- 1School of Biological and Chemical Engineering, NingboTech University, Ningbo, China
- 2College of Biosystems Engineering and Food Science, Zhejiang University, Hangzhou, China
Bayberry leaves proanthocyanidins (BLPs) were distributed in natural plant food, considered to have the potential for metabolic syndrome. In this study, we raised Drosophila melanogaster on high sugar diet (HSD) from the egg stage to induce hyperglycemia, and the ameliorative effect of BLPs was assessed based on this model. Phenotypical, biochemical, and molecular analyses related to diabetes mellitus pathogenesis were measured. Flies exposed to BLPs were found to suppress the HSD-induced high glucose and high triglycerides levels. Moreover, BLPs showed an inhibitory effect on carbohydrate digestive enzymes (α-amylase and α-glucosidase) activity and mRNA expression, exhibiting the potential for carbohydrate digestion retardation. Transcriptional levels of key genes associated with glycolipid metabolism were further evaluated, including dilp, InR, and downstream dAKT-dFOXO-PEPCK, together with E78, SREBP, FAS, and LSD genes, were all downregulated after BLPs-exposure, suggesting the ameliorative effect of BLPs on dysbiosis associated with the insulin signaling pathway. This study provided a new functional compound, which is beneficial to further antidiabetic therapy studies.
Introduction
Diabetes mellitus is a chronic metabolic syndrome as well as an incapacitating disease. It has been reported that millions of adults suffer from diabetes mellitus, and 90% of those are type 2 diabetes mellitus (T2DM) (Lingvay et al., 2022). T2DM, the target cells of insulin fail to respond to the hormone, is often accompanied by a host of pathologies, including obesity, fatty liver, cardiovascular disease, and nephropathy. The imbalance of glucose homeostasis with insulin resistance is one of the hallmarks of T2DM (Roden and Shulman, 2019).
Proanthocyanidins were widely found in many plants and their derived foods, which had a beneficial effect on T2DM, largely free from side effects (Zeng, et al., 2020). Our previous studies found that proanthocyanidins from bayberry (Myrica rubra Sieb. et Zucc.) leaves (BLPs) belonged to prodelphinidins with a potent EGCG unit and a mean degree of polymerization (mDP) of about 6.5, which were different with the structural characteristics of those common procyanidins (Tao et al., 2020). Furthermore, in-vitro experiments have been conducted to evaluate the hypoglycemic potential of BLPs, which were reported to have an advantage in inhibiting α-glucosidase activity and lowering glucose consumption (Wang et al., 2019) (Zhang et al., 2017). BLPs can also effectively reduce the risk related to metabolic disorders in high-fat diet induced obese mice (Zhang et al., 2022). However, the antidiabetic activity of BLPs and their underlying molecular mechanism is necessary to study.
D. melanogaster was increasingly used as a valuable invertebrate model for understanding T2DM (Álvarez-Rendón et al., 2018). Drosophila has organs of the heart, brain, kidney (nephrocytes, Malpighian tubules), liver, fat tissue, gastrointestinal tract, and blood (hemolymph), which contribute to the conserved effect and mechanism in energy metabolism and glucose homeostasis analogous to humans (Pendse et al., 2013). The insulin signaling pathways are closely related to maintaining the glucose homeostasis of Drosophila, which is highly conserved during evolution (Rulifson et al., 2002). Insulin-like peptides in Drosophila (dilps) are equivalent to the vertebrate insulin-like growth factor, affecting the insulin signaling pathway and regulating growth and glucose homeostasis. And insulin signaling in flies follows the same canonical pathway as mammals: insulin-receptor (InR) activation stimulates the downstream AKT-TOR-FOXO signaling (Semaniuk et al., 2021). In addition, Drosophila offers a simpler animal system that allows the molecular mechanisms of gene function to be readily manipulated throughout the lifecycle in comparison to vertebrate models. Therefore, the low cost and rapid generation time of Drosophila make an efficient contribution to the in-vivo investigation.
Recently, high-calorie diet feeding was usually used to induce metabolic disorders (such as insulin resistance or obesity) in Drosophila, which was close to the actual situation. Musselman et al. (2011) found that Drosophila larvae reared on excess sugar (maltose) diet, elicited hyperglycemic and insulin-resistant phenotypes and upregulated expression of genes involved in lipogenesis, gluconeogenesis, and β-oxidation. Based on such a high sugar diet (HSD) or high fat diet (HFD) challenged Drosophila model, the role of some dietary food in anti-diabetic and anti-obesity therapies were evaluated, such as tea polyphenols (Kayashima et al., 2015), Syzygium cumini and Bauhinia forficate (Ecker et al., 2017), Flos Chrysanthemi Indici extract (Bai et al., 2018), vitamin B6 (Mascolo et al., 2022), and so on.
In this study, HSD feeding was applied to induce hyperglycemic Drosophila flies. HSD-fed flies were subsequently exposed to a BLPs-supplemented diet at two stages, to investigate whether BLPs had an ameliorative effect on T2DM-like phenotypes. We hope to provide a new therapy of BLPs for a dietary challenged model with disruption of glucose homeostasis.
2 Materials and methods
Fly stock and culture
The D. melanogaster (w1118 strain) was obtained from the Core Facility of Drosophila Resource and Technology, Shanghai Institute of Biochemistry and Cell Biology, CAS. The flies were cultured on normal diet (ND) containing 10.5% (w/v) corn meal, 7.5% (w/v) sucrose, 4% (w/v) yeast, 0.75% (w/v) agar, and 1% (v/v) propionic acid and maintained in an incubator (25 ± 1°C; relative humidity of 60%; 12-h dark/light cycle). The high-sugar diet (HSD) was prepared made up of 10.5% (w/v) corn meal, 30% (w/v) sucrose, 4% (w/v) yeast, 0.75% (w/v) agar, and 1% (v/v) in distilled water.
Bayberry leaves proanthocyanidins preparation
BLPs were extracted from dried bayberry leaves powder (Cixi, Zhejiang Province, China) and purified by HPD-500 column and Sephadex LH-20 column according to our laboratory methods (Zhang et al., 2017), the structural information of which were shown in Supplementary Materials (Supplementary Figure S1; Supplementary Table S1).
Experiment design
In this study, we exposed HSD-fed flies to BLPs in two ways, including pre-treatment and post-treatment (Figure 1). At the pre-treatment stage, 24 h-newly eggs were treated with the following diets for 21 days passing through embryonic and larval stages to adult flies: ND, HSD, HSD supplemented with 1 mg/ml BLPs (0.1% BLPs/HSD), HSD supplemented with 2 mg/ml BLPs (0.2% BLPs/HSD), HSD supplemented with 5 mg/ml BLPs (0.5% BLPs/HSD), respectively. Thereafter, at the post-treatment stage, ND flies and HSD flies were treated with the following diets for another 21 days: ND flies reared on ND (ND+ND), HSD flies reared on ND (HSD+ND), HSD flies reared on ND supplemented with 1 mg/ml BLPs (HSD+0.1% BLPs/ND), HSD flies reared on ND supplemented with 2 mg/ml BLPs (HSD+0.2% BLPs/ND), HSD flies reared on ND supplemented with 5 mg/ml BLPs (HSD+0.5% BLPs/ND), respectively.
