- 1College of Chinese Materia Medica and Yunnan Key Laboratory of Southern Medicinal Utilization, Yunnan University of Chinese Medicine, Kunming, Yunnan, China
- 2Center for Life Sciences, School of Life Sciences, Yunnan University, Kunming, Yunnan, China
As the body’s critical metabolic organ, the liver plays an essential role in maintaining proper body homeostasis. However, as people’s living standards have improved and the number of unhealthy lifestyles has increased, the liver has become overburdened. These have made liver disease one of the leading causes of death worldwide. Under the influence of adverse factors, liver disease progresses from simple steatosis to hepatitis, to liver fibrosis, and finally to cirrhosis and cancer, followed by increased mortality. Until now, there has been a lack of accepted effective treatments for liver disease. Based on current research, antisense oligonucleotide (ASO), as an alternative intervention for liver diseases, is expected to be an effective treatment due to its high efficiency, low toxicity, low dosage, strong specificity, and additional positive characteristics. In this review, we will first introduce the design, modification, delivery, and the mechanisms of ASO, and then summarize the application of ASO in liver disease treatment, including in non-alcoholic fatty liver disease (NAFLD), hepatitis, liver fibrosis, and liver cancer. Finally, we discuss challenges and perspectives on the transfer of ASO drugs into clinical use. This review provides a current and comprehensive understanding of the integrative and systematic functions of ASO for its use in liver disease.
1 Introduction
The liver is the largest “chemical factory” in the human body. It plays a crucial role in nutrient metabolism, energy generation, detoxification, hormone inactivation, bile production, regulation of blood coagulation, immune defense, and many other processes. However, with the increasing availability of processed foods and the spread of unhealthy lifestyles, the liver can become overburdened. Liver-related diseases are becoming a global epidemic and are one of the leading causes of death worldwide. According to statistics, in 2017, there were 2.14 million liver-related deaths, representing an 11.4% increase since 2012 (Paik et al., 2020).
Numerous predisposing factors contribute to the development of liver disease, such as viral infections, alcohol use, autoimmune diseases, cholestasis, drug use, and metabolic diseases. Liver diseases follow a progression of severity, from hepatic steatosis, to hepatitis, liver fibrosis, and eventually culminating in liver cirrhosis and liver cancer (Anstee et al., 2019). Currently, major treatments for liver-related diseases include lifestyle interventions, drug therapy, and liver transplantation. Of the three, drug therapy has been the dominant approach, compared to the limitations of lifestyle interventions and liver transplantation. Drugs, including small molecule compounds, antibody drugs, polymeric DNA and small interfering RNA nucleic acid drugs, etc., administered alone or in combination, have been approved by the U.S. Food and Drug Administration (FDA) for the treatment of primary biliary cholangitis (PBC), acute hepatic porphyria, parenteral nutrition-associated cholestasis, hepatic veno-occlusive disease, hepatitis C (HCV), hepatitis B (HBV), and liver cancer. However, thus far there is no FDA- approved drug treatment for fatty liver, non-alcoholic steatohepatitis (NASH), and liver fibrosis. Therefore, new drug discovery and development for liver-related diseases is extremely urgent due to unmet clinical needs.
In 1978, Stephenson and Zamecnik (1978) first used Antisense oligonucleotide (ASO) for therapeutic purposes, and subsequently developed multiple ASO for the treatment of diseases. In a landmark move in 1998, the FDA approved Fomivirsen, the first ASO drug, for the treatment of cytomegalovirus (CMV) retinitis. Over the last two decades, the use of ASO drugs has become an increasingly important therapeutic strategy. Prior to 2009, only two ASO drugs were approved. From 2010 to 2021, the FDA approved a total of eight ASO drugs (Table 1) (Hair et al., 2013; Syed, 2016; Hoy, 2017; Keam, 2018; Dhillon, 2020; Heo, 2020; Brown and Wobst, 2021; Shirley, 2021). Among these, Defibrotide, the first ASO drug approved for the treatment of liver-related disease (Choy, 2016), has been used for the treatment of adult or pediatric patients with hepatic veno-occlusive disease (VOD). In recent years, research on ASO therapy for liver disease has been active as never before. Improved ASO design and optimized synthetic nucleic acid chemistry, combined with the development of highly selective and efficient conjugate delivery technology platforms, have established and validated ASO as a new class of drugs.
2 Antisense oligonucleotide
ASO typically consists of 10–30 single-stranded DNA nucleotides (Opalinska and Gewirtz, 2002), that are complementary to the target mRNA. Through the principle of Watson-Crick base pairing, ASO can accurately target the transcription product of this specific gene, thereby exhibiting a precise function in inhibiting gene expression (Crooke et al., 2021). Due to its strong specificity, high efficiency, low toxicity and low dosage, ASO could potentially serve as a new therapeutic direction for drug development in liver diseases. It is important to note that ASO drugs have different absorption and metabolism pathways than traditional small molecule drugs due to their unique properties. ASO drugs are mainly administered by intravenous and subcutaneous routes (Tables 1, 2). When they enter the body, they are mainly degraded by endonucleases and exonucleases in the blood and target organs. In addition, they are not metabolized by the liver and hepatic microsomes, as they are not substrates of the hepatic drug enzyme P450s. When ASOs are metabolized to short oligonucleotides of various sizes, they are mainly excreted into the urethra by membrane leakage, vesicle release, or exosome release (Migliorati et al., 2022). It can be seen that pharmacokinetic parameters such as absorption, distribution, metabolism and excretion of ASO drugs have an essential influence on the dose and toxicity of ASO drugs, and future studies should pay more attention to this aspect.
2.1 The mechanism of action of antisense oligonucleotide
ASO functions mainly through four mechanisms (Figure 1). First, ASO can affect mRNA maturation by entering the nucleus and acting on the precursor mRNA, inhibiting the formation of the 5′ end cap or blocking the polyadenylation of the 3′ end (Gheibi-Hayat and Jamialahmadi, 2021). Second, ASO can selectively regulate the splicing of precursor mRNA. ASO can selectively correct the mis-splicing process through exon skipping and exon inclusion (Pagani and Baralle, 2004; Siva et al., 2014; van der Wal et al., 2017; Yang et al., 2019). Approved drugs using this mechanism include Eteplirsen for exon 51 skipping, Golodirsen and Viltolarsen for exon 53 skipping, and Casimersen for exon 45 skipping in Dystrophin mRNA. Nusinersen targets the precursor mRNA of the survival motor neuron 2 (SMN2), resulting in the inclusion of exon 7 (Wurster and Ludolph, 2018) (Table 1). Third, ASO can activate the RNase H enzyme, which specifically cleaves RNA-DNA hybrid molecules, thus inhibiting the production of proteins (Cerritelli et al., 2009; Lai et al., 2020). Fomivirsen targeting CMV IE2 mRNA, Mipomersen targeting apolipoprotein B (ApoB) mRNA, and Inotersen targeting transthyretin (TTR) mRNA all use this mechanism (Table 1). Finally, ASO can prevent the interaction of RNA and ribosome through steric hindrance, thereby blocking the translation process (Melton, 1985; Gheibi-Hayat and Jamialahmadi, 2021). There are also a few ASO drugs that act through other mechanisms. For example, Pegaptanib is a nucleic acid aptamer that acts by targeting the receptor of the vascular endothelial growth factor (VEGF) (Stein and Castanotto, 2017). Defibrotide is a natural product of DNA depolymerization, and its mechanism of action has not yet been fully elucidated (Stein and Castanotto, 2017; Alhamadani et al., 2022).
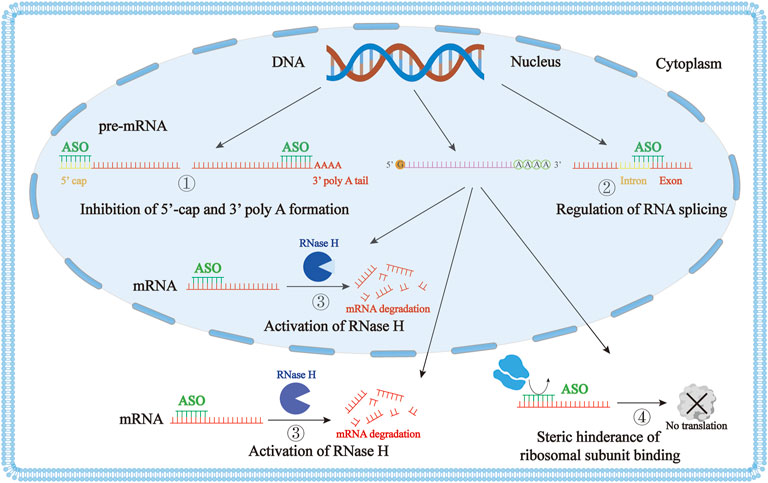
FIGURE 1. The mechanism of action of antisense oligonucleotide (ASO). ① ASO affects mRNA maturation by inhibition of the 5′ end cap formation or by blocking the polyadenylation of the 3′ end. ② ASO can selectively regulate the splicing of precursor mRNA. ③ ASO activates the RNase H enzyme to degrade DNA-mRNA duplexes through cleavage. ④ ASO can prevent the interaction of RNA and ribosomes through steric hindrance, blocking the translation process.
2.2 Design of antisense oligonucleotide
Several key issues need to be carefully considered to ensure that ASO has maximum benefit and minimum harm as a drug. These include sequence design, base modification, delivery, toxicity, pharmacodynamics, pharmacokinetics, and other factors. The sequence design of an ASO is the first and most critical step, as the ASO sequence determines its specificity. Therefore, the following factors must be considered: First, prediction of the secondary structure of the target RNA (Vickers et al., 2000; Andronescu et al., 2005). It can perform this task using software such as mfold, sfold, RNAfold or RNAstructure (Zuker, 2003; Ding et al., 2004; Dowell and Eddy, 2004; Mathews et al., 2004; Mathews et al., 2016). Second, we must consider the identification of locally conserved regions in the RNA secondary structure that can be targeted for ASO hybridization. Third, we must consider the identification of activity-enhanced motifs in locally conserved regions of RNA, such as CCAC, TCCC, ACTC, GCCA, CTCT, etc., which increases antisense efficiency (Matveeva et al., 2000). Also, it has shown that higher GC content in the ASO sequence produces a better effect (Ho et al., 1996). Finally, we must consider the binding energy prediction, where the thermodynamic binding energy between ASO and mRNA can be predicted using RNAstructure software. When the binding energy between ASO and mRNA is greater than or equal to −8 kcal/mol, the effect of the ASO is better (Matveeva et al., 2000). Following this basic process of ASO design can help to identify and create efficient ASO sequences. Nevertheless, experimental validation is still required to screen the overall effectiveness of the newly designed ASO.
2.3 Modification of antisense oligonucleotide
ASO needs to be modified to avoid degradation by nucleases in the organism. Based on the type of modification, ASO has been divided into three generations. The first generation uses a phosphorothioate (PS) modified backbone, that is, the non-bridge oxygen atom on the 3′-phosphate bond is replaced with a sulfur atom, which can enhance the resistance of ASO to nucleases, but reduce their affinity to the target RNA (Deleavey and Damha, 2012; Eckstein, 2014; Juliano, 2016). Among the approved ASO drugs, Fomivirsen, Mipomersen, Nusinersen, and Inotersen fall under this type of modification. Compared to unmodified ASO, PS-ASO is mainly distributed in the liver, kidney, and spleen after systemic administration (Dhuri et al., 2020). The second generation uses a 2′-alkyl modification of the ribose sugar, which improves the affinity between ASO and target RNA and has a high resistance to nucleases as well (Kurreck et al., 2002; Prakash et al., 2008). However, this modification does not support RNase H-mediated mRNA degradation, thus reducing the effectiveness of these ASOs (Crooke, 2004). Approved ASO drugs such as Mipomersen, Nusinersen, and Inotersen all have the 2′-O-methoxyethyl (MOE) modification. To overcome these limitations, researchers have combined both generation-1 and generation-2 modifications to produce chimeric molecules, termed gapmer, to enhance the ASO effect. The third generation of ASO possesses a variety of chemical modifications of the nucleotide furanose ring (Yamamoto et al., 2011). Peptide nucleic acid (PNA), locked nucleic acid (LNA), and phosphoramidate morpholino oligomer (PMO) are the most dominant third-generation ASOs (Kurreck, 2003; Gleave and Monia, 2005). PNA is a synthetic nucleic acid mimetic in which the deoxyribose phosphate backbone is replaced by polyamide (Nielsen and PNA, 2004). LNA refers to the fact that there is a methylene bridge connecting the 2′ oxygen and 4′ carbon of ribose (Fluiter et al., 2009). PMO refers to the substitution of its pentose sugar by a morpholine ring and phosphodiester bonds by phosphoramide bonds (Summerton and Weller, 1997). The four approved PMO-modified drugs (Eteplirsen, Golodirsen, Viltolarsen, Casimersen) are all for the treatment of Duchenne muscular dystrophy (DMD). These three modifications further enhance target affinity, nuclease resistance, biostability, and pharmacokinetics (Yamamoto et al., 2011). In fact, we often use three generations of modifications in combination for better ASO effects.
The enzyme RNase H specifically cleaves RNA-DNA duplexes. For the RNA-ASO complex to be a substrate for the RNase H enzyme, at least five adjacent deoxynucleotides must be present in the ASO, and the enzyme activity is optimal when there are 8–10 adjacent nucleotides in the ASO (Crooke et al., 2021). Thus, the gapmer ASO is composed of 8–10 adjacent phosphorothioate-modified nucleotides in the middle, flanked (5′ and 3′ directions) by several 2′-alkylated modified nucleotides (Chan et al., 2006; Crooke et al., 2021). With the development of third-generation modified ASO, gapmer ASO also includes the unmodified nucleotide in the middle and the third-generation modification (such as the locking nucleotide) at both ends (Dhuri et al., 2020). While most of the above types of modification are well tolerated, some modifications have been reported to have pro-inflammatory, hepatotoxicity, nephrotoxicity, and thrombocytopenia toxicity (Frazier, 2015). For example, LNA and constrained ethyl (cEt) modifications are predisposed to hepatotoxicity (Burel et al., 2016; Kakiuchi-Kiyota et al., 2016; Kamola et al., 2017). However, as research deepens, hepatotoxicity will improve correspondingly. It has been reported that the addition of the 2′-O-methyl (2′-OMe) at position 2 of 3-10-3 cEt gapmer can reduce hepatotoxicity by reducing protein interactions (Shen et al., 2019). Recently, it was furthermore reported that PS substitution with mesyl-phosphoramidate (MsPA) at gap positions 2 and 3 improves RNase H cleavage, which not only does not affect ASO efficacy but also reduces hepatotoxicity (Zhang et al., 2022). It is important to note that when ASO binds to a specific ligand, it can be well delivered to a specific organ. For example, N-acetylgalactosamine (GalNAc) coupled to ASO allows it to achieve liver-specific delivery by binding to the asialoglycoprotein receptor (ASGPR) of hepatocytes (Crooke et al., 2019; Debacker et al., 2020).
