- Department of Pharmaceutics, JSS College of Pharmacy, JSS Academy of Higher Education and Research, Mysuru, India
Breast cancer is the second leading cancer among all types of cancers. It accounts for 12% of the total cases of cancers. The complex and heterogeneous nature of breast cancer makes it difficult to treat in advanced stages. The expression of various enzymes and proteins is regulated by several molecular pathways. Oxidative stress plays a vital role in cellular events that are generally regulated by nuclear factor erythroid 2-related factor 2 (Nrf2). The exact mechanism of Nrf2 behind cytoprotective and antioxidative properties is still under investigation. In healthy cells, Nrf2 expression is lower, which maintains antioxidative stress; however, cancerous cells overexpress Nrf2, which is associated with various phenomena, such as the development of drug resistance, angiogenesis, development of cancer stem cells, and metastasis. Aberrant Nrf2 expression diminishes the toxicity and potency of therapeutic anticancer drugs and provides cytoprotection to cancerous cells. In this article, we have discussed the attributes associated with Nrf2 in the development of drug resistance, angiogenesis, cancer stem cell generation, and metastasis in the specific context of breast cancer. We also discussed the therapeutic strategies employed against breast cancer exploiting Nrf2 signaling cascades.
1 Introduction
Breast cancer is a deadly disease affecting the majority of the female population. In 2020, approximately 2.26 million cases were recorded globally, and 6,85,000 deaths were reported. Out of 2,81,591 reported cases, 48,407 deaths were observed in the United States, while with a similar number (2,54,881) of cases in India, 1,24,975 deaths were reported (https://www.who.int/cancer/country-profiles/en/). According to GLOBOCAN (2020), 2.2 million cases were reported, and it is the second most prevalent cancer among women. Breast cancer is diagnosed in one in four women globally (Morphology). According to immunohistochemical markers, breast cancer has five subtypes that differ in prognosis and therapeutic targets: 1) luminal A (ER positive and/or PR positive and HER2 negative), 2) luminal B (estrogen receptor positive and/or progesterone receptor positive and HER2 positive), 3) HER2 overexpressing (estrogen receptor and progesterone receptor negative and HER2 positive), 4) basal like (estrogen receptor/progesterone receptor/HER2 negative, cytokeratin 5/6 positive, and/or epidermal growth factor receptor positive), and 5) normal breast like. TNBC is a type of breast cancer in which estrogen receptor (ER), progesterone receptor (PR), and human epidermal growth factor receptor-2 (HER2) are not expressed. Gene expression in triple-negative breast cancer often classifies it as a subtype of basal-like breast cancer (Kumar and Aggarwal, 2016). Approximately 15–20% of cases represent TNBC, which has a more aggressive phenotype, rapid onset of metastasis, shorter response duration to therapies, and worse prognosis (Yin et al., 2020).
The complex and heterogeneous nature of breast cancer and the contribution of Nrf2 are currently under investigation, and limited data are available to justify the major role of Nrf2 in breast cancer progression (Oshi et al., 2020). Several studies have proposed a link between the enhanced activity of Nrf2 and the potentiation of breast cancer metastasis. Nrf2 is present in the cytoplasm, where it binds to Kelch-like ECH-associated protein 1 to form a complex with Cul3 and Rbx1, which degrades the Nrf2 proteasomal enzyme (Bellezza et al., 2018). However, the stable Nrf2 heterodimerizes with Maf proteins in nuclei, enhancing the antioxidant property to protect the target gene (Pandey et al., 2017). Nrf2 is also associated with Notch pathways, which enhance the survival, invasion, and chemoresistance associated with tumor cells with abnormal expression of Nrf2 (Lamy et al., 2017). Moreover, Nrf2 has a key role in the activation of HIF1α, which is followed by enhanced glycolysis, which enhances breast cancer progression (Zhang et al., 2018).
Redox mechanism-based therapy is known to play an important role in cancer treatment; however, its utility is compromised with the inherent tendency to develop resistance over time. Here, Nrf2 is responsible for the regulation of antioxidant and cytoprotective properties through the activation of several genes involved in glutathione (GSH) synthesis and chemoresistance (Raghunath et al., 2018). The recent literature is flooded with multiple outputs by various researchers on the role of Nrf2 and its exact involvement in biological functions. In this article, we discuss the role of Nrf2 in the specific context of breast cancer, its development, angiogenesis, chemoresistance, stem cell generation, and metastasis. The effective treatment strategies are also elaborated and explained here for the treatment of breast cancer with abnormal Nrf2 expression.
2 Nuclear Factor Erythroid 2-Related Factor 2: General Mechanism/Pathways
Nrf2 is an omnipresent transcription factor that is essential for maintaining cellular homeostasis. It promotes the activity of cytoprotective genes such as glutamate cysteine ligase (GCS) and NAD(P)H:quinone oxidoreductase-1 (NQO1). Generally, Nrf2 is a transcription factor of the Cap n Collar (CNC) family and contains a basic leucine zipper region (bZip). Nrf2 is made up of 650 amino acid residues and has a molecular weight of 96–118 kDa because of posttranslational changes such as phosphorylation (Moi et al., 1994; Pi et al., 2007). Nrf2 tends to promote the transcription of genes by heterodimerizing with Maf proteins or other homologs to a cis-acting DNA transcriptional regulator, specifically the antioxidant response element (ARE) (Zhu et al., 2016). Nrf2 contains seven Neh domains (Neh1-Neh7) that are considered crucial for its action and suppression (Itoh et al., 1999). Neh2 and Neh6 are degron sections that are targeted by Keap1 and TrCP via 29DLG31/79ETGE82 motifs and 343DSGIS347/382DSAPGS387 motifs, respectively (McMahon et al., 2004; Chowdhry et al., 2013). Ablation or diminution with time is associated with an increase in oxidative stress and cellular death (Lee et al., 2003; Suh et al., 2004; Silva-Islas and Maldonado, 2018).
Nrf2 is considered the key regulator of the oxidative cellular state through the interaction of the proteins CHD6, CBP, and RAC3; Neh3, Neh4, and Neh5 are transactivation domains (Katoh et al., 2001; Nioi et al., 2005; Kim et al., 2013) Finally, the Neh7 domain is linked to the RXRα protein for Nrf2 suppression (Wang et al., 2013). Nrf2 is expressed everywhere (Moi et al., 1994) and regulates the expression of approximately 1,055 genes (Malhotra et al., 2010) that contain the cis-acting antioxidant response element (ARE, 5′-GTGACNNNGC-3′) (Rushmore et al., 1991). They ARE sequence is located in the regulatory regions of genes involved in cellular growth, oxidation and detoxifying response, metabolic, immunologic response, cell survival, signaling, and cellular cycle (Figure 1).
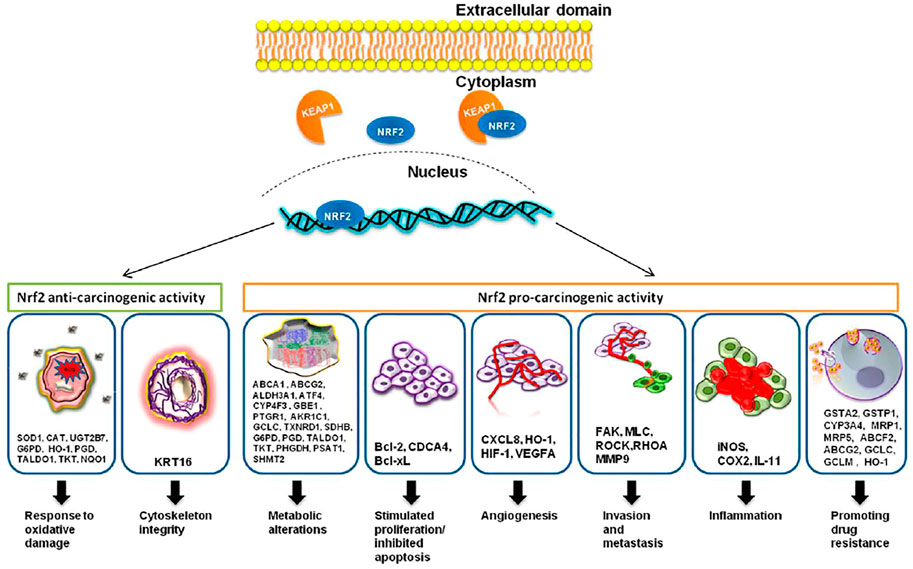
FIGURE 1. Nrf2, through its targeted genes, has an anti-carcinogenic role in the case of normal cells and a pro-carcinogenic effect in the case of transformed malignant cells. The image was acquired from (Zimta et al., 2019). Under creativecommons.org/licenses/by/4.0/.
Chemopreventive drugs activate Nrf2 (Fahey et al., 2002; Iida et al., 2004; Sussan et al., 2009) and pharmacological stimulation of Nrf2 has been extensively supported as a primary method for cancer and other illness prevention (Kwak et al., 2004; Zhang et al., 2004). Moreover, recent research reveals that Nrf2 activity may be elevated in cancer cells, and its cytoprotective action may promote cancer cell survival and proliferation, implying that inhibition of Nrf2 during cancer treatment may be essential (Lau et al., 2008; Kang et al., 2020). The mechanism by which chemopreventive drugs activate Nrf2 is, however, poorly understood. While most research has shown that chemopreventive drugs activate Nrf2 by preventing its protein degradation, there is also some research implying that Nrf2 gene transcription may be promoted (Kwak et al., 2002; Pi et al., 2003).
Keap1 reactive cysteine residues have a negative impact on Keap1-mediated Nrf2 enzymatic activity, which results in Nrf2 accumulation/activation and cytoprotection by enhanced ARE transcriptional genes (Dinkova-Kostova et al., 2005a). Furthermore, chemical alteration of Keap1 cysteines has been found to cause its own ubiquitination and destruction, sparing Nrf2 from destruction (Hong et al., 2005). Nonetheless, some studies suggest that chemically changing Keap1 cysteines are inadequate to interrupt the Nrf2 interaction with Keap1 (D. D. et al., 2004; Eggler et al., 2005), while others state that phosphorylation of Nrf2 (at Ser40) by protein kinase C or extracellular protein kinase PERK increases Keap1 dissociation (Huang et al., 2002).
Nrf2 has a key role in redox homeostasis through NADPH and ROS regeneration and glutathione (GSH) and thioredoxin (TXN) antioxidant synthesis. The regulation and maintenance of GSH synthesis is controlled by Nrf2 and by the expression of two types of subunits, the catalytic subunit (Gclc) and the modifier subunit (Gclm), which help in the synthesis of glutamate-cysteine ligase (Gcl) (Moinova and Mulcahy, 1999; Dinkova-Kostova and Abramov, 2015). Nrf2 regulates several GSHs (Gsta1/2/3/5, Gstam1/2/3, and Gstp1) and other ROS detoxifying enzymes (Thimmulappa et al., 2002). The TXN-based antioxidation system is also controlled and regulated by Nrf2. Nrf2 controls thioredoxin reductase 1 (Txnrd1) and sulfaredoxin, which are essential for the oxidized protein thiol reduction mechanism. The NADPH enzyme is an important factor for cytoprotection. Nrf2 positively regulates NADPH-generating enzymes, such as 6-phosphogluconate dehydrogenase (Pgd), isocitrate dehydrogenase 1 (Idh1), glucose-6-phosphate dehydrogenase (G6PD), and malic enzyme 1 (Me1) (Tonelli et al., 2018). Moreover, Nrf2 is also associated with the regulation of other cytoprotective enzymes. Hmox1 is a part of the heme oxygenase enzyme, which is involved in ferritin production by oxidizing free Fe2+ into Fe3+. Nrf2 regulates the expression of genes that are especially encoded ferritin complex constitutions (Wu et al., 2011). Nrf2 plays a major role in the cellular defense system by controlling xenobiotic and oxidative stress conditions by controlling the expression of antioxidants and detoxifying genes in normal cells (Tonelli et al., 2018).
The cellular Nrf2 level is quite low in normal unstressed situations, but it substantially increases when exposed to electrophilic compounds or reactive oxygen species (ROS) (Itoh et al., 1997). In Keap1, electrophiles alter reactive cysteine residues (Dinkova-Kostova et al., 2005b). Murine Keap1 has 25 cysteine residues, which are classified into different classes based on their reactivity to different electrophiles (Zhang and Hannink, 2003).
Cysteine 151 (C151) and C288, for example, have been proven to sense definite sets of electrophiles generated endogenously or exogenously (Eggler et al., 2008). Although particular cysteine residues changed by ROS have yet to be identified, oxidative alteration of Keap1 has been reported to reduce its binding to Nrf2 or CUL3. These electrophilic and oxidative changes inactivate Keap1, allowing Nrf2 to be stabilized. As a result, the rise in Nrf2 in response to electrophiles and ROS is not a precise induction but rather a process known as depression (from rapid degradation-based repression) (Taguchi and Yamamoto, 2017) (Figure 2).
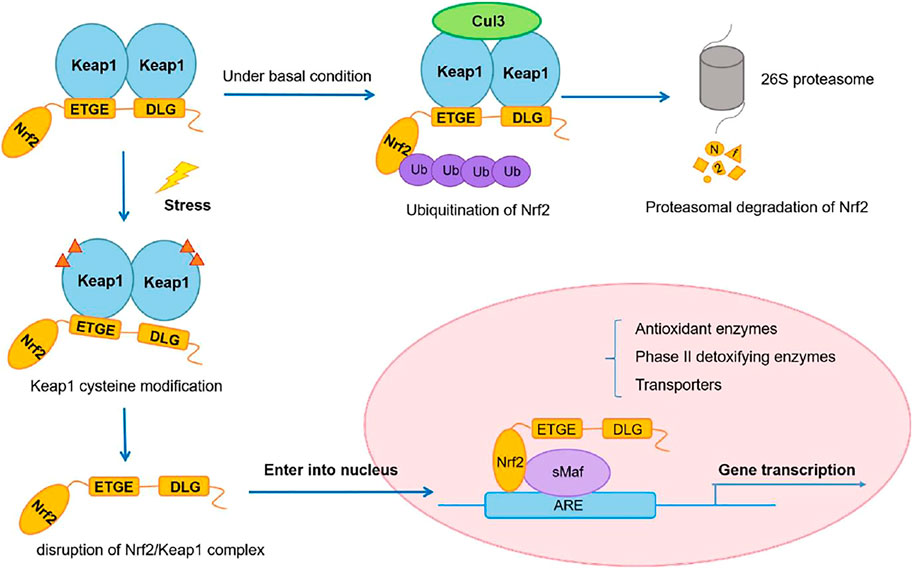
FIGURE 2. Nrf2/Keap1 signaling pathway. Adapted from (Wu et al., 2019). Under © 2019 The Authors. Cancer Medicine published by John Wiley & Sons Ltd. https://creativecommons.org/licenses/by/4.0/.
3 Nuclear Factor Erythroid 2-Related Factor 2 in Breast Cancer
Nuclear factor erythroid 2-related factor 2 (Nrf2) is primarily responsible for the cytoprotection of normal cells by detoxifying mechanisms through oxidation, electrophilic stress, or xenobiotic processes. The abnormal expression of Nrf2 in cancer cells leads to a pro-oncogenic program that stimulates the malignancy of cancerous cells/tissue. An increased level of Nrf2 expression resulted in lower survival and increased cancerous cell progression and proliferation in breast cancer patients (Almeida et al., 2020). Convening evidence from recent decades indicates that Nrf2 can exert chemopreventive properties on normal cells through an ROS-dependent oxidation process. However, aberrant expression in breast cancer imparts cytoprotective effects to cancerous cells by suppressing ROS-dependent DNA damage and carcinogenicity.
