- College of Veterinary Medicine, Northwest A&F University, Yangling, China
Spermatogenesis directly determines the reproductive capacity of male animals. With the development of society, the increasing pressure on people’s lives and changes in the living environment, male fertility is declining. The leaf of Eucommia ulmoides Oliv. (Eucommiae Folium, EF) was recorded in the 2020 Chinese Pharmacopoeia and was used in traditional Chinese medicine as a tonic. In recent years, EF has been reported to improve spermatogenesis, but the mechanisms of EF remain was poorly characterized. In this study, the effect of EF ethanol extract (EFEE) on spermatogenesis was tested in mice. Chemical components related to spermatogenesis in EF were predicted by network pharmacology. The biological activity of the predicted chemical components was measured by the proliferation of C18-4 spermatogonial stem cells (SSCs) and the testosterone secretion of TM3 leydig cells. The biological activity of chlorogenic acid (CGA), the active compound in EF, was tested in vivo. The cell cycle was analysed by flow cytometry. Testosterone secretion was detected by ELISA. RNA interference (RNAi) was used to detect the effect of key genes on cell biological activity. Western blotting, qRT–PCR and immunofluorescence staining were used to analyse the molecular mechanism of related biological activities. The results showed that EFEE and CGA could improve spermatogenesis in mice. Furthermore, the main mechanism was that CGA promoted SSC proliferation, self-renewal and Leydig cell testosterone secretion by promoting the expression of SHP2 and activating the downstream signaling pathways involved in these biological processes. This study provided strong evidence for elucidating the mechanism by which EF promotes the spermatogenesis in mice and a new theoretical basis for dealing with the decrease in male reproductive capacity.
Introduction
Spermatogenesis is a process from SSC to mature sperm through mitosis and meiosis in the seminiferous tubules (Haseeb et al., 2019; Yang and Yang, 2020). The process involves autocrine, paracrine, and other hormonal stimuli and nutrients that are supportive of the germ cells development (Ehmcke and Schlatt, 2006; Dube and Cyr, 2012). The maintenance of spermatogenesis relies on the production of testosterone and follicle stimulating hormone (FSH) (Ramaswamy and Weinbauer, 2014). Testosterone is the cornerstone of spermatogenesis, secondary sexual characteristics and functions (Huhtaniemi, 2018). FSH enhances testosterone action by maintaining the supporting function of Sertoli cells on spermatogenesis (Mclachlan et al., 2002). Testosterone is considered the master switch of spermatogenesis, FSH is known to contribute to the quality and quantity of the sperm (Oduwole and Huhtaniemi, 2014).
In leydig cell, luteinizing hormone (LH) binds luteinizing hormone recepter (LHR) and stimulates cAMP pathway. The cAMP pathway, through protein kinase A (PKA), is essential for regulating the expression of steroidogenic acute regulatory protein (StAR) which acts at the mitochondria to trigger cholesterol movement across the membranes (Papadopoulos and Miller, 2012; Clark, 2016). After cholesterol is transferred from the outer to the inner mitochondrial membrane, it is converted to testosterone by steroidogenic enzymes: CYP11A1, 3β-HSD, CYP17A1 and 17β-HSD (Payne and Hales, 2004). In addition to the well-established regulation of testosterone synthesis by PKA, several regulators were identified. These include the signaling molecules PDGF and DHH; the kinases MAPK, PKG, CAMKI, and AMPK; and the transcription factors NUR77, MEF2, and GATA4 (Tremblay, 2015).
The SSC self-renewal, which encompasses cell division and cell survival, maintains the stem cell pool. Glial cell line-derived neurotrophic factor (GDNF) is a key factor for maintenance of SSC self-renewal. GDNF acts through different signaling pathways to induce target genes that promote SSC self-renewal, such as PI3K/AKT, SFK, and MAPK signaling pathways (Lee et al., 2007; Oatley et al., 2007; Takashima et al., 2015). The well studied GDNF-inducible self-renewal genes include Etv5, Bcl6b, Lhx1, Pou3f1 and Id4. Moreover, there are many GDNF-independent and SSC-derived factors such as PLZF, FOXO1, GILZ and TAF4B that also contribute to regulate the self-renewal of SSC (Song and Wilkinson, 2014).
SHP2 is a non-receptor protein tyrosine phosphatase that is encoded by protein tyrosine phosphatase non-receptor type 11 gene (Ptpn11). A core component of receptor tyrosine kinases (RTKs), cytokines, and G protein-coupled receptor signal transduction, SHP2 shows ubiquitous expression and plays critical roles in cellular growth, survival, proliferation, and migration. In testis, SHP2 played a critical rule for the proliferation and self-renewal of SSC in the process of spermatogenesis (Hu et al., 2015). Meanwhile, SHP2 could support the steroidogenesis in leydig cells leading to testosterone production and maintain the blood testis barrier, which provided a stable environment for spermatogenesis (Puri and Walker, 2016).
Spermatogenesis directly determines the reproductive capacity of male animals. With the development of society, the increasing pressure on people’s lives and changes in the living environment, male fertility is declining (Agarwal et al., 2021). Traditional Chinese medicine (TCM) is commonly used to improve spermatogenesis in China, such as Morindae officinalis Radix (Chen and Wang, 2015), Eucommiae Folium (Fu et al., 2019) Cynomorii Herba (Yang et al., 2010) and Epimedii Folium (Park et al., 2017).
In the monotypic genus Eucommia, Eucommia ulmoides Oliv. is known as Dù-zhòng (Chinese:杜仲), Tuchong (in Japanese), and Chinese rubber tree (Cronquist, 1981). According to the Chinese Pharmacopoeia and the Shennong’s Herbal Classic of Materia Medica, the leaf and bark of this plant have the similar efficacy, as a famous botanical tonics, have been widely used for long time. They can be used alone or mixed with other herbs in the prescription of TCM to treat impotence, spermatorrhoea, prospermia, kidney deficiency pain etc. (He et al., 2014). Ethnopharmacological studies have shown that EFEE could improve the reproductive capacity and the testosterone levels in male rats (Fu et al., 2019). Du zhongkangcha (consist of Eucommiae Folium, Psoraleae Fructus and Lycii Fructus) significantly increased the sexual capacity in male rats (Zhang et al., 1999). Yougui Pill (consist of Radix Rehmanniae Praeparata, Cuscutae Semen, Lycii Fructus, Eucommiae Cortex etc.) could effectively improve the spermatogenic dysfunction in male patients (Qiu, 2020; Zhai et al., 2020). Through network pharmacological analysis, Yougui Pill was involved in the treatment of sexual dysfunction through regulating MAPK signaling pathway (Wang Y. et al., 2019).
EF mainly contains lignans, iridoids, phenylpropanoids and flavonoids. The chemical components include aucubin, eucommiol, pinoresinol, quercetin, rutin, CGA and kaempferol (Zhang et al., 2013). However, the mechanism of its specific compounds, which are involved in spermatogenesis, is not clear. Studies have shown that quercetin could promote the expression of the Star gene, associated with testosterone secretion in the testicular leydig tumour cell line MA-10 (Cormier et al., 2017). Rutin could improve stem cell proliferation by enhancing the phosphorylation of the PI3K/AKT/mTOR signaling pathway (Zhao et al., 2020). Kaempferol promoted stem cell proliferation through the Wnt signaling pathway (Nie et al., 2020). CGA could increase the number of sperm in rat testis (Park and Han, 2013). Moreover, CGA could effectively enhance the expression of the Shp2 gene in stem cells and then promoted the expression of downstream cell proliferation-related genes (Zhou et al., 2016).
The pharmacological action of TCM is mainly related to its chemical components. However, very few studies have investigated the major components of EF associated with spermatogenesis. The uncertainty of chemical components related to spermatogenesis in EF limits its effectiveness in clinical applications. Therefore, the clarification of the main spermatogenic components of EF is of great significance for the further development.
In this study, the main active components related to spermatogenesis in EF and their signaling pathways were predicted by network pharmacology. The C18-4 cell proliferation assay and TM3 cell testosterone secretion assay were used to screen the active compounds in EF, and the active substances that could promote SSC proliferation and leydig cell testosterone secretion were selected. The regulation of spermatogenesis in mice was investigated by detecting the expression of key proteins in the signaling pathways related to SSC proliferation and self-renewal, Leydig cell testosterone secretion and cell proliferation. Our study will provide a theoretical basis for revealing the biochemical mechanism of spermatogenesis regulation by compounds in EF.
Material and Methods
Cell and Culture Materials
C18-4 cells were established by stably transfecting type A spermatogonia from 6-day-old mice with the large T antigen gene under the control of a ponasterone A-driven promoter (Hofmann et al., 2005; He et al., 2009), and they were cultured in DMEM/F12 supplemented with 10% FBS and maintained in a 5% CO2 atmosphere.
TM3 cells were purchased from the American Type Tissue Culture Collection (ATCC, Manassas, VA, United States) and maintained in a 5% CO2 atmosphere in DMEM/F12 (HyClone, United States) supplemented with 2.5% FBS (HyClone, United States), 5% HS (Solarbio Beijing, China) and 1% P/S solution (Solarbio Beijing, China).
Cell Viability Assay
C18-4 cells were cultured in 96-well plates and treated with different concentrations of CGA (Cat. No. YZ-110753, HPLC ≥96.1%), quercetin (Cat. No. SQ8030, HPLC ≥98%), kaempferol (Cat. No. SK8030, HPLC ≥98%) and rutin (Cat. No. SR8250, HPLC ≥98%) (0–100 μM) (Solarbio Beijing, China). Thirty-six hours after treatment, 10 μl of WST-1 (Beyotime Biotechnology, Shanghai, China) solution per well was added and the plate was incubated for 1 h at 37°C. The absorbance of each well was measured at 450 nm by a microplate spectrophotometer. The cell viability was calculated using the following formula: Cell viability= (ODtreated group−ODblank group)/(ODcontrol group−ODblank group).
Extraction and Isolation
EF (voucher specimen number: TCVM-15082501) was collected from Lueyang, Shaanxi Province (33°07′55″N, 105°42′31″E) and stored in the specimen room of Traditional Chinese Veterinary Medicine (TCVM) of the College of Veterinary Medicine. EF powder (160.0851 g) was refluxed for 30 min, at 97°C, with 1600 ml 50% ethanol solution (v/v). After repeated extraction for four times and filtration, the filtrate was concentrated at 90°C and dried to a constant weight by vacuum freeze dryer. The extract was 66.0413 g and the yield of the extract was 41.25% (w/w) (Hou et al., 2016). The content of CGA in EFEE was analysis by HPLC (Supplementary Material).
