- 1School of Life Sciences, Ludong University, Yantai, China
- 2Department of Microbiology, School of Public Health, Cheeloo College of Medicine, Shandong University, Jinan, China
- 3Shandong Provincial Key Laboratory of Quality Safty Monitoring and Risk Assessment for Animal Products, Jinan, China
- 4Yantai Key Laboratory of Animal Pathogenetic Microbiology and Immunology, Yantai, China
- 5Shandong Aquaculture Environmental Control Engineering Laboratory, Yantai, China
Lactobacillus plantarum can interact with macrophages against bacterial enteropathy due to its potential ability to modulate macrophage polarization. However, this mechanism is not completely understood. TLR2 can recognize microbial components and trigger macrophage cytokine responses to different gram-positive strains. The aim of this study was to investigate whether probiotic Lactobacillus plantarum RS-09 can induce macrophage polarization against Salmonella Typhimurium infection via TLR2 signalling. BALB/c mice were preadministered RS-09 continuously for 7 days and then infected with Salmonella Typhimurium ATCC14028. Mouse RAW264.7 mononuclear macrophages were stimulated with RS-09 and coincubated with ATCC14028 or PBS controls. The results of the in vivo study indicated that RS-09 could relieve S. Typhimurium-induced splenomegaly, body weight loss and death rate. RS-09 also limited the colonization and translocation of S. Typhimurium in the gastrointestinal tract and thereby protected against infection. We also observed that RS-09 upregulated the production of M1 macrophage characteristics (e.g., CD11c and IL-6) against S. Typhimurium. Furthermore, RS-09 induced the expression of TLR2 in macrophages. In an in vitro study, treatment of RAW264.7 cells with RS-09 either concurrently with or before S. Typhimurium challenge enhanced the secretion of Reactive oxygen species and Nitric oxide. This effect was related to TLR2 and NF-κB activation. Based on these findings, Lactobacillus plantarum RS-09 was shown to modulate M1 macrophage polarization and induce TLR2-linked NF-κB signalling activity in the innate immune response to S. Typhimurium infection.
Introduction
Salmonella enterica Typhimurium (S. Typhimurium) is an important foodborne pathogen that causes enteric diseases in humans and animals (Dougan et al., 2011; Broz et al., 2012; Riddle and Porter, 2012). The innate immune system is a primary defence mechanism against pathogen infection involving mononuclear phagocytes, such as macrophages, neutrophils, dendritic cells, and monocytes. Macrophages play an essential role in the host response to S. Typhimurium infection. Accumulated evidence has shown that S. Typhimurium can survive and replicate in macrophages to evade the host response. S. Typhimurium can influence the macrophage phenotype and polarize M1 to M2-like macrophages, thus escaping the immune system (Brumell et al., 2001; Antunes et al., 2011). Therefore, the role of the M1 phenotype is in favour of antimicrobial activity in controlling infection.
Increasing evidence has demonstrated that the immune response of macrophages is initiated after pathogen recognition through Toll-like receptors (TLRs), including phagocytosis, pro- and anti-inflammatory cytokine release, oxidative burst activation and M1/M2 macrophage polarization (Atri et al., 2018). Toll-like receptor 2 (TLR2), a member of the Toll-like receptor family expressed in macrophages, is critical for triggering innate immune responses by recognizing microbe-associated molecular patterns (MAMPs, bacterial cell wall components) (Shida et al., 2009; Kaji et al., 2010; Rocha-Ramirez et al., 2020). Furthermore, the TLR2 signalling pathway plays a prominent role in modulating macrophage differentiation to either M1 or M2 after pathogenic bacterial infection. Previous experimental data showed that bacteria can induce M1 macrophages to produce a large amount of proinflammatory mediators, including Tumor necrosis factor-α (TNF-α), Interleukin-1(IL-1), and Nitric oxide (NO), via the TLR2 signalling pathway during the early and acute stages of bacterial infection (Khan et al., 2015). Even though pathogens are recognized by macrophages through TLR2, pathogens may utilize TLR2 as part of their survival mechanism. When the infection persists, macrophages polarize to the M2 phenotype to survive in the hostile environment created by macrophages (Yoshida et al., 2009; Lim et al., 2016; Kewcharoenwong et al., 2018). This makes TLR2 an intriguing molecule to study to understand its role in the regulation of the immune response.
Probiotics have been shown to have a wide range of biological activities, including immune regulation (Sánchez et al., 2017), improvement in the intestinal environment (Collins and Gibson, 1999), and direct antimicrobial activity against pathogens through direct contact with immune cells (Dunne et al., 1999; Corr et al., 2007). Probiotics also have MAMPs that can be recognized by TLR2 on macrophages to trigger the immune response, such as peptidoglycan, lipoteichoic acids and the cell wall (Takeda and Akira, 2004). For instance, Bacillus amyloliquefaciens (Ba) can facilitate polarization of M1 macrophages and increase the expression of Interleukin-1β (IL-1β), Inducible Nitric Oxide synthase (iNOS), TNF-α and Interleukin-6 (IL-6) (Ji et al., 2013). Lactobacillus rhamnosus R0011 (LrF) induced Interleukin-10 (IL-10) and IL-1β production and the expression of CD206, markers of immunoregulatory M2 macrophage polarization (Jeffrey et al., 2020). Lactobacillus rhamnosus GG (LGG) induced M1 macrophage polarization and M1-related cytokine expression against pathogen invasion (Baikui Wang et al., 2020). Thus, when the body encounters microbes or their components, the immune response following MAMP-TLR2 recognition is diversified and dependent on the biochemical characteristics of both the bacterial and host cells.
Among probiotic strains, Lactobacillus plantarum (L. plantarum) is known for its potential probiotic properties, such as human safety, immunoregulatory activity and anti-inflammatory effects. Accumulated evidence has shown that L. plantarum can enhance host immune responses mainly by modulating cytokine production and manipulating macrophage phenotypes to exert immunoregulatory effects (Wang et al., 2008; Sichetti et al., 2018; Kim et al., 2020). However, whether L. plantarum activates the macrophage-polarizing effect against Salmonella infections through TLR2 signalling is not well established. To address this question, we investigated the protective effect of L. plantarum RS-09 challenged with pathogenic S. Typhimurium on the polarization of macrophages and explored the involved signalling pathways.
Materials and Methods
Strains and Growth Conditions
The probiotic L. plantarum RS-09 was isolated from fermented apple and kept at the China General Microbiological Culture Collection Center (CGMCC No. 17118). S. Typhimurium ATCC 14028 (Sty14028) was preserved in our laboratory. From frozen stocks (−80 °C), L. plantarum RS-09 was suspended in Man-Rogosa-Sharpe liquid medium (MRS broth; Solarbio, Beijing, China), cultured anaerobically at 37 °C for 48 h in fresh MRS broth (three–four OD at 600 nm), and harvested by centrifuging at 4,000×g for 15 min. S. Typhimurium (Sty14028) was grown in Luria-Bertani medium (LB broth; Solarbio, Beijing, China), cultured at 37°C for 24 h (1–1.5 OD at 600 nm), and harvested by centrifugation at 6,000×g for 20 min.
For the in vitro study, after three washes with phosphate-buffered saline (PBS, pH = 7.2; BBI, Shanghai, China), the bacteria were resuspended in DMEM (antibiotics-free; Gibco, CA, United States) for preincubation or infection. For the in vivo study, the bacteria were administered orally to mice in 1 ml of PBS.
Cell Culture
The leukaemia cells of the mouse mononuclear macrophage RAW264.7 cell line were cultured in DMEM (Gibco, CA, United States) supplemented with 10% foetal bovine serum (fetal bovine serum, FBS; Gibco, CA, United States) in a humidified incubator at 37°C and 5% CO2. RAW264.7 cells were subcultured every 2-3 days.
Animals
Female SPF-grade BALB/c mice (4-5 weeks old) were obtained from HFK Bioscience Inc., (Beijing, China) and maintained in the Experimental Animal Center of Ludong University. All animal experiments were conducted according to the guidelines and approval of the Laboratory Animal Welfare and Ethics Committee of Ludong University. All mice were raised in an air-conditioned and specific pathogen-free environment (60% RH, 22°C, light exposure from 8:00 to 21:00) with free access to sterilized food and water.
Bacterial Infection Assay in Mice
Figure 1 shows the experimental design. Twenty-four mice were randomly divided into four groups (six mice per group): normal control group treated with PBS alone, control group treated with Sty14028 alone, one group treated with L. plantarum RS-09, and one group treated with L. plantarum RS-09 with S. typhimurium 14,028. The RS-09 group and RS-09 with Sty14028 group received an intragastric administration of 200 μL of a suspension containing 5 × 109 CFU/mouse of live RS-09 once daily for 7 consecutive days via a feeding needle. On the 8th day of RS-09 in the Sty14028 group, mice were infected with a 200 μL median lethal dose of S. typhimurium 14,028 (1 × 105 CFU/mouse). All animal weights and survival rates were monitored daily, and mice were euthanized 8 days post-challenge.
Determination of the Spleen and Liver Index
The spleen and liver were harvested from the mice in each group. The whole spleen and liver were weighed immediately, and the organ index was expressed as the ratio of the organ weight to body weight (mg/g) (Habil et al., 2011).
