- State Key Laboratory of Southwestern Chinese Medicine Resources, Key Laboratory of Standardization for Chinese Herbal Medicine, Ministry of Education, School of Pharmacy, Chengdu University of Traditional Chinese Medicine, Chengdu, China
Polygonum multiflorum Thunb. (He-shou-wu in Chinese), a Chinese botanical drug with a long history, is widely used to treat a variety of chronic diseases in clinic, and has been given the reputation of “rejuvenating and prolonging life” in many places. 2,3,4′,5-tetrahydroxystilbene-2-O-β-D-glucoside (TSG, C20H22O9) is the main and unique active ingredient isolated from Polygonum multiflorum Thunb., which has extensive pharmacological activities. Modern pharmacological studies have confirmed that TSG exhibits significant activities in treating various diseases, including inflammatory diseases, neurodegenerative diseases, cardiovascular diseases, hepatic steatosis, osteoporosis, depression and diabetic nephropathy. Therefore, this review comprehensively summarizes the pharmacological and pharmacokinetic properties of TSG up to 2021 by searching the databases of Web of Science, PubMed, ScienceDirect and CNKI. According to the data, TSG shows remarkable anti-inflammation, antioxidation, neuroprotection, cardiovascular protection, hepatoprotection, anti-osteoporosis, enhancement of memory and anti-aging activities through regulating multiple molecular mechanisms, such as NF-κB, AMPK, PI3K-AKT, JNK, ROS-NO, Bcl-2/Bax/Caspase-3, ERK1/2, TGF-β/Smad, Nrf2, eNOS/NO and SIRT1. In addition, the toxicity and pharmacokinetics of TSG are also discussed in this review, which provided direction and basis for the further development and clinical application of TSG.
1 Introduction
Polygonum multiflorum Thunb. (PM), a famous traditional Chinese botanical drug, is often used as a tonic or anti-aging agent in some regions of Asia (Ling and Xu, 2016). For its clinical application, raw and prepared PM are differentiated, and both of them are collected in the Chinese Pharmacopoeia 2020. It has been reported that raw PM has the efficacies of detoxification, eliminating carbuncle, and relaxing bowel, while prepared PM can tonify the liver and kidney, boost essence blood, strengthen muscles and bones, and eliminate turbid blood and reduce lipids. In fact, PM is often utilized in compound preparations, such as Shouwu Pills, Yangxue Anshen Tablets, Yangxue Shengfa Capsules, and Shenbao Tablets. These preparations and health products containing PM are not only sold in the Chinese market, but also available far from foreign markets. Current research has showed that PM contains multiple components such as anthraquinones, stilbene glycosides, flavonoids, phospholipids, and phenolics (Liu et al., 2018). Among them, 2,3,5,4′-tetrahydroxystilbene-2-O-β-D-glucoside (TSG) is the most unique and important water-soluble ingredient of PM.
Modern pharmacological research has shown that TSG has significant pharmacological effects, including anti-inflammation (Chin et al., 2016; Li et al., 2018), antioxidation (Wen et al., 2020; Wu et al., 2020), neuroprotection (Gao et al., 2020; Tong et al., 2020), cardiovascular protection (Hu et al., 2019; Jia et al., 2019), hepatoprotection (Wang et al., 2020a; Zhang et al., 2020), anti-osteoporosis (Zheng et al., 2017; Zhang et al., 2018a), memory enhancement (Hou et al., 2011; Chen et al., 2016), and anti-aging (Zhang et al., 2013c; Fan et al., 2021), which largely contribute to the prevention and treatment of various diseases, such as inflammatory diseases, neurodegenerative diseases, cardiovascular diseases, liver injury, osteoporosis, and diabetic nephropathy. More importantly, nuclear factor kappa-B (NF-κB) (Lin et al., 2015b), Adenosine 5′-monophosphate (AMP)-activated protein kinase (AMPK) (Ning et al., 2018), Phosphatidylinositide 3-kinase (PI3K)-protein kinase B (AKT) (Zhang et al., 2013b), reactive oxygen species (ROS)-nitric oxide (NO) (Tao et al., 2011), B-cell lymphoma-2 (Bcl-2)/Bcl-2-associated X protein (Bax)/Caspase-3 (Zhou et al., 2020), and transforming growth factor-β (TGF-β)/Smad signaling pathways (Yao et al., 2015), have been shown to mediate the therapeutic effects of TSG. However, there is a lack of a comprehensive summary of TSG currently. Therefore, this article provides a systematic review about the physical/chemical properties, pharmacological effects, toxicity, and pharmacokinetic properties of TSG up to 2021 through searching the Web of Science, PubMed, ScienceDirect and CNKI databases, with the aim to provide guidance and evidence for the further development and clinical application of TSG.
2 Physical and Chemical Properties of TSG
TSG (C20H22O9, Figure 1) is a polyhydroxy stilbene compound extracted from the traditional tonic TCM PM (Lin et al., 2015a). It was first discovered from the tuberous roots of PM by Japanese scientists in 1975 (Hata et al., 1975). Since then, there has been increased attention and research on TSG. More importantly, as the most predominant and unique active ingredient in PM, TSG is considered as a quality detection index in Chinese Pharmacopoeia 2020. It is specified that the TSG content in raw PM should not be less than 1.00% and that in prepared PM less than 0.70% (Wang et al., 2020b). TSG is white amorphous powder with a molecular weight of 406.3833 and a density of 1.593 g/cm3. Some physical and chemical properties of TSG are shown in Table 1 (The data in the table are referred to https://baike.baidu.com).
3 Pharmacological Properties of TSG
Many studies have confirmed that TSG has various pharmacological properties through modulating multiple signaling transduction pathways, such as NF-κB, PI3K-AKT, ERK1/2, AMPK, Nrf2, Bcl-2/Bax/Caspase-3, ROS-NO, TGF-β/Smad, MAPK, and SIRT1. The pharmacological properties of TSG are shown in Table 2. The molecular pathways involved in the pharmacological action of TSG are presented in Figure 2 and Figure 3.
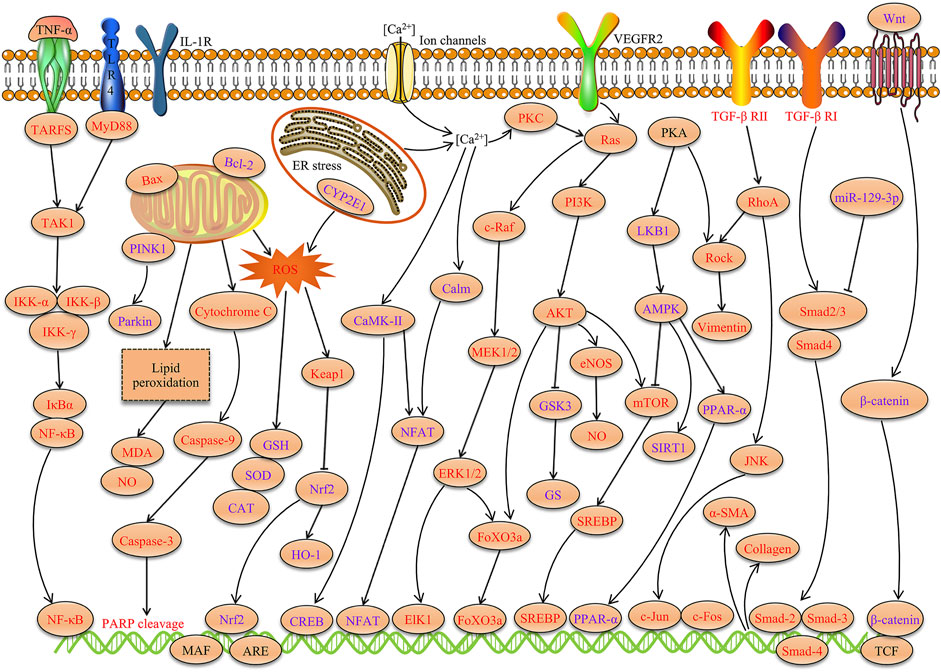
FIGURE 3. Molecular pathways involved in the pharmacological properties of TSG. TSG exerts significant anti-inflammation, antioxidant, neuroprotection, cardiovascular protection, hepatoprotection, anti-osteoporosis, enhancement of memory and anti-aging activities through modulating these signaling transduction pathways (Red represents the inhibitory effect of TSG and purple represents the promotion effect of TSG).
3.1 Anti-Inflammatory Effects
Inflammation is a defensive response to various harmful physiological stimuli (such as infection, tissue injury, tissue stress, and dysfunction), which can activate various immune cells and tissues, including macrophages, lymphocytes, monocytes, and neutrophils (Medzhitov, 2008). Inflammation is not only an important component of the immune system, but also the basis of various physiological and pathological processes, which is closely associated with the occurrence of many chronic diseases, such as cardiovascular disease, neurodegeneration, inflammatory bowel disease, diabetes, and cancer (Yeung et al., 2018). A study has shown that NO and prostaglandin E2 (PGE2), controlled by the expression of inducible nitric oxide synthase (iNOS), endothelial nitric oxide synthase (eNOS) and cyclooxygenase-2 (COX-2), are responsible for the development of inflammation (Yun et al., 2008). In addition, lipopolysaccharide (LPS), a bacterial endotoxin, can activate endothelial cells and promote the release of a large number of inflammatory cytokines, ultimately leading to the activation of inflammatory signaling cascade and immune dysfunction (Dauphinee and Karsan, 2006; Shi et al., 2014). Interestingly, a large number of recent experimental studies have reported the therapeutic effect of TSG on various inflammatory models.
In LPS-induced RAW264.7 cells, TSG (120, 240, 480 μM) inhibited macrophage activation and inflammation by attenuating the secretion of pro-inflammatory cytokines, including interleukin-6 (IL-6) and tumor necrosis factor-α (TNF-α) (Yu et al., 2017). Similarly, in high glucose-induced podocytes of mice, TSG (0.1, 1, 10 μM) protected podocytes from apoptosis by decreasing NLRP3 inflammasome and interleukin-1β (IL-1β) expression (Li et al., 2018). Moreover, in a Porphyromonas gingivalis-induced inflammation model in human gingival fibroblasts and a ligature-induced periodontitis model in rats, TSG showed anti-inflammatory effects by enhancing the AMPK activation and sirtuin 1(SIRT1) expression to suppress the production of inflammatory cytokines, such as TNF-α, IL-1β and IL-6, which was attributed to the activation of extracellular signal-regulated kinase 1/2 (ERK1/2) and AMPK as well as the inactivation of the NF-κB signaling pathway (Chin et al., 2016). Taken together, TSG may serve as a potential drug for the treatment of inflammatory diseases.
In acetic acid-induced acute colitis in mice, TSG (60, 120 mg/kg) significantly ameliorated the colonic injury by reducing the levels of myeloperoxidase (MPO), malonaldehyde (MDA) and NO as well as increasing superoxide dismutase (SOD) activity (Wang et al., 2008). Similarly, in mitomycin C-induced chronic colitis in mice, TSG (60 mg/kg) significantly ameliorated the colonic injury by modulating the above indicators (Wang et al., 2008). The beneficial effects of TSG on acute and chronic experimental colitis are mainly attributed to attenuating oxygen and nitrogen radical levels and down-regulating iNOS expression (Wang et al., 2008). Interestingly, the study of Zeng et al. (2011) also confirmed the protective effects of TSG (10, 30, 60 mg/kg) against acetic acid-induced experimental colitis in mice. Specifically, TSG significantly attenuated acetic acid-induced colonic injury, such as reversing weight loss and ameliorating histopathological changes (Zeng et al., 2011). In addition, TSG can decrease the expression of the inflammatory mediators such as TNF-α, IL-6 and cyclooxygenase-2 (COX-2) as well as the MDA content. The protective mechanisms mainly involved the up-regulation of peroxisome proliferator-activated receptor γ (PPAR-γ) and the inhibition of NF-κB pathway (Zeng et al., 2011). These suggest that TSG may be a promising candidate for the treatment of inflammatory bowel diseases.
In recent years, neuroinflammation has been increasingly implicated in the pathogenesis of neurological diseases, including trauma, stroke and neurodegenerative disorders (González et al., 2014). Neuroglia cells are considered to be the marker of neuroinflammation, particularly the activation of microglia (Voet et al., 2019). Once activated, microglia produce large amounts of proinflammatory cytokines, such as IL-1β, TNF-α, NO and reactive oxygen species (ROS), thus leading to neuronal damage (Voet et al., 2019; Shields et al., 2020). Therefore, inhibiting the activation of microglia may have an ameliorative effect on neuroinflammation-associated diseases. An earlier study showed that TSG (20, 40, 80 μM) inhibited microglia activation by reducing LPS-induced release of proinflammatory factors such as TNF-α, IL-1β and NO in microglia, as well as attenuating LPS-induced NADPH oxidase activation and ROS generation, which further attenuated neuroinflammation (Zhang et al., 2013a). The anti-inflammatory effect was associated with the diminished activation of NADPH oxidase and NF-κB signaling pathway (Zhang et al., 2013a). Similarly, the study by Park et al. (2016) showed that TSG (10, 20, 40 μM) could inhibit the NF-κB and activate the AMPK/Nrf2 pathway to attenuate LPS-induced neuroinflammatory responses in microglia, thereby exerting an anti-inflammatory effect. In addition, Fan et al. (2018a) showed that TSG (50, 100, 200 mg/kg) also alleviated chronic inflammatory pain induced by complete Freund’s adjuvant in mice by inhibiting the neuronal apoptosis, microglial activation, and GluN2B overexpression in the anterior cingulate cortex. The protective effect is mainly attributed to the inhibition of p38/NF-κB signaling pathway (Fan et al., 2018a). Therefore, TSG is expected to be a new anti-inflammatory and analgesic drug.
3.2 Antioxidant Effects
Oxidative stress refers to the excessive production of highly reactive molecules such as ROS and reactive nitrogen, and the degree of oxidation exceeds the ability of cells to scavenge oxides, resulting in the disruption of redox signaling and control, and/or molecular damage (Sies et al., 2017). As we all know, the oxidation reaction of ROS is considered to be a key factor in triggering oxidative stress (Kuwabara et al., 2008). When ROS accumulates excessively in the body, it will produce serious cytotoxicity because of its damage on DNA, protein, and other macromolecules as well as lipid peroxidation (Dixon and Stockwell, 2014). At present, oxidative stress is considered as an important factor of aging and age-related diseases, such as type 2 diabetes, cardiovascular disease, neurodegenerative disease, and cancer (Valko et al., 2006; Luo et al., 2020). The indicators of the damage caused by oxidative free radicals mainly include antioxidant enzymes, such as SOD, catalase (CAT), glutathione peroxidase (GSH-Px), and lipid peroxidation products, such as MDA, 4-hydroxynonenoic acid (Sehirli et al., 2008). Interestingly, a large amount of literature showed that TSG had antioxidant activity by regulating the expression of the oxidative damage markers, which suggested that TSG might be an effective antioxidant.
In human dermal fibroblasts, TSG (20, 100, 500 μM) showed protective effects on UV-B-induced stress premature senescence by up-regulating SOD and inhibiting MDA (Meijiao and Yifeng, 2019). Similarly, in human brain microvascular endothelial cells, TSG (50, 100 μM) exhibited protective effects on hydrogen peroxide-caused oxidative stress by increasing the activity of SOD and GSH, as well as reducing the content of MDA and ROS (Jiang et al., 2017). Furthermore, in 6-hydroxydopamine-induced rat adrenal pheochromocytoma PC12 cells, TSG (10, 20, 50 μM) showed antioxidant effects by inhibiting intracellular ROS and NO overproduction and decreasing apoptotic cells in a dose-dependent manner, which was attributed to the regulation of the ROS-NO signaling pathway (Tao et al., 2011). These indicate that TSG can provide effective protection for oxidative stress-related diseases.
In addition, in gentamicin-induced mouse cochlear UB/OC-2 cells, TSG (5, 10, 20 μM) inhibited the ototoxicity by decreasing the ROS production and activating the antioxidant enzyme SOD (Wen et al., 2020). Nuclear factor erythroid 2-related factor 2 (Nrf2) is a key transcription factor in the cellular anti-oxidative stress system (Vomhof-Dekrey and Picklo, 2012). The Nrf2 signaling pathway regulates oxidative stress mainly by regulating the transcription of various detoxification genes, which plays an important role in the mechanism of resisting endogenous or exogenous oxidative stress (Bellezza et al., 2018). When the redox balance is destroyed, Nrf2 enters the nucleus and activates the transcription of a variety of antioxidant genes, reducing the cell damage caused by ROS and electrophilic substances, which maintains the physiological balance of oxidation-antioxidation in the body (Stępkowski and Kruszewski, 2011; Bellezza et al., 2018). In H2O2-induced oxidative stress of UB/OC-2 cells, TSG (5, 10, 20, 40 μM) showed a strong ability of antioxidant stress by increasing the level of antioxidant/detoxification enzymes such as heme oxygenase 1 (HO-1), NQO1, glutathione-s-transferase (GST), and UGT, as well as inhibiting the excessive production of ROS, apoptosis, and autophagy, which was attributed to the activation of the Nrf2 pathway (Wu et al., 2020). These results suggest that TSG-mediated activation of the Nrf2 signaling pathway may be an important mechanism of enhancing endogenous antioxidant defense system.
