (M de-Brito et al., 2020). Overall, both impaired oxygen delivery and high oxygen cellular metabolic demand contribute to the prevalence of hypoxia in solid tumors.
1.2 Significance of Tumor Hypoxia
Experimental and clinical studies support the fact that hypoxia has detrimental consequences for both cancer progression and response to therapies.
1.2.1 Cellular Response to Hypoxia
In response to the stress caused by hypoxia, cells undergo a large variety of molecular responses (Harris, 2002; Vaupel and Mayer, 2016; Lee et al., 2020; Sørensen and Horsman, 2020). The predominant hypoxia-mediated intracellular signaling pathway is controlled by a family of transcription factors, the hypoxia inducible factors (HIFs) (Semenza and Wang, 1992; Wang and Semenza, 1993; Semenza, 2000; Harris, 2002; Semenza, 2003; Muz et al., 2015; Vaupel and Mayer, 2016; Pugh and Ratcliffe, 2017; Lee et al., 2020) (Figure 2). HIFs are heterodimeric proteins that consist of two proteins, HIF-α and HIF-β. HIF-α stability is the principal key factor for the regulation of HIF activity. HIF-α has three closely related homologues, HIF-1α, HIF-2α, and HIF-3α (Semenza, 2003; Loboda et al., 2010; Muz et al., 2015; Albadari et al., 2019; Codony and Tavassoli, 2021). In normoxia, HIF-α undergoes proteasomal degradation by a mechanism that involves hydroxylation of proline residues on HIF-α by prolyl hydroxylases (PHDs) and subsequent ubiquitination by the pVHL (von Hippel Lindau) protein E3 ubiquitin ligase system. In hypoxia, the PHDs lose their activity, the hydroxylation of the HIF-α subunit is inhibited without subsequent degradation. The non-hydroxylated, stabilized HIF-α subunits translocate to the nucleus where they dimerize with constitutively expressed HIF-β subunit, and bind to DNA to initiate gene transcription (Semenza, 2003; Loboda et al., 2010; Muz et al., 2015; Albadari et al., 2019; Codony and Tavassoli, 2021). Of note, the expression of HIF-1α could also be achieved in a hypoxia-independent manner, including by ROS and by growth factors through receptor tyrosine kinases (Semenza, 2003; Muz et al., 2015). The oxygen-independent HIF regulation is mediated by several signaling pathways including NFκB, PI3K/AKT/mTOR, and MAPK/ERK. These pathways are additionally regulated by hypoxia, which results in multiple levels of HIF-α stimulation, both hypoxic and normoxic (Harris, 2002; Semenza, 2003; Muz et al., 2015; Lee et al., 2020). Genes that are involved in tumor progression are transcriptionally activated by HIF-1 (Figure 2). Among them, target genes included those involved in angiogenesis (VEGF, VEGF-R1, VEGF-R2, PDGF, Ang-1, Ang-2, MMPs), invasion and metastasis (LOX, MMPs, integrins), epithelial-to-mesenchymal transition (EMT) modulation (cadherins, vimentin), cell proliferation (cyclin G2, IGF-BPs), cell survival (ADM, IGF2, IGF-BPs, TGF-β), apoptosis and autophagy (BNIP, NOX), metabolism (GLUT1, GAPDH, PDK, LDHA,PKM), regulation of tumor acidosis (CAIX), and tumor immunity (TGF-β, PD-L1) (Semenza, 2000; Harris, 2002; Feldman et al., 2005; Horsman and Vaupel, 2016; Lee et al., 2020; Codony and Tavassoli, 2021). It should be emphasized that this list of target genes is illustrative rather than exhaustive.
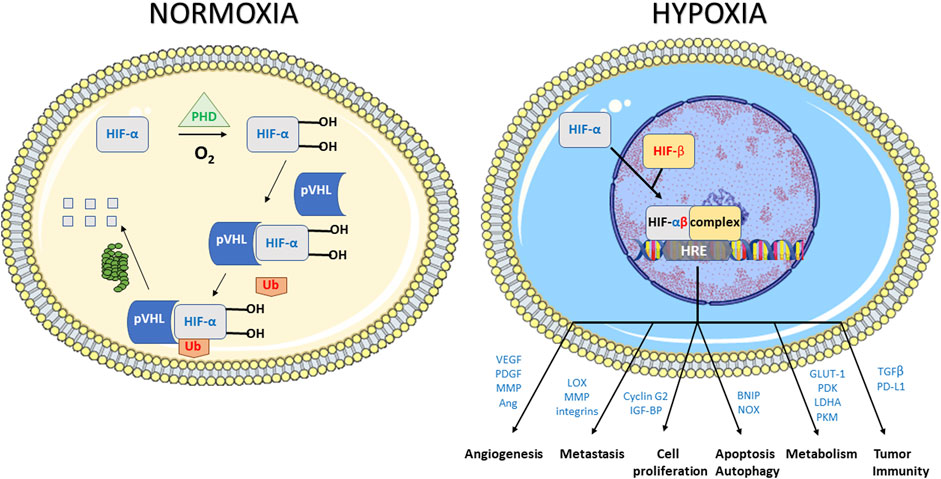
FIGURE 2. Response to hypoxic stress mediated by the hypoxia inducible factors (HIFs). HIF1s are heterodimeric proteins that consist of two proteins, HIF1-α and HIF1-β. In normoxia, HIF1-α undergoes proteasomal degradation by a mechanism that involves hydroxylation of proline residues on HIF1-α by prolyl hydroxylases (PHDs) and subsequent ubiquitination by the pVHL (von Hippel Lindau) system. In hypoxia, the PHDs lose their activity, the hydroxylation of the HIF1-α subunit is inhibited without subsequent degradation. The non-hydroxylated, stabilized HIF1-α subunits translocate to the nucleus where they dimerize with constitutively expressed HIF1-β subunit, and bind to DNA to initiate gene transcription. Illustrative genes that are transcriptionally activated by HIF-1 included those involved in angiogenesis, invasion and metastasis cell proliferation, apoptosis and autophagy, metabolism and tumor immunity.
Under severe hypoxia, one of the stress responses (HIF-independent) is the unfolded protein response (UPR) activated in response to ER (endoplasmic reticulum) stress (Feldman et al., 2005; Horsman and Vaupel, 2016; Lee et al., 2020). ER stress induces cytoprotective functions by activating signaling pathways to keep cellular homeostasis. However, if the stress remains unresolved, signaling pathways will activate apoptosis. The UPR is mediated through the activation of ER transmembrane stress sensors: pancreatic ER kinase (PKR)-like ER kinase (PERK), activating transcription factor 6 (ATF6) and inositol-requiring enzyme 1 (IRE1). These proteins are in an inactive state through a physical interaction between their ER lumen domains and GRP78 (a chaperone glucose-regulated protein of 78 kDa). If the level of unfolded proteins increases in the ER, GRP78 will be redirected to these unfolded proteins, with a release and activation of the ER stress sensors, launching the UPR (Horsman and Vaupel, 2016).
Differential consequences can be actually observed depending on the cell type exposed, the degree of hypoxia and the exposure time to hypoxia (Michiels et al., 2016; Vaupel and Mayer, 2016). Both chronic and acute hypoxia may foster tumor progression. However, it has been suggested in preclinical tumor models that cycling hypoxia through specific signaling pathways, genomic instability and enhanced ROS production may lead to even greater tumor aggressiveness (Cairns et al., 2001; Cairns and Hill, 2004; Dewhirst et al., 2008; Martinive et al., 2009; Miao et al., 2014; Michiels et al., 2016; Vaupel and Mayer, 2016).
1.2.2 Hypoxia as Factor of Resistance to Therapy
While long-term exposure to severe hypoxic conditions is lethal for many cells, subpopulations of tumor cells could adapt to hypoxic conditions and become resistant to radiotherapy, chemotherapy and immunotherapy (Höckel and Vaupel, 2001; Cosse and Michiels, 2008; Rohwer and Cramer, 2011; Muz et al., 2015).
One of the most studied resistance to therapy linked to tumor hypoxia is the resistance to radiation therapy. The potential involvement of oxygen as a modulator of response to irradiation was published already more than 100 years ago. G. Schwarz described the effect of hypoxia as protector from radiation (Schwarz, 1909). He observed that skin compression and reduction in skin blood flow decreased the radiosensitivity (actually reduced radiation burn) (Schwarz, 1909). About 70 years ago, the seminal studies of L.H. Gray and R.H. Thomlinson suggested the importance of microregional structures and associated oxygen gradient for the response to irradiation (Gray et al., 1953; Thomlinson and Gray, 1955). Since these early studies, several thousands of manuscripts have been published on this thematic research [on 28 December 2021, 8,321 references found in Pubmed for a research associating (hypoxia) and (radiation)]. The mechanism responsible for the enhancement of radiation damage by oxygen is generally referred to as the oxygen-fixation hypothesis (Horsman et al., 2009). DNA is generally considered as the ultimate target leading to a mitotic catastrophe (clonogenic death). Damages to DNA may be produced directly or indirectly through the water radiolysis and production of highly reactive free radicals which ultimately produced in DNA a transient radical R• from RH. When oxygen is present, R• can immediately react with O2 to produce ROO• to further produce ROOH. In other words, oxygen is “fixing” the DNA damage with a change in the chemical structure of DNA. In the absence of oxygen, the unstable R• molecules have a longer half-life and can react with H•, thus chemically restoring their original form (Horsman et al., 2009). The “oxygen enhancement ratio” (OER, the ratio of doses required to obtain the same cell survival under hypoxic and aerobic conditions) varies from 2.5 to 3.0, indicating that hypoxic tumor cells will require a dose 2.5–3 times higher to be killed than normoxic cells (Brown, 2007; Horsman et al., 2009). From literature, it appears that OER is dramatically increasing when pO2 is rising from 1 to 10 mmHg (Whillans and Hunt, 1982; Koch et al., 1984; Wouters and Brown, 1997; Brown, 2007; Horsman et al., 2009). Above this value of 10 mmHg, further increase in pO2 does not further enhance the radiosensitivity (Figure 3). The effect of tumor hypoxia on the response to a treatment by ionizing radiation has been demonstrated in a multitude of experimental preclinical studies [reviewed in (Vaup el et al., 2021) and (Gallez, 2021)]. In a series of clinical studies in the early nineties, using pO2 measurements with microelectrodes, P. Vaupel and others definitively demonstrated that tumor hypoxia was predicting the response of tumors to radiation therapy (Gatenby et al., 1988; Höckel et al., 1993a; Höckel et al., 1993b; Okunieff et al., 1993; Stone et al., 1993; Höckel et al., 1996; Fyles et al., 1998; Knocke et al., 1999; Höckel and Vaupel, 2001; Rudat et al., 2001; Nordsmark et al., 2005; Vaupel et al., 2007; Vaupel and Mayer, 2007; Vaup el et al., 2021). Although tumor hypoxia is acknowledged as the major factor of resistance of solid tumors to radiation therapy, the clinical practice actually does not yet fully integrate on a routine basis this factor in the definition of radiation protocols. Possible strategies for improving the curative effect of radiotherapy on hypoxic cells include the alleviation of tumor hypoxia at the time of irradiation and/or the redistribution of the radiation dose integrating the presence of hypoxic areas. From a meta-analysis gathering 10,108 patients from 86 randomized trials designed to modify tumor hypoxia in patients treated with curative attempted primary radiation (Overgaard, 2007), J. Overgaard concluded that “Ample data exist to support a high level of evidence for the benefit of hypoxic modification. However, hypoxic modification still has no impact on general clinical practice” (Overgaard, 2007). In a second meta-analysis, he analyzed the results from clinical trials that included 4,805 patients suffering from squamous cell carcinoma of the head and neck (HNSCC) with attempts to modify the hypoxic radioresistance (by applying normobaric oxygen, carbogen breathing, hyperbaric oxygen or hypoxic radiosensitizers) (Overgaard, 2011). Again, he demonstrated the added value of adding hypoxic modification to radiotherapy in HNSCC patients (Overgaard, 2011). In discussing the results from these meta-analyses, J. Overgaard pointed that the lack of proper identification of the patients with hypoxic tumors requiring adapted treatment was an obstacle to a routine clinical use of hypoxia-targeted interventions (Overgaard, 2007).
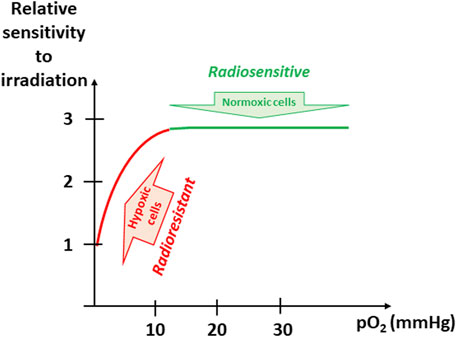
FIGURE 3. Evolution of sensitivity to irradiation as a function of pO2. The “oxygen enhancement ratio” (OER, the ratio of doses required to obtain the same cell survival under hypoxic and aerobic conditions) varies from 2.5 to 3.0, indicating that hypoxic tumor cells will require a dose 2.5–3 times higher to be killed than normoxic cells. The OER is dramatically increasing when pO2 is rising from 1 to 10 mmHg (found in hypoxic tumors). Above this value of 10 mmHg, further increase in pO2 does not further enhance the radiosensitivity.
Tumor hypoxia is also very likely a key detrimental factor for the resistance to anti-cancer chemotherapy. Contrarily to the clinical evidence existing in the field of radiation therapy, there is no direct existing clinical proof linking the level of tumor hypoxia and the (absence of) response to specific chemotherapeutic agent. This is due to the fact that the use of chemotherapy for treating solid tumors is always part of a combined strategy together with surgery and/or radiation therapy without possibility to isolate the role of hypoxia on the sole drug response. However, a compelling body of experimental evidence demonstrates that hypoxia may alter the response to different chemotherapeutic agents. We have already discussed that HIF-mediated cellular processes may alter cell apoptosis, autophagy and tumor stemness which can have a direct impact on the drug response (Cosse and Michiels, 2008; Das et al., 2008; Rohwer and Cramer, 2011). In addition, tumor cells divide at a reduced rate as a result of decline in nutrient and oxygen availability. Consequently, the effect of drugs whose activity is selective for rapidly dividing cell populations is decreased. A large difference in proliferation rate between cells located in perivascular areas and those adjacent to necrotic regions has been clearly demonstrated (Tannock, 1968; Olive, 1989). In addition to hypoxia-induced cellular adaptations, it has been demonstrated that low oxygen level may alter the response to platinum complexes (Fadejeva et al., 2017), doxorubicin (Frederiksen et al., 2003), etoposide (Cosse et al., 2007; Cosse et al., 2009; Sermeus et al., 2013), bleomycin (Roizin-Towle and Hall, 1979). Of note, hypoxia may also affect the expression and activity of drug efflux pump such as p-glycoprotein (P-gp) and therefore contribute to a lower intracellular concentration of active drug (Thews et al., 2008; Abraham et al., 2015). Finally, considering that tumor hypoxia is associated with an abnormal vascularization, the impaired delivery of drugs also contributes as mechanism of resistance to the response to chemotherapy (Jain, 1991; Eskey et al., 1994; Netti et al., 1995; Jain, 1996; Jain, 1997; Tong et al., 2004; Ansiaux et al., 2006a; Martinive et al., 2006; Segers et al., 2006; Cron et al., 2008; Goel et al., 2011; Chauhan et al., 2013).
Tumor hypoxia is also a critical factor involved in the response to immunotherapy through multiple mechanisms (Noman et al., 2015; Lequeux et al., 2019; Noman et al., 2019; Chouaib, 2020; Multhoff and Vaupel, 2020; Terry et al., 2020; Fu et al., 2021; You et al., 2021). Low oxygen tension in tumors may act by reducing survival, cytolytic and migratory activity of immunostimulatory effector cells such as CD4+ cells, CD8+ cytotoxic T cells, natural killer-like T (NKT) cells and natural killer (NK) cells (Kumar and Gabrilovich, 2014; Samanta and Semenza, 2018; Multhoff and Vaupel, 2020). The stabilization of HIF-1α upregulates the expression of Programmed death-ligand 1 (PD-L1) in hypoxic tumor cells as well as the immune checkpoint V-Domain Ig suppressor of T cell activation (VISTA) in hypoxic myeloid-derived suppressor cells (MDSCs). The increased expression of PD-L1 and VISTA results in an inhibition of T cell proliferation and T cell mediated lysis (No man et al., 2014; Deng et al., 2019; Noman et al., 2019). HIF-1α is also involved in the upregulation of the macrophage immune checkpoint CD47 on the surface of tumor cells inducing tumor cell escape from phagocytosis (Noman et al., 2015; Zhang et al., 2015). Hypoxia-induced autophagy also impairs tumor cell susceptibility to CTL and NK-mediated lysis (Viry et al., 2014; Noman et al., 2015). Finally, hypoxia upregulates the expression of immunosuppressive HLA-G on the surface of tumor cells. The immunosuppressive functions of HLA-G depend on the binding to ILT2, ILT4, and KIR2DL4 expressed by several immune cells, including B cells, T cells, NK cells, dendritic cells, monocytes, and macrophages. As a consequence, the hypoxic-dependent overexpression of HLA-G also contributes to tumor escape from immune surveillance (Noman et al., 2015; Garziera et al., 2017). This hypoxia-mediated immunosuppression has stimulated the research for interventions to improve immunotherapy responsiveness, including alleviation of tumor hypoxia, the use of hypoxia-activated drugs and HIF inhibitors (Noman et al., 2015; Fu et al., 2021).
2 Treatments Targeting Hypoxia
The treatments targeting tumor hypoxia can be classified in three main categories: 1) attempts to alleviate tumor hypoxia in order to optimize the response to radiation therapy; 2) prodrugs that are activated to become toxic selectively in hypoxic cells; 3) inhibitors of molecular targets involved in hypoxic cell survival. It should be emphasized that these strategies have been tested mostly in preclinical models. The attempts that have been translated into the clinic are also compiled in the next sections.
2.1 Alleviation of Tumor Hypoxia at the Time of Radiation Therapy
As most solid tumors contain hypoxic regions that can be resistant to irradiation, the alleviation of tumor hypoxia could lead to a therapeutic benefit when combined with radiation therapy. A transient increase in tumor oxygenation at the time of irradiation is generally considered as a safe approach because it will directly impact the radioresistant hypoxic (tumor) cells without affecting the well oxygenated (normal) tissues (Figure 3). It has been described that tumor hypoxia may be considerably influenced by “physical treatments.” The most known example relies on the early changes in oxygenation observed after irradiation itself. The hypothesis of a tumor reoxygenation has been established several decades ago as part of the rule of the 4 Rs or 6 Rs (Radiosensitivity, Repair, Repopulation, Redistribution, Reoxygenation, and Reactivation of anti-tumor immune response) describing the response to an irradiation (Rakotomalala et al., 2021). Experimental evidences with quantitative and dynamic assessments of tumor oxygenation have demonstrated the occurrence of these effects and their contributing factors suggesting that appropriate scheduling may be exploited to potentiate the efficacy of radiation therapy (Olive, 1994; Goda et al., 1995; O'Hara et al., 1995; Goda et al., 1996; O'Hara et al., 1998; Sonveaux et al., 2002; Crokart et al., 2005a; Cron et al., 2005; Hou et al., 2013). Modulations in tumor oxygenation have been also observed after change in temperature (hyper- or hypothermia) (Moon et al., 2010; Neveu et al., 2017) or application of photodynamic therapy using verteporfin or redaporfin as photosensitizer (Pogue et al., 2002; Pogue et al., 2003; Karwicka et al., 2019). Another efficient method to increase tumor oxygenation is to provide a gas enriched in oxygen, for example 100% oxygen or carbogen (i.e., mixture of 95% O2 and 5% CO2 or 98% O2 and 2% CO2) (Siemann et al., 1977; Rojas et al., 1990; Falk et al., 1992; Grau et al., 1992; Horsman et al., 1994; Hoskin et al., 1997; Hill et al., 1998; Kaanders et al., 1998; Powell et al., 1999; Kaanders et al., 2002; Overgaard, 2007; Hoskin et al., 2009; Khan et al., 2009; Hoskin et al., 2010; Khan et al., 2010; Overgaard, 2011; Janssens et al., 2012; Thews and Vaupel, 2016; Song et al., 2021). Hyperbaric oxygen has also demonstrated a beneficial effect to reoxygenate tumors (Henk et al., 1977; Haffty et al., 1999; Becker et al., 2002; Thews and Vaupel, 2015; Chen et al., 2021). While breathing oxygen or carbogen is efficient in alleviating tumor hypoxia, some pharmacological approaches have led to a better response in sensitizing tumors to irradiation because they may act by several mechanisms including intrinsic radiosensitizing properties.
Most attempts of alleviation of tumor hypoxia by pharmacological agents have been assessed in the context of improving the response to irradiation. Conceptually, the strategies to increase the tumor oxygenation are comparable with the filling of a bath. To rise the water level in a bathtub, you may either increase the water supply by playing with the faucet or decrease the opening of the draining plug (Figure 4). In a similar manner, pharmacological strategies aimed at increasing the tumor oxygenation are targeting either the oxygen delivery (through an increase in perfusion, a decrease in blood viscosity or a better release of oxygen from hemoglobin) or the oxygen consumption (through the decrease of metabolic activity of the tumor cells).
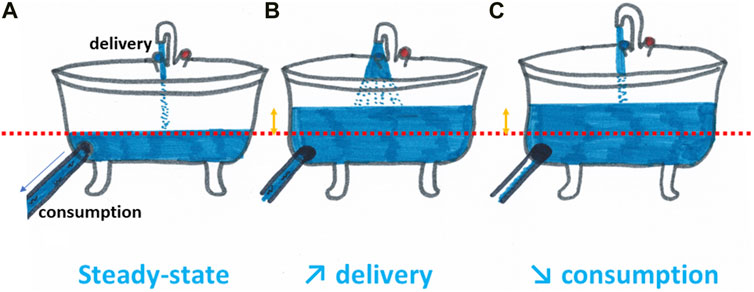
FIGURE 4. Increasing tumor oxygenation can be compared with the filling of a bath. To rise the water level in a bathtub compared to the steady state (A), you may either increase the water supply by playing with the faucet (B) or decrease the opening of the draining plug (C). In a similar manner, pharmacological strategies aimed at increasing the tumor oxygenation are targeting either the oxygen delivery (through an increase in perfusion, a decrease in blood viscosity or a better release of oxygen from hemoglobin) or the oxygen consumption (through the decrease of metabolic activity of the tumor cells).
2.1.1 Decreasing Tumor Hypoxia by Improving the Oxygen Delivery
Historically, it has been thought that increasing the perfusion (and consequently the oxygenation) could be elusive because of the lack of autoregulation by tumor blood vessels. Indeed, immature vessels derived from the angiogenic processes do not function as normal contractile cells (Jain, 1988; Lübbe and Huhnt, 1994; Eberhard et al., 2000; Sonveaux, 2008). However, the tumor vasculature also contains vessels that are able to regulate blood flow (Lübbe and Huhnt, 1994; Bergers and Benjamin, 2003; Julien et al., 2004; Sonveaux, 2008), including coopted preexisting vessels with contractile properties, vessels closed to the tumor margin, and vessels that gain structural maturity to acquire vasoactive capabilities (Peterson and Matts on, 1984; Sonveaux, 2008). Agents acting on the vasomotion of these vessels could therefore be used to increase blood flow and oxygenation. Thanks to methods that allow longitudinal quantitative measurements of tumor oxygenation such as Electron Paramagnetic Resonance (EPR) oximetry (Gallez et al., 2004; Khan et al., 2007; Ahmad and Kuppusamy, 2010), experimental evidences have shown the validity of such approaches. In a very large screening among 34 vasoactive agents (including angiotensin-converting enzyme inhibitors, alpha antagonists, beta-blockers, potassium channel openers, calcium antagonists, NO donors, and peripheral vasoactive agents), it was found that 24 compounds induced a significant increase in tumor oxygenation (Gallez et al., 1999a). Several compounds had profound effect on tumor oxygenation status and were further characterized for their effect on tumor hemodynamics and potential radiosensitizing properties (Gallez, 2021). The increase in tumor oxygenation observed after pre-treatment with nicotinate derivatives (xanthinol nicotinate or benzylnicotinate) and nitrosocaptopril significantly increased the tumor response to irradiation (Hou et al., 2010; Jordan et al., 2010; Segers et al., 2010). It was also found that diphteria toxin decreased the interstitial fluid pressure in solid tumors (Padera et al., 2004). The local administration of botulin neurotoxin was also found to significantly increase the tumor perfusion and oxygenation through an inhibition of neurotransmitter release and neurogenic contraction (Ansiaux et al., 2006a; Ansiaux and Gallez, 2007; Cron et al., 2008). The opening of the vascular bed induced by the local delivery of botulin neurotoxin led to an increase in the response of tumors to radio- and chemotherapy (Ansiaux et al., 2006a; Cron et al., 2008). It was also described that the endothelin receptor A (ETA) antagonist BQ123 decreased the vascular tone of tumor arterioles and increased tumor perfusion and oxygenation with a consequent improved response to radiation therapy and chemotherapy (Sonveaux et al., 2004; Martinive et al., 2006). Still, the success of these approaches playing on the vascular tone will definitely depend on the proportion of vasoreactive vessels compared to immature vessels present in the tumors. In this regard, the ability to measure the impact of those treatments on tumor perfusion and oxygenation by functional imaging will be crucial for a successful personalized treatment.
Another concept that has received particular intention is the normalization of tumor vasculature at the early phase of antiangiogenic treatments (Jain, 2001; Tong et al., 2004; Ansiaux et al., 2005; Jain, 2005; Segers et al., 2006; Batchelor et al., 2007; Mazzone et al., 2009; Carmeliet and Jain, 2011; Goel et al., 2011; Chauhan et al., 2012; Huang et al., 2012; Karroum et al., 2012; Crokart et al., 2013; Huang et al., 2013; Cantelmo et al., 2016; Peterson et al., 2016; Martin et al., 2019; Mpekris et al., 2020). While the long-term effect of antiangiogenic treatments should lead to a deprivation of tumors from oxygen and nutrients, a transient normalization of the tumor vasculature (after the early pruning of immature vessels) occurs that can be exploited to potentiate the response to irradiation (by the reoxygenation of the tumor) (Ansiaux et al., 2005; Karroum et al., 2012; Crokart et al., 2013), to chemotherapy (by increasing the delivery of drugs) (Tong et al., 2004; Segers et al., 2006; Chauhan et al., 2012; Cantelmo et al., 2016) and to immunotherapy (Huang et al., 2012; Huang et al., 2013; Mpekris et al., 2020). The success of these combinations definitely requires the identification in individual tumors of the time window of increase in perfusion and/or oxygenation. It has been indeed demonstrated that the application of co-treatments (irradiation or administration of chemotherapeutic agent) outside the normalization window led to an absence of effect or even a decrease in therapeutic efficacy (Ansiaux et al., 2005; Segers et al., 2006).
Another strategy to increase the oxygen delivery without altering the perfusion is to promote the release of oxygen by hemoglobin (Hb). It has been shown that allosteric effectors (such as efaproxiral or myo-inositol trispyrophosphate) binding to Hb results in a decreased hemoglobin-oxygen (Hb-O2) affinity and an increased tumor oxygenation (Teicher et al., 1998; Khandelwal et al., 1999; Amorino et al., 2001; Hou et al., 2004; Hou et al., 2005; Suh et al., 2006; Hou et al., 2007; Scott et al., 2007; Aprahamian et al., 2011; Limani et al., 2016; Tran et al., 2019; Cao-Pham et al., 2020). The increases in regulated oxygen-releasing capacity of red blood cells has been shown to potentiate the response to irradiation (Teicher et al., 1998; Khandelwal et al., 1999; Amorino et al., 2001; Suh et al., 2006; Hou et al., 2007; Scott et al., 2007; Tran et al., 2019). It has been suggested that the beneficial aspect of allosteric effectors could also be mediated by the suppression of HIF-1α and to down-regulation of HIF-inducible genes such as VEGF (Aprahamian et al., 2011).
Additional oxygen can put in circulation by biocompatible perfluorochemical emulsions or nanoplatforms sometimes used as blood substitutes. These interventions are often combined with oxygen or carbogen breathing in order to potentiate anti-cancer therapy (Krafft, 2020). It has been described that these approaches with an increase blood oxygen-carrying capacity may lead to an increase in response to radiation therapy (Teicher et al., 1991; Koch et al., 2002; Song et al., 2017; Zhou et al., 2018), photodynamic therapy (Fingar et al., 1988; Cheng et al., 2015; Tang et al., 2017; Song et al., 2018; Wang et al., 2018; Hu et al., 2019; Kv et al., 2020; Fang et al., 2021), sonodynamic therapy (Zeng et al., 2020; Guo et al., 2021), chemotherapy (Teicher et al., 1987; Teic her et al., 1990; Teicher, 1994; Song et al., 2019; Wu et al., 2020), and immunotherapy (Jiang et al., 2021; Yang et al., 2021).
Compounds acting on tumor blood flow may also counteract fluctuating hypoxia. In this respect, nicotinamide (vitamin B3) has received particular attention (Horsman et al., 1988; Horsman et al., 1989; Chaplin et al., 1990; Kelleher and Vaupel, 1993; Siemann et al., 1994; Hill and Chaplin, 1995; Thomas et al., 1995; Powell et al., 1997; Baudelet et al., 2004). Nicotinamide improves microcirculatory function and the homogeneity of microregional blood flow (Powell et al., 1997). Nicotinamide was part of the large clinical trials together with carbogen breathing in the ARCON and BCON protocols (Kaanders et al., 1998; Kaanders et al., 2002; Hoskin et al., 2009; Janssens et al., 2012; Song et al., 2021). Alleviation of tumor acute hypoxia has also been reported using drugs that improve fluidity of red blood cells such as pentoxifylline (Lee et al., 1992) and flunarizine (Baudelet et al., 2004). The anti-angiogenic agent sunitinib during the early phase of normalization of the tumor vasculature has also been described to decrease cycling hypoxia (Matsumoto et al., 2011).
2.1.2 Decreasing Tumor Hypoxia by Decreasing the Oxygen Consumption
The second major approach to increase tumor oxygenation is to modulate the metabolism (Gulledge and Dewhirst, 1996; Dewhirst et al., 2007; Danhier et al., 2013; Lin and Maity, 2015; Galle z et al., 2017; Dewhirst, 2018). The mathematical model described by Secomb suggested that a decrease in oxygen consumption should be much more efficient than an increase in oxygen delivery in order to alleviate tumor hypoxia (Secomb et al., 1995). In his computer simulations, he compared the effects of increasing blood pO2 or blood flow rate with a decrease in oxygen consumption rate. He showed that hypoxia can be abolished by a reduction in consumption rate by thirty percent, while it would require an increase in flow rate by a factor four or an increase in arterial pO2 by a factor of eleven or more (Secomb et al., 1995). Overall, this model suggested that decreasing oxygen consumption rate should be more effective than increasing blood flow or oxygen content to alleviate tumor hypoxia.
It is known that the individual basal metabolism is strongly dependent on hormones including thyroid hormones (Oppenheimer et al., 1987) with a potential impact in cancer management (Hercbergs, 1996). In tumor models, it has been demonstrated that the thyroid status was strongly impacting both tumor oxygenation (due to profound changes in tumor oxygen consumption rates) and response to irradiation. Tumors implanted in hypothyroid mice (treated with propylthiouracil) were much less hypoxic than in euthyroid or in hyperthyroid mice (treated with thyroxin) and, consequently, more responsive to irradiation (Jordan et al., 2007). General metabolic suppression (typically during mammalian hibernation) is in part under the control of hydrogen sulfide H2S, the last endogenous gas transmitter identified (Blackstone et al., 2005; Wagner et al., 2009). Taking benefit of the local acidic pH in tumors, it was found that the administration of the prodrug sodium hydrogenosulfide NaHS alleviated tumor hypoxia and radiosensitized tumors, an effect that was due to the inhibition of the mitochondrial respiration by tumor cells (De Preter et al., 2016b).
Besides general effect, other inhibitors of the tumor cell mitochondrial respiratory chain have been also described for their effect on tumor cell respiration, and tumor hypoxia with direct impact on the radiosensitivity. This effect has been described using non-steroidal anti-inflammatory drugs (piroxicam, diclofenac, indomethacin, NS398) (Crokart et al., 2005b), glucocorticoids (dexamethasone, hydrocortisone, prednisolone) (Crokart et al., 2007), metformin (Zannella et al., 2013), atovaquone (Ashton et al., 2016) and papaverine (Benej et al., 2018). Other compounds including some anti-angiogenic agents (Ansiaux et al., 2006b; Ansiaux et al., 2009; Crokart et al., 2013), MAPK inhibitors (Karroum et al., 2012) and EGFR inhibitor (Karroum et al., 2013) were also (unexpectedly) found to inhibit mitochondrial respiration in tumors cells and to potentiate radiation therapy.
Interestingly, cytotoxic agents may also contribute to an oxygen effect by decreasing the utilization of oxygen (Galle z et al., 2017). For example, it was shown that multiple factors contributed to the tumor reoxygenation induced by taxol and paclitaxel-loaded micelles (Milas et al., 1995a; Milas et al., 1995b; Danhier et al., 2012). It was found that the decrease in cell number affected the oxygen respiration (killed cells do not breath), an effect that added to the decrease in OCR affecting alive cells (Galle z et al., 2017). Paclitaxel-loaded micelles also induced an increase in tumor perfusion because of a decrease in the compression of venous vessels by cancer cells. As a consequence, the observed decrease in interstitial fluid pressure was correlated to an increase in tumor perfusion, and a consequent increase in oxygen delivery to tumor cells (Danhier et al., 2012). A comparable effect was observed after ranpirnase treatment, a cytotoxic amphibian ribonuclease (Kim et al., 2007; Lee et al., 2007). Of note, arsenic trioxide also induced an inhibition in tumor cell respiration at low non-cytotoxic concentration in pre-clinical tumor models, contributing to an increase in tumor oxygenation and a dramatic increase in tumor response to irradiation (Diepart et al., 2012).
Finally, it is essential to highlight the major interest for drugs that are releasing or stimulating the production of the free radical nitric oxide (NO). Nitric oxide presents a multi-faceted role in favoring the response to radiation therapy (Jordan et al., 2004; Sonveaux et al., 2009). First, nitric oxide is regulating mitochondrial respiration by virtue of reversible interactions with cytochrome c oxidase (complex IV in the mitochondrial respiratory chain) (Clementi et al., 1999). By inhibiting the oxygen utilization, the level of oxygen is increasing in solid tumors. Another important factor relies on the vasoactive properties of nitric oxide contributing to the increase in oxygen delivery. Finally, it should be emphasized that nitric oxide has intrinsic radiosensitizing properties comparable to oxygen through the fixation of DNA damages (Mitchell et al., 1993; Mitchell et al., 1996; Jordan et al., 2004). The release of nitric oxide can be achieved through the use of NO donors (Gallez et al., 1999a; Jordan et al., 2000; Jordan et al., 2003; Jordan et al., 2010), conversion of nitrite (Frérart et al., 2008), or stimulation of eNOS or iNOS (Jordan et al., 2002; Jordan et al., 2006a; Jiang et al., 2010). These multiple effects render nitric oxide release approaches highly efficient to potentiate radiation therapy.
2.2 Prodrugs Activated in Hypoxic Cells
Hypoxia-activated prodrugs (HAPs) are bioreductive drugs that are selectively reduced by reductases under hypoxic conditions to form cytotoxic compounds that kill hypoxic tumor cells (Denny, 2000; Denny, 2010; Hunter et al., 2016; Phillips, 2016; Baran and Konopleva, 2017; Mistry et al., 2017; Jackson et al., 2019; Li et al., 2021a; Anduran et al., 2021; Li et al., 2021b; Codony and Tavassoli, 2021). This bio-reductive process is inhibited by oxygen preventing the complete reduction of the compound into its active form (Vilaplana-Lopera et al., 2021). In other words, HAPs should not be toxic for normal oxygenated tissues. As radiation therapy is very efficient in killing non-hypoxic cells and as HAPs are selectively killing hypoxic cells, there is a major interest for combining both approaches for a maximal response (Figure 5). As recently reviewed (Li et al., 2021b), several classes of HAPs have been developed and evaluated, including nitromidazoles, quinones, aliphatic and heteroaromatic N-oxides. A few illustrative examples of compounds that have received particular attention are presented hereafter.
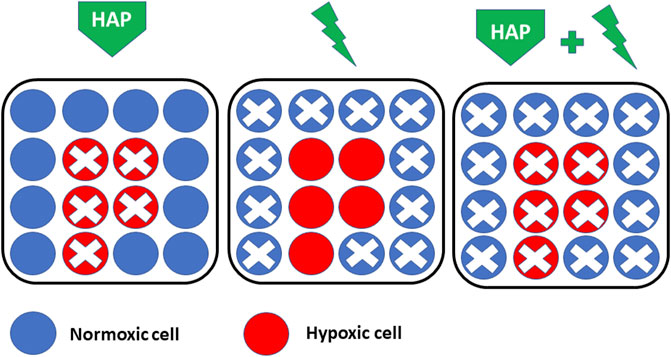
FIGURE 5. Rationale for combining HAP (hypoxia-activated prodrugs) with radiation therapy. HAPs are selectively killing hypoxic cells (Left) while radiation therapy is efficient in killing non-hypoxic cells (Middle). There is a major interest for combining both approaches for a maximal response (Right).
Metronidazole and misonidazole were the first nitroaromatic drugs tested as potential radiosensitizers (Foster and Willson, 1973; Begg et al., 1974; Denekamp et al., 1974; Adams et al., 1976; McNally et al., 1978). While efficient in pre-clinical models, unexpected toxicity and absence of significant therapeutic benefit was observed in clinical trials combining these compounds with radiotherapy (Schwade et al., 1984; Coleman, 1985; Dische, 1985). A second generation of nitroimidazoles (including etanidazole, pimonidazole, and nimorazole) with improved pharmacokinetic properties and reduced toxicity was developed and evaluated clinically. While pimonidazole is nowadays largely used for assessing tumor hypoxia by immunohistochemical staining, pimonidazole and etanidazole did not demonstrate any clinical benefit when associated with radiation therapy (Dische et al., 1993; Eschwège et al., 1997; Urtasun et al., 1998). By contrast, nimorazole has been so far the only nitroimidazole that was demonstrated to improve the response in head and neck squamous cell carcinoma (HNSCC) treated by radiation therapy without increase in toxicity (Overgaard et al., 1998; Henk et al., 2003; Metwally et al., 2014; Hassan Metwally et al., 2015). Intriguingly, nimorazole has become a standard treatment for HNSCC in association with radiation therapy only in Denmark, and not in other countries. Evofosfamide (TH-302) is the last generation nitroimidazole compound linked to bromo-iso-phosphoramide mustard (Br-IPM). TH-302 is a substrate for cellular reductases that generate a radical anion through 1-electron reduction. Under normoxia, the free radical anions are oxidized back to the original prodrug. However, in hypoxia, the free radical anions are further reduced, leading to the release of Br-IPM (Duan et al., 2008; Li et al., 2021a; Li et al., 2021b). TH-302 has been found efficient in combination with irradiation in pre-clinical models (Lohse et al., 2016; Hajj et al., 2017; Nytko et al., 2017; Takakusagi et al., 2018a). TH-302 has been introduced in a series of clinical trials with several chemotherapeutic agents [see (Li et al., 2021a) for a review], but not yet together with radiation therapy.
Tirapazamine (TPZ, also known as SR-4233 or WIN 59075) is another HAP that can generate a reactive free radical through one-electron reduction (Zeman et al., 1986; Brown and Lemmon, 1990; Holden et al., 1992; Wang et al., 1992; Brown, 1993; Brown, 2000; Li et al., 2021b). TPZ was found highly efficient in increasing the effect of radiation in vitro and in pre-clinical models. The addition of TPZ to conventional chemoradiation protocols showed promising results in Phase II clinical trials in delaying recurrence and improving survival (Lee et al., 1998; Treat et al., 1998; Rischin et al., 2005). However, the Phase III trials did not confirm the benefit of using TPZ in association with radiation therapy (Williamson et al., 2005; Rischin et al., 2010), hampering the continuation of clinical trials. Of note, deficiencies in compliance with the initial protocols in these clinical trials were suggested to have contributed to the poor outcome observed in the association chemoradiation + TPZ (Peters et al., 2010).
