- 1School of Life Science, Qinghai Normal University, Xining, China
- 2College of Pharmacy, Jinan University, Guangzhou, China
- 3Northwest Institute of Plateau Biology, Chinese Academy of Sciences, Xining, China
Bufadienolide, an essential member of the C-24 steroid family, is characterized by an α-pyrone positioned at C-17. As the predominantly active constituent in traditional Chinese medicine of Chansu, bufadienolide has been prescribed in the treatment of numerous ailments. It is a specifically potent inhibitor of Na+/K+ ATPase with excellent anti-inflammatory activity. However, the severe side effects triggered by unbiased inhibition of the whole-body cells distributed α1-subtype of Na+/K+ ATPase, restrict its future applicability. Thus, researchers have paved the road for the structural alteration of desirable bufadienolide derivatives with minimal adverse effects via biotransformation. In this review, we give priority to the present evidence for structural diversity, MS fragmentation principles, anti-inflammatory efficacy, and structure modification of bufadienolides derived from toads to offer a scientific foundation for future in-depth investigations and views.
Introduction
Bufadienolide, an essential member of the C-24 steroid family, is characterized by an α-pyrone (six-membered lactone) ring positioned at C-17 (Gao et al., 2011). In 1933, the first bufadienolide, namely Scillaren A, was isolated and identified from Egyptian squill (Stoll et al., 1933). Since then, this class of steroid has become a research hotspot, piquing the interest of researchers worldwide, to promote the isolation and identification of bufadienolide with structural diversity (Steyn and van Heerden 1998). Currently, an increasing number of bufadienolides were originally characterized from the traditional Chinese medicine of ChanSu which is one of the richest sources of bufadienolides (Rodríguez et al., 2017; Wei et al., 2019; Pearson and Tarvin 2022). Another notable source is venom from toads (Bufonidae), such as Bufo bufo gargarizans Cantor (Zhan et al., 2020), Rhinella marina (Hayes et al., 2009), and Bufo melanostictus Schneider (Verpoorte and Svendsen 1979; Verpoorte and Svendsen 1980).
Remarkable progress, in terms of pharmacology and the potential of bufadienolide as a therapeutic agent, has been achieved in recent years. Importantly, bufadienolide exhibited significant antitumor and anti-inflammatory activity (Ogawa et al., 2009; Kanai et al., 2013). The pharmacological mechanism behind bufadienolide was the alteration of intracellular calcium concentration, which was triggered by its potent inhibition of Na+/K+ ATPase (Holmgren et al., 2000). When calcium ion accumulates within the appropriate concentration range in the body, bufadienolide displays the desired biological properties, such as anticancer and anti-inflammatory activity. However, if the calcium ion accumulates in a manner far beyond the threshold concentration, it might result in rather severe toxicity. Brilliantly, some creatures utilized the toxic bufadienolide as chemical defense weapons to highlight its ecological value, which was given by its powerful Na+/K+ ATPase inhibitory activity. Unarmed animals, such as fireflies and Asian snakes, have been found to sequester and store numerous bufadienolides for defensive purposes (Dobler et al., 2012; Petschenka and Agrawal 2015; Ujvari et al., 2015; Mohammadi et al., 2016; Smedley et al., 2017; Bókony et al., 2018).
Promisingly, an increasing number of researchers have concentrated on the anti-inflammatory properties of bufadienolide in recent years. In the 1960s, the anti-inflammatory activity of bufadienolide was firstly documented (Lancaster and Vegad 1967). After that, numerous investigations have verified the anti-inflammatory effect of bufadienolide, as demonstrated by a reduction in inflammatory symptoms in various animal models of acute and chronic inflammation (Esposito 1985; de Vasconcelos et al., 2011; Fürst et al., 2017). To discover the underlying mechanisms, the majority of studies have focused on leukocytes. It was found that bufadienolide exhibited anti-inflammation activity by primarily inhibiting cell proliferation and the secretion of proinflammatory cytokines (Forshammar et al., 2011; Forshammar et al., 2013).
However, some nonnegligible drawbacks, including high toxicity, low bioavailability, and poor water solubility of bufadienolide, severely restrict its further applications (Deng et al., 2017; Fu et al., 2019; Shao et al., 2021). Hence, it is particularly essential to find effective ways for structural modification of bufadienolide with the potential to both lower toxicity and increase or at least keep activity. Although chemical synthesis is usually the go-to option (Michalak et al., 2017; Shimizu et al., 2020; Wu et al., 2020), it is severely hindered by factors beyond the control, such as chemical waste, tedious protection and deprotection steps, unsatisfied synthesis yield accompanied with undesired by-products. Biosynthesis, on the other hand, has been emerged and recognized as an outstanding alternative to conquer these obstacles due to its eco-friendly and cost-effective qualities (Heasley 2012; Huang et al., 2019; Hoshino and Gaucher 2021; Pavesi et al., 2021). In the field of biosynthesis, glycosylation may be a mainstream method for bufadienolide structure modification (Thorson and Vogt 2003; Zhou et al., 2012). Bufadienolide glycosylation generally refers to the enzyme catalysis process to attach glycans at their C-3 position. It was not until 2007 that Thorson performed the first successful enzyme catalytic glycosylation of cardiac steroids (Williams et al., 2007; Gantt et al., 2008). An actinomycete-sourced glycosyltransferase OleD and its practical mutant ASP with substrate heterogeneity were discovered through high-throughput screening to perform cardiac steroid glycosylation. Accordingly, Wen et al. further discovered that C-3 glycosylation of bufadienolide could be accomplished by a glycosyltransferase called UGT74AN1 in an environmentally friendly and highly effective manner (Wen et al., 2018).
The characters, in terms of attractive structure diversity and promising biological activity, make bufadienolide a hot research topic. In the present review, we primarily analyzed and reviewed the current evidence for structure diversity, MS fragmentation principles, anti-inflammatory activity and structure modification of bufadienolides originated from toad source to provide a scientific basis for future in-depth studies and perspectives of potential drug candidates discovery.
Structure diversity
Structurally, toad-sourced bufadienolide could be classified into two groups: free bufadienolide and conjugated bufadienolide. The steroid core of free bufadienolide is replaced with a variable amount of hydroxyl groups. For conjugated bufadienolide, it is defined by esterification of hydroxyl group at C-3 position with specific groups, including argininyl side chain, lactic acid and sulfonic acid. The typical structures of free bufadienolide and conjugated bufadienolide were shown in Figure 1A.
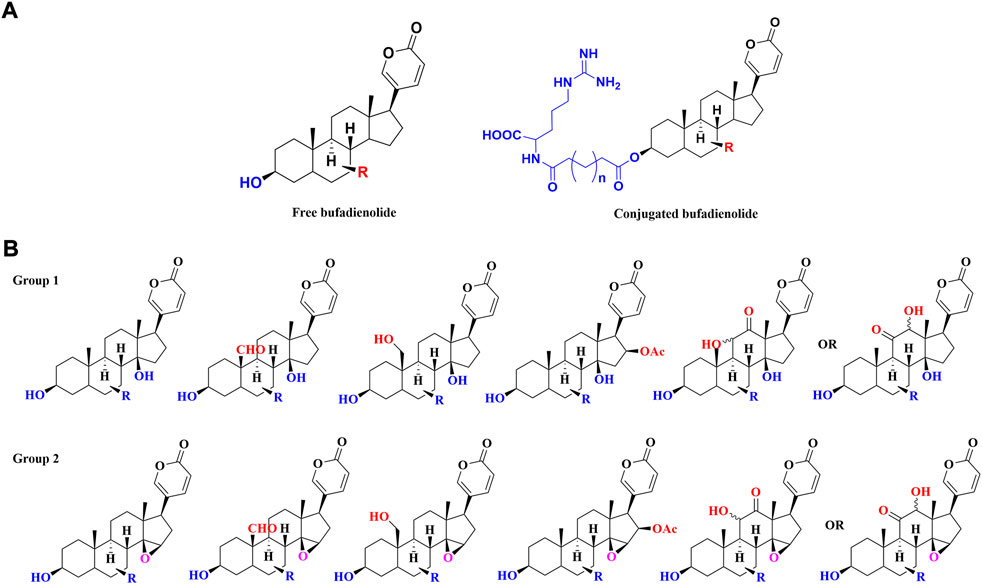
FIGURE 1. The classification of toad-sourced bufadienolide: (A) The typical structures of free bufadienolide and conjugated bufadiendolide; (B) The typical structures of five sub-categories of free bufadienolide according to the contained substituents.
Free bufadienolide
Unsubstituted α or β hydroxyl groups at the C-3 position are the distinguishing features of free bufadienolide. Depending on whether the C-14 position is substituted by a β-OH or the C-14, 15 positions form a β-epoxy structure, free bufadienolide could be divided into two categories (Group 1 and Group 2 in Figure 1B), which could be further classified into five sub-categories based on the contained function groups (hydroxy, aldehyde, carbonyl, etc.) (Figure 1B). It was reported that at least 75 free bufadienolides (1–75) have been identified (Li et al., 2015; Rodríguez et al., 2017; Wei et al., 2019; Zhan et al., 2020), whose chemical structures were shown in Figure 2.
