- 1The First Affiliated Hospital of Zhejiang Chinese Medical University (Zhejiang Provincial Hospital of Traditional Chinese Medicine), Hangzhou, China
- 2School of Pharmaceutical Sciences, Zhejiang Chinese Medical University, Hangzhou, China
The incidence of non-alcoholic fatty liver disease (NAFLD) is increasing rapidly worldwide; however, there are currently limited treatments for NAFLD. The disease spectrum includes simple fatty liver, non-alcoholic steatohepatitis (NASH), fibrosis, cirrhosis, and progression to hepatocellular carcinoma (NASH-HCC). The therapeutic effects of NAFLD remain controversial. Although researchers have conducted studies on the pathogenesis of NAFLD, its pathogenesis and anti-NAFLD mechanisms have not been fully elucidated. Previous studies have found that flavonoids, as natural substances with extensive pharmacological activity and good therapeutic effects, have excellent antioxidant, anti-inflammatory, metabolic disease improvement, anti-tumor, and other properties and can significantly alleviate NAFLD. Flavonoids could be further developed as therapeutic drugs for NAFLD. In this paper, the pathogenesis of NAFLD and the mechanisms of flavonoids against NAFLD are summarized to provide a theoretical basis for screening flavonoids against non-alcoholic liver injury.
Introduction
Non-alcoholic fatty liver disease (NAFLD) is a chronic liver disease characterized by excessive fat deposition in hepatocytes, which is not caused by alcohol or other clear liver-damaging factors (Cobbina and Akhlaghi, 2017). The global incidence rate of NAFLD is approximately 25%, particularly in patients with diabetes and obesity (Mundi et al., 2020). NAFLD is the most common chronic liver disease worldwide and is expected to be the main cause of liver transplantation in the future (Younossi et al., 2016b). NAFLD encompasses a wide range of liver disorders, including simple fat accumulation in the liver cells, non-alcoholic steatohepatitis (NASH), fibrosis through the final stages of cirrhosis, and NASH-HCC (Cobbina and Akhlaghi, 2017). The incidence of NAFLD and NASH is related to sedentary lifestyle and excess dietary energy (Farrell et al., 2013). To date, the Food and Drug Administration has not approved any drugs for the treatment of NASH (Eduardo et al., 2015). Currently, NAFLD can be effectively alleviated only through non-drug management approaches, such as healthy lifestyle, diet, and moderate physical activity (Guillaume et al., 2015). Given the limited clinical treatment for NAFLD, the development of drugs that can effectively alleviate NAFLD is of great significance.
Pathogenesis of non-alcoholic fatty liver disease
The pathogenesis of NAFLD remains unclear so far. However, recent studies have suggested a bidirectional association between NAFLD and metabolic syndrome, with type 2 diabetes increasing the risk of cirrhosis and related complications (Powell et al., 2021). Insulin resistance, diabetes mellitus, and genetic variations in transmembrane 6 superfamily member 2 (TM6SF2) and patatin-like phospholipase domain containing 3 (PNPLA3) play important roles in NAFLD progression (Cobbina and Akhlaghi, 2017). NAFLD is characterized by excessive fatty accumulation in the liver, while simple steatosis is considered pathologically benign. NASH generally indicates liver damage that can progress to severe pathology (Zhang et al., 2018).
The “two-hit” pathogenesis of NAFLD/NASH was widely accepted in the early stage (Chi, 2017). The “first hit” is characterized by an increase in hepatic fat, especially accumulation of hepatic triglycereides and insulin resistance. Once the accumulation of hepatic fat exceeds 5%, it corresponds to hepatic steatosis (Fang et al., 2018). The most direct cause of NAFLD is abnormal liver lipid metabolism, and a large quantity of free fatty acids and triglycerides that accumulate in liver cells (Xiaxia et al., 2019). The “second hit” is that reactive oxygen species (ROS) triggers an inflammatory cascade of liver parenchymal cells and fibrosis (Xiaxia et al., 2019). These effects include high levels of inflammatory cytokines, mitochondrial dysfunction, and oxidative stress. Necrotizing inflammation and fibrosis can progress and eventually lead to cirrhosis (Chi, 2017). However, the widely accepted theory is the “multiple-hit” pathogenesis (Ayonrinde et al., 2015). Changes due to the interaction of genetic and environmental factors, as well as the interactions between different organs and tissues, pancreas, gut, and liver, and broader metabolic dysfunction, are involved (Berardis and Sokal, 2014; Chi, 2017; Vlad et al., 2018). Moreover, scholars believe that environmental and genetic factors and the change in gut microbes in the induction of NAFLD in genetic predisposition, as well as intestinal flora changes lead to intestinal fatty acid, further activate the inflammatory pathways and release proinflammatory factors. Inflammatory cytokines increase liver inflammation and lipid accumulation, and the formation of gut-liver axis to a vicious cycle (Buzzetti et al., 2016; Xiaxia et al., 2019).
In recent years, the functional activity of key genes that synthesize proteins has been decisive in NAFLD. The PNPLA3 variant has been identified as the main genetic determinant of NAFLD. Variants with moderate effect sizes in TM6SF2, membrane bound O-acyltransferase domain containing 7 (MBOAT7), and glucokinase regulator (GCKR) were also shown to contribute significantly (Bellentani et al., 2004). PNPLA3, an enzyme that encodes I148M, is involved in the hydrolysis of triglycerides in adipocytes (Romeo et al., 2008). The lipid TM6SF2 is located in the endoplasmic reticulum and encodes E167K (rs58542926C/T), resulting in the loss of protein function, which in turn increases triglyceride deposition in the liver (Dongiovanni et al., 2015). Natural candidate genes are significantly involved in glucose and lipid metabolism during NAFLD development. Among the single nucleotide polymorphisms (SNPs) that lead to coding region mutations, such as PNPLA3 and TM6SF2, it is reasonable to infer that these defective proteins may be involved. For example, TM6SF2 mutants reduce liver production of very low-density lipoprotein (VLDL), thereby increasing the triglyceride (TG) content in the liver (Bonora et al., 2010).
Some studies have suggested that NAFLD progression follows the process of steatosis, lipotoxicity, and inflammation (Jou et al., 2008). The development of steatosis involves the interaction of many factors, such as dietary habits, gut flora, and genetic factors (Romeo et al., 2008; Jiang et al., 2015; Kirpich et al., 2015). Fat regenesis occurs through upregulation of adipogenic transcription factors, including sterol regulatory binding protein-1c (SREBP1c), carbohydrate-responsive element-binding protein (chREBP), and peroxisome proliferator-activated receptor gamma (PPAR-γ) (Anderson and Borlak, 2008). Fatty acids are mainly stored in the adipose tissue in the form of triacylglycerol. A previousstudy found that fatty acids in obese volunteers seemed to migrate from normal storage organs to the bone and liver tissue. Notably, FAT/CD36 (fatty acid translocation enzymes) promote fatty acid uptake by bone and liver tissues, which are significantly elevated in patients with obesity and NAFLD (Greco et al., 2008; Fabbrini et al., 2009). The accumulation of fat in the liver can lead to lipotoxicity and dysfunction of organelles, such as the mitochondria and endoplasmic reticulum (Browning and Horton, 2004; Bell et al., 2008). Steatosis further leads to the activation of IKKβ, which leads to increased signaling of the transcription factor nuclear factor kappa β (NF-κβ). Activation of NF-κβ induces the production of pro-inflammatory factors. These include tumour necrosis factor-alpha (TNF-α), interleukin 6 (IL-6), and interleukin-1beta (IL-1β) levels. These inflammatory factors can promote aggregation and activation of resident hepatic macrophages to further promote NASH inflammation (Ramadori and Armbrust, 2001; Fabbrini et al., 2009).
