- Department of Pharmaceutics, Poona College of Pharmacy, Bharti Vidyapeeth Deemed University, Pune, India
Drug-resistant species of tuberculosis (TB), which spread faster than traditiona TB, is a severely infectious disease. The conventional drug therapy used in the management of tuberculosis has several challenges linked with adverse effects. Hence, nanotherapeutics served as an emerging technique to overcome problems associated with current treatment. Nanotherapeutics helps to overcome toxicity and poor solubility issues of several drugs used in the management of tuberculosis. Due to their diameter and surface chemistry, nanocarriers encapsulated with antimicrobial drugs are readily taken up by macrophages. Macrophages play a crucial role as they serve as target sites for active and passive targeting for nanocarriers. The surface of the nanocarriers is coated with ligand-specific receptors, which further enhances drug concentration locally and indicates the therapeutic potential of nanocarriers. This review highlights tuberculosis’s current facts, figures, challenges associated with conventional treatment, different nanocarrier-based systems, and its application in vaccine development.
Introduction
Tuberculosis as an Infectious Disease
Tuberculosis (TB) is a disease caused by the bacteria Mycobacterium tuberculosis (Mtb), which is passed from person to person through droplets generated by infected people (Sinha et al., 2016; WHO, 2020).
Current Facts and Figures as per the World Health Organization
According to the World Health Organization’s (WHO) annual 2020 global TB report, an estimated 10 million people were infected with tuberculosis in 2019, with 1.2 million fatalities, making it one of the world’s most infectious diseases (WHO, 2020).
Challenges in Tuberculosis Diagnosis and Treatment
The current treatments are restricted because of low effectiveness, more toxicity (hepatotoxicity), extended duration, patient non-compliance, development of multi-drug resistance (MDR), and extensively drug resistance (XDR) tuberculosis. MDR is the resistance of Mtb strains to at least rifampicin and isoniazid, which are considered cornerstone compounds for managing TB. XDR is TB that occurs due to Mtb strains that fulfil the definition of MDR/RR (Rifampicin resistance)-TB (WHO, 2021).
Hence, targeting either the host or pathogen decreases the treatment duration and limits the appearance of antibiotic resistance in Mtb (Li and Nikaido, 2004; Khan et al., 2017). Additionally, the development of novel drug delivery methods could serve as promising systems in treating MDR/XDR tuberculosis (Dua et al., 2018). The challenges associated with tuberculosis treatment and current facts and figures are as shown in Supplementary Figure S1.
Nanocarrier-Based Systems for Treatment of Tuberculosis
The conventional treatment of tuberculosis consists of administering first, second, and third-line drugs for a prolonged period. The efficient treatment needs a minimum of a 6 months course comprising a combination of antibiotics along with at least three bactericidal compounds (Patil, 2016). This is a leading contributory parameter in the relapses of the infection and the advent of MDR-TB and XDR-TB (Chan and Iseman, 2008). The marketed products available for conventional therapy are linked with numerous challenges like poor penetrability, metabolic instability, poor solubility, and associated adverse effects (Shivangi and Meena, 2018; Raza et al., 2019). Thus, these problems of conventional therapy resulted in a paradigm shift to nano carrier-based drug delivery systems (Patil et al., 2015; Costa et al., 2018). The different types of nanocarriers explored for management of tuberculosis are shown in Figure 1.
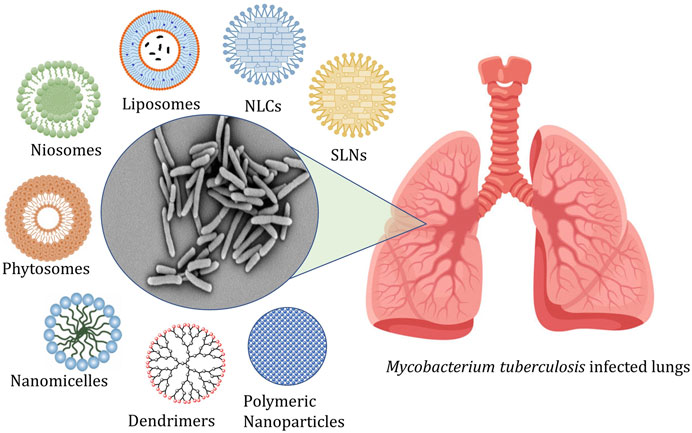
FIGURE 1. Represents the targeting of alveolar macrophages and granulomas using nanocarriers administered by different routes.
Role of Nanocarriers
Nanomedicine is considered the most recent invention in biomedical science, impacting diagnosis, management, and deterrence of several infectious diseases, including tuberculosis. Nanomedicine aids in the delivery of hydrophilic and hydrophobic compounds, macromolecules (intracellularly), and site-specific delivery of actives to cells or tissues (McAllister et al., 2007).
Advantages of Nanocarriers
Nanocarriers provide several benefits like significant decrease in size, high surface volume ratio, site-specificity, and being functionalized to alter the therapeutic potential of drugs (Costa-Gouveia et al., 2017; Patil et al., 2018). Moreover, the specific nanocarriers own intrinsic antimicrobial properties and act as a vehicle for different anti-tubercular substances, thus aiding in decreasing dosing regimen and related adverse effects (Costa-Gouveia et al., 2017). Various researchers have explored several nanocarriers for tuberculosis management and are exemplified below.
Lipid-Based Systems
Solid Lipid Nanoparticles
SLN is a nano-diameter lipid particulate system that has attracted more focus due to its specific distribution, biocompatibility, enhanced encapsulation of drugs, controlled delivery, and increment in the bioavailability of entrapped compounds (Pandey et al., 2005).
Singh et al. developed streptomycin sulfate-laden SLN for enhancement of oral bioavailability. Streptomycin sulfate (STRS)-laden SLN formulated using cold homogenization technique showed particle diameter (218.1 ± 15.46 nm), drug loading (30%), encapsulation efficiency (51.17 ± 0.95%), and mucus penetration characteristics. Compared to pure STRS, STR-SLNs showed a 3-fold decrease in the minimum inhibitory concentration (MIC) against Mtb H37RV. It offered better action against the other two species, namely M. bovis BCG and Mtb H37RV, than pure STRS. The acute cytotoxicity studies indicated the safety of developed formulation both in vitro and in vivo. Finally, a pharmacokinetics study of developed formulation in rats showed a significant increase (160–710%) in oral absorption and bioavailability compared to pure STRS (Singh et al., 2021).
Nanostructured Lipid Carrier
Nanostructured lipid carrier (NLC) is the next generation of lipid carrier systems mainly designed to surpass the shortcomings associated with SLN. NLC combines solid and liquid lipids, thus reducing crystallinity and a loosely arranged matrix system (Garg et al., 2016a; Pinheiro et al., 2016).
