- 1Department of Critical Care Medicine, Sichuan Academy of Medical Science, Chengdu, China
- 2Department of Rheumatology and Immunology, Sichuan Academy of Medical Science and Sichuan Provincial People’s Hospital, University of Electronic Science and Technology of China, Chengdu, China
- 3Clinical Immunology Translational Medicine Key Laboratory of Sichuan Province, Sichuan Provincial People’s Hospital, University of Electronic Science and Technology of China, Chengdu, China
Bladder cancer (BC) is the most frequent type of urinary system cancer. The prognosis of BC is poor due to high metastasis rates and multidrug resistance. Hence, development of novel therapies targeting BC cell death is urgently needed. As a novel cell death type with strong antitumor potential, ferroptosis has been investigated by many groups for its potential in BC treatment. As an iron-dependent cell death process, ferroptosis is characterized by excessive oxidative phospholipids. The molecular mechanisms of ferroptosis include iron overload and the system Xc-GSH-GPX4 signaling pathway. A recent study revealed that ferroptosis is involved in the metastasis, treatment, and prognosis of BC. Herein, in this review, we comprehensively summarize the mechanism of ferroptosis, address newly identified targets involved in ferroptosis, and discuss the potential of new clinical therapies targeting ferroptosis in BC.
1 Introduction
As a genitourinary system tumor, bladder cancer (BC) is the most frequent type of urinary system cancer, accounting for over 570,000 new patients and 210,000 deaths globally in 2020 (Sung et al., 2021). According to the depth of invasion, bladder cancer can be subdivided into two categories: nonmuscle-invasive BC (NMIBC) and muscle-invasive BC (MIBC). BC patients have a comparatively high risk of mortality without proper treatment. In Europe, the standard relative five-year survival rate for BC patients is less than 60%, and the five-year survival rate decreases to 5.5% following metastasis (Richters et al., 2020; Witjes et al., 2021). Therefore, it is crucial to investigate novel cell death signaling pathways to reduce drug resistance and provide more therapeutic options.
Ferroptosis is a type of regulated cell death that differs from necrosis or apoptosis. It is characterized by iron dependence. An imbalance in the generation and degradation of intracellular reactive oxygen species (ROS) results in reduced cellular antioxidant capacity, unrestricted lipid peroxidation, and plasma membrane rupture, finally causing ferroptosis (Aschner et al., 2022; Ozkan and Bakar-Ates, 2022). During ferroptosis, mitochondria are characterized by shrinking, absence of cristae, and increased membrane density. Compared with normal cells, tumor cells require more iron to support their proliferation. This means that tumor cells are more susceptible to iron-catalyzed necrosis. Therefore, ferroptosis has drawn increasing attention because it provides a promising avenue for oncotherapy (Bano et al., 2022; Bi et al., 2022). In the current review, we comprehensively summarize ferroptosis-related mechanisms (Figure 1), discuss newly identified targets involved in ferroptosis, and assess the potential of new clinical therapies targeting ferroptosis in BC.
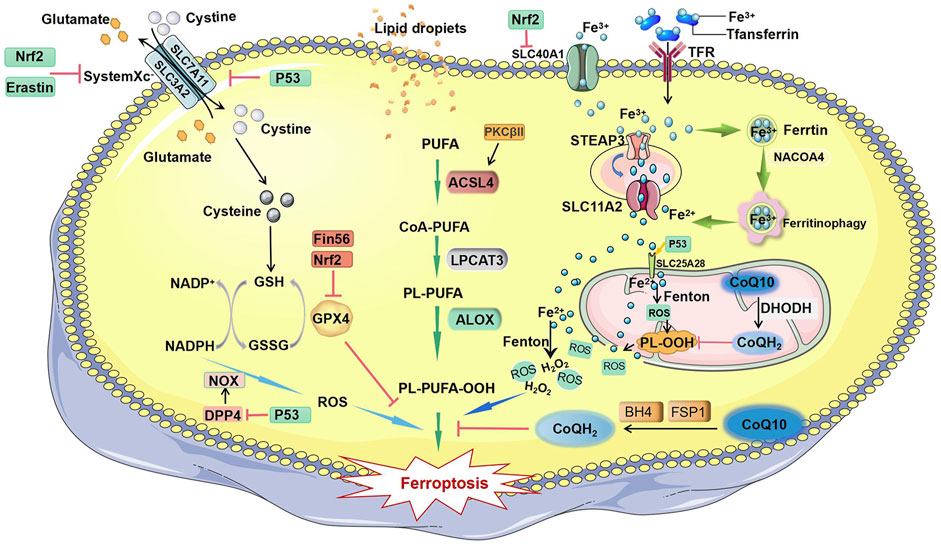
FIGURE 1. Mechanisms of ferroptosis. The initiation of ferroptosis requires two key signals, lipid peroxidation and accumulation of free iron. The generation of polyunsaturated phospholipids (by ACSL4 and LPCAT3) or PUFA-ePLs (by peroxisomal enzymes) and subsequent activation of ALOX have a major role in promoting lipid peroxidation, which can be inhibited by GPX4 and PKCβII. Extracellular iron enters the cell through TFR and facilitates the Fenton reaction to promote PUFA-PL oxidation. Some free iron stored in ferritin is released by ferritinophagy-mediated ferritin degradation. Iron can promote FSP1-CoQ10-NADPH pathways, and BH4 can block the propagation of phospholipid peroxidation and ferroptosis. Abbreviations: ALOX, lipoxygenase; ACSL4, acyl-CoA synthetase long chain family member 4; BH4, tetrahydrobiopterin; CoA-PUFA, coenzyme A-polyunsaturated fatty acid; CoQ10, coenzyme Q10; CoQH2, ubiquinol; DPP4, dipeptidyl peptidase 4; DHODH, dihydroorotate dehydrogenase; Fin56, ferroptosis inducer 56; FSP1, ferroptosis suppressor protein 1; GPX4, glutathione peroxidase 4; GSH, glutathione; GSSG, oxidized glutathione; H2O2, hydrogen peroxide; LPCAT3, lysophosphatidylcholine acyltransferase 3; Nrf2, nuclear factor erythroid 2-related factor 2; NADPH, nicotinamide adenine dinucleotide phosphate; NADP+, nicotinamide adenine dinucleotide phosphate; NACOA4, nuclear receptor coactivator 4; NOX, NADPH oxidase; PKCβII, protein kinase C beta type isoform; PUFA, polyunsaturated fatty acid; PL-PUFA, phospholipid-containing polyunsaturated fatty acid; PL-PUFA-OOH, phospholipid with a peroxidized polyunsaturated fatty acyl tail; P53, protein 53; PL-OOH, phospholipid hydroperoxide; ROS, reactive oxygen species; Slc7A11, solute carrier family 7 member 11; SLC11A2, solute carrier family 11 member 2; SLC25A28, solute carrier family 25 member 28; SLC40A1, solute carrier family 40 member 1; STEAP3, six transmembrane epithelial antigen of the prostate 3; TFR, transferrin receptor.
2 Molecular mechanisms of ferroptosis
Lipid peroxidation and iron overload in cells are two key signals that induce ferroptosis. Among them, excessive lipid peroxidation is the core driving mechanism of ferroptosis. Polyunsaturated fatty acids (PUFAs) are important components in the formation of cell membranes (Kagan et al., 2017; Wenzel et al., 2017).
