- 1Department of Pharmacy, Baotou Medical College, Baotou, China
- 2Inner Mongolia Maternal and Child Health Care Hospital, Hohhot, China
- 3Institute of Bioactive Substance and Function of Mongolian Medicine and Chinese Materia Medica, Baotou Medical College, Baotou, China
Amygdalus mongolica oil is rich in unsaturated fatty acids such as inoleic acid (47.11%) and oleic acid (23.81%). Our research demonstrates that it exerts a protective effect on rat models of pulmonary fibrosis, however, little is known regarding the underlying mechanism of action. This study aimed to characterize the therapeutic mechanism of action of A. mongolica oil on bleomycin-induced pulmonary fibrosis in rats. A. mongolica oil appears to regulate the levels of potential key serum biomarkers which include tetrahydrobiopterin, L-serine, citrulline and estradiol to participate in folate biosynthesis, glycine, serine and threonine metabolism, arginine biosynthesis and steroid hormone biosynthesis. And it also enriched intestinal microbial abundance, homogeneity and modulated the abundance of Duncaniell, Desulfovibrio, Peptococcaceae_unclassified, Dubosiella, Tyzzerella, Lachnospiraceae_NK4A136_group, Lactobacillus, Clostridiales_unclassified to exert a protective effect against pulmonary fibrosis. A. mongolica oil appears to confer protective effects against pulmonary fibrosis by affecting the level of pulmonary fibrosis metabolites and the abundance of related intestinal flora through multiple targets, as evidenced by our untargeted LC-MS/MS metabonomics evaluation and 16S rDNA sequencing technology.
1 Introduction
Pulmonary fibrosis (PF) is a destructive lung disease with a myriad of etiologies and clinicopathologic features. It is characterized by alveolar epithelial cell injury, excessive proliferation of fibroblasts, the accumulation of a large amount of extracellular matrix accompanied by inflammatory damage, destruction of lung tissue structure, and eventually culminates in respiratory failure as a result of impaired gas exchange and lung function (Prasse et al., 2010; Chanda et al., 2019). Common clinical manifestations are cough, chest tightness, asthma, fatigue, and palpitations (Song et al., 2010). The etiology of PF is largely unknown, and the pathogenesis is complex, with multiple signaling pathways intertwined and possibly including chronic inflammatory responses and cytokine actions. During the development of pulmonary fibrosis disease, the damaged alveolar epithelial cells produce and release large amounts of growth factors and cytokines to stimulate inflammatory cells, and eventually leads to excessive proliferation and migration of fibroblasts (Hu et al., 2021a). In Chinese medicine, pulmonary fibrosis belongs to the category of “lung impotence” and “lung paralysis”. The causes of the disease are mostly external evil attack and mismanagement, and the pathogenesis is mostly deficiency of lung and kidney, and mutual stagnation of phlegm and stasis (Wang et al., 2021). Many different diseases may cause PF. Its affects approximately three million people around the world, and its prevalence sharply increases with age (Martinez et al., 2017). At present, Novel Coronavirus Pneumonia is threatening human health in some countries and most patients still have symptoms such as lung inflammation, pulmonary fibrosis, and dyspnea in the prognosis (Zhan et al., 2020). Therefore, there is an urgent need to discover novel therapeutic drugs of treating and improving the prognosis of PF. Current pharmaceutical agents used to treat PF encompass glucocorticoids, immunosuppressants and cytotoxic drugs, all of which are not efficacious in the long-term and cause several adverse effects. In recent years, Chinese medicine has emerged as a potential candidate in protecting against and treating PF (Liu et al., 2019).
Metabonomics studies the response of metabolites to pathological stimuli and drug treatments in the context of complex biological systems. It can be used to predict the level of metabolites in the body and to guide diagnosis as well as intervention (Wu et al., 2019). Metabonomics adopts a “top-down” strategy to derive the functional status of an organism based on its metabolic networks. Understanding how an intervention changes the metabolism of an organism is very much in line with traditional Chinese medicine dogma (Zhang et al., 2012). Therefore, the application of metabonomics in traditional Chinese medicine has important practical significance for understanding the pharmacodynamics, mechanism of action, guiding the treatment course, as well as in researching traditional Chinese medicine germplasm resources and preclinical safety (Lyu et al., 2018).
Intestinal flora possesses a symbiotic relationship with its human host. Given its astonishing complexity, intestinal microbiota is considered an entire metabolic organ on its own that has the ability to influence human health and participate in the occurrence and development of various diseases (Gosalbes et al., 2012). Intestinal flora is closely related to the respiratory tract, with specific flora possessing the ability to affect the pathogenesis of respiratory diseases (Dumas et al., 2018). The interaction between gut microbes and the lungs is called the gut-lung axis (He et al., 2017). Intestinal flora and lungs interact in pathological states, and many pulmonary diseases such as asthma and lung infections are accompanied by gastrointestinal symptoms. However, the lung diseases can also cause changes in the structure and diversity of the intestinal flora of patients (Tan et al., 2020). The specific flora can help the body maintain a stable immune system balance by reducing the inflammatory response and help the lungs fight infection (Shi et al., 2020). The gut microbiome has the potential to become a powerful diagnostic tool, which can either be modified to create personalized treatment or be monitored over time to aid in diagnosing various diseases early. This will have a profound impact on the improvement of human health and the development of precision medicine (Zhou et al., 2020).
The integration of metabonomics and intestinal flora revealed significant interactions between bacteria and host (Clos-Garcia et al., 2020). Today, intestinal microbiome analysis combined with serum metabonomics has been applied to the pathogenesis and treatment of many diseases such as fibromyalgia, colorectal cancer, hyperuricemia and renal fibrosis (Weir et al., 2013; Clos-Garcia et al., 2019; Hu et al., 2020; Wang et al., 2020). The integrated application of multiple-omics has become an important means of comprehensively using systems biology to study drugs and diseases. The characteristics of multi-component, multi-target, multi-level, and multi-metabolic pathways in traditional Chinese medicine are consistent with the overall, systematic, and comprehensive nature in multi-omics. Using intestinal flora and metabonomics technology, it is possible to carry out a comprehensive analysis of outcomes, and to understand the principles and mechanisms of Chinese medicine in depth, which greatly promotes the research of Chinese medicine and is conducive to the modernization of Chinese medicine (Zhu et al., 2008; Liu et al., 2012).
Amygdalus mongolica (Maxim.) Ricker belongs to the Subgen. Amygdalus of Rosaceae Amygdalus, and is known as Wulian-Buyilesi in Inner Mongolia and is unique to the Gobi Desert (Yue et al., 2020). It is rich in alkaloids, total flavonoids, polysaccharides, fatty acids, amygdalin, vitamin E, protein, and other medicinal and nutritional ingredients (Shi et al., 2013; Su et al., 2013; Zhu et al., 2013; Bai et al., 2015; Zhou et al., 2015; Jia et al., 2018; Yang et al., 2018). The seeds of A. mongolica are a substitute for “Yu Liren” (Pruni semen) in traditional Chinese medicine, and is thought to enter the lung meridian to relieve cough and asthma, mainly used to treat dry throat, dry cough, bronchitis, yin deficiency constipation, and edema (Zhao, 1995; Liu et al., 2017). We found that petroleum ether extract of A. mongolica can effectively fight liver, lung and kidney fibrosis (Chang et al., 2020; Jia et al., 2020; Quan et al., 2020; Chang et al., 2021; Wu et al., 2021). A. mongolica is an important woody oil-bearing tree species, with its kernels consisting of more than 50% oil (Zhang et al., 2016a). The oil is rich in mineral elements such as zinc, iron, calcium, as well as 16 different fatty acids (primarily oleic and inoleic acid, which make up 98% of all its fatty acids) (Wang et al., 2012). It meets all the requirements of the national edible oil grade three quality standard and is a substance with high developmental value. The unsaturated fatty acids in A. mongolica oil can play a role in repairing fatty liver damage by enhancing the vitality of liver tissue antioxidant enzymes and antioxidants (Zheng et al., 2017). It can also improve the body’s antioxidant capacity and reduce the degree of renal disease (Hao et al., 2020). Preliminary experimental studies have shown that A. mongolica oil can significantly reduce the content of collagen Ⅲ (COL-III), collagen Ⅳ (COL-Ⅳ), hyaluronic acid (HA), laminin (LN), interleukin-1β (IL-1β), interleukin-6 (IL-6), and hydroxyproline in lung tissues, regulate the TGF-β1/Smad signaling pathway, reduce extracellular matrix deposition, and inhibit collagen fiber proliferation to reduce alveolar inflammation and PF (Li et al., 2021).
Therefore, our experiment combined metabolomics and intestinal microflora research to more completely characterize the protective mechanism of A. mongolica oil on PF rat models. This investigation provides experimental and scientific evidence on the protective effect of A. mongolica oil on the lungs, while also providing new ideas for the rational development and utilization of A. mongolica oil resources in the pharmaceutical and food industries.
2 Materials and methods
2.1 Preparation of A. mongolica oil
The seeds of A. mongolica were collected from the RuoRigong Alxa in Inner Mongolia. The dry mature seeds were identified by Professor Songli Shi of the Inner Mongolia University of Science and Technology Medical College of Baotou. The seeds are dehulled and crushed into granules. Using the solvent extraction method, the seed kernels are wrapped in filter paper and placed in a soxhlet extractor. Petroleum ether (analytical pure, Tianjin Kaitong Chemical Reagent Co., Ltd, China) is then added. The seeds were subjected to the extraction procedure twice (1 time/2 h) in a water bath. The extract was filtered and dried in order to further concentrate it. The concentrated solution was placed in an oven at 105°C to dry for 2 h, and the supernatant was left to cool to obtain the A. mongolica oil. The extraction rate was approximately 87.51%. The oil is then stored in a refrigerator at 4°C until later use. Using GC/MS analysis, we report that the oil content of unsaturated fatty acids comprised of inoleic acid (47.11%), oleic acid (23.81%), palmitic acid (21.37%), palmitoleic acid (2.37%), 8,11 -Octadenoic acid (1.65%), and arachidonic acid (1.25%) (Zhao et al., 2016).
