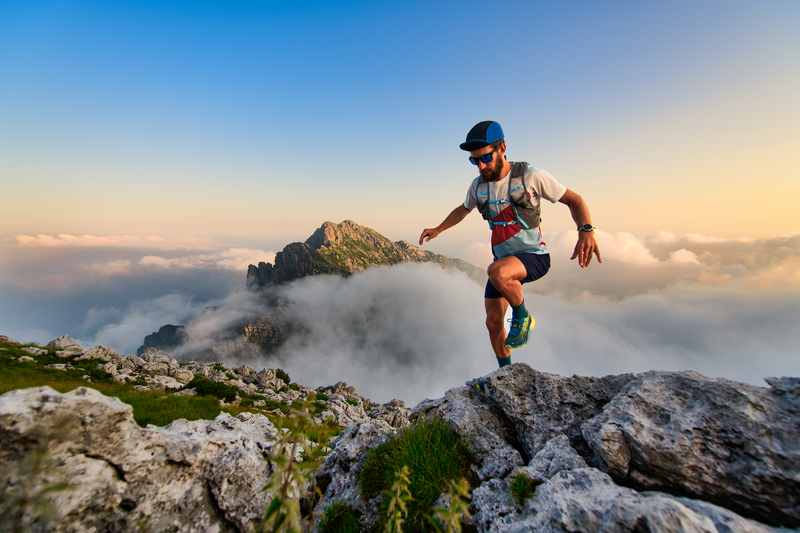
95% of researchers rate our articles as excellent or good
Learn more about the work of our research integrity team to safeguard the quality of each article we publish.
Find out more
REVIEW article
Front. Pharmacol. , 08 November 2022
Sec. Neuropharmacology
Volume 13 - 2022 | https://doi.org/10.3389/fphar.2022.1020918
This article is part of the Research Topic Mechanism and Pharmacodynamic Material Basis of Neurodegenerative Disease Therapies View all 11 articles
Stroke is a common disease in clinical practice, which seriously endangers people’s physical and mental health. The neurovascular unit (NVU) plays a key role in the occurrence and development of ischemic stroke. Different from other classical types of cell death such as apoptosis, necrosis, autophagy, and pyroptosis, ferroptosis is an iron-dependent lipid peroxidation-driven new form of cell death. Interestingly, the function of NVU and stroke development can be regulated by activating or inhibiting ferroptosis. This review systematically describes the NVU in ischemic stroke, provides a comprehensive overview of the regulatory mechanisms and key regulators of ferroptosis, and uncovers the role of ferroptosis in the NVU and the progression of ischemic stroke. We further discuss the latest progress in the intervention of ferroptosis as a therapeutic target for ischemic stroke and summarize the research progress and regulatory mechanism of ferroptosis inhibitors on stroke. In conclusion, ferroptosis, as a new form of cell death, plays a key role in ischemic stroke and is expected to become a new therapeutic target for this disease.
Stroke is a common disease in clinical practice, which seriously endangers people’s health and is mainly divided into two subtypes, including ischemic and hemorrhagic stroke (Peisker et al., 2017). Current evidence suggests that ischemic stroke accounts for approximately 85% of the morbidity of stroke (Benjamin et al., 2018), mainly due to cerebral blood circulation disorder, localized brain tissue necrosis or softening caused by ischemia and hypoxia, leading to corresponding nervous system function defects (Sacco et al., 2013). Ischemic stroke is also a serious disease with high mortality, and the resulting severe cognitive and motor impairments can significantly burden families and society (Owolabi et al., 2015; Donkor, 2018). The post-ischemic brain is characterized by the accumulation of amyloid plaques and neurofibrillary tangles, followed by the development of dementia (Yang et al., 2019). Therefore, ischemic stroke increases the neurological deficits in dementia patients (Owolabi et al., 2015).
It is widely thought that the neurovascular unit (NVU) plays a crucial role in the occurrence and development of ischemic stroke (Iadecola, 2017), as well as in the remodeling of blood vessels and nerves after stroke (Leigh et al., 2018). The past decade has witnessed significant inroads in pathological research on ischemic stroke with the discovery of a new form of cell death in the NVU of ischemic stroke, namely ferroptosis (Doll et al., 2017; Magtanong and Dixon, 2018; Zhou et al., 2021).
In recent years, ferroptosis has become a research hotspot (Dixon et al., 2012). During ferroptosis, a high abundance of unsaturated fatty acids on the cell membrane undergo lipid peroxidation under ferrous iron or ester oxygenase, thereby inducing cell death (Yang et al., 2016). The occurrence and execution of ferroptosis depend on the interaction of amino acid, lipid and iron metabolism (Ursini and Maiorino, 2020), and its sensitivity is also regulated by several key pathways and processes (Chen et al., 2021a). Ferroptosis is associated with various diseases such as Parkinson’s disease (Mahoney-Sanchez et al., 2021), tumor (Kim et al., 2016), and renal failure (Adedoyin et al., 2018), and the development of these diseases can be intervened by activating or inhibiting ferroptosis.
In ischemic stroke, pathological changes are closely related to ferroptosis, such as iron metabolism disorder, lipid peroxidation, and increased ROS (Hu et al., 2019; Ren et al., 2020). An increasing body of evidence from recently published studies substantiates the correlation between ferroptosis and stroke (Hu et al., 2019; Ren et al., 2020). This review provides a comprehensive overview of the NVU of ischemic stroke and the role of ferroptosis in ischemic stroke, providing new insights into the application of ferroptosis in treating ischemic stroke.
Although significant progress has been made in better understanding the mechanism of neuron injury and repair after ischemia (Figure 1), there is still a lack of effective treatment for this disease (Chan, 1996; Hu et al., 2017; Yang et al., 2017). In the past, research on cerebral ischemic injury was mostly limited to neurons (Yang et al., 2019) or different cell groups and structures in the brain (Yang et al., 2017; Qin et al., 2019), ignoring the integrity of brain function and the interaction between different structures. Recently, the concept of the neurovascular unit as a new protective target for ischemic brain injury has been proposed (Cai et al., 2017; Zhao et al., 2020a).
FIGURE 1. Schematic diagram of the pathological mechanism of ischemic stroke. Ischemic stroke triggers cascades of complex events that cause oxidative stress and excitotoxicity due to the accumulation of ROS and calcium (Ca2+), blood-brain-barrier (BBB) breakdown and activated inflammatory responses. Excessive ROS and Ca2+ lead to mitochondrial dysfunction and activation of apoptotic factors, ultimately leading to apoptosis and necrotic cell death.
The neurovascular unit is mainly composed of neurons, glial cells (including astrocytes, microglia, oligodendrocytes), and the blood-brain barrier (BBB, including vascular endothelial cells, astrocytic end-foot processes, basal lamina and pericytes) to maintain homeostasis of the central system (Pardridge, 1991), and extracellular matrix that maintains the integrity of the brain tissue environment (Lo and Rosenberg, 2009; Iadecola, 2017). The NVU maintains the normal physiological function of neurons and the repair of damaged neurons, which emphasizes the importance of the interconnection and mutual influence between neurons, glial cells and cerebrovascular (Stamatovic et al., 2008; Lo and Rosenberg, 2009) and provides the foothold for further study of neuron injury and protection mechanism. Overall, the NVU plays a key role in the clinical treatment of ischemic stroke and is increasingly valued by researchers and clinicians (Iadecola, 2017).
It is well-established that neurons are most vulnerable to cerebral ischemia-reperfusion injury (CIRI) (Lo and Rosenberg, 2009) and are continuously affected by several pathological reactions such as inflammation, excitatory amino acid toxicity, and oxidative stress after CIRI (Rohnert et al., 2012). Among them, excitatory amino acids include glutamate and aspartate (Brann and Mahesh, 1994), glutamate is the main excitatory neurotransmitter in the mammalian central nervous system, which can have long-term effects on the structure and function of neurons, and glutamate-mediated excitatory signal transduction can affect mammalian brain functions, including cognition, memory and learning. The release of a large number of excitatory amino acids will activate plenty of ion channels and lead to persistent intracellular Ca2+ level increase, cell damage and death, which is called excitatory amino acid toxicity (Gillessen et al., 2002). While, this excitotoxicity caused by excitatory amino acids is one of the earliest and widely recognized molecular mechanisms of CIRI (Lai et al., 2014). When the brain is in a state of ischemia and hypoxia, the release of excitatory neurotransmitters is increased and reuptake is impaired due to metabolic disorders, and eventually the level of excitatory neurotransmitters in the ischemic region increases rapidly that leads to aberrant activation of many Ca2+-dependent pathways and initiation of apoptosis, necroptosis and autophagy processes in the brain (Shen et al., 2022). Current evidence suggests that neuronal death accounts for the poor prognosis in ischemic stroke (Chen et al., 2020). Indeed, assessing the severity of an ischemic stroke and the cause of death depends largely on the number of neurons dying in the affected brain area (Lazarov and Hollands, 2016).
Microglia are innate immune cells in the brain, accounting for approximately 5–20% of glial cells (Benveniste, 1997). The main functions of microglia are to recognize pathogens, phagocytose necrotic or apoptotic cells, remove damaged neurons, tissue fragments, small and inactive synapses, infected small molecules and macromolecules, regulate T cell response, and induce inflammatory process (Nimmerjahn et al., 2005). In addition, microglia have extensive connections with other NVU cells (Thurgur and Pinteaux, 2019), which can regulate the microenvironmental homeostasis of NVU, and have positive significance for maintaining the barrier function of BBB (Abdullahi et al., 2018). When an ischemic stroke occurs, neurons activate microglia to differentiate into M1-and M2-phenotypes by releasing certain soluble factors and intracellular components (Akhmetzyanova et al., 2019; Qin et al., 2019). It is well-established that M1-type microglia have a pro-inflammatory and deleterious effect on the ischemic brain (Yu et al., 2022), while M2-type microglia can reduce the inflammatory response and exert neuroprotective effects (Varin and Gordon, 2009; Soehnlein and Lindbom, 2010).
In addition, microglia can affect the activity of neurons by releasing ATP and stimulating astrocytes to release glutamate to increase the excitatory postsynaptic potential (Barakat and Redzic, 2016; Illes et al., 2021). After cerebral ischemia, microglia release many inflammatory factors (Soehnlein and Lindbom, 2010), destroy the normal function of neurons and damage vascular endothelial cells, thereby destroying the BBB structure and aggravating brain edema (Yenari et al., 2010).
Oligodendrocytes are the myelinating cells in the central nervous system (CNS) and originate from oligodendrocyte progenitor cells (OPCs). OPCs can differentiate into oligodendrocytes or astrocytes according to the environment (Hughes et al., 1988). Endothelial cells promote the proliferation of OPCs by releasing trophic factors such as brain-derived neurotrophic factor (BDNF) and basic fibroblast growth factor (bFGF) (Dugas et al., 2008) and release vascular endothelial growth factor A (VEGF-A) to promote oligodendrocyte migration (Guo et al., 2008; Arai and Lo, 2009). The main function of oligodendrocytes is to form an insulating myelin sheath wrapping the axons in the CNS, assist in the efficient transmission of bioelectrical signals, and maintain and protect the normal function of neurons (Baumann and Pham-Dinh, 2001; Kuhn et al., 2019). Abnormalities in oligodendrocytes not only lead to demyelinating lesions of the CNS but also cause neuronal damage, psychiatric diseases, and even brain tumors (Kuhn et al., 2019). Under ischemic conditions, the expression of Nogo-A in oligodendrocytes is upregulated (Kern et al., 2013), thereby inhibiting axonal remodeling, impairing neuronal function, and triggering the early breakdown of BBB by secreting matrix metalloproteinase-9 (Mandai et al., 1997; Dewar et al., 2003).
Vascular endothelial cells (VECs) constitute a monolayer of specialized cells strategically positioned between the vascular wall and the bloodstream (Kruger-Genge et al., 2019). Ischemic stroke results from a combination of factors such as platelet adhesion and aggregation and related release reactions (Del Zoppo, 1998), fibrin protease activation, and fibrin formation after vascular endothelial injury (Zhou et al., 2020). Under normal conditions, certain active factors released by VECs play a protective role in regulating vascular tension, coagulation, fibrinolysis, and maintaining normal blood pressure and hemodynamics (Sandoo et al., 2010). Once the vascular endothelium is damaged, the exposed subendothelial layer can cause platelet adhesion and aggregation, leading to thrombosis (Chen and Lopez, 2005). The stimulated VECs can also release tissue factors to promote the extrinsic coagulation process involving coagulation factor XII and accelerate thrombosis (Lopes-Bezerra and Filler, 2003). Notably, prolonged ischemia-hypoxia and ischemia-reperfusion (I/R) can damage VECs (Zhou et al., 2020). In addition, ischemia and hypoxia can induce the expression of VEGF (Ramakrishnan et al., 2014), which can promote the proliferation of VECs and participate in angiogenesis, thereby suppressing ischemic stroke and playing a neuroprotective role (Lopes-Bezerra and Filler, 2003; Zhou et al., 2020).
In glial cells, astrocytes perform multiple homeostatic functions to maintain the survival and stability of the NVU (Becerra-Calixto and Cardona-Gomez, 2017), exerting neuroprotective, angiogenic, immunomodulatory, neurogenic, and antioxidant effects with the ability to modulate synaptic function (Daneman and Prat, 2015; Becerra-Calixto and Cardona-Gomez, 2017). Under physiological conditions, astrocytes release various neurotrophic factors, which can repair damage to neurons and VECs (Ye et al., 2018). During cerebral ischemia, the energy supply of brain cells is insufficient, resulting in the dysregulation of intracellular calcium and sodium pumps (Peng et al., 2019). Because of the extensive gap junctions and hemichannels in astrocytes, the gap junctions are destroyed, and Ca2+ and toxic substances are rapidly transmitted, causing astrocytes to release a large amount of glutamate to aggravate the excitatory amino acid toxicity (Lim et al., 2021). On the other hand, astrocytes are overactivated in the acute phase of ischemia, eventually forming a glial scar that hinders the repair of neurons (Sofroniew, 2009).
Brain pericytes are located in the center of the NVU and respond by receiving, integrating and processing signals from neighboring cells (Hamilton et al., 2010; Armulik et al., 2011; Winkler et al., 2011). They are critical in maintaining the normal function of the CNS and are involved in the formation and maintenance of BBB, cerebral blood flow (CBF) regulation, immunoregulation, angiogenesis, and stability. Overwhelming evidence substantiates that the dysfunction and loss of pericytes play a key role in the pathogenesis of various cerebrovascular diseases (Winkler et al., 2011). It has been shown that after ischemia, pericytes begin to detach from the cerebral microvessels (Zhou et al., 2022), which causes the destruction of tight junctions between cells, resulting in the destruction and leakage of the BBB (Bergers and Song, 2005). The platelet-derived growth factor receptor β (PDGFRβ) on pericytes is upregulated after cerebral ischemia and can combine with PDGFβ secreted by VECs to promote the recruitment and migration of pericytes for neovascularization to promote maturation (Makihara et al., 2015; Hutter-Schmid and Humpel, 2016). It has been shown that pericytes begin to secrete VEGF within 24 h after ischemic stroke, which promotes angiogenesis in the peri-infarct area by activating VEGFR in endothelial cells (Shibuya, 2011; Yang et al., 2017; Zhou et al., 2022). Under ischemic and hypoxic conditions, pericytes can exhibit pluripotent stem cell properties and participate in immune responses (Davidoff, 2019; Fernandez-Morales et al., 2019). A recent study found that TNF-α could promote the release of IL-6 from pericytes, which contributed to the activation of microglia (Matsumoto et al., 2018a).
This NVU theory emphasizes the important connection between neurons, glial cells and microvessels after cerebral ischemia, corroborating that all components of NVU are involved in the pathological process of cerebral ischemia injury (Stamatovic et al., 2008; Mcconnell et al., 2017). This connection is realized through cell-cell and cell-matrix interaction. There are many chemicals involved in the regulation of BBB permeability in different cell types of NVU. These regulatory chemicals fall into different categories, such as proinflammatory cytokines (e.g., TNF-α, IL-1, IL-6), neurotransmitters (e.g., NO), ROS and other substances (Table 1). Comprehensive treatment of NVU can effectively combat ischemic stroke. Therefore, NVU provides a theoretical basis for the current research and treatment of neurological diseases, and targeting NVU from a global perspective may bring new opportunities for treating ischemic stroke.
Programmed cell death plays an important role in homeostasis and disease development. Among them, ferroptosis is a newly discovered form first proposed by Stockwell et al., in 2012 (Dixon et al., 2012). Ferroptosis is significantly different from other types of cell death such as apoptosis, necrosis, autophagy, and pyroptosis at the morphological, biochemical, and genetic levels (Dixon et al., 2012). The morphological features of ferroptosis are mainly manifested in mitochondria, including reduced volume, increased membrane density, and reduced numbers or absence (Chen et al., 2021a; Jiang et al., 2021). Regarding biochemical characteristics, ferroptosis manifests as glutathione depletion, glutathione peroxidase 4 (GPX4) inactivation, and lipid peroxide accumulation (Chen et al., 2021a; Jiang et al., 2021).
It has been confirmed that ferroptosis is closely related to neurodegenerative diseases, tumors, cardiovascular and cerebrovascular diseases, and acute kidney injury (AKI) (Ward et al., 2014; Fang et al., 2019; Bao et al., 2021), and its inhibitors can effectively delay disease progression and improve clinical symptoms (Angeli et al., 2017). However, the regulatory mechanism of ferroptosis has not yet been fully elucidated. With significant progress achieved in the study of ferroptosis, various regulatory factors and mechanisms have been discovered, suggesting that it is mainly related to iron metabolism disorder, amino acid antioxidant system imbalance, and lipid peroxide accumulation (Figure 2) (Dixon et al., 2012; Chen et al., 2021a). When iron metabolism disorder causes the increase of intracellular free iron, iron catalyzes the production of ROS through the Fenton reaction, and ROS further promotes lipid peroxidation, causing the accumulation of lipid peroxides and inducing ferroptosis (Dixon et al., 2012; Stockwell et al., 2017; Chen et al., 2021a). Indeed, an in-depth study and elucidation of the pathophysiological mechanism of ferroptosis can provide new ideas and treatment methods for ferroptosis-related diseases.