Animal Experiments and Ethics Statement
All experiments were performed on 5-week-old healthy male KM mice. These mice were purchased from Chengdu DOSSY Experimental Animals Co., Ltd. The mice were housed in wire cages at 25°C under a 12 h light-dark cycle with 70% humidity.
EFEE spermatogenic activity assay. Fifty mice were divided into five groups: a negative control group, three experimental groups and a positive control group. The negative control group was intragastrically administered with water, the experimental groups were intragastrically administered with 0.4, 0.8 and 1.2 g/kg EFEE, and the positive control group was intraperitoneally injected with 5 mg/kg testosterone propionate. Each group of mice was given intragastric administration or injection once a day for 10 days.
CGA spermatogenic activity assay. The experimental method was the same as above. The experimental groups were intragastrically administered with 20, 40 and 80 mg/kg CGA.
After 10 days of administration, the mice were anaesthetized, the serum isolated from blood that was collected through heart punctures, and the testes and epididymides were collected. Testosterone and FSH were detected by ELISA kits (Shanghai Enzyme-Linked Biology Company Shanghai, China) according to the instructions. The testes were carefully removed and fixed with 10% formalin solution. After dehydration procedures, the sections were embedded in paraffin. The tissues were after that cut (with a microtome) to produce 4–5 μm sections, transferred to slides and subsequently stained using the traditional hematoxylin and eosin (H&E) stain. The testicular organ coefficient was calculated using the following formula: Testicular organ coefficient = Testicular weight/Body weight. The sperm were collected from the cauda epididymides. The cauda epididymides were minced with scissors and placed in 5 ml of 0.9% saline at 37°C for 30 min. After filtration, 10 μl aliquots of these mixtures were placed on a hemocytometer, and sperm numbers were determined by counting under an optical microscope (Park and Han, 2013; El-Khadragy et al., 2021).
FACS Analysis of Cell Cycle
For all experiments, logarithmic growth phase C18-4 cells were plated in six-well cell culture plates and treated with 0, 10, 50 and 100 μM CGA for 36 h. Logarithmic growth phase TM3 cells were plated in six-well cell culture plates and treated with 0, 0.5, 1 and 10 μM of CGA for 36 h. Then, the cells were resuspended as single cells, washed in precooled PBS and incubated using a Cell Cycle Kit (LianKeBiology, Hangzhou, China) with 1 ml DNA staining solution and 10 μl permeabilization solution for 30 min. Cell cycle analysis was performed with a flow cytometer (Cao et al., 2012).
Quantitative Real-Time PCR (qRT–PCR)
Total RNA was reverse-transcribed to cDNA using the HiScript III RT SuperMix reverse transcriptase reagent kit according to the reagent manual (DiNing, Beijing, China). qRT–PCR was performed on a CFX96 real-time PCR detection system (Bio–Rad, CA 94547) according to the manual for the ChamQTM Universal SYBR qPCR Master Mix kit (DiNing, Beijing, China). For more details on the qRT–PCR protocol, please refer to Wu et al. (2013). The relative expression levels of target genes and differentially expressed miRNAs were normalized to Gapdh expression for each sample respectively. The relative expression levels were calculated using 2−ΔΔCt (Wu et al., 2014). The verified primers of mRNAs are listed in Supplementary Table S1.
Cell Transfection
TM3 and C18-4 cells were transfected with shRNA (Shp2-mus-405: 5ʹ-GCTGAACTGGTTCAGTATTACTTCA AGAGAGTAATACTGAACCAGTTCAGCTT-3ʹ) and NC (5ʹ-TTCTCCGAACGTGTCACGTTTCAAGAGAAC GTGACACGTTCGGAGAATT-3ʹ) (Genepharma Co., Shanghai, China) in a 24-well plate. After 24 h, two kinds of transfected cell were respectively treated with G418 (400 μg/ml) and hygromycin (100 μg/ml). The stably transfected cell clusters were screened by drugs and cultured. These cells were detected by qRT–PCR and Western blot.
Immunofluorescence Staining
The protocol of immunofluorescence staining was performed according to Yu et al. (2014). Briefly, C18-4 cells and Shp2 knockdown cells, which were cultured in a 48-well plate after treatment with CGA, were fixed with 4% formaldehyde for 15 min and washed with PBS three times for 3 min each. The cells were permeabilized with by 0.1% Triton X-100 (Solarbio, Beijing, China) for 15 min and blocked for 30 min with 1% BSA at 37°C. Then, the cells were incubated with primary antibodies specific against PLZF (1:200, Santa Cruz Biotechnology, California), SHP2 (1:100, Abways, Shanghai, China) and C-KIT (1:300, Biolegend, San Diego) for 12 h at 4°C. Alexa-488 (1:500, Beyotime Biotechnology, Shanghai, China) secondary antibodies were used to incubate cells for 1 h at 37°C. The negative control was stained with conjugated secondary antibodies alone: goat anti-rabbit IgG and goat anti-mouse IgG. The nuclei of cells were stained with DAPI (Beyotime Biotechnology, Shanghai, China) (Niu et al., 2016).
Western Blot Analysis
The cells were treated with different doses of CGA for 36 h. The proteins were extracted using a protein extraction reagent kit (Solarbio, Beijing, China). Protein quantification was performed using a BCA quantification kit (Solarbio Beijing, China). 10% separation gel was prepared. Each well was loaded with 30 μg of the protein. The denatured proteins were separated by SDS–PAGE (80 V, 30 min; 120 V, 60 min) and transferred onto PVDF membranes (80 V, 135 min). The membranes were blocked for 2 h at 37°C in TBST containing 5% skim milk and then incubated for 12 h at 4°C in TBST containing specific primary antibodies (SHP2, ERK1/2, pERK1/2, StAR, or β-actin, 1:1000) (Abways, Shanghai, China). After four washes with TBST, the membranes were incubated with HRP-conjugated secondary antibody (Solarbio Beijing, China) for 1.5 h at 37°C. After washing the membranes with TBST three times, the signals were visualized using ECL (DiNing, Beijing, China), and the membranes were exposed on X-ray films. The quantification of protein bands were analyzed by ImageJ version 1.51.
Network Pharmacology Analysis
The main components and their related genes in EF were collected by summarizing the research work on chemical components of EF (He et al., 2014; Wang C.Y. et al., 2019) and searching TCMSP (Ru et al., 2014) (http://tcmspnw.com), Pharm Mapper (Wang et al., 2017) (http://www.lilab-ecust.cn/pharmmapper/) and other traditional Chinese medicine components databases. The selected genes were analysed by literature research (Paz et al., 2016; Puri and Walker, 2016; Zhou et al., 2016), Kegg (Kanehisa et al., 2017) (https://www.kegg.jp/kegg/) and KOBAS (http://kobas.cbi.pku.edu.cn/) enrichment to investigate the possible biological functions of the potential targets and the biological pathways involved in spermatogenesis. Cytoscape version 3.5.1 was used to draw the network diagram of “component-target-pathway-function” (Shannon et al., 2003; Killcoyne et al., 2009) (Supplementary Material).
Statistical Analysis
Data analysis was performed using IBM SPSS statistical software (version 23.0). One-way analysis of variance with a post hoc test was used for multiple comparisons. The histograms were drawn using GraphPad Prism 7. The results were considered significant or extremely significant at a level of p < 0.05 or p < 0.01.
Results
The Effects of EF Extracts on Spermatogenesis in Mice
As shown in Figures 1A,B, compared with the control group, the serum testosterone and FSH levels of 0.8 and 1.2 g/kg EFEE groups were significantly increased (p < 0.01, p < 0.05). Similarly, the testicular tissue coefficient of mice in the medium-dose groups was significantly higher (p < 0.05) than that in the control group (Figure 1C). The sperm count of the 0.8 and 1.2 g/kg EFEE groups were higher (p < 0.05) than that in the control group (Figures 1D,E). Therefore, EFEE could promote the secretion of spermatogenic hormones and sperm production in mice.
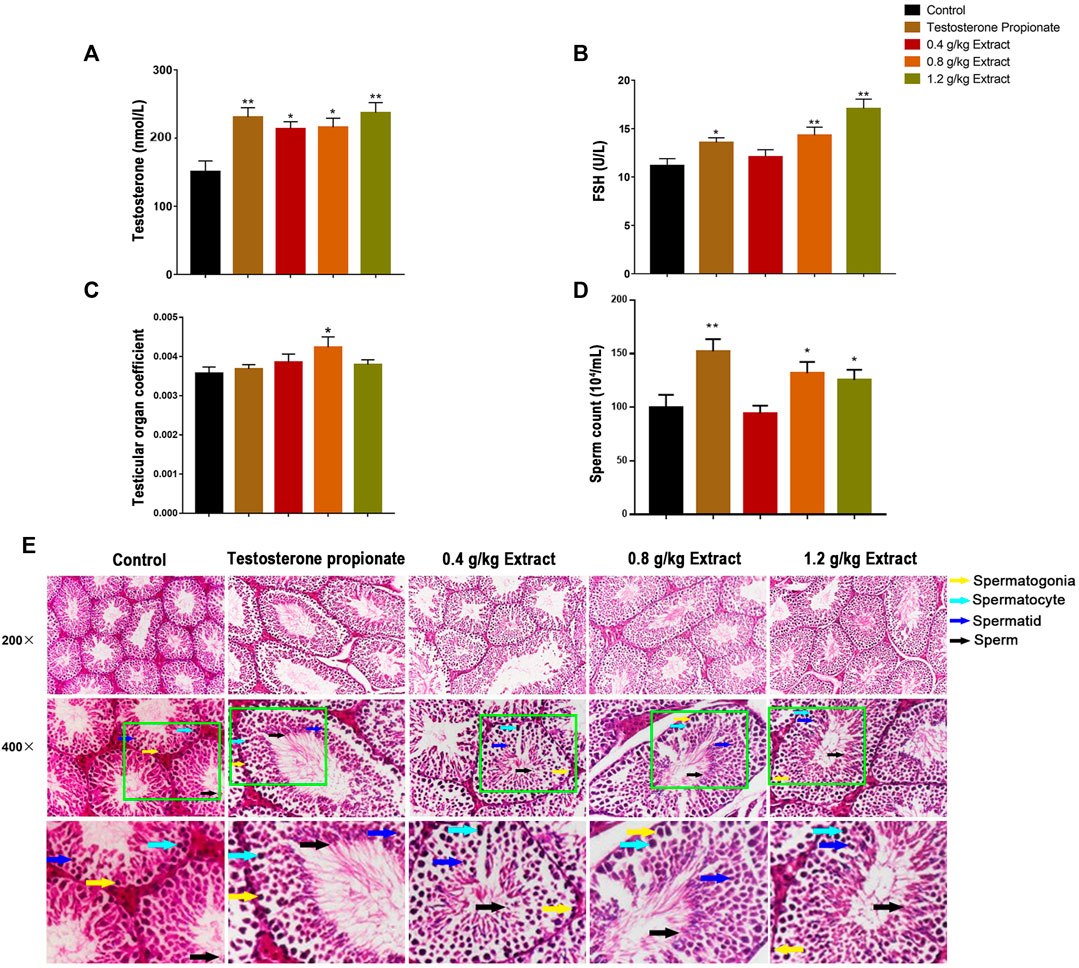
FIGURE 1. The effects of EF extracts on spermatogenesis in mice (Control: water; Testosterone Proplonate: 5 mg/kg; Extracts: 0.4, 0.8, 1.2 g/kg). (A) The concentration of serum testosterone was analysed by ELISA. (B) The concentration of serum FSH was analysed by ELISA. (C) Testicular organ coefficient analysis of testes. (D) The sperm count analysis of epididymis. (E) Haematoxylin and eosin (HE) staining analysis of testes treated with different doses of EFEE. Values are expressed as the mean ± SD (n = 10).*p < 0.05, **p < 0.01 vs control.