2.2 Chemicals and reagents
Pentobarbital sodium was purchased from Merck, Ltd (Germany). Bleomycin powder for injection was purchased from Invitrogen (France). ELISA kit (COL-III, COL-IV, HA, LN, IL-6, IL-1β) was purchased from Jiangsu Immunoenzyme Laboratory Co., LTD (China). Methanol, acetonitrile, and formic acid (Chromatographically pure) were purchased from Fisher (America). E. Z.N.A.®Stool DNA Kit (D4015) was purchased from Omega, Inc (America). AMPure XT beads were purchased from Beckman Coulter Genomics (America). A Qubit dsDNA HSA ssay Kit were purchased from Invitrogen (America).
2.3 Model construction and treatment
Sixty male SPF grade male Sprague-Dawley rats (170–200 g) were purchased from the Department of Medical Sciences of Peking University [Department of Experimental Animal Science, licence number SCXK (Beijing) 2017-0005]. All animals were maintained under a 12 h light/dark cycle at a constant temperature and humidity. Each animal had free access to food and water. The experimental procedure was approved by the Medical Ethics Committee of Baotou Medical College, Inner Mongolia University of Science and Technology (20170317).
A mature, stable, and reproducible one-time tracheal instillation of bleomycin was used to prepare a rat PF model in this experiment (Zhang et al., 2016b). The rats are slowly administered bleomycin through a space between the tracheal cartilage rings into the trachea and lungs to establish a PF model. Sixty rats were randomly divided into six groups according to their body weight: normal control (CON) group, model control (MOD) group, positive control prednisone acetate (PAY) groups, the high (OIL-H), medium (OIL-M) and low (OIL-L) dose A. mongolica oil groups. The CON group and the MOD group were treated with normal saline at a dose of 4 ml/kg daily. The dosage of PAY was 1.5 mg/kg daily, and that of OIL-H, OIL-M and OIL-L were 2.5, 5, and 7.5 ml/kg daily, respectively (Zheng et al., 2018). After 28 days, the rats were anesthetized with 3% sodium pentobarbital via intraperitoneal injection after the last administration to 24 h. The lung tissues were taken out for biochemical indexes and histopathological analysis. Blood samples were collected from abdominal aorta and processed for serum extraction. Serum samples were stored at −80°C until further metabolomics research. The cecums of the rats were removed immediately, and the contents were collected and stored in a refrigerator at −80°C for DNA extraction for intestinal flora research.
2.4 Determination of biochemical indexes and pathological analysis
The COL-III, COL-IV, HA, LN, IL-6, and IL-1β contents in the tissue homogenates were determined by the enzyme-linked immunosorbent assay. Paraffin-embedded lung tissue was cut into 3–4 μm-thick sections and stained with HE and Masson to observe pathological changes.
2.5 Metabolomics analysis
2.5.1 LC-MS/MS acquisitions
All samples were acquired by the LC-MS system followed machine orders. Firstly, all chromatographic separations were performed using an ultra performance liquid chromatography (UPLC) system (SCIEX, United Kingdom). An ACQUITY UPLC T3 column (100 mm*2.1 mm, 1.8 µm, Waters, United Kingdom) was used for the reversed phase separation. The mobile phase consisted of solvent A (water, 0.1% formic acid) and solvent B (Acetonitrile, 0.1% formic acid). Gradient elution conditions were set as follows: 0–0.5 min, 5% B; 0.5–7 min, 5%–100% B; 7–8 min, 100% B; 8–8.1 min, 100%–5% B; 8.1–10 min, 5% B. The injection volume for each sample was 4 µL. The column oven was maintained at 35°C and the flow rate was 0.4 ml/min.
A high-resolution tandem mass spectrometer TripleTOF5600plus (SCIEX, United Kingdom) was used to detect metabolites eluted form the column. The Q-TOF operates in positive and negative ion mode respectively. The curtain gas was set 30 PSI, lon source gas1 and gas2 were set 60 PSI and an interface heater temperature was 650°C. For positive and negative ion mode, the lonspray floating voltage were set at 5000 V and 4500 V, respectively. The mass spectrometry data were acquired using the IDA mode. The TOF mass ranged from 60 to 1200 Da. The survey scans were acquired at 150 ms and total cycle time was fixed to 0.56 s. Pulser frequency value of 11 kHz and dynamic exclusion was set for 4 s. The particle signals from each scan are recorded four times in four channels and then combined and converted into data. Furthermore, in order to evaluate the stability of the LC-MS during the whole acquisition, a quality control sample (Pool of all samples) was acquired after every 10 samples.
2.5.2 Data analysis of metabolomics
The Proteowizard’s MSConvert software was used to convert the raw mass spectrometer data into readable data format mzXML. The XCMS software is used for peak extraction and quality control, and CAMERA was used for additive ion annotation of the extracted substances. The MetaX software was used to identify metabolites using primary spectrometry information for database matching identification. Candidate identified substances were matched against HMDB, KEGG, and other databases for metabolite annotation to explain the physicochemical properties and biological functions of the selected metabolites. The MetaX software was used to quantify metabolites and screen differential metabolites. QC data were used for internal normalization in our study, and metabolic features with a QC relative standard deviation (RSD) > 30% were removed. The principal component analysis (PCA) and partial least squares discriminant analysis (PLS-DA) analyses were used to screen different substances for each group of serum samples. In this experiment, metabolites with variable importance in the projection (VIP) > 1 and p < 0.05 were screened as candidate differential metabolites for the anti-pulmonary fibrosis effect of A. mongolica oil. The MetaboAnalyst software 5.0 was used to analyze the metabolic pathway MetPA (http://metpa.metabolomics.ca), the pathways with a metabolic pathway impact value >0.02 and metabolic pathway significance level −Log (P) > 2 were identified as the key metabolic pathways affecting PF. Based on the differential metabolites involved in these key metabolic pathways, key biomarkers of PF were also identified. Observation of key biomarker changes after A. mongolica oil administration intervention.
2.6.16S rDNA high throughput sequencing
2.6.1 DNA extraction
DNA from different samples of cecums contents was extracted using the E. Z.N.A.®Stool DNA Kit according to manufacturer’s instructions. The total DNA was eluted in 50 μl of Elution buffer and stored at −80°C until further PCR assessment by LC-Bio Technology Co., Ltd., Hang Zhou, Zhejiang Province, China.
2.6.2 PCR amplification and 16S rDNA sequencing
The 5′ ends of the primers were tagged with specific barcodes per sample and sequencing universal primers. The primers of 515F (5′-GTGYCAGCMGCCGCGGTAA-3′), 806R (5′-GGACTACHVGGGTWTCTAAT-3′) are used. PCR amplification was performed in a total volume of 25 μl reaction mixture containing 25 ng of template DNA, 12.5 μl PCR Premix and 2.5 μl of each primer. The PCR conditions to amplify the prokaryotic 16S fragments consisted of an initial denaturation at 98°C (30 s), 32 cycles of denaturation at 98°C (10 s), annealing at 54°C (30 s), extension at 72°C (45 s) and final extension at 72°C (10 min). The PCR products were confirmed with 2% agarose gel electrophoresis. The PCR products were purified and quantified using AMPure XT beads and Qubit, separately. The size and quantity of the amplicon library were assessed with an Agilent 2100 Bioanalyzer and with the Library Quantification Kit for Illumina. The libraries were sequenced on NovaSeq PE250 platform.
2.6.3 Data analysis of intestinal flora
Quality filtering of the raw reads using fqtrim (v0.94) allowed us to obtain high-quality clean tags. Chimeric sequences were filtered using the Vsearch software (v2.3.4). After dereplication using DADA2, we obtained a feature table and feature sequence. Alpha diversity and beta diversity were calculated by normalizing the data to the same sequences randomly. Alpha and beta diversity was obtained using QIIME2. Analysis of gut microbial composition and differences between groups was performed to screen for dominant flora.
2.7 Statistical analysis
The software SPSS19.0 was used to analyze the experimental data and the experimental results are expressed as means ± SD. The differences between all groups were analyzed using one-way analysis of variance (ANOVA). Variations between groups were considered to be significant if p values less than or equal to 0.05.
3 Results
3.1 Determination of biochemical indicators and histopathological analysis
As shown in Figure 1A, the histopathological scores used to assess the severity of pulmonary fibrosis increased significantly in the MOD group and decreased significantly after A. mongolica oil administration. HE and Masson staining showed that the lung tissue structure of CON group was clear and normal. Compared with the CON group, the alveolar structure of the MOD group disappeared, interstitial edema of lung tissue, obvious infiltration of inflammatory cells, serious collagen deposition and obvious fibrosis appeared. Compared with the MOD group, the alveolar wall of rats in PAY group, OIL-L, OIL-M, and OIL-H groups became thinner, a small amount of inflammatory cell infiltration and pulmonary interstitial collagen deposition were observed, and interstitial edema was reduced. Among them, the pulmonary interstitial collagen deposition and fibrosis degree in OIL-L and OIL-M groups were significantly reduced, the alveolar structure was relatively complete, and the area of pulmonary consolidation was small.