Nrf2 has dual roles as a pro-oncogenic and anti-oncogenic in breast cancer cells and healthy cells, respectively. The dual role contributed by the transcription factors depends on metabolic adaptation, cell proliferation, and induction of Nrf2 (Lee et al., 2018; Aliyev et al., 2021). For example, De Blasio and his co-workers demonstrated that Nrf2 upgraded both the proliferation and antioxidant capacity in triple-negative breast cancer (TNBC) cells by downmodulating miR-29b-1-5p expression. miR-29b-1-5p is a prognostic biomarker in basal-like breast cancer that produces cytotoxic events through decreased levels of p-AKT and p-Nrf2 and inhibition of N-methyltransferase expression. Thus, it helps to reduce cell proliferation and invasion. They showed in their research that the activation of miR-29b-1-5p expression inhibits the expression of AKT, which could suppress Nrf2 (De Blasio et al., 2020). Nrf2 could be one of the major hallmarks in the development and regulation of breast cancer. It was also suggested that Nrf2 is highly expressed in ER-negative breast cancer. Nrf2 downregulates CXCL13, which suppresses breast cancer proliferation. The increased level of CXCL13/CXCR5 coarticulation in ER (+) breast cancer cells with lower Nrf2 levels helps advance tumor intrusion and metastasis (Aliyev et al., 2021).
The involvement of Nrf2 and its role in different subtypes in breast cancer are still under investigation. To date, no data have been published explaining the level of Nrf2 expression in different subtypes of breast cancer. However, few published studies have shown that the Nrf2 and keap-1 pathways are more highly activated in breast cancer, which has ER, PR, and HER receptor positivity, than in TNBC (Karihtala et al., 2011). The increased level of Nrf2 causes lower overall survival and disease-free survival in all breast cancer patients. However, normal cells can exert chemopreventive effects via Nrf2. Due to the dual role of Nrf2 (pro-oncogenic and anti-oncogenic) in cancer patients, other factors, such as metabolic genes, proliferative genes, and angiogenesis genes, should also be considered for inhibiting Nrf2 through Nrf2 inhibitors (Almeida et al., 2020; Smolková et al., 2020; Aliyev et al., 2021).
4 Role of Nuclear Factor Erythroid 2-Related Factor 2 in Breast Cancer Resistance
Nrf2 is a leucine zipper protein transcription factor that positively regulates the expression of antioxidant genes, such as GPX4, HO-1, SLC7A11, and NAD(P)H quinone oxidoreductase (Qiao et al., 2020). As discussed above, Nrf2 regulates oxidative stress and protects cancer cells by the toxic effect of therapeutic drugs/anticancer drugs. Nrf2 is a central transcriptional activator with an active role in cellular defense mechanisms against electrophilic or oxidative stress (Uruno and Motohashi, 2011) (Figure 3). Studies have reported that Keap1 constantly degrades Nrf2, which downregulates Nrf2 by the ubiquitin–proteasome pathway under normal cellular conditions. At the somatic mutation stage, Keap1 is inactivated, which enhances Nrf2 expression in the nucleus and induces cytoprotective genes such as heme oxygenase-1 [HO-1 (HMOX1)] and NAD(P)H:quinone oxidoreductase 1 (NQO1) (Baird and Dinkova-Kostova, 2011). Nrf2 overexpression in the protein–protein interaction region compromised the Keap1 checkpoint, resulting in epigenetic and posttranscriptional modification and enhancing proto-oncogenes (Sporn and Liby, 2012). Moreover, Nrf2 phosphorylation and polymorphisms in Nrf2 cause poor prognosis in breast cancer (Hartikainen et al., 2012; Ishikawa, 2014).
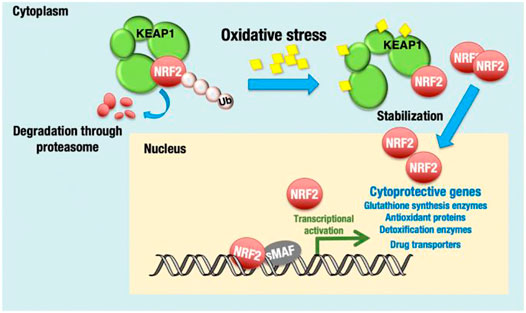
FIGURE 3. KEAP1-NRF2 system for oxidative stress response. NRF2 is a transcription activator and regulates many cytoprotective genes. Under unstressed conditions, Nrf2 is bound by KEAP1 and ubiquitinated for degradation. Adapted from (Okazaki et al., 2020). Under © 2019 The Authors. https://creativecommons.org/licenses/by/4.0/.
Nrf2 overexpression in breast cancer also improves the excess expression of P53 in an inhibitor protein for stimulating apoptosis, also known as Rel-A inhibitor, which promotes cancer development and tumor-associated drug resistance. The higher P53 level in breast cancer cells restricts the binding of free Keap1 to free Nrf2; therefore, the level of free Nrf2 is higher in the nucleus and induces chemoresistance (Ge et al., 2017). Few more studies have suggested that other proteins, such as p21 or p62, also restrict the Keap1–Nrf2 interaction and induce Nrf2-associated drug resistance in tumor cells (Chen et al., 2009; Lau et al., 2010).
5 Role of Nuclear Factor Erythroid 2-Related Factor 2 in Metastasis
In response to various oxidative-driven transcriptional processes, Nrf2 combines physiological stress signals by interacting with antioxidant response domains within the regulatory regions of Nrf2-controlled genes (Itoh et al., 1999; Jaiswal, 2004; Nguyen et al., 2009). Because of its cytoprotective role, Nrf2 has been identified as a tumor suppressor, and its activity can also prevent tumor growth. For example, sulforaphane, a Nrf2 inducer, has also been found to prevent the development and metastasis of effectively supported implanted breast cancer cells in female athymic mice as well as to reduce the growth of human breast cancer cells (Kanematsu et al., 2011). Moreover, several researchers have demonstrated abnormally active Nrf2 in several breast cancer cells (Nioi and Nguyen, 2007; Syed Alwi et al., 2012; Zhong et al., 2013), and recent genetic investigations of breast cancers revealed the crucial role of Nrf2 in oncogenesis (Hayes and McMahon, 2009; Denicola et al., 2011).
In breast cancer, Nrf2 activation enhances Rho expression and downstream proteins such as focal adhesion kinase 1 (FAK), modulator of volume-regulated anion channel current 1 (MLC), and Rho-associated coiled-coil-containing protein kinase 1 (ROCK), whereas it lowers estrogen-related receptor (ERR1) expression. Nrf2 has a direct interaction with the BRCA1 susceptibility protein, resulting in enhanced BRCA protein stability. Estrogen improves Nrf2 activation in the absence of BRCA expression, leading to reduced ROS production and enhanced cytoprotection (Gorrini et al., 2013). Exogenous antioxidants such as phospholipid hydroperoxide glutathione peroxidase (PHGPx) or pro-oxidant 15-lipoxygenase (15-LOX) decrease the levels of vascular cell adhesion molecule (VCAM) through an interaction with Nrf2 in the gene promoter of this locus (Zimta et al., 2019).
RhoA is a member of the Ras superfamily, which regulates cell migration and invasion of cancer cells (Pertz et al., 2006; Vega and Ridley, 2008). RhoA GTPases regulate the formation of actin stress fibers and limit the size of the lamellipodium through their downstream effectors mDIA and ROCKs, which cycle between an inactive GDP-bound and an active GTP-bound form (Riento and Ridley, 2003; Worthylake and Burridge, 2003). The RhoA regulatory effect is controlled to the extent of protein stability and deterioration (Nethe and Hordijk, 2010). However, no constitutively active Rho GTPase mutations have been found in human cancers (Rihet et al., 2001; Fritz et al., 2002), and clinical and experimental studies show a link between enhanced RhoA expression and poor clinical outcome in breast cancer (Bellizzi et al., 2008; Chan et al., 2010; Ma et al., 2010). High levels of Nrf2 are associated with tumorigenesis and poor prognosis, and it promotes RhoA expression by interacting with and silencing the ERR1 gene, allowing breast cancer cells to proliferate and metastasize. In conjunction with published reports, deactivating Nrf2 could be advantageous in breast cancer treatment in the clinical stage (Zhang C. et al., 2016).
6 Role of Nuclear Factor Erythroid 2-Related Factor 2 in Tumor Angiogenesis
Due to excessive ROS generation, by interacting with the Notch/delta-like 4 (Dll4) system, Nrf2 promotes vascular sprouting by reducing the impairment of vascular signal transduction and angiogenesis and regulating the production of tip cells (Wei et al., 2013). Its role in microvascular epithelial cell migration and blood vessel generation has been verified, which suggests the development of a VEGF-Nrf2 positive loop (Li et al., 2016). The positive regulation of endothelin receptor B by nitro-oleic acid (OA-NO2) was substantially regulated by Nrf2, suggesting that Nrf2 silencing enhanced endothelin-1 levels in the circulation (Kansanen et al., 2017). ROS have been demonstrated to be proangiogenic mediators at adequate levels through a different pathway, including increasing VEGF and angiopoietin-1/Tie-2 signaling (Harel et al., 2017; Zou et al., 2019). Under oxidative stress, Nrf2 plays a direct or indirect role in angiogenesis control (Guo and Mo, 2020).
Disturbances in proliferation and apoptosis have an important role in tumor and angiogenesis. Past studies indicate that Nrf2 is required for angiogenesis of normal vasculature. Valcarcel-Ares et al. published that short interfering RNA (siRNA) and Keap1 were involved in silencing the expression of Nrf2 in coronary arterial endothelial cells, leading to cellular proliferative capacity impairment, improper adhesion to extracellular matrix proteins, reduced migration, and impaired capillary formation (Valcarcel-Ares et al., 2012). It is believed that the Nrf2 in breast tumor angiogenesis affects the biological behavior of intratumoral endothelial cells (Zhou et al., 2012).
Nrf2 regulates redox homeostasis and is associated with cellular growth and malignancy (Denicola et al., 2011; Mitsuishi et al., 2012). Neovascularization is essential for organ regeneration, tissue repair, and embryogenesis (Hoeben et al., 2004). Tumor growth is linked to angiogenesis, and in malignant tumors, the exchange of oxygen/carbon dioxide and nutrients/waste products depends on blood vessels. Angiogenesis plays a critical role in the migration and invasion of primary malignancies to distant areas of the body (Carmeliet, 2005). Oxidative stress affects angiogenesis in atherosclerosis, ocular diseases, and tumorigenesis (Chung and Ferrara, 2011; Coso et al., 2012).
Another important factor in angiogenesis is hypoxia, as it activates angiogenesis mediators such as HIF and VEGF transcription factors, which are interlinked to tumor dissemination, invasion, and metastasis (Lee et al., 2009). In addition, elevated levels of peroxides also trigger tumor angiogenesis (Szatrowski and Nathan, 1991). Various studies have shown that Nrf2 participates in angiogenesis regulation. Hypoxia triggers the Nrf2/ARE pathway, which promotes tumor blood vessel development. The forceful blocking of HIF-1 signaling in the absence of Nrf2 can result in a decrease in capillary density (Zhang et al., 2013). Kweider et al. (2011) explored the role of VEGF in cancer cell proliferation. Another study found a link between VEGF and Nrf2 activation, demonstrating that VEGF increased Nrf2 expression in an ERK1/2-dependent manner (Kweider et al., 2011). Shao et al. reported that curcumin upregulates Nrf2 and GSH and causes ROS scavenging, reduces the expression of VEGF, and inhibits hepatocarcinoma angiogenesis and invasion (Shao et al., 2019). As a result, VEGF and Nrf2/HIF-1 facilitate tumor angiogenesis.
The activation of HIF-1α through Nrf2 also enhances the angiogenesis and progression of breast cancer. HIF-1α is the major transcription factor responsible for adaptive hypoxic conditions and regulates metabolic genes (such as GLUT1, HK2, and PGK1), angiogenesis genes (such as VEGF and FGF), and apoptosis genes (e.g., Bax, BCL2, and P53). The inhibition of the aberrant expression of Nrf2 could be effective in breast cancer treatment. This experiment was performed by Zhang and his coworkers in 2018, who found higher levels of Nrf2 and HIF-1α mRNA and proteins in MCF-7 and MDA-MB-231 breast cancer cells than in normal breast cells (MCF-10A). The knockdown of Nrf2 overexpression decreased HIF-1α mRNA levels and reduced breast cancer cell proliferation (Zhang et al., 2018).
7 Role of Nuclear Factor Erythroid 2-Related Factor 2 in Breast Cancer Stem Cells
The studies found and identified Nrf2 as a major regulator of chemoresistance in cancer stem cell (CSC)-enriched breast cancers (Achuthan et al., 2011; Ryoo et al., 2015) as well as the activation of Nrf2-associated antioxidant genes such as HO-1, NQO1, Prx1, and others that leads to radioresistance in several other cancer cells (Zhou et al., 2013). Because breast cancer stem cells (BCSCs) have low ROS levels and increased antioxidant defense (Lobo et al., 2009), the involvement of the Nrf2 pathway in BCSC radioresistance requires further investigation.
For example, an enhancement in ALDH levels in BCSCs causes higher radioresistance, carcinogenesis, decreased apoptosis, and regulation of signaling pathways that enhance mesenchymal–epithelial transition and migration. Moreover, following fractionated irradiation, tumorigenicity was increased. Further examination of the involvement of Nrf2 in radioresistance revealed that following irradiation, Nrf2 and its related genes HO-1 and NQO1 were significantly elevated. All of the foregoing pathways of radioresistance in BCSCs were reduced by shRNA-mediated knockdown of Nrf2 expression. The process of Nrf2 activation was reported to be regulated by Keap1 silencing, as there was no change in GSK-3 or Bach1, a negative regulator of Nrf2. We also found no change in the methylation status of the Keap1 promoter; however, we identified a substantial rise in the expression of miR200a. This suggests that miR200a might be a mechanism for Keap1 silencing. This work offers data for the significance of Nrf2 and its downstream genes in radioresistance in BCSCs and identifies processes by which the Nrf2/Keap1 pathway influences radioresistance in BCSCs (Kamble et al., 2021).
Evidence gathered to date suggests that the upregulation of Nrf2 promotes tumor growth and survival by creating a favorable environment for cancer stem cells. The direct involvement of Nrf2 in cellular ROS regulation and anticancer drug resistance is a potential contribution of Nrf2 to CSC biology. Wu et al. (2015) recently demonstrated that Nrf2 activation was associated with CSC-enriched spheroid breast cells (Wu et al., 2015). Another published report demonstrated that Nrf2 activation is characterized in a CD44-overexpressing breast CSC-like system and investigated the direct link of Nrf2 with the CSC phenotype (Ryoo et al., 2018).
Similarly, in another work, it was suggested that intracellular ROS generation is low and is upregulated by Nrf2-induced GCLC expression, which allows the self-renewal of CSCs through the Fork head box O3a-Bmil-axis (Kim et al., 2020). Furthermore, it has also been demonstrated that hypoxia-driven CSC enrichment in breast cancer originates from a dedifferentiation process and that hypoxia-inducible factors (HIFs) are essential for chemotherapy resistance in breast CSCs (Iriondo et al., 2015). Surprisingly, differentiating CSCs exhibit multidrug resistance (MDR) because of the PERK-Nrf2 signaling pathway (Del Vecchio et al., 2014).
