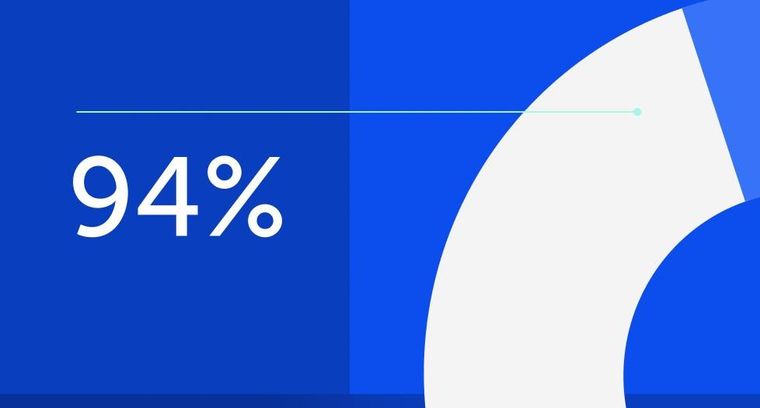
94% of researchers rate our articles as excellent or good
Learn more about the work of our research integrity team to safeguard the quality of each article we publish.
Find out more
REVIEW article
Front. Pharmacol., 13 October 2022
Sec. Inflammation Pharmacology
Volume 13 - 2022 | https://doi.org/10.3389/fphar.2022.1020447
This article is part of the Research TopicFerroptosis as a Novel Therapeutic Target for Inflammation-Related DiseasesView all 8 articles
Ferroptosis is a novel programmed cell death form characterized by iron-mediated reactive oxygen species-induced lipid peroxidation and subsequent cell damage that is distinct from apoptosis, necroptosis, pyroptosis, and autophagy. Most studies on ferroptosis are based on its function and mechanism, but there have been relatively few studies on the effects of drugs, especially anaesthetics, on ferroptosis. Therefore, we summarized the recent literature on the effects of anaesthetics on ferroptosis to understand the underlying mechanism. In particular, we focused on the targets of various anaesthetics in different mechanisms of ferroptosis and the effects of ferroptosis induction or inhibition by narcotics on various diseases. The aims of this review are to provide a relatively reasonable drug regimen for clinicians, to explore potential ferroptosis protection drugs and targets, to reduce perioperative complications and to improve the postoperative performance of patients, especially those who are critically ill.
In 2012, a new regulatory cell death (RCD) method was first proposed and named ferroptosis (Dixon et al., 2012). Since then, a series of studies have focused on ferroptosis. Ferroptosis is a novel programmed cell death form driven by iron-mediated reactive oxygen species (ROS)-induced lipid peroxidation and subsequent cell damage, which is different from other RCDs, such as apoptosis, necroptosis, pyroptosis, and autophagy at biochemical, morphological and genetic levels (Bergsbaken et al., 2009; Linkermann et al., 2014; Skommer et al., 2010; Yang and Stockwell, 2008; Zhang et al., 2015) (Table 1). At the subcellular and cellular levels, ferroptotic cells under the influence of anaesthetics exhibit a characteristic round morphology prior to cell death, resembling necroptotic cells, but without organelle swelling or plasma membrane permeabilization (Dixon et al., 2012; Wu et al., 2020). In ferroptotic cells, the nuclear structure is intact, and there is no chromatin condensation and rupture, nuclear pyknosis rupture, nucleolus disappearance or apoptotic body formation, which are characteristic features of apoptosis (Liang et al., 2019). Furthermore, morphological features such as intense membrane blebbing and loss of plasma membrane integrity in pyroptosis and double-membrane wrapping, autophagolysosome formation from autophagic cells were not observed in ferroptotic cells (Liang et al., 2019). The only unique morphological features of ferroptosis are mitochondrial shrinkage, reduction or disappearance of mitochondrial cristae, and increased bilayer density (Yagoda et al., 2007; Zhao et al., 2022). Although different types of mechanisms regulating cell death have different morphological and biochemical characteristics, there is still some interference between regulators and the components of these different processes. For example, the degradation of ferritin by autophagy can promote ferroptosis (Ma et al., 2016). By observing the apoptosis induced by Tumour necrosis factor related apoptosis-inducing ligand (TRAIL), it can be confirmed that the combination of ferroptosis inducers and TRAIL can significantly improve the killing effect on tumour cells (Lee et al., 2018).
TABLE 1. The main morphological and biochemical features, key genes, inducers and inhibitors of ferroptosis, apoptosis, autophagy, necroptosis, and pyroptosis. And the effect of anaesthetics on cell death.
Most anaesthetics, in addition to anaesthesia, also have anti-tumour effects, such as bupivacaine, lidocaine and propofol, which can promote cell apoptosis and inhibit cancer cell proliferation (Mirshahidi et al., 2021; Tan et al., 2021). At clinically relevant concentrations, most anaesthetics are safe and have significance in protecting important organs. For example, clinical doses of propofol can block the pyroptosis of alveolar macrophages in renal ischemia/reperfusion (rI/R) rats and improve acute lung injury (ALI) caused by rI/R (Liu et al., 2020). However, long-term ketamine-induced bladder injury is usually associated with autophagy, and ketamine can upregulate autophagy-related proteins in bladder smooth muscle tissue and alter bladder angiogenesis (Li et al., 2021a). For oxidative stress, dexmedetomidine (DEX) can alleviate H2O2-induced oxidative stress and necroptosis, thereby protecting the body from damage (Yin et al., 2020). The discovery of ferroptosis broadened the exploration of anaesthetics for RCD. In a sepsis model, DEX can reduce ferroptosis and protect important organ damage by enhancing glutathione peroxidase 4 (GPX4) expression (Wang et al., 2020a). Etomidate inhibits ischaemia/reperfusion (I/R)-induced ferroptosis through the Nrf2 pathway and alleviates myocardial injury, which may supply a fresh idea for clinical reperfusion therapy (Lv et al., 2021). For cancerous cells, anaesthetics such as bupivacaine, propofol, and ketamine can induce ferroptosis and play an anticancer effect (He et al., 2021; Hao et al., 2022; Liu et al., 2021a). In an ALI model, both sevoflurane and DEX inhibited cellular ferroptosis and protected lung function (Liu et al., 2021b; Lin et al., 2022). However, ferroptosis is also involved in inhaled anaesthetic-induced neurotoxicity, and antiferroptosis treatment may provide new preventive ideas for perioperative anaesthesia complications (Zhang et al., 2022a).
Ferroptosis is regulated by various cellular metabolic pathways, including redox homeostasis (Stockwell et al., 2017), iron metabolism (Li et al., 2017), mitochondrial activity (Gao et al., 2019), and the metabolism of amino acids, lipids, and sugars (Yao et al., 2021), and is involved in the pathological mechanisms of various disease signalling pathways (Chen et al., 2020a; Tian et al., 2021; Yang et al., 2014). Ferroptosis plays an essential role in cancer, kidney injury, lung injury, nervous system disease, and ischaemia-reperfusion injury (IRI) (Chen et al., 2021a; Han et al., 2020) (Figure 1).
FIGURE 1. Diseases and narcotics are linked to ferroptosis. These diseases include acute lung injury, acute kidney injury, cancer, cardiovascular disease, neurodegenerative disease, and liver disease, among others. MIRI, myocardial ischaemia reperfusion injury; LIRI, lung ischaemia reperfusion injury; ALI, acute lung injury. “↑” represents promotion, “↓” represents inhibition.
As shown in Figure 2, the regulatory mechanism of ferroptosis is mainly related to the regulation of iron metabolism, cystine-glutamate transporter (System xc-), glutathione (GSH), and GPX4 regulation, and lipid metabolism. I/R, iron overload, ionizing radiation, cytokines (such as INF-γ), certain drugs (for example, sulfasalazine, artemisinin, statins), and experimental reagents (such as erastin) can induce cell ferroptosis (Du et al., 2022; Su et al., 2020; Tang and Kroemer, 2020). Iron chelators, such as deferiprone (DFP), deferoxamine (DFO), ciclopirox (CPX), and 2,2-bipyridine (2,2-BP) (Dixon et al., 2012; Masaldan et al., 2018), and antioxidants, such as vitamin E, ferrostatin-1 (Fer-1), and liproxstatin-1 (Lip-1), showed inhibitory effects on ferroptosis (Kajarabille and Latunde-Dada, 2019).
FIGURE 2. Molecular regulatory mechanism of ferroptosis. Ferroptosis is primarily initiated through two pathways: exogenous (transferrin dependent) pathways and endogenous (enzymatically regulated) pathways. The extrinsic pathway is initiated by cell membrane transporters such as cystine/glutamate transporter or by ferroportin, transferrin and lactoferrin activation. The endogenous pathway is activated by blocking intracellular antioxidant enzymes such as GPX4. The background color is from light to dark, with the light color representing exogenous pathways and the dark color representing endogenous pathways. TFR1, transferrin receptor 1; STEAP3, the six-transmembrane epithelial antigen of the prostate 3; NCOA4, nuclear receptor coactivator 4; DMT1, divalent metal transporter 1; ROS, reactive oxygen species; ACSL4, acyl-CoA synthetase long-chain family member 4; LPCAT3, lysophosphatidylcholine acyltransferase 3; ALOXs, arachidonic acid lipoxygenases; PUFA, polyunsaturated fatty acids; PUFA-CoA, polyunsaturated fatty acyl CoA; PL-PUFA, phospholipid-bound polyunsaturated fatty acids; PLOOH, phospholipid hydroperoxides; LPO, lipid peroxidation; Nrf2, nuclear factor erythroid 2-related factor 2; GLS, Glutaminase; γ-GSC, γ-glutamyl cysteinyl synthetase; γ Glu-Cys, γ glutamyl-cysteine; GSH, glutathione; GSS, glutathione synthetase; GSR, glutathione-disulfide reductase; GSSG, glutathione oxidized; GPX4, glutathione peroxidase 4; ACSL3, acyl-CoA synthetase long-chain family member 3; MUFA, monounsaturated fatty acids; MUFA-CoA, monounsaturated fatty acyl CoA; PL-MUFA, phospholipid-bound monounsaturated fatty acids; PPARα, peroxisome proliferator-activated receptor α; NADPH, nicotinamide adenine dinucleotide phosphate; NADP+, the oxidized form of NADPH; FSP-1, ferroptosis-suppressor-protein 1. “⊥” represents inhibition.
In recent years, with the continuous development of anaesthesiology, the demand for anaesthesia in various clinical departments has been increasing. Anaesthesia is not limited to providing good surgical conditions and painlessness but also pays more attention to the regulation and maintenance of patients’ vital functions, such as stress response control. Its scope of work extends from the operating room to the ward, outpatient clinic, emergency room and other places. For anaesthesiologists, it is of great significance to improve anaesthesia management to reduce the burden of disease. The way anaesthesia is administered and the type of anaesthetic drug chosen in the hours during anaesthesia management may affect cancer recurrence months or years later (Sessler and Riedel, 2019). Anaesthetics can be divided into general anaesthetics and local anaesthetics. General anaesthetics are divided into inhalation anaesthetics and intravenous anaesthetics according to the route of administration. Commonly used inhalation anaesthetics include sevoflurane (Sev), isoflurane, and desflurane. Intravenous anaesthetics include sedatives (propofol, etomidate, etc.), analgesics (fentanyl, sufentanil, remifentanil, etc.). Commonly used local anaesthetics include lidocaine, bupivacaine, and ropivacaine. They all have different dosages and indications (Miller et al., 2005) (Table 2). Here, we review the effects of various anaesthetic drugs on ferroptosis-related molecular mechanisms and signalling pathways, aiming to provide a theoretical basis for targeting ferroptosis to prevent perioperative complications and improve postoperative outcomes for patients.
TABLE 2. Dosages, indications and potential effects on ferroptosis of some commonly used anaesthetics in the perioperative period.
The System xc-/GSH/GPX4 signalling pathway is the most important signalling pathway related to ferroptosis and plays an important role in intracellular lipid peroxidation. System xc- is a heterodimer consisting of the xCT light chain (encoded by the solute carrier family 7 members 11 gene; SLC7A11) and the 4F2 heavy chain (encoded by the SLC3A2 gene) (Sato et al., 1999). SLC3A2 is a single transmembrane protein that maintains the stability and proper membrane localization of the SLC7A11 protein (Nakamura et al., 1999). The 4F2 heavy chain is a ubiquitously expressed cell surface component shared with several other amino acid transport systems (Kandasamy et al., 2018). The xCT light chain consists of 12 specific transmembrane domains linked to the 4F2 heavy chain by extracellular disulfide bonds, selective for cystine and glutamate. System xc- exchanges intracellular glutamate with extracellular cystine at a 1:1 ratio (Bridges et al., 2012). Cystine in cells can be further converted into cysteine, and then glutathione (GSH) is synthesized, so System xc- plays a key role in maintaining intracellular GSH levels. The classic ferroptosis inducer erastin induces ferroptosis by inhibiting the activity of System xc-(26).
