- 1Heilongjiang Key Laboratory for Laboratory Animals and Comparative Medicine, College of Veterinary Medicine, Northeast Agriculture University, Harbin, China
- 2College of Veterinary Medicine, Northeast Agricultural University, Harbin, China
With the gradual deepening of understanding of systemic health and quality of life, the factors affecting osteoarthritis (OA) are not limited to mechanical injury, metabolic abnormality, age and obesity, etc., but circadian rhythm, which plays a non-negligible role in human daily life. The purpose of this study was to explore the molecular mechanism of chronic circadian rhythm disturbance (CRD) inducing cartilage OA-like degeneration. Rats with the anterior cruciate ligament excision transection (ACLT) were used to establish the early-stage OA model (6-week). The light/dark (LD) cycle shifted 12 h per week for 22 weeks in order to establish a chronic CRD model. BMAL1 knockdown (KD) and Wnt/β-catenin pathway inhibition were performed in chondrocytes. The contents of proinflammatory factors and OA biomarkers in serum and chondrocyte secretions were detected by ELISA. Pathological and immunohistochemical staining of articular cartilage indicated the deterioration of cartilage. WB and qPCR were used to evaluate the relationship between matrix degradation and the activation of Wnt/β-catenin signaling pathway in chondrocytes. We found that chronic CRD could cause OA-like pathological changes in knee cartilage of rats, accelerating cartilage matrix degradation and synovial inflammation. The expression of MMP-3, MMP-13, ADAMTS-4, and β-catenin increased significantly; BMAL1, Aggrecan, and COL2A1 decreased significantly in either LD-shifted cartilage or BMAL1-KD chondrocytes. The expression of β-catenin and p-GSK-3β elevated, while p-β-catenin and GSK-3β diminished. The inhibitor XAV-939 was able to mitigated the increased inflammation produced by transfected siBMAL1. Our study demonstrates that chronic CRD disrupts the balance of matrix synthesis and catabolic metabolism in cartilage and chondrocytes, and it is related to the activation of the canonical Wnt/β-catenin signaling pathway.
Introduction
Environmental factors can significantly affect body metabolism, especially light, temperature and humidity. Light/dark (LD) cycle is the most important environmental zeitgeber that affects circadian homeostasis. Irregular LD cycles can disrupt the body’s circadian rhythm, even metabolic disorders, especially in shift workers (Wright et al., 2013). Previous studies have suggested that shift work increased the risk of chronic metabolic-related diseases, such as cardiovascular disease and type 2 diabetes (Hublin et al., 2010; Shan et al., 2018), and one common risk factor shared by these pathologies is inflammation (Castanon-Cervantes et al., 2010). Chronic circadian rhythm disturbance (CRD) could significantly affect the inflammatory response, manifested as the abnormal diurnal variation of proinflammatory factors, such as TNF-α and IL-6 (Vgontzas et al., 2004). Zhou et al. has proposed that shift work was an independent risk factor for knee osteoarthritis (OA) (Zhou et al., 2020). A recent study found that 24 h continuous light induced an inflammatory microenvironment in osteoarthritic joints, and even developed osteoporosis (Hong et al., 2019). Moreover, Kc et al. found OA-like pathological changes in the mouse knee joint by conducting long-term (22 weeks) disruption of the circadian environment (Kc et al., 2015; Kc et al., 2015). These suggest that the occurrence and development of OA are closely related to the biological clock system.
Clock genes are involved in the metabolic pathways of bone and cartilage, and many studies have confirmed the rhythmic changes in cartilage metabolism (Kaoru et al., 2013; Andersson et al., 2006; Kong et al., 2006). As a core clock protein, the expression of brain and muscle ARNT-like 1/Arntl (BMAL1) in OA joints is low, and is inversely proportional to the severity and progression of OA (Dudek et al., 2016; Akagi et al., 2017). Samsa et al. (2016) demonstrated progressive joint injury and systemic low bone mass in BMAL1−/− mouse joints. Additionally, BMAL1 can regulate the activity of chondrocytes through HIF1α-VEGF and TGF-β signaling pathways (Akagi et al., 2017; Ma et al., 2019). The canonical Wnt/β-catenin signaling pathway, as one of the most important pathways related to cell proliferation and development, is also critical to the occurrence and development of OA. The classical Wnt/β-catenin pathway was activated in the chondrocytes of OA patients, resulting in increased expression of matrix metalloproteinases (MMPs) and A disintegrin and metalloproteinase with thrombospondin motifs (ADAMTS), inducing degradation of extracellular matrix (ECM), and exacerbating cartilage damage (Ning et al., 2012). OA-like changes were also found in transgenic mice with high β-catenin specific expression, which directly demonstrated the role of Wnt/β-catenin pathway in OA (Zhu et al., 2009).
Numerous studies have shown that clock genes are involved in activation and inhibition of the Wnt/β-catenin pathway (Guo et al., 2012; He et al., 2013; Lin et al., 2013; Li et al., 2017; Li et al., 2021). Knockdown Period 3 (PER3) accumulated BMAL1 and p-β-catenin protein, and promoted tumor formation. After overexpressing BMAL1 in NIH-3T3 cells, Lin et al. found a significant increase in the expression of β-catenin, suggesting that the activation of the Wnt pathway may be the mechanism by which the clock gene BMAL1 promotes cell proliferation. Additionally, BMAL1 gene plays a synergistic role with the Wnt/β-catenin signaling pathway in adipogenic and osteogenic differentiation of mesenchymal stem cells (MSCs). However, these illustrate an interesting phenomenon that these theories are contrary to the previously proposed expression of BMAL1 and the Wnt pathway in OA cartilage. Therefore, can BMAL1 still cooperates with Wnt/β-catenin pathway in OA? Or whether it’s relevant? In this study, we established chronic CRD models in rats and chondrocytes, in order to study the effects of CRD on rat articular cartilage and the relation between clock proteins and the Wnt/β-catenin pathway.
Materials and Methods
Antibodies
Antibodies used for western blotting (WB) and immunostaining included mouse anti- COL2A1 (Novus; United States), rabbit anti-β-catenin (ABclonal; China), rabbit anti-BMAL1 (Absin; China), rabbit anti-MMP-3 (CST; United States), rabbit anti-MMP-13 (ABclonal; China), rabbit anti-ADAMTS-4 (ABclonal; United States), rabbit anti-Cyclooxygenase-2 (COX-2) (CST; United States), rabbit anti-β-catenin (ABclonal; China), rabbit anti-GSK-3β (ABclonal; China), mouse anti-p-β-catenin (SANTA; United States), mouse anti-p-GSK-3β (SANTA; United States), mouse anti-GAPDH (Zsbio; China), Alexa Fluor® 488-conjugated goat anti-mouse immunoglobulin G (IgG) (H + L) (Servicebio; China), Cy3 conjugated goat anti-rabbit IgG (H + L) (Servicebio; China), Horseradish Peroxidase (HRP) goat anti-mouse IgG (H + L) (Zsbio; China), and HRP goat anti-rabbit IgG (H + L) (Zsbio; China).
Cell Culture and Small Interfering RNAs Transfection
Primary chondrocytes were isolated from tibial platform of rats under sterile conditions, released by treating with 0.25% Trypsin-EDTA and 0.2% Type II collagenase (Gibco Company, United States) at 37°C (5% CO2) in Dulbecco’s Modified Eagle’s Medium (DMEM)/Hams F-12, supplemented with 10% fetal bovine serum (FBS) (BI, Israel) and 1% Penicillin/Streptomycin. After passage, a second generation of chondrocytes was used for the experiment. For cell transfection, chondrocytes in logarithmic growth phase were taken and transfected when the density reached 60–80%. GP-Transfect-Mate medium mixture (serum-free medium + GP-Transfect-Mate) and siRNA medium mixture (serum-free medium + siBMAL1 (20 μM)) (Genepharma, China) were prepared and transfected. Sequences for negative control and siBMAL1s are listed in Table 1. DMEM/F-12 containing 10% FBS was replaced after 4 h, and the result was tested by WB or qPCR at 72 h after transfection.
Immunofluorescence Assay
The secondary generation chondrocytes were diluted to 2×104 cells/ml, and cultured in 6-well plates. Cells were fixed with 4% paraformaldehyde, permeabilized, and then blocked with 3% bovine serum albumin (BSA) at room temperature for 30 min. After removing the blocking solution, chondrocytes were incubated with primary antibody overnight at 4°C. COL2A1 (1:100) and DAPI for chondrocyte identification, β-catenin (1:100) for nuclear translocation. The cell climbing slides were washed and covered with fluorescently labeled secondary antibody for 50 min at room temperature. DAPI was incubated for 10 min at dark. Fluorescence was observed under a fluorescence microscope (Nikon Eclipse Ti-S; Japan) at 545 nm (red) and 340 nm (blue).
