- 1Department of Microbiology, Obafemi Awolowo University, Ile-Ife, Nigeria
- 2Microbiology Unit, Department of Applied Sciences, Osun State College of Technology, Esa-Oke, Nigeria
- 3Department of Molecular Medicine, Morsani College of Medicine, University of South Florida, Tampa, FL, United States
- 4Department of Immunology, Moffit Cancer Center, Tampa, FL, United States
- 5Department of Medicinal and Biological Chemistry, University of Toledo, Toledo, OH, United States
- 6Department of Microbiology and Immunology, Louisiana State University Health Sciences Center, Shreveport, LA, United States
- 7Department of Pharmaceutical and Biomedical Sciences, University of Georgia, Athens, GA, United States
- 8Advanced Space Technology Applications Laboratory, Cooperative Information Network, National Space Research and Development Agency, Ile-Ife, Nigeria
- 9Food Security and Safety Focus Area, Faculty of Natural and Agricultural Sciences, North-West University, Mmabatho, South Africa
- 10Department of Pharmacology, Faculty of Pharmacy, Obafemi Awolowo University, Ile-Ife, Nigeria
The worldwide burden of cancers is increasing at a very high rate, including the aggressive and resistant forms of cancers. Certain levels of breakthrough have been achieved with the conventional treatment methods being used to treat different forms of cancers, but with some limitations. These limitations include hazardous side effects, destruction of non-tumor healthy cells that are rapidly dividing and developing, tumor resistance to anti-cancer drugs, damage to tissues and organs, and so on. However, oncolytic viruses have emerged as a worthwhile immunotherapeutic option for the treatment of different types of cancers. In this treatment approach, oncolytic viruses are being modeled to target cancer cells with optimum cytotoxicity and spare normal cells with optimal safety, without the oncolytic viruses themselves being killed by the host immune defense system. Oncolytic viral infection of the cancer cells are also being genetically manipulated (either by removal or addition of certain genes into the oncolytic virus genome) to make the tumor more visible and available for attack by the host immune cells. Hence, different variants of these viruses are being developed to optimize their antitumor effects. In this review, we examined how grave the burden of cancer is on a global level, particularly in sub-Saharan Africa, major conventional therapeutic approaches to the treatment of cancer and their individual drawbacks. We discussed the mechanisms of action employed by these oncolytic viruses and different viruses that have found their relevance in the fight against various forms of cancers. Some pre-clinical and clinical trials that involve oncolytic viruses in cancer management were reported. This review also examined the toxicity and safety concerns surrounding the adoption of oncolytic viro-immunotherapy for the treatment of cancers and the likely future directions for researchers and general audience who wants updated information.
1 Introduction
Cancer is a global-leading cause of death which accounted for estimated 10 million deaths in the year 2020, meaning that cancer is responsible for the death of one in every six dead persons in the world (Ferlay et al., 2020; World Health Organization, 2022). This death toll is expected to continue to rise with a predicted 13.1 million deaths in the year 2030 alone (Bray et al., 2018; Ferlay et al., 2020; World Health Organization, 2022). To show how grievous cancer is, the deaths caused by tuberculosis, malaria and HIV/AIDS put together is still lesser than the number of deaths caused by cancer alone (Bray et al., 2018; Ferlay et al., 2020). The World Health Organization has listed lung, colon, stomach and breast cancers as the most common in terms of new cases and the most common causes of death due to cancer (World Health Organization, 2022). In the developed countries of the world like the United States of America, cancer is the second disease with the most number of deaths. The number of new cases and deaths in 2022 alone has been predicted to be 1,918,030 and 609,360, respectively, while about 350 deaths have been estimated to happen for lung cancer every day in the year 2022 (Siegel et al., 2022). Between 2017 and 2019, about 167,000 people died of cancer in the United Kingdom; that’s 89,200 males and 78,000 females. This statistics from the UK between 2017 and 2019 showed that about 460 people died daily and one person died every 4 min (Cancer Research UK, 2019).
In the developing nations, cancer is among the top three causes of deaths in adults (Wong et al., 2018). Prior this time, in 2002 precisely, about 6.7 million deaths were recorded to have been caused by cancer, but the death toll in sub-Saharan Africa accounted for less than 5% of these deaths (Torre et al., 2015). Exactly 10 years ago (2012) in the sub-Saharan Africa, new cases of cancer were estimated to be 626, 400 and number of deaths were recorded to be 447,700 (Plummer et al., 2016; Sharma et al., 2022). However, the cancer death toll in sub-Saharan Africa has continued to rise (Olaleye and Ekrikpo, 2017; Sharma et al., 2022). The continuous rise in the incidence and mortality of cancer in sub-Saharan Africa has been linked to late presentation and diagnosis, poor access to treatment facilities and poor outcomes in cases where access to treatment was granted. It has been estimated that 80%–90% of advanced stage cancer cases result to death due to insufficient access to treatment facilities and necessary infrastructure (Bray et al., 2018; Ferlay et al., 2020).
In Nigeria, there are 102,000 new cases of cancer every year, while about 72,000 people die of cancer annually (Federal Ministry of Health, 2018; Fatiregun et al., 2020). In a more recent study, the incidence of cancer in Nigeria was estimated to be between 118,101 and 131,911, with death toll ranging between 74,234 and 83,857 (Sharma et al., 2022). The pattern of cancer incidence in Nigeria has continued to increase, but the cancer data collection in Nigeria is poor (Federal Ministry of Health, 2018). Hence, there is not much information on the annual cancer mortality trends and patterns, particularly for different states in Nigeria. Cancer, among other complex diseases, has emerged to require critical health care. There is need to direct global efforts to reduce the number of new cancer cases and provide adequate treatment to reduce the mortality rates as fast as possible, particularly in sub-Saharan Africa (Sharma et al., 2022).
1.1 Conventional approaches to the treatment of cancer and their limitations
Over the years, different approaches have been used to treat cancer with some level of successes achieved, however, not without their limitations. It is interesting to know that more than 50% of all global clinical trials in the world are targeted on cancer therapy (Abbas and Rehman, 2018; Ferlay et al., 2020). Some of the prominent conventional methods used for treating various forms of cancer include surgical operations, radiotherapy with x-rays and chemotherapy which involves the use of anti-cancer drugs either to cure cancer, lessen the severity of the symptoms or extend the life of the patient (Arruebo et al., 2011; Mondal et al., 2014). Chemotherapy could be used singly or in synergy with radiotherapy, and it has been reported to be the most globally used and most effective treatment in cancer therapy (El-Hussein et al., 2020). Chemotherapeutic drugs target and destroy the tumor cells by the production of reactive oxygen species, a phenomenon tagged genotoxicity (El-Hussein et al., 2020; Debela et al., 2021). However, surgical operation is still the most effective treatment therapy for the removal of cancers at the early stage of disease development (El-Hussein et al., 2020; Debela et al., 2021).
Some of the limitations or drawbacks of these conventional cancer therapies include damage to non-tumor healthy cells, tissues or organs, which is very common with radiotherapy. Almost all the available chemotherapeutic anti-cancer drugs have negative impact on cells that are dividing and developing swiftly, but that are not cancerous cells (El-Hussein et al., 2020; Debela et al., 2021). However, the main issue with the chemotherapeutic approach is the inability of an anti-cancer drug which was once effective in suppressing some cancer cells to become ineffective against the same cancer cells; a phenomenon referred to as drug resistance. This development of drug resistance by cancer cells has been attributed to increase in drug efflux and decrease in drug uptake (Shapira et al., 2011; El-Hussein et al., 2020). Other drawbacks associated with chemotherapy are; fast drug metabolism, dangerous side effects, absence of specificity and difficulty in selection of dosage (Mondal et al., 2014). The result from surgery cannot be effective at the advanced stage and it is unfortunate that only few registered cases are discovered at the early stage of disease development, with over 60% discovered at the advanced stage (Damyanov et al., 2018). The success of the surgical operation is also dependent on the skillfulness of the medical surgeon, but in cases where there is high standard surgery, some micro-tumor cells are not discoverable during the surgery and via diagnostic tools too, so, such cells could progress in the future to become full blown tumor cells. Some other limitations associated with surgical removal of cancer include complications from poor anesthesia, infections, cancer cells distributed in the blood flow and immune system suppression. The last two limitations have been linked to metastases distribution in the body of patients who have undergone surgery (Demicheli et al., 2008; El-Hussein et al., 2020).
In recent times, several approaches are being developed to improve on the various limitations of conventional therapy which include; use of natural antioxidants and nanoparticles, targeted drug therapy (monoclonal antibodies, small molecule inhibitors and ablation cancer therapy), stem cell therapy, sonodynamic therapy, chemodynamic therapy, ferroptosis-based therapy and gene therapy (Abbas and Rehman, 2018; Debela et al., 2021). These approaches have focused on producing efficient and safe cancer therapies. Among these, gene therapy stands out in preventing cancer progression by the insertion of a defective gene into the genome to lyse the tumor cells directly. Gene therapy includes the use of oncolytic viruses, Rexin-G, Kymriah, Zalmoxis, Genicine, among many others being developed (Abbas and Rehman, 2018; Debela et al., 2021).
1.2 The role of oncolytic viruses in cancer immunotherapy
An integral quality of viruses is their ability to selectively replicate and induce cytopathic effects; these qualities, among others have made them well suited for cancer immunotherapy. The viral genome is easily adaptable to changes that boost their affinity (viral tropism) for neoplastic cells (Kaufman et al., 2013; Engeland, 2020). Oncolytic viruses (OVs) are gaining popularity in tumor treatment because they elicit T cell responses and in turn anti-tumor immunity; they are therefore immunogenic in nature, hence their ability to trigger an anti-tumor immune response (Fukuhara et al., 2016; Guo et al., 2017). Following the success of immunotherapy using immune checkpoint inhibitors (Gujar et al., 2018; Vijayakumar et al., 2020), oncolytic viral immunotherapy may represent the next significant advancement in the fight against cancer. Tumor cells through their manipulation thrive in the “harsh environment” of the immune system. They minimize the expression of their neo-antigens and prevent infiltration of effector cells to the tumor bed, paralyzing innate and adaptive immune responses (Russell et al., 2012; Engeland, 2020). Studies have shown that the tumor microenvironment (TME) reconditions their environment to escape immunosurveillance and promote tumor growth (Jiang et al., 2019; Wang et al., 2022).
The principle by which oncolytic viruses act are multimodal and provide a strong rationale for their use in cancer immunotherapy (Lichty et al., 2014; Engeland, 2020; Heidbuechel and Engeland, 2021). They possess activated cell signaling pathways that encourage tumor cell proliferation, while promoting the growth and propagation of viruses within the malignancy (Engeland, 2020; Zeng et al., 2021). Interestingly, tumor cells are limited in their ability to defensively respond to viral infections compared to normal tissues (Lichty et al., 2014; Engeland, 2020). OVs utilize this limitation to their advantage by targeting and destroying the tumor. Their restriction to the tumor site stems from their dependence on the hallmarks of cancer (tumor-specific changes) including defects in antiviral response and altered receptor expression, hence, healthy tissues are unharmed (Engeland, 2020; Heidbuechel and Engeland, 2021).
In this review article, we examined the relevance of oncolytic viro-immunotherapy in the treatment of cancer to improve on the setbacks of the conventional treatment therapies. The mechanism behind this promising and novel anti-cancer approach was also presented in details. With comprehensive explanation, we described the different classes of oncolytic viruses that have found their application in the treatment of different forms of cancers, both at the experimental and clinical trial phases (Tables 1–4). The possible safety and toxicity concerns surrounding the application of oncolytic viro-immunotherapy in cancer treatment were considered and the areas where research efforts should be channeled in the future to better fortify the resource of oncolytic viruses as immunotherapeutic agents in the global fight against cancer were clearly presented as well.
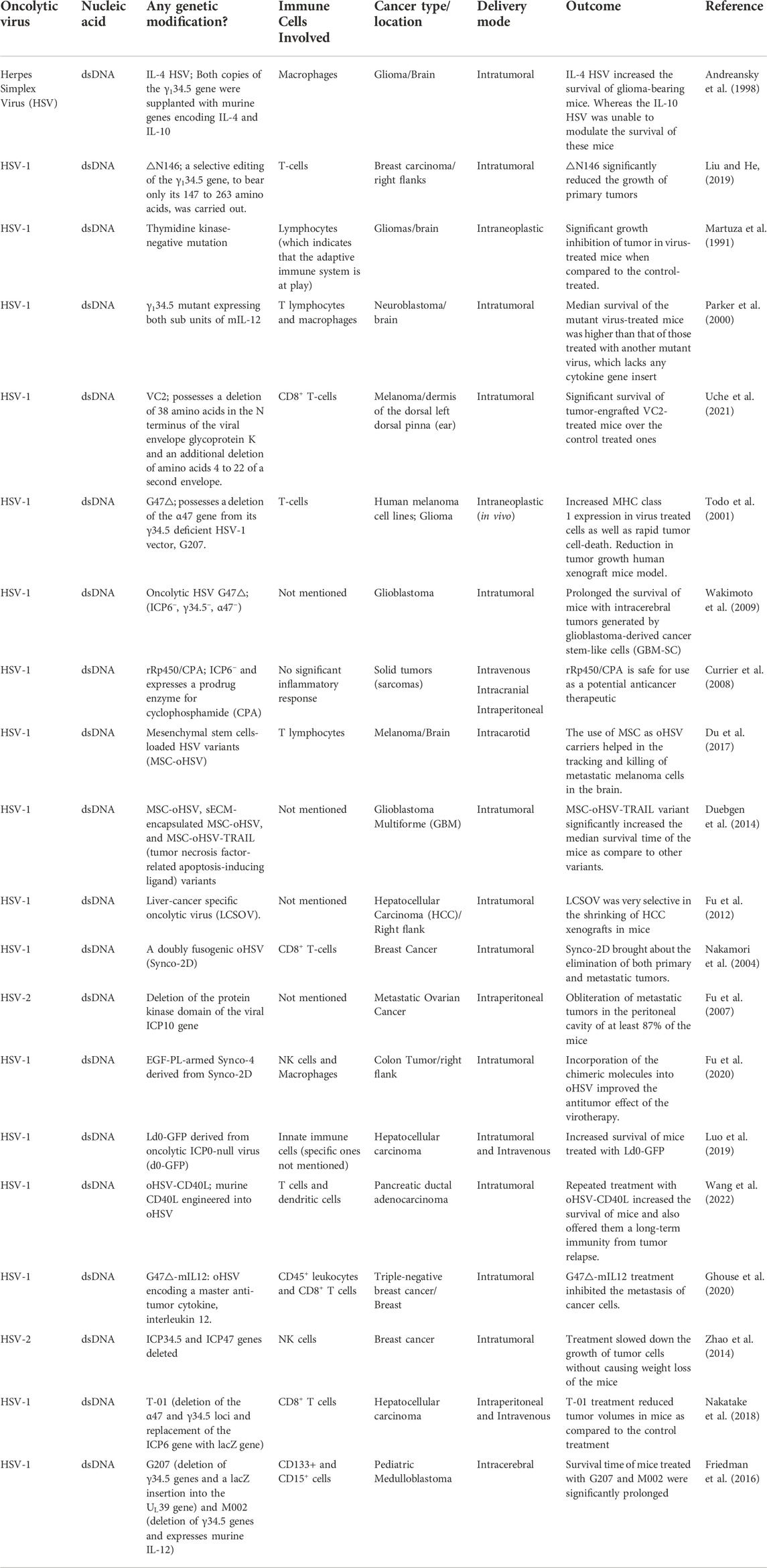
TABLE 1. Summary of herpesviruses implicated as oncolytic viro-immunotherapeutic agents against cancer.
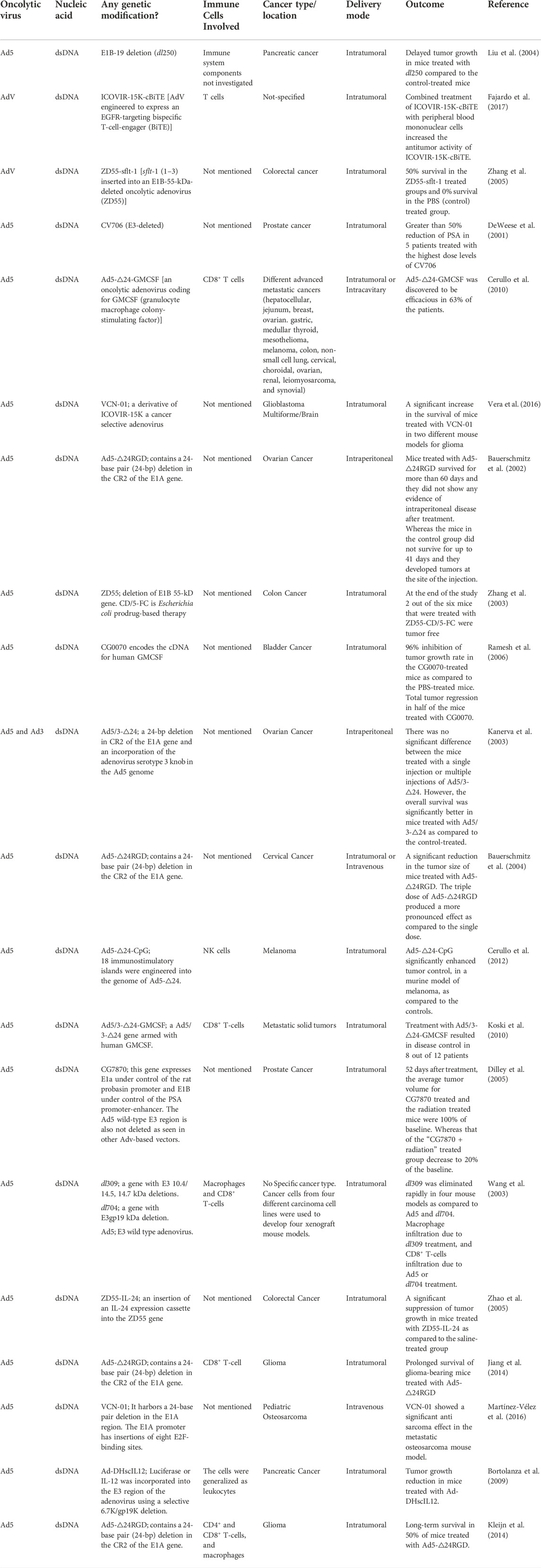
TABLE 2. Summary of adenoviruses implicated as oncolytic viro-immunotherapeutic agents against cancer.
