- 1Pharmaceutical Sciences Research Center, Department of Pharmacy, Children’s Hospital of Nanjing Medical University, Nanjing, China
- 2School of Basic Medicine and Clinical Pharmacy, China Pharmaceutical University, Nanjing, China
- 3Neonatal Intensive Care Unit, Children’s Hospital of Nanjing Medical University, Nanjing, China
Caffeine is the globally consumed psychoactive substance and the drug of choice for the treatment of apnea of prematurity (AOP), but its therapeutic effects are highly variable among preterm infants. Many of the molecular underpinnings of the marked individual response have remained elusive yet. Interestingly, the significant association between Clock gene polymorphisms and the response to caffeine therapy offers an opportunity to advance our understanding of potential mechanistic pathways. In this review, we delineate the functions and mechanisms of human circadian rhythms. An up-to-date advance of the formation and ontogeny of human circadian rhythms during the perinatal period are concisely discussed. Specially, we summarize and discuss the characteristics of circadian rhythms in preterm infants. Second, we discuss the role of caffeine consumption on the circadian rhythms in animal models and human, especially in neonates and preterm infants. Finally, we postulate how circadian-based therapeutic initiatives could open new possibilities to promote precision caffeine therapy for the AOP management in preterm infants.
1 Introduction
Caffeine, one of the bioactive methylxanthines that exist in a variety of natural and processed foods and beverages, is the most frequently consumed psychoactive substance in the world (Gonzalez de Mejia and Ramirez-Mares, 2014; van Dam et al., 2020; Rodak et al., 2021). Studies have confirmed that ingested caffeine has profound effects on the function and health of various systems in the human body through the combination of several molecular mechanisms including the antagonism of adenosine receptors, inhibition of phosphodiesterase, and mobilization of intracellular calcium (Nehlig et al., 1992; Cappelletti et al., 2015; Rodak et al., 2021; Yang et al., 2021). Among these effects of caffeine, the most well-known are those on the central nervous system, such as the regulation of sleep-wake states, learning-memory functions, cognitive-behavioral performances, attention-alertness functions, and mood-consciousness states (Nehlig et al., 1992; Snel and Lorist, 2011; Spaeth et al., 2014; Urry and Landolt, 2015). Therefore, it is no surprise that many people are accustomed to taking caffeinated beverages or foods to combat sleep deprivation induced fatigue and circadian rhythm sleep disorder caused by shift work or rapid transmeridian travel (Landolt, 2015; Clark and Landolt, 2017; Arendt, 2018), while some other people intentionally avoid caffeine in their daily life so as not to interfere with regular sleep habits (Snel and Lorist, 2011).
On the other hand, the therapeutic use of caffeine is very common in clinical practice. Caffeine acts as a potent analgesic adjuvant and is often added to a variety of over-the-counter and prescription analgesics due to its anti-inflammatory and vasoconstrictive effects (Cappelletti et al., 2015; van Dam et al., 2020; Rodak et al., 2021). More strikingly, caffeine is the drug of choice for the treatment of apnea of prematurity (AOP) (Eichenwald et al., 2016; Kumar and Lipshultz, 2019; Moschino et al., 2020; Long et al., 2021) and becomes one of the most commonly prescribed medications in the neonatal intensive care unit (NICU) (Hsieh et al., 2014; Krzyżaniak et al., 2016), evidenced by its short-term and long-term efficacy and safety in reducing apnea, facilitating extubation, preventing bronchopulmonary dysplasia, ameliorating retinopathy of prematurity, reducing patent ductus arteriosus, and improving neurodevelopmental outcome that have been demonstrated in the Caffeine for Apnea of Prematurity (CAP) trial (Schmidt et al., 2006; Schmidt et al., 2007). Assuredly, compared with other methylxanthines, caffeine has higher therapeutic index, longer half-life, and better tolerability (Henderson-Smart and De Paoli, 2010; Henderson-Smart and Steer, 2010; Abdel-Hady et al., 2015). Inspiringly, caffeine has been clinically applied in the treatment of AOP for nearly 50 years, which has created a typical successful story in pediatrics (Kreutzer and Bassler, 2014; Dobson and Hunt, 2018; Williamson et al., 2021).
Recently, the association between caffeine and circadian rhythms has attracted widespread attentions (Landolt, 2015). Many intriguing phenomena occurred, and the underlying mechanisms have been tentatively investigated by several studies conducted in adults and animals (Spaeth et al., 2014), but we still know very little about the truth. Fortunately, however, our previous study revealed that the circadian rhythms in premature infants might play a sophisticated role in determining the efficacy of caffeine therapy (Guo et al., 2022). Therefore, it will be very interesting to summarize the current relevant studies to know about the progress of this research field.
To the best of our knowledge, there is no comprehensive summary of the most recent advances in the circadian rhythms in preterm infants and caffeine therapy. Thus, to fill this knowledge gap, in this review, we begin by introducing the coexistence of tough challenges and new insights in the current caffeine therapy for AOP. Then, our novel findings (Guo et al., 2022) push us to delineate the functions and mechanisms of human circadian rhythms first for better understanding the deep theoretical logic underlying those clinical phenotypes. As a key part of circadian development, an up-to-date knowledge of the formation and ontogeny of human circadian rhythms during the perinatal period are also concisely discussed. Undoubtedly, what attracts our attention the most is the research progress on the effects of caffeine on human circadian rhythms, especially for premature infants, and the progress on the sophisticated roles of circadian rhythms in the response to caffeine therapy for those babies with AOP. Therefore, based on the increasing evidence, a new possibility opens up in this area of research in light of the circadian rhythms.
2 Tough challenges and new findings in current caffeine therapy for AOP
To be honest, the tough challenges are always there for the current AOP therapy with caffeine. The optimal dose regimen, timing and duration of therapy, necessity of therapeutic drug monitoring, and variable clinical outcomes of caffeine in preterm infants remain controversial (Gentle et al., 2018; Davis, 2020; Saroha and Patel, 2020). Impressively, however, those problems related to the clinical use of caffeine in preterm infants have been widely concerned and discussed as the continuous deepening of research, especially as the application of several innovative research technologies, such as artificial intelligence, predictive modeling, and machine learning (Koch et al., 2017; Shirwaikar, 2018; Faramarzi et al., 2021; Dai et al., 2022). Interestingly, several novel findings in those studies provide valuable references for determining the optimal initial dose, tailoring the maintenance dose, enhancing clinical decision making, and then for promoting the achievement of consensus on those tough challenges (Abdel-Hady et al., 2015; Eichenwald, 2020; Moschino et al., 2020).
The clinical response bears the brunt. The most tough and urgent problem is the significant interindividual variability in response to caffeine therapy (He et al., 2021). It remains unclear why some preterm infants have well-controlled outcomes while others have not. To make matters worse, the frequent episodes of apnea among those lacking efficacy cannot be well controlled by solely increasing the dose of caffeine (Saroha and Patel, 2020).
Tentatively to explore the underlying factors that determine the interindividual response to caffeine therapy, a single-center and retrospective study was conducted by our team (He et al., 2021; Guo et al., 2022). In line with previous study (Saroha and Patel, 2020), the plasma concentration of caffeine could not explain the variable efficacy for preterm infants yet (He et al., 2021). Arguably, such highly variable response could not be explained either by the genetic polymorphisms of various genes encoding the metabolic enzymes and transporters (Guo et al., 2022). However, genetic polymorphisms involved in caffeine’s target receptors, directly and indirectly, and quite unexpectedly, in regulation of circadian rhythms were significantly associated with the variable response to caffeine therapy (Guo et al., 2022). Such novel finding bears good clinical significance and is inspirational for future studies to delve into the biological mechanisms.
3 The functions and mechanisms of human circadian rhythms
Due to the rotation of Earth, almost all life forms on the planet have evolved a biological timer to adapt to the daily changes in the environment (Du Pre et al., 2014; Dong et al., 2020; Jha et al., 2021). The endogenous biological clock is commonly called as the circadian (from Latin, meaning “about a day”) rhythms (Dong et al., 2020; Ruan et al., 2021). It is proven that the inherent period of the human pacemaker clock is close to 25 h in most people (Ohdo et al., 2019; Dong et al., 2020). However, because of the entrainment by environmental time signals, or so-called zeitgebers (from German, meaning “time givers”) (Bicker et al., 2020; Ruan et al., 2021), the inherited circadian pacemaker manifests itself in a 24-h pattern (Ohdo et al., 2019; Dong et al., 2020).
3.1 The functions of human circadian rhythms
Circadian rhythms regulate various behavioral, physiological, psychological, and endocrine functions in humans (Froy, 2007; Ribas-Latre and Eckel-Mahan, 2016; Allada and Bass, 2021; Kinouchi et al., 2021; Thosar and Shea, 2021; Zhang and Jain, 2021). One can imagine that circadian dysfunction would cause multiple negative impacts, both short term and long term, which lead to the increased susceptibility to many diseases, decreased quality of life, and even reduced life expectancy (Froy and Miskin, 2007; Jagannath et al., 2013; Roenneberg and Merrow, 2016; Valenzuela et al., 2016; Logan and McClung, 2019; Xu and Lu, 2019; Allada and Bass, 2021). Interestingly, the onsets and symptoms of many diseases, such as stroke, asthma, and depression, also display clear circadian characteristics (Jagannath et al., 2013; Hsieh et al., 2018; Cederroth et al., 2019; Dobrek, 2021; Ruan et al., 2021), which are called as the circadian pathology signs (Cederroth et al., 2019). Speaking of pharmacology, circadian rhythms affect the absorption, distribution, metabolism, and excretion (ADME) or called the pharmacokinetic processes as well as the efficacy and adverse effects of many drugs, which is well known as the chronopharmacology or chronotherapy (Dallmann et al., 2016; Ohdo et al., 2019; Dong et al., 2020; Dobrek, 2021; Nahmias and Androulakis, 2021). Given the importance of circadian rhythms, three researchers who discovered the basic of biological clock in studies of Drosophila were awarded the Nobel Prize in 2017 (Dobrek, 2021; Ruan et al., 2021).
3.2 The mechanisms of human circadian rhythms
Back in the 1990s, the discovery of several circadian clock genes, such as Clock, Bmal1, Per, and Cry (Takahashi, 2004), proved that almost all human cells express these genes and have the capacity to generate circadian oscillations (Du Pre et al., 2014; Takahashi, 2017), which thwarted the previous neuro-centric view that the master clock is located only in the brain (Takahashi, 2017). As is generally believed and well understood, at the systemic level, the human circadian system consists of the inputs, circadian oscillators, and outputs (Figure 1) (Takahashi, 2017; Cederroth et al., 2019; Huang et al., 2020; Ruan et al., 2021), while at the cellular level, it consists of several cell-autonomous molecular oscillators that composed of three transcriptional-translational feedback loops that are widespread throughout the body (Figure 2) (Du Pre et al., 2014; Takahashi, 2017; Logan and McClung, 2019; Huang et al., 2020; Sumova and Cecmanova, 2020; Ruan et al., 2021).
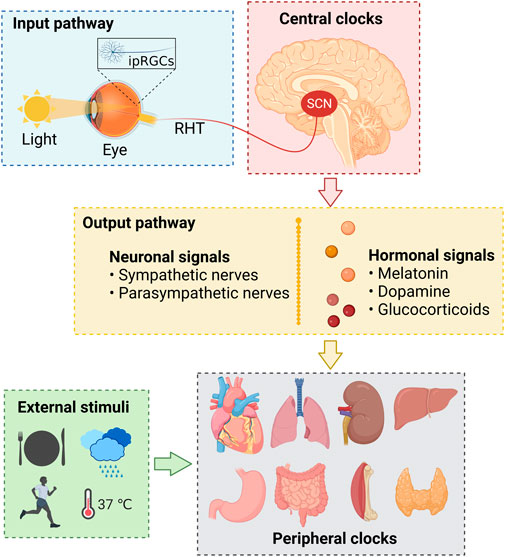
FIGURE 1. The physiological basis of human circadian rhythms. ipRGCs, intrinsically photosensitive retinal ganglion cells; RHT, retinohypothalamic tract; SCN, suprachiasmatic nuclei.
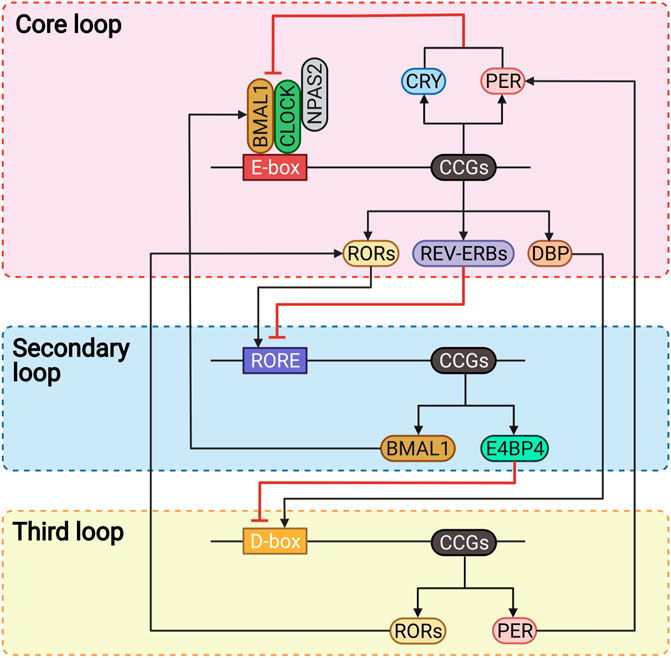
FIGURE 2. The molecular mechanism of human circadian rhythms. BMAL1, brain and muscle ARNT-like 1; CCGs, clock-controlled genes; CLOCK, circadian locomotor output cycles kaput; CRY, cryptochrome; DBP, D-box binding protein; E4BP4, E4 promoter-binding protein 4; NPAS2, neuronal PAS domain-containing protein 2; PER, period; RORE, ROR/REV-ERB response elements; RORs, retinoic acid receptor-related orphan receptors.
3.2.1 Physiological basis
The regulation and maintenance of human circadian rhythms depend on the synergy of the input pathways, central and peripheral clocks, and output pathways (Figure 1) (Huang et al., 2020). The input pathway senses and transmits the environmental rhythm signals to the central circadian clocks (Ruan et al., 2021), which act as the biological rhythm pacemaker to transmit the generated rhythm signals to the periphery through the output pathway (Du Pre et al., 2014; Jha et al., 2021), and then cooperate with the endogenous clock systems of peripheral tissues and organs to regulate the gene expression, cellular function, physiological activity, and metabolism of the body (Huang et al., 2020).
Light, the major input signal in the suprachiasmatic nuclei (SCN) of the circadian system, is perceived by the intrinsically photosensitive retinal ganglion cells (ipRGCs) (Zele et al., 2011), which express the photopigment melanopsin and are modulated by the rods and cones in the retina (Van Cruchten et al., 2017). Then, the ipRGCs generated and transmitted electric rhythm signals to the central clock system that located in the SCN of the hypothalamus through a neural pathway called the retinohypothalamic tract (RHT) (Logan and McClung, 2019; Dong et al., 2020; Jha et al., 2021).
The SCN is comprised of neurons that express the neuropeptide arginine vasopressin (AVP) and vasoactive intestinal polypeptide (VIP), which are essential for the circadian light transduction (Ono et al., 2021). The AVP and VIP neurons in the SCN master pacemaker are also regulated by the neurotransmitters released by the ipRGCs, such as excitatory glutamate and pituitary adenylate cyclase-activating polypeptide (PACAP) (Dong et al., 2020; Jha et al., 2021; Ruan et al., 2021). Subsequently, the SCN transmits such perceived rhythm information via neuronal and hormonal signals (Logan and McClung, 2019), and coordinates other oscillators in extra-SCN brain regions and peripheral tissues and organs, such as heart, lung, liver, and kidney (Takahashi, 2017; Huang et al., 2020).
It is worth mentioning that in addition to be regulated by the SCN master pacemaker, the peripheral clocks could also directly and SCN-independently receive external stimuli, such as food intake, exercise, temperature, and humidity (Figure 1) (Du Pre et al., 2014; Xu and Lu, 2019; Huang et al., 2020).
3.2.2 Molecular mechanism
Three interlocked transcriptional feedback loops constitute the complex molecular clock networks at the cellular level (Figure 2) (Takahashi, 2017; Ruan et al., 2021). The core loop regulates human circadian rhythms with a period of approximately 24-h through a negative feedback mechanism (Huang et al., 2020; Allada and Bass, 2021). The circadian locomotor output cycles kaput (CLOCK) or neuronal PAS domain-containing protein 2 (NPAS2) forms heterodimers with the brain and muscle ARNT-like 1 (BMAL1) via binding to the E-box elements to regulate the transcription of clock-controlled genes (CCGs), including those encoding the period (PER) and cryptochrome (CRY) proteins (Takahashi, 2017; Logan and McClung, 2019; Dong et al., 2020). PER and CRY proteins accumulate in the cytoplasm in the morning (Ruan et al., 2021), then heterodimerize and translocate into the nucleus as negative regulators directly interact with CLOCK-BMAL1 to suppress their transcriptional activity in the late afternoon or evening (Takahashi, 2017; Xu and Lu, 2019). As the suppression progresses, PER and CRY proteins are gradually degraded via the ubiquitination through specific E3 ligase complexes and via the proteasome (Takahashi, 2017). At the same time, the transcription activity of CLOCK-BMAL1 is restored, and a new cycle will restart over the next morning (Ruan et al., 2021).
Besides, another two families of nuclear receptors, REV-ERBs and retinoic acid receptor-related orphan receptors (RORs), are also the direct targets of CLOCK-BMAL1 that stabilize the core loop, regulate the transcription in a distinct phase, and thus form the secondary or called the stabilization loop (Xu and Lu, 2019). The REV-ERBs inhibit the transcription of BMAL1 by competitively binding to the ROR/REV-ERB response elements (RORE) (Hsieh et al., 2018; Ruan et al., 2021). Conversely, the RORs are the positive regulators that bind to the RORE to promote the transcription of BMAL1 (Logan and McClung, 2019; Huang et al., 2020).
The third loop involves the proline- and acidic amino acid-rich basic leucine zipper (PAR-bZIP) factors, such as the D-box binding protein (DBP) and the repressor E4 promoter-binding protein 4 (E4BP4), which competitively bind to the D-box elements, and are driven by the core loop and stabilization loop, respectively (Takahashi, 2017; Ruan et al., 2021). DBP and E4BP4 contribute to circadian robustness by synergistically regulating the expression of RORs and PER proteins (Takahashi, 2017; Dong et al., 2020; Ruan et al., 2021).
Collectively, these three interactive feedback loops regulate the transcription and translation of CCGs by binding to the cis-elements, including E-box, RORE, and D-box, in their gene promoter and enhancer element regions (Dong et al., 2020; Ruan et al., 2021). In addition to these three transcriptional-translational feedback loops, several post-transcriptional and post-translational mechanisms, such as phosphorylation, acetylation, and ubiquitination of circadian proteins, also play important roles in regulating the circadian rhythms (Figure 2) (Takahashi, 2017; Xu and Lu, 2019; Huang et al., 2020).
4 The formation and development of human circadian rhythms
The physiological and molecular mechanisms of human circadian rhythms have been well described, but the formation and development during ontogenesis remain poorly understood (Astiz and Oster, 2020; Sumova and Cecmanova, 2020). Moreover, most studies were performed in rodents and non-human primates, which hinders our understanding of the developmental circadian physiology for humans (Rivkees, 2003; Sumova and Cecmanova, 2020). Nevertheless, the existing evidence reveals that the formation and development of circadian rhythms are the continuously morphological, structural, and functional maturation processes of tissues and organs with ontogenesis (Rivkees, 2003; Seron-Ferre et al., 2012).
4.1 The formation of circadian rhythms: Does fetus have circadian rhythms?
As early as 1975, a rat study (Deguchi, 1975) found, for the first time, that the mammalian fetal clock oscillators could be detected already at or before birth and be entrained by the mother. Subsequent studies have revealed that the fetus of rat, hamster, sheep, baboon, and other mammalians exhibited the circadian rhythms of metabolic activity (Reppert, 1992; Serón-Ferré et al., 1993; Mirmiran and Lunshof, 1996; Seron-Ferre et al., 2012) and the expressions of canonical clock genes (Seron-Ferre et al., 2007; Du Pre et al., 2014; Sumova and Cecmanova, 2020).
In human fetus, circadian rhythms in several physiological and endocrine functions, including heart rate (Lunshof et al., 1998), breathing patterns (Patrick and Challis, 1980), limb movements (Einspieler et al., 2021), sleep-wake states (Peirano et al., 2003; Bennet et al., 2018), and hormone levels (Serón-Ferré et al., 2001a) have been detected at different stages of pregnancy (Seron-Ferre et al., 2007; Du Pre et al., 2014; Wong et al., 2022). Impressively, Frigato et al. (2009), first observed the rhythmic expression of clock genes such as Per2 in the HTR-8/SVneo cells derived from human extravillous trophoblast. As part of a series of important discoveries, Perez et al. (2015) went on to find the rhythmic expression of various circadian genes, including Clock, Bmal1, Per2, and Cry1 in human full-term placenta.
It is incredible that no obvious circadian rhythms were found in the anencephalic fetus despite an intact maternal circadian rhythms were detected through the 24-h period fetal heart rate monitoring for anencephaly (Mirmiran and Lunshof, 1996), which demonstrated that the fetal brain, especially in the SCN, is required for the generation of fetal circadian rhythms (Mirmiran and Lunshof, 1996). It is still unclear when the fetal SCN clock first appeared morphologically, yet through the in vitro autoradiography by 125I-labeled melatonin, the SCN is apparent as discrete nuclei in the human fetus and already has melatonin receptors at 18 weeks of gestational age (GA) (Reppert et al., 1988). Besides, it has been demonstrated that the VIP and AVP neurons were first observed at 31 weeks of GA in the ventrolateral part of the fetal SCN (Swaab et al., 1990; Swaab et al., 1994). Therefore, it is currently recognized that the circadian rhythms in humans are formed and developed during the perinatal period (Rivkees, 1997; Sumova and Cecmanova, 2020), while the components of the circadian system like the SCN are established and functional early in human fetus (Serón-Ferré et al., 1993).
4.2 Prenatal circadian rhythms: Complex interaction of maternal, placental, and fetal circadian systems
Pregnancy presents an unusual circadian physiology pattern in which the fetal circadian system is completely embodied within that of the mother (Mark et al., 2017), and the two systems are connected by the placenta and interact with each other through this interface (Mark et al., 2017; Astiz and Oster, 2020; Bates and Herzog, 2020). Generally, placenta is responsible for the bidirectional transference of nutrients, hormones, metabolites, and gases (i.e., oxygen and carbon dioxide) between the mother and fetus (Seron-Ferre et al., 2012; Valenzuela et al., 2015; Astiz and Oster, 2020). Meanwhile, the placenta conveys the maternal circadian timing cues, such as physical activity, feeding behavior, temperature, heart rate, blood pressure, and hormonal levels, to the fetus (Serón-Ferré et al., 2001a; Seron-Ferre et al., 2012). In particular, multiple hormones produced by the mother, including melatonin, dopamine, glucocorticoids, estrogen, and progesterone, have profound effects on the development and entrainment of the fetal circadian rhythms (Mirmiran and Lunshof, 1996; Rivkees, 1997; Seron-Ferre et al., 2007; Mark et al., 2017). In addition, hormones such as human chorionic gonadotropin (hCG), secreted by the placenta, also exhibit obvious circadian characteristics (Waddell et al., 2012; Mark et al., 2017; Bates and Herzog, 2020). It will be very interesting to know how those hormones affect the formation of the fetal circadian rhythms.