2.4 Delivery of antisense oligonucleotide
ASO needs to traverse the biofilm system in order to bind to RNA and exert its effects. It is well known that biofilm systems are lipophilic, and although single-stranded ASO is amphiphilic (Crooke et al., 2018), delivery is also challenging. Cells can take up ASO through pinocytosis, adsorption and receptor-mediated endocytosis, with the specific pathways utilized being related to the chemical structure of the ASO. To address this obstacle, many delivery methods have been developed. As mentioned above, GalNAc-conjugated ASO can achieve targeting effect in hepatocytes, delivered by binding to the receptor (Debacker et al., 2020). The application of GalNAc-conjugated ASO to liver disease research is described below. In addition, many delivery systems based on polymeric nanoparticles, such as poly (lactic-co-glycolic acid) (PLGA), and polyethyleneimine (PEI), have been developed for ASO delivery (Schiffelers et al., 2004; Zhu et al., 2015). Among them, PLGA, which is biocompatible and FDA-approved, is widely used as a nanoparticle carrier for the delivery of ASOs (Danhier et al., 2012; Cai et al., 2017). Finally, several lipid-based delivery systems, lipid complexes, liposomes, and lipid nanoparticle (LNP) are also widely used to deliver ASOs (ur Rehman et al., 2013; Mahmoodi Chalbatani et al., 2019). LNPs are commonly coated with cholesterol, phospholipids, and polyethylene glycol (PEG), which can increase the stability and membrane fusion of LNP, contribute to encapsulation efficiency and endosomal escape, and minimize aggregation and immune cell opsonization (Zhang et al., 2021). In addition, extracellular vesicles are also studied and applied in ASO delivery as natural liver accumulation drug-delivery vehicles (Zhang et al., 2020).
3 Application of antisense oligonucleotide in liver diseases
Due to the robust development of ASO technology, ASOs are being investigated as promising drugs for liver-related diseases. In the next section, we provide an overview of recent developments in the field of ASO therapeutics for liver diseases, including non-alcoholic fatty liver disease (NAFLD), hepatitis, fibrosis, and cancer. We also summarize the current preclinical trials in progress for these different stages of liver diseases (Figure 2; Table 2). The ASOs described below, except for Bcl-x, which acts by inducing alternative splicing, all act by reducing mRNA or protein levels, thereby improving the corresponding disease indicators.
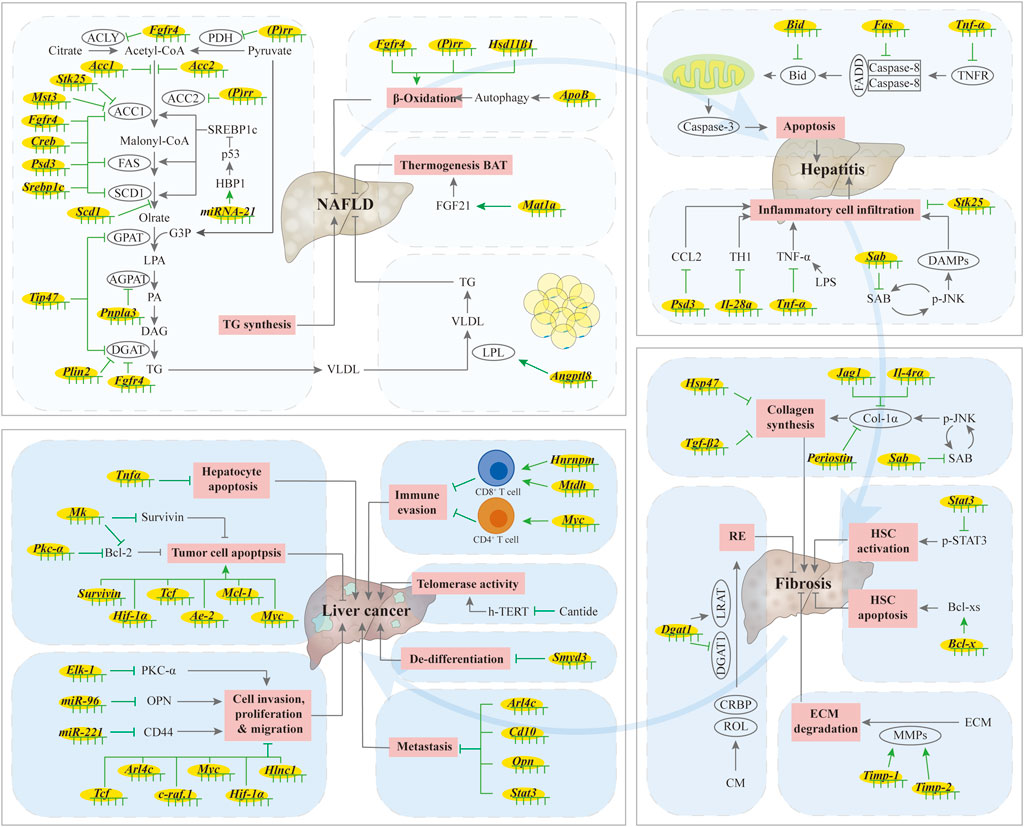
FIGURE 2. Summary of ASO in liver diseases. When the liver is under stress, including viral infection, alcohol abuse, autoimmune disease, drug use, and metabolic diseases, hepatic lipid synthesis increases, fatty acid oxidation decreases, and communication with adipose tissue is blocked, resulting in increased hepatic lipid accumulation and simple hepatic steatosis. Excessive accumulation of lipids in the liver leads to lipotoxicity, leading to ballooning degeneration of hepatocytes, infiltration of inflammatory cells, excessive secretion of inflammatory factors, and death of hepatocytes, which causes hepatitis. The activation of hepatic stellate cells (HSCs) is an important step in liver fibrosis. When hepatic fibrosis occurs, the synthesis and accumulation of extracellular matrix proteins, including collagen, increases, and the production of retinol ester (RE) by HSCs stops. The up-regulation of telomerase activity and hepatocyte apoptosis can lead to the occurrence of liver cancer, while the proliferation, migration, invasion and liver metastasis of tumor cells, as well as the de-differentiation of tumor cells, can enhance the progression of liver cancer. However, ASOs can alleviate the occurrence and development of liver diseases by regulating these processes. ↑/⊥: indicates the promoting or inhibiting effect of ASOs.
3.1 Application of antisense oligonucleotide in non-alcoholic fatty liver disease
The current common fatty liver condition is termed NAFLD (Allison, 2004). It is caused by factors other than excessive alcohol use. NAFLD is a metabolic disorder associated with excessive accumulation of triglycerides and other lipids in the liver. It is becoming the leading cause of chronic liver disease and liver transplantation worldwide, with a prevalence as high as about 25% (Younossi et al., 2019). We consider it to be a complex disease in which the interaction between the environment and the susceptible genetic background determines the symptoms and influences the progression of the disease. The first line of treatment is lifestyle changes, especially dietary changes and exercise, but the effectiveness of this approach varies from person to person (Reinehr et al., 2009; Koot et al., 2011; Ramon-Krauel et al., 2013). With increased demand, the development of fatty liver therapy drugs is advancing rapidly, and ASO-based drugs are being strongly considered.
Several genome-wide associations and large candidate gene studies have identified the I148M patatin-like phospholipase domain protein 3 (PNPLA3) variation as the main co-genetic determinant of NAFLD (Romeo et al., 2008; Trépo et al., 2016; Eslam et al., 2018). Two studies confirmed that Pnpla3-ASO could alleviate hepatic steatosis in mice and rats (Kumashiro et al., 2013; Lindén et al., 2019). Pnpla3-ASO can decrease the level of 1-acylglycerol-3-phosphate O-acyltransferase (AGPAT) to affect the esterification of fatty acids, thereby reducing the synthesis of phosphatidic acid (PA) (Kumashiro et al., 2013). A mutation of leucine 186 to threonine in Pleckstrin and sec7 domain-containing 3 (PSD3) has been reported reducing the risk of fatty liver (Mancina et al., 2022). As predicted, Psd3-ASO downregulates the expression of PSD3 in mice induced by a NASH diet, and decreases the expression of the lipogenic enzymes acetyl-CoA carboxylase (ACC), fatty acid synthase (FAS), and stearoyl-CoA desaturase 1 (SCD1), which consequently alleviates hepatic steatosis (Mancina et al., 2022). Whole genome-wide and exome studies have found that genetic variants near the γ-secretase subunit nicastrin (Ncst) are associated with HDL cholesterol (HDL-c) and LDL cholesterol (LDL-c) levels. Treatment with Ncst-ASO lowers triglyceride (TG) and cholesterol levels, thereby alleviating liver steatosis (Kim et al., 2018; Kim et al., 2020).
Effective regulation of lipid synthesis can alleviate hepatic steatosis. In this process, sterol regulatory element binding proteins (SREBPs) mainly regulate the expression of liver lipid synthesis genes such as FAS, ACC, and SCD1 (Postic and Girard, 2008). Accordingly, Srebp-1c-ASO treatment reduced the accumulation of hepatic triglycerides and improved hepatic steatosis in mice with NAFLD (Frederico et al., 2011). Diacylglycerol acyltransferase (DGAT) is an enzyme required for the last step of triglyceride synthesis and it includes two isoforms: DGAT1 and DGAT2. Studies have found that Dgat2-ASO affects the synthesis of liver fat and alleviates liver fat in obese mice (Yu et al., 2005; Choi et al., 2007). In addition, DGAT2 also has its corresponding antisense drug IONIS-DGAT2RX (ClinicalTrials.gov NCT03334214), which is currently in phase 2 of clinical trials for the treatment of NASH (Loomba et al., 2020). The cAMP-response element binding protein (CREB) is a key transcription factor in regulating the expression of multiple gluconeogenesis genes, and its expression in the liver has a regulatory effect on liver lipid production. Creb-ASO improves liver fat degeneration in type 2 diabetic rats by inhibiting the expression of lipid synthesis related genes (Erion et al., 2009).
The accumulation of lipid droplets (LDs) increases in the fatty liver, and some LD proteins, such as perilipin 2 (PLIN2, also called ADRP) and perilipin 3 (PLIN3, also called TIP47), regulate LDs enlargement (Brasaemle, 2007; Buers et al., 2009). Adrp/Plin2-ASO improves diet-induced insulin resistance and inhibits the expression of lipid synthesis-related genes, thereby decreasing liver fat accumulation (Varela et al., 2008; Imai et al., 2012). Similarly, Tip47/Plin3-ASO affects liver LDs and improves liver steatosis (Carr et al., 2012). Overexpression of serine/threonine protein kinase (STK) 24, also known as MST3, increases the number and size of LDs in the liver (Cansby et al., 2019a). Its mRNA expression level was also positively correlated with liver-related disease. Targeting MST3 using Mst3-ASO reduces the levels of ACC in vivo, thus reducing liver lipids and slowing the progression of diet-induced NAFLD (Caputo et al., 2021). Similarly, Stk25-ASO modified by GalNAc can ameliorate steatosis by regulating the expression of lipid synthesis genes, and reducing the infiltration of macrophages to improve hepatic steatohepatitis (Nuñez-Durán et al., 2018; Cansby et al., 2019b).
MicroRNAs (miRNAs) are small non-coding RNAs that regulate gene expression at the post-transcriptional level by inhibiting mRNA translation or promoting mRNA degradation (Correia de Sousa et al., 2019). A total of 23 miRNAs were observed to be underexpressed or overexpressed in NASH (Cheung et al., 2008). Treatment with miRNA-21-ASO improves steatosis by increasing the expression of its target gene HBP1, a transcriptional activator of p53, which then blocks downstream lipid biosynthetic genes (Wu et al., 2016a).
In addition to reducing lipid synthesis, increasing fatty acid oxidation also alleviates fatty liver. Acc-ASO, fibroblastic growth factor receptor 4 (Fgfr4)-ASO and prorenin receptor ([P]rr)-ASO can ameliorate hepatic steatosis by regulating fatty acid oxidation and lipid synthesis (Savage et al., 2006; Yu et al., 2013; Ren et al., 2018). In addition, hydroxysteroid 11-beta dehydrogenase 1 (Hsd11β1)-ASO decreases de novo lipid synthesis while increasing fatty acid oxidation (Li et al., 2011a). Treatment with Scd1-ASO not only reduces the expression of genes related to lipid synthesis (FAS, ACC1, Srebp-1) but also increases the expression of genes (such as carnitine palmitoyltransferase 1, cpt-1) related to fatty acid oxidation (Jiang et al., 2005). Treatment with ApoB-ASO increases endoplasmic reticulum stress and autophagy, resulting in increased fatty acid oxidation (Conlon et al., 2016). In sum, all three treatments ameliorated hepatic steatosis.
The intercommunication between adipose tissue and liver tissue is critical for maintaining proper physiological homeostasis. In mice, treatment with Angptl8-ASO increases the amount of lipid absorption and prevents the ectopic accumulation of fat in the liver, thus improving liver steatosis (Vatner et al., 2018). Application of Mat1a-ASO promotes brown adipose tissue (BAT) thermogenesis and improves fatty liver in both ob/ob and high-fat diet (HFD) induced mice (Sáenz de Urturi et al., 2022). Fatty acid binding protein (FABP) 3 has been shown to be involved in HFD-induced liver steatosis in zebrafish, and Fabp3-ASO reduces liver lipid accumulation (Shimada et al., 2015). Taken all together, ASOs can prevent fatty liver by regulating hepatic lipid metabolism, the crosstalk between adipose tissue and the liver, and other processes (Figure 2).