Banoxantrone (AQ4N) is an aliphatic N-oxide that is activated under hypoxic conditions into AQ4 through a two-electron reduction mediated by cytochromes with a DNA affinity and cytotoxic potency about one thousand times higher compared to its prodrug (McKeown et al., 1995; Hejmadi et al., 1996). Banoxantrone has shown promises in pre-clinical models in association with radiation therapy and/or chemotherapy (Patterson et al., 2000; Patterson and McKeown, 2000; Gallagher et al., 2001). This compound has been used in Phase I clinical trial without providing any obvious benefit deserving further clinical trial so far (Steward et al., 2007; Albertella et al., 2008; Papadopoulos et al., 2008).
2.3 Inhibitors of Molecular Targets Involved in Hypoxic Cell Survival
As described previously, the activation of transcriptional factors HIFs regulates the expression of hundreds of genes involved in angiogenesis, invasion and metastasis, cell proliferation, cell survival, cell metabolism and tumor immunity (Semenza and Wang, 1992; Wang and Semenza, 1993; Semenza, 2000; Semenza, 2003; Loboda et al., 2010; Muz et al., 2015; Pugh and Ratcliffe, 2017; Albadari et al., 2019; Lee et al., 2020; Ban et al., 2021; Codony and Tavassoli, 2021; Ivan et al., 2021; Sebestyen et al., 2021). Consequently, the inhibition of HIF pathway could be useful in reversing hypoxia-induced effects and treating aggressive cancers (Semenza, 2006; Ban et al., 2021; Ivan et al., 2021; Sebestyen et al., 2021). HIF inhibitors may have different modes of action: they may interfere with HIF protein synthesis, they may promote HIF degradation and/or dimerization, and they may change DNA binding and transcriptional activity of HIF-1 and/or HIF-2 (Yu et al., 2017). Illustrative agents are described hereafter.
As HIFα mRNA is a limiting factor in the rate of protein synthesis, the antisense oligodeoxynucleotide EZN-2968 has been shown to downregulate the expression of HIF-1α protein in human biopsies of treated patients (Greenberger et al., 2008; Jeong et al., 2014). Topoisomerase 1 inhibitors (such as camptothecin, irinotecan, topotecan), were also shown to inhibit the expression of HIF-1α (Bertozzi et al., 2014). PX-478, a compound derived from melphalan by oxidation of the nitrogen mustard moiety, reduced the expression of HIF-1α mRNA and protein in human tumor xenografts, reduced the expression of HIF-1α target genes, and consequently decreased tumor progression and sensitized tumors to radiation therapy (Welsh et al., 2004; Jordan et al., 2005; Schwartz et al., 2009; Jacoby et al., 2010; Lee and Kim, 2011). It has been found that 2-methoxyoestradiol (that is also acting on microtubules) is also an inhibitor of the synthesis of HIF-1α and HIF-2α, and suppresses their transcriptional activity (Ma et al., 2014; Yu et al., 2017).
Other small molecules used in clinical trials such as the Hsp90 inhibitors geldanamycin and tanespimycin (17-AAG) have been shown to promote HIFα degradation (Isaacs et al., 2002; Mabjeesh et al., 2002; Neckers, 2002; Alqawi et al., 2006; Cao et al., 2008). Vorinostat, a histone deacetylase (HDAC) inhibitor is also suppressing the hypoxia signaling by promoting HIF degradation and modulating nuclear translocation of HIF-1α (Zhang et al., 2017). Several compounds also have been found to inhibit HIF dimerization such as acriflavine and PT2385 and were found to be active in a variety of cancer cell lines (Wong et al., 2012; Shay et al., 2014; Chen et al., 2016; Courtney et al., 2018; Montigaud et al., 2018; Courtney et al., 2020; Lequeux et al., 2021).
Another way to inhibit the HIF signaling cascade is to inhibit binding to DNA and interfere with the transcriptional activity. Echinomycin was shown to inhibit the binding of HIF-1 to the Hypoxia-Responsive Element (HRE) sequences (Kong et al., 2005; Vlaminck et al., 2007; Wang et al., 2014). The classical anti-cancer agents daunorubicin and doxorubicin (anthracyclines) also inhibit the binding of HIF-1 to the HRE sequences of the target genes (Kung et al., 2004; Yu et al., 2017). Of course, as these anthracyclines present pleiotropic effects, it is difficult to isolate the contribution of the HIF pathway to the tumor response to treatments.
It is also crucial to remind that HIF regulation could be mediated by several signaling pathways including NFκB, PI3K/AKT/mTOR, and MAPK/ERK. Inhibitors which target these upstream pathways not only impact their own targets but also the HIF pathway (DeBerardinis et al., 2008; Agani and Jiang, 2013; Aoki and Fujishita, 2017). Again, the isolation of sole contribution of HIF in tumor response is elusive, and the beneficial therapeutic observed is obviously coming from hitting multiple targets.
Hypoxia also activates unfolded protein response (UPR) signaling pathways in the endoplasmic reticulum (ER), which tries to restore ER homeostasis and function. Essentially, two main strategies can be used to target the UPR: 1) the inhibition of actors of the UPR (PERK, IRE1) so tumor cells can no longer adapt to the stressful environment thereby leading to cell death; 2) the exacerbation of the UPR stress so the already activated UPR is overloaded, thereby driving the cells towards the death pathway. (Healy et al., 2009; Di Fazio et al., 2012; Shapiro et al., 2016; Ojha and Amaravadi, 2017; Grandjean and Wiseman, 2020).
The inhibition of UPR components can be achieved through PERK inhibition (Healy et al., 2009; Axten, 2017; Ojha and Amaravadi, 2017). GSK2606414 and GSK2656157 are two PERK inhibitors that were found active in tumor models (Axten et al., 2012; Axten et al., 2013). More compounds have been developed to block the IRE1α-XBP1 pathway, including irestatin, toyocamycin, salicylaldimines, hydroxy-aryl-aldehyde, as illustrative examples (Li et al., 2011; Volkmann et al., 2011; Ri et al., 2012; Sanches et al., 2014; Ojha and Amaravadi, 2017). Available compounds that target IRE1α activity have shown potential for anti-cancer treatment in combination with other conventional chemotherapy (Mimura et al., 2012; Ri et al., 2012; Tang et al., 2014; Ojha and Amaravadi, 2017). On the side of drugs that exacerbate the UPR stress, thapsigargin and brefeldin A have been reported to activate all three branches of the UPR (Salles et al., 2004; Denmeade and Isaacs, 2005; Healy et al., 2009; Rajamahanty et al., 2010; Markouli et al., 2020).
3 Assessment of Tumor Hypoxia
The ideal clinical biomarker for assessing tumor hypoxia should combine the following characteristics: able to distinguish normoxia/hypoxia/anoxia/necrosis; able to distinguish between perfusion-related and diffusion-related hypoxia; able to reflect cellular oxygenation in preference to vascular oxygenation; being non-invasive; being applicable to any tumor site; being applicable in pre-clinical models and in patients; being simple to perform and non-toxic; allowing repeated measurements in longitudinal studies; providing maps or hypoxic regions; sensitive at pO2 relevant to tumor therapies; able to monitor the effect of treatments; predictive of the outcome. Despite intense research efforts in the development and validation of hypoxia biomarkers, we should admit that the optimal item does not (yet) exist. However, even with limitations, some approaches could be very useful to guide hypoxia-targeted interventions. In the next paragraphs, we will present a critical overview of different approaches to assess tumor hypoxia. Understanding their main characteristics will allow to define their potential interest as companion diagnostic for pharmacological interventions (see Table 1). The oxygen biomarkers may be categorized into methods providing real direct oxygen measurements and methods that are indirectly reflecting the presence of hypoxic regions.
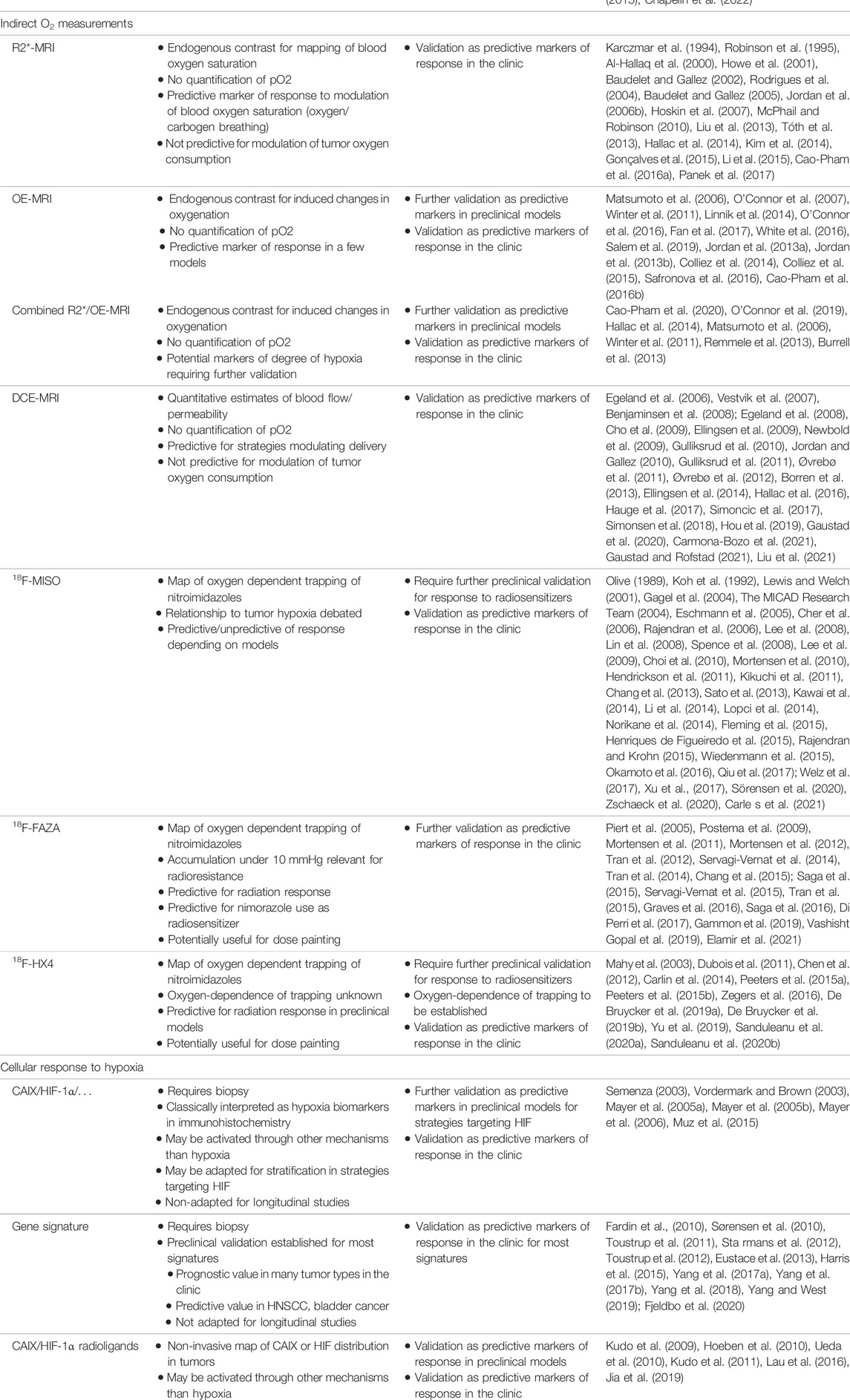
TABLE 1. Key features of technologies for their use as hypoxia biomarkers and challenges for future validation as companion diagnostics.
3.1 Direct Oxygen Measurements
The methods allowing direct oxygen measurements are those where a physicochemical property is directly dependent on the partial pressure of oxygen or the oxygen concentration in a tissue. In this category, we can find oxygen electrodes, optical measurements based on fluorescence quenching by oxygen, EPR oximetry and NMR fluorine relaxometry.
3.1.1 Electrode Measurements
Micro-electrodes can be inserted directly into tissues to measure the pO2. These methods are derived from the seminal work of LC Clark to assess oxygen tension in the blood (Clark, 1956; Clark and Lyons, 1962). The reduction of oxygen at the cathode extremity generates a current proportional to the pO2. The Eppendorf® pO2 histography system has a computerized driver that moves the electrode through the tissue minimizing compression and consumption of oxygen by the electrode (Kallinowski et al., 1990; Dewhirst et al., 2000). This system has been considered as the “gold standard” for assessing tumor oxygenation (Vaupel et al., 2007). This main achievement of this technology has been to definitely demonstrate that tumor hypoxia is a common feature of many solid tumors. Moreover, the method definitely established tumor hypoxia as a predictive marker of tumor outcome after different types of anti-cancer therapy (Höckel et al., 1993a; Höckel et al., 1993b; Okunieff et al., 1993; Stone et al., 1993; Höckel et al., 1996; Fyles et al., 1998; Knocke et al., 1999; Rudat et al., 2001; Nordsmark et al., 2005; Vaupel et al., 2007; Vaup el et al., 2021). In a critical evaluation of pO2 histography (Vaupel et al., 2007), P. Vaupel pointed as main advantages that the method provides absolute pO2 values with a precision around 1 mmHg within tissue micro-areas, provides several quantitative descriptive parameters and pO2 histograms within a tumor. However, the method is invasive and restricted to accessible tumors (such as head and neck, breast, or cervix cancer). While providing a distribution of pO2 along the electrode tracks, the method does not provide oxygen maps within the tumors (hypoxic regions cannot be excluded distant from the tracks). This means that pO2 histography may classify an individual tumor as likely hypoxic and may estimate its hypoxic fraction, but will not be useful for strategies of redistribution of radiation doses in treatment planning. Indeed, hypoxia-based dose painting is strongly dependent on the possibility to visualize and deliver appropriate radiation dose to hypoxic foci in tumors (Malinen et al., 2006; Grégoire et al., 2007; Sovik et al., 2007; Thorwarth et al., 2007; Lee and Le, 2008; Petit et al., 2009; Thorwarth and Alber, 2010; Bentzen and Gregoire, 2011; Toma-Dasu et al., 2012; Clausen et al., 2013; Geets et al., 2013; Hosk in, 2015; Servagi-Vernat et al., 2015; Welz et al., 2017; Gregoire et al., 2018). Another default of the method relies in its inability to differentiate between tumor and normal tissues and to discriminate measurements done in viable or necrotic regions (Vaupel et al., 2007). Finally, as the method is invasive, it is difficult to repeat measurements on the same tumor, for example to monitor the effect of treatments designed to alleviate tumor hypoxia in clinical longitudinal studies. At the pre-clinical level, the monitoring of drug effect using microelectrodes has been applied only acutely after application of a treatment or in different cohorts of tumors (treated vs. control) for chronic treatments (Horsman et al., 1989; Lee et al., 1993; Horsman et al., 1994; Hill and Chaplin, 1995; Horsman et al., 1998; Kelleher et al., 1998). Of note, the Eppendorf® pO2 histograph is no more commercially available.
3.1.2 Fiber-Optic Devices
Another way to assess tumor oxygenation is to use fiber-optic oxygen-sensing devices (such as the OxyLite®). In this system, photodiodes stimulate a fluorophore incorporated in a silicon polymer at the end of the tip, and the lifetime of the fluorescence is inversely proportional to the oxygen tension at the probe tip (Griffiths and Robinson, 1999). Compared to microelectrodes, the main advantage is that the measurement does not consume oxygen allowing the device to stay in place to monitor dynamic changes in oxygenation even in condition of extreme hypoxia. Pre-clinical studies have shown comparable measurements with microelectrodes, but differences in sampling volumes were noted (the OxyLite averages pO2 over a larger area than microelectrodes) (Griffiths and Robinson, 1999; Braun et al., 2001; Seddon et al., 2001). This device has been applied in a series of pre-clinical studies to monitor drug-induced changes in oxygenation (Jordan et al., 2002; Blackwell et al., 2003; Jorda n et al., 2003; Jordan et al., 2003; Wachsberger et al., 2005) and to assess the value of other hypoxia imaging modalities (Baudelet and Gallez, 2002; Demeure et al., 2002; Baudelet and Gallez, 2004; Zanzonico et al., 2004; Elas et al., 2006; Vikram et al., 2007; Jordan et al., 2009; Tran et al., 2012; Frank et al., 2015). As noted for the microelectrodes, the invasiveness, the need for repositioning the probe and the absence of spatial information limit their application for longitudinal studies. Of note, these probes do not possess CE or FDA regulatory approval for use in human subjects.
3.1.3 Electron Paramagnetic Resonance Oximetry
Quantitative assessments of tumor oxygenation can be obtained with EPR oximetry (spectroscopy and/or imaging) (Swartz and Clarkson, 1998; Gallez et al., 1999a; Subramanian et al., 2002; Dunn and Swartz, 2003; Gallez et al., 2004; Gallez and Swartz, 2004; Khan et al., 2007; Ahmad and Kuppusamy, 2010; Hyodo et al., 2010; Epel et al., 2011; Subramanian et al., 2012; Epel et al., 2014; Epel and Halpern, 2015; Gallez, 2021). EPR or equivalently ESR (Electron Spin Resonance) is a magnetic resonance method that detects species containing unpaired electron(s) (paramagnetic compounds). Molecular oxygen is paramagnetic, but no EPR spectra can be recorded from oxygen in tissues in physiological conditions. EPR oximetry methods are actually using the relaxing properties of oxygen which decreases the relaxation times of other paramagnetic compounds (Swartz and Clarkson, 1998; Gallez et al., 1999a; Dunn and Swartz, 2003; Gallez et al., 2004; Khan et al., 2007; Ahmad and Kuppusamy, 2010; Epel and Halpern, 2015; Gallez, 2021). T1 and T2 based measurements of paramagnetic reporters introduced in a biological system provide a direct indication of the oxygenation status (Gallez, 2021). Two classes of paramagnetic compounds can be used as oxygen reporters: soluble materials and insoluble particulate materials (Gallez et al., 2004; Gallez, 2021). Soluble materials include nitroxides (Halpern et al., 1994; Gallez et al., 1996a; Hyodo et al., 2009) and triarylmethyl (trityl) stable free radicals (Ardenkjaer-Larsen et al., 1998; Elas et al., 2006; Charlier et al., 2009; Krishna et al., 2012; Khramtsov, 2018; Chen et al., 2019; Nel et al., 2019; Sanzhaeva et al., 2020). The narrow EPR linewidth of trityl radicals is particularly suitable to obtain oxygen mapping with a high spatial resolution. The soluble EPR sensors present the inconvenience to be rapidly cleared from a tissue, requiring multiple administration if longitudinal oximetry studies are needed (Gallez, 2021). None of the soluble EPR reporters have been approved so far for clinical studies. Compared to soluble materials, particulate materials present two main advantages: they provide much more sensitive measurements of pO2 (variations of less than 1 mmHg can be detected) and, once introduced inside a tissue, they are reporting oxygenation from the same site over very long periods of time making them ideal probes for longitudinal studies (Gallez et al., 2004; Khan et al., 2007; Gallez, 2021). Particulate oxygen sensors include lithium phthalocyanine and derivatives (Liu et al., 1993; Ilangovan et al., 2002; Pandian et al., 2003), as well as paramagnetic carbon materials such as chars, coals, and carbon blacks (Vahidi et al., 1994; James et al., 1997; Jordan et al., 1998; Lan et al., 2004; Desmet et al., 2019). These oxygen paramagnetic reporters have been included in stable pharmaceutical suspensions or oxygen-permeable polymers to insure their biocompatibility (Gallez et al., 1996b; Gallez et al., 1998; Gallez et al., 1999b; Gallez and Mader, 2000; He et al., 2001; Charlier et al., 2004; Dinguizli et al., 2006; Meenakshisundaram et al., 2009a; Meenakshisundaram et al., 2009b; Meenakshisundaram et al., 2010; Hou et al., 2018). The unique capability of EPR oximetry to provide quantitative measurement of tumor oxygenation over time has been exploited in numerous preclinical studies [see (Gallez, 2021) for a review] after application of pharmacological challenges (Gallez et al., 1999a; Jordan et al., 2000; Jordan et al., 2002; Pogue et al., 2002; Jordan et al., 2003; Pogue et al., 2003; Hou et al., 2004; Jordan et al., 2004; Crokart et al., 2005b; Hou et al., 2005; Ansiaux et al., 2006a; Jordan et al., 2006a; Ansiaux et al., 2006b; Martinive et al., 2006; Segers et al., 2006; Crokart et al., 2007; Frérart et al., 2008; Ansiaux et al., 2009; Hou et al., 2010; Jordan et al., 2010; Segers et al., 2010; Matsumoto et al., 2011; Diepart et al., 2012; Karroum et al., 2012; Karroum et al., 2013; Matsumoto et al., 2014; De Preter et al., 2016b; Takakusagi et al., 2018b), carbogen/oxygen breathing challenges (Khan et al., 2009; Khan et al., 2010), or to measure the evolution of tumor oxygenation after irradiation (Goda et al., 1995; O'Hara et al., 1995; Goda et al., 1996; O'Hara et al., 1998; Sonveaux et al., 2002; Crokart et al., 2005a; Cron et al., 2005). EPR oxygen spectroscopy/imaging has been demonstrated as a valuable tool to predict the response to radiation therapy after alleviation of tumor hypoxia by most pharmacological challenges cited before. The identification of the temporal window of reoxygenation allows to propose a rationale for irradiation timing in order to optimize the response to treatment (Figure 6). EPR oxygen imaging also demonstrated its predictive value for tumor control according to tumor oxygenation level and radiation dose (Elas et al., 2008; Elas et al., 2013; Epel et al., 2019). So far, a limited number of studies have been applied in humans to measure by EPR the oxygen level in superficial tumors (Swartz et al., 2014; Swartz et al., 2016; Flood et al., 2018; Jeong et al., 2019; Flood et al., 2020; Schaner et al., 2021).
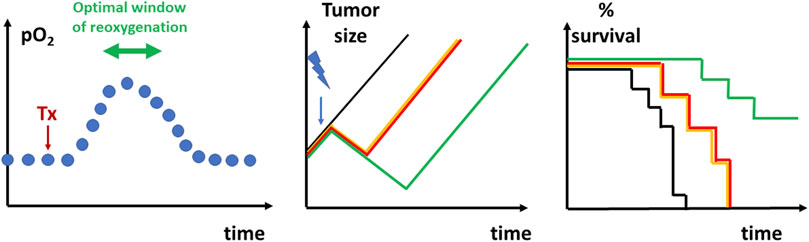
FIGURE 6. Graphical depiction of the identification of reoxygenation timing to radiosensitize tumors. Left: Longitudinal measurements of oxygenation (for example, using EPR oximetry) allows to define the window of reoxygenation after a pharmacological treatment (Tx). Depending of the treatment used and designed to alleviate tumor hypoxia, the window of reoxygenation may occur minutes, hours or days after initiation of a treatment. Middle: tumor regrowth delay experiment. Non-treated tumors (black) will progress regularly over time. Irradiated tumors (yellow) using suboptimal dose will typically present a transient decrease in tumor size due to the cytotoxic effect in a fraction of tumor cells before regrowing. The combination of a treatment together with irradiation administered outside the window of reoxygenation (red) will not lead to an increase in regrowth delay. The combination of the treatment with irradiation in the optimal timing of reoxygenation (green) is increasing the regrowth delay as more cells are killed by the irradiation. Right: Kaplan Meier curve representing the surviving fraction as a function of time depending on the treatment (colors represent the same groups than in the middle panel).
3.1.4 Fluorine-NMR Relaxometry
19F relaxometry is a non-invasive magnetic resonance imaging (MRI) method providing quantitative maps of tumor oxygenation after the injection of a perfluorocarbon emulsion (Fishman et al., 1989; Mason et al., 1991; Mason et al., 1993; Mason and Antich, 1994; Mason et al., 1996; Hunjan et al., 1998; van der Sanden et al., 1999; Jordan et al., 2009; Shi et al., 2013). Calibration curves of the longitudinal relaxation rate (R1 or 1/T1) as a function of pO2 can be acquired for a given temperature and a given perfluorocarbon, and can be used to map tumor oxygenation quantitatively. This method has been used to measure the acute effect of pharmacological interventions or respiratory challenges designed to modulate tumor oxygenation (Hees and Sotak, 1993; Zhao et al., 2001; Zhao et al., 2002; Mason et al., 2003; Nöth et al., 2004; Zhao et al., 2005; Diepart et al., 2011; Zhou et al., 2015). Fluorine relaxometry has also been used to anticipate the response of tumors to irradiation (Zhao et al., 2003; Bourke et al., 2007; Chapelin et al., 2022) and to map spontaneous fluctuations in tumor oxygenation (cycling hypoxia) (Magat et al., 2010). For longitudinal studies, multiple injections are required and it has been shown the interest for using highly biocompatible perfluoro sensors (Mignion et al., 2013). Clinical applications of 19F MRI tumor oximetry measurement have not yet be implemented (Chapelin et al., 2022).
3.2 Indirect Oxygen Measurements
Numerous studies have been performed during the two last decades to develop and evaluate non-invasive imaging biomarkers of tumor hypoxia, including PET radiotracers and different sources of contrast in MRI. These developments have been comprehensively reviewed elsewhere (Horsman et al., 2012; Colliez et al., 2017; Price et al., 2013; Wijsman et al., 2013; Kelada and Carlson, 2014; Fleming et al., 2015; O'Connor et al., 2019; Busk et al., 2020; Huang et al., 2021; Matsumoto et al., 2021; Lopes et al., 2021; Padhani et al., 2007). Here, we summarize the principles of the principal approaches that have been developed, their added value and their limitations in the context of therapeutic guidance.
3.2.1 PET Radiotracers of Tumor Hypoxia
Hypoxia PET imaging requires the intravenous injection of a radiotracer (e.g., a nitroimidazole). While the initial distribution is flow dependent, the nitroimidazole is able to diffuse into cells and is reduced intracellularly. This process is reversible under normoxic conditions leading to an equilibrium of the nitroimidazoles between the intra- and extracellular compartment. However, if cells are hypoxic, the radiotracer is further reduced and trapped by reacting with cellular macromolecules (Figure 7) (Padhani et al., 2007; Kelada and Carlson, 2014; Fleming et al., 2015; Colliez et al., 2017). The reduction is under control of reductases that are only present in viable hypoxic cells. As a consequence, the accumulation of the hypoxic radiotracers is increased in hypoxic viable cells regions and not in necrotic cells. You should note that the process of accumulation of the hypoxia radiotracer is analog to the process of activation of prodrugs selectively killing hypoxic cells described in the section “2.2”. For PET imaging, the radiotracers have mostly been labeled with the 18F positron emitter (half-life: 110 min). Several 18F-nitroimidazoles have been developed, the most cited in the literature being 18F-FMISO, 18F-FAZA and 18F-HX4 (Figure 7).
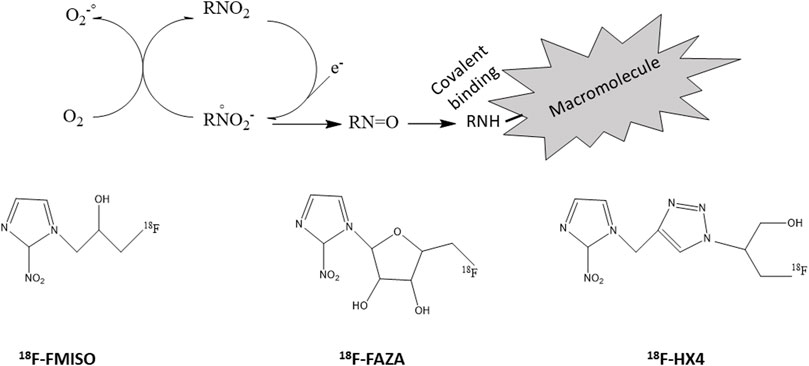
FIGURE 7. Radiolabelled nitroimidazoles. Top: Inside cells, nitroimidazoles (RNO2) are metabolized by reduction. This process is reversible under normoxic conditions leading to an equilibrium of the nitroimidazoles between the intra- and extracellular compartment. However, if cells are hypoxic, the radiotracer is further reduced and trapped by reacting with cellular macromolecules. Bottom: structures of commonly used radiolabeled nitroimidazoles (18F-FMISO, 18F-FAZA, 18F-HX4).
18F-FMISO (18F-Fluoromisonidazole) is the most commonly used hypoxia radiotracer and was the first to be used in the clinic (Koh et al., 1992; Lewis and Welch, 2001; The MICAD Research Team, 2004; Wijsman et al., 2013; Li et al., 2014; Lopci et al., 2014; Fleming et al., 2015; Rajendran and Krohn, 2015; Xu et al., 2017). Due to its rather high lipophilicity, this compound easily crosses the cell membranes and is trapped in hypoxic cells. The cellular clearance of 18F-FMISO is rather slow in normoxic tissues, thereby hampering the contrast between normoxic tissues and moderate hypoxic tumor tissues. The tumor-to-background ratio (TBR) to define a hypoxic region is low (generally defined as 1.2–1.6) 2 h after the injection of 18F-FMISO (Koh et al., 1992; Li et al., 2014; Rajendran and Krohn, 2015). Despite its very large use, puzzling conflictual results were published in the literature regarding the relationship between its accumulation in tissues and the real level of hypoxia. For example, Gagel reported a significant correlation between tumor-to-muscle ratio of 18F-FMISO and parameters of hypoxic fraction in head and neck tumors as measured by pO2 histography (Gagel et al., 2004) while no correlation was found in another study (Mortensen et al., 2010). Xu et al. (2017) reviewed the few studies exploring the correlation between 18F-FMISO uptake and immunohistochemical expressions of HIF-1α and VEGF. 18F-FMISO PET uptake was correlated with HIF-1α expression in oral squamous cell carcinoma (Sato et al., 2013) while the correlation was weak in head and neck cancer (Norikane et al., 2014) and absent in gliomas (Cher et al., 2006; Spence et al., 2008; Kawai et al., 2014). Efforts have been made to assess the feasibility of using 18F-FMISO images for radiation therapy treatment planning and dose distribution according to the presence of hypoxic foci (Thorwarth et al., 2007; Lee et al., 2008; Lin et al., 2008; Choi et al., 2010; Thorwarth and Alber, 2010; Hendrickson et al., 2011; Chang et al., 2013; Henriques de Figueiredo et al., 2015; Qiu et al., 2017; Welz et al., 2017). These studies suggested that dose painting in hypoxic volumes was feasible. Several clinical studies in head and neck cancer observed that the uptake of 18F-FMISO as observed in PET imaging was predictive of the outcome after radiation therapy (Eschmann et al., 2005; Rajendran et al., 2006; Kikuchi et al., 2011; Sörensen et al., 2020; Zschaeck et al., 2020; Carle s et al., 2021) while another study did not observe such predictive value (Lee et al., 2009). Of note, the lack of standardized protocol to define hypoxia on the basis on 18F-FMISO uptake renders difficult the comparison between all these studies. Interestingly, the application of several 18F-FMISO PET acquisitions during the course of radiation therapy revealed a decrease in radiotracer uptake early after starting the treatment, an observation that is consistent with the reoxygenation of the tumors (Wiedenmann et al., 2015; Okamoto et al., 2016).
18F-fluoroazomycin-arabinofuranoside (18F-FAZA) is another nitroimidazole that is more hydrophilic than 18F-FMISO (Piert et al., 2005; Postema et al., 2009). As a consequence, 18F-FAZA displays a faster clearance from the blood and the normal tissues than the more lipophilic 18F-FMISO. The delineation of tumor hypoxia with 18F-FAZA is obtained with a higher signal-to-noise ratio providing a better contrast imaging compared to 18F-FMISO. In a preclinical study, the prognostic value of hypoxia measured by 18F-FAZA or the Eppendorf oxygen electrode was assessed in a mammary carcinoma tumor model (Mortensen et al., 2011). 18F-FAZA PET showed that the accumulation of the radiotracer was predictive of response to irradiation similarly to the Eppendorf pO2 histography. In another preclinical study on rhabdomyosarcoma model, the 18F-FAZA uptake was compared to real pO2 values measured by EPR oximetry (Tran et al., 2012). A clear correlation between 18F-FAZA PET image intensities and tumor oxygenation was established: the accumulation of the radiotracer in vivo dramatically increased wen the pO2 was lower than 10 mmHg (Figure 8) (Tran et al., 2012). In another study, 18F-FAZA was found predictive of the response to radiation therapy (Tran et al., 2014). For 9L-gliomas, a significant correlation between 18F-FAZA tumor-to-background ratio (T/B) and tumor growth delay was found (Figure 8). In addition, carbogen breathing dramatically improved the tumor response to irradiation in this model. Rhabdomyosarcomas that were less responsive to hyperoxic challenge took advantage from dose escalation (Tran et al., 2014). 18F-FAZA PET was also found effective in guiding the use of nimorazole as radiosensitizer. The uptake of the radiotracer identified a subgroup of more hypoxic tumors that benefit from this combined treatment RT + nimorazole (Figure 8) (Tran et al., 2015). Pre-clinical studies also showed that 18F-FAZA PET could be used as a marker of response to treatments targeting tumor hypoxia trough the inhibition of mitochondrial respiration (Chang et al., 2015; Gammon et al., 2019; Vashisht Gopa l et al., 2019). In clinical studies, the treatment outcome was better for patients with non-hypoxic HNSCC tumors than for patients with hypoxic tumors as identified by 18F-FAZA PET (Mortensen et al., 2012; Graves et al., 2016; Saga et al., 2016; Zschaeck et al., 2020). In patients with advanced non-small-cell lung carcinomas (NSCLC), FAZA uptake in lymph nodes, but not in primary lesions, was predictive of treatment outcome (Saga et al., 2015). A PET study during radiation therapy revealed a decrease in 18F-FAZA uptake early after initiation of the treatment in HNSCCs (Servagi-Vernat et al., 2014), but not in NSCLCs (Di Perri et al., 2017). The feasibility of using 18F-FAZA PET for hypoxia-guided adaptive radiation dose escalation in hypoxic volumes has also been assessed in head and neck tumors and pancreatic cancer (Servagi-Vernat et al., 2015; Elamir et al., 2021).
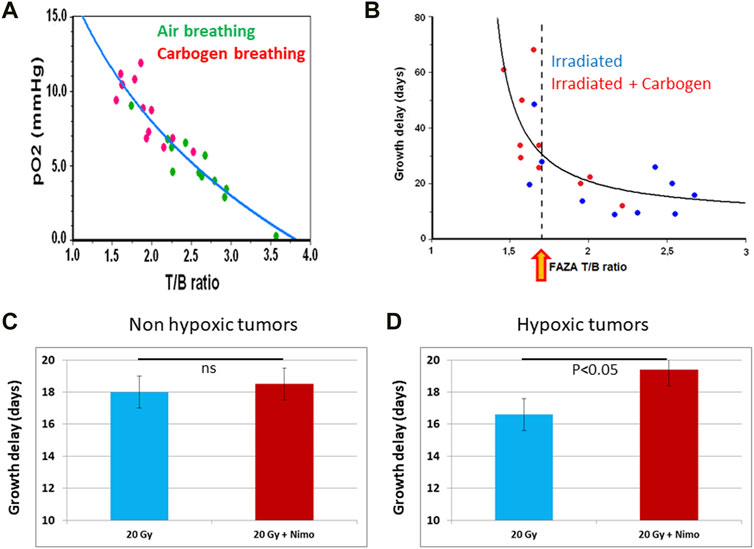
FIGURE 8. 18F-FAZA as predictor of tumor response to radiation therapy. (A) In vivo calibration of 18F-FAZA tumor accumulation (measured by microPET) as a function of tumor pO2 (measured by EPR oximetry) in the same rhabdomyosarcoma tumors. (B) Growth time delay as a function of tumor uptake of 18F-FAZA (measured by microPET) in cohorts of animals breathing air or carbogen. The yellow arrow indicates a tumor-to-background ratio (T/B) corresponding to 10 mmHg (higher T/B means more hypoxic than this value while lower T/B means less hypoxic). (C,D) Value of 18F-FAZA tumor accumulation to predict the outcome of a treatment combining nimorazole together with irradiation. (C) for non-hypoxic tumors, no significant benefit (p > 0.05) was observed when tumors were treated by a combination of irradiation together with nimorazole (n = 7) compared to tumors treated with irradiation alone (n = 7). (D) for hypoxic tumors, a significant benefit was observed when tumors were treated by a combination of irradiation together with nimorazole (n = 9) compared to tumors treated with irradiation alone (n = 5). The figures are built with data from (Tran et al., 2012), (Tran et al., 2014), and (Tran et al., 2015).
18F-flortanidazole (18F-HX4) is another hydrophilic nitroimidazole that quickly clears from normoxic tissues allowing imaging 90 min after the radiotracer administration. This compound demonstrated promising preclinical and clinical results and a high TBR in hypoxic tumors (Sanduleanu et al., 2020a). The compound accumulates in regions with high hypoxic fraction as measured by pimonidazole (Dubois et al., 2011) and CAIX staining (Carlin et al., 2014). In a preclinical model of rhabdomyosarcoma, the uptake of 18F-HX4 was increased in animals breathing a gas with low oxygen content (7%) and decreased after carbogen/nicotinamide treatment (Dubois et al., 2011; Peeters et al., 2015a). However, contrarily to 18F-FAZA and EF5 for which tracer uptake was correlated to quantitative estimates of tumor oxygenation by microelectrodes or EPR oximetry (Mahy et al., 2003; Mortensen et al., 2011; Tran et al., 2012), the critical pO2 values at which 18F-HX4 is trapped in a tumor remain unknown. In a study where tumor rats were treated by irradiation, it was found that a higher 18F-HX4 uptake at baseline was associated with a worse prognosis (Yu et al., 2019). A preclinical study showed that the treatment efficacy of the HAP evofosfamide (TH-302) was dependent on tumor oxygenation as assessed by 18F-HX4: increasing the tumor oxygenation abolished the effect of evofosfamide, whereas enhancing the hypoxic fraction enlarged its therapeutic effect (Peeters et al., 2015b). Using metformin (an inhibitor of mitochondrial electron transport chain) to alleviate tumor hypoxia in NSCLC and colorectal cancer models, 18F-HX4 revealed a reduction in radiotracer uptake (De Bruycker et al., 2019a; De Bruycker et al., 2019b). The clinical trials with 18F-HX4 have been reviewed recently (Sanduleanu et al., 2020a): the assessment of tumor hypoxia with this radiotracer was favorable in NSCLCs, HNSCCs, and pancreatic cancer (Chen et al., 2012; Klaassen et al., 2015; van Elmpt et al., 2016; Zegers et al., 2016; Sanduleanu et al., 2020b). Establishing the clinical prognostic and predictive value will require more studies (Sanduleanu et al., 2020b).
We cannot conclude this section on PET radiotracers of tumor hypoxia without giving a few words on the continuously cited radiotracer 60/62/64Cu-ATSM (copper(II)diacetyl-bis(N4-methylthiosemicarbazone) using 2-deoxy-d-glucose). This compound has been and still continues to be suggested as a non-invasive PET biomarker of tumor hypoxia (Fujibayashi et al., 1997; Lewis et al., 1999; Chao et al., 2001; Dehdashti et al., 2003; Dehdashti et al., 2008; Holland et al., 2009; Sato et al., 2014; Pérès et al., 2019). However, its mechanism of retention in cells is highly controversial (Colombié et al., 2015; Liu et al., 2020). The initially proposed mechanism of retention in hypoxic cells is based on a pO2-dependence of one-electron reduction that is the discriminating factor which controls the reversibility of cellular uptake (Fujibayashi et al., 1997; Holland et al., 2009). However, the selective hypoxia dependence of the uptake and intracellular trapping has been questioned in numerous studies. It has been shown that Cu-ATSM accumulation was not dependent on hypoxic conditions in several tumor models (Yuan et al., 2006; Matsumoto et al., 2007). In addition, it was found a mismatch between Cu-ATSM accumulation and classical markers of hypoxia, for example Cu-ATSM showed the highest radiotracer uptake in regions with the low nitroimidazole uptake and CAIX staining (Carlin et al., 2014; Yuan et al., 2006; O'Don oghue et al., 2005; Valtorta et al., 2013; McCall et al., 2012; Hansen et al., 2012). It was also observed that tumor accumulation does not depend only on hypoxia but also on redox status and correlates with NADH/NADPH content, depends on MDR1 expression and on fatty acid synthase expression (Vave re and Lewis, 2008; Liu et al., 2009; Yoshii et al., 2012). For example, 64Cu-ATSM accumulated in cells with overreduced states due to mitochondrial dysfunction even under normoxia (Yoshii et al., 2012). It was also found that the uptake is cell line dependent (Burgman et al., 2005). Finally, it was also observed that the distribution of radiocopper from 64Cu-ATSM in tumors mirrors that of 64Cu-acetate suggesting that copper metabolism may also play a role in the mechanism of uptake (Hueting et al., 2014). Overall, despite its extensive use (likely due to its simple preparation and accessibility), Cu-ATSM should be considered as a non-reliable hypoxia biomarker considering the diversity of factors contributing to its retention in tissues.
