- 1Longhua Hospital, Shanghai University of Traditional Chinese Medicine, Shanghai, China
- 2Department of Oncology, Shenzhen (Fu Tian) Hospital, Guangzhou University of Chinese Medicine, Guangdong, China
- 3State Key Laboratory of Pharmaceutical Biotechnology and Department of Pharmacology and Pharmacy, The University of Hong Kong, Hong Kong, Hong Kong SAR, China
Metabolic disturbance, particularly of glucose metabolism, is a hallmark of tumors such as non-small cell lung cancer (NSCLC). Cancer cells tend to reprogram a majority of glucose metabolism reactions into glycolysis, even in oxygen-rich environments. Although glycolysis is not an efficient means of ATP production compared to oxidative phosphorylation, the inhibition of tumor glycolysis directly impedes cell survival and growth. This review focuses on research advances in glycolysis in NSCLC and systematically provides an overview of the key enzymes, biomarkers, non-coding RNAs, and signaling pathways that modulate the glycolysis process and, consequently, tumor growth and metastasis in NSCLC. Current medications, therapeutic approaches, and natural products that affect glycolysis in NSCLC are also summarized. We found that the identification of appropriate targets and biomarkers in glycolysis, specifically for NSCLC treatment, is still a challenge at present. However, LDHB, PDK1, MCT2, GLUT1, and PFKM might be promising targets in the treatment of NSCLC or its specific subtypes, and DPPA4, NQO1, GAPDH/MT-CO1, PGC-1α, OTUB2, ISLR, Barx2, OTUB2, and RFP180 might be prognostic predictors of NSCLC. In addition, natural products may serve as promising therapeutic approaches targeting multiple steps in glycolysis metabolism, since natural products always present multi-target properties. The development of metabolic intervention that targets glycolysis, alone or in combination with current therapy, is a potential therapeutic approach in NSCLC treatment. The aim of this review is to describe research patterns and interests concerning the metabolic treatment of NSCLC.
Introduction
Today, cancer is the greatest worldwide public health problem after cardiovascular and cerebral vascular disease (Bray et al., 2021). According to the latest epidemiological statistics from 2020, the global incidence of cancer is 19.3 million and mortality is 9.9 million, with lung cancer occupying the second-highest incidence rate (11.4%) and the highest mortality rate (18%) (Sung et al., 2021). Lung cancer has been divided into two types, small cell lung cancer (SCLC) and non-small cell lung cancer (NSCLC), according to pathological diagnosis. Eighty-five percent of lung cancer cases are NSCLC (Gridelli et al., 2015), which includes three subtypes: adenocarcinoma, squamous cell carcinoma, and large cell carcinoma. Although NSCLC generally has better prognosis and slower rate of progression than SCLC, about 50% of patients are diagnosed with local progression or metastasis (Molina et al., 2008; Niu et al., 2016; Rudin et al., 2021). Despite the development of various therapeutic approaches—including classical chemotherapy, targeting therapy, and immunotherapy—the five-year survival rate of metastatic NSCLC in the United States was still under 5% over the past decade (Arbour and Riely, 2019). The discovery of new drugs for NSCLC treatment thus remains an urgent issue.
Emerging evidence indicates that cancer is a metabolic-associated disease. Metabolic disturbances involving glucose, glutamine, and ketone bodies, and particularly energy metabolism supplied by glucose, have been reported as features of tumors progression (Phan et al., 2014; Seyfried et al., 2014; Reinfeld et al., 2021). Targeting metabolic reprogramming (e.g., energy metabolism) is a novel rationale for metabolic drug development in cancers, including in NSCLC (Wu et al., 2015; Park et al., 2020). Glucose is the main substance in intracellular energy metabolism during glycolysis, which produces CO2 or lactate under aerobic or anaerobic conditions, respectively, with glycolysis generally referring to anaerobic glycolysis (Lunt and Vander Heiden, 2011). In normal physiology, cells only exhibit glycolysis under oxygen-limited conditions, while cancer cells tend to exhibit glycolysis even under aerobic conditions; this is called the Warburg effect or aerobic glycolysis and distinguishes cancer cells from normal cells (Vander Heiden et al., 2009; Phan et al., 2014; Bose and Le, 2018). Briefly, although aerobic glycolysis is not an efficient means of producing adenosine triphosphate (ATP) compared to oxidative phosphorylation, cancer cells still reprogram the metabolism into glycolysis to meet the high demands of proliferation (Vaupel et al., 2019). Aerobic glycolysis has been considered the fundamental feature of tumor metabolism disturbance and not simply the result of a passive response to mitochondrial damage (DeBerardinis et al., 2008; Wise and Thompson, 2010; Santos and Schulze, 2012). This metabolic reprogramming facilitates tumor survival, which can be viewed as an essential hallmark of cancer (Ward and Thompson, 2012). Glycolysis is critical for energy supply in tumors, as it is the most preferential approach toward energy production (Moreno-Sánchez et al., 2007). Furthermore, glycolysis promotes acidification of the tumor microenvironment (TME), leading to drug resistance (Bose and Le, 2018). Similarly, enhanced metabolism of aerobic glycolysis has been observed in tumors by measurement of intra-operative 13C-glucose infusions in NSCLC patients (Hensley et al., 2016). Therefore, targeting the inhibition of glycolytic metabolism could be a potential therapeutic strategy in cancers like NSCLC (Ganapathy-Kanniappan and Geschwind, 2013).
In the current review, we focus on advances in the glycolytic metabolism of NSCLC and provide an overview of key enzymes, biomarkers, and related pathways, as well as the impact of current treatments and candidates in glycolysis.
Glycolytic metabolism in tumor cells
Glucose is the major source of energy metabolism and biomass synthesis (Lunt and Vander Heiden, 2011). Extracellular glucose crosses the cell membrane with the help of glucose transporter 1 (GLUT1), which also determines the production of glucose 6-phosphate in the first central metabolism pathway mediated by hexokinase 1/2 (HK1/2) (Rajas et al., 2019). Glucose metabolism results in the production of two three-carbon pyruvate molecules from each glucose molecule through a series of enzyme-catalyzed reactions. Pyruvate participates in various physiological metabolic pathways and has several metabolic fates, such as fermentation, cellular respiration, and fatty acid synthesis (Prochownik and Wang, 2021). In normal cells, when oxygen is sufficient, pyruvate is the substrate of acetyl-CoA synthesis, which generates 36 molecules of ATP during the TCA cycle via oxidative phosphorylation in mitochondria. Nicotinamide adenine dinucleotide (NADH) is generated from the TCA cycle, and ATP is produced most efficiently through the phosphorylation of ADP, accompanied with the transformation of NADH to NAD+ through the electron transfer chain (ETC) in the mitochondrial inner membrane. This process consumes oxygen and produces CO2 from the breakdown of pyruvate. When the oxygen supply is insufficient, pyruvate tends to be metabolized to lactate in anaerobic glycolysis; this process generates lactate and two molecules of ATP, which is independent on the TCA cycle during mitochondrial respiration (Sieow et al., 2018). In tumor cells, aerobic glycolysis occurs and is characterized by 5% oxidative phosphorylation and 85% glycolysis under oxygen-sufficient conditions (Vander Heiden et al., 2009; Fan et al., 2019), and most of the energy supplied is powered by anaerobic glycolysis instead of oxidative phosphorylation (Zheng, 2012). Unlike anaerobic glycolysis in normal cells, aerobic glycolysis is not the passive consequence of hypoxic stress but is rather due to the active metabolic reprogramming of tumor cells (Xu et al., 2020) (Figure 1). Although aerobic glycolysis is not the most efficient means of supplying energy, the proliferation and survival of tumor cells is benefited by glycolysis, which relies on the consumption of intracellular glucose with access to a variety of materials for cell growth, such as nucleotides, amino acids, and lipids (Pavlova and Thompson, 2016; Schiliro and Firestein, 2021). Although glycolysis appears to have weaker energy productivity compared to oxidative phosphorylation, the ATP/ADP and NADH/NAD+ ratios usually remain high in tumor cells, indicating sufficient ATP supply in glycolytic tumor metabolism (Martínez-Reyes and Chandel, 2021). The accumulation of lactate facilitates acidification in the TME. The imbalance of nutrient partitioning, as in glutamine metabolism, further enhances the degree of glycolysis, leading to a repeating cycle (Damiani et al., 2017; Reinfeld et al., 2021).
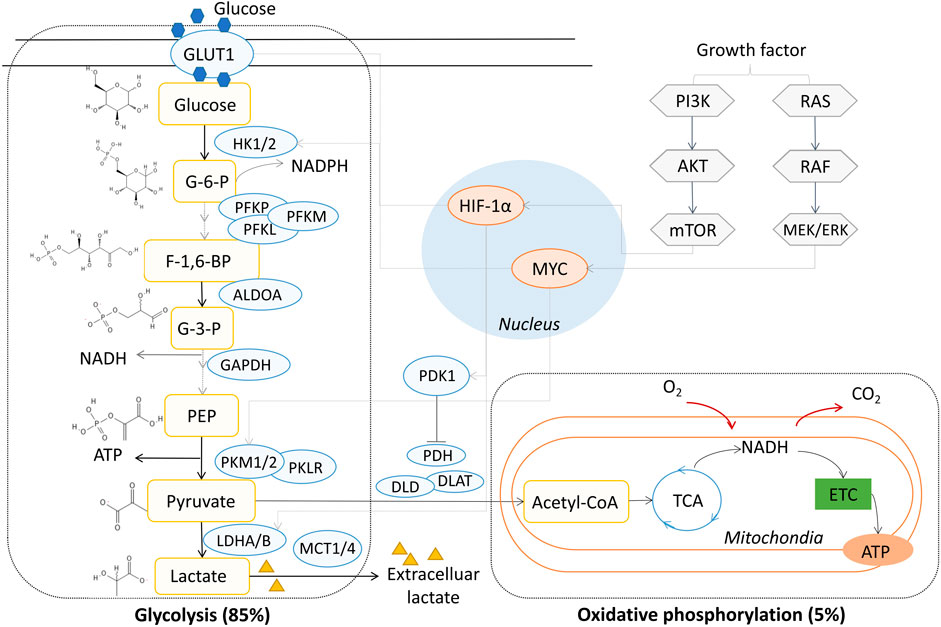
FIGURE 1. Aerobic glycolysis in NSCLC. GLUT1, glucose transporter 1; HK, hexokinase; PFKL, phosphofructokinase, liver type; PFKM, phosphofructokinase, muscle type; PFKP, phosphofructokinase, platelet type; PKM2, pyruvate kinase M2; PKM1, muscle isoform pyruvate kinase M1; PKLR, red cell/liver pyruvate kinase; LDH, lactate dehydrogenase; MCT1-4, monocarboxylate transporter 1-4; PDK1, pyruvate dehydrogenase kinase 1; PDH, pyruvate dehydrogenase; DLD, dihydrolipoamide dehydrogenase; DLAT, dihydrolipoamide acetyltransferase; ATP, adenosine triphosphate; NADH, nicotinamide adenine dinucleotide; PI3K, phosphoinositide 3-kinase; TCA, tricarboxylic acid; ETC, electron transfer chain; MYC, MYC proto-oncogene; HIF-1α, hypoxia-inducible transcription factor-1 alpha; G-6-P, glucose 6-phosphate; F-1,6-BP, fructose 1,6-phosphate; G-3-P, glyceraldehyde 3-phosphate; PEP, phosphoenolpyruvate.
Key glycolysis enzymes in NSCLC
Glucose metabolism reprograming, as evident in the dependence on glycolysis, is a metabolic hallmark and prognosis parameter of NSCLC, as higher levels of whole-body metabolic tumor volume (MTV) and whole-body total lesion glycolysis (TLG) have been found in NSCLC patients with poor survival rates, based on F-18 fluorodeoxyglucose positron emission tomography (FDG-PET) (Chen et al., 2012; Vanhove et al., 2018). In addition, a high level of TLG was the most accurate risk factor for recurrence in a stage-I postoperative NSCLC patient as compared with the standardized uptake value index (SUV index) and MTV (Melloni et al., 2013). A variety of glycolytic enzymes is aberrantly activated during glycolytic metabolism in NSCLC. We reviewed most of the key enzymes in this study.
Glucose transporter
Glucose transporter 1 (GLUT1) functions in extracellular glucose uptake and has been proposed as a promising therapeutic target in cancer (Sizemore et al., 2018). In NSCLC, the heterogeneity of GLUT1 is displayed in its subtypes. FDG-PET shows higher MTV and TLG values in GLUT1-positive adenocarcinomas patients as compared to negative cases. Koh et al. found that high expression of GLUT1 presented in 99% of squamous cell carcinoma and 50% of adenocarcinoma patients (Koh et al., 2017a). Human NSCLC samples, patient-derived xenografts, mouse models, and The Cancer Genome Atlas (TCGA) have all consistently showed high expression levels of GLUT1 in squamous cell carcinoma (Goodwin et al., 2017; Smolle et al., 2020). These studies indicate that high expression of GLUT1 is a risk factor for low survival rate in squamous cell carcinoma and adenocarcinoma in NSCLC. In addition, AKT-GLUT1/HKII-targeted microRNA miR-124 overexpression inhibits proliferation, glycolysis, and energy metabolism in A549 cells (Zhao et al., 2017). The Th2-related cytokine IL-33 has been shown to promote patient-derived NSCLC cell growth and metastasis in a nude mice model, with activation of GLUT1 and enhancement of glycolysis (Wang et al., 2016).
