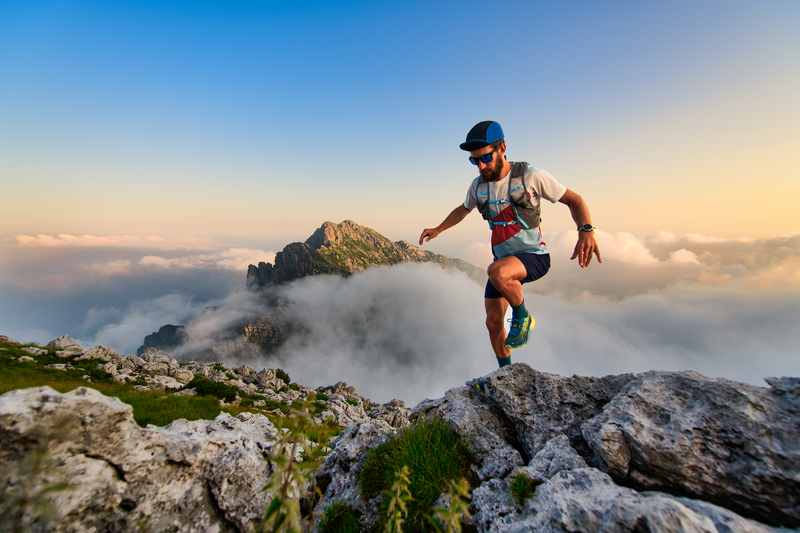
95% of researchers rate our articles as excellent or good
Learn more about the work of our research integrity team to safeguard the quality of each article we publish.
Find out more
REVIEW article
Front. Pharmacol. , 08 November 2022
Sec. Pharmacology of Infectious Diseases
Volume 13 - 2022 | https://doi.org/10.3389/fphar.2022.1025160
Despite the development of effective combined antiretroviral therapy (cART), the neurocognitive impairments associated with human immunodeficiency virus (HIV) remain challenging. The presence of the blood-brain barrier (BBB) and blood-cerebrospinal fluid barrier (BCFB) impedes the adequate penetration of certain antiretroviral drugs into the brain. In addition, reports have shown that some antiretroviral drugs cause neurotoxicity resulting from their interaction with nervous tissues due to long-term systemic exposure. Therefore, the research into the effective therapeutic modality that would cater for the HIV-associated neurocognitive disorders (HAND) and ART toxicity is now receiving broad research attention. Thus, this review explores the latest information in managing HAND using a nanoparticle drug delivery system (NDDS). We discussed the neurotoxicity profile of various approved ART. Also, we explained the applications of silver nanoparticles (AgNPs) in medicine, their different synthesis methods and their interaction with nervous tissues. Lastly, while proposing AgNPs as useful nanoparticles in properly delivering ART to enhance effectiveness and minimize neurocognitive disorders, we hypothesize that the perceived toxicity of AgNPs could be minimized by taking appropriate precautions. One such precaution is using appropriate reducing and stabilizing agents such as trisodium citrate to reduce silver ion Ag + to ground state Ag0 during the synthesis. Also, the usage of medium-sized, spherical-shaped AgNPs is encouraged in AgNPs-based drug delivery to the brain due to their ability to deliver therapeutic agents across BBB. In addition, characterization and functionalization of the synthesized AgNPs are required during the drug delivery approach. Putting all these factors in place would minimize toxicity and enhance the usage of AgNPs in delivering therapeutic agents across the BBB to the targeted brain tissue and could cater for the HIV-associated neurocognitive disorders and neurotoxic effects of antiretroviral drugs (ARDs).
The concept of nanotechnology was first introduced in 1959 by an American physicist called Richard Feynman and is one of the most promising technologies in recent times (Bayda et al., 2019). The term “nanotechnology,” first used and defined by a Japanese scientist, Norio Taniguchi, consists of the separation, consolidation or breaking down of materials by one atom or molecule (Taniguchi, 1974).
The advancement in technology has brought about nanotechnology, the application of technology to harness the use of nanomaterials. Nanotechnology is an appealing field with broad potential and wide applications, having contributed to the fabrication of more potent and sophisticated materials, wastewater recycling, aspects of drug development, diagnosis and treatment of diseases, and advanced information and communication tools (Benelmekki, 2015; Cheon et al., 2019). Nanotechnology deals with the manufacturing and manipulation of nano-sized materials known as nanoparticles. A branch of nanotechnology that involves diagnosing, treating, and preventing diseases with nanoparticles and molecular devices to enhance human well-being is known as nanomedicine (Hosseini et al., 2016; Vahedifard and Chakravarthy, 2021). Nanomedicine entails using nanoparticles to diagnose and ensure targeted delivery and controlled release of therapeutic agents and active materials in living cells (Patra et al., 2018).
Nanoparticles (NPs) are nano-size particles that range between 1 and 100 nm in size. NPs exhibit unique properties due to their dimensions, making them suitable candidates for fabricating other complex nanoconjugates (Benelmekki, 2015). Thus, they recently received broad research attention and biomedical application. NPs are mostly complex particulates with three layers arranged from the innermost to the outermost (Khan et al., 2019), while some are single-layer particulate (Chundi et al., 2020). The innermost core layer represents the central part of the NPs. The middle shell layer is chemically different from other layers, and the outmost surface layer undergoes functionalisation with various macromolecules (Shin et al., 2016).
Nanoparticles are classified into various classes based on their physicochemical property, morphology, and size. On this account, there are organic and inorganic nanoparticles. The organic nanoparticles are lipid-based, polymeric and carbon-based, while inorganic nanoparticles are grouped into ceramic, metallic and semiconductor nanoparticles, as shown in Figure 1 (Patra et al., 2018; Khan et al., 2019).
The most common polymeric NPs are ferritin, chitosan, dendrimers, xanthan gum, dextran, liposomes, cellulose, micelles, and alginate (Patra et al., 2018). Carbon-based are well known for excessive strength, versatility, electron affinity and good electrical conductivity and have received significant attention in biomedical applications (Astefanei et al., 2015; Maiti et al., 2018). Carbon-based NPs include graphene, carbon black, fullerenes, carbon nanotube, and carbon nanofibers (Anu Mary Ealia & Saravanakumar, 2017). The lipid-based NPs have lipid moieties as a solid core, a matrix filled with lipophilic molecules and an outer core stabilized by emulsifiers and surfactants (Rawat et al., 2011; Khan et al., 2019). Examples of lipid-based NPs are solid lipid nanoparticles, liposomes, nanostructured lipid carriers and nano-emulsions (Garcia-Pinel et al., 2019).
Ceramic-based materials are inorganic-based NPs with high thermal resistance and chemical inactivity (Khan et al., 2019). These NPs exhibit various forms like porous, amorphous, dense and polycrystalline. They are usually produced by heating carbides, phosphates, oxides and carbonates, followed by cooling (Bhardwaj et al., 2021). Semiconductor NPs represent other inorganic nanoparticles with smaller sizes, high resistivity, luminescence, quantum size effects, less weight, high surface area, and nonlinear optical activity (Tiwari and Rohiwal, 2019; Ishtiaq et al., 2020). These NPs are found in the periodic table groups II, III, IV, V, and VI. They include Gallium nitride (GaN), silicon, Cadmium telluride (CdTe), Indium phosphide (InP), zinc oxide (ZnO), indium arsenide (InAs), germanium, Cadmium sulfide (CdS), Gallium phosphide (GaP), zinc sulfide (ZnS) and cadmium selenide (CdSe) (Goodilin et al., 2019). Metallic nanoparticles are derived from metals, alkali metals, alkali earth metals and noble metals. These NPs possess great optical and excellent electrical properties, high surface area to volume ratio, small size and localised surface plasmon resonance (LSPR) (Khan et al., 2019). Examples of metallic nanoparticles are aluminosilicates, zinc (Zn), cerium (Ce), silica (Si), titanium dioxide (Ti), manganese (Mn), nickel (Ni), iron (Fe), aluminium (Al), gold (Au), copper (Cu) quantum dots and silver (Ag) (Loomba & Scarabelli, 2013; Khan et al., 2019).
Nanoparticles are swiftly transforming the field of nanotechnology with various applications owing to their special sizes and many other properties such as optical, chemical, mechanical and large surface area (Bhattacharyya et al., 2011; Khan et al., 2019). Among the variety of applications of nanoparticles are in wastewater treatment, prevention of environmental pollution, material remediation, mechanical and electronics, manufacturing, food industry, and medicine.
Nanoparticles that have attracted interest in wastewater treatment are carbon nanotubes, nanocomposites, zero-valent metal nanoparticles, and metal oxide nanoparticles (Oghyanous, 2021). This is due to their unique size and large surface area with catalytic, reactivity and adsorption activities (Schodek et al., 2009; Oghyanous, 2021). Similarly, NPs prevent environmental pollution due to their tunable shape and small size, which are suitable for sensing, preventing, and degrading environmental pollutants (Mehndiratta et al., 2013). Recently, nanocarriers have been used to deliver food additives to products without morphological alterations (Singh et al., 2017). In addition, nanoparticles were utilised as nanosensors to detect the presence of microbes and contaminants in food products (Bratovčić et al., 2015). Organic, inorganic and metallic nanoparticles have wide applications in drug delivery, management and prevention of diseases (Hassan et al., 2017; Rezaei et al., 2019). However, much attention has been directed to FDA-approved nanoparticles such as dendrimers, nanocrystals, liposomes, micelles, proteins, and polymers (Ventola, 2017).
The treatment of various neurological disorders has been difficult due to the blood-brain barrier (BBB) and different side effects (Warren, 2018). The BBB is a barrier between cerebral capillary blood and the brain’s interstitial fluid. It composes of basement membrane and endothelial cells, neuroglial membrane, and the projections of astrocytes. These three (3) components impede the entry of various substances into the central nervous system (Dotiwala et al., 2020). Most viruses, including HIV, can navigate BBB shortly after the primary infection (E. Rahimy et al., 2017). However, BBB impedes antiretroviral drug penetration, resulting in a poor outcome in treating HIV-associated neurocognitive disorders (HAND), as illustrated in Figure 2 (Osborne et al., 2020). The ability of nanoparticles to cross the BBB has been identified as a therapeutic approach to reducing CNS diseases (Cena and Jativa, 2018). Similarly, antiretroviral drugs (ARDs) coupled with nanoparticles have been identified as a solution for reducing HIV in the CNS due to their ability to improve the ARDs tissue distribution and bioavailability, leading to reduced adverse and toxic effects of HIV (Bowen et al., 2020).
FIGURE 2. Illustration of penetration of silver nanoparticles (AgNPs), HIV and Highly Active Antiretroviral Therapy (HAART) + AgNPs (AgNPs + HAART) through the blood-brain barrier (BBB) and their possible mechanism of action within the brain. This figure shows that HIV and infected macrophages traverse the BBB into the brain interstitial space to infect microglia, leading to HIV replication. Furthermore, HAART and AgNPs + HAART traverse the BBB to block or inhibit HIV replication. Lastly, AgNPs alone can also cross BBB into the brain. Within the brain, AgNPs get oxidised to release Ag+. The infected microglia, astrocyte, and Ag+ cause neuroinflammation and increased reactive oxygen species (ROS) generation, leading to neurodegeneration and HIV-associated neurocognitive disorders. While HAART inhibits HIV replication, it also causes neurodegeneration via neuroinflammation, ROS generation and autophagy. On the contrary, AgNPs + HAART show a better output by preventing HIV replication with reduced neurocognitive disorders. Adapted from (Lawal et al., 2022a; Lawal et al., 2021; Lawal et al., 2022b).
Various methods have been developed to synthesise NPs, but these approaches are majorly grouped into two (2): bottom-up and top-down processes. The bottom-up method involves building up atomic constituents, while the top-down approach requires breaking bulk materials into minute components (Rajput, 2015). These approaches are further divided into biological, chemical, and physical methods with subdivisions such as gas condensation, electrodeposition, sol-gel technique, vacuum deposition/vapourisation, mechanical attrition, chemical precipitation, and chemical vapour condensation (Rajput, 2015; Sakhare et al., 2022). Of these techniques, metallic and inorganic NPs are usually prepared by chemical methods, gas condensation, and the sol-gel process. Also, semiconductor NPs and nanocrystalline are typically synthesised using chemical vapour condensation, while ceramics and alloys are manufactured using mechanical attrition (Rajput, 2015). Furthermore, the synthesis of metallic nanoparticles is broadly classified into top-down approaches by utilising physical, biological or chemical methods and bottom-up approaches, as shown in Figure 3 (Thakkar et al., 2010).
Various approaches to synthesising AgNPs, such as physical, chemical, microemulsion, sonoelectrochemistry, photoinduced reduction, electrochemical synthetic, irradiation method, UV-initiated photoreduction, and microwave-assisted techniques were described (Iravani et al., 2014). The physical method of preparing AgNPs utilises physical energies with resultant AgNPs of narrow size dimensions. Also, this technique synthesises a large amount of AgNPs during a single run (Singh and Kaur, 2019). The physical approach involves lithography, laser ablation, gamma irradiation, evaporation-condensation and electrical irradiation, which is broadly employed in preparing AgNPs (Güzel and Erdal, 2018).
Biosynthesis of AgNPs is another method that has attracted significant research interest and wide application because they are eco-friendly, easy to produce, and cost-effective. Biosynthesis (green synthesis) involves using medicinal plant extracts, herbs, and microorganisms like fungi, bacteria, yeast and algae to prepare AgNPs (Güzel and Erdal, 2018). The ready availability of medicinal plants and various essential phytochemicals and functional constituents that can reduce the silver ions was described as another reason the green synthesis is receiving broad attention. In addition, most of these medicinal plants contain active compounds such as amino acids, saponins, polysaccharides, flavones, proteins, tannins, enzymes, terpenoids and vitamins (Güzel and Erdal, 2018).
Chemical reduction is the most widely applied in preparing silver nanoparticles. In this technique, the precursor (silver nitrate, AgNO3) is reduced to Ag0 by using reducing agents like sodium citrate, hydrogen, tollens, trisodium citrate, ascorbate, ethylene glycol-block copolymers, sodium borohydride (NaBH4), and N, N-dimethylformamide (DMF) (Iravani et al., 2014). Also, materials like sodium carboxyl methylcellulose (NaCMC), starch, polyvinyl pyrrolidone (PVP K 30), polysaccharides, bovine serum albumin, peptide, starch, and chitosan have been employed as the stabilising or capping agents in preparing AgNPs (Zewde et al., 2016; Patel et al., 2017). The production of metallic silver precedes the agglomeration of metallic silver into oligomeric clusters, and subsequently, the metallic silver nanoparticle is formed (Iravani et al., 2014). The reducing and stabilising agents play an essential function in the stability of the manufactured AgNPs and the shape and size of AgNPs (Iravani et al., 2014). It is important to note that chemical methods of preparing AgNPs have synthesised different shapes ranging from cylinders, wires, cubic, rods, triangular, bars, pyramidal, prisms and spherical (Gamboa et al., 2019).
Recent studies have utilised silver nitrate (AgNO3) and trisodium citrate to successfully synthesise the AgNPs (Lawal et al., 2021). Briefly, an aqueous solution of silver nitrate (AgNO3) was prepared from AgNO3 crystal. Then, a desired stock aqueous solution of trisodium citrate (TSC) was prepared from crystal trisodium citrate and used as a reducing and stabilising agent. Then, the synthesized AgNPs were characterized with various concentrations (from 0.5 to 2 M) of TSC and mixed with AgNO3. The solution was stirred continuously for 5 min at 90°C. The resultant solution of the mixtures was adjusted with concentrated NaOH at a pH of 10.5. A colour change from colourless to amber-yellow was used to indicate a successful AgNPs synthesis.
The first step is to prepare AgNPs using the chemical method, where the precursor will be silver nitrate and trisodium citrate as stabilising, reducing and capping agents. A small quantity of silver nitrate as low as 0.03 mol/dm3 will be mixed with 0.1 mol/dm3 of trisodium citrate and heated for 90 min.
The mixture will be centrifuged at 12,000–15,000 rpm for about 15–30 min, and the supernatant will be discarded. The mixture will then be redispersed in an aqueous solution and stored at pH 10.5 in NaOH. The colour change to an amber colour will indicate the formation of AgNPs.
After successfully synthesising AgNPs, the characterisation will follow using HR-TEM and HR-SEM for shape and size. Absorption peak by UV-vis spectroscopy. Energy dispersive X-ray analysis to examine the elemental composition. Raman Spectroscopy to show chemical structure, crystallinity and molecular interactions and zeta potential to show the surface charges.
Shortly after that, the appropriate amount of the antiretroviral drugs (powdered form) will be dissolved in the appropriate medium (water or alcohol, depending on the nature of the drugs) and mixed with the synthesised AgNPs. The mixture will be continuously stirred in ultra-sonication and centrifuge at 4,500 rpm for about 40–50 min at 40°C.