In recent years, the finding of free bufadienolide with 3α-OH configuration in toad bile, the epimer of the ubiquitously disseminated free bufadienolides with 3β-OH configuration, has merited our attention (Zou et al., 2018). Generally, free bufadienolide with 3α-OH configuration originated from microbial transformation or animal metabolism (Zhang et al., 2011; Bhatti and Khera 2012; Hegazy et al., 2015; Wei et al., 2022), whose natural occurrence is quite rare. Our group isolated and identified eleven free bufadienolides (76–86) with 3α-OH configuration from the bile of Bufo gargarizans (unpublished data) (Figure 2).
With the development of chromatography technology and unremitting efforts of researchers (Guillarme et al., 2010), novel structures or new backbone type of free bufadienolide have continued to be discovered. Specifically, six free bufadienolides of new backbone type (Matsukawa et al., 1996; Matsukawa et al., 1997; Tian et al., 2010; Tian et al., 2017), namely Bufogargarizins A, Bufogargarizins B, Bufogargarizins C, Bufospirostenin A, Marinosin and Marinosin acid (87–92), were discovered and isolated from Bufo sinensis and Cane toad. Meanwhile, a rare series of 20, 21-epoxybufenolides (Nogawa et al., 2001; Kamano et al., 2002; Chen et al., 2015; Chen et al., 2018), namely 20S, 21-epoxyresibufogenin, 20R, 21-epoxyresibufogenin and 20S, 21R-epoxymarinobufagin (93–95), were discovered and isolated from the Brazilian toad Rhinella schneideri (Figure 2).
Conjugated bufadienolide
The substitution of C-3 hydroxyl group is the defining property of conjugated bufadienolide, which often appears in a form of ester (Figure 3), including sulfonic acid, amino acid, fatty acid and arginine diacyl chain esterification (Gao et al., 2010; Zhou et al., 2017; Zhou et al., 2021).
Eight conjugated bufadienolides that C-3 position esterized with fatty acid from the egg of Bufo bufo gargarizans were isolated and identified by Zhang et al. (96–103) (Zhang et al., 2016). Besides, three unusual conjugated bufadienolides were separated from the ovaries of Bufo marinus (104–106) (Matsukawa et al., 1998). Zhou et al. isolated and identified three pairs of epimers in which the C-3 hydroxyl groups were substituted by lactic acid from the egg of Bufo bufo gargarizans (107–112) (Zhou et al., 2017). It was the first time to reveal the racemization of lactate in amphibians.
Gao et al. isolated and identified five sulfonated bufadienolides from the venom of Bufo Melanosticus by the joint use of LC-DAD-MSn and LC-SPE-NMR (113–117) (Gao et al., 2010). From the bile of the Cane toad, three sulfonated bufadienolides (118–120) were also characterized by Lee et al. (Lee et al., 1994). The sulfonated bufadienolide exhibited better water solubility compared to the corresponding free bufadienolide. Bufadienolide sulfonation may be one of the metabolism and detoxification mechanisms of bufadienolide in animals and it may also indicate that the toxicity of sulfonated bufadienolide could be significantly reduced.
In addition to fatty acid and sulfonic acid conjugated bufadienolide, arginine diacyl ester is the predominant form of conjugated bufadienolide. It is characterized by the esterification of C-3 hydroxyl group with an arginine diacyl group, and the variable length of the arginine diacyl chain leads to the structure diversity of this kind of conjugated bufadienolide. Zhou et al. identified a series of arginine diacyl chain conjugated bufadienolides from Bufo Bufoni by UPLC-QQQ-MS analysis (121–131) (Zhou et al., 2015). Furthermore, at least 55 arginine diacyl chain conjugated bufadienolides have been isolated and identified from Chansu (132–186) (Gao et al., 2011; Wei et al., 2019; Zhan et al., 2020; Li et al., 2021), and their chemical structures were shown in Figure 3.
It was worth mentioning that Argentine researchers discovered a high concentration of arginine diacyl chain conjugated bufadienolides (187–276) in the venom of Argentine toad by MALDI-MS analysis (Table 1) (Petroselli et al., 2018). Furthermore, the characteristic fragment ions indicated that there may still exist numerous arginine diacyl chain conjugated bufadienolides polymerized as dimers or multimers remained to be discovered.
Bufadienolide MS fragmentation principles
In the realm of natural products, the ion response of bufadienolide is more sensitive in the positive ion mode of electrospray ionization (ESI) than in the negative ion mode. The MS/MS spectra of bufadienolide could be divided into two regions: the steroid core region is located at m/z 50-250, while the functional group region spans m/z 250-500. In the functional group region, it is common to observe the fragment ions resulting from the loss of a series of neutral molecules, such as H2O and CO, which correspond to the fragmentation of certain functional groups including hydroxyl, aldehyde, and carbonyl et al. For the steroidal core region, the fragment ions are acquired primarily by the Retro-Diels-Alder (RDA) reaction, induced cleavage, and hydrogenation rearrangement (Tureček and Hanuš 1984; Silva and Lopes 2015). Based on the MS/MS fragmentation of bufadienoldide standards and literature reports (Han et al., 2016; Meng et al., 2016; Han et al., 2017), the general MS/MS fragmentation principles for bufadienolide are summarized as follows (Figure 4).
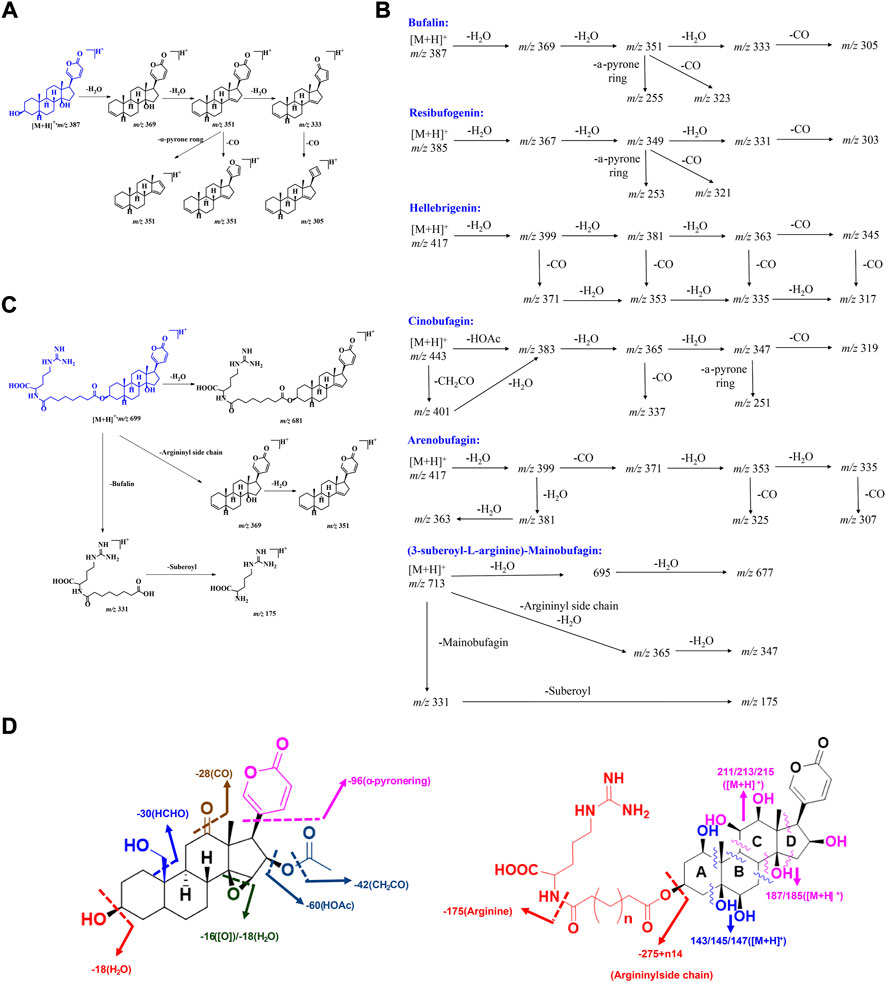
FIGURE 4. The MS fragmentation principles of bufadienolide: (A–C) The typical fragmentation pathways of free and conjugated bufadienolide; (D) The summarized fragmentation patterns of free and conjugated bufadienolide.
Bufalin and its polyhydroxy derivatives
Bufalin produced an obvious [M + H]+ ion at m/z 387.2515. After Collision-Induced Dissociation (CID), a series of MS/MS ions, including [M + H-18]+ (m/z, 369.2414), [M + H-36]+ (m/z, 351.2309), [M + H-54]+ (m/z, 333.2214) and [M + H-36-96]+ (m/z, 255.2100), were produced, which were evoked by successive losses of H2O and CO. Meanwhile, a strong intensity ion at m/z 255.2100 (a neutral loss of 96 Da) was observed, and it was initiated by the elimination of α-pyrone ring at C-17 position. The possible fragmentation pathway of bufalin is shown in Figures 4A,B. The polyhydroxy derivatives of bufalin showed similar fragmentation patterns to bufalin: successive losses of H2O and CO, which are evoked by the elimination of specific functional groups. They are followed by a neutral loss of 96 Da triggered by the elimination of α-pyrone ring at the C-17 position.