Oxidative stress may play an important role in NAFLD progression, and under normal physiological conditions, mitochondrial oxidation is the main oxidation pathway of fatty acid deposition. When ROS are overproduced during fatty acid oxidation, hydrogen polyunsaturated fatty acids are extracted from the liver, resulting in mass production of malondialdehyde (MDA) (Esterbauer et al., 1991). MDA can spread from its original site to other cells both inside and outside the cell, causing damage (Esterbauer et al., 1991). Catalase and glutathione levels decrease when ROS levels are elevated, and oxidative stress is exacerbated (Hongming et al., 2018). Lipid peroxidation increases collagen synthesis and cell death, which promotes steatosis and fibrosis (Huang et al., 2018).
Fatty acid outflow from the diet increases, and new fat formation releases free fatty acids from adipose tissue, contributing to TG accumulation in the liver, although to varying degrees (Yeh and Brunt, 2014). However, TG accumulation in the liver itself is not pathological, and may be protective in some cases. Hepatic diacylglycerol acyltransferase 2 (DGAT2) inactivation catalyzes TG synthase and reduces hepatic TG content but increases hepatitis and balloon-like changes (Brunt et al., 1999). This may seem paradoxical, but highlights the importance of liver fat in metabolic function. One possible mechanism for NASH-associated dysfunction involves a shift from minimal to substantial edema. This increase can be achieved by reducing the phosphatidylcholine (PC) levels (Machado et al., 2006) or lipid droplets coated with proteins (Soderberg et al., 2010; Angulo et al., 2013). Total PC levels were reduced in patients with both NAFLD and NASH (Ekstedt et al., 2006), which may be attributable to choline intake associated with NASH rather than choline deficiency (Richardson et al., 2007). In summary, NAFLD is a multifactorial disease with a complex pathogenesis. The prevention and treatment of NAFLD require further clinical and basic research.
Classification of flavonoids
Some studies have confirmed that flavonoid intake is inversely related to the risk of NAFLD (Mm et al., 2019). The mechanisms by which flavonoids exert anti-NAFLD effects are mainly through ameliorating inflammation, oxidative stress, and lipid metabolism, and regulating intestinal microbiota imbalance and the related gut liver axis. Flavonoids are natural polyphenol compounds that exist widely in all types of natural plants. Now, more than 9,000 kinds of flavonoids have been identified with a structure of a two phenolic hydroxyl benzene ring (A- and B-loops) interconnected through the central three carbon atoms. The basic parent nucleus is called a 2- phenylchromone (Tsuji et al.), biosynthesis from acetic acid and phenylalanine in plants (Weston and Mathesius, 2013). Flavonoids can be divided into flavonoids, flavonols, orange ketones, isoflavones, anthocyanins, chalcones, and dihydrogen derivatives according to the difference in the three-carbon atomic structure of the linked A and B rings, such as whether the ring is formed, oxidized, or replaced (Tsuchiya, 2010). The types of flavonoids from different sources and their anti-NAFLD mechanisms of action are listed in Table 1.
The main targets of flavonoids
Flavonoids have a variety of pharmacological effects, including antitumor, antioxidant, antibacterial, antiviral, anti-inflammatory, and analgesic effects (Maleki et al., 2019; Makunga, 2020). Interestingly, flavonoids have positive effects on various NAFLD pathways, such as regulating lipid metabolism, insulin resistance, inflammation, and oxidative stress (Wier et al., 2015). Based on the above advantages, finding new anti-NAFLD drugs derived from plant flavonoids is a hot topic in current research (Figure 1).
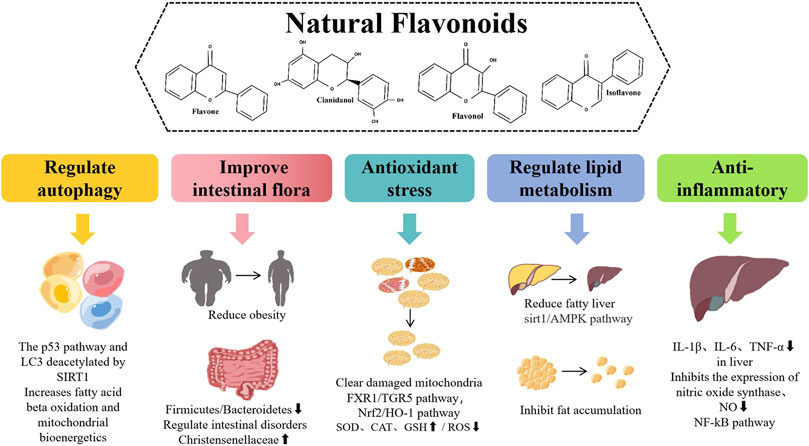
FIGURE 1. Different pharmacological effects and different mechanisms of natural flavonoids for alleviating NAFLD.
Improve the intestinal flora
Intestinal microbiota is involved in the pathogenesis of obesity, NAFLD, and metabolic syndrome (Abu-Shanab and Quigley, 2010). In NAFLD, changes in the gut microbiome and increased intestinal permeability lead to exposure of the liver to bacterial products from the gut, leading to chronic endotoxemia (Aron-Wisnewsky et al., 2013). Porras D found that quercetin could regulate intestinal microflora dysregulation in high fat diet (HFD)-induced NAFLD mice and reverse HFD-induced inhibition of short-chain fatty acids (SCFAs) production and related intestinal barrier dysfunction (Yin et al., 2017). Some scholars have pointed out through animal experiments that the use of flavonols can make mice intestinal Firmicutes/Bacteroidetes (F/B) ratio significantly reduced (Li, 2018). The F/B ratio is an indicator of intestinal health, and lowering it can reduce the risk of diabetes and obesity (Vebø et al., 2016). This suggests that flavonol protection of the intestinal flora can be achieved by reducing the F/B ratio. In addition, flavonol protection of the intestinal flora can also improve intestinal barrier function by increasing the expression of butyric acid receptors and conjunction in the intestinal mucosa (Chen et al., 2019). Anthocyanins can be digested by various intestinal structures to form metabolites that are transmitted throughout the body and exert positive biological effects (Aedín and Anne-Marie, 2017). Some studies have confirmed the results of in vitro microbial experiments. Anthocyanins can increase the growth rate of probiotics, such as Lactobacillus acidophilusor Bifidobacterium, and inhibit the growth of harmful bacteria, such as Staphylococcus aureus and Salmonella typhimurium (Hanju et al., 2018). Lima et al. (2019) confirmed through experimental studies that long-term supplementation of hesperidin and citra can effectively protect intestinal flora because the number and reproduction rate of Bifidobacteria and Lactobacillus in the intestinal tract are regulated by their influence, thus increasing the content of SCFAs to protect intestinal flora. Researchers studied the effects of flavonoids on intestinal microbes and found that when the dosage reached a certain concentration, it could significantly inhibit the reproduction of Escherichia coli, Candida albicans, Staphylococcus aureus, and Bacillus (Madheshwar and Perumal, 2017). Pure total flavonoids from citrus can regulate intestinal flora disorders, particularly Christensenellaceae, to attenuate NAFLD (He et al., 2021). Raw bowel tea polyphenols can reduce the level of Firmicutes in the feces of NAFLD mice, increase the minimum levels of Bacteroidetes and Akkermansia, and reduce the F/B ratio, acting as a regulator of the gut microbiome (Liu et al., 2019). Vine tea polyphenol reduced the F/B ratio and increased the relative abundance of Akkermansia in NAFLD mice (Xie et al., 2020).
