- 1Centre for Interdisciplinary Research in Basic Sciences, Jamia Millia Islamia, New Delhi, India
- 2Pre-Clinical Research Unit, King Fahd Medical Research Center, King Abdulaziz University, Jeddah, Saudi Arabia
- 3Department of Medical Laboratory Sciences, Faculty of Applied Medical Sciences, King Abdulaziz University, Jeddah, Saudi Arabia
- 4Department of Computer Science, Jamia Millia Islamia, New Delhi, India
- 5Chemistry Department, Faculty of Science, King Abdulaziz University, Jeddah, Saudi Arabia
- 6Center of Excellence for Advanced Materials Research, King Abdulaziz University, Jeddah, Saudi Arabia
- 7Centre for Pharmaceutical Nanotechnology, Department of Pharmaceutics, National Institute of Pharmaceutical Education and Research, SAS Nagar Mohali, India
- 8Department of Biology, College of Science, University of Hail, Hail, Saudi Arabia
- 9Department of Biomedical Sciences and Therapeutics, Faculty of Medicine and Health Sciences, Universiti Malaysia Sabah, Kota Kinabalu, Malaysia
- 10Department of Biochemistry, Faculty of Medicine and Health Sciences, Abdurrab University, Pekanbaru, Indonesia
- 11Centre for International Collaboration and Research, Reva University, Bangalore, India
Caffeic acid (CA) has been present in many herbs, vegetables, and fruits. CA is a bioactive compound and exhibits various health advantages that are linked with its anti-oxidant functions and implicated in the therapy and prevention of disease progression of inflammatory diseases and cancer. The anti-tumor action of CA is attributed to its pro-oxidant and anti-oxidant properties. CA’s mechanism of action involves preventing reactive oxygen species formation, diminishing the angiogenesis of cancer cells, enhancing the tumor cells’ DNA oxidation, and repressing MMP-2 and MMP-9. CA and its derivatives have been reported to exhibit anti-carcinogenic properties against many cancer types. CA has indicated low intestinal absorption, low oral bioavailability in rats, and pitiable permeability across Caco-2 cells. In the present review, we have illustrated CA’s therapeutic potential, pharmacokinetics, and characteristics. The pharmacological effects of CA, the emphasis on in vitro and in vivo studies, and the existing challenges and prospects of CA for cancer treatment and prevention are discussed in this review.
Introduction
Caffeic acid (CA) is a phenolic derivative generally found in green tea, red wine, fruits, vegetables, and coffee (Chen et al., 2019; Meinhart et al., 2019; Zhang et al., 2019). CA (3,4-dihydroxycinnamic acid) exhibits anti-bacterial and anti-inflammatory effects and participates in significant functions of the human system (Kępa et al., 2018; Bounegru and Apetrei, 2020). It demonstrates anti-cancer, anti-oxidant, anti-proliferative, and anti-inflammatory properties. CA plays a pro-oxidant role in tumor cells and an anti-oxidant role in healthy cells. The oxidative DNA injury induced by the pro-oxidant property and its downstream pathway stimulate cell death by apoptosis (Kanimozhi and Prasad, 2015). CA has been frequently found as quinic acid ester called chlorogenic acid (Chen and Ho, 1997; Verma and Hansch, 2004; Silva et al., 2014).
CA plays in the protection machinery of plants against infections, predators, and pests, inhibiting the growth and survival of insects, fungi, and bacteria (Tošović, 2017). Polyphenols are organic compounds distinguished by huge manifolds of phenol structural parts that perform as the bases of exclusive chemical, biological, and physical functions to individual constituents of the class. This significant structural variety deeply influences their bioavailability (Birková et al., 2020). The defensive effect of CA on the human system is elucidated because of its anti-oxidant functions that are endorsed for its chemical structure. The chemical features of CA molecules permit the removal of free radicals and inhibit reactive oxygen species (ROS) formation; thus, it has useful impacts on human health (Birková et al., 2020).
CA and its derivatives have been identified with anti-oxidant, anti-viral, anti-inflammatory, and anti-cancer activities (Prasad et al., 2011; Yang W. S. et al., 2013).; Yang et al., 2013b). CA acts as an inhibitor of low-density lipoprotein oxidative alteration that is considered engaged in the pathogenesis of atherosclerosis (Wang and Yang, 2012). CA blocks STAT3 action, and this, in turn, down-triggers HIF-1α action. It is a promising inhibitor of STAT3 and represses cancer angiogenesis via blocking the action of STAT3 and the expression of VEGF and HIF-1α (Jung et al., 2007). Moreover, the mRNA levels of iNOS, COX-2, and TNF-α were less regulated via CA. CA strongly suppresses the nuclear translocation of AP-1 member proteins (Yang W. S. et al., 2013) and may simultaneously repress the activation of NF-κB, NFAT, and AP-1 (Feng et al., 2005).
Based on the broad effects of CA, here we discuss its therapeutic potential in cancer by emphasizing its function in cancer signaling. This review focuses on CA’s chemical and pharmacological effects by representing its mechanism of action, bioavailability, and pharmacokinetic characteristics for expressing the promising therapeutic function in tumors, with emphasis on in vitro and in vivo studies. To conclude, CA’s current challenges and prospects for cancer management are also discussed.
Structural Features of Caffeic Acid
Phenolic compounds offer defense against diseases by regulating cellular mechanisms at different levels, such as enzyme inhibition, protein phosphorylation, and alteration of gene expression (Naz et al., 2017; Naz F. et al., 2018; Naz H. et al., 2018; Ishtikhar et al., 2018; Mohammad et al., 2019; Mohammad et al., 2020; Kasprzak-Drozd et al., 2021). An enhancement in phenolic compounds may change their health advantages (Saibabu et al., 2015; Anwar et al., 2016). However, more than 8,000 phenolic compounds might be categorized into two major groups, such as flavonoids and non-flavonoids (Kumar and Pandey, 2013).
Phenolic acids (PAs) are non-flavonoid phenolic compounds, including a single phenyl group alternated through a carboxylic and one or more OH groups (Leonard et al., 2021). PAs are again categorized by the extent of the chain, which encloses the carboxylic group, including hydroxycinnamic acids (HCs), hydroxyphenyl acids, and hydroxybenzoic acids (Has). HC has a C6–C3 fundamental skeleton. The existence of a CH2 = CH–COOH set in cinnamic acids guarantees a superior anti-oxidant capability than the COOH set in benzoic acid. Hence, one of the main HCs is CA (Göçer and Gülçin, 2011; Vinayagam et al., 2016; Filipe et al., 2018). Moreover, the potential therapeutic prospective of CA examinations has exhibited that the clean shape of CA has the accessibility to be absorbed in the intestines and consequently interfaces with the intention tissue (Sato et al., 2011).
Bioavailability and Metabolism of Caffeic Acid
CA’s partition coefficient fluctuates between 1.0 and 1.3, and its molecular mass is 180.16 g/mol (Wu et al., 2007; Kudugunti et al., 2010). Monocarboxylic acid transporters are responsible for CA absorption in the gastro-intestinal tract (Konishi et al., 2004; Konishi and Kobayashi, 2004). CA metabolism is also linked to the gut microbiota. CA undergoes decarboxylation under an anaerobic condition which passes via bacteria with the production of an analog [3-(3-hydroxyphenyl)-propionic acid] and thus exhibits better anti-oxidant action as compared to CA (Shen et al., 2020). After absorption, CA undergoes widespread metabolic pathways in the liver and kidney (Ito et al., 2005; Lafay et al., 2006). CA has disclosed an excellent safety summary in phase 1 clinical trial.
Hydrolyzation is an incredibly chief step in the human metabolism of CA in the intestine because of esterases, enzymes that are able to hydrolyze chlorogenic acid to make CA. CA is found in its ester in extremely tough foods to get absorbed. The ingestion of CA starts in the stomach, where a little amount is absorbed. Following the action of microbial esterases in the colon, CA gets sliced in free appearance and 95% gets absorbed via the intestinal mucosa (Birková et al., 2020) by active transport induced through monocarboxylic acid transporters. Hence, the highest CA plasma concentration has been detected to reduce 1 h after food ingestion. The detoxification method instantly creates more hydrophilicity after absorption, decreasing its toxic effect and facilitating its removal. The small intestine is the probable location of feruoylquinic acid cleavage into CA and ferulic acid, CA metabolism into 3-O-sulfate and 4-O-sulfate, as well as CA methylation, resulting in the formation of isoferulic acid followed by 3-O-sulfation and glucuronidation. CA is emitted mainly by urine, with calculated urinary secretion being between 5.9 and 27% (Olthof et al., 2001; Manach et al., 2004; Espíndola et al., 2019).
Role of Caffeic Acid in Human Physiology
CA is HA with a C6–C3 skeleton and with a transethylene wire connecting an aromatic ring with a carboxylic acid (Magnani et al., 2014; Silva et al., 2014). Plant CA biosynthesis occurs via a pathway which makes AAA from glucose (Silva et al., 2014; Rodrigues et al., 2015). Beginning with shikimic acid, it undergoes three enzymatic reactions. The first reaction is shikimate kinase-induced phosphorylation, followed by the conjugation of phosphoenolpyruvate induced by 5-EPSP synthase and lastly by chorismate synthetase, producing one of the vital conciliator metabolites of this signaling, chorismic acid (Silva et al., 2014; Ishtikhar et al., 2015; Rodrigues et al., 2015). Chorismic acid is converted by chorismate mutase into prephenic acid.
Furthermore, L-phenylalanine production is induced as a coenzyme by deamination through pyridoxal 5-phosphate and as an electron exchanger through nicotinamide adenine dinucleotide (Silva et al., 2014; Rodrigues et al., 2015). The deamination of L-phenylalanine through phenylalanine ammonia lyase forms cinnamic acid, which is transformed into p-coumaric acid through C4H and CA through the enzyme C3H (Rodrigues et al., 2015). CA is attained from plants by solvent extraction at the highest temperature, though its yield is extremely low, involving huge amounts of botanical substance to obtain a considerable yield (Lin and Yan, 2012; Rodrigues et al., 2015). This compound can be achieved in huge quantities through organic synthesis (Touaibia and Guay, 2011). Genetics alter microorganisms—for example, Escherichia coli strains (Kawaguchi et al., 2017; Hernández-Chávez et al., 2019).
There are several beneficial effects of CA and its derivatives, including anti-bacterial (Matejczyk et al., 2018; Mitani et al., 2018), anti-viral (Langland et al., 2018; Shen et al., 2018), anti-oxidant (Shiozawa et al., 2018; Kfoury et al., 2019), anti-inflammatory (Zaitone et al., 2019; Lima et al., 2020), immune-stimulatory (Krifa et al., 2013; Coleman et al., 2016), antidiabetic (Chiou et al., 2017; Bounegru and Apetrei, 2020), cardioprotective (Agunloye et al., 2019; Salau et al., 2021), anti-proliferative (Pelinson et al., 2019), hepatoprotective (Abdelhafez et al., 2018; Saleem et al., 2019), anti-cancer (Zeng et al., 2018; Martini et al., 2019; Pelinson et al., 2019), and so on. CA plays a central function in the human system because of its many beneficial effects. It can be found in pharmaceuticals (Katsarova et al., 2017; Li et al., 2017).
Several studies showed that high doses of CA might cause considerable side effects, which inhibit the implantation of embryos (Liu et al., 2019) or cancerous effects (Hagiwara et al., 1991). The information is verified; the small intestine absorbs a significant amount of CA, which goes into the bloodstream in a large proportion (Wang et al., 2017). CA can support the progression of squamous cell carcinomas in the kidneys and stomach of mice and rats (Hagiwara et al., 1991). A toxicity study of CA has been performed to understand the reproductive role and the progression of offspring in female mice. However, female mice have incessantly been exposed to different dosages via gavage in the 3-segment analysis. Two CA doses (5 and 150 mg/kg/day) were reported to affect embryo implantation when administered before the sixth day of gestation. Additionally, the CA dose of 150 mg/kg/day influenced the fetal weight to be achieved (Liu et al., 2019).
Pharmacological Effects of Caffeic Acid
Anti-cancer Properties
CA and its derivatives have been recognized for their anti-inflammatory, anti-bacterial, and anti-carcinogenic functions that could be associated with its anti-oxidant action (Genaro-Mattos et al., 2015). CA treatment has increased the ROS levels and changed matrix metalloproteinases (MMP) in ME-180 and HeLa tumor cells. Enhanced apoptotic morphological alterations have been observed in CA-treated cells in ME-180 and HeLa cells (Kanimozhi and Prasad, 2015). Hence, a pro-survival result of CA mediated by the NF-κB pathway has been expressed in lung tumor cells treated with paclitaxel (Lin et al., 2012). The accurate function of ROS in intracellular activity remains less understood and probably depends on particular situations.
New studies show that CA exerts anti-tumor properties by AMPK activation, and a mechanism has been identified in colon tumor cells in vitro (Murad et al., 2015). CA demonstrates a potent anti-tumor effect in the HT-1080 cell line, which might be utilized as an anti-cancer drug (Prasad et al., 2011; Alam et al., 2022). It prevented breast tumor cell proliferation, influencing cell cycle development and downstream effectors. The maximum impact of CA has been observed in MCF-7 cells, where it suppressed the proliferation and survival of breast tumor cells (Rosendahl et al., 2015).