Hexokinase
Hexokinase (HK) plays a vital role in tumor glycolytic metabolism and includes HK1 and HK2 isoforms. It phosphorylates the sixth carbon site of glucose, producing glucose 6-phosphate, and is primary in the pentose phosphate pathway and in glycogen metabolism (Ciscato et al., 2021). The HK1 and HK2 proteins are both highly expressed in KRAS-mutant mouse lung tumors, while the activation of HK2 but not HK1 is inevitable for tumor initiation and progression (Patra et al., 2013). In addition, HK2 has been adopted as an essential metabolic marker for the prognostic spectrum of NSCLC, as detected in circulating tumor cells (CTCs) in NSCLC patients (Ma et al., 2020). HK2 is closely associated with survival in NSCLC, as it is involved in the primary step of glucose metabolism. HK2 is required for tumor initiation in the KRAS-driven NSCLC mouse model, and the deletion of HK2 prolongs the survival of mice (Patra et al., 2013).
Rate-limiting enzyme phosphofructokinase
Phosphofructokinase (PFK) is the rate-limiting enzyme converting fructose 6-phosphate to fructose 1,6-bisphosphate. There are three types of phosphofructokinases: muscle type (PFKM), liver type (PFKL), and platelet type (PFKP). In NSCLC, Wang F. et al. (2021) found that PFKP expression was correlated with lymph node metastasis and that high expression of PFKP reduced apoptosis and promoted glycolysis and cell proliferation in H1299 cells. Shen et al. found PFKP to be highly expressed in tissue samples of NSCLC patients and in the PC-9, NCI-H1650, NCI-H520, NCI-H460, H1975, HCC827, and A549 cell lines. The silencing of PFKP inhibited cell proliferation and cell-cycle progress in NCI-H1650 and A549 cell lines (Shen et al., 2020). Additionally, PFKM is prognostic predictor in postoperative NSCLC patients, according to genetic polymorphism research (Lee et al., 2016).
Pyruvate kinase
Pyruvate is an essential metabolite in glycolysis and has various metabolic fates. The blockage of pyruvate kinase slows the transformation of phosphoenolpyruvate to pyruvate. Pyruvate kinases include the muscle isoforms pyruvate kinase M1 (PKM1) and pyruvate kinase M2 (PKM2), and the liver and red blood cell isoform (PKLR). PKM2, which acts as a nuclear factor and participates in a metabolic loop with GLUT1 (Pan et al., 2019), is highly expressed in NSCLC and is potentially a specific target in treatment of NSCLC, according to data from nine patient-derived cell lines, two established cell lines (H1299 and H358), and nude mice (Suzuki et al., 2019). Hypoxia exacerbates the resistance effects of cisplatin in A549 cells by transmitting exosomal PKM2 to sensitive cells (Wang D. et al., 2021). Pyruvate dehydrogenase (PDH) is one of the key enzyme complexes responsible for the oxidative decarboxylation of pyruvate. Pyruvate dehydrogenase kinase 1 (PDK1), which could inactivate PDH and is an independent risk factor for NSCLC, is highly expressed in tumor tissues of NSCLC patients, and its overexpression promotes the proliferation and metastasis of NSCLC (Liu and Yin, 2017).
Glyceraldehyde 3-phosphate dehydrogenase
Although glyceraldehyde 3-phosphate dehydrogenase (GAPDH) is assumed to be a housekeeping gene, it is a common enzyme with uncommon functions, including glycolysis (Nicholls et al., 2012). GAPDH, an irreversible metabolic switch in glycolysis, catalyzes the conversion of glyceraldehyde 3-phosphate to 1,3-bisphospoglycerate, accompanied by the production of NADH (Liao et al., 2019). GAPDH transcription was upregulated in an NSCLC patient cohort and correlated with the glycolysis and gluconeogenesis pathways (Wang et al., 2013). Silencing of GAPDH by RNA interference induced the senescence of A549 cells and enhanced the therapeutic effects of antimetabolite drugs (Phadke et al., 2013). The ratio of GAPDH to mitochondrially encoded cytochrome c oxidase I (GAPDH/MT-CO1) and the ratio of mitochondrial metabolism transcriptional coactivator to peroxisome proliferator-activated receptor-gamma coactivator (PGC)-1 alpha have been considered as biomarkers for the Warburg effect for evaluating relative drug usage benefits in stage-Ⅲ NSCLC patients (Cruz-Bermúdez et al., 2017).
Lactate dehydrogenase
Lactate dehydrogenase (LDH), which is classified into the two isoforms LDHA and LDHB, is responsible for the mutual transformation between pyruvate and lactate. Intracellular pyruvate is reduced to lactate by LDHA, and then lactate is transported extracellularly, resulting in an acidic TME. On the other hand, LDHB catalyzes the oxidation of lactate to pyruvate (Brisson et al., 2016), which partially promotes the recruitment of intracellular pyruvate. In a meta-analysis study, Deng et al. (2018) found that in lung cancer patients, a high blood LDH concentration was correlated with poor overall survival rate. However, serum LDHB-positive NSCLC patients presented higher recurrence-free survival rates than did LDHB-negative cases, particularly those of the squamous cell carcinoma subtype (Koh et al., 2017b). Deletion of the LDHB gene inhibited tumor initiation and progression through mitochondrial DNA damage in a genetically engineered NSCLC mouse model with combined p53 knockout and KRAS (G12D) overexpression (Deng et al., 2022; Stine et al., 2022).
Monocarboxylate transporter
Monocarboxylate transporters (MCTs) belong to the SLC16A family. MCT1-4 facilitates the membrane-crossing exchange of lactate (Payen et al., 2020). MCT1 and MCT4 are the main functional isoforms of MCTs in cancer and are associated with tumor invasion and metastasis (Sun X. et al., 2020). In NSCLC, overexpressed MCT4 was detected in P29mtB82M human cancer cells (Takenaga et al., 2021), and MCT4-neutralizing antibodies significantly inhibited cell proliferation in glycolysis-preference CL1-5 and Hop62 cells in a concentration-dependent manner (Kuo et al., 2020). Additionally, MCT2 and GLUT1 were reported to be significantly co-overexpressed in adenocarcinomas relative to other NSCLC subtypes (Giatromanolaki et al., 2017).
In summary, key glycolytic enzymes play critical roles in cell proliferation, invasion, and metastasis in NSCLC. Most of the key glycolytic enzymes anomalously expressed in NSCLC could be potential drug development targets. In particular, LDHB may serve as a specific prognostic predictor in the squamous cell carcinomas subtype of NSCLC. The function of LDHB remains ambiguous in other tumor cells, such as triple-negative breast cancer (TNBC) (Naik and Decock, 2020). In addition, targeting both MCT2 and GLUT1 might be promising in the treatment of the adenocarcinoma subtype of NSCLC. Furthermore, PFKM might be a prognostic predictor in postoperative NSCLC patients.
The regulation and biomarkers of glycolysis in NSCLC
Since glycolysis metabolism reprogramming is common in cancers including NSCLC, the identification of biomarkers and regulators associated with key glycolytic enzymes contributes to the discovery and evaluation of effective therapeutic approaches, particularly biomarkers correlated with the diagnosis and outcome prediction of NSCLC.
Glycolytic enzyme-regulated biomarkers
GFPT2
The glucose uptake-related gene glutamine-fructose-6-phosphate transaminase 2 (GFPT2) encodes the rate-limiting enzyme glutamine-fructose-6-phosphate aminotransferase 2 (GFAT2) in the hexosamine biosynthesis pathway. GFPT2 is a GLUT1-independent prognostic predictor in NSCLC patients and functions as a key glucose-uptake mediator. Overexpressed GFPT2 has been detected in cancer-associated fibroblasts in lung adenocarcinoma and, specifically, regulates metabolic reprogramming in this NSCLC subtype (Zhang et al., 2018).
SIX1
Sine oculis homeobox homolog 1 (SIX1) is a transcription factor associated with aerobic glycolysis during tumor growth. SIX1 has been shown to be highly expressed in A549 cells, and SIX1 knockdown in mouse embryos has resulted in the decreasing protein activities of various glycolysis-related enzymes such as HK2, GLUT1, and LDHA (Li et al., 2018a).
IL-33
Overexpression of IL-33, which has been suggested to correlate with tumor progression in humans, facilitates tumor growth and metastasis by upregulating GLUT1 and, consequently, enhancing glycolysis in NSCLC nude mice (Wang et al., 2016).
DPPA4
Developmental pluripotency-associated 4 (DPPA4) was found to be a poor prognostic factor in NSCLC patients due to the enhancement of glycolytic metabolism via increased activity of LDHB, HK2, and GLUT4 (Li L. et al., 2019).
NQO1
NADPH quinone oxidoreductase 1 (NQO1) was reported to be a poor prognostic biomarker based on metabolomic analysis in NSCLC A549 cells (Cheng et al., 2018). NQO1 was overexpressed in NSCLC, and the knockdown of the NQO1 gene expression by its specific siRNA inhibited cell proliferation and tumor glycolysis metabolism in A549 cells by downregulating HK2 expression (Cheng et al., 2018).
OCT-1
The high expression of octamer transcription factor-1 (OCT-1) is a key feature associated with poor survival rate in NSCLC patients. Glycolysis metabolism was shown to be facilitated by the overexpression of OCT-1 and activation of HK2 in H1299 cells (Li et al., 2021).
CD147/basigin
CD147 or basigin (BSG) acts as a molecular chaperone and is responsible for the transport of lactate from the cytoplasm to the plasma membrane, followed by MCT-mediated transport to extracellular matrix. The MCT1/4 expression level and intracellular glycolysis rate were reduced in CD147/BSG-knockout A549, H1975, and H292 cell lines (Granja et al., 2015).
Transcription factors MYC and HIF-1α and their related molecules
Hypoxia-inducible transcription factor-1 alpha (HIF-1α) is a transcription factor overexpressed in multiple cancer types and plays an important role in lung cancer metabolism by promoting tumor survival (Jun et al., 2017). MYC proto-oncogene (MYC) is a pan-cancer oncogene and is aberrantly amplified in lung cancer (Massó-Vallés et al., 2020). Most of the key glycolytic enzymes are affected by MYC and HIF-1α in tumor glycolysis metabolism (Stine et al., 2015; Soni and Padwad, 2017).
TRAF6
Tumor necrosis factor receptor-associated factor 6 (TRAF6) is a biomarker linked with poor prognosis in NSCLC patients (Sun et al., 2019). Downregulation of TRAF6 gene expression by specific shRNA-mediated RNA interference produced anti-tumor effects in the NSCLC cell lines A549 and NCI-H358 and in A549-transplanted BALB/ca nude mice through the activation of the Akt-HIFα pathway (Feng et al., 2021). In TRAF6-knockdown cells, HK2 decreased and deficient HIF-1α was induced, and intracellular glycolysis metabolism was attenuated, accompanied by glucose consumption and lactate production (Feng et al., 2021).
KLF5
Knockdown of Krüppel-like factor 5 (KLF5) gene expression in NSCLC cell lines H1299 and A549 relieved chemotherapy (cisplatin) resistance mediated by hypoxia, in which HIF-1α was suppressed, via inhibition of the phosphoinositide 3-kinase (PI3K)/AKT/mammalian target of rapamycin (mTOR) signaling pathway (Gong et al., 2018).
NOX4
NADPH oxidase 4 (NOX4) was highly expressed in A549 cells and promoted c-Myc-dependent glycolysis via the activation of the reactive oxygen species (ROS)/PI3K/Akt signaling pathway and the pentose phosphate pathway (Zeng et al., 2016).
RFP180
Ring finger protein 180 (RFP180) is a potential anti-tumor target, and low expression of RFP 180 indicates poor survival rates in NSCLC patients (Ding et al., 2022). Upregulation of RFP180 impaired glycolysis and proliferation in H292 cells, and similar anti-tumor effects of RFP180 were also shown in the H358 cell-injected xenograft nude mice model (Ding et al., 2022). The fundamental basis of RFP180’s anti-tumor effect could be attributed to the ubiquitin-dependent degradation of c-Myc (Ding et al., 2022).
EHD1
Downregulation of Eps15 homology domain 1 (EHD1) attenuated glycolysis and tumor growth in A549 cells, NCI-H1299 cells, and a xenograft mouse model. EHD1 promoted tumor progression through the activation of 14-3-3ζ/β-catenin/c-Myc signaling (Abuduwaili et al., 2022).
Oncogenes EGFR and KRAS and their related molecules
Although EGFR and KRAS are associated with many tumors and have been shown to play important roles in NSCLC, they are also very important in glycolysis regulation.
EGFR
Epidermal growth factor receptor (EGFR) has been identified as an oncogene in recent decades. About 20% EGFR activation mutations have been identified in advanced NSCLC cases (Dogan et al., 2012). Autophagy caused by c-Jun N-terminal kinase (JNK) induces EGFR degradation, suggesting the therapeutic potential of the mitogen-activated protein kinase (MAPK)/JNK pathway in EGFR-activated NSCLC (Kim et al., 2018). The metabolic activity parameters MTV and TLG were used as predictors for TKI drug sensitivity and treatment outcomes in EGFR-mutant NSCLC patients (Jiang et al., 2022).
ALDOA
Aldolase A (ALDOA) presents high expression levels in NSCLC, particularly in squamous cell carcinoma. Fu et al. (2018) found that ALDOA promoted cell proliferation by increasing aerobic glycolysis through the activation of the EGFR/MAPK signaling pathway in H157 and H1299 cells.
