- 1Department of Medical Biotechnology, School of Advanced Technologies, Shahrekord University of Medical Sciences, Shahrekord, Iran
- 2Cellular and Molecular Research Center, Basic Health Sciences Institute, Shahrekord University of Medical Sciences, Shahrekord, Iran
- 3Department of Pathology, Isfahan University of Medical Sciences, Kashan, Iran
- 4Institute of Nanoscience and Nanotechnology, University of Kashan, Kashan, Iran
- 5Faculty of Medicine, Mashhad University of Medical Sciences, Mashhad, Iran
- 6Department of Medical Biotechnology, Faculty of Medicine, Mashhad University of Medical Sciences, Mashhad, Iran
- 7Research Center for Biochemistry and Nutrition in Metabolic Diseases, Institute for Basic Sciences, Kashan University of Medical Sciences, Kashan, Iran
- 8Laser Research Centre, Faculty of Health Science, University of Johannesburg, 2028 Doornfontein, Johannesburg, South Africa
- 9Department of Medical Biotechnology, National Institute of Genetic Engineering and Biotechnology, Tehran, Iran
- 10Pharmaceutical Research Center, Pharmaceutical Technology Institute, Mashhad University of Medical Sciences, Mashhad, Iran
- 11Department of Biology, Ardabil Branch, Islamic Azad University, Ardabil, Iran
- 12Student Research Committee, Kashan University of Medical Sciences, Kashan, Iran
Cancer is the main cause of morbidity and mortality worldwide, excluding infectious disease. Because of their lack of specificity in chemotherapy agents are used for cancer treatment, these agents have severe systemic side effects, and gradually lose their therapeutic effects because most cancers become multidrug resistant. Platinum nanoparticles (PtNPs) are relatively new agents that are being tested in cancer therapy. This review covers the various methods for the preparation and physicochemical characterization of PtNPs. PtNPs have been shown to possess some intrinsic anticancer activity, probably due to their antioxidant action, which slows tumor growth. Targeting ligands can be attached to functionalized metal PtNPs to improve their tumor targeting ability. PtNPs-based therapeutic systems can enable the controlled release of drugs, to improve the efficiency and reduce the side effects of cancer therapy. Pt-based materials play a key role in clinical research. Thus, the diagnostic and medical industries are exploring the possibility of using PtNPs as a next-generation anticancer therapeutic agent. Although, biologically prepared nanomaterials exhibit high efficacy with low concentrations, several factors still need to be considered for clinical use of PtNPs such as the source of raw materials, stability, solubility, the method of production, biodistribution, accumulation, controlled release, cell-specific targeting, and toxicological issues to human beings. The development of PtNPs as an anticancer agent is one of the most valuable approaches for cancer treatment. The future of PtNPs in biomedical applications holds great promise, especially in the area of disease diagnosis, early detection, cellular and deep tissue imaging, drug/gene delivery, as well as multifunctional therapeutics.
Introduction
Cancer is the main cause of human mortality excluding infectious disease (Siegel et al., 2021; Sung et al., 2021). Conventional chemotherapeutic agents and anti-cancer drugs such as anti-metabolites and alkylating agents have many toxic effects on patients. Therefore, researchers are interested in utilizing targeted therapy to decrease their side effects (Shafabakhsh et al., 2019; Gao and Dong, 2020). The use of nanomaterials is a new approach to control the release of anti-cancer drugs and increase their in vivo efficacy. Nanoparticles (NPs) can be used as anti-cancer drug carriers. Metal-based NPs can have intrinsic anti-tumor effects, including an antioxidant action (Yu et al., 2022). Furthermore, the anti-cancer activity of NPs can be improved by the use of external energy sources, for example hyperthermia can be produced by applying magnetic fields or infrared radiation (Tseng et al., 2014; Bloch et al., 2021). When NPs are stimulated with external energy, they can produce reactive oxygen species which can destroy the cancer cells and also interact with the tumor environment, including stroma and blood vessels, and inhibit tumor development (Kankala et al., 2020; Bloch et al., 2021; Saitoh et al., 2021). Metallic NPs are increasingly being used in biomedical research. In the case of cancer, they can be designed for therapeutic as well as diagnostic applications (Fu et al., 2020; Kankala et al., 2020; Mukherjee et al., 2020; Bloch et al., 2021; Saitoh et al., 2021; Yu et al., 2021). Metallic and metal oxide NPs can be produced and modified with a variety of chemical functional groups as needed. They can be conjugated with biological molecules (antibodies, nucleic acids or peptides), targeting ligands, and anticancer drugs using appropriate functionalization strategies (Fu et al., 2020; Oliveira et al., 2020; Patel et al., 2020; Zeng et al., 2020). Herein, we summarize the preparation, characterization, and anti-cancer effects of platinum nanoparticles (PtNPs). Moreover, we highlight the use of PtNPs as delivery systems in cancer therapy.
PtNPs Preparation Methods
The overall synthetic approaches for PtNPs include physical methods, chemical methods, and biological methods as shown in Figure 1.
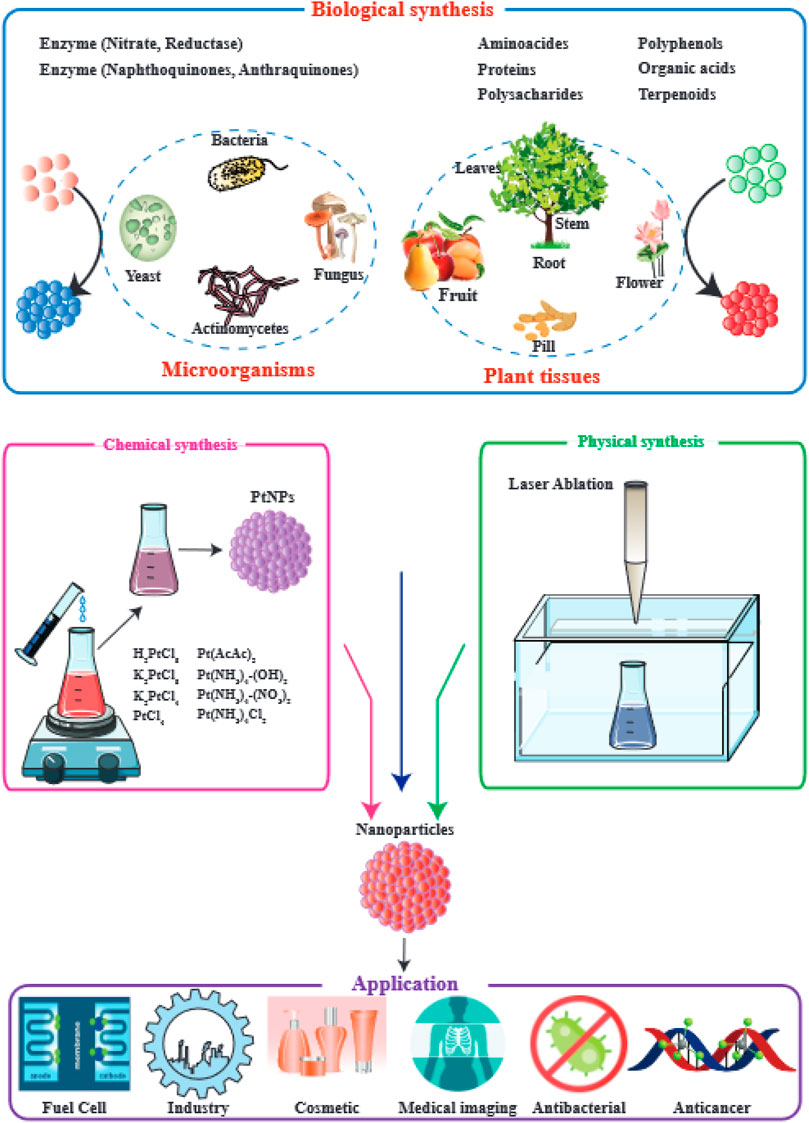
FIGURE 1. Applications of PtNPs and their methods of preparation. This figure adapted from Jeyaraj et al. (2019).
Physical Methods
In physical methods a top-down approach is used. In the top-down approach, various physical techniques can generate NPs through mechanical degradation of the structure of bulk metallic materials. This approach requires energy and is an overall economic process but also takes a long time. However, it may be the best way to regulate the size range of NPs and control their morphologies. The top-down method starts with the bulk material, which is progressively degraded, leading to the generation of fine NPs. For mass manufacture of NPs, a variety of physical techniques are used, including photolithography, milling techniques, anodization, and electron beam lithography (Fichtner et al., 2020; Garlyyev et al., 2020; Kuriganova et al., 2020).
Top down production of PtNPs involves the administration of thermal energy, electrical energy, high energy radiation, or mechanical pressure, leading to the abrasion of bulk metal, or melting, evaporation and condensation in order to synthesize NPs. Thermal techniques to produce PtNPs involve heating in a tube furnace at high air pressure, leading to evaporation followed by condensation. Physical methods have the following advantages: rapidity and purity, lack of toxic chemical reagents, uniform size and shape; however, they have the disadvantages of low productivity, high cost, radiation exposure, high pressure, high temperature, energy consumption, lower thermal stability, significant waste products, high dilution, inadequate control of shape and size. This technique is ineffective for producing NPs with controllable and uniform shapes and sizes, and it significantly alters the physicochemical architecture and the surface of the NPs. There are different types of physical methods which have been used, including; laser ablation, arc discharge, flame spray pyrolysis, ball milling, melt mixing, vapor deposition, and sputter deposition (Dhand et al., 2015).
Laser Ablation
Laser ablation is a simple but expensive process that involves irradiating a solid (or occasionally a liquid) surface with a laser beam to gradually remove material and can be used as an alternative to electrical heating. Laser ablation involves high costs to produce sufficient energy, but its overall energy efficiency is good. Materials exposed to a laser flux absorb the incident laser energy, turning the material to plasma. Laser ablation of solid metal has problems with aggregation and inadequate degradation, therefore in previous studies many researchers have shown generation of PtNPs by PLAL (pulsed laser ablation in liquid) (Waag et al., 2021; Fedorova et al., 2020; N Madlum et al., 2021). The milling process involves gradually decreasing the particle size and mixing them into new phases (Jan et al., 2021; Mathew et al., 2021).
Solvothermal Processes
In solvothermal processes, reactions are carried out at a lower temperature because the reactants become more soluble. It is a low-temperature method that uses polar solvents at high pressure and at temperatures greater than their normal boiling points (Jameel et al., 2021; Mathiesen et al., 2021).
Inert Gas Condensation
IGC methods are employed to evaporate metals in vacuum chambers containing an inert gas at a usual pressure of 100 Pa. IGC is a very efficient process for producing high-quality silver NPs and PtNPs (Maicu et al., 2014). Collisions between gas atoms and metal atoms occur in IGC, and the vaporized metal atoms lose kinetic energy and undergo condensation to produce tiny crystals that accumulate in liquid nitrogen. The same method is also employed for the preparation of gold NPs.
Chemical Methods
The production of metal NPs in chemical solutions via diverse chemical reactions is defined as chemical preparation. The bottom-up method is used in the chemical production of NPs. The bottom-up approach allows individual atoms and molecules to self assemble into various sizes of NPs (Fahmy et al., 2020a; Dang‐Bao et al., 2021). The following are examples of the bottom-up approach (Sung et al., 2021): self-assembly of monomer/polymer molecules (Siegel et al., 2021); chemical or electrochemical nanostructured precipitation (Gao and Dong, 2020); sol–gel formation (Shafabakhsh et al., 2019); laser pyrolysis (Yu et al., 2022); chemical vapor deposition (CVD) (Bloch et al., 2021); plasma or flame spraying synthesis (Tseng et al., 2014); bio-assisted synthesis (Nichols et al., 2006).
One chemical process is known as nucleation, and it primarily requires the use of water-soluble metal cations as a precursor that can be reduced to form metal atoms (Quinson and Jensen, 2020). The process of capping stops the reducing agent, and blocks the growth of the reduced metal atom cluster at the NP scale. Chemical reduction is particularly employed to generate colloidal metal NPs, where chemical agents reduce the metallic ions, leading to formation of metallic NPs (Figure 2). Particles may become thermally stable once their shape has reached a specific size (Quinson and Jensen, 2020).
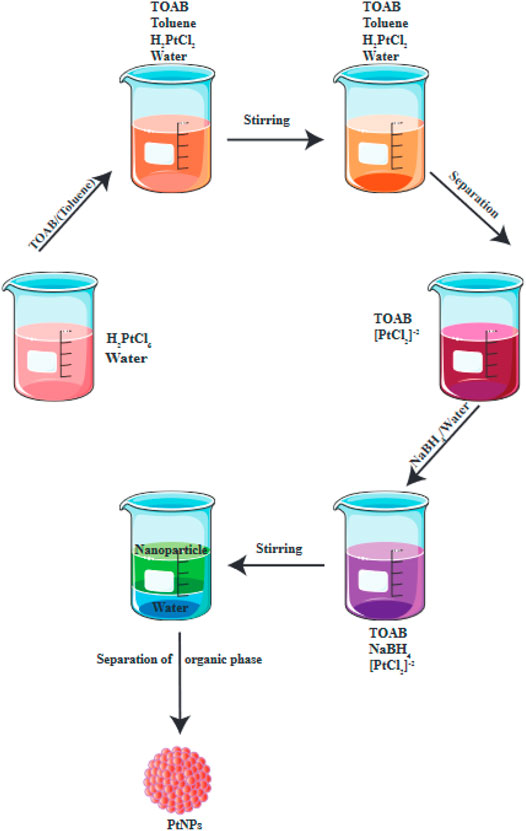
FIGURE 2. Chemical reduction is used for the production of PtNPs. This figure adapted from Jeyaraj et al. (2019).
For the production of PtNPs, a variety of conditions must be optimized, including appropriate temperature, reducing/capping agent, as well as size and shape (Brown et al., 2021). Many studies have optimized the reagents and conditions for the production of PtNPs (Shukla et al., 2007; Du et al., 2008; Yamada et al., 2015). A reducing agent, a capping/stabilizing agent and the metal precursor comprise the three essential components for PtNP preparation. Moreover, aggregation should also be avoided in the approach. Chemical techniques have the following advantages: low cost, good surface chemistry flexibility, simple functionalization, high yields, good control of size, thermal stability, decreased dissipation. However the disadvantages are: low purity, use of toxic chemical reagents and organic solvents that may be harmful to humans and the environment (Quinson and Jensen, 2020; Jan et al., 2021; Mathiesen et al., 2021).
Reduction in Nonpolar Solvents
Chemical reduction of metal ions inside reversed micelles in a nonpolar solvent is the most well-known technique to generate metal NPs (MNPs) (Stepanov et al., 2014). A metal salt dissolved in water is first incorporated in reverse micelles, and is then chemically reduced to produce MNPs. Because size of the particles is important, the volume of the reverse micelles and also the ratio of water to solvent should be carefully controlled.
Fusion Approach
In the early 1920s, Adam and his colleagues produced bulk type PtO2 by a fusion approach at 450°C (Adams, 1974). The material was then modified into PtNPs using a variety of techniques. However these techniques often use toxic chemicals.
Wet Chemical Reduction
Wet chemical reduction is frequently employed to better regulate the size of particles. Numerous chemical reducing agents can be used, including methoxy polyethylene glycol, sodium borohydride, trisodium citrate dihydrate, potassium bitartrate, elemental hydrogen, and ascorbate. The size and shape of the produced NPs depends on the temperature (Leong et al., 2014), the reducing agent (Tao et al., 2008; Chen et al., 2009; Lim et al., 2010; Miyabayashi et al., 2011), and the concentration of the precursor platinum compound (Figure 3).
Other Chemical Methods
A wide range of other chemical synthesis methods have been used, including sol–gel process, pyrolysis, chemical vapor deposition (CVD), microemulsion, hydrothermal, polyol synthesis, electrochemical process, photochemical reduction, hydrolysis, thermal decomposition, confined reaction, sono-decomposition, chemical vapor deposition, and plasma-enhanced chemical vapor deposition (Bönnemann and Richards, 2001), (Liu et al., 2002; Zeng et al., 2006; Kim et al., 2013) (Abdolhosseinzadeh et al., 2018), (Meltzer et al., 2001) (Reetz and Koch, 1999), (Du et al., 2006), (Fujimoto et al., 2001), (Okitsu et al., 2000), (Saminathan et al., 2009), (Shafiei et al., 2010).
Biological Methods
Green Synthesis of PtNPs Using Plant Extracts
Plant derivatives include a variety of primary and secondary compounds that can act as natural reducing agents and capping agents (Bhattacharya and Gupta, 2005; Mandal et al., 2006; Ahmad et al., 2010; Mukunthan and Balaji, 2012; Kharissova et al., 2013; Puja and Kumar, 2019; Castillo-Henríquez et al., 2020). Plant derivatives have been used in the green synthesis of various MNPs, according to several reports (Shankar et al., 2004; Kasthuri et al., 2009; Azzazy et al., 2012). Biosynthesis of MNPs mediated by plants is a simple and rapid process that involves a combination of a plant derivatives and a solution of metal ions at optimal pH and temperature values. A color change in the reaction medium indicates the formation of the NPs (Marslin et al., 2018). Temperature, pH and reaction should all be adjusted to regulate the average shape, size and surface charge of the MNPs (Sathishkumar et al., 2010; Castro et al., 2011; Sankar et al., 2014; Wang et al., 2020). Despite the fact that the reaction mechanism of the biosynthesis of MNPs using plant derivatives needs to be comprehensively explored, a bottom-up mechanism has been postulated (Durán et al., 2011; Keat et al., 2015). There are four essential steps in this hypothesized mechanism: (Sung et al., 2021) the initial activation step (bio-reduction), where metal ions undergo reduction to produce a zero-oxidation state (Malik et al., 2014); (Siegel et al., 2021) the second step involves development and aggregation of these tiny particles into NPs with higher thermal stability (Gao and Dong, 2020); the third stage (termination), in which the MNPs are stabilized and capped to produce NPs with a controlled range of shape and average size (Malik et al., 2014; Fahmy et al., 2020b); (Shafabakhsh et al., 2019) the final stage which involves washing and purification of the MNPs often by centrifugation (Fahmy et al., 2020b).