3.2 Application of antisense oligonucleotide in hepatitis
NASH affects the health and quality of life for 3%–5% of the global population (Povsic et al., 2019). Inflammation and apoptosis both accompany hepatitis, alcoholic liver disease, liver fibrosis, and cirrhosis. The most severe forms of inflammation result in extensive hepatocyte death, severe liver damage, and ultimately death, with a severely elevated mortality rate due to the lack of specific treatment. Fortunately, the use of ASO as an alternative therapy has been reported as a viable treatment for hepatitis.
In most cases, inflammation is caused by the activation of T cells and macrophages, which directly affect hepatocytes or secrete pro-inflammatory factors such as interleukins (IL-1, IL-6, IL-12) and tumor necrosis factors (TNF-α) (Martich et al., 1991; Vergani et al., 2002). Tumor necrosis factor receptor-related proteins (Fas and TNFR1, etc.) play an essential role in this process. TNF-α-induced apoptosis is an early result of liver failure and liver injury (Leist et al., 1995; Kawaguchi et al., 1999). Treatment with Tnf-α-ASO (TJU-2755), which is delivered into kupffer cells by galactosylated low molecular weight chitosan, alleviates the symptoms of severe hepatitis in mice (Dong et al., 2009). Moreover, treatment with Tnf-α-ASO also improves lipopolysaccharide (LPS)-induced hepatitis (Mochizuki and Sakurai, 2011). Fas, a TNF receptor family protein, also plays a role in abnormal apoptosis, and treatment with modified-Fas-ASO (Ionis 22023) protects mice from severe hepatitis (Zhang et al., 2000). Among the apoptosis proteins, the Bcl-2 protein family, members of which either inhibit (such as Bcl-2, Bcl-xL, Bcl-w, A1, Mcl-1, and Boo, etc.) or promote (such as Bax, Bak, Bok, Bik, Bad, and Bid, etc.) apoptosis, has been adequately studied. Accordingly, Bcl-xL-ASO (Ionis 16009) increases Fas-induced hepatocyte apoptosis and increases the lethality of severe hepatitis in mice. Conversely, Bid-ASO (Ionis 119935) protects mice from death and also protects mice from Fas-induced hepatocyte apoptosis when both genes are silenced simultaneously (Zhang et al., 2003). T cell-mediated explosive hepatitis is a potentially life-threatening condition. IL-28A (IFN-λ2) plays a regulatory role in explosive hepatitis, and Il-28a-ASO can also reduce T cell-mediated hepatitis in Con A-induced hepatitis (Siebler et al., 2007).
Treatment with Jnk1-ASO can improve hepatitis induced by HFD (Singh et al., 2009). Mitochondrial SH3 homology associated BTK binding protein (SH3BP5, also named SAB), as a JNK target, plays a role in high-fat, high-calorie, high-fructose (HFHC) diet induced NASH. Treatment with Sab-ASO modified by GalNAc reduces inflammatory cell infiltration into the liver, alleviates NASH-related symptoms, reduces collagen deposition and diminishes liver fibrosis-related symptoms (Win et al., 2021). Treatment with Psd3-ASO reduces the hepatic lobule inflammation score, and has a certain remission effect on steatohepatitis in a mice model for NASH (Mancina et al., 2022). STK25 also has a regulatory role in hepatic steatohepatitis. Stk25-ASO reduces macrophage infiltration and improves the condition of hepatitis to a certain extent (Cansby et al., 2019b). Taken altogether, the use of ASOs can ameliorate severe hepatitis by affecting the apoptosis of hepatocytes in steatohepatitis (Figure 2).
3.3 Application of antisense oligonucleotide in liver fibrosis
Liver fibrosis is a wound healing response characterized by excessive deposition of extracellular matrix proteins, including collagen, in the liver. The activation of hepatic stellate cells (HSCs) is a marker of liver fibrosis. Fibrosis is an advanced stage in the progression of various chronic liver diseases to cirrhosis and cancer. When effectively treated at the fibrosis stage, chronic liver disease can still be prevented from becoming a more severe instance. Therefore, it is particularly critical to develop therapeutic ASO drugs for this phase.
Myriad cellular regulators contribute to the process of liver fibrosis. Matrix metalloproteinases (MMPs) are the main components of hydrolyzing the extracellular matrix. Their activities are regulated by matrix metalloproteinase inhibitors (TIMPs). The role of TIMPs in liver fibrosis has long been studied and TIMP-1 and TIMP-2 have been identified as being the most prominent. Treatment with Timp-1 and Timp-2-ASO reduce their mRNA levels as well as collagen accumulation, and consequently improve liver pathology and liver function (Nie et al., 2001; Nie et al., 2010). Heat shock protein 47 (HSP47) is a specific molecular chaperone necessary for collagen synthesis, and its expression increases in parallel with the mRNA level of procollagen during liver fibrogenesis (Masuda et al., 1994). Based on this observation, Brown et al. (2005) used Hsp47-ASO to inhibit HSP47 expression, and found that this could reduce procollagen synthesis. This indicates that Hsp47-ASO can be used as an anti-fibrotic treatment (Brown et al., 2005). TGF-β2 is a member of the TGF-β family and plays a role in liver fibrosis, and Tgf-β2-ASO decreases collagen deposition to alleviate liver fibrosis (Dropmann et al., 2020).
Aberrant Notch activity in hepatocytes has been shown to be crucial for the development of NASH-induced liver fibrosis, and increased expression of the Notch ligand Jag1 has been closely associated with Notch activation in human liver biopsies and in mouse NASH models. In mice, treatment with Jag1-ASO alleviates NASH diet-induced liver fibrosis (Yu et al., 2021). IL-4Rα is activated by the regulation of IL-4 and IL-13, and is the central switch in the transition of M1 macrophages into the M2 type. IL-4Rα activation regulates the functional polarization of macrophages and promotes the development of fibrosis. Treatment with Il-4rα-ASO is effective in attenuating liver fibrosis (Weng et al., 2018).
Targeting HSCs is a modern perspective regarding the treatment of liver fibrosis. Periostin is a matrix-secreting protein with multiple roles in tissue development and regeneration. It activates HSCs by interacting with the αv integrin, and reduces hepatic fibrosis in hepatotoxicity and cholestatic liver injury when it is absent (Lu et al., 2014; Sugiyama et al., 2016). Treatment with Periostin-ASO reduces liver fibrosis in mice, which is reflected in the reduction of the alpha-smooth muscle actin (α-SMA), collagen type I, and other fibrotic markers (Kobayashi et al., 2020). The signal transducer, activator of transcription 3 (STAT3), drives the activation of HSCs (Xiang et al., 2018). Targeting STAT3 in HSCs by using exosomes to carry the Stat3-ASO significantly reduces the level of STAT3 mRNA, thereby inhibiting the activation of HSCs and thus improving liver fibrosis (Tang et al., 2021). It has been shown that the apoptosis of HSCs is related to the regression of hepatic fibrosis (De Minicis et al., 2012). Remarkably, treatment with a splicing ASO against Bcl-x transforms anti-apoptotic Bcl-xL to pro-apoptotic Bcl-xS, thus inducing apoptosis of HSCs and improving liver fibrosis (Wu et al., 2016b).
The production of retinol esters (RE), which is catalyzed by lecithin-retinol acyltransferase (LRAT) and acyl-CoA retinol acyltransferase (ARAT), disappears in HSC activation and liver fibrosis. DGAT1, an enzyme that catalyzes the last step of triglyceride biosynthesis, exhibits additional ARAT activity in vitro. Treatment with Dgat1-ASO inhibits the activation of HSCs in mice and rats, increases the expression of LRAT, promotes the accumulation of RE in the liver, and alleviates liver fibrosis (Yamaguchi et al., 2008).
Taken together, ASOs can ameliorate liver fibrosis by reducing the synthesis of ECM protein components, increasing their degradation, affecting HSCs activation, regulating lipid synthesis in HSCs, as well as by regulating inflammation (Figure 2).
3.4 Application of antisense oligonucleotide in liver cancer
Liver cancer is a global health challenge with increasing incidence worldwide (Villanueva, 2019). It is estimated that by 2025, one million people will develop liver cancer each year. Liver cancer mainly consists of primary liver cancer and secondary liver cancer. Primary liver cancers include hepatocellular carcinoma (HCC) and intrahepatic cholangiocarcinoma, as well as other fewer common types. Among various cancers, HCC is the most common aggressive malignancy in the world. Regarding the treatment of liver cancer, there are currently no interventions to prevent the development of HCC in high-risk patients, and the effectiveness of different treatment options may be limited due to genetic variability among patients. Therefore, it is necessary to find a personalized treatment plan, and ASO drugs provide excellent potential options.
The application of ASO in liver cancer has been explored for a long time. STAT3 is a convergence point of numerous oncogenic signals. Treatment with Stat3-ASO reduces the circulating vascular endothelial growth factor, basic fibroblast growth factor, tumor volume and weight in HCC, and also prolongs survival in mice (Li et al., 2006). In the process of cancer treatment, radiotherapy is a commonly used approach. However, during radiotherapy, radiation usually causes the apoptosis of hepatocytes and subsequent liver damage, which limits the efficacy of this treatment (Terashima et al., 1995). TNFR1 is an influential factor in the apoptosis signaling pathway. Treatment with Tnfr1-ASO before radiation reduced radiation-induced apoptosis in hepatocytes as well as micronucleus formation (Huang et al., 2006).
Telomerase is a unique ribonucleoprotein complex that plays an important role in cell immortality and tumorigenesis (Holt and Shay, 1999). Studies have shown that telomerase is activated in human tumors, but not in adjacent normal cells (Shay and Bacchetti, 1997), suggesting that telomerase is a target for cancer diagnosis and treatment. Human telomerase reverse transcriptase (h-TERT) is the catalytic subunit of a telomerase. Studies have found that 90% of examined tumors show a relation between telomerase activity and the expression of the activity-limiting component h-TERT (Counter et al., 1998; Yang et al., 2002). Cantide, the h-TERT antisense nucleic acid drug, inhibits tumor cell growth and reduces h-TERT mRNA expression and telomerase activity (Du et al., 2003). Cantide also significantly inhibits the growth of in situ tumors and prevents intrahepatic tumor recurrence and lung metastasis (Lin et al., 2005). In addition, treatment with Tert-ASO also inhibits the invasion and tumor progression of liver cancer caused by TERT activation (Ningarhari et al., 2021). Promoting apoptosis of tumor cells is an important strategy in the treatment of liver cancer. PKC-α is highly expressed in rat hepatoma cells, and treatment with Pkc-α-ASO reduces the expression of anti-apoptotic protein Bcl-2, promotes cell apoptosis, and inhibits the growth of tumor cells (Lin et al., 2000). Mcl-1 and survivin are members of the class of anti-apoptotic proteins associated with cell apoptosis and the cell cycle, and are highly expressed in malignant cells. Treatment with Mcl-1-ASO and Survivin-ASO (LY218308) promote hepatoma carcinoma cell apoptosis (Sieghart et al., 2006; He et al., 2007; Carrasco et al., 2011; Dai et al., 2012; Wang et al., 2021). Heparin binding factor midkine (MK) is also highly expressed in a variety of malignant tumors and treatment with Mk-ASO inhibits the proliferation of tumor cells, reduced the expression of anti-apoptotic proteins survivin and Bcl-2, and promoted apoptosis in tumor cells (Dai et al., 2006; Dai et al., 2007; Dai et al., 2009). Furthermore, antisense therapy using Hif-1α (hypoxia-inducible factor-1), or Ae-2 (anion exchanger 2) has also been found to induce apoptosis (WeiXing et al., 2008; Hwang et al., 2009).
Inhibiting the migration and invasion of tumor cells is critical to stopping the spread of cancer. The ETs protein family of transcription factors regulates the expression of oncogenes and tumor suppressor genes, and plays an important role in the proliferation, survival, and differentiation of normal cells (Oikawa, 2004). ETs-like transcription factor 1 (Elk-1) is a nuclear transcriptional co-activator regulating the expression of ETs protein family genes, and the activation of Elk-1 is necessary for tumor progression (Treisman, 1992; Price et al., 1995; Xiao et al., 2002). Inhibiting the expression of Elk-1 reduces the expression of PKC-α, as well as tumor migration and invasion in HCC (Hsieh et al., 2006). Transfection of Elk-1-ASO reduces cell proliferation and inhibits tumor growth in mice (Ying et al., 2008).
CD44 is one of the essential tumor-initiation cell markers in HCC (Yang et al., 2008; Zhu et al., 2010) and it is involved in regulating the invasion and migration of HCC cells. miR-221 regulates the expression of CD44 in HCC, and treatment with miR-221-ASO downregulates CD44 protein expression (Kim et al., 2017). Osteopontin (OPN) is associated with liver cancer metastasis and invasion, and treatment with Opn-ASO inhibits liver cancer metastasis (Chen et al., 2011). In addition, treatment with miR-96-ASO decreases the expression of OPN, thus preventing the invasion of liver tumors (Chen et al., 2012). MYC promotes tumorigenesis by inducing proliferation, blocking differentiation, and promoting self-renewal (Dang, 2012; Gabay et al., 2014). Thus, treatment with Myc-ASO reduces cell proliferation and induces apoptosis, thus reducing tumor growth and invasion (Li et al., 2011b; Yuan et al., 2014; Dhanasekaran et al., 2020). ADP-ribosylation factor-like 4C (ARL4C) is a member of the small GTP-binding protein family and plays a role in tumor genesis and development. Treatment with Arl4c-ASO inhibits the proliferation and metastasis of primary and metastatic liver tumors (Harada et al., 2019). Long non-coding RNA-HLNC1 antisense therapy inhibits tumor growth (Qian et al., 2020), and HIF-1α, c-raf.1 antisense therapy inhibits cell proliferation (WeiXing et al., 2008; Das et al., 2010).
Immune escape also plays an essential role in the development of tumors. The heterogeneous nuclear ribonucleoprotein M (HNRNPM) is involved in tumor immune escape and is elevated in liver cancer. Treatment with Hnrnpm-ASO promotes activation of CD8+ T cells, thus blocking immune escape and improving tumor treatment (Zhu et al., 2022). Metadherin (MTDH) is expressed in a variety of malignancies (Hu et al., 2009; Yang et al., 2017), and treatment with Mtdh-ASO increases CD8+ T cells and enhances tumor immunity (Wan et al., 2022). MYC oncogenes also promote tumor formation by inducing immune escape, and treatment with Myc-ASO increases the number of CD4+ T cells and reshapes the tumor microenvironment (Dhanasekaran et al., 2020).