Network Pharmacological Analysis of EF Active Components and Their Mechanism
Through network pharmacological analysis, chemical compounds in EF related to spermatogenesis mainly involved MAPK, RAS, PI3K-Akt and regulating the pluripotency of the stem cell signaling pathway. These signaling pathways were involved in the biological processes of SSC proliferation, self-renewal and Leydig cell testosterone secretion. The corresponding compounds were screened to obtain quercetin, kaempferol, CGA and rutin. The targets of these compounds were mainly concentrated in four signaling pathways (Figure 2A). These results suggested that quercetin, kaempferol, CGA and rutin were the main active components in EF that may be involved in spermatogenesis.
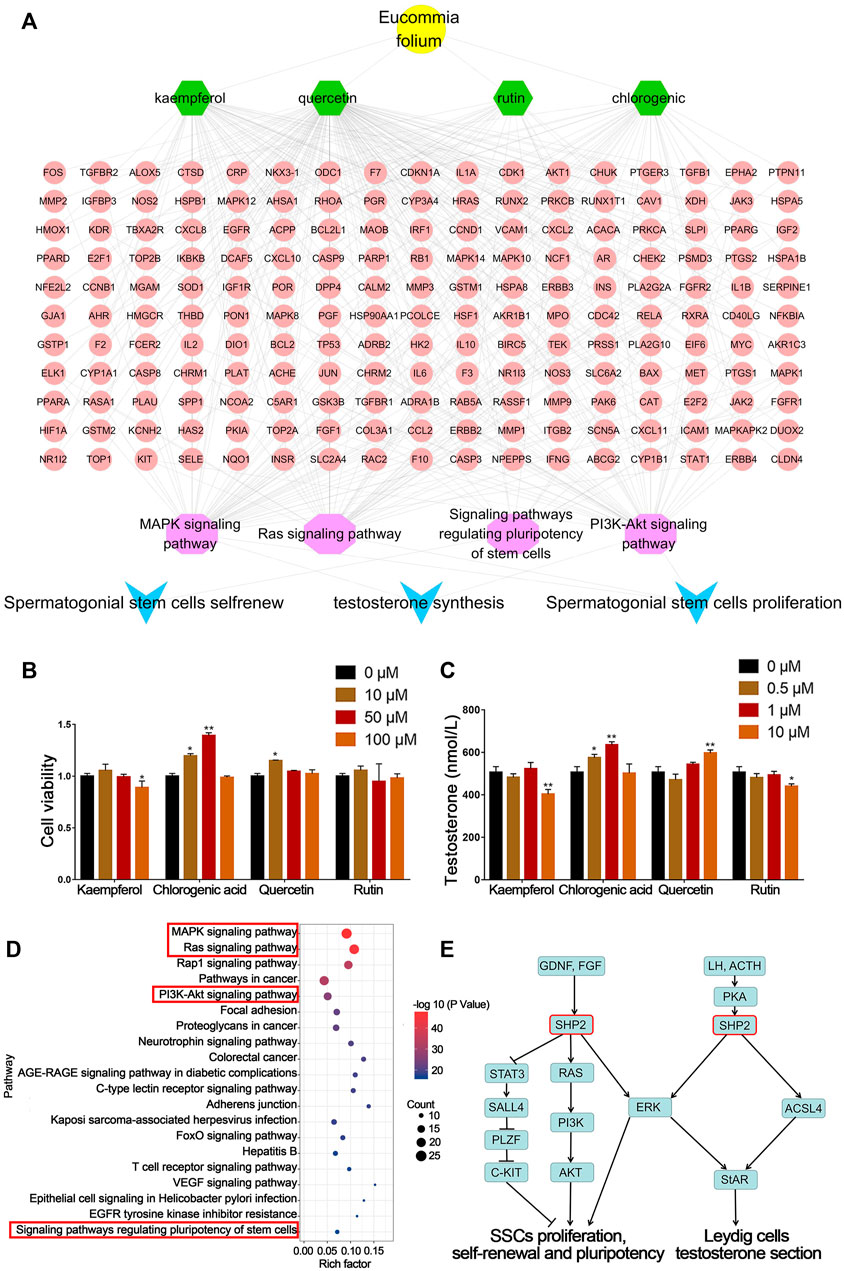
FIGURE 2. Network pharmacology prediction and validation of spermatogenesis related compounds in EF. (A) Network pharmacology analysis of EF active compounds and their mechanism. (B) C18-4 cell viability. (C) Testosterone secretion in TM3 cells. (D,E) KEGG pathway analysis of putative target genes of CGA. Values are expressed as the mean ± SD (n = 3). *p < 0.05, **p < 0.01 vs 0 μM.
Effects of Screened Compounds on Spermatogenesis
As shown in Figure 2B, the four compounds screened above were tested for their biological activities in promoting SSC proliferation and testosterone secretion. Compared with the control group (0 μM), C18-4 cell proliferation in the CGA groups (10 and 50 μM) below their safe concentrations was significantly increased (p < 0.05, p < 0.01). In addition, quercetin treatment (10 μM) also significantly promoted cell proliferation (p < 0.05).
As shown in Figure 2C, the CGA (0.5 and 1 μM) and quercetin (10 μM) treatment groups also significantly enhanced (p < 0.01, p < 0.05) testosterone secretion in TM3 cells, and the CGA groups exhibited stronger activity at low concentrations. The content of CGA in EFEE was 2.58% (w/w) by HPLC (Supplementary Figure S1). These results indicated that CGA was the main compound of EFEE and involved in spermatogenesis. Therefore, CGA was selected for further experiments.
The Effects of CGA on Spermatogenesis in Mice
In order to determine the effect of CGA on spermatogenesis in vivo, the mice were intragastrically administered with 20, 40 and 80 mg/kg CGA. As shown in Figures 3A,B, compared with the control group, the serum testosterone and FSH levels in groups treated with 20 and 40 mg/kg of CGA were significantly increased (p < 0.05). Similarly, the testicular tissue coefficient of mice in the medium-dose groups was significantly higher (p < 0.05) than that in the control group (Figure 3C). The sperm count of 20 and 40 mg/kg CGA groups were higher (p < 0.01, p < 0.05) than that in the control group (Figures 3D,E). The results suggested that CGA could promote the secretion of spermatogenic hormones and sperm production in mice.
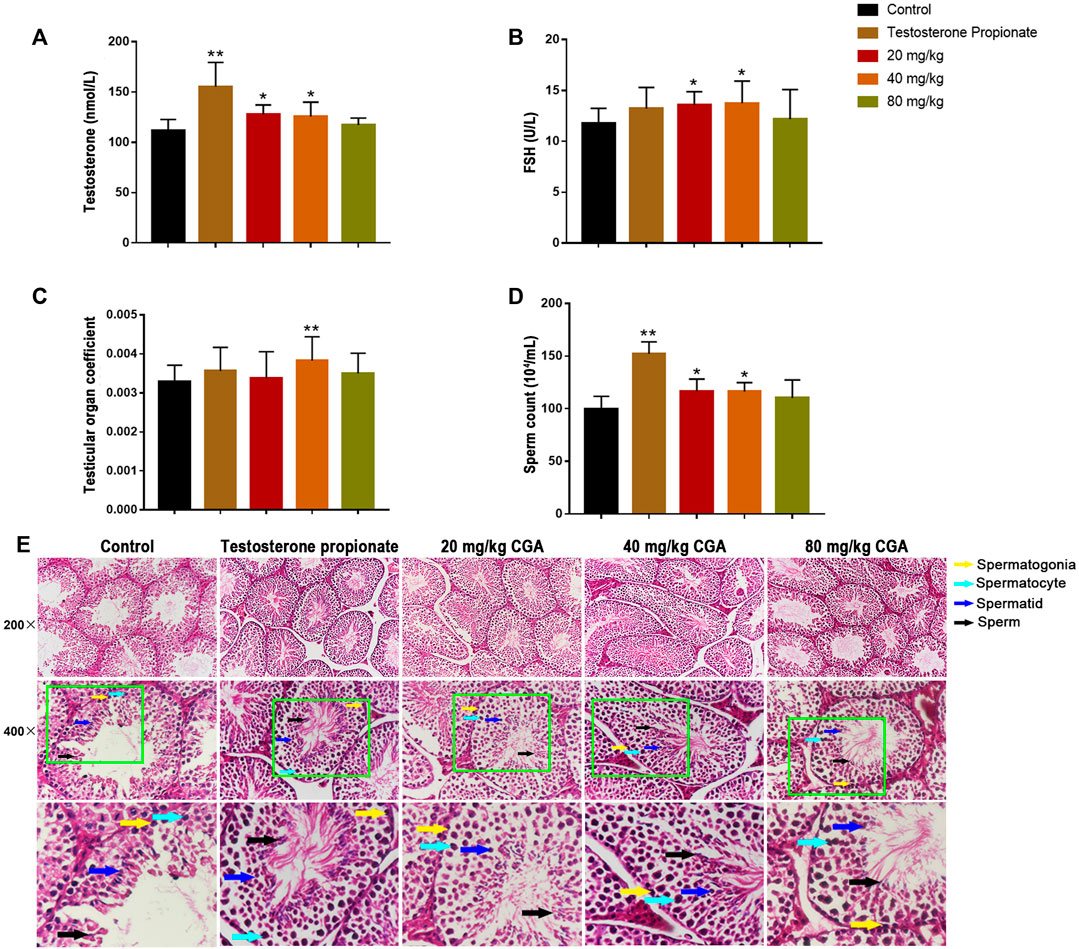
FIGURE 3. The effects of CGA on spermatogenesis in mice (Control: water; Testosterone Proplonate: 5 mg/kg; CGA: 20, 40, 80 mg/kg). (A) The concentration of serum testosterone was analysed by ELISA. (B) The concentration of serum FSH was analysed by ELISA. (C) Testicular organ coefficient analysis of testes. (D) The sperm count analysis of epididymis. (E) Haematoxylin and eosin (HE) staining analysis of testes treated with different doses of EFEE. Values are expressed as the mean ± SD (n = 10).*p < 0.05, **p < 0.01 vs control.
