- 1Hong Kong Traditional Chinese Medicine Phenome Research Center, School of Chinese Medicine, Hong Kong Baptist University, Hong Kong, China
- 2Shanghai Jiao Tong University Affiliated Sixth People’s Hospital, Shanghai, China
Osteoporosis (OP), a systemic bone disease that causes structural bone loss and bone mass loss, is often associated with fragility fractures. Extracellular vesicles (EVs) generated by mammalian and gut bacteria have recently been identified as important mediators in the intercellular signaling pathway that may play a crucial role in microbiota-host communication. EVs are tiny membrane-bound vesicles, which range in size from 20 to 400 nm. They carry a variety of biologically active substances across intra- and intercellular space. These EVs have developed as a promising research area for the treatment of OP because of their nanosized architecture, enhanced biocompatibility, reduced toxicity, drug loading capacity, ease of customization, and industrialization. This review describes the latest development of EVs derived from mammals and bacteria, including their internalization, isolation, biogenesis, classifications, topologies, and compositions. Additionally, breakthroughs in chemical sciences and the distinctive biological features of bacterial extracellular vesicles (BEVs) allow for the customization of modified BEVs for the therapy of OP. In conclusion, we give a thorough and in-depth summary of the main difficulties and potential future of EVs in the treatment of OP, as well as highlight innovative uses and choices for the treatment of osteoarthritis (OA).
1 Introduction
Osteoporosis (OP), a systemic bone disease that causes increased bone fragility and fracture susceptibility, is characterized by a decrease in bone mass and the loss of bone microstructure (Prestwood et al., 1995; Brown, 2017; Liu et al., 2022a). The two most prevalent kinds of primary OP are postmenopausal OP, caused by an inadequate estrogen supply and senile OP brought on by aging (Ensrud and Crandall, 2017). Around 200 million individuals worldwide have OP, and the most prevalent problem, OP fracture, affects 20% of men and 50% of postmenopausal women during their lifespan. This significantly reduces patients’ lifestyle quality and puts a heavy economic strain on society (Black and Rosen, 2016; Chen et al., 2022a). In recent decades, numerous efforts have been made in the treatment of OP owing to its widespread occurrence and complications (Li et al., 2020). The dynamic equilibrium between bone synthesis (osteoblasts) and bone resorption (osteoclasts) has an impact on bone mass the most (Cui et al., 2020). Consequently, anabolic medications (such as synthetic parathyroid hormone) and antiresorptive therapies (such as calcitonin, bisphosphonates, denosumab, and estrogens) are being used to treat OP (Muraca and Cappariello, 2020; Hu et al., 2021). However, the potential applications of these drugs have been constrained by their bioavailability and long-term toxicity. Thus, it is an urgent requirement to generate and develop a more reliable and efficient approach to overcome OP.
2 Extracellular Vesicles (EVs): An innovative strategy to reverse osteoporosis
Pharmacological treatments for OP target to sustain homeostasis of the bone remodeling unit. Bone anti-resorptive and bone anabolic agents are available in clinical management.
2.1 Properties of EVs
EVs, a heterogeneous family of membrane-limited vesicles originating from the endosome or plasma membrane, can be divided into three types according to size (Abels and Breakefield, 2016). Apoptotic bodies, ranging from 800 to 5,000 nm, are produced by shedding cells during apoptosis, while microvesicles with a 200–2000 diameter are produced by the plasma membrane in a budding manner. Besides, exosomes (40–200 nm) are secreted from intracellular multivesicular bodies fused with the cytoplasmic membrane (He et al., 2021; Zhang et al., 2022). Among these EVs, exosome is the most critical one because of the therapeutic potential of exosomes from certain cell types (Lu et al., 2021a).
2.2 Epigenetic regulation and EVs
Epigenetics is the regulation of gene expression or phenotype without altering the sequence of the structural DNA and involves histone modifications, DNA methylation, and RNA-based mechanisms (Tzouvelekis and Kaminski, 2015; Yang et al., 2015; Yang et al., 2020a). Non-coding (nc) RNAs, including microRNAs (miRNAs), long non-coding (lnc) RNAs, and circular (circ) RNAs, are reported to play a significant role in epigenetic modifications (Muraca and Cappariello, 2020; Li et al., 2021). And ncRNAs can affect bone metabolism by regulating cell processes, including proliferation, differentiation, apoptosis, and autophagy. A study revealed that 260 circRNAs, 70 lncRNAs, and 13 miRNAs were differentially expressed between patients with postmenopausal OP and healthy controls (Jin et al., 2018). In addition, recent studies declared that ncRNAs could play an essential role in OP after delivering to recipient cells using EVs as a carrier (Cao et al., 2019; Tu et al., 2019; Zhang et al., 2022).
2.3 Different cell-derived EVs and osteoporosis
2.3.1 MSC-derived EVs
Recently, mesenchymal stem cells (MSCs) have been regarded as potential approaches to treat different diseases based on their capacity for self-renewal, paracrine production, and immunomodulation. MSCs can be isolated from bone marrow, peripheral blood, adipose tissue, umbilical cord, dental pulp, endothelial polyps, placenta, and Wharton’s jelly (Seshareddy et al., 2008; Can and Balci, 2011; Abbaszadeh et al., 2020a; Abbaszadeh et al., 2020b; Huldani et al., 2022). Ge et al. revealed the osteogenic induction and osteoporotic effect of human umbilical cord MSC (HucMSC)-derived exosomes and found two critical miRNA (hsa-miR-2110 and hsa-miR-328-3p) which associated with bone differentiation (Yahao and Xinjia, 2021). According to Qi’s study, exosomes secreted by MSCs derived from human induced pluripotent stem cells (hiPSC-MSC-Exos) could increase mRNA and protein expression of (Osteoblast) OB-related genes in bone marrow MSCs derived from ovariectomized rats. Moreover, hiPSC-MSC-Exos significantly enhanced bone regeneration and angiogenesis in ovariectomized rats (Qi et al., 2016). Based on RNA sequencing results, Wharton’s jelly-MSC-EVs enriched miR-21, miR-29, miR-221, and let-7a, which were corrected to BMP and PI3K/AKT signaling pathways for osteoporotic treatment (Lu et al., 2021b). In addition, MSCs could regulate immune cells’ function, inflammation, and the microenvironment (Malekpour et al., 2022).
In patients with OP, sarcopenia is often associated with (Dai et al., 2022; da Silva et al., 2017; Yoshimura et al., 2017). Bone marrow mesenchymal stem cells (BMSCs), as multipotent cells with self-renewing activities, can differentiate along have osteogenic lineage in response to stimulation by environmental factors. In addition, the senescence and decreased osteogenic abilities of BMSCs play critical roles in OP (Zhang et al., 2020a; Dai et al., 2022). EVs are regarded as an intrinsic systematic delivery vehicle by transporting cargos of ncRNAs, lipids, and proteins, especially miRNAs which are important for various biological processes such as bone formation, resorption, remodeling, and bone cell differentiation (Zhang et al., 2020a; Zhang et al., 2020b; Dai et al., 2022).
According to previous studies, miR-22-3p delivered by BMSC-derived EVs could induce inhibition of the MYC/PI3K/AKT pathway to promote osteogenic differentiation (Zhang et al., 2020a). Peng et al. also found that BMSC-EVs could deliver miR-196a to enhance osteoblastic differentiation by activating the Wnt/β-catenin pathway (Peng et al., 2021). In addition, miR-935, miR-29a, miR-34a, miR-34c, miR-31a-5p, and miR150-3p could also alleviate OP progression (Xu et al., 2018; Sadanand Fulzele et al., 2019; Yang et al., 2019; Lu et al., 2020; Zhang et al., 2021a; Qiu et al., 2021).
2.3.2 OC- and OB-derived EVs
OP, as a metabolic disorder, is induced by the imbalance of bone remodeling (Cao, 2011; Deng et al., 2015; Yuan et al., 2018). Bone remodeling is accomplished via the precise coordination of the activities of the two specific cells: bone-forming osteoblasts and bone-resorbing osteoclasts (Yuan et al., 2018). The crosstalk between (osteoclasts) OCs and (osteoblasts) OBs is critical in the regulation of bone homeostasis (Yuan et al., 2018). Previous studies revealed that OC could regulate OB activity either by direct cell-cell contact or the secretion of cytokines. Thus, in recent years, many researchers have also put focused on OB and OC derived EVs (Holliday et al., 2017; Chen et al., 2018; Yuan et al., 2018; Zhu et al., 2020a).
A study demonstrated that EVs isolated from OCs are paracrine regulators of osteoclastogenesis. OCs express RANKL on their surface. And RANKL binds with RANK on the surface of monocytes to stimulate the differentiation of monotype into OCs. Whereas OBs express OPG, which binds RANKL to inhibit its binding to RANK (Huynh et al., 2016). Deng et al. showed that RANKL enriched-EVs are generated from OCs and stimulated OC formation by engagement of RNAK (Deng et al., 2015). Yang et al. showed that OC-derived miR-23a-5p-containing exosomes could efficiently suppress osteogenic differentiation by inhibiting Runx2 and promoting YAP1-mediated MT1DP (Yang et al., 2020b). Sun et al. reported that OCs secrete microRNA-enriched exosomes, by which miR-214 is transferred into OBs to inhibit their function. In addition, OC-specific miR-214 transgenic mice expressed higher circulating miR-214 and downregulated OC activity (Sun et al., 2016). Moreover, another study also confirmed that OC-derived exosomal miR-214-3p could mediate osteoclast-to-osteoblast communication by inhibiting osteoblastic bone formation (Li et al., 2016a).
For EVs from OBs, Wei et al. demonstrated that EVs released by OBs at the mid-to-late differentiation stage markedly enhanced osteogenesis (Wei et al., 2019). Cui et al. demonstrated that mineralizing OB-derived exosomes could obviously influence miRNA profile and partially cause a change in the expression of miRNA in recipient ST2 cells (Wang et al., 2021). Importantly, Luo et al. found that senescent OB-derived exosome-mediated miR-139-5p regulated endothelial cell function (including upregulation of senescence and apoptosis and inhibition of proliferation and migration) via the exosomal pathway (Lu et al., 2021c).
2.3.3 Osteocyte-derived EVs
Except for BMSCs, OCs, and OBs, bone-derived EVs can also be secreted by osteocytes (Lyu et al., 2020). Osteocytes, as mechanosensitive cells, have regulatory effects on loading-induced bone formation via the secretion of paracrine factors (Eichholz et al., 2020). Another research showed that exosomes secreted by MLO-Y4 osteocyte cells exposed to mechanical strain (Exosome-MS) contributed to HPDLSC proliferation and osteogenic differentiation through PTEN/AKT and BMP2/Runx2 pathways. Moreover, these exosomes expressed higher miR181b-5p which is closely related to cell proliferation, apoptosis, and immune inflammation (Liu et al., 2019; Wang et al., 2019; Lv et al., 2020). Similarly, Eichholz et al. illustrated that osteocyte-derived EVs with pro-osteogenic potential could enhance bone regeneration and repair in diseases such as OP (Eichholz et al., 2020).
2.3.4 Macrophage-derived EVs
Macrophages (Mφs), as an important part of innate immunity, depending on the environment, can be divided into two types: the anti-inflammatory phenotype (M2) and the pro-inflammatory phenotype (M1). As we know, chronic inflammation is one of the direct inducers of OP (Ginaldi et al., 2005; Kong et al., 2019; Liu et al., 2021a; Yu et al., 2021). According to one study, osteolysis occurs in mice when Mφs become polarised toward the M1 phenotype (Gao et al., 2021). Based on microarray analysis, miR-98 is a candidate cargo of the M1 Mφs-EXOs and led to bone loss and OP progression through the DUSP1/JNK pathway (Yu et al., 2021). There is research revealed that all Mφ subtypes (M0, M1, and M2) can promote the osteogenic differentiation of BMSCs, and M1 Mφs may be critical to the early phases of osteo-induction and bone regeneration, while M2 Mφs may foster continued bone regeneration (Chen et al., 2020a; Kang et al., 2020; Liu et al., 2021a; Song et al., 2022a).