FIGURE 2. Regulatory mechanisms of ferroptosis. The primary metabolism involved in ferroptosis can be roughly divided into three categories: iron metabolism, System Xc−/GSH/GPX4 pathway, and lipid peroxidation. Besides, the FSP1-CoQ10-NAD(P)H pathway, which exists as an independent parallel system with GPX4 and GSH, inhibits phospholipid peroxidation and ferroptosis.
Iron metabolism disorders, especially iron overload, are key to ferroptosis (Dixon et al., 2012). Fe3+ in the blood circulation is combined with transferrin and transported to the cell through transferrin receptor 1 (TFR1) on the cell membrane surface (Beguin et al., 2014). Then Fe3+ is reduced to Fe2+ and released into the labile iron pool (LIP) in the cytoplasm (Kakhlon and Cabantchik, 2002), while excess iron is stored in ferritin (Jacobs et al., 1972). During this process, nuclear receptor coactivator 4 (NCOA4) acts as an adaptor protein to mediate the targeted transport of ferritin to lysosomes for autophagic degradation, thereby releasing free Fe2+, a process called ferritinophagy, mainly responsible for iron release and recovery (Mancias et al., 2014). Part of Fe2+ is transported out of cells through ferroportin1 (FPN1) on the cell membrane to ensure that the intracellular iron concentration is not excessively high under physiological conditions (Zhang et al., 2011; Zhang et al., 2012). Current evidence suggests that iron metabolism disorders can increase intracellular LIP and cause an increase in intracellular free iron (Ravingerova et al., 2020).
Due to the instability and high reactivity of Fe2+, hydroxyl radicals can be generated through the Fenton reaction (Thomas et al., 2009), which can directly react with polyunsaturated fatty acids (PUFAs) in the plasma membrane to generate a large amount of lipid ROS (Magtanong et al., 2016), and further promote lipid peroxidation and peroxide accumulation, inducing cell ferroptosis (Stockwell et al., 2017).
The cystine-glutamate antiporter System Xc− is widely distributed in the phospholipid bilayer of biological cells (Bannai, 1986; Sasaki et al., 2002; Bridges et al., 2012). It is a heterodimer composed of light chain solute carrier family seven member 11 (SLC7A11) and heavy chain solute carrier family three member 2 (SLC3A2) (Sato et al., 1999; Broer and Wagner, 2002; Verrey et al., 2004). The System Xc− can export intracellular glutamate to the extracellular space while importing cystine into the cytoplasm, where cystine is reduced to cysteine, which is involved in glutathione (GSH) synthesis (Seib et al., 2011). Glutathione peroxidase (GPX), whose active center is selenocysteine, catalyzes the conversion of reduced GSH to oxidized glutathione (GSSG), converting toxic peroxides into hydroxyl compounds to protect cell membranes from oxidative stress damage (Guan et al., 2017).
GPX4 is the only enzyme found in the GPX family that can reduce peroxides in lipid membranes, and its antioxidant effect is significantly higher than other family members (Margis et al., 2008). In particular, GPX4 can degrade hydrogen peroxide and other small molecule peroxides induced by iron overload in cells, preventing ferroptosis caused by the accumulation of ROS (Borchert et al., 2018). The antioxidant activity of GPX4 depends on GSH, which acts as an electron donor and converts toxic lipid hydroperoxide into non-toxic lipid alcohol (L-OH) (Fei et al., 2020). When System Xc− is blocked, glutamate and cystine cannot be exchanged, resulting in the accumulation of intracellular glutamate, decreased GSH synthesis and GPX4 activity, thereby increasing ROS in lipids and inducing cell ferroptosis (Forcina and Dixon, 2019).
Lipid peroxidation refers to the loss of hydrogen atoms of lipids under the action of free radicals or lipid peroxidase, resulting in the oxidation, fragmentation and shortening of lipid carbon chains and the production of lipid free radicals, lipid hydroperoxides (LOOH) and reactive aldehydes (such as malondialdehyde and 4-hydroxynonenal) and other cytotoxic substances, eventually cause lipid oxidative degradation reactions that damage cells (Ayala et al., 2014). ROS are a group of molecules with partially reduced oxygen, including peroxides, superoxides, singlet oxygen, free radicals, etc., which cause cell death by damaging DNA, RNA and lipid molecules (Su et al., 2019; Villalpando-Rodriguez and Gibson, 2021). As a member of intracellular ROS, lipid peroxides are the ultimate executors of ferroptosis (Cheng et al., 2021). The deleterious effect of lipid peroxidation is mainly reflected in the oxidative degradation of two important biofilm components, including phosphatidylethanolamines (PEs) and PUFAs (Gaschler and Stockwell, 2017).
PUFA is the main component of phospholipids in cell and organelle membranes and is also an important substrate for the synthesis of PE (Stubbs and Smith, 1984). PUFA has a high affinity for free radicals, and the hydrogen atoms between its double bonds are easily oxidized by free radicals (Cunnane, 1994). The lipid peroxidation reaction of PUFA is roughly divided into two stages (Kanner et al., 1987; Girotti, 1998; Ayala et al., 2014). First, ROS acquire hydrogen atoms in PUFA to generate lipid radicals (Yin et al., 2011); subsequently, lipid radicals interact with oxygen molecules to generate lipid peroxyl radicals (LOO-) (Chamulitrat and Mason, 1989). LOO- can reportedly abstract hydrogen atoms from other PUFAs to form lipid radicals and lipid hydroperoxides (Chamulitrat and Mason, 1989; Girotti, 1998). Moreover, LOO- participates in the oxidation process of PUFAs, which ensures that the lipid peroxidation of PUFAs exhibits the characteristics of a cascade reaction (Yin et al., 2011).
However, the affinity between PE and free radicals is not high, and oxidation sites need to be formed under the action of two enzymes before lipid peroxidation occurs (Pratt et al., 2011). First, long-chain acyl-Coa synthetase-4 (ACSL4) utilizes arachidonic acid (AA) and adrenic acid (AdA) to synthesize arachidonoyl-CoA (AA-COA) and adrenoyl-CoA (AdA-COA) (Ma et al., 2021); then, AA/AdA-COA combines with PE to form PE-AA/AdA under the catalytic action of lysophosphatidylcholine acyltransferase 3 (LPCAT3) (Kagan et al., 2017).
PE-AA/AdA is easily oxidized to cytotoxic PE-AA/AdA-OOH by free radicals or Arachidonate 15-Lipoxygenase (ALOX15), which promotes ferroptosis (Kagan et al., 2017). LOX-mediated lipid hydroperoxide production has been suggested to be involved in ferroptosis (Shintoku et al., 2017). The accumulation of lipid peroxides, especially phospholipid peroxides, is a hallmark event of ferroptosis (Shintoku et al., 2017). High levels of ACSL4 and LPCAT3 have been detected in various tumors, such as renal and liver cancer cells (Luo et al., 2021). At present, the expression of these two enzymes has been used to assess the sensitivity of various tumor cells to ferroptosis (Yuan et al., 2016a; Doll et al., 2017; Kagan et al., 2017; Magtanong and Dixon, 2018).
Nuclear factor-E2-related factor 2 (Nrf2) is a transcription factor with a leucine zipper structure, which plays a key anti-oxidation role (Jaiswal, 2004). The activity of Nrf2 is strictly regulated by Kelch-like ECH-associated protein 1 (Keap1) (Kobayashi and Yamamoto, 2006). Under normal conditions, Nrf2 binds to Keap1 and is inactivated with ubiquitination and degradation in the proteasome (Zhang et al., 2004). Once in a state of oxidative stress, Keap1 is degraded by autophagy to release Nrf2 (Kaspar et al., 2009). Free Nrf2 rapidly translocates to the nucleus, which binds to antioxidant response elements (AREs) in the promoter region to drive antioxidant gene expression, balance oxidative stress and maintain cellular redox homeostasis (Kwak et al., 2007).
It is well-established that Nrf2 can regulate a variety of antioxidant enzymes, such as superoxide dismutase (SOD), catalase (CAT), glutathione peroxidase (GPX), glutathione reductase (GR), NAD(P)H quinine oxidoreductase (NQO1) and so on (Dhakshinamoorthy et al., 2000; Zhu et al., 2005). Therefore, Nrf2 is considered an important regulator of ferroptosis and a therapeutic target for tumors and neurodegenerative diseases highly associated with oxidative stress (Abdalkader et al., 2018; Song and Long, 2020).
p53 has attracted much interest as a tumor suppressor molecule since its discovery (Harris, 1996). The p53 molecule can induce apoptosis and cell cycle arrest, exerting a strong tumor suppressor effect (Chen, 2016). In 2015, Jiang et al. linked p53 to ferroptosis for the first time (Jiang et al., 2015) and demonstrated that mutation of p53 can inhibit the activity of System Xc−, downregulate the expression of SLC7A11 (Jiang et al., 2015), and reduce the activity of GPX4, thereby promoting lipid peroxidation and inducing ferroptosis (Jiang et al., 2015). It is widely thought that p53 is at the core of a powerful signaling network; it regulates the sensitivity of cells to ferroptosis in different cell types and under different stress factors through several independent signaling pathways (Harris and Levine, 2005; Huang, 2021).
In addition to increasing sensitivity to ferroptosis, p53 appears to have an opposing effect (Liu et al., 2020a). When cells undergo cysteine deprivation, another signaling pathway is activated, with increased expression of wild-type p53 to induce p21 transcription or inhibit DPP4 binding to NOX1, ultimately inhibiting cell susceptibility to ferroptosis (Wang et al., 2012; Badgley et al., 2020). These two functions seem contradictory, but they are unified in cells. On the one hand, ferroptosis, as a form of regulated cell death, has physiological significance in the evolution of species, and p53 can achieve the purpose of removing abnormal cells and inhibiting tumorigenesis by increasing the sensitivity of cells to ferroptosis (Jiang et al., 2015; Liu et al., 2020a); on the other hand, when metabolic stress occurs, p53 can reduce the sensitivity of cells to ferroptosis by enhancing the ability to regulate ROS level to help normal cells survive the damage induced by various stress factors and promote cell survival (Tarangelo et al., 2018). Overwhelming evidence substantiates that cell types and p53 mutation sites may influence the mechanism of p53 in regulating cell ferroptosis (Wynford-Thomas and Blaydes, 1998; Wang et al., 2008; Ji et al., 2022), although the specific underlying mechanism warrants further study.
Previous studies suggested that GPX4 and free radical antioxidants regulate ferroptosis. Recently, Marcus Conrad and José Pedro Friedmann Angeli’s team used a clonal expression strategy to screen for genes that can inhibit ferroptosis caused by loss of GPX4 in human cancer cells and found that flavoprotein apoptosis-inducing factor mitochondria-related 2 (AIFM2) is an anti-ferroptosis gene and renamed AIFM2 to ferroptosis suppressor protein 1 (FSP1). FSP1, originally described as a pro-apoptotic gene, showed the ability to inhibit ferroptosis induced by GPX4 knockout (Doll et al., 2019). In addition, the researchers found that the main mechanism of FSP1 in inhibiting ferroptosis is to reduce Coenzyme Q10 (CoQ10) with NAD(P)H, inhibit lipid peroxidation, and resist the occurrence of ferroptosis (Doll et al., 2019). The FSP1-CoQ10-NAD(P)H pathway exists as an independent parallel system, which, together with GPX4 and glutathione, inhibits phospholipid peroxidation and ferroptosis (Bersuker et al., 2019; Doll et al., 2019). Their anti-ferroptosis properties have been widely used in the study of anti-tumor therapy (Bersuker et al., 2019). Growing evidence suggests that when GPX4 is inactivated, FSP1 can continue to maintain tumor growth in vivo, while deletion of GPX4 and FSP1 can inhibit tumor growth (Doll et al., 2019). As a novel ferroptosis inhibitor, FSP1 provides a new direction for disease research.
ACSL4 is a lipid metabolism enzyme required for lipid peroxidation and belongs to the ACSL family (Lopes-Marques et al., 2013). Current research suggests that PUFAs can be used as substrates for lipid peroxidation (Ayala et al., 2014), and their accumulation is a marker of ferroptosis; thus, the intracellular PUFAs content determines the development of ferroptosis and increased content can promote the progression of lipid peroxidation-induced ferroptosis (Das, 2019; Kuang et al., 2020). ACSL4 can activate free PUFAs and then complete the peroxidation process of membrane phospholipids with the participation of the other key enzymes, LPCAT3 and ALOX15 (Lee et al., 2021). Therefore, the ACSL4/LPCAT3/ALOX15 pathway can promote lipid peroxidation-induced ferroptosis (Lee et al., 2021). Zhang et al. showed that PKCβII, one of the isoforms of PKC (Steinberg, 2008), is an important lipid peroxidation sensor activated by lipid peroxidation during ferroptosis that can phosphorylate ACSL4 to amplify the effect of lipid peroxidation, eventually inducing ferroptosis (Zhang et al., 2022). This study also confirmed that the PKCβII-ACSL4 mechanism affects the efficacy of cancer immunotherapy by regulating ferroptosis (Zhang et al., 2022).
Heat shock proteins (HSP) are a class of highly conserved molecular chaperones expressed in response to environmental stress and render cells resistant to different types of cell death (Feder and Hofmann, 1999). For example, HSPB1 can inhibit ferroptosis by reducing iron uptake (Sun et al., 2015); HSPA5 binds to and stabilizes GPX4, thereby indirectly avoiding the damage of lipid peroxidation in ferroptosis (Zhu et al., 2017); however, the HSP9 inhibitor CDDO can inhibit ferroptosis in tumor cells, suggesting that HSP90 may play a different role in ferroptosis (Qin et al., 2015).
Mitochondria have been reported to participate in the pathogenesis of ferroptosis (Gao et al., 2019). CDGSH iron-sulfur domain 1 (CISD1) is a class of mitochondrial iron-exporting proteins that inhibit ferroptosis by preventing the accumulation of iron and the production of ROS in mitochondria (Yuan et al., 2016b). In addition, voltage-dependent anion channels (VDACs) located in the mitochondrial outer membrane play an important regulatory role in ferroptosis (Lemasters, 2017). It has been shown that Erastin, a ferroptosis inducer (Shibata et al., 2019; Zhao et al., 2020b), can act on VDAC to promote the release of a large number of oxides, causing ROS-dependent mitochondrial dysfunction and bioenergy exhaustion to induce ferroptosis (Zhao et al., 2020b), while the reduction of VDAC expression can reduce the occurrence of Erastin-induced ferroptosis (Yang et al., 2020).
Epigenetics is a key factor in regulating gene expression, and more and more research results show that epigenetic regulation (e.g., DNA methylation, histone modification and miRNA) plays an important role in ferroptosis (Jaenisch and Bird, 2003; Wu et al., 2020). DNA methylation is the most widely studied epigenetic modification. Increased DNA methylation may lead to gene silencing, while decreased methylation activates gene expression (Newell-Price et al., 2000), and DNA methylation is closely related to iron metabolism and can control the expression of ferroptosis-related genes (e.g., GPX4 and SLC7A11) to regulate ferroptosis (Zhao et al., 2022). Currently, DNA methylation is widely used as a diagnostic, predictive, and prognostic biomarker for multiple cancers (Huo et al., 2019). According to the histone modification studies, it was found that reducing histone 2A ubiquitination (H2Aub) on the SLC7A11 promoter can downregulate SLC7A11 and prevent ferroptosis (Zhang et al., 2019a); the histone 2B monoubiquitination (H2Bub1) modification is significantly down-regulated during the induction of ferroptosis, and artificial inhibition of endogenous H2Bub1 can significantly increase the sensitivity of cells to the ferroptosis inducer Erastin (Wang et al., 2019); in addition, histone deacetylase (HDAC) can regulate iron metabolism by inhibiting HAMP gene expression (Sukiennicki et al., 2019). There are also many studies reporting that a large number of microRNAs (miRNAs) can regulate ROS metabolism and ferroptosis (Zhang et al., 2020a). Mitofusin (Mfn) is a key protein that maintains mitochondrial morphology, regulates cellular lipid metabolism, endoplasmic reticulum stress and ROS generation (Papanicolaou et al., 2011), and plays a potential role in ferroptosis (Wei et al., 2020). miR-195, miR-125a and miR-761 have all been reported to regulate the mitochondrial function and metabolism of breast cancer cells, pancreatic cancer cells and liver cancer cells by targeting Mfn2, respectively, and affect the growth of tumor cells (Guo et al., 2017; Pan et al., 2018; Purohit et al., 2019); miR-137 can negatively regulate ferroptosis by directly targeting glutamine transporter SLC1A5 in melanoma cells (Luo et al., 2018). These further reveal the miRNA regulation role in ferroptosis, which contributes to an in-depth understanding of the mechanism of ferroptosis.
An increasing body of evidence from recently published studies suggests that ferroptosis is closely related to various diseases, such as tumor and neurological diseases (Ward et al., 2014; Fang et al., 2019; Bao et al., 2021). On the one hand, ferroptosis inducers can induce ferroptosis in abnormal cells, and tumor cells are highly sensitive to ferroptosis (Wu et al., 2019). Accordingly, ferroptosis can be induced in tumor cells to treat tumors. Non-targeted strategies based on nanoparticles have been designed to deliver iron, peroxides and other toxic substances to kill tumor cells (Zhang et al., 2019b; Raj et al., 2021).
The discovery of various enzymes regulating ferroptosis has enabled the targeted therapy of tumors, the most prominent target being GPX4, which is expressed in most tumor cells and is important for cell survival (Zhang et al., 2020b). GPX4-deficient cancer cells can be efficiently eliminated by the FSP-specific inhibitor iFSP1, while in GPX4-expressing cancer cells, iFSP1 cooperates with RSL3 to induce cancer cell ferroptosis (Gaschler et al., 2018). Therefore, FSP1 inhibitors may have clinical applications, especially for treating drug-resistant tumors or tumors that exhibit de-differentiation characteristics (Wang et al., 2021a). In addition, pharmacological or genetic inhibition of system Xc− to prevent the development and metastasis of various tumors has yielded good efficacy and low toxicity in mouse models (Zhu et al., 2019).
