- 1Department of Orthopedics, Shanghai Sixth People’s Hospital, Shanghai Jiaotong University, Shanghai, China
- 2Department of Orthopedics, The Second Affiliated Hospital of Chongqing Medical University, Chongqing, China
Osteosarcoma (OS), the most common malignant tumor in the musculoskeletal system, mainly occurs in adolescents. OS results in high mortality and disability rates due to a fatal metastatic tendency and subsequent iatrogenic damage caused by surgery, radiotherapy and chemotherapy. Recently, immunotherapies have resulted in promising prognoses with reduced side effects compared with traditional therapies. Immune checkpoint inhibitors (ICIs), which are a representative immunotherapy for OS, enhance the antitumor effects of immune cells. ICIs have shown satisfactory outcomes in other kinds of malignant tumors, especially hemopoietic tumors. However, there is still a high percentage of failures or severe side effects associated with the use of ICIs to treat OS, leading to far worse outcomes. To reveal the underlying mechanisms of drug resistance and side effects, recent studies elucidated several possible reasons, including the activation of other inhibitory immune cells, low immune cell infiltration in the tumor microenvironment, different immune properties of OS subtypes, and the involvement of osteogenesis and osteolysis. According to these mechanisms, researchers have developed new methods to overcome the shortcomings of ICIs. This review summarizes the recent breakthroughs in the use of ICIs to treat OS. Although numerous issues have not been solved yet, ICIs are still the most promising treatment options to cure OS in the long run.
Introduction
Osteosarcoma (OS), one of the most common malignant bone tumors, tends to affect children and adolescents with a median age of 16 years (Siegel et al., 2018). OS mainly occurs in the long bones of the extremities, such as the tibia, femur and humerus. For nonmetastatic OS patients, a combination of traditional therapies, including wide resection, radiotherapy, and chemotherapy, leads to a 60%–70% 5-year survival rate (Anderson, 2016). Unfortunately, for metastatic patients, a high recurrence rate and low survival rate of nearly 20% make OS treatment challenging (Saraf et al., 2018; Wang et al., 2019).
Treating OS patients surgically with tumor-free resection is a traditional but effective method. According to the size and invasiveness of the tumor, typical operative plans include amputation, rotationplasty and limb-salvage surgery. Traditionally, radical resection of the primary tumor has a higher opportunity to thoroughly remove malignant tumor cells. Therefore, radical resection surgeries such as amputation and rotationplasty are supposed to result in higher survival rates, even though these treatments seriously worsen quality of life (Bläsius et al., 2022). However, recent studies have reached the opposite conclusion. Han et al. (2016) conducted a meta-analysis comparing the effect of limb-salvage surgery and amputation, and the results showed that limb-salvage surgery led to a comparable survival rate with much better quality of life. In addition to surgery, chemotherapy is another method to treat malignant tumors. Commonly used chemotherapeutic drugs for OS are methotrexate, doxorubicin, cisplatin, ifosfamide, and adriamycin (Piperno-Neumann et al., 2016). For localized nonmetastatic OS, tumor resection surgery combined with chemotherapy had a 5-year survival rate of approximately 60%–70%, while the survival rate for metastatic cases was approximately 20% (Senerchia et al., 2017; Ferrari et al., 2018). Tumor resection and adjuvant chemotherapy are the standard treatment for OS at present, but for metastatic patients with chemotherapeutic drug resistance, radiotherapy is another palliative option to extend patient lifespan. Unfortunately, for these palliative therapy patients, radiotherapy had an average survival of only approximately 6 months (Rahn et al., 2015). Radiotherapy can also be used as adjuvant therapy after resection surgery. Reports have shown that radiotherapy can decrease the possibility of local recurrence but does not increase the overall survival rate (Tinkle et al., 2019; Heng et al., 2020).
Immunotherapy has attracted attention from clinicians and researchers for its increased efficacy and reduced side effects (Dongye et al., 2022). Frequently used immunotherapies for OS include immune checkpoint inhibitors (ICIs), cytokines, adoptive T-cell therapy and cancer vaccines. These procedures can activate the restricted immune system in OS patients by targeting different kinds of immune cells (Lu et al., 2022). Among them, ICIs such as cytotoxic T-lymphocyte antigen 4 (CTLA-4) inhibitors, programmed cell death protein-1 (PD-1) and programmed death-ligand 1 (PD-L1) have shown great therapeutic effects on various kinds of malignant tumors with fewer side effects than traditional therapy, especially in treating melanoma and hematologic malignancies (Luke et al., 2022; Mei et al., 2022). However, for solid tumors such as OS, the prognosis of ICI intervention is not satisfactory (Meftahpour et al., 2022). This review introduces the outcomes of ICI interventions for OS and the related mechanisms, summarizes the current breakthroughs, and predicts the developmental direction of immunotherapies for OS in the future.
Mechanisms of immune checkpoint inhibitors
CTLA-4, an immune checkpoint receptor protein that is highly expressed on the surface of T cells, plays a predominant role in inhibiting the functions of T cells (Lindsten et al., 1993). In detail, CTLA-4 is a type 1 transmembrane glycoprotein in the Ig superfamily that is composed of four domains: a signal peptide, an extracellular ligand-binding domain, a transmembrane domain, and a short cytoplasmic tail (Brunet et al., 1987; Ostrov et al., 2000). CTLA-4 and CD28 are both expressed on the surface of T cells and share the ligand B7, but they have opposite biological functions. Interactions between CD28 and the ligand B7 activate T cells and promote proliferation (Wang et al., 2016), while interactions between CTLA-4 and B7, including B7-1 (CD80) and B7-2 (CD86), have the opposite effects (Darlington et al., 2005). CTLA-4 has a higher affinity for B7 than CD28. Studies have suggested that CTLA-4 has an inhibitory effect on T cells through competitive binding to the ligand (Parry et al., 2005; Schneider et al., 2006). In addition, CTLA-4 can remove CD80 and CD86 on antigen-presenting cells (APCs) by preventing the binding of CD80 and CD86 with CD28 and trans-endocytosis, making T cells unable to accept immune signals (Qureshi et al., 2011). In Th-cell-specific CTLA-4 conditional-knockdown mice, CD80 and CD86 are highly expressed on APCs, indicating that CTLA-4 could inhibit the activation of T cells by restricting APCs. CTLA-4 could also reduce the activity of the transcription factors activator protein-1 (AP-1), nuclear factor of activated T cells (NFAT) and nuclear factor-κB (NF-κB), further decreasing the production of interleukin-2 (IL-2) (Fraser et al., 1999). IL-2 plays an important role in the interaction between CD28 and capZIP, a regulator of the actin cytoskeleton, interfering with the activation of T cells (Tian et al., 2015). In addition, CTLA-4 upregulates the activity of regulatory T cells (Tregs) and decreases helper T (Th) cells (Peggs et al., 2009). CTLA-4 is the direct target of forkhead box p3 (Foxp3), the linage-specifying transcription factor of Tregs (Marson et al., 2007). Tregs can reverse transmit signals to dendritic cells (DCs), inducing the expression of the tryptophan-catabolizing enzyme indoleamine 2,3-dioxygenase (IDO), consuming tryptophan and preventing the activation and proliferation of T cells (Fallarino et al., 2003). Recruitment of the serine/threonine phosphatase protein phosphatase 2A (PP2A) is mediated by CTLA-4 and inhibits the Akt signaling pathway, decreasing CD28-mediated glucose uptake by T cells and activating the PI3K pathway, promoting the proliferation of anergic T cells (Frauwirth et al., 2002; Intlekofer and Thompson, 2013). The detailed mechanisms of CTLA-4 are shown in Figure 1.
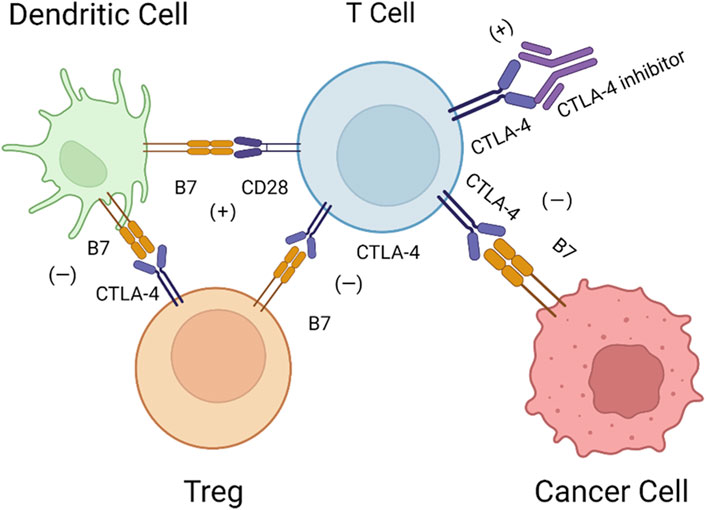
FIGURE 1. Mechanisms of CTLA-4. DCs activate T cells via B7-CD28, while CTLA-4 can bind with B7 on Tregs and cancer cells with higher affinity, and Tregs can inversely inhibit DCs via B7-CTLA-4. Therefore, CTLA-4 inhibitors may block this pathway to restore the activation of T cells.