Exosomes can be released by Mφs cells during the regulation of inflammatory responses. Liu et al. demonstrated that both M1 and M2 Mφ-exosomes upregulated osteogenesis of BMSCs (Liu et al., 2021a). One miRNA sequencing analysis of the exosomes showed that Mφs-derived exosomal miR-3473e plays a pivotal role in the promotion of osteo-/angio-genesis via upregulation of the Akt1 gene (Wang et al., 2022a). Interestingly, Zhang et al. found the miR-144-5p levels were highly enriched in exosomes derived from bone marrow-derived macrophages in type 2 diabetes, and it could be transferred into BMSCs to regulate bone regeneration via Smad1 (Zhang et al., 2021b).
M1 Mφ-exosomes promote osteogenesis of BMSCs through microRNA-21a-5p at the early stage of inflammation (Liu et al., 2021a). Moreover, miR-5106, miR-26a-5p, and miR-22-3p derived from M2 Mφ-exosomes are also reported as targets to promote osteogenic differentiation in BMSCs and MSCs (Xiong et al., 2020; Bin-Bin et al., 2022; Liu et al., 2022b). Besides, Mg2+-mediated Mφs could promote the osteogenic differentiation of BMSCs via the autophagy pathway by reducing miR-381 in macrophage-derived exosomes (Zhu et al., 2022).
2.3.5 Endothelial cell-derived EVs
Research show abnormal angiogenesis and excessive osteoclastic activity would encourage aberrant bone resorption that is connected to OP (Kusumbe et al., 2014; Ramasamy et al., 2014; Fan et al., 2017; Farr et al., 2017; Song et al., 2019). It was reported that there is a positive correlation between blood vessel density and osteogenesis adversely associated with bone resorption (Sivaraj and Adams, 2016; Huang et al., 2018). Endothelial cells (ECs) are located in the inner layer of the vascular vessel and are frequently secreting substances due to their active properties (Seton-Rogers, 2014). Studies have further shown that EC-exos contain a variety of chemicals relevant to cell migration, proliferation, and vascular formation. EC-exos could suppress OP progression via delivering miR-155 (Todorova et al., 2017; Song et al., 2019). Wilner et al. showed that EVs containing miR-31 were secreted from senescent ECs and presented an inhibitory effect on osteogenic differentiation by counteracting the Frizzled-3 gene (Weilner et al., 2016). In addition, EC-Exos delayed the progression of glucocorticoid-induced OP by inhibiting apoptosis and ferroptosis in dexamethasone-stimulated OBs (Yang et al., 2021).
As reported, endothelial progenitor cells (EPCs) can also influence osteoclastogenesis via EVs. Evs derived from EPCs could prevent glucocorticoid-induced OP in mice by suppressing the ferroptosis pathway in OBs (Cui et al., 2019; Lu et al., 2019). Besides, Cui et al. illustrated that EPC-exos promoted osteoclastogenesis by the Lnc-MALAT-1/miR-124 pathway (Cui et al., 2019).
3 Gut microbiota
In recent years, the study of microbial EVs has also begun to receive attention. The skin and mucosal surfaces of the human body are colonized by a large number of microorganisms, which is particularly important in the gut. Our understanding of the connection between gut microbiota (GM) and human health has considerably increased because of the National Institutes of Health-funded Human Microbiome Project (Lloyd-Price et al., 2017). Along the length of the gastrointestinal tract, a complex, dynamic microbial community, including bacteria, viruses, archaea, and eukaryotes, reaches its highest density in the colon (Lozupone et al., 2012). In the human stomach, 1,150 different bacterial species have been found, with 160 different species on average for each person (Lozupone et al., 2012). More than 70% of the gut bacteria belong to the two phyla Bacteroidetes and Firmicutes, other phyla such as Proteobacteria, Verrucomicrobia, Fusobacteria, and Actinobacteria are only present in small amounts (Eckburg et al., 2005; Sultan et al., 2021).
The gut flora has a substantial impact on human health. They play a crucial part in the growth of the immune system (Lee and Mazmanian, 2010). Additionally, they break down indigestible plant fibers to produce vital metabolites like short-chain fatty acids (SCFAs) (Tremaroli and Backhed, 2012). Clostridial clusters IV and X1Va, Bacteroides, and Bifidobacterium, dominate the SCFA production (Martens et al., 2011; Sultan et al., 2021). The three main SCFAs produced by the microbiota are propionate, acetate, and butyrate. In colonocytes, butyrate serves as the predominant energy source, while acetate and propionate are substrates for lipogenesis and gluconeogenesis in peripheral tissues (Wolever et al., 1991; Mottawea et al., 2016). SCFAs also regulate how well the gut barrier is functioning. For instance, butyrate increases the production of proteins linked to tight junctions (Bordin et al., 2004). In addition to the colonic fermentation of dietary fibers, the control of the metabolism of choline, bile acid metabolism, and insulin resistance are a few other host metabolic pathways that the GM interacts with (Tremaroli and Backhed, 2012).
The host senses bacterial metabolites as part of the microbiota-host interaction. Importantly, studies demonstrate that human commensal EV mediates immunological control and disease prevention (Shen et al., 2012). We highlight what is currently known about the roles of bacterial extracellular vesicles (BEVs) on the health of the host as a vehicle for moving bioactive payload (such as miRNA, DNA, mRNA, proteins, lipids, and carbohydrates), as well as their potential function as molecular pathways in Figure 1.
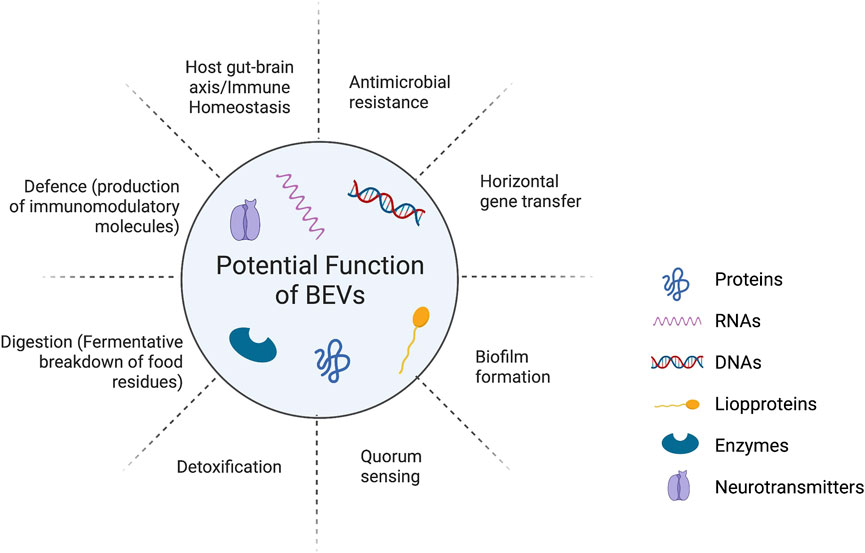
FIGURE 1. The roles of bacterial extracellular vesicles (BEVs) on the health of the host as a vehicle for moving bioactive payload (such as miRNA, DNA, mRNA, proteins, lipids, and carbohydrates) and their potential function.
4 Bacterial EVs (BEVs)
In the intestinal ecosystem, the two-way communication between microbiota and host does not involve direct cellular contact. Both microbiota and host-derived EVs are key players in this interkingdom crosstalk. There is now growing evidence that bacterially secreted vesicles mediate microbiota function by translocating and delivering effector molecules that regulate host signaling pathways and cellular processes into host cells. Emerging evidence suggests that GM is essential for maintaining bone homeostasis and preventing the onset of OP (Xu et al., 2017). According to their contribution to preserving human health, gut microorganisms can be classified as harmful bacteria (probiotics), beneficial bacteria, and neutral bacteria (Gentile and Weir, 2018). Probiotics are recognized as live microorganisms by the Food and Agriculture Organization of the United Nations (FAO) and the World Health Organization (WHO). They are beneficial for the host when supplied in sufficient numbers. For example, probiotics have been demonstrated to reduce OP (Song et al., 2022b). Notably, direct cell interactions are not necessary for probiotics and hosts to communicate. There is mounting evidence that these probiotic EVs modify host signaling pathways and deliver bioactive materials to host cells to control the function of distant organs (Liu et al., 2022a; Chen et al., 2022b; Liu et al., 2022c; Liu et al., 2022d).
BEVs are non-reproducible phospholipid bilayer nanocarriers that are released by most bacteria. They provide communication between bacteria and their hosts by providing various compounds involving metabolites, proteins, lipids, and nucleic acids. As a result, they have a significant impact on the regulation of physiological and pathological processes (Zihao Ou et al., 2022). Owing to their nanosized dimensions, drug loading capacity, cell-free systems, low toxicity, and superior biocompatibility, BEVs, particularly probiotic EVs, have developed into an effective platform for biological applications (Witwer et al., 2021). The advantages of rapid bacterial growth and maturation in high-density cultures (fed-batch fermentation) allow for large-scale manufacture of BEVs, compared to the low extraction rates of mammalian EVs (MEVs), which are very low (Liu et al., 2018; Liu et al., 2020a; Liu et al., 2020b). Additionally, breakthroughs in synthetic biology and the distinctive biological pathways of BEVs allow for the customization of modified BEVs for the therapy of OP (Service, 2014; Toyofuku et al., 2019). Thus, the gut-bone axis system may be significantly impacted by the use of both natural and engineered BEVs, which would effectively manage the onset and progression of OP. At the same time, there are some difficulties with the clinical application of EVs, mainly in terms of yield and targeting. It is difficult to obtain pure exosomes from natural sources, and there is a wide range of exosome sources. Whether all exosomes from various sources can be obtained by a continuous extrusion of cells to obtain exosome-mimicking nanovesicles and whether their structural integrity and physiological activities are the same as those of natural exosomes still need to be further investigated. Although progress has been made in targeting modifications, the in vivo environment is complex, and it is uncertain whether the modified exosomes will still have the desired targeting properties once they enter the body. Therefore, targeting modifications of exosomes is still a major focus of research.
BEVs are lipid nanostructures (about 25–300 nm) derived from parental bacterial cells and are found not only in prokaryotes but also in all living domains, such as fungi, protozoa, and plant cells. We illustrate the biogenesis and composition of EVs derived from eukaryotic host cells (host EVs), gram-negative bacteria (outer membrane vesicles, OMVs), and gram-positive bacteria (membrane vesicles). Generation of EVs by host cells can occur via outward budding of the plasma membrane, resulting in microvesicles. Alternatively, inward budding of the endosomal membrane results in multivesicular endosomes (MVEs) with intraluminal vesicles (Jung et al., 2021). They contain a variety of molecular components such as nucleic acids (DNA, RNA), proteins, lipids, and other organic substances. More recent studies have shown that the production of BEVs can be achieved by different biogenetic mechanisms, with some EVs possibly originating from blistering cells and others being secreted during cell lysis. Each of these groups of EVs contains a “cargo” from the cell’s source: a membrane-rich cargo in the blistering form and a membrane- or cytoplasm-rich cargo in the lysed form. In this way, the primary mechanism for the biogenesis of BEVs is regulated by the cellular response to the environment and therefore affects the composition of BEVs. Table 1 summarizes the overlapping and distinguishing characteristics of these EV populations.
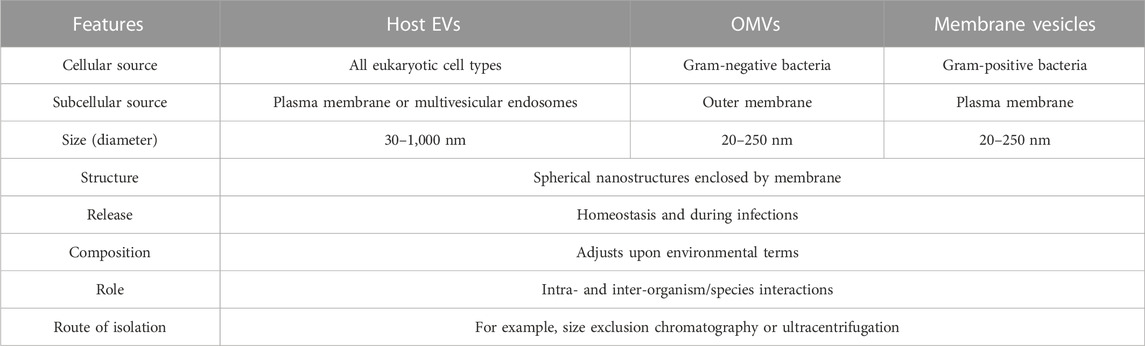
TABLE 1. Overlapping and distinguishing characteristics of EV populations (Jung et al., 2021).
