- 1Hainan General Hospital and Hainan Affiliated Hospital of Hainan Medical University, Haikou, China
- 2Key Laboratory of Tropical Medicinal Plant Chemistry of Ministry of Education, College of Chemistry and Chemical Engineering, Hainan Normal University, Haikou, China
- 3Key Laboratory of Tropical Medicinal Plant Chemistry of Hainan Province, College of Chemistry and Chemical Engineering, Hainan Normal University, Haikou, China
The initial responses to standard chemotherapies among prostate cancer (PCa) patients are usually significant, while most of them will finally develop drug resistance, rendering them with limited therapies. To discover new regimens for the treatment of PCa including resistant PCa, natural products, the richest source of bioactive compounds, can serve as a library for screening and identifying promising candidates, and flavones such as apigenin and genistein have been used in lab and clinical trials for treating PCa over decades. In this mini-review, we take a look into the progress of apigenin and genistein, which are isomers, in treating PCa in the past decade. While possessing very similar structure, these two isomers can both target the same signaling pathways; they also are found to work differently in PCa cells. Given that more combinations are being developed and tested, genistein appears to be the more promising option to be approved. The anticancer efficacies of these two flavones can be confirmed by in-vitro and in-vivo studies, and their applications remain to be validated in clinical trials. Information gained in this work may provide important information for new drug development and the potential application of apigenin and genistein in treating PCa.
Introduction
Prostate cancer (PCa) is the most commonly diagnosed cancer and the second leading cause of cancer death in men (do Pazo and Webster, 2021; Whitaker et al., 2020; Komura et al., 2018; Dunn, 2017). There will be an estimate of 248,530 cases and 34,130 deaths in the United States in 2021, posing a serious burden on health care in the United States and worldwide. The initial response rate of androgen deprivation therapy is usually high, while a significant proportion (around 90%) of PCa patients develops cartration-resistant PCa (CRPC) (Thoma, 2016; Huang et al., 2018; Makino et al., 2021; Welti et al., 2021). And even worse, the vast majority of CRPC patients will eventually become resistant to the first-line treatment docetaxel and enzalutamide (Scott, 2018; Lin et al., 2020; Moussa et al., 2020; Peery et al., 2020). In addition, death is usually not caused by the primary tumor but by the formation of distinct metastatic tumors that show resistant properties to varied therapies (Shore, 2020; Sayegh et al., 2021). There are unmet clinical needs to tackle drug resistance in PCa through discovering novel chemical entities or certain combinations.
Natural active products serve as a rich resource for the identification of hit compounds that can be used for the consequent structural modification and optimization, accounting for approximately 40% of FDA-approved drugs (Cui et al., 2018; Newman and Cragg, 2020). Among all those various active components found in traditional herbals, flavone is one of the most studied both in lab and in clinical trials (Amawi et al., 2017; Bondonno et al., 2019; Bisol et al., 2020). In addition, of roughly 3,000 flavonoids (Ye et al., 2019), apigenin and genistein, two isomers as shown in Figure 1, are particularly interesting to us.
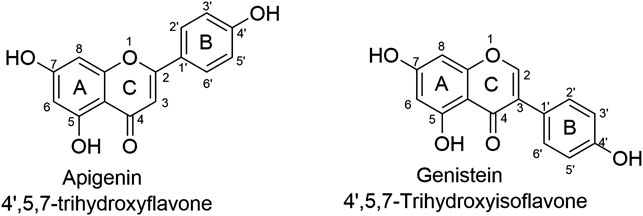
FIGURE 1. The chemical structures of isomers apigenin and genistein. Apigenin and genistein share the same formulate but structurally differ at the linking position of ring B and C. Such minor difference has led to significant different pharmacological profiles.
Apigenin, 4′, 5, 7-trihydroxyflavone, is abundant in vegetables and certain foods that have been used as medicinal plants worldwide for centuries (Salehi et al., 2019; Su et al., 2020; Xu et al., 2021). Genistein, 4′, 5, 7-trihydroxyisoflavone, was first reported in 1899, and its structure was identified in 1926 (Jung et al., 2020). Apigenin and genistein both target dozens of pharmacological targets, such as estrogen receptors, ABC transporters, membrane proteins, mitochondria-associated proteins, cell cycle-associated proteins, epigenetic regulators, cytokines, and many signaling pathways including NF-κB, MAPK/ERK, JAK/STAT, PI3K/Akt and Wnt/β-catenin pathways, exhibiting therapeutic applications in cardiovascular diseases, neurodegenerative diseases, cancers, etc. (Mukund et al., 2017; Jaiswal et al., 2019; Kim and Park, 2020; Javed et al., 2021). Apigenin and genistein have been launched into market as dietary supplements for decades; meanwhile, they are also actively tested in clinical trials, especial for genistein as more clinical trials use it as a candidate for the treatment of various diseases including malignant cancers (Yu et al., 2021). Thus, in the current mini-review, we attempted to have an overview of the progress made in the past decade of the studies (2012–2021) of apigenin and genistein in the treatment of PCa, including resistant forms of PCa, as flavonoids have also shown promising therapeutic application in resistant cancers (Ye et al., 2019). We would like to have a brief discussion of the differences except their structures, including acting modes, targets, and the signaling pathways network.
Apigenin and Genistein Show Great Potentials in Treating PCa
One thing we need to bear in mind is that these two flavones are multi-targeted or multi-functional compounds, meaning they exert their anticancer activities via multiple mechanisms. We attempted to interpret the pharmacological effects including inhibiting cancer cells proliferation and metastasis, enhancing the sensitivity of certain chemotherapeutics.
Apigenin
Inhibiting IκB Kinase α (IKKα)
IKKα functions to activate NF-κB that works as a critical mediator to regulate the crosstalk of inflammation and cancer initiation and progression, serving as a druggable target (Lv et al., 2020; Mo et al., 2021).
Shukla et al. (2015) confirmed that apigenin could directly bind IKKα. Apigenin (2.5–20 μM) attenuated IKKα kinase activity and suppresses the activation of NF-κB/p65 in human PCa PC-3 and 22Rv1 cells. Apigenin caused cell cycle arrest similar to the effects induced by the knockdown of IKKα. In-vivo studies of xenograft mouse model indicate that apigenin (20 and 50 μg/day, gavage) suppressed tumor growth, lowered cancer cells proliferation, and enhanced apoptosis, mediated with the inhibition of p-IKKα, NF-κB/p65 (Shukla et al., 2015). This study suggested that apigenin inhibited cancer growth via suppressing IKKα and its downstream targets NF-κB (Shukla et al., 2015).
In the same group, Shukla et al. (2014a) identified another mechanism related to IKKα/β in apigenin-induced cytotoxicity in PCa (Shukla et al., 2014a). Forkhead box O (FoxO) transcription factors play an important role as tumor suppressors in human malignancies (Yadav et al., 2018). Disruption of FoxO activity due to loss of phosphatase and tensin homolog and the activation of phosphatidylinositol-3 kinase (PI3K)/Akt are frequently observed in PCa. In a model of TRAMP (transgenic adenocarcinoma of the mouse prostate) mice, Apigenin (20 and 50 μg/day, 6 days/week for 20 weeks) treatment suppressed the tumor growth and metastasis (Shukla et al., 2014a). Apigenin, via increasing nuclear retention and decreasing the binding of FoxO3a with 14-3-3, reduced the p-Akt (Ser473) and FoxO3a (Ser253) as confirmed by histologic analyses. Similar results were also observed in human PCa LNCaP and PC-3 cells, as apigenin (10 and 20 μM) increased the binding of FoxO3a with p27/Kip1, leading to cell arrest at G1 phase. In-silico molecular modeling study confirmed a strong affinity of apigenin to either IKKα or IKKβ, which was then validated by in-vitro ELISA assay (Shukla et al., 2014a). Further study showed that apigenin can preferably bind IKKα over IKKβ, leading to the inactivation of NF-κB in PC-3 and 22Rv1 cells, providing convincing evidence that apigenin suppressed PCa progression by targeting the IKK/NF-κB pathway (Shukla et al., 2014a).
