- 1Department of Biomedical and Biotechnological Sciences, School of Medicine, University of Catania, Catania, Italy
- 2Department of Ophthalmology, Fondation Asile des Aveugles, Jules Gonin Eye Hospital, University of Lausanne, Lausanne, Switzerland
- 3Department of Surgical Sciences, University of Torino, Torino, Italy
- 4Center for Research in Ocular Pharmacology–CERFO, University of Catania, Catania, Italy
Age-related macular degeneration (AMD) is a degenerative retinal disease and one of major causes of irreversible vision loss. AMD has been linked to several pathological factors, such as oxidative stress and inflammation. Moreover, Aβ (1–42) oligomers have been found in drusen, the extracellular deposits that accumulate beneath the retinal pigmented epithelium in AMD patients. Hereby, we investigated the hypothesis that treatment with 1,25(OH) 2D3 (vitamin D3) and meso-zeaxathin, physiologically present in the eye, would counteract the toxic effects of three different insults on immortalized human retinal pigmented epithelial cells (ARPE-19). Specifically, ARPE-19 cells have been challenged with Aβ (1–42) oligomers, H2O2, LPS, and TNF-α, respectively. In the present study, we demonstrated that the combination of 1,25(OH)2D3 and meso-zeaxanthin significantly counteracted the cell damage induced by the three insults, at least in these in vitro integrated paradigms of AMD. These results suggest that combination of 1,25(OH)2D3 and meso-zeaxathin could be a useful approach to contrast pathological features of AMD, such as retinal inflammation and oxidative stress.
Introduction
Age-related macular degeneration (AMD) is a progressive neurodegenerative and multifactorial disease that if not treated or managed can impair irreversibly the visual function (Cascella et al., 2014; Pennington and DeAngelis, 2016) in the elderly population (usually older than 60 years) (Nowak, 2006). AMD affects the macula, that is, the central portion of the retina, which is highly sensitive to visual stimuli due to the high density of retinal photoreceptors. In the macula of AMD patients, between the retinal pigment epithelium (RPE) and Bruch’s membrane, lesions named drusen have been found. These lesions are characterized by accumulation of extracellular material, lipid, and protein aggregates. Moreover, the number and size of drusen, along with the presence of choroidal neovascularization, have been found to correlate with the stage of AMD (early, intermediate, or advanced) (Zajaç-Pytrus et al., 2015). Generally, AMD is classified into atrophic (dry or non-exudative form) and neovascular or exudative forms (wet form). Wet AMD is characterized by overexpression of the vascular endothelial growth factor (VEGF-A), which leads to the breakdown of the blood–retinal barrier and choroidal neovascularization (Kauppinen et al., 2016). Retinal degeneration in wet AMD is tightly linked to choroidal neovascularization (CNV) and growth of leaky blood vessels under the macula, due to overproduction of pro-angiogenic factors (VEGF family) and inflammatory cytokines. Dry AMD can progress to the severe stage, wet AMD, which if not managed can lead to macular edema, retinal detachment, and then to irreversible blindness. Actually, only patients with the wet form (neovascular AMD) can be benefitted from pharmacological therapy, specifically the intravitreal administration of anti-vascular endothelial growth factors (anti-VEGF) (Holekamp, 2019), although anti-VEGF agents, used in clinical practice, such as ranibizumab, bevacizumab, and aflibercept, are considerably different in terms of molecular interactions when they bind with VEGF (Giurdanella et al., 2015; Platania et al., 2015). Currently, one of the main unmet medical needs in AMD management is the lack of effective pharmacological treatment for the dry AMD, which represents the 90% of AMD cases (Buschini et al., 2015). Moreover, the pathophysiology of the AMD is only partially understood, considering that it is the result of the interaction between environmental, metabolic, and genetic factors. Main hallmarks of AMD are represented by tissue dysfunctions (RPE, Bruch’s membrane, and choriocapillaris), associated to chronic oxidative stress, autophagy decline, inflammation (Levy et al., 2015; Eandi et al., 2016; Guillonneau et al., 2017), and angiogenesis (Kauppinen et al., 2016; Layana et al., 2017). Several studies highlighted that inflammation is one of the main driving factors of AMD pathogenesis. In fact, drusen deposits contain numerous inflammation-related factors, along with lipids, amyloid-β (Aβ) aggregates, and oxidation by-products (Bucolo et al., 1999; Wang et al., 2009; Krohne et al., 2010). Furthermore, it has been demonstrated that the formation of drusen is induced by chronic low-level inflammation and complement activation, as a result of the activation of inflammatory pathways, such as NFκB (Hageman et al., 2001; D.H. et al., 2002; Johnson et al., 2011). Moreover, the activation of the inflammasome, by amyloid-β, was reported to contribute to RPE dysfunction during AMD (Anderson et al., 2013; Liu et al., 2013). Macrophages, attracted by drusen to the sub-RPE space, release tumor necrosis factor α (TNF-α) that binds tumor necrosis factor receptor 1 (TNFR1), and then stimulate RPE cells’ inflammatory response. AMD is also known as the “dementia of the eye,” due to the age-dependent accumulation of amyloid beta oligomers in drusen deposits. Several studies demonstrated that Aβ-related damage is common to both the retina and brain, as well as the disruption of the tight junctions in the blood–brain barrier (BRB) and the blood–retinal barrier (BRB) (Parks et al., 2004; Bruban et al., 2009; Biron et al., 2011). Together with inflammation and Aβ-related damage, reactive oxygen species (ROS) have a central role in AMD (Kohen and Nyska, 2002). The altered cellular homeostasis in RPE cells, related to ROS overproduction, can be induced by several factors, such as, aging process, light exposure, diet, and cigarette smoking.
Indeed, because of the multifactorial pathophysiology of both dry and wet AMD, we designed an integrated in vitro model of AMD, stimulating RPE cells with three different challenges: Aβ oligomers, hydrogen peroxide (H2O2), and inflammatory stimuli (LPS and TNF-α), and testing the effects of in vitro treatment with anti-inflammatory, anti-angiogenic, and antioxidant molecules: 1,25(OH)2D3 (vitamin D3), meso-zeaxanthin (MZ), and their combination. Specifically, vitamin D3 is a secosteroid able to modulate cell differentiation, homeostasis, and apoptosis through direct and indirect mechanisms of action. The first one is activated by the binding of the active form of vitamin D3 to its receptor (VDR), a transcriptional factor. VDR is expressed in most human cells, supporting the hypothesis that vitamin D3 has a pleiotropic effect. Moreover, anti-inflammatory and anti-angiogenic effects of vitamin D3 have been widely elucidated both in in vitro and in vivo studies (Majewski et al., 1996; Albert et al., 2007; Maj et al., 2018; Almeida Moreira Leal et al., 2020). Interestingly, the vitamin D3 receptor is expressed in the RPE layer, which along with enzymes is able to convert the inactive form into the active form. The rationale of this in vitro study came from previous reports that have shown a tight link between vitamin D3 serum levels and AMD progression. In fact, it has been found that a low vitamin D3 level in serum can be a risk factor for the progression of AMD (Parekh et al., 2007; Millen et al., 2011; Annweiler et al., 2016; Merle et al., 2017; Kan et al., 2020). These findings could be linked to the activation of macrophages phagocytosis of Aβ deposits, along with anti-inflammatory and antioxidant action exerted by vitamin D3 (Lee et al., 2012).
Meso-zeaxanthin [(3R, 30S)-b, b-carotene-3, 30-diol, MZ] is one of the three xanthophyll carotenoids localized in the macula lutea. Carotenoids are lipid-soluble yellow–orange–red pigments with antioxidant and immunomodulatory activity; reduction in carotenoid levels has been linked to increased risk of cardiovascular disease, diabetes, and cancer (Sesso et al., 2004; Hozawa et al., 2006; Eliassen et al., 2015). In particular, MZ is one of the powerful antioxidant carotenoids found in the RPE cell layer. Basically, the source of meso-zeaxanthin in the eye is represented by the endogenous conversion of lutein in the retinal pigment epithelium (Shyam et al., 2017; Green-Gomez et al., 2020). A specific carotenoid-binding protein (Z-binding protein) regulates the retinal uptake from blood of lutein, which can be converted into meso-zeaxanthin (Thurnham et al., 2008; Nolan et al., 2013).
Given these premises on vitamin D3 and meso-zeaxanthin activities, we tested the efficacy of these two compounds and their combination in three different in vitro models of AMD. We found that their combination significantly counteracted the damage induced by Aβ-amyloid oligomers, H2O2, and inflammatory stimuli in immortalized human RPE (ARPE-19) cells. Moreover, a bioinformatic analysis evidenced that the combination of these compounds effectively covers the pathways associated with the three stimuli, resembling the AMD multifactorial pathology.
Methods
Human retinal pigment epithelial cells (ARPE-19) were purchased from ATCC® (Manassas, Virginia, USA). Cells were cultured at 37 °C (humidified atmosphere with 5% CO2) in ATCC-formulated DMEM:F12 medium (ATCC number 30–2006) with 100 U/ml penicillin, 100 μg/ml streptomycin, and 10% fetal bovine serum (FBS). After reaching confluence (70%), ARPE-19 cells were pretreated for 24 h with 50 nM of 1,25(OH)2D3 (Sigma Aldrich, D1530-1mg, St. Louis, MO), 0.1 µM of meso-zeaxanthin (MZ) (Sigma Aldrich, USP reference standard #1733119, St. Louis, MO), and the combination (combo) of 1,25(OH)2D3 (50 nM) and meso-zeaxanthin (MZ, 0.1 µM). Both pretreatment and treatment were performed in medium supplemented with 5% FBS to starve cells. After pretreatment, ARPE-19 cells were challenged with four different stimuli: amyloid-β oligomers (1 and 2.5 µM; amyloid β-protein 1–42 HFIP-treated, Bachem H-7442.0100) (Calafiore et al., 2012; Caruso et al., 2021), hydrogen peroxide (400 µM H2O2), LPS (150 ng/ml and 10 μg/ml, Enzo ALX-581–010-L001, Farmingdale, NY), and tumor necrosis-alpha (TNF-α) (10 ng/ml, Thermo Fisher Scientific, Carlsbad, CA), in order to simulate retinal degeneration, retinal oxidative stress, and early and late inflammation, respectively. 1,25(OH)2D3, MZ, and the combo were also added to the medium containing negative stimuli.
Cell Viability
The 3-[4,5-dimethylthiazol-2-yl]-2,5-diphenyl tetrasodium bromide (MTT; Chemicon, Temecula, CA) was used to assess cell viability after Aβ (1–42) and H2O2 challenge. Optimal cell density was obtained by seeding 3 × 104 cells/well in 96-well plates (Costar, Corning, NY, United States). After pretreatment, ARPE-19 cells were subjected to co-treatment in a fresh medium for 48 h with Aβ (1–42) (1 µM) and for 6 and 24 h with H2O2 (400 µM). At the end of the treatment, ARPE-19 cells were incubated at 37°C with MTT (0.5 mg/ml) for 3 h; then DMSO was added, and absorbance was measured at 570 nm in a plate reader (VariosKan, Thermo Fisher Scientific, Waltham, MA, United States). Graphs were built converting absorbance (abs) to viability (=% of control) using the following equation (absx ÷ absctrl−) × 100, where absx is absorbance in the x well, and absctrl− is the average absorbance of negative control cells (untreated cells).