By regulating the temperature, reaction time, and pH, researchers can optimize the size, morphology. and crystallinity of the MNPs (Feldheim and Foss, 2002; Shahverdi et al., 2011). MNPs in general and PtNPs in particular, are characterized using UV/Vis spectrophotometry, scanning electron microscopy, TEM (transmission electron microscopy), FTIR (Fourier transform infrared spectroscopy), and powder XRD (X-ray diffraction) (Kumar et al., 2004; Nath and Banerjee, 2013; Malik et al., 2014; Mashwani et al., 2016; Khan et al., 2017; Nadimpalli et al., 2018; Aygun et al., 2020). Various plant species have been used in the green production of PtNPs as shown in Figure 4.
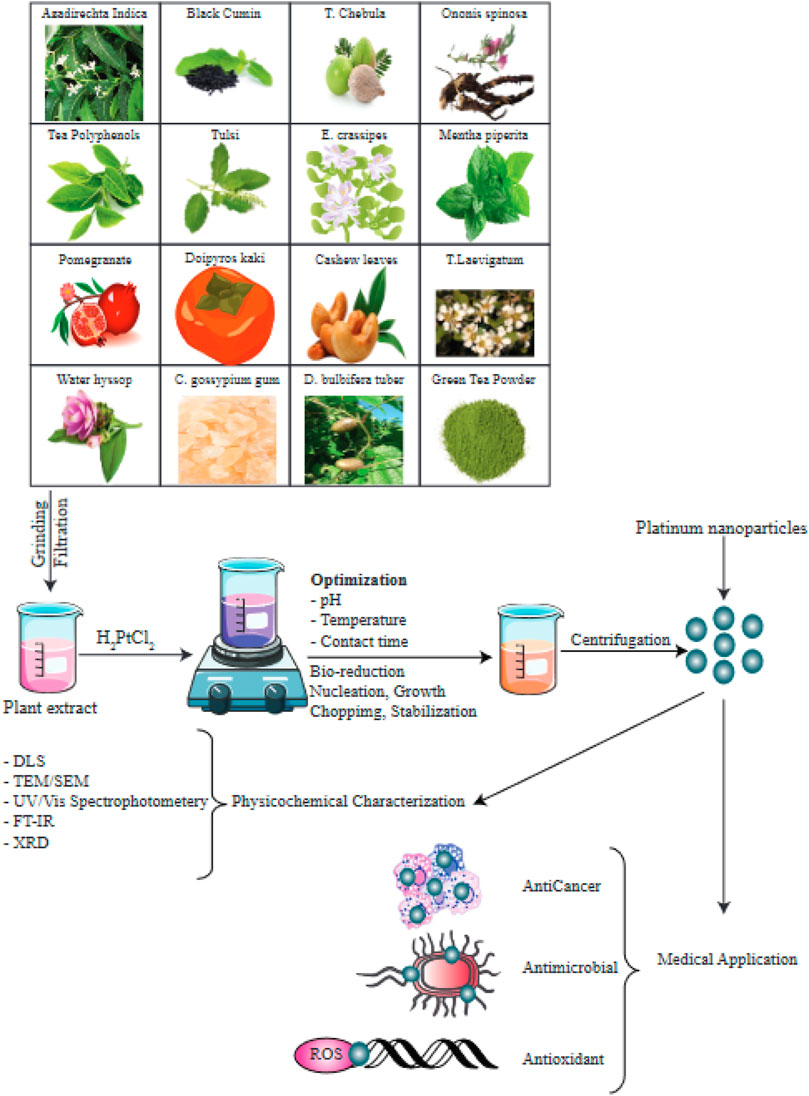
FIGURE 4. Numerous species of plants have been used in bioreduction procedures to produce PtNPs. The factors optimized as well as the methods used to characterize the PtNPs are depicted in this diagram. This figure adapted from Jeyaraj et al. (2019).
Synthesis of PtNPs Using Microorganisms
Both single-celled and multicellular organisms are capable of producing inorganic materials either extracellularly or intracellularly (Nath and Banerjee, 2013). Overall, MNPs could be generated by bacteria using a reduction process governed by intracellular signaling pathways, in which bacterial enzymes converting metal ions into MNPs. The simplicity of handling is one of the main benefits of bacteria-based NP production. Genetic engineering methods can also be used to modify the bacterial cells for specific objectives, such as reducing the toxicity and allowing long-term NP production in cultures. This approach also has certain drawbacks, such as being a time-consuming procedure, high costs, needs downstream processing, and a lack of control over the size and shape of the product. Metal NPs such as silver, for example, have been produced as external bacterial products or as intracellular extracts from bacteria (Kalimuthu et al., 2008; Kalishwaralal et al., 2008). The downstream procedure required for the separation of AgNPs from cell-derived extracts takes a long time. In a study by Riddin et al. the biosynthesis of geometric PtNPs employing soluble, cell-free protein derivatives from a bacterial strain with sulfate-reducing capability was compared to the use of whole cells of the same culture, leading to production of amorphous Pt(0) NPs (Riddin et al., 2010). PtNPs have been synthesized by bacteria with sulfate-reducing capability such as Desulfovibrio desulfuricans (Martins et al., 2017) or Acinetobacter calcoaceticus (Gaidhani et al., 2014). These sulfate-reducing bacteria have the potential to successfully reduce platinum (IV) ions into platinum(0) NPs produced over 24 h, at temperatures below 30°C and pH 7.0 showed the highest production yield. The NPs showed a cuboidal morphology with a size of 2–3.5 nm. These PtNPs were precipitated from Shewanella algae (a bacterial strain with metal-ion reducing potential), and the remainder of the PtNPs were deposited by adding the reducing ion PtCl62− to produce elemental platinum at a neutral pH and room temperature. NPs with a size of 5 nm were shown to be located within the periplasmic space of S. algae cells. This biological process could have two main mechanisms, uptake and deposition or else incorporation (Konishi et al., 2007).
Various fungal species have been employed in the biosynthesis of MNPs. In comparison to plants or prokaryocytes, the use of fungi in the production of MNPs has some advantages, since NPs with a more uniform shape and size, and well-defined dimensions are generated. In addition, fungi grow in a relatively simple medium, allowing easy downstream processing, and scale-up production. Fungi create an easy-to-handle biomass and can secrete significant amounts of protein (Riddin et al., 2006; Govender et al., 2009; Syed and Ahmad, 2012) so that reducing enzymes can generate the NPs (Mohanpuria et al., 2008). Subsequently, NPs with high stability are generated and their aggregation may be prevented (Mukherjee et al., 2008; Narayanan and Sakthivel, 2010). In conclusion, researchers have investigated the use of fungi as an appropriate source of nanomaterials. The majority of fungi generate MNPs via either extracellular or intracellular pathways. NPs produced via an extracellular process, are non-toxic and allow adequate commercial availability.
PtNPs as Therapeutic Agents in Cancer Therapy
PtNPs have several uses, including cancer diagnosis and therapy, as well as overcoming multi-drug resistance in bacteria (Jabir et al., 2012). The biomedical applications of nanotechnology may not only overcome bacterial antibiotic resistance, but can also help in the management of tumors (Taylor and Webster, 2011). By including hydrophilic polymers which provide a suitable surface for opsonization, MNPs can be used in cancer treatment (Jabir et al., 2012).
Platinum itself has a strong anticancer effect (Reedijk, 1987). PtNPs behave differently compared to platinum-containing compounds, but they possess similarly efficient anticancer activity. After entering the cell through passive diffusion or by endocytosis, PtNPs exert cytotoxicity depending on their size, concentration and incubation time, This is mainly caused by the introduction of strand breaks in the chromosomal DNA (Figure 5) (Porcel et al., 2010; Johnstone et al., 2016; Kutwin et al., 2017). DNA damage leads to the inhibition of replication, and the induction of cell cycle arrest and apoptosis. Another possible mechanism of action of PtNPs involves inhibition of cellular metabolic activity, generation of hydroxyl radicals, and release of active free Pt2+ ions, a strategy which is currently used in radiotherapy. At certain concentrations, PtNPs can also act as antioxidants (Asharani et al., 2010; Gehrke et al., 2011; Prasek et al., 2013). In addition, some reports have proposed the potential use of PtNPs as coatings for sensors to detect glucose or other biomolecules (Taurino et al., 2015). Moreover, PtNPs can augment the host anti-tumor immune response through enhanced antigen presentation and T cells activation (Yu et al., 2022).
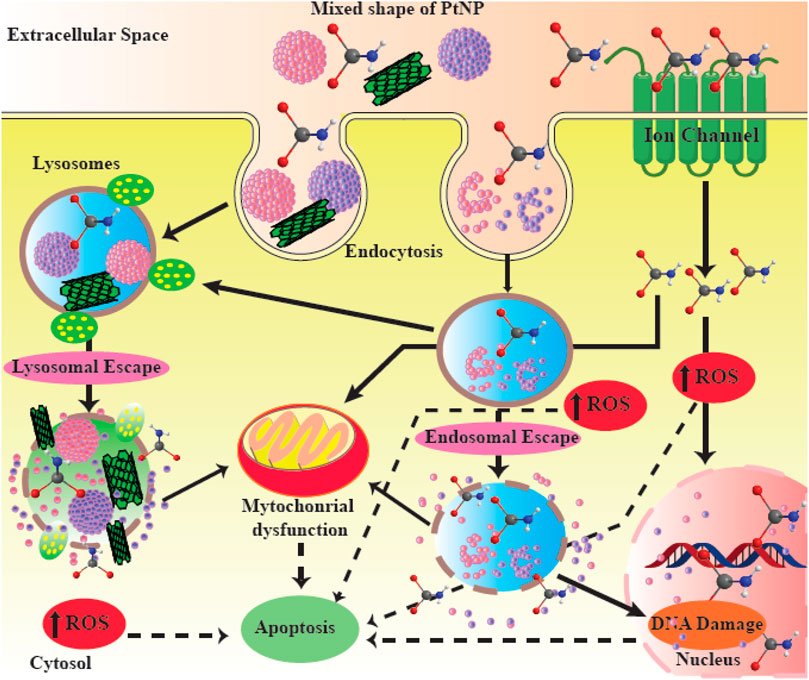
FIGURE 5. Possible mechanisms of action of PtNPs. PtNPs are able to induce apoptosis and DNA damage in cancer cells. This figure adapted from Jeyaraj et al. (2019).
PtNPs can be also used for diagnosis and imaging. For instance they exhibit good photostability after multiphoton stimulation inside cells, therefore they can be used for imaging and microscopy (Botchway et al., 2008). In multiphoton imaging, the depth is improved by utilizing light with a longer wavelength and a lower energy level in the visible or near infrared spectral regions (Botchway et al., 2008). Platinum NPs are a good alternative because of their reduced toxicity and favorable imaging properties (Bendale et al., 2017).
A unique silver (Ag)-platinum (Pt) NP dendritic assembly was prepared by López Ruiz et al. (2020a). TEM analysis was used to characterize the NPs, and EDX was used to confirm their composition. Gram-negative Pseudomonas aeruginosa, Gram-negative multi-drug resistant Escherichia coli and Gram-positive Staphylococcus aureus were all employed to test the antibacterial activity. To assess anticancer activity, researchers used two different cancer cell lines, glioblastoma and melanoma. Finally, cytotoxicity was assessed using normal fibroblasts. The TEM examination showed the AgPtNPs had a dendrimeric NP shape with a mean size of 42 ± 11 nm. The presence of both Ag and Pt metals was confirmed by EDX analysis of the elemental composition. Medically relevant pathogenic bacteria P. aeruginosa, E. coli, and S. aureus were substantially inhibited by the AgPt NPs, which were more effective than silver NPs. The MTS test demonstrated a dose-dependent (10–250 g/ml concentration range) and selective anti-tumor activity of AgPt NPs against melanoma and glioblastoma cell lines. At doses ranging from 10 to 50 g/ml, cytotoxicity tests with normal fibroblasts did not show any harmful effects of NPs on normal cells. These AgPtNPs warrant further investigation as potential anticancer and antibacterial agents (López Ruiz et al., 2020a).
Baskaran et al. reported the use of Streptomyces spp to synthesize PtNPs in an environmentally friendly manner (Baskaran et al., 2017). The generated PtNPs were found to have a face centered cubic system. The involvement of protein amino acids, which act as a crucial reductant for the production of PtNPs, was shown by the FT infrared spectrum. Topographical images of field emission scanning electron microscopy and atomic force microscopy showed the spherical shape of PtNPs with an average size of 20–50 nm. The higher purity of the PtNPs was confirmed by X-ray fluorescence spectra. PtNP size was verified using transmission electron microscopy, and the particles were found to have a uniform size range. PtNPs also exhibited a typical surface plasmon resonance peak at 262 nm. Dynamic light scattering showed that 97.2% of particles were less than 100 nm, with an average particle diameter of 45 nm. Using the 3-(4, 5-dimethyl-2-thiazolyl)-2, 5-diphenyl-tetrazolium (MTT) assay in vitro, PtNPs exhibited an IC50 of about 31.2 μg/ml against MCF-7 breast cancer cells (Baskaran et al., 2017).
Alshatwi et al. used tea polyphenol (TPP) as a surface modification and reducing agent to generate PtNPs (Alshatwi et al., 2015). TEM and XRD were utilized to evaluate the shape and crystalline nature of the TPP-functionalized PtNPs (TPP@Pt). The TPP@Pt had a crystalline nature with a face-centered cubic structure, according to the XRD results. TTP@Pt were flower-shaped, according to TEM imaging, with a well-dispersed 30–60 nm-sized structure. TPP@Pt was then used to treat cervical cancer cells (SiHa) at various doses. Cell cycle progression, nuclear shape, and cell viability were measured. TPP@Pt reduced the growth of SiHa cells, according to a cell culture experiment. Nuclear staining with propidium iodide revealed that TPP@Pt caused chromatin condensation and nuclear fragmentation. Treatment with TPP@Pt increased the proportion of cells in the G2/M stage, indicating cell cycle arrest, as well as an increased number of cells the sub-G0 cell death stage. These data suggested that TPP@Pt might be used to treat cervical cancer (Alshatwi et al., 2015).
Satraplatin, is a platinum-based medicinal drug, previously shown to cause apoptosis by arresting the cell cycle at the G2/M phase (Kalimutho et al., 2011). Shingu et al. also created a platinum compound with two Pt atoms connected together by a spermine linker that led to G2/M cell cycle arrest followed by apoptosis (Shingu et al., 2010). According to previous studies, cell cycle arrest at G2/M phase following DNA injury can result in different outcomes, such as cellular apoptosis, longer permanent arrest, or recovery after DNA damage by repair or adaptation to the damage, which restarts cell cycle progression, while the DNA damage is still present (Passalaris et al., 1999). Cells that undergo G2 arrest may continue and progress their cell cycle or die by apoptosis (Sorenson et al., 1990).
Gurunathan et al., explored the effects of PtNPs on the exosomal and cellular functions in A549 (human lung epithelial adenocarcinoma cells) (Gurunathan et al., 2021a). In A549 cells, PtNPs altered several physiological processes leading to the increased secretion of exosomes. Moreover, in A549 cells cultured with PtNPs, the overall amount of protein biosynthesis and exosome secretion due to platinum-mediated oxidative stress were promoted. Enhanced generation of oxidative stress and activation of the ceramide pathway resulted in increased release of exosomes prompted by PtNPs. The fact that PtNPs promoted the biosynthesis and release of exosomes may contribute to our knowledge about exosomal release and biosynthesis. PtNPs could be novel agents to enhance the production of exosomes in A549 cells. The release of exosomes could have a role in the prevention and treatment of tumors. In conclusion, PtNPs may boost exosome formation via increased activity of the ceramide pathway and generation of oxidative stress (Gurunathan et al., 2021a).
One of the benefits of NPs in the treatment of tumors, could be the use of combined strategies to counteract MDR using formulations of anti-tumor drugs combined with NPs. PtNPs are thermo-plasmonic, thermally stable, effective and non-toxic agents. Additionally, PtNPs can be produced from naturally occurring biologic molecules in a non-toxic and environmental-friendly manner.
Doxorubicin (DOX) is a common chemotherapeutic agent used in clinical proactice, which is often investigated for combination therapy (Frederick et al., 1990; Zhao et al., 2015). One of the benefits of administering a combination therapy of chemotherapeutic drugs and NPs is that they affect more than one pathways, giving a synergistic benefit produced by two different molecules in the treatment of cancer, thus overcoming drug resistance and reducing side effects. Previous studies have shown that a combination of salinomycine (a polyether ionophore antibiotic) and AgNPs promoted cellular autophagy and apoptosis in ovarian cancer cells (Zhang and Gurunathan, 2016). It was also shown that AgNPs could enhance gemcitabine-mediated apoptosis in human ovarian cancer cells (Yuan and Gurunathan, 2017). Moreover, a recent study showed that cellular apoptosis in human breast cancer was enhanced by a combination of tubastatin-A (a histone deacetylase inhibitor) and PdNPs (palladium NPs) via affecting multiple cellular pathways (Yuan and Gurunathan, 2017). Another histone deacetylase inhibitor MS-375 plus AgNPs was reported to stimulate the accumulation of autophagosomes, cause mitochondrial dysfunction, activate caspase 9/3, as well as downregulate anti-apoptotic genes and upregulate pro-apoptotic genes in human basal epithelial alveoli cells (Gurunathan et al., 2018). In a study by Gurunathan et al., the anti-tumor activity of a combination of DOX and PtNPs in human bone osteosarcoma epithelial cells was assessed (Gurunathan et al., 2019a). They prepared the PtNPs using the natural product tangeretin (a pentamethoxyflavone) and demonstrated their thermal stability, non-toxicity, and thermoplasmonic properties. The combination benefits of DOX and PtNPs on multiple variables were assessed, including cell proliferation, viability and morphology, generation of ROS (reactive oxygen species), nitric oxide production, lipid peroxidation, antioxidants, protein carbonyl content, MMP (mitochondrial membrane potential), ATP levels, expression of antiapoptotic and proapoptotic genes, and genes involved in DNA damage and repair. The DOX and PtNPs combination remarkably suppressed proliferation and viability of U2OS cells in a dose-dependent manner, and increased lactate dehydrogenase release. The production of malondialdehyde and ROS, carbonylated protein and nitric oxide levels were all increased. Mitochondrial dysfunction was shown by lower levels of MMP and ATP, as well as higher expression of pro-apoptotic genes and lower expression of anti-apoptotic genes. The fact that oxidative stress plays a key role in both genotoxicity and cytotoxicity was confirmed by lower levels of various antioxidants. Moreover, DOX plus PtNPs enhanced levels of 8-oxo-G and 8-oxo-dG in addition to provoking DNA damage and expression of DNA repair genes (Gurunathan et al., 2019a).
