- 1Student Research Committee, Ahvaz Jundishapur University of Medical Sciences, Ahvaz, Iran
- 2Nutrition and Metabolic Diseases Research Center, Clinical Research Institute, Ahvaz Jundishapur University of Medical Sciences, Ahvaz, Iran
- 3Department of Nutrition, School of Allied Medical Sciences, Ahvaz Jundishapur University of Medical Sciences, Ahvaz, Iran
- 4Cardiovascular Research Center, Tabriz University of Medical Sciences, Tabriz, Iran
- 5Endocrine Research Center, Tabriz University of Medical Sciences, Tabriz, Iran
- 6Faculty of Veterinary Medicine, University of Tabriz, Tabriz, Iran
- 7Stem Cell and Regenerative Medicine Institute, Tabriz University of Medical Sciences, Tabriz, Iran
Cardiovascular diseases (CVD) are major causes of death worldwide. Recently, new roles for intestinal microbiota in pathology and treatment of CVD have been proposed. Butyrate, a bacterial metabolite, is synthesized in the gut and performs most of its functions in there. However, researchers have discovered that butyrate could enter to portal vein and interact with various organs. Butyrate exhibits a broad range of pharmacological activities, including microbiome modulator, anti-inflammatory, anti-obesity, metabolic pathways regulator, anti-angiogenesis, and antioxidant. In this article we review evidence supporting a potentially therapeutic role for butyrate in CVD and the mechanisms and pathways involved in the cardio-protective effects of butyrate from the gut and circulation to the nervous system. In summary, although butyrate exhibits a wide variety of biological activities in different pathways including energy homeostasis, glucose and lipid metabolism, inflammation, oxidative stress, neural signaling, and epigenetic modulation in experimental settings, it remains unclear whether these findings are clinically relevant and whether the molecular pathways are activated by butyrate in humans.
Introduction
Cardiovascular diseases (CVD) are disorders that affect the heart and blood vessels mainly including heart failure (HF), stroke, atherosclerosis, and hypertension (Ahmad et al., 2019). CVD are at the top of the life-threatening ailments list globally (Hulten et al., 2017). Based on the report of the World Health Organization (WHO), 17.9 million deaths were attributed to CVD in 2019, which accounted for 32% of all deaths (Roth et al., 2020).
Despite advances in primary prevention, CVD prevalence has risen in recent years (Tsivgoulis et al., 2018). The proven conditions that increase the risk of CVD including hypertension, dyslipidemia, obesity, and insulin resistance (IR) are concomitantly increased with CVD (Roth et al., 2020). In order to improve CVD prevention and treatment, it is essential to investigate unknown parts of the pathophysiology as well as to identify novel agents affecting risk factors (Singh et al., 2016; Warmbrunn et al., 2020). Dysbiosis of gut microbiota is one of the newest factors which is known to be involved in the development of CVD (Lau et al., 2017). Dysbiosis is characterized as alterations in microbial composition and their metabolites (Serino et al., 2014). An increasing body of evidence in CVD indicates alterations in microbial composition and their metabolites that may play a role in the pathogenesis and progression of these diseases (Brown and Hazen, 2015; Witkowski et al., 2020). The gut microbiota produces a wide variety of metabolites as a result of the anaerobic fermentation of undigested foods (Warmbrunn et al., 2020). Short-chain fatty acids (SCFAs) including acetate, propionate and butyrate, are main metabolites that may provide important protection.
Butyrate is a four-carbon SCFA, mainly known as a fuel for colonocytes. In addition to dietary fibers especially resistant starch, which is an indirect source of butyrate, some types of cheese, butter, and milk also contain small amounts of butyrate. Researches showed that butyrate can absorb into the portal vein and interact with the host body’s important processes like glucose homeostasis, lipid metabolism and gut inflammation. Several in vitro and in vivo studies have demonstrated that butyrate exerts anti-inflammatory, anti-oxidant, anti-obesity and metabolic regulation effects. The aim of the present study was to comprehensively review the therapeutic efficacy of butyrate in CVD as well as the mechanisms of action of butyrate on CVD risk factors.
Butyrate Producing Bacteria and Alterations in CVD
Gut microbiota is made up trillions of microorganisms including bacteria, viruses, fungi and protozoa (Ley et al., 2006). A variety of dietary substrates are used by these microbes to produce a range of metabolites, some of which are beneficial to the host (Scott et al., 2013). The production of butyrate is widespread among diverse phyla of human colon (Louis and Flint, 2009). Firmicutes and Bacteroides are the main butyrate producing phyla (Chen et al., 2020). The identification of butyrate producing spices has been done using sequence based detection algorithms such as metagenomics and 16S ribosomal RNA sequencing (Louis and Flint, 2009). All butyrate-producing bacteria are shown in Figure 1. The most prominent butyrogenic bacteria groups are Faecalibacterium prausnitzii, Butyrivibriocrossotus and Roseburia intestinalis which several studies have reported the depletion of these bacteria in atherosclerosis (Chen et al., 2020). Kasahara et al. demonstrated that the abundance of Roseburia intestinalis is negatively correlated with the size of atherosclerotic lesions in the mouse model of atherosclerosis (Kasahara et al., 2018). In that study, when Roseburia intestinalis was taken along with a high-fiber diet, aortic atherosclerotic plaques were reduced in size. This study suggests that the butyrate, a microbial metabolite, mediates these effects (Kasahara et al., 2018). A research conducted by zeng et al. illustrated that bacteria with capacity of butyrate production, Lachnospiraceae and Ruminococcaceae, were depleted in individuals at a high risk of stroke. Fecal butyrate concentrations also were low in these people (Zeng et al., 2019). Additionally, metagenomes analysis of individuals with symptomatic carotid atherosclerosis showed that gene expression of butyrate-synthesizing enzyme (butyrate-ace- toacetate CoA-transferase) were inversely correlated with C reactive protein (CRP) levels (Karlsson et al., 2012).
Several studies have reported that patients with HF have a decrease in butyrate-producing bacteria, especially, Lachnospiracea and Ruminococcacea families (Trøseid, 2020). Remarkably, reduction of the butyrate-producing Eubacterium Halli and Lachnospiracea is correlated with increased inflammation, severity of disease, heart damage and mortality (Kummen et al., 2018). Moreover, there is evidence that dysbiosis is related to low butyrate production in different HF cohorts (Trøseid, 2020). Likewise, animal and human studies reported decrease in bacteria with capacity of butyrate production in hypertension (Mell et al., 2015; Yang et al., 2015; Gomez-Arango et al., 2016; Yan et al., 2017). According to these studies, amount of butyrate producer bacteria is negatively associated with blood pressure (BP) (Santisteban et al., 2017).
Overall, the evidence suggests that decrease in butyrate-producing bacteria abundance, down regulation of genes involved in butyrate synthesis, and low butyrate levels are associated with sensitivity of developing CVD.
Mechanisms of Action of Butyrate in CVD and CVD Risk Factors
Major Mechanisms
The key aspects of butyrate mechanism of action can be listed as follows. First, butyrate has reported to be an epigenetic modifier by acting as a histone deacetylases (HDACs) inhibitor (Chang et al., 2014). HDACs are chromatin-modifying enzymes that alter genes transcription accessibility by removing acetylate from histones and non-histone proteins (Shakespear et al., 2011). The abnormal regulation of gene expression underlies many human metabolic disorders such as CVD. By inhibiting HDACs, butyrate causes hyper-acetylation of transcription factors and regulates gene expression patterns (Cleophas et al., 2019).Second, butyrate influences cellular responses by binding and activating specific receptors named free fatty acid receptor 2 (FFAR2) and FFAR3 (Previously these receptors were called G protein-coupled receptors (GPRs), GRP 43 and 41 respectively) (Brown et al., 2003).
Butyrate can contribute in widespread cardiovascular-related functions through inducing intracellular signaling pathways by interacting with FFARs on target cells or causing epigenetic changes by inhibiting HDAC.
