- 1University of South Florida, Division of Allergy and Immunology, Department of Internal Medicine, College of Medicine, Tampa, FL, United States
- 2Washington University in St. Louis, Brown School, St. Louis, MO, United States
- 3University of South Florida, Department of Molecular Medicine, College of Medicine, Tampa, FL, United States
- 4Department of Zoology, Yogi Vemana University, Kadapa, India
Acute lung injury (ALI) and its severe manifestation, acute respiratory distress syndrome (ARDS), are treated with high concentrations of supplementary oxygen. However, prolonged exposure to high oxygen concentrations stimulates the production of reactive oxygen species (ROS), which damages the mitochondria and accumulates misfolded proteins in the endoplasmic reticulum (ER). The mitochondrial protein A-kinase anchoring protein 1 (Akap1) is critical for mitochondrial homeostasis. It is known that Akap1 deficiency results in heart damage, neuronal development impairment, and mitochondrial malfunction in preclinical studies. Our laboratory recently revealed that deleting Akap1 increases the severity of hyperoxia-induced ALI in mice. To assess the role of Akap1 deletion in ER stress in lung injury, wild-type and Akap1−/− mice were exposed to hyperoxia for 48 h. This study indicates that Akap1−/− mice exposed to hyperoxia undergo ER stress, which is associated with an increased expression of BiP, JNK phosphorylation, eIF2α phosphorylation, ER stress-induced cell death, and autophagy. This work demonstrates that deleting Akap1 results in increased ER stress in the lungs of mice and that hyperoxia exacerbates ER stress-related consequences.
Introduction
The most common treatment for human acute respiratory distress syndrome is supplemental oxygen (Kallet and Matthay, 2013). However, prolonged exposure to high concentrations of supplementary oxygen leads to increased production of reactive oxygen species (ROS). This prolonged exposure induces hyperoxic acute lung injury in rodent models (Galam et al., 2015), leading to death (Kwak et al., 2006; Kallet and Matthay, 2013). This hyperoxia-induced stress (Fukumoto et al., 2013) can cause protein misfolding in the ER and trigger unfolded protein response (UPR) (Gewandter et al., 2009). The endoplasmic reticulum (ER) possesses receptors to alleviate stress by activation of PERK (protein kinase-like ER kinase), activation of transcription factor 6 (ATF6), and inositol requiring enzyme 1 (IRE1α) (Gewandter et al., 2009). The binding immunoglobulin protein (BiP) also promotes proper folding of proteins (Sano and Reed, 2013). ER stress leads to C-junction N-terminal kinase (JNK) phosphorylation, eukaryotic initiation factor (eIF2α) phosphorylation, ER stress-induced cell death, and autophagy (Sano and Reed, 2013; Lee et al., 2015; Hoffman et al., 2016; Cnop et al., 2017). The rodent hyperoxia model mimics the clinical presentation of ALI by augmenting oxidative stress (Kallet and Matthay, 2013) and exacerbating respiratory failure through ER stress, events for which there are no viable treatments (Gewandter et al., 2009). Therefore, investigating the pathways and mechanisms of acute lung injury in rodents caused by hyperoxia is crucial to finding an effective treatment for acute lung injury in humans. Likewise, the mechanistic role of mitochondrial proteins in hyperoxia-induced ALI needs clarification.
Mitochondria play an essential role in oxidative metabolism, ROS generation, cell cycle progression, and other key biological pathways (McBride et al., 2006; Nagar et al., 2018). Mitochondrial integrity is affected by hyperoxia as it enhances ROS production and thus the levels of lipid peroxidation byproducts, including the reactive aldehyde 4-hydroxy-2-nonenal (4-HNE) (Kolliputi and Waxman, 2009a; Galam et al., 2015; Breitzig et al., 2016; Narala et al., 2018). The resultant mitochondrial disturbances are associated with ER stress due to cross talk between mitochondria and the ER (Malhotra and Kaufman, 2011; van Vliet and Agostinis, 2018; Chu et al., 2019). ER stress is also associated with neurodegenerative diseases, ophthalmological disorders, cancer, inflammation, and metabolic diseases (Sano and Reed, 2013). The cross-link between mitochondria and the ER suggests that targeting ER stress can offer novel insights into the treatment of acute lung injury.
Within mitochondria, Akap1, a scaffolding protein, plays an important role in PKA regulation, cAMP signaling, and maintenance of mitochondrial homeostasis (Carlucci et al., 2008a; Merrill and Strack, 2014). Akap1 is abundantly expressed in the liver, heart, brain, kidney, and skeletal muscles and has three isoforms: AKAP121 (mouse), AKAP149 (human), and AKAP84 (alternative splicing) (Merrill and Strack, 2014). The downregulation of Akap1 causes mitochondrial damage, cardiac dysfunction, lung injury, and neuronal death (Perrino et al., 2010; Merrill and Strack, 2014; Narala et al., 2018). Akap1 deletion is associated with autophagy, mitophagy, and apoptosis in murine studies (Merrill and Strack, 2014; Narala et al., 2018). The significance of Akap1 has been extensively studied in hypoxia-induced cardiac disease. Our laboratory has studied the role of Akap1 in hyperoxic lung injury. However, the role of Akap1 concerning ER stress during hyperoxia is unknown. It is hypothesized that Akap1 deletion will exacerbate ER stress associated with hyperoxia.
Materials and Methods
Mice
All animal procedures were approved by the Institutional Animal Care and Use Committee of the University of South Florida. Dr. Stanley McKnight (University of Washington) generated the Akap1−/− mice (Newhall et al., 2006) and donated them to Dr. Stefan Strack (The University of Iowa). The Akap1−/− mice were generously donated by Dr. Stefan Strack. All the mice aged 7–9 weeks were accommodated in individually ventilated cages and maintained under similar conditions of a dark–light cycle, humidity (60 ± 5%), and temperature (22 ± 1°C). The mice were fed a regular diet ad libitum.
Genotyping
The Akap1 genotype was determined by PCR, and primers were used according to a previous study (Flippo et al., 2018).
In Vivo Hyperoxia Exposure
Wild-type (Wt) and Akap1−/− mice were kept in cages within an airtight hyperoxia cabinet (75 × 50 × 50 cm) and exposed to 100% oxygen for 48 h in a specific pathogen-free environment. The oxygen concentration was measured by using a proOx p100 sensor (Biospherix, New York, NY) as described previously (Kolliputi and Waxman, 2009a).