3.2.2 Magnetic Resonance ImagingUsing Endogenous Contrast
The endogenous contrast in MRI (without need of administration of a contrast agent) is mainly dependent on proton concentration, water motion and relaxation times (the time constants characterizing the return of the magnetization to its initial values after radiofrequency pulse excitation). Relaxations times include T1, T2 and T2*. The spin-lattice (or longitudinal) relaxation time T1 quantifies the rate of transfer of energy from the nuclear spin system to the neighboring molecules (the lattice). T1 is the time constant that characterizes the return kinetics of the magnetization along the longitudinal (z) axis. Spin-spin (or transverse) relaxation time T2 quantifies the decay rate of the magnetization within the xy plane (perpendicular to the applied magnetic field). After a 90° RF pulse, the nuclear spins become coherent in the transverse plane. The T2 characterizes the gradual loss in phase coherence. The combination of T2 relaxation and magnetic field inhomogeneity is referred to as the dephasing time or T2* (also called effective transverse relaxtion time). The inverse of relaxation times is referred to as relation rate R1 (=1/T1), R2 (=1/T2) and R2* (=1/T2*). The oxygen‐sensitive endogenous MRI contrasts R1 and R2* are potential imaging biomarkers of tumor hypoxia.
R2* uses the endogenous paramagnetic contrast agent deoxyhemoglobin (dHB) as a source of contrast that is at the origin of blood oxygen level-dependent (BOLD) contrast mechanism (Thulborn et al., 1982; Ogawa et al., 1990; Ogawa and Lee, 1990). dHb enhances the R2* effective transverse relaxation rates of water in blood and in the tissue surrounding the blood vessels. R2* is sensitive to the concentration of deoxyhemoglobin [dHb] per volume of tissue. A decrease in [dHb], due to an increase in blood oxygen saturation, results in a decrease in R2*. It should be emphasized that R2* is not only sensitive to the change in blood oxygenation through the change in [dHb], but is also sensitive to factors unrelated to the change in oxygenation, such as vascular volume, hematocrit, flow and vessel density (Howe et al., 2001; Baudelet and Gallez, 2005). In preclinical studies, T2* changes have been demonstrated to tackle changes in oxygenation levels during hyperoxic challenges (Karczmar et al., 1994; Robinson et al., 1995; Al-Hallaq et al., 2000; Hallac et al., 2014). Using simultaneous MRI measurements of the evolution of R2* together with direct oxygenation measurements by MR compatible fibre optics during oxygen/carbogen breathing challenges, no correlation has been established between R2* and absolute values of pO2: large differences in pO2 were observed for same R2* values, large differences in R2* were observed for same pO2 values (Baudelet and Gallez, 2002). Changes in R2* should therefore be considered as an indicator of changes in tumor oxygenation (measured in the vascular compartment) rather than a quantitative marker of the level of oxygenation. Studies compared R2* together with pimonidazole staining. A positive correlation was found in prostate cancer in patients (Hoskin et al., 2007) while an inverse correlation was observed rat mammary tumors (McPhail and Robinson, 2010). Attempts were also made to correlate R2* values with expression of HIF-1α: the mean R2* value correlated moderately with the level of HIF-1α in breast invasive ductal carcinoma (Liu et al., 2013), in human glioma (Tóth et al., 2013), but no correlation was found in renal carcinoma (Li et al., 2015). Using pharmacological manipulation of tumor oxygenation, it was found that the evolution of R2* was consistent with changes in tumor oxygenation after isosorbide dinitrate administration (Jordan et al., 2000) and the hemoglobin modifier ITTP (Cao-Pham et al., 2020). For the latter, the increase in R2* resulted from an increase in oxygen release from blood, inducing an increase in [dHb] (Cao-Pham et al., 2020). However, the evolution of R2* was not predictive of evolution of tumor oxygenation (as measured by EPR oximetry) after administration of inhibitors of oxygen consumption (insulin and AINS) (Jordan et al., 2006b) or after the RSR13 hemolobin modifier (Hou et al., 2004). The prognostic value of R2* as marker of response to irradiation was investigated in several preclinical studies. R2* was found predictive of outcome in rats exposed to carbogen challenges in GH3 prolactinomas (Rodrigues et al., 2004), in rat prostate tumors (Hallac et al., 2014), in 9L-gliomas but not in rhabdomyosarcoma (Cao-Pham et al., 2016a). The value of R2* as a predictor of therapeutic response was evaluated in thirthy cervical cancer patients undergoing concurrent chemoradiotherapy. This study showed that tumour R2* values were negatively correlated with final tumour size response, but not final tumor volume response (Kim et al., 2014). The application of serial BOLD MRI measurements has been used to provide non-invasive mapping of spontaneous fluctuations in tumor hypoxia (cycling hypoxia) with a high spatial and temporal resolution in tumor models (Baudelet et al., 2004; Baudelet et al., 2006; Gonçalves et al., 2015). The method demonstrated that treatment with cinnarizine or carbogen/nicotinamide led to a decrease in spontaneous fluctuations of oxygenation and blood flow (Baudelet et al., 2004). The method has also been translated into the clinic in head and neck cancer (Panek et al., 2017). The R2* fluctuation fraction was higher in the non-responding patient group, suggesting that the presence of such fluctuations may predict the outcome following treatment (Panek et al., 2017).
The dissolved paramagnetic molecular oxygen in tissue fluid and in blood plasma increases the spin lattice relaxation rate R1. It should be noted that the basal R1 of a tissue is influenced by many factors (i.e., components of the tissue including macromolecules and endogenous paramagnetic enzymes, mobility, … ) and therefore cannot be interpreted as marker of the tissue oxygenation (O'Connor et al., 2019). However, when switching the inhaled gas from air to an oxygen-enriched gas (100% oxygen or carbogen), the change in dissoved arterial oxygen may lead to a change in tumor oxygenation and consequently to a change in R1 (Matsumoto et al., 2006; O'Connor et al., 2007; Winter et al., 2011). This protocol is sometimes referred to as oxygen-enhanced MRI (OE-MRI) or tissue oxygenation level dependent (TOLD) contrast (O'Connor et al., 2019; Hallac et al., 2014; O'Connor et al., 2007). While changes in R1 in a voxel are resulting from changes in the vascular and tissue oxygen content, it is generally considered that the main effect is coming from the oxygen dissolved in the tissue, contrarily to changes in R2* that are reflecting changes in the vascular compartment. It was found in pre-clinical models that tumor regions with low response to OE-MRI were correlated to hypoxic regions evaluated by pimonidazole staining (Linnik et al., 2014; O'Connor et al., 2016; Fan et al., 2017). The response in OE-MRI was predictive of the response to radiation therapy (White et al., 2016). OE-MRI was also able to detect radiation-induced changes in tumor oxygenation in NSCLCs and glioma preclinical models as well as in NSCLCs in patients (Salem et al., 2019). To improve the sensitivity of oxygen enhanced R1 imaging, measurements of R1 in lipids was proposed to increase the sensitivity of the method (Jordan et al., 2013a; Jordan et al., 2013b). by exploiting the higher solubility of oxygen in lipids (as compared with water). The method provided highly sensitive and quantitative measurements of oxygenation in lipid-rich mammary cancer models (Colliez et al., 2014). However, while sensitive to changes in tissue oxygen content, the lipid R1 turned out to be no more sensitive than water R1 in tissues with lower lipid content, an observation that was done in preclinical models and in patients (Colliez et al., 2015; Cao-Pham et al., 2016b; Safronova et al., 2016).
ΔR1 and ΔR2* predictors of tumor oxygen evolution have been combined in a series of studies (Matsumoto et al., 2006; Winter et al., 2011; Burrell et al., 2013; Remmele et al., 2013; Hallac et al., 2014; Cao-Pham et al., 2020). Models were built thanks to combined measurements of ΔR1 and ΔR2* in order to discriminate different regional responses to oxygen/carbogen breathing challenges, including normoxia, mild hypoxia, severe hypoxia or vascular steal (Cao-Pham et al., 2020; O'Connor et al., 2019). While endogenous MRI contrast is particularly attractive due to non-invasiveness and large accessibility, clinical validation of ΔR1 and ΔR2* remains the main goal to achieve for considering them as reliable biomarkers for tumor-hypoxia targeted treatments.
3.2.3 Magnetic Resonance Imaging With Exogenous Contrast
Dynamic contrast enhanced MRI (DCE-MRI) has been suggested to be a marker of tumor oxygenation in pre-clinical models (Egeland et al., 2006; Vestvik et al., 2007; Ege land et al., 2008; Ellingsen et al., 2009; Hauge et al., 2017; Hou et al., 2019; Gaustad et al., 2020) and in patients (Newbold et al., 2009; Simonsen et al., 2018; Gaustad and Rofstad, 2021; Liu et al., 2021). It has also been suggested to be a predictive marker of response to radiation therapy in pre-clinical models (Gulliksrud et al., 2011; Øvrebø et al., 2011; Øvrebø et al., 2012; Ellingsen et al., 2014; Hallac et al., 2016). It was also shown that hypoxia estimated by 18F-FMISO-PET correlated negatively with Ktrans from DCE-MRI in animal models, in head and neck cancers and in breast tumors, supporting the use of DCE-MRI to measure perfusion-driven hypoxia (Cho et al., 2009; Simoncic et al., 2017; Carmona-Bozo et al., 2021). However, several important limitations were also noted (Benjaminsen et al., 2008; Gulliksrud et al., 2010; Jordan and Gallez, 2010; Borren et al., 2013), including the absence of correlation of DCE-MRI parameters with the expression of HIF-1α and HIF-2α (Øvrebø et al., 2012). It should be emphasized that DCE-MRI takes only into account the perfusion without consideration to the oxygen consumption that plays also an important role in the establishment of tumor hypoxia. The interplay existing between tumor metabolism, oxygen consumption and oxygen delivery/availability may of course influence the occurrence of hypoxia. As a matter of fact, in a restrospective analysis evaluating the reliability of imaging biomarkers to predict changes in tumor oxygenation and improvement in tumor response to irradiation, DCE-MRI was predictive for strategies designed to increase the oxygen delivery through blood perfusion, but DCE-MRI was not predictive for strategies designed to modulate the tumor metabolism and oxygen consumption (Jordan and Gallez, 2010).
3.3 Biomarkers of the Cellular Response to Hypoxia
As discussed in section 1.2.1, cells exposed to hypoxia undergo a large variety of molecular responses, the predominant hypoxia-mediated intracellular signaling pathway being controlled by the transcription factors HIFs (Semenza and Wang, 1992; Wang and Semenza, 1993; Semenza, 2000; Harris, 2002; Semenza, 2003; Muz et al., 2015; Vaupel and Mayer, 2016; Pugh and Ratcliffe, 2017; Lee et al., 2020; Sørensen and Horsman, 2020). A possible way to identify hypoxic tumors is to focus not on oxygen itself but rather on the consequences of hypoxia exposure. Genes that are up- or downregulated in response to hypoxia reflect the hypoxic phenotype and therefore can provide an indirect measure of hypoxia. Their expression can be assessed in biopsies at the protein level using immunohistochemistry, or at the mRNA level, using gene expression arrays (Bussink et al., 2003; Vordermark and Brown, 2003; Mayer et al., 2006; Jubb et al., 2010; Toustrup et al., 2012; Adams et al., 2013; Harris et al., 2015; Swartz et al., 2015; Yang and West, 2019; Thiru thaneeswaran et al., 2021). Expression of HIF-1α and CA IX are classically analyzed by immunohistochemistry and used as markers of hypoxia. While there is no doubt that hypoxia leads to an induction of HIF-1α and CA IX in vitro, we should keep in mind that the expression of HIF-1α and downstream targets could also be achieved in a hypoxia-independent manner (Semenza, 2003; Vordermark and Brown, 2003; Mayer et al., 2005a; Mayer et al., 2005b; Mayer et al., 2006; Muz et al., 2015). It is also established that mutations of the von Hippel-Lindau gene result in normoxic stabilization of HIF-1α (Maxwell et al., 1999). Gene expression microarrays can also be used to determine a global transcriptional response to hypoxia. Genes found to be significantly upregulated, or exceeding a defined threshold from baseline normoxic expression, are typically grouped together and have been referred to as a “hypoxia gene expression signature” (Harris et al., 2015). As reviewed in (Toustrup et al., 2012; Harris et al., 2015; Yang and West, 2019), more than 30 hypoxia gene expression signatures have been published. As illustrative example, we may cite the development of a gene signature for hypoxia in head and neck cancer. A subset of genes upregulated under hypoxia were identified in vitro in several tumor cell lines (three oral carcinoma, one hypopharyngeal and one cervical carcinoma) (Sørensen et al., 2010), and then validated in vivo in xenograft HNSCC tumors by comparison with the FAZA nitroimidazole (Toustrup et al., 2011). The authors finally generated a gene expression classifier containing 15 genes (ADM, ALDOA, ANKRD37, BNIP3, BNIP3L, C3orf28, EGLN3, KCTD11, LOX, NDRG1, P4HA1, P4HA2, PDK1, PFKFB3, SLC2A1) that was validated in several hundreds of patients with HNSCC randomized for nimorazole or placebo in combination with radiotherapy (Toustrup et al., 2011). Tumors categorized as hypoxic on the basis of the classifier were associated with a significantly poorer clinical outcome than nonhypoxic tumors, and the outcome was equalized to the nonhypoxic tumors by addition of nimorazole to radiation therapy (Toustrup et al., 2011). Another 26-gene hypoxia signature has been found predictive for tumors in oropharynx treated by a combination of carbogen/nicotinamide together with accelerated radiation therapy (ARCON protocol) (Eustace et al., 2013). However, this signature was not predictive in bladder cancer treated by irradiation together with carbogen/nicotinamide (Eustace et al., 2013). Another 24-gene hypoxia signature has been found to be prognostic and predictive for muscle-invasive bladder cancer patients as the signature was able to identify patients likely to benefit from the addition of carbogen and nicotinamide to radiotherapy (Yang et al., 2017a). Other illustrative examples of hypoxia gene expression signatures include those found prognostic (but not yet tested for predictivity) in neuroblastomas (Fardin et al., 2010), prostate cancer (Yang et al., 2018), breast cancer (Sta rmans et al., 2012), and sarcomas (Yang et al., 2017b). Interestingly, a recent report suggested the benefit from the combination of imaging and gene expression signature to assess hypoxia-related treatment resistance and thereby enable more information about the disease before treatment-decision (Fjeldbo et al., 2020). Another interesting development relies on the use of miRNA signature. It has been recently shown that a 14-miRNA hypoxia signature can be used with an mRNA hypoxia signature to identify bladder cancer patients benefitting most from having carbogen and nicotinamide with radiotherapy (Khan et al., 2021).
Overall, these molecular markers of response to hypoxia coming from biopsies may definitely help in tumor characterization, prognostic and stratification in treatments targeting hypoxia. However, it is clear that, as for all non-imaging modalities, these markers cannot be used for dose painting in radiation treatment planning. As the method is invasive, it cannot be repeated in longitudinal studies for monitoring tumor hypoxia evolution and evaluate the efficacy of treatments designed to alleviate tumor hypoxia. In this regard, imaging biomarkers targeting CAIX and HIF have attracted attention. An antibody directed against CAIX and labeled with 89Zr (a positron emitter) 89Zr-labeled cG250-F(ab′)2 has been developed. In preclinical studies, this antibody accumulated in head and neck carcinoma xenografts with a spatial distribution correlated to CAIX expression and pimonidazole staining (Hoeben et al., 2010). Benzenesulfonamides radiolabeled with 68Ga selectively recognizing CAIX have also been developed and evaluated in pre-clinical tumor models (Lau et al., 2016). Another radiotracer 18F-CA IX-P1-4-10 has been developed: in preclinical studies, the distribution has been correlated with CA IX expression (Jia et al., 2019). It has been postulated that a probe containing the oxygen-dependent degradation domain (ODD) and inducing degradation in a similar manner as HIF-1α could be useful to evaluate HIF-1 activity in vivo. The protein transduction domain PTD and the ODD was fused with monomeric streptavidin (SAV) to produce a chimeric protein, PTD-ODD-SAV (POS). Radiolabeled biotin derivatives 123I-IBB and 18F-IBB were synthesized. POS was degraded in an oxygen-dependent manner and the accumulation of radiolabeled IBB-conjugated POS in the tumor was found to correlate with the HIF-1 activity (Kudo et al., 2009; Ueda et al., 2010; Kudo et al., 2011). There is a clear need for further validation in terms of prognostic and predictive values in preclinical models and in the clinic before considering these imaging biomarkers as potential companion diagnostic in hypoxia-targeted treatments. (Biomarkers Definitions Working Group, 2001).
4 Current Research Gaps and Potential Perspectives
The successful application of hypoxia-targeted interventions in patients is a challenging task that is will definitely benefit form the use of biomarkers used as companion diagnostics (Tatum et al., 2006; Parkinson et al., 2012; O'Connor et al., 2017; McAteer et al., 2021). As described earlier, there are existing numerous treatment approaches to counteract hypoxia or its consequences. On the other hand, numerous biomarkers have been described and are in the process of qualification or validation in preclinical and clinical trials. The biomarker may help at different levels in the pipeline of the research and development. In preclinical studies, the use of biomarker may help to confirm that the pharmacological agent is actually hitting its target and/or playing a role on the tumor microenvironment, may help in the selection of the lead compound among different candidates, may help to define the appropriate dosing and the time window of action. From preclinical studies, several potential companion diagnostics may be defined by cross-validation with other markers including invasive ones and post-mortem analyses of tissues. At early stages of clinical trials (Phase I/II), the value of companion diagnostics should be qualified as likely predictor of response. It will be then used for appropriate patient stratification in phase III clinical trials. If validated at this later phase, it should become an integrated part of the personalized medicine. Several key research gaps should be filled for future successful targeted-hypoxia interventions, including research needs in qualification/validation of biomarkers and appropriate coupling of companion diagnostics with the selected therapeutic target.
4.1 Hypoxia Biomarkers: Key Features and Challenges for Future Validation as Companion Diagnostics
In our description regarding possible ways to assess hypoxia, we have started by stating that the ideal hypoxia biomarker does not exist yet. That does not mean that existing biomarkers are not helpful. In this regard, some comparison may be helpful to understand their merits and flaws for their convenient application as companion diagnostic. Let’s compare the measurement of hypoxia with the measurement of temperature in a sauna (Figure 9). The most obvious way to measure the temperature is to use a thermometer directly in the sauna. Translated in the hypoxia world, it corresponds to direct measurements of tissue oxygenation (pO2 histography, optic fibres, EPR oximetry, 19F-relaxometry). However, most direct methods are invasive and/or not (or not easily) accessible in the clinic. Another approach is to measure the temperature of the circulating water in the heater. It may help, but we should be cautious as we do not know if the door is open. In other words, we have an information on the delivery of calories, but not on their consumption and the real temperature observed in the sauna remains unknown. This approach is quite analog to the measurements done using the oxygen blood saturation (R2* or BOLD-MRI). While not providing information on oxygen per se, it may be helpful for checking the effect of strategies designed to modify the blood oxygen saturation (oxygen or carbogen breathing) or release from hemoglobin. We may also measure the flow of the circulating water in the heater. It may help, but we should be cautious as we do not know if the door is open and if the circulating water is hot. It is comparable with DCE-MRI. As the method only considers the delivery, no information is provided regarding the oxygenation. However, it could be helpful to assess the effect of treatments designed to modulate the tumor perfusion. Finally, we may look for the presence of naked (or almost) people in the room. While we do not know the temperature, their presence likely means a hot temperature inside the sauna. This situation is analog to the accumulation of nitroimidazoles inside hypoxic cells. We do not know the real oxygenation of the tissue, but it is likely that related compounds that are trapped by the same mechanisms (such as the radiosensitizer nimorazole) can also accumulate in the same cells.
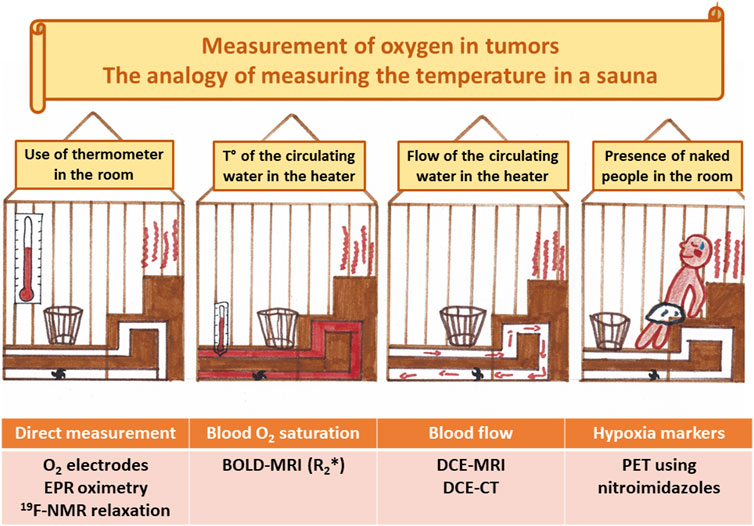
FIGURE 9. Comparison between measuring tumor oxygenation with hypoxia biomarkers and measuring temperature in a sauna. The direct way to measure the temperature is to use a thermometer. Translated in the hypoxia world, it corresponds to direct measurements of tissue oxygenation (pO2 histography, optic fibres, EPR oximetry, 19F-relaxometry). Another approach is to measure the temperature of the circulating water in the heater. Caution: we do not know if the door is open. This approach is analog to the measurements done using the oxygen blood saturation (R2* or BOLD-MRI). It provides information on the oxygen delivery through the blood. Another approach is to measure the flow of the circulating water in the heater. Caution: we do not know if the door is open and if the circulating water is hot. It is comparable with DCE-MRI. The method only considers the delivery, no information is provided regarding the oxygenation. Finally, we may look for the presence of naked (or almost) people in the room. While we do not know the temperature, their presence likely means a hot temperature inside the sauna. This situation is analog to the accumulation of nitroimidazoles inside hypoxic cells. We do not know the real oxygenation of the tissue, but it is likely hypoxic.
In the Table 1, we have summarized the current status and the challenges for future validation of the different biomarkers as companion diagnostics.
4.2 Questions/Answers on the Selection of the Hypoxia Biomarker
Knowing that no hypoxic biomarker is ideal, the choice of a biomarker should be closely related to the scientific question to be addressed. That means that a biomarker may change or evolve during the research progression and phase of development. In the next paragraphs, we provide a pragmatic/practical approach and critical guidance for defining the couple “biomarker/hypoxic targeted intervention” in diverse situations. These suggestions are of course based on the present status of development and qualification of technologies. There is no doubt that the choice will evolve as the technologies and their validation progress. Besides the guidance advices, the purpose is to stimulate the critical thinking before using an approach.
I am radiation oncologist. Is this tumor hypoxic? Quantitative mesurements are not likely available except in specialized centers that still possess the pO2 histography Eppendorf® system that can provide absolute pO2 measurements. Clinical EPR oxygen measurements are still at an early stage of development. The most straightforward, even indirect, way to get the answer is to request CAIX and HIF-1α from biopsies and, if possible, hypoxic gene expression and/or miRNA signatures. However, we should keep in mind that these biomarkers are not fully validated for the purpose. These biomarkers suffer from possible HIF-1α activation through a hypoxia-independent pathway in some circumstances. The addition of a PET scan with nitroimidazoles should be considered. The value of 18F-FMISO is debated regarding conflictual cross validation with other traditional hypoxia biomarkers while 18F-FAZA has been shown to accumulate in tumors under 10 mmHg, a meaningful level regarding radiosensitivity/radioresistance.
I am radiation oncologist. Which biomarker is adapted for dose painting? Knowing that a tumor is hypoxic thanks immunochemistry or gene signature assays is obviously not sufficient for therapeutic guidance in this context. Irradiation treatment adaptation strongly relies on the availability of reliable tumor oxygen mapping. EPR imaging is the only method that provides quantitative oxygen maps, but the technology is still under development and in validation at the preclinical stage. PET images obtained from radiolabeled nitroimidazoles can potentially be useful for radiation therapy treatment planning and dose distribution according to the presence of hypoxic regions. While treatment planning has been established with different tracers, only 18F-FAZA has been tested in vivo for comparison between radiotracer trapping and real pO2 values, showing a dramatic increase in accumulation under 10 mmHg. At the present stage of development, MRI approaches (OE-MRI, R2*-MRI, DCE-MRI) have not been validated for this purpose.
I am radiation oncologist. Which biomarker is adapted for selecting a radiosensitizing approach? The response is dependent on the radiosensitizing approach. Let’s first consider HAP (hypoxia activated prodrugs). This approach requires a go/no go decision for its use in a specific patient based on the presence of hypoxia. Phase III clinical trials have demonstrated that hypoxic gene expression signature is useful as predictor of response for the association nimorazole together with radiation therapy for treating head and neck cancer. The translation to other types of tumor and/other gene signature obviously requires a similar validation. An alternative is to consider PET scan with radiolabeled nitroimidazoles for this purpose. As most HAPs are trapped inside tumor cells using a mechanism similar to nitroimidazoles, this may be considered as a potentially useful biomarker for this application, of course requiring validation according to tumor type and nitroimidazole that will be used. For approaches using interventions designed to alleviate hypoxia by oxygen/carbogen breathing challenges, OE-MRI and R2*-MRI could be used as pharmodynamic biomarker of response in individual patients. However, as these MRI methods do not provide access to tissue oxygenation levels, it has to be combined with information provided by immunohistochemistry staining and/or gene signature regarding hypoxia at the basal level. The advantage of MRI is to provide in a simple challenge a dynamic evolution of parameters related to oxygen in vascular and tissue compartments, as well as the presence or absence of response to a specific challenge.
I develop a pharmacological intervention to alleviate tumor hypoxia. Which biomarker is useful during pre-clinical development and clinical development? At a preclinical stage, direct quantitative measurements can be applied in small animals. To increase statistical power and decrease the number of animals included in the studies, preference should be given to non-invasive longitudinal studies on the same tumor. Dynamic real-time changes in tumor oxygenation from the same sites can be achieved with EPR oximetry. A few centers offer this possibility and EPR systems adapted for the purpose are now commercially available (O2M technologies, Chicago, IL, United States; Novilet, Poznan, Poland; Bruker, Rheinstetten, Germany). In a small cohort of animals, the appropriate dosing and identification of time window of effect can be quickly identified and extended to a series of tumor models. Based on these generated robust data, other biomarkers could be tested as the ambition is to have a natural prolongation up to clinical trials. The other biomarkers should be selected on the basis of the mechanism of action of the pharmacological intervention. For strategies based on changes in oxygen delivery through an increase in blood oxygen saturation or change in hemoglobin saturation curve, the recommendation is to look for the value of combined OE-MRI and R2*-MRI. For strategies designed to facilitate the tumor perfusion, DCE-MRI could be added in the evaluation. For strategies designed to decrease the oxygen consumption, OE-MRI, R2*-MRI and DCE-MRI are not valuable methods, and 17O-MRI could be considered. For strategies that are rapidly changing tumor oxygenation, it is unlikely that PET with nitroimidazoles will gain any value as dynamic changes in oxygenation cannot be tackled considering the long distribution and elimination time of the radiotracers. Except for EPR, all these methods do not provide real pO2 measurements. For appropriate stratification in the clinic, combining these predictors together with immunohistochemistry and/or gene signature may be valuable to assess tumor hypoxia before treatment.
I develop a new strategy targeting HIF or downstream signaling cascades. Which biomarker is useful during clinical development? The identification of potential candidates for a treatment targeting HIF can be based on immunohistochemistry from biopsies revealing the overexpression of HIF-1α or using a gene signature focused of HIF activated genes. Ideally, after validation at the preclinical stage and in early clinical trials (Phase I/II), it could be used as a stratification tool for the enrollment of patients in Phase III, and later when applied routinely in the clinic. The problem is that the biomarker based on biopsy is a snapshot taken only before treatment, and nothing will be known on the possible evolution of expression of HIF over time. This is an appeal to further develop radiotracers targeting HIF that could be used for longitudinal studies. Of course, this will require full validation as predictive marker of response to be considered as potential companion diagnostic in HIF-targeted treatments.
Author Contributions
BG wrote the article.
Conflict of Interest
The authors declare that the research was conducted in the absence of any commercial or financial relationships that could be construed as a potential conflict of interest.
Publisher’s Note
All claims expressed in this article are solely those of the authors and do not necessarily represent those of their affiliated organizations, or those of the publisher, the editors and the reviewers. Any product that may be evaluated in this article, or claim that may be made by its manufacturer, is not guaranteed or endorsed by the publisher.
Acknowledgments
I wish to express my gratitude to my daughter, Fanny Gallez, for drawing the Figures 1–9 after having spent some time to teach her the basics of tumor hypoxia. During my 30 years experience in working on tumor hypoxia, its assessment and its manipulation, I have worked with many brilliant collaborators from my university who all have been key players for the progress in this field. They are all acknowledged for their outstanding contribution: R. Ansiaux, C. Baudelet, C. Buyse, T. Cao-Pham, N. Charlier, F. Colliez, J. Conq, N. Crokart, P. Danhier, C. Desmet, G. De Preter, D. d’Hose, C. Diepart, B. Driesschaert, O. Feron, A.C. Fruytier, Q. Godechal, V. Gregoire, J. He, B. Jordan, N. Joudiou, M. Lan, J. Magat, B. Mathieu, L. Karmani, O. Karroum, P. Leveque, J. Nel, M.A. Neveu, K. Radermacher, S. Scheinok, N. Schleich, C. Schoonjans, P. Sonveaux, L.B.A. Tran.
References
Abraham, J., Salama, N. N., and Azab, A. K. (2015). The Role of P-Glycoprotein in Drug Resistance in Multiple Myeloma. Leuk. Lymphoma 56, 26–33. doi:10.3109/10428194.2014.907890
Adams, A., van Brussel, A. S., Vermeulen, J. F., Mali, W. P., van der Wall, E., van Diest, P. J., et al. (2013). The Potential of Hypoxia Markers as Target for Breast Molecular Imaging-Aa Systematic Review and Meta-Analysis of Human Marker Expression. BMC Cancer 13, 538. doi:10.1186/1471-2407-13-538
Adams, G. E., Dische, S., Fowler, J. F., and Thomlinson, R. H. (1976). Hypoxic Cell Sensitisers in Radiotherapy. Lancet 1, 186–188. doi:10.1016/s0140-6736(76)91285-x
Agani, F., and Jiang, B. H. (2013). Oxygen-independent Regulation of HIF-1: Novel Involvement of PI3K/AKT/mTOR Pathway in Cancer. Curr. Cancer Drug Targets 13, 245–251. doi:10.2174/1568009611313030003
Ahmad, R., and Kuppusamy, P. (2010). Theory, Instrumentation, and Applications of Electron Paramagnetic Resonance Oximetry. Chem. Rev. 110, 3212–3236. doi:10.1021/cr900396q
Al-Hallaq, H. A., Zamora, M., Fish, B. L., Farrell, A., Moulder, J. E., and Karczmar, G. S. (2000). MRI Measurements Correctly Predict the Relative Effects of Tumor Oxygenating Agents on Hypoxic Fraction in Rodent BA1112 Tumors. Int. J. Radiat. Oncol. Biol. Phys. 47, 481–488. doi:10.1016/s0360-3016(00)00445-4
Albadari, N., Deng, S., and Li, W. (2019). The Transcriptional Factors HIF-1 and HIF-2 and Their Novel Inhibitors in Cancer Therapy. Expert Opin. Drug Discov. 14, 667–682. doi:10.1080/17460441.2019.1613370
Albertella, M. R., Loadman, P. M., Jones, P. H., Phillips, R. M., Rampling, R., Burnet, N., et al. (2008). Hypoxia-selective Targeting by the Bioreductive Prodrug AQ4N in Patients with Solid Tumors: Results of a Phase I Study. Clin. Cancer Res. 14, 1096–1104. doi:10.1158/1078-0432.CCR-07-4020
Alqawi, O., Moghaddas, M., and Singh, G. (2006). Effects of Geldanamycin on HIF-1alpha Mediated Angiogenesis and Invasion in Prostate Cancer Cells. Prostate Cancer Prostatic Dis. 9, 126–135. doi:10.1038/sj.pcan.4500852
Amorino, G. P., Lee, H., Holburn, G. E., Paschal, C. B., Hercules, S. K., Shyr, Y., et al. (2001). Enhancement of Tumor Oxygenation and Radiation Response by the Allosteric Effector of Hemoglobin, RSR13. Radiat. Res. 156, 294–300. doi:10.1667/0033-7587(2001)156[0294:eotoar]2.0.co;2
Anduran, E., Dubois, L. J., Lambin, P., and Winum, J.-Y. (2021). Hypoxia-activated Prodrug Derivatives of Anti-cancer Drugs: a Patent Review 2006 - 2021. Expert Opin. Ther. Pat. 32, 1–12. doi:10.1080/13543776.2021.1954617
Ansiaux, R., Baudelet, C., Cron, G. O., Segers, J., Dessy, C., Martinive, P., et al. (2006). Botulinum Toxin Potentiates Cancer Radiotherapy and Chemotherapy. Clin. Cancer Res. 12, 1276–1283. doi:10.1158/1078-0432.CCR-05-1222
Ansiaux, R., Baudelet, C., Jordan, B. F., Beghein, N., Sonveaux, P., De Wever, J., et al. (2005). Thalidomide Radiosensitizes Tumors through Early Changes in the Tumor Microenvironment. Clin. Cancer Res. 11, 743–750.
Ansiaux, R., Baudelet, C., Jordan, B. F., Crokart, N., Martinive, P., DeWever, J., et al. (2006). Mechanism of Reoxygenation after Antiangiogenic Therapy Using SU5416 and its Importance for Guiding Combined Antitumor Therapy. Cancer Res. 66, 9698–9704. doi:10.1158/0008-5472.CAN-06-1854
Ansiaux, R., Dewever, J., Grégoire, V., Feron, O., Jordan, B. F., and Gallez, B. (2009). Decrease in Tumor Cell Oxygen Consumption after Treatment with Vandetanib (ZACTIMA; ZD6474) and its Effect on Response to Radiotherapy. Radiat. Res. 172, 584–591. doi:10.1667/RR1744.1
Ansiaux, R., and Gallez, B. (2007). Use of Botulinum Toxins in Cancer Therapy. Expert Opin. Investig. Drugs 16, 209–218. doi:10.1517/13543784.16.2.209
Aoki, M., and Fujishita, T. (2017). Oncogenic Roles of the PI3K/AKT/mTOR Axis. Curr. Top. Microbiol. Immunol. 407, 153–189. doi:10.1007/82_2017_6
Aprahamian, M., Bour, G., Akladios, C. Y., Fylaktakidou, K., Greferath, R., Soler, L., et al. (2011). Myo-InositolTrisPyroPhosphate Treatment Leads to HIF-1α Suppression and Eradication of Early Hepatoma Tumors in Rats. Chembiochem 12, 777–783. doi:10.1002/cbic.201000619
Ardenkjaer-Larsen, J. H., Laursen, I., Leunbach, I., Ehnholm, G., Wistrand, L. G., Petersson, J. S., et al. (1998). EPR and DNP Properties of Certain Novel Single Electron Contrast Agents Intended for Oximetric Imaging. J. Magn. Reson 133, 1–12. doi:10.1006/jmre.1998.1438
Ashton, T. M., Fokas, E., Kunz-Schughart, L. A., Folkes, L. K., Anbalagan, S., Huether, M., et al. (2016). The Anti-malarial Atovaquone Increases Radiosensitivity by Alleviating Tumour Hypoxia. Nat. Commun. 7, 12308. doi:10.1038/ncomms12308
Axten, J. M., Medina, J. R., Feng, Y., Shu, A., Romeril, S. P., Grant, S. W., et al. (2012). Discovery of 7-methyl-5-(1- {[3-(trifluoromethyl)phenyl]acetyl}-2,3-Dihydro-1h-Indol-5-Yl)-7h-P Yrrolo[2,3-D]pyrimidin-4- Amine (GSK2606414), a Potent and Selective First-In-Class Inhibitor of Protein Kinase R (PKR)-like Endoplasmic Reticulum Kinase (PERK). J. Med. Chem. 55, 7193–7207. doi:10.1021/jm300713s
Axten, J. M. (2017). Protein Kinase R(PKR)-like Endoplasmic Reticulum Kinase (PERK) Inhibitors: a Patent Review (2010-2015). Expert Opin. Ther. Pat. 27, 37–48. doi:10.1080/13543776.2017.1238072
Axten, J. M., Romeril, S. P., Shu, A., Ralph, J., Medina, J. R., Feng, Y., et al. (2013). Discovery of GSK2656157: An Optimized PERK Inhibitor Selected for Preclinical Development. ACS Med. Chem. Lett. 4, 964–968. doi:10.1021/ml400228e
Bader, S. B., Dewhirst, M. W., and Hammond, E. M. (2020). Cyclic Hypoxia: An Update on its Characteristics, Methods to Measure it and Biological Implications in Cancer. Cancers 13, 23. doi:10.3390/cancers13010023
Ban, H. S., Uto, Y., and Nakamura, H. (2021). Hypoxia-inducible Factor (HIF) Inhibitors: a Patent Survey (2016-2020). Expert Opin. Ther. Pat. 31, 387–397. doi:10.1080/13543776.2021.1874345
Baran, N., and Konopleva, M. (2017). Molecular Pathways: Hypoxia-Activated Prodrugs in Cancer Therapy. Clin. Cancer Res. 23, 2382–2390. doi:10.1158/1078-0432.CCR-16-0895
Batchelor, T. T., Sorensen, A. G., di Tomaso, E., Zhang, W. T., Duda, D. G., Cohen, K. S., et al. (2007). AZD2171, a Pan-VEGF Receptor Tyrosine Kinase Inhibitor, Normalizes Tumor Vasculature and Alleviates Edema in Glioblastoma Patients. Cancer Cell 11, 83–95. doi:10.1016/j.ccr.2006.11.021
Baudelet, C., Ansiaux, R., Jordan, B. F., Havaux, X., Macq, B., and Gallez, B. (2004). Physiological Noise in Murine Solid Tumours Using T2*-Weighted Gradient-Echo Imaging: a Marker of Tumour Acute Hypoxia? Phys. Med. Biol. 49, 3389–3411. doi:10.1088/0031-9155/49/15/006
Baudelet, C., Cron, G. O., Ansiaux, R., Crokart, N., DeWever, J., Feron, O., et al. (2006). The Role of Vessel Maturation and Vessel Functionality in Spontaneous Fluctuations of T2*-Weighted GRE Signal within Tumors. NMR Biomed. 19, 69–76. doi:10.1002/nbm.1002
Baudelet, C., and Gallez, B. (2005). Current Issues in the Utility of Blood Oxygen Level Dependent MRI for the Assessment of Modulations in Tumor Oxygenation. Curr. Med. Imag. Rev. 1, 229–243. doi:10.2174/157340505774574754
Baudelet, C., and Gallez, B. (2004). Effect of Anesthesia on the Signal Intensity in Tumors Using BOLD-MRI: Comparison with Flow Measurements by Laser Doppler Flowmetry and Oxygen Measurements by Luminescence-Based Probes. Magn. Reson Imaging 22, 905–912. doi:10.1016/j.mri.2004.02.005
Baudelet, C., and Gallez, B. (2002). How Does Blood Oxygen Level-dependent (BOLD) Contrast Correlate with Oxygen Partial Pressure (pO2) inside Tumors? Magn. Reson Med. 48, 980–986. doi:10.1002/mrm.10318
Bayer, C., Shi, K., Astner, S. T., Maftei, C. A., and Vaupel, P. (2011). Acute versus Chronic Hypoxia: Why a Simplified Classification Is Simply Not Enough. Int. J. Radiat. Oncol. Biol. Phys. 80, 965–968. doi:10.1016/j.ijrobp.2011.02.049
Bayer, C., and Vaupel, P. (2012). Acute versus Chronic Hypoxia in Tumors: Controversial Data Concerning Time Frames and Biological Consequences. Strahlenther Onkol. 188, 616–627. doi:10.1007/s00066-012-0085-4
Becker, A., Kuhnt, T., Liedtke, H., Krivokuca, A., Bloching, M., and Dunst, J. (2002). Oxygenation Measurements in Head and Neck Cancers during Hyperbaric Oxygenation. Strahlenther Onkol. 178, 105–108. doi:10.1007/s00066-002-0892-0
Begg, A. C., Sheldon, P. W., and Foster, J. L. (1974). Demonstration of Radiosensitization of Hypoxic Cells in Solid Tumours by Metronidazole. Br. J. Radiol. 47, 399–404. doi:10.1259/0007-1285-47-559-399
Benej, M., Hong, X., Vibhute, S., Scott, S., Wu, J., Graves, E., et al. (2018). Papaverine and its Derivatives Radiosensitize Solid Tumors by Inhibiting Mitochondrial Metabolism. Proc. Natl. Acad. Sci. U. S. A. 115, 10756–10761. doi:10.1073/pnas.1808945115
Benjaminsen, I. C., Melås, E. A., Mathiesen, B. S., and Rofstad, E. K. (2008). Limitations of Dynamic Contrast-Enhanced MRI in Monitoring Radiation-Induced Changes in the Fraction of Radiobiologically Hypoxic Cells in Human Melanoma Xenografts. J. Magn. Reson Imaging 28 (5), 1209–1218. doi:10.1002/jmri.21602
Bentzen, S. M., and Gregoire, V. (2011). Molecular Imaging-Based Dose Painting: a Novel Paradigm for Radiation Therapy Prescription. Semin. Radiat. Oncol. 21, 101–110. doi:10.1016/j.semradonc.2010.10.001
Bergers, G., and Benjamin, L. E. (2003). Tumorigenesis and the Angiogenic Switch. Nat. Rev. Cancer 3, 401–410. doi:10.1038/nrc1093
Bertozzi, D., Marinello, J., Manzo, S. G., Fornari, F., Gramantieri, L., and Capranico, G. (2014). The Natural Inhibitor of DNA Topoisomerase I, Camptothecin, Modulates HIF-1α Activity by Changing miR Expression Patterns in Human Cancer Cells. Mol. Cancer Ther. 13, 239–248. doi:10.1158/1535-7163.MCT-13-0729
Biomarkers Definitions Working Group (2001). Biomarkers and Surrogate Endpoints: Preferred Definitions and Conceptual Framework. Clin. Pharmacol. Ther. 69, 89–95. doi:10.1067/mcp.2001.113989
Blackstone, E., Morrison, M., and Roth, M. B. (2005). H2S Induces a Suspended Animation-like State in Mice. Science 308, 518. doi:10.1126/science.1108581
Blackwell, K. L., Kirkpatrick, J. P., Snyder, S. A., Broadwater, G., Farrell, F., Jolliffe, L., et al. (2003). Human Recombinant Erythropoietin Significantly Improves Tumor Oxygenation Independent of its Effects on Hemoglobin. Cancer Res. 63, 6162–6165.