GPX4 is a key enzyme and a unique member of the mammalian selenium-dependent GPX family, which can degrade small molecule peroxides and certain lipid peroxides, inhibit lipid peroxidation, maintain lipid oxidation balance, and effectively repair lipid peroxidative damage (Brigelius-Flohé and Flohé, 2020). GSH is required as a substrate during ferroptosis, simultaneously converting reduced GSH to oxidized GSH (glutathione oxidized; GSSG) and converting toxic lipid hydroperoxides into nontoxic lipid alcohols, thereby reducing oxidative stress damage without affecting normal levels of cysteine or glutathione (Seibt et al., 2019), which is a negative regulator of ferroptosis. With reduced NADPH acting as the electron donor, GSH can be regenerated by reducing GSSG using glutathione reductase (GSR). Studies have shown that the regulation of cytosolic NADPH levels through metazoan spot homologue 1 (MESH1) can regulate ferroptosis (Ding et al., 2020). Therefore, GSH is considered to be an important factor in maintaining GPX4 activity. Moreover, RSL3 can irreversibly target selenocysteine binding to the active site of GPX4, thereby inhibiting the activity of GPX4 and inducing ferroptosis (Liang et al., 2019).
p53 is a tumour suppressor gene that is generally associated with cell cycle arrest, apoptosis, and senescence (Brady et al., 2011; Li et al., 2012). Jiang et al. (2015) identified the relationship between p53 and ferroptosis for the first time, and the results showed that p53 can sensitize cells to ferroptosis. p53 not only inhibits the transcription of SLC7A11 through the p53 response element in the 5′ flanking region (Jiang et al., 2015) but also downregulates the expression of SLC7A11 by regulating USP7 and H2Bub1 (Wang et al., 2019). P53 also inhibits the cellular response to cystine uptake, resulting in decreased glutathione peroxidase activity, reduced cellular antioxidant capacity, and enhanced cellular susceptibility to ferroptosis (Guan et al., 2020). Nevertheless, activation of DNA damage by p53 has a dual role in promoting or inhibiting ferroptosis, depending on its gene target or binding protein (Kang et al., 2019; Zhang et al., 2022b) (Figure 3). Some metabolism-related genes, such as GLS2 (Gao et al., 2015), SAT1 (Ou et al., 2016), FDXR (Zhang et al., 2017) and ALOX12 (Chu et al., 2019), can act as p53 targets in different situations to promote ferroptosis. At the same time, p53 can also regulate CDKN1A, DPP4 and PARK2 to inhibit ferroptosis (Kang et al., 2019). Interestingly, p53 3KR (K117R, K161R, and K162R) acetylation-deficient mutants cannot induce cell cycle arrest, apoptosis, and senescence, but fully retain the ability to induce ferroptosis (Jiang et al., 2015). Another acetylation-deficient mutant, p53 4KR (KR98), failed to inhibit SLC7A11 expression or induce ferroptosis (Wang et al., 2016). Mechanistic analysis revealed that the combined removal of K117/161/162 and K98 acetylation blocked p53-mediated transcriptional regulation of TIGAR and GLS2, which is closely related to ferroptosis (Wang et al., 2016).
FIGURE 3. Positive and negative regulation of P53 to ferroptosis. ALOX12, arachidonic acid 12 lipoxygenases; ALOX15, arachidonic acid 15 lipoxygenases; CDKN1A/P21, cyclin-dependent kinase inhibitor; DPP4, dipeptidyl peptidase-4; FDXR, ferredoxin reductase; GLS2, glutaminases; PARK2, Parkinson disease 2; PTGS2, prostaglandin-endoperoxide synthase 2; SAT1, spermidine/spermine N1-acetyltransferase. “⊥” represents inhibition, “→“represents promotion.
Iron is one of the essential nutrients for organisms and plays an important role in life. Iron in food is mainly Fe3+, which is absorbed by intestinal epithelial cells (Das et al., 2020), bound to transferrin (TF), transported through transferrin receptor 1 (TFR1) on the cell membrane surface, and enters cells by endocytosis (Cheng et al., 2004). In an acidic environment, Fe3+ is released from the conjugate, reduced to Fe2+ by the six transmembrane epithelial antigens of prostate 3 (STEAP3), and translocated into the cytoplasm by divalent metal transporter 1 (DMT1) (Torti and Torti, 2013). A small amount of Fe2+ forms an unstable iron pool (LIP) (Zanninelli et al., 2002), excess iron is mainly stored in ferritin in a redox-inactive form, and part of Fe2+ is transported out of the cell by the action of ferroportin 1 (FPN1). Changes in any of these steps may have an impact on iron metabolism. In general, the balance of intracellular iron is reflected in the balance between iron input, storage, and output (Ganz, 2013). Too much iron is harmful. A certain amount of free iron is necessary for ferroptosis to occur. On the one hand, iron can increase the activity of metabolic enzymes such as arachidonic acid lipoxygenase (ALOX) and promote ferroptosis by stimulating the production of lipid ROS (Chen et al., 2020b). On the other hand, iron is able to act as a ferrous ion and react with H2O2 to generate hydroxyl radicals OH and ROS in the Fenton reaction (Toyokuni, 2002), leading to cell death through peroxide damage on DNA, protein and membrane lipids. In conclusion, abnormal iron metabolism induces ferroptosis, which is a necessary pathway for ferroptosis.
Lipid metabolome genes have shown that during ferroptosis, polyunsaturated fatty acids (PUFAs) such as epinephrine (ADA) and arachidonic acid (AA), are most susceptible to peroxidation, damaging lipid bilayers and affecting cell membrane function (Kagan et al., 2017). PUFAs contain easily extractable bisallyl hydrogen atoms and are susceptible to lipid peroxidation (Yang et al., 2016). Acyl-CoA synthetase long-chain family member 4 (ACSL4) belongs to the long-chain acyl-CoA synthetase family and is a crucial enzyme controlling fatty acid metabolism (Kuwata and Hara, 2019). ACSL4 and lysophosphatidylcholine acyltransferase 3 (LPCAT3) promote the incorporation of PUFAs into phospholipids to form phospholipid-bound polyunsaturated fatty acids (PL-PUFA). PL-PUFA is prone to ALOX-induced lipid peroxidation, which is oxidized by LOXs or POR to form lipid peroxides, which ultimately leads to lipid bilayer disruption, thereby promoting ferroptosis by affecting cell membrane function (Du et al., 2022). ACSL4 is considered a key regulator of ferroptosis (Kagan et al., 2017; Yuan et al., 2016). The key enzymes in the synthesis of the unsaturated phospholipids ACSL4 and LPCAT3 are associated with RSL3-induced ferroptosis (Hadian and Stockwell, 2020). At the same time, PUFAs are competitively affected by monounsaturated fatty acids (MUFAs), and MUFAs do not have bisallyl sites, so they are not prone to peroxidation, which means that exogenous MUFAs may produce ferroptosis resistance. While MUFA-induced ferroptosis is dependent on ACSL3, ACSL3 converts MUFAs to acyl-CoA esters that bind to membrane phospholipids and reduce the sensitivity of plasma membrane lipids to lethal oxidation, thereby protecting cancer cells from ferroptosis attack (Magtanong et al., 2019).
Other major regulatory mechanisms of ferroptosis include the voltage-dependent anion channels (VDACs) pathway and the ferroptosis suppressor protein 1 (FSP1)/coenzyme Q10 (CoQ10) signalling pathway. The FSP1/CoQ10 signalling pathway is a new pathway independent of the System xc-/GSH/GPX4 signalling pathway discovered in recent years (Bersuker et al., 2019). FSP1 is an oxidoreductase, which can reduce CoQ10 (also known as ubiquinone) to reduced CoQ10 (also known as ubiquinol) under the action of NADPH, capture lipid peroxy radicals that mediate lipid peroxidation, and inhibit lipid peroxidation. Propagation of oxides inhibits ferroptosis (Doll et al., 2019). VDACs are a class of porin ion channels located in the outer mitochondrial membrane that mainly transport ions and metabolites between the cytoplasm and mitochondria (Kim et al., 2019; Shoshan-Barmatz et al., 2010). Many researchers have found that erastin can act on VDACs to keep them open, thereby enhancing mitochondrial respiration and increasing ROS production and promoting ferroptosis (Yagoda et al., 2007; Yang et al., 2020; Zhao et al., 2020). Interestingly, Yagoda et al. (2007) found that downregulation of VDAC2 and VDAC3 protein levels can reduce mitochondrial ROS production and lead to the development of tolerance to erastin-induced ferroptosis. Mitochondria are the regulatory centres of cellular metabolism and energy metabolism and are constantly remodelled through fission and fusion. Mitochondria are also involved in cellular functions such as ATP production, intracellular calcium regulation, and ROS production (Roy et al., 2019). All of the above evidence demonstrates the indispensable role of mitochondria in the regulation of ferroptosis.
Propofol, etomidate, and ketamine are commonly used intravenous anaesthetics in clinical practice. Among them, propofol is an alkylphenol compound due to its fast induction, rapid recovery, and lack of easy accumulation after continuous infusion. Propofol also has specific nonanaesthetic effects, such as antiemetic, anti-inflammatory, antioxidant, antitumour, neuroprotective, and antiplatelet aggregation effects (Bateman and Kesselheim, 2015; Jiang et al., 2018). As one of the most commonly used intravenous anaesthetics, propofol is widely applied for anaesthesia induction and intraoperative maintenance. Similar to the endogenous antioxidant vitamin E, the molecular structure of propofol contains phenolic hydroxyl groups, so it can directly scavenge ROS and inhibit lipid peroxidation (Wilson and Gelb, 2002). At the same time, propofol can inhibit inflammation (Wu et al., 2018), reduce oxidative stress and ameliorate IRI (85), but I/R can induce ferroptosis. In a mouse cardiac IRI model, treatment with Fer-1 effectively reduced ferroptosis-induced myocardial infarction, improved left ventricular contractility, and reduced left ventricular remodelling (Li et al., 2019a). In intestinal I/R, ACSL4 is critical in this consistent process, and Fer-1 attenuates damage caused by intestinal ischaemia (Li et al., 2019b). These all suggest that ferroptosis is related to I/R. Studies have shown that propofol can increase the levels of ferritin heavy chain-1 (FTH1), xCT, or GPX4, improve the antioxidant capacity of cardiomyocytes via iron level reduction and inhibit cell ferroptosis through the AKT/P53 signalling pathway (Li et al., 2022). AKT is a serine/threonine-specific protein kinase essential for regulating the response to myocardial I/R injury (Yin et al., 2013). Phosphorylation of AKT can promote the binding of murine double minute 2 (MDM2) to p53, leading to p53 degradation (Wang et al., 2020b) and protecting myocardial cells from myocardial ischaemia‒reperfusion-induced ferroptosis. Therefore, propofol can protect some cells from ferroptosis. Propofol also protects neurons by reducing ferroptosis, as propofol (50 μM) reduces erastin-induced high iron concentrations, lipid peroxides, and excess reactive oxygen species (Xuan et al., 2022). However, animal experiments and in vitro cell experiments consistently show that propofol has the potential to inhibit the malignancy of primary cancers (Sessler and Riedel, 2019), promoting ferroptosis in cancer cells. Clinically relevant concentrations (5 µg/ml, 10 µg/ml, 20 µg/ml) of propofol can inhibit the viability of cervical cancer cells in vitro and enhance the morphological changes related to ferroptosis and mitochondrial atrophy in HeLa cells induced by paclitaxel (Zhao et al., 2022). It is manifests as increased intracellular Fe2+ concentrations, inhibition of the SLC7A11/GPX4 and ubiquinol/FSP1/CoQ10 pathways, upregulation of the expression of the ferroptosis regulators ACSL4, TFRC, etc., to promote ferroptosis in C-33A and HeLa cells. However, another in vitro study showed that pretreatment with 10 µg/ml propofol could induce ferroptosis and inhibit the proliferation of MDA-MB-231 breast cancer cells. Propofol induced ferroptosis through the P53-SCL7A11-GPX4 pathway but not the ubiquinone-FSP1-ubiquinol axis, even though propofol downregulates FSP1 expression (Sun et al., 2022). These results are based on in vitro experiments and require further verification in animal models. In addition, unlike directly administering propofol in in vitro cell experiments, the common preparation in clinical practice is a propofol emulsion, which may lead to different antitumour effects and needs to be further explored. Propofol at clinically relevant concentrations promotes ferroptosis in cancer cells, which is inconsistent with previous claims and exhibits antiferroptotic effects in ischemia-reperfusion injury disease. The possible reason for this contradiction is that propofol acts on different disease models and the final response results are different. What controls the ferroptosis signaling pathway network to show different outcomes in different diseases are still unclear, which needs to be further answered in the future. Studies have shown that different propofol concentrations may have both cytoprotective and cytotoxic effects with prolonged use (Lin et al., 2015). However, whether high concentrations and prolonged use of propofol lead to ferroptosis, especially exposure duration, still needs further study.
Etomidate is also a commonly used intravenous anaesthetic, with few side effects on blood circulation, and rapid recovery. Etomidate can reduce the expression of myocardial fibrosis-related proteins (α-smooth muscle actin and collagen II) and the secretion of inflammatory factors (IL-6, IL-1β, and TNF-α) in myocardial ischaemia reperfusion injury (MIRI) rats, reducing superoxide dismutase content, glutathione activity, and GPX4 expression while upregulating malondialdehyde, iron, acyl-CoA and synthase long-chain family member 4 levels and alleviating I/R-induced cardiac ferroptosis (Lv et al., 2021). Experimental studies have shown that the effects of etomidate on I/R-induced myocardial fibrosis and inflammation can be reversed by erastin (Lv et al., 2021), a well-established inducer of ferroptosis (DeHart et al., 2018). It can be seen that etomidate has both anti-inflammatory and anti-ferroptosis effects on the IRI model. The relationship between ferroptosis and inflammation is intricate, and the specific mechanism still needs to be further explored.
