- 1Department of Pharmacy, The Second Xiangya Hospital, Central South University, Changsha, China
- 2Institute of Clinical Pharmacy, Central South University, Changsha, China
Currently, herbal and dietary supplements have been widely applied to prevent and treat various diseases. However, the potential toxicities and adverse reactions of herbal and dietary supplements have been increasingly reported, and have gradually attracted widespread attention from clinical pharmacists and physicians. Metabolic activation of specific natural products from herbal and dietary supplements is mediated by hepatic cytochrome P450 or intestinal bacteria, and generates chemical reactive/toxic metabolites that bind to cellular reduced glutathione or macromolecules, and form reactive metabolites-glutathione/protein/DNA adducts, and these protein/DNA adducts can result in toxicities. The present review focuses on the relation between metabolic activation and toxicities of natural products, and provides updated, comprehensive and critical comment on the toxic mechanisms of reactive metabolites. The key inductive role of metabolic activation in toxicity is highlighted, and frequently toxic functional groups of toxic natural products were summarized. The biotransformation of drug cytochrome P450 or intestinal bacteria involved in metabolic activation were clarified, the reactive metabolites-protein adducts were selected as biomarkers for predicting toxicity. And finally, further perspectives between metabolic activation and toxicities of natural products from herbal and dietary supplements are discussed, to provide a reference for the reasonable and safe usage of herbal and dietary supplements.
1 Introduction
Herbal and dietary supplements are widely used to treat and prevent various diseases for thousands of years worldwide, particularly in Asian countries, including China (Liu et al., 2016), Japan (Watanabe et al., 2011; Hayasaka et al., 2012), North Korea (Jang et al., 2017) and India (Phondani et al., 2010). The usage of herbal and dietary supplements and their preparations or formulation has increased rapidly worldwide over the past three decades. Currently, the curative effects of medicinal plants are obtaining the approval of clinicians in Europe and North American, and the application of herbal remedies has been continuously increasing in complementary and alternative medicine. Approximately 18% of American adults would choose natural drug preparations for treating illnesses (Ekor, 2013). Concomitantly, an increasing number of toxic herbal and dietary supplements are being discovered, reported and verified at the animal and clinical level. Nevertheless, the potential toxicities and adverse reactions greatly limit the reasonable and safe usage of herbal and dietary supplements in clinical.
Hepatotoxicity and nephrotoxicity are two major risk factors to cause synthetic drugs or natural drugs withdraw from the market. Moreover, the prevalence of multiple toxicity caused by herbal and dietary supplements is increasing in the worldwide. The hepatotoxicity (Teschke et al., 2015) and nephrotoxicity (Feng et al., 2018) related to clinical cases caused by traditional Chinese medicine also exist extensively in China, owing to inappropriate use of Chinese medicinal herb (large dosages and/or long-term usage). In addition, herbal and dietary supplements induced liver injury now accounts for 20% of cases of hepatotoxicity in the United States (Navarro et al., 2016). Differ from synthetic drug, natural products from herbal medicines and dietary supplements are more complex and mostly uncertain. The variety of natural products, complexity of multi-ingredient supplements, and unknown concentrations, as well as additional unlabeled substances make the diagnosis and prognostication of toxicity challenging and require further research and attention by the scientific community.
Natural products are widely distributed in herbal and dietary supplements. natural products researcher have isolated and identified a large number of toxic or pre-toxic natural products from medicinal plants or diet (Bunchorntavakul and Reddy, 2012; Posadzki et al., 2013), which usually lead to reversible or irreversible acute organs injury towards animal and humans, and even death. In general, these pre-toxic natural products usually exhibit chemical inertness, and require metabolic activation to form reactive metabolites, which elicit their toxic effects to a certain extent. Extensive research has demonstrated that drug metabolizing enzymes or intestinal bacteria, especially cytochrome P450s (CYP450s), mediate the majority of metabolic activation process, and play a vital catalytic action in formatting reactive intermediates/metabolites. Phase II Enzymes glucuronosyltransferase (UGTs), glutathione S-transferase (GSTs) and sulfotransferases (SULT) mediate the minority of metabolic activation, which closely related to toxicities. Sulfotransferases involve in the metabolic activation of estrogenic (Reinen and Vermeulen, 2015) and aloe-emodin (Li et al., 2019a). UGTs 1A1, 1A9, and 2B7 metabolic activation of rhein responsible for reactive metabolites (Yuan et al., 2016). These reactive intermediates/metabolites are chemically active and electrophilic, if not quenched timely by endogenous nucleophiles, such as antidote glutathione (GSH), or cysteine (Cys). Overdose reactive intermediates/reactive metabolites are also liable to covalently bind to cellular proteins or DNA (Yan et al., 2008), and eventually initiate and trigger a series of toxic effects, including protein abnormal modification, enzyme inactivation, DNA crosslink, formation of immunogenic species, cell death, or oncogene activation, organ injury (Liu et al., 2016), Therefore, metabolic activations is the critical initiating factor responsible for toxicity, and these intermediates/reactive metabolites are usually toxic metabolites of natural products.
The present review focuses on the toxicity of natural products, analyzing the key role of metabolic activation in their toxicities. According to the different catalysis of metabolic activation, they are divided into CYP450s mediated metabolic activation and intestinal bacteria mediated metabolic activation. Moreover, CYP450s mediated metabolic activation are categorized into three categories, pyrrolizidine alkaloids, furan derivatives, epoxy diterpenoids, anthraquinones aristolochic acids, bisbenzylisoquinoline, alkenylbenzenes, based on the types of potentially toxic natural products.
2 CYP450s Mediated Metabolic Activation of Natural Products Leading to Toxicity
2.1 Metabolic Activation of Pyrrolizidine Alkaloids Leading to Hepatotoxicity, Phototoxicity and Pulmonary Toxicity
Pyrrolizidine alkaloids (PAs) are the class of common toxic natural products widely distributed in over 600 herbals around the world, and are found in approximately 3% of the world’s flowering plants (Robertson and Stevens, 2017). Multiple PAs mainly exist in species of the plant families Boraginaceae (all genera), Compositae (tribes Senecionae and Eupatoriae) and Leguminosae (genus Crotalaria) (Watanabe et al., 2011), and they are also could be isolated from multiple medicinal plants, including crotalaria mucronata, crotalaria sessiiflora, senecio scandens, gynura japonica, tussilago farfara, eupatorium japonicum, heliotropium europaeum, eupatorium fortunei, arnebia euchroma, tephroseris kirilowii, lithospermum erythrorhizon. They are also frequently applied in clinical practice of traditional Chinese medicine (Tang and Hattori, 2011). Moreover, consumers can be easily exposed to these hepatotoxic PAs through consumption of PAs contained in herbal and dietary supplements, for instance, herbal tea (Merz and Schrenk, 2016), and dietary components such as milk and honey (Griffin et al., 2015). Currently, PAs-caused hepatic damage has become a world-wide problem of drugs and food safety, and seriously threatened people’s health.
More than 600 PAs and PA N-oxide derivatives have been isolated from medicinal plants at present, and the great majority of them exhibit obvious hepatic damage towards human and animal. The PAs with an α,β-unsaturated necine skeleton in the structure can exert multiple toxic effects, such as hepatotoxicity (Neuman et al., 2015), genotoxicity (Fu et al., 2004), cytotoxicity (Forsch et al., 2017), phototoxicity, and photogenotoxicity (Wang et al., 2014a). Therefore, the unsaturated necine base is toxic functional group of hepatotoxic PAs. PAs exert hepatotoxicity through metabolic activation by hepatic CYP450s to generate reactive intermediates. Unsaturated necine core type of PAs with a double bond in the base can show potent hepatotoxicity, owing to metabolic activation (Ruan et al., 2014a), whereas these natural products with saturated necine moiety does not cause liver damage (Ruan et al., 2014b). The formation of hepatotoxicity was assigned to the metabolic activation of PAs in the liver and generated the reactively toxic metabolites. Metabolic activation of PAs needs three steps: 1) Oxidation (dehydrogenation or aromatization) of otonecine-type, heliotricline-type and retronecine-type, then produces reactive intermediates of pyrrolic esters (didehydro-pyrrolizidine, DHP esters) mediated by CYP450s. Particularly, CYP3A4 can catalyze the oxidation reaction of PAs to form toxic metabolites. Molecular docking also simulated the role of CYP3A4, which displayed that the C3 of lasiocarpine and retrorsine and C26 of senkirkin were closest to the catalytic heme Fe (CYP3A4 active site); 2) Hydrolysis of DHP esters forms didehydro-pyrrolizidine (DHP) (necines and necic acids); 3) N-oxidation of PAs can produce PAs N-oxides. The formation of reactive pyrrolic intermediates (DHP, metabolic pyrrole) has been commonly regarded as the critical step for PAs-caused toxicities (Figure 1). Once formed, DHP tends to capture intracellular nucleophilic biomolecules rapidly, such as capture reaction with GSH to form GSH conjugates (Chen et al., 2016), or attack macromolecules DNA or proteins to form pyrrole-DNA conjugates (Xia et al., 2015) and pyrrole-protein adducts. It is generally accepted that most GSH addition reaction are a self-defending action against toxicities of PAs. Moreover, this reactive DHP can irreversibly bind to hepatic proteins, resulting in hepatotoxicity. Pyrrole-protein adducts were reported to have potential value as the non-invasive biomarkers of PAs -induced hepatotoxicity (Xia et al., 2016). Pyrrole-hemoglobin adducts, a more feasible potential biomarker of pyrrolizidine alkaloid exposure, was discovered (Ma et al., 2019).
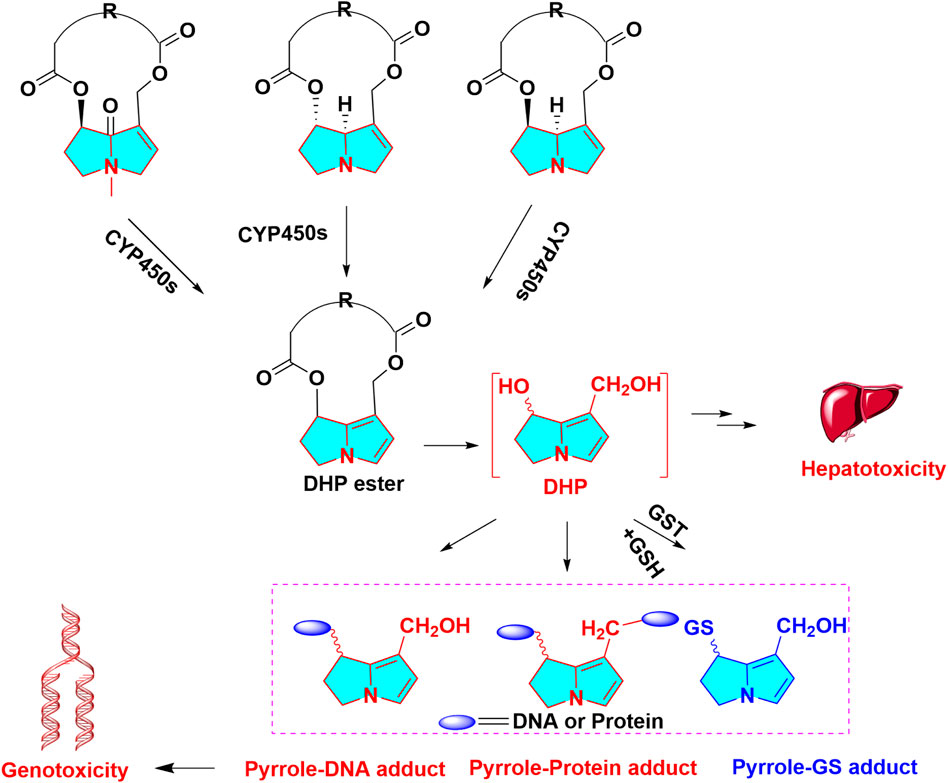
FIGURE 1. Metabolic activation in hepatotoxicity of PAs, otonecine-type PA, heliotridine-type PA and retronecine-type PA are oxidized to dehydro PAs, then dehydro PAs are hydrolyzed to DHP, reactive metabolites can capture GSH, protein, DNA, forming GSH, protein, DNA adduct, respectively.