The Signaling Pathways of CGA Regulate Spermatogenesis by Network Pharmacology Analysis
KEGG enrichment analysis showed that CGA might affected the SSC proliferation, self-renewal, and leydig cell testosterone secretion by regulating MAPK, RAS, PI3K-Akt and Pluripotency of stem cells signaling pathways (Figure 2D). As shown in Figure 2E, SHP2 was involved in these signaling pathways above. These results indicated that Shp2 is a key gene in CGA regulation of spermatogenesis. Therefore, the subsequent studies mainly focused on the regulation of CGA on the expression of SHP2 and its downstream genes.
The Effect of CGA on SSC Proliferation
SHP2 is a widely expressed protein tyrosine phosphatase that is necessary for signal transduction from multiple cell surface receptors (Puri and Walker, 2016). Compared with the control group (0 μM), CGA (10 and 50 μM) significantly increased (p < 0.05) the S phase and reduced the G1 phase of the C18-4 cell cycle (Figures 4A,B). This indicated that CGA could enhance the C18-4 cells proliferation. The inhibition of SHP2 expression significantly decreased the proportion of cells in S phase and increased the proportion of cells in G1 phase (p < 0.01). Moreover, CGA had no significant effect on the cell cycle of Shp2 knockdown C18-4 cells. These results suggested that the Shp2 gene plays a key role in the promotion of C18-4 cells proliferation by CGA.
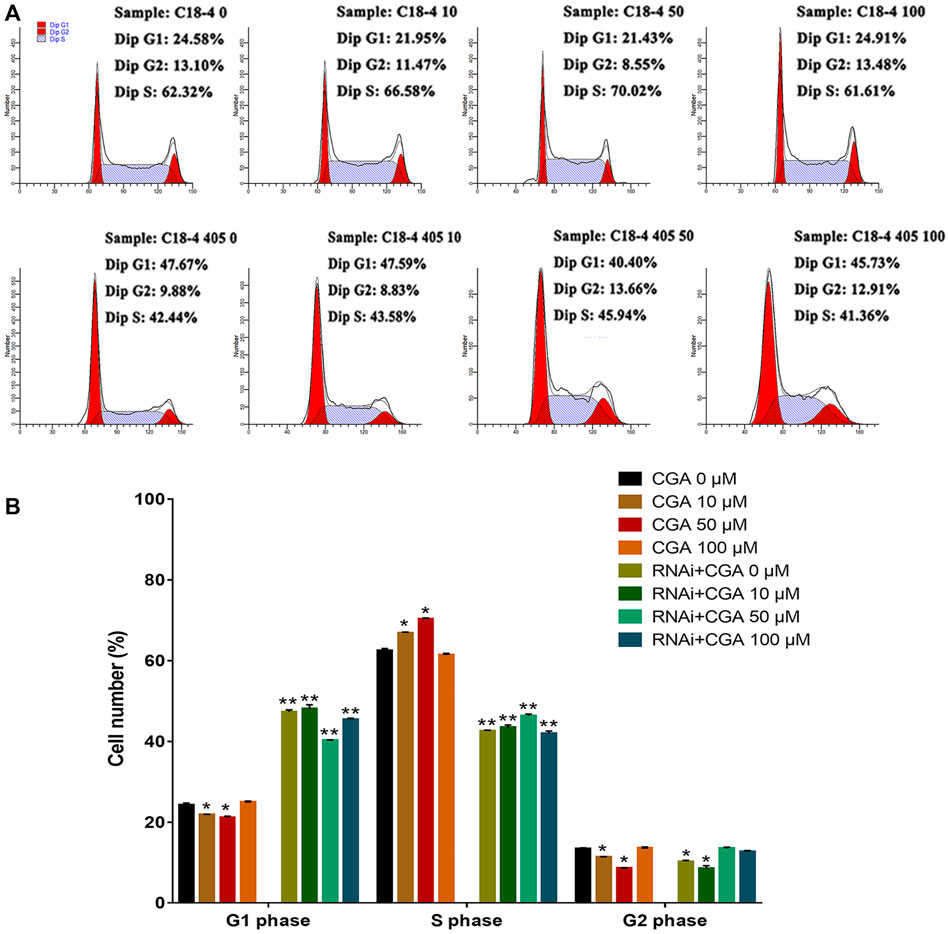
FIGURE 4. The effect of CGA on SSCs proliferation. C18-4 cells and Shp2 knockdown C18-4 cells (RNAi) were cultured with CGA (10, 50, and 100 μM) for 36 h, and the cell cycle was determined using FACS. (A) FACS analysis of the cell cycle. (B) The results of statistical analyses. Values are expressed as the mean ± SD (n = 3)*p < 0.05, **p < 0.01 vs CGA 0 μM.
CGA Activated the SHP2-MAPK Signaling Pathway
The SHP2-MAPK signaling pathway is required for maintaining SSC proliferation and self renewal, and spermatogenesis (Puri et al., 2014). As shown in Figures 5A,B, CGA (1, 10, 20 and 50 μM) treatment induced the SHP2 expression (p < 0.05, p < 0.01) and ERK1/2 phosphorylation (p < 0.05, p < 0.01) in C18-4 cells. After inhibiting Shp2 gene expression, ERK1/2 phosphorylation was significantly reduced compared with that in the control group (0 μM). Meanwhile, CGA could not significantly promote ERK1/2 phosphorylation in Shp2 knockdown cells. These results indicated that CGA could induce SHP2-MAPK signaling pathway activation in SSCs.
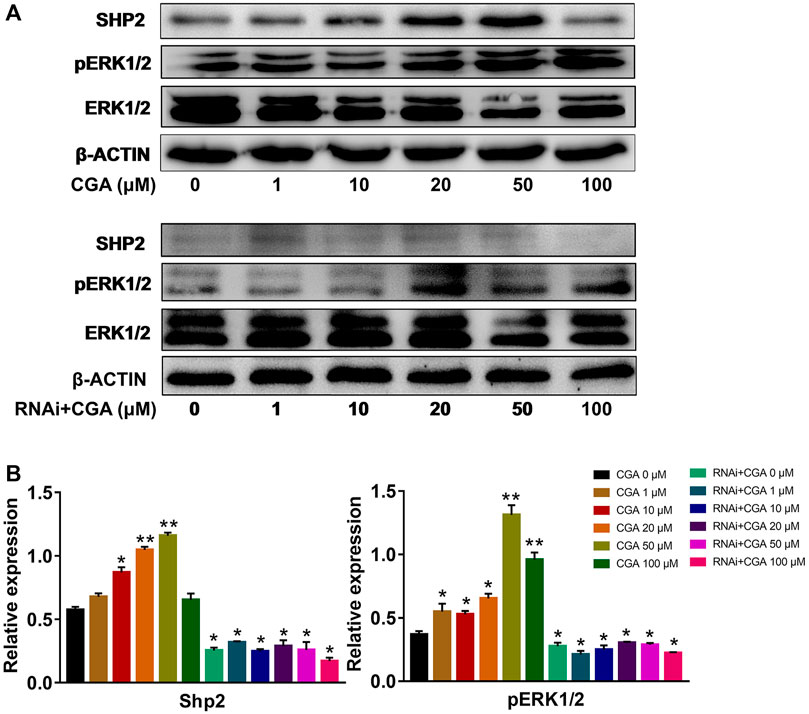
FIGURE 5. CGA activated SHP2-MAPK signaling pathway. C18-4 cells and Shp2 knockdown C18-4 cell (RNAi) were cultured with CGA (1, 10, 20, 50 and 100 μM) for 36 h, and gene expression was determined using Western blotting. (A) Western blot analysis of the effect of CGA on the SHP2-MAPK signaling pathway. (B) The results of statistical analyses. Values are expressed as the mean ± SD (n = 3) *p < 0.05, **p < 0.01 vs CGA 0 μM.
The Effect of CGA on SSC Self-Renewal
SSCs play an essential role in maintaining highly productive spermatogenesis by self-renewal and the continuous generation of daughter spermatogonia that differentiate into spermatozoa, transmitting genetic information to the next generation (Subash and Kumar, 2021). GFRA1 and PLZF are required for the regulation of SSC self-renewal (Buaas et al., 2004; Costoya et al., 2004), and C-KIT is a critical factor in SSC differentiation (Zhang et al., 2014). As shown in Figures 6A,B, the C18-4 cells treated with CGA (10 and 50 μM) induced the expression of SHP2 and PLZF, but decreased C-KIT expression (p < 0.05, p < 0.01). The inhibition of SHP2 expression reduced PLZF expression and promoted C-KIT expression (p < 0.05, p < 0.01). These results suggested that CGA could promote the self-renewal of C18-4 cells and maintain their homeostasis.
