- 1Lambert Initiative for Cannabinoid Therapeutics, The University of Sydney, Sydney, NSW, Australia
- 2Discipline of Pharmacology, Sydney Pharmacy School, Faculty of Medicine and Health, The University of Sydney, Sydney, NSW, Australia
- 3Brain and Mind Centre, The University of Sydney, Sydney, NSW, Australia
- 4Macquarie Medical School, Macquarie University, Sydney, NSW, Australia
- 5School of Psychology, Faculty of Science, The University of Sydney, Sydney, NSW, Australia
Introduction: Cannabidiol (CBD) has been clinically approved for intractable epilepsies, offering hope that novel anticonvulsants in the phytocannabinoid class might be developed. Looking beyond CBD, we have recently reported that a series of biosynthetic precursor molecules found in cannabis display anticonvulsant properties. However, information on the pharmacological activities of these compounds on CNS drug targets is limited. The current study aimed to fill this knowledge gap by investigating whether anticonvulsant phytocannabinoids affect T-type calcium channels, which are known to modulate neuronal excitability, and may be relevant to the anti-seizure effects of this class of compounds.
Materials and methods: A fluorescence-based assay was used to screen the ability of the phytocannabinoids to inhibit human T-type calcium channels overexpressed in HEK-293 cells. A subset of compounds was further examined using patch-clamp electrophysiology. Alphascreen technology was used to characterise selected compounds against G-protein coupled-receptor 55 (GPR55) overexpressed in HEK-293 cells, as GPR55 is another target of the phytocannabinoids.
Results: A single 10 µM concentration screen in the fluorescence-based assay showed that phytocannabinoids inhibited T-type channels with substantial effects on Cav3.1 and Cav3.2 channels compared to the Cav3.3 channel. The anticonvulsant phytocannabinoids cannabigerovarinic acid (CBGVA) and cannabidivarinic acid (CBDVA) had the greatest magnitudes of effect (≥80% inhibition against Cav3.1 and Cav3.2), so were fully characterized in concentration-response studies. CBGVA and CBDVA had IC50 values of 6 μM and 2 µM on Cav3.1 channels; 2 μM and 11 µM on Cav3.2 channels, respectively. Biophysical studies at Cav3.1 showed that CBGVA caused a hyperpolarisation shift of steady-state inhibition. Both CBGVA and CBDVA had a use-dependent effect and preferentially inhibited Cav3.1 current in a slow inactivated state. CBGVA and CBDVA were also shown to antagonise GPR55.
Conclusion and implications: These findings show that CBGVA and CBDVA inhibit T-type calcium channels and GPR55. These compounds should be further investigated to develop novel therapeutics for treating diseases associated with dysfunctional T-type channel activity.
1 Introduction
Plant-derived cannabinoids may provide a new class of anticonvulsant drugs as the phytocannabinoid cannabidiol (CBD, Epidiolex®) was recently approved to treat intractable epilepsies including Dravet syndrome (Marini et al., 2011; Anwar et al., 2019). Cannabis contains over 100 phytocannabinoids, and our laboratory has been investigating whether additional phytocannabinoids display anticonvulsant properties (Anderson et al., 2019; Anderson et al., 2021a; Anderson et al., 2021b).
Using the Scn1a+/– mouse model of Dravet syndrome, we have found several plant cannabinoids with novel anti-seizure effects. Interestingly, we have observed a common theme of active compounds being acidic biosynthetic precursor molecules, which are formed via enzymatic synthesis in the plant. These acidic phytocannabinoids are abundant in raw cannabis or cold-extracted products and undergo decarboxylation when cannabis is heated. Notably, we found cannabichromenic acid (CBCA), cannabichrovarinic acid (CBCVA), cannabigerolic acid (CBGA), cannabigerovarinic acid (CBGVA), and cannabidivarinic acid (CBDVA) were all anticonvulsant against hyperthermia-induced seizures in the Scn1a+/– mice (Anderson et al., 2019; Anderson et al., 2021a; Anderson et al., 2021b).
There exists a paucity of data on the molecular pharmacology of these acidic phytocannabinoids, particularly at epilepsy-relevant CNS drug targets. We have recently reported that phytocannabinoids Δ9-tetrahydrocannabinolic acid (THCA) and CBGA inhibited the voltage-gated T-type calcium (Cav3) channels (Mirlohi et al., 2021, 2022). Cav3 channels, which comprise three subtypes, Cav3.1, Cav3.2, and Cav3.3, are expressed in neuronal cells where they are activated by small membrane depolarisations and are considered neuronal pacemakers (Cribbs et al., 1998) Dysfunction of Cav3 channels is associated with various medical conditions, including epilepsy, sleep disturbances and pain (Bourinet et al., 2005; Tringham et al., 2012). Inhibition of Cav3.1 has been suggested as a novel target for Dravet syndrome as knockout of Cacna1g, which encodes Cav3.1, diminished spontaneous seizures and improved survival of Scn1a+/– mice (Calhoun et al., 2017). Dicer small interfering (Dsi) RNA knockdown of Cacna1h, which encodes Cav3.2, significantly shortened convulsions in the Genetic Absence Epilepsy Rats from Strasbourg (GAERS) model of absence seizures (Cain et al., 2018). Moreover, several anticonvulsant drugs, including ethosuximide and zonisamide, block T-type calcium channels (Baulac et al., 2012). CBD’s anticonvulsant mechanism of action might involve T-type channel inhibition because it inhibits peak Cav3.1 and Cav3.2 currents at clinically relevant concentrations (IC50 values = approximately 1 µM) (Ross et al., 2008). In the present study, we aimed to extend our prior research by examining whether a series of phytocannabinoids recently shown to exhibit anti-seizure properties inhibit T-type calcium channels.
2 Methods
2.1 Compounds
CBDA was purchased from THC Pharm GmbH (Frankfurt, Germany), and CBGV was purchased from Toronto Research Chemicals Inc. (Ontario, CAN). CBDVA, CBGVA, CBCA, and CBCVA were synthesised by the laboratory of Professor Michael Kassiou at the University of Sydney. CBCV was synthesised in-house. NNC 55-0396 and ML-186 were purchased from Cayman Chemical (Michigan, United States) and Enamine (New Jersey, United States) respectively. For patch clamp electrophysiology, CBGVA and CBDVA were purchased from Cerilliant Corporation (Texas, United States). Unless otherwise stated, all drugs were prepared as stock solutions in DMSO and diluted in HEPES-buffered low potassium Hanks Balanced Salt Solution (HBSS) with 0.01% bovine serum albumin (BSA, Sigma, Castle Hill, Australia). HBSS comprises (mM) NaCl 145, HEPES 22, Na2HPO4 0.338, NaHCO3 4.17, KH2PO4 0.441, MgSO4 0.407, MgCl2 0.493, Glucose 5.56, CaCl2 1.26; (pH7.4, osmolarity 315 ± 15 mOsmol). Final DMSO concentrations ranged between 0.1%–0.2%.