On the other hand, ferroptosis inhibitors can inhibit ferroptosis in normal cells and can be used to prevent or treat neurological diseases (Angeli et al., 2017). Studies have found that ferroptosis is associated with Parkinson’s disease (Mahoney-Sanchez et al., 2021). Ferroptosis is an important cell death pathway of dopaminergic neurons (Do Van et al., 2016), and the ferroptosis inhibitor ferrostatin-1 can reportedly inhibit neuronal cell death in vitro and in vivo (Reichert et al., 2020). Interestingly, it has been reported that many pathological features of Alzheimer’s disease are associated with an imbalance in iron homeostasis, and iron overload in the brain may be responsible for the rapid cognitive decline in Alzheimer’s patients (Belaidi and Bush, 2016). Water maze experiments showed that mice with GPX4 knockout in the cerebral cortex and hippocampal neurons showed significant cognitive impairment and degeneration of hippocampal neurons. The degree of neurodegeneration was reduced after treatment with Vitamin E or the ferroptosis inhibitor Liproxstatin-1 (Hambright et al., 2017; Lane et al., 2018). These studies suggest that ferroptosis is widely involved in regulating the functions of neurons related to learning and memory.
It has been established that the levels of intracellular lipid peroxides and Fe2+ are increased during stroke, and ferroptosis inhibitors can upregulate the levels of GSH, GPX4 or system Xc− to alleviate brain damage, indicating that ferroptosis affects the progression of stroke (Magtanong and Dixon, 2018; Zhang et al., 2021b). Studies have shown that ferroptosis inhibitors protect mice from ischemia-reperfusion injury in a middle cerebral artery occlusion (MCAO) model (Tuo et al., 2017), suggesting that ferroptosis can lead to neuronal death and NVU damage after ischemic stroke (Xu et al., 2022). Understanding the roles of iron metabolism, amino acid metabolism, and lipid metabolism of ferroptosis in ischemic stroke provides theoretical support for treating this disease (Jiang et al., 2021). The following content specifically discusses the role of ferroptosis in ischemic stroke (Figure 3).
FIGURE 3. The mechanisms of ferroptosis in ischemic stroke. Following ischemic stroke, the BBB is disrupted, which allows Fe3+ in the blood to be released into cells through TF and TFR1, then stored in the endosome, where Fe3+ is converted into Fe2+ and transported to the cytoplasm by DMT1 with the cooperation of STEAP3. The excess Fe2+ generates ROS and participates in the synthesis of PUFA lipid peroxides (L-OOH), which can induce ferroptosis; System Xc− is simultaneously impaired, which inhibits cystine-glutamate exchange and reduces the generation of GSH and GPX4, thereby inhibiting lipid alcohol (L-OH) production, ultimately leading to ferroptosis. Additionally, the Nrf2 pathway can inhibit ferroptosis and alleviate ischemic stroke injury by inducing GSH, SLC7A11, and GPX4 transcription.
It is well-established that iron homeostasis in the brain is disrupted after ischemic stroke, which impedes NVU recovery (Hu and Song, 2017). Intracellular iron overload is the main mechanism for inducing ferroptosis after cerebral ischemia, and the inhibition of iron overload can suppress ferroptosis in ischemic stroke and reduce damage (Fang et al., 2018). Under pathological conditions of ischemia and hypoxia, the expression of ferritin, transferrin, and TFR1 in the brain is increased, resulting in increased iron uptake by neurons and oligodendrocytes in the NVU (Kawabata, 2019; Ng et al., 2019). The acidic environment of ischemia and hypoxia can cause the overexpression of divalent metal-ion transporter 1 (DMT1) in microglia (Rotin et al., 1986; Chang et al., 2006), resulting in increased brain iron content (Cheli et al., 2018); meanwhile, ischemia can upregulate TFR1 levels, resulting in increased iron uptake (Chen et al., 2019; Tang et al., 2021).
There is a rich literature available suggesting that serum hepcidin and iron concentrations are elevated in patients with ischemic stroke, indicating that hepcidin is critical in cerebral ischemic iron overload (Davalos et al., 1994; Petrova et al., 2016). During ischemic stroke, the expression of interleukin-6 (IL-6) in cells increases hepcidin through the JAK/STAT3 pathway (Cojocaru et al., 2009), which causes FPN1 degradation, resulting in reduced iron release and thus intensified iron accumulation in cells (Cojocaru et al., 2009; Zhou et al., 2017). Therefore, the rational application of iron metabolism inhibitors, such as deferoxamine and iron chelators, to reduce the iron content in the brain after an ischemic stroke can reduce neuronal death and promote the recovery of NVU function after ischemic stroke (Jones et al., 2020; Roemhild et al., 2021; Yang et al., 2021).
In 2017, Tuo et al. revealed the relationship between Tau and ferroptosis and the role of ferroptosis in CIRI using the MCAO mouse model (Tuo et al., 2017). Tau can promote neuronal iron efflux and inhibit ferroptosis, which may be related to the reduction of tau caused by cerebral ischemia. Meanwhile, ferroptosis inhibitors liproxstatin-1 (Lip-1) or ferrostatin-1 (Fer-1) can significantly reduce neurological damage, indicating that ferroptosis can aggravate CIRI.
After ischemic stroke, the BBB is destroyed, leading to cerebral edema and aggravating brain tissue damage and neurological dysfunction (Abdullahi et al., 2018). Numerous studies have shown that systemic iron pools are transferred to neurons in the brain parenchyma when the BBB is disrupted, thereby exacerbating ferroptosis. Accordingly, changes in iron content in brain tissue reflect the degree of BBB dysfunction (Degregorio-Rocasolano et al., 2019). Iron accumulation accompanies the entire pathological process, and iron metabolism is considered an important pathophysiological factor involved in secondary injury after ischemic stroke (Waldvogel-Abramowski et al., 2014; Roemhild et al., 2021).
As the brain’s most abundant excitatory neurotransmitter, glutamate is a critical regulator in maintaining neural function (Zhou and Danbolt, 2014). NVU damage and death caused by excessively high extracellular glutamate concentration is known as excitotoxicity (Yang et al., 2019). In ischemic stroke, when the brain is in a state of ischemia and hypoxia due to metabolic disorders, glutamate release is increased, and reuptake is hindered, resulting in a rapid increase in glutamate levels in the ischemic area of the brain (Castillo et al., 1996). Subsequent activation of glutamate receptors leads to abnormal activation of several signaling pathways and iron deposition to stimulate cell death (Griesmaier and Keller, 2012; Willard and Koochekpour, 2013). Therefore, glutamate excitotoxicity is widely thought to be one of the mechanisms of ferroptosis (Zhang et al., 2021c). Indeed, glutamate excitotoxicity after cerebral ischemia, described as ferroptosis, can be effectively suppressed by the ferroptosis inhibitor Fer-1 (Xie et al., 2022).
As mentioned above, system Xc− has a positive effect on inhibiting ferroptosis; however, the increase of extracellular glutamate content caused by cerebral ischemia is mainly caused by system Xc− (Ikeda et al., 1989), and inhibiting the expression of system Xc− can hinder ferroptosis, thereby reducing cerebral ischemia damage (Vespa et al., 1998). Domercq et al. showed that system Xc− was upregulated in astrocytes and microglia in a rat model of stroke, while its inhibition reduced inflammation and attenuated CIRI (Matute et al., 2006; Martin et al., 2018). It can be concluded that the increased expression of System Xc− during cerebral ischemia does not inhibit but promote the occurrence of ferroptosis, which may be due to the upregulated expression of System Xc−, leading to the excitotoxic effect caused by glutamate release exceeding its own antioxidant protective effect (Polewski et al., 2016).
In addition, GSH, as an endogenous inhibitor of ferroptosis, is reportedly related to ischemic stroke (Zhang et al., 2021b). Enhancing the expression of GPX4 and GSH synthesis can inhibit ferroptosis and reduce ischemic stroke injury (Zhang et al., 2021b). Increased lipid peroxidation levels and decreased GSH levels have been detected in an MCAO model (Liu et al., 2020b). Moreover, Edaravone has been proposed to counteract ferroptosis in various conditions, especially in cystine deficiency leading to decreased GSH content, and has been clinically approved for the treatment of acute ischemic stroke (Kikuchi et al., 2009; Matsumoto et al., 2018b). In addition, selenium (Se) can effectively enhance and maintain the activity of GPX4 (Ferguson et al., 2012; Liu et al., 2021). After ischemic stroke, Se supplementation can effectively inhibit GPX4-dependent ferroptosis and endoplasmic reticulum (ER) stress-induced cell death and improve NVU function by promoting GPX4 expression (Alim et al., 2019). In recent years, much emphasis has been placed on understanding the direct effects of GPX4 and GSH on stroke.
Lipid peroxidation is a key driver of ferroptosis, stimulated by oxidative stress (Lee et al., 2021). ROS can accumulate to toxic levels during oxidative stress, leading to cellular damage and dysfunction, whereas antioxidants can prevent cellular damage by converting ROS into harmless molecules (Conrad et al., 2018). ROS are produced in large quantities during ischemic stroke, along with decreased levels of endogenous antioxidants, leading to oxidative stress (Cadenas, 2018). For example, the 1,2,4-triazole derivative compound 11 can exert a neuroprotective effect by promoting the expression of Nrf2 and SOD to induce an antioxidant effect (Lao et al., 2022). During a stroke, AA and AdA on the cell membrane can generate lipid peroxides through a series of reactions (Mccall et al., 1987; Nishizawa et al., 2021). Growing evidence suggests that ACSL4 and LOX, especially 12/15-LOX, are increased in ischemic stroke (Jin et al., 2008; Singh and Rao, 2019).
LOX is a key enzyme that causes lipid peroxidation and induces ferroptosis (Shintoku et al., 2017). It has been reported that 12/15-Lox gene deletion can reduce the infarct size after stroke (Singh and Rao, 2019). Additionally, 12/15-LOX were highly expressed in a cerebral ischemia model, and their inhibitors could inhibit the damage of ferroptosis to NVU cells (Jin et al., 2008). For example, the specific inhibitor ML351 has been reported to exert a protective effect against CIRI (Tourki et al., 2021). Moreover, ACSL4 participates in the synthesis and remodeling of PEs, thus affecting the synthesis of lipid peroxides, and upregulation of its expression can contribute to ferroptosis (Kuwata et al., 2019). It has been shown that in ischemic stroke, ACSL4 is upregulated and mediates neuronal death, ultimately leading to stroke injury (Li et al., 2019). Moreover, the ACSL4 inhibitor rosiglitazone can inhibit ferroptosis and protect brain function (Li et al., 2019). Cui et al. found that knockout of ACSL4 protects mice from cerebral ischemia, whereas its overexpression can exacerbate brain damage (Cui et al., 2021). Other studies have found that the accumulation of Fe2+ and ROS decreased, the expression of ACSL4 and TFR decreased, and GPX4 and FTH1 increased in MCAO model cells treated with safflower flavin thus inhibiting neuronal ferroptosis in MCAO (Li et al., 2021).
These findings suggest that both ACSL4 and LOX involved in lipid metabolism can serve as innovative therapeutic targets for ischemic stroke (Cui et al., 2021), inhibiting ferroptosis by reducing ROS accumulation and lipid peroxidation, providing new ideas for the treatment of ischemic stroke (Li et al., 2021).
Additionally, mounting evidence suggests that Nrf2 is an important regulator of the cellular antioxidant defense system (Abdalkader et al., 2018), and its moderate activation is beneficial in alleviating cerebral ischemic injury (Lao et al., 2022). Although little evidence is available that changes in Nrf2 levels directly affect ferroptosis in stroke, several studies have suggested that the Nrf2 pathway can alleviate stroke injury (Shih et al., 2005; Liu et al., 2018; Lao et al., 2022). It has also been shown that Epicatechin can regulate oxidative stress through the Nrf2 pathway via penetrating the BBB, thus protecting against transient cerebral ischemic injury (Chang et al., 2014).
With the gradual recognition of the role of ferroptosis in ischemic stroke, treating ischemic stroke by inhibiting ferroptosis has become a research hotspot. As the pathological stimulus signals of ischemic stroke, ischemia, hypoxia and hypertension can all lead to brain damage (Khoshnam et al., 2017), during which local brain tissue metabolism changes, such as the reduction of GSH, GPX4 and tau proteins, and the increase of lipoxygenase (LOX) and BBB permeability, specifically manifested as iron overload and the enhancement of lipid peroxidation, that boost the generation of ROS and ultimately trigger ferroptosis-related cell death (Magtanong and Dixon, 2018). In addition, the restoration of normal blood circulation after ischemic stroke for a period of time will lead to CIRI (Lo and Rosenberg, 2009). Cerebral ischemia-reperfusion will lead to the activation of a variety of cell death pathways, including ferroptosis (Chen et al., 2021b). The phenomenon of increased lipid peroxidation and increased intracellular iron levels contribute to amplify the cerebral oxidative stress and inflammatory response, that further aggravate neuronal injury during reperfusion. Therefore, ferroptosis mediates and aggravates ischemic stroke injury (Figure 4). Major therapeutic advances include ferroptosis inhibitors, iron homeostasis regulators, lipid peroxidation pathway inhibitors, and ROS generation inhibitors. Table 2 summarized some key factors related to regulating ferroptosis, as well as therapeutic reagents in stroke and their functional mechanisms.
FIGURE 4. Possible molecular mechanisms of ferroptosis and potential therapeutic targets in ischemic stroke. The decrease of GSH, GPX4, tau protein, and the increase of lipoxygenase (LOX), and BBB permeability, can lead to the occurrence of ferroptosis in ischemic stroke. Iron chelators like deferoxamine (DFO), ciclopirox (CPX) and 2,2-bipyridyl (2,2-BP) can inhibit ferroptosis; Lipoxygenase inhibitors like Baicalein, Vitamin E, ML351 and Zileuton can suppress LOXs activity to rescue cells from ferroptosis; Ferrostatin-1 (Fer-1) and Liproxstatin-1 (Lip-1) inhibit radical-trapping antioxidants which activate LOXs to prevent ferroptosis in cells.
Current evidence suggests that ferroptosis inhibitors Fer-1 or Lip-1 can effectively reduce brain damage after reperfusion in a mouse model of ischemic stroke (Tuo et al., 2017; Feng et al., 2019), and Edaravone can be used to treat patients with acute ischemic stroke (Enomoto et al., 2019; Kobayashi et al., 2019). Mechanistic studies have suggested that Edaravone can scavenge free radicals and inhibit lipid peroxidation, thereby inhibiting oxidative damage (Kikuchi et al., 2013). Moreover, the intravenous administration of Edaravone after I/R in rats prevented the progression of cerebral edema and cerebral infarction, alleviated the accompanying neurological symptoms, and inhibited delayed neuronal death (Watanabe et al., 2018). In recent years, iron chelation therapy has been shown to suppress ferroptosis in a CIRI model (Cappellini et al., 2006; Grignano et al., 2020). Deferoxamine (DFO), a high-affinity iron chelator with the ability to bind to free iron ions to form stable complexes that weaken the Fenton response, has been approved by the U.S. Food and Drug Administration (FDA) for the treatment of iron overload-related diseases (Hanson et al., 2009; Tevlin et al., 2021). The ferroptosis inhibitor deferoxamine, which also acts as an iron chelator, improved cognitive impairment after stroke in diabetic rats with MCAO (Hanson et al., 2009). In addition, CoQ10 was found to possess beneficial effects in a rat MCAO model and improved the prognosis of neurological impairment in patients with acute ischemic stroke (Nasoohi et al., 2019). Therefore, as an endogenous lipid-soluble antioxidant, CoQ10 can effectively inhibit lipid peroxidation and is expected to be a drug that hinders ferroptosis (Littarru and Tiano, 2007; Rizzardi et al., 2021). Another drug inhibiting ferroptosis during ischemic stroke is Se (Ramezani et al., 2021). Alim et al. found that Se supplementation activates GPX4 homeostatic transcription in vivo, inhibiting cellular ferroptosis and improving neurological function (Alim et al., 2019). Octreotide has anti-inflammatory and antioxidant effects and protects the brain from cerebral ischemic injury by activating the Nrf2/ARE pathway (Wang et al., 2015). In patients with cerebral ischemic injury, melatonin has been reported to reduce nerve cell ferroptosis by increasing Nrf2, and significantly improve the learning, memory and cognitive abilities (Koh, 2008).
Intriguingly, several traditional Chinese herbal medicines have also been shown to inhibit ferroptosis in ischemic stroke. The monoterpenoid phenol carvacrol has been reported to effectively reduce ROS expression, reduce iron deposition and elevate GPX4 levels, thereby protecting hippocampal neurons from CIRI (Friedman, 2014; Li et al., 2016). Galangin inhibition of ferroptosis by activating SLC7A11/GPX4 can reportedly attenuate CIRI (Guan et al., 2021). In addition, Naotaifang extract has been reported to inhibit neuronal ferroptosis by downregulating TFR1/DMT1 and upregulating the SCL7A11/GPX4 pathway, thereby improving neurological function in post-ischemic rats (Lan et al., 2020).
Although these traditional Chinese medicines have not been clinically validated to improve the condition of ischemic stroke patients, their safety and lack of toxicity may facilitate their clinical translation (Fung and Linn, 2015).
Ischemic stroke is a common disease that seriously endangers human health, and its incidence has increased in recent years (Sacco et al., 2013). Its complex pathological process and related mechanisms have become a research hotspot (Peisker et al., 2017; Yang et al., 2019). Thrombolytic therapy for cerebral ischemic injury has been limited by the narrow therapeutic time window, CIRI induction, and a high risk of hemorrhagic transformation, emphasizing the need for new treatments (Ringleb et al., 2002; Schellinger and Warach, 2004).