CPS1
Pham-Danis et al. (2019) found that inhibition of the urea cycle enzyme CPS1 synergistically enhanced the suppressing effects of EGFR inhibitor on glycolysis and tumor growth in EGFR-mutant PC9 and HCC4006 cell lines.
PFKFB3
6-Phosphofructo-2-kinase/fructose-2,6-bisphosphatase 3 (PFKFB3), a regulator of EGFR signaling, is overexpressed in EGFR-mutant cells in PC9 and HCC827. The exposure of EGFR tyrosine kinase inhibitor (TKI) in H522 and PC9 cells led to the upregulation of PFKFB3 expression. The inhibition of both EGFR (TKI) and PFKFB3 (PFK158) enhanced anti-tumor efficacy in NSCLC (Lypova et al., 2019). Thus, the combination of PFKFB3 inhibitor and EGFR TKI is a prospective treatment strategy in NSCLC.
PDK1
Similarly, the pyruvate dehydrogenase kinase 1 (PDK1) inhibitor Cpd64, when combined with EGFR TKI, improved anti-cancer effects in EGFR-mutant NCI-H1975 and NCI-H1650 cell lines and in a xenograft mouse model via the improvement of oxidative phosphorylation and mitochondrial respiration (Wang F. et al., 2021).
KRAS
KRAS mutations have been discovered in multiple cancer types, including NSCLC, and are related to cancer metabolism reprogramming (e.g., promotion of glutaminolysis and glycolysis) (Kawada et al., 2017). KRAS (G12D) mutation significantly increased glycolysis-associated gene expression and resulted in enhanced glucose uptake and lactate generation in mouse embryonic fibroblasts (Kerr et al., 2016). Kim et al. reported that KRAS and tumor suppressor serine/threonine kinase 11 (LKB1/STK11) co-mutations had high risks of metastasis and metabolic rewiring through activation of the hexosamine biosynthesis pathway, according to data from metabolome and transcriptome profiles in mouse and human tumors. Furthermore, the silencing of GFPT2 expression inhibited the cell growth and tumor survival in KRAS/LKB1-co-mutant H460 and H2122 cells and co-mutant mice (Kim et al., 2020). Emanuela Pupo et al. found that KRAS mutants presented distinctive metabolic profiles compared with wild types in tumors (Pupo et al., 2019). Therefore, glycolysis metabolism-related enzymes are potential therapeutic targets for KRAS-mutant NSCLC.
Immune- and inflammation-related molecules
PD-1/PD-L1
PD-1/PD-L1 was detected to be overexpressed in NSCLC patients, and high expression of F-FDG PET/CT and PD-L1 was positively correlated with poor disease free survival (DFS) (Grizzi et al., 2019; Wang et al., 2020). In addition, high expression of PD-1/PD-L1 in cancer cells and tumor infiltrating lymphocytes (TILs) was negative-correlated with lactate dehydrogenase 5 (LDH5) and positive-correlated with HK2 and monocarboxylate transporter 2 (MCT2) Thus, PD-1/PDL-1 accompanied with glycolysis related markers, such as LDH5, act as prognostic and immunotherapy-outcome predictors in NSCLC (Giatromanolaki et al., 2019).
ISLR
High expression of leucine-rich repeat (ISLR) was associated with lower survival rates in NSCLC patients. The silencing of ISLR suppressed glycolysis, proliferation, invasion, and migration in A549 cells by activating the IL-6/JAK/STAT3 signaling pathway (Zhang P. et al., 2021).
NALP3
Inflammasome activator NLR family pyrin domain containing 3 (NALP3) depletion switched glucose metabolism from aerobic glycolysis to oxidative phosphorylation through interaction with DNA methyltransferase 1 associated protein 1 (DMAP1), which modulated transcription regulator DNA (cytosine-5)-methyltransferase 1 (DNMT1) in H1299 and A549 cells (He et al., 2020).
Cell survival-related molecules
ENO1
The high expression of glycolytic enzyme alpha-enolase (ENO1), a key biomarker in tumor glycolytic metabolism, dramatically promoted cell growth and migration in A549 cells by activating the FAK/PI3K/AKT signaling pathway (Fu et al., 2015).
Barx2
The expression of Human BarH-like homeobox 2 (Barx2), a tumor suppressor linked to the Wnt/β-catenin signaling pathway, was relatively lower in tumor samples than in adjacent samples in NSCLC patients, and low Barx2 expression is associated with poor prognosis. Moreover, aerobic glycolysis was enhanced by the downregulation of Barx2, leading to increased cell proliferation, migration, and invasion in A549 cells (Chen et al., 2018).
TRIM59
The downregulation of the oncogene tripartite motif-containing 59 (TRIM59) reduced glycolysis and reversed cisplatin resistance in A549 cells via the suppression of the PTEN ubiquitination and the inhibition of AKT/HK2 activities (He and Liu, 2020).
Hsp27
Argpyrimidine is an advanced glycation end product resulting from the high glycolysis rate in tumor cells. Argpyrimidine-modified heat shock protein 27 (Hsp27) facilitated apoptosis evasion in SW1573 cells (van Heijst et al., 2006).
Ubiquitin-related molecules
JOSD2
Krassikova et al. (2021) found that Josephin domain containing 2 (JOSD2) facilitated the proliferation of A549 cells by deubiquitinating the metabolic enzyme complex of ALDOA, phosphofructokinases PFK-1 and PFKL, and phosphoglycerate dehydrogenase (PHGDH).
OTUB2
Similarly, the deubiquitinating enzyme OTU deubiquitinase, ubiquitin aldehyde binding 2 (OTUB2) is a glycolytic stimulator which indicates poor survival outcome in NSCLC patients. Overexpression of OTUB2 enhanced glycolysis and promoted cell growth, migration, and invasion via the AKT/mTOR signaling pathway in A549 and H1299 cells and a xenograft mouse model (Li J. et al., 2019).
Others
Apart from the diverse biomarkers described earlier, a variety of additional targets and underlying mechanisms have been reported to be correlated with glycolysis in NSCLC. Protein kinase cAMP-activated catalytic subunit alpha (PRKACA) enhances glycolysis by activating triosephosphate isomerase (TPI) serine 58 (Ser58) (Duan et al., 2021). Ji et al. (2022) found that UDP-glycosyltransferase 8 (UGT8) enhanced tumor growth by promoting glycolysis in A549 cells, H460 cells, and a xenograft mouse model, and the depletion of UGT8 diminished tumor malignancy in vitro and in vivo. The depletion of PTEN-induced putative kinase 1 (PINK1) via shRNA in A549 cells suppressed tumor malignancy and increased the sensitivity of glycolysis inhibitor 3-BP by disrupting ATP production, promoting ROS generation, and inducing apoptosis (Dai et al., 2019). The glycolysis-related biomarkers reported earlier are summarized in Table 1 and Figure 2.
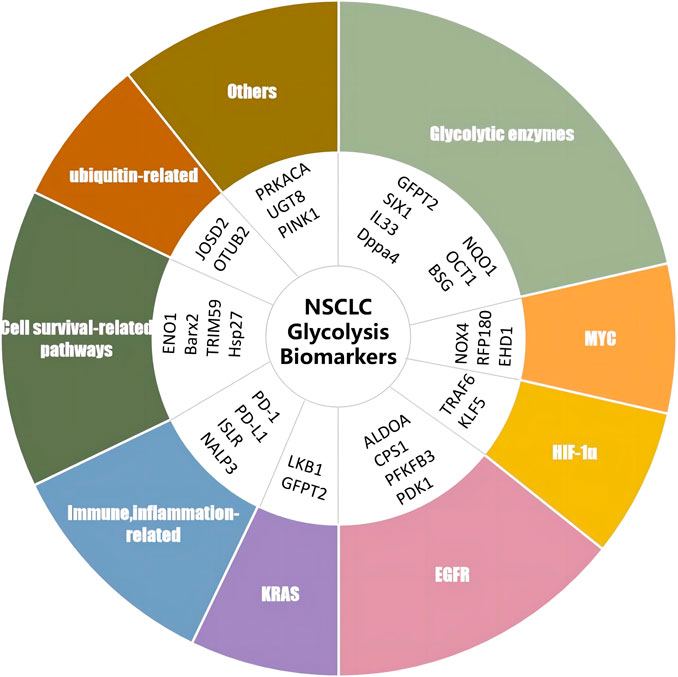
FIGURE 2. Biomarkers of glycolysis in NSCLC. RNF180, ring finger protein 180; UGT8, UDP-glycosyltransferase 8; SIX1, sine oculis homeobox homolog 1; TRAF6, TNF receptor-associated factor 6; EHD1, Eps15 homology domain 1; OCT-1, octamer-transcription factor-1; ISLR, leucine-rich repeat; JOSD2, Josephin domain containing 2; PRKACA, protein kinase cAMP-activated catalytic subunit alpha; PFKP, phosphofructokinase; TRIM59, tripartite motif-containing 59; NALP3, NLR family pyrin domain containing 3; CPS1, carbamoyl phosphate synthetase I; PFKFB3, 6-phosphofructo-2-kinase/fructose-2,6-bisphosphatase; PD-1, programmed cell death protein 1; PD-l1, programmed death-ligand 1; OTUB2, OTU deubiquitinase, ubiquitin aldehyde binding 2; DPPA4, developmental pluripotency-associated 4; PINK1, PTEN-induced putative kinase 1; KLF5, factor 5; ALDOA, aldolase A; EGFR, epidermal growth factor receptor; GFPT2, glutamine-fructose-6-phosphate transaminase 2; NQO1, NAD(P)H quinone oxidoreductase 1; PDK1, pyruvate dehydrogenase kinase 1; BARX2, BarH-like homeobox 2; PGC-1alpha, pPARG coactivator 1 alpha; NOX4, NADPH oxidase 4; IL-33, interleukin 33; ENO1, alpha-enolase; BSG, cD147/basigin; KRAS, Kirsten-RAS; GAPDH, glyceraldehyde-3-phosphate dehydrogenase; FBP1, fructose-1,6-bisphosphatase; HIF-1α, hypoxia-inducible factor 1 subunit alpha; Hsp27, heat shock protein 27.
Current treatments and candidates targeting glycolysis in NSCLC
Despite the insufficiencies of metabolic treatment in NSCLC, various current treatments and candidates—including chemotherapy, targeting therapy, anti-tumor small molecules, biopharmaceutical therapy, and natural products—present potent interventions in glycolysis. We summarize relevant details drawn from previous studies in Tables 2-4 and Figure 3.
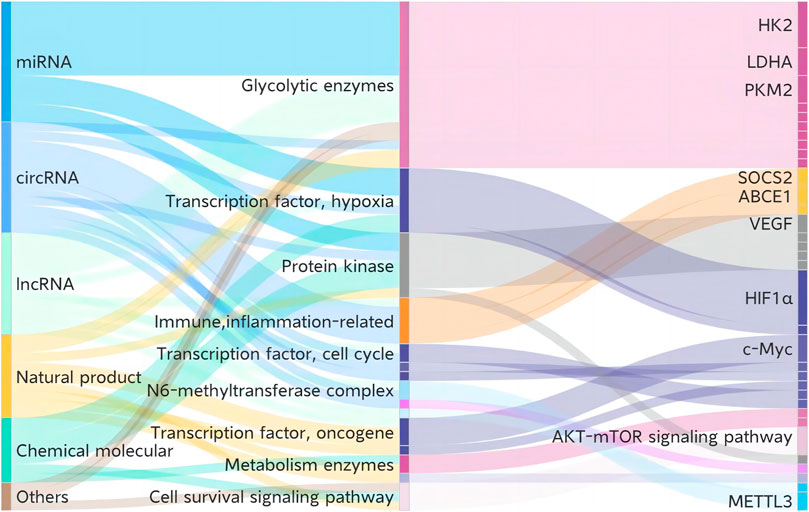
FIGURE 3. Current treatments and candidates targeting glycolysis in NSCLC. HK2, hexokinase 2; PKM2, pyruvate kinase M2; LDHA, lactate dehydrogenase A; SOCS2, suppressor of cytokine signaling 2; ABCE1, ATP-binding cassette subfamily E member 1; VEGF, vascular endothelial growth factor; HIF-1α, hypoxia-inducible factor 1 subunit alpha; c-Myc, MYC proto-oncogene; METTL3, methyltransferase 3.
Chemotherapy and its resistance
Chemotherapy is the mainstream treatment approach in most cancer types due to its significant effectiveness. Glycolysis is revealed to be correlated with the clinical outcomes of chemotherapy. According to gene polymorphism research in NSCLC patients, the glycolysis-related genes phosphofructokinase liver type (PFKL) and glucose phosphate isomerase (GPI) are correlated with outcomes of patients treated with first-line paclitaxel–cisplatin therapy, and genetic polymorphism in ATP-binding cassette subfamily B member 1 (ABCB1) and HIF-1α predicted the efficacy of paclitaxel-carboplatin therapy (Park et al., 2016; Choi et al., 2020). Galactose-conjugate (trans-R,R-cyclohexane-1,2-diamine)-2-chloromalonato-platinum (II) complex (Gal-Pt) outperformed oxaliplatin and cisplatin in antitumor activity by affecting the glucose uptake target GLUTs in H460 cells and a xenograft model (Wu et al., 2016). These studies indicate that glycolysis-related genes could be adopted as prediction factors for chemotherapy outcomes and as potential targets for the enhancement of drug effectiveness.