Butyrate as a PPARs Agonist
Butyrate is known to be a pleiotropic molecule that as well as binding to FFARs, also has the ability to bind peroxisome proliferator-activated receptors (PPARs) (Are et al., 2008; Alex et al., 2013; Korecka et al., 2013; Mattace Raso et al., 2013; Den Besten et al., 2015; Chitrala et al., 2017). PPARs are a family of ligand-activated transcription factors that recognized to have a significant impact on metabolism related pathways. Due to several regulatory roles in metabolic function and energy hemostasis, they are now one of the most often proposed therapeutic targets for metabolic disorders (Oh et al., 2019). Butyrate could induce adipogenesis by activating PPARγ, which is supported by several studies (Toscani et al., 1990; Li et al., 2014; Yan and Ajuwon, 2015). Adipogenesis is associated with reduced inflammatory and oxidative molecules production in adipose tissue, organs lipotoxicity and IR (Hafidi et al., 2019). In diet-induced obese Apo E−/− mice, oral administration of sodium butyrate (SB) (10 ml/kg diet) increased vascular endothelial growth factor (VEGF) mediated vascularization via upregulating PPARγ contributing to improvement of angiogenesis, inflammation and insulin sensitivity (Aguilar et al., 2018). Anti-angiogenic properties of butyrate have also been proposed both in vitro and in vivo, which is associated with upregulation of anti-angiogenic VEGF (Ciura and Jagodziński, 2010). Moreover, upregulation of PPARγ by butyrate modulates nuclear factor-κB (NF-κB) pathway, resulting in improvements in insulin signaling and inflammation (Aguilar et al., 2018).
On the other hand, SB may activate AMP kinase (AMPK), which result in upregulation of PPARγ coactivator (PGC)-1α, PPARα and γ (Mattace Raso et al., 2013; Hong et al., 2016). Activation of PPARs induces mitochondria biogenesis through upregulating uncoupling proteins (UCPs) and increases fatty acid oxidation. It is speculated that this is an adiponectin-mediated pathway and recruitment of this pathway starts with upregulation of adiponectin receptors as a result of butyrate supplementation (Hong et al., 2016).
The Effects of Butyrate on CVD
Butyrate and Atherosclerosis
An increasing number of studies have found that butyrate can exert protective effects in atherosclerosis. Aguilar et al. first found that supplementation of diet with 1% butyrate could reduce atherosclerotic lesions in ApoE knockout mice by decreasing adhesion molecules production and reducing migration of macrophage to the lesion site (Aguilar et al., 2018). Further in vivo research has shown that incubation of macrophage and endothelial cells with butyrate (0.5 mM) for 2 h can increase interleukin-10 (IL-10) production meanwhile can decrease pro-inflammatory cytokines including tumor necrosis factor α (TNFα), IL-1β and IL-6 mainly through suppressing NF-κB pathway. The uptake of oxidized-low density lipoprotein (oxLDL) was also decreased by butyrate treated cells (Aguilar et al., 2018). In Kasahara et al. study, the mice group fed with a 6% tributyrin (TB)-supplemented diet had lower lipid deposition and macrophage accumulation in the lesion. It was also reported that butyrate resulted in improvement of gut permeability (Kasahara et al., 2018).
In high-fat diet-fed ApoE−/− mice, butyrate administration (200 and 400 mg/kg) influenced the microbial composition of the gut and improved diversity in favor of increasing Firmicutes specially Bacteroidetes (Du et al., 2020). Additionally, butyrate ameliorated atherosclerosis by downregulating genes involved in lipid metabolism including acyl-CoA thioesterase1 (Acot1), Acot2, Perilipin2 (Plin2), Plin5, Cytochrome4a (10,14 and 31 isoforms) (Du et al., 2020). Results of this study showed that, cytochrome P450 7A1 (CYP7A1) is upregulated in butyrate treated mice and negatively correlated with atherosclerotic lesions (Du et al., 2020). CYP7A1 controls bile acid biosynthesis and helps for the elimination of cholesterol in the liver. This evidence suggests a potential role of butyrate in bile acid metabolism (Li et al., 2013). In the same study, in vivo, ex vivo and in vitro investigations illustrated that butyrate induces ATP-binding cassette subfamily A member 1 (ABCA1) activity in both hepatocytes and macrophages via transcription factor specific protein 1 (Sp1), which causes reduced total cholesterol (TC) and also cholesterol deposition in plaque (Du et al., 2020). ABCA1 is a crucial transporter that contributes to cholesterol efflux toward the biosynthesis of high-density lipoprotein-cholesterol (HDL-C) (Sahoo et al., 2004). Previous studies have shown ABCA1 constrains the formation of foam cells, which are involved in the development of atherosclerosis (Chawla et al., 2001; Joyce et al., 2002).
Inflammation and oxidative stress are well-known in the pathogenesis of atherosclerosis (Ma et al., 2016). In Wang et al. study, butyrate (100 and 200 μM) showed anti-inflammatory and antioxidants effects on TNF-α induced human umbilical vein endothelial cells (HUVECs) by decreasing adhesion molecules (vascular cell adhesion molecule-1 (VCAM-1) and E-selectin) and subsequent THP-1 monocytes attachment, reducing pro-inflammatory cytokines including monocyte chemoattractant protein-1(MCP-1) and IL-8, attenuating oxidants ROS and 4-hydroxy nonenal (4-HNE), improving the protective function of kruple factor 2 (KLF2) via the extracellular-signal-regulated kinase 5 (ERK5) pathway (Wang et al., 2020).
Taken together, these findings indicate that athero-protective effects of butyrate are accompanied by regulating the expression of genes related to lipid and glucose metabolism, improving gut microbiota diversity, suppressing a wide range of inflammatory and oxidative processes, rescuing protective KLF2, and enhancing vascular health. Summary of the studies about the effects of butyrate on atherosclerosis are shown in Table 1.
Butyrate and Heart Failure
HF is a condition in which the heart’s ability to fill or evacuate blood is compromised (Jin et al., 2020). HF can be caused by any problem that affects the anatomical and/or functional integrity of the heart, such as valve, coronary, or myocardial disease (Luedde et al., 2017).
In a recent study by Mollar et al., butyrate was negatively associated with area under the concentration curve (AUC-H2) in patients with HF, implying that butyrate is lower in patients with higher exhaled hydrogen test (Mollar et al., 2021). Although hydrogen breath tests are not the gold standard for diagnosing small intestinal bacterial overgrowth (SIBO), the results of the aforementioned study suggest that dysbiosis followed by reduced levels of butyrate could play a role in the pathology of HF (Ghoshal, 2011; Mollar et al., 2021). The findings of both in vivo and in vitro experiments show that butyrate exerts histological cardio-protective effects. Badejogbin et al. examined the impact of SB (200 mg/kg) on heart tissue damage in rats fed either chow or high fat diet (HFD) (Badejogbin et al., 2019). In that research, butyrate significantly ameliorated HFD induced cardio-metabolic abnormalities including hyperlipidemia and glucose dysmetabolism as well as elevated plasma malondialdehyde, corticosterone, and lactate dehydrogenase (Badejogbin et al., 2019). Furthermore, the histological study revealed that butyrate improved cardiac tissue infarction, infiltration, and fibrosis. Butyrate has been shown to protect cardiac tissue architecture and integrity by lowering uric acid (plasma and cardiac tissue) and increasing glutathione antioxidant defenses (Badejogbin et al., 2019).
The incubation of endothelin-1 (ET1) induced cardiomyocytes derived from neonatal rats with butyrate (1–4 mM) inhibited hypertrophic growth of cardiomyocytes by epigenetic gene expression alterations (Umei, 2020). In the same study, transcriptome analysis demonstrated that FFARs didn’t have an expression in cardiomyocytes therefore protective anti-hypertrophic action is related to HDAC inhibitory role of butyrate.
In a study conducted by Jiang et al., intraperitoneal butyrate administration (7.5 mg/kg) in rats with myocardial infarction reduced the region of infarction and increased cardiac function by enhancing M2 macrophage polarization, downregulating the expression of inflammatory response-related genes, and suppressing sympathetic nerve remodeling (Jiang et al., 2020). Moreover, SCFAs have been found to influence sympathetic neurons, with the effects mostly relying on the vagus nerve. It seems gut and brain are interconnected mostly via the vagus nerve (Yu et al., 2020).