Inhibiting Inhibitor of Apoptosis Proteins
IAP family members, including XIAP, c-IAP1/2 and survivin, are historically regarded as the major regulators of the apoptosis pathway due to their ability to inhibit pro-apoptotic caspases, highlighting that IAPs can serve as potential therapeutic targets in cancers (Peery et al., 2017; Dizdar et al., 2018; Lalaoui and Vaux, 2018; Rada et al., 2018; Hrdinka and Yabal, 2019).
Shukla et al. (2014b) found that in human PCa PC-3 and DU145 cells that are androgen-refractory, apigenin (5–40 μM) treatment decreased cell viability and induced significant apoptosis mediated by the up-regulation of cleaved caspase 3/9 and PARP (poly [ADP-ribose] polymerase), accompanied with down-regulated levels of IAP members including XIAP, c-IAP1/2, and survivin, as well as the decreased levels of Bcl-xL and Bcl-2 and the increased cytochrome C, a key player in executing apoptosis. Importantly, pretreatment of the inhibitors of caspase 9 (z-LEHD-fmk) and caspase 3 (z-DEVD-fmk) was able to alleviate apigenin-induced apoptosis. Further study indicated that the increased Bax was mediated by the inhibition of histone deacetylases (HDACs) induced by apigenin, which then caused the Ku70 acetylation that lead to the dissociation of Bax from Ku70. In addition, apigenin (20, 50 μg/day, gavage) also showed dose-dependent tumor growth inhibition in PC-3 cells xenograft mouse model, strengthening its role as an anticancer agent (Shukla et al., 2014b).
Targeting Adenine Nucleotide Translocase-2
ANT2 is a mitochondria-associated protein that functions to exchange ADP and ATP (Jang et al., 2008; Lee et al., 2019), serving as a therapeutic target that can be regulated by apigenin but not genistein as confirmed by Oishi et al. (2013). Apigenin was able to bind and inhibit ANT2, leading to the increased death receptor 5 (DR5) and improved apoptosis induced by Apo2L/TRAIL. Meanwhile, these effects induced by apigenin could be attenuated by the ANT2 knockdown, verifying that its mechanism involved ANT2 (Oishi et al., 2013).
Suppressing Epithelial-to-Mesenchymal Transition
Apeginin at a wide range of concentrations/doses has been found to repress cancer cells migration. EMT is a process that cancer cells initiate the migration and invasion, playing critical roles in cancer migration and drug resistance (Brabletz et al., 2018; Georgakopoulos-Soares et al., 2020).
Zhu et al. (2015) found that apigenin (10–80 μM) suppressed the proliferation of the DU145 cells via inducing G2/M phase cell cycle arrest. Aigenin (5–20 μM) inhibited the migration and invasive potentials as shown in the wound healing assay. Western blot analysis showed that upon apigenin (5, 10, 20 μM) treatment, E-cadherin, an essential component to reverse EMT, was significantly up-regulated (Zhu et al., 2015).
Later, Chien et al. (2019) found that Apigenin (0–80 μM) inhibited cell viabilities of four human PCa cell lines including metastatic hormone-sensitive cell line (LNCap) and metastatic CRPC cell lines (DU145, PC-3, and PC-3 M) in both time- and dose-dependent manners, and it reduced colony formation numbers of all 4 cell lines, suggesting it is a broad-spectrum anticancer agent. Apigenin (20 and 40 μM) significantly suppressed the characteristics of migration and invasion of PC-3 M cells. More importantly, apigenin (3 mg/kg/day, IP) significantly reduced tumor metastasis to the lung, liver, pancreas, spine, bone, and brain in an intracardiac injection model constructed by PC-3 M cells, leading to a prolonged survival rate. SPOCK1 (Sparc/osteonectin, cwcv, and kazal-like domains proteoglycan 1) is a crucial modulator in prompting EMT, which is overexpressed in PC-3 M cells. Apigenin (10, 20, 40 μM in-vitro and 3 mg/kg/day in-vivo) induced apoptosis and inhibited metastasis through down-regulating SPOCK1, as well as Snail1/2, which can be reversed by exotic overexpression of SPOCK1, suggesting a pharmacological targeting effect of apigenin (Chien et al., 2019).
Suppressing Cancer Stem Cells
CSCs, a subset of cancer cells that show self-renewing and differentiation properties, are regarded as key players that are responsible for chemoresistance, tumor relapse, and metastasis (Sancho et al., 2016; Cui et al., 2017; Luo et al., 2018; Carvalho et al., 2021). Apigenin has been shown to negatively regulate CSCs, resulting in the re-sensitization of certain chemotherapeutics.
Erdogan et al. (2017) tested apgenin’s effect towards CD44+ PCa stem cells that were isolated from human androgen-independent PC-3 PCa cells. Apigenin (15 μM) significantly enhanced cisplatin’s (7.5 μM) cytotoxic and apoptosis-inducing and migration-suppressing effects through the down-regulation of anti-apoptotic Bcl-2, sharpin and survivin, and the up-regulation of pro-apoptotic members such as caspase-8, Apaf-1 (apoptotic protease activating factor-1), and p53 mRNA expression. The combined therapy down-regulated p-PI3K, p-Akt, NF-κB, and arrested cell cycle at G2/M and S phases (Erdogan et al., 2017).
A similar study by the same group (2016) showed that apigenin (0–100 μM) inhibited CSCs (CD44+ PCa stem cells) and PC-3 cell survival, with a significant increase of p21 and p27, both of which are anti-apoptotic proteins. It appeared that apigenin induced apoptosis via an extrinsic caspase-dependent pathway mediated by up-regulating the mRNA expressions of caspases-8, -3, and TNF-α, but failed to trigger the intrinsic pathway as determined by the levels of pro-apoptotic Bax, cytochrome C, and Apaf-1 in CSCs. While in contrast in PC-3 cells, apigenin exerted cytotoxic effects via intrinsic apoptosis pathway. Apigenin (25 μM) suppressed the migration of CSCs via down-regulating matrix metallopeptidases-2, -9, (MMP2/9), and Snail1/2. Similarly, the expressions of NF-κB p105/p50, p-PI3K, and p-Akt were all decreased after apigenin treatment. Moreover, apigenin treatment significantly reduced the protein expression of pluripotency marker Oct3/4, indicating apigenin’s strong potential in suppressing CSCs, warranting further in-vivo study (Erdogan et al., 2016).
Genistein
Genistein in some cases shows similar or even the same mechanism as apigenin toward certain targets, such as CSCs (Zhang et al., 2012), PI3K/Akt signaling pathway (Song et al., 2020), MMP2 and apoptosis-related proteins including caspase 3 (Shafiee et al., 2020), while genistein appears to regulate more targets than apigenin.