3.3 Neuroprotective Effects
Currently, inflammatory response and oxidative stress are well recognized by the medical community as key events during the development of neurodegenerative diseases (Amor et al., 2014; Singh et al., 2019). Fortunately, in recent years, there have been a great deal of literature reporting that TSG shows neuroprotective effects in several neurodegenerative diseases, such as Alzheimer’s disease (AD), Parkinson’s disease (PD), and cerebral ischemic injury, specifically manifested by inhibiting microglia-mediated neuroinflammation, enhancing mitochondrial function, decreasing oxidative stress, inhibiting neuronal apoptosis, reducing α-synuclein and amyloid β-protein (Aβ) production, and increasing neurotrophic factors (Zhang et al., 2013b; Xie et al., 2018; Yu et al., 2019). TSG may exert neuroprotective effects through the following molecular mechanisms and show synergistic biological effects through their anti-inflammatory and antioxidant activities.
3.3.1 Bcl-2/Bax/Caspase-3
In 1-methyl-4-phenylpyridinium (MPP+)- or glutamate-induced cytotoxicity or apoptosis models, TSG significantly reduced the markers of oxidative stress and exerted neuroprotective effects through multiple pathways due to its excellent antioxidant activity (Zhang et al., 2017a; Lee et al., 2017). On the one hand, TSG significantly enhances the activities of antioxidant enzymes in cells, including SOD, CAT and GSH-Px, as well as decreases MDA content (Sun et al., 2011; Zhang et al., 2017a). On the other hand, TSG significantly up-regulates the Bcl-2/Bax ratio, reverses cytochrome c release, and inhibits Caspase-3 activation (Zhang et al., 2017b; Lee et al., 2017). In addition, TSG also significantly inhibits the activation of the p38 mitogen-activated protein kinase (MAPK) signaling pathway, whereas ERK phosphorylation is unaffected (Zhang et al., 2017a). Taken together, the mechanisms of the neuroprotective effect of TSG may be related to ameliorating mitochondrial dysfunction, reducing intracellular oxidative stress, and ultimately inhibiting apoptosis by regulating the Bcl-2/Bax/Caspase-3 signaling pathway (Sun et al., 2011; Zhang et al., 2017a; Lee et al., 2017).
α-synuclein, a soluble protein expressed in the presynaptic and perinuclear of central nervous system (CNS), is critically involved in the pathogenesis of PD and the associated dysfunction (Ozansoy and Başak, 2013). The study of Zhang et al. (2017b) showed that TSG inhibited the overexpression and aggregation of α-synuclein to achieve neuroprotection. In addition, in the middle cerebral artery occlusion (MCAO) model, TSG attenuated cerebral ischemia/reperfusion injury and alleviated neurological deficit symptoms by decreasing neurological scores, reducing cerebral infarct volume, ameliorating neuronal damage in the ischemic cortex and hippocampus, and inhibiting the expression of NOX4, activated Caspase-3 (9), and Beclin 1 proteins (Yu et al., 2019). Its protective mechanism may involve inhibiting oxidative stress, and reducing neuronal apoptosis and autophagy (Yu et al., 2019).
3.3.2 Inflammatory Signaling Pathway
Neuroinflammation is considered to be a key event in the development of neurodegenerative diseases, and is mainly characterized by microglia activation (Baune, 2015; Spagnuolo et al., 2018). Therefore, the development of drugs that can antagonize microglial activation and reduce the release of pro-inflammatory cytokines becomes a potentially important target for the treatment of neurodegenerative diseases. One study showed that TSG (20, 40, 80 μM) reduced LPS-induced release of proinflammatory factors (IL-1β, TNFα and NO) in microglia, the activation of NADPH oxidase, and ROS generation (Zhang et al., 2013a). The further study suggested that the neuroprotective effect of TSG may involve the NF-κB signaling pathway activation (Zhang et al., 2013a). Similarly, TSG (10, 50 mg/kg) significantly attenuated dopamine neuron loss in the substantia nigra in a model of rats with LPS-induced dopamine neuron injury (Zhou et al., 2018). Specifically, TSG can double-regulate glial cells by ameliorating microglia-mediated neuroinflammation and enhancing the secretion of astrocyte-derived neurotrophic factors, thus protecting dopamine neurons from LPS-induced neurotoxicity (Zhou et al., 2018).
Aβ deposition is regarded as a key pathogenic event in the progression of AD, so the inhibition of Aβ-induced microglial activation is considered to be an effective strategy for the treatment of AD (Borderud et al., 2014). In Aβ-induced microglial model, TSG attenuated Aβ-induced microglial activation and inflammation, and allowed the differentiation of microglia toward the M2 phenotype (Jiao et al., 2018). Specifically, TSG could significantly inhibit the production of inflammatory molecules, including iNOS, NO, COX-2, and PGE2, as well as increase the levels of M2 markers, including IL-10, brain-derived neurotrophic factors, glial cell line derived neurotrophic factors, and arginase-1 (Jiao et al., 2018). Furthermore, TSG (10, 50 mg/kg) significantly protected dopamine neurons from 6-hydroxydopamine-(6-OHDA)-induced neurotoxicity in a dopamine neuron injury model (Huang et al., 2018). Similarly, in a co-culture model of primary glial cells, TSG (20, 40, 80 μM) also showed similar neuroprotective effects (Huang et al., 2018). A subsequent study showed that TSG-mediated neuroprotection was closely related to the inhibition of microglia activation, the subsequent release of pro-inflammatory factors, and the inactivation of MAPK signaling pathway (Huang et al., 2018). Taken together, the neuroprotective effect of TSG is associated with the regulation of microglia, which is mediated by NF-κB and MAPK signaling pathways.
3.3.3 PI3K-AKT
A large number of recent studies have reported that the PI3K/AKT pathway ensures an active state of the neural defense system, thereby exerting a neuroprotective effect by preventing apoptosis and neuroinflammation (Nakano et al., 2017). Therefore, the search and development of drugs that can modulate the PI3K/AKT pathway is crucial for the prevention and treatment of neurodegenerative diseases. A study showed that TSG (0.1, 1, 10 μM) showed significant neuroprotective effects against MPP+-induced PC12 cell injury and apoptosis (Yin et al., 2018). The effect may be partially mediated by the PI3K/AKT signaling pathway (Yin et al., 2018). Two years apart, the team demonstrated the potential neuroprotective effects of TSG (20, 40 mg/kg) in a mouse model of PD (Zhang et al., 2013b). The results showed that TSG could promote the survival of dopamine neurons in vivo, and also reconfirmed that TSG-induced neuroprotection was mediated by the PI3K/AKT signaling pathway (Zhang et al., 2013b).
Studies have reported that Glutamate transporter-1 (GLT-1) deficiency has an important impact on neuronal damage in excitotoxicity-related diseases, such as cerebral ischemia and AD (Mookherjee et al., 2011; Sun et al., 2014). Therefore, the drugs which can enhance GLT-1 protein expression may have beneficial effects on neuronal toxicity-associated diseases. Given that TSG had good neuroprotective effects in a series of experimental models, Chen et al. (2017) evaluated the effects of TSG on GLT-1 protein expression in primary astrocytes of mice. Their results showed that TSG (10, 30, 80 μM) significantly increased GLT-1 protein expression in a dose-dependent manner (Chen et al., 2017). More importantly, they found that the increase of GLT-1 protein levels was achieved by the activation of AKT but not ERK1/2 (Chen et al., 2017). Moreover, Yin et al. (2018) also confirmed the neuroprotective effect of TSG on amyloid precursor protein expression by in vivo (APP/PS1 mice) and in vitro (HEK-293FT cells and SH-SY5Y cells) experiments. The specific mechanisms may be activating the AKT-GSK3β signaling pathway and subsequently attenuating the splicing activity of alternative splicing factors to reduce Aβ deposition (Yin et al., 2018).
3.3.4 JNK
Recently, it has been shown that the Jun N-terminal kinase (JNK) signaling pathway is involved in the occurrence and development of several neuronal diseases, such as cerebral ischemia, intracerebral hemorrhage, AD and PD (Graczyk, 2013). Therefore, the current medical community is trying to develop chemical inhibitors of this pathway to treat neurodegenerative diseases. A previous study showed that TSG (25, 50 μM) could reverse neuronal injury, elevation of intracellular ROS, and dissipation of mitochondrial membrane potential induced by oxygen-glucose deprivation followed by reperfusion in an in vitro ischemia model (Wang et al., 2009b). In an in vivo cerebral ischemia injury model of mice, TSG (15, 40 mg/kg) could significantly reduce the cerebral infarct volume and the number of positive cells in the cerebral cortex (Wang et al., 2009b). With more in-depth studies, we found that the protective effects of TSG against cerebral ischemia/reperfusion injury mainly involved JNK, SIRT1, and NF-κB pathway (Wang et al., 2009b). Similarly, Li et al. (2010b) showed that in the MPP+-induced apoptosis model of PC12 cells, TSG (1, 5, 10 μM) exerted a neuroprotective effect by inhibiting ROS production and regulating JNK activation.
3.3.5 Wnt/β-Catenin
In recent years, cell replacement therapy has been considered as an alternative option for the treatment of neurodegenerative diseases of the CNS (Zhu et al., 2016). In addition, it has been reported that regulating Wnt/β-catenin signaling may improve the cell replacement therapy for PD (L’Episcopo et al., 2014). Interestingly, a recent study confirmed that TSG had a promoting effect on the differentiation of mesencephalic neural stem cells into dopaminergic neurons (Zhang and Yang, 2021). TSG can not only increase the proportion of tyrosine hydroxylase-positive cells and dopamine transporter-positive neurons, the late markers of mature dopaminergic neurons, but also enhance the expression of nuclear receptor-associated factor 1, a specific transcription factor for the development and maintenance of midbrain dopaminergic neurons (Zhang and Yang, 2021). In addition, TSG can also up-regulate the expression of Wnt/β-catenin signaling molecules, including Wnt1, Wnt3a, Wnt5a, and β-catenin (Zhang and Yang, 2021). These findings suggest that TSG can promote neuronal differentiation of neural stem cells in mice by up-regulating the Wnt/β-catenin signaling (Zhang and Yang, 2021). These also suggest that TSG may contribute to the PD treatment by neural stem cell transplantation.
3.3.6 Keap1-Nrf2
Although the etiology and pathogenesis of AD are not fully understood at present in the medical community, a large body of literature has suggested that oxidative stress is an important component of AD’s pathological processes (Wang et al., 2014; Tönnies and Trushina, 2017). In brief, oxidative stress participates in the initiation and progression of AD by promoting Aβ deposition, tau hyperphosphorylation, synaptic dysfunction, and neuronal loss (Chen and Zhong, 2014). Therefore, given the relationship between oxidative stress and AD, antioxidants may be helpful in AD treatment. A study showed that TSG (30, 60, 120 mg/kg) reversed Aβ1-42-induced alterations of cognitive behavior, biochemical changes, and oxidative damage in mice. Specifically, TSG significantly reduced MDA and GSSG levels, and increased GSH, CAT, and SOD activities in the hippocampus and cortex (Xie et al., 2018). In addition, TSG also increases Nrf2 and HO-1 protein expression as well as decreases Keap1 protein expression in a dose-dependent manner (Xie et al., 2018). These beneficial effects of TSG are mainly attributed to the inhibition of Keap1/Nrf2 pathway in hippocampal and cerebral cortical tissues (Xie et al., 2018). Meanwhile, it also suggests us that TSG can be used as a natural agent for the treatment of AD.
3.3.7 AMPK/PINK1/Parkin
AMPK is generally activated upon a decline in energy supply and is closely associated with autophagy especially mitophagy (Hang et al., 2019). The expression of PTEN-induced putative kinase 1 (PINK1) on the outer membrane of dysfunctional mitochondria is promoted by mitophagy, and synchronously elevates Parkin, an E3 ubiquitin ligase (Devi et al., 2017). This suggests that the AMPK/PINK1/Parkin pathway may be a new and attractive target for the treatment of AD. Gao et al. (2020) showed that TSG (0.1, 1, 10 μM) had neuroprotective effects on LPS/ATP- and Aβ25-35-induced microglial and neuronal inflammation. Specifically, TSG treatment significantly reduced inflammatory cytokine secretion and the NLRP3 inflammasome activation, and regulated mitophagy (Gao et al., 2020). More importantly, the team also found that the protective effect of TSG was abolished when PINK1 or parkin was knocked down by the siRNA or the CRISPR/Cas9 system (Gao et al., 2020). These results suggest that the neuroprotective effects of TSG may be achieved by enhancing AMPK/PINK1/Parkin-dependent mitophagy to attenuate inflammatory damage (Gao et al., 2020).
3.3.8 Others
In cerebral ischemic injury, nerve growth factor (NGF) plays an important role on reducing neuronal injury, improving survival rate, and repairing injury (Allen et al., 2013). Therefore, it is of great significance for the protection of neuronal injury after cerebral ischemia to adopt therapeutic measures that can continuously promote NGF expression. A study showed that TSG (60, 120 mg/kg) had a certain neuroprotective effect on rats with cerebral ischemia-reperfusion (Jie et al., 2010). According to the experimental results of biochemical pharmacology, the neuroprotective mechanisms mainly involved up-regulating the NCF protein expression, activating the PKA pathway, and increasing the growth associated protein-43 (GAP-43) expression, a marker of axon regeneration (Jie et al., 2010). In addition, in the rat model with sodium azide-induced mitochondrial dysfunction, TSG (60, 120 mg/kg) was proved to slow the AD progression (Zhang et al., 2018c). The mechanisms mainly included increasing mitochondrial COX activity, reducing Aβ expression by inhibiting the production of amyloidogenic β-amyloid precursor protein (APP), β-site APP cleaving enzyme 1 (BACE1) and presenilin 1 (PS1), and increasing the expression of neurotrophic factors including NGF, brain-derived neurotrophic factor (BDNF), and its receptor tropomyosin-related kinase B (TrkB) (Zhang et al., 2018c). The latest research showed that long-term TSG (50, 100 mg/kg) treatment significantly improved the cognitive impairment by reducing the deposition of Aβ plaques in the hippocampus and cortex in the APP/PS1 AD mouse model, thereby preventing AD (Gao et al., 2021). In summary, these findings raise the possibility that TSG becomes a new drug for the treatment of neurodegenerative diseases such as AD.
3.4 Cardiovascular Protection Effects
The morbidity and mortality of cardiovascular diseases are increasing year by year globally, which have been a major public health problem (Feng et al., 2016). According to epidemiological statistics, approximately 17.8 million deaths due to cardiovascular diseases occurred worldwide in 2017 (Jagannathan et al., 2019). There is clear evidence that some medicines can improve the clinical prognosis of patients with stable cardiovascular diseases, including statins, aspirin, β-receptor blockers and ACE-I/angiotensin receptor blockers, which are even better than surgical treatment (Leong et al., 2017). However, therapeutic affordability and accessibility remain major challenges for cardiovascular disease treatment. Therefore, there is no delay to develop safe, effective, and economical drugs for the treatment of cardiovascular diseases. Fortunately, a large number of studies have shown that TSG is a potential therapeutic agent for cardiovascular diseases, mainly in hypolipidemia, protecting endothelial cells, anti-atherosclerosis, inhibiting vascular smooth muscle cell (VSMC) proliferation, and protecting the myocardium (Xu et al., 2011; Yao et al., 2013; Peng et al., 2016; Chen et al., 2018b; Qi et al., 2020). The signaling pathways involved in treating cardiovascular diseases by TSG are summarized as follows.
3.4.1 TGF-β/Smad
TGF-β/Smad signaling pathway is closely related to cardiovascular diseases, and plays an important role in the pathogenesis of hypertension, atherosclerosis, coronary heart disease, heart failure, myocardial infarction, and other diseases (Zeng et al., 2016). A study showed that TSG (50, 100 µM) protected human umbilical vein endothelial cells (HUVECs) from oxidized low-density lipoprotein (oxLDL)-induced endothelial dysfunction by inhibiting the expression and cleavage of vimentin, and the expression of adhesion molecules and their co-localization with vimentin (Yao et al., 2014). The protective effect may be closely related to blocking the TGF-β/Smad signaling pathway and activating Caspase-3 (Yao et al., 2014). Subsequently, in the model of TNF-α-treated HUVECs, the research team again confirmed that TSG (50, 100 µM) could inhibit the vimentin expression by blocking the TGF-β/Smad signaling pathway, thereby protecting HUVECs from TNF-α-induced cell damage (Yao et al., 2015).
