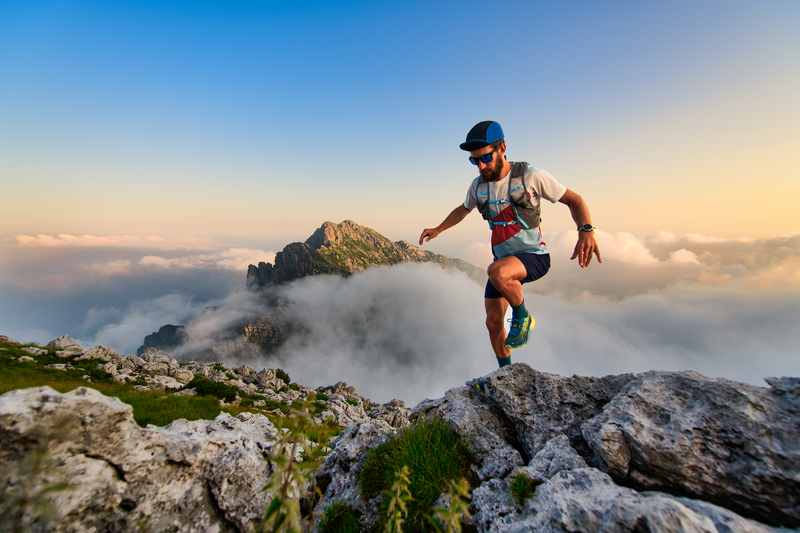
95% of researchers rate our articles as excellent or good
Learn more about the work of our research integrity team to safeguard the quality of each article we publish.
Find out more
MINI REVIEW article
Front. Pharmacol. , 15 December 2021
Sec. Cardiovascular and Smooth Muscle Pharmacology
Volume 12 - 2021 | https://doi.org/10.3389/fphar.2021.758929
This article is part of the Research Topic Medicinal Plants in the Treatment of Myocardial Injury and Vascular Diseases View all 16 articles
Atherosclerosis poses serious health problems and increases the risk of various cardiovascular diseases, including myocardial infarction, heart failure, ischemic stroke, and peripheral arterial disease. Atherosclerosis patients require long-term medications to prevent complications, some of which are costly and may result in unwanted adverse reactions. Natural products have emerged as potential sources of bioactive compounds that provide health benefits in cardiovascular diseases. Increased inflammation and vascular remodeling have been associated with atherosclerosis pathogenesis. The molecules involved in signaling pathways are considered valuable targets for new treatment approaches. Therefore, this review aimed to summarize the available evidence of the anti-inflammatory effects of thymoquinone, the major active compound isolated from Nigella sativa L., via inflammatory signaling pathways in atherosclerosis. Specifically, nuclear factor-κB and mitogen-activated protein kinase signaling pathways were considered. Furthermore, the potential toxic effects elicited by thymoquinone were addressed. These findings suggest a potential role of thymoquinone in managing atherosclerosis, and further studies are required to ascertain its effectiveness and safety profile.
Atherosclerosis is a major cause of cardiovascular disease (CVD) worldwide, including myocardial infarction, heart failure, ischemic stroke, and peripheral arterial disease. According to the Global Burden of Cardiovascular Diseases and Risk Factors (Roth et al., 2020), CVD prevalence has increased from 271 million to 523 million from 1990 to 2019. The CVD mortality had a relative increase of 6.5% in 2019, reaching 18.6 million deaths. It is estimated that 23.6 million people globally will die from CVDs by 2030 (WHO, 2013). The rising burden of CVDs on individuals and the healthcare system warrants research on atherosclerotic diseases and implementation of preventive measures.
There are several theories on atherosclerosis pathogenesis, including lipid theory, oxidative theory, response to injury theory, and inflammatory theory (Minelli et al., 2020). Various inflammatory cells and inflammatory mediators are responsible for fatty streak formation, progression, and rupture of atheromatous plaques (Libby, 2021). The major signaling pathways that mediate inflammation include nuclear factor-κB (NF-κB) and mitogen-activated protein kinase (MAPK). Hence, modulating these inflammatory signaling pathways to produce anti-inflammatory actions may serve as potential therapeutic targets for atherosclerosis management.
There has been increasing interest in medicinal herbs or plants for the treatment and prevention of various diseases, including atherosclerosis. Plant-based traditional medicines have attracted considerable attention owing to their availability, cost, safety, and efficacy. The World Health Organization (WHO) reported that approximately 60–80% of the population use traditional medicines or herbal remedies for their primary health care, particularly in developing countries. It is recommended that the WHO Traditional Medicine Strategy 2014–2023 is implemented for national traditional medicine programs. This strategy aims to explore the potential use of traditional medicine for health and wellness, in addition to encouraging its safe and effective use (Zhang, 2018).
Nigella sativa L., also known as black seed or black cumin, is a plant traditionally used for medicinal purposes in the Middle East, India, Northern Africa, and Europe. N. sativa L. has been used to treat various ailments, including asthma, hypertension, diabetes, inflammation, cough, headache, eczema, fever, and dizziness (Salehi et al., 2021). N. sativa L. is a flowering plant belonging to the family Ranunculaceae. The fruit contains angular-shaped black seeds, which are regarded as the most important component in view of their beneficial health effects (Tavakkoli et al., 2017).