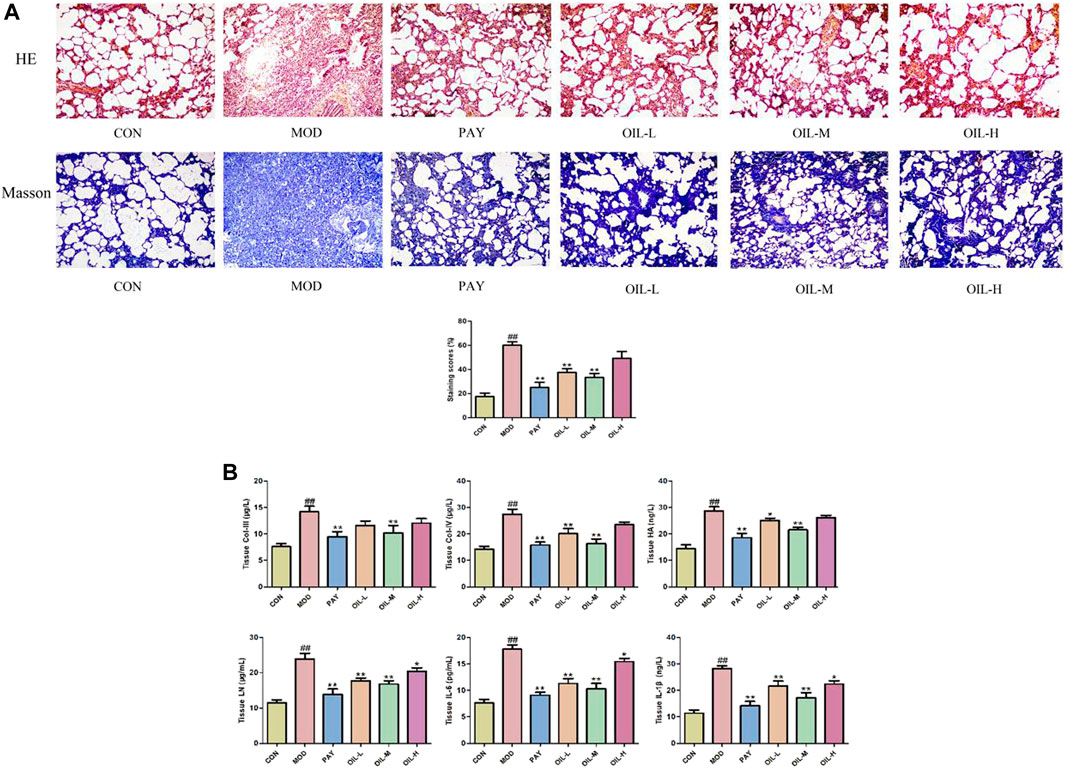
FIGURE 1. The effect of A. mongolica oil on histopathology of pulmonary fibrosis rats (HE, Masson×100) (A). Effects of A. mongolica oil on tissue biochemical indices in rats with pulmonary fibrosis (B). **p < 0.01,*p < 0.05 vs. model group; ##p < 0.01, #p < 0.05 vs. control group.
As shown in Figure 1B, Compared with the CON group, All indexes of lung tissue in MOD group were significantly increased (p < 0.01). Compared with the MOD group, the contents of all biochemical indexes in lung tissue of PAY group were significantly decreased (p < 0.01); The contents of COL-Ⅳ, LN, IL-6, and IL-1β in lung tissue of rats in OIL-L group were significantly decreased (p < 0.01), and the content of HA decreased (p < 0.05); The contents of all biochemical indexes in lung tissue of rats in the OIL-M group decreased significantly (p < 0.01); The contents of LN, IL-6, and IL-1β in lung tissue of rats with OIL-H decreased significantly (p < 0.05).
3.2 Metabolomics analysis
3.2.1 Screening for differential metabolites
According to biochemical indexes and histopathological results, the medium dose of Mongolian almond oil was selected for metabonomic study. The quality control curves in the total ion flow chromatogram of the quality control samples in positive and negative ion modes were basically overlapped, indicating good stability of the detection system. PLS-DA was used as a supervised multivariate statistic to maximize the separation of samples. PLS-DA analysis of the CON and MOD groups in positive and negative ion mode showed significant separation and suggested that there is variability between these two groups of metabolites (Figures 2A,B). The PLS-DA models were verified using the replacement test diagram as shown in Figures 2C,D, which showed that R2 and Q2 were lower than the original value on the far right from left to right and Q2 intersected with Y axis in the negative half axis, indicating good fitting and reliable results. A total of 42 putative identifications were obtained by screening and identifying with VIP >1 and p < 0.05 according to the Volcano Plot (V-plot) (Figures 2E,F and Table 1).
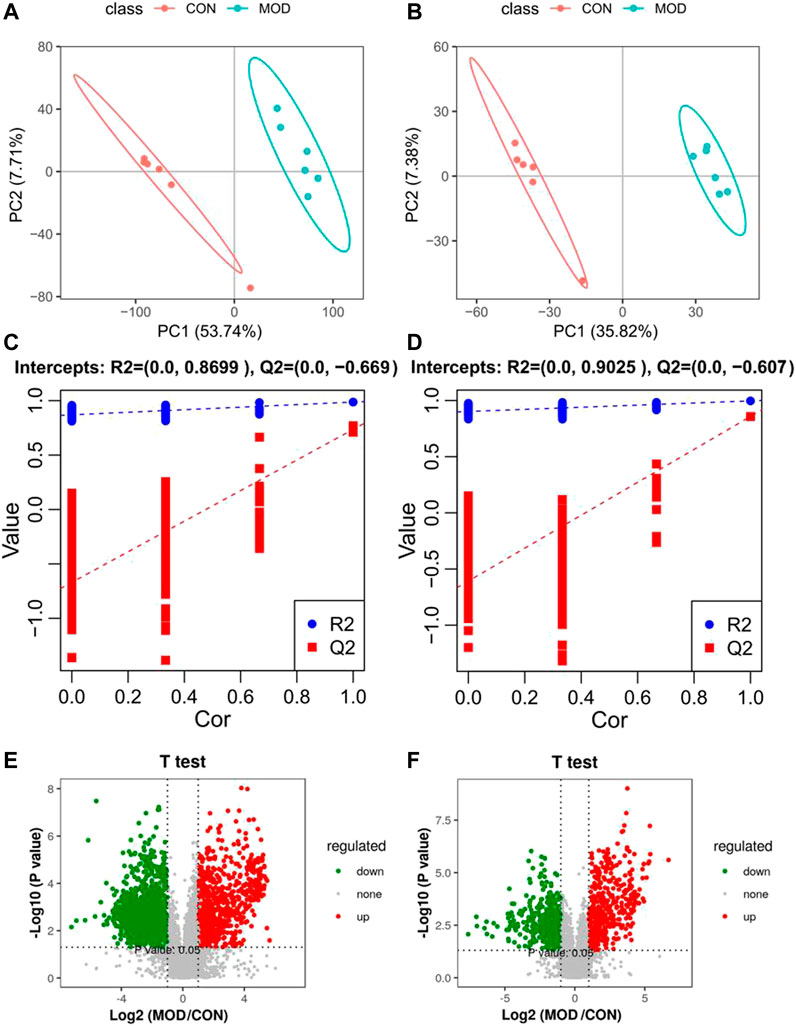
FIGURE 2. PLS-DA score diagram (A,B), corresponding model verification diagram (C,D) and V-plot score chart (E,F) of control group and model group in positive and negative ion mode.
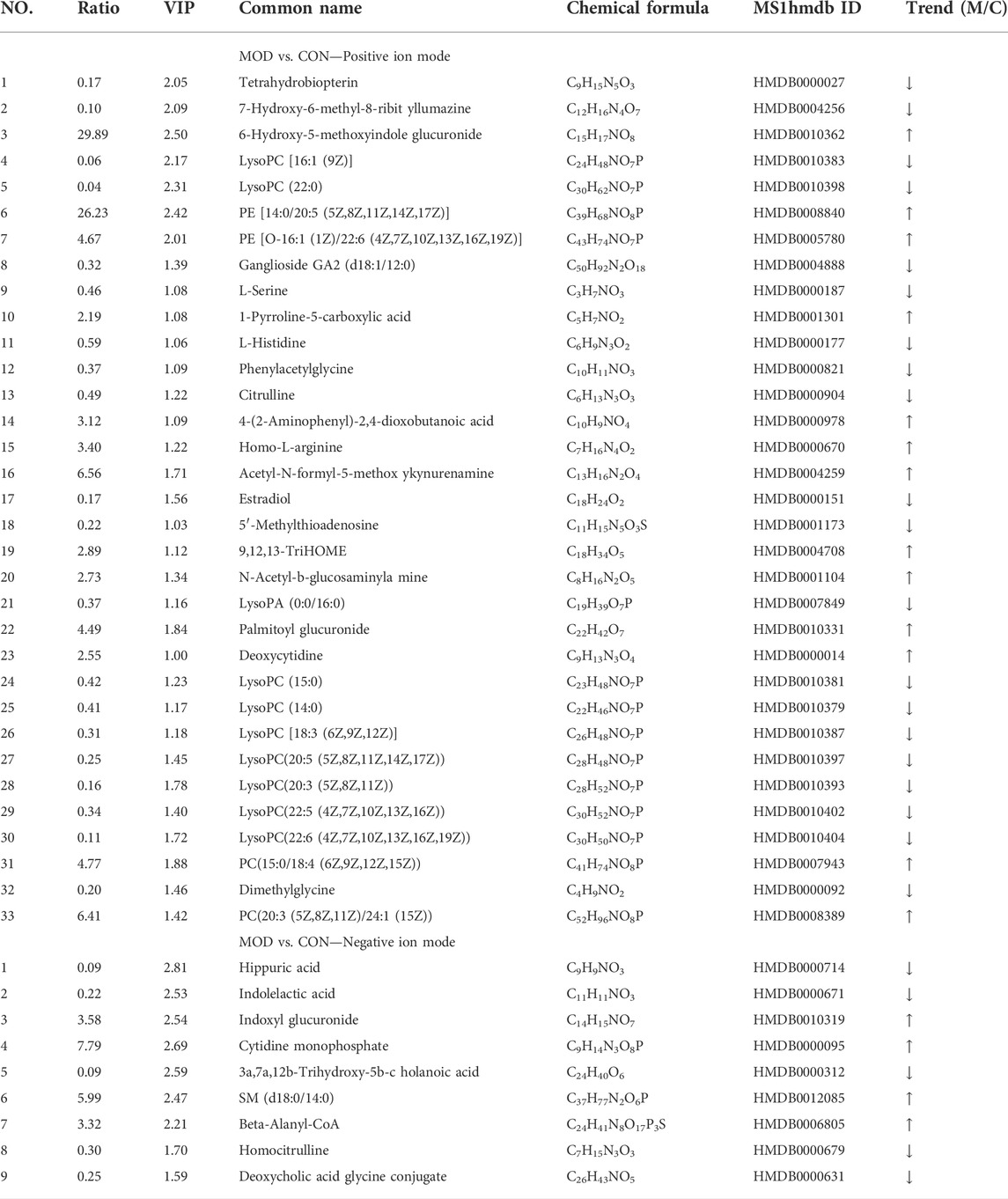
TABLE 1. Differential metabolites and their identification results of serum from the control and model groups.
