- 1Drug Discovery and Development Division, Patanjali Research Institute, Haridwar, Uttarakhand, India
- 2Department of Allied and Applied Sciences, University of Patanjali, Haridwar, Uttarakhand, India
- 3Special Centre for Systems Medicine, Jawaharlal Nehru University, New Delhi, India
The herbo-mineral formulation, Divya-Swasari-Vati (DSV), is a well-known Ayurvedic medication for respiratory ailments. In a recent pre-clinical study, DSV rescued humanized zebrafish from SARS-CoV-2 S-protein-induced pathologies. This merited for an independent evaluation of DSV as a SARS-CoV-2 entry inhibitor in the human host cell and its effectiveness in ameliorating associated cytokine production. The ELISA-based protein-protein interaction study showed that DSV inhibited the interactions of recombinant human ACE 2 with three different variants of S proteins, namely, Smut 1 (the first reported variant), Smut 2 (W436R variant) and Smut 3 (D614G variant). Entry of recombinant vesicular stomatitis SARS-CoV-2 (VSVppSARS-2S) pseudovirus, having firefly luciferase and EGFP reporters, was assessed through luciferase assay and fluorescent microscopy. DSV exhibited dose-dependent inhibition of VSVppSARS-2S pseudovirus entry into human lung epithelial A549 cells and also suppressed elevated levels of secreted pro-inflammatory cytokines such as interleukin-6 (IL-6), interleukin-1β (IL-1β) and tumor necrosis factor-α (TNF-α) induced by viral infection mimicking Poly I:C-, S-protein- and VSVppSARS-2S pseudovirus. In human immune cells, DSV also moderated TNF-α-mediated NF-κB induction, in a dose-dependent manner. The observed anti-viral effect of DSV against SARS-CoV-2 is attributable to the presence of different metabolites Summarily, the observations from this study biochemically demonstrated that DSV interfered with the interaction between SARS-CoV-2 S-protein and human ACE 2 receptor which consequently, inhibited viral entry into the host cells and concomitant induction of inflammatory response.
Introduction
The SARS-CoV-2 virus-led COVID-19 pandemic has dramatically impacted global demographics, causing ∼0.56 billion confirmed cases and ∼6.3 million deaths as of 15 July 2022 (World Health Organization, 2022). It emerged as the most menacing global healthcare catastrophe since the 1918 influenza pandemic. Acute COVID-19 cases involved severe clinical manifestations that involve hospitalization and mortality (Nalbandian et al., 2021). A gamut of factors such as highly contagious nature, rapid genetic evolution through mutations, and limited early testing facility contributed to the far greater epidemiological spread of the SARS-CoV-2 compared to its predecessors, SARS-CoV and MERS-CoV back in 2002 and 2012 respectively (Hu et al., 2021). The lack of treatment makes the management of COVID-19 very challenging (Gandhi et al., 2020). Mild to moderate COVID-19 symptoms, such as fever, cough, dyspnoea and fatigue, overlap with several non-lethal ailments (Fara et al., 2020). Thus, the management of asymptomatic and mild to moderate COVID-19 primarily relied on molecular diagnostics such as RT-PCR for viral identification. This formidable situation, barely managed by social distancing and enforcement of personal and community hygiene, underlines the unmet need for developing effective anti-viral therapeutics.
SARS-CoV-2 is an enveloped, positive-sense single-stranded RNA virus that belongs to the Coronaviridae family. Like the influenza virus, it is also zoonotic and invades alveolar epithelial and endothelial cells and inflicts severe respiratory illness on affected individuals (Hoffmann et al., 2020b). To understand the molecular mechanism behind this infection, the interaction between SARS-CoV-2 spike surface glycoprotein (S protein) and host ACE 2 receptor studied through atomic force microscopy (AFM) has been reported previously (Yang J. et al., 2020). The host-viral interaction was also explored through molecular dynamic simulation (Ali and Vijayan, 2020). The ability of the SARS-CoV-2 S protein to detect and interact with ACE 2 receptors of the target host cell and the functions of proteases like TMPRSS2 for the viral invasion have been reported in several other studies (Hoffmann et al., 2020b; Hu et al., 2021; Jackson et al., 2022). The predisposition of this virus to cause upper and lower respiratory tract infections are well known now. An abundance of ACE 2 receptors in pneumocytes (type I and type II epithelial cells), alveolar macrophages, and enterocytes make these cells favorable primary targets for SARS-CoV-2 infection. The virus enters and replicates, and mature virions are then released to infect new target cells. Besides human lung cells, SARS-CoV-2 can infect other non-human mammalian cells such as mucosal cells of intestines, tubular epithelial cells of kidneys, epithelial cells of renal tubules, cerebral neurons, and immune cells, where ACE 2 receptors are expressed (Fara et al., 2020). There are two subunits of S protein (S1 and S2) with an arginine-rich furin cleavage site in between. The host-cell mediated proteasomal cleavage of this site is indispensable for viral entry into human lung cells and subsequent manifestation of infection (Hoffmann et al., 2020a). The S1 subunit at the N-terminal of the S protein harbours the Receptor Binding Domain (RBD) that enables the S protein to interact with the host cell ACE 2 receptor, whereas the S2 subunit mediates viral-host cell membrane fusion. This receptor-mediated endocytic route of viral entry into the host cell also requires priming of the S protein by host serine protease TMPRSS2, cathepsin L, and furin (Du et al., 2009). However, targeting host proteases involved in the viral entry is likely to cause undesirable side effects. Therefore, specifically limiting S protein-ACE 2 interaction would be a more appropriate method to hinder viral entry into the cell.
The SARS-CoV-2 infection triggers a dramatic host inflammatory response. It involves an overproduction of soluble pro-inflammatory cytokines, such as IL-1β, IL-6, IL-12, type I and II interferons, IFN-γ, TNF-α, monocyte chemoattractant protein-1 (MCP-1), and macrophage inflammatory protein-1A (MIP-1A). A cascade of biochemical events sparks downstream activation of (JAK)-STAT3, JAK-SHP-2-MAP kinase and NF-κB-dependent signalling pathways. This uncontrolled hyper-inflammatory response accelerates toward cytokine storm syndrome (CSS), an apparent hallmark of the COVID-19 malady. Although intended for viral clearance, this overdriven host immune response results in self-annihilation leading to an acute respiratory distress syndrome (ARDS) in the case of severe COVID-19 patients. The severity of COVID-19 prognosis begins with classical signs of inflammation followed by apoptosis of epithelial cells, reduced peripheral oxygen saturation, hemorrhage, vascular leakages, tissue damage, elevated C-reactive proteins and multiple-organ malfunctioning. The aforementioned unregulated clinical manifestations mimic the state of transient autoimmune disorder (Fara et al., 2020; Tang et al., 2020). To combat SARS-CoV-2 induced hypercytokinemia and CSS associated with severe COVID-19, inhibitors of pro-inflammatory STAT1 and NF-κB signaling pathways, several anti-inflammatory drugs, and immunomodulatory agents, like, IL-1β, IL-6 antagonists and TNF-α blockers, were widely used to control the infection (Mehta et al., 2020; Ye et al., 2020; Rabaan et al., 2021). However, indiscriminate use of these agents weakened the host immune system to such an extent that COVID-19-associated mucormycosis (CAM), a fungal endemic was reported in India in the wake of the second wave of the viral pandemic (Patel et al., 2021).
Based on the understanding gained on COVID-19 etiology through previous investigations, it is postulated that a potential anti-COVID therapeutic strategy should aim for developing a drug with multi-targeted functionality. The potential therapeutic approaches are anticipated to disrupt the viral pathway/s as well as block SARS-CoV-2 from fusing with or entering the host cells, hence reducing the overall invading viral load and associated virus-induced CSS in the host cells. In case some infective virions get inside the host cell, the drug should ameliorate viral infection-induced acute pathophysiological conditions by downregulating undesirable boost in cytokine production. Such delayed immune response will rescue host cells from the detrimental autoimmune impact of the CSS caused by a viral infection which inherently minimizes the risk of unhindered proliferation and spread of the infection and alleviates patients from hospitalization (Felsenstein et al., 2020). Several publications, based on computational studies, speculated on the promising potential of metabolites against SARS-CoV-2, resulting in a shift in attention to different traditional systems of medicines. Ethnomedicinal herbs containing natural bioactive molecules of renowned broad spectrum medicinal significance with no side effects could be explored to fill this void in SARS-CoV-2 therapeutic stratagems (Abubakar et al., 2021).
Ayurveda, the system of Traditional Indian Medicine (TIM), has recommended formulations for different types of ailments. Divya-Swasari-Vati (DSV), consisting of various parts of nine herbs and seven classical mineral preparations, called “bhasma” (Table 1), is an Ayurvedic herbo-mineral formulation prescribed for respiratory ailments, such as cold, cough, bronchitis, and asthma. In order to ensure reproducibility of the subsequent pharmacological studies on DSV as required by the “Consensus statement on the Phytochemical Characterisation of Medicinal Plant extracts” (ConPhyMP) (Heinrich et al., 2022), a thorough process optimization has been done for consistent detection of marker metabolites in DSV across different batches (Balkrishna et al., 2021c; Balkrishna et al., 2021d). DSV is rich in several metabolites, such as eugenol, piperine, glycyrrhizin, gallic acid, 6-gingerol, methyl gallate, ellagic acid, glabridin, cinnamic acid, protocatechuic acid and coumarin (Table 2) (Balkrishna et al., 2020c; Balkrishna et al., 2021c). The pharmacological significance of the metabolites present in DSV, particularly their anti-inflammatory properties, are well documented (Semaming et al., 2015; Semwal et al., 2015; Correa et al., 2016; Graebin, 2018; Rios et al., 2018; Kahkeshani et al., 2019; Ruwizhi and Aderibigbe, 2020; Li C.-X. et al., 2021; Nisar et al., 2021; Sharifi-Rad et al., 2021; Tripathi et al., 2022). Recently, DSV has also been reported to ameliorate S protein-induced inflammatory response in A549 xenotransplanted zebrafish by reducing IL-6 and TNF-α, pro-inflammatory cytokine levels and rescuing fever-driven swimming behavioural patterns (Balkrishna et al., 2020c). Converging evidence warrant mechanistic investigation of the anti-viral and immunomodulatory activities of DSV against SARS-CoV-2 pathologies under in vitro experimental setup.
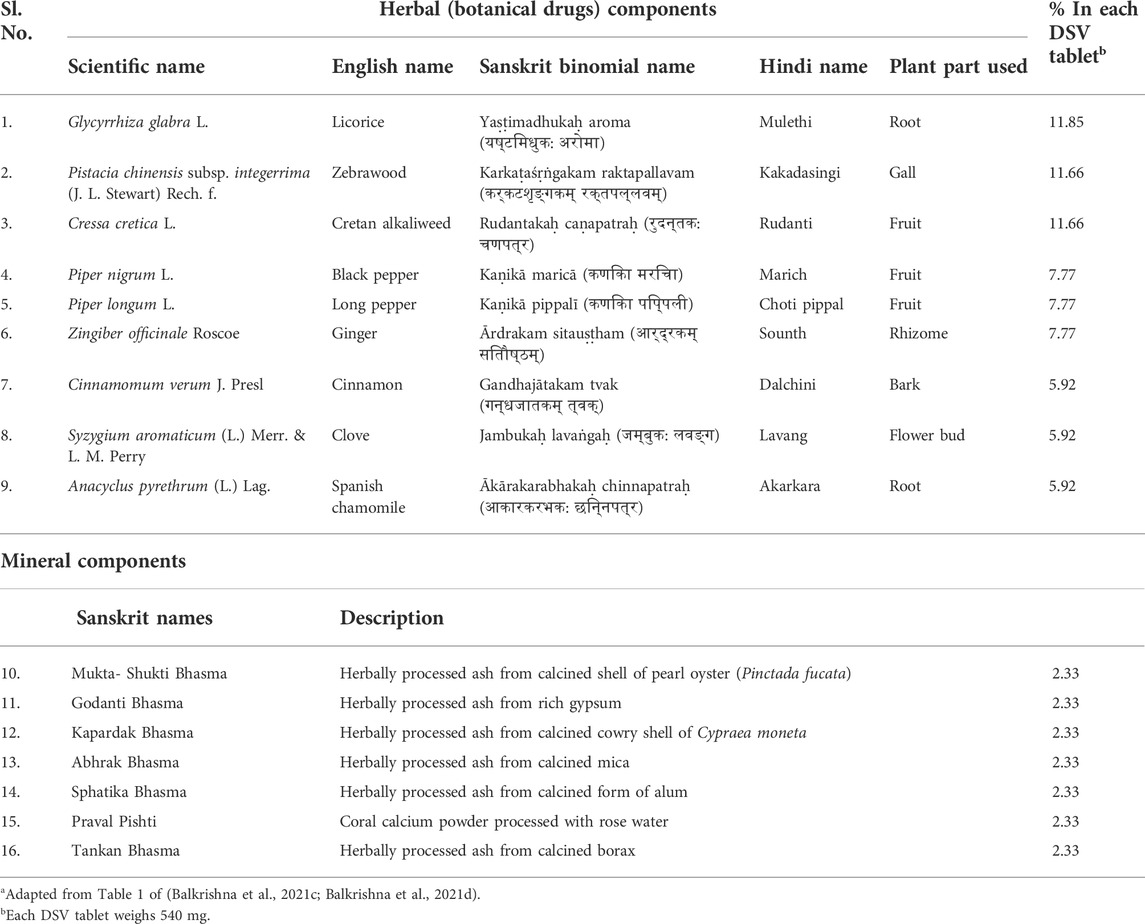
TABLE 1. Different components (botanical drugs and minerals) used in Divya-Swasari-Vati (DSV) tablet formulationa.