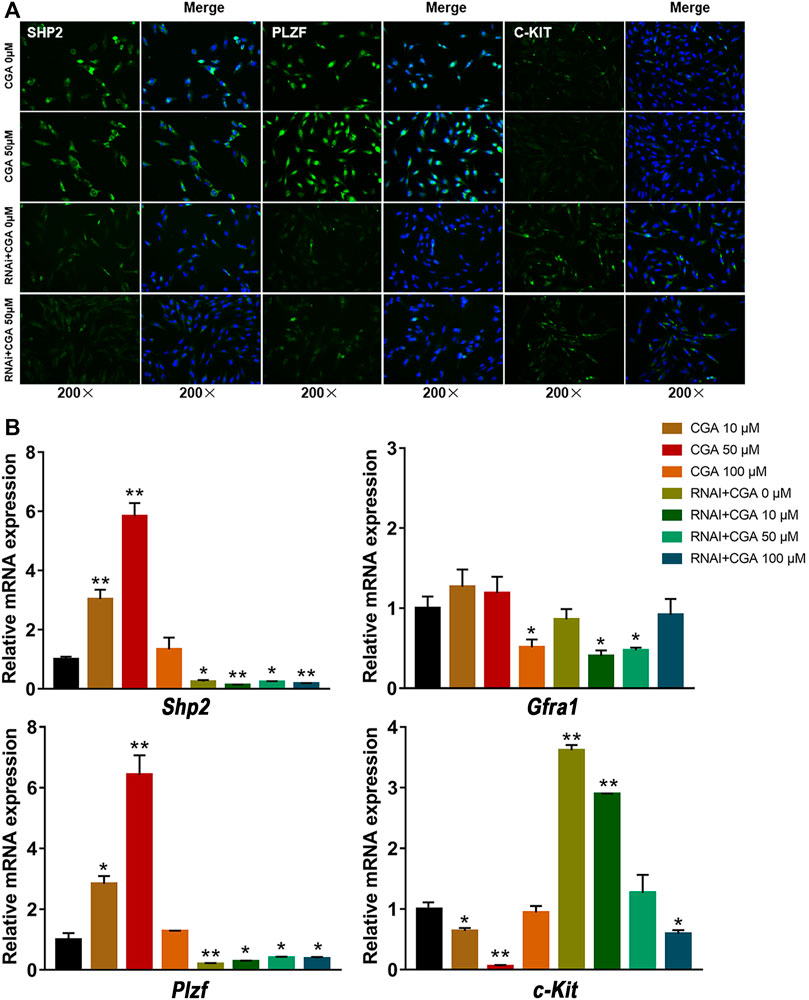
FIGURE 6. The effect of CGA on SSC self-renewal. C18-4 cells and Shp2 knockdown C18-4 cells (RNAi) were cultured with CGA (10, 50, and 100 μM) for 36 h, and gene expression was measured by immunofluorescence immunofluorescence staining and qRT–PCR. (A) Immunofluorescence staining analysis of the effect CGA on SHP2, PLZF and C-KIT expression. (B) qRT–PCR analysis of the effect of CGA on Shp2, Plzf, c-Kit and Gfra1 expression. Values are expressed as the mean ± SD (n = 3) *p < 0.05, **p < 0.01 vs CGA 0 μM.
Effect of CGA on Testosterone Secretion in Leydig Cells
Compared with the control group, CGA (0.5 and 1 μM) effectively promoted testosterone secretion in TM3 cells (p < 0.01). Testosterone secretion in TM3 cells was significantly reduced after the Shp2 gene was inhibited (p < 0.05) (Figure 7C). Furthermore, CGA (0.5 and 1 μM) also significantly increased the proportion of TM3 cells in the S phase of the cell cycle (p < 0.01, p < 0.05). The knockdown of Shp2 gene expression significantly reduced the proportion of cells in S phase compared with that in the control group (p < 0.01, p < 0.05) (Figures 7A,B). These results suggested that CGA could promote testosterone secretion and proliferation in TM3 cells.
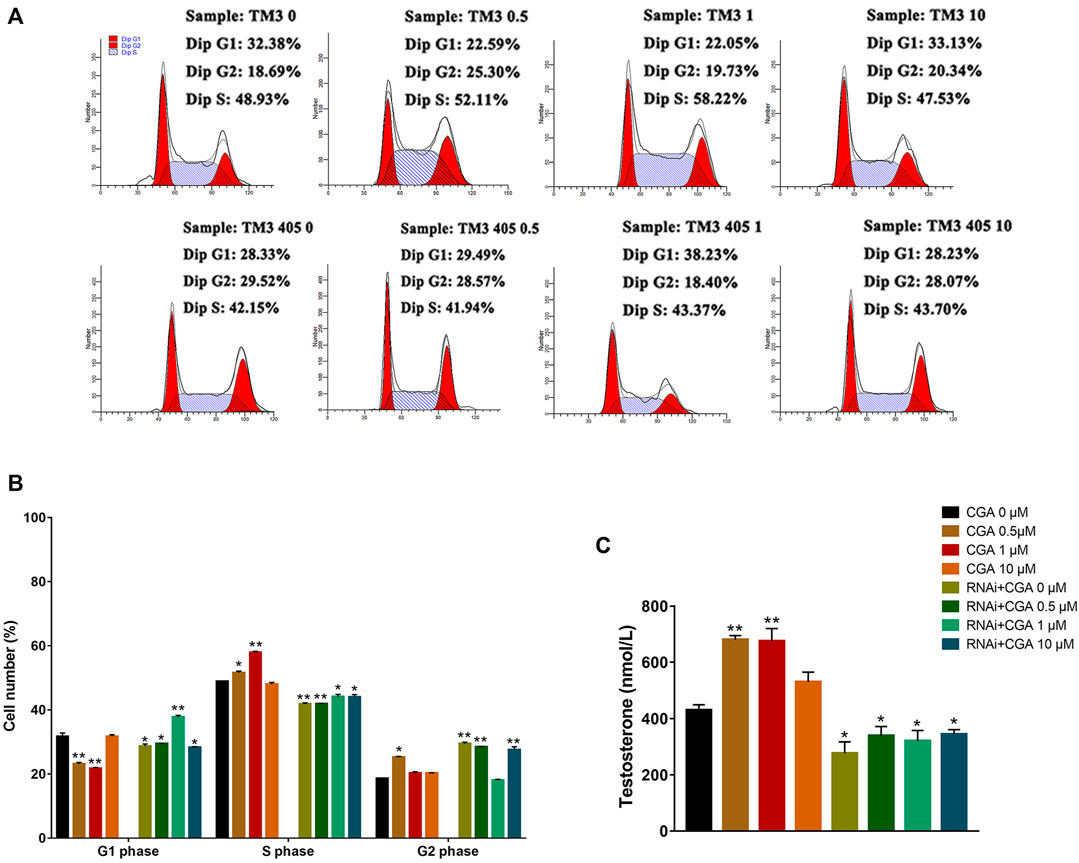
FIGURE 7. Effect of CGA on testosterone secretion in leydig cells. TM3 cells and Shp2 knockdown TM3 cells (RNAi) were cultured with CGA (0.5, 1, and 10 μM) for 36 h; the cell cycle was measured by FACS, and testosterone secretion was measured by ELISA. (A) FACS analysis of the cell cycle. (B) The results of statistical analyses. (C) ELISA analysis of the effect of CGA on testosterone secretion. Values are expressed as the mean ± SD (n = 3). *p < 0.05, **p < 0.01 vs CGA 0 μM.
CGA Increased the Expression of StAR
SHP2 regulates the expression of the downstream Star gene, which is a rate-limiting enzyme involved in testosterone secretion in leydig cells (Cooke et al., 2011). As shown in Figures 8A,B, CGA (0.5 and 1 μM) induced SHP2 expression (p < 0.01) and promoted StAR expression in TM3 cells (p < 0.01). The inhibition of Shp2 gene expression significantly reduced the expression of StAR compared with that in the control group (p < 0.01, p < 0.05), and CGA did not promote Star gene expression in Shp2 knockdown TM3 cells. These results suggested that CGA could promote the testosterone secretion by improving the expression of SHP2 and StAR.
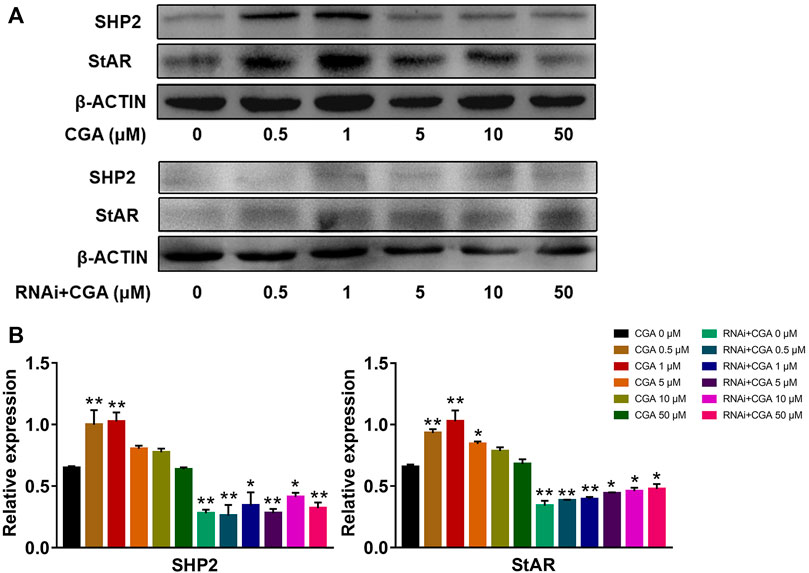
FIGURE 8. CGA activated the SHP2-StAR signaling pathway. TM3 cells and Shp2 knockdown TM3 cells (RNAi) were cultured with CGA (0.5, 1, 5, 10 and 50 μM) for 36 h, and gene expression was measured by Western blot. (A) Western blot analysis of the effect of CGA on the SHP2-StAR signaling pathway. (B) The results of statistical analyses. Values are expressed as the mean ± SD (n = 3). *p < 0.05, **p < 0.01 vs CGA 0 μM.
Discussion
The current study demonstrated that EFEE could promote the spermatogenesis and serum testosterone levels in mice. These changes induced by EFEE may be explained by CGA promoting the proliferation of SSCs and testosterone secretion in leydig cells. Moreover, CGA could promote the spermatogenesis and serum testosterone levels in vivo. The promotion of SSC proliferation was induced by the activation of the SHP2-MAPK signaling pathway after treatment with CGA. Meanwhile, CGA significantly enhanced the expression of SHP2 and StAR, and induced testosterone secretion in leydig cells.