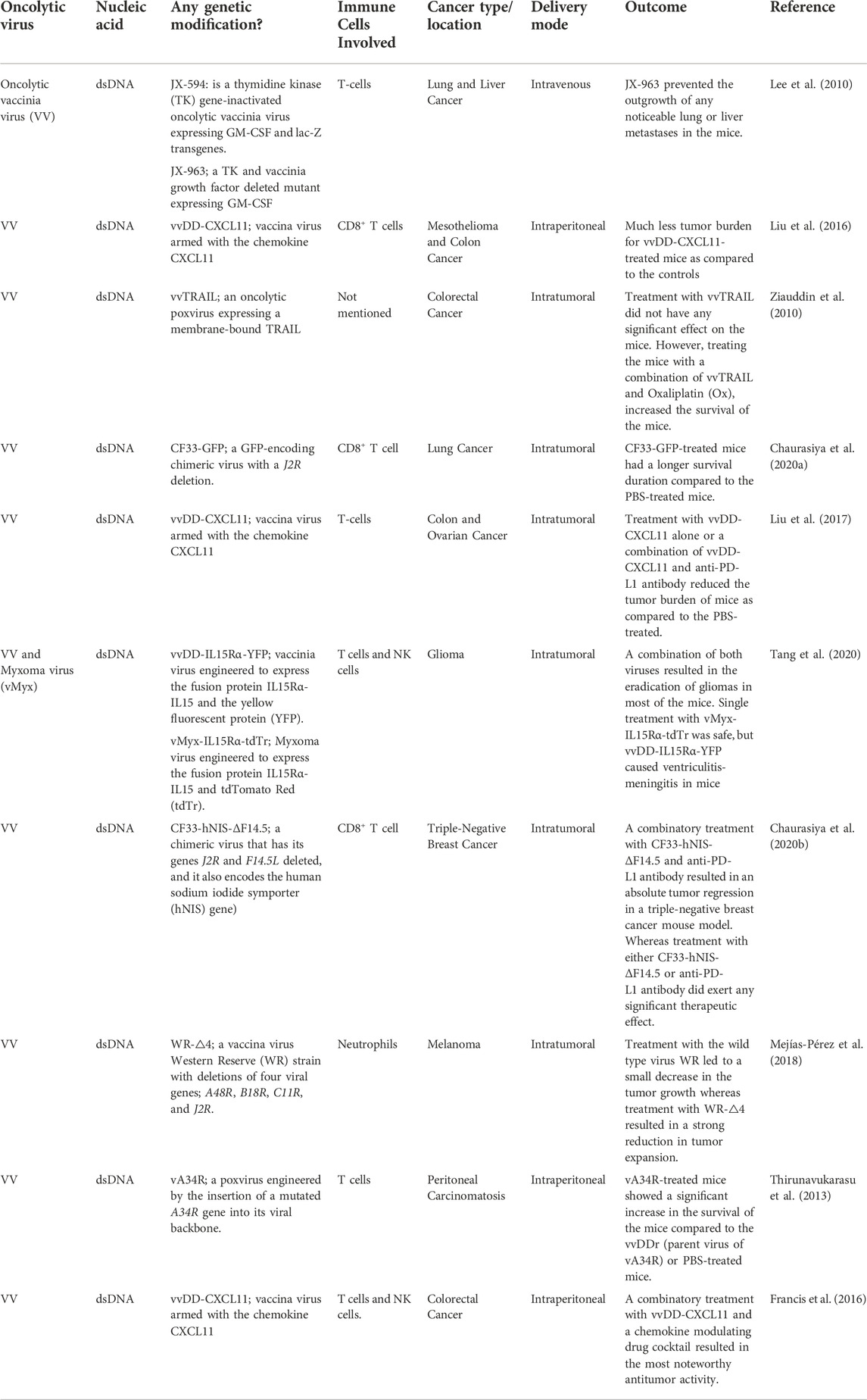
TABLE 3. Summary of poxviruses implicated as oncolytic viro-immunotherapeutic agents against cancer.
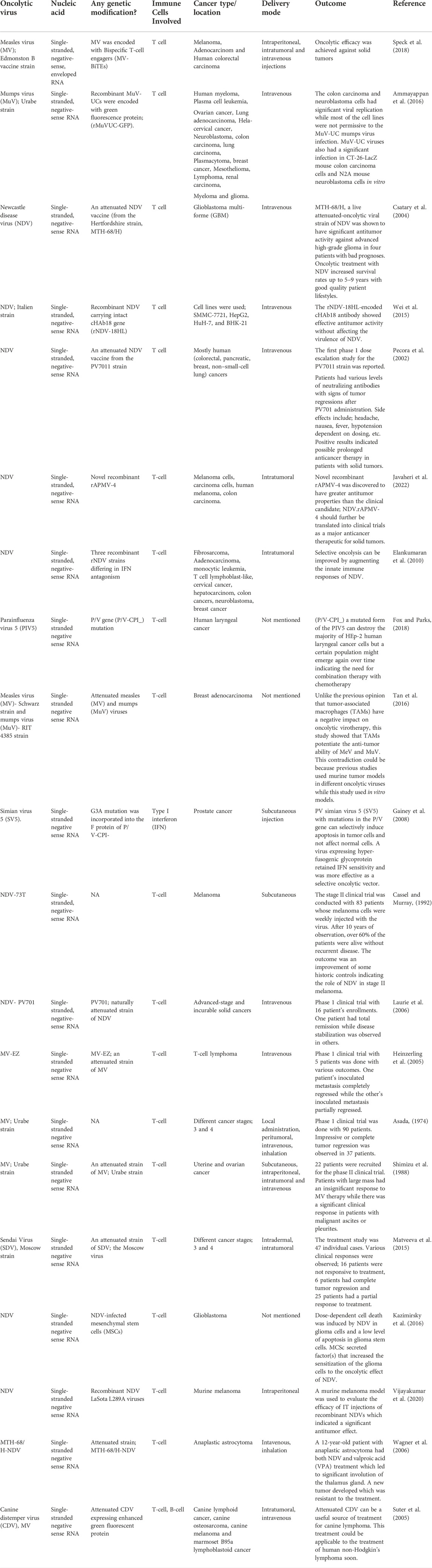
TABLE 4. Summary of paramyxoviruses implicated as oncolytic viro-immunotherapeutic agents against cancer.
2 The principle of oncolytic viro-immunotherapy in cancer treatment
Oncolytic viruses can preferentially infect and destroy tumor cells while stimulating and engaging the immune system through different mechanisms which include: modulation of the TME (that is, converting cold tumors to hot), directly lysing tumor cells or combining therapeutically with cancer immunotherapies. This principle is summarized by the illustrations in Figure 1.
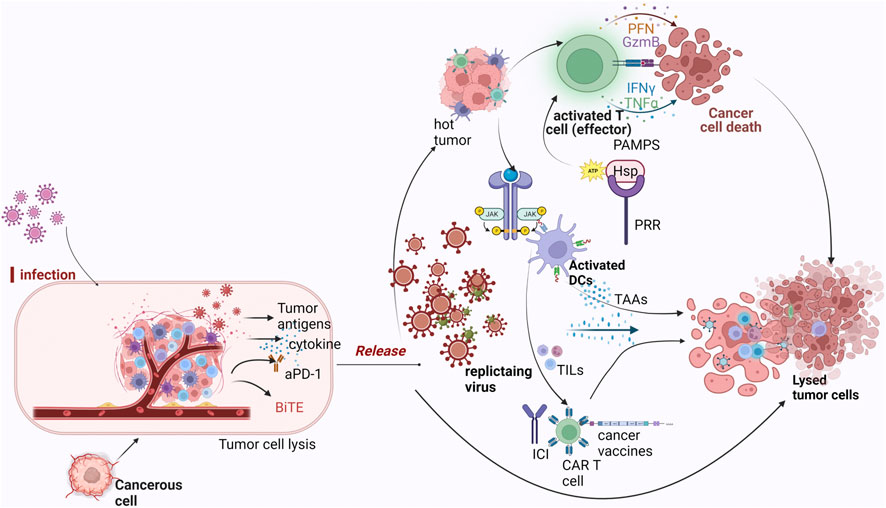
FIGURE 1. Mechanism of oncolytic viruses as immunotherapeutic agents against tumor cells. Legend: Oncolytic viruses infect tumor cells and recondition the tumor microenvironment for the effector cells to be activated. Once they start replicating within the tumor, direct tumor lysis occurs which causes the release of DAMPS and PAMPS which are recognized by PRR expressed on immune subsets like DCs, NK cells and so on. As a result of this interaction, inflammatory cytokines are recruited, hence, attracting other immune cells. Replication of the virus also initiates the expression of TAA which are captured by DCs for presentation to T cells and traffics T cells to the tumor causing ICD. Oncolytic viruses when combined with any of CAR-T therapy, checkpoint inhibitors or cancer vaccines further ensure favorable response, with the presence of TAA and TIL’s enhancing their effects.
2.1 Reconditioning/modulating the TME (converting cold tumors to hot)
The immunological phenotype and landscape of the TME is an important factor in determining disease prognosis and therapeutic efficacy (Achard et al., 2018). Cold tumors are characterized by the inability of effector cells to infiltrate the tumor. Oncolytic viruses make cold tumors hot by taking advantage of cancer’s telltale signs, that is, hallmarks of cancer (Engeland, 2020). Some of these cancer telltale signs include invasion and metastasis, sustained proliferation, induction of angiogenesis, resistance to apoptosis, and evasion of immune surveillance and growth suppressors. These attributes make the tumor microenvironment a dynamic and complex one comprising of not just individual malignant cells but also vascular endothelial cells, fibroblasts, tumor-resident or migratory immune cells, stroma and vasculature; all of which contribute to their immunosuppressive capabilities (Gujar et al., 2018). Reciprocal crosstalk between the tumor and stroma further promotes their invasiveness and metastasis. Attempts by the host to initiate an immune response against the tumor lead to an oppositional response by recruiting immunosuppressive cells to the tumor milieu, hence building an impenetrable fortress (Achard et al., 2018). They stop the infiltration of T cells to the tumor site making the tumor microenvironment cold. Since most current immunotherapies involve harnessing the immune T cell responses to fight cancer, it is only logical to introduce factors that would stimulate their activity. Introducing OVs to the tumor milieu helps reshape the tumor milieu by inducing an acute viral infection that could potentially stimulate inflammation and immune cell infiltration to the tumor site (Samson et al., 2018). For example, oncolytic vaccinia and vesicular stomatitis virus (VSV) can cut off tumor blood supply, enhance T cell infiltration and consequently inhibit tumor progression by targeting the tumor vasculature (Shi et al., 2020). Achard et al. (2018) describe this as the ability of OV to wake up the tumors from an immunological coma. T cell infiltration into the tumor bed makes the environment hot and appropriate for other immunotherapies to function. Once OVs successfully enter the tumor bed, they initiate immunogenic cell death by direct lysis of these OV-infected tumor cells. They can reverse the immunosuppressive environment in the tumor milieu and enable recognition of tumor associated antigens (TAA) by the T cells. This process awakens the immune system within the TME and is just the first step that results in a prolonged antitumor immune response. For example, studies have shown that vesicular stomatitis virus or reovirus primes the adaptive immune response eliciting T cell mediated immunity with evident signs of tumor regression (Diaz et al., 2007).
2.2 Direct lysis of tumor cells
A limitation of cancer cells is their flawed antiviral response pathways, and this defect makes them even more susceptible to OVs. Some of these signaling pathways involved in viral clearance include toll-like receptor (TLR), Janus kinase-signal transducer and activator of transcription (JAK-STAT), protein kinase RNA-activated (PKR) pathways, which are either absent or inhibited in cancer cells; this is exploited by OVs to recondition the tumor milieu (Guo et al., 2017). Direct lysis of OV-infected tumor causes the release of pathogen-associated molecular patterns (PAMPS) such as viral nucleic acids and proteins, and damage-associated molecular patterns (DAMPS) such as heat-shock proteins (HSP), adenosine triphosphate (ATP), ecto-calreticulin, High mobility group box protein 1 (HMGB1) (Lichty et al., 2014). These PAMPS are recognized by pattern-recognition receptors (PRR) expressed on various immune subsets like natural killer (NK) Cells, dendritic cells (DCs) and macrophages within the tumor bed (Diaz et al., 2007). With this recognition, inflammatory cytokines like IFN-α, IFN-γ, IL-12, IL-6 are produced which leads to the recruitment of other immune cells from peripheral organs thereby eliciting anti-viral and anti-tumor immune responses leading to Immunogenic cell death (ICD) (Diaz et al., 2007; Kroemer et al., 2013). For example, the oncolytic MeV (a reovirus with dsRNA genome) activates DCs via PKR signaling, induces Toll-like receptors (TLR) and/or RIG-I-like receptors (RLR) and secrets some of these pro-inflammatory cytokines like IFN-γ to induce anti-tumor immunity (Errington et al., 2008; Achard et al., 2017). In addition, tumor associated antigens (TAA), or tumor-specific antigens (TSA) are released within the tumor as a result of selective replication and tumor lysis, serving as adjuvants for adaptive immunity. Once at the site, DCs capture these TAA for cross-presentation to T cells. This leads to the priming cum activation, proliferation and trafficking of antigen-specific polyclonal T cells (Errington et al., 2008). These activated antigen specific CD8+ and CD4+ T cells through their cytotoxic effect causes immunogenic cell death in the tumor cells. In numerous preclinical experiments, this mechanism has been verified (Shi et al., 2020). Release of OV into the tumor can also induce in situ vaccination as a result of their spread and uptake by DCs (Lichty et al., 2014). This in situ vaccination is a result of the induction of specific TAA-specific adaptive immune responses and is an important component of the success of OV immunotherapy. An example is the T-VEC, an oncolytic HSV-1 which was licensed by the FDA for the treatment of metastatic melanoma (Bastin et al., 2016). It has deletions in the genes ICP34.5, ICP47 and expresses GM-CSF (Granulocyte-macrophage colony-stimulating factor) (Guo et al., 2017). Loss in ICP34.5 and ICP47 confers selectivity to cancer cells and enhances antigen presentation respectively, while GM-CSF aids APC maturation, activation and in turn trafficking of immune cells and induction of systemic antitumor immunity (Kaufman et al., 2013; Guo et al., 2017).
2.3 Combination therapy with cancer immunotherapies
Dwelling on the mechanisms explained above, OVs are suited for combinational therapy approaches to enhance anti-tumor immunity. Therefore, OVs are being explored as antigen-agonists, increasing the action of checkpoint inhibitors, or adoptive cell treatments and cancer vaccines due to their significant potential to combat cancer (Cockle et al., 2016). They have the leverage of being ideal agents that may be used in synergy with available cancer immunotherapies to further augment positive treatment effects in several cancer types (Figure 2).
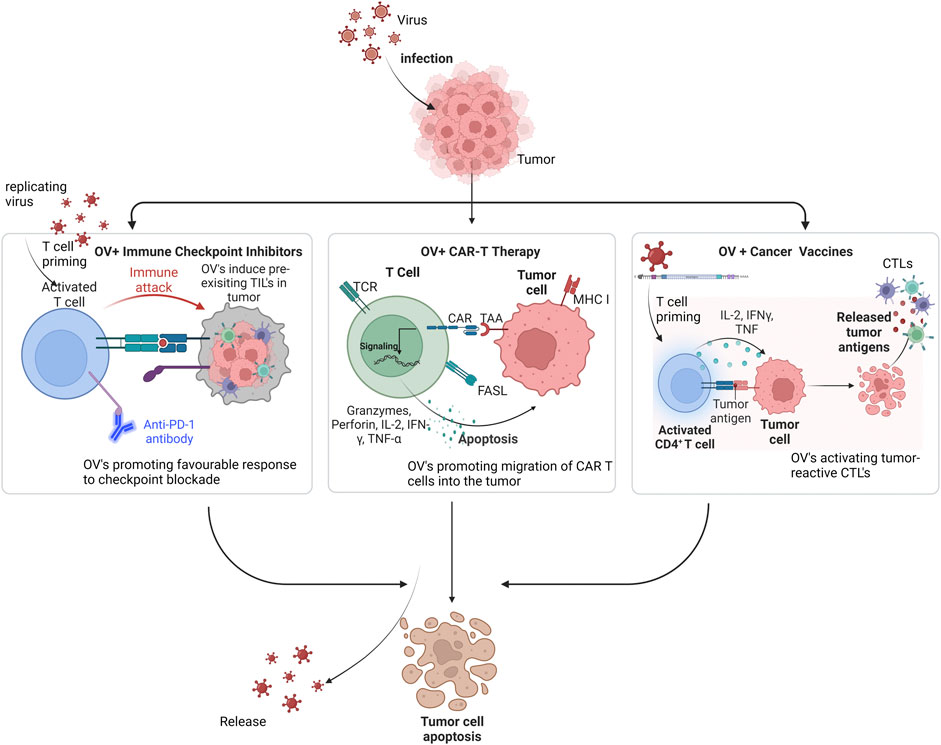
FIGURE 2. Mechanism of oncolytic viruses in combination with other immunotherapies against tumor cells. Legend: Incorporating OV’s with Checkpoint inhibitors primes T cells and makes tumor-infiltrating lymphocytes (TILs) readily available, thereby increasing response rate to this therapy. With CAR T therapy, OV’s deliver cytokines and chemokines to drive migration of CAR T cells into the tumor, thereby, synergistically inducing viral infection and immunogenic cell death. In cancer vaccines, OV’s recruit cytokines that activate tumor-reactive CTLs like the T helper cells via the released tumor antigens.