Apart from hepatotoxicity, approximately half of the 660 PAs and PA N-oxides that have been characterized are cytotoxic (Forsch et al., 2017), genotoxic (Fu et al., 2004), tumorigenic (Neuman et al., 2015; Fu, 2017), phototoxic and photogenotoxic (Wang et al., 2014a). Metabolic activation pathways of PAs can lead to obvious liver tumor initiation. PAs can induce DNA adduction, DNA damage, and activation of tumorigenic hepatic progenitor cells, which initiate hepatocarcinogenesis. Pyrrolizidine alkaloid-protein adducts were detected in 32% of surgically resected specimens from 34 patients with liver cancer in Hong Kong (He et al., 2021a). Hepatic cytochrome P450s mediated metabolic activation induced acute pulmonary injury (He et al., 2021b).
2.2 Metabolic Activation of Furan Derivatives-Induced Hepatotoxicity
Furan derivatives are important aromatic heterocyclic compounds. Early reports indicated that natural furan derivatives were present in numerous processed food, with the highest contents found in coffee. Teucrium chamaedrys, a traditional food and medicinal plant, rich in furan derivatives have been reported to exhibit multiple toxicities, especially for liver injury (Lekehal et al., 1996; Gori et al., 2011; Nencini et al., 2014). As potentially hepatotoxic natural products, furanoterpenoids are widely distributed in food, beverages, medicinal plants, and even marine organisms. Multiple furanoterpenoids, can be classified into three types of furanomonoterpenes, furanoditerpenes and furanotriterpenes (Huang et al., 2020). Reported hepatotoxic furanomonoterpenes majorly included 4-ipomeanol (Parkinson et al., 2016), (-)-ngaione (Ng, 1983), teucrin A, teuchamaedryn A (Lekehal et al., 1996), menthofuran (Lassila et al., 2016). Reported hepatotoxic furanoditerpenes contained teucrin A, teuchamaedryn A (Lekehal et al., 1996), diosbulbin D and diosbulbin E, columbin. Reported hepatotoxic furanotriterpenes contained toosendanin (Yu et al., 2014), rutaevin (Liu et al., 2020), and nomilin (Zhang et al., 2020a), distributed in Meliaceae medicinal plants.
As a medicinal plant, the rhizome of Dioscorea bulbifera, has been extensively used to treat tumors and struma in East Asia (Yonemitsu et al., 1993). Numerous clinical cases of liver injury have been reported after administration of D. bulbifera and its formulation (Jiang et al., 2004). Animal studies also revealed that oral administration of ethanol extracts of D. bulbifera could cause the significant liver injury, together with increased lipid peroxide levels in liver (Wang et al., 2010). Belonging to clane-type diterpene lactone with furane ring, diosbulbin B, diosbulbin D, and 8-epidiosbulbin E Acetate are principal constituents of D. bulbifera, which were verified to cause obvious liver toxicity towards on rat or mouse, respectively (Lin et al., 2015; Lin et al., 2016a; Lin et al., 2016b). Although there is no furan in the structure of pulegone, it can be initially biotransformed to menthofuran after metabolism (Thomassen et al., 1990), and menthofuran can further generate toxic metabolites, after metabolic activation (Ravindranath et al., 1984; Ravindranath et al., 1986). Generally, most furan derivatives are usually hepatoxic and/or carcinogenic. Metabolic activation of these pre-toxic furan derivatives is initially biotransformed by epoxidation, and generate a cis-enedione intermediate. These reactive intermediates can attack cellular nucleophiles (protein or DNA) to trigger toxicities, further leading to hepatotoxicity (Peterson, 2013). Diosbulbin B was elected as a representative case in this section to illustrate the role of metabolic activation. Diosbulbin B can cause obvious liver jury, while the furan ring of diosbulbin B was chemically reduced to a tetrahydrofuran moiety, no obvious liver damage was observed in animals after administration of tetrahydro-diosbulbin B. Therefore, the structure-toxicities relationship of furanoterpenoids revealed that unsaturated furan ring moiety is the hepatoxic functional group (Table 1). Meanwhile, CYP450s-mediated epoxidation occurred in the furan of diosbulbin B was regarded as metabolic activation, forming a reactive intermediate of cis-enedial (Yang et al., 2014; Lin et al., 2016a). The formation of cis-enedial intermediate in liver microsomes (Lin et al., 2014), and CYP3A4-transfected primary rat hepatocytes, HepG2 and L02 cells (Jiang et al., 2017) were significantly inhibited by the potent CYP3A inhibitor of ketoconazole (Lin et al., 2014; Jiang et al., 2017).
Further study disclosed the cis-enedial intermediate is substantially nucleophilic, which was liable to be trapped by N-acetyl lysine, N-acetyl cysteine (NAC) or GSH in rat and human liver. The covalent bind with free glutamyl-amine of GSH is conducted in Schiff-base manner to form N-linked conjugates. The exhaustion of hepatic GSH was also observed in diosbulbin B treated animals (Lin et al., 2014) (Figure 2). The sensitive mass spectrometry strategy has been built to detect furans reactive metabolites plus GSH conjugates, such as neutral loss scanning of 290.0573 Da in the positive ionization mode, and precursor ion scanning of m/z 143.0462+ in the negative ionization mode (Wang et al., 2014b). Moreover, cis-enedial-protein adduct can be analyzed, cysteine (Cys) and lysine (Lys) residue of protein could be easily captured by cis-enedial to form three kinds of protein abnormal modification, through Cys adduction, Schiff’s base, or Cys/Lys crosslink (Wang et al., 2017a), respectively. In addition, the protein adductions of reactive metabolites of furans were determinated as Cys-and Lys-based protein adductions with the reactive metabolites (Wang et al., 2015). Reactive metabolite of Teucrin A-protein adduct was used to identification of the protein targets of the Teucrin A, the protein targets was origin from mitochondrial and endoplasmic reticulum origin (Druckova et al., 2007).
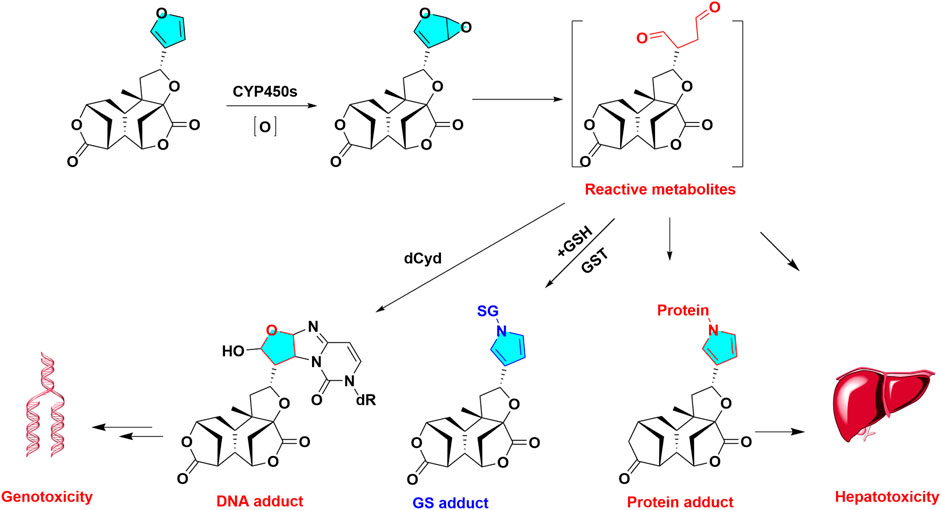
FIGURE 2. Metabolic activation diosbulbin B induced hepatotoxicity. Diosbulbin B is epioxidized and hydrolyzed to reactive metabolites of cis-Enedial, reactive metabolites can capture GSH, protein, DNA, forming GSH, protein, DNA adduct, respectively.
Apart from hepatotoxicity, furan has been classified as “possibly carcinogenic to human” by IARC, a great concern has been addressed to the detection of this substance naturally-occurring in food. Metabolic activation of diosbulbin B and 8-epidiosbulbin E acetate resulted in DNA adduction. In addition, furan ring is an essential toxic structural alert responsible for furan alkaloids of dictamnine which can cause hepatotoxicity, and epoxy of furan ring was also discovered in the metabolism of dictamnine. Furoquinoline alkaloid dictamnine, a furoquinoline alkaloid of the Rutaceae plant family, can resulted in carcinogenicity, cytotoxicity, and genotoxicity via CYP450s mediated metabolic activation. Moreover, a variety of furanocoumarins, including 8-methoxypsoralen and other furanocoumarins, can cause mechanism-based inactivation of CYP 450 (Koenigs and Trager, 1998).
2.3 Metabolic Activation of Epoxy Diterpenoids-Induced Hepatotoxicity
Belonging to the Celastraceae family, Tripterygium wilfordii Hook has been used for numerous centuries in traditional Chinese medicines for treatment of rheumatoid arthritis (Tao et al., 2002), immune complex nephritis and systemic lupus erythematosus (Kao et al., 2010). Previous study revealed the total epoxy diterpenes of T. wilfordii exhibited significant liver injury in clinical (Chai et al., 2011). Triptolide (TP) is an abietane type diterpene with triepoxy ring, one of many toxic ingredients of T. wilfordii. Although TP is a promising lead compound for treatment of rheumatoid arthritis, cancer, and erythema atrophicans, its clinical efficacy and safety are greatly limited by its obvious multiple toxicity, including hepatotoxicity and nephrotoxicity (Wang et al., 2017b).
Epoxy group are a toxic functional group. Owing to triepoxy ring, triptolide (parents) exhibit chemically reactive activity. The biotransformation of triptolide were hydrolysis and hydroxylation reactions in vivo metabolism (Peng et al., 2012; Liu et al., 2013). Total 8 NAC metabolites of triptolide were observed in rat urine. The formation of NAC or GSH conjugates indicated the metabolic activation of epoxy may occur in the metabolic metabolism (Du et al., 2011).The hepatotoxicity of triptolide can be affected by multiple factors, particularly drug metabolic enzymes and transporters. Previous report demonstrated that CYP450s-mediated metabolic activation played the key role in triptolide-induced hepatic damage towards rat hepatocyte (Zhuang et al., 2013). Triptolide induced liver injury could be attenuated by CYP450s broad spectrum inhibitor, 1-aminobenzotriazole (Zhuang et al., 2013). In addition, knockout of hepatic CYP 450 reductase could exacerbate triptolide -induced toxicity in mice (Xue et al., 2011), while pretreatment with CYP3A inducer of dexamethasone could protect against triptolide originated liver injury in rat (Ye et al., 2010), suggested CYP 450s mediated the metabolic activation of triptolide, leading to hepatotoxicity.
Metabolic epoxidation is a critical step in the progress of specifying xenobiotics-induced hepatotoxicity (Wang et al., 2017c). Triptolide possesses multiple obvious potent toxicities, which may be related to the bioactivation of structural alerts (triepoxy) in the metabolism. Owing to the remarkable toxicity, herbal prescriptions containing T. wilfordii or T. hypoglaucum should be carefully administered in clinical.
2.4 Metabolic Activation of Anthraquinones-Induced Hepatotoxicity
Anthraquinones are a class of functionally diverse natural products structurally related to anthracene, which widely exist in Polygonaceae medicinal plants, including Fallopia multiflora, Rheum palmatum and Aloe vera, these medicinal plants are massively consumed in the world. Anthracene possesses significant liver injury, anthraquinone is chemically similar to anthracene, there is a potential risk of hepatotoxicity underlie the application of anthraquinones. These anthraquinones may generate reactive metabolites in the metabolic activation process. The toxic anthraquinones and their metabolic activation are clearly shown in Table 1.