Analysis of biodistribution of PtNPs showed that no Pt was detected in plasma 24 h after injection indicating that PtNPs had been either excreted from the system or deposited in organs or tissues. Organ accumulation data shows a high deposition of Pt in the liver and spleen after 24-h exposure and an increase after 3 weeks. This doubling of Pt in the liver would indicate that the micelles are deposited or accumulated in other organs or tissues, and then transported to the liver over the course of weeks, where they are unable to be metabolically processed for excretion or removal. The increase in total Pt in the spleen, a portion of the lymphatic system, suggests that some particles are scavenged though the lymph system and trafficked to the spleen. Tumor-bearing mice, analyzed 24 h post-injection, show lower accumulation amounts relative to non-tumored mice. The tumors accumulated approximately 3% of the injected Pt, which may account for the lower distributions in the remaining organs (Brown et al., 2018).
Table 1 shows some reports of the anti-tumor effects of PtNPs in several malignancies.
PtNPs as Delivery System
Treatment of cancer with non-targeted drugs encounters huge difficulty since the majority of chemotherapeutic drugs are disseminated all over the body, which results in systemic toxicity and patient intolerance, therefore the treatment is often discontinued (Neha and Momin, 2021).
Tumor cell targeting is an important characteristic of the nanocarriers used for drug delivery, because it enhances treatment effectiveness while avoiding damage to healthy non-cancerous cells and tissues. Various studies have been performed to investigate drug delivery with targeted NPs. It is important to understand how tumors and cancer cells can interact with nano-carriers at a mechanistic level in order to rationally design efficient drug carriers. Mechanisms of targeting can be classified into two distinct categories, active and passive targeting (Figure 6).
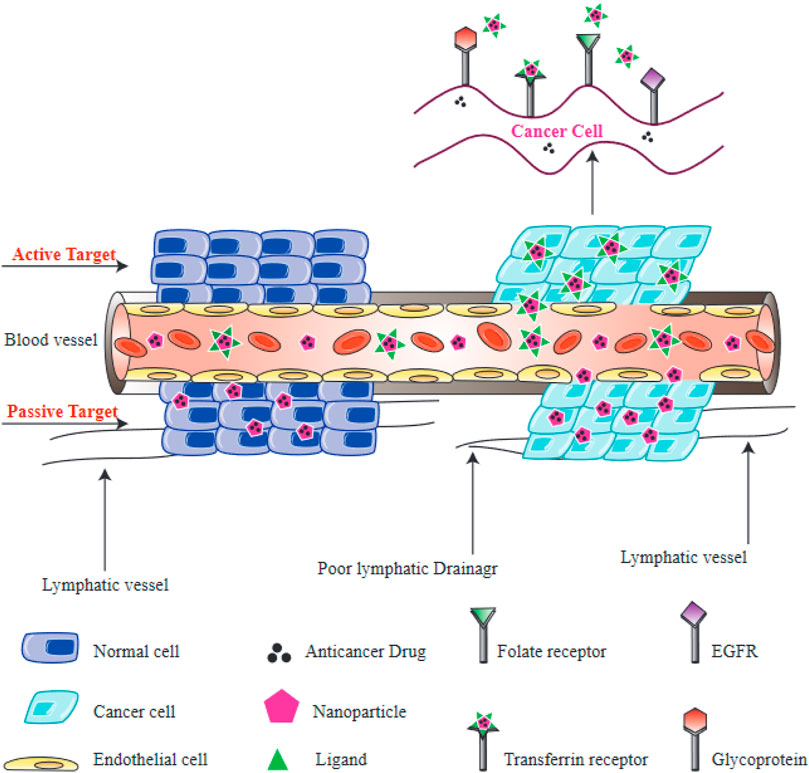
FIGURE 6. Active and passive targeting of NPs to tumors. Passive targeting of NPs is primarily mediated by the enhanced permeability and retention (EPR) effect, which depends on leaky vasculature and defective lymphatic drainage of tumors. Active targeting of NPs is mediated by interactions between receptors on cells and ligands on NPs. Folate receptors, transferrin receptors, epidermal growth factor receptor (EGFR), and glycoprotein receptors (lectins) are all found on the surface of cancer cells.
Active targeting relies on a direct interaction occurring between a receptor and a ligand, to selectively recognize tumor cells. NPs can be equipped with certain ligands on their surface, which recognize aberrantly expressed biomolecules on the tumor cells, and therefore normal cells can be distinguished from targeted cancer cells (Shi et al., 2011; Kamaly et al., 2012). The interaction between receptors located on the surface of tumor cells and the functionalized NPs results in endocytosis, which allows the release of therapeutic agents from the internalized NPs (Farokhzad and Langer, 2009). Consequently, the process of active targeting is considered to be particularly beneficial for the delivery of macromolecular drugs, such as siRNAs or proteins. Targeting moieties which can be used to recognize these receptors, include amino acids, vitamins, peptides, monoclonal antibodies and carbohydrates (Danhier et al., 2010). Some receptors located on the surface of target cells that have been used for targeting include, glycoproteins recognized by lectins, transferrin receptor recognized by transferrin, the epidermal growth factor receptor (EGFR) recognized by epidermal growth factor, and folate receptor recognized by folic acid.
Passive targeting takes advantage of the physical properties of NPs (size, shape, and surface charge) which can interact with properties of healthy or diseased tissues. Tumor cells can rapidly proliferate, which requires the formation of new blood vessels. These form a porous network, meaning that macromolecules and NPs can leak out into the tumor, compared with healthy blood vessels in normal tissues (Carmeliet and Jain, 2000). The leaky neovasculature means that injected NPs will concentrate in tumor microenvironment after an appropriate time. Additionally, cancer tissues generally have poorly developed lymphatic drainage, resulting in the retention of NPs inside the tumor tissue and the subsequent release of their drugs into the tumor cells. Altogether, these processes lead to the enhanced permeability and retention (EPR) effect, which underlies passive targeting (Maeda, 2001). The size of the NPs may affect the EPR effect, since various studies have found that NPs with smaller sizes are more likely to leak out of tumor blood vessels compared to normal blood vessels (Torchilin, 2005; Carita et al., 2018). On the contrary, larger NPs are more likely to be removed by the immune response and the reticuloendothelial system (RES) (Sykes et al., 2014). The cancer microenvironment is also another major factor in the passive delivery of NPs. Glycolysis is considered as a typical metabolic features of tumor cells, and provides a substantial energy source for the proliferation of cancer cells (Pelicano et al., 2006). The acidic environment created by the glycolysis lowers the pH of the tumor microenvironment. Therefore, various NPs have been designed to be sensitive to pH, and can be triggered by a minimum pH level in the tumor microenvironment (Lim et al., 2018). Nevertheless, there are some drawbacks to passive targeting, including non-specific drug distribution, variations in vascular permeability in different tumors, leading to unpredictable EPR targeting (Jain, 1994).
PtNPs as Delivery Vehicles for Anti-Cancer Agents
Multifunctional NPs have been investigated to enhance the treatment efficiency of malignant bone tumors such as osteosarcoma (Schroeder et al., 2011; Cole et al., 2016; Jiang et al., 2017). Nanocarriers have been designed to improve the accumulation of therapeutic compounds within the vicinity of malignant bone tumors for more effective treatment (Bansal et al., 2005; Thamake et al., 2012; Heller et al., 2013; Morton et al., 2014; Zhou et al., 2016). Recent studies have shown that numerous ligands can be used to target bone tumors, such as bisphosphonates (Ramanlal Chaudhari et al., 2012; Cole et al., 2016; Yin et al., 2016), aspartic acid-related oligopeptides (Zhang et al., 2012; Wang et al., 2014), or aptamers (Liang et al., 2015a). Nevertheless, there are still issues remaining involving the safety and complex synthesis of these agents (Wang et al., 2007; Woodward et al., 2011). For example, although bisphosphonates have been commonly applied for the treatment of bone tumors and osteoporosis (Vachal et al., 2006; Body et al., 2010), they have numerous side effects such as esophageal cancer, and, atypical fractures of the femur following prolonged administration (McClung et al., 2013). As a result, developing new targeting ligands with favorable biocompatibility, simple chemistry, and more efficacy is critical for efficient treatment of malignant bone tumors. Phytic acid (PA) is a polyphosphorylated carbohydrate which is present in human diets rich in fibers, and is found in legumes and cereals (Chen, 2004; Vucenik and Shamsuddin, 2006) and is also present in the majority of mammalian species (Wilson et al., 2013). As a component of everyday foodstuffs, it has been shown to be extremely biocompatible (Chen et al., 2014). Structurally, PA contains six phosphate groups, indicating its potential bone-targeting ability similar to bisphosphonates. Moreover, PA also displays an intrinsic anti-tumor activity (Shamsuddin et al., 1997; Shamsuddin, 1999; Vucenik and Shamsuddin, 2006) (Figure 7).
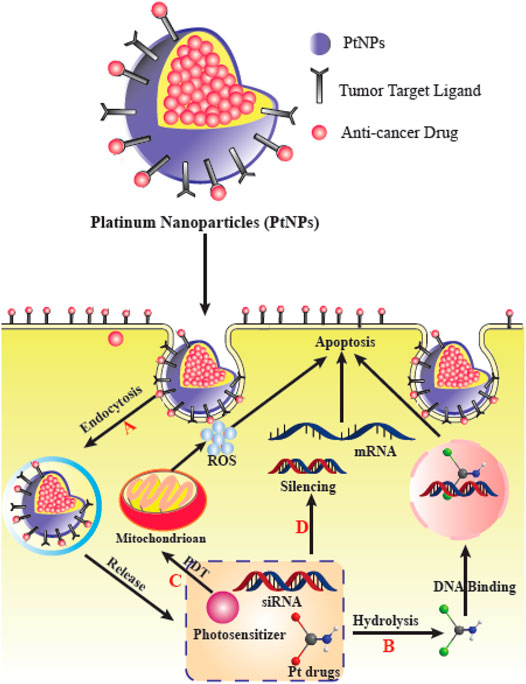
FIGURE 7. PtNPs for delivery of various therapeutic agents and their effects in cancer cells. (A) PtNPs improve cellular uptake via endocytosis to circumvent the limit of transporters. (B) PtNPs with other chemotherapy drugs to achieve a synergistic effect. (C) PtNPs with photodynamic therapy (PDT). (D) Co-delivery PtNPs with siRNA can silence the drug-resistance-related genes. This figure adapted from Xie et al. (2021).
Zhou et al. fabricated PtNPs coated with PA (PA/PtNPs) for bone-targeting, and investigated a combination of PTT (photothermal therapy) plus PA anticancer activity in malignant bone cancers (Zhou et al., 2019). As shown by in vitro assays, the PA/PtNPs displayed a binding affinity for hydroxyapatite and bone fragments, in addition to their intrinsic anti-tumor activity. PA was shown to remarkably promote the accumulation of PA/PtNPs in cancer-related bone lesions as shown by in vivo biodistribution analysis. They found that combination therapy with PA/PtNPs could effectively inhibit bone cancers and decrease osteolysis. This new and effective method to generate bone-targeted NPs with intrinsic anti-tumor activity could be used in the combined therapy of malignant bone cancers (Zhou et al., 2019).
Mesoporous PtNPs have been synthesized using surfactants as agents to control the formation of nanostructures (Teng et al., 2006; Cheong et al., 2009; Wang and Yamauchi, 2009; Wang et al., 2010; Wang et al., 2011). These NPs possess multiple binding sites for various guest molecules, thereby offering a novel alternative in cancer treatment. NP-based combination therapy with chemotherapeutic drugs plus photothermal therapy has been widely investigated. Typically, hyperthermia can be produced by laser activation of the NPs (photothermal effect) while chemotherapeutic drugs can be simultaneously delivered to the tumor in order to enhance the treatment. In one recent review, Li et al. summarized the progress in NP-based combinations of chemotherapy and photothermal therapy, including organic-based, carbon-based, and metal-based nanomaterials (Li et al., 2019b). Due to their capacity to be loaded with high amounts of drugs and good photothermal conversion, mesoporous metal nanomaterials have gained considerable interest.
Fu et al. prepared mesoporous PtNPs using the F127 surfactant (Fu et al., 2020). Next, PEG (polyethylene glycol) was coated on the platinum surface (PEG@Pt), and DOX was loaded by electrostatic adsorption to produce PEG@Pt/Dox. The PEG@Pt/Dox release profile was examined in PBS at pH 5.5 and 7.4. The distribution and uptake of PEG@Pt/Dox in MCF-7/ADR cells was measured by flow cytometry, TEM, and confocal imaging. The photothermal properties of PEG@Pt/Dox were investigated, and in vitro studies using MCF-7/ADR cells treated with PEG@Pt/Dox and irradiated with an 808 nm laser showed cytotoxicity. Dox was released predictably from PEG@Pt/Dox in PBS at pH 5.5. Confocal imaging showed that PEG@Pt/Dox was taken up by Dox-resistant breast tumor cells (MCF-7/ADR). Dox was in the cytoplasm after 1 or 12 h, and in the cell nucleus after a 24-h incubation period. Flow cytometry and TEM also confirmed cell uptake. When irradiated with an 808 nm laser, the antitumor effect was increased, and roughly 84% of tumor cells were destroyed at Dox concentrations of 8 μg/ml or more. Combination therapy was more efficient in MCF-7/ADR cells compared to the wild type MCF-7 cells. Combined therapy with photothermal and chemotherapeutic agents against drug resistant cancer cells could be provided by the multifunctional nanoplatform PEG@Pt/Dox (Fu et al., 2020).
Platinum-based anticancer drugs as well as PtNPs can both cause breakage of DNA strands inside cells (Yamagishi et al., 2013; Dasari and Tchounwou, 2014; Johnstone et al., 2014). However, different from soluble Pt-based drugs, PtNPs can also effectively scavenge hydrogen peroxide and superoxide showing an anti-oxidant activity, and can function in cancer theranostics because of their imaging capability (Kajita et al., 2007; Pelka et al., 2009; Asharani et al., 2010; Park et al., 2010). On the other hand, PtNPs have been shown to exhibit cytotoxicity, which limits their widespread use in medicine and healthcare (Gehrke et al., 2011; Yamagishi et al., 2013). Consequently, designing and testing more biocompatible PtNPs for cancer treatment remains a major issue. Moreover, the efficiency of nanomedicine for cancer may be limited by poor circulation times, and limited persistence of nano-drug conjugates and NPs within the tumor tissue. Typically, nanoconjugates are usually removed from body shortly after being recognized by human immune system. To overcome this problem, NPs are often pegylated by attachment of the Food and Drug Administration (FDA) approved PEG, thereby increasing the circulation and retention time, and reducing non-specific serum protein adsorption to avoid rapid clearance by the RES (Treuel et al., 2014; Pelaz et al., 2015).
Mukherjee et al., used the borohydride reduction technique to generate PEGylated PtNPs in colloidal form at room temperature (Mukherjee et al., 2020). The PtNPs were stable in storage for longer than 2 years, and for roughly 1 week in PBS pH 7.4 and serum. The PtNPs were biocompatible in various normal cell lines in vitro, and a chicken egg embryo model. They then developed a PtNP-based delivery system for DOX (PtNPs-DOX). Various analytical methods were used to characterize PtNPs-DOX and the PtNPs. Numerous in vitro assays demonstrated that PtNPs-DOX suppressed growth of tumor cells (B16F10 and A549). Annexin-V staining confirmed that PtNPs-DOX stimulated apoptosis in tumor cells. When administered intraperitonealy (IP) in a mouse model of subcutaneous melanoma, PtNPs-DOX significantly inhibited tumor proliferation in comparison with the free drug. The anti-tumor activity was confirmed by the decreased expression of Ki-67 and SOX2 proliferation markers, and increased expression of the tumor suppressor protein p53 in malignant melanoma as shown by Western blotting and immunofluorescence. The study suggested that a drug delivery system based on PtNPs should be further investigated in anticancer nanomedicine applications (Mukherjee et al., 2020).
Numerous ligands including, peptides, antibodies, and DNA/RNA aptamers have been used to prepare targeted nanocarriers. Despite widespread use of an anti-EGFR monoclonal antibody to prepare tumor-targeted NPs, its large molecular weight and size, limits the penetration of this monoclonal antibody into the tumor microenvironment (Nida et al., 2005; Wu et al., 2006; Melancon et al., 2008; Patra et al., 2008; Yang et al., 2008; Peng et al., 2011). Meanwhile, aptamers have some advantages compared to antibodies as cancer targeting ligands, such as decreased toxicity, lack of immunogenicity, adequate tissue penetration, and better thermal stablility (Li et al., 2013). Compared to other ligands, the structure of aptamers can be easily altered to abolish undesirable interactions with proteins, to improve the blood circulation time (Farokhzad et al., 2004; McNamara et al., 2006; Javier et al., 2008; Dassie et al., 2009). Moreover, aptamers have been extensively used in the diagnosis and treatment of cancers (Ireson and Kelland, 2006; Cerchia and De Franciscis, 2010; Lee et al., 2010). The use of aptamers to transport chemotherapeutic drugs may improve the efficacy of cancer therapy. The aptamers interact with receptors located on the surface of the tumor cells in an analogous manner to antibodies. Recent research into the use of aptamers as ligands for targeted delivery of chemotherapeutic drugs has yielded promising results (Dobrovolskaia et al., 2008; Guo et al., 2013).
SPIONs (superparamagnetic iron oxide NPs) have been utilized as experimental contrast agents for MRI (magnetic resonance imaging) to diagnose tumors (Lin et al., 2008; Mailänder and Landfester, 2009; Shubayev et al., 2009; Zhang et al., 2010). Two types of SPION contrast agents, Feridex® and Resovist® have received approval by the FDA (Gandhi et al., 2006; Mailänder and Landfester, 2009). Despite the fact that SPIONs can increase contrast in MRI images, ,radioisotope-based imaging techniques including positron emission tomography usually produce stronger contrast enhancement (Tsukada, 2011). Noninvasive diagnosis of tumors requires greater signal enhancement, which can be provided by more powerful MRI contrast agents. One example is SIPPs (superparamagnetic iron platinum NPs), which were previously shown to be more effective MRI contrast agents compared to SPIONs (Taylor et al., 2011; Taylor and Webster, 2011). One study investigated the use SIPPs as MRI magnetic NP-based contrast agents. They showed that SIPP particles could be encapsulated into micelles formed from PEGylated phospholipids. These particles could selectively target prostate tumor cell lines using an anti-PSMA (prostate-specific membrane antigen) antibody in vitro (Taylor et al., 2011).