4.2.1 Melatonin
Melatonin, known as the hormone of night (Seron-Ferre et al., 2007), can be secreted by various organs, including the pineal gland, ovary, and placenta (Itoh et al., 1999; Lanoix et al., 2008; Reiter et al., 2013; Reiter et al., 2014). However, melatonin is not synthesized by the fetal pineal gland or other organs (Mark et al., 2017), so the fetus must rely on the maternal melatonin for photoperiodic information since the unaltered melatonin readily crosses the placenta and distributes to the fetal tissues (Waddell et al., 2012; Reiter et al., 2014; Valenzuela et al., 2015). During normal human gestation, the nighttime peak melatonin level decreases slightly between the first and second trimesters, but begins to increase after 24 weeks, then increases to significantly high levels after 32 weeks, thereafter reaches its peak at the end of pregnancy, and finally returns to the pre-pregnancy level on the day after parturition (Nakamura et al., 2001; Mark et al., 2017). Late in human pregnancy, uterine contractions become intensest during the night as melatonin levels are at their highest (Nakamura et al., 2001), and the peak melatonin at the end of pregnancy is thought to promote uterine contractions that necessary for delivery (McCarthy et al., 2019).
Studies have demonstrated that the onset of human term delivery is more commonly between the late night and the early morning (Glattre and Bjerkedal, 1983; Cooperstock et al., 1987). Similar circadian characteristics of delivery were also observed in preterm labors after 28 weeks of GA (Lindow et al., 2000; Iams et al., 2002), but not in those before 28 weeks (Vatish et al., 2010), which might be explained by the immaturity of fetal circadian system or other pathological factors that bypass the physiological circadian process of labor (Vatish et al., 2010). Interestingly, studies revealed that the elevated nocturnal levels of melatonin synergized with oxytocin to trigger and maintain the uterine contractions during labor and that melatonin sensitized the human uterine to oxytocin (Reiter et al., 2014; Carlomagno et al., 2018; Chuffa et al., 2019). Consistently, women who engage in shift work during pregnancy have an increased incidence of spontaneous miscarriages, preterm deliveries, and low birth weight infants (Zhu et al., 2004; Croteau et al., 2006). Disruptions of the melatonin rhythms due to the shift work might be responsible for these adverse pregnancy outcomes (Reiter et al., 2014). In addition, as a free radical scavenger and an antioxidant, melatonin plays an important role in protecting the fetus and placenta from oxidative stress to promote the embryonic development and to treat the preeclampsia, intrauterine growth restriction, and the undernourished pregnancy (Reiter et al., 2014; Valenzuela et al., 2015; Rodrigues Helmo et al., 2018; Chuffa et al., 2019).
4.2.2 Dopamine
As the antiphase and functionally antagonistic of melatonin, dopamine has been proposed as a “light-phase” entrainment signal of the circadian systems (Iuvone and Gan, 1995; Astiz and Oster, 2020). Plasma dopamine levels in humans peak around the waking time (about 08:00) and drop to a nadir in the middle of sleep (about 03:00) (Sowers and Vlachakis, 1984). Increased dopamine concentrations were detected in women’s amniotic fluid between the second and third trimesters, and were significantly higher than those in maternal and fetal plasma (Peleg et al., 1986), because dopamine could freely cross through the placenta into the fetal circulatory system (Watanabe et al., 1990). Furthermore, D1-dopamine receptors could be detected in the fetal SCN as early as 22 weeks of GA (Rivkees and Lachowicz, 1997). However, it remains unknown when and how the maternal dopamine entrains the circadian rhythms in fetus during the pregnancy (Bates and Herzog, 2020).
4.2.3 Glucocorticoids
Cortisol, the glucocorticoid stress hormone, is regulated by the circadian of the hypothalamic-pituitary-adrenal (HPA) axis (Mark et al., 2017; Oster et al., 2017; McCarthy et al., 2019). During gestation, cortisol levels in maternal plasma peak in the early morning (from 07:30 to 08:30) and drop to a nadir at night (from 18:30 to 01:30) (Patrick et al., 1980). The maternal plasma cortisol levels increase progressively between 11 and 22 weeks of GA and then stay high until the initiation of delivery (Patrick et al., 1980; Carr et al., 1981). Such elevated maternal cortisol is critical for fetal tissue development, especially the maturation of the brain and lung (Matthews et al., 2004), and helpful for dampening the maternal stress signals to protect the fetus (McCarthy et al., 2019). Conversely, excessive cortisol level is detrimental for the fetal development that delaying the fetal and placental growth and increasing the risk of behavioral and mental disorders later in life (Busada and Cidlowski, 2017; Van den Bergh et al., 2020).
The placental glucocorticoid barrier regulates the glucocorticoids’ passage from the mother to the fetus via the enzyme 11β-hydroxysteroid dehydrogenase type 2 (11β-HSD2) that converts the biologically active glucocorticoids (i.e., cortisol and corticosterone) to their inactive forms (i.e., cortisone and 11-dehydrocorticosterone) (Edwards et al., 1996; Burton and Waddell, 1999). In humans, the glucocorticoids passage from the maternal to fetal circulation is gradually reduced due to the increasing placental 11β-HSD2 expression with advancing gestation (Burton and Waddell, 1999; McTernan et al., 2001). Impressively, glucocorticoid receptors have been identified in the fetal circulation, and maternal glucocorticoids could entrain fetal circadian rhythms through binding to these receptors (Bates and Herzog, 2020). Moreover, studies have found that the suppression of maternal adrenal function with glucocorticoid treatment resulted in a temporary disappearance of the fetal heart rate, breathing, and limb movement rhythms (Verdurmen et al., 2013). Interestingly, these inhibitory effects were dependent on the GA when the glucocorticoid therapy was started and disappeared with the restoration of the maternal HPX axis (Mulder et al., 2004), indicating the fetal rhythms depended on the maternal adrenal functions (Koenen et al., 2005).
4.2.4 Sex hormones
The effects of sex hormones on the entrainments of fetal circadian rhythms are still under investigation. Estrogen and progesterone are two sex hormones that are essential for the successful pregnancy (Mark et al., 2017). During the first 3 months of pregnancy, estrogen and progesterone are synthesized and secreted by the ovary. After that, the placenta replaces the corpus luteum to secrete these two hormones, and estrogen is also produced by the uterus (McCarthy et al., 2019). The levels of estrogen and progesterone increase steadily over the human gestation due to an increased secretion from the ovary and placenta (Mark et al., 2017). During gestation, estrogen levels in maternal plasma peak in the morning and become lowest at midnight (Patrick et al., 1979; Challis et al., 1980), whereas a significant antiphase oscillation of the estrogen occur in the progesterone levels (Junkermann et al., 1982; Magiakou et al., 1996), which might be regulated by the circadian of placental glucocorticoids (Serón-Ferré et al., 1993).
Estrogen promotes the synthesis of progesterone (Babischkin et al., 1997), which regulates maternal immunity to facilitate implantation (Hardy et al., 2006), maintains uterine quiescence during pregnancy (Peters et al., 2017), and causes myometrial contractions to trigger labor at the end of pregnancy (Brown et al., 2004). Interestingly, these two hormones were found to inhibit the expression of 11β-HSD2 in human placental extracts, which possibly increased the transport of glucocorticoids from the mother to the fetus (Sun et al., 1998), thereby indirectly influencing the fetal circadian rhythms.
Collectively, much less is known about other rhythmic signals such as leptin, placental lactogen, prolactin, or hCG that generated by the mother or placenta on the development and entrainment of fetal circadian rhythms (Astiz and Oster, 2020; Bates and Herzog, 2020). Because the interactions among maternal, placental, and fetal circadian systems are critical to the establishment, maintenance, and success of pregnancy, and the interactions also affect the growth, development, and even postpartum life of the fetus (Mark et al., 2017; Bates and Herzog, 2020), further studies are still needed to elucidate the complex interactions among the three circadian systems and to bridge the above knowledge gaps.
4.3 Postnatal circadian rhythms: Progressive maturation along with ontogenesis
After birth, neonates immediately begin to establish their own physical and physiological independence while losing the protect of the maternal-placental barrier (Joseph et al., 2015; Wong et al., 2022). From now on, the ontogenesis of the newborn begins to be greatly affected by the external environment (Brooks and Canal, 2013; Hazelhoff et al., 2021). Increasing evidence indicates that human postnatal circadian rhythms gradually mature along with the ontogenesis (Rivkees and Hao, 2000; Rivkees, 2007; Bueno and Menna-Barreto, 2016), in which the external environment, especially the light, plays an important role in the development and maturation (Mirmiran and Ariagno, 2000; Nishihara et al., 2002; Challet, 2007). Particularly, it should be pointed out that, in early infancy, the maternal entrainment factors and maternal-fetal interactions retained during pregnancy are more important than the external environment (Löhr and Siegmund, 1999; Rivkees, 2001; Nishihara et al., 2002; Sumova et al., 2012).
4.3.1 Maternal effects
The first thing to be discussed is the role of hormones. During the first few weeks of life, circadian rhythms in human neonates occur as the retentions of the maternal influence in utero, but the endogenous rhythms appear only later (Rivkees, 1997; Rivkees and Hao, 2000; Brooks and Canal, 2013). For example, an antiphase oscillation of maternal cortisol circadian rhythms (i.e., the peak of cortisol levels occurred between 12:00 and 16:00) was found in the umbilical artery but not the umbilical vein of the term fetus (Serón-Ferré et al., 2001b), which reflects the activation of the intrinsic fetal HPA axis in response to the falling maternal transport of glucocorticoids during the nadir of the maternal rhythms (Mark et al., 2017). Moreover, the neonatal salivary cortisol levels were higher at night than in the morning within the first 8 weeks of postnatal age (PNA) (Iwata et al., 2013; Kinoshita et al., 2016), which were in consonance with the fetal cortisol rhythms (Serón-Ferré et al., 2001b), reflecting the preservation of fetal adrenal rhythms.
Neonates begin to exhibit the circadian salivary cortisol rhythms analogous to that of adults (i.e., higher cortisol levels in the morning than at night) until 2–3 months of PNA (Price et al., 1983; Spangler, 1991; Mantagos et al., 1998; Joseph et al., 2015). However, an adult-type salivary cortisol circadian of term infants appears to be established actually at 1 month and remains stable throughout the first year of life (Ivars et al., 2015). All in all, these studies prove that the fetal cortisol circadian rhythms are preserved in the first few weeks of life, until the adult-type circadian rhythms are established.
The rhythm of melatonin is another example. (Muñoz-Hoyos et al., 1992; Muñoz-Hoyos et al., 1998) found that the adult-type circadian melatonin rhythms occurred in both the umbilical artery and vein for neonates at birth, which depended on the maternal melatonin crossing the placenta, as melatonin levels in the umbilical artery are positively correlated to those in the maternal serum and a similar correlation between the maternal and neonatal melatonin levels in the first voided urine after delivery (Kivelä et al., 1990). Besides, although the increasing amounts of melatonin and its metabolite 6-sulfatoxymelatonin were detected in the urine of the term neonates during the first week of life (Kivelä et al., 1990; Muñoz-Hoyos et al., 1993), the stable circadian melatonin rhythms were not developed until approximately 9–12 weeks of PNA (Attanasio et al., 1986; Kennaway et al., 1992; Kennaway et al., 1996; Joseph et al., 2015).
The second thing to be discussed is the maternal care, primarily maternal feeding, but it is still the roles of hormones in nature (Löhr and Siegmund, 1999; Nishihara et al., 2002; Park et al., 2020). Various hormones in breast milk, such as glucocorticoids and melatonin, can be absorbed and transferred into the neonatal circulation through the gastrointestinal tract (Arslanoglu et al., 2012; Wong et al., 2022). Interestingly, the cortisol and cortisone concentrations in breast milk follow the circadian of maternal HPA axis activity (van der Voorn et al., 2016; Italianer et al., 2020). Moreover, the cortisone rhythm in human breast milk at 1 month postpartum was associated with the nighttime sleep states of newborns at the age of 3 months (Toorop et al., 2020). Similarly, studies have also demonstrated the presence of pronounced circadian melatonin rhythms in the maternal breast milk (Illnerová et al., 1993; Katzer et al., 2016), which might contribute to the synchronization of postnatal circadian rhythms for neonates and their mothers.
One more thing needs to be pointed out is that, in addition to the maternal influence on the neonatal circadian rhythms, the maternal circadian rhythms are in turn affected by the development of the neonatal circadian rhythms (Nishihara and Horiuchi, 1998; Nishihara et al., 2000; Nishihara et al., 2002). For example, the ultradian rhythms (i.e., rhythms with period lengths much less than 24 h) (Rivkees, 1997) of rest-activity states were already detected as early as the third week of life for term infants, and the amplitude of this rhythm gradually increased from the 6th to 12th week, then formed circadian rhythms with a 24-h monophasic pattern (Nishihara et al., 2002). During this period, as neonates develop their own circadian rest-activity rhythms, the mothers’ rhythms would inevitably be affected by their interrupted sleep at night to take care of their babies (Nishihara and Horiuchi, 1998; Nishihara et al., 2000).
4.3.2 Environmental effects
In the late postnatal period, environmental time cues replace the maternal effects and begin to play a critical role in the development of neonatal circadian rhythms (Rivkees, 1997; Rivkees, 2004; Brooks and Canal, 2013). Light is the most dominant zeitgeber (Löhr and Siegmund, 1999; Challet, 2007; Wong et al., 2022), so the importance of light cannot be overemphasized. The light entrainments are functionally affected by the maturity of the eyes, RHT, and SCN (Brooks and Canal, 2013; Hazelhoff et al., 2021).
For term infants, the structural development of the eyes occurs as early as in utero, with the first structure of the eyes beginning to form at 17 days of GA (Van Cruchten et al., 2017), while the development of pupil starts approximately at 17 weeks of GA (Hazelhoff et al., 2021), and thereafter the pupillary light reflex already present at 34 weeks of GA (Robinson and Fielder, 1990). As the sole photoreceptive area in humans (Brooks and Canal, 2013), major classes of photoreceptors in the retina including the ipRGCs, rods, cones, and melanopsin all emerge and develop in the first trimester (Van Cruchten et al., 2017; Hazelhoff et al., 2021).
Covering the eyes of term neonates during the phototherapy for neonatal hyperbilirubinemia would result in significantly increased plasma melatonin levels during the first 72 h of life, indicating the sensitivity of the neonatal pineal glands to the changes of environmental illumination and the functional maturation of the neonatal eyes in transmitting the ambient light cues (Jaldo-Alba et al., 1993). However, it remains unclear when human ipRGCs transmit the light cues to the SCN, but the melanopsin-dependent ipRGCs in mice could provide light signals to the SCN already on the day of birth (Sekaran et al., 2005), and even earlier in late gestation before birth (Rao et al., 2013).
Honestly, only several studies reported the developmental process of human RHT and SCN. RHT has been identified in neonates at 36 weeks of GA (Rivkees, 2004; Rivkees, 2007). On the other hand, it has been found that the SCN of baboons born at term was already responsive to light and could be entrained by the low-intensity (200 lux) lighting (Rivkees et al., 1997). Interestingly, the SCN in preterm baboons functionally responded to light from a stage that was equivalent to 24 weeks of GA for human infants (Hao and Rivkees, 1999). Theoretically, the ambient light signals might be projected from the ipRGCs on retina to the SCN via the RHT at least after birth for term neonates (Hazelhoff et al., 2021). Further maturations of the human SCN continues after birth (Rivkees, 2004; Rivkees, 2007).
The numbers of AVP neurons and total neurons in the SCN of term neonates at birth are only 13% and 20% of those in adults, respectively (Swaab et al., 1990). After birth, these nerve cells increase rapidly to a peak at 1–2 years of age, then decrease gradually to the adult levels (Swaab, 1995). However, the development of VIP neurons in the SCN is slower and does not reach the adult levels until about 3 years of age (Swaab et al., 1994). Interestingly, there is a clear sex difference (i.e., 2-fold higher in males than that in females) in the number of VIP neurons after 10 years of age (Swaab et al., 1994), which suggested a possibility that the SCN involves not only in the timing of circadian rhythms, but also in the temporal organization of sexually dimorphic reproductive functions (Swaab, 1995; Hofman, 1997).
The impact of light on the clock gene expression is also a research progress worthy of special attention. The light affects the expression of clock genes, such as Per1, Per2, and Cry1, in the SCN of rodents at different developmental stages after birth (Kováciková et al., 2005; Ciarleglio et al., 2011). Moreover, it is the cycled light rather than the constant light that promotes the development of their biological clocks (Abraham et al., 2006; Ohta et al., 2006; Bode et al., 2011). Impressively, human neonates, especially the preterm neonates who exposed to cycled light would have better weight gains (Mann et al., 1986; Brandon et al., 2002; Vasquez-Ruiz et al., 2014; Brandon et al., 2017), less crying and fussing behaviors (Guyer et al., 2012), less hospital stay (Vasquez-Ruiz et al., 2014; Brandon et al., 2017), earlier rest-activity rhythms (Rivkees, 2004; Rivkees et al., 2004), longer nighttime sleep duration (Guyer et al., 2015), and even more robust salivary melatonin rhythms (Vasquez-Ruiz et al., 2014) compared to those exposed to continuous light or darkness. Systematic reviews also witnessed the beneficial effects of cycled light over continuous bright light or darkness for preterm neonates (Morag and Ohlsson, 2016; Liao et al., 2018). Therefore, as early as the 1990s, the guidelines for perinatal care that proposed by the American College of Obstetricians and Gynecologists and American Academy of Pediatrics were recommended to introduce a regular day-night cycled light into the NICU and neonatal nursery (Mirmiran et al., 2003a; Guyer et al., 2015).
Besides the light cues, studies have pointed out that the environmental noise disrupted the neurodevelopment of newborns and thus affected the development of their circadian rhythms (Wachman and Lahav, 2011; Kuhn et al., 2013). However, music therapy did improve the heart rate, breathing, and sleep of newborns (Arnon et al., 2006; Loewy et al., 2013), which might exert a positive impact on the well-being and quality of life for neonates, especially for preterm infants in the NICU (Yue et al., 2021). Other environmental factors, such as ambient temperature (Tourneux et al., 2008), comforting touch (Smith et al., 2014), remodeling mattress (Deiriggi, 1990; Visscher et al., 2015), and nursing measures (Collins et al., 2015; Lan et al., 2018) were also found to affect the neonatal rhythms of several physiological parameters, but their roles on the development of circadian rhythms in neonates have not been extensively studied yet (Liao et al., 2018; Gogou et al., 2019).
5 The characteristics of circadian rhythms in preterm infants
Preterm birth is defined as a live birth that occurs before 37 completed weeks of GA (Walani, 2020), which causes the fetus to detach prematurely from the natural protective environment of the uterus (Vohr, 2013; Hazelhoff et al., 2021) and puts an early end of fetal development in the uterus, especially for the brain and lung, which are critical to the neonates’ survival after birth (Saigal and Doyle, 2008). Preterm infants have an increased risk of short-term and long-term morbidities (Deng et al., 2021), like the neurological and respiratory conditions (Vogel et al., 2018). Unfortunately, those babies continue to contribute disproportionately to neonatal mortality and even the childhood morbidity, which puts a heavy burden on health resources (Saigal and Doyle, 2008; Vohr, 2013).
Impressively, circadian rhythms in premature infants primarily occur as ultradian or irregular rhythms (Mirmiran et al., 2003a; Rivkees, 2007; Koch et al., 2021). It is hypothesized that the rhythms in preterm neonates appeared to be closely related to their GA (Begum et al., 2006; Darnall et al., 2006), due to the development of the fetal brain is related to the stages of pregnancy (Andescavage et al., 2017). On the other hand, the continuous active brain maturation occurs after birth (Matthews et al., 2018), so their endogenously-driven rhythms also change with the postmenstrual age (PMA) (Mirmiran et al., 2003a; Darnall et al., 2006). However, due to the remarkable heterogeneity in terms of methodological designs, the characteristics of the circadian rhythms in preterm infants have not been consistently described, and some studies have even found conflicting results (Mirmiran et al., 2003a). For comprehensively and precisely understanding the circadian rhythms in preterm infants, relevant advances are summarized in Tables 1–4 and discussed as follows:
5.1 Sleep-wake rhythms
It is well established that the sleep is essential for normal brain development and health throughout the whole life (Peirano et al., 2003; Gogou et al., 2019). Premature newborns spend more than 70% of their first several weeks sleeping after birth (Ardura et al., 1995; Wong et al., 2022), thereby maintaining the proper sleep homeostasis is even more important for their neurological development and functional maturation (Bennet et al., 2018; Uchitel et al., 2021). The direct behavioral observations, parental sleep questionnaires, video recordings, polysomnography, actigraphy, and electroencephalography (EEG) (Table 1) have been developed to investigate the sleep-wake states of neonates (Mirmiran et al., 2003a; Collins et al., 2015; Gogou et al., 2019).
Based on the behavioral, cardiopulmonary, and EEG patterns (Darnall et al., 2006; Dereymaeker et al., 2017), the sleep states of preterm infants are generally classified as: active sleep (AS), the precursor of adult rapid eye movement (REM) sleep; quiet sleep (QS), the precursor of adult non-REM sleep; and indeterminate sleep (IS), the transition between AS and QS patterns (Mirmiran et al., 2003a; Liao et al., 2018). More specifically, the AS could promote the synapse formation, neuronal differentiation and migration, and the development of brain functional connectivity networks (Kurth et al., 2017; Gogou et al., 2019), whilst the QS promote the myelination, replenishment of energy reserves, and cognitive development in premature infants (Liao et al., 2018; Gogou et al., 2019).
As summarized in Table 1, Curzi-Dascalova et al. (1993), found the AS and QS states can be discerned in preterm infants as early as 27 weeks of GA. The results varied due to the different GA of the enrolled cases, but most studies revealed that preterm infants experienced more total sleep time and AS, while less QS than term ones (Anders and Keener, 1985; Ardura et al., 1995; Sahni et al., 1995; Hoppenbrouwers et al., 2005; Guyer et al., 2015; Georgoulas et al., 2021), which might reflect the accelerated neurological maturation of preterm infants (Mirmiran et al., 2003a; Bennet et al., 2018). Besides, preterm infants had fewer total arousals and, more specifically, fewer arousals in the AS (Guyon et al., 2022), which seemed to cause a higher risk of sudden infant death syndrome (Mirmiran et al., 2003a; Bennet et al., 2018).
With developmental maturity, preterm infants have more sleep during nighttime but less during daytime (Antonini et al., 2000; Korte et al., 2001; Guyer et al., 2015; Lan et al., 2019; Guyon et al., 2022). Meanwhile, as the PMA increased, the AS proportion comes out of a decreasing trend, but it is not true for the QS, IS, wakefulness, and activity, which all experience an increasing trend (Anders and Keener, 1985; Curzi-Dascalova et al., 1988; Curzi-Dascalova et al., 1993; Borghese et al., 1995; Sahni et al., 1995; Ingersoll and Thoman, 1999; Mirmiran et al., 2003b; Holditch-Davis et al., 2004; Hoppenbrouwers et al., 2005; Foreman et al., 2008; Dorn et al., 2014; Guyer et al., 2015; Lan et al., 2019; Cailleau et al., 2020; Park et al., 2020; Georgoulas et al., 2021; Guyon et al., 2022). In addition, other factors like sex, illness severity, body weight, ventilatory support, maternal smoking, and ambient temperature also affect the sleep-wake patterns (Bach et al., 2000; Hoppenbrouwers et al., 2005; Foreman et al., 2008; Lan et al., 2019).