Moreover, glycolysis is associated with chemotherapy resistance. The molecule 5-Fu, a pyrimidine analog for pan-cancer treatment, activated glycose metabolism in resistant A549 cells, while cisplatin-resistant cells suppressed glycose metabolism (Zhao et al., 2014). Hypoxia-induced cisplatin-resistant A549 cells featured a high expression of PKM2, which contributed to tumor glycose metabolism reprogramming and promoted acidic TME and cell proliferation in NSCLC cells A549, accelerating cisplatin resistance effects (Wang D. et al., 2021).
Targeting therapy
Various small molecules or antibodies which specifically target the biomarkers of glycolysis have been investigated as NSCLC-targeting treatments. Gefitinib, a tyrosine kinase inhibitor (TKI), suppressed NSCLC cell growth by impairing cellular glycolysis and inhibiting the PI3K-Akt-mTOR signaling pathway in A549 and H1975 cells (Zhou et al., 2020). The efficacy of the anaplastic lymphoma kinase (ALK) inhibitor crizotinib was suspended by HK2-mediated glycolysis, and the inhibition of glycolysis and AKT/mTOR signaling pathway dramatically improved crizotinib sensitivity in both ALK (+) H3122 and H2228 cells (Lin et al., 2021). As an oncogene, AKT kinase was overexpressed in multiple tumors, including NSCLC, and facilitated glycolysis metabolism in tumor cells (Elstrom et al., 2004). The combination of AKT inhibitor and microtubule-targeting agents (MTAs) caused a synergic anti-tumor effect in multiple paclitaxel-resistant or anti-EGFR-resistant NSCLC cell lines (A549, A549/EpoB40, HCC827, H1650, and H1975 cells) and human NSCLC xenografts (Le Grand et al., 2017). The combination of glycolysis inhibitor 2-deoxy-d-glucose (2DG) with the EGFR TKI afatinib enhanced the sensitivity of H1975 and PC9-GR cells with an EGFR T790M mutation by inhibiting glycolysis through suppression of the AMPK/mTOR/Mcl-1 signaling pathway (Kim et al., 2013). The molecule 5-(n-(8-methoxy-4-quinolyl) amino) pentyl nitrate (5MPN), a selective inhibitor of glycolytic regulatory enzyme 6-phosphofructo-2-kinase/fructose-2,6-bisphosphatase 4 (PFKFB4), effectively attenuated glycolysis and tumor growth in C57BL mice, with high oral bioavailability (Chesney et al., 2015). THZ1, a cyclin-dependent kinase 7 (CDK7) inhibitor, induced cell cycle arrest and apoptosis in H1299, A549, H292, and H23 NSCLC cells, which is attributed to the blockage of glycolysis-related pathways (Cheng et al., 2019). These studies indicate the promising therapeutic efficacy of cancer metabolism intervention, along with targeting therapy.
Anti-tumor small molecules
Methyl N-(6-phenylsulfanyl-1H-benzimidazol-2-yl) carbamate (fenbendazole, FZ), a microtubule-interfering agent, significantly inhibited the glycose uptake genes GLUT and HK2 in A549 cells and impeded the growth of human xenografts in a nu/nu mice model (Dogra et al., 2018). Albendazole (ABZ), a broad-spectrum benzimidazole carbamate anthelminthic and a microtubule inhibitor, presented similar anti-glycolysis effects with FZ by intervening in HIF-1α-dependent glycolysis. ABZ suppressed A549 and H1299 NSCLC cell growth and decreased VEGF and HIF-1α expression in an A549 xenograft mouse model (Zhou et al., 2017). These studies confirm the critical role of glycolysis intervention and tumor-microenvironment metabolism in NSCLC treatment.
The metabolic agent dichloroacetate (DCA), an inhibitor of pyruvate dehydrogenase, has demonstrated therapeutic potential in NSCLC. Aerobic glycolysis was ameliorated in DCA-treated A549 and H1299 cells, with decreased lactate generation and glucose consumption (Allen et al., 2015). The combination of angiogenesis inhibitor 2-methoxyestradiol (2-ME) with DCA showed synergistic anti-tumor effects in A549 cells by reducing the signaling transduction of HIF-3α and, partially, of HIF-1α (Romero et al., 2020). The combination of the energy metabolism inhibitor 3-bromopyruvate (3-BrPA) with the mTOR inhibitor rapamycin synergistically suppressed cell growth in H1299 and H23 cell lines and tumor growth in an A/J mouse model, respectively, indicating the promising therapeutic potential of both mTOR signaling and glycolysis inhibition in NSCLC (Zhang et al., 2015). Therefore, multiple anti-tumor small molecules increase their effects in NSCLC by directly or indirectly targeting the regulation of tumor glycolysis metabolism. Intervention in glycolysis could not only induce cell apoptosis but also reverse drug resistance. The anti-tumor small molecules discussed earlier are listed in Table 2.
Biopharmaceutical therapy
The development of biotechnologies and biopharmaceutics for cancer treatment has grown rapidly in recent years. Ad-apoptin is an oncolytic adenovirus that impairs AMPK-dependent glycolysis in NSCLC. Ad-apoptin inhibited tumor cell invasion and migration in A549 and NCI-H23 cells, as well as suppressing tumor growth in nude mice. The mechanism underlying the anti-NSCLC effect of ad-apoptin is the inhibition of glucose uptake and lactate generation through suppression of the AMPK/mTOR signaling pathway (Song et al., 2021).
Zhang et al. developed an effective TME biomimetic nanoplatform for NSCLC treatment, which was a hybrid nanovesicle loaded with siRNA against glycolytic regulator phosphoglycerate mutase 1 (PGAM1) and the chemotherapeutic drug docetaxel (DTX). This pH-driven siRNA and DTX hybrid nanoplatform ensured precise drug release and synergistic glycolysis impairment in A549 cells and impeded tumor growth in A549-cell tumor-bearing nude BALB/c mice (Zhang W. et al., 2021). Moreover, non-coding RNAs including microRNA (miRNA), circular RNA (circRNA), and long non-coding RNA (lncRNA) have been widely studied for the impairment of glycolysis in NSCLC. Most miRNAs have targeted key glycolytic enzymes such as HK2 (miR-143 and miR-206) (Fang et al., 2012; Jia et al., 2020), LDHA (miR-449a and miR-16-5p) (Li et al., 2018b; Arora et al., 2022), and HIF1α (miR-21, miR-182, and miR-199a) (Ding et al., 2013; Jiang et al., 2016; Wang et al., 2018). The circRNA and lncRNA target sequences have presented diverse glycolytic biomarkers under various molecular mechanisms in NSCLC. For instance, the activation of circ_0008797 showed anti-glycolysis effects in A549 and H1229 cells, as well as in a nude mouse model, by sponging miR-301a-3p and targeting suppressor of cytokine signaling 2 (SOCS2) (Abuduwaili et al., 2022). The lncRNA HOXA transcript antisense RNA myeloid-specific 1 (HOTAIRM1), which sponges the miR-498/ATP-binding cassette subfamily E member 1 (ABCE1), attenuated glycolysis metabolism in NCI-H1299 and A549 cells (Chen et al., 2021). Additionally, the regulation of miR-498 might reverse the resistance effects of osimertinib through the inhibition of the key glycolytic enzymes GLUT1, HK2, and LDHA in NCI-H1299 and A549 cells (Ma et al., 2020). Thus, various biologics have been reported to be correlated with the regulation of intracellular glycolytic metabolism. Although most of the previous research studies have been conducted in vitro, biopharmaceutics targeting glycolysis are a promising strategy for cancer treatment. The information detailed earlier is listed in Table 3.
Natural products
Natural compounds or extracts from animals, plants, or minerals, have been shown to have multiple medicinal and pharmacological activities. Natural products are a precious library for anti-tumor drug discovery, which has been a cutting-edge research field in the recent decades. In research into NSCLC drug candidate discovery, various natural products have been identified with potential for NSCLC treatment via their anti-glycolysis activities. Shikonin, an active ingredient derived from Lithospermum erythrorhizon, dampened intracellular glycolysis in A549 and PC9 cells. Shikonin improved the sensitivity of cisplatin in NSCLC mice by inhibiting the key glycolytic enzyme PKM2. The combination of shikonin with cisplatin synergistically inhibited PKM2 and GLUT1, according to data from immune-histological assays of tumor tissues. Furthermore, shikonin affected the glycolysis regulator PFKFB2 at the transcriptome level in A549 and H446 cells (Sha et al., 2021; Dai et al., 2022). Alpha-hederin is derived from the Pulsatilla chinensis (Bunge) Regel (Ranunculaceae). Fang et al. (2021) found a-hederin to demonstrate anti-proliferation effects in A549, NCI-H460, and NCI-H292 cells and in a xenograft mouse model by suppressing sirtuin 6 (SIRT6)-correlated glycolysis. The natural flavonoid deguelin is extracted from Derris trifoliata Lour, while resveratrol is an active ingredient derived from Veratrum grandiflorum. Deguelin and resveratrol have been studied in multiple cancer types for years and are well-known anti-tumor compounds with inhibitory properties against AKT1 and HK2-mediated glycolysis in the human NSCLC cell lines H460, H1650, and HCC827 (Li et al., 2016; Li et al., 2017). Jolkinolide B (JB), triptolide, and oroxylin A are bioactive compounds extracted from Euphorbia fischeriana Steud, Tripterygium wilfordii Hook F, and Scutellariae radix, respectively. These three natural compounds exhibited anti-metastasis effects in H1299, NCI- H460, and A549 cells and in nude mice, and the underlying mechanism is their intervention in intracellular glycolysis and the AKT/mTOR pathway (Wei et al., 2013; Gao and Han, 2018; Hamdi et al., 2018). Ligustilide, a derivative of Angelica sinensis, inhibited cell proliferation in H1299 and A549 and attenuated tumor growth in nude mice via inhibition of the PTEN/AKT signaling pathway (Jiang et al., 2021). The antimalarial drug dihydroartemisinin (DHA), which is extracted from Artemisia annua, showed cytotoxicity in diverse tumor models, including NSCLC. DHA inhibited glucose uptake and glycolysis in NCI-H358, A549, and PC-9 cells by downregulating GLUT1, mTOR, and S6 ribosomal protein through suppression of the ERK/c-Myc signaling pathway (Mi et al., 2015; Zhang et al., 2022). Beta-elemene, which is an essential oil of Curcuma wenyujin, inhibited miR-301a-3p-induced Warburg effects in NSCLC cell line NCI-H1650 by activating adenosine monophosphate-activated protein kinase a (AMPKα) (Li et al., 2020). Tanshinone IIA (Tan IIA), the active ingredient of Salvia miltiorrhiza, exhibited antitumor effects in NSCLC cells A549 and H292 and xenograft BALB/c-nu/nu nude mice by targeting the glycolytic mediator sine oculis homeobox homolog 1 (SIX1), which is a poor-prognosis predictor in NSCLC (Qi et al., 2022). The natural compound piperlongumine, an active component of Piper longum L, suppressed cell proliferation and colony formation in NSCLC cells HCC827 and H1975 by inhibiting cellular glycolysis and activating the apoptosis pathway. Additionally, the blockage of HK2 and AKT1 was essential for the anti-tumor effects of piperlongumine in a xenograft mouse model (Zhou et al., 2019).
Natural extracts have also presented effective anti-cancer effects in NSCLC through inhibition of glycolytic metabolism. Calderón-Montaño et al. (2013) found that hydroalcoholic extract from the leaves of Nerium oleander, a plant toxic to livestock, demonstrated anti-tumor effects in A549 cells through suppression of glycolysis. Lee et al. (2018) detected that water-extracted branch from Cinnamomum cassia Blume, which is widely used as a food additive or spice, suppressed aerobic glycolysis in A549 and H1299 cells via inhibiting pyruvate dehydrogenase kinase (PDHK), resulting in increased ROS and mitochondrial damage and consequently inducing cell apoptosis. Shenmai injection (SMI), which was developed from the medicinal Chinese herbs Radix Ginseng Rubra and Radix Ophiopogonis, is a commercially available drug for treating cardiovascular diseases. Sun et al. found that the combination of SMI with cisplatin increased the cisplatin sensitivity of resistant A549/DDP cells by suppressing glycolysis through inhibition of the AKT-mTOR-c-Myc signaling pathway (Sun Y. et al., 2020). Thus, natural products have shown inhibitory effects on glycolysis metabolism in cancer for multiple targets. However, the anti-tumor effects of most natural products against NSCLC have been determined in vitro. The combination of natural compounds with current therapies is a promising direction of studies, as well as in vivo study, and clinical study is needed in the future. Detailed information is given in Table 4.
Conclusion and future perspectives
NSCLC is the leading cause of death in cancer. Glycolytic metabolism heterogeneity is a critical feature of tumor metabolism that can distinguish cancer cells from normal cells. The reprogramming of glucose metabolism is common in multiple tumors, including NSCLC. As in other tumors, glycolysis represents the main metabolic process in NSCLC, instead of oxidative phosphorylation. Thus, glycolysis is vital for tumor cell survival, and targeting the inhibition of glycolysis is promising in the treatment of NSCLC.
However, most of the key glycolytic enzymes are involved in the progression of NSCLC, which means that the scope of target selection is broad and its specificity difficult to guarantee. The identification of appropriate targets and biomarkers in glycolysis that are specific for NSCLC treatment is still a challenge at present. However, high expression of PDK1 is an independent prognostic factor in NSCLC. LDHB is a potential specific target since it has been reported as a positive survival predictor in NSCLC, although the exact process of the lactate metabolism is complicated and LDHB has also been revealed to be a risk factor in other tumor cell types. Targeting both MCT2 and GLUT1 might be promising in the treatment of the adenocarcinoma subtype of NSCLC. PFKM might also be a prognostic predictor in postoperative NSCLC patients. DPPA4, NQO1, GAPDH/MT-CO1, PGC-1α, OTUB2, ISLR, Barx2, OTUB2, and RFP180 might be biomarkers or prognostic predictors of NSCLC. In addition, natural compounds or extracts may be promising therapeutic approaches in targeting the multiple steps of glycolysis metabolism since natural products always present multi-target properties.