PD-1 is an immune checkpoint coinhibitory receptor protein that is highly expressed on the surface of T cells (Ishida et al., 1992). In a PD-1 defective animal model, researchers observed delayed-onset, organ-specific autoimmune diseases, including lupus-like syndrome and autoimmune-dilated cardiomyopathy, indicating the lymphocytes inhibit the functions of PD-1 (Nishimura et al., 1999; Okazaki et al., 2003). Similar to CTLA-4, PD-1 is also a type 1 transmembrane protein in the Ig superfamily. PD-1 has three parts: an extracellular N-terminal IgV-like domain, a transmembrane domain, and a cytoplasmic tail (Zhang et al., 2004). The immunoreceptor tyrosine-based inhibitory motif (ITIM) and immunoreceptor tyrosine-based switch motif (ITSM) at the cytoplasmic tail can inhibit the activation of T cells through the phosphorylation of src family kinases, recruiting SHP-1 and SHP-2 protein tyrosine phosphates (Zak et al., 2015). In contrast to CTLA-4, PD-1 is highly expressed on B cells and natural killer (NK) cells (Fanoni et al., 2011; Terme et al., 2011). PD-1 inhibits the activity of peripheral T cells and other autoimmune reactions when the body responds to inflammation, especially chronic inflammation (Keir et al., 2006; Topalian et al., 2016). In malignant tumors, PD-1 inhibits the activity of effector T cells, which is one of the main mechanisms by which tumor cells resist the immune system (Blank et al., 2004).
Furthermore, PD-1 has two ligands: PD-L1 (also known as B7-H1 and CD274) and PD-L2 (also known as B7-DC and CD273) (Freeman et al., 2000; Latchman et al., 2001). PD-L1 is composed of IgV- and IgC-like extracellular domains, a transmembrane domain, and a short cytoplasmic tail. PD-L1 interacts with the extracellular domain of PD-1, changing its conformation (Lin et al., 2008). PD-L1 can be detected on the surface of hematopoietic cells, including DCs, macrophages, T cells, and B cells, and nonhematopoietic cells, including endothelial cells and keratinocytes. PD-L2 can be detected on macrophages and DCs (Liang et al., 2003). PD-L1 and PD-L2 partially share sequence homology, and both can bind to the coinhibitory receptor on T cells (Shin et al., 2005). The detailed mechanisms of PD-1-PD-L1/PD-L2 are shown in Figure 2.
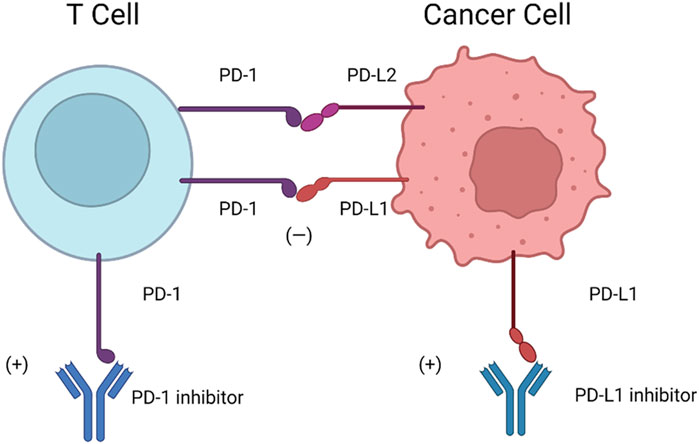
FIGURE 2. Mechanisms of PD-1-PD-L1/PD-L2. Cancer cells are highly capable of PD-L1 expression, which can inhibit the immune function of T cells in response to PD-L1 and PD-1 binding. Therefore, anti-PD-1 and anti-PD-L1 are considered promising strategies to stimulate “exhausted” T cells and eliminate tumors. PD-L2 is similar to PD-L1 in structure, has some sequence homology inhibits the function of T cell inhibition via PD-L2-PD-1.
Immune checkpoint and osteosarcoma
Regarding primary and metastatic OS tumor sites, a previous study reported that PD-1 expression was increased in CD4+ and CD8+ T cells in peripheral blood (Zheng et al., 2015). IL-21 is required for the immune response of CD8+ T cells and is mainly secreted by circulating CD4+ T cells. Compared with those in healthy patients, circulating CD4+ T cells in OS patients have less capacity to secrete IL-21 due to the PD-1 and PD-L1 interactions of follicular helper T cells (Gao et al., 2017). In addition to T cells, the PD-1 and PD-L1 interaction also affects the cytotoxicity of NK cells. Blocking the PD-1/PD-L1 axis enhanced the ability of NK cells to lyse OS tumor cells by secreting granzyme B (Zhang et al., 2019). PD-L2 is also the ligand of the PD-1 receptor. PD-L2 protein was detected in primary OS and was increased in lung metastatic patients. According to the mechanism, the expression of PD-L2 is thought to enhance tumor growth and metastasis. In vitro experiments indicated that PD-L2 knockdown attenuated tumor growth and metastasis by inhibiting the RhoA-ROCK-LIMK2 pathway and autophagy (Ren et al., 2019).
In addition, many studies have reported a close relationship between the expression of PD-1/PD-L1 and the overall survival of OS patients. Koirala et al. (2016) found that PD-L1 expression at the OS tumor site was negatively related to 5-year event-free survival. However, after the immune cell composition was analyzed, the results indicated that PD-L1 expression was associated with the presence of T cells, DCs and NK cells. Hashimoto et al. (2020) reported that in OS, the tumor size was larger in PD-L1-negative cases than in PD-L1-positive cases, but the expression of PD-1 or PD-L1 did not alter the prognosis of patients who received chemotherapy and wide resection. Moreover, Yoshida et al. (2019) found that the expression of PD-L1 was related to early metastasis of OS, and PD-1 and PD-L1 expression were both negatively related to the prognosis of OS after the relationship between the expression levels and survival data of 62 patients were analyzed (Zheng et al., 2018). Consistently, a meta-analysis of eight studies and 413 OS patients was performed, and the pooled results showed that overexpression of PD-1 and PD-L1 led to an increased rate of metastasis and total mortality risk (Huang et al., 2018).
The binding of immune checkpoint receptors and their ligands inhibits T-cell function and other immune system components. Therefore, drugs targeting receptors and ligands are designed to prevent their binding, thus inhibiting the immune system. Researchers have conducted several animal experiments to evaluate the therapeutic effects of ICIs on OS. Yoshida et al. (2020) found that an anti-PD-1 antibody decreased OS tumor volume by decreasing Tregs in the tumor microenvironment (TME) and increasing tumor-infiltrating lymphocytes. Blockade of Blocking the PD-1/PD-L1 interaction decreased the tumor burden and extended survival in an OS mouse model by improving OS-reactive cytotoxic T lymphocytes (Lussier et al., 2015b). In addition, Dhupkar et al. (2018) found that an anti-PD-1 antibody decreased OS tumor cells in lung metastases by inducing apoptosis and inhibiting proliferation. Anti-PD-1 therapy increased the infiltration of NK cells and macrophages and the number of antitumor M1 macrophages and decreased M2 subsets. Interestingly, the PD-1 inhibitor nivolumab could inhibit lung metastasis of OS by increasing CD4+ and CD8+ lymphocytes and upregulating the cytotoxicity of CD8+ lymphocytes in the lung, but it was not effective for primary OS growth (Zheng et al., 2018).
Clinical effects of immune checkpoint inhibitors on osteosarcoma
To date, several clinical trials have been carried out to examine the therapeutic effects of ICIs on OS (Table 1). The effects of PD-1 inhibitors, including pembrolizumab, nivolumab, and camrelizumab, the PD-L1 inhibitor atezolizumab, and the CTLA-4 inhibitor ipilimumab, on OS have been examined. Ipilimumab was used to treat 17 pediatric OS patients, and the results showed that it did not improve patient prognosis (Merchant et al., 2016). Pembrolizumab was used to treat 22 OS patients, and only one metastatic patient responded to this intervention (Tawbi et al., 2017). Clinicians treated 13 OS patients with nivolumab, and the results showed that the drug was well tolerated by children, but the therapeutic effect of this single agent was not observed (Davis et al., 2020). The PD-L1 inhibitor atezolizumab was used to treat 12 refractory or relapsed OS patients, and the results indicated that this drug was also tolerated by children but was ineffective (Geoerger et al., 2020). In addition, Le Cesne et al. (2019) reported that 17 advanced OS patients were treated with pembrolizumab and only one patient with a PD-L1-negative tumor had a partial response to the treatment.