S. Typhimurium Colonization in the Spleen, Liver and Intestinal Segments
The spleen and liver were cut and homogenized using a tissue tearer (TIANGEN, Beijing, China) with 5 ml of PBS. The contents of different intestinal segments were harvested in 5 ml PBS. Serial dilutions were plated onto XLT-4 agar (Hopebiol, Qingdao, China) and incubated at 37°C for 12 h, and the bacterial colonies were recorded as logarithm base 10 (log10 CFU/g).
Blood Sampling
For each mouse, 1.0 ml of blood from the caudal vein was collected. The blood samples were divided into two parts. One was treated using sodium citrate to obtain anticoagulated whole blood to detect routine histopathology by an automatic blood cell analyser (Rayto RT-7600S, Shenzhen, China), and the other was stored at 4°C for 4 h to collect serum for cytokine measurements.
Cytokine Quantification
Cytokines, including mouse IFN-γ, IL-6, and Interleukin-12 (IL-12), were quantified in serum using enzyme-linked immunosorbant assays (ELISA) following the manufacturer’s protocols (R&D Systems, MN, United States). ELISA for IL-10 (Cat. no 430603) using serum was also performed following the manufacturer’s protocols (BioLegend, CA, United States). All ELISAs were performed using 96-well high-binding ELISA plates in ELISA kits, and plates were read at a wavelength of 450 nm using a Synergy HTTR microplate reader (Bio-Tek Instrumentation, VT, United States).
Flow Cytometry (FCM) Analysis of Macrophage Surface TLR2 Expression
To detect the cell surface expression of TLR2, cells were incubated with antibodies against mouse TLR2-FITC, CD11c-PE and CD206-APC for 30 min at room temperature, washed and analysed in a FACS Calibur flow cytometer (BD Biosciences, New York, United States).
Macrophage Stimulation in Vitro
For macrophage polarization assays, RS-09 and Sty14028 were collected by centrifugation and washed three times with PBS. RAW264.7 cells were seeded into a 24-well culture plate at a concentration of 2 × 106 per well. RS-09 was then applied to infect macrophages at an MOI of 20:1 (4 × 107 CFU: 2 × 106 RAW264.7) for 12 h (n = 3 for each group). RAW264.7 cells were then washed with PBS twice. Sty14028 was then applied to infect the macrophages at an MOI of 20:1 (4 × 107 CFU: 2 × 106 RAW264.7). After incubation for 1 h, the cells were washed three times and treated with 200 μL of gentamicin sulfate (100 μg/ml) for 1 h. RAW264.7 cells were then washed with PBS twice and incubated for 12 h with DMEM (including 20 μg/ml of gentamicin sulfate). The cells were harvested for the detection of macrophage phenotype, reactive oxygen species (ROS), and proteins with altered expression, and culture supernatants were simultaneously assayed for NO.
Flow Cytometry (FCM) Analysis of Macrophage Polarization
CD11c and CD206 are markers of the M1 and M2 macrophage phenotypes, respectively. To analyse the activation effects of RS-09 on macrophages, treated RAW264.7 cells were incubated with fluorochrome-conjugated antibodies against mouse CD11c-PE and CD206-APC. CD206-APC was demonstrated by intracellular labelling. Cells were treated with FIX&PERM Kit MEDIUM A (MultiSciences, Hangzhou, China) for 15 min at room temperature and then incubated with FIX&PERM Kit MEDIUM B and CD206-APC for 30 min at room temperature followed by two washes in PBS. The percentages of CD11c+ cells (M1 macrophages) and CD206+ cells (M2 macrophages) were analysed on a FACS Calibur flow cytometer (BD Biosciences, New York, United States).
Determination of Intracellular ROS Levels
The formation of intracellular ROS in bacteria-treated RAW264.7 cells was measured using an intracellular ROS detection kit (Beyotime, Shanghai, China) according to the manufacturer’s instructions. Briefly, cells were stained with dichlorodihydrofluorescein-diacetate (DCFH-DA, 10 μM) and incubated for 30 min at 37°C in a 5% CO2 humidified incubator. After washing twice with PBS, the cells were stained with 4,6-diamidino-2-phenylindole (DAPI) for 10 min at room temperature. RAW264.7 cells were then washed with PBS thrice. Cells were then immediately visualized through a fluorescence microscope and photographed (400×). The formation of intracellular ROS was also measured by a flow cytometer (FACS calibur; BD Biosciences, New York, United States) equipped with an argon laser, yielding a 488-nm primary emission line.
Detection of NO
Cell supernatants were collected to determine the expression of NO by a nitrate/nitrite assay kit (Beyotime, Shanghai, China). Briefly, supernatants (60 μL) were collected and mixed with an equal volume of Griess reagent (1% sulfanilamide and 0.1% N-(1- naphthyl) ethylenediamine dihydrochloride in 5% phosphoric acid) at room temperature for 15 min. After mixing with Griess reagent Ⅰ and Griess reagent Ⅱ for 10 min at room temperature, supernatants were detected at 550 nm using a microplate reader (Biotek, VT, United States), and the actual concentration was calculated using a standard curve with serial dilutions of NaNO2 (Zhang et al., 2009).
Western Blot
After treatment with bacteria, cells were washed with ice-cold PBS and lysed in RIPA lysis buffer containing 1 mMPMSF. With centrifugation at 12,000 rpm and 4°C for 20 min, the protein concentration was determined by the BCA protein quantitative method (Thermo, MA, United States). Equal amounts of protein (50 μg per lane) were separated by 12% sodium dodecyl sulfate–polyacrylamide gel electrophoresis (SDS–PAGE) and transferred to nitrocellulose membranes on ice. The membrane was blocked with 5% skimmed milk (Cell Signalling Technology, MA, United States) for 2 h at room temperature and incubated with iNOS (1:1,000, Cell Signalling Technology, MA, United States) and β-Actin (1:1000, Proteintech, Chicago, United States) antibodies at 4°C overnight. The protein was detected with horseradish peroxidase (HRP)-conjugated secondary antibody (1:2500, Cell Signalling Technology, Mass, United States) for 1 h aat room temperature. Finally, an ECL kit (SuperSignal™ West Pico PLUS, Thermo Scientific, MA, United States) was used to observe the protein bands using a gel imaging analytic system (Bio–Rad, CA, United States). The image grey value was analysed using AlphaEaseFC software (Alpha Innotech, CA, United States).
Statistical Analysis
Graphs were analysed using GraphPad Prism Software version 6 (GraphPad Software, CA, United States) and are presented as the means ± SD of at least three independent experiments. Comparisons between pairs of data were assessed using 2-tailed Student’s t test and between multiple groups of data using one-way analysis of variance (ANOVA). Differences were considered statistically significant for p values <0.05.
Results
Effects of Pretreatment of Mice With L. plantarum RS-09 on S. Typhimurium Infection
Pre-treatment of mice with L. plantarum RS-09 was shown to improve the protective efficacy after S. typhimurium infection. Mice were treated with a dose of RS-09 (5 × 109 CFU/mouse) for 7 days prior to infection. Then, mice were infected with one dose of S. typhimurium 14,028 (Sty14028, 105CFU/mouse) in the presence of RS-09. Figure 2A shows that all of the mice survived RS-09 treatment, whereas 40% of the mice survived after only oral administration of 1 × 105 CFU of Sty14028 (p < 0.05). None of mice pretreated with RS- 09 experienced body weight loss, and all survived Sty14028 infection, indicating that protection by RS-09 treatment was significantly improved (p < 0.05) (Figure 2B). In contrast, the spleen size and the ratio of the spleen weight to body weight of Sty14028-infected mice exhibited more severe splenic hypertrophy than the mice pre-treated with RS-09 (p < 0.05) (Figures 2C,E). Moreover, compared with the control group, the liver/body weight ratio for infected mice showed no significant increase (Figure 2D).
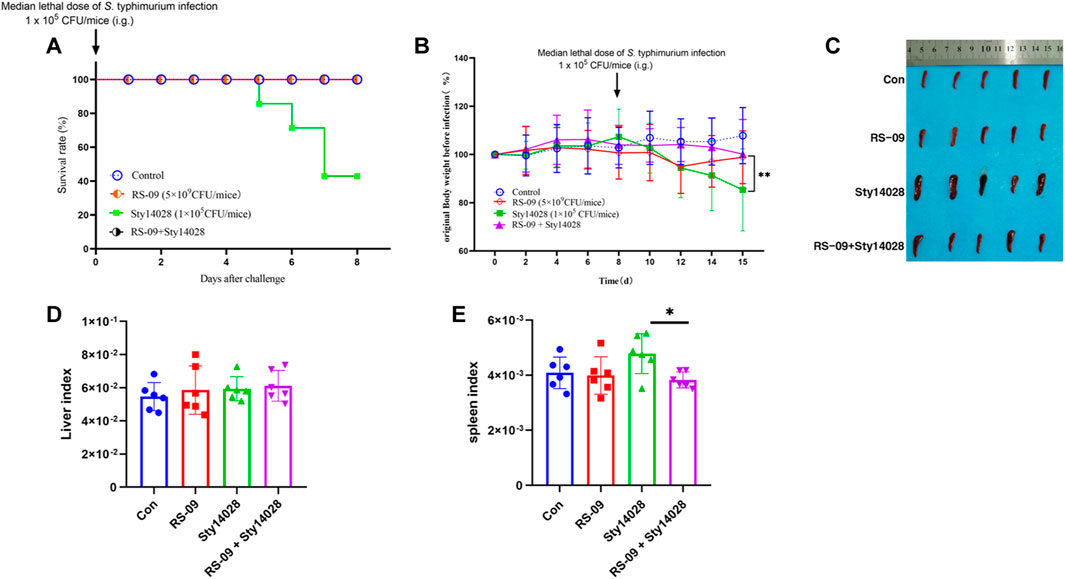
FIGURE 2. Pretreatment with RS-09 protects mice from S. Typhimurium infection. (A) Survival rates of mice following RS-09 pretreatment and challenge with pathogenic S. Typhimurium. RS-09 was administered to mice for 7 days, and they were then orally injected with a sublethal dose of Sty14028 suspension (105 CFU/mouse). The survival of mice was recorded daily. (B) Comparison of body weight loss (current body weight/original body weight before infection) over 8 days post-infection daily. (C) RS-09 relieved Sty14028-induced splenomegaly (the scale is shown in the diagram). (D) The liver weight index (liver weight/body weight) (E) The splenic weight index (spleen weight/body weight). Data are the means ± SD (n = 6). *p < 0.05.