Lactate Dehydrogenase Cell Release
Lactate dehydrogenase (LDH) cell release was measured using the Cytotoxicity Detection KitPLUS (LDH) (ROCHE, Mannheim, Germany). ARPE-19 cells were seeded at 2 × 104 cells/well in 96-well plates (Costar, Corning, NY, United States). After pretreatment, cells were co-treated for 48 h with Aβ (1–42) (1 µM) and for 6 and 24 h in the oxidative stress model (H2O2 400 µM). In control groups, only fresh medium was added. After these time points, according to manufacturer’s protocol, lysis solution was added to positive control wells (non-treated cells) for 15 min. After transferring 100 µl of medium in a new multi-well plate, 100 µl of working solution was added. After 10–15 min at room temperature, at last, 50 µl of stop solution was added. The absorbance values were measured at 490 nm using a plate reader (VarioSkan, Thermo Fisher Scientific, Waltham, MA, United States). LDH release is reported as LDH (% control) (absx ÷ absctrl+) × 100. In the equation, absx is absorbance in the x well and absctrl+ is the average absorbance of positive control cells (untreated lysed cells). Absorbance values were corrected by subtracting medium absorbance.
Reactive Oxygen Species Production
ROS were measured by a 2′,7′-dichlorofluoresceindiacetate (DCFDA)–Cellular Reactive Oxygen Species Detection Assay Kit (Abcam, Cambridge, United Kingdom). DCFDA, a cell permeable fluorogenic dye, is deacetylated by cellular esterases to a non-fluorescent compound and later oxidized by ROS to highly fluorescent 2′,7′-dichlorofluorescein (DCF); fluorescence intensity is proportional to cell ROS concentration. Optimal cell density was obtained by seeding 20 × 103 cells/well in 96-well plates (Costar, Corning, NY, United States). After reaching confluence (70%), ARPE-19 cells were pretreated with 1,25(OH)2D3, mesozeaxanthin, and the combo for 24 h. Subsequently, cells were submitted to co-treatment for 48 h in amyloid-β challenge (1 µM). After treatment, media were aspirated and cells were washed by adding 100 µl/well of 1X buffer, according to manufacturer’s protocol; after washing, ARPE-19 cells were stained by adding 100 µl/well of the diluted DCDFA solution (25 µM). Cells were also incubated with this solution for 45 min at 37°C in the dark. After removing DCDFA solution, 100 µl/well of 1X buffer was added, and ROS concentration was measured immediately by detection of DCF fluorescence (λex = 495 nm, λem = 529 nm) with a Varioskan™ Flash Multimode Reader. According to manufacturer’s protocol, for treatment lower than or equal to 6 h, it is possible to treat cells after adding DCDFA solution. Thus, after 24 h of pretreatment with drug formulations, ARPE-19 cells were washed and stained with DCDFA for 45 min. After removing DCDFA solution and washing again, ARPE-19 cells underwent co-treatment for 6 h in H2O2 challenge (400 µM). At the end of time point, ROS concentration was measured immediately without washing. Results were reported as percentage of control after background subtraction; to determine total ROS formation, the fluorescence was normalized to the fluorescent intensity of control cells (untreated cells).
Extraction of Total Ribonucleic Acid and cDNA Synthesis
Extraction of total RNA, from ARPE-19 cells, was performed with a TRIzol Reagent (Invitrogen, Life Technologies, Carlsbad, CA, United States). The A260/A280 ratio of optical density of RNA samples (measured with Multimode Reader Flash di Varioskan™) was 1.95–2.01; this RNA purity was confirmed with the electrophoresis in non-denaturing 1% agarose gel (in TAE). cDNA was synthesized from 2 µg RNA with a reverse transcription kit (SuperScript™ II Reverse transcriptase, Invitrogen, Thermo Fisher Scientific, Carlsbad, CA, United States).
Real-Time Reverse Transcriptase–Polymerase Chain Reaction
Real-time PCR was carried out with the Rotor-Gene Q (Qiagen). The amplification reaction mix included the Master Mix Qiagen (10 µl) (Qiagen QuantiNova SYBR Green Real-Time PCR Kit) and cDNA (1 µL, 100 ng). Forty-five amplification cycles were carried out for each sample. Results were analyzed with the 2−ΔΔCt method. Quantitative PCR experiments followed the MIQE guidelines. Gene expression levels were normalized with levels of two housekeeping genes (18S and GAPDH). Primers were purchased from Eurofins Genomics (Milan, Italy) and Qiagen (Milan, Italy). Forward and reverse primer sequences (for human genes) and the catalog number are herein listed: human IL-1β (forward: 5′-AGCTACGAATCTCCGACCAC-3'; reverse: 5′-CGTTATCCCATGTGTCGAAGAA-3′), human IL-6 (Catalog Number QT00083720), human TNF-α (forward 5′-AGCCCATGTTGTAGCAAACC-3'; reverse 5′-TGAGGTACAGGCCCTCTGAT-3′), human MMP-9 (forward 5′-CTTTGAGTCCGGTGGACGAT-3'; reverse 5′-TCGCCAGTACTTCCCATCCT-3′), human VEGF-A (forward 5′-AGGGCAGAATCATCACGAAG-3'; reverse 5′-ATCCGCATAATCTGCATGGT-3′), human 18S (forward 5′-AGTCCCTGCCCTTTG-3'; reverse 5′-GATCCGAGGGCCTCACTAAAC-3′), and human GAPDH (forward 5′-CTGCACCACCAACTGCTTAG-3'; reverse 5′-AGGTCCACCACTGACACGTT-3′).
Western Blot
ARPE-19 cells were cultured in 60-mm petri dishes at a density of 1.3 × 106. After 24 h of pretreatment with drugs and co-treatment with different stimuli (400 µM of H2O2 for 4 h, 10 μg/ml of LPS for 2 h, amyloid-β oligomers 2.5 µM for 48 h, and TNF-α 10 ng/ml for 2 h), cytoplasmic and nuclear proteins were extracted by using the CER/NER kit (NE-PER, Invitrogen, Life Technologies, Carlsbad, USA), according to the manufacturer’s protocol. The protein content was determined by the BCA Assay Kit (Pierce™ BCA Protein Assay Kit, Invitrogen, Life Technologies, Carlsbad, United States). Extracted proteins (20 μg) were loaded on a NuPAGE ™ 10% Bis-Tris mini protein gel (Invitrogen, Life Technologies, Carlsbad, CA, United States). After electrophoresis, proteins were transferred to a nitrocellulose membrane (Invitrogen, Life Technologies, Carlsbad, CA, United States). Membranes were blocked with milk, 5% Tris-buffered saline, and 0.2% Tween 20 (TBST) for 1 h at room temperature. Membranes were incubated overnight (4°C) with appropriate primary phospho-NFκB p65 (Ser536; mouse mAb #3036 Cell Signaling Technology, MA, United States, 1:500 dilution), anti-GAPDH (Rabbit mAb #2118 Cell Signaling Technology, MA, United States; 1:1,000 dilution), and anti-lamin B (Mouse monoclonal IgG2b, sc-365214 Santa Cruz Biotechnology; 1:1,000 dilution) antibodies. After overnight incubation, the membranes were then incubated with secondary chemiluminscent antibodies (ECL anti-mouse, NA931 and ECL anti-rabbit, NA934, 1:2000 dilution) for 1 h at room temperature. After secondary antibody, the membranes were incubated with ECL (SuperSignal™ West Pico PLUS Chemiluminescent Substrate, Thermo Fisher Scientific, Carlsbad, CA, United States) and were detected through I-Bright™ 1500 (Invitrogen, Life Technologies, Carlsbad, CA, United States) by using chemiluminescence. Densitometry analyses of blots were performed at non-saturating exposures and analyzed using ImageJ software (NIH, Bethesda, MD). Values were normalized to GAPDH and lamin B, which were used as housekeeping control for cytoplasmic and nuclear fraction, respectively.
Bioinformatics
The STITCH compound app of Cytoscape v. 3.7.0 was used to build an integrated network resembling all the experimental results obtained with our integrated in vitro model. Inputs were (i.e., query terms) β amyloid, LPS, TNF-α, H2O2, meso-zeaxanthin, vitamin D3, IL-6, Il-1β, VEGF-A, and MMP-9. The number of interactors was limited to 15, and the default confidence score was set to 0.40. Enrichment of information was included in the analysis. A centrality metrics analysis was carried out treating the network as an indirect graph (Platania et al., 2015, 2018). Functional clusters were identified with Cytoscape using specific terms: β amyloid, H2O2, LPS, TNF-α, vitamin D3, and meso-zeaxanthin.
Statistical Analysis
Statistical analysis was performed with GraphPad Prism 7 (GraphPad software, La Jolla, California). All experiments were repeated five times (n = 5), and the data are reported as mean ± SD. One-way analysis of variance (ANOVA) was carried out, and Tukey’s post hoc test was used for multiple comparisons. Differences between groups were considered statistically significant for p-values < 0.05.
Results
Aβ-Oligomers Damage
In this study, we tested the protective effect of 1,25(OH)2D3, meso-zeaxanthin (MZ), and their combination against Aβ (1–42) oligomer-induced cytotoxicity, through measurement of ARPE-19 cell viability, after challenge with Aβ (Figure 1). Preliminary studies were carried out with the MTT assay to evaluate Aβ-oligomer toxicity on ARPE-19 cells, and we found that 1 μM Aβ-oligomers for 48 h induced roughly 17% cell death. Indeed, 1 μM Aβ-oligomers concentration was used also for LDH and ROS assays. In preliminary studies, ARPE-19 cells were pretreated with different concentrations of 1,25(OH)2D3, MZ, and their combination for 24 h. Therefore, cells were incubated with 1 μM Aβ for 48 h, the most effective compound concentrations were 50 nM and 0.1 μM for 1,25(OH)2D3 and MZ, respectively; indeed we used these concentrations also in the combination of the two compounds [combo: 1,25(OH)2D3 50 nM + MZ 0.1 μM]. 1,25(OH)2D3 and the combo pretreament significantly (p < 0.05) counteracted cell toxicity induced by challenge with Aβ (MTT assay, Figure 1A). Moreover, LDH release was significantly increased (p < 0.05) after treatment with Aβ; the tested compounds 1,25(OH)2D3 and MZ, and their combination (combo), induced a significant (p < 0.05) reduction of cell damage after 48 h (Figure 1B). Finally, we analyzed the antioxidant activity of tested compounds. After 48 h of exposure, Aβ-oligomer insult significantly increased (p < 0.05) ROS release in ARPE-19 cells. Only the combination of 1,25(OH)2D3 and MZ was able to significantly reduce the amount of ROS after 48 h (p < 0.05) (Figure 1C), compared to Aβ-positive control cells.