5 BEVs and immune homeostasis
The gut microbiota is considered a “hidden organ” because the products encoded by the microbiota actively contribute to many essential host functions. In addition to its role in nutrition, metabolism and energy production, the gut microbiota also regulates immune homeostasis. EVs (especially BEVs) generated from GM are crucial for preserving gut immunological homeostasis. A cascade of immune signaling is triggered by the interaction of BEVs with pattern recognition receptors like NOD1, NOD2, and TLR on immune cells. BEVs contain various copies of microorganism-related molecular patterns, such as LPS, RNA, DNA, periplasmic proteins, and peptidoglycan (Kaparakis et al., 2010; Soult et al., 2013; Bielaszewska et al., 2018; Meganathan et al., 2020). Notably, the interaction between immune cells and EVs cargo is related to virulence and the parent stain. Proteomic research has shown that the TLR 2 lipoprotein agonist is only present in EVs from virulent mycobacterium strains (Prados-Rosales et al., 2011). Additionally, the TLR-EV interaction is receptor-specific. For instance, the cellular responses of TLR 2/1 and TLR 4 were upregulated, but those of TLR 2/6 were suppressed, while TLR 5 was unaffected by the EVs generated by the Lactobacillus and Bifidobacterium genera (van Bergenhenegouwen et al., 2014). Besides, EVs’ sRNA and miRNA content have the potential to suppress the immune system; in the instance of sRNA from the fungus Botrytis cinerea, which silences genes to reduce plant immunity (Weiberg et al., 2013). Additionally, anopheline mosquito-produced microRNA (miRNA) may interfere with host miRNA and modulate some immune responses (Arca et al., 2019), suggesting that EVs would be used as a strategy by pathogens to suppress the host immune system (Lee, 2019).
6 The use of natural BEVs in OP treatment
Over the past 10 years, the interaction between GM and OP has drawn a lot of attention (Zhang et al., 2021c; Wang et al., 2022b). The GM, in particular probiotics, has been identified as a potential target for the treatment of OP, such as Lactobacillus rhamnosus GG, Lactobacillus reuteri, Lactobacillus paracasei, Bifidobacterium longum, and Akkermansia muciniphila (AKK) (Thomas et al., 2012; Ohlsson et al., 2014; Parvaneh et al., 2015; Li et al., 2016b; Liu et al., 2021b). Given the importance of GM in maintaining bone homeostasis, examining the ways in which it interacts with bone can serve as a therapeutic platform for translational medicine. Recently, it has been demonstrated that the secretion of BEVs, which may safely carry numerous bioactive chemicals to distant tissues/cells, is essential for the communication of signals between bacterial and mammalian cells (Chen et al., 2019; Chen et al., 2020b; Chen et al., 2022b). As a result, researchers have focused more on determining if BEV made from GM is an important mechanism for the GM-induced regulation of OP.
Excitingly, a novel treatment approach called Fecal Microbiota Transplantation (FMT) delays the progression of degenerative and chronic disorders (Hanssen et al., 2021). According to Xie et al., replacing aged GM (EGM) with young GM (CGM) prevented the loss of bone strength and bone mass in ovariectomy (OVX)-induced OP mice (Liu et al., 2021b). Transplanting CGM dramatically enhanced the amount of AKK in OP mice, according to 16S rRNA sequencing. Additionally, AKK given directly to OVX-induced mice dramatically increased OP. Further investigation revealed that administration of GW4869, a neutral sphingomyelinase inhibitor, interferes with the secretion of BEVs, and the anti-osteoporotic effects of AKK were dramatically reduced.
Accordingly, the intervention of OVX mice with BEVs derived from AKK can have effects on OBs that are similar to those of their parent bacteria, promoting OB growth and inhibiting OC activity, suppressing the loss of bone mass, strength and the deterioration of the bone microarchitecture (Figure 2). Although Xie et al. did not examine the precise essential mechanisms by which BEVs produced from AKK enhance OP, multi-omics sequencing of BEVs could help to solve this problem and modifying the development of future modified BEVs.
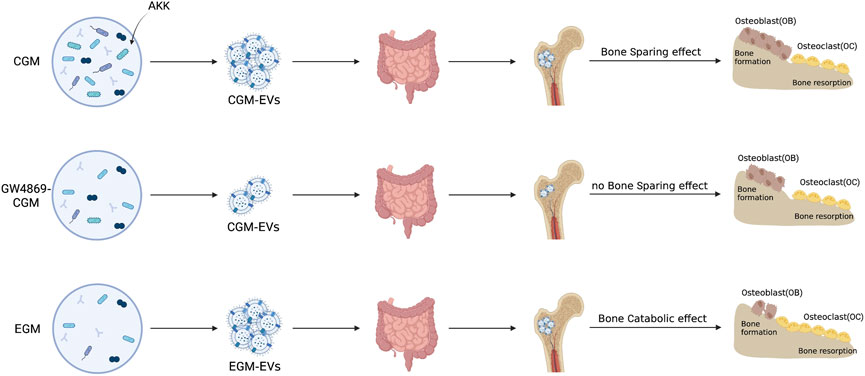
FIGURE 2. OP is treated with natural BEVs. The direct intervening OVX animals shown in the schematic diagram may operate similarly to their parent bacteria in promoting osteoblast growth and suppressing osteoclast activity, suppressing the loss of bone mass and strength, and the degeneration of the bone microarchitecture. Inhibitors of neutral sphingomyelinases, such as GW4896, are used to treat the gut microbiota of youngsters and the elderly (Liu et al., 2021b).
Nandakumar et al. recently showed that BEVs produced from the intestinal strain Proteus mirabilis (PM) suppress osteoclastogenesis and bone resorption (Wang et al., 2022c). In particular, miRNA and mRNA sequencing showed that BEV from PM significantly affected apoptotic signaling pathways and mitochondrial function. Specifically, the expression of Bax, B-cell lymphoma-2, cytochrome C, and caspase-3 was upregulated, and miR-96-5p was downregulated, while intracellular ROS levels and mitochondrial membrane potential were increased. Moreover, OVX-induced bone loss in OP mice was reduced by PM-derived BEVs (Figure 3). The molecular mechanism of novel BEVs with GM osteoprotective activity was demonstrated by histological sequencing and applied to the clinical treatment of OP patients.
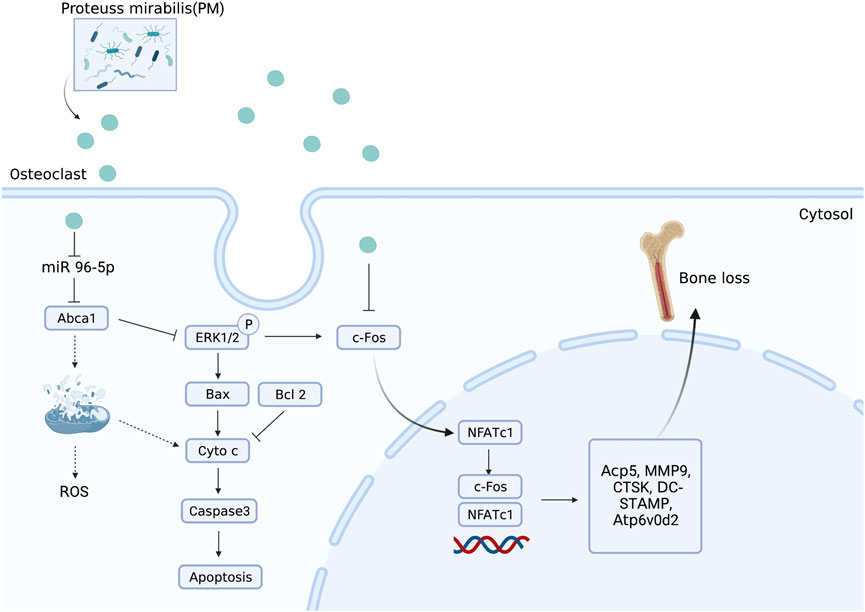
FIGURE 3. BEVs produced from Proteus mirabilis alleviate bone loss in OP. Administration of PM-derived BEVs reduced bone loss in OVX-induced OP mice via the regulation of miR-96-5p/Abca1 pathways.
These findings revealed a novel way of gut-bone axis mediation by intestinal bacteria, providing the potential for the use of transgenes (particularly probiotics) and their functional BEVs for the treatment of OP and OP fractures. In addition, the usage of naturally occurring BEVs created by genetic engineering will grow in popularity as a field of study. Further enhancing the treatment efficacy of these BEVs for OP by the application of genetic engineering approaches.
6.1 BEVs future perspectives
The possibility of treating OP through natural BEVs was mentioned above, and the benefit of BEVs in the treatment of systemic skeletal disorders like OP has shown tremendous promise. BEV-based therapies are a more favorable strategy for OP than parental bacteria, and probiotics, in particular, have been shown to control the onset and disease progression. This is because of the nanosized structures, great biocompatibility, unique cell-free system, and non-reproducible properties of BEVs. By controlling the endocrine, immunological, and intestinal metabolites, the gut-bone axis has been identified as a possible target for the therapy of OP (Tyagi et al., 2012; Sharon et al., 2014; Zhang et al., 2021c). For the gut-bone axis, BEVs have steadily evolved into a very important communication tool. In light of this, probiotics such as AKK, Bifidobacterium spp., and Lactobacillus spp. have been demonstrated to work well against OP and release BEVs that may be more suitable for OP treatment.
Both organic and synthetic BEVs are possibilities for OP treatment. BEVs have developed gradually into a critical communication tool for the gut-bone axis. As a result, using naturally occurring BEVs made from these bacteria is a favored method for treating OP in addition to the documented microorganisms, particularly probiotics. Furthermore, two engineering techniques can be used to create therapeutic BEVs: parental engineering strains and engineering natural BEVs. The improved features of these modified BEVs, such as low toxicity, high yield, bone targeting capability, and bone healing capacity, enable a wider range of treatment options for OP.
These organic BEVs offer a great foundation for the management of OP. However, BEVs have several drawbacks, such as limited therapeutic efficiency and poor targeting capacity, which may necessitate substantial dosages in order to ensure efficacy. Thanks to developments in synthetic biology, it is now possible to adapt multifunctional strains to produce extremely effective BEVs for OP treatment. In addition to engineering the parental strains, BEV-based engineering approaches also provide a wide range of functionalities for BEVs. It is possible to create BEVs with a variety of capabilities (high productivity, low toxicity, bone-targeting ability, and bone-therapeutic ability), which is a viable platform for OP treatment, according to the engineering approaches for parental strains and BEVs. Currently, intravenous injection is the major method used to treat systemic disorders caused by BEVs (Chen et al., 2020c; Chen et al., 2022b; Li et al., 2022), and the only route of administration is rare (Liu et al., 2021b). Oral delivery is typically a safer therapeutic option than an intravenous injection, with higher patient compliance and reduced medical expenses (Taddio et al., 2012; Vela Ramirez et al., 2017). The complicated gastric environment limits the oral delivery of BEVs. Dopamine polymerization-mediated decoration was created by Liu et al. (Pan et al., 2021) to shield probiotics from bile salts and stomach acid. Similar decorating platforms can be created to guarantee BEVs’ oral effectiveness and increase their effectiveness in treating OP.
Interestingly, a team of researchers demonstrated an oral symbiotic bacterial approach based on BEVs (Yue et al., 2022). They found that by interacting with immune cells in the lamina propria, BEVs produced from intestinal bacteria can cross the intestinal epithelial barrier and result in immunomodulation. After oral treatment of intestinal commensal bacteria (with araBAD promoter) and arabinose, the bacteria in the intestine controlled the synthesis of BEVs with target antigens (araBAD promoter inducer). These modified BEVs distributed the stimulant chemicals after crossing the gut epithelial barrier. Similar research also showed that OP mice had significantly lower levels of immune cells called Treg, which have been shown to can improve bone mass and prevent OC development by downregulating the creation of RANKL (Zhu et al., 2020b; Ren et al., 2021), an important target in the development and activation of OCs (Wan et al., 2018; Chen et al., 2020d). Therefore, the administration of oral commensal bacteria approaches based on BEVs to give stimulatory compounds to increase Treg proliferation is an effective way for OP treatments (Campbell et al., 2020; Fu et al., 2022).
