- 1Pharmaceutical Sciences Research Center, Health Institute, Kermanshah University of Medical Sciences, Kermanshah, Iran
- 2Department of Pharmacognosy, School of Pharmacy and Pharmaceutical Sciences, Isfahan University of Medical Sciences, Isfahan, Iran
- 3Departamento de Ciencias del Ambiente, Facultad de Química y Biología, Universidad de Santiago de Chile, Santiago, Chile
Growing studies are revealing the critical manifestations of influenza, dengue virus (DENV) infection, Zika virus (ZIKV) disease, and Ebola virus disease (EVD) as emerging infectious diseases. However, their corresponding mechanisms of major complications headed for neuronal dysfunction are not entirely understood. From the mechanistic point of view, inflammatory/oxidative mediators are activated during emerging infectious diseases towards less cell migration, neurogenesis impairment, and neuronal death. Accordingly, the virus life cycle and associated enzymes, as well as host receptors, cytokine storm, and multiple signaling mediators, are the leading players of emerging infectious diseases. Consequently, chemokines, interleukins, interferons, carbohydrate molecules, toll-like receptors (TLRs), and tyrosine kinases are leading orchestrates of peripheral and central complications which are in near interconnections. Some of the resulting neuronal manifestations have attracted much attention, including inflammatory polyneuropathy, encephalopathy, meningitis, myelitis, stroke, Guillain-Barré syndrome (GBS), radiculomyelitis, meningoencephalitis, memory loss, headaches, cranial nerve abnormalities, tremor, and seizure. The complex pathophysiological mechanism behind the aforementioned complications urges the need for finding multi-target agents with higher efficacy and lower side effects. In recent decades, the natural kingdom has been highlighted as promising neuroprotective natural products in modulating several dysregulated signaling pathways/mediators. The present study provides neuronal manifestations of some emerging infectious diseases and underlying pathophysiological mechanisms. Besides, a mechanistic-based strategy is developed to introduce candidate natural products as promising multi-target agents in combating major dysregulated pathways towards neuroprotection in influenza, DENV infection, ZIKV disease, and EVD.
Introduction
As emerging infectious diseases, influenza, dengue virus (DENV) infection, Zika virus (ZIKV) disease, and Ebola virus disease (EVD) demonstrate various peripheral and central complications. Studies have shown that neurological manifestations have been a critical part of the aforementioned emerging infections (Billioux et al., 2016), through a direct infection and obliteration of neuronal cells. In this line, glial cells, neurons, and progenitors are major targets of the viruses, leading to less cell migration, neurogenesis impairment, and death (Russo et al., 2017). Among the neuronal complications of emerging infectious diseases, major manifestations are encephalopathy, meningitis, myelitis, stroke, Guillain-Barré syndrome (GBS), radiculomyelitis, meningoencephalitis, memory loss, headaches, cranial nerve abnormalities, tremor, and seizure (Munoz et al., 2018). There are multiple signaling mediators behind the neuronal signs of emerging viral infections, including inflammatory mediators and oxidative pathways. Consequently, interleukins (ILs), interferons (IFNs), toll-like receptor (TLR), nuclear factor-kappa B (NF-κB), mitogen-activated protein kinase (MAPK), inducible nitric oxide synthase (iNOS), Tyro3/Axl/Mer (TAM), and aquaporins (AQP) are pivotal dysregulated factors in the pathogenesis of general complications in emerging infectious diseases towards neuronal manifestations. Additionally, various steps of the virus life cycle are of great importance towards associated neuronal signs (Mohammadi Pour et al., 2019).
Despite advances in revealing the pathophysiology of emerging infectious diseases, underlying neuronal mechanisms require further investigation (Russo et al., 2017). On the other hand, considering multiple dysregulated pathways behind the aforementioned neuronal signs, no specific drug has been found to treat neuronal symptoms of emerging infections. Recent developments in providing novel molecular and cellular mechanisms of virus invasion/replication/proliferation have shown alternative effective and innovative therapeutic strategies (Mohammadi Pour et al., 2019). Phytochemicals are promising multi-target agents with promising antiviral potentials for the treatment of infection. These metabolites are auspicious sources of novel chemical classes of drugs and pharmacological mechanisms with profitable biological activities and health benefits (Naseri et al., 2019). These secondary metabolites have attracted particular attention and have opened a new road in treating neuronal manifestations of infectious diseases by targeting inflammation, oxidative stress, and several other signaling mediators (Fakhri et al., 2020a).