Real-Time PCR
Ribonucleic acid (RNA) in the chondrocytes was extracted at 4°C using SV total RNA isolation system (Promega, United States), the concentration was measured at 260 and 280 nm, and then reverse transcribed blending with GoScript™ Reverse Transcription Mix, Oligo (dT) (Promega, United States) into cDNA (1 μg/ml). The mixture of 10 μl GoTaq®qPCR Master Mix, 0.6 μl forward and reverse primers, 2.5 μl cDNA and 6.3 μl RNase-free ddH2O (Promega, United States) were subjected to 40 amplification cycles. Primer sequences are listed in Table 2. The intensity of fluorescence signal accumulation during the entire PCR process was monitored using LightCycler®480 (Roche, Germany) to obtain the cycle threshold (Ct).
Sodium Dodecyl Sulfate -Polyacrylamide Gel Electrophoresis and WB
Total protein samples of chondrocytes were extracted following the instructions (Beyotime; China). The mixture of radio immunoprecipitation assay (RIPA) and Phenylmethylsulfonyl fluoride (PMSF) fully lysed the cells. Then equal 20 μg amounts of protein were separated onto 10% SDS-PAGE and transferred to nitrocellulose (NC) filter membrane. The membranes were blocked in 5% nonfat milk dissolved in Tris Buffered Saline with Tween®20 (TBST) buffer and probed with primary antibodies for BMAL1 (1:1,500), MMP-3 (1:1,000), MMP-13 (1:1,000), ADAMTS-4 (1:1,500), COX-2 (1:1,000), β-catenin (1:2,000), GSK-3β (1:1,500), p-β-catenin (1:1,000), p-GSK-3β (1:1,000), and GAPDH (1:3,000) at 4°C overnight followed by secondary antibody coupled with horseradish peroxidase at room temperature for 2 h. The blots were developed using the ECL system (Tanon-5200; China) and the gray value was quantified with Image J software (1.53c; United States).
In vivo Animals and OA Induction
Adolescent (8–10 week old) Sprague Dawley rats were purchased from Liaoning Changsheng biotechnology co.,Ltd. The applicable Johns Hopkins Bloomberg School of Public Health Guidelines for the care and use of animals were followed. The ARRIVE (Animal Research: Reporting In Vivo Experiments) guidelines to improve standards of reporting of animal experiments was used. The experiment was divided into four groups: Control group; OA group; Shift group; and Non-shift group.
For OA model, the anterior cruciate ligament excision transection (ACLT) to the experimental rats was used according to the modeling method summarized by Teeple et al. (2013). Rats were narcotized with isoflurane and immobilized in the supine position. An incision of 1–1.5 cm in length was cut in the longitudinal direction of the surgical department to expose articular cavity and the ACL was broken by micro-shearing without damaging the articular cartilage under a microsurgical microscope. After performing a drawer experiment to confirm the surgery, the joint capsule and skin were sutured. The control (sham) group only cut the skin and subcutaneous fascia in the surgical department, did not expose the joint cavity, and then sutured. Mice were sacrificed at the end of the sixth week. The entire knee joints were harvested and submitted for histological analyses. The serum was collected for subsequent ELISA tests.
Chronic Environmental Disruption of Circadian Rhythm Protocol
The OA model was not performed in the chronic shift group and the non-shift group. The modeling method of chronic CRD summarized by Preuss et al. (Preuss et al., 2008) was adopted. The non-shift (control) group maintained a constant L/D (12/12) (L: 8 AM-8 PM; D: 8 PM-8 AM) cycle during the entire experiment. The chronic shift model group also maintained a constant L/D (12/12) cycle, but with a 12 h phase shift per week for 22 weeks (Figure 1A). The rats were constantly entering new LD cycles, resulting in chronic CRD. The rat knee joints were harvested at the end of the 22nd week after sacrificed. Subsequent studies were consistent with those of OA rats.
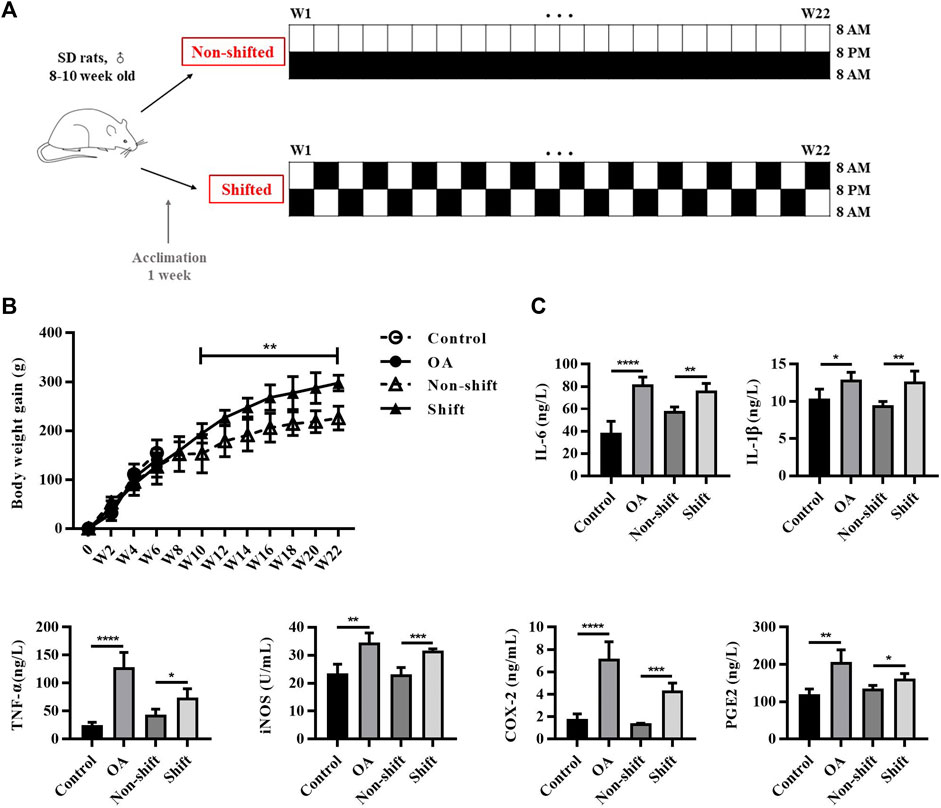
FIGURE 1. Protocol of CRD-rat model and inflammatory response in rats after OA and CRD modeling. (A) Protocol of CRD-rat model and the control group. (B) Alterations of body weight gain of rats in different groups. Results are expressed as mean ± SD; n = 4–6. **p < 0.01 between the shift and non-shift group. (C) Levels of IL-1β, TNF-α, IL-6, iNOS, COX-2, and PGE2 in rat serum in different groups. Results are expressed as mean ± SD; n = 4–6. *p < 0.05, **p < 0.01, ***p < 0.001 and ****p < 0.0001.
Histological Staining and Immunohistochemical Analysis
Bones were dehydrated and waxed in a gradient alcohol. After the wax-soaked tissues were blocked, sliced the trimmed wax block in a paraffin section, 4 μm. The water is roasted with dry wax and stored at room temperature. Paraffin slides dewaxed as follows: Two changes of pure xylene-two changes of pure ethanol-75% ethanol. For special staining, the slides stained in fast green dye solution for 1–5 min, washed away the excess dye solution until the cartilage was colorless, and soaked in 1% hydrochloric acid and alcohol for 10 s. Then staining with saffron dye solution for 1–5 s, and rapid dehydration in absolute ethanol. The slides were immersed in xylene to transparent, sealing with neutral resin. Red or orange-red cartilage and green bone formation were observed under microscope (Nikon E100; Japan), and took images for analysis. The scoring criteria for OA cartilage pathology were based on the assessment system issued by Osteoarthritis Research Society International (OARSI) (Pritzker et al., 2006).
For immunohistochemical analysis, deparaffinzing and rehydrating paraffin sections were performed following by antigen retrieval, blocking endogenous peroxidase activity and serum sealing. Incubations were performed overnight at 4°C with primary antibodies for BMAL1 (1:100), MMP-3 (1:100), MMP-13 (1:100), ADAMTS-4 (1:200), β-catenin (1:100), Aggrecan (1:100), and COL2A1 (1:20) prepared with PBS. Then the tissues were covered with secondary antibody (HRP labeled) and incubated at room temperature for 1.5 h. After Diaminobenzidine (DAB) chromogenic reaction and nucleus counterstaining with hematoxylin stain solution, the slices were dehydrated and sealed following by examined under a microscope (Nikon E100; Japan) and analyzed.
Enzyme-Linked Immunosorbent Assay of Serum and Extracellular Secretions
The serum and extracellular secretions (ECS) was extracted after centrifugation (2,500 rpm/min, 20 min). ELISA was performed following the instructions strictly by using rat tumor necrosis factor α (TNF-α) (MEIMIAN, China), Interleukin-6 (IL-6) (MEIMIAN, China), C-terminal crosslinking telopeptide of type Ⅱ collagen (CTX-Ⅱ) (MEIMIAN, China), cartilage oligomeric matrix protein (COMP) (MEIMIAN, China), inducible Nitric Oxide synthase (iNOS), prostaglandin E2 (PGE2), and cyclooxygenase-2 (COX-2) (NJJCBIO, China) ELISA kit. The absorbance of each tube was measured with a microplate reader at 450 nm reflecting the content and activity. ELISAcalc software was used to calculate the regression equation of the standard curve linear according to the concentration and OD value and four-parameter logistic curve was selected for fitting model.