Notably, BEVs will be an effective tool for controlling OP fracture. Based on clinical findings, the research team proposed a “three-in-one” therapy strategy consisting of active anti-OP nanocarriers to optimize bone healing and improve bone implantation (Chen et al., 2022c). In addition to serving as anti-OP nanocarriers, BEVs can work in conjunction with various biomaterials to hasten the healing of OP fractured bones, including metal scaffolds, hydrogels, and mesoporous inorganic biomaterials (Mora-Raimundo et al., 2019; Mao et al., 2021; Zou et al., 2021). Despite ongoing difficulties, more studies into BEV-based treatments will likely result in cutting-edge treatments for OP and its side effects, speeding up their clinical translation use.
6.2 The use of exosomes in OA treatment
In the same way as the OP, Osteoarthritis (OA) is also a prevalent orthopaedic disease associated with aging. Recent studies have shown that they share similarities in pathological features and pathogenesis. Subchondral bone loss is a feature of OP and is also present in the early stages of OA, which suggests that OA can be attempted in the same way as OP to suppress the course of the disease (Bultink and Lems, 2013; Lafeber and van Laar, 2013). Therefore, as with OP, treatments for OA mediated by EVs show great potential. In the last few years, there has been increasing evidence that miRNA can also regulate genes in a non-cell-autonomous manner. Exosomes are in vivo-derived EVs that contain intact, mature 21 nt-miRNAs that act in intercellular communication. Exosome biogenesis is upregulated by various signals, and their secretion results from the passive activation of different mechanisms. Several parallel pathways can activate exosome biogenesis which releases from the passive activation of various signaling pathways. OCs secrete miR-214-rich exosomes for delivery to OBs in bone. Importantly, in human OA, miRNA is an important regulator of molecular pathophysiological processes in synovium and cartilage.
A new study by Liu et al. shows that OC-derived miRNA is upregulated during surgically induced OA formation in mice in vivo (Liu et al., 2021c). They used an OC-targeted delivery system that disrupted miRNA biogenesis and exosome production in OC by eliminating Rab27a and the Dicer enzyme that controls miRNA production, significantly delaying OA progression (Figure 4). Nevertheless, questions remain about the mechanism of how OC exosomes affect chondrocytes. The authors showed that the expression level of miR-214 in OC was negatively correlated with the expression of metalloproteinase inhibitors in chondrocytes. Mechanistically, exosomal transfer of OC-miRNAs into chondrocytes reduces cartilage resistance to matrix degeneration, osteochondral angiogenesis, and sensory nerves during OA progression by inhibiting TIMP-2/3. The study by Liu et al. clearly shows that in injury-induced OA pathology, exosomes and miRNA play an important role in OC-chondrocyte crosstalk. Therefore, the transfer of exosomes of OC-miRNAs to chondrocytes is thought to be a viable treatment approach to alleviate the development of OA (Figure 5).
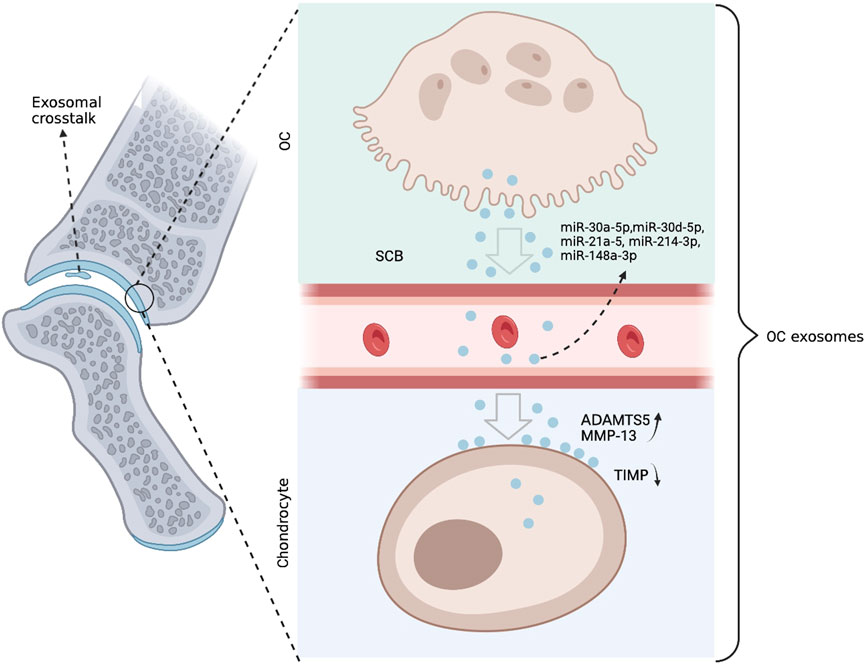
FIGURE 4. Anterior cruciate ligament transection (ACLT) was performed on mice to create the OA phenotype. Combined with in vitro culture models, small extracellular vesicles rich in coding miRNAs are secreted by OCs. These miRNAs are translocated to chondrocytes, where they suppress genes like TIMP that alleviate matrix deterioration, increasing the activities of ADAMTS5 and MMP-13. The concentration of OC miRNAs in circulating exosomes increases, and they appear to leave the SCB and re-enter the chondrocytes.
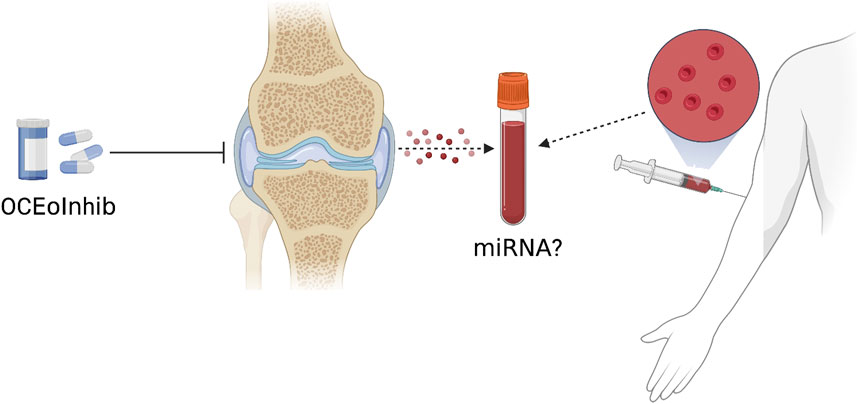
FIGURE 5. A new OC-targeted exosome inhibitor (OCEoInhib) to block Rab27-mediated OC exosome release. The measurement of OC miRNA in circulating exosomes could be used to diagnose illnesses and monitor their treatments.
6.3 The future perspective of exosomes in OA treatment
The pathology of OA involves cartilage, subchondral bone, synovial tissues, and exosomes can act as hubs for their delivery pathways. Synovial fibroblast-derived exosomes can induce OA progression, and serum- and synovial-derived exosomes can contribute to the diagnosis of OA by increasing chondrocyte proliferation and suppressing inflammation. Remarkably Mφs-EXOs can repair joints or alleviate disease progression. While it is also true that these effects of exosomes will greatly facilitate research into the mechanisms, diagnosis, and treatment of OA, there are also several challenges. Firstly, exosomes contain many proteins and non-coding RNAs. Although they are involved in or regulate the development of OA, it is difficult to identify the exact molecules that contribute. Future studies could therefore focus on the functional dissection of a single component and the network of interactions between them. Secondly, both Mφs-EXOs and the modified exosomes act only in small animals, and the exact mechanisms are not yet understood. Therefore, their safety and efficacy in vivo need to be further investigated. In the future, they could be further tested on large animals.
7 Conclusion
Growing evidence points to mammalian and bacteria-derived EVs’ possibility of acting as nano messengers for communications between the host and the microbiota. BEVs represent their parental microbes in a range of interpersonal interactions. Contrary to their origin, they are less likely to enter circulation. They can transfer their contents to locations distant from the intestine, such as the bones. Instead of individual metabolites and secreted proteins, the contents of EVs are encased in bilayers as a means of protecting them from lysozyme and RNase in the extracellular environment and facilitating their diffusion to distant organs (Al-Nedawi et al., 2015; Choi et al., 2015). EVs are still underutilized as a means of connecting with the host.
Earlier investigations have examined the characterization of their proteome and/or RNA contents or the connection of EVs from a particular microorganism with certain bodily reactions (Perez-Burgos et al., 2013; Al-Nedawi et al., 2015; Emery et al., 2017; Zakharzhevskaya et al., 2017; Zhang et al., 2018; Lee et al., 2020). This might be explained by the absence of standardized techniques for isolating and identifying BEV components as well as by the absence of clear-cut biomarkers extracted from BEVs. Furthermore, host EVs and BEVs are not separated using the current techniques. Recent studies have revealed several methods for separating BEVs from bodily fluids using size exclusion chromatography, density gradient centrifugation, and ultrafiltration (Tulkens et al., 2020).
Moreover, the lack of accurate ways to determine the parental bacterial origin of various EVs is another challenge, BEVs or their observed content in different microbial communities, such as transgenic (Nahui Palomino et al., 2021). Future studies are necessary to demonstrate the relationship between the variability of the parent microbiota and the variability of BEV production and composition. Besides, more investigation is needed to determine how BEVs are packaged by microbial cells and the reasons behind the packaging of these specific molecules. Questions such as whether they target specific cells or host cells, whether they can cross biological barriers, including the blood-brain barrier and the intestinal barrier, how they target host cells, and how they release their contents remain to be investigated.
Furthermore, the study on targeting exosome-mediated pathogenesis of other bone disorders like OA is unique in its molecular penetration, clearly demonstrating that exosomes and miRNAs have an important role in OC-chondrocyte crosstalk in injury-induced OA pathology (Meulenbelt et al., 2021). It will be important to investigate in the future whether there is an association between exosome crosstalk on the development of age-related OA. From experiments in mice, it is shown that tissue-targeted inhibition of disease expression by exosomes is feasible. It could lead to breakthroughs in the treatment of OA and other orthopedic diseases. Although the observation of elevated serum exosome levels in patients with severe knee injuries increases the translational significance of this finding, the pathological process of OA characterized by ACLT in young mice is not similar to that of age-related OA in humans.
The recent developments discussed in this review give us a glimpse of host and bacteria EVs’ evolving role as mediators of host-microbiota interaction, despite the various challenges that must be overcome before they may possibly be exploited as a platform for the delivery of biologic therapeutics to particular body sites.
Author contributions
KC, MJ, CX, and JW wrote the article. KC, MJ, CX, and JW prepared the figures and edited the article. All authors reviewed and approved of the manuscript.
Funding
This work was supported by the HKBU Strategic development fund (SDF 19-1216-P03).
Conflict of interest
The authors declare that the research was conducted in the absence of any commercial or financial relationships that could be construed as a potential conflict of interest.
Publisher’s note
All claims expressed in this article are solely those of the authors and do not necessarily represent those of their affiliated organizations, or those of the publisher, the editors and the reviewers. Any product that may be evaluated in this article, or claim that may be made by its manufacturer, is not guaranteed or endorsed by the publisher.