Interactions between flavonoids and the microbiome contribute significantly to human health. The ability of flavonoids to regulate microbes also holds promise for dietary therapies that can be used to treat a variety of diseases associated with microbial disorders.
Regulate lipid metabolism
Quercetin is widely distributed in photosynthetic plants, such as cereals, vegetables, fruit, tea leaves, and Chinese medicinal materials, and is the most abundant foodborne natural flavonoid (Martinon et al., 2002). Yang et al. (2019) established Type 2 diabetes mellitus (T2DM)-induced NAFLD and quercetin treatment models in vivo and in vitro, and found that quercetin reduced serum transaminase levels and significantly reduced liver histological changes. Wang (2021) found that mice fed a high-fat diet exhibited severe fat accumulation in their livers, and a large number of red fat droplets appeared in their visual field. After total flavonoids of Broussonetia papyrifera (TFBP) treatment, the fat content in the liver cells of mice decreased significantly and finally reached the levels observed in normal liver. These results indicated that TFBP had the ability toreduce fat accumulation in hepatocytes. Chian-jiunliou et al. staining with the fluorescent dye BODIPY 493/503 showed that incubating HepG2 cells with oleic acid-induced lipid accumulation and licorice chalcone significantly inhibited the aggregation of lipid droplets and confirmed that licorice chalcone promoted the Sirtuin1/AMP-activated protein kinase (Sirt1/AMPK) pathway in the liver in vivo and in vitro. It effectively inhibited adipogenesis and increased lipid decomposition and fatty acid β-oxidation in NAFLD mice (Liou et al., 2019). Luteolin, lycopene, and their combinations indirectly activate the SIRT1/AMPK pathway in vivo and in vitro, which in turn inhibits lipogenesis and increases β-oxidation, defending against the “two-hit” in NAFLD (Zhu et al., 2020).
Antioxidant stress
Flavonoids may inhibit oxidative stress by regulating malondialdehyde (MDA), superoxide dismutase (SOD), and catalase (CAT). Wang (2021) found that total flavonoids from the leaves of Broussonetia papyrifera (TFBP) effectively inhibited the production of ROS, reduced the content of myeloperoxidase, improved the activity of SOD, and reduced injury to the body by oxidative stress. Western blot results showed that TFBP could regulate oxidative stress depending on the nuclear factor erythroid 2-related factor 2/heme oxygenase 1 (Nrf2/HO-1) signaling pathway, and promote Nrf2 entry into the nucleus of mouse liver cells and HO-1 production, thus improving the body’s ability to resist oxidative stress. Other researchers have concluded that theaflavins significantly reduce ROS production in steatotic hepatocytes and TNF-α production in LPS-stimulated RAW264.7 cells (Luo et al., 2012).
Cyanidin-3-O-glucoside is the most abundant anthocyanidin in the flavonoid family. Li et al. found that centaulin-3-O-glucoside eliminated damaged mitochondria to maintain mitochondrial homeostasis and alleviate oxidative stress (Yin et al., 2017). These results suggest that cybernin-3-O-glucoside alleviates NAFLD by activating PTEN-induced kinase 1 (PINK1)-mediated mitochondrial phagocytosis. In a NASH cell model, the levels of MDA and ROS were significantly increased significantly, while the levels of SOD, CAT, and GSH were significantly decreased. After stimulation with different concentrations of alpha-naphthoflavone (ANF), the level of SOD in the cells was decreased, but the level of SOD was significantly increased. Furthermore, MDA and ROS levels in the liver tissues of HFD-fed mice with different concentrations of ANF were significantly lower than those inthe model group (Xia et al., 2019). Yang et al. (2019) found that quercetin restored the levels of superoxide dismutase, catalase, and glutathione in the liver of NAFLD mice. By activating the farnesoid X receptor 1 (FXR1)/TGR5 signaling pathway, quercetin eliminated lipid droplets and restored total cholesterol and triglyceride levels in HepG2 cells co-cultured with high d-glucose and free fatty acids. Wang et al. (2021) found that hyperoside can regulate bile acids (BAs) in the liver, reduce unconjugated BAs, and increase liver-conjugated BA levels. The expression of FXR in the liver is increased, leading to the promotion of free fatty acid β-oxidation.
Regulate autophagy
Autophagy is a conserved self-digestion process that brings unnecessary or potentially dangerous cytoplasmic materials, such as damaged organelles and misfolded or unfolded proteins, to lysosomes for degradation. Lipid oxidation mainly occurs in the mitochondria, and oxidative stress produces a large amount of ROS, which leads to mitochondrial dysfunction and may inhibit autophagy because autophagy is generated in the mitochondria (Tang et al., 2017). Studies have shown that epigallocatechin-3-gallate (a flavonoid 3-alcohol phenolic compound) can increase the proliferation and autophagy of the liver in HFD-fed mice but reduce apoptosis. This may alleviate HFD-induced NAFLD by inhibiting apoptosis and promoting autophagy (Wu et al., 2021). Galangin is a flavonol and a curcumin derivative. Recent studies confirmed that galangin induces autophagy. Previous studies have reported that galangin mediates autophagy through the p53 pathway, and SIRT1 deacetylates LC3 in HepG2 cells (Zhang et al., 2021). Similarly, apigenin has been found to improve liver lipid deposition by activating mitochondrial autophagyto increase fatty acid β-oxidation and mitochondrial bioenergetics (Hsu et al., 2021).
Anti-inflammatory effect
Oxidative stress-mediated inflammatory responses are an important pathological mechanism of NAFLD. When the level of oxidative stress increases, it can promote IL-6, IL-1β, and TNF-α expressionand induce liver injury (Xiao et al., 2018). The anti-inflammatory effect of flavonoids occurs mainly through the inhibition of the NF-κβ pathway (González et al., 2011). Flavonoids inhibit the phosphorylation of inhibitor of nuclear factor kappaB (IKB) and the inhibitor of nuclear factor kappaB kinase (IKK) complex (Kim et al., 2005) and the activity of regulatory enzymes, such asphospholipid oxygenase and protein tyrosine kinase (Manthey, 2009). Wang et al. found that the levels of IL-1β, IL-6, and TNF-α in the liver tissue of rats in the NAFLD model group were significantly increased, and total flavonoids of Scutellaria baicalensis could reduce these inflammatory factors, suggesting that total flavonoids in Scutellaria baicalensis could reduce the inflammatory response in the liver of rats in the NAFLD model group (Mengmeng et al., 2022). NO leads to highly destructive formation of peroxynitrite under oxidative stress conditions. Flavonoids inhibit inducible nitric oxide synthase (iNOS) expression and NO production (González-Gallego et al., 2010). In addition, flavonoids prevent the degeneration of the anti-inflammatory effects of the glucocorticoid cortisol. Oxidative stress worsens the anti-inflammatory effects of cortisol by eliminating these effects and creating cortisol resistance (Ruijters et al., 2014). Luteolin can significantly reduce a variety of inflammatory factors in NAFLD rats, which indicates that, in addition to its antioxidant effect, luteolin has also a very good anti-inflammatory effect (Abu-Elsaad and El-Karef, 2019). This suggests that NAFLD progression is often accompanied by inflammation and oxidative stress.
