- 1Department of Neuroscience and Behavior, Stony Brook University, Stony Brook, NY, United States
- 2Department of Neuroscience, University of Texas at Austin, Austin, TX, United States
- 3Psychology Department, Florida Atlantic University, Boca Raton, FL, United States
- 4Picower Institute for Learning and Memory, Massachusetts Institute of Technology, Cambridge, MA, United States
- 5Center for Brains Minds and Machines, Massachusetts Institute of Technology, Boston, MA, United States
- 6Department of Anesthesia, Critical Care and Pain Medicine, Massachusetts General Hospital, Harvard Medical School, Boston, MA, United States
- 7Department of Psychiatry, Massachusetts General Hospital, Harvard Medical School, Boston, MA, United States
- 8Athinoula A. Martinos Center for Biomedical Imaging, Charlestown, MA, United States
Clinical populations have memory deficits linked to sleep oscillations that can potentially be treated with sleep medications. Eszopiclone and zolpidem (two non-benzodiazepine hypnotics) both enhance sleep spindles. Zolpidem improved sleep-dependent memory consolidation in humans, but eszopiclone did not. These divergent results may reflect that the two drugs have different effects on hippocampal ripple oscillations, which correspond to the reactivation of neuronal ensembles that represent previous waking activity and contribute to memory consolidation. We used extracellular recordings in the CA1 region of rats and systemic dosing of eszopiclone and zolpidem to test the hypothesis that these two drugs differentially affect hippocampal ripples and spike activity. We report evidence that eszopiclone makes ripples sparser, while zolpidem increases ripple density. In addition, eszopiclone led to a drastic decrease in spike firing, both in putative pyramidal cells and interneurons, while zolpidem did not substantially alter spiking. These results provide an explanation of the different effects of eszopiclone and zolpidem on memory in human studies and suggest that sleep medications can be used to regulate hippocampal ripple oscillations, which are causally linked to sleep-dependent memory consolidation.
Introduction
Noninvasive interventions of sleep oscillations can be used to normalize or enhance memory function (Malkani and Zee, 2020; Manoach et al., 2020; Winkelman and Lecea, 2020). Sleep-dependent memory consolidation relies on oscillations of non-rapid eye movement (NREM) sleep: hippocampal sharp-wave ripples, thalamocortical spindles, and cortical slow oscillations (Staresina et al., 2015; Maingret et al., 2016; Latchoumane et al., 2017; Klinzing et al., 2019; Ngo et al., 2020; Varela and Wilson, 2020). Ripples are particularly crucial because these brief, high-frequency oscillations (100–275 Hz), correspond to the reactivation of hippocampal neuronal ensembles that contribute to memory (Wilson and McNaughton, 1994; Girardeau et al., 2009; Ego-Stengel and Wilson, 2010). Therapeutics that enhance ripples could be valuable to treat memory deficits in several disorders.
Eszopiclone and zolpidem, two non-benzodiazepine sedative hypnotics that are prescribed to treat insomnia (Richey and Krystal, 2011; Matheson and Hainer, 2017; Liang et al., 2019; Krystal, 2020), are among the candidates to normalize sleep oscillations and memory (Kaestner et al., 2013). Zolpidem binds preferentially to GABA (A) receptors with the α1 subunit, while eszopiclone has a broad affinity (Hanson et al., 2008; Dixon et al., 2015); these differences in pharmacology can translate into differential effects at the cellular and network level (Jia et al., 2009). Eszopiclone increased spindles in humans but failed to enhance overnight memory consolidation (Wamsley et al., 2013; Mylonas et al., 2020). In contrast, recent findings suggest that zolpidem improves memory in humans (Niknazar et al., 2015; Zhang et al., 2020). Rodent studies looked at the effect of eszopiclone on cortical oscillations but not ripples (Xi and Chase, 2008; Hambrecht-Wiedbusch et al., 2010; Huang et al., 2010; Methippara et al., 2010; Fox et al., 2013), and there are few studies of zolpidem in rodents (Ponomarenko et al., 2004; Koniaris et al., 2011; Berdyyeva et al., 2014). We hypothesized that the contrasting effects of these drugs on memory consolidation were due to differential effects in hippocampal function.
Here we describe results from extracellular recordings in rats that suggest that eszopiclone disrupts neuronal spiking and ripple occurrence in the hippocampus, while zolpidem increases ripple occurrence. The results could contribute to the contrasting effects of eszopiclone and zolpidem in human studies.
Materials and Methods
We investigated the effect of eszopiclone and zolpidem using microdrive arrays with tetrodes (Kloosterman et al., 2009; Nguyen et al., 2009) and an intraperitoneal tube for drug dosing chronically implanted in Long-Evans male rats (400–500 g; n = 4; purchased from Charles River Laboratories). The electrodes were lowered to the CA1 cell layer to record local field potentials (LFPs; sampled at 600 Hz) and spikes during natural sleep. Rats were housed individually, provided with food and water ad libitum, and monitored in a temperature-controlled room with a 12 h light/dark cycle (lights on/off at 7:00 am/7:00 pm). Experiments were approved by the Committee on Animal Care at the Massachusetts Institute of Technology and conform to US National Institutes of Health guidelines for the care and use of laboratory animals.
All rats received drug and vehicle infusions (n = 4 rats). Eszopiclone was tested in three rats and zolpidem in 2 (the sequence of injections per rat is included in Supplementary Table S2). In each session, rats were placed in a quiet area and their sleep and wakefulness were monitored through the LFP from CA1 and the retrosplenial cortex. Before injection, we recorded up to 1 hour to obtain baseline hippocampal activity; we injected eszopiclone (10 mg/kg), zolpidem (10 mg/kg) or vehicle and recorded the effect for 4–6 h. Dosing was performed between 11.00am and 12.30pm with the exception of one injection of zolpidem that was performed at 2.45pm but we did not find differences in its effect. For both drugs we selected a high i. p. dosage (10 mg/kg) based on dose-response rodent studies (Gauthier et al., 1997; Xi and Chase, 2008; Huang et al., 2010; Methippara et al., 2010). Drug injections were separated by at least 1 week to allow for drug clearance. Electrode location was confirmed in brain sections and with LFP signatures of cortex (delta and spindle oscillations) and hippocampus (ripples).
Surgical Implantation
Aseptic surgery procedures were performed to implant tetrode arrays and an intraperitoneal (i.p.) polyurethane infusion catheter. The microdrive implant was secured to the skull with dental cement and bone screws. The infusion catheter (ID 0.027 in, OD 0.047 in; Harvard Apparatus) was implanted in the peritoneal cavity, and the excess tubing run subcutaneously to the back of the neck, where it was secured by dental cement and remained closed until dosing. Animals were preemptively injected with analgesics (Buprenorphine, 0.5–1 mg/kg, subcutaneous), and monitored for 3 days post-surgery.
Drug Preparation
Eszopiclone and zolpidem were purchased from Sigma in powder form and prepared within 48 h of their application by dissolving in sodium acetate buffer 50 mM pH 4, filtered through a 0.22 µm filter; zolpidem was first dissolved in 5% DMSO, then mixed with the filtered sodium acetate buffer. The vehicle consisted of sodium acetate buffer 50 mM pH 4 (for eszopiclone) and sodium acetate buffer 50 mM pH 4 with DMSO (for zolpidem). The drugs were stored at 4°C; on the experimental day, the solution was drawn into a sterile syringe for immediate administration.
Sleep Detection
We detected NREM sleep and ripples as described before (Ji and Wilson, 2007; Varela and Wilson, 2020). Briefly, we looked for periods of high cortical delta (1–4 Hz) and CA1 ripple (100–275 Hz) power in the LFP, or for periods of high cortical delta (ripple-independent sleep detection; Supplementary Figure S3C-D). Sleep periods of less than 120 s were disregarded, as were the 10 mins preceding and following injections to avoid interference from the procedure. Ripples were detected from the squared filtered (100–275 Hz) CA1 LFP as periods with power greater than 3 standard deviations above the mean ripple power for at least 20 ms.