References
Abbaszadeh, H., Ghorbani, F., Derakhshani, M., Movassaghpour, A. A., Yousefi, M., Talebi, M., et al. (2020). Regenerative potential of wharton's jelly-derived mesenchymal stem cells: A new horizon of stem cell therapy. J. Cell Physiol. 235 (12), 9230–9240. doi:10.1002/jcp.29810
Abbaszadeh, H., Ghorbani, F., Derakhshani, M., Movassaghpour, A., and Yousefi, M. (2020). Human umbilical cord mesenchymal stem cell-derived extracellular vesicles: A novel therapeutic paradigm. J. Cell Physiol. 235 (2), 706–717. doi:10.1002/jcp.29004
Abels, E. R., and Breakefield, X. O. (2016). Introduction to extracellular vesicles: Biogenesis, RNA cargo selection, content, release, and uptake. Cell Mol. Neurobiol. 36 (3), 301–312. doi:10.1007/s10571-016-0366-z
Al-Nedawi, K., Mian, M. F., Hossain, N., Karimi, K., Mao, Y. K., Forsythe, P., et al. (2015). Gut commensal microvesicles reproduce parent bacterial signals to host immune and enteric nervous systems. FASEB J. 29 (2), 684–695. doi:10.1096/fj.14-259721
Arca, B., Colantoni, A., Fiorillo, C., Severini, F., Benes, V., Di Luca, M., et al. (2019). MicroRNAs from saliva of anopheline mosquitoes mimic human endogenous miRNAs and may contribute to vector-host-pathogen interactions. Sci. Rep. 9 (1), 2955. doi:10.1038/s41598-019-39880-1
Bielaszewska, M., Marejkova, M., Bauwens, A., Kunsmann-Prokscha, L., Mellmann, A., and Karch, H. (2018). Enterohemorrhagic Escherichia coli O157 outer membrane vesicles induce interleukin 8 production in human intestinal epithelial cells by signaling via Toll-like receptors TLR4 and TLR5 and activation of the nuclear factor NF-κB. Int. J. Med. Microbiol. 308 (7), 882–889. doi:10.1016/j.ijmm.2018.06.004
Bin-Bin, Z., Da-Wa, Z. X., Chao, L., Lan-Tao, Z., Tao, W., Chuan, L., et al. (2022). M2 macrophagy-derived exosomal miRNA-26a-5p induces osteogenic differentiation of bone mesenchymal stem cells. J. Orthop. Surg. Res. 17 (1), 137. doi:10.1186/s13018-022-03029-0
Black, D. M., and Rosen, C. J. (2016). Clinical practice. Postmenopausal osteoporosis. N. Engl. J. Med. 374 (3), 254–262. doi:10.1056/NEJMcp1513724
Bordin, M., D'Atri, F., Guillemot, L., and Citi, S. (2004). Histone deacetylase inhibitors up-regulate the expression of tight junction proteins. Mol. Cancer Res. 2 (12), 692–701. doi:10.1158/1541-7786.692.2.12
Bultink, I. E., and Lems, W. F. (2013). Osteoarthritis and osteoporosis: What is the overlap? Curr. Rheumatol. Rep. 15 (5), 328. doi:10.1007/s11926-013-0328-0
Campbell, C., McKenney, P. T., Konstantinovsky, D., Isaeva, O. I., Schizas, M., Verter, J., et al. (2020). Bacterial metabolism of bile acids promotes generation of peripheral regulatory T cells. Nature 581 (7809), 475–479. doi:10.1038/s41586-020-2193-0
Can, A., and Balci, D. (2011). “Isolation, culture, and characterization of human umbilical cord stroma-derived mesenchymal stem cells,” in Mesenchymal stem cell assays and applications (Springer), 51–62.
Cao, Q., Guo, Z., Yan, Y., Wu, J., and Song, C. (2019). Exosomal long noncoding RNAs in aging and age-related diseases. IUBMB Life 71 (12), 1846–1856. doi:10.1002/iub.2141
Cao, X. (2011). Targeting osteoclast-osteoblast communication. Nat. Med. 17 (11), 1344–1346. doi:10.1038/nm.2499
Chen, X., Wang, Z., Duan, N., Zhu, G., Schwarz, E. M., and Xie, C. (2018). Osteoblast-osteoclast interactions. Connect. Tissue Res. 59 (2), 99–107. doi:10.1080/03008207.2017.1290085
Chen, C. Y., Rao, S. S., Tan, Y. J., Luo, M. J., Hu, X. K., Yin, H., et al. (2019). Extracellular vesicles from human urine-derived stem cells prevent osteoporosis by transferring CTHRC1 and OPG. Bone Res. 7, 18. doi:10.1038/s41413-019-0056-9
Chen, K., Jiao, Y., Liu, L., Huang, M., He, W., Hou, J., et al. (2020). Communications between bone marrow macrophages and bone cells in bone remodeling. Front. Cell Dev. Biol. 8, 598263. doi:10.3389/fcell.2020.598263
Chen, C. Y., Du, W., Rao, S. S., Tan, Y. J., Hu, X. K., Luo, M. J., et al. (2020). Extracellular vesicles from human urine-derived stem cells inhibit glucocorticoid-induced osteonecrosis of the femoral head by transporting and releasing pro-angiogenic DMBT1 and anti-apoptotic TIMP1. Acta Biomater. 111, 208–220. doi:10.1016/j.actbio.2020.05.020
Chen, Q., Bai, H., Wu, W., Huang, G., Li, Y., Wu, M., et al. (2020). Bioengineering bacterial vesicle-coated polymeric nanomedicine for enhanced cancer immunotherapy and Metastasis prevention. Nano Lett. 20 (1), 11–21. doi:10.1021/acs.nanolett.9b02182
Chen, H., Fang, C., Zhi, X., Song, S., Gu, Y., Chen, X., et al. (2020). Neobavaisoflavone inhibits osteoclastogenesis through blocking RANKL signalling-mediated TRAF6 and c-Src recruitment and NF-κB, MAPK and Akt pathways. J. Cell Mol. Med. 24 (16), 9067–9084. doi:10.1111/jcmm.15543
Chen, S., Chen, X., Geng, Z., and Su, J. (2022). The horizon of bone organoid: A perspective on construction and application. Bioact. Mater 18, 15–25. doi:10.1016/j.bioactmat.2022.01.048
Chen, C. Y., Rao, S. S., Yue, T., Tan, Y. J., Yin, H., Chen, L. J., et al. (2022). Glucocorticoid-induced loss of beneficial gut bacterial extracellular vesicles is associated with the pathogenesis of osteonecrosis. Sci. Adv. 8 (15), eabg8335. doi:10.1126/sciadv.abg8335
Chen, X., Hu, Y., Geng, Z., and Su, J. (2022). The "three in one" bone repair strategy for osteoporotic fractures. Front. Endocrinol. (Lausanne) 13, 910602. doi:10.3389/fendo.2022.910602
Choi, Y., Kwon, Y., Kim, D. K., Jeon, J., Jang, S. C., Wang, T., et al. (2015). Gut microbe-derived extracellular vesicles induce insulin resistance, thereby impairing glucose metabolism in skeletal muscle. Sci. Rep. 5, 15878. doi:10.1038/srep15878
Cui, Y., Fu, S., Sun, D., Xing, J., Hou, T., and Wu, X. (2019). EPC-derived exosomes promote osteoclastogenesis through LncRNA-MALAT1. J. Cell Mol. Med. 23 (6), 3843–3854. doi:10.1111/jcmm.14228
Cui, J., Li, X., Wang, S., Su, Y., Chen, X., Cao, L., et al. (2020). Triptolide prevents bone loss via suppressing osteoclastogenesis through inhibiting PI3K-AKT-NFATc1 pathway. J. Cell Mol. Med. 24 (11), 6149–6161. doi:10.1111/jcmm.15229
da Silva, A. P., Matos, A., RibeiRo, R., Gil, A., Valente, A., BichoM., , et al. (2017). Sarcopenia and osteoporosis in Portuguese centenarians. Eur. J. Clin. Nutr. 71 (1), 56–63. doi:10.1038/ejcn.2016.174
Dai, H., Zheng, W., Luo, J., Yu, G., Song, C., Wu, Y., et al. (2022). Inhibiting uptake of extracellular vesicles derived from senescent bone marrow mesenchymal stem cells by muscle satellite cells attenuates sarcopenia. J. Orthop. Transl. 35, 23–36. doi:10.1016/j.jot.2022.06.002
Deng, L., Wang, Y., Peng, Y., Wu, Y., Ding, Y., Jiang, Y., et al. (2015). Osteoblast-derived microvesicles: A novel mechanism for communication between osteoblasts and osteoclasts. Bone 79, 37–42. doi:10.1016/j.bone.2015.05.022
Eckburg, P. B., Bik, E. M., Bernstein, C. N., Purdom, E., Dethlefsen, L., Sargent, M., et al. (2005). Diversity of the human intestinal microbial flora. Science 308 (5728), 1635–1638. doi:10.1126/science.1110591
Eichholz, K. F., Woods, I., Riffault, M., Johnson, G. P., Corrigan, M., Lowry, M. C., et al. (2020). Human bone marrow stem/stromal cell osteogenesis is regulated via mechanically activated osteocyte-derived extracellular vesicles. Stem Cells Transl. Med. 9 (11), 1431–1447. doi:10.1002/sctm.19-0405
Emery, D. C., Shoemark, D. K., Batstone, T. E., Waterfall, C. M., Coghill, J. A., Cerajewska, T. L., et al. (2017). 16S rRNA next generation sequencing analysis shows bacteria in alzheimer's post-mortem brain. Front. Aging Neurosci. 9, 195. doi:10.3389/fnagi.2017.00195
Ensrud, K. E., and Crandall, C. J. (2017). Osteoporosis. Ann. Intern Med. 167 (3), ITC17–ITC32. doi:10.7326/AITC201708010
Fan, Y., Hanai, J. I., Le, P. T., Bi, R., Maridas, D., DeMambro, V., et al. (2017). Parathyroid hormone directs bone marrow mesenchymal cell fate. Cell Metab. 25 (3), 661–672. doi:10.1016/j.cmet.2017.01.001
Farr, J. N., Xu, M., Weivoda, M. M., Monroe, D. G., Fraser, D. G., Onken, J. L., et al. (2017). Targeting cellular senescence prevents age-related bone loss in mice. Nat. Med. 23 (9), 1072–1079. doi:10.1038/nm.4385
Fu, H., Wang, L., Bao, Q., Ni, D., Hu, P., and Shi, J. (2022). Acid neutralization and immune regulation by calcium-aluminum-layered double hydroxide for osteoporosis reversion. J. Am. Chem. Soc. 144 (20), 8987–8999. doi:10.1021/jacs.2c00749
Gao, X. R., Ge, J., Li, W. Y., Zhou, W. C., Xu, L., and Geng, D. Q. (2021). miR-34a carried by adipocyte exosomes inhibits the polarization of M1 macrophages in mouse osteolysis model. J. Biomed. Mater Res. A 109 (6), 994–1003. doi:10.1002/jbm.a.37088
Gentile, C. L., and Weir, T. L. (2018). The gut microbiota at the intersection of diet and human health. Science 362 (6416), 776–780. doi:10.1126/science.aau5812
Ginaldi, L., Di Benedetto, M. C., and De Martinis, M. (2005). Osteoporosis, inflammation and ageing. Immun. Ageing 2, 14. doi:10.1186/1742-4933-2-14
Hanssen, N. M. J., de Vos, W. M., and Nieuwdorp, M. (2021). Fecal microbiota transplantation in human metabolic diseases: From a murky past to a bright future? Cell Metab. 33 (6), 1098–1110. doi:10.1016/j.cmet.2021.05.005
He, Y., Wuertz-Kozak, K., Kuehl, L. K., and Wippert, P. M. (2021). Extracellular vesicles: Potential mediators of psychosocial stress contribution to osteoporosis? Int. J. Mol. Sci. 22 (11), 5846. doi:10.3390/ijms22115846
Holliday, L. S., McHugh, K. P., Zuo, J., Aguirre, J. I., Neubert, J. K., and Rody, W. J. (2017). Exosomes: Novel regulators of bone remodelling and potential therapeutic agents for orthodontics. Orthod. Craniofac Res. 20 (1), 95–99. doi:10.1111/ocr.12165
Hu, Y., Li, X., Zhi, X., Cong, W., Huang, B., Chen, H., et al. (2021). RANKL from bone marrow adipose lineage cells promotes osteoclast formation and bone loss. EMBO Rep. 22 (7), e52481. doi:10.15252/embr.202152481
Huang, J., Yin, H., Rao, S. S., Xie, P. L., Cao, X., Rao, T., et al. (2018). Harmine enhances type H vessel formation and prevents bone loss in ovariectomized mice. Theranostics 8 (9), 2435–2446. doi:10.7150/thno.22144
Huldani, H., Abdalkareem Jasim, S., Olegovich Bokov, D., Abdelbasset, W. K., Nader Shalaby, M., Thangavelu, L., et al. (2022). Application of extracellular vesicles derived from mesenchymal stem cells as potential therapeutic tools in autoimmune and rheumatic diseases. Int. Immunopharmacol. 106, 108634. doi:10.1016/j.intimp.2022.108634
Huynh, N., VonMoss, L., Smith, D., Rahman, I., Felemban, M. F., Zuo, J., et al. (2016). Characterization of regulatory extracellular vesicles from osteoclasts. J. Dent. Res. 95 (6), 673–679. doi:10.1177/0022034516633189
Jin, D., Wu, X., Yu, H., Jiang, L., Zhou, P., Yao, X., et al. (2018). Systematic analysis of lncRNAs, mRNAs, circRNAs and miRNAs in patients with postmenopausal osteoporosis. Am. J. Transl. Res. 10 (5), 1498–1510.