A growing body of evidence suggests that in addition to butyrate’s action on anatomical features of the heart, butyrate may also act through gut-brain neurological processes especially vagal afferent pathway (Li et al., 2018; Onyszkiewicz et al., 2019; Muller et al., 2020; Yu et al., 2020). In this context, li et al. research showed that SB supplementation (5% w/w) improved energy metabolism in HFD fed rats, through the gut-brain neural circuit and these effects diminished after vagotomy (Li et al., 2018). Yu et al. investigated the effect of SB (200 mmol/L) on reperfusion injury in rats underwent vagotomy + myocardial ischemia/reperfusion (I/R) injury or I/R injury alone (Yu et al., 2021). According to previous studies, reperfusion can reduce cardiac function and is associated with an increased risk of HF (Goel et al., 2013). Butyrate significantly reduced infarct size and myocardial damage indicators (plasma lactate dehydrogenase (LDH), creatine kinase (CK), and CK-MB levels). Likewise, butyrate treated rats showed a decrease in I/R-induced oxidative stress, inflammation, and apoptosis. Nonetheless, these effects reversed with a vagotomy (Yu et al., 2021). It is speculated that butyrate improves myocardial I/R injury through the gut-brain neural circuit, and this cardio-protective effect is probably mediated by suppressing sympathetic nervous system. Summary of the studies about the effects of butyrate on HF are shown in Table 2.
Butyrate, Hypertension, and Vascular Health
It is hypothesized that butyrate’s modulatory effects on BP occur through its interaction with the circulatory system. In Sprague-Dawley rats, intramedullary butyrate treatment decreased angiotensin II (Ang II) ‐induced mean arterial pressure via suppression of (pro) renin receptor (PRR) and its subsequent intrarenal renin-angiotensin system (Wang et al., 2017).
Zhang et al. showed that SB administration (1 g/kg/d) inhibited the activation of the cyclooxygenase-2 (COX-2)/prostaglandin E2 (PGE2) pathway in a HDAC5/HDAC6-dependent manner, contributing to reducing Ang II-induced heart hypertrophy, mean arterial pressure and inflammation (Zhang et al., 2019). Robles-Vera et al. investigated the cardiovascular effects of butyrate (0.5 mg kg day) in spontaneously hypertensive rats (SHR) and control Wistar Kyoto (WKY) rats (Robles‐Vera et al., 2020). Butyrate decreased both systolic and diastolic BP and returned T-helper 17 (Th17)/regulatory T cells (Treg) balance in the SHR to WKY rat levels. These effects are mediated by lowering endotoxemia and increasing Treg cells in the vasculature (Robles‐Vera et al., 2020). Butyrate in the blood circulation stimulates FFAR3 which is found in veins and contributes to vascular tone. FFAR3 is a hypotensive protein, dilates resistance vessels in an endothelium-dependent manner (Natarajan et al., 2016). Furthermore, FFAR2 and FFAR3 have expression in nerves and evidence revealed their expression is higher in WKY than in SHR rats, therefore, intra brain butyrate administration in WKY had a higher drop in BP than SHR (Toral et al., 2019; Yang et al., 2019). Onyszkiewicz et al. showed that butyrate administration (1.4, 2.8, and 5.8 mmol/kg, intracolonic) caused dose-dependent BP reduction in rats fed a standard diet. It seems these hypotensive effects are mediated via afferent colonic vagus nerve vasorelaxation signaling and FFAR2/3 (Onyszkiewicz et al., 2019).
Nutting and Mortesen studies on arties showed that butyrate causes endothelial-dependent vasodilation in the arteries by increasing cyclic AMP (cAMP) levels (Mortensen et al., 1990; Nutting et al., 1991). In Morikawa et al. study, butyrate administration (1 mM) enhanced nitric oxide (NO) production in interferon treated vascular endothelial cells via increasing expression of inducible NO synthase (iNOS) (Morikawa et al., 2004). It is well known that NO stimulates vasodilation, reduces inflammation, and lowers BP (Napoli et al., 2010). Summary of the studies about the effects of butyrate on hypertension and vascular health are shown in Table 3.
Butyrate and CVD Risk Factors
Butyrate and Obesity
Several scientific studies confirm obesity as an independent risk factor for CVD, as well as one of the factors increasing the risk of diseases associated with CVD such as dyslipidemia, IR, hypertension, and atherosclerosis (Cercato and Fonseca, 2019). In a recent review by Bridgeman et al., out of 14 studies that examined the effects of butyrate on obesity in HFD fed animals, butyrate significantly was reduced weight gain in 10 studies (Bridgeman et al., 2020). Butyrate exerts its anti-obesity effects mainly through contributing in energy balance equation. First, it reduces calorie intake by reducing appetite and preventing food intake. Second, butyrate increases energy expenditure by affecting metabolic pathways. According to the previous studies, butyrate reduces appetite by increasing anorexic hormones like peptide YY (PYY), glucagon-like peptide 1 (GLP1) through activating FFARs (Lin et al., 2012; Steinert et al., 2017). In a study by li et al., acute and chronic oral butyrate administration (5% (w/w) SB) by attenuating hypothalamic neuronal signaling decreased food intake in HFD mice (Li et al., 2018). However, butyrate had no effect on food intake after vagotomy in mice, suggesting the gut-brain neural circuit involvement. Since it is shown that GLP-1 contributed in the satiety, it may affect the vagal nerve (Li et al., 2018). In addition, butyrate induces the secretion of leptin and adiponectin from adipocytes which are involved in appetite and food intake control (Hong et al., 2016; Yu et al., 2017; Hafidi et al., 2019).
Evidence shows that butyrate increases thermogenesis and promotes fat oxidation by activating brown adipose tissue (BAT), thereby enhancing energy expenditure in the body. Li and hong et al. studies reported that rats consuming butyrate in their diet increased expression of UCPs in BAT and skeletal muscles (Hong et al., 2016; Li et al., 2018). UCPs are mitochondrial proteins and involved in facilitating heat production (thermogenesis) (Ricquier and Bouillaud, 2000). Moreover, the level of tyrosine hydroxylase protein was elevated in BAT by butyrate, which is linked to sympathetic nervous system activity. Therefore, butyrate-induced appetite reduction and BAT activation may depend on gut-brain neural circuitry and vagal nerve signaling (Li et al., 2018).
However, there are no randomized clinical trials that confirm the anti-obesity effect of butyrate and still need to be studied. There is no doubt that weight management strategies can help to combat obesity-related diseases including CVDs (Ebbert et al., 2014). As a result, butyrate supplementation can be considered as an emerging anti-obesogenic agent in the prevention and treatment of obesity and cardio-metabolic disease.
Butyrate and Dyslipidemia
Several studies have demonstrated that butyrate has beneficial effects on dyslipidemia and may be helpful for lowering the risk of CVD, particularly atherosclerosis. We will review the effects of butyrate on triglycerides and cholesterol. The effect of butyrate on triglyceride levels was inconsistent in different studies. Du and Hong et al. reported that butyrate supplementation did not change triglyceride levels (Hong et al., 2016; Du et al., 2020), where as in Li and khan studies butyrate significantly decreased triglyceride levels (Khan and Jena, 2016; Li et al., 2018). Evidence suggests that butyrate has effects on adipogenesis, lipogenesis, and lipolysis (Toscani et al., 1990; Lu et al., 2012; Li et al., 2014; Rumberger et al., 2014; Yan and Ajuwon, 2015; Yu et al., 2017). The positive effect of butyrate on adipogenesis has been reported to be more pronounced. As previously discussed, butyrate promotes adipogenesis by activating the PPAR pathway, thereby reducing circulating fatty acids and their accumulation in vital organs (Hafidi et al., 2019). Furthermore, in Aguilar et al. study, remodeling and proliferation markers such as matrix metalloproteinases (MMP2 and MMP9) and proliferating cell nuclear antigen (PCNA) increased following the increase in the expression of PPAR (Aguilar et al., 2018). MMPs and PCNA are required components for adipogenesis (Blaut and Clavel, 2007; Bauters et al., 2015). Hence, butyrate prevents metabolic disorders such as IR, dyslipidemia, and fatty liver by increasing adipogenesis.
The effect of butyrate on lipogenesis and lipolysis is contradictory and seems to be due to differences in the dose, duration of butyrate treatment, and cell phenotype in the in vitro studies. Therefore, since butyrate increases lipolysis in a number of studies and inhibits lipolysis in others, the definitive conclusion about the effect of butyrate on lipid metabolism in adipocytes requires further studies.