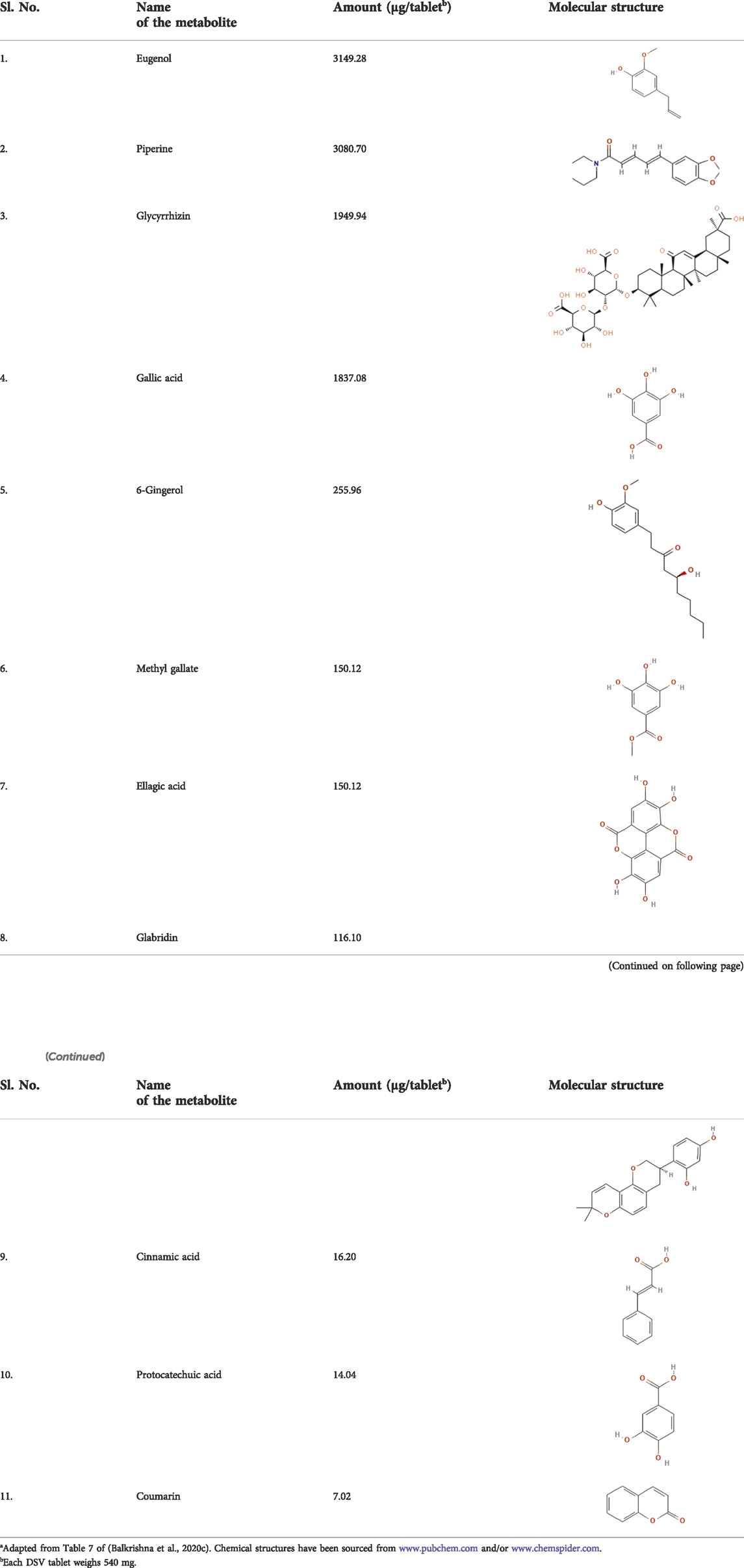
TABLE 2. Metabolites present in Divya-Swasari-Vati (DSV) tablet formulationa.
Therefore, in the present study, DSV was evaluated for its inhibitory potency against SARS-CoV-2 pseudovirus entry into human lung cells and its concomitant immune response. The present investigation was designed to evaluate the prospects of DSV in curbing human ACE 2 and S-protein interaction through an ELISA-based biochemical assay. Furthermore, the efficacy of DSV inhibiting the entry of SARS-CoV-2 S protein pseudotyped virus into ACE 2 expressing human alveolar epithelial A549 cells was also investigated. Furthermore, the molecular pathway targeted by DSV to ameliorate COVID-19 pathologies was explored using reporter cell lines. The effectivity of DSV as an anti-viral, in general, was investigated in Poly I:C-induced THP-1 (human leukemia monocytic cells). The immunomodulatory effect of DSV was confirmed on SARS-CoV-2 S protein- and pseudovirus-induced cytokine response in human alveolar epithelial cells.
Materials and methods
Chemicals, reagents, cell lines and cell culture
DSV (Batch No. #A-SWV047, expiry May 2023) was obtained from Divya Pharmacy (Haridwar, Uttarakhand, India). Dulbecco’s Modified Eagle’s Medium (DMEM) (Catalog #12100046), Roswell Park Memorial Institute Medium 1640 (RPMI 1640) (Catalog #31800022) and Lipofectamine 3000 transfection kit (Catalog #L3000008) were procured from Thermo Fisher Scientific (Waltham, MA, United States). Fetal Bovine Serum (FBS) (Catalog #RM9955), Trypsin Phosphate Versene Glucose (TPVG) solution and Alamar Blue reagent were purchased from HiMedia Laboratories Pvt. Ltd. (Mumbai, Maharashtra, India). Penicillin-Streptomycin mixture (Catalog #P4333) and Poly I:C (Catalog #P1530) were obtained from Sigma-Aldrich (St. Louis, MO, United States). ELISA kits for interleukin-6 (IL-6) (Catalog #555220), interleukin-1 beta (IL-1β) (Catalog #557953), and tumor necrosis factor-alpha (TNF α) (Catalog #555212) were procured from BD Biosciences (San Jose, CA, United States). Purified SARS-CoV-2 Spike (S) proteins and purified human ACE 2 protein were obtained from Sino Biological Inc. (Beijing, China). ELISA-based SARS-CoV-2 inhibitor screening kit (Catalog #EP-105) was purchased from AcroBiosytems (Newark, DE, United States). Streptavidin conjugated horse-radish peroxidase (streptavidin-HRP) along with 3.3′,5,5′-Tetramethylbenzidine (TMB) (Catalog number #555214) were obtained from BD bioscience (San Diego, CA, United States). Luciferase assay substrate (Catalog #E151A), Luciferase assay buffer (Catalog #E152A) and 5X cell culture lysis reagent (Catalog #E153A) were purchased from Promega Corp. (Madison, WI, United States). All other chemicals and reagents were from Sigma-Aldrich (St. Louis, MO, United States) unless otherwise stated. Human alveolar epithelial (A549), human leukemia monocytic cell line (THP-1) and human kidney epithelial (HEK293) cell lines were procured from the American Type Culture Collection (ATCC) licensed cell repository at the National Centre for Cell Science (NCCS, Pune, India), whereas THP1-Blue NF-κB reporter cell line was obtained from InvivoGen (San Diego, CA, United States). A549 and HEK293 cells were cultured in DMEM supplemented with 10% (v/v) FBS, penicillin-streptomycin mixture (100 μg/ml) at 37°C under a humidified atmosphere containing 5% CO2 in a cell culture incubator. NF-κB reporter cells were cultured in RPMI 1640 medium under similar growth conditions as described above.
Cell viability assay
Cytosafety of DSV was analysed through in vitro cell viability assay through Alamar Blue staining. In brief, 3 × 104 PMA differentiated THP-1 cells were seeded in 96 well plate in triplicate and grown overnight. Cells were further incubated for 24 h in the presence of different concentrations of DSV (1, 3, 10, 30, 100 and 300 μg/ml) in a total volume of 100 μl in each well. DSV-untreated control samples were also included. Alamar Blue (10 μl) at a final concentration of 0.15 mg/ml was added to each well and incubated for a period of ∼3 h for the characteristic pink colour to develop in the control samples as per the manufacturer’s protocol. Fluorescence was measured at an emission of 590 nm and excitation of 560 nm, using an Envision microplate reader (PerkinElmer, Waltham, MA, United States), followed by the calculation of percent (%) cell viability considering 100% viability in the control sample.
Quantification of poly I:C-induced cytokine activation in human leukemia monocytic cell line
For this assay, Poly I:C stock was prepared in molecular grade water and heated at 70°C for 10 min in PCR blocks and gradually allowed to cool. Meanwhile, PMA differentiated THP-1 cells were seeded at a cell density of 3 × 104 cells/well. Poly I:C (10 μg/ml) was added to THP-1 cells, which were pre-incubated for 16 h, with varying concentrations of DSV (1, 3, 10, 30 μg/ml). Poly I:C-uninduced and induced THP-1 cells without DSV treatment were considered normal and disease controls, respectively. After 24 h co-treatment with Poly I:C and DSV, levels of secreted pro-inflammatory cytokines, namely IL-6 and TNF-α were estimated in the supernatants of the cultured THP-1 cells by using specific ELISA kits for each of these cytokines, as per the manufacturer’s protocol. Absorbance was measured at 450 nm using the Envision microplate reader (Perkin Elmer, United States).
Enzyme-linked immunoassay-based biochemical assessment to detect the interaction between SARS-CoV-2 spike protein and human angiotensin converting enzyme 2
Three different variants of SARS-CoV-2 spike (S) proteins namely, Smut1 (Catalog #40592-V08B), Smut2 (Catalog #40592-V08H9), and Smut3 (Catalog #40591-V08H3) were analyzed quantitatively for their interactions with recombinant human ACE 2 protein (hACE 2) (Catalog #10108- H08H-B), through an ELISA-based SARS-CoV-2 inhibitor screening kit following the manufacturer’s protocol as reported earlier (Balkrishna et al., 2021a). Briefly, each well of the ELISA plate was incubated separately with 25 ng of different variants of S proteins for coating. Thereafter, following thorough washings, 62.5 ng of purified biotinylated human ACE 2 protein was added to each well in a total volume of 100 μl in the presence of different concentrations of DSV (0.001, 0.003, 0.01, 0.03, 0.1, 0.3, 1.0, 3.0, 10, and 30 μg/ml). Subsequently, samples were incubated for 30 min. Negative control included only ACE 2 and S proteins without any exposure to DSV. Positive control was supplied along with the kit and included a chemical inhibitor (Catalog # AC2-NA005; AcroBiosytems, Newark, DE, United States) against ACE 2-S protein interaction. With the subsequent addition of streptavidin-HRP along with the substrate, TMB helped in quantifying the amount of ACE 2 interacting with S proteins. The absorbance of the oxidized TMB was measured at 450 nm using an Envision microplate reader (Perkin Elmer, United States). ACE 2 and S protein interactions were calculated as percentages (%) with respect to the untreated/negative control group showing 100% interaction.
Estimation of the cytokine response induced by different S proteins in alveolar epithelial A549 cells
Cytokine responses elicited by different variants of S protein in A549 cells were determined as mentioned earlier (Balkrishna et al., 2021a). A549 cells were grown to 50–60% confluent. The cells were then exposed to 8,000 ng/ml of Smut1 and Smut2 and 20,000 ng/ml of Smut3 for 48 h, after which the levels of the pro-inflammatory cytokines, IL-6, TNF-α, and IL-1β secreted in the supernatants of cultured cells were measured by using specific ELISA kits for each of these cytokines, following manufacturer’s protocol. The Envision microplate reader (Perkin Elmer, United States) measured absorbance at 450 nm.
Vesicular stomatitis virus pseudotyping with SARS-CoV-2 S protein
SARS-CoV-2 S protein pseudotyping of vesicular stomatitis virus (VSV) was conducted according to previous reports (Whitt, 2010; Hoffmann et al., 2020b). Briefly, 24 h before infection with replication-deficient VSV*ΔG-FLuc viruses, HEK293 cells were co-transfected with helper plasmids encoding VSV N, P, and L proteins of VSV and SARS-CoV-2S protein expressing pCG1-SARS-2S plasmid. VSV*ΔG-FLuc viruses, set of helper and pCG1-SARS-2S plasmids were kind gifts from Prof. Stefan Pöhlmann (Deutsches Primatenzentrum GmbH, Leibniz-Institut für Primatenforschung, Kellnerweg 4, 37,077 Göttingen, Germany), and received with approval from the Institutional Biosafety Committee of Patanjali Research Institute (vide approval # IBSC/PRI/0221/P015). After 36 h of incubation, the supernatant containing SARS-CoV-2S S protein pseudotyped VSV viruses (VSVppSARS-2S) was harvested and kept at −80°C for use in later investigations.
Evaluation of VSVppSARS-2S entry into A549 cells through luciferase assay and fluorescence imaging
The effect of DSV treatment on the entry of VSVppSARS-2S pseudoviruses into A549 cells was assessed according to previously published protocols, luciferase assay was performed with a few minor modifications (Berger Rentsch and Zimmer, 2011; Hoffmann et al., 2020b) as per approval from the Institutional Biosafety Committee of Patanjali Research Institute (vide approval # IBSC/PRI/0221/P014). DSV pre-treated (1, 3, 10, and 30 μg/ml for 16 h) 70–80 percent confluent A549 cells were infected with VSVppSARS-2S [at a multiplicity of infection (m.o.i.) of 10] at 37°C for 6 h. Subsequently, after removing the medium, cell lysates were prepared in 50 μl of 1X cell culture lysis reagent. Firefly luciferase activity was measured in an Envision microplate reader (Perkin Elmer, United States) immediately after mixing 30 μl of the luciferase substrate, reconstituted in luciferase assay buffer, and 20 μl of the lysate. The extent of VSVppSARS-2S entry into the cells was reflected by the luciferase activity, which was expressed as “x-fold over background”. Uninfected and infected cells without DSV treatment were included as normal and disease controls, respectively. VSVppSARS-2S pseudovirus infected cells treated with 100 μM of camostat mesylate (Hoffmann et al., 2020b) were taken as the positive control. In a parallel experimental setup, A549 cells were infected with VSVppSARS-2S for 6 h (as mentioned above) for fluorescence imaging of EGFP-expressing cells. After fixing with 0.4% paraformaldehyde for 10 min at room temperature, the cells were washed thoroughly with sterile PBS. The cells were then imaged under bright and fluorescent (excitation at ∼445–495 nm) fields using an inverted microscope (Juli, GFP 10X-Smart Fluorescent Cell Analyser, NanoEntake, South Korea). The number of EGFP-expressing A549 cells were counted from images taken from six different fields for each sample.