Quantitative Real-Time PCR
Wt and Akap1−/− mice lungs were collected after normoxia and hyperoxia (48 h) exposure. Total RNA was extracted from the lungs by using RNeasy kit (Qiagen, Hilden, Germany) and reverse-transcribed by using the iScript cDNA synthesis kit (Biorad Laboratories, Hercules, CA) and 1 ug of total RNA was used. qRT-PCR was performed for PERK, IRE1α, and ATF6 by using the SsoFast EvaGreen Supermix kit as per the manufacturer’s instructions (Bio-Rad). The primer sequences for PERK (Saito et al., 2011), IRE1α (Tsuru et al., 2016), ATF6α (Egawa et al., 2011), and 18s (Chen et al., 2009) were obtained from previous studies; and 18s was used as an internal calibrator. The experiment was performed using the Bio-Rad CFX96 real-time system (C1000 Thermal Cycler) as per the manufacturer’s guidelines. A relative fold change was analyzed by CFX Manager software (Bio-Rad) based on the ΔΔCT method. The sequences of the primers are as follows.
PERK forward: 5′- TCTTGGTTGGGTCTGATGAAT -3’.
PERK reverse: 5′- GATGTTCTTGCTGTAGTGGGGG -3’.
Ire1α forward: 5′-GCCGAAGTTCAGATGGAATC-3'.
Ire1α reverse: 5′-ATCAGCAAAGGCCGATGA-3'.
Atf6α forward: 5′-TTATCAGCATACAGCCTGCG-3’.
Atf6α reverse: 5′- CTTGGGACTTTGAGCCTCTG-3’.
18s forward primer: 5′-GGCCCTGTAATTGGAATGAGTC-3’.
18s reverse primer: 5′-CCAAGATCCAACTACGAGCTT-3’.
Western Blotting
Wild-type (Wt) and Akap1−/− mice following normoxia and hyperoxia (48 h) exposure were euthanized, and the lungs were collected, snap-frozen, and stored in liquid nitrogen. The lungs were pulverized and lysed in lysis buffer (20 mM Tris–HCl, pH 7.4, 150 mM NaCl, and 0.5%, Triton X-100), and the supernatant was collected by centrifugation at 21,000 rpm for 15 min at 4°C. The amount of protein was assessed by using the BCA assay kit (Pierce, Rockford, Waltham, MA), and equal amounts of protein (5 µg) were separated by using the SDS-PAGE, followed by transfer onto PVDF membranes. The membranes were blocked in 5% BSA and washed with TBST, followed by incubation with specific primary and secondary antibodies, respectively. The primary antibodies used were BiP, JNK, p-JNK, eIF2α, p-eIF2α, Atg12, Beclin-1, CHOP (C/EBP homologous protein), Lc3b, β-actin (Cell Signaling Technology, Danvers, MA), and Erp57 (Stressgen, Farmingdale, NY), and the secondary antibodies used were anti-rabbit and anti-mouse conjugated to HRP (Jackson ImmunoResearch, West Grove, PA). The proteins were visualized by using Kwik quant ECL solution (Kindle Biosciences, Greenwich, CT) and quantified by using ImageJ (NIH, Bethesda, MD). The ratio of protein to its loading control (β-actin) was recorded. A master mix was prepared by diluting lung lysates to get a protein concentration of 1 µg/µl. From this master mix, equal amount of protein (5 µg) was separated on SDS-PAGE and probed with anti-β-Actin HRP conjugated antibody. The same set of β-Actin control image is shown in multiple figures as it represents identical set of lung lysates used for western blot analyses.
Immunohistochemistry
After euthanizing the mice, the left lung was fixed in 4% paraformaldehyde; immunohistochemistry (IHC) was performed on paraffin-embedded lung tissue sections. In brief, the paraffin-embedded lung sections were deparaffinized with xylene, and the tissue sections were subjected to heat-induced antigen retrieval in a Tris buffer (10 mM Tris–HCl buffer at pH 9). Furthermore, endogenous peroxidase activity was quenched by incubating the sections in 3% hydrogen peroxide for 20 min, and the tissue sections were blocked with 10% goat serum for 20 min. The sections were incubated with specific primary antibodies (BiP, p-JNK, Erp57, and Lc3b) overnight at 4°C. The following day, the sections were probed with goat anti-rabbit antibodies (HRP-conjugated). Finally, detection of protein was carried out by using the Immpact VIP peroxidase substrate kit (Vector Laboratories, Burlingame, CA) (Fukumoto et al., 2019). The sections were imaged by using a microscope (Olympus BX43, Tokyo, Japan), attached to an Olympus DP21. The images were processed using Adobe Photoshop ver C56.
Statistical Analysis
The data are represented as mean ± S.E.M. Statistical analysis was carried out using GraphPad Prism (ver 10, San Diego, CA). Comparisons of multiple groups utilized one-way ANOVA, followed by Tukey’s post hoc test, for normally distributed data, and p < 0.05 was considered statistically significant.
Results
Genotyping and Expression of Akap1 Mice
The genotyping was confirmed by PCR. The agarose gel shows the Wt band (600 bp) and the Akap1 KO band (400 bp) (Supplementary Figure S1). The basal protein levels of Akap1 were previously demonstrated by Western blot (Narala et al., 2018).
Effect of Akap1 Deletion on ER Stress Receptor Transcripts After Hyperoxia
Wt and Akap1−/− mice were exposed to normoxia and hyperoxia as described in earlier studies to investigate the impact of Akap1 deletion on ER stress receptors (Kolliputi and Waxman, 2009b; Kolliputi et al., 2010). The lung samples were subjected to qRT-PCR analysis in Akap1−/− versus Wt mice under normoxia, and the data show a 1.35-, 1.36-, and 1.35-fold increase in PERK, IRE1α, and ATF6α, respectively (Figures 1A–C) However, no significant increase was observed under hyperoxia exposure, suggesting that Akap1−/− may cause slight upregulation of unfolded protein response (UPR) at the transcript level under normoxia.
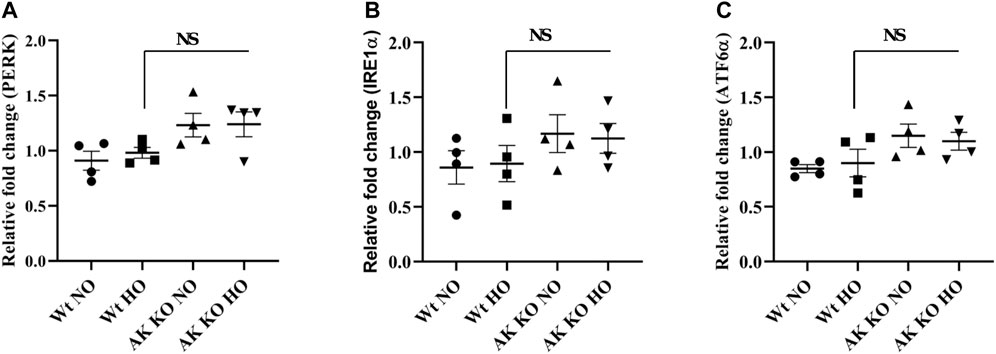
FIGURE 1. Effect of Akap1 genetic deletion on ER stress-related receptor transcripts: Wt and Akap1 mice were exposed to normoxia and hyperoxia for 48 h. After hyperoxia, the mice were euthanized, and total RNA was isolated from the lungs and subjected to qRT-PCR analysis and quantified using the ΔΔCt method. Relative fold change in (A) PERK, (B) IRE1α, and (C) ATF6α is shown. Data are expressed as mean ± SEM (n = 4 mice per group). NS, not significant; NO, normoxia; HO, hyperoxia; AK, Akap1; KO: Knockout.