Resibufogenin and its polyhydroxy derivatives
The MS/MS spectrum of resibufogenin exhibited very similar patterns to those of bufalin. Obvious fragment ions, including [M + H-18]+ (m/z, 367.2263), [M + H-36]+ (m/z, 349.2155), [M + H-18-28]+ (m/z, 339.2310) and [M + H-36-96]+ (m/z, 253.1948), were abundant in the MS/MS spectrum. The above ions were given by successive losses of H2O and CO by elimination of hydroxyl groups, C-14/15 epoxy ring, and α-pyrone ring fragmentation (Figure 4B). Their MS/MS fragment ions generally could be correlated with the number of equipped hydroxyl groups. The whole hydroxyl groups, including C-14/15 epoxy ring, tended to be eliminated to give a stable fragment ion.
Bufadienolide with aldehyde substituent
This group of bufadienolide is characterized by an aldehyde group positioned at C-10. Hellebrigenin is a typical bufadienolide with acetoxyl substituent. It produced an obvious [M + H]+ ion at m/z 417.2257. After CID, hellebrigenin gave a series of MS/MS ions including [M + H-18]+ (m/z, 399.2165), [M + H-36]+ (m/z, 381.2068), [M + H-54]+ (m/z, 363.1952), [M + H-18-28]+ (m/z, 371.2221), [M + H-36-28]+ (m/z, 353.2109), [M + H-54-28]+ (m/z, 335.1996) and [M + H-54-28-96]+ (m/z, 239.1793). Obviously, a series of MS/MS ions characterized by the loss of 28 (CO) were clearly more abundant for bufadienolide with aldehyde substituent than other types of bufadienolide. These ions presumably resulted from the elimination of CO from aldehyde group positioned at C-10 (Figure 4B).
Bufadienolide with acetoxyl substituent
Bufadienolide with acetoxyl substituent is usually equipped with an acetoxyl group at the C-16 position, giving a characteristic fragmentation pattern. The obvious and abundant MS/MS fragment ions of Cinobufalin, a typical bufadienolide with acetoxyl substituent, usually included [M + H-42]+ (m/z, 417.2273), [M + H-60]+ (m/z, 399.2175), [M + H-60-18]+ (m/z, 381.2063), [M + H-60-36]+ (m/z, 363.1952), [M + H-60-54]+ (m/z, 345.1855). In general, the specific ions, namely [M + H-42]+ and [M + H-60]+, are the characteristic ions for this kind of bufadienolide. They are produced by the elimination of acetoxyl group to trigger the initial loss of 42 (CH2CO) or 60 (HOAc) Da (Figure 4B). The remaining ions are produced by consecutive losses of H2O and CO evoked by the specific functional group fragmentation, as with the above three groups of bufadienolide.
Bufadienolide with carbonyl substituent
This group of bufadienolide contains a carbonyl group at the C-11 or C-12 position. A typical bufadienolide belonging to this group is arenobufagin, which contains a carbonyl group at the C-11 position. The MS/MS fragment ions of arenobufagin typically consisted of [M + H-18]+ (m/z, 399.2150), [M + H-36]+ (m/z, 381.2060), [M + H-54]+ (m/z, 363.1954), [M + H-18-28]+ (m/z, 371.2220), [M + H-36-28]+ (m/z, 353.2115), [M + H-54-28]+ (m/z, 335.2003) and [M + H-54-28-96]+ (m/z, 239.1797). Characteristically, the existence of a carbonyl group leads to the neutral loss of 28 Da (CO) to generate the corresponding diagnostic ions (Figure 4B). Importantly, the relative intensity of diagnostic ions, in terms of the ions at m/z 399 generated by the elimination of C-3 hydroxyl group and its subsequent CO eliminated ions, could be utilized to distinguish between isomers of bufadienolides with the carbonyl group substituted at C-11 and C-12 position.
Conjugated bufadienolide (substituted with arginine diacyl chain)
For conjugated bufadienolide, strong arginine diacyl chain ions and a series of fragment ions formed by the subsequent fragmentation of theses diacyl chains were given due to the strong ability of N to stabilize the positive charge. Meanwhile, the bufadienolide part, giving rather weak ion peaks in MS/MS spectrum, was eliminated as a neutral molecular (Figures 4B,C). (3-suberoyl-L-arginine)-Mainobufagin is a typical conjugated bufadienolide with an obvious [M + H]+ ion at m/z 713.4089 in its MS spectrum. After CID, a series of MS/MS fragment ions, including [M + H-18]+ (m/z, 695.3990), [M + H-36]+ (m/z, 677.3921), [M + H-382]+ (m/z, 331.1958), and [M + H-382-156]+ (m/z, 175.1188), were produced. When employing negative ion mode and increasing the induced collision voltage in MS/MS, the ion intensity of bufadienolide part could be significantly increased. However, it is still rather weak compared to that of arginine diacyl chain fragmentation.
MS fragmentation principles of bufadienolide could be primarily and briefly summarized as follows: 1) Bufadienolide containing solely hydroxyl groups can be detected by consecutive losses of H2O and CO, and the amount of hydroxyl groups is generally associated with the resulting ion profile. Unfortunately, determination the hydroxyl substitution location solely based on MS/MS data is difficult or impossible. Meanwhile, the achievement of the 14-OH and 14/15-epoxy ring distinguishment is also impossible just by the MS/MS profile; 2) CO elimination is informative. It may indicate the presence of a C-10 aldehyde group and a carbonyl group at C-11 or C-12 position; 3) The specific loss of 30 Da (HCHO) indicates the presence of a C-19 hydroxyl group; 4) The characteristic loss of 42 Da (CH2CO) and 60 Da (HOAc) indicates the presence of an acetoxyl group at the C-16 position; 5) Strong arginine diacyl chain ion peaks, accompanied by a series of fragment ions formed by the subsequent elimination of these chains, indicate the presence of arginine diacyl chains for conjugated bufadienolide (Figure 4D).
Anti-inflammatory efficacy
On account of the molecular level regulatory ability and specific Na+/K+ ATPase inhibitory activity, bufadienolide exhibited excellent bioactivities, including cardiotonic, anti-inflammatory, anti-tumor, immunoregulatory, NO production, renal sodium excretion and blood pressure stimulating (Prassas and Diamandis 2008; de Vasconcelos et al., 2011; Gao et al., 2011; Wen et al., 2014). Of the whole activities defined for bufadienolide, anti-inflammatory activity may be one of the most promising and interesting research topics.
Specific inhibition of Na+/K+-ATPase
Na+/K+ ATPase was discovered in 1957 by the Danish scientist Jens Christian Skou, who was awarded a Nobel Prize for this milestone discovery in 1997 (Boyer et al., 1997). The predominant function of Na+/K+ ATPase is to transport K+ and Na+ in a manner of reverse gradient using the energy fueled by ATP hydrolysis. For a detailed transport procedure, two K+ are transported into the cell, while three Na+ are transferred out of the cell. By cooperating with ion free diffusion, cell resting potential could be maintained to a state that electrochemical gradient keeps equal on both sides of the cell membrane (Figure 5A). This discovery marked an important step forward in the understanding of how ions get into and out of cells (Jørgensen 1982; Nyblom et al., 2013).
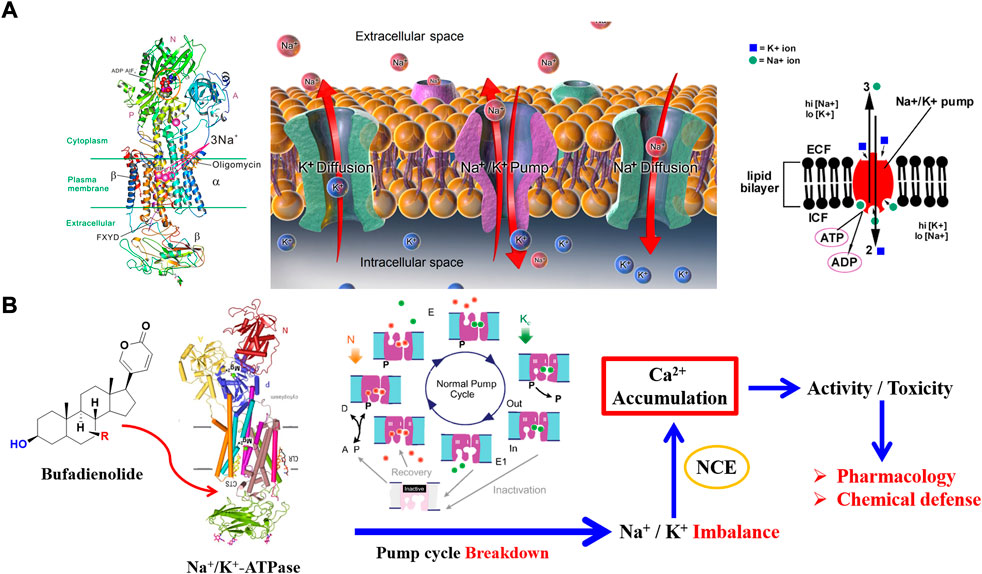
FIGURE 5. Specific inhibition of bufadienolide against Na+/K+ ATPase: (A) The crystal structure and function of Na+/K+ ATPase; (B) The primary act mechanism of bufadienolide against Na+/K+ ATPase.
Structurally, three subunits (α subunit, β subunit, and FXYD subunit) compose the structure of Na+/K+ ATPase (Figure 5A). It is the distribution and expression difference of the three subunits in various tissue that achieves the functional diversity of Na+/K+ ATPase (Morth et al., 2007; Kanai et al., 2013). There exist four subtypes of α subunit, namely α1, α2, α3, and α4 subunit, that have been discovered in human beings (Sweadner 1989). Of the four subtypes of α subunit, α1 subtype is predominant and is found in almost all the body cells. In contrast, α2 subtype, α3 subtype, and α4 subtype mainly distribute in cardiomyocytes, nerve cells, and testis tissue, respectively.