Possible mechanisms of unsatisfactory outcomes
The prognosis of clinical trials for the treatment of OS is unfavorable. Researchers have determined several possible mechanisms to explain these frustrating outcomes. Immune checkpoint receptors are mainly expressed on T cells, and inhibitors can activate these cells. However, there are other kinds of inhibitory cells in the TME that facilitate the immune escape of OS cells. The TME of OS is a combination of various immune cells (DCs, macrophages, T cells, B cells, etc.), stromal cells (mesenchymal stem/stromal cells, fibroblasts), and surrounding mineralized extracellular matrix (ECM). The components and status of the TME determine the proliferation and metastasis of OS.
Macrophages are a type of mature monocyte circulating in the peripheral blood and are a subset of white blood cells derived from hematopoietic stem cells in the bone marrow (Shi and Pamer, 2011). Macrophages are able to assimilate debris, apoptotic cells and pathogens to maintain internal homeostasis (Murray and Wynn, 2011). According to their properties and functions, macrophages are classified into two categories: classically activated (M1) and alternatively activated (M2) macrophages (Shapouri-Moghaddam et al., 2018). In the early stage of OS formation, M1 macrophages play a dominant role in the TME, activating the immune system and inflammatory reactions to eliminate tumor cells. Related cytokines, including IL-1β, tumor necrosis factor-α (TNF-α), and IL-12, are locally released to increase the antitumor reaction (Hu et al., 2022). To avoid severe tissue and organ impairment caused by M1 macrophages, M2 macrophages, which are induced by IL-4/IL-10/IL-13, are designed to exert anti-inflammatory, profibrotic and proangiogenic effects (Wang et al., 2022). The switch from M1 macrophages to M2 macrophages is called polarization (Zhao et al., 2022). M2 macrophages in the TME are known as tumor-associated macrophages (TAMs) (Hasan et al., 2022). In the late stage of OS proliferation and metastasis, TAMs play a dominant role in the TME to support cancer cell growth and suppress immune reactions (Christofides et al., 2022). Based on these mechanisms, drugs targeting TAMs have been evaluated for their effects on OS patients. Drugs inhibiting TAM polarization and depleting or reprogramming TAMs are able to terminate immunosuppression in OS, such as mifamurtide, zoledronic acid, all-trans retinoic acid, and dihydroxycoumarin (Kimura and Sumiyoshi, 2015; Zhou et al., 2017; Lv et al., 2020; Barnes et al., 2022).
DCs are APCs that link innate and adaptive immunity (Mildner and Jung, 2014). In tumor immunity, DCs can capture malignant cell antigens and present them to T cells to start the expansion of tumor-specific T cells (Galluzzi et al., 2017). DCs are responsible for immunosurveillance; impairments in this mechanism are commonly observed in OS patients and lead to less effective therapeutic results and worse outcomes (Kroemer et al., 2013). DCs are distributed in nearly all tissues, and their functions depend on their population and maturation stages. Some subsets (such as CD103+ DCs) act as typical immune cells and present tumor antigens to T cells and other immune cells, such as NK and B cells. Some subsets (such as CD208+ DCs) predict an unfavorable prognosis (Bruni et al., 2020). Tumor cells can secrete several cytokines to inhibit the maturation of DCs, so researchers have attempted to treat malignant tumors using cancer vaccines targeting DCs (Michielsen et al., 2011). DCs can be isolated from peripheral blood mononuclear cells (PBMCs) and stimulated with tumor antigen ex vivo. Then, the cultured cells are injected back into patients. A DC cancer vaccine stimulated with MAGE-A1, MAGE-A3, and NY-ESO-1 was used to treat OS patients, and the results showed that the therapy was tolerated, but the effect was uncertain due to the limited number of recruited patients (Krishnadas et al., 2015).
Myeloid-derived suppressor cells (MDSCs) are another type of immunosuppressive immune cell in the TME (Lim et al., 2014). MDSCs are dramatically increased in the peripheral blood of OS patients (Shi et al., 2019). For these lung metastatic OS patients, the accumulation and activation of polymorphonuclear MDSCs can be detected at the metastasis (Ligon et al., 2021). MDSCs inhibit immune reactions through several methods. For example, MDSCs decrease the lymph node homing of CD4+ and CD8+ T cells to suppress the functions of T cells and NK cells (Najjar and Finke, 2013). MDSCs can also recruit and induce immunosuppressive Tregs (Dysthe and Parihar, 2020). In OS animal models, the level of IL-18 is positively correlated with the number of MDSCs in peripheral blood (Guan et al., 2017). The accumulation of MDSCs in the OS TME is also related to the activation of the PI3Kδ/γ and SDF-1/CXCR4 pathways (Jiang et al., 2019; Shi et al., 2019). All-trans retinoic acid can decrease the number of MDSCs in the OS TME (Long et al., 2016).
In addition to the immunosuppressive effects of the aforementioned immune cells, OS is a “cold tumor” compared with other kinds of tumors. PD-L1 is highly expressed on the surface of OS cells, suppressing the antitumor effects of immune cells. OS cells express nonimmunogenic properties due to the lack of specific antigens (Tsukahara et al., 2008; Bunnell et al., 2010). Genetic alterations were discovered in OS, which exhibits high copy number loss, especially in low immune infiltration conditions, revealing strong immunosuppression (Wu et al., 2020). Recent studies have reported that the expression of HER2 at low levels indicates the possibility of CAR-T therapy, which may turn a “cold tumor” into a “hot tumor” (Rainusso et al., 2012).
Compared with malignant tumors in the viscera or superficial soft tissue, OS is also closely related to the status of bone. The development of OS is correlated with osteolysis, and most OS patients were also diagnosed with fragility fractures. Osteoblasts, osteoclasts and osteocytes are involved in osteolysis. Osteoclasts are overactivated in the OS site, and the underlying mechanism is the binding of RANK and RANKL. However, the combination of chemotherapy and the RANKL inhibitor denosumab or biphosphates did not lead to a better prognosis (Cathomas et al., 2015; Piperno-Neumann et al., 2016). Therefore, treating OS by inhibiting osteolysis has not achieved a positive prognosis and needs further research. In addition, OS is not homogenous for all patients, and it can be classified into three subtypes: osteoblastic, chondroblastic, and fibroblastic (Gill and Gorlick, 2021). The expression of PD-L1 varies in different subtypes and in primary or metastatic tumors, indicating that OS is heterogenous (Lussier et al., 2015b). Therefore, the treatment of OS should be personalized according to the properties of the tumor.
New therapeutic methods
To overcome the drug resistance of current ICI therapy, clinicians and researchers have been trying many new methods to enhance treatment efficacy.
Precision therapy
ICI treatment is effective for some OS patients but ineffective for others. Finding the similarities between these ICI-responsive cases and selecting suitable patients before treatment could lead to a better prognosis. Starzer et al. (2021) recruited eight OS patients and analyzed the DNA methylation profile related to the immunology of tumor cells and the response to anti-PD-1 therapy. The most predominant differences in the DNA methylation profiles of responders and nonresponders were related to Rap1 signaling, adherens junctions, and focal adhesion. Patients with these methylation properties were more responsive to anti-PD-1 therapy.
Synergistic application of immune checkpoint inhibitors
Single-agent use of ICIs seems unsatisfactory for treating OS, so synergistic use of multiple ICIs may lead to improved outcomes. Clinical trials have assessed the therapeutic effects of multiple ICIs. D'Angelo et al. (2018) reported combining the PD-1 inhibitor nivolumab and the CTLA-4 inhibitor ipilimumab to treat OS. Compared with the nivolumab-only group, patients who received the combination therapy had higher rates of response. In addition, animal studies evaluated the effect of synergistic treatment. Lussier et al. (2015a) alcombined anti-PD-1 therapy and anti-CTLA-4 therapy to treat mice with metastatic OS, and the results showed that the combination immunotherapy prevented the immune escape of tumor cells and led to complete control of metastatic OS.
Combination with chemotherapy or radiotherapy
However, traditional therapies such as chemotherapy and radiotherapy exhibited unsatisfactory effects on OS. Recent studies showed that the combination of chemotherapy or radiotherapy with immunotherapy showed a better prognosis than a single application.
