- Department of Anesthesiology, Shanghai General Hospital, Shanghai Jiao Tong University School of Medicine, Shanghai, China
Insulin resistance (IR) is a feature of type 2 diabetes (T2DM) accompanied by reduced glucose uptake and glucose transporter 4 (GLUT4) translocation by skeletal muscle. Neuregulin-1β (NRG-1β) is essential for myogenesis and the regulation of skeletal muscle metabolism. Neuregulin-1β increases insulin sensitivity, promotes glucose uptake and glucose translocation in normal skeletal muscle. Here, we explored whether Neuregulin-1β increased glucose uptake and GLUT4 translocation in palmitate (PA)-treated C2C12 myotubes. After C2C12 myoblasts differentiated into myotubes, we used palmitate to induce cellular insulin resistance. Cells were incubated with or without Neuregulin-1β and glucose uptake was determined using the 2-NBDG assay. The expression level of glucose transporter 4 (GLUT4) was measured via immunofluorescence and Western blotting. MK2206, an inhibitor of AKT, was employed to reveal the important role played by AKT signaling in PA-treated C2C12 myotubes. We then established an animal model with T2DM and evaluated the effects of Neuregulin-1β on body weight and the blood glucose level. The GLUT4 level in the gastrocnemius of T2DM mice was also measured. NRG-1β not only increased glucose uptake by PA-treated myotubes but also promoted GLUT4 translocation to the plasma membrane. The effect of NRG-1β on PA-treated C2C12 myotubes was associated with AKT activation. In T2DM mice, Neuregulin-1β not only improved diabetes-induced weight loss and diabetes-induced hyperglycemia, but also promoted GLUT4 translocation in the gastrocnemius. In summary, Neuregulin-1β increased glucose uptake and promoted translocation of GLUT4 to the plasma membrane in PA-treated C2C12 myotubes by activating the PI3K/AKT signaling pathway.
1 Introduction
The incidence of type 2 diabetes (T2DM) is increasing in both developing and developed countries. The International Diabetes Federation (IDF) Diabetes Atlas indicates that the global diabetes prevalence in those aged 20–79 years in 2021 was 10.5% (536.6 million people), and is expected to rise to 12.2% (783.2 million) in 2045 (Sun et al., 2022). T2DM is closely associated with insulin resistance (IR), a pathophysiological condition characterized by impaired insulin action on insulin-sensitive tissues, including skeletal muscle, liver and adipose tissue (Al-Sulaiti et al., 2019; Hagman et al., 2019). The principal features of IR in insulin-sensitive tissues (including skeletal muscle) are decreases in glucose uptake and glucose transporter 4 (GLUT4) translocation to the plasma membrane (Luo et al., 2019; Sylow et al., 2021). Skeletal muscle accounts for 40% of body weight, and is responsible for up to 70%–90% of insulin-mediated glucose disposal under normal physiological conditions (Liu et al., 2020; Merz and Thurmond, 2020; Feraco et al., 2021). Indeed, skeletal muscle IR is considered as the most important extra-pancreatic factor in T2DM pathogenesis (Song et al., 2013; Yaribeygi et al., 2019; Galicia-Garcia et al., 2020). Thus, improving the insulin sensitivity of skeletal muscle is important to control T2DM progression.
Neuregulin-1 (NRG-1) is a member of a large family of epidermal growth factor (EGF) proteins. Many different isoforms are known; all share an EGF-like domain that mediates biological activity (Falls, 2003). Different NRG-1 isoforms may have distinct tissue specificity and receptor affinity, and NRG-1β was used to study the effects of NRG-1 on skeletal muscle cell in previous studies (Suárez et al., 2001; Canto et al., 2004; Cantó et al., 2006). NRG-1β plays an important role in cell survival, proliferation, migration and differentiation (Falls, 2003; Guma et al., 2010). NRG-1β increased insulin sensitivity, promoted glucose uptake and glucose transporter translocation in normal skeletal muscle (independent of the insulin level) (Suárez et al., 2001; Canto et al., 2004; Cantó et al., 2007; Ennequin et al., 2017). However, there are few reports on whether insulin sensitivity can still be improved in the IR environment by NRG-1β. NRG-1β increases glucose uptake by activating the PI3K/AKT pathway in cardiomyocyte (Pentassuglia et al., 2016; Heim et al., 2020), which can promote GLUT4 translocation (Zhu et al., 2013; Sharma et al., 2015; Li et al., 2016). Hence, NRG-1β may activate the PI3K/AKT pathway in palmitate (PA)-treated C2C12 myotubes.
In our study, we explored whether NRG-1β treatment protected against IR in PA-treated C2C12 myotubes and the molecular mechanism in play. We hypothesized that NRG-1β would increase glucose uptake and promote GLUT4 translocation by activating the PI3K/AKT pathway in PA-treated C2C12 myotubes. To verify this, we investigated the effect of NRG-1β on glucose uptake and GLUT4 translocation in PA-treated C2C12 myotubes, and the relationship between NRG-1β action and PI3K/AKT signaling. We found that NRG-1β treatment increased glucose uptake and GLUT4 translocation via the PI3K/AKT signaling pathway in PA-treated C2C12 myotubes.
2 Materials and methods
2.1 Cell culture, differentiation, and treatment
Mouse skeletal muscle cell lines (C2C12 myoblasts) were obtained from Shanghai FuHeng Biology (Shanghai, China). The cells were cultured in Dulbecco’s modified Eagle’s medium (DMEM; Gibco Co., Ltd., Grand Island, NY, United States) containing 10% (v/v) fetal bovine serum (Gibco) and 1% (w/v) penicillin–streptomycin (P/S; NCM Biotech, Suzhou, China) in a humidified incubator under 5% (v/v) CO2 at 37°C. To initiate differentiation, cells were grown to 70%–80% confluence, and then incubated with DMEM with 2% (v/v) heat-inactivated horse serum (Gibco) and 1% (w/v) P/S for 4–6 days. The medium was changed daily. The fully differentiated myotubes were used in the following experiments.
2.2 Cell viability assessment
C2C12 myoblasts were seeded in 96-well plates and grown for differentiation. After the formation of myotubes, 0, .25, .5, .75 or 1 mM PA was added for 24 h, and then cell viability was measured using cell counting kit-8 (Beyotime) according to the manufacturer’s protocol. Next, the absorbance was measured at a wavelength of 450 nm using Microplate Reader (Thermo Fisher Scientific). Cell viability was expressed as a percentage of the optical density of each treatment group relative to the control group.