Akap1 Deletion Enhances BiP Levels After Hyperoxia
Wt and Akap1−/− mice were exposed to hyperoxia or kept in normoxia to evaluate the interaction between Akap1 deletion and BiP. A significant increase of 3.28-fold occurred in the BiP expression (Figure 2) in Akap1−/− versus Wt mice exposed to hyperoxia in the lung lysates subjected to Western blot analysis. There was a non-significant 1.61- and 2.25-fold increase in BiP expression in Akap1−/− mice under normoxia versus Wt normoxia and Wt hyperoxia, respectively. Enhanced BiP signals in the lung samples as evaluated by IHC were observed in the alveolar and peribronchial regions in Akap1−/− versus Wt mice exposed to hyperoxia (Supplementary Figures S2A,B).
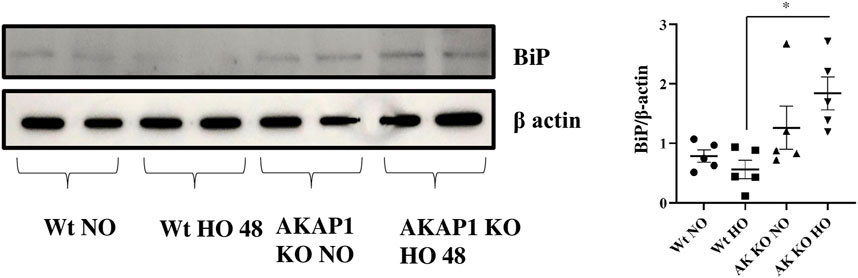
FIGURE 2. Akap1 genetic deletion activates BiP after hyperoxic exposure: Wt and Akap1 mice were exposed to normoxia and hyperoxia for 48 h. After hyperoxia, the mice were killed, and protein from the lungs was extracted and subjected to Western blot analysis to evaluate the expression of BiP, and β-actin was used as a loading control. Densitometry analysis of protein was carried out, and fold change was calculated after normalizing to β-Actin. Data are shown as mean ± S.E.M (*p < 0.05) (n = 5 mice per group), NO, normoxia; HO, hyperoxia; AK, Akap1; KO: Knockout.
Akap1 Deletion Activates JNK After Hyperoxia
Wt and Akap1−/− mice were exposed to normoxia or hyperoxia to investigate the relationship between Akap1 deletion and JNK. The immunoblot analysis showed a 4.1-fold significant increase in the expression of the p-JNK (Figure 3) in Akap1−/− versus Wt mice exposed to hyperoxia. There was no difference under normoxia between Wt and Akap1−/− mice. The expression of p-JNK was evaluated by IHC in the lung samples. Increased p-JNK signals (Supplementary Figure.S3) were observed in the peribronchial region of Akap1−/− versus Wt mice exposed to hyperoxia.
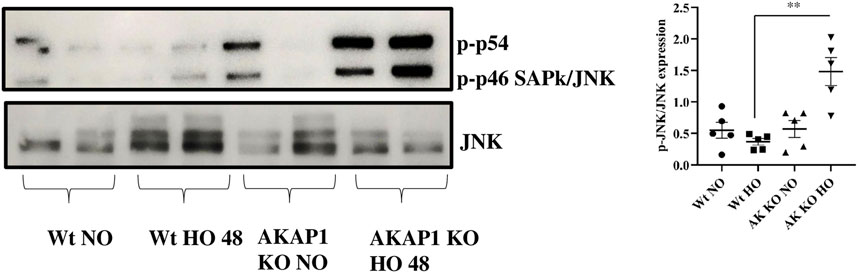
FIGURE 3. Akap1 genetic deletion activates JNK after hyperoxic exposure: Wt and Akap1 mice were exposed to normoxia and hyperoxia for 48 h. After hyperoxia, the mice were killed, and protein from the lungs was extracted and subjected to Western blot analysis to evaluate the expression of JNK and p-JNK. Densitometry analysis was carried out, and after normalization with β-actin, the results were expressed in fold change. Data are shown as mean ± S.E.M (**p < 0.01) (n = 5 mice per group), NO, normoxia; HO, hyperoxia; AK, Akap1; KO: Knockout.
Akap1 Deletion Causes Phosphorylation of Eukaryotic Initiation Factor
Wt and Akap1−/− mice were exposed to normoxia or hyperoxia to investigate the interaction between Akap1 deletion and eIF2α. Immunoblot results showed a 4-fold significant increase in the expression of p-eIF2α (Figure 4) in Akap1−/− versus Wt mice in normoxia and a 2-fold significant increase in Akap1−/− versus Wt following hyperoxia.
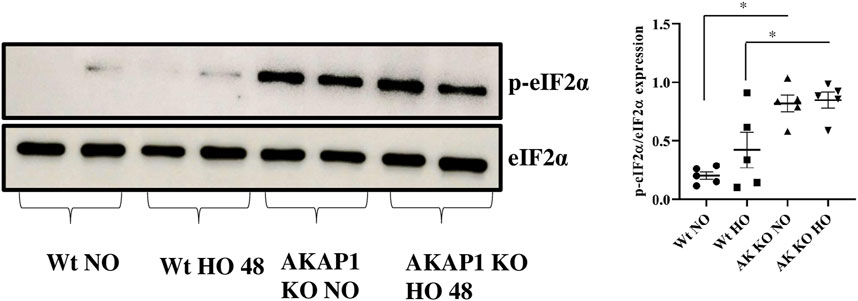
FIGURE 4. Akap1 genetic deletion causes phosphorylation of eukaryotic initiation factor (eIF2α): Wt and Akap1 mice were exposed to normoxia and hyperoxia for 48 h. After hyperoxia, the mice were killed, and protein was extracted from the lungs and subjected to Western blot analysis. Following densitometry analysis and normalization with β-actin, the ratio of p-eIF2α to eIF2α protein levels was evaluated. Results are expressed in fold change. Data are shown as mean ± SEM (*p < 0.05) (n = 5 mice per group). NO, normoxia; HO, hyperoxia; AK, Akap1; KO: Knockout.