Spermatogenesis is a very complicated process that requires the involvement of a variety of cells, hormones, paracrine factors, genes and epigenetic regulators (Neto et al., 2016). As the origin of spermatogenesis, SSCs were located at the base of seminiferous tubules in the testes of male animals. Most SSCs die during the process of spermatogenesis, but one SSC can still produce hundreds of sperm (Kanatsu-Shinohara et al., 2019), so hundreds of millions of sperm can be found in the testis (Law et al., 2019). The development of SSCs into mature sperm is regulated by a variety of proteins and hormones. Testosterone, which is secreted by leydig cells in the testis, is required for at least four critical processes during spermatogenesis: meiosis, sertoli-spermatid adhesion, maintenance of the BTB and sperm release (Smith and Walker, 2014). These studies indicate that the proliferation of SSCs and testosterone secretion in leydig cells are critical processes in spermatogenesis. Therefore, the SSC proliferation and testosterone secretion were selected as the conditions for studying the active spermatogenic compounds of EF.
In recent years, network pharmacology has been widely used in the mechanism of TCM research as a method to predict compounds of TCM and their targets (Luo et al., 2020). In order to investigate the mechanism of which EF promotes spermatogenesis, network pharmacology was used to predict the active compounds in EF. CGA, quercetin, rutin, and kaempferol in EF were predicted to be related to SSC proliferation and testosterone secretion, and the main component CGA was screened to have strong activity. Meanwhile, combined with previous literature reports (Paz et al., 2016; Puri and Walker, 2016; Zhou et al., 2016) and the network pharmacology analysis showed that Shp2 might be a key gene related in the CGA regulation of spermatogenesis. Furthermore, HPLC result showed that the content of CGA in EFEE was more than 2.58% (w/w) (Supplementary Figure S1). These results indicate that CGA was the main active component of EFEE in promoting spermatogenesis. Previous studies showed that CGA could increase the number of sperm in rat testis, moreover, CGA abated tamoxifen-mediated reproductive toxicities and improved the testosterone secretion and sperm motility in male rats (Park and Han, 2013; Owumi et al., 2021). These studies indicate that CGA could promote the spermatogenesis, and our study was consistent with results above. CGA can not only promote the proliferation of various cells but also inhibit the apoptosis of cells (Zhang and Hu, 2016; Liu and Li, 2021). These biological functions of CGA play an important role in spermatogenesis.
In germ cells of the testis, Src homology phosphotyrosyl phosphatase 2 (SHP2) is expressed in most immature A-single spermatogonia (As, SSC) and A-aligned (Aal) spermatogonia cells (Puri and Walker, 2016). Studies have shown that spermatogenic cells at all levels of the tubules were absent after Shp2 knockout. This result suggested that SHP2 is essential for SSCs to maintain fertility (Hu et al., 2015; Puri et al., 2014). Moreover, SHP2 could also promote the activation of the MAPK signaling pathway during SSC proliferation (Li et al., 2020). CGA, the main active compound in EF, could promote the expression of the Shp2 gene (Zhou et al., 2016). The results of the present study were consistent with these studies. In addition, the phosphorylation level of ERK1/2 in SSCs treated with CGA was significantly increased and further promoted their proliferation. After Shp2 knockdown, ERK1/2 phosphorylation was significantly reduced and cell proliferation was inhibited. Moreover, CGA had no significant effect on ERK1/2 phosphorylation or the cell cycle in the Shp2 knockdown SSCs. These results suggested that CGA could enhance the phosphorylation of ERK1/2 by promoting Shp2 expression and further accelerate SSC proliferation.
Approximately 35,000 SSCs are present in a mouse testis, comprising approximately about 0.035% of all germ cells (Tegelenbosch and de Rooij, 1993). In rodents, stem cell activity is exhibited in a subpopulation of undifferentiated spermatogonia that are present as As. As cells can divide to produce separated As cells that retain stem cell activity, or they can divide to produce pairs (Apr) and undifferentiated Aal spermatogonia that increasingly lose stem cell activity (Nakagawa et al., 2010; Hara et al., 2014). Aal cells proliferate further by mitotic divisions and enter a differentiation program that results in the formation of preleptotene spermatocytes that undergo meiosis to produce haploid round spermatids that mature and elongate to form spermatozoa. Markers of undifferentiated spermatogonia that are conserved from rodents to nonhuman primates to humans include GFRa1, UTF1, PLZF, SALL4 and LIN28. C-KIT is a conserved marker of differentiated spermatogonia (Fayomi and Orwig, 2018). In the present study, we detected the differentiation of SSCs by analysing mRNAs and proteins of the marker genes Gfra1, Plzf and c-Kit. The result indicated that, in both of mRNA and protein levels, CGA could promote the expression of PLZF and that C-KIT was inhibited. After Shp2 knockdown, the PLZF expression was significantly decreased and C-KIT was promoted. These results suggested that CGA inhibits SSC differentiation and maintains self-renewal by regulating SHP2.
As one of the important sex hormones in mammals, testosterone plays an important role in maintaining the normal reproductive function of males. Testosterone affects sex differentiation in the embryonic stage after initial oestrus, regulates spermatogenesis and maintains male secondary sexual characteristics (Smith and Walker, 2014; Skurikhin et al., 2017; Rey, 2021). StAR assists cholesterol in entering the mitochondria and this process is a rate-limiting step in testosterone secretion (Hasegawa et al., 2000). In this study, CGA induced the expression of SHP2 and StAR in leydig cells and further enhanced testosterone secretion. After Shp2 gene knockdown, CGA did not promote StAR expression or testosterone secretion. In conclusion, CGA could promote the expression of StAR through SHP2 and further enhance the transport of cholesterol to improve the secretion of testosterone.
By flow cytometry, we found that CGA could promote the proliferation of leydig cells, while cell proliferation was inhibited with the knockdown of Shp2. These results indicated that the effect of CGA on promoting the proliferation of leydig cells were related to the Shp2 gene, but the specific mechanism is still unclear, and is worthy of further study.
Conclusion
In this study, we found that EF could promote the spermatogenesis of mice in vivo. Furthermore, network pharmacological prediction and bioactivity screening were applied to obtain the main compounds that could promote spermatogenesis. In subsequent studies, CGA, one of the main spermatogenic active compounds of EF, could promoted spermatogenesis in vivo, and promote SSC proliferation and Leydig cell testosterone secretion through Shp2 gene-mediated corresponding signaling pathways. Our study provided strong evidence for elucidating the mechanism by which EF promotes spermatogenesis in mice and a new theoretical basis for dealing with the decrease in male reproductive capacity.
Data Availability Statement
The original contributions presented in the study are included in the article/Supplementary Material, further inquiries can be directed to the corresponding author.
Ethics Statement
The animal study was reviewed and approved by Animal Ethical and Welfare Committee, Northwest A&F University, P.R. China.
Author Contributions
HM performed the experiments, wrote the manuscript, and analyzed the data; SL and ST contributed to the plant extraction; BC and ZL contributed to the medicinal plant collection; YF and YL contributed to cell experiments; XS and WM supervised and reviewed the manuscript; MF and WZ helped to use equipment and instruments. All authors approved the final manuscript.
Funding
This work was supported by the Special Fund for Basic Research of the Ministry of Science and Technology, China (grant number 2013FY110600).
Conflict of Interest
The authors declare that the research was conducted in the absence of any commercial or financial relationships that could be construed as a potential conflict of interest.
Publisher’s Note
All claims expressed in this article are solely those of the authors and do not necessarily represent those of their affiliated organizations, or those of the publisher, the editors and the reviewers. Any product that may be evaluated in this article, or claim that may be made by its manufacturer, is not guaranteed or endorsed by the publisher.
Acknowledgments
We are grateful to the staff at the Institute of TCVM of Northwest A&F University for their experimental assistance.
Supplementary Material
The Supplementary Material for this article can be found online at: https://www.frontiersin.org/articles/10.3389/fphar.2022.851930/full#supplementary-material
References
Agarwal, A., Baskaran, S., Parekh, N., Cho, C. L., Henkel, R., Vij, S., et al. (2021). Male Infertility. Lancet 397 (10271), 319–333. doi:10.1016/S0140-6736(20)32667-2
Buaas, F. W., Kirsh, A. L., Sharma, M., Mclean, D. J., Morris, J. L., Griswold, M. D., et al. (2004). Plzf Is Required in Adult Male Germ Cells for Stem Cell Self-Renewal. Nat. Genet. 36 (6), 647–652. doi:10.1038/ng1366
Cao, H., Chu, Y., Lv, X., Qiu, P., Liu, C., Zhang, H., et al. (2012). GSK3 Inhibitor-BIO Regulates Proliferation of Immortalized Pancreatic Mesenchymal Stem Cells (iPMSCs). PLoS One 7 (2), e31502. doi:10.1371/journal.pone.0031502
Chen, T. J., and Wang, W. (2015). Morinda Officinalis Extract Repairs Cytoxan-Impaired Spermatogenesis of Male Rats. Zhonghua Nan Ke Xue 21 (5), 436–442. (In Chinese). doi:10.13263/j.cnki.nja.2015.05.011
Clark, B. J. (2016). ACTH Action on StAR Biology. Front. Neurosci. 10, 547. doi:10.3389/fnins.2016.00547
Cooke, M., Mele, P., Maloberti, P., Duarte, A., Poderoso, C., Orlando, U., et al. (2011). Tyrosine Phosphatases as Key Regulators of StAR Induction and Cholesterol Transport: SHP2 as a Potential Tyrosine Phosphatase Involved in Steroid Synthesis. Mol. Cell Endocrinol. 336 (1-2), 63–69. doi:10.1016/j.mce.2010.11.030
Cormier, M., Ghouili, F., Roumaud, P., Martin, L. J., and Touaibia, M. (2017). Influence of Flavonols and Quercetin Derivative Compounds on MA-10 Leydig Cells Steroidogenic Genes Expressions. Toxicol. Vitro 44, 111–121. doi:10.1016/j.tiv.2017.06.027
Costoya, J. A., Hobbs, R. M., Barna, M., Cattoretti, G., Manova, K., Sukhwani, M., et al. (2004). Essential Role of Plzf in Maintenance of Spermatogonial Stem Cells. Nat. Genet. 36 (6), 653–659. doi:10.1038/ng1367
Cronquist, A. (1981). An Integrated System of Classification of Flowering Plants. New York, New York, USA: Columbia University Press.