The role of CA-targeting gene amplified in squamous cell carcinoma 1 (GASC1) has been established recently. GASC1 is a recently reported oncogene in various cancer types, including esophageal cancer (Sun et al., 2013; Jia et al., 2020). The anti-cancer effect of CA has been studied in advanced esophageal squamous cell cancer (ESCC), and clinical trials are being conducted (ClinicalTrials.gov identifier: NCT04648917). CA was used as a drug for thrombocytopenia when the patient received chemotherapy. A recent clinical trial has been done to see the effectiveness of oral CA tablets in managing primary immune thrombocytopenia. Scientists have observed a very effective role of CA in immune thrombocytopenia patients, with few and mild side effects, thus suggesting a potential therapeutic role of CA (Qin et al., 2015).
The anti-carcinogenic functions of CA have magnetized the consideration of the scientific society (Da Cunha et al., 2004; Damasceno et al., 2017; Sidoryk et al., 2018). Reports have revealed that the utilization of foods rich in CA causes a defensive action in carcinogenesis by inhibiting the creation of nitro compounds, the pathology’s chief inducers (Touaibia et al., 2011; Damasceno et al., 2017). However, these effects of CA are generally linked with its anti-oxidant (Stagos et al., 2012; Magnani et al., 2014) and pro-oxidant abilities (Li et al., 2000; Zhang et al., 2008), which are attributed to their chemical structure. Initially, the existence of free phenolic hydroxyls is probably to reduce the enthalpy of OH-bond dissociation that enhances the transfer speed of H atoms to peroxyl radicals and the number and location on the phenyl ring. Hence, the existence of a double bond in the carbon chain enhances the constancy of the phenolic radical (Son and Lewis, 2002; Touaibia et al., 2011; Magnani et al., 2014). Thus, chemical features connected with CA molecules permit the removal of free radicals, inhibiting the creation of ROS and the initiation of DNA oxidation of tumor cells (Silva et al., 2014; Sidoryk et al., 2018).
Anti-oxidant Activity
CA is an anti-oxidant which may decrease the oxidative stress present in the body because of free radicals. Hence, oxidative stress is described as an inequity between the making of ROS and anti-oxidant protection (Birková et al., 2020). Consequently to this inequity, oxidative stress frequently results in the progression of many diseases in humans, including cancer (Ahmed et al., 2015; Bjørklund and Chirumbolo, 2017). Anti-oxidants inhibit the effects regulated via free radicals and oxidizing compounds (Soares et al., 2005). The grouping of CA with other products, including chlorogenic and caffeic acids, is explained by the high potent anti-oxidant action in different systems (Meyer et al., 1998; Fukumoto and Mazza, 2000). CA is considered as a potential photo-protective agent present in skincare products because of its anti-oxidant action (Yamada et al., 2006). Depending on the exposure time, wavelength, exposed area, and dose, UV radiation may cause premature skin aging, skin burns, skin cell DNA injury, and skin tumor (Scharffetter-Kochanek et al., 1997; Matsumura and Ananthaswamy, 2002).
Based on the mechanisms of CA, it acts in cancer via its promising anti-oxidant ability that inhibits the creation of ROS, thus decreasing oxidative stress, which is incredibly general in disease (Silva et al., 2014; Sidoryk et al., 2018). CA performs as a primary and pro-oxidant (secondary). A primary anti-oxidant performs via disrupting the creation of free radicals via preventing the chain reactions with a different molecule (Angelo and Jorge, 2007; Damasceno et al., 2017). This procedure happens when CA donates hydrogen/electrons for free radicals, changing them into thermodynamically constant products. Hence, these products present better constancy because of the electron delocalization in the aromatic ring of CA (Damasceno et al., 2017). A secondary anti-oxidant performs as a chelating agent and makes complexes with metals (copper and iron), preventing the decomposition of peroxides, decreasing the creation of free radicals and their assault on amino acids, lipids, and bases of DNA, and consequently evading the making of lesions and failure of cellular integrity (Angelo and Jorge, 2007; Damasceno et al., 2017). However, CA has a huge potential effect for decreasing metals because of its structural chemical features; the compound is vulnerable to auto-oxidation and oxidation caused via other biological agents (Medina et al., 2012; Damasceno et al., 2017).
Pro-oxidant Activity
The utilization of foods rich in CA has been revealed to protect against carcinogenesis due to its anti-oxidant and pro-oxidant functions. CA displays pro-oxidative roles in cancer cells, which are correlated with oxidative DNA injury and, pursued by its consequent pathway, the induction of cell death in tumor cells (Birková et al., 2020). This anti-tumor effect of CA by pro-oxidative functions has been first identified by scientists in 2015. They detected enhanced apoptotic morphological alterations in tumor cells treated with CA, where CA enhanced the lipid peroxidation (LPO) markers in ME-180 and HeLa cells. Hence, they detected elevated levels of ROS and modified MMP (Kanimozhi and Prasad, 2015). CA may turn into a pro-oxidant by its capability to chelate metals like copper and stimulate LPO, causing injury on the DNA of tumor cells through oxidation or creation of covalent adducts with DNA (Zheng et al., 2008; Damasceno et al., 2017). CA holds the capability to cap the endogenous Cu ions of human lymphocytes to form CA-Cu (II) (Zheng et al., 2008). CA undertakes deprotonation relative to Cu, producing an oxygen center by high electronic density (Zheng et al., 2008; Damasceno et al., 2017). However, this complex ensures oxygen to make the semiquinone radical anion with Cu (I) (Zheng et al., 2008; Damasceno et al., 2017). CA deprotonation takes place to form a phenoxide wherever the Cu (I) ion should be bound as a bidentate linker (Zheng et al., 2008; Damasceno et al., 2017). Pro-oxidant acts play an anti-tumor effect due to the induction of cell death in the cancer cells (Yang et al., 2012).
Anti-inflammatory Properties
CA exhibits a cardio-protective effect against hypercoagulability, dyslipidemia, inflammation, and oxidative stress in diabetic mice. The dietary supplementation of CA and ellagic acid increased the levels of lipid metabolism and glycemic control in diabetic mice (Birková et al., 2020; Sharifi-Rad et al., 2020). Eventually, both compounds explained the anti-inflammatory, anti-oxidative, and anti-coagulatory defense for the heart of diabetic mice (Chao et al., 2009). CA might defend the cardiac tissue against diabetes-linked hypercoagulability, dyslipidemia, inflammation, and oxidative stress. The inflammatory reaction in the brain is a coordinated regulatory machinery of specialized cells in the CNS known as microglial cells. However, the activation of cells under pathological conditions was revealed to contribute to the progression of numerous neurodegenerative disorders. The discharge of pro-inflammatory mediators in the brain stimulated through various stimulants, including Aβ, was exhibited to account for the inflammatory constituent of neuronal loss in Alzheimer's disease (AD) (Ito et al., 1998; Verri et al., 2012; Prokop et al., 2013).
CA and its derivatives possess anti-inflammatory effects and thus are implicated in AD through these agent candidates. In addition, the activation of Nrf2 has been explained for inhibiting inflammatory gene expression (Chen et al., 2006; Alam et al., 2021a) by signaling the crosstalk linking the HO-1 (Kapturczak et al., 2004). Kim et al. (Kim and Jang, 2014) have illustrated that the Nrf2-induced HO-1 introduction of CAPE is correlated with its anti-inflammatory and anti-oxidant mechanisms. The anti-inflammatory result of other CA esters in microglial cells was connected to the induction of HO-1 (Lu et al., 2013; Alam et al., 2021c). Hence, their multifunctional results indicate the relationship between the CA derivatives’ anti-oxidant and anti-inflammatory effect and their therapeutic potential for AD. Liang et al. (2015) have confirmed that the enhanced NF-κB, p65, and 5-LOX expression correlated with the global cerebral ischemia–reperfusion neuronal injury and memory loss in rats is inverted through CA (10–50 mg/kg) treatment. CA (50 mg/kg) also improved neuronal loss and infarct volume 24 h after ischemia (Zhou et al., 2006). Together with the common anti-inflammatory effect of CA derivatives, these effects are all pertinent mechanisms that might feature anti-AD potential.
Induction of Apoptosis
CA induces apoptosis through inhibiting Bcl-2 action, resulting in the liberation of cyt-c and the consequent activation of caspase-3, representing the fact that CA stimulates apoptosis by the intrinsic apoptotic pathway (Chang et al., 2010; Alam et al., 2019; Alam et al., 2021b). Their anti-oxidant results are arbitrated through modulating pathways, including MAPK, NF-κB, and Akt. Furthermore, they stimulate cell cycle arrest and increase cell death in tongue, neck, and mouth cancer (Srinivasulu et al., 2018). In Ht-29 cells, 5-caffeoylquinic acid and CA decreased cell viability by endorsing specific cell cycle modifications and stimulating cell death in a time- and dose-dependent way (Murad et al., 2015; Anantharaju et al., 2016). CA demonstrated a vital function in inhibiting cancer progression by reducing cell viability and cell death induction. The treatment with CA caused the modulation of the cell cycle, prevention of colony formation, and alteration in caspase expression (Pelinson et al., 2019). CA attenuated cancer stem cell-like functions by the prevention of TGFβ-SMAD2 signaling induced via microRNA-148a in vivo as well as in vitro (Li et al., 2015). The contrast in the anti-tumor results of CA and CAPE showed the exposure time and dose-dependent capability of CAPE to be highly promising in the treatment of tumor cells with its action induced through stimulating cell death and cell cycle arrest in MDA-MB-231 cells (Kabała-Dzik et al., 2017) and decreasing the migration of MCF-7 (Kleczka et al., 2020).
Inhibition of Vascularization and Invasiveness
CA efficiently blocked the VEGF-mediated proliferation and survival of retinal endothelial cells in a concentration-dependent way (Figure 1A). Additionally, the tube formation of cells and VEGF-mediated migration have been presented (Kim et al., 2009; Alam and Mishra, 2020). CA might also perform hepatocellular carcinoma (HCC) cells’ angiogenesis by decreasing the JNK-1 phosphorylation through the reduction of HIF-1α activation. This causes the decline of vascularization mediated through VEGF and represses cancer growth (Gu et al., 2016). HCC is an extremely vascularized tumor whose main distinctive characteristic is angiogenesis, and its major resource of blood supply is the hepatic artery (Dhanasekaran et al., 2016; Gu et al., 2016; Klungboonkrong et al., 2017). This cancer is rich in vascularization; hypoxia is very frequent because of the quick proliferation of cancer cells and, accordingly, the making of huge solid tumor masses, hindering and squeezing the blood vessels about it (Dhanasekaran et al., 2016; Gu et al., 2016; Klungboonkrong et al., 2017). Cancer cells seek to adapt to hypoxia through activating HIF-1 by the JNK-1 pathway, which stimulates numerous proangiogenic factors, including VEGF. When better expressed, VEGF causes extravasation of blood from the cancer blood vessels, leading to hepatic bleeding (Zhu et al., 2011; Gu et al., 2016), a significant factor for cancer survival (Zhu et al., 2011; Dhanasekaran et al., 2016; Gu et al., 2016; Klungboonkrong et al., 2017). CA drastically repressed the retinal neovascularization in oxygen-mediated retinopathy like the animal model of ROP with no retinal cytotoxicity (Kim et al., 2009).
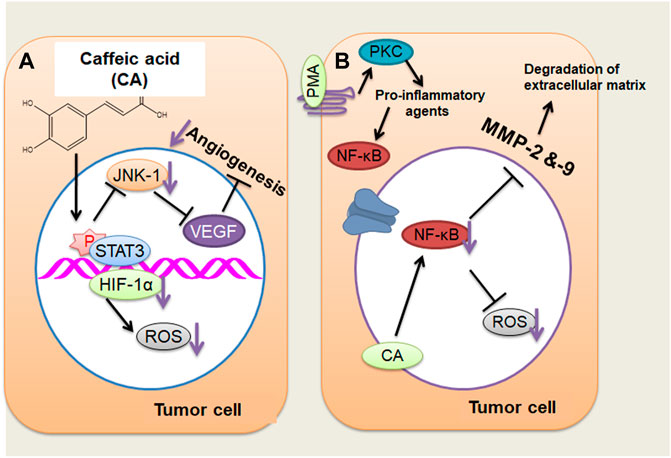
FIGURE 1. (A) Caffeic acid (CA) actions on the angiogenesis of cancer cells through decreasing the JNK-1 phosphorylation and reducing the HIF-1α activation that cause the decline of vascularization mediated by vascular endothelial growth factor. (B) CA may act on tumor cells by repressing MMP-2 and MMP-9 expressions, which, in turn, inhibits the activation of NF-κB stimulated through PMA (activating protein 1) in tumor cells, thus reducing cancer invasiveness and growth. ↓, decrease. (Adapted from Espíndola et al., 2019)
An additional mechanism of action suggested that CA represses MMP-2 and MMP-9 expression in HCC. MMP-2 and MMP-9 are expressed in cancer cells that degrade the extracellular matrix (ECM) type IV collagen during tumor metastasis and invasion (Chung et al., 2004; Lee et al., 2008; Yeh et al., 2012; Pramanik et al., 2016). Phorbol 12-myristate 13-acetate (PMA) can stimulate PKC; once activated, it endorses the induction of pro-inflammatory cytokines, including IL-6 and TNF-a (Jiang and Fleet, 2012). These pro-inflammatory mediators stimulate the activation of NF-κB by c-Src/ERK/NIK/IKK (Touaibia et al., 2011; Kim et al., 2014; Alam and Mishra, 2021). NF-κB produces enhanced MMP-2 and MMP-9 expression that leads to the metastasis and invasion of hepatic cells through the degradation of ECM (Chung et al., 2004; Lee et al., 2008; Yeh et al., 2012; Pramanik et al., 2018). The repressive result of CA on MMP-2 and MMP-9 is connected with the obstruction of NF-κB activation, as identified in liver tumor cells stimulated via PMA, leading to a reduction in cancer invasiveness and growth (Chung et al., 2004; Lee et al., 2008; Alam et al., 2017) (Figure 1B). CA has been noted of its anti-oxidant results via repressing the making of ROS and superoxide dismutase and inhibiting tumor development and migration by reducing cell adhesion via a decreased connection to the ECM in A549 and HT29-D4 cells (Anantharaju et al., 2016; Zhu, 2016).