8 Strategies to Overcome Breast Cancer Resistance
8.1 Endogenous Molecule Inhibitors
Various endogenous molecules, such as E-cadherin (Kim WD. et al., 2012), activating transcription factor-3 (ATF3) (Brown et al., 2008), tumor protein P53 (Faraonio et al., 2006), BTB and CNC homology 1 (bach1) (Dhakshinamoorthy et al., 2005), caveolin-1 (Li et al., 2012), and GSK3 (Chowdhry et al., 2013), play a role in the downregulation of Nrf2. These molecules disrupt the Nrf2/ARE pathway and its expression levels. Specifically, E-cadherin has a role in chemoresistance and enables Keap1 to reduce endogenous Nrf2 levels by recruiting Nrf2 via β-catenin. P53 activation causes downregulation of Nrf2 and generates higher levels of ROS, which promotes apoptosis (Faraonio et al., 2006). These molecules are generally required for normal cell homeostasis; therefore, disruption of the signaling pathway would be based on a thorough understanding of cellular biology and events before beginning this therapy.
8.2 Exogenous Natural Inhibitors
The complex tumor microenvironment limits the application of endogenous inhibitors; hence, exogenous inhibitors are emerging molecules. Some exogenous natural inhibitors not only inhibit Nrf2 but also promote anticancer drug sensitivity in resistant cancerous cells. For example, vitamin C could decrease oxidative stress in the tumor microenvironment, which suppresses the translocation of Nrf2 to the nucleus from the cytoplasm. Ascorbic acid (vitamin C) is a reducing agent that binds to ARE, inhibits Nrf2 translocation to DNA, and decreases the levels of g-GCSl mRNA and GSH. Furthermore, vitamin C in combination helped in the reversal of drug resistance associated with imatinib treatment (Tarumoto et al., 2004). Similarly, Xiu et al. (2007) reported that all-trans retinoic acid (ATRA) prevents binding of Nrf2 to ARE by forming a complex with RARa (Xiu et al., 2007). In another example, trigonelline, a coffee-derived alkaloid, also lowers Nrf2 levels in drug-resistant pancreatic cancer cell lines by blocking Nrf2-dependent proteasomal gene expression of s5a/psmd4 and a5/psma5 and reducing proteasome activities. Trigonelline also reduced basal and tert-butylhydroquinone-induced Nrf2 activity and reduced the drug resistance induced by higher levels of Nrf2 (Arlt et al., 2013).
Nrf2-resistant cancer cells were observed to have higher levels of Nrf2 and other associated genes, such as NQO1, MRP-1, HO-1, CGLM, and CGLC. The expression of these genes is highly linked with the development of drug resistance in lung cancer. Cryptotanshinone treatment was administered in combination with cisplatin to the cells, and it was found that Nrf2 and its associated target gene expression were diminished in cisplatin-resistant lung cancer. Furthermore, it was also observed that cryptotanshinone affects other signaling pathways, such as the MAPK, Akt, and Stat3 (Xia et al., 2015). Luteolin, a vegetable-derived flavonoid, dramatically induces Nrf2 mRNA degradation and other downstream ARE-driven genes, such as NQO1, HO-1, and AKR1C (Tang et al., 2011). As a result, luteolin induced cell death when used in combination with oxaliplatin, bleomycin, and DOX. In TNBC cells, luteolin-loaded nanoparticles reduced the Nrf2, HO1, and MDR1 mRNA expression levels. In addition, luteolin nanoparticles improved doxorubicin sensitivity in MDA-MB-231 cells (Sabzichi et al., 2014).
A few other flavonoids, such as chrysin (Gao et al., 2013a), apigenin (Gao et al., 2013b), wogonin, and 30, 40, 50, 5,7-pentamethoxyflavone (PMF) (Hou et al., 2015), can also inhibit Nrf2 expression in cancerous cells and produce apoptotic effects. Flavonoids are known for their antioxidant and cytoprotective properties, and their Nrf2-inducible effect has been observed in a few studies (Bai et al., 2016). Nanocarriers entrapping flavonoids were developed for targeted drug administration, enhancing the bioavailability of poorly water-soluble medications and delivering macromolecules to the cell’s site of action. Moreover, by combining therapeutic agents with imaging tools that can visualize the drug delivery location and coadministration of two or more medicines, ADRs can be reduced, and nanotechnology plays a key role in this (Kumar et al., 2016; Khan et al., 2021).
8.3 Inhibitors of Nuclear Factor Erythroid 2-Related Factor 2 in Cancer Therapy
Nrf2 regulates genes such as transporters, phase II detoxifying enzymes, and endogenous antioxidants by controlling cellular defense response mechanisms. The literature shows the role of Nrf2 in chemoresistance, and its expression has been identified in many types of cancer (Samadi et al., 2014). High-throughput screening (HTS) in combination with cell-based assays has proven to be a potential approach to discover new anticancer drugs and to identify therapeutic uses of compounds that are approved by the FDA. Plant extracts and other phytochemicals have anticancer activity and are under treatment regimens or in clinical trial investigations.
Procyanidin CCE lowers the levels of Nrf2 expression and inhibits cell growth in the case of cancer. Another compound based on a flavonoid, luteolin, present in fruits and vegetables, inhibits Nrf2 in cancer cells (Choudhari et al., 2020). Similarly, trigonelline, an alkaloid present in hemp seed, coffee beans, oats, garden peas, and fenugreek seed, shows high basal Nrf2 activity that protects against etoposide- or TRAIL-induced apoptosis by elevating proteasomal gene expression (Panieri and Saso, 2019). Brusatol, a quassinoid Brucea javanica plant extract, shows antitumor activity (Yu et al., 2020). Chrysin, a bioflavonoid, protects against carcinogenesis by decreasing the mRNA and protein levels of Nrf2 (Wang et al., 2018). Apigenin, a dietary flavonoid present in fruits and vegetables, is said to exhibit anticancer effects in vitro and in vivo (Yan et al., 2017). Oridonin, a diterpenoid derivative, possesses anticancer effects in solid and hematologic tumors (Lu et al., 2018). Similarly, Honokiol, a lignan isolated from Magnolia, produces toxicity in lymphoid cancer cell lines. Honokiol lowers NF-κB activity and Nrf2 proteins, resulting in higher ROS production and apoptosis (Ong et al., 2019). Halofuginone inhibits and activates Nrf2 constitutively in resistant cancer cells (Panieri and Saso, 2019). Another anticancer agent, plumbagin, a naphthoquinone, induces oxidative stress-dependent Nrf2 activity in cancer cells (Kapur et al., 2018). Berberine is an alkaloid found in various medicinal plants. By inducing oxidative stress, berberine has anticancer properties in breast cancer (Lu et al., 2012). Parthenolide, a sesquiterpene lactone found in medicinal plants, shows anticancer effects by modulating ROS (Mathema et al., 2012). Wogonin, a flavonoid obtained from Scutellaria baicalensis Georgi, also reduced Nrf2 nuclear content (Zhong et al., 2013).
The extract of chestnut leaf works by suppressing the Nrf2-mediated antioxidant system and may increase ROS production and thereby promote paclitaxel-induced apoptotic cell death. The results showed that treatment with chestnut leaf extract reduced the expression ratio of Bcl-2 and Bax, and an increase in the amount of cleaved PARP and paclitaxel-treated CSCs resulted in significant mitochondrial damage compared to untreated or extract-treated CSCs. This result suggests that the combination of paclitaxel with chestnut leaf extract can effectively eliminate paclitaxel-induced CSCs. As a result, when chestnut leaf extract and paclitaxel are in combination, synergistic effects are produced; however, to determine possible adverse effects, such as counteraction or additive toxicity of the drug because of chestnut leaf extract and paclitaxel, further studies need to be performed (Scarpa and Ninfali, 2015; Woo et al., 2017).
The therapeutic role of various polyphenols in breast cancer has also been established. Curcumin activates Nrf2, which promotes the expression of antioxidative enzymes such as NQO1, HO-1, GST, and glutathione reductase (GR) and induces cellular senescence (Das and Vinayak, 2015). Curcumin’s activation of Nrf2 relies on the thiol modulation of KEAP1 (Shin et al., 2020). Similar to curcumin, other polyphenols induce Nrf2 and downstream genes, primarily phase II detoxification enzymes (Foygel et al., 2015). In MCF-7 and MDA-MB-231 breast cancer cells, NRF2 induction by EGCG was examined using Western blot analysis (Hu et al., 2010). The biphasic effects of the grapefruit polyphenol resveratrol are well known. Resveratrol therapy increases cell growth in breast cancer cells at low doses, while it causes cytotoxicity at higher concentrations. Similarly, resveratrol exhibits antioxidant properties at low concentrations while exhibiting a prooxidant profile at larger doses. Rai et al. used resveratrol in the 50–400 M concentration range to treat MCF-7 and MDA-MB-231 cells, which displayed high cytotoxicity in a dose-dependent manner (Rai et al., 2016).
The therapeutic role of melatonin in breast cancer is also known. Melatonin is an indole pineal hormone. Melatonin synthesis and secretion have been shown to be substantial contributing factors for breast cancer growth and progression (Jasser et al., 2006; Hill et al., 2015). Melatonin’s involvement in the protection and treatment of breast cancer, particularly the control of oxidative stress, has been widely researched (Gurer-Orhan et al., 2018), and the regulation of miRNAs is linked to apoptosis, cellular senescence, and proliferative genes (Chuffa et al., 2020). According to oxidative stress–mediated physiological responses, melatonin activates Nrf2 by upregulating cellular mediators such as PKC (Li et al., 2018), SIRT1 (Shi et al., 2019), and PI3K/AKT (Zhang et al., 2017).
Specific to breast cancer, metformin has been shown to target miRNAs, proteins involved in miRNA biogenesis, and target genes in CSCs. Metformin suppresses breast cancer cell proliferation by downregulating miR-27a (Zhao et al., 2016) and upregulating miR-193 (miR-193a-3p and miR-193b), which increases AMPK and decreases FASN levels, respectively (Wahdan-Alaswad et al., 2014). It also increases the expression of let-7a (a tumor suppressor miRNA) while decreasing TGF-induced miR-181a (an oncogenic miRNA) production in MCF7 cells (Oliveras-Ferraros et al., 2011). Metformin’s anticancer activities in renal and breast cancer cells have been linked to the overexpression of miR-34a, which lowers cell proliferation and the Sirt1/Pgc1/Nrf2 pathway, respectively (Do et al., 2014; Xie et al., 2017; Saini and Yang, 2018).
9 Roles of Nuclear Factor Erythroid 2-Related Factor 2 Inhibitors and Inducers in Breast Cancer
In order to enable the readers to understand the anti-oncogenic and pro-oncogenic mechanisms of Nrf2 with various compounds, the information from various references been collated in Tables 1–3. The information has been segregated under subheadings of in vitro/in vivo/clinical studies.
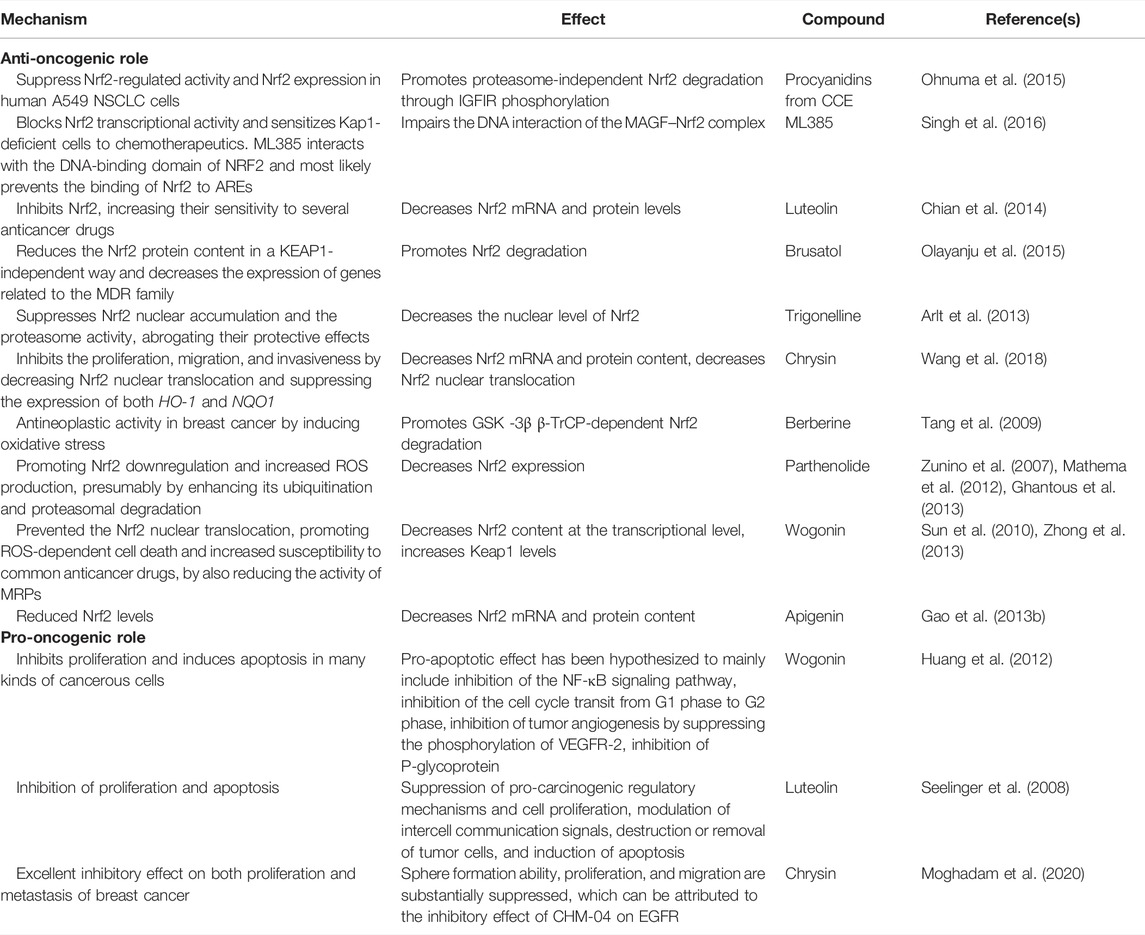
TABLE 1. Anti-oncogenic and pro-oncogenic mechanisms of Nrf2 with various compounds: in vitro studies.