2.2 Cell culture
Human embryonic kidney 293 (HEK) Flp-In T-REx cells stably expressing T-type Cav3.1, Cav 3.2, or Cav 3.3 channels were used (Bladen et al., 2021; Mirlohi et al., 2022). HEK 293 GPR55 cells were generously provided by Dr Julia Kargl (University of Graz, AUT) (Kargl et al., 2012). The cells were cultured in T75 flasks using Dulbecco’s modified Eagle’s medium (DMEM) (supplemented with 10% foetal bovine serum (FBS) and 1% penicillin-streptomycin (P/S; 100 U/ml), and incubated in a humidified atmosphere with 5% CO2 at 37°C. Blasticidin (10 μg/ml, Invivogen) and hygromycin (80 μg/ml) were used as the selection antibiotics for cells expressing Cav3.1, Cav 3.2 or Cav 3.3, while G418 (400 μg/ml) was used for cells expressing GPR55. Cells were passaged at 80% confluency and used for assays at 90% confluency for up to 15 passages.
2.3 FLIPR calcium 5 assay
Calcium 5 dye (Molecular Devices, United States ) was used to investigate compound activities at T-type calcium channels using Flexstation III. HEK293 cells overexpressing Cav3.1, Cav 3.2, and Cav 3.3 cells were resuspended in Leibovitz’s L-15 media containing 1% FBS, 1% P/S and 15 mM glucose. 90 µl of the resuspended cells (120,000 cells/well) were plated in a black-walled, 96-well microplate (Corning, NY, United States ) and incubated overnight in humidified air at 37°C. Channel expression was induced with tetracycline (2 μg/ml, Sigma-Aldrich) at plating. On the day of the assay, 90 µl of HBSS containing 5% Calcium dye (bulk kit) and 2.5 mM probenecid (Invitrogen, United States ) was added to each well and incubated for 1 h at 37°C. Change in fluorescence was measured in a FlexStation III plate reader every 2 s (ʎexcitation = 485 nm, ʎemission = 525 nm) for the duration of the experiment at 37°C. After 2 min baseline recording, drugs (20 µL) were added and read for 5 min, followed by 10 mM CaCl2 (20 µl) and read for a further 3 min.
2.4 Alphascreen ERK phosphorylation assay
GPR55 cells were trypsinised and resuspended in Leibovitz’s (L-15) media containing 1% FBS, 1% P/S and 15 mM glucose. 110,000 cells/well were plated in a 96-well clear flat bottom CellBind™ microplate (Corning, NY, United States ) and incubated overnight for at least 21 h in humidified air at 37°C. L-15 was aspirated and replaced with 50 µl phenol red-free DMEM/F12 (Gibco, United States) and incubated in 5% CO2 at 37°C for 2 h. Each well was treated with 50 µl of drugs (prepared twice the final concentration) for 20 min at 37°C. Media was aspirated, 50 µL/well lysis buffer from the Alphascreen Surefire Kit was added, and the plate was placed on an orbital shaker at 500 rpm for 10 min. According to the manufacturer’s instructions, phosphorylated ERK was measured in 384-well white Proxiplate plates (Perkin Elmer, Australia). Briefly, 5 µl of lysate was incubated with 7 µl of Alphascreen reaction mixture in the dark for 3.5 h and read with a CLARIOstar reader (BMG Labtech) using standard AlphaScreen-compatible filters (excitation 680–40 nm, emission 570–100 nm).
2.5 Whole-cell voltage-clamp electrophysiology
All whole-cell voltage-clamp recordings from HEK293 Flp-In T-REx cells stably transfected with human Cav3.1, Cav 3.2 and Cav 3.3 were performed at room temperature. At least 24 h prior to experiments, cells were detached from flasks using trypsin/EDTA and plated into 10 cm sterile tissue culture dishes containing 10 ml of supplemented DMEM and 10–15 glass coverslips (12 mm diameter, ProScitech, QLD, Australia). Culture dishes were then kept overnight in same conditions as flasks to allow cells to adhere to coverslips. They were then transferred to a 30°C/5% CO2 incubator to inhibit cell proliferation until ready to be used for electrophysiology experiments. At this stage, channel expression was induced with tetracycline (2 μg/ml, Sigma-Aldrich) 8–24 h prior to recording cells.
External recording solutions contained (in mM): 114 CsCl, 5 BaCl2, 1 MgCl2, 10 HEPES, 10 glucose, adjusted to pH 7.4 with CsOH. The internal patch pipette solution contained (in mM): 126.5 CsMeSO4, 2 MgCl2, 11 EGTA, 10 HEPES adjusted to pH 7.3 with CsOH. Internal solution was supplemented with 0.6 mM GTP and 2 mM ATP and mixed thoroughly just before use. Liquid junction potentials for the above solutions were calculated before experiments using pClamp 10 software and corrected during experiments. Compounds were prepared daily from 30 mM DMSO stocks and diluted into an external solution just before use. Compounds were then applied rapidly and locally to the cells using a custom-built gravity-driven micro-perfusion system (Feng et al., 2003). Initial vehicle experiments were performed to ensure that 0.1% DMSO did not affect current amplitudes or channel kinetics (data not shown), and all subsequent experiments contained 0.1% DMSO in control external solutions. Currents were elicited from a holding potential of -100 mV and were measured by conventional whole-cell patch clamp techniques using an Axopatch 200B amplifier in combination with Clampex 9.2 software. (Molecular Devices, Sunnyvale, CA). After establishing whole cell configuration, cellular capacitance was minimised using the amplifier’s built-in analogue compensation. Series resistance was kept to <10 MΩ and was compensated to at least 85% in all experiments. All data were digitised at 10 kHz with a Digidata 1,320 interface (Molecular Devices) and filtered at 1 kHz (8-pole Bessel filter). Raw and online leak-subtracted data were both collected simultaneously, P/N4 leak subtraction was performed using opposite polarity and after the protocol sweep.