It is well understood that the sleep homeostasis in humans are regulated by two independent but synergistic processes (Borbély, 1982; Deboer, 2018): a Clock-dependent circadian process (Process C), controlled by the SCN circadian oscillator, determines the alternation of different sleep propensity (Cremer et al., 2016); and a Sleep-dependent homeostatic process (Process S) that is determined by the prior sleep pressure, which comes from the adenosine buildup in the basal forebrain during wakefulness (Deboer, 2018; Wong et al., 2022). However, due to the immature development of the central nervous system, especially the SCN, Process C and Process S are not stably present in preterm infants or even in term ones (Salzarulo and Fagioli, 1992; Schwichtenberg et al., 2016). As a result, preterm infants experience many sleep and wake episodes within the 24-h period, and those ultradian sleep-wake rhythms persist for several months until the Process C and Process S are gradually developed (Mirmiran et al., 2003a; Cremer et al., 2016).
As shown in Table 1, preterm infants exhibit ultradian or irregular sleep-wake rhythms with different periods in the early postnatal life (Mirmiran et al., 1990; Hayes et al., 1993; Borghese et al., 1995; Shimada et al., 1999; Scher et al., 2005; Dorn et al., 2014; Koch et al., 2021), which might be explained by the environmental factors, such as feeding patterns (Glotzbach et al., 1995; Thomas, 2000; Bueno and Menna-Barreto, 2016) and respiratory states (Palmu et al., 2013). As for when the sleep-wake rhythms begin to occur and entrain, Scher et al. (2005), observed the ultradian sleep-wake rhythms as early as 25 weeks of PMA. Mirmiran and Kok, (1991) found the circadian sleep-wake rhythms began to appear after 29 weeks of PMA. However, McMillen et al. (1991), found that the entrainment of circadian sleep-wake rhythms did not occur in 50% of the preterm infants at 47 weeks of PMA, and all cases did not begin to develop the circadian rhythms until approximately 54 weeks of PMA.
Besides, several studies also demonstrated that a definite sleep-wake cycling existed in preterm infants with the advanced GA and became more prominent as the PMA increased (Sisman et al., 2005; Soubasi et al., 2009; Lee et al., 2010). Therefore, it could be concluded that with the continuous development of the brain and neural functions, circadian sleep-wake rhythms in preterm infants are consolidated and eventually developed to a 24-h pattern, just as those in adults (Mirmiran et al., 2003a; Bennet et al., 2018).
5.2 Cardiorespiratory rhythms
Many physiological biomarkers of the cardiopulmonary system in adults, such as the heart rate, blood pressure, and respiratory rate, exhibit distinct circadian rhythms (Elstad et al., 2018). A complex network that composed of the brainstem respiratory center, autonomic nervous system, and a variety of central and peripheral chemoreceptors and mechanoreceptors is responsible for regulating the rhythmic oscillations of the cardiorespiratory system (Darnall et al., 2006; Longin et al., 2006). Due to the immaturity of this network (Hunt, 2006), cardiorespiratory events like apnea, periodic breathing, and bradycardia are common in premature infants (Hodgman et al., 1990; Darnall et al., 2006), which leads to the erratic cardiopulmonary rhythms with marked individual differences (Begum et al., 2006). Clinically, the incidence and duration of cardiorespiratory events are associated with the GA and PMA (Hellmeyer et al., 2012; Fairchild et al., 2016; Patel et al., 2016).
As shown in Table 2, some, but not all, preterm infants experienced circadian or ultradian rhythms for the heart rate, pulse rate, respiratory rate, blood pressure, and oxygen consumption at the first few weeks after birth (Begum et al., 2006; Mirmiran and Kok, 1991; Bauer et al., 2009; D'Souza et al., 1992; Updike et al., 1985). Interestingly, unlike the ultradian sleep-wake rhythms gradually grew into circadian rhythms after birth, these cardiopulmonary rhythms in premature infants appeared and disappeared erratically (Tenreiro et al., 1991; Dimitriou et al., 1999), e.g., presence on day 2 but absence on day 7 after birth for the heart rate rhythms, which might be caused by the residual of maternal effects (Dimitriou et al., 1999). Tenreiro et al. (1991) also proposed that the circadian components of these cardiopulmonary rhythms gradually and erratically came into phases with one another, while the regular light-dark and feeding patterns seemed to promote the presence of the dominant circadian rhythms, which developed as the increased coupling between the component oscillators.
In addition, the well-developed laryngeal reflexes and coordination of pharyngoesophageal-cardiorespiratory (PECR) responses are essential for the development and maintenance of cardiorespiratory rhythms (Gewolb and Vice, 2006; Hasenstab-Kenney et al., 2020). As shown in Table 2, pharyngeal stimulations cause a decrease of heart rate in premature infants with uncoordinated suck-swallow-respiration rhythms due to the immature laryngeal reflexes and PECR responses, which would aggravate the disturbance of cardiac and respiratory rhythms (Hasenstab et al., 2019; Hasenstab-Kenney et al., 2020). (Gewolb et al., 2001; Gewolb and Vice, 2006) found that the development and establishment of suck-swallow rhythms were associated with their PMA. The swallow rhythms appeared at 32 weeks of PMA first (Gewolb et al., 2001), followed by the stabilization of suck and suck-swallow rhythms between 36 and 40 weeks of PMA (Gewolb and Vice, 2006), then the suck-swallow-respiration rhythms began to coordinate and to integrate as the adaptation of feeding patterns and the maturation of neurodevelopment (Darnall et al., 2006).
5.3 Body temperature rhythms
The human body temperature is precisely regulated by a network that consists of the skin thermal sensors, hypothalamic thermoregulatory center, autonomic nervous system, and several thermoregulation effector systems including brown adipose tissue, peripheral vasomotricity, and sweat glands (Bach et al., 1996; Jost et al., 2017). Due to the immaturity of the regulatory network, especially the dysfunction of the autonomic nervous system, their body temperature during the first few days of life is susceptible to the rapidly changed external environment temperature (Jost et al., 2017). Therefore, premature infants are typically nursed in the incubators to treat the autonomic dysregulation of body temperature (Thomas, 2001). Interestingly, Bueno and Menna-Barreto (2016), found a positive correlation between the wrist temperature and environment temperature inside the incubator, but no significant association between the period or potency for them. Similarly, Thomas (2001) demonstrated that the circadian of incubator temperature did not appear to be the primary determinant of the body temperature rhythms.
As summarized in Table 3, due to the heterogeneity of the body temperature monitoring, the GA of preterm infants, and sample size, the body temperature rhythms have not yet been consistently described. Several studies observed the ultradian body temperature rhythms within the first few days of life (Glotzbach et al., 1995; Mirmiran et al., 2003b; Koch et al., 2021), and the circadian rhythms by approximately 1–3 months of PNA (Mirmiran et al., 2003b; Bueno and Menna-Barreto, 2016). Interestingly, Thomas and Burr (2002), found that the acrophase of circadian abdominal skin temperature rhythms was related to the parental co-sleeping and length of hospital stay for preterm infants at 44–46 weeks of PMA. However, some studies demonstrated that the body temperature rhythms were only found in some, but not all preterm infants (Mirmiran et al., 1990; Mirmiran and Kok, 1991; D'Souza et al., 1992; Updike et al., 1985; Thomas, 2001). For example, Tenreiro et al. (1991) found that the ultradian and circadian rhythms of skin temperature appeared and disappeared erratically during 6–17 weeks of PNA, which was similar to the cardiopulmonary rhythms.
5.4 Hormonal rhythms
As summarized in Table 4, due to the difficulties in sample collection and analysis, studies on hormonal rhythms in preterm infants are still very limited until now, and nearly all focused on the cortisol and melatonin rhythms. With regard to the cortisol, due to the immature of HPA axis (Bolt et al., 2002), no significant circadian or ultradian rhythms were observed during the early postnatal periods (Economou et al., 1993; Jett et al., 1997; Kidd et al., 2005; Dorn et al., 2014). Nevertheless, studies have found that healthy preterm infants had higher nighttime cortisol levels than daytime at birth, and that cortisol levels tended to decrease gradually after birth (Kidd et al., 2005; Dorn et al., 2014). Impressively, premature infants with perinatal stress like respiratory distress experienced higher cortisol levels at nighttime after birth compared with those healthy preterm and term neonates (Economou et al., 1993; Gunes et al., 2006).
It remains unclear when premature infants develop the circadian cortisol rhythms. Antonini et al. (2000) found the salivary cortisol circadian rhythms emerged and persisted at approximately 8–12 weeks of PNA, which was in line with term infants. However, Ivars et al. (2017) found that the cortisol rhythms were established by 1 month of corrected age, persisted throughout the first year of life, but delayed by topical corticosteroid medication. In addition, Ivars et al. (2017) also suggested that the establishment of cortisol rhythms was related to the GA rather than PNA, because the maturation of adrenal cortex was depend on the GA of preterm infants (Bolt et al., 2002).
Circadian melatonin rhythms could not be detected in preterm infants under different ambient illumination conditions during the early postnatal life (Commentz et al., 1996; Mantagos et al., 1996; Biran et al., 2019). Several studies demonstrated that the blood melatonin and urine 6-sulfatoxymelatonin levels were positively correlated with the GA (Biran et al., 2019) and birth weight of preterm infants (Muñoz-Hoyos et al., 2007), but the serum melatonin levels and urine 6-sulfatoxymelaton excretion increased during the first 7 days and even 52 weeks of PNA (Kennaway et al., 1992; Commentz et al., 1996; Muñoz-Hoyos et al., 2007), which might be attributed to the gradual maturation of the pineal gland where the melatonin is mainly synthesized (Commentz et al., 1997).
However, Commentz et al. (1996) found the urine melatonin and 6-hydroxymelatonin sulfate excretion in male preterm infants during 2–7 days of PNA were negatively associated with the GA, indicating that the melatonin levels might be related to the sex. As for the establishment of circadian melatonin rhythms, Kennaway et al. (1992) observed the appearance of urine 6-sulfatoxymelaton circadian rhythms was approximately at 18–21 weeks of PNA, which was delayed by 9 weeks than those term infants and 2–3 weeks after correcting for GA.
6 The effects and mechanisms of caffeine on circadian rhythms
The potential association between caffeine consumption and circadian rhythms has attracted extensive attention in the past decades (Landolt, 2015). However, the underlying mechanisms remain largely elusive. Various research attempts in the non-human field also reinforce this impression (Spaeth et al., 2014). In this section, we briefly introduce the up-to-date progress that achieved in human and non-human mammals, while the effects on premature infants will be delineated in the next section.
6.1 The effects of caffeine on circadian rhythms
In humans, several clinical observational studies with small sample size have witnessed the alterations of circadian sleep-wake (Landolt et al., 1995a; Landolt et al., 1995b; McHill et al., 2014; Weibel et al., 2021), body temperature (Wright et al., 1997; Wright et al., 2000; McHill et al., 2014), blood pressure (Green and Suls, 1996; Guessous et al., 2014), heart rates (Green and Suls, 1996; Kohler et al., 2006; Crooks et al., 2019), melatonin (Wright et al., 1997; Wright et al., 2000; Burke et al., 2015), and cortisol rhythms (Lovallo et al., 2005; Rieth et al., 2016) in adults who consumed caffeine by comparison with placebo controls.
In rodents, caffeine disrupted the mesors, amplitudes, and acrophases of the circadian heart rate, temperature, motor activity, and sleep-wake rhythms (Pelissier et al., 1999; Pelissier-Alicot et al., 2002; Vivanco et al., 2013; Panagiotou et al., 2019). Caffeine also potentiated the light-induced phase shift, which responded to the rest-activity circadian rhythms, indicating that caffeine enhanced the clock sensitivity to light (Antle et al., 2001; Vivanco et al., 2013; van Diepen et al., 2014; Jha et al., 2017; Ruby et al., 2018). In addition, caffeine lengthened the period and amplitude of circadian clocks in mammalian cells in vitro and in mice ex vivo and in vivo (Oike et al., 2011; Narishige et al., 2014; Burke et al., 2015). At the cellular level, caffeine also altered the expression of circadian clock genes, such as Clock, Bmal1, and Per1 in the liver and jejunum of mice under ad libitum feeding conditions (Sherman et al., 2011).
6.2 The mechanisms of caffeine on circadian rhythms
Caffeine influences the circadian rhythms by modulating the endogenous cAMP/Ca2+ signaling pathway, the core components of the mammalian circadian pacemaker (Harvey et al., 2020; O'Neill et al., 2008), through a variety of complex mechanisms (Aguilar-Roblero et al., 2007; Narishige et al., 2014; Burke et al., 2015; Landolt, 2015; Jagannath et al., 2021) (Figure 3). Basically, caffeine antagonizes all types of adenosine receptors (A1, A2A, A2B, and A3 receptors) and mainly functions by non-specifically antagonizing the A1 and A2A receptors (Nehlig et al., 1992; Cappelletti et al., 2015; Rodak et al., 2021; Yang et al., 2021). The blockade of adenosine receptors indirectly regulates the production of cAMP by inhibition (A1 and A3 receptors) or stimulation (A2A and A2B receptors) of adenylate cyclase (Nehlig et al., 1992; Kumar and Lipshultz, 2019; Yang et al., 2021). Caffeine also prevents the degradation and increases the intracellular cAMP levels by non-selectively inhibiting phosphodiesterase (Nehlig et al., 1992; Cappelletti et al., 2015; Kumar and Lipshultz, 2019; Yang et al., 2021). In addition, caffeine mobilizes intracellular Ca2+ transmission from the endoplasmic reticulum through activating the ryanodine receptor channels (Aguilar-Roblero et al., 2007; Kumar and Lipshultz, 2019) and the inositol triphosphate receptors (Yang et al., 2021).
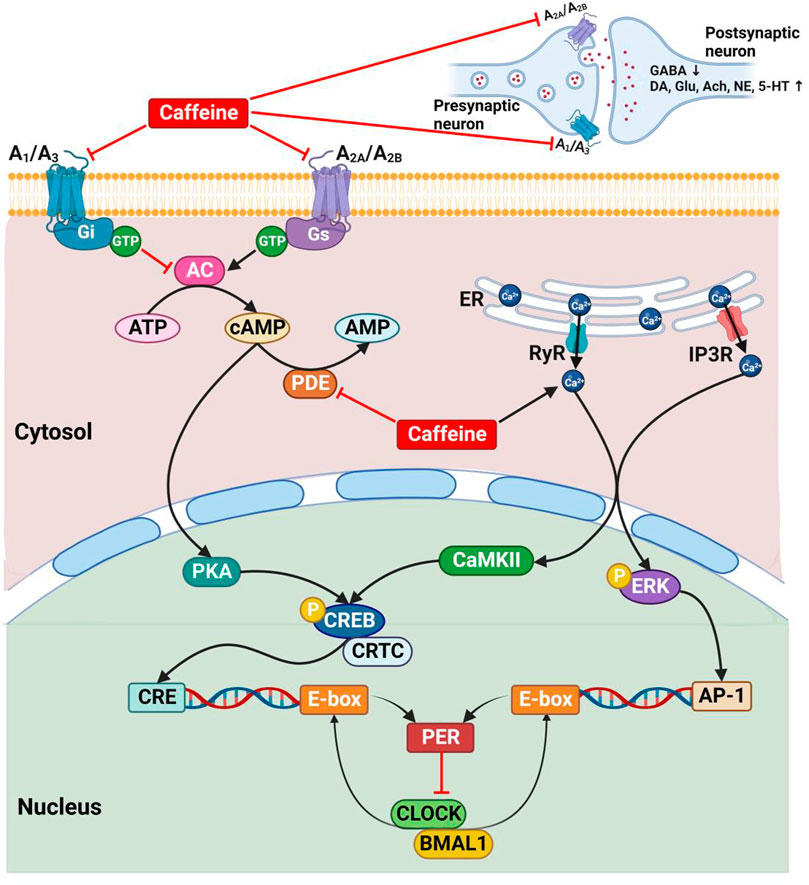
FIGURE 3. The mechanisms of caffeine on circadian rhythms. A1, A3, A2A, and A2B, adenosine receptors; AC, adenylate cyclase; Ach, acetylcholine; AMP, adenosine monophosphate; ATP, adenosine triphosphate; AP-1, activator protein 1; BMAL1, brain and muscle ARNT-like 1; CaMKⅡ, Ca2+/calmodulin-dependent protein kinase Ⅱ; cAMP, cyclic adenosine monophosphate; CLOCK, circadian locomotor output cycles kaput; CRE, cAMP response element; CREB, cAMP responsive element binding protein; CRTC, CREB regulated transcription coactivator; DA, dopamine; ER, endoplasmic reticulum; ERK, extracellular regulated protein kinases; GABA, γ-aminobutyric acid; Gi, inhibitory adenylate cyclase G protein; Glu, glutamate; Gs, stimulating adenylate cyclase G protein; GTP, guanosine triphosphate; IP3R, inositol triphosphate receptor; NE, norepinephrine; P, phosphorylation; PDE, phosphodiesterase; PER, period; PKA, protein kinase A; RyR, ryanodine receptor; 5-HT, serotonin.
The increased cytosolic cAMP/Ca2+ activates the protein kinase A (PKA) and Ca2+/calmodulin-dependent protein kinase Ⅱ (CaMKⅡ), thereby leading to the phospho-dependent activation of cAMP response element binding protein (CREB), which in concert with its coactivators to activate the cAMP response element (CRE) (Narishige et al., 2014; Harvey et al., 2020; Reichert et al., 2022). Besides, the increased intracellular Ca2+ levels also result in the phosphorylation of extracellular regulated protein kinases (ERK), which drives to form the activator protein 1 (AP-1) transcription factor (Jagannath et al., 2021). Then, interestingly, CRE and AP-1 together drive the Per gene transcription (Narishige et al., 2014; Jagannath et al., 2021), which in turn participates in the transcriptional feedback loops that regulate circadian rhythms (Figure 3).
In addition, caffeine affects the release of neurotransmitters, such as γ-aminobutyric acid, dopamine, glutamate, acetylcholine, norepinephrine, and serotonin, between synaptic neurons in almost all brain areas by blocking the adenosine receptors (Nehlig et al., 1992; Cappelletti et al., 2015; Yang et al., 2021) (Figure 3), thereby significantly influencing the sleep-wake rhythms (Kumar and Lipshultz, 2019).
7 The effects of caffeine on circadian rhythms in preterm infants
Caffeine is widely prescribed to treat or prevent the AOP (Eichenwald, 2020; van Dam et al., 2020) and has recently been attempted to prevent the encephalopathy (Williamson et al., 2021; Yang et al., 2021) for preterm neonates in the NICU. Therefore, studies on the caffeine treatment in preterm infants mainly focus on the respiratory and neurodevelopmental outcomes (Schmidt et al., 2006; Schmidt et al., 2007), while less attention has been paid to its effects on their circadian rhythms.
In fact, the ultradian or irregular circadian rhythms due to the neurodevelopmental immaturity of preterm infants with different GA during the early postnatal life (Begum et al., 2006; Darnall et al., 2006) are more likely to mask caffeine’s effects. Moreover, preterm infants with different PNA and/or PMA experience different circadian characteristics (Mirmiran et al., 2003a; Darnall et al., 2006), so whether the response to caffeine therapy are partly related to the maturation of the circadian system in preterm infants remains to be explored.
Thus, relevant advances are summarized here to delineate those effects of caffeine on the circadian rhythms in preterm infants. Besides, theophylline and aminophylline, another two methylxanthines and fully metabolized in the body to produce the main metabolite caffeine (Bory et al., 1979; Pacifici, 2014), are also commonly used in the treatment of AOP (Henderson-Smart and De Paoli, 2010; Henderson-Smart and Steer, 2010; Eichenwald et al., 2016). The real effects of theophylline and aminophylline are thus thought to be related to caffeine in nature (Bory et al., 1979). Collectively, studies involving the effects of caffeine, theophylline, and aminophylline on the circadian rhythms in preterm infants are summarized in Tables 5, 6 and described as follows:
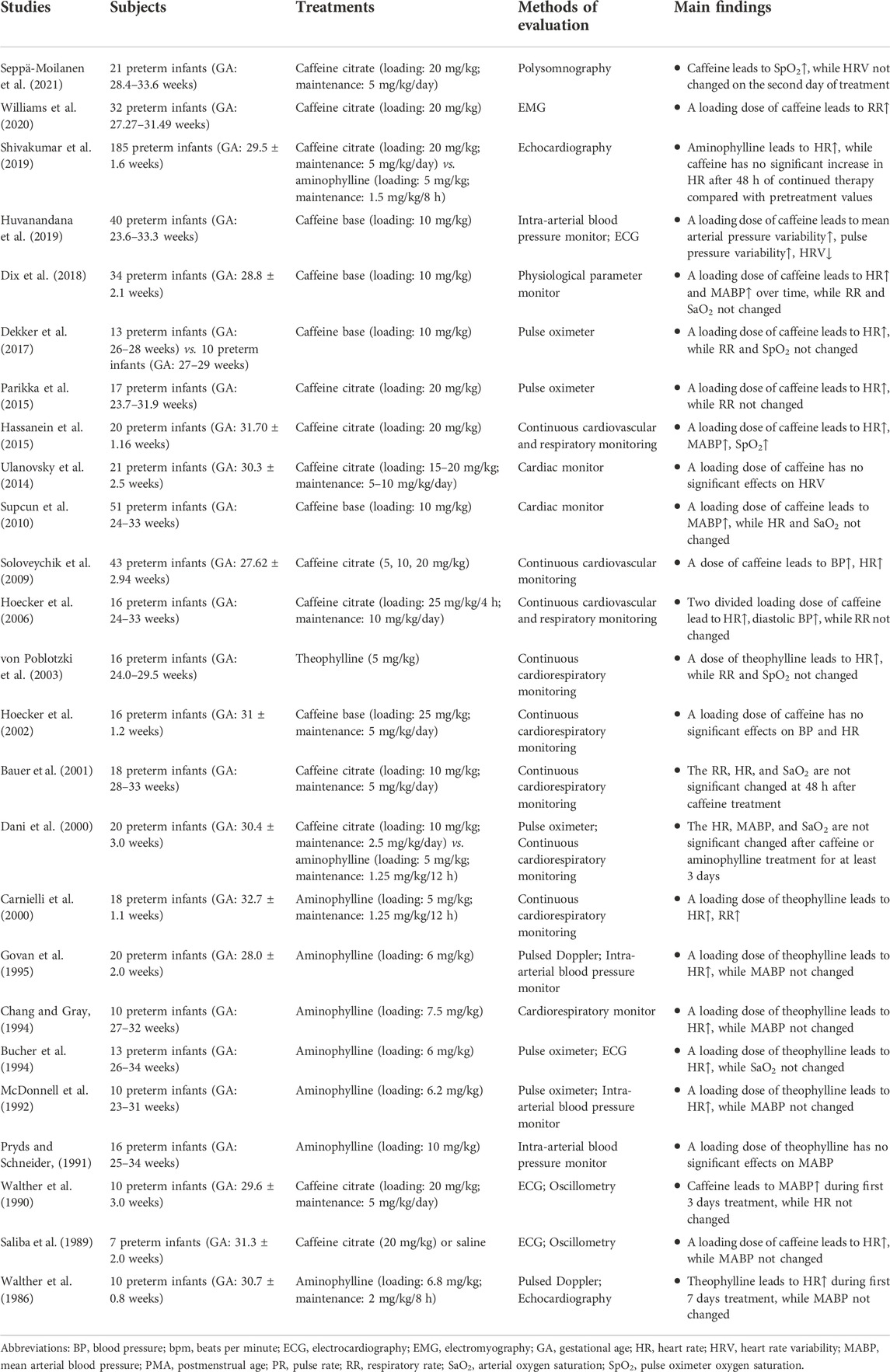
TABLE 6. Studies about the effects of methylxanthine on cardiorespiratory rhythms in preterm infants.
7.1 The effects on sleep-wake rhythms
The well-studied effects of caffeine on sleep-wake rhythms in preterm infants are still limited as the sample sizes were small and the study designs were heterogeneous (Table 5). Some studies revealed that the sleep-wake patterns were not significantly changed after short-term treatment with caffeine or theophylline during short observation periods (Gabriel et al., 1978; Curzi-Dascalova et al., 2002; Chardon et al., 2004; Seppä-Moilanen et al., 2021).