N. sativa L. contains various bioactive compounds, including thymoquinone (TQ), dithymoquinone, thymol, and thymohydroquinone. Among the isolated compounds, TQ was the most abundant. Hence, the extensive therapeutic benefits exerted by N. sativa L. may be attributed to TQ (Alagawany et al., 2021). Previous studies have shown that TQ possesses various pharmacological properties, including antioxidant (Abd-Elkareem et al., 2021), antimicrobial (Mouwakeh et al., 2018), antihypertensive (Enayatfard et al., 2018), antidiabetic (Bule et al., 2020), lipid-lowering (Majdalawieh et al., 2021), neuroprotective (Abulfadl et al., 2018), gastroprotective (Bukar et al., 2017), anticancer (Edris, 2021), and anti-inflammatory (Alkharfy et al., 2018; Ahmad et al., 2020). Given the potential health benefits of TQ, the present study aimed to examine the available evidence on its anti-inflammatory effects in atherosclerosis via signaling pathway modulation, and to highlight its potential toxicity.
NF-κB pathway activation is regulated by inhibitory proteins of the κB family (IκB) kinase through IκB phosphorylation (Christian et al., 2016), which causes its degradation by the proteasome, leading to the release of NF-κB for nuclear translocation and gene transcription activation. This pathway regulates inflammatory cytokine production and inflammatory cell recruitment, which contribute to the inflammatory response.
MAPKs consist of three members: extracellular signal-regulated kinases (ERKs), p38 MAPK, and c-Jun N-terminal kinases (JNKs). ERKs are generally activated by mitogens and differentiation signals (Sun et al., 2015), while p38 MAPK and JNK are activated by inflammatory stimuli and stress (Chan et al., 2017). MAPK activation leads to phosphorylation and activation of transcription factors, which are responsible for inflammatory response regulation (Chen et al., 2018).
Studies involving signaling pathways have documented that cytokine-mediated inflammation is a crucial element in atherosclerosis pathogenesis. Hence, inflammatory response regulation is a fundamental aspect in atherosclerosis prevention and treatment (Liu et al., 2017; Sun et al., 2018). The proposed atheroprotective effects of TQ via NF-κB and MAPK pathway modulation are shown in Figure 1.
FIGURE 1. Proposed antiatherogenic effects of thymoquinone in atherosclerosis via modulation of NF-κB and MAPK signaling pathways. IL, interleukin; LOX-1, lectin-like oxidized low-density lipoprotein receptor 1; MAPK, mitogen-activated protein kinase; MMP-2, matrix metalloproteinase 2; NF-κB, nuclear factor κB; ox-LDL, oxidized low-density lipoprotein; NLRP3, NOD-like receptor protein 3; p-ERK, phosphorylation of extracellular signal-regulated kinase; p-p38, phosphorylation of p38; TNF-α, tumor necrosis factor alpha; VSMC, vascular smooth muscle cell; ⊥, suppress. Adapted from “Suppression of Inflammasome by IRF4 and IRF8 is Critical for T Cell Priming”, by BioRender.com (2021). Retrieved from https://app.biorender.com/biorender-templates
Vascular cell adhesion molecule 1, intercellular adhesion molecule 1, chemokines interleukin 8 (IL-8), and monocyte chemoattractant protein 1 (MCP-1) are major molecules that recruit circulating mononuclear leukocytes to the arterial intima. This process is important in atherosclerosis and is mediated by NF-κB activation (Mussbacher et al., 2019). Amartey et al. (2019) reported that concurrent treatment with TQ (6.25 μg/ml) showed a tendency to reduce inflammatory response by suppressing IL-6 and IL-8 protein levels in human vascular endothelial cells (HVECs) exposed to lipopolysaccharides (LPS, 100 ng/ml) at 24 h. Furthermore, TQ downregulated the mRNA expression of important inflammation regulators vascular endothelial growth factor (VEGF) and MCP-1 in LPS-treated cells. VEGF mediates angiogenesis, whereas MCP-1 is involved in endothelial monocyte activation.
Furthermore, the expression of NOD-like receptor protein 3 (NLRP3) inflammasome and IL-1β was attenuated by TQ in HVECs exposed to LPS for 24 h. In the presence of inflammation, ten-eleven translocation 2 (TET-2) gene expression increased with concurrent administration of TQ (Amartey et al., 2019). The role of TET-2 in atherosclerosis has been elucidated by Fuster et al. (2017). Macrophages with TET-2 deficiency led to increased pro-inflammatory cytokine IL-1β secretion, which is dependent on the action of NLRP3 (Grebe et al., 2018). According to these findings, TQ plays a regulatory role in inflammation and monocyte recruitment, and modulates NLRP3 and TET-2 in vitro. However, no positive controls were used in this study. Media-treated cells were used as a control to differentiate the effects of LPS and TQ. Further studies on multiple cell lines and in vivo studies are required to confirm the anti-inflammatory effect of TQ against atherosclerosis. This study did not investigate the mechanism of action of TQ. The anti-inflammatory action of TQ could be due to NF-κB suppression in view of its regulatory role in NRLP3 and pro-inflammatory cytokines such as the IL-1 family (Liu et al., 2017).