Inactivating DNA Repair Pathways
Homologous recombination (HR) and the nonhomologous end joining (NHEJ) pathways are two DNA repair pathways that are activated by PCa cells when treated by certain chemotherapeutics, resulting in drug resistance (Sharda et al., 2020; Xiang et al., 2020; Filsinger et al., 2021). Genistein can work as chemotherapy sensitizer via impacting HR and NHEJ. Tang et al. (2018) developed a triple combination therapy composed with genistein (30 μM), AG1024 (10 μM), and radiotherapy. As shown in PC-3 and DU145 cells, the pretreatment of genistein and AG1024 could markedly enhance the inhibition of cells proliferation and induction of apoptosis induced by radiotherapy, which was mediated by the up-regulated Bax and cleaved caspase 3, leading to reduced colony formation (Tang et al., 2018). The triple combination induced a cell arrest at the S phase and decreased the G2/M phases. Further study indicated that this combination could induce double-strand breaks mediated by the inactivation of HR and NHEJ pathways, evidenced by the down-regulation of DNA-PKcs (Thr2609), Rad51, and Ku70. The in-vivo study also confirmed that this triple combination (100 mg/kg/day of genistein and AG1024, plus X-irradiation) surpassed the efficacies of other dual therapies, without showing any obvious adverse effects (Tang et al., 2018).
Genistein was also shown to regulate another key player in DNA repair, which was the APE1 (apurinic/apyrimidinic endonuclease1) that served as an oxidative DNA repair enzyme (Maayah et al., 2015; Pekhale et al., 2017; Lebedeva et al., 2020). In order to reduce the severely toxic effects toward normal tissues of doxorubicin (DOX), a nanoparticles system named as DOX-NPs, loaded with DOX and genistein, was synthesized by Wang et al. (2018) and evaluated in-vitro and in-vivo. Not only could genistein, when worked as an APE1 inhibitor, enhance DOX-induced cell death mediated by overproduced ROS (reactive oxygen species), it can also alleviate DOX-induced toxic effects. DOX-NPs showed a strong activity in suppressing distant metastasis in the in-vivo model (Wang et al., 2018).
Inhibiting Glucose Transporter 1
Glut-1 functions to the transportation of glucose across cell membrane, facilitating ATP production (Almahmoud et al., 2019; Pragallapati and Manyam, 2019; Qamar et al., 2019; Ahopelto et al., 2020). Enhanced Glut-1 protein as well as COX-2 (cyclooxygenase-2) is reported as one of the major driving factors, leading to the initiation and progress of PCa (Carreño et al., 2019; Gasinska et al., 2020; King et al., 2020; Joshi et al., 2021). Tian et al. (2019) synthesized a liposomal system composed with COX-2 inhibitor celecoxib and genistein (100 μM celecoxib and 10 μM genistein), and this liposomal system exhibited selective cytotoxic effects towards PC-3 and LNCaP cells over non-cancer cells via inducing apoptosis as confirmed by up-regulated cleaved caspase 3, and it suppressed PC-3 cells migration. This liposomal system could induce ROS production, decrease GSH level, and inhibit COX-2 and Glut-1 receptors simultaneously, leading to decreased glucose uptake in PC-3 and LNCaP cells, indicating a dual targeting (Tian et al., 2019). Further models are clearly needed to elucidate its safety and efficacy.
Demethylating Effect
Similar as apigenin, genistein can also regulate epigenetic proteins. Methylation of certain genes can be hijacked by cancer cells to promote its tumorigenesis (Wu et al., 2020; Zhao et al., 2020; Tolkach et al., 2021), while genistein was shown to down-regulate methylation.
ER-β (Estrogen receptor β) promoter hyper-methylation has a tumor inducing effect in PCa. Mahmoud et al. (2015) found that genistein (0.5–10 μM) significantly reduced the methylation of ER-β promoter accompanied with corresponding dose-dependent increased of ER-β expression in LNCaP and LAPC-4 but not in PC-3 cells with lower basal levels of ER-β promoter methylation. Genistein (0.5–10 μM) could down-regulate DNA methyl transferases (DNMTs) and increase the phosphorylation, nuclear translocation, and transcriptional activity of ER-β (working as a cancer suppressor) in all three PCa cell lines. Inhibitory effects of genistein on LAPC-4 and PC-3 cell proliferation were diminished using a specific ER-β antagonist, suggesting an ER-β-mediated mechanism (Mahmoud et al., 2015).
Inhibiting Insulin-like Growth Factor-1 Receptor
IGF-1, an essential player in anabolism, is found to be elevated in several different cancers, including PCa (Uzunlulu et al., 2016; Mancarella et al., 2017; Larsson et al., 2020; Ohishi et al., 2020). The inhibition of IGF-1 and its downstream signaling pathways may have therapeutic implications (Nordstrand et al., 2013; Nordstrand et al., 2017).
Lee at al. (2012) found that genistein treatment caused a significant inhibition of IGF-1-stimulated PC-3 cells growth through decreasing the proliferation of IGF-1-stimulated cells and inducing cell arrest at G0/G1 phase (Lee et al., 2012). Genistein effectively inhibited the phosphorylation of IGF-1R and the phosphorylation of its downstream targets, such as Src, Akt, and GSK-3β (glycogen synthase kinase-3β), and it greatly attenuated IGF-1-induced β-catenin signaling and cyclin D1 levels in PC-3 cells (Phillip et al., 2012).
Modulating miRNAs and Long Non-coding RNAs
Different with apigenin, genistein could regulate miRNAz and lncRNAs to achieve its treatment efficacies in PCa.
Hirata et al. (2014) found that miRNA-1260b expression was significantly decreased by genistein (25 μM for 4 days) in PC-3 and DU-145 cells; meanwhile, the knockdown of miR-1260b suppressed cell proliferation, invasion, migration, and TCF reporter activity in PC cells, similar phenomenon as genistein treatment (Hirata et al., 2014).
Chiyomaru et al. (2013a) found that genistein may impact the axis of lncRNAs and miRNAs as confirmed by microarray assay which showed that lncRNA HOTAIR and miR-34a were regulated by genistein (25 µM) in PC-3 and DU145 cells (Chiyomaru et al., 2013a). By this same group (2013), they reported another finding that genistein (25 and 50 µM) might up-regulate tumor suppressor miR-574-3p in PC-3 cells and in PCa tissues (Chiyomaru et al., 2013b). In addition, Chiyomaru et al. (2012) also identified another key player, oncogenic miR-151, which can be down-regulated by genistein (25 µM) in PC-3 and DU145 cells (Chiyomaru et al., 2012).
Certain Combinations That Induced Apoptosis
Genistein could work as a chemosensitizer.
Zhang et al. (2013) found that genistein (5 and 10 μg/ml) when combined with cabazitaxel (5–100 nM) could enhance the sensitivity of cabazitaxel to metastatic CRPC cells in-vitro and in-vivo via increasing the expression of pro-apoptotic Bax and cleaved caspase 3 (Zhang et al., 2013). In a PC-3-luciferase xenograft model, the combined treatment with genistein (100 mg/kg) and cabazitaxel (5 mg/kg) significantly retarded the growth of mCRPC when compared to vehicle control, cabazitaxel, or genistein (Zhang et al., 2013).
Another study showed that the combination of topotecan and genistein exhibited better efficacy in LNCaP and PCa cells than either monotherapy, which was mediated by inducing apoptosis activated by caspase-3 and -9 and by ROS generation (Hörmann et al., 2012).
Another genistein nano-suspension (named as BIO 300) was synthesized by Jackson et al. (2019). BIO 300 positively synergized with radiation, resulting in tumor growth delay and prolonged survival as confirmed in hormone-sensitive and insensitive prostate tumor-bearing mice (Jackson et al., 2019). Their data also strongly support the clinical translation of BIO 300 for mitigation of ED (erectile dysfunction) among PCa patients (Jackson et al., 2019).