Jung, A. L., Schmeck, B., Wiegand, M., Bedenbender, K., and Benedikter, B. J. (2021). The clinical role of host and bacterial-derived extracellular vesicles in pneumonia. Adv. Drug Deliv. Rev. 176, 113811. doi:10.1016/j.addr.2021.05.021
Kang, M., Huang, C. C., Lu, Y., Shirazi, S., Gajendrareddy, P., Ravindran, S., et al. (2020). Bone regeneration is mediated by macrophage extracellular vesicles. Bone 141, 115627. doi:10.1016/j.bone.2020.115627
Kaparakis, M., Turnbull, L., Carneiro, L., Firth, S., Coleman, H. A., Parkington, H. C., et al. (2010). Bacterial membrane vesicles deliver peptidoglycan to NOD1 in epithelial cells. Cell Microbiol. 12 (3), 372–385. doi:10.1111/j.1462-5822.2009.01404.x
Kong, L., Wang, Y., Smith, W., and Hao, D. (2019). Macrophages in bone homeostasis. Curr. Stem Cell Res. Ther. 14 (6), 474–481. doi:10.2174/1574888X14666190214163815
Kusumbe, A. P., Ramasamy, S. K., and Adams, R. H. (2014). Coupling of angiogenesis and osteogenesis by a specific vessel subtype in bone. Nature 507 (7492), 323–328. doi:10.1038/nature13145
Lafeber, F. P., and van Laar, J. M. (2013). Strontium ranelate: Ready for clinical use as disease-modifying osteoarthritis drug? Ann. Rheum. Dis. 72 (2), 157–161. doi:10.1136/annrheumdis-2012-202453
Lee, H. J. (2019). Microbe-host communication by small RNAs in extracellular vesicles: Vehicles for transkingdom RNA transportation. Int. J. Mol. Sci. 20 (6), 1487. doi:10.3390/ijms20061487
Lee, K. E., Kim, J. K., Han, S. K., Lee, D. Y., Lee, H. J., Yim, S. V., et al. (2020). The extracellular vesicle of gut microbial Paenalcaligenes hominis is a risk factor for vagus nerve-mediated cognitive impairment. Microbiome 8 (1), 107. doi:10.1186/s40168-020-00881-2
Lee, Y. K., and Mazmanian, S. K. (2010). Has the microbiota played a critical role in the evolution of the adaptive immune system? Science 330 (6012), 1768–1773. doi:10.1126/science.1195568
Li, D., Liu, J., Guo, B., Liang, C., Dang, L., Lu, C., et al. (2016). Osteoclast-derived exosomal miR-214-3p inhibits osteoblastic bone formation. Nat. Commun. 7, 10872. doi:10.1038/ncomms10872
Li, J. Y., Chassaing, B., Tyagi, A. M., Vaccaro, C., Luo, T., Adams, J., et al. (2016). Sex steroid deficiency-associated bone loss is microbiota dependent and prevented by probiotics. J. Clin. Invest. 126 (6), 2049–2063. doi:10.1172/JCI86062
Li, X., Wang, L., Huang, B., Gu, Y., Luo, Y., Zhi, X., et al. (2020). Targeting actin-bundling protein L-plastin as an anabolic therapy for bone loss. Sci. Adv. 6 (47), eabb7135. doi:10.1126/sciadv.abb7135
Li, Z., Xue, H., Tan, G., and Xu, Z. (2021). Effects of miRNAs, lncRNAs and circRNAs on osteoporosis as regulatory factors of bone homeostasis (Review). Mol. Med. Rep. 24 (5), 788. doi:10.3892/mmr.2021.12428
Li, Y., Zhang, K., Wu, Y., Yue, Y., Cheng, K., Feng, Q., et al. (2022). Antigen capture and immune modulation by bacterial outer membrane vesicles as in situ vaccine for cancer immunotherapy post-photothermal therapy. Small 18 (14), e2107461. doi:10.1002/smll.202107461
Liu, H., Fang, G., Wu, H., Li, Z., and Ye, Q. (2018). L-cysteine production in Escherichia coli based on rational metabolic engineering and modular strategy. Biotechnol. J. 13 (5), e1700695. doi:10.1002/biot.201700695
Liu, B., Guo, Z., and Gao, W. (2019). miR-181b-5p promotes proliferation and inhibits apoptosis of hypertrophic scar fibroblasts through regulating the MEK/ERK/p21 pathway. Exp. Ther. Med. 17 (3), 1537–1544. doi:10.3892/etm.2019.7159
Liu, H., Hou, Y., Wang, Y., and Li, Z. (2020). Enhancement of sulfur conversion rate in the production of l-cysteine by engineered Escherichia coli. J. Agric. Food Chem. 68 (1), 250–257. doi:10.1021/acs.jafc.9b06330
Liu, H., Wang, Y., Hou, Y., and Li, Z. (2020). Fitness of chassis cells and metabolic pathways for l-cysteine overproduction in Escherichia coli. J. Agric. Food Chem. 68 (50), 14928–14937. doi:10.1021/acs.jafc.0c06134
Liu, K., Luo, X., Lv, Z. Y., Zhang, Y. J., Meng, Z., Li, J., et al. (2021). Macrophage-derived exosomes promote bone mesenchymal stem cells towards osteoblastic fate through microRNA-21a-5p. Front. Bioeng. Biotechnol. 9, 801432. doi:10.3389/fbioe.2021.801432
Liu, J. H., Chen, C. Y., Liu, Z. Z., Luo, Z. W., Rao, S. S., Jin, L., et al. (2021). Extracellular vesicles from child gut microbiota enter into bone to preserve bone mass and strength. Adv. Sci. (Weinh) 8 (9), 2004831. doi:10.1002/advs.202004831
Liu, J., Wu, X., Lu, J., Huang, G., Dang, L., Zhang, H., et al. (2021). Exosomal transfer of osteoclast-derived miRNAs to chondrocytes contributes to osteoarthritis progression. Nat. Aging 1 (4), 368–384. doi:10.1038/s43587-021-00050-6
Liu, H., Zhao, Y., Zheng, Q., Yang, T., Zhao, H., Zou, H., et al. (2022). Identification and quantification of bu shen yi sui capsule by UPLC-LTQ-orbitrap-MSn and UPLC-QTOF-MS/MS. Chem. Eng. J. 60, 450–457. doi:10.1093/chromsci/bmab091
Liu, C., Liang, T., Zhang, Z., Chen, J., Xue, J., Zhan, X., et al. (2022). Transfer of microRNA-22-3p by M2 macrophage-derived extracellular vesicles facilitates the development of ankylosing spondylitis through the PER2-mediated Wnt/β-catenin axis. Cell Death Discov. 8 (1), 269. doi:10.1038/s41420-022-00900-1
Liu, H., Zhang, Q., Wang, S., Weng, W., Jing, Y., and Su, J. (2022). Bacterial extracellular vesicles as bioactive nanocarriers for drug delivery: Advances and perspectives. Bioact. Mater 14, 169–181. doi:10.1016/j.bioactmat.2021.12.006
Liu, H., Geng, Z., and Su, J. (2022). Engineered mammalian and bacterial extracellular vesicles as promising nanocarriers for targeted therapy. Extracell. Vesicles Circulating Nucleic Acids 3 (1), 63–86. doi:10.20517/evcna.2022.04
Lloyd-Price, J., Mahurkar, A., Rahnavard, G., Crabtree, J., Orvis, J., Hall, A. B., et al. (2017). Strains, functions and dynamics in the expanded human microbiome Project. Nature 550 (7674), 61–66. doi:10.1038/nature23889
Lozupone, C. A., Stombaugh, J. I., Gordon, J. I., Jansson, J. K., and Knight, R. (2012). Diversity, stability and resilience of the human gut microbiota. Nature 489 (7415), 220–230. doi:10.1038/nature11550
Lu, J., Yang, J., Zheng, Y., Chen, X., and Fang, S. (2019). Extracellular vesicles from endothelial progenitor cells prevent steroid-induced osteoporosis by suppressing the ferroptotic pathway in mouse osteoblasts based on bioinformatics evidence. Sci. Rep. 9 (1), 16130. doi:10.1038/s41598-019-52513-x
Lu, G. D., Cheng, P., Liu, T., and Wang, Z. (2020). BMSC-derived exosomal miR-29a promotes angiogenesis and osteogenesis. Front. Cell Dev. Biol. 8, 608521. doi:10.3389/fcell.2020.608521
Lu, C. H., Chen, Y. A., Ke, C. C., and Liu, R. S. (2021). Mesenchymal stem cell-derived extracellular vesicle: A promising alternative therapy for osteoporosis. Int. J. Mol. Sci. 22 (23), 12750. doi:10.3390/ijms222312750
Lu, C. H., Chen, Y. A., Ke, C. C., Chiu, S. J., Jeng, F. S., Chen, C. C., et al. (2021). Multiplexed molecular imaging strategy integrated with RNA sequencing in the assessment of the therapeutic effect of wharton's jelly mesenchymal stem cell-derived extracellular vesicles for osteoporosis. Int. J. Nanomedicine 16, 7813–7830. doi:10.2147/IJN.S335757
Lu, Q., Qin, H., Tan, H., Wei, C., Yang, X., He, J., et al. (2021). Senescence osteoblast-derived exosome-mediated miR-139-5p regulates endothelial cell functions. BioMed Res. Int. 2021, 5576023. doi:10.1155/2021/5576023
Lv, P. Y., Gao, P. F., Tian, G. J., Yang, Y. Y., Mo, F. F., Wang, Z. H., et al. (2020). Osteocyte-derived exosomes induced by mechanical strain promote human periodontal ligament stem cell proliferation and osteogenic differentiation via the miR-181b-5p/PTEN/AKT signaling pathway. Stem Cell Res. Ther. 11 (1), 295. doi:10.1186/s13287-020-01815-3
Lyu, H., Xiao, Y., Guo, Q., Huang, Y., and Luo, X. (2020). The role of bone-derived exosomes in regulating skeletal metabolism and extraosseous diseases. Front. Cell Dev. Biol. 8, 89. doi:10.3389/fcell.2020.00089
Malekpour, K., Hazrati, A., Zahar, M., Markov, A., Zekiy, A. O., Navashenaq, J. G., et al. (2022). The potential use of mesenchymal stem cells and their derived exosomes for orthopedic diseases treatment. Stem Cell Rev. Rep. 18 (3), 933–951. doi:10.1007/s12015-021-10185-z
Mao, Y., Han, M., Chen, C., Wang, X., Han, J., Gao, Y., et al. (2021). A biomimetic nanocomposite made of a ginger-derived exosome and an inorganic framework for high-performance delivery of oral antibodies. Nanoscale 13 (47), 20157–20169. doi:10.1039/d1nr06015e
Martens, E. C., Lowe, E. C., Chiang, H., Pudlo, N. A., Wu, M., McNulty, N. P., et al. (2011). Recognition and degradation of plant cell wall polysaccharides by two human gut symbionts. PLoS Biol. 9 (12), e1001221. doi:10.1371/journal.pbio.1001221
Meganathan, V., Moyana, R., Natarajan, K., Kujur, W., Kusampudi, S., Mulik, S., et al. (2020). Bacterial extracellular vesicles isolated from organic dust induce neutrophilic inflammation in the lung. Am. J. Physiol. Lung Cell Mol. Physiol. 319 (6), L893–L907. doi:10.1152/ajplung.00107.2020
Meulenbelt, I., Ramos, Y. F. M., Baglio, S. R., and Pegtel, D. M. (2021). Censoring exosomal crosstalk in osteoarthritis. Nat. Aging 1 (4), 332–334. doi:10.1038/s43587-021-00052-4
Mora-Raimundo, P., Lozano, D., Manzano, M., and Vallet-Regi, M. (2019). Nanoparticles to knockdown osteoporosis-related gene and promote osteogenic marker expression for osteoporosis treatment. ACS Nano 13 (5), 5451–5464. doi:10.1021/acsnano.9b00241
Mottawea, W., Chiang, C. K., Muhlbauer, M., Starr, A. E., Butcher, J., Abujamel, T., et al. (2016). Altered intestinal microbiota-host mitochondria crosstalk in new onset Crohn's disease. Nat. Commun. 7, 13419. doi:10.1038/ncomms13419
Muraca, M., and Cappariello, A. (2020). The role of extracellular vesicles (EVs) in the epigenetic regulation of bone metabolism and osteoporosis. Int. J. Mol. Sci. 21 (22), 8682. doi:10.3390/ijms21228682
Nahui Palomino, R. A., Vanpouille, C., Costantini, P. E., and Margolis, L. (2021). Microbiota-host communications: Bacterial extracellular vesicles as a common language. PLoS Pathog. 17 (5), e1009508. doi:10.1371/journal.ppat.1009508