Butyrate supplementation has been shown to lower total serum cholesterol in a number of animal studies (Gao et al., 2009; Mattace Raso et al., 2013; Khan and Jena, 2016; Mollica et al., 2017; Zhao et al., 2017; Hu et al., 2018) Cholesterol in the body has two sources, dietary cholesterol and endogenous cholesterol (Kapourchali et al., 2016). According to studies, butyrate can affect both pathways of endogenous cholesterol biosynthesis and dietary cholesterol uptake. Butyrate treatment (200 and 400 mg kg) in HFD fed mice caused reduction in non-high-density lipoprotein cholesterol (non-HDL-C), low-density lipoprotein cholesterol (LDL-C), and total cholesterol (TC) (Du et al., 2020). It is noteworthy that regression analyze revealed that non-HDL-C, LDL-C and TC were positively correlated with percentage of aortic lesions, suggesting that butyrate by down-regulating fatty acid synthesis genes, modifies serum lipid levels and inhibits the progression of atherosclerosis (Du et al., 2020). Alvaro and Marcil reported butyrate decreased 3-hydroxy-3-methyl-glutaryl-coenzyme A (HMG-CoA) reductase gene expression and activity in Caco-2 cells (Marcil et al., 2003; Alvaro et al., 2008). The effect of butyrate on cholesterol biosynthesis is mainly through the downregulation of genes involved in cholesterol synthesis such as isopentenyl diphosphate isomerase, dimethylallyl/geranyl trans-transferase and farnesyl-diphosphatase farnesyltransferase. In addition, butyrate prevents intestinal absorption of cholesterol and lowers cholesterol levels. Chen et al. reported that butyrate downregulated Niemann-Pick C1-Like 1 which is involved in intestinal cholesterol uptake (Chen et al., 2018).
Butyrate and Insulin Resistance
There is considerable evidence that both IR and its clinical manifestation, metabolic syndrome, are linked to CVDs (Abdul-Ghani et al., 2019). Numerous studies have shown butyrate can cause an increase in insulin sensitivity by increasing activity of insulin receptors through enhancing insulin receptor substrates. As a result of increased insulin signaling, glucose uptake by cells increases and hyperglycemia alleviates (Yan and Ajuwon, 2015). An increase in glucose transporters (GLUTs) including GLUT2 and GLUT4 after treatment by butyrate has been reported which is further support this hypothesis (Mollica et al., 2017). As mentioned earlier butyrate is also involved in improving insulin sensitivity by increasing adipogenesis (Hafidi et al., 2019). Furthermore, there is evidence indicating that butyrate is effective in reducing hyperglycemia by reducing the expression of gluconeogenesis related genes (Mihaylova et al., 2011; Khan and Jena, 2016). On the other hand, oxidative stress can cause IR with disrupting insulin signaling (Evans et al., 2005). SB administration has been found to reduce oxidative stress in a variety of tissues and cells (Sun et al., 2019). SB induces nuclear factor E2-related factor 2 (Nrf2) and increases expression of downstream antioxidant enzymes, thus contributes in the amelioration of oxidative stress and IR (Sun et al., 2019).
Knowledge Gaps and Future Directions
Numerous in vivo and in vitro studies have been conducted exploring the direct and indirect cardiovascular protective capacities of butyrate. The existing body of research on butyrate efficacy suggests that butyrate health promoting effects are mainly due to its two main properties being HDAC inhibitor and activating FFARs. However, in most studies it is not clear exactly, these positive effects are related to which feature. In addition, recent studies have shown new ability such as binding to PPARs that require further study.
However, there is a paucity of clinical trials evaluating efficacy of butyrate supplementation in CVD prevention and treatment. Hence, the main knowledge gap is the lack of human clinical trials to investigate therapeutic benefits of butyrate in CVD. Certainly, large-scale clinical trials with detailed insights of the mechanisms involved are required to confirm the promising effects of butyrate in the management of CVD.
Based on evidence, small amounts of butyrate reaches systemic circulation due to high hepatic clearance (van der Beek et al., 2015). Consequently, placebo-controlled trials of butyrate supplements and butyrate generating bacteria are recommended to determine whether they are effective at increasing systemic butyrate levels. Moreover, Clinical trials are needed to examine the effects of butyrate on AMPK signaling pathway factors like adiponectin receptors, PGC-1α and UCPs which are contributed in energy hemostasis and lipid metabolism.
Conclusion
Butyrate has been shown favorable effects in the animal models of CVD as well as CVD-related risk factors such as obesity, dyslipidemia and IR. The beneficial results of butyrate go beyond the gut and it affects various organs as shown in Figure 2. Although butyrate exhibits a wide variety of biological activities in different pathways including energy homeostasis, glucose and lipid metabolism, inflammation, oxidative stress, neural signaling, and epigenetic modulation in experimental settings, it remains unclear whether these findings are clinically relevant and whether the molecular pathways are activated by butyrate in humans. Considering several factors that may contribute to cardio-protective activities of butyrate, further well-designed studies are needed to focus on these factors. Understanding exact mechanisms of butyrate in humans will facilitate the application of butyrate as a safe supplement in prevention and management of CVD.
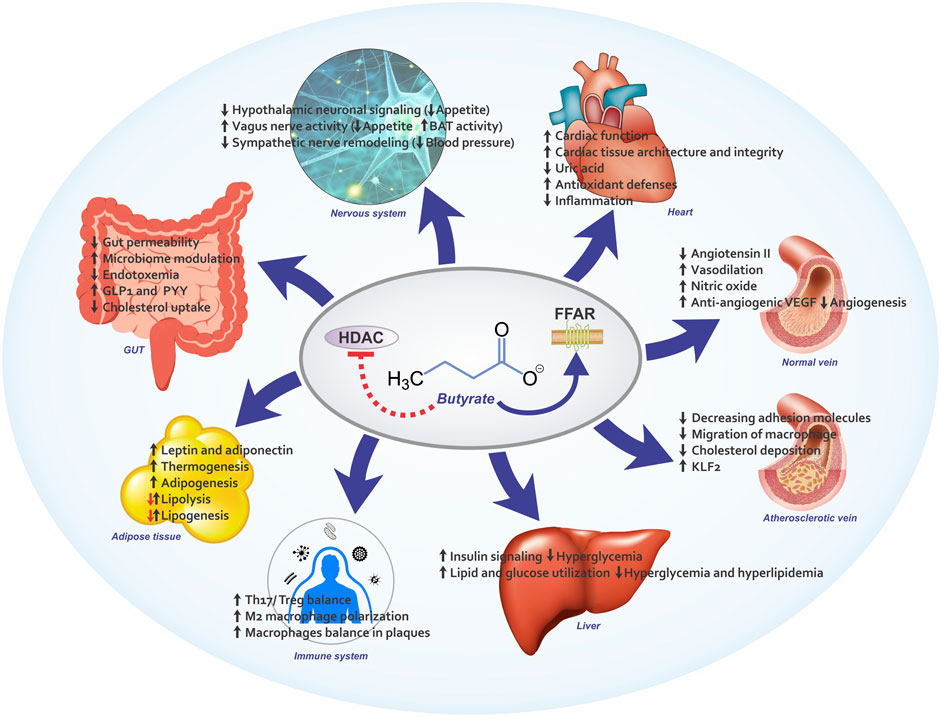
FIGURE 2. An overview of butyrate’s protective effects in CVD and CVD risk factors. FFAR: free fatty acid receptor, HDAC: Histone deacetylase, KLF2: Kruppel Like Factor 2,VEGF: vascular endothelial growth factor, GLP-1: glucagon-like peptide 1, PYY, peptide YY.
Author Contributions
The authors’ responsibilities were as follows PA and NR: wrote the original manuscript and contributed to the conception of the article; EM, SA, and HT: contributed to data collection and figures designing; SH and ShG: provided advice and consultation; SaG: contributed to the final revision of the manuscript, and all authors: read and approved the final manuscript.
Conflict of Interest
The authors declare that the research was conducted in the absence of any commercial or financial relationships that could be construed as a potential conflict of interest.
Publisher’s Note
All claims expressed in this article are solely those of the authors and do not necessarily represent those of their affiliated organizations, or those of the publisher, the editors and the reviewers. Any product that may be evaluated in this article, or claim that may be made by its manufacturer, is not guaranteed or endorsed by the publisher.