Hyperlipidemia has been reported to accelerate lipid accumulation, atherosclerosis, and chronic inflammation in apolipoprotein E knockout (ApoE−/-) or low-density lipoprotein receptor-deficient (LDL-R−/−) mice (Zhao et al., 2018). ApoE−/- and LDL-R−/− mice are two models commonly used in atherosclerosis research that require hypercholesterolemia induction. Their mechanisms of enhancing atherosclerosis development and the involved lipoproteins are different (Getz and Reardon, 2016). ApoE deficiency in macrophages may contribute to hypercholesterolemia, while the lack of LDL-R in hepatocytes is responsible for hypercholesterolemia in ApoE−/- and LDL-R−/− models. The following studies utilized normal diet-fed mice as the control group.
Xu et al. (2018) reported that concurrent treatment with TQ (oral, 25 mg/kg/day for 8 weeks) decreased serum high-sensitivity C-reactive protein levels in high-cholesterol diet-fed adult male ApoE−/- mice. Additionally, TQ suppressed the upregulation of tumor necrosis factor α (TNF-α) and IL-6 expression in cardiac tissues isolated from high-cholesterol diet-fed mice. Similar results were reported by Pei et al. (2020) in LDL-R−/− mice. Pei et al. (2020) documented that a high-cholesterol diet supplemented with TQ (oral, 50 mg/kg/day for 8 weeks) reduced TNF-α and IL-6 serum levels and gene expression in mice. Cluster of differentiation 68 markers, which are highly expressed in macrophages, were reduced following TQ administration, indicating a reduction in macrophage numbers in the cardiac tissue of high-cholesterol diet-fed LDL-R−/− mice. In addition, TQ administration downregulated the increased protein and gene expression of NLRP3, caspase-1, IL-1β, and IL-18 induced by a high-cholesterol diet. Decreased NF-κB protein expression was observed following concurrent high-cholesterol diet with TQ supplementation in LDL-R−/− mice (Pei et al., 2020). Pyroptosis, a programmed cell death mechanism mediated by NLRP3 activation, has been associated with hyperlipidemia development. NLRP3 activation stimulates caspase-1, an IL-1 converting enzyme that cleaves precursors of the inflammatory cytokines IL-1β and IL-18. Subsequently, the release of pro-inflammatory cytokines is enhanced, leading to pyroptosis (Borges et al., 2017).
Oxidized low-density lipoprotein (ox-LDL) contributes to atherosclerosis-associated inflammation (Rhoads and Major, 2018). Ox-LDL causes endothelial dysfunction, leading to adhesion molecule expression and monocyte recruitment in the subendothelial space. Ox-LDL is taken up by macrophages via lectin-like ox-LDL receptor 1 (LOX-1). LOX-1 expression is upregulated by ox-LDL (Barreto et al., 2021). Additionally, ox-LDL promotes the growth and migration of smooth muscle cells, monocytes, and macrophages (Pirillo et al., 2013).
Xu et al. (2018) revealed that ApoE−/- mice receiving a high-cholesterol diet concurrent with TQ (oral, 25 mg/kg/day) for 8 weeks had reduced LOX-1 protein and gene expression in cardiac tissues. Lipid deposition, foam cell formation, and ERK phosphorylation (p-ERK) are regulated by protein kinases (Lin et al., 2012). Upregulated LOX-1 expression was suppressed by ERK inhibitors, suggesting that MAPK pathway activation is a crucial signaling event in LOX-1 gene regulation (Zhang et al., 2017). p-ERK was significantly reduced in ApoE−/- mice receiving TQ and a high-cholesterol diet than in mice without TQ supplementation (Xu et al., 2018). Therefore, TQ may regulate LOX-1 via the p-ERK pathway. ERK inhibition may exert potential antiatherosclerotic effects, as indicated by reduced uptake of ox-LDL and foam cell formation in hypercholesteremic TQ-supplemented ApoE−/- mice (Xu et al., 2018).
Pei et al. (2020) investigated the effect of TQ on hyperlipidemia-induced cardiac damage in male LDL-R−/−mice. It was demonstrated that concurrent treatment with TQ (oral, 50 mg/kg/day) reduced total cholesterol and LDL-cholesterol levels in addition to the pro-inflammatory cytokines in mice fed a high-cholesterol diet for 8 weeks. There was a reduction in lipid accumulation and inflammatory cell infiltration in the cardiac tissue of TQ-administered mice compared to that in the non-supplemented mice. TQ decreased p38 and p-ERK levels in high-cholesterol diet-fed mice. These findings suggest that TQ suppresses high-cholesterol diet-induced inflammation and cardiac damage via p38 and ERK pathway inhibition.
Various pathological events are involved in vascular remodeling in response to vascular damage, including endothelial dysfunction, vascular smooth muscle cell (VSMC) proliferation and migration, arterial calcification, and extracellular matrix remodeling (Wang and Khalid, 2018; Zhang et al., 2021). Such injury-induced vascular remodeling is primarily due to excessive proliferation and migration of VSMCs and medial VSMC invasion into the intimal space, eventually leading to neointimal formation.