Cell dedifferentiation is also a hallmark of liver cancer. Dedifferentiation processes in cancer are pivotal for the generation and expansion of cancer stem cells, which play a role in cancer cell renewal and metastatic potential, and correlate with the level of aggressiveness and drug resistance of the tumor (Nikolaou et al., 2015; Atashzar et al., 2020; Schulte et al., 2020). The oncogenic function of suppressor of variegation, enhancer of zeste, and Myeloid-Nervy-DEAF1-domain family methyltransferase Smyd3 has been implicated in various malignancies, including HCC. Treatment with Smyd3-ASO blocks the cell dedifferentiation process and reduces the burden of DEN-induced liver cancer (Kontaki et al., 2021).
Secondary liver cancer is due to liver metastasis caused by other cancers, a process in which tumor cells from other organs migrate to the body and colonize new locations, including adenocarcinoma liver metastasis, colorectal liver metastasis, etc. The expression of CD10 has been correlated with liver metastasis of colorectal cancer (Fujimoto et al., 2005; Fujita et al., 2007) and treatment with Cd10-ASO inhibits CD10 expression and reduces the number and size of liver metastases in nude mice (Luo et al., 2009).
In summary, ASO can regulate the proliferation, migration, and invasion of tumor cells, as well as regulate immunity and liver metastasis by affecting multiple genes and proteins, and may also improve therapeutic effects of radiotherapy by mitigating side effects during the treatment process (Figure 2).
4 Challenges and perspectives of antisense oligonucleotide in liver diseases
Liver diseases are rapidly becoming widespread, and their treatment is a clinical challenge. The use of ASO, as a potential therapeutic for liver diseases, has attracted great attention in recent years. ASO has superior tissue distribution in the liver after intraperitoneal and subcutaneous administration (Geary et al., 2015). The use of GalNAc-coupled ASO enables the targeting of ASO in the liver, which is also a major advance in the field of ASO delivery. Thus, the concentration and persistence of ASO in the liver after treatment produces a high tissue distribution, giving ASO potential applications in both the early and late stages of liver-related diseases.
Despite these positive outlooks, the withdrawal of Fomivirsen and Mipomersen from the market indicates the potential for drug toxicity and side effects in ASO drugs. Therefore, several issues must be kept in mind when developing ASO drugs for liver diseases. First, ASO-mediated hepatotoxicity is a primary challenge. Studies have shown that locked nucleic acid modification can enhance the biological effect of the ASO, but can also bring about significant hepatotoxicity (Swayze et al., 2007; Burdick et al., 2014; Kasuya et al., 2016). In addition, GAP-modified ASO (Gapmer) has also been found to cause severe hepatotoxicity due to the RNA off-target cleavage effect (Kasuya and Kugimiya, 2018). Second, nephrotoxicity was also found in the use of ASO (Geary et al., 2015; Moisan et al., 2017). Fortunately, GalNAc-coupled ASO shows reduced nephrotoxicity (Sewing et al., 2019). In addition, the double coupling of cholesterol and GalNAc reduced the distribution of ASO in the kidney, which also lowered nephrotoxicity (Wada et al., 2018). The third issue we need to be mindful of is that ASO is readily degraded in plasma and is prone to off-target effects (Kulkarni et al., 2021). By constructing liver-specific or whole-body monoacylglycerol acyltransferase 1 (Mogat1) knockout mice, Lutkewitte et al. (2021) found that Mogat1 knockout did not affect glucose tolerance, but Mogat1-ASO treatment could improve glucose tolerance in wild-type and Mogat1 knockout mice, suggesting that Mogat1-ASO treatment had an off-target effect. Meanwhile, hybridization-dependent toxicity also mediates off-target effects in splice-switching ASOs (Scharner et al., 2020). When the ASO binds to the RNA, RNase H recognizes the presence of the DNA-RNA duplex and then degrades the RNA strand. The cleavage requires merely five continuous base pairs (Wu et al., 1999), which can result in the knock-down of partially hybridized targets. The presence of locked nucleic acid and restricted ethyl nucleotide modifications in Gapmers is also prone to an off-target effect (Kamola et al., 2015; Hagedorn et al., 2017). Luckily, this effect can be reduced by extending the length of Gapmers (Yasuhara et al., 2022). Although spatial blocking oligonucleotides have the potential to bind to nearby complementary sites in the transcriptome, leading to off-target effects (Holgersen et al., 2021), this property can be avoided by RNA-Seq experimental screening (Holgersen et al., 2021). Moreover, off-target effects can be reduced during the design of ASOs. As genome research expands and continues to grow, as bioinformatics continues to improve and information technology platforms continue to evolve, target genes can be more accurately modeled in the preliminary design process for ASOs. With the aid of such information, unwanted toxic reactions due to off-target effects can be minimized.
Finally, Defibrotide was the first ASO drug approved for the treatment of VOD (Choy, 2016). There are also several ongoing clinical trials of antisense oligonucleotides for liver disease. One such is bepirovirsen, a second-generation 2′-O-methoxyethyl (MOE)-modified antisense phosphorothioate oligonucleotide (ClinicalTrials.gov NCT02981602) (Yuen et al., 2021), another is GSK3389404, which is formed by covalent binding of bepirovirsen to three GalNAcs (ClinicalTrials.gov NCT03020745) (Yuen et al., 2022), and finally, RO7062931, a GalNAc conjugated single stranded locked nucleic acid oligonucleotide (ClinicalTrials.gov NCT03038113) (Gane et al., 2021) for the treatment of HBV. In addition, the aforementioned DGAT2 and its corresponding antisense drug IONIS-DGAT2RX (ClinicalTrials.gov NCT03334214) are currently undergoing phase 2 clinical trials for the treatment of NASH (Loomba et al., 2020). While all relevant applications of ASOs to liver disease reviewed in this paper are from animal model systems, various human cell lines have been used to investigate the application of human ASOs in these animal models. An example is the human-derived cells used in the ectopic naked mouse model in Section 3.4 to study liver cancer. In summary, the development of ASO drugs for the treatment of liver diseases has great potential. There is an urgent need to move ASOs from the animal level into clinical trials.
5 Summary
Liver-related diseases are a global epidemic and one of the leading causes of death. The discovery and development of drugs for liver-related diseases is extremely urgent. ASO, as a precise targeted drug, has great potential for the treatment of liver-related diseases.
Author contributions
KL offered ideas, KL and QF conceived and wrote the original draft. XZ provided advice and supervision, and finally revised the manuscript. All authors contributed to the article and approved the submitted version.
Funding
This work was supported by the National Natural Science Foundation of China (81700520, U1702288, 31671230, 91857113, 31860323), the Ministry of Science and Technology of the People’s Republic of China (2018YFA0800700), the Yunnan Applied Basic Research Projects (2017FA007, 2018FB117, 2019FY003021).
Conflict of interest
The authors declare that the research was conducted in the absence of any commercial or financial relationships that could be construed as a potential conflict of interest.
Publisher’s note
All claims expressed in this article are solely those of the authors and do not necessarily represent those of their affiliated organizations, or those of the publisher, the editors and the reviewers. Any product that may be evaluated in this article, or claim that may be made by its manufacturer, is not guaranteed or endorsed by the publisher.
References
Alhamadani, F., Zhang, K., Parikh, R., Wu, H., Rasmussen, T. P., Bahal, R., et al. (2022). Adverse drug reactions and toxicity of the food and drug administration-approved antisense oligonucleotide drugs. Drug Metab. Dispos. 50 (6), 879–887. doi:10.1124/dmd.121.000418
Andronescu, M., Zhang, Z. C., and Condon, A. (2005). Secondary structure prediction of interacting RNA molecules. J. Mol. Biol. 345 (5), 987–1001. doi:10.1016/j.jmb.2004.10.082
Anstee, Q. M., Reeves, H. L., Kotsiliti, E., Govaere, O., and Heikenwalder, M. (2019). From NASH to HCC: Current concepts and future challenges. Nat. Rev. Gastroenterol. Hepatol. 16 (7), 411–428. doi:10.1038/s41575-019-0145-7
Atashzar, M. R., Baharlou, R., Karami, J., Abdollahi, H., Rezaei, R., Pourramezan, F., et al. (2020). Cancer stem cells: A review from origin to therapeutic implications. J. Cell. Physiol. 235 (2), 790–803. doi:10.1002/jcp.29044
Brasaemle, D. L. (2007). Thematic review series: Adipocyte biology. The perilipin family of structural lipid droplet proteins: Stabilization of lipid droplets and control of lipolysis. J. Lipid Res. 48 (12), 2547–2559. doi:10.1194/jlr.R700014-JLR200
Brown, D. G., and Wobst, H. J. (2021). A decade of FDA-approved drugs (2010-2019): Trends and future directions. J. Med. Chem. 64 (5), 2312–2338. doi:10.1021/acs.jmedchem.0c01516
Brown, K. E., Broadhurst, K. A., Mathahs, M. M., Brunt, E. M., and Schmidt, W. N. (2005). Expression of HSP47, a collagen-specific chaperone, in normal and diseased human liver. Lab. Invest. 85 (6), 789–797. doi:10.1038/labinvest.3700271
Buers, I., Robenek, H., Lorkowski, S., Nitschke, Y., Severs, N. J., and Hofnagel, O. (2009). TIP47, a lipid cargo protein involved in macrophage triglyceride metabolism. Arterioscler. Thromb. Vasc. Biol. 29 (5), 767–773. doi:10.1161/ATVBAHA.108.182675
Burdick, A. D., Sciabola, S., Mantena, S. R., Hollingshead, B. D., Stanton, R., Warneke, J. A., et al. (2014). Sequence motifs associated with hepatotoxicity of locked nucleic acid-modified antisense oligonucleotides. Nucleic Acids Res. 42 (8), 4882–4891. doi:10.1093/nar/gku142
Burel, S. A., Hart, C. E., Cauntay, P., Hsiao, J., Machemer, T., Katz, M., et al. (2016). Hepatotoxicity of high affinity gapmer antisense oligonucleotides is mediated by RNase H1 dependent promiscuous reduction of very long pre-mRNA transcripts. Nucleic Acids Res. 44 (5), 2093–2109. doi:10.1093/nar/gkv1210
Cai, C., Xie, Y., Wu, L., Chen, X., Liu, H., Zhou, Y., et al. (2017). Author correction: PLGA-based dual targeted nanoparticles enhance miRNA transfection efficiency in hepatic carcinoma. Sci. Rep. 7, 6913. doi:10.1038/s41598-020-63754-6
Cansby, E., Kulkarni, N. M., Magnusson, E., Kurhe, Y., Amrutkar, M., Nerstedt, A., et al. (2019). Protein kinase MST3 modulates lipid homeostasis in hepatocytes and correlates with nonalcoholic steatohepatitis in humans. Faseb J. 33 (9), 9974–9989. doi:10.1096/fj.201900356RR
Cansby, E., Nunez-Duran, E., Magnusson, E., Amrutkar, M., Booten, S. L., Kulkarni, N. M., et al. (2019). Targeted delivery of Stk25 antisense oligonucleotides to hepatocytes protects mice against nonalcoholic fatty liver disease. Cell. Mol. Gastroenterol. Hepatol. 7 (3), 597–618. doi:10.1016/j.jcmgh.2018.12.004