Statistical Analysis
Data are presented as mean ± SD in vivo and mean ± S.E.M in vitro. Unpaired t test was performed between the two groups. When three or more groups are compared, IBM SPSS Statistics 25.0 with one-way ANOVA and Tukey test for post hoc test were used. Graphs were drawn using the GraphPad Prism (version 8). Values with p < 0.05 were considered statistically significant, and p < 0.01 extremely significant.
Results
Chronic CRD can Induce Inflammation Similar to OA in Rats
Epidemiological investigation showed that long-term shift workers were more prone to obesity (Kubo et al., 2011), and obesity is also one of the major causes of OA. In this study, we monitored the body weight of rats, and found abnormal increase in shift group. No significant difference in body weight gain was found between control and OA group in 6 weeks. However, from week 10 to week 22, the weight gain of shift group was significantly higher than that of non-shift group (p < 0.01) (Figure 1B). By evaluating the changes of pro-inflammatory cytokines in circulating blood, the levels of IL-1β, IL-6, TNF-α, iNOS, COX-2 and PGE2 of OA rats were significantly increased compared with the control group (p < 0.05, p < 0.01, and p < 0.0001). Similarly, compared with non-shift rats, the shift group showed a similar upward trend of these inflammatory markers (p < 0.05, p < 0.01, and p < 0.001) (Figure 1C). It suggests that CRD can induce inflammation in rats.
Chronic CRD Alters Matrix Degradation of Cartilage and Clock Protein Expression
To assess the effect of CRD on the articular cartilage, histological staining and immunological assays of the tibial plateau were performed. As shown in Figure 2A, the surface of cartilage in the control and non-shift group was smooth, and the matrix was evenly red stained. In the OA and Shift groups, the surface layer was rough, and red staining was decreased (asterisk), indicating the reduction of cartilage matrix synthesis or increase of degradation. However, hypertrophic and vacuolar chondrocytes could be seen (arrows) in OA cartilage but not in shift group. Additionally, OA and shift rats showed significant hyperplasia of synovium lining cell layer compared with control and non-shift rats, and inflammatory cells accumulated in small quantities (Figure 2B). Meanwhile, the OA biomarkers COMP and CTX-Ⅱ were significantly increased in OA rats and shift rats (Figure 2C). These indicate that the OA-like pathological changes of cartilage can be induced by altering the LD cycle for a long time.
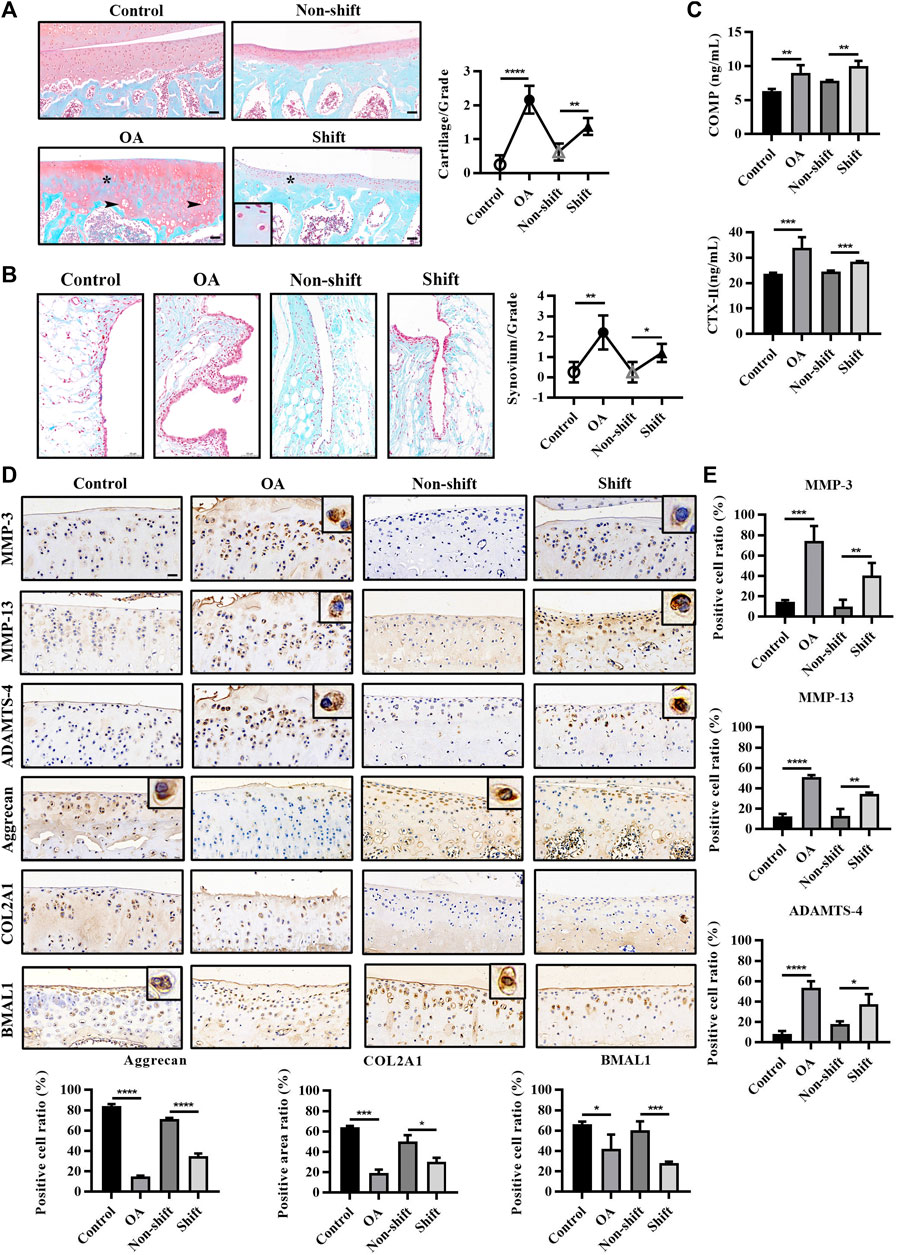
FIGURE 2. Pathological changes and immunological detection of knee cartilage in each group. (A) Safranine O and fast green staining of rat tibia cartilage and OARSI cartilage score. Arrows, vacuolar and hypertrophic chondrocytes; asterisk, absence of cationic staining. Magnification (Mag), 200×. Scale bar, 50 μm. Results are expressed as mean ± SD; n = 4–6. (B) Safranine O and fast green staining of rat knee synovium. Mag, 200×. Scale bar, 50 μm. Results are expressed as mean ± SD; n = 3–6. (C) Levels of COMP and CTX-Ⅱ in rat serum in each group. Results are expressed as mean ± SD; n = 4–6. (D,E) Immunohistochemical staining of MMP-3, MMP-13, ADAMTS-4, Aggrecan, COL2A1, and BMAL1 in chondrocytes. Mag, 200×. Scale bar, 50 μm. Results are expressed as mean ± SD; n = 4. *p < 0.05, **p < 0.01, ***p < 0.001, and ****p < 0.0001.
Additionally, the significant increase of some hydrolytic proteases (MMP-3, MMP-13 and ADAMTS-4) (p < 0.001 and p < 0.0001) and the significant decrease of matrix fibers (Aggrecan and COL2A1) (p < 0.001 and p < 0.0001) in OA cartilage indicated that the inflammatory response of OA cartilage was severe. Interestingly, similar changes in protein expression were observed in the shift group (Figures 2D,E) (p < 0.05, p < 0.01, p < 0.001, and p < 0.0001), which suggested that LD cycle disturbance bias the rate of cartilage degradation over matrix synthesis. Both ACLT and CRD significantly reduced the expression of BMAL1 in cartilage (Figures 2D,E) (p < 0.05 and p < 0.001), indicating the dysregulation of cartilage clock.
Knockdown-BMAL1 Stimulates an Inflammatory Response in Chondrocytes
In order to further explore the underlying mechanism of cartilage clock disorder, we knocked down the BMAL1 protein in second-generation chondrocytes (Supplementary Figure S1). All three siBMAL1s significantly reduced the expression of BMAL1 protein in chondrocytes, with interference efficiency between 30 and 40% (Supplementary Figure S2A). And compared with the control group, no significant change of BMAL1 expression in negative control (mock) group was found. In order to improve the efficiency of siRNA interference, a hybrid siBMAL1 interference (siRNA614:siRNA1083:siRNA1125 = 1:1:1) was performed. Protein silencing efficiency was about 50% (p < 0.0001) (Supplementary Figure S2B), and gene silencing efficiency was about 80% (p < 0.0001) (Supplementary Figure S2C). In vitro OA chondrocyte model was established by treating chondrocytes with 10 ng/ml IL-1β (Wu et al., 2018). The secretion of IL-6, TNF-α, and PGE2 was significantly increased after treatment with IL-1β and siBMAL1 compared with the control group (Figure 3A). It is suggested that inhibition of BMAL1 expression can produce an inflammatory response similar to IL-1β stimulation.