Developmental rate and pupation morphology
Growth behaviors of HSD-fed Drosophila in the periods of egg-adult were recorded using photographs every day, and the first time observed larvae, pre-pupa, pupa, and flies were recorded as well. Meanwhile, the pupas climbed up the walls were collected using a brush and then mounted onto the slide using glycerin for microscope photography (Jun et al., 2016).
Body weight of flies
At the end of each experiment, flies were transferred into a pre-weighed EP tube under brief anesthesia. The total body weight of 10 flies per group was assessed using the microbalance.
Glucose and triglyceride level
Glucose, triglyceride (TAG) and protein levels were estimated using Glucose Assay Kit (GOPOD format) (Megazyme, Ireland), Triglyceride Assay Kit (GPO-PAP format) (Nanjing Jiancheng Bioengineering Institute, China), and BCA protein Assay Kit (Beyotime Institute of Biotechnology, China) following manufacturer’s protocols. Sample preparation was followed by the previous report with some modifications (Westfall et al., 2018). For homogenate extraction, five flies were liquid nitrogen frozen and homogenized in 300 μl PBS. After centrifuging at 12,000 rpm for 10 min, 2 μl of supernatant was obtained for TAG content assay and 20 μl of supernatant was used for protein content determination. Following, the homogenate was heat-treated for 20 min at 70°C to remove any complexes. After centrifuging, the body glucose content was obtained and analyzed. Quantities of glucose and triglyceride were both standardized against the corresponding protein content to account for variations in fly mass.
Activity assay of α-amylase and α-glucosidase
The α-amylase and α-glucosidase activity assays were carried out according to the previous report (Oboh et al., 2019). Flies were liquid nitrogen frozen, homogenized in PBS, and centrifuged (12,000 rpm, 10 min) to obtain supernatant. The supernatant was pre-incubated with the substrate (starch paste or pNPG), and the enzymatic reaction began immediately once the enzyme solution was added. After incubation at 37°C for 5 min, the absorbance was measured. The digestive enzyme activity was standardized against the protein content.
Real-time quantitative PCR assay
Total RNA was extracted from frozen flies with Trizol reagent (GenStar Biosolutions Co., Ltd, China) following the manufacturer’s protocol (DeAngelis and Johnson, 2019). The purity and content of RNA were measured by NanoDrop spectrophotometer (Thermo Fisher Scientific Inc., United States). Reverse transcription was then carried out from isolated RNA using PrimeScript RT reagent Kit with gDNA Eraser Kit (Takara Bio Inc., China). Synthesized cDNA was used as the template for expression profiling, together with specific primers and PowerUp SYBR Green Master Mix (Applied Biosystems, United States) through Quant Studio 3 Real-Time PCR System (Applied Biosystems, United States). The relative transcription level of candidate genes was calculated using the 2−ΔΔCt equation and rp49 (internal control). Specific primers in this study were listed in Table 1.
Statistical analysis
Data were expressed as the mean value ±standard deviation. Analysis of variance (ANOVA) with Duncan’s difference analysis was applied to all data using Version 20.0 SPSS Statistics (SPSS Inc., Chicago, IL).
Results and discussion
High sugar diet induced T2DM-like phenotype of drosophila
In this study, Drosophila offspring were reared on a high-sugar diet (HSD) starting from eggs to simulate T2DM. To explore the appropriate concentration of HSD (20%–40% sucrose), growth behavior including developmental rate and pupation morphology of Drosophila were observed.
Ordinarily, in the fly life cycle, eggs laid by adult flies hatch into larvae and grow into first-instar and second-instar larvae in 5–6 days. At the stage of the third instar, larvae begin to leave the food and “wander” to the wall. After about 11–12 days, third-instar larvae metamorphose into pre-pupa and pupa and then adult flies (Abrat et al., 2018). In the present study, we observed that the growth process of normal diet (ND) fed flies was broadly consistent with expectations, but that of 20% or 30% HSD fed flies had a delay of 2–3 days whether in the larval stage or pupa stage. Meanwhile, a sugar tolerance with growth retardation happened in 40% HSD intake, which was observed to stop growing at the stage of third-instar larvae and even cannot reach the pupal state (Supplementary Figure S2). Furthermore, pupa treated with 30% HSD were observed to show a more “transparent-like” appearance (Figure 2), which tended to be in the pre-pupa stage, indicating that 30% of sucrose induced a more severe growth-deficiency phenotype during the growth and development period of Drosophila.
Besides developmental delay, 30% HSD fed-flies exhibited an evident increase in body glucose and triglyceride level (Supplementary Figure S3), as well as carbohydrate digestive enzymes activities (Supplementary Figure S4). Subsequently, the mRNA levels of genes involved in glucose and lipid metabolism significantly altered in flies with HSD consumption (Supplementary Figure S5). Our results revealed that 30%HSD intake led to the pathophysiological and transcriptional changes in Drosophila, which are consistent with the T2DM phenotype as previously reported (Meshrif et al., 2022) (Eickelberg et al., 2022). In the present study, HSD participated the growth and development stages of Drosophila, therefore affecting the organ development and insulin action, which in turn influenced the glucose homeostasis and insulin sensitivity of flies (Cassim et al., 2018).
Physiological markers associated with hyperglycemia in high sugar diet-fed drosophila exposed to bayberry leaves proanthocyanidins
To examine the ameliorative effect of BLPs on physiological markers associated with hyperglycemia in Drosophila, the body weight, and the levels of glucose and triglyceride were determined (Figure 3).