L. plantarum RS-09 Reduces Enteric Pathogenic Bacteria After S. Typhimurium Infection
Previous works proved that L. plantarum RS-09 has antibacterial activity on S. Typhimurium obviously by utilizing oxford cup ( Supplementary Figure 3S). We next evaluated whether RS-09 in hosts can prevent S. Typhimurium colonization in the intestine of mice. The intestinal contents of different intestinal sections from each group were placed on XLT-4 agar plates. Sty14028 loads in the intestine (p < 0.05) were lower in the group pre-treated with RS-09 than the Sty14028 group, except in the duodenum and ileum (Figure 3). Furthermore, we did not observe Sty14028 in the intestine of the control or RS-09 groups. Our data suggest that pre-administration of RS-09 can restrain the growth of S. Typhimurium in vivo.
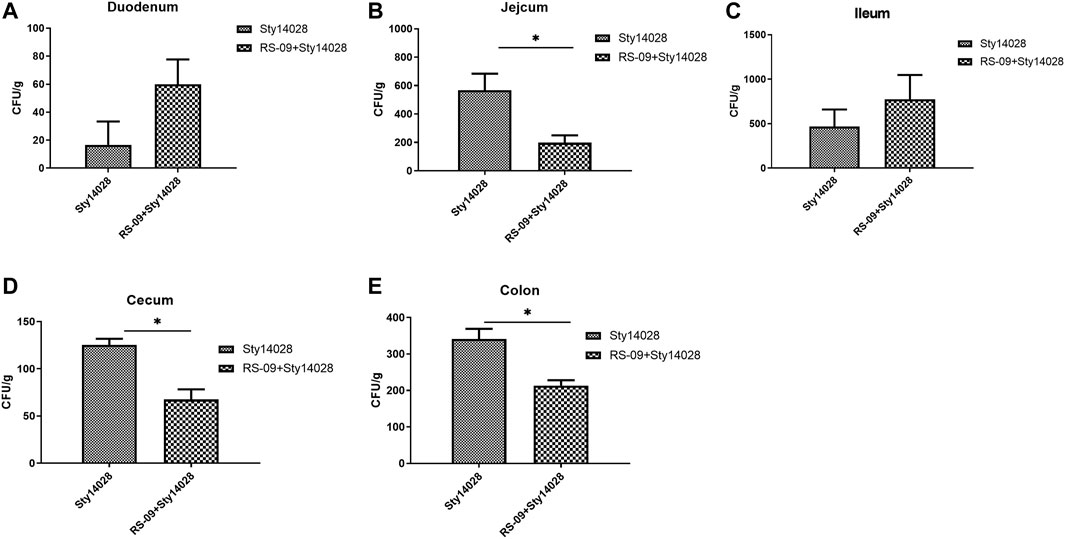
FIGURE 3. Effect of RS-09 on bacterial load during infection with S. Typhimurium. At the indicated times postinfection, dilutions were plated onto XLT-4 agar plates. (A–E) Sty14028 loads in the duodenum, jejunum, ileum, caecum and colon for the Sty14028 and RS-09 + Sty14028 groups. The bactericidal activity is shown as the surviving bacterial numbers. Data are the means ± SD (n = 6). *p < 0.05.
Effects of L. plantarum RS-09 on Haematological Parameters
Given the demonstrated in vivo effect of bacteria on immune cells, we measured haematological parameters during murine infection with S. Typhimurium. At the end of the experiment, animals were sacrificed, and blood was collected for haematological analysis, the results of which are shown in Figure 4. Our findings demonstrated no significant changes in the cellular constituents of blood between the control and RS-09 alone treatment groups, including total white blood cell (WBC), polymorphonuclear neutrophil (PMN), lymphocyte and monocyte counts (Figures 4A,C,D). Following infection, the Sty14028 mice had significantly higher absolute counts, especially WBC (median of 10.13×109 versus 4.73×109 cells/L), neutrophils (median of 6.11×108 versus 1.05×108 cells/L) and lymphocytes (median of 8.87×109 versus 4.38×109 cells/L), compared to control mice blood (Figures 4A–C). In addition, pre-treatment of RS-09 mice showed an increase in monocyte counts throughout infection (Figure 4D). Taken together, these results suggest that the haematological parameters reflect the progression of infection in mice and suggest that pre-treated RS-09 mice are in a recovery phase of Salmonella infection compared to Sty14028 mice.
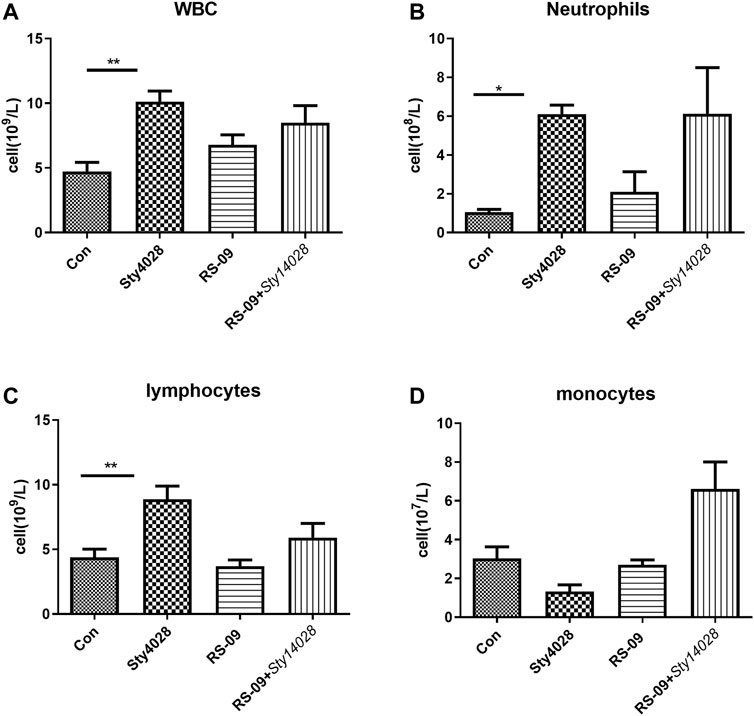
FIGURE 4. Haematological analysis of mice following an intragastric challenge with S. Typhimurium. The total numbers of WBCs (A), as well as the levels of circulating neutrophils (B), lymphocytes (C), and monocytes (D), were evaluated in different groups. Data are the means ± SD (n = 3). *p < 0.05; **p < 0.01.
L. plantarum RS-09 Modulates Cytokine Production in S. Typhimurium-Infected Mice
Inflammatory cytokines are known to be associated with S. Typhimurium infection. To better understand the beneficial effects of RS-09 treatment, cytokine levels were measured in the serum of mice by ELISA. The levels of IFN-γ and IL-10 in the serum of infected mice were significantly higher than those in the serum of the PBS mice (p < 0.05) (Figures 5A,D). The infected mice fed RS-09 revealed increased levels of IFN-γ and IL-6 compared to S. Typhimurium-infected group (Figures 5A,B). However, IL-12 production was not significantly different in the serum of mice across groups (Figure 5C).
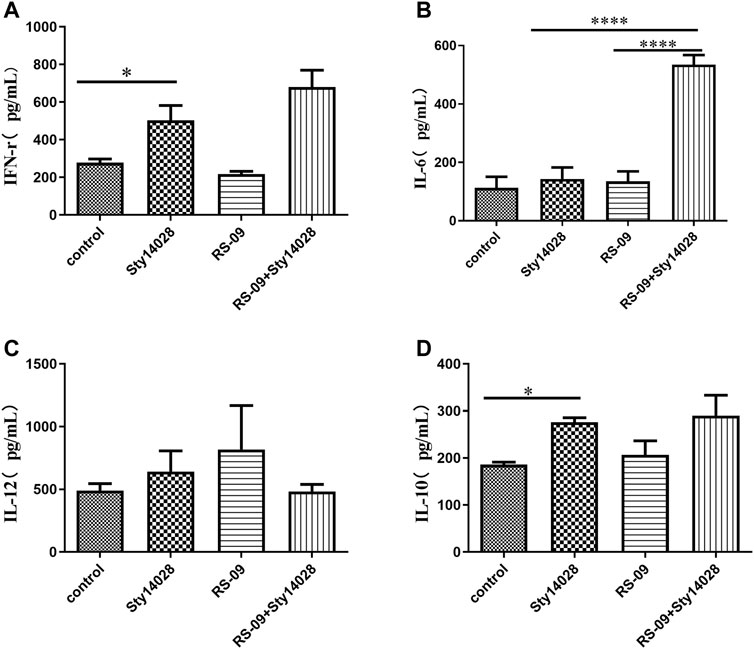
FIGURE 5. RS-09 modulates cytokine production in mice challenged with pathogenic S. Typhimurium. RS-09 (5 × 109 CFU/mouse) was orally administered to mice for 7 days, and they were then orally administered a sublethal dose of Sty14028 suspension (105 CFU/mouse). At 8 d postinfection, serum was collected from the blood to evaluate the expression of (A) IFN-γ, (B) IL-6, (C) IL-12 and (D) IL-10. The data are the means ± SD (n = 3). p values were calculated by ANOVA. *p < 0.05; ****p < 0.0001.