Reference
Abdul-Ghani, M. A., Jayyousi, A., DeFronzo, R. A., Asaad, N., and Al-Suwaidi, J. (2019). Insulin Resistance the Link between T2DM and CVD: Basic Mechanisms and Clinical Implications. Curr. Vasc. Pharmacol. 17 (2), 153–163. doi:10.2174/1570161115666171010115119
Aguilar, E. C., da Silva, J. F., Navia-Pelaez, J. M., Leonel, A. J., Lopes, L. G., Menezes-Garcia, Z., et al. (2018). Sodium Butyrate Modulates Adipocyte Expansion, Adipogenesis, and Insulin Receptor Signaling by Upregulation of PPAR-γ in Obese Apo E Knockout Mice. Nutrition 47, 75–82. doi:10.1016/j.nut.2017.10.007
Ahmad, A. F., Dwivedi, G., O'Gara, F., Caparros-Martin, J., and Ward, N. C. (2019). The Gut Microbiome and Cardiovascular Disease: Current Knowledge and Clinical Potential. Am. J. Physiol. Heart Circ. Physiol. 317 (5), H923–H938. doi:10.1152/ajpheart.00376.2019
Alex, S., Lange, K., Amolo, T., Grinstead, J. S., Haakonsson, A. K., Szalowska, E., et al. (2013). Short-chain Fatty Acids Stimulate Angiopoietin-like 4 Synthesis in Human colon Adenocarcinoma Cells by Activating Peroxisome Proliferator-Activated Receptor γ. Mol. Cel Biol 33 (7), 1303–1316. doi:10.1128/MCB.00858-12
Alvaro, A., Solà, R., Rosales, R., Ribalta, J., Anguera, A., Masana, L., et al. (2008). Gene Expression Analysis of a Human Enterocyte Cell Line Reveals Downregulation of Cholesterol Biosynthesis in Response to Short-Chain Fatty Acids. IUBMB life 60 (11), 757–764. doi:10.1002/iub.110
Are, A., Aronsson, L., Wang, S., Greicius, G., Lee, Y. K., Gustafsson, J. A., et al. (2008). Enterococcus faecalis from Newborn Babies Regulate Endogenous PPARgamma Activity and IL-10 Levels in Colonic Epithelial Cells. Proc. Natl. Acad. Sci. U S A. 105 (6), 1943–1948. doi:10.1073/pnas.0711734105
Badejogbin, C., Areola, D. E., Olaniyi, K. S., Adeyanju, O. A., and Adeosun, I. O. (2019). Sodium Butyrate Recovers High-Fat Diet-Fed Female Wistar Rats from Glucose Dysmetabolism and Uric Acid-Associated Cardiac Tissue Damage. Naunyn Schmiedebergs Arch. Pharmacol. 392 (11), 1411–1419. doi:10.1007/s00210-019-01679-2
Bauters, D., Scroyen, I., Van Hul, M., and Lijnen, H. R. (2015). Gelatinase A (MMP-2) Promotes Murine Adipogenesis. Biochim. Biophys. Acta 1850 (7), 1449–1456. doi:10.1016/j.bbagen.2015.04.003
Blaut, M., and Clavel, T. (2007). Metabolic Diversity of the Intestinal Microbiota: Implications for Health and Disease. J. Nutr. 137 (3), 751S–5S. doi:10.1093/jn/137.3.751S
Bridgeman, S. C., Northrop, W., Melton, P. E., Ellison, G. C., Newsholme, P., and Mamotte, C. D. S. (2020). Butyrate Generated by Gut Microbiota and its Therapeutic Role in Metabolic Syndrome. Pharmacol. Res. 160, 105174. doi:10.1016/j.phrs.2020.105174
Brown, A. J., Goldsworthy, S. M., Barnes, A. A., Eilert, M. M., Tcheang, L., Daniels, D., et al. (2003). The Orphan G Protein-Coupled Receptors GPR41 and GPR43 Are Activated by Propionate and Other Short Chain Carboxylic Acids. J. Biol. Chem. 278 (13), 11312–11319. doi:10.1074/jbc.M211609200
Brown, J. M., and Hazen, S. L. (2015). The Gut Microbial Endocrine Organ: Bacterially Derived Signals Driving Cardiometabolic Diseases. Annu. Rev. Med. 66, 343–359. doi:10.1146/annurev-med-060513-093205
Cercato, C., and Fonseca, F. A. (2019). Cardiovascular Risk and Obesity. Diabetol. Metab. Syndr. 11 (1), 74–15. doi:10.1186/s13098-019-0468-0
Chang, P. V., Hao, L., Offermanns, S., and Medzhitov, R. (2014). The Microbial Metabolite Butyrate Regulates Intestinal Macrophage Function via Histone Deacetylase Inhibition. Proc. Natl. Acad. Sci. U S A. 111 (6), 2247–2252. doi:10.1073/pnas.1322269111
Chawla, A., Boisvert, W. A., Lee, C. H., Laffitte, B. A., Barak, Y., Joseph, S. B., et al. (2001). A PPAR Gamma-LXR-ABCA1 Pathway in Macrophages Is Involved in Cholesterol Efflux and Atherogenesis. Mol. Cel 7 (1), 161–171. doi:10.1016/s1097-2765(01)00164-2
Chen, W., Zhang, S., Wu, J., Ye, T., Wang, S., Wang, P., et al. (2020). Butyrate-producing Bacteria and the Gut-Heart axis in Atherosclerosis. Clin. Chim. Acta 507, 236–241. doi:10.1016/j.cca.2020.04.037
Chen, Y., Xu, C., Huang, R., Song, J., Li, D., and Xia, M. (2018). Butyrate from Pectin Fermentation Inhibits Intestinal Cholesterol Absorption and Attenuates Atherosclerosis in Apolipoprotein E-Deficient Mice. J. Nutr. Biochem. 56, 175–182. doi:10.1016/j.jnutbio.2018.02.011
Chitrala, K. N., Guan, H., Singh, N. P., Busbee, B., Gandy, A., Mehrpouya-Bahrami, P., et al. (2017). CD44 Deletion Leading to Attenuation of Experimental Autoimmune Encephalomyelitis Results from Alterations in Gut Microbiome in Mice. Eur. J. Immunol. 47 (7), 1188–1199. doi:10.1002/eji.201646792
Ciura, J., and Jagodziński, P. P. (2010). Butyrate Increases the Formation of Anti-angiogenic Vascular Endothelial Growth Factor Variants in Human Lung Microvascular Endothelial Cells. Mol. Biol. Rep. 37 (8), 3729–3734. doi:10.1007/s11033-010-0026-1
Cleophas, M. C., Ratter, J. M., Quintin, K., Schraa, J. K., Stroes, E. S., Netea, M. G., et al. (2019). Effects of Oral Butyrate Supplementation on Inflammatory Potential of Circulating Peripheral Blood Mononuclear Cells in Healthy and Obese Males. Scientific Rep. (Nature Publ. Group) 9 (1).