The nanoconjugate will then be evaluated for proper conjugation by UV-Vis spectroscopy. Fourier transform infrared spectroscopy will be used to examine the absorption and functional groups, while the elemental composition of the nanoconjugates will be determined by Energy dispersive X-ray. Also, Raman Spectroscopy to show chemical structure, crystallinity and molecular interactions (Lawal et al., 2021). More importantly, any retroviral drugs can be used with this new approach. This is based on the fact that AgNPs exhibit a large surface area to volume ratio, one of the essential features in the drug delivery system. For Example, Tenofovir disoproxil fumarate was coupled successfully with silver nanoparticles, and their effects were investigated in animal model experiments (Lawal et al., 2022a; Olojede et al., 2022). However, before considering loading any retroviral drugs using this new proposed approach, characterisation of the AgNPs to examine the morphology, shape, size, and elemental composition are all essential.
Silver is a fundamental and non-toxic element that forms the precursor of silver nanoparticles (AgNPs) with unique electrical and thermal properties (Chen and Schluesener, 2008). AgNPs are nanoparticles of sizes ranging from 1 to 100 nm on the nanoscale (Klaus et al., 1999). Silver nanoparticles represent one of the essential metallic nanoparticles with appealing characteristics that fuelled their wide application in biomedical sciences. AgNPs have been extensively used to diagnose, treat, and prevent diseases in nanomedicine. For example, AgNPs have received broad attention and great success in cancer detection and management (Zink et al., 2016).
Silver nanoparticles exhibit excellent physicochemical properties such as effective electrical conductivity, unique optical properties, potent thermal properties, and promising biological activities (Zhang et al., 2016a). These outstanding characteristics of AgNPs were ascribed to their large surface area to volume ratio, small size, and tunable shapes (Li et al., 2001; Sharma et al., 2009; Calderón-Jiménez et al., 2017; Ivanova et al., 2018). In addition, one of the optical properties of AgNPs is surface plasmon resonance (SPR) which entails the ability of AgNPs to receive and scatter light effectively. This process (SPR) occurs when electrons on the metal surface are excited and go through oscillation to absorb and disperse (Kim et al., 2007; Bansal et al., 2014; Keat et al., 2015). Interestingly, this property has been more significant in AgNPs than in other metals like copper and gold (Xia and Halas, 2005).
Silver nanoparticles are metallic nanoparticles ranging between 1 and 100 nm in size, with higher loading efficacy (Mahajan et al., 2012). Despite the benefits of silver nanoparticles, their toxicity is also well-documented (Lekamge et al., 2018; Ferdous and Nemmar, 2020). Studies have attributed AgNPs toxicity to one or both releases of silver ions in the cell or the method used in the AgNPs synthesis (Akter et al., 2018; Smith et al., 2018). The physiochemical properties of AgNPs (e.g., size, shape, concentration, agglomeration, or interaction with the biological system) are major contributors to their toxicity. These properties have been associated with mitochondrial dysfunction and oxidative injury (Akter et al., 2018). AgNPs toxicity mechanism is linked with the generation of excessive reactive oxygen species (ROS), cellular mitochondrial dysfunction, inflammatory response and cell apoptosis (Yu et al., 2020). Recently, a study reported that cardiac cells treated with AgNPs generate ROS and mitochondrial dysfunction, and mitochondrial ATP was reduced as implicated in metabolic disorders (Khan et al., 2021). Another study observed that neural cells can uptake AgNPs of 3–5 nm in size, as indicated by the expression of inflammatory genes and neurodegenerative disorders in murine brain astrocytes and microglia (Huang et al., 2015). AgNPs cross the BBB irrespective of the size and concentration to influence the brain cells, as observed after 28 days of 5 mg kg−1 BW AgNPs (20 and 200 nm) injection in the experimental Wistar rats (Tang et al., 2010). Interestingly, literature has reported that not all silvers are toxic. AgNPs ranging from 12 to 20 nm were not toxic in rats’ tissue. However, as the dose and concentration increased, harmful effects were observed (Sung et al., 2011; Smith et al., 2018).
AgNPs have received significant attention in diagnostics, drug delivery, the pharmaceutical industry, the food industry, cosmetics, nutraceuticals, and the manufacturing of biosensors (Chernousova and Epple, 2013). AgNPs are utilised in biomedical research to deliver therapeutic agents such as antiviral, antibacterial, antifungal, antidiabetic, anti-inflammatory, and antioxidant agents, as illustrated in Figure 4 (Alkaladi et al., 2014; Bedlovicova et al., 2020; Kwon and Koh, 2020). Of all the applications of silver nanoparticles, the antiviral, antidiabetic, anti-inflammatory and antioxidant properties are keys to alleviating HIV-associated neurocognitive disorders (HAND) and ART toxicity.
Conventional antiviral drugs have been used to manage viral infections, but some agents may lead to physiological toxicity due to systemic exposure (Jeevanandam et al., 2022). Recently, silver nanoparticles have demonstrated an inhibitory property against pathogenic microbes, including viruses and bacteria (Jeevanandam et al., 2022). Several biomedical research studies have reported silver nanoparticles’ ability to effectively manage the viral infection and antiviral resistance (Das et al., 2020; Ratan et al., 2021). Silver nanoparticles exhibit unique properties such as the large surface area to volume ratio, surface plasmon resonance, size and shape-controlled production, which give them an advantage for coupling therapeutic agents to their surface (Khan et al., 2019; Loiseau et al., 2019).
A study has reported that the antimicrobial activities of silver nanoparticles are due to the presence of positive Ag ions (Ag+) that inhibit the growth of microorganisms via interaction with DNA, thereby causing denaturation of DNA and interruption of cell division (Kumar et al., 2016). Silver nanoparticles are used as an antiviral agent against HIV-1, monkeypox virus, Hepatitis virus, influenza, herpes simplex virus and respiratory syncytial virus (Khandelwal et al., 2014).
Several studies have experimented with using AgNPs as an antiviral agent and their cell toxicity profile. An in vitro experiment showed that the non-cytotoxic concentration of AgNPs exerted antiviral activities against HIV-1 (Sun et al., 2005). Another study reported that AgNPs interact with HIV-1 by biding to glycoprotein 120 knobs in a size-dependent manner (Elechiguerra et al., 2005). The antiviral properties of AgNPs are reported to be size-dependent. Previous studies have shown that smaller AgNPs of sizes between 5–20 nm can inhibit the replication of HIV (Khandelwal et al., 2014; Suganya et al., 2015; Ratan et al., 2021). Furthermore, small-medium AgNPs sized between 1 and 10 nm act on HIV-1 via binding to the disulfide bond regions of the CD4 domain located on the gp120 glycoprotein of the viral envelope (Elechiguerra et al., 2005). This shows that the size of silver nanoparticles influences the potency of their antiviral property.
A recent study has reported the potential benefits of using AgNPs as a treatment against SARS-CoV-2 because the metallic aspect of the nanoparticles interferes with the structural protein of SARS-CoV-2, thereby inhibiting it from binding to genetic material and preventing its replication (Das et al., 2020; Almanza-Reyes et al., 2021).
AgNPs have been reported to demonstrate robust antidiabetic activity via effective inhibition against carbohydrate digestive and key enzymes of diabetes, including α-amylase and α-glucosidase (Balan et al., 2016). Furthermore, a study has reported that the AgNPs significantly inhibited non-enzymatic glycosylation compared with the aqueous leaf extract of Pouteria sapota (Prabhu et al., 2018). A similar study revealed that AgNPs possess antidiabetic activity by showing maximum inhibition of α-amylase at 78.84% and α-glucosidase at 58.86% at 100 μg/ml concentration (R and Scleeva P 2021). Several studies have reported that small-medium biosynthesised AgNPs control glycaemic index by significantly reducing the blood glucose levels in experimental rats (Agarwal et al., 2018; Alkaladi et al., 2014; S. Lawal et al., 2022b; Lawal et al., 2021). The mechanism of action of AgNPs on glycaemic control is linked with an increase in insulin secretion, which enhances hepatic glycogenesis and a significant increase in the expression of GLUT-2 levels in hepatic tissues (Alkaladi et al., 2014). Green synthesis of AgNPs with natural products such as medicinal plants has been evaluated for the potential antidiabetic activities and synergistic effects. Also, synthesised herbal-mediated AgNPs are the therapeutic target in treating type 2 diabetes due to their ability to inhibit protein tyrosine phosphatase 1B (PTP1B), which is the major negative regulator of the insulin signalling pathway (Shanker et al., 2017). Another study revealed Pterocarpus marsupium loaded with AgNPs was more effective for antidiabetic activity than Pterocarpus marsupium only (Bagyalakshmi and Haritha, 2017).
Recent studies have demonstrated that AgNPs alone or in combination with therapeutic agents have excellent anti-inflammatory activity (Fehaid et al., 2020; Tyavambiza et al., 2021). AgNPs possess unique properties, such as a large surface area to volume ratio, which offer them an advantage of blocking the inflammation-enhancers like cytokines and inflammation-assisting enzymes (Agarwal et al., 2019). Green synthesis of AgNPs from leaf extracts has demonstrated an anti-inflammatory action in both in vitro and in vivo experiments (Agarwal et al., 2019). The mechanism of action of AgNPs green synthesis is attributed to their ability to reduce inflammatory cytokines such as tumour necrosis factor (TNF-α), interleukin 6 (IL-6) and interleukin 1β (IL-1β) (David et al., 2014). Furthermore, antiretroviral drugs coupled with AgNPs have been reported to offer an advantage in reducing the inflammatory effects of the drugs via reducing the inflammatory cytokines produced during the metabolism of the drugs (S. Lawal et al., 2022a; Olojede et al., 2021).
Antioxidants play a major role in controlling oxidative reactions by balancing the free radicals which attack macromolecules (such as lipids, proteins and nucleic acids), thereby preventing cell damage (Bratovcic, 2020). The accumulation of reactive species in the body is linked with diverse chronic pathologies, such as diabetes mellitus and neurological disorders (Volpe et al., 2018). Literature has reported the antioxidant activity of AgNPs in vivo and in vitro experiments (Jadczak et al., 2020; Keshari et al., 2020). A study reported a significant increase in antioxidant activities by AgNPs compared with Vitamin C (Keshari et al., 2020). The mechanism by which AgNPs act as antioxidant agents is not fully understood. However, studies have demonstrated that AgNPs activate the synthesis of intracellular enzymes such as superoxide dismutase (SOD), superoxide reductases (SOR), catalase (CAT) and reduced glutathione (GSH) which prevent oxidative damage in the body cell and tissues (Bedlovicova et al., 2020; Lawal et al., 2021). Moreover, AgNPs inhibit the reactive oxygen species by scavenging the free radicals via a decrease in hydroxyl and superoxide radicals (Guntur et al., 2018; Bedlovicova et al., 2020).
A great feature of nanoparticles is their large surface area which can be affected by several factors, including their size (1–100 nm) and aggregation, thereby affecting their activity. Studies have shown that the effect/activity of AgNPs depends on their size. For example, a study showed that AgNPs of the smallest size (10 nm) were more toxic to cells than larger sizes of 20–80 nm (Ivask et al., 2014). AgNPs attenuated the morphological switch and biofilm formation of some opportunistic pathogenic yeasts in a size-dependent manner. However, the smallest (7 nm)-sized AgNPs caused the highest attenuation rate (Szerencsés et al., 2020). In terms of the nanoparticle delivery system, the particle size is the essential factor that influences AgNPs delivery into the body because the size affects AgNPs cellular uptake, transportation and accumulation (Wu et al., 2019). In this regard, Wu et al. demonstrated that AgNP entry into B16 cells is size and time-dependent. Five-nanometer (5 nm) AgNPs were detected at 30 min, while 20 and 50 nm were detected in the cytoplasm and nucleus after 2 h. On the contrary, 100 nm AgNPs were only observed in the nucleus 12 h after incubating them with B16 in vitro (Wu et al., 2019).
Furthermore, Wu et al. (2019) experiments also showed that while AgNPs of lesser size entered the cell cytoplasm and nucleus earlier than the larger sizes, the uptake efficiencies (partly measured by the rate of entry and exit of the AgNPs through the cell) were 40.3 ± 7.6%, 22.0 ± 1.5%, 52.3 ± 4.7%, and 76.2 ± 8.0% for 5, 20, 50, and 100 nm AgNPs, respectively at 12 h. At 24 h, the efficiency was 58.5% ± 8.5%, 34.2% ± 8.3%, 57.9% ± 2.5% and 66.1% ± 9.7% for 5, 20, 50 and 100 nm AgNPs, respectively. At 5 nm, the AgNPs were more toxic to the cells, while at 100 nm, the AgNPs might not be able to enter the cellular nucleus and cause the expected regulation of cellular functions.
In another research, Bélteky et al. (2021) concluded that although smaller particles might have greater biological activity, they might become ineffective soon as they exit the cell. In comparison, larger particles might have a long-lasting effect. Therefore, aiming for the smallest possible nanoparticles might not be the best option (Bélteky et al., 2021). In the same vein, studies have shown that nuclear pore complexes have diameters ∼20–50 nm (Wente, 2000; Fahrenkrog & Aebi, 2003) and that nanoparticles with <50 nm size can enter the nucleus while those <35 nm can pass through the blood-brain barrier (Dawson et al., 2009; Hackenberg et al., 2011). Furthermore, maximum uptake efficiency has been reported for nanoparticles of sizes 30–50 nm (Huang et al., 2010; Varela et al., 2012; Gliga et al., 2014), and AgNPs of size 20–30 nm also showed no adverse effects and organ toxicity in animal studies (Lara et al., 2010; Recordati et al., 2015). Lastly, our previous studies showed that AgNPs with size 20–35 nm successfully delivered antiretroviral drugs to the brain and could be used as an appropriate nanodelivery method that can alleviate the effect of prolonged use of antiretroviral medications (Lawal et al., 2022a; Lawal et al., 2022b). Thus, one can suggest that optimal uptake efficiency or delivery for AgNPs could be achieved at sizes between 20 and 50 nm.
Apart from size, concentration is another factor affecting the activities of AgNPs. The work by Lau et al. showed that bacterial viability decreased as the concentration of AgNPs increased (Lau et al., 2017). Also, the Graphene oxide (GO) silver nanoparticles composite (GO–Ag) concentrations of 0.1, 0.5, 1.0 and 1.5 μg/ml showed no antibacterial activity against Pseudomonas aeruginosa (P. aeruginosa) bacteria. Thus, AgNPs concentrations of 2.5 and 5.0 μg/ml, which caused a significant decrease in cellular viability, were considered optimal biocidal concentrations for P. aeruginosa (de Faria et al., 2014). In producing AgNPs, the concentration should be kept low because the low concentration can achieve a therapeutic effect with little to no toxicity to human and animal cells. This is because even at low concentrations (0.0035 μg/ml 20 nm AgNPs and 0.22 μg/ml 80 nm AgNPs), AgNPs were taken up by retinal cells and disrupted retinal structure (Söderstjerna et al., 2014). Furthermore, soil enzymes were inhibited at higher AgNPs concentrations (Mishra et al., 2021), while low concentrations (0.06–0.12 mM) enhanced Horseradish Peroxidase activity (Karim et al., 2012).
Apart from size, the shape of the nanoparticles also affects their delivery and biological activity. The spherical shape is mostly favoured (Ghiuță and Cristea, 2020; Lawal et al., 2022b).
Dating back to the 1980s, HIV has continued to exist as a global pandemic for nearly 40 years (Ash et al., 2021), affecting nearly 38 million people worldwide (Osborne et al., 2020). HIV is an infection that targets the body’s immune system, specifically the CD4 white blood cells, destroying these cells and weakening a person’s immunity against opportunistic infections (Vidya Vijayan et al., 2017). Significant progress has been made in the treatment of individuals living with HIV. However, the cure remains elusive to scientists and clinicians due to the yet-to-be-defined latent HIV reservoirs (stable accumulations of replication-competent forms of the virus in a myriad of anatomical sites throughout the body) (Ash et al., 2021; Blankson et al., 2002).
Some precise anatomical locations have been identified to harbour HIV infection. Those sites include the gut (oesophagus, duodenum, colon, etc.) and central nervous system (CNS), especially the brain (Chun et al., 2008; Wong and Yukl, 2016). The compartmentalisation of HIV within the brain allows for virus evolution with the possibility of evading clearance mechanisms and exhibiting a unique phenotype that only permits viral replication contingent upon immunosuppression (Bednar et al., 2015). The brain is not immune to HIV infection. Studies have identified that HIV invades the brain within 1–2 weeks of infection (Roberts et al., 2004; Valcour et al., 2012). In addition, the brain can subsequently serve as a sanctuary for ongoing HIV replication, even after systemic viral suppression has been achieved (Saylor et al., 2016). The perivascular macrophages and microglial cells are the productively infected cell types by HIV in vivo (Cosenza et al., 2002; Atluri et al., 2015). The neuro-invasion of HIV occurs both dependently and independently of a breakdown in the BBB through the frequent compromise of the BBB and infecting immune cells that can traffic into the brain by crossing the BBB, respectively (Osborne et al., 2020; Ash et al., 2021).