Evaluation of pseudotyped virus (VSVppSARS-2S)-induced cytokine response in A549 cells
50%–60% confluent A549 cells were infected with VSVppSARS-2S pseudoviruses. After 48 h of incubation, the quantities of pro-inflammatory cytokines, namely, IL-6, IL-1β, and TNF-α that were released in the supernatants of cultured A549 cells were estimated by using ELISA, as previously described (Balkrishna et al., 2021a).
Secreted embryonic alkaline phosphatase based NF-κB/AP-1 reporter assay
THP1-Blue NF-κB reporter cells were used to monitor the level of NF-κB activation. Briefly, cells with a density of 5 × 105/ml were seeded in a 96-well plate (Thermo Fisher Scientific, MA, United States) and co-treated with 10 ng/ml TNF-α (Catalogue #300-01A-50UG; Peprotech, Cranbury,NJ, United States) and DSV (1, 3,10 and 30 μg/ml) for 24 h. Post-incubation, SEAP was quantified using QUANTI-Blue Solution (InvivoGen, CA, United States) as per the manufacturer’s instructions. The optical density was read at 630 nm using an Envision (PerkinElmer, MA, United States) multimode plate reader.
Statistical analysis
All experiments were carried out in triplicate at least three times independently and data were represented with mean ± standard error (SEM). Statistical significance of the variations observed between the mean of different groups and data points were evaluated through one-way ANOVA with Dunnett multiple comparison. GraphPad Prism software 8.0.2 (GraphPad Inc., San Diego, CA, United States) was used for statistical analyses and graphical representations of the quantitative data.
Results
DSV ameliorated poly I:C induced pro-inflammatory cytokine response in THP-1 cells at cyto-safe doses
In the in vitro cytotoxicity assay, treatment of THP-1 cells with various concentrations of DSV (0–300 μg/ml) for 24 h revealed no significant effects on the cell viability even when the cells were exposed to the highest concentration used for the assay as evident in Figure 1A. Up to 300 μg/ml under in vitro conditions, DSV was considered to be cytosafe for THP-1 cells. A much lower range of DSV concentrations (1–30 μg/ml) was used for subsequent in vitro assays. Elicitation of pro-inflammatory cytokine levels in THP-1 macrophage cells as a host defence mechanism against infections is a common phenomenon. In the event of SARS-CoV-2 infection, immune cells can develop the CSS condition with hyper-expressed pro-inflammatory cytokines. This condition was mimicked in vitro by incubating THP-1 macrophage cells with an inducer such as Poly I:C. Polyinosinic:polycytidylic acid (Poly I:C) is a synthetic immunostimulant that mimics double-stranded RNA, a molecular pattern associated with viral infections and easily recognized by TLR3 of the host innate immune system. By mimicking viral infection, exposure to Poly I:C can stimulate virus-induced cytokine and interferon production in mammalian cells (Tamir et al., 2022). For this assay, 24 h of exposure to 10 μg/ml Poly I:C significantly raised the secreted levels of IL-6 (Figure 1B) and TNF-α (Figure 1C) in cell culture supernatants as compared to the negative control where the inducing agent, as well as DSV exposure, were absent. DSV was competent enough to reduce Poly I:C-induced IL-6 and TNF-α in a dose-dependent manner. Interestingly, 30 μg/ml DSV was shown to reduce Poly I:C-induced cytokine levels up to 50%.
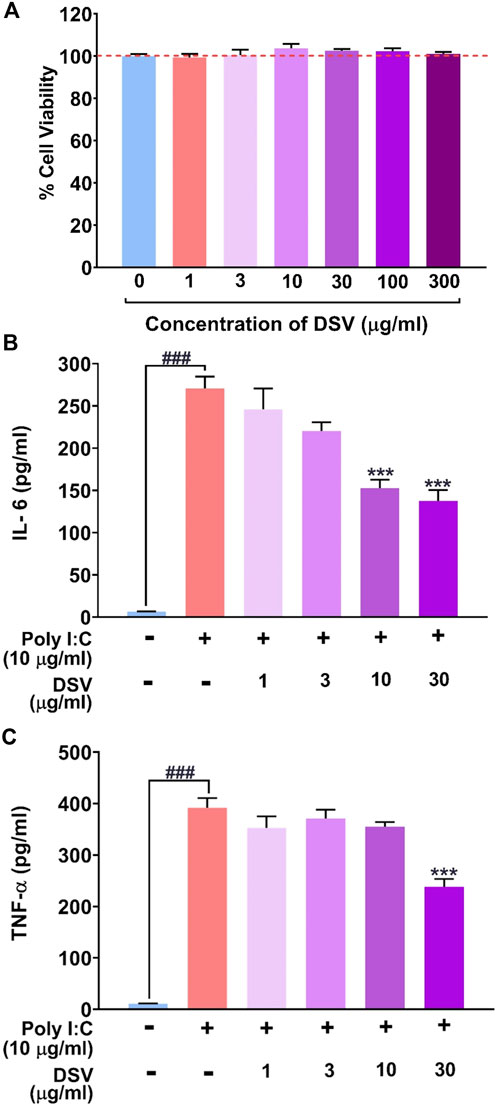
FIGURE 1. DSV attenuated Poly I:C induced pro-inflammatory cytokine response in THP-1 macrophage cells. (A) Cytotoxicity of DSV on THP-1 cells expressed as % cell viability. Similar cell viabilities in untreated and DSV treated cells are indicated with the broken red line. (B,C) THP-1 cells were pre-treated with DSV for 16h followed by its co-treatment with Poly I:C for 24 h and assayed for the secretion of cytokines, IL-6 (B) and TNF-α (C) using Enzyme-Linked Immunosorbent Assay (ELISA). Data on the levels of secreted cytokines are represented as mean ± SEM from three independent experiments. The statistical significance of the differences observed between the means was analysed through one-way ANOVA followed by Dunnett’s multiple comparison test and represented as ### for p < 0.001 when compared to the normal cells without DSV and Poly I:C treatment and as *** for p < 0.001 compared to poly I:C-induced cells without DSV treatment.
DSV interfered with human ACE 2 receptor and SARS-CoV-2 S protein interaction
In light of the early findings from the previous experiment, it was intriguing to look closely at the molecular docking studies and experimental results obtained by independent research groups on the constituent herb/s or active phytocomponent/s present in the DSV formulation such as Pistacia chinensis subsp. integerrima (Kumar Paul et al., 2022). Glycyrrhizin/liquorice extract (Luo et al., 2020) and coumarins (Abdelmohsen et al., 2021) have been shown to block molecular interaction between S-protein and hACE 2. These previous converging reports act as a foundation for establishing DSV as a potent anti-SARS-CoV-2 agent. Therefore, an ELISA-based biochemical assay was conducted to decipher the effect of DSV in restricting the interaction between hACE 2 and viral S protein. For this assay, we have tested the interaction of three different viral SARS-CoV-2 S protein mutants namely, Smut1 (the one identified at the beginning of the pandemic), Smut2 (W436R) and Smut3 (D614G), immobilized on an ELISA plate with biotinylated purified human ACE 2 protein. The pharmacological inhibitor of ACE 2-S protein interaction provided in the kit served as a positive control, whereas the reaction mixture without any control or experimental inhibitor served as the negative control yielding complete ACE 2-S protein/s interaction. Consequently, the inhibition compared to the positive control caused by different concentrations of DSV was calculated. As evident from (Figures 2A–C), DSV exerted a dose-dependent inhibitory effect on the interactions between ACE 2 and all variants of S proteins, although there is some variation in the strength of the inhibition across all S protein mutants. The experimental setup found a 30%–50% DSV-driven inhibition of the ACE 2-S protein interaction. To compare the inhibitory effect of DSV among these S protein mutant variants, the IC20 values of DSV against each variant were calculated. They were found to be 23.33, 2.28, and 7.78 μg/ml for viral Smut1, Smut2, and Smut3 respectively. DSV exhibited a more effective inhibitory potency when Smut2 interacted with the ACE 2 receptor. These findings unambiguously depicted the potency of DSV in preventing viral S proteins from communicating with human ACE 2 receptors at the molecular level.
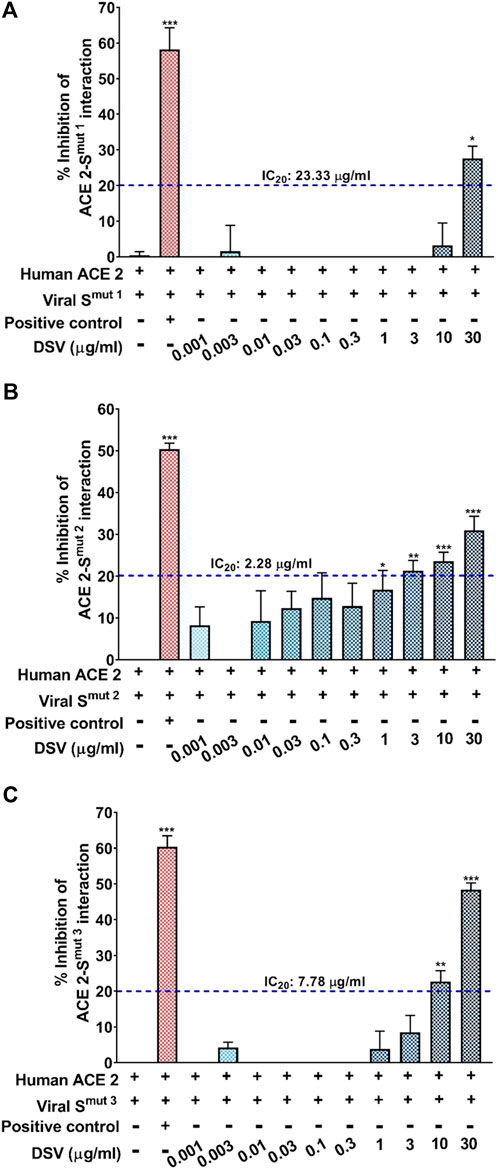
FIGURE 2. DSV inhibited interaction between human ACE 2 receptor and SARS-CoV-2 S proteins. (A–C) ELISA-based assay to quantify the dose-dependent effect of DSV on the interactions between human ACE 2 receptors and different types of SARS-CoV-2 S proteins, namely, Smut1 (A), Smut2 (B) and Smut3 (C) and represented as percentage (%) inhibition relative to the extent of ACE 2-S protein interactions observed in the reaction mix without any inhibitor. Data represented as mean ± SEM from three independent experiments. The statistical significance of the observed differences in the means compared to the no inhibitor group [first column in each case from (A–C)] was analysed through one-way ANOVA followed by Dunnett’s multiple comparison test and represented as *, ** or *** depending on whether the calculated p value was <0.05, <0.01 or <0.001. IC20 concentrations of DSV against ACE 2 interaction with each variant of S protein were determined and indicated.
DSV moderated levels of viral S protein-induced inflammatory cytokines in A549 cells
ACE 2 receptor expression makes the alveolar type II (AT- II) A549 epithelial cells a favourable target for SARS-CoV-2 infection. Therefore, the aforementioned cell line is chosen for testing our formulation in vitro studies (Foster et al., 1998). In continuation with our earlier observations where DSV downregulated Poly I:C induced cytokines in THP-1 and restricted the ACE 2-S protein interaction, it was further conceived that the DSV could downregulate S-protein-induced cytokines in A549. To assess this premise, A549 lung epithelial cells were pre-treated with DSV at doses of 1, 3, 10 and 30 μg/ml for 12 h, and subsequently, the activation and release of three immune-reactive pro-inflammatory cytokines (TNF-α, IL-6, and IL-1β) in response to the introduction of viral S-protein variants (used earlier) were examined (Figure 3A). Cells unexposed to S proteins and untreated with DSV were taken as normal control, while those incubated with S-proteins but not treated with DSV were included as disease control. Smut3 (Figure 3D) does not have the same effect on any of the measured cytokines as Smut1 (Figure 3B) or Smut2 (Figure 3C), which showed significantly high levels of all three immune-reactive cytokines in the disease control. Interestingly, these cytokines were decreased in cells treated with a gradient of DSV at increasing concentrations. However, only a few instances of dosage dependence were observed. As shown in (Figure 3B), levels of both TNF-α and IL-1β in the cells were elevated by about five folds in response to Smut1. These cytokine levels were restored almost to normal by DSV treatment in a dose-dependent manner. Notably, a steeper fall in the Smut1-induced cytokines level after DSV-treatment on A549 cells is observed for IL-1β compared to TNF-α. As an illustration, 1 μg/ml of DSV treatment lowered Smut1-induced TNF-α and IL-1β on A549 cells by ∼1.4 and ∼2.5 folds respectively.