Probiotic RS-09 Promotes M1 Macrophage Polarization and TLR2 Expression
M1 macrophages promote inflammatory responses and clear pathogens (Italiani and Boraschi, 2014). Macrophages treated with Sty14028 showed a tendency toward M1 polarization. Flow cytometry showed that M1 expression in the Sty14028 group was significantly higher than that in the LPS + IFN-γ group (p < 0.01), and the M2 expression level was similar to that in the IL-4 group (Figures 6A,B). We subsequently attempted to identify Sty14028-infected macrophage cell subtypes after RS-09 pre-treatment. RS-09 treatment of RAW264.7 cells resulted in increases in CD11c+ populations by 114-fold compared with the control (Figure 6A). We also detected the proportion of M1 macrophages in the mesenteric lymph nodes. The results showed RS-09 did induce M1 macrophage polarization compared with control group in vivo ( Supplementary Figure 6S). Therefore, increases in M1 macrophage polarization by RS-09 might contribute to improving protection against Salmonella infection.
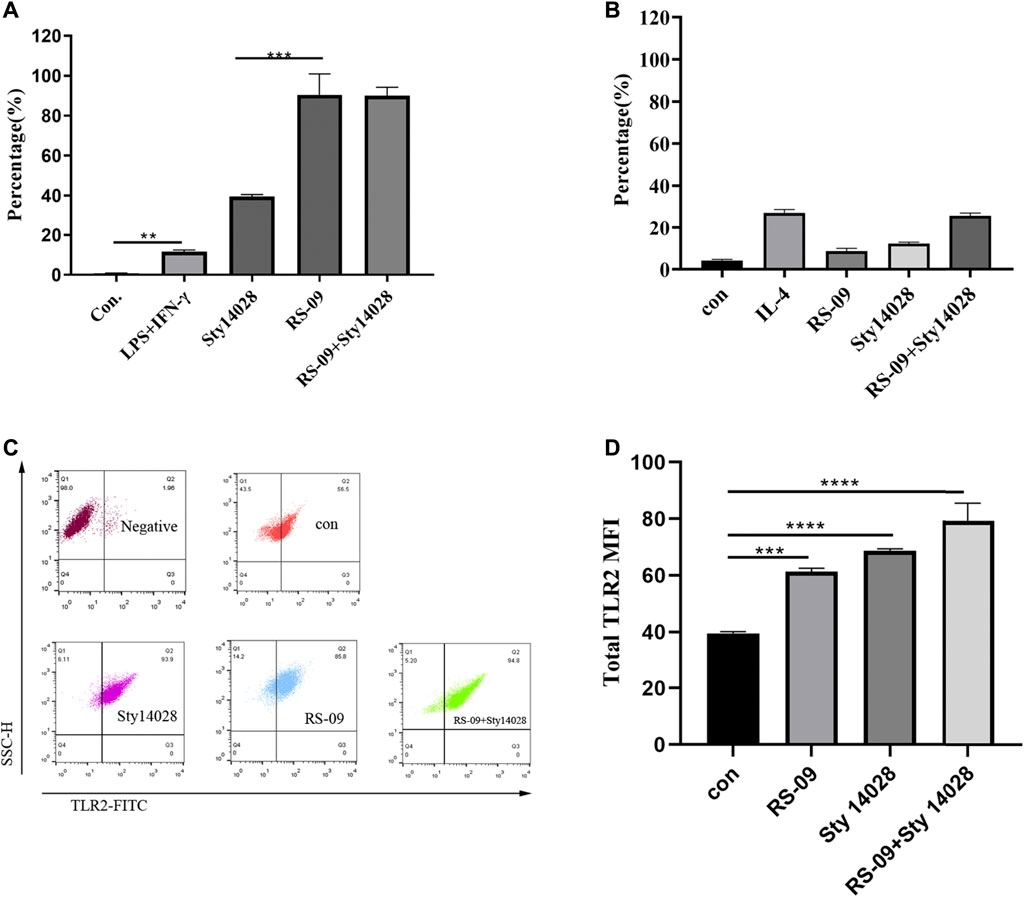
FIGURE 6. RS-09 stimulates M1 polarization in vitro. RAW264.7 macrophages were differentiated and pretreated with RS-09 for 12 h and then treated with Sty14028 for 1 h. (A) Representative plot showing the effect of RS-09 on the expression of the M1 marker CD11c+CD206− in RAW264.7 macrophages. RAW264.7 cells were treated with LPS (100 ng/ml) plus IFN-γ (20 ng/ml) to differentiate into M1 (M1 positive control) macrophages. (B) Detection of M2 macrophage polarization-related markers by flow cytometry. RAW264.7 cells were treated with IL-4 (40 ng/ml) to differentiate into M2 macrophages (M2 positive control). (C) Representative plot showing the expression of TLR2 in RAW264.7 macrophages. (D) Quantitation of TLR2-positive cells derived from RAW264.7 cells after different combination treatments for 12 h **p < 0.01; ***p < 0.001; ***p < 0.0001. Data are the means ± SD of three independent experiments.
TLRs in the cell membrane of macrophages contribute to the early recognition of pathogen-associated molecular patterns (PAMPs) of external pathogens (Kawai and Akira, 2011). In addition, TLR signalling has been reported to induce the activation of macrophage inflammatory responses to fight external pathogens (Fitzgerald and Kagan, 2020). Thus, we investigated the expression of TLR2 by FCM analysis. As shown in Figure 6C, compared with the control group, both RS-09 and Sty14028 exposure significantly upregulated the expression of TLR2.
Effect of RS-09 on S. Typhimurium-Induced ROS Production in RAW264.7 Cells
Macrophages with ROS production can protect against microbial pathogens. With this in mind, we next detected whether pre-treatment with RS-09 was able to induce infected RAW264.7 macrophages to produce ROS by the fluorescent probe DCFH-DA (green) with immunofluorescence microscopic studies. As demonstrated in Figure 7, RAW264.7 cells infected with the Sty14028 strain and stained with DCFH-DA displayed significantly higher levels of fluorescence, whereas Sty14028-induced ROS generation was significantly improved by RS-09 pre-treatment. We also analyzed the quantitative fluorescence of ROS (Supplementary Figure 7S). The ROS levels of pre-treatment with RS-09 cells were significantly higher than that in the Sty14028 group (p < 0.0001). This reconfirmed that the RS-09 strain could induce ROS production during resistance to Salmonella infection.
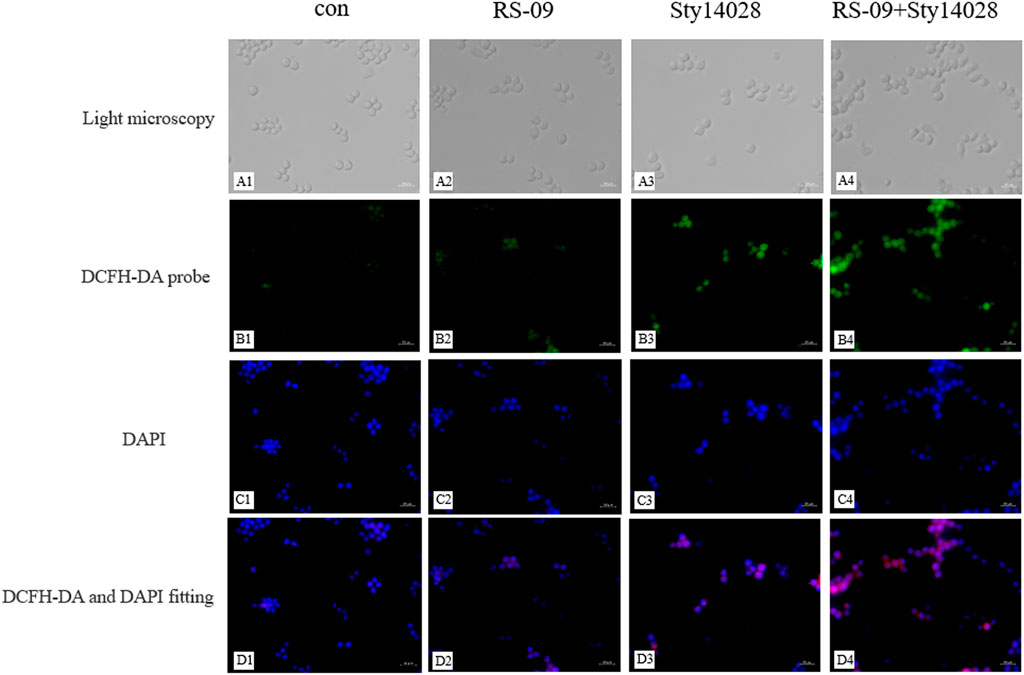
FIGURE 7. Effect of RS-09 on Sty14028-induced ROS generation in RAW264.7 macrophages. RAW264.7 cells were cultured in DMEM for 12 h and then incubated with the probe DCFH-DA (green) for 30 min. DNA was stained with DAPI (blue) for 10 min (A1–A4) The cell morphology was observed by light microscopy. (B1–B4) ROS detection was performed using fluorescence macroscopy (C1–C4) Treated cells were stained with DAPI and observed under fluorescence microscopy (D1–D4) Merged image of DCFH-DA and DAPI. These experiments were repeated independently 3 times (400×).