2.3.1 Oncolytic viruses with checkpoint inhibitors
Checkpoint inhibitors prevent checkpoint proteins from binding/interacting with their partner proteins with the purpose of interrupting immunosuppression by tumor signals. T cells express checkpoint proteins like CLA4, PD-1, which help keep the immune system in check while cancer cells express PD-L1, PDL-2, VISTA (Pardoll, 2012; Topalian et al., 2014). Cancer cells unfortunately hijack these mechanisms to hinder anti-tumor immunity. There are antibodies that have been developed to target these checkpoint inhibitors; pembrolizumab and nivolumab targeting PD-1, ipilimumab targeting CTLA-4 (Patnaik et al., 2015). These monoclonal antibodies have shown encouraging results in several solid tumors (Wolchok et al., 2017). However, a common shortcoming is the low response rate due to minimal levels of tumor-infiltrating lymphocytes (TILs) (Jacquelot et al., 2017). Pre-clinical and clinical studies have emphasized the importance of pre-existing TILs in the TME because it promotes favorable response to checkpoint blockade (Wolchok et al., 2017). Incorporating OV has the prospects of overcoming these deficiencies. In numerous clinical trials, the interaction of OVs with immunological checkpoints is being studied, with PD-1/PD-L1 and CTLA-4 combinations making the most progress. For example, Samson et al. (2018), in a pre-clinical study published in 2018 reported a significant increase in cytotoxic CD8+ T cell infiltration in TNBC mouse model after treatment with oncolytic Maraba rhabdovirus and reovirus in combination with PD-1 inhibitors. An increase in response rate of about 33% was reported in advanced melanoma after combinational treatment with TVEC and ipilimumab in a phase I clinical trial when compared with either treatment alone (Ribas et al., 2017; Samson et al., 2018). Other examples of current studies in clinical trials include Phase I clinical trial of oncolytic virus injection RT-01 and PD-1 combination therapy (NCT05228119); Phase II clinical trials of Pexa-vec oncolytic virus (vaccinia virus) in combination with Tremelimunab (binds CTLA-4) or Durvalumab (binds PD-L1) in patients with refractory metastatic colorectal cancer (NCT03206073). Other studies have also shown that OVs can effectively act as neoadjuvant to prime the tumor microenvironment for immune checkpoint therapy (Bourgeois-Daigneault et al., 2018). While promising results are seen with this combinatorial therapy, a caveat is the possibility of abrogating OV replication and tumor infection due to excessive priming of systemic antiviral responses (Shi et al., 2020).
2.3.2 Oncolytic viruses with chimeric antigen receptor T cell therapy
Adoptive therapy is hinged on the premise of ex vivo expansion of the patients’ T cells and reinfusion of these expanded, tumor-reactive T cells. In CAR-T cell therapy, the T cells can recognize tumor antigens through the CAR structure on T cells. These CAR-T cells infiltrate the tumor cells and eliminate them based on their antigens (Houot et al., 2015), with the ability to recognize TAA independently of MHC being their strong point. So far, they have shown promising effects and have been approved by FDA for the treatment of B-cell malignancies (Lichtman and Dotti, 2017). However, they have shown limited progress in solid tumors owing to the restricted trafficking of T cells into the tumor (Sauter et al., 2019). The ability of oncolytic viruses to induce viral infection and immunogenic cell death can be exploited in synergy with CAR-T cell therapy (Ajina and Maher, 2017). The possibility of engineering oncolytic viruses to deliver T cell chemokines and cytokinesis is increasingly gaining recognition and can be used to promote stimulation and migration of CAR-T cells into the tumor. For example, an engineered vaccinia virus that produces CXCL11 can increase T cell trafficking into a subcutaneous tumor. This enhanced recruitment of antigen-specific T cells after CAR-T cell administration significantly improved anti-tumor immunity (Moon et al., 2018). OVs can also be engineered with EGFR-targeting bispecific T cell engagers (BiTE) or re-wired to produce antibodies against checkpoint inhibitors to enhance CAR-T cell therapy. BiTEs are fusion proteins and they guide polyclonal T lymphocytes towards tumor cells without the aid of MHC. Because of this, they are able to elicit anti-tumor responses at low dosages (Heidbuechel and Engeland, 2021). They have shown promising results in the treatment of hematological malignancies (Viardot et al., 2016). A study by Wing et al. (2018) reported that oncolytic adenovirus engineered with EGFR-targeting BiTE improved the activation and proliferation of CAR-T cells, improving survival in the mouse model. In another study, some researchers generated an oncolytic virus that expresses TNFα- and IL-2 (Ad-mTNFα-mIL2) and in combination with CAR-T cell therapy, they were able to treat human pancreatic adenocarcinoma-xenograft immunodeficient mice. They discovered that the combination therapy enhanced T cell trafficking into the immunosuppressive milieu leading to DC maturation and M1 macrophage polarization (Watanabe et al., 2018).
2.3.3 Oncolytic viruses with cancer vaccines
Cancer vaccines are designed with the intent to induce immune responses (cellular and humoral) in vivo for immunological memory to prevent and control tumor growth. Cancer vaccines include peptide, DNA, RNA and DC vaccines, all of which are in clinical trials for treatment of different solid malignancies like melanoma, glioma and colon carcinoma. A major drawback to this treatment option is their low recruitment profile of T helper cells and major histocompatibility complex II (MHC II) epitope on the surface of DC leading to less efficiency of the T cell anti-tumor effects. Thus, combining OVs with cancer vaccines has the potential to activate tumor reactive cytotoxic T lymphocytes (CTLs) (Li et al., 2017). The potential of this combinatorial therapy in booting priming of T cell responses has been reported. An example is the chemokine CCR5-expressing oncolytic vaccinia virus vvCCR5 which when mixed with DC1 (type-1-polarized DCs) triggered chemo taxis of lymphocytes in vitro and in vivo (Li et al., 2011). For the most part, most of the OV-cancer vaccines target the TAAs and are very promising; they can utilize the mechanism of turning the cold tumors into hot in order to elicit anti T cell responses (Shi et al., 2020).
3 Different oncolytic viruses applied as Immunotherapeutic agents in cancer treatment
Various oncolytic viruses have been exploited to treat different forms of cancers which include; herpesviruses, adenoviruses, poxviruses, rhabdoviruses, paramyxoviruses and reoviruses. The activities of some of these types of viruses in the fight against different forms of cancer both at the experimental and clinical stages are discussed below:
3.1 Herpesviruses
Herpesviruses are DNA viruses which are capable of establishing lytic and latent modes of infection in their hosts. Among herpesviruses, the utility of herpes simplex virus-1 (HSV-1) as an oncolytic agent has been explored the most (Koch et al., 2020; Saravanan et al., 2022). The large genome capacity of HSV-1 allows its use as a vector for delivering choice transgenes to cancer cells (Burton et al., 2002; Saravanan et al., 2022). Talimogene laherparepvec (T-VEC) which is derived from JS1, a primary isolate of HSV-1, was the first oncolytic virus to be approved for public use in the US for the treatment of advanced melanoma (Pol et al., 2016).
The selectivity of oncolytic HSV (oHSV) for cancer cells is achieved through viral tropism and replicative fitness. The deletion of ICP34.5—a leaky late gene of HSV-1 in T-VEC relieves protein kinase R (PKR)-induced block to cellular protein synthesis, which is caused by the inactivation of the eukaryotic translation initiation factor, eIF2α. Since cancer cells often have a dysfunctional PKR, T-VEC selectively kills tumor cells but not normal cells as shown in Figure 3 (Liu et al., 2003; Saravanan et al., 2022). In addition, the deletion of ICP47 and the enhanced expression of US11 in T-VEC were shown to promote tumor clearance (Liu et al., 2003). The defectivity in the cyclic GMP–AMP synthase (cGAS)–stimulator of interferon genes (STING) signaling pathway in some cancer cells also provides an Achille’s heel for enhancing viral oncolysis (Xia et al., 2016; de Queiroz et al., 2019).
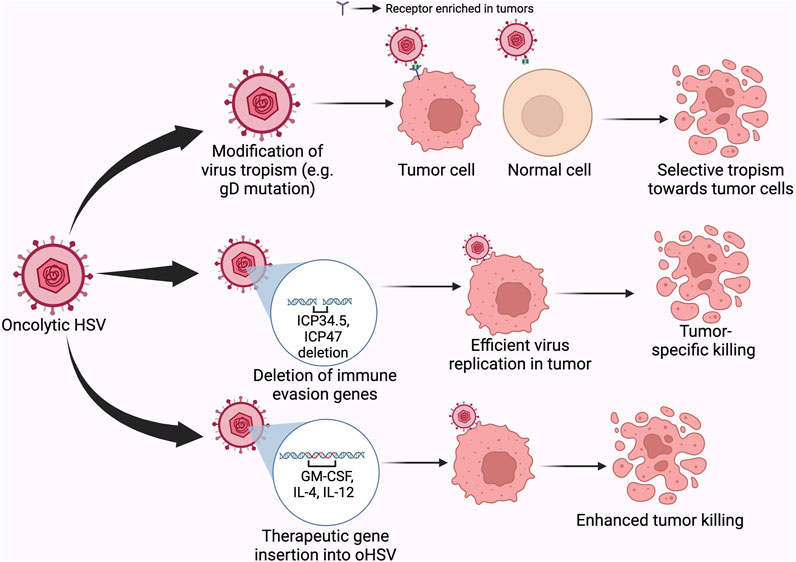
FIGURE 3. Different mechanisms by which oncolytic HSV achieve tumor killing. Legend: Modifications within the viral glycoprotein D to redirect tropism or to other regions of the genome to alter the interaction of the virus with intrinsic antiviral pathways in the cell, or the introduction of therapeutic genes within the genome of OVs provide mechanisms for achieving or improving targeted-killing of cancer cells.
HSV-1 entry is facilitated by the interaction of the viral glycoprotein D (gD) with different cellular receptors, including nectin-1, herpes virus entry mediator and 3-O sulfated heparan sulfate (Shibata et al., 2016). Since some tumors have decreased expression of nectin-1, to enhance infectivity as well as selectivity, the residues on gD necessary for interaction with nectin-1 were replaced with a single chain antibody targeting epithelial cell adhesion activating molecule (EpCAM), thereby detargeting the virus from nectin-1 and retargeting virus tropism towards cells with surface expression of EpCAM (Shibata et al., 2016). Of note, EpCAM has long been identified as a prognostic factor for many human cancers (Baeuerle and Gires, 2007). Similar mutations have been used to direct virus tropism towards tumor cells expressing HER2 and EGFR to achieve oncolysis (Menotti et al., 2006; Uchida et al., 2013). Loss of the virus-encoded thymidine kinase (TK) gene also allows for tumor-specific virus replication (Martuza et al., 1991), but this mutation might hinder responsiveness to acyclovir (the replication of HSV-1 is naturally suppressed by acyclovir) under potential situations of uncontrolled virus replication or replication in unwanted sites (Gilbert et al., 2002).
To enhance the immunological response against tumors, T-VEC was engineered to express granulocyte-macrophage colony-stimulating factor (GM-CSF), thereby promoting the recruitment of T cells to the site of the tumor (Liu et al., 2003). Since ICP47 normally prevents antigen presentation, the loss of ICP47 in T-VEC proved a useful mutation in suppressing tumor growth. Among several mechanisms for immune escape, some tumors express programmed death ligand 1 (PD-L1), thereby inhibiting the activation of T cells infiltrating the tumor microenvironment (TME) (Jiang et al., 2019). Co-administration of T-VEC with anti-PD-1 antibody, or engineering oHSV to express a single chain antibody against PD-1 (programmed cell death protein 1) was shown to suppress tumor growth (Ribas et al., 2017; Passaro et al., 2019).
Interleukin-4 (IL-4)-expressing oHSV promotes tumor clearance, whereas, the expression of IL-10 suppresses survival of the mice bearing the tumor, lending credence to the importance of the nature of the immune response at the site of the tumor in promoting or preventing tumor clearance (Andreansky et al., 1998). In another study, encoding IL-12, a cytokine that promotes the killing ability of natural killer cells and cytotoxic T cells within the genome of oHSV similarly improves the cytolytic activity of the immune cells (Parker et al., 2000). Besides the primary immune response elicited following treatment with oHSV, primary infection with oHSV was also shown to prevent against subsequent exposure to the tumor (Liu et al., 2003). Different studies that have applied herpesviruses as immunotherapeutic agents in the fight against cancer are presented in Table 1.
In addition to T-VEC, other oHSVs have been objects of clinical trials including G207, 1716, HF10, and ND1020 (Koch et al., 2020). HSV-1716 (Seprehvir) was the first oHSV to be administered via intravenous route in humans (Streby et al., 2019). Intravenous delivery of Seprehvir was well tolerated, but the virus was not recovered from the tumor. Since the patients recruited for this study also received other therapies besides Seprehvir, it was difficult to determine the contribution of Seprehvir alone in the survival of the patients (Streby et al., 2019). This study indicates a need for more research in understanding the factors contributing to efficient biodistribution of oHSV, as this would prove useful in targeting metastatic tumors.
Engineering oHSVs to express reporter genes allows easy tracking of the virus within animal hosts and provides a mechanism for detecting the virus in case of spread to sites outside the tumor (Mineta et al., 1995; Peters and Rabkin, 2015). This consideration becomes especially important given reports of possibilities for virus replication in non-tumor sites in mice after receiving oHSVs (Kesari et al., 1998). In the future, combining the beneficial mutations seen in different oHSVs with the transgenic delivery of therapeutic genes in single oHSV vectors may further improve the efficacy of oHSVs against human tumors. For example, designing single oHSVs with tumor-specific tropism, selective replication in tumors, and improved ability to promote tumor infiltration and sustained anti-tumor activities of immune cells at the TME would significantly improve the oncolytic potential of oHSVs against several human cancers.
3.2 Adenoviruses
Adenoviruses (AdVs) are small, non-enveloped DNA viruses with genome size of ∼36 kb. The viral genome is divided into early and late transcription units. The genome is enclosed by an icosahedral capsid with penton borne fibers projecting from each of the 12 vertices of the icosahedron. The fiber knob at the terminus of the fiber interacts with cellular receptors to dictate the tropism of AdV types (Barnett et al., 2002). Secondary interactions between the penton base proteins and integrin are also important for virus internalization (Stepanenko and Chekhonin, 2018). AdV type 5 (Ad5) is the most common AdV utilized for oncolytic virotherapy as evident from the findings in Table 2. The cellular receptor for Ad5, coxsackie virus and adenovirus receptor (CAR), is expressed at low levels in some tumors (Reeh et al., 2013), thereby reducing the tropism of the virus for cancer cells. However, generating a chimera of AdV types carrying knobs derived from other AdV types on the background of Ad5 allows for enhanced transduction and selectivity of oncolytic AdVs (oAdVs) into tumors (Koodie et al., 2019; Gao et al., 2020; Zafar et al., 2021). Other mechanisms for enhancing tropism of oAdVs are reviewed here (Stepanenko and Chekhonin, 2018). Interestingly, the loss of the CAR and/or integrin binding was shown to promote the hematogenous distribution of oAdV, suggesting that this mechanism may be exploited for targeting metastatic tumors (Akiyama et al., 2004).
The early gene products, E1A and E1B target the retinoblastoma protein (pRb) and p53 respectively to enforce S phase entry and virus replication (Tessier et al., 2021). An E1B-55 kDa-deficient AdV mutant (ONYX-015) was shown to replicate in, and lyse only p53-deficient cells, thereby providing selectivity between tumors and normal cells (Bischoff et al., 1996). Subsequent studies revealed that the mechanism driving the selectivity of ONYX-015 might be more complex than was previously thought (Kirn, 2001; Edwards et al., 2002). ONYX-015 became the first engineered oncolytic virus to be administered to humans, demonstrating safety at high doses independent of the route of administration in clinical trials (Kirn, 2001). Nevertheless, ONYX-015 by itself failed to promote tumor regression, but showed promise in combinational approaches with chemotherapy (Kirn, 2001; Garber, 2006). Others have also shown that tumor selectivity can also be achieved by the deletion of E1B-19 kDa (Liu et al., 2004). Another oAdV, H101, is similar to ONYX-015 except it carries a mutation in the E3 gene region which is associated with immune evasion (Zhang et al., 2021). H101 was well tolerated and demonstrated anti-tumoral activity in patients (Lu et al., 2004). It was eventually approved for human use in China in 2005 (Garber, 2006).