Summary and prospect
The incidence of NAFLD increases each year, similar to clinical stress. Currently, NAFLD has an estimated annual medical and social cost of $292 billion (Younossi et al., 2016a). The different manifestations of NAFLD complicate the diagnosis, which ignores the true condition. The medical system is facing a severe challenge incombating this growing liver disease. Flavonoids have been proven to have very strong pharmacological activity and have excellent alleviating effects on NAFLD and NASH. Flavonoids may ameliorate NAFLD by regulating lipid metabolism, intestinal flora, and autophagy. Therefore, natural flavonoids have huge potential for the clinical development of NAFLD drugs in the future.
Author contributions
PT, LJ, XQ, and BH participated in drafting the manuscript. All of the authors read and approved the final manuscript. Author XQ contributed equally to this work.
Funding
This study was supported by the Zhejiang Provincial Natural Science Foundation of China (Nos. LGF22H290001), the Fund of State Administration of Traditional Chinese Medicine of Zhejiang Province (No. 2022ZB120).
Conflict of interest
The authors declare that the research was conducted in the absence of any commercial or financial relationships that could be construed as a potential conflict of interest.
Publisher’s note
All claims expressed in this article are solely those of the authors and do not necessarily represent those of their affiliated organizations, or those of the publisher, the editors and the reviewers. Any product that may be evaluated in this article, or claim that may be made by its manufacturer, is not guaranteed or endorsed by the publisher.
References
Abu-Elsaad, N., and El-Karef, A. (2019). Protection against nonalcoholic steatohepatitis through targeting IL-18 and IL-1alpha by luteolin. Pharmacol. Rep. 71 (4), 688–694. doi:10.1016/j.pharep.2019.03.009 | |
Abu-Shanab, A., and Quigley, E. M. M. (2010). The role of the gut microbiota in nonalcoholic fatty liver disease. Nat. Rev. Gastroenterol. Hepatol. 7, 691–701. doi:10.1038/nrgastro.2010.172 | |
Aedín, C., and Anne-Marie, M. (2017). The role of metabolism (and the microbiome) in defining the clinical efficacy of dietary flavonoids. Am. J. Clin. Nutr. 105, 10–22. |
Anderson, N., and Borlak, J. (2008). Molecular mechanisms and therapeutic targets in steatosis and steatohepatitis. Pharmacol. Rev. 60, 311–357. doi:10.1124/pr.108.00001 | |
Angulo, P., Bugianesi, E., Bjornsson, E. S., Charatcharoenwitthaya, P., Mills, P. R., Barrera, F., et al. (2013). Simple noninvasive systems predict long-term outcomes of patients with nonalcoholic fatty liver disease. Gastroenterology 145, 782–e4. doi:10.1053/j.gastro.2013.06.057 | |
Aron-Wisnewsky, J., Gaborit, B., Dutour, A., and Clement, (2013). Gut microbiota and non-alcoholic fatty liver disease: New insights. Elsternwick, VIC, Australia: Clinical Microbiology & Infection.
Ayonrinde, O. T., Olynyk, J. K., Marsh, J. A., Beilin, L. J., Mori, L. A., Oddy, W. H., et al. (2015). Childhood adiposity trajectories and risk of nonalcoholic fatty liver disease in adolescents. J. Gastroenterol. Hepatol. 30, 163–171. doi:10.1111/jgh.12666 | |
Bell, M., Wang, H., Chen, H., Mclenithan, J. C., Gong, D. W., Yang, R. Z., et al. (2008). Consequences of lipid droplet coat protein downregulation in liver cells: Abnormal lipid droplet metabolism and induction of insulin resistance. Diabetes 57, 2037–2045. doi:10.2337/db07-1383 | |
Bellentani, S., Bedogni, G., Miglioli, L., and Tiribelli, C. (2004). The epidemiology of fatty liver. Eur. J. Gastroenterol. Hepatol. 16, 1087–1093. doi:10.1097/00042737-200411000-00002 | |
Berardis, S., and Sokal, E. (2014). Pediatric non-alcoholic fatty liver disease: An increasing public health issue. Eur. J. Pediatr. 173. doi:10.1007/s00431-013-2157-6 | |
Bonora, E., Zoppini, G., Padovani, R., Zenari, L., Sorgato, C., Bertolini, L., et al. (2010). Prevalence of non-alcoholic fatty liver disease and its association with cardiovascular disease in patients with type 1 diabetes. J. Hepatol. 53 (4), 713–718. doi:10.1016/j.jhep.2010.04.030 | |
Browning, J. D., and Horton, J. D. (2004). Molecular mediators of hepatic steatosis and liver injury. J. Clin. Invest. 114, 147–152. doi:10.1172/JCI22422 | |
Brunt, E. M., Janney, C. G., Bisceglie, A. M., Neuschwander-Tetri, B. A., and Bacon, B. R. (1999). Nonalcoholic steatohepatitis: A proposal for grading and staging the histological lesions. Am. J. Gastroenterology 94, 2467–2474. doi:10.1111/j.1572-0241.1999.01377.x | |
Buzzetti, E., Tsochatzis, E., and Pinzani, M. (2016). The multiple-hit pathogenesis of non-alcoholic fatty liver disease (NAFLD). Philadelphia, PA, United States: Metabolism Clinical & Experimental.
Chen, J., Xuan, Y. H., Luo, M. X., Ni, X. G., and Li, H. (2019a). Kaempferol alleviates acute alcoholic liver injury in mice by regulating intestinal tight junction proteins and butyrate receptors and transporters. Toxicology 429, 152338. doi:10.1016/j.tox.2019.152338 | |
Chen, Y. J., Tang, Z. Z., Du, L., Liu, Y., Lu, Q., Ma, T. F., et al. (2019b). A novel compound AB-38b improves diabetes-associated cognitive decline in mice via activation of Nrf2/ARE pathway. Brain Res. Bull. 150, 160–167. doi:10.1016/j.brainresbull.2019.05.010 | |
Chi, Z. C. (2017). Pathogenesis of non-alcoholic fatty liver disease. World Chin. J. Dig. 25, 670. doi:10.11569/wcjd.v25.i8.670 |
Choi, H. N., Shin, J. Y., and Kim, J. I. (2021). Ameliorative effect of myricetin on nonalcoholic fatty liver disease in ob/ob mice. J. Med. Food 24, 1092–1099. doi:10.1089/jmf.2021.K.0090 | |
Cobbina, E., and Akhlaghi, F. (2017). Non-alcoholic fatty liver disease (NAFLD) – pathogenesis, classification, and effect on drug metabolizing enzymes and transporters. Drug Metab. Rev. 49 (2), 197–211. doi:10.1080/03602532.2017.1293683 | |
Cremonini, E., Iglesias, D. E., Matsukuma, K. E., Hester, S. N., Wood, S. M., Bartlett, M., et al. (2022). Supplementation with cyanidin and delphinidin mitigates high fat diet-induced endotoxemia and associated liver inflammation in mice. Food Funct. 13, 781–794. doi:10.1039/d1fo03108b | |
Dongiovanni, P., Petta, S., Maglio, C., Fracanzani, A. L., Pipitone, R., Mozzi, E., et al. (2015). Transmembrane 6 superfamily member 2 gene variant disentangles nonalcoholic steatohepatitis from cardiovascular disease. Hepatology 61, 506–514. doi:10.1002/hep.27490 | |
Eduardo, V. G., Yadina, M. P., Luis, C. B., Ana, T. G., Bienvenido, G. O., Licet, G. F., et al. (2015). Weight loss through lifestyle modification significantly reduces features of nonalcoholic steatohepatitis. Gastroenterology 149, 367–e315. |