Macrophage-derived foam cell formation is an important event in the development of atherosclerosis (Chistiakov et al., 2017). In the U937 cell model induced by phorbol-12-myristate-13-acetate (PMA) and oxLDL, TSG (50, 100 µM) treatment significantly inhibited PMA-induced cell differentiation, and oxLDL-induced macrophage apoptosis and foam cell formation (Yao et al., 2016a). More importantly, TSG also attenuated PMA- and OXLDL-induced Caspase-3 activation and adhesion molecule levels (Yao et al., 2016a). The specific mechanisms may be interrupting TGF-β1/Smad signaling and Caspase-3 activation to inhibit the expression and degradation of vimentin (Yao et al., 2016a). In addition, increased proliferation and migration of vascular smooth muscle cells (VSMC) is also a key step in the formation of atherosclerotic lesions (Grootaert et al., 2018). Yao et al. (2016b) showed that TSG (50, 100 µM) significantly inhibited TNF-α-induced migration of VSMCs. At the same time, it could also inhibit the expression of TGF-β1 and TGF-βR1, the phosphorylation of TGF-βR1 and Smad2/3, and the nuclear translocation of Smad4 (Yao et al., 2016b). The effect of TSG may be achieved by blocking the TGF-β/Smad signaling pathway to inhibit the redistribution and expression of vimentin.
3.4.2 eNOS/NO
Abnormal metabolism of NO has been found to be a predisposing factor for a variety of cardiovascular diseases (Bryan, 2018). Moreover, endothelial function is mainly based on the function and activity of eNOS, a rate limiting enzyme of NO synthesis (Daiber et al., 2019). Therefore, eNOS/NO signaling pathway has an important regulatory effect on cardiovascular function. A study showed that TSG (30, 60, 120 mg/kg) attenuated intimal hyperplasia and improved endothelial function in rats with atherosclerosis (Zhang et al., 2009b). The molecular mechanisms might be related to preventing the changes of eNOS and iNOS gene and protein expression as well as the consequent increased NO production (Zhang et al., 2009b). It is well known that VSMC proliferation is a critical step in the development of atherosclerosis, which is closely associated with other cellular processes such as inflammation, apoptosis, and matrix alterations (Bennett et al., 2016). Therefore, the inhibition of VSMC proliferation may have beneficial effects on atherosclerosis. Two years apart, the team confirmed again that TSG (100 μM) had an inhibitory effect on platelet derived growth factor (PDGF)-BB-stimulated VSMC proliferation (Xu et al., 2012). The molecular mechanisms may involve the NO/cGMP/PKG signaling pathway (Xu et al., 2012).
3.4.3 ERK1/2
ERK1/2 are involved in the Ras/Raf/MEK/ERK signal transduction cascade, which is involved in the regulation of several processes, including cell adhesion, cell cycle progression, cell survival, cell proliferation, cell differentiation, gene transcription, and so on (Wortzel and Seger, 2011; Roskoski, 2012). Therefore, ERK1/2 play an important role in the pathogenesis and pathophysiology of several diseases. Xu et al. (2011) showed that TSG (10, 25, 50 μM) could dose-dependently inhibit PDGF-BB-induced VSMC proliferation. Its anti-proliferative effects may be mediated by preventing cell cycle progression, regulating protein expression of cell cycle regulators, and inhibiting ERK1/2 phosphorylation (Xu et al., 2011). Similarly, TSG (10, 50, 100 μM) also had a concentration-dependent inhibitory effect on angiotensin II (Ang II)-induced VSMC proliferation (Xu et al., 2015). According to the results of biochemical experimental studies such as flow cytometry and Western blotting, the effect might be related to the down-regulation of intracellular ROS and the inhibition of the Src-MEK1/2-ERK1/2 signaling pathway, which blocked cell cycle progression (Xu et al., 2015). A subsequent study showed that TSG (30, 60, 120 mg/kg) reduced the cardiac remodeling in pressure-overloaded rats in an abdominal aortic banding-induced cardiac remodeling model (Xu et al., 2014). With subsequent in-depth studies, the protective mechanisms may be related to reducing Ang II levels, enhancing SOD and GSH-Px activities in serum and cardiac tissue, and inhibiting TGF-β1 protein expression as well as ERK1/2 and p38 phosphorylation (Xu et al., 2014).
3.4.4 VEGF/ICAM-1
Studies have reported that high concentrations of vascular endothlial growth factor (VEGF) -A are detected in some cardiovascular diseases and are often associated with poor prognosis and disease severity (ErŽen et al., 2014). Therefore, controlling angiogenesis and VEGF-A may improve the life quality and longevity of patients with cardiovascular diseases. A study showed that TSG could promote reendothelialization by increasing the levels of serum VEGF and the expression of CD34 in the vessel wall (Hu et al., 2019). In addition, intercellular cell adhesion molecule-1 (ICAM-1), also known as CD54, is an important adhesion molecule mediating adhesive responses (Bui et al., 2020). Enhanced expression of ICAM-1 is considered as an important marker of atherosclerotic lesions (Watanabe and Fan, 1998). Thus, ICAM-1 can be exploited to identify the development and prognosis of atherosclerosis. Yang et al. (2005) showed that stilbene glycoside from PM had anti-atherosclerotic effects by inhibiting ICAM-1 and VEGF expression in foam cells. Subsequently, the study by Wang et al. (2013) further confirmed that TSG could inhibit the expression of adhesion molecules (ICAM-1/VCAM-1) in both in vitro (oxLDL-induced U937 cells) and in vivo (the aortic wall of rats with diet-induced atherosclerosis) models. However, the mechanism by which TSG orchestrates this effect is unknown, remaining more thorough experimental research.
3.4.5 RhoA/ROCK
Accumulating evidence from basic and clinical studies suggests a pivotal role of RhoA/ROCK signaling in the pathogenesis of a variety of cardiovascular diseases, including essential hypertension, congestive heart failure, coronary heart disease, and atherosclerosis (Cai et al., 2016). Among them, increased ROCK activity mediates VSMC hypercontractility, endothelial dysfunction, inflammatory cell recruitment, and vascular remodeling (Surma et al., 2011). A recent study showed that TSG (25, 50, 100 μM) could ameliorate LPS-induced endothelial dysfunction by inhibiting RhoA/ROCK signaling (Qi et al., 2020). It is well known that the reorganization of F-actin in endothelial cells is an important pathological factor leading to increased endothelial permeability and endothelial dysfunction (Shi et al., 2013). Interestingly, the team also found that TSG regulated F-actin cytoskeletal rearrangement by inhibiting the RhoA/ROCK signaling pathway, thus inhibiting the formation of contractile ring and the changes of cell morphology (Qi et al., 2020).
3.4.6 Autophagy
Under normal conditions, the level of autophagic activity plays an important role in maintaining the homeostasis and function of cells. In particular, autophagy contributes to the maintenance of intracellular homeostasis in most cardiovascular cells, including cardiomyocytes, endothelial cells, and arterial smooth muscle cells (Bravo-San Pedro et al., 2017). However, defective or excessive autophagy may contribute to cardiovascular diseases such as atherosclerosis, heart failure, and hypertension (Mialet-Perez and Vindis, 2017). Dong et al. (2016) reported that TSG could inhibit excessive autophagy and improve microvascular endothelial dysfunction in a rat model of spontaneous hypertension. The protective effect was mainly attributed to the restoration of microvascular endothelial dysfunction by activating the AKT/mTOR pathway, which inhibited autophagy (Dong et al., 2016). This suggests that TSG can be applied to protect vascular function against subclinical changes in prehypertension.
3.4.7 MicroRNAs
It is reported that microRNA plays a key regulatory role in some important cellular pathways and the occurrence and development of various diseases (Liu et al., 2014). In cardiovascular diseases, it can play a cardiovascular protective role by regulating cardiomyocytes, fibroblasts and endothelial cells (Kalayinia et al., 2021). For example, a study showed that miR-129-3p may be involved in angiotensin II-mediated cardiac biology and diseases (Jeppesen et al., 2011). In addition, a genome-wide expression study of circulating microRNAs in patients with heart failure showed that the level of miR-129-3p in serum was significantly reduced (Cakmak et al., 2015). These results suggested that miR-129-3p might be a susceptible gene associated with cardiovascular disease, which was inhibited under the pathological conditions of myocardial cells. In one study, TSG (0.4 mM) treatment could reduce the palmitic acid-induced myocardial cells inflammation and apoptosis (Zou and Kong, 2019). According to the results of biochemical pharmacology and bioinformatics, its protective effect on cardiomyocytes may be mediated by targeting the miR-129-3p/Smad3 signaling pathway, including up-regulating the expression of miR-129-3p and inhibiting the expression of p-Smad3 (Zou and Kong, 2019). These results show that microRNA, as an important regulatory factor in the body, can regulate different molecular networks by interfering with the target genes, thus showing the effect of cardiovascular protection.
3.4.8 Others
Doxorubicin, also known as adriamycin, is an anthracycline antibiotic commonly used to treat a variety of cancers (Rivankar, 2014). However, severe side effects, such as cardiomyopathy and heart failure, have been observed in patients receiving doxorubicin treatment, which greatly limit its clinical application (Octavia et al., 2012). Interestingly, a study showed that TSG could reduce doxorubicin-induced cardiotoxicity in vitro (cardiomyocytes from neonatal Wistar rats) and in vivo (Kunming male mice) (Zhang et al., 2009a). With more in-depth studies, it has been shown that its cardioprotective effects are mainly achieved by inhibiting ROS production, the increase of intracellular Ca2+, and apoptosis-related signaling pathways (Zhang et al., 2009a). From these aspects, TSG may be a promising agent combined with doxorubicin to attenuate cardiotoxicity and improve its clinical efficacy. In addition, Peng et al. (2016) showed that TSG (120 mg/kg) also had beneficial effects on pressure overload-induced cardiac fibrosis. The protective effect on the heart was mainly attributed to the up-regulation of endogenous PPAR-γ, a potent endogenous antifibrotic factor (Peng et al., 2016).
Furthermore, Yao et al. (2013) investigated the protective effects of TSG on atherosclerotic rats by proteomic analysis. The results showed that a total of 21 proteins were involved in the anti-atherosclerotic effects of TSG, including HSP70, lipocortin 1, Apo A-I, calreticulin, vimentin, and so on, which were closely related to inflammation, cholesterol transport, apoptosis, and adhesion (Yao et al., 2013). However, the specific molecular mechanisms by which TSG regulated the expression of these proteins are not well defined. Subsequently, the study of Chen et al. (2018b) confirmed that TSG could also attenuate atherosclerosis in apolipoprotein E-deficient mice by promoting reverse cholesterol transport. Specifically, TSG achieved its protective effect on arteriolosclerosis by promoting the cholesterol efflux of macrophages and SR-BI-mediated hepatic cholesterol uptake, increasing the secretion of cholesterol from ABCG5 into bile, and improving cholesterol metabolism via CYP7A1 (Chen et al., 2018b).
3.5 Hepatoprotective Effects
TSG has been reported for the treatment of various liver diseases, such as alcoholic fatty liver disease, non-alcoholic fatty liver disease (NAFLD) and non-alcohol steatohepatitis (NASH). For example, TSG (25, 50, 100 μg/ml) significantly improved high cholesterol diet-induced NAFLD in zebrafish larvae (Wang et al., 2020a). According to the results of biochemical pharmacology, the mechanisms of its anti-NAFLD effect are mainly attributed to the regulation of the lipid metabolism-related pathways, the anti-inflammatory pathway IKKβ/NF-κB and the antioxidant pathway Keap1-Nrf2 (Wang et al., 2020a). Similarly, TSG (50, 100 mg/kg) was first demonstrated to significantly improve NASH and atherosclerosis in the mouse models with high fat diet (HFD)-induced metabolic syndrome and NASH (Xu et al., 2019). Its hepatoprotective effect was at least partly dependent on modulating the key regulators of lipid metabolism, inflammation, fibrosis and oxidative stress (Xu et al., 2019). In addition, Zhang et al. (2020) found TSG had a good ameliorative effect on alcoholic hepatic steatosis by in vitro (THP-1 macrophages) and in vivo (C57BL/6 mice) experiments. Specifically, TSG inhibits P2X7R-NLRP3 signaling in macrophages, and is subsequently unperturbed by activated macrophages, thus inhibiting the lipid accumulation in hepatocytes to attenuate alcoholic hepatic steatosis (Zhang et al., 2020). In conclusion, the regulation of alcoholic hepatic steatosis by TSG may be achieved by targeting the crosstalk between macrophages and hepatocytes.
In recent years, increasing evidence has demonstrated that gut microbiota plays a crucial role in the pathogenesis of liver diseases (Albhaisi et al., 2020). Briefly, when gut microbiota is disrupted or dysregulated, the bacteria in the gut and their metabolites such as endotoxin and LPS, can be transferred through the damaged intestinal tight junctions in the portal circulation, thereby activating cytokines via toll-like receptors and releasing IL-8 to stimulate the inflammatory response, which may contribute to liver inflammation and fibrosis (Henao-Mejia et al., 2012; Stevens et al., 2018). Interestingly, the study by Lin et al. (2015b) showed that, in HFD-induced NAFLD rats, TSG (12, 24, 48 mg/kg) could improve intestinal mucosal barrier function by regulating the balance of intestinal flora as well as increasing the protein expression of ZO-1 and Occludin, and then reverse the occurrence and development of NAFLD. These results suggest that TSG may serve as a promising drug candidate for the prevention and intervention of NAFLD/NASH.
3.6 Anti-Osteoporosis Effects
Despite the availability of drugs to treat osteoporosis, osteoporosis currently remains a huge and growing public health problem due to the certain rare side effects of these drugs and the lack of clear evidence supporting their long-term efficacy (Khosla and Hofbauer, 2017). Therefore, new drugs with no side effect and long-term anabolic effects on bone are need urgently. In recent years, there have been research showing that TSG has the ability to promote bone formation as well as protect osteoblasts, with a greater potential in the treatment of osteoporosis. Zhang et al. (2012) showed that TSG (0.1, 1, 10 μM) pretreatment for 24 h protected against H2O2-induced dysfunction and oxidative stress in osteoblastic MC3T3-E1 cells. Its protective effect is mainly mediated by inhibiting the release of bone resorption mediators and oxidative damage to cells (Zhang et al., 2012). Similarly, in MC3T3-E1 cells and bone tissue of BALB/c mouse model, TSG may also achieve preventive and protective effects against osteoporosis via PI3K/AKT, Wnt/FoxO3a, immune system and chemokine signaling pathway (Zhang et al., 2018a; Fan et al., 2018b).
Emerging evidence suggests that an increase in the activity of the skeletal renin-angiotensin system (RAS) is detrimental to bone tissue during hyperglycemia (Yamamoto et al., 2015; Zhang et al., 2016). In addition, it has been shown that TSG blocks the activity of tissue RAS, which plays a key role in the development of diabetic osteoporosis (Skov et al., 2014). Interestingly, the study of Zhang et al. (2019) confirmed that TSG (10, 40 mg/kg) had a protective effect on streptozotocin-induced diabetic osteoporosis in hyperglycemic mice, which might be attributed to its regulation of bone formation and bone resorption by inhibiting local RAS and acting on osteoblasts and osteoclasts (Zhang et al., 2019). However, whether TSG can ameliorate other tissue damage caused by local activation of RAS or directly act on RAS require further investigation. Furthermore, in bone marrow mesenchymal stem cells of rats and the dexamethasone-induced zebrafish model of osteoporosis, different doses of TSG were shown to promote osteoblast differentiation, as indicated by enhanced alkaline phosphatase activity, increased osteocalcin content, and increased nodule area (Zheng et al., 2017). Taken together, TSG may serve as a good drug candidate for the treatment of osteoporosis.
3.7 Memory-Enhancing Effects
It has been reported that TSG (1, 5, 10 μM) can improve and modify neuronal networks by increasing the activity of CaMKII and ERK1/2 in the hippocampus of normal mice and promoting hippocampal long-term potentiation (LTP), which enhances their learning and memory abilities (Wang et al., 2011). Similarly, the study by Zhou et al. (2012) suggested that the protective effect of TSG (50 mg/kg) on learning and memory impairment might be achieved by improving the structure and function of neuronal synapses through the up-regulation of SRC and p-NR2B. In addition, the study of Chen et al. (2016) showed that TSG (20, 40, 80 mg/kg) could promote hippocampal memory and synaptic plasticity in normal mice. Specifically, TSG enhances hippocampus-dependent contextual fear memory and novel object recognition, promoting hippocampal LTP and increasing dendritic spine density in the CA1 region (Chen et al., 2016). According to their further research, the mechanism that TSG enhanced memory might be related to the phosphorylation of ERK1/2, CaMKII, cAMP-response element binding protein (CREB) in the hippocampus, the up-regulation of BDNF expression, and the activation of the SIRT1/miR-134 signaling pathway (Chen et al., 2016).