Clinical Evidence
Many ongoing clinical studies of different stages have also revealed that genistein may benefit PCa patients via either inhibiting metastasis or suppressing tumor growth (Pavese et al., 2014). Retrospective clinical studies have shown that high consumption of soybean products (genistein as its major active component) has been associated with a low incidence of PCa (Hwang et al., 2009). Wu et al. (2015) reported a preliminarily evaluation of the associations among plasma genistein and PCa in a Chinese population composed with 100 men aged over 40 and diagnosed with PCa (Zhu et al., 2015) or PCa-free (Zhang et al., 2012), and analyzed the physiological data before and after certain doses of genistein dietary intake. Their data showed that cancer-free patients possessed higher plasma genistein concentration than PCa patients, and the plasma genistein concentration negatively correlated to PCa (Wu et al., 2015). Bilir et al. (2017) invested the effects of genistein on Norwegian patients who received 30 mg genistein or placebo capsules daily for 3–6 weeks before prostatectomy. Microarrays and qPCR data showed that several differentially methylated sites and expressed genes between placebo and genistein groups. Importantly, the MYC activity reduced and PTEN activity increased in patients receiving genistein, highlighting the Pca-preventive of genistein (Bilir et al., 2017).
While some clinical studies showed unfavorable results towards the remission of PCa (Jarrard et al., 2016), or the plasma genistein concentration was not associated with PCa risk in large cohort of European men (Travis et al., 2012), more clinical trials with well-filtered qualified PCa patients are warranted.
Summary and Brief Discussion
The above information strongly supports the potential application of apigenin and genistein in treating PCa including resistant forms of PCa as shown in Table 1.
While apigenin and genistein are isomers, they appear to treat PCa via a slight different mechanism. Both of them can regulate CSCs (Zhang et al., 2012; Erdogan et al., 2016), PI3K/Akt (Shukla et al., 2014a; Song et al., 2020), MMP2, and apoptosis-related proteins including caspase 3 (Shafiee et al., 2020) and DNA damage-associated repair pathway (Phillip et al., 2012; Sharma et al., 2014; Tang et al., 2018). While apigenin was able to inhibit IAP members, genistein seems to impact membrane-associated proteins, such as certain receptors including Glut-1 and IGF-1 (Lee et al., 2012; Tian et al., 2019) as summarized in Figure 2.
Genistein appears to be more useful in combination than apigenin that only was shown to sensitize cisplatin. Several combinations regimens, such as genistein + celecoxib (Tian et al., 2019), genistein + cabazitaxel (Zhang et al., 2013), genistein + topotecan (Hörmann et al., 2012), genistein + vorinostat, as well as triple combination of genistein + AG1024 + radiotherapy (Jackson et al., 2019), were optimized and tested in cell-based assay and in animal models, strongly supporting its potential as chemotherapy in PCa.
Furthermore, these two flavones can serve as leading compound that undergo structural modifications to achieve higher activities but lower toxic effects (Chen et al., 2015; Xiong et al., 2015; George et al., 2018).
Conclusion
Flavones isomers apigenin and genistein, by mono-therapy or combinational therapy, exhibited great potentials in the treatment of PCa including resistant PCa.
Author Contributions
XJ, KL, and QY designed this review; XJ, KL, QL, QS, and FH wrote the manuscript; QY and CZ revised the manuscript. All authors read and approved the final manuscript. All authors contributed to the article and approved the submitted version. XJ and KL have contributed equally to this work.
Funding
We are grateful to the support from the National Natural Science Foundation of China (Grant number: 82160735) and Hainan Normal University 2021 Annual Graduate Innovation and Scientific Research Project (Grant number: hsyx 2021-4).
Conflict of Interest
The authors declare that the research was conducted in the absence of any commercial or financial relationships that could be construed as a potential conflict of interest.
Publisher’s Note
All claims expressed in this article are solely those of the authors and do not necessarily represent those of their affiliated organizations, or those of the publisher, the editors, and the reviewers. Any product that may be evaluated in this article, or claim that may be made by its manufacturer, is not guaranteed or endorsed by the publisher.
References
Ahopelto, K., Laitinen, A., Hagström, J., Böckelman, C., and Haglund, C. (2020). Transketolase-Like Protein 1 and Glucose Transporter 1 in Gastric Cancer. Oncology 98 (9), 643–652. doi:10.1159/000507350
Almahmoud, S., Wang, X., Vennerstrom, J. L., and Zhong, H. A. (2019). Conformational Studies of Glucose Transporter 1 (GLUT1) as an Anticancer Drug Target. Molecules 24 (11), 2159. doi:10.3390/molecules24112159
Amawi, H., Ashby, C. R., and Tiwari, A. K. (2017). Erratum to: Cancer Chemoprevention through Dietary Flavonoids: What's Limiting. Chin. J. Cancer 36 (1), 70. doi:10.1186/s40880-017-0237-0
Bilir, B., Sharma, N. V., Lee, J., Hammarstrom, B., Svindland, A., Kucuk, O., et al. (2017). Effects of Genistein Supplementation on Genomewide DNA Methylation and Gene Expression in Patients with Localized Prostate Cancer. Int. J. Oncol. 51 (1), 223–234. doi:10.3892/ijo.2017.4017
Bisol, Â., de Campos, P. S., and Lamers, M. L. (2020). Flavonoids as Anticancer Therapies: A Systematic Review of Clinical Trials. Phytother. Res. 34 (3), 568–582. doi:10.1002/ptr.6551
Bondonno, N. P., Dalgaard, F., Kyrø, C., Murray, K., Bondonno, C. P., Lewis, J. R., et al. (2019). Flavonoid Intake Is Associated with Lower Mortality in the Danish Diet Cancer and Health Cohort. Nat. Commun. 10 (1), 3651. doi:10.1038/s41467-019-11622-x
Brabletz, T., Kalluri, R., Nieto, M. A., and Weinberg, R. A. (2018). EMT in Cancer. Nat. Rev. Cancer 18 (2), 128–134. doi:10.1038/nrc.2017.118
Carreño, D., Corro, N., Torres-Estay, V., Véliz, L. P., Jaimovich, R., Cisternas, P., et al. (2019). Fructose and Prostate Cancer: toward an Integrated View of Cancer Cell Metabolism. Prostate Cancer Prostatic Dis. 22 (1), 49–58. doi:10.1038/s41391-018-0072-7