For tonic inhibition of T-type currents, membrane potential was stepped from −100 mV to −30 mV for 200 ms and then allowed to recover for 12 s (1 sweep). A minimum of 10 sweeps were collected under vehicle control external perfusion to allow for control peak current to equilibrate. The drug was then continuously perfused, and sweeps were recorded until no further inhibition was seen (minimum of 3 sweeps with the same amplitude). In current-voltage relation studies, the membrane potential was held at −100 mV and cells were depolarised from −70 to 50 mV in 10 mV increments. For steady-state inactivation studies, a 3.6 s conditioning pre-pulse of various magnitudes (initial holding at −110 mV) was followed by a depolarising pulse to −30 mV. Individual sweeps were separated by 12 s to permit recovery from inactivation between conditioning pulses. The duration of the test pulse was typically 20 ms, and the current amplitude obtained from each test pulse was normalised to that observed at the holding potential of −100 mV.
2.6 Data analysis
Unless otherwise stated, five independent experiments were performed for each condition, with each experiment conducted in duplicate. For the calcium assay, change in fluorescence by 10 mM Ca in the presence of drugs was normalised to change in fluorescence caused by 10 mM Ca in the presence of vehicle only to allow for comparison. In the Alphascreen assay, results were normalised to EC80 of ML-186 after subtraction of basal ERK phosphorylation (presence of a vehicle and 0.2% DMSO) to allow for easy comparison between phytocannabinoid activities. We fitted all concentration-response curves to a 4-parameter sigmoidal inhibitory response curve in GraphPad Prism (Version 6 GraphPad Software Inc., CA, United States) to derive IC50 and Emax values. All statistical analyses were conducted with GraphPad Prism. Two-tailed, unpaired t-tests were used to compare two data points and one-way ANOVA for more than two data points with Tukey post-hoc analysis. p-values < 0.05 were considered statistically significant.
3 Results
3.1 Phytocannabinoids inhibit T-type Ca channels based on FLIPR assays
Our investigation of T-type channels began with a single-point screen, as described by Bladen et al. (2021). We screened the inhibitory effect of 10 µM phytocannabinoids on the change in fluorescence elicited by the addition of 10 mM Ca2+ in cells expressing Cav3.1, Cav3.2 or Cav3.3 channels (Xie et al., 2007; Bladen et al., 2021). Compounds that displayed ≥80% inhibition of the 10 mM Ca2+ response were then further characterised with concentration-response curves (Bladen et al., 2021).
10 µM CBGVA and CBDVA inhibited calcium entry in cells expressing Cav 3.1 channels by 82 ± 6% and 80 ± 6%, respectively, which was similar in magnitude to the effect of 10 µM NNC550396 (82 ± 8%), an established non-selective T-type Ca channel inhibitor (McGivern, 2008). CBGV, CBCVA, CBCV, and CBCA showed <80% inhibition of calcium entry (Table 1).
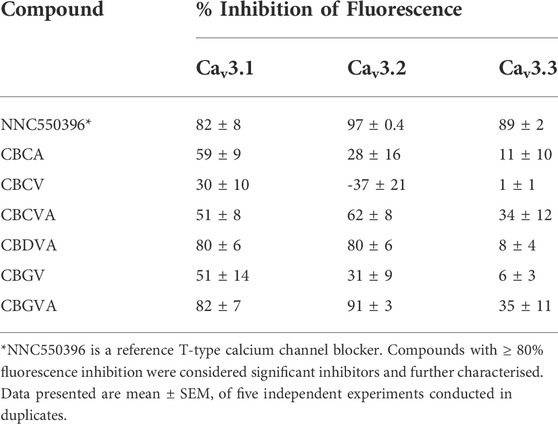
TABLE 1. % inhibition of 10 mM Ca-induced fluorescence by phytocannabinoids (10 µM) at T-type calcium channels using FLIPR assay in HEK293 cells.
CBGVA and CBDVA inhibited fluorescence in cells expressing Cav3.2 by 91 ± 3% and 80 ± 6%, respectively. NNC550396 inhibited calcium entry by 89 ± 2% while CBGV, CBCVA and CBCA showed <80% inhibition. However, we observed that CBCV appeared to increase the influx of Ca2+ ions in cells expressing Cav3.2 channels. At Cav3.3, NNC550396 inhibited calcium influx by 89 ± 2%, but no tested phytocannabinoid showed ≥80% inhibition (Table 1).
We next determined the concentration-response of CBGVA and CBDVA in cells expressing Cav3.1 or Cav3.2 channels. We did not determine the concentration-response of any phytocannabinoid on Cav 3.3 as no compound met the criterion for the single-point screen. CBGVA and CBDVA inhibited the change in fluorescence in cells expressing Cav3.1 with IC50 values of 6 µM and 2 μM, respectively (Figures 1A,B). We observed similar potency in cells expressing Cav3.2, with CBGVA and CBDVA having IC50 values of 2 µM and 11 μM, respectively (Figures 1C,D). Both phytocannabinoids were less potent than NNC550396, which exhibited IC50 values of 730 nM and 945 nM at Cav3.1 and Cav3.2 channels, respectively.
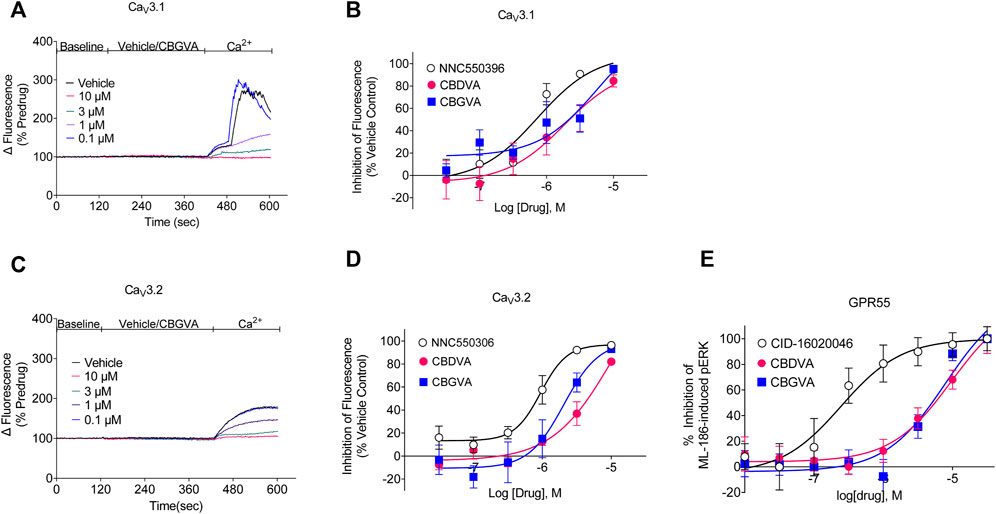
FIGURE 1. CBGVA and CBDVA inhibit Cav3.1 and Cav3.2 channels and GPR55 receptors. (A) A representative trace of the change in fluorescence caused by vehicle control and CBGVA in HEK 293 Cav3.1 cells. After 2 min of baseline reading, vehicle or CBGVA were added for 5 min, followed by adding 10 mM Ca2+ and read for a further 3 min. (B) Concentration-response curves for NNC550396, CBGVA and CBDVA inhibition of Cav3.1 response. (C) A representative trace of the change in fluorescence caused by vehicle control and CBGVA in HEK 293 Cav3.2 cells. (D) Concentration-response curves for NNC550396, CBGVA and CBDVA inhibition of Cav3.1 response. (E) Concentration-response curves of CBDVA and CBGVA against 0.45 µM ML-186 -induced ERK phosphorylation in HEK 293 cells expressing GPR55 receptors. All data presented are mean ± SEM of five independent experiments conducted in duplicates. Some data points do not have visible error bars due to low variability.