As the concept of NVU was proposed, researchers began to assess the feasibility of treating ischemic stroke from multiple approaches and perspectives (Cai et al., 2017; Zhao et al., 2020a). Stroke caused by different causes was regarded as a reactive injury process involving brain NVU (Yenari et al., 2010; Hu et al., 2017; Iadecola, 2017). In the treatment after brain injury, it has been transformed from single neuron protection to more comprehensive and in-depth protection of NVU (Wang et al., 2021b). The pathophysiological changes of NVU are typically characterized by tissue hypoxia, inflammation, activation of angiogenesis, and complex interactions between various components of NVU, which together lead to increased BBB permeability, brain edema, neuronal dysfunction and injury (Stanimirovic and Friedman, 2012). Cerebral ischemic injury is an inflammatory stimulus response, and all cellular components and matrix components of NVU are involved and make related responses (Ishikawa et al., 2004). The research and development of traditional drugs is limited to a certain pathological link in the pathological process of NVU, so the effect is not ideal. Therefore, during the treatment, the overall structure of NVU should be targeted, and the dynamic changes of each component should be coordinated to reduce nerve damage and promote repair. Ferroptosis is a new cell death mode discovered in recent years, with the continuous research, it has been recognized that ferroptosis plays an important role in a wide range of biological processes, including normal physiology and various pathological conditions (Stockwell et al., 2017). At present, the morphology, biology, and mechanism pathways of ferroptosis are partially understood, but the process of ferroptosis involves a variety of mechanisms, which are precisely regulated by signaling pathways. Questions remain as to how ferroptosis is related to the occurrence of diseases and whether it is associated with other modes of cell death to mediate the progression of diseases. Therefore, further in-depth study of the mechanism of ferroptosis and its role in different disease types is of great significance for finding therapeutic targets for related diseases and the development of targeted drugs.
The pathogenesis of ischemic stroke is complicated, current evidence suggests that ferroptosis plays an important role in the progression of ischemic stroke, inhibiting ferroptosis can alleviate ischemic stroke injury (Li et al., 2019; Liu et al., 2020b; Zhang et al., 2021b). When ischemic stroke occurs, iron ion aggregation in neurons leads to iron overload, which causes ROS aggregation through Fenton reaction, and GSH level is significantly reduced and lipid peroxidation increase in ischemic stroke mouse model (Liu et al., 2020b), indicating that ferroptosis is also a way of neurons death during the pathophysiological process of ischemic stroke. Ferroptosis inhibitors and iron chelators can effectively reduce the damage of neurons during ischemic brain death, suggesting that there are potential targets in the ferroptosis pathway to regulate ischemic stroke and its inhibition during ischemia has huge prospects for clinical application (Zhou et al., 2021).
However, investigating the role of ferroptosis in ischemic stroke is still in its preliminary stages, and many questions remain to be answered. For example, during ischemia and hypoxia, different cell types of NVU in brain tissue (Wang et al., 2021b), including neurons, microglia, astrocytes, oligodendrocytes, etc., are stimulated and damaged (Cai et al., 2017), and ferroptosis occurs in these different cell types, although its role remains to be elucidated (Doll et al., 2017; Jiang et al., 2021; Lee et al., 2021; Nishizawa et al., 2021), warranting further studies. In the field of basic research, there is a lack of effective biomarkers for ferroptosis, such as caspase activation in apoptosis or autolysosome formation in autophagy (Earnshaw et al., 1999; Hundeshagen et al., 2011). Accordingly, exploring specific biomarkers of ferroptosis is urgent. Over the years, studies of the molecular mechanisms of ferroptosis in ischemic stroke have mostly focused on cell and animal models, indicating that more clinical studies on patients with ischemic stroke are required. In addition, drug development targeting ferroptosis in ischemic stroke is an important aspect of research. Among the ferroptosis inhibitors, only one drug, Edaraavone, is used to treat patients with acute ischemic stroke (Enomoto et al., 2019; Kobayashi et al., 2019). Other drugs have been found to be effective in animal and cell models of stroke (Hanson et al., 2009; Narayan et al., 2021). Therefore, there is an urgent need to develop and validate effective drugs in clinical treatment, including traditional Chinese medicine.
Although ferroptosis, as a new mode of cell death, plays a key role in ischemic stroke and is expected to become a new therapeutic target to improve the outcomes of this patient population, ischemic stroke is regulated by a variety of cell death pathways. Both ferroptosis and other programmed cell death modes (including cell apoptosis, necroptosis and autophagy) play an important role in the pathological process of ischemic stroke. More and more evidence has shown that there are interacting signaling pathways between these cell death modes with similar initial signals and molecular regulators. For example, p53, not only induces apoptosis, but also regulates ferroptosis (Hong et al., 2017). In the relationship between ferroptosis and necroptosis, iron overload leads to the opening of the mitochondrial permeability transition pore (MPTP), which exacerbates RIP1 phosphorylation and leads to cell necroptosis (Tian et al., 2020); HSP90 induces necroptosis and ferroptosis by promoting RIP1 phosphorylation and inhibiting GPX4 activation (Wang et al., 2018). In addition, research have shown that ferroptosis is an autophagic cell death process (Gao et al., 2016). Knockdown of autophagy-related Atg5 and Atg7 genes can limit Erastin-induced ferroptosis by reducing intracellular iron and lipid peroxidation, and knockdown of NCOA4 can inhibit ferritin degradation and prevent ferroptosis (Lu et al., 2017), however, the specific mechanism of autophagy mediated ferroptosis needs to be further explored. This evidence suggests a strong crosslink between them, combination therapy targeting different cell death pathways may be the most effective strategy for the treatment of ischemic stroke.
ZW, YX, MW, QF, and YX conceptualized and wrote the manuscript and created Figures. ZW, HZ, and KR contributed to the writing of the manuscript. ZW, MW, KR, QF, and YX reviewed and modified the manuscript. All authors approved the final version of the manuscript.
This work was supported by the National Natural Science Foundation of China (82204389), the Natural Science Foundation of Henan Province (222300420350) and the Henan Medical Science and Technique Foundation (LHGJ20200310, LHGJ20210351, LHGJ20190265), the Key R&D and promotion Special Projects of Henan Province (212102310194) and the Medical Science and Technology Research Project of Henan Province (SBGJ202102145).
The authors declare that the research was conducted in the absence of any commercial or financial relationships that could be construed as a potential conflict of interest.
All claims expressed in this article are solely those of the authors and do not necessarily represent those of their affiliated organizations, or those of the publisher, the editors and the reviewers. Any product that may be evaluated in this article, or claim that may be made by its manufacturer, is not guaranteed or endorsed by the publisher.
Abdalkader, M., Lampinen, R., Kanninen, K. M., Malm, T. M., and Liddell, J. R. (2018). Targeting Nrf2 to suppress ferroptosis and mitochondrial dysfunction in neurodegeneration. Front. Neurosci. 12, 466. doi:10.3389/fnins.2018.00466
Abdullahi, W., Tripathi, D., and Ronaldson, P. T. (2018). Blood-brain barrier dysfunction in ischemic stroke: Targeting tight junctions and transporters for vascular protection. Am. J. Physiol. Cell Physiol. 315 (3), C343–C356. doi:10.1152/ajpcell.00095.2018
Adedoyin, O., Boddu, R., Traylor, A., Lever, J. M., Bolisetty, S., George, J. F., et al. (2018). Heme oxygenase-1 mitigates ferroptosis in renal proximal tubule cells. Am. J. Physiol. Ren. Physiol. 314 (5), F702–F714. doi:10.1152/ajprenal.00044.2017
Akhmetzyanova, E., Kletenkov, K., Mukhamedshina, Y., and Rizvanov, A. (2019). Different approaches to modulation of microglia phenotypes after spinal cord injury. Front. Syst. Neurosci. 13, 37. doi:10.3389/fnsys.2019.00037
Alim, I., Caulfield, J. T., Chen, Y., Swarup, V., Geschwind, D. H., Ivanova, E., et al. (2019). Selenium drives a transcriptional adaptive program to block ferroptosis and treat stroke. Cell 177 (5), 1262–1279. doi:10.1016/j.cell.2019.03.032
Angeli, J. P. F., Shah, R., Pratt, D. A., and Conrad, M. (2017). Ferroptosis inhibition: Mechanisms and opportunities. Trends Pharmacol. Sci. 38 (5), 489–498. doi:10.1016/j.tips.2017.02.005
Anilkumar, U., Khacho, M., Cuillerier, A., Harris, R., Patten, D. A., Bilen, M., et al. (2020). MCL-1(Matrix) maintains neuronal survival by enhancing mitochondrial integrity and bioenergetic capacity under stress conditions. Cell Death Dis. 11 (5), 321. doi:10.1038/s41419-020-2498-9
Arai, K., and Lo, E. H. (2009). An oligovascular niche: Cerebral endothelial cells promote the survival and proliferation of oligodendrocyte precursor cells. J. Neurosci. 29 (14), 4351–4355. doi:10.1523/JNEUROSCI.0035-09.2009
Armulik, A., Genove, G., and Betsholtz, C. (2011). Pericytes: Developmental, physiological, and pathological perspectives, problems, and promises. Dev. Cell 21 (2), 193–215. doi:10.1016/j.devcel.2011.07.001
Awooda, H. A., Lutfi, M. F., Sharara, G. G., and Saeed, A. M. (2015). Oxidative/nitrosative stress in rats subjected to focal cerebral ischemia/reperfusion. Int. J. Health Sci. 9 (1), 17–24. doi:10.12816/0024679
Ayala, A., Munoz, M. F., and Arguelles, S. (2014). Lipid peroxidation: Production, metabolism, and signaling mechanisms of malondialdehyde and 4-hydroxy-2-nonenal. Oxid. Med. Cell. Longev. 2014, 360438. doi:10.1155/2014/360438
Badgley, M. A., Kremer, D. M., Maurer, H. C., DelGiorno, K. E., Lee, H. J., Purohit, V., et al. (2020). Cysteine depletion induces pancreatic tumor ferroptosis in mice. Science 368 (6486), 85–89. doi:10.1126/science.aaw9872
Bannai, S. (1986). Exchange of cystine and glutamate across plasma membrane of human fibroblasts. J. Biol. Chem. 261 (5), 2256–2263. doi:10.1016/s0021-9258(17)35926-4
Bao, W. D., Pang, P., Zhou, X. T., Hu, F., Xiong, W., Chen, K., et al. (2021). Loss of ferroportin induces memory impairment by promoting ferroptosis in Alzheimer's disease. Cell Death Differ. 28 (5), 1548–1562. doi:10.1038/s41418-020-00685-9
Barakat, R., and Redzic, Z. (2016). The role of activated microglia and resident macrophages in the neurovascular unit during cerebral ischemia: Is the jury still out? Med. Princ. Pract. 25, 3–14. doi:10.1159/000435858
Baumann, N., and Pham-Dinh, D. (2001). Biology of oligodendrocyte and myelin in the mammalian central nervous system. Physiol. Rev. 81 (2), 871–927. doi:10.1152/physrev.2001.81.2.871
Becerra-Calixto, A., and Cardona-Gomez, G. P. (2017). The role of astrocytes in neuroprotection after brain stroke: Potential in cell therapy. Front. Mol. Neurosci. 10, 88. doi:10.3389/fnmol.2017.00088
Beguin, Y., Aapro, M., Ludwig, H., Mizzen, L., and Osterborg, A. (2014). Epidemiological and nonclinical studies investigating effects of iron in carcinogenesis--a critical review. Crit. Rev. Oncol. Hematol. 89 (1), 1–15. doi:10.1016/j.critrevonc.2013.10.008
Belaidi, A. A., and Bush, A. I. (2016). Iron neurochemistry in alzheimer's disease and Parkinson's disease: Targets for therapeutics. J. Neurochem. 139, 179–197. doi:10.1111/jnc.13425
Benjamin, E. J., Virani, S. S., Callaway, C. W., Chamberlain, A. M., Chang, A. R., Cheng, S., et al. (2018). Heart disease and stroke statistics-2018 update: A report from the American heart association. Circulation 137 (12), e67–e492. doi:10.1161/CIR.0000000000000558
Benveniste, E. N. (1997). Role of macrophages/microglia in multiple sclerosis and experimental allergic encephalomyelitis. J. Mol. Med. 75 (3), 165–173. doi:10.1007/s001090050101
Bergers, G., and Song, S. (2005). The role of pericytes in blood-vessel formation and maintenance. Neuro. Oncol. 7 (4), 452–464. doi:10.1215/S1152851705000232
Bersuker, K., Hendricks, J. M., Li, Z., Magtanong, L., Ford, B., Tang, P. H., et al. (2019). The CoQ oxidoreductase FSP1 acts parallel to GPX4 to inhibit ferroptosis. Nature 575 (7784), 688–692. doi:10.1038/s41586-019-1705-2
Bonnans, C., Chou, J., and Werb, Z. (2014). Remodelling the extracellular matrix in development and disease. Nat. Rev. Mol. Cell Biol. 15 (12), 786–801. doi:10.1038/nrm3904
Borchert, A., Kalms, J., Roth, S. R., Rademacher, M., Schmidt, A., Holzhutter, H. G., et al. (2018). Crystal structure and functional characterization of selenocysteine-containing glutathione peroxidase 4 suggests an alternative mechanism of peroxide reduction. Biochim. Biophys. Acta. Mol. Cell Biol. Lipids 1863 (9), 1095–1107. doi:10.1016/j.bbalip.2018.06.006
Brann, D. W., and Mahesh, V. B. (1994). Excitatory amino acids: Function and significance in reproduction and neuroendocrine regulation. Front. Neuroendocrinol. 15 (1), 3–49. doi:10.1006/frne.1994.1002
Bridges, R. J., Natale, N. R., and Patel, S. A. (2012). System xc(-) cystine/glutamate antiporter: An update on molecular pharmacology and roles within the CNS. Br. J. Pharmacol. 165 (1), 20–34. doi:10.1111/j.1476-5381.2011.01480.x
Broer, S., and Wagner, C. A. (2002). Structure-function relationships of heterodimeric amino acid transporters. Cell biochem. Biophys. 36 (2-3), 155–168. doi:10.1385/CBB:36:2-3:155
Cadenas, S. (2018). ROS and redox signaling in myocardial ischemia-reperfusion injury and cardioprotection. Free Radic. Biol. Med. 117, 76–89. doi:10.1016/j.freeradbiomed.2018.01.024
Cai, W., Zhang, K., Li, P., Zhu, L., Xu, J., Yang, B., et al. (2017). Dysfunction of the neurovascular unit in ischemic stroke and neurodegenerative diseases: An aging effect. Ageing Res. Rev. 34, 77–87. doi:10.1016/j.arr.2016.09.006
Cappellini, M. D., Cohen, A., Piga, A., Bejaoui, M., Perrotta, S., Agaoglu, L., et al. (2006). A phase 3 study of deferasirox (ICL670), a once-daily oral iron chelator, in patients with beta-thalassemia. Blood 107 (9), 3455–3462. doi:10.1182/blood-2005-08-3430
Castillo, J., Davalos, A., Naveiro, J., and Noya, M. (1996). Neuroexcitatory amino acids and their relation to infarct size and neurological deficit in ischemic stroke. Stroke 27 (6), 1060–1065. doi:10.1161/01.str.27.6.1060
Cekanaviciute, E., and Buckwalter, M. S. (2016). Astrocytes: Integrative regulators of neuroinflammation in stroke and other neurological diseases. Neurotherapeutics 13 (4), 685–701. doi:10.1007/s13311-016-0477-8
Chamulitrat, W., and Mason, R. P. (1989). Lipid peroxyl radical intermediates in the peroxidation of polyunsaturated fatty acids by lipoxygenase. J. Biol. Chem. 264 (35), 20968–20973. doi:10.1016/s0021-9258(19)30031-6
Chan, P. H. (1996). Role of oxidants in ischemic brain damage. Stroke 27 (6), 1124–1129. doi:10.1161/01.str.27.6.1124
Chang, Y. Z., Ke, Y., Du, J. R., Halpern, G. M., Ho, K. P., Zhu, L., et al. (2006). Increased divalent metal transporter 1 expression might be associated with the neurotoxicity of L-DOPA. Mol. Pharmacol. 69 (3), 968–974. doi:10.1124/mol.105.017756
Chang, C. F., Cho, S., and Wang, J. (2014). (-)-Epicatechin protects hemorrhagic brain via synergistic Nrf2 pathways. Ann. Clin. Transl. Neurol. 1 (4), 258–271. doi:10.1002/acn3.54
Cheli, V. T., Santiago Gonzalez, D. A., Marziali, L. N., Zamora, N. N., Guitart, M. E., Spreuer, V., et al. (2018). The divalent metal transporter 1 (DMT1) is required for iron uptake and normal development of oligodendrocyte progenitor cells. J. Neurosci. 38 (43), 9142–9159. doi:10.1523/JNEUROSCI.1447-18.2018
Chen, J., and Lopez, J. A. (2005). Interactions of platelets with subendothelium and endothelium. Microcirculation 12 (3), 235–246. doi:10.1080/10739680590925484
Chen, J. (2016). The cell-cycle arrest and apoptotic functions of p53 in tumor initiation and progression. Cold Spring Harb. Perspect. Med. 6 (3), a026104. doi:10.1101/cshperspect.a026104
Chen, C., Liu, P., Duan, X., Cheng, M., and Xu, L. X. (2019). Deferoxamine-induced high expression of TfR1 and DMT1 enhanced iron uptake in triple-negative breast cancer cells by activating IL-6/PI3K/AKT pathway. Onco. Targets. Ther. 12, 4359–4377. doi:10.2147/OTT.S193507
Chen, Y. C., Ma, N. X., Pei, Z. F., Wu, Z., Do-Monte, F. H., Keefe, S., et al. (2020). A NeuroD1 AAV-based gene therapy for functional brain repair after ischemic injury through in vivo astrocyte-to-neuron conversion. Mol. Ther. 28 (1), 217–234. doi:10.1016/j.ymthe.2019.09.003
Chen, X., Li, J., Kang, R., Klionsky, D. J., and Tang, D. (2021). Ferroptosis: Machinery and regulation. Autophagy 17 (9), 2054–2081. doi:10.1080/15548627.2020.1810918
Chen, Y., Fan, H., Wang, S., Tang, G., Zhai, C., and Shen, L. (2021). Ferroptosis: A novel therapeutic target for ischemia-reperfusion injury. Front. Cell Dev. Biol. 9, 688605. doi:10.3389/fcell.2021.688605
Cheng, Y., Song, Y., Chen, H., Li, Q., Gao, Y., Lu, G., et al. (2021). Ferroptosis mediated by lipid reactive oxygen species: A possible causal link of neuroinflammation to neurological disorders. Oxid. Med. Cell. Longev. 2021, 5005136. doi:10.1155/2021/5005136
Cojocaru, I. M., Cojocaru, M., Tanasescu, R., Iliescu, I., Dumitrescu, L., and Silosi, I. (2009). Expression of IL-6 activity in patients with acute ischemic stroke. Rom. J. Intern Med. 47 (4), 393–396.