In a study by Taylor et al., multifunctional SIPP-PTX micelles (SPMs) were produced from biotin, fluorescein, and PEG functionalized phospholipids. They were conjugated to an antibody against PSMA and loaded with paclitaxel. These multifunctional SIPP-PTX micelles were tested for the treatment of prostate tumors in a mouse xenograft model, with the advantages of MRI and selective targeting (Taylor and Sillerud, 2012). The average diameter of the SPMs was 45.4 ± 24.9 nm and they contained 247.0 ± 33.4 μg/ml platinum, 702.6 ± 206 μg/ml paclitaxel, and 160.7 ± 22.9 μg/ml iron. Measurement of drug release, showed that approximately 50% of paclitaxel was released in serum after 30.2 h, while in saline it took twice as long. SPMs conjugated to anti-PSMA could selectively bind to C4-2 human prostate tumor cells in a binding assay, and release paclitaxel into the tumor cells in vitro. Free paclitaxel was shown to be more cytotoxic compared to SPMs in C4-2 cells, after 48 and 24 h incubation periods (1.6 times and 2.2 times, respectively). However, after 72 h, SPMs and paclitaxel displayed similar cytotoxicity. The MRI transverse relaxivity of SPMs was 389 ± 15.5 Hz/mM iron. SIPP micelles could generate contrast enhancement in MRI regardless of the presence of drug in vivo. In nude mice, the formation and proliferation of C4-2 prostate tumor xenografts was specifically inhibited by paclitaxel loaded PSMA-targeted SPMs. Besides, nude mice injected with PSMA-targeted SPMs, showed higher concentrations of both platinum and paclitaxel in the cancer tissue in comparison with nude mice injected with non-targeted paclitaxel and SPMs (Taylor and Sillerud, 2012).
Table 2 summarizes some reports of platinum NPs which have been used to deliver different drugs to different tumors.
Conclusion
Platinum-based biomolecules have attracted much interest for several years. For example, platinum-derived therapeutic drugs have been extensively investigated to destroy tumor cells. However, these chemotherapeutic agents also have several problems, as they cause significant dose-dependent acute and chronic side effects due to their non-selective action on both tumor and healthy cells.
Recently, the development of nanomaterials with eco-friendly properties has played a major role in many different fields, such as energy applications, electronics, biomedical engineering, and drug delivery. Platinum NPs are one type of metal NPs, which have many advantages, such as anti-tumor and anti-bacterial activity, making them appropriate candidates for a wide range of pharmaceutical applications. Because PtNPs display remarkable behavior in a variety of applications, methods for their synthesis and characterization have become increasingly important. Numerous synthetic routes for PtNPs have been described, such as microemulsion, colloidal systems, sol–gel, reduction, sonochemical methods, electrochemical synthesis, and methods based on microbial and plant extracts. Because of their shape and size-dependent catalytic and optical properties, functional PtNPs have attracted considerable attention in recent years as theranostic and therapeutic platforms. These features have the potential to circumvent some drawbacks of conventional pharmaceuticals, such as unfavorable side effects, imperfect selectivity, and undesirable pharmacokinetics. Some current efforts were done to synthesize more biocompatible platinum nanoparticles which are hemocompatible (Mukherjee et al., 2021).
The vast amount of basic research on platinum coordination complexes has produced. Over the past years, several thousand new molecules for preclinical screening and 28 compounds which have entered clinical development. The goals of these research activities have been to identify compounds with superior efficacy, reduced toxicity, lack of cross-resistance or improved pharmacological characteristics as compared with the parent compound, cisplatin. Whereas carboplatin is an analogue with an improved therapeutic index over that of cisplatin, new compounds clearly more active than or non-cross-resistant with cisplatin have not yet been identified (Lebwohl and Canetta, 19901998). Although, there have been a few platinum-based nanomaterials, which have been suggested to be useful in cancer therapy. In addition, functional PtNPs have been shown to trigger apoptosis in cancer cells via a range of target-specific pathways. The development of new enzyme-free sensors for platinum-based nanomaterials with good selectivity, high sensitivity, portability, in vivo detection, and commercial application, is therefore the key direction of future research and development.
Author Contributions
HM and SD involved in conception, design, statistical analysis and drafting of the manuscript. AA, MD, MK, MS, MM, MH, MRH, SAM, HS, and FFD contributed in data collection and manuscript drafting. All authors approved the final version for submission.
Conflict of Interest
The authors declare that the research was conducted in the absence of any commercial or financial relationships that could be construed as a potential conflict of interest.
Publisher’s Note
All claims expressed in this article are solely those of the authors and do not necessarily represent those of their affiliated organizations, or those of the publisher, the editors and the reviewers. Any product that may be evaluated in this article, or claim that may be made by its manufacturer, is not guaranteed or endorsed by the publisher.
References
Abdolhosseinzadeh, S., Sadighikia, S., and Alkan Gürsel, S. (2018). Scalable Synthesis of Sub-nanosized Platinum-Reduced Graphene Oxide Composite by an Ultraprecise Photocatalytic Method. ACS Sustainable Chem. Eng. 6 (3), 3773–3782. doi:10.1021/acssuschemeng.7b04148
Adams, D. M. (1974). Inorganic Solids: An Introduction to Concepts in Solid-State Structural Chemistry. John Wiley & Sons.
Ahmad, N., Sharma, S., Alam, M. K., Singh, V. N., Shamsi, S. F., Mehta, B. R., et al. (2010). Rapid Synthesis of Silver Nanoparticles Using Dried Medicinal Plant of Basil. Colloids Surf. B Biointerfaces 81 (1), 81–86. doi:10.1016/j.colsurfb.2010.06.029
Al-Fahdawi, M. Q., Al-Doghachi, F. A. J., Abdullah, Q. K., Hammad, R. T., Rasedee, A., Ibrahim, W. N., et al. (2021). Oxidative Stress Cytotoxicity Induced by Platinum-Doped Magnesia Nanoparticles in Cancer Cells. Biomed. Pharmacother. 138, 111483. doi:10.1016/j.biopha.2021.111483
Almarzoug, M. H. A., Ali, D., Alarifi, S., Alkahtani, S., and Alhadheq, A. M. (2020). Platinum Nanoparticles Induced Genotoxicity and Apoptotic Activity in Human normal and Cancer Hepatic Cells via Oxidative Stress-Mediated Bax/Bcl-2 and Caspase-3 Expression. Environ. Toxicol. 35 (9), 930–941. doi:10.1002/tox.22929
Alshatwi, A. A., Athinarayanan, J., and Vaiyapuri Subbarayan, P. (2015). Green Synthesis of Platinum Nanoparticles that Induce Cell Death and G2/M-phase Cell Cycle Arrest in Human Cervical Cancer Cells. J. Mater. Sci. Mater. Med. 26 (1), 5330. doi:10.1007/s10856-014-5330-1
Armada-Moreira, A., Taipaleenmäki, E., Baekgaard-Laursen, M., Schattling, P. S., Sebastião, A. M., Vaz, S. H., et al. (2017). Platinum Nanoparticle-Based Microreactors as Support for Neuroblastoma Cells. ACS Appl. Mater. Inter. 10 (9), 7581–7592. doi:10.1021/acsami.7b10724
Asharani, P. V., Xinyi, N., Hande, M. P., and Valiyaveettil, S. (2010). DNA Damage and P53-Mediated Growth Arrest in Human Cells Treated with Platinum Nanoparticles. Nanomedicine (Lond) 5 (1), 51–64. doi:10.2217/nnm.09.85
Aygun, A., Gülbagca, F., Ozer, L. Y., Ustaoglu, B., Altunoglu, Y. C., Baloglu, M. C., et al. (2020). Biogenic Platinum Nanoparticles Using Black Cumin Seed and Their Potential Usage as Antimicrobial and Anticancer Agent. J. Pharm. Biomed. Anal. 179, 112961. doi:10.1016/j.jpba.2019.112961
Azzazy, H. M., Mansour, M. M., Samir, T. M., and Franco, R. (2012). Gold Nanoparticles in the Clinical Laboratory: Principles of Preparation and Applications. Clin. Chem. Lab. Med. 50 (2), 193–209. doi:10.1515/CCLM.2011.732
Bansal, G., Wright, J. E., Kucharski, C., and Uludağ, H. (2005). A Dendritic Tetra(bisphosphonic Acid) for Improved Targeting of Proteins to Bone. Angew. Chem. Int. Ed. Engl. 44 (24), 3710–3714. doi:10.1002/anie.200500350
Bao, Y. W., Hua, X. W., Chen, X., and Wu, F. G. (2018). Platinum-doped Carbon Nanoparticles Inhibit Cancer Cell Migration under Mild Laser Irradiation: Multi-Organelle-Targeted Photothermal Therapy. Biomaterials 183, 30–42. doi:10.1016/j.biomaterials.2018.08.031
Baskaran, B., Muthukumarasamy, A., Chidambaram, S., Sugumaran, A., Ramachandran, K., and Rasu Manimuthu, T. (2017). Cytotoxic Potentials of Biologically Fabricated Platinum Nanoparticles from Streptomyces Sp. On MCF-7 Breast Cancer Cells. IET Nanobiotechnol 11 (3), 241–246. doi:10.1049/iet-nbt.2016.0040
Bendale, Y., Bendale, V., and Paul, S. (2017). Evaluation of Cytotoxic Activity of Platinum Nanoparticles against normal and Cancer Cells and its Anticancer Potential through Induction of Apoptosis. Integr. Med. Res. 6 (2), 141–148. doi:10.1016/j.imr.2017.01.006
Bhattacharya, D., and Gupta, R. K. (2005). Nanotechnology and Potential of Microorganisms. Crit. Rev. Biotechnol. 25 (4), 199–204. doi:10.1080/07388550500361994
Blank, H., Schneider, R., Gerthsen, D., Gehrke, H., Jarolim, K., and Marko, D. (2014). Application of Low-Energy Scanning Transmission Electron Microscopy for the Study of Pt-Nanoparticle Uptake in Human colon Carcinoma Cells. Nanotoxicology 8 (4), 433–446. doi:10.3109/17435390.2013.796535
Bloch, K., Pardesi, K., Satriano, C., and Ghosh, S. (2021). Bacteriogenic Platinum Nanoparticles for Application in Nanomedicine. Front. Chem. 9 (32), 624344. doi:10.3389/fchem.2021.624344
Body, J. J., Lipton, A., Gralow, J., Steger, G. G., Gao, G., Yeh, H., et al. (2010). Effects of Denosumab in Patients with Bone Metastases with and without Previous Bisphosphonate Exposure. J. Bone Miner Res. 25 (3), 440–446. doi:10.1359/jbmr.090810
Bönnemann, H., and Richards, R. M. (2001). Nanoscopic Metal Particles− Synthetic Methods and Potential Applications. Eur. J. Inorg. Chem. 2001 (10), 2455–2480.
Bortot, B., Mongiat, M., Valencic, E., Dal Monego, S., Licastro, D., Crosera, M., et al. (2020). Nanotechnology-Based Cisplatin Intracellular Delivery to Enhance Chemo-Sensitivity of Ovarian Cancer. Int. J. Nanomedicine 15, 4793–4810. doi:10.2147/IJN.S247114
Botchway, S. W., Charnley, M., Haycock, J. W., Parker, A. W., Rochester, D. L., Weinstein, J. A., et al. (2008). Time-resolved and Two-Photon Emission Imaging Microscopy of Live Cells with Inert Platinum Complexes. Proc. Natl. Acad. Sci. U S A. 105 (42), 16071–16076. doi:10.1073/pnas.0804071105
Brown, A. L., Kai, M. P., DuRoss, A. N., Sahay, G., and Sun, C. (2018). Biodistribution and Toxicity of Micellar Platinum Nanoparticles in Mice via Intravenous Administration. Nanomaterials (Basel) 8 (6), 410. doi:10.3390/nano8060410
Brown, R., Lönn, B., Pfeiffer, R., Frederiksen, H., and Wickman, B. (2021). Plasma-Induced Heating Effects on Platinum Nanoparticle Size during Sputter Deposition Synthesis in Polymer and Ionic Liquid Substrates. Langmuir 37 (29), 8821–8828. doi:10.1021/acs.langmuir.1c01190
Carita, A. C., Eloy, J. O., Chorilli, M., Lee, R. J., and Leonardi, G. R. (2018). Recent Advances and Perspectives in Liposomes for Cutaneous Drug Delivery. Curr. Med. Chem. 25 (5), 606–635. doi:10.2174/0929867324666171009120154
Carmeliet, P., and Jain, R. K. (2000). Angiogenesis in Cancer and Other Diseases. nature 407 (6801), 249–257. doi:10.1038/35025220
Castillo-Henríquez, L., Alfaro-Aguilar, K., Ugalde-Álvarez, J., Vega-Fernández, L., Montes de Oca-Vásquez, G., and Vega-Baudrit, J. R. (2020). Green Synthesis of Gold and Silver Nanoparticles from Plant Extracts and Their Possible Applications as Antimicrobial Agents in the Agricultural Area. Nanomaterials 10 (9), 1763.
Castro, L., Blázquez, M. L., Muñoz, J. A., González, F., García-Balboa, C., and Ballester, A. (2011). Biosynthesis of Gold Nanowires Using Sugar Beet Pulp. Process Biochem. 46 (5), 1076–1082. doi:10.1016/j.procbio.2011.01.025
Cerchia, L., and De Franciscis, V. (2010). Targeting Cancer Cells with Nucleic Acid Aptamers. Trends Biotechnol. 28 (10), 517–525. doi:10.1016/j.tibtech.2010.07.005
Chaturvedi, V. K., Yadav, N., Rai, N. K., Bohara, R. A., Rai, S. N., Aleya, L., et al. (2021). Two Birds with One Stone: Oyster Mushroom Mediated Bimetallic Au-Pt Nanoparticles for Agro-Waste Management and Anticancer Activity. Environ. Sci. Pollut. Res. Int. 28 (11), 13761–13775. doi:10.1007/s11356-020-11435-2
Chen, J., Lim, B., Lee, E. P., and Xia, Y. (2009). Shape-controlled Synthesis of Platinum Nanocrystals for Catalytic and Electrocatalytic Applications. Nano Today 4 (1), 81–95. doi:10.1016/j.nantod.2008.09.002
Chen, Q. (2004). Determination of Phytic Acid and Inositol Pentakisphosphates in Foods by High-Performance Ion Chromatography. J. Agric. Food Chem. 52 (15), 4604–4613. doi:10.1021/jf035294x
Chen, Y., Wang, J., Wang, J., Wang, L., Tan, X., Tu, K., et al. (2016). Aptamer Functionalized Cisplatin-Albumin Nanoparticles for Targeted Delivery to Epidermal Growth Factor Receptor Positive Cervical Cancer. J. Biomed. Nanotechnol 12 (4), 656–666. doi:10.1166/jbn.2016.2203
Chen, Y., Zhao, S., Liu, B., Chen, M., Mao, J., He, H., et al. (2014). Corrosion-controlling and Osteo-Compatible Mg Ion-Integrated Phytic Acid (Mg-PA) Coating on Magnesium Substrate for Biodegradable Implants Application. ACS Appl. Mater. Inter. 6 (22), 19531–19543. doi:10.1021/am506741d
Cheong, S., Watt, J., Ingham, B., Toney, M. F., and Tilley, R. D. (2009). In Situ and Ex Situ Studies of Platinum Nanocrystals: Growth and Evolution in Solution. J. Am. Chem. Soc. 131 (40), 14590–14595. doi:10.1021/ja9065688
Cole, L. E., Vargo-Gogola, T., and Roeder, R. K. (2016). Targeted Delivery to Bone and mineral Deposits Using Bisphosphonate Ligands. Adv. Drug Deliv. Rev. 99 (Pt A), 12–27. doi:10.1016/j.addr.2015.10.005
Daneshvar, F., Salehi, F., Karimi, M., Vais, R. D., Mosleh-Shirazi, M. A., and Sattarahmady, N. (2020). Combined X-ray Radiotherapy and Laser Photothermal Therapy of Melanoma Cancer Cells Using Dual-Sensitization of Platinum Nanoparticles. J. Photochem. Photobiol. B 203, 111737. doi:10.1016/j.jphotobiol.2019.111737
Dang‐Bao, T., Favier, I., and Gómez, M. (2021). Metal Nanoparticles in Polyols: Bottom‐up and Top‐down Syntheses and Catalytic Applications. Nanoparticles Catal. Adv. Synth. Appl. 1, 99–122.