Although current therapies including chemotherapy, targeting therapy, and biopharmaceutical therapy have shown dramatic effectiveness in the treatment of NSCLC, partially through glycolysis suppression, the occurrence of drug resistance during treatment is a crucial concern. Targeting the enhancement of tumor glycolytic metabolism inhibition may also be beneficial in reversing drug resistance and enhancing the anti-tumor effects of current treatments in NSCLC. Thus, the combination of natural products, small molecules, and biopharmaceutics systems targeting the inhibition of glycolysis with current therapeutic approaches might be a promising strategy as in vivo and clinical studies accumulate in the future.
In conclusion, targeting glycolysis might be a potential therapeutic approach in NSCLC treatment, although finding specific targets and biomarkers is still challenging at present. The combination of glycolysis intervention with current therapeutic approaches might increase treatment efficacy in NSCLC patients.
Author contributions
J-QX, Y-LF, and Z-YZ conducted the manuscript writing; JZ, JM, and Z-YZ revised the manuscript; K-YZ and JM collected the references; and J-YT and Z-WZ organized the project.
Funding
This study was supported by the National Health Commission of Shanghai (GWIV-28, ZY-(2018-2020)-FWTX-8001) and the Shanghai Committee of Science and Technology (22ZR1462200 and 21ZR1463200).
Conflict of interest
The authors declare that the research was conducted in the absence of any commercial or financial relationships that could be construed as a potential conflict of interest.
Publisher’s note
All claims expressed in this article are solely those of the authors and do not necessarily represent those of their affiliated organizations, or those of the publisher, the editors, and the reviewers. Any product that may be evaluated in this article, or claim that may be made by its manufacturer, is not guaranteed or endorsed by the publisher.
References
Abuduwaili, K., Zhu, X., Shen, Y., Lu, S., and Liu, C. (2022). circ_0008797 attenuates non-small cell lung cancer proliferation, metastasis, and aerobic glycolysis by sponging miR-301a-3p/SOCS2. Environ. Toxicol. 37 (7), 1697–1710. doi:10.1002/tox.23518
Allen, K. T., Chin-Sinex, H., DeLuca, T., Pomerening, J. R., Sherer, J., Watkins, J. B., et al. (2015). Dichloroacetate alters Warburg metabolism, inhibits cell growth, and increases the X-ray sensitivity of human A549 and H1299 NSC lung cancer cells. Free Radic. Biol. Med. 89, 263–273. doi:10.1016/j.freeradbiomed.2015.08.006
Arbour, K. C., and Riely, G. J. (2019). Systemic therapy for locally advanced and metastatic non-small cell lung cancer: A review. Jama 322 (8), 764–774. doi:10.1001/jama.2019.11058
Arora, S., Singh, P., Tabassum, G., Dohare, R., and Syed, M. A. (2022). miR-16-5p regulates aerobic glycolysis and tumorigenesis of NSCLC cells via LDH-A/lactate/NF-κB signaling. Life Sci. 304, 120722. doi:10.1016/j.lfs.2022.120722
Bose, S., and Le, A. (2018). Glucose metabolism in cancer. Adv. Exp. Med. Biol. 1063, 3–12. doi:10.1007/978-3-319-77736-8_1
Bray, F., Laversanne, M., Weiderpass, E., and Soerjomataram, I. (2021). The ever-increasing importance of cancer as a leading cause of premature death worldwide. Cancer 127 (16), 3029–3030. doi:10.1002/cncr.33587
Brisson, L., Bański, P., Sboarina, M., Dethier, C., Danhier, P., Fontenille, M. J., et al. (2016). Lactate dehydrogenase B controls lysosome activity and autophagy in cancer. Cancer Cell 30 (3), 418–431. doi:10.1016/j.ccell.2016.08.005
Calderón-Montaño, J. M., Burgos-Morón, E., Orta, M. L., Mateos, S., and López-Lázaro, M. (2013). A hydroalcoholic extract from the leaves of Nerium oleander inhibits glycolysis and induces selective killing of lung cancer cells. Planta Med. 79 (12), 1017–1023. doi:10.1055/s-0032-1328715
Chen, D., Li, Y., Wang, Y., and Xu, J. (2021). LncRNA HOTAIRM1 knockdown inhibits cell glycolysis metabolism and tumor progression by miR-498/ABCE1 axis in non-small cell lung cancer. Genes Genomics 43 (2), 183–194. doi:10.1007/s13258-021-01052-9
Chen, H. H., Chiu, N. T., Su, W. C., Guo, H. R., and Lee, B. F. (2012). Prognostic value of whole-body total lesion glycolysis at pretreatment FDG PET/CT in non-small cell lung cancer. Radiology 264 (2), 559–566. doi:10.1148/radiol.12111148
Chen, H., Zhang, M., Zhang, W., Li, Y., Zhu, J., Zhang, X., et al. (2018). Downregulation of BarH-like homeobox 2 promotes cell proliferation, migration and aerobic glycolysis through Wnt/β-catenin signaling, and predicts a poor prognosis in non-small cell lung carcinoma. Thorac. Cancer 9 (3), 390–399. doi:10.1111/1759-7714.12593
Cheng, X., Liu, F., Liu, H., Wang, G., and Hao, H. (2018). Enhanced glycometabolism as a mechanism of NQO1 potentiated growth of NSCLC revealed by metabolomic profiling. Biochem. Biophys. Res. Commun. 496 (1), 31–36. doi:10.1016/j.bbrc.2017.12.160
Cheng, Z. J., Miao, D. L., Su, Q. Y., Tang, X. L., Wang, X. L., Deng, L. B., et al. (2019). THZ1 suppresses human non-small-cell lung cancer cells in vitro through interference with cancer metabolism. Acta Pharmacol. Sin. 40 (6), 814–822. doi:10.1038/s41401-018-0187-3
Chesney, J., Clark, J., Lanceta, L., Trent, J. O., and Telang, S. (2015). Targeting the sugar metabolism of tumors with a first-in-class 6-phosphofructo-2-kinase (PFKFB4) inhibitor. Oncotarget 6 (20), 18001–18011. doi:10.18632/oncotarget.4534
Choi, S. H., Jin, C. C., Do, S. K., Lee, S. Y., Choi, J. E., Kang, H. G., et al. (2020). Polymorphisms in glycolysis-related genes are associated with clinical outcomes of paclitaxel-cisplatin chemotherapy in non-small cell lung cancer. Oncology 98 (7), 468–477. doi:10.1159/000504175
Ciscato, F., Ferrone, L., Masgras, I., Laquatra, C., and Rasola, A. (2021). Hexokinase 2 in cancer: A prima donna playing multiple characters. Int. J. Mol. Sci. 22 (9), 4716. doi:10.3390/ijms22094716
Cruz-Bermúdez, A., Vicente-Blanco, R. J., Laza-Briviesca, R., García-Grande, A., Laine-Menéndez, S., Gutiérrez, L., et al. (2017). PGC-1alpha levels correlate with survival in patients with stage III NSCLC and may define a new biomarker to metabolism-targeted therapy. Sci. Rep. 7 (1), 16661. doi:10.1038/s41598-017-17009-6
Dai, K., Radin, D. P., and Leonardi, D. (2019). PINK1 depletion sensitizes non-small cell lung cancer to glycolytic inhibitor 3-bromopyruvate: Involvement of ROS and mitophagy. Pharmacol. Rep. 71 (6), 1184–1189. doi:10.1016/j.pharep.2019.08.002
Dai, Y., Liu, Y., Li, J., Jin, M., Yang, H., and Huang, G. (2022). Shikonin inhibited glycolysis and sensitized cisplatin treatment in non-small cell lung cancer cells via the exosomal pyruvate kinase M2 pathway. Bioengineered 13 (5), 13906–13918. doi:10.1080/21655979.2022.2086378
Damiani, C., Colombo, R., Gaglio, D., Mastroianni, F., Pescini, D., Westerhoff, H. V., et al. (2017). A metabolic core model elucidates how enhanced utilization of glucose and glutamine, with enhanced glutamine-dependent lactate production, promotes cancer cell growth: The WarburQ effect. PLoS Comput. Biol. 13 (9), e1005758. doi:10.1371/journal.pcbi.1005758
DeBerardinis, R. J., Lum, J. J., Hatzivassiliou, G., and Thompson, C. B. (2008). The biology of cancer: Metabolic reprogramming fuels cell growth and proliferation. Cell Metab. 7 (1), 11–20. doi:10.1016/j.cmet.2007.10.002
Deng, H., Gao, Y., Trappetti, V., Hertig, D., Karatkevich, D., Losmanova, T., et al. (2022). Targeting lactate dehydrogenase B-dependent mitochondrial metabolism affects tumor initiating cells and inhibits tumorigenesis of non-small cell lung cancer by inducing mtDNA damage. Cell. Mol. Life Sci. 79 (8), 445. doi:10.1007/s00018-022-04453-5
Deng, T., Zhang, J., Meng, Y., Zhou, Y., and Li, W. (2018). Higher pretreatment lactate dehydrogenase concentration predicts worse overall survival in patients with lung cancer. Med. Baltim. 97 (38), e12524. doi:10.1097/md.0000000000012524
Ding, G., Huang, G., Liu, H. D., Liang, H. X., Ni, Y. F., Ding, Z. H., et al. (2013). MiR-199a suppresses the hypoxia-induced proliferation of non-small cell lung cancer cells through targeting HIF1α. Mol. Cell. Biochem. 384 (1-2), 173–180. doi:10.1007/s11010-013-1795-3
Ding, Y., Lu, Y., Xie, X., Cao, L., and Zheng, S. (2022). Ring finger protein 180 suppresses cell proliferation and energy metabolism of non-small cell lung cancer through downregulating C-myc. World J. Surg. Oncol. 20 (1), 162. doi:10.1186/s12957-022-02599-x
Dogan, S., Shen, R., Ang, D. C., Johnson, M. L., D'Angelo, S. P., Paik, P. K., et al. (2012). Molecular epidemiology of EGFR and KRAS mutations in 3, 026 lung adenocarcinomas: Higher susceptibility of women to smoking-related KRAS-mutant cancers. Clin. Cancer Res. 18 (22), 6169–6177. doi:10.1158/1078-0432.Ccr-11-3265
Dogra, N., Kumar, A., and Mukhopadhyay, T. (2018). Fenbendazole acts as a moderate microtubule destabilizing agent and causes cancer cell death by modulating multiple cellular pathways. Sci. Rep. 8 (1), 11926. doi:10.1038/s41598-018-30158-6
Duan, Y., Li, J., Wang, F., Wei, J., Yang, Z., Sun, M., et al. (2021). Protein modifications throughout the lung cancer proteome unravel the cancer-specific regulation of glycolysis. Cell Rep. 37 (12), 110137. doi:10.1016/j.celrep.2021.110137
Elstrom, R. L., Bauer, D. E., Buzzai, M., Karnauskas, R., Harris, M. H., Plas, D. R., et al. (2004). Akt stimulates aerobic glycolysis in cancer cells. Cancer Res. 64 (11), 3892–3899. doi:10.1158/0008-5472.can-03-2904
Fan, T., Sun, G., Sun, X., Zhao, L., Zhong, R., and Peng, Y. (2019). Tumor energy metabolism and potential of 3-bromopyruvate as an inhibitor of aerobic glycolysis: Implications in tumor treatment. Cancers (Basel) 11 (3), E317. doi:10.3390/cancers11030317
Fang, C., Liu, Y., Chen, L., Luo, Y., Cui, Y., Zhang, N., et al. (2021). α-Hederin inhibits the growth of lung cancer A549 cells in vitro and in vivo by decreasing SIRT6 dependent glycolysis. Pharm. Biol. 59 (1), 11–20. doi:10.1080/13880209.2020.1862250
Fang, R., Xiao, T., Fang, Z., Sun, Y., Li, F., Gao, Y., et al. (2012). MicroRNA-143 (miR-143) regulates cancer glycolysis via targeting hexokinase 2 gene. J. Biol. Chem. 287 (27), 23227–23235. doi:10.1074/jbc.M112.373084
Feng, L., Feng, S., Nie, Z., Deng, Y., Xuan, Y., Chen, X., et al. (2021). TRAF6 promoted tumor glycolysis in non-small-cell lung cancer by activating the akt-hifα pathway. Biomed. Res. Int. 2021, 3431245. doi:10.1155/2021/3431245
Fu, H., Gao, H., Qi, X., Zhao, L., Wu, D., Bai, Y., et al. (2018). Aldolase A promotes proliferation and G(1)/S transition via the EGFR/MAPK pathway in non-small cell lung cancer. Cancer Commun. 38 (1), 18. doi:10.1186/s40880-018-0290-3