Another oAdV, ZD55-sflt-1 which was constructed on the ONYX-015 backbone, expressed an anti-angiogenic factor and further decreased survival of tumor cells when compared to ONYX-015 (Zhang et al., 2005). Engineering oAdv to encode vehicle genes under the regulation of tumor-specific promoters provided another means for achieving tumor selectivity (Abudoureyimu et al., 2019). The safety profile of ONYX-015 and the efficacy of H101 even at high doses prove the suitability of oAdVs as viro-immunotherapeutic agents and support the need for further investigations to improve their efficacy against different human tumors. The mechanism of cancer immunotherapy by adenoviruses is presented in Figure 4.
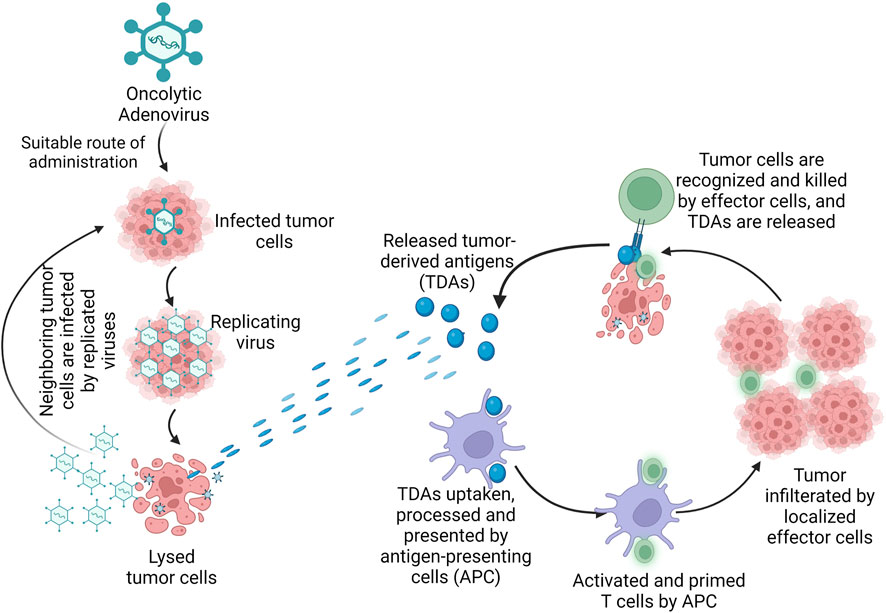
FIGURE 4. Mechanism of oncolytic adenovirus in cancer immunotherapy. Legend: The oncolytic adenovirus once administered by suitable route, infects the tumor cells and replicates in the tumor, causing direct lysis of the tumor. Once the tumor is lysed, tumor-derived antigens (TDAs) are released and the cells of the immune system are activated. The TDAs are uptaken, processed and presented by antigen-presenting cells (APC); the APC activates and primes the T cells which result in tumor-specific killing by the effector cells of the host immune system.
3.3 Poxviruses
Poxviruses (POXVs) are large DNA viruses with linear, double stranded genome. They replicate entirely in the cytoplasm of infected cells in structures called viral factories (Schramm and Locker, 2005). POXVs package within their particles, RNA polymerases as well as transcription factors required for early gene expression. The virus encodes its own RNA and DNA polymerases as well as other factors required for viral gene expression and replication in the cytoplasm (Wittek, 1982). The genome comprises of a central conserved region and terminal regions that are variable among POXVs, and which encodes factors for evading the host immune response (Wittek, 1982). Variola virus is the etiologic agent for smallpox in humans. A related POXV, vaccinia virus (VV) was used in its live, attenuated form as the vaccine for smallpox in humans.
The fast replication kinetics of VV, their efficient mechanism for cell-to-cell spread, their suitability for intravenous dissemination, and their long history of use as a vaccine agent make them ideal candidates for oncolytic virotherapy (Doceul et al., 2010; Irwin and Evans, 2012). Moreover, the large genome of POXVs generally allows them to serve as vectors for the expression of therapeutic genes. VV is the most common POXV applied in viral oncolysis. VV encodes a TK gene whose loss is associated with decreased virulence (Buller et al., 1985). Unlike normal cells, cancer cells produce abnormally high levels of a human homolog, TK1 (Bitter et al., 2020). Hence, the deletion of TK in oncolytic vaccinia virus (oVV) is often used to achieve selectivity for cancer cells. Different oncolytic poxviruses that have been used in the treatment of various cancer types are presented in Table 3.
One of the oVVs that has made it to clinical trials is JX-594 (Kim et al., 2006). JX-594 is gene-inactivated for TK and is also engineered to encode GM-CSF (Kim et al., 2006). The illustration showing the mechanism of action of oncolytic vaccinia virus is shown in Figure 5. Phase I application of JX-594 via intratumoral administration against liver and skin cancers demonstrated safety and anti-tumor activity with hyperbilirubinemia as a dose-limiting symptom (Mastrangelo et al., 1999; Park et al., 2008). Other POXVs that have been investigated as oncolytic agents include GLC-1h68, VV-FCU1, JX-795, JX-963, and vvDD which are all defective for TK expression, in addition to other unique mutations or insertions (McCart et al., 2001; Kirn et al., 2007; Thorne et al., 2007; Foloppe et al., 2008; Zhang et al., 2009). Expressing HSV-derived, truncated TK in oVV renders the virus susceptible to treatment with ganciclovir without affecting tumor selectivity, thereby strengthening the safety profile of oVV (Islam et al., 2020). oVVs deleted for virus-encoded immune evasion genes also hold potential as agents for driving tumoral selectivity in future studies, leaving intact the viral TK gene (Ho et al., 2021).
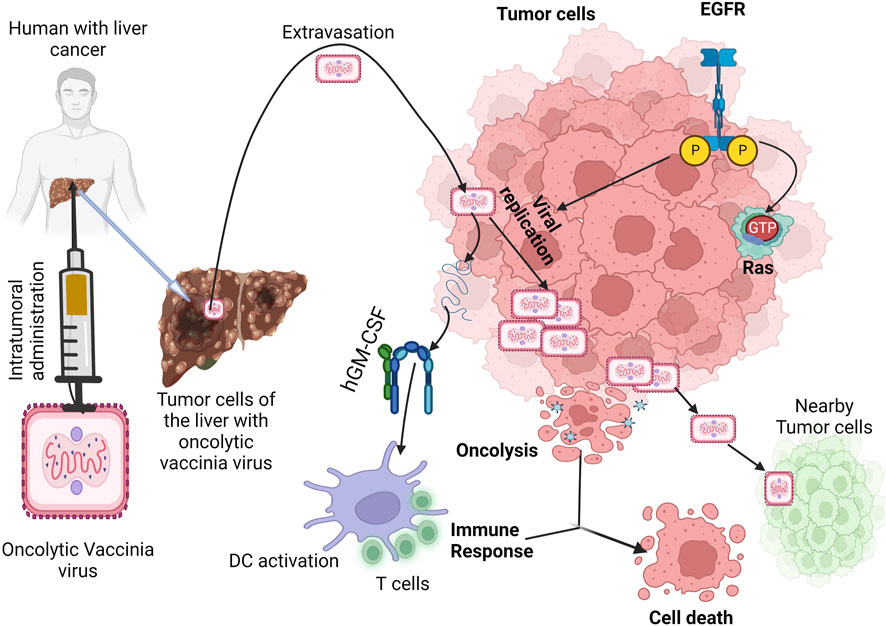
FIGURE 5. Mechanism of oncolytic vaccinia virus in cancer immunotherapy. Legend: The oncolytic vaccinia virus replicates in the tumor cell via active pathway of EGFR-RAS. Once the replication process is completed, tumor cell death occurs by viral-induced oncolysis and GM-CSF is expressed simultaneously to activate immune-induced cell death (Hernandez-Gea et al., 2013).
As one of the mechanisms to dampen the immune response at the TME, cancer cells in solid tumors outcompete lymphocytes for nutrients, resulting in metabolic insufficiency (Siska and Rathmell, 2015; Rivadeneira et al., 2019). Leptin-encoding oVV overcomes this effect, increasing T cell activity and memory as well as promoting tumor regression in mice (Rivadeneira et al., 2019). Cytokines secreted by stromal cells in the TME are known to participate in promoting tumor initiation and progression (Pearl et al., 2019). Consequently, reprogramming the TME through vvDD-IL-12-FG-mediated local delivery of IL-12 to the tumor promoted elevated the IFN-γ levels, increased the infiltration of CD8+ T cells, decreased the T cell exhaustion; consequently resulting in increased tumor clearance (Ge et al., 2020). Of note, the combination of vvDD-IL-12-FG with a PD-1 inhibitor dramatically enhanced the survival of mice bearing advanced tumors. It would be interesting to investigate the outcome of this combinational therapy in clinical trials (Ge et al., 2020).
3.4 Paramyxoviruses
The paramyxoviruses (PVs) can be described as viruses causing diseases in both humans and animals and belong to the members of the Paramyxoviridae. They are non-segmented negative-sense RNA viruses with envelopes and a diameter of 100–300 nm (Javaheri et al., 2022). Examples of PV include morbillivirus, measles, Newcastle disease virus, and so on (Keshavarz et al., 2019). A polycistronic gene that encodes two or more overlapping open reading frames (ORFs) is a popular genetic feature shared by viruses belonging to the paramyxoviruses (Horvath, 2004). Oncolytic PVs have an impressive affinity to cancerous cells that have viral receptors on their surface. For example, cancer cells with overexpression of sialoglycoproteins receptors can be highly and selectively bonded by NDV and MuV (Matveeva et al., 2018). Various danger signals can be activated by the PVs to establish excellent anti-cancer innate and adaptive immune responses (Figure 6). PVs are strong inducers of interferons (IFN) and other immuno-stimulating cytokines. A great advantage of PVs is the ability to trigger syncytium formation (Matveeva et al., 2015). T cell targeted therapy is becoming popular in clinical cancer treatment. The single chain variable fragments (scFv) of two antibodies of the bispecific T cell engagers (BiTEs) can be used to channel T cells to destroy target cells (Huang et al., 2021). A great example was the genetic engineering of the measles virus with BiTEs measles virus encoding bispecific T cell engagers (MV-BiTEs) and tested against solid tumors. In vitro models and in vivo models were both tested to determine the oncolytic functionality of MV-BiTEs. MV engineering with BiTE cassettes did not attenuate the oncolytic or replicative efficacy against solid tumors nor did it induce toxicity. The functionality is based on the ability to bind to antigens, specificity in T cell activation, and inducing T cell cytotoxicity. The delivery of some oncolytic agents remains a challenging area and this was reflected with MV-BiTEs serum levels being below the serum detection limit between two to 24 h after MV-BiTEs treatment both in syngeneic and patient-derived models. MV-BiTEs can also enable long-term immunity against tumor growths (Speck et al., 2018). Table 4 is a summary of various studies that have applied paramyxoviruses in cancer therapy.
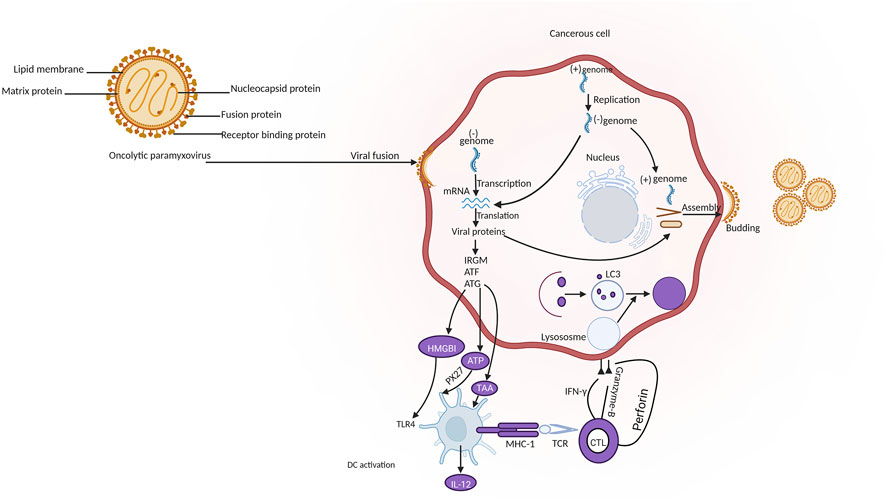
FIGURE 6. Mechanism of oncolytic paramyxovirus in cancer immunotherapy. Legend: The pathway involves the release of tumor-associated antigen (TAA) and damage-associated molecular patterns (DAMPs) by the tumor cells after the replication of oncolytic paramyxovirus. This is followed by the recognition by pattern recognition receptors (PRRs) on antigen presenting cells (APCs) and the release of inflammatory cytokines. TAAs can also be introduced by mature APCs through major histocompatibility complex-I (MHC-I) to cluster of differentiation 8+ (CD8+) T cancerous cells which ultimately leads to antitumor immune response and the lysis of cancerous cells through the release of granzyme B, perforin and interferon-gamma (IFN-γ) (Keshavarz et al., 2019).
Another interesting oncolytic virus is the mumps virus which has been shown to have a cytopathic effect (CPE). The antineoplastic efficacy of the wild-type of mumps virus had been previously illustrated and it had been revealed that intertumoral administration was better than systemic administration (Asada, 1974; Okuno et al., 1978), but Ammayappan et al. (2016) proved the oncolytic activity of the recombinant MuV-UCs (rMuV-UCs) encoded with human sodium iodide symporter (MV-NIS). In their study, Mumps Virus; Urabe strain (MuV-US) was developed with a reverse genetics platform based on the nucleotide sequence. The recombinant MuV showed a better growth rate than the parent virus. The different infectivity rates observed among the various cell lines can be attributed to a couple of possible factors including various cellular factors, interferon pathway-related genes or receptors and co-receptors expression levels. The colon carcinoma and neuroblastoma cells had significant viral replication while most of the cell lines were not permissive to the MuV-UC mumps virus infection. MuV-UC viruses also had a significant infection in CT-26-LacZ mouse colon carcinoma cells and N2A mouse neuroblastoma cells in vitro. It was hypothesized and proven that the green fluorescence protein (GFP) could affect the replication of the virus in vivo (Ammayappan et al., 2016). The use of tumor-associated macrophages (TAMs) illustrates a different outlook on the therapeutic role of TAMs in oncolytic virotherapy. This is because TAMs can acquire an anti-tumor phenotype to enhance the anti-tumor effect of the viruses (Tan et al., 2016).
Also, the Newcastle disease virus (NDV) of the PVs class can serve as an oncolytic agent. Wei et al., 2015 genetically engineered a recombinant NDV carrying intact cHAb18 gene (rNDV-18HL). The cHAb18 antibody-engineered NDV served as a new strategy for anti-tumor therapy without attenuating the viral replication of NDV (Wei et al., 2015). Another group discovered members of the avian avulaviruses groups with inherent antitumor activity. Various avian paramyxoviruses (APMVs) were studied to identify the ones with oncolytic capacity. APMV-4 Duck (Hong Kong/D3/1975 OV), new cancer therapeutic was discovered to have greater antitumor properties than the clinical candidate (NDV) and should be clinically translated for the treatment of solid tumors (Javaheri et al., 2022). Studies have shown that NDV is not selectively cytotoxic to normal cells due to a lack in the interferon (IFN) antiviral responses of tumor cells (Elankumaran et al., 2010; Wei et al., 2015; Kazimirsky et al., 2016). Various genetic engineered strains of NDV were studied to understand their potential toxicities and it was observed that the differential regulation of IFN-α and downstream antiviral genes induced by IFN-α determines the tumor-selective replication of rNDV (Elankumaran et al., 2010). Some clinical trials where oncolytic therapy has been applied include the following; attenuated NDV vaccine from the Hertfordshire strain, MTH-68/H clinical trial showed increased survival rate and good quality patient lifestyles (Csatary et al., 2004), and attenuated NDV vaccine from the PV7011 strain also yielded remarkable levels of neutralizing antibodies with signs of tumor regressions after PV701 administration (Pecora et al., 2002).
Among the paramyxoviruses generally, wild-type (WT) parainfluenza virus 5 (PIV5) has been established to poorly induce host cell responses in some human cell types and to be highly non-cytopathic to a lot of in vitro cell types. A study showed that the mutated form (P/V-CPI-) is highly cytopathic and can destroy HEp-2 human laryngeal cancer cells but some cells might emerge again over time. A proposed solution would be the combination of oncolytic viruses with chemotherapies, and this has found grounds in some ongoing clinical trials. For example, a phase II clinical trial outcome showed that combination therapy of 5-fluorouracil, cisplatin, and oncolytic adenovirus ONYX-015 was more effective than individual therapies in persistent head and neck cancer cases (Fox and Parks, 2018).