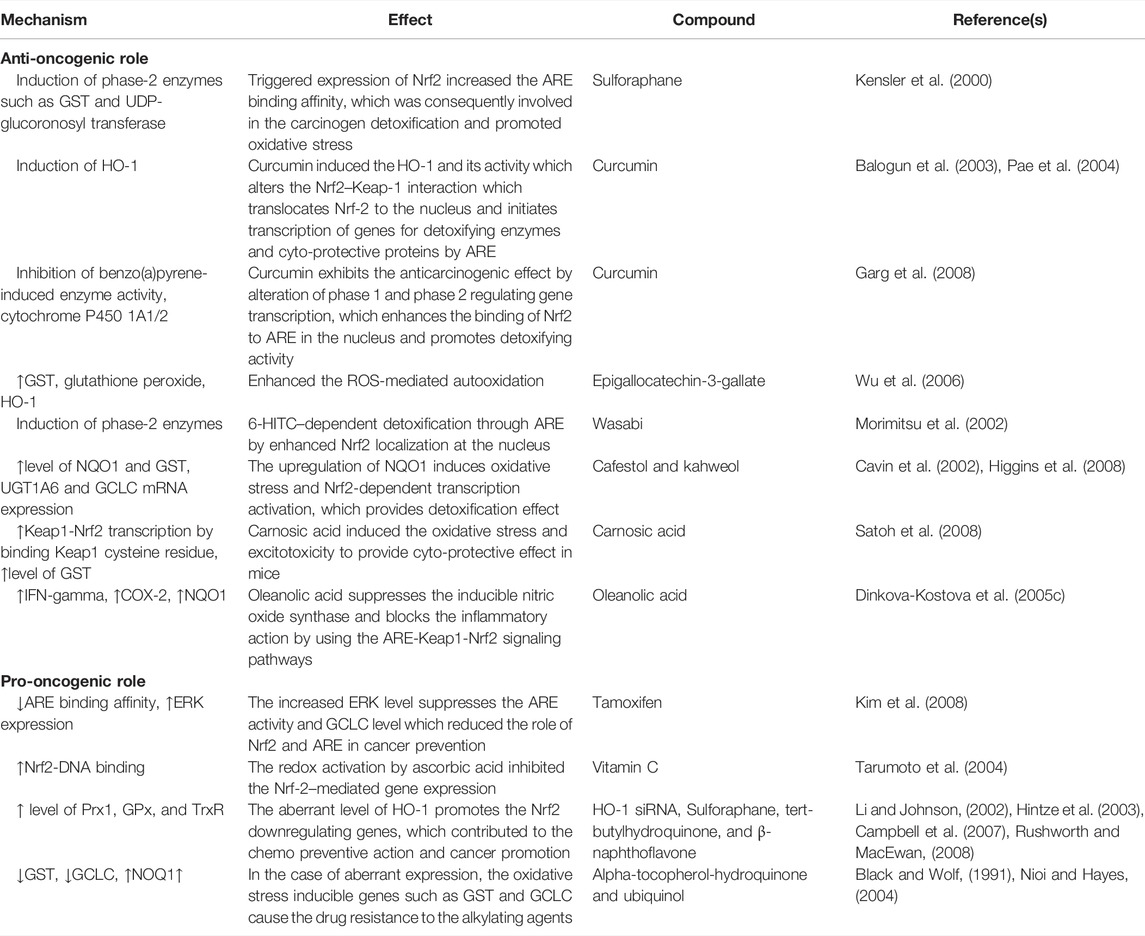
TABLE 2. Anti-oncogenic and pro-oncogenic mechanisms of Nrf2 with various compounds: in vivo studies.
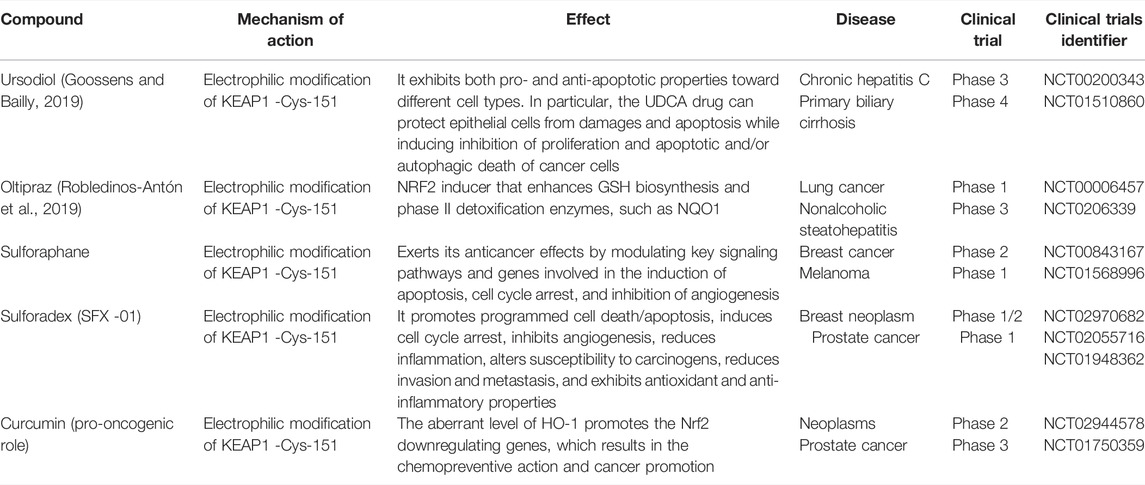
TABLE 3. Anti-oncogenic and pro-oncogenic mechanisms of Nrf2 with various compounds: clinical studies (Su et al., 2018; Goossens and Bailly, 2019; Robledinos-Antón et al., 2019; Nandini et al., 2020).
9.1 Nuclear Factor Erythroid 2-Related Factor 2 Inhibitor
Nrf2 exhibits dual roles, such as being pro-oncogenic and anti-oncogenic. Hence, Nrf2 is involved in the inhibition of cancer development, and aberrant Nrf2 expression is also involved in cancer progression. In metastatic cancer, the contribution of Nrf2 to cancer progression could be minimized or eliminated by exploring some Nrf2 inhibitors. Vitamin C is a potent Nrf2 inhibitor and is used in the suppression of Nrf2 translocation (Mostafavi-Pour et al., 2017). Following the treatment of breast cancer cells with vitamin C, there was a substantial decrease in the expression of Nrf2 mRNA and protein levels. The nuclear/cytosolic Nrf2 ratio was lowered by 1.7-fold in MDA-MB-231 cells, 2-fold in MDA-MB 468 cells, 1.4-fold in MCF-7 cells, and 1.2-fold in A549 cells after treatment with vitamin C. In a dose-dependent manner, sequential treatment with vitamin C reduced endogenous ROS generation (p = 0.027). The findings suggested that vitamin C treatment could be developed as an adjuvant for cancer patients with Nrf2 overexpression (Mostafavi-Pour et al., 2017).
Brusatol, another class of Nrf2 inhibitors, diminishes the protein levels of Nrf2 in MDA-MB-231 breast tumor cell lines (Ren et al., 2011). In recent studies, it was observed that in mammospheres obtained from breast tumor cell lines, brusatol diminishes the protein levels of Nrf2 and deposition of intracellular ROS due to the increased cytotoxicity of Taxol (Yuan et al., 2017; Muralimanoharan et al., 2018). Berberine has recently been discovered to have anticancer properties in breast cancer by causing oxidative stress (Tang et al., 2009; Kim S. et al., 2012). Zhang and colleagues focused on BT-474 and AU-565 breast cancer cells that were resistant to lapatinib, a new tyrosine kinase inhibitor of HER2/EGFR (epidermal growth factor receptor) that was used in the study to treat breast cancer that is HER2-positive. Liquid nanocrystalline nanoparticles were developed to improve the solubility and anticancer properties of berberine in MCF-7 breast cancer cells (Zhang R. et al., 2016).
Parthenolide is a sesquiterpene class of Nrf2 inhibitor with antitumor and anti-inflammatory activities based on the control of reactive oxygen species (ROS) (Zunino et al., 2007; Mathema et al., 2012). By increasing Nrf2 downregulation and enhancing ROS generation, parthenolide (PN) and its soluble counterpart dimethyl amino parthenolide (DMPN) have been demonstrated to diminish mammosphere development in triple-negative breast cancer (TNBC) cell lines, as well as the survival of mammosphere-derived CSCs, most likely through increased ubiquitination and proteasome degradation (Carlisi et al., 2016). A combination of parthenolide and vinorelbine stealthy liposomes was developed for the suppression of breast cancer. Plumbagin, a member of the naphthoquinone class of Nrf2 inhibitors, is well known for its antitumor properties and redox impairment facilitated by plumbagin, which leads to ROS-dependent cell death in tumor cells. Loading of plumbagin in transferrin-bearing liposomes dramatically increased plumbagin uptake by tumor cells, resulting in improved anti-proliferative and antiapoptotic activity (Kapur et al., 2018).
9.2 Nuclear Factor Erythroid 2-Related Factor 2 Inducers
Activation of Nrf2 has primarily been reported as therapeutic, but a recent study has indicated that depending on the status of Nrf2 activation, the process can also be pro-oncogenic. According to a recent study, oncogenic signaling may influence Nrf2 activity by raising its mRNA levels. The oncogenic activation of K-RAS and B-RAF, depending on this, is sufficient to raise Nrf2 mRNA levels and enable ROS detoxification in human cancer cells. Curcumin, sulforaphane, and oltipraz, which have been identified as Nrf2 activators, have been discovered to be non–target-specific and may raise the risk of “off-target” toxicity due to their potential to interact with the cysteines of other enzymes and proteins. Molecular instability, decreased membrane permeability, and poor bioavailability of several Nrf2 modulators are also of significant concern (Telkoparan-Akillilar et al., 2021). Several Nrf2 inducers are used to elevate and translocate Nrf2 into the nucleus and activate the ARE mechanism for the detoxification of the cells.
10 Conclusion
Nrf2 plays a crucial role in cellular redox homeostasis in healthy as well as cancerous cells. In the absence of Nrf2, ROS production is upregulated, which leads to DNA damage and tumor development. Nrf2 is directly involved in managing the expression of GSH, TXN, and NADPH and controls the level of ROS. Interestingly, under oxidative stress, not only does Nrf2 regulate NADPH but ROS can also produce NADPH oxidase, which further activates Nrf2. These findings suggest the role of Nrf2 as an oncogenic factor. The variety of molecules that can be utilized to create better treatment options for breast cancer involve Nrf2-associated events. Therefore, a better understanding of cellular events and signaling cascades would enable finding a correct therapeutic regimen against breast cancer.
Author Contributions
HK—writing and editing, RMK—writing, DB—writing, PS—writing and editing, and VJ—writing, editing, and supervision.
Conflict of Interest
The authors declare that the research was conducted in the absence of any commercial or financial relationships that could be construed as a potential conflict of interest.
Publisher’s Note
All claims expressed in this article are solely those of the authors and do not necessarily represent those of their affiliated organizations, or those of the publisher, the editors, and the reviewers. Any product that may be evaluated in this article, or claim that may be made by its manufacturer, is not guaranteed or endorsed by the publisher.
Acknowledgments
VJ acknowledges the Science and Engineering Research Board (SERB), Department of Science and Technology (DST), New Delhi, India, for providing research funding (EMR/2016/006338).
References
Achuthan, S., Santhoshkumar, T. R., Prabhakar, J., Nair, S. A., and Pillai, M. R. (2011). Drug-induced Senescence Generates Chemoresistant Stemlike Cells with Low Reactive Oxygen Species. J. Biol. Chem. 286, 37813–37829. doi:10.1074/jbc.M110.200675
Almeida, M., Soares, M., Ramalhinho, A. C., Moutinho, J. F., Breitenfeld, L., and Pereira, L. (2020). The Prognostic Value of NRF2 in Breast Cancer Patients: a Systematic Review with Meta-Analysis. Breast Cancer Res. Treat. 179, 523–532. doi:10.1007/s10549-019-05494-4
Arlt, A., Sebens, S., Krebs, S., Geismann, C., Grossmann, M., Kruse, M. L., et al. (2013). Inhibition of the Nrf2 Transcription Factor by the Alkaloid Trigonelline Renders Pancreatic Cancer Cells More Susceptible to Apoptosis through Decreased Proteasomal Gene Expression and Proteasome Activity. Oncogene 32, 4825–4835. doi:10.1038/onc.2012.493
Bai, X., Chen, Y., Hou, X., Huang, M., and Jin, J. (2016). Emerging Role of NRF2 in Chemoresistance by Regulating Drug-Metabolizing Enzymes and Efflux Transporters. Drug Metab. Rev. 48, 541–567. doi:10.1080/03602532.2016.1197239
Baird, L., and Dinkova-Kostova, A. T. (2011). The Cytoprotective Role of the Keap1-Nrf2 Pathway. Arch. Toxicol. 85, 241–272. doi:10.1007/s00204-011-0674-5
Balogun, E., Hoque, M., Gong, P., Killeen, E., Green, C. J., Foresti, R., et al. (2003). Curcumin Activates the Haem Oxygenase-1 Gene via Regulation of Nrf2 and the Antioxidant-Responsive Element. Biochem. J. 371, 887–895. doi:10.1042/bj20021619
Bellezza, I., Giambanco, I., Minelli, A., and Donato, R. (2018). Nrf2-Keap1 Signaling in Oxidative and Reductive Stress. Biochim. Biophys. Acta Mol. Cell Res 1865, 721–733. doi:10.1016/j.bbamcr.2018.02.010
Bellizzi, A., Mangia, A., Chiriatti, A., Petroni, S., Quaranta, M., Schittulli, F., et al. (2008). RhoA Protein Expression in Primary Breast Cancers and Matched Lymphocytes Is Associated with Progression of the Disease. Int. J. Mol. Med. 22, 25–31. Available at: http://ovidsp.ovid.com/ovidweb.cgi?T=JS&PAGE=reference&D=emed10&NEWS=N&AN=352512114. doi:10.3892/ijmm.22.1.25
Black, S. M., and Wolf, C. R. (1991). The Role of Glutathione-dependent Enzymes in Drug Resistance. Pharmacol. Ther. 51, 139–154. doi:10.1016/0163-7258(91)90044-M
Brown, S. L., Sekhar, K. R., Rachakonda, G., Sasi, S., and Freeman, M. L. (2008). Activating Transcription Factor 3 Is a Novel Repressor of the Nuclear Factor Erythroid-Derived 2-related Factor 2 (Nrf2)-Regulated Stress Pathway. Cancer Res. 68, 364–368. doi:10.1158/0008-5472.CAN-07-2170
Campbell, L., Howie, F., Arthur, J. R., Nicol, F., and Beckett, G. (2007). Selenium and Sulforaphane Modify the Expression of Selenoenzymes in the Human Endothelial Cell Line EAhy926 and Protect Cells from Oxidative Damage. Nutrition 23, 138–144. doi:10.1016/j.nut.2006.10.006
Carlisi, D., Buttitta, G., Di Fiore, R., Scerri, C., Drago-Ferrante, R., Vento, R., et al. (2016). Parthenolide and DMAPT Exert Cytotoxic Effects on Breast Cancer Stem-like Cells by Inducing Oxidative Stress, Mitochondrial Dysfunction and Necrosis. Cell Death Dis 7, e2194. doi:10.1038/cddis.2016.94
Carmeliet, P. (2005). Angiogenesis in Life, Disease and Medicine. Nature 438, 932–936. doi:10.1038/nature04478
Cavin, C., Holzhaeuser, D., Scharf, G., Constable, A., Huber, W. W., and Schilter, B. (2002). Cafestol and Kahweol, Two Coffee Specific Diterpenes with Anticarcinogenic Activity. Food Chem. Toxicol. 40, 1155–1163. doi:10.1016/S0278-6915(02)00029-7
Chan, C. H., Lee, S. W., Li, C. F., Wang, J., Yang, W. L., Wu, C. Y., et al. (2010). Deciphering the Transcriptional Complex Critical for RhoA Gene Expression and Cancer Metastasis. Nat. Cell Biol. 12, 457–467. doi:10.1038/ncb2047
Chen, W., Sun, Z., Wang, X. J., Jiang, T., Huang, Z., Fang, D., et al. (2009). Direct Interaction between Nrf2 and p21(Cip1/WAF1) Upregulates the Nrf2-Mediated Antioxidant Response. Mol. Cell 34, 663–673. doi:10.1016/j.molcel.2009.04.029
Chian, S., Thapa, R., Chi, Z., Wang, X. J., and Tang, X. (2014). Luteolin Inhibits the Nrf2 Signaling Pathway and Tumor Growth In Vivo. Biochem. Biophys. Res. Commun. 447, 602–608. doi:10.1016/j.bbrc.2014.04.039
Choudhari, A. S., Mandave, P. C., Deshpande, M., Ranjekar, P., and Prakash, O. (2020). Phytochemicals in Cancer Treatment: From Preclinical Studies to Clinical Practice. Front. Pharmacol. 10, 1614. doi:10.3389/fphar.2019.01614
Chowdhry, S., Zhang, Y., McMahon, M., Sutherland, C., Cuadrado, A., and Hayes, J. D. (2013). Nrf2 Is Controlled by Two Distinct β-TrCP Recognition Motifs in its Neh6 Domain, One of Which Can Be Modulated by GSK-3 Activity. Oncogene 32, 3765–3781. doi:10.1038/onc.2012.388
Chuffa, L. G. A., Carvalho, R. F., Justulin, L. A., Cury, S. S., Seiva, F. R. F., Jardim-Perassi, B. V., et al. (2020). A Meta-Analysis of microRNA Networks Regulated by Melatonin in Cancer: Portrait of Potential Candidates for Breast Cancer Treatment. J. Pineal Res. 69, e12693. doi:10.1111/jpi.12693