Polygoni multiflori radix is a popular medicinal plant, extensively used in China, Japan and Korea, which show anti-aging effect and health care value. However, polygoni multiflora and their total anthraquinones were reported to cause hepatotoxicity clinically (Furukawa et al., 2010; Kyoung Ah et al., 2011). Emodin is a common anthraquinone component widely distributed in Polygonaceae plants, such as polygoni multiflori and polygonum multiflorum. Three phase I metabolites of emodin, including 2-hydroxyemodin, 5-hydroxyemodin, and ω-hydroxyemodin, were observed in CYP1A2 and CYP2C19 incubations. Three hydroxylated metabolites of emodin were found to be electrophilic species, reactive to NAC and GSH (Qin et al., 2016). Another anthraquinone compound, physcion, and its oxidative metabolites were also reported to be conjugated with NAC and GSH after metabolic activation (Qin et al., 2018). Based on structure-toxicity relationship analysis, the para-quinone was confirmed as toxic moiety in the structure of anthraquinones. CYP450s mediated metabolic activation was closely associated with the toxicity of anthraquinones.
Para-Quinone was characterized as toxic functional group and structural alert of anthraquinones. Owing to the chemically reactive activity of para-quinone, epoxidation firstly occurs in the metabolism of anthraquinones and forms reactive metabolites, which can further conjugate with nucleophilic substance, including NAC and GSH. The depletion of GSH would aggravate the anthraquinones induced hepatotoxicity (Figure 3). Currently, the related data about mechanism of anthraquinones-caused hepatotoxicity and nephrotoxicity is limited, which need further research.
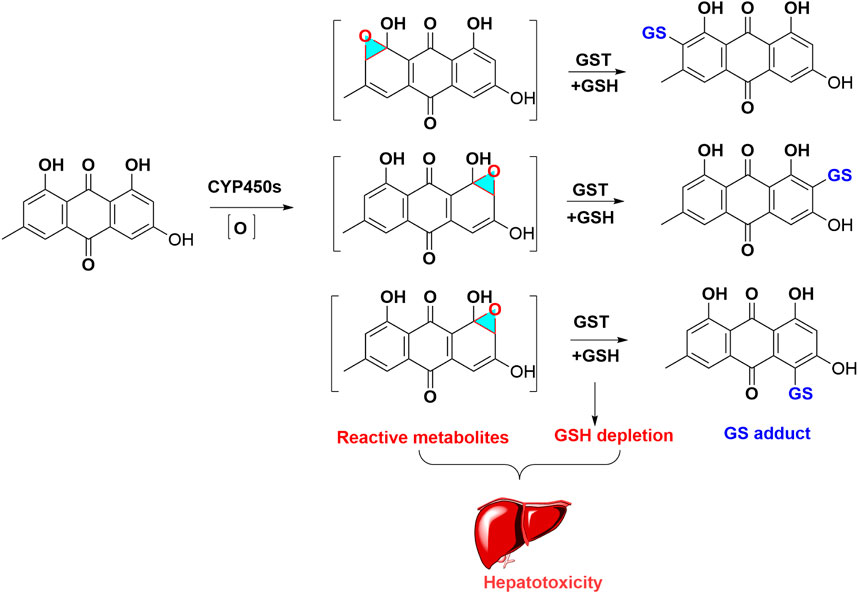
FIGURE 3. Metabolic activation of emodin induced hepatotoxicity. Emodin formed oxidated intermediates metabolites, these reactive metabolites captured with GSH, and produced GSH adduct, which led to GSH exhaustion.
2.5 Metabolic Activation of Bisbenzylisoquinoline-Induced Pulmonary Toxicity
Bisbenzylisoquinoline alkaloids (BBI) are a large kind of natural product, which usually consist of two benzylisoquinoline moieties by the linker of carbon–carbon bridge or ether bridge in their structure. This class of alkaloids extensively distributed in multiple herbs, majorly existed in plants of Annonaceae, Berberidaceae, Menispermaceae, Ranunculaceae, and Magnoliaceae family (Gao and Xiao, 1998). Numerous medicinal plants and diets were reported to contain this type alkaloids, which have been used as traditional medicines in East Asia, such as Menispermum dauricum, Mahonia fortune, Stephania japonica, Stephaniae tetrandrae and so on. Reported pharmacological activities of bis-benzylisoquinoline alkaloids majorly including antimalarial, anti-inflammatory, anticancer, immunosuppression and anti-hepatitis activities (Gao and Xiao, 1998).
Numerous toxic bisbenzylisoquinoline alkaloids and their metabolic activation are summarized in Table 1. As a typical case, dauricine is discussed in this section to illustrate the role of metabolic activation. Dauricine, a bisbenzylisoquinoline alkaloid, is the major bioactive component of Menispermum dauricum. As regards to its metabolism, four GSH conjugates of dauricine were detected in rat bile or HLMs incubations with supplemented NADPH and GSH. The reacted sites of GSH addition were elucidated as 6-position of phenol moiety, demonstrating that metabolic activation occurred in phenol ring, which generated reactive quinone intermediates via oxidation. Recombinant human CYP450 enzymes revealed CYP3A4 was the major metabolic enzyme responsible for the bioactivation of dauricine (Wang et al., 2008). Moreover, a reactive quinone methide metabolite of dauricine was observed and identified in MLMs, which spontaneously captured by GSH (Figure 4), and its GSH adducts can be suppressed by CYP3A inhibitor ketoconazole (Jin et al., 2010). Moreover, ketoconazole could counteract the increased lactate dehydrogenase activity induced by dauricine (Jin et al., 2010), and reverse pulmonary cellular GSH depletion and cell apoptosis in the pulmonary injury caused by dauricine (Jin et al., 2012). The GSH depletory agent of l-buthionine sulfoximine showed potentiating effect on cytotoxicity and apoptosis caused by dauricine (Jin et al., 2012), demonstrating the pulmonary toxicity was associated with the CYP3A mediated metabolic activation. The reactive quinone methide intermediate of dauricine can covalently medicated protein, and form quinone methide-protein adduct, which has been detected by liquid chromatography-mass spectrometry (LC-MS/MS) (Xie et al., 2016).
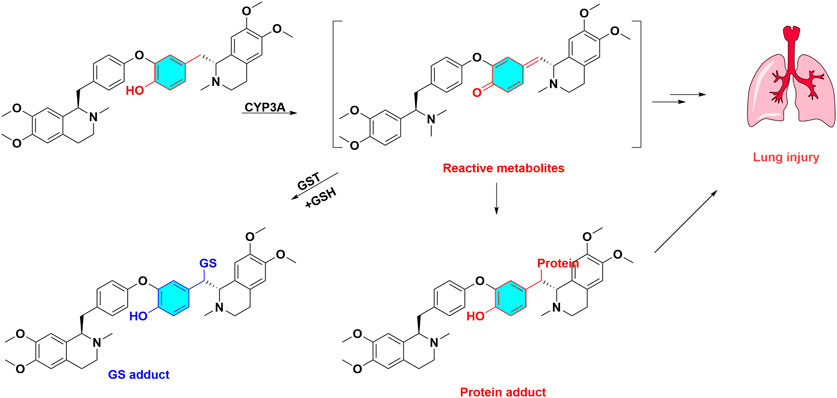
FIGURE 4. Metabolic activation and toxicity of dauricine. Dauricine are biotransformed quinone methide intermediate catalyzed by CYP3A, these reactive metabolites capture GSH and protein, then produce GSH adduct, protein adduct, at last led to lung injury.
Apart from bisbenzylisoquinoline alkaloids, toxic bisbenzylisoquinolines also included berbamine, tetrandrine and neferine (Table 1). The quinone methide intermediate of berbamine in vitro and in vivo metabolism were detected by LC-MS/MS, which can be covalently bind with NAC to generate NAC-derived adducts. CYP3A4 also played a key role in the metabolic activation process of berbamine (Sun et al., 2017). Metabolic activation of tetrandrine can lead to pulmonary toxicity toward CD-1 mice (Jin et al., 2011), and CYP3A5 mediated bioactivation was also closely associated with cytotoxicity of tetrandrine (Tian et al., 2016). CYP3A4 predominantly catalyzed the formation of neferine-GSH conjugates, and GSH depletion significantly aggravated neferine-induced cytotoxicity (Shen et al., 2014). Taken together, para-methylene phenol is key toxic structural alerts of bisbenzylisoquinoline alkaloids, and activated para-quinone methides bio transforming from para -methylene phenol is a pivotal step.
2 6 Metabolic Activation of Alkenylbenzenes-Induced Carcinogenicity
Dietary alkenylbenzenes are a class of aromatic natural products, which are presented in diversified vegetables, spices and medicinal plants, such as cinnamon, clove nutmeg, pepper, fennel, anise and basil. The common natural alkenylbenzenes majorly include estragole, safrole, methyleugenol, elemicin and myristicin. There are substantial evidences supporting for the genotoxicity and carcinogenicity of allylalkoxybenzenes (Rietjens et al., 2014).
The genotoxic alkenylbenzenes and their metabolic activation are listed in Table 1. The initial toxic metabolites of alkenylbenzenes were their 1′-hydroxy derivatives, such as 1′-hydroxysafrole, and their ultimate carcinogenic metabolites are their 1′-sulfooxy derivatives, such as 1′-sulfooxysafrole (Borchert et al., 1973; Boberg et al., 1983), which can be degraded to alkylating carbocations intermediates. These intermediates are electrophilic and reactive, which can conjugate DNA, leading to genotoxicity and carcinogenicity. Therefore, 1′-sulfooxyalkenylbenzenes were elucidated as tumor-initiating metabolites. Overall, the bioactivation of alkenylbenzenes underwent three steps: 1) Hydroxylation reaction at the alkene side chain 1′ site, alkenylbenzenes can be transformed into 1′-hydorxyalkenylbenzenes, therefore, allyl is toxic functional group of alkenylbenzenes; 2) Sulfation reaction, 1′-hydroxyalkenylbenzenes can be sulfated into 1′-sulfooxyalkenylbenzenes. These sulfated metabolites were confirmed as the ultimate electrophilic metabolites. 3) DNA addition, 1′-sulfooxyalkenylbenzenes can eliminate from sulfonate ion, and form an intermediate of carbocation. Carbocation subsequently covalently binds with adenine or guanine base to form DNA adducts, as depicted in Figure 5. Metabolic activation of the alkenylbenzenes to their ultimate carcinogens require the key catalysis of both CYP450s and sulfotransferases. In addition, reactive 1′-hydroxymyristicin showed chemical reactive activity which can react with NAC.
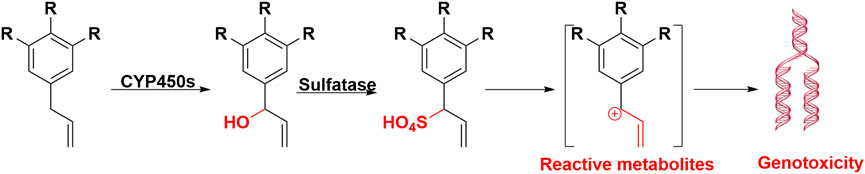
FIGURE 5. Metabolic activation of alkenylbenzenes induced carcinogenicity. After hydroxylation and solidification, alkenylbenzenes were formed carbocation intermediate, at last produced DNA adducts, and led to carcinogenicity.
2.7 Metabolic Activation of Flavonoids-Induced Cytotoxicity
Flavonoids are natural phenolic acid components in the diet and medicinal plants. Dietary flavonoids are the most abundant secondary metabolites in the plant kingdom and they play a regulating or preventing action in many disorders or diseases for a long period of time. Currently, owing to their extensively biochemical and pharmacological effects, flavonoids obtain the burgeoning interest in complementary and alternative medicine.