Numerous studies on animals and humans have shown that cognitive decline is related to the accumulation of neurotoxin Aβ in the aging brain, which is produced by the proteolytic cleavage of APP (Kikuchi et al., 2011). Therefore, APP cleaving enzymes such as a disintegrin and metalloprotease (ADAM) 10 and 17, may be effective targets for reducing Aβ peptide formation and function and ameliorating the cognitive deficits associated with aging (Chow et al., 2010). Interestingly, the study by Hou et al. (2011) confirmed that TSG (50 mg/kg) could improve learning and memory ability of rats via the APP pathway. The further study showed that the effect is at least partly mediated by selectively promoting ADAM10 expression (Hou et al., 2011). Moreover, in APP695V717I-transgenic mice, TSG (100 mg/kg) could also alleviate Aβ neurotoxin-induced functional impairment of the endoplasmic reticulum by reducing the expression of Beclin-1 and LC3-Ⅱ in autophagy pathway, which improves the learning, memory, and spatially oriented behavior in mice (Luo et al., 2015).
Furthermore, Zhang et al. (2006) showed that TSG (120, 240 μM/kg) not only prevented the learning and memory deficits at the early stage of AD-like model, but also reversed them at the late stage. Seven years later, the team found that the protective effect of TSG might be closely related to inhibiting the overexpression of α-synuclein in APP-transgenic mice (Zhang et al., 2013c). Because TSG can not only inhibit α-synuclein overexpression at an early stage, but also reverse the increased expression, which inhibits its subsequent aggregation (Zhang et al., 2013c). Taken together, these results suggest that TSG supplementation may have clinical implications for the treatment of the diseases associated with cognitive deficits.
3.8 Anti-Aging Effects
Cellular senescence refers to the growth arrest due to various cellular stresses, including DNA damage, inadequate mitochondrial function, activated oncogenes, and oxidative stress (Bellei and Picardo, 2020). Meanwhile, cellular senescence is also an important mechanism to protect cells from oncogenic stress (Campisi and d'Adda di Fagagna, 2007). Among them, skin aging is a typical manifestation in aging mammals or humans. Its extent depends on a high content of collagen fibers, natural elastic fibers, and a slowly thinning dermal layer, which all lead to the skin aging symptoms such as wrinkling and skin laxity (Quan et al., 2012; Velarde et al., 2012). A report showed that TSG (180 mg/kg) treatment increased collagen fibers, thickened dermal layers, and decreased insulin and insulin-like growth factor-1 (IGF-1) levels in the skin of aging mice (Zhou et al., 2014). Further studies have shown that the mechanism of its anti-cutaneous aging effect is mainly through inhibiting the insulin/IGF-1 signaling pathway (Zhou et al., 2014). In the angiotensin II-induced senescence model of HUVECs, TSG (20, 40 μg/ml) treatment decreased the percent of senescence-associated-β-galactosidase positive cells and the expression levels of cellular senescence biomarkers, p53 and PAI-1 protein (Fan et al., 2021). Its anti-aging mechanism is mainly attributed to the regulation of SIRT1 activity (Fan et al., 2021). Similarly, in the mouse model established by high calorie diet, TSG delayed the aging of aged mice consuming excess calories (Ning et al., 2018). The mechanism may be related to regulating the AMPK/SIRT1/PGC-1α signaling pathway to improve motor function, bone mineral density, and organ protection in the aging mice (Ning et al., 2018).
It has been reported that enhancing the expression of Klotho, a type I transmembrane protein closely related to aging, can retard the aging process (Kuro-o, 2008). Interestingly, Zhou et al. (2015) demonstrated that TSG (2, 20, 50 μM) had an anti-aging effect on senescence-accelerated mouse prone 8 (SAMP8). According to the findings of biochemical pharmacology, the protective mechanism may be associated with the up-regulation of neural Klotho and down-regulation of neural insulin or IGF-1 levels (Zhou et al., 2015). In addition, the study by Shen et al. (2015) showed that TSG (50, 100, 200 mg/kg) could also significantly improve memory and motor function in aged mice. Specifically, TSG treatment significantly protected synaptic ultrastructure, increased the number of synaptic connections and the levels of synapse-related proteins (synaptophysin, phosphorylated synaptophysin I, and postsynaptic density protein 95), and suppressed the overexpression of α-synuclein in the hippocampus, striatum, and cerebral cortex of aged mice (Shen et al., 2015). Therefore, TSG may serve as a potential candidate for preventing aging and treating aging-related neurodegenerative diseases.
3.9 Other Pharmacological Effects
TSG (10, 20 mg/kg) significantly reduced TC, TG, blood urea nitrogen, creatinine, 24 h urinary protein, and MDA levels, as well as significantly increased SOD and GSH-Px activities in rats with diabetic nephropathy (Li et al., 2010a). Moreover, TSG treatment also significantly suppressed the overexpression of TGF-β1 and COX-2 in diabetic rats, and restored the decrease of SIRT1 expression (Li et al., 2010a). Its protective mechanisms against diabetic nephropathy may involve attenuating oxidative stress damage, inhibiting inflammation, and regulating SIRT1 and TGF-β1 signaling pathway (Li et al., 2010a). A recent study showed that TSG (12 mg/kg) also ameliorated traumatic brain injury by attenuating oxidative stress, inflammation and apoptosis in the mouse model (Cao et al., 2020). The protective effect may be achieved by inhibiting the Ras/JNK signaling pathway (Cao et al., 2020).
It was reported that TSG (10, 20, 40, 80 mg/kg) could prolong sleep duration and prevent insomnia in mice (Wei et al., 2017). The effect may be closely related to the regulation of sleep time by affecting the activities of lactate dehydrogenase and salivary amylase (Wei et al., 2017). Furthermore, Wang et al. (2017) used forced swim test, suspended tail test, and chronic social defeat stress depression model to explore the antidepressant effect of TSG. The results showed that TSG (30, 60 mg/kg) exerted antidepressant-like effects in mice by enhancing the hippocampal BDNF system (Wang et al., 2017). Interestingly, it has also been shown that TSG (200 μM) has a significant hair-regeneration effect (Chen et al., 2018a). Because TSG could inhibit the p53-, Fas-, and Bax-induced apoptotic signaling pathways, thus preventing hair follicles from entering the catagen phase (Chen et al., 2018a).
In addition, the study of Lin et al. (2018) showed that TSG enhanced the self-renewal of human dental pulp stem cells (HDPSCs). Specifically, TSG (10 μM) treatment not only enhanced cell viability, colony formation rate, and telomerase activity of HDPSCs, but also significantly increased the mRNA expression of proliferation markers, including the NAD+-dependent histone deacetylase SIRT1, ribonucleotide reductase subunit M2, proliferating cell nuclear antigen, and cyclin D1 (Lin et al., 2018). In addition, TSG treatment could also increase the expression of proliferation-related proteins and pluripotent stem cell markers, including AMPK, ERK, and so on. (Lin et al., 2018). The beneficial effect may be achieved by enhancing the renewal capacity and proliferative potential of HDPSCs through the AMPK/ERK/SIRT1 axis (Lin et al., 2018). The effects of TSG on enhancing the osteodifferentiation potential and molecular regulation of HDPSCs deserve more in-depth investigation, as it may provide a novel autologous cell-based therapeutic strategy for regenerative dentistry.
4 Toxicity of TSG
At present, most experiments have proved that TSG is not obviously toxic to cells or experimental animals. For example, the study by Yu et al. (2011) showed that TSG (20–300 μM) had no cytotoxicity for the human hepatocyte cell line LO2 in terms of cell proliferation, cell membrane integrity and enzyme secretion. Although TSG with a single use was not significantly toxic, a few studies have shown that TSG enhances the toxic effects of other drugs. For example, emodin, which has been shown to have significant hepatotoxicity, is metabolically eliminated in vivo mainly through a phase II metabolic reaction. However, the study by Ma et al. (2013) showed that TSG (117 mg/kg) inhibited emodin elimination in vivo by down-regulating UDP glucuronosyltransferase 1A8, which leaded to emodin accumulation, thus exhibiting liver toxicity. Similarly, the study by Xu et al. (2017) showed that TSG (100–800 mg/kg) could exacerbate acetaminophen-induced hepatotoxicity because it increased the expression and catalytic activity of hepatic CYP2E1, CYP3A4, and CYP1A2. In contrast, the serum ALT/AST activity assay and liver histopathology result showed that the single use of TSG (100–800 mg/kg) did not induce significant pathological changes (Xu et al., 2017). This suggested that there might be interactions between different ingredients, which affected the related drug metabolizing enzymes. Therefore, it is important to pay special attention to the combined use of TSG with other drugs in clinic. Moreover, a comprehensive and systematic toxicology study is needed in order to access the toxicity and mechanism of action of TSG.
5 Pharmacokinetic Study of TSG
Nowadays, pharmacokinetic research is in an equally important position as pharmacodynamic and toxicological research, having become an important part of preclinical and clinical research of drugs. At the same time, it plays an important role in the creation of new drugs, the improvement of dosage forms, and the study of the mechanism of dosage forms (Li et al., 2015). The method of current pharmacokinetic studies on TSG is mainly using high performance liquid chromatography tandem mass spectrometry (HPLC-MS/MS) and ultra performance liquid chromatography tandem mass spectrometry (UPLC-MS/MS) to analyze plasma samples from rats (Zhang et al., 2018b; Shen et al., 2018; Ma et al., 2021). A comparison of the pharmacokinetic parameters of TSG from 5 studies is shown in Table 3.
The plasma concentration-time profile of TSG after its oral and intravenous administration was fitted to a two-compartment pharmacokinetic model in rats (Zhao et al., 2013). The study by Zhao et al. (2013) showed that TSG was rapidly absorbed and eliminated in rats, as it was detected in plasma at 2 min. Similarly, it was also confirmed in the study of Shen et al. (2018). After oral administration of 100 mg/kg TSG to rats for 30 min, the heart (1,324 μg/g) and kidney (1,155 μg/g) are the main tissues with preferential distribution of TSG, followed by liver, lung, and stomach (295–654 μg/g), with the least distribution in brain and intestine (Zhao et al., 2013; Li et al., 2020a). Differently, after intravenous administration of 10 mg/kg TSG for 10 min, the liver is the main organ with preferential distribution of TSG (89.75 μg/g), followed by heart, lung, spleen, kidney, stomach, small intestine, and brain (7.56–37.15 μg/g), with the least distribution in testis (Zhao et al., 2013; Li et al., 2020a). The Cmax values of TSG after oral administration (50, 100 mg/kg) were 5.7 ± 1.6 and 21.9 ± 2.5 μg/ml respectively, whereas they were 22.8 ± 2.6 and 64.2 ± 3.6 μg/ml respectively after intravenous administration (10, 20 mg/kg), which indicated that the plasma level of TSG was much higher after intravenous administration than oral administration (Zhao et al., 2013). Recent preclinical studies have shown that phase II metabolism is the main metabolic pathway of TSG (Li et al., 2020a). After incubating TSG with liver microsomes of rats for 1 h, only TSG glucuronide was determined (Wang et al., 2009a). After oral administration of TSG in rats, its glucuronidation metabolites were also identified as the main metabolites (Zhao et al., 2013). In addition, a study showed that the absolute oral bioavailabilities of TSG with a low dose (50 mg/kg) and a high dose (100 mg/kg) were 24.2 and 36.5% respectively (Zhao et al., 2013). Taken together, these studies about pharmacokinetics and bioavailability suggest that TSG is rapidly absorbed and eliminated, and may have a wide biological distribution after oral administration in normal rats.
Due to the poor stability in vitro, poor absorption in the intestine, and only short-term storage in tissues, some researchers focus on improving the stability and bioavailability of TSG through numerous experiments. For example, Sun et al. (2018) showed that Ophiopogon japonicus (Thunb.) Ker Gawl. polysaccharides (OJPs) could significantly improve the water solubility and stability of TSG. Significantly, the Tmax, Cmax, and AUC(0-tn) values of TSG-OJP were respectively 3.5-, 1.45-, and 2.32-fold higher than those of TSG, indicating that OJPs can be used to improve the biopharmaceutic properties of TSG as well as enhance its pharmacological effects (Sun et al., 2018). In conclusion, it is expected that follow-up pharmacokinetic studies of TSG can be performed in multiple animals, which may provide useful information for the development of potential new dosage forms and the clinical use of drugs.
6 Discussion and Future Perspective
PM, one of the most widely used traditional Chinese medicines for thousands of years, is often used clinically to treat hyperlipidemia, coronary heart disease, stroke, alopecia as well as aging disorders such as aged white hair, tooth loss, and senile plaques. As the main and unique active index ingredient of PM, TSG has various pharmacological effects, including anti-inflammation, antioxidation, neuroprotection, cardiovascular protection, hepatoprotection, anti-osteoporosis, memory enhancement, antidepressant, and anti-aging. Therefore, TSG plays an important role in the treatment of many diseases involving inflammatory diseases, neurodegenerative diseases, cardiovascular diseases, liver injury, osteoporosis, diabetic nephropathy, depression, and aging.
For inflammatory diseases, TSG regulates the NF-κB, AMPK/Nrf2, NLRP3 inflammasome, p38 MAPK, and ERK1/2 signaling pathways to exhibit significant anti-inflammatory effects on various inflammatory models. In addition, TSG has significant scavenging ability for free radicals and can exert excellent antioxidant effects by regulating oxidative damage markers (SOD, CAT, GSH-Px), inhibiting the overproduction of ROS, NO, and lipid peroxidation products, decreasing apoptosis, alleviating autophagy, and enhancing the endogenous antioxidant defense system by activating the Nrf2 antioxidant pathway. Interestingly, due to its significant anti-inflammatory and antioxidant activities, TSG exerts neuroprotective and cardiovascular protective effects through multiple molecular mechanisms. In terms of the neuroprotective effects, through modulating the Bcl-2/Bax/Caspase-3, NF-κB, MAPK, PI3K-AKT, JNK, Wnt/β-Catenin, keap1-Nrf2, and AMPK/PINK1/Parkin signaling pathways, TSG shows excellent neuroprotective effects against neurodegenerative diseases such as AD, PD, and cerebral ischemic injury. Regarding its cardiovascular protective effects, TSG shows significant protective effects against various cardiovascular diseases by regulating the TGF-β/Smad, eNOS/NO, Src-MEK1/2-ERK1/2, NO/cGMP/PKG, VEGF/ICAM-1, and RhoA/ROCK signaling pathways as well as autophagy and microRNAs.
The anti-inflammatory and antioxidant pharmacological activities of TSG also contribute to its hepatoprotective effects, making TSG a promising candidate for the treatment of liver diseases, such as alcoholic fatty liver disease, NAFLD, and NASH. This is attributed primarily to the regulation on lipid metabolism, IKKβ/NF-κB, keap1-Nrf2, and P2X7R-NLRP3 signaling pathways as well as gut microbiota and intestinal mucosal barrier function. Furthermore, TSG achieves preventive and protective effects against osteoporosis by modulating the PI3K/AKT, Wnt/FoxO3a, immune system, and chemokine signaling pathways as well as blocking the tissue RAS activity. Interestingly, TSG can also enhance learning and memory abilities by promoting hippocampal long-term potentiation, improving the structure and function of neuronal synapses, decreasing the expression of Beclin-1 and LC3-II in the autophagy pathway, and alleviating Aβ neurotoxin-induced functional impairment of the endoplasmic reticulum. More importantly, TSG can serve as a potential candidate for aging prevention and the treatment of aging-associated diseases, which is mainly attributed to regulating the insulin/IGF-1 and AMPK/SIRT1/PGC-1α signaling pathways. In addition, TSG shows protective effects against diabetic nephropathy, traumatic brain injury, depression, and alopecia. In summary, TSG has broad and significant pharmacological activities, and is a potential compound for the treatment of many diseases.