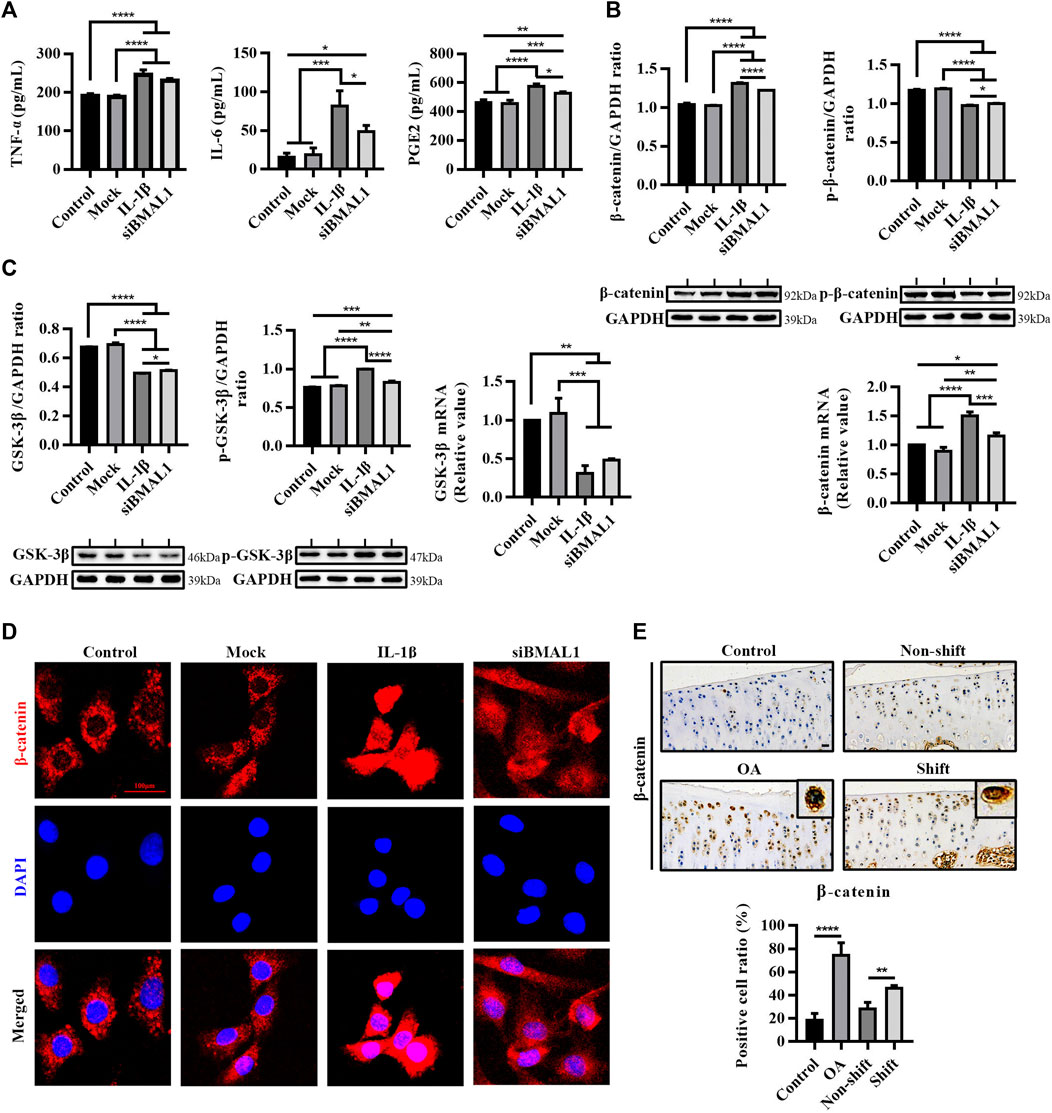
FIGURE 3. Inflammatory response and activation of the WNT/β-catenin pathway in vitro chondrocytes stimulated by IL-1β and siBMAL1. (A) Levels of TNF-α, IL-6, and PGE2 in ECS. Results are expressed as mean ± SEM; n = 3–4. (B) Protein expression changes of β-catenin and p-β-catenin, and mRNA levels of β-catenin in chondrocytes in each group. All experiments were performed in triplicate, and the results are expressed as the mean ± SEM. (C) Protein expression changes of GSK-3β and p-GSK-3β, and mRNA levels of GSK-3β in chondrocytes in each group. All experiments were performed in triplicate, and the results are expressed as the mean ± SEM. (D) Nuclear translocation of β-catenin in chondrocytes stimulated with IL-1β and transfected with siBMAL1. β-catenin was highly expressed in the cytoplasm of control chondrocytes, yet mostly transferred into the nucleus after IL-1β stimulation. A considerable number of β-catenin protein were also transferred to the nucleus in KD-BMAL1 chondrocytes. Mag, 400×. Scale bar, 100 μm. (E) Expression changes of β-catenin in tibial plateau cartilage of rats in each group. Mag, 200×. Scale bar, 50 μm. Results are expressed as mean ± SD; n = 4. *p < 0.05, **p < 0.01, ***p < 0.001, and ****p < 0.0001.
Inhibition of BMAL1 Activates the Wnt/β-Catenin Pathway in Chondrocytes
Two studies have shown that IL-1β activates the Wnt/β-catenin signaling pathway in chondrocytes (Wu et al., 2018; Xi et al., 2020). In this study, the expression of β-catenin protein and mRNA in chondrocytes treated with IL-1β were significantly increased (p < 0.0001), and the expression of p-β-catenin was significantly decreased (p < 0.0001), compared with the control group. Similarly, a similar trend occurred after silencing BMAL1 (p < 0.0001) (Figure 3B). The protein and mRNA contents of GSK-3β were significantly dampened after IL-1β stimulation (p < 0.0001), BMAL1-KD also accelerated the phosphorylation of GSK-3β (p < 0.01) (Figure 3C). Moreover, IL-1β and siBMAL1 both induced different degrees of nuclear translocation of β-catenin in chondrocytes (Figure 3D). These indicate that the down-regulation of BMAL1 expression activates the Wnt/β-catenin signaling pathway.
In vivo, the percentage of β-catenin positive cells in the OA and Shift groups was extremely significantly ascended compared to the control group (Figure 3E). It is suggested that the disturbance of the cartilage clock may induce the expression of a series of downstream inflammatory factors and matrix degradation enzymes by activating the Wnt/β-catenin signaling pathway, thus accelerating the degradation of cartilage.
Suppression of Wnt/β-Catenin Pathway Alleviates the Degradation of Chondrocyte Matrix Caused by siBMAL1
As can be seen from Figures 4A,B, compared with the control group and mock group, the expression levels of MMP-3, MMP-13, ADAMTS-4, and COX-2 protein and corresponding mRNA levels in chondrocytes treated with IL-1β and siBMAL1 were significantly increased (p < 0.01, p < 0.001, and p < 0.0001). This corresponds to the results in vivo. The Wnt/β-catenin pathway specific inhibitor XAV-939 has been shown to be significantly effective in improving an OA-mouse model (Lietman et al., 2018). After co-treatment with XAV-939, the expression levels of these proteins and mRNAs were significantly decreased compared with siBMAL1 group (p < 0.05 and p < 0.0001) (Figures 4A,B). XAV-939 also significantly dampened the increase of COMP caused by siBMAL1 (p < 0.05), but had no obvious effect on CTX-Ⅱ (Figure 4C). These findings suggest that the canonical Wnt/β-catenin pathway plays an important role in the mechanism of accelerated matrix degradation caused by disruption of cartilage clock.