3.2 CBGVA and CBDVA block ML-186-induced ERK phosphorylation in GPR55 cells
We have recently shown that a major biosynthetic precursor molecule in cannabis, CBGA, displays anticonvulsant properties (Anderson et al., 2021b). CBGA inhibits T-type calcium channels but affects other targets, including antagonising GPR55. GPR55 is thought to contribute to the anticonvulsant mechanism of action of CBD (Kaplan et al., 2017). To determine whether CBGVA and CBDVA antagonise GPR55, we conducted concentration-response curves for CBGVA and CBDVA in the presence of the EC80 of ML-186 (Figure 1E). We found that CBGVA and CBDVA were low potency antagonists (IC50 = 8 µM and 10 μM, respectively) compared to the established GPR55 antagonist CID-16020046 (IC50 = 245 nM).
3.3 Single-cell patch-clamp electrophysiology shows CBGVA and CBDVA tonically inhibit Cav3.1 currents
Cav3.1 is an emerging target of interest for treating intractable epilepsies, as it has been shown that genetic deletion of Cacna1g, which encodes Cav3.1, reduces spontaneous seizure frequency in a mouse model of Dravet syndrome (Calhoun et al., 2017). Thus we explored further the inhibitory effects of CBGVA and CBDVA on Cav3.1 channels using single-cell electrophysiology. We measured tonic inhibition by repetitively stepping the cell membrane potential from −100 mV to −30 mV to elicit peak amplitude while superfusing concentrations of the tested phytocannabinoids. We found that CBGVA and CBDVA inhibited Cav3.1 current amplitude in a concentration-dependent manner with IC50 values of 3 µM and 7 μM, respectively (Figure 2A).
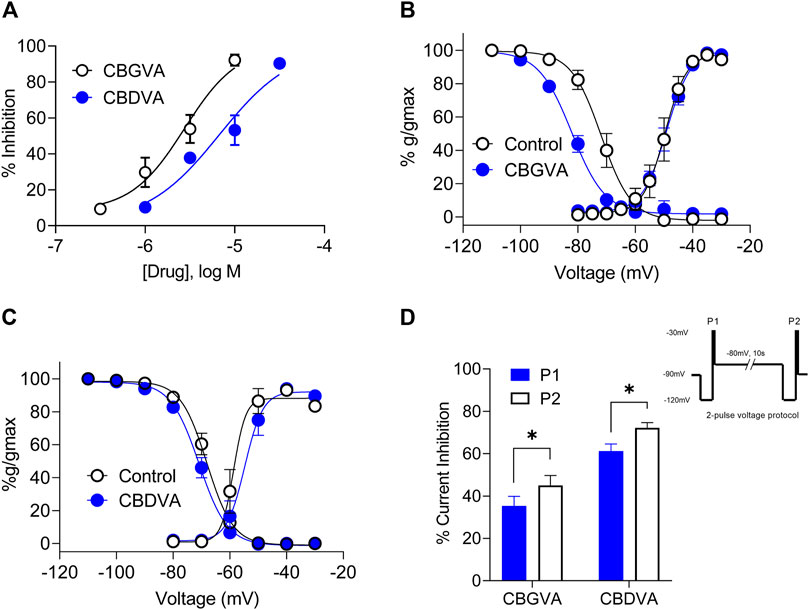
FIGURE 2. CBGVA and CBDVA inhibit Cav3.1 channel currents. (A) Concentration-response curve for CBGVA and CBDVA inhibition of Cav3.1 channels. Whole-cell patch-clamp recordings were made from HEK T-rex Cav3.1 channel currents evoked by stepping −100 mv to −30 mv every 12 s. Steady-state activation and inactivation curves of HEK Cav3.1 in the absence (control) and presence of (B) CBGVA (5 µM) (C) CBDVA (5 µM). Data presented are mean ± SEM of 10–11 cells. (D) Cav3.1 channel slow inactivated state was elicited by a 2-pulse voltage protocol (inset). This elicited a significant increase in peak current inhibition by CBGVA and CBDVA. Data presented are mean ± SEM of % inhibition of peak current of vehicle control for 9 cells. Statistical analysis was performed using a student T-test with * denoting p < 0.05. Some data points do not have visible error bars due to low variability.
3.4 The effect of CBGVA and CBDVA on activation and inactivation states of Cav3.1 channels
We next investigated whether CBGVA and CBDVA affected Cav3.1 channel gating by examining the effect of each compound (5 µM) on steady-state inactivation (SSI), the voltage dependence of activation and cumulative slow inactivation of Cav3.1. We found that CBGVA significantly shifted SSI curves towards more hyperpolarised membrane potentials with a half-inactivation (V0.5) of −82.1 ± 0.8 mV; control −71.8 ± 0.9 mV. CBDVA had a negligible effect on steady-state inactivation (−70.9 ± 0.5 mV; control −68.1 ± 0.5 mV) (Figures 2B,C).
Both CBGVA (V0.5 value of −49.1 ± 0.5 mV; control −49.6 ± 0.9 mV) and CBDVA (V0.5 value of −54.7 ± 1.2 mV; control −58.6 ± 1.5 mV) did not significantly alter voltage dependence of Cav3.1 activation (Figures 2B,C).
The effect of CBGVA and CBDVA (5 µM each) on slow inactivation was tested using a 2-pulse voltage protocol as described in Bladen et al., (2021). Briefly, cells expressing Cav3.1 were initially depolarised with a 200 ms test pulse from -120 mV to −30 mV to generate peak current (P1) and followed by a 10 s pre-conditioning pulse to −80 mV to reverse fast inactivation. Cells were further depolarised with another 200 ms test pulse from −120 to −30 mV (P2) (Figure 2 inset). We found that CBGVA inhibition of Cav3.1 current significantly increased from 61 ± 3% to 72 ± 3%, and CBDVA inhibition increased from 35 ± 5% to 45 ± 5% (Figure 2D), suggesting that both compounds more significantly inhibit Cav3.1 channels in the slow inactivated state than a fast inactivated state.