Besides, limited studies reported natural secondary metabolites and candidate phytochemicals as promising agents for the prevention/treatment of emerging infections. This is the first comprehensive review on neurological manifestations of emerging infectious diseases and associated dysregulated pathways as well as the modulatory effects of candidate phytochemicals on the associated signaling pathways.
Study Design
Scopus, Medline, PubMed, Cochrane, and Web of Science were employed as electronic databases to conduct the comprehensive review. Besides, related articles in other sources were included. Accordingly, keywords (“Influenza” OR “Dengue” OR “Zika” OR “Ebola”) AND (“neurological sign” OR “neurological manifestation” OR “neuron” OR “nerve” OR “CNS” OR “central nervous system” OR “brain” OR “neuropathy” OR “neurology” OR “stroke” OR “multiple sclerosis” OR “encephalitis” OR “encephalopathy” OR “Alzheimer’s disease” OR “Parkinson’s disease” OR “pain” OR “Huntington’s disease” OR “multiple sclerosis” OR “autism” OR “aging” OR “depression”) (title/abstract/keywords) were used. All the phytochemicals possessing both the antiviral and neuroprotective activities within the classes (“Alkaloid” OR “Polyphenol” OR “Flavonoid” OR “Terpenoids”) were also searched in the whole text. Overall, the entire plant-derived secondary metabolites with neuroprotective and antiviral effects, modulating neurological complications of emerging infectious diseases, were included. Data were collected without date and language restrictions until March 2021. The screening procedure of retrieved articles was also performed on the reference citation/lists. Regarding completing the search on electronic databases, hand searching also was provided relying on the authors’ expertise on the neuronal pathophysiological mechanisms of emerging infectious diseases and candidate phytochemicals.
Neurological Manifestations of Emerging Infectious Diseases
As provided, influenza, DENV infection, ZIKV, and EVD show neurological manifestations passing through multiple signaling pathways.
Neurological Manifestations of Influenza Virus
Influenza virus, belonging to the RNA viruses in the Orthomyxoviridae family, is categorized into four virus types based on antigenically distinct of A, B, C, and D. However, influenza virus types C and D are not considered as health threats (Francis et al., 2019). In a retrospective study conducted by Takia and coworkers, neurological manifestations of influenza A (H1N1) were reported during the 2019 outbreak (Takia et al., 2020). The neurological manifestations include altered sensorium, cerebrospinal fluid (CSF) pleocytosis, and seizures (Takia et al., 2020). Based on neuroimaging evidence, acute necrotizing encephalopathy, diffuse cerebral edema of childhood, elevation in intracranial pressure (ICP), and acute disseminated encephalomyelitis were also reported as H1N1 neuronal complications (Takia et al., 2020).
Based on the previous reports, all types of influenza, such as seasonal H1N1 and associated pandemic in 2009, displayed a meaningful impact on both the central nervous system (CNS) and peripheral nervous system (PNS) (Blut, 2009; Paksu et al., 2018). Accordingly, other neurological symptoms in patients with H1N1 are sensory polyneuropathy with flaccid tetraparesis, somnolence, coordination disorder, stupor, confusion, language, and behavior disorders. Besides, disorientation, memory dysfunction, nystagmus, positive Kernig’s sign (Radzišauskienė et al., 2021), febrile convulsion, encephalopathy, acute encephalitis, aseptic meningitis, acute cerebellar ataxia, and myelitis are among major neuronal complications during H1N1. Consequently, GBS, acute mental status change, acute disseminated encephalomyelitis (ADEM), and cerebrovascular illness (e.g., cerebral infarction) are other H1N1-associated neuronal signs (Chen et al., 2020).