The most attractive point of bufadienolide is cardiotonic activity, which is triggered by the specific inhibition of Na+/K+ ATPase. For a detailed action mechanism, the specific inhibition of Na+/K+ ATPase leads to an increase of intracellular Na+ concentration and a decrease of the K+ concentration. The above ions concentration change will activate Na+/Ca2+ exchanger on the sarcoplasmic reticulum to regulate the Ca2+ concentration for a balanced cell electrochemical gradient. Eventually, a positive inotropic activity is achieved via promoting the contraction of troponin in cardiomyocytes triggered by the upregulated Ca2+ concentration (Ogawa et al., 2009; Laursen et al., 2015). However, serious toxicity would be obtained if the intracellular calcium ion is excessively accumulated (Figure 5). As mentioned above, both the cell membrane of the whole tissue in the body almost distributes the α1 subtype of Na+/K+ ATPase, which will result in a narrow therapeutic safety window. Consequently, the therapeutic dose has reached the level of 60% of the toxic dose due to its double-edged sword property (Huang et al., 2019). Thus, it is emergency to discover Na+/K+ ATPase inhibitors with high selectivity that can regulate the activity of specific subtypes of Na+/K+ ATPase present in targeted tissues.
Anti-inflammatory activity
Generally, anti-inflammatory activity is closely linked with anticancer activity. Bufadienolide has been comprehensively studied for its anticancer activity in diverse types of cancer, such as breast cancer, lung carcinoma, human leukemia, gastrointestinal, hepatoma et al. The underlying anticancer mechanisms were always linked with anti-inflammatory pathway (Prassas and Diamandis 2008; Gao et al., 2011; Deng et al., 2021).
It was revealed that chronic inflammation could result in tissue damage accompanied by an increased risk of cancer development. Generally, the microenvironment with inflammation is the prerequisite for cancer progression. Cancer tissues would frequently produce inflammation without the occurrence of precancerous inflammation (Coussens and Werb 2002). For cancer-involved inflammation, the expression level of numerous inflammatory products is dramatically affected by a predominant transcription factor namely NF-κB (Liu et al., 2017). Consequently, inflammatory diseases, such as rheumatoid arthritis, could be treated by some anticancer drugs. In turn, it provides a promising approach for cancer prevention and therapy by inflammation elimination (Todoric et al., 2016).
Bufadienolide has been long reported for anti-inflammatory activity, especially for cancer-involved inflammation (Gao et al., 2011; Qi et al., 2014; Rong et al., 2014). Ye found that bufalin exhibited anti-inflammatory activity by blocking TNF (tumor necrosis factor), which was mediated via the NF-κB nuclear translocation. Jiang found that NF-κB expression and phosphorylation could be suppressed by bufalin (Jiang et al., 2010), which supported the previous conclusion. An investigation led by Chen revealed that phosphoinisitide-3-kinase (PI3K) and phosphorylation of AKT could be attenuated by bufalin in the SK-Hep1 cell line. Importantly, this attenuation was triggered by the decreased expression level of NF-κB (Chen et al., 2015). Furthermore, Dong demonstrated that the expression of NF-κB p65 protein could be dramatically suppressed by cinobufagin in the HepG2 cell line (Dong et al., 2010).
Meanwhile, NO, a key inflammatory factor, was found to be a predominant signaling molecule regulated by bufadienolide in the tumorigenesis process. Bhuiyan further confirmed the NO regulatory ability of bufalin (Bhuiyan et al., 2003). Their work also showed an interesting phenomenon: NO production was significantly up-regulated by a low concentration of bufalin, while bufalin with a high concentration gave the opposite result. In another study led by Kim via the BV2 microglial cell line, it was found that the expression of two key inflammatory-related enzymes, namely iNOS and COX-2, was significantly inhibited by Chansu. At the same time, it also suppressed the production of NO and PGE2 (Kim et al., 2008). Wang further discovered the powerful anti-inflammatory activity of Huachansu. It was found that TNF-α mediated NF-κB and COX-2 activation were inhibited, and the expression levels of two pro-inflammatory cytokines, namely IL-6 and IL-8, were decreased by bufadienolide in Huchansu (Wang et al., 2019).
Structure modification
Despite the excellent pharmacological activity of bufadienolide, (cardio)toxicity is a non-negligible drawback (Li et al., 2015). In addition, as a specific inhibitor of Na+/K+ ATPase, bufadienolide would result in serious side effects, including cardiac dysfunction, conduction block, and arrhythmia (Bagrov et al., 2009). It is this serious side effect that severely limits the further pharmacological application of bufadienolide. Therefore, researchers have lit a way to discover bufadienolide derivatives with reserved activity and reduced side effects by performing structure modification via biosynthesis.
Plant suspension culture cell transformation
It is a promising way to employ plant suspension culture cells to perform desired structure modification. A series of works have been reported for bufadienolide structural modification via plant suspension culture cells. Ye performed biotransformation of cinobufagin via suspension culture cells originated from Catharanthus roseus and Platycodon grandiflorum. Three cinobufagin derivatives, namely 3-epi-desacetylcinobufagin, desacetylcinobufagin and 1-hydroxyyl desacetylcinobufagin, were obtained (Ye et al., 2003). Xue also modified telocinobufagin by utilizing Catharanthus roseus suspension culture cells, and a new bufadienolide namely 3-epi-telocinobufagin was produced. Three bufadienolides, including bufatalin, gamabufalin and telocinobufagin, were biotranformed by Zhang via Saussurea involucrate suspension culture cells. Fortunately, eleven bufadienolide derivatives were obtained, which strongly supported the practicability of plant suspension culture cell transformation for bufadienolide structure modification (Zhang et al., 2011).
Microbial transformation
Besides the plant suspension culture cell approach, microbial transformation is also a promising alternative. Alternaria alternata was employed by Ye et al. to perform the biotransformation of cinobufagin (Ye and Guo 2008), and six cinobufagin derivatives were obtained. After reaction condition optimization, cinobufagin could be totally converted to the major transformation product 12β-hydroxy-cinobufagin. Importantly, Ma discovered that Fusarium solani could achieve an isomerization reaction for bufadienolide. In detail, it could convert bufadienolide with 3β-hydroxyl configuration to 3α-hydroxyl configuration, and selectively oxidize the 3β-hydroxyl, instead of 3α-hydroxyl, to ketone (Ma et al., 2007).
The nature of the above two approaches is to employ the corresponding enzymes therein with satisfied substrate heterogeneity for bufadienolide structure modification. The operation simplicity and mildness of reaction conditions made them effective approaches to replace chemical synthesis. Nonetheless, there existed obvious drawbacks of these transformation approaches. Of which the most annoying aspect is that these transformations did not exhibit any selectivity to the desired targets due to too many enzymes involving the transformation. This also resulted in the relatively low yield of desired products. For example, only 2% of yield was obtained for cinobufagin biotransformation via plant suspension cells. Therefore, both the above two approaches exhibit some limitations and could not be applied to an industrial scale (Yue et al., 2016).
Enzyme-catalyzed glycosylation
To obtain structurally modified bufadienolides with reduced toxicity, glycosylation may be the first choice with a bright future. In comparison to conventional chemical synthesis, laborious protection/deprotection processes are avoided, and the process is more eco-friendly. Meanwhile, in contrast to plant suspension cell and microbial transformation, enzyme catalytic glycosylation is more efficient and exhibits perfect regional or stereoselectivity, allowing it to be directly exploited to produce structure-modified bufadienolides with the desired property (Zhang et al., 2006). Currently, enzyme catalytic glycosylation has become a focal point of study for bufadienolide structure modification (Elshahawi et al., 2015).
In 2007, Thorson completed the first enzyme catalyzed glycosylation of cardiac steroids. They discovered an actinomycete-sourced glycosyltransferase OleD. After high-throughput screening, a mutant named ASP with high substrate heterogeneity was further discovered (Williams et al., 2007). At the same time, Thorson dug the catalysis property of glycosyltransferase OleD/ASP. It was found that OleD/ASP did not exhibit steroid core site selectivity, and both C-3 and C-12 positions could be glycosylated without any differentiation. Accordingly, Zhu skillfully employed a pair of bufadienolides with different configuration of hydroxyl group at the C-3 position to further explore the stereoselectivity of OleD/ASP. It was revealed that OleD/ASP exhibited highly stereoselectivity: bufadienolide with 3β-OH configuration could be glycosylated, while it was powerless to the bufadienolide with 3α-OH configuration (Zhu et al., 2018).
Promisingly, Wen excavated a glycosyltransferase named UGT74AN1 from Asclepias curassavica, and it could conduct the glycosylation of bufadienolide specifically at C-3 position (Wen et al., 2018) (Figures 6A,B). UGT74AN1 is the first reported steroid glycosyltransferase to generate 3-O-β-D-glucoside of bufadienolide with high efficiency and regiospecificity. Furthermore, Huang developed a one-pot enzymatic system coupling UGT74AN3 and CGTase with high efficiency to generate bufadienolides linked with sugar chains of varying lengths at the C-3 position (Huang et al., 2019) (Figure 6C). Importantly, the glycosylation products displayed improved inhibitory efficacy with specificity for the Na+/K+ ATPase α2 subunit.