Akap1 Deletion Causes ER Stress-Induced Cell Death After Hyperoxia
Wt and Akap1−/− mice were exposed to normoxia or hyperoxia to investigate the connection between Akap1 deletion and ER stress-induced cell death. Western blot analysis indicated that there were significant increases in the expression of ERp57 (3.13-fold) and CHOP (2.56-fold) (Figure 5) in Akap1−/− versus Wt mice exposed to hyperoxia for 48 h. Furthermore, mouse lung tissue samples were subjected to IHC to confirm ER stress-induced cell death. ERp57 signals (Supplementary Figures S4A,B) were more abundant in the alveolar and peribronchial regions of Akap1−/− versus Wt mice exposed to hyperoxia for 48 h.
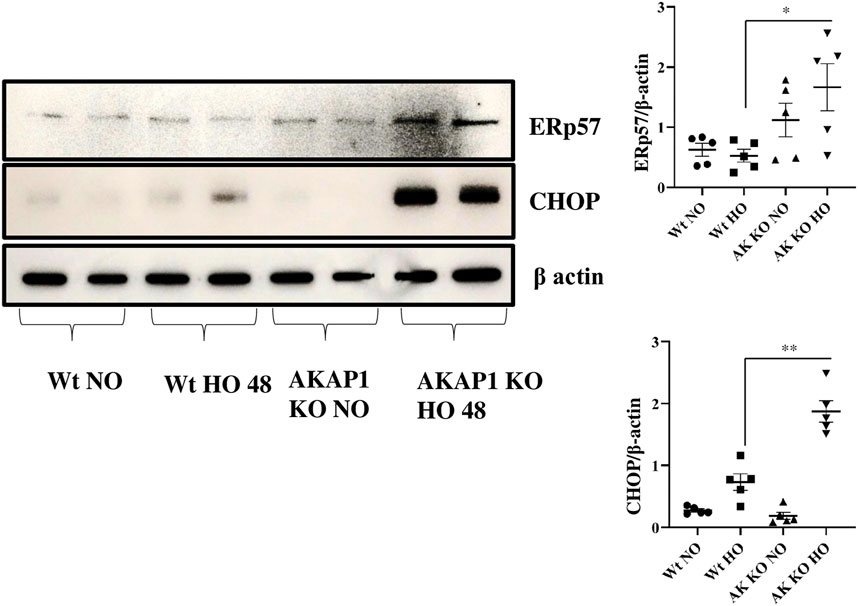
FIGURE 5. Akap1 genetic deletion enhances ER stress-induced cell death after hyperoxic exposure: Wt and Akap1 mice were exposed to normoxia and hyperoxia for 48 h. After hyperoxia, the mice were killed, and protein was extracted from the lungs and subjected to Western blot analysis to evaluate the expression of CHOP and Erp57. β-actin was used as a loading control. Densitometry analysis of CHOP and Erp57 protein was followed by normalization to β-actin. Data are expressed as mean ± SEM (*p < 0.05, **p < 0.005) (n = 5 mice per group). NO, normoxia; HO, hyperoxia; AK, Akap1; KO: Knockout.
Akap1 Deletion Causes Autophagy After Hyperoxia
The protein autophagy-related gene 12 (ATG12) plays a key role in the formation of the autophagosome (Otomo et al., 2013), and the Beclin-1 and Lc3b proteins induce autophagy (Wirawan et al., 2012; Satyavarapu et al., 2018; Vega-Rubín-de-Celis, 2019). Wt and Akap1−/− mice were exposed to normoxia or hyperoxia to investigate the effect of Akap1 deletion on autophagy. Immunoblot results indicate a significant 3.42- and 1.67-fold increase in the expression of ATG12 and Beclin-1 (Figure 6), respectively, in Akap1−/− versus Wt mice exposed to hyperoxia for 48 h. Mouse tissue samples were subjected to IHC analysis to investigate the effects on autophagy. The Lc3b (Supplementary Figures S5A,B) was highly expressed in the alveolar and peribronchial regions of Akap1−/− versus Wt mice exposed to hyperoxia for 48 h.
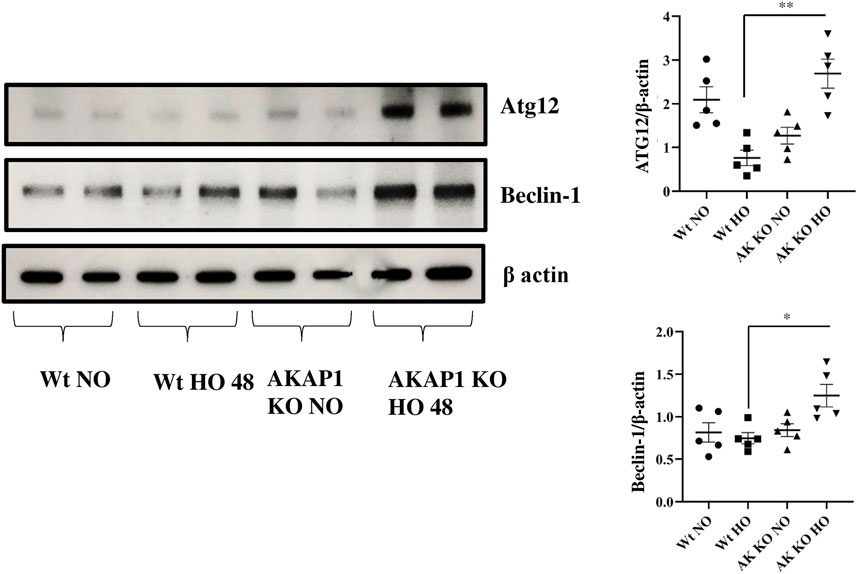
FIGURE 6. Akap1 genetic deletion enhances autophagy after hyperoxic exposure: Wt and Akap1 mice were exposed to normoxia and hyperoxia for 48 h. After hyperoxia, the mice were killed, and protein was extracted from the lungs and subjected to Western blot analysis to evaluate the protein expression of Atg12 and Beclin-1. β-actin was used as a loading control. Densitometry analysis of Atg12 and Beclin-1 was followed by normalization with β-actin. Data are shown as mean ± S.E.M (*p < 0.05, **p < 0.01) (n = 5 mice per group). NO, normoxia; HO, hyperoxia; AK, Akap1; KO: Knockout.
Discussion
These studies indicate that Akap1 genetic deletion exacerbates ER stress associated with hyperoxia. Akap1 deletion results in the following: 1) partial activation of the ER stress receptors under normoxia, 2) BiP activation after hyperoxic exposure, 3) JNK phosphorylation following hyperoxic exposure, 4) phosphorylation of eukaryotic initiation factor, 5) an increase in ER stress-induced cell death, and 6) increased autophagy.