Fu, Q. F., Liu, Y., Fan, Y., Hua, S. N., Qu, H. Y., Dong, S. W., et al. (2015). Alpha-enolase promotes cell glycolysis, growth, migration, and invasion in non-small cell lung cancer through FAK-mediated PI3K/AKT pathway. J. Hematol. Oncol. 8, 22. doi:10.1186/s13045-015-0117-5
Ganapathy-Kanniappan, S., and Geschwind, J. F. (2013). Tumor glycolysis as a target for cancer therapy: Progress and prospects. Mol. Cancer 12, 152. doi:10.1186/1476-4598-12-152
Gao, X., and Han, H. (2018). Jolkinolide B inhibits glycolysis by downregulating hexokinase 2 expression through inactivating the Akt/mTOR pathway in non-small cell lung cancer cells. J. Cell. Biochem. 119 (6), 4967–4974. doi:10.1002/jcb.26742
Giatromanolaki, A., Koukourakis, I. M., Balaska, K., Mitrakas, A. G., Harris, A. L., and Koukourakis, M. I. (2019). Programmed death-1 receptor (PD-1) and PD-ligand-1 (PD-L1) expression in non-small cell lung cancer and the immune-suppressive effect of anaerobic glycolysis. Med. Oncol. 36 (9), 76. doi:10.1007/s12032-019-1299-4
Giatromanolaki, A., Sivridis, E., Arelaki, S., and Koukourakis, M. I. (2017). Expression of enzymes related to glucose metabolism in non-small cell lung cancer and prognosis. Exp. Lung Res. 43 (4-5), 167–174. doi:10.1080/01902148.2017.1328714
Gong, T., Cui, L., Wang, H., Wang, H., and Han, N. (2018). Knockdown of KLF5 suppresses hypoxia-induced resistance to cisplatin in NSCLC cells by regulating HIF-1α-dependent glycolysis through inactivation of the PI3K/Akt/mTOR pathway. J. Transl. Med. 16 (1), 164. doi:10.1186/s12967-018-1543-2
Goodwin, J., Neugent, M. L., Lee, S. Y., Choe, J. H., Choi, H., Jenkins, D. M. R., et al. (2017). The distinct metabolic phenotype of lung squamous cell carcinoma defines selective vulnerability to glycolytic inhibition. Nat. Commun. 8, 15503. doi:10.1038/ncomms15503
Granja, S., Marchiq, I., Le Floch, R., Moura, C. S., Baltazar, F., and Pouysségur, J. (2015). Disruption of BASIGIN decreases lactic acid export and sensitizes non-small cell lung cancer to biguanides independently of the LKB1 status. Oncotarget 6 (9), 6708–6721. doi:10.18632/oncotarget.2862
Gridelli, C., Rossi, A., Carbone, D. P., Guarize, J., Karachaliou, N., Mok, T., et al. (2015). Non-small-cell lung cancer. Nat. Rev. Dis. Prim. 1, 15009. doi:10.1038/nrdp.2015.9
Grizzi, F., Castello, A., Qehajaj, D., Toschi, L., Rossi, S., Pistillo, D., et al. (2019). Independent expression of circulating and tissue levels of PD-L1: Correlation of clusters with tumor metabolism and outcome in patients with non-small cell lung cancer. Cancer Immunol. Immunother. 68 (9), 1537–1545. doi:10.1007/s00262-019-02387-9
Hamdi, A. M., Jiang, Z. Z., Guerram, M., Yousef, B. A., Hassan, H. M., Ling, J. W., et al. (2018). Biochemical and computational evaluation of Triptolide-induced cytotoxicity against NSCLC. Biomed. Pharmacother. 103, 1557–1566. doi:10.1016/j.biopha.2018.04.198
He, R., and Liu, H. (2020). TRIM59 knockdown blocks cisplatin resistance in A549/DDP cells through regulating PTEN/AKT/HK2. Gene 747, 144553. doi:10.1016/j.gene.2020.144553
He, Z. F., Jin, X. R., Lin, J. J., Zhang, X., Liu, Y., Xu, H. L., et al. (2020). NALP3 orchestrates cellular bioenergetics to facilitate non-small cell lung cancer cell growth. Life Sci. 241, 117165. doi:10.1016/j.lfs.2019.117165
Hensley, C. T., Faubert, B., Yuan, Q., Lev-Cohain, N., Jin, E., Kim, J., et al. (2016). Metabolic heterogeneity in human lung tumors. Cell 164 (4), 681–694. doi:10.1016/j.cell.2015.12.034
Ji, J., Xie, M., Qian, Q., Xu, Y., Shi, W., Chen, Z., et al. (2022). SOX9-mediated UGT8 expression promotes glycolysis and maintains the malignancy of non-small cell lung cancer. Biochem. Biophys. Res. Commun. 587, 139–145. doi:10.1016/j.bbrc.2021.11.099
Jia, K. G., Feng, G., Tong, Y. S., Tao, G. Z., and Xu, L. (2020). miR-206 regulates non-small-cell lung cancer cell aerobic glycolysis by targeting hexokinase 2. J. Biochem. 167 (4), 365–370. doi:10.1093/jb/mvz099
Jiang, M., Zhang, X., Chen, Y., Chen, P., Guo, X., Ma, L., et al. (2022). A review of the correlation between epidermal growth factor receptor mutation status and (18)F-fdg metabolic activity in non-small cell lung cancer. Front. Oncol. 12, 780186. doi:10.3389/fonc.2022.780186
Jiang, S., Wang, R., Yan, H., Jin, L., Dou, X., and Chen, D. (2016). MicroRNA-21 modulates radiation resistance through upregulation of hypoxia-inducible factor-1α-promoted glycolysis in non-small cell lung cancer cells. Mol. Med. Rep. 13 (5), 4101–4107. doi:10.3892/mmr.2016.5010
Jiang, X., Zhao, W., Zhu, F., Wu, H., Ding, X., Bai, J., et al. (2021). Ligustilide inhibits the proliferation of non-small cell lung cancer via glycolytic metabolism. Toxicol. Appl. Pharmacol. 410, 115336. doi:10.1016/j.taap.2020.115336
Jun, J. C., Rathore, A., Younas, H., Gilkes, D., and Polotsky, V. Y. (2017). Hypoxia-inducible factors and cancer. Curr. Sleep. Med. Rep. 3 (1), 1–10. doi:10.1007/s40675-017-0062-7
Kawada, K., Toda, K., and Sakai, Y. (2017). Targeting metabolic reprogramming in KRAS-driven cancers. Int. J. Clin. Oncol. 22 (4), 651–659. doi:10.1007/s10147-017-1156-4
Kerr, E. M., Gaude, E., Turrell, F. K., Frezza, C., and Martins, C. P. (2016). Mutant Kras copy number defines metabolic reprogramming and therapeutic susceptibilities. Nature 531 (7592), 110–113. doi:10.1038/nature16967
Kim, J. H., Nam, B., Choi, Y. J., Kim, S. Y., Lee, J. E., Sung, K. J., et al. (2018). Enhanced glycolysis supports cell survival in EGFR-mutant lung adenocarcinoma by inhibiting autophagy-mediated EGFR degradation. Cancer Res. 78 (16), 4482–4496. doi:10.1158/0008-5472.Can-18-0117
Kim, J., Lee, H. M., Cai, F., Ko, B., Yang, C., Lieu, E. L., et al. (2020). The hexosamine biosynthesis pathway is a targetable liability in KRAS/LKB1 mutant lung cancer. Nat. Metab. 2 (12), 1401–1412. doi:10.1038/s42255-020-00316-0
Kim, S. M., Yun, M. R., Hong, Y. K., Solca, F., Kim, J. H., Kim, H. J., et al. (2013). Glycolysis inhibition sensitizes non-small cell lung cancer with T790M mutation to irreversible EGFR inhibitors via translational suppression of Mcl-1 by AMPK activation. Mol. Cancer Ther. 12 (10), 2145–2156. doi:10.1158/1535-7163.Mct-12-1188
Koh, Y. W., Lee, S. J., and Park, S. Y. (2017a). Differential expression and prognostic significance of GLUT1 according to histologic type of non-small-cell lung cancer and its association with volume-dependent parameters. Lung Cancer 104, 31–37. doi:10.1016/j.lungcan.2016.12.003
Koh, Y. W., Lee, S. J., and Park, S. Y. (2017b). Prognostic significance of lactate dehydrogenase B according to histologic type of non-small-cell lung cancer and its association with serum lactate dehydrogenase. Pathol. Res. Pract. 213 (9), 1134–1138. doi:10.1016/j.prp.2017.07.006
Krassikova, L., Zhang, B., Nagarajan, D., Queiroz, A. L., Kacal, M., Samakidis, E., et al. (2021). The deubiquitinase JOSD2 is a positive regulator of glucose metabolism. Cell Death Differ. 28 (3), 1091–1109. doi:10.1038/s41418-020-00639-1
Kuo, T. C., Huang, K. Y., Yang, S. C., Wu, S., Chung, W. C., Chang, Y. L., et al. (2020). Monocarboxylate transporter 4 is a therapeutic target in non-small cell lung cancer with aerobic glycolysis preference. Mol. Ther. Oncolytics 18, 189–201. doi:10.1016/j.omto.2020.06.012
Le Grand, M., Berges, R., Pasquier, E., Montero, M. P., Borge, L., Carrier, A., et al. (2017). Akt targeting as a strategy to boost chemotherapy efficacy in non-small cell lung cancer through metabolism suppression. Sci. Rep. 7, 45136. doi:10.1038/srep45136
Lee, E. J., Chung, T. W., Lee, J. H., Kim, B. S., Kim, E. Y., Lee, S. O., et al. (2018). Water-extracted branch of Cinnamomum cassia promotes lung cancer cell apoptosis by inhibiting pyruvate dehydrogenase kinase activity. J. Pharmacol. Sci. 138 (2), 146–154. doi:10.1016/j.jphs.2018.10.005
Lee, S. Y., Jin, C. C., Choi, J. E., Hong, M. J., Jung, D. K., Do, S. K., et al. (2016). Genetic polymorphisms in glycolytic pathway are associated with the prognosis of patients with early stage non-small cell lung cancer. Sci. Rep. 6, 35603. doi:10.1038/srep35603
Li, J., Cheng, D., Zhu, M., Yu, H., Pan, Z., Liu, L., et al. (2019a). OTUB2 stabilizes U2AF2 to promote the Warburg effect and tumorigenesis via the AKT/mTOR signaling pathway in non-small cell lung cancer. Theranostics 9 (1), 179–195. doi:10.7150/thno.29545
Li, L., Liang, Y., Kang, L., Liu, Y., Gao, S., Chen, S., et al. (2018a). Transcriptional regulation of the warburg effect in cancer by SIX1. Cancer Cell 33 (3), 368–385. e367. doi:10.1016/j.ccell.2018.01.010
Li, L., Liu, H., Du, L., Xi, P., Wang, Q., Li, Y., et al. (2018b). miR-449a suppresses LDHA-mediated glycolysis to enhance the sensitivity of non-small cell lung cancer cells to ionizing radiation. Oncol. Res. 26 (4), 547–556. doi:10.3727/096504017x15016337254605
Li, L., Wang, Y., Wang, Q., Qu, J., Wei, X., Xu, J., et al. (2019b). High developmental pluripotency-associated 4 expression promotes cell proliferation and glycolysis, and predicts poor prognosis in non-small-cell lung cancer. Mol. Med. Rep. 20 (1), 445–454. doi:10.3892/mmr.2019.10272
Li, L., Zhao, D., Cheng, G., Li, Q., Chu, Y., Chu, H., et al. (2020). β-elemene suppresses Warburg effect in NCI-H1650 non-small-cell lung cancer cells by regulating the miR-301a-3p/AMPKα axis. Biosci. Rep. 40 (6), BSR20194389. doi:10.1042/bsr20194389
Li, W., Gao, F., Ma, X., Wang, R., Dong, X., and Wang, W. (2017). Deguelin inhibits non-small cell lung cancer via down-regulating Hexokinases II-mediated glycolysis. Oncotarget 8 (20), 32586–32599. doi:10.18632/oncotarget.15937
Li, W., Ma, X., Li, N., Liu, H., Dong, Q., Zhang, J., et al. (2016). Resveratrol inhibits Hexokinases II mediated glycolysis in non-small cell lung cancer via targeting Akt signaling pathway. Exp. Cell Res. 349 (2), 320–327. doi:10.1016/j.yexcr.2016.11.002
Li, Z., Su, J., Sun, M., Song, J., Sun, H., Fan, J., et al. (2021). Octamer transcription factor-1 induces the Warburg effect via up-regulation of hexokinase 2 in non-small cell lung cancer. Mol. Cell. Biochem. 476 (9), 3423–3431. doi:10.1007/s11010-021-04171-9
Liao, S. T., Han, C., Xu, D. Q., Fu, X. W., Wang, J. S., and Kong, L. Y. (2019). 4-Octyl itaconate inhibits aerobic glycolysis by targeting GAPDH to exert anti-inflammatory effects. Nat. Commun. 10 (1), 5091. doi:10.1038/s41467-019-13078-5
Lin, C., Chen, H., Han, R., Li, L., Lu, C., Hao, S., et al. (2021). Hexokinases II-mediated glycolysis governs susceptibility to crizotinib in ALK-positive non-small cell lung cancer. Thorac. Cancer 12 (23), 3184–3193. doi:10.1111/1759-7714.14184
Liu, T., and Yin, H. (2017). PDK1 promotes tumor cell proliferation and migration by enhancing the Warburg effect in non-small cell lung cancer. Oncol. Rep. 37 (1), 193–200. doi:10.3892/or.2016.5253