A small quantity of tumor-infiltrating immune cells decreased the efficacy of ICIs on OS. Deng et al. (2020) reported that neoadjuvant chemotherapy increased the numbers of CD3+, CD8+, and Ki67+ CD8+ T cells and PD-L1+ immune cells and decreased the numbers of MDSCs in the TME, converting OS from “cold” to “hot”.
In addition, although radiotherapy alone is insufficient for treating OS, several studies have reported that combining radiotherapy with ICIs could overcome the shortcomings of monotherapy. In 2018, Xia et al. (2018) first reported that in a mouse model, radiotherapy could enhance the efficacy of PD-1 inhibition on brain metastatic OS, increasing the number of CD8+ T cells in the TME. Katsuki et al. (2022) alfound a similar outcome of combination therapy in a mouse OS model. Callaghan et al. (2020) combined pembrolizumab with stereotactic body radiation to treat chondroblastic OS. Although the sample size was limited, the outcome proved that the combination therapy was well tolerated and that the prognosis was favorable.
Furthermore, carbon ion radiotherapy has been suggested to be more efficient for many kinds of malignant tumors than traditional radiotherapy (Kamada et al., 2015). Several recent studies showed that carbon ion radiotherapy could alleviate the drug resistance of PD-1 blockade therapy on OS. Permata et al. (2021) found that carbon ion radiotherapy upregulated the expression of PD-L1 on OS cells in a manner that was dependent on ATR kinase activity. Zhou et al. (2022) found that carbon ion radiotherapy triggered more immunogenic tumor cell death and increased the infiltration of CD4+ and CD8+ T cells, improving the efficacy of PD-1 blockade therapy for OS. Helm et al. (2021) treated OS mice with carbon ion radiotherapy combined with PD-1 and CTLA-4 inhibitors. ICIs or radiotherapy alone could not alleviate the progression of tumors, and the combination of the two treatments inhibited lung metastasis by increasing CD8+ T cells.
Oncolytic viruses
Oncolytic viruses exert antitumor effects by directly lysing tumor cells. Mochizuki et al. (2021) designed the telomerase-specific oncolytic adenovirus OBP-502, which induces lytic tumor cell death by binding to integrins. Intratumoral injection of OBP-502 abrogated the restriction of PD-1 blockade on OS by enhancing tumor-infiltrating CD8+ T cells. In addition, Christie et al. (2021) genetically engineered myxoma virus to express TNF, and peripheral blood monocytes that were preloaded with the bioengineered virus increased the immune reaction and had an effective synergistic effect with anti-PD-1, anti-PD-L1, and anti-CTLA4 therapy.
Others
In addition to the drugs and methods mentioned previously, many other drugs and interventions also have synergistic effects on OS when combined with ICIs. The growth and metastasis of tumors are accompanied by the formation of blood vessels. Vascular endothelial growth factor (VEGF) is an angiogenic factor that is essential for the formation of new blood vessels (Apte et al., 2019). Apatinib is a competitive inhibitor of VEGFR2 that is capable of inhibiting angiogenesis and carcinogenesis (Zhao et al., 2021). Xie et al. (2020) combined apatinib with the PD-1 inhibitor camrelizumab to treat advanced OS patients and followed up for 48 weeks. Combination therapy resulted in a better prognosis than the use of apatinib alone, especially for patients with PD-L1 overexpression or with lung metastasis.
In addition, MDSCs heavily infiltrate the TME of OS. Jiang et al. (2019) found that activation of the SDF-1/CXCR4 axis reduced MDSC apoptosis, upregulating the functions of Tregs. CXCR4 antagonists have synergistic effects with PD-1/PD-L1 inhibitors for treating OS. Sunitinib, a multitargeted receptor tyrosine kinase inhibitor, can activate the immune reaction by changing immune cell subsets. In OS, sunitinib reduced the population of Tregs and led to the DC-based cross-priming of IFN-γ-producing effector T cells. Sunitinib had a synergistic effect with nivolumab-mediated PD-1 blockade to treat OS (Ocadlikova et al., 2021). Secreted frizzled-related protein 2 (SFRP2) promotes the migration of tumor cells and tube formation in endothelial cells, which are associated with OS metastasis. An in vitro study showed that a monoclonal antibody against SFRP2 combined with a PD-1 antibody synergistically inhibited the metastasis of OS (Nasarre et al., 2021). Recruitment of MDSCs to the TME inhibits the effect of anti-PD1 therapy. Shi et al. (2019) reported that SNA, a specific inhibitor of PI3Kδ/γ, could inhibit the function of MDSCs and had a synergistic therapeutic effect with anti-PD1 therapy on OS tumor-bearing mice.
Conclusion
OS is the most common primary musculoskeletal malignant tumor. Traditional therapies, such as surgery, chemotherapy, and radiotherapy, result in barely satisfactory prognoses for OS, and their side effects are serious. Immunotherapy is used to activate the inhibited immune system of OS patients, and ICIs have attracted the attention of researchers, clinicians, and patients. PD-1, PD-L1, and CTLA-4 inhibitors are representative ICIs. Their therapeutic effects on OS are not as good as those on hematopoietic tumors. We elucidated the possible underlying mechanisms and summarized the current research on alleviating ICI drug resistance in OS. By further determining the underlying mechanisms and developing drug design and drug administration technology, the therapeutic effects of ICIs on OS will be dramatically improved in the future.
Author contributions
ZZ investigated the literature. ZZ and XT wrote the manuscript, and HW and ZJ revised the figures. HY guided and revised the overall structure and content of the review.
Funding
This work was supported by the National Natural Science Foundation of China (Nos 81702133 and 81974320) and the Excellent Youth Training Program of Shanghai Jiaotong University Affiliated Sixth People’s Hospital (No. ynyq202102).
Conflict of interest
The authors declare that the research was conducted in the absence of any commercial or financial relationships that could be construed as a potential conflict of interest.
Publisher’s note
All claims expressed in this article are solely those of the authors and do not necessarily represent those of their affiliated organizations, or those of the publisher, the editors and the reviewers. Any product that may be evaluated in this article, or claim that may be made by its manufacturer, is not guaranteed or endorsed by the publisher.