In a retrospective study, neurologic complications of the influenza virus were also evaluated. The findings showed that 4% of patients displayed associated neurological manifestations. In this line, the most reiterated complication was influenza-associated encephalitis (IAE) in 65% of patients, and 13% were categorized as having neurological residuals. In addition, 16% showed epileptic seizures, 5% demonstrated acute inflammatory demyelinating polyneuropathy (AIDP), and 14% were classified as having an infection-associated stroke (Mylonaki et al., 2020). One of the most frequent and severe reported neurological manifestations directly related to influenza is IAE, mainly caused by H1N1. However, rare case reports were presented about neurological complications associated with influenza B (Mylonaki et al., 2020). Miller Fischer syndrome, stupor, infection-associated stroke, multiple ischemic strokes at the moment of admittance fever, tetraparesis with right hemispasticity, delirium, and convulsive status epilepticus, consistent with acute hemorrhagic leukoencephalitis, are some other neurologic manifestations of influenza virus (Mylonaki et al., 2020). In one case studied by Mylonaki et al., following developed cerebral edema, secondary cerebral hemorrhage, multiple organ failure (MOF), and cardiopulmonary arrest also occurred (Mylonaki et al., 2020). The neurological complications such as seizures, focal deficit, and altered sensorium are probably associated with febrile seizures, deterioration of preexisting neurological disease secondary to acute illness, sepsis, hypoxia, and MOF. However, a number of patients demonstrate neurological complications in their absence (Jain and Lodha, 2020).
Neurological Manifestations of DENV
DENV, belonging to the arthropod-borne flavivirus family, is one of the fast-growing tropical infections (Pathak and Mohan, 2019). In Murthy et al. study, the neurological involvement of DENV, in the form of atypical cases, was discussed and based on pathogenic mechanisms was categorized into metabolic disturbance (e.g., encephalopathy), viral invasion (e.g., meningitis, encephalitis, myelitis, and myositis), and immune-mediated reactions, inclusive of neuromyelitis optica, myelitis, ADEM, optic neuritis, encephalopathy, neuroophthalmic complications, and GBS (Murthy, 2010). The new classification was categorized into the DENV associated involvement of CNS, PNS, and post-DENV immune-mediated syndromes (Solbrig and Perng, 2015). Panda et al. indicated that DENV encephalopathy, transverse myelitis, and cranial nerve palsies are other neural manifestations of DENV disease. Besides, parkinsonism secondary to DENV infection is of other uncommon neural presentations. In a case study, a 13-year-old premorbidly normal boy was described with bradyphonia, bradykinesia, mask face, and cogwheel rigidity (Panda et al., 2020). In another case report that was conducted by Ho and coworkers, a case of DENV fever with unrelated neuropathies was highlighted. Consequently, mononeuritis multiplex, full resolution of diplopia, and partial resolution of left foot drop were manifested (Ho et al., 2020). In another report, uncommon manifestations of DENV disease were reported by Tun and coworkers, including photophobia, anxiety, irritability, generalized fits, loss of consciousness, confusion, respiratory failure (assisted ventilation), irritability, lethargy, alteration of mental status, and brain stem dysfunction symptoms. Additionally, confusion, neck stiffness, disorientation, hallucinations, transverse myelitis, and numbness in extremities of the upper arm as well as affecting weakness and picking pain were also reported as DENV-neuronal signs (Tun et al., 2020).