Danhier, F., Feron, O., and Préat, V. (2010). To Exploit the Tumor Microenvironment: Passive and Active Tumor Targeting of Nanocarriers for Anti-cancer Drug Delivery. J. Control. Release 148 (2), 135–146. doi:10.1016/j.jconrel.2010.08.027
Dasari, S., and Tchounwou, P. B. (2014). Cisplatin in Cancer Therapy: Molecular Mechanisms of Action. Eur. J. Pharmacol. 740, 364–378. doi:10.1016/j.ejphar.2014.07.025
Dassie, J. P., Liu, X. Y., Thomas, G. S., Whitaker, R. M., Thiel, K. W., Stockdale, K. R., et al. (2009). Systemic Administration of Optimized Aptamer-siRNA Chimeras Promotes Regression of PSMA-Expressing Tumors. Nat. Biotechnol. 27 (9), 839–849. doi:10.1038/nbt.1560
Depciuch, J., Stec, M., Klebowski, B., Baran, J., and Parlinska-Wojtan, M. (2019). Platinum-gold Nanoraspberries as Effective Photosensitizer in Anticancer Photothermal Therapy. J. Nanobiotechnology 17 (1), 107–112. doi:10.1186/s12951-019-0539-2
Dhand, C., Dwivedi, N., Loh, X. J., Jie Ying, A. N., Verma, N. K., Beuerman, R. W., et al. (2015). Methods and Strategies for the Synthesis of Diverse Nanoparticles and Their Applications: a Comprehensive Overview. RSC Adv. 5 (127), 105003–105037. doi:10.1039/c5ra19388e
Dhar, S., Gu, F. X., Langer, R., Farokhzad, O. C., and Lippard, S. J. (2008). Targeted Delivery of Cisplatin to Prostate Cancer Cells by Aptamer Functionalized Pt(IV) Prodrug-PLGA-PEG Nanoparticles. Proc. Natl. Acad. Sci. U S A. 105 (45), 17356–17361. doi:10.1073/pnas.0809154105
Dhar, S., Kolishetti, N., Lippard, S. J., and Farokhzad, O. C. (2011). Targeted Delivery of a Cisplatin Prodrug for Safer and More Effective Prostate Cancer Therapy In Vivo. Proc. Natl. Acad. Sci. U S A. 108 (5), 1850–1855. doi:10.1073/pnas.1011379108
Ding, F., Zhang, L., Chen, H., Song, H., Chen, S., and Xiao, H. (2020). Enhancing the Chemotherapeutic Efficacy of Platinum Prodrug Nanoparticles and Inhibiting Cancer Metastasis by Targeting Iron Homeostasis. Nanoscale Horiz 5 (6), 999–1015. doi:10.1039/d0nh00148a
Dobrovolskaia, M. A., Aggarwal, P., Hall, J. B., and McNeil, S. E. (2008). Preclinical Studies to Understand Nanoparticle Interaction with the Immune System and its Potential Effects on Nanoparticle Biodistribution. Mol. Pharm. 5 (4), 487–495. doi:10.1021/mp800032f
Dobrucka, R., Romaniuk-Drapała, A., and Kaczmarek, M. (2019). Evaluation of Biological Synthesized Platinum Nanoparticles Using Ononidis Radix Extract on the Cell Lung Carcinoma A549. Biomed. Microdevices 21 (3), 75–10. doi:10.1007/s10544-019-0424-7
Du, H-Y., Wang, C-H., Hsu, H-C., Chang, S-T., Chen, U-S., Yen, S., et al. (2008). Controlled Platinum Nanoparticles Uniformly Dispersed on Nitrogen-Doped Carbon Nanotubes for Methanol Oxidation. Diamond Relat. Mater. 17 (4-5), 535–541. doi:10.1016/j.diamond.2008.01.116
Du, Y. K., Yang, P., Mou, Z. G., Hua, N. P., and Jiang, L. (2006). Thermal Decomposition Behaviors of PVP Coated on Platinum Nanoparticles. J. Appl. Polym. Sci. 99 (1), 23–26. doi:10.1002/app.21886
Durán, N., Marcato, P. D., Durán, M., Yadav, A., Gade, A., and Rai, M. (2011). Mechanistic Aspects in the Biogenic Synthesis of Extracellular Metal Nanoparticles by Peptides, Bacteria, Fungi, and Plants. Appl. Microbiol. Biotechnol. 90 (5), 1609–1624.
E. Taylor, and T. J. Webster (Editors) (2011). “Reducing Infections through Nanotechnology,” The Twenty-first International Offshore and Polar Engineering Conference, Maui, HI, United States, June 19–24, 2011. (International Society of Offshore and Polar Engineers).
Fahmy, S. A., Preis, E., Bakowsky, U., and Azzazy, H. M. E. (2020). Palladium Nanoparticles Fabricated by Green Chemistry: Promising Chemotherapeutic, Antioxidant and Antimicrobial Agents. Materials (Basel) 13 (17), 3661. doi:10.3390/ma13173661
Fahmy, S. A., Preis, E., Bakowsky, U., and Azzazy, H. M. E. (2020). Platinum Nanoparticles: green Synthesis and Biomedical Applications. Molecules 25 (21), 4981. doi:10.3390/molecules25214981
Farokhzad, O. C., Jon, S., Khademhosseini, A., Tran, T. N., LaVan, D. A., and Langer, R. (2004). Nanoparticle-aptamer Bioconjugates: a New Approach for Targeting Prostate Cancer Cells. Cancer Res. 64 (21), 7668–7672. doi:10.1158/0008-5472.CAN-04-2550
Farokhzad, O. C., and Langer, R. (2009). Impact of Nanotechnology on Drug Delivery. ACS nano 3 (1), 16–20. doi:10.1021/nn900002m
Fedorova, E. A., Stadnichenko, A. I., Slavinskaya, E. M., Kibis, L. S., Stonkus, O. A., Svintsitskiy, D. A., et al. (2020). A Study of Pt/Al2O3 Nanocomposites Obtained by Pulsed Laser Ablation to Be Used as Catalysts of Oxidation Reactions. J. Struct. Chem. 61 (2), 316–329. doi:10.1134/s0022476620020171
Feldheim, D., and Foss, C. (2002). Metal Nanoparticles. Synthesis, Characterization and Applications. New York: Basel Marsel Dekker. Inc.
Feldmann, D. P., Heyza, J., Zimmermann, C. M., Patrick, S. M., and Merkel, O. M. (2020). Nanoparticle-Mediated Gene Silencing for Sensitization of Lung Cancer to Cisplatin Therapy. Molecules 25 (8), 1994. doi:10.3390/molecules25081994
Fichtner, J., Watzele, S., Garlyyev, B., Kluge, R. M., Haimerl, F., El-Sayed, H. A., et al. (2020). Tailoring the Oxygen Reduction Activity of Pt Nanoparticles through Surface Defects: a Simple Top-Down Approach. ACS Catal. 10 (5), 3131–3142. doi:10.1021/acscatal.9b04974
Frederick, C. A., Williams, L. D., Ughetto, G., Van der Marel, G. A., Van Boom, J. H., Rich, A., et al. (1990). Structural Comparison of Anticancer Drug-DNA Complexes: Adriamycin and Daunomycin. Biochemistry 29 (10), 2538–2549. doi:10.1021/bi00462a016
Fu, B., Dang, M., Tao, J., Li, Y., and Tang, Y. (2020). Mesoporous Platinum Nanoparticle-Based Nanoplatforms for Combined Chemo-Photothermal Breast Cancer Therapy. J. Colloid Interf. Sci 570, 197–204. doi:10.1016/j.jcis.2020.02.051
Fujimoto, T., Terauchi, S.-y., Umehara, H., Kojima, I., and Henderson, W. (2001). Sonochemical Preparation of Single-Dispersion Metal Nanoparticles from Metal Salts. Chem. Mater. 13 (3), 1057–1060. doi:10.1021/cm000910f
Gaidhani, S. V., Yeshvekar, R. K., Shedbalkar, U. U., Bellare, J. H., and Chopade, B. A. (2014). Bio-reduction of Hexachloroplatinic Acid to Platinum Nanoparticles Employing Acinetobacter Calcoaceticus. Process Biochem. 49 (12), 2313–2319. doi:10.1016/j.procbio.2014.10.002
Gandhi, S. N., Brown, M. A., Wong, J. G., Aguirre, D. A., and Sirlin, C. B. (2006). MR Contrast Agents for Liver Imaging: what, when, How. Radiographics 26 (6), 1621–1636. doi:10.1148/rg.266065014
Gao, X., and Dong, Q. Z. (2020). Advance in Metabolism and Target Therapy in Breast Cancer Stem Cells. World J. Stem Cell 12 (11), 1295–1306. doi:10.4252/wjsc.v12.i11.1295
Garlyyev, B., Watzele, S., Fichtner, J., Michalička, J., Schökel, A., Senyshyn, A., et al. (2020). Electrochemical Top-Down Synthesis of C-Supported Pt Nano-Particles with Controllable Shape and Size: Mechanistic Insights and Application. Nano Res. 1, 1–8. doi:10.1007/s12274-020-3281-z
Gehrke, H., Pelka, J., Hartinger, C. G., Blank, H., Bleimund, F., Schneider, R., et al. (2011). Platinum Nanoparticles and Their Cellular Uptake and DNA Platination at Non-cytotoxic Concentrations. Arch. Toxicol. 85 (7), 799–812. doi:10.1007/s00204-010-0636-3
Govender, Y., Riddin, T., Gericke, M., and Whiteley, C. G. (2009). Bioreduction of Platinum Salts into Nanoparticles: a Mechanistic Perspective. Biotechnol. Lett. 31 (1), 95–100. doi:10.1007/s10529-008-9825-z
Gu, T., Wang, Y., Lu, Y., Cheng, L., Feng, L., Zhang, H., et al. (2019). Platinum Nanoparticles to Enable Electrodynamic Therapy for Effective Cancer Treatment. Adv. Mater. 31 (14), e1806803. doi:10.1002/adma.201806803
Guo, S., Wang, Y., Miao, L., Xu, Z., Lin, C. M., Zhang, Y., et al. (2013). Lipid-coated Cisplatin Nanoparticles Induce Neighboring Effect and Exhibit Enhanced Anticancer Efficacy. ACS nano 7 (11), 9896–9904. doi:10.1021/nn403606m
Gurunathan, S., Jeyaraj, M., Kang, M. H., and Kim, J. H. (2020). Anticancer Properties of Platinum Nanoparticles and Retinoic Acid: Combination Therapy for the Treatment of Human Neuroblastoma Cancer. Int. J. Mol. Sci. 21 (18), 6792. doi:10.3390/ijms21186792
Gurunathan, S., Jeyaraj, M., Kang, M. H., and Kim, J. H. (2019). Tangeretin-Assisted Platinum Nanoparticles Enhance the Apoptotic Properties of Doxorubicin: Combination Therapy for Osteosarcoma Treatment. Nanomaterials (Basel) 9 (8), 1089. doi:10.3390/nano9081089
Gurunathan, S., Jeyaraj, M., Kang, M. H., and Kim, J. H. (2019). Tangeretin-assisted Platinum Nanoparticles Enhance the Apoptotic Properties of Doxorubicin: Combination Therapy for Osteosarcoma Treatment. Nanomaterials (Basel) 9 (8), 1089. doi:10.3390/nano9081089
Gurunathan, S., Jeyaraj, M., La, H., Yoo, H., Choi, Y., Do, J. T., et al. (2020). Anisotropic Platinum Nanoparticle-Induced Cytotoxicity, Apoptosis, Inflammatory Response, and Transcriptomic and Molecular Pathways in Human Acute Monocytic Leukemia Cells. Int. J. Mol. Sci. 21 (2), 440. doi:10.3390/ijms21020440
Gurunathan, S., Kang, M. H., Jeyaraj, M., and Kim, J. H. (2021). Platinum Nanoparticles Enhance Exosome Release in Human Lung Epithelial Adenocarcinoma Cancer Cells (A549): Oxidative Stress and the Ceramide Pathway Are Key Players. Int. J. Nanomedicine 16, 515–538. doi:10.2147/IJN.S291138
Gurunathan, S., Kang, M.-H., Jeyaraj, M., and Kim, J.-H. (2021). Platinum Nanoparticles Enhance Exosome Release in Human Lung Epithelial Adenocarcinoma Cancer Cells (A549): Oxidative Stress and the Ceramide Pathway Are Key Players. Ijn Vol. 16, 515–538. doi:10.2147/ijn.s291138
Gurunathan, S., Kang, M.-h., and Kim, J.-H. (2018). Combination Effect of Silver Nanoparticles and Histone Deacetylases Inhibitor in Human Alveolar Basal Epithelial Cells. Molecules 23 (8), 2046. doi:10.3390/molecules23082046
Gusti-Ngurah-Putu, E.-P., Huang, L., and Hsu, Y.-C. (2019). Effective Combined Photodynamic Therapy with Lipid Platinum Chloride Nanoparticles Therapies of Oral Squamous Carcinoma Tumor Inhibition. Jcm 8 (12), 2112. doi:10.3390/jcm8122112
Heller, D. A., Levi, Y., Pelet, J. M., Doloff, J. C., Wallas, J., Pratt, G. W., et al. (2013). Modular 'click-In-Emulsion' Bone-Targeted Nanogels. Adv. Mater. 25 (10), 1449–1454. doi:10.1002/adma.201202881
Ireson, C. R., and Kelland, L. R. (2006). Discovery and Development of Anticancer Aptamers. Mol. Cancer Ther. 5 (12), 2957–2962. doi:10.1158/1535-7163.MCT-06-0172
Jabir, N. R., Tabrez, S., Ashraf, G. M., Shakil, S., Damanhouri, G. A., and Kamal, M. A. (2012). Nanotechnology-based Approaches in Anticancer Research. Int. J. Nanomedicine 7, 4391–4408. doi:10.2147/IJN.S33838
Jain, R. K. (1994). Barriers to Drug Delivery in Solid Tumors. Sci. Am. 271 (1), 58–65. doi:10.1038/scientificamerican0794-58
Jameel, M. S., Aziz, A. A., and Dheyab, M. A. (2021). Impacts of Various Solvents in Ultrasonic Irradiation and green Synthesis of Platinum Nanoparticle. Inorg. Chem. Commun. 128, 108565. doi:10.1016/j.inoche.2021.108565
Jan, H., Gul, R., Andleeb, A., Ullah, S., Shah, M., Khanum, M., et al. (2021). A Detailed Review on Biosynthesis of Platinum Nanoparticles (PtNPs), Their Potential Antimicrobial and Biomedical Applications. J. Saudi Chem. Soc. 25, 101297. doi:10.1016/j.jscs.2021.101297
Javier, D. J., Nitin, N., Levy, M., Ellington, A., and Richards-Kortum, R. (2008). Aptamer-targeted Gold Nanoparticles as Molecular-specific Contrast Agents for Reflectance Imaging. Bioconjug. Chem. 19 (6), 1309–1312. doi:10.1021/bc8001248
Jawaid, P., Rehman, M. U., Hassan, M. A., Zhao, Q. L., Li, P., Miyamoto, Y., et al. (2016). Effect of Platinum Nanoparticles on Cell Death Induced by Ultrasound in Human Lymphoma U937 Cells. Ultrason. Sonochem. 31, 206–215. doi:10.1016/j.ultsonch.2015.12.013
Jeyaraj, M., Gurunathan, S., Qasim, M., Kang, M. H., and Kim, J. H. (2019). A Comprehensive Review on the Synthesis, Characterization, and Biomedical Application of Platinum Nanoparticles. Nanomaterials (Basel) 9 (12), 1719. doi:10.3390/nano9121719
Jia, Y. Y., Zhang, J. J., Zhang, Y. X., Wang, W., Li, C., Zhou, S. Y., et al. (2020). Construction of Redox-Responsive Tumor Targeted Cisplatin Nano-Delivery System for Effective Cancer Chemotherapy. Int. J. Pharm. 580, 119190. doi:10.1016/j.ijpharm.2020.119190
Jiang, C., Wang, Y., Wang, J., Song, W., and Lu, L. (2017). Achieving Ultrasensitive In Vivo Detection of Bone Crack with Polydopamine-Capsulated Surface-Enhanced Raman Nanoparticle. Biomaterials 114, 54–61. doi:10.1016/j.biomaterials.2016.11.007
Johnstone, T. C., Park, G. Y., and Lippard, S. J. (2014). Understanding and Improving Platinum Anticancer Drugs-Pphenanthriplatin. Anticancer Res. 34 (1), 471–476.