References
Anderson, M. E. (2016). Update on survival in osteosarcoma. Orthop. Clin. North Am. 47 (1), 283–292. doi:10.1016/j.ocl.2015.08.022
Apte, R. S., Chen, D. S., and Ferrara, N. (2019). VEGF in signaling and disease: Beyond discovery and development. Cell 176 (6), 1248–1264. doi:10.1016/j.cell.2019.01.021
Barnes, D. J., Dutton, P., Bruland, Ø., Gelderblom, H., Faleti, A., Bühnemann, C., et al. (2022). Outcomes from a mechanistic biomarker multi-arm and randomised study of liposomal MTP-PE (Mifamurtide) in metastatic and/or recurrent osteosarcoma (EuroSarc-Memos trial). BMC Cancer 22 (1), 629. doi:10.1186/s12885-022-09697-9
Blank, C., Brown, I., Peterson, A. C., Spiotto, M., Iwai, Y., Honjo, T., et al. (2004). PD-L1/B7H-1 inhibits the effector phase of tumor rejection by T cell receptor (TCR) transgenic CD8+ T cells. Cancer Res. 64 (3), 1140–1145. doi:10.1158/0008-5472.can-03-3259
Bläsius, F., Delbrück, H., Hildebrand, F., and Hofmann, U. K. (2022). Surgical treatment of bone sarcoma. Cancers (Basel) 14 (11), 2694. doi:10.3390/cancers14112694
Boye, K., Longhi, A., Guren, T., Lorenz, S., Næss, S., Pierini, M., et al. (2021). Pembrolizumab in advanced osteosarcoma: Results of a single-arm, open-label, phase 2 trial. Cancer Immunol. Immunother. 70 (9), 2617–2624. doi:10.1007/s00262-021-02876-w
Brunet, J. F., Denizot, F., Luciani, M. F., Roux-Dosseto, M., Suzan, M., Mattei, M. G., et al. (1987). A new member of the immunoglobulin superfamily-CTLA-4. Nature 328 (6127), 267–270. doi:10.1038/328267a0
Bruni, D., Angell, H. K., and Galon, J. (2020). The immune contexture and Immunoscore in cancer prognosis and therapeutic efficacy. Nat. Rev. Cancer 20 (11), 662–680. doi:10.1038/s41568-020-0285-7
Bunnell, B. A., Betancourt, A. M., and Sullivan, D. E. (2010). New concepts on the immune modulation mediated by mesenchymal stem cells. Stem Cell Res. Ther. 1 (5), 34. doi:10.1186/scrt34
Callaghan, C. M., Seyedin, S. N., Mohiuddin, I. H., Hawkes, K. L., Petronek, M. S., Anderson, C. M., et al. (2020). The effect of concurrent stereotactic body radiation and anti-PD-1 therapy for recurrent metastatic sarcoma. Radiat. Res. 194 (2), 124–132. doi:10.1667/rade-20-00017
Cathomas, R., Rothermundt, C., Bode, B., Fuchs, B., von Moos, R., and Schwitter, M. (2015). RANK ligand blockade with denosumab in combination with sorafenib in chemorefractory osteosarcoma: A possible step forward? Oncology 88 (4), 257–260. doi:10.1159/000369975
Christie, J. D., Appel, N., Canter, H., Achi, J. G., Elliott, N. M., de Matos, A. L., et al. (2021). Systemic delivery of TNF-armed myxoma virus plus immune checkpoint inhibitor eliminates lung metastatic mouse osteosarcoma. Mol. Ther. Oncolytics 22, 539–554. doi:10.1016/j.omto.2021.07.014
Christofides, A., Strauss, L., Yeo, A., Cao, C., Charest, A., and Boussiotis, V. A. (2022). The complex role of tumor-infiltrating macrophages. Nat. Immunol. 23 (8), 1148–1156. doi:10.1038/s41590-022-01267-2
D'Angelo, S. P., Mahoney, M. R., Van Tine, B. A., Atkins, J., Milhem, M. M., Jahagirdar, B. N., et al. (2018). Nivolumab with or without ipilimumab treatment for metastatic sarcoma (alliance A091401): Two open-label, non-comparative, randomised, phase 2 trials. Lancet. Oncol. 19 (3), 416–426. doi:10.1016/s1470-2045(18)30006-8
Darlington, P. J., Kirchhof, M. G., Criado, G., Sondhi, J., and Madrenas, J. (2005). Hierarchical regulation of CTLA-4 dimer-based lattice formation and its biological relevance for T cell inactivation. J. Immunol. 175 (2), 996–1004. doi:10.4049/jimmunol.175.2.996
Davis, K. L., Fox, E., Merchant, M. S., Reid, J. M., Kudgus, R. A., Liu, X., et al. (2020). Nivolumab in children and young adults with relapsed or refractory solid tumours or lymphoma (ADVL1412): A multicentre, open-label, single-arm, phase 1-2 trial. Lancet. Oncol. 21 (4), 541–550. doi:10.1016/s1470-2045(20)30023-1
Deng, C., Xu, Y., Fu, J., Zhu, X., Chen, H., Xu, H., et al. (2020). Reprograming the tumor immunologic microenvironment using neoadjuvant chemotherapy in osteosarcoma. Cancer Sci. 111 (6), 1899–1909. doi:10.1111/cas.14398
Dhupkar, P., Gordon, N., Stewart, J., and Kleinerman, E. S. (2018). Anti-PD-1 therapy redirects macrophages from an M2 to an M1 phenotype inducing regression of OS lung metastases. Cancer Med. 7 (6), 2654–2664. doi:10.1002/cam4.1518
Dongye, Z., Li, J., and Wu, Y. (2022). Toll-like receptor 9 agonists and combination therapies: Strategies to modulate the tumour immune microenvironment for systemic anti-tumour immunity. Br. J. Cancer, 1–11. doi:10.1038/s41416-022-01876-6
Dysthe, M., and Parihar, R. (2020). Myeloid-derived suppressor cells in the tumor microenvironment. Adv. Exp. Med. Biol. 1224, 117–140. doi:10.1007/978-3-030-35723-8_8
Fallarino, F., Grohmann, U., Hwang, K. W., Orabona, C., Vacca, C., Bianchi, R., et al. (2003). Modulation of tryptophan catabolism by regulatory T cells. Nat. Immunol. 4 (12), 1206–1212. doi:10.1038/ni1003
Fanoni, D., Tavecchio, S., Recalcati, S., Balice, Y., Venegoni, L., Fiorani, R., et al. (2011). New monoclonal antibodies against B-cell antigens: Possible new strategies for diagnosis of primary cutaneous B-cell lymphomas. Immunol. Lett. 134 (2), 157–160. doi:10.1016/j.imlet.2010.09.022
Ferrari, S., Bielack, S. S., Smeland, S., Longhi, A., Egerer, G., Sundby Hall, K., et al. (2018). EURO-B.O.S.S.: A European study on chemotherapy in bone-sarcoma patients aged over 40: Outcome in primary high-grade osteosarcoma. Tumori 104 (1), 30–36. doi:10.5301/tj.5000696
Fraser, J. H., Rincón, M., McCoy, K. D., and Le Gros, G. (1999). CTLA4 ligation attenuates AP-1, NFAT and NF-kappaB activity in activated T cells. Eur. J. Immunol. 29 (3), 838–844. doi:10.1002/(SICI)1521-4141(199903)29:03<838::AID-IMMU838>3.0.CO;2-P
Frauwirth, K. A., Riley, J. L., Harris, M. H., Parry, R. V., Rathmell, J. C., Plas, D. R., et al. (2002). The CD28 signaling pathway regulates glucose metabolism. Immunity 16 (6), 769–777. doi:10.1016/s1074-7613(02)00323-0
Freeman, G. J., Long, A. J., Iwai, Y., Bourque, K., Chernova, T., Nishimura, H., et al. (2000). Engagement of the PD-1 immunoinhibitory receptor by a novel B7 family member leads to negative regulation of lymphocyte activation. J. Exp. Med. 192 (7), 1027–1034. doi:10.1084/jem.192.7.1027
Galluzzi, L., Buqué, A., Kepp, O., Zitvogel, L., and Kroemer, G. (2017). Immunogenic cell death in cancer and infectious disease. Nat. Rev. Immunol. 17 (2), 97–111. doi:10.1038/nri.2016.107
Gao, W., Zhou, J., and Ji, B. (2017). Evidence of interleukin 21 reduction in osteosarcoma patients due to PD-1/PD-L1-mediated suppression of follicular helper T cell functionality. DNA Cell Biol. 36 (9), 794–800. doi:10.1089/dna.2017.3669