3.5 CBGVA and CBDVA inhibition of Cav3.1 is frequency-dependent
THC and CBD cause substantial frequency-dependent inhibition of Cav3.2 and Nav1.8 channels, respectively (Ross et al., 2008; Bladen et al., 2021; Zhang and Bean, 2021). Therefore, we explored a possible use-dependent effect of CBGVA and CBDVA on Cav3.1 action potentials delivered in 50 sweeps at frequencies of 0.4, 0.8 and 2 Hz. CBGVA caused a higher frequency-dependent reduction than CBDVA across all tested frequencies (Figures 3A–F). At the 50th action potential, 5 µM CBGVA reduced Cav3.1 relative current to 73 ± 3%; 88 ± 1% and 77 ± 1% of that evoked by the first action potential during 0.4 Hz, 0.8 Hz and 2.0 Hz stimulation, respectively. 5 µM CBDVA also reduced Cav3.1 current to 90 ± 1%; 93 ± 1%, and 83 ± 1% when stimulated at 0.4 Hz, 0.8 Hz and 2.0 Hz. CBGVA and CBDVA produce a modest use-dependent reduction of Cav3.1 currents.
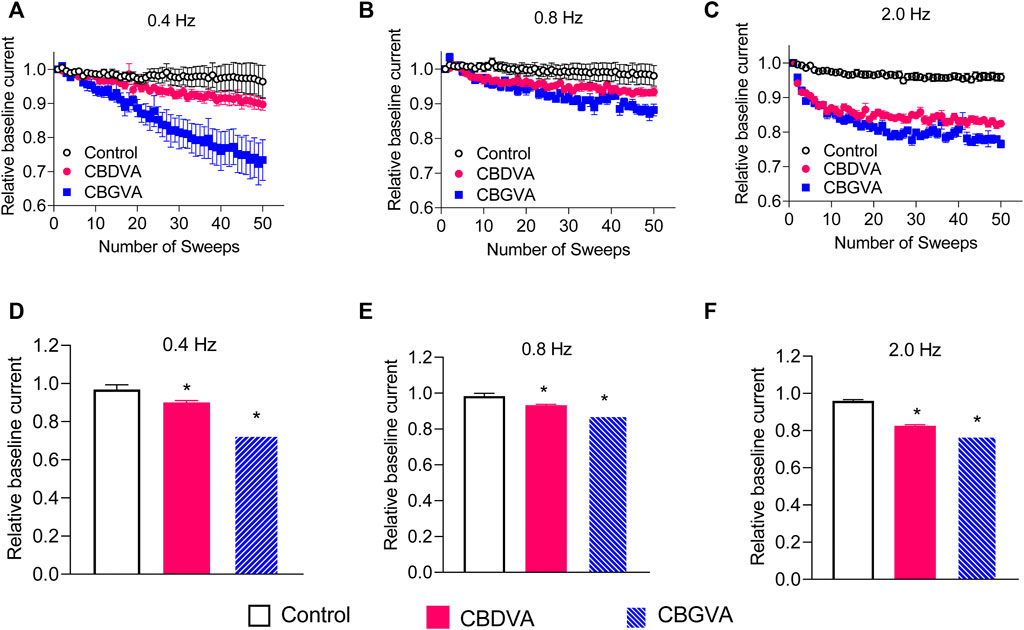
FIGURE 3. CBGVA and CBDVA block Cav3.1 channels in a frequency-dependent manner. In the absence (control) or presence of 5 µM CBGVA and CBDVA, cells were held at −100 mV and stepped at three different frequencies (A) 0.4 Hz (B) 0.8 Hz, (C) 2.0 Hz to −30 mV with intersweep holding potential of −100 mV. Data presented are mean ± SEM of peak currents of each sweep after normalisation to the average peak current of the baseline sweeps (first three sweeps) in 5–11 cells. Summary data of the relative current of CBDVA and CBGVA at (D) 0.4 Hz, (E) 0.8 Hz (F) 2.0 Hz. Data presented are mean ± SEM of the last three sweeps in 5–11 cells. Statistical analysis was performed using a student t-test with * denoting p < 0.05.
4 Discussion
We have recently reported that a series of acidic biosynthetic precursor molecules found in cannabis display anticonvulsant properties in the Scn1a+/− mouse model of Dravet syndrome, including CBGVA and CBDVA (Anderson et al., 2019; Anderson et al., 2021a; Anderson et al., 2021b). However, the molecular pharmacology of these acidic compounds is poorly understood. The present investigation provides evidence that these two anticonvulsant phytocannabinoids inhibit T-type calcium channels (Cav3.1 and Cav3.2) and GPR55 receptors at low micromolar concentrations. Given that Cav3.1 is an emerging target for treating Dravet syndrome, we conducted more detailed patch-clamp electrophysiology studies to illuminate the biophysical properties of the inhibitory effects at this channel. We found that at 5 μM, CBGVA negatively shifted the half-inactivation voltage of Cav3.1, while CBDVA did not. Both CBDVA and CBGVA did not significantly alter the half activation of Cav3.1 channels. Interestingly, both compounds displayed use-dependent blockade of Cav3.1 current and higher inhibition of Cav3.1 current in a slow than a fast inactivated state.
Our findings that the minor phytocannabinoids CBDVA and CBGVA inhibit T-type calcium channels extend upon research showing that phytocannabinoids and synthetic cannabinoids inhibit these channels (Ross et al., 2008; Mirlohi et al., 2021, 2022). We recently reported that the structurally related phytocannabinoid CBGA, which is also anticonvulsant, inhibited Cav3.1 and Cav3.2 channels (Mirlohi et al., 2022). Here CBDVA and CBGVA displayed lower potency than CBGA using an identical fluorescent reporter assay. Moreover, CBGVA and CBDVA affected the biophysical properties of the channel distinctly to CBGA. At concentrations similar to the EC50 of tonic inhibition, CBGA caused a significant negative shift of both fast inactivation and activation of Cav3.1 current (Mirlohi et al., 2022). Whereas in this study, CBGVA negatively shifted fast inactivation but not activation, and CBDVA did not affect either state. The preferential shift of the fast inactivation curve in Cav3.1 channels by CBGVA is similar to CBD, CBG and CBDV at Cav3.1 channels (Ross et al., 2008; Mirlohi et al., 2022). Hyperpolarising shifts of steady-state fast inactivation suggest preferential binding of CBGVA to the inactivated state of the Cav3.1 channels (Freeze et al., 2006; Eckle and Todorovic, 2010). Future studies should investigate the effect of CBGVA and similar phytocannabinoids on the rate of Cav3.1 recovery from inactivation because drugs that stabilise inactive states of channels are often simultaneously slowing the recovery from inactivation due to slow drug dissociation (Burgess et al., 2002; Talavera et al., 2004; Ghovanloo et al., 2022).