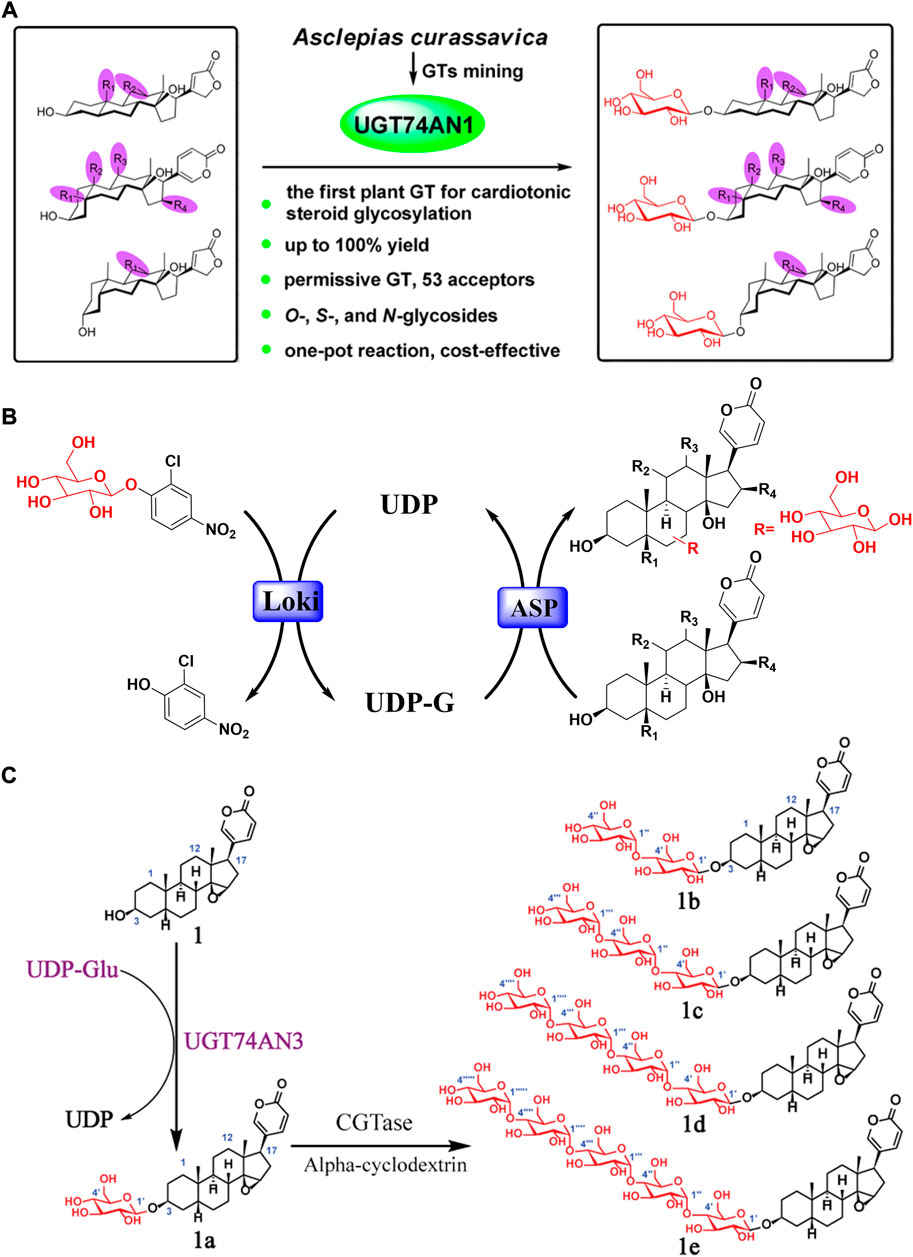
FIGURE 6. Glycosylation structure modification of bufadienolide: (A) UGT74AN1 catalyzed glycosylation of bufadienolide with regiospecificity; (B) The typical one-pot glycosylation system for bufadienolide; (C) Bufadienolide derivatives linked with sugar chains of varied length at the C-3 position obtained by enzyme catalyzed glycosylation.
In addition, Ye modified bufadienlide at the C-3 position via glycosyltransferase Yjic1. It gave a mono-glycoside (bufalin 3-O-β-D-glucoside) and a di-glycoside (bufalin 3-O-β-D-glccosyl (1→2)-β-D-glucoside) with satisfying yields. Compared to the substrate, the solubility of corresponding glycosides was increased by 25-fold and 102-fold, respectively (Li et al., 2017). Brilliantly, Fu first reported the glycosylation of bufadienolide mixtures by glycosyltransferase Yjic1. The subsequent zebrafish embryo toxicity experiment revealed that the toxicity of glycosylated bufadienolides were much less than the corresponding aglycone (Fu et al., 2019).
Conclusion
The review has highlighted toad-sourced bufadienolides and their anti-inflammatory activity. Based on whether the C-3 hydroxyl group is substituted or not, two types of toad-derived bufadienolide (free or conjugated bufadienolide) were distinguished. And the structural diversity, as well as their MS fragmentation principles, have also been primarily summarized, laying a foundation for future analysis. In addition, the remarkable Na+/K+ ATPase inhibitory and anti-inflammatory efficacy reported for bufadienolide were also briefly highlighted. During the research process, the serious toxicity pushed the development of structural modification of bufadienolide via biotransformation for side effects reduction. It lighted a promising way for broadening the structural diversity and enhancing the pharmacological activity of bufadienolide. The current evidence of bufadienolide we summarized would provide a scientific basis for future in-depth studies and perspectives of potential drug candidate discovery.
Author contributions
DZ conceived, wrote and revised the article. QW, YL, LH, and DL wrote and revised the article. TC, DS, TY, YW, MG, and FH participated in the work of references selection and organization.
Funding
This work was supported by the National Natural Science Foundation of China (No. 32260129), the General Program of Natural Science Foundation of Qinghai Province (2021-ZJ-976Q), General Program of Qinghai Normal University (2020QZR016).
Conflict of interest
The authors declare that the research was conducted in the absence of any commercial or financial relationships that could be construed as a potential conflict of interest.
Publisher’s note
All claims expressed in this article are solely those of the authors and do not necessarily represent those of their affiliated organizations, or those of the publisher, the editors and the reviewers. Any product that may be evaluated in this article, or claim that may be made by its manufacturer, is not guaranteed or endorsed by the publisher.
References
Bagrov, A. Y., Shapiro, J. I., and Fedorova, O. V. (2009). Endogenous cardiotonic steroids: Physiology, pharmacology, and novel therapeutic targets. Pharmacol. Rev. 61 (1), 9–38. doi:10.1124/pr.108.000711
Bhatti, H. N., and Khera, R. A. (2012). Biological transformations of steroidal compounds: A review. Steroids 77 (12), 1267–1290. doi:10.1016/j.steroids.2012.07.018
Bhuiyan, M. B. A., Fant, M. E., and Dasgupta, A. (2003). Study on mechanism of action of Chinese medicine chan su: Dose-dependent biphasic production of nitric oxide in trophoblastic BeWo cells. Clin. Chim. Acta. 330 (1-2), 179–184. doi:10.1016/S0009-8981(03)00047-0
Bókony, V., Üveges, B., Móricz, Á. M., and Hettyey, A. (2018). Competition induces increased toxin production in toad larvae without allelopathic effects on heterospecific tadpoles. Funct. Ecol. 32 (3), 667–675. doi:10.1111/1365-2435.12994
Boyer, P. D., Walker, J. E., and Skou, J. C. (1997). Nobel prize in chemistry 1997. Med. (B Aires. 58, 107.
Chen, H., Meng, Y.-H., Guo, D.-A., Liu, X., Liu, J.-H., and Hu, L.-H. (2015). New cytotoxic 19-norbufadienolide and bufogargarizin isolated from Chan Su. Fitoterapia 104, 1–6. doi:10.1016/j.fitote.2015.05.011
Chen, Y.-l., Bian, X.-l., Guo, F.-j., Wu, Y.-c., and Li, Y.-m. (2018). Two new 19-norbufadienolides with cardiotonic activity isolated from the venom of Bufo bufo gargarizans. Fitoterapia 131, 215–220. doi:10.1016/j.fitote.2018.10.023
Chen, Y. Y., Lu, H. F., Hsu, S. C., Kuo, C. L., Chang, S. J., Lin, J. J., et al. (2015). Bufalin inhibits migration and invasion in human hepatocellular carcinoma SK‐Hep1 cells through the inhibitions of NF-kB and matrix metalloproteinase-2/-9-signaling pathways. Environ. Toxicol. 30 (1), 74–82. doi:10.1002/tox.21896
Coussens, L. M., and Werb, Z. (2002). Inflammation and cancer. Nature 420 (6917), 860–867. doi:10.1038/nature01322
de Vasconcelos, D. I. B., Leite, J. A., Carneiro, L. T., Piuvezam, M. R., de Lima, M. R. V., de Morais, L. C. L., et al. (2011). Anti-inflammatory and antinociceptive activity of ouabain in mice. Mediat. Inflamm. 2011, 912925. doi:10.1155/2011/912925
Deng, L.-J., Lei, Y.-H., Quan, J.-Y., Li, B.-J., Zhang, D.-M., Tian, H.-Y., et al. (2021). 1β–OH–arenobufagin induces mitochondrial apoptosis in hepatocellular carcinoma through the suppression of mTOR signaling pathway. J. Ethnopharmacol. 266, 113443. doi:10.1016/j.jep.2020.113443
Deng, L.-J., Wang, L.-H., Peng, C.-K., Li, Y.-B., Huang, M.-H., Chen, M.-F., et al. (2017). Fibroblast activation protein α activated tripeptide bufadienolide antitumor prodrug with reduced cardiotoxicity. J. Med. Chem. 60 (13), 5320–5333. doi:10.1021/acs.jmedchem.6b01755
Dobler, S., Dalla, S., Wagschal, V., and Agrawal, A. A. (2012). Community-wide convergent evolution in insect adaptation to toxic cardenolides by substitutions in the Na, K-ATPase. Proc. Natl. Acad. Sci. U. S. A. 109 (32), 13040–13045. doi:10.1073/pnas.120211110910.1073/pnas.1202111109
Dong, Y., Ma, W., Gu, J., and Zheng, W. (2010). Effect of cinobufagin on nuclear factor-kappaB pathway in HepG2 cells. Nan Fang. Yi Ke Da Xue Xue Bao 30 (1), 137–139.