Previous studies indicated that quercetin was mutagenic without microsomal activation (Bjeldanes and Chang, 1977). Recently, metabolic activation of quercetin majorly including initially enzymatic or chemical oxidation of quercetin, formation of quercetin ortho-quinone, followed by isomerisation of the ortho-quinone to quinone methides. These quinone methides were reported to be the alkylating DNA-reactive intermediates (Vrijsen et al., 1990). In addition to mutagenicity, multiple flavonoids can potentiate their cytotoxicity toward breast cancer cells after CYP1A1 and CYP1B1 mediated metabolic activation. And CYP1-mediated metabolic activation of dietary flavonoids enhanced their toxicity in breast cancer cells (Androutsopoulos et al., 2008; Androutsopoulos et al., 2009). Compared to other flavonoids, 4′-hydoxyl-flavonoids can easily transform to quinone methide, and showed higher cytotoxicity in Figure 6. It was reported that metabolic activation of numerous other flavonoids was involved in or aggravated their toxicities (Table 1). Taken together, quinone intermediate including quinone methides and ortho‐benzoquinone, were usually formed in the metabolism of most pre-toxic flavonoids, which can further result in the depletion of cellular GSH and DNA damage.
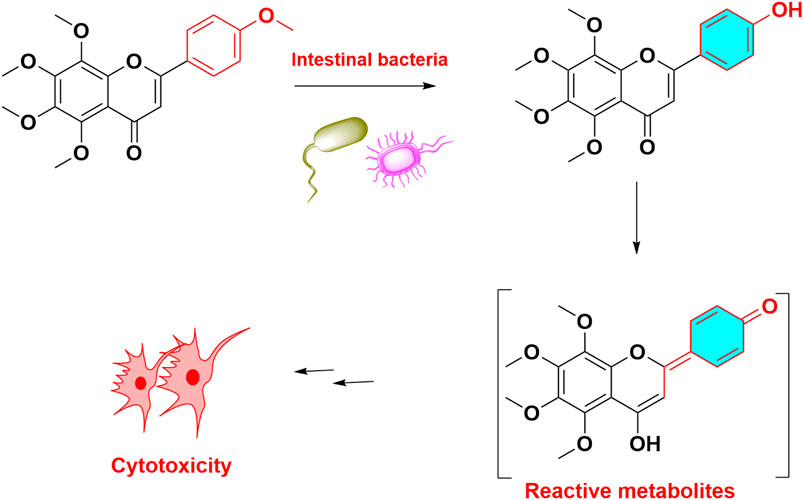
FIGURE 6. Metabolic activation and toxicity of tangeretin. Tangeretin demethylated and formed quinone methide, led to cytotoxicit.
3 Intestinal Flora Mediated Metabolic Activation of Natural Products Leading to Toxicity
Gut flora extensively implicates in the metabolism of multiple medicinal drugs, consequences for interpersonal variation in drug or xenobiotics toxicity (Zimmermann et al., 2019; Wilson and Nicholson, 2017; Zheng et al., 2013; Alexander et al., 2017). Dietary natural products can be extensively metabolized in the gut, not only by digestive and intestinal mucosal enzymes, but also by the gut microbiota. Microbiome-driven drug metabolism can lead to adverse consequences and toxicity. Similar to CYP450s mediated metabolic activation, the intestinal microflora also can form reactive metabolites and significantly aggravate xenobiotic-induced toxicities via metabolic activation (Table 1). Additionally, the gut microbiota can affect drug metabolism and toxicity indirectly, such as competition of bacterial-derived metabolites for xenobiotic metabolism pathways or the modulation of host metabolic systems (Wilson et al., 2015).
Gut microbiota involves in many metabolic reactions, such as demethylation, dehydroxylation, deacylation, decarboxylation, and hydrolysis reactions as well as acetylation (Wilson et al., 2015). Hydrolysis products of glucosides can form their aglycones in the intestine, which are easily absorbed into blood, and circulate throughout the human body. In particular, intestinal bacterial secretes β-glucuronidase, which hydrolyzes glucuronidated metabolites to their toxic aglycones in intestines and results in intestinal damage. For example, intestinal microbiota-mediated geniposide bio-transform to genipin dialdehyde intermediate, leading to hepatotoxicity in rats (Li et al., 2019b). Pre-toxic arbutin can be hydrolyzed into toxic hydroquinone with the aid of intestinal flora transformation (Kang et al., 2011).
4 Metabolic Bioactivation of Natural Products Leading to Mechanism-Based Inactivation of CYP450s
CYP450s are the most common phase I metabolic enzymes, and involve in the majority of the metabolism of clinical drugs and natural products. Inhibition of CYP450s is by far the most common factor leading to drug-drug interaction (DDI). CYP450s inhibition can be classed as reversible (competitive or non-competitive) or irreversible (mechanism-based inactivation). In particular, mechanism-based inactivation (MBI) often involves metabolic bioactivation of natural products by drug metabolizing enzyme to an electrophilic reactive intermediate, which covalently modifies an active site amino acid residue and/or coordinates to the heme prosthetic group, and leads to quasi-irreversible or irreversible inactivation (Appiah-Opong et al., 2009; Kamel and Harriman, 2013). Compared to reversible inhibition, irreversible inhibition more frequently results in unfavorable DDIs as the inactivated P450 enzyme has to be replaced by newly synthesized protein. Natural flavonoids, phenylpropanoids, terpenoids, quinones, and alkaloids are mechanisms -based inactivators, may trigger herb-drug or food-drug interactions. thiophene, furan, alkylamines are common latent functional groups responsible for reactive metabolites induced MBI (Mirzaei et al., 2021). The risks for intake of naturally occurring irreversible P450 enzyme inhibitors have been rising, owing to the rapid growth of the global consumption of natural products (Zhang et al., 2020b). Mechanism-based inactivation, the structure of reactive metabolites−MBI relationships, should be applied in clinical to mitigate the risk of idiosyncratic drug toxicity (Orr et al., 2012).
5 Novel Analytical Techniques Used for Detection of the Reactive Metabolites of Natural Products
Metabolic activation of a natural product resulting in reactive metabolite(s) that can covalently modify proteins is considered an initial step that may lead to drug-induced organ toxicities, therefore, detecting and characterizing of reactive metabolites will provide a useful clue for predicting metabolic activation mediated toxicity. Ultra-high liquid chromatography coupled with mass spectrometry (UPLC‐MS) plays a key role as the predominant analytical platform for analysis and detection of reactive metabolites. However, it is difficult to detect most reactive metabolites directly. Reactive metabolites are liable to form stable adducts by covalent combination with trapping reagents, reactive metabolites related adducts make the reactive metabolites detectable. Trapping assays, especially glutathione trapping, are usually performed to detect reactive metabolites that can contribute to drug toxicity. These trapping reactions are often performed in liver microsomes with NADPH and appropriate nucleophilic trapping agents, such as thiols (glutathione (GSH), its ethyl ester derivative, or N-acetylcysteine), amines (semicarbazide and methoxylamine), or cyanide anion. In the UPLC–MS/MS, a neutral loss scan or a precursor ion scan mode are usually applied to detect of GSH-trapped reactive metabolites, however, the sensitivity and selectivity in the scan mode are sometimes poor owing to the interference of endogenous biological matrices derived from HLM incubation. In addition, the selectivity of GSH adduct in conventional reversed-C18 phase liquid phase separations is not sufficient, and needs to be improved to minimize false positive and/or negative results. It remains a challenge to analyze these reactive metabolites adducts. Glutathione labeled with a fluorescence tag of dansyl (dGSH) can be applied as a trapping agent for the fluorescent quantification and identification of hard reactive metabolites (Gan et al., 2005; Nishijo et al., 2020).On one hand, dGSH can increase the detection sensitivity of trapped reactive metabolites; On the other hand, dGSH captures only soft electrophilic reactive metabolites. CysGlu-Dan labeled cystine have been built to detect soft and hard electrophilic reactive metabolites (Shibazaki et al., 2021). Thus, a high‐throughput sensitive and selective GSH trapping assay using the combination of stable isotope‐labeled GSH and UPLC-MS system for identification and characterization of reactive metabolite “all‐in‐one” is recommended.
Metabolomics-based toxicology can evaluate toxicity and identify toxicological biomarker of natural product, which is helpful to guide clinical medication and reduce adverse drug reactions. UPLC-MS-based metabolomic approach is a sensitive, effective and unbiased tool for profiling of drug metabolism and metabolic activation (Li et al., 2011; Li et al., 2012; Hanhineva et al., 2017) to eliminate complex matrix interferences. It was reported that natural cocaine can form reactive metabolites, leading to hepatotoxicity. These reactive metabolites of cocaine were easily screened and analyzed by metabolomics (Yao et al., 2013). In addition, reactive metabolites-proteins adducts are thought to be a principal factor in natural drug-induced liver injury. targeted proteomics approach to the identification of peptides modified by reactive metabolites, which is generally suitable for the identification and characterization of modified proteins and metabolite structures involved in covalent binding and may serve as a valuable tool to link protein targets with clinically relevant toxicities (Tzouros and Pähler, 2009). Quantitative chemical proteomic profiled the in vivo toxic targets of reactive drug metabolites (Whitby et al., 2017).
6 Concluding and Perspective
The review provides a reference for the reasonable and safe usage of herbal and dietary natural products. In recent years, the toxicity of natural drugs has attracted widespread concerns around the world, especially for inappropriate dosage or overdose use. The toxicity of natural drugs largely affected their applications clinically. Metabolic activation of natural products can initiate or aggravate their hepatotoxicity, nephrotoxicity, and pulmonary toxicities.
Although some toxic components were discovered from natural herbal and dietary, there are still many unknown toxic compounds to be identified. Firstly, rapid high throughput approach screening and assessing the toxic component in natural drugs should be established. Considering that complex biological factors can affect the evaluation of metabolic activation leading to toxicity, the sensitive and specific method, UPLC-MS/MS, should be developed for exclusively detecting reactive metabolites and their GSH conjugates. Moreover, metabolic activation of many different natural products formats chemically reactive/toxic metabolites, that can result in toxicity through binding to macromolecular targets (proteins or DNA). Reactive metabolites -protein adducts can be selected as toxic marker for predicting and evaluating metabolic activation leading to toxicity, and nontargeted identification of reactive metabolite protein adducts is desirable. Methods for qualitative and quantitative detection of reactive metabolites-protein adducts should be developed and applied for the clinical diagnosis of toxic natural products exposure and toxic natural products-induced liver injury. The determination of reactive metabolites-protein adducts using mass spectrometry is an emerging area which allows comprehensive understanding of the underlying mechanisms involved in toxicity and reveal potential biomarkers of exposure or toxic response. More specific fluorescent probes for analysis and detection reactive metabolites should be designed for diagnosis the toxicities of natural products. Standard drug discovery and development strategies should be applied to natural products.
Although the molecular machinery underlying toxicity remains largely unclear, more toxic action should be clarified from the perspective of molecular biology or systematic biology in further study. It is essential to establish the diagnostic strategies to detect drug-induced toxicities clinically, which is also very important to find the strategy of detoxification to decrease the toxicity of natural drugs, and more comprehensive understandings of toxicity are urgently required.
Considering the multitude of potentially toxic natural products in multi-ingredient supplements, the unknown concentrations, and missing or inappropriate labels, as well as the absorption, distribution, metabolism and excretion in the host, the varying phenotypic presentation and unpredictable spectrum of toxicity, making the diagnosis challenging, these above unaddressed issues warrant further study. The current toxic raw data of most natural drugs and products are too limited to utilize. Therefore, the toxic database for herbal and dietary natural products should be built to record the side/toxic effects of natural drugs and their clinical formulation, and determine toxic components for the further investigation. The known potentially toxic indigents and their toxic effects should be labeled on the package of herbal and dietary supplements.