Ekstedt, M., Franzen, L. E., Mathiesen, U. L., Thorelius, L., Holmqvist, M., Bodemar, G., et al. (2006). Long-term follow-up of patients with NAFLD and elevated liver enzymes. Hepatology 44, 865–873. doi:10.1002/hep.21327 | |
Esterbauer, H., Schaur, R. J., and Zollner, H. (1991). Chemistry and biochemistry of 4-hydroxynonenal, malonaldehyde and related aldehydes. Free Radic. Biol. Med. 11, 81–128. doi:10.1016/0891-5849(91)90192-6 | |
Fabbrini, E., Magkos, F., Mohammed, B. S., Pietka, T., Abumrad, N. A., Patterson, B. W., et al. (2009). Intrahepatic fat, not visceral fat, is linked with metabolic complications of obesity. Proc. Natl. Acad. Sci. U. S. A. 106, 15430–15435. doi:10.1073/pnas.0904944106 | |
Fan, H., Ma, X., Lin, P., Kang, Q., Zhao, Z., Wang, L., et al. (2017). Scutellarin prevents nonalcoholic fatty liver disease (NAFLD) and hyperlipidemia via PI3K/AKT-Dependent activation of nuclear factor (Erythroid-Derived 2)-like 2 (Nrf2) in rats. Med. Sci. Monit. 23, 5599–5612. doi:10.12659/msm.907530 | |
Fang, Y. L., Chen, H., Wang, C. L., Liang, L., and Pediatrics, D. O. (2018). Pathogenesis of non-alcoholic fatty liver disease in children and adolescence: From "two hit theory" to "multiple hit model. World J. Gastroenterology Engl. Ed. Electron. Ed. 24, 10. doi:10.3748/wjg.v24.i27.2974 | |
Farrell, G. C., Wong, W. S., and Chitturi, S. (2013). NAFLD in Asia—As common and important as in the west. Nat. Rev. Gastroenterology Hepatology 10, 307–318. doi:10.1038/nrgastro.2013.34 | |
Feng, X., Yu, W., Li, X., Zhou, F., Zhang, W., Shen, Q., et al. (2017). Biochemical pharmacology. Kansas City, KS, United States: S0006295217302319. doi:10.1016/j.bcp.2017.04.014Apigenin, a modulator of PPARγ, attenuates HFD-induced NAFLD by regulating hepatocyte lipid metabolism and oxidative stress via Nrf2 activation. |
Gan, L., Cao, Y., and Yuan, J. (2021). Effects of (+)-catechin and epigallocatechin gallate on alcoholic fatty liver in mice models. Zhonghua Yu Fang. Yi Xue Za Zhi 55, 1305–1310. doi:10.3760/cma.j.cn112150-20201102-01264 | |
Geng, Y., Wu, Z., Buist-Homan, M., Blokzijl, H., and Moshage, H. (2020). Hesperetin protects against palmitate-induced cellular toxicity via induction of GRP78 in hepatocytes. Toxicol. Appl. Pharmacol. 404, 115183. doi:10.1016/j.taap.2020.115183 | |
González, R., Ballester, I., López-Posadas, R., Suárez, M., and Medina, F. (2011). Effects of flavonoids and other polyphenols on inflammation. Crit. Rev. food Sci. Nutr. 51, 331–362. |
González-Gallego, J., García-Mediavilla, M., Sánchez-Campos, S., and Tuón, M. J. (2010). Fruit polyphenols, immunity and inflammation. Br. J. Nutr. 104 (3), S15–S27. doi:10.1017/S0007114510003910 | |
Greco, D., Kotronen, A., Westerbacka, J., Puig, O., and Yki-Järvinen, H. (2008). Gene expression in human NAFLD. Am. J. Physiol. Gastrointest. Liver Physiol. 294, G1281. doi:10.1152/ajpgi.00074.2008 | |
Guillaume, L., Robert, C., David, B., Marie, P., Hélène, V., Julien, L., et al. (2015). Bariatric surgery reduces features of nonalcoholic steatohepatitis in morbidly obese patients. Gastroenterology 149, 379–e316. doi:10.1053/j.gastro.2015.04.014 | |
Hanju, S., Zhang, P., Zhu, Y., Lou, Q., and He, S. (2018). Antioxidant and prebiotic activity of five peonidin-based anthocyanins extracted from purple sweet potato (Ipomoea batatas (L.) Lam.). Sci. Rep. 8 (1), 5018. doi:10.1038/s41598-018-23397-0 | |
He, B., Jiang, J., Shi, Z., Wu, L., Yan, J., Chen, Z., et al. (2021). Pure total flavonoids from citrus attenuate non-alcoholic steatohepatitis via regulating the gut microbiota and bile acid metabolism in mice. Biomed. Pharmacother. 135, 111183. doi:10.1016/j.biopha.2020.111183 | |
Hongming, S., Yuting, L., Dongwen, H., Lianghua, X., Huihui, K., Xiaodong, Z., et al. (2018). Procyanidin B2 ameliorates free fatty acids-induced hepatic steatosis through regulating TFEB-mediated lysosomal pathway and redox state. Free Radic. Biol. Med. 126. doi:10.1016/j.freeradbiomed.2018.08.024 |
Hsu, M. C., Guo, B. C., Chen, C. H., Hu, P. A., and Lee, T. S. (2021). Apigenin ameliorates hepatic lipid accumulation by activating the autophagy-mitochondria pathway. J. Food Drug Anal. 29, 240–254. doi:10.38212/2224-6614.3269 | |
Huang, B., Bao, J., Cao, Y-R., Gao, H-F., and Jin, Y. (2018). Cytochrome P450 1A1 (CYP1A1) catalyzes lipid peroxidation of oleic acid-induced HepG2 cells. Biochemistry 83, 595–602. doi:10.1134/S0006297918050127 | |
Jiang, W., Wu, N., Wang, X., Chi, Y., Zhang, Y., Qiu, X., et al. (2015). Dysbiosis gut microbiota associated with inflammation and impaired mucosal immune function in intestine of humans with non-alcoholic fatty liver disease. Sci. Rep. 5, 8096. doi:10.1038/srep08096 | |
Jou, J., Choi, S. S., and Diehl, A. M. (2008). Mechanisms of disease progression in nonalcoholic fatty liver disease. Semin. Liver Dis. 28, 370–379. doi:10.1055/s-0028-1091981 | |
Ke, J. Y., Kliewer, K. L., Hamad, E. M., Cole, R. M., Powell, K. A., Andridge, R. R., et al. (2015). The flavonoid, naringenin, decreases adipose tissue mass and attenuates ovariectomy-associated metabolic disturbances in mice. Nutr. Metab. (Lond) 12, 1. doi:10.1186/1743-7075-12-1 | |
Kim, H., Kong, H., Choi, B., Yang, Y., Kim, Y., Lim, M. J., et al. (2005). Metabolic and pharmacological properties of rutin, a dietary quercetin glycoside, for treatment of inflammatory bowel disease. Pharm. Res. 22, 1499–1509. doi:10.1007/s11095-005-6250-z | |
Kim, M. H., Park, J. S., Jung, J. W., Byun, K. W., Kang, K. S., and Lee, Y. S. (2011). Daidzein supplementation prevents non-alcoholic fatty liver disease through alternation of hepatic gene expression profiles and adipocyte metabolism. Int. J. Obes. 35 (8), 1019–1030. doi:10.1038/ijo.2010.256 | |
Kirpich, I. A., Marsano, L. S., and Mcclain, C. J. (2015). Gut-liver axis, nutrition, and non-alcoholic fatty liver disease. Clin. Biochem. 48, 923–930. doi:10.1016/j.clinbiochem.2015.06.023 | |
Lascala, A., Martino, C., Parafati, M., Salerno, R., Oliverio, M., Pellegrino, D., et al. (2018). Analysis of proautophagic activities of Citrus flavonoids in liver cells reveals the superiority of a natural polyphenol mixture over pure flavones. J. Nutr. Biochem. 58, 119–130. doi:10.1016/j.jnutbio.2018.04.005 | |
Li, F. (2018). Quality evaluation of traditional Chinese medicine rattan tea and its related research on the metabolism of its active ingredient. Chongqing, China: dihydromyricetin. 硕士, Huazhong University of Science and Technology.