Borren, A., Groenendaal, G., van der Groep, P., Moman, M. R., Boeken Kruger, A. E., van der Heide, U. A., et al. (2013). Expression of Hypoxia-Inducible Factor-1α and -2α in Whole-Mount Prostate Histology: Relation with Dynamic Contrast-Enhanced MRI and Gleason Score. Oncol. Rep. 29, 2249–2254. doi:10.3892/or.2013.2392
Bourke, V. A., Zhao, D., Gilio, J., Chang, C. H., Jiang, L., Hahn, E. W., et al. (2007). Correlation of Radiation Response with Tumor Oxygenation in the Dunning Prostate R3327-AT1 Tumor. Int. J. Radiat. Oncol. Biol. Phys. 67, 1179–1186. doi:10.1016/j.ijrobp.2006.11.037
Braun, R. D., Lanzen, J. L., and Dewhirst, M. W. (1999). Fourier Analysis of Fluctuations of Oxygen Tension and Blood Flow in R3230Ac Tumors and Muscle in Rats. Am. J. Physiol. 277, H551–H568. H551–H568. doi:10.1152/ajpheart.1999.277.2.H551
Braun, R. D., Lanzen, J. L., Snyder, S. A., and Dewhirst, M. W. (2001). Comparison of Tumor and Normal Tissue Oxygen Tension Measurements Using OxyLite or Microelectrodes in Rodents. Am. J. Physiol. Heart Circ. Physiol. 280, H2533–H2544. doi:10.1152/ajpheart.2001.280.6.H2533
Brown, J. M. (1979). Evidence for Acutely Hypoxic Cells in Mouse Tumours, and a Possible Mechanism of Reoxygenation. Br. J. Radiol. 52, 650–656. doi:10.1259/0007-1285-52-620-650
Brown, J. M. (2000). Exploiting the Hypoxic Cancer Cell: Mechanisms and Therapeutic Strategies. Mol. Med. Today 6, 157–162. doi:10.1016/s1357-4310(00)01677-4
Brown, J. M., and Lemmon, M. J. (1990). Potentiation by the Hypoxic Cytotoxin SR 4233 of Cell Killing Produced by Fractionated Irradiation of Mouse Tumors. Cancer Res. 50, 7745–7749.
Brown, J. M. (1993). SR 4233 (Tirapazamine): a New Anticancer Drug Exploiting Hypoxia in Solid Tumours. Br. J. Cancer 67, 1163–1170. doi:10.1038/bjc.1993.220
Brown, J. M. (2007). Tumor Hypoxia in Cancer Therapy. Methods Enzymol. 435, 297–321. doi:10.1016/S0076-6879(07)35015-5
Burgman, P., O'Donoghue, J. A., Lewis, J. S., Welch, M. J., Humm, J. L., and Ling, C. C. (2005). Cell Line-dependent Differences in Uptake and Retention of the Hypoxia-Selective Nuclear Imaging Agent Cu-ATSM. Nucl. Med. Biol. 32, 623–630. doi:10.1016/j.nucmedbio.2005.05.003
Burrell, J. S., Walker-Samuel, S., Baker, L. C., Boult, J. K., Jamin, Y., Halliday, J., et al. (2013). Exploring ΔR2* and ΔR1 as Imaging Biomarkers of Tumor Oxygenation. J. Magn. Reson Imaging 38, 429–434. doi:10.1002/jmri.23987
Busk, M., and Horsman, M. R. (2013). Relevance of Hypoxia in Radiation Oncology: Pathophysiology, Tumor Biology and Implications for Treatment. Q. J. Nucl. Med. Mol. Imaging 57, 219–234.
Busk, M., Overgaard, J., and Horsman, M. R. (2020). Imaging of Tumor Hypoxia for Radiotherapy: Current Status and Future Directions. Semin. Nucl. Med. 50, 562–583. doi:10.1053/j.semnuclmed.2020.05.003
Bussink, J., Kaanders, J. H., and van der Kogel, A. J. (2003). Tumor Hypoxia at the Micro-regional Level: Clinical Relevance and Predictive Value of Exogenous and Endogenous Hypoxic Cell Markers. Radiother. Oncol. 67, 3–15. doi:10.1016/s0167-8140(03)00011-2
Cairns, R. A., and Hill, R. P. (2004). Acute Hypoxia Enhances Spontaneous Lymph Node Metastasis in an Orthotopic Murine Model of Human Cervical Carcinoma. Cancer Res. 64, 2054–2061. doi:10.1158/0008-5472.can-03-3196
Cairns, R. A., Kalliomaki, T., and Hill, R. P. (2001). Acute (Cyclic) Hypoxia Enhances Spontaneous Metastasis of KHT Murine Tumors. Cancer Res. 61, 8903–8908.
Cantelmo, A. R., Conradi, L. C., Brajic, A., Goveia, J., Kalucka, J., Pircher, A., et al. (2016). Inhibition of the Glycolytic Activator PFKFB3 in Endothelium Induces Tumor Vessel Normalization, Impairs Metastasis, and Improves Chemotherapy. Cancer Cell 30, 968–985. doi:10.1016/j.ccell.2016.10.006
Cao, X., Bloomston, M., Zhang, T., Frankel, W. L., Jia, G., Wang, B., et al. (2008). Synergistic Antipancreatic Tumor Effect by Simultaneously Targeting Hypoxic Cancer Cells with HSP90 Inhibitor and Glycolysis Inhibitor. Clin. Cancer Res. 14, 1831–1839. doi:10.1158/1078-0432.CCR-07-1607
Cao-Pham, T. T., Tran, L. B., Colliez, F., Joudiou, N., El Bachiri, S., Gregoire, V., et al. (2016). Monitoring Tumor Response to Carbogen Breathing by Oxygen-Sensitive Magnetic Resonance Parameters to Predict the Outcome of Radiation Therapy: a Preclinical Study. Int. J. Radiat. Oncol. Biol. Phys. 96, 149–160. doi:10.1016/j.ijrobp.2016.04.029
Cao-Pham, T. T., Tran, L. B., Colliez, F., Joudiou, N., El Bachiri, S., Grégoire, V., et al. (2016). Monitoring Tumor Response to Carbogen Breathing by Oxygen-Sensitive Magnetic Resonance Parameters to Predict the Outcome of Radiation Therapy: A Preclinical Study. Int. J. Radiat. Oncol. Biol. Phys. 96, 149–160. doi:10.1016/j.ijrobp.2016.04.029
Cao-Pham, T. T., Tran-Ly-Binh, A., Heyerick, A., Fillée, C., Joudiou, N., Gallez, B., et al. (2020). Combined Endogenous MR Biomarkers to Assess Changes in Tumor Oxygenation Induced by an Allosteric Effector of Hemoglobin. NMR Biomed. 33, e4181. doi:10.1002/nbm.4181
Cárdenas-Navia, L. I., Mace, D., Richardson, R. A., Wilson, D. F., Shan, S., and Dewhirst, M. W. (2008). The Pervasive Presence of Fluctuating Oxygenation in Tumors. Cancer Res. 68, 5812–5819. doi:10.1158/0008-5472.CAN-07-6387
Carles, M., Fechter, T., Grosu, A. L., Sörensen, A., Thomann, B., Stoian, R. G., et al. (2021). 18F-FMISO-PET Hypoxia Monitoring for Head-And-Neck Cancer Patients: Radiomics Analyses Predict the Outcome of Chemo-Radiotherapy. Cancers 13, 3449. doi:10.3390/cancers13143449
Carlin, S., Zhang, H., Reese, M., Ramos, N. N., Chen, Q., and Ricketts, S. A. (2014). A Comparison of the Imaging Characteristics and Microregional Distribution of 4 Hypoxia PET Tracers. J. Nucl. Med. 55, 515–521. doi:10.2967/jnumed.113.126615
Carmeliet, P., and Jain, R. K. (2011). Principles and Mechanisms of Vessel Normalization for Cancer and Other Angiogenic Diseases. Nat. Rev. Drug Discov. 10, 417–427. doi:10.1038/nrd3455
Carmona-Bozo, J. C., Manavaki, R., Woitek, R., Torheim, T., Baxter, G. C., Caraco, C., et al. (2021). Hypoxia and Perfusion in Breast Cancer: Simultaneous Assessment Using PET/MR Imaging. Eur. Radiol. 31, 333–344. doi:10.1007/s00330-020-07067-2
Chang, E., Liu, H., Unterschemmann, K., Ellinghaus, P., Liu, S., Gekeler, V., et al. (2015). 18F-FAZA PET Imaging Response Tracks the Reoxygenation of Tumors in Mice upon Treatment with the Mitochondrial Complex I Inhibitor BAY 87-2243. Clin. Cancer Res. 21, 335–346. doi:10.1158/1078-0432.CCR-14-0217
Chang, J. H., Wada, M., Anderson, N. J., Lim Joon, D., Lee, S. T., Gong, S. J., et al. (2013). Hypoxia-targeted Radiotherapy Dose Painting for Head and Neck Cancer Using 18F-FMISO PET: a Biological Modeling Study. Acta Oncol. 52, 1723–1729. doi:10.3109/0284186X.2012.759273
Chao, K. S., Bosch, W. R., Mutic, S., Lewis, J. S., Dehdashti, F., Mintun, M. A., et al. (2001). A Novel Approach to Overcome Hypoxic Tumor Resistance: Cu-ATSM-Guided Intensity-Modulated Radiation Therapy. Int. J. Radiat. Oncol. Biol. Phys. 49, 1171–1182. doi:10.1016/s0360-3016(00)01433-4
Chapelin, F., Gedaly, R., Sweeney, Z., and Gossett, L. J. (2022). Prognostic Value of Fluorine-19 MRI Oximetry Monitoring in Cancer. Mol. Imaging Biol. 24, 220. doi:10.1007/s11307-021-01678-x
Chaplin, D. J., Horsman, M. R., and Trotter, M. J. (1990). Effect of Nicotinamide on the Microregional Heterogeneity of Oxygen Delivery within a Murine Tumor. J. Natl. Cancer Inst. 82, 672–676. doi:10.1093/jnci/82.8.672
Chaplin, D. J., Olive, P. L., and Durand, R. E. (1987). Intermittent Blood Flow in a Murine Tumor: Radiobiological Effects. Cancer Res. 47, 597–601.
Charlier, N., Beghein, N., and Gallez, B. (2004). Development and Evaluation of Biocompatible Inks for the Local Measurement of Oxygen Using In Vivo EPR. NMR Biomed. 17, 303–310. doi:10.1002/nbm.902
Charlier, N., Driesschaert, B., Wauthoz, N., Beghein, N., Préat, V., Amighi, K., et al. (2009). Nano-emulsions of Fluorinated Trityl Radicals as Sensors for EPR Oximetry. J. Magn. Reson 197, 176–180. doi:10.1016/j.jmr.2008.12.013
Chauhan, V. P., Martin, J. D., Liu, H., Lacorre, D. A., Jain, S. R., Kozin, S. V., et al. (2013). Angiotensin Inhibition Enhances Drug Delivery and Potentiates Chemotherapy by Decompressing Tumour Blood Vessels. Nat. Commun. 4, 2516. doi:10.1038/ncomms3516
Chauhan, V. P., Stylianopoulos, T., Martin, J. D., Popović, Z., Chen, O., Kamoun, W. S., et al. (2012). Normalization of Tumour Blood Vessels Improves the Delivery of Nanomedicines in a Size-dependent Manner. Nat. Nanotechnol. 7, 383–388. doi:10.1038/nnano.2012.45
Chen, L., Zhang, Z., Kolb, H. C., Walsh, J. C., Zhang, J., and Guan, Y. (2012). 1⁸F-HX4 Hypoxia Imaging with PET/CT in Head and Neck Cancer: a Comparison with 1⁸F-FMISO. Nucl. Med. Commun. 33, 1096–1102. doi:10.1097/MNM.0b013e3283571016
Chen, N. T., Barth, E. D., Lee, T. H., Chen, C. T., Epel, B., Halpern, H. J., et al. (2019). Highly Sensitive Electron Paramagnetic Resonance Nanoradicals for Quantitative Intracellular Tumor Oxymetric Images. Int. J. Nanomedicine 14, 2963–2971. doi:10.2147/IJN.S194779
Chen, S. Y., Tsuneyama, K., Yen, M. H., Lee, J. T., Chen, J. L., and Huang, S. M. (2021). Hyperbaric Oxygen Suppressed Tumor Progression through the Improvement of Tumor Hypoxia and Induction of Tumor Apoptosis in A549-Cell-Transferred Lung Cancer. Sci. Rep. 11, 12033. doi:10.1038/s41598-021-91454-2
Chen, W., Hill, H., Christie, A., Kim, M. S., Holloman, E., Pavia-Jimenez, A., et al. (2016). Targeting Renal Cell Carcinoma with a HIF-2 Antagonist. Nature 539, 112–117. doi:10.1038/nature19796
Cheng, Y., Cheng, H., Jiang, C., Qiu, X., Wang, K., Huan, W., et al. (2015). Perfluorocarbon Nanoparticles Enhance Reactive Oxygen Levels and Tumour Growth Inhibition in Photodynamic Therapy. Nat. Commun. 6, 8785. doi:10.1038/ncomms9785
Cher, L. M., Murone, C., Lawrentschuk, N., Ramdave, S., Papenfuss, A., Hannah, A., et al. (2006). Correlation of Hypoxic Cell Fraction and Angiogenesis with Glucose Metabolic Rate in Gliomas Using 18F-Fluoromisonidazole, 18F-FDG PET, and Immunohistochemical Studies. J. Nucl. Med. 47, 410–418.
Cho, H., Ackerstaff, E., Carlin, S., Lupu, M. E., Wang, Y., Rizwan, A., et al. (2009). Noninvasive Multimodality Imaging of the Tumor Microenvironment: Registered Dynamic Magnetic Resonance Imaging and Positron Emission Tomography Studies of a Preclinical Tumor Model of Tumor Hypoxia. Neoplasia 11, 247–259. doi:10.1593/neo.81360
Choi, W., Lee, S. W., Park, S. H., Ryu, J. S., Oh, S. J., Im, K. C., et al. (2010). Planning Study for Available Dose of Hypoxic Tumor Volume Using Fluorine-18-Labeled Fluoromisonidazole Positron Emission Tomography for Treatment of the Head and Neck Cancer. Radiother. Oncol. 97, 176–182. doi:10.1016/j.radonc.2010.04.012
Chouaib, S. (2020). The Antitumor Cytotoxic Response: If the Killer Cells Play the Music, the Microenvironmental Hypoxia Plays the Tune. Crit. Rev. Immunol. 40, 157–166. doi:10.1615/CritRevImmunol.2020033492
Clark, L. C., and Lyons, C. (1962). Electrode Systems for Continuous Monitoring in Cardiovascular Surgery. Ann. N. Y. Acad. Sci. 102, 29–45. doi:10.1111/j.1749-6632.1962.tb13623.x
Clark, L. C. (1956). Monitor and Control of Blood and Tissue O2 Tensions. Trans. Am. Soc. Artif. Intern Organs 2, 41–48.
Clausen, M. M., Hansen, A. E., Af Rosenschold, P. M., Kjaer, A., Kristensen, A. T., McEvoy, F. J., et al. (2013). Dose Escalation to High-Risk Sub-volumes Based on Non-invasive Imaging of Hypoxia and Glycolytic Activity in Canine Solid Tumors: a Feasibility Study. Radiat. Oncol. 8, 262. doi:10.1186/1748-717X-8-262
Clementi, E., Brown, G. C., Foxwell, N., and Moncada, S. (1999). On the Mechanism by Which Vascular Endothelial Cells Regulate Their Oxygen Consumption. Proc. Natl. Acad. Sci. U. S. A. 96, 1559–1562. doi:10.1073/pnas.96.4.1559
Codony, V. L., and Tavassoli, M. (2021). Hypoxia-induced Therapy Resistance: Available Hypoxia-Targeting Strategies and Current Advances in Head and Neck Cancer. Transl. Oncol. 14, 101017. doi:10.1016/j.tranon.2021.101017
Coleman, C. N. (1985). Hypoxic Cell Radiosensitizers: Expectations and Progress in Drug Development. Int. J. Radiat. Oncol. Biol. Phys. 11, 323–329. doi:10.1016/0360-3016(85)90154-3
Colliez, F., Gallez, B., and Jordan, B. F. (2017). Assessing Tumor Oxygenation for Predicting Outcome in Radiation Oncology: A Review of Studies Correlating Tumor Hypoxic Status and Outcome in the Preclinical and Clinical Settings. Front. Oncol. 7, 10. doi:10.3389/fonc.2017.00010
Colliez, F., Neveu, M. A., Magat, J., Cao Pham, T. T., Gallez, B., and Jordan, B. F. (2014). Qualification of a Noninvasive Magnetic Resonance Imaging Biomarker to Assess Tumor Oxygenation. Clin. Cancer Res. 20, 5403–5411. doi:10.1158/1078-0432.CCR-13-3434
Colliez, F., Safronova, M. M., Magat, J., Joudiou, N., Peeters, A. P., Jordan, B. F., et al. (2015). Oxygen Mapping within Healthy and Acutely Infarcted Brain Tissue in Humans Using the NMR Relaxation of Lipids: A Proof-Of-Concept Translational Study. PLoS One 10, e0135248. doi:10.1371/journal.pone.0135248
Colombié, M., Gouard, S., Frindel, M., Vidal, A., Chérel, M., Kraeber-Bodéré, F., et al. (2015). Focus on the Controversial Aspects of (64)Cu-ATSM in Tumoral Hypoxia Mapping by PET Imaging. Front. Med. 2, 58. doi:10.3389/fmed.2015.00058
Corbet, C., and Feron, O. (2017). Cancer Cell Metabolism and Mitochondria: Nutrient Plasticity for TCA Cycle Fueling. Biochim. Biophys. Acta Rev. Cancer 1868, 7–15. doi:10.1016/j.bbcan.2017.01.002
Cosse, J. P., and Michiels, C. (2008). Tumour Hypoxia Affects the Responsiveness of Cancer Cells to Chemotherapy and Promotes Cancer Progression. Anticancer Agents Med. Chem. 8, 790–797. doi:10.2174/187152008785914798
Cosse, J. P., Ronvaux, M., Ninane, N., Raes, M. J., and Michiels, C. (2009). Hypoxia-induced Decrease in P53 Protein Level and Increase in C-Jun DNA Binding Activity Results in Cancer Cell Resistance to Etoposide. Neoplasia 11, 976–986. doi:10.1593/neo.09632
Cosse, J. P., Sermeus, A., Vannuvel, K., Ninane, N., Raes, M., and Michiels, C. (2007). Differential Effects of Hypoxia on Etoposide-Induced Apoptosis According to the Cancer Cell Lines. Mol. Cancer 6, 61. doi:10.1186/1476-4598-6-61
Courtney, K. D., Infante, J. R., Lam, E. T., Figlin, R. A., Rini, B. I., Brugarolas, J., et al. (2018). Phase I Dose-Escalation Trial of PT2385, a First-In-Class Hypoxia-Inducible Factor-2α Antagonist in Patients with Previously Treated Advanced Clear Cell Renal Cell Carcinoma. J. Clin. Oncol. 36, 867–874. doi:10.1200/JCO.2017.74.2627
Courtney, K. D., Ma, Y., Diaz de Leon, A., Christie, A., Xie, Z., Woolford, L., et al. (2020). HIF-2 Complex Dissociation, Target Inhibition, and Acquired Resistance with PT2385, a First-In-Class HIF-2 Inhibitor, in Patients with Clear Cell Renal Cell Carcinoma. Clin. Cancer Res. 26, 793–803. doi:10.1158/1078-0432.CCR-19-1459
Crokart, N., Danhier, F., Daugimont, L., Gonçalves, N., Jordan, B. F., Grégoire, V., et al. (2013). Potentiation of Radiotherapy by a Localized Antiangiogenic Gene Therapy. Radiother. Oncol. 107, 252–258. doi:10.1016/j.radonc.2013.03.018
Crokart, N., Jordan, B. F., Baudelet, C., Ansiaux, R., Sonveaux, P., Grégoire, V., et al. (2005). Early Reoxygenation in Tumors after Irradiation: Determining Factors and Consequences for Radiotherapy Regimens Using Daily Multiple Fractions. Int. J. Radiat. Oncol. Biol. Phys. 63, 901–910. doi:10.1016/j.ijrobp.2005.02.038
Crokart, N., Jordan, B. F., Baudelet, C., Cron, G. O., Hotton, J., Radermacher, K., et al. (2007). Glucocorticoids Modulate Tumor Radiation Response through a Decrease in Tumor Oxygen Consumption. Clin. Cancer Res. 13, 630–635. doi:10.1158/1078-0432.CCR-06-0802
Crokart, N., Radermacher, K., Jordan, B. F., Baudelet, C., Cron, G. O., Grégoire, V., et al. (2005). Tumor Radiosensitization by Antiinflammatory Drugs: Evidence for a New Mechanism Involving the Oxygen Effect. Cancer Res. 65, 7911–7916. doi:10.1158/0008-5472.CAN-05-1288
Cron, G. O., Beghein, N., Ansiaux, R., Martinive, P., Feron, O., and Gallez, B. (2008). 19F NMR In Vivo Spectroscopy Reflects the Effectiveness of Perfusion-Enhancing Vascular Modifiers for Improving Gemcitabine Chemotherapy. Magn. Reson Med. 59, 19–27. doi:10.1002/mrm.21469
Cron, G. O., Beghein, N., Crokart, N., Chavée, E., Bernard, S., Vynckier, S., et al. (2005). Changes in the Tumor Microenvironment during Low-Dose-Rate Permanent Seed Implantation Iodine-125 Brachytherapy. Int. J. Radiat. Oncol. Biol. Phys. 63, 1245–1251. doi:10.1016/j.ijrobp.2005.07.971
Danhier, F., Danhier, P., Magotteaux, N., De Preter, G., Ucakar, B., Karroum, O., et al. (2012). Electron Paramagnetic Resonance Highlights that the Oxygen Effect Contributes to the Radiosensitizing Effect of Paclitaxel. PLoS One 7, e40772. doi:10.1371/journal.pone.0040772
Danhier, P., De Saedeleer, C. J., Karroum, O., De Preter, G., Porporato, P. E., Jordan, B. F., et al. (2013). Optimization of Tumor Radiotherapy with Modulators of Cell Metabolism: toward Clinical Applications. Semin. Radiat. Oncol. 23, 262–272. doi:10.1016/j.semradonc.2013.05.008
Das, B., Tsuchida, R., Malkin, D., Koren, G., Baruchel, S., and Yeger, H. (2008). Hypoxia Enhances Tumor Stemness by Increasing the Invasive and Tumorigenic Side Population Fraction. Stem Cells 26, 1818–1830. doi:10.1634/stemcells.2007-0724
De Bruycker, S., Vangestel, C., Staelens, S., Wyffels, L., Detrez, J., Verschuuren, M., et al. (2019). Effects of Metformin on Tumor Hypoxia and Radiotherapy Efficacy: a [18 F]HX4 PET Imaging Study in Colorectal Cancer Xenografts. EJNMMI Res. 9, 74. doi:10.1186/s13550-019-0543-4
De Bruycker, S., Vangestel, C., Van den Wyngaert, T., Pauwels, P., Wyffels, L., Staelens, S., et al. (2019). 18 F-Flortanidazole Hypoxia PET Holds Promise as a Prognostic and Predictive Imaging Biomarker in a Lung Cancer Xenograft Model Treated with Metformin and Radiotherapy. J. Nucl. Med. 60, 34–40. doi:10.2967/jnumed.118.212225
De Preter, G., Deriemaeker, C., Danhier, P., Brisson, L., Cao Pham, T. T., Grégoire, V., et al. (2016). A Fast Hydrogen Sulfide-Releasing Donor Increases the Tumor Response to Radiotherapy. Mol. Cancer Ther. 15, 154–161. doi:10.1158/1535-7163.MCT-15-0691-T
De Preter, G., Neveu, M. A., Danhier, P., Brisson, L., Payen, V. L., Porporato, P. E., et al. (2016). Inhibition of the Pentose Phosphate Pathway by Dichloroacetate Unravels a Missing Link between Aerobic Glycolysis and Cancer Cell Proliferation. Oncotarget 7, 2910–2920. doi:10.18632/oncotarget.6272
DeBerardinis, R. J., Lum, J. J., Hatzivassiliou, G., and Thompson, C. B. (2008). The Biology of Cancer: Metabolic Reprogramming Fuels Cell Growth and Proliferation. Cell Metab. 7, 11–20. doi:10.1016/j.cmet.2007.10.002
Dehdashti, F., Grigsby, P. W., Lewis, J. S., Laforest, R., Siegel, B. A., and Welch, M. J. (2008). Assessing Tumor Hypoxia in Cervical Cancer by PET with 60Cu-Labeled Diacetyl-bis(N4-Methylthiosemicarbazone). J. Nucl. Med. 49, 201–205. doi:10.2967/jnumed.107.048520
Dehdashti, F., Grigsby, P. W., Mintun, M. A., Lewis, J. S., Siegel, B. A., and Welch, M. J. (2003). Assessing Tumor Hypoxia in Cervical Cancer by Positron Emission Tomography with 60Cu-ATSM: Relationship to Therapeutic Response-A Preliminary Report. Int. J. Radiat. Oncol. Biol. Phys. 55, 1233–1238. doi:10.1016/s0360-3016(02)04477-2
Demeure, R. J., Jordan, B. F., Yang, Q. X., Beghein, N., Smith, M. B., Grégoire, V., et al. (2002). Removal of Local Field Gradient Artefacts in BOLD Contrast Imaging of Head and Neck Tumours. Phys. Med. Biol. 47, 1819–1825. doi:10.1088/0031-9155/47/10/315
Denekamp, J., Michael, B. D., and Harris, S. R. (1974). Hypoxic Cell Radiosensitizers: Comparative Tests of Some Electron Affinic Compounds Using Epidermal Cell Survival In Vivo. Radiat. Res. 60, 119–132. doi:10.2307/3574011
Deng, J., Li, J., Sarde, A., Lines, J. L., Lee, Y. C., Qian, D. C., et al. (2019). Hypoxia-Induced VISTA Promotes the Suppressive Function of Myeloid-Derived Suppressor Cells in the Tumor Microenvironment. Cancer Immunol. Res. 7, 1079–1090. doi:10.1158/2326-6066.CIR-18-0507
Denmeade, S. R., and Isaacs, J. T. (2005). The SERCA Pump as a Therapeutic Target: Making a “Smart Bomb” for Prostate Cancer. Cancer Biol. Ther. 4, 14–22. doi:10.4161/cbt.4.1.1505
Denny, W. A. (2010). Hypoxia-activated Prodrugs in Cancer Therapy: Progress to the Clinic. Future Oncol. 6, 419–428. doi:10.2217/fon.10.1
Denny, W. A. (2000). The Role of Hypoxia-Activated Prodrugs in Cancer Therapy. Lancet Oncol. 1, 25–29. doi:10.1016/S1470-2045(00)00006-1
Desmet, C. M., Tran, L. B. A., Danhier, P., and Gallez, B. (2019). Characterization of a Clinically Used Charcoal Suspension for In Vivo EPR Oximetry. MAGMA 32, 205–212. doi:10.1007/s10334-018-0704-x
Dewhirst, M. W. (2018). A Potential Solution for Eliminating Hypoxia as a Cause for Radioresistance. Proc. Natl. Acad. Sci. U. S. A. 115, 10548–10550. doi:10.1073/pnas.1814212115
Dewhirst, M. W., Cao, Y., and Moeller, B. (2008). Cycling Hypoxia and Free Radicals Regulate Angiogenesis and Radiotherapy Response. Nat. Rev. Cancer 8, 425–437. doi:10.1038/nrc2397
Dewhirst, M. W., Kimura, H., Rehmus, S. W., Braun, R. D., Papahadjopoulos, D., Hong, K., et al. (1996). Microvascular Studies on the Origins of Perfusion-Limited Hypoxia. Br. J. Cancer Suppl. 27, S247–S251.
Dewhirst, M. W., Klitzman, B., Braun, R. D., Brizel, D. M., Haroon, Z. A., and Secomb, T. W. (2000). Review of Methods to Study Oxygen Transport at the Microcirculatory Level. Int. J. Cancer 90, 237–255. doi:10.1002/1097-0215(20001020)90:5<237::aid-ijc1>3.0.co;2-t
Dewhirst, M. W., Navia, I. C., Brizel, D. M., Willett, C., and Secomb, T. W. (2007). Multiple Etiologies of Tumor Hypoxia Require Multifaceted Solutions. Clin. Cancer Res. 13, 375–377. doi:10.1158/1078-0432.CCR-06-2629
Dewhirst, M. W., Ong, E. T., Braun, R. D., Smith, B., Klitzman, B., Evans, S. M., et al. (1999). Quantification of Longitudinal Tissue pO2 Gradients in Window Chamber Tumours: Impact on Tumour Hypoxia. Br. J. Cancer 79, 1717–1722. doi:10.1038/sj.bjc.6690273
Dewhirst, M. W., Secomb, T. W., Ong, E. T., Hsu, R., and Gross, J. F. (1994). Determination of Local Oxygen Consumption Rates in Tumors. Cancer Res. 54, 3333–3336.
Di Fazio, P., Ocker, M., and Montalbano, R. (2012). New Drugs, Old Fashioned Ways: ER Stress Induced Cell Death. Curr. Pharm. Biotechnol. 13, 2228–2234. doi:10.2174/138920112802501962
Di Perri, D., Lee, J. A., Bol, A., Hanin, F. X., Janssens, G., Labar, D., et al. (2017). Evolution of [(18)F]fluorodeoxyglucose and [(18)F]fluoroazomycin Arabinoside PET Uptake Distributions in Lung Tumours during Radiation Therapy. Acta Oncol. 56, 516–524. doi:10.1080/0284186X.2017.1287943
Diepart, C., Karroum, O., Magat, J., Feron, O., Verrax, J., Calderon, P. B., et al. (2012). Arsenic Trioxide Treatment Decreases the Oxygen Consumption Rate of Tumor Cells and Radiosensitizes Solid Tumors. Cancer Res. 72, 482–490. doi:10.1158/0008-5472.CAN-11-1755
Diepart, C., Magat, J., Jordan, B. F., and Gallez, B. (2011). In Vivo mapping of Tumor Oxygen Consumption Using 19F MRI Relaxometry. NMR Biomed. 24, 458–463. doi:10.1002/nbm.1604
Dinguizli, M., Jeumont, S., Beghein, N., He, J., Walczak, T., Lesniewski, P. N., et al. (2006). Development and Evaluation of Biocompatible Films of Polytetrafluoroethylene Polymers Holding Lithium Phthalocyanine Crystals for Their Use in EPR Oximetry. Biosens. Bioelectron. 21, 1015–1022. doi:10.1016/j.bios.2005.03.009
Dische, S., Chassagne, D., Hope-Stone, H. F., Dawes, P. J. D. K., Roberts, J. T., Yosef, H., et al. (1993). A Trial of Ro 03-8799 (Pimonidazole) in Carcinoma of the Uterine Cervix: an Interim Report from the Medical Research Council Working Party on Advanced Carcinoma of the Cervix. Radiother. Oncol. 26, 93–103. doi:10.1016/0167-8140(93)90089-q
Dische, S. (1985). Chemical Sensitizers for Hypoxic Cells: a Decade of Experience in Clinical Radiotherapy. Radiother. Oncol. 3, 97–115. doi:10.1016/s0167-8140(85)80015-3
Duan, J. X., Jiao, H., Kaizerman, J., Stanton, T., Evans, J. W., Lan, L., et al. (2008). Potent and Highly Selective Hypoxia-Activated Achiral Phosphoramidate Mustards as Anticancer Drugs. J. Med. Chem. 51, 2412–2420. doi:10.1021/jm701028q
Dubois, L. J., Lieuwes, N. G., Janssen, M. H., Peeters, W. J., Windhorst, A. D., Walsh, J. C., et al. (2011). Preclinical Evaluation and Validation of [18F]HX4, a Promising Hypoxia Marker for PET Imaging. Proc. Natl. Acad. Sci. U. S. A. 108, 14620–14625. doi:10.1073/pnas.1102526108
Dunn, J. F., and Swartz, H. M. (2003). In Vivo electron Paramagnetic Resonance Oximetry with Particulate Materials. Methods 30, 159–166. doi:10.1016/s1046-2023(03)00077-x
Eberhard, A., Kahlert, S., Goede, V., Hemmerlein, B., Plate, K. H., and Augustin, H. G. (2000). Heterogeneity of Angiogenesis and Blood Vessel Maturation in Human Tumors: Implications for Antiangiogenic Tumor Therapies. Cancer Res. 60, 1388–1393.
Egeland, T. A., Gaustad, J. V., Benjaminsen, I. C., Hedalen, K., Mathiesen, B., and Rofstad, E. K. (2008). Assessment of Fraction of Hypoxic Cells in Human Tumor Xenografts with Necrotic Regions by Dynamic Contrast-Enhanced MRI. Radiat. Res. 169, 689–699. doi:10.1667/RR1311.1
Egeland, T. A., Gaustad, J. V., Vestvik, I. K., Benjaminsen, I. C., Mathiesen, B., and Rofstad, E. K. (2006). Assessment of Fraction of Radiobiologically Hypoxic Cells in Human Melanoma Xenografts by Dynamic Contrast-Enhanced MRI. Magn. Reson Med. 55, 874–882. doi:10.1002/mrm.20852
Elamir, A. M., Stanescu, T., Shessel, A., Tadic, T., Yeung, I., Letourneau, D., et al. (2021). Simulated Dose Painting of Hypoxic Sub-volumes in Pancreatic Cancer Stereotactic Body Radiotherapy. Phys. Med. Biol. 66, 18. doi:10.1088/1361-6560/ac215c
Elas, M., Ahn, K. H., Parasca, A., Barth, E. D., Lee, D., Haney, C., et al. (2006). Electron Paramagnetic Resonance Oxygen Images Correlate Spatially and Quantitatively with Oxylite Oxygen Measurements. Clin. Cancer Res. 12, 4209–4217. doi:10.1158/1078-0432.CCR-05-0446
Elas, M., Bell, R., Hleihel, D., Barth, E. D., McFaul, C., Haney, C. R., et al. (2008). Electron Paramagnetic Resonance Oxygen Image Hypoxic Fraction Plus Radiation Dose Strongly Correlates with Tumor Cure in FSa Fibrosarcomas. Int. J. Radiat. Oncol. Biol. Phys. 71, 542–549. doi:10.1016/j.ijrobp.2008.02.022
Elas, M., Magwood, J. M., Butler, B., Li, C., Wardak, R., DeVries, R., et al. (2013). EPR Oxygen Images Predict Tumor Control by a 50% Tumor Control Radiation Dose. Cancer Res. 73, 5328–5335. doi:10.1158/0008-5472.CAN-13-0069
Ellingsen, C., Egeland, T. A., Gulliksrud, K., Gaustad, J. V., Mathiesen, B., and Rofstad, E. K. (2009). Assessment of Hypoxia in Human Cervical Carcinoma Xenografts by Dynamic Contrast-Enhanced Magnetic Resonance Imaging. Int. J. Radiat. Oncol. Biol. Phys. 73, 838–845. doi:10.1016/j.ijrobp.2008.10.062
Ellingsen, C., Hompland, T., Galappathi, K., Mathiesen, B., and Rofstad, E. K. (2014). DCE-MRI of the Hypoxic Fraction, Radioresponsiveness, and Metastatic Propensity of Cervical Carcinoma Xenografts. Radiother. Oncol. 110, 335–341. doi:10.1016/j.radonc.2013.10.018
Epel, B., Bowman, M. K., Mailer, C., and Halpern, H. J. (2014). Absolute oxygen R1e imaging In Vivo with pulse electron paramagnetic resonance. Magn. Reson Med. 72, 362–368. doi:10.1002/mrm.24926
Epel, B., and Halpern, H. J. (2015). In Vivo pO2 Imaging of Tumors: Oxymetry with Very Low-Frequency Electron Paramagnetic Resonance. Methods Enzymol. 564, 501–527. doi:10.1016/bs.mie.2015.08.017
Epel, B., Maggio, M. C., Barth, E. D., Miller, R. C., Pelizzari, C. A., Krzykawska-Serda, M., et al. (2019). Oxygen-Guided Radiation Therapy. Int. J. Radiat. Oncol. Biol. Phys. 103, 977–984. doi:10.1016/j.ijrobp.2018.10.041
Epel, B., Sundramoorthy, S. V., Barth, E. D., Mailer, C., and Halpern, H. J. (2011). Comparison of 250 MHz Electron Spin Echo and Continuous Wave Oxygen EPR Imaging Methods for In Vivo Applications. Med. Phys. 38, 2045–2052. doi:10.1118/1.3555297
Eschmann, S. M., Paulsen, F., Reimold, M., Dittmann, H., Welz, S., Reischl, G., et al. (2005). Prognostic Impact of Hypoxia Imaging with 18F-Misonidazole PET in Non-small Cell Lung Cancer and Head and Neck Cancer before Radiotherapy. J. Nucl. Med. 46, 253–260.