In this study, CBGVA and CBDVA more effectively inhibited Cav3.1 channels in a slow inactivation state, similar to CBD (Bladen et al., 2021). CBD is also reported to preferentially bind the slow inactivated state of Nav1.8 channels (Zhang and Bean, 2021). Up to 90% of Nav1.8 channels can be in a slow inactivated state at depolarised potentials (Tripathi et al., 2006), and a slow inactivated state of Cav3.1 can reduce calcium currents by up to 50% (Hering et al., 2004). Given that T-type calcium channels critically determine the threshold of Na+ channel all or none firing, it can be posited that CBD’s preferential binding to slow inactivated states may be a major contributory mechanism that reduces neuronal excitability in epilepsy. This could also apply to CBDVA and CBGVA’s anticonvulsant properties, but it is unclear whether they inhibit Na+ channels, which could be addressed in future research.
Phytocannabinoids tend to be multi-modal and affect numerous drug targets, which may contribute to their efficacy as potential therapeutic agents (Brodie et al., 2015). To advance knowledge on the molecular pharmacology of CBDVA and CBGVA, we also demonstrated here that these compounds antagonise GPR55. GPR55 is thought to contribute to the anticonvulsant effects of CBD (Gray and Whalley, 2020; Kaplan et al., 2017), and a growing list of anticonvulsant phytocannabinoids such as CBGA and CBDV share this property (Anavi-Goffer et al., 2012; Anderson et al., 2021b). Future studies in preclinical epilepsy models are needed to assess whether GPR55 and T-type calcium channels are necessary for the anticonvulsant effect of these phytocannabinoids. It would also be interesting to explore the effects of these phytocannabinoids in other disease models in which T-type channels and GPR55 play a role, such as in models of insomnia, pain, and gastrointestinal disorders (Harding and Zamponi, 2022). Given the promiscuity of the phytocannabinoids, additional cellular pharmacology studies are needed to address whether CBGVA and CBDVA affect other phytocannabinoid targets such as cannabinoid receptors and transient receptor potential (TRP) channels. Notably, recent studies reported that CBDVA did not alter excitatory neurotransmission in autaptic hippocampal neurons, which predominantly express cannabinoid CB1 receptors (Straiker et al., 2021). Further, CBDVA did not alter calcium responses in dorsal root ganglion neurons (which express a mixed population of TRP channels). CBDVA has been shown to inhibit the endocannabinoid synthetic enzyme diacylglycerol lipase at a relatively high concentration (de Petrocellis et al., 2011).
An important question arises as to whether the effects we observed here are relevant to the levels of exposure associated with the anticonvulsant effects of CBDVA and CBGVA in preclinical models (Anderson et al., 2021b). Peak plasma concentrations of CBGVA and CBDVA at anticonvulsant doses in mice are approximately 1,200 µM and 239 μM, respectively (Anderson et al., 2021b). The brain-plasma ratios of CBGVA and CBDVA are 4% and 2%, respectively (Anderson et al., 2019). Thus, anticonvulsant doses would be expected to achieve brain concentrations of 48 μM and 5 µM for CBGVA and CBDVA, respectively. Therefore, the effects of CBGVA and CBDVA on GPR55 receptors and T-type calcium channels might be relevant to the anticonvulsant effects of these compounds. However, the limited brain uptake of these molecules would need to be surmounted for these compounds to be advanced as therapeutics for CNS disorders.
It is unlikely that the effects observed in this study will be experienced after using medicinal cannabis products because these products generally contain negligible concentrations of these phytocannabinoids (Hanuš et al., 2016; Suraev et al., 2018). However, it is noteworthy that CBDVA was the most abundant phytocannabinoid found in a newly characterised Chinese accession (G-309) of a hemp form of cannabis with low THC content (Muscarà et al., 2021). This highlights that such phytocannabinoids might not necessarily be “minor” in some cannabis strains. Moreover, a new enzymatic bioreactor technology can synthesise CBGVA and CBDVA at scale with high purity (Valliere et al., 2019). This reiterates the significance of investigating the activities of minor phytocannabinoids because they could be used in new cannabinoid formulations.
5 Conclusion
Here we report that the understudied minor phytocannabinoids CBDVA and CBGVA, which are biosynthetic precursor molecules found in the cannabis plant, inhibit both T-type calcium channels and GPR55 receptors in vitro. Our data suggest that these compounds could be further explored for therapeutic potential in disease states which involve these channels or receptors, such as epilepsy, insomnia, pain and gastrointestinal disorders.
Data availability statement
The original contributions presented in the study are included in the article/Supplementary Material, further inquiries can be directed to the corresponding authors.
Author contributions
MU and JA conceived the study. MU, CB, MH, VJ, LA, and JA designed the experiments. JL synthesised compounds used in the study. MU, MH, and CB performed the experiments and analysed the data. MU and JA drafted the manuscript. All authors reviewed the manuscript and approved its final form.
Funding
This research was supported by the Lambert Initiative for Cannabinoid Therapeutics, a philanthropically-funded centre for medicinal cannabis research at the University of Sydney, and the Australian National Health and Medical Research Council (NHMRC GNT1161571).
Acknowledgments
The authors gratefully acknowledge Barry and Joy Lambert for their continued support of the Lambert Initiative for Cannabinoid Therapeutics. In addition, we thank Professor Mark Connor for his helpful comments on this study.
Conflict of interest
JA and IM have served as expert witnesses in various medicolegal cases involving cannabis and cannabinoids. JA, LA, and IM hold patents on cannabinoid therapies (PCT/AU2018/05,089 and PCT/AU2019/050,554). JA has received consulting fees from Creo Inc. And Medicinal Cannabis Industry Australia (MCIA). IM acts as a consultant to Kinoxis Therapeutics and has received honoraria from Janssen. He has also received consulting fees from MCIA. CB is a founder and shareholder of Zymedyne Therapeutics. Zymedyne is not a publicly traded entity. The remaining authors have no conflicts of interest.
The remaining authors declare that the research was conducted in the absence of any commercial or financial relationships that could be construed as a potential conflict of interest.