3.2.2 Analysis of serum metabolic profile under A. mongolica oil intervention
The PCA results (Figures 3A,B) of the serum metabolic profile of rats in each group showed significant separation between the MOD and CON groups in both positive and negative ion modes. All metabolites separated and clustered well. Moreover, there was a good degree of separation between the A. mongolica oil group (OIL) and the MOD group, with OIL group eventually returned to the CON group. This indicates that A. mongolica oil has a positive regulatory effect on the endogenous metabolites of PF rats. In order to better distinguish the influence of each group on the metabolic profile, the PLS-DA is used to analyze the metabolic profile to the maximum extent (Figures 3C,D). The results showed that the metabolites of the model and the CON groups were significantly different. The OIL group was further separated from the MOD group and trended towards the CON group. The PLS-DA models were verified using class permutation tests as shown in Figures 3E,F, indicating that these models are of good fit. The predictive R2 and Q2 values were 0.91 and 0.06 in the positive mode and 0.70 and 0.27 in the negative mode, respectively.
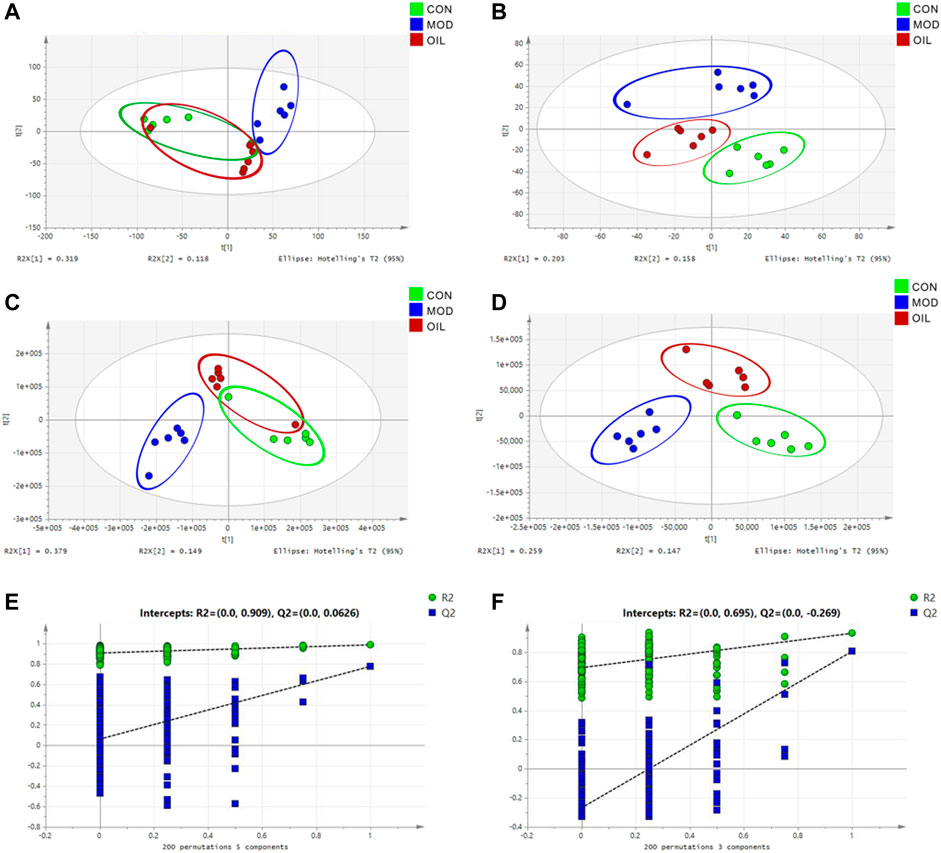
FIGURE 3. PCA score plot (A,B), PLS-DA score plot (C,D) and validation plot of the PLS-DA model (E,F) of control group, model group and A. mongolica oil group in positive and negative ion mode.
3.2.3 Effects of A. mongolica oil on differential metabolites
In order to explore the influence of A. mongolica oil on 42 putative identifications, a heat map cluster analysis was performed on the change trend of each group of differential metabolites, as shown in Figure 4A. The metabolites expression in model group was significantly changed compared with the normal group, and the OIL group could callback the levels of these differential metabolites which were altered by the MOD group. The p value heat map (Figure 4B) shows a significant difference between the model and the CON groups, as well as between the OIL group and the MOD group. Our analysis showed that compared with CON group, a total of 17 differential metabolites were significantly up-regulated and 25 were significantly down-regulated in MOD group, while seven of them were down-regulated and 11 were up-regulated in OIL group (p < 0.05).
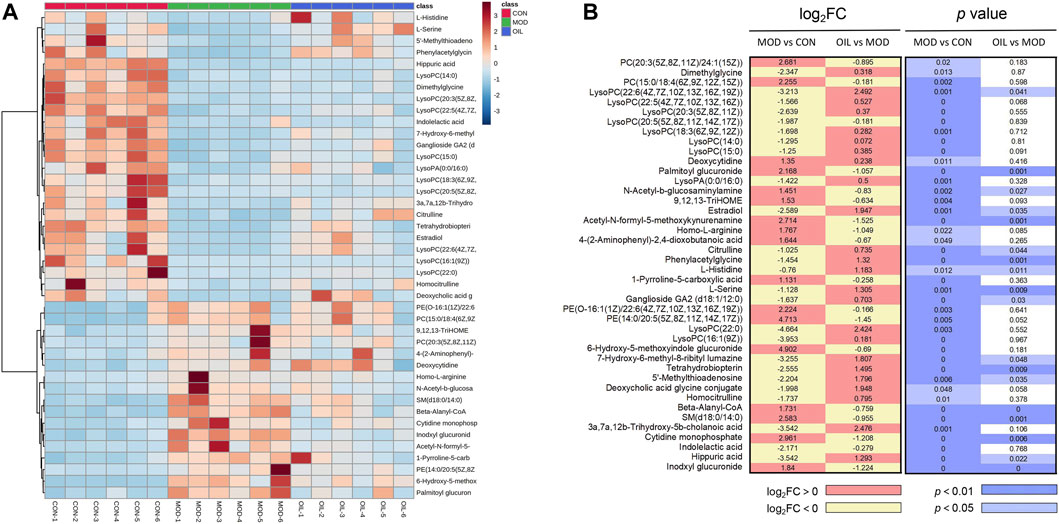
FIGURE 4. Heat map of the differentially abundant metabolites in all groups (A). The degree of colour saturation indicates the metabolite expression with blue and red respectively indicating the lowest and highest expression. p-Value heat map of the differential abundance of metabolites in all groups (B). The degree of colour saturation indicates intergroup differences in metabolite expression values with white and blue indicating non-significant and significant differences, and pink and yellow indicating upregulation and downregulation, respectively.
3.2.4 Effect of A. mongolica oil on metabolic pathways and key biomarkers
The 42 different metabolites initially identified in the serum of the model and CON groups were analyzed using the MetPA metabolic pathway. A total of seven key differential metabolic pathways were selected based on -Log (P) > 2 and Impact value >0.02 (Figure 5A): pentose and glucuronate interconversions, arginine and proline metabolism, folate biosynthesis, glycine, serine and threonine metabolism, arginine biosynthesis, steroid hormone biosynthesis and glycerophospholipid metabolism. The screening of corresponding metabolites in the pathways are the key potential biomarkers affecting PF in Table 2. The changes in key biomarkers content after administration of A. mongolica oil were shown in Figure 5B. Compared to the MOD group, A. mongolica oil significantly regulated the levels of tetrahydrobiopterin, L-serine, citrulline and estradiol based on ANOVA analysis (p < 0.05).
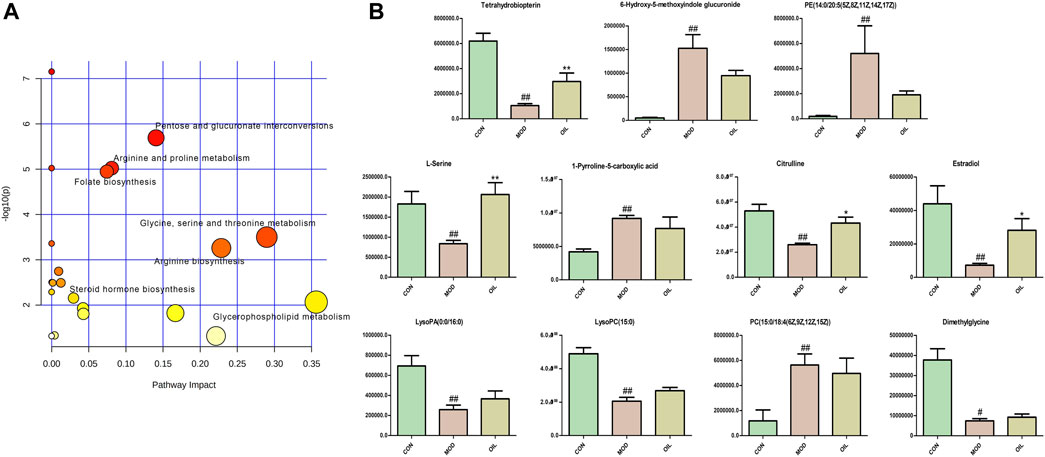
FIGURE 5. Metabolic pathway analysis of crucial biomarkers (A). The size and colour of each circle indicate the pathway impact value and p-value respectively. Statistical significance of the 11 potential biomarkers were calculated using ANOVA after administration of A. mongolica oil (B). *p < 0.05 and **p < 0.01 compared to the model control group. #p < 0.05 and ##p < 0.01 compared to the control group.
3.3 Analysis of intestinal flora
3.3.1 Sequence diversity evaluation
The Venn diagram in Figure 6A shows that the CON and the MOD groups jointly detected 688 common OTU numbers. The number of OTUs shared by the OIL group and the CON group is more than the number of OTUs shared by the MOD group and CON group. It shows that the OTU of the OIL and the CON group are highly similar. PCA was used to analyze the difference of intestinal flora in each group. Figure 6B shows a significant separation between the MOD group and the CON group, indicating that there was a remarkable difference in microbial composition. The OIL group was significantly separated from the MOD group. However, the OIL group partially overlapped with the CON group, indicating that the microbial composition and structure between the samples were more similar and the difference was small. The evaluation of flora diversity mainly reflects the abundance and uniformity through Chao1, Observed species, shannon, Simpson and other indexes. The abundance and uniformity indices of the MOD group were lower than those of the CON group. But these indices increased upon treatment with A. mongolica oil, and simpson index was significant. (Figure 6C, p < 0.05).