2.1 Lipid peroxidation in ferroptosis
PUFAs are components of the cell membrane and regulate many biological functions, including immunity, inflammation, and cellular growth, and also play a crucial role in promoting ferroptosis (Yin et al., 2011; Magtanong et al., 2019). The biosynthesis and transformation of PUFAs in cell membranes require acyl coenzyme A (CoA) synthetase long-chain family member 4 (ACSL4) and hemolytic phosphatidylcholine acyl transferase 3 (LPCAT3) enzymes (Kagan et al., 2017; Liu et al., 2022a). Under the catalysis of ACSL4, long-chain PUFAs (such as free arachidonic acid or epinephrine) combine with coenzyme A to form PUFA-CoA, which is then promoted by lysophosphatidylcholine acyl transferase 3 (LPCAT3). The esterification reaction produces the membrane phospholipid of polyunsaturated fatty acids (PUFA-PL). Exogenous administration of the monounsaturated fatty acid (MUFA) oleic acid (OA, C18:1) can compete with PUFAs for incorporation into phospholipids (PLs) and therefore inhibit erastin-induced ferroptosis (Yin et al., 2011). Because of the presence of bisallylmoieties in PUFAs, PUFA-PLs are prone to peroxidation to form PL-PUFA-OOH (Yagoda et al., 2007; Doll et al., 2017; Sung et al., 2021). After oxidation by lipoxygenases (ALOXs) or cytochrome P450 oxidoreductases (PORs), harmful lipid peroxidation products (phospholipid hydroperoxides, PLOOHs) are formed, causing rapid and irreparable damage to cell membranes and eventually leading to cell death (Li et al., 2021). Some studies have found that lipid peroxides can activate protein kinase βII (PKCβII) and that the activated PKCβII can phosphorylate the T328 site of ACSL4, promoting activation of ACSL4. The active ACSL4 promotes the synthesis of unsaturated fatty acid phospholipids and induces the production of lipid peroxides (Liu et al., 2021) (Liu et al., 2022a).
2.2 Iron overload in ferroptosis
Iron overload in cells is also an important cause of ferroptosis. The Fe3+ in the blood circulation is bound to transferrin (Tf). Subsequently, the transferrin receptor (TFRC) recognizes Fe3+ and transports it into the cytoplasm. Some of the iron is stored in ferritin, and the rest is reduced to divalent iron ions by an endosomal membrane protein, namely, STEAP3, and released into the cytoplasm through solute carrier family 11 member 2 (SLC11A2) (Gao et al., 2015; Li et al., 2021). When iron homeostasis is unbalanced in the body, nuclear receptor coactivator 4 (NCOA4) transports ferritin to lysosomes where it undergoes autophagic degradation, releasing free iron ions (Santana-Codina et al., 2021; Zhou et al., 2022). Fe2+ is unstable and easily oxidized and can interact with H202 produced by mitochondria. Hydroxyl radicals are generated through the Fenton reaction, which further causes PUFA-PL peroxidation and triggers ferroptosis (Hou et al., 2016; Zheng and Conrad, 2020). Increased levels of intracellular iron can enhance the sensitivity of cells to ferroptosis. Recent research has revealed that mitochondria play a crucial role in regulation of ferroptosis and are also the main source of reactive oxygen species (ROS) (Takashi et al., 2020). In the early process of erastin-induced ferroptosis, intracellular lipid oxygen species localize to mitochondria and subsequently appear in other cellular regions, such as the cell membrane. Mitochondria produce ROS and convert them to H2O2 via superoxide dismutase. H2O2 reacts with labile iron through the Fenton reaction to generate hydroxyl radicals, thereby promoting PUFA-PL peroxidation (Zheng and Conrad, 2020; Lei et al., 2022). In the absence of cysteine, glutamine changes the electron transport activity of protein complexes in the inner mitochondrial membrane by regulating the mitochondrial tricarboxylic acid cycle, causing the mitochondrial membrane potential to hyperpolarize, accelerating GSH depletion, promoting lipid ROS accumulation, and inducing ferroptosis (Gao et al., 2019).
2.3 System xc-GSH-GPX4 signaling pathway in ferroptosis
The system Xc-GSH-GPX4 pathway is the predominant signaling pathway for ferroptosis. System Xc belongs to the heterodimeric amino acid transporter family. It is a heterodimer that includes solute carrier family 3 member 2 (SLC3A2) and solute carrier family 7 member 11 (SLC7A11) linked through disulfide bonds. SLC3A2 and SLC7A11 are both embedded in the cell membrane. SLC7A11, the major functional subunit in system Xc-, is responsible for transportation of cystine into cells (Koppula et al., 2021). Cystine is reduced to cysteine after entering cells (Koppula et al., 2018; Liu et al., 2020). Cysteine is a key amino acid building block for glutathione (GSH), limiting the biosynthesis of reduced GSH (Meister, 1995). Glutathione peroxidase 4 (GPX4), which is also named phospholipid hydrogen peroxide glutathione peroxidase (PHGPx), is the major enzyme responsible for catalyzing the reduction of PLOOHS in mammalian cells (Ursini et al., 1982; Seibt et al., 2019). Through the catalytic selenocysteine residue of GPX4 and two electrons provided by glutathione (GSH), GPX4 can reduce phospholipids and cholesterol hydroperoxides to their corresponding alcohols. Erastin can prevent cystine import in a Gpx4 knockout mouse model, leading to PLOOH accumulation and causing unrepairable and rapid damage to membranes and ultimately cell death (Maiorino et al., 2018).
2.4 The role of p53 in ferroptosis
Gaurav et al. found that p53 is the most common genetic mutation in the population, with a mutation rate of 35% (Mendiratta et al., 2021). As a critical tumor suppressor gene, p53 is located at the short arm of chromosome 17 and encodes the p53 protein. P53 can be induced by many stress signals, bind to DNA in the form of tetramers, and participate in cell cycle regulation, DNA repair, cell senescence, and death (Liu et al., 2019). The SLC7A11 gene is a known target of p53, and the activation of p53 leads to transcriptional repression of SLC7A11 (Jiang et al., 2015). P53 has also been reported to inhibit the uptake of cystine by downregulating SCL7A11 expression (Liu and Gu, 2022). This ultimately results in a decrease in the activity of glutathione peroxidase, a reduction in the antioxidant capacity of cells, and an enhancement of cell resistance to ferroptosis sensitivity (Liu and Gu, 2021; He et al., 2022; Hu et al., 2022). In an analysis of the expression levels of ferroptosis genes, GPX4, SLC7A11, and GSS were found to be highly expressed in some bladder cancer patients, and ACSL1 and ACSL4 were expressed at low levels. This means that bladder cancer cells can evade ferroptosis (Tang et al., 2022). The p53-SLC7A11 pathway has been reported to stimulate ferroptosis in a GSH-independent manner. Lipid oxidase ALOX12 is a key regulator of p53-dependent ferroptosis. ALOX12 is located on human chromosome 17p13.1 and is a popular site for heavy monoallelic deletions in human cancers. It has been reported that free ALOX12 oxidizes the polyunsaturated fatty acid chains of cell membrane phospholipids, leading to cell iron death. SLC7A11 can directly bind to free ALOX12 and limit its function. When p53 downregulates SLC7A11 expression, ALOX12 is released to induce ferroptosis (Chu et al., 2019). A p53 inducer can directly regulate the level of iPLA2β, but this response is very sensitive to the treatment time and concentration of the p53 inducer. The phospholipase iPLA2β can specifically hydrolyze the sn-2 acyl bonds of phospholipids, scavenge peroxidized phospholipids, and inhibit ROS-induced ferroptosis, independent of GPX4 and FSP1 (Li et al., 2021). P53 in mitochondria can bind with solute carrier family 25 member 28 (SLC25A28) to form a complex (p53-SLC25A28). Therefore, SLC25A28 activity is greatly enhanced, which leads to redox-active iron accumulation and ferroptosis (Zhang Z. et al., 2020). P53 can also directly bind to DPP4, preventing it from entering the cytoplasm and binding to NOX1, thereby promoting ferroptosis (Xie et al., 2017). In addition, the ferroptosis marker molecule PTGS2 was also confirmed to be a target gene of p53 (Yagoda et al., 2007; Doll et al., 2017; Guan et al., 2020; Sung et al., 2021). Numerous studies indicate that p53 promotes ferroptosis.