Patil et al. developed a clofazimine-loaded NLC to improve its bioavailability and safety in TB (Patil and Deshpande, 2021). In vitro hemocompatibility tests, cell viability tests on macrophage J774 cell lines, and in vivo acute inhalation toxicity analysis were used to investigate the safety profile of clofazimine-NLC and mannosylated-clofazimine-NLC. Acute toxicity data collected in vivo for 14 days showed no physiological or behavioral impairments and no mortality. The pharmacokinetic results indicated the highest amount of clofazimine (Cmax) of 35.44 ± 0.34 μg/g from mannosylated-clofazimine NLC posts 48 h. Mannosylated-clofazimine NLC exhibited a maximum (AUC0–∞) of 2,691.83 h μg/ml in the lungs, suggesting two times more bioavailability than clofazimine dispersion. As a result, mannosylated NLC can be a safe and effective system for delivery clofazimine in TB. Supplementary Figure S2 showed lipidic nanocarriers used in Mtb targeting.
Lipid-Drug Conjugate
Lipid drug conjugate (LDC) are lipidic prodrugs (Lambert, 2000) that consist of drugs bound to the lipidic moiety like fatty acid, phosphoglyceride, or a diglyceride bound together covalently or non-covalently (Adhikari et al., 2017).
Pandit et al. developed and studied intracellular trafficking of lipid-drug conjugate nanoparticles containing isoniazid (Pandit et al., 2020). LDC containing isoniazid was formulated using cold homogenization technique and characterized for various parameters. The developed LDC had a drug load of 92.73 ± 6.31% w/w and a monodisperse particle size of 124.60 ± 5.56 nm. Furthermore, the LDC revealed the ability to promote intracellular trafficking into endosomal and lysosomal vesicles, showing that CD63, LAMP-2, EEA1, and Rab 11 are involved in Mtb pathogenesis. Thus, prepared LDC showed the capability to enhance the intracellular transport of a highly water-soluble molecule like isoniazid (Pandit et al., 2020).
Lipid-polymer Hybrid Nanoparticles
Lipid polymer hybrid nanoparticle (LPHNPs) acts as potential drug delivery system that uses the drug delivery action of liposomes and polymeric nanoparticles to overcome their shortcomings (Garg et al., 2017; Garg et al., 2018; Jadon et al., 2021).
Bhardwaj et al. developed LPHNPs loaded with isoniazid using the spray drying technique. The formulated isoniazid LPHNPs were spherical with 111.81 ± 1.2 nm particle size and 0.189 ± 1.4 polydispersity index (PDI), suggesting their uptake by alveolar macrophages. The developed formulation was proven to have more uptake potential by J774A.1 cells than free isoniazid. Finally, an in vivo study in mice using dry powder insufflator containing isoniazid was more clearly detected in the plasma post 24 h administration of isoniazid loaded LPHNPs than free isoniazid (not traced post 24 h). Thus, isoniazid-loaded LPHNPs serve as a suitable carrier for pulmonary delivery in the management of tuberculosis (Bhardwaj et al., 2016).
Emulsion-Based Systems
Microemulsion
Microemulsions are transparent and isotropic drug delivery systems that are thermodynamically stable. They consist of a surfactant and co-surfactant that alter at least two mutually incompatible solvents, classically water and oil (Talmon and Prager, 1977; You et al., 2017).
Kaur et al. (2015) developed Brig 96 microemulsion containing both hydrophobic and hydrophilic drugs for synergistic action. The microemulsion was prepared to entrap anti-tubercular compounds to overcome the stability problems of rifampicin (RIF) in the presence of isoniazid (INH) and pyrazinamide (PZA). According to the release kinetics investigation, INH and PZA followed a diffusional (Fickian) while RIF followed an anomalous release mechanism. The antimicrobial study showed that compounds when used in combination provided potential antimicrobial activity. Thus, the study suggested that microemulsions serve as an excellent carrier in multiple drug therapy.
Nanoemulsion
Nanoemulsion is a potential carrier for enhancing the bioavailability of anti-tubercular compounds mainly via the oral route. The nanoemulsion laden with anti-tubercular molecules can freely transport across the biological barriers and therefore decrease the load of Mtb (Patil et al., 2018).
Henostroza et al. developed rifampicin-loaded cationic nanoemulsion with specific surface alteration using chitosan and polymyxin B. Rifampicin nanoemulsion containing chitosan and rifampicin nanoemulsion containing polymyxin B have both shown particle size around 150 nm and zeta potential values of +51.3 mV and +5.5 mV, respectively. In vitro mucoadhesion study results reported an electrostatic interaction among the cationic nanoemulsion and negatively charged mucin. This results in enhancing the effectiveness of the developed preparation by improving its residence time at the target site for treating ocular tuberculosis (Bazán Henostroza et al., 2020).
Vesicular Drug Delivery Systems
Liposomes
Liposomes are biocompatible, spherical, lipid bilayers vesicles assembled with an inner core comprising an aqueous phase. The reported liposomal carriers have shown effectiveness against multi-drug and extensively drug-resistant strains of Mycobacterium (Bekraki, 2020). Liposomes have also been a helpful system for vaccine delivery in managing tuberculosis (Tyagi et al., 2015; Tyagi et al., 2016; Diogo et al., 2019; Tandel et al., 2020; Chaudhari et al., 2021).
Tian et al. formulated and evaluated the pCMFO antigen-loaded dimethyl-dioctadecyl-ammonium (DDA) liposome with and without mono-phosphoryl lipid A (MPLA) and trehalose 6,6′-dibehenate (TDB). The in vivo studies on experimental mice were performed with different treatments: pCMFO, pCMFO/DDA, or pCMFO/DMT. The immunogenicity and protective efficiency of BCG-vaccinated mice against Mtb infection were also compared and analyzed. The dimethyl-dioctadecyl-ammonium liposome alone, or the addition of TDB or MPLA to the DDA liposome, elicited early interleukin-17 and interferon responses in vaccinated mice. Thus, the controlled release function of the DMT liposome may be connected to the enhanced efficacy of DMT (adjuvant) vaccination against TB (Tian et al., 2018).
Niosomes
Niosomes exhibit pharmaceutically acceptable properties such as non-toxicity, stability, sustained action, biodegradability, modification of drug distribution, and potential for improved drug bioavailability (Khan and Irchhaiya, 2016; Khoee and Yaghoobian, 2017).
Ethionamide and D-Cycloserine dual drug-loaded self-assembled niosomes have been produced by Kulkarni et al. for the successful treatment of multidrug-resistant tuberculosis. The niosomes were optimized by Box Behnken design and characterized for atomic force microscopy, in vitro hemodialysis, osmotic shock, and antimicrobial studies. The hemodialysis studies proved to offer safe intravenous administration of dual drug-loaded niosomes. The MIC of dual drug-loaded niosomes was the lowest vis-a-vis pure drug and single drug-loaded niosomes. This effectiveness was observed due to the slow release of lipophilic ethambutol and the initial burst release of D-Cycloserine. Thus, the synergetic effect of a dual drug embedded in niosomes was an effective treatment for tuberculosis (Kulkarni et al., 2019).
Figure 1 represents the targeting of alveolar macrophages and granulomas using nanocarriers administered by different routes, and Table 1 shows a list of nanocarriers for tuberculosis management.