2.3 Palmitate-induced insulin resistance in C2C12 myotubes
Firstly, palmitate (33.40mg, Sigma, St. Louis, MO, United States) was dissolved in 3 mL double distilled water (v/v) with heating at 75°C. Next, preparation 40% free fatty acid-free bovine serum albumin solution (BSA), fatty acid free BSA powder (1.2 g, Beyotime, Shanghai, China) dissolved in phosphate-buffered saline (PBS), centrifugated at room temperature 8,000 rpm for 15 min until BSA completely dissolved, and then fixed to 3 mL with PBS. Thirdly, mixing the two liquids together to make a 20 mM solution and then sterilized by passing through a .22-μm-pore-sized filter, and this solution was stored at −20°C and used within 2 weeks. Fully differentiated myotubes were treated with .25 mM PA for 24 h (Petersen and Shulman, 2018; Shen et al., 2019). Control cells were treated with the same volume of PBS–BSA DMEM with or without insulin. PA-treated C2C12 myotubes were serum-depleted for 4.5 h and then incubated with or without 10 ng/mL NRG-1β (PeproTech, Rocky Hill, NJ, United States) for 1.5 h (Suárez et al., 2001; Canto et al., 2004; Heim et al., 2020). After 1 h treatment of NRG-1β, cells were then incubated with or without insulin (100 nM) for 30 min.
2.4 Glucose uptake assay
Glucose uptake by differentiated C2C12 myotubes was measured using the 2-NBDG assay that employs a fluorescent D-glucose analogue (Zou et al., 2005; Bala et al., 2021). After incubation with or without NRG-1β, cells were incubated with 80 µM 2-NBDG (APExBIO, Houston, TX, United States) at 37°C for 30 min 2-NBDG uptake was stopped by removing the incubation medium and washing the cells with 1×PBS followed to remove free 2-NBDG. And then discarding the PBS, the cells were subsequently resuspended in pre-cool RIPA (Beyotime) and transferred to 96-well culture plates (Xu et al., 2018). Next, 2-NBDG levels were determined using a fluorescence microplate (excitation 485 nm, emission 535 nm; Thermo Fisher Scientific, Massachusetts, MA, United States). And we were very careful to avoid light during the experiment.
2.5 Animal model
The animal protocol was approved (Protocol number: 2019-A005-1, Shanghai, China) by the Shanghai General Hospital Clinical Center Laboratory Animal Welfare and Ethics Committee. Thirty pathogen-free 3–4-week-old male C57BL/6J wild-type mice (SLAC, Shanghai, China) (18–20 g) were used. The animals were housed in a temperature-controlled (25°C ± 2°C) room under a 12 h light/dark cycle with a relative humidity of 50%–70%. T2DM was induced as described previously (Hamza et al., 2011; Kleinert et al., 2018; Yang et al., 2020). The mice were randomly divided into a control group (CON, n = 10), a T2DM + PBS group (DM, n = 10) and a T2DM + NRG-1β group (DM + NRG-1β, n = 10). All mice were acclimatized for 1 week prior to experimentation. The CON group was fed normal chow; the DM and the DM + NRG-1β groups were fed a high-fat diet [high fat (60FDC) Purified Rodent Diet; D12492; Research Diets, New Brunswick, NJ, United States) for five consecutive weeks. Then, after 12 h of fasting, the DM and DM + NRG-1β groups were intraperitoneally injected with 50 mg/kg streptozocin (STZ) (S0130; Sigma, St. Louis, MO, United States) daily for 3 days. The CON group was intraperitoneally injected with isometric citric acid buffer. The blood glucose levels were measured 7 days after the final injection. When that level was >16.7 mmol/L, T2DM was considered established (Kleinert et al., 2018; Yang et al., 2020). DM + NRG-1β group and DM group received 30 μg/kg NRG-1β (via intraperitoneal injection) or the same volume of PBS weekly for 4 weeks, respectively (Ennequin et al., 2015; Wu et al., 2019; Liu et al., 2020). After a week final treatment of NRG-1β, mice were anesthetized with 1% (w/v) pentobarbital sodium and euthanized by decapitation. The gastrocnemius tissues were dissected, weighed, and soaked overnight at 4°C in 4% (v/v) paraformaldehyde (PFA) and then in 30% (w/v) sucrose at 4°C for 48 h to immunofluorescence analysis or frozen in liquid nitrogen and stored at −80°C for later biochemical analysis.
2.6 Western blotting
After washing with ice-cold PBS, cells or gastrocnemius tissues were homogenized. Total protein was extracted into pre-cooled RIPA lysis buffer (Beyotime) with 1% (w/v) of a protease/phosphatase inhibitor cocktail (NCM Biotech) at 4°C for 15 min, and the protein concentration was measured using a BCA protein assay kit (Beyotime). Protein loading buffer (5X) (NCM Biotech) was added to the lysates followed by protein denaturation by placing the tubes in boiling water for 10 min.
Membrane proteins (including GLUT4) were obtained using Membrane and Cytosol Protein Extraction Kit (Beyotime), according to the manufacturer’s instructions. Western blotting was employed to determine GLUT4 expression levels on plasma membranes. The membrane marker Na+-K+-ATPase served as a control.
Proteins (20–30 μg/lane) were subjected to 10% (v/v) sodium dodecyl sulphate polyacrylamide gel electrophoresis (Epizyme, Suzhou, China) and transferred to polyvinylidene fluoride membranes (Immobilon P; Millipore, Billerica, MA, United States) and the blots saturated with blocking buffer (NCM Biotech) for 15 min at room temperature and then incubated overnight at 4°C with antibodies against β-actin (1:5,000; Proteintech, Wuhan, China), myosin heavy chain (MHC; 1:1,000; Proteintech), Phosphor-Akt (ser 473) (1:1,000; Cell Signaling Technology, Massachusetts, MA, United States), AKT (1:1,000; Proteintech), GLUT4 (1:1,000; Cell Signaling Technology) and Na+-K+-ATPase (1:1,000; Cell Signaling Technology). The gray intensity of protein was measured using ImageJ software (United States National Institutes of Health). Three independent experiments were performed and the data averaged.
2.7 Immunofluorescence
C2C12 myoblasts were cultured on 35-mm-diameter confocal dishes and allowed to differentiate for 4–5 days. After treatment with NRG-1β, the myotubes were fixed in 4% (v/v) PFA for 15 min, permeabilized by adding a buffer containing saponin (Beyotime) for 10 min at room temperature, and then incubated in PBS with 3% (w/v) BSA for 1 h at room temperature, followed by incubation with anti-GLUT4 (1:300; Affinity, Suzhou, China) overnight at 4°C. The cells were washed three times with PBS (10 min each time) and then incubated with Alexa Fluor 594-conjugated goat anti-mouse secondary antibody for 2 h at room temperature. Detection was performed using the confocal microscope (TCS SP8 X; Leica Heidelberg, Germany).