Publisher’s note
All claims expressed in this article are solely those of the authors and do not necessarily represent those of their affiliated organizations, or those of the publisher, the editors and the reviewers. Any product that may be evaluated in this article, or claim that may be made by its manufacturer, is not guaranteed or endorsed by the publisher.
References
Anavi-Goffer, S., Baillie, G., Irving, A. J., Gertsch, J., Greig, I. R., Pertwee, R. G., et al. (2012). Modulation of L-α-lysophosphatidylinositol/GPR55 mitogen-activated protein kinase (MAPK) signaling by cannabinoids. J. Biol. Chem. 287 (1), 91–104. doi:10.1074/jbc.M111.296020
Anderson, L. L., Ametovski, A., Lin Luo, J., Everett-Morgan, D., McGregor, I. S., Banister, S. D., et al. (2021a). Cannabichromene, related phytocannabinoids, and 5-Fluoro-cannabichromene have anticonvulsant properties in a mouse model of Dravet syndrome. ACS Chem. Neurosci. 12 (2), 330–339. doi:10.1021/acschemneuro.0c00677
Anderson, L. L., Heblinski, M., Absalom, N. L., Hawkins, N. A., Bowen, M., Benson, M. J., et al. (2021b). Cannabigerolic acid, a major biosynthetic precursor molecule in cannabis, exhibits divergent effects on seizures in mouse models of epilepsy. Br. J. Pharmacol. 178 (24), 4826–4841. doi:10.1111/BPH.15661),
Anderson, L. L., Low, I. K., Banister, S. D., McGregor, I. S., and Arnold, J. C. (2019). Pharmacokinetics of phytocannabinoid acids and anticonvulsant effect of cannabidiolic acid in a mouse model of Dravet syndrome. J. Nat. Prod. 82 (11), 3047–3055. doi:10.1021/acs.jnatprod.9b00600
Anwar, A., Saleem, S., Patel, U. K., Arumaithurai, K., and Malik, P. (2019). Dravet syndrome: an overview. Cureus 11 (6), e5006. doi:10.7759/CUREUS.5006
Baulac, M., Brodie, M. J., Patten, A., Segieth, J., and Giorgi, L. (2012). Efficacy and tolerability of zonisamide versus controlled-release carbamazepine for newly diagnosed partial epilepsy: a phase 3, randomised, double-blind, non-inferiority trial. Lancet. Neurol. 11 (7), 579–588. doi:10.1016/S1474-4422(12)70105-9
Bladen, C., Mirlohi, S., Santiago, M., Longworth, M., Kassiou, M., Banister, S., et al. (2021). Modulation of human T-type calcium channels by synthetic cannabinoid receptor agonists in vitro. Neuropharmacology 187, 108478. doi:10.1016/J.NEUROPHARM.2021.108478
Bourinet, E., Alloui, A., Monteil, A., Barrère, C., Couette, B., Poirot, O., et al. (2005). Silencing of the Cav3.2 T-type calcium channel gene in sensory neurons demonstrates its major role in nociception. EMBO J. 24 (2), 315–324. doi:10.1038/sj.emboj.7600515
Brodie, J. S., di Marzo, V., and Guy, G. W. (2015). Polypharmacology shakes hands with complex aetiopathology. Trends Pharmacol. Sci. 36 (12), 802–821. doi:10.1016/J.TIPS.2015.08.010
Burgess, D. E., Crawford, O., Delisle, B. P., and Satin, J. (2002). Mechanism of inactivation gating of human T-type (low-voltage activated) calcium channels. Biophys. J. 82 (4), 1894–1906. doi:10.1016/S0006-3495(02)75539-2
Cain, S. M., Tyson, J. R., Choi, H.-B., Ko, R., Lin, P. J. C., LeDue, J. M., et al. (2018). Ca V 3.2 drives sustained burst-firing, which is critical for absence seizure propagation in reticular thalamic neurons. Epilepsia 59 (4), 778–791. doi:10.1111/epi.14018
Calhoun, J. D., Hawkins, N. A., Zachwieja, N. J., and Kearney, J. A. (2017). Cacna1g is a genetic modifier of epilepsy in a mouse model of Dravet syndrome. Epilepsia 58 (8), e111–e115. doi:10.1111/epi.13811
Cribbs, L. L., Lee, J.-H., Yang, J., Satin, J., Zhang, Y., Daud, A., et al. (1998). Cloning and characterization of alpha1H from human heart, a member of the T-type Ca2+ channel gene family. Circ. Res. 83 (1), 103–109. doi:10.1161/01.RES.83.1.103
de Petrocellis, L., Ligresti, A., Moriello, A. S., Allarà, M., Bisogno, T., Petrosino, S., et al. (2011). Effects of cannabinoids and cannabinoid-enriched Cannabis extracts on TRP channels and endocannabinoid metabolic enzymes. Br. J. Pharmacol. 163 (7), 1479–1494. doi:10.1111/J.1476-5381.2010.01166.X
Eckle, V. S., and Todorovic, S. M. (2010). Mechanisms of inhibition of CaV3.1 T-type calcium current by aliphatic alcohols. Neuropharmacology 59 (1–2), 58–69. doi:10.1016/J.NEUROPHARM.2010.03.016
Feng, Z.-P., Doering, C. J., Winkfein, R. J., Beedle, A. M., Spafford, J. D., and Zamponi, G. W. (2003). Determinants of inhibition of transiently expressed voltage-gated calcium channels by ω-conotoxins GVIA and MVIIA. J. Biol. Chem. 278 (22), 20171–20178. doi:10.1074/jbc.M300581200
Freeze, B. S., McNulty, M. M., and Hanck, D. A. (2006). State-dependent verapamil block of the cloned human Cav3.1 T-type Ca2+ channel. Mol. Pharmacol. 70 (2), 718–726. doi:10.1124/MOL.106.023473
Ghovanloo, M., Estacion, M., Higerd‐Rusli, G. P., Zhao, P., Dib‐Hajj, S., and Waxman, S. G. (2022). Inhibition of sodium conductance by cannabigerol contributes to a reduction of dorsal root ganglion neuron excitability. Br. J. Pharmacol. 179, 4010–4030. doi:10.1111/bph.15833
Gray, R. A., and Whalley, B. J. (2020). The proposed mechanisms of action of CBD in epilepsy. Epileptic Disorders: International Epilepsy J. Videotape 22 (S1), 10–15. doi:10.1684/epd.2020.1135
Hanuš, L. O., Meyer, S. M., Muñoz, E., Taglialatela-Scafati, O., and Appendino, G. (2016). Phytocannabinoids: a unified critical inventory. Nat. Product. Rep. 33 (12), 1357–1392. doi:10.1039/c6np00074f