2.5 Other inhibitors of ferroptosis
Nuclear factor erythroid 2 (Nrf2), which plays a key role in antioxidant activity, is considered to be an important regulatory factor in ferroptosis. On one hand, Nrf2 can regulate the expression of GPX4 protein, while GPX4 overexpression results in ferroptosis resistance (Fan et al., 2017; Cui et al., 2021; Gao et al., 2022). On the other hand, Nrf2 can directly bind with the antioxidant-responsive element (ARE) sequence of the SLC7A11 subunit promoter, increasing the expression of SLC7A11 and increasing the GSH level to inhibit ferroptosis (Carpi-Santos and Calaza, 2018; Dong et al., 2020; Chen et al., 2022). Nrf2 regulates the intracellular free iron content by activating iron metabolism-related genes (SLC40A1). SLC40A1 can transport iron ions out of cells and inhibit the occurrence of ferroptosis (Chen et al., 2021) (Wu et al., 2017). The ferroptosis suppressor protein 1 (FSP1)-coenzyme Q10 (CoQ10) signaling pathway is another important ferroptosis inhibitory pathway. FSP1 is a flavoprotein oxidoreductase. FSP1 overexpression can suppress ferroptosis caused by GPX4 inhibition (Bersuker et al., 2019; Yan et al., 2021). Further studies found that FSP1 can act as an oxidoreductase to reduce COQ to COQ-H2 on the plasma membrane (Shukla and Dubey, 2018). CoQ, a lipophilic metabolite composed of a redox-active quinone head group and a long polyisoprenoid lipid tail, plays an essential role as a reversible redox carrier in the Golgi apparatus membrane and in plasma membrane electron transport. Its fully reduced form CoQH2 can act as a free-radical-trapping antioxidant to reduce lipid peroxidation free radicals (Stocker et al., 1991; Morre and Morre, 2011) (Gao et al., 2019) (Liu et al., 2022b). DHODH is the rate-limiting enzyme in the pyrimidine synthesis pathway and is an iron-containing flavin-dependent enzyme located on the inner mitochondrial membrane. One study found that a mitochondrial enzyme, dihydroorotate dehydrogenase (DHODH), on the outer surface of the inner mitochondrial membrane, can reduce coenzyme Q (CoQ) to ubiquinol (CoQH2) (Mao et al., 2021; Wang and Min, 2021). Tetrahydrobiopterin (BH4) is an alternative ferroptosis defense system independent of the GPX4-independent inhibitor of ferroptosis. BH4 is a powerful free radical-trapping antioxidant in cell membranes that promotes regeneration of CoQH2 and alpha-tocopherol to combat lipid peroxidation and ferroptosis (Crabtree et al., 2009; Kraft et al., 2020; Soula et al., 2020). BH4 is regenerated from its oxidized form boron dihydride (BH2) by dihydrofolate reductase (DHFR). Thus, DHFR inactivation significantly increases cellular susceptibility to ferroptosis (Hadian, 2020; Kraft et al., 2020).
3 The role of ferroptosis in bladder cancer
Abnormal expression of multiple ferroptosis-related proteins (FRPs), long noncoding RNAs (lncRNAs), microRNAs (miRNAs), and circular RNAs (circRNAs) has been found in bladder cancer specimens, indicating that ferroptosis plays an important role in the occurrence of BC (Zhang Y. et al., 2020). At the same time, FRPs and lncRNAs show good predictive value for prognosis and drug resistance in bladder cancer.
3.1 Predictive role of ferroptosis-related genes
Ferroptosis gene expression levels and the clinical data of BC patients were analyzed in The Cancer Genome Atlas (TCGA) and Gene Expression Omnibus (GEO) databases. It was found that a number of ferroptosis-related genes were abnormally expressed, such as SLC7A11, GPX4, TFRC, NCOA4, ACSL4, ALOX15, glucose-6-phosphate dehydrogenase (G6PD), and DPP4(66). Based on these ferroptosis regulator genes, various prognostic signatures have been established that can predict not only disease progression but also patient response to programmed death 1 (PD-1) and programmed death 1 (PD-L1) immunotherapy (Yi et al., 2021; Liu et al., 2022c; Gui et al., 2022). By analyzing data downloaded from multiple databases, Yue found that 23 FRGs were abnormally expressed and that low expression of recombinant heat shock 70 kDa protein 5 (HSPA5) and high expression of CDGSH iron sulfur domain 1 (CISD1) were associated with poor 1-, 3-, and 5-year overall survival (Yang et al., 2022). Xia used the Consensus Cluster Plus R package to divide the validated ferroptosis genes (VFGs) into four VFG clusters and found that there were differences in the prognosis of patients with different VGF clusters and tumor clinical manifestations. Scoring based on these phenotype-related genes revealed that VFR Cluster A had the highest score and was related to a worse response to PD-1 blockade immunotherapy (Xia et al., 2022).
3.2 Predictive role of lncRNAs
Accumulating evidence has demonstrated that multiple ferroptosis-related lncRNAs can be used to predict the prognosis and drug resistance of BC patients (Liang et al., 2021; Zhou et al., 2021; Li et al., 2022a; Liu et al., 2022a). LncRNAs, which are noncoding RNAs approximately 200 nucleotides in length, play a variety of roles in cancer immune responses and the tumor microenvironment (Yu et al., 2018; Yu et al., 2021). Liu found that lncRNAs were closely related to the tumor microenvironment and immunotherapy response. High-risk groups were associated with a poor prognosis and lower expression of certain proteins, including PD-1, PD-L1, and cytotoxic T lymphocyte-associated antigen-4 (CTLA-4), which indicate a poorer treatment response to immunotherapy (Liu et al., 2022a; Liu et al., 2022d). By analyzing ferroptosis-related lncRNA pairs and constructing a Cox proportional hazards model, it was found that multiple ferroptosis-related signaling pathways were altered in BC (Wang et al., 2022; Hou et al., 2022). The study showed high ferroptosis risk scores were correlated with high expression levels of the gene encoding PD-1, with a lower half-maximal inhibitory concentration (IC50) value for docetaxel, cisplatin, and pazopanib. This suggests that the combined use of ferroptosis-related drugs with immune checkpoint inhibitors in the high-risk group would benefit patients (Li et al., 2022b).