LFP and Spike Analyses
We analyzed both LFPs (which represent input into a brain region) and spikes, which represent the output from CA1 (Herreras, 2016). Spectrograms were calculated with a moving window of 20 s using the mtspecgramc function in the Chronux package, and power spectra using the pspectrum function in MATLAB. To obtain a metric of LFP ripple activity independent of ripple detection methods, we quantified changes in ripple power in the spectrograms relative to the baseline. We then detected ripples as described in previous work (Ji and Wilson, 2007; Varela and Wilson, 2020) and calculated ripple density as the number of ripples per second. Spike rates were calculated as spikes per second; to study spikes during ripples, we calculated peri-ripple histograms by averaging the spike counts (bin = 5 ms) in a 200 ms window around ripples. In most sessions, we normalized spike rates to the rate in the sleep before dosing. In three sessions with less than 15 min of sleep before injection, we normalized the firing rate using baseline recordings (session with no injection) from the same rat. We further normalized by the average rate within the baseline session and by its duration. This took into account variations in spike counts and session length in different days.
Spike Waveform Classification
To separate spikes from putative pyramidal cells and interneurons, we used a threshold on the peak-to-trough waveform duration at 0.35 ms (Csicsvari et al., 1999a; Nowak et al., 2003; Le Van Quyen et al., 2008). Confirmation of spike type was done by examining the autocorrelation of spike times within each cluster and by comparing the resulting clusters to spikes sorted based on amplitude projections by an expert user.
Statistics
Given the multi-variate nature of the data, which varies as a function of time, drug, and inter- and intra-rat conditions, we tested significance by fitting the data to a linear mixed model with session as the experimental unit and rat as a random effect, and calculated p-values through an F-test that compares the linear regression model coefficients. The model was computed using MATLAB’s function fitlme and the p-values were extracted using coefTest. Statistical results are reported for each analysis as the size of the effect (percent change), the statistical value, the degrees of freedom, and the p value; statistics are summarized in Supplementary Table S1.
Results
Decrease in CA1 Ripple Power After Eszopiclone and Increase After Zolpidem
To assess the effect of the two drugs on ripples, we first calculated spectrograms of the CA1 LFP for drug and vehicle sessions (Figure 1A; power spectra and spectrograms for all drug sessions and sample vehicle sessions in Supplementary Figures S1,2). The spectral power after dosing was normalized to the average baseline sleep power for each frequency. Dosing was performed through the intraperitoneal cannula and is considered time 0 (t0) in all sessions.
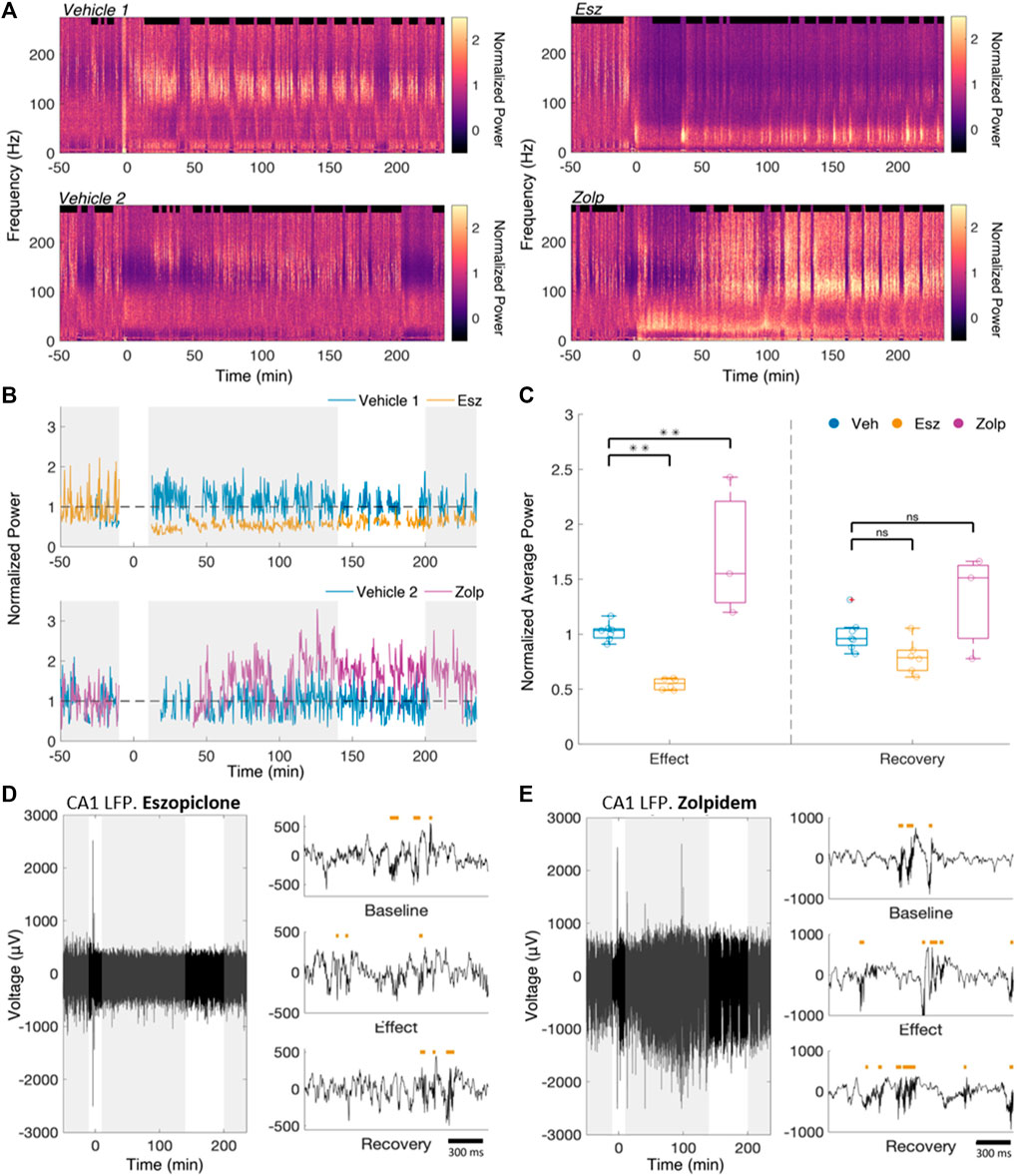
FIGURE 1. Eszopiclone and zolpidem have differential effects on CA1 power in the ripple band. (A) Raw spectrogram of the CA1 LFP for a pair of vehicle and eszopiclone sessions (top) and vehicle and zolpidem sessions (bottom) from the same rat. Drug (10 mg/kg) or vehicle was injected at time 0. (B) Average normalized ripple power (100–200 Hz) during sleep for the spectrograms in Figure 1A. Gray boxes indicate time windows of interest (from left to right: Baseline, Effect, Recovery). Effects near the injection (t = 0) were ignored. (C) Boxplots (each circle one session) of the ratio of average normalized sleep ripple power (100–200 Hz), within “Effect” and “Recovery” relative to the “Baseline”. Significance was determined using an F-test on a fitted mixed linear model (n = 4 rats; see Supplementary Table S2 for details). (D) Example raw CA1 LFP recordings from a session in which eszopiclone was injected, along with detected ripples (red bars) within the three time windows of interest (top, Baseline; middle, Effect; bottom, Recovery). (E) Same for zolpidem session.