Den Besten, G., Bleeker, A., Gerding, A., van Eunen, K., Havinga, R., van Dijk, T. H., et al. (2015). Short-Chain Fatty Acids Protect against High-Fat Diet-Induced Obesity via a PPARγ-dependent Switch from Lipogenesis to Fat Oxidation. Diabetes 64 (7), 2398–2408. doi:10.2337/db14-1213
Du, Y., Li, X., Su, C., Xi, M., Zhang, X., Jiang, Z., et al. (2020). Butyrate Protects against High-Fat Diet-Induced Atherosclerosis via Up-Regulating ABCA1 Expression in Apolipoprotein E-Deficiency Mice. Br. J. Pharmacol. 177 (8), 1754–1772. doi:10.1111/bph.14933
Ebbert, J. O., Elrashidi, M. Y., and Jensen, M. D. (2014). Managing Overweight and Obesity in Adults to Reduce Cardiovascular Disease Risk. Curr. Atheroscler. Rep. 16 (10), 445. doi:10.1007/s11883-014-0445-x
Evans, J. L., Maddux, B. A., and Goldfine, I. D. (2005). The Molecular Basis for Oxidative Stress-Induced Insulin Resistance. Antioxid. Redox Signal. 7 (7-8), 1040–1052. doi:10.1089/ars.2005.7.1040
Gao, Z., Yin, J., Zhang, J., Ward, R. E., Martin, R. J., Lefevre, M., et al. (2009). Butyrate Improves Insulin Sensitivity and Increases Energy Expenditure in Mice. Diabetes 58 (7), 1509–1517. doi:10.2337/db08-1637
Ghoshal, U. C. (2011). How to Interpret Hydrogen Breath Tests. J. Neurogastroenterol Motil. 17 (3), 312–317. doi:10.5056/jnm.2011.17.3.312
Goel, K., Pinto, D. S., and Gibson, C. M. (2013). Association of Time to Reperfusion with Left Ventricular Function and Heart Failure in Patients with Acute Myocardial Infarction Treated with Primary Percutaneous Coronary Intervention: a Systematic Review. Am. Heart J. 165 (4), 451–467. doi:10.1016/j.ahj.2012.11.014
Gomez-Arango, L. F., Barrett, H. L., McIntyre, H. D., Callaway, L. K., Morrison, M., and Dekker Nitert, M. (2016). Increased Systolic and Diastolic Blood Pressure Is Associated with Altered Gut Microbiota Composition and Butyrate Production in Early Pregnancy. Hypertension 68 (4), 974–981. doi:10.1161/HYPERTENSIONAHA.116.07910
Hafidi, M. E., Buelna-Chontal, M., Sánchez-Muñoz, F., and Carbó, R. (2019). Adipogenesis: a Necessary but Harmful Strategy. Int. J. Mol. Sci. 20 (15), 3657. doi:10.3390/ijms20153657
Hong, J., Jia, Y., Pan, S., Jia, L., Li, H., Han, Z., et al. (2016). Butyrate Alleviates High Fat Diet-Induced Obesity through Activation of Adiponectin-Mediated Pathway and Stimulation of Mitochondrial Function in the Skeletal Muscle of Mice. Oncotarget 7 (35), 56071–56082. doi:10.18632/oncotarget.11267
Hu, Y., Liu, J., Yuan, Y., Chen, J., Cheng, S., Wang, H., et al. (2018). Sodium Butyrate Mitigates Type 2 Diabetes by Inhibiting PERK-CHOP Pathway of Endoplasmic Reticulum Stress. Environ. Toxicol. Pharmacol. 64, 112–121. doi:10.1016/j.etap.2018.09.002
Hulten, E. A., Bittencourt, M. S., Preston, R., Singh, A., Romagnolli, C., Ghoshhajra, B., et al. (2017). Obesity, Metabolic Syndrome and Cardiovascular Prognosis: from the Partners Coronary Computed Tomography Angiography Registry. Cardiovasc. Diabetol. 16 (1), 14–11. doi:10.1186/s12933-017-0496-8
Jiang, X., Huang, X., Tong, Y., and Gao, H. (2020). Butyrate Improves Cardiac Function and Sympathetic Neural Remodeling Following Myocardial Infarction in Rats. Can. J. Physiol. Pharmacol. 98 (6), 391–399. doi:10.1139/cjpp-2019-0531
Jin, L., Shi, X., Yang, J., Zhao, Y., Xue, L., Xu, L., et al. (2020). Gut Microbes in Cardiovascular Diseases and Their Potential Therapeutic Applications. Protein & Cell 12, 1–14. doi:10.1007/s13238-020-00785-9
Joyce, C. W., Amar, M. J., Lambert, G., Vaisman, B. L., Paigen, B., Najib-Fruchart, J., et al. (2002). The ATP Binding Cassette Transporter A1 (ABCA1) Modulates the Development of Aortic Atherosclerosis in C57BL/6 and apoE-Knockout Mice. Proc. Natl. Acad. Sci. U S A. 99 (1), 407–412. doi:10.1073/pnas.012587699
Kapourchali, F. R., Surendiran, G., Goulet, A., and Moghadasian, M. H. (2016). The Role of Dietary Cholesterol in Lipoprotein Metabolism and Related Metabolic Abnormalities: a Mini-Review. Crit. Rev. Food Sci. Nutr. 56 (14), 2408–2415. doi:10.1080/10408398.2013.842887
Karlsson, F. H., Fåk, F., Nookaew, I., Tremaroli, V., Fagerberg, B., Petranovic, D., et al. (2012). Symptomatic Atherosclerosis Is Associated with an Altered Gut Metagenome. Nat. Commun. 3 (1), 1245–1248. doi:10.1038/ncomms2266
Kasahara, K., Krautkramer, K. A., Org, E., Romano, K. A., Kerby, R. L., Vivas, E. I., et al. (2018). Interactions between Roseburia Intestinalis and Diet Modulate Atherogenesis in a Murine Model. Nat. Microbiol. 3 (12), 1461–1471. doi:10.1038/s41564-018-0272-x
Khan, S., and Jena, G. (2016). Sodium Butyrate Reduces Insulin-Resistance, Fat Accumulation and Dyslipidemia in Type-2 Diabetic Rat: a Comparative Study with Metformin. Chem. Biol. Interact 254, 124–134. doi:10.1016/j.cbi.2016.06.007
Korecka, A., de Wouters, T., Cultrone, A., Lapaque, N., Pettersson, S., Doré, J., et al. (2013). ANGPTL4 Expression Induced by Butyrate and Rosiglitazone in Human Intestinal Epithelial Cells Utilizes Independent Pathways. Am. J. Physiol. Gastrointest. Liver Physiol. 304 (11), G1025–G1037. doi:10.1152/ajpgi.00293.2012
Kummen, M., Mayerhofer, C. C. K., Vestad, B., Broch, K., Awoyemi, A., Storm-Larsen, C., et al. (2018). Gut Microbiota Signature in Heart Failure Defined from Profiling of 2 Independent Cohorts. J. Am. Coll. Cardiol. 71 (10), 1184–1186. doi:10.1016/j.jacc.2017.12.057
Lau, K., Srivatsav, V., Rizwan, A., Nashed, A., Liu, R., Shen, R., et al. (2017). Bridging the gap between Gut Microbial Dysbiosis and Cardiovascular Diseases. Nutrients 9 (8), 859. doi:10.3390/nu9080859
Ley, R. E., Peterson, D. A., and Gordon, J. I. (2006). Ecological and Evolutionary Forces Shaping Microbial Diversity in the Human Intestine. Cell 124 (4), 837–848. doi:10.1016/j.cell.2006.02.017
Li, G., Yao, W., and Jiang, H. (2014). Short-chain Fatty Acids Enhance Adipocyte Differentiation in the Stromal Vascular Fraction of Porcine Adipose Tissue. J. Nutr. 144 (12), 1887–1895. doi:10.3945/jn.114.198531
Li, T., Francl, J. M., Boehme, S., and Chiang, J. Y. (2013). Regulation of Cholesterol and Bile Acid Homeostasis by the Cholesterol 7α-Hydroxylase/steroid Response Element-Binding Protein 2/microRNA-33a axis in Mice. Hepatology 58 (3), 1111–1121. doi:10.1002/hep.26427
Li, Z., Yi, C. X., Katiraei, S., Kooijman, S., Zhou, E., Chung, C. K., et al. (2018). Butyrate Reduces Appetite and Activates Brown Adipose Tissue via the Gut-Brain Neural Circuit. Gut 67 (7), 1269–1279. doi:10.1136/gutjnl-2017-314050
Lin, H. V., Frassetto, A., Kowalik, E. J., Nawrocki, A. R., Lu, M. M., Kosinski, J. R., et al. (2012). Butyrate and Propionate Protect against Diet-Induced Obesity and Regulate Gut Hormones via Free Fatty Acid Receptor 3-independent Mechanisms. PloS one 7 (4), e35240. doi:10.1371/journal.pone.0035240
Louis, P., and Flint, H. J. (2009). Diversity, Metabolism and Microbial Ecology of Butyrate-Producing Bacteria from the Human Large Intestine. FEMS Microbiol. Lett. 294 (1), 1–8. doi:10.1111/j.1574-6968.2009.01514.x
Lu, H., Su, S., and Ajuwon, K. M. (2012). Butyrate Supplementation to Gestating Sows and Piglets Induces Muscle and Adipose Tissue Oxidative Genes and Improves Growth Performance. J. Anim. Sci. 90 Suppl 4 (Suppl. l_4), 430–432. doi:10.2527/jas.53817
Luedde, M., Winkler, T., Heinsen, F. A., Rühlemann, M. C., Spehlmann, M. E., Bajrovic, A., et al. (2017). Heart Failure Is Associated with Depletion of Core Intestinal Microbiota. ESC Heart Fail. 4 (3), 282–290. doi:10.1002/ehf2.12155