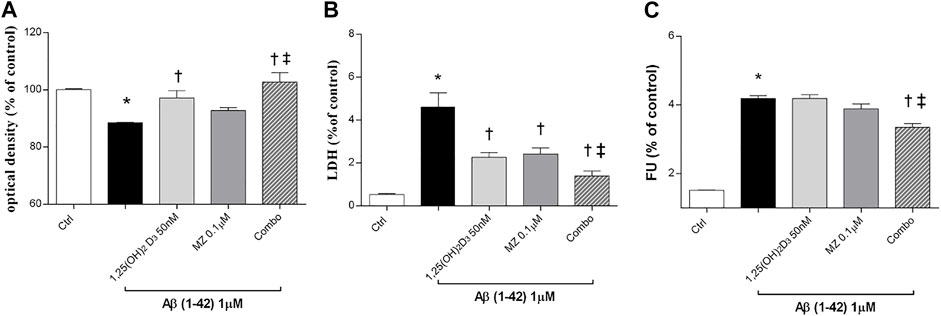
FIGURE 1. 1,25(OH)2D3, meso-zeaxanthin (MZ), and their combination show protective effect in ARPE-19 cells treated with Aβ (1–42). Cells were pretreated for 24 h with tested compounds and for 48 h with Aβ insult. At the end of treatment were carried out MTT (A), LDH (B), and the ROS assay (C). Values are reported as mean ± SD (n = 5). Data were analyzed by one-way ANOVA and Tukey’s post hoc test for multiple comparisons. *p < 0.05 vs. control; †p < 0.05 vs. Aβ, ‡p < 0.05 vs. 50 nM 1,25(OH)2D3 or 0.1 µM MZ.
After 24h, Aβ oligomers exposure (1 µM) significantly (p < 0.05) increased mRNA expression of IL-1β, IL-6, and TNF-α (Figures 2A–C) in ARPE-19 cells. The treatment with 1,25 (OH)2D3, meso-zeaxanthin (MZ), and their combination significantly decreased IL-1β (Figure 2A) and IL-6 (Figure 2B), while only 1,25(OH)2D3 and the combo significantly reduced TNF-α mRNA expression (Figure 2C). Furthermore, Aβ treatment significantly (p < 0.05) increased nuclear translocation of p-NFκB p65 after 48 h of insult (Figure 2D). On the other hand, pretreatment for 24 h with 1,25(OH)2D3, MZ, and combo significantly (p < 0.05) reduced the translocation p-NFκB p65, confirming the anti-inflammatory effect of these two compounds and their combination, in retinal pigmented epithelial cells, challenged with Aβ oligomers (Figures 2D,E).
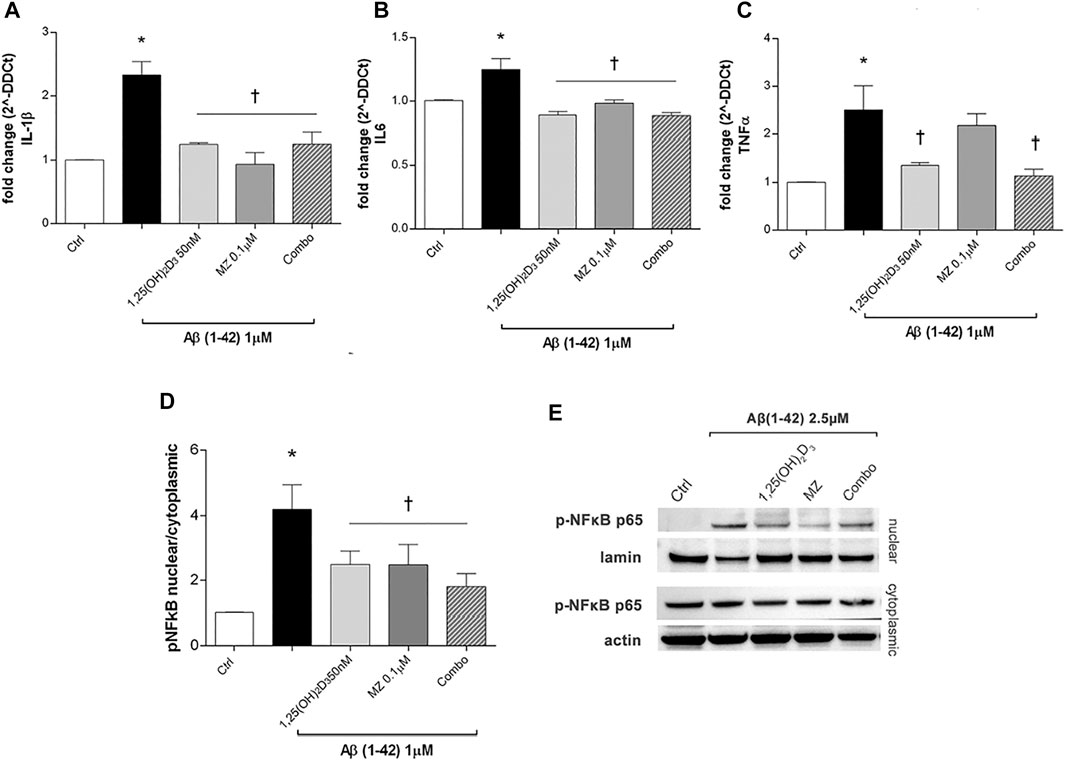
FIGURE 2. Treatment of ARPE-19 cells with 1,25(OH)2D3, meso-zeaxanthin (MZ), and their combination (combo) counteract inflammation after Aβ (1–42) exposure. The treatment with 1,25(OH)2D3, MZ, and their combo reduced IL-1β (A), IL-6 (B), and TNF-α (C) mRNA expression. The mRNA levels were evaluated by qPCR. (D) Western blot analysis. Densitometry analysis of each band (ratio of nuclear p-NFκB p-65/lamin B and cytoplasmic p-NFκB p-65/actin) was carried out with the ImageJ program. (E) Representative blots of nuclear and cytoplasmic extracted proteins from control and treated cells. Each bar represents the mean value ±SD (n = 5; each run in triplicate). One-way ANOVA and Tukey’s post hoc test for multiple comparisons were carried out. *p < 0.05 vs. control; †p < 0.05 vs. Aβ.
Oxidative Stress
Preliminary studies on ARPE-19 cells were carried out to assess the best H2O2 concentration and time of exposure to oxidative stress able to elicit roughly 15% cell death. Therefore, human retinal pigmented epithelial cells were pretreated for 24 h with 1,25(OH)2D3 (50 nM), meso-zeaxanthin (MZ) (0.1 µM), and combo (1,25(OH)2D3 50 nM, MZ 0.1 µM), and then cells were incubated in 400 µM H2O2 for 6 h (Figure 3A) and 24 h (Figure 3B). After 6 h of challenge, 1,25(OH)2D3 was not able to counteract H2O2-induced cell damage, instead after 24 h both compounds and their combination significantly restored cell viability. Moreover, H2O2 significantly (p < 0.05) increased LDH levels in ARPE-19 cells, and the pretreatment with tested compounds induced a significant reduction of cell damage (Figure 3C). Furthermore, we evaluated the effect of the tested compounds and their combination in terms of ROS production on ARPE-19 cells after H2O2 exposure. After 6h, H2O2 significantly increased (p < 0.05) ROS in ARPE-19 cells, compared to control cells (Figure 3D). Pretreatment with 1,25(OH)2D3, MZ, and their combination significantly (p < 0.05) counteracted oxidative stress in retinal cells, reducing ROS release.
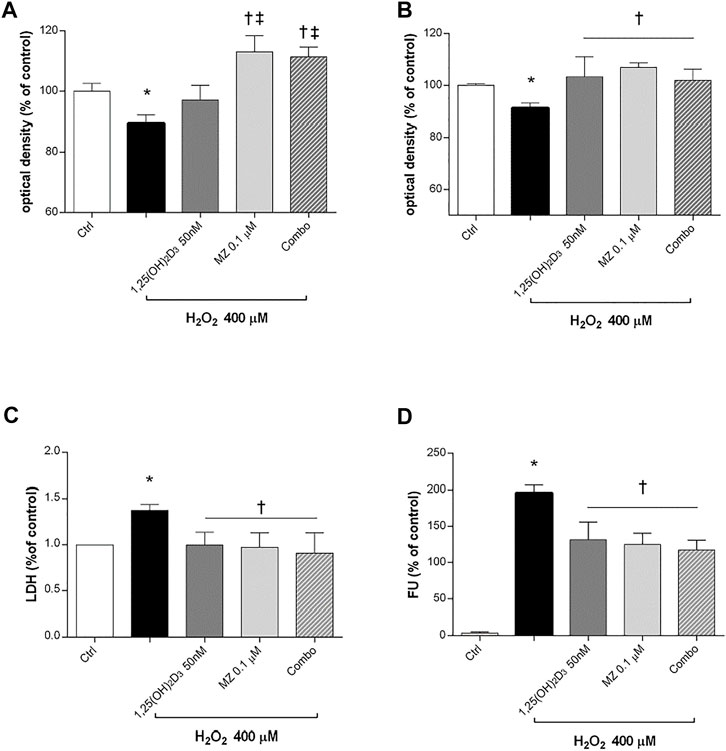
FIGURE 3. 1,25(OH)2D3, meso-zeaxanthin (MZ), and their combination protect ARPE-19 cells from oxidative damage. ARPE-19 cells were pretreated for 24 h with 1,25(OH)2D3 (50 nM), MZ (0.1 µM), and their combo (1,25(OH)2D3 50 nM, MZ 0.1 µM), and then treated with H2O2 (400 µM) for the MTT assay at 6 (A) and 24 h (B). (C) LDH release of ARPE-19 cells treated for 24 h with H2O2 (400 µM). (D) Pretreatment with 1,25(OH)2D3 (50 nM), MZ (0.1 µM), and their combination decreased ROS (fluorescent units, FU) production in ARPE-19 cells, challenged for 6 h with 400 µM H2O2. The results are expressed as mean ± SD (n = 5; each run in triplicate). Data were analyzed by one-way ANOVA and Tukey’s post hoc test for multiple comparisons. *p < 0.05 vs. ctrl; †p < 0.05 vs. H2O2; ‡p < 0.05 vs. 1,25(OH)2D3.
Furthermore, we analyzed IL-1β and TNF-α mRNA levels to assess the effect of 1,25(OH)2D3 and meso-zeaxanthin (MZ) in modulation of inflammatory response, in ARPE-19 cells challenged with H2O2 (400 µM) for 6 h. H2O2 challenge led to significant (p < 0.05) increase in IL-1β and TNF-α mRNA expression (Figures 4A,B). Treatment with 1,25(OH)2D3 (50 nM), MZ (0.1 µM), and their combination reverted the effect of H2O2 (Figures 4A,B). Furthermore, we assessed effects of those compounds in reducing MMP-9 and VEGF-A mRNA levels, both involved in retinal angiogenesis and neovascularization. H2O2 treatment induced a significant (p < 0.05) upregulation of both factors (Figures 4C,D). The MMP-9 mRNA levels were significantly (p < 0.05) reduced by 1,25(OH)2D3, MZ, and their combination, compared to H2O2-treated cells (Figure 4C). Only the combination of 1,25(OH)2D3 and MZ significantly reduced VEGF-A mRNA levels, in comparison to cells exposed to H2O2 (p < 0.05) (Figure 4D). Furthermore, we assessed the effect of tested compounds in terms of p65-NFκB nuclear translocation. H2O2 challenge led to a higher (p < 0.05) p-p65 nuclear translocation after 4 h. This process was significantly (p < 0.05) counteracted by pretreatment with 1,25(OH)2D3 (50 nM), MZ (0.1 µM), and their combination. Particularly, the combo significantly inhibited p65-NFκB translocation, compared to tested compounds and H2O2-exposed cells (Figures 4E,F).