Mice were anesthetized with 1% (w/v) pentobarbital sodium and perfused with saline for a few minutes, followed by perfusion with 4% (v/v) PFA in PBS. The gastrocnemius tissues were dissected and soaked overnight at 4°C in 4% (v/v) PFA and then in 30% (w/v) sucrose at 4°C for 48 h. Gastrocnemius slices (20 μm) were prepared and immunofluorescence detected as described above.
2.8 Statistical analysis
All experiments were repeated at least three times. Data are presented as means ± standard error of the mean (SEM) and were compared via one-way analysis of variance. p-values <.05 were considered statistically significant.
3 Results
3.1 NRG-1β increased glucose uptake in PA-treated C2C12 myotubes
C2C12 myoblasts differentiated into myotubes, as confirmed by the development of the myotube-like multi-nuclear structure (Supplementary Figures S1A, B) and a structure protein marker MHC (Supplementary Figures S1C, D). To explore the effects of NRG-1β on glucose uptake and GLUT4 translocation in IR environment, we reproduced the classic cellular model in the C2C12 myotubes (Nieuwoudt et al., 2017; Petersen and Shulman, 2018). Glucose uptake was measured with the aid of the 2-NBDG assay (Zou et al., 2005; Bala et al., 2021). PA at .25 mM did not affect C2C12 myotube cell viability (Figure 1A). And then, C2C12 myotubes were incubated with various concentrations of NRG-1β for 1.5 h (Suárez et al., 2001; Canto et al., 2004). NRG-1β at 10 ng/mL optimally increased glucose uptake (Figure 1B). Glucose uptake was decreased in PA-treated (plus insulin) C2C12 myotubes compared to BSA (plus insulin) group, but NRG-1β rescued the uptake (Figure 1C). Thus, NRG-1β increased glucose uptake in PA-treated C2C12 myotubes.
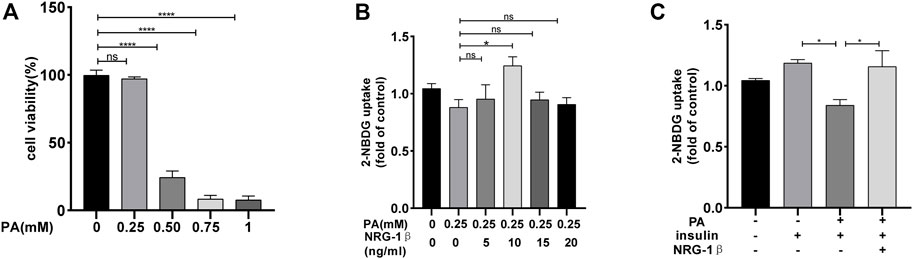
FIGURE 1. NRG-1β increased glucose uptake in PA-treated C2C12 myotubes. C2C12 myotubes were incubated with different concentrations of PA for 24 h and cell viability measured using the CCK8 assay (A). C2C12 myotubes were serum depleted for 4.5 h and then incubated for 1.5 h with different concentrations of NRG-1β (0, 5, 10, 15, and 20 ng/mL) (B). C2C12 myotubes were incubated in the presence or absence of the indicated concentration (10 ng/mL) of NRG-1β for 1.5 h, the cells were incubated with 80 μM 2-NBDG for 30 min. The 2-NBDG uptake was determined as described in the Methods (C). The data are presented as the mean ± SEM. ns, p > .05; *p < .05; ****p < .0001.
3.2 NRG-1β promoted GLUT4 translocation in PA-treated C2C12 myotubes
To explore the effects of NRG-1β on GLUT4 translocation in PA-treated C2C12 myotubes, we used immunofluorescence staining to detect GLUT4. The GLUT4 levels were significantly decreased in PA-treated (plus insulin) C2C12 myotubes compared BSA (plus insulin) group; however, NRG-1β rescued the decrease (Figures 2A, B). Next, we examined cell membrane GLUT4 levels in the presence or absence of NRG-1β. GLUT4 membrane translocation decreased in PA-treated C2C12 myotubes, but NRG-1β rescued the fall (Figures 2C, D). Therefore, NRG-1β promoted GLUT4 translocation in PA-treated C2C12 myotubes.
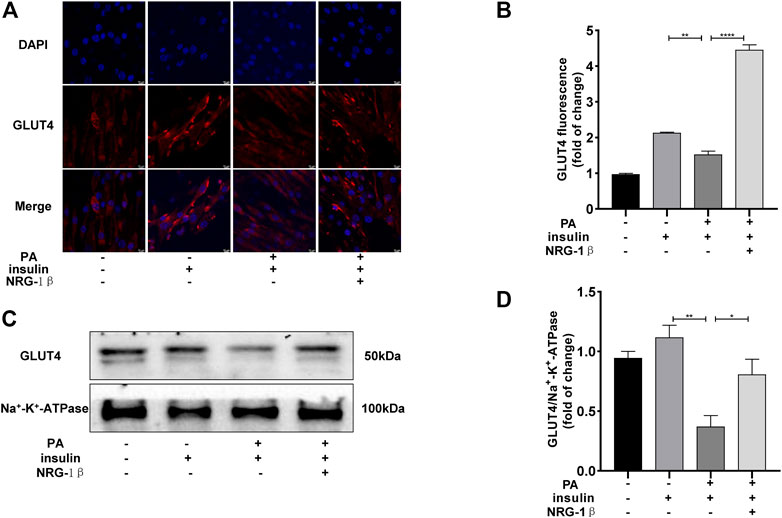
FIGURE 2. NRG-1β promoted the translocation of GLUT4 in PA-treated C2C12 myotubes. Effects of NRG-1β on GLUT4 translocation in PA-treated (plus insulin) C2C12 myotubes, GLUT4 was detected by using a fluorescent anti-GLUT4 antibody (A,B) (Scale bar: 10 μm) and Western blotting (C,D). The data are presented as the mean ± SEM. *p < .05; **p < .01; ****p < .0001.
3.3 AKT played an essential role in NRG-1β improving IR
The PI3K/AKT pathway triggers the GLUT4 translocation that is essential for glucose uptake and metabolism (Zhu et al., 2013; Li et al., 2017; Sharma and Dey, 2021). To explore the molecular mechanisms in play, we measured the AKT and phosphorylation of AKT protein levels. Phosphor-Akt (ser 473) expression was significantly decreased in PA-treated (plus insulin) C2C12 myotubes compared to the BSA (plus insulin) group, but NRG-1β increased the level significantly (Figures 3A, B), indicating that NRG-1β activated PI3K/AKT signaling in PA-treated C2C12 myotubes. Next, we determined the effects of pretreatment with MK2206 (an AKT inhibitor) on glucose uptake and translocation of GLUT4 to the plasma membrane of PA-treated C2C12 myotubes. Pretreatment with MK2206 significantly reduced the effects of NRG-1β on glucose uptake (Figure 3C) and GLUT4 translocation to the plasma membrane (Figure 4). These data suggested that NRG-1β increased glucose uptake and GLUT4 translocation in PA-treated C2C12 myotubes by activating PI3K/AKT signaling.