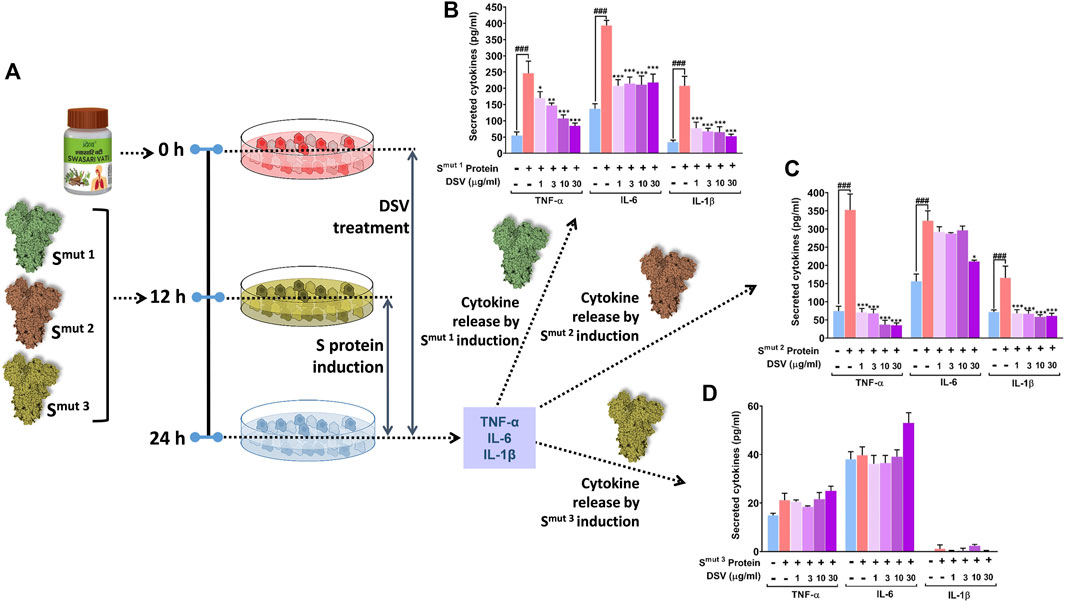
FIGURE 3. DSV attenuated viral S protein-induced cytokine activation in A549 cells. (A) Schematic representation of the experimental plan. (B–D) Activation of pro-inflammatory cytokines in response to SARS-CoV-2 S protein variants, Smut1 (B), Smut2 (C) and Smut3 (D) in alveolar epithelial A549 cells and its dose-dependent amelioration upon DSV treatment were measured by ELISA. Observations are depicted as levels of secreted cytokines. Data represented as mean ± SEM from three independent experiments. The statistical significance of the differences observed between the means was analysed through one-way ANOVA followed by Dunnett’s multiple comparison test and represented as ### for p < 0.001 when compared to normal cells without DSV treatment and exposure to S protein and as *, **, and *** for p < 0.05, 0.01, and 0.001, respectively, when compared to cells exposed to S protein induction but not treated with DSV.
IL-6 in A549 cells was found to increase by about 3.5-folds in response to Smut1. DSV treatment significantly reduces this elevated cytokine level to nearly half compared to the cytokine level obtained for disease control. However, no dose-dependent effect of DSV was observed in the case of this mutant. Compared to control groups, TNF-α, IL-6 and IL-1 levels elevated almost 5, 2.2, and 2.5-fold in the disease control groups of Smut2 protein-treated cells, respectively (Figure 3C). DSV demonstrated high resilience to these elevated cytokine levels, effectively restoring TNF-α, and IL-1β to normal control at a dose as low as 1 μg/ml, without any apparent dose-dependency. Treatment with 30 μg/ml DSV is required for a significant reduction of IL-6 that brings down its level closer to the normal control. While analysing Smut3 protein-treated cells, we did not find any significant change in any of the cytokines studied, when compared to normal control groups (Figure 3D). Besides, a negligible amount of IL-1β levels were detected in the case of Smut3 variant. Since no induction in the cytokine levels was observed in Smut3 treated cells, the discernible effect of DSV treatment was unapparent. In summary, it is evident that all purified S protein mutant variants, except Smut3, elicited in vitro cytokine response in pulmonary epithelial cells and DSV was found to mitigate that response mostly restoring the cytokine levels to normalcy with varying efficiency based on the type of mutation that exists in the viral S protein.
DSV inhibited VSVppSARS-2S entry into alveolar epithelial cells
DSV effectively moderated the S protein-mediated rise in pro-inflammatory cytokines. Therefore, it can be conceived as a rational approach to study the effect of DSV on hindering the interaction of S protein expressing SARS-CoV-2 virus with A549 cells expressing hACE 2 receptors. To eliminate the need for specialized containment facilities to work on SARS-CoV-2, replication-defective, S protein pseudotyped infective virus particles were generated (Whitt, 2010). Host A549 cells were pre-treated with DSV at different concentrations (1, 3, 10, and 30 μg/ml) for 16 h before exposing them to VSVpp2S viruses for another 24 h in the presence of DSV. Thereafter, viral entry was assessed through luciferase assay and fluorescence imaging (Figure 4A). Untreated cells that had been pseudovirus transduced and uninfected cells that had not been transduced were used as disease and normal controls, respectively. The expression of reporter genes that have been stably integrated into the VSVppSARS-2S genome by infected host cells allowed the measurement of the amount of VSVppSARS-2S that entered the lung epithelial A549 cells. These reporters include the firefly luciferase gene (FLuc) and EGFP, which produced luciferase activity and green fluorescence in the infected cells, respectively. As demonstrated in (Figure 4B), compared to uninfected cells (considered as background), pseudovirus-transduced cells displayed about 80 times as much virus entry. An almost 3.5-fold decline in virus entry was observed in the infected cells treated with camostat mesylate, a serine protease inhibitor effective against TMPRSS 2 (Hoffmann et al., 2020b). Remarkably, a significant dose-dependent reduction of the virus entry in transduced cells, as compared to untreated cells, was observed upon DSV (1, 3, 10 and 30 μg/ml) pre-treatment. The reduction in viral entry into the host cell due to DSV treatment was comparable to the observed camostat mesylate effect. The results obtained by luciferase assay corroborated with the microscopic analysis of EGFP-expressing cells (Figure 4C) and were subsequently represented in quantifiable form (Figure 4D). Altogether, the results acquired through these assays establish the ability of DSV to prevent the entry of SARS-CoV-2 into host cells.
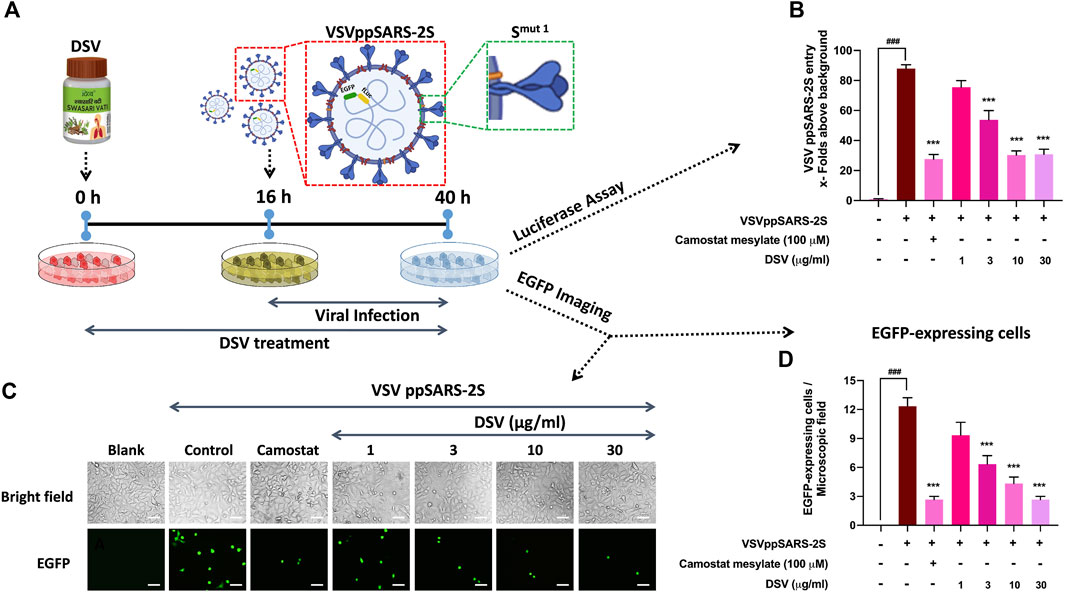
FIGURE 4. DSV inhibited entry of VSVppSARS-2S in alveolar epithelial cells. (A) Schematic representation of the experimental plan. (B) VSVppSARS-2S entry into the transduced A549 cells and the effect thereupon due to treatment with DSV and positive control Camostat mesylate, assessed through luciferase assay, were represented as fold change in luminescence units relative to the normal cells, taken as background. (C) Representative fluorescent micrographs of uninfected cells, VSVppSARS-2S virus-infected cells, infected cells treated with positive control Camostat mesylate and different concentration of DSV. Scale bar: 50 μm (D) Average number of EGFP positive cells in uninfected A549 cells, virus-infected cells, infected cells treated with positive control Camostat mesylate and DSV, determined from six different fields. Data represented as mean ± SEM from three independent experiments. The statistical significance of the differences observed between the means was analysed through one-way ANOVA followed by Dunnett’s multiple comparison test and represented as ### for p < 0.001 when compared to normal cells without DSV treatment and exposure to S protein and as *** for p < 0.001, when compared to cells infected with VSVppSARS-2S virus but not treated with DSV.
DSV subdued VSVppSARS-2S-induced cytokine upsurge in alveolar epithelial cells
Previous results indicated efficient management of S protein-evoked cytokines by DSV and its ability to inhibit viral entry. SARS-CoV-2 is known to trigger the immune system and cause CSS (Moore and June, 2020). Owing to the presence of functional S protein in a replication-defective, non-pathogenic VSVppSARS-2S mimics infection mechanism and pathophysiology of an infective SARS-CoV-2 virus that involves an increase in inflammatory cytokine (cytokine storm) secretion (Fara et al., 2020). Thus, ELISA was used to evaluate the amounts of immune-reactive pro-inflammatory cytokines secreted by VSVppSARS-2S-transduced A549 cells with and without DSV treatment. Uninfected cells without DSV treatment were used as normal control, whereas VSVppSARS-2S transduced A549 cells were used as disease control (Figures 5A–C). A549 cells infected with the VSVppSARS-2S virus underwent 4, 3, and 5-fold increases in TNF-α, IL-6, and IL-1β levels compared to the normal control, respectively. Pre-treatment and co-treatment of the infected cells with varying concentrations of DSV (1, 3, 10, and 30 μg/ml) dramatically lowered, TNF-α, IL-6, and IL-1β levels by 50, 33 and 60%, respectively, compared to the disease control. The reduction in the levels of secreted cytokines was noticeable with 1 μg/ml of DSV treatment, and it nearly reached the usual normal control level (uninfected) for all tested cytokines with the highest DSV concentration (30 μg/ml) taken for the assay. A decrease in IL-6 level in response to DSV treatment exhibited a dose-dependent trend. Overall, these observations ascertained the potential of DSV to counteract viral infection-induced cytokine release syndrome.
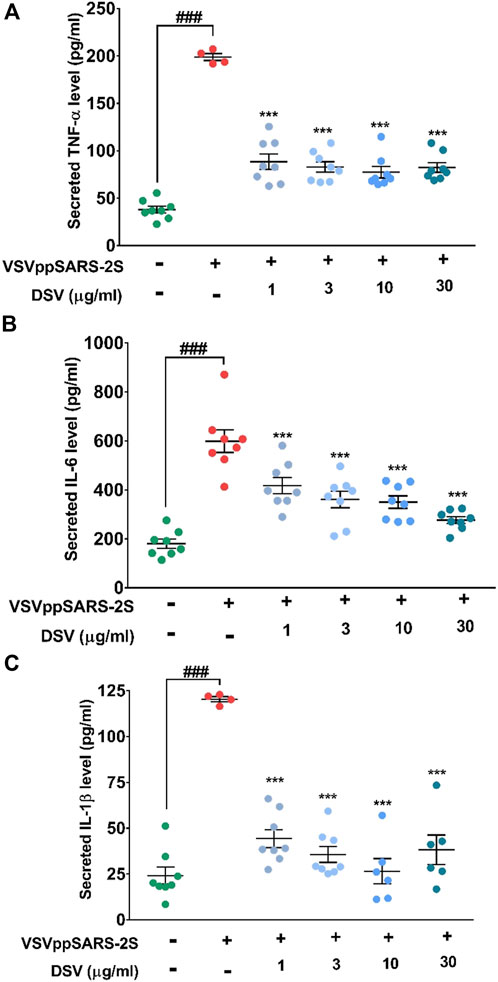
FIGURE 5. DSV reduced pseudovirus elicited cytokine response in A549 cells. (A–C) Cells were pre-treated with DSV followed by co-treatment with pseudovirus infection with VSVppSARS-2S for 24 h and assayed for the secretion of pro-inflammatory cytokines, TNF-α (A), IL-6 (B) and IL-1β (C) by ELISA. Measured levels show the evoked cytokine responses and their reduction upon treatment with DSV. Results from three independent experiments were represented as mean ± SEM levels of secreted cytokines. The statistical significance of the differences observed between the means was analysed through one-way ANOVA and represented as ### for p < 0.001 when compared to non-transduced cells and as *** for p < 0.001 when compared to untreated virus-transduced cells.