Ma, S., Tian, X. Y., Zhang, Y., Mu, C., Shen, H., Bismuth, J., et al. (2016). E-selectin-targeting Delivery of microRNAs by Microparticles Ameliorates Endothelial Inflammation and Atherosclerosis. Sci. Rep. 6 (1), 22910–22911. doi:10.1038/srep22910
Marcil, V., Delvin, E., Garofalo, C., and Levy, E. (2003). Butyrate Impairs Lipid Transport by Inhibiting Microsomal Triglyceride Transfer Protein in Caco-2 Cells. J. Nutr. 133 (7), 2180–2183. doi:10.1093/jn/133.7.2180
Mattace Raso, G., Simeoli, R., Russo, R., Iacono, A., Santoro, A., Paciello, O., et al. (2013). Effects of Sodium Butyrate and its Synthetic Amide Derivative on Liver Inflammation and Glucose Tolerance in an Animal Model of Steatosis Induced by High Fat Diet. PloS one 8 (7), e68626. doi:10.1371/journal.pone.0068626
Mell, B., Jala, V. R., Mathew, A. V., Byun, J., Waghulde, H., Zhang, Y., et al. (2015). Evidence for a Link between Gut Microbiota and Hypertension in the Dahl Rat. Physiol. Genomics 47 (6), 187–197. doi:10.1152/physiolgenomics.00136.2014
Mihaylova, M. M., Vasquez, D. S., Ravnskjaer, K., Denechaud, P. D., Yu, R. T., Alvarez, J. G., et al. (2011). Class IIa Histone Deacetylases Are Hormone-Activated Regulators of FOXO and Mammalian Glucose Homeostasis. Cell 145 (4), 607–621. doi:10.1016/j.cell.2011.03.043
Mollar, A., Marrachelli, V. G., Núñez, E., Monleon, D., Bodí, V., Sanchis, J., et al. (2021). Bacterial Metabolites Trimethylamine N-Oxide and Butyrate as Surrogates of Small Intestinal Bacterial Overgrowth in Patients with a Recent Decompensated Heart Failure. Sci. Rep. 11 (1), 6110–6116. doi:10.1038/s41598-021-85527-5
Mollica, M. P., Mattace Raso, G., Cavaliere, G., Trinchese, G., De Filippo, C., Aceto, S., et al. (2017). Butyrate Regulates Liver Mitochondrial Function, Efficiency, and Dynamics in Insulin-Resistant Obese Mice. Diabetes 66 (5), 1405–1418. doi:10.2337/db16-0924
Morikawa, A., Sugiyama, T., Koide, N., Mori, I., Mu, M. M., Yoshida, T., et al. (2004). Butyrate Enhances the Production of Nitric Oxide in Mouse Vascular Endothelial Cells in Response to Gamma Interferon. J. Endotoxin Res. 10 (1), 32–38. doi:10.1179/096805104225003852
Mortensen, F. V., Nielsen, H., Mulvany, M. J., and Hessov, I. (1990). Short Chain Fatty Acids Dilate Isolated Human Colonic Resistance Arteries. Gut 31 (12), 1391–1394. doi:10.1136/gut.31.12.1391
Muller, P. A., Schneeberger, M., Matheis, F., Wang, P., Kerner, Z., Ilanges, A., et al. (2020). Author Correction: Microbiota Modulate Sympathetic Neurons via a Gut-Brain Circuit. Nature 585 (7816), E2–E446. doi:10.1038/s41586-020-2657-2
Napoli, C., Lerman, L. O., Balestrieri, M. L., and Ignarro, L. J. (2010). “Nitric Oxide in Vascular Damage and Regeneration,” in Nitric Oxide (Elsevier), 629–672. doi:10.1016/b978-0-12-373866-0.00020-4
Natarajan, N., Hori, D., Flavahan, S., Steppan, J., Flavahan, N. A., Berkowitz, D. E., et al. (2016). Microbial Short Chain Fatty Acid Metabolites Lower Blood Pressure via Endothelial G Protein-Coupled Receptor 41. Physiol. Genomics 48 (11), 826–834. doi:10.1152/physiolgenomics.00089.2016
Nutting, C. W., Islam, S., and Daugirdas, J. T. (1991). Vasorelaxant Effects of Short Chain Fatty Acid Salts in Rat Caudal Artery. Am. J. Physiol. 261 (2), H561–H567. doi:10.1152/ajpheart.1991.261.2.H561
Oh, H. Y. P., Visvalingam, V., and Wahli, W. (2019). The PPAR-Microbiota-Metabolic Organ Trilogy to fine-tune Physiology. FASEB J. 33 (9), 9706–9730. doi:10.1096/fj.201802681RR
Onyszkiewicz, M., Gawrys-Kopczynska, M., Konopelski, P., Aleksandrowicz, M., Sawicka, A., Koźniewska, E., et al. (2019). Butyric Acid, a Gut Bacteria Metabolite, Lowers Arterial Blood Pressure via colon-vagus Nerve Signaling and GPR41/43 Receptors. Pflugers Arch. 471 (11), 1441–1453. doi:10.1007/s00424-019-02322-y
Ricquier, D., and Bouillaud, F. (2000). Mitochondrial Uncoupling Proteins: from Mitochondria to the Regulation of Energy Balance. J. Physiol. 529 (1), 3–10. doi:10.1111/j.1469-7793.2000.00003.x
Robles‐Vera, I., Toral, M., de la Visitación, N., Sánchez, M., Gómez-Guzmán, M., Romero, M., et al. (2020). Probiotics Prevent Dysbiosis and the Rise in Blood Pressure in Genetic Hypertension: Role of Short‐chain Fatty Acids. Mol. Nutr. Food Res. 64 (6), 1900616. doi:10.1002/mnfr.201900616
Roth, G. A., Mensah, G. A., Johnson, C. O., Addolorato, G., Ammirati, E., Baddour, L. M., et al. (2020). Global Burden of Cardiovascular Diseases and Risk Factors, 1990-2019: Update from the GBD 2019 Study. J. Am. Coll. Cardiol. 76 (25), 2982–3021. doi:10.1016/j.jacc.2020.11.010
Rumberger, J. M., Arch, J. R. S., and Green, A. (2014). Butyrate and Other Short-Chain Fatty Acids Increase the Rate of Lipolysis in 3T3-L1 Adipocytes. Butyrate and other short-chain fatty acids increase the rate of lipolysis in 3T3-L1 adipocytesPeerJ 2, e611. doi:10.7717/peerj.611
Sahoo, D., Trischuk, T. C., Chan, T., Drover, V. A., Ho, S., Chimini, G., et al. (2004). ABCA1-dependent Lipid Efflux to Apolipoprotein A-I Mediates HDL Particle Formation and Decreases VLDL Secretion from Murine Hepatocytes. J. Lipid Res. 45 (6), 1122–1131. doi:10.1194/jlr.M300529-JLR200
Santisteban, M. M., Qi, Y., Zubcevic, J., Kim, S., Yang, T., Shenoy, V., et al. (2017). Hypertension-linked Pathophysiological Alterations in the Gut. Circ. Res. 120 (2), 312–323. doi:10.1161/CIRCRESAHA.116.309006
Scott, K. P., Gratz, S. W., Sheridan, P. O., Flint, H. J., and Duncan, S. H. (2013). The Influence of Diet on the Gut Microbiota. Pharmacol. Res. 69 (1), 52–60. doi:10.1016/j.phrs.2012.10.020
Serino, M., Blasco-Baque, V., Nicolas, S., and Burcelin, R. (2014). Far from the Eyes, Close to the Heart: Dysbiosis of Gut Microbiota and Cardiovascular Consequences. Curr. Cardiol. Rep. 16 (11), 540. doi:10.1007/s11886-014-0540-1
Shakespear, M. R., Halili, M. A., Irvine, K. M., Fairlie, D. P., and Sweet, M. J. (2011). Histone Deacetylases as Regulators of Inflammation and Immunity. Trends Immunol. 32 (7), 335–343. doi:10.1016/j.it.2011.04.001
Singh, V., Yeoh, B. S., and Vijay-Kumar, M. (2016). Gut Microbiome as a Novel Cardiovascular Therapeutic Target. Curr. Opin. Pharmacol. 27, 8–12. doi:10.1016/j.coph.2016.01.002
Steinert, R. E., Feinle-Bisset, C., Asarian, L., Horowitz, M., Beglinger, C., and Geary, N. (2017). Ghrelin, CCK, GLP-1, and PYY(3-36): Secretory Controls and Physiological Roles in Eating and Glycemia in Health, Obesity, and after RYGB. Physiol. Rev. 97 (1), 411–463. doi:10.1152/physrev.00031.2014
Sun, B., Jia, Y., Yang, S., Zhao, N., Hu, Y., Hong, J., et al. (2019). Sodium Butyrate Protects against High-Fat Diet-Induced Oxidative Stress in Rat Liver by Promoting Expression of Nuclear Factor E2-Related Factor 2. Br. J. Nutr. 122 (4), 400–410. doi:10.1017/S0007114519001399
Toral, M., Robles-Vera, I., de la Visitación, N., Romero, M., Yang, T., Sánchez, M., et al. (2019). Critical Role of the Interaction Gut Microbiota - Sympathetic Nervous System in the Regulation of Blood Pressure. Front. Physiol. 10, 231. doi:10.3389/fphys.2019.00231
Toscani, A., Soprano, D. R., and Soprano, K. J. (1990). Sodium Butyrate in Combination with Insulin or Dexamethasone Can Terminally Differentiate Actively Proliferating Swiss 3T3 Cells into Adipocytes. J. Biol. Chem. 265 (10), 5722–5730. doi:10.1016/s0021-9258(19)39423-2
Trøseid, M. (2020). The Gut Microbiome in Coronary Artery Disease and Heart Failure: Current Knowledge and Future Directions. EBioMedicine 52, 102649.