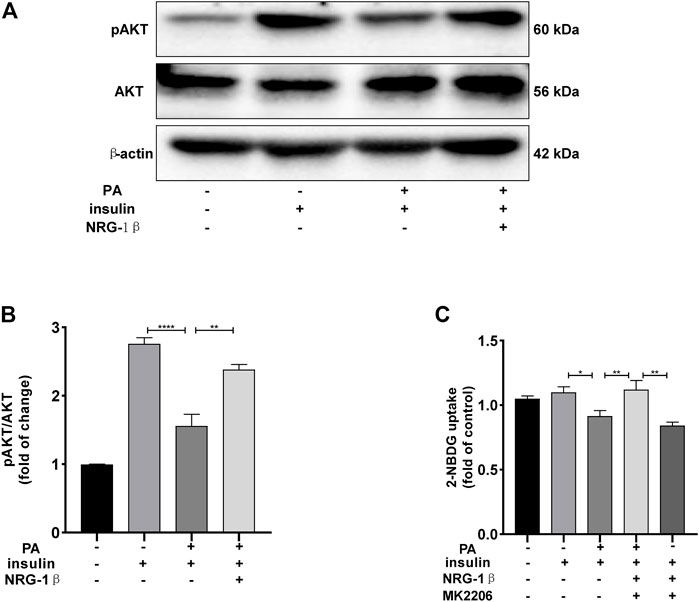
FIGURE 3. NRG-1β increased glucose uptake in PA-treated C2C12 myotubes by activating the PI3K/AKT signaling pathway. Effects of NRG-1β on levels of AKT and Phosphor-Akt (ser 473) in PA-treated (plus insulin) C2C12 myotubes were detected via Western blotting (A,B). Dimethyl sulphoxide (DMSO) or 10 μM MK2206 (an AKT inhibitor) in DMSO was added before culturing .25 mM PA-treated (plus insulin) C2C12 myotubes, effects of MK2206 on glucose uptake using the 2-NBDG assay (C). The data are presented as the mean ± SEM. *p < .05; **p < .01; ****p < .0001.
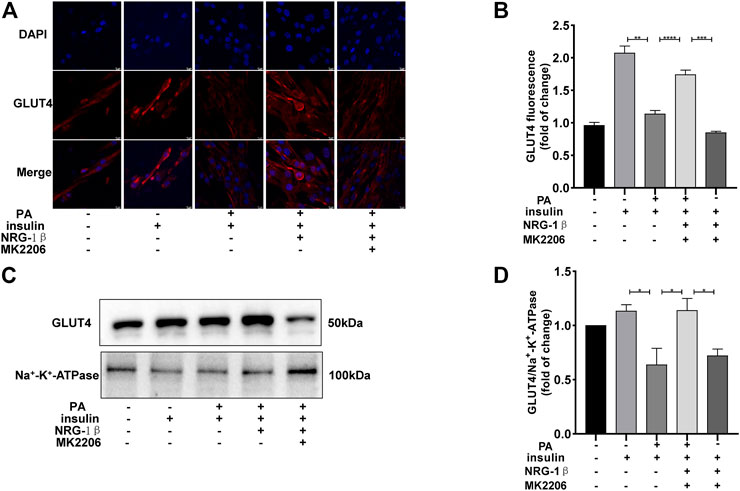
FIGURE 4. NRG-1β promoted the translocation of GLUT4 in PA-treated C2C12 myotubes by activating the PI3K/AKT signaling pathway. Effects of MK2206 of GLUT4 translocation in PA-treated C2C12 myotubes, GLUT4 was detected by using a fluorescent anti-GLUT4 antibody (Scale bar: 10 μm) (A,B) and Western blotting (C,D). The data are presented as the mean ± SEM. *p < .05; **p < .01; ***p < .001; ****p < .0001.
3.4 NRG-1β improved diabetes-induced weight loss and attenuated diabetes-induced hyperglycemia in T2DM mice
We established a T2DM animal model by combing high-fat diet with a low dose of STZ to evaluate the effects of NRG-1β. Seven days after the final intraperitoneal injection of STZ, establishment was successful (the blood glucose level was >16.7 mmol/L) (Hamza et al., 2011; Kleinert et al., 2018; Yang et al., 2020). The body weight of the DM group began to decrease after STZ injection, in contrast to the constant weight gain of the CON group, but NRG-1β improved diabetes-induced weight loss (Figure 5A). The blood glucose level remained high after STZ injection in the DM group; NRG-1β attenuated diabetes-induced hyperglycemia significantly (Figure 5B).
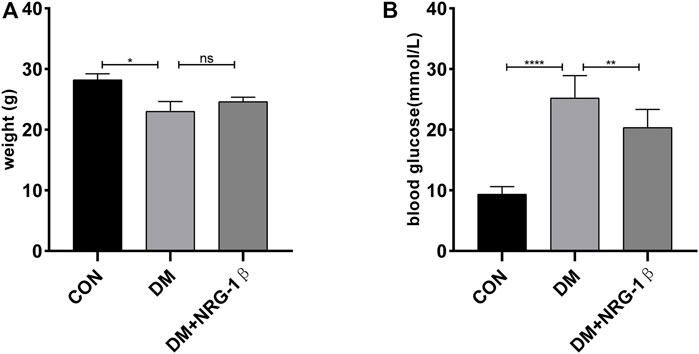
FIGURE 5. NRG-1β improved diabetes-induced weight loss and attenuated diabetes-induced hyperglycemia in T2DM mice. Effects of NRG-1β on the body weights (A) and blood glucose levels (B). The data are presented as the mean ± SEM. ns, p > .05; *p < .05; **p < .01; ****p < .0001.
3.5 NRG-1β promoted the translocation of GLUT4 in the skeletal muscle of T2DM mice
T2DM induced a remarkable change in the distribution of GLUT4 in skeletal muscle tissue (Fang et al., 2017; Fujiwara et al., 2017). After treatment with 30 μg/kg NRG-1β once weekly for 4 weeks, we examined the translocation of GLUT4 in gastrocnemius tissues in C57BL/6J and T2DM mice. The GLUT4 fluorescence intensity of T2DM mice were significantly lower than those of C57BL/6J mice of the same age; however, NRG-1β inhibited the reduction (Figures 6A, B). These observations were confirmed by Western blotting (Figures 6C, D).