Ketamine is a fast-acting anaesthetic with a short half-life and mild respiratory depression. Ketamine may lead to abuse; otherwise, it is an ideal anaesthetic. In addition to anaesthetic effects, ketamine also showed anti-inflammatory, anti-anxiety, anti-depressant, analgesic, and other effects (Roytblat et al., 1998; Wolff and Winstock, 2006; Zarate et al., 2006) and potential antitumour properties. Ketamine was applied to treat breast cancer, and the results showed that ketamine could increase lipid ROS, malondialdehyde (MDA), and Fe2+ in breast cancer cells, inhibiting the expression of the ferroptosis factor GPX4 by attenuating KAT5 on the GPX4 promoter region and reducing histone H3 lysine 27 acetylation (H3K27ac) and the enrichment of RNA polymer II (RNA pol II), ultimately leading to breast cancer cell ferroptosis (Li et al., 2021b). In ketamine- or Sev-induced neurotoxicity, ketamine or Sev exposure caused upregulation of excitatory N-methyl-D aspartate receptor (NMDAR) subunits (Wu et al., 2020), which may be a compensatory mechanism (Haseneder et al., 2013; Liu et al., 2013; Sinner et al., 2015). In addition, both Sev and ketamine led to the upregulation of RASD1, which can form a ternary complex with DMT1 and PAP7, thereby enhancing the capability of NMDARs to activate DMT1 for iron uptake (Cheah et al., 2006; Chen et al., 2015a). DMT1-mediated iron influx promotes iron overload and stimulates lysosome iron release (White et al., 2016), causing iron-induced cellular damage. These results suggest that some non-narcotic effects of ketamine are closely related to ferroptosis.
Currently, ferroptosis-related inhalation anaesthetics mainly include Sev and isoflurane. There is growing evidence showing that inhaled anaesthetics induce neuronal cell death, especially in older patients, and increase the risk of postoperative cognitive dysfunction (POCD) (Hudson and Hemmings, 2011). Iron is essential for neural development, neurotransmitter synthesis, and mitochondrial function (Singh et al., 2014). Excess iron can lead to neurotoxicity and cognitive impairment. In neurons, ferroptosis was induced by Sev through iron regulatory protein (IRP2)- and transferrin (TFR1)-mediated iron overload (Wu et al., 2020). In Sev-exposed neurons, anaesthesia increased MIB2 expression in mouse hippocampal neurons and tissues; MIB2 knockout attenuated Sev-induced neuronal ferroptosis, while GPX4 knockout reversed this effect (Zhao et al., 2021). MIB2 can directly bind to GPX4 and regulate its stability and ubiquitination, thus regulating ferroptosis in mouse hippocampal neurons (Figure 4). ACSL4 is also involved in Sev-induced neuronal ferroptosis. Sev-induced ferroptosis is inhibited by ACSL4 downregulation through the 5′ AMP-activated protein kinase (AMPK)/mammalian target of rapamycin (mTOR) signalling pathway (Cheng et al., 2021). Therefore, targeting ACSL4 may be a potential therapeutic strategy to alleviate Sev-induced POCD. Another study confirmed that Sev could regulate the development of various tumours and exert an antitumour effect on ovarian cancer (Zhang et al., 2019a), colorectal cancer (Fan et al., 2019), lung cancer (Liang et al., 2012), glioma (Xu et al., 2022), etc. Sev treatment also induced ferroptosis in glioma cells. Basic experiments have shown that Sev can inhibit cell viability in a dose-dependent manner in U87 and U251 glioma cells, increase ROS levels and Fe2+ concentrations, upregulate the expression of transferrin, ferritin, and Beclin-1, and induce glial tumour cell death. According to Sev transcriptional sequencing, the expression of ferroptosis- and mitophagy-related gene-activating transcription factor 4 (ATF4) was enhanced, and Sev induced ferroptosis in glioma cells by activating the ATF4-CHAC1 pathway (Xu et al., 2022). In lipopolysaccharide (LPS)-induced ALI, in BEAS-2B cells, Sev inhibited ferroptosis via haem oxygenase-1 (HO-1) upregulation (Liu et al., 2021b). HO-1, as the initiating and rate-limiting enzyme of haem catabolism, can catalyse the conversion of haem to biliverdin, carbon monoxide, and iron (Zhang et al., 2018a), so HO-1 may be a potential source of intracellular iron on which ferroptosis depends and is beneficial for cancer therapy (Adedoyin et al., 2018). However, in the study by LIU, the increased HO-1 after Sev treatment did not cause iron overload or GPX4 overexpression. This may be because HO-1 also has anti-ferroptosis properties (Su et al., 2019), which are particularly reflected in its protective effect against lung injury (Dong et al., 2020; Zampetaki et al., 2003). This result may be due to the different sensitivities of drugs in different organs and the different expression of related genes in different physiological states of the body, and further research is needed in the future.
FIGURE 4. Sevoflurane increases MIB2 expression in the mouse hippocampus. MIB2 can directly bind to GPX4, regulate its stability and ubiquitination, and regulate ferroptosis in mouse hippocampal neurons. “↑” represents promotion.
Several animal and clinical studies have confirmed that isoflurane and other anaesthetics (including sevoflurane, midazolam, etc.) can cause neurotoxicity in the brain. However, the neurotoxicity caused by isoflurane is limited to the developing brain and has not been found in the adult brain (Andropoulos, 2018; McCann and Soriano, 2019). A study by Liu et al. (2021c) showed that isoflurane induces ferroptosis in hippocampal neurons in a time- and dose-dependent manner. Isoflurane downregulated SLC7A11 protein levels, resulting in cellular cystine, cysteine, and GSH depletion, which disrupted cellular redox homeostasis and ultimately led to ferroptosis. Meanwhile, isoflurane exposure also increased the activity of cytochrome c oxide/complex IV in the mitochondrial electron transport chain (ETC), further promoting cysteine deprivation-induced lipid ROS accumulation and ultimately resulting in ferroptosis. Isoflurane-induced ferroptosis could be reversed by a ferroptosis inhibitor (ferrostatin-1) and a mitochondrial protectant (dimethyl fumarate; DMF). Similar results were obtained in another basic study. Isoflurane upregulated the phosphorylation of Beclin1, which formed the Beclin1-SLC7A11 complex, affected the activity of the cystine/glutamate transporter, and regulated ferroptosis in SH-SY5Y neuroblastoma cells by inhibiting the release of glutamate and decreasing intracellular GSH activity (Liu et al., 2019). In conclusion, neuronal ferroptosis is closely related to neurotoxicity after inhalation general anaesthesia in elderly patients. We speculate that anti-ferroptosis preconditioning may improve postoperative learning and memory, and further clinical research is needed.
Lidocaine is a cationic lipophilic molecule with anaesthetic and antiarrhythmic properties. As a commonly used local anaesthetic in clinical practice, it exerts its effect by interacting with lipid membranes (Santa-Maria et al., 2019). An in vitro study found that the expression levels of FTH1 and GPX4 were significantly reduced by hypoxia/reoxygenation (H/R) in A549 cells. Administration of lidocaine reversed the expression levels of FTH1 and GPX4 to inhibit ferroptosis (Ma et al., 2022). Interestingly, 5 mM lidocaine appeared to have similar effects on ferroptosis markers and claudin as 10 mM lidocaine. However, 10 mM lidocaine had stronger effects on cell viability, the inflammatory response, and oxidative stress than 5 mM lidocaine. This may be due to the different susceptibilities of lidocaine to the biological activity of different cells. Lidocaine can promote ferroptosis in ovarian and breast cancer cells by enhancing the expression of microRNA-382-5p (miR-382-5p) in cells and downregulating SLC7A11 (123). However, it may just be one of the downstream mechanisms by which lidocaine inhibits cancer progression, so further research is needed. The effect of lidocaine on ferroptosis is not absolute. It not only exerts a ferroptotic effect on tumour cell lines but also exerts an antiferroptotic effect on I/R-induced cell damage. This may be because lidocaine has different responses based on the genomes of different cell lines, and it is necessary to delve deeper into its mechanism to find a common pathway. Furthermore, whether ferroptosis is a necessary regulatory pathway for lidocaine is still unclear, and more in vivo experiments are required for future investigation.
Bupivacaine, a commonly used clinical local anaesthetic with odium channel blocking properties, is administered through local infiltration and epidural and intrathecal anaesthesia. At the same time, bupivacaine can also inhibit the growth of tumour cells (Dan et al., 2018). In an in vitro study, the Fe2+ concentration in T24 and 5637 cells was upregulated by bupivacaine in a concentration-dependent manner. At the protein level, bupivacaine inhibited the expression of solute carrier family 7 members 11 (SLC7A11; also commonly known as xCT) and GPX4 while increasing ROS levels. Further results showed that bupivacaine decreased mitochondrial membrane potential, decreased GSH, increased MDA levels, and attenuated the phosphorylation of PI3K, Akt, and mTOR (Hao et al., 2022). These data suggest that bupivacaine promotes ferroptosis in bladder cancer cells.
Levobupivacaine (L-bupivacaine), a new long-acting amide local anaesthetic, is an isomer of bupivacaine and has potential antitumour properties. Over the past few decades, local anaesthetics such as levobupivacaine, bupivacaine, and lidocaine have been shown to affect the progression of a variety of cancers, including gastric, breast, and lung cancers (Dan et al., 2018; Li et al., 2018). Levobupivacaine can inhibit the proliferation, invasion and migration of non-small cell lung cancer (NSCLC) cells and induce their apoptosis (Meng et al., 2021). Meanwhile, levobupivacaine treatment enhanced erastin-induced growth inhibition of NSCLC cells, significantly increased the levels of ROS, iron and Fe2+ in NSCLC cells, and promoted tumour cell ferroptosis. Above studies suggest that the antitumor properties of local anaesthetics are associated with ferroptosis. However, such research is still very lacking and needs further studies.
As a novel, highly selective, and particular α2 adrenergic receptor (α2-AR) agonist with sedative, analgesic, anxiolytic and hypnotic effects, DEX is widely used in intensive care and anaesthesia adjuvants (Sun et al., 2019a). In vivo studies showed that intraperitoneal injection of DEX 20 µg/kg 1 h before surgery alleviated LPS-induced ALI in mice and reversed LPS-induced upregulation of MDA and downregulation of GPX4 in the lung. It has been suggested that DEX can protect ALI well through ferroptosis (Lin et al., 2022). Another study reached similar conclusions; She et al. (2021a) observed the mitochondrial structure of pulmonary veins in septic rats induced by caecal ligation and puncture (CLP) with transmission electron microscopy. The results showed that mitochondrial fission was excessive, and the morphology was fragmented, which seriously affected mitochondrial function. However, DEX treatment significantly improved mitochondrial morphology and significantly reduced the frequency of mitochondrial fission. DEX inhibited mitochondrial fission by regulating the translocation of dynamin-related protein 1 (Drp1) while reducing ER-MITO contacts in vascular endothelial cells (VECs) through actin polymerization blockade (She et al., 2021a). Hierarchical clustering heatmaps and radar maps were applied to analyse the influence of DEX administration. The results showed that γ-glutamylcysteine, D-ribose 5-phosphate, L-malate and L-glutamate generation were significantly promoted, suggesting metabolic reprogramming from glycolysis to the pentose phosphate pathway (PPP). Ferroptosis was inhibited by DEX through the PPP metabolic, G6PD/Nrf2 (nuclear factor erythroid 2-related factor 2), GSH, free radical scavenging, and lipid peroxidation pathways (She et al., 2021b). Nrf2 is a redox-sensitive transcription factor. When prooxidant levels are elevated, Nrf2 can no longer be sequestered by Keap-1 and subsequently translocates into the nucleus, where it binds to promoters and activates the transcription of target genes. At present, the mechanism by which DEX activates Nrf2 is still unclear. Xu et al. (2021) reported that Nrf2 could bind to α2-AR so DEX-induced α2-AR activation may be responsible for the upregulation of Nrf2. However, the detailed mechanism requires further investigation. Subsequent members of this group (Wang et al., 2020a) further found that CLP surgery-induced cardiomyocyte injury in sepsis was alleviated by DEX through regulation of HO-1 iron concentration and GPX4. In ischaemia-reperfusion models, DEX can regulate the import and export of iron through the JNK/SP1 and STST4/SP1 signalling pathways, prevent iron efflux by reducing FPN1 expression or by reducing its influx of TFR1 and DMT1, maintain iron homeostasis and protect cells from ferroptosis (Qiu et al., 2020). Meanwhile, DEX can also alleviate ferroptosis-mediated renal ischemia/reperfusion injury and inflammation through α2-AR inhibition of ACSL4 signalling (Tao et al., 2022). In general, DEX, as a commonly used adjuvant drug for anaesthesia, has anti-ferroptosis effect on inflammatory diseases and has protective significance for the body. However, more research is needed to focus on the regulatory mechanism of DEX in inflammatory diseases via ferroptosis.