Li, J., Wang, T., Liu, P., Yang, F., Wang, X., Zheng, W., et al. (2021). Hesperetin ameliorates hepatic oxidative stress and inflammation via the PI3K/AKT-Nrf2-ARE pathway in oleic acid-induced HepG2 cells and a rat model of high-fat diet-induced NAFLD. Food & Funct. 12. doi:10.1039/d0fo02736g |
Lima, A. C. D., Cecatti, C., Fidélix, M. P., Adorno, M. a. T., Sakamoto, I. K., Cesar, T. B., et al. (2019). Effect of daily consumption of orange juice on the levels of blood glucose, lipids, and gut microbiota metabolites: Controlled clinical trials. J. Med. Food 22 (2), 202–210. doi:10.1089/jmf.2018.0080 | |
Liou, C. J., Lee, Y. K., Ting, N. C., Chen, Y. L., Shen, S. C., Wu, S. J., et al. (2019). Protective effects of licochalcone A ameliorates obesity and non-alcoholic fatty liver disease via promotion of the sirt-1/AMPK pathway in mice fed a high-fat diet. Cells 8. doi:10.3390/cells8050447 | |
Liu, B., Zhang, J., Sun, P., Yi, R., Han, X., and Zhao, X. (2019). Raw bowl tea (tuocha) polyphenol prevention of nonalcoholic fatty liver disease by regulating intestinal function in mice. Biomolecules 9. doi:10.3390/biom9090435 | |
Luo, X. Y., Takahara, T., Hou, J., Kawai, K., Sugiyama, T., Tsukada, K., et al. (2012). Theaflavin attenuates ischemia-reperfusion injury in a mouse fatty liver model. Biochem. Biophys. Res. Commun. 417, 287–293. doi:10.1016/j.bbrc.2011.11.102 | |
Lv, Y., Gao, X., Fan, W., Shen, T., and Yan, L. (2019). Apigenin ameliorates HFD-induced NAFLD through regulation of the XO/NLRP3 pathways. J. Nutr. Biochem. 71. doi:10.1016/j.jnutbio.2019.05.015 | |
Machado, M., Marques-Vidal, P., and Cortez-Pinto, H. (2006). Hepatic histology in obese patients undergoing bariatric surgery. J. Hepatol. 45, 600–606. doi:10.1016/j.jhep.2006.06.013 | |
Madheshwar, R. V., and Perumal, T. (2017). Dual organisms lichens"-parmotrema perlatum.Evaluation of phytochemical analysis and antithrombolytic activities of
Makunga, N. P. (2020). LC-MS-Based metabolomics for the chemosystematics of Kenyan dodonaea viscosa jacq (sapindaceae) populations. Molecules 25.
Maleki, S. J., Crespo, J. F., and Cabanillas, B. (2019). Anti-inflammatory effects of flavonoids. Food Chem. 299, 125124–125121. doi:10.1016/j.foodchem.2019.125124 | |
Manthey, J. A. (2009). Biological properties of flavonoids pertaining to inflammation. Microcirculation 7, S29–S34. |
Martinon, F., Burns, K., and Tschopp, J. (2002). The inflammasome: A molecular platform triggering activation of inflammatory caspases and processing of proIL-beta. Mol. Cell 10, 417–426. doi:10.1016/s1097-2765(02)00599-3 | |
Mengmeng, W., Xue, Q., Qionglian, F., Shengnan, F., Xinping, L., Feng, H., et al. (2022). Effect of different polar extracts of scutellaria baicalensis total flavonoids on non-alcoholic fatty liver model rats. Chin. Pharm. 33, 1338–1342.
Mm, A., Nk, B., and Mbcd, E. (2019). A higher flavonoid intake is associated with less likelihood of nonalcoholic fatty liver disease: Results from a multiethnic study. J. Nutr. Biochem. 65, 66–71. |
Mundi, M. S., Velapati, S., Patel, J., Kellogg, T. A., Abu Dayyeh, B. K., and Hurt, R. T. (2020). Evolution of NAFLD and its management. Nutr. Clin. Pract. 35, 72–84. doi:10.1002/ncp.10449 | |
Porras, D., Esther, L., Martínez-Flórez, M., Pisonero-Vaqueror, S., Olcoz, J. S., and Jover, R. (2017). Protective effect of quercetin on high-fat diet-induced non-alcoholic fatty liver disease in mice is mediated by modulating intestinal microbiota imbalance and related gut-liver axis activation. Free Radic. Biol. Med. Official J. Oxyg. Soc. 102, 188–202. doi:10.1016/j.freeradbiomed.2016.11.037 | |
Powell, E. E., Wong, V. W., and Rinella, M. (2021). Non-alcoholic fatty liver disease. Lancet 397, 2212–2224. doi:10.1016/S0140-6736(20)32511-3 | |
Ramadori, G., and Armbrust, T. (2001). Cytokines in the liver. Eur. J. Gastroenterology Hepatology 13, 777–784. doi:10.1097/00042737-200107000-00004 | |
Richardson, M. M., Jonsson, T. R., Powell, E. E., Brunt, E. M., Neuschwander-Tetri, B. A., Bhathal, P. S., et al. (2007). progressive fibrosis IN NON-alcoholic steatohepatitis - association with altered regeneration and a ductular reaction. Gastroenterology 133 (1), 80–90. doi:10.1016/s0168-8278(07)61711-5 | |
Romeo, S., Kozlitina, J., Xing, C., Pertsemlidis, A., Cox, D., Pennacchio, L. A., et al. (2008). Genetic variation in PNPLA3 confers susceptibility to nonalcoholic fatty liver disease. Nat. Genet. 40, 1461–1465. doi:10.1038/ng.257 | |
Ruijters, E., Haenen, G., Weseler, A. R., and Bast, A. (2014). The cocoa flavanol (-)-epicatechin protects the cortisol response. Pharmacol. Res. 79, 28–33. doi:10.1016/j.phrs.2013.11.004 | |
Sasaki, G. Y., Li, J., Cichon, M. J., Kopec, R. E., and Bruno, R. S. (2021). Catechin-rich green tea extract and the loss-of-TLR4 signaling differentially alter the hepatic metabolome in mice with nonalcoholic steatohepatitis. Mol. Nutr. Food Res. 65, e2000998. doi:10.1002/mnfr.202000998 | |
Soderberg, C., Stal, P., Askling, J., Glaumann, H., Lindberg, G., Marmur, J., et al. (2010). Decreased survival of subjects with elevated liver function tests during a 28-year follow-up. Hepatology 51, 595–602. doi:10.1002/hep.23314 | |
Su, H., Li, Y., Hu, D., Xie, L., Ke, H., Zheng, X., et al. (2018). Procyanidin B2 ameliorates free fatty acids-induced hepatic steatosis through regulating TFEB-mediated lysosomal pathway and redox state. Free Radic. Biol. Med. 126, 269–286. doi:10.1016/j.freeradbiomed.2018.08.024 | |
Sun, W., Liu, P., Wang, T., Wang, X., Zheng, W., and Li, J. (2020). Baicalein reduces hepatic fat accumulation by activating AMPK in oleic acid-induced HepG2 cells and high-fat diet-induced non-insulin-resistant mice. Food Funct. 11, 711–721. doi:10.1039/c9fo02237f | |
Sun, W. L., Li, X. Y., Dou, H. Y., Wang, X. D., Li, J. D., Shen, L., et al. (2021). Myricetin supplementation decreases hepatic lipid synthesis and inflammation by modulating gut microbiota. Cell Rep. 36, 109641. doi:10.1016/j.celrep.2021.109641 | |
Tang, Q., Zheng, G., Feng, Z., Chen, Y., Lou, Y., Wang, C., et al. (2017). Trehalose ameliorates oxidative stress-mediated mitochondrial dysfunction and ER stress via selective autophagy stimulation and autophagic flux restoration in osteoarthritis development. Cell Death Dis. 8, e3081. doi:10.1038/cddis.2017.453 | |
Tsuchiya, H. (2010). Structure-dependent membrane interaction of flavonoids associated with their bioactivity. Food Chem. 120, 1089–1096. doi:10.1016/j.foodchem.2009.11.057 |
Tsuji, P. A., Stephenson, K. K., Wade, K. L., Liu, H., and Fahey, J. W. Structure-activity analysis of flavonoids: Direct and indirect antioxidant, and antiinflammatory potencies and toxicities. doi:10.1080/01635581.2013.809127
Vebø, H. C., Karlsson, M. K., Avershina, E., Finnby, L., and Rudi, K. (2016). Bead-beating artefacts in the Bacteroidetes to Firmicutes ratio of the human stool metagenome. J. Microbiol. Methods 129, 78–80. doi:10.1016/j.mimet.2016.08.005 | |
Vlad, R., Stefano, B., Helena, C. P., Chris, D., and Giulio, M. (2018). A position statement on NAFLD/NASH based on the EASL 2009 special conference.