Synergistic Effect of Caffeic Acid With Anti-cancer Agents
Combination therapy is the treatment and management move toward two or more agents/drugs with the target of achieving equivalent efficiency levels with minor toxicities and at doses lesser than normal and having superior influence with synergistic/additive effects (Chen et al., 2016; Bayat Mokhtari et al., 2017). A combined treatment approach with natural products might inhibit the source of acquired drug resistance, including chemotherapy. However, successful combinations of potent therapeutic drugs/agents with products may attain the desired conclusion but with lesser toxicity (Bukowska et al., 2015; Das et al., 2018). CA and Metformin (Met) was identified to have additive/synergistic effects while combined with anti-tumor therapies, mainly for HTB-34 cells (Tyszka-Czochara et al., 2017b). CA induces cytotoxicity by necrosis for SiHa cancer cells but, combined with Met and its cytotoxicity machinery, moved toward cell death without disturbing healthy human fibroblasts. However, the combination for controlling mitochondrial metabolism that stimulated ROS making in metastatic cancer cells has been found. Hence, incubating tumor cells with CA and Met caused a remarkable move to the G0 from the G1 stage. Studies continue to explore the mechanism of anti-cancer function of the combination of CA and Met (Tyszka-Czochara et al., 2017a).
CA and cisplatin illustrated a potent anti-tumor action in cancer. Furthermore, cisplatin-sensitive cells, while exposed to a combination therapy of 50 μM CA and 5 μM cisplatin, quickly enhanced the action of apoptotic cascade through enhanced caspase action (1.7 folds) in contrast to the administration of 5 μM cisplatin only. A study on A2780cisR cells confirmed that combining 5:50 μM (cisplatin/CA) increases the caspase action by 4:3 folds through 60% cell viability (Sirota et al., 2017). An analysis has been performed wherein the combination of CA with cisplatin has been checked to stop the resistance progression in tumor treatment. CA is an inhibitor of glutathione S-transferase and glutathione reductase that are the catalytic enzymes of GTH (Figure 2) (Siddik, 2003; Islam et al., 2017). Hence, there is a scope of testing the combined result of CA and CAF to reveal their potential therapeutic effect against tumor to evaluate the molecular mechanisms of the combination with a multi-target (Figure 2). However, CA combined with cisplatin enhanced its therapeutic effect, leading to the prevention of cell growth and survival of CaSki and HeLa cells that might be elucidated via a synergistic effect. Hence, this combination has been correlated with enhancing the expression of caspase-3, caspase-7, and caspase-9 (Koraneekit et al., 2018).
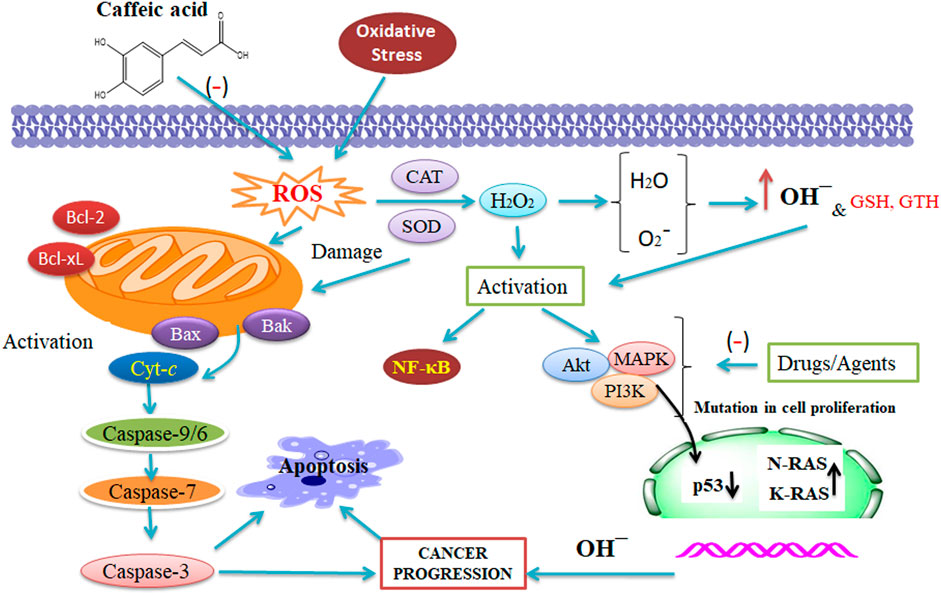
FIGURE 2. Synergistic action of caffeic acid (CA) with anti-tumor therapy that affects the reactive oxygen species, catalase, and superoxide dismutase. The combined result of CA and CAF reveals their potential against tumors and evaluates the molecular mechanisms of the combination with a several-target approach (Maity et al., 2021 and Alam et al., 2022). This figure was drown by ChemBioDraw.
Clinical Significance of Caffeic Acid
A study has explained that CAPE (50 μM) considerably enhanced the apoptosis induced via tumor necrosis factor-related apoptosis-inducing ligand (TRAIL) through the positive regulation of DR5 induced via CHOP in Hep3B HCC cells. However, TRAIL is a ligand with anti-tumor functions able to stimulate cell death in tumor cells (Mongkolsapaya et al., 1998; Dilshara et al., 2016). This act happens via its binding with DR5, which interrelates with Fas through recruiting caspase-8 and caspase-3 and stimulating cell death (Siegmund et al., 2001; Dilshara et al., 2016). CAPE found in the bee propolis extract potentiated TRAIL-induced apoptosis, motivating the CHOP protein expression being dependable for DR5 regulation (Dilshara et al., 2016). One experiment identified CAPE (30 μg/ml) to potentiate TRAIL-mediated cell death (30 ng/ml) by DR5 regulation through p38 and repression of JNK in SK-Hep1 cells (Yang SY. et al., 2013; Kim et al., 2014). However, the combination of TRAIL and CAPE has generated apoptosis by the intrinsic pathway and through the extrinsic pathway (Yang SY. et al., 2013). In intrinsic CAPE and TRAIL signaling, mitochondrial membrane depolarization stimuli have been enhanced, consequential in the liberation of cyt-c and the making of the apoptosome and resulting in the activation of apoptosis-stimulating caspase 9 (Nicholson, 1999; Yang SY. et al., 2013). Alternatively, CAPE and TRAIL endorsed p38 activation via the extrinsic signaling pathway by enhancing the expression of apoptosis, stimulating DR5, and blocking the JNK phosphorylation, which contributes to TRAIL resistance and, accordingly, reduced the DR5 expression (Lu et al., 2011; Yang SY. et al., 2013).
CA (1 mM) inhibited cell proliferation and survival in HCC cells extracted from marmots (Wilkins et al., 2017). Hence, the compound’s activity is connected with its participation in the failure of mitochondrial integrity, resulting in cyt-c liberation, apoptosome making, and caspase-9 activation, thus inducing apoptosis. CAPE (12.5 μM) blocked the invasion and MMP-2 and MMP-9 expression in SK-Hep1 cells that obstruct NF-κB (Lee et al., 2008). CA (200 μg/ml) blocked cancer regression and invasion in HepG2 and Huh7 cells via reducing pro-inflammatory cytokines, including TNF-a, IL-1b, and IL-8, and anti-inflammatory cytokines, including IL-10 (Guerriero et al., 2011). A neurotoxicity examination using PC12 cells treated with 10 μM Aβ (Sul et al., 2009; Lee et al., 2011; Kim J. H. et al., 2015; Yang et al., 2015) for 24 h confirmed that CA holds a cytoprotective effect in a dose-dependent way (10 and 20 μg/ml) when added 1 h earlier to Aβ (Sul et al., 2009). The pre-treatment of PC12 cell lines with chlorogenic acid (CGA) was proven to protect them from Aβ-mediated cell death together with attenuation of calcium levels and a decrease in the level of cell death-associated proteins such as caspase-3, Bax, and Bcl-2 (Lee et al., 2011). The defensive action of CA from Aβ (Sul et al., 2009; Lee et al., 2011; Kim J. H. et al., 2015; Yang et al., 2015) and LPS-mediated neuronal cell injury and neuronal inflammation has also been evaluated in C6 glial cells (Kim J. H. et al., 2015). However, the result exhibited a positive conclusion, but considering the greater dose (5 mM CA) utilized in the study, the result is not believed to be of therapeutic application until an action at a lesser dose is exhibited (Kim J. H. et al., 2015). In Aβ-mediated axonal atrophy in cultured cortical neurons of mice (Sul et al., 2009; Lee et al., 2011; Kim J. H. et al., 2015; Yang et al., 2015), CA 4-O-glucoside was shown to induce considerable axonal elongation results on Aβ-mediated atrophy (Sul et al., 2009; Lee et al., 2011; Kim J. H. et al., 2015; Yang et al., 2015).
The activity of CA was determined (100 mg/kg) on the structural alterations caused by HCC in the rat microbiota, showing that the compound decreases and alters the markers of liver damage during exposure to HCC, including transaminase, aspartate, alanine, aminotransferase, phosphatase, and total cholesterol. Hence, a possible machinery by CA actions is associated in the prevention of survival of malefic bacteria (Del Chierico et al., 2017; Zhang et al., 2017; Ali et al., 2021) and the introduction of the growth and survival of microbiota-beneficial bacteria during HCC progression (Li et al., 2016; Zhang et al., 2017). CA possesses anti-oxidant functions, making it capable of eliminating oxygen radicals and which facilitates the growth of beneficial bacteria, which are anaerobic and grow extremely without oxygen (Zhao et al., 2014; Zhang et al., 2017). Compounds possess an anti-microbial action in removing malefic bacteria of the microbiota, supporting the control of markers of liver injury (Pinho et al., 2015; Zhang et al., 2017).
CA (1 mM) improved the efficiency of Tris-acetate-EDTA (TAE) in rats with tumors. Hence, TAE is a therapeutic process utilized in patients with HCC to promote ischemia because of occlusion of the arterial blood supply, resulting in the obstruction of nutrients and oxygen for cancer (Johnson et al., 2016; Wilkins et al., 2017). The chief nutrient for HCC is lactate, which is created by glycolytic metabolism that is dependable for enhancing vascular growth factor expression in vasculogenesis (Mathupala et al., 2007; Wilkins et al., 2017). This effect is probably because of the anti-cancer, anti-inflammatory, and anti-oxidant properties that produce ROS and fragment DNA, which causes apoptosis in tumor cells (Wilkins et al., 2017). CA can stimulate the intrinsic pathway of cell death by changing the mitochondria’s membrane potential (Prasad et al., 2011; Wilkins et al., 2017).
CA is an extremely flexible compound with various biological actions impacting the human system, including anti-tumor, anti-oxidant, anti-microbial, anti-inflammatory (Verma and Hansch, 2004; Yang SY. et al., 2013; Genaro-Mattos et al., 2015). This information seems to favor its activity in the HCC, as in vitro and in vivo investigations already exhibited its act by numerous mechanisms of action in the battle against diseases, including ROS prevention (Silva et al., 2014; Sidoryk et al., 2018), angiogenesis (Gu et al., 2016), and repression of MMP-2 and MMP-9 (Chung et al., 2004; Gu et al., 2016), thus explaining the diversities in the effects found. Kim et al. (2015) examined the defensive capabilities of CA in an Aβ-injected (Sul et al., 2009; Lee et al., 2011; Kim J. H. et al., 2015; Yang et al., 2015) AD mouse model through the administration of 10–50 mg/kg/day for 2 weeks. CA, in a dose-dependent way, blocked LPO and nitric oxide creation in the kidney, liver, and brain, while this was contrasted with the Aβ-injected (Sul et al., 2009; Lee et al., 2011; Kim J. H. et al., 2015; Yang et al., 2015) healthy group. In kainic acid-mediated cognitive dysfunction in rats, CA demonstrated a considerable enhancement in memory presentation, oxidative stress parameters, and a mitochondrial role compared to the control group (Kumar et al., 2012). By utilizing the global cerebral ischemia–reperfusion damage analysis model in rats, a study (Liang et al., 2015) has examined the effect of CA on memory wherever the bilateral carotid artery has been occluded for 20 min as pursued via reperfusion. The analysis discovered that CA (10–50 mg/kg) noticeably decreased the escape latency, reassured hippocampal neuron injury, and enhanced the neuronal count compared to that in untreated rats.