Johnstone, T. C., Suntharalingam, K., and Lippard, S. J. (2016). The Next Generation of Platinum Drugs: Targeted Pt(II) Agents, Nanoparticle Delivery, and Pt(IV) Prodrugs. Chem. Rev. 116 (5), 3436–3486. doi:10.1021/acs.chemrev.5b00597
Kajita, M., Hikosaka, K., Iitsuka, M., Kanayama, A., Toshima, N., and Miyamoto, Y. (2007). Platinum Nanoparticle Is a Useful Scavenger of Superoxide Anion and Hydrogen Peroxide. Free Radic. Res. 41 (6), 615–626. doi:10.1080/10715760601169679
Kalimutho, M., Minutolo, A., Grelli, S., Formosa, A., Sancesario, G., Valentini, A., et al. (2011). Satraplatin (JM-216) Mediates G2/M Cell Cycle Arrest and Potentiates Apoptosis via Multiple Death Pathways in Colorectal Cancer Cells Thus Overcoming Platinum Chemo-Resistance. Cancer Chemother. Pharmacol. 67 (6), 1299–1312. doi:10.1007/s00280-010-1428-4
Kalimuthu, K., Suresh Babu, R., Venkataraman, D., Bilal, M., and Gurunathan, S. (2008). Biosynthesis of Silver Nanocrystals by Bacillus Licheniformis. Colloids Surf. B Biointerfaces 65 (1), 150–153. doi:10.1016/j.colsurfb.2008.02.018
Kalishwaralal, K., Deepak, V., Ramkumarpandian, S., Nellaiah, H., and Sangiliyandi, G. (2008). Extracellular Biosynthesis of Silver Nanoparticles by the Culture Supernatant of Bacillus Licheniformis. Mater. Lett. 62 (29), 4411–4413. doi:10.1016/j.matlet.2008.06.051
Kamaly, N., Xiao, Z., Valencia, P. M., Radovic-Moreno, A. F., and Farokhzad, O. C. (2012). Targeted Polymeric Therapeutic Nanoparticles: Design, Development and Clinical Translation. Chem. Soc. Rev. 41 (7), 2971–3010. doi:10.1039/c2cs15344k
Kang, S., Kang, K., Chae, A., Kim, Y. K., Jang, H., and Min, D. H. (2019). Fucoidan-coated Coral-like Pt Nanoparticles for Computed Tomography-Guided Highly Enhanced Synergistic Anticancer Effect against Drug-Resistant Breast Cancer Cells. Nanoscale 11 (32), 15173–15183. doi:10.1039/c9nr04495g
Kankala, R. K., Liu, C.-G., Yang, D.-Y., Wang, S.-B., and Chen, A.-Z. (2020). Ultrasmall Platinum Nanoparticles Enable Deep Tumor Penetration and Synergistic Therapeutic Abilities through Free Radical Species-Assisted Catalysis to Combat Cancer Multidrug Resistance. Chem. Eng. J. 383, 123138. doi:10.1016/j.cej.2019.123138
Kasthuri, J., Kathiravan, K., and Rajendiran, N. (2009). Phyllanthin-assisted Biosynthesis of Silver and Gold Nanoparticles: a Novel Biological Approach. J. Nanopart Res. 11 (5), 1075–1085. doi:10.1007/s11051-008-9494-9
Keat, C. L., Aziz, A., Eid, A. M., and Elmarzugi, N. A. (2015). Biosynthesis of Nanoparticles and Silver Nanoparticles. Bioresour. Bioproc. 2 (1), 47. doi:10.1186/s40643-015-0076-2
Khan, Z. U. H., Khan, A., Chen, Y., Shah, N. S., Muhammad, N., Khan, A. U., et al. (2017). Biomedical Applications of green Synthesized Nobel Metal Nanoparticles. J. Photochem. Photobiol. B 173, 150–164. doi:10.1016/j.jphotobiol.2017.05.034
Kharissova, O. V., Dias, H. V., Kharisov, B. I., Pérez, B. O., and Pérez, V. M. (2013). The Greener Synthesis of Nanoparticles. Trends Biotechnol. 31 (4), 240–248. doi:10.1016/j.tibtech.2013.01.003
Kim, K. T., Jin, S.-H., Chang, S.-C., and Park, D.-S. (2013). Green Synthesis of Platinum Nanoparticles by Electroreduction of a K2PtCl6Solid-State Precursor and its Electrocatalytic Effects on H2O2Reduction. Bull. Korean Chem. Soc. 34 (12), 3835–3839. doi:10.5012/bkcs.2013.34.12.3835
Kim, M. I., Kim, M. S., Woo, M. A., Ye, Y., Kang, K. S., Lee, J., et al. (2014). Highly Efficient Colorimetric Detection of Target Cancer Cells Utilizing superior Catalytic Activity of Graphene Oxide-Magnetic-Platinum Nanohybrids. Nanoscale 6 (3), 1529–1536. doi:10.1039/c3nr05539f
Klebowski, B., Depciuch, J., Stec, M., Krzempek, D., Komenda, W., Baran, J., et al. (2020). Fancy-Shaped Gold-Platinum Nanocauliflowers for Improved Proton Irradiation Effect on Colon Cancer Cells. Ijms 21 (24), 9610. doi:10.3390/ijms21249610
Konishi, Y., Ohno, K., Saitoh, N., Nomura, T., Nagamine, S., Hishida, H., et al. (2007). Bioreductive Deposition of Platinum Nanoparticles on the Bacterium Shewanella Algae. J. Biotechnol. 128 (3), 648–653. doi:10.1016/j.jbiotec.2006.11.014
Kumar, A., Joshi, H. M., Mandale, A. B., Srivastava, R., Adyanthaya, S. D., Pasricha, R., et al. (2004). Phase Transfer of Platinum Nanoparticles from Aqueous to Organic Solutions Using Fatty Amine Molecules. J. Chem. Sci. 116 (5), 293–300. doi:10.1007/bf02708280
Kuriganova, A., Faddeev, N., Gorshenkov, M., Kuznetsov, D., Leontyev, I., and Smirnova, N. (2020). A Comparison of "Bottom-Up" and "Top-Down" Approaches to the Synthesis of Pt/C Electrocatalysts. Processes 8 (8), 947. doi:10.3390/pr8080947
Kutwin, M., Sawosz, E., Jaworski, S., Hinzmann, M., Wierzbicki, M., Hotowy, A., et al. (2017). Investigation of Platinum Nanoparticle Properties against U87 Glioblastoma Multiforme. Arch. Med. Sci. 13 (6), 1322–1334. doi:10.5114/aoms.2016.58925
Kutwin, M., Sawosz, E., Jaworski, S., Wierzbicki, M., Strojny, B., Grodzik, M., et al. (2019). Nanocomplexes of Graphene Oxide and Platinum Nanoparticles against Colorectal Cancer colo205, HT-29, HTC-116, SW480, Liver Cancer HepG2, Human Breast Cancer MCF-7, and Adenocarcinoma LNCaP and Human Cervical Hela B Cell Lines. Materials (Basel) 12 (6), 909. doi:10.3390/ma12060909
Lebwohl, D., and Canetta, R. (19901998)., 34. Oxford, England, 1522–1534. doi:10.1016/s0959-8049(98)00224-xClinical Development of Platinum Complexes in Cancer Therapy: an Historical Perspective and an UpdateEur. J. Cancer10
Lee, D. Y., Kim, J. Y., Lee, Y., Lee, S., Miao, W., Kim, H. S., et al. (2017). Black Pigment Gallstone Inspired Platinum-Chelated Bilirubin Nanoparticles for Combined Photoacoustic Imaging and Photothermal Therapy of Cancers. Angew. Chem. Int. Ed. Engl. 56 (44), 13684–13688. doi:10.1002/anie.201707137
Lee, J. H., Yigit, M. V., Mazumdar, D., and Lu, Y. (2010). Molecular Diagnostic and Drug Delivery Agents Based on Aptamer-Nanomaterial Conjugates. Adv. Drug Deliv. Rev. 62 (6), 592–605. doi:10.1016/j.addr.2010.03.003
Leong, G. J., Schulze, M. C., Strand, M. B., Maloney, D., Frisco, S. L., Dinh, H. N., et al. (2014). Shape-directed Platinum Nanoparticle Synthesis: Nanoscale Design of Novel Catalysts. Appl. Organometal. Chem. 28 (1), 1–17. doi:10.1002/aoc.3048
Li, X., Zhao, Q., and Qiu, L. (2013). Smart Ligand: Aptamer-Mediated Targeted Delivery of Chemotherapeutic Drugs and siRNA for Cancer Therapy. J. Control. Release 171 (2), 152–162. doi:10.1016/j.jconrel.2013.06.006
Li, Y., Yun, K. H., Lee, H., Goh, S. H., Suh, Y. G., and Choi, Y. (2019). Porous Platinum Nanoparticles as a High-Z and Oxygen Generating Nanozyme for Enhanced Radiotherapy In Vivo. Biomaterials 197, 12–19. doi:10.1016/j.biomaterials.2019.01.004
Li, Z., Chen, Y., Yang, Y., Yu, Y., Zhang, Y., Zhu, D., et al. (2019). Recent Advances in Nanomaterials-Based Chemo-Photothermal Combination Therapy for Improving Cancer Treatment. Front. Bioeng. Biotechnol. 7, 293. doi:10.3389/fbioe.2019.00293
Liang, C., Guo, B., Wu, H., Shao, N., Li, D., Liu, J., et al. (2015). Aptamer-functionalized Lipid Nanoparticles Targeting Osteoblasts as a Novel RNA Interference-Based Bone Anabolic Strategy. Nat. Med. 21 (3), 288–294. doi:10.1038/nm.3791
Liang, S., Zhou, Q., Wang, M., Zhu, Y., Wu, Q., and Yang, X. (2015). Water-soluble L-Cysteine-Coated FePt Nanoparticles as Dual MRI/CT Imaging Contrast Agent for Glioma. Int. J. Nanomedicine 10, 2325–2333. doi:10.2147/IJN.S75174
Lim, E. K., Chung, B. H., and Chung, S. J. (2018). Recent Advances in pH-Sensitive Polymeric Nanoparticles for Smart Drug Delivery in Cancer Therapy. Curr. Drug Targets 19 (4), 300–317. doi:10.2174/1389450117666160602202339
Lim, S. I., Ojea-Jiménez, I., Varon, M., Casals, E., Arbiol, J., and Puntes, V. (2010). Synthesis of Platinum Cubes, Polypods, Cuboctahedrons, and Raspberries Assisted by Cobalt Nanocrystals. Nano Lett. 10 (3), 964–973. doi:10.1021/nl100032c
Lin, M. M., Kim, D. K., El Haj, A. J., and Dobson, J. (2008). Development of Superparamagnetic Iron Oxide Nanoparticles (SPIONS) for Translation to Clinical Applications. IEEE Trans. Nanobioscience 7 (4), 298–305. doi:10.1109/TNB.2008.2011864
Liu, Z., Lee, J. Y., Han, M., Chen, W., and Gan, L. M. (2002). Synthesis and Characterization of PtRu/C Catalysts from Microemulsions and Emulsions. J. Mater. Chem. 12 (8), 2453–2458. doi:10.1039/b200875k
López Ruiz, A., Bartomeu Garcia, C., Navarro Gallón, S., and Webster, T. J. (2020). Novel Silver-Platinum Nanoparticles for Anticancer and Antimicrobial Applications. Int. J. Nanomedicine. 15, 169–179. doi:10.2147/IJN.S176737
López Ruiz, A., Bartomeu Garcia, C., Navarro Gallón, S., and Webster, T. J. (2020). Novel Silver-Platinum Nanoparticles for Anticancer and Antimicrobial Applications. Int. J. Nanomedicine 15, 169–179. doi:10.2147/IJN.S176737
López, T., Figueras, F., Manjarrez, J., Bustos, J., Alvarez, M., Silvestre-Albero, J., et al. (2010). Catalytic Nanomedicine: a New Field in Antitumor Treatment Using Supported Platinum Nanoparticles. In Vitro DNA Degradation and In Vivo Tests with C6 Animal Model on Wistar Rats. Eur. J. Med. Chem. 45 (5), 1982–1990. doi:10.1016/j.ejmech.2010.01.043
Ma, H., Zhang, X., Li, X., Li, R., Du, B., and Wei, Q. (2015). Electrochemical Immunosensor for Detecting Typical Bladder Cancer Biomarker Based on Reduced Graphene Oxide-Tetraethylene Pentamine and Trimetallic AuPdPt Nanoparticles. Talanta 143, 77–82. doi:10.1016/j.talanta.2015.05.029
Ma, Y., Zhang, D. Y., Peng, Z., Guan, S., and Zhai, J. (2020). Delivery of Platinum(IV) Prodrugs via Bi2Te3 Nanoparticles for Photothermal-Chemotherapy and Photothermal/Photoacoustic Imaging. Mol. Pharm. 17 (9), 3403–3411. doi:10.1021/acs.molpharmaceut.0c00458
Maeda, H. (2001). The Enhanced Permeability and Retention (EPR) Effect in Tumor Vasculature: the Key Role of Tumor-Selective Macromolecular Drug Targeting. Adv. Enzyme Regul. 41, 189–207. doi:10.1016/s0065-2571(00)00013-3
Maicu, M., Schmittgens, R., Hecker, D., Glöß, D., Frach, P., and Gerlach, G. (2014). Synthesis and Deposition of Metal Nanoparticles by Gas Condensation Process. J. Vacuum Sci. Technology A: Vacuum, Surf. Films 32 (2), 02B113. doi:10.1116/1.4859260
Mailänder, V., and Landfester, K. (2009). Interaction of Nanoparticles with Cells. Biomacromolecules 10 (9), 2379–2400. doi:10.1021/bm900266r
Malik, P., Shankar, R., Malik, V., Sharma, N., and Mukherjee, T. K. (2014). Green Chemistry Based Benign Routes for Nanoparticle Synthesis. J. Nanoparticles 2014, 1. doi:10.1155/2014/302429
Mandal, D., Bolander, M. E., Mukhopadhyay, D., Sarkar, G., and Mukherjee, P. (2006). The Use of Microorganisms for the Formation of Metal Nanoparticles and Their Application. Appl. Microbiol. Biotechnol. 69 (5), 485–492. doi:10.1007/s00253-005-0179-3
Manikandan, M., Hasan, N., and Wu, H. F. (2013). Platinum Nanoparticles for the Photothermal Treatment of Neuro 2A Cancer Cells. Biomaterials 34 (23), 5833–5842. doi:10.1016/j.biomaterials.2013.03.077
Marslin, G., Siram, K., Maqbool, Q., Selvakesavan, R. K., Kruszka, D., Kachlicki, P., et al. (2018). Secondary Metabolites in the green Synthesis of Metallic Nanoparticles. Materials (Basel) 11 (6), 940. doi:10.3390/ma11060940
Martins, M., Mourato, C., Sanches, S., Noronha, J. P., Crespo, M. T. B., and Pereira, I. A. C. (2017). Biogenic Platinum and Palladium Nanoparticles as New Catalysts for the Removal of Pharmaceutical Compounds. Water Res. 108, 160–168. doi:10.1016/j.watres.2016.10.071
Mashwani, Z. U., Khan, M. A., Khan, T., and Nadhman, A. (2016). Applications of Plant Terpenoids in the Synthesis of Colloidal Silver Nanoparticles. Adv. Colloid Interf. Sci 234, 132–141. doi:10.1016/j.cis.2016.04.008
Mathew, G., Narayanan, N., Abraham, D. A., De, M., and Neppolian, B. (2021). Facile Green Approach for Developing Electrochemically Reduced Graphene Oxide-Embedded Platinum Nanoparticles for Ultrasensitive Detection of Nitric Oxide. ACS omega 6 (12), 8068–8080. doi:10.1021/acsomega.0c05644
Mathiesen, J. K., Quinson, J., Dworzak, A., Vosch, T., Juelsholt, M., Kjær, E. T. S., et al. (2021). Insights from In Situ Studies on the Early Stages of Platinum Nanoparticle Formation. J. Phys. Chem. Lett. 12 (12), 3224–3231. doi:10.1021/acs.jpclett.1c00241
McClung, M., Harris, S. T., Miller, P. D., Bauer, D. C., Davison, K. S., Dian, L., et al. (2013). Bisphosphonate Therapy for Osteoporosis: Benefits, Risks, and Drug holiday. Am. J. Med. 126 (1), 13–20. doi:10.1016/j.amjmed.2012.06.023
McNamara, J. O., Andrechek, E. R., Wang, Y., Viles, K. D., Rempel, R. E., Gilboa, E., et al. (2006). Cell Type-specific Delivery of siRNAs with Aptamer-siRNA Chimeras. Nat. Biotechnol. 24 (8), 1005–1015. doi:10.1038/nbt1223
Medhat, A., Mansour, S., El-Sonbaty, S., Kandil, E., and Mahmoud, M. (2017). Evaluation of the Antitumor Activity of Platinum Nanoparticles in the Treatment of Hepatocellular Carcinoma Induced in Rats. Tumour Biol. 39 (7), 1010428317717259. doi:10.1177/1010428317717259
Melancon, M. P., Lu, W., Yang, Z., Zhang, R., Cheng, Z., Elliot, A. M., et al. (2008). In Vitro and In Vivo Targeting of Hollow Gold Nanoshells Directed at Epidermal Growth Factor Receptor for Photothermal Ablation Therapy. Mol. Cancer Ther. 7 (6), 1730–1739. doi:10.1158/1535-7163.MCT-08-0016
Meltzer, S., Resch, R., Koel, B. E., Thompson, M. E., Madhukar, A., Requicha, A. A. G., et al. (2001). Fabrication of Nanostructures by Hydroxylamine Seeding of Gold Nanoparticle Templates. Langmuir 17 (5), 1713–1718. doi:10.1021/la001170s
Miyabayashi, K., Nakamura, S., and Miyake, M. (2011). Synthesis of Small Platinum Cube with Less Than 3 Nm by the Control of Growth Kinetics. Cryst. Growth Des. 11 (10), 4292–4295. doi:10.1021/cg200937u
Mohanpuria, P., Rana, N. K., and Yadav, S. K. (2008). Biosynthesis of Nanoparticles: Technological Concepts and Future Applications. J. Nanopart Res. 10 (3), 507–517. doi:10.1007/s11051-007-9275-x
Morton, S. W., Shah, N. J., Quadir, M. A., Deng, Z. J., Poon, Z., and Hammond, P. T. (2014). Osteotropic Therapy via Targeted Layer-By-Layer Nanoparticles. Adv. Healthc. Mater. 3 (6), 867–875. doi:10.1002/adhm.201300465
Mukherjee, P., Roy, M., Mandal, B. P., Dey, G. K., Mukherjee, P. K., Ghatak, J., et al. (2008). Green Synthesis of Highly Stabilized Nanocrystalline Silver Particles by a Non-pathogenic and Agriculturally Important Fungus T. Asperellum. Nanotechnology 19 (7), 075103. doi:10.1088/0957-4484/19/7/075103
Mukherjee, S., Kotcherlakota, R., Haque, S., Bhattacharya, D., Kumar, J. M., Chakravarty, S., et al. (2020). Improved Delivery of Doxorubicin Using Rationally Designed PEGylated Platinum Nanoparticles for the Treatment of Melanoma. Mater. Sci. Eng. C Mater. Biol. Appl. 108, 110375. doi:10.1016/j.msec.2019.110375
Mukherjee, S., Bollu, V. S., Roy, A., Nethi, S. K., Madhusudana, K., Kumar, J. M., et al. (2021). Acute Toxicity, Biodistribution, and Pharmacokinetics Studies of Pegylated Platinum Nanoparticles in Mouse Model. Adv. Nanobio Res. 1 (7), 2000082. doi:10.1002/anbr.202000082
Mukunthan, K. S., and Balaji, S. (2012). Cashew Apple Juice (Anacardium occidentaleL.) Speeds up the Synthesis of Silver Nanoparticles. Int. J. Green Nanotechnology 4 (2), 71–79. doi:10.1080/19430892.2012.676900
N Madlum, K., Jasim Khamees, E., Abdulridha Ahmed, S., and Akram Naji, R. (2021). Antimicrobial and Cytotoxic Activity of Platinum Nanoparticles Synthesized by Laser Ablation Technique. J. Nanostructures 11 (1), 13–19.