Zhu et al. (2019) reported that TQ (10, 12.5, 15 μmol/L) suppressed platelet-derived growth factor (PDGF, 40 ng/ml)-induced VSMC proliferation at 24 h. Furthermore, TQ decreased α-smooth muscle actin and Ki-67-positive cells, confirming the antiproliferative effect of TQ on VSMCs. Additionally, TQ (5–15 μmol/L) attenuated PDGF-stimulated VSMC migration, and TQ (15 μmol/L) blocked the activity and expression of matrix metalloproteinase 2 (MMP-2) in VSMCs at 24 h (Zhu et al., 2019). MMP-2 is involved in VSMC migration via extracellular matrix degradation (Xiao et al., 2018). Inhibition of p38 activation also blocked MMP-2 expression (Zhu et al., 2019). Hence, p38 might be responsible for the inhibitory effect of TQ on MMP-2 expression. TQ treatment increased the number of apoptotic VSMCs in the presence of reactive oxygen species (Zhu et al., 2019). The results showed that TQ abolished the upregulation of B-cell lymphoma 2 (Bcl-2), cleaved caspase 3, and cleaved poly (ADP-ribose) polymerase, and blocked Bcl-2-associated X protein (Bax) downregulation. It has been suggested that the pro-apoptotic effect of TQ is mediated via the mitochondria-dependent apoptosis pathway. Zhu et al. (2019) also documented that 8 mg/kg and 16 mg/kg TQ stopped the increase in neointimal area and neointima/media ratio, and attenuated neointimal formation in atherosclerosis at 14 days using a rat carotid artery ligation model. Therefore, the inhibitory activity of TQ on VSMC proliferation and migration may be attributed to the blockade of p38 MAPK activation.
A single intraperitoneal (i.p.) dose of TQ was administered to BALB/c mice at doses ranging from 10 to 80 mg/kg body weight to test the oxidative effect of TQ after 24 h (Table 1). TQ at 40 and 80 mg/kg caused a considerable increase in malondialdehyde levels and catalase activity in the kidneys and liver (Harzallah et al., 2012). Oral acute toxicity of TQ from doses 0.5–3 g/kg was evaluated in male Swiss albino mice (Badary et al., 1998). Death occurred within the first 3 h associated with hypoactivity and respiratory problems, particularly with 2 or 3 g/kg TQ. No mortality was reported until 24 h. There was an increase of plasma activity of alanine aminotransferase, lactate dehydrogenase, creatinine phosphokinase, and increased plasma concentrations of urea and creatinine with 2 or 3 g/kg TQ. Besides, a reduction of reduced glutathione levels was reported (Table 1).
Al-Ali et al. (2008) showed that the LD50 values for TQ in albino mice were 104.7 and 870.9 mg/kg after i. p. and oral administration, respectively. Furthermore, LD50 values for i. p. injection and oral ingestion of TQ in Wistar rats were recorded as 57.5 and 794.3 mg/kg, respectively. Abukhader (2012) revealed that the maximum tolerated doses (MTDs) for i. p. TQ injection were 22.5 and 15 mg/kg in male and female rats, respectively, whereas the MTD for oral TQ was 250 mg/kg in both male and female rats. Thus, TQ is regarded as a reasonably safe drug, particularly when administered orally.
Acute toxicity was compared between encapsulated TQ in a nanostructured lipid carrier (TQNLC) and TQ in female BALB/c mice (Ong et al., 2016). Mice administered with 300 mg/kg TQ died within 24 h. In contrast, a mouse treated with 300 mg/kg TQNLC died after 24 h. In the subacute toxicity study (Ong et al., 2016), oral administration of 100 mg/kg TQNLC or TQ for 28 days did not cause mortality in either male or female mice.
A single injection of 25 mg/kg TQNLC was administered to the tail of female Sprague-Dawley rats (Yazan et al., 2019). The same dose was administered to the other rats at 48 h intervals. Intravenous administration of 25 mg/kg TQNLC did not induce toxicity in rats during the 14-days observation period. Male and female Sprague-Dawley rats were observed for 14 days after receiving a single dose of TQ-rich fraction nano-emulsion at 20 ml/kg (Tubesha et al., 2013). The animals appeared normal and healthy throughout the study (Table 1).
In summary, the route of administration can influence the severity of TQ-induced toxicity. Oral administration has a better safety profile than i. p. injections. Compared to that of TQ alone, the use of TQ together with nanostructured lipid carriers or nano-emulsions has less evidence of toxicity, suggesting their potential use during TQ administration.
Male Swiss albino mice were administered 30, 60, or 90 mg/kg TQ for 90 days via drinking water (Badary et al., 1998). No signs of toxicity were noted (Table 1).
Decreased maternal body weight and complete fetal resorption were reported after a single i. p. injection of 35 mg/kg or 50 mg/kg TQ to pregnant rats on day 11 of gestation (Abukhader et al., 2013). Administration of 50 mg/kg TQ on day 14 resulted in a higher incidence of fetal resorption, and viable fetuses did not show malformations (Table 1). Complete pregnancy loss was reported in pregnant Wistar rats administered 80 mg/kg TQ orally at the second gestational week for 7 days (Abdollahzade Fard et al., 2021). Reduced offspring body weight was recorded on postnatal days 14 and 21 by TQ (oral, 40 mg/kg). However, pregnant rats treated with TQ at gestation week 3 did not show such toxicity. In conclusion, i. p. injection of TQ between 35 mg/kg and 50 mg/kg during gestation has exhibited teratogenicity, suggesting that doses lower than 35 mg/kg could be safer to avoid fetal abnormalities or deformities. Moreover, failed pregnancy is associated with TQ administered orally at 80 mg/kg and at gestation week 2. Therefore, prenatal TQ administration should be carefully assessed.