Dubé, E., and Cyr, D. G. (2012). The Blood-Epididymis Barrier and Human Male Fertility. Adv. Exp. Med. Biol. 763, 218–236. doi:10.1007/978-1-4614-4711-5_11
Ehmcke, J., and Schlatt, S. (2006). A Revised Model for Spermatogonial Expansion in Man: Lessons from Non-human Primates. Reproduction 132 (5), 673–680. doi:10.1530/rep.1.01081
El-Khadragy, M. F., Al-Megrin, W. A., Alomar, S., Alkhuriji, A. F., Metwally, D. M., Mahgoub, S., et al. (2021). Chlorogenic Acid Abates Male Reproductive Dysfunction in Arsenic-Exposed Mice via Attenuation of Testicular Oxido-Inflammatory Stress and Apoptotic Responses. Chem. Biol. Interact 333, 109333. doi:10.1016/j.cbi.2020.109333
Fayomi, A. P., and Orwig, K. E. (2018). Spermatogonial Stem Cells and Spermatogenesis in Mice, Monkeys and Men. Stem Cel Res 29, 207–214. doi:10.1016/j.scr.2018.04.009
Fu, H., Bai, X., Le, L., Tian, D., Gao, H., Qi, L. X., et al. (20192019). Eucommia Ulmoides Oliv. Leaf Extract Improves Erectile Dysfunction in Streptozotocin-Induced Diabetic Rats by Protecting Endothelial Function and Ameliorating Hypothalamic-Pituitary-Gonadal Axis Function. Evid. Based Complement. Alternat Med. 2019, 1782953. doi:10.1155/2019/1782953
Hara, K., Nakagawa, T., Enomoto, H., Suzuki, M., Yamamoto, M., Simons, B. D., et al. (2014). Mouse Spermatogenic Stem Cells Continually Interconvert between Equipotent Singly Isolated and Syncytial States. Cell Stem Cell 14 (5), 658–672. doi:10.1016/j.stem.2014.01.019
Haseeb, A., Bai, X., Vistro, W. A., Tarique, I., Chen, H., Yang, P., et al. (2019). Characterization of In Vivo Autophagy during Avian Spermatogenesis1. Poult. Sci. 98 (10), 5089–5099. doi:10.3382/ps/pez320
Hasegawa, T., Zhao, L., Caron, K. M., Majdic, G., Suzuki, T., Shizawa, S., et al. (2000). Developmental Roles of the Steroidogenic Acute Regulatory Protein (StAR) as Revealed by StAR Knockout Mice. Mol. Endocrinol. 14 (9), 1462–1471. doi:10.1210/mend.14.9.0515
He, X., Wang, J., Li, M., Hao, D., Yang, Y., Zhang, C., et al. (2014). Eucommia Ulmoides Oliv.: Ethnopharmacology, Phytochemistry and Pharmacology of an Important Traditional Chinese Medicine. J. Ethnopharmacol 151 (1), 78–92. doi:10.1016/j.jep.2013.11.023
He, Z., Jiang, J., Kokkinaki, M., and Dym, M. (2009). Nodal Signaling via an Autocrine Pathway Promotes Proliferation of Mouse Spermatogonial Stem/progenitor Cells through Smad2/3 and Oct-4 Activation. Stem Cells 27 (10), 2580–2590. doi:10.1002/stem.198
Hofmann, M. C., Braydich-Stolle, L., Dettin, L., Johnson, E., and Dym, M. (2005). Immortalization of Mouse Germ Line Stem Cells. Stem Cells 23 (2), 200–210. doi:10.1634/stemcells.2003-0036doi:10.1634/stemcell.2003-0036
Hou, W. F., Guo, C., Fan, Y. P., Ma, W. R., and Song, X. P. (2016). Response Surface Methodology Was Used to Optimize the Preparation Process of Eucommiae Folium Extract. Prog. Vet. Med. 37 (11), 74–77. doi:10.16437/j.cnki.1007-5038.2016.11.015
Hu, X., Tang, Z., Li, Y., Liu, W., Zhang, S., Wang, B., et al. (2015). Deletion of the Tyrosine Phosphatase Shp2 in Sertoli Cells Causes Infertility in Mice. Sci. Rep. 5, 12982. doi:10.1038/srep12982
Huhtaniemi, I. (2018). Mechanisms in Endocrinology: Hormonal Regulation of Spermatogenesis: Mutant Mice Challenging Old Paradigms. Eur. J. Endocrinol. 179 (3), R143–R150. doi:10.1530/EJE-18-0396
Kanatsu-Shinohara, M., Yamamoto, T., Toh, H., Kazuki, Y., Kazuki, K., Imoto, J., et al. (2019). Aging of Spermatogonial Stem Cells by Jnk-Mediated Glycolysis Activation. Proc. Natl. Acad. Sci. U S A. 116 (33), 16404–16409. doi:10.1073/pnas.1904980116
Kanehisa, M., Furumichi, M., Tanabe, M., Sato, Y., and Morishima, K. (2017). KEGG: New Perspectives on Genomes, Pathways, Diseases and Drugs. Nucleic Acids Res. 45 (D1), D353–D361. doi:10.1093/nar/gkw1092
Killcoyne, S., Carter, G. W., Smith, J., and Boyle, J. (2009). Cytoscape: a Community-Based Framework for Network Modeling. Methods Mol. Biol. 563, 219–239. doi:10.1007/978-1-60761-175-2_12
Law, N. C., Oatley, M. J., and Oatley, J. M. (2019). Developmental Kinetics and Transcriptome Dynamics of Stem Cell Specification in the Spermatogenic Lineage. Nat. Commun. 10 (1), 2787. doi:10.1038/s41467-019-10596-0
Lee, J., Kanatsu-Shinohara, M., Inoue, K., Ogonuki, N., Miki, H., Toyokuni, S., et al. (2007). Akt Mediates Self-Renewal Division of Mouse Spermatogonial Stem Cells. Development 134 (10), 1853–1859. doi:10.1242/dev.003004
Li, Y., Liu, W. S., Yi, J., Kong, S. B., Ding, J. C., Zhao, Y. N., et al. (2020). The Role of Tyrosine Phosphatase Shp2 in Spermatogonial Differentiation and Spermatocyte Meiosis. Asian J. Androl. 22 (1), 79–87. doi:10.4103/aja.aja_49_19
Liu, Z.-H., and Li, B. (2021). Chlorogenic Acid and β-glucan from highland Barley Grain Ameliorate β-cell Dysfunction via Inhibiting Apoptosis and Improving Cell Proliferation. Food Funct. 12 (20), 10040–10052. doi:10.1039/d1fo01532j
Luo, T. T., Lu, Y., Yan, S. K., Xiao, X., Rong, X. L., and Guo, J. (2020). Network Pharmacology in Research of Chinese Medicine Formula: Methodology, Application and Prospective. Chin. J. Integr. Med. 26 (1), 72–80. doi:10.1007/s11655-019-3064-0
Mclachlan, R. I., O'Donnell, L., Meachem, S. J., Stanton, P. G., de Kretser, D. M., Pratis, K., et al. (2002). Identification of Specific Sites of Hormonal Regulation in Spermatogenesis in Rats, Monkeys, and Man. Recent Prog. Horm. Res. 57, 149–179. doi:10.1210/rp.57.1.149
Nakagawa, T., Sharma, M., Nabeshima, Y., Braun, R. E., and Yoshida, S. (2010). Functional Hierarchy and Reversibility within the Murine Spermatogenic Stem Cell Compartment. Science 328 (5974), 62–67. doi:10.1126/science.1182868
Neto, F. T., Bach, P. V., Najari, B. B., Li, P. S., and Goldstein, M. (2016). Spermatogenesis in Humans and its Affecting Factors. Semin. Cel Dev Biol 59, 10–26. doi:10.1016/j.semcdb.2016.04.009
Nie, F., Zhang, W., Cui, Q., Fu, Y., Li, H., and Zhang, J. (2020). Kaempferol Promotes Proliferation and Osteogenic Differentiation of Periodontal Ligament Stem Cells via Wnt/β-Catenin Signaling Pathway. Life Sci. 258, 118143. doi:10.1016/j.lfs.2020.118143
Niu, B., Wu, J., Mu, H., Li, B., Wu, C., He, X., et al. (2016). miR-204 Regulates the Proliferation of Dairy Goat Spermatogonial Stem Cells via Targeting to Sirt1. Rejuvenation Res. 19 (2), 120–130. doi:10.1089/rej.2015.1719
Oatley, J. M., Avarbock, M. R., and Brinster, R. L. (2007). Glial Cell Line-Derived Neurotrophic Factor Regulation of Genes Essential for Self-Renewal of Mouse Spermatogonial Stem Cells Is Dependent on Src Family Kinase Signaling. J. Biol. Chem. 282 (35), 25842–25851. doi:10.1074/jbc.M703474200
Oduwole, O. O., and Huhtaniemi, I. T. (2014). Feasibility of Male Hormonal Contraception: Lessons from Clinical Trials and Animal Experiments. Curr. Mol. Pharmacol. 7 (2), 109–118. doi:10.2174/1874467208666150126154732
Owumi, S. E., Anaikor, R. A., Arunsi, U. O., Adaramoye, O. A., and Oyelere, A. K. (2021). Chlorogenic Acid Co-administration Abates Tamoxifen-Mediated Reproductive Toxicities in Male Rats: An Experimental Approach. J. Food Biochem. 45 (2), e13615. doi:10.1111/jfbc.13615
Papadopoulos, V., and Miller, W. L. (2012). Role of Mitochondria in Steroidogenesis. Best Pract. Res. Clin. Endocrinol. Metab. 26 (6), 771–790. doi:10.1016/j.beem.2012.05.002
Park, H. J., Koo, Y. K., Park, M. J., Hwang, Y. K., Hwang, S. Y., and Park, N. C. (2017). Restoration of Spermatogenesis Using a New Combined Herbal Formula of Epimedium Koreanum Nakai and Angelica gigas Nakai in an Luteinizing Hormone-Releasing Hormone Agonist-Induced Rat Model of Male Infertility. World J. Mens Health 35 (3), 170–177. doi:10.5534/wjmh.17031
Park, J. S., and Han, K. (2013). The Spermatogenic Effect of Yacon Extract and its Constituents and Their Inhibition Effect of Testosterone Metabolism. Biomol. Ther. (Seoul) 21 (2), 153–160. doi:10.4062/biomolther.2012.093