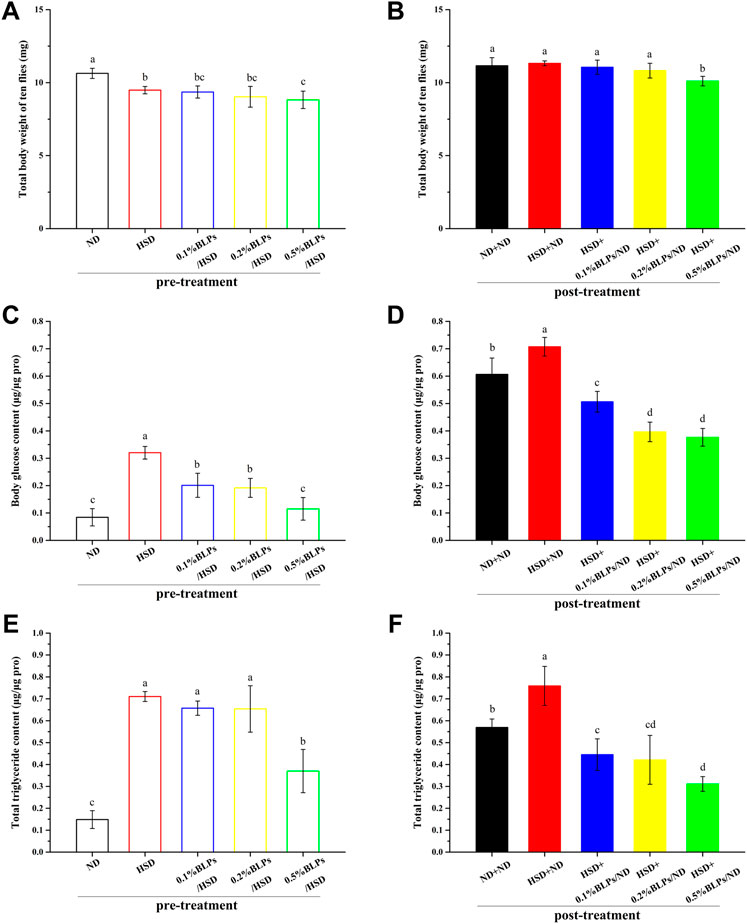
FIGURE 3. Body weight (ten flies) (A,B), body glucose content (C,D), and total triglyceride content (E,F) of those flies fed on a diet containing BLPs at different concentrations in pre-treatment or post-treatment, respectively. Values with different letters (A–D) represent significant differences among the groups (p < 0.05).
As can be observed in Figure 3A, flies exposed to BLPs supplemented with HSD appeared a slight weight loss compared to HSD-fed counterparts in pre-treatment. In post-treatment (Figure 3B), a high dosage of 0.5% BLPs consumption led to a 10.73% weight loss in HSD flies. A similar result was observed in green tea polyphenols (GTP), which were reported to reduce the size and weight of Drosophila at the 2.5–10 mg/ml doses of GTP, was the result of reduced cell size or cell numbers (Lopez et al., 2016). Previous findings illustrated that body weight and visceral index in HFD mice decreased after a 4-week intervention of BLPs (Zhou et al., 2017).
Moreover, we observed that the elevated total body glucose and triglyceride levels in HSD-fed flies were diminished with the administration of BLPs. In detail, those flies treated with BLPs supplemented with HSD had a great reduction in body glucose level by 64.08% (Figure 3C) and in triglyceride level by 47.92% occurring at 0.5% BLPs (Figure 3E). In post-treatment, HSD induction was no longer continued, and the hyperglycemia and accumulation of lipid have improved but remained. HSD+ND group had 1.17-fold higher levels of body glucose and 1.33-fold higher levels of total triglyceride compared to the ND+ND group. After BLPs intervention, flies exhibited hypoglycemic and lipid-lowering effects, with a reduction in body glucose level by 46.78% (Figure 3D) and in triglyceride level by 59.01% occurring at 0.5% BLPs (Figure 3F). The HSD-induced glucose and triglyceride levels were reported to significantly lowered in w1118 flies exposed to HSD supplemented with Solanum anguivi Lam. fruit (Nakitto et al., 2021) and Avens root extract (Günther et al., 2021), respectively.
Our findings indicated that the administrated BLPs whether in pre-treatment or post-treatment had an alleviative effect on HSD-induced accumulation of glucose and triglyceride, considered an anti-diabetic property (Alfa and Kim, 2016). It was consistent with the previous reports in rodent models that BLPs supplementation significantly reduced the blood glucose and AUC of OGTT (Zhang et al., 2022), as well as serum total cholesterol (TG) and triglyceride (TC) content in high-fat-fed rats (Zhou et al., 2017). To further investigate the hypoglycemic mechanism of BLPs, we focused on the role of BLPs in starch digestion and the glucose metabolism process, contributing to glucose homeostasis in HSD-fed flies.
Activity and mRNA expression of digestive enzymes in high sugar diet-induced drosophila exposed to bayberry leaves proanthocyanidins
α-Amylase and α-glucosidase are considered the major carbohydrate hydrolyzing enzymes in Drosophila, same as human beings. The starch and other polysaccharides in the diet were hydrolyzed to disaccharides by pancreatic α-amylase, followed by being digested to glucose by α-glucosidase in the small intestine. Therefore, the activity of these enzymes is relevant to the glucose release from carbohydrates in the diet (Sun and Miao, 2020).
The activity of two typical carbohydrate digestive enzymes (α-amylase and α-glucosidase) in flies was shown in Figure 4. Our findings showed that HSD feeding significantly simulated α-glucosidase activity. Similar results were observed in the report of Oyeniran et al. (2020), which indicated that flies fed with a diet supplemented with 30% sucrose obviously increased the digestive enzyme activity. This report was inclined to the view that the changes in enzyme activity reflected a change in enzyme quantity rather than a change in catalytic efficiency in the presence of high sugar. In this study, HSD caused a relative increase in α-glucosidase activity in response to the increase in sucrose (as substrate) amount. Moreover, carbohydrate digestive enzyme activities were closely associated with glucose homeostasis in Drosophila flies (Bezzar-Bendjazia et al., 2017). The higher activities of digestive enzymes after HSD-feeding could be one explanation for the dysglycemia as above mentioned.
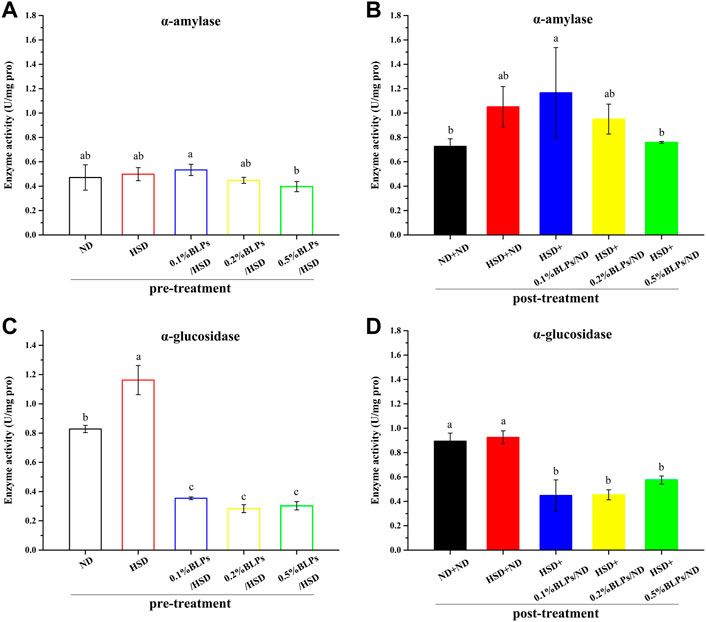
FIGURE 4. α-Amylase activity (A,B) and α-glucosidase activity (C,D) of those flies fed on a diet containing BLPs at different concentrations in pre-treatment or post-treatment, respectively. Values with different letters (A–D) represent significant differences among the groups (p < 0.05).