However, some other studies observed significant effects of caffeine on the sleep-wake rhythms, although these effects were not entirely consistent (Dietrich et al., 1978; Thoman et al., 1985; Hayes et al., 2007; Hassanein et al., 2015; Koch et al., 2020). For example, Koch et al. (2020), found that the AS decreased while the wakefulness increased but QS unchanged as caffeine concentrations and the PNA increased over the first 5 days of life in preterm infants more than 28 weeks of GA, but no clear effects on the sleep-wake states were found in preterm infants less than 28 weeks of GA, and no such PNA effects were found in no-caffeine cohort. Hassanein et al. (2015) also detected significant decreases in the AS, QS, and drowsiness, while increases in the quite alert, active alert, and crying in preterm infants half an hour after caffeine administration. Similar methylxanthine-induced changes in the AS and wakefulness states were also observed in studies conducted by Hayes et al. (2007) and by Thoman et al. (1985). However, Dietrich et al. (1978) found the AS and wakefulness increased while the QS and IS decreased during theophylline therapy.
In addition, Lee et al. (2010) discovered that the appearance of sleep-wake cycling was associated with the aminophylline use and more prominent. However, in the prospective follow-up study of the CAP trial (Marcus et al., 2014), no significant differences in sleep states were found in preterm infants aged 5–12 years who had been treated with caffeine after birth compared with the placebo group, which possibly due to the apparent discrepancy in total recording and sleep time between the two groups.
This is also true for some animal studies. Denenberg et al. (1982) found that theophylline reduced the AS, while increased wakefulness, delayed the development of QS, and affected the intermediate states of sleep-wake and AS-QS transitions in newborn rabbits. Montandon et al. (2009) also discovered that the sleep time was reduced, sleep onset latency was increased, and non-REM sleep was fragmented in adult rats treated with caffeine compared to controls during the neonatal period.
Due to the heterogeneous designs and inconsistent results of the above studies, it is difficult to draw clear conclusions. Nonetheless, it can be summarized that caffeine affects the sleep patterns in preterm infants, especially the AS and wakefulness, and the effects might persist into the childhood and even the adulthood. If this hypothesis holds true, then the inhibition of adenosine receptors by caffeine would exactly explain the altered sleep-wake states in preterm infants, as the association between caffeine, adenosine, and sleep has been well documented in adults (Huang et al., 2011; Porkka-Heiskanen and Kalinchuk, 2011; Huang et al., 2014a; Urry and Landolt, 2015; Reichert et al., 2022). In addition, the alteration of sleep-wake patterns might be partially responsible for the caffeine-induced increase in cerebral cortical activity (Supcun et al., 2010; Hassanein et al., 2015) and decrease in apneic episodes (Dietrich et al., 1978; Montandon et al., 2009; Seppä-Moilanen et al., 2019; Seppä-Moilanen et al., 2021).
7.2 The effects on cardiorespiratory rhythms
Current studies have confirmed that caffeine acts both peripherally and centrally to stimulate respiration mainly via inhibiting the adenosine A1 and A2A receptors (Abdel-Hady et al., 2015; Eichenwald et al., 2016; Dobson and Hunt, 2018). Caffeine activates the medullary respiratory center, improves sensitivity to carbon dioxide, increases respiratory muscle strength, enhances diaphragmatic contractility, and induces bronchodilation (Kassim et al., 2009; Parikka et al., 2015; Dekker et al., 2017; Sanchez-Solis et al., 2020; Williams et al., 2020), which synergistically cause the increased minute ventilation and oxygen consumption, while cause the decreased apnea, periodic breathing, and intermittent hypoxia (Seppä-Moilanen et al., 2021; Seppä-Moilanen et al., 2019; Dobson et al., 2017; Rhein et al., 2014; von Poblotzki et al., 2003; Bauer et al., 2001; Carnielli et al., 2000).
In addition, caffeine or theophylline therapy increases the cardiac output, stroke volume, and metabolic rate (Walther et al., 1986; Walther et al., 1990; Carnielli et al., 2000; Bauer et al., 2001; Soloveychik et al., 2009; Shivakumar et al., 2019), but decreases blood flow velocities in cerebral and intestinal arteries (Pryds and Schneider, 1991; McDonnell et al., 1992; Bucher et al., 1994; Chang and Gray, 1994; Govan et al., 1995; Lundstrøm et al., 1995; Lane et al., 1999; Hoecker et al., 2002; Hoecker et al., 2006; Dix et al., 2018; Hwang et al., 2018; Abdel Wahed et al., 2019) for preterm infants, which appeared to be related to the enhanced endothelial function through antagonism of adenosine receptors, inhibition of phosphodiesterase, and through promotion of intracellular calcium concentrations (Higashi, 2019). Although the clinical significance remains unclear, this reduced perfusion activity was a reminder that caffeine might have adverse effects on the developing brain and gastrointestinal tract (McDonnell et al., 1992; Lane et al., 1999; Hoecker et al., 2002; Hoecker et al., 2006; Atik et al., 2017; Abdel Wahed et al., 2019).
Unlike the cardiopulmonary system, the effects of caffeine on the cardiorespiratory rhythms in preterm infants have not been specifically studied. Nonetheless, the effects of caffeine on the heart rate, respiratory rate, blood pressure, and oxygen saturation have been examined. As summarized in Table 6, some studies found that a loading of caffeine or theophylline increases the heart rate (Hassanein et al., 2015; Dekker et al., 2017; Parikka et al., 2015; von Poblotzki et al., 2003; Carnielli et al., 2000; Soloveychik et al., 2009; Dix et al., 2018; Govan et al., 1995; Chang and Gray, 1994; Bucher et al., 1994; McDonnell et al., 1992; Saliba et al., 1989), blood pressure (Soloveychik et al., 2009; Supcun et al., 2010; Hassanein et al., 2015; Dix et al., 2018; Huvanandana et al., 2019), respiratory rate (Williams et al., 2020), and oxygen saturation (Hassanein et al., 2015), which were in line with those studies with multiple caffeine dosing (Walther et al., 1986; Walther et al., 1990; Hoecker et al., 2006; Shivakumar et al., 2019). Those findings reflected the complex effects, directly or indirectly like the enhanced autonomic nervous system responsiveness (Huvanandana et al., 2019), of caffeine on the cardiopulmonary system. However, several other studies did not find similar effects (Pryds and Schneider, 1991; Dani et al., 2000; Bauer et al., 2001; Hoecker et al., 2002; Ulanovsky et al., 2014).
Unfortunately, no research has touched this area yet in premature infants until now. It is worth mentioning that neonatal caffeine treatment upregulates adenosine receptors in cardiorespiratory related nuclei of the rat brain (Gaytan et al., 2006; Gaytan and Pasaro, 2012), and this effect persists into the adulthood (Bairam et al., 2009), which underscores the urgent to study the potential long-term effects of caffeine on the cardiorespiratory system in preterm infants (Montandon et al., 2008). In view of the complex and profound effects of caffeine in this field, systematic and in-depth research is still necessary.
7.3 The effects on other rhythms
Two studies recorded the body temperature of preterm infants and incubator temperature during short-term caffeine administration. Chardon et al. (2004) found that caffeine has no significant effect on the skin temperature and incubator temperature. However, Bauer et al. (2001) observed that a lower incubator temperature was sufficient to maintain a normal body temperature for preterm infants after caffeine treatment, which might be related to the increased metabolism caused by methylxanthines (Bucher et al., 1994; Carnielli et al., 2000; Bauer et al., 2001). However, the effects of caffeine on circadian body temperature rhythms have not been extensively studied. Similarly, although caffeine has been shown to affect melatonin (Wright et al., 1997; Wright et al., 2000; Burke et al., 2015) and cortisol (Lovallo et al., 2005; Rieth et al., 2016) rhythms in adults, these effects in premature infants still need to be addressed.
Collectively, the relevant research on the circadian rhythms in premature infants receiving caffeine therapy is still scarce. Although existing studies have suggested the possible effects of caffeine on the circadian rhythms, heterogeneity in study designs and inconsistency in conclusions weaken the power of those evidence. More research is needed in the future to confirm the effects of caffeine and the underlying mechanisms. The story should not end here.
8 Circadian-based caffeine therapeutic strategies for AOP: New possibility opens up
It is estimated that more than 15 million neonates are born preterm globally each year, and the preterm birth appears to be increasing in most countries (Vogel et al., 2018; Walani, 2020; Deng et al., 2021). Premature babies may have various problems like AOP. Unfortunately, the tough challenges are always there for the current AOP therapy, such as significant interindividual variability in the response to caffeine (Saroha and Patel, 2020; He et al., 2021). Intriguingly, one most recent study revealed that the Clock gene polymorphisms were significantly associated with the response to caffeine therapy in preterm infants (Guo et al., 2022). Although the molecular action mechanism through which there is a better response is unknown, these results show that the circadian rhythms might play a critical role in response to the therapy. In this way, a new possibility opens up in this area of research (Figure 4), and we tentatively propose three initiatives.
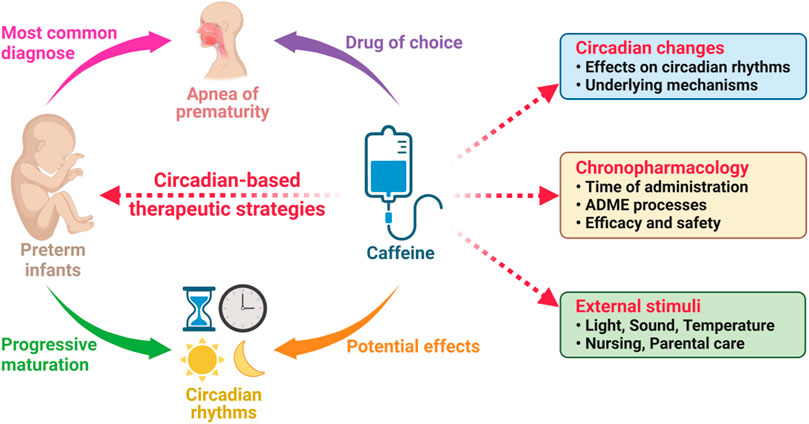
FIGURE 4. The circadian-based therapeutic strategies of caffeine in preterm infants with apnea of prematurity. ADME, absorption, distribution, metabolism, and excretion.
8.1 Considering the circadian changes
As discussed above, the efficacy of caffeine appeared to interact with the circadian rhythms in premature infants. Studies have demonstrated the significant effects and underlying mechanisms of caffeine in adults and in animals (Landolt, 2015), but it remains unclear whether the similar mechanisms also exist in those preterm infants. The effects of caffeine on the circadian rhythms, especially the sleep-wake rhythms, are advised to be considered into the strategy of the caffeine therapy (Figure 4).
In addition, studies have revealed that several circadian-related problems like sleep, breathing, and blood pressure in premature infants may persist into childhood and even adulthood (Weisman et al., 2011; Huang et al., 2014b; Sipola-Leppanen et al., 2015; Caravale et al., 2017; Durankus et al., 2020). Based on the existing evidence, it is feasible to propose that caffeine’s effects on circadian rhythms may ameliorate those problems and promote the maturation of circadian rhythms in preterm infants to the level of normal term infants.
8.2 Considering the chronopharmacology
The concept of chronopharmacology holds that the ADME processes and the sensitivity of a biological target to a drug are determined by the endogenous biological circadian oscillations (Ohdo et al., 2019; Bicker et al., 2020; Dong et al., 2020; Dobrek, 2021). Variable efficacy and safety profiles would be exhibited for many drugs if they are administered at different times of the day (Dallmann et al., 2016; Cederroth et al., 2019; Nahmias and Androulakis, 2021). For preterm infants, interestingly, several circadian-related gene polymorphisms were found to be significantly associated with the response to caffeine therapy for AOP (Guo et al., 2022). It remains unclear whether caffeine administrated at different times of the day would cause changes in the ADME processes and the therapeutic effects, but it really opens a possibility to applicate the chronopharmacology in the NICU.
Although less research is currently available, there are rare but thought-provoking reports that arouse our strong interests (Smolensky et al., 1987; Pelissier-Alicot et al., 2002), which will lead us into a wonderland in the future. For examples, Pelissier-Alicot et al. (2002) found that the pharmacokinetic profiles of caffeine in rats, such as the clearance, volume of distribution, and area under the plasma concentration-time curve (AUC), depended strongly on the time of day of administration, while the daily rhythmicity of heart rate, body temperature, and locomotor activity in rats also changed with the dosing time of caffeine. Similarly, Smolensky et al. (1987) demonstrated that the pharmacokinetic profiles and therapeutic effects of theophylline in asthmatic children varied with the dosing time. These findings attract us that the circadian rhythms might play a critical role in the ADME processes as well as the efficacy and safety of caffeine therapy in preterm infants.
Currently, caffeine is now commonly administered once daily in preterm infants (Long et al., 2021). The question is whether we are willing to make positive attempts to tailor the dosing time according to the principles of chronopharmacology. If the significant association between circadian-related gene polymorphisms and response to caffeine therapy in preterm infants (Guo et al., 2022) were true and phenotypically manifested, then the administration at different time points of the day is more likely to witness those potentially altered pharmacokinetics of and clinical response to caffeine.
Maintaining normal circadian rhythms are necessary to stay health. Essentially, caffeine interferes with these rhythms to a certain extent, and its arousal effects are very important for the AOP management among various pharmacological mechanisms. Therefore, whether to apply caffeine in accordance with the circadian rhythms to maintain the stabilities of these rhythms as much as possible, or to subtly counteract these rhythms to amplify its arousal effect and achieve a better therapeutic effect, all these aspects deserve our in-depth consideration (Figure 4).
8.3 Considering the other external stimuli
If the homeostasis of circadian rhythms were necessary for health, then correcting the possible adverse effects due to preterm birth is a matter that needs to be taken seriously in the NICU, including the effects on the treatment drugs being used. As discussed above, several external stimuli or known as zeitgebers, such as light, sound, temperature, nursing, and parental care, etc., play important roles in the maturation of circadian rhythms. Cycled light (Abraham et al., 2006; Ohta et al., 2006; Bode et al., 2011), music therapy (Arnon et al., 2006; Loewy et al., 2013), appropriate incubator temperature (Tourneux et al., 2008), comfortable nursing (Collins et al., 2015; Lan et al., 2018), and even the adequate parental care (Löhr and Siegmund, 1999; Nishihara et al., 2002; Park et al., 2020) are helpful for the development and maturation of the circadian rhythms in neonates.
Therefore, the beneficial effects of those external stimuli on the circadian rhythms for premature infants cannot be ignored in the NICU, taking the application of caffeine to manage the AOP for example (Figure 4). Coordinating all treatment strategies with the principles of circadian rhythms will be a constructive attempt to improve the disease management and care for premature infants. Assuredly, we have to admit that only rare evidence is available currently, and the realization of the therapeutic strategies cannot be achieved overnight. However, any kind of discussions, attempts, and efforts in this field should well be encouraged in the future.
9 Conclusion
Due to the tough challenges and potential role of circadian rhythms in the response to current caffeine therapy for the AOP management, a comprehensive review was conducted here. Studies have revealed that the human circadian system begins to form in early pregnancy, receives the maternal circadian signals through the placenta before birth, and progressively matures under the influence of the external cues and the mother after birth. Preterm infants experience the ultradian or irregular rhythms during the early postnatal life, which are progressively developed into circadian rhythms as the maturation of neurodevelopment. Caffeine alters the circadian rhythms in humans and animals, and its promising role in preterm infants has also been revealed. The proposed novel circadian-based therapeutic strategies could open new possibilities in the clinical practice to promote the precision caffeine therapy. Arguably, as studies going on, it is believed that in the near future, these initiatives will remain powerful approaches to enhance our biological understanding of the relationship between preterm infants, circadian rhythms, and caffeine therapy.
Author contributions
H-RD, FC: Conceptualization. H-RD, H-LG, Y-HH: data curation. H-RD: Writing—original draft. JX, X-SD, RC, FC: Supervision, Writing—review and editing. FC: Project administration, Funding acquisition. All authors read and approved the final manuscript.
Funding
This study was supported by the Specially Appointed Medical Expert Project of the Jiangsu Commission of Health (2019) and Special Fund for Clinical Research of the Wu Jieping Medical Foundation (320.6750.2020-04-07). This study was also supported by the Scientific Research Support Foundation for Top Young Scholars at the Children’s Hospital of Nanjing Medical University (2020).
Acknowledgments
Figures were created with BioRender (https://app.biorender.com/).
Conflict of interest
The authors declare that the research was conducted in the absence of any commercial or financial relationships that could be construed as a potential conflict of interest.
Publisher’s note
All claims expressed in this article are solely those of the authors and do not necessarily represent those of their affiliated organizations, or those of the publisher, the editors and the reviewers. Any product that may be evaluated in this article, or claim that may be made by its manufacturer, is not guaranteed or endorsed by the publisher.