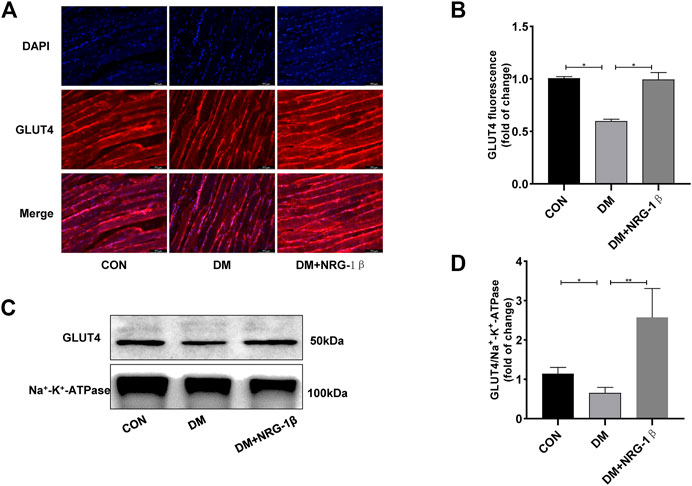
FIGURE 6. NRG-1β promoted the translocation of GLUT4 in the skeletal muscle of T2DM mice. Effects of NRG-1β on gastrocnemius GLUT4 translocation, GLUT4 was detected by using a fluorescent anti-GLUT4 antibody (Scale bar: 100 μm) (A,B) and Western blotting (C,D). The data are presented as the mean ± SEM. *p < .05; **p < .01.
4 Discussion
The principal function of insulin in the skeletal muscle is to promote cellular glucose uptake, a process controlled by GLUT4 translocation (Petersen and Shulman, 2018; Luo et al., 2019; Sylow et al., 2021). When skeletal muscle occurred IR, the action of insulin was impaired. In addition, skeletal muscle IR plays a central role in the pathogenesis of T2DM (Song et al., 2013). NRG-1β plays a key role in the development of skeletal muscle; it not only increased mitochondrial oxidative capacity and insulin sensitivity in normal skeletal muscle cells, but also improved complex 2-mediated mitochondrial respiration in the gastrocnemius of both control and diabetic mice (Suárez et al., 2001; Canto et al., 2004; Cantó et al., 2007; Ennequin et al., 2017). We initially studied the effects of NRG-1β on glucose uptake and GLUT4 translocation in PA-treated skeletal muscle cells, and then in T2DM mice. NRG-1β increased glucose uptake and promoted the translocation of GLUT4 in PA-treated C2C12 myotubes via the PI3K/AKT signaling pathway. Moreover, NRG-1β not only improved diabetes-induced weight loss and diabetes-induced hyperglycemia, but also increased the translocation of GLUT4 in the gastrocnemius in T2DM.
It has become increasingly apparent that elevated levels of plasma free fatty acids play an essential role in the impairment of insulin sensitivity characteristic of T2DM (Merz and Thurmond, 2020). Specifically, saturated fatty acids change the insulin biology and high consumption of PA (a saturated fatty acid) is associated with IR development. PA potently induces IR in cultured myocytes by impairing glucose uptake and reducing GLUT4 translocation in skeletal muscle, and PA treatment is commonly used to establish IR phenotype in vitro (Miller et al., 2009; Petersen and Shulman, 2018; Feraco et al., 2021; Sánchez-Alegría et al., 2021). We found that C2C12 myotubes treated with .25 mM PA remained fully viable, but evidenced decreases in glucose uptake and translocation of GLUT4 to the plasma membrane, as reported previously (Liu et al., 2020; Feraco et al., 2021). GLUT4 is a major mediator of glucose removal from the circulation to cell and a key regulator of whole-body glucose homeostasis (Huang and Czech, 2007). In the absence of stimulation, GLUT4 resides in cytoplasmic vesicles in a non-active state; only ∼1% of cellular GLUT4 is in the plasma membrane to implement transport function (Sadler et al., 2013; Yaribeygi et al., 2019). In response to stimulation, GLUT4 translocates to the cell membrane and facilitates glucose entry into cells, greatly lowering the blood glucose (Sadler et al., 2013; Yaribeygi et al., 2019). Reductions of GLUT4 translocation and activity affect glucose uptake, triggering IR; enhanced expression and translocation of GLUT4 improve IR in T2DM models (Watson and Pessin, 2001; Ariga et al., 2008; Yang et al., 2014). The GLUT4 level on the surface of muscle cells provoked systemic changes in glucose disposal in vivo (Huang and Czech, 2007). We thus explored the effect of NRG-1β on the cell surface GLUT4 level; NRG-1β rescued the reduction in GLUT4 translocation caused by PA. This is the first study to describe the effect of NRG-1β on glucose uptake and GLUT4 translocation in PA-treated C2C12 myotubes (Suárez et al., 2001; Canto et al., 2004; Pentassuglia et al., 2016; Heim et al., 2020).
Activation of the PI3K/AKT pathway (which is associated with GLUT4 translocation) is essential for glucose uptake and metabolism (Zhu et al., 2013; Sharma et al., 2015; Li et al., 2017; Miyata et al., 2017). AKT plays the predominant role in regulating glucose uptake by skeletal muscle; AKT knockdown decreases GLUT4 translocation, ultimately causing IR (Sharma and Dey, 2021; Sylow et al., 2021). In skeletal myotubes, PA affects the insulin-mediated activation of AKT (Schmitz-Peiffer et al., 1999). Thus, we explored whether NRG-1β improved IR in PA-treated C2C12 myotubes via the PI3K/AKT signaling pathway. We found that NRG-1β increased the expression of phosphor-Akt in PA-treated C2C12 myotubes. We used the AKT inhibitor MK2206 to explore the role played by AKT signaling. As predicted, pretreatment with MK2206 significantly reduced the effects of NRG-1β on glucose uptake and GLUT4 translocation in PA-treated C2C12 myotubes. In another study, NRG-1 did not affect the phosphorylation level of AKT in L6E9 myotubes (Canto et al., 2004). However, we found that NRG-1β significantly increased AKT phosphorylation in PA-treated C2C12 myotubes. It was reported that ceramides promoted IR in vitro by suppressing C2C12 and L6 myotube activities in different ways, probably reflecting differences in cell membrane structures or compositions (Mahfouz et al., 2014). However, further work is needed. In our study, we found that NRG-1β increased glucose uptake and GLUT4 translocation in PA-treated C2C12 myotubes via the PI3K/AKT signaling pathway.