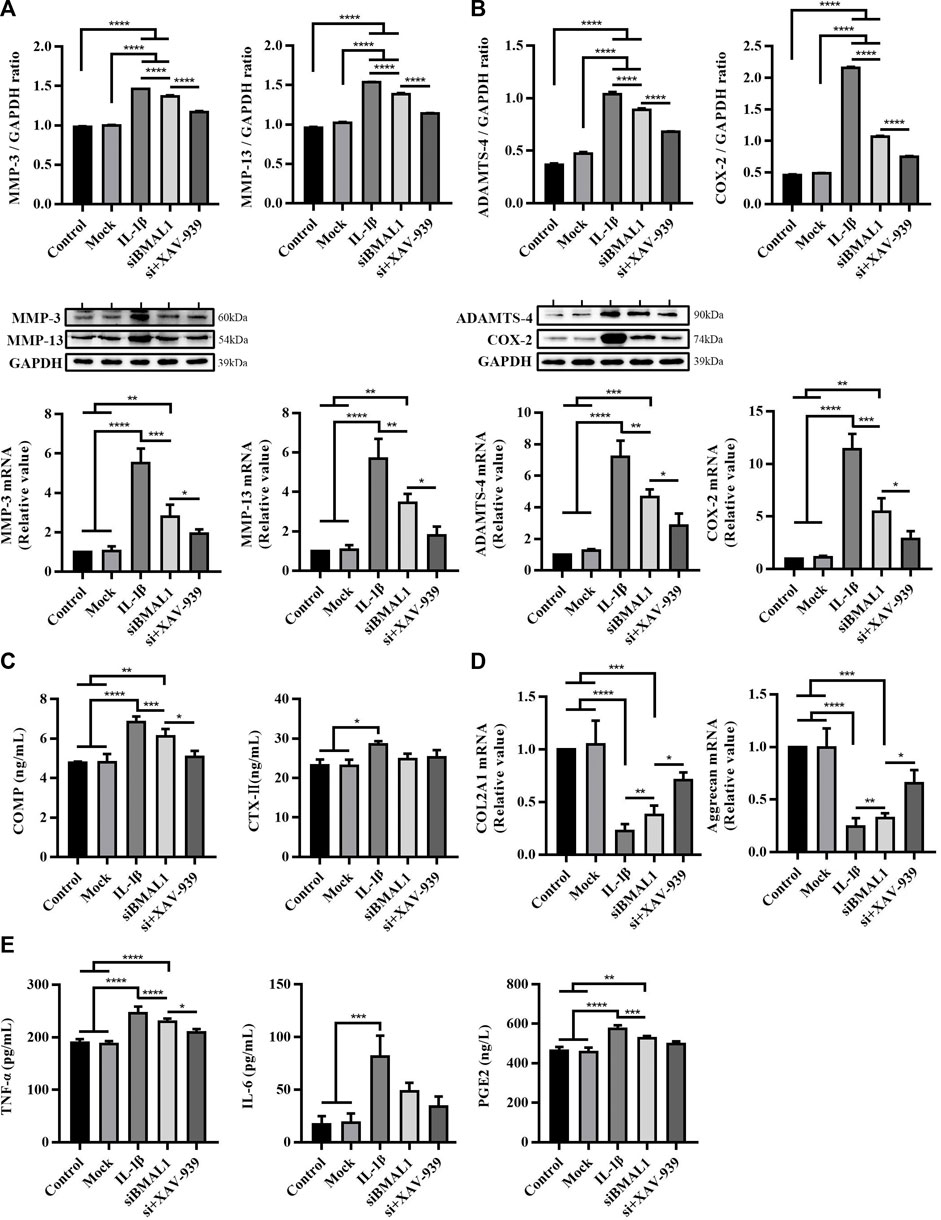
FIGURE 4. Cartilage matrix degradation and inflammation response in chondrocytes after stimulating with IL-1β, transfecting with siBMAL1 and suppressing β-catenin pathway. (A) Protein expression and mRNA level changes of MMP-3 and MMP-13 in chondrocytes in each group. (B) Protein expression and mRNA levels changes of ADAMTS-4 and COX-2 in chondrocytes in each group. (C) Levels of COMP and CTX-Ⅱ in ECS. (D) mRNA level changes of COL2A1 and Aggrecan in chondrocytes in different groups. (E) Levels of TNF-α, IL-6, and PGE2 in ECS. All experiments were performed in triplicate, and the results are expressed as the mean ± SEM. *p < 0.05, **p < 0.01, ***p < 0.001, and ****p < 0.0001.
Additionally, the contents of COL2A1 and Aggrecan mRNA in cells were extremely decreased after stimulation of IL-1β and transfection of siBMAL1 (p < 0.001 and p < 0.0001). XAV-939 significantly recovered the reduced COL2A1 and Aggrecan mRNA (p < 0.05) (Figure 4D), promoting chondrocyte matrix synthesis. In addition, levels of pro-inflammatory factors in ECS following inhibition of the Wnt/β-catenin pathway were also detected. The results showed that XAV-939 significantly reduced TNF-α secretion, but was not sensitive to IL-6 and PGE2 secretion (Figure 4E).
Discussion
Exposure to long-term LD cycle phase shifts can lead to immune system disruptions and increased levels of chemicals associated with inflammation and oxidative stress (Wright et al., 2015; Amara et al., 2019; Schilperoort et al., 2020). Disruption of circadian rhythms can also lead to an increase in circulating monocytes and macrophages, which can lead to increased systemic or peripheral tissue inflammation (McAlpine et al., 2016). In this study, we found that chronic CRD can cause OA-like pathological changes in knee cartilage, which is similar to other studies. In a study of temporomandibular joint osteoarthritis (TMJ-OA), Chen et al. (2020) found that interference with circadian rhythms with an improved multiplatform approach in Wistar rats resulted in OA-like lesions in mandibular cartilage. Meng et al. found similar OA-like pathological changes in knee cartilage of wild-type C57BL/6J mice, with cartilage surface fibrosis (Kc et al., 2015). However, the mechanism of the inflammation increase has not been clarified. Some studies proposed that CRD may exacerbate mice colitis by promoting mucosal immune dysregulation or intestinal necroptosis through dysactivation of the NF-κB pathway (Preuss et al., 2008; Pagel et al., 2017). Therefore, further studies in vivo or KEGG enrichment analysis are needed.
Core clock genes dominate the rhythm balance of tissues, among which BMAL1 can regulate the existence of several other clock genes, and plays a vital role in maintaining the normal function and integrity of body (Koronowski and Sassone-Corsi, 2021). Cartilage has its own peripheral biological clock, and is regulated by the central circadian clock. BMAL1 is stably expressed in healthy cartilage, but abnormally decreased in OA cartilage (Dudek et al., 2016; Akagi et al., 2017), which has become one of the characteristics of OA. On the contrary, abnormal expression of rhythm genes can also be one of the inducements to the occurrence of OA. This study found that both ACLT and CRD reduced the expression of BMAL1 in chondrocytes, which is also consistent with the research conclusion of Chen et al. (2020). They found that disruption of circadian rhythm resulted in decreased expression of BMAL1 in mandibular cartilage, which may be one of the causes of MMPs/TIMPs regulation imbalance. Systemic or cartilage-specific elimination of BMAL1 could impair the circadian rhythm of postpartum mice, showing significant loss of prosodic expression of several genes associated with cartilage, and distinctly elevated expression of MMP-3, MMP-13, VEGF, and Runx2 (Zhao et al., 2018; Ma et al., 2019). This suggests that inflammation and external stimuli disrupt the cartilage clock, possibly accelerating matrix degradation by activating some inflammatory pathways. In this experiment, the expression of MMP-3, MMP-13 and ADAMTS-4 in OA and CRD cartilage was significantly increased along with the decrease of BMAL1 protein expression, which proved the anabolism disorder of cartilage. Kc et al. already proposed that the OA-like pathological feature in mouse cartilage caused by CRD may be related to the activation of PKCδ-Erk-Runx2/NFκB and β-catenin signaling pathways (Kc et al., 2015). Based on this, we conducted in vitro studies targeting the β-catenin signaling pathway.
Wnt/β-catenin signaling pathway, one of the most studied pathways in OA, is closely related to chondrocyte differentiation, proliferation and apoptosis, and plays a crucial role in cartilage catabolism and regeneration (Onuora, 2017). Zhou et al. (2013) in their study of the therapeutic effect of tetrandrine on OA found that inhibiting the Wnt/β-catenin signaling pathway effectively reduced the expression of MMPs, and increased the expression of TIMP-1. Therefore, inhibiting the activation of Wnt/β-catenin may be an effective treatment for OA (Li et al., 2014). We demonstrated the dramatical increase of β-catenin expression in OA rat cartilage, and the pathway was significantly activated in cultured chondrocytes stimulated with IL-1β, highlighting the role of this pathway in OA. After XAV-939 inhibited this pathway, MMPs and inflammatory factors in chondrocytes were significantly reduced, indicating that inflammation of chondrocytes was dampened. One study found that β-catenin-induced degradation of PER2 increased CRD in intestinal mucosa of ApcMin/+ mice (Yang et al., 2009); in exudative age-related macular degeneration (AMD) and autism spectrum disorder (ASD), the increase of the canonical WNT/β-catenin pathway is enhanced by the dysregulation of circadian rhythms (Vallée et al., 2020; Vallée et al., 2020). These evidences show that Wnt/β-catenin signaling pathway is regulated by circadian rhythm/circadian rhythm genes in many diseases. Specially, some studies have shown that BMAL1 can regulate Wnt/β-catenin pathway (Karantanos et al., 2014; Mao et al., 2020). Inhibition of BMAL1 expression leads to abnormal fat regulation through Wnt/β-catenin signaling pathway, resulting in increased adipogenesis in mice (Guo et al., 2012). Various Wnt-related proteins, such as Wnt10α, decreased in BMAL1−/− mice (Janich et al., 2011). Combined with the previous description, BMAL1 interacts synergistically with the Wnt/β-catenin signaling pathway in most diseases. Interestingly, an opposite result was obtained in our experiment. We found that OA and CRD significantly reduced the expression of BMAL1 in cartilage, but dramatically activated the Wnt/β-catenin signaling pathway. The in vitro studies also confirmed the results.