Phytosomes
Phytosomes are commonly used to enhance the bioavailability of herbal extracts (Sharma and Roy, 2010; Awasthi et al., 2011). The anti-tubercular herbal extract of Glycyrrhiza glabra was embedded into the inhalable liposomes and showed significant inhibition of mycobacteria in tuberculosis-induced mice (Viswanathan et al., 2019).
Transferosomes
Transferosomes are ultra-deformable elastic vesicles composed of phospholipids and an edge activator (Rajan et al., 2011; Fernández-García et al., 2020).
Van et al. formulated the lipidic vesicles of artemisone, clofazimine, and decoquinate for the topical treatment of cutaneous tuberculosis. The vesicles comprising 1% each of active pharmaceutical ingredients were formulated by thin-film hydration technique. The various studies like hot stage microscopy and differential scanning isothermal calorimeter have shown minimal incompatibility among the APIs and other components of vesicles. Finally, developed formulation effectiveness was studied against the Mtb H37Rv laboratory strain and showed highest percentage inhibition with niosomes containing 1% clofazimine (van Zyl and Viljoen, 2019).
Other Nanocarriers
Dendrimers
Dendrimers are nanosized and symmetrically ordered branched polymers with several functional groups significantly used for targeted drug delivery. Due to their unique structure, dendrimers are suitable for encapsulating anti-tubercular drugs (Ghavami and Sardari, 2011).
Ahmed et al. developed and explored the effectiveness of a surface-modified 4.0 G PAMAM dendrimer as an innovative drug delivery system loaded with rifampicin to manage tuberculosis. The rifampicin was encapsulated into dendrimers using a simple dissolution solvent evaporation technique and evaluated for different parameters. The percentage coverage of 4.0 G PAMAM dendrimer peripheral with PEG was attained between 38–100%. The entrapment efficiency of native dendrimer was found to be 7.5% (W/W), and PEGylated dendrimer was >60% (w/w). The PEGylated dendrimers indicated a slow-release rate compared to the native formulation and free drug. The PEGylation of a dendrimer showed negligible toxicity for formulation with 100% functionalization. Thus, formulated PEGylated 4.0 G PAMAM dendrimers are proven to be the choice of drug carrier with high loading, negligible toxicity, and extended-release pattern for rifampicin (Ahmed et al., 2021).
An isoniazid embedded dendrimer complexation with copper nanoclusters showed synergism in dose reduction on the Mycobacterium tuberculosis H37Ra strain. This nanocluster could offer a novel alternative in treating tuberculosis (Rodrigues and Shende, 2020). Bellini et al. implemented molecular dynamics simulations to investigate rifampicin conjugation with a G4-PAMAM, a fourth-generation poly-amido-amine dendrimer for effective drug delivery, emphasizing physiological stability and pH-dependent release (Bellini et al., 2015).
Carbon Nanotubes
Carbon nanotubes (CNTs) are hollow tubular/cylindrical nanosized carbon atom structures that are hexagonally organized (Popov, 2004; Grumezescu, 2016). Many studies explored the possibility of using carbon nanotubes as effective carriers for delivering anti-tubercular compounds and overcoming tuberculosis’s antibacterial resistance (Praphakar et al., 2018).
Chen et al. (2019) developed and characterized isoniazid-chitosan-carbon nanotubes. In the in vitro studies, optimized drug-loaded nanotubes effectively inhibited Mycobacterium tuberculosis colonies. The isoniazid-chitosan-carbon nanotubes significantly healed tuberculosis ulcers which were induced in experimental guinea pigs. The immunohistochemistry showed the lowest number of CD4+T cells concerning pure isoniazid. The constructed isoniazid chitosan CNTs prolonged the release of isoniazid and reduced cytotoxicity and inflammation.
Nanomicelles
Nanomicelles are versatile nanocarriers constructed from amphiphilic monomers. The polymeric nanomicelles can encapsulate both hydrophilic and lipophilic drugs, thus providing a platform for solubility enhancement of poorly soluble drugs (Cholkar et al., 2012; Trinh et al., 2017).
Praphakar et al. developed the rifampicin-loaded chitosan nanomicelles and characterized the ex vivo cellular uptake using U937 cells and internalization of the multi-drug micelles in the zebrafish model, which showed excellent results (Amarnath Praphakar et al., 2019). Fluorescence micrographs of nanomicelles showed significant entry into A549 cell lines and thus proved its therapeutic effectiveness for the management of tuberculosis (Praphakar et al., 2017).
Application of Different Nanocarriers in Vaccine Development
Vaccines as a preventive measure have been formulated using killed or live attenuated microorganisms or components (like DNA or RNA) of a pathogen (Homma et al., 2013). Many vaccines are under development in different phases of clinical trials, namely AEC/BC02, MTBVAC, ID 93+GLA-SE, VPM 1002, RUTI, H56:IC31, Ad5Ag85A, M72 + AS01, DAR-901 booster, and TB-FLU-04L (Hussain et al., 2019). The BCG vaccine is made from a weakened strain of mycobacteria, and it uses the immune system to protect against the most severe forms of tuberculosis by stimulating T cell responses (Doherty and Andersen, 2005). VPM1002, a recombinant BCG (rBCG) vaccine, is one of the most advanced tuberculosis vaccines being tested in clinical trials.
The nanocarriers play a pivotal role in developing a vaccine that enhances the cellular and humoral immunological responses. The use of nanocarriers in vaccine development enhances antigen stability and immunogenicity and improves targeted delivery of vaccines. The nanocarriers, such as polymeric nanoparticles, liposomes, nanotubes, micelles, and virus-like particles, have been shown to be good vehicles for delivering antigens and acting as adjuvants (Hajizade et al., 2014; Mehdi et al., 2020).
The liposomes act as an immunological adjuvant and are extensively studied in vaccine development. The clinical trials have been conducted on many liposomal vaccines and received approval for effective tuberculosis management (Kim et al., 2014).
Mansury et al. formulated and evaluated the immunogenicity of the liposomes composed of the fusion protein (FP) of Mtb. It was observed that the optimized liposomal administration post-BCG vaccine contributed to the enhancement of γ interferon and interleukin-12, which confirms the enhancement of the BCG vaccine’s effectiveness (Mansury et al., 2019). Cationic liposomes can achieve the stability of subunit TB vaccines at the injection site for an extended period; it also provides solid electrostatic interactions with antigen-presenting sites and enhances immune responses (Khademi et al., 2018).
Recent Developments and Future Prospects
The recent updates in the management of tuberculosis includes three drugs, namely bedaquiline (2012), delamanid (2014), and pretomanid (2019), with a novel mechanism of action that have received conditional regulatory approval for the treatment of MDR and XDR tuberculosis. WHO reported newer anti-tubercular drugs in clinical trial phases: contezoid, delpazolid, mcaozinone, pretomanid, sutezolid, OPC-167832, Q203, SQ109, TBA-7371, and TBI-166 (Hussain et al., 2019).