MicroRNAs (miRNAs) are defined as endogenous short noncoding RNAs. miRNAs typically function by interacting with mRNAs of target genes and hindering the successful translation of their proteins, and miRNA regulation can occur in various biological processes during tumour development, such as growth, angiogenesis, and metastasis (Lee and Dutta, 2009). Wang et al. (He et al., 2021) proposed that ketamine-induced ferroptosis in hepatoma cells in vivo or in vitro was related to the miRNA profile, which was manifested as inhibiting GPX4 expression by reducing lncRNA PVT1 and promoting ferroptosis by upregulating miR-214-3p. A luciferase reporter assay revealed a direct interaction between miR-214-3p and the sponge of the GPX4 3′UTR, and lncPVT1 promoted GPX4 expression by sponging miR-214-3p. Currently, miR-214-3p is an inhibitory miRNA that has been extensively studied in cancer and has been proposed as a diagnostic and prognostic biomarker for several cancers (Fang et al., 2019a; Han et al., 2019; Lu et al., 2020). Propofol inhibited the expression of STAT3 by upregulating miR-125b-5p in gastric cancer cells, and STAT3 overexpression reversed the accumulation of Fe2+, ROS, MDA levels in erastin-treated SGC7901 and BGC823 cells by propofol, thereby demonstrating that propofol can enhance the ferroptosis of gastric cancer cells (Liu et al., 2021a). Additionally, in SGC7901 gastric cancer cells, levobupivacaine treatment elevated the level of miR-489-3p and further acted as a sponge for SLC7A11 to inhibit its expression, thereby promoting ferroptosis in gastric cancer cells (Mao et al., 2021). Transfer RNA‐derived small RNAs (tsRNAs) are an abundant type of noncoding small RNAs that play multiple roles in different physiological processes (Kim et al., 2017; Wang et al., 2020c). There is increasing evidence that tsRNA is capable of affecting gene expression at the posttranscriptional level by targeting the 3′UTR, showing a mechanism similar to that of miRNAs (Canella et al., 2010; Haussecker et al., 2010). Recent evidence has shown that DEX reverses LPS-induced ferroptosis in LPS-induced ALI. RNA sequencing was performed to explore aberrantly expressed tsRNAs between the LPS and LPS + DEX groups in lung tissue. The results showed that tsRNA-1018, tsRNA-3045b, tsRNA-5021a, and tsRNA-1020 were downregulated, and tsRNA-3025b was upregulated (Lin et al., 2022). Sev induces ferroptosis in a variety of diseases, including glioma. In addition to regulating the expression of mRNAs, Sev was also found to influence the expression of a few lncRNAs in glioma cells (Xu et al., 2022).
LncRNAs are noncoding RNAs over 200 nt in length that can interact with RNA, proteins, and DNA. The lncRNA TMEM161B-AS1 was discovered to promote certain malignant behaviours and temozolomide resistance by enhancing the expression of multiple ferroptosis-related genes (Chen et al., 2021b). However, the disadvantage is that although the above studies have shown that the effect of anaesthetics on ferroptosis is related to noncoding RNA, the related epigenetic regulation has not been discussed, and further exploration may be required in the future. Taken together, these findings contribute to further understanding of the mechanisms underlying the antitumour properties of anaesthetics. It is demonstrated that anaesthetics may act as tumour suppressors to inhibit cancer growth through ferroptosis-related noncoding RNAs and could be a new therapeutic strategy for cancer.
Accumulating clinical data suggest that human disease is linked to ferroptosis-related modulators (activators or inhibitors) and pathways (Jiang et al., 2021; Tang et al., 2021). Therefore, targeting ferroptosis by pharmacological modulators may represent a possible approach to the treatment of multiple pathologies. In surgical operations, the selection and application of anaesthetics is likely to affect the development of the patient’s postoperative condition. Although the physiological function of ferroptosis remains unclear, its role in a large number of human diseases, such as cancer, neurodegeneration, IRI, and other pathological conditions, has been widely documented. As discussed in Table 3, the induction or inhibition of ferroptosis by anaesthesia drugs is associated with a variety of disease mechanisms, which further shows that the use of anaesthesiologists in each choice of narcotics is crucial Here, we will try to illustrate the role of ferroptosis in diseases through several good examples.
Bupivacaine, levobupivacaine, lidocaine, sevoflurane, propofol and ketamine can induce ferroptosis in cancer cells and exert an antitumour effect. Different types of cancer cells have different susceptibilities to ferroptosis. Among the eight different tissue types used in the NCI (National Cancer Institute) Developmental Therapy Program (DTP), renal cancer cells and diffuse large B-cell lymphoma (DLBCL) are more sensitive to erastin and more susceptible to ferroptosis than cancer cells from the other six tissues (central nervous system, lung, breast, ovary, melanocytes, and colon) (Xie et al., 2016). In many cases, ferroptosis inducers may be beneficial, and the combination of the ferroptosis inducer erastin with certain chemotherapeutic agents (cisplatin, doxorubicin/adriamycin, cytarabine/ara-C and temozolomide) enhances its antitumour activity and has a favourable prognosis in patients treated with chemotherapy alone (Chen et al., 2015b; Yu et al., 2015). In terms of specific tumour therapy, the System xc-inhibitor imidazole-ketone-erastin (IKE) was effective in xenografts of DLBCL, and it upregulated ferroptosis markers in xenografts (Zhang et al., 2019b). Ovarian cancer is a common and deadly gynecological cancer (Sung et al., 2021). Among them, high-grade serous ovarian cancer (HGSOC) is the most common subtype, with a low 5-year survival rate of only 9–34% (Vang et al., 2009). Although clinically relevant treatments have certain curative effects, most patients will develop cancer cell metastasis, develop drug resistance, and eventually develop into end-stage cancer. However, ovarian cancer cells are susceptible to ferroptosis due to their tumour-initiating cells (TICs) iron addiction, which reduces the level of the iron efflux pump (FPN) and overexpresses the iron importer (TFR1) (Basuli et al., 2017). Therefore, effective targeting of TIC therapy may improve the difficult problem of ovarian cancer treatment. Some experiments have shown that anaesthetics can induce ferroptosis. Experimental studies by Sun et al. indicated that lidocaine could inhibit SLC7A11 expression and enhance ferroptosis in ovarian cancer cells in a dose-dependent and time-dependent manner. We speculate whether local anaesthetics also have auxiliary functions in treating specific tumours, and further research is needed. Despite of significant advances in tumour therapy, tumour drug resistance remains a serious problem. Viswanathan et al. (Viswanathan et al., 2017)found that the treatment resistance state of cancer cells is related to the lipid peroxidase pathway regulated by GPX4, and ketamine can inhibit GPX4 expression. ACSL4-mediated lipid metabolism is also related to tumour metastasis and invasion. Xinyu Wu et al. found that ACSL4 promoted the invasion and migration of prostate and breast cancer cells (Wu et al., 2013; Wu et al., 2015), while LPO generated by ACSL4-mediated lipid metabolism promoted ferroptosis. This finding suggests that ferroptosis can be used as an effective treatment to inhibit cancer metastasis and invasion, whereas propofol can promote ACSL4 expression, and anaesthetics can also enhance the effect of certain chemotherapeutic drugs, such as propofol enhances the ferroptosis of cervical cancer cells induced by paclitaxel. To sum up, it further shows that the selection of perioperative anaesthetics has a certain significance for the prognosis of cancer. The anti-tumour effect of anaesthetics has once again broadened the range of drugs for clinicians, and opened up a new option for cancer treatment.
Ferroptosis is also involved in sevoflurane- and isoflurane-induced neurodegenerative diseases. Anaesthesia-related neurodegenerative diseases are mainly POCD, which is particularly severe in elderly patients, manifesting as impairments in memory, concentration, and information processing, as well as changes in personality, which can cause serious problems for patients and caregivers to trouble (Batistaki et al., 2017). In addition, POCD is irreversible in many cases, can persist for years after surgery, and increases the overall morbidity and mortality (Subramaniyan and Terrando, 2019). Fer-1 can significantly reduce learning and memory impairment induced by inhalation anaesthetics, and fer-1 preconditioning may be a potential clinical intervention for neuroprotection (Xia et al., 2018), with significant protection from surgery in paediatric and elderly patients. However, increasing evidence suggests a link between the pathogenesis of AD and POCD (Wang et al., 2022). Iron chelators and fer-1 have been shown to prevent AD development by protecting the stability of HIF1A in the brain, thereby inhibiting neuronal death, including ferroptosis (Tang et al., 2021; Zhang et al., 2018b).
Rational use of dexmedetomidine, lidocaine, propofol, and etomidate can reduce the expression of ferroptosis and protect organs from IRI. Inflammation and massive cell death are caused by I/R in affected organs, leading to ischaemic heart disease, stroke, intestinal injury, renal failure, etc. Afterwards, it was confirmed that ferroptosis was involved in the progression of I/R (Friedmann Angeli et al., 2014; Gao et al., 2015; Li et al., 2019b; Tuo et al., 2017). Remarkably, ischaemic heart disease without effective treatment results in the highest mortality rate worldwide (Benjamin et al., 2017). Although the mechanism of ischaemia-induced cell death is elusive, there are still no therapies that act by preventing ischaemic organ injury (IOI)-related cell death. However, over the past few years, multiple studies have identified ferroptosis as a major contributor to the associated cell death in IOI, highlighting the potential value of ferroptosis-targeted treatment in ischaemic heart disease. This was fully confirmed in an isolated mouse heart model mimicking I/R, as well as in in vivo experiments, where pharmacological inhibition of ferroptosis by both glutaminase inhibitors and iron chelators significantly reduced cardiac injury (Fang et al., 2019b; Gao et al., 2015).
Lung and liver fibrosis (Yu et al., 2020; Zhang et al., 2018c), chronic obstructive pulmonary disease from smoking (Park et al., 2019; Yoshida et al., 2019), tissue necrosis from tuberculosis (Amaral et al., 2019), and a rare genetic disorder called Pelizaeus-Merzbacher disease (Nobuta et al., 2019) have also been linked to ferroptosis. There are still gaps in the research of anaesthetic drugs on these diseases, which can be further explored in the future.
Ferroptosis plays an important role in the pathological process of tumours, I/R, ALI, and neurodegenerative disorders. Therefore, research on ferroptosis protection is significant for preventing and treating acute diseases. Anaesthetic drugs protect against ferroptosis or promote tumour cell ferroptosis through the System xc-/GPX4 pathways, iron metabolism, lipid metabolism and other pathways. The choice of anaesthetics is of great significance to postoperative disease outcomes. However, along with increasing exposure time and concentration, sevoflurane, lidocaine, and bupivacaine lead to ferroptosis, while the effect of other anaesthetics on ferroptosis has not been reported and needs to be further explored. Whether different analgesics also affect cell ferroptosis has not yet been reported. The gene profile epigenetic regulation of ferroptosis by anaesthetics also needs to be further studied.
Furthermore, the exact molecular mechanism and pathophysiology of drug-related ferroptosis remain to be further investigated, and some conflicting results remain to be further discussed. For example, at the same clinically relevant concentrations, propofol appears to inhibit ferroptosis in the I/R model, whereas for tumour cells, propofol appears to promote ferroptosis. Summarizing the above and Table 3, we found that in the I/R model, the effect of narcotics is anti-ferroptosis, which protects the body. For cancer cells, the effect of anaesthetic drugs is to promote ferroptosis. This may be due to the different responses of anaesthetics to different disease models and microenvironments. Different models show different effects under different conditions, further evidence is needed in the future. Meanwhile, inflammatory cytokines are often increased in ferroptosis. While some anaesthetics, such as ketamine, are anti-inflammatory, they appear to promote ferroptosis in cancer cells. We found none of the experiments showing that ketamine induces both ferroptosis and anti-inflammatory effects. We speculate that the mechanism of action of anaesthetics varies in different experiments and in different cellular systems. It may exhibit anti-inflammatory and anti-ferroptosis effects in inflammatory diseases such as IRI, which requires further validation. We also found that for the same disease model, different anaesthetics can exhibit the same effect, especially in terms of lung protection. For example, in the ALI disease model, the effects of relevant concentrations of DEX, Sev, and lidocaine were shown to be antiferroptotic. Whether other drugs have similar effects needs to be further expanded in the future. In addition, since the mechanisms underlying the action of different anaesthetics on ferroptosis are not precisely identical, it is necessary to have a deeper understanding of the action of different anaesthetics on ferroptosis to provide a theoretical basis for exploring the potential targets of intraoperative anaesthesia to protect cells from ferroptosis. We propose that studying the interaction of anaesthetics and ferroptosis in humans will be an active area of research in the anaesthesia field in the next few years.
XZ collected the literature and wrote the manuscript. JL revised the manuscript. FY and RX designed, wrote, edited and prepared the manuscript for submission. All authors read and approved the final manuscript.
This study was supported by grant 2021CFB210 from the Science and Technology Research Project of Hubei Province and the National Natural Science Foundation of China (No. 82004020).
The authors declare that the research was conducted in the absence of any commercial or financial relationships that could be construed as a potential conflict of interest.
All claims expressed in this article are solely those of the authors and do not necessarily represent those of their affiliated organizations, or those of the publisher, the editors and the reviewers. Any product that may be evaluated in this article, or claim that may be made by its manufacturer, is not guaranteed or endorsed by the publisher.