4 Toxicity and safety concerns surrounding the adoption of oncolytic viruses as immunotherapeutic agents in cancer treatment
Different immunotherapeutic studies with oncolytic viruses have been initiated in the past 10–15 years (Forbes et al., 2018; Torres-Domínguez and McFadden, 2019; Zheng et al., 2019). These studies have taught us a lot about the processes of cancer vaccination and how to choose the best individual oncolytic viruses. To date, just few oncolytic viruses have been authorized for use in cancer therapies, however many more are still in the process of being approved. The approved oncolytic viruses for use against different forms of cancers are described herewith. ECHO-7 (RIGVIR®), an unmodified Picornaviridae family virus strain was approved in the Republic of Latvia by the State Agency of Medicines in 2004 to treat skin melanoma, subcutaneous melanoma metastases, and prevent relapse cum metastases upon surgery (Doniņa et al., 2015). ONYX-015 (H101 or Oncorine), a genetically-modified Adenovirus type 5 strain was approved in China by the State Food and Drug Administration in 2005 to treat nasopharyngeal carcinoma (Jiang et al., 2015). Talimogene laherparepvec (T-VEC) or IMLYGIC®, a genetically-modified herpes simplex virus I (HSV-1) strain was approved in the United States by the Food and Drug Administration (FDA) in 2015 to treat unresectable cutaneous, subcutaneous and nodal lesions in melanoma patients (Pol et al., 2016). Pelareorep (REOLYSIN®), an unmodified reovirus type 3 strain was granted an orphan drug designation to treat malignant glioma, pancreatic, gastric, peritoneal, tube and ovarian cancer in 2015 and fast track designation to treat metastatic breast cancer in 2017, by the United States FDA (Terrível et al., 2020; U.S. Food and Drug Administration, 2022). Also, orphan drug designation was granted to Toca-511 by the United States FDA to treat glioblastoma, but it is still in clinical trial (Rusell and Peng, 2018; Terrível et al., 2020). Some of the oncolytic viruses in clinical trial and awaiting approval include; JX-594 (NCT02630368 for solid tumors, soft tissue sarcoma, breast cancer), DNX-2401 (NCT03896568 for anaplastic astrocytoma, glioblastoma, gliosarcoma, malignant glioma), ColoAd1 or Enadenotucirev (NCT03916510 for locally advanced rectal cancer), GL-ONC1 (NCT05281471 for endometrioid, high-grade serous, platinum-resistant and platinum-refractory ovarian cancer, peritoneal cancer, fallopian tube cancer), H-1PV (NCT01301430 for glioblastoma multiforme; NCT02653313 for carcinoma, pancreatic ductal), ADV-TK (NCT02768363 for prostate cancer; NCT04495153 for non-small cell lung cancer; NCT02446093 for pancreatic adenocarcinoma; NCT03541928 for prostate cancer), Adenovirus/PSA Vaccine (NCT00583024 for refractory prostate cancer) (Terrível et al., 2020; Li et al., 2022; Clinical Trials Database, 2022). However, some oncolytic viruses have been abandoned for cancer therapy due to ineffectiveness, intolerable toxicity and severe safety concerns (Lauer and Beil, 2022).
Few adverse effects and limited fatality have been linked to replicating viruses in many oncolytic viruses used for human trials (Terrível et al., 2020). When compared to the toxicity of standard cytotoxic medicines, the toxicity of oncolytic viruses in viro-immunotherapy is satisfactory. In all, off-target effects and viral mutation/transmission remain the hallmarks of their safety issues (Forbes et al., 2018). The interactions of these engineered oncolytic replicating viruses with both host and environment are far more difficult to predict, because these interactions require oncolytic viruses to be thoroughly structured to have enough virulence needed to significantly decrease and lessen the tumor mass so as to avoid causing adverse effects in a patient receiving such treatment (Shilpa et al., 2014; Reale et al., 2019).
4.1 Safety concerns for the host
While it is true that natural and engineered selectivity has reduced pathogenicity, there is still the chance of off-target effects and genetic modification which may result in unintended toxic effects (Hemminki et al., 2020). Viruses have a high possibility of evolving each time the original virus is replicated, resulting in the proliferation of new viral lineages owing to viral polymerase defects. As a result, one major concern with the use of oncolytic viruses is the propensity of these viruses to acquire new cell tropisms or lose restriction factors (Lawler et al., 2017).
According to Lauer and Beil (2022), genetically modified oncolytic viruses were adopted in almost two-thirds of documented oncolytic virus clinical studies rather than natural viruses. Despite the special consideration given to engineered oncolytic viruses and the acceptance of newer oncolytic viruses with low seroprevalence and the ability to avoid neutralizing antibodies, there has been an increased risk of viral spread with less favorable safety profiles documented for the majority of assessed genetically engineered oncolytic viruses (Chaurasiya et al., 2021).
The prospect of oncolytic viruses harboring immunostimulatory genes causing excessive production of immune modulators and, as a result, immune system overreaction, should also be addressed, although such detrimental consequences are yet to be expressively described. In contrast, overexpression of immune responses may improve the success of oncolytic viral treatment, in which viruses that produce immunostimulatory proteins are used (Zeyaullah et al., 2012). Uncontrolled reproduction and viral transmission have been addressed in known oncolytic viruses by including suicide genes (HSV-TK) utilizing drugs; nevertheless, this cannot be confirmed for all oncolytic viruses, particularly newer oncolytic viruses. Incorporating suicide genes (HSV-TK) into drugs to treat viral cells that are out of control may allow the virus to evade immune-mediated elimination, potentially overcoming the immunological barrier to undesirable viral spread and infections (Prestwich et al., 2008; Thomas and Bartee, 2022). Immunity to microbial agents may develop in response to systemic administration because human systems have evolved to combat infections. Serum neutralization and hepatotoxicity are significant challenges in the development of systemic administration. A neutralizing antibody response has been reported in nearly every virus-treated patient, although it has not been linked to a response or shortage thereof (Forbes et al., 2018).
Oncolytic viruses’ toxicity and safety problems may be due to the increased volume necessary to eliminate and prevent relapses of malignant cells. According to Tadesse and Bekuma (2018), it has been challenging to understand how oncolytic viruses travel to the tumor site since most investigations require injecting large viral titers directly into the tumor site. This might have resulted in the serious safety issues mentioned earlier.
4.2 Safety concerns for the environment
Following treatment, individuals may shed live replicating viruses, boosting the likelihood of transmission to healthy individuals. Given the fast mutation rate of viruses, particularly RNA viruses, there is a possibility of transmission when released into the environment via waste products (Prestwich et al., 2008). For instance, NDV has been isolated from urine for up to 3 weeks following therapy. Although the virus delivered may not be a human pathogen, it may evolve to acquire pathogenic traits and effectively infect the normal host tissues of a victim. Similarly, mutated viruses can revert to wild-type or integrate with wild-type viruses. All these safety issues continue to influence the development of oncolytic viral therapy and its wide acceptance in cancer treatment, even with their potency as immunotherapeutic agents.
5 Future perspectives
Over the years, the usage of oncolytic viruses as a sole anti-cancer agent both at the preclinical and clinical stages has proven effective, but with some limitations emerging as a result of the dynamic nature of tumor cells. There is possibility for the cancer to reoccur and metastasize after being treated with the oncolytic virus. As with the case of HSV-1716 (Seprehvir) which was not recovered from the tumor after administration, there is need to establish the factors that contribute to the biodistribution of oHSV to aid in targeted therapy against metastatic tumors. Since oncolytic viruses stimulate both the innate and adaptive immune system, efforts need to be channeled towards the adequate understanding of the interaction between the oncolytic virus, the tumor and the immune system of the host. This will help in creating better strategies, based on the findings of these studies, to fight against various cancer cells.
Researchers need to focus on exploring other types of unexplored viruses, but with oncolytic property, particularly in clinical trials. These viruses will serve as alternative viruses when there is diminished efficacy of a particular oncolytic virus as a result of repetitive administrations. It has become very clear that there is need for synergistic therapy of oncolytic viruses with other cancer therapies. As highlighted in this review, there are already studies combining oncolytic viro-immunotherapy with other methods like chemotherapy and radiotherapy, but this space is yet to be fully maximized. An example is the better outcome achieved with 5-fluorouracil, cisplatin, and oncolytic adenovirus ONYX-015 in head and neck cancer clinical trial phase II than observed when the oncolytic adenovirus ONYX-015 was applied (Fox and Parks, 2018). When vvDD-IL-12-FG was combined with a PD-1 inhibitor, it radically increased the survival of the model mice used. This promising synergistic therapy also needs to proceed to clinical trial phases (Ge et al., 2020). In order to maximize this combinational approach, researchers need to put some factors into consideration; the mode/method and route of delivery (intratumoral, intraneoplastic, intravenous, intracranial, intraperitoneal, intracarotid, intracerebral, intracavitary, intradermal, peritumoral, inhalation, among others), location of the cancer cells, toxicity to non-tumor healthy cells, tissues or organs of the host, specificity in selection of dosage/titer and the specific immune responses elicited. This will greatly empower the arsenal of oncolytic viruses as immunotherapeutic agents against different types of cancer, and appreciably lessen the global burden of the disease.
6 Conclusion
The efficacy of oncolytic viruses as immunotherapeutic agents for the treatment of different forms of cancers has been well established with improvement in their safety, production, selectivity, potency and methods of delivery. It is interesting to know that oncolytic viruses do not only lyse cells, but can also stimulate different types of immune responses, depending on the group of virus used as the oncolytic agent. It is more interesting to know that the genetic activities of these oncolytic viruses could be modified or manipulated to prevent these viruses from being killed by the cells of the host immune system and to enhance their therapeutic potential against tumor cells. In the future, the success of oncolytic viruses in cancer immunotherapy will depend largely on approaches to combine them with conventional cancer therapies and to understand the specific interaction between the tumor and immune status of individual patients.
Author contributions
RO: Conceptualization, literature search, wrote the first draft, contributed to illustrations, reviewed and edited the manuscript. OO: literature search, wrote the first draft, contributed to illustrations. MA: literature search, contributed to tables. JE: literature search, wrote the first draft, contributed to illustrations. EA: literature search, wrote the first draft, contributed to tables and illustrations. OD: literature search, wrote the first draft, text editing. YA: literature search, wrote the first draft. TO: literature search. NT: reviewed and edited the manuscript. MA: literature search and referencing. OA: reviewed and edited the manuscript. OS: literature search and referencing.
Funding
This review received assistance from the Open Access Publication Fee Support 2022 provided by Frontiers.
Acknowledgments
Figures are created with biorender.com.
Conflict of interest
The authors declare that the research was conducted in the absence of any commercial or financial relationships that could be construed as a potential conflict of interest.
Publisher’s note
All claims expressed in this article are solely those of the authors and do not necessarily represent those of their affiliated organizations, or those of the publisher, the editors and the reviewers. Any product that may be evaluated in this article, or claim that may be made by its manufacturer, is not guaranteed or endorsed by the publisher.
References
Abbas, Z., and Rehman, S. (2018). “An overview of cancer treatment modalities,” in Neoplasm. Editor H. N. Shahzad (London: InTech). doi:10.5772/intechopen.76558
Abudoureyimu, M., Lai, Y., Tian, C., Wang, T., Wang, R., and Chu, X. (2019). Oncolytic adenovirus—a nova for gene-targeted oncolytic viral therapy in HCC. Front. Oncol. 9, 1182. doi:10.3389/fonc.2019.01182
Achard, C., Guillerme, J.-B., Bruni, D., Boisgerault, N., Combredet, C., Tangy, F., et al. (2017). Oncolytic measles virus induces tumor necrosis factor-related apoptosis-inducing ligand (TRAIL)-mediated cytotoxicity by human myeloid and plasmacytoid dendritic cells. OncoImmunology 6, e1261240. doi:10.1080/2162402X.2016.1261240
Achard, C., Surendran, A., Wedge, M.-E., Ungerechts, G., Bell, J., and Ilkow, C. S. (2018). Lighting a fire in the tumor microenvironment using oncolytic immunotherapy. EBioMedicine 31, 17–24. doi:10.1016/j.ebiom.2018.04.020
Ajina, A., and Maher, J. (2017). Prospects for combined use of oncolytic viruses and CAR T-cells. J. Immunother. Cancer 5, 90. doi:10.1186/s40425-017-0294-6
Akiyama, M., Thorne, S., Kirn, D., Roelvink, P. W., Einfeld, D. A., King, C. R., et al. (2004). Ablating CAR and integrin binding in adenovirus vectors reduces nontarget organ transduction and permits sustained bloodstream persistence following intraperitoneal administration. Mol. Ther. 9, 218–230. doi:10.1016/j.ymthe.2003.10.010
Ammayappan, A., Russell, S. J., and Federspiel, M. J. (2016). Recombinant mumps virus as a cancer therapeutic agent. Mol. Ther. Oncolytics 3, 16019. S2372770517300128. doi:10.1038/mto.2016.19
Andreansky, S., He, B., van Cott, J., McGhee, J., Markert, J., Gillespie, G., et al. (1998). Treatment of intracranial gliomas in immunocompetent mice using herpes simplex viruses that express murine interleukins. Gene Ther. 5, 121–130. doi:10.1038/sj.gt.3300550
Arruebo, M., Vilaboa, N., Sáez-Gutierrez, B., Lambea, J., Tres, A., Valladares, M., et al. (2011). Assessment of the evolution of cancer treatment therapies. Cancers 3, 3279–3330. doi:10.3390/cancers3033279
Asada, T. (1974). Treatment of human cancer with mumps virus. Cancer 34, 1907–1928. doi:10.1002/1097-0142(197412)34:6<1907::AID-CNCR2820340609>3.0
Baeuerle, P. A., and Gires, O. (2007). EpCAM (CD326) finding its role in cancer. Br. J. Cancer 96, 417–423. doi:10.1038/sj.bjc.6603494
Barnett, B. G., Crews, C. J., and Douglas, J. T. (2002). Targeted adenoviral vectors. Biochim. Biophys. Acta 1575, 1–14. doi:10.1016/S0167-4781(02)00249-X
Bastin, D., Walsh, S., Al Saigh, M., and Wan, Y. (2016). Capitalizing on cancer specific replication: Oncolytic viruses as a versatile platform for the enhancement of cancer immunotherapy strategies. Biomedicines 4, 21. doi:10.3390/biomedicines4030021
Bauerschmitz, G. J., Kanerva, A., Wang, M., Herrmann, I., Shaw, D. R., Strong, T. V., et al. (2004). Evaluation of a selectively oncolytic adenovirus for local and systemic treatment of cervical cancer. Int. J. Cancer 111, 303–309. doi:10.1002/ijc.20217
Bauerschmitz, G. J., Lam, J. T., Kanerva, A., Suzuki, K., Nettelbeck, D. M., Dmitriev, I., et al. (2002). Treatment of ovarian cancer with a tropism modified oncolytic adenovirus. Cancer Res. 62, 1266–1270.
Bischoff, J. R., Kirn, D. H., Williams, A., Heise, C., Horn, S., Muna, M., et al. (1996). An adenovirus mutant that replicates selectively in p53- deficient human tumor cells. Science 274, 373–376. doi:10.1126/science.274.5286.373
Bitter, E. E., Townsend, M. H., Erickson, R., Allen, C., and O’Neill, K. L. (2020). Thymidine kinase 1 through the ages: A comprehensive review. Cell Biosci. 10, 138. doi:10.1186/s13578-020-00493-1
Bortolanza, S., Bunuales, M., Otano, I., Gonzalez-Aseguinolaza, G., Ortiz-de-Solorzano, C., Perez, D., et al. (2009). Treatment of pancreatic cancer with an oncolytic adenovirus expressing interleukin-12 in Syrian hamsters. Mol. Ther. 17, 614–622. doi:10.1038/mt.2009.9
Bourgeois-Daigneault, M.-C., Roy, D. G., Aitken, A. S., El Sayes, N., Martin, N. T., Varette, O., et al. (2018). Neoadjuvant oncolytic virotherapy before surgery sensitizes triple-negative breast cancer to immune checkpoint therapy. Sci. Transl. Med. 10, eaao1641. doi:10.1126/scitranslmed.aao1641
Bray, F., Ferlay, J., Soerjomataram, I., Siegel, R. L., Torre, L. A., and Jemal, A. (2018). Global cancer statistics 2018: GLOBOCAN estimates of incidence and mortality worldwide for 36 cancers in 185 countries. Ca. Cancer J. Clin. 68, 394–424. doi:10.3322/caac.21492
Buller, R. M. L., Smith, G. L., Cremer, K., Notkins, A. L., and Moss, B. (1985). Decreased virulence of recombinant vaccinia virus expression vectors is associated with a thymidine kinase-negative phenotype. Nature 317, 813–815. doi:10.1038/317813a0
Burton, E. A., Fink, D. J., and Glorioso, J. C. (2002). Gene delivery using herpes simplex virus vectors. DNA Cell Biol. 21, 915–936. doi:10.1089/104454902762053864
Cancer Research Uk, (2019). Cancer statistics for the UK. Accessed at: https://www.cancerresearchuk.org/health-professional/cancer-statistics-for-the-uk#heading-One 15/10/2022.