The BBB, as the name implies, is the barrier between the brain capillary blood and interstitial fluid of the brain (cells and other components that make up the brain tissue), ensuring a homeostatic environment (Banks, 2009; Dotiwala et al., 2020). The BBB came into existence in the late 19th century when Paul Ehrlich, a German Physician, noticed that a peripherally infused dye infiltrated all tissues except the brain and spinal cord. Thus, the BBB plays a vital role in controlling the influx and efflux of biological substances essential for the brain’s metabolic activity and neuronal function (Kadry et al., 2020). The BBB comprises capillary endothelial cells, basement membrane, neurological membrane, and glial podocyte (Dotiwala et al., 2020). The development of this barrier begins with angiogenesis, whereby pre-existing vessels, guided by vascular endothelial growth factor (VEGF), invade a developing neuro-ectoderm and give rise to new vessels that exhibit many BBB properties (Dotiwala et al., 2018). Unlike other endothelial cells, endothelial cells in the brain cavity present distinctive morphological, structural, and functional characteristics. These characteristics include the expression of tight junctions that seals the paracellular pathways between adjacent endothelial cells, permitting only a few selected types of substances between the cells. Other characteristics are the absence of fenestrations and lack of pinocytic activity, and expression of active transport mechanism (Ballabh et al., 2004; Dotiwala et al., 2018).
HIV habitually compromises the integrity of the BBB and infects the central nervous system in the early stages of infection (Osborne et al., 2020). In a study that used an in vitro BBB model, HIV infection of leukocytes caused an increase in the transmigration of HIV-infected cells across the BBB in response to the chemokine ligand 2 due to increased permeability, reduced tight junction proteins such as zonula occludens-1, claudin-1, and occludin in the BBB cells, and upregulation of matrix metalloproteinases-2 and -9 (Eugenin et al., 2006). Magnetic resonance imaging scans have revealed brain tissue alterations such as the expansion of the brain stem and third ventricle, loss of white matter integrity, and mild neurocognitive deficit within the first several months of CNS HIV infection (∼100 days) (Ragin et al., 2015). The persistent CNS-HIV infection and inflammation probably contribute to the development spectrum of neurologic or neurodegenerative diseases commonly referred to as HIV-associated neurocognitive disorders (HAND), which may affect more than 30% of people living with HIV regardless of virological suppression (Clifford and Ances, 2013). HAND can be categorised into three, each associated with an increasing level of impairment: 1) Asymptomatic neurocognitive impairment (30% prevalence)- shows HIV-associated impairment in cognitive function, but everyday functioning is not affected; 2) Mild neurocognitive disorder (20%–30% prevalence)- characterised by HIV-associated impairment in a cognitive function where interference in everyday functioning is displayed; and 3) HIV-associated dementia (2%–8% prevalence)- shows marked impairment in cognitive function, especially in learning of new information, information processing, and attention or concentration (Robertson et al., 2010; Eggers et al., 2017). Nonetheless, the incidence of HAND remains in the range of 20%–50% for people living with HIV (Eggers et al., 2017; Ash et al., 2021).
The blood-brain barrier (BBB) plays a vital role in protecting the brain from pathogens, toxins and other harmful blood components, thereby maintaining the homeostasis between the blood and central nervous system (Le Guennec et al., 2019). The presence of biological barriers such as the blood-brain barrier and blood-cerebrospinal fluid barrier in the central nervous system has been proven to allow the penetration of HIV and other viruses but impede the adequate penetration of certain antiretroviral drugs (Rahimy et al., 2017; Warren, 2018). The BBB is formed by the astrocyte, pericyte, and endothelial cells linked together by tight junction proteins, which restrict the entry of specific molecules into the brain cells (Ortiz et al., 2014). The tight junction is regulated by signalling factors from blood microvascular endothelial cells as well as astrocyte and pericytes (Keaney and Campbell, 2015). The essential nutrients, such as amino acids, nucleotides, and carbohydrates, cross the BBB into CNS through the transport system, such as carriers and receptors (Ghalamfarsa et al., 2016). Also, more giant molecules cross the BBB through active transport systems such as P-glycoprotein, multi-resistance-associated proteins, L-transporters, and concentrative nucleoside transporter (Donaghue et al., 2014). Alteration in the BBB and increases in its permeability have been strongly linked with pathological acute and chronic CNS diseases (Yang et al., 2015). Likewise, HIV penetration and its replication in the CNS have been associated with various neurological disorders, such as behavioural, motor, and cognitive impairments (Qosa et al., 2015). HIV infiltrates CNS by viral transcytosis across the BBB endothelium and infects the target cells. This infection leads to neuroinflammation and brain damage, causing neurological disorders, as illustrated in Figure 2 (Lorin et al., 2020).
In the past, HIV was considered a terminal illness, not until the development of Antiretroviral drugs (ARVDs). The availability of ARVDs to HIV patients has allowed them to live normally (Vance, 2010; Costagliola and AIDS, 2014). Over 40 ARVDs have been approved for use as of 2017 by the United States Food and Drug Administration (FDA). While the developing of new compounds has been slow for over a decade, many traditional compounds have made it to phase III clinical trials (Zhang, 2018). In the early years of HIV drug development, the available compounds could reduce viral load and increase CD4+ count, but a major challenge included drug toxicity (Vaidya et al., 2016; Zhang, 2018).
Treating HIV with ARVDs requires lifelong drug administration, predisposing the patients to develop certain pathologies, including neurological diseases, as listed in Table 1 (Bhatia and Chow, 2016). HIV-AIDS leads to progressive neurodegeneration of the immune and nervous systems with significant economic and health burdens (Ahmadu et al., 2022). HIV targets the central nervous system (CNS), where it replicates and may persist for a long among untreated seropositive patients (Varatharajan and Thomas, 2009). The prevalence of neurocognitive impairment associated with HIV varies between 25% and 70%, depending on the profile of the study population and the neurocognitive impairment (NCI) definition (Heaton et al., 2011; Wright et al., 2015; Santos et al., 2019).
ARVDs, which have a high capacity to penetrate the CNS, would serve as a promising treatment strategy to control HIV replication while reducing the risk of developing NCI (Santos et al., 2019). CNS penetration-effectiveness score (CPE Score) is used to assess the capacity of ARVDs to penetrate the CNS, inhibiting viral replication. Therefore, a high CPE score favours a reduction in CSF HIV RNA (Letendre et al., 2008; Cusini et al., 2013). Although a high CPE has been associated with reduced CNS HIV RNA replication (Letendre et al., 2008; Cusini et al., 2013), some authors have found a negative association or no association between CPE scores and NCI (Marra et al., 2009; Simioni et al., 2010; Caniglia et al., 2014; Baker et al., 2015). A negative association between CPE scores and NCI has been attributed to the neurotoxic effect of some ARVDs (Robertson et al., 2012). Despite the systemic effects of ARVDs, there is an increased presence of HIV in the CNS. The increased presence might be due to the inefficiency of ARVDs in crossing the BBB (Cunningham et al., 2000; Ene et al., 2011). Besides, the efficiency of ARVDs is only determined by plasma HIV RNA level (Varatharajan and Thomas, 2009; Ene et al., 2011).
The development and introduction of HAART have led to a significant reduction in neurological comorbidity associated with AIDS (Ganau et al., 2012; Sacktor et al., 2016). Although some of these diseases can result from HIV infection associated with prolonged inflammation, there is evidence that ARVDs toxicity can also contribute to the development of these comorbidities (Bertrand et al., 2016). Several side effects have been linked to ARVDs use, including peripheral neuropathy and mental disorders (Chen et al., 2013; Margolis et al., 2014). For instance, there is evidence of inflammation and neurotoxicity following non-nucleoside reverse transcriptase inhibitors (NNRTIs) use (Diaz-Delfin et al., 2011).
Also, HAART has been linked with mitochondrial damage and subsequently increases the risk of neuropathy and neuroinflammation (Lin et al., 2018). Chronic administration of HAART to HIV-positive patients has been reported to cause neuroinflammation, changes in astrocyte mitochondrial membrane and mitochondrial ROS production (Cohen et al., 2017). The continuous use of HAART to prevent a viral rebound in people living with HIV and diabetes causes neuroinflammation with detrimental effects on CNS astrocytes. This contributes significantly to neuropathologies aetiology (Yang et al., 2015; Cohen et al., 2017; Yirong). Excessive production of pro-inflammatory cytokines during neuroinflammation has been implicated in cognitive deficits and anxiety disorders (Charlton and Starkey, 2018; Li et al., 2019). Interestingly, in the post-HAART era, people living with HIV have experienced an improvement in motor skills and verbal fluency, but executive functions and anxiety-like behaviour remain dominant impairments (Heaton et al., 2011; Checa et al., 2020). In addition, the prevalence of anxiety and depression among the patient receiving HAART remains high (Rabkin et al., 2000). The most used components of HAART (Efavirenz and Tenofovir) have been reported to cross the blood-brain barrier, causing mitochondrial dysfunction and some neurological-related adverse effects like depression and anxiety (Chen et al., 2019; Checa et al., 2020). In contrast, another study that investigated the effect of HAART (Efavirenz and Ritonavir) on HIV-negative patients found no significant effect of the drugs on functional connectivity or cerebral blood flow. The authors concluded that functional connectivity and cerebral blood flow in HIV-positive patients receiving HAART are not due to the drugs but to the virus (Brier et al., 2015). In addition, HIV patients also have a higher incidence of cerebrovascular diseases when compared to non-infected patients (Ovbiagele and Nath, 2011; Benjamin et al., 2012). HIV-infected patients with stroke often exhibit different characteristics from others with lesser symptoms, e.g., high blood pressure (Chow et al., 2012). In Wistar rats, Bakshi and colleagues showed that Nevirapine had a neuroprotective effect against ischemic stroke in middle cerebral artery occlusion (MCAO) (Pathakala et al., 2016).
Furthermore, a recent study found that the administration of Nevirapine increased the number of line crossings in the open field test (OFT), resulting in locomotor activities in experimental mice (Ahmadu et al., 2022). An increase in locomotor activity in the context of the OFT suggests anxiolytic effects (Iorjiim et al., 2020). Similarly, a previous study found that Nevirapine administration reversed MCAO-induced reduction in open-field exploration (Pathakala et al., 2016). In another study where cART (Emtricitabine + Tenofovir disoproxil fumarate) was administered for 8 weeks, no changes in exploratory activity were observed (Zulu et al., 2021). A recent preclinical study found that administering Nevirapine increased other gross behaviour, including piloerection, the Straub-tail phenomenon and grooming (Ahmadu et al., 2022). There is evidence of delayed Efavirenz neurotoxicity (Sarma et al., 2022). Sarma and colleagues recently studied an HIV patient placed on HAART (Tenofovir 300 mg, Lamivudine 150 mg and Efavirenz 600 mg) for about 3 years. When they applied Naranjo’s algorithm, they found a score of 6, indicating Efavirenz’s toxicity (Naranjo et al., 1981; Sarma et al., 2022). They later considered Efavirenz-induced encephalopathy and cerebellar ataxia with HIV-related polyneuropathy as possible diagnosis (Sarma et al., 2022). The underlying mechanism of action of the neurotoxic effect of Efavirenz is unclear. However, oxidative stress has been suggested to contribute to its adverse effects (Lanman et al., 2021). Also, sleep dysfunction has been associated with ARVDs in rodents. A recent study by Ahmadu and colleagues showed that the administration of Nevirapine delayed sleep onset and decreased sleep duration in diazepam-induced sleep in mice (Ahmadu et al., 2022).
Moreover, there is evidence of cognitive deficits following ARVD administration. A previous study by Bakshi and colleagues found that Nevirapine administration reversed middle cerebral artery occlusion (MCAO)-induced working memory deficits in rats (Pathakala et al., 2016). In another study where cART (Emtricitabine + Tenofovir disoproxil fumarate) was administered for 8 weeks, it was observed that cART increased escape latencies in the Morris water maze test for spatial memory, indicating cognitive impairment (Zulu et al., 2021). A recent study by Lawal and colleagues found no effect of HAART (a fixed-dose combination of Efavirenz, emtricitabine, and tenofovir disoproxil fumarate) administration on spatial and working memory in rats (Lawal et al., 2021). In another study by Lawal and colleagues, they also found that the administration of Tenofovir disoproxil fumarate (TDF) alone did not alter working memory in rats as tested in Y-maze. However, TDF administration worsened the working memory of diabetic rats (S. Lawal et al., 2022b).
In addition, several neurochemical changes have been observed following ARVD administration. In a preclinical study of ischemic stroke, Nevirapine administration offered neuroprotective effects by reducing hippocampal levels of acetylcholinesterase (AChE) and glutamate following MCAO in rats (Pathakala et al., 2016). Zulu et al. (2018) administered either Nevirapine or Tenofovir disoproxil fumarate for 8 weeks and observed that Nevirapine or Tenofovir disoproxil fumarate administered separately increased the number of GFAP-positive cells, IL-1β concentration and TNF-α expression in the hippocampi of mice. However, Nevirapine but not Tenofovir disoproxil fumarate reduced BDNF expression (Zulu et al., 2018). In another study by Zulu et al. (2021), it was also found that prolonged cART treatment induced oxidative damage by increasing 4-hydroxynonenal concentration in the hippocampus of mice. Also, treatment with cART promoted autophagy via increased expression levels of LC3B-II and reduced hippocampal neuroplasticity via downregulation of BDNF and synaptophysin (Zulu et al., 2021). Nevirapine administration also offered antioxidant effects by increasing brain levels of catalase and GPx following MCAO in rats (Pathakala et al., 2016). In contrast, it was observed that while TDF administration did not alter the Malondialdehyde (MDA, a marker of lipid peroxidation) and interleukin-1 beta (IL-1β, a pro-inflammatory cytokine) in the prefrontal cortex of normal rats, TDF increased MDA and IL-1β levels in the prefrontal cortex of diabetic rats when compared to rats that did not receive TDF (S. Lawal et al., 2022a). In the same study, an ultrastructural assessment of the prefrontal cortex revealed that diabetic rats that received TDF had reduced GFAP-positive astrocytes and increased mitochondrial damage (S. Lawal et al., 2022b).
The blood-brain barrier is an effective mechanism of the brain to regulate the influx and efflux of substances, hence protecting the brain from toxins and pathogens reaching the periphery (Qosa et al., 2015). The BBB consists of endothelial cells, astrocytes and pericytes, which work together to interact with the neurons. The endothelial cells are composed of tight junctions that control the passage of molecules in and out of the neurons (Daneman and Prat, 2015; Keaney and Campbell, 2015). Several acute and chronic diseases can impair the BBB, allowing immune cells into the CNS, inducing inflammation, neuronal apoptosis, and the influx of other pathogens. Dysregulated influx of molecules in the CNS can lead to the development of Alzheimer’s Disease or Stroke (Obermeier et al., 2013).
Of all the diseases associated with HIV, cerebrovascular diseases are the leading cause of death (Aldaz et al., 2011; Cima et al., 2016). A study that examined the role of NNRTIs on BBB integrity found that out of all the NNRTIs tested, only Efavirenz altered endothelial integrity by downregulating claudin-5 expression. Furthermore, they showed that this impaired BBB exacerbated tissue damage in stroke (Bertrand et al., 2016). Thus, evidence suggests that the choice of ARVDs is essential to avoid neurotoxicity, eventually leading to a decline in treatment outcomes (Bertrand et al., 2016).
Despite a relationship between CPE scores and NCI, a high CPE does not necessarily correlate with reduced neurocognitive impairment (NCI) (Peluso et al., 2012). While some authors found that a high CPE score was associated with reduced NCI (Ciccarelli et al., 2013; Vassallo et al., 2014; Carvalhal et al., 2016), others found no association, including a negative association (Marra et al., 2009; Simioni et al., 2010; Caniglia et al., 2014; Baker et al., 2015) between CPE scores and NCI.
Nevirapine belongs to the class of non-nucleoside reverse transcriptase inhibitors (NNRTIs) of ARVDs and has the highest CPE score (Antinori et al., 2005; Nwogu et al., 2016). Nevirapine acts by inhibiting the reverse transcriptase enzyme responsible for the reverse transcription of HIV RNA to DNA (Ahmadu et al., 2022).
The application of nanoparticles (NPs) to diagnose, treat and control various diseases represents a tremendous advancement in nanotechnology and nanomedicine (Mohamed et al., 2022). Notably, a nanoparticle drug delivery system (NDDS) is a remarkable advancement in nanomedicine that employs nano-size particles to diagnose and control the delivery of various curative agents to the target cell/tissue (Patra et al., 2018). The nanoparticle delivery system ensures targeted and sustained delivery, enhanced drug bioavailability, and improved drug pharmaco-efficiency, as illustrated in Figure 5. Thus, its road application in delivering immunotherapeutic, chemotherapeutic, and biological agents in managing diseases (Ventola, 2017; Patra et al., 2018; Naidu et al., 2021; Mohamed et al., 2022).