Although N. sativa L. has long been used for treating diseases and enhancing general health, research into its therapeutic potential and mechanisms of action has just begun. Metabolomics is a useful technology for analyzing the chemical composition of N. sativa L. to allow its authentication and to ensure uniformity in bioactivity for quality control (Farag et al., 2021). Limited studies have investigated the anti-inflammatory effects of TQ in atherosclerosis. No positive controls were used in the available published studies. The comparative anti-inflammatory effects of TQ cannot be appreciated. Hence, future studies should incorporate positive controls to validate the effectiveness of TQ as an anti-inflammatory agent. Previous studies have indicated the possible involvement of the NF-κB and MAPK pathways in mediating the anti-inflammatory effects of TQ. However, its direct involvement in such signaling pathways requires exploration. Further investigation is warranted to identify the associated pathways and to determine the molecular targets that mediate the protective effects of TQ in atherosclerosis.
TQ has been shown to be toxic in vitro and in vivo studies, indicating the requirement for more in-depth research to provide a more complete toxicological profile for TQ before considering this promising natural product as a therapeutic agent for human use. The TQ dosage required to achieve optimal anti-inflammatory benefits in humans remains unknown and requires further investigation. Moreover, the protective effects of TQ have yet to be verified in clinical trials, and more safety assessments are needed to determine the potential toxicities of TQ for long-term use in humans. Therefore, more research is required to confirm its traditional use as a therapy for atherosclerosis.
X-FL and KC designed, wrote and revised the manuscript. AA wrote the manuscript. All authors read and approved the final version of the manuscript.
The authors declare that the research was conducted in the absence of any commercial or financial relationships that could be construed as a potential conflict of interest.
The handling editor declared a shared affiliation, though no other collaboration, with one of the authors XL.
All claims expressed in this article are solely those of the authors and do not necessarily represent those of their affiliated organizations, or those of the publisher, the editors and the reviewers. Any product that may be evaluated in this article, orclaim that may be made by its manufacturer, is not guaranteed or endorsed by the publisher.
The authors would like to thank Universiti Kebangsaan Malaysia, Geran Penyelidikan Khas (GPK): 600-RMC/GPK 5/3 (269/2020) from Universiti Teknologi MARA and Universiti Sains Islam Malaysia for supporting this work.
Abd-Elkareem, M., Abd El-Rahman, M. A. M., Khalil, N. S. A., and Amer, A. S. (2021). Antioxidant and Cytoprotective Effects of Nigella Sativa L. Seeds on the Testis of Monosodium Glutamate Challenged Rats. Sci. Rep. 11, 13519. doi:10.1038/s41598-021-92977-4
Abdollahzade Fard, A., Saboory, E., Tahmazi, Y., Rasmi, Y., Dindarian, S., and Parsamanesh, N. (2021). Effect of Orally-Administrated Thymoquinone during Pregnancy on Litter Size, Pentylenetetrazol-Induced Seizure, and Body Weight in Rat Offspring. Iran J. Basic Med. Sci. 24, 30–37. doi:10.22038/ijbms.2020.47479.10930
Abukhader, M. M., Khater, S. H., and Al-Matubsi, H. Y. (2013). Acute Effects of Thymoquinone on the Pregnant Rat and Embryo-Fetal Development. Drug Chem. Toxicol. 36, 27–34. doi:10.3109/01480545.2011.648326
Abukhader, M. M. (2012). The Effect of Route of Administration in Thymoquinone Toxicity in Male and Female Rats. Indian J. Pharm. Sci. 74, 195–200. doi:10.4103/0250-474X.106060
Abulfadl, Y. S., El-Maraghy, N. N., Ahmed, A. A. E., Nofal, S., and Badary, O. A. (2018). Protective Effects of Thymoquinone on D-Galactose and Aluminum Chloride Induced Neurotoxicity in Rats: Biochemical, Histological and Behavioral Changes. Neurol. Res. 40, 324–333. doi:10.1080/01616412.2018.1441776
Ahmad, A., Alkharfy, K. M., Jan, B. L., Ahad, A., Ansari, M. A., Al-Jenoobi, F. I., et al. (2020). Thymoquinone Treatment Modulates the Nrf2/HO-1 Signaling Pathway and Abrogates the Inflammatory Response in an Animal Model of Lung Fibrosis. Exp. Lung Res. 46, 53–63. doi:10.1080/01902148.2020.1726529
Al-Ali, A., Alkhawajah, A. A., Randhawa, M. A., and Shaikh, N. A. (2008). Oral and Intraperitoneal LD50 of Thymoquinone, an Active Principle of Nigella Sativa, in Mice and Rats. J. Ayub. Med. Coll. Abbottabad. 20, 25–27.