We classified NREM as periods of high delta (1–4 Hz) power in cortex and high ripple power in CA1 (Ji and Wilson, 2007; Davidson et al., 2009; Varela and Wilson, 2020). We saw no significant difference in the average sleep bout duration between vehicle, eszopiclone or zolpidem sessions (10.98, 12.46 and 11.55 min respectively; vehicle/eszopiclone, F (1,13) = 1.46; p = 0.25; vehicle/zolpidem, F (1,13) = 0.26, p = 0.62), nor in the average number of bouts per hour (2.92 bouts/hour, 2.93 bouts/hour and 3.01 bouts/hour respectively; vehicle/eszopiclone, F (1,13) = 1.41; p = 0.26; vehicle/zolpidem, F (1,13) = 1.96, p = 0.19; Supplementary Figure S3A). We also obtained no significant drug effects on sleep time (vehicle/eszopiclone, F (1,13) = 0.89; p = 0.36; vehicle/zolpidem, F (1,13) = 0.09, p = 0.77) or sleep bouts (vehicle/eszopiclone, F (1,13) = 0.06; p = 0.81; vehicle/zolpidem, F (1,13) = 0.002, p = 0.96) with a classification of NREM based only on cortical delta power (thus independent of CA1 ripple power; Supplementary Figure S3B). However, we observed a drop in ripple power (Figure 1B) following the injection of eszopiclone, and an increase following zolpidem.
We defined three time windows based on their pharmacokinetics in rats (Gaillot et al., 1983; Trenque et al., 1994): baseline (50–10 min pre-injection), effect (10–140 min post-injection), and recovery (200–235 min post-injection). We took the ratio of the sleep ripple power during effect and recovery to the baseline power (normalized as described in Methods; Figure 1C). If there were less than 15 min of detected sleep within the baseline (3 sessions), we instead used the first hour of a different recording session from the same rat in which there was no injection. During the effect, eszopiclone decreased ripple power by 44%, whereas zolpidem increased it by 97% (vehicle/eszopiclone, F (1,13) = 11.11; p = 0.005; vehicle/zolpidem, F (1,13) = 15.83, p = 0.002; Supplementary Table S1). The normalized sleep ripple power during recovery was not significantly different compared to vehicle (19% below baseline in eszopiclone and 50% above baseline with zolpidem; vehicle/eszopiclone, F (1,13) = 2.53; p = 0.14; vehicle/zolpidem, F (1,13) = 3.77, p = 0.07). These results suggest that eszopiclone has an acute adverse effect on ripple power, while zolpidem increases hippocampus ripple activity.
Eszopiclone Decreases and Zolpidem Increases CA1 Ripple Density
Noting a change in ripple power, we analyzed whether eszopiclone and zolpidem modulate the number of ripples (Figures 1D,E). The cumulative ripple count showed that eszopiclone decreased, while zolpidem increased, the number of ripples (Figure 2A). Specifically, the ripple density (ripples per second) significantly decreased after eszopiclone (by an average of 60%; n = 3 rats) and increased after zolpidem [by 102%; n = 2 rats; vehicle/eszopiclone, F (1,13) = 43.66; p = 1.7e-5; vehicle/zolpidem, F (1,13) = 52.46, p = 6.5e-6; Figure 2B]. To resolve the time course of the effect, we looked at the ripple density every 30 min and found that eszopiclone reached a maximum effect within 30 min of injection while the increase in ripples induced by zolpidem had slower dynamics (Supplementary Figure S4). With both drugs, the ripple density did not fully recover to baseline levels within the recorded period (up to 6 h), suggesting that they can have a prolonged effect on hippocampal ripples.
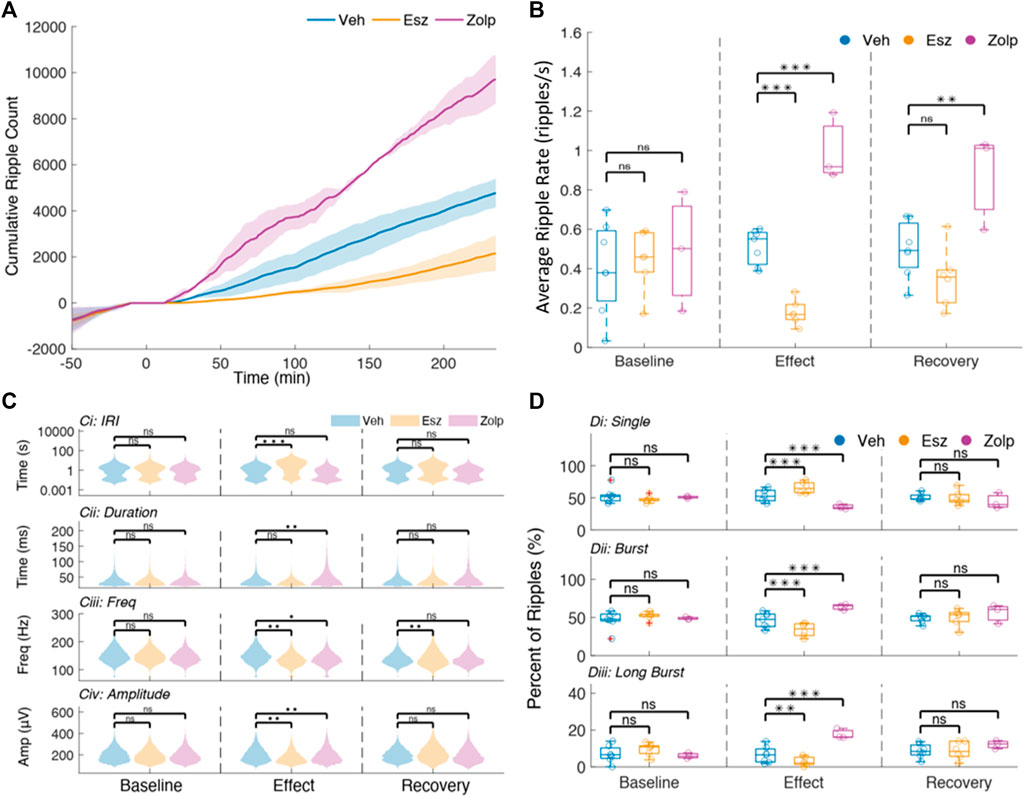
FIGURE 2. Ripple sparsity increases after eszopiclone and decreases with zolpidem. (A) Mean cumulative ripple counts across sessions with shaded standard deviations for vehicle, Eszopiclone and Zolpidem (n = 4 rats; see Supplementary Table S2 for details). Time 0 indicates injection. (B) Mean ripple rate (per second) within each time window of interest in all sessions (each circle one session). Significance was determined using an F-test on a fitted mixed linear model. (C) Violin plots of Inter-Ripple-Intervals (top row, y-axis log scaled), Ripple duration (second row), Intra-ripple frequency (third row) and normalized ripple amplitude (bottom row), comparing Vehicle (blue), Esz (red) and Zolp (purple) across time windows: Baseline (left), Effect (middle), and Recovery (right). (D) Distribution of the proportion of “single” ripples (Di), ripple bursts (Dii) and “long” ripple bursts (bursts with four ripples or more; Diii) for all sessions during Baseline, Effect and Recovery.
The generation of ripples relies on interactions between hippocampus sub-regions (Buzsáki et al., 1983; Carr et al., 2012; Csicsvari et al., 1999b; Yamamoto and Tonegawa, 2017), while the morphology of the ripple oscillation depends on interactions between CA1 pyramidal cells and interneurons (Klausberger and Somogyi, 2008; Stark et al., 2014; Ylinen et al., 1995). To gain insight on the mechanisms by which eszopiclone and zolpidem affect ripples, we studied several ripple features: inter-ripple-interval, duration, intrinsic ripple frequency and peak-to-trough amplitude (Figure 2C; Supplementary Table S1). Ripples often occur in bursts (Davidson et al., 2009; Yamamoto and Tonegawa, 2017), and we also quantified the effect of the drugs on ripple bursts (Figure 2D).
The baseline inter-ripple interval (IRI) distributions were bimodal (Figure 2C), with the first peak indicating ripple bursts, while the second implies ‘single’ events. Eszopiclone shifted the mean to longer IRIs (from an average 2°s IRI in baseline to 6°s during the effect) thus increasing ripple sparsity, while zolpidem shifted the mean to shorter IRIs (from 2 to 1°s). These shifts do not by themselves entail a change in the proportion of ripple bursts and single ripples; to estimate that, we calculated the percent of “single” ripples and ripples in bursts (based on the IRI at the minimum between the two modes for each session). In eszopiclone, ripple bursts dropped significantly to 34% (compared to 52% during Baseline) and the fraction of single ripples increased proportionally. Zolpidem increased ripple bursts to 64% (from 49% in Baseline). To confirm the effect, we used a more stringent classification of bursts by detecting “long” bursts (4 ripples or more) and again found a significant decrease of long bursts with eszopiclone and a significant increase with zolpidem. These results (Figure 2D; Supplementary Table S1) suggest that the two drugs change ripple density by disrupting (eszopiclone) or enhancing (zolpidem) the grouping of ripples [vehicle/eszopiclone, F (1,13) = 33.84; p = 6e-5; vehicle/zolpidem, F (1,13) = 1.42; p = 0.26].