Growing research evidence has revealed the blood-brain barrier (BBB) as a significant obstacle in managing neurological disorders because it hinders major neurological therapeutic agents (Zhu et al., 2019). As a result of this, various classes of the US Food and Drug Administration (FDA) approved NPs have been investigated in animal studies and clinical trials for their ability to cross the BBB and ensure therapeutic efficiency, as shown in Figure 6 (Bobo et al., 2016; Wen et al., 2017; Van Norman, 2019). Of NP classes, some polymeric- and lipid-based NPs have received approval from FDA, some are undergoing approval processes, and others are available for clinical use (Sainz et al., 2015; Bobo et al., 2016; Ventola, 2017). In clinical investigations, examples of the approved liposome-based nano-drug are liposomal vincristine for general use, protectant alfa for respiratory distress syndrome, liposomal cytarabine for lymphomatous meningitis, and doxorubicin HCl liposome injection for managing Karposi’s sarcoma (Bobo et al., 2016; Caster et al., 2017; Ventola, 2017). Other liposome-based nanodrugs are liposomal amphoteric B and B complex for managing fungal infections and liposomal morphine sulphate for managing postoperative analgesia. In addition, liposomal Irinotecan, liposomal verteporfin and liposomal daunorubicin were approved for managing pancreatic cancer, ocular histoplasmosis, and acute myelogenous leukaemia (Ventola, 2017).
Previously, Chen et al. (2016) formulated transferrin-based liposomes to deliver α-Mangostin across the blood-brain barrier and improve the existence of cerebral cortical neurons in rats. This formulated α-Mangostin was delineated as a possible therapeutic agent in managing Alzheimer’s disease (Chen et al., 2016). More so, a liposome-modified agent was intravenously delivered and crossed BBB to selectively bind Amyloid-β plaque deposits in APP/PSEN1 Transgenic Mice (Tanifum et al., 2012).
Polymer micelles represent other FDA-approved NPs for drug delivery purposes. In recent times, synthetic polymers such as poly (N-isopropyl, bio-absorbable polymers, dendritic polymers, acrylamide), poly (2-hydroxyethyl methacrylate), biodegradable, and poly (ethylenimine) have received great attention in drug delivery (Sung and Kim, 2020). Likewise, several natural polymers that include dextrin, polysaccharides, hyaluronic acid, chitosan, poly (lactic acid), arginine, and poly (glycolic acid) have been employed to load and deliver therapeutic agents (Sung and Kim, 2020; Damiri et al., 2022). Polymeric NPs are characterised by targeted delivery, sustained release, and enhanced drug effectiveness (Patel et al., 2013). More so, advancement in formulating vehicle-enhanced transportation of nanoparticle-loaded drugs across the biological barrier like the BBB is gaining wide priority and has provided a clue on the targeted delivery to various parts of the brain (Cacciatore et al., 2016; Teleanu et al., 2018).
Dendrimer nanoparticles represent other NPs currently attracting attention in drug delivery systems due to their high solubility, multivalency, chemical stability, electrostatic interactivity, uniform size, and low cytotoxicity (Abbasi et al., 2014). Recently, a poly (amido) amine dendrimer conjugated streptavidin and poly-amidoamine dendrimers were formulated and investigated on murine and mouse models. These conjugates demonstrated an excellent permeation into the central nervous system to deliver the therapeutic agent in managing neurological disorders (Srinageshwar et al., 2017; Moscariello et al., 2018). Moreso, remarkable results have been obtained in managing Parkinson’s and Alzheimer’s diseases by using dendrimer tesaglitazar composite to induce a phenotype interaction of glia cells and improved β-amyloid phagocytosis (DeRidder et al., 2021).
Interestingly, inorganic/metallic nanoparticles have been widely investigated for their unique properties, which gives them an edge to be used in delivering therapeutic agents across BBB and ensuring effective management of neurological disorders. Tisch et al. (2013) reported fast, cost-effective and dependable biomarkers for Parkinson’s disease (PD) and Alzheimer’s disease (AD) using functionalised carbon nanotubes and gold nanoparticle-based sensors. In a similar study, Sonawane and colleagues fabricated protein-capped metal NPs (PC-CdS and PC-Fe2O4) and explored their potential therapeutics against protein accumulation in AD (Sonawane et al., 2019). Their findings, especially with PC-CdS, revealed excellent inhibition of Tau aggregation and suggested a potent AD therapeutic delivery.
The limitations and the circumstances surrounding the conventional therapeutics of neurological disorders such as epilepsy, AD, PD and Huntington’s disease have directed the research attention and biomedical application to the usage of metallic NPs to detect and manage these disorders (Haque and Patra, 2022). Of these classes of NPs, gold NPs bioconjugates have been extensively used to deliver drugs to the brain in attempts to manage neurological disorders (Mirsadeghi et al., 2015; Soursou et al., 2015; Sivanesan and Rajeshkumar, 2019; Talebpour and Ghahghaei, 2020; Xu et al., 2021). Also, Parveen et al. (2022) reported an exceptional therapeutic efficacy of biosynthesised silver NPs (AgNPs) against PD and AD. However, despite their unique characteristics, there is a paucity of attention and application to AgNPs in neurological therapeutics delivery.
The upsurge in neurological disorders is becoming a public health issue of grave concern and requires biomedical researchers’ attention (WHO, 2007; Naqvi et al., 2020). Previously, many therapeutic modalities have been investigated and employed, but the effectiveness of these treatment regimens could not provide a solution to these neurodegenerative disorders (Ghosh and De, 2020). The central nervous system-associated biological barriers that limit the efficacy of these therapeutic modalities include; the blood-cerebrospinal fluid barrier and BBB (Naqvi et al., 2020). These barriers regulate the influx and efflux of drugs and substances in and out of the brain (De Boer et al., 2003; Löscher and Potschka, 2005). For this reason, the selectively permeable nature of the BBB has been described as a significant setback in the treatment of neurodegenerative disorders (Lawal et al., 2018).
As the research into the effective therapeutic modality for neurodegenerative disorders intensifies, the nanoparticle drug delivery system (NDDS) is now receiving broad research attention. Growing research evidence has shown that nanoparticle-loaded drugs have higher drug bioavailability, better penetration of biological barriers, better distribution, and higher metabolism (Kaur et al., 2016). AgNPs serve as a candidate to deliver therapeutic agents through BBB to reach the brain effectively. AgNPs can effectively deliver therapeutic agents through BBB to the brain based on their size, sustained drug delivery, and physicochemical antioxidant, anti-inflammatory and antimicrobial properties (Al-Obaidi et al., 2018; Jiang et al., 2018; Karthik et al., 2018; Selvan et al., 2018).
Previously, the effects of biosynthesised AgNPs against amyloid build-ups were explored, and significant inhibition of amyloid fibril build-ups was reported, suggesting an effective treatment modality in managing amyloidosis diseases (Dehvari and Ghahghaei, 2018). The mechanistic understanding of the pathogenesis of most neurological disorders was linked to the upregulation of the amyloid-beta (Aβ) aggregation. Aβ represents peptide amino acids, the derivatives of the amyloid precursor proteins (APP), and their upregulation causes neurological disorders like AD (Dong et al., 2012). We hypothesise that since AgNPs have been shown to inhibit Aβ aggregation, they could be useful in delivering therapeutic agents across BBB and cater for neurological disorders.
Despite many attempts to use metallic NPs to deliver drugs to the CNS with outstanding results, the safety of these metallic NPs, especially AgNPs, has raised concerns as experts advise about their potential toxicities. The cause of the AgNPs’ toxicity could be the ease at which they undergo oxidation by oxygen and other substances in the body system or environment, leading to the release of silver ion (Ag+), a well-known toxic ion. Ag + has been described as a significant cause of AgNPs’ toxicity (Bar-Ilan et al., 2009; McShan et al., 2014). Also, previous studies have revealed that the toxicity of AgNPs is size-dependent. For example, more cytotoxicity was observed at the smaller size (2–4 nm) of AgNPs in macrophage cells compared to medium size (5–7 nm) and larger size (20–40 nm) (Yen et al., 2009). Also, embryonic zebrafish exposed to smaller-sized (3 nm) AgNPs displayed higher malformation and mortality than those that were exposed to a larger size (10–50 nm) (Bar-Ilan et al., 2009). In addition, a study revealed that smaller size (5 nm) AgNPs were cytotoxic to 4 cell lines, SGC-7901, HepG2, A549 and MCF-7, than 20–50 nm AgNPs (Liu et al., 2010).
Another factor contributing to the AgNPs’ toxicity is their shape. Based on existing evidence, spherical shape AgNPs are mainly non-cytotoxic. A study that compared wire AgNP and spherical AgNPs in A549 cells showed no deleterious effect of spherical AgNP but cytotoxicity of wire AgNP (Mahmuda Akter et al., 2018; Stoehr et al., 2011). The route of administration of the synthesised AgNPs represents another factor responsible for various toxicity profiles of AgNPs. There are diverse routes of NPs’ entry into the body system. NPs can be inhaled, ingested, transdermally applied, intraperitoneally or intravenously injected (De Matteis, 2017). However, studies have shown that intraperitoneal, intravenous and subcutaneous administration of AgNPs allows AgNPs to gain direct access to systemic circulation and has thus been the most adopted route in AgNP-based therapeutic vehicles (Ferdous and Nemmar, 2020). Notably, the characterisation of AgNPs is an essential step that involves the evaluation of the size, shape, forms, charge, ion release, level of aggregation, functional groups, particle dissolution, and the elemental composition of the synthesised AgNPs. Several studies have ascribed the proper biological activity of AgNPs to the characterisation (Froggett et al., 2014; Wei et al., 2015; Zhang H., 2016; Kim et al., 2017).
The mechanism for the AgNPs toxicity has alluded to the ability of Ag + to generate free radicals in the brain, which serves as the primary target of metallic NPs upon entering the systemic circulation with potential neurotoxicity through apoptosis, inflammation, and free radicals radical-induced oxidative stress (Song et al., 2016). The summary of the factors that could minimize the toxicity profile of silver nanoparticles is illustrated in Figure 7.
Despite the development of effective ART, the neurocognitive impairment associated with HIV remains a challenge, and there is still a dearth of information regarding the neuropharmacological effects of ARVDs (Ahmadu et al., 2022). Furthermore, many side effects of ARVDs, such as sleep disturbance or prolonged wakefulness, reported as feedback from clinicians are mainly unpublished. Similarly, there is underreporting of patients’ experiences following ARVDs administration (Ahmadu et al., 2022), which challenges the development of targeted HIV/AIDS therapies. It is imperative to manage and reduce neurological complications of AIDS in HIV patients. Although there is an ongoing development of better therapeutic strategies to improve HIV treatment, therapies targeted at neurological disorders are insufficient. Therefore, nanoparticle-targeted drug delivery could reduce the neurological effects of ARVDs and improve their efficiency. AgNPs have been widely used in drug delivery, but their use has been subjected to scientific deliberations due to their perceived toxicity.
We hypothesise that the toxicity of AgNPs could be minimised by using appropriate reducing and stabilising agents such as trisodium citrate to reduce silver ion Ag + to ground state Ag0 during the synthesis. More so, usage of medium size, spherical-shaped AgNPs is encouraged in AgNPs-based drug delivery to the brain due to their ability to deliver therapeutic agents across BBB. In addition, characterisation and functionalisation of the synthesised AgNPs are required during the drug delivery approach. In addition, understanding the pharmacokinetics and cellular uptake of AgNPs as well as how the properties of AgNPs affect the pharmacokinetics of the therapeutic agents would be a major breakthrough in drug delivery. Putting all these factors in place would minimise toxicity and enhance the usage of AgNPs in delivering therapeutic agents across the BBB to the targeted brain tissue and could cater for the HIV-associated neurocognitive disorders and neurotoxic effects of ARVDs.
SKL: Conceptualisation, methodology, investigation, formal analysis, writing original draft, and funding acquisition. SOO: Conceptualisation, writing review and editing. OSF: Methodology, validation, and writing original draft. OSA: Methodology, validation, investigation, formal analysis, and writing original draft. MM: Conceptualisation, writing review and editing. SOS: Writing review and editing formal analysis, and validation. ECSN: Resources and visualisation, writing review and editing and supervision. COR: Resources, supervision, project administration, writing review and editing and funding acquisition. OOA: Conceptualisation, writing review, editing and supervision.
This work was partially supported by the College of Health Sciences, University of KwaZulu-Natal, as part of a PhD scholarship awarded to the corresponding author.
The authors appreciate the Biomedical Research Unit (BRU) staff and the school of chemistry and physics, the natural product laboratory of the University of KwaZulu-Natal, for their technical support.
The authors declare that the research was conducted in the absence of any commercial or financial relationships that could be construed as a potential conflict of interest.
All claims expressed in this article are solely those of the authors and do not necessarily represent those of their affiliated organizations, or those of the publisher, the editors and the reviewers. Any product that may be evaluated in this article, or claim that may be made by its manufacturer, is not guaranteed or endorsed by the publisher.
Abbasi, E., Aval, S. F., Akbarzadeh, A., Milani, M., Nasrabadi, H. T., Joo, S. W., et al. (2014). Dendrimers: Synthesis, applications, and properties. Nanoscale Res. Lett. 9 (1), 247. doi:10.1186/1556-276X-9-247
Agarwal, H., Kumar, S. V., and Rajeshkumar, S. (2018). Antidiabetic effect of silver nanoparticles synthesized using lemongrass (cymbopogon citratus) through conventional heating and microwave irradiation approach. J. Microbiol. Biotechnol. Food Sci. 7 (3), 371–376. doi:10.15414/jmbfs.2018.7.4.371-376
Agarwal, H., Nakara, A., and Shanmugam, V. K. (2019). Anti-inflammatory mechanism of various metal and metal oxide nanoparticles synthesized using plant extracts: A review. Biomed. Pharmacother. 109, 2561–2572. doi:10.1016/j.biopha.2018.11.116
Ahmadu, P. U., Victor, E., and Ameh, F. S. (2022). Studies on some neuropharmacological properties of Nevirapine in mice. IBRO Neurosci. Rep. 12, 12–19. doi:10.1016/j.ibneur.2021.11.002
Akter, M., Sikder, M. T., Rahman, M. M., Ullah, A. A., Hossain, K. F. B., Banik, S., et al. (2018). A systematic review on silver nanoparticles-induced cytotoxicity: Physicochemical properties and perspectives. J. Adv. Res. 9, 1–16. doi:10.1016/j.jare.2017.10.008
Al-Obaidi, H., Kalgudi, R., and Zariwala, M. G. (2018). Fabrication of inhaled hybrid silver/ciprofloxacin nanoparticles with synergetic effect against Pseudomonas aeruginosa. Eur. J. Pharm. Biopharm. 128, 27–35. doi:10.1016/j.ejpb.2018.04.006
Aldaz, P., Moreno-Iribas, C., Egues, N., Irisarri, F., Floristan, Y., Sola-Boneta, J., et al. (2011). Mortality by causes in HIV-infected adults: Comparison with the general population. BMC Public Health 11, 300. doi:10.1186/1471-2458-11-300
Alkaladi, A., Abdelazim, A. M., and Afifi, M. (2014). Antidiabetic activity of zinc oxide and silver nanoparticles on streptozotocin-induced diabetic rats. Int. J. Mol. Sci. 15 (2), 2015–2023. doi:10.3390/ijms15022015
Almanza-Reyes, H., Moreno, S., Plascencia-Lopez, I., Alvarado-Vera, M., Patron-Romero, L., Borrego, B., et al. (2021). Evaluation of silver nanoparticles for the prevention of SARS-CoV-2 infection in health workers: In vitro and in vivo. PLoS One 16 (8), e0256401. doi:10.1371/journal.pone.0256401
Antinori, A., Perno, C. F., Giancola, M. L., Forbici, F., Ippolito, G., Hoetelmans, R. M., et al. (2005). Efficacy of cerebrospinal fluid (CSF)-penetrating antiretroviral drugs against HIV in the neurological compartment: Different patterns of phenotypic resistance in CSF and plasma. Clin. Infect. Dis. 41 (12), 1787–1793. doi:10.1086/498310
Anu Mary Ealia, S., and Saravanakumar, M. P. (2017). A review on the classification, characterisation, synthesis of nanoparticles and their application. IOP Conf. Ser. Mat. Sci. Eng. 263, 032019. doi:10.1088/1757-899x/263/3/032019
Ash, M. K., Al-Harthi, L., and Schneider, J. R. (2021). HIV in the brain: Identifying viral reservoirs and addressing the challenges of an HIV cure. Vaccines (Basel) 9 (8), 867. doi:10.3390/vaccines9080867
Astefanei, A., Nunez, O., and Galceran, M. T. (2015). Characterisation and determination of fullerenes: A critical review. Anal. Chim. Acta 882, 1–21. doi:10.1016/j.aca.2015.03.025
Atluri, V. S., Hidalgo, M., Samikkannu, T., Kurapati, K. R., Jayant, R. D., Sagar, V., et al. (2015). Effect of human immunodeficiency virus on blood-brain barrier integrity and function: An update. Front. Cell. Neurosci. 9, 212. doi:10.3389/fncel.2015.00212
Bagyalakshmi, J., and Haritha, H. (2017). Green synthesis and characterization of silver nanoparticles using Pterocarpus marsupium and assessment of its in vitro Antidiabetic activity. Am. J. Adv. Drug Deliv. 1, 1. doi:10.21767/2321-547x.1000019
Baker, L. M., Paul, R. H., Heaps-Woodruff, J. M., Chang, J. Y., Ortega, M., Margolin, Z., et al. (2015). The effect of central nervous system penetration effectiveness of highly active antiretroviral therapy on neuropsychological performance and neuroimaging in HIV infected individuals. J. Neuroimmune Pharmacol. 10 (3), 487–492. doi:10.1007/s11481-015-9610-4
Balan, K., Qing, W., Wang, Y., Liu, X., Palvannan, T., Wang, Y., et al. (2016). Antidiabetic activity of silver nanoparticles from green synthesis using Lonicera japonica leaf extract. RSC Adv. 6 (46), 40162–40168. doi:10.1039/c5ra24391b
Ballabh, P., Braun, A., and Nedergaard, M. (2004). The blood-brain barrier: An overview: Structure, regulation, and clinical implications. Neurobiol. Dis. 16 (1), 1–13. doi:10.1016/j.nbd.2003.12.016
Banks, W. A. (2009). Characteristics of compounds that cross the blood-brain barrier. BMC Neurol. 9 (1), S3. doi:10.1186/1471-2377-9-S1-S3
Bansal, A., Sekhon, J. S., and Verma, S. (2014). Scattering efficiency and LSPR tunability of bimetallic Ag, Au, and Cu nanoparticles. Plasmonics 9 (1), 143–150. doi:10.1007/s11468-013-9607-x
Bar‐Ilan, O., Albrecht, R. M., Fako, V. E., and Furgeson, D. Y. (2009). Toxicity assessments of multisized gold and silver nanoparticles in zebrafish embryos. Small 5 (16), 1897–1910. doi:10.1002/smll.200801716
Bayda, S., Adeel, M., Tuccinardi, T., Cordani, M., and Rizzolio, F. (2019). The history of nanoscience and nanotechnology: From chemical-physical applications to nanomedicine. Molecules 25 (1), E112. doi:10.3390/molecules25010112
Bedlovicova, Z., Strapac, I., Balaz, M., and Salayova, A. (2020). A brief overview on antioxidant activity determination of silver nanoparticles. Molecules 25 (14), E3191. doi:10.3390/molecules25143191
Bednar, M. M., Sturdevant, C. B., Tompkins, L. A., Arrildt, K. T., Dukhovlinova, E., Kincer, L. P., et al. (2015). Compartmentalization, viral evolution, and viral latency of HIV in the CNS. Curr. HIV/AIDS Rep. 12 (2), 262–271. doi:10.1007/s11904-015-0265-9
Bélteky, P., Rónavári, A., Zakupszky, D., Boka, E., Igaz, N., Szerencsés, B., et al. (2021). Are smaller nanoparticles always better? Understanding the biological effect of size-dependent silver nanoparticle aggregation under biorelevant conditions. Int. J. Nanomedicine 16, 3021–3040. doi:10.2147/IJN.S304138
Benelmekki, M. (2015). “An introduction to nanoparticles and nanotechnology,” in Designing hybrid nanoparticles. (Williston, VT: Morgan & Claypool Publishers).