Pharmacokinetics of Caffeic Acid
CA occurs in esterified and free forms, indicating around 75–100% of the entire content of hydroxycinnamic acid in fruits (Lee et al., 1995). CA is tricky to be absorbed through the body (Lee et al., 1995; Scalbert and Williamson, 2000; Kolodziejczyk-Czepas et al., 2015). To be absorbed, this compound requires to be hydrolyzed via the colonic microflora in the intestine since human tissues and biological fluids do not have enzymes, known as esterases, able to hydrolyze the CGA to liberate CA (Lee et al., 1995; Scalbert and Williamson, 2000; Kolodziejczyk-Czepas et al., 2015). Accordingly, the pharmacokinetic procedure starts with the ingestion of CA inward in the stomach, after which a little part is absorbed. Hence, in the colon, the microbial esterases slice the ester piece of CA. It is absorbed through the intestinal mucosa (Oliveira and Bastos, 2011). The transmembrane run of CA into the intestinal cells is via active transport mediated through MCT (Oliveira and Bastos, 2011). The highest plasma concentration of the compound has been detected merely 1 h after ingestion of foods, including coffee, and subsequently, the plasma concentration quickly reduced, requiring reiterated doses every 2 h to sustain the high concentrations (Lee et al., 1995; Scalbert and Williamson, 2000; Kolodziejczyk-Czepas et al., 2015). Instantly after absorption, CA is subjected to three major procedures of enzymatic conjugation—methylation, sulphation, and glucuronidation—by the action of UDP-glucosyltransferases and catechol-o-methyltransferases. Hence, this creates a high level of hydrophilicity, decreasing its toxicity and assisting its exclusion (Oliveira and Bastos, 2011). The emission of CA (5.9–27%) takes place mainly in urine (Lee et al., 1995).
Toxicity and Limitations of Caffeic Acid
CA has exhibited selective toxicity in HCC (Brautigan et al., 2018). In a study, a group of 15 male hamsters was supplemented with 1% (10 g/kg diet) CA (98% pure) for 5 months. Later, the urinary bladders and stomach were examined by histopathology and radiography (Shikov et al., 2014). Moderate epithelial hyperplasia was detected in 14 animals, 1 was severe, and 7 were untreated. An increase in the number of labeled cells was detected in the forestomach and pyloric region when H-thymidine incorporation was assessed in the infected hamsters. It is not statistically significant.
Another study revealed carcinogenic activity in male and female F344 rats and C57BL/6N × C3H/HeN F1 mice in the squamous cell epithelium in the forestomach (Hagiwara et al., 1991). However, successful combinations of potent therapeutic drugs/agents with products may attain the desired conclusion but with lesser toxicity (Bukowska et al., 2015; Das et al., 2018). CA has been believed to be a toxic compound of anaerobic wastewater treatment (Hernandez and Edyvean, 2018). However, CA can act differently in terms of digestibility or toxicity in some anaerobic situations—for example, CA undergoes autoxidation in aqueous systems with oxygen draws, resulting in aggregates with adsorptive properties (Rochelle, 2001). CA is carcinogenic towards animals, but no sufficient evidence exists for humans. To date, the accurate mechanism of the toxic effect of CA remains unknown.
Conclusion and Future Directions
Phytochemicals are presently huge achievements in cancer prevention and treatment as they possess anti-oxidant, anti-proliferative, anti-angiogenic, pro-apoptotic, and anti-tumor properties. CA is a potential chemotherapeutic agent/drug which demonstrates anti-oxidant, anti-inflammatory, anti-proliferative, anti-microbial, and anti-tumor functions and has an anti-oxidant role in normal cells and a pro-oxidant role in tumor cells. It leads to pro-oxidant-induced oxidative DNA injury, and its downstream pathway stimulates apoptotic tumor cell death. The anti-tumor action of CA appears to be correlated with its promising anti-oxidant and pro-oxidant action attributed to its chemical structure and free phenolic hydroxyls. CA denotes a potent anti-tumor effect in several tumor cells and thus might be used as an anti-tumor agent.
CA plays a central role in preventing cancer development through reduced cell viability and apoptosis induction. CA treatment leads to cell cycle modulation, prevention of cancer progression, and alteration of caspase expression. However, CA stimulates apoptosis by blocking Bcl-2 action, which leads to liberating cyt-c and the consequent activation of caspase-3, showing that CA stimulates apoptosis through the intrinsic apoptotic pathway. CA reveals action against numerous cancers, inhibiting the exaggerated creation of ROS and supporting cancer cell destruction via DNA oxidation and angiogenesis by acting to decrease VEGF-mediated vascularization and repression of MMP-2 and MMP-9. The repressive result of CA on MMP-2 and MMP-9 is connected with the obstruction of NF-κB activation as verified in cancer cells induced via PMA, which reduces cancer invasiveness and growth.
The anti-carcinogenic action of CA has been established, and the mechanism of action has been extensively studied. This opens an important scope for prospective investigations of CA in combination with chemical moieties/drugs in authorized animal models. Such studies might lead to the improvement of CA as a potential clinical aspirant in diverse cancer circumstances as combination therapy. CA, both in its free appearance and when conjugated with other moieties, generates its considerable pharmacological effect.
Combination therapy is an attractive alternative to drug development in pharmaceutical manufacturing to resolve drug resistance, decrease unfavorable drug reactions, and enhance drug efficiency. Multiple disease situations primarily need a combination therapy because of their difficult pathophysiology and progression. However, drug development research needs more data on suitable efficacy for a superior translational result in clinical trials.
CA possesses a strong anti-cancer effect and can be an effective chemotherapeutic agent. Further detailed clinical trials on CA will be significant in developing novel anti-cancer drugs. The experimental and clinical findings reveal the various anti-tumor properties of CA against several cancers, which might sensitize cancer cells and decrease cancer growth and survival. CA has a broad range of biological activities. However, CA alone or in combination with other chemotherapeutic agents/drugs might be suggested to treat and manage cancer.
Author Contributions
MA contributed to conceptualization, writing—original draft preparation, data curation, investigation, and methodology. GA contributed to conceptualization, investigation, supervision, and writing of the manuscript. KS contributed to validation, investigation, and writing—review and editing. AK contributed to conceptualization, investigation, supervision, and final editing. SA contributed to investigation and writing—review and editing. MdA contributed to investigation and writing—review and editing. MA contributed to investigation, supervision, and writing of the manuscript. VIP contributed to investigation, supervision, and final editing. MIH contributed to conceptualization, writing—original draft preparation, investigation, supervision, and project administration.
Funding
The authors extend their appreciation to the Deputyship for Research and Innovation, Ministry of Education in Saudi Arabia, for funding this research work through project number IFPRP-586-141-1442 and to King Abdulaziz University, DSR, Jeddah, Saudi Arabia.
Conflict of Interest
The authors declare that the research was conducted in the absence of any commercial or financial relationships that could be construed as a potential conflict of interest.
Publisher’s Note
All claims expressed in this article are solely those of the authors and do not necessarily represent those of their affiliated organizations, or those of the publisher, the editors, and the reviewers. Any product that may be evaluated in this article or claim that may be made by its manufacturer is not guaranteed or endorsed by the publisher.
Acknowledgments
MA thanks the Indian Council of Medical Research for financial support (grant no. 45/6/2020-DDI/BMS). MIH expresses gratitude to the Department of Science and Technology, the Government of India, for the FIST support (FIST program no. SR/FST/LSII/2020/782).
Abbreviations
AAA, aromatic amino acids; CA, caffeic acid; CAPE, caffeic acid phenethyl ester; CGA, chlorogenic acid; CGA, chlorogenic acid; CLL, chronic lymphocytic leukemia; COX-2, cyclooxygenase 2; ECM, extracellular matrix; EGFR, epidermal growth factor receptor; ERK, extracellular signal-regulated kinase; H2O2, hydrogen peroxide; HCs, hydroxycinnamic acids; JNK, c-Jun N-terminal kinase; MCT, monocarboxylic acid transporters; MMP, matrix metalloproteinases; NAD, nicotinamide adenine dinucleotide; NF-κB, nuclear factor kappa B; PKC, kinase C protein; PLP, pyridoxal 5-phosphate; ROS, reactive oxygen species; STAT3, transcription factor and signal translation 3; TAL, tyrosine ammonium lyase; TNF, tumor necrosis factor; VEGF, vascular endothelial growth factor
References
Abdelhafez, O. H., Fawzy, M. A., Fahim, J. R., Desoukey, S. Y., Krischke, M., Mueller, M. J., et al. (2018). Hepatoprotective Potential of Malvaviscus Arboreus against Carbon Tetrachloride-Induced Liver Injury in Rats. PLoS One 13, e0202362. doi:10.1371/journal.pone.0202362
Agunloye, O. M., Oboh, G., Ademiluyi, A. O., Ademosun, A. O., Akindahunsi, A. A., Oyagbemi, A. A., et al. (2019). Cardio-protective and Antioxidant Properties of Caffeic Acid and Chlorogenic Acid: Mechanistic Role of Angiotensin Converting Enzyme, Cholinesterase and Arginase Activities in Cyclosporine Induced Hypertensive Rats. Biomed. Pharmacother. 109, 450–458. doi:10.1016/j.biopha.2018.10.044
Ahmed, A. A., Fedail, J. S., Musa, H. H., Kamboh, A. A., Sifaldin, A. Z., and Musa, T. H. (2015). Gum Arabic Extracts Protect against Hepatic Oxidative Stress in Alloxan Induced Diabetes in Rats. Pathophysiology 22, 189–194. doi:10.1016/j.pathophys.2015.08.002
Alam, M., Ali, S., Ahmed, S., Elasbali, A. M., Adnan, M., Islam, A., et al. (2021a). Therapeutic Potential of Ursolic Acid in Cancer and Diabetic Neuropathy Diseases. Int. J. Mol. Sci. 22, 12162. doi:10.3390/ijms222212162
Alam, M., Ali, S., Ashraf, G. M., Bilgrami, A. L., Yadav, D. K., and Hassan, M. I. (2022). Epigallocatechin 3-Gallate: From Green Tea to Cancer Therapeutics. Food Chem. 379, 132135. doi:10.1016/j.foodchem.2022.132135
Alam, M., Ali, S., Mohammad, T., Hasan, G. M., Yadav, D. K., and Hassan, M. I. (2021b). B Cell Lymphoma 2: A Potential Therapeutic Target for Cancer Therapy. Int. J. Mol. Sci. 22, 10442. doi:10.3390/ijms221910442
Alam, M., Kashyap, T., Mishra, P., Panda, A. K., Nagini, S., and Mishra, R. (2019). Role and Regulation of Proapoptotic Bax in Oral Squamous Cell Carcinoma and Drug Resistance. Head Neck 41, 185–197. doi:10.1002/hed.25471
Alam, M., Kashyap, T., Pramanik, K. K., Singh, A. K., Nagini, S., and Mishra, R. (2017). The Elevated Activation of NFκB and AP-1 Is Correlated with Differential Regulation of Bcl-2 and Associated with Oral Squamous Cell Carcinoma Progression and Resistance. Clin. Oral Investig. 21, 2721–2731. doi:10.1007/s00784-017-2074-6
Alam, M., Hasan, G. M., and Hassan, M. I. (2021c). A Review on the Role of TANK-Binding Kinase 1 Signaling in Cancer. Int. J. Biol. Macromolecules 183, 2364–2375. doi:10.1016/j.ijbiomac.2021.06.022
Alam, M., and Mishra, R. (2021). Bcl-xL Expression and Regulation in the Progression, Recurrence, and Cisplatin Resistance of Oral Cancer. Life Sci. 280, 119705.
Alam, M., and Mishra, R. (2020). Role of PI3K and EGFR in Oral Cancer Progression and Drug Resistance. Ijrasb 7, 85–89. doi:10.31033/ijrasb.7.6.14
Ali, S., Alam, M., Hasan, G. M., and Hassan, M. I. (2021). Potential Therapeutic Targets of Klebsiella pneumoniae: a Multi-Omics Review Perspective. Brief. Funct. genomics, elab038. doi:10.1093/bfgp/elab038
Anantharaju, P. G., Gowda, P. C., Vimalambike, M. G., and Madhunapantula, S. V. (2016). An Overview on the Role of Dietary Phenolics for the Treatment of Cancers. Nutr. J. 15, 99–16. doi:10.1186/s12937-016-0217-2
Angelo, P. M., and Jorge, N. (2007). Compostos Fenólicos Em Alimentos-uma Breve Revisão. Revista do Instituto Adolfo Lutz (Impresso) 66, 01–09.