DSV attenuated TNF-α- induced transcriptional activity of NF-κB
A significant pathogenic trait of the SARS-CoV-2 infection is the production of a cytokine storm characterized by a massive increase in pro-inflammatory cytokines (Coperchini et al., 2020). NF-κB is the master regulator involved in lung inflammation (Barnes, 2006), and TNF-α is a very effective inducer of the NF-κB signalling pathway (Hayden and Ghosh, 2014). TNF-α-induced NF-κB signaling pathway associated with neuroinflammation (CIPN) is well known (Fumagalli et al., 2020; Zhang P. et al., 2020). Besides, the NF-κB pathway has been identified as a potential therapeutic target in the treatment of COVID-19 (Kircheis et al., 2020). Our earlier observations showing the pro-inflammatory cytokine modulating effect of DSV, together with these converging evidence, encouraged us to assess the effect of DSV on TNF-α-mediated NF-κB induction. Accordingly, TNF-α-induced activation of NF-κB promoter was assessed through transactivation assay using THP1-Blue™ NF- κB reporter system. THP1-Blue™ is a stable cell line engineered to express SEAP enzyme from an NF-κB regulatable promoter. The addition of TNF-α triggers NF-κB mediated SEAP expression, which is quantifiable through an enzymatic reaction. This quantification indirectly reflected the treatment-mediated modulation of TNF-α-facilitated NF-κB induction (Figure 6A). Cells exposed to TNF-α with no DSV treatment are the disease control, while cells without TNF-α and DSV treatment act as normal control where NF-κB transcriptional activity is absent. The addition of 10 ng/ml TNF-α induced an ∼14 -fold increase in NF-κB promoter induction activity compared to the normal control, while co-treatment of these reporter cells with DSV significantly inhibited the TNF-α induced NF-κB transcriptional activation in a dose-dependent manner (Figure 6B). At the highest DSV concentration (30 μg/ml) used for the assay, the TNF-α-induced NF-κB transcriptional activation falls to nearly 50% of the untreated disease control. Therefore, taken together, these observations corroborate the anti-inflammatory properties of DSV and demonstrate the potential of DSV in moderating the undesirable cytokine-storm (TNF-α)-induced NF-κB induction. These observations strongly implicate that DSV is not only capable of countering the upsurge of cytokines during SARS-CoV-2 infection, but also has the potential to prevent the inflammatory response from going into an overdrive by targeting the NF-κB pathway.
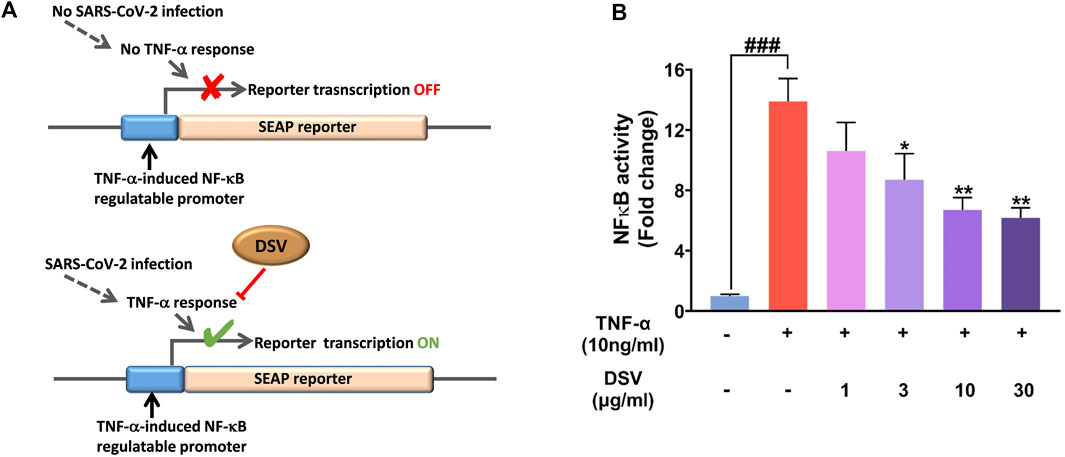
FIGURE 6. DSV inhibited TNF-α induced NF-κB transcriptional activity. (A) Schematic representation of the experimental rationale. (B) THP1 Blue cells stably expressing the NF-κB inducible SEAP reporter gene were pre-treated with various doses of DSV followed by treatment with TNF-α and SEAP activity measured after 24 h. Results from three independent experiments were represented as mean ± SEM levels of secreted cytokines. The statistical significance of the differences observed between the means was analysed through one-way ANOVA and represented as ### for p < 0.001 when compared to normal cells without DSV and TNF-α treatment and as * and ** for p < 0.05 and 0.01, respectively, when compared to cells without DSV treatment but exposed to TNF-α.
Discussion
SARS-CoV-2 infection is contagious, with a high mortality rate. Catastrophic prognosis includes suppressed immunity, cytokine storm, organ failure and susceptibility to pneumonia (Ragab et al., 2020). During the crisis, a concerted effort by the global scientific community was made towards repurposing certain already available medications, such as umifenovir, camostat mesylate, hydroxychloroquine, soluble recombinant hACE 2 that induced a drop in the levels of S protein bound to the cell membrane mACE 2, S protein-specific monoclonal antibodies or fusion inhibitors like arbidol, immunosuppressants (like, dexamethasone, IL6 inhibitory monoclonal antibody tocilizumab, IL-1β receptor antagonist anakinra and sarilumab) (Hu et al., 2021), viral replication inhibitors (remdesivir, favilavir, ribavirin, lopinavir, and ritonavir) (Beigel et al., 2020). Combinatorial treatment regimens were also considered keeping the pharmacokinetics of the medicines and the patient’s risk profile in perspective (Felsenstein et al., 2020). However, the efficacy of none of these treatment options was found to be optimal against COVID-19, let alone the severe side effects, especially for immunocompromised cancer and diabetic patients (Di Lorenzo et al., 2020). During the initial phase of the pandemic, several in silico studies reported potentially effective metabolites against SARS-CoV-2 infection (Balkrishna et al., 2021a; Balkrishna et al., 2021b; Kumar V. et al., 2021; Kumar et al., 2022). Several herbal plants target immune responses by reducing cytokine levels (Li S. et al., 2021). During the COVID-19 pandemic, the knowledge base of traditional systems of medicines from India and China proved quite effective. Traditional Chinese Medicine (TCM) has been successfully applied to combat the SARS-CoV-2 pandemic (Yang Y. et al., 2020). In fact, as emergency measures, governments in India and China approved traditional medicines (based on medicinal herbs and natural products) as supporting measures to manage COVID-19 during the peak of the pandemic. Traditional medicinal concoctions of natural products contain bioactive components in a well-defined functional matrix capable of multi-targeting a disease etiology. The concept of poly-herbalism in formulating medicinal concoctions to achieve greater therapeutic efficacy with reduced toxicity is highlighted in Ayurvedic literature called Sarangdhar Samhita (Parasuraman et al., 2014). Clinical trials of anti-viral drugs such as hydroxychloroquine (Boulware et al., 2020) and remdesivir (Beigel et al., 2020) yielded ineffective results against the SARS-CoV-2 virus. In contrast, promising SARS-CoV-2 clearance and fast recovery with no adverse effects were observed for a placebo-controlled pilot-scale pre-clinical trial carried out with ayurvedic treatment regime on COVID-19 positive patients (Devpura et al., 2021). DSV is a classical Ayurvedic formulation that has been used to treat ailments of the respiratory system, including asthma, for thousands of years. This, along with the ability of DSV to ameliorate the pathology induced by the SARS-CoV-2 S protein in zebrafish (Balkrishna et al., 2020c) warranted a detailed study to gain mechanistic insight into the mode of action of this formulation as an anti-SARS-CoV-2 agent. Therefore, the current study was designed to evaluate the anti-SARS-CoV-2 activity of DSV by monitoring its effect on viral-host interaction levels.
DSV is a herbo-mineral Ayurvedic formulation whose individual metabolites are reported to have anti-viral, anti-inflammatory and immunomodulatory properties. The anti-SARS-CoV-2 property was also shown by another major DSV metabolite, glycyrrhizin, a triterpene saponin that has a long history of medical use. It can not only bind ACE 2 to prevent SARS-CoV-2 infection (Luo et al., 2020), but can also inhibit SARS-CoV-2 replication by blocking COVID-19 main protease Mpro (Van De Sand et al., 2021). Besides, glycyrrhizin is effective against many other viruses such as hepatitis, dengue and influenza (Bailly and Vergoten, 2020). Glabridin is capable of binding at the active site of Mpro, suggesting an enzyme inhibitory effect (Srivastava et al., 2022). 6-Gingerol and eugenol, two other metabolites present in DSV, are shown to have a high binding affinity for Mpro active sites. Apart from SARS-CoV-2, 6-Gingerol was reported to exhibit potent anti-viral activity against chikungunya virus (CHIKV) infection of human hepatocyte HepG2 cells (Hayati et al., 2021) and human respiratory syncytial virus (HRSV) in respiratory upper and lower tract cell lines, HEp-2 and A549, respectively (Chang et al., 2013; Rathinavel et al., 2020; Chandra Manivannan et al., 2022). Eugenol was found to bind SARS-CoV-2 S1 protein to inhibit the host-pathogen interaction between human ACE 2 receptor and viral S protein (Paidi et al., 2021). Gallic and ellagic acids also showed potential binding with RNA-dependent RNA polymerase of SARS-CoV-2 in molecular docking studies (El Sohaimy et al., 2021). Besides, gallic and ellagic acids, together with protocatechuic acid, were also found to target 3CLpro of the SARS-CoV-2 virus (Arokiyaraj et al., 2020). Molecular docking studies with coumarin, also one of the identified marker metabolites present in DSV, revealed good docking scores indicating strong binding with RBD of S-protein, which could inhibit the viral attachment and entry into human host cells (Abdelmohsen et al., 2021). It is the major bioactive molecule of Cressa cretica, one of the nine medicinal herbs present in DSV that is effective as an anti-viral agent against a wide range of viruses such as influenza, dengue, chikungunya and enterovirus. Coumarin is known to inhibit NF-κB signaling, which is crucial for viral entry and replication (Mishra et al., 2020). In silico studies by Kumar G. et al. (2021) had shown that piperine from Piper nigrum and curcumin from Curcuma longa effectively bind to the ACE 2 receptors of the host cell. Similarly, a study by Pandey et al. (2020) demonstrated that the metabolites present in Piper nigrum, Syzygium aromaticum and Zingiber officinale could target the main protease of SARS-CoV-2. Methyl gallate (Correa et al., 2020) and cinnamic acid (Yakhchali et al., 2021) are very good anti-inflammatory agents with strong immune-modulatory potentials, which are likely to attune the over-driven inflammatory responses during SARS-CoV-2 infection. Pistacia chinensis subsp. integerrima, one of the most abundant herbal components of DSV, contains 28-dimethyl-β-amyrone, 24-Noroleana-3,12-diene, and stigmasterol. These metabolites, although not detected in DSV formulations per se, have demonstrated strong binding with S protein RBD of SARS-CoV-2, forming stable complexes, thereby attenuating its interaction with the host cell (Kumar Paul et al., 2022).
Our study focused on studying the effect of DSV in ameliorating viral infection-induced pro-inflammatory cytokines upsurge. The inflammatory response may occur due to an infestation of pulmonary pathogens such as Mycoplasma pneumoniae (Yang et al., 2002), bacterial endotoxin like liposaccharide (Crestani et al., 1994; Lin et al., 2020) or viruses such as H1N1 infection (Wang et al., 2015) and respiratory syncytial virus (Levitz et al., 2012). Several previous reports have indicated a sudden violent outburst of systemic cytokine levels in SARS-CoV-2 infected individuals, instigating a cascade of detrimental autoimmune consequences known as CSS (Mehta et al., 2020). The key perpetrating pro-inflammatory cytokines which were targeted to manage the symptoms of COVID-19 are IL6 and TNF-α (Costela-Ruiz et al., 2020; Moore and June, 2020). The primary source of pathogenic IL-6 induced by SARS-CoV-2 infection appears to be myeloid cells (Gubernatorova et al., 2020). Moreover, several inflammatory cytokines associated with NF-κB signaling pathways (Su et al., 2021), JAK/STAT and MAPK/ERK are also involved in the progression of the cytokine storm (Dosch et al., 2009). The primary goal of an anti-COVID therapeutic strategy is to alleviate excess production of cytokines. According to a report, immunosuppressant compounds derived from plant sources like curcumin, luteolin, piperine, and resveratrol are known to inhibit the production and release of pro-inflammatory cytokines and chemokines (Peter et al., 2020). Medicinal plants present in DSV, like P. chinensis subsp. integerrima reportedly subdued the expressions of inflammatory molecules, TNF-α, IL-4, and IL-5 in the mouse model of ovalbumin (OVA) induced allergic asthma, thereby alleviating pulmonary edema. This in vivo immunomodulatory activity can be helpful in the treatment of asthma and cough (Rana et al., 2016). Consistent with this observation, Divya-Swasari-Ras (DSR) demonstrated suppression of pro-inflammatory cytokine response in the mouse model with ovalbumin (OVA)-induced allergic asthma (Balkrishna et al., 2020a). Furthermore, P. chinensis subsp. integerrima exhibited high anti-oxidant activity. Glycyrrhizin, a marker metabolite of DSV, displayed diverse pharmacological properties, including anti-cancerous, anti-thrombotic, anti-mutagenic, anti-ulcerogenic (Isbrucker and Burdock, 2006) anti-microbial, anti-parasitic, anti-allergenic, anti-tussive (Hasan et al., 2021), hepatoprotective, anti-oxidative, and anti-inflammatory properties attributed to its inhibitory effect on NF-κB, that helps in abating pro-inflammatory cytokines. Reactive oxygen species (ROS) and Respiratory Distress Syndrome (RDS) are induced by viral infections (Ghosh et al., 2011; El-Saber Batiha et al., 2020). Other metabolites present in DSV, like piperine, exhibit a strong anti-oxidant and immunomodulatory effects (Miryan et al., 2021). In addition to herbal components, DSV also contains seven different bhasmas that maintain optimal alkalinity and potential anti-inflammatory activity (Balkrishna et al., 2020c). It was alluring to assess the vast computational discoveries and numerous experimental evidence indicating the broad spectrum of anti-viral capabilities of herbal constituents of DSV that complements our current endeavour.