Author Contributions
Y-KW: Conceptualization, Writing—original draft, Visualization, Software. WL: Writing—original draft, (table), Visualization, Software. SX: Writing—original draft, (table). LG: Writing—original draft, (table). YM, B-KZ: Conceptualization, Writing—review and, editing, Supervision.
Funding
This work was supported by the National Natural Science Foundation of China (Grant Nos. 81974532 and 1803830) and Science and Technology Department of Hunan Province, China (Grant No. 2017SK1030).
Conflict of Interest
The authors declare that the research was conducted in the absence of any commercial or financial relationships that could be construed as a potential conflict of interest.
Publisher’s Note
All claims expressed in this article are solely those of the authors and do not necessarily represent those of their affiliated organizations, or those of the publisher, the editors and the reviewers. Any product that may be evaluated in this article, or claim that may be made by its manufacturer, is not guaranteed or endorsed by the publisher.
References
Alajlouni, A. M., Al Malahmeh, A. J., Kiwamoto, R., Wesseling, S., Soffers, A. E., Al-Subeihi, A. A., et al. (2016). Mode of Action Based Risk Assessment of the Botanical Food-Borne Alkenylbenzene Apiol from Parsley Using Physiologically Based Kinetic (PBK) Modelling and Read-Across from Safrole. Food Chem. Toxicol. 89, 138–150. doi:10.1016/j.fct.2016.01.018
Alexander, J. L., Wilson, I. D., Teare, J., Marchesi, J. R., Nicholson, J. K., and Kinross, J. M. (2017). Gut Microbiota Modulation of Chemotherapy Efficacy and Toxicity. Nat. Rev. Gastroenterol. Hepatol. 14 (6), 356–365. doi:10.1038/nrgastro.2017.20
Androutsopoulos, V., Arroo, R. R., Hall, J. F., Surichan, S., and Potter, G. A. (2008). Antiproliferative and Cytostatic Effects of the Natural Product Eupatorin on MDA-MB-468 Human Breast Cancer Cells Due to CYP1-Mediated Metabolism. Breast Cancer Res. 10 (3), R39. doi:10.1186/bcr2090
Androutsopoulos, V., Wilsher, N., Arroo, R. R., and Potter, G. A. (2009). Bioactivation of the Phytoestrogen Diosmetin by CYP1 Cytochromes P450. Cancer Lett. 274 (1), 54–60. doi:10.1016/j.canlet.2008.08.032
Appiah-Opong, R., Commandeur, J. N., Istyastono, E., Bogaards, J. J., and Vermeulen, N. P. (2009). Inhibition of Human Glutathione S-Transferases by Curcumin and Analogues. Xenobiotica 39 (4), 302–311. doi:10.1080/00498250802702316
Baer, B. R., Rettie, A. E., and Henne, K. R. (2005). Bioactivation of 4-ipomeanol by CYP4B1: Adduct Characterization and Evidence for an Enedial Intermediate. Chem. Res. Toxicol. 18 (5), 855–864. doi:10.1021/tx0496993
Bjeldanes, L. F., and Chang, G. W. (1977). Mutagenic Activity of Quercetin and Related Compounds. Science 197 (4303), 577–578. doi:10.1126/science.327550
Boberg, E. W., Miller, E. C., Miller, J. A., Poland, A., and Liem, A. (1983). Strong Evidence from Studies with Brachymorphic Mice and Pentachlorophenol that 1'-sulfoöxysafrole Is the Major Ultimate Electrophilic and Carcinogenic Metabolite of 1'-hydroxysafrole in Mouse Liver. Cancer Res. 43 (11), 5163–5173.
Borchert, P., Miller, J. A., Miller, E. C., and Shires, T. K. (1973). 1'-Hydroxysafrole, a Proximate Carcinogenic Metabolite of Safrole in the Rat and Mouse. Cancer Res. 33 (3), 590–600.
Buckpitt, A. R., and Boyd, M. R. (1982). Metabolic Activation of 4-ipomeanol by Avian Tissue Microsomes. Toxicol. Appl. Pharmacol. 65 (1), 53–62. doi:10.1016/0041-008X(82)90361-1
Bunchorntavakul, C., and Reddy, K. R. (2012). Review Article: Herbal and Dietary Supplement Hepatotoxicity. Aliment. Pharmacol. Ther. 37 (1), 3–17. doi:10.1111/apt.12109
Chai, Z., Zhou, W. J., Gao, L., Wang, Y. H., Fan, H. J., and Zhou, R. (2011). Experimental Progress of Liver Toxicity of Tripterygium Wilfordii and its Mechanism[J]. Chinese J Experi Traditional Medical Formula 7, 243–246.
Chen, M., Li, L., Zhong, D., Shen, S., Zheng, J., and Chen, X. (2016). 9-Glutathionyl-6,7-dihydro-1-hydroxymethyl-5H-pyrrolizine Is the Major Pyrrolic Glutathione Conjugate of Retronecine-type Pyrrolizidine Alkaloids in Liver Microsomes and in Rats. Chem. Res. Toxicol. 29 (2), 180–189. doi:10.1021/acs.chemrestox.5b00427
Druckova, A., and Marnett, L. J. (2006). Characterization of the Amino Acid Adducts of the Enedial Derivative of Teucrin A. Chem. Res. Toxicol. 19 (10), 1330–1340. doi:10.1021/tx060143k
Druckova, A., Mernaugh, R. L., Ham, A. J., and Marnett, L. J. (2007). Identification of the Protein Targets of the Reactive Metabolite of Teucrin A In Vivo in the Rat. Chem. Res. Toxicol. 20 (10), 1393–1408. doi:10.1021/tx7001405
Du, F., Liu, T., Liu, T., Wang, Y., Wan, Y., and Xing, J. (2011). Metabolite Identification of Triptolide by Data-dependent Accurate Mass Spectrometric Analysis in Combination with Online Hydrogen/deuterium Exchange and Multiple Data-Mining Techniques. Rapid Commun. Mass. Spectrom. 25 (20), 3167–3177. doi:10.1002/rcm.5211
Ekor, M. (2013). The Growing Use of Herbal Medicines: Issues Relating to Adverse Reactions and Challenges in Monitoring Safety. Front. Pharmacol. 4 (4), 177. doi:10.3389/fphar.2013.00177
Feng, X., Fang, S. N., Gao, Y. X., Liu, J. P., and Chen, W. (2018). Current Research Situation of Nephrotoxicity of Chinese Herbal Medicine. Zhongguo Zhong Yao Za Zhi 43 (3), 417–424. doi:10.19540/j.cnki.cjcmm.2018.0009
Forsch, K., Siewert, B., Disch, L., Unger, M., and Drewe, J. (2017). In Vitro screening of Acute Hepatic Cytotoxicity of Pyrrolizidine Alkaloids in Human and Rodent Hepatic Cell Lines. Toxicol. Lett. 280 (S 01), S197. doi:10.1016/j.toxlet.2017.07.859
Fu, P. P., Xia, Q., Lin, G., and Chou, M. W. (2004). Pyrrolizidine Alkaloids-Ggenotoxicity, Metabolism Enzymes, Metabolic Activation, and Mechanisms. Drug Metab. Rev. 36 (1), 1–55. doi:10.1081/dmr-120028426
Fu, P. P. (2017). Pyrrolizidine Alkaloids: Metabolic Activation Pathways Leading to Liver Tumor Initiation. Chem. Res. Toxicol. 30 (1), 81–93. doi:10.1021/acs.chemrestox.6b00297
Furukawa, M., Kasajima, S., Nakamura, Y., Shouzushima, M., Nagatani, N., Takinishi, A., et al. (2010). Toxic Hepatitis Induced by show-Wu-pian, a Chinese Herbal Preparation. Intern. Med. 49 (15), 1537–1540. doi:10.2169/internalmedicine.49.3509
Gan, J., Harper, T. W., Hsueh, M. M., Qu, Q., and Humphreys, W. G. (2005). Dansyl Glutathione as a Trapping Agent for the Quantitative Estimation and Identification of Reactive Metabolites. Chem. Res. Toxicol. 18 (5), 896–903. doi:10.1021/tx0496791
Gao, G. Y., and Xiao, P. G. (1998). Review of Studies of Bisbenzylisoquinoline Alkaloid (BBI) on Distribution in Higher Plant and Physiological Activities[J]. Nat. Prod. Res. Dev. 11 (3), 96–103.
Gori, L., Galluzzi, P., Mascherini, V., Gallo, E., Lapi, F., Menniti-Ippolito, F., et al. (2011). Two Contemporary Cases of Hepatitis Associated with Teucrium Chamaedrys L. Decoction Use: Case Reports and Review of Literature. Basic Clin. Pharmacol. Toxicol. 109 (6), 521–526. doi:10.1111/j.1742-7843.2011.00781.x
Griffin, C. T., O’Mahony, J., Danaher, M., and Furey, A. (2015). Liquid Chromatography Tandem Mass Spectrometry Detection of Targeted Pyrrolizidine Alkaloids in Honeys Purchased within Ireland. Food Anal. Methods 8 (1), 18–31. doi:10.1007/s12161-014-9855-1
Hanhineva, K., and Pasanen, M. (2017). “Metabolomics Applications in Herbal Medicine,” in Toxicology of Herbal Products. Editors O. Pelkonen, P. Duez, P. M. Vuorela, and H. Vuorela (Cham: Springer International Publishing), 165–178. doi:10.1007/978-3-319-43806-1_8
Hayasaka, S., Kodama, T., and Ohira, A. (2012). Traditional Japanese Herbal (Kampo) Medicines and Treatment of Ocular Diseases: A Review. Am. J. Chin. Med. 40 (5), 887–904. doi:10.1142/S0192415x12500668
He, Y., Shi, M., Wu, X., Ma, J., Ng, K. T. P., Xia, Q., et al. (2021). Mutational Signature Analysis Reveals Widespread Contribution of Pyrrolizidine Alkaloid Exposure to Human Liver Cancer. Hepatology 74, 264–280. doi:10.1002/hep.31723
He, Y., Lian, W., Ding, L., Fan, X., Ma, J., Zhang, Q.-Y., et al. (2021). Lung Injury Induced by Pyrrolizidine Alkaloids Depends on Metabolism by Hepatic Cytochrome P450s and Blood Transport of Reactive Metabolites. Arch. Toxicol. 95 (1), 103–116. doi:10.1007/s00204-020-02921-0
Huang, L., Li, Y., Pan, H., Lu, Y., Zhou, X., and Shi, F. (2020). Cortex Dictamni-Induced Liver Injury in Mice: The Role of P450-Mediated Metabolic Activation of Furanoids. Toxicol. Lett. 330, 41–52. doi:10.1016/j.toxlet.2020.05.004
Huijzer, J. C., Adams, J. D., and Yost, G. S. (1987). Decreased Pneumotoxicity of Deuterated 3-methylindole: Bioactivation Requires Methyl C-H Bond Breakage. Toxicol. Appl. Pharmacol. 90 (1), 60–68. doi:10.1016/0041-008X(87)90306-1
Jang, S., Kim, K. H., Sun, S. H., Go, H. Y., Lee, E. K., Jang, B. H., et al. (2017). Characteristics of Herbal Medicine Users and Adverse Events Experienced in South Korea: A Survey Study. Evid. Based Complement. Alternat Med. 2017 (1), 4089019–9. doi:10.1155/2017/4089019
Jeurissen, S. M., Bogaards, J. J., Awad, H. M., Boersma, M. G., Brand, W., Fiamegos, Y. C., et al. (2004). Human Cytochrome P450 Enzyme Specificity for Bioactivation of Safrole to the Proximate Carcinogen 1'-hydroxysafrole. Chem. Res. Toxicol. 17 (9), 1245–1250. doi:10.1021/tx040001v
Jeurissen, S. M., Bogaards, J. J., Boersma, M. G., ter Horst, J. P., Awad, H. M., Fiamegos, Y. C., et al. (2006). Human Cytochrome P450 Enzymes of Importance for the Bioactivation of Methyleugenol to the Proximate Carcinogen 1'-hydroxymethyleugenol. Chem. Res. Toxicol. 19 (1), 111–116. doi:10.1021/tx050267h
Jeurissen, S. M., Punt, A., Boersma, M. G., Bogaards, J. J., Fiamegos, Y. C., Schilter, B., et al. (2007). Human Cytochrome P450 Enzyme Specificity for the Bioactivation of Estragole and Related Alkenylbenzenes. Chem. Res. Toxicol. 20 (5), 798–806. doi:10.1021/tx700012d
Jiang, M., Xiong, N. N., Liu, S. L., Wei-Min, F. U., and Bo, Y. E. (2004). Adverse Drug Reaction of Compound Prescription of Huangyaozi(Dioscorea Bulbifera L.) in Clinical Trial and its Management. Chin. J Evid-Based Med. 257–258.