Carvalho, L. S., Gonçalves, N., Fonseca, N. A., and Moreira, J. N. (2021). Cancer Stem Cells and Nucleolin as Drivers of Carcinogenesis. Pharmaceuticals (Basel) 14 (1), 60. doi:10.3390/ph14010060
Chen, Q. H., Yu, K., Zhang, X., Chen, G., Hoover, A., Leon, F., et al. (2015). A New Class of Hybrid Anticancer Agents Inspired by the Synergistic Effects of Curcumin and Genistein: Design, Synthesis, and Anti-proliferative Evaluation. Bioorg. Med. Chem. Lett. 25 (20), 4553–4556. doi:10.1016/j.bmcl.2015.08.064
Chien, M. H., Lin, Y. W., Wen, Y. C., Yang, Y. C., Hsiao, M., Chang, J. L., et al. (2019). Targeting the SPOCK1-Snail/slug axis-mediated Epithelial-To-Mesenchymal Transition by Apigenin Contributes to Repression of Prostate Cancer Metastasis. J. Exp. Clin. Cancer Res. 38 (1), 246. doi:10.1186/s13046-019-1247-3
Chiyomaru, T., Yamamura, S., Fukuhara, S., Hidaka, H., Majid, S., Saini, S., et al. (2013). Genistein Up-Regulates Tumor Suppressor microRNA-574-3p in Prostate Cancer. PLoS One 8 (3), e58929. doi:10.1371/journal.pone.0058929
Chiyomaru, T., Yamamura, S., Fukuhara, S., Yoshino, H., Kinoshita, T., Majid, S., et al. (2013). Genistein Inhibits Prostate Cancer Cell Growth by Targeting miR-34a and Oncogenic HOTAIR. PLoS One 8 (8), e70372. doi:10.1371/journal.pone.0070372
Chiyomaru, T., Yamamura, S., Zaman, M. S., Majid, S., Deng, G., Shahryari, V., et al. (2012). Genistein Suppresses Prostate Cancer Growth through Inhibition of Oncogenic microRNA-151. PLoS One 7 (8), e43812. doi:10.1371/journal.pone.0043812
Cui, Q., Wen, S., and Huang, P. (2017). Targeting Cancer Cell Mitochondria as a Therapeutic Approach: Recent Updates. Future Med. Chem. 9 (9), 929–949. doi:10.4155/fmc-2017-0011
Cui, Q., Yang, D. H., and Chen, Z. S. (2018). Special Issue: Natural Products: Anticancer and beyond. Molecules 23 (6), 1246–1249. doi:10.3390/molecules23061246
Dizdar, L., Jünemann, L. M., Werner, T. A., Verde, P. E., Baldus, S. E., Stoecklein, N. H., et al. (2018). Clinicopathological and Functional Implications of the Inhibitor of Apoptosis Proteins Survivin and XIAP in Esophageal Cancer. Oncol. Lett. 15 (3), 3779–3789. doi:10.3892/ol.2018.7755
do Pazo, C., and Webster, R. M. (2021). The Prostate Cancer Drug Market. Nat. Rev. Drug Discov. 20 (9), 663–664. doi:10.1038/d41573-021-00111-w
Dunn, M. W. (2017). Prostate Cancer Screening. Semin. Oncol. Nurs. 33 (2), 156–164. doi:10.1016/j.soncn.2017.02.003
Erdogan, S., Doganlar, O., Doganlar, Z. B., Serttas, R., Turkekul, K., Dibirdik, I., et al. (2016). The Flavonoid Apigenin Reduces Prostate Cancer CD44(+) Stem Cell Survival and Migration through PI3K/Akt/NF-Κb Signaling. Life Sci. 162, 77–86. doi:10.1016/j.lfs.2016.08.019
Erdogan, S., Turkekul, K., Serttas, R., and Erdogan, Z. (2017). The Natural Flavonoid Apigenin Sensitizes Human CD44+ Prostate Cancer Stem Cells to Cisplatin Therapy. Biomed. Pharmacother. 88, 210–217. doi:10.1016/j.biopha.2017.01.056
Filsinger, G. T., Wannier, T. M., Pedersen, F. B., Lutz, I. D., Zhang, J., Stork, D. A., et al. (2021). Characterizing the Portability of Phage-Encoded Homologous Recombination Proteins. Nat. Chem. Biol. 17 (4), 394–402. doi:10.1038/s41589-020-00710-5
Gasinska, A., Jaszczynski, J., Rychlik, U., Łuczynska, E., Pogodzinski, M., and Palaczynski, M. (2020). Prognostic Significance of Serum PSA Level and Telomerase, VEGF and GLUT-1 Protein Expression for the Biochemical Recurrence in Prostate Cancer Patients after Radical Prostatectomy. Pathol. Oncol. Res. 26 (2), 1049–1056. doi:10.1007/s12253-019-00659-4
Georgakopoulos-Soares, I., Chartoumpekis, D. V., Kyriazopoulou, V., and Zaravinos, A. (2020). EMT Factors and Metabolic Pathways in Cancer. Front. Oncol. 10, 499. doi:10.3389/fonc.2020.00499
George, A., Raji, I., Cinar, B., Kucuk, O., and Oyelere, A. K. (2018). Design, Synthesis, and Evaluation of the Antiproliferative Activity of Hydantoin-Derived Antiandrogen-Genistein Conjugates. Bioorg. Med. Chem. 26 (8), 1481–1487. doi:10.1016/j.bmc.2018.01.009
Hirata, H., Hinoda, Y., Shahryari, V., Deng, G., Tanaka, Y., Tabatabai, Z. L., et al. (2014). Genistein Downregulates Onco-miR-1260b and Upregulates sFRP1 and Smad4 via Demethylation and Histone Modification in Prostate Cancer Cells. Br. J. Cancer 110 (6), 1645–1654. doi:10.1038/bjc.2014.48
Hörmann, V., Kumi-Diaka, J., Durity, M., and Rathinavelu, A. (2012). Anticancer Activities of Genistein-Topotecan Combination in Prostate Cancer Cells. J. Cel. Mol. Med. 16 (11), 2631–2636. doi:10.1111/j.1582-4934.2012.01576.x
Hrdinka, M., and Yabal, M. (2019). Inhibitor of Apoptosis Proteins in Human Health and Disease. Genes Immun. 20 (8), 641–650. doi:10.1038/s41435-019-0078-8
Huang, Y., Jiang, X., Liang, X., and Jiang, G. (2018). Molecular and Cellular Mechanisms of Castration Resistant Prostate Cancer. Oncol. Lett. 15 (5), 6063–6076. doi:10.3892/ol.2018.8123
Hwang, Y. W., Kim, S. Y., Jee, S. H., Kim, Y. N., and Nam, C. M. (2009). Soy Food Consumption and Risk of Prostate Cancer: a Meta-Analysis of Observational Studies. Nutr. Cancer 61 (5), 598–606. doi:10.1080/01635580902825639
Jackson, I. L., Pavlovic, R., Alexander, A. A., Connors, C. Q., Newman, D., Mahmood, J., et al. (2019). BIO 300, a Nanosuspension of Genistein, Mitigates Radiation-Induced Erectile Dysfunction and Sensitizes Human Prostate Cancer Xenografts to Radiation Therapy. Int. J. Radiat. Oncol. Biol. Phys. 105 (2), 400–409. doi:10.1016/j.ijrobp.2019.05.062
Jaiswal, N., Akhtar, J., Singh, S. P., Ahsan, F., and Ahsan, F. (2019). An Overview on Genistein and its Various Formulations. Drug Res. (Stuttg) 69 (6), 305–313. doi:10.1055/a-0797-3657
Jang, J. Y., Choi, Y., Jeon, Y. K., and Kim, C. W. (2008). Suppression of Adenine Nucleotide Translocase-2 by Vector-Based siRNA in Human Breast Cancer Cells Induces Apoptosis and Inhibits Tumor Growth In Vitro and In Vivo. Breast Cancer Res. 10 (1), R11. doi:10.1186/bcr1857
Jarrard, D., Konety, B., Huang, W., Downs, T., Kolesar, J., Kim, K. M., et al. (2016). Phase IIa, Randomized Placebo-Controlled Trial of Single High Dose Cholecalciferol (Vitamin D3) and Daily Genistein (G-2535) versus Double Placebo in Men with Early Stage Prostate Cancer Undergoing Prostatectomy. Am. J. Clin. Exp. Urol. 4 (2), 17–27.