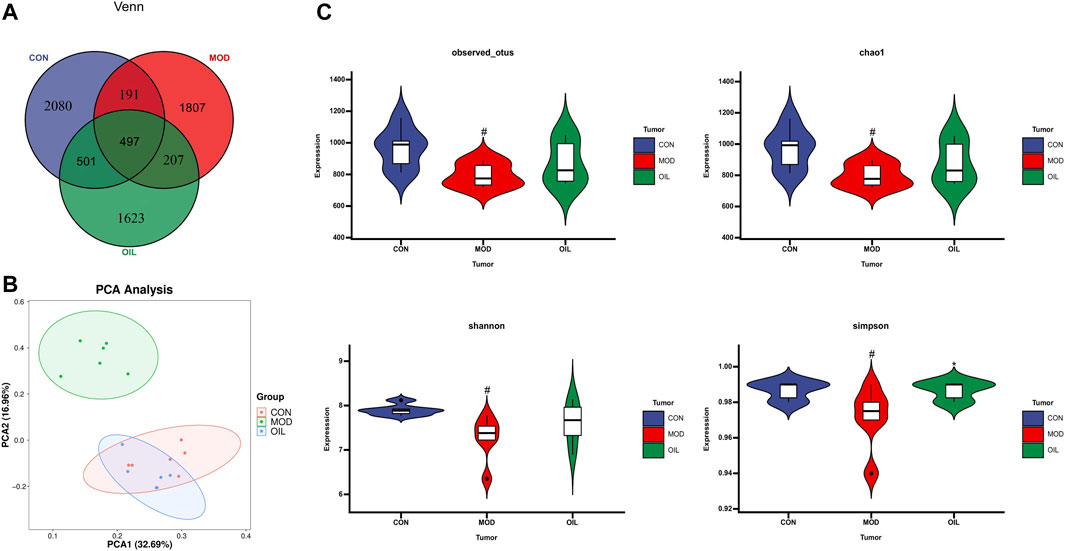
FIGURE 6. Venn diagram depicting the distribution of OTUs among different groups (A). PCA score plot of control group, model group and Amygdalus mongolica oil group (B). Intestinal microbial Alpha diversity of Chao1, Simpson, Shannon and Observed species in all groups (C). Statistical significance was calculated with ANOVA. *p < 0.05 and **p < 0.01 compared to the model control group. #p < 0.05 and ##p < 0.01 compared to the control group.
3.3.2 Analysis of intestinal microbe composition
The highest abundance was selected to form the dominant intestinal flora at the genus level as shown in Figure 7A. There are Muribaculaceae_unclassified, Lachnospiraceae_NK4A136_group, Firmicutes_unclassified, Lachnospiraceae_unclassified, Intestinimonas, Eubacterium]_coprostanoligenes_group, Helicobacter, Desulfovibrio, Duncaniella, Ruminococcaceae_UCG-005 and so on. They belong to the Firmicutes, Bacteroidetes, Proteobacteria, Epsilonbacteraeota and other, srespectively (Figure 7B). Clustering according to the similarity between the root samples is presented using a heat map as shown in Figure 7C. The results show that the MOD group is significantly separated from the CON group. The OIL group is clustered close to the CON group, and has a callback effect on the altered bacterial genera in the MOD group.
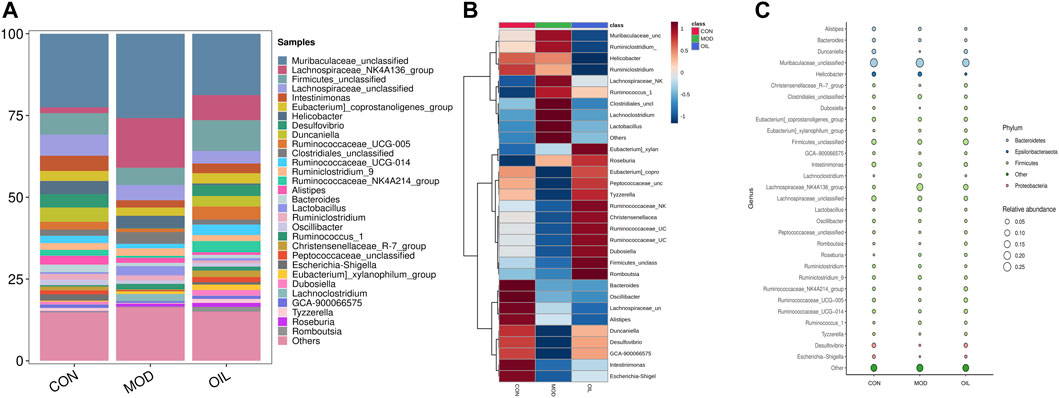
FIGURE 7. Species composition abundance map at the genus level (A). Abundance clustering heat map of the genera (B). The closer to blue, the lower the abundance, and the closer to red, the higher the abundance. Buble plot at the genus level (C). The color represents the phylum respectively.
3.3.3 Analysis of differences between groups
To further explore differences in the gut microbiota among the CON group, the MOD group and OIL group, we used LDA Effect Size (LEfSe) to recognize the specific altered bacterial phenotypes at each phylogenetic level. As Figure 8A shows that Screened the rank sum test p < 0.05 and LDA >3.0 were the biomarkers that were statistically different. At the genus level, the following microbiota was the most abundant in the A. mongolica oil: Christensenellaceae_R-7_group, Eubacterium]_xylanophilum_group, Peptococcaceae_unclassified, Dubosiella, Ruminococcaceae_NK4A214_group and so on. The changes in the microbiota among the three groups were also investigated using the ANOVA at genus level as shown in Figure 8B. Compared with the CON group, the abundance of Lachnospiraceae_NK4A136_group, Clostridiales_unclassified, Lactobacillus, Ruminococcus_1, Eubacterium]_xylanophilum_group and Lachnoclostridium in the MOD group were significantly increased (p < 0.05). On the other hand, the abundance of Intestinimonas, Desulfovibrio, Duncaniella, Alistipes, Bacteroides, Christensenellaceae_R-7_group, Escherichia-Shigella and Dubosiella were significantly reduced (p < 0.05). However, the abundance of Lachnospiraceae_NK4A136_group, Desulfovibrio, Duncaniella, Clostridiales_unclassified, Lactobacillus, Christensenellaceae_R-7_group, Peptococcaceae_unclassified, Dubosiella and Lachnoclostridium were close to the CON group significantly after the administration of A. mongolica oil (p < 0.05).
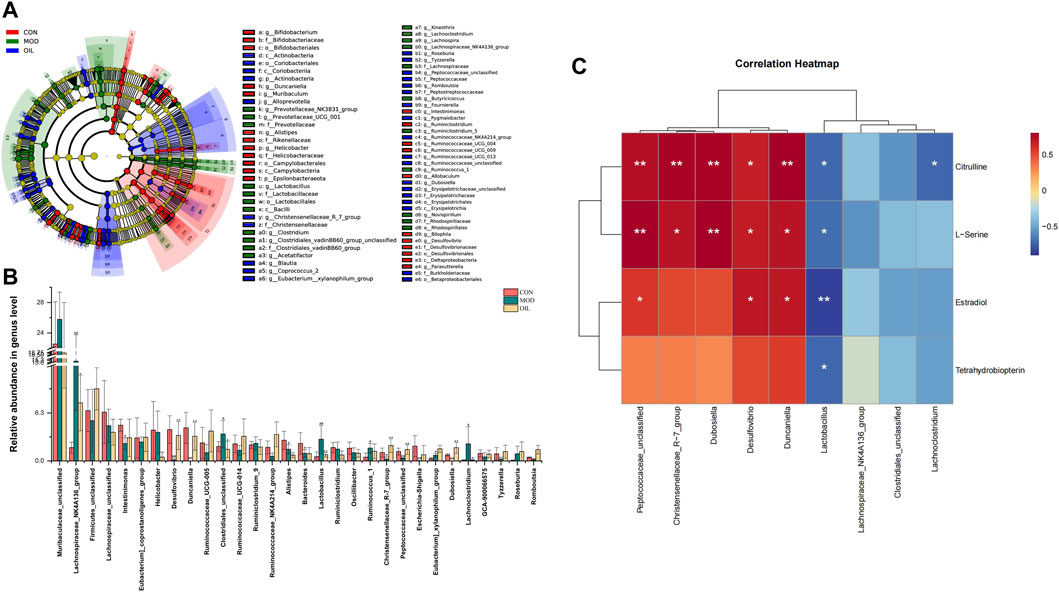
FIGURE 8. Intestinal microbial LEfSe from domain to species (A). Statistical significance of the intestinal contents were calculated using ANOVA after administration of A. mongolica oil at the genus level (B). *p < 0.05 and **p < 0.01 compared to the model control group. #p < 0.05 and ##p < 0.01 compared to the control group. Spearman analysis of potential biomarkers and key difference gut microbes (C).
3.4 Correlation analysis
Correlation clustering was used to analyze the interaction between serum metabolites and intestinal flora. Spearman correlation was used to calculate the differences about the four key metabolites and nine key gut microbes which varied significantly were found in the callback to the CON group after the administration of A. mongolica oil. As Figure 8C shows that we can see significant positive and negative associations between identified metabolites and gut microbiota were shown as a correlation work.
4 Discussion
In this study, untargeted LC-MS/MS metabolomics and 16S rDNA sequencing technology were used to determine the effect of A. mongolica oil treatment on the intestinal flora and serum metabolite profiles of bleomycin-induced PF rat models. The results indicated for the serum metabolite profiles and intestinal flora species of rats were affected by A. mongolica oil treatment. Rats in the MOD group were first screened and a total 11 potential key serum biomarkers were identified: 6-Hydroxy-5-methoxyindole glucuronide, 1-Pyrroline-5-carboxylic acid, Tetrahydrobiopterin, Dimethylglycine, L-Serine, Citrulline, Estradiol, LysoPA (0:0/16:0), PC(15:0/18:4 (6Z,9Z,12Z,15Z)), PE (14:0/20:5 (5Z,8Z,11Z,14Z,17Z)) and LysoPC(15:0). These metabolites participated in Pentose and glucuronate interconversions, Arginine and proline metabolism, Folate biosynthesis, Glycine, serine and threonine metabolism, Arginine biosynthesis, Steroid hormone biosynthesis and Glycerophospholipid metabolism. Tetrahydrobiopterin, L-Serine, Citrull A. mongolica and Estradiol demonstrated significant callback compared to controls after oil treatment. And the abundance of Lachnospiraceae_NK4A136_group, Desulfovibrio, Duncaniella, Clostridiales_unclassified, Lactobacillus, Christensenellaceae_R-7_group, Peptococcaceae_unclassified, Dubosiella and Lachnoclostridium were close to the CON group significantly after the administration of A. mongolica oil. The results show that A. mongolica oil affected the levels of PF metabolites and the abundance of certain intestinal flora through multiple targets, thereby improving PF.