Cassel, W. A., and Murray, D. R. (1992). A ten-year follow-up on stage II Malignant Melanoma patients treated postsurgically with newcastle disease virus oncolysate. Med. Oncol. Tumor Pharmacother. 9, 169–171. doi:10.1007/BF02987752
Cerullo, V., Diaconu, I., Romano, V., Hirvinen, M., Ugolini, M., Escutenaire, S., et al. (2012). An oncolytic adenovirus enhanced for toll-like receptor 9 stimulation increases antitumor immune responses and tumor clearance. Mol. Ther. 20, 2076–2086. doi:10.1038/mt.2012.137
Cerullo, V., Pesonen, S., Diaconu, I., Escutenaire, S., Arstila, P. T., Ugolini, M., et al. (2010). Oncolytic adenovirus coding for granulocyte macrophage colony-stimulating factor induces antitumoral immunity in cancer patients. Cancer Res. 70, 4297–4309. doi:10.1158/0008-5472.CAN-09-3567
Chaurasiya, S., Chen, N. G., Lu, J., Martin, N., Shen, Y., Kim, S.-I., et al. (2020a). A chimeric poxvirus with J2R (thymidine kinase) deletion shows safety and anti-tumor activity in lung cancer models. Cancer Gene Ther. 27, 125–135. doi:10.1038/s41417-019-0114-x
Chaurasiya, S., Kim, S.-I., O’Leary, M., Park, A. K., Lu, J., Kang, S., et al. (2021). Toward comprehensive imaging of oncolytic viroimmunotherapy. Mol. Ther. Oncolytics 23, 303–310. doi:10.1016/j.omto.2021.06.010
Chaurasiya, S., Yang, A., Kang, S., Lu, J., Kim, S.-I., Park, A. K., et al. (2020b). Oncolytic poxvirus CF33-hNIS-ΔF14.5 favorably modulates tumor immune microenvironment and works synergistically with anti-PD-L1 antibody in a triple-negative breast cancer model. OncoImmunology 9, 1729300. doi:10.1080/2162402X.2020.1729300
Clinical Trials Database (2022). ClinicalTrials.gov is a database of privately and publicly funded clinical studies conducted around the world. Available at: https://clinicaltrials.gov. (Accessed November 22, 2022)
Cockle, J. V., Rajani, K., Zaidi, S., Kottke, T., Thompson, J., Diaz, R. M., et al. (2016). Combination viroimmunotherapy with checkpoint inhibition to treat glioma, based on location-specific tumor profiling. Neuro. Oncol. 18, 518–527. doi:10.1093/neuonc/nov173
Csatary, L. K., Gosztonyi, G., Szeberenyi, J., Fabian, Z., Liszka, V., Bodey, B., et al. (2004). MTH-68/H oncolytic viral treatment in human high-grade gliomas. J. Neurooncol. 67, 83–93. doi:10.1023/B:NEON.0000021735.85511.05
Currier, M. A., Gillespie, R. A., Sawtell, N. M., Mahller, Y. Y., Stroup, G., Collins, M. H., et al. (2008). Efficacy and safety of the oncolytic herpes simplex virus rRp450 alone and combined with cyclophosphamide. Mol. Ther. 16, 879–885. doi:10.1038/mt.2008.49
Damyanov, C. A., Maslev, I. K., Pavlov, V. S., and Avramov, L. (2018). Conventional treatment of cancer realities and problems. Ann. Complement. Altern. Med. 1 (1), 1002.
de Queiroz, N. M. G. P., Xia, T., Konno, H., and Barber, G. N. (2019). Ovarian cancer cells commonly exhibit defective STING signaling which affects sensitivity to viral oncolysis. Mol. Cancer Res. 17, 974–986. doi:10.1158/1541-7786.MCR-18-0504
Debela, D. T., Muzazu, S. G., Heraro, K. D., Ndalama, M. T., Mesele, B. W., Haile, D. C., et al. (2021). New approaches and procedures for cancer treatment: Current perspectives. SAGE Open Med. 9, 20503121211034366. doi:10.1177/20503121211034366
Demicheli, R., Retsky, M. W., Hrushesky, W. J. M., Baum, M., and Gukas, I. D. (2008). The effects of surgery on tumor growth: A century of investigations. Ann. Oncol. 19, 1821–1828. doi:10.1093/annonc/mdn386
DeWeese, T. L., van der Poel, H., Li, S., Mikhak, B., Drew, R., Goemann, M., et al. (2001). A phase I trial of CV706, a replication-competent, PSA selective oncolytic adenovirus, for the treatment of locally recurrent prostate cancer following radiation therapy. Cancer Res. 61, 7464–7472.
Diaz, R. M., Galivo, F., Kottke, T., Wongthida, P., Qiao, J., Thompson, J., et al. (2007). Oncolytic immunovirotherapy for melanoma using vesicular stomatitis virus. Cancer Res. 67, 2840–2848. doi:10.1158/0008-5472.CAN-06-3974
Dilley, J., Reddy, S., Ko, D., Nguyen, N., Rojas, G., Working, P., et al. (2005). Oncolytic adenovirus CG7870 in combination with radiation demonstrates synergistic enhancements of antitumor efficacy without loss of specificity. Cancer Gene Ther. 12, 715–722. doi:10.1038/sj.cgt.7700835
Doceul, V., Hollinshead, M., van der Linden, L., and Smith, G. L. (2010). Repulsion of superinfecting virions: A mechanism for rapid virus spread. Science 327, 873–876. doi:10.1126/science.1183173
Doniņa, S., Strēle, I., Proboka, G., Auziņš, J., Alberts, P., Jonsson, B., et al. (2015). Adapted ECHO-7 virus Rigvir immunotherapy (oncolytic virotherapy) prolongs survival in melanoma patients after surgical excision of the tumour in a retrospective study. Melanoma Res. 25, 421–426. doi:10.1097/CMR.0000000000000180
Du, W., Seah, I., Bougazzoul, O., Choi, G., Meeth, K., Bosenberg, M. W., et al. (2017). Stem cell-released oncolytic herpes simplex virus has therapeutic efficacy in brain metastatic melanomas. Proc. Natl. Acad. Sci. U. S. A. 114, E6157–E6165. doi:10.1073/pnas.1700363114
Duebgen, M., Martinez-Quintanilla, J., Tamura, K., Hingtgen, S., Redjal, N., Wakimoto, H., et al. (2014). Stem cells loaded with multimechanistic oncolytic herpes simplex virus variants for brain tumor therapy. J. Natl. Cancer Inst. 106, dju090. doi:10.1093/jnci/dju090
Edwards, S. J., Dix, B. R., Myers, C. J., Dobson-Le, D., Huschtscha, L., Hibma, M., et al. (2002). Evidence that replication of the antitumor adenovirus ONYX-015 is not controlled by the p53 and p14 ARF tumor suppressor genes. J. Virol. 76, 12483–12490. doi:10.1128/JVI.76.24.12483-12490.2002
El-Hussein, A., Manoto, S. L., Ombinda-Lemboumba, S., Alrowaili, Z. A., and Mthunzi-Kufa, P. (2020). A review of chemotherapy and photodynamic therapy for lung cancer treatment. Anticancer. Agents Med. Chem. 21, 149–161. doi:10.2174/1871520620666200403144945
Elankumaran, S., Chavan, V., Qiao, D., Shobana, R., Moorkanat, G., Biswas, M., et al. (2010). Type I interferon-sensitive recombinant newcastle disease virus for oncolytic virotherapy. J. Virol. 84, 3835–3844. doi:10.1128/JVI.01553-09
Engeland., C. E. (2020). Oncolytic viruses. New York, NY: Springer New York. doi:10.1007/978-1-4939-9794-7
Errington, F., Steele, L., Prestwich, R., Harrington, K. J., Pandha, H. S., Vidal, L., et al. (2008). Reovirus activates human dendritic cells to promote innate antitumor immunity. J. Immunol. 180, 6018–6026. doi:10.4049/jimmunol.180.9.6018
Fajardo, C. A., Guedan, S., Rojas, L. A., Moreno, R., Arias-Badia, M., de Sostoa, J., et al. (2017). Oncolytic adenoviral delivery of an EGFR-targeting T-cell engager improves antitumor efficacy. Cancer Res. 77, 2052–2063. doi:10.1158/0008-5472.CAN-16-1708
Fatiregun, O. A., Bakare, O., Ayeni, S., Oyerinde, A., Sowunmi, A. C., Popoola, A., et al. (2020). 10-Year mortality pattern among cancer patients in lagos state university teaching hospital, ikeja, lagos. Front. Oncol. 10, 573036. doi:10.3389/fonc.2020.573036
Federal Ministry of Health (2018). Nigeria national cancer control plan 2018 – 2022. Available at: https://www.iccp-portal.org/system/files/plans/NCCP_Final%5B1%5D.pdf.
Ferlay, J., Ervik, M., Lam, F., Colombet, M., Mery, L., Piñeros, M., et al. (2020). Global cancer observatory: Cancer today. Lyon: International Agency for Research on Cancer.
Foloppe, J., Kintz, J., Futin, N., Findeli, A., Cordier, P., Schlesinger, Y., et al. (2008). Targeted delivery of a suicide gene to human colorectal tumors by a conditionally replicating vaccinia virus. Gene Ther. 15, 1361–1371. doi:10.1038/gt.2008.82
Forbes, N. S., Coffin, R. S., Deng, L., Evgin, L., Fiering, S., Giacalone, M., et al. (2018). White paper on microbial anti-cancer therapy and prevention. J. Immunother. Cancer 6, 78. doi:10.1186/s40425-018-0381-3
Fox, C. R., and Parks, G. D. (2018). Parainfluenza virus infection sensitizes cancer cells to DNA-damaging agents: Implications for oncolytic virus therapy. J. Virol. 92, e01948-17. doi:10.1128/JVI.01948-17
Francis, L., Guo, Z. S., Liu, Z., Ravindranathan, R., Urban, J. A., Sathaiah, M., et al. (2016). Modulation of chemokines in the tumor microenvironment enhances oncolytic virotherapy for colorectal cancer. Oncotarget 7, 22174–22185. doi:10.18632/oncotarget.7907
Friedman, G. K., Moore, B. P., Nan, L., Kelly, V. M., Etminan, T., Langford, C. P., et al. (2016). Pediatric medulloblastoma xenografts including molecular subgroup 3 and CD133+ and CD15+ cells are sensitive to killing by oncolytic herpes simplex viruses. Neuro. Oncol. 18, 227–235. doi:10.1093/neuonc/nov123
Fu, X., Rivera, A., Tao, L., De Geest, B., and Zhang, X. (2012). Construction of an oncolytic herpes simplex virus that precisely targets hepatocellular carcinoma cells. Mol. Ther. 20, 339–346. doi:10.1038/mt.2011.265
Fu, X., Tao, L., Wu, W., and Zhang, X. (2020). Arming HSV-based oncolytic viruses with the ability to redirect the host’s innate antiviral immunity to attack tumor cells. Mol. Ther. Oncolytics 19, 33–46. doi:10.1016/j.omto.2020.09.002
Fu, X., Tao, L., and Zhang, X. (2007). An oncolytic virus derived from type 2 herpes simplex virus has potent therapeutic effect against metastatic ovarian cancer. Cancer Gene Ther. 14, 480–487. doi:10.1038/sj.cgt.7701033
Fukuhara, H., Ino, Y., and Todo, T. (2016). Oncolytic virus therapy: A new era of cancer treatment at dawn. Cancer Sci. 107, 1373–1379. doi:10.1111/cas.13027
Gainey, M. D., Manuse, M. J., and Parks, G. D. (2008). A hyperfusogenic F protein enhances the oncolytic potency of a paramyxovirus simian virus 5 P/V mutant without compromising sensitivity to type I interferon. J. Virol. 82, 9369–9380. doi:10.1128/JVI.01054-08
Gao, J., Zhang, W., Mese, K., Bunz, O., Lu, F., and Ehrhardt, A. (2020). Transient chimeric ad5/37 fiber enhances NK-92 carrier cell-mediated delivery of oncolytic adenovirus type 5 to tumor cells. Mol. Ther. Methods Clin. Dev. 18, 376–389. doi:10.1016/j.omtm.2020.06.010
Garber, K. (2006). China approves world’s first oncolytic virus therapy for cancer treatment. J. Natl. Cancer Inst. 98, 298–300. doi:10.1093/jnci/djj111
Ge, Y., Wang, H., Ren, J., Liu, W., Chen, L., Chen, H., et al. (2020). Oncolytic vaccinia virus delivering tethered IL-12 enhances antitumor effects with improved safety. J. Immunother. Cancer 8, e000710. doi:10.1136/jitc-2020-000710
Ghouse, S. M., Nguyen, H.-M., Bommareddy, P. K., Guz-Montgomery, K., and Saha, D. (2020). Oncolytic herpes simplex virus encoding IL12 controls triple-negative breast cancer growth and metastasis. Front. Oncol. 10, 384. doi:10.3389/fonc.2020.00384
Gilbert, C., Bestman-Smith, J., and Boivin, G. (2002). Resistance of herpesviruses to antiviral drugs: Clinical impacts and molecular mechanisms. Drug resist. updat. 5, 88–114. doi:10.1016/S1368-7646(02)00021-3
Gujar, S., Pol, J. G., and Kroemer, G. (2018). Heating it up: Oncolytic viruses make tumors ‘hot’ and suitable for checkpoint blockade immunotherapies. OncoImmunology 7, e1442169. doi:10.1080/2162402X.2018.1442169
Guo, Z. S., Liu, Z., Kowalsky, S., Feist, M., Kalinski, P., Lu, B., et al. (2017). Oncolytic immunotherapy: Conceptual evolution, current strategies, and future perspectives. Front. Immunol. 8, 555. doi:10.3389/fimmu.2017.00555
Heidbuechel, J. P. W., and Engeland, C. E. (2021). Oncolytic viruses encoding bispecific T cell engagers: A blueprint for emerging immunovirotherapies. J. Hematol. Oncol. 14, 63. doi:10.1186/s13045-021-01075-5
Heinzerling, L., Künzi, V., Oberholzer, P. A., Kündig, T., Naim, H., and Dummer, R. (2005). Oncolytic measles virus in cutaneous T-cell lymphomas mounts antitumor immune responses in vivo and targets interferon-resistant tumor cells. Blood 106, 2287–2294. doi:10.1182/blood-2004-11-4558
Hemminki, O., dos Santos, J. M., and Hemminki, A. (2020). Oncolytic viruses for cancer immunotherapy. J. Hematol. Oncol. 13, 84. doi:10.1186/s13045-020-00922-1
Hernandez-Gea, V., Alsinet, C., and Llovet, J. M. (2013). Oncolytic immunotherapeutic virus in HCC: Can it compete with molecular therapies?. J. Hematol. 59, 882–884. doi:10.1016/j.jhep.2013.05.006
Ho, T. Y., Mealiea, D., Okamoto, L., Stojdl, D. F., and McCart, J. A. (2021). Deletion of immunomodulatory genes as a novel approach to oncolytic vaccinia virus development. Mol. Ther. Oncolytics 22, 85–97. doi:10.1016/j.omto.2021.05.007
Horvath, C. M. (2004). Silencing STATs: Lessons from paramyxovirus interferon evasion. Cytokine Growth Factor Rev. 15, 117–127. doi:10.1016/j.cytogfr.2004.02.003
Houot, R., Schultz, L. M., Marabelle, A., and Kohrt, H. (2015). T-cell–based immunotherapy: Adoptive cell transfer and checkpoint inhibition. Cancer Immunol. Res. 3, 1115–1122. doi:10.1158/2326-6066.CIR-15-0190
Huang, Q., Cai, W.-Q., Han, Z.-W., Wang, M.-Y., Zhou, Y., Cheng, J.-T., et al. (2021). Bispecific T cell engagers and their synergistic tumor immunotherapy with oncolytic viruses. Am. J. Cancer Res. 11, 2430–2455.