Javed, Z., Sadia, H., Iqbal, M. J., Shamas, S., Malik, K., Ahmed, R., et al. (2021). Apigenin Role as Cell-Signaling Pathways Modulator: Implications in Cancer Prevention and Treatment. Cancer Cel Int 21 (1), 189. doi:10.1186/s12935-021-01888-x
Joshi, S. N., Murphy, E. A., Olaniyi, P., and Bryant, R. J. (2021). The Multiple Effects of Aspirin in Prostate Cancer Patients. Cancer Treat. Res. Commun. 26, 100267. doi:10.1016/j.ctarc.2020.100267
Jung, Y. S., Rha, C. S., Baik, M. Y., Baek, N. I., and Kim, D. O. (2020). A Brief History and Spectroscopic Analysis of Soy Isoflavones. Food Sci. Biotechnol. 29 (12), 1605–1617. doi:10.1007/s10068-020-00815-6
Kim, J. K., and Park, S. U. (2020). Recent Insights into the Biological Functions of Apigenin. EXCLI J. 19, 984–991. doi:10.17179/excli2020-2579
King, L., Christie, D., Arora, D., and Anoopkumar-Dukie, S. (2020). Cyclooxygenase-2 Inhibitors Delay Relapse and Reduce Prostate Specific Antigen (PSA) Velocity in Patients Treated with Radiotherapy for Nonmetastatic Prostate Cancer: a Pilot Study. Prostate Int. 8 (1), 34–40. doi:10.1016/j.prnil.2019.10.004
Komura, K., Sweeney, C. J., Inamoto, T., Ibuki, N., Azuma, H., and Kantoff, P. W. (2018). Current Treatment Strategies for Advanced Prostate Cancer. Int. J. Urol. 25 (3), 220–231. doi:10.1111/iju.13512
Lalaoui, N., and Vaux, D. L. (2018). Recent Advances in Understanding Inhibitor of Apoptosis Proteins. F1000Res 7, F1000. doi:10.12688/f1000research.16439.1
Larsson, S. C., Carter, P., Vithayathil, M., Kar, S., Mason, A. M., and Burgess, S. (2020). Insulin-like Growth Factor-1 and Site-specific Cancers: A Mendelian Randomization Study. Cancer Med. 9 (18), 6836–6842. doi:10.1002/cam4.3345
Lebedeva, N. A., Rechkunova, N. I., Endutkin, A. V., and Lavrik, O. I. (2020). Apurinic/Apyrimidinic Endonuclease 1 and Tyrosyl-DNA Phosphodiesterase 1 Prevent Suicidal Covalent DNA-Protein Crosslink at Apurinic/Apyrimidinic Site. Front Cel Dev Biol 8, 617301. doi:10.3389/fcell.2020.617301
Lee, C. H., Kim, M. J., Lee, H. H., Paeng, J. C., Park, Y. J., Oh, S. W., et al. (2019). Adenine Nucleotide Translocase 2 as an Enzyme Related to [18F] FDG Accumulation in Various Cancers. Mol. Imaging Biol. 21 (4), 722–730. doi:10.1007/s11307-018-1268-x
Lee, J., Ju, J., Park, S., Hong, S. J., and Yoon, S. (2012). Inhibition of IGF-1 Signaling by Genistein: Modulation of E-Cadherin Expression and Downregulation of β-catenin Signaling in Hormone Refractory PC-3 Prostate Cancer Cells. Nutr. Cancer 64 (1), 153–162. doi:10.1080/01635581.2012.630161
Lin, J. Z., Wang, W. W., Hu, T. T., Zhu, G. Y., Li, L. N., Zhang, C. Y., et al. (2020). FOXM1 Contributes to Docetaxel Resistance in Castration-Resistant Prostate Cancer by Inducing AMPK/mTOR-mediated Autophagy. Cancer Lett. 469, 481–489. doi:10.1016/j.canlet.2019.11.014
Luo, M., Shang, L., Brooks, M. D., Jiagge, E., Zhu, Y., Buschhaus, J. M., et al. (2018). Targeting Breast Cancer Stem Cell State Equilibrium through Modulation of Redox Signaling. Cell Metab 28 (1), 69–e6. doi:10.1016/j.cmet.2018.06.006
Lv, S., Wang, F., Wang, K., Fan, Y., Xu, J., Zheng, J., et al. (2020). IκB Kinase α: an Independent Prognostic Factor that Promotes the Migration and Invasion of Oral Squamous Cell Carcinoma. Br. J. Oral Maxillofac. Surg. 58 (3), 296–303. doi:10.1016/j.bjoms.2019.11.025
Maayah, Z. H., Ghebeh, H., Alhaider, A. A., El-Kadi, A. O., Soshilov, A. A., Denison, M. S., et al. (2015). Metformin Inhibits 7,12-Dimethylbenz[a]anthracene-Induced Breast Carcinogenesis and Adduct Formation in Human Breast Cells by Inhibiting the Cytochrome P4501A1/aryl Hydrocarbon Receptor Signaling Pathway. Toxicol. Appl. Pharmacol. 284 (2), 217–226. doi:10.1016/j.taap.2015.02.007
Mahmoud, A. M., Al-Alem, U., Ali, M. M., and Bosland, M. C. (2015). Genistein Increases Estrogen Receptor Beta Expression in Prostate Cancer via Reducing its Promoter Methylation. J. Steroid Biochem. Mol. Biol. 152, 62–75. doi:10.1016/j.jsbmb.2015.04.018
Makino, T., Izumi, K., and Mizokami, A. (2021). Undesirable Status of Prostate Cancer Cells after Intensive Inhibition of AR Signaling: Post-AR Era of CRPC Treatment. Biomedicines 9 (4), 414. doi:10.3390/biomedicines9040414
Mancarella, C., Casanova-Salas, I., Calatrava, A., García-Flores, M., Garofalo, C., Grilli, A., et al. (2017). Insulin-like Growth Factor 1 Receptor Affects the Survival of Primary Prostate Cancer Patients Depending on TMPRSS2-ERG Status. BMC Cancer 17 (1), 367. doi:10.1186/s12885-017-3356-8
Mo, D., Zhu, H., Wang, J., Hao, H., Guo, Y., Wang, J., et al. (2021). Icaritin Inhibits PD-L1 Expression by Targeting Protein IκB Kinase α. Eur. J. Immunol. 51 (4), 978–988. doi:10.1002/eji.202048905
Moussa, M., Papatsoris, A., Abou Chakra, M., Sryropoulou, D., and Dellis, A. (2020). Pharmacotherapeutic Strategies for Castrate-Resistant Prostate Cancer. Expert Opin. Pharmacother. 21 (12), 1431–1448. doi:10.1080/14656566.2020.1767069