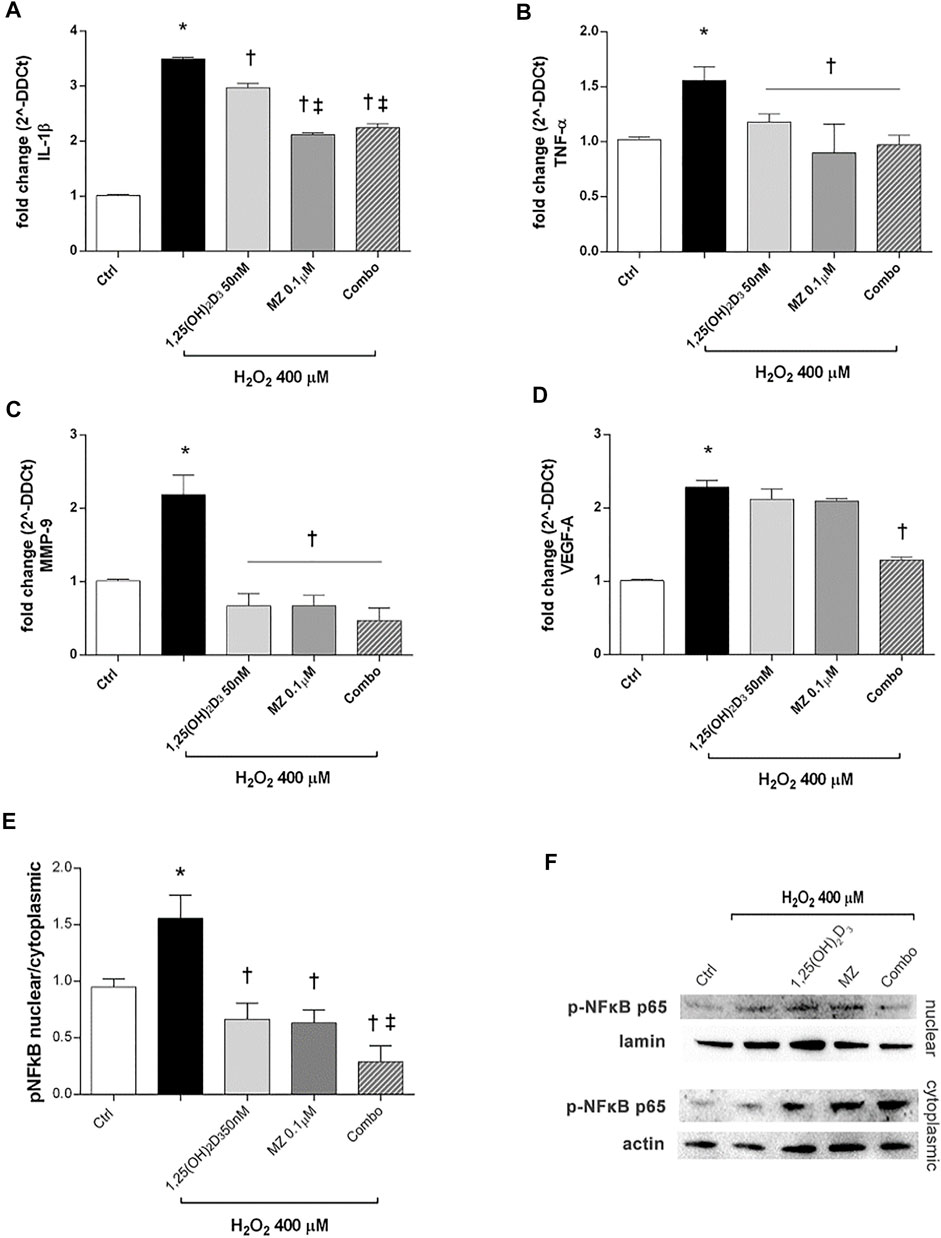
FIGURE 4. 1,25(OH)2D3, MZ, and their combination attenuate H2O2-induced inflammation. 1,25(OH)2D3, MZ, and the combination reduced IL-1β (A), TNF-α (B), and MMP-9 (C) mRNA expression. The combo decreased VEGF-A mRNA expression induced after 6 h of H2O2 treatment (D). ARPE-19 cells were pretreated for 24 h with 1,25(OH)2D3 (50 nM), MZ (0.1 µM), and their combo (1,25(OH)2D3 50 nM + meso-zeaxanthin 0.1 µM), and then challenged with H2O2 (400 µM) for 6 h. The mRNA levels were evaluated by qPCR. (E) Western blot analysis. Densitometry analysis of each band (ratio of nuclear p-NFκB p-65/lamin B and cytoplasmic p-NFκB p-65/actin) was carried out with ImageJ program. (F) Representative blots of nuclear and cytoplasmic proteins. Each bar represents mean value ±SD (n = 5; each run in triplicate). Data were analyzed by one-way ANOVA and Tukey’s post hoc test for multiple comparisons. *p < 0.05 vs. control; †p < 0.05 vs. H2O2; ‡p < 0.05 vs. 1,25(OH)2D3 and MZ.
LPS Insult
ARPE-19 cells were pretreated with 1,25(OH)2D3 (50 nM), meso-zeaxanthin (MZ, 0.1 µM), and their combination (combo: 1,25(OH)2D3 50 nM + MZ 0.1 µM) for 24h, and then exposed to LPS (150 ng/ml) for 2 h. IL-1β, IL-6, and TNF-α mRNA levels were significantly increased in the LPS-stimulated cells, compared to control cells (p < 0.05). Both compounds and their combination (p < 0.05) significantly reduced cytokine mRNA levels (Figures 5A–C). MZ significantly reduced TNF-α mRNA expression, compared to 50 nM 1,25(OH)2D3 and the combo (50 nM 1,25(OH)2D3 + 0.1 µM MZ). Furthermore, LPS treatment significantly induced the upregulation of VEGF-A mRNA (p < 0.05) (Figure 5D), and the treatment with 1,25(OH)2D3, MZ, and their combo significantly reduced the expression of the latter (p < 0.05). After 2 h exposure, LPS (10 μg/ml) led to a significant increase of p-NFκB p65 nuclear translocation, in comparison to control cells (p < 0.05) (Figures 5E,F). The treatment with 1,25(OH)2D3, MZ, and their combo significantly inhibited this translocation, leading to a reduction in p-p65 nuclear protein amount (p < 0.05) (Figures 5E,F).
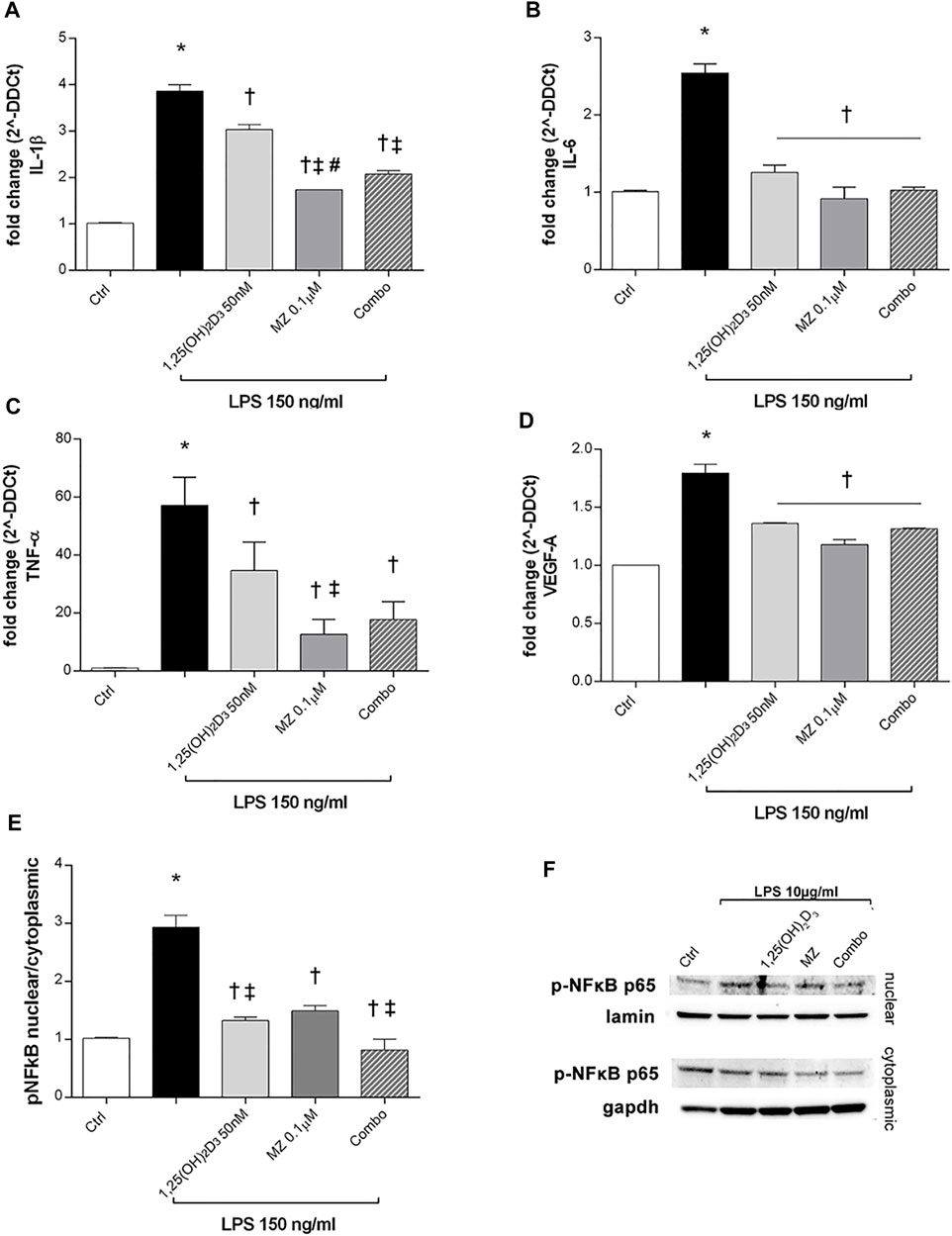
FIGURE 5. 1,25(OH)2D3, meso-zeaxanthin (MZ), and their combination protect ARPE-19 cells from LPS-induced damage. 1,25(OH)2D3, meso-zeaxanthin (MZ), and their combo reduced IL-1β (A), IL-6 (B), TNF-α (C), and VEGF-A (D) mRNA expression. ARPE-19 cells were pretreated for 24 h with 1,25(OH)2D3 (50 nM), MZ (0.1 µM), and their combo (1,25(OH)2D3 50 nM + MZ 0.1 µM), and then challenged with LPS (150 ng/ml) for 2 h. The mRNA levels were evaluated by qPCR. (E) Densitometry of p-NFκB p65 nuclear translocation in treated cells. ARPE-19 cells were pretreated for 24 h with 1,25(OH)2D3 (50 nM), MZ (0.1 µM), and their combo (1,25(OH)2D3 50 nM + MZ 0.1 µM), and then challenged with LPS (10 μg/ml) for 2 h. (F) Representative images of blots of nuclear and cytoplasmic protein. Each bar represents the mean value ±SD (n = 5; each run in triplicate). Data were analyzed by one-way ANOVA and Tukey’s post hoc test for multiple comparisons. *p < 0.05 vs. control; †p < 0.05 vs. LPS; ‡p < 0.05 vs. 1,25(OH)2D3 and MZ; #p < 0.05 vs. combo.