Effect of RS-09 on NO Production by RAW264.7 Cells
NO is an important biological effector molecule and the main catabolite in M1 macrophages, which has cytotoxic effects on invading pathogenic microorganisms (Li et al., 2015). To investigate the ability of RS-09 to produce NO in RAW264.7 macrophages, we examined the NO levels in the culture supernatants of cells stimulated with RS-09 (4 × 107 CFU) for 12 h and those stimulated with Sty14028 (4 × 107 CFU) for 1 h. As shown in Figure 8, compared with the control group, Sty14028 significantly stimulated NO production in RAW264.7 cells (p < 0.0001). RS-09 also induced NO production in RAW264.7 cells. However, after pretreatment with RS-09, the NO levels of infected cells increased to 49.65 μM, which was significantly higher than that in the Sty14028 group (p < 0.0001). This increased production of NO suggests that RS-09 may activate the bactericidal activities of macrophages and potentiate the M1 polarity of RAW264.7 macrophages.
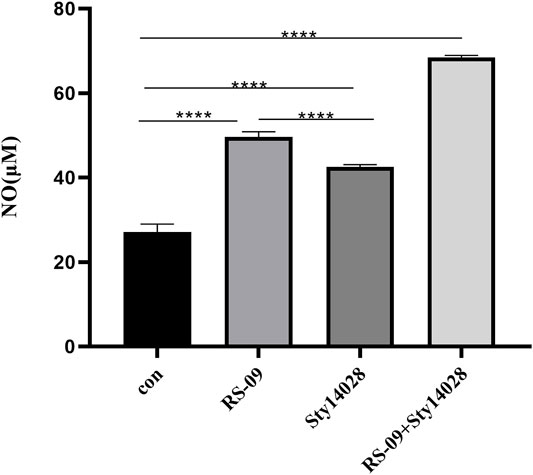
FIGURE 8. Effect of RS-09 on nitrite (NO) production in bacteria-stimulated RAW264.7 macrophages. The content of NO in the culture supernatant was measured by the Griess reaction assay. The values represent the means ± SD of three independent experiments. ****p < 0.0001.
RS-09 Modulates TLR2/NF-κB Signalling to Clear S. Typhimurium in M1 Macrophages
NF-κB is a key member of the signalling pathway responsible for the activation of inflammatory cytokine secretion by monocytes-macrophages. It has also been reported that nuclear factor-κB (NF-κB) activated by the stimulation of TLRs is an essential master regulatory signalling pathway that contributes to the production of immunomodulators by macrophages and other innate immune cells against external pathogens (Kawai and Akira, 2007; Dorrington and Fraser, 2019). TLRs, among PRRs of macrophages, contribute to the early recognition of external pathogens (Kawai and Akira, 2011), and TLR signalling has been reported to induce the secretion of various immunomodulators (Strieter et al., 2003). To elucidate the molecular mechanism of RS-09-induced M1 polarization in vitro, we analysed the TLR2/NF-κB pathway by immunoblot analysis. We found that the iNOS level of M1 macrophage-related markers increased after treatment with RS-09 and Sty14028 (Figure 9A). Analysis of intracellular proteins showed that the group infected with Sty14028 exhibited higher levels of NF-κB expression than the control group (Figure 9B) (p < 0.001). Notably, stimulation of RS-09-treated Raw264.7 cells combined with Sty14028 significantly increased NF-κB levels (p < 0.001). However, concurrent treatment with RS-09 and Sty14028 resulted in higher NF-κB levels than treatment with RS-09 or Sty14028 alone (p < 0.001; p < 0.05). In line with the change in the level of NF-κB expression, the protein levels of iNOS and TLR2 in RAW246.7 macrophages were also upregulated after RS-09 treatment. Based on these results, RS-09 can promote the inflammatory response of Sty14028-infected M1 macrophages through the TLR2-mediated NF-κB signalling pathway.
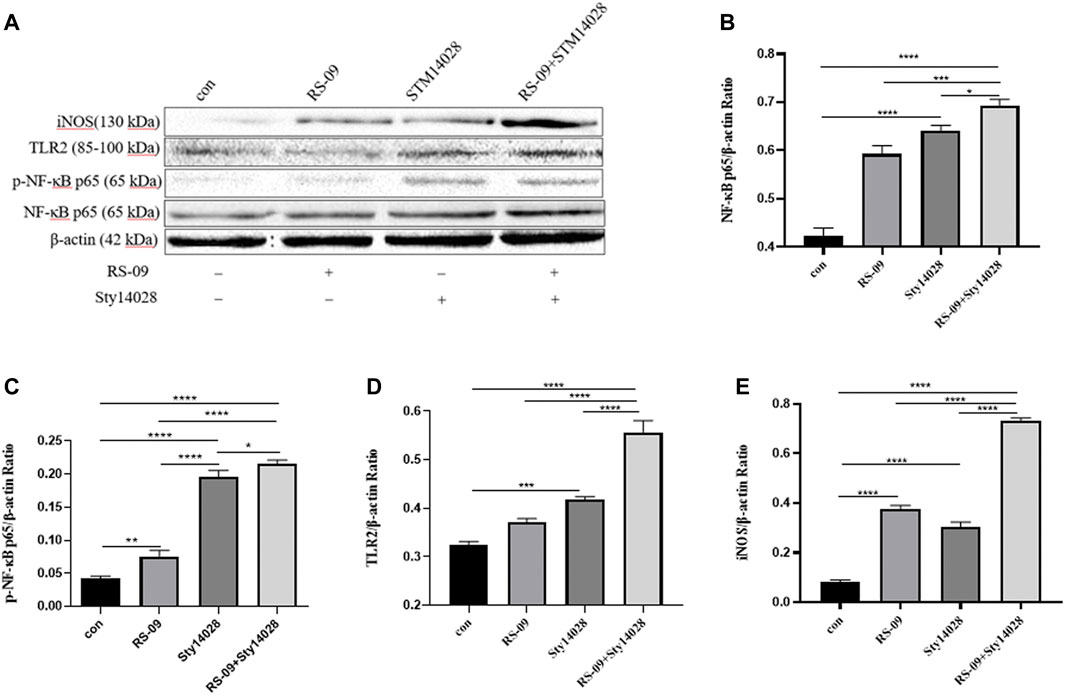
FIGURE 9. RS-09 promotes the expression of NF-κB in M1-type macrophages. Raw264.7 cells were stimulated with RS-09 alone, Sty14028 alone, or both RS-09 and Sty14028 for 12 h. (A) Levels of iNOS, TLR2, p-NF-κB and NF-κB were detected using Western blotting (B–D) The quantified values of NF-κB, p-NF-κB, TLR2 and iNOS (E) expression in RAW264.7 cells were determined during 12 h of incubation. The image grey value was analysed using AlphaEaseFC software (Alpha Innotech, CA, United States). All values are expressed as the means ± S.D (n = 3). *p < 0.05; **p < 0.01; ***p < 0.001; ****p < 0.0001.
Discussion
Salmonella-mediated inflammation is dependent on an intricate series of host-pathogen interactions affected by the immunologic status of the host. In this study, we evaluated the protective capacity of the immune response by L. plantarum RS-09 against a challenge with S. Typhimurium. The findings indicated that RS-09 leads to anti-inflammatory effects by reducing S. Typhimurium colonization in the intestine, upregulating the expression of proinflammatory cytokines and ameliorating the oxidative stress response.
S. Typhimurium is first observed in intestinal tissues at the beginning of infection and then crosses the lining of intestinal epithelial cells, translocates to extraintestinal organs such as the spleen and liver, and survives in host immune cells (such as macrophages and dendritic cells). As such, colonization by S. Typhimurium in different intestinal tissues was inconsistent in the present study. The number of S. Typhimurium duodenal and ileac colonies in the RS-09 + Sty14028 group was higher than in the Sty14028 group. The S. Typhimurium colonies in other intestinal sections after RS-09 treatment were reduced compared with the Sty14028 group. Brewer reported less Salmonella enterica colonization in the ileum of probiotic-supplemented calves than control calves (Brewer et al., 2014). According to Finlay and Brumell (Finlay and Brumell, 2000), the effect of probiotics on the colonization of S. Typhimurium in gastrointestinal tracts is highly dependent on adhesion and persistence (Duary et al., 2011). In our previous investigation, we evaluated probiotic strains of L. plantarum RS-09 for their ability to adhere to HT-29 cells. On comparative evaluation based on adhesion score, L. plantarum RS-09 was found to be the most adhesive strain and could therefore inhibit S. Typhimurium adhesion to HT-29 cells. Similarly, other workers have also reported the competitive exclusion of pathogens by lactobacilli (Collado et al., 2007; Pham et al., 2009; Zhang et al., 2009). In our results, the competitive profile of S. Typhimurium by L. plantarum RS-09 strains was different in the gut, indicating that the pathogen inhibition capacity of probiotic strains was possibly due to different intestinal section surface characteristics. This suggests that the mechanism involved in inhibition is complicated and that many factors may be involved.