Eschwège, F., Sancho-Garnier, H., Chassagne, D., Brisgand, D., Guerra, M., Malaise, E. P., et al. (1997). Results of a European Randomized Trial of Etanidazole Combined with Radiotherapy in Head and Neck Carcinomas. Int. J. Radiat. Oncol. Biol. Phys. 39, 275–281. doi:10.1016/s0360-3016(97)00327-1
Eskey, C. J., Wolmark, N., McDowell, C. L., Domach, M. M., and Jain, R. K. (1994). Residence Time Distributions of Various Tracers in Tumors: Implications for Drug Delivery and Blood Flow Measurement. J. Natl. Cancer Inst. 86, 293–299. doi:10.1093/jnci/86.4.293
Eustace, A., Mani, N., Span, P. N., Irlam, J. J., Taylor, J., Betts, G. N., et al. (2013). A 26-gene Hypoxia Signature Predicts Benefit from Hypoxia-Modifying Therapy in Laryngeal Cancer but Not Bladder Cancer. Clin. Cancer Res. 19, 4879–4888. doi:10.1158/1078-0432.CCR-13-0542
Fadejeva, I., Olschewski, H., and Hrzenjak, A. (2017). MicroRNAs as Regulators of Cisplatin-Resistance in Non-small Cell Lung Carcinomas. Oncotarget 8, 115754–115773. doi:10.18632/oncotarget.22975
Falk, S. J., Ward, R., and Bleehen, N. M. (1992). The Influence of Carbogen Breathing on Tumour Tissue Oxygenation in Man Evaluated by Computerised P02 Histography. Br. J. Cancer 66, 919–924. doi:10.1038/bjc.1992.386
Fan, Q., Tang, C. Y., Gu, D., Zhu, J., Li, G., Wu, Y., et al. (2017). Investigation of Hypoxia Conditions Using Oxygen-Enhanced Magnetic Resonance Imaging Measurements in Glioma Models. Oncotarget 8, 31864–31875. doi:10.18632/oncotarget.16256
Fang, H., Gai, Y., Wang, S., Liu, Q., Zhang, X., Ye, M., et al. (2021). Biomimetic Oxygen Delivery Nanoparticles for Enhancing Photodynamic Therapy in Triple-Negative Breast Cancer. J. Nanobiotechnol 19, 81. doi:10.1186/s12951-021-00827-2
Fardin, P., Barla, A., Mosci, S., Rosasco, L., Verri, A., Versteeg, R., et al. (2010). A Biology-Driven Approach Identifies the Hypoxia Gene Signature as a Predictor of the Outcome of Neuroblastoma Patients. Mol. Cancer 9, 185. doi:10.1186/1476-4598-9-185
Feldman, D. E., Chauhan, V., and Koong, A. C. (2005). The Unfolded Protein Response: a Novel Component of the Hypoxic Stress Response in Tumors. Mol. Cancer Res. 3, 597–605. doi:10.1158/1541-7786.MCR-05-0221
Fingar, V. H., Mang, T. S., and Henderson, B. W. (1988). Modification of Photodynamic Therapy-Induced Hypoxia by Fluosol-DA (20%) and Carbogen Breathing in Mice. Cancer Res. 48, 3350–3354.
Fishman, J. E., Joseph, P. M., Carvlin, M. J., Saadi-Elmandjra, M., Mukherji, B., and Sloviter, H. A. (1989). In Vivo measurements of Vascular Oxygen Tension in Tumors Using MRI of a Fluorinated Blood Substitute. Invest Radiol. 24, 65–71. doi:10.1097/00004424-198901000-00014
Fjeldbo, C. S., Hompland, T., Hillestad, T., Aarnes, E. K., Günther, C. C., Kristensen, G. B., et al. (2020). Combining Imaging- and Gene-Based Hypoxia Biomarkers in Cervical Cancer Improves Prediction of Chemoradiotherapy Failure Independent of Intratumour Heterogeneity. EBioMedicine 57, 102841. doi:10.1016/j.ebiom.2020.102841
Fleming, I. N., Manavaki, R., Blower, P. J., West, C., Williams, K. J., Harris, A. L., et al. (2015). Imaging Tumour Hypoxia with Positron Emission Tomography. Br. J. Cancer 112, 238–250. doi:10.1038/bjc.2014.610
Flood, A. B., Schaner, P. E., Vaupel, P., Williams, B. B., Gallez, B., Chen, E. Y., et al. (2020). Clinical and Statistical Considerations when Assessing Oxygen Levels in Tumors: Illustrative Results from Clinical EPR Oximetry Studies. Adv. Exp. Med. Biol. 1232, 155–168. doi:10.1007/978-3-030-34461-0_20
Flood, A. B., Wood, V. A., Schreiber, W., Williams, B. B., Gallez, B., and Swartz, H. M. (2018). Guidance to Transfer 'Bench-Ready' Medical Technology into Usual Clinical Practice: Case Study - Sensors and Spectrometer Used in EPR Oximetry. Adv. Exp. Med. Biol. 1072, 233–239. doi:10.1007/978-3-319-91287-5_37
Foster, J. L., and Willson, R. L. (1973). Radiosensitization of Anoxic Cells by Metronidazole. Br. J. Radiol. 46, 234–235. doi:10.1259/0007-1285-46-543-234
Frank, J., Gündel, D., Drescher, S., Thews, O., and Mäder, K. (2015). Injectable LiNc-BuO Loaded Microspheres as In Vivo EPR Oxygen Sensors after Co-implantation with Tumor Cells. Free Radic. Biol. Med. 89, 741–749. doi:10.1016/j.freeradbiomed.2015.10.401
Frederiksen, L. J., Siemens, D. R., Heaton, J. P., Maxwell, L. R., Adams, M. A., and Graham, C. H. (2003). Hypoxia Induced Resistance to Doxorubicin in Prostate Cancer Cells Is Inhibited by Low Concentrations of Glyceryl Trinitrate. J. Urol. 170, 1003–1007. doi:10.1097/01.ju.0000081126.71235.e0
Frérart, F., Sonveaux, P., Rath, G., Smoos, A., Meqor, A., Charlier, N., et al. (2008). The Acidic Tumor Microenvironment Promotes the Reconversion of Nitrite into Nitric Oxide: towards a New and Safe Radiosensitizing Strategy. Clin. Cancer Res. 14, 2768–2774. doi:10.1158/1078-0432.CCR-07-4001
Fu, Z., Mowday, A. M., Smaill, J. B., Hermans, I. F., and Patterson, A. V. (2021). Tumour Hypoxia-Mediated Immunosuppression: Mechanisms and Therapeutic Approaches to Improve Cancer Immunotherapy. Cells 10, 1006. doi:10.3390/cells10051006
Fujibayashi, Y., Taniuchi, H., Yonekura, Y., Ohtani, H., Konishi, J., and Yokoyama, A. (1997). Copper-62-ATSM: a New Hypoxia Imaging Agent with High Membrane Permeability and Low Redox Potential. J. Nucl. Med. 38, 1155–1160.
Fyles, A. W., Milosevic, M., Wong, R., Kavanagh, M. C., Pintilie, M., Sun, A., et al. (1998). Oxygenation Predicts Radiation Response and Survival in Patients with Cervix Cancer. Radiother. Oncol. 48, 149–156. doi:10.1016/s0167-8140(98)00044-9
Gagel, B., Reinartz, P., Dimartino, E., Zimny, M., Pinkawa, M., Maneschi, P., et al. (2004). pO2 Polarography versus Positron Emission Tomography ([18F] Fluoromisonidazole, [18F]-2-Fluoro-2'-Deoxyglucose). An Appraisal of Radiotherapeutically Relevant Hypoxia. Strahlenther Onkol. 180, 616–622. doi:10.1007/s00066-004-1229-y
Gallagher, R., Hughes, C. M., Murray, M. M., Friery, O. P., Patterson, L. H., Hirst, D. G., et al. (2001). The Chemopotentiation of Cisplatin by the Novel Bioreductive Drug AQ4N. Br. J. Cancer 85, 625–629. doi:10.1054/bjoc.2001.1975
Gallez, B., Neveu, M. A., Danhier, P., and Jordan, B. F. (2017). Manipulation of Tumor Oxygenation and Radiosensitivity through Modification of Cell Respiration. A Critical Review of Approaches and Imaging Biomarkers for Therapeutic Guidance. Biochim. Biophys. Acta Bioenerg. 1858, 700–711. doi:10.1016/j.bbabio.2017.01.002
Gallez, B., Bacic, G., Goda, F., Jiang, J., O'Hara, J. A., Dunn, J. F., et al. (1996). Use of Nitroxides for Assessing Perfusion, Oxygenation, and Viability of Tissues: In Vivo EPR and MRI Studies. Magn. Reson Med. 35, 97–106. doi:10.1002/mrm.1910350113
Gallez, B., Baudelet, C., and Jordan, B. F. (2004). Assessment of Tumor Oxygenation by Electron Paramagnetic Resonance: Principles and Applications. NMR Biomed. 17, 240–262. doi:10.1002/nbm.900
Gallez, B., Debuyst, R., Dejehet, F., Liu, K. J., Walczak, T., Goda, F., et al. (1998). Small Particles of Fusinite and Carbohydrate Chars Coated with Aqueous Soluble Polymers: Preparation and Applications for In Vivo EPR Oximetry. Magn. Reson Med. 40, 152–159. doi:10.1002/mrm.1910400120
Gallez, B., Debuyst, R., Liu, K. J., Demeure, R., Dejehet, F., and Swartz, H. M. (1996). Development of Biocompatible Implants of Fusinite for In Vivo EPR Oximetry. MAGMA 4, 71–75. doi:10.1007/BF01759782
Gallez, B., Jordan, B. F., and Baudelet, C. (1999). Microencapsulation of Paramagnetic Particles by Pyrroxylin to Preserve Their Responsiveness to Oxygen when Used as Sensors for In Vivo EPR Oximetry. Magn. Reson Med. 42, 193–196. doi:10.1002/(sici)1522-2594(199907)42:1<193::aid-mrm25>3.0.co;2-c
Gallez, B., Jordan, B. F., Baudelet, C., and Misson, P. D. (1999). Pharmacological Modifications of the Partial Pressure of Oxygen in Murine Tumors: Evaluation Using In Vivo EPR Oximetry. Magn. Reson Med. 42, 627–630. doi:10.1002/(sici)1522-2594(199910)42:4<627::aid-mrm2>3.0.co;2-m
Gallez, B., and Mader, K. (2000). Accurate and Sensitive Measurements of pO2In Vivo Using Low Frequency EPR Spectroscopy: How to Confer Biocompatibility to the Oxygen Sensors. Free Radic. Biol. Med. 29, 1078–1084. doi:10.1016/s0891-5849(00)00405-6
Gallez, B., and Swartz, H. M. (2004). In Vivo EPR: when, How and Why? NMR Biomed. 17, 223–225. doi:10.1002/nbm.913
Gallez, B. (2021). Oxygenation Status in Normal Tissues, Pathological Tissues and Malignant Tumors: A pO2 Database Based on Electron Paramagnetic Resonance (EPR) Oximetry Measurements. Appl. Magn. Reson 52, 1395–1450. doi:10.1007/s00723-021-01358-7
Gammon, S. T., Pisaneschi, F., Bandi, M. L., Smith, M. G., Sun, Y., Rao, Y., et al. (2019). Mechanism-Specific Pharmacodynamics of a Novel Complex-I Inhibitor Quantified by Imaging Reversal of Consumptive Hypoxia with [(18)F]FAZA PET In Vivo. Cells 8, 1487. doi:10.3390/cells8121487
Garziera, M., Scarabel, L., and Toffoli, G. (2017). Hypoxic Modulation of HLA-G Expression through the Metabolic Sensor HIF-1 in Human Cancer Cells. J. Immunol. Res. 2017, 4587520. doi:10.1155/2017/4587520
Gatenby, R. A., Kessler, H. B., Rosenblum, J. S., Coia, L. R., Moldofsky, P. J., Hartz, W. H., et al. (1988). Oxygen Distribution in Squamous Cell Carcinoma Metastases and its Relationship to Outcome of Radiation Therapy. Int. J. Radiat. Oncol. Biol. Phys. 14, 831–838. doi:10.1016/0360-3016(88)90002-8
Gaustad, J. V., Hauge, A., Wegner, C. S., Simonsen, T. G., Lund, K. V., Hansem, L. M. K., et al. (2020). DCE-MRI of Tumor Hypoxia and Hypoxia-Associated Aggressiveness. Cancers 12, 1979. doi:10.3390/cancers12071979
Gaustad, J. V., and Rofstad, E. K. (2021). Assessment of Hypoxic Tissue Fraction and Prediction of Survival in Cervical Carcinoma by Dynamic Contrast-Enhanced MRI. Front. Oncol. 11, 668916. doi:10.3389/fonc.2021.668916
Geets, X., Grégoire, V., and Lee, J. A. (2013). Implementation of Hypoxia PET Imaging in Radiation Therapy Planning. Q. J. Nucl. Med. Mol. Imaging 57, 271–282.
Goda, F., Bacic, G., O'Hara, J. A., Gallez, B., Swartz, H. M., and Dunn, J. F. (1996). The Relationship between Partial Pressure of Oxygen and Perfusion in Two Murine Tumors after X-Ray Irradiation: a Combined Gadopentetate Dimeglumine Dynamic Magnetic Resonance Imaging and In Vivo Electron Paramagnetic Resonance Oximetry Study. Cancer Res. 56, 3344–3349.
Goda, F., O'Hara, J. A., Rhodes, E. S., Liu, K. J., Dunn, J. F., Bacic, G., et al. (1995). Changes of Oxygen Tension in Experimental Tumors after a Single Dose of X-Ray Irradiation. Cancer Res. 55, 2249–2252.
Goel, S., Duda, D. G., Xu, L., Munn, L. L., Boucher, Y., Fukumura, D., et al. (2011). Normalization of the Vasculature for Treatment of Cancer and Other Diseases. Physiol. Rev. 91, 1071–1121. doi:10.1152/physrev.00038.2010
Gonçalves, M. R., Johnson, S. P., Ramasawmy, R., Pedley, R. B., Lythgoe, M. F., and Walker-Samuel, S. (2015). Decomposition of Spontaneous Fluctuations in Tumour Oxygenation Using BOLD MRI and Independent Component Analysis. Br. J. Cancer 113, 1168–1177. doi:10.1038/bjc.2015.270
Grandjean, J. M. D., and Wiseman, R. L. (2020). Small Molecule Strategies to Harness the Unfolded Protein Response: where Do We Go from Here? J. Biol. Chem. 295, 15692–15711. doi:10.1074/jbc.REV120.010218
Grau, C., Horsman, M. R., and Overgaard, J. (1992). Improving the Radiation Response in a C3H Mouse Mammary Carcinoma by Normobaric Oxygen or Carbogen Breathing. Int. J. Radiat. Oncol. Biol. Phys. 22, 415–419. doi:10.1016/0360-3016(92)90844-8
Graves, E. E., Hicks, R. J., Binns, D., Bressel, M., Le, Q. T., Peters, L., et al. (2016). Quantitative and Qualitative Analysis of [(18)F]FDG and [(18)F]FAZA Positron Emission Tomography of Head and Neck Cancers and Associations with HPV Status and Treatment Outcome. Eur. J. Nucl. Med. Mol. Imaging 43, 617–625. doi:10.1007/s00259-015-3247-7
Gray, L. H., Conger, A. D., Ebert, M., Hornsey, S., and Scott, O. C. (1953). The Concentration of Oxygen Dissolved in Tissues at the Time of Irradiation as a Factor in Radiotherapy. Br. J. Radiol. 26, 638–648. doi:10.1259/0007-1285-26-312-638
Greenberger, L. M., Horak, I. D., Filpula, D., Sapra, P., Westergaard, M., Frydenlund, H. F., et al. (2008). A RNA Antagonist of Hypoxia-Inducible Factor-1alpha, EZN-2968, Inhibits Tumor Cell Growth. Mol. Cancer Ther. 7, 3598–3608. doi:10.1158/1535-7163.MCT-08-0510
Grégoire, V., Haustermans, K., Geets, X., Roels, S., and Lonneux, M. (2007). PET-Based Treatment Planning in Radiotherapy: a New Standard? J. Nucl. Med. 48, 68S–77S.
Gregoire, V., Thorwarth, D., and Lee, J. A. (2018). Molecular Imaging-Guided Radiotherapy for the Treatment of Head-And-Neck Squamous Cell Carcinoma: Does it Fulfill the Promises? Semin. Radiat. Oncol. 28, 35–45. doi:10.1016/j.semradonc.2017.08.003
Griffiths, J. R., and Robinson, S. P. (1999). The OxyLite: a Fibre-Optic Oxygen Sensor. Br. J. Radiol. 72, 627–630. doi:10.1259/bjr.72.859.10624317
Gulledge, C. J., and Dewhirst, M. W. (1996). Tumor Oxygenation: a Matter of Supply and Demand. Anticancer Res. 16, 741–749.
Gulliksrud, K., Mathiesen, B., Galappathi, K., and Rofstad, E. K. (2010). Quantitative Assessment of Hypoxia in Melanoma Xenografts by Dynamic Contrast-Enhanced Magnetic Resonance Imaging: Intradermal versus Intramuscular Tumors. Radiother. Oncol. 97, 233–238. doi:10.1016/j.radonc.2010.09.005
Gulliksrud, K., Øvrebø, K. M., Mathiesen, B., and Rofstad, E. K. (2011). Differentiation between Hypoxic and Non-hypoxic Experimental Tumors by Dynamic Contrast-Enhanced Magnetic Resonance Imaging. Radiother. Oncol. 98, 360–364. doi:10.1016/j.radonc.2010.12.016
Guo, R., Xu, N., Liu, Y., Ling, G., Yu, J., and Zhang, P. (2021). Functional Ultrasound-Triggered Phase-Shift Perfluorocarbon Nanodroplets for Cancer Therapy. Ultrasound Med. Biol. 47, 2064–2079. doi:10.1016/j.ultrasmedbio.2021.04.003
Haffty, B. G., Hurley, R., and Peters, L. J. (1999). Radiation Therapy with Hyperbaric Oxygen at 4 Atmospheres Pressure in the Management of Squamous Cell Carcinoma of the Head and Neck: Results of a Randomized Clinical Trial. Cancer J. Sci. Am. 5, 341–347.
Hajj, C., Russell, J., Hart, C. P., Goodman, K. A., Lowery, M. A., Haimovitz-Friedman, A., et al. (2017). A Combination of Radiation and the Hypoxia-Activated Prodrug Evofosfamide (TH-302) Is Efficacious against a Human Orthotopic Pancreatic Tumor Model. Transl. Oncol. 10, 760–765. doi:10.1016/j.tranon.2017.06.010
Hallac, R. R., Zhou, H., Pidikiti, R., Song, K., Solberg, T., Kodibagkar, V. D., et al. (2016). A Role for Dynamic Contrast-Enhanced Magnetic Resonance Imaging in Predicting Tumour Radiation Response. Br. J. Cancer 114, 1206–1211. doi:10.1038/bjc.2016.110
Hallac, R. R., Zhou, H., Pidikiti, R., Song, K., Stojadinovic, S., Zhao, D., et al. (2014). Correlations of Noninvasive BOLD and TOLD MRI with pO2 and Relevance to Tumor Radiation Response. Magn. Reson Med. 71, 1863–1873. doi:10.1002/mrm.24846
Halpern, H. J., Yu, C., Peric, M., Barth, E., Grdina, D. J., and Teicher, B. A. (1994). Oxymetry Deep in Tissues with Low-Frequency Electron Paramagnetic Resonance. Proc. Natl. Acad. Sci. U. S. A. 91, 13047–13051. doi:10.1073/pnas.91.26.13047
Hansen, A. E., Kristensen, A. T., Jørgensen, J. T., McEvoy, F. J., Busk, M., van der Kogel, A. J., et al. (2012). (64)Cu-ATSM and (18)FDG PET Uptake and (64)Cu-ATSM Autoradiography in Spontaneous Canine Tumors: Comparison with Pimonidazole Hypoxia Immunohistochemistry. Radiat. Oncol. 7, 89. doi:10.1186/1748-717X-7-89
Harris, A. L. (2002). Hypoxia--a Key Regulatory Factor in Tumour Growth. Nat. Rev. Cancer 2, 38–47. doi:10.1038/nrc704
Harris, B. H. L., Barberis, A., West, C. M. L., and Buffa, F. M. (2015). Gene Expression Signatures as Biomarkers of Tumour Hypoxia. Clin. Oncol. 27, 547–560. doi:10.1016/j.clon.2015.07.004
Hassan Metwally, M. A., Ali, R., Kuddu, M., Shouman, T., Strojan, P., Iqbal, K., et al. (2015). IAEA-HypoX. A Randomized Multicenter Study of the Hypoxic Radiosensitizer Nimorazole Concomitant with Accelerated Radiotherapy in Head and Neck Squamous Cell Carcinoma. Radiother. Oncol. 116, 15–20. doi:10.1016/j.radonc.2015.04.005
Hauge, A., Wegner, C. S., Gaustad, J. V., Simonsen, T. G., Andersen, L. M. K., and Rofstad, E. K. (2017). DCE-MRI of Patient-Derived Xenograft Models of Uterine Cervix Carcinoma: Associations with Parameters of the Tumor Microenvironment. J. Transl. Med. 15, 225. doi:10.1186/s12967-017-1331-4
He, J., Beghein, N., Ceroke, P., Clarkson, R. B., Swartz, H. M., and Gallez, B. (2001). Development of Biocompatible Oxygen-Permeable Films Holding Paramagnetic Carbon Particles: Evaluation of Their Performance and Stability in EPR Oximetry. Magn. Reson Med. 46, 610–614. doi:10.1002/mrm.1234
Healy, S. J., Gorman, A. M., Mousavi-Shafaei, P., Gupta, S., and Samali, A. (2009). Targeting the Endoplasmic Reticulum-Stress Response as an Anticancer Strategy. Eur. J. Pharmacol. 625, 234–246. doi:10.1016/j.ejphar.2009.06.064
Hees, P. S., and Sotak, C. H. (1993). Assessment of Changes in Murine Tumor Oxygenation in Response to Nicotinamide Using 19F NMR Relaxometry of a Perfluorocarbon Emulsion. Magn. Reson Med. 29, 303–310. doi:10.1002/mrm.1910290305
Hejmadi, M. V., McKeown, S. R., Friery, O. P., McIntyre, I. A., Patterson, L. H., and Hirst, D. G. (1996). DNA Damage Following Combination of Radiation with the Bioreductive Drug AQ4N: Possible Selective Toxicity to Oxic and Hypoxic Tumour Cells. Br. J. Cancer 73 (4), 499–505. doi:10.1038/bjc.1996.87
Hendrickson, K., Phillips, M., Smith, W., Peterson, L., Krohn, K., and Rajendran, J. (2011). Hypoxia Imaging with [F-18] FMISO-PET in Head and Neck Cancer: Potential for Guiding Intensity Modulated Radiation Therapy in Overcoming Hypoxia-Induced Treatment Resistance. Radiother. Oncol. 101, 369–375. doi:10.1016/j.radonc.2011.07.029
Henk, J. M., Bishop, K., and Shepherd, S. F. (2003). Treatment of Head and Neck Cancer with CHART and Nimorazole: Phase II Study. Radiother. Oncol. 66, 65–70. doi:10.1016/s0167-8140(02)00284-0
Henk, J. M., Kunkler, P. B., and Smith, C. W. (1977). Radiotherapy and Hyperbaric Oxygen in Head and Neck Cancer. Final Report of First Controlled Clinical Trial. Lancet 2, 101–103. doi:10.1016/s0140-6736(77)90116-7
Henriques de Figueiredo, B., Zacharatou, C., Galland-Girodet, S., Benech, J., De Clermont-Gallerande, H., Lamare, F., et al. (2015). Hypoxia Imaging with [18F]-FMISO-PET for Guided Dose Escalation with Intensity-Modulated Radiotherapy in Head-And-Neck Cancers. Strahlenther Onkol. 191, 217–224. doi:10.1007/s00066-014-0752-8
Hercbergs, A. (1996). The Thyroid Gland as an Intrinsic Biologic Response-Modifier in Advanced Neoplasia-Aa Novel Paradigm. In Vivo 10, 245–247.
Hill, S. A., and Chaplin, D. J. (1995). The Effect of Nicotinamide on Microregional Blood Flow within Tumours Assessed Using Laser Doppler Probes. Acta Oncol. 34, 401–404. doi:10.3109/02841869509093997
Hill, S. A., Collingridge, D. R., Vojnovic, B., and Chaplin, D. J. (1998). Tumour Radiosensitization by High-Oxygen-Content Gases: Influence of the Carbon Dioxide Content of the Inspired Gas on PO2, Microcirculatory Function and Radiosensitivity. Int. J. Radiat. Oncol. Biol. Phys. 40, 943–951. doi:10.1016/s0360-3016(97)00892-4
Höckel, M., Knoop, C., Schlenger, K., Vorndran, B., Baussmann, E., Mitze, M., et al. (1993). Intratumoral pO2 Predicts Survival in Advanced Cancer of the Uterine Cervix. Radiother. Oncol. 26, 45–50. doi:10.1016/0167-8140(93)90025-4
Höckel, M., Schlenger, K., Mitze, M., Schäffer, U., and Vaupel, P. (1996). Hypoxia and Radiation Response in Human Tumors. Semin. Radiat. Oncol. 6, 3–9. doi:10.1053/SRAO0060003
Höckel, M., and Vaupel, P. (2001). Tumor Hypoxia: Definitions and Current Clinical, Biologic, and Molecular Aspects. J. Natl. Cancer Inst. 93, 266–276.
Höckel, M., Vorndran, B., Schlenger, K., Baussmann, E., and Knapstein, P. G. (1993). Tumor Oxygenation: a New Predictive Parameter in Locally Advanced Cancer of the Uterine Cervix. Gynecol. Oncol. 51, 141–149. doi:10.1006/gyno.1993.1262
Hoeben, B. A., Kaanders, J. H., Franssen, G. M., Troost, E. G., Rijken, P. F., Oosterwijk, E., et al. (2010). PET of Hypoxia with 89Zr-Labeled cG250-F(ab')2 in Head and Neck Tumors. J. Nucl. Med. 51, 1076–1083. doi:10.2967/jnumed.109.073189
Holden, S. A., Teicher, B. A., Ara, G., Herman, T. S., and Coleman, C. N. (1992). Enhancement of Alkylating Agent Activity by SR-4233 in the FSaIIC Murine Fibrosarcoma. J. Natl. Cancer Inst. 84, 187–193. doi:10.1093/jnci/84.3.187
Holland, J. P., Lewis, J. S., and Dehdashti, F. (2009). Assessing Tumor Hypoxia by Positron Emission Tomography with Cu-ATSM. Q. J. Nucl. Med. Mol. Imaging 53, 193–200.
Hompland, T., Fjeldbo, C. S., and Lyng, H. (2021). Tumor Hypoxia as a Barrier in Cancer Therapy: Why Levels Matter. Cancers (Basel) 13, 499. doi:10.3390/cancers13030499
Horsman, M. R., Brown, J. M., Hirst, V. K., Lemmon, M. J., Wood, P. J., Dunphy, E. P., et al. (1988). Mechanism of Action of the Selective Tumor Radiosensitizer Nicotinamide. Int. J. Radiat. Oncol. Biol. Phys. 15, 685–690. doi:10.1016/0360-3016(88)90312-4
Horsman, M. R., Chaplin, D. J., and Brown, J. M. (1989). Tumor Radiosensitization by Nicotinamide: a Result of Improved Perfusion and Oxygenation. Radiat. Res. 118, 139–150. doi:10.2307/3577429
Horsman, M. R., Ehrnrooth, E., Ladekarl, M., and Overgaard, J. (1998). The Effect of Combretastatin A-4 Disodium Phosphate in a C3H Mouse Mammary Carcinoma and a Variety of Murine Spontaneous Tumors. Int. J. Radiat. Oncol. Biol. Phys. 42, 895–898. doi:10.1016/s0360-3016(98)00299-5
Horsman, M. R., Mortensen, L. S., Petersen, J. B., Busk, M., and Overgaard, J. (2012). Imaging Hypoxia to Improve Radiotherapy Outcome. Nat. Rev. Clin. Oncol. 9, 674–687. doi:10.1038/nrclinonc.2012.171
Horsman, M. R., Nordsmark, M., Khalil, A. A., Hill, S. A., Chaplin, D. J., Siemann, D. W., et al. (1994). Reducing Acute and Chronic Hypoxia in Tumours by Combining Nicotinamide with Carbogen Breathing. Acta Oncol. 33, 371–376. doi:10.3109/02841869409098431
Horsman, M. R., and Vaupel, P. (2016). Pathophysiological Basis for the Formation of the Tumor Microenvironment. Front. Oncol. 6, 66. doi:10.3389/fonc.2016.00066
Horsman, M., Wouters, B., Joiner, M., and Overgaard, J. (2009). “The Oxygen Effect and Fractionated Radiotherapy,” in Basic Clinical Radiobiology. Editors M. Joiner (London, UK: Hodder Arnold), 207–216. doi:10.1201/b13224-16
Hoskin, P. J. (2015). Hypoxia Dose Painting in Prostate and Cervix Cancer. Acta Oncol. 54, 1259–1262. doi:10.3109/0284186X.2015.1061692
Hoskin, P. J., Carnell, D. M., Taylor, N. J., Smith, R. E., Stirling, J. J., Daley, F. M., et al. (2007). Hypoxia in Prostate Cancer: Correlation of BOLD-MRI with Pimonidazole Immunohistochemistry-Initial Observations. Int. J. Radiat. Oncol. Biol. Phys. 68, 1065–1071. doi:10.1016/j.ijrobp.2007.01.018
Hoskin, P. J., Rojas, A. M., Bentzen, S. M., and Saunders, M. I. (2010). Radiotherapy with Concurrent Carbogen and Nicotinamide in Bladder Carcinoma. J. Clin. Oncol. 28, 4912–4918. doi:10.1200/JCO.2010.28.4950
Hoskin, P. J., Rojas, A. M., Saunders, M. I., Bentzen, S. M., and Motohashi, K. J. (2009). Carbogen and Nicotinamide in Locally Advanced Bladder Cancer: Early Results of a Phase-III Randomized Trial. Radiother. Oncol. 91, 120–125. doi:10.1016/j.radonc.2008.10.001
Hoskin, P. J., Saunders, M. I., Phillips, H., Cladd, H., Powell, M. E., Goodchild, K., et al. (1997). Carbogen and Nicotinamide in the Treatment of Bladder Cancer with Radical Radiotherapy. Br. J. Cancer 76, 260–263. doi:10.1038/bjc.1997.372
Hou, H., Abramovic, Z., Lariviere, J. P., Sentjurc, M., Swartz, H., and Khan, N. (2010). Effect of a Topical Vasodilator on Tumor Hypoxia and Tumor Oxygen Guided Radiotherapy Using EPR Oximetry. Radiat. Res. 173, 651–658. doi:10.1667/RR1947.1
Hou, H., Khan, N., Gohain, S., Kuppusamy, M. L., and Kuppusamy, P. (2018). Pre-clinical Evaluation of OxyChip for Long-Term EPR Oximetry. Biomed. Microdevices 20, 29. doi:10.1007/s10544-018-0272-x
Hou, H., Khan, N., Grinberg, O. Y., Yu, H., Grinberg, S. A., Lu, S., et al. (2007). The Effects of Efaproxyn (Efaproxiral) on Subcutaneous RIF-1 Tumor Oxygenation and Enhancement of Radiotherapy-Mediated Inhibition of Tumor Growth in Mice. Radiat. Res. 168, 218–225. doi:10.1667/RR0962.1
Hou, H., Khan, N., O'Hara, J. A., Grinberg, O. Y., Dunn, J. F., Abajian, M. A., et al. (2005). Increased Oxygenation of Intracranial Tumors by Efaproxyn (Efaproxiral), an Allosteric Hemoglobin Modifier: In Vivo EPR Oximetry Study. Int. J. Radiat. Oncol. Biol. Phys. 61, 1503–1509. doi:10.1016/j.ijrobp.2004.12.077
Hou, H., Khan, N., O'Hara, J. A., Grinberg, O. Y., Dunn, J. F., Abajian, M. A., et al. (2004). Effect of RSR13, an Allosteric Hemoglobin Modifier, on Oxygenation in Murine Tumors: an In Vivo Electron Paramagnetic Resonance Oximetry and Bold MRI Study. Int. J. Radiat. Oncol. Biol. Phys. 59, 834–843. doi:10.1016/j.ijrobp.2004.02.039
Hou, H., Mupparaju, S. P., Lariviere, J. P., Hodge, S., Gui, J., Swartz, H. M., et al. (2013). Assessment of the Changes in 9L and C6 Glioma pO2 by EPR Oximetry as a Prognostic Indicator of Differential Response to Radiotherapy. Radiat. Res. 179, 343–351. doi:10.1667/RR2811.1
Hou, W., Xue, Y., Tang, W., Pan, H., Xu, M., Li, X., et al. (2019). Evaluation of Tumor Hypoxia in C6 Glioma Rat Model with Dynamic Contrast-Enhanced Magnetic Resonance Imaging. Acad. Radiol. 26, e224–e232. doi:10.1016/j.acra.2018.09.011
Howe, F. A., Robinson, S. P., McIntyre, D. J., Stubbs, M., and Griffiths, J. R. (2001). Issues in Flow and Oxygenation Dependent Contrast (FLOOD) Imaging of Tumours. NMR Biomed. 14, 497–506. doi:10.1002/nbm.716
Hu, H., Yan, X., Wang, H., Tanaka, J., Wang, M., You, W., et al. (2019). Perfluorocarbon-based O2 Nanocarrier for Efficient Photodynamic Therapy. J. Mater Chem. B 7, 1116–1123. doi:10.1039/c8tb01844h
Huang, Y., Fan, J., Li, Y., Fu, S., Chen, Y., and Wu, J. (2021). Imaging of Tumor Hypoxia with Radionuclide-Labeled Tracers for PET. Front. Oncol. 11, 731503. doi:10.3389/fonc.2021.731503
Huang, Y., Goel, S., Duda, D. G., Fukumura, D., and Jain, R. K. (2013). Vascular Normalization as an Emerging Strategy to Enhance Cancer Immunotherapy. Cancer Res. 73, 2943–2948. doi:10.1158/0008-5472.CAN-12-4354
Huang, Y., Yuan, J., Righi, E., Kamoun, W. S., Ancukiewicz, M., Nezivar, J., et al. (2012). Vascular Normalizing Doses of Antiangiogenic Treatment Reprogram the Immunosuppressive Tumor Microenvironment and Enhance Immunotherapy. Proc. Natl. Acad. Sci. U. S. A. 109, 17561–17566. doi:10.1073/pnas.1215397109
Hueting, R., Kersemans, V., Cornelissen, B., Tredwell, M., Hussien, K., Christlieb, M., et al. (2014). A Comparison of the Behavior of (64)Cu-Acetate and (64)Cu-ATSM In Vitro and In Vivo. J. Nucl. Med. 55, 128–134. doi:10.2967/jnumed.113.119917
Hughes, V. S., Wiggins, J. M., and Siemann, D. W. (2019). Tumor Oxygenation and Cancer Therapy-Then and Now. Br. J. Radiol. 92, 20170955. doi:10.1259/bjr.20170955
Hunjan, S., Mason, R. P., Constantinescu, A., Peschke, P., Hahn, E. W., and Antich, P. P. (1998). Regional Tumor Oximetry: 19F NMR Spectroscopy of Hexafluorobenzene. Int. J. Radiat. Oncol. Biol. Phys. 41, 161–171. doi:10.1016/s0360-3016(98)00020-0
Hunter, F. W., Wouters, B. G., and Wilson, W. R. (2016). Hypoxia-activated Prodrugs: Paths Forward in the Era of Personalised Medicine. Br. J. Cancer 114, 1071–1077. doi:10.1038/bjc.2016.79
Hyodo, F., Matsumoto, S., Devasahayam, N., Dharmaraj, C., Subramanian, S., Mitchell, J. B., et al. (2009). Pulsed EPR Imaging of Nitroxides in Mice. J. Magn. Reson 197, 181–185. doi:10.1016/j.jmr.2008.12.018
Hyodo, F., Matsumoto, S., Hyodo, E., Matsumoto, A., Matsumoto, K., and Krishna, M. C. (2010). In Vivo measurement of Tissue Oxygen Using Electron Paramagnetic Resonance Spectroscopy with Oxygen-Sensitive Paramagnetic Particle, Lithium Phthalocyanine. Methods Mol. Biol. 610, 29–39. doi:10.1007/978-1-60327-029-8_2
Ilangovan, G., Manivannan, A., Li, H., Yanagi, H., Zweier, J. L., and Kuppusamy, P. (2002). A Naphthalocyanine-Based EPR Probe for Localized Measurements of Tissue Oxygenation. Free Radic. Biol. Med. 32, 139–147. doi:10.1016/s0891-5849(01)00784-5
Isaacs, J. S., Jung, Y. J., Mimnaugh, E. G., Martinez, A., Cuttitta, F., and Neckers, L. M. (2002). Hsp90 regulates a von Hippel Lindau-independent hypoxia inducible factor-1 alpha-degradative pathway. J. Biol. Chem. 277, 29936–29944. doi:10.1074/jbc.M204733200
Ivan, M., Fishel, M. L., Tudoran, O. M., Pollok, K. E., Wu, X., and Smith, P. J. (2021). Hypoxia Signaling: Challenges and Opportunities for Cancer Therapy. Semin. Cancer Biol. 2021. doi:10.1016/j.semcancer.2021.10.002
Jackson, R. K., Liew, L. P., and Hay, M. P. (2019). Overcoming Radioresistance: Small Molecule Radiosensitisers and Hypoxia-Activated Prodrugs. Clin. Oncol. R. Coll. Radiol. 31, 290–302. doi:10.1016/j.clon.2019.02.004
Jacoby, J. J., Erez, B., Korshunova, M. V., Williams, R. R., Furutani, K., Takahashi, O., et al. (2010). Treatment with HIF-1alpha Antagonist PX-478 Inhibits Progression and Spread of Orthotopic Human Small Cell Lung Cancer and Lung Adenocarcinoma in Mice. J. Thorac. Oncol. 5, 940–949. doi:10.1097/JTO.0b013e3181dc211f
Jain, R. K. (1997). Delivery of Molecular and Cellular Medicine to Solid Tumors. Adv. Drug Deliv. Rev. 26, 71–90. doi:10.1016/s0169-409x(97)00027-6
Jain, R. K. (1996). Delivery of Molecular Medicine to Solid Tumors. Science 271, 1079–1080. doi:10.1126/science.271.5252.1079
Jain, R. K. (1991). Haemodynamic and Transport Barriers to the Treatment of Solid Tumours. Int. J. Radiat. Biol. 60, 85–100. doi:10.1080/09553009114551621
Jain, R. K. (2005). Normalization of Tumor Vasculature: an Emerging Concept in Antiangiogenic Therapy. Science 307, 58–62. doi:10.1126/science.1104819
Jain, R. K. (2001). Normalizing Tumor Vasculature with Anti-angiogenic Therapy: a New Paradigm for Combination Therapy. Nat. Med. 7, 987–989. doi:10.1038/nm0901-987
James, P. E., Grinberg, O. Y., Goda, F., Panz, T., O'Hara, J. A., and Swartz, H. M. (1997). Gloxy: an Oxygen-Sensitive Coal for Accurate Measurement of Low Oxygen Tensions in Biological Systems. Magn. Reson Med. 38, 48–58. doi:10.1002/mrm.1910380109
Janssens, G. O., Rademakers, S. E., Terhaard, C. H., Doornaert, P. A., Bijl, H. P., van den Ende, P., et al. (2012). Accelerated Radiotherapy with Carbogen and Nicotinamide for Laryngeal Cancer: Results of a Phase III Randomized Trial. J. Clin. Oncol. 30, 1777–1783. doi:10.1200/JCO.2011.35.9315
Jeong, J. J., Liu, T., Yang, X., Torres, M., Lin, J., Schreiber, W., et al. (2019). Clinical Measurements of Oxygen via Electron Paramagnetic Resonance (EPR) during and after Breast Radiation Therapy: Preliminary Results of Baseline Evaluations and Response to Hyperoxygenation. J. Radiol. Radiat. Ther. 7, 1082.