Caputo, M., Kurhe, Y., Kumari, S., Cansby, E., Amrutkar, M., Scandalis, E., et al. (2021). Silencing of STE20-type kinase MST3 in mice with antisense oligonucleotide treatment ameliorates diet-induced nonalcoholic fatty liver disease. Faseb J. 35 (5), e21567. doi:10.1096/fj.202002671RR
Carr, R. M., Patel, R. T., Rao, V., Dhir, R., Graham, M. J., Crooke, R. M., et al. (2012). Reduction of TIP47 improves hepatic steatosis and glucose homeostasis in mice. Am. J. Physiol. Regul. Integr. Comp. Physiol. 302 (8), R996–R1003. doi:10.1152/ajpregu.00177.2011
Carrasco, R. A., Stamm, N. B., Marcusson, E., Sandusky, G., Iversen, P., and Patel, B. K. R. (2011). Antisense inhibition of survivin expression as a cancer therapeutic. Mol. Cancer Ther. 10 (2), 221–232. doi:10.1158/1535-7163.MCT-10-0756
Cerritelli, S. M., Crouch, R. J., and Ribonuclease, H. (2009). Ribonuclease H: The enzymes in eukaryotes. Febs J. 276 (6), 1494–1505. doi:10.1111/j.1742-4658.2009.06908.x
Chan, J. H., Lim, S., and Wong, W. S. (2006). Antisense oligonucleotides: From design to therapeutic application. Clin. Exp. Pharmacol. Physiol. 33 (5-6), 533–540. doi:10.1111/j.1440-1681.2006.04403.x
Chen, R. X., Xia, Y. H., Xue, T. C., and Ye, S. L. (2012). Suppression of microRNA-96 expression inhibits the invasion of hepatocellular carcinoma cells. Mol. Med. Rep. 5 (3), 800–804. doi:10.3892/mmr.2011.695
Chen, R. X., Xia, Y. H., Xue, T. C., Zhang, H., and Ye, S. L. (2011). Down-regulation of osteopontin inhibits metastasis of hepatocellular carcinoma cells via a mechanism involving MMP-2 and uPA. Oncol. Rep. 25 (3), 803–808. doi:10.3892/or.2010.1116
Cheung, O., Puri, P., Eicken, C., Contos, M. J., Mirshahi, F., Maher, J. W., et al. (2008). Nonalcoholic steatohepatitis is associated with altered hepatic MicroRNA expression. Hepatology 48 (6), 1810–1820. doi:10.1002/hep.22569
Choi, C. S., Savage, D. B., Kulkarni, A., Yu, X. X., Liu, Z. X., Morino, K., et al. (2007). Suppression of diacylglycerol acyltransferase-2 (DGAT2), but not DGAT1, with antisense oligonucleotides reverses diet-induced hepatic steatosis and insulin resistance. J. Biol. Chem. 282 (31), 22678–22688. doi:10.1074/jbc.M704213200
Conlon, D. M., Thomas, T., Fedotova, T., Hernandez-Ono, A., Di Paolo, G., Chan, R. B., et al. (2016). Inhibition of apolipoprotein B synthesis stimulates endoplasmic reticulum autophagy that prevents steatosis. J. Clin. Invest. 126 (10), 3852–3867. doi:10.1172/JCI86028
Correia de Sousa, M., Gjorgjieva, M., Dolicka, D., Sobolewski, C., and Foti, M. (2019). Deciphering miRNAs' action through miRNA editing. Int. J. Mol. Sci. 20 (24), 6249. doi:10.3390/ijms20246249
Counter, C. M., MeyersonM., , Eaton, E. N., Ellisen, L. W., Caddle, S. D., Haber, D. A., et al. (1998). Telomerase activity is restored in human cells by ectopic expression of hTERT (hEST2), the catalytic subunit of telomerase. Oncogene 16 (9), 1217–1222. doi:10.1038/sj.onc.1201882
Crooke, S. T., Baker, B. F., Crooke, R. M., and Liang, X. H. (2021). Antisense technology: An overview and prospectus. Nat. Rev. Drug Discov. 20 (6), 427–453. doi:10.1038/s41573-021-00162-z
Crooke, S. T., Baker, B. F., Xia, S., Yu, R. Z., Viney, N. J., Wang, Y., et al. (2019). Integrated assessment of the clinical performance of GalNAc(3)-conjugated 2'-O-methoxyethyl chimeric antisense oligonucleotides: I. Human volunteer experience. Nucleic Acid. Ther. 29 (1), 16–32. doi:10.1089/nat.2018.0753
Crooke, S. T. (2004). Progress in antisense technology. Annu. Rev. Med. 55, 61–95. doi:10.1146/annurev.med.55.091902.104408
Crooke, S. T., Witztum, J. L., Bennett, C. F., and Baker, B. F. (2018). RNA-targeted therapeutics. Cell Metab. 27 (4), 714–739. doi:10.1016/j.cmet.2018.03.004
Dai, D., Liang, Y., Xie, Z., Fu, J., Zhang, Y., and Zhang, Z. (2012). Survivin deficiency induces apoptosis and cell cycle arrest in HepG2 hepatocellular carcinoma cells. Oncol. Rep. 27 (3), 621–627. doi:10.3892/or.2011.1544
Dai, L. C., Wang, X., Yao, X., Lu, Y. l., Ping, J. l., and He, J. f. (2006). Antisense oligonucleotides targeting midkine induced apoptosis and increased chemosensitivity in hepatocellular carcinoma cells. Acta Pharmacol. Sin. 27 (12), 1630–1636. doi:10.1111/j.1745-7254.2006.00459.x
Dai, L. C., Wang, X., Yao, X., Lu, Y. L., Ping, J. L., and He, J. F. (2007). Enhanced therapeutic effects of combined chemotherapeutic drugs and midkine antisense oligonucleotides for hepatocellular carcinoma. World J. Gastroenterol. 13 (13), 1989–1994. doi:10.3748/wjg.v13.i13.1989
Dai, L. C., Yao, X., Wang, X., Niu, S. Q., Zhou, L. F., Fu, F. F., et al. (2009). In vitro and in vivo suppression of hepatocellular carcinoma growth by midkine-antisense oligonucleotide-loaded nanoparticles. World J. Gastroenterol. 15 (16), 1966–1972. doi:10.3748/wjg.15.1966
Danhier, F., Ansorena, E., Silva, J. M., Coco, R., Le Breton, A., and Preat, V. (2012). PLGA-Based nanoparticles: An overview of biomedical applications. J. Control. Release 161 (2), 505–522. doi:10.1016/j.jconrel.2012.01.043
Das, T., Patra, F., and Mukherjee, B. (2010). Effect of antisense oligomer in controlling c-raf.1 overexpression during diethylnitrosamine-induced hepatocarcinogenesis in rat. Cancer Chemother. Pharmacol. 65 (2), 309–318. doi:10.1007/s00280-009-1035-4
De Minicis, S., Candelaresi, C., Agostinelli, L., Taffetani, S., Saccomanno, S., Rychlicki, C., et al. (2012). Endoplasmic Reticulum stress induces hepatic stellate cell apoptosis and contributes to fibrosis resolution. Liver Int. 32 (10), 1574–1584. doi:10.1111/j.1478-3231.2012.02860.x
Debacker, A. J., Voutila, J., Catley, M., Blakey, D., and Habib, N. (2020). Delivery of oligonucleotides to the liver with GalNAc: From research to registered therapeutic drug. Mol. Ther. 28 (8), 1759–1771. doi:10.1016/j.ymthe.2020.06.015
Deleavey, G. F., and Damha, M. J. (2012). Designing chemically modified oligonucleotides for targeted gene silencing. Chem. Biol. 19 (8), 937–954. doi:10.1016/j.chembiol.2012.07.011
Dhanasekaran, R., Park, J., Yevtodiyenko, A., Bellovin, D. I., Adam, S. J., Kd, A. R., et al. (2020). MYC ASO impedes tumorigenesis and elicits oncogene addiction in autochthonous transgenic mouse models of HCC and RCC. Mol. Ther. Nucleic Acids 21, 850–859. doi:10.1016/j.omtn.2020.07.008
Dhillon, S. (2020). Viltolarsen: First approval. Drugs 80 (10), 1027–1031. doi:10.1007/s40265-020-01339-3
Dhuri, K., Bechtold, C., Quijano, E., Pham, H., Gupta, A., Vikram, A., et al. (2020). Antisense oligonucleotides: An emerging area in drug discovery and development. J. Clin. Med. 9 (6), 2004. doi:10.3390/jcm9062004
Ding, Y., Chan, C. Y., and Lawrence, C. E. (2004). Sfold web server for statistical folding and rational design of nucleic acids. Nucleic Acids Res. 32, W135–W141. (Web Server issue). doi:10.1093/nar/gkh449
Dong, L., Zuo, L., Xia, S., Gao, S., Zhang, C., Chen, J., et al. (2009). Reduction of liver tumor necrosis factor-alpha expression by targeting delivery of antisense oligonucleotides into Kupffer cells protects rats from fulminant hepatitis. J. Gene Med. 11 (3), 229–239. doi:10.1002/jgm.1293
Dowell, R. D., and Eddy, S. R. (2004). Evaluation of several lightweight stochastic context-free grammars for RNA secondary structure prediction. BMC Bioinforma. 5, 71. doi:10.1186/1471-2105-5-71
Dropmann, A., Dooley, S., Dewidar, B., Hammad, S., Dediulia, T., Werle, J., et al. (2020). TGF-β2 silencing to target biliary-derived liver diseases. Gut 69 (9), 1677–1690. doi:10.1136/gutjnl-2019-319091
Du, Q. Y., Wang, X. B., Chen, X. J., Zheng, W., and Wang, S. Q. (2003). Antitumor mechanism of antisense cantide targeting human telomerase reverse transcriptase. World J. Gastroenterol. 9 (9), 2030–2035. doi:10.3748/wjg.v9.i9.2030
Eckstein, F. (2014). Phosphorothioates, essential components of therapeutic oligonucleotides. Nucleic Acid. Ther. 24 (6), 374–387. doi:10.1089/nat.2014.0506
Erion, D. M., Ignatova, I. D., Yonemitsu, S., Nagai, Y., Chatterjee, P., Weismann, D., et al. (2009). Prevention of hepatic steatosis and hepatic insulin resistance by knockdown of cAMP response element-binding protein. Cell Metab. 10 (6), 499–506. doi:10.1016/j.cmet.2009.10.007
Eslam, M., Valenti, L., and Romeo, S. (2018). Genetics and epigenetics of NAFLD and NASH: Clinical impact. J. Hepatol. 68 (2), 268–279. doi:10.1016/j.jhep.2017.09.003
Fluiter, K., Mook, O. R., and Baas, F. (2009). The therapeutic potential of LNA-modified siRNAs: Reduction of off-target effects by chemical modification of the siRNA sequence. Methods Mol. Biol. 487, 189–203. doi:10.1007/978-1-60327-547-7_9
Frazier, K. S. (2015). Antisense oligonucleotide therapies: The promise and the challenges from a toxicologic pathologist's perspective. Toxicol. Pathol. 43 (1), 78–89. doi:10.1177/0192623314551840
Frederico, M. J., Vitto, M. F., Cesconetto, P. A., Engelmann, J., De Souza, D. R., Luz, G., et al. (2011). Short-term inhibition of SREBP-1c expression reverses diet-induced non-alcoholic fatty liver disease in mice. Scand. J. Gastroenterol. 46 (11), 1381–1388. doi:10.3109/00365521.2011.613945
Fujimoto, Y., Nakanishi, Y., Sekine, S., Yoshimura, K., Akasu, T., Moriya, Y., et al. (2005). CD10 expression in colorectal carcinoma correlates with liver metastasis. Dis. Colon Rectum 48 (10), 1883–1889. doi:10.1007/s10350-005-0141-6
Fujita, S., Yamamoto, S., Akasu, T., Moriya, Y., Taniguchi, H., and Shimoda, T. (2007). Quantification of CD10 mRNA in colorectal cancer and relationship between mRNA expression and liver metastasis. Anticancer Res. 27 (5), 3307–3311.
Gabay, M., Li, Y., and Felsher, D. W. (2014). MYC activation is a hallmark of cancer initiation and maintenance. Cold Spring Harb. Perspect. Med. 4 (6), a014241. doi:10.1101/cshperspect.a014241
Gane, E., Yuen, M. F., Kim, D. J., Chan, H. L. Y., Surujbally, B., Pavlovic, V., et al. (2021). Clinical study of single-stranded oligonucleotide RO7062931 in healthy volunteers and patients with chronic hepatitis B. Hepatology 74 (4), 1795–1808. doi:10.1002/hep.31920
Geary, R. S., Norris, D., Yu, R., and Bennett, C. F. (2015). Pharmacokinetics, biodistribution and cell uptake of antisense oligonucleotides. Adv. Drug Deliv. Rev. 87, 46–51. doi:10.1016/j.addr.2015.01.008
Gheibi-Hayat, S. M., and Jamialahmadi, K. (2021). Antisense oligonucleotide (AS-ODN) technology: Principle, mechanism and challenges. Biotechnol. Appl. Biochem. 68 (5), 1086–1094. doi:10.1002/bab.2028
Gleave, M. E., and Monia, B. P. (2005). Antisense therapy for cancer. Nat. Rev. Cancer 5 (6), 468–479. doi:10.1038/nrc1631
Hagedorn, P. H., Hansen, B. R., Koch, T., and Lindow, M. (2017). Managing the sequence-specificity of antisense oligonucleotides in drug discovery. Nucleic Acids Res. 45 (5), 2262–2282. doi:10.1093/nar/gkx056
Hair, P., Cameron, F., and McKeage, K. (2013). Mipomersen sodium: First global approval. Drugs 73 (5), 487–493. doi:10.1007/s40265-013-0042-2
Harada, T., Matsumoto, S., Hirota, S., Kimura, H., Fujii, S., Kasahara, Y., et al. (2019). Chemically modified antisense oligonucleotide against ARL4C inhibits primary and metastatic liver tumor growth. Mol. Cancer Ther. 18 (3), 602–612. doi:10.1158/1535-7163.MCT-18-0824