References
Abdel Wahed, M. A., Issa, H. M., Khafagy, S. M., and Abdel Raouf, S. M. (2019). Effect of caffeine on superior mesenteric artery blood flow velocities in preterm neonates. J. Matern. Fetal. Neonatal Med. 32 (3), 357–361. doi:10.1080/14767058.2017.1378337
Abdel-Hady, H., Nasef, N., Shabaan, A. E., and Nour, I. (2015). Caffeine therapy in preterm infants. World J. Clin. Pediatr. 4 (4), 81–93. doi:10.5409/wjcp.v4.i4.81
Abraham, D., Dallmann, R., Steinlechner, S., Albrecht, U., Eichele, G., and Oster, H. (2006). Restoration of circadian rhythmicity in circadian clock-deficient mice in constant light. J. Biol. Rhythms 21 (3), 169–176. doi:10.1177/0748730406288040
Aguilar-Roblero, R., Mercado, C., Alamilla, J., Laville, A., and Díaz-Muñoz, M. (2007). Ryanodine receptor Ca2+-release channels are an output pathway for the circadian clock in the rat suprachiasmatic nuclei. Eur. J. Neurosci. 26 (3), 575–582. doi:10.1111/j.1460-9568.2007.05679.x
Allada, R., and Bass, J. (2021). Circadian mechanisms in medicine. N. Engl. J. Med. 384 (6), 550–561. doi:10.1056/NEJMra1802337
Anders, T. F., and Keener, M. (1985). Developmental course of nighttime sleep-wake patterns in full-term and premature infants during the first year of life. I. Sleep 8 (3), 173–192. doi:10.1093/sleep/8.3.173
Andescavage, N. N., du Plessis, A., McCarter, R., Serag, A., Evangelou, I., Vezina, G., et al. (2017). Complex trajectories of brain development in the healthy human fetus. Cereb. Cortex 27 (11), 5274–5283. doi:10.1093/cercor/bhw306
Antle, M. C., Steen, N. M., and Mistlberger, R. E. (2001). Adenosine and caffeine modulate circadian rhythms in the Syrian hamster. Neuroreport 12 (13), 2901–2905. doi:10.1097/00001756-200109170-00029
Antonini, S. R., Jorge, S. M., and Moreira, A. C. (2000). The emergence of salivary cortisol circadian rhythm and its relationship to sleep activity in preterm infants. Clin. Endocrinol. 52 (4), 423–426. doi:10.1046/j.1365-2265.2000.00935.x
Ardura, J., Andrés, J., Aldana, J., and Revilla, M. A. (1995). Development of sleep-wakefulness rhythm in premature babies. Acta Paediatr. 84 (5), 484–489. doi:10.1111/j.1651-2227.1995.tb13679.x
Arendt, J. (2018). Approaches to the pharmacological management of jet lag. Drugs 78 (14), 1419–1431. doi:10.1007/s40265-018-0973-8
Arnon, S., Shapsa, A., Forman, L., Regev, R., Bauer, S., Litmanovitz, I., et al. (2006). Live music is beneficial to preterm infants in the neonatal intensive care unit environment. Birth 33 (2), 131–136. doi:10.1111/j.0730-7659.2006.00090.x
Arslanoglu, S., Bertino, E., Nicocia, M., and Moro, G. E. (2012). WAPM working group on nutrition: potential chronobiotic role of human milk in sleep regulation. J. Perinat. Med. 40 (1), 1–8. doi:10.1515/jpm.2011.134
Astiz, M., and Oster, H. (2020). Feto-maternal crosstalk in the development of the circadian clock system. Front. Neurosci. 14, 631687. doi:10.3389/fnins.2020.631687
Atik, A., Harding, R., De Matteo, R., Kondos-Devcic, D., Cheong, J., Doyle, L. W., et al. (2017). Caffeine for apnea of prematurity: Effects on the developing brain. Neurotoxicology 58, 94–102. doi:10.1016/j.neuro.2016.11.012
Attanasio, A., Rager, K., and Gupta, D. (1986). Ontogeny of circadian rhythmicity for melatonin, serotonin, and N-acetylserotonin in humans. J. Pineal Res. 3 (3), 251–256. doi:10.1111/j.1600-079x.1986.tb00747.x
Babischkin, J. S., Grimes, R. W., Pepe, G. J., and Albrecht, E. D. (1997). Estrogen stimulation of P450 cholesterol side-chain cleavage activity in cultures of human placental syncytiotrophoblasts. Biol. Reprod. 56 (1), 272–278. doi:10.1095/biolreprod56.1.272
Bach, V., Telliez, F., Krim, G., and Libert, J. P. (1996). Body temperature regulation in the newborn infant: interaction with sleep and clinical implications. Neurophysiol. Clin. 26 (6), 379–402. doi:10.1016/s0987-7053(97)89152-6
Bach, V., Telliez, F., Leke, A., and Libert, J. P. (2000). Gender-related sleep differences in neonates in thermoneutral and cool environments. J. Sleep. Res. 9 (3), 249–254. doi:10.1046/j.1365-2869.2000.00206.x
Bairam, A., Joseph, V., Lajeunesse, Y., and Kinkead, R. (2009). Altered expression of adenosine A1 and A2A receptors in the carotid body and nucleus tractus solitarius of adult male and female rats following neonatal caffeine treatment. Brain Res. 1287, 74–83. doi:10.1016/j.brainres.2009.06.064
Bates, K., and Herzog, E. D. (2020). Maternal-fetal circadian communication during pregnancy. Front. Endocrinol. 11, 198. doi:10.3389/fendo.2020.00198
Bauer, J., Janecke, A., Gerss, J., Masjosthusmann, K., Werner, C., and Hoffmann, G. (2009). Circadian variation on oxygen consumption in preterm infants. J. Perinat. Med. 37 (4), 413–417. doi:10.1515/JPM.2009.067
Bauer, J., Maier, K., Linderkamp, O., and Hentschel, R. (2001). Effect of caffeine on oxygen consumption and metabolic rate in very low birth weight infants with idiopathic apnea. Pediatrics 107 (4), 660–663. doi:10.1542/peds.107.4.660
Begum, E., Bonno, M., Obata, M., Yamamoto, H., Kawai, M., and Komada, Y. (2006). Emergence of physiological rhythmicity in term and preterm neonates in a neonatal intensive care unit. J. Circadian Rhythms 4, 11. doi:10.1186/1740-3391-4-11
Bennet, L., Walker, D. W., and Horne, R. S. C. (2018). Waking up too early - the consequences of preterm birth on sleep development. J. Physiol. 596 (23), 5687–5708. doi:10.1113/JP274950
Bicker, J., Alves, G., Falcao, A., and Fortuna, A. (2020). Timing in drug absorption and disposition: the past, present, and future of chronopharmacokinetics. Br. J. Pharmacol. 177 (10), 2215–2239. doi:10.1111/bph.15017
Biran, V., Decobert, F., Bednarek, N., Boizeau, P., Benoist, J. F., Claustrat, B., et al. (2019). Melatonin levels in preterm and term infants and their mothers. Int. J. Mol. Sci. 20 (9), 2077. doi:10.3390/ijms20092077
Bode, B., Taneja, R., Rossner, M. J., and Oster, H. (2011). Advanced light-entrained activity onsets and restored free-running suprachiasmatic nucleus circadian rhythms in per2/dec mutant mice. Chronobiol. Int. 28 (9), 737–750. doi:10.3109/07420528.2011.607374
Bolt, R. J., Van Weissenbruch, M. M., Popp-Snijders, C., Sweep, F. G., Lafeber, H. N., and Delemarre-van de Waal, H. A. (2002). Maturity of the adrenal cortex in very preterm infants is related to gestational age. Pediatr. Res. 52 (3), 405–410. doi:10.1203/00006450-200209000-00017
Borghese, I. F., Minard, K. L., and Thoman, E. B. (1995). Sleep rhythmicity in premature infants: implications for development status. Sleep 18 (7), 523–530. doi:10.1093/sleep/18.7.523
Bory, C., Baltassat, P., Porthault, M., Bethenod, M., Frederich, A., and Aranda, J. V. (1979). Metabolism of theophylline to caffeine in premature newborn infants. J. Pediatr. 94 (6), 988–993. doi:10.1016/s0022-3476(79)80246-2
Brandon, D. H., Holditch-Davis, D., and Belyea, M. (2002). Preterm infants born at less than 31 weeks' gestation have improved growth in cycled light compared with continuous near darkness. J. Pediatr. 140 (2), 192–199. doi:10.1067/mpd.2002.121932
Brandon, D. H., Silva, S. G., Park, J., Malcolm, W., Kamhawy, H., and Holditch-Davis, D. (2017). Timing for the introduction of cycled light for extremely preterm infants: A randomized controlled trial. Res. Nurs. Health 40 (4), 294–310. doi:10.1002/nur.21797
Brooks, E., and Canal, M. M. (2013). Development of circadian rhythms: role of postnatal light environment. Neurosci. Biobehav. Rev. 37 (4), 551–560. doi:10.1016/j.neubiorev.2013.02.012
Brown, A. G., Leite, R. S., and Strauss, J. F. (2004). Mechanisms underlying "functional" progesterone withdrawal at parturition. Ann. N. Y. Acad. Sci. 1034, 36–49. doi:10.1196/annals.1335.004
Bucher, H. U., Wolf, M., Keel, M., von Siebenthal, K., and Duc, G. (1994). Effect of aminophylline on cerebral haemodynamics and oxidative metabolism in premature infants. Eur. J. Pediatr. 153 (2), 123–128. doi:10.1007/BF01959223
Bueno, C., and Menna-Barreto, L. (2016). Development of sleep/wake, activity and temperature rhythms in newborns maintained in a neonatal intensive care unit and the impact of feeding schedules. Infant Behav. Dev. 44, 21–28. doi:10.1016/j.infbeh.2016.05.004
Burke, T. M., Markwald, R. R., McHill, A. W., Chinoy, E. D., Snider, J. A., Bessman, S. C., et al. (2015). Effects of caffeine on the human circadian clock in vivo and in vitro. Sci. Transl. Med. 7 (305), 305ra146. doi:10.1126/scitranslmed.aac5125
Burton, P. J., and Waddell, B. J. (1999). Dual function of 11beta-hydroxysteroid dehydrogenase in placenta: modulating placental glucocorticoid passage and local steroid action. Biol. Reprod. 60 (2), 234–240. doi:10.1095/biolreprod60.2.234
Busada, J. T., and Cidlowski, J. A. (2017). Mechanisms of glucocorticoid action during development. Curr. Top. Dev. Biol. 125, 147–170. doi:10.1016/bs.ctdb.2016.12.004
Cailleau, L., Weber, R., Cabon, S., Flamant, C., Roue, J. M., Favrais, G., et al. (2020). Quiet sleep organization of very preterm infants is correlated with postnatal maturation. Front. Pediatr. 8, 559658. doi:10.3389/fped.2020.559658
Cappelletti, S., Piacentino, D., Sani, G., and Aromatario, M. (2015). Caffeine: cognitive and physical performance enhancer or psychoactive drug? Curr. Neuropharmacol. 13 (1), 71–88. doi:10.2174/1570159X13666141210215655
Caravale, B., Sette, S., Cannoni, E., Marano, A., Riolo, E., Devescovi, A., et al. (2017). Sleep characteristics and temperament in preterm children at two years of age. J. Clin. Sleep. Med. 13 (9), 1081–1088. doi:10.5664/jcsm.6728
Carlomagno, G., Minini, M., Tilotta, M., and Unfer, V. (2018). From implantation to birth: Insight into molecular melatonin functions. Int. J. Mol. Sci. 19 (9), 2802. doi:10.3390/ijms19092802
Carnielli, V. P., Verlato, G., Benini, F., Rossi, K., Cavedagni, M., Filippone, M., et al. (2000). Metabolic and respiratory effects of theophylline in the preterm infant. Arch. Dis. Child. Fetal Neonatal Ed. 83 (1), F39–F43. doi:10.1136/fn.83.1.f39
Carr, B. R., Parker, C. R., Madden, J. D., MacDonald, P. C., and Porter, J. C. (1981). Maternal plasma adrenocorticotropin and cortisol relationships throughout human pregnancy. Am. J. Obstet. Gynecol. 139 (4), 416–422. doi:10.1016/0002-9378(81)90318-5
Cederroth, C. R., Albrecht, U., Bass, J., Brown, S. A., Dyhrfjeld-Johnsen, J., Gachon, F., et al. (2019). Medicine in the fourth dimension. Cell Metab. 30 (2), 238–250. doi:10.1016/j.cmet.2019.06.019
Challet, E. (2007). Minireview: Entrainment of the suprachiasmatic clockwork in diurnal and nocturnal mammals. Endocrinology 148 (12), 5648–5655. doi:10.1210/en.2007-0804
Challis, J. R., Patrick, J. E., Campbell, K., Natale, R., and Richardson, B. (1980). Diurnal changes in maternal plasma oestrone and oestradiol at 30 to 31, 34 to 35 and 38 to 39 weeks gestational age. Br. J. Obstet. Gynaecol. 87 (11), 983–988. doi:10.1111/j.1471-0528.1980.tb04462.x
Chang, J., and Gray, P. H. (1994). Aminophylline therapy and cerebral blood flow velocity in preterm infants. J. Paediatr. Child. Health 30 (2), 123–125. doi:10.1111/j.1440-1754.1994.tb00594.x
Chardon, K., Bach, V., Telliez, F., Cardot, V., Tourneux, P., Leke, A., et al. (2004). Effect of caffeine on peripheral chemoreceptor activity in premature neonates: interaction with sleep stages. J. Appl. Physiol. 96 (6), 2161–2166. doi:10.1152/japplphysiol.01160.2003
Chuffa, L. G. A., Lupi, L. A., Cucielo, M. S., Silveira, H. S., Reiter, R. J., and Seiva, F. R. F. (2019). Melatonin promotes uterine and placental health: Potential molecular mechanisms. Int. J. Mol. Sci. 21 (1), 300. doi:10.3390/ijms21010300
Ciarleglio, C. M., Axley, J. C., Strauss, B. R., Gamble, K. L., and McMahon, D. G. (2011). Perinatal photoperiod imprints the circadian clock. Nat. Neurosci. 14 (1), 25–27. doi:10.1038/nn.2699
Clark, I., and Landolt, H. P. (2017). Coffee, caffeine, and sleep: a systematic review of epidemiological studies and randomized controlled trials. Sleep. Med. Rev. 31, 70–78. doi:10.1016/j.smrv.2016.01.006
Collins, C. L., Barfield, C., Davis, P. G., and Horne, R. S. (2015). Randomized controlled trial to compare sleep and wake in preterm infants less than 32weeks of gestation receiving two different modes of non-invasive respiratory support. Early Hum. Dev. 91 (12), 701–704. doi:10.1016/j.earlhumdev.2015.09.011
Commentz, J. C., Henke, A., Dammann, O., Hellwege, H. H., and Willig, R. P. (1996). Decreasing melatonin and 6-hydroxymelatonin sulfate excretion with advancing gestational age in preterm and term newborn male infants. Eur. J. Endocrinol. 135 (2), 184–187. doi:10.1530/eje.0.1350184
Commentz, J. C., Uhlig, H., Henke, A., Hellwege, H. H., and Willig, R. P. (1997). Melatonin and 6-hydroxymelatonin sulfate excretion is inversely correlated with gonadal development in children. Horm. Res. 47 (3), 97–101. doi:10.1159/000185442
Cooperstock, M., England, J. E., and Wolfe, R. A. (1987). Circadian incidence of premature rupture of the membranes in term and preterm births. Obstet. Gynecol. 69 (6), 936–941.
Cremer, M., Jost, K., Gensmer, A., Pramana, I., Delgado-Eckert, E., Frey, U., et al. (2016). Immediate effects of phototherapy on sleep in very preterm neonates: an observational study. J. Sleep. Res. 25 (5), 517–523. doi:10.1111/jsr.12408
Crooks, E., Hansen, D. A., Satterfield, B. C., Layton, M. E., and Van Dongen, H. P. A. (2019). Cardiac autonomic activity during sleep deprivation with and without caffeine administration. Physiol. Behav. 210, 112643. doi:10.1016/j.physbeh.2019.112643
Croteau, A., Marcoux, S., and Brisson, C. (2006). Work activity in pregnancy, preventive measures, and the risk of delivering a small-for-gestational-age infant. Am. J. Public Health 96 (5), 846–855. doi:10.2105/AJPH.2004.058552
Curzi-Dascalova, L., Aujard, Y., Gaultier, C., and Rajguru, M. (2002). Sleep organization is unaffected by caffeine in premature infants. J. Pediatr. 140 (6), 766–771. doi:10.1067/mpd.2002.124383
Curzi-Dascalova, L., Figueroa, J. M., Eiselt, M., Christova, E., Virassamy, A., d'Allest, A. M., et al. (1993). Sleep state organization in premature infants of less than 35 weeks' gestational age. Pediatr. Res. 34 (5), 624–628. doi:10.1203/00006450-199311000-00013
Curzi-Dascalova, L., Peirano, P., and Morel-Kahn, F. (1988). Development of sleep states in normal premature and full-term newborns. Dev. Psychobiol. 21 (5), 431–444. doi:10.1002/dev.420210503
D'Souza, S. W., Tenreiro, S., Minors, D., Chiswick, M. L., Sims, D. G., and Waterhouse, J. (1992). Skin temperature and heart rate rhythms in infants of extreme prematurity. Arch. Dis. Child. 67 (7), 784–788. doi:10.1136/adc.67.7_spec_no.784
Dai, H. R., Liu, Y., Lu, K. Y., He, X., Guo, H. L., Hu, Y. H., et al. (2022). Population pharmacokinetic modeling of caffeine in preterm infants with apnea of prematurity: New findings from concomitant erythromycin and AHR genetic polymorphisms. Pharmacol. Res. 184, 106416. doi:10.1016/j.phrs.2022.106416
Dallmann, R., Okyar, A., and Levi, F. (2016). Dosing-time makes the poison: Circadian regulation and pharmacotherapy. Trends Mol. Med. 22 (5), 430–445. doi:10.1016/j.molmed.2016.03.004
Dani, C., Bertini, G., Reali, M. F., Tronchin, M., Wiechmann, L., Martelli, E., et al. (2000). Brain hemodynamic changes in preterm infants after maintenance dose caffeine and aminophylline treatment. Biol. Neonate 78 (1), 27–32. doi:10.1159/000014243
Darnall, R. A., Ariagno, R. L., and Kinney, H. C. (2006). The late preterm infant and the control of breathing, sleep, and brainstem development: a review. Clin. Perinatol. 33 (4), 883–914. doi:10.1016/j.clp.2006.10.004
Davis, P. G. (2020). When to start and stop caffeine and why respiratory status matters. Semin. Fetal Neonatal Med. 25 (6), 101175. doi:10.1016/j.siny.2020.101175
Deboer, T. (2018). Sleep homeostasis and the circadian clock: Do the circadian pacemaker and the sleep homeostat influence each other's functioning? Neurobiol. Sleep. Circadian Rhythms 5, 68–77. doi:10.1016/j.nbscr.2018.02.003
Deguchi, T. (1975). Ontogenesis of a biological clock for serotonin:acetyl coenzyme A N-acetyltransferase in pineal gland of rat. Proc. Natl. Acad. Sci. U. S. A. 72 (7), 2814–2818. doi:10.1073/pnas.72.7.2814
Deiriggi, P. M. (1990). Effects of waterbed flotation on indicators of energy expenditure in preterm infants. Nurs. Res. 39 (3), 140–146. doi:10.1097/00006199-199005000-00003
Dekker, J., Hooper, S. B., van Vonderen, J. J., Witlox, R., Lopriore, E., and Te Pas, A. B. (2017). Caffeine to improve breathing effort of preterm infants at birth: a randomized controlled trial. Pediatr. Res. 82 (2), 290–296. doi:10.1038/pr.2017.45
Denenberg, V. H., Zeidner, L. P., Thoman, E. B., Kramer, P., Rowe, J. C., Philipps, A. F., et al. (1982). Effects of theophylline on behavioral state development in the newborn rabbit. J. Pharmacol. Exp. Ther. 221 (3), 604–608.
Deng, K., Liang, J., Mu, Y., Liu, Z., Wang, Y., Li, M., et al. (2021). Preterm births in China between 2012 and 2018: an observational study of more than 9 million women. Lancet. Glob. Health 9 (9), e1226–e1241. doi:10.1016/S2214-109X(21)00298-9
Dereymaeker, A., Pillay, K., Vervisch, J., De Vos, M., Van Huffel, S., Jansen, K., et al. (2017). Review of sleep-EEG in preterm and term neonates. Early Hum. Dev. 113, 87–103. doi:10.1016/j.earlhumdev.2017.07.003
Dietrich, J., Krauss, A. N., Reidenberg, M., Drayer, D. E., and Auld, P. A. (1978). Alterations in state in apneic pre-term infants receiving theophylline. Clin. Pharmacol. Ther. 24 (4), 474–478. doi:10.1002/cpt1978244474
Dimitriou, G., Greenough, A., Kavvadia, V., and Mantagos, S. (1999). Blood pressure rhythms during the perinatal period in very immature, extremely low birthweight neonates. Early Hum. Dev. 56 (1), 49–56. doi:10.1016/s0378-3782(99)00034-1
Dix, L. M. L., van Bel, F., Baerts, W., and Lemmers, P. M. A. (2018). Effects of caffeine on the preterm brain: An observational study. Early Hum. Dev. 120, 17–20. doi:10.1016/j.earlhumdev.2018.03.008
Dobrek, L. (2021). Chronopharmacology in therapeutic drug monitoring-dependencies between the rhythmics of pharmacokinetic processes and drug concentration in blood. Pharmaceutics 13 (11), 1915. doi:10.3390/pharmaceutics13111915
Dobson, N. R., and Hunt, C. E. (2018). Caffeine: an evidence-based success story in VLBW pharmacotherapy. Pediatr. Res. 84 (3), 333–340. doi:10.1038/s41390-018-0089-6
Dobson, N. R., Rhein, L. M., Darnall, R. A., Corwin, M. J., Heeren, T. C., Eichenwald, E., et al. (2017). Caffeine decreases intermittent hypoxia in preterm infants nearing term-equivalent age. J. Perinatol. 37 (10), 1135–1140. doi:10.1038/jp.2017.82
Dong, D., Yang, D., Lin, L., Wang, S., and Wu, B. (2020). Circadian rhythm in pharmacokinetics and its relevance to chronotherapy. Biochem. Pharmacol. 178, 114045. doi:10.1016/j.bcp.2020.114045
Dorn, F., Wirth, L., Gorbey, S., Wege, M., Zemlin, M., Maier, R. F., et al. (2014). Influence of acoustic stimulation on the circadian and ultradian rhythm of premature infants. Chronobiol. Int. 31 (9), 1062–1074. doi:10.3109/07420528.2014.948183
Du Pre, B. C., Van Veen, T. A., Young, M. E., Vos, M. A., Doevendans, P. A., and Van Laake, L. W. (2014). Circadian rhythms in cell maturation. Physiol. (Bethesda) 29 (1), 72–83. doi:10.1152/physiol.00036.2013
Durankus, F., Aladag Ciftdemir, N., Vatansever Ozbek, U., Duran, R., and Acunas, B. (2020). Comparison of sleep problems between term and preterm born preschool children. Sleep. Med. 75, 484–490. doi:10.1016/j.sleep.2020.09.013
Economou, G., Andronikou, S., Challa, A., Cholevas, V., and Lapatsanis, P. D. (1993). Cortisol secretion in stressed babies during the neonatal period. Horm. Res. 40 (5-6), 217–221. doi:10.1159/000183798
Edwards, C. R., Benediktsson, R., Lindsay, R. S., and Seckl, J. R. (1996). 11 beta-hydroxysteroid dehydrogenases: key enzymes in determining tissue-specific glucocorticoid effects. Steroids 61 (4), 263–269. doi:10.1016/0039-128x(96)00033-5
Eichenwald, E. C., Watterberg, K. L., Aucott, S., Benitz, W. E., Cummings, J. J., Goldsmith, J., et al. (2016). Apnea of prematurity. Pediatrics 137 (1), e20153757. doi:10.1542/peds.2015-3757
Eichenwald, E. C. (2020). National and international guidelines for neonatal caffeine use: are they evidenced-based? Semin. Fetal Neonatal Med. 25 (6), 101177. doi:10.1016/j.siny.2020.101177
Einspieler, C., Prayer, D., and Marschik, P. B. (2021). Fetal movements: the origin of human behaviour. Dev. Med. Child. Neurol. 63 (10), 1142–1148. doi:10.1111/dmcn.14918
Elstad, M., O'Callaghan, E. L., Smith, A. J., Ben-Tal, A., and Ramchandra, R. (2018). Cardiorespiratory interactions in humans and animals: rhythms for life. Am. J. Physiol. Heart Circ. Physiol. 315 (1), H6–H17. doi:10.1152/ajpheart.00701.2017
Fairchild, K., Mohr, M., Paget-Brown, A., Tabacaru, C., Lake, D., Delos, J., et al. (2016). Clinical associations of immature breathing in preterm infants: part 1-central apnea. Pediatr. Res. 80 (1), 21–27. doi:10.1038/pr.2016.43
Faramarzi, F., Shiran, M., Rafati, M., Farhadi, R., Salehifar, E., and Nakhshab, M. (2021). Prediction of pharmacokinetic values of two various dosages of caffeine in premature neonates with apnea. Indian J. Pharmacol. 53 (2), 108–114. doi:10.4103/ijp.IJP_504_19