Most experiments have confirmed that TSG is not obviously toxic to cells and experimental animals, but its combined use with other toxic drugs may affect the related drug metabolizing enzymes, thus leading to highly toxic side effects that should be noted. It is worth mentioning that TSG exists as cis- and trans-isomers. Although TSG mostly exists in trans-structure in nature, TSG is optically sensitive, and the conversion of trans-structure to cis-structure occurs during traditional processing steps or under UV irradiation, leading to an increased content of cis TSG. Trans-TSG and cis-TSG have been reported to differ in their pharmacological effects, toxicity, and pharmacokinetic properties. However, most of the published articles on TSG do not clearly indicate its specific structure in research, which makes it difficult to distinguish and compare the activities of the two isomers. Therefore, it is necessary to remind other scholars studying TSG to clearly indicate the specific structure of TSG when publishing their articles, which may provide direction and basis for the further development and clinical application of TSG.
The pharmacokinetic results of TSG vary because of the different route and dosage of administration, and different experimental models. In general, pharmacokinetic studies showed that the absorption and elimination of TSG in vivo is very rapid and has a wide biological distribution. Although TSG has been proved to be a promising compound with multiple pharmacological effects, it also has some disadvantages, such as poor stability in vitro, poor intestinal absorption, and short-term storage in tissues. Therefore, we propose the following views. First of all, in subsequent studies, we can combine omics research methods, such as transcriptomics/proteomics/metabolomics, to screen out the differentially expressed genes/proteins/metabolites, and further clarify the molecular mechanism by which TSG exerts multiple pharmacological effects as well as the specific targets. Secondly, it is suggested to add more randomized controlled clinical trials on TSG in the future to fully evaluate its actual clinical efficacy and its safety in clinical application. In addition, in view of the poor stability and low bioavailability of TSG, in the future, we should focus on modifying its chemical structure and developing its new dosage forms, so as to improve its stability and bioavailability, which provides a wider range for the clinic use of TSG. It is worth mentioning that, in recent years, there are many reports that liposomes and exosomes can effectively improve the targeting effect and bioavailability of drugs (Liao et al., 2019; Guimarães et al., 2021). Therefore, in follow-up studies, we can further explore how to increase the stability and bioavailability of TSG through the targeted delivery of liposomes and exosomes in vivo. In summary, this study provides a comprehensive review and summary of the physical/chemical properties, pharmacological activity, toxicity, and pharmacokinetic properties of TSG, with a view to providing a theoretical basis for the development and clinical application of TSG.
Author Contributions
CW, LG, and YXL designed the paper and recommended a structure for the review. CW, SD, CM, YFL, and HZ wrote the initial draft and prepared figures. LG, KF, and YXL helped to revise the manuscript.
Funding
The work was supported by National Natural Science Foundation of China (No: 81891012, 81630101, and U19A2010), Sichuan Province Science and Technology Program (No: 2021JDRC0041), and Xinglin Scholar Research Premotion Project of Chengdu University of Traditional Chinese Medicine (No: CXTD2018019).
Conflict of Interest
The authors declare that the research was conducted in the absence of any commercial or financial relationships that could be construed as a potential conflict of interest.
Publisher’s Note
All claims expressed in this article are solely those of the authors and do not necessarily represent those of their affiliated organizations, or those of the publisher, the editors and the reviewers. Any product that may be evaluated in this article, or claim that may be made by its manufacturer, is not guaranteed or endorsed by the publisher.
References
Albhaisi, S. A. M., Bajaj, J. S., and Sanyal, A. J. (2020). Role of Gut Microbiota in Liver Disease. Am. J. Physiol. Gastrointest. Liver Physiol. 318 (1), G84–G98. doi:10.1152/ajpgi.00118.2019
Allen, S. J., Watson, J. J., Shoemark, D. K., Barua, N. U., and Patel, N. K. (2013). GDNF, NGF and BDNF as Therapeutic Options for Neurodegeneration. Pharmacol. Ther. 138 (2), 155–175. doi:10.1016/j.pharmthera.2013.01.004
Amor, S., Peferoen, L. A., Vogel, D. Y., Breur, M., van der Valk, P., Baker, D., et al. (2014). Inflammation in Neurodegenerative Diseases-Aan Update. Immunology 142 (2), 151–166. doi:10.1111/imm.12233
Baune, B. T. (2015). Inflammation and Neurodegenerative Disorders: Is There Still hope for Therapeutic Intervention? Curr. Opin. Psychiatry 28 (2), 148–154. doi:10.1097/yco.0000000000000140
Bellei, B., and Picardo, M. (2020). Premature Cell Senescence in Human Skin: Dual Face in Chronic Acquired Pigmentary Disorders. Ageing Res. Rev. 57, 100981. doi:10.1016/j.arr.2019.100981
Bellezza, I., Giambanco, I., Minelli, A., and Donato, R. (2018). Nrf2-Keap1 Signaling in Oxidative and Reductive Stress. Biochim. Biophys. Acta Mol. Cel Res 1865 (5), 721–733. doi:10.1016/j.bbamcr.2018.02.010
Bennett, M. R., Sinha, S., and Owens, G. K. (2016). Vascular Smooth Muscle Cells in Atherosclerosis. Circ. Res. 118 (4), 692–702. doi:10.1161/circresaha.115.306361
Borderud, S. P., Li, Y., Burkhalter, J. E., Sheffer, C. E., and Ostroff, J. S. (2014). Electronic Cigarette Use Among Patients with Cancer: Characteristics of Electronic Cigarette Users and Their Smoking Cessation Outcomes. Cancer 120 (22), 3527–3535. doi:10.1002/cncr.28811
Bravo-San Pedro, J. M., Kroemer, G., and Galluzzi, L. (2017). Autophagy and Mitophagy in Cardiovascular Disease. Circ. Res. 120 (11), 1812–1824. doi:10.1161/circresaha.117.311082
Bryan, N. S. (2018). Functional Nitric Oxide Nutrition to Combat Cardiovascular Disease. Curr. Atheroscler. Rep. 20 (5), 21. doi:10.1007/s11883-018-0723-0
Bui, T. M., Wiesolek, H. L., and Sumagin, R. (2020). ICAM-1: A Master Regulator of Cellular Responses in Inflammation, Injury Resolution, and Tumorigenesis. J. Leukoc. Biol. 108 (3), 787–799. doi:10.1002/jlb.2mr0220-549r
Cai, A., Li, L., and Zhou, Y. (2016). Pathophysiological Effects of RhoA and Rho-Associated Kinase on Cardiovascular System. J. Hypertens. 34 (1), 3–10. doi:10.1097/hjh.0000000000000768
Cakmak, H. A., Coskunpinar, E., Ikitimur, B., Barman, H. A., Karadag, B., Tiryakioglu, N. O., et al. (2015). The Prognostic Value of Circulating microRNAs in Heart Failure: Preliminary Results from a Genome-wide Expression Study. J. Cardiovasc. Med. (Hagerstown) 16 (6), 431–437. doi:10.2459/jcm.0000000000000233
Campisi, J., and d'Adda di Fagagna, F. (2007). Cellular Senescence: when Bad Things Happen to Good Cells. Nat. Rev. Mol. Cel Biol 8 (9), 729–740. doi:10.1038/nrm2233
Cao, Y., Chen, Y., Wang, F., Wang, Y., and Long, J. (2020). PARP1 Might Enhance the Therapeutic Effect of Tetrahydroxystilbene Glucoside in Traumatic Brain Injury via Inhibition of Ras/JNK Signalling Pathway. Folia Neuropathol. 58 (1), 45–56. doi:10.5114/fn.2020.94006
Chen, L., Duan, H., Xie, F., Gao, Z., Wu, X., Chen, F., et al. (2018a). Tetrahydroxystilbene Glucoside Effectively Prevents Apoptosis Induced Hair Loss. Biomed. Res. Int. 2018, 1380146. doi:10.1155/2018/1380146
Chen, T., Yang, Y. J., Li, Y. K., Liu, J., Wu, P. F., Wang, F., et al. (2016). Chronic Administration Tetrahydroxystilbene Glucoside Promotes Hippocampal Memory and Synaptic Plasticity and Activates ERKs, CaMKII and SIRT1/miR-134 In Vivo. J. Ethnopharmacol 190, 74–82. doi:10.1016/j.jep.2016.06.012
Chen, X., Hu, W., Lu, X., Jiang, B., Wang, J., Zhang, W., et al. (2017). Mechanism of 2,3,4',5-Tetrahydroxystilbene 2-O-β-D-Glucoside-Induced Upregulation of Glutamate Transporter 1 Protein Expression in Mouse Primary Astrocytes. Pharmacology 99, 153–159. doi:10.1159/000452672
Chen, X., Tang, K., Peng, Y., and Xu, X. (2018b). 2,3,4',5-tetrahydroxystilbene-2-O-β-d-glycoside Attenuates Atherosclerosis in apolipoprotein E-Deficient Mice: Role of Reverse Cholesterol Transport. Can. J. Physiol. Pharmacol. 96 (1), 8–17. doi:10.1139/cjpp-2017-0474
Chen, Z., and Zhong, C. (2014). Oxidative Stress in Alzheimer's Disease. Neurosci. Bull. 30 (2), 271–281. doi:10.1007/s12264-013-1423-y
Chin, Y. T., Hsieh, M. T., Lin, C. Y., Kuo, P. J., Yang, Y. C., Shih, Y. J., et al. (2016). 2,3,5,4'-Tetrahydroxystilbene-2-O-β-glucoside Isolated from Polygoni Multiflori Ameliorates the Development of Periodontitis. Mediators Inflamm. 2016, 6953459. doi:10.1155/2016/6953459
Chistiakov, D. A., Melnichenko, A. A., Myasoedova, V. A., Grechko, A. V., and Orekhov, A. N. (2017). Mechanisms of Foam Cell Formation in Atherosclerosis. J. Mol. Med. (Berl) 95 (11), 1153–1165. doi:10.1007/s00109-017-1575-8
Chow, V. W., Savonenko, A. V., Melnikova, T., Kim, H., Price, D. L., Li, T., et al. (2010). Modeling an Anti-amyloid Combination Therapy for Alzheimer's Disease. Sci. Transl Med. 2 (13), 13ra1. doi:10.1126/scitranslmed.3000337
Daiber, A., Xia, N., Steven, S., Oelze, M., Hanf, A., Kröller-Schön, S., et al. (2019). New Therapeutic Implications of Endothelial Nitric Oxide Synthase (eNOS) Function/Dysfunction in Cardiovascular Disease. Int. J. Mol. Sci. 20 (1), 187. doi:10.3390/ijms20010187
Dauphinee, S. M., and Karsan, A. (2006). Lipopolysaccharide Signaling in Endothelial Cells. Lab. Invest. 86 (1), 9–22. doi:10.1038/labinvest.3700366
Devi, T. S., Somayajulu, M., Kowluru, R. A., and Singh, L. P. (2017). TXNIP Regulates Mitophagy in Retinal Müller Cells under High-Glucose Conditions: Implications for Diabetic Retinopathy. Cell Death Dis 8 (5), e2777. doi:10.1038/cddis.2017.190
Dixon, S. J., and Stockwell, B. R. (2014). The Role of Iron and Reactive Oxygen Species in Cell Death. Nat. Chem. Biol. 10 (1), 9–17. doi:10.1038/nchembio.1416
Dong, Q., Xing, W., Fu, F., Liu, Z., Wang, J., Liang, X., et al. (2016). Tetrahydroxystilbene Glucoside Inhibits Excessive Autophagy and Improves Microvascular Endothelial Dysfunction in Prehypertensive Spontaneously Hypertensive Rats. Am. J. Chin. Med. 44 (7), 1393–1412. doi:10.1142/s0192415x16500786
Eržen, B., Šilar, M., and Šabovič, M. (2014). Stable Phase post-MI Patients Have Elevated VEGF Levels Correlated with Inflammation Markers, but Not with Atherosclerotic burden. BMC Cardiovasc. Disord. 14, 166. doi:10.1186/1471-2261-14-166
Fan, W., Guo, Y., Cao, S., Cao, S., Xie, Y., Liu, X., et al. (2021). Tetrahydroxystilbene Glucoside Alleviates Angiotensin II Induced HUVEC Senescence via SIRT1. Can. J. Physiol. Pharmacol. 99 (4), 389–394. doi:10.1139/cjpp-2020-0202
Fan, Y. F., Guan, S. Y., Luo, L., Li, Y. J., Yang, L., Zhou, X. X., et al. (2018a). Tetrahydroxystilbene Glucoside Relieves the Chronic Inflammatory Pain by Inhibiting Neuronal Apoptosis, Microglia Activation, and GluN2B Overexpression in Anterior Cingulate Cortex. Mol. Pain 14, 1744806918814367. doi:10.1177/1744806918814367
Fan, Y. S., Li, Q., Hamdan, N., Bian, Y. F., Zhuang, S., Fan, K., et al. (2018b). Tetrahydroxystilbene Glucoside Regulates Proliferation, Differentiation, and OPG/RANKL/M-CSF Expression in MC3T3-E1 Cells via the PI3K/Akt Pathway. Molecules 23 (9), 2306. doi:10.3390/molecules23092306
Feng, Y. M., Verfaillie, C., and Yu, H. (2016). Vascular Diseases and Metabolic Disorders. Stem Cell Int 2016, 5810358. doi:10.1155/2016/5810358
Gao, D., Chen, C., Huang, R., Yang, C. C., Miao, B. B., Li, L., et al. (2021). Tetrahydroxy Stilbene Glucoside Ameliorates Cognitive Impairments and Pathology in APP/PS1 Transgenic Mice. Curr. Med. Sci. 41 (2), 279–286. doi:10.1007/s11596-021-2344-z
Gao, Y., Li, J., Li, J., Hu, C., Zhang, L., Yan, J., et al. (2020). Tetrahydroxy Stilbene Glycoside Alleviated Inflammatory Damage by Mitophagy via AMPK Related PINK1/Parkin Signaling Pathway. Biochem. Pharmacol. 177, 113997. doi:10.1016/j.bcp.2020.113997
González, H., Elgueta, D., Montoya, A., and Pacheco, R. (2014). Neuroimmune Regulation of Microglial Activity Involved in Neuroinflammation and Neurodegenerative Diseases. J. Neuroimmunol 274, 1–13. doi:10.1016/j.jneuroim.2014.07.012
Graczyk, P. P. (2013). JNK Inhibitors as Anti-inflammatory and Neuroprotective Agents. Future Med. Chem. 5 (5), 539–551. doi:10.4155/fmc.13.34
Grootaert, M. O. J., Moulis, M., Roth, L., Martinet, W., Vindis, C., Bennett, M. R., et al. (2018). Vascular Smooth Muscle Cell Death, Autophagy and Senescence in Atherosclerosis. Cardiovasc. Res. 114 (4), 622–634. doi:10.1093/cvr/cvy007
Guimarães, D., Cavaco-Paulo, A., and Nogueira, E. (2021). Design of Liposomes as Drug Delivery System for Therapeutic Applications. Int. J. pharmaceutics 601, 120571. doi:10.1016/j.ijpharm.2021.120571