The Nix-TB is the first TB clinical trial to test a new drug combination; pretomanid, bedaquiline, and linezolid. It is also collectively known as the BPal regimen, predicated to cure XDR-TB in six to 9 months (Conradie et al., 2020). Multiple active targeting of nanocarriers to the macrophages using various ligands is one of the research strategies recently explored by different researchers (Baranyai et al., 2021). Further, advances in drug loading, characterization, and scalability are needed to develop optimized and scalable formulations for tuberculosis treatment. A multidisciplinary approach is required for effective and focused tuberculosis treatment (Doherty and Andersen, 2005).
Conclusion
Tuberculosis is the most common cause of death around the world. More prevalence is due to the infectious process’ ability to grow and multiply within macrophages, leading to multi-drug resistance (MDR) and extensive drug resistance (XDR) tuberculosis. Nanotechnology offers novel avenues for tuberculosis diagnosis, treatment, and prevention, the most potential output being developing various nano carrier-based drug delivery systems and vaccines. Nanocarrier-based drug delivery systems improve bioavailability and biodistribution by reducing the dose and severity of accompanying adverse effects. Thus, to attain ground-breaking development and ensure the pulmonary administration of nanocarrier-based drug delivery systems for non-invasive clinical trials, multidisciplinary techniques are needed; nanotechnology, medicine, and engineering should work together.
Author Contributions
AR and SM contributed in terms of writing the review and VP serve as moderator.
Conflict of Interest
The authors declare that the research was conducted in the absence of any commercial or financial relationships that could be construed as a potential conflict of interest.
Publisher’s Note
All claims expressed in this article are solely those of the authors and do not necessarily represent those of their affiliated organizations, or those of the publisher, the editors and the reviewers. Any product that may be evaluated in this article, or claim that may be made by its manufacturer, is not guaranteed or endorsed by the publisher.
Supplementary Material
The Supplementary Material for this article can be found online at: https://www.frontiersin.org/articles/10.3389/fphar.2021.749945/full#supplementary-material
Abbreviations
CNTs, carbon nanotubes; DDA, dimethyl octadecyl-ammonium; DOT, direct observation therapy; FP, fusion protein; INH, isoniazid; LDC, lipid drug conjugate; LP, liposomes; MDR, multi-drug resistance; ME, microemulsion; MIC, minimum inhibitory concentration; Mtb, Mycobacterium tuberculosis; MWCNTs, multiwalled carbon nanotubes; NE, nanoemulsion; NLC, nanostructured lipid carrier; NMP, nano-microparticles; NPs, nanoparticles; PZA, pyrazinamide; RIF, rifampicin; RR, rifampicin resistance; SLM, solid lipid microparticles; STRS, streptomycin sulphate; SWCNTs, single-walled carbon nanotubes; TB, Tuberculosis; TDB, Trehalose 6,6-dibehenate; TEM, transmission electron microscopy; TPG, tocopheryl polyethylene glycol succinate; WHO, world health organization; XDR, extensive drug resistance; ZrO2, zirconia.
References
Adhikari, P., Pal, P., Das, A. K., Ray, S., Bhattacharjee, A., and Mazumder, B. (2017). Nano Lipid-Drug Conjugate: An Integrated Review. Int. J. Pharm. 529 (1), 629–641. doi:10.1016/j.ijpharm.2017.07.039
Ahmad Khan, F., Salim, M. A. H., du Cros, P., Casas, E. C., Khamraev, A., Sikhondze, W., et al. (2017). Effectiveness and Safety of Standardised Shorter Regimens for Multidrug-Resistant Tuberculosis: Individual Patient Data and Aggregate Data Meta-Analyses. Eur. Respir. J. 50 (1). doi:10.1183/13993003.00061-2017
Ahmed, R., Aucamp, M., Ebrahim, N., and Samsodien, H. (2021). Supramolecular Assembly of Rifampicin and PEGylated PAMAM Dendrimer as a Novel Conjugate for Tuberculosis. J. Drug Deliv. Sci. Technology 66, 102773. doi:10.1016/j.jddst.2021.102773
Amarnath Praphakar, R., Sam Ebenezer, R., Vignesh, S., Shakila, H., and Rajan, M. (2019). Versatile pH-Responsive Chitosan-G-Polycaprolactone/Maleic Anhydride-Isoniazid Polymeric Micelle to Improve the Bioavailability of Tuberculosis Multidrugs. ACS Appl. Bio Mater. 2 (5), 1931–1943. doi:10.1021/acsabm.9b00003
Awasthi, R., Kulkarni, G., and Pawar, V. K. (2011). Phytosomes: an Approach to Increase the Bioavailability of Plant Extracts. Int. J. Pharm. Pharm. Sci. 3 (2), 1–3.
Baranyai, Z., Soria‐Carrera, H., Alleva, M., Millán‐Placer, A. C., Lucía, A., Martín‐Rapún, R., et al. (2021). Nanotechnology‐Based Targeted Drug Delivery: An Emerging Tool to Overcome Tuberculosis. Adv. Therap. 4 (1), 2000113. doi:10.1002/adtp.202000113
Bazán Henostroza, M. A., Curo Melo, K. J., Nishitani Yukuyama, M., Löbenberg, R., and Araci Bou-Chacra, N. (2020). Cationic Rifampicin Nanoemulsion for the Treatment of Ocular Tuberculosis. Colloids Surf. A: Physicochemical Eng. Aspects 597, 124755. doi:10.1016/j.colsurfa.2020.124755
Bellini, R. G., Guimarães, A. P., Pacheco, M. A., Dias, D. M., Furtado, V. R., de Alencastro, R. B., et al. (2015). Association of the Anti-tuberculosis Drug Rifampicin with a PAMAM Dendrimer. J. Mol. Graph Model. 60, 34–42. doi:10.1016/j.jmgm.2015.05.012
Bhardwaj, A., Mehta, S., Yadav, S., Singh, S. K., Grobler, A., Goyal, A. K., et al. (2016). Pulmonary Delivery of Antitubercular Dugs Using spray-dried Lipid-Polymer Hybrid Nanoparticles. Artif. Cell Nanomed Biotechnol 44 (6), 1544–1555. doi:10.3109/21691401.2015.1062389