Nadimpalli, N. K. V., Bandyopadhyaya, R., and Runkana, V. (2018). Thermodynamic Analysis of Hydrothermal Synthesis of Nanoparticles. Fluid Phase Equilibria 456, 33–45. doi:10.1016/j.fluid.2017.10.002
Narayanan, K. B., and Sakthivel, N. (2010). Biological Synthesis of Metal Nanoparticles by Microbes. Adv. Colloid Interf. Sci 156 (1), 1–13. doi:10.1016/j.cis.2010.02.001
Nascimento, A. V., Singh, A., Bousbaa, H., Ferreira, D., Sarmento, B., and Amiji, M. M. (2015). Combinatorial-designed Epidermal Growth Factor Receptor-Targeted Chitosan Nanoparticles for Encapsulation and Delivery of Lipid-Modified Platinum Derivatives in Wild-type and Resistant Non-small-cell Lung Cancer Cells. Mol. Pharm. 12 (12), 4466–4477. doi:10.1021/acs.molpharmaceut.5b00642
Nath, D., and Banerjee, P. (2013). Green Nanotechnology - a new hope for Medical Biology. Environ. Toxicol. Pharmacol. 36 (3), 997–1014. doi:10.1016/j.etap.2013.09.002
Neha, D., and Momin, M. Metallic Nanoparticles as Drug Delivery System for the Treatment of Cancer. 2021:1–30.doi:10.1080/17425247.2021.1912008
Nichols, W. T., Sasaki, T., and Koshizaki, N. (2006). Laser Ablation of a Platinum Target in Water. I. Ablation Mechanisms. J. Appl. Phys. 100 (11), 114911. doi:10.1063/1.2390640
Nida, D. L., Rahman, M. S., Carlson, K. D., Richards-Kortum, R., and Follen, M. (2005). Fluorescent Nanocrystals for Use in Early Cervical Cancer Detection. Gynecol. Oncol. 99 (3), S89–S94. doi:10.1016/j.ygyno.2005.07.050
Okitsu, K., Yue, A., Tanabe, S., and Matsumoto, H. (2000). Sonochemical Preparation and Catalytic Behavior of Highly Dispersed Palladium Nanoparticles on Alumina. Chem. Mater. 12 (10), 3006–3011. doi:10.1021/cm0001915
Oliveira, B. L., Stenton, B. J., Unnikrishnan, V. B., de Almeida, C. R., Conde, J., Negrão, M., et al. (2020). Platinum-triggered Bond-Cleavage of Pentynoyl Amide and N-Propargyl Handles for Drug-Activation. J. Am. Chem. Soc. 142 (24), 10869–10880. doi:10.1021/jacs.0c01622
Paraskar, A., Soni, S., Basu, S., Amarasiriwardena, C. J., Lupoli, N., Srivats, S., et al. (2011). Rationally Engineered Polymeric Cisplatin Nanoparticles for Improved Antitumor Efficacy. Nanotechnology 22 (26), 265101. doi:10.1088/0957-4484/22/26/265101
Paraskar, A., Soni, S., Roy, B., Papa, A. L., and Sengupta, S. (2012). Rationally Designed Oxaliplatin-Nanoparticle for Enhanced Antitumor Efficacy. Nanotechnology 23 (7), 075103. doi:10.1088/0957-4484/23/7/075103
Paraskar, A. S., Soni, S., Chin, K. T., Chaudhuri, P., Muto, K. W., Berkowitz, J., et al. (2010). Harnessing Structure-Activity Relationship to Engineer a Cisplatin Nanoparticle for Enhanced Antitumor Efficacy. Proc. Natl. Acad. Sci. U S A. 107 (28), 12435–12440. doi:10.1073/pnas.1007026107
Park, E. J., Kim, H., Kim, Y., and Park, K. (2010). Intratracheal Instillation of Platinum Nanoparticles May Induce Inflammatory Responses in Mice. Arch. Pharm. Res. 33 (5), 727–735. doi:10.1007/s12272-010-0512-y
Passalaris, T. M., Benanti, J. A., Gewin, L., Kiyono, T., and Galloway, D. A. (1999). The G(2) Checkpoint Is Maintained by Redundant Pathways. Mol. Cel Biol 19 (9), 5872–5881. doi:10.1128/mcb.19.9.5872
Patel, P., Nadar, V. M., Umapathy, D., Manivannan, S., Venkatesan, R., Joseph Arokiyam, V. A., et al. (2020). Doxorubicin-Conjugated Platinum Theranostic Nanoparticles Induce Apoptosis via Inhibition of a Cell Survival (PI3K/AKT) Signaling Pathway in Human Breast Cancer Cells. ACS Appl. Nano Mater. 4 (1), 198–210. doi:10.1021/acsanm.0c02521
Patra, C. R., Bhattacharya, R., Wang, E., Katarya, A., Lau, J. S., Dutta, S., et al. (2008). Targeted Delivery of Gemcitabine to Pancreatic Adenocarcinoma Using Cetuximab as a Targeting Agent. Cancer Res. 68 (6), 1970–1978. doi:10.1158/0008-5472.CAN-07-6102
Pelaz, B., del Pino, P., Maffre, P., Hartmann, R., Gallego, M., Rivera-Fernández, S., et al. (2015). Surface Functionalization of Nanoparticles with Polyethylene Glycol: Effects on Protein Adsorption and Cellular Uptake. ACS Nano 9 (7), 6996–7008. doi:10.1021/acsnano.5b01326
Pelicano, H., Martin, D. S., Xu, R. H., and Huang, P. (2006). Glycolysis Inhibition for Anticancer Treatment. Oncogene 25 (34), 4633–4646. doi:10.1038/sj.onc.1209597
Pelka, J., Gehrke, H., Esselen, M., Türk, M., Crone, M., Bräse, S., et al. (2009). Cellular Uptake of Platinum Nanoparticles in Human colon Carcinoma Cells and Their Impact on Cellular Redox Systems and DNA Integrity. Chem. Res. Toxicol. 22 (4), 649–659. doi:10.1021/tx800354g
Peng, X. H., Wang, Y., Huang, D., Wang, Y., Shin, H. J., Chen, Z., et al. (2011). Targeted Delivery of Cisplatin to Lung Cancer Using ScFvEGFR-Heparin-Cisplatin Nanoparticles. Acs Nano 5 (12), 9480–9493. doi:10.1021/nn202410f
Porcel, E., Liehn, S., Remita, H., Usami, N., Kobayashi, K., Furusawa, Y., et al. (2010). Platinum Nanoparticles: a Promising Material for Future Cancer Therapy? Nanotechnology 21 (8), 85103. doi:10.1088/0957-4484/21/8/085103
Prasek, M., Sawosz, E., Jaworski, S., Grodzik, M., Ostaszewska, T., Kamaszewski, M., et al. (2013). Influence of Nanoparticles of Platinum on Chicken Embryo Development and Brain Morphology. Nanoscale Res. Lett. 8 (1), 251–259. doi:10.1186/1556-276X-8-251
Puja, P., and Kumar, P. (2019). A Perspective on Biogenic Synthesis of Platinum Nanoparticles and Their Biomedical Applications. Spectrochim Acta A. Mol. Biomol. Spectrosc. 211, 94–99. doi:10.1016/j.saa.2018.11.047
Quarta, A., Amorín, M., Aldegunde, M. J., Blasi, L., Ragusa, A., Nitti, S., et al. (2019). Novel Synthesis of Platinum Complexes and Their Intracellular Delivery to Tumor Cells by Means of Magnetic Nanoparticles. Nanoscale 11 (48), 23482–23497. doi:10.1039/c9nr07015j
Quinson, J., and Jensen, K. M. Ø. (2020). From Platinum Atoms in Molecules to Colloidal Nanoparticles: a Review on Reduction, Nucleation and Growth Mechanisms. Adv. Colloid Interf. Sci 286, 102300. doi:10.1016/j.cis.2020.102300
Ramanlal Chaudhari, K., Kumar, A., Megraj Khandelwal, V. K., Ukawala, M., Manjappa, A. S., Mishra, A. K., et al. (2012). Bone Metastasis Targeting: a Novel Approach to Reach Bone Using Zoledronate Anchored PLGA Nanoparticle as Carrier System Loaded with Docetaxel. J. Control. Release 158 (3), 470–478. doi:10.1016/j.jconrel.2011.11.020
Reedijk, J. (1987). The Mechanism of Action of Platinum Antitumor Drugs. Pure Appl. Chem. 59 (2), 181–192. doi:10.1351/pac198759020181
Reetz, M. T., and Koch, M. G. (1999). Water-soluble Colloidal Adams Catalyst: Preparation and Use in Catalysis. J. Am. Chem. Soc. 121 (34), 7933–7934. doi:10.1021/ja9906498
Riddin, T., Gericke, M., and Whiteley, C. G. (2010). Biological Synthesis of Platinum Nanoparticles: Effect of Initial Metal Concentration. Enzyme Microb. Technol. 46 (6), 501–505. doi:10.1016/j.enzmictec.2010.02.006
Riddin, T. L., Gericke, M., and Whiteley, C. G. (2006). Analysis of the Inter- and Extracellular Formation of Platinum Nanoparticles by Fusarium Oxysporum F. Sp. Lycopersici Using Response Surface Methodology. Nanotechnology 17 (14), 3482–3489. doi:10.1088/0957-4484/17/14/021
Sadhukha, T., and Prabha, S. (2014). Encapsulation in Nanoparticles Improves Anti-cancer Efficacy of Carboplatin. Aaps Pharmscitech 15 (4), 1029–1038. doi:10.1208/s12249-014-0139-2
Şahin, B., Aygün, A., Gündüz, H., Şahin, K., Demir, E., Akocak, S., et al. (2018). Cytotoxic Effects of Platinum Nanoparticles Obtained from Pomegranate Extract by the green Synthesis Method on the MCF-7 Cell Line. Colloids Surf. B: Biointerfaces 163, 119–124.
Saitoh, Y., Kawasaki, N., Eguchi, N., and Ikeshima, M. (2021). Combined Treatment with Dissolved Hydrogen Molecule and Platinum Nanocolloid Exerts Carcinostatic/carcinocidal Effects by Increasing Hydrogen Peroxide Generation and Cell Death in the Human Gastric Cancer Cell Line NUGC-4. Free Radic. Res. 55, 1–10. doi:10.1080/10715762.2021.1902514
Samadi, A., Klingberg, H., Jauffred, L., Kjær, A., Bendix, P. M., and Oddershede, L. B. (2018). Platinum Nanoparticles: a Non-toxic, Effective and Thermally Stable Alternative Plasmonic Material for Cancer Therapy and Bioengineering. Nanoscale 10 (19), 9097–9107. doi:10.1039/c8nr02275e
Saminathan, K., Kamavaram, V., Veedu, V., and Kannan, A. M. (2009). Preparation and Evaluation of Electrodeposited Platinum Nanoparticles on In Situ Carbon Nanotubes Grown Carbon Paper for Proton Exchange Membrane Fuel Cells. Int. J. Hydrogen Energ. 34 (9), 3838–3844. doi:10.1016/j.ijhydene.2009.03.009
Sánchez-Ramírez, D. R., Domínguez-Ríos, R., Juárez, J., Valdés, M., Hassan, N., Quintero-Ramos, A., et al. (2020). Biodegradable Photoresponsive Nanoparticles for Chemo-, Photothermal-And Photodynamic Therapy of Ovarian Cancer. Mater. Sci. Eng. C. 116, 111196.
Sankar, R., Manikandan, P., Malarvizhi, V., Fathima, T., Shivashangari, K. S., and Ravikumar, V. (2014). Green Synthesis of Colloidal Copper Oxide Nanoparticles Using Carica Papaya and its Application in Photocatalytic Dye Degradation. Spectrochim Acta A. Mol. Biomol. Spectrosc. 121, 746–750. doi:10.1016/j.saa.2013.12.020
Sathishkumar, M., Sneha, K., and Yun, Y. S. (2010). Immobilization of Silver Nanoparticles Synthesized Using Curcuma Longa Tuber Powder and Extract on Cotton Cloth for Bactericidal Activity. Bioresour. Technol. 101 (20), 7958–7965. doi:10.1016/j.biortech.2010.05.051
Schroeder, A., Heller, D. A., Winslow, M. M., Dahlman, J. E., Pratt, G. W., Langer, R., et al. (2011). Treating Metastatic Cancer with Nanotechnology. Nat. Rev. Cancer 12 (1), 39–50. doi:10.1038/nrc3180
Setua, S., Ouberai, M., Piccirillo, S. G., Watts, C., and Welland, M. (2014). Cisplatin-tethered Gold Nanospheres for Multimodal Chemo-Radiotherapy of Glioblastoma. Nanoscale 6 (18), 10865–10873. doi:10.1039/c4nr03693j
Shafabakhsh, R., Pourhanifeh, M. H., Mirzaei, H. R., Sahebkar, A., Asemi, Z., and Mirzaei, H. (2019). Targeting Regulatory T Cells by Curcumin: A Potential for Cancer Immunotherapy. Pharmacol. Res. 147, 104353. doi:10.1016/j.phrs.2019.104353
Shafiei, M., Riahi, A. R., Sen, F. G., and Alpas, A. T. (2010). Improvement of Platinum Adhesion to Carbon Surfaces Using PVD Coatings. Surf. Coat. Technology 205 (2), 306–311. doi:10.1016/j.surfcoat.2010.06.050
Shahverdi, A.-R., Shakibaie, M., and Nazari, P. (2011). “Basic and Practical Procedures for Microbial Synthesis of Nanoparticles,” in Metal Nanoparticles in Microbiology (Springer), 177–195. doi:10.1007/978-3-642-18312-6_8
Shamsuddin, A. M. (1999). Metabolism and Cellular Functions of IP6: a Review. Anticancer Res. 19 (5), 3733–3736.
Shamsuddin, A. M., Vucenik, I., and Cole, K. E. (1997). IP6: a Novel Anti-cancer Agent. Life Sci. 61 (4), 343–354. doi:10.1016/s0024-3205(97)00092-1
Shankar, S. S., Rai, A., Ankamwar, B., Singh, A., Ahmad, A., and Sastry, M. (2004). Biological Synthesis of Triangular Gold Nanoprisms. Nat. Mater. 3 (7), 482–488. doi:10.1038/nmat1152
Shi, C., Yu, H., Sun, D., Ma, L., Tang, Z., Xiao, Q., et al. (2015). Cisplatin-loaded Polymeric Nanoparticles: Characterization and Potential Exploitation for the Treatment of Non-small Cell Lung Carcinoma. Acta Biomater. 18, 68–76. doi:10.1016/j.actbio.2015.02.009
Shi, H., Xu, M., Zhu, J., Li, Y., He, Z., Zhang, Y., et al. (2020). Programmed Co-delivery of Platinum Nanodrugs and Gemcitabine by a Clustered Nanocarrier for Precision Chemotherapy for NSCLC Tumors. J. Mater. Chem. B 8 (2), 332–342. doi:10.1039/c9tb02055a
Shi, J., Xiao, Z., Kamaly, N., and Farokhzad, O. C. (2011). Self-assembled Targeted Nanoparticles: Evolution of Technologies and Bench to Bedside Translation. Acc. Chem. Res. 44 (10), 1123–1134. doi:10.1021/ar200054n
Shin, S. S., Noh, D. H., Hwang, B., Lee, J. W., Park, S. L., Park, S. S., et al. (2018). Inhibitory Effect of Au@Pt-NSs on Proliferation, Migration, and Invasion of EJ Bladder Carcinoma Cells: Involvement of Cell Cycle Regulators, Signaling Pathways, and Transcription Factor-Mediated MMP-9 Expression. Int. J. Nanomedicine 13, 3295–3310. doi:10.2147/IJN.S158463
Shingu, T., Chumbalkar, V. C., Gwak, H. S., Fujiwara, K., Kondo, S., Farrell, N. P., et al. (2010). The Polynuclear Platinum BBR3610 Induces G2/M Arrest and Autophagy Early and Apoptosis Late in Glioma Cells. Neuro Oncol. 12 (12), 1269–1277. doi:10.1093/neuonc/noq095
Shoshan, M. S., Vonderach, T., Hattendorf, B., and Wennemers, H. (2019). Peptide-Coated Platinum Nanoparticles with Selective Toxicity against Liver Cancer Cells. Angew. Chem. Int. Ed. Engl. 58 (15), 4901–4905. doi:10.1002/anie.201813149
Shubayev, V. I., Pisanic, T. R., and Jin, S. (2009). Magnetic Nanoparticles for Theragnostics. Adv. Drug Deliv. Rev. 61 (6), 467–477. doi:10.1016/j.addr.2009.03.007
Shukla, N., Svedberg, E. B., and Ell, J. (2007). Surfactant Isomerization and Dehydrogenation on FePt Nanoparticles. Colloids Surf. A: Physicochemical Eng. Aspects 301 (1-3), 113–116. doi:10.1016/j.colsurfa.2006.12.031
Siegel, R. L., Miller, K. D., Jemal, A., and Jemal, A. (2021). Cancer Statistics, 2017. CA Cancer J. Clin. 67 (1), 7–30. doi:10.3322/caac.21387
Sorenson, C. M., Barry, M. A., and Eastman, A. (1990). Analysis of Events Associated with Cell Cycle Arrest at G2 Phase and Cell Death Induced by Cisplatin. J. Natl. Cancer Inst. 82 (9), 749–755. doi:10.1093/jnci/82.9.749
Stepanov, A., Golubev, A., Nikitin, S., and Osin, Y. (2014). A Review on the Fabrication and Properties of Platinum Nanoparticles. Rev. Adv. Mater. Sci. 38 (2), 160–175.
Sun, H., Chen, X., Chen, D., Dong, M., Fu, X., Li, Q., et al. (2012). Influences of Surface Coatings and Components of FePt Nanoparticles on the Suppression of Glioma Cell Proliferation. Int. J. Nanomedicine 7, 3295–3307. doi:10.2147/IJN.S32678
Sun, Y., Miao, H., Ma, S., Zhang, L., You, C., Tang, F., et al. (2018). FePt-Cys Nanoparticles Induce ROS-dependent Cell Toxicity, and Enhance Chemo-Radiation Sensitivity of NSCLC Cells In Vivo and In Vitro. Cancer Lett. 418, 27–40. doi:10.1016/j.canlet.2018.01.024
Sung, H., Ferlay, J., Siegel, R. L., Laversanne, M., Soerjomataram, I., Jemal, A., et al. (2021). Global Cancer Statistics 2020: GLOBOCAN Estimates of Incidence and Mortality Worldwide for 36 Cancers in 185 Countries. CA Cancer J. Clin. 71 (3), 209–249. doi:10.3322/caac.21660
Syed, A., and Ahmad, A. (2012). Extracellular Biosynthesis of Platinum Nanoparticles Using the Fungus Fusarium Oxysporum. Colloids Surf. B Biointerfaces 97, 27–31. doi:10.1016/j.colsurfb.2012.03.026
Sykes, E. A., Chen, J., Zheng, G., and Chan, W. C. (2014). Investigating the Impact of Nanoparticle Size on Active and Passive Tumor Targeting Efficiency. ACS nano 8 (6), 5696–5706. doi:10.1021/nn500299p
Tanaka, M., Okinaga, T., Iwanaga, K., Matsuo, K., Toyono, T., Sasaguri, M., et al. (2019). Anticancer Effect of Novel Platinum Nanocomposite Beads on Oral Squamous Cell Carcinoma Cells. J. Biomed. Mater. Res. B Appl. Biomater. 107 (7), 2281–2287. doi:10.1002/jbm.b.34320
Tao, A. R., Habas, S., and Yang, P. (2008). Shape Control of Colloidal Metal Nanocrystals. small 4 (3), 310–325. doi:10.1002/smll.200701295
Taurino, I., Sanzó, G., Mazzei, F., Favero, G., De Micheli, G., and Carrara, S. (2015). Fast Synthesis of Platinum Nanopetals and Nanospheres for Highly-Sensitive Non-enzymatic Detection of Glucose and Selective Sensing of Ions. Sci. Rep. 5 (1), 15277. doi:10.1038/srep15277
Taylor, R. M., Huber, D. L., Monson, T. C., Ali, A. M., Bisoffi, M., and Sillerud, L. O. (2011). Multifunctional Iron Platinum Stealth Immunomicelles: Targeted Detection of Human Prostate Cancer Cells Using Both Fluorescence and Magnetic Resonance Imaging. J. Nanopart Res. 13 (10), 4717–4729. doi:10.1007/s11051-011-0439-3
Taylor, R. M., and Sillerud, L. O. (2012). Paclitaxel-loaded Iron Platinum Stealth Immunomicelles Are Potent MRI Imaging Agents that Prevent Prostate Cancer Growth in a PSMA-dependent Manner. Int. J. Nanomedicine 7, 4341–4352. doi:10.2147/IJN.S34381
Teng, X., Liang, X., Maksimuk, S., and Yang, H. (2006). Synthesis of Porous Platinum Nanoparticles. Small 2 (2), 249–253. doi:10.1002/smll.200500244
Thamake, S. I., Raut, S. L., Gryczynski, Z., Ranjan, A. P., and Vishwanatha, J. K. (2012). Alendronate Coated Poly-Lactic-Co-Glycolic Acid (PLGA) Nanoparticles for Active Targeting of Metastatic Breast Cancer. Biomaterials 33 (29), 7164–7173. doi:10.1016/j.biomaterials.2012.06.026
Torchilin, V. P. (2005). Recent Advances with Liposomes as Pharmaceutical Carriers. Nat. Rev. Drug Discov. 4 (2), 145–160. doi:10.1038/nrd1632
Treuel, L., Brandholt, S., Maffre, P., Wiegele, S., Shang, L., and Nienhaus, G. U. (2014). Impact of Protein Modification on the Protein corona on Nanoparticles and Nanoparticle-Cell Interactions. ACS Nano 8 (1), 503–513. doi:10.1021/nn405019v
Tsai, H. I., Jiang, L., Zeng, X., Chen, H., Li, Z., Cheng, W., et al. (2018). DACHPt-Loaded Nanoparticles Self-Assembled from Biodegradable Dendritic Copolymer Polyglutamic Acid-B-D-α-Tocopheryl Polyethylene Glycol 1000 Succinate for Multidrug Resistant Lung Cancer Therapy. Front. Pharmacol. 9, 119. doi:10.3389/fphar.2018.00119
Tsai, M. H., Pan, C. H., Peng, C. L., and Shieh, M. J. (2017). Panitumumab-Conjugated Pt-Drug Nanomedicine for Enhanced Efficacy of Combination Targeted Chemotherapy against Colorectal Cancer. Adv. Healthc. Mater. 6 (13), 1700111. doi:10.1002/adhm.201700111
Tseng, C.-L., Chang, K.-C., Yeh, M.-C., Yang, K.-C., Tang, T.-P., and Lin, F.-H. (2014). Development of a Dual-Functional Pt-Fe-HAP Magnetic Nanoparticles Application for Chemo-Hyperthermia Treatment of Cancer. Ceramics Int. 40 (4), 5117–5127. doi:10.1016/j.ceramint.2013.09.137
Tsukada, H. (2011). Application of Pre-clinical PET Imaging for Drug Development. Nihon Shinkei Seishin Yakurigaku Zasshi 31 (5-6), 231–237.