Irwin, C. R., and Evans, D. H. (2012). Modulation of the myxoma virus plaque phenotype by vaccinia virus protein F11. J. Virol. 86, 7167–7179. doi:10.1128/JVI.06936-11
Islam, S. M. B. U., Lee, B., Jiang, F., Kim, E.-K., Ahn, S. C., and Hwang, T.-H. (2020). Engineering and characterization of oncolytic vaccinia virus expressing truncated herpes simplex virus thymidine kinase. Cancers 12, 228. doi:10.3390/cancers12010228
Jacquelot, N., Roberti, M. P., Enot, D. P., Rusakiewicz, S., Ternès, N., Jegou, S., et al. (2017). Predictors of responses to immune checkpoint blockade in advanced melanoma. Nat. Commun. 8, 592. doi:10.1038/s41467-017-00608-2
Javaheri, A., Bykov, Y., Mena, I., García-Sastre, A., and Cuadrado-Castano, S. (2022). Avian paramyxovirus 4 antitumor activity leads to complete remissions and long-term protective memory in preclinical melanoma and colon carcinoma models. Cancer Res. Commun. 2, 602–615. doi:10.1158/2767-9764.CRC-22-0025
Jiang, H., Clise-Dwyer, K., Ruisaard, K. E., Fan, X., Tian, W., Gumin, J., et al. (2014). Delta-24-RGD oncolytic adenovirus elicits anti-glioma immunity in an immunocompetent mouse model. PLoS ONE 9, e97407. doi:10.1371/journal.pone.0097407
Jiang, H., Gomez-Manzano, C., Rivera-Molina, Y., Lang, F. F., Conrad, C. A., and Fueyo, J. (2015). Oncolytic adenovirus research evolution: From cell-cycle checkpoints to immune checkpoints. Curr. Opin. Virol. 13, 33–39. doi:10.1016/j.coviro.2015.03.009
Jiang, X., Wang, J., Deng, X., Xiong, F., Ge, J., Xiang, B., et al. (2019). Role of the tumor microenvironment in PD-L1/PD-1-mediated tumor immune escape. Mol. Cancer 18, 10. doi:10.1186/s12943-018-0928-4
Kanerva, A., Zinn, K. R., Chaudhuri, T. R., Lam, J. T., Suzuki, K., Uil, T. G., et al. (2003). Enhanced therapeutic efficacy for ovarian cancer with a serotype 3 receptor-targeted oncolytic adenovirus. Mol. Ther. 8, 449–458. doi:10.1016/S1525-0016(03)00200-4
Kaufman, H., Goldufsky, J., SivendranHarcharik, S., Pan, M., Bernardo, S., Stern, R. H., et al. (2013). Oncolytic virus therapy for cancer. Oncolytic Virother. 31, 31–46. doi:10.2147/OV.S38901
Kazimirsky, G., Jiang, W., Slavin, S., Ziv-Av, A., and Brodie, C. (2016). Mesenchymal stem cells enhance the oncolytic effect of Newcastle disease virus in glioma cells and glioma stem cells via the secretion of TRAIL. Stem Cell Res. Ther. 7, 149. doi:10.1186/s13287-016-0414-0
Keshavarz, M., Solaymani-Mohammadi, F., Miri, S. M., and Ghaemi, A. (2019). Oncolytic paramyxoviruses-induced autophagy; a prudent weapon for cancer therapy. J. Biomed. Sci. 26, 48. doi:10.1186/s12929-019-0542-9
Kesari, S., Lasner, T. M., Balsara, K. R., Randazzo, B. P., Lee, V. M., and Trojanowski, J. Q. (1998). A neuroattenuated ICP34.5-deficient herpes simplex virus type 1 replicates in ependymal cells of the murine central nervous system. J. Gen. Virol. 79 (Pt 3), 525–536. doi:10.1099/0022-1317-79-3-525
Kim, J. H., Oh, J. Y., Park, B. H., Lee, D. E., Kim, J. S., Park, H. E., et al. (2006). Systemic armed oncolytic and immunologic therapy for cancer with JX-594, a targeted poxvirus expressing GM-CSF. Mol. Ther. 14, 361–370. doi:10.1016/j.ymthe.2006.05.008
Kirn, D. (2001). Clinical research results with dl1520 (Onyx-015), a replication-selective adenovirus for the treatment of cancer: What have we learned? Gene Ther. 8, 89–98. doi:10.1038/sj.gt.3301377
Kirn, D. H., Wang, Y., Le Boeuf, F., Bell, J., and Thorne, S. H. (2007). Targeting of interferon-beta to produce a specific, multi-mechanistic oncolytic vaccinia virus. PLoS Med. 4, e353. doi:10.1371/journal.pmed.0040353
Kleijn, A., Kloezeman, J., Treffers-Westerlaken, E., Fulci, G., Leenstra, S., Dirven, C., et al. (2014). The in vivo therapeutic efficacy of the oncolytic adenovirus delta24-RGD is mediated by tumor-specific immunity. PLoS ONE 9, e97495. doi:10.1371/journal.pone.0097495
Koch, M., Lawler, S., and Chiocca, E. (2020). HSV-1 oncolytic viruses from bench to bedside: An overview of current clinical trials. Cancers 12, 3514. doi:10.3390/cancers12123514
Koodie, L., Robertson, M. G., Chandrashekar, M., Ruth, G., Dunning, M., Bianco, R. W., et al. (2019). Rodents versus pig model for assessing the performance of serotype chimeric ad5/3 oncolytic adenoviruses. Cancers 11, 198. doi:10.3390/cancers11020198
Koski, A., Kangasniemi, L., Escutenaire, S., Pesonen, S., Cerullo, V., Diaconu, I., et al. (2010). Treatment of cancer patients with a serotype 5/3 chimeric oncolytic adenovirus expressing GMCSF. Mol. Ther. 18, 1874–1884. doi:10.1038/mt.2010.161
Kroemer, G., Galluzzi, L., Kepp, O., and Zitvogel, L. (2013). Immunogenic cell death in cancer therapy. Annu. Rev. Immunol. 31, 51–72. doi:10.1146/annurev-immunol-032712-100008
Lauer, U. M., and Beil, J. (2022). Oncolytic viruses: Challenges and considerations in an evolving clinical landscape. Future Oncol. 18, 2713–2732. doi:10.2217/fon-2022-0440
Laurie, S. A., Bell, J. C., Atkins, H. L., Roach, J., Bamat, M. K., O’Neil, J. D., et al. (2006). A phase 1 clinical study of intravenous administration of PV701, an oncolytic virus, using two-step desensitization. Clin. Cancer Res. 12, 2555–2562. doi:10.1158/1078-0432.CCR-05-2038
Lawler, S. E., Speranza, M.-C., Cho, C.-F., and Chiocca, E. A. (2017). Oncolytic viruses in cancer treatment: A review. JAMA Oncol. 3, 841–849. doi:10.1001/jamaoncol.2016.2064
Lee, J.-H., Roh, M.-S., Lee, Y.-K., Kim, M.-K., Han, J.-Y., Park, B.-H., et al. (2010). Oncolytic and immunostimulatory efficacy of a targeted oncolytic poxvirus expressing human GM-CSF following intravenous administration in a rabbit tumor model. Cancer Gene Ther. 17, 73–79. doi:10.1038/cgt.2009.50
Li, J., O’Malley, M., Urban, J., Sampath, P., Guo, Z. S., Kalinski, P., et al. (2011). Chemokine expression from oncolytic vaccinia virus enhances vaccine therapies of cancer. Mol. Ther. 19, 650–657. doi:10.1038/mt.2010.312
Li, K., Zhao, Y., Hu, X., Jiao, J., Wang, W., and Yao, H. (2022). Advances in the clinical development of oncolytic viruses. Am. J. Transl. Res. 14, 4192–4206.
Li, L., Goedegebuure, S. P., and Gillanders, W. E. (2017). Preclinical and clinical development of neoantigen vaccines. Ann. Oncol. 28, xii11–xii17. doi:10.1093/annonc/mdx681
Lichtman, E. I., and Dotti, G. (2017). Chimeric antigen receptor T-cells for B-cell malignancies. Transl. Res. 187, 59–82. doi:10.1016/j.trsl.2017.06.011
Lichty, B. D., Breitbach, C. J., Stojdl, D. F., and Bell, J. C. (2014). Going viral with cancer immunotherapy. Nat. Rev. Cancer 14, 559–567. doi:10.1038/nrc3770
Liu, B. L., Robinson, M., Han, Z.-Q., Branston, R. H., English, C., Reay, P., et al. (2003). ICP34.5 deleted herpes simplex virus with enhanced oncolytic, immune stimulating, and anti-tumour properties. Gene Ther. 10, 292–303. doi:10.1038/sj.gt.3301885
Liu, T.-C., Hallden, G., Wang, Y., Brooks, G., Francis, J., Lemoine, N., et al. (2004). An E1B-19 kDa gene deletion mutant adenovirus demonstrates tumor necrosis factor-enhanced cancer selectivity and enhanced oncolytic potency. Mol. Ther. 9, 786–803. doi:10.1016/j.ymthe.2004.03.017
Liu, X., and He, B. (2019). Selective editing of herpes simplex virus 1 enables interferon induction and viral replication that destroy malignant cells. J. Virol. 93, e01761-18. doi:10.1128/JVI.01761-18
Liu, Z., Ravindranathan, R., Kalinski, P., Guo, Z. S., and Bartlett, D. L. (2017). Rational combination of oncolytic vaccinia virus and PD-L1 blockade works synergistically to enhance therapeutic efficacy. Nat. Commun. 8, 14754. doi:10.1038/ncomms14754
Liu, Z., Ravindranathan, R., Li, J., Kalinski, P., Guo, Z. S., and Bartlett, D. L. (2016). CXCL11-Armed oncolytic poxvirus elicits potent antitumor immunity and shows enhanced therapeutic efficacy. OncoImmunology 5, e1091554. doi:10.1080/2162402X.2015.1091554
Lu, W., Zheng, S., Li, X. F., Huang, J. J., Zheng, X., and Li, Z. (2004). Intra-tumor injection of H101, a recombinant adenovirus, in combination with chemotherapy in patients with advanced cancers: A pilot phase II clinical trial. World J. Gastroenterol. 10, 3634–3638. doi:10.3748/wjg.v10.i24.3634
Luo, Y., Lin, C., Ren, W., Ju, F., Xu, Z., Liu, H., et al. (2019). Intravenous injections of a rationally selected oncolytic herpes virus as a potent virotherapy for hepatocellular carcinoma. Mol. Ther. Oncolytics 15, 153–165. doi:10.1016/j.omto.2019.09.004
Martínez-Vélez, N., Xipell, E., Vera, B., Acanda de la Rocha, A., Zalacain, M., Marrodán, L., et al. (2016). The oncolytic adenovirus VCN-01 as therapeutic approach against pediatric osteosarcoma. Clin. Cancer Res. 22, 2217–2225. doi:10.1158/1078-0432.CCR-15-1899
Martuza, R. L., Malick, A., Markert, J. M., Ruffner, K. L., and Coen, D. M. (1991). Experimental therapy of human glioma by means of a genetically engineered virus mutant. Science 252, 854–856. doi:10.1126/science.1851332
Mastrangelo, M. J., Maguire, H. C., Eisenlohr, L. C., Laughlin, C. E., Monken, C. E., McCue, P. A., et al. (1999). Intratumoral recombinant GM-CSF-encoding virus as gene therapy in patients with cutaneous melanoma. Cancer Gene Ther. 6, 409–422. doi:10.1038/sj.cgt.7700066
Matveeva, O. V., Guo, Z. S., Shabalina, S. A., and Chumakov, P. M. (2015). Oncolysis by paramyxoviruses: Multiple mechanisms contribute to therapeutic efficiency. Mol. Ther. Oncolytics 2, 15011. doi:10.1038/mto.2015.11
Matveeva, O. V., Kochneva, G. V., Zainutdinov, S. S., Ilyinskaya, G. V., and Chumakov, P. M. (2018). Oncolytic paramyxoviruses: Mechanism of action, preclinical and clinical studies. Mol. Biol. 52, 360–379. doi:10.7868/S0026898418030023
McCart, J. A., Ward, J. M., Lee, J., Hu, Y., Alexander, H. R., Libutti, S. K., et al. (2001). Systemic cancer therapy with a tumor-selective vaccinia virus mutant lacking thymidine kinase and vaccinia growth factor genes. Cancer Res. 61, 8751–8757.
Mejías-Pérez, E., Carreño-Fuentes, L., and Esteban, M. (2018). Development of a safe and effective vaccinia virus oncolytic vector WR-d4 with a set of gene deletions on several viral pathways. Mol. Ther. Oncolytics 8, 27–40. doi:10.1016/j.omto.2017.12.002
Menotti, L., Cerretani, A., and Campadelli-Fiume, G. (2006). A herpes simplex virus recombinant that exhibits a single-chain antibody to HER2/neu enters cells through the mammary tumor receptor, independently of the gD receptors. J. Virol. 80, 5531–5539. doi:10.1128/JVI.02725-05
Mineta, T., Rabkin, S. D., Yazaki, T., Hunter, W. D., and Martuza, R. L. (1995). Attenuated multi–mutated herpes simplex virus–1 for the treatment of malignant gliomas. Nat. Med. 1, 938–943. doi:10.1038/nm0995-938
Mondal, J., Panigrahi, A. K., and Khuda-Bukhsh, A. R. (2014). Conventional chemotherapy: Problems and scope for combined therapies with certain herbal products and dietary supplements. Austin J. Mol. Cell Biol. 1, 10.
Moon, E. K., Wang, L.-C. S., Bekdache, K., Lynn, R. C., Lo, A., Thorne, S. H., et al. (2018). Intra-tumoral delivery of CXCL11 via a vaccinia virus, but not by modified T cells, enhances the efficacy of adoptive T cell therapy and vaccines. OncoImmunology 7, e1395997. doi:10.1080/2162402X.2017.1395997
Nakamori, M., Fu, X., Rousseau, R., Chen, S.-Y., and Zhang, X. (2004). Destruction of nonimmunogenic mammary tumor cells by a fusogenic oncolytic herpes simplex virus induces potent antitumor immunity. Mol. Ther. 9, 658–665. doi:10.1016/j.ymthe.2004.02.019
Nakatake, R., Kaibori, M., Nakamura, Y., Tanaka, Y., Matushima, H., Okumura, T., et al. (2018). Third-generation oncolytic herpes simplex virus inhibits the growth of liver tumors in mice. Cancer Sci. 109, 600–610. doi:10.1111/cas.13492
Okuno, Y., Asada, T., Yamanishi, K., Otsuka, T., Takahashi, M., Tanioka, T., et al. (1978). Studies on the use of mumps virus for treatment of human cancer. doi:10.18910/82571
Olaleye, O., and Ekrikpo, U. (2017). “Epidemiology of cancers in sub-saharan Africa,” in Cancer in sub-saharan Africa. Editor O. A. Adedeji (Cham: Springer International Publishing). 3–19. doi:10.1007/978-3-319-52554-9_1
Pardoll, D. M. (2012). The blockade of immune checkpoints in cancer immunotherapy. Nat. Rev. Cancer 12, 252–264. doi:10.1038/nrc3239
Park, B.-H., Hwang, T., Liu, T.-C., Sze, D. Y., Kim, J.-S., Kwon, H.-C., et al. (2008). Use of a targeted oncolytic poxvirus, JX-594, in patients with refractory primary or metastatic liver cancer: a phase I trial. Lancet. Oncol. 9, 533–542. doi:10.1016/S1470-2045(08)70107-4
Parker, J. N., Gillespie, G. Y., Love, C. E., Randall, S., Whitley, R. J., and Markert, J. M. (2000). Engineered herpes simplex virus expressing IL-12 in the treatment of experimental murine brain tumors. Proc. Natl. Acad. Sci. U. S. A. 97, 2208–2213. doi:10.1073/pnas.040557897
Passaro, C., Alayo, Q., DeLaura, I., McNulty, J., Grauwet, K., Ito, H., et al. (2019). Arming an oncolytic herpes simplex virus type 1 with a single-chain fragment variable antibody against PD-1 for experimental glioblastoma therapy. Clin. Cancer Res. 25, 290–299. doi:10.1158/1078-0432.CCR-18-2311
Patnaik, A., Kang, S. P., Rasco, D., Papadopoulos, K. P., Elassaiss-Schaap, J., Beeram, M., et al. (2015). Phase I study of pembrolizumab (MK-3475; anti–PD-1 monoclonal antibody) in patients with advanced solid tumors. Clin. Cancer Res. 21, 4286–4293. doi:10.1158/1078-0432.CCR-14-2607
Pearl, T. M., Markert, J. M., Cassady, K. A., and Ghonime, M. G. (2019). Oncolytic virus-based cytokine expression to improve immune activity in brain and solid tumors. Mol. Ther. Oncolytics 13, 14–21. doi:10.1016/j.omto.2019.03.001
Pecora, A. L., Rizvi, N., Cohen, G. I., Meropol, N. J., Sterman, D., Marshall, J. L., et al. (2002). Phase I trial of intravenous administration of PV701, an oncolytic virus, in patients with advanced solid cancers. J. Clin. Oncol. 20, 2251–2266. doi:10.1200/JCO.2002.08.042
Peters, C., and Rabkin, S. D. (2015). Designing herpes viruses as oncolytics. Mol. Ther. Oncolytics 2, 15010. doi:10.1038/mto.2015.10
Plummer, M., de Martel, C., Vignat, J., Ferlay, J., Bray, F., and Franceschi, S. (2016). Global burden of cancers attributable to infections in 2012: A synthetic analysis. Lancet. Glob. Health 4, e609–e616. doi:10.1016/S2214-109X(16)30143-7
Pol, J., Kroemer, G., and Galluzzi, L. (2016). First oncolytic virus approved for melanoma immunotherapy. OncoImmunology 5, e1115641. doi:10.1080/2162402X.2015.1115641
Prestwich, R. J., Errington, F., Harrington, K. J., Pandha, H. S., Selby, P., and Melcher, A. (2008). Oncolytic viruses: Do they have a role in anti-cancer therapy? Clin. Med. Oncol. 2, 83–96. doi:10.4137/CMO.S416
Ramesh, N., Ge, Y., Ennist, D. L., Zhu, M., Mina, M., Ganesh, S., et al. (2006). CG0070, a conditionally replicating granulocyte macrophage colony-stimulating factor–armed oncolytic adenovirus for the treatment of bladder cancer. Clin. Cancer Res. 12, 305–313. doi:10.1158/1078-0432.CCR-05-1059
Reale, A., Vitiello, A., Conciatori, V., Parolin, C., Calistri, A., and Palù, G. (2019). Perspectives on immunotherapy via oncolytic viruses. Infect. Agent. Cancer 14, 5. doi:10.1186/s13027-018-0218-1
Reeh, M., Bockhorn, M., Görgens, D., Vieth, M., Hoffmann, T., Simon, R., et al. (2013). Presence of the coxsackievirus and adenovirus receptor (CAR) in human neoplasms: A multitumour array analysis. Br. J. Cancer 109, 1848–1858. doi:10.1038/bjc.2013.509
Ribas, A., Dummer, R., Puzanov, I., VanderWalde, A., Andtbacka, R. H. I., Michielin, O., et al. (2017). Oncolytic virotherapy promotes intratumoral T cell infiltration and improves anti-PD-1 immunotherapy. Cell 170, 1109–1119. doi:10.1016/j.cell.2017.08.027
Rivadeneira, D. B., DePeaux, K., Wang, Y., Kulkarni, A., Tabib, T., Menk, A. V., et al. (2019). Oncolytic viruses engineered to enforce leptin expression reprogram tumor-infiltrating T cell metabolism and promote tumor clearance. Immunity 51, 548–560. doi:10.1016/j.immuni.2019.07.003
Russell, L., and Peng, K.-W. (2018). The emerging role of oncolytic virus therapy against cancer. Chin. Clin. Oncol. 7, 16. doi:10.21037/cco.2018.04.04
Russell, S. J., Peng, K.-W., and Bell, J. C. (2012). Oncolytic virotherapy. Nat. Biotechnol. 30, 658–670. doi:10.1038/nbt.2287
Samson, A., Scott, K. J., Taggart, D., West, E. J., Wilson, E., Nuovo, G. J., et al. (2018). Intravenous delivery of oncolytic reovirus to brain tumor patients immunologically primes for subsequent checkpoint blockade. Sci. Transl. Med. 10, eaam7577. doi:10.1126/scitranslmed.aam7577
Saravanan, M., Kolade Omole, R., and Torimiro, N. (2022). Oncogenic virus in oral squamous cell carcinoma and the role of oncolytic HSV-1 viro-immunotherapy as an emerging treatment. Oral Oncol. 133, 106021. doi:10.1016/j.oraloncology.2022.106021
Sauter, C. S., Senechal, B., Rivière, I., Ni, A., Bernal, Y., Wang, X., et al. (2019). CD19 CAR T cells following autologous transplantation in poor-risk relapsed and refractory B-cell non-Hodgkin lymphoma. Blood 134, 626–635. doi:10.1182/blood.2018883421
Schramm, B., and Locker, J. K. (2005). Cytoplasmic organization of POXvirus DNA replication: Vaccinia virus host interactions. Traffic 6, 839–846. doi:10.1111/j.1600-0854.2005.00324.x
Shapira, A., Livney, Y. D., Broxterman, H. J., and Assaraf, Y. G. (2011). Nanomedicine for targeted cancer therapy: Towards the overcoming of drug resistance. Drug resist. updat. 14, 150–163. doi:10.1016/j.drup.2011.01.003
Sharma, R., Aashima,, , Nanda, M., Fronterre, C., Sewagudde, P., Ssentongo, A. E., et al. (2022). Mapping cancer in Africa: A comprehensive and comparable characterization of 34 cancer types using estimates from globocan 2020. Front. Public Health 10, 839835. doi:10.3389/fpubh.2022.839835
Shi, T., Song, X., Wang, Y., Liu, F., and Wei, J. (2020). Combining oncolytic viruses with cancer immunotherapy: Establishing a new generation of cancer treatment. Front. Immunol. 11, 683. doi:10.3389/fimmu.2020.00683
Shibata, T., Uchida, H., Shiroyama, T., Okubo, Y., Suzuki, T., Ikeda, H., et al. (2016). Development of an oncolytic HSV vector fully retargeted specifically to cellular EpCAM for virus entry and cell-to-cell spread. Gene Ther. 23, 479–488. doi:10.1038/gt.2016.17
Shilpa, P., Kaul, R., Sultana, N., Pandeshwar, P., and Bhat, S. (2014). Oncolytic viruses in head and neck cancer: A new ray of hope in the management protocol. Ann. Med. Health Sci. Res. 4, 178–S184. doi:10.4103/2141-9248.141953
Shimizu, Y., Hasumi, K., Okudaira, Y., Yamanishi, K., and Takahashi, M. (1988). Immunotherapy of advanced gynecologic cancer patients utilizing mumps virus. Cancer detect. Prev. 12, 487–495.