Colonna, M., and Butovsky, O. (2017). Microglia function in the central nervous system during health and neurodegeneration. Annu. Rev. Immunol. 35, 441–468. doi:10.1146/annurev-immunol-051116-052358
Conrad, M., Kagan, V. E., Bayir, H., Pagnussat, G. C., Head, B., Traber, M. G., et al. (2018). Regulation of lipid peroxidation and ferroptosis in diverse species. Genes Dev. 32 (9-10), 602–619. doi:10.1101/gad.314674.118
Cregan, S. P., Maclaurin, J. G., Craig, C. G., Robertson, G. S., Nicholson, D. W., Park, D. S., et al. (1999). Bax-dependent caspase-3 activation is a key determinant in p53-induced apoptosis in neurons. J. Neurosci. 19 (18), 7860–7869. doi:10.1523/jneurosci.19-18-07860.1999
Cui, Y., Zhang, Y., Zhao, X., Shao, L., Liu, G., Sun, C., et al. (2021). ACSL4 exacerbates ischemic stroke by promoting ferroptosis-induced brain injury and neuroinflammation. Brain Behav. Immun. 93, 312–321. doi:10.1016/j.bbi.2021.01.003
Cunnane, S. C. (1994). Antioxidants, free radicals and PUFA. Prostagl. Leukot. Essent. Fat. Acids 50 (6), 363–364. doi:10.1016/0952-3278(94)90248-8
Daneman, R., and Prat, A. (2015). The blood-brain barrier. Cold Spring Harb. Perspect. Biol. 7 (1), a020412. doi:10.1101/cshperspect.a020412
Das, U. N. (2019). Saturated fatty acids, MUFAs and PUFAs regulate ferroptosis. Cell Chem. Biol. 26 (3), 309–311. doi:10.1016/j.chembiol.2019.03.001
Davalos, A., Fernandez-Real, J. M., Ricart, W., Molins, A., Planas, E., and Genis, D. (1994). Iron-related damage in acute ischemic stroke. Stroke 25 (8), 1543–1546. doi:10.1161/01.str.25.8.1543
Davidoff, M. S. (2019). The pluripotent microvascular pericytes are the adult stem cells even in the testis. Adv. Exp. Med. Biol. 1122, 235–267. doi:10.1007/978-3-030-11093-2_13
Degregorio-Rocasolano, N., Marti-Sistac, O., and Gasull, T. (2019). Deciphering the iron side of stroke: Neurodegeneration at the crossroads between iron dyshomeostasis, excitotoxicity, and ferroptosis. Front. Neurosci. 13, 85. doi:10.3389/fnins.2019.00085
Del Zoppo, G. J. (1998). The role of platelets in ischemic stroke. Neurology 51, S9–S14. doi:10.1212/wnl.51.3_suppl_3.s9
Dewar, D., Underhill, S. M., and Goldberg, M. P. (2003). Oligodendrocytes and ischemic brain injury. J. Cereb. Blood Flow. Metab. 23 (3), 263–274. doi:10.1097/01.WCB.0000053472.41007.F9
Dhakshinamoorthy, S., Long, D. J., and Jaiswal, A. K. (2000). Antioxidant regulation of genes encoding enzymes that detoxify xenobiotics and carcinogens. Curr. Top. Cell. Regul. 36, 201–216. doi:10.1016/s0070-2137(01)80009-1
Dixon, S. J., Lemberg, K. M., Lamprecht, M. R., Skouta, R., Zaitsev, E. M., Gleason, C. E., et al. (2012). Ferroptosis: An iron-dependent form of nonapoptotic cell death. Cell 149 (5), 1060–1072. doi:10.1016/j.cell.2012.03.042
Do Van, B., Gouel, F., Jonneaux, A., Timmerman, K., Gele, P., Petrault, M., et al. (2016). Ferroptosis, a newly characterized form of cell death in Parkinson's disease that is regulated by PKC. Neurobiol. Dis. 94, 169–178. doi:10.1016/j.nbd.2016.05.011
Doll, S., Freitas, F. P., Shah, R., Aldrovandi, M., da Silva, M. C., Ingold, I., et al. (2019). FSP1 is a glutathione-independent ferroptosis suppressor. Nature 575 (7784), 693–698. doi:10.1038/s41586-019-1707-0
Doll, S., Proneth, B., Tyurina, Y. Y., Panzilius, E., Kobayashi, S., Ingold, I., et al. (2017). ACSL4 dictates ferroptosis sensitivity by shaping cellular lipid composition. Nat. Chem. Biol. 13 (1), 91–98. doi:10.1038/nchembio.2239
Donkor, E. S. (2018). Stroke in the 21(st) century: A snapshot of the burden, epidemiology, and quality of life. Stroke Res. Treat. 2018, 3238165. doi:10.1155/2018/3238165
Dugas, J. C., Mandemakers, W., Rogers, M., Ibrahim, A., Daneman, R., and Barres, B. A. (2008). A novel purification method for CNS projection neurons leads to the identification of brain vascular cells as a source of trophic support for corticospinal motor neurons. J. Neurosci. 28 (33), 8294–8305. doi:10.1523/JNEUROSCI.2010-08.2008
Earnshaw, W. C., Martins, L. M., and Kaufmann, S. H. (1999). Mammalian caspases: Structure, activation, substrates, and functions during apoptosis. Annu. Rev. Biochem. 68, 383–424. doi:10.1146/annurev.biochem.68.1.383
Enomoto, M., Endo, A., Yatsushige, H., Fushimi, K., and Otomo, Y. (2019). Clinical effects of early edaravone use in acute ischemic stroke patients treated by endovascular reperfusion therapy. Stroke 50 (3), 652–658. doi:10.1161/STROKEAHA.118.023815
Fang, S., Yu, X., Ding, H., Han, J., and Feng, J. (2018). Effects of intracellular iron overload on cell death and identification of potent cell death inhibitors. Biochem. Biophys. Res. Commun. 503 (1), 297–303. doi:10.1016/j.bbrc.2018.06.019
Fang, X., Wang, H., Han, D., Xie, E., Yang, X., Wei, J., et al. (2019). Ferroptosis as a target for protection against cardiomyopathy. Proc. Natl. Acad. Sci. U. S. A. 116 (7), 2672–2680. doi:10.1073/pnas.1821022116
Feder, M. E., and Hofmann, G. E. (1999). Heat-shock proteins, molecular chaperones, and the stress response: Evolutionary and ecological physiology. Annu. Rev. Physiol. 61, 243–282. doi:10.1146/annurev.physiol.61.1.243
Fei, W., Chen, D., Tang, H., Zheng, W., Chen, F., Chen, F., et al. (2020). Targeted GSH-exhausting and hydroxyl radical self-producing manganese-silica nanomissiles for MRI guided ferroptotic cancer therapy. Nanoscale 12 (32), 16738–16754. doi:10.1039/d0nr02396e
Feng, Y., Madungwe, N. B., Imam Aliagan, A. D., Tombo, N., and Bopassa, J. C. (2019). Liproxstatin-1 protects the mouse myocardium against ischemia/reperfusion injury by decreasing VDAC1 levels and restoring GPX4 levels. Biochem. Biophys. Res. Commun. 520 (3), 606–611. doi:10.1016/j.bbrc.2019.10.006
Ferguson, L. R., Karunasinghe, N., Zhu, S., and Wang, A. H. (2012). Selenium and its' role in the maintenance of genomic stability. Mutat. Res. 733 (1-2), 100–110. doi:10.1016/j.mrfmmm.2011.12.011
Fernandez-Morales, J. C., Hua, W., Yao, Y., and Morad, M. (2019). Regulation of Ca(2+) signaling by acute hypoxia and acidosis in cardiomyocytes derived from human induced pluripotent stem cells. Cell Calcium 78, 1–14. doi:10.1016/j.ceca.2018.12.006
Forcina, G. C., and Dixon, S. J. (2019). GPX4 at the crossroads of lipid homeostasis and ferroptosis. Proteomics 19 (18), e1800311. doi:10.1002/pmic.201800311
Friedman, M. (2014). Chemistry and multibeneficial bioactivities of carvacrol (4-isopropyl-2-methylphenol), a component of essential oils produced by aromatic plants and spices. J. Agric. Food Chem. 62 (31), 7652–7670. doi:10.1021/jf5023862
Fung, F. Y., and Linn, Y. C. (2015). Developing traditional Chinese medicine in the era of evidence-based medicine: Current evidences and challenges. Evid. Based. Complement. Altern. Med. 2015, 425037. doi:10.1155/2015/425037
Gao, M., Monian, P., Pan, Q., Zhang, W., Xiang, J., and Jiang, X. (2016). Ferroptosis is an autophagic cell death process. Cell Res. 26 (9), 1021–1032. doi:10.1038/cr.2016.95
Gao, M., Yi, J., Zhu, J., Minikes, A. M., Monian, P., Thompson, C. B., et al. (2019). Role of mitochondria in ferroptosis. Mol. Cell 73 (2), 354–363. doi:10.1016/j.molcel.2018.10.042
Gaschler, M. M., Andia, A. A., Liu, H., Csuka, J. M., Hurlocker, B., Vaiana, C. A., et al. (2018). FINO2 initiates ferroptosis through GPX4 inactivation and iron oxidation. Nat. Chem. Biol. 14 (5), 507–515. doi:10.1038/s41589-018-0031-6
Gaschler, M. M., and Stockwell, B. R. (2017). Lipid peroxidation in cell death. Biochem. Biophys. Res. Commun. 482 (3), 419–425. doi:10.1016/j.bbrc.2016.10.086
Gillessen, T., Budd, S. L., and Lipton, S. A. (2002). Excitatory amino acid neurotoxicity. Adv. Exp. Med. Biol. 513, 3–40. doi:10.1007/978-1-4615-0123-7_1
Girotti, A. W. (1998). Lipid hydroperoxide generation, turnover, and effector action in biological systems. J. Lipid Res. 39 (8), 1529–1542. doi:10.1016/s0022-2275(20)32182-9
Griesmaier, E., and Keller, M. (2012). Glutamate receptors - prenatal insults, long-term consequences. Pharmacol. Biochem. Behav. 100 (4), 835–840. doi:10.1016/j.pbb.2011.04.011
Grignano, E., Birsen, R., Chapuis, N., and Bouscary, D. (2020). From iron chelation to overload as a therapeutic strategy to induce ferroptosis in leukemic cells. Front. Oncol. 10, 586530. doi:10.3389/fonc.2020.586530
Guan, T., Song, J., Wang, Y., Guo, L., Yuan, L., Zhao, Y., et al. (2017). Expression and characterization of recombinant bifunctional enzymes with glutathione peroxidase and superoxide dismutase activities. Free Radic. Biol. Med. 110, 188–195. doi:10.1016/j.freeradbiomed.2017.06.005
Guan, X., Li, Z., Zhu, S., Cheng, M., Ju, Y., Ren, L., et al. (2021). Galangin attenuated cerebral ischemia-reperfusion injury by inhibition of ferroptosis through activating the SLC7A11/GPX4 axis in gerbils. Life Sci. 264, 118660. doi:10.1016/j.lfs.2020.118660
Guo, S., Kim, W. J., Lok, J., Lee, S. R., Besancon, E., Luo, B. H., et al. (2008). Neuroprotection via matrix-trophic coupling between cerebral endothelial cells and neurons. Proc. Natl. Acad. Sci. U. S. A. 105 (21), 7582–7587. doi:10.1073/pnas.0801105105
Guo, G. C., Wang, J. X., Han, M. L., Zhang, L. P., and Li, L. (2017). microRNA-761 induces aggressive phenotypes in triple-negative breast cancer cells by repressing TRIM29 expression. Cell. Oncol. 40 (2), 157–166. doi:10.1007/s13402-016-0312-6
Hambright, W. S., Fonseca, R. S., Chen, L., Na, R., and Ran, Q. (2017). Ablation of ferroptosis regulator glutathione peroxidase 4 in forebrain neurons promotes cognitive impairment and neurodegeneration. Redox Biol. 12, 8–17. doi:10.1016/j.redox.2017.01.021
Hamel, E. (2006). Perivascular nerves and the regulation of cerebrovascular tone. J. Appl. Physiol. (1985) 100 (3), 1059–1064. doi:10.1152/japplphysiol.00954.2005
Hamilton, N. B., Attwell, D., and Hall, C. N. (2010). Pericyte-mediated regulation of capillary diameter: A component of neurovascular coupling in health and disease. Front. Neuroenergetics 2, 5. doi:10.3389/fnene.2010.00005
Hanson, L. R., Roeytenberg, A., Martinez, P. M., Coppes, V. G., Sweet, D. C., Rao, R. J., et al. (2009). Intranasal deferoxamine provides increased brain exposure and significant protection in rat ischemic stroke. J. Pharmacol. Exp. Ther. 330 (3), 679–686. doi:10.1124/jpet.108.149807
Harris, C. C. (1996). p53 tumor suppressor gene: from the basic research laboratory to the clinic--an abridged historical perspective. Carcinogenesis 17 (6), 1187–1198. doi:10.1093/carcin/17.6.1187
Harris, S. L., and Levine, A. J. (2005). The p53 pathway: Positive and negative feedback loops. Oncogene 24 (17), 2899–2908. doi:10.1038/sj.onc.1208615
Henke, N., Schmidt-Ullrich, R., Dechend, R., Park, J. K., Qadri, F., Wellner, M., et al. (2007). Vascular endothelial cell-specific NF-kappaB suppression attenuates hypertension-induced renal damage. Circ. Res. 101 (3), 268–276. doi:10.1161/CIRCRESAHA.107.150474
Hong, S. H., Lee, D. H., Lee, Y. S., Jo, M. J., Jeong, Y. A., Kwon, W. T., et al. (2017). Molecular crosstalk between ferroptosis and apoptosis: Emerging role of ER stress-induced p53-independent PUMA expression. Oncotarget 8 (70), 115164–115178. doi:10.18632/oncotarget.23046
Hori, S., Ohtsuki, S., Hosoya, K., Nakashima, E., and Terasaki, T. (2004). A pericyte-derived angiopoietin-1 multimeric complex induces occludin gene expression in brain capillary endothelial cells through Tie-2 activation in vitro. J. Neurochem. 89 (2), 503–513. doi:10.1111/j.1471-4159.2004.02343.x
Hoshi, O., and Ushiki, T. (2004). Neutrophil extravasation in rat mesenteric venules induced by the chemotactic peptide N-formyl-methionyl-luecylphenylalanine (fMLP), with special attention to a barrier function of the vascular basal lamina for neutrophil migration. Arch. Histol. Cytol. 67 (1), 107–114. doi:10.1679/aohc.67.107
Hu, H. J., and Song, M. (2017). Disrupted ionic homeostasis in ischemic stroke and new therapeutic targets. J. Stroke Cerebrovasc. Dis. 26 (12), 2706–2719. doi:10.1016/j.jstrokecerebrovasdis.2017.09.011
Hu, X., De Silva, T. M., Chen, J., and Faraci, F. M. (2017). Cerebral vascular disease and neurovascular injury in ischemic stroke. Circ. Res. 120 (3), 449–471. doi:10.1161/CIRCRESAHA.116.308427
Hu, X., Wu, D., He, X., Zhao, H., He, Z., Lin, J., et al. (2019). circGSK3β promotes metastasis in esophageal squamous cell carcinoma by augmenting β-catenin signaling. Mol. Cancer 18 (1), 160. doi:10.1186/s12943-019-1095-y
Huang, J. (2021). Current developments of targeting the p53 signaling pathway for cancer treatment. Pharmacol. Ther. 220, 107720. doi:10.1016/j.pharmthera.2020.107720
Hughes, S. M., Lillien, L. E., Raff, M. C., RoHrer, H., and SendtnerM., (1988). Ciliary neurotrophic factor induces type-2 astrocyte differentiation in culture. Nature 335 (6185), 70–73. doi:10.1038/335070a0
Hundeshagen, P., Hamacher-Brady, A., Eils, R., and Brady, N. R. (2011). Concurrent detection of autolysosome formation and lysosomal degradation by flow cytometry in a high-content screen for inducers of autophagy. BMC Biol. 9, 38. doi:10.1186/1741-7007-9-38
Huo, X., Sun, H., Cao, D., Yang, J., Peng, P., Yu, M., et al. (2019). Identification of prognosis markers for endometrial cancer by integrated analysis of DNA methylation and RNA-Seq data. Sci. Rep. 9 (1), 9924. doi:10.1038/s41598-019-46195-8
Hutter-Schmid, B., and Humpel, C. (2016). Platelet-derived growth factor receptor-beta is differentially regulated in primary mouse pericytes and brain slices. Curr. Neurovasc. Res. 13 (2), 127–134. doi:10.2174/1567202613666160219120411
Iadecola, C. (2017). The neurovascular unit coming of age: A journey through neurovascular coupling in health and disease. Neuron 96 (1), 17–42. doi:10.1016/j.neuron.2017.07.030
Ikeda, M., Nakazawa, T., Abe, K., Kaneko, T., and Yamatsu, K. (1989). Extracellular accumulation of glutamate in the hippocampus induced by ischemia is not calcium dependent--in vitro and in vivo evidence. Neurosci. Lett. 96 (2), 202–206. doi:10.1016/0304-3940(89)90058-x
Ikeshima-Kataoka, H. (2016). Neuroimmunological implications of AQP4 in astrocytes. Int. J. Mol. Sci. 17 (8), E1306. doi:10.3390/ijms17081306
Illes, P., Verkhratsky, A., and Tang, Y. (2021). Surveilling microglia dampens neuronal activity: Operation of a purinergically mediated negative feedback mechanism. Signal Transduct. Target. Ther. 6 (1), 160. doi:10.1038/s41392-021-00586-4