Benjamin, L. A., Bryer, A., Emsley, H. C. A., Khoo, S., Solomon, T., and Connor, M. D. (2012). HIV infection and stroke: Current perspectives and future directions. Lancet. Neurol. 11 (10), 878–890. doi:10.1016/s1474-4422(12)70205-3
Bertrand, L., Dygert, L., and Toborek, M. (2016). Antiretroviral treatment with efavirenz disrupts the blood-brain barrier integrity and increases stroke severity. Sci. Rep. 6, 39738. doi:10.1038/srep39738
Bhardwaj, P., Singh, B., and Behera, S. P. (2021). Green approaches for nanoparticle synthesis: Emerging trends, Nanomaterials. 1, 167–193.
Bhattacharyya, A., Datta, P., Chaudhuri, P., Barik, B., Blankson, J. N., Persaud, D., et al. (2011). The challenge of viral reservoirs in HIV-1 infection. Annu. Rev. Med. 53, 557–593. doi:10.1146/annurev.med.53.082901.104024
Bhatia, N. S., and Chow, F. C. (2016). Neurologic Complications in Treated HIV-1 Infection. Curr. Neurol Neurosci Rep.16 (7), 62.
Blankson, J. N., Persaud, D., and Siliciano, R. F. (2022). The challenge of viral reservoirs in HIV-1 infection. Annu. Rev. Med. 53, 557–593.
Bobo, D., Robinson, K. J., Islam, J., Thurecht, K. J., and Corrie, S. R. (2016). Nanoparticle-based medicines: A review of FDA-approved materials and clinical trials to date. Pharm. Res. 33 (10), 2373–2387. doi:10.1007/s11095-016-1958-5
Bowen, A., Sweeney, E. E., and Fernandes, R. (2020). Nanoparticle-based immunoengineered approaches for combating HIV. Front. Immunol. 11, 789. doi:10.3389/fimmu.2020.00789
Bratovcic, A. (2020). Antioxidant enzymes and their role in preventing cell damage. Act. Scie. Nutr. 4 (3), 01–07. doi:10.31080/asnh.2020.04.0659
Bratovčić, A., Odobašić, A., Ćatić, S., and Šestan, I. (2015). Application of polymer nanocomposite materials in food packaging. Croat. J. Food Sci. Technol. 7 (2), 86–94. doi:10.17508/cjfst.2015.7.2.06
Brier, M. R., Wu, Q., Tanenbaum, A. B., Westerhaus, E. T., Kharasch, E. D., and Ances, B. M. (2015). Effect of HAART on brain organization and function in HIV-negative subjects. J. Neuroimmune Pharmacol. 10 (4), 517–521. doi:10.1007/s11481-015-9634-9
Cacciatore, I., Ciulla, M., Fornasari, E., Marinelli, L., and Di Stefano, A. (2016). Solid lipid nanoparticles as a drug delivery system for the treatment of neurodegenerative diseases. Expert Opin. Drug Deliv. 13 (8), 1121–1131. doi:10.1080/17425247.2016.1178237
Calderón-Jiménez, B., Johnson, M. E., Montoro Bustos, A. R., Murphy, K. E., Winchester, M. R., and Vega Baudrit, J. R. (2017). Silver nanoparticles: Technological advances, societal impacts, and metrological challenges. Front. Chem. 5, 6. doi:10.3389/fchem.2017.00006
Caniglia, E. C., Cain, L. E., Justice, A., Tate, J., Logan, R., Sabin, C., et al. (2014). Antiretroviral penetration into the CNS and incidence of AIDS-defining neurologic conditions. Neurology 83 (2), 134–141. doi:10.1212/WNL.0000000000000564
Carvalhal, A., Gill, M. J., Letendre, S. L., Rachlis, A., Bekele, T., Raboud, J., et al. (2016). Central nervous system penetration effectiveness of antiretroviral drugs and neuropsychological impairment in the Ontario HIV Treatment Network Cohort Study. J. Neurovirol. 22 (3), 349–357. doi:10.1007/s13365-015-0404-5
Caster, J. M., Patel, A. N., Zhang, T., and Wang, A. (2017). Investigational nanomedicines in 2016: A review of nanotherapeutics currently undergoing clinical trials. WIREs Nanomed. Nanobiotechnol. 9 (1), e1416. doi:10.1002/wnan.1416
Cena, V., and Jativa, P. (2018). Nanoparticle crossing of blood-brain barrier: A road to new therapeutic approaches to central nervous system diseases. Nanomedicine (Lond) 13 (13), 1513–1516. doi:10.2217/nnm-2018-0139
Charlton, S. G., and Starkey, N. J. (2018). Memory for everyday driving. Transp. Res. Part F Traffic Psychol. Behav. 57, 129–138. doi:10.1016/j.trf.2017.06.007
Checa, A., Castillo, A., Camacho, M., Tapia, W., Hernandez, I., and Teran, E. (2020). Depression is associated with efavirenz-containing treatments in newly antiretroviral therapy initiated HIV patients in Ecuador. AIDS Res. Ther. 17 (1), 47. doi:10.1186/s12981-020-00303-1
Chen, H., Clifford, D. B., Deng, L., Wu, K., Lee, A. J., Bosch, R. J., et al. (2013). Peripheral neuropathy in ART-experienced patients: Prevalence and risk factors. J. Neurovirol. 19 (6), 557–564. doi:10.1007/s13365-013-0216-4
Chen, X., and Schluesener, H. J. (2008). Nanosilver: A nanoproduct in medical application. Toxicol. Lett. 176 (1), 1–12. doi:10.1016/j.toxlet.2007.10.004
Chen, Y. F., Stampley, J. E., Irving, B. A., and Dugas, T. R. (2019). Chronic nucleoside reverse transcriptase inhibitors disrupt mitochondrial homeostasis and promote premature endothelial senescence. Toxicol. Sci. 172 (2), 445–456. doi:10.1093/toxsci/kfz203
Chen, Z.-L., Huang, M., Wang, X.-R., Fu, J., Han, M., Shen, Y.-Q., et al. (2016). Transferrin-modified liposome promotes α-mangostin to penetrate the blood–brain barrier. Nanomedicine 12 (2), 421–430. doi:10.1016/j.nano.2015.10.021
Cheon, J., Chan, W., and Zuhorn, I. (2019). The future of nanotechnology: Cross-disciplined progress to improve health and medicine. Acc. Chem. Res. 52 (9), 2405. doi:10.1021/acs.accounts.9b00423
Chernousova, S., and Epple, M. (2013). Silver as antibacterial agent: Ion, nanoparticle, and metal. Angew. Chem. Int. Ed. Engl. 52 (6), 1636–1653. doi:10.1002/anie.201205923
Chow, F. C., Regan, S., Feske, S., Meigs, J. B., Grinspoon, S. K., and Triant, V. A. (2012). Comparison of ischemic stroke incidence in HIV-infected and non-HIV-infected patients in a US health care system. J. Acquir. Immune Defic. Syndr. 60 (4), 351–358. doi:10.1097/QAI.0b013e31825c7f24
Chun, T. W., Nickle, D. C., Justement, J. S., Meyers, J. H., Roby, G., Hallahan, C. W., et al. (2008). Persistence of HIV in gut-associated lymphoid tissue despite long-term antiretroviral therapy. J. Infect. Dis. 197 (5), 714–720. doi:10.1086/527324
Chundi, N., Das, B., Kolli, C. S. R., Madiwala, S. P., Koppoju, S., Ramasamy, E., et al. (2020). Single layer hollow MgF2 nanoparticles as high-performance omnidirectional broadband antireflective coating for solar application. Sol. Energy Mater. Sol. Cells 1, 110680. doi:10.1016/j.solmat.2020.110680
Ciccarelli, N., Fabbiani, M., Colafigli, M., Trecarichi, E. M., Silveri, M. C., Cauda, R., et al. (2013). Revised central nervous system neuropenetration-effectiveness score is associated with cognitive disorders in HIV-infected patients with controlled plasma viraemia. Antivir. Ther. 18 (2), 153–160. doi:10.3851/IMP2560
Cima, M., Parker, R. D., Ahmed, Y., Cook, S., Dykema, S., Dukes, K., et al. (2016). Cause of death in HIV-infected patients in South Carolina (2005-2013). Int. J. STD AIDS 27 (1), 25–32. doi:10.1177/0956462415571970
Clifford, D. B., and Ances, B. M. (2013). HIV-associated neurocognitive disorder. Lancet. Infect. Dis. 13 (11), 976–986. doi:10.1016/s1473-3099(13)70269-x
Cohen, J., D'Agostino, L., Wilson, J., Tuzer, F., and Torres, C. (2017). Astrocyte senescence and metabolic changes in response to HIV antiretroviral therapy drugs. Front. Aging Neurosci. 9, 281. doi:10.3389/fnagi.2017.00281
Cosenza, M. A., Zhao, M. L., Si, Q., and Lee, S. C. (2002). Human brain parenchymal microglia express CD14 and CD45 and are productively infected by HIV-1 in HIV-1 encephalitis. Brain Pathol. 12 (4), 442–455. doi:10.1111/j.1750-3639.2002.tb00461.x
Costagliola, D. J. C. O. i. H.AIDS (2014). Demographics of HIV and aging. Curr. Opin. HIV AIDS 9 (4), 294–301. doi:10.1097/coh.0000000000000076
Cunningham, P. H., Smith, D. G., Satchell, C., Cooper, D. A., and Brew, B. (2000). Evidence for independent development of resistance to HIV-1 reverse transcriptase inhibitors in the cerebrospinal fluid. AIDS 14 (13), 1949–1954. doi:10.1097/00002030-200009080-00010
Cusini, A., Vernazza, P. L., Yerly, S., Decosterd, L. A., Ledergerber, B., Fux, C. A., et al. (2013). Higher CNS penetration-effectiveness of long-term combination antiretroviral therapy is associated with better HIV-1 viral suppression in cerebrospinal fluid. J. Acquir. Immune Defic. Syndr. 62 (1), 28–35. doi:10.1097/QAI.0b013e318274e2b0
Damiri, F., Kommineni, N., Ebhodaghe, S. O., Bulusu, R., Jyothi, V. G. S., Sayed, A. A., et al. (2022). Microneedle-based natural polysaccharide for drug delivery systems (DDS): Progress and challenges. Pharmaceuticals 15 (2), 190. doi:10.3390/ph15020190
Daneman, R., and Prat, A. (2015). The blood-brain barrier. Cold Spring Harb. Perspect. Biol. 7 (1), a020412. doi:10.1101/cshperspect.a020412
Das, C., Paul, S. S., Saha, A., Singh, T., Saha, A., Im, J., et al. (2020). Silver-based nanomaterials as therapeutic agents against coronaviruses: A review. Int. J. Nanomedicine 15, 9301–9315. doi:10.2147/IJN.S280976
David, L., Moldovan, B., Vulcu, A., Olenic, L., Perde-Schrepler, M., Fischer-Fodor, E., et al. (2014). Green synthesis, characterization and anti-inflammatory activity of silver nanoparticles using European black elderberry fruits extract. Colloids Surf. B Biointerfaces 122, 767–777. doi:10.1016/j.colsurfb.2014.08.018
Dawson, K. A., Salvati, A., and Lynch, I. (2009). Nanotoxicology: Nanoparticles reconstruct lipids. Nat. Nanotechnol. 4 (2), 84–85. doi:10.1038/nnano.2008.426
De Boer, A., Van Der Sandt, I., and Gaillard, P. (2003). The role of drug transporters at the blood-brain barrier. Annu. Rev. Pharmacol. Toxicol. 43 (1), 629–656. doi:10.1146/annurev.pharmtox.43.100901.140204
de Faria, A. F., Martinez, D. S. T., Meira, S. M. M., de Moraes, A. C. M., Brandelli, A., Filho, A. G. S., et al. (2014). Anti-adhesion and antibacterial activity of silver nanoparticles supported on graphene oxide sheets. Colloids Surf. B Biointerfaces 113, 115–124. doi:10.1016/j.colsurfb.2013.08.006
De Matteis, V. (2017). Exposure to inorganic nanoparticles: Ro.utes of entry. Immune Response, Biodistribution and. Vitro/In Vivo.
Dehvari, M., and Ghahghaei, A. (2018). The effect of green synthesis silver nanoparticles (AgNPs) from Pulicaria undulata on the amyloid formation in α-lactalbumin and the chaperon action of α-casein. Int. J. Biol. Macromol. 108, 1128–1139. doi:10.1016/j.ijbiomac.2017.12.040
DeRidder, L., Sharma, A., Liaw, K., Sharma, R., John, J., Kannan, S., et al. (2021). Dendrimer–tesaglitazar conjugate induces a phenotype shift of microglia and enhances β-amyloid phagocytosis. Nanoscale 13 (2), 939–952. doi:10.1039/d0nr05958g
Diaz-Delfin, J., del Mar Gutierrez, M., Gallego-Escuredo, J. M., Domingo, J. C., Gracia Mateo, M., Villarroya, F., et al. (2011). Effects of nevirapine and efavirenz on human adipocyte differentiation, gene expression, and release of adipokines and cytokines. Antivir. Res. 91 (2), 112–119. doi:10.1016/j.antiviral.2011.04.018
Donaghue, I. E., Tam, R., Sefton, M. V., and Shoichet, M. S. J. J. o. C. R. (2014). Cell and biomolecule delivery for tissue repair and regeneration in the central nervous system. J. Control. Release 190, 219–227. doi:10.1016/j.jconrel.2014.05.040
Dong, S., Duan, Y., Hu, Y., and Zhao, Z. (2012). Advances in the pathogenesis of alzheimer’s disease: A re-evaluation of amyloid cascade hypothesis. Transl. Neurodegener. 1 (1), 18–12. doi:10.1186/2047-9158-1-18
Dotiwala, A. K., McCausland, C., and Samra, N. S. (2018). Anatomy, head and neck, blood brain barrier Florida, USA: In: StatPearls. StatPearls Publishing, Treasure Island.