Lunt, S. Y., and Vander Heiden, M. G. (2011). Aerobic glycolysis: Meeting the metabolic requirements of cell proliferation. Annu. Rev. Cell Dev. Biol. 27, 441–464. doi:10.1146/annurev-cellbio-092910-154237
Lypova, N., Telang, S., Chesney, J., and Imbert-Fernandez, Y. (2019). Increased 6-phosphofructo-2-kinase/fructose-2, 6-bisphosphatase-3 activity in response to EGFR signaling contributes to non-small cell lung cancer cell survival. J. Biol. Chem. 294 (27), 10530–10543. doi:10.1074/jbc.RA119.007784
Ma, J., Qi, G., and Li, L. (2020). A novel serum exosomes-based biomarker hsa_circ_0002130 facilitates osimertinib-resistance in non-small cell lung cancer by sponging miR-498. Onco. Targets. Ther. 13, 5293–5307. doi:10.2147/ott.S243214
Martínez-Reyes, I., and Chandel, N. S. (2021). Cancer metabolism: Looking forward. Nat. Rev. Cancer 21 (10), 669–680. doi:10.1038/s41568-021-00378-6
Massó-Vallés, D., Beaulieu, M. E., and Soucek, L. (2020). MYC, MYCL, and MYCN as therapeutic targets in lung cancer. Expert Opin. Ther. Targets 24 (2), 101–114. doi:10.1080/14728222.2020.1723548
Melloni, G., Gajate, A. M., Sestini, S., Gallivanone, F., Bandiera, A., Landoni, C., et al. (2013). New positron emission tomography derived parameters as predictive factors for recurrence in resected stage I non-small cell lung cancer. Eur. J. Surg. Oncol. 39 (11), 1254–1261. doi:10.1016/j.ejso.2013.07.092
Mi, Y. J., Geng, G. J., Zou, Z. Z., Gao, J., Luo, X. Y., Liu, Y., et al. (2015). Dihydroartemisinin inhibits glucose uptake and cooperates with glycolysis inhibitor to induce apoptosis in non-small cell lung carcinoma cells. PLoS One 10 (3), e0120426. doi:10.1371/journal.pone.0120426
Molina, J. R., Yang, P., Cassivi, S. D., Schild, S. E., and Adjei, A. A. (2008). Non-small cell lung cancer: Epidemiology, risk factors, treatment, and survivorship. Mayo Clin. Proc. 83 (5), 584–594. doi:10.4065/83.5.584
Moreno-Sánchez, R., Rodríguez-Enríquez, S., Marín-Hernández, A., and Saavedra, E. (2007). Energy metabolism in tumor cells. Febs J. 274 (6), 1393–1418. doi:10.1111/j.1742-4658.2007.05686.x
Naik, A., and Decock, J. (2020). Lactate metabolism and immune modulation in breast cancer: A focused review on triple negative breast tumors. Front. Oncol. 10, 598626. doi:10.3389/fonc.2020.598626
Nicholls, C., Li, H., and Liu, J. P. (2012). Gapdh: A common enzyme with uncommon functions. Clin. Exp. Pharmacol. Physiol. 39 (8), 674–679. doi:10.1111/j.1440-1681.2011.05599.x
Niu, F. Y., Zhou, Q., Yang, J. J., Zhong, W. Z., Chen, Z. H., Deng, W., et al. (2016). Distribution and prognosis of uncommon metastases from non-small cell lung cancer. BMC Cancer 16, 149. doi:10.1186/s12885-016-2169-5
Pan, Y., Zheng, Q., Ni, W., Wei, Z., Yu, S., Jia, Q., et al. (2019). Breaking glucose transporter 1/pyruvate kinase M2 glycolytic loop is required for cantharidin inhibition of metastasis in highly metastatic breast cancer. Front. Pharmacol. 10, 590. doi:10.3389/fphar.2019.00590
Park, H. S., Lim, S. M., Shin, H. J., Cho, A., Shin, J. G., Lee, M. G., et al. (2016). Pharmacogenetic analysis of advanced non-small-cell lung cancer patients treated with first-line paclitaxel and carboplatin chemotherapy. Pharmacogenet. Genomics 26 (3), 116–125. doi:10.1097/fpc.0000000000000196
Park, J. H., Pyun, W. Y., and Park, H. W. (2020). Cancer metabolism: Phenotype, signaling and therapeutic targets. Cells 9 (10), E2308. doi:10.3390/cells9102308
Patra, K. C., Wang, Q., Bhaskar, P. T., Miller, L., Wang, Z., Wheaton, W., et al. (2013). Hexokinase 2 is required for tumor initiation and maintenance and its systemic deletion is therapeutic in mouse models of cancer. Cancer Cell 24 (2), 213–228. doi:10.1016/j.ccr.2013.06.014
Pavlova, N. N., and Thompson, C. B. (2016). The emerging hallmarks of cancer metabolism. Cell Metab. 23 (1), 27–47. doi:10.1016/j.cmet.2015.12.006
Payen, V. L., Mina, E., Van Hée, V. F., Porporato, P. E., and Sonveaux, P. (2020). Monocarboxylate transporters in cancer. Mol. Metab. 33, 48–66. doi:10.1016/j.molmet.2019.07.006
Phadke, M., Krynetskaia, N., and Krynetskiy, E. (2013). Cytotoxicity of chemotherapeutic agents in glyceraldehyde-3-phosphate dehydrogenase-depleted human lung carcinoma A549 cells with the accelerated senescence phenotype. Anticancer. Drugs 24 (4), 366–374. doi:10.1097/CAD.0b013e32835e3378
Pham-Danis, C., Gehrke, S., Danis, E., Rozhok, A. I., Daniels, M. W., Gao, D., et al. (2019). Urea cycle sustains cellular energetics upon EGFR inhibition in EGFR-mutant NSCLC. Mol. Cancer Res. 17 (6), 1351–1364. doi:10.1158/1541-7786.Mcr-18-1068
Phan, L. M., Yeung, S. C., and Lee, M. H. (2014). Cancer metabolic reprogramming: Importance, main features, and potentials for precise targeted anti-cancer therapies. Cancer Biol. Med. 11 (1), 1–19. doi:10.7497/j.issn.2095-3941.2014.01.001
Prochownik, E. V., and Wang, H. (2021). The metabolic fates of pyruvate in normal and neoplastic cells. Cells 10 (4), 762. doi:10.3390/cells10040762
Pupo, E., Avanzato, D., Middonti, E., Bussolino, F., and Lanzetti, L. (2019). KRAS-driven metabolic rewiring reveals novel actionable targets in cancer. Front. Oncol. 9, 848. doi:10.3389/fonc.2019.00848
Qi, H., Chen, Z., Qin, Y., Wang, X., Zhang, Z., and Li, Y. (2022). Tanshinone IIA inhibits cell growth by suppressing SIX1-induced aerobic glycolysis in non-small cell lung cancer cells. Oncol. Lett. 23 (6), 184. doi:10.3892/ol.2022.13304
Rajas, F., Gautier-Stein, A., and Mithieux, G. (2019). Glucose-6 phosphate, A central hub for liver carbohydrate metabolism. Metabolites 9 (12), E282. doi:10.3390/metabo9120282
Reinfeld, B. I., Madden, M. Z., Wolf, M. M., Chytil, A., Bader, J. E., Patterson, A. R., et al. (2021). Cell-programmed nutrient partitioning in the tumour microenvironment. Nature 593 (7858), 282–288. doi:10.1038/s41586-021-03442-1
Romero, Y., Castillejos-López, M., Romero-García, S., Aguayo, A. S., Herrera, I., Garcia-Martin, M. O., et al. (2020). Antitumor therapy under hypoxic microenvironment by the combination of 2-methoxyestradiol and sodium dichloroacetate on human non-small-cell lung cancer. Oxid. Med. Cell. Longev. 2020, 3176375. doi:10.1155/2020/3176375
Rudin, C. M., Brambilla, E., Faivre-Finn, C., and Sage, J. (2021). Small-cell lung cancer. Nat. Rev. Dis. Prim. 7 (1), 3. doi:10.1038/s41572-020-00235-0
Santos, C. R., and Schulze, A. (2012). Lipid metabolism in cancer. Febs J. 279 (15), 2610–2623. doi:10.1111/j.1742-4658.2012.08644.x
Schiliro, C., and Firestein, B. L. (2021). Mechanisms of metabolic reprogramming in cancer cells supporting enhanced growth and proliferation. Cells 10 (5), 1056. doi:10.3390/cells10051056
Seyfried, T. N., Flores, R. E., Poff, A. M., and D'Agostino, D. P. (2014). Cancer as a metabolic disease: Implications for novel therapeutics. Carcinogenesis 35 (3), 515–527. doi:10.1093/carcin/bgt480
Sha, L., Lv, Z., Liu, Y., Zhang, Y., Sui, X., Wang, T., et al. (2021). Shikonin inhibits the Warburg effect, cell proliferation, invasion and migration by downregulating PFKFB2 expression in lung cancer. Mol. Med. Rep. 24 (2), 560. doi:10.3892/mmr.2021.12199
Shen, J., Jin, Z., Lv, H., Jin, K., Jonas, K., Zhu, C., et al. (2020). PFKP is highly expressed in lung cancer and regulates glucose metabolism. Cell. Oncol. 43 (4), 617–629. doi:10.1007/s13402-020-00508-6
Sieow, J. L., Gun, S. Y., and Wong, S. C. (2018). The sweet surrender: How myeloid cell metabolic plasticity shapes the tumor microenvironment. Front. Cell Dev. Biol. 6, 168. doi:10.3389/fcell.2018.00168
Sizemore, S. T., Zhang, M., Cho, J. H., Sizemore, G. M., Hurwitz, B., Kaur, B., et al. (2018). Pyruvate kinase M2 regulates homologous recombination-mediated DNA double-strand break repair. Cell Res. 28 (11), 1090–1102. doi:10.1038/s41422-018-0086-7
Smolle, E., Leko, P., Stacher-Priehse, E., Brcic, L., El-Heliebi, A., Hofmann, L., et al. (2020). Distribution and prognostic significance of gluconeogenesis and glycolysis in lung cancer. Mol. Oncol. 14 (11), 2853–2867. doi:10.1002/1878-0261.12780
Song, G., Fang, J., Shang, C., Li, Y., Zhu, Y., Xiu, Z., et al. (2021). Ad-apoptin inhibits glycolysis, migration and invasion in lung cancer cells targeting AMPK/mTOR signaling pathway. Exp. Cell Res. 409 (2), 112926. doi:10.1016/j.yexcr.2021.112926
Soni, S., and Padwad, Y. S. (2017). HIF-1 in cancer therapy: Two decade long story of a transcription factor. Acta Oncol. 56 (4), 503–515. doi:10.1080/0284186x.2017.1301680
Stine, Z. E., Schug, Z. T., Salvino, J. M., and Dang, C. V. (2022). Targeting cancer metabolism in the era of precision oncology. Nat. Rev. Drug Discov. 21 (2), 141–162. doi:10.1038/s41573-021-00339-6
Stine, Z. E., Walton, Z. E., Altman, B. J., Hsieh, A. L., and Dang, C. V. (2015). MYC, metabolism, and cancer. Cancer Discov. 5 (10), 1024–1039. doi:10.1158/2159-8290.Cd-15-0507
Sun, J., Zhao, B., Du, K., and Liu, P. (2019). TRAF6 correlated to invasion and poor prognosis of glioblastoma via elevating MMP9 expression. Neuroreport 30 (2), 127–133. doi:10.1097/wnr.0000000000001171
Sun, X., Wang, M., Wang, M., Yao, L., Li, X., Dong, H., et al. (2020a). Role of proton-coupled monocarboxylate transporters in cancer: From metabolic crosstalk to therapeutic potential. Front. Cell Dev. Biol. 8, 651. doi:10.3389/fcell.2020.00651
Sun, Y., Chen, Y., Xu, M., Liu, C., Shang, H., and Wang, C. (2020b). Shenmai injection supresses glycolysis and enhances cisplatin cytotoxicity in cisplatin-resistant A549/DDP cells via the AKT-mTOR-c-myc signaling pathway. Biomed. Res. Int. 2020, 9243681. doi:10.1155/2020/9243681
Sung, H., Ferlay, J., Siegel, R. L., Laversanne, M., Soerjomataram, I., Jemal, A., et al. (2021). Global cancer statistics 2020: GLOBOCAN estimates of incidence and mortality worldwide for 36 cancers in 185 countries. Ca. Cancer J. Clin. 71 (3), 209–249. doi:10.3322/caac.21660
Suzuki, A., Puri, S., Leland, P., Puri, A., Moudgil, T., Fox, B. A., et al. (2019). Subcellular compartmentalization of PKM2 identifies anti-PKM2 therapy response in vitro and in vivo mouse model of human non-small-cell lung cancer. PLoS One 14 (5), e0217131. doi:10.1371/journal.pone.0217131
Takenaga, K., Koshikawa, N., Akimoto, M., Tatsumi, Y., Lin, J., Itami, M., et al. (2021). MCT4 is induced by metastasis-enhancing pathogenic mitochondrial NADH dehydrogenase gene mutations and can be a therapeutic target. Sci. Rep. 11 (1), 13302. doi:10.1038/s41598-021-92772-1
van Heijst, J. W., Niessen, H. W., Musters, R. J., van Hinsbergh, V. W., Hoekman, K., and Schalkwijk, C. G. (2006). Argpyrimidine-modified heat shock protein 27 in human non-small cell lung cancer: A possible mechanism for evasion of apoptosis. Cancer Lett. 241 (2), 309–319. doi:10.1016/j.canlet.2005.10.042