In addition, we found a slight but significant decrease in intra-ripple frequency with eszopiclone [10% during effect; F (1,13) = 16.95; p = 0.001, and 8% during recovery; F (1,13) = 16.68; p = 0.001] and a slight increase in intra-ripple frequency during the effect of zolpidem [1%; F (1,13) = 5.4; p = 0.04]. We also found that ripple duration increased during zolpidem [31%; F (1,13) = 15.45; p = 0.002], and saw a significant decrease [11% decrease; F (1,13) = 14.58; p = 0.002] in peak-to-trough amplitude with eszopiclone and a significant increase with zolpidem [8%, F (1,13) = 10.89; p = 0.01].
These results suggest that eszopiclone and zolpidem mainly affect the density of hippocampal ripples, with slight effects on the frequency, duration, and amplitude of the ripple oscillation. Both drugs had a strong but opposite effect on the density of ripple bursts, eszopiclone made ripples sparser while zolpidem made ripples denser.
Decrease of Spike Activity in CA1 With Eszopiclone and Moderate Change Following Zolpidem
We next examined the effect on multi-unit spikes within the hippocampus (MUA, all spikes recorded by each tetrode; Figure 3A). We normalized the firing rate in each tetrode (4 CA1 tetrodes per session on average) to the average rate during baseline sleep. If there were less than 15 min of sleep within baseline, as before, we normalized using the first 60 min of sleep within a recording session with no injection. We looked at spikes in the three windows of interest (baseline, effect and recovery; results for all tetrodes in Supplementary Figure S5), and at the average spike activity every 30 min post-injection. Figure 3B shows the mean normalized firing rate in CA1 for all tetrodes (top) and broken down into 30 min intervals (bottom). Eszopiclone produced the highest drop in firing rate 10–40 min after injection [60%; F (1,47) = 137.22; p = 1.53e-15], followed by a slow recovery to baseline (27% decrease by 190–200 min; not different from vehicle). Zolpidem did not produce significant changes in spiking.
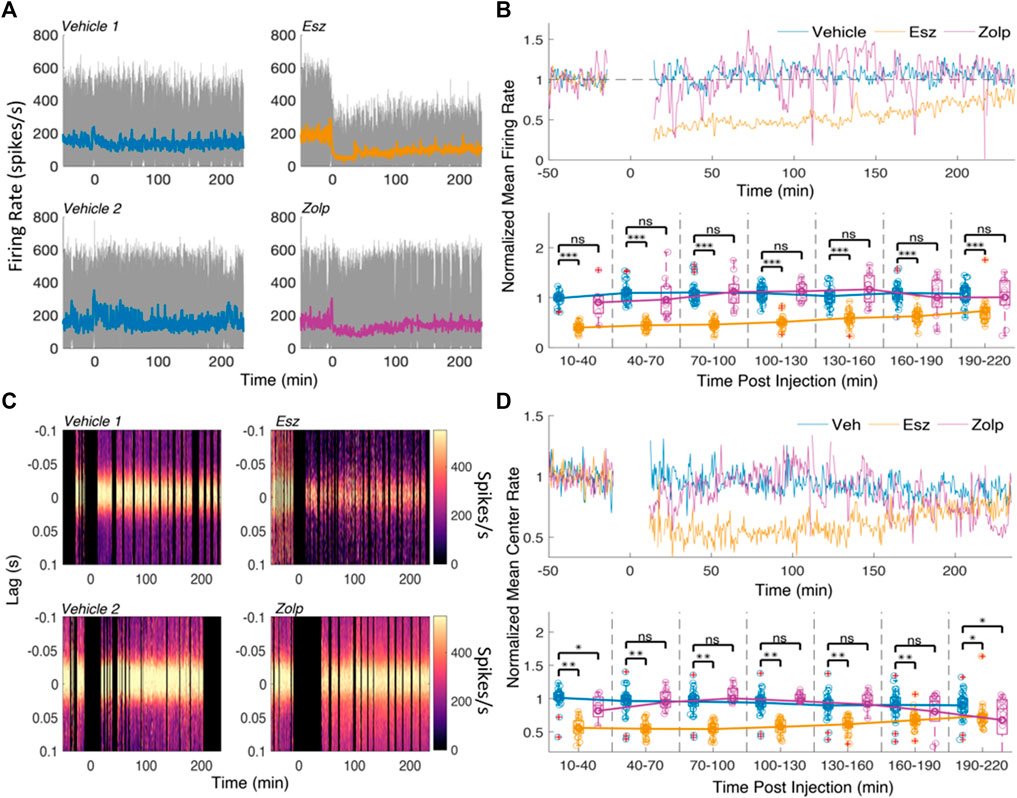
FIGURE 3. Spike rate decreases following eszopiclone and does not change significantly with zolpidem. (A) Multi-unit (MUA) firing rates for a pair of vehicle and eszopiclone sessions (top), and a pair of vehicle and zolpidem sessions (bottom) from the same rat. Color line shows the mean rate over time. (B) Top: average firing rate across all sessions (n = 4; see Supplementary Table S2 for details), normalized by the mean baseline activity; spiking near injection (t = 0) was ignored. Bottom: Boxplots of the normalized mean rates in 30-minute time intervals post-injection with line connecting the means of each drug type (each circle corresponds to the spike rate in one CA1 electrode; red crosses indicate outliers). Significance was determined using an F-test on a fitted mixed linear model. (C) Examples of peri-ripple histograms of the MUA spikes over time for a pair of vehicle and eszopiclone sessions (top) and a pair of vehicle and zolpidem sessions (bottom). Lag 0 corresponds to ripple events and color scheme shows the spike rate. Black vertical areas indicate periods with no sleep and the 10 minutes around injection. (D) Top: Mean MUA spike rate at the center (40 ms around lag 0) of the peri-ripple histograms, normalized by the average baseline activity, across all sessions; bottom: boxplots showing individual sessions in 30 min bins post-injection with lines connecting the means (each circle one tetrode). Significance was determined using an F-test on a fitted mixed linear model.
Although zolpidem did not change the overall firing rate, it is possible that spike firing is affected during ripples. To find out, we analyzed the effect of eszopiclone and zolpidem on the peak (40 ms around lag 0) of peri-ripple histograms of MUA. Figure 3C shows the peri-ripple histograms for the sessions in Figure 3A, and Figure 3D shows average (top) and binned (bottom) spike rates at the peak (40 ms around lag 0) of the peri-ripple histogram across all sessions.
Eszopiclone decreased ripple-associated spike rate through the session [45% 10–40 min post-injection; F (1,47) = 61.317; p = 4.57e-10]; zolpidem produced a small drop in ripple-associated spike rate [17% 10–40 min post-injection; F (1,47) = 6.51; p = 0.01], which was significant in the first and last 30 min bins. These results demonstrate that eszopiclone strongly decreases CA1 firing. The smaller drop with zolpidem, at the start and end of the recording, may reflect a dose-dependent effect on spike firing.
We tested one further possibility, which is that the drug effects could be specific to neuronal type. For example, zolpidem could enhance GABA currents in inhibitory interneurons (Gao and Fritschy, 1994; Klausberger et al., 2002; Baude et al., 2007), and disinhibit excitatory pyramidal cells; such an effect could explain the small changes in MUA, which includes both neurons. Instead, eszopiclone may affect both excitatory and inhibitory neurons due to its broad affinity for different GABA (A) receptors (Hanson et al., 2008). A spike width less than 350 μs was used to identify putative interneuron spikes (pInt), otherwise the waveform was classified as a putative pyramidal cell (pPy). Manual spike sorting and autocorrelograms similar to published data (Le Van Quyen et al., 2008) were used to validate the classification (Supplementary Figure S6).