Chung, A. S., and Ferrara, N. (2011). Developmental and Pathological Angiogenesis. Annu. Rev. Cell Dev. Biol. 27, 563–584. doi:10.1146/annurev-cellbio-092910-154002
Coso, S., Harrison, I., Harrison, C. B., Vinh, A., Sobey, C. G., Drummond, G. R., et al. (2012). NADPH Oxidases as Regulators of Tumor Angiogenesis: Current and Emerging Concepts. Antioxid. Redox Signal 16, 1229–1247. doi:10.1089/ars.2011.4489
Das, L., and Vinayak, M. (2015). Long Term Effect of Curcumin in Restoration of Tumour Suppressor P53 and Phase-II Antioxidant Enzymes via Activation of Nrf2 Signalling and Modulation of Inflammation in Prevention of Cancer. PLoS One 10, e0124000. doi:10.1371/journal.pone.0124000
De Blasio, A., Di Fiore, R., Pratelli, G., Drago-Ferrante, R., Saliba, C., Baldacchino, S., et al. (2020). A Loop Involving NRF2, miR-29b-1-5p and AKT, Regulates Cell Fate of MDA-MB-231 Triple-Negative Breast Cancer Cells. J. Cell. Physiol. 235, 629–637. doi:10.1002/jcp.29062
Del Vecchio, C. A., Feng, Y., Sokol, E. S., Tillman, E. J., Sanduja, S., Reinhardt, F., et al. (2014). De-Differentiation Confers Multidrug Resistance via Noncanonical PERK-Nrf2 Signaling. Plos Biol. 12, e1001945. doi:10.1371/journal.pbio.1001945
Denicola, G. M., Karreth, F. A., Humpton, T. J., Gopinathan, A., Wei, C., Frese, K., et al. (2011). Oncogene-induced Nrf2 Transcription Promotes ROS Detoxification and Tumorigenesis. Nature 475, 106–109. doi:10.1038/nature10189
Dhakshinamoorthy, S., Jain, A. K., Bloom, D. A., and Jaiswal, A. K. (2005). Bach1 Competes with Nrf2 Leading to Negative Regulation of the Antioxidant Response Element (ARE)-mediated NAD(P)H:quinone Oxidoreductase 1 Gene Expression and Induction in Response to Antioxidants. J. Biol. Chem. 280, 16891–16900. doi:10.1074/jbc.M500166200
Dinkova-Kostova, A. T., and Abramov, A. Y. (2015). The Emerging Role of Nrf2 in Mitochondrial Function. Free Radic. Biol. Med. 88, 179–188. doi:10.1016/j.freeradbiomed.2015.04.036
Dinkova-Kostova, A. T., Holtzclaw, W. D., and Kensler, T. W. (2005a). The Role of Keap1 in Cellular Protective Responses. Chem. Res. Toxicol. 18, 1779–1791. doi:10.1021/tx050217c
Dinkova-Kostova, A. T., Holtzclaw, W. D., and Wakabayashi, N. (2005b). Keap1, the Sensor for Electrophiles and Oxidants that Regulates the Phase 2 Response, Is a Zinc Metalloprotein. Biochemistry 44, 6889–6899. doi:10.1021/bi047434h
Dinkova-Kostova, A. T., Liby, K. T., Stephenson, K. K., Holtzclaw, W. D., Gao, X., Suh, N., et al. (2005c). Extremely Potent Triterpenoid Inducers of the Phase 2 Response: Correlations of protection against Oxidant and Inflammatory Stress. Proc. Natl. Acad. Sci. U S A. 102, 4584–4589. doi:10.1073/pnas.0500815102
Do, M. T., Kim, H. G., Choi, J. H., and Jeong, H. G. (2014). Metformin Induces microRNA-34a to Downregulate the Sirt1/Pgc-1α/Nrf2 Pathway, Leading to Increased Susceptibility of Wild-type P53 Cancer Cells to Oxidative Stress and Therapeutic Agents. Free Radic. Biol. Med. 74, 21–34. doi:10.1016/j.freeradbiomed.2014.06.010
Eggler, A. L., Liu, G., Pezzuto, J. M., Van Breemen, R. B., and Mesecar, A. D. (2005). Modifying Specific Cysteines of the Electrophile-Sensing Human Keap1 Protein Is Insufficient to Disrupt Binding to the Nrf2 Domain Neh2. Proc. Natl. Acad. Sci. U. S. A. 102, 10070–10075. doi:10.1073/pnas.0502402102
Eggler, A. L., Luo, Y., van Breemen, R. B., and Mesecar, A. D. (2008). Identification of the Highly Reactive Cysteine 151 in the Chemopreventive Agent-Sensor Keap1 Protein Is Method-dependent. Chem. Res. Toxicol. 21, 556. doi:10.1021/tx800015c
Fahey, J. W., Haristoy, X., Dolan, P. M., Kensler, T. W., Scholtus, I., Stephenson, K. K., et al. (2002). Sulforaphane Inhibits Extracellular, Intracellular, and Antibiotic-Resistant Strains of Helicobacter pylori and Prevents Benzo[a]pyrene-Induced Stomach Tumors. Proc. Natl. Acad. Sci. U. S. A. 99, 7610–7615. doi:10.1073/pnas.112203099
Faraonio, R., Vergara, P., Di Marzo, D., Pierantoni, M. G., Napolitano, M., Russo, T., et al. (2006). p53 Suppresses the Nrf2-dependent Transcription of Antioxidant Response Genes. J. Biol. Chem. 281, 39776–39784. doi:10.1074/jbc.M605707200
Foygel, K., Sekar, T. V., and Paulmurugan, R. (2015). Monitoring the Antioxidant Mediated Chemosensitization and ARE-Signaling in Triple Negative Breast Cancer Therapy. PLoS One 10, e0141913. doi:10.1371/journal.pone.0141913
Fritz, G., Brachetti, C., Bahlmann, F., Schmidt, M., and Kaina, B. (2002). Rho GTPases in Human Breast Tumours: Expression and Mutation Analyses and Correlation with Clinical Parameters. Br. J. Cancer 87, 635–644. doi:10.1038/sj.bjc.6600510
Gao, A. M., Ke, Z. P., Shi, F., Sun, G. C., and Chen, H. (2013a). Chrysin Enhances Sensitivity of BEL-7402/ADM Cells to Doxorubicin by Suppressing PI3K/Akt/Nrf2 and ERK/Nrf2 Pathway. Chem. Biol. Interact. 206, 100–108. doi:10.1016/j.cbi.2013.08.008
Gao, A. M., Ke, Z. P., Wang, J. N., Yang, J. Y., Chen, S. Y., and Chen, H. (2013b). Apigenin Sensitizes Doxorubicin-Resistant Hepatocellular Carcinoma BEL-7402/ADM Cells to Doxorubicin via Inhibiting PI3K/Akt/Nrf2 Pathway. Carcinogenesis 34, 1806–1814. doi:10.1093/carcin/bgt108
Garg, R., Gupta, S., and Maru, G. B. (2008). Dietary Curcumin Modulates Transcriptional Regulators of Phase I and Phase II Enzymes in Benzo[a]pyrene-Treated Mice: Mechanism of its Anti-initiating Action. Carcinogenesis 29, 1022–1032. doi:10.1093/carcin/bgn064
Ge, W., Zhao, K., Wang, X., Li, H., Yu, M., He, M., et al. (2017). iASPP Is an Antioxidative Factor and Drives Cancer Growth and Drug Resistance by Competing with Nrf2 for Keap1 Binding. Cancer Cell 32, 561–e6. doi:10.1016/j.ccell.2017.09.008
Ghantous, A., Sinjab, A., Herceg, Z., and Darwiche, N. (2013). Parthenolide: From Plant Shoots to Cancer Roots. Drug Discov. Today 18, 894–905. doi:10.1016/j.drudis.2013.05.005
GLOBOCAN (2020). GLOBOCAN 2020: New Global Cancer Data. Morphology, T. C. Available at: https://www.uicc.org/news/globocan-2020-new-global-cancer-data.
Goossens, J. F., and Bailly, C. (2019). Ursodeoxycholic Acid and Cancer: From Chemoprevention to Chemotherapy. Pharmacol. Ther. 203, 107396. doi:10.1016/j.pharmthera.2019.107396
Gorrini, C., Baniasadi, P. S., Harris, I. S., Silvester, J., Inoue, S., Snow, B., et al. (2013). BRCA1 Interacts with Nrf2 to Regulate Antioxidant Signaling and Cell Survival. J. Exp. Med. 210, 1529–1544. doi:10.1084/jem.20121337
Guo, Z., and Mo, Z. (2020). Keap1-Nrf2 Signaling Pathway in Angiogenesis and Vascular Diseases. J. Tissue Eng. Regen. Med. 14, 869–883. doi:10.1002/term.3053
Gurer-Orhan, H., Ince, E., Konyar, D., Saso, L., and Suzen, S. (2018). The Role of Oxidative Stress Modulators in Breast Cancer. Curr. Med. Chem. 25, 4084–4101. doi:10.2174/0929867324666170711114336
Harel, S., Mayaki, D., Sanchez, V., and Hussain, S. N. A. (2017). NOX2, NOX4, and Mitochondrial-Derived Reactive Oxygen Species Contribute to Angiopoietin-1 Signaling and Angiogenic Responses in Endothelial Cells. Vascul. Pharmacol. 92, 22–32. doi:10.1016/j.vph.2017.03.002
Hartikainen, J. M., Tengström, M., Kosma, V. M., Kinnula, V. L., Mannermaa, A., and Soini, Y. (2012). Genetic Polymorphisms and Protein Expression of NRF2 and Sulfiredoxin Predict Survival Outcomes in Breast Cancer. Cancer Res. 72, 5537–5546. doi:10.1158/0008-5472.CAN-12-1474
Hayes, J. D., and McMahon, M. (2009). NRF2 and KEAP1 Mutations: Permanent Activation of an Adaptive Response in Cancer. Trends Biochem. Sci. 34, 176–188. doi:10.1016/j.tibs.2008.12.008
Higgins, L. G., Cavin, C., Itoh, K., Yamamoto, M., and Hayes, J. D. (2008). Induction of Cancer Chemopreventive Enzymes by Coffee Is Mediated by Transcription Factor Nrf2. Evidence that the Coffee-specific Diterpenes Cafestol and Kahweol Confer protection against Acrolein. Toxicol. Appl. Pharmacol. 226, 328–337. doi:10.1016/j.taap.2007.09.018
Hill, S. M., Belancio, V. P., Dauchy, R. T., Xiang, S., Brimer, S., Mao, L., et al. (2015). Melatonin: An Inhibitor of Breast Cancer. Endocr. Relat. Cancer 22, R183–R204. doi:10.1530/ERC-15-0030
Hintze, K. J., Wald, K. A., Zeng, H., Jeffery, E. H., and Finley, J. W. (2003). Thioredoxin Reductase in Human Hepatoma Cells Is Transcriptionally Regulated by Sulforaphane and Other Electrophiles via an Antioxidant Response Element. J. Nutr. 133, 2721–2727. doi:10.1093/jn/133.9.2721
Hoeben, A., Landuyt, B., Highley, M. S., Wildiers, H., Van Oosterom, A. T., and De Bruijn, E. A. (2004). Vascular Endothelial Growth Factor and Angiogenesis. Pharmacol. Rev. 56, 549–580. doi:10.1124/pr.56.4.3
Hong, F., Sekhar, K. R., Freeman, M. L., and Liebler, D. C. (2005). Specific Patterns of Electrophile Adduction Trigger Keap1 Ubiquitination and Nrf2 Activation. J. Biol. Chem. 280, 31768–31775. doi:10.1074/jbc.M503346200
Hou, X., Bai, X., Gou, X., Zeng, H., Xia, C., Zhuang, W., et al. (2015). 3',4',5',5,7-pentamethoxyflavone Sensitizes Cisplatin-Resistant A549 Cells to Cisplatin by Inhibition of Nrf2 Pathway. Mol. Cells 38, 396–401. https://www.who.int/cancer/country-profiles/en/. doi:10.14348/molcells.2015.2183
Hu, L., Miao, W., Loignon, M., Kandouz, M., and Batist, G. (2010). Putative Chemopreventive Molecules Can Increase Nrf2-Regulated Cell Defense in Some Human Cancer Cell Lines, Resulting in Resistance to Common Cytotoxic Therapies. Cancer Chemother. Pharmacol. 66, 467–474. doi:10.1007/s00280-009-1182-7
Huang, H. C., Nguyen, T., and Pickett, C. B. (2002). Phosphorylation of Nrf2 at Ser-40 by Protein Kinase C Regulates Antioxidant Response Element-Mediated Transcription. J. Biol. Chem. 277, 42769–42774. doi:10.1074/jbc.M206911200
Huang, K. F., Zhang, G. D., Huang, Y. Q., and Diao, Y. (2012). Wogonin Induces Apoptosis and Down-Regulates Survivin in Human Breast Cancer MCF-7 Cells by Modulating PI3K-AKT Pathway. Int. Immunopharmacol. 12, 334–341. doi:10.1016/j.intimp.2011.12.004
Iida, K., Itoh, K., Kumagai, Y., Oyasu, R., Hattori, K., Kawai, K., et al. (2004). Nrf2 Is Essential for the Chemopreventive Efficacy of Oltipraz against Urinary Bladder Carcinogenesis. Cancer Res. 64, 6424–6431. doi:10.1158/0008-5472.CAN-04-1906
Iriondo, O., Rábano, M., Domenici, G., Carlevaris, O., López-Ruiz, J. A., Zabalza, I., et al. (2015). Distinct Breast Cancer Stem/progenitor Cell Populations Require Either HIF1α or Loss of PHD3 to Expand under Hypoxic Conditions. Oncotarget 6, 31721–31739. doi:10.18632/oncotarget.5564
Ishikawa, T. (2014). Genetic Polymorphism in the NRF2 Gene as a Prognosis Marker for Cancer Chemotherapy. Front. Genet. 5, 383. doi:10.3389/fgene.2014.00383
Itoh, K., Chiba, T., Takahashi, S., Ishii, T., Igarashi, K., Katoh, Y., et al. (1997). An Nrf2/small Maf Heterodimer Mediates the Induction of Phase II Detoxifying Enzyme Genes through Antioxidant Response Elements. Biochem. Biophys. Res. Commun. 236, 313–322. doi:10.1006/bbrc.1997.6943
Itoh, K., Wakabayashi, N., Katoh, Y., Ishii, T., Igarashi, K., Engel, J. D., et al. (1999). Keap1 Represses Nuclear Activation of Antioxidant Responsive Elements by Nrf2 through Binding to the Amino-Terminal Neh2 Domain. Genes Dev. 13, 76–86. doi:10.1101/gad.13.1.76