Dotiwala, A. K., McCausland, C., and Samra, N. S. (2020). Anatomy, head and neck, blood brain barrier. StatPearls [Internet].
Eggers, C., Arendt, G., Hahn, K., Husstedt, I. W., Maschke, M., Neuen-Jacob, E., et al. (2017). HIV-1-associated neurocognitive disorder: Epidemiology, pathogenesis, diagnosis, and treatment. J. Neurol. 264 (8), 1715–1727. doi:10.1007/s00415-017-8503-2
Elechiguerra, J. L., Burt, J. L., Morones, J. R., Camacho-Bragado, A., Gao, X., Lara, H. H., et al. (2005). Interaction of silver nanoparticles with HIV-1. J. Nanobiotechnology 3, 6. doi:10.1186/1477-3155-3-6
Ene, L., Duiculescu, D., and Ruta, S. M. (2011). How much do antiretroviral drugs penetrate into the central nervous system? J. Med. Life 4 (4), 432–439.
Eugenin, E. A., Osiecki, K., Lopez, L., Goldstein, H., Calderon, T. M., and Berman, J. W. (2006). CCL2/monocyte chemoattractant protein-1 mediates enhanced transmigration of human immunodeficiency virus (HIV)-infected leukocytes across the blood-brain barrier: A potential mechanism of HIV-CNS invasion and NeuroAIDS. J. Neurosci. 26 (4), 1098–1106. doi:10.1523/JNEUROSCI.3863-05.2006
Fahrenkrog, B., and Aebi, U. (2003). The nuclear pore complex: Nucleocytoplasmic transport and beyond. Nat. Rev. Mol. Cell Biol. 4 (10), 757–766. doi:10.1038/nrm1230
Fehaid, A., Fujii, R., Sato, T., and Taniguchi, A. (2020). Silver nanoparticles affect the inflammatory response in a lung epithelial cell line. Open Biotechnol. J. 14 (1), 113–123. doi:10.2174/1874070702014010113
Ferdous, Z., and Nemmar, A. (2020). Health impact of silver nanoparticles: A review of the biodistribution and toxicity following various routes of exposure. Int. J. Mol. Sci. 21 (7), 2375. doi:10.3390/ijms21072375
Froggett, S. J., Clancy, S. F., Boverhof, D. R., and Canady, R. A. (2014). A review and perspective of existing research on the release of nanomaterials from solid nanocomposites. Part. Fibre Toxicol. 11 (1), 17–28. doi:10.1186/1743-8977-11-17
Gamboa, S., Rojas, E., Martínez, V., and Vega-Baudrit, J. (2019). Synthesis and characterization of silver nanoparticles and their application as an antibacterial agent. Int. J. Biosens. Bioelectron. 5, 166–173. doi:10.15406/ijbsbe.2019.05.00172
Ganau, M., Prisco, L., Pescador, D., and Ganau, L. (2012). Challenging new targets for CNS-HIV infection. Front. Neurol. 3, 43. doi:10.3389/fneur.2012.00043
Garcia-Pinel, B., Porras-Alcala, C., Ortega-Rodriguez, A., Sarabia, F., Prados, J., Melguizo, C., et al. (2019). Lipid-based nanoparticles: Application and recent advances in cancer treatment. Nanomater. (Basel) 9 (4), E638. doi:10.3390/nano9040638
Ghalamfarsa, G., Hojjat-Farsangi, M., Mohammadnia-Afrouzi, M., Anvari, E., Farhadi, S., Yousefi, M., et al. (2016). Application of nanomedicine for crossing the blood-brain barrier: Theranostic opportunities in multiple sclerosis. J. Immunotoxicol. 13 (5), 603–619. doi:10.3109/1547691X.2016.1159264
Ghiuță, I., and Cristea, D. (2020). “Silver nanoparticles for delivery purposes,” in Nanoengineered biomaterials for advanced drug delivery (Elsevier), 347–371. doi:10.1016/B978-0-08-102985-5.00015-2
Ghosh, P., and De, P. (2020). Modulation of amyloid protein fibrillation by synthetic polymers: Recent advances in the context of neurodegenerative diseases. ACS Appl. Bio Mat. 3 (10), 6598–6625. doi:10.1021/acsabm.0c01021
Gliga, A. R., Skoglund, S., Odnevall Wallinder, I., Fadeel, B., and Karlsson, H. L. (2014). Size- dependent cytotoxicity of silver nanoparticles in human lung cells: The role of cellular uptake, agglomeration and Ag release. Part. Fibre Toxicol. 11 (1), 11. doi:10.1186/1743-8977-11-11
Goodilin, E. A., Weiss, P. S., and Gogotsi, Y. (2019). Nanotechnology facets of the periodic table of elements. ACS Nano 13 (10), 10879–10886. doi:10.1021/acsnano.9b06998
Guntur, S. R., Kumar, N. S., Hegde, M. M., and Dirisala, V. R. (2018). In vitro studies of the antimicrobial and free-radical scavenging potentials of silver nanoparticles biosynthesized from the extract of desmostachya bipinnata. Anal. Chem. Insights 13, 1. doi:10.1177/1177390118782877
Güzel, R., and Erdal, G. (2018). Synthesis of silver nanoparticles. London, United Kingdom: IntechOpen doi:10.5772/intechopen.74372
Hackenberg, S., Scherzed, A., Kessler, M., Hummel, S., Technau, A., Froelich, K., et al. (2011). Silver nanoparticles: Evaluation of DNA damage, toxicity and functional impairment in human mesenchymal stem cells. Toxicol. Lett. 201 (1), 27–33. doi:10.1016/j.toxlet.2010.12.001
Haque, S., and Patra, C. R. (2022). “Metal nanoparticles for neurodegenerative diseases,” in Nanomedical drug delivery for neurodegenerative diseases (Elsevier), 183–206.
Hassan, S., Prakash, G., Ozturk, A. B., Saghazadeh, S., Sohail, M. F., Seo, J., et al. (2017). Evolution and clinical translation of drug delivery nanomaterials. Nano today 15, 91–106. doi:10.1016/j.nantod.2017.06.008
Heaton, R. K., Franklin, D. R., Ellis, R. J., McCutchan, J. A., Letendre, S. L., Leblanc, S., et al. (2011). HIV-Associated neurocognitive disorders before and during the era of combination antiretroviral therapy: Differences in rates, nature, and predictors. J. Neurovirol. 17 (1), 3–16. doi:10.1007/s13365-010-0006-1
Hosseini, M., Haji-Fatahaliha, M., Jadidi-Niaragh, F., Majidi, J., and Yousefi, M. (2016). The use of nanoparticles as a promising therapeutic approach in cancer immunotherapy. Artif. Cells Nanomed. Biotechnol. 44 (4), 1051–1061. doi:10.3109/21691401.2014.998830
Huang, C. L., Hsiao, I. L., Lin, H. C., Wang, C. F., Huang, Y. J., and Chuang, C. Y. (2015). Silver nanoparticles affect on gene expression of inflammatory and neurodegenerative responses in mouse brain neural cells. Environ. Res. 136, 253–263. doi:10.1016/j.envres.2014.11.006
Huang, J., Bu, L., Xie, J., Chen, K., Cheng, Z., Li, X., et al. (2010). Effects of nanoparticle size on cellular uptake and liver MRI with polyvinylpyrrolidone-coated iron oxide nanoparticles. ACS Nano 4 (12), 7151–7160. doi:10.1021/nn101643u
Iorjiim, W. M., Omale, S., Etuh, M. A., Bagu, G. D., Ogwu, S. O., Gyang, S. S., et al. (2020). EFVb-HAART increases mortality, locomotor deficits and reduces reproductive capacity in Drosophila melanogaster. J. Adv. biol. biotechnol.23 (1), 26–38. doi:10.9734/JABB/2020/v23i130136
Iravani, S., Korbekandi, H., Mirmohammadi, S. V., and Zolfaghari, B. (2014). Synthesis of silver nanoparticles: Chemical, physical and biological methods. Res. Pharm. Sci. 9 (6), 385–406.
Ishtiaq, M., al-Rashida, M., Alharthy, R. D., and Hameed, A. (2020). “Ionic liquid–based colloidal nanoparticles: Applications in organic synthesis,” in Metal nanoparticles for drug delivery and diagnostic applications (Elsevier), 279–299.
Ivanova, N., Gugleva, V., Dobreva, M., Pehlivanov, I., Stefanov, S., and Andonova, V. (2018). “Silver nanoparticles as multi-functional drug delivery systems,” in Nanomedicines (IntechOpen.
Ivask, A., Kurvet, I., Kasemets, K., Blinova, I., Aruoja, V., Suppi, S., et al. (2014). Size-dependent toxicity of silver nanoparticles to bacteria, yeast, algae, Crustaceans and mammalian cells in vitro. PLoS ONE 9 (7), e102108. doi:10.1371/journal.pone.0102108
Jadczak, P., Kulpa, D., Drozd, R., Przewodowski, W., and Przewodowska, A. (2020). Effect of AuNPs and AgNPs on the antioxidant system and antioxidant activity of lavender (lavandula angustifolia mill.) from in vitro cultures. Molecules 25 (23), E5511. doi:10.3390/molecules25235511
Jeevanandam, J., Krishnan, S., Hii, Y. S., Pan, S., Chan, Y. S., Acquah, C., et al. (2022). Synthesis approach-dependent antiviral properties of silver nanoparticles and nanocomposites. J. Nanostructure Chem. 1, 809–831. doi:10.1007/s40097-021-00465-y
Jiang, Q., Yu, S., Li, X., Ma, C., and Li, A. (2018). Evaluation of local anesthetic effects of Lidocaine-Ibuprofen ionic liquid stabilized silver nanoparticles in Male Swiss mice. J. Photochem. Photobiol. B 178, 367–370. doi:10.1016/j.jphotobiol.2017.11.028
Kadry, H., Noorani, B., and Cucullo, L. (2020). A blood-brain barrier overview on structure, function, impairment, and biomarkers of integrity. Fluids Barriers CNS 17 (1), 69. doi:10.1186/s12987-020-00230-3
Karim, Z., Adnan, R., and Ansari, M. S. (2012). Low concentration of silver nanoparticles not only enhances the activity of horseradish peroxidase but alter the structure also. PLoS ONE 7 (7), e41422. doi:10.1371/journal.pone.0041422
Karthik, C., Manukumar, H., Ananda, A., Nagashree, S., Rakesh, K., Mallesha, L., et al. (2018). Synthesis of novel benzodioxane midst piperazine moiety decorated chitosan silver nanoparticle against biohazard pathogens and as potential anti-inflammatory candidate: A molecular docking studies. Int. J. Biol. Macromol. 108, 489–502. doi:10.1016/j.ijbiomac.2017.12.045
Kaur, P., Garg, T., Rath, G., and Goyal, A. K. (2016). In situ nasal gel drug delivery: A novel approach for brain targeting through the mucosal membrane. Artif. Cells Nanomed. Biotechnol. 44 (4), 1167–1176. doi:10.3109/21691401.2015.1012260
Keaney, J., and Campbell, M. (2015). The dynamic blood-brain barrier. FEBS J. 282 (21), 4067–4079. doi:10.1111/febs.13412
Keat, C., Aziz, A., Eid, A., and Elmarzugi, N. (2015). Biosynthesis of nanoparticles and silver nanoparticles. Bioresour. Bioprocess. 2 (1), 47. doi:10.1186/s40643-015-0076-2
Keshari, A. K., Srivastava, R., Singh, P., Yadav, V. B., and Nath, G. (2020). Antioxidant and antibacterial activity of silver nanoparticles synthesized by Cestrum nocturnum. J. Ayurveda Integr. Med. 11 (1), 37–44. doi:10.1016/j.jaim.2017.11.003
Khan, A. A., Alanazi, A. M., Alsaif, N., Al-Anazi, M., Sayed, A. Y. A., and Bhat, M. A. (2021). Potential cytotoxicity of silver nanoparticles: Stimulation of autophagy and mitochondrial dysfunction in cardiac cells. Saudi J. Biol. Sci. 28 (5), 2762–2771. doi:10.1016/j.sjbs.2021.03.021
Khan, I., Saeed, K., and Khan, I. (2019). Nanoparticles: Properties, applications and toxicities. Arabian J. Chem. 12 (7), 908–931. doi:10.1016/j.arabjc.2017.05.011
Khandelwal, N., Kaur, G., Kumar, N., and Tiwari, A. (2014). Application of silver nanoparticles in viral inhibition: A new hope for antivirals. Dig. J. Nanomater. Biostructures (DJNB) 9 (1).
Kim, D. H., Park, J. C., Jeon, G. E., Kim, C. S., and Seo, J. H. (2017). Effect of the size and shape of silver nanoparticles on bacterial growth and metabolism by monitoring optical density and fluorescence intensity. Biotechnol. Bioprocess Eng. 22 (2), 210–217. doi:10.1007/s12257-016-0641-3
Kim, J. S., Kuk, E., Yu, K. N., Kim, J.-H., Park, S. J., Lee, H. J., et al. (2007). Antimicrobial effects of silver nanoparticles. Nanomedicine 3 (1), 95–101. doi:10.1016/j.nano.2006.12.001
Klaus, T., Joerger, R., Olsson, E., and Granqvist, C.-G. (1999). Silver-based crystalline nanoparticles, microbially fabricated. Proc. Natl. Acad. Sci. U. S. A. 96 (24), 13611–13614. doi:10.1073/pnas.96.24.13611
Kumar, N., Das, S., Jyoti, A., and Kaushik, S. (2016). Synergistic effect of silver nanoparticles with doxycycline against Klebsiella pneumoniae. Int. J. Pharm. Pharm. Sci. 8 (7), 183–186.