Jeong, W., Rapisarda, A., Park, S. R., Kinders, R. J., Chen, A., Melillo, G., et al. (2014). Pilot Trial of EZN-2968, an Antisense Oligonucleotide Inhibitor of Hypoxia-Inducible Factor-1 Alpha (HIF-1α), in Patients with Refractory Solid Tumors. Cancer Chemother. Pharmacol. 73, 343–348. doi:10.1007/s00280-013-2362-z
Jia, L., Li, X., Cheng, D., and Zhang, L. (2019). Fluorine-18 Click Radiosynthesis and microPET/CT Evaluation of a Small Peptide-A Potential PET Probe for Carbonic Anhydrase IX. Bioorg Med. Chem. 27, 785–789. doi:10.1016/j.bmc.2019.01.014
Jiang, H., De Ridder, M., Verovski, V. N., Sonveaux, P., Jordan, B. F., Law, K., et al. (2010). Activated Macrophages as a Novel Determinant of Tumor Cell Radioresponse: the Role of Nitric Oxide-Mediated Inhibition of Cellular Respiration and Oxygen Sparing. Int. J. Radiat. Oncol. Biol. Phys. 76, 1520–1527. doi:10.1016/j.ijrobp.2009.10.047
Jiang, M., Qin, B., Luo, L., Li, X., Shi, Y., Zhang, J., et al. (2021). A Clinically Acceptable Strategy for Sensitizing Anti-PD-1 Treatment by Hypoxia Relief. J. Control Release 335, 408–419. doi:10.1016/j.jconrel.2021.06.001
Jordan, B. F., Sonveaux, P., Feron, O., Grégoire, V., Beghein, N., and Gallez, B. (2003). Nitric Oxide-Mediated Increase in Tumor Blood Flow and Oxygenation of Tumors Implanted in Muscles Stimulated by Electric Pulses. Int. J. Radiat. Oncol. Biol. Phys. 55, 1066–1073. doi:10.1016/s0360-3016(02)04505-4
Jordan, B. F., Baudelet, C., and Gallez, B. (1998). Carbon-centered Radicals as Oxygen Sensors for In Vivo Electron Paramagnetic Resonance: Screening for an Optimal Probe Among Commercially Available Charcoals. MAGMA 7, 121–129. doi:10.1007/BF02592236
Jordan, B. F., Beghein, N., Aubry, M., Grégoire, V., and Gallez, B. (2003). Potentiation of Radiation-Induced Regrowth Delay by Isosorbide Dinitrate in FSaII Murine Tumors. Int. J. Cancer 103, 138–141. doi:10.1002/ijc.10786
Jordan, B. F., Beghein, N., Crokart, N., Baudelet, C., Grégoire, V., and Gallez, B. (2006). Preclinical Safety and Antitumor Efficacy of Insulin Combined with Irradiation. Radiother. Oncol. 81, 112–117. doi:10.1016/j.radonc.2006.08.023
Jordan, B. F., Christian, N., Crokart, N., Grégoire, V., Feron, O., and Gallez, B. (2007). Thyroid Status Is a Key Modulator of Tumor Oxygenation: Implication for Radiation Therapy. Radiat. Res. 168, 428–432. doi:10.1667/RR0931.1
Jordan, B. F., Crokart, N., Baudelet, C., Cron, G. O., Ansiaux, R., and Gallez, B. (2006). Complex Relationship between Changes in Oxygenation Status and Changes in R2*: the Case of Insulin and NS-398, Two Inhibitors of Oxygen Consumption. Magn. Reson Med. 56, 637–643. doi:10.1002/mrm.20963
Jordan, B. F., Cron, G. O., and Gallez, B. (2009). Rapid Monitoring of Oxygenation by 19F Magnetic Resonance Imaging: Simultaneous Comparison with Fluorescence Quenching. Magn. Reson Med. 61, 634–638. doi:10.1002/mrm.21594
Jordan, B. F., and Gallez, B. (2010). Surrogate MR Markers of Response to Chemo- or Radiotherapy in Association with Co-treatments: a Retrospective Analysis of Multi-Modal Studies. Contrast Media Mol. Imaging 5, 323–332. doi:10.1002/cmmi.397
Jordan, B. F., Grégoire, V., Demeure, R. J., Sonveaux, P., Feron, O., O'Hara, J., et al. (2002). Insulin Increases the Sensitivity of Tumors to Irradiation: Involvement of an Increase in Tumor Oxygenation Mediated by a Nitric Oxide-dependent Decrease of the Tumor Cells Oxygen Consumption. Cancer Res. 62, 3555–3561.
Jordan, B. F., Magat, J., Colliez, F., Ozel, E., Fruytier, A. C., Marchand, V., et al. (2013). Mapping of Oxygen by Imaging Lipids Relaxation Enhancement: a Potential Sensitive Endogenous MRI Contrast to Map Variations in Tissue Oxygenation. Magn. Reson Med. 70, 732–734. doi:10.1002/mrm.24511
Jordan, B. F., Magat, J., Colliez, F., Ozel, E., Fruytier, A. C., Marchand, V., et al. (2013). Application of MOBILE (Mapping of Oxygen by Imaging Lipids Relaxation Enhancement) to Study Variations in Tumor Oxygenation. Adv. Exp. Med. Biol. 789, 281–288. doi:10.1007/978-1-4614-7411-1_38
Jordan, B. F., Misson, P., Demeure, R., Baudelet, C., Beghein, N., and Gallez, B. (2000). Changes in Tumor Oxygenation/perfusion Induced by the NO Donor, Isosorbide Dinitrate, in Comparison with Carbogen: Monitoring by EPR and MRI. Int. J. Radiat. Oncol. Biol. Phys. 48, 565–570. doi:10.1016/s0360-3016(00)00694-5
Jordan, B. F., Peeterbroeck, J., Karroum, O., Diepart, C., Magat, J., Grégoire, V., et al. (2010). Captopril and S-Nitrosocaptopril as Potent Radiosensitizers: Comparative Study and Underlying Mechanisms. Cancer Lett. 293, 213–219. doi:10.1016/j.canlet.2010.01.016
Jordan, B. F., Runquist, M., Raghunand, N., Baker, A., Williams, R., Kirkpatrick, L., et al. (2005). Dynamic Contrast-Enhanced and Diffusion MRI Show Rapid and Dramatic Changes in Tumor Microenvironment in Response to Inhibition of HIF-1alpha Using PX-478. Neoplasia 7, 475–485. doi:10.1593/neo.04628
Jordan, B. F., Sonveaux, P., Feron, O., Grégoire, V., Beghein, N., Dessy, C., et al. (2004). Nitric Oxide as a Radiosensitizer: Evidence for an Intrinsic Role in Addition to its Effect on Oxygen Delivery and Consumption. Int. J. Cancer 109, 768–773. doi:10.1002/ijc.20046
Jubb, A. M., Buffa, F. M., and Harris, A. L. (2010). Assessment of Tumour Hypoxia for Prediction of Response to Therapy and Cancer Prognosis. J. Cell Mol. Med. 14, 18–29. doi:10.1111/j.1582-4934.2009.00944.x
Julien, C., Payen, J. F., Troprès, I., Farion, R., Grillon, E., Montigon, O., et al. (2004). Assessment of Vascular Reactivity in Rat Brain Glioma by Measuring Regional Blood Volume during Graded Hypoxic Hypoxia. Br. J. Cancer 91, 374–380. doi:10.1038/sj.bjc.6601908
Kaanders, J. H., Pop, L. A., Marres, H. A., Bruaset, I., van den Hoogen, F. J., Merkx, M. A., et al. (2002). ARCON: Experience in 215 Patients with Advanced Head-And-Neck Cancer. Int. J. Radiat. Oncol. Biol. Phys. 52, 769–778. doi:10.1016/s0360-3016(01)02678-5
Kaanders, J. H., Pop, L. A., Marres, H. A., Liefers, J., van den Hoogen, F. J., van Daal, W. A., et al. (1998). Accelerated Radiotherapy with Carbogen and Nicotinamide (ARCON) for Laryngeal Cancer. Radiother. Oncol. 48, 115–122. doi:10.1016/s0167-8140(98)00043-7
Kallinowski, F., Zander, R., Hockel, M., and Vaupel, P. (1990). Tumor Tissue Oxygenation as Evaluated by Computerized-pO2-Histography. Int. J. Radiat. Oncol. Biol. Phys. 19, 953–961. doi:10.1016/0360-3016(90)90018-f
Karczmar, G. S., River, J. N., Li, J., Vijayakumar, S., Goldman, Z., and Lewis, M. Z. (1994). Effects of Hyperoxia on T2* and Resonance Frequency Weighted Magnetic Resonance Images of Rodent Tumours. NMR Biomed. 7, 3–11. doi:10.1002/nbm.1940070103
Karroum, O., Kengen, J., Danhier, P., Magat, J., Mignion, L., Bouzin, C., et al. (2012). Tumor Reoxygenation Following Administration of Mitogen-Activated Protein Kinase Inhibitors: a Rationale for Combination with Radiation Therapy. Radiother. Oncol. 105, 64–71. doi:10.1016/j.radonc.2012.05.005
Karroum, O., Kengen, J., Grégoire, V., Gallez, B., and Jordan, B. F. (2013). Tumor Reoxygenation Following Administration of the EGFR Inhibitor, Gefitinib, in Experimental Tumors. Adv. Exp. Med. Biol. 789, 265–271. doi:10.1007/978-1-4614-7411-1_36
Karwicka, M., Pucelik, B., Gonet, M., Elas, M., and Dąbrowski, J. M. (2019). Effects of Photodynamic Therapy with Redaporfin on Tumor Oxygenation and Blood Flow in a Lung Cancer Mouse Model. Sci. Rep. 9, 12655. doi:10.1038/s41598-019-49064-6
Kawai, N., Lin, W., Cao, W. D., Ogawa, D., Miyake, K., Haba, R., et al. (2014). Correlation between 18F-Fluoromisonidazole PET and Expression of HIF-1alpha and VEGF in Newly Diagnosed and Recurrent Malignant Gliomas. Eur. J. Nucl. Med. Mol. Imaging 41, 1870–1878. doi:10.1007/s00259-014-2776-9
Kelada, O. J., and Carlson, D. J. (2014). Molecular Imaging of Tumor Hypoxia with Positron Emission Tomography. Radiat. Res. 181, 335–349. doi:10.1667/RR13590.1
Kelleher, D. K., Thews, O., and Vaupel, P. (1998). Can Erythropoietin Improve Tumor Oxygenation? Strahlenther Onkol. 174 (Suppl. 4), 20–23.
Kelleher, D. K., and Vaupel, P. W. (1993). Nicotinamide Exerts Different Acute Effects on Microcirculatory Function and Tissue Oxygenation in Rat Tumors. Int. J. Radiat. Oncol. Biol. Phys. 26, 95–102. doi:10.1016/0360-3016(93)90178-x
Khan, M. T., Irlam-Jones, J. J., Pereira, R. R., Lane, B., Valentine, H. R., Aragaki, K., et al. (2021). A miRNA Signature Predicts Benefit from Addition of Hypoxia-Modifying Therapy to Radiation Treatment in Invasive Bladder Cancer. Br. J. Cancer 125, 85–93. doi:10.1038/s41416-021-01326-9
Khan, N., Li, H., Hou, H., Lariviere, J. P., Gladstone, D. J., Demidenko, E., et al. (2009). Tissue pO2 of Orthotopic 9L and C6 Gliomas and Tumor-specific Response to Radiotherapy and Hyperoxygenation. Int. J. Radiat. Oncol. Biol. Phys. 73, 878–885. doi:10.1016/j.ijrobp.2008.10.025
Khan, N., Mupparaju, S., Hekmatyar, S. K., Hou, H., Lariviere, J. P., Demidenko, E., et al. (2010). Effect of Hyperoxygenation on Tissue pO2 and its Effect on Radiotherapeutic Efficacy of Orthotopic F98 Gliomas. Int. J. Radiat. Oncol. Biol. Phys. 78, 1193–1200. doi:10.1016/j.ijrobp.2010.05.045
Khan, N., Williams, B. B., Hou, H., Li, H., and Swartz, H. M. (2007). Repetitive Tissue pO2 Measurements by Electron Paramagnetic Resonance Oximetry: Current Status and Future Potential for Experimental and Clinical Studies. Antioxid. Redox Signal 9, 1169–1182. doi:10.1089/ars.2007.1635
Khandelwal, S. R., Kavanagh, B. D., Lin, P. S., Truong, Q. T., Lu, J., Abraham, D. J., et al. (1999). RSR13, an Allosteric Effector of Haemoglobin, and Carbogen Radiosensitize FSAII and SCCVII Tumours in C3H Mice. Br. J. Cancer 79, 814–820. doi:10.1038/sj.bjc.6690130
Khramtsov, V. V. (2018). In Vivo Molecular Electron Paramagnetic Resonance-Based Spectroscopy and Imaging of Tumor Microenvironment and Redox Using Functional Paramagnetic Probes. Antioxid. Redox Signal 28, 1365–1377. doi:10.1089/ars.2017.7329
Kikuchi, M., Yamane, T., Shinohara, S., Fujiwara, K., Hori, S. Y., Tona, Y., et al. (2011). 18F-fluoromisonidazole Positron Emission Tomography before Treatment Is a Predictor of Radiotherapy Outcome and Survival Prognosis in Patients with Head and Neck Squamous Cell Carcinoma. Ann. Nucl. Med. 25, 625–633. doi:10.1007/s12149-011-0508-9
Kim, C. K., Park, S. Y., Park, B. K., Park, W., and Huh, S. J. (2014). Blood Oxygenation Level-dependent MR Imaging as a Predictor of Therapeutic Response to Concurrent Chemoradiotherapy in Cervical Cancer: a Preliminary Experience. Eur. Radiol. 24, 1514–1520. doi:10.1007/s00330-014-3167-0
Kim, D. H., Kim, E. J., Kalota, A., Gewirtz, A. M., Glickson, J., Shogen, K., et al. (2007). Possible Mechanisms of Improved Radiation Response by Cytotoxic RNase, Onconase, on A549 Human Lung Cancer Xenografts of Nude Mice. Adv. Exp. Med. Biol. 599, 53–59. doi:10.1007/978-0-387-71764-7_8
Kimura, H., Braun, R. D., Ong, E. T., Hsu, R., Secomb, T. W., Papahadjopoulos, D., et al. (1996). Fluctuations in Red Cell Flux in Tumor Microvessels Can Lead to Transient Hypoxia and Reoxygenation in Tumor Parenchyma. Cancer Res. 56, 5522–5528.
Klaassen, R., Bennink, R. J., van Tienhoven, G., Bijlsma, M. F., Besselink, M. G., van Berge Henegouwen, M. I., et al. (2015). Feasibility and Repeatability of PET with the Hypoxia Tracer [(18)F]HX4 in Oesophageal and Pancreatic Cancer. Radiother. Oncol. 116, 94–99. doi:10.1016/j.radonc.2015.05.009
Knocke, T. H., Weitmann, H. D., Feldmann, H. J., Selzer, E., and Pötter, R. (1999). Intratumoral pO2-Measurements as Predictive Assay in the Treatment of Carcinoma of the Uterine Cervix. Radiother. Oncol. 53, 99–104. doi:10.1016/s0167-8140(99)00139-5
Koch, C. J., Oprysko, P. R., Shuman, A. L., Jenkins, W. T., Brandt, G., and Evans, S. M. (2002). Radiosensitization of Hypoxic Tumor Cells by Dodecafluoropentane: a Gas-phase Perfluorochemical Emulsion. Cancer Res. 62, 3626–3629.
Koch, C. J., Stobbe, C. C., and Bump, E. A. (1984). The Effect on the Km for Radiosensitization at 0 Degree C of Thiol Depletion by Diethylmaleate Pretreatment: Quantitative Differences Found Using the Radiation Sensitizing Agent Misonidazole or Oxygen. Radiat. Res. 98, 141–153. doi:10.2307/3576058
Koh, W. J., Rasey, J. S., Evans, M. L., Grierson, J. R., Lewellen, T. K., Graham, M. M., et al. (1992). Imaging of Hypoxia in Human Tumors with [F-18]fluoromisonidazole. Int. J. Radiat. Oncol. Biol. Phys. 22, 199–212. doi:10.1016/0360-3016(92)91001-4
Konerding, M. A., Malkusch, W., Klapthor, B., van Ackern, C., Fait, E., Hill, S. A., et al. (1999). Evidence for Characteristic Vascular Patterns in Solid Tumours: Quantitative Studies Using Corrosion Casts. Br. J. Cancer 80, 724–732. doi:10.1038/sj.bjc.6690416
Kong, D., Park, E. J., Stephen, A. G., Calvani, M., Cardellina, J. H., Monks, A., et al. (2005). Echinomycin, a Small-Molecule Inhibitor of Hypoxia-Inducible Factor-1 DNA-Binding Activity. Cancer Res. 65, 9047–9055. doi:10.1158/0008-5472.CAN-05-1235
Krafft, M. P. (2020). Alleviating Tumor Hypoxia with Perfluorocarbon-Based Oxygen Carriers. Curr. Opin. Pharmacol. 53, 117–125. doi:10.1016/j.coph.2020.08.010
Krishna, M. C., Matsumoto, S., Yasui, H., Saito, K., Devasahayam, N., Subramanian, S., et al. (2012). Electron Paramagnetic Resonance Imaging of Tumor pO₂. Radiat. Res. 177, 376–386. doi:10.1667/rr2622.1
Kudo, T., Ueda, M., Konishi, H., Kawashima, H., Kuge, Y., Mukai, T., et al. (2011). PET Imaging of Hypoxia-Inducible Factor-1-Active Tumor Cells with Pretargeted Oxygen-dependent Degradable Streptavidin and a Novel 18F-Labeled Biotin Derivative. Mol. Imaging Biol. 13, 1003–1010. doi:10.1007/s11307-010-0418-6
Kudo, T., Ueda, M., Kuge, Y., Mukai, T., Tanaka, S., Masutani, M., et al. (2009). Imaging of HIF-1-Active Tumor Hypoxia Using a Protein Effectively Delivered to and Specifically Stabilized in HIF-1-Active Tumor Cells. J. Nucl. Med. 50, 942–949. doi:10.2967/jnumed.108.061119
Kumar, V., and Gabrilovich, D. I. (2014). Hypoxia-inducible Factors in Regulation of Immune Responses in Tumour Microenvironment. Immunology 143, 512–519. doi:10.1111/imm.12380
Kung, A. L., Zabludoff, S. D., France, D. S., Freedman, S. J., Tanner, E. A., Vieira, A., et al. (2004). Small Molecule Blockade of Transcriptional Coactivation of the Hypoxia-Inducible Factor Pathway. Cancer Cell 6, 33–43. doi:10.1016/j.ccr.2004.06.009
Kv, R., Liu, T. I., Lu, I. L., Liu, C. C., Chen, H. H., Lu, T. Y., et al. (2020). Tumor Microenvironment-Responsive and Oxygen Self-Sufficient Oil Droplet Nanoparticles for Enhanced Photothermal/photodynamic Combination Therapy against Hypoxic Tumors. J. Control Release 328, 87–99. doi:10.1016/j.jconrel.2020.08.038
Lan, M., Beghein, N., Charlier, N., and Gallez, B. (2004). Carbon Blacks as EPR Sensors for Localized Measurements of Tissue Oxygenation. Magn. Reson Med. 51, 1272–1278. doi:10.1002/mrm.20077
Lau, J., Zhang, Z., Jenni, S., Kuo, H. T., Liu, Z., Vullo, D., et al. (2016). PET Imaging of Carbonic Anhydrase IX Expression of HT-29 Tumor Xenograft Mice with 68Ga-Labeled Benzenesulfonamides. Mol. Pharm. 13, 1137–1146. doi:10.1021/acs.molpharmaceut.5b00934
Lee, C. T., Boss, M. K., and Dewhirst, M. W. (2014). Imaging Tumor Hypoxia to Advance Radiation Oncology. Antioxid. Redox Signal 21, 313–337. doi:10.1089/ars.2013.5759
Lee, D. J., Trotti, A., Spencer, S., Rostock, R., Fisher, C., von Roemeling, R., et al. (1998). Concurrent Tirapazamine and Radiotherapy for Advanced Head and Neck Carcinomas: a Phase II Study. Int. J. Radiat. Oncol. Biol. Phys. 42, 811–815. doi:10.1016/s0360-3016(98)00310-1
Lee, I., Kim, D. H., Sunar, U., Magnitsky, S., and Shogen, K. (2007). The Therapeutic Mechanisms of Ranpirnase-Induced Enhancement of Radiation Response on A549 Human Lung Cancer. In Vivo 21, 721–728.
Lee, I., Kim, J. H., Levitt, S. H., and Song, C. W. (1992). Increases in Tumor Response by Pentoxifylline Alone or in Combination with Nicotinamide. Int. J. Radiat. Oncol. Biol. Phys. 22, 425–429. doi:10.1016/0360-3016(92)90846-a
Lee, I., Levitt, S. H., and Song, C. W. (1993). Improved Tumour Oxygenation and Radiosensitization by Combination with Nicotinamide and Pentoxifylline. Int. J. Radiat. Biol. 64, 237–244. doi:10.1080/09553009314551351
Lee, K., and Kim, H. M. (2011). A Novel Approach to Cancer Therapy Using PX-478 as a HIF-1α Inhibitor. Arch. Pharm. Res. 34, 1583–1585. doi:10.1007/s12272-011-1021-3
Lee, N., Nehmeh, S., Schöder, H., Fury, M., Chan, K., Ling, C. C., et al. (2009). Prospective Trial Incorporating Pre-/mid-treatment [18F]-Misonidazole Positron Emission Tomography for Head-And-Neck Cancer Patients Undergoing Concurrent Chemoradiotherapy. Int. J. Radiat. Oncol. Biol. Phys. 75, 101–108. doi:10.1016/j.ijrobp.2008.10.049
Lee, N. Y., and Le, Q. T. (2008). New Developments in Radiation Therapy for Head and Neck Cancer: Intensity-Modulated Radiation Therapy and Hypoxia Targeting. Semin. Oncol. 35, 236–250. doi:10.1053/j.seminoncol.2008.03.003
Lee, N. Y., Mechalakos, J. G., Nehmeh, S., Lin, Z., Squire, O. D., Cai, S., et al. (2008). Fluorine-18-labeled Fluoromisonidazole Positron Emission and Computed Tomography-Guided Intensity-Modulated Radiotherapy for Head and Neck Cancer: a Feasibility Study. Int. J. Radiat. Oncol. Biol. Phys. 70, 2–13. doi:10.1016/j.ijrobp.2007.06.039
Lee, P., Chandel, N. S., and Simon, M. C. (2020). Cellular Adaptation to Hypoxia through Hypoxia Inducible Factors and beyond. Nat. Rev. Mol. Cell Biol. 21, 268–283. doi:10.1038/s41580-020-0227-y
Lequeux, A., Noman, M. Z., Xiao, M., Sauvage, D., Van Moer, K., Viry, E., et al. (2019). Impact of Hypoxic Tumor Microenvironment and Tumor Cell Plasticity on the Expression of Immune Checkpoints. Cancer Lett. 458, 13–20. doi:10.1016/j.canlet.2019.05.021
Lequeux, A., Noman, M. Z., Xiao, M., Van Moer, K., Hasmim, M., Benoit, A., et al. (2021). Targeting HIF-1 Alpha Transcriptional Activity Drives Cytotoxic Immune Effector Cells into Melanoma and Improves Combination Immunotherapy. Oncogene 40, 4725–4735. doi:10.1038/s41388-021-01846-x
Lewis, J. S., McCarthy, D. W., McCarthy, T. J., Fujibayashi, Y., and Welch, M. J. (1999). Evaluation of 64Cu-ATSM In Vitro and In Vivo in a Hypoxic Tumor Model. J. Nucl. Med. 40, 177–183.
Li, D., Wang, X., Wang, S., and Cheng, J. (2015). Correlation between BOLD-MRI and HIF Expression Level in Renal Carcinoma. Int. J. Clin. Exp. Pathol. 8, 13759–13763.
Li, F., Joergensen, J. T., Hansen, A. E., and Kjaer, A. (2014). Kinetic Modeling in PET Imaging of Hypoxia. Am. J. Nucl. Med. Mol. Imaging 4, 490–506.
Li, X., Zhang, K., and Li, Z. (2011). Unfolded Protein Response in Cancer: the Physician's Perspective. J. Hematol. Oncol. 4, 8. doi:10.1186/1756-8722-4-8
Li, Y., Zhao, L., and Li, X. F. (2021). Targeting Hypoxia: Hypoxia-Activated Prodrugs in Cancer Therapy. Front. Oncol. 11, 700407. doi:10.3389/fonc.2021.700407
Li, Y., Zhao, L., and Li, X. F. (2021). The Hypoxia-Activated Prodrug TH-302: Exploiting Hypoxia in Cancer Therapy. Front. Pharmacol. 12, 636892. doi:10.3389/fphar.2021.636892
Limani, P., Linecker, M., Kron, P., Samaras, P., Pestalozzi, B., Stupp, R., et al. (2016). Development of OXY111A, a Novel Hypoxia-Modifier as a Potential Antitumor Agent in Patients with Hepato-Pancreato-Biliary Neoplasms - Protocol of a First Ib/IIa Clinical Trial. BMC Cancer 16, 812. doi:10.1186/s12885-016-2855-3
Lin, A., and Maity, A. (2015). Molecular Pathways: A Novel Approach to Targeting Hypoxia and Improving Radiotherapy Efficacy via Reduction in Oxygen Demand. Clin. Cancer Res. 21, 1995–2000. doi:10.1158/1078-0432.CCR-14-0858
Lin, Z., Mechalakos, J., Nehmeh, S., Schoder, H., Lee, N., Humm, J., et al. (2008). The Influence of Changes in Tumor Hypoxia on Dose-Painting Treatment Plans Based on 18F-FMISO Positron Emission Tomography. Int. J. Radiat. Oncol. Biol. Phys. 70, 1219–1228. doi:10.1016/j.ijrobp.2007.09.050
Linnik, I. V., Scott, M. L., Holliday, K. F., Woodhouse, N., Waterton, J. C., O'Connor, J. P., et al. (2014). Noninvasive Tumor Hypoxia Measurement Using Magnetic Resonance Imaging in Murine U87 Glioma Xenografts and in Patients with Glioblastoma. Magn. Reson Med. 71, 1854–1862. doi:10.1002/mrm.24826
Liu, J., Hajibeigi, A., Ren, G., Lin, M., Siyambalapitiyage, W., Liu, Z., et al. (2009). Retention of the Radiotracers 64Cu-ATSM and 64Cu-PTSM in Human and Murine Tumors Is Influenced by MDR1 Protein Expression. J. Nucl. Med. 50, 1332–1339. doi:10.2967/jnumed.109.061879
Liu, K. J., Gast, P., Moussavi, M., Norby, S. W., Vahidi, N., Walczak, T., et al. (1993). Lithium Phthalocyanine: a Probe for Electron Paramagnetic Resonance Oximetry in Viable Biological Systems. Proc. Natl. Acad. Sci. U. S. A. 90, 5438–5442. doi:10.1073/pnas.90.12.5438
Liu, L., Hu, L., Zeng, Q., Peng, D., Chen, Z., Huang, C., et al. (2021). Dynamic Contrast-Enhanced MRI of Nasopharyngeal Carcinoma: Correlation of Quantitative Dynamic Contrast-Enhanced Magnetic Resonance Imaging (DCE-MRI) Parameters with Hypoxia-Inducible Factor 1α Expression and Tumor Grade/stage. Ann. Palliat. Med. 10, 2238–2253. doi:10.21037/apm-21-303
Liu, M., Guo, X., Wang, S., Jin, M., Wang, Y., Li, J., et al. (2013). BOLD-MRI of Breast Invasive Ductal Carcinoma: Correlation of R2* Value and the Expression of HIF-1α. Eur. Radiol. 23, 3221–3227. doi:10.1007/s00330-013-2937-4
Liu, T., Karlsen, M., Karlberg, A. M., and Redalen, K. R. (2020). Hypoxia Imaging and Theranostic Potential of [ 64 Cu][Cu(ATSM)] and Ionic Cu(II) Salts: a Review of Current Evidence and Discussion of the Retention Mechanisms. EJNMMI Res. 10, 33. doi:10.1186/s13550-020-00621-5
Loboda, A., Jozkowicz, A., and Dulak, J. (2010). HIF-1 and HIF-2 Transcription Factors-Ssimilar but Not Identical. Mol. Cells 29, 435–442. doi:10.1007/s10059-010-0067-2
Lohse, I., Rasowski, J., Cao, P., Pintilie, M., Do, T., Tsao, M. S., et al. (2016). Targeting Hypoxic Microenvironment of Pancreatic Xenografts with the Hypoxia-Activated Prodrug TH-302. Oncotarget 7, 33571–33580. doi:10.18632/oncotarget.9654
Lopci, E., Grassi, I., Chiti, A., Nanni, C., Cicoria, G., Toschi, L., et al. (2014). PET Radiopharmaceuticals for Imaging of Tumor Hypoxia: a Review of the Evidence. Am. J. Nucl. Med. Mol. Imaging 4, 365–384.
Lopes, S., Ferreira, S., and Caetano, M. (2021). PET/CT in the Evaluation of Hypoxia for Radiotherapy Planning in Head and Neck Tumors: Systematic Literature Review. J. Nucl. Med. Technol. 49, 107–113. doi:10.2967/jnmt.120.249540
Lübbe, A. S., and Huhnt, W. (1994). Microvessel Diameters of Human Colon Adenocarcinoma during Acute Treatment with Serotonin. Int. J. Microcirc. Clin. Exp. 14, 218–225. doi:10.1159/000178832
M de-Brito, N., Duncan-Moretti, J., C da-Costa, H., Saldanha-Gama, R., Paula-Neto, H. A., G Dorighello, G., et al. (2020). Aerobic Glycolysis Is a Metabolic Requirement to Maintain the M2-like Polarization of Tumor-Associated Macrophages. Biochim. Biophys. Acta Mol. Cell Res. 1867, 118604. doi:10.1016/j.bbamcr.2019.118604
Ma, L., Li, G., Zhu, H., Dong, X., Zhao, D., Jiang, X., et al. (2014). 2-methoxyestradiol Synergizes with Sorafenib to Suppress Hepatocellular Carcinoma by Simultaneously Dysregulating Hypoxia-Inducible Factor-1 and -2. Cancer Lett. 355, 96–105. doi:10.1016/j.canlet.2014.09.011
Mabjeesh, N. J., Post, D. E., Willard, M. T., Kaur, B., Van Meir, E. G., Simons, J. W., et al. (2002). Geldanamycin Induces Degradation of Hypoxia-Inducible Factor 1alpha Protein via the Proteasome Pathway in Prostate Cancer Cells. Cancer Res. 62, 2478–2482.
Magat, J., Jordan, B. F., Cron, G. O., and Gallez, B. (2010). Noninvasive Mapping of Spontaneous Fluctuations in Tumor Oxygenation Using 19F MRI. Med. Phys. 37, 5434–5441. doi:10.1118/1.3484056
Mahy, P., De Bast, M., Gallez, B., Gueulette, J., Koch, C. J., Scalliet, P., et al. (2003). In Vivo colocalization of 2-nitroimidazole EF5 Fluorescence Intensity and Electron Paramagnetic Resonance Oximetry in Mouse Tumors. Radiother. Oncol. 67, 53–61. doi:10.1016/s0167-8140(03)00028-8
Malinen, E., Sovik, A., Hristov, D., Bruland, O. S., and Olsen, D. R. (2006). Adapting Radiotherapy to Hypoxic Tumours. Phys. Med. Biol. 51, 4903–4921. doi:10.1088/0031-9155/51/19/012
Marchetti, P., Fovez, Q., Germain, N., Khamari, R., and Kluza, J. (2020). Mitochondrial Spare Respiratory Capacity: Mechanisms, Regulation, and Significance in Non-transformed and Cancer Cells. FASEB J. 34, 13106–13124. doi:10.1096/fj.202000767R
Markouli, M., Strepkos, D., Papavassiliou, A. G., and Piperi, C. (2020). Targeting of Endoplasmic Reticulum (ER) Stress in Gliomas. Pharmacol. Res. 157, 104823. doi:10.1016/j.phrs.2020.104823
Martin, J. D., Seano, G., and Jain, R. K. (2019). Normalizing Function of Tumor Vessels: Progress, Opportunities, and Challenges. Annu. Rev. Physiol. 81, 505–534. doi:10.1146/annurev-physiol-020518-114700
Martinive, P., De Wever, J., Bouzin, C., Baudelet, C., Sonveaux, P., Grégoire, V., et al. (2006). Reversal of Temporal and Spatial Heterogeneities in Tumor Perfusion Identifies the Tumor Vascular Tone as a Tunable Variable to Improve Drug Delivery. Mol. Cancer Ther. 5, 1620–1627. doi:10.1158/1535-7163.MCT-05-0472
Martinive, P., Defresne, F., Quaghebeur, E., Daneau, G., Crokart, N., Grégoire, V., et al. (2009). Impact of Cyclic Hypoxia on HIF-1alpha Regulation in Endothelial Cells-Nnew Insights for Anti-tumor Treatments. FEBS J. 276, 509–518. doi:10.1111/j.1742-4658.2008.06798.x
Mason, R. P., and Antich, P. P. (1994). Tumor Oxygen Tension: Measurement Using Oxygent as a 19F NMR Probe at 4.7 T. Artif. Cells Blood Substit. Immobil. Biotechnol. 22, 1361–1367. doi:10.3109/10731199409138838
Mason, R. P., Constantinescu, A., Ran, S., and Thorpe, P. E. (2003). Oxygenation in a Human Tumor Xenograft: Manipulation through Respiratory Challenge and Antibody-Directed Infarction. Adv. Exp. Med. Biol. 530, 197–204. doi:10.1007/978-1-4615-0075-9_19
Mason, R. P., Nunnally, R. L., and Antich, P. P. (1991). Tissue Oxygenation: a Novel Determination Using 19F Surface Coil NMR Spectroscopy of Sequestered Perfluorocarbon Emulsion. Magn. Reson Med. 18, 71–79. doi:10.1002/mrm.1910180109
Mason, R. P., Rodbumrung, W., and Antich, P. P. (1996). Hexafluorobenzene: a Sensitive 19F NMR Indicator of Tumor Oxygenation. NMR Biomed. 9, 125–134. doi:10.1002/(SICI)1099-1492(199605)9:3<125::AID-NBM405>3.0.CO;2-F
Mason, R. P., Shukla, H., and Antich, P. P. (1993). In Vivo oxygen Tension and Temperature: Simultaneous Determination Using 19F NMR Spectroscopy of Perfluorocarbon. Magn. Reson Med. 29, 296–302. doi:10.1002/mrm.1910290304
Matsumoto, K., Bernardo, M., Subramanian, S., Choyke, P., Mitchell, J. B., Krishna, M. C., et al. (2006). MR Assessment of Changes of Tumor in Response to Hyperbaric Oxygen Treatment. Magn. Reson Med. 56, 240–246. doi:10.1002/mrm.20961
Matsumoto, K., Szajek, L., Krishna, M. C., Cook, J. A., Seidel, J., Grimes, K., et al. (2007). The Influence of Tumor Oxygenation on Hypoxia Imaging in Murine Squamous Cell Carcinoma Using [64Cu]Cu-ATSM or [18F]Fluoromisonidazole Positron Emission Tomography. Int. J. Oncol. 30, 873–881. doi:10.3892/ijo.30.4.873
Matsumoto, K. I., Mitchell, J. B., and Krishna, M. C. (2021). Multimodal Functional Imaging for Cancer/Tumor Microenvironments Based on MRI. EPRI, PET. Mol. 26, 1614. doi:10.3390/molecules26061614
Matsumoto, S., Batra, S., Saito, K., Yasui, H., Choudhuri, R., Gadisetti, C., et al. (2011). Antiangiogenic Agent Sunitinib Transiently Increases Tumor Oxygenation and Suppresses Cycling Hypoxia. Cancer Res. 71, 6350–6359. doi:10.1158/0008-5472.CAN-11-2025
Matsumoto, S., Saito, K., Takakusagi, Y., Matsuo, M., Munasinghe, J. P., Morris, H. D., et al. (2014). In Vivo imaging of Tumor Physiological, Metabolic, and Redox Changes in Response to the Anti-angiogenic Agent Sunitinib: Longitudinal Assessment to Identify Transient Vascular Renormalization. Antioxid. Redox Signal 21, 1145–1155. doi:10.1089/ars.2013.5725
Matsumoto, S., Yasui, H., Mitchell, J. B., and Krishna, M. C. (2010). Imaging Cycling Tumor Hypoxia. Cancer Res. 70, 10019–10023. doi:10.1158/0008-5472.CAN-10-2821
Maxwell, P. H., Wiesener, M. S., Chang, G. W., Clifford, S. C., Vaux, E. C., Cockman, M. E., et al. (1999). The Tumour Suppressor Protein VHL Targets Hypoxia-Inducible Factors for Oxygen-dependent Proteolysis. Nature 399, 271–275. doi:10.1038/20459
Mayer, A., Höckel, M., and Vaupel, P. (2005). Carbonic Anhydrase IX Expression and Tumor Oxygenation Status Do Not Correlate at the Microregional Level in Locally Advanced Cancers of the Uterine Cervix. Clin. Cancer Res. 11, 7220–7225. doi:10.1158/1078-0432.CCR-05-0869
Mayer, A., Höckel, M., and Vaupel, P. (2006). Endogenous Hypoxia Markers in Locally Advanced Cancers of the Uterine Cervix: Reality or Wishful Thinking? Strahlenther Onkol. 182, 501–510. doi:10.1007/s00066-006-1525-9
Mayer, A., Höckel, M., Wree, A., and Vaupel, P. (2005). Microregional Expression of Glucose Transporter-1 and Oxygenation Status: Lack of Correlation in Locally Advanced Cervical Cancers. Clin. Cancer Res. 11, 2768–2773. doi:10.1158/1078-0432.CCR-04-2344
Mazzone, M., Dettori, D., de Oliveira, R. L., Loges, S., Schmidt, T., Jonckx, B., et al. (2009). Heterozygous Deficiency of PHD2 Restores Tumor Oxygenation and Inhibits Metastasis via Endothelial Normalization. Cell 136, 839–851. doi:10.1016/j.cell.2009.01.020
McAteer, M. A., O'Connor, J. P. B., Koh, D. M., Leung, H. Y., Doran, S. J., Jauregui-Osoro, M., et al. (2021). Introduction to the National Cancer Imaging Translational Accelerator (NCITA): a UK-wide Infrastructure for Multicentre Clinical Translation of Cancer Imaging Biomarkers. Br. J. Cancer 125, 1462–1465. doi:10.1038/s41416-021-01497-5
McCall, K. C., Humm, J. L., Bartlett, R., Reese, M., and Carlin, S. (2012). Copper-64-diacetyl-bis(N(4)-methylthiosemicarbazone) Pharmacokinetics in FaDu Xenograft Tumors and Correlation with Microscopic Markers of Hypoxia. Int. J. Radiat. Oncol. Biol. Phys. 84, e393–9. doi:10.1016/j.ijrobp.2012.05.005
McKeown, S. R., Hejmadi, M. V., McIntyre, I. A., McAleer, J. J., and Patterson, L. H. (1995). AQ4N: An Alkylaminoanthraquinone N-Oxide Showing Bioreductive Potential and Positive Interaction with Radiation In Vivo. Br. J. Cancer 72, 76–81. doi:10.1038/bjc.1995.280
McNally, N. J., Denekamp, J., Sheldon, P. W., and Flockhart, I. R. (1978). Hypoxic Cell Sensitization by Misonidazole In Vivo and In Vitro. Br. J. Radiol. 51, 317–318. doi:10.1259/0007-1285-51-604-317
McPhail, L. D., and Robinson, S. P. (2010). Intrinsic Susceptibility MR Imaging of Chemically Induced Rat Mammary Tumors: Relationship to Histologic Assessment of Hypoxia and Fibrosis. Radiology 254, 110–118. doi:10.1148/radiol.2541090395
Meenakshisundaram, G., Eteshola, E., Pandian, R. P., Bratasz, A., Lee, S. C., and Kuppusamy, P. (2009). Fabrication and Physical Evaluation of a Polymer-Encapsulated Paramagnetic Probe for Biomedical Oximetry. Biomed. Microdevices 11, 773–782. doi:10.1007/s10544-009-9292-x
Meenakshisundaram, G., Eteshola, E., Pandian, R. P., Bratasz, A., Selvendiran, K., Lee, S. C., et al. (2009). Oxygen Sensitivity and Biocompatibility of an Implantable Paramagnetic Probe for Repeated Measurements of Tissue Oxygenation. Biomed. Microdevices 11, 817–826. doi:10.1007/s10544-009-9298-4
Meenakshisundaram, G., Pandian, R. P., Eteshola, E., Lee, S. C., and Kuppusamy, P. (2010). A Paramagnetic Implant Containing Lithium Naphthalocyanine Microcrystals for High-Resolution Biological Oximetry. J. Magn. Reson 203, 185–189. doi:10.1016/j.jmr.2009.11.016
Metwally, M. A., Frederiksen, K. D., and Overgaard, J. (2014). Compliance and Toxicity of the Hypoxic Radiosensitizer Nimorazole in the Treatment of Patients with Head and Neck Squamous Cell Carcinoma (HNSCC). Acta Oncol. 53, 654–661. doi:10.3109/0284186X.2013.864050
Miao, Z. F., Zhao, T. T., Wang, Z. N., Xu, Y. Y., Mao, X. Y., Wu, J. H., et al. (2014). Influence of Different Hypoxia Models on Metastatic Potential of SGC-7901 Gastric Cancer Cells. Tumour Biol. 35, 6801–6808. doi:10.1007/s13277-014-1928-7
Michiels, C., Tellier, C., and Feron, O. (2016). Cycling Hypoxia: A Key Feature of the Tumor Microenvironment. Biochim. Biophys. Acta 1866, 76–86. doi:10.1016/j.bbcan.2016.06.004
Mignion, L., Magat, J., Schakman, O., Marbaix, E., Gallez, B., and Jordan, B. F. (2013). Hexafluorobenzene in Comparison with Perfluoro-15-Crown-5-Ether for Repeated Monitoring of Oxygenation Using 19F MRI in a Mouse Model. Magn. Reson Med. 69, 248–254. doi:10.1002/mrm.24245
Milas, L., Hunter, N., Mason, K. A., Milross, C., and Peters, L. J. (1995). Tumor Reoxygenation as a Mechanism of Taxol-Induced Enhancement of Tumor Radioresponse. Acta Oncol. 34, 409–412. doi:10.3109/02841869509093999
Milas, L., Hunter, N. R., Mason, K. A., Milross, C. G., Saito, Y., and Peters, L. J. (1995). Role of Reoxygenation in Induction of Enhancement of Tumor Radioresponse by Paclitaxel. Cancer Res. 55, 3564–3568.