Jaiswal, A. K. (2004). Nrf2 Signaling in Coordinated Activation of Antioxidant Gene Expression. Free Radic. Biol. Med. 36, 1199–1207. doi:10.1016/j.freeradbiomed.2004.02.074
Jasser, S. A., Blask, D. E., and Brainard, G. C. (2006). Light during Darkness and Cancer: Relationships in Circadian Photoreception and Tumor Biology. Cancer Causes Control 17, 515–523. doi:10.1007/s10552-005-9013-6
Kamble, D., Mahajan, M., Dhat, R., and Sitasawad, S. (2021). Keap1-Nrf2 Pathway Regulates ALDH and Contributes to Radioresistance in Breast Cancer Stem Cells. Cells 10, 83. doi:10.3390/cells10010083
Kanematsu, S., Yoshizawa, K., Uehara, N., Miki, H., Sasaki, T., Kuro, M., et al. (2011). Sulforaphane Inhibits the Growth of KPL-1 Human Breast Cancer Cells In Vitro and Suppresses the Growth and Metastasis of Orthotopically Transplanted KPL-1 Cells in Female Athymic Mice. Oncol. Rep. 26, 603–608. doi:10.3892/or.2011.1311
Kang, J. S., Nam, L. B., Yoo, O. K., and Keum, Y. S. (2020). Molecular Mechanisms and Systemic Targeting of NRF2 Dysregulation in Cancer. Biochem. Pharmacol. 177, 114002. doi:10.1016/j.bcp.2020.114002
Kansanen, E., Kuosmanen, S. M., Ruotsalainen, A. K., Hynynen, H., and Levonen, A. L. (2017). Nitro-oleic Acid Regulates Endothelin Signaling in Human Endothelial Cells. Mol. Pharmacol. 92, 481–490. doi:10.1124/mol.117.109751
Kapur, A., Beres, T., Rathi, K., Nayak, A. P., Czarnecki, A., Felder, M., et al. (2018). Oxidative Stress via Inhibition of the Mitochondrial Electron Transport and Nrf-2-Mediated Anti-oxidative Response Regulate the Cytotoxic Activity of Plumbagin. Sci. Rep. 8, 1073. doi:10.1038/s41598-018-19261-w
Karihtala, P., Kauppila, S., Soini, Y., and Vuorinen, A-J. (2011). Oxidative Stress and Counteracting Mechanisms in Hormone Receptor Positive, Triple-Negative and Basal-like Breast Carcinomas. BMC Cancer 11, 262. doi:10.1186/1471-2407-11-262
Katoh, Y., Itoh, K., Yoshida, E., Miyagishi, M., Fukamizu, A., and Yamamoto, M. (2001). Two Domains of Nrf2 Cooperatively Bind CBP, a CREB Binding Protein, and Synergistically Activate Transcription. Genes Cells 6, 857–868. doi:10.1046/j.1365-2443.2001.00469.x
Kensler, T. W., Curphey, T. J., Maxiutenko, Y., and Roebuck, B. D. (2000). Chemoprotection by Organosulfur Inducers of Phase 2 Enzymes: Dithiolethiones and Dithiins. Drug Metabol. Drug Interact. 17, 3–22. doi:10.1515/DMDI.2000.17.1-4.3
Khan, H., Ullah, H., Martorell, M., Valdes, S. E., Belwal, T., Tejada, S., et al. (2021). Flavonoids Nanoparticles in Cancer: Treatment, Prevention and Clinical Prospects. Semin. Cancer Biol. 69, 200–211. doi:10.1016/j.semcancer.2019.07.023
Kim, D. H., Jang, J. H., Kwon, O. S., Cha, H. J., Youn, H. J., Chun, K. S., et al. (2020). Nuclear Factor Erythroid-Derived 2-Like 2-Induced Reductive Stress Favors Self-Renewal of Breast Cancer Stem-like Cells via the FoxO3a-Bmi-1 Axis. Antioxid. Redox Signal 32, 1313–1329. doi:10.1089/ars.2019.7730
Kim, J. H., Yu, S., Chen, J. D., and Kong, A. N. (2013). The Nuclear Cofactor RAC3/AIB1/SRC-3 Enhances Nrf2 Signaling by Interacting with Transactivation Domains. Oncogene 32, 514–527. doi:10.1038/onc.2012.59
Kim, S., Han, J., Lee, S. K., Choi, M. Y., Kim, J., Lee, J., et al. (2012). Berberine Suppresses the TPA-Induced MMP-1 and MMP-9 Expressions through the Inhibition of PKC-α in Breast Cancer Cells. J. Surg. Res. 176, e21–9. doi:10.1016/j.jss.2011.11.1041
Kim, S. K., Yang, J. W., Kim, M. R., Roh, S. H., Kim, H. G., Lee, K. Y., et al. (2008). Increased Expression of Nrf2/ARE-dependent Anti-oxidant Proteins in Tamoxifen-Resistant Breast Cancer Cells. Free Radic. Biol. Med. 45, 537–546. doi:10.1016/j.freeradbiomed.2008.05.011
Kim, W. D., Kim, Y. W., Cho, I. J., Lee, C. H., and Kim, S. G. (2012). E-cadherin Inhibits Nuclear Accumulation of Nrf2: Implications for Chemoresistance of Cancer Cells. J. Cell Sci. 125, 1284–1295. doi:10.1242/jcs.095422
Kumar, P., and Aggarwal, R. (2016). An Overview of Triple-Negative Breast Cancer. Arch. Gynecol. Obstet. 293, 247–269. doi:10.1007/s00404-015-3859-y
Kumar, S., Meena, R., and Rajamani, P. (2016). Fabrication of BSA-Green Tea Polyphenols-Chitosan Nanoparticles and Their Role in Radioprotection: A Molecular and Biochemical Approach. J. Agric. Food Chem. 64, 6024–6034. doi:10.1021/acs.jafc.6b02068
Kwak, M. K., Wakabayashi, N., and Kensler, T. W. (2004). Chemoprevention Through the Keap1-Nrf2 Signaling Pathway by Phase 2 Enzyme Inducers. Mutat. Res., 555 (1–2), 133–148. doi:10.1016/j.mrfmmm.2004.06.041
Kwak, M. K., Itoh, K., Yamamoto, M., and Kensler, T. W. (2002). Enhanced Expression of the Transcription Factor Nrf2 by Cancer Chemopreventive Agents: Role of Antioxidant Response Element-like Sequences in the Nrf2 Promoter. Mol. Cell. Biol. 22, 2883–2892. doi:10.1128/mcb.22.9.2883-2892.2002
Kweider, N., Fragoulis, A., Rosen, C., Pecks, U., Rath, W., Pufe, T., et al. (2011). Interplay between Vascular Endothelial Growth Factor (VEGF) and Nuclear Factor Erythroid 2-related Factor-2 (Nrf2): Implications for Preeclampsia. J. Biol. Chem. 286, 42863–42872. doi:10.1074/jbc.M111.286880
Lamy, M., Ferreira, A., Dias, J. S., Braga, S., Silva, G., and Barbas, A. (2017). Notch-out for Breast Cancer Therapies. N. Biotechnol. 39, 215–221. doi:10.1016/j.nbt.2017.08.004
Lau, A., Villeneuve, N. F., Sun, Z., Wong, P. K., and Zhang, D. D. (2008). Dual Roles of Nrf2 in Cancer. Pharmacol. Res. 58, 262–270. doi:10.1016/j.phrs.2008.09.003
Lau, A., Wang, X. J., Zhao, F., Villeneuve, N. F., Wu, T., Jiang, T., et al. (2010). A Noncanonical Mechanism of Nrf2 Activation by Autophagy Deficiency: Direct Interaction between Keap1 and P62. Mol. Cell. Biol. 30, 3275–3285. doi:10.1128/mcb.00248-10
Lee, J. M., Calkins, M. J., Chan, K., Kan, Y. W., and Johnson, J. A. (2003). Identification of the NF-E2-Related Factor-2-dependent Genes Conferring protection against Oxidative Stress in Primary Cortical Astrocytes Using Oligonucleotide Microarray Analysis. J. Biol. Chem. 278, 12029–12038. doi:10.1074/jbc.M211558200
Lee, S. B., Sellers, B. N., and Denicola, G. M. (2018). The Regulation of NRF2 by Nutrient-Responsive Signaling and its Role in Anabolic Cancer Metabolism. Antioxid. Redox Signal 29, 1774–1791. doi:10.1089/ars.2017.7356
Lee, S. L., Rouhi, P., Dahl Jensen, L., Zhang, D., Ji, H., Hauptmann, G., et al. (2009). Hypoxia-induced Pathological Angiogenesis Mediates Tumor Cell Dissemination, Invasion, and Metastasis in a Zebrafish Tumor Model. Proc. Natl. Acad. Sci. U. S. A. 106, 19485–19490. doi:10.1073/pnas.0909228106
Li, B., Feng, X. J., Hu, X. Y., Chen, Y. P., Sha, J. C., Zhang, H. Y., et al. (2018). Effect of Melatonin on Attenuating the Isoflurane-Induced Oxidative Damage Is Related to PKCα/Nrf2 Signaling Pathway in Developing Rats. Brain Res. Bull. 143, 9–18. doi:10.1016/j.brainresbull.2018.09.018
Li, J., and Johnson, J. A. (2002). Time-dependent Changes in ARE-Driven Gene Expression by Use of a Noise-Filtering Process for Microarray Data. Physiol. Genomics 9, 137–144. doi:10.1152/physiolgenomics.00003.2002
Li, L., Pan, H., Wang, H., Li, X., Bu, X., Wang, Q., et al. (2016). Interplay between VEGF and Nrf2 Regulates Angiogenesis Due to Intracranial Venous Hypertension. Sci. Rep. 6, 37338. doi:10.1038/srep37338
Li, W., Liu, H., Zhou, J. S., Cao, J. F., Zhou, X. B., Choi, A. M., et al. (2012). Caveolin-1 Inhibits Expression of Antioxidant Enzymes through Direct Interaction with Nuclear Erythroid 2 P45-Related Factor-2 (Nrf2). J. Biol. Chem. 287, 20922–20930. doi:10.1074/jbc.M112.352336
Lobo, N. A., Joshua, B., Kalisky, T., Somlo, G., Shen, J., Dorie, M. J., et al. (2009). Association of Reactive Oxygen Species Levels and Radioresistance in Cancer Stem Cells. Nature 458, 780–783. Available at:http://www.nature.com/doifinder/10.1038/nature07733%0Afile:///Files/f2/f23456c6-7956-47eb-aee5-552a20158ed3.pdf%0Apapers3://publication/doi/10.1038/nature07733. doi:10.1038/nature07733
Lu, J. J., Bao, J. L., Chen, X. P., Huang, M., and Wang, Y. T. (20122012). Alkaloids Isolated from Natural Herbs as the Anticancer Agents. Evid. Based. Complement. Alternat. Med. 2012, 485042. doi:10.1155/2012/485042
Lu, Y., Sun, Y., Zhu, J., Yu, L., Jiang, X., Zhang, J., et al. (2018). Oridonin Exerts Anticancer Effect on Osteosarcoma by Activating PPAR-γ and Inhibiting Nrf2 Pathway. Cell Death Dis 9, 15. doi:10.1038/s41419-017-0031-6
Ma, L., Liu, Y. P., Geng, C. Z., Wang, X. L., Wang, Y. J., and Zhang, X. H. (2010). Over Expression of Rhoa Is Associated with Progression in Invasive Breast Duct Carcinoma. Breast J. 16, 105–107. doi:10.1111/j.1524-4741.2009.00860.x
Malhotra, D., Portales-Casamar, E., Singh, A., Srivastava, S., Arenillas, D., Happel, C., et al. (2010). Global Mapping of Binding Sites for Nrf2 Identifies Novel Targets in Cell Survival Response through Chip-Seq Profiling and Network Analysis. Nucleic Acids Res. 38, 5718–5734. doi:10.1093/nar/gkq212
Mathema, V. B., Koh, Y. S., Thakuri, B. C., and Sillanpää, M. (2012). Parthenolide, a Sesquiterpene Lactone, Expresses Multiple Anti-cancer and Anti-inflammatory Activities. Inflammation 35, 560–565. doi:10.1007/s10753-011-9346-0
McMahon, M., Thomas, N., Itoh, K., Yamamoto, M., and Hayes, J. D. (2004). Redox-regulated Turnover of Nrf2 Is Determined by at Least Two Separate Protein Domains, the Redox-Sensitive Neh2 Degron and the Redox-Insensitive Neh6 Degron. J. Biol. Chem. 279, 31556–31567. doi:10.1074/jbc.M403061200
Mitsuishi, Y., Taguchi, K., Kawatani, Y., Shibata, T., Nukiwa, T., Aburatani, H., et al. (2012). Nrf2 Redirects Glucose and Glutamine into Anabolic Pathways in Metabolic Reprogramming. Cancer Cell 22, 66–79. doi:10.1016/j.ccr.2012.05.016
Moghadam, E. R., Ang, H. L., Asnaf, S. E., Zabolian, A., Saleki, H., Yavari, M., et al. (2020). Broad-Spectrum Preclinical Antitumor Activity of Chrysin: Current Trends and Future Perspectives. Biomolecules 10, 1374. doi:10.3390/biom10101374
Moi, P., Chan, K., Asunis, I., Cao, A., and Kan, Y. W. (1994). Isolation of NF-E2-Related Factor 2 (Nrf2), a NF-E2-like Basic Leucine Zipper Transcriptional Activator that Binds to the Tandem NF-E2/ap1 Repeat of the Beta-Globin Locus Control Region. Proc. Natl. Acad. Sci. U. S. A. 91, 9926–9930. doi:10.1073/pnas.91.21.9926
Moinova, H. R., and Mulcahy, R. T. (1999). Up-regulation of the Human Gamma-Glutamylcysteine Synthetase Regulatory Subunit Gene Involves Binding of Nrf-2 to an Electrophile Responsive Element. Biochem. Biophys. Res. Commun. 261, 661–668. doi:10.1006/bbrc.1999.1109
Morimitsu, Y., Nakagawa, Y., Hayashi, K., Fujii, H., Kumagai, T., Nakamura, Y., et al. (2002). A Sulforaphane Analogue that Potently Activates the Nrf2-dependent Detoxification Pathway. J. Biol. Chem. 277, 3456–3463. doi:10.1074/jbc.M110244200
Mostafavi-Pour, Z., Ramezani, F., Keshavarzi, F., and Samadi, N. (2017). The Role of Quercetin and Vitamin C in Nrf2-dependent Oxidative Stress Production in Breast Cancer Cells. Oncol. Lett. 13, 1965–1973. doi:10.3892/ol.2017.5619
Muralimanoharan, S., Kwak, Y. T., and Mendelson, C. R. (2018). Redox-Sensitive Transcription Factor NRF2 Enhances Trophoblast Differentiation via Induction of miR-1246 and Aromatase. Endocrinology 159, 2022–2033. doi:10.1210/en.2017-03024
Nandini, D. B., Rao, R. S., Deepak, B. S., and Reddy, P. B. (2020). Sulforaphane in Broccoli: The green Chemoprevention!! Role in Cancer Prevention and Therapy. J. Oral Maxillofac. Pathol. 24, 405. doi:10.4103/jomfp.JOMFP_126_19