We examined the effect of the drugs on the two spike types by looking at their normalized average firing rate over time (Figure 4A). Eszopiclone produced a stark decrease in spike rate in both putative pyramidal cells and interneurons; with zolpidem we saw an initial decrease that increased and overshot the baseline rate, then recovered to near baseline. We saw no difference between the pyramidal and interneuron waveforms in vehicle, eszopiclone or zolpidem (Figure 4B). The strongest effect was with eszopiclone [pPy: 35%; F (1,63) = 98.41; p = 1.7e-14, pInt: 21%; F (1,63) = 53.91; p = 5.05e-10, during the first 10–30 min] and the effect was about half the size with zolpidem [pPy: 14%; F (1,63) = 13.1; p = 5.9e-4, pInt: 16%; F (1,63) = 10.43; p = 0.002].
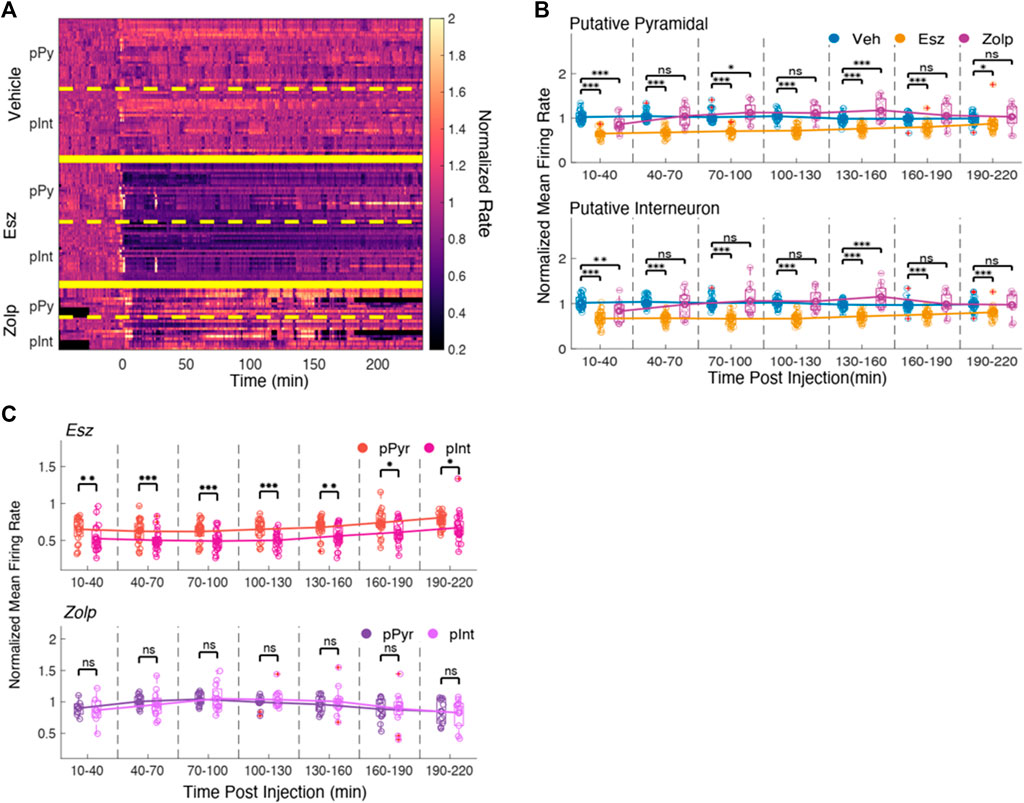
FIGURE 4. Eszopiclone and zolpidem have similar effects on spikes from putative pyramidal cells and interneurons. (A) Smoothed histograms of spike rate normalized by average rate during the ‘baseline’ time period for putative pyramidal cell spikes (pPy) and putative interneuron spikes (pInt). Rate matrix is divided into vehicle (top), eszopiclone (middle) and zolpidem (bottom), and further separated into putative pyramidal cells and putative interneurons (n = 4 rats). (B) Boxplots of the normalized mean spike rates for putative pyramidal cell (top) and putative interneuron spikes (bottom) in 30-minute time intervals post-injection with line connecting the means of each drug type. Each circle one tetrode; red crosses indicate outliers. Significance was determined using an F-test on a fitted mixed linear model. (C) Boxplots comparing side by side the effect on the peri-ripple histograms for putative pyramidal and putative interneuron spikes in eszopiclone (top) and zolpidem sessions (bottom). Peri-ripple rates (normalized to baseline) are binned every 30 min post-injection with lines connecting the means. Significance was determined using an F-test on a fitted mixed linear model.
Stronger Disruption of Interneuron Ripple Correlations
Although we observed similar effects on the firing rates of putative pyramidal cells and interneurons, these two types of neurons contribute differently to ripples (Klausberger and Somogyi, 2008), and it is possible that their participation in ripples is differentially affected by eszopiclone or zolpidem. We therefore compared the spike rate at the center of the peri-ripple histogram for the putative pyramidal cells and interneurons in eszopiclone and zolpidem (Figure 4C). Only after eszopiclone the peri-ripple histogram of interneuron spikes was significantly lower relative to the peri-ripple histogram of putative pyramidal neurons [10–30 min: F (1,36) = 11.04; p = 0.002, 130–160 min: F (1,48) = 11.01; p = 0.002, 190–220 min: F (1,48) = 4.85; p = 0.03], indicating that eszopiclone may produce a stronger disruption of the timing between ripples and interneuron spikes.
Discussion
Eszopiclone and zolpidem produced opposite effects on sleep-dependent memory consolidation in humans. To gain mechanistic insight on how these drugs could produce opposing effects on memory, we investigated their effect on ripples and spikes in rat CA1 during sleep. We found that eszopiclone significantly decreased ripple density while zolpidem significantly increased ripple density and their grouping in bursts. The drugs had small effects on the ripple oscillation: both drugs reduced intra-ripple frequency slightly, and zolpidem increased ripple duration; ripple amplitude was smaller after eszopiclone and larger after zolpidem. In addition, eszopiclone strongly reduced spiking in putative pyramidal cells and interneurons, whereas zolpidem had small effects on CA1 spikes. The different effects on ripples and cell firing may help explain why zolpidem (but not eszopiclone) had beneficial effects on memory consolidation in human studies.
Pharmacological Regulation of Ripple Density
Eszopiclone and zolpidem changed ripple density without substantial changes to the ripple waveform. Both drugs affected primarily ripple bursts, suggesting an effect on the circuits that initiate and group ripples (CA3; entorhinal cortex -EC-). Three mechanisms could explain the effects on ripple density: changes in CA3 neuron excitability, which produced increases and decreases in ripple density (Schlingloff et al., 2014). Modulation of the pathway from medial EC to CA1, which correlated with greater incidence of ripple bursts (Yamamoto and Tonegawa, 2017). Lastly, ripple density was associated with neuronal activity in the raphe (Logothetis et al., 2012; Wang et al., 2015), which could be affected by activation of GABA (A) receptors.
Eszopiclone has a broad GABA (A) receptor affinity (Hanson et al., 2008) and could produce a general enhancement of GABA (A) currents and decrease spike activity directly in CA1, or indirectly by increasing GABA (A) currents in neuromodulatory centers (Hasselmo and McGaughy, 2004; Olsen and Sieghart, 2009; Dixon et al., 2015). Zolpidem binds preferentially to GABA (A) receptors with α1 subunits (Hanson et al., 2008; Fitzgerald et al., 2014; Che Has et al., 2016), which mediate inhibition from parvalbumin interneurons (Gao and Fritschy, 1994; Klausberger et al., 2002; Bacci et al., 2003; Baude et al., 2007). A more restricted cell population target could explain the subtler effects on spike rates we observed with zolpidem, which are consistent with mild effects of zolpidem on hippocampal theta (Yoshimoto et al., 1999).