Geoerger, B., Zwaan, C. M., Marshall, L. V., Michon, J., Bourdeaut, F., Casanova, M., et al. (2020). Atezolizumab for children and young adults with previously treated solid tumours, non-hodgkin lymphoma, and hodgkin lymphoma (iMATRIX): A multicentre phase 1-2 study. Lancet. Oncol. 21 (1), 134–144. doi:10.1016/s1470-2045(19)30693-x
Gill, J., and Gorlick, R. (2021). Advancing therapy for osteosarcoma. Nat. Rev. Clin. Oncol. 18 (10), 609–624. doi:10.1038/s41571-021-00519-8
Guan, Y., Zhang, R., Peng, Z., Dong, D., Wei, G., and Wang, Y. (2017). Inhibition of IL-18-mediated myeloid derived suppressor cell accumulation enhances anti-PD1 efficacy against osteosarcoma cancer. J. Bone Oncol. 9, 59–64. doi:10.1016/j.jbo.2017.10.002
Han, G., Bi, W. Z., Xu, M., Jia, J. P., and Wang, Y. (2016). Amputation versus limb-salvage surgery in patients with osteosarcoma: A meta-analysis. World J. Surg. 40 (8), 2016–2027. doi:10.1007/s00268-016-3500-7
Hasan, M. N., Capuk, O., Patel, S. M., and Sun, D. (2022). The role of metabolic plasticity of tumor-associated macrophages in shaping the tumor microenvironment immunity. Cancers (Basel) 14 (14), 3331. doi:10.3390/cancers14143331
Hashimoto, K., Nishimura, S., and Akagi, M. (2020). Characterization of PD-1/PD-L1 immune checkpoint expression in osteosarcoma. Diagn. (Basel) 10 (8), E528. doi:10.3390/diagnostics10080528
Helm, A., Tinganelli, W., Simoniello, P., Kurosawa, F., Fournier, C., Shimokawa, T., et al. (2021). Reduction of lung metastases in a mouse osteosarcoma model treated with carbon ions and immune checkpoint inhibitors. Int. J. Radiat. Oncol. Biol. Phys. 109 (2), 594–602. doi:10.1016/j.ijrobp.2020.09.041
Heng, M., Gupta, A., Chung, P. W., Healey, J. H., Vaynrub, M., Rose, P. S., et al. (2020). The role of chemotherapy and radiotherapy in localized extraskeletal osteosarcoma. Eur. J. Cancer 125, 130–141. doi:10.1016/j.ejca.2019.07.029
Hu, J., Yang, Q., Zhang, W., Du, H., Chen, Y., Zhao, Q., et al. (2022). Cell membrane-anchored and tumor-targeted IL-12 (attIL12)-T cell therapy for eliminating large and heterogeneous solid tumors. J. Immunother. Cancer 10 (1), e003633. doi:10.1136/jitc-2021-003633
Huang, X., Zhang, W., Zhang, Z., Shi, D., Wu, F., Zhong, B., et al. (2018). Prognostic value of programmed cell death 1 ligand-1 (PD-L1) or PD-1 expression in patients with osteosarcoma: A meta-analysis. J. Cancer 9 (14), 2525–2531. doi:10.7150/jca.25011
Intlekofer, A. M., and Thompson, C. B. (2013). At the bench: Preclinical rationale for CTLA-4 and PD-1 blockade as cancer immunotherapy. J. Leukoc. Biol. 94 (1), 25–39. doi:10.1189/jlb.1212621
Ishida, Y., Agata, Y., Shibahara, K., and Honjo, T. (1992). Induced expression of PD-1, a novel member of the immunoglobulin gene superfamily, upon programmed cell death. Embo J. 11 (11), 3887–3895. doi:10.1002/j.1460-2075.1992.tb05481.x
Jiang, K., Li, J., Zhang, J., Wang, L., Zhang, Q., Ge, J., et al. (2019). SDF-1/CXCR4 axis facilitates myeloid-derived suppressor cells accumulation in osteosarcoma microenvironment and blunts the response to anti-PD-1 therapy. Int. Immunopharmacol. 75, 105818. doi:10.1016/j.intimp.2019.105818
Kamada, T., Tsujii, H., Blakely, E. A., Debus, J., De Neve, W., Durante, M., et al. (2015). Carbon ion radiotherapy in Japan: An assessment of 20 years of clinical experience. Lancet. Oncol. 16 (2), e93–e100. doi:10.1016/s1470-2045(14)70412-7
Katsuki, S., Takahashi, Y., Tamari, K., Minami, K., Takenaka, W., Ibuki, Y., et al. (2022). Radiation therapy enhances systemic antitumor efficacy in PD-L1 therapy regardless of sequence of radiation in murine osteosarcoma. PLoS One 17 (7), e0271205. doi:10.1371/journal.pone.0271205
Keir, M. E., Liang, S. C., Guleria, I., Latchman, Y. E., Qipo, A., Albacker, L. A., et al. (2006). Tissue expression of PD-L1 mediates peripheral T cell tolerance. J. Exp. Med. 203 (4), 883–895. doi:10.1084/jem.20051776
Kimura, Y., and Sumiyoshi, M. (2015). Antitumor and antimetastatic actions of dihydroxycoumarins (esculetin or fraxetin) through the inhibition of M2 macrophage differentiation in tumor-associated macrophages and/or G1 arrest in tumor cells. Eur. J. Pharmacol. 746, 115–125. doi:10.1016/j.ejphar.2014.10.048
Koirala, P., Roth, M. E., Gill, J., Piperdi, S., Chinai, J. M., Geller, D. S., et al. (2016). Immune infiltration and PD-L1 expression in the tumor microenvironment are prognostic in osteosarcoma. Sci. Rep. 6, 30093. doi:10.1038/srep30093
Krishnadas, D. K., Shusterman, S., Bai, F., Diller, L., Sullivan, J. E., Cheerva, A. C., et al. (2015). A phase I trial combining decitabine/dendritic cell vaccine targeting MAGE-A1, MAGE-A3 and NY-ESO-1 for children with relapsed or therapy-refractory neuroblastoma and sarcoma. Cancer Immunol. Immunother. 64 (10), 1251–1260. doi:10.1007/s00262-015-1731-3
Kroemer, G., Galluzzi, L., Kepp, O., and Zitvogel, L. (2013). Immunogenic cell death in cancer therapy. Annu. Rev. Immunol. 31, 51–72. doi:10.1146/annurev-immunol-032712-100008
Latchman, Y., Wood, C. R., Chernova, T., Chaudhary, D., Borde, M., Chernova, I., et al. (2001). PD-L2 is a second ligand for PD-1 and inhibits T cell activation. Nat. Immunol. 2 (3), 261–268. doi:10.1038/85330
Le Cesne, A., Marec-Berard, P., Blay, J. Y., Gaspar, N., Bertucci, F., Penel, N., et al. (2019). Programmed cell death 1 (PD-1) targeting in patients with advanced osteosarcomas: Results from the PEMBROSARC study. Eur. J. Cancer 119, 151–157. doi:10.1016/j.ejca.2019.07.018
Liang, S. C., Latchman, Y. E., Buhlmann, J. E., Tomczak, M. F., Horwitz, B. H., Freeman, G. J., et al. (2003). Regulation of PD-1, PD-L1, and PD-L2 expression during normal and autoimmune responses. Eur. J. Immunol. 33 (10), 2706–2716. doi:10.1002/eji.200324228
Ligon, J. A., Choi, W., Cojocaru, G., Fu, W., Hsiue, E. H., Oke, T. F., et al. (2021). Pathways of immune exclusion in metastatic osteosarcoma are associated with inferior patient outcomes. J. Immunother. Cancer 9 (5), e001772. doi:10.1136/jitc-2020-001772
Lim, H. X., Hong, H. J., Cho, D., and Kim, T. S. (2014). IL-18 enhances immunosuppressive responses by promoting differentiation into monocytic myeloid-derived suppressor cells. J. Immunol. 193 (11), 5453–5460. doi:10.4049/jimmunol.1401282
Lin, D. Y., Tanaka, Y., Iwasaki, M., Gittis, A. G., Su, H. P., Mikami, B., et al. (2008). The PD-1/PD-L1 complex resembles the antigen-binding Fv domains of antibodies and T cell receptors. Proc. Natl. Acad. Sci. U. S. A. 105 (8), 3011–3016. doi:10.1073/pnas.0712278105
Lindsten, T., Lee, K. P., Harris, E. S., Petryniak, B., Craighead, N., Reynolds, P. J., et al. (1993). Characterization of CTLA-4 structure and expression on human T cells. J. Immunol. 151 (7), 3489–3499.