TNF-α Insult
To evaluate ARPE-19 cells response to TNF-α challenge (10 μg/ml), we analyzed TNF-α, IL-6, and IL-1β mRNA levels. After 2 h, those cytokines were significantly increased by TNF-α treatment (10 ng/ml) (p < 0.05) and were strongly downregulated by 1,25(OH)2D3, meso-zeaxanthin (MZ), and the combo pretreatments (p < 0.05) (Figures 6A–C). We confirmed the anti-inflammatory effects of tested compounds against TNF-α exposure also through evaluation of the p-NFκB p65 nuclear translocation (Figures 6D,E). TNF-α challenge significantly increased the nuclear translocation of p-NFκB p65 (p < 0.05). Only the combination of 1,25(OH)2D3 and MZ significantly reduced the amount of nuclear p-NFκB p65 (p < 0.05) (Figures 6D,E).
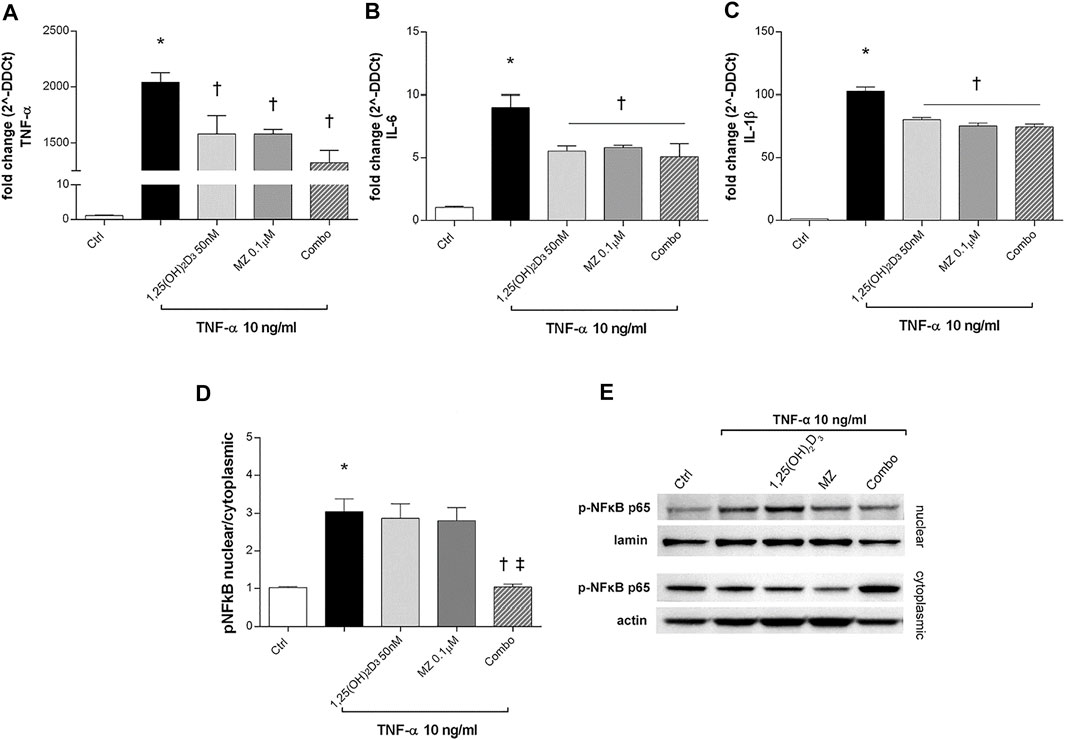
FIGURE 6. 1,25(OH)2D3, meso-zeaxanthin (MZ), and the combination inhibit LPS-induced inflammation. 1,25(OH)2D3, meso-zeaxanthin (MZ), and their combo reduced TNF-α (A), IL-6 (B), and IL-1β (C) mRNA expression. ARPE-19 cells were pretreated for 24 h with 1,25(OH)2D3 (50 nM), MZ (0.1 µM), and their combo (1,25(OH)2D3 50 nM + MZ 0.1 µM), and then challenged with TNF-α (10 ng/ml) for 2h, both for mRNA and protein analyses. The mRNA levels were evaluated by qPCR. (D) Densitometric analysis of each band (ratio of nuclear p-NFκB p65/lamin B and cytoplasmic p-NFκB p65/actin) was carried out with the ImageJ program. (E) Representative images of nuclear and cytoplasmic proteins. Each bar represents the mean value ±SD (n = 5; each run in triplicate). Data were analyzed by one-way ANOVA and Tukey’s post hoc test for multiple comparisons. *p < 0.05 vs. control; †p < 0.05 vs. TNF-α; ‡p < 0.05 vs. 1,25(OH)2D3 and MZ.
Bioinformatic Analysis
We built the protein–compound interaction network that resembled our integrated in vitro model of AMD through the STITCH compound app of Cytoscape v. 3.7.0, according to the approach described in the Methods section. The network was characterized by 136 nodes and 463 edges; a centrality metrics analysis was carried out treating the network as an indirect graph. Nodes with highest betweenness centrality have represented using a color scale (blue < red) (Figure 7), and the following nodes were characterized by the highest betweenness centrality and the average shortest path: APP > TLR4> IL6> TNF-α> PSEN1> H2O2> CAT > IL-1β, VEGF-A. We identified in this network functional clusters associated to the in vitro models used in our study: amyloid β (Supplementary Figure S1), H2O2 (Supplementary Figure S2), and inflammation, that is, LPS (Supplementary Figure S3) and TNF-α challenge (Supplementary Figure S4).
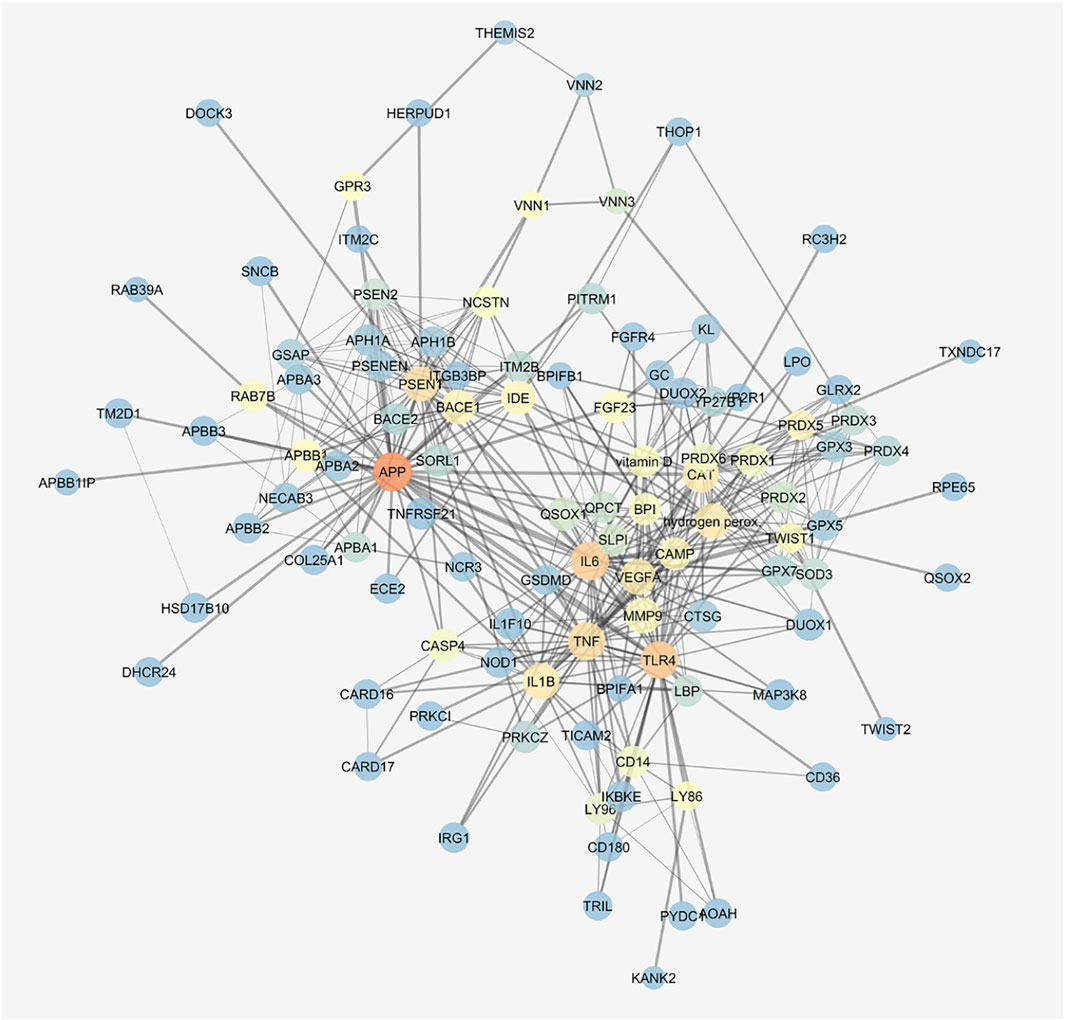
FIGURE 7. Gene activated by amyloid β, H2O2, and LPS are connected. STITCH protein–compound network representing the in vitro models. Nodes are represented on the basis of betweenness centrality values (color scale blue < red) and closeness centrality values (node dimension); edge thickness is proportional to edge betweenness values.
The cluster related to vitamin D3 covered most of the network (Figure 8), but meso-zeaxanthin was linked only to RPE65 and VEGF-A. This last result would be linked to lack of literature data on meso-zeaxanthin, beyond compound antioxidant properties, and the documented RPE65 “lutein to meso-zeaxanthin” isomerase activity (Shyam et al., 2017).
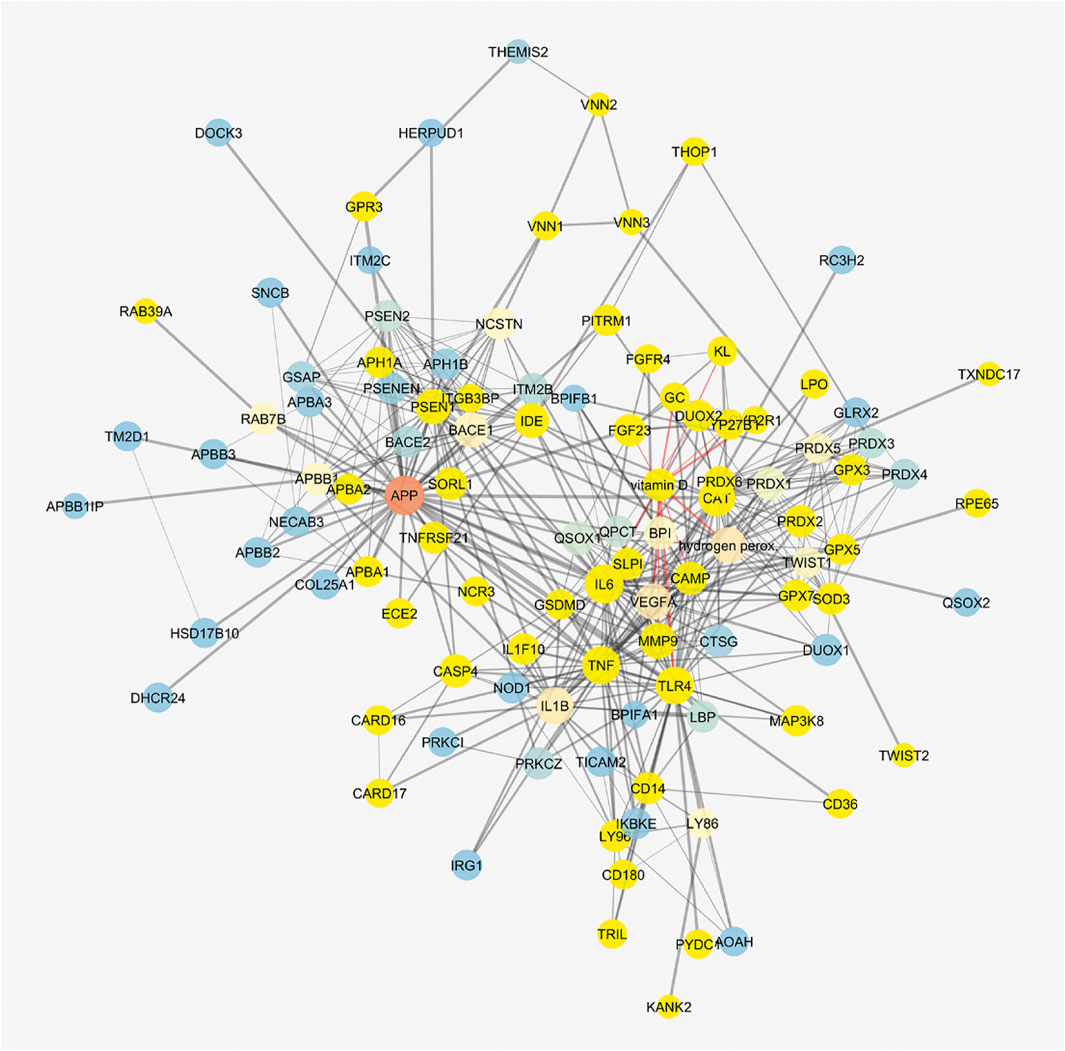
FIGURE 8. Vitamin D3–interacting nodes cover most of the STITCH protein–compound network. Red edges highlight direct interactions with vitamin D3, and yellow nodes represent direct and indirect interactors of vitamin D3.