Mukund, V., Mukund, D., Sharma, V., Mannarapu, M., and Alam, A. (2017). Genistein: Its Role in Metabolic Diseases and Cancer. Crit. Rev. Oncol. Hematol. 119, 13–22. doi:10.1016/j.critrevonc.2017.09.004
Newman, D. J., and Cragg, G. M. (2020). Natural Products as Sources of New Drugs over the Nearly Four Decades from 01/1981 to 09/2019. J. Nat. Prod. 83 (3), 770–803. doi:10.1021/acs.jnatprod.9b01285
Nordstrand, A., Bergström, S. H., Thysell, E., Bovinder-Ylitalo, E., Lerner, U. H., Widmark, A., et al. (2017). Inhibition of the Insulin-like Growth Factor-1 Receptor Potentiates Acute Effects of Castration in a Rat Model for Prostate Cancer Growth in Bone. Clin. Exp. Metastasis 34 (3-4), 261–271. doi:10.1007/s10585-017-9848-8
Nordstrand, A., Lundholm, M., Larsson, A., Lerner, U. H., Widmark, A., and Wikström, P. (2013). Inhibition of the Insulin-like Growth Factor-1 Receptor Enhances Effects of Simvastatin on Prostate Cancer Cells in Co-culture with Bone. Cancer Microenviron 6 (3), 231–240. doi:10.1007/s12307-013-0129-z
Ohishi, T., Abe, H., Sakashita, C., Saqib, U., Baig, M. S., Ohba, S. I., et al. (2020). Inhibition of Mitochondria ATP Synthase Suppresses Prostate Cancer Growth through Reduced Insulin-like Growth Factor-1 Secretion by Prostate Stromal Cells. Int. J. Cancer 146 (12), 3474–3484. doi:10.1002/ijc.32959
Oishi, M., Iizumi, Y., Taniguchi, T., Goi, W., Miki, T., and Sakai, T. (2013). Apigenin Sensitizes Prostate Cancer Cells to Apo2L/TRAIL by Targeting Adenine Nucleotide Translocase-2. PLoS One 8 (2), e55922. doi:10.1371/journal.pone.0055922
Pavese, J. M., Krishna, S. N., and Bergan, R. C. (2014). Genistein Inhibits Human Prostate Cancer Cell Detachment, Invasion, and Metastasis. Am. J. Clin. Nutr. 100 (Suppl. 1), 431S–6S. doi:10.3945/ajcn.113.071290
Peery, R., Kyei-Baffour, K., Dong, Z., Liu, J., de Andrade Horn, P., Dai, M., et al. (2020). Synthesis and Identification of a Novel Lead Targeting Survivin Dimerization for Proteasome-dependent Degradation. J. Med. Chem. 63 (13), 7243–7251. doi:10.1021/acs.jmedchem.0c00475
Peery, R. C., Liu, J. Y., and Zhang, J. T. (2017). Targeting Survivin for Therapeutic Discovery: Past, Present, and Future Promises. Drug Discov. Today 22 (10), 1466–1477. doi:10.1016/j.drudis.2017.05.009
Pekhale, K., Haval, G., Perween, N., Antoniali, G., Tell, G., Ghaskadbi, S., et al. (2017). DNA Repair Enzyme APE1 from Evolutionarily Ancient Hydra Reveals Redox Activity Exclusively Found in Mammalian APE1. DNA Repair (Amst) 59, 44–56. doi:10.1016/j.dnarep.2017.09.005
Phillip, C. J., Giardina, C. K., Bilir, B., Cutler, D. J., Lai, Y. H., Kucuk, O., et al. (2012). Genistein Cooperates with the Histone Deacetylase Inhibitor Vorinostat to Induce Cell Death in Prostate Cancer Cells. BMC Cancer 12, 145. doi:10.1186/1471-2407-12-145
Pragallapati, S., and Manyam, R. (2019). Glucose Transporter 1 in Health and Disease. J. Oral Maxillofac. Pathol. 23 (3), 443–449. doi:10.4103/jomfp.JOMFP_22_18
Qamar, S., Fatima, S., Rehman, A., Khokhar, M. A., Mustafa, Z., and Awan, N. (2019). Glucose Transporter 1 Overexpression in Oral Squamous Cell Carcinoma. J. Coll. Physicians Surg. Pak 29 (8), 724–727. doi:10.29271/jcpsp.2019.08.724
Rada, M., Nallanthighal, S., Cha, J., Ryan, K., Sage, J., Eldred, C., et al. (2018). Inhibitor of Apoptosis Proteins (IAPs) Mediate Collagen Type XI Alpha 1-driven Cisplatin Resistance in Ovarian Cancer. Oncogene 37 (35), 4809–4820. doi:10.1038/s41388-018-0297-x
Salehi, B., Venditti, A., Sharifi-Rad, M., Kręgiel, D., Sharifi-Rad, J., Durazzo, A., et al. (2019). The Therapeutic Potential of Apigenin. Int. J. Mol. Sci. 20 (6), 1305. doi:10.3390/ijms20061305
Sancho, P., Barneda, D., and Heeschen, C. (2016). Hallmarks of Cancer Stem Cell Metabolism. Br. J. Cancer 114 (12), 1305–1312. doi:10.1038/bjc.2016.152
Sayegh, N., Swami, U., and Agarwal, N. (2021). Recent Advances in the Management of Metastatic Prostate Cancer. JCO Oncol. Pract. 18, 45–55. doi:10.1200/OP.21.00206
Scott, L. J. (2018). Enzalutamide: A Review in Castration-Resistant Prostate Cancer. Drugs 78 (18), 1913–1924. doi:10.1007/s40265-018-1029-9
Shafiee, G., Saidijam, M., Tayebinia, H., and Khodadadi, I. (2020). Beneficial Effects of Genistein in Suppression of Proliferation, Inhibition of Metastasis, and Induction of Apoptosis in PC3 Prostate Cancer Cells. Arch. Physiol. Biochem.. 1–9. doi:10.1080/13813455.2020.1717541
Sharda, M., Badrinarayanan, A., and Seshasayee, A. S. N. (2020). Evolutionary and Comparative Analysis of Bacterial Nonhomologous End Joining Repair. Genome Biol. Evol. 12 (12), 2450–2466. doi:10.1093/gbe/evaa223
Sharma, H., Kanwal, R., Bhaskaran, N., and Gupta, S. (2014). Plant Flavone Apigenin Binds to Nucleic Acid Bases and Reduces Oxidative DNA Damage in Prostate Epithelial Cells. PLoS One 9 (3), e91588. doi:10.1371/journal.pone.0091588
Shore, N. D. (2020). Current and Future Management of Locally Advanced and Metastatic Prostate Cancer. Rev. Urol. 22 (3), 110–123.