Jiang, J. Z., Yang, B. H., Ji, L. L., Yang, L., Mao, Y. C., Hu, Z. H., et al. (2017). Metabolic-induced Cytotoxicity of Diosbulbin B in CYP3A4-Expressing Cells. Toxicol. Vitro 38, 59–66. doi:10.1016/j.tiv.2016.11.006
Jiang, L. L., Jiang, Y., Zhao, D. S., Fan, Y. X., Yu, Q., Li, P., et al. (2018). CYP3A Activation and Glutathione Depletion Aggravate Emodin-Induced Liver Injury. Chem. Res. Toxicol. 31 (10), 1052–1060. doi:10.1021/acs.chemrestox.8b00117
Jin, H., Dai, J., Chen, X., Liu, J., Zhong, D., Gu, Y., et al. (2010). Pulmonary Toxicity and Metabolic Activation of Dauricine in CD-1 Mice. J. Pharmacol. Exp. Ther. 332 (3), 738–746. doi:10.1124/jpet.109.162297
Jin, H., Li, L., Zhong, D., Liu, J., Chen, X., and Zheng, J. (2011). Pulmonary Toxicity and Metabolic Activation of Tetrandrine in CD-1 Mice. Chem. Res. Toxicol. 24 (12), 2142–2152. doi:10.1021/tx200290s
Jin, H., Shen, S., Chen, X., Zhong, D., and Zheng, J. (2012). CYP3A-mediated Apoptosis of Dauricine in Cultured Human Bronchial Epithelial Cells and in Lungs of CD-1 Mice. Toxicol. Appl. Pharmacol. 261 (3), 248–254. doi:10.1016/j.taap.2012.03.025
Kamel, A., and Harriman, S. (2013). Inhibition of Cytochrome P450 Enzymes and Biochemical Aspects of Mechanism-Based Inactivation (MBI). Drug Discov. Today Technol. 10 (1), e177–89. doi:10.1016/j.ddtec.2012.09.011
Kang, M. J., Ha, H. W., Kim, H. G., Lee, D. H., Kong, M. J., Ahn, Y. T., et al. (2011). Role of Metabolism by Intestinal Bacteria in Arbutin-Induced Toxicity In Vitro. Arch. Pharm. Res. 34 (4), 687–693. doi:10.1007/s12272-011-0420-9
Kang, M. J., Khanal, T., Kim, H. G., Lee, D. H., Yeo, H. K., Lee, Y. S., et al. (2012). Role of Metabolism by Human Intestinal Microflora in Geniposide-Induced Toxicity in HepG2 Cells. Arch. Pharm. Res. 35 (4), 733–738. doi:10.1007/s12272-012-0418-y
Kao, N. L., Richmond, G. W., and Moy, J. N. (2010). Resolution of Severe Lupus Nephritis Associated with Tripterygium Wilfordii Hook F Ingestion. Arthritis Rheum. 36 (12), 1751–1752. doi:10.1002/art.1780361217
Kim, D. H., Yu, K. U., Bae, E. A., and Han, M. J. (1998). Metabolism of Puerarin and Daidzin by Human Intestinal Bacteria and Their Relation to In Vitro Cytotoxicity. Biol. Pharm. Bull. 21 (6), 628–630. doi:10.1248/bpb.21.628
Koenigs, L. L., and Trager, W. F. (1998). Mechanism-Based Inactivation of Cytochrome P450 2B1 by 8-Methoxypsoralen and Several Other Furanocoumarins. Biochemistry 37 (38), 13184–13193. doi:10.1021/bi981198r
Kyoung Ah, J., Hyun Ju, M., Seung Suk, Y., Jun, K. H., Nyoung, C. S., Yoon, H. C., et al. (2011). Drug-Induced Liver Injury: Twenty Five Cases of Acute Hepatitis Following Ingestion of Polygonum Multiflorum Thunb. Gut Liver 5 (4), 493–499. doi:10.5009/gnl.2011.5.4.493
Lassila, T., Mattila, S., Turpeinen, M., Pelkonen, O., and Tolonen, A. (2016). Tandem Mass Spectrometric Analysis of S- and N-Linked Glutathione Conjugates of Pulegone and Menthofuran and Identification of P450 Enzymes Mediating Their Formation. Rapid Commun. Mass. Spectrom. 30 (7), 917–926. doi:10.1002/rcm.7518
Lekehal, M., Pessayre, D., Lereau, J. M., Moulis, C., Fourasté, I., and Fau, D. (1996). Hepatotoxicity of the Herbal Medicine Germander: Metabolic Activation of its Furano Diterpenoids by Cytochrome P450 3A Depletes Cytoskeleton-Associated Protein Thiols and Forms Plasma Membrane Blebs in Rat Hepatocytes. Hepatology 24 (1), 212–218. doi:10.1002/hep.510240134
Li, F., Lu, J., and Ma, X. (2011). Profiling the Reactive Metabolites of Xenobiotics Using Metabolomic Technologies. Chem. Res. Toxicol. 24 (5), 744–751. doi:10.1021/tx200033v
Li, F., Gonzalez, F. J., and Ma, X. (2012). LC-MS-Based Metabolomics in Profiling of Drug Metabolism and Bioactivation. Acta Pharm. Sin. B 2 (2), 118–125. doi:10.1016/j.apsb.2012.02.010
Li, R., Li, W., You, Y., Guo, X., Peng, Y., and Zheng, J. (2019). Metabolic Activation and Cytotoxicity of Aloe-Emodin Mediated by Sulfotransferases. Chem. Res. Toxicol. 32 (6), 1281–1288. doi:10.1021/acs.chemrestox.9b00081
Li, Y., Pan, H., Li, X., Jiang, N., Huang, L., Lu, Y., et al. (2019). Role of Intestinal Microbiota-Mediated Genipin Dialdehyde Intermediate Formation in Geniposide-Induced Hepatotoxicity in Rats. Toxicol. Appl. Pharmacol. 377, 114624. doi:10.1016/j.taap.2019.114624
Lin, D., Li, C., Peng, Y., Gao, H., and Zheng, J. (2014). Cytochrome P450-Mediated Metabolic Activation of Diosbulbin B. Drug Metab. Dispos 42 (10), 1727–1736. doi:10.1124/dmd.114.059261
Lin, D., Guo, X., Gao, H., Cheng, L., Cheng, M., Song, S., et al. (2015). In Vitro and In Vivo Studies of the Metabolic Activation of 8-Epidiosbulbin E Acetate. Chem. Res. Toxicol. 28 (9), 1737–1746. doi:10.1021/acs.chemrestox.5b00174
Lin, D., Wang, K., Guo, X., Gao, H., Peng, Y., Zheng, J., et al. (2016). Lysine- and Cysteine-Based Protein Adductions Derived from Toxic Metabolites of 8-Epidiosbulbin E Acetate. Toxicol. Lett. 264, 20–28. doi:10.1016/j.toxlet.2016.10.007
Lin, D., Li, W., Peng, Y., Jiang, C., Xu, Y., Gao, H., et al. (2016). Role of Metabolic Activation in 8-Epidiosbulbin E Acetate-Induced Liver Injury: Mechanism of Action of the Hepatotoxic Furanoid. Chem. Res. Toxicol. 29 (3), 359–366. doi:10.1021/acs.chemrestox.5b00501
Lin, D., Li, W., Tian, X., Peng, Y., and Zheng, J. (2019). In Vitro DNA Adduction Resulting from Metabolic Activation of Diosbulbin B and 8-Epidiosbulbin E Acetate. Chem. Res. Toxicol. 32 (1), 38–48. doi:10.1021/acs.chemrestox.8b00071
Liu, J., Li, L., Zhou, X., Chen, X., Huang, H., Zhao, S., et al. (2013). Metabolite Profiling and Identification of Triptolide in Rats. J. Chromatogr. B Analyt Technol. Biomed. Life Sci. 939 (22), 51–58. doi:10.1016/j.jchromb.2013.08.015
Liu, C., Fan, H., Li, Y., and Xiao, X. (2016). Research Advances on Hepatotoxicity of Herbal Medicines in China. Biomed. Res. Int. 2016, 7150391. doi:10.1155/2016/7150391
Liu, Y., Liu, C., Liu, Y., Ge, Q., and Sun, C. (2020). Cytochrome P450 Mediated Bioactivation of Rutaevin, a Bioactive and Potentially Hepatotoxic Component of Evodia Rutaecarpa. Chem. Res. Toxicol. 33 (12), 3054–3064. doi:10.1021/acs.chemrestox.0c00475
Ma, J., Ruan, J., Chen, X., Li, D., Yao, S., Fu, P. P., et al. (2019). Pyrrole-Hemoglobin Adducts, a More Feasible Potential Biomarker of Pyrrolizidine Alkaloid Exposure. Chem. Res. Toxicol. 32 (6), 1027–1039. doi:10.1021/acs.chemrestox.8b00369
Merz, K. H., and Schrenk, D. (2016). Interim Relative Potency Factors for the Toxicological Risk Assessment of Pyrrolizidine Alkaloids in Food and Herbal Medicines. Toxicol. Lett. 263, 44–57. doi:10.1016/j.toxlet.2016.05.002
Mirzaei, M. S., Ivanov, M. V., Taherpour, A. A., and Mirzaei, S. (2021). Mechanism-Based Inactivation of Cytochrome P450 Enzymes: Computational Insights. Chem. Res. Toxicol. 34 (4), 959–987. doi:10.1021/acs.chemrestox.0c00483
Morgan, R. W., and Hoffmann, G. R. (1983). Cycasin and its Mutagenic Metabolites. Mutat. Res. 114 (1), 19–58. doi:10.1016/0165-1110(83)90018-0
Navarro, V. J., Khan, I., Björnsson, E., Seeff, L. B., Serrano, J., and Hoofnagle, J. H. (2016). Liver Injury from Herbal and Dietary Supplements. Hepatology 65, 363–373. doi:10.1002/hep.28813
Nencini, C., Galluzzi, P., Pippi, F., Menchiari, A., and Micheli, L. (2014). Hepatotoxicity of Teucrium Chamaedrys L. Decoction: Role of Difference in the Harvesting Area and Preparation Method. Indian J. Pharmacol. 46 (2), 181–184. doi:10.4103/0253-7613.129313
Neuman, M. G., Cohen, L., Opris, M., Nanau, R. M., and Hyunjin, J. (2015). Hepatotoxicity of Pyrrolizidine Alkaloids. J. Pharm. Pharm. Sci. 18 (4), 825–843. doi:10.18433/j3bg7j
Ng, J. C. (1983). Metabolism of (-)-Ngaione, a Toxic Furanoid Ketone from Myoporum Species. Queensland University of Technology.