These present a question that whether the interaction mechanisms of BMAL1 and Wnt pathways are different in different microenvironments? And the Wnt/β-catenin pathway plays a different role? There are several possible explanations for the current contradictory findings. Firstly, The environment of cell proliferation is different. Some injuries, such as mechanical injury OA, compensatory cell proliferation is intense; The Wnt/β-catenin pathway is also significantly activated during vigorous periods of normal or abnormal proliferation of tumor or stem cells. Clock genes may play different roles in different contexts. In addition, the mechanism of Wnt/β-catenin pathway in OA is still unclear. Liu et al. presented a novel therapy in alleviating OA by activating the Wnt/β-catenin signaling pathway (Liu et al., 2019). Therefore, we temporarily speculated that the purpose of cell proliferation is discrepancy in the early and late stages of OA development, which may not be significantly related to the abnormal decrease of clock genes in OA. Furthermore, according to the phenomenon that low expression of BMAL1 in chondrocytes can also activate the Wnt/β-catenin pathway, it may be related to tissue or cell specificity. We have previously hypothesized that the clock is cell-specific (Song et al., 2021), therefore it is possible that clock genes may be abnormally expressed differently in different environments stimulated by inflammation or other cytokines for different cells (chondrocytes or tumor cells). In conclusion, the interaction between BMAL1 and Wnt/β-catenin signaling pathway needs further studies in vitro (Figure 5).
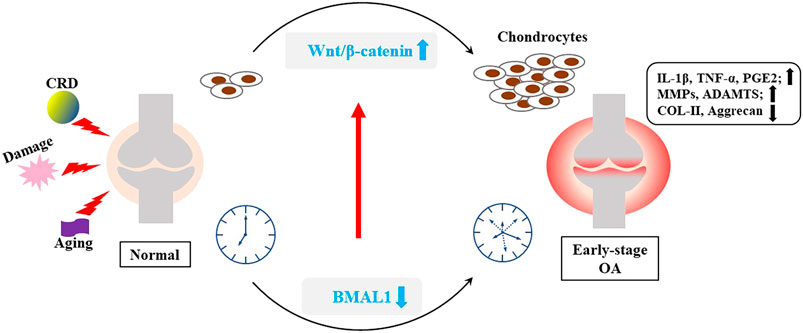
FIGURE 5. Interaction between BMAL1 and Wnt/β-catenin. Some internal and external factors, such as mechanical injury, aging and CRD, can stimulate inflammation of the knee cartilage, and disrupt the cartilage clock and matrix metabolism.
Additionally, we found that long-term CRD significantly accelerated weight gain in rats from Week 10, which was consistent with the results of Kc et al. (2015). Studies have shown a parallel relationship between increased nighttime lighting and obesity and metabolic disorders (Fonken and Nelson, 2014). CRD leads to abnormal hormone secretion, and then causes imbalance of the generation and decomposition of fat (Fonken and Nelson, 2014), indicating that CRD is one of the important causes of obesity. Different patterns of chronic jet lag (CJL) had diverse effects on rodent growth. A mount of systematic reviews has evaluated that shift work carried an extremely high risk of obesity (Sun et al., 2018; Zhang et al., 2020). The reason was that disruption of circadian rhythm would lead to a series of metabolic disorders such as hyperglycemia, hyperlipidemia, and hypoinsulinemia (Bass and Takahashi, 2010; Maury, 2019; McHill and Wright et al., 2017).
Data Availability Statement
The original contributions presented in the study are included in the article/Supplementary Material, further inquiries can be directed to the corresponding author.
Ethics Statement
The animal study was reviewed and approved by The Tab of Laboratory Animal Welfare and Ethics Committee of Northeast Agricultural University.
Author Contributions
All authors were involved in the conception and design of the study, or in acquisition analysis and interpretation of data, and in revising it critically for important intellectual content. The experiments were designed by XS and HH. The experiments were performed by XS, TM, HH, MZ, XW, and LL. TL, XS and XX collected and analyzed data. HB and XZ interpreted the data. XS and TM wrote and edited the manuscript. LG approved the publishment of the manuscript. All authors critically reviewed the content and approved final version for publication.
Funding
This work was supported by the funding from The National Key Research and Development Program of China (Project No. 2017YFD0502200) and Applied Technology Research and Development Plan of Heilongjiang, China (GA18B203).
Conflict of Interest
The authors declare that the research was conducted in the absence of any commercial or financial relationships that could be construed as a potential conflict of interest.
Publisher’s Note
All claims expressed in this article are solely those of the authors and do not necessarily represent those of their affiliated organizations, or those of the publisher, the editors and the reviewers. Any product that may be evaluated in this article, or claim that may be made by its manufacturer, is not guaranteed or endorsed by the publisher.
Acknowledgments
The authors would like to thank the Department of Veterinary Surgery at Northeast Agricultural University for technical support during the study.
Supplementary Material
The Supplementary Material for this article can be found online at: https://www.frontiersin.org/articles/10.3389/fphar.2021.760988/full#supplementary-material
Supplementary Figure S1 | Chondrocyte morphology and siBMAL1 transfection identification. (A) Toluidine blue staining of the secondary generation chondrocytes. Mag, 100×. (B) Immunofluorescent staining of COL2A1 in secondary generation chondrocytes. COL2A1, red; DAPI, blue. Mag, 400×. (C) Results of transfection of siBMAL1 into chondrocytes. Mag, 200×.
Supplementary Figure S2 | Efficiency of silencing BMAL1 by siRNA. (A) Protein expression levels of BMAL1 in chondrocytes after transfecting three siBMAL1s. (B) Protein expression levels of BMAL1 in chondrocytes after transfecting the hybrid siBMAL1. (C) mRNA levels of BMAL1 in chondrocytes after transfecting the hybrid siBMAL1. All experiments are performed in triplicate, and the results are expressed as the mean±SEM. **p<0.01 vs. control group.
References
Akagi, R., Akatsu, Y., Fisch, K. M., Alvarez-Garcia, O., Teramura, T., Muramatsu, Y., et al. (2017). Dysregulated Circadian Rhythm Pathway in Human Osteoarthritis: NR1D1 and BMAL1 Suppression Alters TGF-β Signaling in Chondrocytes. Osteoarthritis Cartilage 25 (6), 943–951. doi:10.1016/j.joca.2016.11.007
Amara, J., Saliba, Y., Hajal, J., Smayra, V., Bakhos, J. J., Sayegh, R., et al. (2019). Circadian Rhythm Disruption Aggravates DSS-Induced Colitis in Mice with Fecal Calprotectin as a Marker of Colitis Severity. Dig. Dis. Sci. 64 (11), 3122–3133. doi:10.1007/s10620-019-05675-7
Andersson, M. L., Petersson, I. F., Karlsson, K. E., Jonsson, E. N., Månsson, B., Heinegård, D., et al. (2006). Diurnal Variation in Serum Levels of Cartilage Oligomeric Matrix Protein in Patients with Knee Osteoarthritis or Rheumatoid Arthritis. Ann. Rheum. Dis. 65 (11), 1490–1494. doi:10.1136/ard.2005.051292
Bass, J., and Takahashi, J. S. (2010). Circadian Integration of Metabolism and Energetics. Science 330, 1349–1354. doi:10.1126/science.1195027
Castanon-Cervantes, O., Wu, M., Ehlen, J. C., Paul, K., Gamble, K. L., Johnson, R. L., et al. (2010). Dysregulation of Inflammatory Responses by Chronic Circadian Disruption. J. Immunol. 185 (10), 5796–5805. doi:10.4049/jimmunol.1001026