Elshahawi, S. I., Shaaban, K. A., Kharel, M. K., and Thorson, J. S. (2015). A comprehensive review of glycosylated bacterial natural products. Chem. Soc. Rev. 44 (21), 7591–7697. doi:10.1039/C4CS00426D
Esposito, A. L. (1985). Digoxin disrupts the inflammatory response in experimental pneumococcal pneumonia. J. Infect. Dis. 152 (1), 14–23. doi:10.1093/infdis/152.1.14
Forshammar, J., Block, L., Lundborg, C., Biber, B., and Hansson, E. (2011). Naloxone and ouabain in ultralow concentrations restore Na+/K+-ATPase and cytoskeleton in lipopolysaccharide-treated astrocytes. J. Biol. Chem. 286 (36), 31586–31597. doi:10.1074/jbc.M111.247767
Forshammar, J., Jörneberg, P., Björklund, U., Westerlund, A., Lundborg, C., Biber, B., et al. (2013). Anti-inflammatory substances can influence some glial cell types but not others. Brain Res. 1539, 34–40. doi:10.1016/j.brainres.2013.09.052
Fu, Z.-H., Wen, C., Ye, Q.-M., Huang, W., Liu, X.-M., and Jiang, R.-W. (2019). An efficient strategy for the glycosylation of total bufadienolides in venenum bufonis. ACS Omega 4 (4), 6819–6825. doi:10.1021/acsomega.9b00386
Fürst, R., Zündorf, I., and Dingermann, T. (2017). New knowledge about old drugs: The anti-inflammatory properties of cardiac glycosides. Planta Med. 83 (12/13), 977–984. doi:10.1055/s-0043-105390
Gantt, R. W., Goff, R. D., Williams, G. J., and Thorson, J. S. (2008). Probing the aglycon promiscuity of an engineered glycosyltransferase. Angew. Chem. Int. Ed. Engl. 47 (46), 8889–8892. doi:10.1002/anie.200803508
Gao, H., Popescu, R., Kopp, B., and Wang, Z. (2011). Bufadienolides and their antitumor activity. Nat. Prod. Rep. 28 (5), 953–969. doi:10.1039/C0NP00032A
Gao, H., Zehl, M., Kaehlig, H., Schneider, P., Stuppner, H., Moreno, L., et al. (2010). Rapid structural identification of cytotoxic bufadienolide sulfates in toad venom from Bufo melanosticus by LC-DAD-MS n and LC-SPE-NMR. J. Nat. Prod. 73 (4), 603–608. doi:10.1021/np900746k
Guillarme, D., Ruta, J., Rudaz, S., and Veuthey, J.-L. (2010). New trends in fast and high-resolution liquid chromatography: A critical comparison of existing approaches. Anal. Bioanal. Chem. 397 (3), 1069–1082. doi:10.1007/s00216-009-3305-8
Han, L., Si, N., Gao, B., Wang, H., Zhou, Y., Yang, J., et al. (2017). Metabolites analysis of 4 arenobufagin structure-related bufadienolides in human liver microsomes using UHPLC-ESI-Orbitrap mass. Int. J. Mass Spectrom. 422, 88–93. doi:10.1016/j.ijms.2017.08.011
Han, L., Wang, H., Si, N., Ren, W., Gao, B., Li, Y., et al. (2016). Metabolites profiling of 10 bufadienolides in human liver microsomes and their cytotoxicity variation in HepG2 cell. Anal. Bioanal. Chem. 408 (10), 2485–2495. doi:10.1007/s00216-016-9345-y
Hayes, R. A., Crossland, M. R., Hagman, M., Capon, R. J., and Shine, R. (2009). Ontogenetic variation in the chemical defenses of cane toads (Bufo marinus): Toxin profiles and effects on predators. J. Chem. Ecol. 35 (4), 391–399. doi:10.1007/s10886-009-9608-6
Heasley, B. (2012). Chemical synthesis of the cardiotonic steroid glycosides and related natural products. Chemistry 18 (11), 3092–3120. doi:10.1002/chem.201103733
Hegazy, M.-E. F., Mohamed, T. A., ElShamy, A. I., Abou-El-Hamd, H. M., Mahalel, U. A., Reda, E. H., et al. (2015). Microbial biotransformation as a tool for drug development based on natural products from mevalonic acid pathway: A review. J. Adv. Res. 6 (1), 17–33. doi:10.1016/j.jare.2014.11.009
Holmgren, M., Wagg, J., Bezanilla, F., Rakowski, R. F., De Weer, P., and Gadsby, D. C. (2000). Three distinct and sequential steps in the release of sodium ions by the Na+/K+-ATPase. Nature 403 (6772), 898–901. doi:10.1038/35002599
Hoshino, Y., and Gaucher, E. A. (2021). Evolution of bacterial steroid biosynthesis and its impact on eukaryogenesis. Proc. Natl. Acad. Sci. U. S. A. 118 (25), e2101276118. doi:10.1073/pnas.2101276118
Huang, W., Wen, C., Zhou, Z. R., Fu, Z. H., Katz, A., Plotnikov, A., et al. (2019). An efficient one‐pot enzymatic synthesis of cardiac glycosides with varied sugar chain lengths. Adv. Synth. Catal. 361 (13), 3114–3119. doi:10.1002/adsc.201900227
Jiang, Y., Zhang, Y., Luan, J., Duan, H., Zhang, F., Yagasaki, K., et al. (2010). Effects of bufalin on the proliferation of human lung cancer cells and its molecular mechanisms of action. Cytotechnology 62 (6), 573–583. doi:10.1007/s10616-010-9310-0
Jørgensen, P. L. (1982). Mechanism of the Na+, K+ pump protein structure and conformations of the pure (Na++ K+)-ATPase. Biochim. Biophys. Acta 694 (1), 27–68. doi:10.1016/0304-4157(82)90013-2
Kamano, Y., Nogawa, T., Yamashita, A., Hayashi, M., Inoue, M., Drašar, P., et al. (2002). Isolation and structure of a 20, 21-epoxybufenolide series from “ch'an su”. J. Nat. Prod. 65 (7), 1001–1005. doi:10.1021/np0200360
Kanai, R., Ogawa, H., Vilsen, B., Cornelius, F., and Toyoshima, C. (2013). Crystal structure of a Na+-bound Na+, K+-ATPase preceding the E1P state. Nature 502 (7470), 201–206. doi:10.1038/nature12578
Kim, M.-H., Lyu, J.-H., Lyu, S., Hong, S.-H., Kim, W.-I., Yoon, H.-J., et al. (2008). Inhibitory effect of Chan-Su on the secretion of PGE2 and NO in LPS-stimulated BV2 microglial cells. J. Physiology Pathology Korean Med. 22 (5), 1315–1321.