Siegel, R. L., Miller, K. D., Fuchs, H. E., and Jemal, A. (2022). Cancer statistics, 2022. Ca. Cancer J. Clin. 72, 7–33. doi:10.3322/caac.21708
Siska, P. J., and Rathmell, J. C. (2015). T cell metabolic fitness in antitumor immunity. Trends Immunol. 36, 257–264. doi:10.1016/j.it.2015.02.007
Speck, T., Heidbuechel, J. P. W., Veinalde, R., Jaeger, D., von Kalle, C., Ball, C. R., et al. (2018). Targeted BiTE expression by an oncolytic vector augments therapeutic efficacy against solid tumors. Clin. Cancer Res. 24, 2128–2137. doi:10.1158/1078-0432.CCR-17-2651
Stepanenko, A. A., and Chekhonin, V. P. (2018). Tropism and transduction of oncolytic adenovirus 5 vectors in cancer therapy: Focus on fiber chimerism and mosaicism, hexon and pIX. Virus Res. 257, 40–51. doi:10.1016/j.virusres.2018.08.012
Streby, K. A., Currier, M. A., Triplet, M., Ott, K., Dishman, D. J., Vaughan, M. R., et al. (2019). First-in-Human intravenous Seprehvir in young cancer patients: A phase 1 clinical trial. Mol. Ther. 27, 1930–1938. doi:10.1016/j.ymthe.2019.08.020
Suter, S. E., Chein, M. B., von Messling, V., Yip, B., Cattaneo, R., Vernau, W., et al. (2005). In vitro canine distemper virus infection of canine lymphoid cells: A prelude to oncolytic therapy for lymphoma. Clin. Cancer Res. 11, 1579–1587. doi:10.1158/1078-0432.CCR-04-1944
Tadesse, T., and Bekuma, A. (2018). A Promising Modality of Oncolytic Virotherapy for Cancer Treatment, National Agricultural Library. 5.
Tan, D. Q., Zhang, L., Ohba, K., Ye, M., Ichiyama, K., and Yamamoto, N. (2016). Macrophage response to oncolytic paramyxoviruses potentiates virus-mediated tumor cell killing. Eur. J. Immunol. 46, 919–928. doi:10.1002/eji.201545915
Tang, B., Guo, Z. S., Bartlett, D. L., Yan, D. Z., Schane, C. P., Thomas, D. L., et al. (2020). Synergistic combination of oncolytic virotherapy and immunotherapy for glioma. Clin. Cancer Res. 26, 2216–2230. doi:10.1158/1078-0432.CCR-18-3626
Terrível, M., Gromicho, C., and Matos, A. M. (2020). Oncolytic viruses: What to expect from their use in cancer treatment. Microbiol. Immunol. 64, 477–492. doi:10.1111/1348-0421.12753
Tessier, T. M., Dodge, M. J., MacNeil, K. M., Evans, A. M., Prusinkiewicz, M. A., and Mymryk, J. S. (2021). Almost famous: Human adenoviruses (and what they have taught us about cancer). Tumour Virus Res. 12, 200225. doi:10.1016/j.tvr.2021.200225
Thirunavukarasu, P., Sathaiah, M., Gorry, M. C., O’Malley, M. E., Ravindranathan, R., Austin, F., et al. (2013). A rationally designed A34R mutant oncolytic poxvirus: Improved efficacy in peritoneal carcinomatosis. Mol. Ther. 21, 1024–1033. doi:10.1038/mt.2013.27
Thomas, R. J., and Bartee, E. (2022). The use of oncolytic virotherapy in the neoadjuvant setting. J. Immunother. Cancer 10, e004462. doi:10.1136/jitc-2021-004462
Thorne, S. H., Hwang, T.-H. H., O’Gorman, W. E., Bartlett, D. L., Sei, S., Kanji, F., et al. (2007). Rational strain selection and engineering creates a broad-spectrum, systemically effective oncolytic poxvirus, JX-963. J. Clin. Invest. 117, 3350–3358. doi:10.1172/JCI32727
Todo, T., Martuza, R. L., Rabkin, S. D., and Johnson, P. A. (2001). Oncolytic herpes simplex virus vector with enhanced MHC class I presentation and tumor cell killing. Proc. Natl. Acad. Sci. U. S. A. 98, 6396–6401. doi:10.1073/pnas.101136398
Topalian, S. L., Sznol, M., McDermott, D. F., Kluger, H. M., Carvajal, R. D., Sharfman, W. H., et al. (2014). Survival, durable tumor remission, and long-term safety in patients with advanced melanoma receiving nivolumab. J. Clin. Oncol. 32, 1020–1030. doi:10.1200/JCO.2013.53.0105
Torre, L. A., Bray, F., Siegel, R. L., Ferlay, J., Lortet-Tieulent, J., and Jemal, A. (2015). Global cancer statistics, 2012: Global cancer statistics, 2012. Ca. Cancer J. Clin. 65, 87–108. doi:10.3322/caac.21262
Torres-Domínguez, L. E., and McFadden, G. (2019). Poxvirus oncolytic virotherapy. Expert Opin. Biol. Ther. 19, 561–573. doi:10.1080/14712598.2019.1600669
Uche, I. K., Fowlkes, N., Vu, L., Watanabe, T., Carossino, M., Nabi, R., et al. (2021). Novel oncolytic herpes simplex virus 1 VC2 promotes long-lasting, systemic anti-melanoma tumor immune responses and increased survival in an immunocompetent B16F10-derived mouse melanoma model. J. Virol. 95, e01359-20. doi:10.1128/JVI.01359-20
Uchida, H., Marzulli, M., Nakano, K., Goins, W. F., Chan, J., Hong, C.-S., et al. (2013). Effective treatment of an orthotopic xenograft model of human glioblastoma using an EGFR-retargeted oncolytic herpes simplex virus. Mol. Ther. 21, 561–569. doi:10.1038/mt.2012.211
U.S. Food and Drug Administration (2022). Search orphan drug designations and approvals for reolysin. Available at: https://www.accessdata.fda.gov/scripts/opdlisting/oopd/listResult.cfm. [Accessed November 20, 2022].
Vera, B., Martínez-Vélez, N., Xipell, E., Acanda de la Rocha, A., Patiño-García, A., Saez-Castresana, J., et al. (2016). Characterization of the antiglioma effect of the oncolytic adenovirus VCN-01. PLoS ONE 11, e0147211. doi:10.1371/journal.pone.0147211
Viardot, A., Goebeler, M.-E., Hess, G., Neumann, S., Pfreundschuh, M., Adrian, N., et al. (2016). Phase 2 study of the bispecific T-cell engager (BiTE) antibody blinatumomab in relapsed/refractory diffuse large B-cell lymphoma. Blood 127, 1410–1416. doi:10.1182/blood-2015-06-651380
Vijayakumar, G., McCroskery, S., and Palese, P. (2020). Engineering newcastle disease virus as an oncolytic vector for intratumoral delivery of immune checkpoint inhibitors and immunocytokines. J. Virol. 94, e01677-19. doi:10.1128/JVI.01677-19
Wagner, S., Csatary, C. M., Gosztonyi, G., Koch, H.-C., Hartmann, C., Peters, O., et al. (2006). Combined treatment of pediatric high-grade glioma with the oncolytic viral strain MTH-68/H and oral valproic acid.: Case report. Apmis 114, 731–743. doi:10.1111/j.1600-0463.2006.apm_516.x
Wakimoto, H., Kesari, S., Farrell, C. J., Curry, W. T., Zaupa, C., Aghi, M., et al. (2009). Human glioblastoma–derived cancer stem cells: Establishment of invasive glioma models and treatment with oncolytic herpes simplex virus vectors. Cancer Res. 69, 3472–3481. doi:10.1158/0008-5472.CAN-08-3886
Wang, R., Chen, J., Wang, W., Zhao, Z., Wang, H., Liu, S., et al. (2022). CD40L-armed oncolytic herpes simplex virus suppresses pancreatic ductal adenocarcinoma by facilitating the tumor microenvironment favorable to cytotoxic T cell response in the syngeneic mouse model. J. Immunother. Cancer 10, e003809. doi:10.1136/jitc-2021-003809
Wang, Y., Hallden, G., Hill, R., Anand, A., Liu, T.-C., Francis, J., et al. (2003). E3 gene manipulations affect oncolytic adenovirus activity in immunocompetent tumor models. Nat. Biotechnol. 21, 1328–1335. doi:10.1038/nbt887
Watanabe, K., Luo, Y., Da, T., Guedan, S., Ruella, M., Scholler, J., et al. (2018). Pancreatic cancer therapy with combined mesothelin-redirected chimeric antigen receptor T cells and cytokine-armed oncolytic adenoviruses. JCI Insight 3, e99573. doi:10.1172/jci.insight.99573
Wei, D., Li, Q., Wang, X.-L., Wang, Y., Xu, J., Feng, F., et al. (2015). Oncolytic Newcastle disease virus expressing chimeric antibody enhanced anti-tumor efficacy in orthotopic hepatoma-bearing mice. J. Exp. Clin. Cancer Res. 34, 153. doi:10.1186/s13046-015-0271-1
Wing, A., Fajardo, C. A., Posey, A. D., Shaw, C., Da, T., Young, R. M., et al. (2018). Improving CART-cell therapy of solid tumors with oncolytic virus–driven production of a bispecific T-cell engager. Cancer Immunol. Res. 6, 605–616. doi:10.1158/2326-6066.CIR-17-0314
Wittek, R. (1982). Organization and expression of the poxvirus genome. Experientia 38, 285–297. doi:10.1007/BF01949349
Wolchok, J. D., Chiarion-Sileni, V., Gonzalez, R., Rutkowski, P., Grob, J.-J., Cowey, C. L., et al. (2017). Overall survival with combined nivolumab and ipilimumab in advanced melanoma. N. Engl. J. Med. 377, 1345–1356. doi:10.1056/NEJMoa1709684
Wong, M. C. S., Fung, F. D. H., Leung, C., Cheung, W. W. L., Goggins, W. B., and Ng, C. F. (2018). The global epidemiology of bladder cancer: A joinpoint regression analysis of its incidence and mortality trends and projection. Sci. Rep. 8, 1129. doi:10.1038/s41598-018-19199-z
World Health Organization (2022). Available at: https://www.who.int/news-room/fact-sheets/detail/cancer.[Accessed November 20, 2022].
Xia, T., Konno, H., and Barber, G. N. (2016). Recurrent loss of STING signaling in melanoma correlates with susceptibility to viral oncolysis. Cancer Res. 76, 6747–6759. doi:10.1158/0008-5472.CAN-16-1404
Zafar, S., Quixabeira, D. C. A., Kudling, T. V., Cervera-Carrascon, V., Santos, J. M., Grönberg-Vähä-Koskela, S., et al. (2021). Ad5/3 is able to avoid neutralization by binding to erythrocytes and lymphocytes. Cancer Gene Ther. 28, 442–454. doi:10.1038/s41417-020-00226-z
Zeng, J., Li, X., Sander, M., Zhang, H., Yan, G., and Lin, Y. (2021). Oncolytic viro-immunotherapy: An emerging option in the treatment of gliomas. Front. Immunol. 12, 721830. doi:10.3389/fimmu.2021.721830
Zeyaullah, Md., Patro, M., Ahmad, I., Ibraheem, K., Sultan, P., Nehal, M., et al. (2012). Oncolytic viruses in the treatment of cancer: A review of current strategies. Pathol. Oncol. Res. 18, 771–781. doi:10.1007/s12253-012-9548-2
Zhang, Q., Liang, C., Yu, Y. A., Chen, N., Dandekar, T., and Szalay, A. A. (2009). The highly attenuated oncolytic recombinant vaccinia virus GLV-1h68: Comparative genomic features and the contribution of F14.5L inactivation. Mol. Genet. Genomics 282, 417–435. doi:10.1007/s00438-009-0475-1
Zhang, R., Cui, Y., Guan, X., and Jiang, X. (2021). A recombinant human adenovirus type 5 (H101) combined with chemotherapy for advanced gastric carcinoma: A retrospective cohort study. Front. Oncol. 11, 752504. doi:10.3389/fonc.2021.752504
Zhang, Z. L., Zou, W. G., Luo, C. X., Li, B. H., Wang, J. H., Sun, L. Y., et al. (2003). An armed oncolytic adenovirus system, ZD55-gene, demonstrating potent antitumoral efficacy. Cell Res. 13, 481–489. doi:10.1038/sj.cr.7290191
Zhang, Z., Zou, W., Wang, J., Gu, J., Dang, Y., Li, B., et al. (2005). Suppression of tumor growth by oncolytic adenovirus-mediated delivery of an antiangiogenic gene, Soluble Flt-1. Mol. Ther. 11, 553–562. doi:10.1016/j.ymthe.2004.12.015
Zhao, L., Gu, J., Dong, A., Zhang, Y., Zhong, L., He, L., et al. (2005). Potent antitumor activity of oncolytic adenovirus expressing mda-7/IL-24 for colorectal cancer. Hum. Gene Ther. 16, 845–858. doi:10.1089/hum.2005.16.845
Zhao, Q., Zhang, W., Ning, Z., Zhuang, X., Lu, H., Liang, J., et al. (2014). A novel oncolytic herpes simplex virus type 2 has potent anti-tumor activity. PLoS ONE 9, e93103. doi:10.1371/journal.pone.0093103
Zheng, M., Huang, J., Tong, A., and Yang, H. (2019). Oncolytic viruses for cancer therapy: Barriers and recent advances. Mol. Ther. Oncolytics 15, 234–247. doi:10.1016/j.omto.2019.10.007
Keywords: cancer, viro-immunotherapy, oncolytic virus, tumor microenvironment, treatment, anti-cancer, immunology, targeted killing
Citation: Omole RK, Oluwatola O, Akere MT, Eniafe J, Agboluaje EO, Daramola OB, Ayantunji YJ, Omotade TI, Torimiro N, Ayilara MS, Adeyemi OI and Salinsile OS (2022) Comprehensive assessment on the applications of oncolytic viruses for cancer immunotherapy. Front. Pharmacol. 13:1082797. doi: 10.3389/fphar.2022.1082797
Received: 28 October 2022; Accepted: 24 November 2022;
Published: 08 December 2022.
Edited by:
Yugal Kishore Mohanta, University of Science and Technology, Meghalaya, IndiaReviewed by:
Ramzan Ahmed, University of Science and Technology, Meghalaya, IndiaBibhudutta Mishra, All India Institute of Medical Sciences, India
Debasis Nayak, North Orissa University, India
Copyright © 2022 Omole, Oluwatola, Akere, Eniafe, Agboluaje, Daramola, Ayantunji, Omotade, Torimiro, Ayilara, Adeyemi and Salinsile. This is an open-access article distributed under the terms of the Creative Commons Attribution License (CC BY). The use, distribution or reproduction in other forums is permitted, provided the original author(s) and the copyright owner(s) are credited and that the original publication in this journal is cited, in accordance with accepted academic practice. No use, distribution or reproduction is permitted which does not comply with these terms.
*Correspondence: Richard Kolade Omole, cmljaGFyZG9tb2xlQGdtYWlsLmNvbQ==