Ishikawa, M., Zhang, J. H., Nanda, A., and Granger, D. N. (2004). Inflammatory responses to ischemia and reperfusion in the cerebral microcirculation. Front. Biosci. 9, 1339–1347. doi:10.2741/1330
Jacobs, A., Miller, F., Worwood, M., and Wardrop, C. A. (1972). Ferritin in the serum of normal subjects and patients with iron deficiency and iron overload. Br. Med. J. 4 (5834), 206–208. doi:10.1136/bmj.4.5834.206
Jaenisch, R., and Bird, A. (2003). Epigenetic regulation of gene expression: How the genome integrates intrinsic and environmental signals. Nat. Genet. 33, 245–254. doi:10.1038/ng1089
Jaiswal, A. K. (2004). Nrf2 signaling in coordinated activation of antioxidant gene expression. Free Radic. Biol. Med. 36 (10), 1199–1207. doi:10.1016/j.freeradbiomed.2004.02.074
Ji, H., Wang, W., Li, X., Han, X., Zhang, X., Wang, J., et al. (2022). p53: A double-edged sword in tumor ferroptosis. Pharmacol. Res. 177, 106013. doi:10.1016/j.phrs.2021.106013
Jiang, L., Kon, N., Li, T., Wang, S. J., Su, T., Hibshoosh, H., et al. (2015). Ferroptosis as a p53-mediated activity during tumour suppression. Nature 520 (7545), 57–62. doi:10.1038/nature14344
Jiang, X., Stockwell, B. R., and Conrad, M. (2021). Ferroptosis: Mechanisms, biology and role in disease. Nat. Rev. Mol. Cell Biol. 22 (4), 266–282. doi:10.1038/s41580-020-00324-8
Jin, G., Arai, K., Murata, Y., Wang, S., Stins, M. F., Lo, E. H., et al. (2008). Protecting against cerebrovascular injury: Contributions of 12/15-lipoxygenase to edema formation after transient focal ischemia. Stroke 39 (9), 2538–2543. doi:10.1161/STROKEAHA.108.514927
Jones, G., Goswami, S. K., Kang, H., Choi, H. S., and Kim, J. (2020). Combating iron overload: A case for deferoxamine-based nanochelators. Nanomedicine (Lond) 15, 1341–1356. doi:10.2217/nnm-2020-0038
Kagan, V. E., Mao, G., Qu, F., Angeli, J. P. F., Doll, S., Croix, C. S., et al. (2017). Oxidized arachidonic and adrenic PEs navigate cells to ferroptosis. Nat. Chem. Biol. 13 (1), 81–90. doi:10.1038/nchembio.2238
Kakhlon, O., and Cabantchik, Z. I. (2002). The labile iron pool: Characterization, measurement, and participation in cellular processes(1). Free Radic. Biol. Med. 33 (8), 1037–1046. doi:10.1016/s0891-5849(02)01006-7
Kang, R., Gamdzyk, M., Lenahan, C., Tang, J., Tan, S., and Zhang, J. H. (2020). The dual role of microglia in blood-brain barrier dysfunction after stroke. Curr. Neuropharmacol. 18 (12), 1237–1249. doi:10.2174/1570159X18666200529150907
Kanner, J., German, J. B., and Kinsella, J. E. (1987). Initiation of lipid peroxidation in biological systems. Crit. Rev. Food Sci. Nutr. 25 (4), 317–364. doi:10.1080/10408398709527457
Kaspar, J. W., Niture, S. K., and Jaiswal, A. K. (2009). Nrf2:INrf2 (Keap1) signaling in oxidative stress. Free Radic. Biol. Med. 47 (9), 1304–1309. doi:10.1016/j.freeradbiomed.2009.07.035
Kawabata, H. (2019). Transferrin and transferrin receptors update. Free Radic. Biol. Med. 133, 46–54. doi:10.1016/j.freeradbiomed.2018.06.037
Kern, F., Stanika, R. I., Sarg, B., Offterdinger, M., Hess, D., Obermair, G. J., et al. (2013). Nogo-A couples with Apg-1 through interaction and co-ordinate expression under hypoxic and oxidative stress. Biochem. J. 455 (2), 217–227. doi:10.1042/BJ20130579
Khoshnam, S. E., Winlow, W., Farzaneh, M., Farbood, Y., and Moghaddam, H. F. (2017). Pathogenic mechanisms following ischemic stroke. Neurol. Sci. 38 (7), 1167–1186. doi:10.1007/s10072-017-2938-1
Kikuchi, K., Tancharoen, S., Matsuda, F., Biswas, K. K., Ito, T., Morimoto, Y., et al. (2009). Edaravone attenuates cerebral ischemic injury by suppressing aquaporin-4. Biochem. Biophys. Res. Commun. 390 (4), 1121–1125. doi:10.1016/j.bbrc.2009.09.015
Kikuchi, K., Tancharoen, S., Takeshige, N., Yoshitomi, M., Morioka, M., Murai, Y., et al. (2013). The efficacy of edaravone (radicut), a free radical scavenger, for cardiovascular disease. Int. J. Mol. Sci. 14 (7), 13909–13930. doi:10.3390/ijms140713909
Kim, S. E., Zhang, L., Ma, K., Riegman, M., Chen, F., Ingold, I., et al. (2016). Ultrasmall nanoparticles induce ferroptosis in nutrient-deprived cancer cells and suppress tumour growth. Nat. Nanotechnol. 11 (11), 977–985. doi:10.1038/nnano.2016.164
Kim, S. U., and De Vellis, J. (2005). Microglia in health and disease. J. Neurosci. Res. 81 (3), 302–313. doi:10.1002/jnr.20562
Kobayashi, M., and Yamamoto, M. (2006). Nrf2-Keap1 regulation of cellular defense mechanisms against electrophiles and reactive oxygen species. Adv. Enzyme Regul. 46, 113–140. doi:10.1016/j.advenzreg.2006.01.007
Kobayashi, S., Fukuma, S., Ikenoue, T., and Fukuhara, S. (2019). Effect of edaravone on neurological symptoms in real-world patients with acute ischemic stroke. Stroke 50 (7), 1805–1811. doi:10.1161/STROKEAHA.118.024351
Koh, P. O. (2008). Melatonin attenuates the focal cerebral ischemic injury by inhibiting the dissociation of pBad from 14-3-3. J. Pineal Res. 44 (1), 101–106. doi:10.1111/j.1600-079X.2007.00495.x
Kruger-Genge, A., Blocki, A., Franke, R. P., and Jung, F. (2019). Vascular endothelial cell biology: An update. Int. J. Mol. Sci. 20 (18), E4411. doi:10.3390/ijms20184411
Kuang, F., Liu, J., Tang, D., and Kang, R. (2020). Oxidative damage and antioxidant defense in ferroptosis. Front. Cell Dev. Biol. 8, 586578. doi:10.3389/fcell.2020.586578
Kuhn, S., Gritti, L., Crooks, D., and Dombrowski, Y. (2019). Oligodendrocytes in development, myelin generation and beyond. Cells 8 (11), E1424. doi:10.3390/cells8111424
Kuwata, H., Nakatani, E., Shimbara-Matsubayashi, S., Ishikawa, F., Shibanuma, M., Sasaki, Y., et al. (2019). Long-chain acyl-CoA synthetase 4 participates in the formation of highly unsaturated fatty acid-containing phospholipids in murine macrophages. Biochim. Biophys. Acta. Mol. Cell Biol. Lipids 1864 (11), 1606–1618. doi:10.1016/j.bbalip.2019.07.013
Kwak, M. K., Cho, J. M., Huang, B., Shin, S., and Kensler, T. W. (2007). Role of increased expression of the proteasome in the protective effects of sulforaphane against hydrogen peroxide-mediated cytotoxicity in murine neuroblastoma cells. Free Radic. Biol. Med. 43 (5), 809–817. doi:10.1016/j.freeradbiomed.2007.05.029
Lai, T. W., Zhang, S., and Wang, Y. T. (2014). Excitotoxicity and stroke: Identifying novel targets for neuroprotection. Prog. Neurobiol. 115, 157–188. doi:10.1016/j.pneurobio.2013.11.006
Lan, B., Ge, J. W., Cheng, S. W., Zheng, X. L., Liao, J., He, C., et al. (2020). Extract of Naotaifang, a compound Chinese herbal medicine, protects neuron ferroptosis induced by acute cerebral ischemia in rats. J. Integr. Med. 18 (4), 344–350. doi:10.1016/j.joim.2020.01.008
Lane, D. J. R., Ayton, S., and Bush, A. I. (2018). Iron and alzheimer's disease: An update on emerging mechanisms. J. Alzheimers Dis. 64, S379–S395. doi:10.3233/JAD-179944
Lao, Y., Wang, Y., Chen, J., Huang, P., Su, R., Shi, J., et al. (2022). Synthesis and biological evaluation of 1, 2, 4-triazole derivatives as potential Nrf2 activators for the treatment of cerebral ischemic injury. Eur. J. Med. Chem. 236, 114315. doi:10.1016/j.ejmech.2022.114315
Lazarov, O., and Hollands, C. (2016). Hippocampal neurogenesis: Learning to remember. Prog. Neurobiol. 138-140, 1–18. doi:10.1016/j.pneurobio.2015.12.006
Lee, J. Y., Kim, W. K., Bae, K. H., Lee, S. C., and Lee, E. W. (2021). Lipid metabolism and ferroptosis. Biol. (Basel) 10 (3), 184. doi:10.3390/biology10030184
Leigh, R., Knutsson, L., Zhou, J., and van Zijl, P. C. (2018). Imaging the physiological evolution of the ischemic penumbra in acute ischemic stroke. J. Cereb. Blood Flow. Metab. 38 (9), 1500–1516. doi:10.1177/0271678X17700913
Lemasters, J. J. (2017). Evolution of voltage-dependent anion channel function: From molecular sieve to governator to actuator of ferroptosis. Front. Oncol. 7, 303. doi:10.3389/fonc.2017.00303
Li, Z., Hua, C., Pan, X., Fu, X., and Wu, W. (2016). Carvacrol exerts neuroprotective effects via suppression of the inflammatory response in middle cerebral artery occlusion rats. Inflammation 39 (4), 1566–1572. doi:10.1007/s10753-016-0392-5
Li, Y., Feng, D., Wang, Z., Zhao, Y., Sun, R., Tian, D., et al. (2019). Ischemia-induced ACSL4 activation contributes to ferroptosis-mediated tissue injury in intestinal ischemia/reperfusion. Cell Death Differ. 26 (11), 2284–2299. doi:10.1038/s41418-019-0299-4
Li, X., Ma, N., Xu, J., Zhang, Y., Yang, P., Su, X., et al. (2021). Targeting ferroptosis: Pathological mechanism and treatment of ischemia-reperfusion injury. Oxid. Med. Cell. Longev. 2021, 1587922. doi:10.1155/2021/1587922
Lim, S., Kim, T. J., Kim, Y. J., Kim, C., Ko, S. B., and Kim, B. S. (2021). Senolytic therapy for cerebral ischemia-reperfusion injury. Int. J. Mol. Sci. 22 (21), 11967. doi:10.3390/ijms222111967
Littarru, G. P., and Tiano, L. (2007). Bioenergetic and antioxidant properties of coenzyme Q10: Recent developments. Mol. Biotechnol. 37 (1), 31–37. doi:10.1007/s12033-007-0052-y
Liu, L., Vollmer, M. K., Fernandez, V. M., Dweik, Y., Kim, H., and Dore, S. (2018). Korean red ginseng pretreatment protects against long-term sensorimotor deficits after ischemic stroke likely through Nrf2. Front. Cell. Neurosci. 12, 74. doi:10.3389/fncel.2018.00074
Liu, J., Zhang, C., Wang, J., Hu, W., and Feng, Z. (2020). The regulation of ferroptosis by tumor suppressor p53 and its pathway. Int. J. Mol. Sci. 21 (21), E8387. doi:10.3390/ijms21218387
Liu, J., Guo, Z. N., Yan, X. L., Huang, S., Ren, J. X., Luo, Y., et al. (2020). Crosstalk between autophagy and ferroptosis and its putative role in ischemic stroke. Front. Cell. Neurosci. 14, 577403. doi:10.3389/fncel.2020.577403
Liu, L., Wang, M., Gong, N., Tian, P., and Deng, H. (2021). Se improves GPX4 expression and SOD activity to alleviate heat-stress-induced ferroptosis-like death in goat mammary epithelial cells. Anim. Cells Syst. 25 (5), 283–295. doi:10.1080/19768354.2021.1988704
Lo, E. H., and Rosenberg, G. A. (2009). The neurovascular unit in health and disease: Introduction. Stroke 40 (3), S2–S3. doi:10.1161/STROKEAHA.108.534404
Lopes-Bezerra, L. M., and Filler, S. G. (2003). Endothelial cells, tissue factor and infectious diseases. Braz J. Med. Biol. Res. 36 (8), 987–991. doi:10.1590/s0100-879x2003000800004
Lopes-Marques, M., Cunha, I., Reis-Henriques, M. A., Santos, M. M., and Castro, L. F. C. (2013). Diversity and history of the long-chain acyl-CoA synthetase (Acsl) gene family in vertebrates. BMC Evol. Biol. 13, 271. doi:10.1186/1471-2148-13-271
Lu, B., Chen, X. B., Ying, M. D., He, Q. J., Cao, J., and Yang, B. (2017). The role of ferroptosis in cancer development and treatment response. Front. Pharmacol. 8, 992. doi:10.3389/fphar.2017.00992
Luo, M., Wu, L., Zhang, K., Wang, H., Zhang, T., Gutierrez, L., et al. (2018). miR-137 regulates ferroptosis by targeting glutamine transporter SLC1A5 in melanoma. Cell Death Differ. 25 (8), 1457–1472. doi:10.1038/s41418-017-0053-8
Luo, W., Wang, J., Dai, X., Zhang, H., Qu, Y., Xiao, W., et al. (2021). ACSL4 expression is associated with CD8+ T cell infiltration and immune response in bladder cancer. Front. Oncol. 11, 754845. doi:10.3389/fonc.2021.754845
Ma, Y., Zhang, X., Alsaidan, O. A., Yang, X., Sulejmani, E., Zha, J., et al. (2021). Long-chain acyl-CoA synthetase 4-mediated fatty acid metabolism sustains androgen receptor pathway-independent prostate cancer. Mol. Cancer Res. 19 (1), 124–135. doi:10.1158/1541-7786.MCR-20-0379
Magtanong, L., and Dixon, S. J. (2018). Ferroptosis and brain injury. Dev. Neurosci. 40 (5-6), 382–395. doi:10.1159/000496922
Magtanong, L., Ko, P. J., and Dixon, S. J. (2016). Emerging roles for lipids in non-apoptotic cell death. Cell Death Differ. 23 (7), 1099–1109. doi:10.1038/cdd.2016.25
Mahoney-Sanchez, L., Bouchaoui, H., Ayton, S., Devos, D., Duce, J. A., and Devedjian, J. C. (2021). Ferroptosis and its potential role in the physiopathology of Parkinson's Disease. Prog. Neurobiol. 196, 101890. doi:10.1016/j.pneurobio.2020.101890
Makihara, N., Arimura, K., Ago, T., Tachibana, M., Nishimura, A., Nakamura, K., et al. (2015). Involvement of platelet-derived growth factor receptor beta in fibrosis through extracellular matrix protein production after ischemic stroke. Exp. Neurol. 264, 127–134. doi:10.1016/j.expneurol.2014.12.007
Mancias, J. D., Wang, X., Gygi, S. P., Harper, J. W., and Kimmelman, A. C. (2014). Quantitative proteomics identifies NCOA4 as the cargo receptor mediating ferritinophagy. Nature 509 (7498), 105–109. doi:10.1038/nature13148
Mandai, K., Matsumoto, M., Kitagawa, K., Matsushita, K., OhTsuki, T., Mabuchi, T., et al. (1997). Ischemic damage and subsequent proliferation of oligodendrocytes in focal cerebral ischemia. Neuroscience 77 (3), 849–861. doi:10.1016/s0306-4522(96)00517-9
Marcelo, K. L., Goldie, L. C., and Hirschi, K. K. (2013). Regulation of endothelial cell differentiation and specification. Circ. Res. 112 (9), 1272–1287. doi:10.1161/CIRCRESAHA.113.300506
Margis, R., Dunand, C., Teixeira, F. K., and Margis-Pinheiro, M. (2008). Glutathione peroxidase family - an evolutionary overview. FEBS J. 275 (15), 3959–3970. doi:10.1111/j.1742-4658.2008.06542.x
Martin, A., Domercq, M., and Matute, C. (2018). Inflammation in stroke: The role of cholinergic, purinergic and glutamatergic signaling. Ther. Adv. Neurol. Disord. 11, 1756286418774267. doi:10.1177/1756286418774267
Matsumoto, J., Dohgu, S., Takata, F., Machida, T., Bolukbasi Hatip, F. F., Hatip-Al-Khatib, I., et al. (2018). TNF-α-sensitive brain pericytes activate microglia by releasing IL-6 through cooperation between IκB-NFκB and JAK-STAT3 pathways. Brain Res. 1692, 34–44. doi:10.1016/j.brainres.2018.04.023
Matsumoto, S., Murozono, M., Kanazawa, M., Nara, T., Ozawa, T., and Watanabe, Y. (2018). Edaravone and cyclosporine A as neuroprotective agents for acute ischemic stroke. Acute Med. Surg. 5 (3), 213–221. doi:10.1002/ams2.343
Matute, C., Domercq, M., and Sanchez-Gomez, M. V. (2006). Glutamate-mediated glial injury: Mechanisms and clinical importance. Glia 53 (2), 212–224. doi:10.1002/glia.20275
Mccall, J. M., Braughler, J. M., and Hall, E. D. (1987). Lipid peroxidation and the role of oxygen radicals in CNS injury. Acta Anaesthesiol. belg. 38 (4), 373–379.