Carneiro, S. P., Carvalho, K. V., de Oliveira Aguiar Soares, R. D., Carneiro, C. M., de Andrade, M. H. G., Duarte, R. S., et al. (2019). Functionalized Rifampicin-Loaded Nanostructured Lipid Carriers Enhance Macrophages Uptake and Antimycobacterial Activity. Colloids Surf. B Biointerfaces 175, 306–313. doi:10.1016/j.colsurfb.2018.12.003
Chan, E. D., and Iseman, M. D. (2008). Multidrug-resistant and Extensively Drug-Resistant Tuberculosis: a Review. Curr. Opin. Infect. Dis. 21 (6), 587–595. doi:10.1097/QCO.0b013e328319bce6
Changsan, N., Chan, H. K., Separovic, F., and Srichana, T. (2009). Physicochemical Characterization and Stability of Rifampicin Liposome Dry Powder Formulations for Inhalation. J. Pharm. Sci. 98 (2), 628–639. doi:10.1002/jps.21441
Chaudhari, R., Tandel, N., Sahu, K., Negi, S., Bashir, H., Rupareliya, A., et al. (2021). Transdermal Immunization of Elastic Liposome-Laden Recombinant Chimeric Fusion Protein of P. Falciparum (PfMSP-Fu24) Mounts Protective Immune Response. Nanomaterials 11 (2), 406. doi:10.3390/nano11020406
Chen, G., Wu, Y., Yu, D., Li, R., Luo, W., Ma, G., et al. (2019). Isoniazid-loaded Chitosan/carbon Nanotubes Microspheres Promote Secondary Wound Healing of Bone Tuberculosis. J. Biomater. Appl. 33 (7), 989–996. doi:10.1177/0885328218814988
Cholkar, K., Patel, A., Vadlapudi, A. D., and Mitra, A. K. (2012). Novel Nanomicellar Formulation Approaches for Anterior and Posterior Segment Ocular Drug Delivery. Recent Pat Nanomed 2 (2), 82–95. doi:10.2174/1877912311202020082
Conradie, F., Diacon, A. H., Ngubane, N., Howell, P., Everitt, D., Crook, A. M., et al. (2020). Treatment of Highly Drug-Resistant Pulmonary Tuberculosis. N. Engl. J. Med. 382 (10), 893–902. doi:10.1056/NEJMoa1901814
Costa, A., Sarmento, B., and Seabra, V. (2018). Mannose-functionalized Solid Lipid Nanoparticles Are Effective in Targeting Alveolar Macrophages. Eur. J. Pharm. Sci. 114, 103–113. doi:10.1016/j.ejps.2017.12.006
Costa-Gouveia, J., Aínsa, J. A., Brodin, P., and Lucía, A. (2017). How Can Nanoparticles Contribute to Antituberculosis Therapy? Drug Discov. Today 22 (3), 600–607. doi:10.1016/j.drudis.2017.01.011
De Matteis, L., Jary, D., Lucía, A., García-Embid, S., Serrano-Sevilla, I., Pérez, D., et al. (2018). New Active Formulations against M. tuberculosis: Bedaquiline Encapsulation in Lipid Nanoparticles and Chitosan Nanocapsules. Chem. Eng. J. 340, 181–191. doi:10.1016/j.cej.2017.12.110
Diogo, G. R., Hart, P., Copland, A., Kim, M. Y., Tran, A. C., Poerio, N., et al. (2019). Immunization with Mycobacterium tuberculosis Antigens Encapsulated in Phosphatidylserine Liposomes Improves Protection Afforded by BCG. Front. Immunol. 10, 1349. doi:10.3389/fimmu.2019.01349
Doherty, T. M., and Andersen, P. (2005). Vaccines for Tuberculosis: Novel Concepts and Recent Progress. Clin. Microbiol. Rev. 18 (4), 687–702. doi:10.1128/CMR.18.4.687-702.2005
Dua, K., Rapalli, V. K., Shukla, S. D., Singhvi, G., Shastri, M. D., Chellappan, D. K., et al. (2018). Multi-drug Resistant Mycobacterium tuberculosis & Oxidative Stress Complexity: Emerging Need for Novel Drug Delivery Approaches. Biomed. Pharmacother. 107, 1218–1229. doi:10.1016/j.biopha.2018.08.101
El-Ridy, M. S., Mostafa, D. M., Shehab, A., Nasr, E. A., and Abd El-Alim, S. (2007). Biological Evaluation of Pyrazinamide Liposomes for Treatment of Mycobacterium tuberculosis. Int. J. Pharm. 330 (1-2), 82–88. doi:10.1016/j.ijpharm.2006.09.017
Fernández-García, R., Lalatsa, A., Statts, L., Bolás-Fernández, F., Ballesteros, M. P., and Serrano, D. R. (2020). Transferosomes as Nanocarriers for Drugs across the Skin: Quality by Design from Lab to Industrial Scale. Int. J. Pharm. 573, 118817. doi:10.1016/j.ijpharm.2019.118817
Garg, N. K., Singh, B., Tyagi, R. K., Sharma, G., and Katare, O. P. (2016a). Effective Transdermal Delivery of Methotrexate through Nanostructured Lipid Carriers in an Experimentally Induced Arthritis Model. Colloids Surf. B Biointerfaces 147, 17–24. doi:10.1016/j.colsurfb.2016.07.046
Garg, N. K., Tandel, N., Jadon, R. S., Tyagi, R. K., and Katare, O. P. (2018). Lipid-polymer Hybrid Nanocarrier-Mediated Cancer Therapeutics: Current Status and Future Directions. Drug Discov. Today 23 (9), 1610–1621. doi:10.1016/j.drudis.2018.05.033
Garg, N. K., Tyagi, R. K., Sharma, G., Jain, A., Singh, B., Jain, S., et al. (2017). Functionalized Lipid-Polymer Hybrid Nanoparticles Mediated Codelivery of Methotrexate and Aceclofenac: A Synergistic Effect in Breast Cancer with Improved Pharmacokinetics Attributes. Mol. Pharm. 14 (6), 1883–1897. doi:10.1021/acs.molpharmaceut.6b01148
Garg, T., Rath, G., and Goyal, A. K. (2016b). Inhalable Chitosan Nanoparticles as Antitubercular Drug Carriers for an Effective Treatment of Tuberculosis. Artif. Cell Nanomed Biotechnol 44 (3), 997–1001. doi:10.3109/21691401.2015.1008508
Ghavami, G., and Sardari, S. (2011). New Chimeric Anti-tubercular Dendrimers with Self-Delivering Property. Afr. J. Microbiol. Res. 5 (18), 2575–2582.
Grumezescu, A. (2016). Surface Chemistry of Nanobiomaterials: Applications of Nanobiomaterials. Amsterdam, Netherlands: William Andrew, 528.
Hajizade, A., Ebrahimi, F., Salmanian, A.-H., Arpanaei, A., and Amani, J. (2014). Nanoparticles in Vaccine Development. J. Appl. Biotechnol. Rep. 1 (4), 125–134.