Chen, G., Zhao, H., Ma, S., Chen, L., Wu, G., Zhu, Y., et al. (2020). Circadian Rhythm Protein Bmal1 Modulates Cartilage Gene Expression in Temporomandibular Joint Osteoarthritis via the MAPK/ERK Pathway. Front. Pharmacol. 11, 527744. doi:10.3389/fphar.2020.527744
Chen, Y., Zhu, D., Yuan, J., Han, Z., Wang, Y., Qian, Z., et al. (2016). CLOCK-BMAL1 Regulate the Cardiac L-type Calcium Channel Subunit CACNA1C through PI3K-Akt Signaling Pathway. Can. J. Physiol. Pharmacol. 94 (9), 1023–1032. doi:10.1139/cjpp-2015-0398
Dudek, M., Gossan, N., Yang, N., Im, H. J., Ruckshanthi, J. P., Yoshitane, H., et al. (2016). The Chondrocyte Clock Gene Bmal1 Controls Cartilage Homeostasis and Integrity. J. Clin. Invest. 126 (1), 365–376. doi:10.1172/JCI82755
Fonken, L. K., and Nelson, R. J. (2014). The Effects of Light at Night on Circadian Clocks and Metabolism. Endocr. Rev. 35 (4), 648–670. doi:10.1210/er.2013-1051
Guo, B., Chatterjee, S., Li, L., Kim, J. M., Lee, J., Yechoor, V. K., et al. (2012). The Clock Gene, Brain and Muscle Arnt-like 1, Regulates Adipogenesis via Wnt Signaling Pathway. FASEB J. 26 (8), 3453–3463. doi:10.1096/fj.12-205781
He, Y., Chen, Y., Zhao, Q., and Tan, Z. (2013). Roles of Brain and Muscle ARNT-like 1 and Wnt Antagonist Dkk1 during Osteogenesis of Bone Marrow Stromal Cells. Cell Prolif 46 (6), 644–653. doi:10.1111/cpr.12075
Hong, Y., Lee, S., Choi, J., Jin, Y., Won, J., and Hong, Y. (2019). Conditional Controlled Light/Dark Cycle Influences Exercise-Induced Benefits in a Rat Model with Osteoarthritis: In Vitro and In Vivo Study. J. Clin. Med. 8 (11), 1855. doi:10.3390/jcm8111855
Huang, K., and Wu, L. D. (2018). Dehydroepiandrosterone: Molecular Mechanisms and Therapeutic Implications in Osteoarthritis. J. Steroid Biochem. Mol. Biol. 183, 27–38. doi:10.1016/j.jsbmb.2018.05.004
Hublin, C., Partinen, M., Koskenvuo, K., Silventoinen, K., Koskenvuo, M., and Kaprio, J. (2010). Shift-work and Cardiovascular Disease: a Population-Based 22-year Follow-Up Study. Eur. J. Epidemiol. 25 (5), 315–323. doi:10.1007/s10654-010-9439-3
Igarashi, K., Saeki, S., and Shinoda, H. (2013). Diurnal Rhythms in the Incorporation and Secretion of 3H-Proline and 3H-Galactose by Cartilage Cells and Osteoblasts in Various Bone-Forming Sites in Growing Rats. Orthod. Waves 72 (1), 11–15. doi:10.1016/j.odw.2012.09.001
Janich, P., Pascual, G., Merlos-Suárez, A., Batlle, E., Ripperger, J., Albrecht, U., et al. (2011). The Circadian Molecular Clock Creates Epidermal Stem Cell Heterogeneity. Nature 480 (7376), 209–214. doi:10.1038/nature10649
Karantanos, T., Theodoropoulos, G., Pektasides, D., and Gazouli, M. (2014). Clock Genes: Their Role in Colorectal Cancer. World J. Gastroenterol. 20 (8), 1986–1992. doi:10.3748/wjg.v20.i8.1986
Kc, R., Li, X., Forsyth, C. B., Voigt, R. M., Summa, K. C., Vitaterna, M. H., et al. (2015). Osteoarthritis-like Pathologic Changes in the Knee Joint Induced by Environmental Disruption of Circadian Rhythms Is Potentiated by a High-Fat Diet. Sci. Rep. 5 (5), 16896. doi:10.1038/srep16896
Kc, R., Li, X., Voigt, R. M., Ellman, M. B., Summa, K. C., Vitaterna, M. H., et al. (2015). Environmental Disruption of Circadian Rhythm Predisposes Mice to Osteoarthritis-like Changes in Knee Joint. J. Cel Physiol 230 (9), 2174–2183. doi:10.1002/jcp.24946
Kaoru, I., Shuichi, S., and Hisashi, S. (2013). Diurnal Rhythms in the Incorporation and Secretion of 3H-Proline and 3H-Galactose by Cartilage Cells and Osteoblasts in Various Bone-Forming Sites in Growing Rats. Orthod. Waves 72 (1), 11–15. doi:10.1016/j.odw.2012.09.001
Kong, S. Y., Stabler, T. V., Criscione, L. G., Elliott, A. L., Jordan, J. M., and Kraus, V. B. (2006). Diurnal Variation of Serum and Urine Biomarkers in Patients with Radiographic Knee Osteoarthritis. Arthritis Rheum. 54 (8), 2496–2504. doi:10.1002/art.21977
Koronowski, K. B., and Sassone-Corsi, P. (2021). Communicating Clocks Shape Circadian Homeostasis. Science 371 (6530), eabd0951. doi:10.1126/science.abd0951
Kubo, T., Oyama, I., Nakamura, T., Shirane, K., Otsuka, H., Kunimoto, M., et al. (2011). Retrospective Cohort Study of the Risk of Obesity Among Shift Workers: Findings from the Industry-Based Shift Workers' Health Study, Japan. Occup. Environ. Med. 68 (5), 327–331. doi:10.1136/oem.2009.054445
Li, J., Zhou, X. D., Yang, K. H., Fan, T. D., Chen, W. P., Jiang, L. F., et al. (2014). Hinokitiol Reduces Matrix Metalloproteinase Expression by Inhibiting Wnt/β-Catenin Signaling In Vitro and In Vivo. Int. Immunopharmacol 23 (1), 85–91. doi:10.1016/j.intimp.2014.08.012
Li, Q., Xia, D., Wang, Z., Liu, B., Zhang, J., Peng, P., et al. (2021). Circadian Rhythm Gene PER3 Negatively Regulates Stemness of Prostate Cancer Stem Cells via WNT/β-Catenin Signaling in Tumor Microenvironment. Front Cel Dev Biol 9, 656981. doi:10.3389/fcell.2021.656981
Li, X., Liu, N., Gu, B., Hu, W., Li, Y., Guo, B., et al. (2018). BMAL1 Regulates Balance of Osteogenic-Osteoclastic Function of Bone Marrow Mesenchymal Stem Cells in Type 2 Diabetes Mellitus through the NF-Κb Pathway. Mol. Biol. Rep. 45 (6), 1691–1704. doi:10.1007/s11033-018-4312-7
Li, X., Liu, N., Wang, Y., Liu, J., Shi, H., Qu, Z., et al. (2017). Brain and Muscle Aryl Hydrocarbon Receptor Nuclear Translocator-like Protein-1 Cooperates with Glycogen Synthase Kinase-3β to Regulate Osteogenesis of Bone-Marrow Mesenchymal Stem Cells in Type 2 Diabetes. Mol. Cel Endocrinol 440, 93–105. doi:10.1016/j.mce.2016.10.001
Lietman, C., Wu, B., Lechner, S., Shinar, A., Sehgal, M., Rossomacha, E., et al. (2018). Inhibition of Wnt/β-Catenin Signaling Ameliorates Osteoarthritis in a Murine Model of Experimental Osteoarthritis. JCI Insight 3 (3), e96308. doi:10.1172/jci.insight.96308
Lin, F., Chen, Y., Li, X., Zhao, Q., and Tan, Z. (2013). Over-expression of Circadian Clock Gene Bmal1 Affects Proliferation and the Canonical Wnt Pathway in NIH-3T3 Cells. Cell Biochem Funct 31 (2), 166–172. doi:10.1002/cbf.2871
Liu, X., Wang, L., Ma, C., Wang, G., Zhang, Y., and Sun, S. (2019). Exosomes Derived from Platelet-Rich Plasma Present a Novel Potential in Alleviating Knee Osteoarthritis by Promoting Proliferation and Inhibiting Apoptosis of Chondrocyte via Wnt/β-Catenin Signaling Pathway. J. Orthop. Surg. Res. 14 (1), 470. doi:10.1186/s13018-019-1529-7
Ma, Z., Jin, X., Qian, Z., Li, F., Xu, M., Zhang, Y., et al. (2019). Deletion of Clock Gene Bmal1 Impaired the Chondrocyte Function Due to Disruption of the HIF1α-VEGF Signaling Pathway. Cell Cycle 18 (13), 1473–1489. doi:10.1080/15384101.2019.1620572
Mao, X., Li, X., Hu, W., Hao, S., Yuan, Y., Guan, L., et al. (2020). Expression of Concern: Downregulated Brain and Muscle Aryl Hydrocarbon Receptor Nuclear Translocator-like Protein-1 Inhibits Osteogenesis of BMSCs through P53 in Type 2 Diabetes Mellitus. Biol. Open 9 (7), bio051482. doi:10.1242/bio.05148210.1242/bio.055525
Maury, E. (2019). Off the Clock: From Circadian Disruption to Metabolic Disease. Int. J. Mol. Sci. 20 (7), 1597. doi:10.3390/ijms20071597
McAlpine, C. S., and Swirski, F. K. (2016). Circadian Influence on Metabolism and Inflammation in Atherosclerosis. Circ. Res. 119 (1), 131–141. doi:10.1161/CIRCRESAHA.116.308034