Vander Heiden, M. G., Cantley, L. C., and Thompson, C. B. (2009). Understanding the warburg effect: The metabolic requirements of cell proliferation. Science 324 (5930), 1029–1033. doi:10.1126/science.1160809
Vanhove, K., Mesotten, L., Heylen, M., Derwael, R., Louis, E., Adriaensens, P., et al. (2018). Prognostic value of total lesion glycolysis and metabolic active tumor volume in non-small cell lung cancer. Cancer Treat. Res. Commun. 15, 7–12. doi:10.1016/j.ctarc.2017.11.005
Vaupel, P., Schmidberger, H., and Mayer, A. (2019). The warburg effect: Essential part of metabolic reprogramming and central contributor to cancer progression. Int. J. Radiat. Biol. 95 (7), 912–919. doi:10.1080/09553002.2019.1589653
Wang, C., Chen, Z., Bu, X., Han, Y., Shan, S., Ren, T., et al. (2016). IL-33 signaling fuels outgrowth and metastasis of human lung cancer. Biochem. Biophys. Res. Commun. 479 (3), 461–468. doi:10.1016/j.bbrc.2016.09.081
Wang, D., Moothart, D. R., Lowy, D. R., and Qian, X. (2013). The expression of glyceraldehyde-3-phosphate dehydrogenase associated cell cycle (GACC) genes correlates with cancer stage and poor survival in patients with solid tumors. PLoS One 8 (4), e61262. doi:10.1371/journal.pone.0061262
Wang, D., Zhao, C., Xu, F., Zhang, A., Jin, M., Zhang, K., et al. (2021a). Cisplatin-resistant NSCLC cells induced by hypoxia transmit resistance to sensitive cells through exosomal PKM2. Theranostics 11 (6), 2860–2875. doi:10.7150/thno.51797
Wang, F., Li, L., and Zhang, Z. (2021b). Platelet isoform of phosphofructokinase promotes aerobic glycolysis and the progression of non-small cell lung cancer. Mol. Med. Rep. 23 (1), 74. doi:10.3892/mmr.2020.11712
Wang, M., Wang, W., Wang, J., and Zhang, J. (2018). MiR-182 promotes glucose metabolism by upregulating hypoxia-inducible factor 1α in NSCLC cells. Biochem. Biophys. Res. Commun. 504 (2), 400–405. doi:10.1016/j.bbrc.2018.06.035
Wang, Y., Zhao, N., Wu, Z., Pan, N., Shen, X., Liu, T., et al. (2020). New insight on the correlation of metabolic status on (18)F-FDG PET/CT with immune marker expression in patients with non-small cell lung cancer. Eur. J. Nucl. Med. Mol. Imaging 47 (5), 1127–1136. doi:10.1007/s00259-019-04500-7
Ward, P. S., and Thompson, C. B. (2012). Metabolic reprogramming: A cancer hallmark even warburg did not anticipate. Cancer Cell 21 (3), 297–308. doi:10.1016/j.ccr.2012.02.014
Wei, L., Dai, Q., Zhou, Y., Zou, M., Li, Z., Lu, N., et al. (2013). Oroxylin A sensitizes non-small cell lung cancer cells to anoikis via glucose-deprivation-like mechanisms: c-Src and hexokinase II. Biochim. Biophys. Acta 1830 (6), 3835–3845. doi:10.1016/j.bbagen.2013.03.009
Wise, D. R., and Thompson, C. B. (2010). Glutamine addiction: A new therapeutic target in cancer. Trends biochem. Sci. 35 (8), 427–433. doi:10.1016/j.tibs.2010.05.003
Wu, M., Li, H., Liu, R., Gao, X., Zhang, M., Liu, P., et al. (2016). Galactose conjugated platinum(II) complex targeting the Warburg effect for treatment of non-small cell lung cancer and colon cancer. Eur. J. Med. Chem. 110, 32–42. doi:10.1016/j.ejmech.2016.01.016
Wu, R., Galan-Acosta, L., and Norberg, E. (2015). Glucose metabolism provide distinct prosurvival benefits to non-small cell lung carcinomas. Biochem. Biophys. Res. Commun. 460 (3), 572–577. doi:10.1016/j.bbrc.2015.03.071
Xu, C., Gu, L., Kuerbanjiang, M., Wen, S., Xu, Q., and Xue, H. (2020). Thrombospondin 2/toll-like receptor 4 Axis contributes to HIF-1α-Derived glycolysis in colorectal cancer. Front. Oncol. 10, 557730. doi:10.3389/fonc.2020.557730
Zeng, C., Wu, Q., Wang, J., Yao, B., Ma, L., Yang, Z., et al. (2016). NOX4 supports glycolysis and promotes glutamine metabolism in non-small cell lung cancer cells. Free Radic. Biol. Med. 101, 236–248. doi:10.1016/j.freeradbiomed.2016.10.500
Zhang, P., Li, Z., and Yang, G. (2021a). Silencing of ISLR inhibits tumour progression and glycolysis by inactivating the IL-6/JAK/STAT3 pathway in non-small cell lung cancer. Int. J. Mol. Med. 48 (6), 222. doi:10.3892/ijmm.2021.5055
Zhang, Q., Pan, J., Lubet, R. A., Komas, S. M., Kalyanaraman, B., Wang, Y., et al. (2015). Enhanced antitumor activity of 3-bromopyruvate in combination with rapamycin in vivo and in vitro. Cancer Prev. Res. 8 (4), 318–326. doi:10.1158/1940-6207.Capr-14-0142
Zhang, W., Bouchard, G., Yu, A., Shafiq, M., Jamali, M., Shrager, J. B., et al. (2018). GFPT2-Expressing cancer-associated fibroblasts mediate metabolic reprogramming in human lung adenocarcinoma. Cancer Res. 78 (13), 3445–3457. doi:10.1158/0008-5472.Can-17-2928
Zhang, W., Gong, C., Chen, Z., Li, M., Li, Y., and Gao, J. (2021b). Tumor microenvironment-activated cancer cell membrane-liposome hybrid nanoparticle-mediated synergistic metabolic therapy and chemotherapy for non-small cell lung cancer. J. Nanobiotechnology 19 (1), 339. doi:10.1186/s12951-021-01085-y
Zhang, Y., Wang, Y., Li, Y., Huang, C., Xiao, X., Zhong, Z., et al. (2022). Dihydroartemisinin and artesunate inhibit aerobic glycolysis via suppressing c-Myc signaling in non-small cell lung cancer. Biochem. Pharmacol. 198, 114941. doi:10.1016/j.bcp.2022.114941
Zhao, J. G., Ren, K. M., and Tang, J. (2014). Overcoming 5-Fu resistance in human non-small cell lung cancer cells by the combination of 5-Fu and cisplatin through the inhibition of glucose metabolism. Tumour Biol. 35 (12), 12305–12315. doi:10.1007/s13277-014-2543-3
Zhao, X., Lu, C., Chu, W., Zhang, B., Zhen, Q., Wang, R., et al. (2017). MicroRNA-124 suppresses proliferation and glycolysis in non-small cell lung cancer cells by targeting AKT-GLUT1/HKII. Tumour Biol. 39 (5), 1010428317706215. doi:10.1177/1010428317706215
Zheng, J. (2012). Energy metabolism of cancer: Glycolysis versus oxidative phosphorylation (Review). Oncol. Lett. 4 (6), 1151–1157. doi:10.3892/ol.2012.928
Zhou, F., Du, J., and Wang, J. (2017). Albendazole inhibits HIF-1α-dependent glycolysis and VEGF expression in non-small cell lung cancer cells. Mol. Cell. Biochem. 428 (1-2), 171–178. doi:10.1007/s11010-016-2927-3
Zhou, L., Li, M., Yu, X., Gao, F., and Li, W. (2019). Repression of hexokinases II-mediated glycolysis contributes to piperlongumine-induced tumor suppression in non-small cell lung cancer cells. Int. J. Biol. Sci. 15 (4), 826–837. doi:10.7150/ijbs.31749
Zhou, Q., Li, J., Pang, J., Fan, F., Li, S., and Liu, H. (2020). Gefitinib inhibits glycolysis and induces programmed cell death in non-small cell lung cancer cells. Nan Fang. Yi Ke Da Xue Xue Bao 40 (6), 884–892. doi:10.12122/j.issn.1673-4254.2020.06.17
Glossary
ABCB1 ATP-binding cassette subfamily B member 1
ABCE1 ATP-binding cassette subfamily E member 1
ABZ albendazole
AChE acetylcholinesterase
ADP adenosine diphosphate
ALDOA aldolase A
ALK anaplastic lymphoma kinase
AMPK adenosine monophosphate-activated protein kinase
AMPKα adenosine monophosphate-activated protein kinase a
ATP adenosine triphosphate
Barx2 BarH-like homeobox 2
BSG basigin
CDK7 cyclin-dependent kinase 7
circRNA circular RNA
CPS1 carbamoyl phosphate synthetase I
CTC circulating tumor cell
DCA dichloroacetate
DHA dihydroartemisinin
DPPA4 developmental pluripotency-associated 4
DTX docetaxel
EGFR epidermal growth factor receptor
EHD1 eps15 homology domain 1
ENO1 alpha-enolase
ETC electron transfer chain
FBP1 fructose-1,6-bisphosphatase
FDG-PET F-fluorodeoxyglucose positron emission tomography
5MPN 5-(n-(8-methoxy-4-quinolyl) amino) pentyl nitrate
Gal-Pt galactose-conjugate (trans-R,R-cyclohexane-1,2-diamine)-2- chloromalonato-platinum (II) complex
GAPDH glyceraldehyde-3-phosphate dehydrogenase
GFPT2 glutamine-fructose-6-phosphate transaminase 2
GLUT1 glucose transporter 1
GPI glucose phosphate isomerase
HIF-1α hypoxia-inducible transcription factor-1 alpha
HK hexokinase
Hsp27 heat shock protein 27
IL-33 interleukin 33
ISLR leucine-rich repeat
JB jolkinolide B
JNK c-Jun N-terminal kinase
JOSD2 Josephin domain containing 2
KLF5 Krũppel-like factor 5
KRAS Kirsten RAS
LDH lactate dehydrogenase
lncRNA long non-coding RNA
MAPK mitogen-activated protein kinase
MCT1-4 monocarboxylate transporter 1-4
miRNA microRNA
MTAs microtubule-targeting agents
MTH1 MutT homolog 1
mTOR mammalian target of rapamycin
MTV metabolic tumor volume
NADH nicotinamide adenine dinucleotide
NALP3 NLR family pyrin domain containing 3
NOX4 NADPH oxidase 4
NQO1 NADPH quinone oxidoreductase 1
NSCLC non-small cell lung cancer
OCT1 octamer-transcription factor 1
OTUB2 OTU deubiquitinase, ubiquitin aldehyde binding 2
PD-1 programmed cell death protein 1
PDK1 pyruvate dehydrogenase kinase 1
PFKFB3 6-phosphofructo-2-kinase/fructose-2,6-bisphosphatase 3
PFKL phosphofructokinase, liver type
PFKM phosphofructokinase, muscle type
PFKP phosphofructokinase
RFP180 ring finger protein 180
PGAM1 phosphoglycerate mutase 1
PINK1 PTEN-induced putative kinase 1
PI3K phosphoinositide 3-kinase
PKLR liver and red blood cell isoform
PKM1 muscle isoform pyruvate kinase M1
PKM2 pyruvate kinase M2
PRKACA protein kinase cAMP-activated catalytic subunit alpha
ROS reactive oxygen species
SCLC small cell lung cancer
SIX1 sine oculis homeobox homolog 1
SOCS2 suppressor of cytokine signaling 2
STK11 serine/threonine kinase 11
Tan IIA tanshinone IIA
TCA tricarboxylic acid
TKI tyrosine kinase inhibitor
TLG total lesion glycolysis
TME tumor microenvironment
TRAF6 TNF receptor-associated factor 6
TRIM59 tripartite motif-containing 59
2DG 2-deoxy-d-glucose
2-ME 2-methoxyestradiol
3-BrPA 3-bromopyruvate
UGT8 UDP-glycosyltransferase 8
VEGF vascular endothelial cell growth factor
Keywords: metastasis, tumor microenvironment, aerobic glycolysis, glucose transporter, pyruvate kinase M2, natural product, chemotherapy resistance, biopharmaceutical therapy
Citation: Xu J-Q, Fu Y-L, Zhang J, Zhang K-Y, Ma J, Tang J-Y, Zhang Z-W and Zhou Z-Y (2022) Targeting glycolysis in non-small cell lung cancer: Promises and challenges. Front. Pharmacol. 13:1037341. doi: 10.3389/fphar.2022.1037341
Received: 05 September 2022; Accepted: 04 November 2022;
Published: 30 November 2022.
Edited by:
Weiwei Liu, Shenyang Pharmaceutical University, ChinaCopyright © 2022 Xu, Fu, Zhang, Zhang, Ma, Tang, Zhang and Zhou. This is an open-access article distributed under the terms of the Creative Commons Attribution License (CC BY). The use, distribution or reproduction in other forums is permitted, provided the original author(s) and the copyright owner(s) are credited and that the original publication in this journal is cited, in accordance with accepted academic practice. No use, distribution or reproduction is permitted which does not comply with these terms.
*Correspondence: Zhong-Yan Zhou, Ymlvenp5QDEyNi5jb20=; Zhi-Wei Zhang, Mzc0NTYzNDNAcXEuY29t; Jing-Yi Tang, ZHJfdGFuZ0AxNjMuY29t
†These authors have contributed equally to this work