He, S. Q., Rehman, H., Gong, M. G., Zhao, Y. Z., Huang, Z. Y., Li, C. H., et al. (2007). Inhibiting survivin expression enhances TRAIL-induced tumoricidal activity in human hepatocellular carcinoma via cell cycle arrest. Cancer Biol. Ther. 6 (8), 1247–1257. doi:10.4161/cbt.6.8.4444
Heo, Y. A. (2020). Golodirsen: First approval. Drugs 80 (3), 329–333. doi:10.1007/s40265-020-01267-2
Ho, S. P., Britton, D. H., Stone, B. A., Behrens, D. L., Leffet, L. M., Hobbs, F. W., et al. (1996). Potent antisense oligonucleotides to the human multidrug resistance-1 mRNA are rationally selected by mapping RNA-accessible sites with oligonucleotide libraries. Nucleic Acids Res. 24 (10), 1901–1907. doi:10.1093/nar/24.10.1901
Holgersen, E. M., Gandhi, S., Zhou, Y., Kim, J., Vaz, B., Bogojeski, J., et al. (2021). Transcriptome-wide off-target effects of steric-blocking oligonucleotides. Nucleic Acid. Ther. 31 (6), 392–403. doi:10.1089/nat.2020.0921
Holt, S. E., and Shay, J. W. (1999). Role of telomerase in cellular proliferation and cancer. J. Cell. Physiol. 180 (1), 10–18. doi:10.1002/(SICI)1097-4652(199907)180:1<10::AID-JCP2>3.0.CO;2-D
Hoy, S. M. (2017). Nusinersen: First global approval. Drugs 77 (4), 473–479. doi:10.1007/s40265-017-0711-7
Hsieh, Y. H., Wu, T. T., Tsai, J. H., Huang, C. Y., and Liu, J. Y. (2006). PKCalpha expression regulated by Elk-1 and MZF-1 in human HCC cells. Biochem. Biophys. Res. Commun. 339 (1), 217–225. doi:10.1016/j.bbrc.2005.11.015
Hu, G., Chong, R. A., Yang, Q., Wei, Y., Blanco, M. A., Li, F., et al. (2009). MTDH activation by 8q22 genomic gain promotes chemoresistance and metastasis of poor-prognosis breast cancer. Cancer Cell 15 (1), 9–20. doi:10.1016/j.ccr.2008.11.013
Huang, X. W., Yang, J., Dragovic, A. F., Zhang, H., Lawrence, T. S., and Zhang, M. (2006). Antisense oligonucleotide inhibition of tumor necrosis factor receptor 1 protects the liver from radiation-induced apoptosis. Clin. Cancer Res. 12 (9), 2849–2855. doi:10.1158/1078-0432.CCR-06-0360
Hwang, J. M., Kao, S. H., Hsieh, Y. H., Li, K. L., Wang, P. H., Hsu, L. S., et al. (2009). Reduction of anion exchanger 2 expression induces apoptosis of human hepatocellular carcinoma cells. Mol. Cell. Biochem. 327 (1-2), 135–144. doi:10.1007/s11010-009-0051-3
Imai, Y., Boyle, S., Varela, G. M., Caron, E., Yin, X., Dhir, R., et al. (2012). Effects of perilipin 2 antisense oligonucleotide treatment on hepatic lipid metabolism and gene expression. Physiol. Genomics 44 (22), 1125–1131. doi:10.1152/physiolgenomics.00045.2012
Jiang, G., Li, Z., Liu, F., Ellsworth, K., Dallas-Yang, Q., Wu, M., et al. (2005). Prevention of obesity in mice by antisense oligonucleotide inhibitors of stearoyl-CoA desaturase-1. J. Clin. Invest. 115 (4), 1030–1038. doi:10.1172/JCI23962
Juliano, R. L. (2016). The delivery of therapeutic oligonucleotides. Nucleic Acids Res. 44 (14), 6518–6548. doi:10.1093/nar/gkw236
Kakiuchi-Kiyota, S., Whiteley, L. O., Ryan, A. M., and Mathialagan, N. (2016). Development of a method for profiling protein interactions with LNA-modified antisense oligonucleotides using protein microarrays. Nucleic Acid. Ther. 26 (2), 93–101. doi:10.1089/nat.2015.0576
Kamola, P. J., Kitson, J. D. A., Turner, G., Maratou, K., Eriksson, S., Panjwani, A., et al. (2015). In silico and in vitro evaluation of exonic and intronic off-target effects form a critical element of therapeutic ASO gapmer optimization. Nucleic Acids Res. 43 (18), 8638–8650. doi:10.1093/nar/gkv857
Kamola, P. J., Maratou, K., Wilson, P. A., Rush, K., Mullaney, T., McKevitt, T., et al. (2017). Strategies for in vivo screening and mitigation of hepatotoxicity associated with antisense drugs. Mol. Ther. Nucleic Acids 8, 383–394. doi:10.1016/j.omtn.2017.07.003
Kasuya, T., Hori, S. I., Watanabe, A., Nakajima, M., Gahara, Y., Rokushima, M., et al. (2016). Ribonuclease H1-dependent hepatotoxicity caused by locked nucleic acid-modified gapmer antisense oligonucleotides. Sci. Rep. 6, 30377. doi:10.1038/srep30377
Kasuya, T., and Kugimiya, A. (2018). Role of computationally evaluated target specificity in the hepatotoxicity of gapmer antisense oligonucleotides. Nucleic Acid. Ther. 28 (5), 312–317. doi:10.1089/nat.2018.0724
Kawaguchi, K., Kikuchi, S., Hasegawa, H., Maruyama, H., Morita, H., and Kumazawa, Y. (1999). Suppression of lipopolysaccharide-induced tumor necrosis factor-release and liver injury in mice by naringin. Eur. J. Pharmacol. 368 (2-3), 245–250. doi:10.1016/s0014-2999(98)00867-x
Keam, S. J. (2018). Inotersen: First global approval. Drugs 78 (13), 1371–1376. doi:10.1007/s40265-018-0968-5
Kim, J., Jiang, J., Badawi, M., and Schmittgen, T. D. (2017). miR-221 regulates CD44 in hepatocellular carcinoma through the PI3K-AKT-mTOR pathway. Biochem. Biophys. Res. Commun. 487 (3), 709–715. doi:10.1016/j.bbrc.2017.04.121
Kim, K., Goldberg, I. J., Graham, M. J., Sundaram, M., Bertaggia, E., Lee, S. X., et al. (2018). γ-Secretase inhibition lowers plasma triglyceride-rich lipoproteins by stabilizing the LDL receptor. Cell Metab. 27 (4), 816–827. doi:10.1016/j.cmet.2018.02.010
Kim, K., Yu, J., Kang, J. K., Morrow, J. P., and Pajvani, U. B. (2020). Liver-selective γ-secretase inhibition ameliorates diet-induced hepatic steatosis, dyslipidemia and atherosclerosis. Biochem. Biophys. Res. Commun. 527 (4), 979–984. doi:10.1016/j.bbrc.2020.04.157
Kobayashi, T., Kanno, K., Nguyen, P. T., Sugiyama, A., Kawahara, A., Otani, Y., et al. (2020). Periostin antisense oligonucleotide prevents hepatic steatosis and fibrosis in a mouse model of non-alcoholic steatohepatitis. J. Gastroenterol. Hepatol. 35 (12), 2140–2150. doi:10.1111/jgh.15088
Kontaki, H., Koukaki, M., Vasilarou, M., Giakountis, A., Deligianni, E., Luo, X., et al. (2021). Targeting Smyd3 by next-generation antisense oligonucleotides suppresses liver tumor growth. iScience 24 (5), 102473. doi:10.1016/j.isci.2021.102473
Koot, B. G., van der Baan-Slootweg, O. H., Tamminga-Smeulders, C. L. J., Rijcken, T. H. P., Korevaar, J. C., van Aalderen, W. M., et al. (2011). Lifestyle intervention for non-alcoholic fatty liver disease: Prospective cohort study of its efficacy and factors related to improvement. Arch. Dis. Child. 96 (7), 669–674. doi:10.1136/adc.2010.199760
Kulkarni, J. A., Witzigmann, D., Thomson, S. B., Chen, S., Leavitt, B. R., Cullis, P. R., et al. (2021). The current landscape of nucleic acid therapeutics. Nat. Nanotechnol. 16 (6), 630–643. doi:10.1038/s41565-021-00898-0
Kumashiro, N., Yoshimura, T., Cantley, J. L., Majumdar, S. K., Guebre-Egziabher, F., Kursawe, R., et al. (2013). Role of patatin-like phospholipase domain-containing 3 on lipid-induced hepatic steatosis and insulin resistance in rats. Hepatology 57 (5), 1763–1772. doi:10.1002/hep.26170
Kurreck, J. (2003). Antisense technologies. Improvement through novel chemical modifications. Eur. J. Biochem. 270 (8), 1628–1644. doi:10.1046/j.1432-1033.2003.03555.x
Kurreck, J., Wyszko, E., Gillen, C., and Erdmann, V. A. (2002). Design of antisense oligonucleotides stabilized by locked nucleic acids. Nucleic Acids Res. 30 (9), 1911–1918. doi:10.1093/nar/30.9.1911
Lai, F., Damle, S. S., Ling, K. K., and Rigo, F. (2020). Directed RNase H cleavage of nascent transcripts causes transcription termination. Mol. Cell 77 (5), 1032–1043. doi:10.1016/j.molcel.2019.12.029
Leist, M., GantnerF., , Jilg, S., and Wendel, A. (1995). Activation of the 55 kDa TNF receptor is necessary and sufficient for TNF-induced liver failure, hepatocyte apoptosis, and nitrite release. J. Immunol. 154 (3), 1307–1316.
Li, G., Hernandez-Ono, A., Crooke, R. M., Graham, M. J., and Ginsberg, H. N. (2011). Effects of antisense-mediated inhibition of 11β-hydroxysteroid dehydrogenase type 1 on hepatic lipid metabolism. J. Lipid Res. 52 (5), 971–981. doi:10.1194/jlr.M013748
Li, W. C., Ye, S. L., Sun, R. X., Liu, Y. K., Tang, Z. Y., Kim, Y., et al. (2006). Inhibition of growth and metastasis of human hepatocellular carcinoma by antisense oligonucleotide targeting signal transducer and activator of transcription 3. Clin. Cancer Res. 12 (23), 7140–7148. doi:10.1158/1078-0432.CCR-06-0484
Li, Z. R., Wu, Y. F., Nie, S. D., Mao, X. H., and Shi, Y. Z. (2011). Down-regulation of c-Myc expression inhibits the invasion of bile duct carcinoma cells. Cell Biol. Int. 35 (8), 799–802. doi:10.1042/CBI20110099
Lin, R. X., Tuo, C. w., Lu, Q. j., Zhang, W., and Wang, S. q. (2005). Inhibition of tumor growth and metastasis with antisense oligonucleotides (Cantide) targeting hTERT in an in situ human hepatocellular carcinoma model. Acta Pharmacol. Sin. 26 (6), 762–768. doi:10.1111/j.1745-7254.2005.00762.x
Lin, S. B., Wu, L. C., Huang, S. L., Hsu, H. L., Hsieh, S. H., Chi, C. W., et al. (2000). In vitro and in vivo suppression of growth of rat liver epithelial tumor cells by antisense oligonucleotide against protein kinase C-alpha. J. Hepatol. 33 (4), 601–608. doi:10.1034/j.1600-0641.2000.033004601.x
Lindén, D., Ahnmark, A., Pingitore, P., Ciociola, E., Ahlstedt, I., Andreasson, A. C., et al. (2019). Pnpla3 silencing with antisense oligonucleotides ameliorates nonalcoholic steatohepatitis and fibrosis in Pnpla3 I148M knock-in mice. Mol. Metab. 22, 49–61. doi:10.1016/j.molmet.2019.01.013
Loomba, R., Morgan, E., Watts, L., Xia, S., Hannan, L. A., Geary, R. S., et al. (2020). Novel antisense inhibition of diacylglycerol O-acyltransferase 2 for treatment of non-alcoholic fatty liver disease: A multicentre, double-blind, randomised, placebo-controlled phase 2 trial. Lancet. Gastroenterol. Hepatol. 5 (9), 829–838. doi:10.1016/S2468-1253(20)30186-2
Lu, Y., Liu, X., Jiao, Y., Xiong, X., Wang, E., Wang, X., et al. (2014). Periostin promotes liver steatosis and hypertriglyceridemia through downregulation of PPARα. J. Clin. Invest. 124 (8), 3501–3513. doi:10.1172/JCI74438
Luo, Y., Fujii, K., Ohmori, H., Sasahira, T., Moriwaka, Y., Isobe, M., et al. (2009). Antisense phosphorothioate oligodeoxynucleic acid for CD10 suppresses liver metastasis of colorectal cancer. Pathobiology 76 (5), 267–273. doi:10.1159/000228903
Lutkewitte, A. J., Singer, J. M., Shew, T. M., Martino, M. R., Hall, A. M., He, M., et al. (2021). Multiple antisense oligonucleotides targeted against monoacylglycerol acyltransferase 1 (Mogat1) improve glucose metabolism independently of Mogat1. Mol. Metab. 49, 101204. doi:10.1016/j.molmet.2021.101204
Mahmoodi Chalbatani, G., Dana, H., Gharagouzloo, E., Grijalvo, S., Eritja, R., Logsdon, C. D., et al. (2019). Small interfering RNAs (siRNAs) in cancer therapy: A nano-based approach. Int. J. Nanomedicine 14, 3111–3128. doi:10.2147/IJN.S200253
Mancina, R. M., Sasidharan, K., Lindblom, A., Wei, Y., Ciociola, E., Jamialahmadi, O., et al. (2022). PSD3 downregulation confers protection against fatty liver disease. Nat. Metab. 4 (1), 60–75. doi:10.1038/s42255-021-00518-0
Martich, G. D., Danner, R. L., CeskaM., , and Suffredini, A. F. (1991). Detection of interleukin 8 and tumor necrosis factor in normal humans after intravenous endotoxin: The effect of antiinflammatory agents. J. Exp. Med. 173 (4), 1021–1024. doi:10.1084/jem.173.4.1021
Masuda, H., FukuMotoM., , Hirayoshi, K., and Nagata, K. (1994). Coexpression of the collagen-binding stress protein HSP47 gene and the alpha 1(I) and alpha 1(III) collagen genes in carbon tetrachloride-induced rat liver fibrosis. J. Clin. Invest. 94 (6), 2481–2488. doi:10.1172/JCI117617