Hang, L., Thundyil, J., Goh, G. W. Y., and Lim, K. L. (2019). AMP Kinase Activation Is Selectively Disrupted in the Ventral Midbrain of Mice Deficient in Parkin or PINK1 Expression. Neuromolecular Med. 21 (1), 25–32. doi:10.1007/s12017-018-8517-7
Hata, K., Kozawa, M., and Baba, K. (1975). [A New Stilbene Glucoside from Chinese Crude Drug "Heshouwu," the Roots of Polygonum Multiflorum Thunb (Author's Transl)]. Yakugaku Zasshi 95 (2), 211–213. doi:10.1248/yakushi1947.95.2_211
Henao-Mejia, J., Elinav, E., Jin, C., Hao, L., Mehal, W. Z., Strowig, T., et al. (2012). Inflammasome-mediated Dysbiosis Regulates Progression of NAFLD and Obesity. Nature 482 (7384), 179–185. doi:10.1038/nature10809
Hou, Y., Yang, Q., Zhou, L., Du, X., Li, M., Yuan, M., et al. (2011). Tetrahydroxystilbene Glucoside Improves Learning and (Or) Memory Ability of Aged Rats and May Be Connected to the APP Pathway. Can. J. Physiol. Pharmacol. 89 (11), 801–809. doi:10.1139/y11-081
Hu, A., Huang, J., Li, S., Gao, Y., Wu, L., Deng, J., et al. (2019). Involvement of Stromal Cell-Derived Factor-1α (SDF-1α), Stem Cell Factor (SCF), Fractalkine (FKN) and VEGF in TSG protection against Intimal Hyperplasia in Rat Balloon Injury. Biomed. Pharmacother. 110, 887–894. doi:10.1016/j.biopha.2018.12.030
Huang, C., Lin, F., Wang, G., Lu, D., Wu, Q., Liu, J., et al. (2018). Tetrahydroxystilbene Glucoside Produces Neuroprotection against 6-OHDA-Induced Dopamine Neurotoxicity. Oxid Med. Cel Longev 2018, 7927568. doi:10.1155/2018/7927568
Jagannathan, R., Patel, S. A., Ali, M. K., and Narayan, K. M. V. (2019). Global Updates on Cardiovascular Disease Mortality Trends and Attribution of Traditional Risk Factors. Curr. Diab Rep. 19 (7), 44. doi:10.1007/s11892-019-1161-2
Jeppesen, P. L., Christensen, G. L., Schneider, M., Nossent, A. Y., Jensen, H. B., Andersen, D. C., et al. (2011). Angiotensin II Type 1 Receptor Signalling Regulates microRNA Differentially in Cardiac Fibroblasts and Myocytes. Br. J. Pharmacol. 164 (2), 394–404. doi:10.1111/j.1476-5381.2011.01375.x
Jia, M., Zhou, X. X., Qin, Q., Wang, F., Li, J., Xu, C. B., et al. (2019). Tetrahydroxystilbene Glucoside-Induced Relaxation of the superior Mesenteric Artery via Both Endothelium-dependent and Endothelium-independent Mechanisms. Microvasc. Res. 123, 42–49. doi:10.1016/j.mvr.2018.10.007
Jiang, Z., Wang, W., and Guo, C. (2017). Tetrahydroxy Stilbene Glucoside Ameliorates H2O2-Induced Human Brain Microvascular Endothelial Cell Dysfunction In Vitro by Inhibiting Oxidative Stress and Inflammatory Responses. Mol. Med. Rep. 16 (4), 5219–5224. doi:10.3892/mmr.2017.7225
Jiao, C., Gao, F., Ou, L., Yu, J., Li, M., Wei, P., et al. (2018). Tetrahydroxystilbene Glycoside Antagonizes β-amyloid-induced Inflammatory Injury in Microglia Cells by Regulating PU.1 Expression. Neuroreport 29 (10), 787–793. doi:10.1097/wnr.0000000000001032
Jie, Y., Zhiwen, Z., Qidong, Y., Lijun, H., and Jin, Z. (2010). Neuroprotective Mechanism of Tetrahydroxystilbene Glucoside on Rats after Cerebral Ischemia-Reperfusion. J. Cent. South. Univ. (Med Sci. 35 (04), 321–328. doi:10.3969/j.issn.1672-7347.2010.04.007
Kalayinia, S., Arjmand, F., Maleki, M., Malakootian, M., and Singh, C. P. (2021). MicroRNAs: Roles in Cardiovascular Development and Disease. Cardiovasc. Pathol. 50, 107296. doi:10.1016/j.carpath.2020.107296
Khosla, S., and Hofbauer, L. C. (2017). Osteoporosis Treatment: Recent Developments and Ongoing Challenges. Lancet Diabetes Endocrinol. 5 (11), 898–907. doi:10.1016/s2213-8587(17)30188-2
Kikuchi, M., Hirosawa, T., Yokokura, M., Yagi, S., Mori, N., Yoshikawa, E., et al. (2011). Effects of Brain Amyloid Deposition and Reduced Glucose Metabolism on the Default Mode of Brain Function in normal Aging. J. Neurosci. 31 (31), 11193–11199. doi:10.1523/jneurosci.2535-11.2011
Kuro-o, M. (2008). Klotho as a Regulator of Oxidative Stress and Senescence. Biol. Chem. 389 (3), 233–241. doi:10.1515/bc.2008.028
Kuwabara, M., Asanuma, T., Niwa, K., and Inanami, O. (2008). Regulation of Cell Survival and Death Signals Induced by Oxidative Stress. J. Clin. Biochem. Nutr. 43 (2), 51–57. doi:10.3164/jcbn.2008045
L'Episcopo, F., Tirolo, C., Testa, N., Caniglia, S., Morale, M. C., Serapide, M. F., et al. (2014). Wnt/β-catenin Signaling Is Required to rescue Midbrain Dopaminergic Progenitors and Promote Neurorepair in Ageing Mouse Model of Parkinson's Disease. Stem Cells 32 (8), 2147–2163. doi:10.1002/stem.1708
Lee, S. Y., Ahn, S. M., Wang, Z., Choi, Y. W., Shin, H. K., and Choi, B. T. (2017). Neuroprotective Effects of 2,3,5,4'-Tetrahydoxystilbene-2-O-β-D-Glucoside from Polygonum Multiflorum against Glutamate-Induced Oxidative Toxicity in HT22 Cells. J. Ethnopharmacol 195, 64–70. doi:10.1016/j.jep.2016.12.001
Leong, D. P., Joseph, P. G., McKee, M., Anand, S. S., Teo, K. K., Schwalm, J. D., et al. (2017). Reducing the Global Burden of Cardiovascular Disease, Part 2: Prevention and Treatment of Cardiovascular Disease. Circ. Res. 121 (6), 695–710. doi:10.1161/circresaha.117.311849
Li, C., Cai, F., Yang, Y., Zhao, X., Wang, C., Li, J., et al. (2010a). Tetrahydroxystilbene Glucoside Ameliorates Diabetic Nephropathy in Rats: Involvement of SIRT1 and TGF-Β1 Pathway. Eur. J. Pharmacol. 649, 382–389. doi:10.1016/j.ejphar.2010.09.004
Li, D., Yang, M., and Zuo, Z. (2020a). Overview of Pharmacokinetics and Liver Toxicities of Radix Polygoni Multiflori. Toxins (Basel) 12 (11), 729. doi:10.3390/toxins12110729
Li, F., Zhang, T., He, Y., Gu, W., Yang, X., Zhao, R., et al. (2020b). Inflammation Inhibition and Gut Microbiota Regulation by TSG to Combat Atherosclerosis in ApoE-/- Mice. J. Ethnopharmacol 247, 112232. doi:10.1016/j.jep.2019.112232
Li, J., Wang, B., Zhou, G., Yan, X., and Zhang, Y. (2018). Tetrahydroxy Stilbene Glucoside Alleviates High Glucose-Induced MPC5 Podocytes Injury through Suppression of NLRP3 Inflammasome. Am. J. Med. Sci. 355 (6), 588–596. doi:10.1016/j.amjms.2018.03.005
Li, X., Li, Y., Chen, J., Sun, J., Li, X., Sun, X., et al. (2010b). Tetrahydroxystilbene Glucoside Attenuates MPP+-induced Apoptosis in PC12 Cells by Inhibiting ROS Generation and Modulating JNK Activation. Neurosci. Lett. 483 (1), 1–5. doi:10.1016/j.neulet.2010.07.027
Li, Y., Wang, Y., Tai, W., Yang, L., Chen, Y., Chen, C., et al. (2015). Challenges and Solutions of Pharmacokinetics for Efficacy and Safety of Traditional Chinese Medicine. Curr. Drug Metab. 16 (9), 765–776. doi:10.2174/138920021609151201114223
Liao, W., Du, Y., Zhang, C., Pan, F., Yao, Y., Zhang, T., et al. (2019). Exosomes: The Next Generation of Endogenous Nanomaterials for Advanced Drug Delivery and Therapy. Acta Biomater. 86, 1–14. doi:10.1016/j.actbio.2018.12.045
Lin, C. Y., Chin, Y. T., Kuo, P. J., Lee, H. W., Huang, H. M., Lin, H. Y., et al. (2018). 2,3,5,4'-Tetrahydroxystilbene-2-O-β-glucoside Potentiates Self-Renewal of Human Dental Pulp Stem Cells via the AMPK/ERK/SIRT1 axis. Int. Endod. J. 51 (10), 1159–1170. doi:10.1111/iej.12935
Lin, L., Ni, B., Lin, H., Zhang, M., Li, X., Yin, X., et al. (2015a). Traditional Usages, Botany, Phytochemistry, Pharmacology and Toxicology of Polygonum Multiflorum Thunb.: a Review. J. Ethnopharmacol 159, 158–183. doi:10.1016/j.jep.2014.11.009
Lin, P., Lu, J., Wang, Y., Gu, W., Yu, J., and Zhao, R. (2015b). Naturally Occurring Stilbenoid TSG Reverses Non-alcoholic Fatty Liver Diseases via Gut-Liver Axis. PloS one 10 (10), e0140346. doi:10.1371/journal.pone.0140346
Ling, S., Duan, J., Ni, R., and Xu, J. W. (2016). 2,3,5,4'-Tetrahydroxystilbene-2-O-β-D-glucoside Promotes Expression of the Longevity Gene Klotho. Oxid Med. Cel Longev 2016, 3128235. doi:10.1155/2016/3128235
Ling, S., and Xu, J. W. (2016). Biological Activities of 2,3,5,4'-Tetrahydroxystilbene-2-O-β-D-Glucoside in Antiaging and Antiaging-Related Disease Treatments. Oxid Med. Cel Longev 2016, 4973239. doi:10.1155/2016/4973239
Liu, B., Wang, J., and Zhao, Y. Q. (2014). Tail Asymptotics of the Waiting Time and the Busy Period for the $${{\varvec{M/G/1/K}}}$$ Queues with Subexponential Service Times. Queueing Syst. 76 (1), 1–19. doi:10.1007/s11134-013-9348-8
Liu, Y., Wang, Q., Yang, J., Guo, X., Liu, W., Ma, S., et al. (2018). Polygonum Multiflorum Thunb.: A Review on Chemical Analysis, Processing Mechanism, Quality Evaluation, and Hepatotoxicity. Front. Pharmacol. 9, 364. doi:10.3389/fphar.2018.00364
Luo, H., Li, Y., Guo, J., Liu, Z., Zhang, Z., Wang, Y., et al. (2015). Tetrahydroxy Stilbene Glucoside Improved the Behavioral Disorders of APP695V717I Transgenic Mice by Inhibiting the Expression of Beclin-1 and LC3-II. J. Tradit Chin. Med. 35 (003), 295–300. doi:10.1016/s0254-6272(15)30100-x
Luo, J., Mills, K., le Cessie, S., Noordam, R., and van Heemst, D. (2020). Ageing, Age-Related Diseases and Oxidative Stress: What to Do Next? Ageing Res. Rev. 57, 100982. doi:10.1016/j.arr.2019.100982
Ma, J., Zheng, L., Deng, T., Li, C. L., He, Y. S., Li, H. J., et al. (2013). Stilbene Glucoside Inhibits the Glucuronidation of Emodin in Rats through the Down-Regulation of UDP-Glucuronosyltransferases 1A8: Application to a Drug-Drug Interaction Study in Radix Polygoni Multiflori. J. Ethnopharmacol 147 (2), 335–340. doi:10.1016/j.jep.2013.03.013
Ma, N., Zhang, Y., Sun, L., Zhao, Y., Ding, Y., and Zhang, T. (2021). Comparative Studies on Multi-Component Pharmacokinetics of Polygonum Multiflorum Thunb Extract after Oral Administration in Different Rat Models. Front. Pharmacol. 12, 655332. doi:10.3389/fphar.2021.655332
Medzhitov, R. (2008). Origin and Physiological Roles of Inflammation. Nature 454 (7203), 428–435. doi:10.1038/nature07201
Meijiao, X., and Yifeng, W. (2019). Stilbene Glucoside Inhibits Ultraviolet Radiation B-Induced Photoaging in Human Skin Fibroblasts. J. Zhejiang Univ. (Medical Sciences) 48 (06), 625–630.
Mialet-Perez, J., and Vindis, C. (2017). Autophagy in Health and Disease: Focus on the Cardiovascular System. Essays Biochem. 61 (6), 721–732. doi:10.1042/ebc20170022
Mookherjee, P., Green, P. S., Watson, G. S., Marques, M. A., Tanaka, K., Meeker, K. D., et al. (2011). GLT-1 Loss Accelerates Cognitive Deficit Onset in an Alzheimer's Disease Animal Model. J. Alzheimers Dis. 26 (3), 447–455. doi:10.3233/jad-2011-110503
Nakano, N., Matsuda, S., Ichimura, M., Minami, A., Ogino, M., Murai, T., et al. (2017). PI3K/AKT Signaling Mediated by G protein-coupled R-eceptors I-s I-nvolved in N-eurodegenerative Parkinson's D-isease (Review). Int. J. Mol. Med. 39 (2), 253–260. doi:10.3892/ijmm.2016.2833
Ning, Z., Li, Y., Liu, D., Owoicho Orgah, J., Zhu, J., Wang, Y., et al. (2018). Tetrahydroxystilbene Glucoside Delayed Senile Symptoms in Old Mice via Regulation of the AMPK/SIRT1/PGC-1α Signaling Cascade. Gerontology 64 (5), 457–465. doi:10.1159/000487360
Octavia, Y., Tocchetti, C. G., Gabrielson, K. L., Janssens, S., Crijns, H. J., and Moens, A. L. (2012). Doxorubicin-induced Cardiomyopathy: from Molecular Mechanisms to Therapeutic Strategies. J. Mol. Cel Cardiol 52 (6), 1213–1225. doi:10.1016/j.yjmcc.2012.03.006
Ozansoy, M., and Başak, A. N. (2013). The central Theme of Parkinson's Disease: α-synuclein. Mol. Neurobiol. 47 (2), 460–465. doi:10.1007/s12035-012-8369-3
Park, S. Y., Jin, M. L., Wang, Z., Park, G., and Choi, Y. W. (2016). 2,3,4',5-tetrahydroxystilbene-2-O-β-d-glucoside Exerts Anti-inflammatory Effects on Lipopolysaccharide-Stimulated Microglia by Inhibiting NF-Κb and Activating AMPK/Nrf2 Pathways. Food Chem. Toxicol. 97, 159–167. doi:10.1016/j.fct.2016.09.010
Peng, Y., Zeng, Y., Xu, J., Huang, X. L., Zhang, W., and Xu, X. L. (2016). PPAR-γ Is Involved in the Protective Effect of 2,3,4',5-Tetrahydroxystilbene-2-O-Beta-D-Glucoside against Cardiac Fibrosis in Pressure-Overloaded Rats. Eur. J. Pharmacol. 791, 105–114. doi:10.1016/j.ejphar.2016.08.025
Qi, Y., Liang, X., Hu, X., He, H., Tang, L., and Yao, W. (2020). Tetrahydroxystilbene Glucoside Protects against LPS-Induced Endothelial Dysfunction via Inhibiting RhoA/ROCK Signaling and F-Actin Remodeling. Gen. Physiol. Biophys. 39 (5), 407–417. doi:10.4149/gpb_2020028
Qin, R., Li, X., Li, G., Tao, L., Li, Y., Sun, J., et al. (2011). Protection by Tetrahydroxystilbene Glucoside against Neurotoxicity Induced by MPP+: the Involvement of PI3K/Akt Pathway Activation. Toxicol. Lett. 202 (1), 1–7. doi:10.1016/j.toxlet.2011.01.001
Quan, T., Qin, Z., Voorhees, J. J., and Fisher, G. J. (2012). Cysteine-rich Protein 61 (CCN1) Mediates Replicative Senescence-Associated Aberrant Collagen Homeostasis in Human Skin Fibroblasts. J. Cel Biochem 113 (9), 3011–3018. doi:10.1002/jcb.24179
Rivankar, S. (2014). An Overview of Doxorubicin Formulations in Cancer Therapy. J. Cancer Res. Ther. 10 (4), 853–858. doi:10.4103/0973-1482.139267
Roskoski, R. (2012). ERK1/2 MAP Kinases: Structure, Function, and Regulation. Pharmacol. Res. 66 (2), 105–143. doi:10.1016/j.phrs.2012.04.005