Although our sample size was small, the results were consistently observed in all sessions and animals, and are supported by a linear mixed-effects models to treat sessions as experimental units and rats as random effects. Future dose-response studies will be important to make sense of the different zolpidem effects among hippocampus studies. Zolpidem increased ripples in hippocampal slices (Koniaris et al., 2011), but a study in Wistar rats found a transient reduction in ripples (Ponomarenko et al., 2004); likewise, work in mice (Berdyyeva et al., 2014) found a decrease in calcium transients (which may correspond to ripples and to subthreshold activity) in CA1 after oral zolpidem dosing. The variability across studies suggest that the effect of zolpidem on hippocampus activity depends on the bioavailability and effective dosage achieved in different preparations. In this respect, we found that zolpidem transiently decreased ripple-associated spikes after injection and in the last 30 min of recording, which may reflect changes in its brain concentration.
Drug Interventions to Regulate Sleep Oscillations and Cognition
In humans, eszopiclone failed to restore sleep-dependent memory consolidation in either healthy people or schizophrenia patients (Wamsley et al., 2013; Mylonas et al., 2020). In contrast, zolpidem enhanced sleep-dependent memory consolidation (Kaestner et al., 2013; Mednick et al., 2013; Niknazar et al., 2015; Zhang et al., 2020). Pharmacology studies of the effect of eszopiclone and zolpidem on memory are more limited in the animal literature. Eszopiclone and zolpidem reduced freezing behavior after contextual fear training in rats, consistent with a disruption of memory consolidation, although reduced freezing may also result from anxiolytic effects (Huang et al., 2010). Rodent work suggests a direct link between ripples, memory reactivation and consolidation (Foster, 2017; Joo and Frank, 2018; Klinzing et al., 2019). Invasive techniques that disrupt or enhance ripples produced, respectively, memory impairment or enhancement (Girardeau et al., 2009; Ego-Stengel and Wilson, 2010; Fernández-Ruiz et al., 2019). A further step to understand the mechanisms of action of eszopiclone and zolpidem will be to determine if the changes in ripple rate translate into opposite effects on memory. The decrease of spike activity with eszopiclone may contribute to additional effects on behavior. Another important aspect will be to investigate the effect of both drugs on the coupling of ripples with spindle and slow oscillations, which is thought to play a crucial role in systems memory consolidation (McClelland et al., 1995; Staresina et al., 2015; Varela and Wilson, 2020).
Data Availability Statement
The raw data supporting the conclusion of this article will be made available by the authors, without undue reservation.
Ethics Statement
The animal study was reviewed and approved by the Committee on Animal Care at the Massachusetts Institute of Technology.
Author Contributions
LAB and CV wrote the code, analyzed the data and wrote the paper; CV, HP, and FF performed the experiments CV, HP, DM, and MW contributed to the conception and design of the experiments. All authors have made substantial intellectual contribution to the conception, design or interpretation of results.
Funding
This work was funded by an MGH-MIT Grand Challenge on Neurosciences Grant and an R01MH092638-07 to DM and MW. CBMM Alumni Seed Grant to CV (NSF STC award CCF-1231216). The authors declare they have no competing financial interests.
Conflict of Interest
The authors declare that the research was conducted in the absence of any commercial or financial relationships that could be construed as a potential conflict of interest.
Publisher’s Note
All claims expressed in this article are solely those of the authors and do not necessarily represent those of their affiliated organizations, or those of the publisher, the editors and the reviewers. Any product that may be evaluated in this article, or claim that may be made by its manufacturer, is not guaranteed or endorsed by the publisher.
Acknowledgments
We thank members of the Manoach and Wilson labs for helpful suggestions. In particular, we thank Dimitrios Mylonas for help setting up the statistical analyses and linear mixed models.
Supplementary Material
The Supplementary Material for this article can be found online at: https://www.frontiersin.org/articles/10.3389/fphar.2021.792148/full#supplementary-material
References
Bacci, A., Rudolph, U., Huguenard, J. R., and Prince, D. A. (2003). Major Differences in Inhibitory Synaptic Transmission onto Two Neocortical Interneuron Subclasses. J. Neurosci. 23, 9664–9674. doi:10.1523/jneurosci.23-29-09664.2003
Baude, A., Bleasdale, C., Dalezios, Y., Somogyi, P., and Klausberger, T. (2007). Immunoreactivity for the GABAA Receptor Alpha1 Subunit, Somatostatin and Connexin36 Distinguishes Axoaxonic, Basket, and Bistratified Interneurons of the Rat hippocampus. Cereb. Cortex 17, 2094–2107. doi:10.1093/cercor/bhl117
Berdyyeva, T., Otte, S., Aluisio, L., Ziv, Y., Burns, L. D., Dugovic, C., et al. (2014). Zolpidem Reduces Hippocampal Neuronal Activity in Freely Behaving Mice: a Large Scale Calcium Imaging Study with Miniaturized Fluorescence Microscope. PLoS ONE 9, e112068. doi:10.1371/journal.pone.0112068
Buzsáki, G., Leung, L. W., and Vanderwolf, C. H. (1983). Cellular Bases of Hippocampal EEG in the Behaving Rat. Brain Res. 287, 139–171.
Carr, M. F., Karlsson, M. P., and Frank, L. M. (2012). Transient Slow Gamma Synchrony Underlies Hippocampal Memory Replay. Neuron 75, 700–713. doi:10.1016/j.neuron.2012.06.014
Che Has, A. T., Absalom, N., van Nieuwenhuijzen, P. S., Clarkson, A. N., Ahring, P. K., and Chebib, M. (2016). Zolpidem Is a Potent Stoichiometry-Selective Modulator of α1β3 GABAA Receptors: Evidence of a Novel Benzodiazepine Site in the α1-α1 Interface. Sci. Rep. 6, 28674. doi:10.1038/srep28674
Csicsvari, J., Hirase, H., Czurkó, A., Mamiya, A., and Buzsáki, G. (1999a). Oscillatory Coupling of Hippocampal Pyramidal Cells and Interneurons in the Behaving Rat. J. Neurosci. 19, 274–287. doi:10.1523/JNEUROSCI.19-01-00274.1999
Csicsvari, J., Hirase, H., Czurkó, A., Mamiya, A., and Buzsáki, G. (1999b). Fast Network Oscillations in the Hippocampal CA1 Region of the Behaving Rat. J. Neurosci. 19, RC20. doi:10.1523/jneurosci.19-16-j0001.1999
Davidson, T. J., Kloosterman, F., and Wilson, M. A. (2009b). Hippocampal Replay of Extended Experience. Neuron 63, 497–507. doi:10.1016/j.neuron.2009.07.027
Dixon, C. L., Harrison, N. L., Lynch, J. W., and Keramidas, A. (2015). Zolpidem and Eszopiclone Prime α1β2γ2 GABAA Receptors for Longer Duration of Activity. Br. J. Pharmacol. 172, 3522–3536. doi:10.1111/bph.13142