Mathews, D. H., Disney, M. D., Childs, J. L., Schroeder, S. J., Zuker, M., and Turner, D. H. (2004). Incorporating chemical modification constraints into a dynamic programming algorithm for prediction of RNA secondary structure. Proc. Natl. Acad. Sci. U. S. A. 101 (19), 7287–7292. doi:10.1073/pnas.0401799101
Mathews, D. H., Turner, D. H., and Watson, R. M. (2016). RNA secondary structure prediction. Curr. Protoc. Nucleic Acid. Chem. 67, 11.2.1–11. doi:10.1002/cpnc.19
Matveeva, O. V., Tsodikov, A. D., GiddingsM., , Freier, S. M., Wyatt, J. R., Spiridonov, A. N., et al. (2000). Identification of sequence motifs in oligonucleotides whose presence is correlated with antisense activity. Nucleic Acids Res. 28 (15), 2862–2865. doi:10.1093/nar/28.15.2862
Melton, D. A. (1985). Injected anti-sense RNAs specifically block messenger RNA translation in vivo. Proc. Natl. Acad. Sci. U. S. A. 82 (1), 144–148. doi:10.1073/pnas.82.1.144
Migliorati, J. M., Liu, S., Liu, A., Gogate, A., Nair, S., Bahal, R., et al. (2022). Absorption, distribution, metabolism, and excretion of US food and drug administration-approved antisense oligonucleotide drugs. Drug Metab. Dispos. 50 (6), 888–897. doi:10.1124/dmd.121.000417
Mochizuki, S., and Sakurai, K. (2011). Dectin-1 targeting delivery of TNF-α antisense ODNs complexed with β-1, 3-glucan protects mice from LPS-induced hepatitis. J. Control. Release 151 (2), 155–161. doi:10.1016/j.jconrel.2011.01.026
Moisan, A., Gubler, M., Zhang, J. D., Tessier, Y., Dumong Erichsen, K., Sewing, S., et al. (2017). Inhibition of EGF uptake by nephrotoxic antisense drugs in vitro and implications for preclinical safety profiling. Mol. Ther. Nucleic Acids 6, 89–105. doi:10.1016/j.omtn.2016.11.006
Nie, Q. H., Cheng, Y. Q., Xie, Y. M., Zhou, Y. X., and Cao, Y. Z. (2001). Inhibiting effect of antisense oligonucleotides phosphorthioate on gene expression of TIMP-1 in rat liver fibrosis. World J. Gastroenterol. 7 (3), 363–369. doi:10.3748/wjg.v7.i3.363
Nie, Q. H., Zhu, C. L., Zhang, Y. F., Yang, J., Zhang, J. C., and Gao, R. T. (2010). Inhibitory effect of antisense oligonucleotide targeting TIMP-2 on immune-induced liver fibrosis. Dig. Dis. Sci. 55 (5), 1286–1295. doi:10.1007/s10620-009-0858-5
Nielsen, P. E., and Pna, Technology. (2004). PNA technology. Mol. Biotechnol. 26 (3), 233–248. doi:10.1385/MB:26:3:233
Nikolaou, K. C., Moulos, P., Chalepakis, G., Hatzis, P., Oda, H., Reinberg, D., et al. (2015). Spontaneous development of hepatocellular carcinoma with cancer stem cell properties in PR-SET7-deficient livers. Embo J. 34 (4), 430–447. doi:10.15252/embj.201489279
Ningarhari, M., Caruso, S., Hirsch, T. Z., Bayard, Q., Franconi, A., Vedie, A. L., et al. (2021). Telomere length is key to hepatocellular carcinoma diversity and telomerase addiction is an actionable therapeutic target. J. Hepatol. 74 (5), 1155–1166. doi:10.1016/j.jhep.2020.11.052
Nuñez-Durán, E., Aghajan, M., Amrutkar, M., Sutt, S., Cansby, E., Booten, S. L., et al. (2018). Serine/threonine protein kinase 25 antisense oligonucleotide treatment reverses glucose intolerance, insulin resistance, and nonalcoholic fatty liver disease in mice. Hepatol. Commun. 2 (1), 69–83. doi:10.1002/hep4.1128
Oikawa, T. (2004). ETS transcription factors: Possible targets for cancer therapy. Cancer Sci. 95 (8), 626–633. doi:10.1111/j.1349-7006.2004.tb03320.x
Opalinska, J. B., and Gewirtz, A. M. (2002). Nucleic-acid therapeutics: Basic principles and recent applications. Nat. Rev. Drug Discov. 1 (7), 503–514. doi:10.1038/nrd837
Pagani, F., and Baralle, F. E. (2004). Genomic variants in exons and introns: Identifying the splicing spoilers. Nat. Rev. Genet. 5 (5), 389–396. doi:10.1038/nrg1327
Paik, J. M., Golabi, P., Younossi, Y., Mishra, A., and Younossi, Z. M. (2020). Changes in the global burden of chronic liver diseases from 2012 to 2017: The growing impact of NAFLD. Hepatology 72 (5), 1605–1616. doi:10.1002/hep.31173
Postic, C., and Girard, J. (2008). Contribution of de novo fatty acid synthesis to hepatic steatosis and insulin resistance: Lessons from genetically engineered mice. J. Clin. Invest. 118 (3), 829–838. doi:10.1172/JCI34275
Povsic, M., Wong, O. Y., Perry, R., and Bottomley, J. (2019). A structured literature review of the epidemiology and disease burden of non-alcoholic steatohepatitis (NASH). Adv. Ther. 36 (7), 1574–1594. doi:10.1007/s12325-019-00960-3
Prakash, T. P., Kawasaki, A. M., Wancewicz, E. V., Shen, L., Monia, B. P., Ross, B. S., et al. (2008). Comparing in vitro and in vivo activity of 2'-O-[2-(methylamino)-2-oxoethyl]- and 2'-O-methoxyethyl-modified antisense oligonucleotides. J. Med. Chem. 51 (9), 2766–2776. doi:10.1021/jm701537z
Price, M. A., Rogers, A. E., and Treisman, R. (1995). Comparative analysis of the ternary complex factors Elk-1, SAP-1a and SAP-2 (ERP/NET). Embo J. 14 (11), 2589–2601. doi:10.1002/j.1460-2075.1995.tb07257.x
Qian, X., Li, S., Yang, Z., and Zhang, J. (2020). The long non-coding RNA HLNC1 potentiates hepatocellular carcinoma progression via interaction with USP49. J. Clin. Lab. Anal. 34 (11), e23462. doi:10.1002/jcla.23462
Ramon-Krauel, M., Salsberg, S. L., Ebbeling, C. B., Voss, S. D., Mulkern, R. V., Apura, M. M., et al. (2013). A low-glycemic-load versus low-fat diet in the treatment of fatty liver in obese children. Child. Obes. 9 (3), 252–260. doi:10.1089/chi.2013.0022
Reinehr, T., Schmidt, C., Toschke, A. M., and Andler, W. (2009). Lifestyle intervention in obese children with non-alcoholic fatty liver disease: 2-year follow-up study. Arch. Dis. Child. 94 (6), 437–442. doi:10.1136/adc.2008.143594
Ren, L., Sun, Y., Lu, H., Ye, D., Han, L., Wang, N., et al. (2018). (Pro)renin receptor inhibition reprograms hepatic lipid metabolism and protects mice from diet-induced obesity and hepatosteatosis. Circ. Res. 122 (5), 730–741. doi:10.1161/CIRCRESAHA.117.312422
Romeo, S., Kozlitina, J., Xing, C., Pertsemlidis, A., Cox, D., Pennacchio, L. A., et al. (2008). Genetic variation in PNPLA3 confers susceptibility to nonalcoholic fatty liver disease. Nat. Genet. 40 (12), 1461–1465. doi:10.1038/ng.257
Sáenz de Urturi, D., Buque, X., Porteiro, B., Folgueira, C., Mora, A., Delgado, T. C., et al. (2022). Methionine adenosyltransferase 1a antisense oligonucleotides activate the liver-brown adipose tissue axis preventing obesity and associated hepatosteatosis. Nat. Commun. 13 (1), 1096. doi:10.1038/s41467-022-28749-z
Savage, D. B., Choi, C. S., Samuel, V. T., Liu, Z. X., Zhang, D., Wang, A., et al. (2006). Reversal of diet-induced hepatic steatosis and hepatic insulin resistance by antisense oligonucleotide inhibitors of acetyl-CoA carboxylases 1 and 2. J. Clin. Invest. 116 (3), 817–824. doi:10.1172/JCI27300
Scharner, J., Ma, W. K., Zhang, Q., Lin, K. T., Rigo, F., Bennett, C. F., et al. (2020). Hybridization-mediated off-target effects of splice-switching antisense oligonucleotides. Nucleic Acids Res. 48 (2), 802–816. doi:10.1093/nar/gkz1132
Schiffelers, R. M., Ansari, A., Xu, J., Zhou, Q., Tang, Q., Storm, G., et al. (2004). Cancer siRNA therapy by tumor selective delivery with ligand-targeted sterically stabilized nanoparticle. Nucleic Acids Res. 32 (19), e149. doi:10.1093/nar/gnh140
Schulte, L. A., Lopez-Gil, J. C., Sainz, B., and Hermann, P. C. (2020). The cancer stem cell in hepatocellular carcinoma. Cancers (Basel) 12 (3), 684. doi:10.3390/cancers12030684
Sewing, S., Gubler, M., Gerard, R., Avignon, B., Mueller, Y., Braendli-Baiocco, A., et al. (2019). GalNAc conjugation attenuates the cytotoxicity of antisense oligonucleotide drugs in renal tubular cells. Mol. Ther. Nucleic Acids 14, 67–79. doi:10.1016/j.omtn.2018.11.005
Shay, J. W., and Bacchetti, S. (1997). A survey of telomerase activity in human cancer. Eur. J. Cancer 33 (5), 787–791. doi:10.1016/S0959-8049(97)00062-2
Shen, W., De Hoyos, C. L., Migawa, M. T., Vickers, T. A., Sun, H., Low, A., et al. (2019). Chemical modification of PS-ASO therapeutics reduces cellular protein-binding and improves the therapeutic index. Nat. Biotechnol. 37 (6), 640–650. doi:10.1038/s41587-019-0106-2
Shimada, Y., Kuninaga, S., Ariyoshi, M., Zhang, B., Shiina, Y., Takahashi, Y., et al. (2015). E2F8 promotes hepatic steatosis through FABP3 expression in diet-induced obesity in zebrafish. Nutr. Metab. 12, 17. doi:10.1186/s12986-015-0012-7
Shirley, M. (2021). Casimersen: First approval. Drugs 81 (7), 875–879. doi:10.1007/s40265-021-01512-2
Siebler, J., Wirtz, S., Weigmann, B., Atreya, I., Schmitt, E., Kreft, A., et al. (2007). IL-28A is a key regulator of T-cell-mediated liver injury via the T-box transcription factor T-bet. Gastroenterology 132 (1), 358–371. doi:10.1053/j.gastro.2006.10.028
Sieghart, W., Losert, D., Strommer, S., Cejka, D., Schmid, K., Rasoul-Rockenschaub, S., et al. (2006). Mcl-1 overexpression in hepatocellular carcinoma: A potential target for antisense therapy. J. Hepatol. 44 (1), 151–157. doi:10.1016/j.jhep.2005.09.010
Singh, R., Wang, Y., Xiang, Y., Tanaka, K. E., Gaarde, W. A., and Czaja, M. J. (2009). Differential effects of JNK1 and JNK2 inhibition on murine steatohepatitis and insulin resistance. Hepatology 49 (1), 87–96. doi:10.1002/hep.22578
Siva, K., Covello, G., and Denti, M. A. (2014). Exon-skipping antisense oligonucleotides to correct missplicing in neurogenetic diseases. Nucleic Acid. Ther. 24 (1), 69–86. doi:10.1089/nat.2013.0461
Stein, C. A., and Castanotto, D. (2017). FDA-approved oligonucleotide therapies in 2017. Mol. Ther. 25 (5), 1069–1075. doi:10.1016/j.ymthe.2017.03.023
Stephenson, M. L., and Zamecnik, P. C. (1978). Inhibition of Rous sarcoma viral RNA translation by a specific oligodeoxyribonucleotide. Proc. Natl. Acad. Sci. U. S. A. 75 (1), 285–288. doi:10.1073/pnas.75.1.285
Sugiyama, A., Kanno, K., Nishimichi, N., Ohta, S., Ono, J., Conway, S. J., et al. (2016). Periostin promotes hepatic fibrosis in mice by modulating hepatic stellate cell activation via α(v) integrin interaction. J. Gastroenterol. 51 (12), 1161–1174. doi:10.1007/s00535-016-1206-0
Summerton, J., and Weller, D. (1997). Morpholino antisense oligomers: Design, preparation, and properties. Antisense Nucleic Acid. Drug Dev. 7 (3), 187–195. doi:10.1089/oli.1.1997.7.187
Swayze, E. E., Siwkowski, A. M., Wancewicz, E. V., Migawa, M. T., Wyrzykiewicz, T. K., Hung, G., et al. (2007). Antisense oligonucleotides containing locked nucleic acid improve potency but cause significant hepatotoxicity in animals. Nucleic Acids Res. 35 (2), 687–700. doi:10.1093/nar/gkl1071
Syed, Y. Y. (2016). Eteplirsen: First global approval. Drugs 76 (17), 1699–1704. doi:10.1007/s40265-016-0657-1
Tang, M., Chen, Y., Li, B., Sugimoto, H., Yang, S., Yang, C., et al. (2021). Therapeutic targeting of STAT3 with small interference RNAs and antisense oligonucleotides embedded exosomes in liver fibrosis. Faseb J. 35 (5), e21557. doi:10.1096/fj.202002777RR
Terashima, M., Ogawa, Y., HamadaN., , NishiokA, A., MesaKi, K., InomaTa, T., et al. (1995). Development of apoptosis induced by whole-body irradiation in murine liver. Nihon Igaku Hoshasen Gakkai Zasshi. 55 (9), 700–702.