Homma, A., Tanuri, A., Duarte, A. J., Marques, E., de Almeida, A., Martins, R., et al. (2013). Vaccine Research, Development, and Innovation in Brazil: a Translational Science Perspective. Vaccine 31, B54–B60. doi:10.1016/j.vaccine.2012.11.084
Hussain, A., Singh, S., Das, S. S., Anjireddy, K., Karpagam, S., and Shakeel, F. (2019). Nanomedicines as Drug Delivery Carriers of Anti-tubercular Drugs: from Pathogenesis to Infection Control. Curr. Drug Deliv. 16 (5), 400–429. doi:10.2174/1567201816666190201144815
Ibrahim Bekraki, A. (2020). “Liposomes-and Niosomes-Based Drug Delivery Systems for Tuberculosis Treatment,” in Nanotechnology Based Approaches for Tuberculosis Treatment (Elsevier), 107–122. doi:10.1016/b978-0-12-819811-7.00007-2
Jadon, R. S., Sharma, G., Garg, N. K., Tandel, N., Gajbhiye, K. R., Salve, R., et al. (2021). Efficient In Vitro and In Vivo Docetaxel Delivery Mediated by pH-Sensitive LPHNPs for Effective Breast Cancer Therapy. Colloids Surf. B Biointerfaces 203, 111760. doi:10.1016/j.colsurfb.2021.111760
Jahagirdar, P. S., Gupta, P. K., Kulkarni, S. P., and Devarajan, P. V. (2020). Intramacrophage Delivery of Dual Drug Loaded Nanoparticles for Effective Clearance of Mycobacterium tuberculosis. J. Pharm. Sci. 109 (7), 2262–2270. doi:10.1016/j.xphs.2020.03.018
Jain, C., Vyas, S., and Dixit, V. (2006). Niosomal System for Delivery of Rifampicin to Lymphatics. Indian J. Pharm. Sci. 68 (5). doi:10.4103/0250-474x.29622
Kaur, G., Mehta, S. K., Kumar, S., Bhanjana, G., and Dilbaghi, N. (2015). Coencapsulation of Hydrophobic and Hydrophilic Antituberculosis Drugs in Synergistic Brij 96 Microemulsions: A Biophysical Characterization. J. Pharm. Sci. 104 (7), 2203–2212. doi:10.1002/jps.24469
Khademi, F., Taheri, R. A., Momtazi-Borojeni, A. A., Farnoosh, G., Johnston, T. P., and Sahebkar, A. (2018). Potential of Cationic Liposomes as Adjuvants/delivery Systems for Tuberculosis Subunit Vaccines. Rev. Physiol. Biochem. Pharmacol. 175, 47–69. doi:10.1007/112_2018_9
Khan, D. H., Bashir, S., Khan, M. I., Figueiredo, P., Santos, H. A., and Peltonen, L. (2020). Formulation Optimization and In Vitro Characterization of Rifampicin and Ceftriaxone Dual Drug Loaded Niosomes with High Energy Probe Sonication Technique. J. Drug Deliv. Sci. Technology 58, 101763. doi:10.1016/j.jddst.2020.101763
Khan, R., and Irchhaiya, R. (2016). Niosomes: a Potential Tool for Novel Drug Delivery. J. Pharm. Invest. 46 (3), 195–204. doi:10.1007/s40005-016-0249-9
Khoee, S., and Yaghoobian, M. (2017). “Niosomes: A Novel Approach in Modern Drug Delivery Systems,” in Nanostructures for Drug Delivery (Elsevier), 207–237. doi:10.1016/b978-0-323-46143-6.00006-3
Kim, M.-G., Park, J. Y., Shon, Y., Kim, G., Shim, G., and Oh, Y.-K. (2014). Nanotechnology and Vaccine Development. Asian J. Pharm. Sci. 9 (5), 227–235. doi:10.1016/j.ajps.2014.06.002
Kulkarni, P., Rawtani, D., and Barot, T. (2019). Formulation and Optimization of Long Acting Dual Niosomes Using Box-Behnken Experimental Design Method for Combinative Delivery of Ethionamide and D-Cycloserine in Tuberculosis Treatment. Colloids Surf. A: Physicochemical Eng. Aspects 565, 131–142. doi:10.1016/j.colsurfa.2019.01.004
Lambert, D. M. (2000). Rationale and Applications of Lipids as Prodrug Carriers. Eur. J. Pharm. Sci. 11, S15–S27. doi:10.1016/s0928-0987(00)00161-5
Li, X. Z., and Nikaido, H. (2004). Efflux-mediated Drug Resistance in Bacteria. Drugs 64, 159–204. doi:10.2165/00003495-200464020-00004
Ma, C., Wu, M., Ye, W., Huang, Z., Ma, X., Wang, W., et al. (2021). Inhalable Solid Lipid Nanoparticles for Intracellular Tuberculosis Infection Therapy: Macrophage-Targeting and pH-Sensitive Properties. Drug Deliv. Transl Res. 11 (3), 1218–1235. doi:10.1007/s13346-020-00849-7
Makled, S., Boraie, N., and Nafee, N. (2021). Nanoparticle-mediated Macrophage Targeting-A New Inhalation Therapy Tackling Tuberculosis. Drug Deliv. Transl Res. 11 (3), 1037–1055. doi:10.1007/s13346-020-00815-3
Manca, M. L., Manconi, M., Valenti, D., Lai, F., Loy, G., Matricardi, P., et al. (2012). Liposomes Coated with Chitosan-Xanthan Gum (Chitosomes) as Potential Carriers for Pulmonary Delivery of Rifampicin. J. Pharm. Sci. 101 (2), 566–575. doi:10.1002/jps.22775
Mani, S., Cheemalapati, S., Chen, S.-M., and Devadas, B. (2015). Anti-tuberculosis Drug Pyrazinamide Determination at Multiwalled Carbon Nanotubes/graphene Oxide Hybrid Composite Fabricated Electrode. Int. J. Electrochem. Sci. 10, 7049–7062.
Mansury, D., Ghazvini, K., Amel Jamehdar, S., Badiee, A., Tafaghodi, M., Nikpoor, A. R., et al. (2019). Enhancement of the Effect of BCG Vaccine against Tuberculosis Using DDA/TDB Liposomes Containing a Fusion Protein of HspX, PPE44, and EsxV. Artif. Cell Nanomed Biotechnol 47 (1), 370–377. doi:10.1080/21691401.2018.1557674
McAllister, C., Ramachandran, R., and Ruetsch, S. (2007). Impact of Nanotechnology on Hair Attributes. ACS Nano 3 (1), 1–7.