Wang, Q. (2021). Therapeutic effect of total flavonoids from Fructus Arganae leaves on non-alcoholic fatty liver disease in mice.
Wang, S., Sheng, F., Zou, L., Xiao, J., and Li, P. (2021). Hyperoside attenuates non-alcoholic fatty liver disease in rats via cholesterol metabolism and bile acid metabolism. J. Adv. Res. 34, 109–122. doi:10.1016/j.jare.2021.06.001 | |
Wang, W., Chen, J., Mao, J., Li, H., Wang, M., Zhang, H., et al. (2018). Genistein ameliorates non-alcoholic fatty liver disease by targeting the thromboxane A2 pathway. J. Agric. Food Chem. 66 (23), 5853–5859. doi:10.1021/acs.jafc.8b01691 | |
Wenji Zhang, R. A., Li, Q., Sun, L., and Lai, X. (2020). Theaflavin TF3 relieves hepatocyte lipid deposition through activating an AMPK signaling pathway by targeting plasma kallikrein. J. Agric. Food Chem. 68, 2673–2683. doi:10.1021/acs.jafc.0c00148 | |
Weston, L. A., and Mathesius, U. (2013). Flavonoids: Their structure, biosynthesis and role in the rhizosphere, including allelopathy. J. Chem. Ecol. 39 (2), 283–297. doi:10.1007/s10886-013-0248-5 | |
Wier, B. V. D., Koek, G. H., Bast, A., and Haenen, G. R. M. M. (2015). The potential of flavonoids in the treatment of non-alcoholic fatty liver disease. C R C Crit. Rev. Food Technol. 57, 834–855. doi:10.1080/10408398.2014.952399 |
Wu, D., Liu, Z., Wang, Y., Zhang, Q., Li, J., Zhong, P., et al. (2021). Epigallocatechin-3-Gallate alleviates high-fat diet-induced nonalcoholic fatty liver disease via inhibition of apoptosis and promotion of autophagy through the ROS/MAPK signaling pathway. Hindawi Limited.
Xia, H., Zhu, X., Zhang, X., Jiang, H., and Jin, Y. (2019). Alpha-naphthoflavone attenuates non-alcoholic fatty liver disease in oleic acid-treated HepG2 hepatocytes and in high fat diet-fed mice. Biomed. Pharmacother. 118, 109287. doi:10.1016/j.biopha.2019.109287 | |
Xia, L. A., Rs, A., Zl, A., Rx, A., Pl, A., Xs, B., et al. (2021). Luteolin alleviates non-alcoholic fatty liver disease in rats via restoration of intestinal mucosal barrier damage and microbiota imbalance involving in gut-liver axis. San Antonio, TX, United States: Archives of Biochemistry and Biophysics.
Xiao, M. L., Chen, G. D., Zeng, F. F., Qiu, R., and Chen, Y. M. (2018). Higher serum carotenoids associated with improvement of non-alcoholic fatty liver disease in adults: A prospective study. Eur. J. Nutr. 58, 721–730. doi:10.1007/s00394-018-1678-1 | |
Xiaxia, Z., Xiaotian, L., Sha, L., Hongqia, H., and Yu, L. (2019). Research progress on the effect of 1,25-dihydroxyvitamin D_3 on nonalcoholic fatty liver disease. J. Pract. Clin. Med. 23, 125–128.