Treisman, R. (1992). The serum response element. Trends biochem. Sci. 17 (10), 423–426. doi:10.1016/0968-0004(92)90013-y
Trépo, E., Romeo, S., Zucman-Rossi, J., and Nahon, P. (2016). PNPLA3 gene in liver diseases. J. Hepatol. 65 (2), 399–412. doi:10.1016/j.jhep.2016.03.011
ur Rehman, Z., Hoekstra, D., and Zuhorn, I. S. (2013). Mechanism of polyplex- and lipoplex-mediated delivery of nucleic acids: Real-time visualization of transient membrane destabilization without endosomal lysis. ACS Nano 7 (5), 3767–3777. doi:10.1021/nn3049494
van der Wal, E., Bergsma, A. J., Pijnenburg, J. M., van der Ploeg, A. T., and Pijnappel, W. W. M. P. (2017). Antisense oligonucleotides promote exon inclusion and correct the common c.-32-13t>G GAA splicing variant in pompe disease. Mol. Ther. Nucleic Acids 7, 90–100. doi:10.1016/j.omtn.2017.03.001
Varela, G. M., Antwi, D. A., Dhir, R., Yin, X., Singhal, N. S., Graham, M. J., et al. (2008). Inhibition of ADRP prevents diet-induced insulin resistance. Am. J. Physiol. Gastrointest. Liver Physiol. 295 (3), G621–G628. doi:10.1152/ajpgi.90204.2008
Vatner, D. F., Goedeke, L., Camporez, J. P. G., Lyu, K., Nasiri, A. R., Zhang, D., et al. (2018). Angptl8 antisense oligonucleotide improves adipose lipid metabolism and prevents diet-induced NAFLD and hepatic insulin resistance in rodents. Diabetologia 61 (6), 1435–1446. doi:10.1007/s00125-018-4579-1
Vergani, D., Choudhuri, K., Bogdanos, D. P., and Mieli-Vergani, G. (2002). Pathogenesis of autoimmune hepatitis. Clin. Liver Dis. 6 (3), 727–737. doi:10.1016/s1089-3261(02)00018-1
Vickers, T. A., Wyatt, J. R., and Freier, S. M. (2000). Effects of RNA secondary structure on cellular antisense activity. Nucleic Acids Res. 28 (6), 1340–1347. doi:10.1093/nar/28.6.1340
Villanueva, A. (2019). Hepatocellular carcinoma. N. Engl. J. Med. 380 (15), 1450–1462. doi:10.1056/NEJMra1713263
Wada, F., Yamamoto, T., Ueda, T., Sawamura, M., Wada, S., Harada-Shiba, M., et al. (2018). Cholesterol-GalNAc dual conjugation strategy for reducing renal distribution of antisense oligonucleotides. Nucleic Acid. Ther. 28 (1), 50–57. doi:10.1089/nat.2017.0698
Wan, J. L., Wang, B., Wu, M. L., Li, J., Gong, R. M., Song, L. N., et al. (2022). MTDH antisense oligonucleotides reshape the immunosuppressive tumor microenvironment to sensitize Hepatocellular Carcinoma to immune checkpoint blockade therapy. Cancer Lett. 541, 215750. doi:10.1016/j.canlet.2022.215750
Wang, H., Guo, M., Wei, H., and Chen, Y. (2021). Targeting MCL-1 in cancer: Current status and perspectives. J. Hematol. Oncol. 14 (1), 67. doi:10.1186/s13045-021-01079-1
WeiXing, C., Tiantian, H., Qun, N., Chaohui, Y., and Ping, X. (2008). Inhibitory effect of hypoxia inducible factor-1 antisense oligonucleotide on growth of human hepatocellular carcinoma cells. Med. Oncol. 25 (1), 88–92. doi:10.1007/s12032-007-0050-8
Weng, S. Y., Wang, X., Vijayan, S., Tang, Y., Kim, Y. O., Padberg, K., et al. (2018). IL-4 receptor alpha signaling through macrophages differentially regulates liver fibrosis progression and reversal. EBioMedicine 29, 92–103. doi:10.1016/j.ebiom.2018.01.028
Win, S., Min, R. W. M., Zhang, J., Kanel, G., Wanken, B., Chen, Y., et al. (2021). Hepatic mitochondrial SAB deletion or knockdown alleviates diet-induced metabolic syndrome, steatohepatitis, and hepatic fibrosis. Hepatology 74 (6), 3127–3145. doi:10.1002/hep.32083
Wu, H., Lima, W. F., and Crooke, S. T. (1999). Properties of cloned and expressed human RNase H1. J. Biol. Chem. 274 (40), 28270–28278. doi:10.1074/jbc.274.40.28270
Wu, H., Ng, R., Chen, X., Steer, C. J., and Song, G. (2016). MicroRNA-21 is a potential link between non-alcoholic fatty liver disease and hepatocellular carcinoma via modulation of the HBP1-p53-Srebp1c pathway. Gut 65 (11), 1850–1860. doi:10.1136/gutjnl-2014-308430
Wu, L., Mao, C., and Ming, X. (2016). Modulation of bcl-x alternative splicing induces apoptosis of human hepatic stellate cells. Biomed. Res. Int. 2016, 7478650. doi:10.1155/2016/7478650
Wurster, C. D., and Ludolph, A. C. (2018). Nusinersen for spinal muscular atrophy. Ther. Adv. Neurol. Disord. 11, 1756285618754459. doi:10.1177/1756285618754459
Xiang, D. M., Sun, W., Ning, B. F., Zhou, T. F., Li, X. F., Zhong, W., et al. (2018). The HLF/IL-6/STAT3 feedforward circuit drives hepatic stellate cell activation to promote liver fibrosis. Gut 67 (9), 1704–1715. doi:10.1136/gutjnl-2016-313392
Xiao, D., Qu, X., and Weber, H. C. (2002). GRP receptor-mediated immediate early gene expression and transcription factor Elk-1 activation in prostate cancer cells. Regul. Pept. 109 (1-3), 141–148. doi:10.1016/s0167-0115(02)00197-0
Yamaguchi, K., Yang, L., McCall, S., Huang, J., Yu, X. X., Pandey, S. K., et al. (2008). Diacylglycerol acyltranferase 1 anti-sense oligonucleotides reduce hepatic fibrosis in mice with nonalcoholic steatohepatitis. Hepatology 47 (2), 625–635. doi:10.1002/hep.21988
Yamamoto, T., Nakatani, M., Narukawa, K., and Obika, S. (2011). Antisense drug discovery and development. Future Med. Chem. 3 (3), 339–365. doi:10.4155/fmc.11.2
Yang, L., He, K., Yan, S., Yang, Y., Gao, X., Zhang, M., et al. (2017). Metadherin/Astrocyte elevated gene-1 positively regulates the stability and function of forkhead box M1 during tumorigenesis. Neuro. Oncol. 19 (3), 352–363. doi:10.1093/neuonc/now229
Yang, Q., Zhao, J., Zhang, W., Chen, D., and Wang, Y. (2019). Aberrant alternative splicing in breast cancer. J. Mol. Cell Biol. 11 (10), 920–929. doi:10.1093/jmcb/mjz033
Yang, Y., Chen, Y., Zhang, C., Huang, H., and Weissman, S. M. (2002). Nucleolar localization of hTERT protein is associated with telomerase function. Exp. Cell Res. 277 (2), 201–209. doi:10.1006/excr.2002.5541
Yang, Z. F., Ngai, P., Ho, D. W., Yu, W. C., Ng, M. N. P., Lau, C. K., et al. (2008). Identification of local and circulating cancer stem cells in human liver cancer. Hepatology 47 (3), 919–928. doi:10.1002/hep.22082
Yasuhara, H., Yoshida, T., Sasaki, K., Obika, S., and Inoue, T. (2022). Reduction of off-target effects of gapmer antisense oligonucleotides by oligonucleotide extension. Mol. Diagn. Ther. 26 (1), 117–127. doi:10.1007/s40291-021-00573-z
Ying, T. H., Hsieh, Y. H., Hsieh, Y. S., and Liu, J. Y. (2008). Antisense oligonucleotide Elk-1 suppresses the tumorigenicity of human hepatocellular carcinoma cells. Cell Biol. Int. 32 (2), 210–216. doi:10.1016/j.cellbi.2007.08.027
Younossi, Z., Tacke, F., Arrese, M., Chander Sharma, B., Mostafa, I., Bugianesi, E., et al. (2019). Global perspectives on nonalcoholic fatty liver disease and nonalcoholic steatohepatitis. Hepatology 69 (6), 2672–2682. doi:10.1002/hep.30251
Yu, J., Zhu, C., Wang, X., Kim, K., Bartolome, A., Dongiovanni, P., et al. (2021). Hepatocyte TLR4 triggers inter-hepatocyte Jagged1/Notch signaling to determine NASH-induced fibrosis. Sci. Transl. Med. 13 (599), eabe1692. doi:10.1126/scitranslmed.abe1692
Yu, X. X., Murray, S. F., Pandey, S. K., Booten, S. L., Bao, D., Song, X. Z., et al. (2005). Antisense oligonucleotide reduction of DGAT2 expression improves hepatic steatosis and hyperlipidemia in obese mice. Hepatology 42 (2), 362–371. doi:10.1002/hep.20783
Yu, X. X., Watts, L. M., Manchem, V. P., Chakravarty, K., Monia, B. P., McCaleb, M. L., et al. (2013). Peripheral reduction of FGFR4 with antisense oligonucleotides increases metabolic rate and lowers adiposity in diet-induced obese mice. PLoS One 8 (7), e66923. doi:10.1371/journal.pone.0066923
Yuan, Y., Cai, H., Yang, X. J., Li, W., He, J., Guo, T. K., et al. (2014). Liposome-mediated induction of apoptosis of human hepatoma cells by c-myc antisense phosphorothioate oligodeoxynucleotide and 5-fluorouracil. Asian pac. J. Cancer Prev. 15 (14), 5529–5533. doi:10.7314/apjcp.2014.15.14.5529
Yuen, M. F., Heo, J., Jang, J. W., Yoon, J. H., Kweon, Y. O., Park, S. J., et al. (2021). Safety, tolerability and antiviral activity of the antisense oligonucleotide bepirovirsen in patients with chronic Hepatitis B: A phase 2 randomized controlled trial. Nat. Med. 27 (10), 1725–1734. doi:10.1038/s41591-021-01513-4
Yuen, M. F., Heo, J., Kumada, H., Suzuki, F., Suzuki, Y., Xie, Q., et al. (2022). Phase IIa, randomised, double-blind study of GSK3389404 in patients with chronic Hepatitis B on stable nucleos(t)ide therapy. J. Hepatol. 77 (4), 967–977. doi:10.1016/j.jhep.2022.05.031
Zhang, G., Huang, X., Xiu, H., Sun, Y., Chen, J., Cheng, G., et al. (2020). Extracellular vesicles: Natural liver-accumulating drug delivery vehicles for the treatment of liver diseases. J. Extracell. Vesicles 10 (2), e12030. doi:10.1002/jev2.12030
Zhang, H., Cook, J., Nickel, J., Yu, R., StecKer, K., Myers, K., et al. (2000). Reduction of liver Fas expression by an antisense oligonucleotide protects mice from fulminant hepatitis. Nat. Biotechnol. 18 (8), 862–867. doi:10.1038/78475
Zhang, H., Taylor, J., Luther, D., Johnston, J., Murray, S., Wyatt, J. R., et al. (2003). Antisense oligonucleotide inhibition of Bcl-xL and Bid expression in liver regulates responses in a mouse model of Fas-induced fulminant hepatitis. J. Pharmacol. Exp. Ther. 307 (1), 24–33. doi:10.1124/jpet.103.050435
Zhang, L., Liang, X. H., De Hoyos, C. L., Migawa, M., Nichols, J. G., Freestone, G., et al. (2022). The combination of mesyl-phosphoramidate inter-nucleotide linkages and 2'-O-methyl in selected positions in the antisense oligonucleotide enhances the performance of RNaseH1 active PS-ASOs. Nucleic Acid. Ther. 32 (5), 401–411. doi:10.1089/nat.2022.0005
Zhang, R., El-Mayta, R., Murdoch, T. J., Warzecha, C. C., Billingsley, M. M., Shepherd, S. J., et al. (2021). Helper lipid structure influences protein adsorption and delivery of lipid nanoparticles to spleen and liver. Biomater. Sci. 9 (4), 1449–1463. doi:10.1039/d0bm01609h
Zhu, G. Q., Wang, Y., Wang, B., Liu, W. R., Dong, S. S., Chen, E. B., et al. (2022). Targeting HNRNPM inhibits cancer stemness and enhances antitumor immunity in wnt-activated hepatocellular carcinoma. Cell. Mol. Gastroenterol. Hepatol. 13 (5), 1413–1447. doi:10.1016/j.jcmgh.2022.02.006
Zhu, X., Xu, Y., Solis, L. M., Tao, W., Wang, L., Behrens, C., et al. (2015). Long-circulating siRNA nanoparticles for validating Prohibitin1-targeted non-small cell lung cancer treatment. Proc. Natl. Acad. Sci. U. S. A. 112 (25), 7779–7784. doi:10.1073/pnas.1505629112
Zhu, Z., Hao, X., Yan, M., Yao, M., Ge, C., Gu, J., et al. (2010). Cancer stem/progenitor cells are highly enriched in CD133+CD44+ population in hepatocellular carcinoma. Int. J. Cancer 126 (9), 2067–2078. doi:10.1002/ijc.24868
Zuker, M. (2003). Mfold web server for nucleic acid folding and hybridization prediction. Nucleic Acids Res. 31 (13), 3406–3415. doi:10.1093/nar/gkg595
Glossary
ASO antisense oligonucleotide
ARL4C ADP-ribosylation factor-like 4c
ARAT acyl-CoA retinol acyltransferase
ApoB apolipoprotein B
ANGPTL8 Angiopoietin-like 8
AMD age-related macular degeneration
AGPAT 1-acylglycerol-3-phosphate O-acyltransferase
AE-2 anion exchanger 2
ADRP adipose differentiation-related protein
ACC acetyl-CoA carboxylase
ASGPR asialoglycoprotein receptor
BAT brown adipose tissue
CREB cAMP-response element binding protein
CMV cytomegalovirus
DMD Duchenne muscular dystrophy
DGAT diacylglycerol acyltransferase
FABP fatty acid binding protein
Elk-1 ETs-like transcription factor 1
FDA U.S. Food and Drug Administration
FAS fatty acid synthase
GalNAc N-acetylgalactosamine
h-TERT human telomerase reverse transcriptase
HSP47 heat shock protein 47
HSC hepatic stellate cells
HNRNPM heterogeneous nuclear ribonucleoprotein M
HFD high-fat diet
HDL-c high-density lipoprotein cholesterol
HCV hepatitis C
HCC hepatocellular carcinoma
HBV hepatitis B
IL-4Rα interleukin 4 receptor A subunit
Ie2 intermediate early region 2
Jag1 jagged-1
LPS lipopolysaccharide
LPL lipoprotein lipase
LDs lipid droplets
LDL-c low-density lipoprotein cholesterol
LNA locked nucleic acid
LNP lipid nanoparticle
LRAT lecithin-retinol acyltransferase
MUFAs monounsaturated fatty acids
MTDH metastasis adhesion protein
MST3 serine/threonine protein kinase 24
MMPs matrix metalloproteinases
MK midkine
Mat1a methionine adenosine transferase 1A
MOE 2′-O-methoxyethyl
NCST nicastrin
OPN osteopontin
PSD3 pleckstrin and sec7 domain-containing 3
PNPLA3 patatin-like phospholipase domain-containing 3
PKCε protein kinase C epsilon
PDH pyruvate dehydrogenase
PBC primary biliary cholangitis
PA phospholipid acid
(P)RR Prorenin receptor
PS phosphorothioate
PNA Peptide nucleic acid
PMO phosphoramidate morpholino oligomer
PEG polyethylene glycol
RE retinol esters
STK25 serine/threonine protein kinase 25
STAT3 signal transducer and activator of transcription 3
SREBP sterol regulatory element binding protein
SMA spinal muscular atrophy
SCD1 stearyl CoA desaturase 1
SAB SH3 homology associated BTK binding protein
SMN2 survival of motor neuron 2
TNF-α tumor necrosis factor-α
TNFR1 tumor necrosis factor receptor 1
TIP47 tail interacting protein 47
TIMPs matrix metalloproteinase inhibitors
TG triglyceride
TTR transthyretin
VOD veno-occlusive disease
VLDL very low density lipoprotein
VEGF vascular endothelial growth factor
Keywords: antisense oligonucleotide (ASO), NAFLD, hepatitis, liver fibrosis, liver cancer
Citation: Lu K, Fan Q and Zou X (2022) Antisense oligonucleotide is a promising intervention for liver diseases. Front. Pharmacol. 13:1061842. doi: 10.3389/fphar.2022.1061842
Received: 05 October 2022; Accepted: 28 November 2022;
Published: 09 December 2022.
Edited by:
Runping Liu, Beijing University of Chinese Medicine, ChinaReviewed by:
Edward N. Harris, University of Nebraska System, United StatesJoonbae Seo, Cincinnati Children’s Hospital Medical Center, United States
Copyright © 2022 Lu, Fan and Zou. This is an open-access article distributed under the terms of the Creative Commons Attribution License (CC BY). The use, distribution or reproduction in other forums is permitted, provided the original author(s) and the copyright owner(s) are credited and that the original publication in this journal is cited, in accordance with accepted academic practice. No use, distribution or reproduction is permitted which does not comply with these terms.
*Correspondence: Xiaoju Zou, eGlhb2p1em91QDE2My5jb20=
†These authors have contributed equally to this work