Turiel-Fernández, D., Gutiérrez-Romero, L., Corte-Rodriguez, M., Bettmer, J., and Montes-Bayón, M. (2021). Ultrasmall Iron Oxide Nanoparticles Cisplatin (IV) Prodrug Nanoconjugate: ICP-MS Based Strategies to Evaluate the Formation and Drug Delivery Capabilities in Single Cells. Analytica Chim. Acta 1159, 338356.
Ullah, S., Ahmad, A., Wang, A., Raza, M., Jan, A. U., Tahir, K., et al. (2017). Bio-fabrication of Catalytic Platinum Nanoparticles and Their In Vitro Efficacy against Lungs Cancer Cells Line (A549). J. Photochem. Photobiol. B 173, 368–375. doi:10.1016/j.jphotobiol.2017.06.018
Vachal, P., Hale, J. J., Lu, Z., Streckfuss, E. C., Mills, S. G., MacCoss, M., et al. (2006). Synthesis and Study of Alendronate Derivatives as Potential Prodrugs of Alendronate Sodium for the Treatment of Low Bone Density and Osteoporosis. J. Med. Chem. 49 (11), 3060–3063. doi:10.1021/jm060398v
Vucenik, I., and Shamsuddin, A. M. (2006). Protection against Cancer by Dietary IP6 and Inositol. Nutr. Cancer 55 (2), 109–125. doi:10.1207/s15327914nc5502_1
Waag, F., Streubel, R., Gökce, B., and Barcikowski, S. (2021). Synthesis of Gold, Platinum, and Gold-Platinum alloy Nanoparticle Colloids with High-Power Megahertz-Repetition-Rate Lasers: the Importance of the Beam Guidance Method. Appl. Nanosci 11 (4), 1303–1312. doi:10.1007/s13204-021-01693-y
Wang, D., Miller, S. C., Shlyakhtenko, L. S., Portillo, A. M., Liu, X. M., Papangkorn, K., et al. (2007). Osteotropic Peptide that Differentiates Functional Domains of the Skeleton. Bioconjug. Chem. 18 (5), 1375–1378. doi:10.1021/bc7002132
Wang, H., Jeong, H. Y., Imura, M., Wang, L., Radhakrishnan, L., Fujita, N., et al. (2011). Shape- and Size-Controlled Synthesis in Hard Templates: Sophisticated Chemical Reduction for Mesoporous Monocrystalline Platinum Nanoparticles. J. Am. Chem. Soc. 133 (37), 14526–14529. doi:10.1021/ja2058617
Wang, H., Qiu, Z., Liu, H., Jayawardhana, A. M. D. S., Yue, Z., Daghlas, H., et al. (2019). Nanoparticles of Metal-Organic Cages Overcoming Drug Resistance in Ovarian Cancer. Front. Chem. 7, 39. doi:10.3389/fchem.2019.00039
Wang, L., and Yamauchi, Y. (2009). Block Copolymer Mediated Synthesis of Dendritic Platinum Nanoparticles. J. Am. Chem. Soc. 131 (26), 9152–9153. doi:10.1021/ja902485x
Wang, L., Wang, H., Nemoto, Y., and Yamauchi, Y. (2010). Rapid and Efficient Synthesis of Platinum Nanodendrites with High Surface Area by Chemical Reduction with Formic Acid. Chem. Mater. 22 (9), 2835–2841. doi:10.1021/cm9038889
Wang, M., Li, H., Li, Y., Mo, F., Li, Z., Chai, R., et al. (2020). Dispersibility and Size Control of Silver Nanoparticles with Anti-algal Potential Based on Coupling Effects of Polyvinylpyrrolidone and Sodium Tripolyphosphate. Nanomaterials (Basel) 10 (6), 1042. doi:10.3390/nano10061042
Wang, X., Chang, Z., Nie, X., Li, Y., Hu, Z., Ma, J., et al. (2019). A Conveniently Synthesized Pt (IV) Conjugated Alginate Nanoparticle with Ligand Self-Shielded Property for Targeting Treatment of Hepatic Carcinoma. Nanomedicine 15 (1), 153–163. doi:10.1016/j.nano.2018.09.012
Wang, X., Yang, Y., Jia, H., Jia, W., Miller, S., Bowman, B., et al. (2014). Peptide Decoration of Nanovehicles to Achieve Active Targeting and Pathology-Responsive Cellular Uptake for Bone Metastasis Chemotherapy. Biomater. Sci. 2 (7), 961–971. doi:10.1039/C4BM00020J
Wawrowicz, K., Majkowska-Pilip, A., Gaweł, D., Chajduk, E., Pieńkowski, T., and Bilewicz, A. (2021). Au@Pt Core-Shell Nanoparticle Bioconjugates for the Therapy of HER2+ Breast Cancer and Hepatocellular Carcinoma. Model Studies on the Applicability of 193mPt and 195mPt Radionuclides in Auger Electron Therapy. Molecules 26 (7), 2051. doi:10.3390/molecules26072051
Wilson, M. S., Livermore, T. M., and Saiardi, A. (2013). Inositol Pyrophosphates: between Signalling and Metabolism. Biochem. J. 452 (3), 369–379. doi:10.1042/BJ20130118
Woodward, E., Jagdev, S., McParland, L., Clark, K., Gregory, W., Newsham, A., et al. (2011). Skeletal Complications and Survival in Renal Cancer Patients with Bone Metastases. Bone 48 (1), 160–166. doi:10.1016/j.bone.2010.09.008
Wu, G., Barth, R. F., Yang, W., Kawabata, S., Zhang, L., and Green-Church, K. (2006). Targeted Delivery of Methotrexate to Epidermal Growth Factor Receptor-Positive Brain Tumors by Means of Cetuximab (IMC-C225) Dendrimer Bioconjugates. Mol. Cancer Ther. 5 (1), 52–59. doi:10.1158/1535-7163.MCT-05-0325
Xie, P., Wang, Y., Wei, D., Zhang, L., Zhang, B., Xiao, H., et al. (2021). Nanoparticle-based Drug Delivery Systems with Platinum Drugs for Overcoming Cancer Drug Resistance. J. Mater. Chem. B 9 (26), 5173–5194. doi:10.1039/d1tb00753j
Xu, C., Yuan, Z., Kohler, N., Kim, J., Chung, M. A., and Sun, S. (2009). FePt Nanoparticles as an Fe Reservoir for Controlled Fe Release and Tumor Inhibition. J. Am. Chem. Soc. 131 (42), 15346–15351. doi:10.1021/ja905938a
Yamada, M., Foote, M., and Prow, T. W. (2015). Therapeutic Gold, Silver, and Platinum Nanoparticles. Wiley Interdiscip. Rev. Nanomed Nanobiotechnol 7 (3), 428–445. doi:10.1002/wnan.1322
Yamagishi, Y., Watari, A., Hayata, Y., Li, X., Kondoh, M., Yoshioka, Y., et al. (2013). Acute and Chronic Nephrotoxicity of Platinum Nanoparticles in Mice. Nanoscale Res. Lett. 8 (1), 395. doi:10.1186/1556-276X-8-395
Yang, C., Wang, M., Zhou, J., and Chi, Q. (2017). Bio-synthesis of Peppermint Leaf Extract Polyphenols Capped Nano-Platinum and Their In-Vitro Cytotoxicity towards colon Cancer Cell Lines (HCT 116). Mater. Sci. Eng. C Mater. Biol. Appl. 77, 1012–1016. doi:10.1016/j.msec.2017.04.020
Yang, J., Eom, K., Lim, E. K., Park, J., Kang, Y., Yoon, D. S., et al. (2008). In Situ detection of Live Cancer Cells by Using Bioprobes Based on Au Nanoparticles. Langmuir 24 (21), 12112–12115. doi:10.1021/la802184m
Yang, Y. T., Shi, Y., Jay, M., and Di Pasqua, A. J. (2014). Enhanced Toxicity of Cisplatin with Chemosensitizer Phenethyl Isothiocyanate toward Non-small Cell Lung Cancer Cells when Delivered in Liposomal Nanoparticles. Chem. Res. Toxicol. 27 (6), 946–948. doi:10.1021/tx5001128
Yin, Q., Tang, L., Cai, K., Tong, R., Sternberg, R., Yang, X., et al. (2016). Pamidronate Functionalized Nanoconjugates for Targeted Therapy of Focal Skeletal Malignant Osteolysis. Proc. Natl. Acad. Sci. U S A. 113 (32), E4601–E4609. doi:10.1073/pnas.1603316113
Yogesh, B., Vineeta, B., Rammesh, N., and Saili, P. (2016). Biosynthesized Platinum Nanoparticles Inhibit the Proliferation of Human Lung-Cancer Cells In Vitro and Delay the Growth of a Human Lung-Tumor Xenograft In Vivo. J. Pharmacopunct 19 (2), 114–121. doi:10.3831/kpi.2016.19.012
Yoshihisa, Y., Zhao, Q. L., Hassan, M. A., Wei, Z. L., Furuichi, M., Miyamoto, Y., et al. (2011). SOD/catalase Mimetic Platinum Nanoparticles Inhibit Heat-Induced Apoptosis in Human Lymphoma U937 and HH Cells. Free Radic. Res. 45 (3), 326–335. doi:10.3109/10715762.2010.532494
Yu, H., Yu, J., Li, L., Zhang, Y., Xin, S., Ni, X., et al. (2021). Recent Progress of the Practical Applications of the Platinum Nanoparticle-Based Electrochemistry Biosensors. Front. Chem. 9. doi:10.3389/fchem.2021.677876
Yu, J., Liu, S., Wang, Y., He, X., Zhang, Q., Qi, Y., et al. (2022). Synergistic Enhancement of Immunological Responses Triggered by Hyperthermia Sensitive Pt NPs via NIR Laser to Inhibit Cancer Relapse and Metastasis. Bioact Mater. 7, 389–400. doi:10.1016/j.bioactmat.2021.05.030
Yuan, Y. G., and Gurunathan, S. (2017). Combination of Graphene Oxide-Silver Nanoparticle Nanocomposites and Cisplatin Enhances Apoptosis and Autophagy in Human Cervical Cancer Cells. Int. J. Nanomedicine 12, 6537–6558. doi:10.2147/IJN.S125281
Zeng, J., Lee, J. Y., and Zhou, W. (2006). Activities of Pt/C Catalysts Prepared by Low Temperature Chemical Reduction Methods. Appl. Catal. A: Gen. 308, 99–104. doi:10.1016/j.apcata.2006.04.019
Zeng, X., Sun, J., Li, S., Shi, J., Gao, H., Sun Leong, W., et al. (2020). Blood-triggered Generation of Platinum Nanoparticle Functions as an Anti-cancer Agent. Nat. Commun. 11 (1), 567. doi:10.1038/s41467-019-14131-z
Zhang, C., Liu, T., Gao, J., Su, Y., and Shi, C. (2010). Recent Development and Application of Magnetic Nanoparticles for Cell Labeling and Imaging. Mini Rev. Med. Chem. 10 (3), 193–202. doi:10.2174/138955710791185073
Zhang, C., Zhao, X., and Guo, H. (2018). Synergic Highly Effective Photothermal-Chemotherapy with Platinum Prodrug Linked Melanin-like Nanoparticles. Artif. Cell Nanomed Biotechnol 46 (Suppl. 2), 356–363. doi:10.1080/21691401.2018.1457536
Zhang, G., Guo, B., Wu, H., Tang, T., Zhang, B. T., Zheng, L., et al. (2012). A Delivery System Targeting Bone Formation Surfaces to Facilitate RNAi-Based Anabolic Therapy. Nat. Med. 18 (2), 307–314. doi:10.1038/nm.2617
Zhang, M., Hagan, C. T., Min, Y., Foley, H., Tian, X., Yang, F., et al. (2018). Nanoparticle Co-delivery of Wortmannin and Cisplatin Synergistically Enhances Chemoradiotherapy and Reverses Platinum Resistance in Ovarian Cancer Models. Biomaterials 169, 1–10. doi:10.1016/j.biomaterials.2018.03.055
Zhang, Q., Kuang, G., Zhou, D., Qi, Y., Wang, M., Li, X., et al. (2020). Photoactivated Polyprodrug Nanoparticles for Effective Light-Controlled Pt(iv) and siRNA Codelivery to Achieve Synergistic Cancer Therapy. J. Mater. Chem. B 8 (27), 5903–5911. doi:10.1039/d0tb01103g
Zhang, R., Ru, Y., Gao, Y., Li, J., and Mao, S. (2017). Layer-by-layer Nanoparticles Co-loading Gemcitabine and Platinum (IV) Prodrugs for Synergistic Combination Therapy of Lung Cancer. Drug Des. Devel Ther. 11, 2631–2642. doi:10.2147/DDDT.S143047
Zhang, X. F., and Gurunathan, S. (2016). Combination of Salinomycin and Silver Nanoparticles Enhances Apoptosis and Autophagy in Human Ovarian Cancer Cells: an Effective Anticancer Therapy. Int. J. Nanomedicine 11, 3655–3675. doi:10.2147/IJN.S111279
Zhao, Y., Chen, F., Pan, Y., Li, Z., Xue, X., Okeke, C. I., et al. (2015). Nanodrug Formed by Coassembly of Dual Anticancer Drugs to Inhibit Cancer Cell Drug Resistance. ACS Appl. Mater. Inter. 7 (34), 19295–19305. doi:10.1021/acsami.5b05347
Zheng, Y., Tang, Y., Bao, Z., Wang, H., Ren, F., Guo, M., et al. (2015). FePt Nanoparticles as a Potential X-ray Activated Chemotherapy Agent for HeLa Cells. Int. J. Nanomedicine 10, 6435–6444. doi:10.2147/IJN.S88458
Zhou, Z., Fan, T., Yan, Y., Zhang, S., Zhou, Y., Deng, H., et al. (2019). One Stone with Two Birds: Phytic Acid-Capped Platinum Nanoparticles for Targeted Combination Therapy of Bone Tumors. Biomaterials 194, 130–138. doi:10.1016/j.biomaterials.2018.12.024
Keywords: platinum nanoparticles, cancer therapy, delivery systems, nanoparticle, cancer
Citation: Abed A, Derakhshan M, Karimi M, Shirazinia M, Mahjoubin-Tehran M, Homayonfal M, Hamblin MR, Mirzaei SA, Soleimanpour H, Dehghani S, Dehkordi FF and Mirzaei H (2022) Platinum Nanoparticles in Biomedicine: Preparation, Anti-Cancer Activity, and Drug Delivery Vehicles. Front. Pharmacol. 13:797804. doi: 10.3389/fphar.2022.797804
Received: 19 October 2021; Accepted: 13 January 2022;
Published: 23 February 2022.
Edited by:
Raquel Montenegro, Federal University of Ceara, BrazilReviewed by:
Hamed Barabadi, Shahid Beheshti University of Medical Sciences, IranSudip Mukherjee, Rice University, United States
Copyright © 2022 Abed, Derakhshan, Karimi, Shirazinia, Mahjoubin-Tehran, Homayonfal, Hamblin, Mirzaei, Soleimanpour, Dehghani, Dehkordi and Mirzaei. This is an open-access article distributed under the terms of the Creative Commons Attribution License (CC BY). The use, distribution or reproduction in other forums is permitted, provided the original author(s) and the copyright owner(s) are credited and that the original publication in this journal is cited, in accordance with accepted academic practice. No use, distribution or reproduction is permitted which does not comply with these terms.
*Correspondence: Hamed Mirzaei, h.mirzaei2002@gmail.com; Sadegh Dehghani, Dehghanis931@mums.ac.ir; Farnaz Farzaneh Dehkordi, farzaneh_farnaz2007@yahoo.com