Foreman, S. W., Thomas, K. A., and Blackburn, S. T. (2008). Individual and gender differences matter in preterm infant state development. J. Obstet. Gynecol. Neonatal Nurs. 37 (6), 657–665. doi:10.1111/j.1552-6909.2008.00292.x
Frigato, E., Lunghi, L., Ferretti, M. E., Biondi, C., and Bertolucci, C. (2009). Evidence for circadian rhythms in human trophoblast cell line that persist in hypoxia. Biochem. Biophys. Res. Commun. 378 (1), 108–111. doi:10.1016/j.bbrc.2008.11.006
Froy, O., and Miskin, R. (2007). The interrelations among feeding, circadian rhythms and ageing. Prog. Neurobiol. 82 (3), 142–150. doi:10.1016/j.pneurobio.2007.03.002
Froy, O. (2007). The relationship between nutrition and circadian rhythms in mammals. Front. Neuroendocrinol. 28 (2-3), 61–71. doi:10.1016/j.yfrne.2007.03.001
Gabriel, M., Witolla, C., and Albani, M. (1978). Sleep and aminophylline treatment of apnea in preterm infants. Eur. J. Pediatr. 128 (3), 145–149. doi:10.1007/BF00444299
Gaytan, S. P., and Pasaro, R. (2012). Neonatal caffeine treatment up-regulates adenosine receptors in brainstem and hypothalamic cardio-respiratory related nuclei of rat pups. Exp. Neurol. 237 (2), 247–259. doi:10.1016/j.expneurol.2012.06.028
Gaytan, S. P., Saadani-Makki, F., Bodineau, L., Frugiere, A., Larnicol, N., and Pasaro, R. (2006). Effect of postnatal exposure to caffeine on the pattern of adenosine A1 receptor distribution in respiration-related nuclei of the rat brainstem. Auton. Neurosci. 126-127, 339–346. doi:10.1016/j.autneu.2006.03.009
Gentle, S. J., Travers, C. P., and Carlo, W. A. (2018). Caffeine controversies. Curr. Opin. Pediatr. 30 (2), 177–181. doi:10.1097/MOP.0000000000000588
Georgoulas, A., Jones, L., Laudiano-Dray, M. P., Meek, J., Fabrizi, L., and Whitehead, K. (2021). Sleep-wake regulation in preterm and term infants. Sleep 44 (1), zsaa148. doi:10.1093/sleep/zsaa148
Gewolb, I. H., and Vice, F. L. (2006). Maturational changes in the rhythms, patterning, and coordination of respiration and swallow during feeding in preterm and term infants. Dev. Med. Child. Neurol. 48 (7), 589–594. doi:10.1017/S001216220600123X
Gewolb, I. H., Vice, F. L., Schwietzer-Kenney, E. L., Taciak, V. L., and Bosma, J. F. (2001). Developmental patterns of rhythmic suck and swallow in preterm infants. Dev. Med. Child. Neurol. 43 (1), 22–27. doi:10.1017/s0012162201000044
Glattre, E., and Bjerkedal, T. (1983). The 24-hour rhythmicity of birth. A populational study. Acta Obstet. Gynecol. Scand. 62 (1), 31–36. doi:10.3109/00016348309155754
Glotzbach, S. F., Edgar, D. M., and Ariagno, R. L. (1995). Biological rhythmicity in preterm infants prior to discharge from neonatal intensive care. Pediatrics 95 (2), 231–237. doi:10.1542/peds.95.2.231
Gogou, M., Haidopoulou, K., and Pavlou, E. (2019). Sleep and prematurity: sleep outcomes in preterm children and influencing factors. World J. Pediatr. 15 (3), 209–218. doi:10.1007/s12519-019-00240-8
Gonzalez de Mejia, E., and Ramirez-Mares, M. V. (2014). Impact of caffeine and coffee on our health. Trends Endocrinol. Metab. 25 (10), 489–492. doi:10.1016/j.tem.2014.07.003
Govan, J. J., Ohlsson, A., Ryan, M. L., Myhr, T., and Fong, K. (1995). Aminophylline and Doppler time-averaged mean velocity in the middle cerebral artery in preterm neonates. J. Paediatr. Child. Health 31 (5), 461–464. doi:10.1111/j.1440-1754.1995.tb00858.x
Green, P. J., and Suls, J. (1996). The effects of caffeine on ambulatory blood pressure, heart rate, and mood in coffee drinkers. J. Behav. Med. 19 (2), 111–128. doi:10.1007/BF01857602
Guessous, I., Eap, C. B., and Bochud, M. (2014). Blood pressure in relation to coffee and caffeine consumption. Curr. Hypertens. Rep. 16 (9), 468. doi:10.1007/s11906-014-0468-2
Gunes, T., Koklu, E., Ozturk, M. A., Koklu, S., and Cetin, N. (2006). Evaluation of serum cortisol levels in a relatively large and mature group of ventilated and nonventilated preterm infants with respiratory distress syndrome. Am. J. Perinatol. 23 (6), 335–339. doi:10.1055/s-2006-948222
Guo, H. L., Long, J. Y., Hu, Y. H., Liu, Y., He, X., Li, L., et al. (2022). Caffeine therapy for apnea of prematurity: Role of the circadian CLOCK gene polymorphism. Front. Pharmacol. 12, 724145. doi:10.3389/fphar.2021.724145
Guyer, C., Huber, R., Fontijn, J., Bucher, H. U., Nicolai, H., Werner, H., et al. (2012). Cycled light exposure reduces fussing and crying in very preterm infants. Pediatrics 130 (1), e145–e151. doi:10.1542/peds.2011-2671
Guyer, C., Huber, R., Fontijn, J., Bucher, H. U., Nicolai, H., Werner, H., et al. (2015). Very preterm infants show earlier emergence of 24-hour sleep-wake rhythms compared to term infants. Early Hum. Dev. 91 (1), 37–42. doi:10.1016/j.earlhumdev.2014.11.002
Guyon, A., Ravet, F., Champavert, A., Thieux, M., Patural, H., Plancoulaine, S., et al. (2022). Maturation of arousals during day and night in preterm infants. Children 9 (2), 223. doi:10.3390/children9020223
Hao, H., and Rivkees, S. A. (1999). The biological clock of very premature primate infants is responsive to light. Proc. Natl. Acad. Sci. U. S. A. 96 (5), 2426–2429. doi:10.1073/pnas.96.5.2426
Hardy, D. B., Janowski, B. A., Corey, D. R., and Mendelson, C. R. (2006). Progesterone receptor plays a major antiinflammatory role in human myometrial cells by antagonism of nuclear factor-kappaB activation of cyclooxygenase 2 expression. Mol. Endocrinol. 20 (11), 2724–2733. doi:10.1210/me.2006-0112
Harvey, J. R. M., Plante, A. E., and Meredith, A. L. (2020). Ion channels controlling circadian rhythms in suprachiasmatic nucleus excitability. Physiol. Rev. 100 (4), 1415–1454. doi:10.1152/physrev.00027.2019
Hasenstab, K. A., Nawaz, S., Lang, I. M., Shaker, R., and Jadcherla, S. R. (2019). Pharyngoesophageal and cardiorespiratory interactions: potential implications for premature infants at risk of clinically significant cardiorespiratory events. Am. J. Physiol. Gastrointest. Liver Physiol. 316 (2), G304–G12. doi:10.1152/ajpgi.00303.2018
Hasenstab-Kenney, K. A., Bellodas Sanchez, J., Prabhakar, V., Lang, I. M., Shaker, R., and Jadcherla, S. R. (2020). Mechanisms of bradycardia in premature infants: Aerodigestive-cardiac regulatory-rhythm interactions. Physiol. Rep. 8 (13), e14495. doi:10.14814/phy2.14495
Hassanein, S. M., Gad, G. I., Ismail, R. I., and Diab, M. (2015). Effect of caffeine on preterm infants' cerebral cortical activity: an observational study. J. Matern. Fetal. Neonatal Med. 28 (17), 2090–2095. doi:10.3109/14767058.2014.978757
Hayes, M. J., Akilesh, M. R., Fukumizu, M., Gilles, A. A., Sallinen, B. A., Troese, M., et al. (2007). Apneic preterms and methylxanthines: arousal deficits, sleep fragmentation and suppressed spontaneous movements. J. Perinatol. 27 (12), 782–789. doi:10.1038/sj.jp.7211820
Hayes, M. J., Plante, L., Kumar, S. P., and Delivoria-Papadopoulos, M. (1993). Spontaneous motility in premature infants: features of behavioral activity and rhythmic organization. Dev. Psychobiol. 26 (5), 279–291. doi:10.1002/dev.420260505
Hazelhoff, E. M., Dudink, J., Meijer, J. H., and Kervezee, L. (2021). Beginning to see the light: Lessons learned from the development of the circadian system for optimizing light conditions in the neonatal intensive care unit. Front. Neurosci. 15, 634034. doi:10.3389/fnins.2021.634034
He, X., Qiu, J. C., Lu, K. Y., Guo, H. L., Li, L., Jia, W. W., et al. (2021). Therapy for apnoea of prematurity: a retrospective study on effects of standard dose and genetic variability on clinical response to caffeine citrate in Chinese preterm infants. Adv. Ther. 38 (1), 607–626. doi:10.1007/s12325-020-01544-2
Hellmeyer, L., Herz, K., Liedtke, B., Wohlmuth, P., Schmidt, S., and Hackeloeer, B. J. (2012). The underestimation of immaturity in late preterm infants. Arch. Gynecol. Obstet. 286 (3), 619–626. doi:10.1007/s00404-012-2366-7
Henderson-Smart, D. J., and De Paoli, A. G. (2010). Methylxanthine treatment for apnoea in preterm infants. Cochrane Database Syst. Rev. (12), CD000140. doi:10.1002/14651858.CD000140.pub2
Henderson-Smart, D. J., and Steer, P. A. (2010). Caffeine versus theophylline for apnea in preterm infants. Cochrane Database Syst. Rev. (1), CD000273. doi:10.1002/14651858.CD000273
Higashi, Y. (2019). Coffee and endothelial function: A coffee paradox? Nutrients 11 (9), 2104. doi:10.3390/nu11092104
Hodgman, J. E., Gonzalez, F., Hoppenbrouwers, T., and Cabal, L. A. (1990). Apnea, transient episodes of bradycardia, and periodic breathing in preterm infants. Am. J. Dis. Child. 144 (1), 54–57. doi:10.1001/archpedi.1990.02150250064032
Hoecker, C., Nelle, M., Beedgen, B., Rengelshausen, J., and Linderkamp, O. (2006). Effects of a divided high loading dose of caffeine on circulatory variables in preterm infants. Arch. Dis. Child. Fetal Neonatal Ed. 91 (1), F61–F64. doi:10.1136/adc.2005.073866
Hoecker, C., Nelle, M., Poeschl, J., Beedgen, B., and Linderkamp, O. (2002). Caffeine impairs cerebral and intestinal blood flow velocity in preterm infants. Pediatrics 109 (5), 784–787. doi:10.1542/peds.109.5.784
Hofman, M. A. (1997). Lifespan changes in the human hypothalamus. Exp. Gerontol. 32 (4-5), 559–575. doi:10.1016/s0531-5565(96)00162-3
Holditch-Davis, D., Scher, M., Schwartz, T., and Hudson-Barr, D. (2004). Sleeping and waking state development in preterm infants. Early Hum. Dev. 80 (1), 43–64. doi:10.1016/j.earlhumdev.2004.05.006
Hoppenbrouwers, T., Hodgman, J. E., Rybine, D., Fabrikant, G., Corwin, M., Crowell, D., et al. (2005). Sleep architecture in term and preterm infants beyond the neonatal period: the influence of gestational age, steroids, and ventilatory support. Sleep 28 (11), 1428–1436. doi:10.1093/sleep/28.11.1428
Hsieh, E. M., Hornik, C. P., Clark, R. H., Laughon, M. M., Benjamin, D. K., Smith, P. B., et al. (2014). Medication use in the neonatal intensive care unit. Am. J. Perinatol. 31 (9), 811–821. doi:10.1055/s-0033-1361933
Hsieh, P. N., Zhang, L., and Jain, M. K. (2018). Coordination of cardiac rhythmic output and circadian metabolic regulation in the heart. Cell. Mol. Life Sci. 75 (3), 403–416. doi:10.1007/s00018-017-2606-x
Huang, S., Jiao, X., Lu, D., Pei, X., Qi, D., and Li, Z. (2020). Recent advances in modulators of circadian rhythms: an update and perspective. J. Enzyme Inhib. Med. Chem. 35 (1), 1267–1286. doi:10.1080/14756366.2020.1772249
Huang, Y. S., Paiva, T., Hsu, J. F., Kuo, M. C., and Guilleminault, C. (2014). Sleep and breathing in premature infants at 6 months post-natal age. BMC Pediatr. 14, 303. doi:10.1186/s12887-014-0303-6
Huang, Z. L., Urade, Y., and Hayaishi, O. (2011). The role of adenosine in the regulation of sleep. Curr. Top. Med. Chem. 11 (8), 1047–1057. doi:10.2174/156802611795347654
Huang, Z. L., Zhang, Z., and Qu, W. M. (2014). Roles of adenosine and its receptors in sleep-wake regulation. Int. Rev. Neurobiol. 119, 349–371. doi:10.1016/B978-0-12-801022-8.00014-3
Hunt, C. E. (2006). Ontogeny of autonomic regulation in late preterm infants born at 34-37 weeks postmenstrual age. Semin. Perinatol. 30 (2), 73–76. doi:10.1053/j.semperi.2006.02.005
Huvanandana, J., Thamrin, C., McEwan, A. L., Hinder, M., and Tracy, M. B. (2019). Cardiovascular impact of intravenous caffeine in preterm infants. Acta Paediatr. 108 (3), 423–429. doi:10.1111/apa.14382
Hwang, J., Kim, Y. S., Shin, J. H., and Choi, B. M. (2018). Hemodynamic effects on systemic blood flow and ductal shunting flow after loading dose of intravenous caffeine in preterm infants according to the patency of ductus arteriosus. J. Korean Med. Sci. 33 (4), e25. doi:10.3346/jkms.2018.33.e25
Iams, J. D., Newman, R. B., Thom, E. A., Goldenberg, R. L., Mueller-Heubach, E., Moawad, A., et al. (2002). Frequency of uterine contractions and the risk of spontaneous preterm delivery. N. Engl. J. Med. 346 (4), 250–255. doi:10.1056/NEJMoa002868
Illnerová, H., Buresová, M., and Presl, J. (1993). Melatonin rhythm in human milk. J. Clin. Endocrinol. Metab. 77 (3), 838–841. doi:10.1210/jcem.77.3.8370707
Ingersoll, E. W., and Thoman, E. B. (1999). Sleep/wake states of preterm infants: stability, developmental change, diurnal variation, and relation with caregiving activity. Child. Dev. 70 (1), 1–10. doi:10.1111/1467-8624.00001
Italianer, M. F., Naninck, E. F. G., Roelants, J. A., van der Horst, G. T. J., Reiss, I. K. M., Goudoever, J. B. V., et al. (2020). Circadian variation in human milk composition, a systematic review. Nutrients 12 (8), 2328. doi:10.3390/nu12082328
Itoh, M. T., Ishizuka, B., Kuribayashi, Y., Amemiya, A., and Sumi, Y. (1999). Melatonin, its precursors, and synthesizing enzyme activities in the human ovary. Mol. Hum. Reprod. 5 (5), 402–408. doi:10.1093/molehr/5.5.402
Iuvone, P. M., and Gan, J. (1995). Functional interaction of melatonin receptors and D1 dopamine receptors in cultured chick retinal neurons. J. Neurosci. 15 (2), 2179–2185. doi:10.1523/jneurosci.15-03-02179.1995
Ivars, K., Nelson, N., Theodorsson, A., Theodorsson, E., Strom, J. O., and Morelius, E. (2015). Development of salivary cortisol circadian rhythm and reference intervals in full-term infants. PLoS One 10 (6), e0129502. doi:10.1371/journal.pone.0129502
Ivars, K., Nelson, N., Theodorsson, A., Theodorsson, E., Strom, J. O., and Morelius, E. (2017). Development of salivary cortisol circadian rhythm in preterm infants. PLoS One 12 (8), e0182685. doi:10.1371/journal.pone.0182685
Iwata, O., Okamura, H., Saitsu, H., Saikusa, M., Kanda, H., Eshima, N., et al. (2013). Diurnal cortisol changes in newborn infants suggesting entrainment of peripheral circadian clock in utero and at birth. J. Clin. Endocrinol. Metab. 98 (1), E25–E32. doi:10.1210/jc.2012-2750
Jagannath, A., Peirson, S. N., and Foster, R. G. (2013). Sleep and circadian rhythm disruption in neuropsychiatric illness. Curr. Opin. Neurobiol. 23 (5), 888–894. doi:10.1016/j.conb.2013.03.008
Jagannath, A., Varga, N., Dallmann, R., Rando, G., Gosselin, P., Ebrahimjee, F., et al. (2021). Adenosine integrates light and sleep signalling for the regulation of circadian timing in mice. Nat. Commun. 12 (1), 2113. doi:10.1038/s41467-021-22179-z
Jaldo-Alba, F., Muñóz-Hoyos, A., Molina-Carballo, A., Molina-Font, J. A., Acuña-Castroviejo, D., and Munoz-Hoyos, A. (1993). Light deprivation increases plasma levels of melatonin during the first 72 h of life in human infants. Acta Endocrinol. 129 (5), 442–445. doi:10.1530/acta.0.1290442
Jett, P. L., Samuels, M. H., McDaniel, P. A., Benda, G. I., Lafranchi, S. H., Reynolds, J. W., et al. (1997). Variability of plasma cortisol levels in extremely low birth weight infants. J. Clin. Endocrinol. Metab. 82 (9), 2921–2925. doi:10.1210/jcem.82.9.4206
Jha, P. K., Bouaouda, H., Gourmelen, S., Dumont, S., Fuchs, F., Goumon, Y., et al. (2017). Sleep deprivation and caffeine treatment potentiate photic resetting of the master circadian clock in a diurnal rodent. J. Neurosci. 37 (16), 4343–4358. doi:10.1523/JNEUROSCI.3241-16.2017
Jha, P. K., Bouaouda, H., Kalsbeek, A., and Challet, E. (2021). Distinct feedback actions of behavioural arousal to the master circadian clock in nocturnal and diurnal mammals. Neurosci. Biobehav. Rev. 123, 48–60. doi:10.1016/j.neubiorev.2020.12.011
Joseph, D., Chong, N. W., Shanks, M. E., Rosato, E., Taub, N. A., Petersen, S. A., et al. (2015). Getting rhythm: how do babies do it? Arch. Dis. Child. Fetal Neonatal Ed. 100 (1), F50–F54. doi:10.1136/archdischild-2014-306104
Jost, K., Pramana, I., Delgado-Eckert, E., Kumar, N., Datta, A. N., Frey, U., et al. (2017). Dynamics and complexity of body temperature in preterm infants nursed in incubators. PLoS One 12 (4), e0176670. doi:10.1371/journal.pone.0176670
Junkermann, H., Mangold, H., Vecsei, P., and Runnebaum, B. (1982). Circadian rhythm of serum progesterone levels in human pregnancy and its relation to the rhythm of cortisol. Acta Endocrinol. (Copenh). 101 (1), 98–104. doi:10.1530/acta.0.1010098
Kassim, Z., Greenough, A., and Rafferty, G. F. (2009). Effect of caffeine on respiratory muscle strength and lung function in prematurely born, ventilated infants. Eur. J. Pediatr. 168 (12), 1491–1495. doi:10.1007/s00431-009-0961-9
Katzer, D., Pauli, L., Mueller, A., Reutter, H., Reinsberg, J., Fimmers, R., et al. (2016). Melatonin concentrations and antioxidative capacity of human breast milk according to gestational age and the time of day. J. Hum. Lact. 32 (4), NP105–NP10. doi:10.1177/0890334415625217
Kennaway, D. J., Goble, F. C., and Stamp, G. E. (1996). Factors influencing the development of melatonin rhythmicity in humans. J. Clin. Endocrinol. Metab. 81 (4), 1525–1532. doi:10.1210/jcem.81.4.8636362
Kennaway, D. J., Stamp, G. E., and Goble, F. C. (1992). Development of melatonin production in infants and the impact of prematurity. J. Clin. Endocrinol. Metab. 75 (2), 367–369. doi:10.1210/jcem.75.2.1639937
Kidd, S., Midgley, P., Nicol, M., Smith, J., and McIntosh, N. (2005). Lack of adult-type salivary cortisol circadian rhythm in hospitalized preterm infants. Horm. Res. 64 (1), 20–27. doi:10.1159/000087324
Kinoshita, M., Iwata, S., Okamura, H., Saikusa, M., Hara, N., Urata, C., et al. (2016). Paradoxical diurnal cortisol changes in neonates suggesting preservation of foetal adrenal rhythms. Sci. Rep. 6, 35553. doi:10.1038/srep35553
Kinouchi, K., Mikami, Y., Kanai, T., and Itoh, H. (2021). Circadian rhythms in the tissue-specificity from metabolism to immunity: insights from omics studies. Mol. Asp. Med. 80, 100984. doi:10.1016/j.mam.2021.100984
Kivelä, A., Kauppila, A., Leppäluoto, J., Vakkuri, O., and Kivela, A. (1990). Melatonin in infants and mothers at delivery and in infants during the first week of life. Clin. Endocrinol. 32 (5), 593–598. doi:10.1111/j.1365-2265.1990.tb00902.x
Koch, G., Datta, A. N., Jost, K., Schulzke, S. M., van den Anker, J., and Pfister, M. (2017). Caffeine citrate dosing adjustments to assure stable caffeine concentrations in preterm neonates. J. Pediatr. 191, 50–56. doi:10.1016/j.jpeds.2017.08.064
Koch, G., Jost, K., Schulzke, S. M., Koch, R., Pfister, M., and Datta, A. N. (2021). The rhythm of a preterm neonate's life: ultradian oscillations of heart rate, body temperature and sleep cycles. J. Pharmacokinet. Pharmacodyn. 48 (3), 401–410. doi:10.1007/s10928-020-09735-8
Koch, G., Schonfeld, N., Jost, K., Atkinson, A., Schulzke, S. M., Pfister, M., et al. (2020). Caffeine preserves quiet sleep in preterm neonates. Pharmacol. Res. Perspect. 8 (3), e00596. doi:10.1002/prp2.596
Koenen, S. V., Mulder, E. J., Wijnberger, L. D., and Visser, G. H. (2005). Transient loss of the diurnal rhythms of fetal movements, heart rate, and its variation after maternal betamethasone administration. Pediatr. Res. 57 (1), 662–666. doi:10.1203/01.PDR.0000159762.50504.1F
Kohler, M., Pavy, A., and van den Heuvel, C. (2006). The effects of chewing versus caffeine on alertness, cognitive performance and cardiac autonomic activity during sleep deprivation. J. Sleep. Res. 15 (4), 358–368. doi:10.1111/j.1365-2869.2006.00547.x
Korte, J., Wulff, K., Oppe, C., and Siegmund, R. (2001). Ultradian and circadian activity-rest rhythms of preterm neonates compared to full-term neonates using actigraphic monitoring. Chronobiol. Int. 18 (4), 697–708. doi:10.1081/cbi-100106082
Kováciková, Z., Sládek, M., Laurinová, K., Bendová, Z., Illnerová, H., Sumová, A., et al. (2005). Ontogenesis of photoperiodic entrainment of the molecular core clockwork in the rat suprachiasmatic nucleus. Brain Res. 1064 (1-2), 83–89. doi:10.1016/j.brainres.2005.10.022
Kreutzer, K., and Bassler, D. (2014). Caffeine for apnea of prematurity: a neonatal success story. Neonatology 105 (4), 332–336. doi:10.1159/000360647
Krzyżaniak, N., Pawłowska, I., and Bajorek, B. (2016). Review of drug utilization patterns in NICUs worldwide. J. Clin. Pharm. Ther. 41 (6), 612–620. doi:10.1111/jcpt.12440
Kuhn, P., Zores, C., Langlet, C., Escande, B., Astruc, D., and Dufour, A. (2013). Moderate acoustic changes can disrupt the sleep of very preterm infants in their incubators. Acta Paediatr. 102 (10), 949–954. doi:10.1111/apa.12330
Kumar, V. H. S., and Lipshultz, S. E. (2019). Caffeine and clinical outcomes in premature neonates. Child. (Basel) 6 (11), 118. doi:10.3390/children6110118
Kurth, S., Riedner, B. A., Dean, D. C., O'Muircheartaigh, J., Huber, R., Jenni, O. G., et al. (2017). Traveling slow oscillations during sleep: A marker of brain connectivity in childhood. Sleep 40 (9), zsx121. doi:10.1093/sleep/zsx121
Lan, H. Y., Yang, L., Hsieh, K. H., Yin, T., Chang, Y. C., and Liaw, J. J. (2018). Effects of a supportive care bundle on sleep variables of preterm infants during hospitalization. Res. Nurs. Health 41 (3), 281–291. doi:10.1002/nur.21865
Lan, H. Y., Yin, T., Chen, J. L., Chang, Y. C., and Liaw, J. J. (2019). Factors associated with preterm infants' circadian sleep/wake patterns at the hospital. Clin. Nurs. Res. 28 (4), 456–472. doi:10.1177/1054773817724960
Landolt, H. P. (2015). CIRCADIAN RHYTHMS. Caffeine, the circadian clock, and sleep. Science 349 (6254), 1289. doi:10.1126/science.aad2958