McHill, A. W., and Wright, K. P. (2017). Role of Sleep and Circadian Disruption on Energy Expenditure and in Metabolic Predisposition to Human Obesity and Metabolic Disease. Obes. Rev. 18 Suppl 1 (Suppl. 1), 15–24. doi:10.1111/obr.12503
Ning, B., Wang, P., Pei, X., Kang, Y., Song, J., Wang, D., et al. (2012). Dual Function of β-catenin in Articular Cartilage Growth and Degeneration at Different Stages of Postnatal Cartilage Development. Int. Orthop. 36 (3), 655–664. doi:10.1007/s00264-011-1315-6
Onuora, S. (2017). Osteoarthritis: Wnt Inhibitor Shows Potential as a DMOAD. Nat. Rev. Rheumatol. 13 (11), 634. doi:10.1038/nrrheum.2017.164
Pagel, R., Bär, F., Schröder, T., Sünderhauf, A., Künstner, A., Ibrahim, S. M., et al. (2017). Circadian Rhythm Disruption Impairs Tissue Homeostasis and Exacerbates Chronic Inflammation in the Intestine. FASEB J. 31 (11), 4707–4719. doi:10.1096/fj.201700141RR
Preuss, F., Tang, Y., Laposky, A. D., Arble, D., Keshavarzian, A., and Turek, F. W. (2008). Adverse Effects of Chronic Circadian Desynchronization in Animals in a "challenging" Environment. Am. J. Physiol. Regul. Integr. Comp. Physiol. 295 (6), R2034–R2040. doi:10.1152/ajpregu.00118.2008
Pritzker, K. P., Gay, S., Jimenez, S. A., Ostergaard, K., Pelletier, J. P., Revell, P. A., et al. (2006). Osteoarthritis Cartilage Histopathology: Grading and Staging. Osteoarthritis Cartilage 14 (1), 13–29. doi:10.1016/j.joca.2005.07.014
Samsa, W. E., Vasanji, A., Midura, R. J., and Kondratov, R. V. (2016). Deficiency of Circadian Clock Protein BMAL1 in Mice Results in a Low Bone Mass Phenotype. Bone 84, 194–203. doi:10.1016/j.bone.2016.01.006
Schilperoort, M., van den Berg, R., Bosmans, L. A., van Os, B. W., Dollé, M. E. T., Smits, N. A. M., et al. (2020). Disruption of Circadian Rhythm by Alternating Light-Dark Cycles Aggravates Atherosclerosis Development in APOE*3-Leiden.CETP Mice. J. Pineal Res. 68 (1), e12614. doi:10.1111/jpi.12614
Shan, Z., Li, Y., Zong, G., Guo, Y., Li, J., Manson, J. E., et al. (2018). Rotating Night Shift Work and Adherence to Unhealthy Lifestyle in Predicting Risk of Type 2 Diabetes: Results from Two Large US Cohorts of Female Nurses. BMJ 363, k4641. doi:10.1136/bmj.k4641
Song, X., Bai, H., Meng, X., Xiao, J., and Gao, L. (2021). Drivers of Phenotypic Variation in Cartilage: Circadian Clock Genes. J. Cel Mol Med 25 (16), 7593–7601. doi:10.1111/jcmm.16768
Sun, M., Feng, W., Wang, F., Li, P., Li, Z., Li, M., et al. (2018). Meta-analysis on Shift Work and Risks of Specific Obesity Types. Obes. Rev. 19 (1), 28–40. doi:10.1111/obr.12621
Teeple, E., Jay, G. D., Elsaid, K. A., and Fleming, B. C. (2013). Animal Models of Osteoarthritis: Challenges of Model Selection and Analysis. AAPS J. 15 (2), 438–446. doi:10.1208/s12248-013-9454-x
Vallée, A., Lecarpentier, Y., Guillevin, R., and Vallée, J. N. (2020). The Influence of Circadian Rhythms and Aerobic Glycolysis in Autism Spectrum Disorder. Transl Psychiatry 10 (1), 400. doi:10.1038/s41398-020-01086-9
Vallée, A., Lecarpentier, Y., Vallée, R., Guillevin, R., and Vallée, J. N. (2020). Circadian Rhythms in Exudative Age-Related Macular Degeneration: The Key Role of the Canonical WNT/β-Catenin Pathway. Int. J. Mol. Sci. 21 (3), 820. doi:10.3390/ijms21030820
Vgontzas, A. N., Zoumakis, E., Bixler, E. O., Lin, H. M., Follett, H., Kales, A., et al. (2004). Adverse Effects of Modest Sleep Restriction on Sleepiness, Performance, and Inflammatory Cytokines. J. Clin. Endocrinol. Metab. 89 (5), 2119–2126. doi:10.1210/jc.2003-031562
Wright, K. P., Drake, A. L., Frey, D. J., Fleshner, M., Desouza, C. A., Gronfier, C., et al. (2015). Influence of Sleep Deprivation and Circadian Misalignment on Cortisol, Inflammatory Markers, and Cytokine Balance. Brain Behav. Immun. 47, 24–34. doi:10.1016/j.bbi.2015.01.004
Wright, K. P., McHill, A. W., Birks, B. R., Griffin, B. R., Rusterholz, T., and Chinoy, E. D. (2013). Entrainment of the Human Circadian Clock to the Natural Light-Dark Cycle. Curr. Biol. 23 (16), 1554–1558. doi:10.1016/j.cub.2013.06.039
Wu, J., Huang, J. F., Qin, X. X., Hu, F., Chen, Z. F., Zheng, Y., et al. (2018). Platelet-rich Plasma Inhibits Wnt/β-Catenin Signaling in Rabbit Cartilage Cells Activated by IL-1β. Int. Immunopharmacol 55, 282–289. doi:10.1016/j.intimp.2017.12.031
Xi, Y., Huang, X., Tan, G., Chu, X., Zhang, R., Ma, X., et al. (2020). Protective Effects of Erdosteine on Interleukin-1β-Stimulated Inflammation via Inhibiting the Activation of MAPK, NF-Κb, and Wnt/β-Catenin Signaling Pathways in Rat Osteoarthritis. Eur. J. Pharmacol. 873, 172925. doi:10.1016/j.ejphar.2020.172925
Yang, X., Wood, P. A., Ansell, C. M., Ohmori, M., Oh, E. Y., Xiong, Y., et al. (2009). Beta-catenin Induces Beta-TrCP-Mediated PER2 Degradation Altering Circadian Clock Gene Expression in Intestinal Mucosa of ApcMin/+ Mice. J. Biochem. 145 (3), 289–297. doi:10.1093/jb/mvn167
Zhao, J., Zhou, X., Tang, Q., Yu, R., Yu, S., Long, Y., et al. (2018). BMAL1 Deficiency Contributes to Mandibular Dysplasia by Upregulating MMP3. Stem Cel Rep. 10 (1), 180–195. doi:10.1016/j.stemcr.2017.11.017
Zhang, Q., Chair, S. Y., Lo, S. H. S., Chau, J. P., Schwade, M., and Zhao, X. (2020). Association between Shift Work and Obesity Among Nurses: A Systematic Review and Meta-Analysis. Int. J. Nurs. Stud. 112, 103757. doi:10.1016/j.ijnurstu.2020.103757
Zhou, M., Yang, S., Guo, Y., Wang, D., Qiu, W., Wang, B., et al. (2020). Shift Work and the Risk of Knee Osteoarthritis Among Chinese Workers: a Retrospective Cohort Study. Scand. J. Work Environ. Health 46 (2), 152–160. doi:10.5271/sjweh.3861
Zhou, X., Li, W., Jiang, L., Bao, J., Tao, L., Li, J., et al. (2013). Tetrandrine Inhibits the Wnt/β -Catenin Signalling Pathway and Alleviates Osteoarthritis: An In Vitro and In Vivo Study. Evid. Based Complement. Alternat Med. 2013, 809579. doi:10.1155/2013/809579
Keywords: circadian rhythm disturbance, osteoarthritis, wnt/β-catenin signaling pathway, cartilage, rat
Citation: Song X, Ma T, Hu H, Zhao M, Bai H, Wang X, Liu L, Li T, Sheng X, Xu X, Zhang X and Gao L (2021) Chronic Circadian Rhythm Disturbance Accelerates Knee Cartilage Degeneration in Rats Accompanied by the Activation of the Canonical Wnt/β-Catenin Signaling Pathway. Front. Pharmacol. 12:760988. doi: 10.3389/fphar.2021.760988
Received: 19 August 2021; Accepted: 11 October 2021;
Published: 11 November 2021.
Edited by:
Galina Sud’ina, Lomonosov Moscow State University, RussiaReviewed by:
Jiapeng Bao, Zhejiang University School of Medicine, ChinaIngrid Y. Liu, Tzu Chi University, Taiwan
Copyright © 2021 Song, Ma, Hu, Zhao, Bai, Wang, Liu, Li, Sheng, Xu, Zhang and Gao. This is an open-access article distributed under the terms of the Creative Commons Attribution License (CC BY). The use, distribution or reproduction in other forums is permitted, provided the original author(s) and the copyright owner(s) are credited and that the original publication in this journal is cited, in accordance with accepted academic practice. No use, distribution or reproduction is permitted which does not comply with these terms.
*Correspondence: Li Gao, Z2FvbGk0MzQ1MEAxNjMuY29t
†These authors have contributed equally to this work and share first authorship