3.3 Potential therapeutic targets of circRNAs
CircRNAs are another type of noncoding endogenous RNAs, and they have circular configurations and stable structures (95). CircST6GAINAC6 was found to be significantly reduced in bladder cancer cells according to second-generation sequencing technology. One study showed that circST6GAINAC6 can bind to the N-terminus of heat shock protein 1, block phosphorylation at Ser15, activate the P38/MAPK pathway, and decrease the levels of SLC7A11 and GPX4, thereby promoting ferroptosis in bladder cells (96).
3.4 Potential therapeutic targets
Ferroptosis has a unique role in anticancer therapeutic strategies (Yi et al., 2021; Liu et al., 2022c; Gui et al., 2022). Treatments for induction of ferroptosis in bladder cancer are shown in Table 1. The system xc-GSH-GPX4 pathway is a classical inhibitory ferroptosis pathway. In recent years, most studies on potential therapeutic drugs or targets have focused on this pathway. SLC7A11 is an important functional component of system Xc-, and regulation of SLC7A11 can affect cell ferroptosis. Iron metabolism disorder is also an important cause of ferroptosis. One study found that RP11-89 mediates miR-129-5p expression and upregulates PROM2. Elevated PROM2 in cells is associated with diminished iron export, multivesicular body formation, and reduced mitochondrial abnormalities. Thus, RP11-89 may serve as a potential biomarker or therapeutic target in bladder cancer (Luo et al., 2021). The expression of ovarian tumor family deubiquitinase 1 (OUTB1) is significantly increased in human bladder cancer. Through in vitro and in vivo experiments, knocking out the OTUB1 gene was found to reduce the level of SLC7A11, inhibit the uptake of cystine by cells, and promote ferroptosis (Shimada et al., 2016). Epithelial membrane protein 1 (EMP1) was found to be downregulated in BC cells. In cells lacking EMP1, the addition of the ferroptosis inducer erastin promoted anti-ferroptosis cell death through upregulation of SLC7A11 expression (Hao et al., 2022). Thus, OUTB1 and EMP1 may be potential therapeutic targets.
4 Potential therapeutic drugs
4.1 Chemotherapy and immunosuppressants
For traditional chemotherapy, cisplatin, gemcitabine, or carboplatin have certain effects in the treatment of bladder cancer (Taber et al., 2020; Pfister et al., 2022). Other chemotherapies, such as quinazolinyl-arylurea derivative 7j, can bind to active GPX4, inhibit the sxc-/GPX4/ROS pathways, and induce ferroptosis (Chen et al., 2020). Immune checkpoint drugs, such as atezolizumab and pembrolizumab, are also effective against several diseases, including bladder cancer (Nadal and Bellmunt, 2019). Although single-drug treatment with immune checkpoint inhibitors (ICIs) has been successful, the long-term persistent remission rate is still very low, and many patients relapse. Early efforts to combine ICIs with traditional chemotherapy have shown a minimal impact on clinical benefits (Nadal and Bellmunt, 2019).
4.2 Traditional Chinese medicine
Traditional Chinese medicine has been used in the treatment of bladder cancer. Huang qin, which is extracted from the roots of S. baicalensis Georgi (Scutellariae Radix), triggers ferroptosis in vitro and in vivo by increasing intracellular chelate iron enrichment and ROS accumulation through overexpression of ferritin heavy chain 1 (FTH-1) (Kong et al., 2021). Erianin, a ferroptosis inducer, can promote ROS accumulation by inactivating nuclear factor E2-related factor (Nrf2) and GSH, thereby inducing ferroptosis in tumor cells (Xiang et al., 2021).
4.3 Nano drugs and gold clusters
A variety of nano drugs have been reported to specifically adhere to bladder cancer cells and deliver iron through endocytosis when exposed to laser irradiation, resulting in ROS generation and accumulation and subsequent GSH and GPX4 depletion, ultimately leading to ferroptosis (Liao et al., 2022). Another approach is to enhance photodynamic therapy (PDT)-chemodynamic therapy (CDT) sensitivity and induce toxicity (Chin et al., 2022; Liao et al., 2022). AuNRs&IONs@Gel is a gel delivery platform with embedded gold nanorods (AuNRs) and iron oxide nanoparticles (IONs). The IONs can be absorbed by bladder tumor cells and activate iron-mediated lipid peroxidation (Guo et al., 2020). The innovative antitumor mechanism of gold complexes has attracted attention. Gold clusters (PAA4 and PAA5) can trigger the rapid release of Au(l) ions from GSH, thus inhibiting thioredoxin reductases, stimulating oxidative reactions, and accelerating ferroptosis (Xiao et al., 2022).
4.4 Other potential therapeutic drugs
Many additional drugs have shown therapeutic prospects. The compound Fin56, a ferroptosis inducer derived from caspase-3/7-independent lethal 56, can induce ferroptosis by inhibiting mTOR-mediated autophagy to degrade GPX4 in BC cells (Shimada et al., 2016; Sun et al., 2021). Bupivacaine (0.25–16 mM), a common local anesthetic, was found to inhibit PI3K/AKT signaling pathway activity by increasing Fe2+ levels and ROS levels. Furthermore, it reduced GSH levels and increased malonaldehyde (MDA) levels in BC cells, which suppressed the growth of xenografted tumors and induced ferroptosis (Hao et al., 2022).
5 Conclusion and perspectives
Bladder cancer has a high recurrence rate and high morbidity and mortality. Current treatments cannot effectively cope with the resistance of cancer cells to existing chemotherapy drugs. With the development of precision medicine, precise disease diagnosis and individualized selection of the best treatment strategy have become a trend in developing treatment strategies. Ferroptosis is a new type of cell death that differs from apoptosis and necrosis. Many studies have demonstrated that ferroptosis is involved in the metastasis, treatment, and prognosis of bladder cancer. The prediction of prognosis and drug resistance based on a ferroptosis-associated gene-based molecular typing model has also received extensive attention. By analyzing the related ferroptosis gene data of bladder cancer patients in The Cancer Genome Atlas (TCGA) and Gene Expression Omnibus (GEO) databases, the constructed risk score model can predict bladder cancer prognosis and sensitivity to chemotherapy drugs. Therefore, based on the precise identification of biomarkers of ferroptosis in bladder cancer patients, it is expected that subsequent molecular targeted precision therapy can be achieved. Some conventional drugs that activate ferroptosis have shown favorable antitumor effects in vivo and in vitro. Through intravesical injection, drug-containing compounds can reach a high concentration in the bladder without entering the bloodstream, which avoids many side effects of systemic medication. At present, a variety of iron-containing nanomedicines have achieved good efficacy in vivo. This will be a new research direction. However, no drug targeting the canonical ferroptosis pathway has undergone clinical trials in bladder cancer. We believe that with more in-depth studies on the mechanisms and targets of action of ferroptosis inhibitors, clinical trials are essential. Clinical trials may become the key for future research. As shown here, targeting ferroptosis in bladder cells could become a new anticancer therapy approach in the future.