The ER plays an important role in the homeostasis of cells by regulating lipid synthesis, protein secretion, calcium homeostasis, and protein folding (Szegezdi et al., 2006). Disturbance in the homeostasis of the ER triggers UPR by activating the ER receptors PERK, IRE1α, and ATF6 (Malhotra and Kaufman, 2007). In this study, Akap1 deletion shows a minimal increase in UPR receptor proteins versus Wt mice under normoxia. In this study, the mice were exposed to hyperoxia for 48 h which did not seem to affect the ER stress receptors (Figures 1A–C). This corroborates with another study which showed that hyperoxia does not impact ER receptors in Wt mice exposed to hyperoxia for 64 h (Gewandter et al., 2009). A short period of hyperoxia exposure (3–6 h) may be required to observe the effect of hyperoxia on ER stress receptor transcripts.
BiP is a hallmark of ER stress response and UPR (Ron and Walter, 2007). The consistent protein aggregation from ER stress causes a transition from pro-survival to pro-apoptotic/ER stress-induced cell death (Lu et al., 2015). Increased BiP levels were found in Akap1−/− versus Wt mice exposed to normoxia and hyperoxia, indicating that Akap1−/− mice are susceptible to ER stress and that hyperoxia exacerbates this stress by causing the aggregation of misfolded proteins (Lu et al., 2015). BiP expression can be increased in cancer, drug-resistant cancer cells, and dormant cancer cells (Li et al., 2011).
PKA protects cells from ER stress and Akap1 regulates PKA (Gewandter et al., 2009; Aguileta et al., 2016). The presence of PKA negatively regulates the JNK protein (Zeitlin et al., 2011). JNK, as with oxidative stress, environmental stress, and the presence of pro-inflammatory cytokines, is phosphorylated under stress conditions (Yamasaki et al., 2017). Our data indicate that Akap1 deletion depletes PKA levels and elevates the phosphorylation of JNK. Hyperoxia treatment also augments p-JNK levels, in agreement with our previous studies (Kolliputi and Waxman, 2009b; Galam et al., 2016). Additionally, hydrogen peroxide treatment also activates JNK for initiating apoptosis (Kolliputi and Waxman, 2009b; Wei et al., 2010). JNK phosphorylation can occur during mitochondrial dysfunction, atherosclerosis, and metabolic diseases (Sano and Reed, 2013).
Prolonged hyperoxia exposure cause UPR and can induce eIF2α phosphorylation under stress conditions (Konsavage et al., 2012; Lu et al., 2015). The eIF2α phosphorylation also inhibits protein translation to induce endoplasmic stress-associated degradation (ERAD) (Ohno, 2014). Akap1 is predominantly a mitochondrial protein. The shorter form of Akap1 (N0) is known to target mitochondria, while the longer form (N1) targets the ER (Konsavage et al., 2010). It is plausible that the ER effects due to the Akap1 deletion may be attributed to the N1 form. As previously observed, deletion of Akap1 alters the size and structure of mitochondria (Schiattarella et al., 2016; Narala et al., 2018; Schiattarella et al., 2018). eIF2α phosphorylation is seen in Parkinson’s disease and Alzheimer’s disease (Sano and Reed, 2013; Ohno, 2014). These results suggest that Akap1 deletion leads to eIF2α phosphorylation under normoxic and hyperoxic conditions. Further studies are required to evaluate the interaction between Akap1 and eIF2α phosphorylation in human cell lines.
CHOP is expressed during ER stress and can lead to apoptosis (Xu et al., 2009; Hoffman et al., 2016). This protein is usually present at low levels but is upregulated and activated by eIF2α phosphorylation during ER stress, which causes DNA damage and growth arrest (Oyadomari and Mori, 2004; Xu et al., 2009; Hoffman et al., 2016). The protein, ERp57, is also expressed during ER stress and causes apoptosis (Xu et al., 2009; Hoffman et al., 2016). The data show an increase in ER stress-induced cell death markers, CHOP and ERp57, in Akap1−/− versus Wt mice exposed to hyperoxia. CHOP expression was seen in viral infection, neurodegenerative disease, atherosclerosis, metabolic disease, inflammation, and ophthalmology disease (Sano and Reed, 2013). The Akap1−/− mice under normoxia have increased expression of eIF2α phosphorylation but do not undergo CHOP mediated cell death. Akap1−/− mice under normoxia display enhanced ERp57 expression. It is possible that Akap1−/− mice may suffer ERp57-mediated cell death via the NFκB or STAT3 pathway (Liu et al., 2019). A wide array of evidence shows that ERp57 dysregulation occurs in melanoma, laryngeal cancer, and leukemia (Liu et al., 2019). ERp57 deletion causes a decrease in inflammatory cells, epidermal growth factor, periostin, and increased airway resistance (Hoffman et al., 2016).
ER stress leads to cellular degradation, apoptosis, inflammation, autophagy, mitophagy, and protein degradation (Senft and Ronai, 2015). The protein ATG12 plays a role in autophagy initiation and causes mitochondrial apoptosis (Rubinstein et al., 2011), while Beclin-1 is a regulator of autophagy, and LC3b is a marker of autophagy (Meyer et al., 2013). The data suggest that Akap1 deletion induces autophagy compared to Wt mice exposed to hyperoxia. The expression of ATG12 in Wt mice is higher than that of Akap1−/− mice under normoxia. This suggests that certain cells undergo the formation of autophagosomes to maintain mitochondrial homeostasis due to damaged mitochondria (Radoshevich et al., 2010). Also, Lc3b−/- mice demonstrate decreased caspase activity (Sauler et al., 2015). Cho et al., 2009 found that Beclin-1 links apoptosis to autophagy in HELA cells mediated by caspases (Cho et al., 2009). Autophagy is also associated with neurodegenerative disease and cancer promotion, suggesting the impact of Akap1−/− on autophagy (Sano and Reed, 2013).
These findings suggest that Akap1 has a crucial role in the lung in the setting of hyperoxic exposure. Akap1-knockout mice exposed to hyperoxia show activation of BiP, JNK phosphorylation, ER stress-induced cell death, autophagy, and eIF2α phosphorylation (even under normoxia). Therefore, Akap1 is a potential therapeutic target in ALI for patients who require supplemental oxygen.
Data Availability Statement
The raw data supporting the conclusions of this article will be made available by the authors, without undue reservation.
Ethics Statement
The animal study was reviewed and approved by the University of South Florida.
Author Contributions
Concept and experimental design: SSP, LG, JF, RS, and MB; experiments performed: SSP, JF, HH-C, RS, and ML; figure preparations and data analyzed: SSP, JF, RS, VRN, and MA; manuscript revision and proofreading: NK, RS, VRN, MA, LG, RL, MB, HH-C, and MA; and final approval of the manuscript: LG and NK.