Discussion
Although several pathogenic mechanisms have been linked to onset and progression of AMD, management, and treatment of AMD is still affected by several unmet medical needs. Specifically, only wet AMD could be therapeutically managed through costly and invasive treatments, such as the anti-VEGF intravitreal injections, which can be ineffective in about 15% of patients (Krebs et al., 2013). Non-responders to intravitreal anti-VEGF treatments can encounter to irreversible vision loss, leading to burden of care linked to direct and indirect costs of blindness. Moreover, no therapy has been already approved for treatment of dry AMD, or for treatment of early phases of the disease.
Multivitamins and mineral supplementation are largely marketed for AMD patients, and clinical trials were carried out regarding specific formulations; the first was the Age-Related Eye Disease Study (2001) formulation, containing vitamins C and E, beta-carotene, and zinc with copper (Age-Related Eye Disease Study Research Group 2001; Kassoff et al., 2001; Chew et al., 2013a, 2014). A second trial “The Age-Related Eye Disease Study 2” (AREDS2) evidenced that substitution of β-carotene with lutein/zeaxanthin was safer for smokers and former smokers. In this AREDS2 study, lutein or zeaxanthin was compared with placebo. The authors found that there was a modest or no effect on AMD progression, but this was not statistically significant since all participants took the AREDS formula, and there was no proper control group (Chew et al., 2013b). On this regard, a systematic review with a meta-analysis evidenced that lutein and zeaxanthin supplements have little or no effect in AMD progression (Evans and Lawrenson, 2017), although this conclusion had a low level of certainty. In the same systematic review, authors evidenced that AMD subjects taking antioxidants multivitamin supplementation, including vitamin D3, were at lower risk of AMD progression, but no evidence on visual acuity was found by meta-analysis. Since, there is no intervention to slow down the progression of the disease, depending on the AMD stage, correct supplementation of antioxidants and vitamins would be of benefit, but up to now, current supplement formulation trials did not provide evidence-based efficacy.
Therefore, in search of an improved formulation of supplements, we hereby explored for the first time, in an integrated in vitro model of AMD, the effects of 1,25(OH)2D3 (vitamin D3) and meso-zeaxanthin combination on several endpoints related to inflammation, oxidative stress, and cellular damage: amyloid β, H2O2, and inflammatory insults, that is, LPS and TNFα. The rationale of these integrated in vitro models of AMD is behind its multifactorial pathogenic etiology (Bucolo et al., 2006; Di Filippo et al., 2014; Fisichella et al., 2016; Platania et al., 2017, 2019; Romano et al., 2017; Giordano et al., 2020; Micera et al., 2021), involving amyloid-β and oxidative stress, has already been mentioned. As regards as, the LPS challenge is widely used as an experimental model of AMD, involving the activation of Toll-like receptor 4 (TLR-4) and the downstream activation of NFκB (Sung et al., 2019; Hikage et al., 2021), and then triggering the expression of inflammatory cytokines. While, the most potent downstream inflammatory cytokine, TNF-α has been found to promote, in ARPE-19 cells, secretion of proteins involved in AMD pathology, such as complement C3 (An et al., 2008). Worthy of note, antioxidant and anti-inflammatory strategies have been largely explored for treatment of ocular diseases (Bucolo et al., 1999; Shafiee et al., 2011).
As shown by our data, vitamin D3 and meso-zeaxanthin combination effectively protected cells from damage induced by β-amyloid, H2O2, LPS, and TNF-α. However, based on analyzed endpoints, we cannot hypothesize an additive or synergistic effect between vitamin D3 and meso-zeaxanthin. Specifically, the combination of vitamin D3 and meso-zeaxanthin was significantly effective, compared to the two single components, in decreasing Il-1β, TNF-α, and VEGF-A (H2O2 insult). Moreover, the combination of 1,25(OH)2D3 + meso-zeaxanthin, compared to the two single components, significantly reduced NFκB nuclear translocation, in ARPE-19 cells challenged with H2O2, LPS, and TNF-α. While in the β-amyloid model, both vitamin D3 and the combination with meso-zeaxanthin inhibited NFκB pathway activation but not the meso-zeaxanthin treatment alone.
Our findings about vitamin D3 activity on ARPE-19 cells challenged with H2O2 and LPS are supported by recent findings on 1,25(OH)2D3 antioxidant and anti-inflammatory activity (Fernandez-Robredo et al., 2020; Hernandez et al., 2021). Preclinical and clinical studies evidenced protective effects of vitamin D3 in Alzheimer disease, an amyloid-β–related pathology (Sultan et al., 2020; McCarty et al., 2021). The link between AMD and AD pathology has been largely documented (Romano et al., 2017), and low-vitamin D3 levels in serum were linked to progression of AMD, however with small effect (i.e., small adjusted odd ratio) (McKay et al., 2017). We proved for the first time that in ARPE-19 cells, vitamin D3, meso-zeaxanthin, and their combination protected cells from damage induced by β-amyloid exposure, oxidative stress, and inflammatory stimuli. Recently, it has been demostrated that lutein and meso-zeaxanthin are taken up by ARPE-19 cells via different mechanisms with preferential uptake of meso-zeaxanthin (Thomas and Harrison, 2016). Additionally, it is known that the enzyme RPE65 converts dietary lutein to meso-zeaxanthin in the retinal pigment epithelium of vertebrates (Shyam et al., 2017). Meso-zeaxanthin is a well-known antioxidant compound that accumulates as other xanthophyll carotenoids in the macula, increasing macular pigments and then protecting pigmented epithelial cells and photoreceptors from photo-oxidative stress (Bone et al., 2007). Up to now, there is an evidence of non-inferiority of meso-zeaxanthin enriched formulation, compared to AREDS2 formulation (Akuffo et al., 2017). On the contrary, non-advanced AMD subjects taking the meso-zeaxanthin–enriched formulation have shown significant higher meso-zeaxanthin and zeaxanthin serum levels and total serum carotenoids, than AREDS2 subjects (Akuffo et al., 2017). Despite large-scale clinical trials that showed the benefits of xanthophyll carotenoids against AMD, recommendations for nutritional interventions are underappreciated by ophthalmologists. Besides the well-known antioxidant activity of meso-zeaxanthin, only few non-ocular studies have reported an anti-inflammatory activity (Firdous et al., 2015; Sahin et al., 2017). Lack of literature findings about meso-zeaxanthin’s anti-inflammatory activity was also emerged in our in silico analysis. Interestingly, meso-zeaxanthin decreased levels of nuclear p-NFκB and TNF-α secretion in the insulin-resistant rodent model (Sahin et al., 2017); this anti-inflammatory activity has been evidenced also in our experimental settings, since the single treatment with meso-zeaxanthin effectively delivered anti-inflammatory effects.
In conclusion, we hereby provided in vitro evidence that vitamin D3 and meso-zeaxanthin association protected retinal pigmented epithelium from several damages that recapitulate the multifactorial pathogenic mechanisms of AMD. With this regard, vitamin D3 and meso-zeaxanthin supplementation would be of value in AMD patients, especially for subject diagnosed with early diagnosis of AMD, as already evidenced by several systematic reviews.
Data Availability Statement
The original contributions presented in the study are included in the article/Supplementary Material; further inquiries can be directed to the corresponding author.
Author Contributions
CB made substantial contributions to conception, design, and interpretation of data. FL, FC, and CP carried out experiments. FL, FC, and CP carried out formal analysis of data. FL, FC, CP, and CB wrote initial draft of the manuscript. CB, CME, and FD reviewed the manuscript critically for important intellectual content and gave final approval of the version to be submitted.
Conflict of Interest
The authors declare that the research was conducted in the absence of any commercial or financial relationships that could be construed as a potential conflict of interest.
Publisher’s Note
All claims expressed in this article are solely those of the authors and do not necessarily represent those of their affiliated organizations, or those of the publisher, the editors, and the reviewers. Any product that may be evaluated in this article, or claim that may be made by its manufacturer, is not guaranteed or endorsed by the publisher.
Supplementary Material
The Supplementary Material for this article can be found online at: https://www.frontiersin.org/articles/10.3389/fphar.2021.778165/full#supplementary-material
References
Age Related Eye Disease Study Research Group) (2001). The Age-Related Eye Disease Study System for Classifying Age-Related Macular Degeneration from Stereoscopic Color Fundus Photographs: The Age-Related Eye Disease Study Report Number. Am. J. Ophthalmol. doi:10.1016/S0002-9394(01)01218-1
Akuffo, K. O., Beatty, S., Peto, T., Stack, J., Stringham, J., Kelly, D., et al. (2017). The Impact of Supplemental Antioxidants on Visual Function in Nonadvanced Age-Related Macular Degeneration: A Head-To-Head Randomized Clinical Trial. Invest. Ophthalmol. Vis. Sci. 58, 5347. doi:10.1167/iovs.16-21192
Albert, D. M., Scheef, E. A., Wang, S., Mehraein, F., Darjatmoko, S. R., Sorenson, C. M., et al. (2007). Calcitriol Is a Potent Inhibitor of Retinal Neovascularization. Invest. Ophthalmol. Vis. Sci. 48, 2327. doi:10.1167/iovs.06-1210
Almeida Moreira Leal, L. K., Lima, L. A., Alexandre de Aquino, P. E., Costa de Sousa, J. A., Jataí Gadelha, C. V., Felício Calou, I. B., et al. (2020). Vitamin D (VD3) Antioxidative and Anti-inflammatory Activities: Peripheral and central Effects. Eur. J. Pharmacol. 879, 173099. doi:10.1016/j.ejphar.2020.173099
An, E., Gordish-Dressman, H., and Hathout, Y. (2008). Effect of TNF-α on Human ARPE-19-Secreted Proteins. Mol. Vis.