Sehirli, O., Tozan, A., Omurtag, G. Z., Cetinel, S., Contuk, G., Gedik, N., et al. (2008). Protective Effect of Resveratrol against Naphthalene-Induced Oxidative Stress in Mice. Ecotoxicol Environ. Saf. 71 (1), 301–308. doi:10.1016/j.ecoenv.2007.08.023
Shen, C., Sun, F. L., Zhang, R. Y., Zhang, L., Li, Y. L., Zhang, L., et al. (2015). Tetrahydroxystilbene Glucoside Ameliorates Memory and Movement Functions, Protects Synapses and Inhibits α-synuclein Aggregation in hippocampus and Striatum in Aged Mice. Restor Neurol. Neurosci. 33 (4), 531–541. doi:10.3233/rnn-150514
Shen, J., Wei, J., Li, L., Ouyang, H., Chang, Y., Chen, X., et al. (2018). Development of a HPLC-MS/MS Method to Determine 11 Bioactive Compounds in Tongmai Yangxin Pill and Application to a Pharmacokinetic Study in Rats. Evidence-Based Complement. Altern. Med. 2018, 1–11. doi:10.1155/2018/6460393
Shi, J., Wu, X., Surma, M., Vemula, S., Zhang, L., Yang, Y., et al. (2013). Distinct Roles for ROCK1 and ROCK2 in the Regulation of Cell Detachment. Cel Death Dis 4, e483. doi:10.1038/cddis.2013.10
Shi, J., Zhao, Y., Wang, Y., Gao, W., Ding, J., Li, P., et al. (2014). Inflammatory Caspases Are Innate Immune Receptors for Intracellular LPS. Nature 514 (7521), 187–192. doi:10.1038/nature13683
Shields, D. C., Haque, A., and Banik, N. L. (2020). Neuroinflammatory Responses of Microglia in central Nervous System Trauma. J. Cereb. Blood Flow Metab. 40, S25–S33. doi:10.1177/0271678x20965786
Sies, H., Berndt, C., and Jones, D. P. (2017). Oxidative Stress. Annu. Rev. Biochem. 86, 715–748. doi:10.1146/annurev-biochem-061516-045037
Singh, A., Kukreti, R., Saso, L., and Kukreti, S. (2019). Oxidative Stress: A Key Modulator in Neurodegenerative Diseases. Molecules 24 (8), 1583. doi:10.3390/molecules24081583
Skov, J., Persson, F., Frøkiær, J., and Christiansen, J. S. (2014). Tissue Renin-Angiotensin Systems: a Unifying Hypothesis of Metabolic Disease. Front. Endocrinol. (Lausanne) 5, 23. doi:10.3389/fendo.2014.00023
Spagnuolo, C., Moccia, S., and Russo, G. L. (2018). Anti-inflammatory Effects of Flavonoids in Neurodegenerative Disorders. Eur. J. Med. Chem. 153, 105–115. doi:10.1016/j.ejmech.2017.09.001
Stępkowski, T. M., and Kruszewski, M. K. (2011). Molecular Cross-Talk between the NRF2/KEAP1 Signaling Pathway, Autophagy, and Apoptosis. Free Radic. Biol. Med. 50 (9), 1186–1195. doi:10.1016/j.freeradbiomed.2011.01.033
Stevens, B. R., Goel, R., Seungbum, K., Richards, E. M., Holbert, R. C., Pepine, C. J., et al. (2018). Increased Human Intestinal Barrier Permeability Plasma Biomarkers Zonulin and FABP2 Correlated with Plasma LPS and Altered Gut Microbiome in Anxiety or Depression. Gut 67 (8), 1555–1557. doi:10.1136/gutjnl-2017-314759
Sun, F. L., Zhang, L., Zhang, R. Y., and Li, L. (2011). Tetrahydroxystilbene Glucoside Protects Human Neuroblastoma SH-Sy5y Cells against MPP+-induced Cytotoxicity. Eur. J. Pharmacol. 660, 283–290. doi:10.1016/j.ejphar.2011.03.046
Sun, L. L., Wang, M., Zhang, H. J., You, G. J., Liu, Y. N., Ren, X. L., et al. (2018). The Influence of Polysaccharides from Ophiopogon Japonicus on 2,3,5,4'-Tetrahydroxy-Stilbene-2-O-β-D-Glucoside about Biopharmaceutical Properties In Vitro and Pharmacokinetics In Vivo. Int. J. Biol. Macromol 119, 677–682. doi:10.1016/j.ijbiomac.2018.07.179
Sun, P., Zhang, S., Li, Y., and Wang, L. (2014). Harmine Mediated Neuroprotection via Evaluation of Glutamate Transporter 1 in a Rat Model of Global Cerebral Ischemia. Neurosci. Lett. 583, 32–36. doi:10.1016/j.neulet.2014.09.023
Surma, M., Wei, L., and Shi, J. (2011). Rho Kinase as a Therapeutic Target in Cardiovascular Disease. Future Cardiol. 7 (5), 657–671. doi:10.2217/fca.11.51
Tao, L., Li, X., Zhang, L., Tian, J., Li, X., Sun, X., et al. (2011). Protective Effect of Tetrahydroxystilbene Glucoside on 6-OHDA-Induced Apoptosis in PC12 Cells through the ROS-NO Pathway. PloS one 6 (10), e26055. doi:10.1371/journal.pone.0026055
Tong, J., Gao, J., Liu, Q., He, C., Zhao, X., Qi, Y., et al. (2020). Resveratrol Derivative Excited Postsynaptic Potentiation Specifically via PKCβ-NMDA Receptor Mediation. Pharmacol. Res. 152, 104618. doi:10.1016/j.phrs.2019.104618
Tönnies, E., and Trushina, E. (2017). Oxidative Stress, Synaptic Dysfunction, and Alzheimer's Disease. J. Alzheimers Dis. 57 (4), 1105–1121. doi:10.3233/jad-161088
Valko, M., Rhodes, C. J., Moncol, J., Izakovic, M., and Mazur, M. (2006). Free Radicals, Metals and Antioxidants in Oxidative Stress-Induced Cancer. Chem. Biol. Interact 160 (1), 1–40. doi:10.1016/j.cbi.2005.12.009
Velarde, M. C., Flynn, J. M., Day, N. U., Melov, S., and Campisi, J. (2012). Mitochondrial Oxidative Stress Caused by Sod2 Deficiency Promotes Cellular Senescence and Aging Phenotypes in the Skin. Aging (Albany NY) 4 (1), 3–12. doi:10.18632/aging.100423
Voet, S., Prinz, M., and van Loo, G. (2019). Microglia in Central Nervous System Inflammation and Multiple Sclerosis Pathology. Trends Mol. Med. 25 (2), 112–123. doi:10.1016/j.molmed.2018.11.005
Vomhof-Dekrey, E. E., and Picklo, M. J. (2012). The Nrf2-Antioxidant Response Element Pathway: a Target for Regulating Energy Metabolism. J. Nutr. Biochem. 23 (10), 1201–1206. doi:10.1016/j.jnutbio.2012.03.005
Wang, C., Hu, N. H., Yu, L. Y., Gong, L. H., Dai, X. Y., Peng, C., et al. (2020a). 2,3,5,4'-tetrahydroxystilbence-2-O-β-D-glucoside Attenuates Hepatic Steatosis via IKKβ/NF-Κb and Keap1-Nrf2 Pathways in Larval Zebrafish. Biomed. Pharmacother. 127, 110138. doi:10.1016/j.biopha.2020.110138
Wang, C., Zhou, Y., Gong, X., Zheng, L., and Li, Y. (2020b). In Vitro and In Situ Study on Characterization and Mechanism of the Intestinal Absorption of 2,3,5,4'-Tetrahydroxy-Stilbene-2-O-β-D-Glucoside. BMC Pharmacol. Toxicol. 21 (1), 7. doi:10.1186/s40360-020-0384-9
Wang, C., Guo, D., Yuan, Z., Feng, X., and Zhang, L. (2009a). Metabolism of Stilbene Glycoside in Rats and In Vitro. Chin. J. Pharm. 40 (02), 120–123.
Wang, H., Zhao, Y., Wang, Y. J., Song, L., Wang, J. L., Huang, C., et al. (2017). Antidepressant-like Effects of Tetrahydroxystilbene Glucoside in Mice: Involvement of BDNF Signaling cascade in the hippocampus. CNS Neurosci. Ther. 23 (7), 627–636. doi:10.1111/cns.12708
Wang, R., Tang, Y., Feng, B., Ye, C., Fang, L., Zhang, L., et al. (2007). Changes in Hippocampal Synapses and Learning-Memory Abilities in Age-Increasing Rats and Effects of Tetrahydroxystilbene Glucoside in Aged Rats. Neuroscience 149 (4), 739–746. doi:10.1016/j.neuroscience.2007.07.065
Wang, T., Gu, J., Wu, P. F., Wang, F., Xiong, Z., Yang, Y. J., et al. (2009b). Protection by Tetrahydroxystilbene Glucoside against Cerebral Ischemia: Involvement of JNK, SIRT1, and NF-kappaB Pathways and Inhibition of Intracellular ROS/RNS Generation. Free Radic. Biol. Med. 47 (3), 229–240. doi:10.1016/j.freeradbiomed.2009.02.027
Wang, T., Yang, Y. J., Wu, P. F., Wang, W., Hu, Z. L., Long, L. H., et al. (2011). Tetrahydroxystilbene Glucoside, a Plant-Derived Cognitive Enhancer, Promotes Hippocampal Synaptic Plasticity. Eur. J. Pharmacol. 650 (1), 206–214. doi:10.1016/j.ejphar.2010.10.002
Wang, X., Wang, W., Li, L., Perry, G., Lee, H. G., and Zhu, X. (2014). Oxidative Stress and Mitochondrial Dysfunction in Alzheimer's Disease. Biochim. Biophys. Acta 1842 (8), 1240–1247. doi:10.1016/j.bbadis.2013.10.015
Wang, X., Zhao, L., Han, T., Chen, S., and Wang, J. (2008). Protective Effects of 2,3,5,4'-Tetrahydroxystilbene-2-O-Beta-D-Glucoside, an Active Component of Polygonum Multiflorum Thunb, on Experimental Colitis in Mice. Eur. J. Pharmacol. 578, 339–348. doi:10.1016/j.ejphar.2007.09.013
Wang, Y. Q., Shen, Y., Li, F., Wang, C. H., and Zhang, W. (2013). 2,3,4',5-tetrahydroxystilbene-2-O-β-D-glucoside Suppresses Expression of Adhesion Molecules in Aortic wall of Dietary Atherosclerotic Rats and Promonocytic U937 Cells. Cell Biochem Biophys 67 (3), 997–1004. doi:10.1007/s12013-013-9595-7
Watanabe, T., and Fan, J. (1998). Atherosclerosis and Inflammation Mononuclear Cell Recruitment and Adhesion Molecules with Reference to the Implication of ICAM-1/LFA-1 Pathway in Atherogenesis. Int. J. Cardiol. 66 (Suppl. 1), S45–S53. doi:10.1016/s0167-5273(98)00147-8
Wei, Q., Ta, G., He, W., Wang, W., and Wu, Q. (2017). Stilbene Glucoside, a Putative Sleep Promoting Constituent from Polygonum Multiflorum Affects Sleep Homeostasis by Affecting the Activities of Lactate Dehydrogenase and Salivary Alpha Amylase. Chem. Pharm. Bull. (Tokyo) 65 (11), 1011–1019. doi:10.1248/cpb.c17-00275
Wen, Y.-H., Lin, J.-N., Wu, R.-S., Yu, S.-H., Hsu, C.-J., Tseng, G.-F., et al. (2020). Otoprotective Effect of 2,3,4′,5-Tetrahydroxystilbene-2-O-β-D-Glucoside on Gentamicin-Induced Apoptosis in Mouse Cochlear UB/OC-2 Cells. MoleculesMolecules (Basel, Switzerland) 225 (3413), 30703070. doi:10.3390/molecules25133070
Wortzel, I., and Seger, R. (2011). The ERK Cascade: Distinct Functions within Various Subcellular Organelles. Genes Cancer 2 (3), 195–209. doi:10.1177/1947601911407328
Wu, T. Y., Lin, J. N., Luo, Z. Y., Hsu, C. J., Wang, J. S., and Wu, H. P. (2020). 2,3,4',5-Tetrahydroxystilbene-2-O-β-D-Glucoside (THSG) Activates the Nrf2 Antioxidant Pathway and Attenuates Oxidative Stress-Induced Cell Death in Mouse Cochlear UB/OC-2 Cells. Biomolecules 10 (3), 465. doi:10.3390/biom10030465
Xie, M., Zhang, G., Yin, W., Hei, X. X., and Liu, T. (2018). Cognitive Enhancing and Antioxidant Effects of Tetrahydroxystilbene Glucoside in Aβ1-42-Induced Neurodegeneration in Mice. J. Integr. Neurosci. 17, 355–365. doi:10.3233/jin-170059
Xu, J., Peng, Y., Zeng, Y., Hua, Y. Q., and Xu, X. L. (2019). 2, 3, 4', 5-Tetrahydroxystilbene-2-0-β-D Glycoside Attenuates Age- and Diet-Associated Non-alcoholic Steatohepatitis and Atherosclerosis in LDL Receptor Knockout Mice and its Possible Mechanisms. Int. J. Mol. Sci. 20 (7), 1617. doi:10.3390/ijms20071617
Xu, S., Liu, J., Shi, J., Wang, Z., and Ji, L. (2017). 2,3,4',5-tetrahydroxystilbene-2-O-β-D-glucoside Exacerbates Acetaminophen-Induced Hepatotoxicity by Inducing Hepatic Expression of CYP2E1, CYP3A4 and CYP1A2. Sci. Rep. 7 (1), 16511. doi:10.1038/s41598-017-16688-5
Xu, X. L., Huang, Y. J., Chen, X. F., Lin, D. Y., and Zhang, W. (2012). 2,3,4',5-tetrahydroxystilbene-2-O-β-D-glucoside Inhibits Proliferation of Vascular Smooth Muscle Cells: Involvement of NO/cGMP/PKG Pathway. Phytother Res. 26 (7), 1068–1074. doi:10.1002/ptr.3691
Xu, X. L., Huang, Y. J., Ling, D. Y., and Zhang, W. (2015). Inhibitory Effects of 2,3,4',5-Tetrahydroxystilbene-2-O-β-D-Glucoside on Angiotensin II-Induced Proliferation of Vascular Smooth Muscle Cells. Chin. J. Integr. Med. 21 (3), 204–210. doi:10.1007/s11655-014-1821-7
Xu, X. L., Huang, Y. J., Wang, Y. Q., Chen, X. F., and Zhang, W. (2011). 2,3,4',5-Tetrahydroxystilbene-2-O-β-d-glucoside Inhibits Platelet-Derived Growth Factor-Induced Proliferation of Vascular Smooth Muscle Cells by Regulating the Cell Cycle. Clin. Exp. Pharmacol. Physiol. 38 (5), 307–313. doi:10.1111/j.1440-1681.2011.05502.x
Xu, X. L., Zhu, Q. Y., Zhao, C., Wang, F., Zhou, Z. Y., Hu, Y. E., et al. (2014). The Effect of 2,3,4',5-Tetrahydroxystilbene-2-O-β-D-Glucoside on Pressure Overload-Induced Cardiac Remodeling in Rats and its Possible Mechanism. Planta Med. 80, 130–138. doi:10.1055/s-0033-1360198
Yamamoto, S., Kido, R., Onishi, Y., Fukuma, S., Akizawa, T., Fukagawa, M., et al. (2015). Use of Renin-Angiotensin System Inhibitors Is Associated with Reduction of Fracture Risk in Hemodialysis Patients. PloS one 10 (4), e0122691. doi:10.1371/journal.pone.0122691
Yang, P. Y., Almofti, M. R., Lu, L., Kang, H., Zhang, J., Li, T. J., et al. (2005). Reduction of Atherosclerosis in Cholesterol-Fed Rabbits and Decrease of Expressions of Intracellular Adhesion Molecule-1 and Vascular Endothelial Growth Factor in Foam Cells by a Water-Soluble Fraction of Polygonum Multiflorum. J. Pharmacol. Sci. 99 (3), 294–300. doi:10.1254/jphs.fp0050333
Yao, W., Fan, W., Huang, C., Zhong, H., Chen, X., and Zhang, W. (2013). Proteomic Analysis for Anti-atherosclerotic Effect of Tetrahydroxystilbene Glucoside in Rats. Biomed. Pharmacother. 67 (2), 140–145. doi:10.1016/j.biopha.2012.10.007
Yao, W., Gu, C., Shao, H., Meng, G., Wang, H., Jing, X., et al. (2015). Tetrahydroxystilbene Glucoside Improves TNF-α-Induced Endothelial Dysfunction: Involvement of TGFβ/Smad Pathway and Inhibition of Vimentin Expression. Am. J. Chin. Med. 43 (1), 183–198. doi:10.1142/s0192415x15500123
Yao, W., Huang, C., Sun, Q., Jing, X., Wang, H., and Zhang, W. (2014). Tetrahydroxystilbene Glucoside Protects against Oxidized LDL-Induced Endothelial Dysfunction via Regulating Vimentin Cytoskeleton and its Colocalization with ICAM-1 and VCAM-1. Cell Physiol Biochem 34 (5), 1442–1454. doi:10.1159/000366349
Yao, W., Huang, L., Sun, Q., Yang, L., Tang, L., Meng, G., et al. (2016a). The Inhibition of Macrophage Foam Cell Formation by Tetrahydroxystilbene Glucoside Is Driven by Suppressing Vimentin Cytoskeleton. Biomed. Pharmacother. 83, 1132–1140. doi:10.1016/j.biopha.2016.08.032