Adedoyin, O., Boddu, R., Traylor, A., Lever, J. M., Bolisetty, S., George, J. F., et al. (2018). Heme oxygenase-1 mitigates ferroptosis in renal proximal tubule cells. Am. J. Physiol. Ren. Physiol. 314 (5), F702–f14. doi:10.1152/ajprenal.00044.2017
Amaral, E. P., Costa, D. L., Namasivayam, S., Riteau, N., Kamenyeva, O., Mittereder, L., et al. (2019). A major role for ferroptosis in Mycobacterium tuberculosis-induced cell death and tissue necrosis. J. Exp. Med. 216 (3), 556–570. doi:10.1084/jem.20181776
Andropoulos, D. B. (2018). Effect of anesthesia on the developing brain: Infant and fetus. Fetal diagn. Ther. 43 (1), 1–11. doi:10.1159/000475928
Basuli, D., Tesfay, L., Deng, Z., Paul, B., Yamamoto, Y., Ning, G., et al. (2017). Iron addiction: A novel therapeutic target in ovarian cancer. Oncogene 36 (29), 4089–4099. doi:10.1038/onc.2017.11
Bateman, B. T., and Kesselheim, A. S. (2015). Propofol as a transformative drug in anesthesia: Insights from key early investigators. Drug Discov. Today 20 (8), 1012–1017. doi:10.1016/j.drudis.2015.04.007
Batistaki, C., Riga, M., Zafeiropoulou, F., Lyrakos, G., Kostopanagiotou, G., and Matsota, P. (2017). Effect of sugammadex versus neostigmine/atropine combination on postoperative cognitive dysfunction after elective surgery. Anaesth. Intensive Care 45 (5), 581–588. doi:10.1177/0310057X1704500508
Benjamin, E. J., Blaha, M. J., Chiuve, S. E., Cushman, M., Das, S. R., Deo, R., et al. (2017). Heart disease and stroke statistics-2017 update: A report from the American heart association. Circulation 135 (10), e146–e603. doi:10.1161/CIR.0000000000000485
Bergsbaken, T., Fink, S. L., and Cookson, B. T. (2009). Pyroptosis: Host cell death and inflammation. Nat. Rev. Microbiol. 7 (2), 99–109. doi:10.1038/nrmicro2070
Bersuker, K., Hendricks, J. M., Li, Z., Magtanong, L., Ford, B., Tang, P. H., et al. (2019). The CoQ oxidoreductase FSP1 acts parallel to GPX4 to inhibit ferroptosis. Nature 575 (7784), 688–692. doi:10.1038/s41586-019-1705-2
Brady, C. A., Jiang, D., Mello, S. S., Johnson, T. M., Jarvis, L. A., Kozak, M. M., et al. (2011). Distinct p53 transcriptional programs dictate acute DNA-damage responses and tumor suppression. Cell 145 (4), 571–583. doi:10.1016/j.cell.2011.03.035
Bridges, R., Lutgen, V., Lobner, D., and Baker, D. A. (2012). Thinking outside the cleft to understand synaptic activity: Contribution of the cystine-glutamate antiporter (system xc-) to normal and pathological glutamatergic signaling. Pharmacol. Rev. 64 (3), 780–802. doi:10.1124/pr.110.003889
Brigelius-Flohé, R., and Flohé, L. (2020). Regulatory phenomena in the glutathione peroxidase superfamily. Antioxid. Redox Signal. 33 (7), 498–516. doi:10.1089/ars.2019.7905
Cai, X., Liu, Y., Hu, Y., Liu, X., Jiang, H., Yang, S., et al. (2018). ROS-mediated lysosomal membrane permeabilization is involved in bupivacaine-induced death of rabbit intervertebral disc cells. Redox Biol. 18, 65–76. doi:10.1016/j.redox.2018.06.010
Canella, D., Praz, V., Reina, J. H., Cousin, P., and Hernandez, N. (2010). Defining the RNA polymerase III transcriptome: Genome-wide localization of the RNA polymerase III transcription machinery in human cells. Genome Res. 20 (6), 710–721. doi:10.1101/gr.101337.109
Cheah, J. H., Kim, S. F., Hester, L. D., Clancy, K. W., Patterson, S. E., Papadopoulos, V., et al. (2006). NMDA receptor-nitric oxide transmission mediates neuronal iron homeostasis via the GTPase Dexras1. Neuron 51 (4), 431–440. doi:10.1016/j.neuron.2006.07.011
Chen, L., Li, X., Liu, L., Yu, B., Xue, Y., and Liu, Y. (2015). Erastin sensitizes glioblastoma cells to temozolomide by restraining xCT and cystathionine-γ-lyase function. Oncol. Rep. 33 (3), 1465–1474. doi:10.3892/or.2015.3712
Chen, Q., Wang, W., Wu, Z., Chen, S., Chen, X., Zhuang, S., et al. (2021). Over-expression of lncRNA TMEM161B-AS1 promotes the malignant biological behavior of glioma cells and the resistance to temozolomide via up-regulating the expression of multiple ferroptosis-related genes by sponging hsa-miR-27a-3p. Cell Death Discov. 7 (1), 311. doi:10.1038/s41420-021-00709-4
Chen, X., Kang, R., Kroemer, G., and Tang, D. (2021). Broadening horizons: The role of ferroptosis in cancer. Nat. Rev. Clin. Oncol. 18 (5), 280–296. doi:10.1038/s41571-020-00462-0
Chen, X., Yu, C., Kang, R., and Tang, D. (2020). Iron metabolism in ferroptosis. Front. Cell Dev. Biol. 8, 590226. doi:10.3389/fcell.2020.590226
Chen, Y., Li, N., Wang, H., Wang, N., Peng, H., Wang, J., et al. (2020). Amentoflavone suppresses cell proliferation and induces cell death through triggering autophagy-dependent ferroptosis in human glioma. Life Sci. 247, 117425. doi:10.1016/j.lfs.2020.117425
Chen, Y., Mathias, L., Falero-Perez, J. M., and Kim, S. F. (2015). PKA-mediated phosphorylation of Dexras1 suppresses iron trafficking by inhibiting S-nitrosylation. FEBS Lett. 589 (20), 3212–3219. doi:10.1016/j.febslet.2015.08.041
Cheng, L., Zhu, X., Liu, Y., Zhu, K., Lin, K., and Li, F. (2021). ACSL4 contributes to sevoflurane-induced ferroptotic neuronal death in SH-SY5Y cells via the 5' AMP-activated protein kinase/mammalian target of rapamycin pathway. Ann. Transl. Med. 9 (18), 1454. doi:10.21037/atm-21-4249
Cheng, Y., Zak, O., Aisen, P., Harrison, S. C., and Walz, T. (2004). Structure of the human transferrin receptor-transferrin complex. Cell 116 (4), 565–576. doi:10.1016/s0092-8674(04)00130-8
Chu, B., Kon, N., Chen, D., Li, T., Liu, T., Jiang, L., et al. (2019). ALOX12 is required for p53-mediated tumour suppression through a distinct ferroptosis pathway. Nat. Cell Biol. 21 (5), 579–591. doi:10.1038/s41556-019-0305-6
Dan, J., Gong, X., Li, D., Zhu, G., Wang, L., and Li, F. (2018). Inhibition of gastric cancer by local anesthetic bupivacaine through multiple mechanisms independent of sodium channel blockade. Biomed. Pharmacother. = Biomedecine Pharmacother. 103, 823–828. doi:10.1016/j.biopha.2018.04.106
Das, N. K., Schwartz, A. J., Barthel, G., Inohara, N., Liu, Q., Sankar, A., et al. (2020). Microbial metabolite signaling is required for systemic iron homeostasis. Cell Metab. 31 (1), 115–130. e6. doi:10.1016/j.cmet.2019.10.005
DeHart, D. N., Fang, D., Heslop, K., Li, L., Lemasters, J. J., and Maldonado, E. N. (2018). Opening of voltage dependent anion channels promotes reactive oxygen species generation, mitochondrial dysfunction and cell death in cancer cells. Biochem. Pharmacol. 148, 155–162. doi:10.1016/j.bcp.2017.12.022
Ding, C. C., Rose, J., Sun, T., Wu, J., Chen, P. H., Lin, C. C., et al. (2020). MESH1 is a cytosolic NADPH phosphatase that regulates ferroptosis. Nat. Metab. 2 (3), 270–277. doi:10.1038/s42255-020-0181-1
Dixon, S. J., Lemberg, K. M., Lamprecht, M. R., Skouta, R., Zaitsev, E. M., Gleason, C. E., et al. (2012). Ferroptosis: An iron-dependent form of nonapoptotic cell death. Cell 149 (5), 1060–1072. doi:10.1016/j.cell.2012.03.042
Doll, S., Freitas, F. P., Shah, R., Aldrovandi, M., da Silva, M. C., Ingold, I., et al. (2019). FSP1 is a glutathione-independent ferroptosis suppressor. Nature 575 (7784), 693–698. doi:10.1038/s41586-019-1707-0
Dong, H., Qiang, Z., Chai, D., Peng, J., Xia, Y., Hu, R., et al. (2020). Nrf2 inhibits ferroptosis and protects against acute lung injury due to intestinal ischemia reperfusion via regulating SLC7A11 and HO-1. Aging 12 (13), 12943–12959. doi:10.18632/aging.103378
Du, H., Ren, X., Bai, J., Yang, W., Gao, Y., and Yan, S. (2022). Research progress of ferroptosis in adiposity-based chronic disease (ABCD). Oxidative Med. Cell. Longev. 2022, 1052699. doi:10.1155/2022/1052699
Fan, L., Wu, Y., Wang, J., He, J., and Han, X. (2019). Sevoflurane inhibits the migration and invasion of colorectal cancer cells through regulating ERK/MMP-9 pathway by up-regulating miR-203. Eur. J. Pharmacol. 850, 43–52. doi:10.1016/j.ejphar.2019.01.025
Fang, X., Wang, H., Han, D., Xie, E., Yang, X., Wei, J., et al. (2019). Ferroptosis as a target for protection against cardiomyopathy. Proc. Natl. Acad. Sci. U. S. A. 116 (7), 2672–2680. doi:10.1073/pnas.1821022116
Fang, Y. Y., Tan, M. R., Zhou, J., Liang, L., Liu, X. Y., Zhao, K., et al. (2019). miR-214-3p inhibits epithelial-to-mesenchymal transition and metastasis of endometrial cancer cells by targeting TWIST1. Onco. Targets. Ther. 12, 9449–9458. doi:10.2147/OTT.S181037
Friedmann Angeli, J. P., Schneider, M., Proneth, B., Tyurina, Y. Y., Tyurin, V. A., Hammond, V. J., et al. (2014). Inactivation of the ferroptosis regulator Gpx4 triggers acute renal failure in mice. Nat. Cell Biol. 16 (12), 1180–1191. doi:10.1038/ncb3064
Ganz, T. (2013). Systemic iron homeostasis. Physiol. Rev. 93 (4), 1721–1741. doi:10.1152/physrev.00008.2013
Gao, M., Monian, P., Quadri, N., Ramasamy, R., and Jiang, X. (2015). Glutaminolysis and transferrin regulate ferroptosis. Mol. Cell 59 (2), 298–308. doi:10.1016/j.molcel.2015.06.011
Gao, M., Yi, J., Zhu, J., Minikes, A. M., Monian, P., Thompson, C. B., et al. (2019). Role of mitochondria in ferroptosis. Mol. Cell 73 (2), 354–363. e3. doi:10.1016/j.molcel.2018.10.042
Guan, Z., Chen, J., Li, X., and Dong, N. (2020). Tanshinone IIA induces ferroptosis in gastric cancer cells through p53-mediated SLC7A11 down-regulation. Biosci. Rep. 40 (8), BSR20201807. doi:10.1042/BSR20201807
Hadian, K., and Stockwell, B. R. (2020). SnapShot: Ferroptosis. Cell 181 (5), 1188–e1. doi:10.1016/j.cell.2020.04.039
Han, C., Liu, Y., Dai, R., Ismail, N., Su, W., and Li, B. (2020). Ferroptosis and its potential role in human diseases. Front. Pharmacol. 11, 239. doi:10.3389/fphar.2020.00239
Han, L. C., Wang, H., Niu, F. L., Yan, J. Y., and Cai, H. F. (2019). Effect miR-214-3p on proliferation and apoptosis of breast cancer cells by targeting survivin protein. Eur. Rev. Med. Pharmacol. Sci. 23 (17), 7469–7474. doi:10.26355/eurrev_201909_18856
Hao, J., Zhang, W., and Huang, Z. (2022). Bupivacaine modulates the apoptosis and ferroptosis in bladder cancer via phosphatidylinositol 3-kinase (PI3K)/AKT pathway. Bioengineered 13 (3), 6794–6806. doi:10.1080/21655979.2022.2036909
Haseneder, R., Starker, L., Berkmann, J., Kellermann, K., Jungwirth, B., Blobner, M., et al. (2013). Sevoflurane anesthesia improves cognitive performance in mice, but does not influence in vitro long-term potentation in hippocampus CA1 stratum radiatum. PloS one 8 (5), e64732. doi:10.1371/journal.pone.0064732