Mimura, N., Fulciniti, M., Gorgun, G., Tai, Y. T., Cirstea, D., Santo, L., et al. (2012). Blockade of XBP1 Splicing by Inhibition of IRE1alpha Is a Promising Therapeutic Option in Multiple Myeloma. Blood 119, 5772–5781. doi:10.1182/blood-2011-07-366633
Mistry, I. N., Thomas, M., Calder, E. D. D., Conway, S. J., and Hammond, E. M. (2017). Clinical Advances of Hypoxia-Activated Prodrugs in Combination with Radiation Therapy. Int. J. Radiat. Oncol. Biol. Phys. 98, 1183–1196. doi:10.1016/j.ijrobp.2017.03.024
Mitchell, J. B., Cook, J. A., Krishna, M. C., DeGraff, W., Gamson, J., Fisher, J., et al. (1996). Radiation Sensitisation by Nitric Oxide Releasing Agents. Br. J. Cancer Suppl. 27, S181–S184.
Mitchell, J. B., Wink, D. A., DeGraff, W., Gamson, J., Keefer, L. K., and Krishna, M. C. (1993). Hypoxic Mammalian Cell Radiosensitization by Nitric Oxide. Cancer Res. 53, 5845–5848.
Montigaud, Y., Ucakar, B., Krishnamachary, B., Bhujwalla, Z. M., Feron, O., Préat, V., et al. (2018). Optimized Acriflavine-Loaded Lipid Nanocapsules as a Safe and Effective Delivery System to Treat Breast Cancer. Int. J. Pharm. 551, 322–328. doi:10.1016/j.ijpharm.2018.09.034
Moon, E. J., Sonveaux, P., Porporato, P. E., Danhier, P., Gallez, B., Batinic-Haberle, I., et al. (2010). NADPH Oxidase-Mediated Reactive Oxygen Species Production Activates Hypoxia-Inducible Factor-1 (HIF-1) via the ERK Pathway after Hyperthermia Treatment. Proc. Natl. Acad. Sci. U. S. A. 107, 20477–20482. doi:10.1073/pnas.1006646107
Mortensen, L. S., Busk, M., Nordsmark, M., Jakobsen, S., Theil, J., Overgaard, J., et al. (2011). Accessing Radiation Response Using Hypoxia PET Imaging and Oxygen Sensitive Electrodes: a Preclinical Study. Radiother. Oncol. 99, 418–423. doi:10.1016/j.radonc.2011.06.034
Mortensen, L. S., Buus, S., Nordsmark, M., Bentzen, L., Munk, O. L., Keiding, S., et al. (2010). Identifying Hypoxia in Human Tumors: A Correlation Study between 18F-FMISO PET and the Eppendorf Oxygen-Sensitive Electrode. Acta Oncol. 49, 934–940. doi:10.3109/0284186X.2010.516274
Mortensen, L. S., Johansen, J., Kallehauge, J., Primdahl, H., Busk, M., Lassen, P., et al. (2012). FAZA PET/CT Hypoxia Imaging in Patients with Squamous Cell Carcinoma of the Head and Neck Treated with Radiotherapy: Results from the DAHANCA 24 Trial. Radiother. Oncol. 105, 14–20. doi:10.1016/j.radonc.2012.09.015
Mpekris, F., Voutouri, C., Baish, J. W., Duda, D. G., Munn, L. L., Stylianopoulos, T., et al. (2020). Combining Microenvironment Normalization Strategies to Improve Cancer Immunotherapy. Proc. Natl. Acad. Sci. U. S. A. 117, 3728–3737. doi:10.1073/pnas.1919764117
Multhoff, G., and Vaupel, P. (2020). Hypoxia Compromises Anti-cancer Immune Responses. Adv. Exp. Med. Biol. 1232, 131–143. doi:10.1007/978-3-030-34461-0_18
Muz, B., de la Puente, P., Azab, F., and Azab, A. K. (2015). The Role of Hypoxia in Cancer Progression, Angiogenesis, Metastasis, and Resistance to Therapy. Hypoxia (Auckl) 3, 83–92. doi:10.2147/HP.S93413
Neckers, L. (2002). Heat Shock Protein 90 Is a Rational Molecular Target in Breast Cancer. Breast Dis. 15, 53–60. doi:10.3233/bd-2002-15106
Nel, J., Desmet, C. M., Driesschaert, B., Saulnier, P., Lemaire, L., and Gallez, B. (2019). Preparation and Evaluation of Trityl-Loaded Lipid Nanocapsules as Oxygen Sensors for Electron Paramagnetic Resonance Oximetry. Int. J. Pharm. 554, 87–92. doi:10.1016/j.ijpharm.2018.11.007
Netti, P. A., Baxter, L. T., Boucher, Y., Skalak, R., and Jain, R. K. (1995). Time-dependent Behavior of Interstitial Fluid Pressure in Solid Tumors: Implications for Drug Delivery. Cancer Res. 55, 5451–5458.
Neveu, M. A., Joudiou, N., De Preter, G., Dehoux, J. P., Jordan, B. F., and Gallez, B. (2017). 17 O MRS Assesses the Effect of Mild Hypothermia on Oxygen Consumption Rate in Tumors. NMR Biomed. 30, e3836. doi:10.1002/nbm.3726
Newbold, K., Castellano, I., Charles-Edwards, E., Mears, D., Sohaib, A., Leach, M., et al. (2009). An Exploratory Study into the Role of Dynamic Contrast-Enhanced Magnetic Resonance Imaging or Perfusion Computed Tomography for Detection of Intratumoral Hypoxia in Head-And-Neck Cancer. Int. J. Radiat. Oncol. Biol. Phys. 74, 29–37. doi:10.1016/j.ijrobp.2008.07.039
Noman, M. Z., Desantis, G., Janji, B., Hasmim, M., Karray, S., Dessen, P., et al. (2014). PD-L1 Is a Novel Direct Target of HIF-1α, and its Blockade under Hypoxia Enhanced MDSC-Mediated T Cell Activation. J. Exp. Med. 211, 781–790. doi:10.1084/jem.20131916
Noman, M. Z., Hasmim, M., Lequeux, A., Xiao, M., Duhem, C., Chouaib, S., et al. (2019). Improving Cancer Immunotherapy by Targeting the Hypoxic Tumor Microenvironment: New Opportunities and Challenges. Cells 8, 1083. doi:10.3390/cells8091083
Noman, M. Z., Hasmim, M., Messai, Y., Terry, S., Kieda, C., Janji, B., et al. (2015). Hypoxia: a Key Player in Antitumor Immune Response. A Review in the Theme: Cellular Responses to Hypoxia. Am. J. Physiol. Cell Physiol. 309, C569–C579. doi:10.1152/ajpcell.00207.2015
Nordsmark, M., Bentzen, S. M., Rudat, V., Brizel, D., Lartigau, E., Stadler, P., et al. (2005). Prognostic Value of Tumor Oxygenation in 397 Head and Neck Tumors after Primary Radiation Therapy. An International Multi-Center Study. Radiother. Oncol. 77, 18–24. doi:10.1016/j.radonc.2005.06.038
Norikane, T., Yamamoto, Y., Maeda, Y., Kudomi, N., Matsunaga, T., Haba, R., et al. (2014). Correlation of (18)F-Fluoromisonidazole PET Findings with HIF-1alpha and P53 Expressions in Head and Neck Cancer: Comparison with (18)F-FDG PET. Nucl. Med. Commun. 35, 30–35. doi:10.1097/MNM.0000000000000010
Nöth, U., Rodrigues, L. M., Robinson, S. P., Jork, A., Zimmermann, U., Newell, B., et al. (2004). In Vivo determination of Tumor Oxygenation during Growth and in Response to Carbogen Breathing Using 15C5-Loaded Alginate Capsules as Fluorine-19 Magnetic Resonance Imaging Oxygen Sensors. Int. J. Radiat. Oncol. Biol. Phys. 60, 909–919. doi:10.1016/j.ijrobp.2004.07.671
Nytko, K. J., Grgic, I., Bender, S., Ott, J., Guckenberger, M., Riesterer, O., et al. (2017). The Hypoxia-Activated Prodrug Evofosfamide in Combination with Multiple Regimens of Radiotherapy. Oncotarget 8, 23702–23712. doi:10.18632/oncotarget.15784
O'Connor, J. P., Aboagye, E. O., Adams, J. E., Aerts, H. J., Barrington, S. F., Beer, A. J., et al. (2017). Imaging Biomarker Roadmap for Cancer Studies. Nat. Rev. Clin. Oncol. 14, 169–186. doi:10.1038/nrclinonc.2016.162
O'Connor, J. P., Boult, J. K., Jamin, Y., Babur, M., Finegan, K. G., Williams, K. J., et al. (2016). Oxygen-Enhanced MRI Accurately Identifies, Quantifies, and Maps Tumor Hypoxia in Preclinical Cancer Models. Cancer Res. 76, 787–795. doi:10.1158/0008-5472.CAN-15-2062
O'Connor, J. P., Jackson, A., Buonaccorsi, G. A., Buckley, D. L., Roberts, C., Watson, Y., et al. (2007). Organ-specific Effects of Oxygen and Carbogen Gas Inhalation on Tissue Longitudinal Relaxation Times. Magn. Reson Med. 58, 490–496. doi:10.1002/mrm.21357
O'Connor, J. P. B., Robinson, S. P., and Waterton, J. C. (2019). Imaging Tumour Hypoxia with Oxygen-Enhanced MRI and BOLD MRI. Br. J. Radiol. 92, 20180642. doi:10.1259/bjr.20180642
O'Donoghue, J. A., Zanzonico, P., Pugachev, A., Wen, B., Smith-Jones, P., Cai, S., et al. (2005). Assessment of Regional Tumor Hypoxia Using 18F-Fluoromisonidazole and 64Cu(II)-diacetyl-bis(N4-methylthiosemicarbazone) Positron Emission Tomography: Comparative Study Featuring microPET Imaging, pO2 Probe Measurement, Autoradiography, and Fluorescent Microscopy in the R3327-AT and FaDu Rat Tumor Models. Int. J. Radiat. Oncol. Biol. Phys. 61, 1493–1502. doi:10.1016/j.ijrobp.2004.12.057
O'Hara, J. A., Goda, F., Demidenko, E., and Swartz, H. M. (1998). Effect on Regrowth Delay in a Murine Tumor of Scheduling Split-Dose Irradiation Based on Direct pO2 Measurements by Electron Paramagnetic Resonance Oximetry. Radiat. Res. 150, 549–556. doi:10.2307/3579872
O'Hara, J. A., Goda, F., Liu, K. J., Bacic, G., Hoopes, P. J., and Swartz, H. M. (1995). The pO2 in a Murine Tumor after Irradiation: an In Vivo Electron Paramagnetic Resonance Oximetry Study. Radiat. Res. 144, 222–229. doi:10.2307/3579262
Ogawa, S., Lee, T. M., Kay, A. R., and Tank, D. W. (1990). Brain Magnetic Resonance Imaging with Contrast Dependent on Blood Oxygenation. Proc. Natl. Acad. Sci. U. S. A. 87, 9868–9872. doi:10.1073/pnas.87.24.9868
Ogawa, S., and Lee, T. M. (1990). Magnetic Resonance Imaging of Blood Vessels at High Fields: In Vivo and In Vitro Measurements and Image Simulation. Magn. Reson Med. 16, 9–18. doi:10.1002/mrm.1910160103
Ojha, R., and Amaravadi, R. K. (2017). Targeting the Unfolded Protein Response in Cancer. Pharmacol. Res. 120, 258–266. doi:10.1016/j.phrs.2017.04.003
Okamoto, S., Shiga, T., Yasuda, K., Watanabe, S., Hirata, K., Nishijima, K. I., et al. (2016). The Reoxygenation of Hypoxia and the Reduction of Glucose Metabolism in Head and Neck Cancer by Fractionated Radiotherapy with Intensity-Modulated Radiation Therapy. Eur. J. Nucl. Med. Mol. Imaging 43, 2147–2154. doi:10.1007/s00259-016-3431-4
Okunieff, P., Hoeckel, M., Dunphy, E. P., Schlenger, K., Knoop, C., and Vaupel, P. (1993). Oxygen Tension Distributions Are Sufficient to Explain the Local Response of Human Breast Tumors Treated with Radiation Alone. Int. J. Radiat. Oncol. Biol. Phys. 26, 631–636. doi:10.1016/0360-3016(93)90280-9
Olive, P. L. (1989). Distribution, Oxygenation, and Clonogenicity of Macrophages in a Murine Tumor. Cancer Commun. 1, 93–100. doi:10.3727/095535489820875273
Olive, P. L. (1994). Radiation-induced Reoxygenation in the SCCVII Murine Tumour: Evidence for a Decrease in Oxygen Consumption and an Increase in Tumour Perfusion. Radiother. Oncol. 32, 37–46. doi:10.1016/0167-8140(94)90447-2
Oppenheimer, J. H., Schwartz, H. L., Mariash, C. N., Kinlaw, W. B., Wong, N. C., and Freake, H. C. (1987). Advances in Our Understanding of Thyroid Hormone Action at the Cellular Level. Endocr. Rev. 8, 288–308. doi:10.1210/edrv-8-3-288
Overgaard, J., Hansen, H. S., Overgaard, M., Bastholt, L., Berthelsen, A., Specht, L., et al. (1998). A Randomized Double-Blind Phase III Study of Nimorazole as a Hypoxic Radiosensitizer of Primary Radiotherapy in Supraglottic Larynx and Pharynx Carcinoma. Results of the Danish Head and Neck Cancer Study (DAHANCA) Protocol 5-85. Radiother. Oncol. 46, 135–146. doi:10.1016/s0167-8140(97)00220-x
Overgaard, J. (2011). Hypoxic Modification of Radiotherapy in Squamous Cell Carcinoma of the Head and Neck-Aa Systematic Review and Meta-Analysis. Radiother. Oncol. 100, 22–32. doi:10.1016/j.radonc.2011.03.004
Overgaard, J. (2007). Hypoxic Radiosensitization: Adored and Ignored. J. Clin. Oncol. 25, 4066–4074. doi:10.1200/JCO.2007.12.7878
Øvrebø, K. M., Gulliksrud, K., Mathiesen, B., and Rofstad, E. K. (2011). Assessment of Tumor Radioresponsiveness and Metastatic Potential by Dynamic Contrast-Enhanced Magnetic Resonance Imaging. Int. J. Radiat. Oncol. Biol. Phys. 81, 255–261. doi:10.1016/j.ijrobp.2011.04.008
Øvrebø, K. M., Hompland, T., Mathiesen, B., and Rofstad, E. K. (2012). Assessment of Hypoxia and Radiation Response in Intramuscular Experimental Tumors by Dynamic Contrast-Enhanced Magnetic Resonance Imaging. Radiother. Oncol. 102, 429–435. doi:10.1016/j.radonc.2011.11.013
Padera, T. P., Stoll, B. R., Tooredman, J. B., Capen, D., di Tomaso, E., and Jain, R. K. (2004). Pathology: Cancer Cells Compress Intratumour Vessels. Nature 427, 695. doi:10.1038/427695a
Padhani, A. R., Krohn, K. A., Lewis, J. S., and Alber, M. (2007). Imaging Oxygenation of Human Tumours. Eur. Radiol. 17, 861–872. doi:10.1007/s00330-006-0431-y
Pandian, R. P., Parinandi, N. L., Ilangovan, G., Zweier, J. L., and Kuppusamy, P. (2003). Novel Particulate Spin Probe for Targeted Determination of Oxygen in Cells and Tissues. Free Radic. Biol. Med. 35, 1138–1148. doi:10.1016/s0891-5849(03)00496-9
Panek, R., Welsh, L., Baker, L. C. J., Schmidt, M. A., Wong, K. H., Riddell, A. M., et al. (2017). Noninvasive Imaging of Cycling Hypoxia in Head and Neck Cancer Using Intrinsic Susceptibility MRI. Clin. Cancer Res. 23, 4233–4241. doi:10.1158/1078-0432.CCR-16-1209
Papadopoulos, K. P., Goel, S., Beeram, M., Wong, A., Desai, K., Haigentz, M., et al. (2008). A Phase 1 Open-Label, Accelerated Dose-Escalation Study of the Hypoxia Activated Prodrug AQ4N in Patients with Advanced Malignancies. Clin. Cancer Res. 14 (21), 7110–7115. doi:10.1158/1078-0432.CCR-08-0483
Parkinson, D. R., Johnson, B. E., and Sledge, G. W. (2012). Making Personalized Cancer Medicine a Reality: Challenges and Opportunities in the Development of Biomarkers and Companion Diagnostics. Clin. Cancer Res. 18, 619–624. doi:10.1158/1078-0432.CCR-11-2017
Patterson, L. H., and McKeown, S. R. (2000). AQ4N: a New Approach to Hypoxia-Activated Cancer Chemotherapy. Br. J. Cancer 83, 1589–1593. doi:10.1054/bjoc.2000.1564
Patterson, L. H., McKeown, S. R., Ruparelia, K., Double, J. A., Bibby, M. C., Cole, S., et al. (2000). Enhancement of Chemotherapy and Radiotherapy of Murine Tumours by AQ4N, a Bioreductively Activated Anti-tumour Agent. Br. J. Cancer 82, 1984–1990. doi:10.1054/bjoc.2000.1163
Peeters, S. G., Zegers, C. M., Biemans, R., Lieuwes, N. G., van Stiphout, R. G., Yaromina, A., et al. (2015). TH-302 in Combination with Radiotherapy Enhances the Therapeutic Outcome and Is Associated with Pretreatment [18F]HX4 Hypoxia PET Imaging. Clin. Cancer Res. 21, 2984–2992. doi:10.1158/1078-0432.CCR-15-0018
Peeters, S. G., Zegers, C. M., Lieuwes, N. G., van Elmpt, W., Eriksson, J., van Dongen, G. A., et al. (2015). A Comparative Study of the Hypoxia PET Tracers [1⁸F]HX4, [1⁸F]FAZA, and [1⁸F]FMISO in a Preclinical Tumor Model. Int. J. Radiat. Oncol. Biol. Phys. 91, 351–359. doi:10.1016/j.ijrobp.2014.09.045
Pérès, E. A., Toutain, J., Paty, L. P., Divoux, D., Ibazizène, M., Guillouet, S., et al. (2019). 64 Cu-ATSM/64 Cu-Cl 2 and Their Relationship to Hypoxia in Glioblastoma: a Preclinical Study. EJNMMI Res. 9, 114. doi:10.1186/s13550-019-0586-6
Peters, L. J., O'Sullivan, B., Giralt, J., Fitzgerald, T. J., Trotti, A., Bernier, J., et al. (2010). Critical Impact of Radiotherapy Protocol Compliance and Quality in the Treatment of Advanced Head and Neck Cancer: Results from TROG 02.02. J. Clin. Oncol. 28, 2996–3001. doi:10.1200/JCO.2009.27.4498
Peterson, H. I., and Mattson, J. (1984). Vasoactive Drugs and Tumor Blood Flow. Biorheology 21, 503–508. doi:10.3233/bir-1984-21409
Peterson, T. E., Kirkpatrick, N. D., Huang, Y., Farrar, C. T., Marijt, K. A., Kloepper, J., et al. (2016). Dual Inhibition of Ang-2 and VEGF Receptors Normalizes Tumor Vasculature and Prolongs Survival in Glioblastoma by Altering Macrophages. Proc. Natl. Acad. Sci. U. S. A. 113, 4470–4475. doi:10.1073/pnas.1525349113
Petit, S. F., Dekker, A. L., Seigneuric, R., Murrer, L., van Riel, N. A., Nordsmark, M., et al. (2009). Intra-voxel Heterogeneity Influences the Dose Prescription for Dose-Painting with Radiotherapy: a Modelling Study. Phys. Med. Biol. 54, 2179–2196. doi:10.1088/0031-9155/54/7/022
Phillips, R. M. (2016). Targeting the Hypoxic Fraction of Tumours Using Hypoxia-Activated Prodrugs. Cancer Chemother. Pharmacol. 77, 441–457. doi:10.1007/s00280-015-2920-7
Piert, M., Machulla, H. J., Picchio, M., Reischl, G., Ziegler, S., Kumar, P., et al. (2005). Hypoxia-specific Tumor Imaging with 18F-Fluoroazomycin Arabinoside. J. Nucl. Med. 46, 106–113.
Pogue, B. W., O'Hara, J. A., Demidenko, E., Wilmot, C. M., Goodwin, I. A., Chen, B., et al. (2003). Photodynamic Therapy with Verteporfin in the Radiation-Induced Fibrosarcoma-1 Tumor Causes Enhanced Radiation Sensitivity. Cancer Res. 63, 1025–1033.
Pogue, B. W., O'Hara, J. A., Goodwin, I. A., Wilmot, C. J., Fournier, G. P., Akay, A. R., et al. (2002). Tumor PO(2) Changes during Photodynamic Therapy Depend upon Photosensitizer Type and Time after Injection. Comp. Biochem. Physiol. A Mol. Integr. Physiol. 132, 177–184. doi:10.1016/s1095-6433(01)00545-1
Postema, E. J., McEwan, A. J., Riauka, T. A., Kumar, P., Richmond, D. A., Abrams, D. N., et al. (2009). Initial Results of Hypoxia Imaging Using 1-Alpha-D: -(5-Deoxy-5-[18f]-Fluoroarabinofuranosyl)-2-Nitroimidazole (18F-FAZA). Eur. J. Nucl. Med. Mol. Imaging 36, 1565–1573. doi:10.1007/s00259-009-1154-5
Powell, M. E., Collingridge, D. R., Saunders, M. I., Hoskin, P. J., Hill, S. A., and Chaplin, D. J. (1999). Improvement in Human Tumour Oxygenation with Carbogen of Varying Carbon Dioxide Concentrations. Radiother. Oncol. 50, 167–171. doi:10.1016/s0167-8140(98)00123-6
Powell, M. E., Hill, S. A., Saunders, M. I., Hoskin, P. J., and Chaplin, D. J. (1997). Human Tumor Blood Flow Is Enhanced by Nicotinamide and Carbogen Breathing. Cancer Res. 57, 5261–5264.
Price, J. M., Robinson, S. P., and Koh, D. M. (2013). Imaging Hypoxia in Tumours with Advanced MRI. Q. J. Nucl. Med. Mol. Imaging 57, 257–270.
Pugh, C. W., and Ratcliffe, P. J. (2017). New Horizons in Hypoxia Signaling Pathways. Exp. Cell Res. 356, 116–121. doi:10.1016/j.yexcr.2017.03.008
Qiu, J., Lv, B., Fu, M., Wang, X., Zheng, X., and Zhuo, W. (2017). 18 F-Fluoromisonidazole Positron Emission tomography/CT-guided Volumetric-Modulated Arc Therapy-Based Dose Escalation for Hypoxic Subvolume in Nasopharyngeal Carcinomas: A Feasibility Study. Head. Neck 39, 2519–2527. doi:10.1002/hed.24925
Rajamahanty, S., Alonzo, C., Aynehchi, S., Choudhury, M., and Konno, S. (2010). Growth Inhibition of Androgen-Responsive Prostate Cancer Cells with Brefeldin A Targeting Cell Cycle and Androgen Receptor. J. Biomed. Sci. 17, 5. doi:10.1186/1423-0127-17-5
Rajendran, J. G., and Krohn, K. A. (2015). F-18 Fluoromisonidazole for Imaging Tumor Hypoxia: Imaging the Microenvironment for Personalized Cancer Therapy. Semin. Nucl. Med. 45, 151–162. doi:10.1053/j.semnuclmed.2014.10.006
Rajendran, J. G., Schwartz, D. L., O'Sullivan, J., Peterson, L. M., Ng, P., Scharnhorst, J., et al. (2006). Tumor Hypoxia Imaging with [F-18] Fluoromisonidazole Positron Emission Tomography in Head and Neck Cancer. Clin. Cancer Res. 12, 5435–5441. doi:10.1158/1078-0432.CCR-05-1773
Rakotomalala, A., Escande, A., Furlan, A., Meignan, S., and Lartigau, E. (2021). Hypoxia in Solid Tumors: How Low Oxygenation Impacts the "Six Rs" of Radiotherapy. Front. Endocrinol. 12, 742215. doi:10.3389/fendo.2021.742215
Remmele, S., Sprinkart, A. M., Müller, A., Träber, F., von Lehe, M., Gieseke, J., et al. (2013). Dynamic and Simultaneous MR Measurement of R1 and R2* Changes during Respiratory Challenges for the Assessment of Blood and Tissue Oxygenation. Magn. Reson Med. 70, 136–146. doi:10.1002/mrm.24458
Ri, M., Tashiro, E., Oikawa, D., Shinjo, S., Tokuda, M., Yokouchi, Y., et al. (2012). Identification of Toyocamycin, an Agent Cytotoxic for Multiple Myeloma Cells, as a Potent Inhibitor of ER Stress- Induced XBP1 mRNA Splicing. Blood Cancer J. 2, e79. doi:10.1038/bcj.2012.26
Rischin, D., Peters, L., Fisher, R., Macann, A., Denham, J., Poulsen, M., et al. (2005). Tirapazamine, Cisplatin, and Radiation versus Fluorouracil, Cisplatin, and Radiation in Patients with Locally Advanced Head and Neck Cancer: a Randomized Phase II Trial of the Trans-tasman Radiation Oncology Group (TROG 98.02). J. Clin. Oncol. 23, 79–87. doi:10.1200/JCO.2005.01.072
Rischin, D., Peters, L. J., O'Sullivan, B., Giralt, J., Fisher, R., Yuen, K., et al. (2010). Tirapazamine, Cisplatin, and Radiation versus Cisplatin and Radiation for Advanced Squamous Cell Carcinoma of the Head and Neck (TROG 02.02, HeadSTART): a Phase III Trial of the Trans-tasman Radiation Oncology Group. J. Clin. Oncol. 28, 2989–2995. doi:10.1200/JCO.2009.27.4449
Robinson, S. P., Howe, F. A., and Griffiths, J. R. (1995). Noninvasive Monitoring of Carbogen-Induced Changes in Tumor Blood Flow and Oxygenation by Functional Magnetic Resonance Imaging. Int. J. Radiat. Oncol. Biol. Phys. 33, 855–859. doi:10.1016/0360-3016(95)00072-1
Rodrigues, L. M., Howe, F. A., Griffiths, J. R., and Robinson, S. P. (2004). Tumor R2* Is a Prognostic Indicator of Acute Radiotherapeutic Response in Rodent Tumors. J. Magn. Reson Imaging 19, 482–488. doi:10.1002/jmri.20024
Rohwer, N., and Cramer, T. (2011). Hypoxia-mediated Drug Resistance: Novel Insights on the Functional Interaction of HIFs and Cell Death Pathways. Drug Resist Updat 14, 191–201. doi:10.1016/j.drup.2011.03.001
Roizin-Towle, L., and Hall, E. J. (1979). The Effect of Bleomycin on Aerated and Hypoxic Cells In Vitro, in Combination with Irradiation. Int. J. Radiat. Oncol. Biol. Phys. 5, 1491–1494. doi:10.1016/0360-3016(79)90756-9
Rojas, A., Carl, U., and Reghebi, K. (1990). Effect of Normobaric Oxygen on Tumor Radiosensitivity: Fractionated Studies. Int. J. Radiat. Oncol. Biol. Phys. 18, 547–553. doi:10.1016/0360-3016(90)90059-s
Rudat, V., Stadler, P., Becker, A., Vanselow, B., Dietz, A., Wannenmacher, M., et al. (2001). Predictive Value of the Tumor Oxygenation by Means of pO2 Histography in Patients with Advanced Head and Neck Cancer. Strahlenther Onkol. 177, 462–468. doi:10.1007/pl00002427
Safronova, M. M., Colliez, F., Magat, J., Joudiou, N., Jordan, B. F., Raftopoulos, C., et al. (2016). Mapping of Global R1 and R2* Values versus Lipids R1 Values as Potential Markers of Hypoxia in Human Glial Tumors: A Feasibility Study. Magn. Reson Imaging 34, 105–113. doi:10.1016/j.mri.2015.10.021
Saga, T., Inubushi, M., Koizumi, M., Yoshikawa, K., Zhang, M. R., Tanimoto, K., et al. (2015). Prognostic Value of 18F-Fluoroazomycin Arabinoside PET/CT in Patients with Advanced Non-small-cell Lung Cancer. Cancer Sci. 106, 1554–1560. doi:10.1111/cas.12771
Saga, T., Inubushi, M., Koizumi, M., Yoshikawa, K., Zhang, M. R., Obata, T., et al. (2016). Prognostic Value of PET/CT with (18)F-Fluoroazomycin Arabinoside for Patients with Head and Neck Squamous Cell Carcinomas Receiving Chemoradiotherapy. Ann. Nucl. Med. 30, 217–224. doi:10.1007/s12149-015-1048-5
Salem, A., Little, R. A., Latif, A., Featherstone, A. K., Babur, M., Peset, I., et al. (2019). Oxygen-enhanced MRI Is Feasible, Repeatable, and Detects Radiotherapy-Induced Change in Hypoxia in Xenograft Models and in Patients with Non-small Cell Lung Cancer. Clin. Cancer Res. 25, 3818–3829. doi:10.1158/1078-0432.CCR-18-3932
Salles, F. T., Hespanhol, A. M., Jaeger, R. G., and Marques, M. M. (2004). Brefeldin-A Induces Apoptosis in Human Adenoid Cystic Carcinoma Cultured Cells. Oral Oncol. 40, 585–590. doi:10.1016/j.oraloncology.2003.12.007
Samanta, D., and Semenza, G. L. (2018). Metabolic Adaptation of Cancer and Immune Cells Mediated by Hypoxia-Inducible Factors. Biochim. Biophys. Acta Rev. Cancer 1870, 15–22. doi:10.1016/j.bbcan.2018.07.002
Sanches, M., Duffy, N. M., Talukdar, M., Thevakumaran, N., Chiovitti, D., Canny, M. D., et al. (2014). Structure and Mechanism of Action of the Hydroxy-Aryl-Aldehyde Class of IRE1 Endoribonuclease Inhibitors. Nat. Commun. 5, 4202. doi:10.1038/ncomms5202
Sanduleanu, S., Hamming-Vrieze, O., Wesseling, F. W. R., Even, A. J. G., Hoebers, F. J., Hoeben, A., et al. (2020). [ 18 F]-HX4 PET/CT Hypoxia in Patients with Squamous Cell Carcinoma of the Head and Neck Treated with Chemoradiotherapy: Prognostic Results from Two Prospective Trials. Clin. Transl. Radiat. Oncol. 23, 9–15. doi:10.1016/j.ctro.2020.04.004
Sanduleanu, S., Wiel, A. M. A. V., Lieverse, R. I. Y., Marcus, D., Ibrahim, A., Primakov, S., et al. (2020). Hypoxia PET Imaging with [18F]-HX4-A Promising Next-Generation Tracer. Cancers 12, 1322. doi:10.3390/cancers12051322
Sanzhaeva, U., Poncelet, M., Tseytlin, O., Tseytlin, M., Gencheva, M., Eubank, T. D., et al. (2020). Synthesis, Characterization, and Application of a Highly Hydrophilic Triarylmethyl Radical for Biomedical EPR. J. Org. Chem. 85, 10388–10398. doi:10.1021/acs.joc.0c00557
Sato, J., Kitagawa, Y., Yamazaki, Y., Hata, H., Okamoto, S., Shiga, T., et al. (2013). 18F-fluoromisonidazole PET Uptake Is Correlated with Hypoxia-Inducible Factor-1alpha Expression in Oral Squamous Cell Carcinoma. J. Nucl. Med. 54, 1060–1065. doi:10.2967/jnumed.112.114355
Sato, Y., Tsujikawa, T., Oh, M., Mori, T., Kiyono, Y., Fujieda, S., et al. (2014). Assessing Tumor Hypoxia in Head and Neck Cancer by PET with ⁶2Cu-diacetyl-bis(N⁴-methylthiosemicarbazone). Clin. Nucl. Med. 39, 1027–1032. doi:10.1097/RLU.0000000000000537
Schaner, P. E., Williams, B. B., Chen, E. Y., Pettus, J. R., Schreiber, W. A., Kmiec, M. M., et al. (2021). First-In-Human Study in Cancer Patients Establishing the Feasibility of Oxygen Measurements in Tumors Using Electron Paramagnetic Resonance with the OxyChip. Front. Oncol. 11, 743256. doi:10.3389/fonc.2021.743256
Schwade, J. G., Kinsella, T. J., Kelly, B., Rowland, J., Johnston, M., and Glatstein, E. (1984). Clinical Experience with Intravenous Misonidazole for Carcinoma of the Esophagus: Results in Attempting Radiosensitization of Each Fraction of Exposure. Cancer Invest 2, 91–95. doi:10.3109/07357908409020291
Schwartz, D. L., Powis, G., Thitai-Kumar, A., He, Y., Bankson, J., Williams, R., et al. (2009). The Selective Hypoxia Inducible Factor-1 Inhibitor PX-478 Provides In Vivo Radiosensitization through Tumor Stromal Effects. Mol. Cancer Ther. 8, 947–958. doi:10.1158/1535-7163.MCT-08-0981
Schwarz, G. (1909). Über Desensibilisierung gegen Röntgen- und Radiumstrahlen. Münch Med. Wschr 56, 1217–1218.
Scott, C., Suh, J., Stea, B., Nabid, A., and Hackman, J. (2007). Improved Survival, Quality of Life, and Quality-Adjusted Survival in Breast Cancer Patients Treated with Efaproxiral (Efaproxyn) Plus Whole-Brain Radiation Therapy for Brain Metastases. Am. J. Clin. Oncol. 30, 580–587. doi:10.1097/COC.0b013e3180653c0d
Sebestyen, A., Kopper, L., Danko, T., and Timar, J. (2021). Hypoxia Signaling in Cancer: From Basics to Clinical Practice. Pathol. Oncol. Res. 27, 1609802. doi:10.3389/pore.2021.1609802
Secomb, T. W., Hsu, R., Ong, E. T., Gross, J. F., and Dewhirst, M. W. (1995). Analysis of the Effects of Oxygen Supply and Demand on Hypoxic Fraction in Tumors. Acta Oncol. 34, 313–316. doi:10.3109/02841869509093981
Seddon, B. M., Honess, D. J., Vojnovic, B., Tozer, G. M., and Workman, P. (2001). Measurement of Tumor Oxygenation: In Vivo Comparison of a Luminescence Fiber-Optic Sensor and a Polarographic Electrode in the P22 Tumor. Radiat. Res. 155, 837–846. doi:10.1667/0033-7587(2001)155[0837:motoiv]2.0.co;2
Segers, J., Crokart, N., Danhier, P., Grégoire, V., Jordan, B. F., and Gallez, B. (2010). Use of Xanthinol Nicotinate as a Co-treatment for Radio- and Chemo-Therapy in Experimental Tumors. Int. J. Cancer 126, 583–588. doi:10.1002/ijc.24724
Segers, J., Di Fazio, V., Ansiaux, R., Martinive, P., Feron, O., Wallemacq, P., et al. (2006). Potentiation of Cyclophosphamide Chemotherapy Using the Anti-angiogenic Drug Thalidomide: Importance of Optimal Scheduling to Exploit the 'normalization' Window of the Tumor Vasculature. Cancer Lett. 244, 129–135. doi:10.1016/j.canlet.2005.12.017
Semenza, G. L. (2006). Development of Novel Therapeutic Strategies that Target HIF-1. Expert Opin. Ther. Targets 10, 267–280. doi:10.1517/14728222.10.2.267
Semenza, G. L. (2000). HIF-1: Mediator of Physiological and Pathophysiological Responses to Hypoxia. J. Appl. Physiol. (1985) 88, 1474–1480. doi:10.1152/jappl.2000.88.4.1474
Semenza, G. L. (2003). Targeting HIF-1 for Cancer Therapy. Nat. Rev. Cancer 3, 721–732. doi:10.1038/nrc1187
Semenza, G. L., and Wang, G. L. (1992). A Nuclear Factor Induced by Hypoxia via De Novo Protein Synthesis Binds to the Human Erythropoietin Gene Enhancer at a Site Required for Transcriptional Activation. Mol. Cell Biol. 12, 5447–5454. doi:10.1128/mcb.12.12.5447
Sermeus, A., Rebucci, M., Fransolet, M., Flamant, L., Desmet, D., Delaive, E., et al. (2013). Differential Effect of Hypoxia on Etoposide-Induced DNA Damage Response and P53 Regulation in Different Cell Types. J. Cell Physiol. 228, 2365–2376. doi:10.1002/jcp.24409
Servagi-Vernat, S., Differding, S., Hanin, F. X., Labar, D., Bol, A., Lee, J. A., et al. (2014). A Prospective Clinical Study of 18F-FAZA PET-CT Hypoxia Imaging in Head and Neck Squamous Cell Carcinoma before and during Radiation Therapy. Eur. J. Nucl. Med. Mol. Imaging 41, 1544–1552. doi:10.1007/s00259-014-2730-x
Servagi-Vernat, S., Differding, S., Sterpin, E., Hanin, F. X., Labar, D., Bol, A., et al. (2015). Hypoxia-guided Adaptive Radiation Dose Escalation in Head and Neck Carcinoma: a Planning Study. Acta Oncol. 54, 1008–1016. doi:10.3109/0284186X.2014.990109
Sevick, E. M., and Jain, R. K. (1989). Geometric Resistance to Blood Flow in Solid Tumors Perfused Ex Vivo: Effects of Tumor Size and Perfusion Pressure. Cancer Res. 49, 3506–3512.