L- Serine participates in the biosynthesis of macromolecules such as protein, nucleic acids, and lipids. It is considered to be the third largest metabolite after glucose and glutamine (Frezza, 2016). It is involved in pathophysiology of diabetes and neuropsychiatric diseases, regulation of tumor development, and inhibition of macrophage and neutrophil-mediated inflammatory response (Amelio et al., 2014; El-Hattab, 2016; He et al., 2019; Holm and Buschaed, 2019). As a non-protein amino acid, Citrulline takes part in peripheral protein homeostasis and is a precursor of de novo arginine synthesis in the kidneys, endothelial cells and immune cells (Bahri et al., 2013). Citrulline has roles in the treatment of rheumatoid arthritis, gastrointestinal function, multiple sclerosis, fibrosis, as well as several other diseases (Crenn et al., 2008; Gudmann et al., 2015; Darrah and Andrade, 2018). Citrulline is able to establish an anti-inflammatory microenvironment by increasing interleukin-10 and reducing IL-1β and interleukin-12 in human proximal tubular cells. It is also able to significantly reduce urinary albumin excretion, renal tubulointerstitial fibrosis, and kidney hypertrophy (Romero et al., 2013). The results of this study show that the A. mongolica oil treatment increases the level of nutritional amino acids with anti-inflammatory and antioxidant properties such as L-serine and citrulline, thereby enhancing the body’s ability to promote the expression of anti-inflammatory and antioxidant related genes to productive against PF. Estradiol is an antioxidant that can inhibit lipid peroxidation, loss of antioxidant enzyme activity and liver fibrosis (Lu et al., 2004). It reduces glomerular sclerosis and tubulointerstitial fibrosis by reducing extracellular matrix synthesis and increasing extracellular matrix degradation, thereby playing a role in renal protection (Mankhey et al., 2007). Tetrahydrobiopterin can improve the vascular endothelial function of patients with cystic fibrosis and those with advanced myocardial hypertrophy and fibrosis. It may reduce PF-induced pulmonary hypertension and pulmonary artery endothelial-mesenchymal transition (Moens et al., 2008; Almudéver et al., 2013; Jeong et al., 2019). A. mongolica oil enhances the levels of estradiol and tetrahydrobiopterin, resulting in reduced extracellular matrix formation in rats with PF.
Studies in humans and other mammals have revealed the effects of intestinal flora profiles on a range of physiologic processes which are affecting energy homeostasis, metabolism and immunologic activity among several other systems (Barko et al., 2017). The effect of the intestinal microbiota on the lungs is known as the “gut-lung” axis, and most of the inflammation involved in the alteration of bacteria or bacterial products crosses the gastrointestinal barrier into the blood vessels (Bingula et al., 2017). Dubosiella as beneficial bacteria, showed a decrease abundances in the PF mice model induced by irradiation of X-ray in Li’s research (Li et al., 2020). This is in accordance with our results. Additional studies demonstrated that Antrodin A from Antrodia camphorata can increase the relative abundance of Dubosiella and restore the composition of the intestinal flora to improve liver fat deposition, oxidative stress and inflammation induced by alcohol exposure and liver injury (Yi et al., 2021). Resistant dextrin can increase the relative abundance of Dubosiella to improved intestinal injury and reduce levels of pro-inflammatory cytokines and intestinal permeability (Zhang et al., 2021). Faecalibacterium prausnitzii can improve microbial imbalance, while higher levels of Dubosiella protects against allergic asthma caused by house dust mites and also works to reduce allergic airway inflammation (Hu et al., 2021b). β-globulin has anti-oxidant, antibacterial, and immunomodulatory properties. Rats fed with β-globulin showed an increased abundance of Duncaniella (Xia et al., 2020). Christensenellaceae_R-7_group may be involved in amino acid, peptide and lipid metabolism (Gong et al., 2020). Continuous probiotic supplementation increases the abundance of Christensenellaceae_R7_group (Khan and Chousalkar, 2020). Our study demonstrates that the abundance of Dubosiella, Duncaniela and Christensenellaceae_R7_group were increased after A. mongolica oil treatment compared to the MOD group and effectively regulated intestinal microbiota while inhibiting the infiltration of inflammatory cells and lung tissue fibrosis (Figure 9).
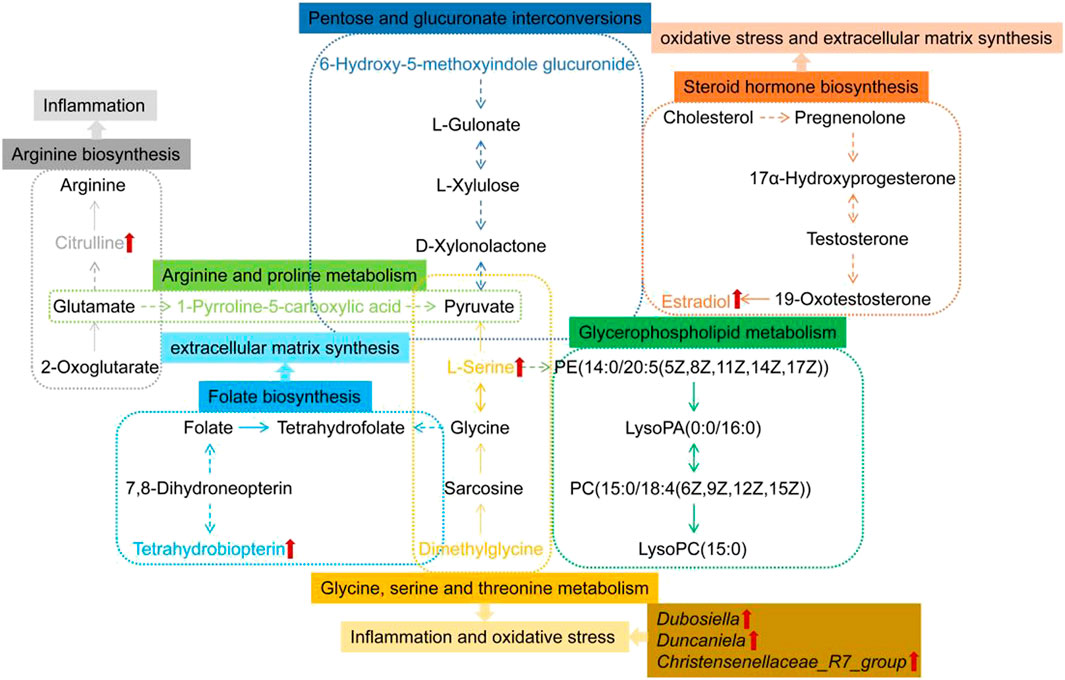
FIGURE 9. Metabolic networks of differential metabolites in response to PF and the preventive effect of A. mongolica oil. Key biomarkers for A. mongolica oil callbacks in red arrow.
5 Conclusion
Using untargeted LC-MS/MS metabolomics and 16S rDNA sequencing technology, we show that the A. mongolica oil can increase the level of Tetrahydrobiopterin, L-Serine, Citrulline and Estradiol to reduce oxidative and inflammatory damage, regulate the levels of extracellular matrix, resulting an overall improvement in rat models of PF. Additionally, it also can increase the abundance of Dubosiella, Duncaniela, Christensenellaceae_R7_group and so on to increase intestinal flora diversity and improve inflammation to improve PF. Following research should aim to verify the relationship between intestinal flora and key metabolic pathways. This study provides experimental and scientific basis supporting the protective effect of A. mongolica oil on fibrotic lungs, while providing new ideas for the rational development and utilization of A. mongolica oil resources in the pharmaceutical and food industries at the same time.
Data availability statement
The data presented in the study are deposited in the Genome Sequence Archive (Genomics, Proteomics & Bioinformatics 2021) in National Genomics Data Center (Nucleic Acids Res 2022), China National Center for Bioinformation/Beijing Institute of Genomics, Chinese Academy of Sciences (GSA: CRA008575) that are publicly accessible at https://ngdc.cncb.ac.cn/gsa.
Ethics statement
The animal study was reviewed and approved by the Baotou Medical college.
Author contributions
QL, HC, and H-BZ contributed to the literature search and writing the manuscript. J-QL, W-FB, JW, Z-JY, and MQ contributed to the table and figures. HC and S-LS contributed to the conception and design of the review. All authors have read and agreed to the published version of the manuscript.
Funding
This work was supported by the National Natural Science Foundation of China (81641137, 81760782, 82060784), the “Grassland Talents” Youth Innovation and Entrepreneurship Talent Project of Inner Mongolia Autonomous Region, China (Q2017046), the 11th “Grassland Talents” Talent Project of Inner Mongolia Autonomous Region, China [(2021)8], the Natural Science Foundation of Inner Mongolia Autonomous Region, China (2019MS08189), the Scientific Research Project of Inner Mongolia Autonomous Region, China (NJZY21048), the Baotou Medical College Doctor Scientific Research Start-up Fund (BSJJ201809), the Project of Baotou Medical College Innovation Team Development, China (bycxtd-02), the Project of Baotou Medical College Bud project, China (2021-56).
Conflict of interest
The authors declare that the research was conducted in the absence of any commercial or financial relationships that could be construed as a potential conflict of interest.
Publisher’s note
All claims expressed in this article are solely those of the authors and do not necessarily represent those of their affiliated organizations, or those of the publisher, the editors and the reviewers. Any product that may be evaluated in this article, or claim that may be made by its manufacturer, is not guaranteed or endorsed by the publisher.