Infection with S. Typhimurium first induces local inflammatory responses in the intestinal mucosa. Additionally, severe infections can cause systemic inflammation, which can influence other organs. A previous study illustrated that rats supplemented with Lactobacillus casei had no difference in either liver or spleen weight compared with control rats (De Waard et al., 2002). In agreement, our study in mice demonstrated no difference in either liver index or spleen index between the L. plantarum RS-09 group and a control group. Moreover, Jain et al. demonstrated no difference in liver weight between a Lactobacillus casei group and a control group after a Salmonella Typhimurium challenge (Jain et al., 2008). This result was consistent with our findings. In addition, the spleen index tended to decrease in the RS-09 + Sty14028 group compared with the Sty14028 group, the difference was significant (p < 0.05).
Additionally, we evaluated the biochemical parameters of mice challenged with S. typhimurium and supplemented with L. plantarum RS-09 inoculum. Significant differences were observed in total WBC, neutrophil and lymphocyte counts after treatment with the S. Typhimurium inoculum. We detected no difference in total monocyte counts between the control and RS-09 groups. Furthermore, the RS-09 + Sty14028 group had an increased Polymorphonuclear Neutrophils (PMN) percentage compared with the Sty14028 group. As reported earlier, an increase in PMN count is one of the first innate immune actions observed in response to Salmonella infection (Wick, 2004). Furthermore, probiotics have been demonstrated to increase the production of immune cell populations, including neutrophils and monocytes (Liang et al., 2020). While there was no significant increase in WBC or lymphocyte concentrations in the RS-09 group compared with the control group, the concentrations were on average reduced in the RS-09 + Sty14028 treatment group compared to Sty14028 mice. Alternatively, the attenuated response of WBCs and lymphocytes after RS-09 + Sty14028 treatment may also indicate that the infection was better controlled locally or by some other component of the immune system.
Salmonella is an intracellular pathogen that elicits an inflammatory response once taken up by intestinal epithelial cells. This action can activate innate immune cells, one pathway being through the bacterial component recognized by TLRs, and results in the release of cytokines, such as IFN-γ, into circulation (Benoun et al., 2018; Gabr et al., 2021). M1 Macrophages can be stimulated by IFN-γ after intracellular pathogen challenge (Ramarathinam et al., 1993). M1 macrophages are pro-inflammatory and produce pro-inflammatory cytokines such as IFN-γ, IL-6 and IL-12, which destroying pathogens (Shapouri-Moghaddam et al., 2018). Similar results were observed in our study, where the IFN-γ level increased in Salmonella Typhimurium-challenged mice. Additionally, the monocyte ratio of RS-09 + Sty14028 mice was significantly increased compared with that of any other group. This observation was supported by Shida et al. (Shida et al., 2006), who observed that a Lactobacillus casei strain could be phagocytosed by human mononuclear cells and then induce the production of IFN-γ and IL-12. Elevation of serum IL-12 levels in the RS-09 group was consistent with our findings. The lower expression of IL-12 in the RS-09 + Sty14028 group may be related to the process of inflammatory reaction. With the inflammation continues, it can cause tissue damage. Therefore, RS-09 secrete amounts of IL-10 to suppress the inflammation, contribute to tissue repair, remodeling and retain homeostasis. This observation was supported by Duan, B (Duan et al., 2021), who observed that Lactobacillus rhamnosus GG strain induced the production of IL-10. When the inflammation improved, it maybe associated with the decreased the expression of Inflammatory cytokine IL-12 in the RS-09 + Sty14028 group.
Accumulated evidence has shown that macrophages can be phenotypically polarized by microbes into two main groups: M1 macrophages and M2 macrophages. The type of polarized phenotype depends on many factors, such as bacterial strain, dose and exposure time (Yang Wang et al., 2020). The M1 phenotype favours vital microbicidal properties. Our current work demonstrated that probiotic RS-09 influences the outcome of S. Typhimurium infection and is associated with M1 macrophage polarization (Martinez et al., 2008). We have shown that the presence of RS-09 promotes the development of a robust proinflammatory response in mice, characterized by the expression of proinflammatory cytokines, ROS, and NO for clearance of the bacteria (Liu et al., 2014). In our study, we found that RS-09 pre-treatment significantly increased the expression of proinflammatory cytokines (IL-6), suggesting that RS-09 may serve as a regulator to improve the proinflammatory response of macrophages. In previous studies, an established probiotic cocktail (LDB-ST) was reported to elevate the expression of proinflammatory macrophage markers (IL-6, IL-1β, IL-12, and TNF-α) in RAW264.7 cells (Guha et al., 2019). As mentioned in previous studies, ROS production has been recognized as one of the earliest host innate immune responses followinng microbial invasion (Li et al., 2019). In agreement with these findings, we found ROS production in RAW264.7 cells in response to RS-09 treatment. NO has been identified as one of the major effector molecules produced by activated macrophages and is the main catabolite of iNOS in M1 macrophages (Kim et al., 2006; Wang et al., 2008; Chiou et al., 2011). Our study demonstrated that RS-09 promotes macrophage antimicrobial activity against S. Typhimurium, which is also consistent with M1 macrophage function.
The inflammatory response is regulated by complex and cross-linked endogenous cellular signalling pathways and their modulators (Takeuchi and Akira, 2010). Evidence has shown that host–microbe interactions depend on pattern recognition receptors; for instance, TLR2 can recognize many different microbial components (Beutler et al., 2006; Takeuchi and Akira, 2010). Many pathways and transcription factors are involved in macrophage polarization, such as nuclear factor NF-κB (Liu et al., 2014). There is evidence that NF-κB signalling pathways are involved in macrophage polarization (Liu et al., 2017; Luo et al., 2020). M1 phenotypic macrophages can be activated by bacterial components via the TLR2/NF-κB signalling pathway. The main role of the TLR2/NF-κB pathway is to induce the expression of inflammatory cytokines (such as iNOS), which are biomarkers of M1 macrophages (Cunha et al., 2016). In the present study, Western blot results showed that RS-09 increased the expression of TLR2, NF-κB and iNOS in RAW264.7 cells. Further studies are needed to define the role of probiotic component interactions with TLR2 receptors and signalling events.
Conclusion
In conclusion, our data demonstrate that probiotic RS-09 induces M1 polarization of macrophages and has potent antimicrobial activity against S. Typhimurium by activating TLR2/NF-κB signalling pathways. These findings are beneficial for a better understanding of the mechanism by which RS-09 modulates the host immune response against pathogen infection, especially the molecular mechanisms of probiotics in regulating M1 polarization.
Data Availability Statement
The original contributions presented in the study are included in the article/Supplementary Material, further inquiries can be directed to the corresponding authors.
Ethics Statement
The animal study was reviewed and approved by the Laboratory Animal Welfare and Ethics Committee of Ludong University.
Author Contributions
LJ and CZ designed the experiments. HC, XZ, WT, and HL carried out the experiments. MW, YL, and JZ analyzed the experimental results. GC, XY, and HZ, wrote the manuscript. XZ reviewed and edited the manuscript.
Funding
This research was financially supported by the Natural Science Foundation of Shandong Province, China (Grant No. ZR2020QC227), Natural Science Foundation of Shandong Province (No. ZR2020KC028), the Key Research and Development Program of Yantai (2020XDRH101), and the Key Research and Development Program of Yantai (2021XDHZ076), and the Cooperation Project of University and Local Enterprise in Yantai of Shandong Province (2020XDRHXMPT34).
Conflict of Interest
The authors declare that the research was conducted in the absence of any commercial or financial relationships that could be construed as a potential conflict of interest.
Publisher’s Note
All claims expressed in this article are solely those of the authors and do not necessarily represent those of their affiliated organizations, or those of the publisher, the editors and the reviewers. Any product that may be evaluated in this article, or claim that may be made by its manufacturer, is not guaranteed or endorsed by the publisher.