Alagawany, M., Elnesr, S. S., Farag, M. R., Abd El-Hack, M. E., Khafaga, A. F., Sharun, K., et al. (2021). ““Health-Promoting Activities of Nigella Sativa Essential Oil,” in Black Cumin (Nigella Sativa),” in Seeds: Chemistry, Technology, Functionality, and Applications. Food Bioactive Ingredients. Editor M. Fawzy Ramadan (Cham: Springer). doi:10.1007/978-3-030-48798-0_29
Alkharfy, K. M., Ahmad, A., Jan, B. L., and Raish, M. (2018). Thymoquinone Reduces Mortality and Suppresses Early Acute Inflammatory Markers of Sepsis in a Mouse Model. Biomed. Pharmacother. 98, 801–805. doi:10.1016/j.biopha.2018.01.028
Amartey, J., Gapper, S., Hussein, N., Morris, K., and Withycombe, C. E. (2019). Nigella Sativa Extract and Thymoquinone Regulate Inflammatory Cytokine and TET-2 Expression in Endothelial Cells. Artery Res. 25, 157–163. doi:10.2991/artres.k.191114.002
Badary, O. A., Al-Shabanah, O. A., Nagi, M. N., Al-Bekairi, A. M., and Elmazar, M. M. A. (1998). Acute and Subchronic Toxicity of Thymoquinone in Mice. Drug Dev. Res. 44, 56–61. doi:10.1002/(sici)1098-2299(199806/07)44:2/3<56:aid-ddr2>3.0.co;2-9
Barreto, J., Karathanasis, S. K., Remaley, A., and Sposito, A. C. (2021). Role of LOX-1 (Lectin-like Oxidized Low-Density Lipoprotein Receptor 1) as a Cardiovascular Risk Predictor: Mechanistic Insight and Potential Clinical Use. Arterioscler. Thromb. Vasc. Biol. 41, 153–166. doi:10.1161/ATVBAHA.120.315421
Borges, P. V., Moret, K. H., Raghavendra, N. M., Maramaldo Costa, T. E., Monteiro, A. P., Carneiro, A. B., et al. (2017). Protective Effect of Gedunin on TLR-Mediated Inflammation by Modulation of Inflammasome Activation and Cytokine Production: Evidence of a Multitarget Compound. Pharmacol. Res. 115, 65–77. doi:10.1016/j.phrs.2016.09.015
Bukar, M. A., Ishaya, H. B., Dibal, N. I., and Attah, M. O. (2017). Gastroprotective Effect of Nigella Sativa Seed on Ethanol-Induced Gastric Ulcer in Rats. Libyan J. Med. Sci. 1, 63–67. doi:10.4103/LJMS.LJMS_23_17
Bule, M., Nikfar, S., Amini, M., and Abdollahi, M. (2020). The Antidiabetic Effect of Thymoquinone: A Systematic Review and Meta-Analysis of Animal Studies. Food Res. Int. 127, 108736. doi:10.1016/j.foodres.2019.108736
Chan, L. P., Liu, C., Chiang, F. Y., Wang, L. F., Lee, K. W., Chen, W. T., et al. (2017). IL-8 Promotes Inflammatory Mediators and Stimulates Activation of P38 MAPK/ERK-NF-κB Pathway and Reduction of JNK in HNSCC. Oncotarget 8, 56375–56388. doi:10.18632/oncotarget.16914
Chen, L., Deng, H., Cui, H., Fang, J., Zuo, Z., Deng, J., et al. (2018). Inflammatory Responses and Inflammation-Associated Diseases in Organs. Oncotarget 9, 7204–7218. doi:10.18632/oncotarget.23208
Christian, F., Smith, E. L., and Carmody, R. J. (2016). The Regulation of NF-Κb Subunits by Phosphorylation. Cells 5, 12. doi:10.3390/cells5010012
Edris, A. E. (2021). in “Thymoquinone: Chemistry and Functionality,” in Black Cumin (Nigella Sativa) Seeds: Chemistry, Technology, Functionality, and Applications. Food Bioactive Ingredients. Editor M. Fawzy Ramadan (Cham: Springer). doi:10.1007/978-3-030-48798-0_8
Enayatfard, L., Mohebbati, R., Niazmand, S., Hosseini, M., and Shafei, M. N. (2018). The Standardized Extract of Nigella Sativa and its Major Ingredient, Thymoquinone, Ameliorates Angiotensin II-Induced Hypertension in Rats. J. Basic Clin. Physiol. Pharmacol. 30, 51–58. doi:10.1515/jbcpp-2018-0074
Farag, M. A., Saad, H. H., and Hegazi, N. M. (2021). “Rediscovering Nigella Seeds Bioactives Chemical Composition Using Metabolomics Technologies,” in ” in Black Cumin (Nigella Sativa) Seeds: Chemistry, Technology, Functionality, and Applications. Food Bioactive Ingredients. Editor M. Fawzy Ramadan (Cham: Springer). doi:10.1007/978-3-030-48798-0_10
Fuster, J. J., MacLauchlan, S., Zuriaga, M. A., Polackal, M. N., Ostriker, A. C., Chakraborty, R., et al. (2017). Clonal Hematopoiesis Associated with TET2 Deficiency Accelerates Atherosclerosis Development in Mice. Science 355, 842–847. doi:10.1126/science.aag1381