Kwon, H. S., and Koh, S. H. (2020). Neuroinflammation in neurodegenerative disorders: The roles of microglia and astrocytes. Transl. Neurodegener. 9 (1), 42. doi:10.1186/s40035-020-00221-2
Lanman, T., Letendre, S., Ma, Q., Bang, A., and Ellis, R. (2021). CNS neurotoxicity of antiretrovirals. J. Neuroimmune Pharmacol. 16 (1), 130–143. doi:10.1007/s11481-019-09886-7
Lara, H. H., Ayala-Nuñez, N. V., Ixtepan-Turrent, L., and Rodriguez-Padilla, C. (2010). PVP-coated silver nanoparticles block the transmission of cell-free and cell-associated HIV-1 in human cervical culture. J. Nanobiotechnology 8 (1), 15. doi:10.1186/1477-3155-8-15
Lau, C. P., Abdul-Wahab, M. F., Jaafar, J., Chan, G. F., and Abdul Rashid, N. A. (2017). Toxic effect of high concentration of sonochemically synthesized polyvinylpyrrolidone-coated silver nanoparticles on Citrobacter sp. A1 and Enterococcus sp. C1. J. Microbiol. Immunol. Infect. 50 (4), 427–434. doi:10.1016/j.jmii.2015.08.004
Lawal, M., Olotu, F. A., and Soliman, M. E. (2018). Across the blood-brain barrier: Neurotherapeutic screening and characterization of naringenin as a novel CRMP-2 inhibitor in the treatment of Alzheimer's disease using bioinformatics and computational tools. Comput. Biol. Med. 98, 168–177. doi:10.1016/j.compbiomed.2018.05.012
Lawal, S. K., Olojede, S. O., Dare, A., Faborode, O. S., Naidu, E. C. S., Rennie, C. O., et al. (2021). Silver nanoparticles conjugate Attenuates highly active antiretroviral therapy-induced hippocampal Nissl substance and cognitive deficits in diabetic rats. J. Diabetes Res. 2021, 2118538. doi:10.1155/2021/2118538
Lawal, S. K., Olojede, S. O., Dare, A., Faborode, O. S., Sulaiman, S. O., Naidu, E. C., et al. (2022b). Highly active antiretroviral therapy-silver nanoparticle conjugate interacts with neuronal and glial cells and alleviates anxiety-like behaviour in streptozotocin-induced diabetic rats. IBRO Neurosci. Rep. 1, 57–68. doi:10.1016/j.ibneur.2022.06.003
Lawal, S., Olojede, S. O., Sulaiman, S. O., Aladeyelu, O. S., Moodley, R., Naidu, E. C. S., et al. (2022a). Tenofovir-silver nanoparticles conjugate ameliorates neurocognitive disorders and protects ultrastructural and cytoarchitectonic properties of the prefrontal cortex in diabetic rats. Bosn. J. Basic Med. Sci. 1, 569–579. doi:10.17305/bjbms.2021.6699
Le Guennec, L., Coureuil, M., Nassif, X., and Bourdoulous, S. (2019). Strategies used by bacterial pathogens to cross the blood–brain barrier. Cell. Microbiol. 22 (1), e13132. doi:10.1111/cmi.13132
Lekamge, S., Miranda, A. F., Abraham, A., Li, V., Shukla, R., Bansal, V., et al. (2018). The toxicity of silver nanoparticles (AgNPs) to three freshwater invertebrates with different life strategies: Hydra vulgaris, Daphnia carinata, and paratya australiensis. Front. Environ. Sci. 6. doi:10.3389/fenvs.2018.00152
Letendre, S., Marquie-Beck, J., Capparelli, E., Best, B., Clifford, D., Collier, A. C., et al. (2008). Validation of the CNS Penetration-Effectiveness rank for quantifying antiretroviral penetration into the central nervous system. Arch. Neurol. 65 (1), 65–70. doi:10.1001/archneurol.2007.31
Li, J., Zhou, Y., Du, G., Qin, X., and Gao, L. (2019). Integration of transcriptomics and network analysis deciphers the mechanisms of baicalein in improving learning and memory impairment in senescence-accelerated mouse prone 8 (SAMP8). Eur. J. Pharmacol. 865, 172789. doi:10.1016/j.ejphar.2019.172789
Li, L.-s., Hu, J., Yang, W., and Alivisatos, A. P. (2001). Band gap variation of size-and shape-controlled colloidal CdSe quantum rods. Nano Lett. 1 (7), 349–351. doi:10.1021/nl015559r
Lin, S. P., Wu, C. Y., Wang, C. B., Li, T. C., Ko, N. Y., and Shi, Z. Y. (2018). Risk of diabetes mellitus in HIV-infected patients receiving highly active antiretroviral therapy: A nationwide population-based study. Med. Baltim. 97 (36), e12268. doi:10.1097/MD.0000000000012268
Liu, W., Wu, Y., Wang, C., Li, H. C., Wang, T., Liao, C. Y., et al. (2010). Impact of silver nanoparticles on human cells: Effect of particle size. Nanotoxicology 4 (3), 319–330. doi:10.3109/17435390.2010.483745
Loiseau, A., Asila, V., Boitel-Aullen, G., Lam, M., Salmain, M., and Boujday, S. (2019). Silver-based plasmonic nanoparticles for and their use in biosensing. Biosens. (Basel) 9 (2), E78. doi:10.3390/bios9020078
Loomba, L., and Scarabelli, T. (2013). Metallic nanoparticles and their medicinal potential. Part II: Aluminosilicates, nanobiomagnets, quantum dots and cochleates. Ther. Deliv. 4 (9), 1179–1196. doi:10.4155/tde.13.74
Lorin, V., Danckaert, A., Porrot, F., Schwartz, O., Afonso, P. V., Mouquet, H., et al. (2020). Antibody neutralization of HIV-1 crossing the blood-brain barrier. mBio 11 (5), 1-11. doi:10.1128/mBio.02424-20
Löscher, W., and Potschka, H. (2005). Blood-brain barrier active efflux transporters: ATP-binding cassette gene family. NeuroRx 2 (1), 86–98. doi:10.1602/neurorx.2.1.86
Mahajan, S. D., Aalinkeel, R., Law, W.-C., Reynolds, J. L., Nair, B. B., Sykes, D. E., et al. (2012). Anti-HIV-1 nanotherapeutics: Promises and challenges for the future. Int. J. Nanomedicine 7, 5301–5314. doi:10.2147/IJN.S25871
Maiti, D., Tong, X., Mou, X., and Yang, K. (2018). Carbon-based nanomaterials for biomedical applications: A recent study. Front. Pharmacol. 9, 1401. doi:10.3389/fphar.2018.01401
Margolis, A. M., Heverling, H., Pham, P. A., and Stolbach, A. (2014). A review of the toxicity of HIV medications. J. Med. Toxicol. 10 (1), 26–39. doi:10.1007/s13181-013-0325-8
Marra, C. M., Zhao, Y., Clifford, D. B., Letendre, S., Evans, S., Henry, K., et al. (2009). Impact of combination antiretroviral therapy on cerebrospinal fluid HIV RNA and neurocognitive performance. AIDS 23 (11), 1359–1366. doi:10.1097/QAD.0b013e32832c4152
McShan, D., Ray, P. C., and Yu, H. (2014). Molecular toxicity mechanism of nanosilver. J. Food Drug Anal. 22 (1), 116–127. doi:10.1016/j.jfda.2014.01.010
Mehndiratta, P., Jain, A., Srivastava, S., and Gupta, N. (2013). Environmental pollution and nanotechnology. Environ. Pollut. (Tor). 2 (2), 49. doi:10.5539/ep.v2n2p49
Mirsadeghi, S., Dinarvand, R., Ghahremani, M. H., Hormozi-Nezhad, M. R., Mahmoudi, Z., Hajipour, M. J., et al. (2015). Protein corona composition of gold nanoparticles/nanorods affects amyloid beta fibrillation process. Nanoscale 7 (11), 5004–5013. doi:10.1039/c4nr06009a
Mishra, P., Xue, Y., Eivazi, F., and Afrasiabi, Z. (2021). Size, concentration, coating, and exposure time effects of silver nanoparticles on the activities of selected soil enzymes. Geoderma 381, 114682. doi:10.1016/j.geoderma.2020.114682
Mohamed, N. A., Marei, I., Crovella, S., and Abou-Saleh, H. (2022). Recent developments in nanomaterials-based drug delivery and upgrading treatment of cardiovascular diseases. Int. J. Mol. Sci. 23 (3), 1404. doi:10.3390/ijms23031404
Moscariello, P., Ng, D. Y., Jansen, M., Weil, T., Luhmann, H. J., and Hedrich, J. (2018). Brain delivery of multifunctional dendrimer protein bioconjugates. Adv. Sci. 5 (5), 1700897. doi:10.1002/advs.201700897
Naidu, E. C. S., Olojede, S. O., Lawal, S. K., Rennie, C. O., and Azu, O. O. (2021). Nanoparticle delivery system, highly active antiretroviral therapy, and testicular morphology: The role of stereology. Pharmacol. Res. Perspect. 9 (3), e00776. doi:10.1002/prp2.776
Naqvi, S., Panghal, A., and Flora, S. (2020). Nanotechnology: A promising approach for delivery of neuroprotective drugs. Front. Neurosci. 14, 494. doi:10.3389/fnins.2020.00494
Naranjo, C. A., Busto, U., Sellers, E. M., Sandor, P., Ruiz, I., Roberts, E. A., et al. (1981). A method for estimating the probability of adverse drug reactions. Clin. Pharmacol. Ther. 30 (2), 239–245. doi:10.1038/clpt.1981.154
Nwogu, J. N., Ma, Q., Babalola, C. P., Adedeji, W. A., Morse, G. D., and Taiwo, B. (2016). Pharmacokinetic, pharmacogenetic, and other factors influencing CNS penetration of antiretrovirals. AIDS Res. Treat. 2016, 2587094. doi:10.1155/2016/2587094
Obermeier, B., Daneman, R., and Ransohoff, R. M. (2013). Development, maintenance and disruption of the blood-brain barrier. Nat. Med. 19 (12), 1584–1596. doi:10.1038/nm.3407
Olojede, S. O., Lawal, S. K., Dare, A., Moodley, R., Rennie, C. O., Naidu, E. C. S., et al. (2021). Highly active antiretroviral therapy conjugated silver nanoparticle ameliorates testicular injury in type-2 diabetic rats. Heliyon 7 (12), e08580. doi:10.1016/j.heliyon.2021.e08580
Olojede, S. O., Lawal, S. K., Faborode, O. S., Dare, A., Aladeyelu, O. S., Moodley, R., et al. (2022). Testicular ultrastructure and hormonal changes following administration of tenofovir disoproxil fumarate-loaded silver nanoparticle in type-2 diabetic rats. Sci. Rep. 12 (1), 9633. doi:10.1038/s41598-022-13321-y
Ortiz, G. G., Pacheco-Moisés, F. P., Macías-Islas, M. Á., Flores-Alvarado, L. J., Mireles-Ramírez, M. A., González-Renovato, E. D., et al. (2014). Role of the blood–brain barrier in multiple sclerosis. Arch. Med. Res. 45 (8), 687–697. doi:10.1016/j.arcmed.2014.11.013
Osborne, O., Peyravian, N., Nair, M., Daunert, S., and Toborek, M. (2020). The paradox of HIV blood-brain barrier penetrance and antiretroviral drug delivery deficiencies. Trends Neurosci. 43 (9), 695–708. doi:10.1016/j.tins.2020.06.007
Ovbiagele, B., and Nath, A. (2011). Increasing incidence of ischemic stroke in patients with HIV infection. Neurology 76 (5), 444–450. doi:10.1212/WNL.0b013e31820a0cfc
Parveen, M., Kumar, A., Khan, M. S., Rahman, R., Furkan, M., Khan, R. H., et al. (2022). Comparative study of biogenically synthesized silver and gold nanoparticles of Acacia auriculiformis leaves and their efficacy against Alzheimer's and Parkinson's disease. Int. J. Biol. Macromol. 1, 292–301. doi:10.1016/j.ijbiomac.2022.01.116
Patel, K., Bharatiya, B., Mukherjee, T., Soni, T., Shukla, A., and Suhagia, B. (2017). Role of stabilizing agents in the formation of stable silver nanoparticles in aqueous solution: Characterization and stability study. J. Dispersion Sci. Technol. 38 (5), 626–631. doi:10.1080/01932691.2016.1185374
Patel, M., Souto, E. B., and Singh, K. K. (2013). Advances in brain drug targeting and delivery: Limitations and challenges of solid lipid nanoparticles. Expert Opin. Drug Deliv. 10 (7), 889–905. doi:10.1517/17425247.2013.784742
Pathakala, N., Begum, N., Ram, C. H. M., and Bakshi, V. (2016). Neuroprotective effect of nevirapine on cerebral ischemic stroke by middle cerebral artery occlusion in wistar rats. Int. J. Appl. Pharm. Sci. Res. 1 (01), 16–24. doi:10.21477/ijapsr.v1i1.9604
Patra, J. K., Das, G., Fraceto, L. F., Campos, E. V. R., Rodriguez-Torres, M. D. P., Acosta-Torres, L. S., et al. (2018). Nano based drug delivery systems: Recent developments and future prospects. J. Nanobiotechnology 16 (1), 71. doi:10.1186/s12951-018-0392-8
Peluso, M. J., Ferretti, F., Peterson, J., Lee, E., Fuchs, D., Boschini, A., et al. (2012). Cerebrospinal fluid HIV escape associated with progressive neurologic dysfunction in patients on antiretroviral therapy with well controlled plasma viral load. AIDS 26 (14), 1765–1774. doi:10.1097/QAD.0b013e328355e6b2
Prabhu, S., Vinodhini, S., Elanchezhiyan, C., and Rajeswari, D. (2018). Evaluation of antidiabetic activity of biologically synthesized silver nanoparticles using Pouteria sapota in streptozotocin-induced diabetic rats. J. Diabetes 10 (1), 28–42. doi:10.1111/1753-0407.12554
Qosa, H., Miller, D. S., Pasinelli, P., and Trotti, D. (2015). Regulation of ABC efflux transporters at blood-brain barrier in health and neurological disorders. Brain Res. 1628 (1), 298–316. doi:10.1016/j.brainres.2015.07.005
Rabkin, J. G., Ferrando, S. J., Lin, S.-H., Sewell, M., and McElhiney, M. (2000). Psychological effects of HAART: A 2-year study. Psychosom. Med. 62 (3), 413–422. doi:10.1097/00006842-200005000-00015
Ragin, A. B., Wu, Y., Gao, Y., Keating, S., Du, H., Sammet, C., et al. (2015). Brain alterations within the first 100 days of HIV infection. Ann. Clin. Transl. Neurol. 2 (1), 12–21. doi:10.1002/acn3.136
Rahimy, E., Li, F.-Y., Hagberg, L., Fuchs, D., Robertson, K., Meyerhoff, D. J., et al. (2017). Blood-brain barrier disruption is initiated during primary HIV infection and not rapidly altered by antiretroviral therapy. J. Infect. Dis. 215 (7), 1132–1140. doi:10.1093/infdis/jix013
Rajput, N. (2015). Methods of preparation of nanoparticles-a review. Int. J. Adv. Eng. Technol. 7 (6), 1806.
Ratan, Z. A., Mashrur, F. R., Chhoan, A. P., Shahriar, S. M., Haidere, M. F., Runa, N. J., et al. (2021). Silver nanoparticles as potential antiviral agents. Pharmaceutics 13 (12), 2034. doi:10.3390/pharmaceutics13122034
Rawat, M. K., Jain, A., and Singh, S. (2011). Studies on binary lipid matrix based solid lipid nanoparticles of repaglinide: In vitro and in vivo evaluation. J. Pharm. Sci. 100 (6), 2366–2378. doi:10.1002/jps.22435
Recordati, C., de Maglie, M., Bianchessi, S., Argentiere, S., Cella, C., Mattiello, S., et al. (2015). Tissue distribution and acute toxicity of silver after single intravenous administration in mice: Nano- specific and size-dependent effects. Part. Fibre Toxicol. 13 (1), 12. doi:10.1186/s12989-016-0124-x
Rezaei, R., Safaei, M., Mozaffari, H. R., Moradpoor, H., Karami, S., Golshah, A., et al. (2019). The role of nanomaterials in the treatment of diseases and their effects on the immune system. Open Access Maced. J. Med. Sci. 7 (11), 1884–1890. doi:10.3889/oamjms.2019.486
Roberts, E. S., Burudi, E. M., Flynn, C., Madden, L. J., Roinick, K. L., Watry, D. D., et al. (2004). Acute SIV infection of the brain leads to upregulation of IL6 and interferon-regulated genes: Expression patterns throughout disease progression and impact on neuroAIDS. J. Neuroimmunol. 157 (1-2), 81–92. doi:10.1016/j.jneuroim.2004.08.030
Robertson, K., Liner, J., and Meeker, R. B. (2012). Antiretroviral neurotoxicity. J. Neurovirol. 18 (5), 388–399. doi:10.1007/s13365-012-0120-3
Robertson, K. R., Su, Z., Margolis, D. M., Krambrink, A., Havlir, D. V., Evans, S., et al. (2010). Neurocognitive effects of treatment interruption in stable HIV-positive patients in an observational cohort. Neurology 74 (16), 1260–1266. doi:10.1212/WNL.0b013e3181d9ed09
Sacktor, N., Skolasky, R. L., Seaberg, E., Munro, C., Becker, J. T., Martin, E., et al. (2016). Prevalence of HIV-associated neurocognitive disorders in the multicenter AIDS cohort study. Neurology 86 (4), 334–340. doi:10.1212/WNL.0000000000002277
Sainz, V., Conniot, J., Matos, A. I., Peres, C., Zupanǒiǒ, E., Moura, L., et al. (2015). Regulatory aspects on nanomedicines. Biochem. Biophys. Res. Commun. 468 (3), 504–510. doi:10.1016/j.bbrc.2015.08.023
Sakhare, K., Prasasvi, K. R., and Palani, S. G. (2022). “Plant and bacteria mediated green synthesis of silver nanoparticles,” in Green functionalized nanomaterials for environmental applications (Elsevier), 155–178.
Santos, G. M. A., Locatelli, I., Metral, M., Calmy, A., Lecompte, T. D., Nadin, I., et al. (2019). Neurocognitive assessment in the, M., & aging cohort study, GCross-sectional and cumulative longitudinal central nervous system penetration effectiveness scores are not associated with neurocognitive impairment in a well treated aging human immunodeficiency virus-positive population in Switzerland. Open Forum Infect. Dis. 6 (7), ofz277. doi:10.1093/ofid/ofz277
Sarma, G., Dsouza, D., Sebastian, S., and Prakash, P. (2022). Efavirenz-induced delayed onset cerebellar ataxia and encephalopathy. Ann. Indian Acad. Neurol. 25 (1), 153–155. doi:10.4103/aian.AIAN_83_21
Schodek, D. L., Ferreira, P., and Ashby, M. F. (2009). Nanomaterials, nanotechnologies and design: An introduction for engineers and architects. Butterworth-Heinemann.