Anwar, S., Fratantonio, D., Ferrari, D., Saija, A., Cimino, F., and Speciale, A. (2016). Berry Anthocyanins Reduce Proliferation of Human Colorectal Carcinoma Cells by Inducing Caspase-3 Activation and P21 Upregulation. Mol. Med. Rep. 14, 1397–1403. doi:10.3892/mmr.2016.5397
Bayat Mokhtari, R., Homayouni, T. S., Baluch, N., Morgatskaya, E., Kumar, S., Das, B., et al. (2017). Combination Therapy in Combating Cancer. Oncotarget 8, 38022–38043. doi:10.18632/oncotarget.16723
Birková, A., Hubková, B., Bolerázska, B., Mareková, M., and Čižmárová, B. (2020). Caffeic Acid: a Brief Overview of its Presence, Metabolism, and Bioactivity. Bioactive Compounds Health Dis. 3, 74–81.
Bjørklund, G., and Chirumbolo, S. (2017). Role of Oxidative Stress and Antioxidants in Daily Nutrition and Human Health. Nutrition 33, 311–321.
Bounegru, A. V., and Apetrei, C. (2020). Voltamperometric Sensors and Biosensors Based on Carbon Nanomaterials Used for Detecting Caffeic Acid-A Review. Int. J. Mol. Sci. 21, 9275. doi:10.3390/ijms21239275
Brautigan, D. L., Gielata, M., Heo, J., Kubicka, E., and Wilkins, L. R. (2018). Selective Toxicity of Caffeic Acid in Hepatocellular Carcinoma Cells. Biochem. Biophys. Res. Commun. 505, 612–617. doi:10.1016/j.bbrc.2018.09.155
Bukowska, B., Gajek, A., and Marczak, A. (2015). Two Drugs Are Better Than One. A Short History of Combined Therapy of Ovarian Cancer. Contemp. Oncol. (Pozn) 19, 350–353. doi:10.5114/wo.2014.43975
Chang, W. C., Hsieh, C. H., Hsiao, M. W., Lin, W. C., Hung, Y. C., and Ye, J. C. (2010). Caffeic Acid Induces Apoptosis in Human Cervical Cancer Cells through the Mitochondrial Pathway. Taiwan J. Obstet. Gynecol. 49, 419–424. doi:10.1016/S1028-4559(10)60092-7
Chao, P. C., Hsu, C. C., and Yin, M. C. (2009). Anti-inflammatory and Anti-coagulatory Activities of Caffeic Acid and Ellagic Acid in Cardiac Tissue of Diabetic Mice. Nutr. Metab. (Lond) 6, 33–38. doi:10.1186/1743-7075-6-33
Chen, J. H., and Ho, C.-T. (1997). Antioxidant Activities of Caffeic Acid and its Related Hydroxycinnamic Acid Compounds. J. Agric. Food Chem. 45, 2374–2378. doi:10.1021/jf970055t
Chen, T.-W., Rajaji, U., Chen, S.-M., Govindasamy, M., Paul Selvin, S. S., Manavalan, S., et al. (2019). Sonochemical Synthesis of Graphene Oxide Sheets Supported Cu2S Nanodots for High Sensitive Electrochemical Determination of Caffeic Acid in Red Wine and Soft Drinks. Composites B: Eng. 158, 419–427. doi:10.1016/j.compositesb.2018.09.099
Chen, X., Ren, B., Chen, M., Wang, Q., Zhang, L., and Yan, G. (2016). NLLSS: Predicting Synergistic Drug Combinations Based on Semi-supervised Learning. Plos Comput. Biol. 12, e1004975. doi:10.1371/journal.pcbi.1004975
Chen, X. L., Dodd, G., Thomas, S., Zhang, X., Wasserman, M. A., Rovin, B. H., et al. (2006). Activation of Nrf2/ARE Pathway Protects Endothelial Cells from Oxidant Injury and Inhibits Inflammatory Gene Expression. Am. J. Physiol. Heart Circ. Physiol. 290, H1862–H1870. doi:10.1152/ajpheart.00651.2005
Chiou, S. Y., Sung, J. M., Huang, P. W., and Lin, S. D. (2017). Antioxidant, Antidiabetic, and Antihypertensive Properties of Echinacea Purpurea Flower Extract and Caffeic Acid Derivatives Using In Vitro Models. J. Med. Food 20, 171–179. doi:10.1089/jmf.2016.3790
Chung, T. W., Moon, S. K., Chang, Y. C., Ko, J. H., Lee, Y. C., Cho, G., et al. (2004). Novel and Therapeutic Effect of Caffeic Acid and Caffeic Acid Phenyl Ester on Hepatocarcinoma Cells: Complete Regression of Hepatoma Growth and Metastasis by Dual Mechanism. FASEB J. 18, 1670–1681. doi:10.1096/fj.04-2126com
Coleman, J. J., Komura, T., Munro, J., Wu, M. P., Busanelli, R. R., Koehler, A. N., et al. (2016). Activity of Caffeic Acid Phenethyl Ester in Caenorhabditis elegans. Future Med. Chem. 8, 2033–2046. doi:10.4155/fmc-2016-0085
Da Cunha, F. M., Duma, D., Assreuy, J., Buzzi, F. C., Niero, R., Campos, M. M., et al. (2004). Caffeic Acid Derivatives: In Vitro and In Vivo Anti-inflammatory Properties. Free Radic. Res. 38, 1241–1253. doi:10.1080/10715760400016139
Damasceno, S. S., Dantas, B. B., Ribeiro-Filho, J., Antônio M Araújo, D., and Galberto M da Costa, J. (2017). Chemical Properties of Caffeic and Ferulic Acids in Biological System: Implications in Cancer Therapy. A Review. Curr. Pharm. Des. 23, 3015–3023. doi:10.2174/1381612822666161208145508
Das, P., Delost, M. D., Qureshi, M. H., Smith, D. T., and Njardarson, J. T. (2018). A Survey of the Structures of US FDA Approved Combination Drugs. J. Med. Chem. 62, 4265–4311. doi:10.1021/acs.jmedchem.8b01610
Del Chierico, F., Nobili, V., Vernocchi, P., Russo, A., De Stefanis, C., Gnani, D., et al. (2017). Gut Microbiota Profiling of Pediatric Nonalcoholic Fatty Liver Disease and Obese Patients Unveiled by an Integrated Meta-Omics-Based Approach. Hepatology 65, 451–464. doi:10.1002/hep.28572
Dhanasekaran, R., Bandoh, S., and Roberts, L. R. (2016). Molecular Pathogenesis of Hepatocellular Carcinoma and Impact of Therapeutic Advances. F1000Research 5, 879. doi:10.12688/f1000research.6946.1
Dilshara, M. G., Jayasooriya, R. G., Park, S. R., Choi, Y. H., Choi, I. W., and Kim, G. Y. (2016). Caffeic Acid Phenethyl Ester Enhances TRAIL-Mediated Apoptosis via CHOP-Induced Death Receptor 5 Upregulation in Hepatocarcinoma Hep3B Cells. Mol. Cell Biochem 418, 13–20. doi:10.1007/s11010-016-2726-x
Espíndola, K. M. M., Ferreira, R. G., Narvaez, L. E. M., Silva Rosario, A. C. R., Da Silva, A. H. M., Silva, A. G. B., et al. (2019). Chemical and Pharmacological Aspects of Caffeic Acid and its Activity in Hepatocarcinoma. Front. Oncol. 9, 541. doi:10.3389/fonc.2019.00541
Feng, R., Lu, Y., Bowman, L. L., Qian, Y., Castranova, V., and Ding, M. (2005). Inhibition of Activator Protein-1, NF-kappaB, and MAPKs and Induction of Phase 2 Detoxifying Enzyme Activity by Chlorogenic Acid. J. Biol. Chem. 280, 27888–27895. doi:10.1074/jbc.M503347200
Filipe, H. A. L., Sousa, C., Marquês, J. T., Vila-Viçosa, D., De Granada-Flor, A., Viana, A. S., et al. (2018). Differential Targeting of Membrane Lipid Domains by Caffeic Acid and its Ester Derivatives. Free Radic. Biol. Med. 115, 232–245. doi:10.1016/j.freeradbiomed.2017.12.002
Fukumoto, L. R., and Mazza, G. (2000). Assessing Antioxidant and Prooxidant Activities of Phenolic Compounds. J. Agric. Food Chem. 48, 3597–3604. doi:10.1021/jf000220w
Genaro-Mattos, T. C., Maurício, Â. Q., Rettori, D., Alonso, A., and Hermes-Lima, M. (2015). Antioxidant Activity of Caffeic Acid against Iron-Induced Free Radical Generation-A Chemical Approach. PLoS One 10, e0129963. doi:10.1371/journal.pone.0129963
Göçer, H., and Gülçin, İ. (2011). Caffeic Acid Phenethyl Ester (CAPE): Correlation of Structure and Antioxidant Properties. Int. J. Food Sci. Nutr. 62, 821–825.
Gu, W., Yang, Y., Zhang, C., Zhang, Y., Chen, L., Shen, J., et al. (2016). Caffeic Acid Attenuates the Angiogenic Function of Hepatocellular Carcinoma Cells via Reduction in JNK-1-Mediated HIF-1α Stabilization in Hypoxia. RSC Adv. 6, 82774–82782. doi:10.1039/c6ra07703j
Guerriero, E., Sorice, A., Capone, F., Costantini, S., Palladino, P., D'ischia, M., et al. (2011). Effects of Lipoic Acid, Caffeic Acid and a Synthesized Lipoyl-Caffeic Conjugate on Human Hepatoma Cell Lines. Molecules 16, 6365–6377. doi:10.3390/molecules16086365
Hagiwara, A., Hirose, M., Takahashi, S., Ogawa, K., Shirai, T., and Ito, N. (1991). Forestomach and Kidney Carcinogenicity of Caffeic Acid in F344 Rats and C57BL/6N X C3H/HeN F1 Mice. Cancer Res. 51, 5655–5660.
Hernandez, J., and Edyvean, R. (2018). Toxicity and Biodegradability of Caffeic Acid in Anaerobic Digesting Sludge. Water SA 44, 27–36. doi:10.4314/wsa.v44i1.04
Hernández-Chávez, G., Martinez, A., and Gosset, G. (2019). Metabolic Engineering Strategies for Caffeic Acid Production in Escherichia coli. Electron. J. Biotechnol. 38, 19–26.
Ishtikhar, M., Ahmad, E., Siddiqui, Z., Ahmad, S., Khan, M. V., Zaman, M., et al. (2018). Biophysical Insight into the Interaction Mechanism of Plant Derived Polyphenolic Compound Tannic Acid with Homologous Mammalian Serum Albumins. Int. J. Biol. Macromol 107, 2450–2464. doi:10.1016/j.ijbiomac.2017.10.136
Ishtikhar, M., Rabbani, G., Khan, S., and Khan, R. H. (2015). Biophysical Investigation of Thymoquinone Binding to 'N' and 'B' Isoforms of Human Serum Albumin: Exploring the Interaction Mechanism and Radical Scavenging Activity. RSC Adv. 5, 18218–18232. doi:10.1039/c4ra09892g
Islam, S., Rahman, I. A., Islam, T., and Ghosh, A. (2017). Genome-wide Identification and Expression Analysis of Glutathione S-Transferase Gene Family in Tomato: Gaining an Insight to Their Physiological and Stress-specific Roles. PLoS One 12, e0187504. doi:10.1371/journal.pone.0187504
Ito, D., Imai, Y., Ohsawa, K., Nakajima, K., Fukuuchi, Y., and Kohsaka, S. (1998). Microglia-specific Localisation of a Novel Calcium Binding Protein, Iba1. Brain Res. Mol. Brain Res. 57, 1–9. doi:10.1016/s0169-328x(98)00040-0
Ito, H., Gonthier, M. P., Manach, C., Morand, C., Mennen, L., Rémésy, C., et al. (2005). Polyphenol Levels in Human Urine after Intake of Six Different Polyphenol-Rich Beverages. Br. J. Nutr. 94, 500–509. doi:10.1079/bjn20051522
Jia, R., Mi, Y., Yuan, X., Kong, D., Li, W., Li, R., et al. (2020). GASC1-adapted Neoadjuvant Chemotherapy for Resectable Esophageal Squamous Cell Carcinoma: A Prospective Clinical Biomarker Trial. J. Oncol. 2020, 1607860. doi:10.1155/2020/1607860
Jiang, Y., and Fleet, J. C. (2012). Effect of Phorbol 12‐myristate 13‐acetate Activated Signaling Pathways on 1α, 25 Dihydroxyvitamin D3 Regulated Human 25‐hydroxyvitamin D3 24‐hydroxylase Gene Expression in Differentiated Caco‐2 Cells. J. Cell. Biochem. 113, 1599–1607.
Johnson, C. G., Sharma, K. V., Levy, E. B., Woods, D. L., Morris, A. H., Bacher, J. D., et al. (2016). Microvascular Perfusion Changes Following Transarterial Hepatic Tumor Embolization. J. Vasc. Interv. Radiol. 27, 133–e3. e133. doi:10.1016/j.jvir.2015.06.036
Jung, J. E., Kim, H. S., Lee, C. S., Park, D. H., Kim, Y. N., Lee, M. J., et al. (2007). Caffeic Acid and its Synthetic Derivative CADPE Suppress Tumor Angiogenesis by Blocking STAT3-Mediated VEGF Expression in Human Renal Carcinoma Cells. Carcinogenesis 28, 1780–1787. doi:10.1093/carcin/bgm130
Kabała-Dzik, A., Rzepecka-Stojko, A., Kubina, R., Jastrzębska-Stojko, Ż., Stojko, R., Wojtyczka, R. D., et al. (2017). Comparison of Two Components of Propolis: Caffeic Acid (CA) and Caffeic Acid Phenethyl Ester (CAPE) Induce Apoptosis and Cell Cycle Arrest of Breast Cancer Cells MDA-MB-231. Molecules 22, 1554.