Funding
LG is supported by the AHA National Scientist Development Grant 17SDG32780002 and NK is supported by the National Institutes of Health R01 HL105932.
Conflict of Interest
The authors declare that the research was conducted in the absence of any commercial or financial relationships that could be construed as a potential conflict of interest.
Publisher’s Note
All claims expressed in this article are solely those of the authors and do not necessarily represent those of their affiliated organizations, or those of the publisher, the editors, and the reviewers. Any product that may be evaluated in this article, or claim that may be made by its manufacturer, is not guaranteed or endorsed by the publisher.
Supplementary Material
The Supplementary Material for this article can be found online at: https://www.frontiersin.org/articles/10.3389/fphar.2022.762840/full#supplementary-material
Abbreviations
AK, Akap1; AV, alveoli; HO, hyperoxia; KO, knockout; NO, normoxia; PB, peribronchial; Wt, wild type.
References
Aguileta, M. A., Rojas-Rivera, D., Goossens, V., Estornes, Y., Van Isterdael, G., Vandenabeele, P., et al. (2016). A siRNA Screen Reveals the Prosurvival Effect of Protein Kinase A Activation in Conditions of Unresolved Endoplasmic Reticulum Stress. Cell Death Differ. 23, 1670–1680. doi:10.1038/cdd.2016.59
Breitzig, M., Bhimineni, C., Lockey, R., and Kolliputi, N. (2016). 4-Hydroxy-2-nonenal: a Critical Target in Oxidative Stress? Am. J. Physiol. Cell Physiol. 311, C537–C543. doi:10.1152/ajpcell.00101.2016
Carlucci, A., Adornetto, A., Scorziello, A., Viggiano, D., Foca, M., Cuomo, O., et al. (2008). Proteolysis of AKAP121 Regulates Mitochondrial Activity during Cellular Hypoxia and Brain Ischaemia. EMBO J. 27, 1073–1084. doi:10.1038/emboj.2008.33
Carlucci, A., Lignitto, L., and Feliciello, A. (2008). Control of Mitochondria Dynamics and Oxidative Metabolism by cAMP, AKAPs and the Proteasome. Trends Cell Biol. 18, 604–613. doi:10.1016/j.tcb.2008.09.006
Chen, C., Wickenheisser, J., Ewens, K. G., Ankener, W., Legro, R. S., Dunaif, A., et al. (2009). PDE8A Genetic Variation, Polycystic Ovary Syndrome and Androgen Levels in Women. Mol. Hum. Reprod. 15, 459–469. doi:10.1093/molehr/gap035
Cho, D. H., Jo, Y. K., Hwang, J. J., Lee, Y. M., Roh, S. A., and Kim, J. C. (2009). Caspase-mediated Cleavage of ATG6/Beclin-1 Links Apoptosis to Autophagy in HeLa Cells. Cancer Lett. 274, 95–100. doi:10.1016/j.canlet.2008.09.004
Chu, Q., Martinez, T. F., Novak, S. W., Donaldson, C. J., Tan, D., Vaughan, J. M., et al. (2019). Regulation of the ER Stress Response by a Mitochondrial Microprotein. Nat. Commun. 10, 4883. doi:10.1038/s41467-019-12816-z
Cnop, M., Toivonen, S., Igoillo-Esteve, M., and Salpea, P. (2017). Endoplasmic Reticulum Stress and eIF2α Phosphorylation: The Achilles Heel of Pancreatic β Cells. Mol. Metab. 6, 1024–1039. doi:10.1016/j.molmet.2017.06.001
Egawa, N., Yamamoto, K., Inoue, H., Hikawa, R., Nishi, K., Mori, K., et al. (2011). The Endoplasmic Reticulum Stress Sensor, ATF6α, Protects against Neurotoxin-Induced Dopaminergic Neuronal Death. J. Biol. Chem. 286, 7947–7957. doi:10.1074/jbc.M110.156430
Flippo, K. H., Gnanasekaran, A., Perkins, G. A., Ajmal, A., Merrill, R. A., Dickey, A. S., et al. (2018). AKAP1 Protects from Cerebral Ischemic Stroke by Inhibiting Drp1-dependent Mitochondrial Fission. J. Neurosci. 38, 8233–8242. doi:10.1523/JNEUROSCI.0649-18.2018
Fukumoto, J., Fukumoto, I., Parthasarathy, P. T., Cox, R., Huynh, B., Ramanathan, G. K., et al. (2013). NLRP3 Deletion Protects from Hyperoxia-Induced Acute Lung Injury. Am. J. Physiol. Cell Physiol. 305, C182–C189. doi:10.1152/ajpcell.00086.2013
Fukumoto, J., Leung, J., Cox, R., Czachor, A., Parthasarathy, P. T., Lagishetty, V., et al. (2019). Oxidative Stress Induces Club Cell Proliferation and Pulmonary Fibrosis in Atp8b1 Mutant Mice. Aging (Albany NY) 11, 209–229. doi:10.18632/aging.101742
Galam, L., Failla, A., Soundararajan, R., Lockey, R. F., and Kolliputi, N. (2015). 4-hydroxynonenal Regulates Mitochondrial Function in Human Small Airway Epithelial Cells. Oncotarget 6, 41508–41521. doi:10.18632/oncotarget.6131
Galam, L., Soundararajan, R., Breitzig, M., Rajan, A., Yeruva, R. R., Czachor, A., et al. (2016). SOCS-1 Rescues IL-1β-mediated Suppression of Epithelial Sodium Channel in Mouse Lung Epithelial Cells via ASK-1. Oncotarget 7, 29081–29091. doi:10.18632/oncotarget.8543
Gewandter, J. S., Staversky, R. J., and O'Reilly, M. A. (2009). Hyperoxia Augments ER-Stress-Induced Cell Death Independent of BiP Loss. Free Radic. Biol. Med. 47, 1742–1752. doi:10.1016/j.freeradbiomed.2009.09.022
Hoffman, S. M., Chapman, D. G., Lahue, K. G., Cahoon, J. M., Rattu, G. K., Daphtary, N., et al. (2016). Protein Disulfide Isomerase-Endoplasmic Reticulum Resident Protein 57 Regulates Allergen-Induced Airways Inflammation, Fibrosis, and Hyperresponsiveness. J. Allergy Clin. Immunol. 137, 822–e7. doi:10.1016/j.jaci.2015.08.018
Kallet, R. H., and Matthay, M. A. (2013). Hyperoxic Acute Lung Injury. Respir. Care 58, 123–141. doi:10.4187/respcare.01963