Long, A. H., Highfill, S. L., Cui, Y., Smith, J. P., Walker, A. J., Ramakrishna, S., et al. (2016). Reduction of MDSCs with all-trans retinoic acid improves CAR therapy efficacy for sarcomas. Cancer Immunol. Res. 4 (10), 869–880. doi:10.1158/2326-6066.Cir-15-0230
Lu, Y., Zhang, J., Chen, Y., Kang, Y., Liao, Z., He, Y., et al. (2022). Novel immunotherapies for osteosarcoma. Front. Oncol. 12, 830546. doi:10.3389/fonc.2022.830546
Luke, J. J., Rutkowski, P., Queirolo, P., Del Vecchio, M., Mackiewicz, J., Chiarion-Sileni, V., et al. (2022). Pembrolizumab versus placebo as adjuvant therapy in completely resected stage IIB or IIC melanoma (KEYNOTE-716): A randomised, double-blind, phase 3 trial. Lancet 399 (10336), 1718–1729. doi:10.1016/s0140-6736(22)00562-1
Lussier, D. M., Johnson, J. L., Hingorani, P., and Blattman, J. N. (2015a). Combination immunotherapy with α-CTLA-4 and α-PD-L1 antibody blockade prevents immune escape and leads to complete control of metastatic osteosarcoma. J. Immunother. Cancer 3, 21. doi:10.1186/s40425-015-0067-z
Lussier, D. M., O'Neill, L., Nieves, L. M., McAfee, M. S., Holechek, S. A., Collins, A. W., et al. (2015b). Enhanced T-cell immunity to osteosarcoma through antibody blockade of PD-1/PD-L1 interactions. J. Immunother. 38 (3), 96–106. doi:10.1097/cji.0000000000000065
Lv, J., Chen, F. K., Liu, C., Liu, P. J., Feng, Z. P., Jia, L., et al. (2020). Zoledronic acid inhibits thyroid cancer stemness and metastasis by repressing M2-like tumor-associated macrophages induced Wnt/β-catenin pathway. Life Sci. 256, 117925. doi:10.1016/j.lfs.2020.117925
Marson, A., Kretschmer, K., Frampton, G. M., Jacobsen, E. S., Polansky, J. K., MacIsaac, K. D., et al. (2007). Foxp3 occupancy and regulation of key target genes during T-cell stimulation. Nature 445 (7130), 931–935. doi:10.1038/nature05478
Meftahpour, V., Aghebati-Maleki, A., Fotouhi, A., Safarzadeh, E., and Aghebati-Maleki, L. (2022). Prognostic significance and therapeutic potentials of immune checkpoints in osteosarcoma. Excli J. 21, 250–268. doi:10.17179/excli2021-4094
Mei, M. G., Lee, H. J., Palmer, J. M., Chen, R., Tsai, N. C., Chen, L., et al. (2022). Response-adapted anti-PD-1-based salvage therapy for Hodgkin lymphoma with nivolumab alone or in combination with ICE. Blood 139 (25), 3605–3616. doi:10.1182/blood.2022015423
Merchant, M. S., Wright, M., Baird, K., Wexler, L. H., Rodriguez-Galindo, C., Bernstein, D., et al. (2016). Phase I clinical trial of ipilimumab in pediatric patients with advanced solid tumors. Clin. Cancer Res. 22 (6), 1364–1370. doi:10.1158/1078-0432.Ccr-15-0491
Michielsen, A. J., Hogan, A. E., Marry, J., Tosetto, M., Cox, F., Hyland, J. M., et al. (2011). Tumour tissue microenvironment can inhibit dendritic cell maturation in colorectal cancer. PLoS One 6 (11), e27944. doi:10.1371/journal.pone.0027944
Mildner, A., and Jung, S. (2014). Development and function of dendritic cell subsets. Immunity 40 (5), 642–656. doi:10.1016/j.immuni.2014.04.016
Mochizuki, Y., Tazawa, H., Demiya, K., Kure, M., Kondo, H., Komatsubara, T., et al. (2021). Telomerase-specific oncolytic immunotherapy for promoting efficacy of PD-1 blockade in osteosarcoma. Cancer Immunol. Immunother. 70 (5), 1405–1417. doi:10.1007/s00262-020-02774-7
Murray, P. J., and Wynn, T. A. (2011). Protective and pathogenic functions of macrophage subsets. Nat. Rev. Immunol. 11 (11), 723–737. doi:10.1038/nri3073
Najjar, Y. G., and Finke, J. H. (2013). Clinical perspectives on targeting of myeloid derived suppressor cells in the treatment of cancer. Front. Oncol. 3, 49. doi:10.3389/fonc.2013.00049
Nasarre, P., Garcia, D. I., Siegel, J. B., Bonilla, I. V., Mukherjee, R., Hilliard, E., et al. (2021). Overcoming PD-1 inhibitor resistance with a monoclonal antibody to secreted frizzled-related protein 2 in metastatic osteosarcoma. Cancers (Basel) 13 (11), 2696. doi:10.3390/cancers13112696
Nishimura, H., Nose, M., Hiai, H., Minato, N., and Honjo, T. (1999). Development of lupus-like autoimmune diseases by disruption of the PD-1 gene encoding an ITIM motif-carrying immunoreceptor. Immunity 11 (2), 141–151. doi:10.1016/s1074-7613(00)80089-8
Ocadlikova, D., Lecciso, M., Broto, J. M., Scotlandi, K., Cavo, M., Curti, A., et al. (2021). Sunitinib exerts in vitro immunomodulatory activity on sarcomas via dendritic cells and synergizes with PD-1 blockade. Front. Immunol. 12, 577766. doi:10.3389/fimmu.2021.577766
Okazaki, T., Tanaka, Y., Nishio, R., Mitsuiye, T., Mizoguchi, A., Wang, J., et al. (2003). Autoantibodies against cardiac troponin I are responsible for dilated cardiomyopathy in PD-1-deficient mice. Nat. Med. 9 (12), 1477–1483. doi:10.1038/nm955
Ostrov, D. A., Shi, W., Schwartz, J. C., Almo, S. C., and Nathenson, S. G. (2000). Structure of murine CTLA-4 and its role in modulating T cell responsiveness. Science 290 (5492), 816–819. doi:10.1126/science.290.5492.816
Parry, R. V., Chemnitz, J. M., Frauwirth, K. A., Lanfranco, A. R., Braunstein, I., Kobayashi, S. V., et al. (2005). CTLA-4 and PD-1 receptors inhibit T-cell activation by distinct mechanisms. Mol. Cell. Biol. 25 (21), 9543–9553. doi:10.1128/mcb.25.21.9543-9553.2005
Peggs, K. S., Quezada, S. A., Chambers, C. A., Korman, A. J., and Allison, J. P. (2009). Blockade of CTLA-4 on both effector and regulatory T cell compartments contributes to the antitumor activity of anti-CTLA-4 antibodies. J. Exp. Med. 206 (8), 1717–1725. doi:10.1084/jem.20082492
Permata, T. B. M., Sato, H., Gu, W., Kakoti, S., Uchihara, Y., Yoshimatsu, Y., et al. (2021). High linear energy transfer carbon-ion irradiation upregulates PD-L1 expression more significantly than X-rays in human osteosarcoma U2OS cells. J. Radiat. Res. 62 (5), 773–781. doi:10.1093/jrr/rrab050
Piperno-Neumann, S., Le Deley, M. C., Rédini, F., Pacquement, H., Marec-Bérard, P., Petit, P., et al. (2016). Zoledronate in combination with chemotherapy and surgery to treat osteosarcoma (OS2006): A randomised, multicentre, open-label, phase 3 trial. Lancet. Oncol. 17 (8), 1070–1080. doi:10.1016/s1470-2045(16)30096-1
Qureshi, O. S., Zheng, Y., Nakamura, K., Attridge, K., Manzotti, C., Schmidt, E. M., et al. (2011). Trans-endocytosis of CD80 and CD86: A molecular basis for the cell-extrinsic function of CTLA-4. Science 332 (6029), 600–603. doi:10.1126/science.1202947
Rahn, D. A., Mundt, A. J., Murphy, J. D., Schiff, D., Adams, J., and Murphy, K. T. (2015). Clinical outcomes of palliative radiation therapy for children. Pract. Radiat. Oncol. 5 (3), 183–187. doi:10.1016/j.prro.2014.08.015
Rainusso, N., Brawley, V. S., Ghazi, A., Hicks, M. J., Gottschalk, S., Rosen, J. M., et al. (2012). Immunotherapy targeting HER2 with genetically modified T cells eliminates tumor-initiating cells in osteosarcoma. Cancer Gene Ther. 19 (3), 212–217. doi:10.1038/cgt.2011.83
Ren, T., Zheng, B., Huang, Y., Wang, S., Bao, X., Liu, K., et al. (2019). Osteosarcoma cell intrinsic PD-L2 signals promote invasion and metastasis via the RhoA-ROCK-LIMK2 and autophagy pathways. Cell Death Dis. 10 (4), 261. doi:10.1038/s41419-019-1497-1
Saraf, A. J., Fenger, J. M., and Roberts, R. D. (2018). Osteosarcoma: Accelerating progress makes for a hopeful future. Front. Oncol. 8, 4. doi:10.3389/fonc.2018.00004
Schneider, H., Downey, J., Smith, A., Zinselmeyer, B. H., Rush, C., Brewer, J. M., et al. (2006). Reversal of the TCR stop signal by CTLA-4. Science 313 (5795), 1972–1975. doi:10.1126/science.1131078
Senerchia, A. A., Macedo, C. R., Ferman, S., Scopinaro, M., Cacciavillano, W., Boldrini, E., et al. (2017). Results of a randomized, prospective clinical trial evaluating metronomic chemotherapy in nonmetastatic patients with high-grade, operable osteosarcomas of the extremities: A report from the Latin American group of osteosarcoma treatment. Cancer 123 (6), 1003–1010. doi:10.1002/cncr.30411