Shapiro, D. J., Livezey, M., Yu, L., Zheng, X., and Andruska, N. (2016). Anticipatory UPR Activation: A Protective Pathway and Target in Cancer. Trends Endocrinol. Metab. 27, 731–741. doi:10.1016/j.tem.2016.06.002
Shay, J. E., Imtiyaz, H. Z., Sivanand, S., Durham, A. C., Skuli, N., Hsu, S., et al. (2014). Inhibition of Hypoxia-Inducible Factors Limits Tumor Progression in a Mouse Model of Colorectal Cancer. Carcinogenesis 35, 1067–1077. doi:10.1093/carcin/bgu004
Shi, Y., Oeh, J., Eastham-Anderson, J., Yee, S., Finkle, D., Peale, F. V., et al. (2013). Mapping In Vivo Tumor Oxygenation within Viable Tumor by 19F-MRI and Multispectral Analysis. Neoplasia 15, 1241–1250. doi:10.1593/neo.131468
Siemann, D. W., Hill, R. P., and Bush, R. S. (1977). The Importance of the Pre-irradiation Breathing Times of Oxygen and Carbogen (5% CO2: 95% O2) on the In Vivo Radiation Response of a Murine Sarcoma. Int. J. Radiat. Oncol. Biol. Phys. 2, 903–911. doi:10.1016/0360-3016(77)90188-2
Siemann, D. W., Horsman, M. R., and Chaplin, D. J. (1994). The Radiation Response of KHT Sarcomas Following Nicotinamide Treatment and Carbogen Breathing. Radiother. Oncol. 31, 117–122. doi:10.1016/0167-8140(94)90391-3
Simoncic, U., Leibfarth, S., Welz, S., Schwenzer, N., Schmidt, H., Reischl, G., et al. (2017). Comparison of DCE-MRI Kinetic Parameters and FMISO-PET Uptake Parameters in Head and Neck Cancer Patients. Med. Phys. 44, 2358–2368. doi:10.1002/mp.12228
Simonsen, T. G., Lund, K. V., Hompland, T., Kristensen, G. B., and Rofstad, E. K. (2018). DCE-MRI-Derived Measures of Tumor Hypoxia and Interstitial Fluid Pressure Predict Outcomes in Cervical Carcinoma. Int. J. Radiat. Oncol. Biol. Phys. 102, 1193–1201. doi:10.1016/j.ijrobp.2018.04.035
Song, D., Beringhs, A. O., Zhuang, Z., Joshi, G., Tran, T. H., Claffey, K. P., et al. (2019). Overcoming Hypoxia-Induced Chemoresistance to Cisplatin through Tumor Oxygenation Monitored by Optical Imaging. Nanotheranostics 3, 223–235. doi:10.7150/ntno.35935
Song, G., Ji, C., Liang, C., Song, X., Yi, X., Dong, Z., et al. (2017). TaOx Decorated Perfluorocarbon Nanodroplets as Oxygen Reservoirs to Overcome Tumor Hypoxia and Enhance Cancer Radiotherapy. Biomaterials 112, 257–263. doi:10.1016/j.biomaterials.2016.10.020
Song, R., Hu, D., Chung, H. Y., Sheng, Z., and Yao, S. (2018). Lipid-Polymer Bilaminar Oxygen Nanobubbles for Enhanced Photodynamic Therapy of Cancer. ACS Appl. Mater Interfaces 10, 36805–36813. doi:10.1021/acsami.8b15293
Song, Y. P., Mistry, H., Irlam, J., Valentine, H., Yang, L., Lane, B., et al. (2021). Long-Term Outcomes of Radical Radiation Therapy with Hypoxia Modification with Biomarker Discovery for Stratification: 10-Year Update of the BCON (Bladder Carbogen Nicotinamide) Phase 3 Randomized Trial (ISRCTN45938399). Int. J. Radiat. Oncol. Biol. Phys. 110, 1407–1415. doi:10.1016/j.ijrobp.2021.03.001
Sonveaux, P., Dessy, C., Brouet, A., Jordan, B. F., Grégoire, V., Gallez, B., et al. (2002). Modulation of the Tumor Vasculature Functionality by Ionizing Radiation Accounts for Tumor Radiosensitization and Promotes Gene Delivery. FASEB J. 16, 1979–1981. doi:10.1096/fj.02-0487fje
Sonveaux, P., Dessy, C., Martinive, P., Havaux, X., Jordan, B. F., Gallez, B., et al. (2004). Endothelin-1 Is a Critical Mediator of Myogenic Tone in Tumor Arterioles: Implications for Cancer Treatment. Cancer Res. 64, 3209–3214. doi:10.1158/0008-5472.can-03-1291
Sonveaux, P., Jordan, B. F., Gallez, B., and Feron, O. (2009). Nitric Oxide Delivery to Cancer: Why and How? Eur. J. Cancer 45, 1352–1369. doi:10.1016/j.ejca.2008.12.018
Sonveaux, P. (2008). Provascular Strategy: Targeting Functional Adaptations of Mature Blood Vessels in Tumors to Selectively Influence the Tumor Vascular Reactivity and Improve Cancer Treatment. Radiother. Oncol. 86, 300–313. doi:10.1016/j.radonc.2008.01.024
Sörensen, A., Carles, M., Bunea, H., Majerus, L., Stoykow, C., Nicolay, N. H., et al. (2020). Textural Features of Hypoxia PET Predict Survival in Head and Neck Cancer during Chemoradiotherapy. Eur. J. Nucl. Med. Mol. Imaging 47, 1056–1064. doi:10.1007/s00259-019-04609-9
Sørensen, B. S., and Horsman, M. R. (2020). Tumor Hypoxia: Impact on Radiation Therapy and Molecular Pathways. Front. Oncol. 10, 562. doi:10.3389/fonc.2020.00562
Sørensen, B. S., Toustrup, K., Horsman, M. R., Overgaard, J., and Alsner, J. (2010). Identifying pH Independent Hypoxia Induced Genes in Human Squamous Cell Carcinomas In Vitro. Acta Oncol. 49, 895–905. doi:10.3109/02841861003614343
Sovik, A., Malinen, E., Bruland, O. S., Bentzen, S. M., and Olsen, D. R. (2007). Optimization of Tumour Control Probability in Hypoxic Tumours by Radiation Dose Redistribution: a Modelling Study. Phys. Med. Biol. 52, 499–513. doi:10.1088/0031-9155/52/2/013
Spence, A. M., Muzi, M., Swanson, K. R., O'Sullivan, F., Rockhill, J. K., Rajendran, J. G., et al. (2008). Regional Hypoxia in Glioblastoma Multiforme Quantified with [18F]fluoromisonidazole Positron Emission Tomography before Radiotherapy: Correlation with Time to Progression and Survival. Clin. Cancer Res. 14, 2623–2630. doi:10.1158/1078-0432.CCR-07-4995
Starmans, M. H., Chu, K. C., Haider, S., Nguyen, F., Seigneuric, R., Magagnin, M. G., et al. (2012). The Prognostic Value of Temporal In Vitro and In Vivo Derived Hypoxia Gene-Expression Signatures in Breast Cancer. Radiother. Oncol. 102, 436–443. doi:10.1016/j.radonc.2012.02.002
Steward, W. P., Middleton, M., Benghiat, A., Loadman, P. M., Hayward, C., Waller, S., et al. (2007). The Use of Pharmacokinetic and Pharmacodynamic End Points to Determine the Dose of AQ4N, a Novel Hypoxic Cell Cytotoxin, Given with Fractionated Radiotherapy in a Phase I Study. Ann. Oncol. 18, 1098–1103. doi:10.1093/annonc/mdm120
Stone, H. B., Brown, J. M., Phillips, T. L., and Sutherland, R. M. (1993). Oxygen in Human Tumors: Correlations between Methods of Measurement and Response to Therapy. Summary of a Workshop Held November 19-20, 1992, at the National Cancer Institute, Bethesda, Maryland. Radiat. Res. 136, 422–434. doi:10.2307/3578556
Subramanian, S., Devasahayam, N., McMillan, A., Matsumoto, S., Munasinghe, J. P., Saito, K., et al. (2012). Reporting of Quantitative Oxygen Mapping in EPR Imaging. J. Magn. Reson 214, 244–251. doi:10.1016/j.jmr.2011.11.013
Subramanian, S., Yamada, K., Irie, A., Murugesan, R., Cook, J. A., Devasahayam, N., et al. (2002). Noninvasive In Vivo Oximetric Imaging by Radiofrequency FT EPR. Magn. Reson Med. 47, 1001–1008. doi:10.1002/mrm.10133
Suh, J. H., Stea, B., Nabid, A., Kresl, J. J., Fortin, A., Mercier, J. P., et al. (2006). Phase III Study of Efaproxiral as an Adjunct to Whole-Brain Radiation Therapy for Brain Metastases. J. Clin. Oncol. 24, 106–114. doi:10.1200/JCO.2004.00.1768
Swartz, H. M., and Clarkson, R. B. (1998). The Measurement of Oxygen In Vivo Using EPR Techniques. Phys. Med. Biol. 43, 1957–1975. doi:10.1088/0031-9155/43/7/017
Swartz, H. M., Flood, A. B., Schaner, P. E., Halpern, H., Williams, B. B., Pogue, B. W., et al. (2020). How Best to Interpret Measures of Levels of Oxygen in Tissues to Make Them Effective Clinical Tools for Care of Patients with Cancer and Other Oxygen-dependent Pathologies. Physiol. Rep. 8, e14541. doi:10.14814/phy2.14541
Swartz, H. M., Williams, B. B., Hou, H., Khan, N., Jarvis, L. A., Chen, E. Y., et al. (2016). Direct and Repeated Clinical Measurements of pO2 for Enhancing Cancer Therapy and Other Applications. Adv. Exp. Med. Biol. 923, 95–104. doi:10.1007/978-3-319-38810-6_13
Swartz, H. M., Williams, B. B., Zaki, B. I., Hartford, A. C., Jarvis, L. A., Chen, E. Y., et al. (2014). Clinical EPR: Unique Opportunities and Some Challenges. Acad. Radiol. 21, 197–206. doi:10.1016/j.acra.2013.10.011
Swartz, J. E., Pothen, A. J., Stegeman, I., Willems, S. M., and Grolman, W. (2015). Clinical Implications of Hypoxia Biomarker Expression in Head and Neck Squamous Cell Carcinoma: a Systematic Review. Cancer Med. 4, 1101–1116. doi:10.1002/cam4.460
Takakusagi, Y., Naz, S., Takakusagi, K., Ishima, M., Murata, H., Ohta, K., et al. (2018). A Multimodal Molecular Imaging Study Evaluates Pharmacological Alteration of the Tumor Microenvironment to Improve Radiation Response. Cancer Res. 78, 6828–6837. doi:10.1158/0008-5472.CAN-18-1654
Takakusagi, Y., Kishimoto, S., Naz, S., Matsumoto, S., Saito, K., Hart, C. P., et al. (2018). Radiotherapy Synergizes with the Hypoxia-Activated Prodrug Evofosfamide: In Vitro and In Vivo Studies. Antioxidants Redox Signal. 28, 131–140. doi:10.1089/ars.2017.7106
Tang, C. H., Ranatunga, S., Kriss, C. L., Cubitt, C. L., Tao, J., Pinilla-Ibarz, J. A., et al. (2014). Inhibition of ER Stress-Associated IRE-1/XBP-1 Pathway Reduces Leukemic Cell Survival. J. Clin. Invest 124, 2585–2598. doi:10.1172/JCI73448
Tang, X., Cheng, Y., Huang, S., Zhi, F., Yuan, A., Hu, Y., et al. (2017). Overcome the Limitation of Hypoxia against Photodynamic Therapy to Treat Cancer Cells by Using Perfluorocarbon Nanodroplet for Photosensitizer Delivery. Biochem. Biophys. Res. Commun. 487, 483–487. doi:10.1016/j.bbrc.2017.03.142
Tannock, I. F. (1968). The Relation between Cell Proliferation and the Vascular System in a Transplanted Mouse Mammary Tumour. Br. J. Cancer 22, 258–273. doi:10.1038/bjc.1968.34
Tatum, J. L., Kelloff, G. J., Gillies, R. J., Arbeit, J. M., Brown, J. M., Chao, K. S., et al. (2006). Hypoxia: Importance in Tumor Biology, Noninvasive Measurement by Imaging, and Value of its Measurement in the Management of Cancer Therapy. Int. J. Radiat. Biol. 82, 699–757. doi:10.1080/09553000601002324
Teicher, B. A., Holden, S. A., al-Achi, A., and Herman, T. S. (1990). Classification of Antineoplastic Treatments by Their Differential Toxicity toward Putative Oxygenated and Hypoxic Tumor Subpopulations In Vivo in the FSaIIC Murine Fibrosarcoma. Cancer Res. 50, 3339–3344.
Teicher, B. A. (1994). Combination of Perfluorochemical Emulsions and Carbogen Breathing with Cancer Chemotherapy. Artif. Cells Blood Substit. Immobil. Biotechnol. 22, 1109–1120. doi:10.3109/10731199409138806
Teicher, B. A., Crawford, J. M., Holden, S. A., and Cathcart, K. N. (1987). Effects of Various Oxygenation Conditions on the Enhancement by Fluosol-DA of Melphalan Antitumor Activity. Cancer Res. 47, 5036–5041.
Teicher, B. A., Herman, T. S., Hopkins, R. E., and Menon, K. (1991). Effect of Oxygen Level on the Enhancement of Tumor Response to Radiation by Perfluorochemical Emulsions or a Bovine Hemoglobin Preparation. Int. J. Radiat. Oncol. Biol. Phys. 21, 969–974. doi:10.1016/0360-3016(91)90737-o
Teicher, B. A., Wong, J. S., Takeuchi, H., Gravelin, L. M., Ara, G., and Buxton, D. (1998). Allosteric Effectors of Hemoglobin as Modulators of Chemotherapy and Radiation Therapy In Vitro and In Vivo. Cancer Chemother. Pharmacol. 42, 24–30. doi:10.1007/s002800050780
Terry, S., Engelsen, A. S. T., Buart, S., Elsayed, W. S., Venkatesh, G. H., and Chouaib, S. (2020). Hypoxia-driven Intratumor Heterogeneity and Immune Evasion. Cancer Lett. 492, 1–10. doi:10.1016/j.canlet.2020.07.004
The MICAD Research Team (2004–2013). “[18F]Fluoromisonidazole,” in Molecular Imaging and Contrast Agent Database (MICAD) [Internet] (Bethesda (MD): National Center for Biotechnology Information).
Thews, O., Gassner, B., Kelleher, D. K., and Gekle, M. (2008). Activity of Drug Efflux Transporters in Tumor Cells under Hypoxic Conditions. Adv. Exp. Med. Biol. 614, 157–164. doi:10.1007/978-0-387-74911-2_19
Thews, O., and Vaupel, P. (2015). Spatial Oxygenation Profiles in Tumors during Normo- and Hyperbaric Hyperoxia. Strahlenther Onkol. 191, 875–882. doi:10.1007/s00066-015-0867-6
Thews, O., and Vaupel, P. (2016). Temporal Changes in Tumor Oxygenation and Perfusion upon Normo- and Hyperbaric Inspiratory Hyperoxia. Strahlenther Onkol. 192, 174–181. doi:10.1007/s00066-015-0916-1
Thiruthaneeswaran, N., Bibby, B. A. S., Yang, L., Hoskin, P. J., Bristow, R. G., Choudhury, A., et al. (2021). Lost in Application: Measuring Hypoxia for Radiotherapy Optimization. Eur. J. Cancer 148, 260–276. doi:10.1016/j.ejca.2021.01.039
Thomas, C. D., Prade, M., and Guichard, M. (1995). Tumour Oxygenation, Radiosensitivity, and Necrosis before And/or after Nicotinamide, Carbogen and Perflubron Emulsion Administration. Int. J. Radiat. Biol. 67, 597–605. doi:10.1080/09553009514550711
Thomlinson, R. H., and Gray, L. H. (1955). The Histological Structure of Some Human Lung Cancers and the Possible Implications for Radiotherapy. Br. J. Cancer 9, 539–549. doi:10.1038/bjc.1955.55
Thorwarth, D., and Alber, M. (2010). Implementation of Hypoxia Imaging into Treatment Planning and Delivery. Radiother. Oncol. 97, 172–175. doi:10.1016/j.radonc.2010.05.012
Thorwarth, D., Eschmann, S. M., Paulsen, F., and Alber, M. (2007). Hypoxia Dose Painting by Numbers: a Planning Study. Int. J. Radiat. Oncol. Biol. Phys. 68, 291–300. doi:10.1016/j.ijrobp.2006.11.061
Thulborn, K. R., Waterton, J. C., Matthews, P. M., and Radda, G. K. (1982). Oxygenation Dependence of the Transverse Relaxation Time of Water Protons in Whole Blood at High Field. Biochim. Biophys. Acta 714, 265–270. doi:10.1016/0304-4165(82)90333-6
Toma-Dasu, I., Uhrdin, J., Antonovic, L., Dasu, A., Nuyts, S., Dirix, P., et al. (2012). Dose Prescription and Treatment Planning Based on FMISO-PET Hypoxia. Acta Oncol. 51, 222–230. doi:10.3109/0284186X.2011.599815
Tong, R. T., Boucher, Y., Kozin, S. V., Winkler, F., Hicklin, D. J., and Jain, R. K. (2004). Vascular Normalization by Vascular Endothelial Growth Factor Receptor 2 Blockade Induces a Pressure Gradient across the Vasculature and Improves Drug Penetration in Tumors. Cancer Res. 64, 3731–3736. doi:10.1158/0008-5472.CAN-04-0074
Tóth, V., Förschler, A., Hirsch, N. M., den Hollander, J., Kooijman, H., Gempt, J., et al. (2013). MR-Based Hypoxia Measures in Human Glioma. J. Neurooncol 115, 197–207.
Toustrup, K., Sørensen, B. S., Alsner, J., and Overgaard, J. (2012). Hypoxia Gene Expression Signatures as Prognostic and Predictive Markers in Head and Neck Radiotherapy. Semin. Radiat. Oncol. 22, 119–127. doi:10.1016/j.semradonc.2011.12.006
Toustrup, K., Sørensen, B. S., Nordsmark, M., Busk, M., Wiuf, C., Alsner, J., et al. (2011). Development of a Hypoxia Gene Expression Classifier with Predictive Impact for Hypoxic Modification of Radiotherapy in Head and Neck Cancer. Cancer Res. 71, 5923–5931. doi:10.1158/0008-5472.CAN-11-1182
Tran, L. B., Bol, A., Labar, D., Jordan, B., Magat, J., Mignion, L., et al. (2012). Hypoxia Imaging with the Nitroimidazole 18F-FAZA PET Tracer: a Comparison with OxyLite, EPR Oximetry and 19F-MRI Relaxometry. Radiother. Oncol. 105, 29–35. doi:10.1016/j.radonc.2012.04.011
Tran, L. B., Bol, A., Labar, D., Cao-Pham, T. T., Jordan, B., Grégoire, V., et al. (2015). Predictive Value of (18)F-FAZA PET Imaging for Guiding the Association of Radiotherapy with Nimorazole: a Preclinical Study. Radiother. Oncol. 114, 189–194. doi:10.1016/j.radonc.2014.12.015
Tran, L. B., Bol, A., Labar, D., Karroum, O., Bol, V., Jordan, B., et al. (2014). Potential Role of Hypoxia Imaging Using (18)F-FAZA PET to Guide Hypoxia-Driven Interventions (Carbogen Breathing or Dose Escalation) in Radiation Therapy. Radiother. Oncol. 113, 204–209. doi:10.1016/j.radonc.2014.09.016
Tran, L. B., Cao-Pham, T. T., Jordan, B. F., Deschoemaeker, S., Heyerick, A., and Gallez, B. (2019). Impact of Myo-Inositol Trispyrophosphate (ITPP) on Tumour Oxygenation and Response to Irradiation in Rodent Tumour Models. J. Cell Mol. Med. 23, 1908–1916. doi:10.1111/jcmm.14092
Treat, J., Johnson, E., Langer, C., Belani, C., Haynes, B., Greenberg, R., et al. (1998). Tirapazamine with Cisplatin in Patients with Advanced Non-small-cell Lung Cancer: a Phase II Study. J. Clin. Oncol. 16, 3524–3527. doi:10.1200/JCO.1998.16.11.3524
Ueda, M., Kudo, T., Kuge, Y., Mukai, T., Tanaka, S., Konishi, H., et al. (2010). Rapid Detection of Hypoxia-Inducible Factor-1-Active Tumours: Pretargeted Imaging with a Protein Degrading in a Mechanism Similar to Hypoxia-Inducible Factor-1alpha. Eur. J. Nucl. Med. Mol. Imaging 37, 1566–1574. doi:10.1007/s00259-010-1467-4
Urtasun, R. C., Palmer, M., Kinney, B., Belch, A., Hewitt, J., and Hanson, J. (1998). Intervention with the Hypoxic Tumor Cell Sensitizer Etanidazole in the Combined Modality Treatment of Limited Stage Small-Cell Lung Cancer. A One-Institution Study. Int. J. Radiat. Oncol. Biol. Phys. 40, 337–342. doi:10.1016/s0360-3016(97)00771-2
Vahidi, N., Clarkson, R. B., Liu, K. J., Norby, S. W., Wu, M., and Swartz, H. M. (1994). In Vivo and In Vitro EPR Oximetry with Fusinite: a New Coal-Derived, Particulate EPR Probe. Magn. Reson Med. 31, 139–146. doi:10.1002/mrm.1910310207
Valtorta, S., Belloli, S., Sanvito, F., Masiello, V., Di Grigoli, G., Monterisi, C., et al. (2013). Comparison of 18F-Fluoroazomycin-Arabinofuranoside and 64Cu-Diacetyl-bis(N4-Methylthiosemicarbazone) in Preclinical Models of Cancer. J. Nucl. Med. 54, 1106–1112. doi:10.2967/jnumed.112.111120
van der Sanden, B. P., Heerschap, A., Simonetti, A. W., Rijken, P. F., Peters, H. P., Stüben, G., et al. (1999). Characterization and Validation of Noninvasive Oxygen Tension Measurements in Human Glioma Xenografts by 19F-MR Relaxometry. Int. J. Radiat. Oncol. Biol. Phys. 44, 649–658. doi:10.1016/s0360-3016(98)00555-0
van Elmpt, W., Zegers, C. M. L., Reymen, B., Even, A. J. G., Dingemans, A. C., Oellers, M., et al. (2016). Multiparametric Imaging of Patient and Tumour Heterogeneity in Non-small-cell Lung Cancer: Quantification of Tumour Hypoxia, Metabolism and Perfusion. Eur. J. Nucl. Med. Mol. Imaging 43, 240–248. doi:10.1007/s00259-015-3169-4
Vashisht Gopal, Y. N., Gammon, S., Prasad, R., Knighton, B., Pisaneschi, F., Roszik, J., et al. (2019). A Novel Mitochondrial Inhibitor Blocks MAPK Pathway and Overcomes MAPK Inhibitor Resistance in Melanoma. Clin. Cancer Res. 25, 6429–6442. doi:10.1158/1078-0432.CCR-19-0836
Vaupel, P., Flood, A. B., and Swartz, H. M. (2021). Oxygenation Status of Malignant Tumors vs. Normal Tissues: Critical Evaluation and Updated Data Source Based on Direct Measurements with pO2 Microsensors. Appl. Magn. Reson 52, 1451–1479. doi:10.1007/s00723-021-01383-6
Vaupel, P., Höckel, M., and Mayer, A. (2007). Detection and Characterization of Tumor Hypoxia Using pO2 Histography. Antioxid. Redox Signal 9, 1221–1235. doi:10.1089/ars.2007.1628
Vaupel, P., Mayer, A., and Höckel, M. (2004). Tumor Hypoxia and Malignant Progression. Methods Enzymol. 381, 335–354. doi:10.1016/S0076-6879(04)81023-1
Vaupel, P., and Mayer, A. (2007). Hypoxia in Cancer: Significance and Impact on Clinical Outcome. Cancer Metastasis Rev. 26, 225–239. doi:10.1007/s10555-007-9055-1
Vaupel, P., and Mayer, A. (2016). Tumor Hypoxia: Causative Mechanisms, Microregional Heterogeneities, and the Role of Tissue-Based Hypoxia Markers. Adv. Exp. Med. Biol. 923, 77–86. doi:10.1007/978-3-319-38810-6_11
Vaupel, P., and Multhoff, G. (2021). Revisiting the Warburg Effect: Historical Dogma versus Current Understanding. J. Physiol. 599, 1745–1757. doi:10.1113/JP278810
Vaupel, P., and Multhoff, G. (2021). The Warburg Effect: Historical Dogma versus Current Rationale. Adv. Exp. Med. Biol. 1269, 169–177. doi:10.1007/978-3-030-48238-1_27
Vaupel, P., and Mayer, A. (2014). Hypoxia in Tumors: Pathogenesis-Related Classification, Characterization of Hypoxia Subtypes, and Associated Biological and Clinical Implications. Adv. Exp. Med. Biol. 812, 19–24. doi:10.1007/978-1-4939-0620-8_3
Vavere, A. L., and Lewis, J. S. (2008). Examining the Relationship between Cu-ATSM Hypoxia Selectivity and Fatty Acid Synthase Expression in Human Prostate Cancer Cell Lines. Nucl. Med. Biol. 35, 273–279.
Vestvik, I. K., Egeland, T. A., Gaustad, J. V., Mathiesen, B., and Rofstad, E. K. (2007). Assessment of Microvascular Density, Extracellular Volume Fraction, and Radiobiological Hypoxia in Human Melanoma Xenografts by Dynamic Contrast-Enhanced MRI. J. Magn. Reson Imaging 26, 1033–1042. doi:10.1002/jmri.21110
Vikram, D. S., Bratasz, A., Ahmad, R., and Kuppusamy, P. (2007). A Comparative Evaluation of EPR and OxyLite Oximetry Using a Random Sampling of pO(2) in a Murine Tumor. Radiat. Res. 168, 308–315. doi:10.1667/RR0854.1
Vilaplana-Lopera, N., Besh, M., and Moon, E. J. (2021). Targeting Hypoxia: Revival of Old Remedies. Biomolecules 11, 1604. doi:10.3390/biom11111604
Viry, E., Baginska, J., Berchem, G., Noman, M. Z., Medves, S., Chouaib, S., et al. (2014). Autophagic Degradation of GZMB/granzyme B: A New Mechanism of Hypoxic Tumor Cell Escape from Natural Killer Cell-Mediated Lysis. Autophagy 10, 173–175. doi:10.4161/auto.26924
Vlaminck, B., Toffoli, S., Ghislain, B., Demazy, C., Raes, M., and Michiels, C. (2007). Dual Effect of Echinomycin on Hypoxia-Inducible Factor-1 Activity under Normoxic and Hypoxic Conditions. FEBS J. 274, 5533–5542. doi:10.1111/j.1742-4658.2007.06072.x
Volkmann, K., Lucas, J. L., Vuga, D., Wang, X., Brumm, D., Stiles, C., et al. (2011). Potent and Selective Inhibitors of the Inositol-Requiring Enzyme 1 Endoribonuclease. J. Biol. Chem. 286, 12743–12755. doi:10.1074/jbc.M110.199737
Vordermark, D., and Brown, J. M. (2003). Endogenous Markers of Tumor Hypoxia Predictors of Clinical Radiation Resistance? Strahlenther Onkol. 179, 801–811. doi:10.1007/s00066-003-1150-9
Wachsberger, P. R., Burd, R., Marero, N., Daskalakis, C., Ryan, A., McCue, P., et al. (2005). Effect of the Tumor Vascular-Damaging Agent, ZD6126, on the Radioresponse of U87 Glioblastoma. Clin. Cancer Res. 11, 835–842.
Wagner, F., Asfar, P., Calzia, E., Radermacher, P., and Szabó, C. (2009). Bench-to-bedside Review: Hydrogen Sulfide-Tthe Third Gaseous Transmitter: Applications for Critical Care. Crit. Care 13, 213. doi:10.1186/cc7700
Wang, G. L., and Semenza, G. L. (1993). General Involvement of Hypoxia-Inducible Factor 1 in Transcriptional Response to Hypoxia. Proc. Natl. Acad. Sci. U. S. A. 90, 4304–4308. doi:10.1073/pnas.90.9.4304
Wang, J., Biedermann, K. A., and Brown, J. M. (1992). Repair of DNA and Chromosome Breaks in Cells Exposed to SR 4233 under Hypoxia or to Ionizing Radiation. Cancer Res. 52, 4473–4477.
Wang, Q., Li, J. M., Yu, H., Deng, K., Zhou, W., Wang, C. X., et al. (2018). Fluorinated Polymeric Micelles to Overcome Hypoxia and Enhance Photodynamic Cancer Therapy. Biomater. Sci. 6, 3096–3107. doi:10.1039/c8bm00852c
Wang, Y., Liu, Y., Tang, F., Bernot, K. M., Schore, R., Marcucci, G., et al. (2014). Echinomycin Protects Mice against Relapsed Acute Myeloid Leukemia without Adverse Effect on Hematopoietic Stem Cells. Blood 124, 1127–1135. doi:10.1182/blood-2013-12-544221
Welsh, S., Williams, R., Kirkpatrick, L., Paine-Murrieta, G., and Powis, G. (2004). Antitumor Activity and Pharmacodynamic Properties of PX-478, an Inhibitor of Hypoxia-Inducible Factor-1alpha. Mol. Cancer Ther. 3, 233–244. doi:10.1158/1535-7163.233.3.3
Welz, S., Mönnich, D., Pfannenberg, C., Nikolaou, K., Reimold, M., La Fougère, C., et al. (2017). Prognostic Value of Dynamic Hypoxia PET in Head and Neck Cancer: Results from a Planned Interim Analysis of a Randomized Phase II Hypoxia-Image Guided Dose Escalation Trial. Radiother. Oncol. 124, 526–532. doi:10.1016/j.radonc.2017.04.004
Whillans, D. W., and Hunt, J. W. (1982). A Rapid-Mixing Comparison of the Mechanisms of Radiosensitization by Oxygen and Misonidazole in CHO Cells. Radiat. Res. 90, 126–141. doi:10.2307/3575801
White, D. A., Zhang, Z., Li, L., Gerberich, J., Stojadinovic, S., Peschke, P., et al. (2016). Developing Oxygen-Enhanced Magnetic Resonance Imaging as a Prognostic Biomarker of Radiation Response. Cancer Lett. 380, 69–77. doi:10.1016/j.canlet.2016.06.003
Wiedenmann, N. E., Bucher, S., Hentschel, M., Mix, M., Vach, W., Bittner, M. I., et al. (2015). Serial [18F]-Fluoromisonidazole PET during Radiochemotherapy for Locally Advanced Head and Neck Cancer and its Correlation with Outcome. Radiother. Oncol. 117, 113–117. doi:10.1016/j.radonc.2015.09.015
Wijsman, R., Kaanders, J. H., Oyen, W. J., and Bussink, J. (2013). Hypoxia and Tumor Metabolism in Radiation Oncology: Targets Visualized by Positron Emission Tomography. Q. J. Nucl. Med. Mol. Imaging 57, 244–256.
Williamson, S. K., Crowley, J. J., Lara, P. N., McCoy, J., Lau, D. H., Tucker, R. W., et al. (2005). Phase III Trial of Paclitaxel Plus Carboplatin with or without Tirapazamine in Advanced Non-small-cell Lung Cancer: Southwest Oncology Group Trial S0003. J. Clin. Oncol. 23, 9097–9104. doi:10.1200/JCO.2005.01.3771
Winter, J. D., Estrada, M., and Cheng, H. L. (2011). Normal Tissue Quantitative T1 and T2* MRI Relaxation Time Responses to Hypercapnic and Hyperoxic Gases. Acad. Radiol. 18, 1159–1167. doi:10.1016/j.acra.2011.04.016
Wong, C. C., Zhang, H., Gilkes, D. M., Chen, J., Wei, H., Chaturvedi, P., et al. (2012). Inhibitors of Hypoxia-Inducible Factor 1 Block Breast Cancer Metastatic Niche Formation and Lung Metastasis. J. Mol. Med. 90, 803–815. doi:10.1007/s00109-011-0855-y
Wouters, B. G., and Brown, J. M. (1997). Cells at Intermediate Oxygen Levels Can Be More Important Than the "hypoxic Fraction" in Determining Tumor Response to Fractionated Radiotherapy. Radiat. Res. 147, 541–550. doi:10.2307/3579620
Wu, P., Zhou, Q., Zhu, H., Zhuang, Y., and Bao, J. (2020). Enhanced Antitumor Efficacy in Colon Cancer Using EGF Functionalized PLGA Nanoparticles Loaded with 5-Fluorouracil and Perfluorocarbon. BMC Cancer 20, 354. doi:10.1186/s12885-020-06803-7
Xu, Z., Li, X. F., Zou, H., Sun, X., and Shen, B. (2017). 18F-Fluoromisonidazole in Tumor Hypoxia Imaging. Oncotarget 8, 94969–94979. doi:10.18632/oncotarget.21662
Yang, L., Forker, L., Irlam, J. J., Pillay, N., Choudhury, A., and West, C. M. L. (2017). Validation of a Hypoxia Related Gene Signature in Multiple Soft Tissue Sarcoma Cohorts. Oncotarget 9, 3946–3955. doi:10.18632/oncotarget.23280
Yang, L., Roberts, D., Takhar, M., Erho, N., Bibby, B. A. S., Thiruthaneeswaran, N., et al. (2018). Development and Validation of a 28-gene Hypoxia-Related Prognostic Signature for Localized Prostate Cancer. EBioMedicine 31, 182–189. doi:10.1016/j.ebiom.2018.04.019
Yang, L., Taylor, J., Eustace, A., Irlam, J. J., Denley, H., Hoskin, P. J., et al. (2017). A Gene Signature for Selecting Benefit from Hypoxia Modification of Radiotherapy for High-Risk Bladder Cancer Patients. Clin. Cancer Res. 23, 4761–4768. doi:10.1158/1078-0432.CCR-17-0038
Yang, L., and West, C. M. (2019). Hypoxia Gene Expression Signatures as Predictive Biomarkers for Personalising Radiotherapy. Br. J. Radiol. 92, 20180036. doi:10.1259/bjr.20180036
Yang, Z., Tao, D., Zhong, W., Liu, Z., Feng, L., and Chen, M. (2021). Perfluorocarbon Loaded Fluorinated Covalent Organic Polymers with Effective Sonosensitization and Tumor Hypoxia Relief Enable Synergistic Sonodynamic-Immunotherapy. Biomaterials 15, 121250.
Yasui, H., Matsumoto, S., Devasahayam, N., Munasinghe, J. P., Choudhuri, R., Saito, K., et al. (2010). Low-field Magnetic Resonance Imaging to Visualize Chronic and Cycling Hypoxia in Tumor-Bearing Mice. Cancer Res. 70, 6427–6436. doi:10.1158/0008-5472.CAN-10-1350
Yoshii, Y., Yoneda, M., Ikawa, M., Furukawa, T., Kiyono, Y., Mori, T., et al. (2012). Radiolabeled Cu-ATSM as a Novel Indicator of Overreduced Intracellular State Due to Mitochondrial Dysfunction: Studies with Mitochondrial DNA-Less ρ0 Cells and Cybrids Carrying MELAS Mitochondrial DNA Mutation. Nucl. Med. Biol. 39, 177–185. doi:10.1016/j.nucmedbio.2011.08.008
You, L., Wu, W., Wang, X., Fang, L., Adam, V., Nepovimova, E., et al. (2021). The Role of Hypoxia-Inducible Factor 1 in Tumor Immune Evasion. Med. Res. Rev. 41, 1622–1643. doi:10.1002/med.21771
Yu, T., Tang, B., and Sun, X. (2017). Development of Inhibitors Targeting Hypoxia-Inducible Factor 1 and 2 for Cancer Therapy. Yonsei Med. J. 58, 489–496. doi:10.3349/ymj.2017.58.3.489
Yu, W., Qiao, F., Su, X., Zhang, D., Wang, H., Jiang, J., et al. (2019). 18F-HX4/18F-FMISO-based Micro PET for Imaging of Tumor Hypoxia and Radiotherapy-Associated Changes in Mice. Biomed. Pharmacother. 119, 109454. doi:10.1016/j.biopha.2019.109454
Yuan, H., Schroeder, T., Bowsher, J. E., Hedlund, L. W., Wong, T., and Dewhirst, M. W. (2006). Intertumoral Differences in Hypoxia Selectivity of the PET Imaging Agent 64Cu(II)-diacetyl-bis(N4-methylthiosemicarbazone). J. Nucl. Med. 47, 989–998.
Zannella, V. E., Dal Pra, A., Muaddi, H., McKee, T. D., Stapleton, S., Sykes, J., et al. (2013). Reprogramming Metabolism with Metformin Improves Tumor Oxygenation and Radiotherapy Response. Clin. Cancer Res. 19, 6741–6750. doi:10.1158/1078-0432.CCR-13-1787
Zanzonico, P., O'Donoghue, J., Chapman, J. D., Schneider, R., Cai, S., Larson, S., et al. (2004). Iodine-124-labeled Iodo-Azomycin-Galactoside Imaging of Tumor Hypoxia in Mice with Serial microPET Scanning. Eur. J. Nucl. Med. Mol. Imaging 31, 117–128. doi:10.1007/s00259-003-1322-y
Zegers, C. M., Hoebers, F. J., van Elmpt, W., Bons, J. A., Öllers, M. C., Troost, E. G., et al. (2016). Evaluation of Tumour Hypoxia during Radiotherapy Using [ 18 F]HX4 PET Imaging and Blood Biomarkers in Patients with Head and Neck Cancer. Eur. J. Nucl. Med. Mol. Imaging 43, 2139–2146. doi:10.1007/s00259-016-3429-y
Zeman, E. M., Brown, J. M., Lemmon, M. J., Hirst, V. K., and Lee, W. W. (1986). Sr-4233: A New Bioreductive Agent with High Selective Toxicity for Hypoxic Mammalian Cells. Int. J. Radiat. Oncol. Biol. Phys. 12, 1239–1242. doi:10.1016/0360-3016(86)90267-1
Zeng, Q., Qiao, L., Cheng, L., Li, C., Cao, Z., Chen, Z., et al. (2020). Perfluorohexane-Loaded Polymeric Nanovesicles with Oxygen Supply for Enhanced Sonodynamic Therapy. ACS Biomater. Sci. Eng. 6, 2956–2969. doi:10.1021/acsbiomaterials.0c00407
Zhang, C., Yang, C., Feldman, M. J., Wang, H., Pang, Y., Maggio, D. M., et al. (2017). Vorinostat Suppresses Hypoxia Signaling by Modulating Nuclear Translocation of Hypoxia Inducible Factor 1 Alpha. Oncotarget 8, 56110–56125. doi:10.18632/oncotarget.18125
Zhang, H., Lu, H., Xiang, L., Bullen, J. W., Zhang, C., Samanta, D., et al. (2015). HIF-1 Regulates CD47 Expression in Breast Cancer Cells to Promote Evasion of Phagocytosis and Maintenance of Cancer Stem Cells. Proc. Natl. Acad. Sci. U. S. A. 112, E6215–E6223. doi:10.1073/pnas.1520032112
Zhao, D., Constantinescu, A., Chang, C. H., Hahn, E. W., and Mason, R. P. (2003). Correlation of Tumor Oxygen Dynamics with Radiation Response of the Dunning Prostate R3327-HI Tumor. Radiat. Res. 159, 621–631. doi:10.1667/0033-7587(2003)159[0621:cotodw]2.0.co;2
Zhao, D., Constantinescu, A., Hahn, E. W., and Mason, R. P. (2002). Differential Oxygen Dynamics in Two Diverse Dunning Prostate R3327 Rat Tumor Sublines (MAT-Lu and HI) with Respect to Growth and Respiratory Challenge. Int. J. Radiat. Oncol. Biol. Phys. 53, 744–756. doi:10.1016/s0360-3016(02)02822-5
Zhao, D., Constantinescu, A., Hahn, E. W., and Mason, R. P. (2001). Tumor Oxygen Dynamics with Respect to Growth and Respiratory Challenge: Investigation of the Dunning Prostate R3327-HI Tumor. Radiat. Res. 156, 510–520. doi:10.1667/0033-7587(2001)156[0510:todwrt]2.0.co;2
Zhao, D., Jiang, L., Hahn, E. W., and Mason, R. P. (2005). Tumor Physiologic Response to Combretastatin A4 Phosphate Assessed by MRI. Int. J. Radiat. Oncol. Biol. Phys. 62, 872–880. doi:10.1016/j.ijrobp.2005.03.009
Zhdanov, A. V., Waters, A. H., Golubeva, A. V., Dmitriev, R. I., and Papkovsky, D. B. (2014). Availability of the Key Metabolic Substrates Dictates the Respiratory Response of Cancer Cells to the Mitochondrial Uncoupling. Biochim. Biophys. Acta 1837, 51–62. doi:10.1016/j.bbabio.2013.07.008
Zhou, H., Hallac, R. R., Lopez, R., Denney, R., MacDonough, M. T., Li, L., et al. (2015). Evaluation of Tumor Ischemia in Response to an Indole-Based Vascular Disrupting Agent Using BLI and 19F MRI. Am. J. Nucl. Med. Mol. Imaging 5, 143–153.
Zhou, Z., Zhang, B., Wang, H., Yuan, A., Hu, Y., and Wu, J. (2018). Two-stage Oxygen Delivery for Enhanced Radiotherapy by Perfluorocarbon Nanoparticles. Theranostics 8, 4898–4911. doi:10.7150/thno.27598
Zschaeck, S., Löck, S., Hofheinz, F., Zips, D., Saksø Mortensen, L., Zöphel, K., et al. (2020). Individual Patient Data Meta-Analysis of FMISO and FAZA Hypoxia PET Scans from Head and Neck Cancer Patients Undergoing Definitive Radio-Chemotherapy. Radiother. Oncol. 149, 189–196. doi:10.1016/j.radonc.2020.05.022
Keywords: tumor hypoxia, oxygen, imaging, biomarker, cancer, predictive marker, therapy, theranostics
Citation: Gallez B (2022) The Role of Imaging Biomarkers to Guide Pharmacological Interventions Targeting Tumor Hypoxia. Front. Pharmacol. 13:853568. doi: 10.3389/fphar.2022.853568
Received: 12 January 2022; Accepted: 23 June 2022;
Published: 15 July 2022.
Edited by:
Patricia Sancho, Universidad de Zaragoza, SpainReviewed by:
Ellen Ackerstaff, Memorial Sloan Kettering Cancer Center, United StatesChristel Vangestel, Antwerp University Hospital, Belgium
Copyright © 2022 Gallez. This is an open-access article distributed under the terms of the Creative Commons Attribution License (CC BY). The use, distribution or reproduction in other forums is permitted, provided the original author(s) and the copyright owner(s) are credited and that the original publication in this journal is cited, in accordance with accepted academic practice. No use, distribution or reproduction is permitted which does not comply with these terms.
*Correspondence: Bernard Gallez, YmVybmFyZC5nYWxsZXpAdWNsb3V2YWluLmJl