Author contributions
YW, QZ, and XH contributed to the conception and design of the work. FZ and QZ drafted the manuscript. NW prepared the figure. YW, QZ, and XH substantively revised the manuscript. All authors read and approved the final manuscript.
Funding
This research was supported by the National Natural Science Foundation of China (81802504 and 81872207), the Sichuan Science and Technology Bureau (2019YFS0439, 2020JDJQ0067, 2020JDRC0118, 2021YJ0564, and 2022YFH0005), the Science and Technology Innovation Project of Chengdu, China (No. 2021-YF05-00225-SN, 2021-YF05-02246-SN), and a Sichuan Medical Association grant (No. Q19037).
Conflict of interest
The authors declare that the research was conducted in the absence of any commercial or financial relationships that could be construed as a potential conflict of interest.
Publisher’s note
All claims expressed in this article are solely those of the authors and do not necessarily represent those of their affiliated organizations, or those of the publisher, the editors, and the reviewers. Any product that may be evaluated in this article, or claim that may be made by its manufacturer, is not guaranteed or endorsed by the publisher.
References
Aschner, M., Skalny, A. V., Martins, A. C., Sinitskii, A. I., Farina, M., Lu, R., et al. (2022). Ferroptosis as a mechanism of non-ferrous metal toxicity. Arch. Toxicol. 96, 2391–2417. doi:10.1007/s00204-022-03317-y
Bano, I., Horky, P., Abbas, S. Q., Majid, M., Bilal, A. H. M., Ali, F., et al. (2022). Ferroptosis: A new road towards cancer management. Molecules 27, 2129. doi:10.3390/molecules27072129
Bersuker, K., Hendricks, J. M., Li, Z., Magtanong, L., Ford, B., Tang, P. H., et al. (2019). The CoQ oxidoreductase FSP1 acts parallel to GPX4 to inhibit ferroptosis. Nature 575, 688–692. doi:10.1038/s41586-019-1705-2
Bi, Q., Sun, Z. J., Wu, J. Y., and Wang, W. (2022). Ferroptosis-mediated formation of tumor-promoting immune microenvironment. Front. Oncol. 12, 868639. doi:10.3389/fonc.2022.868639
Carpi-Santos, R., and Calaza, K. C. (2018). Alterations in system xc(-) expression in the retina of type 1 diabetic rats and the role of Nrf2. Mol. Neurobiol. 55, 7941–7948. doi:10.1007/s12035-018-0961-8
Chen, G. H., Song, C. C., Pantopoulos, K., Wei, X. L., Zheng, H., and Luo, Z. (2022). Mitochondrial oxidative stress mediated Fe-induced ferroptosis via the NRF2-ARE pathway. Free Radic. Biol. Med. 180, 95–107. doi:10.1016/j.freeradbiomed.2022.01.012
Chen, J. N., Li, T., Cheng, L., Qin, T. S., Sun, Y. X., Chen, C. T., et al. (2020). Synthesis and in vitro anti-bladder cancer activity evaluation of quinazolinyl-arylurea derivatives. Eur. J. Med. Chem. 205, 112661. doi:10.1016/j.ejmech.2020.112661
Chen, X., Kang, R., Kroemer, G., and Tang, D. (2021). Broadening horizons: The role of ferroptosis in cancer. Nat. Rev. Clin. Oncol. 18, 280–296. doi:10.1038/s41571-020-00462-0
Chin, Y. C., Yang, L. X., Hsu, F. T., Hsu, C. W., Chang, T. W., Chen, H. Y., et al. (2022). Iron oxide@chlorophyll clustered nanoparticles eliminate bladder cancer by photodynamic immunotherapy-initiated ferroptosis and immunostimulation. J. Nanobiotechnology 20, 373. doi:10.1186/s12951-022-01575-7
Chu, B., Kon, N., Chen, D., Li, T., Liu, T., Jiang, L., et al. (2019). ALOX12 is required for p53-mediated tumour suppression through a distinct ferroptosis pathway. Nat. Cell Biol. 21, 579–591. doi:10.1038/s41556-019-0305-6
Crabtree, M. J., Tatham, A. L., Hale, A. B., Alp, N. J., and Channon, K. M. (2009). Critical role for tetrahydrobiopterin recycling by dihydrofolate reductase in regulation of endothelial nitric-oxide synthase coupling: Relative importance of the de novo biopterin synthesis versus salvage pathways. J. Biol. Chem. 284, 28128–28136. doi:10.1074/jbc.M109.041483
Cui, Y., Zhang, Z., Zhou, X., Zhao, Z., Zhao, R., Xu, X., et al. (2021). Microglia and macrophage exhibit attenuated inflammatory response and ferroptosis resistance after RSL3 stimulation via increasing Nrf2 expression. J. Neuroinflammation 18, 249. doi:10.1186/s12974-021-02231-x
Doll, S., Proneth, B., Tyurina, Y. Y., Panzilius, E., Kobayashi, S., Ingold, I., et al. (2017). ACSL4 dictates ferroptosis sensitivity by shaping cellular lipid composition. Nat. Chem. Biol. 13, 91–98. doi:10.1038/nchembio.2239
Dong, H., Qiang, Z., Chai, D., Peng, J., Xia, Y., Hu, R., et al. (2020). Nrf2 inhibits ferroptosis and protects against acute lung injury due to intestinal ischemia reperfusion via regulating SLC7A11 and HO-1. Aging (Albany NY) 12, 12943–12959. doi:10.18632/aging.103378
Fan, Z., Wirth, A. K., Chen, D., Wruck, C. J., RauhM., , BuchfelderM., , et al. (2017). Nrf2-Keap1 pathway promotes cell proliferation and diminishes ferroptosis. Oncogenesis 6, e371. doi:10.1038/oncsis.2017.65
Gao, M., Monian, P., Quadri, N., Ramasamy, R., and Jiang, X. (2015). Glutaminolysis and transferrin regulate ferroptosis. Mol. Cell 59, 298–308. doi:10.1016/j.molcel.2015.06.011
Gao, M., Yi, J., Zhu, J., Minikes, A. M., Monian, P., Thompson, C. B., et al. (2019). Role of mitochondria in ferroptosis. Mol. Cell 73, 354–363. doi:10.1016/j.molcel.2018.10.042
Gao, Z., Zhang, Z., Gu, D., Li, Y., Zhang, K., Dong, X., et al. (2022). Hemin mitigates contrast-induced nephropathy by inhibiting ferroptosis via HO-1/Nrf2/GPX4 pathway. Clin. Exp. Pharmacol. Physiol. 49, 858–870. doi:10.1111/1440-1681.13673
Guan, Z., Chen, J., Li, X., and Dong, N. (2020). Tanshinone IIA induces ferroptosis in gastric cancer cells through p53-mediated SLC7A11 down-regulation. Biosci. Rep. 40, BSR20201807. doi:10.1042/BSR20201807