Mehdi, K., Mohsen, M., Naser Mohammadpour, D., Mohsen, M., and Alireza, M. (2020). Nanoparticles and Vaccine Development. Pharm. Nanotechnology 8 (1), 6–21. doi:10.2174/2211738507666191024162042
Moazeni, E., Gilani, K., Sotoudegan, F., Pardakhty, A., Najafabadi, A. R., Ghalandari, R., et al. (2010). Formulation and In Vitro Evaluation of Ciprofloxacin Containing Niosomes for Pulmonary Delivery. J. Microencapsul 27 (7), 618–627. doi:10.3109/02652048.2010.506579
Nkanga, C. I., Krause, R. W., Noundou, X. S., and Walker, R. B. (2017). Preparation and Characterization of Isoniazid-Loaded Crude Soybean Lecithin Liposomes. Int. J. Pharm. 526 (1-2), 466–473. doi:10.1016/j.ijpharm.2017.04.074
Pandey, R., Sharma, S., and Khuller, G. K. (2005). Oral Solid Lipid Nanoparticle-Based Antitubercular Chemotherapy. Tuberculosis (Edinb) 85 (5), 415–420. doi:10.1016/j.tube.2005.08.009
Pandit, S., Roy, S., Pillai, J., and Banerjee, S. (2020). Formulation and Intracellular Trafficking of Lipid-Drug Conjugate Nanoparticles Containing a Hydrophilic Antitubercular Drug for Improved Intracellular Delivery to Human Macrophages. ACS Omega 5 (9), 4433–4448. doi:10.1021/acsomega.9b03523
Patil, J. S., Devi, V. K., Devi, K., and Sarasija, S. (2015). A Novel Approach for Lung Delivery of Rifampicin-Loaded Liposomes in Dry Powder Form for the Treatment of Tuberculosis. Lung India 32 (4), 331–338. doi:10.4103/0970-2113.159559
Patil, J. (2016). Significance of Particulate Drug Delivery System in Anti-microbial Therapy. Adv. Pharmacoepidemiol. Drug Saf. 5, 139. doi:10.4172/2167-1052.1000e139
Patil, K., Bagade, S., Bonde, S., Sharma, S., and Saraogi, G. (2018). Recent Therapeutic Approaches for the Management of Tuberculosis: Challenges and Opportunities. Biomed. Pharmacother. 99, 735–745. doi:10.1016/j.biopha.2018.01.115
Patil, T. S., and Deshpande, A. S. (2021). Nanostructured Lipid Carrier-Mediated Lung Targeted Drug Delivery System to Enhance the Safety and Bioavailability of Clofazimine. Drug Dev. Ind. Pharm. 47 (3), 385–393. doi:10.1080/03639045.2021.1892743
Patil-Gadhe, A., and Pokharkar, V. (2014). Single Step spray Drying Method to Develop Proliposomes for Inhalation: a Systematic Study Based on Quality by Design Approach. Pulm. Pharmacol. Ther. 27 (2), 197–207. doi:10.1016/j.pupt.2013.07.006
Pinheiro, M., Ribeiro, R., Vieira, A., Andrade, F., and Reis, S. (2016). Design of a Nanostructured Lipid Carrier Intended to Improve the Treatment of Tuberculosis. Drug Des. Devel Ther. 10, 2467–2475. doi:10.2147/DDDT.S104395
Popov, V. (2004). Carbon Nanotubes: Properties and Application. Mater. Sci. Eng. R: Rep. 43 (3), 61–102. doi:10.1016/j.mser.2003.10.001
Praphakar, R. A., Munusamy, M. A., and Rajan, M. (2017). Development of Extended-Voyaging Anti-oxidant Linked Amphiphilic Polymeric Nanomicelles for Anti-tuberculosis Drug Delivery. Int. J. Pharm. 524 (1-2), 168–177. doi:10.1016/j.ijpharm.2017.03.089
Praphakar, R. A., Shakila, H., Azger Dusthackeer, V. N., Munusamy, M. A., Kumar, S., and Rajan, M. (2018). A Mannose-Conjugated Multi-Layered Polymeric Nanocarrier System for Controlled and Targeted Release on Alveolar Macrophages. Polym. Chem. 9 (5), 656–667. doi:10.1039/c7py02000g
Rajan, R., Jose, S., Mukund, V. P., and Vasudevan, D. T. (2011). Transferosomes - A Vesicular Transdermal Delivery System for Enhanced Drug Permeation. J. Adv. Pharm. Technol. Res. 2 (3), 138–143. doi:10.4103/2231-4040.85524
Rani, N. P., Suriyaprakash, T., and Senthamarai, R. (2010). Formulation and Evaluation of Rifampicin and Gatifloxacin Niosomes on Logarithmic-phase Cultures of Mycobacterium tuberculosis. Int. J. Pharma Bio Sci. 1 (4), 379–387.
Rawal, T., Parmar, R., Tyagi, R. K., and Butani, S. (2017). Rifampicin Loaded Chitosan Nanoparticle Dry Powder Presents an Improved Therapeutic Approach for Alveolar Tuberculosis. Colloids Surf. B Biointerfaces 154, 321–330. doi:10.1016/j.colsurfb.2017.03.044
Rawal, T., Patel, S., and Butani, S. (2018). Chitosan Nanoparticles as a Promising Approach for Pulmonary Delivery of Bedaquiline. Eur. J. Pharm. Sci. 124, 273–287. doi:10.1016/j.ejps.2018.08.038
Raza, A., Sime, F. B., Cabot, P. J., Maqbool, F., Roberts, J. A., and Falconer, J. R. (2019). Solid Nanoparticles for Oral Antimicrobial Drug Delivery: a Review. Drug Discov. Today 24 (3), 858–866. doi:10.1016/j.drudis.2019.01.004
Rodrigues, B., and Shende, P. (2020). Monodispersed Metal-Based Dendrimeric Nanoclusters for Potentiation of Anti-tuberculosis Action. J. Mol. Liquids 304, 112731. doi:10.1016/j.molliq.2020.112731
Rojanarat, W., Nakpheng, T., Thawithong, E., Yanyium, N., and Srichana, T. (2012). Inhaled Pyrazinamide Proliposome for Targeting Alveolar Macrophages. Drug Deliv. 19 (7), 334–345. doi:10.3109/10717544.2012.721144
Shah, S., Ghetiya, R., Soniwala, M., and Chavda, J. (2021). Development and Optimization of Inhalable Levofloxacin Nanoparticles for the Treatment of Tuberculosis. Curr. Drug Deliv. 18, 779–793. doi:10.2174/1567201817999201103194626
Sharma, S., and Roy, R. K. (2010). Phytosomes: an Emerging Technology. Int. J. Pharm. Res. Dev. 2 (5), 1–7.