Ohlsson, C., Engdahl, C., Fak, F., Andersson, A., Windahl, S. H., Farman, H. H., et al. (2014). Probiotics protect mice from ovariectomy-induced cortical bone loss. PLoS One 9 (3), e92368. doi:10.1371/journal.pone.0092368
Pan, C., Li, J., Hou, W., Lin, S., Wang, L., Pang, Y., et al. (2021). Polymerization-mediated multifunctionalization of living cells for enhanced cell-based therapy. Adv. Mater 33 (13), e2007379. doi:10.1002/adma.202007379
Parvaneh, K., Ebrahimi, M., Sabran, M. R., Karimi, G., Hwei, A. N. M., Abdul-Majeed, S., et al. (2015). Probiotics (Bifidobacterium longum) increase bone mass density and upregulate sparc and bmp-2 genes in rats with bone loss resulting from ovariectomy. Biomed. Res. Int. 2015, 897639. doi:10.1155/2015/897639
Peng, Z., Lu, S., Lou, Z., Li, Z., Li, S., Yang, K., et al. (2021). Exosomes from bone marrow mesenchymal stem cells promoted osteogenic differentiation by delivering miR-196a that targeted Dickkopf-1 to activate Wnt/β-catenin pathway. Bioengineered. doi:10.1080/21655979.2021.1996015
Perez-Burgos, A., Wang, B., Mao, Y. K., Mistry, B., McVey Neufeld, K. A., Bienenstock, J., et al. (2013). Psychoactive bacteria Lactobacillus rhamnosus (JB-1) elicits rapid frequency facilitation in vagal afferents. Am. J. Physiol. Gastrointest. Liver Physiol. 304 (2), G211–G220. doi:10.1152/ajpgi.00128.2012
Prados-Rosales, R., Baena, A., Martinez, L. R., Luque-Garcia, J., Kalscheuer, R., Veeraraghavan, U., et al. (2011). Mycobacteria release active membrane vesicles that modulate immune responses in a TLR2-dependent manner in mice. J. Clin. Invest. 121 (4), 1471–1483. doi:10.1172/JCI44261
Prestwood, K. M., Pilbeam, C. C., and Raisz, L. G. (1995). Treatment of osteoporosis. Annu. Rev. Med. 46, 249–256. doi:10.1146/annurev.med.46.1.249
Qi, X., Zhang, J., Yuan, H., Xu, Z., Li, Q., Niu, X., et al. (2016). Exosomes secreted by human-induced pluripotent stem cell-derived mesenchymal stem cells repair critical-sized bone defects through enhanced angiogenesis and osteogenesis in osteoporotic rats. Int. J. Biol. Sci. 12 (7), 836–849. doi:10.7150/ijbs.14809
Qiu, M., Zhai, S., Fu, Q., and Liu, D. (2021). Bone marrow mesenchymal stem cells-derived exosomal MicroRNA-150-3p promotes osteoblast proliferation and differentiation in osteoporosis. Hum. Gene Ther. 32 (13-14), 717–729. doi:10.1089/hum.2020.005
Ramasamy, S. K., Kusumbe, A. P., Wang, L., and Adams, R. H. (2014). Endothelial Notch activity promotes angiogenesis and osteogenesis in bone. Nature 507 (7492), 376–380. doi:10.1038/nature13146
Ren, X., Liu, H., Wu, X., Weng, W., Wang, X., and Su, J. (2021). Reactive oxygen species (ROS)-Responsive biomaterials for the treatment of bone-related diseases. Front. Bioeng. Biotechnol. 9, 820468. doi:10.3389/fbioe.2021.820468
Sadanand Fulzele, B. M., Khayrullin, A., Johnson, M., Kaiser, H., Yutao Liu, C. M. I., Hamrick, M. W., et al. (2019). Muscle-derived miR-34a increases with age in circulating extracellular vesicles and induces senescence of bone marrow stem cells. Aging 11 (6), 1791–1803. doi:10.18632/aging.101874
Service, R. F. (2014). Synthetic biology. Synthetic biologists design 'living materials' that build themselves. Science 343 (6178), 1421. doi:10.1126/science.343.6178.1421
Seshareddy, K., Troyer, D., and Weiss, M. L. (2008). Method to isolate mesenchymal-like cells from Wharton's Jelly of umbilical cord. Methods Cell. Biol. 86, 101–119. doi:10.1016/S0091-679X(08)00006-X
Seton-Rogers, S. (2014). Microenvironment: Endothelial cells create a niche. Nat. Rev. Cancer 14 (5), 298. doi:10.1038/nrc3730
Sharon, G., Garg, N., Debelius, J., Knight, R., Dorrestein, P. C., and Mazmanian, S. K. (2014). Specialized metabolites from the microbiome in health and disease. Cell Metab. 20 (5), 719–730. doi:10.1016/j.cmet.2014.10.016
Shen, Y., Giardino Torchia, M. L., Lawson, G. W., Karp, C. L., Ashwell, J. D., and Mazmanian, S. K. (2012). Outer membrane vesicles of a human commensal mediate immune regulation and disease protection. Cell Host Microbe 12 (4), 509–520. doi:10.1016/j.chom.2012.08.004
Sivaraj, K. K., and Adams, R. H. (2016). Blood vessel formation and function in bone. Development 143 (15), 2706–2715. doi:10.1242/dev.136861
Song, H., Li, X., Zhao, Z., Qian, J., Wang, Y., Cui, J., et al. (2019). Reversal of osteoporotic activity by endothelial cell-secreted bone targeting and biocompatible exosomes. Nano Lett. 19 (5), 3040–3048. doi:10.1021/acs.nanolett.9b00287
Song, X., Xue, Y., Fan, S., Hao, J., and Deng, R. (2022). Lipopolysaccharide-activated macrophages regulate the osteogenic differentiation of bone marrow mesenchymal stem cells through exosomes. PeerJ 10, e13442. doi:10.7717/peerj.13442
Song, S., Guo, Y., Yang, Y., and Fu, D. (2022). Advances in pathogenesis and therapeutic strategies for osteoporosis. Pharmacol. Ther. 237, 108168. doi:10.1016/j.pharmthera.2022.108168
Soult, M. C., Lonergan, N. E., Shah, B., Kim, W. K., Britt, L. D., and Sullivan, C. J. (2013). Outer membrane vesicles from pathogenic bacteria initiate an inflammatory response in human endothelial cells. J. Surg. Res. 184 (1), 458–466. doi:10.1016/j.jss.2013.05.035
Sultan, S., Mottawea, W., Yeo, J., and Hammami, R. (2021). Gut microbiota extracellular vesicles as signaling molecules mediating host-microbiota communications. Int. J. Mol. Sci. 22 (23), 13166. doi:10.3390/ijms222313166
Sun, W., Zhao, C., Li, Y., Wang, L., Nie, G., Peng, J., et al. (2016). Osteoclast-derived microRNA-containing exosomes selectively inhibit osteoblast activity. Cell Discov. 2, 16015. doi:10.1038/celldisc.2016.15
Taddio, A., Ipp, M., Thivakaran, S., Jamal, A., Parikh, C., Smart, S., et al. (2012). Survey of the prevalence of immunization non-compliance due to needle fears in children and adults. Vaccine 30 (32), 4807–4812. doi:10.1016/j.vaccine.2012.05.011
Thomas, C. M., Hong, T., van Pijkeren, J. P., Hemarajata, P., Trinh, D. V., Hu, W., et al. (2012). Histamine derived from probiotic Lactobacillus reuteri suppresses TNF via modulation of PKA and ERK signaling. PLoS One 7 (2), e31951. doi:10.1371/journal.pone.0031951
Todorova, D., Simoncini, S., Lacroix, R., Sabatier, F., and Dignat-George, F. (2017). Extracellular vesicles in angiogenesis. Circ. Res. 120 (10), 1658–1673. doi:10.1161/CIRCRESAHA.117.309681
Toyofuku, M., Nomura, N., and Eberl, L. (2019). Types and origins of bacterial membrane vesicles. Nat. Rev. Microbiol. 17 (1), 13–24. doi:10.1038/s41579-018-0112-2
Tremaroli, V., and Backhed, F. (2012). Functional interactions between the gut microbiota and host metabolism. Nature 489 (7415), 242–249. doi:10.1038/nature11552
Tu, C., He, J., Chen, R., and Li, Z. (2019). The emerging role of exosomal non-coding RNAs in musculoskeletal diseases. Curr. Pharm. Des. 25 (42), 4523–4535. doi:10.2174/1381612825666191113104946
Tulkens, J., De Wever, O., and Hendrix, A. (2020). Analyzing bacterial extracellular vesicles in human body fluids by orthogonal biophysical separation and biochemical characterization. Nat. Protoc. 15 (1), 40–67. doi:10.1038/s41596-019-0236-5
Tyagi, A. M., Srivastava, K., Mansoori, M. N., Trivedi, R., Chattopadhyay, N., and Singh, D. (2012). Estrogen deficiency induces the differentiation of IL-17 secreting Th17 cells: A new candidate in the pathogenesis of osteoporosis. PLoS One 7 (9), e44552. doi:10.1371/journal.pone.0044552
Tzouvelekis, A., and Kaminski, N. (2015). Epigenetics in idiopathic pulmonary fibrosis. Biochem. Cell Biol. 93 (2), 159–170. doi:10.1139/bcb-2014-0126
van Bergenhenegouwen, J., Kraneveld, A. D., Rutten, L., Kettelarij, N., Garssen, J., and Vos, A. P. (2014). Extracellular vesicles modulate host-microbe responses by altering TLR2 activity and phagocytosis. PLoS One 9 (2), e89121. doi:10.1371/journal.pone.0089121
Vela Ramirez, J. E., Sharpe, L. A., and Peppas, N. A. (2017). Current state and challenges in developing oral vaccines. Adv. Drug Deliv. Rev. 114, 116–131. doi:10.1016/j.addr.2017.04.008
Wan, L., Song, H., Chen, X., Zhang, Y., Yue, Q., Pan, P., et al. (2018). A magnetic-field guided interface coassembly approach to magnetic mesoporous silica nanochains for osteoclast-targeted inhibition and heterogeneous nanocatalysis. Adv. Mater 30 (25), e1707515. doi:10.1002/adma.201707515
Wang, X., Sun, H., Liu, H., Ma, L., Jiang, C., Liao, H., et al. (2019). MicroRNA-181b-5p modulates tumor necrosis factor-alpha-induced inflammatory responses by targeting interleukin-6 in cementoblasts. J. Cell Physiol. 234 (12), 22719–22730. doi:10.1002/jcp.28837
Wang, Q., Shen, X., Chen, Y., Chen, J., and Li, Y. (2021). Osteoblasts-derived exosomes regulate osteoclast differentiation through miR-503-3p/Hpse axis. Acta histochem. 123 (7), 151790. doi:10.1016/j.acthis.2021.151790
Wang, Z., Zhao, F., Zhao, Y., Bai, L., and Hang, R. (2022). Simultaneously enhanced osteogenesis and angiogenesis via macrophage-derived exosomes upon stimulation with titania nanotubes. Mater Sci. Eng. C Mater Biol. Appl. 134, 112708. doi:10.1016/j.msec.2022.112708
Wang, N., Ma, S., and Fu, L. (2022). Gut microbiota dysbiosis as one cause of osteoporosis by impairing intestinal barrier function. Calcif. Tissue Int. 110 (2), 225–235. doi:10.1007/s00223-021-00911-7
Wang, T., Mo, L., Ou, J., Fang, Q., Wu, H., Wu, Y., et al. (2022). Proteus mirabilis vesicles induce mitochondrial apoptosis by regulating miR96-5p/abca1 to inhibit osteoclastogenesis and bone loss. Front. Immunol. 13, 833040. doi:10.3389/fimmu.2022.833040
Wei, Y., Tang, C., Zhang, J., Li, Z., Zhang, X., Miron, R. J., et al. (2019). Extracellular vesicles derived from the mid-to-late stage of osteoblast differentiation markedly enhance osteogenesis in vitro and in vivo. Biochem. Biophys. Res. Commun. 514 (1), 252–258. doi:10.1016/j.bbrc.2019.04.029
Weiberg, A., Wang, M., Lin, F. M., Zhao, H., Zhang, Z., Kaloshian, I., et al. (2013). Fungal small RNAs suppress plant immunity by hijacking host RNA interference pathways. Science 342 (6154), 118–123. doi:10.1126/science.1239705