Nethe, M., and Hordijk, P. L. (2010). The Role of Ubiquitylation and Degradation in RhoGTPase Signalling. J. Cell Sci. 123, 4011–4018. doi:10.1242/jcs.078360
Nguyen, T., Nioi, P., and Pickett, C. B. (2009). The Nrf2-Antioxidant Response Element Signaling Pathway and its Activation by Oxidative Stress. J. Biol. Chem. 284, 13291–13295. doi:10.1074/jbc.R900010200
Nioi, P., and Hayes, J. D. (2004). Contribution of NAD(P)H:quinone Oxidoreductase 1 to protection against Carcinogenesis, and Regulation of its Gene by the Nrf2 Basic-Region Leucine Zipper and the Arylhydrocarbon Receptor Basic helix-loop-helix Transcription Factors. Mutat. Res. 555, 149–171. doi:10.1016/j.mrfmmm.2004.05.023
Nioi, P., and Nguyen, T. (2007). A Mutation of Keap1 Found in Breast Cancer Impairs its Ability to Repress Nrf2 Activity. Biochem. Biophys. Res. Commun. 362, 816–821. doi:10.1016/j.bbrc.2007.08.051
Nioi, P., Nguyen, T., Sherratt, P. J., and Pickett, C. B. (2005). The Carboxy-Terminal Neh3 Domain of Nrf2 Is Required for Transcriptional Activation. Mol. Cell. Biol. 25, 10895–10906. doi:10.1128/mcb.25.24.10895-10906.2005
Ohnuma, T., Anzai, E., Suzuki, Y., Shimoda, M., Saito, S., Nishiyama, T., et al. (2015). Selective Antagonization of Activated Nrf2 and Inhibition of Cancer Cell Proliferation by Procyanidins from Cinnamomi Cortex Extract. Arch. Biochem. Biophys. 585, 17–24. doi:10.1016/j.abb.2015.09.007
Okazaki, K., Papagiannakopoulos, T., and Motohashi, H. (2020). Metabolic Features of Cancer Cells in NRF2 Addiction Status. Biophys. Rev. 12, 435–441. doi:10.1007/s12551-020-00659-8
Olayanju, A., Copple, I. M., Bryan, H. K., Edge, G. T., Sison, R. L., Wong, M. W., et al. (2015). Brusatol Provokes a Rapid and Transient Inhibition of Nrf2 Signaling and Sensitizes Mammalian Cells to Chemical Toxicity-Implications for Therapeutic Targeting of Nrf2. Free Radic. Biol. Med. 78, 202–212. doi:10.1016/j.freeradbiomed.2014.11.003
Oliveras-Ferraros, C., Cufí, S., Vazquez-Martin, A., Torres-Garcia, V. Z., Del Barco, S., Martin-Castillo, B., et al. (2011). Micro(mi)RNA Expression Profile of Breast Cancer Epithelial Cells Treated with the Anti-diabetic Drug Metformin: Induction of the Tumor Suppressor miRNA Let-7a and Suppression of the TGFβ-Induced oncomiR miRNA-181a. Cell Cycle 10, 1144–1151. doi:10.4161/cc.10.7.15210
Ong, C. P., Lee, W. L., Tang, Y. Q., and Yap, W. H. (2019). Honokiol: A Review of its Anticancer Potential and Mechanisms. Cancers (Basel) 12, 48. doi:10.3390/cancers12010048
Oshi, M., Angarita, F. A., Tokumaru, Y., Yan, L., Matsuyama, R., Endo, I., et al. (2020). High Expression of Nrf2 Is Associated with Increased Tumor-Infiltrating Lymphocytes and Cancer Immunity in Er-Positive/her2-Negative Breast Cancer. Cancers (Basel) 12, 1–15. doi:10.3390/cancers12123856
Pae, H. O., Choi, B. M., Oh, G. S., Lee, M. S., Ryu, D. G., Rhew, H. Y., et al. (2004). Roles of Heme Oxygenase-1 in the Antiproliferative and Antiapoptotic Effects of Nitric Oxide on Jurkat T Cells. Mol. Pharmacol. 66, 122–128. doi:10.1124/mol.66.1.122
Pandey, P., Singh, A. K., Singh, M., Tewari, M., Shukla, H. S., and Gambhir, I. S. (2017). The See-Saw of Keap1-Nrf2 Pathway in Cancer. Crit. Rev. Oncol. Hematol. 116, 89–98. doi:10.1016/j.critrevonc.2017.02.006
Panieri, E., and Saso, L. (20192019). Potential Applications of NRF2 Inhibitors in Cancer Therapy. Oxidative Med. Cell Longevity 2019, 1–34. doi:10.1155/2019/8592348
Pertz, O., Hodgson, L., Klemke, R. L., and Hahn, K. M. (2006). Spatiotemporal Dynamics of RhoA Activity in Migrating Cells. Nature 440, 1069–1072. doi:10.1038/nature04665
Pi, J., Bai, Y., Reece, J. M., Williams, J., Liu, D., Freeman, M. L., et al. (2007). Molecular Mechanism of Human Nrf2 Activation and Degradation: Role of Sequential Phosphorylation by Protein Kinase CK2. Free Radic. Biol. Med. 42, 1797–1806. doi:10.1016/j.freeradbiomed.2007.03.001
Pi, J., Qu, W., Reece, J. M., Kumagai, Y., and Waalkes, M. P. (2003). Transcription Factor Nrf2 Activation by Inorganic Arsenic in Cultured Keratinocytes: Involvement of Hydrogen Peroxide. Exp. Cell Res. 290, 234–245. doi:10.1016/S0014-4827(03)00341-0
Qiao, J., Chen, Y., Mi, Y., Jin, H., Huang, T., Liu, L., et al. (2020). NR5A2 Synergizes with NCOA3 to Induce Breast Cancer Resistance to BET Inhibitor by Upregulating NRF2 to Attenuate Ferroptosis. Biochem. Biophys. Res. Commun. 530, 402–409. doi:10.1016/j.bbrc.2020.05.069
Raghunath, A., Sundarraj, K., Nagarajan, R., Arfuso, F., Bian, J., Kumar, A. P., et al. (2018). Antioxidant Response Elements: Discovery, Classes, Regulation and Potential Applications. Redox Biol. 17, 297–314. doi:10.1016/j.redox.2018.05.002
Rai, G., Mishra, S., Suman, S., and Shukla, Y. (2016). Resveratrol Improves the Anticancer Effects of Doxorubicin In Vitro and In Vivo Models: A Mechanistic Insight. Phytomedicine 23, 233–242. doi:10.1016/j.phymed.2015.12.020
Ren, D., Villeneuve, N. F., Jiang, T., Wu, T., Lau, A., Toppin, H. A., et al. (2011). Brusatol Enhances the Efficacy of Chemotherapy by Inhibiting the Nrf2-Mediated Defense Mechanism. Proc. Natl. Acad. Sci. U. S. A. 108, 1433–1438. doi:10.1073/pnas.1014275108
Riento, K., and Ridley, A. J. (2003). Rocks: Multifunctional Kinases in Cell Behaviour. Nat. Rev. Mol. Cell Biol. 4, 446–456. doi:10.1038/nrm1128
Rihet, S., Vielh, P., Camonis, J., Goud, B., Chevillard, S., and de Gunzburg, J. (2001). Mutation Status of Genes Encoding RhoA, Rac1, and Cdc42 GTPases in a Panel of Invasive Human Colorectal and Breast Tumors. J. Cancer Res. Clin. Oncol. 127, 733–738. doi:10.1007/s004320100272
Robledinos-Antón, N., Fernández-Ginés, R., Manda, G., and Cuadrado, A. (20192019). Activators and Inhibitors of NRF2: A Review of Their Potential for Clinical Development. Oxid. Med. Cell. Longev. 2019, 9372182–9372220. doi:10.1155/2019/9372182
Rushmore, T. H., Morton, M. R., and Pickett, C. B. (1991). The Antioxidant Responsive Element. Activation by Oxidative Stress and Identification of the DNA Consensus Sequence Required for Functional Activity. J. Biol. Chem. 266, 11632–11639. doi:10.1016/s0021-9258(18)99004-6
Rushworth, S. A., and MacEwan, D. J. (2008). HO-1 Underlies Resistance of AML Cells to TNF-Induced Apoptosis. Blood 111, 3793–3801. doi:10.1182/blood-2007-07-104042
Ryoo, I. G., Choi, B. H., Ku, S. K., and Kwak, M. K. (2018). High CD44 Expression Mediates P62-Associated NFE2L2/NRF2 Activation in Breast Cancer Stem Cell-like Cells: Implications for Cancer Stem Cell Resistance. Redox Biol. 17, 246–258. doi:10.1016/j.redox.2018.04.015
Ryoo, I. G., Choi, B. H., and Kwak, M. K. (2015). Activation of NRF2 by P62 and Proteasome Reduction in Sphere-Forming Breast Carcinoma Cells. Oncotarget 6, 8167–8184. doi:10.18632/oncotarget.3047
Sabzichi, M., Hamishehkar, H., Ramezani, F., Sharifi, S., Tabasinezhad, M., Pirouzpanah, M., et al. (2014). Luteolin-loaded Phytosomes Sensitize Human Breast Carcinoma MDA-MB 231 Cells to Doxorubicin by Suppressing Nrf2 Mediated Signalling. Asian Pac. J. Cancer Prev. 15, 5311–5316. doi:10.7314/APJCP.2014.15.13.5311
Saini, N., and Yang, X. (2018). Metformin as an Anti-cancer Agent: Actions and Mechanisms Targeting Cancer Stem Cells. Acta Biochim. Biophys. Sin (Shanghai) 50, 133–143. doi:10.1093/abbs/gmx106
Samadi, N., Ramezani, F., and Sabzichi, M. (2014). 72 Nrf2 as a Molecular Target in Overwhelming Chemoresistance in Breast Cancer Therapy. Eur. J. Cancer 50, 28. doi:10.1016/s0959-8049(14)70198-4
Satoh, T., Kosaka, K., Itoh, K., Kobayashi, A., Yamamoto, M., Shimojo, Y., et al. (2008). Carnosic Acid, a Catechol-type Electrophilic Compound, Protects Neurons Both In Vitro and In Vivo through Activation of the Keap1/Nrf2 Pathway via S-Alkylation of Targeted Cysteines on Keap1. J. Neurochem. 104, 1116–1131. doi:10.1111/j.1471-4159.2007.05039.x
Scarpa, E. S., and Ninfali, P. (2015). Phytochemicals as Innovative Therapeutic Tools against Cancer Stem Cells. Int. J. Mol. Sci. 16, 15727–15742. doi:10.3390/ijms160715727
Seelinger, G., Merfort, I., Wölfle, U., and Schempp, C. M. (2008). Anti-carcinogenic Effects of the Flavonoid Luteolin. Molecules 13, 2628–2651. doi:10.3390/molecules13102628
Shao, S., Duan, W., Xu, Q., Li, X., Han, L., Li, W., et al. (20192019). Curcumin Suppresses Hepatic Stellate Cell-Induced Hepatocarcinoma Angiogenesis and Invasion through Downregulating CTGF. Oxidative Med. Cell Longevity 2019, 1–12. doi:10.1155/2019/8148510
Shi, S., Lei, S., Tang, C., Wang, K., and Xia, Z. (2019). Melatonin Attenuates Acute Kidney Ischemia/reperfusion Injury in Diabetic Rats by Activation of the SIRT1/Nrf2/HO-1 Signaling Pathway. Biosci. Rep. 39. doi:10.1042/BSR20181614
Shin, J. W., Chun, K. S., Kim, D. H., Kim, S. J., Kim, S. H., Cho, N. C., et al. (2020). Curcumin Induces Stabilization of Nrf2 Protein through Keap1 Cysteine Modification. Biochem. Pharmacol. 173, 113820. doi:10.1016/j.bcp.2020.113820
Silva-Islas, C. A., and Maldonado, P. D. (2018). Canonical and Non-canonical Mechanisms of Nrf2 Activation. Pharmacol. Res. 134, 92–99. doi:10.1016/j.phrs.2018.06.013
Singh, A., Venkannagari, S., Oh, K. H., Zhang, Y. Q., Rohde, J. M., Liu, L., et al. (2016). Small Molecule Inhibitor of NRF2 Selectively Intervenes Therapeutic Resistance in KEAP1-Deficient NSCLC Tumors. ACS Chem. Biol. 11, 3214–3225. doi:10.1021/acschembio.6b00651
Smolková, K., Mikó, E., Kovács, T., Leguina-Ruzzi, A., Sipos, A., and Bai, P. (2020). Nuclear Factor Erythroid 2-related Factor 2 in Regulating Cancer Metabolism. Antioxid. Redox Signaling 33, 966–997. doi:10.1089/ars.2020.8024
Sporn, M. B., and Liby, K. T. (2012). NRF2 and Cancer: The Good, the Bad and the Importance of Context. Nat. Rev. Cancer 12, 564–571. doi:10.1038/nrc3278
Su, X., Jiang, X., Meng, L., Dong, X., Shen, Y., and Xin, Y. (2018). Anticancer Activity of Sulforaphane: The Epigenetic Mechanisms and the Nrf2 Signaling Pathway. Oxid. Med. Cell. Longev. 2018, 5438179–5438210. doi:10.1155/2018/5438179
Suh, J. H., Shenvi, S. V., Dixon, B. M., Liu, H., Jaiswal, A. K., Liu, R. M., et al. (2004). Decline in Transcriptional Activity of Nrf2 Causes Age-Related Loss of Glutathione Synthesis, Which Is Reversible with Lipoic Acid. Proc. Natl. Acad. Sci. U. S. A. 101, 3381–3386. doi:10.1073/pnas.0400282101
Sun, Y., St Clair, D. K., Xu, Y., Crooks, P. A., and St Clair, W. H. (2010). A NADPH Oxidase-dependent Redox Signaling Pathway Mediates the Selective Radiosensitization Effect of Parthenolide in Prostate Cancer Cells. Cancer Res. 70, 2880–2890. doi:10.1158/0008-5472.CAN-09-4572
Sussan, T. E., Rangasamy, T., Blake, D. J., Malhotra, D., El-Haddad, H., Bedja, D., et al. (2009). Targeting Nrf2 with the Triterpenoid CDDO-Imidazolide Attenuates Cigarette Smoke-Induced Emphysema and Cardiac Dysfunction in Mice. Proc. Natl. Acad. Sci. U. S. A. 106, 250–255. doi:10.1073/pnas.0804333106
Syed Alwi, S. S., Cavell, B. E., Donlevy, A., and Packham, G. (2012). Differential Induction of Apoptosis in Human Breast Cancer Cell Lines by Phenethyl Isothiocyanate, a Glutathione Depleting Agent. Cell Stress Chaperones 17, 529–538. doi:10.1007/s12192-012-0329-3
Szatrowski, T. P., and Nathan, C. F. (1991). Production of Large Amounts of Hydrogen Peroxide by Human Tumor Cells. Cancer Res. 51, 794–798.