With the exposure of BLPs, it seemed that BLPs inhibited α-amylase and α-glucosidase activity to some extent, indicating the hypoglycemic potential. In detail, 0.5% of BLPs inhibited α-amylase activity by 20.47% but were not significant in pre-treatment, while greatly inhibited α-amylase activity by 27.79% in post-treatment. Effective inhibition of α-glucosidase activity has been achieved at the low dosage of 0.1% BLPs, around a 73.95% reduction in pre-treatment and a 51.55% reduction in post-treatment. Comparatively, BLPs had a more dramatic inhibition effect on α-glucosidase activity than α-amylase activity. This result was following our previous in-vitro studies, which suggested that BLPs had the inhibitory activity to α-glucosidase of 517.01 mM acarbose equivalents/g extract (Wang et al., 2019) and the inhibitory activity to α-amylase of 2.92 mM acarbose equivalents/g extract (Wang et al., 2020), respectively. The inhibitory effect of BLPs on the activity of these two enzymes was mainly attributed to affecting the conformational structure and micro-environment of the enzymes through BLPs-enzyme interaction.
Further, previous reports suggested that the digestive enzyme activity is involved in the transcription level of multi-duplicated genes. Drosophila genomes involved Amy (Amy-alleles and Amy-genotypes) and ten duplicated Mal genes, and these genes were regulated and associated with carbohydrate changes in the food substrate (Inomata et al., 2019), (Lai et al., 2014). In this study, the expression levels of the main digestive enzymes genes (Amy and Mal-5b) were investigated (Figure 5). mRNA expressions of Amy and Mal-5b in the BLPs-exposed flies were both downregulated, which led to the changes in enzyme activity. The retardation of BLPs on carbohydrate digestion is one of the main ways to achieve hypoglycemic action, especially through inhibiting the α-glucosidase activity.
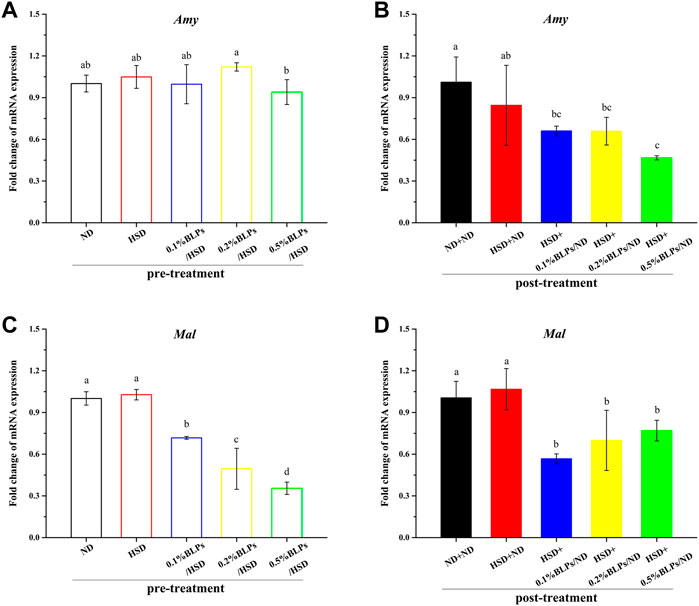
FIGURE 5. mRNA expression of Amy gene (A,B) and Mal-5b gene (C,D) of those flies fed on media containing BLPs at different concentrations in pre-treatment and post-treatment, respectively. Values with different letters (A–D) represent significant differences among the groups (p < 0.05).
Gene markers of glucose metabolism in high sugar diet-induced drosophila exposed to bayberry leaves proanthocyanidins
Regulation of the insulin signaling pathway was closely relevant to glucose metabolism. To further determine whether BLPs made a difference in glucose metabolism in Drosophila, the transcriptional level of some key genes regulating the insulin signaling pathway was accessed (Figure 6).
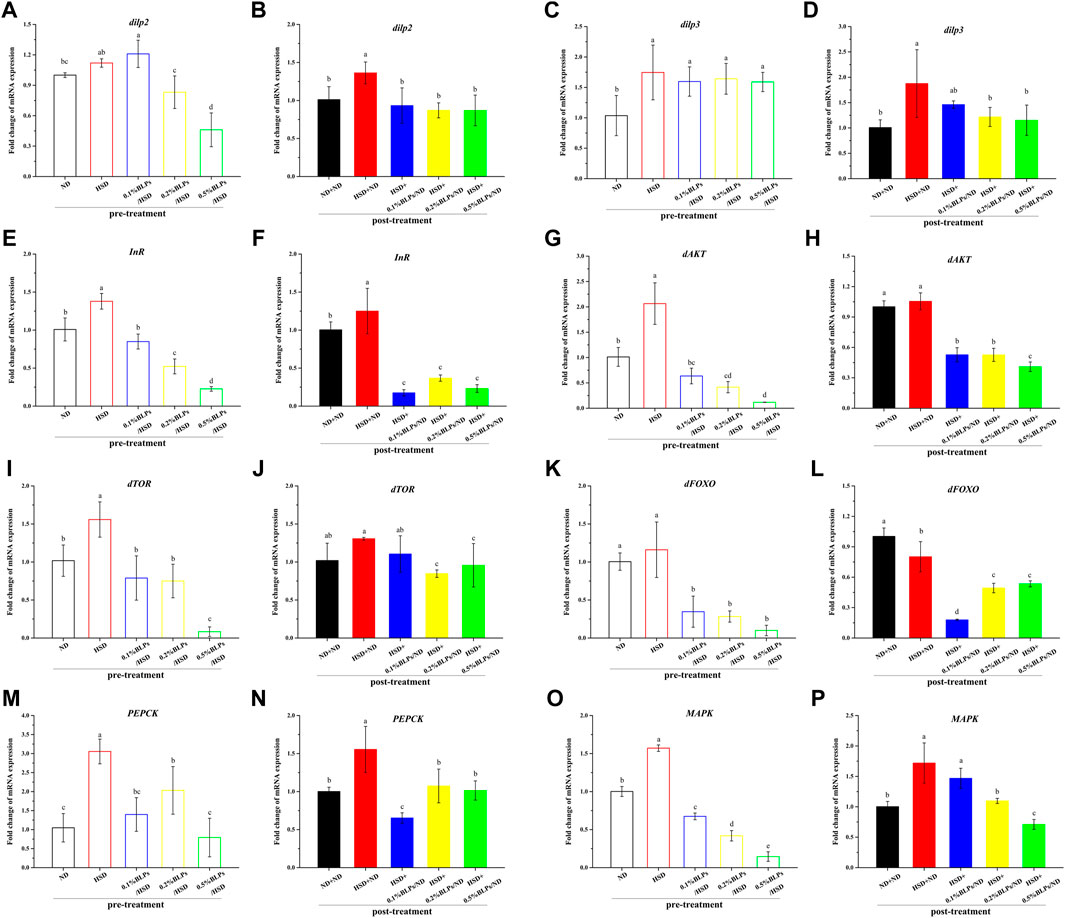
FIGURE 6. mRNA expression of main genes associated with glucose metabolism of those flies fed on media containing BLPs at different concentrations. Values with different letters (A–P) represent significant differences among the groups (p < 0.05).