Payne, A. H., and Hales, D. B. (2004). Overview of Steroidogenic Enzymes in the Pathway from Cholesterol to Active Steroid Hormones. Endocr. Rev. 25 (6), 947–970. doi:10.1210/er.2003-0030
Paz, C., Cornejo Maciel, F., Gorostizaga, A., Castillo, A. F., Maloberti, P. M., et al. (2016). Role of Protein Phosphorylation and Tyrosine Phosphatases in the Adrenal Regulation of Steroid Synthesis and Mitochondrial Function. Front. Endocrinol. (Lausanne) 7, 60. doi:10.3389/fendo.2016.00060
Puri, P., Phillips, B. T., Suzuki, H., Orwig, K. E., Rajkovic, A., Lapinski, P. E., et al. (2014). The Transition from Stem Cell to Progenitor Spermatogonia and Male Fertility Requires the SHP2 Protein Tyrosine Phosphatase. Stem Cells 32 (3), 741–753. doi:10.1002/stem.1572
Puri, P., and Walker, W. H. (2016). The Regulation of Male Fertility by the PTPN11 Tyrosine Phosphatase. Semin. Cel Dev Biol 59, 27–34. doi:10.1016/j.semcdb.2016.01.020
Qiu, G. (2020). Effect of Guipi Decoction and Yougui Pills on Male Sexual Dysfunction. Smart Healthc. 6 (14), 100–101. doi:10.19335/j.cnki.2096-1219.2020.14.039
Ramaswamy, S., and Weinbauer, G. F. (2014). Endocrine Control of Spermatogenesis: Role of FSH and LH/Testosterone. Spermatogenesis 4 (2), e996025. doi:10.1080/21565562.2014.996025
Rey, R. A. (2021). The Role of Androgen Signaling in Male Sexual Development at Puberty. Endocrinology 162 (2), bqaa215. doi:10.1210/endocr/bqaa215
Ru, J., Li, P., Wang, J., Zhou, W., Li, B., Huang, C., et al. (2014). TCMSP: a Database of Systems Pharmacology for Drug Discovery from Herbal Medicines. J. Cheminform 6, 13. doi:10.1186/1758-2946-6-13
Shannon, P., Markiel, A., Ozier, O., Baliga, N. S., Wang, J. T., Ramage, D., et al. (2003). Cytoscape: a Software Environment for Integrated Models of Biomolecular Interaction Networks. Genome Res. 13 (11), 2498–2504. doi:10.1101/gr.1239303
Skurikhin, E. G., Pakhomova, A. V., Pershina, O. V., Krupin, V. A., Ermakova, N. N., Pan, E. S., et al. (2017). Role of Sertoli and Leydig Cells in the Regulation of Spermatogonial Stem Cell and Development of Reproductive Disorders in Male C57Bl/6 Mice with Type 1 Diabetes Mellitus. Bull. Exp. Biol. Med. 164 (2), 127–131. doi:10.1007/s10517-017-3940-6
Smith, L. B., and Walker, W. H. (2014). The Regulation of Spermatogenesis by Androgens. Semin. Cel Dev Biol 30, 2–13. doi:10.1016/j.semcdb.2014.02.012
Song, H. W., and Wilkinson, M. F. (2014). Transcriptional Control of Spermatogonial Maintenance and Differentiation. Semin. Cel Dev Biol 30, 14–26. doi:10.1016/j.semcdb.2014.02.005
Subash, S. K., and Kumar, P. G. (2021). Spermatogonial Stem Cells: A story of Self-Renewal and Differentiation. Front. Biosci. (Landmark Ed. 26, 163–205. doi:10.2741/4891
Takashima, S., Kanatsu-Shinohara, M., Tanaka, T., Morimoto, H., Inoue, K., Ogonuki, N., et al. (2015). Functional Differences between GDNF-dependent and FGF2-dependent Mouse Spermatogonial Stem Cell Self-Renewal. Stem Cel Rep. 4 (3), 489–502. doi:10.1016/j.stemcr.2015.01.010
Tegelenbosch, R. A., and de Rooij, D. G. (1993). A Quantitative Study of Spermatogonial Multiplication and Stem Cell Renewal in the C3H/101 F1 Hybrid Mouse. Mutat. Res. 290 (2), 193–200. doi:10.1016/0027-5107(93)90159-d
Tremblay, J. J. (2015). Molecular Regulation of Steroidogenesis in Endocrine Leydig Cells. Steroids 103, 3–10. doi:10.1016/j.steroids.2015.08.001
Wang, X., Shen, Y., Wang, S., Li, S., Zhang, W., Liu, X., et al. (2017). PharmMapper 2017 Update: a Web Server for Potential Drug Target Identification with a Comprehensive Target Pharmacophore Database. Nucleic Acids Res. 45 (W1), W356–W360. doi:10.1093/nar/gkx374
Wang, C. Y., Tang, L., He, J. W., Li, J., and Wang, Y. Z. (2019). Ethnobotany, Phytochemistry and Pharmacological Properties of Eucommia Ulmoides: A Review. Am. J. Chin. Med. 47 (2), 259–300. doi:10.1142/S0192415X19500137
Wang, Y., Yu, W., Shi, C., Jiao, W., Li, J., Ge, J., et al. (2019). Network Pharmacology of Yougui Pill Combined with Buzhong Yiqi Decoction for the Treatment of Sexual Dysfunction. Evid. Based Complement. Alternat Med. 2019, 1243743. doi:10.1155/2019/1243743
Wu, J., Song, W., Zhu, H., Niu, Z., Mu, H., Lei, A., et al. (2013). Enrichment and Characterization of Thy1-Positive Male Germline Stem Cells (mGSCs) from Dairy Goat (Capra hircus) Testis Using Magnetic Microbeads. Theriogenology 80 (9), 1052–1060. doi:10.1016/j.theriogenology.2013.08.003
Wu, J., Zhu, H., Song, W., Li, M., Liu, C., Li, N., et al. (2014). Identification of Conservative microRNAs in Saanen Dairy Goat Testis through Deep Sequencing. Reprod. Domest. Anim. 49 (1), 32–40. doi:10.1111/rda.12217
Yang, T., and Yang, W. X. (2020). The Dynamics and Regulation of Microfilament during Spermatogenesis. Gene 744, 144635. doi:10.1016/j.gene.2020.144635
Yang, W. M., Kim, H. Y., Park, S. Y., Kim, H. M., Chang, M. S., and Park, S. K. (2010). Cynomorium Songaricum Induces Spermatogenesis with Glial Cell-Derived Neurotrophic Factor (GDNF) Enhancement in Rat Testes. J. Ethnopharmacol 128 (3), 693–696. doi:10.1016/j.jep.2010.02.020
Yu, M., Mu, H., Niu, Z., Chu, Z., Zhu, H., and Hua, J. (2014). miR-34c Enhances Mouse Spermatogonial Stem Cells Differentiation by Targeting Nanos2. J. Cel Biochem 115 (2), 232–242. doi:10.1002/jcb.24655
Zhai, X. Y., Ge, M. Y., and Zhao, J. H. (2020). Clinical Study of Yongui Pill in the Treatment of Diabetic Erectile Dysfunction with Syndrome of Deficiency of Kidney Yin and Yang. Shanghai J. Traditional Chin. Med. 54 (S1), 61–63. (In Chinese). doi:10.16305/j.1007-1334.2020.S1.020
Zhang, G. H., Guo, M. F., Yang, Z. H., Wu, Z. J., Liu, X. S., Song, J. H., et al. (1999). Effect of Du-Zhongkangcha on Sexual Behavior and its Acute Toxicity. J. Hebei Med. Univ. 20 (03), 15–17. (In Chinese). doi:10.1016/j.jep.2013.11.023
Zhang, M., and Hu, X. (2016). Mechanism of Chlorogenic Acid Treatment on Femoral Head Necrosis and its protection of Osteoblasts. Biomed. Rep. 5 (1), 57–62. doi:10.3892/br.2016.679
Zhang, M., Zhou, H., Zheng, C., Xiao, J., Zuo, E., Liu, W., et al. (2014). The Roles of Testicular C-Kit Positive Cells in De Novo Morphogenesis of Testis. Sci. Rep. 4, 5936. doi:10.1038/srep05936
Zhang, Q., Su, Y., and Zhang, J. (2013). Seasonal Difference in Antioxidant Capacity and Active Compounds Contents of Eucommia Ulmoides Oliver Leaf. Molecules 18 (2), 1857–1868. doi:10.3390/molecules18021857
Zhao, B., Xiong, Y., Zhang, Y., Jia, L., Zhang, W., and Xu, X. (2020). Rutin Promotes Osteogenic Differentiation of Periodontal Ligament Stem Cells through the GPR30-Mediated PI3K/AKT/mTOR Signaling Pathway. Exp. Biol. Med. (Maywood) 245 (6), 552–561. doi:10.1177/1535370220903463
Keywords: Eucommiae Folium, spermatogenesis, CGA, SHP2, spermatogonial stem cell, leydig cell
Citation: Mu H, Liu S, Tian S, Chen B, Liu Z, Fan Y, Liu Y, Ma W, Zhang W, Fu M and Song X (2022) Study on the SHP2-Mediated Mechanism of Promoting Spermatogenesis Induced by Active Compounds of Eucommiae Folium in Mice. Front. Pharmacol. 13:851930. doi: 10.3389/fphar.2022.851930
Received: 10 January 2022; Accepted: 07 March 2022;
Published: 22 March 2022.
Edited by:
Ashwell Rungano Ndhlala, University of Limpopo, South AfricaReviewed by:
Bhola Shankar Pradhan, Łukasiewicz Research Network - PORT The Polish Center for Technology Development, PolandLaura O'Hara, University of Edinburgh, United Kingdom
Copyright © 2022 Mu, Liu, Tian, Chen, Liu, Fan, Liu, Ma, Zhang, Fu and Song. This is an open-access article distributed under the terms of the Creative Commons Attribution License (CC BY). The use, distribution or reproduction in other forums is permitted, provided the original author(s) and the copyright owner(s) are credited and that the original publication in this journal is cited, in accordance with accepted academic practice. No use, distribution or reproduction is permitted which does not comply with these terms.
*Correspondence: Xiaoping Song, c3hwdGN2bUAxNjMuY29t