Getz, G. S., and Reardon, C. A. (2016). Do the Apoe-/- and Ldlr-/- Mice Yield the Same Insight on Atherogenesis? Arterioscler. Thromb. Vasc. Biol. 36, 1734–1741. doi:10.1161/ATVBAHA.116.306874
Grebe, A., Hoss, F., and Latz, E. (2018). NLRP3 Inflammasome and the IL-1 Pathway in Atherosclerosis. Circ. Res. 122, 1722–1740. doi:10.1161/CIRCRESAHA.118.311362
Harzallah, H. J., Rjiba, K., Maaloul, A., Abid-Essafi, S., and Mahjoub, T. (2012). Oxidative and Genotoxic Effects of Thymoquinone, Nigella Sativa Active compoundBalb/c Mice. Afr. J. Food Sci. 6, 529–534. doi:10.5897/AJFS12.06610.5897/ajmr11.1073
Libby, P. (2021). Inflammation in Atherosclerosis-No Longer a Theory. Clin. Chem. 67, 131–142. doi:10.1093/clinchem/hvaa275
Lin, C. S., Lin, F. Y., Ho, L. J., Tsai, C. S., Cheng, S. M., Wu, W. L., et al. (2012). PKCδ Signalling Regulates SR-A and CD36 Expression and Foam Cell Formation. Cardiovasc. Res. 95, 346–355. doi:10.1093/cvr/cvs189
Liu, T., Zhang, L., Joo, D., and Sun, S. C. (2017). NF-κB Signaling in Inflammation. Signal. Transduct. Target. Ther. 2, 17023. doi:10.1038/sigtrans.2017.23
Majdalawieh, A. F., Yousef, S. A., and Abu-Yosef, I. A. (2021). Thymoquinone, a Major Constituent in Nigella Sativa Seeds, Is a Potential Preventative and Treatment Option for Atherosclerosis. Eur. J. Pharmacol. 909, 174420. doi:10.1016/j.ejphar.2021.174420
Minelli, S., Minelli, P., and Montinari, M. R. (2020). Reflections on Atherosclerosis: Lesson from the Past and Future Research Directions. J. Multidiscip. Healthc. 13, 621–633. doi:10.2147/JMDH.S254016
Mouwakeh, A., Telbisz, Á., Spengler, G., Mohácsi-Farkas, C., and Kiskó, G. (2018). Antibacterial and Resistance Modifying Activities of Nigella Sativa Essential Oil and its Active Compounds against. Listeria Monocytogenes 32, 737–743. doi:10.21873/invivo.11230210.21873/invivo.11302
Mussbacher, M., Salzmann, M., Brostjan, C., Hoesel, B., Schoergenhofer, C., Datler, H., et al. (2019). Cell Type-specific Roles of NF-Κb Linking Inflammation and Thrombosis. Front. Immunol. 10, 85. doi:10.3389/fimmu.2019.00085
Ong, Y. S., Saiful Yazan, L., Ng, W. K., Noordin, M. M., Sapuan, S., Foo, J. B., et al. (2016). Acute and Subacute Toxicity Profiles of Thymoquinone-Loaded Nanostructured Lipid Carrier in BALB/c Mice. Int. J. Nanomedicine 11, 5905–5915. doi:10.2147/IJN.S114205
Pei, Z. W., Guo, Y., Zhu, H. L., Dong, M., Zhang, Q., and Wang, F. (2020). Thymoquinone Protects against Hyperlipidemia-Induced Cardiac Damage in Low-Density Lipoprotein Receptor-Deficient (LDL-R-/-) Mice via its Anti-inflammatory and Antipyroptotic Effects. Biomed. Res. Int. 2020, 4878704. doi:10.1155/2020/4878704
Pirillo, A., Norata, G. D., and Catapano, A. L. (20132013). LOX-1, OxLDL, and Atherosclerosis. Mediators Inflamm., 152786. doi:10.1155/2013/152786
Rhoads, J. P., and Major, A. S. (2018). How Oxidized Low-Density Lipoprotein Activates Inflammatory Responses. Crit. Rev. Immunol. 38, 333–342. doi:10.1615/CritRevImmunol.2018026483
Roth, G. A., Mensah, G. A., Johnson, C. O., Addolorato, G., Ammirati, E., Baddour, L. M., et al. (2020). Global burden of Cardiovascular Diseases and Risk Factors, 1990-2019: Update from the GBD 2019 Study. J. Am. Coll. Cardiol. 76, 2982–3021. doi:10.1016/j.jacc.2020.11.010
Salehi, B., Quispe, C., Imran, M., Ul-Haq, I., Živković, J., Abu-Reidah, I. M., et al. (2021). Sen S, Nigella Plants - Traditional Uses, Bioactive Phytoconstituents, Preclinical and Clinical Studies. Front. Pharmacol. 12, 625386. doi:10.3389/fphar.2021.625386
Sun, L. F., An, D. Q., Niyazi, G. L., Ma, W. H., Xu, Z. W., and Xie, Y. (2018). Effects of Tianxiangdan Granule Treatment on Atherosclerosis via NF-Κb and P38 MAPK Signaling Pathways. Mol. Med. Rep. 17, 1642–1650. doi:10.3892/mmr.2017.8067