Nishijo, N., Hayama, T., Tomita, R., Yamaguchi, M., and Fujioka, T. (2020). Application of a Fluorous Derivatization Method for Characterization of Glutathione-Trapped Reactive Metabolites with Liquid Chromatography-Tandem Mass Spectrometry Analysis. J. Chromatogr. A. 1622, 461160. doi:10.1016/j.chroma.2020.461160
Orr, S. T., Ripp, S. L., Ballard, T. E., Henderson, J. L., Scott, D. O., Obach, R. S., et al. (2012). Mechanism-based Inactivation (MBI) of Cytochrome P450 Enzymes: Structure-Activity Relationships and Discovery Strategies to Mitigate Drug-Drug Interaction Risks. J. Med. Chem. 55 (11), 4896–4933. doi:10.1021/jm300065h
Parkinson, O. T., Kelly, E. J., Bezabih, E., Whittington, D., and Rettie, A. E. (2012). Bioactivation of 4-Ipomeanol by a CYP4B Enzyme in Bovine Lung and Inhibition by HET0016. J. Vet. Pharmacol. Ther. 35 (4), 402–405. doi:10.1111/j.1365-2885.2011.01339.x
Parkinson, O. T., Liggitt, H. D., Rettie, A. E., and Kelly, E. J. (2013). Generation and Characterization of a Cyp4b1 Null Mouse and the Role of CYP4B1 in the Activation and Toxicity of Ipomeanol. Toxicol. Sci. 134 (2), 243–250. doi:10.1093/toxsci/kft123
Parkinson, O. T., Teitelbaum, A. M., Whittington, D., Kelly, E. J., and Rettie, A. E. (2016). Species Differences in Microsomal Oxidation and Glucuronidation of 4-Ipomeanol: Relationship to Target Organ Toxicity. Drug Metab. Dispos 44 (10), 1598–1602. doi:10.1124/dmd.116.070003
Peng, Z. H., Wang, J. J., Du, P., and Chen, Y. (2012). Identification of In Vivo and In Vitro Metabolites of Triptolide by Liquid Chromatography-Tandem Mass Spectrometry. J. Pharm. Biomed. Anal. 70 (11), 624–630. doi:10.1016/j.jpba.2012.06.026
Peterson, L. A. (2013). Reactive Metabolites in the Biotransformation of Molecules Containing a Furan Ring. Chem. Res. Toxicol. 26 (1), 6–25. doi:10.1021/tx3003824
Phondani, P. C., Maikhuri, R. K., and Kala, C. P. (2010). Ethnoveterinary Uses of Medicinal Plants Among Traditional Herbal Healers in Alaknanda Catchment of Uttarakhand, India. Afr. J. Tradit Complement. Altern. Med. 7 (3), 195–206. doi:10.4314/ajtcam.v7i3.54775
Poór, M., Zrínyi, Z., and Kőszegi, T. (2016). Structure Related Effects of Flavonoid Aglycones on Cell Cycle Progression of HepG2 Cells: Metabolic Activation of Fisetin and Quercetin by Catechol-O-Methyltransferase (COMT). Biomed. Pharmacother. 83, 998–1005. doi:10.1016/j.biopha.2016.08.009
Posadzki, P., Watson, L. K., and Ernst, E. (2013). Adverse Effects of Herbal Medicines: an Overview of Systematic Reviews. Clin. Med. (Lond) 13 (1), 7–12. doi:10.7861/clinmedicine.13-1-7
Qin, B., Xu, Y., Chen, J., Huang, W., Peng, Y., Zheng, J., et al. (2016). Chemical Reactivity of Emodin and its Oxidative Metabolites to Thiols. Chem. Res. Toxicol. 29 (12), 2114–2124. doi:10.1021/acs.chemrestox.6b00191
Qin, X., Peng, Y., and Zheng, J. (2018). In Vitro and In Vivo Studies of the Electrophilicity of Physcion and its Oxidative Metabolites. Chem. Res. Toxicol. 31 (5), 340–349. doi:10.1021/acs.chemrestox.8b00026
Ravindranath, V., Burka, L. T., and Boyd, M. R. (1984). Reactive Metabolites from the Bioactivation of Toxic Methylfurans. Science 224 (4651), 884–886. doi:10.1126/science.6719117
Ravindranath, V., McMenamin, M. G., Dees, J. H., and Boyd, M. R. (1986). 2-Methylfuran Toxicity in Rats-Rrole of Metabolic Activation In Vivo. Toxicol. Appl. Pharmacol. 85 (1), 78–91. doi:10.1016/0041-008X(86)90389-3
Reinen, J., and Vermeulen, N. P. (2015). Biotransformation of Endocrine Disrupting Compounds by Selected Phase I and Phase II Enzymes-Fformation of Estrogenic and Chemically Reactive Metabolites by Cytochromes P450 and Sulfotransferases. Curr. Med. Chem. 22 (4), 500–527. doi:10.2174/0929867321666140916123022
Rietjens, I. M., Cohen, S. M., Fukushima, S., Gooderham, N. J., Hecht, S., Marnett, L. J., et al. (2014). Impact of Structural and Metabolic Variations on the Toxicity and Carcinogenicity of Hydroxy- and Alkoxy-Substituted Allyl- and Propenylbenzenes. Chem. Res. Toxicol. 27 (7), 1092–1103. doi:10.1021/tx500109s
Robertson, J., and Stevens, K. (2017). Pyrrolizidine Alkaloids: Occurrence, Biology, and Chemical Synthesis. Nat. Prod. Rep. 34 (1), 62–89. doi:10.1039/c5np00076a
Ruan, J., Yang, M., Fu, P., Ye, Y., and Lin, G. (2014). Metabolic Activation of Pyrrolizidine Alkaloids: Insights into the Structural and Enzymatic Basis. Chem. Res. Toxicol. 27 (6), 1030–1039. doi:10.1021/tx500071q
Ruan, J., Liao, C., Ye, Y., and Lin, G. (2014). Lack of Metabolic Activation and Predominant Formation of an Excreted Metabolite of Nontoxic Platynecine-type Pyrrolizidine Alkaloids. Chem. Res. Toxicol. 27 (1), 7–16. doi:10.1021/tx4004159
Shen, Q., Zuo, M., Ma, L., Tian, Y., Wang, L., Jiang, H., et al. (2014). Demethylation of Neferine in Human Liver Microsomes and Formation of Quinone Methide Metabolites Mediated by CYP3A4 Accentuates its Cytotoxicity. Chem. Biol. Interact 224, 89–99. doi:10.1016/j.cbi.2014.10.014
Shi, F., Zhao, P., Li, X., Pan, H., Ma, S., and Ding, L. (2015). Cytotoxicity of Luteolin in Primary Rat Hepatocytes: the Role of CYP3A-Mediated Ortho-Benzoquinone Metabolite Formation and Glutathione Depletion. J. Appl. Toxicol. 35 (11), 1372–1380. doi:10.1002/jat.3106
Shibazaki, C., Ohe, T., Takahashi, K., Nakamura, S., and Mashino, T. (2021). Development of Fluorescent-Labeled Trapping Reagents Based on Cysteine to Detect Soft and Hard Electrophilic Reactive Metabolites. Drug Metab. Pharmacokinet. 39, 100386. doi:10.1016/j.dmpk.2021.100386
Skordos, K. W., Skiles, G. L., Laycock, J. D., Lanza, D. L., and Yost, G. S. (1998). Evidence Supporting the Formation of 2,3-Epoxy-3-Methylindoline: a Reactive Intermediate of the Pneumotoxin 3-methylindole. Chem. Res. Toxicol. 11 (7), 741–749. doi:10.1021/tx9702087
Soares, V. C., Varanda, E. A., and Raddi, M. S. (2006). In Vitro basal and Metabolism-Mediated Cytotoxicity of Flavonoids. Food Chem. Toxicol. 44 (6), 835–838. doi:10.1016/j.fct.2005.11.006
Sun, Y., Yao, T., Li, H., Peng, Y., and Zheng, J. (2017). In Vitro and In Vivo Metabolic Activation of Berbamine to Quinone Methide Intermediate. J. Biochem. Mol. Toxicol. 31 (4). doi:10.1002/jbt.21876
Sun, Y., Xin, X., Zhang, K., Cui, T., Peng, Y., and Zheng, J. (2018). Cytochrome P450 Mediated Metabolic Activation of Chrysophanol. Chem. Biol. Interact 289, 57–67. doi:10.1016/j.cbi.2018.04.015
Surichan, S., Androutsopoulos, V. P., Sifakis, S., Koutala, E., Tsatsakis, A., Arroo, R. R., et al. (2012). Bioactivation of the Citrus Flavonoid Nobiletin by CYP1 Enzymes in MCF7 Breast Adenocarcinoma Cells. Food Chem. Toxicol. 50 (9), 3320–3328. doi:10.1016/j.fct.2012.06.030
Surichan, S., Arroo, R. R., Tsatsakis, A. M., and Androutsopoulos, V. P. (2018). Tangeretin Inhibits the Proliferation of Human Breast Cancer Cells via CYP1A1/CYP1B1 Enzyme Induction and CYP1A1/CYP1B1-Mediated Metabolism to the Product 4' Hydroxy Tangeretin. Toxicol. Vitro 50, 274–284. doi:10.1016/j.tiv.2018.04.001
Surichan, S., Arroo, R. R., Ruparelia, K., Tsatsakis, A. M., and Androutsopoulos, V. P. (2018). Nobiletin Bioactivation in MDA-MB-468 Breast Cancer Cells by Cytochrome P450 CYP1 Enzymes. Food Chem. Toxicol. 113, 228–235. doi:10.1016/j.fct.2018.01.047
Tang, J., and Hattori, M. (2011). Pyrrolizidine Alkaloids-Containing Chinese Medicines in the Chinese Pharmacopoeia and Related Safety Concerns. Yao Xue Xue Bao 46 (7), 762–772. doi:10.1631/jzus.B1000135
Tao, X., Younger, J., Fan, F. Z., Wang, B., and Lipsky, P. E. (2002). Benefit of an Extract of Tripterygium Wilfordii Hook F in Patients with Rheumatoid Arthritis: a Double-Blind, Placebo-Controlled Study. Arthritis Rheum. 46 (7), 1735–1743. doi:10.1002/art.10411
Teschke, R., Zhang, L., Long, H., Schwarzenboeck, A., Schmidt-Taenzer, W., Genthner, A., et al. (2015). Traditional Chinese Medicine and Herbal Hepatotoxicity: a Tabular Compilation of Reported Cases. Ann. Hepatol. 14 (1), 7–19. doi:10.1016/s1665-2681(19)30796-3
Thomassen, D., Slattery, J. T., and Nelson, S. D. (1990). Menthofuran-Dependent and Independent Aspects of Pulegone Hepatotoxicity: Roles of Glutathione. J. Pharmacol. Exp. Ther. 253 (2), 567–572.
Tian, Y., Shen, S., Jiang, Y., Shen, Q., Zeng, S., and Zheng, J. (2016). CYP3A5 Mediates Bioactivation and Cytotoxicity of Tetrandrine. Arch. Toxicol. 90 (7), 1737–1748. doi:10.1007/s00204-015-1584-8
Tzouros, M., and Pähler, A. (2009). A Targeted Proteomics Approach to the Identification of Peptides Modified by Reactive Metabolites. Chem. Res. Toxicol. 22 (5), 853–862. doi:10.1021/tx800426x
Verschoyle, R. D., Philpot, R. M., Wolf, C. R., and Dinsdale, D. (1993). CYP4B1 Activates 4-ipomeanol in Rat Lung. Toxicol. Appl. Pharmacol. 123 (2), 193–198. doi:10.1006/taap.1993.1237
Vrijsen, R., Michotte, Y., and Boeyé, A. (1990). Metabolic Activation of Quercetin Mutagenicity. Mutat. Res. 232 (2), 243–248. doi:10.1016/0027-5107(90)90130-V
Wang, Y., Chen, X., and Zhong, D. (2008). “P450-Mediated Metabolic Activation of Dauricine: Identification of Glutathione Conjugates in Human Liver Microsomes and in Rats[C],” in 2nd Asian Pacific Regional ISSX2nd Asian Pacific Regional ISSX Meeting.