The ability of DSV to inhibit hACE-2-S protein interactions was evaluated using an ELISA-based protein-protein interaction assay. For this, a panel of three different SARS-CoV-2 S protein mutants was used, namely, viral Smut1 (one identified at the beginning of the pandemic), viral Smut2 (W436R) and viral Smut3 (D 614G). A global sampling of SARS-CoV-2 indicates rapid mutations in spike protein amino acid sequences, which presumably allow the virus to gain an evolutionary advantage of greater infectivity by evading host anti-viral responses and geographical prevalence by improved transmissibility. The major strain of SARS-CoV-2 predominant in global circulation during pandemic has the Smut3 mutation. This mutation of the S protein has implications in attaining greater viral infectivity, as PCR-based studies revealed that people infected with this variant of SARS-CoV-2 had higher viral loads in the upper respiratory tract (Korber et al., 2020). The greater incorporation of S protein into the virions during packaging accounts for their improved infectivity and transmissivity (Zhang L. et al., 2020). S protein-hACE 2 binding affinity and the severity of disease caused by Smut2 mutation is comparable to other mutant variants of SARS-CoV-2. Apparently, this fact is noticeable as a nearly identical percentage of the inhibitory effect caused by positive control (chemical inhibitor provided with the Elisa kit) during ACE 2-S protein interaction studies with all three S protein mutants. However, the inhibitory effect exerted by DSV on hACE 2–Smut3 is comparatively more than on hACE 2–Smut1. This reflects the ability of DSV to curb the infection caused by a comparatively more infectious/transmissible stain of SARS-CoV-2. Moreover, there was no increase in the levels of released pro-inflammatory cytokines, namely IL-6, TNF-α, and IL-1β in A549 cells after Smut3 protein exposure confirming the clinical evidence that there is no correlation between the illness severity with this mutation (Zhang L. et al., 2020). RBD of S protein plays a crucial role in viral attachment, entry and subsequent infection. Mutations in specific residues of RBD known as “hot-spot” under high positive selection pressure develop mutant strains that displayed enhanced ACE 2 affinity. Smut2 has a RBD hot-spot mutation where incorporation of positively charged arginine enabled S protein to interact strongly with highly negatively charged ACE 2 surface. There are also cold-spot residues where point mutation can result in decreased interactions (Padhi and Tripathi, 2020; Ou et al., 2021). Owing to hot-spot mutation, the ACE 2–Smut2 interaction is stronger than the ACE 2–Smut1 complex. It is noticeable that DSV inhibited hACE 2–Smut2 interactions much more efficiently with the least IC20 value compared to hACE 2–Smut1 and hACE 2–Smut3. Our observations also indicate that Smut2 protein-induced elicitation of secreted pro cytokine response in A549 cells was conspicuous and virtually equivalent to that seen with Smut1 protein, and it gets effectively attenuated by DSV.
SARS-CoV-2 S protein pseudotyped vesicular stomatitis virus (VSV) infection of A549 cells resulted in the expression of the reporter gene (luciferase and EGFP) as well as elicited cytokine response. DSV successfully quelled both of them. These findings are explained collectively by the potency of DSV to excellently restrain the viral entry into the host cell. The ideal model system to examine SARS-CoV-2 entry and the associated inflammatory responses in human alveolar epithelial A549 cells as SARS-CoV-2 infection mostly targets human lung cells that abundantly express ACE 2 protein, a host cell receptor protein playing a vital role in viral attachment and entry. On stimulation by SARS-CoV-2 S proteins, A549 cells also produce a traceable amount of cytokine response. The maximum bioavailability of DSV under in-vitro conditions served as the foundation for our results. However, the effectiveness of an active molecule under in vivo conditions is often determined by its route of administration, blood concentration and binding to plasma protein and tissue (Mueller et al., 2004). Regarding pharmacokinetics, the in vitro experimental setup cannot be directly compared with in vivo circumstances. Thus, it is a common practice to employ drugs at concentrations exceeding the in vitro effective concentrations to obtain measurable activity under in vivo conditions. In the case of DSV, a pre-clinical study in zebrafish exhibiting symptoms of COVID-19 induced by SARS-CoV-2 S protein already established the pharmacological efficacy of this formulation (Balkrishna et al., 2020c). Nonetheless, in the current study, the in vitro pseudovirus and cell-based assays provided a platform to comprehend the mode of action of DSV as an inhibitor of SARS-CoV-2 entrance into host cells.
Our findings demonstrated that DSV blocks the interaction of viral S protein with ACE 2, thereby downregulating the virus infection-induced pro-inflammatory cytokines (IL-6), (IL-1β), and (TNF-α) which are highly elevated instigating the innate anti-viral response. Previous reports from our group had also validated the efficacy of DSV under in vivo experimental design where DSV attenuated pro-inflammatory cytokine response in an induced allergic asthma mouse model (Balkrishna et al., 2020b) and rescued humanized zebrafish (xenotransplanted with human alveolar epithelial A549 cells) from SARS-CoV-2 S-protein-induced pathologies (Balkrishna et al., 2020a). Mounting cytokines in response to viral infection influences each other and triggers other inflammatory pathways, such as NF-κB signaling. TNF-α which is also a major transcriptional regulator, is known to mediate the cytokine response through the action of NF-κB, a downstream effector. We found that TNF-α-mediated NF-κB induction could be moderated in a dose-dependent manner by DSV treatment. This indicated that DSV is endowed with immunomodulatory properties and helps in subduing the detrimental rise in inflammation.
Through our current research findings, we are successful in deciphering the potent anti-SARS-CoV-2 activity of DSV, particularly in terms of blocking the interaction of viral S protein with human ACE 2 receptor, preventing viral entry into the cells and ameliorating the associated immunogenic responses, thereby minimizing transition of innate to adaptive immune response. The likelihood of the adaptive reaction being overdriven is also diminished. This could ultimately reduce the chances of organ failure caused by anti-nucleoprotein antibodies and catastrophic disease consequences. All these can be attributed to the richness of DSV in metabolites that collectively display desirable positive traits.
Conclusion
The current study has provided the mechanistic insight into the anti-viral activity of the herbo-mineral formulation Divya-Swasari-Vati (DSV) against SARS-CoV-2. Interaction between SARS-CoV-2 spike (S) protein and human host ACE 2 receptor is a prime requirement of COVID-19 infection, and concomitant severe inflammatory responses. Our observations from this study revealed that DSV inhibited this biomolecular interaction between viral S protein of different SARS-CoV-2 strains and host ACE 2 receptor, thereby inhibiting the viral entry into the human host lung epithelial A549 cells. DSV also curbed the immunogenic cytokine response mounted during SARS-CoV-2 infection of A549 cells in vitro, implicating its potential to attenuate the overdriven cytokine response, associated with severe COVID-19 infection. DSV moderated TNF-α-induced transcriptional activation of NF-κB signaling. The observed anti-SARS-CoV-2 property of DSV is attributable to its rich repertoire of metabolites. Therefore, taken together, DSV was found to be capable of inhibiting the entry of the SARS-CoV-2 pseudovirus into human lung epithelial cells. Consequently, DSV could also mitigate the host-pathogen interaction and cytokine upsurge. These properties of DSV substantiate its prophylactic and therapeutic potentials against COVID-19.
Data availability statement
The original contributions presented in the study are included in the article, further inquiries can be directed to the corresponding author.
Author contributions
AB: Conceptualization, resources; SG: Methodology, data acquisition, visualization; HS: Methodology, data acquisition, visualization; VG: Methodology, data acquisition, visualization; RD: Methodology, data acquisition, visualization, verification, analysis; SH: Methodology, data acquisition, visualization, verification, analysis; Writing—original draft; AV: Conceptualization, supervision, project administration, writing—review and editing. All authors have critically reviewed the article; gave final approval of the version to be published; have agreed on the journal to which the article has been submitted; and agreed to be accountable for all aspects of the work.
Funding
This presented work has been conducted using internal research funds from Patanjali Research Foundation Trust, Haridwar, India.
Acknowledgments
We express our humble gratitude towards Prof. Stefan Pöhlmann and Dr. Markus Hoffmann (Deutsches Primatenzentrum GmbH, Leibniz-Institut für Primatenforschung, Kellnerweg 4, 37077 Göttingen, Germany) for sharing VSV*ΔG-FLuc viruses and helper plasmids to prepare SARS-CoV-2 pseudotypes. We thank Ms. Meenu Tomer, Mr. Sudeep Verma and Dr. Jyotish Srivastava for analytical chemistry support. We are thankful to Dr. Rajesh Mishra and Dr. Bhaskar Joshi for the Sanskrit names of the botanical drugs present in the test article and classical literature search. We would like to thank Mr. Devendra Kumawat for his excellent assistance with graphics. We extend our gratitude to Ms. Priyanka Kandpal, Mr. Tarun Rajput, Mr. Gagan Kumar and Mr. Lalit Mohan for their swift administrative support.
Conflict of interest
The test article, Divya-Swasari-Vati, was sourced from Divya Pharmacy, Haridwar, Uttarakhand, India. AB is an honorary trustee in Divya Yog Mandir Trust that governs Divya Pharmacy, Haridwar. Besides, providing the test article, Divya Pharmacy was not involved in any aspect of the study. AB also holds an honorary managerial position in Patanjali Ayurved Ltd., Haridwar, India. Divya Pharmacy and Patanjali Ayurveda Ltd. commercially manufacture and sell several ayurvedic products. All other authors, SG, HS, VG, RD, SH, and AV, have been employed at Patanjali Research Institute which is governed by Patanjali Research Foundation Trust (PRFT), Haridwar, Uttarakhand, India, a not-for-profit organization. In addition, AV is an adjunct professor in the Department of Allied and Applied Sciences, University of Patanjali, NH-58, Haridwar-249405, Uttarakhand, India, and in Special Centre for Systems Medicine, Jawaharlal Nehru University, New Delhi 110067, India. All other authors declare that they have no conflict of interest.
Publisher’s note
All claims expressed in this article are solely those of the authors and do not necessarily represent those of their affiliated organizations, or those of the publisher, the editors and the reviewers. Any product that may be evaluated in this article, or claim that may be made by its manufacturer, is not guaranteed or endorsed by the publisher.
Abbreviations
DSV, Divya-Swasari-Vati; ACE 2, Angiotensin Converting Enzyme 2; ATCC, American Type Culture Collection; COVID-19, Coronavirus Disease 2019; DMEM, Dulbecco’s Modified Eagle Medium; ELISA, Enzyme-linked Immunoassay; FBS, Fetal Bovine Serum; HRP, Horse Radish Peroxidase; IL-1β, Interleukin-1β; IL-6, Interleukin-6; MERS, Middle East Respiratory Syndrome; MERS-CoV, Middle East Respiratory Syndrome-related Coronavirus; Mpro, Main Protease; NCCS, National Centre for Cell Science; RBD, Receptor Binding Domain; RPMI, Roswell Park Memorial Institute Medium; S protein, Spike protein; SARS, Severe Acute Respiratory Syndrome; SARS-CoV, Severe Acute Respiratory Syndrome Coronavirus; SARS-CoV-2, Severe Acute Respiratory Syndrome Coronavirus 2; SEAP, Secreted Embryonic Alkaline Phosphatase; TNF-α, Tumor Necrosis Factor-α; VSV, Vesicular Stomatitis Virus; VSVppSARS-2S, SARS-CoV-2S pseudotyped VSV.