Supplementary material
The Supplementary Material for this article can be found online at: https://www.frontiersin.org/articles/10.3389/fphar.2022.1037563/full#supplementary-material
References
Almudéver, P., Milara, J., De Diego, A., Serrano-Mollar, A., Xaubet, A., Perez-Vizcaino, F., et al. (2013). Role of tetrahydrobiopterin in pulmonary vascular remodelling associated with pulmonary fibrosis. Thorax 68 (10), 938–948. doi:10.1136/thoraxjnl-2013-203408
Amelio, I., Markert, E. K., Rufini, A., Antonov, A. V., Sayan, B. S., Tucci, P., et al. (2014). P73 regulates serine biosynthesis in cancer. Oncogene 33 (42), 5039–5046. doi:10.1038/onc.2013.456
Bahri, S., Zerrouk, N., Aussel, C., Moinard, C., Crenn, P., Curis, E., et al. (2013). Citrulline: From metabolism to therapeutic use. Nutrition 29 (3), 479–484. doi:10.1016/j.nut.2012.07.002
Bai, W. F., Shi, S. L., Zhou, H. B., and Zhang, Y. (2015). Comparision of contents of amygdalin in Amygdalus mongolica seed from different producing area. Chin. J. Health Lab. Technol. 25, 315–317.
Barko, P. C., McMichael, M. A., Swanson, K. S., and Williams, D. A. (2017). The gastrointestinal microbiome: A review. J. Vet. Intern. Med. 32 (1), 9–25. doi:10.1111/jvim.14875
Bingula, R., Filaire, M., Radosevic-Robin, N., Bey, M., Berthon, J. Y., Bernalier-Donadille, A., et al. (2017). Desired turbulence? Gut-lung Axis, immunity, and lung cancer. J. Oncol. 2017, 5035371. doi:10.1155/2017/5035371
Chanda, D., Otoupalova, E., Smith, S. R., Volckaert, T., De Langhe, S. P., and Thannickal, V. J. (2019). Developmental pathways in the pathogenesis of lung fibrosis. Mol. Asp. Med. 65, 56–69. doi:10.1016/j.mam.2018.08.004
Chang, H., Bai, W. F., Li, Q., Liu, Q. L., Meng, H. Y., and Shi, S. L. (2021). Dynamic study on protective effects of Amygdala mongolica on hepatic fibrosis based on changes of serum biomarkers. Chin. Tradit. Herb. Drugs. 52, 108–117.
Chang, H., Liu, Q., Bai, W. F., Bai, Y. C., Jia, X. Y., Gao, C., et al. (2020). Protective effects of Amygdalus mongolica on rats with renal fibrosis based on serummetabolomics. J. Ethnopharmacol. 257, 112858. doi:10.1016/j.jep.2020.112858
Clos-Garcia, M., Andrés-Marin, N., Fernández-Eulate, G., Abecia, L., Lavín, J. L., van Liempd, S., et al. (2019). Gut microbiome and serum metabolome analyses identify molecular biomarkers and altered glutamate metabolism in fibromyalgia. EBioMedicine 46, 499–511. doi:10.1016/j.ebiom.2019.07.031
Clos-Garcia, M., Garcia, K., Alonso, C., Iruarrizaga-Lejarreta, M., D'Amato, M., Crespo, A., et al. (2020). Integrative analysis of fecal metagenomics and metabolomics in colorectal cancer. Cancers (Basel) 12 (5), 1142. doi:10.3390/cancers12051142
Crenn, P., Messing, B., and Cynober, L. (2008). Citrulline as a biomarker of intestinal failure due to enterocyte mass reduction. Clin. Nutr. 27 (3), 328–339. doi:10.1016/j.clnu.2008.02.005
Darrah, E., and Andrade, F. (2018). Rheumatoid arthritis and citrullination. Curr. Opin. Rheumatol. 30 (1), 72–78. doi:10.1097/BOR.0000000000000452
Dumas, A., Bernard, L., Poquet, Y., Lugo-Villarino, G., and Neyrolles, O. (2018). The role of the lung microbiota and the gut-lung axis in respiratory infectious diseases. Cell. Microbiol. 20 (12), e12966. doi:10.1111/cmi.12966
El-Hattab, A. W. (2016). Serine biosynthesis and transport defects. Mol. Genet. Metab. 118 (3), 153–159. doi:10.1016/j.ymgme.2016.04.010
Frezza, C. (2016). Cancer metabolism: Addicted to serine. Nat. Chem. Biol. 12 (6), 389–390. doi:10.1038/nchembio.2086
Gong, S., Ye, T., Wang, M., Wang, M., Li, Y., Ma, L., et al. (2020). Traditional Chinese medicine formula kang shuai Lao pian improves obesity, gut dysbiosis, and fecal metabolic disorders in high-fat diet-fed mice. Front. Pharmacol. 11, 297. doi:10.3389/fphar.2020.00297
Gosalbes, M. J., Abellan, J. J., Durbán, A., Pérez-Cobas, A. E., Latorre, A., Moya, A., et al. (2012). Metagenomics of human microbiome: Beyond 16s rDNA. Clin. Microbiol. Infect. 18, 47–49. doi:10.1111/j.1469-0691.2012.03865.x
Gudmann, N. S., Hansen, N. U., Jensen, A. C., Karsdal, M. A., and Siebuhr, A. S. (2015). Biological relevance of citrullinations: Diagnostic, prognostic and therapeutic options. Autoimmunity 48 (2), 73–79. doi:10.3109/08916934.2014.962024
Hao, H. M., Shi, S. L., Zhou, H. B., Liu, Q., Bai, W. F., Chang, H., et al. (2020). Protective effect of Amygdalus Mongolica oil on the kidneys in rats with renal fibrosis. Pharmacol. Clin. Chin. Mat. Med. 36, 105–109.
He, F., Yin, Z., Wu, C., Xia, Y., Wu, M., Li, P., et al. (2019). l-Serine lowers the inflammatory responses during pasteurella multocida infection. Infect. Immun. 87 (12), e00677. doi:10.1128/IAI.00677-19
He, Y., Wen, Q., Yao, F., Xu, D., Huang, Y., and Wang, J. (2017). Gut-lung axis: The microbial contributions and clinical implications. Crit. Rev. Microbiol. 43, 81–95. doi:10.1080/1040841X.2016.1176988
Holm, L. J., and Buschaed, K. (2019). L-Serine: A neglected amino acid with a potential therapeutic role in diabetes. APMIS 127 (10), 655–659. doi:10.1111/apm.12987
Hu, J. B., Li, J., and Yang, J. S. (2021). Advances in the pathogenesis and clinical treatment of idiopathic pulmonary fibrosis. Jilin Med. J. 42 (12), 3020–3023.
Hu, W., Lu, W., Li, L., Zhang, H., Lee, Y. K., Chen, W., et al. (2021). Both living and dead Faecalibacterium prausnitzii alleviate house dust mite-induced allergic asthma through the modulation of gut microbiota and short-chain fatty acid production. J. Sci. Food Agric. 101 (13), 5563–5573. doi:10.1002/jsfa.11207
Hu, X., Xie, Y., Xiao, Y., Zeng, W., Gong, Z., and Du, J. (2020). Longitudinal analysis of fecal microbiome and metabolome during renal fibrotic progression in a unilateral ureteral obstruction animal model. Eur. J. Pharmacol. 886, 173555. doi:10.1016/j.ejphar.2020.173555
Jeong, J. H., Lee, N., Tucker, M. A., Rodriguez-Miguelez, P., Looney, J., Thomas, J., et al. (2019). Tetrahydrobiopterin improves endothelial function in patients with cystic fibrosis. J. Appl. Physiol. 126 (1), 60–66. doi:10.1152/japplphysiol.00629.2018
Jia, X. Y., Gao, C., Liu, Q., Bai, Y. C., and Shi, S. L. (2018). Determination of total alkaloids in Amygdalus mongolica. Guangzhou Chem. Ind. 46, 100–102.
Jia, X. Y., Gao, C., Liu, Q., Shi, S. L., Zhou, H. B., Bai, Y. C., et al. (2020). Screening of effective extraction sites of Amygdalus mongolica medicinalmaterials against rat renal fibrosis. Sci. Technol. Food Ind. 41, 297–308.
Khan, S., and Chousalkar, K. K. (2020). Salmonella Typhimurium infection disrupts but continuous feeding of Bacillus based probiotic restores gut microbiota in infected hens. J. Anim. Sci. Biotechnol. 11, 29. doi:10.1186/s40104-020-0433-7
Li, Q., Bai, W. F., Zhou, H. B., Hao, H. M., Li, X., Chang, H., et al. (2021). Protective effect of Amygdalus Mongolica oil on lung in rats with pulmonary fibrosis. Pharmacol. Clin. Chin. Mate.r Med. 37 (4), 90–96.
Li, W., Lu, L., Liu, B., and Qin, S. (2020). Effects of phycocyanin on pulmonary and gut microbiota in a radiation-induced pulmonary fibrosis model. Biomed. Pharmacother. 132, 110826. doi:10.1016/j.biopha.2020.110826
Liu, G. R., Li, Y., and Tian, Y. G. (2019). Research progress of traditional Chinese medicine on pulmonary fibrosis. Chin. Medi. Mod. Dis. Educ. China. 17, 116–119.
Liu, P., Liu, S., Tian, D., and Wang, P. (2012). The applications and obstacles of metabonomics in traditional Chinese medicine. Evid. Based. Complement. Altern. Med. 2012, 945824. doi:10.1155/2012/945824
Liu, X. J., Zhang, Y. Q., and Li, J. (2017). Research of bencaological study and chemicalconstiutents of Pruni Semen. J. Liaoning Univ. Tradit. Chin. Med. 19, 100–103.