We then studied the effect of NRG-1β on T2DM mice. In our study, NRG-1β improved diabetes-induced weight loss and diabetes-induced hyperglycemia obviously. Glucose is an important fuel for skeletal muscle, entering the cells via GLUT4, which is transferred from intracellular storage depots to the plasma membrane upon muscle contraction (Richter and Hargreaves, 2013). Previous studied reported that NRG-1 increased insulin sensitivity in normal skeletal muscle cells and improved glucose tolerance in db/db mice by liver regulatory action (Cantó et al., 2007; Ennequin et al., 2015; Caillaud et al., 2016; López-Soldado et al., 2016; Ennequin et al., 2020; Gumà et al., 2020). However, they only evaluated systematic IR; neither glucose uptake nor GLUT4 expression/translocation in the gastrocnemius was measured. In our study, we found that NRG-1β promoted the translocation of GLUT4 to the plasma membrane in gastrocnemius tissues of T2DM mice; this may improve the skeletal muscle IR. A further study should explore the mechanism by which NRG-1β affects skeletal muscle IR in T2DM mice.
In conclusion, NRG-1β increased glucose uptake and promoted GLUT4 translocation to the plasma membrane in PA-treated C2C12 myotubes by activating the PI3K/AKT signaling pathway. NRG-1β improved diabetes-induced weight loss and diabetes-induced hyperglycemia of T2DM mice compared to controls. Moreover, NRG-1β increased GLUT4 translocation in the gastrocnemius of T2DM mice.
Data availability statement
The original contributions presented in the study are included in the article/Supplementary Material, further inquiries can be directed to the corresponding authors.
Ethics statement
The animal study was reviewed and approved by Shanghai General Hospital Clinical Center Laboratory Animal Welfare and Ethics Committee.
Author contributions
LC designed the study. MY and SW performed the experimental phase, and collected and analyzed data. MY, CG, and LC drafted the manuscript. MY and SW contributed with reagents, materials, and analysis tools. MY, SW, CG, and LC have collaborated and approved the final manuscript version.
Funding
This work was funded by the National Natural Science Foundation of China (Grant No.81870714).
Conflict of interest
The authors declare that the research was conducted in the absence of any commercial or financial relationships that could be construed as a potential conflict of interest.
Publisher’s note
All claims expressed in this article are solely those of the authors and do not necessarily represent those of their affiliated organizations, or those of the publisher, the editors and the reviewers. Any product that may be evaluated in this article, or claim that may be made by its manufacturer, is not guaranteed or endorsed by the publisher.
Supplementary material
The Supplementary Material for this article can be found online at: https://www.frontiersin.org/articles/10.3389/fphar.2022.1066279/full#supplementary-material
References
Al-Sulaiti, H., Diboun, I., Agha, M. V., Mohamed, F. F. S., Atkin, S., Dömling, A. S., et al. (2019). Metabolic signature of obesity-associated insulin resistance and type 2 diabetes. J. Transl. Med. 17 (1), 348. doi:10.1186/s12967-019-2096-8
Ariga, M., Nedachi, T., Katagiri, H., and Kanzaki, M. (2008). Functional role of sortilin in myogenesis and development of insulin-responsive glucose transport system in C2C12 myocytes. J. Biol. Chem. 283 (15), 10208–10220. doi:10.1074/jbc.M710604200
Bala, M., Gupta, P., Gupta, S., Dua, A., Injeti, E., and Mittal, A. (2021). Efficient and modified 2-NBDG assay to measure glucose uptake in cultured myotubes. J. Pharmacol. Toxicol. methods 109, 107069. doi:10.1016/j.vascn.2021.107069
Caillaud, K., Boisseau, N., Ennequin, G., Chavanelle, V., Etienne, M., Li, X., et al. (2016). Neuregulin 1 improves glucose tolerance in adult and old rats. Diabetes & metabolism 42 (2), 96–104. doi:10.1016/j.diabet.2015.08.003
Cantó, C., Chibalin, A., Barnes, B., Glund, S., Suárez, E., Ryder, J., et al. (2006). Neuregulins mediate calcium-induced glucose transport during muscle contraction. J. Biol. Chem. 281 (31), 21690–21697. doi:10.1074/jbc.M600475200
Cantó, C., Pich, S., Paz, J., Sanches, R., Martínez, V., Orpinell, M., et al. (2007). Neuregulins increase mitochondrial oxidative capacity and insulin sensitivity in skeletal muscle cells. Diabetes 56 (9), 2185–2193. doi:10.2337/db06-1726
Canto, C., Suarez, E., Lizcano, J. M., Grino, E., Shepherd, P. R., Fryer, L. G., et al. (2004). Neuregulin signaling on glucose transport in muscle cells. J. Biol. Chem. 279 (13), 12260–12268. doi:10.1074/jbc.M308554200
Ennequin, G., Boisseau, N., Caillaud, K., Chavanelle, V., Etienne, M., Li, X., et al. (2015). Neuregulin 1 improves glucose tolerance in db/db mice. PloS one 10 (7), e0130568. doi:10.1371/journal.pone.0130568
Ennequin, G., Caillaud, K., Chavanelle, V., Teixeira, A., Etienne, M., Li, X., et al. (2020). Neuregulin 1 treatment improves glucose tolerance in diabetic db/db mice, but not in healthy mice. Archives physiology Biochem. 126 (4), 320–325. doi:10.1080/13813455.2018.1534243
Ennequin, G., Capel, F., Caillaud, K., Chavanelle, V., Etienne, M., Teixeira, A., et al. (2017). Neuregulin 1 improves complex 2-mediated mitochondrial respiration in skeletal muscle of healthy and diabetic mice. Sci. Rep. 7 (1), 1742. doi:10.1038/s41598-017-02029-z
Falls, D. (2003). Neuregulins: Functions, forms, and signaling strategies. Exp. Cell Res. 284 (1), 14–30. doi:10.1016/s0014-4827(02)00102-7