As can be observed in Figures 6A–D, mRNA expression of energy metabolism regulators, insulin like-peptide 2 and 3 (dilp2 and dilp3), were elevated in HSD-fed flies compared to ND-fed flies, while decreased after BLPs exposure. At the dosage of 5 mg/ml, BLPs greatly downregulated dilp2 and dilp3 levels to 0.41-fold and 0.91-fold that of the HSD group in pre-treatment, and to 0.64-fold and 0.61-fold that of the HSD+ND group in post-treatment. Moreover, insulin receptor (InR) transcript level also showed an increase in HSD flies, which had a remarkable down-regulation in BLPs-exposed flies (Figures 6E,F). It exhibited a dose-dependent decrease in pre-treatment, with a maximum drop ratio of 83.60%. However, there was no significant difference between the multiple dosages of BLPs, accompanied by a 70.54%–86.04% decrease.
Correspondingly, transcript levels of insulin-signaling regulators-protein kinase B (dAKT) and protein synthesis regulators-target of rapamycin (dTOR) were significantly upregulated, with a slight increase in Forkhead Box-O (dFOXO) in response to HSD feeding in pre-treatment, whereas dose-dependent downregulated in BLPs-exposed flies. And with a cessation of HSD induction in post-treatment, elevated mRNA expressions of dAKT, dTOR, and dFOXO in HSD flies have been impaired, which were further decreased when flies were reared on a BLPs-supplemented diet (Figure 6G–L). What’s more, phosphoenolpyruvate carboxykinase (PEPCK) is regulated by dFOXO activation. Elevated PEPCK expression in HFD flies significantly downregulated in the presence of BLPs (Figure 6M–N), reflecting inhibition of gluconeogenesis. Mitogen-activated protein kinase (MAPK), a key regulator of energy metabolism, was also reduced in mRNA expression by BLPs.
Insulin resistance is regarded as an over-production of insulin due to the cells’ reduced sensitivity to insulin (Westfall et al., 2018), which is related to the imbalance in insulin signaling pathway regulation. Generally, the insulin signaling pathway in Drosophila involves the following links. Insulin-like peptides (dilps) are secreted by the insulin-producing cells (IPCs), which subsequently bind to the single insulin receptor (InR) to activate the insulin/insulin-like growth factor signaling pathway (IIS) (Tatar et al., 2001). As a result, InR is activated, and thus leads to the stimulation of dAKT along with the activity regulation of the downstream dTOR pathway, and the subsequent sequestration of transcription factor dFOXO activity (Loreto et al., 2021). Considering that the mRNA expression of dilp2, dilp3, InR, and downstream dAKT-dTOR were all upregulated in HSD flies, it conformed to the insulin resistance-like (Nayak and Mishra, 2021). Our results illustrated that HSD feeding increased the signs of glucose metabolic stress in statistics and successfully induced insulin resistance based on mRNA expression whether in pre-treatment or post-treatment. And decreased dilp, InR, dAKT, and dTOR mRNA expression in BLPs-exposed flies were the consequence of reduced insulin signaling, suggesting the ameliorative effect of BLPs on HSD-induced insulin-resistance. Numerous studies have demonstrated the hypoglycemic action of some proanthocyanidins, such as grape seed procyanidins (Montagut et al., 2010), apple procyanidins (Ogura et al., 2016), proanthocyanidins from I. lacteals (Tie et al., 2020). They were reported to alleviate insulin resistance in type 2 diabetes mellitus via modulating second messenger signaling pathways, including PI3K-AKT, MAPK, and JNK signaling. What’s more, BLPs downregulated dFOXO-PEPCK expression, resulting in gluconeogenesis inhibition, which subsequently contributed to a reduction of glucose output (Gu et al., 2018).
Gene markers of lipid metabolism in high sugar diet-induced drosophila exposed to bayberry leaves proanthocyanidins
Dysbiosis associated with the insulin signaling pathway would lead to insulin resistance accompanied by obesity in general (Reynés et al., 2017), so the transcriptional level of some key genes related to obesity was also investigated (Figure 7). E78 as the downstream transcriptional target of peroxisome proliferator-activated receptor-γ (PPARγ), mRNA expression of which was down-regulated with BLPs-exposure (Figure 7A–B). In parallel, as the key lipogenesis regulator, mRNA expression of sterol-regulatory element binding protein (SREBP) was slightly upregulated in HSD-fed flies compared to ND-fed flies in pre-treatment. It was aggravated in post-treatment but improved with BLPs treatment (Figure 7C–D). In terms of fatty acid synthase (FAS) and lipid storage droplet 2 (LSD), their transcript level was elevated with HSD feeding, whereas impaired in flies exposed to BLPs.
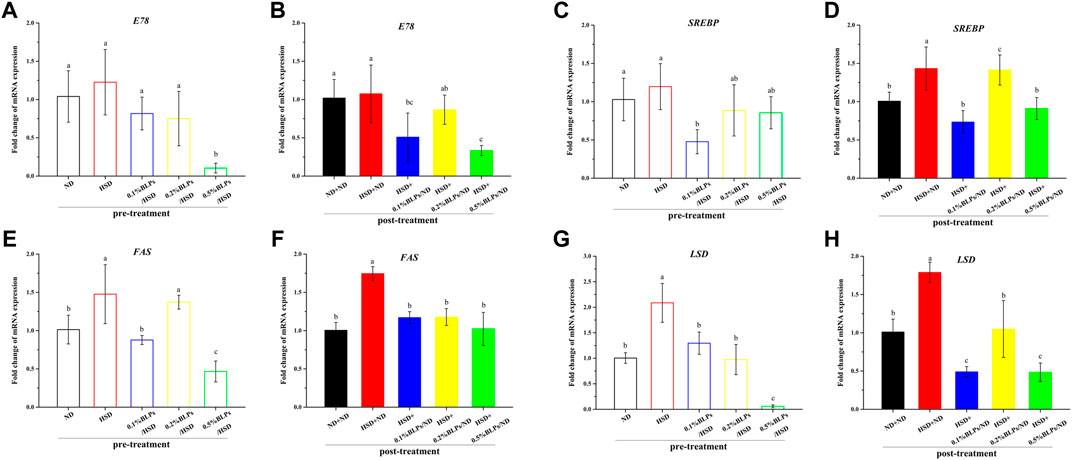
FIGURE 7. mRNA expression of main genes associated with lipid metabolism of those flies fed on media containing BLPs at different concentrations. Values with different letters (A–H) represent significant differences among the groups (p < 0.05).