Haussecker, D., Huang, Y., Lau, A., Parameswaran, P., Fire, A. Z., and Kay, M. A. (2010). Human tRNA-derived small RNAs in the global regulation of RNA silencing. RNA (New York, NY) 16 (4), 673–695. doi:10.1261/rna.2000810
He, G. N., Bao, N. R., Wang, S., Xi, M., Zhang, T. H., and Chen, F. S. (2021). Ketamine induces ferroptosis of liver cancer cells by targeting lncRNA PVT1/miR-214-3p/GPX4. Drug Des. devel. Ther. 15, 3965–3978. doi:10.2147/DDDT.S332847
Hudson, A. E., and Hemmings, H. C. (2011). Are anaesthetics toxic to the brain? Br. J. Anaesth. 107 (1), 30–37. doi:10.1093/bja/aer122
Jiang, L., Kon, N., Li, T., Wang, S. J., Su, T., Hibshoosh, H., et al. (2015). Ferroptosis as a p53-mediated activity during tumour suppression. Nature 520 (7545), 57–62. doi:10.1038/nature14344
Jiang, S., Liu, Y., Huang, L., Zhang, F., and Kang, R. (2018). Effects of propofol on cancer development and chemotherapy: Potential mechanisms. Eur. J. Pharmacol. 831, 46–51. doi:10.1016/j.ejphar.2018.04.009
Jiang, X., Stockwell, B. R., and Conrad, M. (2021). Ferroptosis: Mechanisms, biology and role in disease. Nat. Rev. Mol. Cell Biol. 22 (4), 266–282. doi:10.1038/s41580-020-00324-8
Jing, H., Wang, C., Zhao, L., Cheng, J., Qin, P., and Lin, H. (2021). Propofol protects cardiomyocytes from hypoxia/reoxygenation injury via regulating MALAT1/miR-206/ATG3 axis. J. Biochem. Mol. Toxicol. 35 (10), e22880. doi:10.1002/jbt.22880
Kagan, V. E., Mao, G., Qu, F., Angeli, J. P., Doll, S., Croix, C. S., et al. (2017). Oxidized arachidonic and adrenic PEs navigate cells to ferroptosis. Nat. Chem. Biol. 13 (1), 81–90. doi:10.1038/nchembio.2238
Kajarabille, N., and Latunde-Dada, G. O. (2019). Programmed cell-death by ferroptosis: Antioxidants as mitigators. Int. J. Mol. Sci. 20 (19), E4968. doi:10.3390/ijms20194968
Kandasamy, P., Gyimesi, G., Kanai, Y., and Hediger, M. A. (2018). Amino acid transporters revisited: New views in health and disease. Trends biochem. Sci. 43 (10), 752–789. doi:10.1016/j.tibs.2018.05.003
Kang, R., Kroemer, G., and Tang, D. (2019). The tumor suppressor protein p53 and the ferroptosis network. Free Radic. Biol. Med. 133, 162–168. doi:10.1016/j.freeradbiomed.2018.05.074
Kim, H. K., Fuchs, G., Wang, S., Wei, W., Zhang, Y., Park, H., et al. (2017). A transfer-RNA-derived small RNA regulates ribosome biogenesis. Nature 552 (7683), 57–62. doi:10.1038/nature25005
Kim, J., Gupta, R., Blanco, L. P., Yang, S., Shteinfer-Kuzmine, A., Wang, K., et al. (2019). VDAC oligomers form mitochondrial pores to release mtDNA fragments and promote lupus-like disease. Sci. (New York, NY) 366 (6472), 1531–1536. doi:10.1126/science.aav4011
Kuwata, H., and Hara, S. (2019). Role of acyl-CoA synthetase ACSL4 in arachidonic acid metabolism. Prostagl. Other Lipid Mediat. 144, 106363. doi:10.1016/j.prostaglandins.2019.106363
Lee, Y. S., and Dutta, A. (2009). MicroRNAs in cancer. Annu. Rev. Pathol. 4, 199–227. doi:10.1146/annurev.pathol.4.110807.092222
Lee, Y. S., Lee, D. H., Choudry, H. A., Bartlett, D. L., and Lee, Y. J. (2018). Ferroptosis-induced endoplasmic reticulum stress: Cross-talk between ferroptosis and apoptosis. Mol. Cancer Res. 16 (7), 1073–1076. doi:10.1158/1541-7786.MCR-18-0055
Li, H., Liu, W., Zhang, X., Wu, F., Sun, D., and Wang, Z. (2021). Ketamine suppresses proliferation and induces ferroptosis and apoptosis of breast cancer cells by targeting KAT5/GPX4 axis. Biochem. Biophys. Res. Commun. 585, 111–116. doi:10.1016/j.bbrc.2021.11.029
Li, Q., Han, X., Lan, X., Gao, Y., Wan, J., Durham, F., et al. (2017). Inhibition of neuronal ferroptosis protects hemorrhagic brain. JCI insight 2 (7), e90777. doi:10.1172/jci.insight.90777
Li, R., Xiao, C., Liu, H., Huang, Y., Dilger, J. P., and Lin, J. (2018). Effects of local anesthetics on breast cancer cell viability and migration. BMC cancer 18 (1), 666. doi:10.1186/s12885-018-4576-2
Li, S., Lei, Z., Yang, X., Zhao, M., Hou, Y., Wang, D., et al. (2022). Corrigendum: Propofol protects myocardium from ischemia/reperfusion injury by inhibiting ferroptosis through the AKT/p53 signaling pathway. Front. Pharmacol. 13, 910421. doi:10.3389/fphar.2022.910421
Li, T., Kon, N., Jiang, L., Tan, M., Ludwig, T., Zhao, Y., et al. (2012). Tumor suppression in the absence of p53-mediated cell-cycle arrest, apoptosis, and senescence. Cell 149 (6), 1269–1283. doi:10.1016/j.cell.2012.04.026
Li, W., Feng, G., Gauthier, J. M., Lokshina, I., Higashikubo, R., Evans, S., et al. (2019). Ferroptotic cell death and TLR4/Trif signaling initiate neutrophil recruitment after heart transplantation. J. Clin. Invest. 129 (6), 2293–2304. doi:10.1172/JCI126428
Li, Y., Dong, Z., Wen, G., Ren, X., Ren, W., Yan, L., et al. (2021). Long-term ketamine administration induces bladder damage and upregulates autophagy-associated proteins in bladder smooth muscle tissue. Environ. Toxicol. 36 (12), 2521–2529. doi:10.1002/tox.23365
Li, Y., Feng, D., Wang, Z., Zhao, Y., Sun, R., Tian, D., et al. (2019). Ischemia-induced ACSL4 activation contributes to ferroptosis-mediated tissue injury in intestinal ischemia/reperfusion. Cell Death Differ. 26 (11), 2284–2299. doi:10.1038/s41418-019-0299-4
Liang, C., Zhang, X., Yang, M., and Dong, X. (2019). Recent progress in ferroptosis inducers for cancer therapy. Adv. Mat. 31 (51), e1904197. doi:10.1002/adma.201904197
Liang, H., Gu, M., Yang, C., Wang, H., Wen, X., and Zhou, Q. (2012). Sevoflurane inhibits invasion and migration of lung cancer cells by inactivating the p38 MAPK signaling pathway. J. Anesth. 26 (3), 381–392. doi:10.1007/s00540-011-1317-y
Lin, M. C., Lin, C. F., Li, C. F., Sun, D. P., Wang, L. Y., and Hsing, C. H. (2015). Anesthetic propofol overdose causes vascular hyperpermeability by reducing endothelial glycocalyx and ATP production. Int. J. Mol. Sci. 16 (6), 12092–12107. doi:10.3390/ijms160612092
Lin, Y., Cai, J., Huang, D., Zhou, B., Luo, Z., Yu, S., et al. (2022). Effects of dexmedetomidine on the expression profile of tsRNAs in LPS-induced acute lung injury. J. Clin. Lab. Anal. 36 (1), e24115. doi:10.1002/jcla.24115
Linkermann, A., Skouta, R., Himmerkus, N., Mulay, S. R., Dewitz, C., De Zen, F., et al. (2014). Synchronized renal tubular cell death involves ferroptosis. Proc. Natl. Acad. Sci. U. S. A. 111 (47), 16836–16841. doi:10.1073/pnas.1415518111
Liu, F., Patterson, T. A., Sadovova, N., Zhang, X., Liu, S., Zou, X., et al. (2013). Ketamine-induced neuronal damage and altered N-methyl-D-aspartate receptor function in rat primary forebrain culture. Toxicol. Sci. 131 (2), 548–557. doi:10.1093/toxsci/kfs296
Liu, P., Yuan, J., Feng, Y., Chen, X., Wang, G., and Zhao, L. (2021). Ferroptosis contributes to isoflurane-induced neurotoxicity and learning and memory impairment. Cell Death Discov. 7 (1), 72. doi:10.1038/s41420-021-00454-8
Liu, R., Li, X., and Zhao, G. (2019). Beclin1-mediated ferroptosis activation is associated with isoflurane-induced toxicity in SH-SY5Y neuroblastoma cells. Acta Biochim. Biophys. Sin. 51 (11), 1134–1141. doi:10.1093/abbs/gmz104
Liu, X., Wang, L., Xing, Q., Li, K., Si, J., Ma, X., et al. (2021). Sevoflurane inhibits ferroptosis: A new mechanism to explain its protective role against lipopolysaccharide-induced acute lung injury. Life Sci. 275, 119391. doi:10.1016/j.lfs.2021.119391
Liu, Y. P., Qiu, Z. Z., Li, X. H., and Li, E. Y. (2021). Propofol induces ferroptosis and inhibits malignant phenotypes of gastric cancer cells by regulating miR-125b-5p/STAT3 axis. World J. Gastrointest. Oncol. 13 (12), 2114–2128. doi:10.4251/wjgo.v13.i12.2114
Liu, Z., Meng, Y., Miao, Y., Yu, L., and Yu, Q. (2020). Propofol reduces renal ischemia/reperfusion-induced acute lung injury by stimulating sirtuin 1 and inhibiting pyroptosis. Aging 13 (1), 865–876. doi:10.18632/aging.202191
Lu, T., Yang, Y., Li, Z., and Lu, S. (2020). MicroRNA-214-3p inhibits the stem-like properties of lung squamous cell cancer by targeting YAP1. Cancer Cell Int. 20, 413. doi:10.1186/s12935-020-01506-2
Lv, N., and Li, X. (2020). Isoflurane suppresses lung ischemia-reperfusion injury by inactivating NF-κB and inhibiting cell apoptosis. Exp. Ther. Med. 20 (5), 74. doi:10.3892/etm.2020.9202
Lv, Z., Wang, F., Zhang, X., Zhang, X., Zhang, J., and Liu, R. (2021). Etomidate attenuates the ferroptosis in myocardial ischemia/reperfusion rat model via Nrf2/HO-1 pathway. Shock (Augusta, Ga) 56 (3), 440–449. doi:10.1097/SHK.0000000000001751
Ma, S., Henson, E. S., Chen, Y., and Gibson, S. B. (2016). Ferroptosis is induced following siramesine and lapatinib treatment of breast cancer cells. Cell Death Dis. 7 (7), e2307. doi:10.1038/cddis.2016.208
Ma, X., Yan, W., and He, N. (2022). Lidocaine attenuates hypoxia/reoxygenation-induced inflammation, apoptosis and ferroptosis in lung epithelial cells by regulating the p38 MAPK pathway. Mol. Med. Rep. 25 (5), 150. doi:10.3892/mmr.2022.12666
Magtanong, L., Ko, P. J., To, M., Cao, J. Y., Forcina, G. C., Tarangelo, A., et al. (2019). Exogenous monounsaturated fatty acids promote a ferroptosis-resistant cell state. Cell Chem. Biol. 26 (3), 420–432. e9. doi:10.1016/j.chembiol.2018.11.016
Mao, S. H., Zhu, C. H., Nie, Y., Yu, J., and Wang, L. (2021). Levobupivacaine induces ferroptosis by miR-489-3p/slc7a11 signaling in gastric cancer. Front. Pharmacol. 12, 681338. doi:10.3389/fphar.2021.681338
Masaldan, S., Clatworthy, S. A. S., Gamell, C., Meggyesy, P. M., Rigopoulos, A. T., Haupt, S., et al. (2018). Iron accumulation in senescent cells is coupled with impaired ferritinophagy and inhibition of ferroptosis. Redox Biol. 14, 100–115. doi:10.1016/j.redox.2017.08.015
McCann, M. E., and Soriano, S. G. (2019). Does general anesthesia affect neurodevelopment in infants and children? BMJ Clin. Res. ed) 367, l6459. doi:10.1136/bmj.l6459
Meng, M., Huang, M., Liu, C., Wang, J., Ren, W., Cui, S., et al. (2021). Local anesthetic levobupivacaine induces ferroptosis and inhibits progression by up-regulating p53 in non-small cell lung cancer. Aging 13. doi:10.18632/aging.203138
Miller, R. D., Eriksson, L. I., Fleisher, L. A., Wiener-Kronish, J. P., and Young, W. L. (2005). Miller's anesthesia. Amsterdam, Netherlands: Elsevier Churchill Livingstone Philadelphia.