Ego-Stengel, V., and Wilson, M. A. (2010). Disruption of Ripple-Associated Hippocampal Activity during Rest Impairs Spatial Learning in the Rat. Hippocampus 20, 1–10. doi:10.1002/hipo.20707
Fitzgerald, A. C., Wright, B. T., and Heldt, S. A. (2014). The Behavioral Pharmacology of Zolpidem: Evidence for the Functional Significance of α1-containing GABA(A) Receptors. Psychopharmacology (Berl) 231, 1865–1896. doi:10.1007/s00213-014-3457-x
Fernández-Ruiz, A., Oliva, A., Fermino de Oliveira, E., Rocha-Almeida, F., Tingley, D., and Buzsáki, G. (2019). Long-duration hippocampal sharp wave ripples improve memory. Science 364, 1082–1086. doi:10.1126/science.aax0758
Foster, D. J. (2017). Replay Comes of Age. Annu. Rev. Neurosci. 40, 581–602. doi:10.1146/annurev-neuro-072116-031538
Fox, S. V., Gotter, A. L., Tye, S. J., Garson, S. L., Savitz, A. T., Uslaner, J. M., et al. (2013). Quantitative Electroencephalography within Sleep/wake States Differentiates GABAA Modulators Eszopiclone and Zolpidem from Dual Orexin Receptor Antagonists in Rats. Neuropsychopharmacology 38, 2401–2408. doi:10.1038/npp.2013.139
Gaillot, J., Heusse, D., Hougton, G. W., Marc Aurele, J., and Dreyfus, J. F. (1983). Pharmacokinetics and Metabolism of Zopiclone. Pharmacology 27 Suppl 2 (Suppl. 2), 76–91. doi:10.1159/000137914
Gao, B., and Fritschy, J. M. (1994). Selective Allocation of GABAA Receptors Containing the Alpha 1 Subunit to Neurochemically Distinct Subpopulations of Rat Hippocampal Interneurons. Eur. J. Neurosci. 6, 837–853. doi:10.1111/j.1460-9568.1994.tb00994.x
Gauthier, P., Arnaud, C., Stutzmann, J. M., and Gottesmann, C. (1997). Influence of Zopiclone, a New Generation Hypnotic, on the Intermediate Stage and Paradoxical Sleep in the Rat. Psychopharmacology (Berl) 130, 139–143. doi:10.1007/s002130050221
Girardeau, G., Benchenane, K., Wiener, S. I., Buzsáki, G., and Zugaro, M. B. (2009). Selective Suppression of Hippocampal Ripples Impairs Spatial Memory. Nat. Neurosci. 12, 1222–1223. doi:10.1038/nn.2384
Hambrecht-Wiedbusch, V. S., Gauthier, E. A., Baghdoyan, H. A., and Lydic, R. (2010). Benzodiazepine Receptor Agonists Cause Drug-specific and State-specific Alterations in EEG Power and Acetylcholine Release in Rat Pontine Reticular Formation. Sleep 33, 909–918. doi:10.1093/sleep/33.7.909
Hanson, S. M., Morlock, E. V., Satyshur, K. A., and Czajkowski, C. (2008). Structural Requirements for Eszopiclone and Zolpidem Binding to the Gamma-Aminobutyric Acid Type-A (GABAA) Receptor Are Different. J. Med. Chem. 51, 7243–7252. doi:10.1021/jm800889m
Hasselmo, M. E., and McGaughy, J. (2004). High Acetylcholine Levels Set Circuit Dynamics for Attention and Encoding and Low Acetylcholine Levels Set Dynamics for Consolidation. Prog. Brain Res. 145, 207–231. doi:10.1016/S0079-6123(03)45015-2
Herreras, O. (2016). Local Field Potentials: Myths and Misunderstandings. Front. Neural Circuits 10, 101. doi:10.3389/fncir.2016.00101
Huang, M. P., Radadia, K., Macone, B. W., Auerbach, S. H., and Datta, S. (2010). Effects of Eszopiclone and Zolpidem on Sleep-Wake Behavior, Anxiety-like Behavior and Contextual Memory in Rats. Behav. Brain Res. 210, 54–66. doi:10.1016/j.bbr.2010.02.018
Ji, D., and Wilson, M. A. (2007). Coordinated Memory Replay in the Visual Cortex and hippocampus during Sleep. Nat. Neurosci. 10, 100–107. doi:10.1038/nn1825
Jia, F., Goldstein, P. A., and Harrison, N. L. (2009). The Modulation of Synaptic GABA(A) Receptors in the Thalamus by Eszopiclone and Zolpidem. J. Pharmacol. Exp. Ther. 328, 1000–1006. doi:10.1124/jpet.108.146084
Joo, H. R., and Frank, L. M. (2018). The Hippocampal Sharp Wave-Ripple in Memory Retrieval for Immediate Use and Consolidation. Nat. Rev. Neurosci. 19, 744–757. doi:10.1038/s41583-018-0077-1
Kaestner, E. J., Wixted, J. T., and Mednick, S. C. (2013). Pharmacologically Increasing Sleep Spindles Enhances Recognition for Negative and High-Arousal Memories. J. Cogn. Neurosci. 25, 1597–1610. doi:10.1162/jocn_a_00433
Klausberger, T., and Somogyi, P. (2008). Neuronal Diversity and Temporal Dynamics: the unity of Hippocampal Circuit Operations. Science 321, 53–57. doi:10.1126/science.1149381
Klausberger, T., Roberts, J. D., and Somogyi, P. (2002). Cell Type- and Input-specific Differences in the Number and Subtypes of Synaptic GABA(A) Receptors in the hippocampus. J. Neurosci. 22, 2513–2521. doi:10.1523/JNEUROSCI.22-07-02513.2002
Klinzing, J. G., Niethard, N., and Born, J. (2019). Mechanisms of Systems Memory Consolidation during Sleep. Nat. Neurosci. 22, 1598–1610. doi:10.1038/s41593-019-0467-3
Kloosterman, F., Davidson, T. J., Gomperts, S. N., Layton, S. P., Hale, G., Nguyen, D. P., et al. (2009). Micro-Drive Array for Chronic In Vivo Recording: Drive Fabrication. JoVE, 1094. doi:10.3791/1094
Koniaris, E., Drimala, P., Sotiriou, E., and Papatheodoropoulos, C. (2011). Different Effects of Zolpidem and Diazepam on Hippocampal Sharp Wave-Ripple Activity In Vitro. Neuroscience 175, 224–234. doi:10.1016/j.neuroscience.2010.11.027
Krystal, A. D. (2020). Sleep Therapeutics and Neuropsychiatric Illness. Neuropsychopharmacology 45, 166–175. doi:10.1038/s41386-019-0474-9
Latchoumane, C. V., Ngo, H. V., Born, J., and Shin, H. S. (2017). Thalamic Spindles Promote Memory Formation during Sleep through Triple Phase-Locking of Cortical, Thalamic, and Hippocampal Rhythms. Neuron 95, 424–435.e6. doi:10.1016/j.neuron.2017.06.025
Le Van Quyen, M., Bragin, A., Staba, R., Crépon, B., Wilson, C. L., and Engel, J. (2008). Cell Type-Specific Firing during Ripple Oscillations in the Hippocampal Formation of Humans. J. Neurosci. 28, 6104–6110. doi:10.1523/JNEUROSCI.0437-08.2008
Liang, L., Huang, Y., Xu, R., Wei, Y., Xiao, L., and Wang, G. (2019). Eszopiclone for the Treatment of Primary Insomnia: a Systematic Review and Meta-Analysis of Double-Blind, Randomized, Placebo-Controlled Trials. Sleep Med. 62, 6–13. doi:10.1016/j.sleep.2019.03.016
Logothetis, N. K., Eschenko, O., Murayama, Y., Augath, M., Steudel, T., Evrard, H. C., et al. (2012). Hippocampal-cortical Interaction during Periods of Subcortical Silence. Nature 491, 547–553. doi:10.1038/nature11618
Maingret, N., Girardeau, G., Todorova, R., Goutierre, M., and Zugaro, M. (2016). Hippocampo-cortical Coupling Mediates Memory Consolidation during Sleep. Nat. Neurosci. 19, 959–964. doi:10.1038/nn.4304
Malkani, R. G., and Zee, P. C. (2020). Brain Stimulation for Improving Sleep and Memory. Sleep Med. Clin. 15, 101–115. doi:10.1016/j.jsmc.2019.11.002
Manoach, D. S., Mylonas, D., and Baxter, B. (2020). Targeting Sleep Oscillations to Improve Memory in Schizophrenia. Schizophr. Res. 221, 63–70. doi:10.1016/j.schres.2020.01.010
Matheson, E., and Hainer, B. L. (2017). Insomnia: Pharmacologic Therapy. Am. Fam. Physician 96, 29–35.