PPARγ is known as an important regulator of cholesterol, lipid and glucose metabolism (Han et al., 2019). With the activation of PPARγ, the mRNA expression of the downstream gene (E78) and adipogenic and lipogenic gene (such as SREBP) would be in turn stimulated, therefore leading to lipid storage, fatty acid oxidation, triglyceride synthesis as well as insulin sensitivity (Evans et al., 2013). SREBP is also the master transcriptional regulator of lipogenic enzymes (e.g. FAS), committed steps of cholesterol or fatty acid synthesis (Bertolio et al., 2019). And LSD plays a key role in lipid storage control (Kühnlein, 2012). Our results showed that HSD treatment upregulated the mRNA expression of SREBP, FAS, and LSD, which was the markers of aggravated obesity, including lipid storage, triglyceride synthesis and insulin resistance. As expected, BLPs markedly attenuated these dyslipidemia symptoms induced by HSD consumption. BLPs reducing oleic acid-induced lipid accumulation were previously observed in HepG2 cells, by modulating the expression of proteins related to TAG biosynthesis and sterols (Zhang et al., 2017).
Conclusion
In this study, we sought to utilize Drosophila fed a 30% high sugar diet to induce T2DM-like flies. Our results demonstrated that 30% HSD induced developmental delay, hyperglycemic phenotypes and transcriptional disturbances related to insulin signaling. As the HSD intake from the egg stage was felt throughout the whole life cycle, which was more likely to go through the process of organ development and cell signaling pathways, sequentially affecting glucose levels and insulin sensitivity of adult flies. Furthermore, we raised HSD-fed flies with a concomitant or subsequent intervention of BLPs. All findings showed that BLPs ameliorated the symptoms of HSD-induced dysglycemia involving retardation of carbohydrate digestion and alleviation of insulin resistance. In brief, flies exposed to BLPs had weight loss, decreased body glucose and triglycerides levels, inhibited the activity of carbohydrate digestive enzymes, and restored over-production of insulin based on gene expression, compared with HSD-fed flies (see Figure 8). We hypothesized that BLPs were the potential to be developed as functional foods contributing to preventing and treating the abnormalities in T2DM. Further, the experiments using other animal models remain to be investigated, which helped deeply elucidate the hypoglycemic mechanism of BLPs.
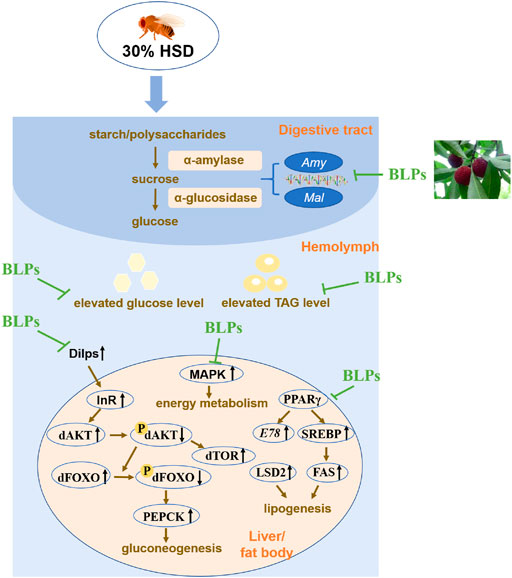
FIGURE 8. Schematic diagram of BLPs regulating T2DM-like phenotypes in HSD-fed flies. In brief, BLPs can effectively retard carbohyrate digestion and modulate the key proteins in glucolipid metabolism pathway, contributing to decrease the elevated glucose and TAG levels in HSD-fed flies. Dilps, Drosophila insulin-like peptides; InR, insulin-receptor; dAKT, protein kinase B in Drosophila; dTOR, target of rapamycin in Drosophila; dFOXO, forkhead box-O in Drosophila; PEPCK, phosphoenolpyruvate carboxykinase; MAPK, mitogen-activated protein kinase; PPARγ, peroxisome proliferator-activated receptor-γ; SREBP, sterol-regulatory element binding protein; FAS, fatty acid synthase; LSD2, lipid storage droplet 2.
Data availability statement
The original contributions presented in the study are included in the article/Supplementary Material, further inquiries can be directed to the corresponding authors.
Ethics statement
The animal study was reviewed and approved by Animal Welfare Committee of Zhejiang University.
Author contributions
MW analyzed the data as well as writted the original draft. HM did part of the experiment and adjusted the format. JC provided conceptualization and constructive suggestions. LQ and JW conceived the project and revised manuscript critically for Writing—review and editing. All authors have read and agreed to the published version of the manuscript.
Funding
This work was supported by the Talent Introduction Fund of NingboTech University (20220322Z0056) and the Natural Science Foundation of Ningbo (202003N4305).
Conflict of interest
The authors declare that the research was conducted in the absence of any commercial or financial relationships that could be construed as a potential conflict of interest.
Publisher’s note
All claims expressed in this article are solely those of the authors and do not necessarily represent those of their affiliated organizations, or those of the publisher, the editors and the reviewers. Any product that may be evaluated in this article, or claim that may be made by its manufacturer, is not guaranteed or endorsed by the publisher.