Kolliputi, N., Shaik, R. S., and Waxman, A. B. (2010). The Inflammasome Mediates Hyperoxia-Induced Alveolar Cell Permeability. J. Immunol. 184, 5819–5826. doi:10.4049/jimmunol.0902766
Kolliputi, N., and Waxman, A. B. (2009). IL-6 Cytoprotection in Hyperoxic Acute Lung Injury Occurs via PI3K/Akt-Mediated Bax Phosphorylation. Am. J. Physiol. Lung Cell Mol. Physiol. 297, L6–L16. doi:10.1152/ajplung.90381.2008
Kolliputi, N., and Waxman, A. B. (2009). IL-6 Cytoprotection in Hyperoxic Acute Lung Injury Occurs via Suppressor of Cytokine Signaling-1-Induced Apoptosis Signal-Regulating Kinase-1 Degradation. Am. J. Respir. Cell Mol. Biol. 40, 314–324. doi:10.1165/rcmb.2007-0287OC
Konsavage, W., Zhang, L., Vary, T., and Shenberger, J. S. (2010). Hyperoxia Inhibits Protein Synthesis and Increases eIF2α Phosphorylation in the Newborn Rat Lung. Am. J. Physiol. Lung Cell Mol. Physiol. 298, L678–L686. doi:10.1152/ajplung.00262.2009
Konsavage, W. M., Zhang, L., Wu, Y., and Shenberger, J. S. (2012). Hyperoxia-induced Activation of the Integrated Stress Response in the Newborn Rat Lung. Am. J. Physiol. Lung Cell Mol. Physiol. 302, L27–L35. doi:10.1152/ajplung.00174.2011
Kwak, D. J., Kwak, S. D., and Gauda, E. B. (2006). The Effect of Hyperoxia on Reactive Oxygen Species (ROS) in Rat Petrosal Ganglion Neurons during Development Using Organotypic Slices. Pediatr. Res. 60, 371–376. doi:10.1203/01.pdr.0000239817.39407.61
Lee, W. S., Yoo, W. H., and Chae, H. J. (2015). ER Stress and Autophagy. Curr. Mol. Med. 15, 735–745. doi:10.2174/1566524015666150921105453
Li, X., Zhang, K., and Li, Z. (2011). Unfolded Protein Response in Cancer: the Physician's Perspective. J. Hematol. Oncol. 4, 8. doi:10.1186/1756-8722-4-8
Liu, Y., Wang, J. X., Nie, Z. Y., Wen, Y., Jia, X. J., Zhang, L. N., et al. (2019). Upregulation of ERp57 Promotes clear Cell Renal Cell Carcinoma Progression by Initiating a STAT3/ILF3 Feedback Loop. J. Exp. Clin. Cancer Res. 38, 439. doi:10.1186/s13046-019-1453-z
Lu, H. Y., Zhang, J., Wang, Q. X., Tang, W., and Zhang, L. J. (2015). Activation of the Endoplasmic Reticulum Stress Pathway Involving CHOP in the Lungs of Rats with Hyperoxia-induced B-ronchopulmonary D-ysplasia. Mol. Med. Rep. 12, 4494–4500. doi:10.3892/mmr.2015.3979
Malhotra, J. D., and Kaufman, R. J. (2011). ER Stress and its Functional Link to Mitochondria: Role in Cell Survival and Death. Cold Spring Harb. Perspect. Biol. 3, a004424. doi:10.1101/cshperspect.a004424
Malhotra, J. D., and Kaufman, R. J. (2007). The Endoplasmic Reticulum and the Unfolded Protein Response. Semin. Cell Dev. Biol. 18, 716–731. doi:10.1016/j.semcdb.2007.09.003
McBride, H. M., Neuspiel, M., and Wasiak, S. (2006). Mitochondria: More Than Just a Powerhouse. Curr. Biol. 16, R551–R560. doi:10.1016/j.cub.2006.06.054
Merrill, R. A., and Strack, S. (2014). Mitochondria: a Kinase Anchoring Protein 1, a Signaling Platform for Mitochondrial Form and Function. Int. J. Biochem. Cell Biol. 48, 92–96. doi:10.1016/j.biocel.2013.12.012
Meyer, G., Czompa, A., Reboul, C., Csepanyi, E., Czegledi, A., Bak, I., et al. (2013). The Cellular Autophagy Markers Beclin-1 and LC3B-II Are Increased during Reperfusion in Fibrillated Mouse Hearts. Curr. Pharm. Des. 19, 6912–6918. doi:10.2174/138161281939131127122510
Nagar, H., Piao, S., and Kim, C. S. (2018). Role of Mitochondrial Oxidative Stress in Sepsis. Acute Crit. Care 33, 65–72. doi:10.4266/acc.2018.00157
Narala, V. R., Fukumoto, J., Hernández-Cuervo, H., Patil, S. S., Krishnamurthy, S., Breitzig, M., et al. (2018). Akap1 Genetic Deletion Increases the Severity of Hyperoxia-Induced Acute Lung Injury in Mice. Am. J. Physiol. Lung Cell Mol. Physiol. 314, L860–L870. doi:10.1152/ajplung.00365.2017
Newhall, K. J., Criniti, A. R., Cheah, C. S., Smith, K. C., Kafer, K. E., Burkart, A. D., et al. (2006). Dynamic Anchoring of PKA Is Essential during Oocyte Maturation. Curr. Biol. 16, 321–327. doi:10.1016/j.cub.2005.12.031
Ohno, M. (2014). Roles of eIF2α Kinases in the Pathogenesis of Alzheimer's Disease. Front. Mol. Neurosci. 7, 22. doi:10.3389/fnmol.2014.00022
Otomo, C., Metlagel, Z., Takaesu, G., and Otomo, T. (2013). Structure of the Human ATG12∼ATG5 Conjugate Required for LC3 Lipidation in Autophagy. Nat. Struct. Mol. Biol. 20, 59–66. doi:10.1038/nsmb.2431
Oyadomari, S., and Mori, M. (2004). Roles of CHOP/GADD153 in Endoplasmic Reticulum Stress. Cell Death Differ 11, 381–389. doi:10.1038/sj.cdd.4401373
Perrino, C., Feliciello, A., Schiattarella, G. G., Esposito, G., Guerriero, R., Zaccaro, L., et al. (2010). AKAP121 Downregulation Impairs Protective cAMP Signals, Promotes Mitochondrial Dysfunction, and Increases Oxidative Stress. Cardiovasc. Res. 88, 101–110. doi:10.1093/cvr/cvq155
Radoshevich, L., Murrow, L., Chen, N., Fernandez, E., Roy, S., Fung, C., et al. (2010). ATG12 Conjugation to ATG3 Regulates Mitochondrial Homeostasis and Cell Death. Cell 142, 590–600. doi:10.1016/j.cell.2010.07.018