Wang, C. J., Hu, C. P., Xu, K. P., Yuan, Q., Li, F. S., Zou, H., et al. (2010). Protective Effect of Selaginellin on Glutamate-Induced Cytotoxicity and Apoptosis in Differentiated PC12 Cells. Naunyn Schmiedebergs Arch. Pharmacol. 381 (1), 73–81. doi:10.1007/s00210-009-0470-4
Wang, C. C., Xia, Q., Li, M., Wang, S., Zhao, Y., Tolleson, W. H., et al. (2014). Metabolic Activation of Pyrrolizidine Alkaloids Leading to Phototoxicity and Photogenotoxicity in Human HaCaT Keratinocytes. J. Environ. Sci. Health C Environ. Carcinog. Ecotoxicol. Rev. 32 (4), 362–384. doi:10.1080/10590501.2014.969980
Wang, K., Zheng, L., Peng, Y., Song, J. E., and Zheng, J. (2014). Selective and Sensitive Platform for Function-Based Screening of Potentially Harmful Furans. Anal. Chem. 86 (21), 10755–10762. doi:10.1021/ac502796x
Wang, K., Li, W., Chen, J., Peng, Y., and Zheng, J. (2015). Detection of Cysteine- and Lysine-Based Protein Adductions by Reactive Metabolites of 2,5-dimethylfuran. Anal. Chim. Acta 896, 93–101. doi:10.1016/j.aca.2015.09.017
Wang, K., Lin, D., Guo, X., Huang, W., Peng, Y., and Zheng, J. (2017). Chemical Identity of Interaction of Protein with Reactive Metabolite of Diosbulbin B In Vitro and In Vivo. Toxins (Basel) 9 (8), 249. doi:10.3390/toxins9080249
Wang, D., Zhao, X. H., Cui, Y., Zhang, T. T., Wang, F., and Hu, Y. H. (2017). Efficacy and Safety of Tripterygium Wilfordii Hook F for CKD in Mainland China: A Systematic Review and Meta-Analysis. Phytother Res. 32 (3), 436–451. doi:10.1002/ptr.5987
Wang, H., Peng, Y., Zhang, T., Lan, Q., Zhao, H., Wang, W., et al. (2017). Metabolic Epoxidation Is a Critical Step for the Development of Benzbromarone-Induced Hepatotoxicity. Drug Metab. Dispos 45 (12), 1354–1363. doi:10.1124/dmd.117.077818
Watanabe, K., Matsuura, K., Gao, P., Hottenbacher, L., Tokunaga, H., Nishimura, K., et al. (2011). Traditional Japanese Kampo Medicine: Clinical Research between Modernity and Traditional Medicine-The State of Research and Methodological Suggestions for the Future. Evid. Based Complement. Alternat Med. 2011, 513842. doi:10.1093/ecam/neq067
Whitby, L. R., Obach, R. S., Simon, G. M., Hayward, M. M., and Cravatt, B. F. (2017). Quantitative Chemical Proteomic Profiling of the In Vivo Targets of Reactive Drug Metabolites. ACS Chem. Biol. 12 (8), 2040–2050. doi:10.1021/acschembio.7b00346
Wilsher, N. E., Arroo, R. R., Matsoukas, M. T., Tsatsakis, A. M., Spandidos, D. A., and Androutsopoulos, V. P. (2017). Cytochrome P450 CYP1 Metabolism of Hydroxylated Flavones and Flavonols: Selective Bioactivation of Luteolin in Breast Cancer Cells. Food Chem. Toxicol. 110, 383–394. doi:10.1016/j.fct.2017.10.051
Wilson, I. D., and Nicholson, J. K. (2017). Gut Microbiome Interactions with Drug Metabolism, Efficacy, and Toxicity. Transl Res. 179, 204–222. doi:10.1016/j.trsl.2016.08.002
Wilson, I. D., and Nicholson, J. K. (2015). “The Modulation of Drug Efficacy and Toxicity by the Gut Microbiome,” in Metabonomics and Gut Microbiota in Nutrition and Disease. Editors S. Kochhar, and F. -P. Martin (London: Springer London), 323–341. doi:10.1007/978-1-4471-6539-2_15
Xia, Q., Ma, L., He, X., Cai, L., and Fu, P. P. (2015). 7-glutathione Pyrrole Adduct: a Potential DNA Reactive Metabolite of Pyrrolizidine Alkaloids. Chem. Res. Toxicol. 28 (4), 615–620. doi:10.1021/tx500417q
Xia, Q., Zhao, Y., Lin, G., Beland, F. A., Cai, L., and Fu, P. P. (2016). Pyrrolizidine Alkaloid-Protein Adducts: Potential Non-invasive Biomarkers of Pyrrolizidine Alkaloid-Induced Liver Toxicity and Exposure. Chem. Res. Toxicol. 29 (8), 1282–1292. doi:10.1021/acs.chemrestox.6b00120
Xie, H., Liu, Y., Peng, Y., Zhao, D., and Zheng, J. (2016). Detection of Protein Adduction Derived from Dauricine by Alkaline Permethylation. Anal. Bioanal. Chem. 408 (15), 4111–4119. doi:10.1007/s00216-016-9505-0
Xu, Y., Mao, X., Qin, B., Peng, Y., and Zheng, J. (2018). In Vitro and In Vivo Metabolic Activation of Rhein and Characterization of Glutathione Conjugates Derived from Rhein. Chem. Biol. Interact 283, 1–9. doi:10.1016/j.cbi.2018.01.001
Xue, X., Gong, L., Qi, X., Wu, Y., Xing, G., Yao, J., et al. (2011). Knockout of Hepatic P450 Reductase Aggravates Triptolide-Induced Toxicity. Toxicol. Lett. 205 (1), 47–54. doi:10.1016/j.toxlet.2011.05.003
Yan, J., Xia, Q., Chou, M. W., and Fu, P. P. (2008). Metabolic Activation of Retronecine and Retronecine N-Oxide - Formation of DHP-Derived DNA Adducts. Toxicol. Ind. Health 24 (3), 181–188. doi:10.1177/0748233708093727
Yang, B., Liu, W., Chen, K., Wang, Z., and Wang, C. (2014). Metabolism of Diosbulbin B In Vitro and In Vivo in Rats: Formation of Reactive Metabolites and Human Enzymes Involved. Drug Metab. Dispos 42 (10), 1737–1750. doi:10.1124/dmd.114.058222
Yao, D., Shi, X., Wang, L., Gosnell, B. A., and Chen, C. (2013). Characterization of Differential Cocaine Metabolism in Mouse and Rat through Metabolomics-Guided Metabolite Profiling. Drug Metab. Dispos 41 (1), 79–88. doi:10.1124/dmd.112.048678
Ye, X., Li, W., Yan, Y., Mao, C., Cai, R., Xu, H., et al. (2010). Effects of Cytochrome P4503A Inducer Dexamethasone on the Metabolism and Toxicity of Triptolide in Rat. Toxicol. Lett. 192 (2), 212–220. doi:10.1016/j.toxlet.2009.10.028
Yockey, O. P., Jha, V., Ghodke, P. P., Xu, T., Xu, W., Ling, H., et al. (2017). Mechanism of Error-free DNA Replication Past Lucidin-Derived DNA Damage by Human DNA Polymerase κ. Chem. Res. Toxicol. 30 (11), 2023–2032. doi:10.1021/acs.chemrestox.7b00227
Yonemitsu, M., Fukuda, N., Kimura, T., and Komori, T. (1993). Diosbulbin-B from the Leaves and Stems of Dioscorea Bulbifera: 1H-1H and 13C-1H COSY NMR Studies. Planta Med. 59 (06), 577. doi:10.1055/s-2006-959772
Yost, G. S. (1989). Mechanisms of 3-methylindole Pneumotoxicity. Chem. Res. Toxicol. 2 (5), 273–279. doi:10.1021/tx00011a001
Yu, J., Deng, P., Zhong, D., and Chen, X. (2014). Identification of Amino Acid and Glutathione N-Conjugates of Toosendanin: Bioactivation of the Furan Ring Mediated by CYP3A4. Chem. Res. Toxicol. 27 (9), 1598–1609. doi:10.1021/tx5002145
Yuan, Y., Zheng, J., Wang, M., Li, Y., Ruan, J., and Zhang, H. (2016). Metabolic Activation of Rhein: Insights into the Potential Toxicity Induced by Rhein-Containing Herbs. J. Agric. Food Chem. 64 (28), 5742–5750. doi:10.1021/acs.jafc.6b01872
Zhang, L., Liu, Q., Pan, Y., Qi, X., Li, Y., Chen, C., et al. (2020). Cytochrome P450 3A4-Mediated Bioactivation and its Role in Nomilin-Induced Hepatotoxicity. Chem. Res. Toxicol. 33 (8), 2208–2217. doi:10.1021/acs.chemrestox.0c00228
Zhang, T., Rao, J., Li, W., Wang, K., and Qiu, F. (2020). Mechanism-based Inactivation of Cytochrome P450 Enzymes by Natural Products Based on Metabolic Activation. Drug Metab. Rev. 52 (4), 1–30. doi:10.1080/03602532.2020.1828910
Zheng, X., Zhao, A., Xie, G., Chi, Y., Zhao, L., Li, H., et al. (2013). Melamine-Induced Renal Toxicity Is Mediated by the Gut Microbiota. Sci. Transl Med. 5 (172), 172ra22. doi:10.1126/scitranslmed.3005114
Zhu, X., Wang, Y. K., Yang, X. N., Xiao, X. R., Zhang, T., Yang, X. W., et al. (2019). Metabolic Activation of Myristicin and its Role in Cellular Toxicity. J. Agric. Food Chem. 67 (15), 4328–4336. doi:10.1021/acs.jafc.9b00893
Zhuang, X. M., Shen, G. L., Xiao, W. B., Tan, Y., Lu, C., and Li, H. (2013). Assessment of the Roles of P-Glycoprotein and Cytochrome P450 in Triptolide-Induced Liver Toxicity in sandwich-cultured Rat Hepatocyte Model. Drug Metab. Dispos 41 (12), 2158–2165. doi:10.1124/dmd.113.054056
Keywords: herbal and dietary supplements-induced liver injury, natural products, metabolic activation, reactive metabolites, toxicity
Citation: Wang Y-K, Li WQ, Xia S, Guo L, Miao Y and Zhang B-K (2021) Metabolic Activation of the Toxic Natural Products From Herbal and Dietary Supplements Leading to Toxicities. Front. Pharmacol. 12:758468. doi: 10.3389/fphar.2021.758468
Received: 14 August 2021; Accepted: 05 October 2021;
Published: 20 October 2021.
Edited by:
Takeo Nakanishi, Takasaki University of Health and Welfare, JapanReviewed by:
Ge Lin, The Chinese University of Hong Kong, ChinaNico P. E. Vermeulen, VU University Amsterdam, Netherlands
Copyright © 2021 Wang, Li, Xia, Guo, Miao and Zhang. This is an open-access article distributed under the terms of the Creative Commons Attribution License (CC BY). The use, distribution or reproduction in other forums is permitted, provided the original author(s) and the copyright owner(s) are credited and that the original publication in this journal is cited, in accordance with accepted academic practice. No use, distribution or reproduction is permitted which does not comply with these terms.
*Correspondence: Yi-Kun Wang, MTU5NzIyOTE1MEBxcS5jb20=; Yan Miao, eWFubWlhb0Bjc3UuZWR1LmNu; Bi-Kui Zhang, emhiazY4QDE2My5jb20=