Kanimozhi, G., and Prasad, N. R. (2015). “Anticancer Effect of Caffeic Acid on Human Cervical Cancer Cells,” in Coffee in Health and Disease Prevention (Elsevier), 655–661. doi:10.1016/b978-0-12-409517-5.00073-5
Kapturczak, M. H., Wasserfall, C., Brusko, T., Campbell-Thompson, M., Ellis, T. M., Atkinson, M. A., et al. (2004). Heme Oxygenase-1 Modulates Early Inflammatory Responses: Evidence from the Heme Oxygenase-1-Deficient Mouse. Am. J. Pathol. 165, 1045–1053. doi:10.1016/S0002-9440(10)63365-2
Kasprzak-Drozd, K., Oniszczuk, T., Stasiak, M., and Oniszczuk, A. (2021). Beneficial Effects of Phenolic Compounds on Gut Microbiota and Metabolic Syndrome. Int. J. Mol. Sci. 22, 3715. doi:10.3390/ijms22073715
Katsarova, M., Dimitrova, S., Lukanov, L., and Sadakov, F. (2017). “Determination of Phenolic Acids, Flavonoids, Terpenes and Ecdysteroids in Medicinal Plant Extracts and Food Supplements,” in Comptes Rendus L’académie Bulg Des Sci (Berlin, Germany: ResearchGate).
Kawaguchi, H., Katsuyama, Y., Danyao, D., Kahar, P., Nakamura-Tsuruta, S., Teramura, H., et al. (2017). Caffeic Acid Production by Simultaneous Saccharification and Fermentation of Kraft Pulp Using Recombinant Escherichia coli. Appl. Microbiol. Biotechnol. 101, 5279–5290. doi:10.1007/s00253-017-8270-0
Kępa, M., Miklasińska-Majdanik, M., Wojtyczka, R. D., Idzik, D., Korzeniowski, K., Smoleń-Dzirba, J., et al. (2018). Antimicrobial Potential of Caffeic Acid against Staphylococcus aureus Clinical Strains. Biomed. Research International 2018, 7413504. doi:10.1155/2018/7413504
Kfoury, M., Geagea, C., Ruellan, S., Greige-Gerges, H., and Fourmentin, S. (2019). Effect of Cyclodextrin and Cosolvent on the Solubility and Antioxidant Activity of Caffeic Acid. Food Chem. 278, 163–169. doi:10.1016/j.foodchem.2018.11.055
Kim, J. H., Lee, B. J., Kim, J. H., Yu, Y. S., and Kim, K. W. (2009). Anti-angiogenic Effect of Caffeic Acid on Retinal Neovascularization. Vascul Pharmacol. 51, 262–267. doi:10.1016/j.vph.2009.06.010
Kim, J. H., Wang, Q., Choi, J. M., Lee, S., and Cho, E. J. (2015a). Protective Role of Caffeic Acid in an Aβ25-35-Induced Alzheimer's Disease Model. Nutr. Res. Pract. 9, 480–488. doi:10.4162/nrp.2015.9.5.480
Kim, J. H., Wang, Q., Lee, S., and Cho, E. J. (2015b). Antioxidant Activity and Protective Effect of Caffeic Acid against Oxidative Stress Induced by Amyloid Beta and LPS in C6 Glial Cells. Korean J. Pharmacognosy 46, 109–115.
Kim, J. K., and Jang, H. D. (2014). Nrf2-mediated HO-1 Induction Coupled with the ERK Signaling Pathway Contributes to Indirect Antioxidant Capacity of Caffeic Acid Phenethyl Ester in HepG2 Cells. Int. J. Mol. Sci. 15, 12149–12165. doi:10.3390/ijms150712149
Kim, S. R., Jung, Y. R., Kim, D. H., An, H. J., Kim, M. K., Kim, N. D., et al. (2014). Caffeic Acid Regulates LPS-Induced NF-Κb Activation through NIK/IKK and C-Src/ERK Signaling Pathways in Endothelial Cells. Arch. Pharm. Res. 37, 539–547. doi:10.1007/s12272-013-0211-6
Kleczka, A., Kubina, R., Dzik, R., Jasik, K., Stojko, J., Cholewa, K., et al. (2020). Caffeic Acid Phenethyl Ester (CAPE) Induced Apoptosis in Serous Ovarian Cancer OV7 Cells by Deregulation of BCL2/BAX Genes. Molecules 25, 3514. doi:10.3390/molecules25153514
Klungboonkrong, V., Das, D., and Mclennan, G. (2017). Molecular Mechanisms and Targets of Therapy for Hepatocellular Carcinoma. J. Vasc. Interv. Radiol. 28, 949–955. doi:10.1016/j.jvir.2017.03.002
Kolodziejczyk-Czepas, J., Szejk, M., Pawlak, A., and Zbikowska, H. (2015). Właściwości Przeciwutleniające Kwasu Kawowego I Jego Pochodnych. Żywność Nauka Technologia Jakość 22, 5–17.
Konishi, Y., Hitomi, Y., and Yoshioka, E. (2004). Intestinal Absorption of P-Coumaric and Gallic Acids in Rats after Oral Administration. J. Agric. Food Chem. 52, 2527–2532. doi:10.1021/jf035366k
Konishi, Y., and Kobayashi, S. (2004). Transepithelial Transport of Chlorogenic Acid, Caffeic Acid, and Their Colonic Metabolites in Intestinal Caco-2 Cell Monolayers. J. Agric. Food Chem. 52, 2518–2526. doi:10.1021/jf035407c
Koraneekit, A., Limpaiboon, T., Sangka, A., Boonsiri, P., Daduang, S., and Daduang, J. (2018). Synergistic Effects of Cisplatin-Caffeic Acid Induces Apoptosis in Human Cervical Cancer Cells via the Mitochondrial Pathways. Oncol. Lett. 15, 7397–7402. doi:10.3892/ol.2018.8256
Krifa, M., Bouhlel, I., Ghedira-Chekir, L., and Ghedira, K. (2013). Immunomodulatory and Cellular Anti-oxidant Activities of an Aqueous Extract of Limoniastrum Guyonianum Gall. J. Ethnopharmacol 146, 243–249. doi:10.1016/j.jep.2012.12.038
Kudugunti, S. K., Vad, N. M., Whiteside, A. J., Naik, B. U., Yusuf, M. A., Srivenugopal, K. S., et al. (2010). Biochemical Mechanism of Caffeic Acid Phenylethyl Ester (CAPE) Selective Toxicity towards Melanoma Cell Lines. Chem. Biol. Interact 188, 1–14. doi:10.1016/j.cbi.2010.05.018
Kumar, A., Prakash, A., Pahwa, D., and Mishra, J. (2012). Montelukast Potentiates the Protective Effect of Rofecoxib against Kainic Acid-Induced Cognitive Dysfunction in Rats. Pharmacol. Biochem. Behav. 103, 43–52. doi:10.1016/j.pbb.2012.07.015
Kumar, S., and Pandey, A. K. (2013). Chemistry and Biological Activities of Flavonoids: an Overview. scientific World J. 2013, 162750. doi:10.1155/2013/162750
Lafay, S., Morand, C., Manach, C., Besson, C., and Scalbert, A. (2006). Absorption and Metabolism of Caffeic Acid and Chlorogenic Acid in the Small Intestine of Rats. Br. J. Nutr. 96, 39–46. doi:10.1079/bjn20061714
Langland, J., Jacobs, B., Wagner, C. E., Ruiz, G., and Cahill, T. M. (2018). Antiviral Activity of Metal Chelates of Caffeic Acid and Similar Compounds towards Herpes Simplex, VSV-Ebola Pseudotyped and Vaccinia Viruses. Antivir. Res 160, 143–150. doi:10.1016/j.antiviral.2018.10.021
Lee, C.-W., Won, T.-J., Kim, H.-R., Lee, D.-H., Hwang, K.-W., and Park, S.-Y. (2011). Protective Effect of Chlorogenic Acid against Aβ-Induced Neurotoxicity. Biomolecules Ther. 19, 181–186. doi:10.4062/biomolther.2011.19.2.181
Lee, K. W., Kang, N. J., Kim, J. H., Lee, K. M., Lee, D. E., Hur, H. J., et al. (2008). Caffeic Acid Phenethyl Ester Inhibits Invasion and Expression of Matrix Metalloproteinase in SK-Hep1 Human Hepatocellular Carcinoma Cells by Targeting Nuclear Factor Kappa B. Genes Nutr. 2, 319–322. doi:10.1007/s12263-007-0067-9
Lee, Y., Howard, L. R., and Villalón, B. (1995). Flavonoids and Antioxidant Activity of Fresh Pepper (Capsicum Annuum) Cultivars. J. Food Sci. 60, 473–476. doi:10.1111/j.1365-2621.1995.tb09806.x
Leonard, W., Zhang, P., Ying, D., and Fang, Z. (2021). Hydroxycinnamic Acids on Gut Microbiota and Health. Compr. Rev. Food Sci. Food Saf. 20, 710–737. doi:10.1111/1541-4337.12663
Li, A. S., Bandy, B., Tsang, S. S., and Davison, A. J. (2000). DNA-breaking versus DNA-Protecting Activity of Four Phenolic Compounds In Vitro. Free Radic. Res. 33, 551–566. doi:10.1080/10715760000301091
Li, J., Sung, C. Y., Lee, N., Ni, Y., Pihlajamäki, J., Panagiotou, G., et al. (2016). Probiotics Modulated Gut Microbiota Suppresses Hepatocellular Carcinoma Growth in Mice. Proc. Natl. Acad. Sci. U S A. 113, E1306–E1315. doi:10.1073/pnas.1518189113
Li, J., Bai, Y., Bai, Y., Zhu, R., Liu, W., Cao, J., et al. (2017). Pharmacokinetics of Caffeic Acid, Ferulic Acid, Formononetin, Cryptotanshinone, and Tanshinone IIA after Oral Administration of Naoxintong Capsule in Rat by HPLC-MS/MS. Evidence-Based Complement. Altern. Med. 2017, 9057238. doi:10.1155/2017/9057238
Li, Y., Jiang, F., Chen, L., Yang, Y., Cao, S., Ye, Y., et al. (2015). Blockage of TGFβ-SMAD2 by Demethylation-Activated miR-148a Is Involved in Caffeic Acid-Induced Inhibition of Cancer Stem Cell-like Properties In Vitro and In Vivo. FEBS Open Bio 5, 466–475. doi:10.1016/j.fob.2015.05.009
Liang, G., Shi, B., Luo, W., and Yang, J. (2015). The Protective Effect of Caffeic Acid on Global Cerebral Ischemia-Reperfusion Injury in Rats. Behav. Brain Funct. 11, 18–10. doi:10.1186/s12993-015-0064-x
Lima, R. D., Brondani, J. C., Dornelles, R. C., Lhamas, C. L., Faccin, H., Silva, C. V., et al. (2020). Anti-inflammatory Activity and Identification of the Verbena Litoralis Kunth Crude Extract Constituents. Braz. J. Pharm. Sci. 56. doi:10.1590/s2175-97902019000417419
Lin, C. L., Chen, R. F., Chen, J. Y., Chu, Y. C., Wang, H. M., Chou, H. L., et al. (2012). Protective Effect of Caffeic Acid on Paclitaxel Induced Anti-proliferation and Apoptosis of Lung Cancer Cells Involves NF-Κb Pathway. Int. J. Mol. Sci. 13, 6236–6245. doi:10.3390/ijms13056236
Lin, Y., and Yan, Y. (2012). Biosynthesis of Caffeic Acid in Escherichia coli Using its Endogenous Hydroxylase Complex. Microb. Cell Fact 11, 42–49. doi:10.1186/1475-2859-11-42
Liu, Y., Qiu, S., Wang, L., Zhang, N., Shi, Y., Zhou, H., et al. (2019). Reproductive and Developmental Toxicity Study of Caffeic Acid in Mice. Food Chem. Toxicol. 123, 106–112. doi:10.1016/j.fct.2018.10.040
Lu, D. Y., Huang, B. R., Yeh, W. L., Lin, H. Y., Huang, S. S., Liu, Y. S., et al. (2013). Anti-neuroinflammatory Effect of a Novel Caffeamide Derivative, KS370G, in Microglial Cells. Mol. Neurobiol. 48, 863–874. doi:10.1007/s12035-013-8474-y
Lu, S., Sun, P., Li, T., Kurtán, T., Mándi, A., Antus, S., et al. (2011). Bioactive Nonanolide Derivatives Isolated from the Endophytic Fungus Cytospora Sp. J. Org. Chem. 76, 9699–9710. doi:10.1021/jo201755v
Magnani, C., Isaac, V. L. B., Correa, M. A., and Salgado, H. R. N. (2014). Caffeic Acid: a Review of its Potential Use in Medications and Cosmetics. Anal. Methods 6, 3203–3210. doi:10.1039/c3ay41807c
Manach, C., Scalbert, A., Morand, C., Rémésy, C., and Jiménez, L. (2004). Polyphenols: Food Sources and Bioavailability. Am. J. Clin. Nutr. 79, 727–747. doi:10.1093/ajcn/79.5.727
Martini, S., Conte, A., and Tagliazucchi, D. (2019). Antiproliferative Activity and Cell Metabolism of Hydroxycinnamic Acids in Human colon Adenocarcinoma Cell Lines. J. Agric. Food Chem. 67, 3919–3931. doi:10.1021/acs.jafc.9b00522
Matejczyk, M., Świsłocka, R., Golonko, A., Lewandowski, W., and Hawrylik, E. (2018). Cytotoxic, Genotoxic and Antimicrobial Activity of Caffeic and Rosmarinic Acids and Their Lithium, Sodium and Potassium Salts as Potential Anticancer Compounds. Adv. Med. Sci. 63, 14–21. doi:10.1016/j.advms.2017.07.003
Mathupala, S. P., Colen, C. B., Parajuli, P., and Sloan, A. E. (2007). Lactate and Malignant Tumors: a Therapeutic Target at the End Stage of Glycolysis. J. Bioenerg. Biomembr 39, 73–77. doi:10.1007/s10863-006-9062-x
Matsumura, Y., and Ananthaswamy, H. N. (2002). Short-term and Long-Term Cellular and Molecular Events Following UV Irradiation of Skin: Implications for Molecular Medicine. Expert Rev. Mol. Med. 4, 1–22. doi:10.1017/S146239940200532X
Medina, I., Undeland, I., Larsson, K., Storrø, I., Rustad, T., Jacobsen, C., et al. (2012). Activity of Caffeic Acid in Different Fish Lipid Matrices: A Review. Food Chem. 131, 730–740. doi:10.1016/j.foodchem.2011.09.032
Meinhart, A. D., Damin, F. M., Caldeirão, L., De Jesus Filho, M., Da Silva, L. C., Da Silva Constant, L., et al. (2019). Chlorogenic and Caffeic Acids in 64 Fruits Consumed in Brazil. Food Chem. 286, 51–63. doi:10.1016/j.foodchem.2019.02.004
Meyer, A. S., Donovan, J. L., Pearson, D. A., Waterhouse, A. L., and Frankel, E. N. (1998). Fruit Hydroxycinnamic Acids Inhibit Human Low-Density Lipoprotein Oxidation In Vitro. J. Agric. Food Chem. 46, 1783–1787. doi:10.1021/jf9708960
Mitani, T., Ota, K., Inaba, N., Kishida, K., and Koyama, H. A. (2018). Antimicrobial Activity of the Phenolic Compounds of Prunus Mume against Enterobacteria. Biol. Pharm. Bull. 41, 208–212. doi:10.1248/bpb.b17-00711
Mohammad, T., Khan, F. I., Lobb, K. A., Islam, A., Ahmad, F., and Hassan, M. I. (2019). Identification and Evaluation of Bioactive Natural Products as Potential Inhibitors of Human Microtubule Affinity-Regulating Kinase 4 (MARK4). J. Biomol. Struct. Dyn. 37, 1813–1829. doi:10.1080/07391102.2018.1468282
Mohammad, T., Siddiqui, S., Shamsi, A., Alajmi, M. F., Hussain, A., Islam, A., et al. (2020). Virtual Screening Approach to Identify High-Affinity Inhibitors of Serum and Glucocorticoid-Regulated Kinase 1 Among Bioactive Natural Products: Combined Molecular Docking and Simulation Studies. Molecules 25. doi:10.3390/molecules25040823
Mongkolsapaya, J., Cowper, A. E., Xu, X. N., Morris, G., Mcmichael, A. J., Bell, J. I., et al. (1998). Lymphocyte Inhibitor of TRAIL (TNF-Related Apoptosis-Inducing Ligand): a New Receptor Protecting Lymphocytes from the Death Ligand TRAIL. J. Immunol. 160, 3–6.