Selvan, D. A., Mahendiran, D., Kumar, R. S., Rahiman, A. K., and Kalilur RahimAn, A. (2018). Garlic, green tea and turmeric extracts-mediated green synthesis of silver nanoparticles: Phytochemical, antioxidant and in vitro cytotoxicity studies. J. Photochem. Photobiol. B 180, 243–252. doi:10.1016/j.jphotobiol.2018.02.014
Shanker, K., Mohan, G. K., Hussain, M. A., Jayarambabu, N., and Pravallika, P. L. (2017). Green biosynthesis, characterization, in vitro antidiabetic activity, and investigational acute toxicity studies of some herbal-mediated silver nanoparticles on animal models. Pharmacogn. Mag. 13 (49), 188–192. doi:10.4103/0973-1296.197642
Sharma, V. K., Yngard, R. A., and Lin, Y. (2009). Silver nanoparticles: Green synthesis and their antimicrobial activities. Adv. Colloid Interface Sci. 145 (1-2), 83–96. doi:10.1016/j.cis.2008.09.002
Shin, W. K., Cho, J., Kannan, A. G., Lee, Y. S., and Kim, D. W. (2016). Cross-linked composite gel polymer electrolyte using mesoporous methacrylate-functionalized SiO2 nanoparticles for lithium-ion polymer batteries. Sci. Rep. 6, 26332. doi:10.1038/srep26332
Simioni, S., Cavassini, M., Annoni, J. M., Rimbault Abraham, A., Bourquin, I., Schiffer, V., et al. (2010). Cognitive dysfunction in HIV patients despite long-standing suppression of viremia. AIDS 24 (9), 1243–1250. doi:10.1097/QAD.0b013e3283354a7b
Singh, A., and Kaur, K. (2019). Biological and physical applications of silver nanoparticles with emerging trends of green synthesis. London, United Kingdom.:Engineered Nanomaterials-Health and Safety. doi:10.5772/intechopen.83105
Singh, T., Shukla, S., Kumar, P., Wahla, V., Bajpai, V. K., and Rather, I. A. (2017). Corrigendum: Application of nanotechnology in food science: Perception and overview. Front. Microbiol. 8, 2517. doi:10.3389/fmicb.2017.02517
Sivanesan, S., and Rajeshkumar, S. (2019). “Gold nanoparticles in diagnosis and treatment of alzheimer’s disease,” in Nanobiotechnology in neurodegenerative diseases (Springer), 289–306.
Smith, J. N., Thomas, D. G., Jolley, H., Kodali, V. K., Littke, M. H., Munusamy, P., et al. (2018). All that is silver is not toxic: Silver ion and particle kinetics reveals the role of silver ion aging and dosimetry on the toxicity of silver nanoparticles. Part. Fibre Toxicol. 15 (1), 47. doi:10.1186/s12989-018-0283-z
Söderstjerna, E., Bauer, P., Cedervall, T., Abdshill, H., Johansson, F., and Johansson, U. E. (2014). Silver and gold nanoparticles exposure to in vitro cultured retina – studies on nanoparticle internalization, apoptosis, oxidative stress, glial- and microglial activity. PLoS ONE 9 (8), e105359. doi:10.1371/journal.pone.0105359
Sonawane, S. K., Ahmad, A., and Chinnathambi, S. (2019). Protein-capped metal nanoparticles inhibit tau aggregation in Alzheimer’s disease. ACS omega 4 (7), 12833–12840. doi:10.1021/acsomega.9b01411
Song, B., Zhang, Y., Liu, J., Feng, X., Zhou, T., and Shao, L. (2016). Is neurotoxicity of metallic nanoparticles the cascades of oxidative stress? Nanoscale Res. Lett. 1 (11), 291–311. doi:10.1186/s11671-016-1508-4
Soursou, G., Alexiou, A., Md Ashraf, G., Ali Siyal, A., Mushtaq, G., and A Kamal, M. (2015). Applications of nanotechnology in diagnostics and therapeutics of Alzheimer’s and Parkinson’s disease. Curr. Drug Metab. 16 (8), 705–712. doi:10.2174/138920021608151107125049
Srinageshwar, B., Peruzzaro, S., Andrews, M., Johnson, K., Hietpas, A., Clark, B., et al. (2017). PAMAM dendrimers cross the blood–brain barrier when administered through the carotid artery in C57BL/6J mice. Int. J. Mol. Sci. 18 (3), 628. doi:10.3390/ijms18030628
Stoehr, L. C., Gonzalez, E., Stampfl, A., Casals, E., Duschl, A., Puntes, V., et al. (2011). Shape matters: Effects of silver nanospheres and wires on human alveolar epithelial cells. Part. Fibre Toxicol. 8 (1), 36–15. doi:10.1186/1743-8977-8-36
Suganya, K. S., Govindaraju, K., Kumar, V. G., Dhas, T. S., Karthick, V., Singaravelu, G., et al. (2015). Size controlled biogenic silver nanoparticles as antibacterial agent against isolates from HIV infected patients. Spectrochim. Acta. A Mol. Biomol. Spectrosc. 144, 266–272. doi:10.1016/j.saa.2015.02.074
Sun, R. W.-Y., Chen, R., Chung, N. P.-Y., Ho, C.-M., Lin, C.-L. S., and Che, C.-M. J. C. c. (2005). Silver nanoparticles fabricated in Hepes buffer exhibit cytoprotective activities toward HIV-1 infected cells, 5059–5061.40
Sung, J. H., Ji, J. H., Song, K. S., Lee, J. H., Choi, K. H., Lee, S. H., et al. (2011). Acute inhalation toxicity of silver nanoparticles. Toxicol. Ind. Health 27 (2), 149–154. doi:10.1177/0748233710382540
Sung, Y. K., and Kim, S. W. (2020). Recent advances in polymeric drug delivery systems. Biomater. Res. 24 (1), 12. doi:10.1186/s40824-020-00190-7
Szerencsés, B., Igaz, N., Tóbiás, Á., Prucsi, Z., Rónavári, A., Bélteky, P., et al. (2020). Size-dependent activity of silver nanoparticles on the morphological switch and biofilm formation of opportunistic pathogenic yeasts. BMC Microbiol. 20 (1), 176. doi:10.1186/s12866-020-01858-9
Talebpour, F., and Ghahghaei, A. (2020). Effect of green synthesis of gold nanoparticles (AuNPs) from Hibiscus sabdariffa on the aggregation of α-lactalbumin. Int. J. Pept. Res. Ther. 26 (4), 2297–2306. doi:10.1007/s10989-020-10023-9
Tang, J., Xiong, L., Zhou, G., Wang, S., Wang, J., Liu, L., et al. (2010). Silver nanoparticles crossing through and distribution in the blood-brain barrier in vitro. J. Nanosci. Nanotechnol. 10 (10), 6313–6317. doi:10.1166/jnn.2010.2625
Tanifum, E. A., Dasgupta, I., Srivastava, M., Bhavane, R. C., Sun, L., Berridge, J., et al. (2012). Intravenous delivery of targeted liposomes to amyloid-β pathology in APP/PSEN1 transgenic mice. PLoS One 7 (10), e48515. doi:10.1371/journal.pone.0048515
Teleanu, D. M., Chircov, C., Grumezescu, A. M., Volceanov, A., and Teleanu, R. I. (2018). Blood-brain delivery methods using nanotechnology. Pharmaceutics 10 (4), 269. doi:10.3390/pharmaceutics10040269
Thakkar, K. N., Mhatre, S. S., and Parikh, R. Y. (2010). Biological synthesis of metallic nanoparticles. Nanomedicine 6 (2), 257–262. doi:10.1016/j.nano.2009.07.002
Tisch, U., Schlesinger, I., Ionescu, R., Nassar, M., Axelrod, N., Robertman, D., et al. (2013). Detection of Alzheimer’s and Parkinson’s disease from exhaled breath using nanomaterial-based sensors. Nanomedicine 8 (1), 43–56. doi:10.2217/nnm.12.105
Tiwari, A., and Rohiwal, S. (2019). “Synthesis and bioconjugation of hybrid nanostructures for biomedical applications,” in Hybrid nanostructures for cancer theranostics (Elsevier), 17–41.
Tyavambiza, C., Elbagory, A. M., Madiehe, A. M., Meyer, M., and Meyer, S. (2021). The antimicrobial and anti-inflammatory effects of silver nanoparticles synthesised from cotyledon orbiculata aqueous extract. Nanomater. (Basel) 11 (5), 1343. doi:10.3390/nano11051343
Vahedifard, F., and Chakravarthy, K. (2021). Nanomedicine for COVID-19: The role of nanotechnology in the treatment and diagnosis of COVID-19. Emergent Mat. 4 (1), 75–99. doi:10.1007/s42247-021-00168-8
Vaidya, K., Kadam, A., and Nema, V. (2016). Anti-retroviral drugs for HIV: Old and new. Austin J. HIV AIDS Res. 3, 1026.
Valcour, V., Chalermchai, T., Sailasuta, N., Marovich, M., Lerdlum, S., Suttichom, D., et al. (2012). Central nervous system viral invasion and inflammation during acute HIV infection. J. Infect. Dis. 206 (2), 275–282. doi:10.1093/infdis/jis326
Van Norman, G. A. (2019). Limitations of animal studies for predicting toxicity in clinical trials: Is it time to rethink our current approach? JACC. Basic Transl. Sci. 4 (7), 845–854. doi:10.1016/j.jacbts.2019.10.008
Vance, D. E. (2010). Aging with HIV: Clinical considerations for an emerging population. Am. J. Nurs. 110 (3), 42–47. quiz 48-49. doi:10.1097/01.NAJ.0000368952.80634.42
Varatharajan, L., and Thomas, S. A. (2009). The transport of anti-HIV drugs across blood-CNS interfaces: Summary of current knowledge and recommendations for further research. Antivir. Res. 82 (2), A99–A109. doi:10.1016/j.antiviral.2008.12.013
Varela, J. A., Bexiga, M. G., Åberg, C., Simpson, J. C., and Dawson, K. A. (2012). Quantifying size- dependent interactions between fluorescently labeled polystyrene nanoparticles and mammalian cells. J. Nanobiotechnology 10 (1), 39. doi:10.1186/1477-3155-10-39
Vassallo, M., Durant, J., Biscay, V., Lebrun-Frenay, C., Dunais, B., Laffon, M., et al. (2014). Can high central nervous system penetrating antiretroviral regimens protect against the onset of HIV-associated neurocognitive disorders? AIDS 28 (4), 493–501. doi:10.1097/QAD.0000000000000096
Ventola, C. L. (2017). Progress in nanomedicine: Approved and investigational nanodrugs. P T. 42 (12), 742–755.
Vidya Vijayan, K. K., Karthigeyan, K. P., Tripathi, S. P., and Hanna, L. E. (2017). Pathophysiology of CD4+ T-cell depletion in HIV-1 and HIV-2 infections. Front. Immunol. 8, 580. doi:10.3389/fimmu.2017.00580
Volpe, C. M. O., Villar-Delfino, P. H., Dos Anjos, P. M. F., and Nogueira-Machado, J. A. (2018). Cellular death, reactive oxygen species (ROS) and diabetic complications. Cell Death Dis. 9 (2), 119. doi:10.1038/s41419-017-0135-z
Warren, K. E. (2018). Beyond the blood:brain barrier: The importance of central nervous system (CNS) pharmacokinetics for the treatment of CNS tumors, including diffuse intrinsic pontine glioma. Front. Oncol. 8, 239. doi:10.3389/fonc.2018.00239
Wei, L., Lu, J., Xu, H., Patel, A., Chen, Z.-S., and Chen, G. (2015). Silver nanoparticles: Synthesis, properties, and therapeutic applications. Drug Discov. Today 20 (5), 595–601. doi:10.1016/j.drudis.2014.11.014
Wen, M. M., El-Salamouni, N. S., El-Refaie, W. M., Hazzah, H. A., Ali, M. M., Tosi, G., et al. (2017). Nanotechnology-based drug delivery systems for Alzheimer's disease management: Technical, industrial, and clinical challenges. J. Control. Release 245, 95–107. doi:10.1016/j.jconrel.2016.11.025
Wente, S. R. (2000). Gatekeepers of the nucleus. Science 288 (5470), 1374–1377. doi:10.1126/science.288.5470.1374
Wong, J. K., and Yukl, S. A. (2016). Tissue reservoirs of HIV. Curr. Opin. HIV AIDS 11 (4), 362–370. doi:10.1097/COH.0000000000000293
Wright, E. J., Grund, B., Cysique, L. A., Robertson, K. R., Brew, B. J., Collins, G., et al. (2015). International network for strategic initiatives in GlobalFactors associated with neurocognitive test performance at baseline: A substudy of the INSIGHT strategic timing of AntiRetroviral treatment (START) trial. HIV Med. 16 (1), 97–108. doi:10.1111/hiv.12238
Wu, M., Guo, H., Liu, L., Liu, Y., and Xie, L. (2019). Size-dependent cellular uptake and localization profiles of silver nanoparticles. Int. J. Nanomedicine 14, 4247–4259. doi:10.2147/IJN.S201107
Xia, Y., and Halas, N. J. (2005). Shape-controlled synthesis and surface plasmonic properties of metallic nanostructures. MRS Bull. 30 (5), 338–348. doi:10.1557/mrs2005.96
Xia, Z. K., Ma, Q. H., Li, S. Y., Zhang, D. Q., Cong, L., Tian, Y. L., et al. (2016). The antifungal effect of silver nanoparticles on Trichosporon asahii. J. Microbiol. Immunol. Infect. 49 (2), 182–188. doi:10.1016/j.jmii.2014.04.013
Xu, X. Y., Tran, T. H. M., Perumalsamy, H., Sanjeevram, D., and Kim, Y.-J. (2021). Biosynthetic gold nanoparticles of Hibiscus syriacus L. callus potentiates anti-inflammation efficacy via an autophagy-dependent mechanism. Mat. Sci. Eng. C Mat. Biol. Appl. 124, 112035. doi:10.1016/j.msec.2021.112035
Yang, Y., Salayandia, V. M., Thompson, J. F., Yang, L. Y., Estrada, E. Y., and Yang, Y. (2015). Attenuation of acute stroke injury in rat brain by minocycline promotes blood-brain barrier remodeling and alternative microglia/macrophage activation during recovery. J. Neuroinflammation 12 (1), 26. doi:10.1186/s12974-015-0245-4
Yen, H. J., Hsu, S. h., and Tsai, C. L. (2009). Cytotoxicity and immunological response of gold and silver nanoparticles of different sizes. Small 5 (13), 1553–1561. doi:10.1002/smll.200900126
Yu, Z., Li, Q., Wang, J., Yu, Y., Wang, Y., Zhou, Q., et al. (2020). Reactive oxygen species-related nanoparticle toxicity in the biomedical field. Nanoscale Res. Lett. 15 (1), 115. doi:10.1186/s11671-020-03344-7
Zewde, B., Ambaye, A., Stubbs, J., and Raghavan, D. (2016). A review of stabilized silver nanoparticles–synthesis, biological properties, characterization, and potential areas of applications. Nanomed 4 (1043), 1–14.
Zhang H, H. (2016). Onivyde for the therapy of multiple solid tumors. Onco. Targets. Ther. 9, 3001–3007. doi:10.2147/OTT.S105587
Zhang, X.-F., Liu, Z.-G., Shen, W., and Gurunathan, S. (2016a). Silver nanoparticles: Synthesis, characterization, properties, applications, and therapeutic approaches. Int. J. Mol. Sci. 17 (9), 1534. doi:10.3390/ijms17091534
Zhang, X. (2018). Anti-retroviral drugs: Current state and development in the next decade. Acta Pharm. Sin. B 8 (2), 131–136. doi:10.1016/j.apsb.2018.01.012
Zhu, Y., Liu, C., and Pang, Z. (2019). Dendrimer-based drug delivery systems for brain targeting. Biomolecules 9 (12), 790. doi:10.3390/biom9120790
Zink, J., Wyrobnik, T., Prinz, T., and Schmid, M. (2016). Physical, chemical and biochemical modifications of protein-based films and coatings: An extensive review. Int. J. Mol. Sci. 17 (9), 1376. doi:10.3390/ijms17091376
Zulu, S. S., Abboussi, O., Simola, N., Mabandla, M. V., and Daniels, W. M. U. (2021). Effects of combination antiretroviral drugs (cART) on hippocampal neuroplasticity in female mice. J. Neurovirol. 27 (2), 325–333. doi:10.1007/s13365-021-00967-z
Keywords: human immunodeficiency virus, blood-brain barrier, neurological disorders, silver nanoparticles, metabolic disorder
Citation: Lawal SK, Olojede SO, Faborode OS, Aladeyelu OS, Matshipi MN, Sulaiman SO, Naidu ECS, Rennie CO and Azu OO (2022) Nanodelivery of antiretroviral drugs to nervous tissues. Front. Pharmacol. 13:1025160. doi: 10.3389/fphar.2022.1025160
Received: 22 August 2022; Accepted: 25 October 2022;
Published: 08 November 2022.
Edited by:
Raveen Parboosing, National Health Laboratory Service (NHLS), South AfricaReviewed by:
Anand Krishnan, University of the Free State, South AfricaCopyright © 2022 Lawal, Olojede, Faborode, Aladeyelu, Matshipi, Sulaiman, Naidu, Rennie and Azu. This is an open-access article distributed under the terms of the Creative Commons Attribution License (CC BY). The use, distribution or reproduction in other forums is permitted, provided the original author(s) and the copyright owner(s) are credited and that the original publication in this journal is cited, in accordance with accepted academic practice. No use, distribution or reproduction is permitted which does not comply with these terms.
*Correspondence: Sodiq Kolawole Lawal, bGF3YWxzb2RpcTZAZ21haWwuY29t
Disclaimer: All claims expressed in this article are solely those of the authors and do not necessarily represent those of their affiliated organizations, or those of the publisher, the editors and the reviewers. Any product that may be evaluated in this article or claim that may be made by its manufacturer is not guaranteed or endorsed by the publisher.
Research integrity at Frontiers
Learn more about the work of our research integrity team to safeguard the quality of each article we publish.