Xie, K., He, X., Chen, K., Sakao, K., and Hou, D. X. (2020). Ameliorative effects and molecular mechanisms of vine tea on Western diet-induced NAFLD. Food Funct. 11, 5976–5991. doi:10.1039/d0fo00795a | |
Xing, Y., Ren, X., Li, X., Sui, L., and Dong, Y. (2021). Baicalein enhances the effect of acarbose on the improvement of nonalcoholic fatty liver disease associated with prediabetes via the inhibition of de novo lipogenesis. J. Agric. Food Chem. 69 (34), 9822–9836. doi:10.1021/acs.jafc.1c04194 | |
Xing, Y., Shi, Z., Zhu, Y., Shen, T., Wang, H., and Shui, G. (2020). Cyanidin-3-O-glucoside improves non-alcoholic fatty liver disease by promoting PINK1-mediated mitophagy in mice. Br. J. Pharmacol. 177 (15), 3591–3607. doi:10.1111/bph.15083 | |
Xu, Y., Ke, H., Li, Y., Xie, L., Su, H., Xie, J., et al. (2021). Malvidin-3-O-Glucoside from blueberry ameliorates nonalcoholic fatty liver disease by regulating transcription factor EB-mediated lysosomal function and activating the Nrf2/ARE signaling pathway. J. Agric. Food Chem. 69, 4663–4673. doi:10.1021/acs.jafc.0c06695 | |
Yang, H., Yang, T., Heng, C., Zhou, Y., Jiang, Z., Qian, X., et al. (2019). Quercetin improves nonalcoholic fatty liver by ameliorating inflammation, oxidative stress, and lipid metabolism in db/db mice. Phytother. Res. 33, 3140–3152. doi:10.1002/ptr.6486 | |
Yang, Y., Wu, Y., Zou, J., Wang, Y. H., Xu, M. X., Huang, W., et al. (2021). Naringenin attenuates non-alcoholic fatty liver disease by enhancing energy expenditure and regulating autophagy via AMPK. Front. Pharmacol. 12, 687095. doi:10.3389/fphar.2021.687095 | |
Yeh, M. M., and Brunt, E. M. (2014). Pathological features of fatty liver disease. Gastroenterology 147, 754–764. doi:10.1053/j.gastro.2014.07.056 | |
Yin, Y., Gao, L. G., Lin, H., Wu, Y., Han, X., Zhu, Y., et al. (2017). Luteolin improves non-alcoholic fatty liver disease in db/db mice by inhibition of liver X receptor activation to down-regulate expression of sterol regulatory element binding protein 1c. Biochem. Biophysical Res. Commun. 482, 720–726. doi:10.1016/j.bbrc.2016.11.101 | |
Younossi, Z. M., Henry, L., Stepanova, M., Younossi, Y., Racila, A., Hunt, S., et al. (2016a). The economic and clinical burden of nonalcoholic fatty liver disease in the United States and Europe. Hepatology 64, S502–S503. doi:10.1002/hep.28785 | |
Younossi, Z. M., Koenig, A. B., Abdelatif, D., Fazel, Y., Henry, L., and Wymer, M. (2016b). Global epidemiology of nonalcoholic fatty liver disease—meta-analytic assessment of prevalence, incidence, and outcomes. Hepatology 64. doi:10.1002/hep.28431 | |
Zhang, L., Yao, Z., and Ji, G. (2018b). Herbal extracts and natural products in alleviating non-alcoholic fatty liver disease via activating autophagy. Front. Pharmacol. 9. doi:10.3389/fphar.2018.01459 | |
Zhang, P., Ji, R., Sun, H., Peng, J., Ma, X., and Wang, C. (2018a). Scutellarin ameliorates nonalcoholic fatty liver disease through the PPARγ/PGC-1α-Nrf2 pathway. Free Radic. Res. 52 (2), 198–211. doi:10.1080/10715762.2017.1422602 | |
Zhang, X., Deng, Y., Xiang, J., Liu, H., Zhang, J., Liao, J., et al. (2020). Galangin improved non-alcoholic fatty liver disease in mice by promoting autophagy. Drug Des. Devel Ther. 14, 3393–3405. doi:10.2147/DDDT.S258187 | |
Zhang, X., Deng, Y., Xiang, J., Liu, H., Zhang, J., Liao, J., et al. (2021). Galangin improved non-alcoholic fatty liver disease in mice by promoting autophagy [corrigendum]. Auckland, New Zealand: Dove Press.
Zhong, H., Liu, H., and Jiang, Z. (2017). Genistein ameliorates fat accumulation through AMPK activation in fatty acid induced BRL cells. J. Food Sci. 82, 2719–2725. doi:10.1111/1750-3841.13856 | |
Zhu, X., Xiong, T., Liu, P., Guo, X., Xiao, L., Zhou, F., et al. (2018). Quercetin ameliorates HFD-induced NAFLD by promoting hepatic VLDL assembly and lipophagy via the IRE1a/XBP1s pathway. Food Chem. Toxicol. 114, 52–60. doi:10.1016/j.fct.2018.02.019 | |
Zhu, X., Yao, P., Liu, J., Guo, X., and Tang, Y. (2019). Baicalein attenuates impairment of hepatic lysosomal acidification induced by high fat diet via maintaining V-ATPase assembly. Food Chem. Toxicol. 136, 110990. doi:10.1016/j.fct.2019.110990 | |
Zhu, Y., Liu, R., Shen, Z., and Cai, G. (2020). Combination of luteolin and lycopene effectively protect against the "two-hit" in NAFLD through Sirt1/AMPK signal pathway. Life Sci. 256, 117990. doi:10.1016/j.lfs.2020.117990 | |
Zou, W., Zhang, C., Gu, X., Li, X., and Zhu, H. (2021). Metformin in combination with malvidin prevents progression of non-alcoholic fatty liver disease via improving lipid and glucose metabolisms, and inhibiting inflammation in type 2 diabetes rats. Drug Des. Devel Ther. 15, 2565–2576. doi:10.2147/DDDT.S307257 | |
Glosarry
ALT alanine aminotransferase
AMPK AMP-activated protein kinase
ANF alpha-naphthoflavone
ARE antioxidant response element
AST aspartate aminotransferase
BP broussonetiapyrifera
C/EBP-α CCAAT/enhancer binding protein alpha
ChREBP carbohydrate-responsive element-binding protein
CPT1 carnitine palmitoyltransferase 1A
CYP7A1 cytochrome P450 7A1
DGAT2 diacylglycerol acyltransferase 2
DNL lysosomal DNA-ase
E167K residue 167
FA fatty acids
FXR1 farnesoid X receptor 1
GCKR glucokinase regulator
HO-1heme hemeoxygenase 1
IKB inhibitor of nuclear factor kappaB
IKK inhibitor of nuclear factor kappaB kinase
IKKβ inhibitor of nuclear factor kappaB kinase beta
IL-1β interleukin-1β
IL-6 interleukin 6
iNOS inducible nitric oxide synthase
IRE1α inositol-requiring enzyme 1 Alpha
LPL lipoProtein lipase
MBOAT7 membrane bound o-acyltransferase domain-containing 7
MDA malondialdehyde
NAFLD non-alcoholic fatty liver disease
NASH non-alcoholic steatohepatitis
NASH-HCC non-alcoholic steatohepatitis-hepatocellular carcinoma
NF-κβ nuclear factor kappa β
NLRP3 nucleotide-binding oligomerization domain, leucine-rich repeat and pyrin domain-containing 3
Nrf2 nuclear factor erythroid 2-related factor 2
PC phosphatidylcholine
PGC-1α peroxisome proliferator-activated receptor-gamma coactivator 1 alpha
PI3K phosphatidylinositol-3-kinase
PINK1 putative kinase 1-mediated
PNPLA3 patatin-like phospholipase domain containing 3
PPAR-α peroxisome proliferator-activated receptor alpha
PPAR-γ peroxisome proliferator-activated receptor gamma
ROS reactive oxygen species
Sirt1 sirtuin 1
SOD speroxide Dismutase
SREBP1c sterol regulatory binding protein-1c
T2DM type 2 diabetes mellitus
TAG triacylglycerol
TC total cholesterol
TFBP total flavonoids of broussonetia papyrifera
TG triglyceride
TM6SF2 transmembrane 6 superfamily member 2
TNF-α tumor necrosis factor-α
TXA2 thromboxane A2
V-ATPase vacuolar proton ATPase
VLDL very low density lipoprotein
XBP1s X-box binding protein 1 spliced
Keywords: non-alcoholic fatty liver disease, natural flavonoids, antioxidant, anti-inflammatory, intestinal flora, oxidat ive stress, inflammtion
Citation: Tan P, Jin L, Qin X and He B (2022) Natural flavonoids: Potential therapeutic strategies for non-alcoholic fatty liver disease. Front. Pharmacol. 13:1005312. doi: 10.3389/fphar.2022.1005312
Received: 28 July 2022; Accepted: 26 August 2022;
Published: 16 September 2022.
Edited by:
Dan Tang, Guangdong Pharmaceutical University, ChinaReviewed by:
Helda Tutunchi, Tabriz University of Medical Sciences, IranAsra Iftikhar, University of Faisalabad, Pakistan
Copyright © 2022 Tan, Jin, Qin and He. This is an open-access article distributed under the terms of the Creative Commons Attribution License (CC BY). The use, distribution or reproduction in other forums is permitted, provided the original author(s) and the copyright owner(s) are credited and that the original publication in this journal is cited, in accordance with accepted academic practice. No use, distribution or reproduction is permitted which does not comply with these terms.
*Correspondence: Beihui He, Z3JhZjMwM0BzaW5hLmNvbQ==
†These authors contributed equally to this work