Weilner, S., Schraml, E., Wieser, M., Messner, P., Schneider, K., Wassermann, K., et al. (2016). Secreted microvesicular miR-31 inhibits osteogenic differentiation of mesenchymal stem cells. Aging Cell 15 (4), 744–754. doi:10.1111/acel.12484
Witwer, K. W., Soekmadji, C., Hill, A. F., Wauben, M. H., Buzas, E. I., Di Vizio, D., et al. (2021). Updating the MISEV minimal requirements for extracellular vesicle studies: Building bridges to reproducibility. J. Extracell. Vesicles 10 (14), 1396823. doi:10.1080/20013078.2017.1396823
Wolever, T. M., Spadafora, P., and Eshuis, H. (1991). Interaction between colonic acetate and propionate in humans. Am. J. Clin. Nutr. 53 (3), 681–687. doi:10.1093/ajcn/53.3.681
Xiong, Y., Chen, L., Yan, C., Zhou, W., Yu, T., Sun, Y., et al. (2020). M2 Macrophagy-derived exosomal miRNA-5106 induces bone mesenchymal stem cells towards osteoblastic fate by targeting salt-inducible kinase 2 and 3. J. Nanobiotechnology 18 (1), 66. doi:10.1186/s12951-020-00622-5
Xu, X., Jia, X., Mo, L., Liu, C., Zheng, L., Yuan, Q., et al. (2017). Intestinal microbiota: A potential target for the treatment of postmenopausal osteoporosis. Bone Res. 5, 17046. doi:10.1038/boneres.2017.46
Xu, R., Shen, X., Si, Y., Fu, Y., Zhu, W., Xiao, T., et al. (2018). MicroRNA-31a-5p from aging BMSCs links bone formation and resorption in the aged bone marrow microenvironment. Aging Cell 17 (4), e12794. doi:10.1111/acel.12794
Yahao, G., and Xinjia, W. (2021). The role and mechanism of exosomes from umbilical cord mesenchymal stem cells in inducing osteogenesis and preventing osteoporosis. Cell Transpl. 30, 9636897211057465. doi:10.1177/09636897211057465
Yang, J. J., Tao, H., Deng, Z. Y., Lu, C., and Li, J. (2015). Non-coding RNA-mediated epigenetic regulation of liver fibrosis. Metabolism 64 (11), 1386–1394. doi:10.1016/j.metabol.2015.08.004
Yang, X, Lei, P., Wen, T., and Wen, T. (2019). LncRNA MALAT1 shuttled by bone marrow-derived mesenchymal stem cells-secreted exosomes alleviates osteoporosis through mediating microRNA-34c/SATB2 axis. Aging 11 (20), 8777–8791. doi:10.18632/aging.102264
Yang, Y., Yujiao, W., Fang, W., Linhui, Y., Ziqi, G., Zhichen, W., et al. (2020). The roles of miRNA, lncRNA and circRNA in the development of osteoporosis. Biol. Res. 53 (1), 40. doi:10.1186/s40659-020-00309-z
Yang, J. X., Xie, P., Li, Y. S., Wen, T., and Yang, X. C. (2020). Osteoclast-derived miR-23a-5p-containing exosomes inhibit osteogenic differentiation by regulating Runx2. Cell Signal 70, 109504. doi:10.1016/j.cellsig.2019.109504
Yang, R. Z., Xu, W. N., Zheng, H. L., Zheng, X. F., Li, B., Jiang, L. S., et al. (2021). Exosomes derived from vascular endothelial cells antagonize glucocorticoid-induced osteoporosis by inhibiting ferritinophagy with resultant limited ferroptosis of osteoblasts. J. Cell Physiol. 236 (9), 6691–6705. doi:10.1002/jcp.30331
Yoshimura, N., Muraki, S., Oka, H., Iidaka, T., Kodama, R., KawagucHi, H., et al. (2017). Is osteoporosis a predictor for future sarcopenia or vice versa? Four-year observations between the second and third ROAD study surveys. Osteoporos. Int. 28 (1), 189–199. doi:10.1007/s00198-016-3823-0
Yu, L., Hu, M., Cui, X., Bao, D., Luo, Z., Li, D., et al. (2021). M1 macrophage-derived exosomes aggravate bone loss in postmenopausal osteoporosis via a microRNA-98/DUSP1/JNK axis. Cell Biol. Int. 45 (12), 2452–2463. doi:10.1002/cbin.11690
Yuan, F. L., Wu, Q. Y., Miao, Z. N., Xu, M. H., Xu, R. S., Jiang, D. L., et al. (2018). Osteoclast-derived extracellular vesicles: Novel regulators of osteoclastogenesis and osteoclast-osteoblasts communication in bone remodeling. Front. Physiol. 9, 628. doi:10.3389/fphys.2018.00628
Yue, Y., Xu, J., Li, Y., Cheng, K., Feng, Q., Ma, X., et al. (2022). Antigen-bearing outer membrane vesicles as tumour vaccines produced in situ by ingested genetically engineered bacteria. Nat. Biomed. Eng. 6 (7), 898–909. doi:10.1038/s41551-022-00886-2
Zakharzhevskaya, N. B., Vanyushkina, A. A., Altukhov, I. A., Shavarda, A. L., Butenko, I. O., Rakitina, D. V., et al. (2017). Outer membrane vesicles secreted by pathogenic and nonpathogenic Bacteroides fragilis represent different metabolic activities. Sci. Rep. 7 (1), 5008. doi:10.1038/s41598-017-05264-6
Zhang, X., Deeke, S. A., Ning, Z., Starr, A. E., Butcher, J., Li, J., et al. (2018). Metaproteomics reveals associations between microbiome and intestinal extracellular vesicle proteins in pediatric inflammatory bowel disease. Nat. Commun. 9 (1), 2873. doi:10.1038/s41467-018-05357-4
Zhang, X., Wang, Y., Zhao, H., Han, X., Zhao, T., Qu, P., et al. (2020). Extracellular vesicle-encapsulated miR-22-3p from bone marrow mesenchymal stem cell promotes osteogenic differentiation via FTO inhibition. Stem Cell Res. Ther. 11 (1), 227. doi:10.1186/s13287-020-01707-6
Zhang, X., Wang, W., Wang, Y., Zhao, H., Han, X., Zhao, T., et al. (2020). Extracellular vesicle-encapsulated miR-29b-3p released from bone marrow-derived mesenchymal stem cells underpins osteogenic differentiation. Front. Cell Dev. Biol. 8, 581545. doi:10.3389/fcell.2020.581545
Zhang, Y., Cao, X., Li, P., Fan, Y., Zhang, L., Ma, X., et al. (2021). microRNA-935-modified bone marrow mesenchymal stem cells-derived exosomes enhance osteoblast proliferation and differentiation in osteoporotic rats. Life Sci. 272, 119204. doi:10.1016/j.lfs.2021.119204
Zhang, D., Wu, Y., Li, Z., Chen, H., Huang, S., Jian, C., et al. (2021). MiR-144-5p, an exosomal miRNA from bone marrow-derived macrophage in type 2 diabetes, impairs bone fracture healing via targeting Smad1. J. Nanobiotechnology 19 (1), 226. doi:10.1186/s12951-021-00964-8
Zhang, Y. W., Li, Y. J., Lu, P. P., Dai, G. C., Chen, X. X., and Rui, Y. F. (2021). The modulatory effect and implication of gut microbiota on osteoporosis: From the perspective of "brain-gut-bone" axis. Food Funct. 12 (13), 5703–5718. doi:10.1039/d0fo03468a
Zhang, W., Huang, P., Lin, J., and Zeng, H. (2022). The role of extracellular vesicles in osteoporosis: A scoping review. Membr. (Basel) 12 (3), 324. doi:10.3390/membranes12030324
Zhu, Y., Li, Z., Zhang, Y., Lan, F., He, J., and Wu, Y. (2020). The essential role of osteoclast-derived exosomes in magnetic nanoparticle-infiltrated hydroxyapatite scaffold modulated osteoblast proliferation in an osteoporosis model. Nanoscale 12 (16), 8720–8726. doi:10.1039/d0nr00867b
Zhu, L., Hua, F., Ding, W., Ding, K., Zhang, Y., and Xu, C. (2020). The correlation between the Th17/Treg cell balance and bone health. Immun. Ageing 17, 30. doi:10.1186/s12979-020-00202-z
Zhu, Y., Zhao, S., Cheng, L., Lin, Z., Zeng, M., Ruan, Z., et al. (2022). Mg(2+) -mediated autophagy-dependent polarization of macrophages mediates the osteogenesis of bone marrow stromal stem cells by interfering with macrophage-derived exosomes containing miR-381. J. Orthop. Res. 40 (7), 1563–1576. doi:10.1002/jor.25189
Zihao Ou, X. H., Li, Q., Cao, N., Gao, M., He, B., Zhang, M., et al. (2022). High-performance tracking of bacterial extracellular vesicles in living systems using an aggregation-induced emission luminogen. Chem. Eng. J. 446, 136847. doi:10.1016/j.cej.2022.136847
Zou, Y., Huang, B., Cao, L., Deng, Y., and Su, J. (2021). Tailored mesoporous inorganic biomaterials: Assembly, functionalization, and drug delivery engineering. Adv. Mater 33 (2), e2005215. doi:10.1002/adma.202005215
Glossary
OP Osteoporosis
EVs Extracellular vesicles
BEVs Bacterial extracellular vesicles
OA Osteoarthritis
GM Gut microbiota
OBs osteoblasts
OCs osteoclasts
MSCs Mesenchymal stem cells
HucMSC Human umbilical cord MSC
hiPSC-MSC human induced pluripotent stem cells
BMP Bone morphogenetic protein
PI3K Phosphoinositide-3 kinase
BMSCs Bone marrow mesenchymal stem cells
RANKL Receptor activator of nuclear factor-κB ligand
OPG Osteoclastogenesis inhibitory factor
RANK Receptor activator of nuclear factor-κB
Runx2 Runt-related transcription factor 2
YAP1 Yes-associated protein 1
MT1DP Metallothionein 1D pseudogene
PTEN phosphatase and tensin homolog
Mφs Macrophages
DUSP1 Ddual specificity phosphatase 1
JNK C-Jun N-terminal kinase
EC Endothelial cells
EPCs Endothelial progenitor cells
MALAT-1 Metastasis associated lung adenocarcinoma transcript 1
SCFAs Short-chain fatty acids
FAO Food and Agriculture Organization of the United Nations
WHO World Health Organization
MEVs Mammalian EVs
TLR Toll-Like Receptors
LGG Lactobacillus rhamnosus GG
AKK Akkermansia muciniphila
FMT Fecal microbiota transplantation
EGM Elderly GM
CGM Children GM
OVX Ovariectomy
PM Proteus mirabilis
Bax BCL2-associated X protein
ROS Reactive oxygen species
TIMP tissue inhibitor of metalloproteinases
SCB subchondral bone
ACLT anterior cruciate ligament transection
ADAMST5 activity of a disintegrin and metalloproteinase with thrombospondin motifs 5
MMp-13 matrix metalloproteinase-13
Keywords: osteoporosis, gut microbiota, microbiota extracellular vesicles, microbiota-host communications, osteoarthritis, exosome
Citation: Cheung KCP, Jiao M, Xingxuan C and Wei J (2023) Extracellular vesicles derived from host and gut microbiota as promising nanocarriers for targeted therapy in osteoporosis and osteoarthritis. Front. Pharmacol. 13:1051134. doi: 10.3389/fphar.2022.1051134
Received: 22 September 2022; Accepted: 21 December 2022;
Published: 06 January 2023.
Edited by:
Paulo Noronha Lisboa-Filho, São Paulo State University, BrazilReviewed by:
Bing Shen, Anhui Medical University, ChinaCláudia M. Deus, University of Coimbra, Portugal
Copyright © 2023 Cheung, Jiao, Xingxuan and Wei. This is an open-access article distributed under the terms of the Creative Commons Attribution License (CC BY). The use, distribution or reproduction in other forums is permitted, provided the original author(s) and the copyright owner(s) are credited and that the original publication in this journal is cited, in accordance with accepted academic practice. No use, distribution or reproduction is permitted which does not comply with these terms.
*Correspondence: Kenneth Chat Pan Cheung, a2NwY2hldW5nQGhrYnUuZWR1Lmhr; Jia Wei, d2VpamlhMUBoa2J1LmVkdS5oaw==
†These authors have contributed equally to this work