Supplementary Material
The Supplementary Material for this article can be found online at: https://www.frontiersin.org/articles/10.3389/fphar.2022.832245/full#supplementary-material
References
Antunes, L. C., Arena, E. T., Menendez, A., Han, J., Ferreira, R. B., Buckner, M. M., et al. (2011). Impact of salmonella Infection on Host Hormone Metabolism Revealed by Metabolomics. Infect. Immun. 79, 1759–1769. doi:10.1128/IAI.01373-10
Atri, C., Guerfali, F. Z., and Laouini, D. (2018). Role of Human Macrophage Polarization in Inflammation during Infectious Diseases. Int. J. Mol. Sci. 19, 1801. doi:10.3390/ijms19061801
Benoun, J. M., Peres, N. G., Wang, N., Pham, O. H., Rudisill, V. L., Fogassy, Z. N., et al. (2018). Optimal protection against Salmonella Infection Requires Noncirculating Memory. Proc. Natl. Acad. Sci. U S A. 115, 10416–10421. doi:10.1073/pnas.1808339115
Beutler, B., Jiang, Z., Georgel, P., Crozat, K., Croker, B., Rutschmann, S., et al. (2006). Genetic Analysis of Host Resistance: Toll-like Receptor Signaling and Immunity at Large. Annu. Rev. Immunol. 24, 353–389. doi:10.1146/annurev.immunol.24.021605.090552
Brewer, M. T., Anderson, K. L., Yoon, I., Scott, M. F., and Carlson, S. A. (2014). Amelioration of Salmonellosis in Pre-weaned Dairy Calves Fed Saccharomyces cerevisiae Fermentation Products in Feed and Milk Replacer. Vet. Microbiol. 172, 248–255. doi:10.1016/j.vetmic.2014.05.026
Broz, P., Ohlson, M. B., and Monack, D. M. (2012). Innate Immune Response to Salmonella typhimurium, a Model Enteric Pathogen. Gut Microbes 3, 62–70. doi:10.4161/gmic.19141
Brumell, J. H., Rosenberger, C. M., Gotto, G. T., Marcus, S. L., and Finlay, B. B. (2001). SifA Permits Survival and Replication of Salmonella typhimurium in Murine Macrophages. Cell Microbiol 3, 75–84. doi:10.1046/j.1462-5822.2001.00087.x
Chiou, W. F., Don, M. J., Liao, J. F., and Wei, B. L. (2011). Psoralidin Inhibits LPS-Induced iNOS Expression via Repressing Syk-Mediated Activation of PI3K-IKK-Iκb Signaling Pathways. Eur. J. Pharmacol. 650, 102–109. doi:10.1016/j.ejphar.2010.10.004
Collado, M. C., Surono, I., Meriluoto, J., and Salminen, S. (2007). Indigenous Dadih Lactic Acid Bacteria: Cell-Surface Properties and Interactions with Pathogens. J. Food Sci. 72, M89–M93. doi:10.1111/j.1750-3841.2007.00294.x
Collins, M. D., and Gibson, G. R. (1999). Probiotics, Prebiotics, and Synbiotics: Approaches for Modulating the Microbial Ecology of the Gut. Am. J. Clin. Nutr. 69, 1052S–1057S. doi:10.1093/ajcn/69.5.1052s
Corr, S. C., Li, Y., Riedel, C. U., O'toole, P. W., Hill, C., and Gahan, C. G. (2007). Bacteriocin Production as a Mechanism for the Antiinfective Activity of Lactobacillus Salivarius UCC118. Proc. Natl. Acad. Sci. U S A. 104, 7617–7621. doi:10.1073/pnas.0700440104
Cunha, C., Gomes, C., Vaz, A. R., and Brites, D. (2016). Exploring New Inflammatory Biomarkers and Pathways during LPS-Induced M1 Polarization. Mediators Inflamm. 2016, 6986175. doi:10.1155/2016/6986175
De Waard, R., Garssen, J., Bokken, G. C., and Vos, J. G. (2002). Antagonistic Activity of Lactobacillus Casei Strain Shirota against Gastrointestinal Listeria Monocytogenes Infection in Rats. Int. J. Food Microbiol. 73, 93–100. doi:10.1016/s0168-1605(01)00699-7
Dorrington, M. G., and Fraser, I. D. C. (2019). NF-κB Signaling in Macrophages: Dynamics, Crosstalk, and Signal Integration. Front. Immunol. 10, 705. doi:10.3389/fimmu.2019.00705
Dougan, G., John, V., Palmer, S., and Mastroeni, P. (2011). Immunity to Salmonellosis. Immunol. Rev. 240, 196–210. doi:10.1111/j.1600-065X.2010.00999.x
Duan, B., Shao, L., Liu, R., Msuthwana, P., Hu, J., and Wang, C. (2021). Lactobacillus Rhamnosus GG Defense against Salmonella enterica Serovar Typhimurium Infection through Modulation of M1 Macrophage Polarization. Microb. Pathog. 156, 104939. doi:10.1016/j.micpath.2021.104939
Duary, R. K., Rajput, Y. S., Batish, V. K., and Grover, S. (2011). Assessing the Adhesion of Putative Indigenous Probiotic Lactobacilli to Human Colonic Epithelial Cells. Indian J. Med. Res. 134, 664–671. doi:10.4103/0971-5916.90992
Dunne, C., Murphy, L., Flynn, S., O'mahony, L., O'halloran, S., Feeney, M., et al. (1999). Probiotics: from Myth to Reality. Demonstration of Functionality in Animal Models of Disease and in Human Clinical Trials. Antonie Van Leeuwenhoek 76, 279–292.
Finlay, B. B., and Brumell, J. H. (2000). Salmonella Interactions with Host Cells: In Vitro to In Vivo. Philos. Trans. R. Soc. Lond. B Biol. Sci. 355, 623–631. doi:10.1098/rstb.2000.0603
Fitzgerald, K. A., and Kagan, J. C. (2020). Toll-like Receptors and the Control of Immunity. Cell 180, 1044–1066. doi:10.1016/j.cell.2020.02.041
Gabr, M. M., Saeed, I., Miles, J. A., Ross, B. P., Shaw, P. N., Hollmann, M. W., et al. (2021). Interaction of Opioids with TLR4-Mechanisms and Ramifications. Cancers (Basel) 13, 5274. doi:10.3390/cancers13215274
Guha, D., Banerjee, A., Mukherjee, R., Pradhan, B., Peneva, M., Aleksandrov, G., et al. (2019). A Probiotic Formulation Containing Lactobacillus Bulgaricus DWT1 Inhibits Tumor Growth by Activating Pro-inflammatory Responses in Macrophages. J. Funct. Foods 56, 232–245. doi:10.1016/j.jff.2019.03.030
Habil, N., Al-Murrani, W., Beal, J., and Foey, A. D. (2011). Probiotic Bacterial Strains Differentially Modulate Macrophage Cytokine Production in a Strain-dependent and Cell Subset-specific Manner. Benef Microbes 2, 283–293. doi:10.3920/BM2011.0027
Italiani, P., and Boraschi, D. (2014). From Monocytes to M1/M2 Macrophages: Phenotypical vs. Functional Differentiation. Front. Immunol. 5, 514. doi:10.3389/fimmu.2014.00514
Jain, S., Yadav, H., Sinha, P. R., Naito, Y., and Marotta, F. (2008). Dahi Containing Probiotic Lactobacillus Acidophilus and Lactobacillus Casei Has a Protective Effect against Salmonella Enteritidis Infection in Mice. Int. J. Immunopathol Pharmacol. 21, 1021–1029. doi:10.1177/039463200802100428
Jeffrey, M. P., Jones Taggart, H., Strap, J. L., Edun, G., and Green-Johnson, J. M. (2020). Milk Fermented with Lactobacillus Rhamnosus R0011 Induces a Regulatory Cytokine Profile in LPS-Challenged U937 and THP-1 Macrophages. Curr. Res. Food Sci. 3, 51–58. doi:10.1016/j.crfs.2020.02.002
Ji, J., Hu, S. L., Cui, Z. W., and Li, W. F. (2013). Probiotic Bacillus Amyloliquefaciens Mediate M1 Macrophage Polarization in Mouse Bone Marrow-Derived Macrophages. Arch. Microbiol. 195, 349–356. doi:10.1007/s00203-013-0877-7
Kaji, R., Kiyoshima-Shibata, J., Nagaoka, M., Nanno, M., and Shida, K. (2010). Bacterial Teichoic Acids Reverse Predominant IL-12 Production Induced by Certain Lactobacillus Strains into Predominant IL-10 Production via TLR2-dependent ERK Activation in Macrophages. J. Immunol. 184, 3505–3513. doi:10.4049/jimmunol.0901569
Kawai, T., and Akira, S. (2007). Signaling to NF-kappaB by Toll-like Receptors. Trends Mol. Med. 13, 460–469. doi:10.1016/j.molmed.2007.09.002
Kawai, T., and Akira, S. (2011). Toll-like Receptors and Their Crosstalk with Other Innate Receptors in Infection and Immunity. Immunity 34, 637–650. doi:10.1016/j.immuni.2011.05.006
Kewcharoenwong, C., Prabowo, S. A., Bancroft, G. J., Fletcher, H. A., and Lertmemongkolchai, G. (2018). Glibenclamide Reduces Primary Human Monocyte Functions against Tuberculosis Infection by Enhancing M2 Polarization. Front. Immunol. 9, 2109. doi:10.3389/fimmu.2018.02109
Khan, J., Sharma, P. K., and Mukhopadhaya, A. (2015). Vibrio cholerae Porin OmpU Mediates M1-Polarization of Macrophages/monocytes via TLR1/TLR2 Activation. Immunobiology 220, 1199–1209. doi:10.1016/j.imbio.2015.06.009