Lu, G., Shimizu, I., Cui, X., Itonaga, M., Tamaki, K., Fukuno, H., et al. (2004). Antioxidant and antiapoptotic activities of idoxifene and estradiol in hepatic fibrosis in rats. Life Sci. 74 (7), 897–907. doi:10.1016/j.lfs.2003.08.004
Lyu, S., Yang, S. L., Rao, Y., and Feng, Y. L. (2018). Application of metabolomics and related technologies in research and development field of traditional Chinese medicine. China. J. Chin. Mat. Med. 43, 4182–4191. doi:10.19540/j.cnki.cjcmm.20180709.005
Mankhey, R. W., Wells, C. C., Bhatti, F., and Maric, C. (2007). 17beta-Estradiol supplementation reduces tubulointerstitial fibrosis by increasing MMP activity in the diabetic kidney. Am. J. Physiol. Regul. Integr. Comp. Physiol. 292 (2), 769–777. doi:10.1152/ajpregu.00375.2006
Martinez, F. J., Collard, H. R., Pardo, A., Raghu, G., Richeldi, L., Selman, M., et al. (2017). Idiopathic pulmonary fibrosis. Nat. Rev. Dis. Prim. 3, 17074. doi:10.1038/nrdp.2017.74
Moens, A. L., Takimoto, E., Tocchetti, C. G., Chakir, K., Bedja, D., Cormaci, G., et al. (2008). Reversal of cardiac hypertrophy and fibrosis from pressure overload by tetrahydrobiopterin: Efficacy of recoupling nitric oxide synthase as a therapeutic strategy. Circulation 117 (20), 2626–2636. doi:10.1161/CIRCULATIONAHA.107.737031
Prasse, A., Holle, J. U., and Müller-Quernheim, J. (2010). Pulmonary fibrosis. Internist (Berl) 51 (1), 6–13. doi:10.1007/s00108-009-2406-y
Quan, B. W., Wu, T., Liu, Q., Gao, C., Zhou, H. B., Bai, Y. C., et al. (2020). Protective effect of different polar parts of Amygdalus mongolica on pulmonary fibrosis rat models induced by bleomycin. Sci. Technol. Food Ind. 41, 305–309.
Romero, M. J., Yao, L., Sridhar, S., Bhatta, A., Dou, H., Ramesh, G., et al. (2013). l-Citrulline protects from kidney damage in type 1 diabetic mice. Front. Immunol. 4, 480. doi:10.3389/fimmu.2013.00480
Shi, C., Lin, L. L., Xie, T., Shen, C. S., Ji, J. J., Zhao, X., et al. (2020). Exploring the influence of pulmonary and intestinal microecology on lung diseases based on the "lung-gut. axis, JNMU. 36 (02), 168–173.
Shi, S. L., Bai, Y. C., Zhou, H. B., and Niu, S. F. (2013). Extraction and determination of content of polysaccharides in Amygdalus mongolica. Lishizhen Med. Mat. Med. Res. 24, 257–258.
Song, J. P., Du, B. R., Zhang, R., Li, S. Y., Zhou, M., Fang, H., et al. (2010). Investigation on clinical manifestations in common of pulmonary fibrosis. Chin. J. Basic Med. TCM. 16, 141–142.
Su, K., Shi, S. L., Zheng, D. H., and Li, J. H. (2013). Determination of alpha tocopherol content in Amygdalus mongolica by HPLC. Chin. J. Exp. Tradit. Med. Formulae 19, 70–72.
Tan, J. Y., Tang, Y. C., and Huang, J. (2020). Gut microbiota and lung injury. Adv. Exp. Med. Biol. 12, 55–72. doi:10.1007/978-981-15-2385-4_5
Wang, L. M., Wang, P., Teka, T., Zhang, Y. C., Yang, W. Z., Zhang, Y., et al. (2020). 1H NMR and UHPLC/Q-Orbitrap-MS-Based metabolomics combined with 16S rRNA gut microbiota analysis revealed the potential regulation mechanism of nuciferine in hyperuricemia rats. J. Agric. Food Chem. 68 (47), 14059–14070. doi:10.1021/acs.jafc.0c04985
Wang, Y. L., Li, Y. H., W, J., Zhu, Q., and Wang, L. (2012). Analysis of nutritional components of three kind of Amygdalus splant. Guangdong agri. Sci. 39, 127–129.
Wang, Q., Zhao, Y. Q., Shen, X. L., and Xiao, Q. L. (2021). Research progress in the treatment of pulmonary fibrosis in Chinese medicine. Hebei Med. 27 (10), 1751–1753.
Weir, T. L., Manter, D. K., Sheflin, A. M., Barnett, B. A., Heuberger, A. L., and Ryan, E. P. (2013). Stool microbiome and metabolome differences between colorectal cancer patients and healthy adults. PLoS One 8 (8), e70803. doi:10.1371/journal.pone.0070803
Wu, G. S., Li, H. K., and Zhang, W. D. (2019). Metabolomics and its application in the treatment of coronary heart disease with traditional Chinese medicine. Chin. J. Nat. Med. 17 (5), 321–330. doi:10.1016/S1875-5364(19)30037-8
Wu, T., Zhou, H. B., Wang, J., Chang, H., Bai, W. F., Quan, B. W., et al. (2021). Effect of different solvent extracts of Amygdalus mongolicaon liver fibrosis rat models induced by carbon tetrachloride and its mechanisms. Sci. Technol. Food Ind. 42 (14), 348–355.
Xia, Y., Fukunaga, M., Kuda, T., Goto, M., Chiaraluce, G., Hoshiba, H., et al. (2020). Detection and isolation of protein susceptible indigenous bacteria affected by dietary milk-casein, albumen and soy-protein in the caecum of ICR mice. Int. J. Biol. Macromol. 144, 813–820. doi:10.1016/j.ijbiomac.2019.09.159
Yang, M. Q., Zhou, H. B., Bai, Y. C., and Shi, S. L. (2018). Determination of flavonoids inleaves of Amygdalus mongolica from 6 different areas. Guangzhou Chem. Ind. 46, 77–79.
Yi, Z., Liu, X., Liang, L., Wang, G., Xiong, Z., Zhang, H., et al. (2021). Antrodin A from Antrodia camphorata modulates the gut microbiome and liver metabolome in mice exposed to acute alcohol intake. Food Funct. 12 (7), 2925–2937. doi:10.1039/d0fo03345f
Yue, X., Li, J. W., Wu, H. Y., Wang, T. B., Si, Q. G. W., and Wang, Z. Z. (2020). Experimental study on container seedling of Amygdalus mongolica as a desert native treeSpecies in WulatPlateau of lnner Mongolia. Inn. Mong. For. Inves. Des. 43, 33–36.
Zhan, X., Liu, B., and Tong, Z. H. (2020). Postinflammatroy pulmonary fibrosis of COVID-19: The current status and perspective. Chin. J. Tub. Res. Dis. 43 (9), 728–732. doi:10.3760/cma.j.cn112147-20200317-00359
Zhang, A., Sun, H., and Wang, X. (2012). Recent highlights of metabolomics for traditional Chinese medicine. Pharmazie 67, 667–675.
Zhang, K., Si, X. P., Huang, J., Han, J., Liang, X., Xu, X. B., et al. (2016). Preventive effects of Rhodiola rosea L. on bleomycin-induced pulmonary fibrosis in rats. Int. J. Mol. Sci. 17 (6), 879. doi:10.3390/ijms17060879
Zhang, X. Q., Se, C. B. T., and Wu, R. G. M. L. (2016). The measurement of the chemical characteristic constants of prunus mongolica oil. J. Jining Norm. Univer. 38, 13–15.
Zhang, Z., Chen, X., and Cui, B. (2021). Modulation of the fecal microbiome and metabolome by resistant dextrin ameliorates hepatic steatosis and mitochondrial abnormalities in mice. Food Funct. 12 (10), 4504–4518. doi:10.1039/d1fo00249j
Zhao, Y. S., Zheng, Q. N., Shi, S. L., Liu, Q. L., Su, K., and Zhou, H. B. (2016). Study on solvent extraction and fatty acids composition of Amygdalus mongolica oil. Food Res. Dev. 37, 34–37.
Zhao, Y. Z. (1995). Study on floristic geographical distribution of Amygdalus mongolica. J. Inn. Mong. Univ. Natur. Sci. Edit.) 6, 713–715.
Zheng, Q. N., Feng, C. M., Wu, T., Zhou, H. B., and Shi, S. L. (2018). Effect of Amygdalus mongolica Oil on the liver and mechanisms in hyperlipidemic rats. Sci. Technol. Food Ind. 39, 286–292.
Zheng, Q. N., Wang, J., Zhou, H. B., Niu, S. F., Liu, Q. L., Yang, Z. J., et al. (2017). Effectiveness of Amygdalus mongolica oil in hyperlipidemic rats and underlying antioxidant processes. J. Toxicol. Environ. Health. A 80 (22), 1193–1198. doi:10.1080/15287394.2017.1367124
Zhou, B., Yuan, Y., Zhang, S., Guo, C., Li, X., Li, G., et al. (2020). Intestinal flora and disease mutually shape the regional immune system in the intestinal tract. Front. Immunol. 11, 575. doi:10.3389/fimmu.2020.00575
Zhou, H. B., Niu, S. F., Shi, S. L., and Wang, Y. C. (2015). Determination of the contents of protein in different parts of Amygdalus mongolica. J. Baotou Med. Coll. 31, 3–4.
Zhu, C., Hu, P., Liang, Q. L., Wang, Y. M., and Luo, G. A. (2008). Integration of metabonomics technology and its application in modernization of traditional Chinese medicine. Pharm. Sin. 43 (7), 683–689.
Keywords: metabolomics, intestinal flora, Amygdalus mongolica oil, pulmonary fibrosis, Amygdalus mongolica
Citation: Li Q, Zhou H-B, Liu J-Q, Bai W-F, Wang J, Yang Z-J, Qiu M, Chang H and Shi S-L (2022) The intervention effect of Amygdalus mongolica oil on the metabolomics and intestinal flora in pulmonary fibrosis. Front. Pharmacol. 13:1037563. doi: 10.3389/fphar.2022.1037563
Received: 06 September 2022; Accepted: 14 October 2022;
Published: 01 November 2022.
Edited by:
Yurong Lai, Faaps, Gilead (United States), United StatesReviewed by:
Anutthaman Parthasarathy, University of Bradford, United KingdomGiovanna Baron, University of Milan, Italy
Copyright © 2022 Li, Zhou, Liu, Bai, Wang, Yang, Qiu, Chang and Shi. This is an open-access article distributed under the terms of the Creative Commons Attribution License (CC BY). The use, distribution or reproduction in other forums is permitted, provided the original author(s) and the copyright owner(s) are credited and that the original publication in this journal is cited, in accordance with accepted academic practice. No use, distribution or reproduction is permitted which does not comply with these terms.
*Correspondence: Hong Chang, Y2hhbmdob25nX2Nvb2xAMTYzLmNvbQ==; Song-Li Shi, c2hpc29uZ2xpMTIyQDEyNi5jb20=
†These authors have contributed equally to this work