Harding, E. K., and Zamponi, G. W. (2022). Central and peripheral contributions of T-type calcium channels in pain. Mol. Brain 15 (1), 39–13. doi:10.1186/s13041-022-00923-w
Hering, J., Feltz, A., and Lambert, R. C. (2004). Slow inactivation of the Ca V 3.1 isotype of T-type calcium channels. J. Physiol. 555 (2), 331–344. doi:10.1113/jphysiol.2003.054361
Kaplan, J. S., Stella, N., Catterall, W. A., and Westenbroek, R. E. (2017). Cannabidiol attenuates seizures and social deficits in a mouse model of Dravet syndrome. Proc. Natl. Acad. Sci. U. S. A. 114 (42), 11229–11234. doi:10.1073/pnas.1711351114
Kargl, J., Balenga, N., Parzmair, G. P., Brown, A. J., Heinemann, A., and Waldhoer, M. (2012). The cannabinoid receptor CB1 modulates the signaling properties of the lysophosphatidylinositol receptor GPR55. J. Biol. Chem. 287, 44234–44248. doi:10.1074/jbc.M112.364109
Marini, C., Scheffer, I. E., Nabbout, R., Suls, A., Guerrini, R., Zara, F., et al. (2011). The genetics of Dravet syndrome. Epilepsia 52 (2), 24–29. doi:10.1111/J.1528-1167.2011.02997.X
McGivern, J. (2008). Pharmacology and drug discovery for T-type calcium channels. CNS Neurol. Disord. Drug Targets 5 (6), 587–603. doi:10.2174/187152706779025535
Mirlohi, S., Bladen, C., Santiago, M., and Connor, M. (2021). Modulation of recombinant human T-type calcium channels by Δ 9 -tetrahydrocannabinolic acid in vitro. Cannabis Cannabinoid Res. 7, 34–45. doi:10.1089/can.2020.0134
Mirlohi, S., Bladen, C., Santiago, M. J., Arnold, J. C., McGregor, I., and Connor, M. (2022). Inhibition of human recombinant T‐type calcium channels by phytocannabinoids in vitro. Br. J. Pharmacol. 179, 4031–4043. doi:10.1111/bph.15842
Muscarà, C., Smeriglio, A., Trombetta, D., Mandalari, G., la Camera, E., Occhiuto, C., et al. (2021). Antioxidant and antimicrobial activity of two standardized extracts from a new Chinese accession of non-psychotropic Cannabis sativa L. Phytother. Res. 35 (2), 1099–1112. doi:10.1002/PTR.6891
Ross, H. R., Napier, I., and Connor, M. (2008). Inhibition of recombinant human T-type calcium channels by Delta9-tetrahydrocannabinol and cannabidiol. J. Biol. Chem. 283 (23), 16124–16134. doi:10.1074/jbc.M707104200
Straiker, A., Wilson, S., Corey, W., Dvorakova, M., Bosquez, T., Tracey, J., et al. (2021). An evaluation of understudied phytocannabinoids and their effects in two neuronal models. Molecules. 26 (17), 1–17. doi:10.3390/molecules26175352
Suraev, A., Lintzeris, N., Stuart, J., Kevin, R. C., Blackburn, R., Richards, E., et al. (2018). Composition and use of cannabis extracts for childhood epilepsy in the Australian community. Sci. Rep. 8 (1), 10154. doi:10.1038/s41598-018-28127-0
Talavera, K., Staes, M., Janssens, A., Droogmans, G., and Nilius, B. (2004). Mechanism of arachidonic acid modulation of the T-type Ca2+ channel alpha1G. J. Gen. Physiol. 124 (3), 225–238. doi:10.1085/JGP.200409050
Tringham, E., Powell, K. L., Cain, S. M., Kuplast, K., Mezeyova, J., Weerapura, M., et al. (2012). T-type calcium channel blockers that attenuate thalamic burst firing and suppress absence seizures. Sci. Transl. Med. 4 (121), 121ra19. doi:10.1126/scitranslmed.3003120
Tripathi, P. K., Trujillo, L., Cardenas, C. A., Cardenas, C. G., de Armendi, A. J., and Scroggs, R. S. (2006). Analysis of the variation in use-dependent inactivation of high-threshold tetrodotoxin-resistant sodium currents recorded from rat sensory neurons. Neuroscience 143 (4), 923–938. doi:10.1016/J.NEUROSCIENCE.2006.08.052
Valliere, M. A., Korman, T. P., Woodall, N. B., Khitrov, G. A., Taylor, R. E., Baker, D., et al. (2019). A cell-free platform for the prenylation of natural products and application to cannabinoid production. Nat. Commun. 10 (1). doi:10.1038/S41467-019-08448-Y
Xie, X. S., Van Deusen, A. L., Vitko, I., Babu, D. A., Davies, L. A., Huynh, N., et al. (2007). Validation of high throughput screening assays against three subtypes of Cav3 T-type channels using molecular and pharmacologic approaches. Assay. Drug Dev. Technol. 5 (2), 191–203. doi:10.1089/adt.2006.054
Keywords: CBDVA, CBGVA, Cav3.1, Cav3.2, GPR55, Phytocannabinoids
Citation: Udoh M, Bladen C, Heblinski M, Luo JL, Janve VS, Anderson LL, McGregor IS and Arnold JC (2022) The anticonvulsant phytocannabinoids CBGVA and CBDVA inhibit recombinant T-type channels. Front. Pharmacol. 13:1048259. doi: 10.3389/fphar.2022.1048259
Received: 19 September 2022; Accepted: 17 October 2022;
Published: 01 November 2022.
Edited by:
Oscar Moran, National Research Council (CNR), ItalyReviewed by:
Marina Sciancalepore, University of Trieste, ItalyMaria Virginia Soldovieri, University of Molise, Italy
Copyright © 2022 Udoh, Bladen, Heblinski, Luo, Janve, Anderson, McGregor and Arnold. This is an open-access article distributed under the terms of the Creative Commons Attribution License (CC BY). The use, distribution or reproduction in other forums is permitted, provided the original author(s) and the copyright owner(s) are credited and that the original publication in this journal is cited, in accordance with accepted academic practice. No use, distribution or reproduction is permitted which does not comply with these terms.
*Correspondence: Chris Bladen, Q2hyaXMuYmxhZGVuQG1xLmVkdS5hdQ==; Jonathon C. Arnold, am9uYXRob24uYXJub2xkQHN5ZG5leS5lZHUuYXU=
†These authors share first authorship