Landolt, H. P., Dijk, D. J., Gaus, S. E., and Borbély, A. A. (1995). Caffeine reduces low-frequency delta activity in the human sleep EEG. Neuropsychopharmacology 12 (3), 229–238. doi:10.1016/0893-133X(94)00079-F
Landolt, H. P., Werth, E., Borbély, A. A., and Dijk, D. J. (1995). Caffeine intake (200 mg) in the morning affects human sleep and EEG power spectra at night. Brain Res. 675 (1-2), 67–74. doi:10.1016/0006-8993(95)00040-w
Lane, A. J., Coombs, R. C., Evans, D. H., and Levin, R. J. (1999). Effect of caffeine on neonatal splanchnic blood flow. Arch. Dis. Child. Fetal Neonatal Ed. 80 (2), F128–F129. doi:10.1136/fn.80.2.f128
Lanoix, D., Beghdadi, H., Lafond, J., and Vaillancourt, C. (2008). Human placental trophoblasts synthesize melatonin and express its receptors. J. Pineal Res. 45 (1), 50–60. doi:10.1111/j.1600-079X.2008.00555.x
Lee, H. J., Kim, H. S., Kim, S. Y., Sim, G. H., Kim, E. S., Choi, C. W., et al. (2010). Effects of postnatal age and aminophylline on the maturation of amplitude-integrated electroencephalography activity in preterm infants. Neonatology 98 (3), 245–253. doi:10.1159/000277936
Liao, J. H., Hu, R. F., Su, L. J., Wang, S., Xu, Q., Qian, X. F., et al. (2018). Nonpharmacological interventions for sleep promotion on preterm infants in neonatal intensive care unit: A systematic review. Worldviews Evid. Based. Nurs. 15 (5), 386–393. doi:10.1111/wvn.12315
Lindow, S. W., Jha, R. R., and Thompson, J. W. (2000). 24 hour rhythm to the onset of preterm labour. BJOG 107 (9), 1145–1148. doi:10.1111/j.1471-0528.2000.tb11114.x
Loewy, J., Stewart, K., Dassler, A. M., Telsey, A., and Homel, P. (2013). The effects of music therapy on vital signs, feeding, and sleep in premature infants. Pediatrics 131 (5), 902–918. doi:10.1542/peds.2012-1367
Logan, R. W., and McClung, C. A. (2019). Rhythms of life: circadian disruption and brain disorders across the lifespan. Nat. Rev. Neurosci. 20 (1), 49–65. doi:10.1038/s41583-018-0088-y
Löhr, B., and Siegmund, R. (1999). Ultradian and circadian rhythms of sleep-wake and food-intake behavior during early infancy. Chronobiol. Int. 16 (2), 129–148. doi:10.3109/07420529909019081
Long, J. Y., Guo, H. L., He, X., Hu, Y. H., Xia, Y., Cheng, R., et al. (2021). Caffeine for the pharmacological treatment of apnea of prematurity in the NICU: dose selection conundrum, therapeutic drug monitoring and genetic factors. Front. Pharmacol. 12, 681842. doi:10.3389/fphar.2021.681842
Longin, E., Gerstner, T., Schaible, T., Lenz, T., and Konig, S. (2006). Maturation of the autonomic nervous system: differences in heart rate variability in premature vs. term infants. J. Perinat. Med. 34 (4), 303–308. doi:10.1515/JPM.2006.058
Lovallo, W. R., Whitsett, T. L., al'Absi, M., Sung, B. H., Vincent, A. S., and Wilson, M. F. (2005). Caffeine stimulation of cortisol secretion across the waking hours in relation to caffeine intake levels. Psychosom. Med. 67 (5), 734–739. doi:10.1097/01.psy.0000181270.20036.06
Lundstrøm, K. E., Larsen, P. B., Brendstrup, L., Skov, L., and Greisen, G. (1995). Cerebral blood flow and left ventricular output in spontaneously breathing, newborn preterm infants treated with caffeine or aminophylline. Acta Paediatr. 84 (1), 6–9. doi:10.1111/j.1651-2227.1995.tb13474.x
Lunshof, S., Boer, K., Wolf, H., van Hoffen, G., Bayram, N., and Mirmiran, M. (1998). Fetal and maternal diurnal rhythms during the third trimester of normal pregnancy: outcomes of computerized analysis of continuous twenty-four-hour fetal heart rate recordings. Am. J. Obstet. Gynecol. 178 (2), 247–254. doi:10.1016/s0002-9378(98)80008-2
Magiakou, M. A., Mastorakos, G., Rabin, D., Margioris, A. N., Dubbert, B., Calogero, A. E., et al. (1996). The maternal hypothalamic-pituitary-adrenal axis in the third trimester of human pregnancy. Clin. Endocrinol. 44 (4), 419–428. doi:10.1046/j.1365-2265.1996.683505.x
Mann, N. P., Haddow, R., Stokes, L., Goodley, S., and Rutter, N. (1986). Effect of night and day on preterm infants in a newborn nursery: randomised trial. Br. Med. J. 293 (6557), 1265–1267. doi:10.1136/bmj.293.6557.1265
Mantagos, S., Moustogiannis, A., Makri, M., Vagenakis, A., and VagenAkis, A. (1996). The effect of light on plasma melatonin levels in premature infants. J. Pediatr. Endocrinol. Metab. 9 (3), 387–392. doi:10.1515/jpem.1996.9.3.387
Mantagos, S., Moustogiannis, A., and Vagenakis, A. G. (1998). Diurnal variation of plasma cortisol levels in infancy. J. Pediatr. Endocrinol. Metab. 11 (4), 549–553. doi:10.1515/jpem.1998.11.4.549
Marcus, C. L., Meltzer, L. J., Roberts, R. S., Traylor, J., Dix, J., D'Ilario, J., et al. (2014). Long-term effects of caffeine therapy for apnea of prematurity on sleep at school age. Am. J. Respir. Crit. Care Med. 190 (7), 791–799. doi:10.1164/rccm.201406-1092OC
Mark, P. J., Crew, R. C., Wharfe, M. D., and Waddell, B. J. (2017). Rhythmic three-Part Harmony: The complex interaction of maternal, placental and fetal circadian systems. J. Biol. Rhythms 32 (6), 534–549. doi:10.1177/0748730417728671
Matthews, L. G., Walsh, B. H., Knutsen, C., Neil, J. J., Smyser, C. D., Rogers, C. E., et al. (2018). Brain growth in the NICU: critical periods of tissue-specific expansion. Pediatr. Res. 83 (5), 976–981. doi:10.1038/pr.2018.4
Matthews, S. G., Owen, D., Kalabis, G., Banjanin, S., Setiawan, E. B., Dunn, E. A., et al. (2004). Fetal glucocorticoid exposure and hypothalamo-pituitary-adrenal (HPA) function after birth. Endocr. Res. 30 (4), 827–836. doi:10.1081/erc-200044091
McCarthy, R., Jungheim, E. S., Fay, J. C., Bates, K., Herzog, E. D., and England, S. K. (2019). Riding the rhythm of melatonin through pregnancy to deliver on time. Front. Endocrinol. 10, 616. doi:10.3389/fendo.2019.00616
McDonnell, M., Ives, N. K., and Hope, P. L. (1992). Intravenous aminophylline and cerebral blood flow in preterm infants. Arch. Dis. Child. 67 (4), 416–418. doi:10.1136/adc.67.4_spec_no.416
McHill, A. W., Smith, B. J., and Wright, K. P. (2014). Effects of caffeine on skin and core temperatures, alertness, and recovery sleep during circadian misalignment. J. Biol. Rhythms 29 (2), 131–143. doi:10.1177/0748730414523078
McMillen, I. C., Kok, J. S., Adamson, T. M., Deayton, J. M., and Nowak, R. (1991). Development of circadian sleep-wake rhythms in preterm and full-term infants. Pediatr. Res. 29 (1), 381–384. doi:10.1203/00006450-199104000-00010
McTernan, C. L., Draper, N., Nicholson, H., Chalder, S. M., Driver, P., Hewison, M., et al. (2001). Reduced placental 11beta-hydroxysteroid dehydrogenase type 2 mRNA levels in human pregnancies complicated by intrauterine growth restriction: an analysis of possible mechanisms. J. Clin. Endocrinol. Metab. 86 (10), 4979–4983. doi:10.1210/jcem.86.10.7893
Mirmiran, M., and Ariagno, R. L. (2000). Influence of light in the NICU on the development of circadian rhythms in preterm infants. Semin. Perinatol. 24 (4), 247–257. doi:10.1053/sper.2000.8593
Mirmiran, M., Baldwin, R. B., and Ariagno, R. L. (2003). Circadian and sleep development in preterm infants occurs independently from the influences of environmental lighting. Pediatr. Res. 53 (6), 933–938. doi:10.1203/01.PDR.0000061541.94620.12
Mirmiran, M., and Kok, J. H. (1991). Circadian rhythms in early human development. Early Hum. Dev. 26 (2), 121–128. doi:10.1016/0378-3782(91)90016-v
Mirmiran, M., Kok, J. H., de Kleine, M. J., Koppe, J. G., Overdijk, J., and Witting, W. (1990). Circadian rhythms in preterm infants: a preliminary study. Early Hum. Dev. 23 (2), 139–146. doi:10.1016/0378-3782(90)90137-8
Mirmiran, M., and Lunshof, S. (1996). Perinatal development of human circadian rhythms. Prog. Brain Res. 111, 217–226. doi:10.1016/s0079-6123(08)60410-0
Mirmiran, M., Maas, Y. G., and Ariagno, R. L. (2003). Development of fetal and neonatal sleep and circadian rhythms. Sleep. Med. Rev. 7 (4), 321–334. doi:10.1053/smrv.2002.0243
Montandon, G., Horner, R. L., Kinkead, R., and Bairam, A. (2009). Caffeine in the neonatal period induces long-lasting changes in sleep and breathing in adult rats. J. Physiol. 587 (22), 5493–5507. doi:10.1113/jphysiol.2009.171918
Montandon, G., Kinkead, R., and Bairam, A. (2008). Adenosinergic modulation of respiratory activity: developmental plasticity induced by perinatal caffeine administration. Respir. Physiol. Neurobiol. 164 (1-2), 87–95. doi:10.1016/j.resp.2008.07.013
Morag, I., and Ohlsson, A. (2016). Cycled light in the intensive care unit for preterm and low birth weight infants. Cochrane Database Syst. Rev. (8), CD006982. doi:10.1002/14651858.CD006982.pub3
Moschino, L., Zivanovic, S., Hartley, C., Trevisanuto, D., Baraldi, E., and Roehr, C. C. (2020). Caffeine in preterm infants: where are we in 2020? ERJ Open Res. 6 (1), 00330-2019. doi:10.1183/23120541.00330-2019
Mulder, E. J., Koenen, S. V., Blom, I., and Visser, G. H. (2004). The effects of antenatal betamethasone administration on fetal heart rate and behaviour depend on gestational age. Early Hum. Dev. 76 (1), 65–77. doi:10.1016/j.earlhumdev.2003.10.007
Muñoz-Hoyos, A., Bonillo-Perales, A., Avila-Villegas, R., González-Ripoll, M., Uberos, J., Florido-Navío, J., et al. (2007). Melatonin levels during the first week of life and their relation with the antioxidant response in the perinatal period. Neonatology 92 (3), 209–216. doi:10.1159/000102957
Muñoz-Hoyos, A., Jaldo-Alba, F., Molina-Carballo, A., Rodríguez-Cabezas, T., Molina-Font, J. A., Acuña-Castroviejo, D., et al. (1993). Absence of plasma melatonin circadian rhythm during the first 72 hours of life in human infants. J. Clin. Endocrinol. Metab. 77 (3), 699–703. doi:10.1210/jcem.77.3.8370692
Muñoz-Hoyos, A., Molina-Carballo, A., Macías, M., Rodríguez-Cabezas, T., Martín-Medina, E., Narbona-López, E., et al. (1998). Comparison between tryptophan methoxyindole and kynurenine metabolic pathways in normal and preterm neonates and in neonates with acute fetal distress. Eur. J. Endocrinol. 139 (1), 89–95. doi:10.1530/eje.0.1390089
Muñoz-Hoyos, A., Rodriguez-Cabezas, T., Molina-Carballo, A., Martinez-Sempere, J. J., Ruiz-Cosano, C., Acuña-Castroviejo, D., et al. (1992). Melatonin concentration in the umbilical artery and vein in human preterm and term neonates and neonates with acute fetal distress. J. Pineal Res. 13 (4), 184–191. doi:10.1111/j.1600-079x.1992.tb00074.x
Nahmias, Y., and Androulakis, I. P. (2021). Circadian effects of drug responses. Annu. Rev. Biomed. Eng. 23, 203–224. doi:10.1146/annurev-bioeng-082120-034725
Nakamura, Y., Tamura, H., Kashida, S., Takayama, H., Yamagata, Y., Karube, A., et al. (2001). Changes of serum melatonin level and its relationship to feto-placental unit during pregnancy. J. Pineal Res. 30 (1), 29–33. doi:10.1034/j.1600-079x.2001.300104.x
Narishige, S., Kuwahara, M., Shinozaki, A., Okada, S., Ikeda, Y., Kamagata, M., et al. (2014). Effects of caffeine on circadian phase, amplitude and period evaluated in cells in vitro and peripheral organs in vivo in PER2::LUCIFERASE mice. Br. J. Pharmacol. 171 (24), 5858–5869. doi:10.1111/bph.12890
Nehlig, A., Daval, J. L., and Debry, G. (1992). Caffeine and the central nervous system: mechanisms of action, biochemical, metabolic and psychostimulant effects. Brain Res. Brain Res. Rev. 17 (2), 139–170. doi:10.1016/0165-0173(92)90012-b
Nishihara, K., and Horiuchi, S. (1998). Changes in sleep patterns of young women from late pregnancy to postpartum: relationships to their infants' movements. Percept. Mot. Ski. 87 (1), 1043–1056. doi:10.2466/pms.1998.87.3.1043
Nishihara, K., Horiuchi, S., Eto, H., and Uchida, S. (2000). Mothers' wakefulness at night in the post-partum period is related to their infants' circadian sleep-wake rhythm. Psychiatry Clin. Neurosci. 54 (3), 305–306. doi:10.1046/j.1440-1819.2000.00689.x
Nishihara, K., Horiuchi, S., Eto, H., and Uchida, S. (2002). The development of infants' circadian rest-activity rhythm and mothers' rhythm. Physiol. Behav. 77 (1), 91–98. doi:10.1016/s0031-9384(02)00846-6
O'Neill, J. S., Maywood, E. S., Chesham, J. E., Takahashi, J. S., and Hastings, M. H. (2008). cAMP-dependent signaling as a core component of the mammalian circadian pacemaker. Science 320 (5878), 949–953. doi:10.1126/science.1152506
Ohdo, S., Koyanagi, S., and Matsunaga, N. (2019). Chronopharmacological strategies focused on chrono-drug discovery. Pharmacol. Ther. 202, 72–90. doi:10.1016/j.pharmthera.2019.05.018
Ohta, H., Mitchell, A. C., and McMahon, D. G. (2006). Constant light disrupts the developing mouse biological clock. Pediatr. Res. 60 (3), 304–308. doi:10.1203/01.pdr.0000233114.18403.66
Oike, H., Kobori, M., Suzuki, T., and Ishida, N. (2011). Caffeine lengthens circadian rhythms in mice. Biochem. Biophys. Res. Commun. 410 (3), 654–658. doi:10.1016/j.bbrc.2011.06.049
Ono, D., Honma, K. I., and Honma, S. (2021). Corrigendum: Roles of neuropeptides, VIP and AVP, in the mammalian central circadian clock. Front. Neurosci. 15, 810796. doi:10.3389/fnins.2021.810796
Oster, H., Challet, E., Ott, V., Arvat, E., de Kloet, E. R., Dijk, D. J., et al. (2017). The functional and clinical significance of the 24-hour rhythm of circulating glucocorticoids. Endocr. Rev. 38 (1), 3–45. doi:10.1210/er.2015-1080
Pacifici, G. M. (2014). Clinical pharmacology of theophylline in preterm infants: effects, metabolism and pharmacokinetics. Curr. Pediatr. Rev. 10 (4), 297–303. doi:10.2174/1573396311666150113213352
Palmu, K., Kirjavainen, T., Stjerna, S., Salokivi, T., and Vanhatalo, S. (2013). Sleep wake cycling in early preterm infants: comparison of polysomnographic recordings with a novel EEG-based index. Clin. Neurophysiol. 124 (9), 1807–1814. doi:10.1016/j.clinph.2013.03.010
Panagiotou, M., Meijer, M., Meijer, J. H., and Deboer, T. (2019). Effects of chronic caffeine consumption on sleep and the sleep electroencephalogram in mice. J. Psychopharmacol. 33 (1), 122–131. doi:10.1177/0269881118806300
Parikka, V., Beck, J., Zhai, Q., Leppasalo, J., Lehtonen, L., and Soukka, H. (2015). The effect of caffeine citrate on neural breathing pattern in preterm infants. Early Hum. Dev. 91 (10), 565–568. doi:10.1016/j.earlhumdev.2015.06.007
Park, J., Silva, S. G., Thoyre, S. M., and Brandon, D. H. (2020). Sleep-wake states and feeding progression in preterm infants. Nurs. Res. 69 (1), 22–30. doi:10.1097/NNR.0000000000000395
Patel, M., Mohr, M., Lake, D., Delos, J., Moorman, J. R., Sinkin, R. A., et al. (2016). Clinical associations with immature breathing in preterm infants: part 2-periodic breathing. Pediatr. Res. 80 (1), 28–34. doi:10.1038/pr.2016.58
Patrick, J., Challis, J., Campbell, K., Carmichael, L., Natale, R., and Richardson, B. (1980). Circadian rhythms in maternal plasma cortisol and estriol concentrations at 30 to 31, 34 to 35, and 38 to 39 weeks' gestational age. Am. J. Obstet. Gynecol. 136 (3), 325–334. doi:10.1016/0002-9378(80)90857-1
Patrick, J., and Challis, J. (1980). Measurement of human fetal breathing movements in healthy pregnancies using a real-time scanner. Semin. Perinatol. 4 (4), 275–286.
Patrick, J., Challis, J., Natale, R., and Richardson, B. (1979). Circadian rhythms in maternal plasma cortisol, estrone, estradiol, and estriol at 34 to 35 weeks' gestation. Am. J. Obstet. Gynecol. 135 (6), 791–798. doi:10.1016/0002-9378(79)90393-4
Peirano, P., Algarı́n, C., and Uauy, R. (2003). Sleep-wake states and their regulatory mechanisms throughout early human development. J. Pediatr. 143 (4), 70–79. doi:10.1067/s0022-3476(03)00404-9
Peleg, D., Munsick, R. A., Diker, D., Goldman, J. A., and Ben-Jonathan, N. (1986). Distribution of catecholamines between fetal and maternal compartments during human pregnancy with emphasis on L-dopa and dopamine. J. Clin. Endocrinol. Metab. 62 (5), 911–914. doi:10.1210/jcem-62-5-911
Pelissier, A. L., Gantenbein, M., and Bruguerolle, B. (1999). Caffeine-induced modifications of heart rate, temperature, and motor activity circadian rhythms in rats. Physiol. Behav. 67 (1), 81–88. doi:10.1016/s0031-9384(99)00038-4
Pelissier-Alicot, A. L., Schreiber-Deturmeny, E., Simon, N., Gantenbein, M., and Bruguerolle, B. (2002). Time-of-day dependent pharmacodynamic and pharmacokinetic profiles of caffeine in rats. Naunyn. Schmiedeb. Arch. Pharmacol. 365 (4), 318–325. doi:10.1007/s00210-001-0527-5
Perez, S., Murias, L., Fernandez-Plaza, C., Diaz, I., Gonzalez, C., Otero, J., et al. (2015). Evidence for clock genes circadian rhythms in human full-term placenta. Syst. Biol. Reprod. Med. 61 (6), 360–366. doi:10.3109/19396368.2015.1069420
Peters, G. A., Yi, L., Skomorovska-Prokvolit, Y., Patel, B., Amini, P., Tan, H., et al. (2017). Inflammatory stimuli increase progesterone receptor-A stability and transrepressive activity in myometrial cells. Endocrinology 158 (1), 158–169. doi:10.1210/en.2016-1537
Porkka-Heiskanen, T., and Kalinchuk, A. V. (2011). Adenosine, energy metabolism and sleep homeostasis. Sleep. Med. Rev. 15 (2), 123–135. doi:10.1016/j.smrv.2010.06.005
Price, D. A., Close, G. C., and Fielding, B. A. (1983). Age of appearance of circadian rhythm in salivary cortisol values in infancy. Arch. Dis. Child. 58 (6), 454–456. doi:10.1136/adc.58.6.454
Pryds, O., and Schneider, S. (1991). Aminophylline reduces cerebral blood flow in stable, preterm infants without affecting the visual evoked potential. Eur. J. Pediatr. 150 (5), 366–369. doi:10.1007/BF01955942
Rao, S., Chun, C., Fan, J., Kofron, J. M., Yang, M. B., Hegde, R. S., et al. (2013). A direct and melanopsin-dependent fetal light response regulates mouse eye development. Nature 494 (7436), 243–246. doi:10.1038/nature11823
Reichert, C. F., Deboer, T., and Landolt, H. P. (2022). Adenosine, caffeine, and sleep-wake regulation: state of the science and perspectives. J. Sleep. Res. 31 (4), e13597. doi:10.1111/jsr.13597
Reiter, R. J., Tan, D. X., Korkmaz, A., and Rosales-Corral, S. A. (2014). Melatonin and stable circadian rhythms optimize maternal, placental and fetal physiology. Hum. Reprod. Update 20 (2), 293–307. doi:10.1093/humupd/dmt054
Reiter, R. J., Tan, D. X., Rosales-Corral, S., and Manchester, L. C. (2013). The universal nature, unequal distribution and antioxidant functions of melatonin and its derivatives. Mini Rev. Med. Chem. 13 (3), 373–384. doi:10.2174/1389557511313030006
Reppert, S. M. (1992). Pre-natal development of a hypothalamic biological clock. Prog. Brain Res. 93, 119–131. doi:10.1016/s0079-6123(08)64568-9
Reppert, S. M., Weaver, D. R., Rivkees, S. A., and Stopa, E. G. (1988). Putative melatonin receptors in a human biological clock. Science 242 (4875), 78–81. doi:10.1126/science.2845576
Rhein, L. M., Dobson, N. R., Darnall, R. A., Corwin, M. J., Heeren, T. C., Poets, C. F., et al. (2014). Effects of caffeine on intermittent hypoxia in infants born prematurely: a randomized clinical trial. JAMA Pediatr. 168 (3), 250–257. doi:10.1001/jamapediatrics.2013.4371
Ribas-Latre, A., and Eckel-Mahan, K. (2016). Interdependence of nutrient metabolism and the circadian clock system: importance for metabolic health. Mol. Metab. 5 (3), 133–152. doi:10.1016/j.molmet.2015.12.006
Rieth, N., Vibarel-Rebot, N., Buisson, C., Jaffre, C., and Collomp, K. (2016). Caffeine and saliva steroids in young healthy recreationally trained women: impact of regular caffeine intake. Endocrine 52 (2), 391–394. doi:10.1007/s12020-015-0780-x
Rivkees, S. A. (2003). Developing circadian rhythmicity in infants. Pediatrics 112 (2), 373–381. doi:10.1542/peds.112.2.373
Rivkees, S. A. (1997). Developing circadian rhythmicity. Basic and clinical aspects. Pediatr. Clin. North Am. 44 (2), 467–487. doi:10.1016/s0031-3955(05)70486-7
Rivkees, S. A. (2004). Emergence and influences of circadian rhythmicity in infants. Clin. Perinatol. 31 (2), 217–228. doi:10.1016/j.clp.2004.04.011
Rivkees, S. A., and Hao, H. (2000). Developing circadian rhythmicity. Semin. Perinatol. 24 (4), 232–242. doi:10.1053/sper.2000.8598
Rivkees, S. A., Hofman, P. L., and Fortman, J. (1997). Newborn primate infants are entrained by low intensity lighting. Proc. Natl. Acad. Sci. U. S. A. 94 (1), 292–297. doi:10.1073/pnas.94.1.292
Rivkees, S. A., and Lachowicz, J. E. (1997). Functional D1 and D5 dopamine receptors are expressed in the suprachiasmatic, supraoptic, and paraventricular nuclei of primates. Synapse 26 (1), 1–10. doi:10.1002/(SICI)1098-2396(199705)26:1<1::AID-SYN1>3.0.CO;2-D
Rivkees, S. A., Mayes, L., Jacobs, H., and Gross, I. (2004). Rest-activity patterns of premature infants are regulated by cycled lighting. Pediatrics 113 (4), 833–839. doi:10.1542/peds.113.4.833
Rivkees, S. A. (2001). Mechanisms and clinical significance of circadian rhythms in children. Curr. Opin. Pediatr. 13 (4), 352–357. doi:10.1097/00008480-200108000-00012
Rivkees, S. A. (2007). The development of circadian rhythms: From animals to humans. Sleep. Med. Clin. 2 (3), 331–341. doi:10.1016/j.jsmc.2007.05.010
Robinson, J., and Fielder, A. R. (1990). Pupillary diameter and reaction to light in preterm neonates. Arch. Dis. Child. 65 (1), 35–38. doi:10.1136/adc.65.1_spec_no.35
Rodak, K., Kokot, I., and Kratz, E. M. (2021). Caffeine as a factor influencing the functioning of the human body-friend or foe? Nutrients 13 (9), 3088. doi:10.3390/nu13093088