Supplementary material
The Supplementary Material for this article can be found online at: https://www.frontiersin.org/articles/10.3389/fphar.2022.1008580/full#supplementary-material
References
Abrat, O. B., Storey, J. M., Storey, K. B., and Lushchak, V. I. (2018). High amylose starch consumption induces obesity in Drosophila melanogaster and metformin partially prevents accumulation of storage lipids and shortens lifespan of the insects. Comp. Biochem. Physiol. A Mol. Integr. Physiol. 215, 55–62. doi:10.1016/j.cbpa.2017.10.011
Alfa, R. W., and Kim, S. K. (2016). Using Drosophila to discover mechanisms underlying type 2 diabetes. Dis. Model. Mech. 9, 365–376. doi:10.1242/dmm.023887
Álvarez-Rendón, J. P., Salceda, R., and Riesgo-Escovar, J. R. (2018). Drosophila melanogaster as a model for diabetes type 2 progression. Biomed. Res. Int. 2018, 1417528. doi:10.1155/2018/1417528
Bai, Y., Li, K., Shao, J., Luo, Q., and Jin, L. H. (2018). Flos Chrysanthemi Indici extract improves a high-sucrose diet-induced metabolic disorder in Drosophila. Exp. Ther. Med. 16, 2564–2572. doi:10.3892/etm.2018.6470
Bertolio, R., Napoletano, F., Mano, M., Maurer-Stroh, S., Fantuz, M., Zannini, A., et al. (2019). Sterol regulatory element binding protein 1 couples mechanical cues and lipid metabolism. Nat. Commun. 10, 1326–1411. doi:10.1038/s41467-019-09152-7
Bezzar-Bendjazia, R., Kilani-Morakchi, S., Maroua, F., and Aribi, N. (2017). Azadirachtin induced larval avoidance and antifeeding by disruption of food intake and digestive enzymes in Drosophila melanogaster (Diptera: Drosophilidae). Pestic. Biochem. Physiol. 143, 135–140. doi:10.1016/j.pestbp.2017.08.006
Cassim, J., Kolkman, F., and Helmer, M. (2018). Drosophila models to investigate insulin action and mechanisms underlying human diabetes mellitus. Adv. Exp. Med. Biol. 1076, 235–256. doi:10.1007/978-981-13-0529-0_13
DeAngelis, M. W., and Johnson, R. I. (2019). Dissection of the Drosophila pupal retina for immunohistochemistry, Western analysis, and RNA isolation. J. Vis. Exp. 145, e59299. doi:10.3791/59299
Ecker, A., Gonzaga, T. K. S. do N., Seeger, R. L., Santos, M. M. dos, Loreto, J. S., Boligon, A. A., et al. (2017). High-sucrose diet induces diabetic-like phenotypes and oxidative stress in Drosophila melanogaster: Protective role of Syzygium cumini and Bauhinia forficata. Biomed. Pharmacother. 89, 605–616. doi:10.1016/j.biopha.2017.02.076
Eickelberg, V., Lüersen, K., Staats, S., and Rimbach, G. (2022). Phenotyping of Drosophila melanogaster—a nutritional perspective. Biomolecules 12, 221. doi:10.3390/biom12020221
Evans, A., Suh, J. M., Hah, N., Liddle, C., Atkins, A. R., Downes, M., et al. (2013). PPARγ signaling and metabolismthe good, the bad & the future. Nat. Med. 19, 3159. doi:10.1038/nm.3159.PPAR
Gu, C. J., Yi, H. H., Feng, J., Zhang, Z. G., Zhou, J., Zhou, L. N., et al. (2018). Intermittent hypoxia disrupts glucose homeostasis in liver cells in an insulin-dependent and independent manner. Cell. Physiol. biochem. 47, 1042–1050. doi:10.1159/000490169
Günther, I., Rimbach, G., Nevermann, S., Neuhauser, C., Stadlbauer, V., Schwarzinger, B., et al. (2021). Avens root (Geum Urbanum L.) extract discovered by target-based screening exhibits antidiabetic activity in the Hen’s egg test model and Drosophila melanogaster. Front. Pharmacol. 12, 794404–794415. doi:10.3389/fphar.2021.794404
Han, T., Lv, Y., Wang, S., Hu, T., Hong, H., and Fu, Z. (2019). PPARγ overexpression regulates cholesterol metabolism in human L02 hepatocytes. J. Pharmacol. Sci. 139, 1–8. doi:10.1016/j.jphs.2018.09.013
Inomata, N., Takahasi, K. R., and Koga, N. (2019). Association between duplicated maltase genes and the transcriptional regulation for the carbohydrate changes in Drosophila melanogaster. Gene 686, 141–145. doi:10.1016/j.gene.2018.11.007
Jun, J. W., Han, G., Yun, H. M., Lee, G. J., and Hyun, S. (2016). Torso, a Drosophila receptor tyrosine kinase, plays a novel role in the larval fat body in regulating insulin signaling and body growth. J. Comp. Physiol. B 186, 701–709. doi:10.1007/s00360-016-0992-2
Kayashima, Y., Murata, S., Sato, M., Matsuura, K., Asanuma, T., Chimoto, J., et al. (2015). Tea polyphenols ameliorate fat storage induced by high-fat diet in Drosophila melanogaster. Biochem. Biophys. Rep. 4, 417–424. doi:10.1016/j.bbrep.2015.10.013
Kühnlein, R. P. (2012). Thematic review series: Lipid droplet synthesis and metabolism: From yeast to man. Lipid droplet-based storage fat metabolism in Drosophila. J. Lipid Res. 53, 1430–1436. doi:10.1194/jlr.R024299
Lai, D., Jin, X., Wang, H., Yuan, M., and Xu, H. (2014). Gene expression profile change and growth inhibition in Drosophila larvae treated with azadirachtin. J. Biotechnol. 185, 51–56. doi:10.1016/j.jbiotec.2014.06.014
Lingvay, I., Sumithran, P., Cohen, R. V., and le Roux, C. W. (2022). Obesity management as a primary treatment goal for type 2 diabetes: Time to reframe the conversation. Lancet 399, 394–405. doi:10.1016/S0140-6736(21)01919-X
Lopez, T. E., Pham, H. M., Barbour, J., Tran, P., Van Nguyen, B., Hogan, S. P., et al. (2016). The impact of green tea polyphenols on development and reproduction in Drosophila melanogaster. J. Funct. Foods 20, 556–566. doi:10.1016/j.jff.2015.11.002
Loreto, J. S., Ferreira, S. A., Ardisson-Araújo, D. M., and Barbosa, N. V. (2021). Human type 2 diabetes mellitus-associated transcriptional disturbances in a high-sugar diet long-term exposed Drosophila melanogaster. Comp. Biochem. Physiol. Part D. Genomics Proteomics 39, 100866. doi:10.1016/j.cbd.2021.100866
Mascolo, E., Volonté, C., Tramonti, A., Liguori, F., Gnocchini, E., Salvo, M. L. Di, et al. (2022). Vitamin B6 rescues insulin resistance and glucose ‐ induced DNA damage caused by reduced activity of Drosophila PI3K. J. Cell. Physiol. 1, 30812. doi:10.1002/jcp.30812
Meshrif, W. S., El Husseiny, I. M., and Elbrense, H. (2022). Drosophila melanogaster as a low-cost and valuable model for studying type 2 diabetes. J. Exp. Zool. A Ecol. Integr. Physiol. 337, 457–466. doi:10.1002/jez.2580
Montagut, G., Onnockx, S., Vaqué, M., Bladé, C., Blay, M., Fernández-Larrea, J., et al. (2010). Oligomers of grape-seed procyanidin extract activate the insulin receptor and key targets of the insulin signaling pathway differently from insulin. J. Nutr. Biochem. 21, 476–481. doi:10.1016/j.jnutbio.2009.02.003
Musselman, L. P., Fink, J. L., Narzinski, K., Ramachandran, P. V., Hathiramani, S. S., Cagan, R. L., et al. (2011). A high-sugar diet produces obesity and insulin resistance in wild-type Drosophila. Dis. Model. Mech. 4, 842–849. doi:10.1242/dmm.007948