Tsivgoulis, G., Safouris, A., Kim, D. E., and Alexandrov, A. V. (2018). Recent Advances in Primary and Secondary Prevention of Atherosclerotic Stroke. J. Stroke 20 (2), 145–166. doi:10.5853/jos.2018.00773
Umei, M. (2020). Protective Action of the Microbial Metabolite Butyrate against Cardiomyocyte Hypertrophy. Eur. Heart J. 41 (Suppl. ment_2), ehaa9463666. doi:10.1093/ehjci/ehaa946.3666
van der Beek, C. M., Bloemen, J. G., van den Broek, M. A., Lenaerts, K., Venema, K., Buurman, W. A., et al. (2015). Hepatic Uptake of Rectally Administered Butyrate Prevents an Increase in Systemic Butyrate Concentrations in Humans. J. Nutr. 145 (9), 2019–2024. doi:10.3945/jn.115.211193
Wang, L., Zhu, Q., Lu, A., Liu, X., Zhang, L., Xu, C., et al. (2017). Sodium Butyrate Suppresses Angiotensin II-Induced Hypertension by Inhibition of Renal (Pro)renin Receptor and Intrarenal Renin-Angiotensin System. J. Hypertens. 35 (9), 1899–1908. doi:10.1097/HJH.0000000000001378
Wang, Y., Xu, Y., Yang, M., Zhang, M., Xiao, M., and Li, X. (2020). Butyrate Mitigates TNF-α-Induced Attachment of Monocytes to Endothelial Cells. J. Bioenerg. Biomembr 52 (4), 247–256. doi:10.1007/s10863-020-09841-9
Warmbrunn, M. V., Herrema, H., Aron-Wisnewsky, J., Soeters, M. R., Van Raalte, D. H., and Nieuwdorp, M. (2020). Gut Microbiota: a Promising Target against Cardiometabolic Diseases. Expert Rev. Endocrinol. Metab. 15 (1), 13–27. doi:10.1080/17446651.2020.1720511
Witkowski, M., Weeks, T. L., and Hazen, S. L. (2020). Gut Microbiota and Cardiovascular Disease. Circ. Res. 127 (4), 553–570. doi:10.1161/CIRCRESAHA.120.316242
Yan, H., and Ajuwon, K. M. (2015). Mechanism of Butyrate Stimulation of Triglyceride Storage and Adipokine Expression during Adipogenic Differentiation of Porcine Stromovascular Cells. PloS one 10 (12), e0145940. doi:10.1371/journal.pone.0145940
Yan, Q., Gu, Y., Li, X., Yang, W., Jia, L., Chen, C., et al. (2017). Alterations of the Gut Microbiome in Hypertension. Front Cel Infect Microbiol 7, 381. doi:10.3389/fcimb.2017.00381
Yang, T., Magee, K. L., Colon-Perez, L. M., Larkin, R., Liao, Y. S., Balazic, E., et al. (2019). Impaired Butyrate Absorption in the Proximal colon, Low Serum Butyrate and Diminished central Effects of Butyrate on Blood Pressure in Spontaneously Hypertensive Rats. Acta Physiol. (Oxf) 226 (2), e13256. doi:10.1111/apha.13256
Yang, T., Santisteban, M. M., Rodriguez, V., Li, E., Ahmari, N., Carvajal, J. M., et al. (2015). Gut Dysbiosis Is Linked to Hypertension. Hypertension 65 (6), 1331–1340. doi:10.1161/HYPERTENSIONAHA.115.05315
Yu, C. D., Xu, Q. J., and Chang, R. B. (2020). Vagal Sensory Neurons and Gut-Brain Signaling. Curr. Opin. Neurobiol. 62, 133–140. doi:10.1016/j.conb.2020.03.006
Yu, S., Ren, E., Xu, J., Su, Y., and Zhu, W. (2017). Effects of Early Intervention with Sodium Butyrate on Lipid Metabolism-Related Gene Expression and Liver Metabolite Profiles in Neonatal Piglets. Livestock Sci. 195, 80–86. doi:10.1016/j.livsci.2016.11.013
Yu, Z., Han, J., Chen, H., Wang, Y., Zhou, L., Wang, M., et al. (2021). Oral Supplementation with Butyrate Improves Myocardial Ischemia/Reperfusion Injury via a Gut-Brain Neural Circuit. Front. Cardiovasc. Med. 8. doi:10.3389/fcvm.2021.718674
Zeng, X., Gao, X., Peng, Y., Wu, Q., Zhu, J., Tan, C., et al. (2019). Higher Risk of Stroke Is Correlated with Increased Opportunistic Pathogen Load and Reduced Levels of Butyrate-Producing Bacteria in the Gut. Front. Cel Infect Microbiol 9, 4. doi:10.3389/fcimb.2019.00004
Zhang, L., Deng, M., Lu, A., Chen, Y., Chen, Y., Wu, C., et al. (2019). Sodium Butyrate Attenuates Angiotensin II-Induced Cardiac Hypertrophy by Inhibiting COX2/PGE2 Pathway via a HDAC5/HDAC6-dependent Mechanism. J. Cel Mol Med 23 (12), 8139–8150. doi:10.1111/jcmm.14684
Keywords: gut microbiota, butyrate, cardiovascular diseases, epigenetic modulation, antioxidant
Citation: Amiri P, Hosseini SA, Ghaffari S, Tutunchi H, Ghaffari S, Mosharkesh E, Asghari S and Roshanravan N (2022) Role of Butyrate, a Gut Microbiota Derived Metabolite, in Cardiovascular Diseases: A comprehensive narrative review. Front. Pharmacol. 12:837509. doi: 10.3389/fphar.2021.837509
Received: 16 December 2021; Accepted: 31 December 2021;
Published: 02 February 2022.
Edited by:
Vadim B. Vasilyev, Institute of Experimental Medicine (RAS), RussiaReviewed by:
Bin Geng, Chinese Academy of Medical Sciences and Peking Union Medical College, ChinaYunping Qiu, Albert Einstein College of Medicine, United States
Copyright © 2022 Amiri, Hosseini, Ghaffari, Tutunchi, Ghaffari, Mosharkesh, Asghari and Roshanravan. This is an open-access article distributed under the terms of the Creative Commons Attribution License (CC BY). The use, distribution or reproduction in other forums is permitted, provided the original author(s) and the copyright owner(s) are credited and that the original publication in this journal is cited, in accordance with accepted academic practice. No use, distribution or reproduction is permitted which does not comply with these terms.
*Correspondence: Neda Roshanravan, bmVkYS5yb3NoYW5yYXZhbjEwQGdtYWlsLmNvbQ==