Gui, C. P., Li, J. Y., Fu, L. M., Luo, C. G., Zhang, C., Tang, Y. M., et al. (2022). Identification of mRNA vaccines and conserved ferroptosis related immune landscape for individual precision treatment in bladder cancer. J. Big Data 9, 88. doi:10.1186/s40537-022-00641-z
Guo, P., Wang, L., Shang, W., Chen, J., Chen, Z., Xiong, F., et al. (2020). Intravesical in situ immunostimulatory gel for triple therapy of bladder cancer. ACS Appl. Mat. Interfaces 12, 54367–54377. doi:10.1021/acsami.0c15176
Hadian, K. (2020). Ferroptosis suppressor protein 1 (FSP1) and coenzyme Q10 cooperatively suppress ferroptosis. Biochemistry 59, 637–638. doi:10.1021/acs.biochem.0c00030
Hao, J., Zhang, W., and Huang, Z. (2022). Bupivacaine modulates the apoptosis and ferroptosis in bladder cancer via phosphatidylinositol 3-kinase (PI3K)/AKT pathway. Bioengineered 13, 6794–6806. doi:10.1080/21655979.2022.2036909
He, C., Wang, C., Liu, H., and Shan, B. (2022). Kayadiol exerted anticancer effects through p53-mediated ferroptosis in NKTCL cells. BMC cancer 22, 724. doi:10.1186/s12885-022-09825-5
Hou, J., Lu, Z., Cheng, X., Dong, R., Jiang, Y., Wu, G., et al. (2022). Ferroptosis-related long non-coding RNA signature predicts the prognosis of bladder cancer. BMC cancer 22, 719. doi:10.1186/s12885-022-09805-9
Hou, W., Xie, Y., Song, X., Sun, X., Lotze, M. T., Zeh, H. J., et al. (2016). Autophagy promotes ferroptosis by degradation of ferritin. Autophagy 12, 1425–1428. doi:10.1080/15548627.2016.1187366
Hu, J., Zhang, R., Chang, Q., Ji, M., Geng, R., Geng, R., et al. (2022). p53: A regulator of ferroptosis induced by galectin-1 derived peptide 3 in MH7A cells. Front. Genet. 13, 920273. doi:10.3389/fgene.2022.920273
Jiang, L., Kon, N., Li, T., Wang, S. J., Su, T., Hibshoosh, H., et al. (2015). Ferroptosis as a p53-mediated activity during tumour suppression. Nature 520, 57–62. doi:10.1038/nature14344
Kagan, V. E., Mao, G., Qu, F., Angeli, J. P. F., Doll, S., Croix, C. S., et al. (2017). Oxidized arachidonic and adrenic PEs navigate cells to ferroptosis. Nat. Chem. Biol. 13, 81–90. doi:10.1038/nchembio.2238
Kong, N., Chen, X., Feng, J., Duan, T., Liu, S., Sun, X., et al. (2021). Baicalin induces ferroptosis in bladder cancer cells by downregulating FTH1. Acta Pharm. Sin. B 11, 4045–4054. doi:10.1016/j.apsb.2021.03.036
Koppula, P., Zhang, Y., Zhuang, L., and Gan, B. (2018). Amino acid transporter SLC7A11/xCT at the crossroads of regulating redox homeostasis and nutrient dependency of cancer. Cancer Commun. 38, 12. doi:10.1186/s40880-018-0288-x
Koppula, P., Zhuang, L., and Gan, B. (2021). Cystine transporter slc7a11/xCT in cancer: Ferroptosis, nutrient dependency, and cancer therapy. Protein Cell 12, 599–620. doi:10.1007/s13238-020-00789-5
Kraft, V. A. N., Bezjian, C. T., Pfeiffer, S., Ringelstetter, L., Muller, C., Zandkarimi, F., et al. (2020). GTP cyclohydrolase 1/tetrahydrobiopterin counteract ferroptosis through lipid remodeling. ACS Cent. Sci. 6, 41–53. doi:10.1021/acscentsci.9b01063
Lei, G., Zhuang, L., and Gan, B. (2022). Targeting ferroptosis as a vulnerability in cancer. Nat. Rev. Cancer 22, 381–396. doi:10.1038/s41568-022-00459-0
Li, N., Shen, J., Qiao, X., Gao, Y., Su, H. B., and Zhang, S. (2022). Long non-coding RNA signatures associated with ferroptosis predict prognosis in colorectal cancer. Int. J. Gen. Med. 15, 33–43. doi:10.2147/IJGM.S331378
Li, X., Huang, J., Chen, J., Zhan, Y., Zhang, R., Lu, E., et al. (2021). A novel prognostic signature based on ferroptosis-related genes predicts the prognosis of patients with advanced bladder urothelial carcinoma. Front. Oncol. 11, 726486. doi:10.3389/fonc.2021.726486
Li, X., Zhou, L., Lu, T., Zhang, L., Li, Y., Xu, J., et al. (2022). Constructing an immune- and ferroptosis-related lncRNA signature to predict the immune landscape of human bladder cancer. J. Clin. Lab. Anal. 36, e24389. doi:10.1002/jcla.24389
Liang, Y., Ye, F., Xu, C., Zou, L., Hu, Y., Hu, J., et al. (2021). A novel survival model based on a Ferroptosis-related gene signature for predicting overall survival in bladder cancer. BMC cancer 21, 943. doi:10.1186/s12885-021-08687-7
Liao, M. Y., Huang, T. C., Chin, Y. C., Cheng, T. Y., and Lin, G. M. (2022). Surfactant-free green synthesis of Au@chlorophyll nanorods for NIR PDT-elicited CDT in bladder cancer therapy. ACS Appl. Bio Mat. 5, 2819–2833. doi:10.1021/acsabm.2c00228
Liu, J., Cui, J., Zhao, S., Wu, M., Wang, J., Zhang, Y., et al. (2022). Ferroptosis-related long noncoding RNAs have excellent predictive ability for multiomic characteristics of bladder cancer. Oxid. Med. Cell. Longev. 2022, 9316847. doi:10.1155/2022/9316847
Liu, J., Kang, R., and Tang, D. (2021). Signaling pathways and defense mechanisms of ferroptosis. FEBS J., 16059. doi:10.1111/febs.16059
Liu, J., Zhang, Z., Liu, X., Zhang, W., Meng, L., Wang, J., et al. (2022). Predictive role of ferroptosis-related long non-coding RNAs in bladder cancer and their association with immune microenvironment and immunotherapy response. World J. Surg. Oncol. 20, 47. doi:10.1186/s12957-022-02514-4
Liu, J., Zhang, Z., Zhang, W., Meng, L., Wang, J., Lv, Z., et al. (2022). Ferroptosis mediation patterns reveal novel tool to implicate immunotherapy and multi-omics characteristics in bladder cancer. Front. Cell Dev. Biol. 10, 791630. doi:10.3389/fcell.2022.791630
Liu, M., Kong, X. Y., Yao, Y., Wang, X. A., Yang, W., Wu, H., et al. (2022). The critical role and molecular mechanisms of ferroptosis in antioxidant systems: A narrative review. Ann. Transl. Med. 10, 368. doi:10.21037/atm-21-6942
Liu, X., Olszewski, K., Zhang, Y., Lim, E. W., Shi, J., Zhang, X., et al. (2020). Cystine transporter regulation of pentose phosphate pathway dependency and disulfide stress exposes a targetable metabolic vulnerability in cancer. Nat. Cell Biol. 22, 476–486. doi:10.1038/s41556-020-0496-x