Shapouri-Moghaddam, A., Mohammadian, S., Vazini, H., Taghadosi, M., Esmaeili, S. A., Mardani, F., et al. (2018). Macrophage plasticity, polarization, and function in health and disease. J. Cell. Physiol. 233 (9), 6425–6440. doi:10.1002/jcp.26429
Shi, C., and Pamer, E. G. (2011). Monocyte recruitment during infection and inflammation. Nat. Rev. Immunol. 11 (11), 762–774. doi:10.1038/nri3070
Shi, X., Li, X., Wang, H., Yu, Z., Zhu, Y., and Gao, Y. (2019). Specific inhibition of PI3Kδ/γ enhances the efficacy of anti-PD1 against osteosarcoma cancer. J. Bone Oncol. 16, 100206. doi:10.1016/j.jbo.2018.11.001
Shin, T., Yoshimura, K., Shin, T., Crafton, E. B., Tsuchiya, H., Housseau, F., et al. (2005). In vivo costimulatory role of B7-DC in tuning T helper cell 1 and cytotoxic T lymphocyte responses. J. Exp. Med. 201 (10), 1531–1541. doi:10.1084/jem.20050072
Siegel, R. L., Miller, K. D., and Jemal, A. (2018). Cancer statistics, 2018. Ca. Cancer J. Clin. 68 (1), 7–30. doi:10.3322/caac.21442
Starzer, A. M., Berghoff, A. S., Hamacher, R., Tomasich, E., Feldmann, K., Hatziioannou, T., et al. (2021). Tumor DNA methylation profiles correlate with response to anti-PD-1 immune checkpoint inhibitor monotherapy in sarcoma patients. J. Immunother. Cancer 9 (3), e001458. doi:10.1136/jitc-2020-001458
Tawbi, H. A., Burgess, M., Bolejack, V., Van Tine, B. A., Schuetze, S. M., Hu, J., et al. (2017). Pembrolizumab in advanced soft-tissue sarcoma and bone sarcoma (SARC028): A multicentre, two-cohort, single-arm, open-label, phase 2 trial. Lancet. Oncol. 18 (11), 1493–1501. doi:10.1016/s1470-2045(17)30624-1
Terme, M., Ullrich, E., Aymeric, L., Meinhardt, K., Desbois, M., Delahaye, N., et al. (2011). IL-18 induces PD-1-dependent immunosuppression in cancer. Cancer Res. 71 (16), 5393–5399. doi:10.1158/0008-5472.Can-11-0993
Tian, R., Wang, H., Gish, G. D., Petsalaki, E., Pasculescu, A., Shi, Y., et al. (2015). Combinatorial proteomic analysis of intercellular signaling applied to the CD28 T-cell costimulatory receptor. Proc. Natl. Acad. Sci. U. S. A. 112 (13), E1594–E1603. doi:10.1073/pnas.1503286112
Tinkle, C. L., Lu, J., Han, Y., Li, Y., McCarville, B. M., Neel, M. D., et al. (2019). Curative-intent radiotherapy for pediatric osteosarcoma: The St. Jude experience. Pediatr. Blood Cancer 66 (8), e27763. doi:10.1002/pbc.27763
Topalian, S. L., Taube, J. M., Anders, R. A., and Pardoll, D. M. (2016). Mechanism-driven biomarkers to guide immune checkpoint blockade in cancer therapy. Nat. Rev. Cancer 16 (5), 275–287. doi:10.1038/nrc.2016.36
Tsukahara, T., Kawaguchi, S., Torigoe, T., Kimura, S., Murase, M., Ichimiya, S., et al. (2008). Prognostic impact and immunogenicity of a novel osteosarcoma antigen, papillomavirus binding factor, in patients with osteosarcoma. Cancer Sci. 99 (2), 368–375. doi:10.1111/j.1349-7006.2008.00695.x
Wang, S. D., Li, H. Y., Li, B. H., Xie, T., Zhu, T., Sun, L. L., et al. (2016). The role of CTLA-4 and PD-1 in anti-tumor immune response and their potential efficacy against osteosarcoma. Int. Immunopharmacol. 38, 81–89. doi:10.1016/j.intimp.2016.05.016
Wang, Z., Wang, Z., Li, B., Wang, S., Chen, T., and Ye, Z. (2019). Innate immune cells: A potential and promising cell population for treating osteosarcoma. Front. Immunol. 10, 1114. doi:10.3389/fimmu.2019.01114
Wang, S., Liu, G., Li, Y., and Pan, Y. (2022). Metabolic reprogramming induces macrophage polarization in the tumor microenvironment. Front. Immunol. 13, 840029. doi:10.3389/fimmu.2022.840029
Wu, C.-C., Beird, H. C., Andrew Livingston, J., Advani, S., Mitra, A., Cao, S., et al. (2020). Immuno-genomic landscape of osteosarcoma. Nat. Commun. 11 (1), 1008. doi:10.1038/s41467-020-14646-w
Xia, L., Wu, H., and Qian, W. (2018). Irradiation enhanced the effects of PD-1 blockade in brain metastatic osteosarcoma. J. Bone Oncol. 12, 61–64. doi:10.1016/j.jbo.2018.05.002
Xie, L., Xu, J., Sun, X., Guo, W., Gu, J., Liu, K., et al. (2020). Apatinib plus camrelizumab (anti-PD1 therapy, SHR-1210) for advanced osteosarcoma (APFAO) progressing after chemotherapy: A single-arm, open-label, phase 2 trial. J. Immunother. Cancer 8 (1), e000798. doi:10.1136/jitc-2020-000798
Yoshida, K., Okamoto, M., Sasaki, J., Kuroda, C., Ishida, H., Ueda, K., et al. (2019). Clinical outcome of osteosarcoma and its correlation with programmed death-ligand 1 and T cell activation markers. Onco. Targets. Ther. 12, 2513–2518. doi:10.2147/ott.S198421
Yoshida, K., Okamoto, M., Sasaki, J., Kuroda, C., Ishida, H., Ueda, K., et al. (2020). Anti-PD-1 antibody decreases tumour-infiltrating regulatory T cells. BMC Cancer 20 (1), 25. doi:10.1186/s12885-019-6499-y
Zak, K. M., Kitel, R., Przetocka, S., Golik, P., Guzik, K., Musielak, B., et al. (2015). Structure of the complex of human programmed death 1, PD-1, and its ligand PD-L1. Structure 23 (12), 2341–2348. doi:10.1016/j.str.2015.09.010
Zhang, X., Schwartz, J. C., Guo, X., Bhatia, S., Cao, E., Lorenz, M., et al. (2004). Structural and functional analysis of the costimulatory receptor programmed death-1. Immunity 20 (3), 337–347. doi:10.1016/s1074-7613(04)00051-2
Zhang, M. L., Chen, L., Li, Y. J., and Kong, D. L. (2019). PD-L1/PD-1 axis serves an important role in natural killer cell-induced cytotoxicity in osteosarcoma. Oncol. Rep. 42 (5), 2049–2056. doi:10.3892/or.2019.7299
Zhao, L., Peng, Y., He, S., Li, R., Wang, Z., Huang, J., et al. (2021). Apatinib induced ferroptosis by lipid peroxidation in gastric cancer. Gastric Cancer 24 (3), 642–654. doi:10.1007/s10120-021-01159-8
Zhao, K., Wang, X., Zhao, D., Lin, Q., Zhang, Y., and Hu, Y. (2022). lncRNA HITT inhibits lactate production by repressing PKM2 oligomerization to reduce tumor growth and macrophage polarization. Res. (Wash D C) 2022, 9854904. doi:10.34133/2022/9854904
Zheng, W., Xiao, H., Liu, H., and Zhou, Y. (2015). Expression of programmed death 1 is correlated with progression of osteosarcoma. Apmis 123 (2), 102–107. doi:10.1111/apm.12311
Zheng, B., Ren, T., Huang, Y., Sun, K., Wang, S., Bao, X., et al. (2018). PD-1 axis expression in musculoskeletal tumors and antitumor effect of nivolumab in osteosarcoma model of humanized mouse. J. Hematol. Oncol. 11 (1), 16. doi:10.1186/s13045-018-0560-1
Zhou, Q., Xian, M., Xiang, S., Xiang, D., Shao, X., Wang, J., et al. (2017). All-trans retinoic acid prevents osteosarcoma metastasis by inhibiting M2 polarization of tumor-associated macrophages. Cancer Immunol. Res. 5 (7), 547–559. doi:10.1158/2326-6066.Cir-16-0259
Keywords: osteosarcoma, immunotherapy, immune checkpoint inhibitor, side effects, mechanism
Citation: Zhang Z, Tan X, Jiang Z, Wang H and Yuan H (2022) Immune checkpoint inhibitors in osteosarcoma: A hopeful and challenging future. Front. Pharmacol. 13:1031527. doi: 10.3389/fphar.2022.1031527
Received: 30 August 2022; Accepted: 07 October 2022;
Published: 17 October 2022.
Edited by:
Satish Ramalingam, SRM Institute of Science and Technology, IndiaReviewed by:
Chiara Focaccetti, Università di Roma Tor Vergata, ItalyStefania Maiello, University of Bologna, Italy
Copyright © 2022 Zhang, Tan, Jiang, Wang and Yuan. This is an open-access article distributed under the terms of the Creative Commons Attribution License (CC BY). The use, distribution or reproduction in other forums is permitted, provided the original author(s) and the copyright owner(s) are credited and that the original publication in this journal is cited, in accordance with accepted academic practice. No use, distribution or reproduction is permitted which does not comply with these terms.
*Correspondence: Hao Wang, doctorwhao@163.com; Hengfeng Yuan, yuanhf@shsmu.edu.cn
†These authors have contributed equally to this work