Anderson, D. H., Mullins, R. F., and Hageman, L V Johnson, G. S. (2002). A Role for Local Inflammation in the Formation of Drusen in the Aging Eye. Am. J. Ophthalmol. 134, 411–431. doi:10.1016/s0002-9394(02)01624-0
Anderson, O. A., Finkelstein, A., and Shima, D. T. (2013). A2E Induces IL-1ß Production in Retinal Pigment Epithelial Cells via the NLRP3 Inflammasome. PLoS One 8, e67263. doi:10.1371/journal.pone.0067263
Annweiler, C., Drouet, M., Duval, G. T., Paré, P.-Y., Leruez, S., Dinomais, M., et al. (2016). Circulating Vitamin D Concentration and Age-Related Macular Degeneration: Systematic Review and Meta-Analysis. Maturitas 88, 101–112. doi:10.1016/j.maturitas.2016.04.002
Biron, K. E., Dickstein, D. L., Gopaul, R., and Jefferies, W. A. (2011). Amyloid Triggers Extensive Cerebral Angiogenesis Causing Blood Brain Barrier Permeability and Hypervascularity in Alzheimer's Disease. PLoS One 6, e23789. doi:10.1371/journal.pone.0023789
Bone, R. A., Landrum, J. T., Cao, Y., Howard, A. N., and Alvarez-Calderon, F. (2007). Macular Pigment Response to a Supplement Containing Meso-Zeaxanthin, Lutein and Zeaxanthin. Nutr. Metab. (Lond) 4, 12. doi:10.1186/1743-7075-4-12
Bruban, J., Glotin, A.-L., Dinet, V., Chalour, N., Sennlaub, F., Jonet, L., et al. (2009). Amyloid-β (1-42) Alters Structure and Function of Retinal Pigmented Epithelial Cells. Aging Cell 8, 162–177. doi:10.1111/j.1474-9726.2009.00456.x
Bucolo, C., Campana, G., Di Toro, R., Cacciaguerra, S., and Spampinato, S. (1999). σ Recognition Sites in Rabbit Iris-Ciliary Body: Topical σ-1-site Agonists Lower Intraocular Pressure. J. Pharmacol. Exp. Ther.
Bucolo, C., Drago, F., Lin, L.-R., and Reddy, V. N. (2006). Sigma Receptor Ligands Protect Human Retinal Cells against Oxidative Stress. Neuroreport 17, 287–291. doi:10.1097/01.wnr.0000199469.21734.e1
Buschini, E., Zola, M., Fea, A. M., Lavia, C. A., Nassisi, M., Pignata, G., et al. (2015). Recent Developments in the Management of Dry Age-Related Macular Degeneration. Opth, 563. doi:10.2147/OPTH.S59724
Calafiore, M., Copani, A., and Deng, W. (2012). DNA Polymerase-β Mediates the Neurogenic Effect of β-amyloid Protein in Cultured Subventricular Zone Neurospheres. J. Neurosci. Res. 90, 559–567. doi:10.1002/jnr.22780
Caruso, G., Benatti, C., Musso, N., Fresta, C. G., Fidilio, A., Spampinato, G., et al. (2021). Carnosine Protects Macrophages against the Toxicity of Aβ1-42 Oligomers by Decreasing Oxidative Stress. Biomedicines 9, 477. doi:10.3390/biomedicines9050477
Cascella, R., Ragazzo, M., Strafella, C., Missiroli, F., Borgiani, P., Angelucci, F., et al. (2014). Age-Related Macular Degeneration: Insights into Inflammatory Genes. J. Ophthalmol. 2014, 1–9. doi:10.1155/2014/582842
Chew, E. Y., Clemons, T. E., Agrón, E., Sperduto, R. D., SanGiovanni, J. P., Davis, M. D., et al. (2014). Ten-Year Follow-Up of Age-Related Macular Degeneration in the Age-Related Eye Disease Study. JAMA Ophthalmol. 132, 272. doi:10.1001/jamaophthalmol.2013.6636
Chew, E. Y., Clemons, T. E., Agrón, E., Sperduto, R. D., Sangiovanni, J. P., Kurinij, N., et al. (2013a). Long-Term Effects of Vitamins C and E, β-Carotene, and Zinc on Age-Related Macular Degeneration. Ophthalmology 120, 1604–1611. doi:10.1016/j.ophtha.2013.01.021
Chew, E. Y., Clemons, T. E., SanGiovanni, J. P., Danis, R., Ferris, F. L., Elman, M., et al. (2013b). Lutein + Zeaxanthin and Omega-3 Fatty Acids for Age-Related Macular Degeneration. Jama 309, 2005. doi:10.1001/jama.2013.4997
Di Filippo, C., Zippo, M. V., Maisto, R., Trotta, M. C., Siniscalco, D., Ferraro, B., et al. (2014). Inhibition of Ocular Aldose Reductase by a New Benzofuroxane Derivative Ameliorates Rat Endotoxic Uveitis. Mediators Inflamm. 2014, 1–9. doi:10.1155/2014/857958
Eandi, C. M., Charles Messance, H., Augustin, S., Dominguez, E., Lavalette, S., Forster, V., et al. (2016). Subretinal Mononuclear Phagocytes Induce Cone Segment Loss via IL-1β. Elife 5. doi:10.7554/eLife.16490
Eliassen, A. H., Liao, X., Rosner, B., Tamimi, R. M., Tworoger, S. S., and Hankinson, S. E. (2015). Plasma Carotenoids and Risk of Breast Cancer over 20 Y of Follow-Up. Am. J. Clin. Nutr. 101, 1197–1205. doi:10.3945/ajcn.114.105080
Evans, J. R., and Lawrenson, J. G. (2017). Antioxidant Vitamin and mineral Supplements for Slowing the Progression of Age-Related Macular Degeneration. Cochrane Database Syst. Rev. 2017. doi:10.1002/14651858.CD000254.pub4
Fernandez-Robredo, P., González-Zamora, J., Recalde, S., Bilbao-Malavé, V., Bezunartea, J., Hernandez, M., et al. (2020). Vitamin D Protects against Oxidative Stress and Inflammation in Human Retinal Cells. Antioxidants 9, 838. doi:10.3390/antiox9090838
Firdous, A. P., Kuttan, G., and Kuttan, R. (2015). Anti-inflammatory Potential of Carotenoidmeso-Zeaxanthin and its Mode of Action. Pharm. Biol. 53, 961–967. doi:10.3109/13880209.2014.950673
Fisichella, V., Giurdanella, G., Platania, C. B. M., Romano, G. L., Leggio, G. M., Salomone, S., et al. (2016). TGF-β1 Prevents Rat Retinal Insult Induced by Amyloid-β (1-42) Oligomers. Eur. J. Pharmacol. 787, 72–77. doi:10.1016/j.ejphar.2016.02.002
Giordano, M., Trotta, M. C., Ciarambino, T., D’Amico, M., Galdiero, M., Schettini, F., et al. (2020). Circulating MiRNA-195-5p and -451a in Diabetic Patients with Transient and Acute Ischemic Stroke in the Emergency Department. Ijms 21, 7615. doi:10.3390/ijms21207615
Giurdanella, G., Anfuso, C. D., Olivieri, M., Lupo, G., Caporarello, N., Eandi, C. M., et al. (2015). Aflibercept, Bevacizumab and Ranibizumab Prevent Glucose-Induced Damage in Human Retinal Pericytes In Vitro, through a PLA2/COX-2/VEGF-A Pathway. Biochem. Pharmacol. 96, 278–287. doi:10.1016/j.bcp.2015.05.017
Green-Gomez, M., Prado-Cabrero, A., Moran, R., Power, T., Gómez-Mascaraque, L. G., Stack, J., et al. (2020). The Impact of Formulation on Lutein, Zeaxanthin, and Meso-Zeaxanthin Bioavailability: A Randomised Double-Blind Placebo-Controlled Study. Antioxidants 9, 767. doi:10.3390/antiox9080767
Guillonneau, X., Eandi, C. M., Paques, M., Sahel, J.-A., Sapieha, P., and Sennlaub, F. (2017). On Phagocytes and Macular Degeneration. Prog. Retin. Eye Res. 61, 98–128. doi:10.1016/j.preteyeres.2017.06.002
Hageman, G. S., Luthert, P. J., Victor Chong, N. H., Johnson, L. V., Anderson, D. H., and Mullins, R. F. (2001). An Integrated Hypothesis that Considers Drusen as Biomarkers of Immune-Mediated Processes at the RPE-Bruch’s Membrane Interface in Aging and Age-Related Macular Degeneration. Prog. Retin. Eye Res. doi:10.1016/S1350-9462(01)00010-6
Hernandez, M., Recalde, S., González-Zamora, J., Bilbao-Malavé, V., Sáenz de Viteri, M., Bezunartea, J., et al. (2021). Anti-inflammatory and Anti-oxidative Synergistic Effect of Vitamin D and Nutritional Complex on Retinal Pigment Epithelial and Endothelial Cell Lines against Age-Related Macular Degeneration. Nutrients 13, 1423. doi:10.3390/nu13051423
Hikage, F., Lennikov, A., Mukwaya, A., Lachota, M., Ida, Y., Utheim, T. P., et al. (2021). NF-κB Activation in Retinal Microglia Is Involved in the Inflammatory and Neovascularization Signaling in Laser-Induced Choroidal Neovascularization in Mice. Exp. Cel. Res. 403, 112581. doi:10.1016/j.yexcr.2021.112581
Holekamp, N. M. (2019). Review of Neovascular Age-Related Macular Degeneration Treatment Options. Am. J. Manag. Care.