Shukla, S., Bhaskaran, N., Babcook, M. A., Fu, P., Maclennan, G. T., and Gupta, S. (2014). Apigenin Inhibits Prostate Cancer Progression in TRAMP Mice via Targeting PI3K/Akt/FoxO Pathway. Carcinogenesis 35 (2), 452–460. doi:10.1093/carcin/bgt316
Shukla, S., Fu, P., and Gupta, S. (2014). Apigenin Induces Apoptosis by Targeting Inhibitor of Apoptosis Proteins and Ku70-Bax Interaction in Prostate Cancer. Apoptosis 19 (5), 883–894. doi:10.1007/s10495-014-0971-6
Shukla, S., Kanwal, R., Shankar, E., Datt, M., Chance, M. R., Fu, P., et al. (2015). Apigenin Blocks IKKα Activation and Suppresses Prostate Cancer Progression. Oncotarget 6 (31), 31216–31232. doi:10.18632/oncotarget.5157
Song, Y. Y., Yuan, Y., Shi, X., and Che, Y. Y. (2020). Improved Drug Delivery and Anti-tumor Efficacy of Combinatorial Liposomal Formulation of Genistein and Plumbagin by Targeting Glut1 and Akt3 Proteins in Mice Bearing Prostate Tumor. Colloids Surf. B Biointerfaces 190, 110966. doi:10.1016/j.colsurfb.2020.110966
Su, T., Huang, C., Yang, C., Jiang, T., Su, J., Chen, M., et al. (2020). Apigenin Inhibits STAT3/CD36 Signaling axis and Reduces Visceral Obesity. Pharmacol. Res. 152, 104586. doi:10.1016/j.phrs.2019.104586
Tang, Q., Ma, J., Sun, J., Yang, L., Yang, F., Zhang, W., et al. (2018). Genistein and AG1024 Synergistically Increase the Radiosensitivity of Prostate Cancer Cells. Oncol. Rep. 40 (2), 579–588. doi:10.3892/or.2018.6468
Thoma, C. (2016). Prostate Cancer: Targeting Apoptosis Resistance in CRPC. Nat. Rev. Urol. 13 (11), 631. doi:10.1038/nrurol.2016.200
Tian, J., Guo, F., Chen, Y., Li, Y., Yu, B., and Li, Y. (2019). Nanoliposomal Formulation Encapsulating Celecoxib and Genistein Inhibiting COX-2 Pathway and Glut-1 Receptors to Prevent Prostate Cancer Cell Proliferation. Cancer Lett. 448, 1–10. doi:10.1016/j.canlet.2019.01.002
Tolkach, Y., Zarbl, R., Bauer, S., Ritter, M., Ellinger, J., Hauser, S., et al. (2021). DNA Promoter Methylation and ERG Regulate the Expression of CD24 in Prostate Cancer. Am. J. Pathol. 191 (4), 618–630. doi:10.1016/j.ajpath.2020.12.014
Travis, R. C., Allen, N. E., Appleby, P. N., Price, A., Kaaks, R., Chang-Claude, J., et al. (2012). Prediagnostic Concentrations of Plasma Genistein and Prostate Cancer Risk in 1,605 Men with Prostate Cancer and 1,697 Matched Control Participants in EPIC. Cancer Causes Control 23 (7), 1163–1171. doi:10.1007/s10552-012-9985-y
Uzunlulu, M., Telci Caklili, O., and Oguz, A. (2016). Association between Metabolic Syndrome and Cancer. Ann. Nutr. Metab. 68 (3), 173–179. doi:10.1159/000443743
Wang, G., Zhang, D., Yang, S., Wang, Y., Tang, Z., and Fu, X. (2018). Co-administration of Genistein with Doxorubicin-Loaded Polypeptide Nanoparticles Weakens the Metastasis of Malignant Prostate Cancer by Amplifying Oxidative Damage. Biomater. Sci. 6 (4), 827–835. doi:10.1039/c7bm01201b
Welti, J., Sharp, A., Brooks, N., Yuan, W., McNair, C., Chand, S. N., et al. (2021). Targeting the P300/CBP Axis in Lethal Prostate Cancer. Cancer Discov. 11 (5), 1118–1137. doi:10.1158/2159-8290.CD-20-0751
Whitaker, H., Tam, J. O., Connor, M. J., and Grey, A. (2020). Prostate Cancer Biology & Genomics. Transl Androl. Urol. 9 (3), 1481–1491. doi:10.21037/tau.2019.07.17
Wu, A., Cremaschi, P., Wetterskog, D., Conteduca, V., Franceschini, G. M., Kleftogiannis, D., et al. (2020). Genome-wide Plasma DNA Methylation Features of Metastatic Prostate Cancer. J. Clin. Invest. 130 (4), 1991–2000. doi:10.1172/JCI130887
Wu, Y., Zhang, L., Na, R., Xu, J., Xiong, Z., Zhang, N., et al. (2015). Plasma Genistein and Risk of Prostate Cancer in Chinese Population. Int. Urol. Nephrol. 47 (6), 965–970. doi:10.1007/s11255-015-0981-5
Xiang, C., Wu, X., Zhao, Z., Feng, X., Bai, X., Liu, X., et al. (2020). Nonhomologous End Joining and Homologous Recombination Involved in Luteolin-Induced DNA Damage in DT40 Cells. Toxicol. Vitro 65, 104825. doi:10.1016/j.tiv.2020.104825
Xiong, P., Wang, R., Zhang, X., DeLa Torre, E., Leon, F., Zhang, Q., et al. (2015). Design, Synthesis, and Evaluation of Genistein Analogues as Anti-cancer Agents. Anticancer Agents Med. Chem. 15 (9), 1197–1203. doi:10.2174/1871520615666150520142437
Xu, L., Zaky, M. Y., Yousuf, W., Ullah, A., Abdelbaset, G. R., Zhang, Y., et al. (2021). The Anticancer Potential of Apigenin via Immunoregulation. Curr. Pharm. Des. 27 (4), 479–489. doi:10.2174/1381612826666200713171137
Yadav, R. K., Chauhan, A. S., Zhuang, L., and Gan, B. (2018). FoxO Transcription Factors in Cancer Metabolism. Semin. Cancer Biol. 50, 65–76. doi:10.1016/j.semcancer.2018.01.004
Ye, Q., Liu, K., Shen, Q., Li, Q., Hao, J., Han, F., et al. (2019). Reversal of Multidrug Resistance in Cancer by Multi-Functional Flavonoids. Front. Oncol. 9, 487. doi:10.3389/fonc.2019.00487
Yu, T., Huang, D., Wu, H., Chen, H., Chen, S., and Cui, Q. (2021). Navigating Calcium and Reactive Oxygen Species by Natural Flavones for the Treatment of Heart Failure. Front. Pharmacol. 12, 718496. doi:10.3389/fphar.2021.718496
Zhang, L., Li, L., Jiao, M., Wu, D., Wu, K., Li, X., et al. (2012). Genistein Inhibits the Stemness Properties of Prostate Cancer Cells through Targeting Hedgehog-Gli1 Pathway. Cancer Lett. 323 (1), 48–57. doi:10.1016/j.canlet.2012.03.037
Zhang, S., Wang, Y., Chen, Z., Kim, S., Iqbal, S., Chi, A., et al. (2013). Genistein Enhances the Efficacy of Cabazitaxel Chemotherapy in Metastatic Castration-Resistant Prostate Cancer Cells. Prostate 73 (15), 1681–1689. doi:10.1002/pros.22705
Zhao, S. G., Chen, W. S., Li, H., Foye, A., Zhang, M., Sjöström, M., et al. (2020). The DNA Methylation Landscape of Advanced Prostate Cancer. Nat. Genet. 52 (8), 778–789. doi:10.1038/s41588-020-0648-8
Keywords: prostate cancer, resistance, flavone, apigenin, genistein
Citation: Ji X, Liu K, Li Q, Shen Q, Han F, Ye Q and Zheng C (2022) A Mini-Review of Flavone Isomers Apigenin and Genistein in Prostate Cancer Treatment. Front. Pharmacol. 13:851589. doi: 10.3389/fphar.2022.851589
Received: 10 January 2022; Accepted: 20 January 2022;
Published: 11 March 2022.
Edited by:
Qingbin Cui, University of Toledo, United StatesReviewed by:
Fangfang Xu, Guangzhou University of Chinese Medicine, ChinaCopyright © 2022 Ji, Liu, Li, Shen, Han, Ye and Zheng. This is an open-access article distributed under the terms of the Creative Commons Attribution License (CC BY). The use, distribution or reproduction in other forums is permitted, provided the original author(s) and the copyright owner(s) are credited and that the original publication in this journal is cited, in accordance with accepted academic practice. No use, distribution or reproduction is permitted which does not comply with these terms.
*Correspondence: Qingmei Ye, cWluZ21laS15ZUBoYWlubWMuZWR1LmNu; Caijuan Zheng, Y2FpanVhbjIwMDJAMTYzLmNvbQ==
†These authors have contributed equally to this work