Yao, W., Sun, Q., Huang, L., Meng, G., Wang, H., Jing, X., et al. (2016b). Tetrahydroxystilbene Glucoside Inhibits TNF-α-Induced Migration of Vascular Smooth Muscle Cells via Suppression of Vimentin. Can. J. Physiol. Pharmacol. 94 (2), 155–160. doi:10.1139/cjpp-2015-0160
Yeung, Y. T., Aziz, F., Guerrero-Castilla, A., and Arguelles, S. (2018). Signaling Pathways in Inflammation and Anti-inflammatory Therapies. Curr. Pharm. Des. 24 (14), 1449–1484. doi:10.2174/1381612824666180327165604
Yin, X., Chen, C., Xu, T., Li, L., and Zhang, L. (2018). Tetrahydroxystilbene Glucoside Modulates Amyloid Precursor Protein Processing via Activation of AKT-Gsk3β Pathway in Cells and in APP/PS1 Transgenic Mice. Biochem. Biophys. Res. Commun. 495 (1), 672–678. doi:10.1016/j.bbrc.2017.11.059
Yu, F., Xue, W., Dong, L., Hu, X., Huang, D., and Wang, K. (2019). Tetrahydroxystilbene Glucoside Suppresses NAPDH Oxidative Stress to Mitigate Apoptosis and Autophagy Induced by Cerebral Ischemia/Reperfusion Injury in Mice. Evidence-Based Complement. Altern. Med. 2019, 1–9. doi:10.1155/2019/3913981
Yu, J., Xie, J., Mao, X. J., Wang, M. J., Li, N., Wang, J., et al. (2011). Hepatoxicity of Major Constituents and Extractions of Radix Polygoni Multiflori and Radix Polygoni Multiflori Praeparata. J. Ethnopharmacol 137 (3), 1291–1299. doi:10.1016/j.jep.2011.07.055
Yu, W., Zhang, X., Wu, H., Zhou, Q., Wang, Z., Liu, R., et al. (2017). HO-1 Is Essential for Tetrahydroxystilbene Glucoside Mediated Mitochondrial Biogenesis and Anti-inflammation Process in LPS-Treated RAW264.7 Macrophages. Oxid Med. Cel Longev 2017, 1818575. doi:10.1155/2017/1818575
Yun, K. J., Koh, D. J., Kim, S. H., Park, S. J., Ryu, J. H., Kim, D. G., et al. (2008). Anti-inflammatory Effects of Sinapic Acid through the Suppression of Inducible Nitric Oxide Synthase, Cyclooxygase-2, and Proinflammatory Cytokines Expressions via Nuclear Factor-kappaB Inactivation. J. Agric. Food Chem. 56 (21), 10265–10272. doi:10.1021/jf802095g
Zeng, C., Xiao, J. H., Chang, M. J., and Wang, J. L. (2011). Beneficial Effects of THSG on Acetic Acid-Induced Experimental Colitis: Involvement of Upregulation of PPAR-γ and Inhibition of the Nf-Κb Inflammatory Pathway. Molecules 16 (10), 8552–8568. doi:10.3390/molecules16108552
Zeng, L., Dang, T. A., and Schunkert, H. (2016). Genetics Links between Transforming Growth Factor β Pathway and Coronary Disease. Atherosclerosis 253, 237–246. doi:10.1016/j.atherosclerosis.2016.08.029
Zhang, F., Wang, Y. Y., Yang, J., Lu, Y. F., Liu, J., and Shi, J. S. (2013a). Tetrahydroxystilbene Glucoside Attenuates Neuroinflammation through the Inhibition of Microglia Activation. Oxid Med. Cel Longev 2013, 680545. doi:10.1155/2013/680545
Zhang, J., Chen, X., Chen, B., Tong, L., and Zhang, Y. (2019). Tetrahydroxy Stilbene Glucoside Protected against Diabetes-Induced Osteoporosis in Mice with Streptozotocin-Induced Hyperglycemia. Phytother Res. 33 (2), 442–451. doi:10.1002/ptr.6240
Zhang, J., Li, S., Wei, L., Peng, Y., Zheng, Z., Xue, J., et al. (2018a). Protective Effects of 2,3,5,4-Tetrahydroxystilbene-2-O-β-D-Glucoside against Osteoporosis: Current Knowledge and Proposed Mechanisms. Int. J. Rheum. Dis. 21 (8), 1504–1513. doi:10.1111/1756-185x.13357
Zhang, J. K., Yang, L., Meng, G. L., Fan, J., Chen, J. Z., He, Q. Z., et al. (2012). Protective Effect of Tetrahydroxystilbene Glucoside against Hydrogen Peroxide-Induced Dysfunction and Oxidative Stress in Osteoblastic MC3T3-E1 Cells. Eur. J. Pharmacol. 689, 31–37. doi:10.1016/j.ejphar.2012.05.045
Zhang, L., Huang, L., Chen, L., Hao, D., and Chen, J. (2013b). Neuroprotection by Tetrahydroxystilbene Glucoside in the MPTP Mouse Model of Parkinson's Disease. Toxicol. Lett. 222 (2), 155–163. doi:10.1016/j.toxlet.2013.07.020
Zhang, L., Huang, L., Li, X., Liu, C., Sun, X., Wu, L., et al. (2017a). Potential Molecular Mechanisms Mediating the Protective Effects of Tetrahydroxystilbene Glucoside on MPP+-induced PC12 Cell Apoptosis. Mol. Cel Biochem 436, 203–213. doi:10.1007/s11010-017-3169-8
Zhang, L., Xing, Y., Ye, C. F., Ai, H. X., Wei, H. F., and Li, L. (2006). Learning-memory Deficit with Aging in APP Transgenic Mice of Alzheimer's Disease and Intervention by Using Tetrahydroxystilbene Glucoside. Behav. Brain Res. 173 (2), 246–254. doi:10.1016/j.bbr.2006.06.034
Zhang, L., and Yang, H. (2021). Promotive Effects of Tetrahydroxystilbene Glucoside on the Differentiation of Neural Stem Cells from the Mesencephalon into Dopaminergic Neurons. Neurosci. Lett. 742, 135520. doi:10.1016/j.neulet.2020.135520
Zhang, L., Yu, S., Zhang, R., Xing, Y., Li, Y., and Li, L. (2013c). Tetrahydroxystilbene Glucoside Antagonizes Age-Related α-synuclein Overexpression in the hippocampus of APP Transgenic Mouse Model of Alzheimer's Disease. Restor Neurol. Neurosci. 31 (1), 41–52. doi:10.3233/rnn-120260
Zhang, M., Lin, L., Lin, H., Qu, C., Yan, L., and Ni, J. (2018b). Interpretation the Hepatotoxicity Based on Pharmacokinetics Investigated through Oral Administrated Different Extraction Parts of Polygonum Multiflorum on Rats. Front. Pharmacol. 9, 505. doi:10.3389/fphar.2018.00505
Zhang, R., Sun, F., Zhang, L., Sun, X., and Li, L. (2017b). Tetrahydroxystilbene Glucoside Inhibits α-synuclein Aggregation and Apoptosis in A53T α-synuclein-transfected Cells Exposed to MPP. Can. J. Physiol. Pharmacol. 95 (6), 750–758. doi:10.1139/cjpp-2016-0209
Zhang, R. Y., Zhang, L., Zhang, L., Wang, Y. L., and Li, L. (2018c). Anti-amyloidgenic and Neurotrophic Effects of Tetrahydroxystilbene Glucoside on a Chronic Mitochondrial Dysfunction Rat Model Induced by Sodium Azide. J. Nat. Med. 72 (3), 596–606. doi:10.1007/s11418-018-1177-y
Zhang, S. H., Wang, W. Q., and Wang, J. L. (2009a). Protective Effect of Tetrahydroxystilbene Glucoside on Cardiotoxicity Induced by Doxorubicin In Vitro and In Vivo. Acta Pharmacol. Sin 30 (11), 1479–1487. doi:10.1038/aps.2009.144
Zhang, W., Wang, C. H., Li, F., and Zhu, W. Z. (2008). 2,3,4',5-Tetrahydroxystilbene-2-O-beta-D-glucoside Suppresses Matrix Metalloproteinase Expression and Inflammation in Atherosclerotic Rats. Clin. Exp. Pharmacol. Physiol. 35 (3), 310–316. doi:10.1111/j.1440-1681.2007.04824.x
Zhang, W., Xu, X. L., Wang, Y. Q., Wang, C. H., and Zhu, W. Z. (2009b). Effects of 2,3,4',5-tetrahydroxystilbene 2-O-Beta-D-Glucoside on Vascular Endothelial Dysfunction in Atherogenic-Diet Rats. Planta Med. 75 (11), 1209–1214. doi:10.1055/s-0029-1185540
Zhang, Y., Jiang, M., Cui, B. W., Jin, C. H., Wu, Y. L., Shang, Y., et al. (2020). P2X7 Receptor-Targeted Regulation by Tetrahydroxystilbene Glucoside in Alcoholic Hepatosteatosis: A New Strategy towards Macrophage-Hepatocyte Crosstalk. Br. J. Pharmacol. 177 (12), 2793–2811. doi:10.1111/bph.15007
Zhang, Y., Wang, L., Song, Y., Zhao, X., Wong, M. S., and Zhang, W. (2016). Renin Inhibitor Aliskiren Exerts Beneficial Effect on Trabecular Bone by Regulating Skeletal Renin-Angiotensin System and Kallikrein-Kinin System in Ovariectomized Mice. Osteoporos. Int. 27 (3), 1083–1092. doi:10.1007/s00198-015-3348-y
Zhao, Y. Y., Zhang, L., Feng, Y. L., Chen, D. Q., Xi, Z. H., Du, X., et al. (2013). Pharmacokinetics of 2,3,5,4'-Tetrahydroxystilbene-2-O-β-D-Glucoside in Rat Using Ultra-performance LC-Quadrupole TOF-MS. J. Sep. Sci. 36 (5), 863–871. doi:10.1002/jssc.201200668
Zheng, Y., Li, J., Wu, J., Yu, Y., Yao, W., Zhou, M., et al. (2017). Tetrahydroxystilbene Glucoside Isolated from Polygonum Multiflorum Thunb. Demonstrates Osteoblast Differentiation Promoting Activity. Exp. Ther. Med. 14 (4), 2845–2852. doi:10.3892/etm.2017.4915
Zhou, L., Hou, Y., Yang, Q., Du, X., Li, M., Yuan, M., et al. (2012). Tetrahydroxystilbene Glucoside Improves the Learning and Memory of Amyloid-Β(₁₋₄₂)-Injected Rats and May Be Connected to Synaptic Changes in the hippocampus. Can. J. Physiol. Pharmacol. 90 (11), 1446–1455. doi:10.1139/y2012-121
Zhou, X., Ge, L., Yang, Q., Xie, Y., Sun, J., Cao, W., et al. (2014). Thinning of Dermas with the Increasing Age May Be against by Tetrahydroxystilbene Glucoside in Mice. Int. J. Clin. Exp. Med. 7 (8), 2017–2024.
Zhou, X., Yang, Q., Song, F., Bi, L., Yuan, J., Guan, S., et al. (2020). Tetrahydroxystilbene Glucoside Ameliorates Infrasound-Induced Central Nervous System (CNS) Injury by Improving Antioxidant and Anti-inflammatory Capacity. Oxid Med. Cel Longev 2020, 6576718. doi:10.1155/2020/6576718
Zhou, X., Yang, Q., Xie, Y., Sun, J., Hu, J., Qiu, P., et al. (2015). Tetrahydroxystilbene Glucoside Extends Mouse Life Span via Upregulating Neural Klotho and Downregulating Neural Insulin or Insulin-like Growth Factor 1. Neurobiol. Aging 36 (3), 1462–1470. doi:10.1016/j.neurobiolaging.2014.11.002
Zhou, Y., Wang, G., Li, D., Wang, Y., Wu, Q., Shi, J., et al. (2018). Dual Modulation on Glial Cells by Tetrahydroxystilbene Glucoside Protects against Dopamine Neuronal Loss. J. Neuroinflammation 15 (1), 161. doi:10.1186/s12974-018-1194-5
Zhu, B., Caldwell, M., and Song, B. (2016). Development of Stem Cell-Based Therapies for Parkinson's Disease. Int. J. Neurosci. 126 (11), 955–962. doi:10.3109/00207454.2016.1148034
Zou, Y., and Kong, M. (2019). Tetrahydroxy Stilbene Glucoside Alleviates Palmitic Acid-Induced Inflammation and Apoptosis in Cardiomyocytes by Regulating miR-129-3p/Smad3 Signaling. Cell Mol Biol Lett 24, 5. doi:10.1186/s11658-018-0125-x
Glossary
PM Polygonum multiflorum Thunb.
TSG 2,3,5,4′-tetrahydroxystilbene-2-O-β-D-glucoside
NF-κB nuclear factor kappa-B
AMPK adenosine 5′-monophosphate (AMP)-activated protein kinase
PI3K phosphatidyl-inositol-3-kinase
AKT protein kinase B
ROS reactive oxygen species
NO nitric oxide
Bcl-2 B-cell lymphoma-2
Bax Bcl-2-associated X protein
TGF-β transforming growth factor-β
PGE2 prostaglandin E2
iNOS inducible nitric oxide synthase
eNOS endothelial nitric oxide synthase
COX-2 cyclooxygenase-2 cyclooxygenase-2
LPS lipopolysaccharide
IL-6 interleukin-6
TNF-α tumor necrosis factor-α
IL-1β interleukin-1β
SIRT1 sirtuin 1
MPO myeloperoxidase
MDA malonaldehyde
SOD superoxide dismutase
COX-2 cyclooxygenase-2 cyclooxygenase-2
PPAR-γ peroxisome proliferator-activated receptor γ
CAT catalase
GSH-Px glutathione peroxidase
Nrf2 nuclear factor erythroid 2-related factor 2
HO-1 heme oxygenase 1
GST glutathione-s-transferase
AD Alzheimer’s disease
PD Parkinson’s disease
Aβ amyloid β-protein
MPP+ 1-methyl-4-phenylpyridinium
MAPK mitogen-activated protein kinase
CNS central nervous system
MCAO middle cerebral artery occlusion
GLT-1 glutamate transporter-1
JNK Jun N-terminal kinase
PINK1 PTEN induced putative kinase 1
ROCK Rho kinase
NGF nerve growth factor
GAP-43 growth associated protein-43
APP β-amyloid precursor protein
BACE1 β-site APP cleaving enzyme 1
PS1 presenilin 1
BDNF brain-derived neurotrophic factor
TrkB tropomyosin-related kinase B
HUVECs human umbilical vein endothelial cells
oxLDL oxidized low-density lipoprotein
PMA phorbol-12-myristate-13-acetate
VSMC vascular smooth muscle cells
ERK1/2 extracellular signal-regulated kinase 1/2
AngII angiotensin II
VEGF vascular endothlial growth factor
ICAM-1 intercellular cell adhesion molecule-1
NAFLD non-alcoholic fatty liver disease
NASH non-alcohol steatohepatitis
HFD high fat diet
RAS renin-angiotensin system
LTP long-term potentiation
CREB cAMP-response element binding protein
ADAM a disintegrin and metalloprotease
IGF-1 insulin-like growth factor-1
SAMP8 senescence-accelerated mouse prone
HDPSCs human dental pulp stem cells
HPLC-MS/MS high performance liquid chromatography tandem mass spectrometry
UPLC-MS/MS ultra performance liquid chromatography tandem mass spectrometry
Keywords: 2,3,5,4′-tetrahydroxystilbene-2-O-β-D-glucoside, anti-inflammation, antioxidant, neuroprotection, cardiovascular protection, pharmacological and pharmacokinetic properties, toxicity
Citation: Wang C, Dai S, Gong L, Fu K, Ma C, Liu Y, Zhou H and Li Y (2022) A Review of Pharmacology, Toxicity and Pharmacokinetics of 2,3,5,4′-Tetrahydroxystilbene-2-O-β-D-Glucoside. Front. Pharmacol. 12:791214. doi: 10.3389/fphar.2021.791214
Received: 08 October 2021; Accepted: 10 December 2021;
Published: 05 January 2022.
Edited by:
Uraiwan Panich, Mahidol University, ThailandReviewed by:
Zhenzhou Jiang, China Pharmaceutical University, ChinaHaolong Liu, Peking University Health Science Centre, China
Copyright © 2022 Wang, Dai, Gong, Fu, Ma, Liu, Zhou and Li. This is an open-access article distributed under the terms of the Creative Commons Attribution License (CC BY). The use, distribution or reproduction in other forums is permitted, provided the original author(s) and the copyright owner(s) are credited and that the original publication in this journal is cited, in accordance with accepted academic practice. No use, distribution or reproduction is permitted which does not comply with these terms.
*Correspondence: Yunxia Li, bHl4dGd5eGNkdXRjbUAxNjMuY29t
†These authors have contributed equally to this work