Nakitto, A. M. S., Rudloff, S., Borsch, C., and Wagner, A. E. (2021). Solanum anguivi Lam. fruit preparations counteract the negative effects of a high-sugar diet on the glucose metabolism in Drosophila melanogaster. Food Funct. 12, 9238–9247. doi:10.1039/d1fo01363g
Nayak, N., and Mishra, M. (2021). High fat diet induced abnormalities in metabolism, growth, behavior, and circadian clock in Drosophila melanogaster. Life Sci. 281, 119758. doi:10.1016/j.lfs.2021.119758
Oboh, G., Ogunsuyi, O. B., Adegbola, D. O., Ademiluyi, A. O., and Oladun, F. L. (2019). Influence of gallic and tannic acid on therapeutic properties of acarbose in vitro and in vivo in Drosophila melanogaster. Biomed. J. 42, 317–327. doi:10.1016/j.bj.2019.01.005
Ogura, K., Ogura, M., Shoji, T., Sato, Y., Tahara, Y., Yamano, G., et al. (2016). Oral administration of apple procyanidins ameliorates insulin resistance via suppression of pro-inflammatory cytokine expression in liver of diabetic ob/ob mice. J. Agric. Food Chem. 64, 8857–8865. doi:10.1021/acs.jafc.6b03424
Oyeniran, O. H., Ademiluyi, A. O., and Oboh, G. (2020). Modulatory effects of moringa (Moringa oleifera L.) leaves infested with African mistletoe (Tapinanthus bangwensis L.) on the antioxidant, antidiabetic, and neurochemical indices in high sucrose diet-induced diabetic-like phenotype in fruit flies (Drosophila melanogaster M.). J. Food Biochem. 00, e13318. doi:10.1111/jfbc.13318
Pendse, J., Ramachandran, P. V., Na, J., Narisu, N., Fink, J. L., Cagan, R. L., et al. (2013). A Drosophila functional evaluation of candidates from human genome-wide association studies of type 2 diabetes and related metabolic traits identifies tissue-specific roles for dHHEX. BMC Genomics 14, 136. doi:10.1186/1471-2164-14-136
Reynés, B., Palou, M., and Palou, A. (2017). Gene expression modulation of lipid and central energetic metabolism related genes by high-fat diet intake in the main homeostatic tissues. Food Funct. 8, 629–650. doi:10.1039/c6fo01473a
Roden, M., and Shulman, G. I. (2019). The integrative biology of type 2 diabetes. Nature 576, 51–60. doi:10.1038/s41586-019-1797-8
Rulifson, E. J., Kim, S. K., and Nusse, R. (2002). Ablation of insulin-producing neurons in flies: Growth and diabetic phenotypes. Science 296, 1118–1120. doi:10.1126/science.1070058
Semaniuk, U., Strilbytska, O., Malinovska, K., Storey, K. B., Vaiserman, A., Lushchak, V., et al. (2021). Factors that regulate expression patterns of insulin-like peptides and their association with physiological and metabolic traits in Drosophila. Insect biochem. Mol. Biol. 135, 103609. doi:10.1016/j.ibmb.2021.103609
Sun, L., and Miao, M. (2020). Dietary polyphenols modulate starch digestion and glycaemic level: A review. Crit. Rev. Food Sci. Nutr. 60, 541–555. doi:10.1080/10408398.2018.1544883
Tao, W., Wei, C., Shen, S., Wang, M., Chen, S., Ye, X., et al. (2020). Mainly dimers and trimers of Chinese bayberry leaves proanthocyanidins (BLPs) are utilized by gut microbiota: In vitro digestion and fermentation coupled with caco-2 transportation. Molecules 25, 184. doi:10.3390/molecules25010184
Tatar, M., Kopelman, A., Epstein, D., Tu, M. P., Yin, C. M., and Garofalo, R. S. (2001). A mutant Drosophila insulin receptor homolog that extends life-span and impairs neuroendocrine function. Science 292, 107–110. doi:10.1126/science.1057987
Tie, F., Wang, J., Liang, Y., Zhu, S., Wang, Z., Li, G., et al. (2020). Proanthocyanidins ameliorated deficits of lipid metabolism in type 2 diabetes mellitus via inhibiting adipogenesis and improving mitochondrial function. Int. J. Mol. Sci. 21, 2029. doi:10.3390/ijms21062029
Wang, M., Chen, J., Ye, X., and Liu, D. (2020). In vitro inhibitory effects of Chinese bayberry (Myrica rubra Sieb. et Zucc.) leaves proanthocyanidins on pancreatic α-amylase and their interaction. Bioorg. Chem. 101, 104029. doi:10.1016/j.bioorg.2020.104029
Wang, M., Jiang, J., Tian, J., Chen, S., Ye, X., Hu, Y., et al. (2019). Inhibitory mechanism of novel allosteric inhibitor, Chinese bayberry (Myrica rubra Sieb. et Zucc.) leaves proanthocyanidins against α-glucosidase. J. Funct. Foods 56, 286–294. doi:10.1016/j.jff.2019.03.026
Westfall, S., Lomis, N., and Prakash, S. (2018). A polyphenol-rich prebiotic in combination with a novel probiotic formulation alleviates markers of obesity and diabetes in Drosophila. J. Funct. Foods 48, 374–386. doi:10.1016/j.jff.2018.07.012
Zeng, Y., Wang, S., Wei, L., Cui, Y., and Chen, Y. (2020). Proanthocyanidins: Components, pharmacokinetics and biomedical properties. Am. J. Chin. Med. 48, 813–869. doi:10.1142/S0192415X2050041X
Zhang, Y., Chen, S., Wei, C., Chen, J., and Ye, X. (2017). Proanthocyanidins from Chinese bayberry (Myrica rubra Sieb. et Zucc.) leaves regulate lipid metabolism and glucose consumption by activating AMPK pathway in HepG2 cells. J. Funct. Foods 29, 217–225. doi:10.1016/j.jff.2016.12.030
Zhang, Y., Pan, H., Ye, X., and Chen, S. (2022). Proanthocyanidins from Chinese bayberry leaves reduce obesity and associated metabolic disorders in high-fat diet-induced obese mice through a combination of AMPK activation and an alteration in gut microbiota. Food Funct. 13, 2295–2305. doi:10.1039/d1fo04147a
Keywords: bayberry leaves proanthocyanidins, hyperglycemia, drosophila, physiological markers, gene markers
Citation: Wang M, Mao H, Chen J, Qi L and Wang J (2022) Ameliorative effect of bayberry leaves proanthocyanidins on high sugar diet induced Drosophila melanogaster. Front. Pharmacol. 13:1008580. doi: 10.3389/fphar.2022.1008580
Received: 01 August 2022; Accepted: 30 August 2022;
Published: 15 September 2022.
Edited by:
Pukar Khanal, KLE College of Pharmacy, IndiaReviewed by:
Harish Darasaguppe Ramachandra, Indian Council of Medical Research, IndiaVishal Patil, KLE College of Pharmacy, India
Copyright © 2022 Wang, Mao, Chen, Qi and Wang. This is an open-access article distributed under the terms of the Creative Commons Attribution License (CC BY). The use, distribution or reproduction in other forums is permitted, provided the original author(s) and the copyright owner(s) are credited and that the original publication in this journal is cited, in accordance with accepted academic practice. No use, distribution or reproduction is permitted which does not comply with these terms.
*Correspondence: Lili Qi, cWxsQG5idC5lZHUuY24=; Jinbo Wang, d2piQG5idC5lZHUuY24=