Lancaster, M., and Vegad, J. (1967). Suppression of the early inflammatory response in the sheep by strophanthin G. Nature 213 (5078), 840–841. doi:10.1038/213840b0
Laursen, M., Gregersen, J. L., Yatime, L., Nissen, P., and Fedosova, N. U. (2015). Structures and characterization of digoxin-and bufalin-bound Na+, K+-ATPase compared with the ouabain-bound complex. Proc. Natl. Acad. Sci. U. S. A. 112 (6), 1755–1760. doi:10.1073/pnas.1422997112
Lee, S.-S., Derguini, F., Bruening, R. C., Nakanishi, K., Wallick, E. T., Akizawa, T., et al. (1994). Digitalis-like compounds of toad bile: Sulfation and reduction of bufadienolides decrease potency of Na+, K+-ATPase inhibition. Heterocycles 2 (39), 669–686. doi:10.3987/com-94-s(b)59
Li, B.-J., Tian, H.-Y., Zhang, D.-M., Lei, Y.-H., Wang, L., Jiang, R.-W., et al. (2015). Bufadienolides with cytotoxic activity from the skins of Bufo bufo gargarizans. Fitoterapia 105, 7–15. doi:10.1016/j.fitote.2015.05.013
Li, F. J., Hu, J. H., Ren, X., Zhou, C. M., Liu, Q., and Zhang, Y. Q. (2021). Toad venom: A comprehensive review of chemical constituents, anticancer activities, and mechanisms. Arch. Pharm. 354 (7), 2100060. doi:10.1002/ardp.202100060
Li, K., Feng, J., Kuang, Y., Song, W., Zhang, M., Ji, S., et al. (2017). Enzymatic synthesis of bufadienolide O‐glycosides as potent antitumor agents using a microbial glycosyltransferase. Adv. Synth. Catal. 359 (21), 3765–3772. doi:10.1002/adsc.201700777
Li, W., Lin, X., Yang, Z., Zhang, W., Ren, T., Qu, F., et al. (2015). A bufadienolide-loaded submicron emulsion for oral administration: Stability, antitumor efficacy and toxicity. Int. J. Pharm. 479 (1), 52–62. doi:10.1016/j.ijpharm.2014.12.054
Liu, T., Zhang, L., Joo, D., and Sun, S.-C. (2017). NF-κB signaling in inflammation. Signal Transduct. Target. Ther. 2 (1), 17023–17029. doi:10.1038/sigtrans.2017.23
Ma, X.-C., Zheng, J., and Guo, D.-A. (2007). Highly selective isomerization and dehydrogenation of three major bufadienolides at 3-OH by Fusarium solani. Enzyme Microb. Technol. 40 (6), 1585–1591. doi:10.1016/j.enzmictec.2006.11.015
Matsukawa, M., Akizawa, T., Morris, J. F., Butler, J., and Yoshioka, M. (1996). Marinoic acid, a novel bufadienolide-related substance in the skin of the giant toad, Bufo marinus. Chem. Pharm. Bull. 44 (1), 255–257. doi:10.1248/cpb.44.255
Matsukawa, M., Akizawa, T., Ohigashi, M., Morris, J. F., Butler, J., and Yoshioka, M. (1997). A novel bufadienolide, marinosin, in the skin of the giant toad, Bufo marinus. Chem. Pharm. Bull. 45 (2), 249–254. doi:10.1248/cpb.45.249
Matsukawa, M., Mukai, T., Akizawa, T., Miyatake, S., Yoshioka, M., Morris, J. F., et al. (1998). Isolation and characterization of novel endogenous digitalis-like factors in the ovary of the giant toad, Bufo m arinus. J. Nat. Prod. 61 (12), 1476–1481. doi:10.1021/np980189g
Meng, Q., Yau, L.-F., Lu, J.-G., Wu, Z.-Z., Zhang, B.-X., Wang, J.-R., et al. (2016). Chemical profiling and cytotoxicity assay of bufadienolides in toad venom and toad skin. J. Ethnopharmacol. 187, 74–82. doi:10.1016/j.jep.2016.03.062
Michalak, M., Michalak, K., and Wicha, J. (2017). The synthesis of cardenolide and bufadienolide aglycones, and related steroids bearing a heterocyclic subunit. Nat. Prod. Rep. 34 (4), 361–410. doi:10.1039/C6NP00107F
Mohammadi, S., Gompert, Z., Gonzalez, J., Takeuchi, H., Mori, A., and Savitzky, A. H. (2016). Toxin-resistant isoforms of Na+/K+-ATPase in snakes do not closely track dietary specialization on toads. Proc. Biol. Sci. 283 (1842), 20162111. doi:10.1098/rspb.2016.2111
Morth, J. P., Pedersen, B. P., Toustrup-Jensen, M. S., Sørensen, T. L.-M., Petersen, J., Andersen, J. P., et al. (2007). Crystal structure of the sodium–potassium pump. Nature 450 (7172), 1043–1049. doi:10.1038/nature06419
Nogawa, T., Kamano, Y., Yamashita, A., and Pettit, G. R. (2001). Isolation and structure of five new cancer cell growth inhibitory bufadienolides from the Chinese traditional drug Ch'an Su. J. Nat. Prod. 64 (9), 1148–1152. doi:10.1021/np0101088
Nyblom, M., Poulsen, H., Gourdon, P., Reinhard, L., Andersson, M., Lindahl, E., et al. (2013). Crystal structure of Na+, K+-ATPase in the Na+-bound state. Science 342 (6154), 123–127. doi:10.1126/science.1243352
Ogawa, H., Shinoda, T., Cornelius, F., and Toyoshima, C. (2009). Crystal structure of the sodium-potassium pump (Na+, K+-ATPase) with bound potassium and ouabain. Proc. Natl. Acad. Sci. U. S. A. 106 (33), 13742–13747. doi:10.1073/pnas.0907054106
Pavesi, C., Flon, V., Mann, S., Leleu, S., Prado, S., and Franck, X. (2021). Biosynthesis of azaphilones: A review. Nat. Prod. Rep. 38 (6), 1058–1071. doi:10.1039/D0NP00080A
Pearson, K. C., and Tarvin, R. D. (2022). A review of chemical defense in harlequin toads (Bufonidae; Atelopus). Toxicon X, 100092. doi:10.1016/j.toxcx.2022.100092
Petroselli, G., Raices, M., Jungblut, L. D., Pozzi, A. G., and Erra-Balsells, R. (2018). MALDI-MS argininyl bufadienolide esters fingerprint from parotoid gland secretions of Rhinella arenarum: Age, gender, and seasonal variation. J. Mass Spectrom. 53 (6), 465–475. doi:10.1002/jms.4082
Petschenka, G., and Agrawal, A. A. (2015). Milkweed butterfly resistance to plant toxins is linked to sequestration, not coping with a toxic diet. Proc. Biol. Sci. 282 (1818), 20151865. doi:10.1098/rspb.2015.1865
Prassas, I., and Diamandis, E. P. (2008). Novel therapeutic applications of cardiac glycosides. Nat. Rev. Drug Discov. 7 (11), 926–935. doi:10.1038/nrd2682
Qi, J., Tan, C., Hashimi, S. M., Zulfiker, A. H. M., Good, D., and Wei, M. Q. (2014). Toad glandular secretions and skin extractions as anti-inflammatory and anticancer agents. Evid. Based. Complement. Altern. Med. 2014, 312684. doi:10.1155/2014/312684
Rodríguez, C., Rollins-Smith, L., Ibáñez, R., Durant-Archibold, A. A., and Gutiérrez, M. (2017). Toxins and pharmacologically active compounds from species of the family Bufonidae (Amphibia, Anura). J. Ethnopharmacol. 198, 235–254. doi:10.1016/j.jep.2016.12.021
Rong, X., Ni, W., Liu, Y., Wen, J., Qian, C., Sun, L., et al. (2014). Bufalin, a bioactive component of the Chinese medicine chansu, inhibits inflammation and invasion of human rheumatoid arthritis fibroblast-like synoviocytes. Inflammation 37 (4), 1050–1058. doi:10.1007/s10753-014-9828-y
Shao, H., Li, B., Li, H., Gao, L., Zhang, C., Sheng, H., et al. (2021). Novel strategies for solubility and bioavailability enhancement of bufadienolides. Molecules 27 (1), 51. doi:10.3390/molecules27010051
Shimizu, S., Hagiwara, K., Itoh, H., and Inoue, M. (2020). Unified total synthesis of five bufadienolides. Org. Lett. 22 (21), 8652–8657. doi:10.1021/acs.orglett.0c03251
Silva, D. B., and Lopes, N. P. (2015). MALDI-MS of flavonoids: A systematic investigation of ionization and in‐source dissociation mechanisms. J. Mass Spectrom. 50 (1), 182–190. doi:10.1002/jms.3516
Smedley, S. R., Risteen, R. G., Tonyai, K. K., Pitino, J. C., Hu, Y., Ahmed, Z. B., et al. (2017). Bufadienolides (lucibufagins) from an ecologically aberrant firefly (Ellychnia corrusca). Chemoecology 27 (4), 141–153. doi:10.1007/s00049-017-0240-6
Steyn, P. S., and van Heerden, F. R. (1998). Bufadienolides of plant and animal origin. Nat. Prod. Rep. 15 (4), 397–413. doi:10.1039/A815397Y
Stoll, A., Suter, E., Kreis, W., Bussemaker, B. B., and Hofmann, A. (1933). Die herzaktiven Substanzen der Meerzwiebel. Scillaren A. Helv. Chim. Acta 16, 703–733. doi:10.1002/hlca.19330160198
Sweadner, K. J. (1989). Isozymes of the Na+/K+-AtPase. Biochim. Biophys. Acta 988 (2), 185–220. doi:10.1016/0304-4157(89)90019-1
Thorson, J. S., and Vogt, T. (2003). Glycosylated natural products. Carbohydrate-based drug discovery. New York City, NY: John Wiley&Sons, Ltd., 685–711.