Hozawa, A., Jacobs, D. R., Steffes, M. W., Gross, M. D., Steffen, L. M., and Lee, D.-H. (2006). Associations of Serum Carotenoid Concentrations with the Development of Diabetes and with Insulin Concentration: Interaction with Smoking. Am. J. Epidemiol. 163, 929–937. doi:10.1093/aje/kwj136
Johnson, L. V., Forest, D. L., Banna, C. D., Radeke, C. M., Maloney, M. A., Hu, J., et al. (2011). Cell Culture Model that Mimics Drusen Formation and Triggers Complement Activation Associated with Age-Related Macular Degeneration. Proc. Natl. Acad. Sci. 108, 18277–18282. doi:10.1073/pnas.1109703108
Kan, E., Kan, E. K., and Yücel, Ö. E. (2020). The Possible Link between Vitamin D Levels and Exudative Age-Related Macular Degeneration. Oman Med. J. 35, e83. doi:10.5001/omj.2020.01
Kassoff, A., Kassoff, J., Buehler, J., Eglow, M., Kaufman, F., Mehu, M., et al. (2001). A Randomized, Placebo-Controlled, Clinical Trial of High-Dose Supplementation with Vitamins C and E, Beta Carotene, and Zinc for Age-Related Macular Degeneration and Vision Loss. Arch. Ophthalmol. 119, 1417. doi:10.1001/archopht.119.10.1417
Kauppinen, A., Paterno, J. J., Blasiak, J., Salminen, A., and Kaarniranta, K. (2016). Inflammation and its Role in Age-Related Macular Degeneration. Cell Mol. Life Sci. 73, 1765–1786. doi:10.1007/s00018-016-2147-8
Kohen, R., and Nyska, A. (2002). Invited Review: Oxidation of Biological Systems: Oxidative Stress Phenomena, Antioxidants, Redox Reactions, and Methods for Their Quantification. Toxicol. Pathol. 30, 620–650. doi:10.1080/01926230290166724
Krebs, I., Glittenberg, C., Ansari-Shahrezaei, S., Hagen, S., Steiner, I., and Binder, S. (2013). Non-responders to Treatment with Antagonists of Vascular Endothelial Growth Factor in Age-Related Macular Degeneration. Br. J. Ophthalmol. 97, 1443–1446. doi:10.1136/bjophthalmol-2013-303513
Krohne, T. U., Holz, F. G., and Kopitz, J. (2010). Apical-to-basolateral Transcytosis of Photoreceptor Outer Segments Induced by Lipid Peroxidation Products in Human Retinal Pigment Epithelial Cells. Invest. Ophthalmol. Vis. Sci. 51, 553. doi:10.1167/iovs.09-3755
Layana, A., Minnella, A., Garhöfer, G., Aslam, T., Holz, F., Leys, A., et al. (2017). Vitamin D and Age-Related Macular Degeneration. Nutrients 9, 1120. doi:10.3390/nu9101120
Lee, V., Rekhi, E., Hoh Kam, J., and Jeffery, G. (2012). Vitamin D Rejuvenates Aging Eyes by Reducing Inflammation, Clearing Amyloid Beta and Improving Visual Function. Neurobiol. Aging 33, 2382–2389. doi:10.1016/j.neurobiolaging.2011.12.002
Levy, O., Lavalette, S., Hu, S. J., Housset, M., Raoul, W., Eandi, C., et al. (2015). APOE Isoforms Control Pathogenic Subretinal Inflammation in Age-Related Macular Degeneration. J. Neurosci. 35, 13568–13576. doi:10.1523/JNEUROSCI.2468-15.2015
Liu, R. T., Gao, J., Cao, S., Sandhu, N., Cui, J. Z., Chou, C. L., et al. (2013). Inflammatory Mediators Induced by Amyloid-Beta in the Retina and RPE In Vivo: Implications for Inflammasome Activation in Age-Related Macular Degeneration. Invest. Ophthalmol. Vis. Sci. 54, 2225. doi:10.1167/iovs.12-10849
Maj, E., Filip-Psurska, B., Milczarek, M., Psurski, M., Kutner, A., and Wietrzyk, J. (2018). Vitamin3D Derivatives Potentiate the Anticancer and Anti-angiogenic Activity of Tyrosine Kinase Inhibitors in Combination with Cytostatic Drugs in an A549 Non-small Cell Lung Cancer Model. Int. J. Oncol. doi:10.3892/ijo.2017.4228
Majewski, S., Skopinska, M., Marczak, M., Szmurlo, A., Bollag, W., and Jablonska, S. (1996). Vitamin D3 Is a Potent Inhibitor of Tumor Cell-Induced Angiogenesis. J. Investig. Dermatol. Symp. Proc.
McCarty, M. F., Dinicolantonio, J. J., and Lerner, A. (2021). A Fundamental Role for Oxidants and Intracellular Calcium Signals in Alzheimer's Pathogenesis-And How a Comprehensive Antioxidant Strategy May Aid Prevention of This Disorder. Ijms 22, 2140. doi:10.3390/ijms22042140
McKay, G. J., Young, I. S., McGinty, A., Bentham, G. C. G., Chakravarthy, U., Rahu, M., et al. (2017). Associations between Serum Vitamin D and Genetic Variants in Vitamin D Pathways and Age-Related Macular Degeneration in the European Eye Study. Ophthalmology 124, 90–96. doi:10.1016/j.ophtha.2016.09.007
Merle, B. M. J., Silver, R. E., Rosner, B., and Seddon, J. M. (2017). Associations between Vitamin D Intake and Progression to Incident Advanced Age-Related Macular Degeneration. Invest. Ophthalmol. Vis. Sci. 58, 4569. doi:10.1167/iovs.17-21673
Micera, A., Balzamino, B. O., Di Zazzo, A., Dinice, L., Bonini, S., and Coassin, M. (2021). Biomarkers of Neurodegeneration and Precision Therapy in Retinal Disease. Front. Pharmacol. 11. doi:10.3389/fphar.2020.601647
Millen, A. E., Voland, R., Sondel, S. A., Parekh, N., Horst, R. L., Wallace, R. B., et al. (2011). Vitamin D Status and Early Age-Related Macular Degeneration in Postmenopausal Women. Arch. Ophthalmol. 129, 481. doi:10.1001/archophthalmol.2011.48
Nolan, J. M., Meagher, K., Kashani, S., and Beatty, S. (2013). What Is Meso-Zeaxanthin, and where Does it Come from? Eye 27, 899–905. doi:10.1038/eye.2013.98
Nowak, J. Z. (2006). Age-related Macular Degeneration (AMD): Pathogenesis and Therapy. Pharmacol. Rep.
Parekh, N., Chappell, R. J., Millen, A. E., Albert, D. M., and Mares, J. A. (2007). Association between Vitamin D and Age-Related Macular Degeneration in the Third National Health and Nutrition Examination Survey, 1988 through 1994. Arch. Ophthalmol. 125, 661. doi:10.1001/archopht.125.5.661
Parks, W. C., Wilson, C. L., and López-Boado, Y. S. (2004). Matrix Metalloproteinases as Modulators of Inflammation and Innate Immunity. Nat. Rev. Immunol. 4, 617–629. doi:10.1038/nri1418
Pennington, K. L., and DeAngelis, M. M. (2016). Epidemiology of Age-Related Macular Degeneration (AMD): Associations with Cardiovascular Disease Phenotypes and Lipid Factors. Eye Vis. 3. doi:10.1186/s40662-016-0063-5
Platania, C. B. M., Di Paola, L., Leggio, G. M., Leggio, G. L., Drago, F., Salomone, S., et al. (2015). Molecular Features of Interaction between VEGFA and Anti-angiogenic Drugs Used in Retinal Diseases: A Computational Approach. Front. Pharmacol. 6. doi:10.3389/fphar.2015.00248
Platania, C. B. M., Leggio, G. M., Drago, F., Salomone, S., and Bucolo, C. (2018). Computational Systems Biology Approach to Identify Novel Pharmacological Targets for Diabetic Retinopathy. Biochem. Pharmacol. 158, 13–26. doi:10.1016/j.bcp.2018.09.016
Platania, C. B. M., Maisto, R., Trotta, M. C., D'Amico, M., Rossi, S., Gesualdo, C., et al. (2019). Retinal and Circulating miRNAexpression Patterns in Diabetic Retinopathy: An In Silico and In Vivo Approach. Br. J. Pharmacol. doi:10.1111/bph.14665
Platania, C., Fisichella, V., Fidilio, A., Geraci, F., Lazzara, F., Leggio, G., et al. (2017). Topical Ocular Delivery of TGF-Β1 to the Back of the Eye: Implications in Age-Related Neurodegenerative Diseases. Ijms 18, 2076. doi:10.3390/ijms18102076
Romano, G. L., Platania, C. B. M., Drago, F., Salomone, S., Ragusa, M., Barbagallo, C., et al. (2017). Retinal and Circulating miRNAs in Age-Related Macular Degeneration: An In Vivo Animal and Human Study. Front. Pharmacol. 8. doi:10.3389/fphar.2017.00168
Sahin, K., Orhan, C., Akdemir, F., Tuzcu, M., Sahin, N., Yilmaz, I., et al. (2017). Mesozeaxanthin Protects the Liver and Reduces Cardio-Metabolic Risk Factors in an Insulin Resistant Rodent Model. Food Nutr. Res. 61, 1353360. doi:10.1080/16546628.2017.1353360
Sesso, H. D., Buring, J. E., Norkus, E. P., and Gaziano, J. M. (2004). Plasma Lycopene, Other Carotenoids, and Retinol and the Risk of Cardiovascular Disease in Women. Am. J. Clin. Nutr. 79, 47–53. doi:10.1093/ajcn/79.1.47
Shafiee, A., Bucolo, C., Budzynski, E., Ward, K. W., and López, F. J. (2011). In Vivo ocular Efficacy Profile of Mapracorat, a Novel Selective Glucocorticoid Receptor Agonist, in Rabbit Models of Ocular Disease. Invest. Ophthalmol. Vis. Sci. 52, 1422. doi:10.1167/iovs.10-5598
Shyam, R., Gorusupudi, A., Nelson, K., Horvath, M. P., and Bernstein, P. S. (2017). RPE65 Has an Additional Function as the Lutein Tomeso-Zeaxanthin Isomerase in the Vertebrate Eye. Proc. Natl. Acad. Sci. USA 114, 10882–10887. doi:10.1073/pnas.1706332114
Sultan, S., Taimuri, U., Basnan, S. A., Ai-Orabi, W. K., Awadallah, A., Almowald, F., et al. (2020). Low Vitamin D and its Association with Cognitive Impairment and Dementia. J. Aging Res. 2020, 1–10. doi:10.1155/2020/6097820
Sung, I. S., Park, S. Y., Jeong, K.-Y., and Kim, H. M. (2019). Investigation of the Preventive Effect of Calcium on Inflammation-Mediated Choroidal Neovascularization. Life Sci. 233, 116727. doi:10.1016/j.lfs.2019.116727
Thomas, S. E., and Harrison, E. H. (2016). Mechanisms of Selective Delivery of Xanthophylls to Retinal Pigment Epithelial Cells by Human Lipoproteins. J. Lipid Res. 57, 1865–1878. doi:10.1194/jlr.M070193
Thurnham, D. I., Trémel, A., and Howard, A. N. (2008). A Supplementation Study in Human Subjects with a Combination Ofmeso-Zeaxanthin, (3R,3′R)-zeaxanthin and (3R,3′R,6′R)-lutein. Br. J. Nutr. 100, 1307–1314. doi:10.1017/S0007114508971336
Wang, A. L., Lukas, T. J., Yuan, M., Du, N., Tso, M. O., and Neufeld, A. H. (2009). Autophagy and Exosomes in the Aged Retinal Pigment Epithelium: Possible Relevance to Drusen Formation and Age-Related Macular Degeneration. PLoS One 4, e4160. doi:10.1371/journal.pone.0004160
Keywords: 1,25(OH)2D3, meso-zeaxanthin, amyloid beta, inflammation, oxidative stress, cytokines
Citation: Lazzara F, Conti F, Platania CBM, Eandi CM, Drago F and Bucolo C (2021) Effects of Vitamin D3 and Meso-Zeaxanthin on Human Retinal Pigmented Epithelial Cells in Three Integrated in vitro Paradigms of Age-Related Macular Degeneration. Front. Pharmacol. 12:778165. doi: 10.3389/fphar.2021.778165
Received: 16 September 2021; Accepted: 30 September 2021;
Published: 05 November 2021.
Edited by:
Cesare Mancuso, Catholic University of the Sacred Heart, ItalyReviewed by:
Enza Palazzo, Università della Campania Luigi Vanvitelli, ItalyMoreno Paolini, University of Bologna, Italy
Copyright © 2021 Lazzara, Conti, Platania, Eandi, Drago and Bucolo. This is an open-access article distributed under the terms of the Creative Commons Attribution License (CC BY). The use, distribution or reproduction in other forums is permitted, provided the original author(s) and the copyright owner(s) are credited and that the original publication in this journal is cited, in accordance with accepted academic practice. No use, distribution or reproduction is permitted which does not comply with these terms.
*Correspondence: Claudio Bucolo, YnVjb2NsYUB1bmljdC5pdA==