Fang, P., Yu, M., Zhang, L., Wan, D., Shi, M., Zhu, Y., et al. (2017). Baicalin against obesity and insulin resistance through activation of AKT/AS160/GLUT4 pathway. Mol. Cell. Endocrinol. 448, 77–86. doi:10.1016/j.mce.2017.03.027
Feraco, A., Gorini, S., Armani, A., Camajani, E., Rizzo, M., and Caprio, M. (2021). Exploring the role of skeletal muscle in insulin resistance: Lessons from cultured cells to animal models. Int. J. Mol. Sci. 22 (17), 9327. doi:10.3390/ijms22179327
Fujiwara, Y., Tsukahara, C., Ikeda, N., Sone, Y., Ishikawa, T., Ichi, I., et al. (2017). Oleuropein improves insulin resistance in skeletal muscle by promoting the translocation of GLUT4. J. Clin. Biochem. Nutr. 61 (3), 196–202. doi:10.3164/jcbn.16-120
Galicia-Garcia, U., Benito-Vicente, A., Jebari, S., Larrea-Sebal, A., Siddiqi, H., Uribe, K. B., et al. (2020). Pathophysiology of type 2 diabetes mellitus. Int. J. Mol. Sci. 21 (17), 6275. doi:10.3390/ijms21176275
Gumà, A., Díaz-Sáez, F., Camps, M., and Zorzano, A. (2020). Neuregulin, an effector on mitochondria metabolism that preserves insulin sensitivity. Front. physiology 11, 696. doi:10.3389/fphys.2020.00696
Guma, A., Martinez-Redondo, V., Lopez-Soldado, I., Canto, C., and Zorzano, A. (2010). Emerging role of neuregulin as a modulator of muscle metabolism. Am. J. Physiol. Endocrinol. Metab. 298 (4), E742–E750. doi:10.1152/ajpendo.00541.2009
Hagman, E., Besor, O., Hershkop, K., Santoro, N., Pierpont, B., Mata, M., et al. (2019). Relation of the degree of obesity in childhood to adipose tissue insulin resistance. Acta Diabetol. 56 (2), 219–226. doi:10.1007/s00592-018-01285-3
Hamza, N., Berke, B., Cheze, C., Le Garrec, R., Lassalle, R., Agli, A., et al. (2011). Treatment of high fat diet induced type 2 diabetes in C57BL/6J mice by two medicinal plants used in traditional treatment of diabetes in the east of Algeria. J. Ethnopharmacol. 133 (2), 931–933. doi:10.1016/j.jep.2010.11.019
Heim, P., Morandi, C., Brouwer, G., Xu, L., Montessuit, C., and Brink, M. (2020). Neuregulin-1 triggers GLUT4 translocation and enhances glucose uptake independently of insulin receptor substrate and ErbB3 in neonatal rat cardiomyocytes. Biochimica biophysica acta. Mol. Cell Res. 1867 (3), 118562. doi:10.1016/j.bbamcr.2019.118562
Huang, S., and Czech, M. (2007). The GLUT4 glucose transporter. Cell metab. 5 (4), 237–252. doi:10.1016/j.cmet.2007.03.006
Kleinert, M., Clemmensen, C., Hofmann, S. M., Moore, M. C., Renner, S., Woods, S. C., et al. (2018). Animal models of obesity and diabetes mellitus. Nat. Rev. Endocrinol. 14 (3), 140–162. doi:10.1038/nrendo.2017.161
Li, H., Liu, S., Yuan, H., Niu, Y., and Fu, L. (2017). Sestrin 2 induces autophagy and attenuates insulin resistance by regulating AMPK signaling in C2C12 myotubes. Exp. Cell Res. 354 (1), 18–24. doi:10.1016/j.yexcr.2017.03.023
Li, W., Liang, X., Zeng, Z., Yu, K., Zhan, S., Su, Q., et al. (2016). Simvastatin inhibits glucose uptake activity and GLUT4 translocation through suppression of the IR/IRS-1/Akt signaling in C2C12 myotubes. Biomed. Pharmacother. 83, 194–200. doi:10.1016/j.biopha.2016.06.029
Liu, M., Wang, L., Li, X., Wu, Y., Yin, F., and Liu, J. (2020). Trilobatin ameliorates insulin resistance through IRS-AKT-GLUT4 signaling pathway in C2C12 myotubes and ob/ob mice. Chin. Med. 15, 110. doi:10.1186/s13020-020-00390-2
López-Soldado, I., Niisuke, K., Veiga, C., Adrover, A., Manzano, A., Martínez-Redondo, V., et al. (2016). Neuregulin improves response to glucose tolerance test in control and diabetic rats. Am. J. physiology. Endocrinol. metabolism 310 (6), E440–E451. doi:10.1152/ajpendo.00226.2015
Luo, W., Ai, L., Wang, B., and Zhou, Y. (2019). High glucose inhibits myogenesis and induces insulin resistance by down-regulating AKT signaling. Biomed. Pharmacother. = Biomedecine Pharmacother. 120, 109498. doi:10.1016/j.biopha.2019.109498
Mahfouz, R., Khoury, R., Blachnio-Zabielska, A., Turban, S., Loiseau, N., Lipina, C., et al. (2014). Characterising the inhibitory actions of ceramide upon insulin signaling in different skeletal muscle cell models: A mechanistic insight. PLoS One 9 (7), e101865. doi:10.1371/journal.pone.0101865
Merz, K. E., and Thurmond, D. C. (2020). Role of skeletal muscle in insulin resistance and glucose uptake. Compr. Physiol. 10 (3), 785–809. doi:10.1002/cphy.c190029
Miller, T., Icli, B., Cote, G., Lebrasseur, N., Borkan, S., Pimentel, D., et al. (2009). Palmitate alters neuregulin signaling and biology in cardiac myocytes. Biochem. biophysical Res. Commun. 379 (1), 32–37. doi:10.1016/j.bbrc.2008.11.150
Miyata, S., Yada, T., Ishikawa, N., Taheruzzaman, K., Hara, R., Matsuzaki, T., et al. (2017). Insulin-like growth factor 1 regulation of proliferation and differentiation of Xenopus laevis myogenic cells in vitro. Vitro Cell Dev. Biol. Anim. 53 (3), 231–247. doi:10.1007/s11626-016-0099-9
Nieuwoudt, S., Mulya, A., Fealy, C., Martelli, E., Dasarathy, S., Naga Prasad, S., et al. (2017). In vitro contraction protects against palmitate-induced insulin resistance in C2C12 myotubes. Am. J. physiology. Cell physiology 313 (5), C575–C583. doi:10.1152/ajpcell.00123.2017
Pentassuglia, L., Heim, P., Lebboukh, S., Morandi, C., Xu, L., and Brink, M. (2016). Neuregulin-1β promotes glucose uptake via PI3K/Akt in neonatal rat cardiomyocytes. Am. J. physiology Endocrinol. metabolism 310 (9), E782–E794. doi:10.1152/ajpendo.00259.2015
Petersen, M. C., and Shulman, G. I. (2018). Mechanisms of insulin action and insulin resistance. Physiol. Rev. 98 (4), 2133–2223. doi:10.1152/physrev.00063.2017