Murad, L. D., Soares, Nda. C., Brand, C., Monteiro, M. C., and Teodoro, A. J. (2015). Effects of Caffeic and 5-caffeoylquinic Acids on Cell Viability and Cellular Uptake in Human colon Adenocarcinoma Cells. Nutr. Cancer 67, 532–542. doi:10.1080/01635581.2015.1004736
Naz, F., Khan, F. I., Mohammad, T., Khan, P., Manzoor, S., Hasan, G. M., et al. (2018a). Investigation of Molecular Mechanism of Recognition between Citral and MARK4: A Newer Therapeutic Approach to Attenuate Cancer Cell Progression. Int. J. Biol. Macromol 107, 2580–2589. doi:10.1016/j.ijbiomac.2017.10.143
Naz, H., Khan, P., Tarique, M., Rahman, S., Meena, A., Ahamad, S., et al. (2017). Binding Studies and Biological Evaluation of β-carotene as a Potential Inhibitor of Human Calcium/calmodulin-dependent Protein Kinase IV. Int. J. Biol. Macromol 96, 161–170. doi:10.1016/j.ijbiomac.2016.12.024
Naz, H., Tarique, M., Khan, P., Luqman, S., Ahamad, S., Islam, A., et al. (2018b). Evidence of Vanillin Binding to CAMKIV Explains the Anti-cancer Mechanism in Human Hepatic Carcinoma and Neuroblastoma Cells. Mol. Cell Biochem 438, 35–45. doi:10.1007/s11010-017-3111-0
Nicholson, D. W. (1999). Caspase Structure, Proteolytic Substrates, and Function during Apoptotic Cell Death. Cell Death Differ 6, 1028–1042. doi:10.1038/sj.cdd.4400598
Oliveira, D. M. d., and Bastos, D. H. M. (2011). Biodisponibilidade de ácidos fenólicos. Quím. Nova 34, 1051–1056. doi:10.1590/s0100-40422011000600023
Olthof, M. R., Hollman, P. C., and Katan, M. B. (2001). Chlorogenic Acid and Caffeic Acid Are Absorbed in Humans. J. Nutr. 131, 66–71. doi:10.1093/jn/131.1.66
Pelinson, L. P., Assmann, C. E., Palma, T. V., Da Cruz, I. B. M., Pillat, M. M., Mânica, A., et al. (2019). Antiproliferative and Apoptotic Effects of Caffeic Acid on SK-Mel-28 Human Melanoma Cancer Cells. Mol. Biol. Rep. 46, 2085–2092. doi:10.1007/s11033-019-04658-1
Pinho, E., Soares, G., and Henriques, M. (2015). Evaluation of Antibacterial Activity of Caffeic Acid Encapsulated by β-cyclodextrins. J. Microencapsul 32, 804–810. doi:10.3109/02652048.2015.1094531
Pramanik, K. K., Nagini, S., Singh, A. K., Mishra, P., Kashyap, T., Nath, N., et al. (2018). Glycogen Synthase Kinase-3β Mediated Regulation of Matrix Metalloproteinase-9 and its Involvement in Oral Squamous Cell Carcinoma Progression and Invasion. Cell Oncol (Dordr) 41, 47–60. doi:10.1007/s13402-017-0358-0
Pramanik, K. K., Singh, A. K., Alam, M., Kashyap, T., Mishra, P., Panda, A. K., et al. (2016). Reversion-inducing Cysteine-Rich Protein with Kazal Motifs and its Regulation by Glycogen Synthase Kinase 3 Signaling in Oral Cancer. Tumour Biol. 37, 15253–15264. doi:10.1007/s13277-016-5362-x
Prokop, S., Miller, K. R., and Heppner, F. L. (2013). Microglia Actions in Alzheimer’s Disease. Acta Neuropathol. 126, 461–477. doi:10.1007/s00401-013-1182-x
Qin, P., Wei, Y., Hou, M., Zhao, C., and Shen, Z. (2015). A Multicenter Clinical Trial of Caffeic Acid Tablet in Treatment of 103 Primary Immune Thrombocytopenia Patients. Zhonghua Xue Ye Xue Za Zhi 36, 103–106. doi:10.3760/cma.j.issn.0253-2727.2015.02.004
Rajendra Prasad, N., Karthikeyan, A., Karthikeyan, S., and Reddy, B. V. (2011). Inhibitory Effect of Caffeic Acid on Cancer Cell Proliferation by Oxidative Mechanism in Human HT-1080 Fibrosarcoma Cell Line. Mol. Cell Biochem 349, 11–19. doi:10.1007/s11010-010-0655-7
Rochelle, P. A. (2001). Environmental Molecular Microbiology: Protocols and Applications. Horizon Scientific Press.
Rodrigues, J. L., Araújo, R. G., Prather, K. L., Kluskens, L. D., and Rodrigues, L. R. (2015). Heterologous Production of Caffeic Acid from Tyrosine in Escherichia coli. Enzyme Microb. Technol. 71, 36–44. doi:10.1016/j.enzmictec.2015.01.001
Rosendahl, A. H., Perks, C. M., Zeng, L., Markkula, A., Simonsson, M., Rose, C., et al. (2015). Caffeine and Caffeic Acid Inhibit Growth and Modify Estrogen Receptor and Insulin-like Growth Factor I Receptor Levels in Human Breast Cancer. Clin. Cancer Res. 21, 1877–1887. doi:10.1158/1078-0432.CCR-14-1748
Saibabu, V., Fatima, Z., Khan, L. A., and Hameed, S. (2015). Therapeutic Potential of Dietary Phenolic Acids. Adv. Pharmacol. Sci. 2015, 823539. doi:10.1155/2015/823539
Salau, V. F., Erukainure, O. L., and Islam, M. S. (2021). Caffeic Acid Protects against Iron-Induced Cardiotoxicity by Suppressing Angiotensin-Converting Enzyme Activity and Modulating Lipid Spectrum, Gluconeogenesis and Nucleotide Hydrolyzing Enzyme Activities. Biol. Trace Elem. Res. 199, 1052–1061. doi:10.1007/s12011-020-02227-3
Saleem, M., Ali, H. A., Akhtar, M. F., Saleem, U., Saleem, A., and Irshad, I. (2019). Chemical Characterisation and Hepatoprotective Potential of Cosmos Sulphureus Cav. And Cosmos Bipinnatus Cav. Nat. Prod. Res. 33, 897–900. doi:10.1080/14786419.2017.1413557
Sato, Y., Itagaki, S., Kurokawa, T., Ogura, J., Kobayashi, M., Hirano, T., et al. (2011). In Vitro and In Vivo Antioxidant Properties of Chlorogenic Acid and Caffeic Acid. Int. J. Pharm. 403, 136–138. doi:10.1016/j.ijpharm.2010.09.035
Scalbert, A., and Williamson, G. (2000). Chocolate: Modern Science Investigates an Ancient Medicine. J. Med. Food 3, 121–125.
Scharffetter-Kochanek, K., Wlaschek, M., Brenneisen, P., Schauen, M., Blaudschun, R., and Wenk, J. (1997). UV-induced Reactive Oxygen Species in Photocarcinogenesis and Photoaging. Biol. Chem. 378, 1247–1257.