Tian, H.-Y., Ruan, L.-J., Yu, T., Zheng, Q.-F., Chen, N.-H., Wu, R.-B., et al. (2017). Bufospirostenin A and Bufogargarizin C, steroids with rearranged skeletons from the toad Bufo bufo gargarizans. J. Nat. Prod. 80 (4), 1182–1186. doi:10.1021/acs.jnatprod.6b01018
Tian, H. Y., Wang, L., Zhang, X. Q., Wang, Y., Zhang, D. M., Jiang, R. W., et al. (2010). Bufogargarizins A and B: Two novel 19‐norbufadienolides with unprecedented skeletons from the venom of Bufo bufo gargarizans. Chemistry 16 (36), 10989–10993. doi:10.1002/chem.201000847
Todoric, J., Antonucci, L., and Karin, M. (2016). Targeting inflammation in cancer prevention and therapy. Cancer Prev. Res. 9 (12), 895–905. doi:10.1158/1940-6207.CAPR-16-0209
Tureček, F., and Hanuš, V. (1984). Retro-Diels-Alder reaction in mass spectrometry. Mass Spectrom. Rev. 3 (1), 85–152. doi:10.1002/mas.1280030104
Ujvari, B., Casewell, N. R., Sunagar, K., Arbuckle, K., Wüster, W., Lo, N., et al. (2015). Widespread convergence in toxin resistance by predictable molecular evolution. Proc. Natl. Acad. Sci. U. S. A. 112 (38), 11911–11916. doi:10.1073/pnas.1511706112
Verpoorte, R., and Svendsen, A. B. (1979). Chemical constituents of Vietnamese toad venom collected from Bufo melanostictus schneider: Part I. The sterols. J. Ethnopharmacol. 1 (2), 197–202. doi:10.1016/0378-8741(79)90007-2
Verpoorte, R., and Svendsen, A. B. (1980). Chemical constituents of Vietnamese toad venom, collected from Bufo melanostictus Schneider. Part II. The bufadienolides. J. Nat. Prod. 43 (3), 347–352. doi:10.1021/np50009a005
Wang, S.-w., Bai, Y.-f., Weng, Y.-y., Fan, X.-y., Huang, H., Zheng, F., et al. (2019). Cinobufacini ameliorates dextran sulfate sodium–induced colitis in mice through inhibiting M1 macrophage polarization. J. Pharmacol. Exp. Ther. 368 (3), 391–400. doi:10.1124/jpet.118.254516
Wei, W.-L., Hou, J.-J., Wang, X., Yu, Y., Li, H.-J., Li, Z.-W., et al. (2019). Venenum bufonis: An overview of its traditional use, natural product chemistry, pharmacology, pharmacokinetics and toxicology. J. Ethnopharmacol. 237, 215–235. doi:10.1016/j.jep.2019.03.042
Wei, W.-L., Li, H.-J., Yang, W.-Z., Qu, H., Li, Z.-W., Yao, C.-L., et al. (2022). An integrated strategy for comprehensive characterization of metabolites and metabolic profiles of bufadienolides from Venenum Bufonis in rats. J. Pharm. Anal. 12 (1), 136–144. doi:10.1016/j.jpha.2021.02.003
Wen, C., Huang, W., Zhu, X.-L., Li, X.-S., Zhang, F., and Jiang, R.-W. (2018). UGT74AN1, a permissive glycosyltransferase from Asclepias curassavica for the regiospecific steroid 3-O-glycosylation. Org. Lett. 20 (3), 534–537. doi:10.1021/acs.orglett.7b03619
Wen, L., Huang, Y., Xie, X., Huang, W., Yin, J., Lin, W., et al. (2014). Anti-inflammatory and antinociceptive activities of bufalin in rodents. Mediat. Inflamm. 2014, 171839. doi:10.1155/2014/171839
Williams, G. J., Zhang, C., and Thorson, J. S. (2007). Expanding the promiscuity of a natural-product glycosyltransferase by directed evolution. Nat. Chem. Biol. 3 (10), 657–662. doi:10.1038/nchembio.2007.28
Wu, W.-Y., Fan, T.-Y., Li, N.-G., Duan, J.-A., Shi, Z.-H., Chen, M., et al. (2020). Total synthesis, chemical modification and structure-activity relationship of bufadienolides. Eur. J. Med. Chem. 189, 112038. doi:10.1016/j.ejmech.2020.112038
Ye, M., and Guo, D. (2008). A new bufadienolide obtained from the biotransformation of cinobufagin by Alternaria alternata. Nat. Prod. Res. 22 (1), 26–30. doi:10.1080/14786410601130141
Ye, M., Ning, L., Zhan, J., Guo, H., and Guo, D. (2003). Biotransformation of cinobufagin by cell suspension cultures of Catharanthus roseus and Platycodon grandiflorum. J. Mol. Catal. B Enzym. 22 (1-2), 89–95. doi:10.1016/S1381-1177(03)00011-0
Yue, W., Ming, Q.-l., Lin, B., Rahman, K., Zheng, C.-J., Han, T., et al. (2016). Medicinal plant cell suspension cultures: Pharmaceutical applications and high-yielding strategies for the desired secondary metabolites. Crit. Rev. Biotechnol. 36 (2), 215–232. doi:10.3109/07388551.2014.923986
Zhan, X., Wu, H., Wu, H., Wang, R., Luo, C., Gao, B., et al. (2020). Metabolites from Bufo gargarizans (cantor, 1842): A review of traditional uses, pharmacological activity, toxicity and quality control. J. Ethnopharmacol. 246, 112178. doi:10.1016/j.jep.2019.112178
Zhang, C., Griffith, B. R., Fu, Q., Albermann, C., Fu, X., Lee, I.-K., et al. (2006). Exploiting the reversibility of natural product glycosyltransferase-catalyzed reactions. Science 313 (5791), 1291–1294. doi:10.1126/science.1130028
Zhang, P.-W., Tian, H.-Y., Nie, Q.-L., Wang, L., Zhou, S.-W., Ye, W.-C., et al. (2016). Structures and inhibitory activity against breast cancer cells of new bufadienolides from the eggs of toad Bufo bufo gargarizans. RSC Adv. 6 (96), 93832–93841. doi:10.1039/C6RA18676A
Zhang, X., Ye, M., Dong, Y.-h., Hu, H.-b., Tao, S.-j., Yin, J., et al. (2011). Biotransformation of bufadienolides by cell suspension cultures of Saussurea involucrata. Phytochemistry 72 (14-15), 1779–1785. doi:10.1016/j.phytochem.2011.05.004
Zhou, J., Gong, Y., Ma, H., Wang, H., Qian, D., Wen, H., et al. (2015). Effect of drying methods on the free and conjugated bufadienolide content in toad venom determined by ultra-performance liquid chromatography-triple quadrupole mass spectrometry coupled with a pattern recognition approach. J. Pharm. Biomed. Anal. 114, 482–487. doi:10.1016/j.jpba.2015.05.032
Zhou, M., Hou, Y., Hamza, A., Zhan, C.-G., Bugni, T. S., Thorson, J. S., et al. (2012). Probing the regiospecificity of enzyme-catalyzed steroid glycosylation. Org. Lett. 14 (21), 5424–5427. doi:10.1021/ol3024924
Zhou, S.-w., Quan, J.-y., Li, Z.-w., Ye, G., Shang, Z., Chen, Z.-p., et al. (2021). Bufadienolides from the eggs of the toad Bufo bufo gargarizans and their antimelanoma activities. J. Nat. Prod. 84 (5), 1425–1433. doi:10.1021/acs.jnatprod.0c00840
Zhou, S., Zheng, Q., Huang, X., Wang, Y., Luo, S., Jiang, R., et al. (2017). Isolation and identification of l/d-lactate-conjugated bufadienolides from toad eggs revealing lactate racemization in amphibians. Org. Biomol. Chem. 15 (26), 5609–5615. doi:10.1039/C7OB01055A
Zhu, X.-L., Wen, C., Ye, Q.-M., Xu, W., Zou, D.-L., Liang, G.-P., et al. (2018). Probing the stereoselectivity of OleD-catalyzed glycosylation of cardiotonic steroids. RSC Adv. 8 (10), 5071–5078. doi:10.1039/C7RA11979H
Zou, D., Zhu, X., Zhang, F., Du, Y., Ma, J., and Jiang, R. (2018). An efficient strategy based on liquid–liquid extraction with three-phase solvent system and high speed counter-current chromatography for rapid enrichment and separation of epimers of minor bufadienolide from toad meat. J. Agric. Food Chem. 66 (4), 1008–1014. doi:10.1021/acs.jafc.7b05310
Keywords: toad sourced bufadienolide, structure diversity, MS fragmentation principles, anti-inflammatory activity, structure modification
Citation: Zou D, Wang Q, Chen T, Sang D, Yang T, Wang Y, Gao M, He F, Li Y, He L and Longzhu D (2022) Bufadienolides originated from toad source and their anti-inflammatory activity. Front. Pharmacol. 13:1044027. doi: 10.3389/fphar.2022.1044027
Received: 14 September 2022; Accepted: 10 October 2022;
Published: 19 October 2022.
Edited by:
Shuai Wang, Guangzhou University of Chinese Medicine, ChinaReviewed by:
Daijie Wang, Qilu University of Technology, ChinaXiang Luo, Guangzhou University of Chinese Medicine, China
Copyright © 2022 Zou, Wang, Chen, Sang, Yang, Wang, Gao, He, Li, He and Longzhu. This is an open-access article distributed under the terms of the Creative Commons Attribution License (CC BY). The use, distribution or reproduction in other forums is permitted, provided the original author(s) and the copyright owner(s) are credited and that the original publication in this journal is cited, in accordance with accepted academic practice. No use, distribution or reproduction is permitted which does not comply with these terms.
*Correspondence: Denglang Zou, ZGxhbmd6b3VAZm94bWFpbC5jb20=; Yulin Li, bGl5dWxpbkBud2lwYi5jYXMuY24=; Liangliang He, aGVsaWFuZ2xpYW5nNTg3OEAxNjMuY29t; Duojie Longzhu, ODUwMDAyNjMyQHFxLmNvbQ==
†These authors have contributed equally to this work