Sun, Y., Liu, W. Z., Liu, T., Feng, X., Yang, N., and Zhou, H. F. (2015). Signaling Pathway of MAPK/ERK in Cell Proliferation, Differentiation, Migration, Senescence and Apoptosis. J. Recept. Signal. Transduct. Res. 35, 600–604. doi:10.3109/10799893.2015.1030412
Tavakkoli, A., Mahdian, V., Razavi, B. M., and Hosseinzadeh, H. (2017). Review on Clinical Trials of Black Seed (Nigella Sativa) and its Active Constituent, Thymoquinone. J. Pharmacopuncture 20, 179–193. doi:10.3831/KPI.2017.20.021
Tubesha, Z., Imam, M. U., Mahmud, R., and Ismail, M. (2013). Study on the Potential Toxicity of a Thymoquinone-Rich Fraction Nanoemulsion in Sprague Dawley Rats. Molecules 18, 7460–7472. doi:10.3390/molecules18077460
Wang, X., and Khalil, R. A. (2018). Matrix Metalloproteinases, Vascular Remodeling, and Vascular Disease. Adv. Pharmacol. 81, 241–330. doi:10.1016/bs.apha.2017.08.002
Xiao, X. L., Hu, N., Zhang, X. Z., Jiang, M., Chen, C., Ma, R., et al. (2018). Niclosamide Inhibits Vascular Smooth Muscle Cell Proliferation and Migration and Attenuates Neointimal Hyperplasia in Injured Rat Carotid Arteries. Br. J. Pharmacol. 175, 1707–1718. doi:10.1111/bph.14182
Xu, J., Zhu, L., Liu, H., Li, M., Liu, Y., Yang, F., et al. (2018). Thymoquinone Reduces Cardiac Damage Caused by Hypercholesterolemia in Apolipoprotein E-Deficient Mice. Lipids Health Dis. 17, 173. doi:10.1186/s12944-018-0829-y
Yazan, L. S., Mohd Azlan, S. N., Ansar, F. H. Z., and Gopalsamy, B. (2019). Acute Toxicity Study of Intravenous Administration of Thymoquinone-Loaded Nanostructured Lipid Carrier (TQ-NLC) in Sprague Dawley Rat. Mal. J. Med. Health Sci. 15, 51–57.
Zhang, L., Yao, J., Yao, Y., and Boström, K. I. (2021). Contributions of the Endothelium to Vascular Calcification. Front. Cel. Dev. Biol. 9, 620882. doi:10.3389/fcell.2021.620882
Zhang, Q. (2018). Global Situation and WHO Strategy on Traditional Medicine. Tradit. Med. Mod. Med. 1, 11–13. doi:10.1142/S257590001820001X
Zhang, Z., Zhang, D., Du, B., and Chen, Z. (2017). Hyperoside Inhibits the Effects Induced by Oxidized Low-Density Lipoprotein in Vascular Smooth Muscle Cells via oxLDL-LOX-1-ERK Pathway. Mol. Cel. Biochem. 433, 169–176. doi:10.1007/s11010-017-3025-x
Zhao, Y., Yang, Y., Xing, R., Cui, X., Xiao, Y., Xie, L., et al. (2018). Hyperlipidemia Induces Typical Atherosclerosis Development in Ldlr and Apoe Deficient Rats. Atherosclerosis 271, 26–35. doi:10.1016/j.atherosclerosis.2018.02.015
Keywords: atherosclerosis, inflammation, thymoquinone, nuclear factor-kappa B, mitogen-activated protein kinase
Citation: Leong X-F, Choy KW and Alias A (2021) Anti-Inflammatory Effects of Thymoquinone in Atherosclerosis: A Mini Review. Front. Pharmacol. 12:758929. doi: 10.3389/fphar.2021.758929
Received: 16 August 2021; Accepted: 23 November 2021;
Published: 15 December 2021.
Edited by:
Yusof Kamisah, Universiti Kebangsaan Malaysia, MalaysiaReviewed by:
Ajaz Ahmad, King Saud University, Saudi ArabiaCopyright © 2021 Leong, Choy and Alias. This is an open-access article distributed under the terms of the Creative Commons Attribution License (CC BY). The use, distribution or reproduction in other forums is permitted, provided the original author(s) and the copyright owner(s) are credited and that the original publication in this journal is cited, in accordance with accepted academic practice. No use, distribution or reproduction is permitted which does not comply with these terms.
*Correspondence: Xin-Fang Leong, bGVvbmd4aW5mYW5nQHVrbS5lZHUubXk=; Ker Woon Choy, Y2hveWtlcndvb25AdWl0bS5lZHUubXk=
Disclaimer: All claims expressed in this article are solely those of the authors and do not necessarily represent those of their affiliated organizations, or those of the publisher, the editors and the reviewers. Any product that may be evaluated in this article or claim that may be made by its manufacturer is not guaranteed or endorsed by the publisher.
Research integrity at Frontiers
Learn more about the work of our research integrity team to safeguard the quality of each article we publish.