Sharifi-Rad, J., Rodrigues, C. F., Sharopov, F., Docea, A. O., Can Karaca, A., Sharifi-Rad, M., et al. (2020). Diet, Lifestyle and Cardiovascular Diseases: Linking Pathophysiology to Cardioprotective Effects of Natural Bioactive Compounds. Ijerph 17, 2326. doi:10.3390/ijerph17072326
Shen, H., Tong, X., Yang, J., Yu, L., Zhou, H., Wang, Y., et al. (2020). Biotransformation of Natural Hydroxycinnamic Acids by Gut Microbiota from normal and Cerebral Ischemia-Reperfusion Injured Rats: a Comparative Study. Food Funct. 11, 5389–5395. doi:10.1039/d0fo00775g
Shen, J., Wang, G., and Zuo, J. (2018). Caffeic Acid Inhibits HCV Replication via Induction of IFNα Antiviral Response through P62-Mediated Keap1/Nrf2 Signaling Pathway. Antivir. Res 154, 166–173. doi:10.1016/j.antiviral.2018.04.008
Shikov, A. N., Pozharitskaya, O. N., Makarov, V. G., Wagner, H., Verpoorte, R., and Heinrich, M. (2014). Medicinal Plants of the Russian Pharmacopoeia; Their History and Applications. J. Ethnopharmacol 154, 481–536. doi:10.1016/j.jep.2014.04.007
Shiozawa, R., Inoue, Y., Murata, I., and Kanamoto, I. (2018). Effect of Antioxidant Activity of Caffeic Acid with Cyclodextrins Using Ground Mixture Method. Asian J. Pharm. Sci. 13, 24–33. doi:10.1016/j.ajps.2017.08.006
Siddik, Z. H. (2003). Cisplatin: Mode of Cytotoxic Action and Molecular Basis of Resistance. Oncogene 22, 7265–7279. doi:10.1038/sj.onc.1206933
Sidoryk, K., Jaromin, A., Filipczak, N., Cmoch, P., and Cybulski, M. (2018). Synthesis and Antioxidant Activity of Caffeic Acid Derivatives. Molecules 23, 2199. doi:10.3390/molecules23092199
Siegmund, D., Mauri, D., Peters, N., Juo, P., Thome, M., Reichwein, M., et al. (2001). Fas-associated Death Domain Protein (FADD) and Caspase-8 Mediate Up-Regulation of C-Fos by Fas Ligand and Tumor Necrosis Factor-Related Apoptosis-Inducing Ligand (TRAIL) via a FLICE Inhibitory Protein (FLIP)-regulated Pathway. J. Biol. Chem. 276, 32585–32590. doi:10.1074/jbc.M100444200
Silva, T., Oliveira, C., and Borges, F. (2014). Caffeic Acid Derivatives, Analogs and Applications: a Patent Review (2009-2013). Expert Opin. Ther. Pat 24, 1257–1270. doi:10.1517/13543776.2014.959492
Sirota, R., Gibson, D., and Kohen, R. (2017). The Timing of Caffeic Acid Treatment with Cisplatin Determines Sensitization or Resistance of Ovarian Carcinoma Cell Lines. Redox Biol. 11, 170–175. doi:10.1016/j.redox.2016.12.006
Soares, D. G., Andreazza, A. C., and Salvador, M. (2005). Avaliação de compostos com atividade antioxidante em células da levedura Saccharomyces cerevisiae. Rev. Bras. Cienc. Farm. 41, 95–100. doi:10.1590/s1516-93322005000100011
Son, S., and Lewis, B. A. (2002). Free Radical Scavenging and Antioxidative Activity of Caffeic Acid Amide and Ester Analogues: Structure-Activity Relationship. J. Agric. Food Chem. 50, 468–472. doi:10.1021/jf010830b
Srinivasulu, C., Ramgopal, M., Ramanjaneyulu, G., Anuradha, C. M., and Suresh Kumar, C. (2018). Syringic Acid (SA) ‒ A Review of its Occurrence, Biosynthesis, Pharmacological and Industrial Importance. Biomed. Pharmacother. 108, 547–557. doi:10.1016/j.biopha.2018.09.069
Stagos, D., Amoutzias, G. D., Matakos, A., Spyrou, A., Tsatsakis, A. M., and Kouretas, D. (2012). Chemoprevention of Liver Cancer by Plant Polyphenols. Food Chem. Toxicol. 50, 2155–2170. doi:10.1016/j.fct.2012.04.002
Sul, D., Kim, H. S., Lee, D., Joo, S. S., Hwang, K. W., and Park, S. Y. (2009). Protective Effect of Caffeic Acid against Beta-Amyloid-Induced Neurotoxicity by the Inhibition of Calcium Influx and Tau Phosphorylation. Life Sci. 84, 257–262. doi:10.1016/j.lfs.2008.12.001
Sun, L. L., Holowatyj, A., Xu, X. E., Wu, J. Y., Wu, Z. Y., Shen, J. H., et al. (2013). Histone Demethylase GASC1, a Potential Prognostic and Predictive Marker in Esophageal Squamous Cell Carcinoma. Am. J. Cancer Res. 3, 509–517.
Tosovic, J. (2017). Spectroscopic Features of Caffeic Acid: Theoretical Study. Kragujevac J. Sci. 2017, 99–108. doi:10.5937/kgjsci1739099t
Touaibia, M., Jean-François, J., and Doiron, J. (2011). Caffeic Acid, a Versatile Pharmacophore: an Overview. Mini Rev. Med. Chem. 11, 695–713. doi:10.2174/138955711796268750
Touaibia, M., and Guay, M. (2011). Natural Product Total Synthesis in the Organic Laboratory: Total Synthesis of Caffeic Acid Phenethyl Ester (CAPE), a Potent 5-lipoxygenase Inhibitor from Honeybee Hives. J. Chem. Educ. 88, 473–475. doi:10.1021/ed100050z
Tyszka-Czochara, M., Bukowska-Strakova, K., and Majka, M. (2017a). Metformin and Caffeic Acid Regulate Metabolic Reprogramming in Human Cervical Carcinoma SiHa/HTB-35 Cells and Augment Anticancer Activity of Cisplatin via Cell Cycle Regulation. Food Chem. Toxicol. 106, 260–272. doi:10.1016/j.fct.2017.05.065
Tyszka-Czochara, M., Konieczny, P., and Majka, M. (2017b). Caffeic Acid Expands Anti-tumor Effect of Metformin in Human Metastatic Cervical Carcinoma HTB-34 Cells: Implications of AMPK Activation and Impairment of Fatty Acids De Novo Biosynthesis. Int. J. Mol. Sci. 18, 462. doi:10.3390/ijms18020462
Verma, R. P., and Hansch, C. (2004). An Approach towards the Quantitative Structure-Activity Relationships of Caffeic Acid and its Derivatives. ChemBioChem 5, 1188–1195. doi:10.1002/cbic.200400094
Verri, M., Pastoris, O., Dossena, M., Aquilani, R., Guerriero, F., Cuzzoni, G., et al. (2012). Mitochondrial Alterations, Oxidative Stress and Neuroinflammation in Alzheimer's Disease. Int. J. Immunopathol Pharmacol. 25, 345–353. doi:10.1177/039463201202500204
Vinayagam, R., Jayachandran, M., and Xu, B. (2016). Antidiabetic Effects of Simple Phenolic Acids: a Comprehensive Review. Phytother Res. 30, 184–199. doi:10.1002/ptr.5528
Wang, F., and Yang, J. (2012). A Comparative Study of Caffeic Acid and a Novel Caffeic Acid Conjugate SMND-309 on Antioxidant Properties In Vitro. LWT - Food Sci. Technology 46, 239–244. doi:10.1016/j.lwt.2011.09.025
Wang, G., Lei, Z., Zhong, Q., Wu, W., Zhang, H., Min, T., et al. (2017). Enrichment of Caffeic Acid in Peanut Sprouts and Evaluation of its In Vitro Effectiveness against Oxidative Stress-Induced Erythrocyte Hemolysis. Food Chem. 217, 332–341. doi:10.1016/j.foodchem.2016.07.126
Wilkins, L. R., Brautigan, D. L., Wu, H., Yarmohammadi, H., Kubicka, E., Serbulea, V., et al. (2017). Cinnamic Acid Derivatives Enhance the Efficacy of Transarterial Embolization in a Rat Model of Hepatocellular Carcinoma. Cardiovasc. Intervent Radiol. 40, 430–437. doi:10.1007/s00270-016-1515-y
Wu, W., Lu, L., Long, Y., Wang, T., Liu, L., Chen, Q., et al. (2007). Free Radical Scavenging and Antioxidative Activities of Caffeic Acid Phenethyl Ester (CAPE) and its Related Compounds in Solution and Membranes: A Structure-Activity Insight. Food Chem. 105, 107–115. doi:10.1016/j.foodchem.2007.03.049
Yamada, Y., Yasui, H., and Sakurai, H. (2006). Suppressive Effect of Caffeic Acid and its Derivatives on the Generation of UVA-Induced Reactive Oxygen Species in the Skin of Hairless Mice and Pharmacokinetic Analysis on Organ Distribution of Caffeic Acid in ddY Mice. Photochem. Photobiol. 82, 1668–1676. doi:10.1562/2006-03-24-RA-857
Yang, B., Chen, F., Hua, Y., Huang, S.-S., Lin, S., Wen, L., et al. (2012). Prooxidant Activities of Quercetin, P-Courmaric Acid and Their Derivatives Analysed by Quantitative Structure-Activity Relationship. Food Chem. 131, 508–512. doi:10.1016/j.foodchem.2011.09.014
Yang, S. Y., Hong, C. O., Lee, G. P., Kim, C. T., and Lee, K. W. (2013a). The Hepatoprotection of Caffeic Acid and Rosmarinic Acid, Major Compounds of Perilla Frutescens, against T-BHP-Induced Oxidative Liver Damage. Food Chem. Toxicol. 55, 92–99. doi:10.1016/j.fct.2012.12.042
Yang, W. S., Jeong, D., Yi, Y.-S., Park, J. G., Seo, H., Moh, S. H., et al. (2013b). IRAK1/4-targeted Anti-inflammatory Action of Caffeic Acid. Mediators Inflamm. 2013, 518183. doi:10.1155/2013/518183
Yang, Z. Y., Kuboyama, T., Kazuma, K., Konno, K., and Tohda, C. (2015). Active Constituents from Drynaria Fortunei Rhizomes on the Attenuation of Aβ(25-35)-Induced Axonal Atrophy. J. Nat. Prod. 78, 2297–2300. doi:10.1021/acs.jnatprod.5b00290
Yeh, C. B., Hsieh, M. J., Hsieh, Y. H., Chien, M. H., Chiou, H. L., and Yang, S. F. (2012). Antimetastatic Effects of Norcantharidin on Hepatocellular Carcinoma by Transcriptional Inhibition of MMP-9 through Modulation of NF-kB Activity. PLoS One 7, e31055. doi:10.1371/journal.pone.0031055
Zaitone, S. A., Ahmed, E., Elsherbiny, N. M., Mehanna, E. T., El-Kherbetawy, M. K., Elsayed, M. H., et al. (2019). Caffeic Acid Improves Locomotor Activity and Lessens Inflammatory burden in a Mouse Model of Rotenone-Induced Nigral Neurodegeneration: Relevance to Parkinson's Disease Therapy. Pharmacol. Rep. 71, 32–41. doi:10.1016/j.pharep.2018.08.004
Zeng, N., Hongbo, T., Xu, Y., Wu, M., and Wu, Y. (2018). Anticancer Activity of Caffeic Acid N-butyl E-ster against A431 S-kin C-arcinoma C-ell L-ine O-ccurs via I-nduction of A-poptosis and I-nhibition of the mTOR/PI3K/AKT S-ignaling P-athway. Mol. Med. Rep. 17, 5652–5657. doi:10.3892/mmr.2018.8599
Zhang, L., Li, Y., Liang, Y., Liang, K., Zhang, F., Xu, T., et al. (2019). Determination of Phenolic Acid Profiles by HPLC-MS in Vegetables Commonly Consumed in China. Food Chem. 276, 538–546. doi:10.1016/j.foodchem.2018.10.074
Zhang, Z., Wang, D., Qiao, S., Wu, X., Cao, S., Wang, L., et al. (2017). Metabolic and Microbial Signatures in Rat Hepatocellular Carcinoma Treated with Caffeic Acid and Chlorogenic Acid. Sci. Rep. 7, 4508–4510. doi:10.1038/s41598-017-04888-y
Zhang, Z. C., Xu, M., Sun, S. F., Qiao, X., Wang, B. R., Han, J., et al. (2008). Metabolic Analysis of Four Phenolic Acids in Rat by Liquid Chromatography-Tandem Mass Spectrometry. J. Chromatogr. B Analyt Technol. Biomed. Life Sci. 871, 7–14. doi:10.1016/j.jchromb.2008.06.019
Zhao, J., Zhang, Z., Dai, J., wang, L., Zhang, C., Ye, Y., et al. (2014). Synergistic Protective Effect of Chlorogenic Acid, Apigenin and Caffeic Acid against Carbon Tetrachloride-Induced Hepatotoxicity in Male Mice. RSC Adv. 4, 43057–43063. doi:10.1039/c4ra07261h
Zheng, L. F., Dai, F., Zhou, B., Yang, L., and Liu, Z. L. (2008). Prooxidant Activity of Hydroxycinnamic Acids on DNA Damage in the Presence of Cu(II) Ions: Mechanism and Structure-Activity Relationship. Food Chem. Toxicol. 46, 149–156. doi:10.1016/j.fct.2007.07.010
Zhou, Y., Fang, S. H., Ye, Y. L., Chu, L. S., Zhang, W. P., Wang, M. L., et al. (2006). Caffeic Acid Ameliorates Early and Delayed Brain Injuries after Focal Cerebral Ischemia in Rats. Acta Pharmacol. Sin 27, 1103–1110. doi:10.1111/j.1745-7254.2006.00406.x
Keywords: caffeic acid, cancer, anti-cancer, anti-oxidant activity, bioavailability, clinical trials
Citation: Alam M, Ashraf GM, Sheikh K, Khan A, Ali S, Ansari MM, Adnan M, Pasupuleti VR and Hassan MI (2022) Potential Therapeutic Implications of Caffeic Acid in Cancer Signaling: Past, Present, and Future. Front. Pharmacol. 13:845871. doi: 10.3389/fphar.2022.845871
Received: 30 December 2021; Accepted: 26 January 2022;
Published: 09 March 2022.
Edited by:
Letizia Giampietro, University G. d’Annunzio, ItalyReviewed by:
Ehtesham Arif, Medical University of South Carolina, United StatesTooba N. Shamsi, University of Alberta, Canada
Mohd Ishtikhar, Texas A&M University College Station, United States
Copyright © 2022 Alam, Ashraf, Sheikh, Khan, Ali, Ansari, Adnan, Pasupuleti and Hassan. This is an open-access article distributed under the terms of the Creative Commons Attribution License (CC BY). The use, distribution or reproduction in other forums is permitted, provided the original author(s) and the copyright owner(s) are credited and that the original publication in this journal is cited, in accordance with accepted academic practice. No use, distribution or reproduction is permitted which does not comply with these terms.
*Correspondence: Visweswara Rao Pasupuleti, cHZyYW9AdW1zLmVkdS5teQ==; Md. Imtaiyaz Hassan, bWloYXNzYW5Aam1pLmFjLmlu