Ron, D., and Walter, P. (2007). Signal Integration in the Endoplasmic Reticulum Unfolded Protein Response. Nat. Rev. Mol. Cell Biol. 8, 519–529. doi:10.1038/nrm2199
Rubinstein, A. D., Eisenstein, M., Ber, Y., Bialik, S., and Kimchi, A. (2011). The Autophagy Protein Atg12 Associates with Antiapoptotic Bcl-2 Family Members to Promote Mitochondrial Apoptosis. Mol. Cell 44, 698–709. doi:10.1016/j.molcel.2011.10.014
Saito, A., Ochiai, K., Kondo, S., Tsumagari, K., Murakami, T., Cavener, D. R., et al. (2011). Endoplasmic Reticulum Stress Response Mediated by the PERK-eIF2(alpha)-ATF4 Pathway Is Involved in Osteoblast Differentiation Induced by BMP2. J. Biol. Chem. 286, 4809–4818. doi:10.1074/jbc.M110.152900
Sano, R., and Reed, J. C. (2013). ER Stress-Induced Cell Death Mechanisms. Biochim. Biophys. Acta 1833, 3460–3470. doi:10.1016/j.bbamcr.2013.06.028
Satyavarapu, E. M., Das, R., Mandal, C., Mukhopadhyay, A., and Mandal, C. (2018). Autophagy-independent Induction of LC3B through Oxidative Stress Reveals its Non-canonical Role in Anoikis of Ovarian Cancer Cells. Cell Death Dis. 9, 934. doi:10.1038/s41419-018-0989-8
Sauler, M., Zhang, Y., Min, J. N., Leng, L., Shan, P., Roberts, S., et al. (2015). Endothelial CD74 Mediates Macrophage Migration Inhibitory Factor protection in Hyperoxic Lung Injury. FASEB J. 29, 1940–1949. doi:10.1096/fj.14-260299
Schiattarella, G. G., Cattaneo, F., Carrizzo, A., Paolillo, R., Boccella, N., Ambrosio, M., et al. (2018). Akap1 Regulates Vascular Function and Endothelial Cells Behavior. Hypertension 71, 507–517. doi:10.1161/HYPERTENSIONAHA.117.10185
Schiattarella, G. G., Cattaneo, F., Pironti, G., Magliulo, F., Carotenuto, G., Pirozzi, M., et al. (2016). Akap1 Deficiency Promotes Mitochondrial Aberrations and Exacerbates Cardiac Injury Following Permanent Coronary Ligation via Enhanced Mitophagy and Apoptosis. PloS One 11, e0154076. doi:10.1371/journal.pone.0154076
Senft, D., and Ronai, Z. A. (2015). UPR, Autophagy, and Mitochondria Crosstalk Underlies the ER Stress Response. Trends Biochem. Sci. 40, 141–148. doi:10.1016/j.tibs.2015.01.002
Szegezdi, E., Logue, S. E., Gorman, A. M., and Samali, A. (2006). Mediators of Endoplasmic Reticulum Stress-Induced Apoptosis. EMBO Rep. 7, 880–885. doi:10.1038/sj.embor.7400779
Tsuru, A., Imai, Y., Saito, M., and Kohno, K. (2016). Novel Mechanism of Enhancing IRE1α-XBP1 Signalling via the PERK-ATF4 Pathway. Sci. Rep. 6, 24217. doi:10.1038/srep24217
van Vliet, A. R., and Agostinis, P. (2018). Mitochondria-Associated Membranes and ER Stress. Curr. Top. Microbiol. Immunol. 414, 73–102. doi:10.1007/82_2017_2
Vega-Rubín-de-Celis, S. (2019). The Role of Beclin 1-Dependent Autophagy in Cancer. Biology (Basel) 9. doi:10.3390/biology9010004
Wei, H., Li, Z., Hu, S., Chen, X., and Cong, X. (2010). Apoptosis of Mesenchymal Stem Cells Induced by Hydrogen Peroxide Concerns Both Endoplasmic Reticulum Stress and Mitochondrial Death Pathway through Regulation of Caspases, P38 and JNK. J. Cell Biochem. 111, 967–978. doi:10.1002/jcb.22785
Wirawan, E., Lippens, S., Vanden Berghe, T., Romagnoli, A., Fimia, G. M., Piacentini, M., et al. (2012). Beclin1: a Role in Membrane Dynamics and beyond. Autophagy 8, 6–17. doi:10.4161/auto.8.1.16645
Xu, D., Perez, R. E., Rezaiekhaligh, M. H., Bourdi, M., and Truog, W. E. (2009). Knockdown of ERp57 Increases BiP/GRP78 Induction and Protects against Hyperoxia and Tunicamycin-Induced Apoptosis. Am. J. Physiol. Lung Cell Mol. Physiol. 297, L44–L51. doi:10.1152/ajplung.90626.2008
Yamasaki, T., Deki-Arima, N., Kaneko, A., Miyamura, N., Iwatsuki, M., Matsuoka, M., et al. (2017). Age-dependent Motor Dysfunction Due to Neuron-specific Disruption of Stress-Activated Protein Kinase MKK7. Sci. Rep. 7, 7348. doi:10.1038/s41598-017-07845-x
Keywords: ALI, ARDS, ROS, ER stress, Akap1
Citation: Sidramagowda Patil S, Soundararajan R, Fukumoto J, Breitzig M, Hernández-Cuervo H, Alleyn M, Lin M, Narala VR, Lockey R, Kolliputi N and Galam L (2022) Mitochondrial Protein Akap1 Deletion Exacerbates Endoplasmic Reticulum Stress in Mice Exposed to Hyperoxia. Front. Pharmacol. 13:762840. doi: 10.3389/fphar.2022.762840
Received: 23 August 2021; Accepted: 27 January 2022;
Published: 14 March 2022.
Edited by:
Irfan Rahman, University of Rochester, United StatesReviewed by:
Sekhar P. Reddy, University of Illinois at Chicago, United StatesSreerama Shetty, University of Texas at Tyler, United States
Copyright © 2022 Sidramagowda Patil, Soundararajan, Fukumoto, Breitzig, Hernández-Cuervo, Alleyn, Lin, Narala, Lockey, Kolliputi and Galam. This is an open-access article distributed under the terms of the Creative Commons Attribution License (CC BY). The use, distribution or reproduction in other forums is permitted, provided the original author(s) and the copyright owner(s) are credited and that the original publication in this journal is cited, in accordance with accepted academic practice. No use, distribution or reproduction is permitted which does not comply with these terms.
*Correspondence: Narasaiah Kolliputi, bmtvbGxpcHVAdXNmLmVkdQ==; Lakshmi Galam, bGdhbGFtQHVzZi5lZHU=