Mirshahidi, S., Shields, T. G., de Necochea-Campion, R., Yuan, X., Janjua, A., Williams, N. L., et al. (2021). Bupivacaine and lidocaine induce apoptosis in osteosarcoma tumor cells. Clin. Orthop. Relat. Res. 479 (1), 180–194. doi:10.1097/CORR.0000000000001510
Nakamura, E., Sato, M., Yang, H., Miyagawa, F., Harasaki, M., Tomita, K., et al. (1999). 4F2 (CD98) heavy chain is associated covalently with an amino acid transporter and controls intracellular trafficking and membrane topology of 4F2 heterodimer. J. Biol. Chem. 274 (5), 3009–3016. doi:10.1074/jbc.274.5.3009
Nobuta, H., Yang, N., Ng, Y. H., Marro, S. G., Sabeur, K., Chavali, M., et al. (2019). Oligodendrocyte death in pelizaeus-merzbacher disease is rescued by iron chelation. Cell stem Cell 25 (4), 531–541. e6. doi:10.1016/j.stem.2019.09.003
Ou, Y., Wang, S. J., Li, D., Chu, B., and Gu, W. (2016). Activation of SAT1 engages polyamine metabolism with p53-mediated ferroptotic responses. Proc. Natl. Acad. Sci. U. S. A. 113 (44), E6806–E6812. e12. doi:10.1073/pnas.1607152113
Park, E. J., Park, Y. J., Lee, S. J., Lee, K., and Yoon, C. (2019). Whole cigarette smoke condensates induce ferroptosis in human bronchial epithelial cells. Toxicol. Lett. 303, 55–66. doi:10.1016/j.toxlet.2018.12.007
Qiu, L., Ge, L., and Hu, Q. (2020). Dexmedetomidine protects SK-N-sh nerve cells from oxidative injury by maintaining iron homeostasis. Biol. Pharm. Bull. 43 (3), 424–431. doi:10.1248/bpb.b19-00711
Roy, S., Kim, D., and Sankaramoorthy, A. (2019). Mitochondrial structural changes in the pathogenesis of diabetic retinopathy. J. Clin. Med. 8 (9), E1363. doi:10.3390/jcm8091363
Roytblat, L., Talmor, D., Rachinsky, M., Greemberg, L., Pekar, A., Appelbaum, A., et al. (1998). Ketamine attenuates the interleukin-6 response after cardiopulmonary bypass. Anesth. Analg. 87 (2), 266–271. doi:10.1097/00000539-199808000-00006
Santa-Maria, A. R., Walter, F. R., Valkai, S., Brás, A. R., Mészáros, M., Kincses, A., et al. (2019). Lidocaine turns the surface charge of biological membranes more positive and changes the permeability of blood-brain barrier culture models. Biochim. Biophys. Acta. Biomembr. 1861 (9), 1579–1591. doi:10.1016/j.bbamem.2019.07.008
Sato, H., Tamba, M., Ishii, T., and Bannai, S. (1999). Cloning and expression of a plasma membrane cystine/glutamate exchange transporter composed of two distinct proteins. J. Biol. Chem. 274 (17), 11455–11458. doi:10.1074/jbc.274.17.11455
Seibt, T. M., Proneth, B., and Conrad, M. (2019). Role of GPX4 in ferroptosis and its pharmacological implication. Free Radic. Biol. Med. 133, 144–152. doi:10.1016/j.freeradbiomed.2018.09.014
Sessler, D. I., and Riedel, B. (2019). Anesthesia and cancer recurrence: Context for divergent study outcomes. Anesthesiology 130 (1), 3–5. doi:10.1097/ALN.0000000000002506
She, H., Hu, Y., Zhou, Y., Tan, L., Zhu, Y., Ma, C., et al. (2021). Protective effects of dexmedetomidine on sepsis-induced vascular leakage by alleviating ferroptosis via regulating metabolic reprogramming. J. Inflamm. Res. 14, 6765–6782. doi:10.2147/JIR.S340420
She, H., Zhu, Y., Deng, H., Kuang, L., Fang, H., Zhang, Z., et al. (2021). Protective effects of dexmedetomidine on the vascular endothelial barrier function by inhibiting mitochondrial fission via ER/mitochondria contact. Front. Cell Dev. Biol. 9, 636327. doi:10.3389/fcell.2021.636327
Shoshan-Barmatz, V., De Pinto, V., Zweckstetter, M., Raviv, Z., Keinan, N., and Arbel, N. (2010). VDAC, a multi-functional mitochondrial protein regulating cell life and death. Mol. Asp. Med. 31 (3), 227–285. doi:10.1016/j.mam.2010.03.002
Singh, N., Haldar, S., Tripathi, A. K., Horback, K., Wong, J., Sharma, D., et al. (2014). Brain iron homeostasis: From molecular mechanisms to clinical significance and therapeutic opportunities. Antioxid. Redox Signal. 20 (8), 1324–1363. doi:10.1089/ars.2012.4931
Sinner, B., Friedrich, O., Lindner, R., Bundscherer, A., and Graf, B. M. (2015). Long-term NMDA receptor inhibition affects NMDA receptor expression and alters glutamatergic activity in developing rat hippocampal neurons. Toxicology 333, 147–155. doi:10.1016/j.tox.2015.04.017
Skommer, J., Brittain, T., and Raychaudhuri, S. (2010). Bcl-2 inhibits apoptosis by increasing the time-to-death and intrinsic cell-to-cell variations in the mitochondrial pathway of cell death. Apoptosis 15 (10), 1223–1233. doi:10.1007/s10495-010-0515-7
Stockwell, B. R., Friedmann Angeli, J. P., Bayir, H., Bush, A. I., Conrad, M., Dixon, S. J., et al. (2017). Ferroptosis: A regulated cell death nexus linking metabolism, redox biology, and disease. Cell 171 (2), 273–285. doi:10.1016/j.cell.2017.09.021
Su, L., Jiang, X., Yang, C., Zhang, J., Chen, B., Li, Y., et al. (2019). Pannexin 1 mediates ferroptosis that contributes to renal ischemia/reperfusion injury. J. Biol. Chem. 294 (50), 19395–19404. doi:10.1074/jbc.RA119.010949
Su, Y., Zhao, B., Zhou, L., Zhang, Z., Shen, Y., Lv, H., et al. (2020). Ferroptosis, a novel pharmacological mechanism of anti-cancer drugs. Cancer Lett. 483, 127–136. doi:10.1016/j.canlet.2020.02.015
Subramaniyan, S., and Terrando, N. (2019). Neuroinflammation and perioperative neurocognitive disorders. Anesth. Analg. 128 (4), 781–788. doi:10.1213/ANE.0000000000004053
Sun, C., Liu, P., Pei, L., Zhao, M., and Huang, Y. (2022). Propofol inhibits proliferation and augments the anti-tumor effect of doxorubicin and paclitaxel partly through promoting ferroptosis in triple-negative breast cancer cells. Front. Oncol. 12, 837974. doi:10.3389/fonc.2022.837974
Sun, D., Li, Y. C., and Zhang, X. Y. (2021). Lidocaine promoted ferroptosis by targeting miR-382-5p/SLC7A11 Axis in ovarian and breast cancer. Front. Pharmacol. 12, 681223. doi:10.3389/fphar.2021.681223
Sun, L., Ma, W., Gao, W., Xing, Y., Chen, L., Xia, Z., et al. (2019). Propofol directly induces caspase-1-dependent macrophage pyroptosis through the NLRP3-ASC inflammasome. Cell Death Dis. 10 (8), 542. doi:10.1038/s41419-019-1761-4
Sun, Y. B., Zhao, H., Mu, D. L., Zhang, W., Cui, J., Wu, L., et al. (2019). Dexmedetomidine inhibits astrocyte pyroptosis and subsequently protects the brain in in vitro and in vivo models of sepsis. Cell Death Dis. 10 (3), 167. doi:10.1038/s41419-019-1416-5
Sung, H., Ferlay, J., Siegel, R. L., Laversanne, M., Soerjomataram, I., Jemal, A., et al. (2021). Global cancer statistics 2020: GLOBOCAN estimates of incidence and mortality worldwide for 36 cancers in 185 countries. Ca. Cancer J. Clin. 71 (3), 209–249. doi:10.3322/caac.21660
Tan, S. H., Ding, H. J., Mei, X. P., Liu, J. T., Tang, Y. X., and Li, Y. (2021). Propofol suppressed cell proliferation and enhanced apoptosis of bladder cancer cells by regulating the miR-340/CDK2 signal axis. Acta Histochem. 123 (5), 151728. doi:10.1016/j.acthis.2021.151728
Tang, D., Chen, X., Kang, R., and Kroemer, G. (2021). Ferroptosis: Molecular mechanisms and health implications. Cell Res. 31 (2), 107–125. doi:10.1038/s41422-020-00441-1
Tao, W. H., Shan, X. S., Zhang, J. X., Liu, H. Y., Wang, B. Y., Wei, X., et al. (2022). Dexmedetomidine attenuates ferroptosis-mediated renal ischemia/reperfusion injury and inflammation by inhibiting ACSL4 via α2-AR. Front. Pharmacol. 13, 782466. doi:10.3389/fphar.2022.782466
Tian, X., Li, S., and Ge, G. (2021). Apatinib promotes ferroptosis in colorectal cancer cells by targeting ELOVL6/ACSL4 signaling. Cancer Manag. Res. 13, 1333–1342. doi:10.2147/CMAR.S274631
Torti, S. V., and Torti, F. M. (2013). Iron and cancer: More ore to be mined. Nat. Rev. Cancer 13 (5), 342–355. doi:10.1038/nrc3495
Toyokuni, S. (2002). Iron and carcinogenesis: From Fenton reaction to target genes. Redox Rep. 7 (4), 189–197. doi:10.1179/135100002125000596
Tuo, Q. Z., Lei, P., Jackman, K. A., Li, X. L., Xiong, H., Li, X. L., et al. (2017). Tau-mediated iron export prevents ferroptotic damage after ischemic stroke. Mol. Psychiatry 22 (11), 1520–1530. doi:10.1038/mp.2017.171
Vang, R., Shih, Ie M., and Kurman, R. J. (2009). Ovarian low-grade and high-grade serous carcinoma: Pathogenesis, clinicopathologic and molecular biologic features, and diagnostic problems. Adv. Anat. Pathol. 16 (5), 267–282. doi:10.1097/PAP.0b013e3181b4fffa
Viswanathan, V. S., Ryan, M. J., Dhruv, H. D., Gill, S., Eichhoff, O. M., Seashore-Ludlow, B., et al. (2017). Dependency of a therapy-resistant state of cancer cells on a lipid peroxidase pathway. Nature 547 (7664), 453–457. doi:10.1038/nature23007
Wang, C., Yuan, W., Hu, A., Lin, J., Xia, Z., Yang, C. F., et al. (2020). Dexmedetomidine alleviated sepsis-induced myocardial ferroptosis and septic heart injury. Mol. Med. Rep. 22 (1), 175–184. doi:10.3892/mmr.2020.11114
Wang, S. J., Li, D., Ou, Y., Jiang, L., Chen, Y., Zhao, Y., et al. (2016). Acetylation is crucial for p53-mediated ferroptosis and tumor suppression. Cell Rep. 17 (2), 366–373. doi:10.1016/j.celrep.2016.09.022
Wang, T., Mei, J., Li, X., Xu, X., Ma, B., and Li, W. (2020). A novel tsRNA-16902 regulating the adipogenic differentiation of human bone marrow mesenchymal stem cells. Stem Cell Res. Ther. 11 (1), 365. doi:10.1186/s13287-020-01882-6
Wang, W., Qin, J. J., Rajaei, M., Li, X., Yu, X., Hunt, C., et al. (2020). Targeting MDM2 for novel molecular therapy: Beyond oncology. Med. Res. Rev. 40 (3), 856–880. doi:10.1002/med.21637
Wang, Y., Yang, L., Zhang, X., Cui, W., Liu, Y., Sun, Q. R., et al. (2019). Epigenetic regulation of ferroptosis by H2B monoubiquitination and p53. EMBO Rep. 20 (7), e47563. doi:10.15252/embr.201847563
Wang, Y. W., Wang, L., Yuan, S. J., Zhang, Y., Zhang, X., and Zhou, L. T. (2022). Postoperative cognitive dysfunction and alzheimer's disease: A transcriptome-based comparison of animal models. Front. Aging Neurosci. 14, 900350. doi:10.3389/fnagi.2022.900350
White, R. S., Bhattacharya, A. K., Chen, Y., Byrd, M., McMullen, M. F., Siegel, S. J., et al. (2016). Lysosomal iron modulates NMDA receptor-mediated excitation via small GTPase, Dexras1. Mol. Brain 9, 38. doi:10.1186/s13041-016-0220-8
Wilson, J. X., and Gelb, A. W. (2002). Free radicals, antioxidants, and neurologic injury: Possible relationship to cerebral protection by anesthetics. J. Neurosurg. Anesthesiol. 14 (1), 66–79. doi:10.1097/00008506-200201000-00014
Wolff, K., and Winstock, A. R. (2006). Ketamine : From medicine to misuse. CNS drugs 20 (3), 199–218. doi:10.2165/00023210-200620030-00003
Wu, G. J., Lin, Y. W., Tsai, H. C., Lee, Y. W., Chen, J. T., and Chen, R. M. (2018). Sepsis-induced liver dysfunction was ameliorated by propofol via suppressing hepatic lipid peroxidation, inflammation, and drug interactions. Life Sci. 213, 279–286. doi:10.1016/j.lfs.2018.10.038
Wu, J., Yang, J. J., Cao, Y., Li, H., Zhao, H., Yang, S., et al. (2020). Iron overload contributes to general anaesthesia-induced neurotoxicity and cognitive deficits. J. Neuroinflammation 17 (1), 110. doi:10.1186/s12974-020-01777-6