Kim, J. H., Kim, D. H., Baek, S. H., Lee, H. J., Kim, M. R., Kwon, H. J., et al. (2006). Rengyolone Inhibits Inducible Nitric Oxide Synthase Expression and Nitric Oxide Production by Down-Regulation of NF-kappaB and P38 MAP Kinase Activity in LPS-Stimulated RAW 264.7 Cells. Biochem. Pharmacol. 71, 1198–1205. doi:10.1016/j.bcp.2005.12.031
Kim, W., Lee, E. J., Bae, I. H., Myoung, K., Kim, S. T., Park, P. J., et al. (2020). Lactobacillus Plantarum-derived Extracellular Vesicles Induce Anti-inflammatory M2 Macrophage Polarization In Vitro. J. Extracell Vesicles 9, 1793514. doi:10.1080/20013078.2020.1793514
Li, J., Qian, W., Xu, Y., Chen, G., Wang, G., Nie, S., et al. (2015). Activation of RAW 264.7 Cells by a Polysaccharide Isolated from Antarctic Bacterium Pseudoaltermonas Sp. S-5. Carbohydr. Polym. 130, 97–103. doi:10.1016/j.carbpol.2015.04.070
Li, Y., Deng, S. L., Lian, Z. X., and Yu, K. (2019). Roles of Toll-like Receptors in Nitroxidative Stress in Mammals. Cells 8, 576. doi:10.3390/cells8060576
Liang, Y., Hudson, R. E., and Ballou, M. A. (2020). Supplementing Neonatal Jersey Calves with a Blend of Probiotic Bacteria Improves the Pathophysiological Response to an Oral Salmonella enterica Serotype Typhimurium challenge. J. Dairy Sci. 103, 7351–7363. doi:10.3168/jds.2019-17480
Lim, Y. J., Yi, M. H., Choi, J. A., Lee, J., Han, J. Y., Jo, S. H., et al. (2016). Roles of Endoplasmic Reticulum Stress-Mediated Apoptosis in M1-Polarized Macrophages during Mycobacterial Infections. Sci. Rep. 6, 37211. doi:10.1038/srep37211
Liu, C. P., Zhang, X., Tan, Q. L., Xu, W. X., Zhou, C. Y., Luo, M., et al. (2017). NF-κB Pathways Are Involved in M1 Polarization of RAW 264.7 Macrophage by Polyporus Polysaccharide in the Tumor Microenvironment. PLoS One 12, e0188317. doi:10.1371/journal.pone.0188317
Liu, Y. C., Zou, X. B., Chai, Y. F., and Yao, Y. M. (2014). Macrophage Polarization in Inflammatory Diseases. Int. J. Biol. Sci. 10, 520–529. doi:10.7150/ijbs.8879
Luo, Q., Luo, J., and Wang, Y. (2020). YAP Deficiency Attenuates Pulmonary Injury Following Mechanical Ventilation through the Regulation of M1/M2 Macrophage Polarization. J. Inflamm. Res. 13, 1279–1290. doi:10.2147/JIR.S288244
Martinez, F. O., Sica, A., Mantovani, A., and Locati, M. (2008). Macrophage Activation and Polarization. Front. Biosci. 13, 453–461. doi:10.2741/2692
Pham, L. C., Van Spanning, R. J., Röling, W. F., Prosperi, A. C., Terefework, Z., Ten Cate, J. M., et al. (2009). Effects of Probiotic Lactobacillus Salivarius W24 on the Compositional Stability of Oral Microbial Communities. Arch. Oral Biol. 54, 132–137. doi:10.1016/j.archoralbio.2008.09.007
Ramarathinam, L., Niesel, D. W., and Klimpel, G. R. (1993). Ity Influences the Production of IFN-Gamma by Murine Splenocytes Stimulated In Vitro with Salmonella typhimurium. J. Immunol. 150, 3965–3972.
Riddle, M. S., and Porter, C. K. (2012). Detection Bias and the Association between Inflammatory Bowel Disease and Salmonella and Campylobacter Infection. Gut 61, 635. doi:10.1136/gutjnl-2011-300617
Rocha-Ramírez, L. M., Hernández-Ochoa, B., Gómez-Manzo, S., Marcial-Quino, J., Cárdenas-Rodríguez, N., Centeno-Leija, S., et al. (2020). Evaluation of Immunomodulatory Activities of the Heat-Killed Probiotic Strain Lactobacillus Casei IMAU60214 on Macrophages In Vitro. Microorganisms 8, 79. doi:10.3390/microorganisms8010079
Sánchez, B., Delgado, S., Blanco-Míguez, A., Lourenço, A., Gueimonde, M., and Margolles, A. (2017). Probiotics, Gut Microbiota, and Their Influence on Host Health and Disease. Mol. Nutr. Food Res. 61, 1600240. doi:10.1002/mnfr.201600240
Shapouri-Moghaddam, A., Mohammadian, S., Vazini, H., Taghadosi, M., Esmaeili, S. A., Mardani, F., et al. (2018). Macrophage Plasticity, Polarization, and Function in Health and Disease. J. Cel Physiol 233, 6425–6440. doi:10.1002/jcp.26429
Shida, K., Kiyoshima-Shibata, J., Kaji, R., Nagaoka, M., and Nanno, M. (2009). Peptidoglycan from Lactobacilli Inhibits Interleukin-12 Production by Macrophages Induced by Lactobacillus Casei through Toll-like Receptor 2-dependent and Independent Mechanisms. Immunology 128, e858–69. doi:10.1111/j.1365-2567.2009.03095.x
Shida, K., Suzuki, T., Kiyoshima-Shibata, J., Shimada, S., and Nanno, M. (2006). Essential Roles of Monocytes in Stimulating Human Peripheral Blood Mononuclear Cells with Lactobacillus Casei to Produce Cytokines and Augment Natural Killer Cell Activity. Clin. Vaccin. Immunol 13, 997–1003. doi:10.1128/CVI.00076-06
Sichetti, M., De Marco, S., Pagiotti, R., Traina, G., and Pietrella, D. (2018). Anti-inflammatory Effect of Multistrain Probiotic Formulation (L. Rhamnosus, B. Lactis, and B. Longum). Nutrition 53, 95–102. doi:10.1016/j.nut.2018.02.005
Strieter, R. M., Belperio, J. A., and Keane, M. P. (2003). Host Innate Defenses in the Lung: the Role of Cytokines. Curr. Opin. Infect. Dis. 16, 193–198. doi:10.1097/00001432-200306000-00002
Takeda, K., and Akira, S. (2004). Microbial Recognition by Toll-like Receptors. J. Dermatol. Sci. 34, 73–82. doi:10.1016/j.jdermsci.2003.10.002
Takeuchi, O., and Akira, S. (2010). Pattern Recognition Receptors and Inflammation. Cell 140, 805–820. doi:10.1016/j.cell.2010.01.022
Wang, B., Wu, Y., Liu, R., Xu, H., Mei, X., Shang, Q., et al. (2020). Lactobacillus Rhamnosus GG Promotes M1 Polarization in Murine Bone Marrow-Derived Macrophages by Activating TLR2/MyD88/MAPK Signaling Pathway. Anim. Sci. J. 91, e13439. doi:10.1111/asj.13439
Wang, S. Y., Tai, G. X., Zhang, P. Y., Mu, D. P., Zhang, X. J., and Liu, Z. H. (2008). Inhibitory Effect of Activin A on Activation of Lipopolysaccharide-Stimulated Mouse Macrophage RAW264.7 Cells. Cytokine 42, 85–91. doi:10.1016/j.cyto.2008.01.010
Wang, Y., Liu, H., and Zhao, J. (2020). Macrophage Polarization Induced by Probiotic Bacteria: a Concise Review. Probiotics Antimicrob. Proteins 12, 798–808. doi:10.1007/s12602-019-09612-y
Wick, M. J. (2004). Living in the Danger Zone: Innate Immunity to Salmonella. Curr. Opin. Microbiol. 7, 51–57. doi:10.1016/j.mib.2003.12.008
Yoshida, A., Inagawa, H., Kohchi, C., Nishizawa, T., and Soma, G. (2009). The Role of Toll-like Receptor 2 in Survival Strategies of Mycobacterium tuberculosis in Macrophage Phagosomes. Anticancer Res. 29, 907–910.
Keywords: Lactobacillus plantarum, Salmonella typhimurium, macrophage, macrophage polarization, M1 macrophage, TLR2
Citation: Zhao C, Chen H, Liang H, Zhao X, Tang W, Wei M, Li Y, Zhang J, Yu X, Chen G, Zhu H, Jiang L and Zhang X (2022) Lactobacillus plantarum RS-09 Induces M1-Type Macrophage Immunity Against Salmonella Typhimurium Challenge via the TLR2/NF-κB Signalling Pathway. Front. Pharmacol. 13:832245. doi: 10.3389/fphar.2022.832245
Received: 09 December 2021; Accepted: 26 January 2022;
Published: 07 March 2022.
Edited by:
Yuan Zhou, Institute of Hematology and Blood Diseases Hospital (CAMS), ChinaReviewed by:
Manikandan Subramanian, Queen Mary University of London, United KingdomEugenia Bezirtzoglou, Democritus University of Thrace, Greece
Copyright © 2022 Zhao, Chen, Liang, Zhao, Tang, Wei, Li, Zhang, Yu, Chen, Zhu, Jiang and Zhang. This is an open-access article distributed under the terms of the Creative Commons Attribution License (CC BY). The use, distribution or reproduction in other forums is permitted, provided the original author(s) and the copyright owner(s) are credited and that the original publication in this journal is cited, in accordance with accepted academic practice. No use, distribution or reproduction is permitted which does not comply with these terms.
*Correspondence: Linlin Jiang, bGlubGluamlhbmcxOTg2QDE2My5jb20=; Xingxiao Zhang, emhhbmd4aW5neGlhbzIwMTdAMTYzLmNvbQ==