Shivangi, , and Meena, L. S. (2018). A Novel Approach in Treatment of Tuberculosis by Targeting Drugs to Infected Macrophages Using Biodegradable Nanoparticles. Appl. Biochem. Biotechnol. 185 (3), 815–821. doi:10.1007/s12010-018-2695-5
Singh, G., Dwivedi, H., Saraf, S. K., and Saraf, S. A. (2011). Niosomal Delivery of Isoniazid-Development and Characterization. Trop. J. Pharm. Res. 10 (2). doi:10.4314/tjpr.v10i2.66564
Singh, M., Schiavone, N., Papucci, L., Maan, P., Kaur, J., Singh, G., et al. (2021). Streptomycin Sulphate Loaded Solid Lipid Nanoparticles Show Enhanced Uptake in Macrophage, Lower MIC in Mycobacterium and Improved Oral Bioavailability. Eur. J. Pharm. Biopharm. 160, 100–124. doi:10.1016/j.ejpb.2021.01.009
Sinha, P., Gupta, A., Prakash, P., Anupurba, S., Tripathi, R., and Srivastava, G. N. (2016). Differentiation of Mycobacterium tuberculosis Complex from Non-tubercular Mycobacteria by Nested Multiplex PCR Targeting IS6110, MTP40 and 32kD Alpha Antigen Encoding Gene Fragments. BMC Infect. Dis. 16 (1), 123. doi:10.1186/s12879-016-1450-1
Song, X., Lin, Q., Guo, L., Fu, Y., Han, J., Ke, H., et al. (2015). Rifampicin Loaded Mannosylated Cationic Nanostructured Lipid Carriers for Alveolar Macrophage-specific Delivery. Pharm. Res. 32 (5), 1741–1751. doi:10.1007/s11095-014-1572-3
Su, F. Y., Chen, J., Son, H. N., Kelly, A. M., Convertine, A. J., West, T. E., et al. (2018). Polymer-augmented Liposomes Enhancing Antibiotic Delivery against Intracellular Infections. Biomater. Sci. 6 (7), 1976–1985. doi:10.1039/c8bm00282g
Talmon, Y., and Prager, S. (1977). Statistical Mechanics of Microemulsions. Nature 267 (5609), 333–335. doi:10.1038/267333a0
Tandel, N., Joseph, A. Z., Joshi, A., Shrama, P., Mishra, R. P., Tyagi, R. K., et al. (2020). An Evaluation of Liposome-Based Diagnostics of Pulmonary and Extrapulmonary Tuberculosis. Expert Rev. Mol. Diagn. 20 (5), 533–541. doi:10.1080/14737159.2020.1740596
Tian, M., Zhou, Z., Tan, S., Fan, X., Li, L., and Ullah, N. (2018). Formulation in DDA-MPLA-TDB Liposome Enhances the Immunogenicity and Protective Efficacy of a DNA Vaccine against Mycobacterium tuberculosis Infection. Front. Immunol. 9 (310), 310. doi:10.3389/fimmu.2018.00310
Trinh, H. M., Joseph, M., Cholkar, K., Mitra, R., and Mitra, A. K. (2017). “Nanomicelles in Diagnosis and Drug Delivery∗,” in Emerging Nanotechnologies for Diagnostics, Drug Delivery and Medical Devices (Elsevier), 45–58. doi:10.1016/b978-0-323-42978-8.00003-6
Truzzi, E., Nascimento, T. L., Iannuccelli, V., Costantino, L., Lima, E. M., Leo, E., et al. (2020). In Vivo biodistribution of Respirable Solid Lipid Nanoparticles Surface-Decorated with a Mannose-Based Surfactant: a Promising Tool for Pulmonary Tuberculosis Treatment? Nanomaterials (Basel) 10 (3), 568. doi:10.3390/nano10030568
Tyagi, R. K., Garg, N. K., Dalai, S. K., and Awasthi, A. (2016). Transdermal Immunization of P. Falciparum Surface Antigen (MSP-119) via Elastic Liposomes Confers Robust Immunogenicity. Hum. Vaccin. Immunother. 12 (4), 990–992. doi:10.1080/21645515.2015.1116656
Tyagi, R. K., Garg, N. K., Jadon, R., Sahu, T., Katare, O. P., Dalai, S. K., et al. (2015). Elastic Liposome-Mediated Transdermal Immunization Enhanced the Immunogenicity of P. Falciparum Surface Antigen, MSP-119. Vaccine 33 (36), 4630–4638. doi:10.1016/j.vaccine.2015.06.054
van Zyl, L., Viljoen, J. M., Haynes, R. K., Aucamp, M., Ngwane, A. H., and du Plessis, J. (2019). Topical Delivery of Artemisone, Clofazimine and Decoquinate Encapsulated in Vesicles and Their In Vitro Efficacy against Mycobacterium tuberculosis. AAPS PharmSciTech 20 (1), 33. doi:10.1208/s12249-018-1251-5
Vieira, A. C. C., Chaves, L. L., Pinheiro, M., Lima, S. A. C., Ferreira, D., Sarmento, B., et al. (2018). Mannosylated Solid Lipid Nanoparticles for the Selective Delivery of Rifampicin to Macrophages. Artif. Cell Nanomed Biotechnol 46, 653–663. doi:10.1080/21691401.2018.1434186
Viswanathan, V., Pharande, R., Bannalikar, A., Gupta, P., Gupta, U., and Mukne, A. (2019). Inhalable Liposomes of Glycyrrhiza Glabra Extract for Use in Tuberculosis: Formulation, In Vitro Characterization, In Vivo Lung Deposition, and In Vivo Pharmacodynamic Studies. Drug Dev. Ind. Pharm. 45 (1), 11–20. doi:10.1080/03639045.2018.1513025
Vladimirsky, M. A., and Ladigina, G. A. (1982). Antibacterial Activity of Liposome-Entrapped Streptomycin in Mice Infected with Mycobacterium tuberculosis. Biomed. Pharmacother. 36 (8-9), 375–377.
Vyas, S. P., Kannan, M. E., Jain, S., Mishra, V., and Singh, P. (2004). Design of Liposomal Aerosols for Improved Delivery of Rifampicin to Alveolar Macrophages. Int. J. Pharm. 269 (1), 37–49. doi:10.1016/j.ijpharm.2003.08.017
Who, (2021). WHO Announces Updated Definitions of Extensively Drug-Resistant Tuberculosis, January, 27, 2021.
Wiens, T., Redelmeier, T., and Av-Gay, Y. (2004). Development of a Liposome Formulation of Ethambutol. Antimicrob. Agents Chemother. 48 (5), 1887–1888. doi:10.1128/aac.48.5.1887-1888.2004
You, X., Li, L., Liu, J., Wu, L., He, M., and Lyu, X. (2017). Investigation of Particle Collection and Flotation Kinetics within the Jameson Cell Downcomer. Powder Technology 310, 221–227. doi:10.1016/j.powtec.2017.01.002
Zaru, M., Sinico, C., De Logu, A., Caddeo, C., Lai, F., Manca, M. L., et al. (2009). Rifampicin-loaded Liposomes for the Passive Targeting to Alveolar Macrophages: In Vitro and In Vivo Evaluation. J. Liposome Res. 19 (1), 68–76. doi:10.1080/08982100802610835
Keywords: tuberculosis, Mycobacterium tuberculosis, drug delivery systems, nanocarriers, vaccines
Citation: Rajput A, Mandlik S and Pokharkar V (2021) Nanocarrier-Based Approaches for the Efficient Delivery of Anti-Tubercular Drugs and Vaccines for Management of Tuberculosis. Front. Pharmacol. 12:749945. doi: 10.3389/fphar.2021.749945
Received: 30 July 2021; Accepted: 11 October 2021;
Published: 21 December 2021.
Edited by:
Rajeev K. Tyagi, Institute of Microbial Technology (CSIR), IndiaReviewed by:
Rahul Shukla, National Institute of Pharmaceutical Education and Research, IndiaNeeraj Garg, Post Graduate Institute of Medical Education and Research (PGIMER), India
Copyright © 2021 Rajput, Mandlik and Pokharkar. This is an open-access article distributed under the terms of the Creative Commons Attribution License (CC BY). The use, distribution or reproduction in other forums is permitted, provided the original author(s) and the copyright owner(s) are credited and that the original publication in this journal is cited, in accordance with accepted academic practice. No use, distribution or reproduction is permitted which does not comply with these terms.
*Correspondence: Varsha Pokharkar, dmFyc2hhLnBva2hhcmthckBiaGFyYXRpdmlkeWFwZWV0aC5lZHU=