Wu, X., Deng, F., Li, Y., Daniels, G., Du, X., Ren, Q., et al. (2015). ACSL4 promotes prostate cancer growth, invasion and hormonal resistance. Oncotarget 6 (42), 44849–44863. doi:10.18632/oncotarget.6438
Wu, X., Li, Y., Wang, J., Wen, X., Marcus, M. T., Daniels, G., et al. (2013). Long chain fatty Acyl-CoA synthetase 4 is a biomarker for and mediator of hormone resistance in human breast cancer. PloS one 8 (10), e77060. doi:10.1371/journal.pone.0077060
Xia, Y., Sun, X., Luo, Y., and Stary, C. M. (2018). Ferroptosis contributes to isoflurane neurotoxicity. Front. Mol. Neurosci. 11, 486. doi:10.3389/fnmol.2018.00486
Xie, Y., Hou, W., Song, X., Yu, Y., Huang, J., Sun, X., et al. (2016). Ferroptosis: Process and function. Cell Death Differ. 23 (3), 369–379. doi:10.1038/cdd.2015.158
Xu, D., Zhou, C., Lin, J., Cai, W., and Lin, W. (2021). Dexmedetomidine provides protection to neurons against OGD/R-induced oxidative stress and neuronal apoptosis. Toxicol. Mech. Methods 31 (5), 374–382. doi:10.1080/15376516.2021.1888363
Xu, Y., Zhang, N., Chen, C., Xu, X., Luo, A., Yan, Y., et al. (2022). Sevoflurane induces ferroptosis of glioma cells through activating the ATF4-CHAC1 pathway. Front. Oncol. 12, 859621. doi:10.3389/fonc.2022.859621
Xuan, W., Lu, X., Yang, Z., Li, J., Jin, W., and Li, Y. (2022). Propofol protects against erastin-induced ferroptosis in HT-22 cells. J. Mol. Neurosci. 72 (9), 1797–1808. doi:10.1007/s12031-022-02017-7
Yagoda, N., von Rechenberg, M., Zaganjor, E., Bauer, A. J., Yang, W. S., Fridman, D. J., et al. (2007). RAS-RAF-MEK-dependent oxidative cell death involving voltage-dependent anion channels. Nature 447 (7146), 864–868. doi:10.1038/nature05859
Yang, W. S., Kim, K. J., Gaschler, M. M., Patel, M., Shchepinov, M. S., and Stockwell, B. R. (2016). Peroxidation of polyunsaturated fatty acids by lipoxygenases drives ferroptosis. Proc. Natl. Acad. Sci. U. S. A. 113 (34), E4966–E4975. doi:10.1073/pnas.1603244113
Yang, W. S., SriRamaratnam, R., Welsch, M. E., Shimada, K., Skouta, R., Viswanathan, V. S., et al. (2014). Regulation of ferroptotic cancer cell death by GPX4. Cell 156 (1-2), 317–331. doi:10.1016/j.cell.2013.12.010
Yang, W. S., and Stockwell, B. R. (2008). Synthetic lethal screening identifies compounds activating iron-dependent, nonapoptotic cell death in oncogenic-RAS-harboring cancer cells. Chem. Biol. 15 (3), 234–245. doi:10.1016/j.chembiol.2008.02.010
Yang, Y., Luo, M., Zhang, K., Zhang, J., Gao, T., Connell, D. O., et al. (2020). Nedd4 ubiquitylates VDAC2/3 to suppress erastin-induced ferroptosis in melanoma. Nat. Commun. 11 (1), 433. doi:10.1038/s41467-020-14324-x
Yao, X., Li, W., Fang, D., Xiao, C., Wu, X., Li, M., et al. (2021). Emerging roles of energy metabolism in ferroptosis regulation of tumor cells. Adv. Sci. 8 (22), e2100997. doi:10.1002/advs.202100997
Yin, W., Wang, C., Peng, Y., Yuan, W., Zhang, Z., Liu, H., et al. (2020). Dexmedetomidine alleviates H(2)O(2)-induced oxidative stress and cell necroptosis through activating of α2-adrenoceptor in H9C2 cells. Mol. Biol. Rep. 47 (5), 3629–3639. doi:10.1007/s11033-020-05456-w
Yin, Y., Guan, Y., Duan, J., Wei, G., Zhu, Y., Quan, W., et al. (2013). Cardioprotective effect of Danshensu against myocardial ischemia/reperfusion injury and inhibits apoptosis of H9c2 cardiomyocytes via Akt and ERK1/2 phosphorylation. Eur. J. Pharmacol. 699 (1-3), 219–226. doi:10.1016/j.ejphar.2012.11.005
Yoshida, M., Minagawa, S., Araya, J., Sakamoto, T., Hara, H., Tsubouchi, K., et al. (2019). Involvement of cigarette smoke-induced epithelial cell ferroptosis in COPD pathogenesis. Nat. Commun. 10 (1), 3145. doi:10.1038/s41467-019-10991-7
Yu, Y., Jiang, L., Wang, H., Shen, Z., Cheng, Q., Zhang, P., et al. (2020). Hepatic transferrin plays a role in systemic iron homeostasis and liver ferroptosis. Blood 136 (6), 726–739. doi:10.1182/blood.2019002907
Yu, Y., Xie, Y., Cao, L., Yang, L., Yang, M., Lotze, M. T., et al. (2015). The ferroptosis inducer erastin enhances sensitivity of acute myeloid leukemia cells to chemotherapeutic agents. Mol. Cell. Oncol. 2 (4), e1054549. doi:10.1080/23723556.2015.1054549
Yuan, H., Li, X., Zhang, X., Kang, R., and Tang, D. (2016). Identification of ACSL4 as a biomarker and contributor of ferroptosis. Biochem. Biophys. Res. Commun. 478 (3), 1338–1343. doi:10.1016/j.bbrc.2016.08.124
Zampetaki, A., Minamino, T., Mitsialis, S. A., and Kourembanas, S. (2003). Effect of heme oxygenase-1 overexpression in two models of lung inflammation. Exp. Biol. Med. 228 (5), 442–446. doi:10.1177/15353702-0322805-02
Zanninelli, G., Loréal, O., Brissot, P., Konijn, A. M., Slotki, I. N., Hider, R. C., et al. (2002). The labile iron pool of hepatocytes in chronic and acute iron overload and chelator-induced iron deprivation. J. Hepatol. 36 (1), 39–46. doi:10.1016/s0168-8278(01)00222-7
Zarate, C. A., Singh, J. B., Carlson, P. J., Brutsche, N. E., Ameli, R., Luckenbaugh, D. A., et al. (2006). A randomized trial of an N-methyl-D-aspartate antagonist in treatment-resistant major depression. Arch. Gen. Psychiatry 63 (8), 856–864. doi:10.1001/archpsyc.63.8.856
Zhang, C., Wang, B., Wang, X., Sheng, X., and Cui, Y. (2019). Sevoflurane inhibits the progression of ovarian cancer through down-regulating stanniocalcin 1 (STC1). Cancer Cell Int. 19, 339. doi:10.1186/s12935-019-1062-0
Zhang, J., Ng, S., Wang, J., Zhou, J., Tan, S. H., Yang, N., et al. (2015). Histone deacetylase inhibitors induce autophagy through FOXO1-dependent pathways. Autophagy 11 (4), 629–642. doi:10.1080/15548627.2015.1023981
Zhang, L., Hou, N., Chen, B., Kan, C., Han, F., Zhang, J., et al. (2022). Post-translational modifications of p53 in ferroptosis: Novel pharmacological targets for cancer therapy. Front. Pharmacol. 13, 908772. doi:10.3389/fphar.2022.908772
Zhang, P., Chen, Y., Zhang, S., and Chen, G. (2022). Mitochondria-related ferroptosis drives cognitive deficits in neonatal mice following sevoflurane administration. Front. Med. 9, 887062. doi:10.3389/fmed.2022.887062
Zhang, Q., Cui, T., Chang, Y., Zhang, W., Li, S., He, Y., et al. (2018). HO-1 regulates the function of Treg: Association with the immune intolerance in vitiligo. J. Cell. Mol. Med. 22 (9), 4335–4343. doi:10.1111/jcmm.13723
Zhang, Y., Qian, Y., Zhang, J., Yan, W., Jung, Y. S., Chen, M., et al. (2017). Ferredoxin reductase is critical for p53-dependent tumor suppression via iron regulatory protein 2. Genes Dev. 31 (12), 1243–1256. doi:10.1101/gad.299388.117
Zhang, Y., Tan, H., Daniels, J. D., Zandkarimi, F., Liu, H., Brown, L. M., et al. (2019). Imidazole ketone erastin induces ferroptosis and slows tumor growth in a mouse lymphoma model. Cell Chem. Biol. 26 (5), 623–633. e9. doi:10.1016/j.chembiol.2019.01.008
Zhang, Y. H., Wang, D. W., Xu, S. F., Zhang, S., Fan, Y. G., Yang, Y. Y., et al. (2018). α-Lipoic acid improves abnormal behavior by mitigation of oxidative stress, inflammation, ferroptosis, and tauopathy in P301S Tau transgenic mice. Redox Biol. 14, 535–548. doi:10.1016/j.redox.2017.11.001
Zhang, Z., Yao, Z., Wang, L., Ding, H., Shao, J., Chen, A., et al. (2018). Activation of ferritinophagy is required for the RNA-binding protein ELAVL1/HuR to regulate ferroptosis in hepatic stellate cells. Autophagy 14 (12), 2083–2103. doi:10.1080/15548627.2018.1503146
Zhao, L., Gong, H., Huang, H., Tuerhong, G., and Xia, H. (2021). Participation of mind bomb-2 in sevoflurane anesthesia induces cognitive impairment in aged mice via modulating ferroptosis. ACS Chem. Neurosci. 12 (13), 2399–2408. doi:10.1021/acschemneuro.1c00131
Zhao, M. Y., Liu, P., Sun, C., Pei, L. J., and Huang, Y. G. (2022). Propofol augments paclitaxel-induced cervical cancer cell ferroptosis in vitro. Front. Pharmacol. 13, 816432. doi:10.3389/fphar.2022.816432
Zhao, Y., Li, Y., Zhang, R., Wang, F., Wang, T., and Jiao, Y. (2020). The role of erastin in ferroptosis and its prospects in cancer therapy. Onco. Targets. Ther. 13, 5429–5441. doi:10.2147/OTT.S254995
ACSL4 acyl CoA synthase long-chain family member 4
ALI acute lung injury
ALOX arachidonic acid lipoxygenase
AMPK 5′ AMP-activated protein kinase
CLP caecal ligation and puncture
CPX ciclopirox
DFO deferoxamine
DFP deferiprone
DEX dexmedetomidine
DLBCL diffuse large B-cell lymphoma
DMF dimethyl fumarate
DMT1 divalent metal transporter 1
ETC electron transport chain
Fer-1 ferrostatin-1
FTH1 ferritin heavy chain-1
GPX4 glutathione peroxidase 4
GSH glutathione
GSSG glutathione oxidized
HO-1 haem oxygenase-1
H3K27ac histone H3 lysine 27 acetylation
H/R hypoxia/reoxygenation
IKE imidazole-ketone-erastin
IOI ischaemic organ injury
I/R ischaemia/reperfusion
IRI ischaemia-reperfusion injury
IRP2 iron regulatory proteins 2
L-bupivacaine levobupivacaine
Lip-1 liproxstatin-1
LPCAT3 lysophosphatidylcholine acyltransferase 3
LPS lipopolysaccharide
MDA malondialdehyde
MDM2 murine double minute 2
MIRI myocardial ischaemia reperfusion injury
mTOR mammalian target of rapamycin
NMDAR excitatory N-methyl-D-aspartate receptors
Nrf2 nuclear factor erythroid 2-related factor 2
NSCLC Non-small cell lung cancer
POCD postoperative cognitive dysfunction
PL-PUFA phospholipid-bound polyunsaturated fatty acids
PUFA polyunsaturated fatty acids
PPP pentose phosphate pathway
ROS reactive oxygen species
Sev sevoflurane
SLC7A11 solute carrier family 7 members 11
TFR1 transferrin receptor 1
VECs vascular endothelial cells
2,2-BP 2,2-bipyridile
Keywords: ferroptosis, anaesthetic, iron overload, glutathione peroxidase 4, cystine glutamate transporter
Citation: Zeng X, Li J, Yang F and Xia R (2022) The effect of narcotics on ferroptosis-related molecular mechanisms and signalling pathways. Front. Pharmacol. 13:1020447. doi: 10.3389/fphar.2022.1020447
Received: 16 August 2022; Accepted: 30 September 2022;
Published: 13 October 2022.
Edited by:
Lian Xiang Luo, Guangdong Medical University, ChinaReviewed by:
Weiwei Shi, Mayo Clinic, United StatesCopyright © 2022 Zeng, Li, Yang and Xia. This is an open-access article distributed under the terms of the Creative Commons Attribution License (CC BY). The use, distribution or reproduction in other forums is permitted, provided the original author(s) and the copyright owner(s) are credited and that the original publication in this journal is cited, in accordance with accepted academic practice. No use, distribution or reproduction is permitted which does not comply with these terms.
*Correspondence: Fuyuan Yang, eWFuZ2Z1eXVhbkB5YW5ndHpldS5lZHUuY24=; Rui Xia, ODc5NTYwMzUwQHFxLmNvbQ==
Disclaimer: All claims expressed in this article are solely those of the authors and do not necessarily represent those of their affiliated organizations, or those of the publisher, the editors and the reviewers. Any product that may be evaluated in this article or claim that may be made by its manufacturer is not guaranteed or endorsed by the publisher.
Research integrity at Frontiers
Learn more about the work of our research integrity team to safeguard the quality of each article we publish.