Mcconnell, H. L., Kersch, C. N., Woltjer, R. L., and Neuwelt, E. A. (2017). The translational significance of the neurovascular unit. J. Biol. Chem. 292 (3), 762–770. doi:10.1074/jbc.R116.760215
Narayan, S. K., Grace Cherian, S., Babu Phaniti, P., Babu Chidambaram, S., Rachel Vasanthi, A. H., and Arumugam, M. (2021). Preclinical animal studies in ischemic stroke: Challenges and some solutions. Anim. Model. Exp. Med. 4 (2), 104–115. doi:10.1002/ame2.12166
Nasoohi, S., Simani, L., Khodagholi, F., Nikseresht, S., Faizi, M., and Naderi, N. (2019). Coenzyme Q10 supplementation improves acute outcomes of stroke in rats pretreated with atorvastatin. Nutr. Neurosci. 22 (4), 264–272. doi:10.1080/1028415X.2017.1376928
Nave, K. A., and Werner, H. B. (2014). Myelination of the nervous system: Mechanisms and functions. Annu. Rev. Cell Dev. Biol. 30, 503–533. doi:10.1146/annurev-cellbio-100913-013101
Newell-Price, J., Clark, A. J., and King, P. (2000). DNA methylation and silencing of gene expression. Trends Endocrinol. Metab. 11 (4), 142–148. doi:10.1016/s1043-2760(00)00248-4
Ng, S. W., Norwitz, S. G., and Norwitz, E. R. (2019). The impact of iron overload and ferroptosis on reproductive disorders in humans: Implications for preeclampsia. Int. J. Mol. Sci. 20 (13), E3283. doi:10.3390/ijms20133283
Nguyen, B., Bix, G., and Yao, Y. (2021). Basal lamina changes in neurodegenerative disorders. Mol. Neurodegener. 16 (1), 81. doi:10.1186/s13024-021-00502-y
Nimmerjahn, A., Kirchhoff, F., and Helmchen, F. (2005). Resting microglial cells are highly dynamic surveillants of brain parenchyma in vivo. Science 308 (5726), 1314–1318. doi:10.1126/science.1110647
Nishizawa, H., Matsumoto, M., Chen, G., Ishii, Y., Tada, K., Onodera, M., et al. (2021). Lipid peroxidation and the subsequent cell death transmitting from ferroptotic cells to neighboring cells. Cell Death Dis. 12 (4), 332. doi:10.1038/s41419-021-03613-y
Onat, D., Brillon, D., Colombo, P. C., and Schmidt, A. M. (2011). Human vascular endothelial cells: A model system for studying vascular inflammation in diabetes and atherosclerosis. Curr. Diab. Rep. 11 (3), 193–202. doi:10.1007/s11892-011-0182-2
Owolabi, M. O., Akarolo-Anthony, S., Akinyemi, R., Arnett, D., Gebregziabher, M., Jenkins, C., et al. (2015). The burden of stroke in africa: A glance at the present and a glimpse into the future. Cardiovasc. J. Afr. 26, S27–S38. doi:10.5830/CVJA-2015-038
Pan, L., Zhou, L., Yin, W., Bai, J., and Liu, R. (2018). miR-125a induces apoptosis, metabolism disorder and migrationimpairment in pancreatic cancer cells by targeting Mfn2-related mitochondrial fission. Int. J. Oncol. 53 (1), 124–136. doi:10.3892/ijo.2018.4380
Papanicolaou, K. N., Khairallah, R. J., Ngoh, G. A., Chikando, A., Luptak, I., O'Shea, K. M., et al. (2011). Mitofusin-2 maintains mitochondrial structure and contributes to stress-induced permeability transition in cardiac myocytes. Mol. Cell. Biol. 31 (6), 1309–1328. doi:10.1128/MCB.00911-10
Pardridge, W. M. (1991). Advances in cell biology of blood-brain barrier transport. Semin. Cell Biol. 2 (6), 419–426.
Peisker, T., Koznar, B., Stetkarova, I., and Widimsky, P. (2017). Acute stroke therapy: A review. Trends cardiovasc. Med. 27 (1), 59–66. doi:10.1016/j.tcm.2016.06.009
Peng, L., Zhao, Y., Li, Y., Zhou, Y., Li, L., Lei, S., et al. (2019). Effect of DJ-1 on the neuroprotection of astrocytes subjected to cerebral ischemia/reperfusion injury. J. Mol. Med. 97 (2), 189–199. doi:10.1007/s00109-018-1719-5
Petrova, J., Manolov, V., Vasilev, V., Tzatchev, K., and Marinov, B. (2016). Ischemic stroke, inflammation, iron overload - connection to a hepcidin. Int. J. Stroke 11 (1), NP16–17. doi:10.1177/1747493015607509
Plemel, J. R., Keough, M. B., Duncan, G. J., Sparling, J. S., Yong, V. W., Stys, P. K., et al. (2014). Remyelination after spinal cord injury: Is it a target for repair? Prog. Neurobiol. 117, 54–72. doi:10.1016/j.pneurobio.2014.02.006
Polewski, M. D., Reveron-Thornton, R. F., Cherryholmes, G. A., Marinov, G. K., Cassady, K., and Aboody, K. S. (2016). Increased expression of system xc- in glioblastoma confers an altered metabolic state and temozolomide resistance. Mol. Cancer Res. 14 (12), 1229–1242. doi:10.1158/1541-7786.MCR-16-0028
Pratt, D. A., Tallman, K. A., and Porter, N. A. (2011). Free radical oxidation of polyunsaturated lipids: New mechanistic insights and the development of peroxyl radical clocks. Acc. Chem. Res. 44 (6), 458–467. doi:10.1021/ar200024c
Purohit, P. K., Edwards, R., Tokatlidis, K., and Saini, N. (2019). MiR-195 regulates mitochondrial function by targeting mitofusin-2 in breast cancer cells. RNA Biol. 16 (7), 918–929. doi:10.1080/15476286.2019.1600999
Qin, D. J., Tang, C. X., Yang, L., Lei, H., Wei, W., Wang, Y. Y., et al. (2015). Hsp90 is a novel target molecule of CDDO-me in inhibiting proliferation of ovarian cancer cells. PLoS One 10 (7), e0132337. doi:10.1371/journal.pone.0132337
Qin, C., Zhou, L. Q., Ma, X. T., Hu, Z. W., Yang, S., Chen, M., et al. (2019). Dual functions of microglia in ischemic stroke. Neurosci. Bull. 35 (5), 921–933. doi:10.1007/s12264-019-00388-3
Raj, S., Khurana, S., Choudhari, R., Kesari, K. K., Kamal, M. A., Garg, N., et al. (2021). Specific targeting cancer cells with nanoparticles and drug delivery in cancer therapy. Semin. Cancer Biol. 69, 166–177. doi:10.1016/j.semcancer.2019.11.002
Ramakrishnan, S., Anand, V., and Roy, S. (2014). Vascular endothelial growth factor signaling in hypoxia and inflammation. J. Neuroimmune Pharmacol. 9 (2), 142–160. doi:10.1007/s11481-014-9531-7
Ramezani, M., Simani, L., Abedi, S., and Pakdaman, H. (2021). Is selenium supplementation beneficial in acute ischemic stroke? Neurologist 27 (2), 51–55. doi:10.1097/NRL.0000000000000365
Ravingerova, T., Kindernay, L., Bartekova, M., Ferko, M., Adameova, A., Zohdi, V., et al. (2020). The molecular mechanisms of iron metabolism and its role in cardiac dysfunction and cardioprotection. Int. J. Mol. Sci. 21 (21), E7889. doi:10.3390/ijms21217889
Reichert, C. O., De Freitas, F. A., Sampaio-Silva, J., Rokita-Rosa, L., Barros, P. d. L., Levy, D., et al. (2020). Ferroptosis mechanisms involved in neurodegenerative diseases. Int. J. Mol. Sci. 21 (22), E8765. doi:10.3390/ijms21228765
Ren, X., Hu, H., Farooqi, I., and Simpkins, J. W. (2020). Blood substitution therapy rescues the brain of mice from ischemic damage. Nat. Commun. 11 (1), 4078. doi:10.1038/s41467-020-17930-x
Ringleb, P. A., Schellinger, P. D., Schranz, C., and Hacke, W. (2002). Thrombolytic therapy within 3 to 6 hours after onset of ischemic stroke: Useful or harmful? Stroke 33 (5), 1437–1441. doi:10.1161/01.str.0000015555.21285.db
Rizzardi, N., Liparulo, I., Antonelli, G., Orsini, F., Riva, A., Bergamini, C., et al. (2021). Coenzyme Q10 phytosome formulation improves CoQ10 bioavailability and mitochondrial functionality in cultured cells. Antioxidants 10 (6), 927. doi:10.3390/antiox10060927
Roemhild, K., Von Maltzahn, F., Weiskirchen, R., Knuchel, R., von Stillfried, S., and Lammers, T. (2021). Iron metabolism: Pathophysiology and pharmacology. Trends Pharmacol. Sci. 42 (8), 640–656. doi:10.1016/j.tips.2021.05.001
Rohnert, P., Schroder, U. H., Ziabreva, I., Tager, M., Reymann, K. G., and Striggow, F. (2012). Insufficient endogenous redox buffer capacity may underlie neuronal vulnerability to cerebral ischemia and reperfusion. J. Neurosci. Res. 90 (1), 193–202. doi:10.1002/jnr.22754
Rotin, D., Robinson, B., and Tannock, I. F. (1986). Influence of hypoxia and an acidic environment on the metabolism and viability of cultured cells: Potential implications for cell death in tumors. Cancer Res. 46 (6), 2821–2826.
Rustenhoven, J., Jansson, D., Smyth, L. C., and Dragunow, M. (2017). Brain pericytes as mediators of neuroinflammation. Trends Pharmacol. Sci. 38 (3), 291–304. doi:10.1016/j.tips.2016.12.001
Sacco, R. L., Kasner, S. E., Broderick, J. P., Caplan, L. R., Connors, J. J. B., Culebras, A., et al. (2013). An updated definition of stroke for the 21st century: A statement for healthcare professionals from the American heart association/American stroke association. Stroke 44 (7), 2064–2089. doi:10.1161/STR.0b013e318296aeca
Sandoo, A., Van Zanten, J. J., Metsios, G. S., Carroll, D., and Kitas, G. D. (2010). The endothelium and its role in regulating vascular tone. Open cardiovasc. Med. J. 4, 302–312. doi:10.2174/1874192401004010302
Sasaki, H., Sato, H., Kuriyama-Matsumura, K., Maebara, K., Wang, H., Tamba, M., et al. (2002). Electrophile response element-mediated induction of the cystine/glutamate exchange transporter gene expression. J. Biol. Chem. 277 (47), 44765–44771. doi:10.1074/jbc.M208704200
Sato, H., Tamba, M., Ishii, T., and Bannai, S. (1999). Cloning and expression of a plasma membrane cystine/glutamate exchange transporter composed of two distinct proteins. J. Biol. Chem. 274 (17), 11455–11458. doi:10.1074/jbc.274.17.11455
Schellinger, P. D., and Warach, S. (2004). Therapeutic time window of thrombolytic therapy following stroke. Curr. Atheroscler. Rep. 6 (4), 288–294. doi:10.1007/s11883-004-0060-3
Seib, T. M., Patel, S. A., and Bridges, R. J. (2011). Regulation of the system x(C)- cystine/glutamate exchanger by intracellular glutathione levels in rat astrocyte primary cultures. Glia 59 (10), 1387–1401. doi:10.1002/glia.21176
Shen, Z., Xiang, M., Chen, C., Ding, F., Wang, Y., Shang, C., et al. (2022). Glutamate excitotoxicity: Potential therapeutic target for ischemic stroke. Biomed. Pharmacother. 151, 113125. doi:10.1016/j.biopha.2022.113125
Shibata, Y., Yasui, H., Higashikawa, K., Miyamoto, N., and Kuge, Y. (2019). Erastin, a ferroptosis-inducing agent, sensitized cancer cells to X-ray irradiation via glutathione starvation in vitro and in vivo. PLoS One 14 (12), e0225931. doi:10.1371/journal.pone.0225931
Shibuya, M. (2011). Vascular endothelial growth factor (VEGF) and its receptor (VEGFR) signaling in angiogenesis: A crucial target for anti- and pro-angiogenic therapies. Genes Cancer 2 (12), 1097–1105. doi:10.1177/1947601911423031
Shih, A. Y., Li, P., and Murphy, T. H. (2005). A small-molecule-inducible Nrf2-mediated antioxidant response provides effective prophylaxis against cerebral ischemia in vivo. J. Neurosci. 25 (44), 10321–10335. doi:10.1523/JNEUROSCI.4014-05.2005
Shintoku, R., Takigawa, Y., Yamada, K., Kubota, C., Yoshimoto, Y., Takeuchi, T., et al. (2017). Lipoxygenase-mediated generation of lipid peroxides enhances ferroptosis induced by erastin and RSL3. Cancer Sci. 108 (11), 2187–2194. doi:10.1111/cas.13380
Singh, N. K., and Rao, G. N. (2019). Emerging role of 12/15-Lipoxygenase (ALOX15) in human pathologies. Prog. Lipid Res. 73, 28–45. doi:10.1016/j.plipres.2018.11.001
Soehnlein, O., and Lindbom, L. (2010). Phagocyte partnership during the onset and resolution of inflammation. Nat. Rev. Immunol. 10 (6), 427–439. doi:10.1038/nri2779
Sofroniew, M. V. (2009). Molecular dissection of reactive astrogliosis and glial scar formation. Trends Neurosci. 32 (12), 638–647. doi:10.1016/j.tins.2009.08.002
Song, X., and Long, D. (2020). Nrf2 and ferroptosis: A new research direction for neurodegenerative diseases. Front. Neurosci. 14, 267. doi:10.3389/fnins.2020.00267
Stamatovic, S. M., Keep, R. F., and Andjelkovic, A. V. (2008). Brain endothelial cell-cell junctions: How to "open" the blood brain barrier. Curr. Neuropharmacol. 6 (3), 179–192. doi:10.2174/157015908785777210
Stanimirovic, D. B., and Friedman, A. (2012). Pathophysiology of the neurovascular unit: Disease cause or consequence? J. Cereb. Blood Flow. Metab. 32 (7), 1207–1221. doi:10.1038/jcbfm.2012.25
Steinberg, S. F. (2008). Structural basis of protein kinase C isoform function. Physiol. Rev. 88 (4), 1341–1378. doi:10.1152/physrev.00034.2007
Stockwell, B. R., Friedmann Angeli, J. P., Bayir, H., Bush, A. I., Conrad, M., Dixon, S. J., et al. (2017). Ferroptosis: A regulated cell death nexus linking metabolism, redox biology, and disease. Cell 171 (2), 273–285. doi:10.1016/j.cell.2017.09.021
Stubbs, C. D., and Smith, A. D. (1984). The modification of mammalian membrane polyunsaturated fatty acid composition in relation to membrane fluidity and function. Biochim. Biophys. Acta 779 (1), 89–137. doi:10.1016/0304-4157(84)90005-4
Su, L. J., Zhang, J. H., Gomez, H., Murugan, R., Hong, X., Xu, D., et al. (2019). Reactive oxygen species-induced lipid peroxidation in apoptosis, autophagy, and ferroptosis. Oxid. Med. Cell. Longev. 2019, 5080843. doi:10.1155/2019/5080843
Sukiennicki, G. M., Marciniak, W., Muszynska, M., Baszuk, P., Gupta, S., Bialkowska, K., et al. (2019). Iron levels, genes involved in iron metabolism and antioxidative processes and lung cancer incidence. PLoS One 14 (1), e0208610. doi:10.1371/journal.pone.0208610
Sun, X., Ou, Z., Xie, M., Kang, R., Fan, Y., Niu, X., et al. (2015). HSPB1 as a novel regulator of ferroptotic cancer cell death. Oncogene 34 (45), 5617–5625. doi:10.1038/onc.2015.32
Tang, L. J., Zhou, Y. J., Xiong, X. M., Li, N. S., Zhang, J. J., Luo, X. J., et al. (2021). Ubiquitin-specific protease 7 promotes ferroptosis via activation of the p53/TfR1 pathway in the rat hearts after ischemia/reperfusion. Free Radic. Biol. Med. 162, 339–352. doi:10.1016/j.freeradbiomed.2020.10.307
Tarangelo, A., Magtanong, L., Bieging-Rolett, K. T., Li, Y., Ye, J., Attardi, L. D., et al. (2018). p53 suppresses metabolic stress-induced ferroptosis in cancer cells. Cell Rep. 22 (3), 569–575. doi:10.1016/j.celrep.2017.12.077
Tevlin, R., Longaker, M. T., and Wan, D. C. (2021). Deferoxamine to minimize fibrosis during radiation therapy. Adv. Wound Care 11, 548–559. doi:10.1089/wound.2021.0021
Thomas, C., Mackey, M. M., Diaz, A. A., and Cox, D. P. (2009). Hydroxyl radical is produced via the Fenton reaction in submitochondrial particles under oxidative stress: Implications for diseases associated with iron accumulation. Redox Rep. 14 (3), 102–108. doi:10.1179/135100009X392566
Thurgur, H., and Pinteaux, E. (2019). Microglia in the neurovascular unit: Blood-brain barrier-microglia interactions after central nervous system disorders. Neuroscience 405, 55–67. doi:10.1016/j.neuroscience.2018.06.046