Liu, Y., and Gu, W. (2022). p53 in ferroptosis regulation: the new weapon for the old guardian. Cell Death Differ. 29, 895–910. doi:10.1038/s41418-022-00943-y
Liu, Y., and Gu, W. (2021). The complexity of p53-mediated metabolic regulation in tumor suppression. Semin. Cancer Biol. S1044-579X(21)00060-2 doi:10.1016/j.semcancer.2021.03.010
Liu, Y., Tavana, O., and Gu, W. (2019). p53 modifications: exquisite decorations of the powerful guardian. J. Mol. Cell Biol. 11, 564–577. doi:10.1093/jmcb/mjz060
Luo, W., Wang, J., Xu, W., Ma, C., Wan, F., Huang, Y., et al. (2021). LncRNA RP11-89 facilitates tumorigenesis and ferroptosis resistance through PROM2-activated iron export by sponging miR-129-5p in bladder cancer. Cell Death Dis. 12, 1043. doi:10.1038/s41419-021-04296-1
Magtanong, L., Ko, P. J., To, M., Cao, J. Y., Forcina, G. C., Tarangelo, A., et al. (2019). Exogenous monounsaturated fatty acids promote a ferroptosis-resistant cell state. Cell Chem. Biol. 26, 420–432. doi:10.1016/j.chembiol.2018.11.016
Maiorino, M., Conrad, M., and Ursini, F. (2018). GPx4, lipid peroxidation, and cell death: Discoveries, rediscoveries, and open issues. Antioxid. Redox Signal. 29, 61–74. doi:10.1089/ars.2017.7115
Mao, C., Liu, X., Zhang, Y., Lei, G., Yan, Y., Lee, H., et al. (2021). DHODH-mediated ferroptosis defence is a targetable vulnerability in cancer. Nature 593, 586–590. doi:10.1038/s41586-021-03539-7
Meister, A. (1995). Glutathione metabolism. Methods Enzymol. 251, 3–7. doi:10.1016/0076-6879(95)51106-7
Mendiratta, G., Ke, E., Aziz, M., Liarakos, D., Tong, M., and Stites, E. C. (2021). Cancer gene mutation frequencies for the U.S. population. Nat. Commun. 12, 5961. doi:10.1038/s41467-021-26213-y
Morre, D. J., and Morre, D. M. (2011). Non-mitochondrial coenzyme Q. Biofactors 37, 355–360. doi:10.1002/biof.156
Nadal, R., and Bellmunt, J. (2019). Management of metastatic bladder cancer. Cancer Treat. Rev. 76, 10–21. doi:10.1016/j.ctrv.2019.04.002
Ozkan, E., and Bakar-Ates, F. (2022). Ferroptosis: A trusted ally in combating drug resistance in cancer. Curr. Med. Chem. 29, 41–55. doi:10.2174/0929867328666210810115812
Pfister, C., Gravis, G., Flechon, A., Chevreau, C., Mahammedi, H., Laguerre, B., et al. (2022). Dose-dense methotrexate, vinblastine, doxorubicin, and cisplatin or gemcitabine and cisplatin as perioperative chemotherapy for patients with nonmetastatic muscle-invasive bladder cancer: Results of the GETUG-AFU V05 VESPER trial. J. Clin. Oncol. 40, 2013–2022. doi:10.1200/JCO.21.02051
Richters, A., Aben, K. K. H., and Kiemeney, L. (2020). The global burden of urinary bladder cancer: An update. World J. Urol. 38, 1895–1904. doi:10.1007/s00345-019-02984-4
Santana-Codina, N., Gikandi, A., and Mancias, J. D. (2021). The role of NCOA4-mediated ferritinophagy in ferroptosis. Adv. Exp. Med. Biol. 1301, 41–57. doi:10.1007/978-3-030-62026-4_4
Seibt, T. M., Proneth, B., and Conrad, M. (2019). Role of GPX4 in ferroptosis and its pharmacological implication. Free Radic. Biol. Med. 133, 144–152. doi:10.1016/j.freeradbiomed.2018.09.014
Shimada, K., Skouta, R., Kaplan, A., Yang, W. S., Hayano, M., Dixon, S. J., et al. (2016). Global survey of cell death mechanisms reveals metabolic regulation of ferroptosis. Nat. Chem. Biol. 12, 497–503. doi:10.1038/nchembio.2079
Shukla, S., and Dubey, K. K. (2018). CoQ10 a super-vitamin: Review on application and biosynthesis. 3 Biotech. 8, 249. doi:10.1007/s13205-018-1271-6
Soula, M., Weber, R. A., Zilka, O., Alwaseem, H., La, K., Yen, F., et al. (2020). Metabolic determinants of cancer cell sensitivity to canonical ferroptosis inducers. Nat. Chem. Biol. 16, 1351–1360. doi:10.1038/s41589-020-0613-y
Stocker, R., Bowry, V. W., and Frei, B. (1991). Ubiquinol-10 protects human low density lipoprotein more efficiently against lipid peroxidation than does alpha-tocopherol. Proc. Natl. Acad. Sci. U. S. A. 88, 1646–1650. doi:10.1073/pnas.88.5.1646
Sun, Y., Berleth, N., Wu, W., Schlutermann, D., Deitersen, J., Stuhldreier, F., et al. (2021). Fin56-induced ferroptosis is supported by autophagy-mediated GPX4 degradation and functions synergistically with mTOR inhibition to kill bladder cancer cells. Cell Death Dis. 12, 1028. doi:10.1038/s41419-021-04306-2
Sung, H., Ferlay, J., Siegel, R. L., Laversanne, M., Soerjomataram, I., Jemal, A., et al. (2021). Global cancer statistics 2020: GLOBOCAN estimates of incidence and mortality worldwide for 36 cancers in 185 countries. Ca. Cancer J. Clin. 71, 209–249. doi:10.3322/caac.21660
Taber, A., Christensen, E., Lamy, P., Nordentoft, I., Prip, F., Lindskrog, S. V., et al. (2020). Molecular correlates of cisplatin-based chemotherapy response in muscle invasive bladder cancer by integrated multi-omics analysis. Nat. Commun. 11, 4858. doi:10.1038/s41467-020-18640-0
Takashi, Y., Tomita, K., Kuwahara, Y., Roudkenar, M. H., Roushandeh, A. M., Igarashi, K., et al. (2020). Mitochondrial dysfunction promotes aquaporin expression that controls hydrogen peroxide permeability and ferroptosis. Free Radic. Biol. Med. 161, 60–70. doi:10.1016/j.freeradbiomed.2020.09.027
Tang, B., Yan, R., Zhu, J., Cheng, S., Kong, C., Chen, W., et al. (2022). Integrative analysis of the molecular mechanisms, immunological features and immunotherapy response of ferroptosis regulators across 33 cancer types. Int. J. Biol. Sci. 18, 180–198. doi:10.7150/ijbs.64654