Richter, E., and Hargreaves, M. (2013). Exercise, GLUT4, and skeletal muscle glucose uptake. Physiol. Rev. 93 (3), 993–1017. doi:10.1152/physrev.00038.2012
Sadler, J., Bryant, N., Gould, G., and Welburn, C. (2013). Posttranslational modifications of GLUT4 affect its subcellular localization and translocation. Int. J. Mol. Sci. 14 (5), 9963–9978. doi:10.3390/ijms14059963
Sánchez-Alegría, K., Bastián-Eugenio, C. E., Vaca, L., and Arias, C. (2021). Palmitic acid induces insulin resistance by a mechanism associated with energy metabolism and calcium entry in neuronal cells. Faseb J. 35 (7), e21712. doi:10.1096/fj.202100243R
Schmitz-Peiffer, C., Craig, D. L., and Biden, T. J. (1999). Ceramide generation is sufficient to account for the inhibition of the insulin-stimulated PKB pathway in C2C12 skeletal muscle cells pretreated with palmitate. J. Biol. Chem. 274 (34), 24202–24210. doi:10.1074/jbc.274.34.24202
Sharma, B. R., Kim, H. J., and Rhyu, D. Y. (2015). Caulerpa lentillifera extract ameliorates insulin resistance and regulates glucose metabolism in C57BL/KsJ-db/db mice via PI3K/AKT signaling pathway in myocytes. J. Transl. Med. 13, 62. doi:10.1186/s12967-015-0412-5
Sharma, M., and Dey, C. S. (2021). Akt ISOFORMS-AS160-GLUT4: The defining axis of insulin resistance. Rev. Endocr. Metab. Disord. 22 (4), 973–986. doi:10.1007/s11154-021-09652-2
Shen, S., Liao, Q., Zhang, T., Pan, R., and Lin, L. (2019). Myricanol modulates skeletal muscle-adipose tissue crosstalk to alleviate high-fat diet-induced obesity and insulin resistance. Br. J. Pharmacol. 176 (20), 3983–4001. doi:10.1111/bph.14802
Song, R., Peng, W., Zhang, Y., Lv, F., Wu, H. K., Guo, J., et al. (2013). Central role of E3 ubiquitin ligase MG53 in insulin resistance and metabolic disorders. Nature 494 (7437), 375–379. doi:10.1038/nature11834
Suárez, E., Bach, D., Cadefau, J., Palacin, M., Zorzano, A., Gumá, A., et al. (2001). A novel role of neuregulin in skeletal muscle. Neuregulin stimulates glucose uptake, glucose transporter translocation, and transporter expression in muscle cells. J. Biol. Chem. 276 (21), 18257–18264. doi:10.1074/jbc.M008100200
Sun, H., Saeedi, P., Karuranga, S., Pinkepank, M., Ogurtsova, K., Duncan, B., et al. (2022). IDF Diabetes Atlas: Global, regional and country-level diabetes prevalence estimates for 2021 and projections for 2045. Diabetes Res. Clin. Pract. 183, 109119. doi:10.1016/j.diabres.2021.109119
Sylow, L., Tokarz, V. L., Richter, E. A., and Klip, A. (2021). The many actions of insulin in skeletal muscle, the paramount tissue determining glycemia. Cell Metab. 33 (4), 758–780. doi:10.1016/j.cmet.2021.03.020
Watson, R. T., and Pessin, J. E. (2001). Intracellular organization of insulin signaling and GLUT4 translocation. Recent Prog. Horm. Res. 56, 175–193. doi:10.1210/rp.56.1.175
Wu, J., Liu, H., Chu, T., Jiang, P., and Li, S. (2019). Neuregulin-1β attenuates sepsis-induced diaphragm atrophy by activating the PI3K/Akt signaling pathway. J. muscle Res. Cell Motil. 40 (1), 43–51. doi:10.1007/s10974-019-09512-2
Xu, D., Wang, L., Jiang, Z., Zhao, G., Hassan, H., Sun, L., et al. (2018). A new hypoglycemic mechanism of catalpol revealed by enhancing MyoD/MyoG-mediated myogenesis. Life Sci. 209, 313–323. doi:10.1016/j.lfs.2018.08.028
Yang, J., Zhao, P., Wan, D., Zhou, Q., Wang, C., Shu, G., et al. (2014). Antidiabetic effect of methanolic extract from berberis julianae schneid. Via activation of AMP-activated protein kinase in type 2 diabetic mice. Evid. Based Complement. Altern. Med. 2014, 106206. doi:10.1155/2014/106206
Yang, L., Lin, H., Lin, W., and Xu, X. (2020). Exercise ameliorates insulin resistance of type 2 diabetes through motivating short-chain fatty acid-mediated skeletal muscle cell autophagy. Biology 9 (8), 203. doi:10.3390/biology9080203
Yaribeygi, H., Farrokhi, F., Butler, A., and Sahebkar, A. (2019). Insulin resistance: Review of the underlying molecular mechanisms. J. Cell. physiology 234 (6), 8152–8161. doi:10.1002/jcp.27603
Zhu, Y., Pereira, R. O., O'Neill, B. T., Riehle, C., Ilkun, O., Wende, A. R., et al. (2013). Cardiac PI3K-Akt impairs insulin-stimulated glucose uptake independent of mTORC1 and GLUT4 translocation. Mol. Endocrinol. 27 (1), 172–184. doi:10.1210/me.2012-1210
Keywords: Neuregulin-1β, C2C12 myotubes, glucose uptake, GLUT4 translocation, PI3K/AKT
Citation: Yu M, Wu S, Gong C and Chen L (2023) Neuregulin-1β increases glucose uptake and promotes GLUT4 translocation in palmitate-treated C2C12 myotubes by activating PI3K/AKT signaling pathway. Front. Pharmacol. 13:1066279. doi: 10.3389/fphar.2022.1066279
Received: 10 October 2022; Accepted: 26 December 2022;
Published: 10 January 2023.
Edited by:
Jun-Li Liu, McGill University, CanadaReviewed by:
Carlos Puebla, Universidad de O'Higgins, ChileCongshan Sun, Johns Hopkins Medicine, United States
Copyright © 2023 Yu, Wu, Gong and Chen. This is an open-access article distributed under the terms of the Creative Commons Attribution License (CC BY). The use, distribution or reproduction in other forums is permitted, provided the original author(s) and the copyright owner(s) are credited and that the original publication in this journal is cited, in accordance with accepted academic practice. No use, distribution or reproduction is permitted which does not comply with these terms.
*Correspondence: Chao Gong, MTk4M2dieUAxNjMuY29t; Lianhua Chen, bGlhbmh1YWNoZW4xOTk0QGFsaXl1bi5jb20=
†These authors have contributed equally to this work and share first authorship