References
Abdelmohsen, U. R., Albohy, A., Abdulrazik, B. S., Bayoumi, S. a. L., Malak, L. G., Khallaf, I. S. A., et al. (2021). Natural coumarins as potential anti-SARS-CoV-2 agents supported by docking analysis. RSC Adv. 11, 16970–16979. doi:10.1039/d1ra01989a
Abubakar, M. B., Usman, D., El-Saber Batiha, G., Cruz-Martins, N., Malami, I., Ibrahim, K. G., et al. (2021). Natural products modulating Angiotensin converting enzyme 2 (ACE2) as potential COVID-19 therapies. Front. Pharmacol. 12, 629935. doi:10.3389/fphar.2021.629935
Ali, A., and Vijayan, R. (2020). Dynamics of the ACE2-SARS-CoV-2/SARS-CoV spike protein interface reveal unique mechanisms. Sci. Rep. 10, 14214. doi:10.1038/s41598-020-71188-3
Arokiyaraj, S., Stalin, A., Kannan, B. S., and Shin, H. (2020). Geranii herba as a potential inhibitor of SARS-CoV-2 main 3CL(pro), spike RBD, and regulation of unfolded protein response: An in silico approach. Antibiot. (Basel) 9, E863. doi:10.3390/antibiotics9120863
Bailly, C., and Vergoten, G. (2020). Glycyrrhizin: An alternative drug for the treatment of COVID-19 infection and the associated respiratory syndrome? Pharmacol. Ther. 214, 107618. doi:10.1016/j.pharmthera.2020.107618
Balkrishna, A., Haldar, S., Singh, H., Roy, P., and Varshney, A. (2021a). Coronil, a tri-herbal formulation, attenuates spike-protein-mediated SARS-CoV-2 viral entry into human alveolar epithelial cells and pro-inflammatory cytokines production by inhibiting spike protein-ACE-2 interaction. J. Inflamm. Res. 14, 869–884. doi:10.2147/JIR.S298242
Balkrishna, A., Pokhrel, S., Singh, H., Joshi, M., Mulay, V. P., Haldar, S., et al. (2021b). Withanone from Withania somnifera attenuates SARS-CoV-2 RBD and host ACE2 interactions to rescue spike protein induced pathologies in humanized zebrafish model. Drug Des. devel. Ther. 15, 1111–1133. doi:10.2147/DDDT.S292805
Balkrishna, A., Sharma, P., Joshi, M., Srivastava, J., and Varshney, A. (2021c). Development and validation of a rapid high-performance thin-layer chromatographic method for quantification of gallic acid, cinnamic acid, piperine, eugenol, and glycyrrhizin in Divya-Swasari-Vati, an ayurvedic medicine for respiratory ailments. J. Sep. Sci. 44, 3146–3157. doi:10.1002/jssc.202100096
Balkrishna, A., Solleti, S. K., Singh, H., Tomer, M., Sharma, N., and Varshney, A. (2020a). Calcio-herbal formulation, Divya-Swasari-Ras, alleviates chronic inflammation and suppresses airway remodelling in mouse model of allergic asthma by modulating pro-inflammatory cytokine response. Biomed. Pharmacother. 126, 110063. doi:10.1016/j.biopha.2020.110063
Balkrishna, A., Solleti, S. K., Singh, H., Verma, S., Sharma, N., Nain, P., et al. (2020b). Herbal decoction Divya-Swasari-Kwath attenuates airway inflammation and remodeling through Nrf-2 mediated antioxidant lung defence in mouse model of allergic asthma. Phytomedicine 78, 153295. doi:10.1016/j.phymed.2020.153295
Balkrishna, A., Verma, S., Sharma, P., Tomer, M., Srivastava, J., and Varshney, A. (2021d). Comprehensive and rapid quality evaluation method for the ayurvedic medicine divya-swasari-vati using two analytical techniques: UPLC/QToF MS and HPLC-DAD. Pharm. (Basel) 14, 297. doi:10.3390/ph14040297
Balkrishna, A., Verma, S., Solleti, S. K., Khandrika, L., and Varshney, A. (2020c). Calcio-herbal medicine divya-swasari-vati ameliorates SARS-CoV-2 spike protein-induced pathological features and inflammation in humanized zebrafish model by moderating IL-6 and TNF-α cytokines. J. Inflamm. Res. 13, 1219–1243. doi:10.2147/JIR.S286199
Barnes, P. J. (2006). Transcription factors in airway diseases. Lab. 86, 867–872. doi:10.1038/labinvest.3700456
Beigel, J. H., Tomashek, K. M., Dodd, L. E., Mehta, A. K., Zingman, B. S., Kalil, A. C., et al. (2020). Remdesivir for the treatment of covid-19 - final report. N. Engl. J. Med. 383, 1813–1826. doi:10.1056/NEJMoa2007764
Berger Rentsch, M., and Zimmer, G. (2011). A vesicular stomatitis virus replicon-based bioassay for the rapid and sensitive determination of multi-species type I interferon. PLoS One 6, e25858. doi:10.1371/journal.pone.0025858
Boulware, D. R., Pullen, M. F., Bangdiwala, A. S., Pastick, K. A., Lofgren, S. M., Okafor, E. C., et al. (2020). A randomized trial of hydroxychloroquine as postexposure prophylaxis for covid-19. N. Engl. J. Med. 383, 517–525. doi:10.1056/NEJMoa2016638
Chandra Manivannan, A., Malaisamy, A., Eswaran, M., Meyyazhagan, A., Arumugam, V. A., Rengasamy, K. R. R., et al. (2022). Evaluation of clove phytochemicals as potential antiviral drug candidates targeting SARS-CoV-2 main protease: Computational docking, molecular dynamics simulation, and pharmacokinetic profiling. Front. Mol. Biosci. 9, 918101. doi:10.3389/fmolb.2022.918101
Chang, J. S., Wang, K. C., Yeh, C. F., Shieh, D. E., and Chiang, L. C. (2013). Fresh ginger (Zingiber officinale) has anti-viral activity against human respiratory syncytial virus in human respiratory tract cell lines. J. Ethnopharmacol. 145, 146–151. doi:10.1016/j.jep.2012.10.043
Coperchini, F., Chiovato, L., Croce, L., Magri, F., and Rotondi, M. (2020). The cytokine storm in COVID-19: An overview of the involvement of the chemokine/chemokine-receptor system. Cytokine Growth Factor Rev. 53, 25–32. doi:10.1016/j.cytogfr.2020.05.003
Correa, L. B., Padua, T. A., Seito, L. N., Costa, T. E., Silva, M. A., Candea, A. L., et al. (2016). Anti-inflammatory effect of methyl gallate on experimental arthritis: Inhibition of neutrophil recruitment, production of inflammatory mediators, and activation of macrophages. J. Nat. Prod. 79, 1554–1566. doi:10.1021/acs.jnatprod.5b01115
Correa, L. B., Seito, L. N., Manchope, M. F., Verri, W. A., Cunha, T. M., Henriques, M. G., et al. (2020). Methyl gallate attenuates inflammation induced by Toll-like receptor ligands by inhibiting MAPK and NF-Κb signaling pathways. Inflamm. Res. 69, 1257–1270. doi:10.1007/s00011-020-01407-0
Costela-Ruiz, V. J., Illescas-Montes, R., Puerta-Puerta, J. M., Ruiz, C., and Melguizo-Rodriguez, L. (2020). SARS-CoV-2 infection: The role of cytokines in COVID-19 disease. Cytokine Growth Factor Rev. 54, 62–75. doi:10.1016/j.cytogfr.2020.06.001
Crestani, B., Cornillet, P., Dehoux, M., Rolland, C., Guenounou, M., and Aubier, M. (1994). Alveolar type II epithelial cells produce interleukin-6 in vitro and in vivo. Regulation by alveolar macrophage secretory products. J. Clin. 94, 731–740. doi:10.1172/JCI117392
Devpura, G., Tomar, B. S., Nathiya, D., Sharma, A., Bhandari, D., Haldar, S., et al. (2021). Randomized placebo-controlled pilot clinical trial on the efficacy of ayurvedic treatment regime on COVID-19 positive patients. Phytomedicine. 84, 153494. doi:10.1016/j.phymed.2021.153494
Di Lorenzo, G., Di Trolio, R., Kozlakidis, Z., Busto, G., Ingenito, C., Buonerba, L., et al. (2020). COVID 19 therapies and anti-cancer drugs: A systematic review of recent literature. Crit. Rev. Oncol. Hematol. 152, 102991. doi:10.1016/j.critrevonc.2020.102991
Dosch, S. F., Mahajan, S. D., and Collins, A. R. (2009). SARS coronavirus spike protein-induced innate immune response occurs via activation of the NF-kappaB pathway in human monocyte macrophages in vitro. Virus Res. 142, 19–27. doi:10.1016/j.virusres.2009.01.005
Du, L., He, Y., Zhou, Y., Liu, S., Zheng, B. J., and Jiang, S. (2009). The spike protein of SARS-CoV--a target for vaccine and therapeutic development. Nat. Rev. Microbiol. 7, 226–236. doi:10.1038/nrmicro2090
El Sohaimy, S., Abdo, N., Shehata, M., and Moheyeldin, O. (2021). Inhibition of COVID-19 RNA-dependent RNA polymerase by natural bioactive compounds: Molecular docking analysis. Egypt. J. Chem. 64, 1989–2001. doi:10.21608/ejchem.2021.45739.2947
El-Saber Batiha, G., Magdy Beshbishy, A., El-Mleeh, A., Abdel-Daim, M. M., and Prasad Devkota, H. (2020). Traditional uses, bioactive chemical constituents, and pharmacological and toxicological activities of Glycyrrhiza glabra L. (Fabaceae). Biomolecules 10, 352. doi:10.3390/biom10030352
Fara, A., Mitrev, Z., Rosalia, R. A., and Assas, B. M. (2020). Cytokine storm and COVID-19: A chronicle of pro-inflammatory cytokines. Open Biol. 10, 200160. doi:10.1098/rsob.200160
Felsenstein, S., Herbert, J. A., Mcnamara, P. S., and Hedrich, C. M. (2020). COVID-19: Immunology and treatment options. Clin. Immunol. 215, 108448. doi:10.1016/j.clim.2020.108448
Foster, K. A., Oster, C. G., Mayer, M. M., Avery, M. L., and Audus, K. L. (1998). Characterization of the A549 cell line as a type II pulmonary epithelial cell model for drug metabolism. Exp. Cell Res. 243, 359–366. doi:10.1006/excr.1998.4172
Fumagalli, G., Monza, L., Cavaletti, G., Rigolio, R., and Meregalli, C. (2020). Neuroinflammatory process involved in different preclinical models of chemotherapy-induced peripheral neuropathy. Front. Immunol. 11, 626687. doi:10.3389/fimmu.2020.626687
Gandhi, M., Yokoe, D. S., and Havlir, D. V. (2020). Asymptomatic transmission, the achilles' heel of current strategies to control covid-19. N. Engl. J. Med. 382, 2158–2160. doi:10.1056/NEJMe2009758
Ghosh, N., Ghosh, R., Mandal, V., and Mandal, S. C. (2011). Recent advances in herbal medicine for treatment of liver diseases. Pharm. Biol. 49, 970–988. doi:10.3109/13880209.2011.558515
Graebin, C. S. (2018). The pharmacological activities of glycyrrhizinic acid (“Glycyrrhizin”) and glycyrrhetinic acid. Sweeteners 2018, 245–261. doi:10.1007/978-3-319-27027-2_15
Gubernatorova, E. O., Gorshkova, E. A., Polinova, A. I., and Drutskaya, M. S. (2020). IL-6: Relevance for immunopathology of SARS-CoV-2. Cytokine Growth Factor Rev. 53, 13–24. doi:10.1016/j.cytogfr.2020.05.009
Hasan, M. K., Ara, I., Mondal, M. S. A., and Kabir, Y. (2021). Phytochemistry, pharmacological activity, and potential health benefits of Glycyrrhiza glabra. Heliyon 7, e07240. doi:10.1016/j.heliyon.2021.e07240
Hayati, R. F., Better, C. D., Denis, D., Komarudin, A. G., Bowolaksono, A., Yohan, B., et al. (2021). [6]-Gingerol inhibits chikungunya virus infection by suppressing viral replication. Biomed. Res. Int. 2021, 6623400. doi:10.1155/2021/6623400
Hayden, M. S., and Ghosh, S. (2014). Regulation of NF-κB by TNF family cytokines. Semin. Immunol. 26, 253–266. doi:10.1016/j.smim.2014.05.004
Heinrich, M., Jalil, B., Abdel-Tawab, M., Echeverria, J., Kulic, Z., Mcgaw, L. J., et al. (2022). Best Practice in the chemical characterisation of extracts used in pharmacological and toxicological research-The ConPhyMP-Guidelines. Front. Pharmacol. 13, 953205. doi:10.3389/fphar.2022.953205
Hoffmann, M., Kleine-Weber, H., and Pohlmann, S. (2020a). A multibasic cleavage site in the spike protein of SARS-CoV-2 is essential for infection of human lung cells. Mol. Cell 78, 779–784. e775. doi:10.1016/j.molcel.2020.04.022
Hoffmann, M., Kleine-Weber, H., Schroeder, S., Krüger, N., Herrler, T., Erichsen, S., et al. (2020b). SARS-CoV-2 cell entry depends on ACE2 and TMPRSS2 and is blocked by a clinically proven protease inhibitor. Cell 181, 271–280. e278. doi:10.1016/j.cell.2020.02.052
Hu, B., Guo, H., Zhou, P., and Shi, Z. L. (2021). Characteristics of SARS-CoV-2 and COVID-19. Nat. Rev. Microbiol. 19, 141–154. doi:10.1038/s41579-020-00459-7
Isbrucker, R. A., and Burdock, G. A. (2006). Risk and safety assessment on the consumption of Licorice root (Glycyrrhiza sp.), its extract and powder as a food ingredient, with emphasis on the pharmacology and toxicology of glycyrrhizin. Regul. Toxicol. Pharmacol. 46, 167–192. doi:10.1016/j.yrtph.2006.06.002
Jackson, C. B., Farzan, M., Chen, B., and Choe, H. (2022). Mechanisms of SARS-CoV-2 entry into cells. Nat. Rev. Mol. Cell Biol. 23, 3–20. doi:10.1038/s41580-021-00418-x
Kahkeshani, N., Farzaei, F., Fotouhi, M., Alavi, S. S., Bahramsoltani, R., Naseri, R., et al. (2019). Pharmacological effects of gallic acid in health and diseases: A mechanistic review. Iran. J. Basic Med. Sci. 22, 225–237. doi:10.22038/ijbms.2019.32806.7897
Kircheis, R., Haasbach, E., Lueftenegger, D., Heyken, W. T., Ocker, M., and Planz, O. (2020). NF-κB pathway as a potential target for treatment of critical stage COVID-19 patients. Front. Immunol. 11, 598444. doi:10.3389/fimmu.2020.598444
Korber, B., Fischer, W. M., Gnanakaran, S., Yoon, H., Theiler, J., Abfalterer, W., et al. (2020). Tracking changes in SARS-CoV-2 spike: Evidence that D614G increases infectivity of the COVID-19 virus. Cell 182, 812–827. doi:10.1016/j.cell.2020.06.043