Ursini, F., Maiorino, M., Valente, M., Ferri, L., and Gregolin, C. (1982). Purification from pig liver of a protein which protects liposomes and biomembranes from peroxidative degradation and exhibits glutathione peroxidase activity on phosphatidylcholine hydroperoxides. Biochim. Biophys. Acta 710, 197–211. doi:10.1016/0005-2760(82)90150-3
Wang, F., and Min, J. (2021). DHODH tangoing with GPX4 on the ferroptotic stage. Signal Transduct. Target. Ther. 6, 244. doi:10.1038/s41392-021-00656-7
Wang, Y., Zhang, S., Bai, Y., Li, G., Wang, S., Chen, J., et al. (2022). Development and validation of ferroptosis-related LncRNA biomarker in bladder carcinoma. Front. Cell Dev. Biol. 10, 809747. doi:10.3389/fcell.2022.809747
Wenzel, S. E., Tyurina, Y. Y., Zhao, J., St Croix, C. M., Dar, H. H., Mao, G., et al. (2017). PEBP1 wardens ferroptosis by enabling lipoxygenase generation of lipid death signals. Cell 171, 628–641. doi:10.1016/j.cell.2017.09.044
Witjes, J. A., Bruins, H. M., Cathomas, R., Comperat, E. M., Cowan, N. C., Gakis, G., et al. (2021). European association of urology guidelines on muscle-invasive and metastatic bladder cancer: Summary of the 2020 guidelines. Eur. Urol. 79, 82–104. doi:10.1016/j.eururo.2020.03.055
Wu, J., Bao, L., Zhang, Z., and Yi, X. (2017). Nrf2 induces cisplatin resistance via suppressing the iron export related gene SLC40A1 in ovarian cancer cells. Oncotarget 8, 93502–93515. doi:10.18632/oncotarget.19548
Xia, Q. D., Sun, J. X., Liu, C. Q., Xu, J. Z., An, Y., Xu, M. Y., et al. (2022). Ferroptosis patterns and tumor microenvironment infiltration characterization in bladder cancer. Front. Cell Dev. Biol. 10, 832892. doi:10.3389/fcell.2022.832892
Xiang, Y., Chen, X., Wang, W., Zhai, L., Sun, X., Feng, J., et al. (2021). Natural product erianin inhibits bladder cancer cell growth by inducing ferroptosis via NRF2 inactivation. Front. Pharmacol. 12, 775506. doi:10.3389/fphar.2021.775506
Xiao, K., Zhang, N., Li, F., Hou, D., Zhai, X., Xu, W., et al. (2022). Pro-oxidant response and accelerated ferroptosis caused by synergetic Au(I) release in hypercarbon-centered gold(I) cluster prodrugs. Nat. Commun. 13, 4669. doi:10.1038/s41467-022-32474-y
Xie, Y., Zhu, S., Song, X., Sun, X., Fan, Y., Liu, J., et al. (2017). The tumor suppressor p53 limits ferroptosis by blocking DPP4 activity. Cell Rep. 20, 1692–1704. doi:10.1016/j.celrep.2017.07.055
Yagoda, N., von Rechenberg, M., Zaganjor, E., Bauer, A. J., Yang, W. S., Fridman, D. J., et al. (2007). RAS-RAF-MEK-dependent oxidative cell death involving voltage-dependent anion channels. Nature 447, 864–868. doi:10.1038/nature05859
Yan, H. F., Zou, T., Tuo, Q. Z., Xu, S., Li, H., Belaidi, A. A., et al. (2021). Ferroptosis: Mechanisms and links with diseases. Signal Transduct. Target. Ther. 6, 49. doi:10.1038/s41392-020-00428-9
Yang, Y., Hu, H., Chen, L., Zhang, H., and Yang, J. (2022). A new survival model based on ferroptosis-related genes (FRGS) for prognostic prediction in bladder cancer. Actas Urol. Esp. 46, 494–503. doi:10.1016/j.acuroe.2022.06.001
Yi, K., Liu, J., Rong, Y., Wang, C., Tang, X., Zhang, X., et al. (2021). Biological functions and prognostic value of ferroptosis-related genes in bladder cancer. Front. Mol. Biosci. 8, 631152. doi:10.3389/fmolb.2021.631152
Yin, H., Xu, L., and Porter, N. A. (2011). Free radical lipid peroxidation: Mechanisms and analysis. Chem. Rev. 111, 5944–5972. doi:10.1021/cr200084z
Yu, F., Sankaran, V. G., and Yuan, G. C. (2021). CUT&RUNTools 2.0: A pipeline for single-cell and bulk-level CUT&RUN and CUT&Tag data analysis. Bioinformatics 38, 252–254. doi:10.1093/bioinformatics/btab507
Yu, F., Zhang, G., Shi, A., Hu, J., Li, F., Zhang, X., et al. (2018). LnChrom: A resource of experimentally validated lncRNA-chromatin interactions in human and mouse. Oxford: Database.
Zhang, Y., Zhang, Y., Hu, J., Zhang, J., Guo, F., Zhou, M., et al. (2020). scTPA: a web tool for single-cell transcriptome analysis of pathway activation signatures. Bioinformatics 36, 4217–4219. doi:10.1093/bioinformatics/btaa532
Zhang, Z., Guo, M., Shen, M., Kong, D., Zhang, F., Shao, J., et al. (2020). The BRD7-P53-SLC25A28 axis regulates ferroptosis in hepatic stellate cells. Redox Biol. 36, 101619. doi:10.1016/j.redox.2020.101619
Zheng, J., and Conrad, M. (2020). The metabolic underpinnings of ferroptosis. Cell Metab. 32, 920–937. doi:10.1016/j.cmet.2020.10.011
Zhou, H., Zhou, Y. L., Mao, J. A., Tang, L. F., Xu, J., Wang, Z. X., et al. (2022). NCOA4-mediated ferritinophagy is involved in ionizing radiation-induced ferroptosis of intestinal epithelial cells. Redox Biol. 55, 102413. doi:10.1016/j.redox.2022.102413
Keywords: bladder cancer, ferroptosis, iron metabolism, system Xc-GSH-GPX4 signaling pathway, metastasis, treatment
Citation: Zeng F, Lan Y, Wang N, Huang X, Zhou Q and Wang Y (2022) Ferroptosis: A new therapeutic target for bladder cancer. Front. Pharmacol. 13:1043283. doi: 10.3389/fphar.2022.1043283
Received: 13 September 2022; Accepted: 17 October 2022;
Published: 03 November 2022.
Edited by:
Chunpeng (Craig) Wan, Jiangxi Agricultural University, ChinaCopyright © 2022 Zeng, Lan, Wang, Huang, Zhou and Wang. This is an open-access article distributed under the terms of the Creative Commons Attribution License (CC BY). The use, distribution or reproduction in other forums is permitted, provided the original author(s) and the copyright owner(s) are credited and that the original publication in this journal is cited, in accordance with accepted academic practice. No use, distribution or reproduction is permitted which does not comply with these terms.
*Correspondence: Xiaobo Huang, ZHJodWFuZ3hiQDE2My5jb20=; Qiao Zhou, MjI5MjI5MzRAcXEuY29t; Yi Wang, d195aTIwMjJAMTYzLmNvbQ==
†These authors have contributed equally to this work