Tian, Q., Qin, B., Gu, Y., Zhou, L., Chen, S., Zhang, S., et al. (2020). ROS-mediated necroptosis is involved in iron overload-induced osteoblastic cell death. Oxid. Med. Cell. Longev. 2020, 1295382. doi:10.1155/2020/1295382
Tourki, B., Black, L. M., Kain, V., and Halade, G. V. (2021). Lipoxygenase inhibitor ML351 dysregulated an innate inflammatory response leading to impaired cardiac repair in acute heart failure. Biomed. Pharmacother. 139, 111574. doi:10.1016/j.biopha.2021.111574
Tuo, Q. Z., Lei, P., Jackman, K. A., Li, X. L., Xiong, H., Li, X. L., et al. (2017). Tau-mediated iron export prevents ferroptotic damage after ischemic stroke. Mol. Psychiatry 22 (11), 1520–1530. doi:10.1038/mp.2017.171
Ursini, F., and Maiorino, M. (2020). Lipid peroxidation and ferroptosis: The role of GSH and GPx4. Free Radic. Biol. Med. 152, 175–185. doi:10.1016/j.freeradbiomed.2020.02.027
Varin, A., and Gordon, S. (2009). Alternative activation of macrophages: Immune function and cellular biology. Immunobiology 214 (7), 630–641. doi:10.1016/j.imbio.2008.11.009
Verrey, F., Closs, E. I., Wagner, C. A., Palacin, M., Endou, H., and Kanai, Y. (2004). CATs and HATs: The SLC7 family of amino acid transporters. Pflugers Arch. 447 (5), 532–542. doi:10.1007/s00424-003-1086-z
Vespa, P., Prins, M., Ronne-Engstrom, E., CaronM., , Shalmon, E., Hovda, D. A., et al. (1998). Increase in extracellular glutamate caused by reduced cerebral perfusion pressure and seizures after human traumatic brain injury: A microdialysis study. J. Neurosurg. 89 (6), 971–982. doi:10.3171/jns.1998.89.6.0971
Villalpando-Rodriguez, G. E., and Gibson, S. B. (2021). Reactive oxygen species (ROS) regulates different types of cell death by acting as a rheostat. Oxid. Med. Cell. Longev. 2021, 9912436. doi:10.1155/2021/9912436
Waldvogel-Abramowski, S., Waeber, G., Gassner, C., Buser, A., Frey, B. M., Favrat, B., et al. (2014). Physiology of iron metabolism. Transfus. Med. Hemother. 41 (3), 213–221. doi:10.1159/000362888
Wang, Y., Dai, S. D., Qi, F. J., Xu, H. T., and Wang, E. H. (2008). p53 protein expression and genetic mutation in two primary cell types in pulmonary sclerosing haemangioma. J. Clin. Pathol. 61 (2), 192–196. doi:10.1136/jcp.2007.050401
Wang, H., Zhou, W., Zheng, Z., Zhang, P., Tu, B., He, Q., et al. (2012). The HDAC inhibitor depsipeptide transactivates the p53/p21 pathway by inducing DNA damage. DNA Repair (Amst) 11 (2), 146–156. doi:10.1016/j.dnarep.2011.10.014
Wang, J., Sun, Z., Shen, J., Wu, D., Liu, F., Yang, R., et al. (2015). Octreotide protects the mouse retina against ischemic reperfusion injury through regulation of antioxidation and activation of NF-κB. Oxid. Med. Cell. Longev. 2015, 970156. doi:10.1155/2015/970156
Wang, Z., Guo, L. M., Wang, Y., Zhou, H. K., Wang, S. C., Chen, D., et al. (2018). Inhibition of HSP90α protects cultured neurons from oxygen-glucose deprivation induced necroptosis by decreasing RIP3 expression. J. Cell. Physiol. 233 (6), 4864–4884. doi:10.1002/jcp.26294
Wang, Y., Yang, L., Zhang, X., Cui, W., Liu, Y., Sun, Q. R., et al. (2019). Epigenetic regulation of ferroptosis by H2B monoubiquitination and p53. EMBO Rep. 20 (7), e47563. doi:10.15252/embr.201847563
Wang, H., Lin, D., Yu, Q., Li, Z., Lenahan, C., Dong, Y., et al. (2021). A promising future of ferroptosis in tumor therapy. Front. Cell Dev. Biol. 9, 629150. doi:10.3389/fcell.2021.629150
Wang, L., Xiong, X., Zhang, L., and Shen, J. (2021). Neurovascular unit: A critical role in ischemic stroke. CNS Neurosci. Ther. 27 (1), 7–16. doi:10.1111/cns.13561
Ward, R. J., Zucca, F. A., Duyn, J. H., Crichton, R. R., and Zecca, L. (2014). The role of iron in brain ageing and neurodegenerative disorders. Lancet. Neurol. 13 (10), 1045–1060. doi:10.1016/S1474-4422(14)70117-6
Watanabe, K., Tanaka, M., Yuki, S., Hirai, M., and Yamamoto, Y. (2018). How is edaravone effective against acute ischemic stroke and amyotrophic lateral sclerosis? J. Clin. Biochem. Nutr. 62 (1), 20–38. doi:10.3164/jcbn.17-62
Wei, S., Qiu, T., Wang, N., Yao, X., Jiang, L., Jia, X., et al. (2020). Ferroptosis mediated by the interaction between Mfn2 and IREα promotes arsenic-induced nonalcoholic steatohepatitis. Environ. Res. 188, 109824. doi:10.1016/j.envres.2020.109824
Willard, S. S., and Koochekpour, S. (2013). Glutamate, glutamate receptors, and downstream signaling pathways. Int. J. Biol. Sci. 9 (9), 948–959. doi:10.7150/ijbs.6426
Winkler, E. A., Bell, R. D., and Zlokovic, B. V. (2011). Central nervous system pericytes in health and disease. Nat. Neurosci. 14 (11), 1398–1405. doi:10.1038/nn.2946
Wu, J., Minikes, A. M., Gao, M., Bian, H., Li, Y., Stockwell, B. R., et al. (2019). Intercellular interaction dictates cancer cell ferroptosis via NF2-YAP signalling. Nature 572 (7769), 402–406. doi:10.1038/s41586-019-1426-6
Wu, Y., Zhang, S., Gong, X., Tam, S., Xiao, D., Liu, S., et al. (2020). The epigenetic regulators and metabolic changes in ferroptosis-associated cancer progression. Mol. Cancer 19 (1), 39. doi:10.1186/s12943-020-01157-x
Wynford-Thomas, D., and Blaydes, J. (1998). The influence of cell context on the selection pressure for p53 mutation in human cancer. Carcinogenesis 19 (1), 29–36. doi:10.1093/carcin/19.1.29
Xie, Z., Xu, M., Xie, J., Liu, T., Xu, X., Gao, W., et al. (2022). Inhibition of ferroptosis attenuates glutamate excitotoxicity and nuclear autophagy in a CLP septic mouse model. Shock 57 (5), 694–702. doi:10.1097/SHK.0000000000001893
Xu, Y., Liu, Y., Li, K., Yuan, D., Yang, S., Zhou, L., et al. (2022). COX-2/PGE2 pathway inhibits the ferroptosis induced by cerebral ischemia reperfusion. Mol. Neurobiol. 59 (3), 1619–1631. doi:10.1007/s12035-021-02706-1
Yang, W. S., Kim, K. J., Gaschler, M. M., Patel, M., Shchepinov, M. S., and Stockwell, B. R. (2016). Peroxidation of polyunsaturated fatty acids by lipoxygenases drives ferroptosis. Proc. Natl. Acad. Sci. U. S. A. 113 (34), E4966–E4975. doi:10.1073/pnas.1603244113
Yang, S., Jin, H., Zhu, Y., Wan, Y., Opoku, E. N., Zhu, L., et al. (2017). Diverse functions and mechanisms of pericytes in ischemic stroke. Curr. Neuropharmacol. 15 (6), 892–905. doi:10.2174/1570159X15666170112170226
Yang, Q., Huang, Q., Hu, Z., and Tang, X. (2019). Potential neuroprotective treatment of stroke: Targeting excitotoxicity, oxidative stress, and inflammation. Front. Neurosci. 13, 1036. doi:10.3389/fnins.2019.01036
Yang, Y., Luo, M., Zhang, K., Zhang, J., Gao, T., Connell, D. O., et al. (2020). Nedd4 ubiquitylates VDAC2/3 to suppress erastin-induced ferroptosis in melanoma. Nat. Commun. 11 (1), 433. doi:10.1038/s41467-020-14324-x
Yang, F., Wu, Z., Dai, D., Zhang, L., Zhang, X., Zhang, X., et al. (2021). The iron chelator deferoxamine decreases myeloma cell survival. J. Int. Med. Res. 49 (1), 300060520987396. doi:10.1177/0300060520987396
Ye, J., Sun, Z., and Hu, W. (2018). Roles of astrocytes in cerebral infarction and related therapeutic strategies. Zhejiang Da Xue Xue Bao Yi Xue Ban. 47 (5), 493–498. doi:10.3785/j.issn.1008-9292.2018.10.08
Yenari, M. A., Kauppinen, T. M., and Swanson, R. A. (2010). Microglial activation in stroke: Therapeutic targets. Neurotherapeutics 7 (4), 378–391. doi:10.1016/j.nurt.2010.07.005
Yin, H., Xu, L., and Porter, N. A. (2011). Free radical lipid peroxidation: Mechanisms and analysis. Chem. Rev. 111 (10), 5944–5972. doi:10.1021/cr200084z
Yu, S. S., Li, Z. Y., Xu, X. Z., Yao, F., Luo, Y., Liu, Y. C., et al. (2022). M1-type microglia can induce astrocytes to deposit chondroitin sulfate proteoglycan after spinal cord injury. Neural Regen. Res. 17 (5), 1072–1079. doi:10.4103/1673-5374.324858
Yuan, H., Li, X., Zhang, X., Kang, R., and Tang, D. (2016). Identification of ACSL4 as a biomarker and contributor of ferroptosis. Biochem. Biophys. Res. Commun. 478 (3), 1338–1343. doi:10.1016/j.bbrc.2016.08.124
Yuan, H., Li, X., Zhang, X., Kang, R., and Tang, D. (2016). CISD1 inhibits ferroptosis by protection against mitochondrial lipid peroxidation. Biochem. Biophys. Res. Commun. 478 (2), 838–844. doi:10.1016/j.bbrc.2016.08.034
Zhang, D. D., Lo, S. C., Cross, J. V., Templeton, D. J., and Hannink, M. (2004). Keap1 is a redox-regulated substrate adaptor protein for a Cul3-dependent ubiquitin ligase complex. Mol. Cell. Biol. 24 (24), 10941–10953. doi:10.1128/MCB.24.24.10941-10953.2004
Zhang, Z., Zhang, F., An, P., Guo, X., Shen, Y., Tao, Y., et al. (2011). Ferroportin1 deficiency in mouse macrophages impairs iron homeostasis and inflammatory responses. Blood 118 (7), 1912–1922. doi:10.1182/blood-2011-01-330324
Zhang, Z., Zhang, F., Guo, X., An, P., Tao, Y., and Wang, F. (2012). Ferroportin1 in hepatocytes and macrophages is required for the efficient mobilization of body iron stores in mice. Hepatology 56 (3), 961–971. doi:10.1002/hep.25746
Zhang, Y., Koppula, P., and Gan, B. (2019). Regulation of H2A ubiquitination and SLC7A11 expression by BAP1 and PRC1. Cell Cycle 18 (8), 773–783. doi:10.1080/15384101.2019.1597506
Zhang, C., Yan, L., Gu, Z., and Zhao, Y. (2019). Strategies based on metal-based nanoparticles for hypoxic-tumor radiotherapy. Chem. Sci. 10 (29), 6932–6943. doi:10.1039/c9sc02107h
Zhang, X., Wang, L., Li, H., Zhang, L., Zheng, X., and Cheng, W. (2020). Crosstalk between noncoding RNAs and ferroptosis: New dawn for overcoming cancer progression. Cell Death Dis. 11 (7), 580. doi:10.1038/s41419-020-02772-8
Zhang, X., Sui, S., Wang, L., Li, H., Zhang, L., Xu, S., et al. (2020). Inhibition of tumor propellant glutathione peroxidase 4 induces ferroptosis in cancer cells and enhances anticancer effect of cisplatin. J. Cell. Physiol. 235 (4), 3425–3437. doi:10.1002/jcp.29232
Zhang, W., Liu, Y., and Zhang, H. (2021). Extracellular matrix: An important regulator of cell functions and skeletal muscle development. Cell Biosci. 11 (1), 65. doi:10.1186/s13578-021-00579-4
Zhang, Y., Lu, X., Tai, B., Li, W., and Li, T. (2021). Ferroptosis and its multifaceted roles in cerebral stroke. Front. Cell. Neurosci. 15, 615372. doi:10.3389/fncel.2021.615372
Zhang, X., Yu, K., Ma, L., Qian, Z., Tian, X., Miao, Y., et al. (2021). Endogenous glutamate determines ferroptosis sensitivity via ADCY10-dependent YAP suppression in lung adenocarcinoma. Theranostics 11 (12), 5650–5674. doi:10.7150/thno.55482
Zhang, H. L., Hu, B. X., Li, Z. L., Du, T., Shan, J. L., Ye, Z. P., et al. (2022). PKCβII phosphorylates ACSL4 to amplify lipid peroxidation to induce ferroptosis. Nat. Cell Biol. 24 (1), 88–98. doi:10.1038/s41556-021-00818-3
Zhao, Y., Yang, J., Li, C., Zhou, G., Wan, H., Ding, Z., et al. (2020). Role of the neurovascular unit in the process of cerebral ischemic injury. Pharmacol. Res. 160, 105103. doi:10.1016/j.phrs.2020.105103
Zhao, Y., Li, Y., Zhang, R., Wang, F., Wang, T., and Jiao, Y. (2020). The role of erastin in ferroptosis and its prospects in cancer therapy. Onco. Targets. Ther. 13, 5429–5441. doi:10.2147/OTT.S254995
Zhao, Q., Ge, Z., Fu, S., Wan, S., Shi, J., Wu, Y., et al. (2022). DNA methylation plays an important role in iron-overloaded Tibetans. Genes Genet. Syst. 97 (2), 55–66. doi:10.1266/ggs.21-00006
Zhou, S., Du, X., Xie, J., and Wang, J. (2017). Interleukin-6 regulates iron-related proteins through c-Jun N-terminal kinase activation in BV2 microglial cell lines. PLoS One 12 (7), e0180464. doi:10.1371/journal.pone.0180464
Zhou, S., Gao, B., Sun, C., Bai, Y., Cheng, D., Zhang, Y., et al. (2020). Vascular endothelial cell-derived exosomes protect neural stem cells against ischemia/reperfusion injury. Neuroscience 441, 184–196. doi:10.1016/j.neuroscience.2020.05.046
Zhou, Y., Liao, J., Mei, Z., Liu, X., and Ge, J. (2021). Insight into crosstalk between ferroptosis and necroptosis: Novel therapeutics in ischemic stroke. Oxid. Med. Cell. Longev. 2021, 9991001. doi:10.1155/2021/9991001
Zhou, S. Y., Guo, Z. N., Zhang, D. H., Qu, Y., and Jin, H. (2022). The role of pericytes in ischemic stroke: Fom cellular functions to therapeutic targets. Front. Mol. Neurosci. 15, 866700. doi:10.3389/fnmol.2022.866700
Zhou, Y., and Danbolt, N. C. (2014). Glutamate as a neurotransmitter in the healthy brain. J. Neural Transm. 121 (8), 799–817. doi:10.1007/s00702-014-1180-8
Zhu, H., Itoh, K., Yamamoto, M., Zweier, J. L., and Li, Y. (2005). Role of Nrf2 signaling in regulation of antioxidants and phase 2 enzymes in cardiac fibroblasts: Protection against reactive oxygen and nitrogen species-induced cell injury. FEBS Lett. 579 (14), 3029–3036. doi:10.1016/j.febslet.2005.04.058
Zhu, S., Zhang, Q., Sun, X., Zeh, H. J., Lotze, M. T., Kang, R., et al. (2017). HSPA5 regulates ferroptotic cell death in cancer cells. Cancer Res. 77 (8), 2064–2077. doi:10.1158/0008-5472.CAN-16-1979
Keywords: stroke, neurovascular unit (NVU), ferroptosis, inhibitors, therapeutic target
Citation: Wei Z, Xie Y, Wei M, Zhao H, Ren K, Feng Q and Xu Y (2022) New insights in ferroptosis: Potential therapeutic targets for the treatment of ischemic stroke. Front. Pharmacol. 13:1020918. doi: 10.3389/fphar.2022.1020918
Received: 16 August 2022; Accepted: 26 October 2022;
Published: 08 November 2022.
Edited by:
Yi Wang, Zhejiang University, ChinaReviewed by:
Zili Zhang, Nanjing University of Chinese Medicine, ChinaCopyright © 2022 Wei, Xie, Wei, Zhao, Ren, Feng and Xu. This is an open-access article distributed under the terms of the Creative Commons Attribution License (CC BY). The use, distribution or reproduction in other forums is permitted, provided the original author(s) and the copyright owner(s) are credited and that the original publication in this journal is cited, in accordance with accepted academic practice. No use, distribution or reproduction is permitted which does not comply with these terms.
*Correspondence: Kaidi Ren, cmVua2QwMDZAMTYzLmNvbQ==; Qi Feng, ZmVuZ3FpMjAxOUB6enUuZWR1LmNu; Yuming Xu, eHV5dW1pbmdAenp1LmVkdS5jbg==
Disclaimer: All claims expressed in this article are solely those of the authors and do not necessarily represent those of their affiliated organizations, or those of the publisher, the editors and the reviewers. Any product that may be evaluated in this article or claim that may be made by its manufacturer is not guaranteed or endorsed by the publisher.
Research integrity at Frontiers
Learn more about the work of our research integrity team to safeguard the quality of each article we publish.