Kumar, G., Kumar, D., and Singh, N. P. (2021). Therapeutic approach against 2019-nCoV by inhibition of ACE-2 receptor. Drug Res. (Stuttg) 71, 213–218. doi:10.1055/a-1275-0228
Kumar Paul, G., Mahmud, S., Aldahish, A. A., Afroze, M., Biswas, S., Briti Ray Gupta, S., et al. (2022). Computational screening and biochemical analysis of Pistacia integerrima and Pandanus odorifer plants to find effective inhibitors against Receptor-Binding domain (RBD) of the spike protein of SARS-Cov-2. Arab. J. Chem. 15, 103600. doi:10.1016/j.arabjc.2021.103600
Kumar, V., Dhanjal, J. K., Bhargava, P., Kaul, A., Wang, J., Zhang, H., et al. (2022). Withanone and Withaferin-A are predicted to interact with transmembrane protease serine 2 (TMPRSS2) and block entry of SARS-CoV-2 into cells. J. Biomol. Struct. Dyn. 40, 1–13. doi:10.1080/07391102.2020.1775704
Kumar, V., Dhanjal, J. K., Kaul, S. C., Wadhwa, R., and Sundar, D. (2021). Withanone and caffeic acid phenethyl ester are predicted to interact with main protease (Mpro) of SARS-CoV-2 and inhibit its activity. J. Biomol. Struct. Dyn. 39, 3842–3854. doi:10.1080/07391102.2020.1772108
Levitz, R., Wattier, R., Phillips, P., Solomon, A., Lawler, J., Lazar, I., et al. (2012). Induction of IL-6 and CCL5 (RANTES) in human respiratory epithelial (A549) cells by clinical isolates of respiratory syncytial virus is strain specific. Virol. J. 9, 190. doi:10.1186/1743-422X-9-190
Li, C.-X., Li, T.-H., Zhua, M., Lai, J., and Wu, Z.-P. (2021). Pharmacological properties of glabridin (a flavonoid extracted from licorice): A comprehensive review. J. Funct. Foods 85, 104638. doi:10.1016/j.jff.2021.104638
Li, S., Cheng, C. S., Zhang, C., Tang, G. Y., Tan, H. Y., Chen, H. Y., et al. (2021). Edible and herbal plants for the prevention and management of COVID-19. Front. Pharmacol. 12, 656103. doi:10.3389/fphar.2021.656103
Lin, J., Li, J., Shu, M., Wu, W., Zhang, W., Dou, Q., et al. (2020). The rCC16 protein protects against LPS-induced cell apoptosis and inflammatory responses in human lung pneumocytes. Front. Pharmacol. 11, 1060. doi:10.3389/fphar.2020.01060
Luo, P., Liu, D., and Li, J. (2020). Pharmacological perspective: Glycyrrhizin may be an efficacious therapeutic agent for COVID-19. Int. J. Antimicrob. Agents 55, 105995. doi:10.1016/j.ijantimicag.2020.105995
Mehta, P., Mcauley, D. F., Brown, M., Sanchez, E., Tattersall, R. S., Manson, J. J., et al. (2020). COVID-19: Consider cytokine storm syndromes and immunosuppression. Lancet 395, 1033–1034. doi:10.1016/S0140-6736(20)30628-0
Miryan, M., Soleimani, D., Askari, G., Jamialahmadi, T., Guest, P. C., Bagherniya, M., et al. (2021). Curcumin and piperine in COVID-19: A promising duo to the rescue? Adv. Exp. Med. Biol. 1327, 197–204. doi:10.1007/978-3-030-71697-4_16
Mishra, S., Pandey, A., and Manvati, S. (2020). Coumarin: An emerging antiviral agent. Heliyon 6, e03217. doi:10.1016/j.heliyon.2020.e03217
Moore, J. B., and June, C. H. (2020). Cytokine release syndrome in severe COVID-19. Science 368, 473–474. doi:10.1126/science.abb8925
Mueller, M., De La Pena, A., and Derendorf, H. (2004). Issues in pharmacokinetics and pharmacodynamics of anti-infective agents: Kill curves versus MIC. Antimicrob. Agents Chemother. 48, 369–377. doi:10.1128/AAC.48.2.369-377.2004
Nalbandian, A., Sehgal, K., Gupta, A., Madhavan, M. V., Mcgroder, C., Stevens, J. S., et al. (2021). Post-acute COVID-19 syndrome. Nat. Med. 27, 601–615. doi:10.1038/s41591-021-01283-z
Nisar, M. F., Khadim, M., Rafiq, M., Chen, J., Yang, Y., and Wan, C. C. (2021). Pharmacological properties and health benefits of eugenol: A comprehensive review. Oxid. Med. Cell. Longev. 2021, 2497354. doi:10.1155/2021/2497354
Ou, J., Zhou, Z., Dai, R., Zhao, S., Wu, X., Zhang, J., et al. (2021). Emergence of RBD mutations in circulating SARS-CoV-2 strains enhancing the structural stability and human ACE2 receptor affinity of the spike protein. bioRxiv 2003, 2015991844. doi:10.1101/2020.03.15.991844
Padhi, A. K., and Tripathi, T. (2020). Can SARS-CoV-2 accumulate mutations in the S-protein to increase pathogenicity? ACS Pharmacol. Transl. Sci. 3, 1023–1026. doi:10.1021/acsptsci.0c00113
Paidi, R. K., Jana, M., Raha, S., Mckay, M., Sheinin, M., Mishra, R. K., et al. (2021). Eugenol, a component of holy basil (tulsi) and common spice clove, inhibits the interaction between SARS-CoV-2 spike S1 and ACE2 to induce therapeutic responses. J. Neuroimmune Pharmacol. 16, 743–755. doi:10.1007/s11481-021-10028-1
Pandey, P., Singhal, D., Khan, F., and Arif, M. (2020). An in silico screening on Piper nigrum, Syzygium aromaticum and Zingiber officinale roscoe derived compounds against SARS‐CoV‐2: A drug repurposing approach. Biointerface Res. Appl. Chem. 11, 11122–11134. doi:10.33263/briac114.1112211134
Parasuraman, S., Thing, G. S., and Dhanaraj, S. A. (2014). Polyherbal formulation: Concept of ayurveda. Pharmacogn. Rev. 8, 73–80. doi:10.4103/0973-7847.134229
Patel, A., Agarwal, R., Rudramurthy, S. M., Shevkani, M., Xess, I., Sharma, R., et al. (2021). Multicenter epidemiologic study of coronavirus disease-associated mucormycosis, India. Emerg. Infect. Dis. 27, 2349–2359. doi:10.3201/eid2709.210934
Peter, A. E., Sandeep, B. V., Rao, B. G., and Kalpana, V. L. (2020). Calming the storm: Natural immunosuppressants as adjuvants to target the cytokine storm in COVID-19. Front. Pharmacol. 11, 583777. doi:10.3389/fphar.2020.583777
Rabaan, A. A., Al-Ahmed, S. H., Muhammad, J., Khan, A., Sule, A. A., Tirupathi, R., et al. (2021). Immunopathology and Immunomodulatory Drugs to Counter Cytokine Storm, 9. doi:10.3390/vaccines9050436Role of inflammatory cytokines in COVID-19 patients: A review on molecular mechanisms, immune functionsVaccines (Basel)
Ragab, D., Salah Eldin, H., Taeimah, M., Khattab, R., and Salem, R. (2020). The COVID-19 cytokine storm; what we know so far. Front. Immunol. 11, 1446. doi:10.3389/fimmu.2020.01446
Rana, S., Shahzad, M., and Shabbir, A. (2016). Pistacia integerrima ameliorates airway inflammation by attenuation of TNF-alpha, IL-4, and IL-5 expression levels, and pulmonary edema by elevation of AQP1 and AQP5 expression levels in mouse model of ovalbumin-induced allergic asthma. Phytomedicine 23, 838–845. doi:10.1016/j.phymed.2016.04.006
Rathinavel, T., Palanisamy, M., Palanisamy, S., Subramanian, A., and Thangaswamy, S. (2020). Phytochemical 6-Gingerol – a promising Drug of choice for COVID-19. Int. J. Adv. Sci. Eng. Technol. 06, 1482–1489. doi:10.29294/ijase.6.4.2020.1482-1489
Rios, J. L., Giner, R. M., Marin, M., and Recio, M. C. (2018). A pharmacological update of ellagic acid. Planta Med. 84, 1068–1093. doi:10.1055/a-0633-9492
Ruwizhi, N., and Aderibigbe, B. A. (2020). Cinnamic acid derivatives and their biological efficacy. Int. J. Mol. Sci. 21, 5712. doi:10.3390/ijms21165712
Semaming, Y., Pannengpetch, P., Chattipakorn, S. C., and Chattipakorn, N. (2015). Pharmacological properties of protocatechuic acid and its potential roles as complementary medicine. Evid. Based. Complement. Altern. Med. 2015, 593902. doi:10.1155/2015/593902
Semwal, R. B., Semwal, D. K., Combrinck, S., and Viljoen, A. M. (2015). Gingerols and shogaols: Important nutraceutical principles from ginger. Phytochemistry 117, 554–568. doi:10.1016/j.phytochem.2015.07.012
Sharifi-Rad, J., Cruz-Martins, N., Lopez-Jornet, P., Lopez, E. P., Harun, N., Yeskaliyeva, B., et al. (2021). Natural coumarins: Exploring the pharmacological complexity and underlying molecular mechanisms. Oxid. Med. Cell. Longev. 2021, 6492346. doi:10.1155/2021/6492346
Srivastava, V., Yadav, A., and Sarkar, P. (2022). Molecular docking and ADMET study of bioactive compounds of Glycyrrhiza glabra against main protease of SARS-CoV2. Mat. Today. Proc. 49, 2999–3007. doi:10.1016/j.matpr.2020.10.055
Su, C. M., Wang, L., and Yoo, D. (2021). Activation of NF-κB and induction of proinflammatory cytokine expressions mediated by ORF7a protein of SARS-CoV-2. Sci. Rep. 11, 13464. doi:10.1038/s41598-021-92941-2
Tamir, H., Melamed, S., Erez, N., Politi, B., Yahalom-Ronen, Y., Achdout, H., et al. (2022). Induction of innate immune response by TLR3 agonist protects mice against SARS-CoV-2 infection. Viruses 14, 189. doi:10.3390/v14020189
Tang, Y., Liu, J., Zhang, D., Xu, Z., Ji, J., and Wen, C. (2020). Cytokine storm in COVID-19: The current evidence and treatment strategies. Front. Immunol. 11, 1708. doi:10.3389/fimmu.2020.01708
Tripathi, A. K., Ray, A. K., and Mishra, S. K. (2022). Molecular and pharmacological aspects of piperine as a potential molecule for disease prevention and management: Evidence from clinical trials. Beni. Suef. Univ. J. Basic Appl. Sci. 11, 16. doi:10.1186/s43088-022-00196-1
Van De Sand, L., Bormann, M., Alt, M., Schipper, L., Heilingloh, C. S., Steinmann, E., et al. (2021). Glycyrrhizin effectively inhibits SARS-CoV-2 replication by inhibiting the viral main protease. Viruses 13, 609. doi:10.3390/v13040609
Wang, J., Wang, Q., Han, T., Li, Y. K., Zhu, S. L., Ao, F., et al. (2015). Soluble interleukin-6 receptor is elevated during influenza A virus infection and mediates the IL-6 and IL-32 inflammatory cytokine burst. Cell. Mol. Immunol. 12, 633–644. doi:10.1038/cmi.2014.80
Whitt, M. A. (2010). Generation of VSV pseudotypes using recombinant ΔG-VSV for studies on virus entry, identification of entry inhibitors, and immune responses to vaccines. J. Virol. Methods 169, 365–374. doi:10.1016/j.jviromet.2010.08.006
World Health Organization (2022). WHO Coronavirus disease (COVID-19) dashboard. World health organization. Available at: https://covid19.who.int/? (Accessed July 15, 2022).
Yakhchali, M., Taghipour, Z., Mirabzadeh Ardakani, M., Alizadeh Vaghasloo, M., Vazirian, M., and Sadrai, S. (2021). Cinnamon and its possible impact on COVID-19: The viewpoint of traditional and conventional medicine. Biomed. Pharmacother. 143, 112221. doi:10.1016/j.biopha.2021.112221
Yang, J., Hooper, W. C., Phillips, D. J., and Talkington, D. F. (2002). Regulation of proinflammatory cytokines in human lung epithelial cells infected with Mycoplasma pneumoniae. Infect. Immun. 70, 3649–3655. doi:10.1128/IAI.70.7.3649-3655.2002
Yang, J., Petitjean, S. J. L., Koehler, M., Zhang, Q., Dumitru, A. C., Chen, W., et al. (2020). Molecular interaction and inhibition of SARS-CoV-2 binding to the ACE2 receptor. Nat. Commun. 11, 4541. doi:10.1038/s41467-020-18319-6
Yang, Y., Islam, M. S., Wang, J., Li, Y., and Chen, X. (2020). Traditional Chinese medicine in the treatment of patients infected with 2019-new coronavirus (SARS-CoV-2): A review and perspective. Int. J. Biol. Sci. 16, 1708–1717. doi:10.7150/ijbs.45538
Ye, Q., Wang, B., and Mao, J. (2020). The pathogenesis and treatment of the `Cytokine Storm' in COVID-19. J. Infect. 80, 607–613. doi:10.1016/j.jinf.2020.03.037
Zhang, L., Jackson, C. B., Mou, H., Ojha, A., Rangarajan, E. S., Izard, T., et al. (2020). SARS-CoV-2 spike-protein D614G mutation increases virion spike density and infectivity. Nat. Commun. 11, 6013. doi:10.1038/s41467-020-19808-4
Keywords: Divya-Swasari-Vati (DSV), SARS-CoV-2, pseudovirus, spike protein, ACE 2, proinflammatory cytokines
Citation: Balkrishna A, Goswami S, Singh H, Gohel V, Dev R, Haldar S and Varshney A (2022) Herbo-mineral formulation, Divya-Swasari-Vati averts SARS-CoV-2 pseudovirus entry into human alveolar epithelial cells by interfering with spike protein-ACE 2 interaction and IL-6/TNF-α /NF-κB signaling. Front. Pharmacol. 13:1024830. doi: 10.3389/fphar.2022.1024830
Received: 22 August 2022; Accepted: 06 October 2022;
Published: 26 October 2022.
Edited by:
Maria De Lourdes Pereira, University of Aveiro, PortugalReviewed by:
Mahaveer Dhobi, Delhi Pharmaceutical Sciences and Research University, IndiaMithun Rudrapal, Rasiklal M. Dhariwal Institute of Pharmaceutical Education and Research, India
Maria Correia, Universidade Católica Portuguesa, Portugal
Copyright © 2022 Balkrishna, Goswami, Singh, Gohel, Dev, Haldar and Varshney. This is an open-access article distributed under the terms of the Creative Commons Attribution License (CC BY). The use, distribution or reproduction in other forums is permitted, provided the original author(s) and the copyright owner(s) are credited and that the original publication in this journal is cited, in accordance with accepted academic practice. No use, distribution or reproduction is permitted which does not comply with these terms.
*Correspondence: Anurag Varshney, YW51cmFnQHBhdGFuamFsaS5yZXMuaW4=