Taguchi, K., and Yamamoto, M. (2017). The KEAP1-NRF2 System in Cancer. Front. Oncol. 7, 85. doi:10.3389/fonc.2017.00085
Tang, J., Feng, Y., Tsao, S., Wang, N., Curtain, R., and Wang, Y. (2009). Berberine and Coptidis Rhizoma as Novel Antineoplastic Agents: A Review of Traditional Use and Biomedical Investigations. J. Ethnopharmacol. 126, 5–17. doi:10.1016/j.jep.2009.08.009
Tang, X., Wang, H., Fan, L., Wu, X., Xin, A., Ren, H., et al. (2011). Luteolin Inhibits Nrf2 Leading to Negative Regulation of the Nrf2/ARE Pathway and Sensitization of Human Lung Carcinoma A549 Cells to Therapeutic Drugs. Free Radic. Biol. Med. 50, 1599–1609. doi:10.1016/j.freeradbiomed.2011.03.008
Tarumoto, T., Nagai, T., Ohmine, K., Miyoshi, T., Nakamura, M., Kondo, T., et al. (2004). Ascorbic Acid Restores Sensitivity to Imatinib via Suppression of Nrf2-dependent Gene Expression in the Imatinib-Resistant Cell Line. Exp. Hematol. 32, 375–381. doi:10.1016/j.exphem.2004.01.007
Tascioglu Aliyev, A., Panieri, E., Stepanić, V., Gurer-Orhan, H., and Saso, L. (2021). Involvement of NRF2 in Breast Cancer and Possible Therapeutical Role of Polyphenols and Melatonin. Molecules 26, 1853. doi:10.3390/molecules26071853
Telkoparan-Akillilar, P., Panieri, E., Cevik, D., Suzen, S., and Saso, L. (2021). Therapeutic Targeting of the NRF2 Signaling Pathway in Cancer. Molecules 26, 1417. doi:10.3390/molecules26051417
Thimmulappa, R. K., Mai, K. H., Srisuma, S., Kensler, T. W., Yamamoto, M., and Biswal, S. (2002). Identification of Nrf2-Regulated Genes Induced by the Chemopreventive Agent Sulforaphane by Oligonucleotide Microarray. Cancer Res. 62, 5196–5203.
Tonelli, C., Chio, I. I. C., and Tuveson, D. A. (2018). Transcriptional Regulation by Nrf2. Antioxid. Redox Signal 29, 1727–1745. doi:10.1089/ars.2017.7342
Uruno, A., and Motohashi, H. (2011). The Keap1-Nrf2 System as an In Vivo Sensor for Electrophiles. Nitric Oxide 25, 153–160. doi:10.1016/j.niox.2011.02.007
Valcarcel-Ares, M. N., Gautam, T., Warrington, J. P., Bailey-Downs, L., Sosnowska, D., De Cabo, R., et al. (2012). Disruption of Nrf2 Signaling Impairs Angiogenic Capacity of Endothelial Cells: Implications for Microvascular Aging. J. Gerontol. A. Biol. Sci. Med. Sci. 67, 821–829. doi:10.1093/gerona/glr229
Vega, F. M., and Ridley, A. J. (2008). Rho GTPases in Cancer Cell Biology. FEBS Lett. 582, 2093–2101. doi:10.1016/j.febslet.2008.04.039
Wahdan-Alaswad, R. S., Cochrane, D. R., Spoelstra, N. S., Howe, E. N., Edgerton, S. M., Anderson, S. M., et al. (2014). Metformin-Induced Killing of Triple-Negative Breast Cancer Cells Is Mediated by Reduction in Fatty Acid Synthase via miRNA-193b. Horm. Cancer 5, 374–389. doi:10.1007/s12672-014-0188-8
Wang, H., Liu, K., Geng, M., Gao, P., Wu, X., Hai, Y., et al. (2013). RXRα Inhibits the NRF2-ARE Signaling Pathway through a Direct Interaction with the Neh7 Domain of NRF2. Cancer Res. 73, 3097–3108. doi:10.1158/0008-5472.CAN-12-3386
Wang, J., Wang, H., Sun, K., Wang, X., Pan, H., Zhu, J., et al. (2018). Chrysin Suppresses Proliferation, Migration, and Invasion in Glioblastoma Cell Lines via Mediating the ERK/Nrf2 Signaling Pathway. Drug Des. Devel. Ther. 12, 721–733. doi:10.2147/DDDT.S160020
Wang, X. J., Hayes, J. D., Henderson, C. J., and Wolf, C. R. (2007). Identification of Retinoic Acid as an Inhibitor of Transcription Factor Nrf2 through Activation of Retinoic Acid Receptor Alpha. Proc. Natl. Acad. Sci. U. S. A. 104, 19589–19594. doi:10.1073/pnas.0709483104
Wei, Y., Zou, Z., Becker, N., Anderson, M., Sumpter, R., Xiao, G., et al. (2013). EGFR-mediated Beclin 1 Phosphorylation in Autophagy Suppression, Tumor Progression, and Tumor Chemoresistance. Cell 154, 1269–1284. doi:10.1016/j.cell.2013.08.015
Woo, Y., Oh, J., and Kim, J. S. (2017). Suppression of Nrf2 Activity by Chestnut Leaf Extract Increases Chemosensitivity of Breast Cancer Stem Cells to Paclitaxel. Nutrients 9, 760. doi:10.3390/nu9070760
Worthylake, R. A., and Burridge, K. (2003). RhoA and ROCK Promote Migration by Limiting Membrane Protrusions. J. Biol. Chem. 278, 13578–13584. doi:10.1074/jbc.M211584200
Wu, C. C., Hsu, M. C., Hsieh, C. W., Lin, J. B., Lai, P. H., and Wung, B. S. (2006). Upregulation of Heme Oxygenase-1 by Epigallocatechin-3-Gallate via the Phosphatidylinositol 3-kinase/Akt and ERK Pathways. Life Sci. 78, 2889–2897. doi:10.1016/j.lfs.2005.11.013
Wu, K. C., Cui, J. Y., and Klaassen, C. D. (2011). Beneficial Role of Nrf2 in Regulating NADPH Generation and Consumption. Toxicol. Sci. 123, 590–600. doi:10.1093/toxsci/kfr183
Wu, S., Lu, H., and Bai, Y. (2019). Nrf2 in Cancers: A Double-Edged Sword. Cancer Med. 8, 2252–2267. doi:10.1002/cam4.2101
Wu, T., Harder, B. G., Wong, P. K., Lang, J. E., and Zhang, D. D. (2015). Oxidative Stress, Mammospheres and Nrf2-New Implication for Breast Cancer Therapy? Mol. Carcinog. 54, 1494–1502. doi:10.1002/mc.22202
Xia, C., Bai, X., Hou, X., Gou, X., Wang, Y., Zeng, H., et al. (2015). Cryptotanshinone Reverses Cisplatin Resistance of Human Lung Carcinoma A549 Cells through Down-Regulating Nrf2 Pathway. Cell. Physiol. Biochem. 37, 816–824. doi:10.1159/000430398
Xie, W., Wang, L., Sheng, H., Qiu, J., Zhang, D., Zhang, L., et al. (2017). Metformin Induces Growth Inhibition and Cell Cycle Arrest by Upregulating microRNA34a in Renal Cancer Cells. Med. Sci. Monit. 23, 29–37. doi:10.12659/MSM.898710
Yan, X., Qi, M., Li, P., Zhan, Y., and Shao, H. (2017). Apigenin in Cancer Therapy: Anti-cancer Effects and Mechanisms of Action. Cell Biosci 7, 50. doi:10.1186/s13578-017-0179-x
Yin, L., Duan, J. J., Bian, X. W., and Yu, S. C. (2020). Triple-negative Breast Cancer Molecular Subtyping and Treatment Progress. Breast Cancer Res. 22, 61. doi:10.1186/s13058-020-01296-5
Yu, X.-q., ShangShang, X.-y., Huang, X.-x., Yao, G.-d., and jiang, S.-j. (2020). Brusatol: A Potential Anti-tumor Quassinoid from Brucea Javanica. Chin. Herbal Medicines 12, 359–366. doi:10.1016/j.chmed.2020.05.007
Yuan, Z., Zhang, J., Huang, Y., Zhang, Y., Liu, W., Wang, G., et al. (2017). NRF2 Overexpression in Mesenchymal Stem Cells Induces Stem-Cell Marker Expression and Enhances Osteoblastic Differentiation. Biochem. Biophys. Res. Commun. 491, 228–235. doi:10.1016/j.bbrc.2017.07.083
Zhang, C., Wang, H. J., Bao, Q. C., Wang, L., Guo, T. K., Chen, W. L., et al. (2016). NRF2 Promotes Breast Cancer Cell Proliferation and Metastasis by Increasing RhoA/ROCK Pathway Signal Transduction. Oncotarget 7, 73593–73606. doi:10.18632/oncotarget.12435
Zhang, D. D., and Hannink, M. (2003). Distinct Cysteine Residues in Keap1 Are Required for Keap1-dependent Ubiquitination of Nrf2 and for Stabilization of Nrf2 by Chemopreventive Agents and Oxidative Stress. Mol. Cell. Biol. 23, 8137–8151. doi:10.1128/mcb.23.22.8137-8151.2003
Zhang, D. D., Lo, S.-C., Cross, J. V., Templeton, J., T., and Hannink, M., H. (2004). Keap1 Is a Redox-Regulated Substrate Adaptor Protein for a Cul3-dependent Ubiquitin Ligase Complex. Mol. Cell. Biol. 24, 10941–10953. Available at: http://www.embase.com/search/results?subaction=viewrecord&from=export&id=L39603148%0A. doi:10.1128/MCB.24.24.10941-10953.2004
Zhang, H. S., Du, G. Y., Zhang, Z. G., Zhou, Z., Sun, H. L., Yu, X. Y., et al. (2018). NRF2 Facilitates Breast Cancer Cell Growth via HIF1ɑ-Mediated Metabolic Reprogramming. Int. J. Biochem. Cell Biol. 95, 85–92. doi:10.1016/j.biocel.2017.12.016
Zhang, R., Qiao, H., Chen, S., Chen, X., Dou, K., Wei, L., et al. (2016). Berberine Reverses Lapatinib Resistance of HER2-Positive Breast Cancer Cells by Increasing the Level of ROS. Cancer Biol. Ther. 17, 925–934. doi:10.1080/15384047.2016.1210728
Zhang, Y., and Gordon, G. B. (2004). A Strategy for Cancer Prevention: Stimulation of the Nrf2-ARE Signaling Pathway. Mol. Cancer Ther. 3, 885–893. doi:10.1158/1535-7163.885.3.7
Zhang, Y., Wei, Z., Liu, W., Wang, J., He, X., Huang, H., et al. (2017). Melatonin Protects against Arsenic Trioxide-Induced Liver Injury by the Upregulation of Nrf2 Expression through the Activation of PI3K/AKT Pathway. Oncotarget 8, 3773–3780. doi:10.18632/oncotarget.13931
Zhang, Z., Wang, Q., Ma, J., Yi, X., Zhu, Y., Xi, X., et al. (2013). Reactive Oxygen Species Regulate FSH-Induced Expression of Vascular Endothelial Growth Factor via Nrf2 and HIF1α Signaling in Human Epithelial Ovarian Cancer. Oncol. Rep. 29, 1429–1434. doi:10.3892/or.2013.2278
Zhao, W., Zhang, X., Liu, J., Sun, B., Tang, H., and Zhang, H. (2016). miR-27a-mediated Antiproliferative Effects of Metformin on the Breast Cancer Cell Line MCF-7. Oncol. Rep. 36 (6), 3691–3699. doi:10.3892/or.2016.5185
Zhong, Y., Zhang, F., Sun, Z., Zhou, W., Li, Z. Y., You, Q. D., et al. (2013). Drug Resistance Associates with Activation of Nrf2 in MCF-7/DOX Cells, and Wogonin Reverses it by Down-Regulating Nrf2-Mediated Cellular Defense Response. Mol. Carcinog. 52, 824–834. doi:10.1002/mc.21921
Zhou, S., Ye, W., Shao, Q., Zhang, M., and Liang, J. (2013). Nrf2 Is a Potential Therapeutic Target in Radioresistance in Human Cancer. Crit. Rev. Oncol. Hematol. 88, 706–715. doi:10.1016/j.critrevonc.2013.09.001
Zhou, S., Ye, W., Zhang, M., and Liang, J. (2012). The Effects of Nrf2 on Tumor Angiogenesis: A Review of the Possible Mechanisms of Action. Crit. Rev. Eukaryot. Gene Expr. 22, 149–160. doi:10.1615/CritRevEukarGeneExpr.v22.i2.60
Zhu, J., Wang, H., Chen, F., Fu, J., Xu, Y., Hou, Y., et al. (2016). An Overview of Chemical Inhibitors of the Nrf2-ARE Signaling Pathway and Their Potential Applications in Cancer Therapy. Free Radic. Biol. Med. 99, 544–556. doi:10.1016/j.freeradbiomed.2016.09.010
Zimta, A. A., Cenariu, D., Irimie, A., Magdo, L., Nabavi, S. M., Atanasov, A. G., et al. (2019). The Role of Nrf2 Activity in Cancer Development and Progression. Cancers (Basel) 11. doi:10.3390/cancers11111755
Zou, J., Fei, Q., Xiao, H., Wang, H., Liu, K., Liu, M., et al. (2019). VEGF-A Promotes Angiogenesis after Acute Myocardial Infarction through Increasing ROS Production and Enhancing ER Stress-Mediated Autophagy. J. Cell. Physiol. 234, 17690–17703. doi:10.1002/jcp.28395
Keywords: Nrf2, breast cancer, drug resistance, angiogenesis, metastasis
Citation: Kumar H, Kumar RM, Bhattacharjee D, Somanna P and Jain V (2022) Role of Nrf2 Signaling Cascade in Breast Cancer: Strategies and Treatment. Front. Pharmacol. 13:720076. doi: 10.3389/fphar.2022.720076
Received: 03 June 2021; Accepted: 31 March 2022;
Published: 29 April 2022.
Edited by:
Ramkumar Kunka Mohanram, SRM Institute of Science and Technology, IndiaReviewed by:
Khuloud Bajbouj, University of Sharjah, United Arab EmiratesKumar Ganesan, The University of Hong Kong, Hong Kong SAR, China
Copyright © 2022 Kumar, Kumar, Bhattacharjee, Somanna and Jain. This is an open-access article distributed under the terms of the Creative Commons Attribution License (CC BY). The use, distribution or reproduction in other forums is permitted, provided the original author(s) and the copyright owner(s) are credited and that the original publication in this journal is cited, in accordance with accepted academic practice. No use, distribution or reproduction is permitted which does not comply with these terms.
*Correspondence: Vikas Jain, dmlrYXNqYWluQGpzc3VuaS5lZHUuaW4=