McClelland, J. L., McNaughton, B. L., and O'Reilly, R. C. (1995). Why There Are Complementary Learning Systems in the hippocampus and Neocortex: Insights from the Successes and Failures of Connectionist Models of Learning and Memory. Psychol. Rev. 102, 419–457. doi:10.1037/0033-295X.102.3.419
Mednick, S. C., McDevitt, E. A., Walsh, J. K., Wamsley, E., Paulus, M., Kanady, J. C., et al. (2013). The Critical Role of Sleep Spindles in Hippocampal-dependent Memory: a Pharmacology Study. J. Neurosci. 33, 4494–4504. doi:10.1523/JNEUROSCI.3127-12.2013
Methippara, M., Bashir, T., Suntsova, N., Szymusiak, R., and McGinty, D. (2010). Hippocampal Adult Neurogenesis Is Enhanced by Chronic Eszopiclone Treatment in Rats. J. Sleep Res. 19, 384–393. doi:10.1111/j.1365-2869.2010.00833.x
Mylonas, D., Baran, B., Demanuele, C., Cox, R., Vuper, T. C., Seicol, B. J., et al. (2020). The Effects of Eszopiclone on Sleep Spindles and Memory Consolidation in Schizophrenia: a Randomized Clinical Trial. Neuropsychopharmacology 45, 2189–2197. doi:10.1038/s41386-020-00833-2
Ngo, H. V., Fell, J., and Staresina, B. (2020). Sleep Spindles Mediate Hippocampal-Neocortical Coupling during Long-Duration Ripples. Elife 9, e57011 doi:10.7554/eLife.57011
Nguyen, D. P., Layton, S. P., Hale, G., Gomperts, S. N., Davidson, T. J., Kloosterman, F., et al. (2009). Micro-drive Array for Chronic In Vivo Recording: Tetrode Assembly. JoVE, 1098. doi:10.3791/1098
Niknazar, M., Krishnan, G. P., Bazhenov, M., and Mednick, S. C. (2015). Coupling of Thalamocortical Sleep Oscillations Are Important for Memory Consolidation in Humans. PLoS ONE 10, e0144720. doi:10.1371/journal.pone.0144720
Nowak, L. G., Azouz, R., Sanchez-Vives, M. V., Gray, C. M., and McCormick, D. A. (2003). Electrophysiological Classes of Cat Primary Visual Cortical Neurons In Vivo as Revealed by Quantitative Analyses. J. Neurophysiol. 89, 1541–1566. doi:10.1152/jn.00580.2002
Olsen, R. W., and Sieghart, W. (2009). GABA A Receptors: Subtypes Provide Diversity of Function and Pharmacology. Neuropharmacology 56, 141–148. doi:10.1016/j.neuropharm.2008.07.045
Ponomarenko, A. A., Korotkova, T. M., Sergeeva, O. A., and Haas, H. L. (2004). Multiple GABAA Receptor Subtypes Regulate Hippocampal Ripple Oscillations. Eur. J. Neurosci. 20, 2141–2148. doi:10.1111/j.1460-9568.2004.03685.x
Richey, S. M., and Krystal, A. D. (2011). Pharmacological Advances in the Treatment of Insomnia. Curr. Pharm. Des. 17, 1471–1475. doi:10.2174/138161211796197052
Schlingloff, D., Káli, S., Freund, T. F., Hájos, N., and Gulyás, A. I. (2014). Mechanisms of Sharp Wave Initiation and Ripple Generation. J. Neurosci. 34, 11385–11398. doi:10.1523/JNEUROSCI.0867-14.2014
Staresina, B. P., Bergmann, T. O., Bonnefond, M., van der Meij, R., Jensen, O., Deuker, L., et al. (2015). Hierarchical Nesting of Slow Oscillations, Spindles and Ripples in the Human hippocampus during Sleep. Nat. Neurosci. 18, 1679–1686. doi:10.1038/nn.4119
Stark, E., Roux, L., Eichler, R., Senzai, Y., Royer, S., and Buzsáki, G. (2014). Pyramidal Cell-Interneuron Interactions Underlie Hippocampal Ripple Oscillations. Neuron 83, 467–480. doi:10.1016/j.neuron.2014.06.023
Trenque, T., Bustany, P., Lamiable, D., Legros, S., and Choisy, H. (1994). Pharmacokinetics and Brain Distribution of Zolpidem in the Rat after Acute and Chronic Administration. J. Pharm. Pharmacol. 46, 611–613. doi:10.1111/j.2042-7158.1994.tb03868.x
Varela, C., and Wilson, M. A. (2020). mPFC Spindle Cycles Organize Sparse Thalamic Activation and Recently Active CA1 Cells during Non-REM Sleep. eLife 9, e48881. doi:10.7554/eLife.48881
Wamsley, E. J., Shinn, A. K., Tucker, M. A., Ono, K. E., McKinley, S. K., Ely, A. V., et al. (2013). The Effects of Eszopiclone on Sleep Spindles and Memory Consolidation in Schizophrenia: a Randomized Placebo-Controlled Trial. Sleep 36, 1369–1376. doi:10.5665/sleep.2968
Wang, D. V., Yau, H. J., Broker, C. J., Tsou, J. H., Bonci, A., and Ikemoto, S. (2015). Mesopontine Median Raphe Regulates Hippocampal Ripple Oscillation and Memory Consolidation. Nat. Neurosci. 18, 728–735. doi:10.1038/nn.3998
Wilson, M. A., and McNaughton, B. L. (1994). Reactivation of Hippocampal Ensemble Memories during Sleep. Science 265, 676–679. doi:10.1126/science.8036517
Winkelman, J. W., and Lecea, L. (2020). Sleep and Neuropsychiatric Illness. Neuropsychopharmacology 45, 1–2. doi:10.1038/s41386-019-0514-5
Xi, M., and Chase, M. H. (2008). Effects of Eszopiclone and Zolpidem on Sleep and Waking States in the Adult Guinea Pig. Sleep 31, 1043–1051.
Yamamoto, J., and Tonegawa, S. (2017). Direct Medial Entorhinal Cortex Input to Hippocampal CA1 Is Crucial for Extended Quiet Awake Replay. Neuron 96, 217–227.e4. doi:10.1016/j.neuron.2017.09.017
Ylinen, A., Bragin, A., Nádasdy, Z., Jandó, G., Szabó, I., Sik, A., et al. (1995). Sharp Wave-Associated High-Frequency Oscillation (200 Hz) in the Intact hippocampus: Network and Intracellular Mechanisms. J. Neurosci. 15, 30–46. doi:10.1523/jneurosci.15-01-00030.1995
Yoshimoto, M., Higuchi, H., Kamata, M., Yoshida, K., Shimizu, T., and Hishikawa, Y. (1999). The Effects of Benzodiazepine (Triazolam), Cyclopyrrolone (Zopiclone) and Imidazopyridine (Zolpidem) Hypnotics on the Frequency of Hippocampal Theta Activity and Sleep Structure in Rats. Eur. Neuropsychopharmacol. 9, 29–35. doi:10.1016/s0924-977x(97)00102-8
Keywords: hippocampus, sharp-wave ripples, memory, eszopiclone, zolpidem, sleep, insomnia treatment
Citation: Becker LA, Penagos H, Flores FJ, Manoach DS, Wilson MA and Varela C (2022) Eszopiclone and Zolpidem Produce Opposite Effects on Hippocampal Ripple Density. Front. Pharmacol. 12:792148. doi: 10.3389/fphar.2021.792148
Received: 09 October 2021; Accepted: 13 December 2021;
Published: 11 January 2022.
Edited by:
Philippe De Deurwaerdere, Université de Bordeaux, FranceReviewed by:
Kamran Diba, University of Michigan, United StatesRegis Parmentier, Centre National de la Recherche Scientifique (CNRS), France
Copyright © 2022 Becker, Penagos, Flores, Manoach, Wilson and Varela. This is an open-access article distributed under the terms of the Creative Commons Attribution License (CC BY). The use, distribution or reproduction in other forums is permitted, provided the original author(s) and the copyright owner(s) are credited and that the original publication in this journal is cited, in accordance with accepted academic practice. No use, distribution or reproduction is permitted which does not comply with these terms.
*Correspondence: Carmen Varela, Y2FybWVudi53b3JrQGdtYWlsLmNvbQ==