Rodrigues Helmo, F., Etchebehere, R. M., Bernardes, N., Meirelles, M. F., Galvao Petrini, C., Penna Rocha, L., et al. (2018). Melatonin treatment in fetal and neonatal diseases. Pathol. Res. Pract. 214 (12), 1940–1951. doi:10.1016/j.prp.2018.10.016
Roenneberg, T., and Merrow, M. (2016). The circadian clock and human health. Curr. Biol. 26 (10), R432–R443. doi:10.1016/j.cub.2016.04.011
Ruan, W., Yuan, X., and Eltzschig, H. K. (2021). Circadian rhythm as a therapeutic target. Nat. Rev. Drug Discov. 20 (4), 287–307. doi:10.1038/s41573-020-00109-w
Ruby, C. L., Verbanes, N. M., Palmer, K. N., Zisk, C. F., Bunion, D. J., and Marinos, L. N. (2018). Caffeine delays light-entrained activity and potentiates circadian photic phase-resetting in mice. J. Biol. Rhythms 33 (5), 523–534. doi:10.1177/0748730418789236
Sahni, R., Schulze, K. F., Stefanski, M., Myers, M. M., and Fifer, W. P. (1995). Methodological issues in coding sleep states in immature infants. Dev. Psychobiol. 28 (2), 85–101. doi:10.1002/dev.420280203
Saigal, S., and Doyle, L. W. (2008). An overview of mortality and sequelae of preterm birth from infancy to adulthood. Lancet 371 (9608), 261–269. doi:10.1016/S0140-6736(08)60136-1
Saliba, E., Autret, E., Gold, F., Pourcelot, L., and Laugier, J. (1989). Caffeine and cerebral blood flow velocity in preterm infants. Dev. Pharmacol. Ther. 13 (2-4), 134–138. doi:10.1159/000457595
Salzarulo, P., and Fagioli, I. (1992). Post-natal development of sleep organization in man: speculations on the emergence of the 'S process. Neurophysiol. Clin. 22 (2), 107–115. doi:10.1016/s0987-7053(05)80748-8
Sanchez-Solis, M., Garcia-Marcos, P. W., Aguera-Arenas, J., Mondejar-Lopez, P., and Garcia-Marcos, L. (2020). Impact of early caffeine therapy in preterm newborns on infant lung function. Pediatr. Pulmonol. 55 (1), 102–107. doi:10.1002/ppul.24540
Saroha, V., and Patel, R. M. (2020). Caffeine for preterm infants: Fixed standard dose, adjustments for age or high dose? Semin. Fetal Neonatal Med. 25 (6), 101178. doi:10.1016/j.siny.2020.101178
Scher, M. S., Johnson, M. W., and Holditch-Davis, D. (2005). Cyclicity of neonatal sleep behaviors at 25 to 30 weeks' postconceptional age. Pediatr. Res. 57 (6), 879–882. doi:10.1203/01.PDR.0000157678.84132.A8
Schmidt, B., Roberts, R. S., Davis, P., Doyle, L. W., Barrington, K. J., Ohlsson, A., et al. (2006). Caffeine therapy for apnea of prematurity. N. Engl. J. Med. 354 (20), 2112–2121. doi:10.1056/NEJMoa054065
Schmidt, B., Roberts, R. S., Davis, P., Doyle, L. W., Barrington, K. J., Ohlsson, A., et al. (2007). Long-term effects of caffeine therapy for apnea of prematurity. N. Engl. J. Med. 357 (19), 1893–1902. doi:10.1056/NEJMoa073679
Schwichtenberg, A. J., Christ, S., Abel, E., and Poehlmann-Tynan, J. A. (2016). Circadian sleep patterns in toddlers born preterm: Longitudinal associations with developmental and health concerns. J. Dev. Behav. Pediatr. 37 (5), 358–369. doi:10.1097/DBP.0000000000000287
Sekaran, S., Lupi, D., Jones, S. L., Sheely, C. J., Hattar, S., Yau, K. W., et al. (2005). Melanopsin-dependent photoreception provides earliest light detection in the mammalian retina. Curr. Biol. 15 (12), 1099–1107. doi:10.1016/j.cub.2005.05.053
Seppä-Moilanen, M., Andersson, S., and Kirjavainen, T. (2021). Caffeine is a respiratory stimulant without effect on sleep in the short-term in late-preterm infants. Pediatr. Res. 92, 776–782. doi:10.1038/s41390-021-01794-y
Seppä-Moilanen, M., Andersson, S., Rantakari, K., Mikkola, K., and Kirjavainen, T. (2019). Caffeine and supplemental oxygen effectively suppress periodic breathing with only minor effects during long episodes of apnoea in preterm infants. Acta Paediatr. 108 (3), 443–451. doi:10.1111/apa.14541
Serón-Ferré, M., Ducsay, C. A., and Valenzuela, G. J. (1993). Circadian rhythms during pregnancy. Endocr. Rev. 14 (5), 594–609. doi:10.1210/edrv-14-5-594
Seron-Ferre, M., Mendez, N., Abarzua-Catalan, L., Vilches, N., Valenzuela, F. J., Reynolds, H. E., et al. (2012). Circadian rhythms in the fetus. Mol. Cell. Endocrinol. 349 (1), 68–75. doi:10.1016/j.mce.2011.07.039
Serón-Ferré, M., Riffo, R., Valenzuela, G. J., and Germain, A. M. (2001). Twenty-four-hour pattern of cortisol in the human fetus at term. Am. J. Obstet. Gynecol. 184 (6), 1278–1283. doi:10.1067/mob.2001.113322
Serón-Ferré, M., Torres-Farfán, C., Forcelledo, M. L., and Valenzuela, G. J. (2001). The development of circadian rhythms in the fetus and neonate. Semin. Perinatol. 25 (6), 363–370. doi:10.1053/sper.2001.29037
Seron-Ferre, M., Valenzuela, G. J., and Torres-Farfan, C. (2007). Circadian clocks during embryonic and fetal development. Birth Defects Res. C Embryo Today 81 (3), 204–214. doi:10.1002/bdrc.20101
Sherman, H., Gutman, R., Chapnik, N., Meylan, J., le Coutre, J., and Froy, O. (2011). Caffeine alters circadian rhythms and expression of disease and metabolic markers. Int. J. Biochem. Cell Biol. 43 (5), 829–838. doi:10.1016/j.biocel.2011.02.008
Shimada, M., Takahashi, K., Segawa, M., Higurashi, M., Samejim, M., and Horiuchi, K. (1999). Emerging and entraining patterns of the sleep-wake rhythm in preterm and term infants. Brain Dev. 21 (7), 468–473. doi:10.1016/s0387-7604(99)00054-6
Shirwaikar, R. D. (2018). Estimation of caffeine regimens: a machine learning approach for enhanced clinical decision making at a neonatal intensive care unit (NICU). Crit. Rev. Biomed. Eng. 46 (2), 93–115. doi:10.1615/CritRevBiomedEng.2018025933
Shivakumar, M., Nayak, K., Lewis, L. E. S., Kamath, A., and Purkayastha, J. (2019). Acute hemodynamic effects of methylxanthine therapy in preterm neonates: Effect of variations in subgroups. J. Trop. Pediatr. 65 (3), 264–272. doi:10.1093/tropej/fmy044
Sipola-Leppanen, M., Karvonen, R., Tikanmaki, M., Matinolli, H. M., Martikainen, S., Pesonen, A. K., et al. (2015). Ambulatory blood pressure and its variability in adults born preterm. Hypertension 65 (3), 615–621. doi:10.1161/HYPERTENSIONAHA.114.04717
Sisman, J., Campbell, D. E., and Brion, L. P. (2005). Amplitude-integrated EEG in preterm infants: maturation of background pattern and amplitude voltage with postmenstrual age and gestational age. J. Perinatol. 25 (6), 391–396. doi:10.1038/sj.jp.7211291
Smith, J. R., McGrath, J., Brotto, M., and Inder, T. (2014). A randomized-controlled trial pilot study examining the neurodevelopmental effects of a 5-week M Technique intervention on very preterm infants. Adv. Neonatal Care 14 (3), 187–200. doi:10.1097/ANC.0000000000000093
Smolensky, M. H., Scott, P. H., Harrist, R. B., Hiatt, P. H., Wong, T. K., Baenziger, J. C., et al. (1987). Administration-time-dependency of the pharmacokinetic behavior and therapeutic effect of a once-a-day theophylline in asthmatic children. Chronobiol. Int. 4 (3), 435–447. doi:10.3109/07420528709083532
Snel, J., and Lorist, M. M. (2011). Effects of caffeine on sleep and cognition. Prog. Brain Res. 190, 105–117. doi:10.1016/B978-0-444-53817-8.00006-2
Soloveychik, V., Bin-Nun, A., Ionchev, A., Sriram, S., and Meadow, W. (2009). Acute hemodynamic effects of caffeine administration in premature infants. J. Perinatol. 29 (3), 205–208. doi:10.1038/jp.2008.193
Soubasi, V., Mitsakis, K., Nakas, C. T., Petridou, S., Sarafidis, K., Griva, M., et al. (2009). The influence of extrauterine life on the aEEG maturation in normal preterm infants. Early Hum. Dev. 85 (12), 761–765. doi:10.1016/j.earlhumdev.2009.10.004
Sowers, J. R., and Vlachakis, N. (1984). Circadian variation in plasma dopamine levels in man. J. Endocrinol. Invest. 7 (4), 341–345. doi:10.1007/BF03351014
Spaeth, A. M., Goel, N., and Dinges, D. F. (2014). Cumulative neurobehavioral and physiological effects of chronic caffeine intake: individual differences and implications for the use of caffeinated energy products. Nutr. Rev. 72 (S1), 34–47. doi:10.1111/nure.12151
Spangler, G. (1991). The emergence of adrenocortical circadian function in newborns and infants and its relationship to sleep, feeding and maternal adrenocortical activity. Early Hum. Dev. 25 (3), 197–208. doi:10.1016/0378-3782(91)90116-k
Sumova, A., and Cecmanova, V. (2020). Mystery of rhythmic signal emergence within the suprachiasmatic nuclei. Eur. J. Neurosci. 51 (1), 300–309. doi:10.1111/ejn.14141
Sumova, A., Sladek, M., Polidarova, L., Novakova, M., and Houdek, P. (2012). Circadian system from conception till adulthood. Prog. Brain Res. 199, 83–103. doi:10.1016/B978-0-444-59427-3.00005-8
Sun, K., Yang, K., and Challis, J. R. (1998). Regulation of 11beta-hydroxysteroid dehydrogenase type 2 by progesterone, estrogen, and the cyclic adenosine 5'-monophosphate pathway in cultured human placental and chorionic trophoblasts. Biol. Reprod. 58 (6), 1379–1384. doi:10.1095/biolreprod58.6.1379
Supcun, S., Kutz, P., Pielemeier, W., and Roll, C. (2010). Caffeine increases cerebral cortical activity in preterm infants. J. Pediatr. 156 (3), 490–491. doi:10.1016/j.jpeds.2009.10.033
Swaab, D. F. (1995). Development of the human hypothalamus. Neurochem. Res. 20 (5), 509–519. doi:10.1007/BF01694533
Swaab, D. F., Hofman, M. A., and Honnebier, M. B. (1990). Development of vasopressin neurons in the human suprachiasmatic nucleus in relation to birth. Brain Res. Dev. Brain Res. 52 (1-2), 289–293. doi:10.1016/0165-3806(90)90247-v
Swaab, D. F., Zhou, J. N., Ehlhart, T., and Hofman, M. A. (1994). Development of vasoactive intestinal polypeptide neurons in the human suprachiasmatic nucleus in relation to birth and sex. Brain Res. Dev. Brain Res. 79 (2), 249–259. doi:10.1016/0165-3806(94)90129-5
Takahashi, J. S. (2004). Finding new clock components: past and future. J. Biol. Rhythms 19 (5), 339–347. doi:10.1177/0748730404269151
Takahashi, J. S. (2017). Transcriptional architecture of the mammalian circadian clock. Nat. Rev. Genet. 18 (3), 164–179. doi:10.1038/nrg.2016.150
Tenreiro, S., Dowse, H. B., D'Souza, S., Minors, D., Chiswick, M., Simms, D., et al. (1991). The development of ultradian and circadian rhythms in premature babies maintained in constant conditions. Early Hum. Dev. 27 (1-2), 33–52. doi:10.1016/0378-3782(91)90026-y
Thoman, E. B., Davis, D. H., Raye, J. R., Philipps, A. F., Rowe, J. C., and Denenberg, V. H. (1985). Theophylline affects sleep-wake state development in premature infants. Neuropediatrics 16 (1), 13–18. doi:10.1055/s-2008-1052537
Thomas, K. A. (2001). Biological rhythm development in preterm infants: does health status influence body temperature circadian rhythm? Res. Nurs. Health 24 (3), 170–180. doi:10.1002/nur.1020
Thomas, K. A., and Burr, R. (2002). Preterm infant temperature circadian rhythm: possible effect of parental cosleeping. Biol. Res. Nurs. 3 (3), 150–159. doi:10.1177/1099800402003003005
Thomas, K. A. (2000). Differential effects of breast- and formula-feeding on preterm infants' sleep-wake patterns. J. Obstet. Gynecol. Neonatal Nurs. 29 (2), 145–152. doi:10.1111/j.1552-6909.2000.tb02034.x
Thosar, S. S., and Shea, S. A. (2021). Circadian control of human cardiovascular function. Curr. Opin. Pharmacol. 57, 89–97. doi:10.1016/j.coph.2021.01.002
Toorop, A. A., van der Voorn, B., Hollanders, J. J., Dijkstra, L. R., Dolman, K. M., Heijboer, A. C., et al. (2020). Diurnal rhythmicity in breast-milk glucocorticoids, and infant behavior and sleep at age 3 months. Endocrine 68 (3), 660–668. doi:10.1007/s12020-020-02273-w
Tourneux, P., Cardot, V., Museux, N., Chardon, K., Léké, A., Telliez, F., et al. (2008). Influence of thermal drive on central sleep apnea in the preterm neonate. Sleep 31 (4), 549–556. doi:10.1093/sleep/31.4.549
Uchitel, J., Vanhatalo, S., and Austin, T. (2021). Early development of sleep and brain functional connectivity in term-born and preterm infants. Pediatr. Res. 91 (4), 771–786. doi:10.1038/s41390-021-01497-4
Ulanovsky, I., Haleluya, N. S., Blazer, S., and Weissman, A. (2014). The effects of caffeine on heart rate variability in newborns with apnea of prematurity. J. Perinatol. 34 (8), 620–623. doi:10.1038/jp.2014.60
Updike, P. A., Accurso, F. J., and Jones, R. H. (1985). Physiologic circadian rhythmicity in preterm infants. Nurs. Res. 34 (3), 160–163. doi:10.1097/00006199-198505000-00007
Urry, E., and Landolt, H. P. (2015). Adenosine, caffeine, and performance: from cognitive neuroscience of sleep to sleep pharmacogenetics. Curr. Top. Behav. Neurosci. 25, 331–366. doi:10.1007/7854_2014_274
Valenzuela, F. J., Vera, J., Venegas, C., Munoz, S., Oyarce, S., Munoz, K., et al. (2016). Evidences of polymorphism associated with circadian system and risk of pathologies: a review of the literature. Int. J. Endocrinol. 2016, 2746909. doi:10.1155/2016/2746909
Valenzuela, F. J., Vera, J., Venegas, C., Pino, F., and Lagunas, C. (2015). Circadian system and melatonin hormone: Risk factors for complications during pregnancy. Obstet. Gynecol. Int. 2015, 825802. doi:10.1155/2015/825802
Van Cruchten, S., Vrolyk, V., Perron Lepage, M. F., Baudon, M., Voute, H., Schoofs, S., et al. (2017). Pre- and postnatal development of the eye: A species comparison. Birth Defects Res. 109 (19), 1540–1567. doi:10.1002/bdr2.1100
van Dam, R. M., Hu, F. B., and Willett, W. C. (2020). Coffee, caffeine, and health. N. Engl. J. Med. 383 (4), 369–378. doi:10.1056/NEJMra1816604
Van den Bergh, B. R. H., van den Heuvel, M. I., Lahti, M., Braeken, M., de Rooij, S. R., Entringer, S., et al. (2020). Prenatal developmental origins of behavior and mental health: The influence of maternal stress in pregnancy. Neurosci. Biobehav. Rev. 117, 26–64. doi:10.1016/j.neubiorev.2017.07.003
van der Voorn, B., de Waard, M., van Goudoever, J. B., Rotteveel, J., Heijboer, A. C., and Finken, M. J. (2016). Breast-milk cortisol and cortisone concentrations follow the diurnal rhythm of maternal hypothalamus-pituitary-adrenal Axis Activity. J. Nutr. 146 (11), 2174–2179. doi:10.3945/jn.116.236349
van Diepen, H. C., Lucassen, E. A., Yasenkov, R., Groenen, I., Ijzerman, A. P., Meijer, J. H., et al. (2014). Caffeine increases light responsiveness of the mouse circadian pacemaker. Eur. J. Neurosci. 40 (10), 3504–3511. doi:10.1111/ejn.12715
Vasquez-Ruiz, S., Maya-Barrios, J. A., Torres-Narvaez, P., Vega-Martinez, B. R., Rojas-Granados, A., Escobar, C., et al. (2014). A light/dark cycle in the NICU accelerates body weight gain and shortens time to discharge in preterm infants. Early Hum. Dev. 90 (9), 535–540. doi:10.1016/j.earlhumdev.2014.04.015
Vatish, M., Steer, P. J., Blanks, A. M., Hon, M., and Thornton, S. (2010). Diurnal variation is lost in preterm deliveries before 28 weeks of gestation. BJOG 117 (6), 765–767. doi:10.1111/j.1471-0528.2010.02526.x
Verdurmen, K. M., Renckens, J., van Laar, J. O., and Oei, S. G. (2013). The influence of corticosteroids on fetal heart rate variability: a systematic review of the literature. Obstet. Gynecol. Surv. 68 (12), 811–824. doi:10.1097/OGX.0000000000000002
Visscher, M. O., Lacina, L., Casper, T., Dixon, M., Harmeyer, J., Haberman, B., et al. (2015). Conformational positioning improves sleep in premature infants with feeding difficulties. J. Pediatr. 166 (1), 44–48. doi:10.1016/j.jpeds.2014.09.012
Vivanco, P., Studholme, K. M., and Morin, L. P. (2013). Drugs that prevent mouse sleep also block light-induced locomotor suppression, circadian rhythm phase shifts and the drop in core temperature. Neuroscience 254, 98–109. doi:10.1016/j.neuroscience.2013.09.025
Vogel, J. P., Chawanpaiboon, S., Moller, A. B., Watananirun, K., Bonet, M., and Lumbiganon, P. (2018). The global epidemiology of preterm birth. Best. Pract. Res. Clin. Obstet. Gynaecol. 52, 3–12. doi:10.1016/j.bpobgyn.2018.04.003
Vohr, B. (2013). Long-term outcomes of moderately preterm, late preterm, and early term infants. Clin. Perinatol. 40 (4), 739–751. doi:10.1016/j.clp.2013.07.006
von Poblotzki, M., Rieger-Fackeldey, E., and Schulze, A. (2003). Effects of theophylline on the pattern of spontaneous breathing in preterm infants less than 1000 g of birth weight. Early Hum. Dev. 72 (1), 47–55. doi:10.1016/s0378-3782(03)00010-0
Wachman, E. M., and Lahav, A. (2011). The effects of noise on preterm infants in the NICU. Arch. Dis. Child. Fetal Neonatal Ed. 96 (4), F305–F309. doi:10.1136/adc.2009.182014
Waddell, B. J., Wharfe, M. D., Crew, R. C., and Mark, P. J. (2012). A rhythmic placenta? Circadian variation, clock genes and placental function. Placenta 33 (7), 533–539. doi:10.1016/j.placenta.2012.03.008
Walani, S. R. (2020). Global burden of preterm birth. Int. J. Gynaecol. Obstet. 150 (1), 31–33. doi:10.1002/ijgo.13195
Walther, F. J., Erickson, R., and Sims, M. E. (1990). Cardiovascular effects of caffeine therapy in preterm infants. Am. J. Dis. Child. 144 (10), 1164–1166. doi:10.1001/archpedi.1990.02150340110035
Walther, F. J., Sims, M. E., Siassi, B., and Wu, P. Y. (1986). Cardiac output changes secondary to theophylline therapy in preterm infants. J. Pediatr. 109 (5), 874–876. doi:10.1016/s0022-3476(86)80717-x
Watanabe, T., Matsuhashi, K., and Takayama, S. (1990). Placental and blood-brain barrier transfer following prenatal and postnatal exposures to neuroactive drugs: relationship with partition coefficient and behavioral teratogenesis. Toxicol. Appl. Pharmacol. 105 (1), 66–77. doi:10.1016/0041-008x(90)90359-3
Weibel, J., Lin, Y. S., Landolt, H. P., Berthomier, C., Brandewinder, M., Kistler, J., et al. (2021). Regular caffeine intake delays REM sleep promotion and attenuates sleep quality in healthy men. J. Biol. Rhythms 36 (4), 384–394. doi:10.1177/07487304211013995
Weisman, O., Magori-Cohen, R., Louzoun, Y., Eidelman, A. I., and Feldman, R. (2011). Sleep-wake transitions in premature neonates predict early development. Pediatrics 128 (4), 706–714. doi:10.1542/peds.2011-0047
Williams, E. E., Hunt, K. A., Jeyakara, J., Subba-Rao, R., Dassios, T., and Greenough, A. (2020). Electrical activity of the diaphragm following a loading dose of caffeine citrate in ventilated preterm infants. Pediatr. Res. 87 (4), 740–744. doi:10.1038/s41390-019-0619-x
Williamson, M., Poorun, R., and Hartley, C. (2021). Apnoea of prematurity and neurodevelopmental outcomes: Current understanding and future prospects for research. Front. Pediatr. 9, 755677. doi:10.3389/fped.2021.755677
Wong, S. D., Wright, K. P., Spencer, R. L., Vetter, C., Hicks, L. M., Jenni, O. G., et al. (2022). Development of the circadian system in early life: maternal and environmental factors. J. Physiol. Anthropol. 41 (1), 22. doi:10.1186/s40101-022-00294-0
Wright, K. P., Badia, P., Myers, B. L., Plenzler, S. C., and Hakel, M. (1997). Caffeine and light effects on nighttime melatonin and temperature levels in sleep-deprived humans. Brain Res. 747 (1), 78–84. doi:10.1016/s0006-8993(96)01268-1
Wright, K. P., Myers, B. L., Plenzler, S. C., Drake, C. L., and Badia, P. (2000). Acute effects of bright light and caffeine on nighttime melatonin and temperature levels in women taking and not taking oral contraceptives. Brain Res. 873 (2), 310–317. doi:10.1016/s0006-8993(00)02557-9
Xu, T., and Lu, B. (2019). The effects of phytochemicals on circadian rhythm and related diseases. Crit. Rev. Food Sci. Nutr. 59 (6), 882–892. doi:10.1080/10408398.2018.1493678
Yang, L., Yu, X., Zhang, Y., Liu, N., Xue, X., and Fu, J. (2021). Encephalopathy in preterm infants: Advances in neuroprotection with caffeine. Front. Pediatr. 9, 724161. doi:10.3389/fped.2021.724161
Yue, W., Han, X., Luo, J., Zeng, Z., and Yang, M. (2021). Effect of music therapy on preterm infants in neonatal intensive care unit: Systematic review and meta-analysis of randomized controlled trials. J. Adv. Nurs. 77 (2), 635–652. doi:10.1111/jan.14630
Zele, A. J., Feigl, B., Smith, S. S., and Markwell, E. L. (2011). The circadian response of intrinsically photosensitive retinal ganglion cells. PLoS One 6 (3), e17860. doi:10.1371/journal.pone.0017860
Zhang, L., and Jain, M. K. (2021). Circadian regulation of cardiac metabolism. J. Clin. Invest. 131 (15), e148276. doi:10.1172/JCI148276
Keywords: apnea of prematurity, caffeine, chronopharmacology, circadian rhythms, preterm infants
Citation: Dai H-R, Guo H-L, Hu Y-H, Xu J, Ding X-S, Cheng R and Chen F (2022) Precision caffeine therapy for apnea of prematurity and circadian rhythms: New possibilities open up. Front. Pharmacol. 13:1053210. doi: 10.3389/fphar.2022.1053210
Received: 25 September 2022; Accepted: 18 November 2022;
Published: 01 December 2022.
Edited by:
Reza Shirazi, UNSW Sydney, AustraliaReviewed by:
Lourdes Franco, University of Extremadura, SpainSajad Razavi Bazaz, University of Technology Sydney, Australia
Copyright © 2022 Dai, Guo, Hu, Xu, Ding, Cheng and Chen. This is an open-access article distributed under the terms of the Creative Commons Attribution License (CC BY). The use, distribution or reproduction in other forums is permitted, provided the original author(s) and the copyright owner(s) are credited and that the original publication in this journal is cited, in accordance with accepted academic practice. No use, distribution or reproduction is permitted which does not comply with these terms.
*Correspondence: Feng Chen, Y3kuY2hlbjUwOEBnbWFpbC5jb20=