Neurological Manifestations of ZIKV
ZIKV belongs to the RNA virus in the Flaviviridae family, closely related to other flaviviruses, including DENV, West Nile virus, Japanese encephalitis virus, Chikungunya virus, and yellow fever virus (Arora, 2020). Zika infection was closely related to CNS and PNS diseases, in particular stroke or transient ischemic attack, GBS (Ferreira et al., 2020), meningoencephalitis, transverse myelitis (Da Silva et al., 2017), retroorbital pain (Sharma et al., 2020), and myalgia (Bandeira et al., 2020). Evidence has shown a close relationship between Zika infection during pregnancy, congenital abnormalities, and microcephaly (Mlakar et al., 2016). One of the critical impairments of ZIKV in newborns may result in microcephaly and congenital CNS malformation (Pan American Health Organization/World Health Organization (PAHO/WHO), 2016; Kazmi et al., 2020). Of some newborns with congenital ZIKV and normal head circumference, later developed microcephaly is provided (Pereira et al., 2020). A special tropism of ZIKV demonstrates the CNS manifestation such as generating cerebral calcifications, impairing the development of the fetal brain, intrauterine growth restriction, ventriculomegaly, and finally fetal demise in some cases (Ventura and Ventura, 2018; Beckman et al., 2020).
In a report by Pereira et al., some characteristics of congenital Zika syndrome were detected by head computerized tomography (CT) from the junction of white and grey matter (Pereira et al., 2020). The degree of calcifications was variable, from sparse and scant to coalescent and multiple (Pereira et al., 2020). Additionally, cortical dysplasia was identified, in different presentations, from focal and small to diffuse and large lesions in both hemispheres (Pereira et al., 2020). Cortical dysplasia presented as pachygyria or an infinitesimal thin cortex with agyria, closely linked to hydrocephalus or loss of white-matter volume. In a survey by Pereira et al., children demonstrated a reduction in cerebral volume assorting from slight to severe (Pereira et al., 2020). The findings demonstrated cortical dysplasia, subcortical calcifications, and variations in disease involvement of children comprising scant calcification and multiple coalescent foci. Besides, cortical dysplasia was reported to impact both cerebral hemispheres and focal dysplasia in the insular and temporal lobes. Consistently, cortical thickening, diffuse pachygyria, and cerebral parenchymal thinning with agyria, related to obstructive hydrocephalus, are other neuronal complications of ZIKV (Van Den Pol et al., 2017; Pereira et al., 2020).
Neurological Manifestations of Ebola Virus
The Ebola virus (EBOV), an RNA virus from the Filoviridae family, consists of five species, including Reston ebolavirus, Sudan ebolavirus, Taï Forest ebolavirus, Bundibugyo ebolavirus, and Zaire ebolavirus (Rojas et al., 2020). Experimental evidence demonstrates two groups of associated neurological manifestations regarding CNS and PNS. Neurological abnormalities referring to EVD (Inan, 2019) associated with the cerebellar pathways, sensory-peripheral nerves, and subcortical structures were observed in most survivors (Bowen et al., 2016).
The CNS neurological manifestations of EVD include seizures, meningoencephalitis (Billioux et al., 2016), encephalopathy (Mobula et al., 2018), respiratory distress (Mobula et al., 2018), hearing loss (Rowe et al., 1999), aural fullness, tinnitus (Mattia et al., 2016), dizziness (Qureshi et al., 2015), depressed mood (Bowen et al., 2016), and coma (Billioux et al., 2016). Similarly, some participants with EVD showed Parkinson’s syndrome with rigidity, shuffling gait, and retropulsion on examination (Bowen et al., 2016). EVD also caused PNS manifestation including malaise, fatigue (West and Von Saint André-Von Arnim, 2014; Fischer et al., 2015), hiccups, headache (Mobula et al., 2018), muscle weakness (Chertow et al., 2016), frontal release signs, myoclonus, asterixis (involuntary movements), hyperreflexia (sustained clonus), myopathy (generalized weakness) (Billioux et al., 2016), retroorbital pain, and arthralgia (Rowe et al., 1999).
In a study by Bowen and coworkers, a certain degree of objective abnormality was observed on neurologic examination of EVD, such as impairments of either saccades or pursuits, tremors, abnormal sensory manifestations or abnormal reflexes, and frontal signs. Consistent focal deficits along with stroke have been also presented in several survivors of EVD, such as those with homonymous hemiparesis, hemianopia, and cranial nerve palsies (Bowen et al., 2016).
Some other neurological complications may appear after Ebola, including memory loss, seizures, cranial nerve abnormalities, headaches, and tremors (Billioux et al., 2016). The other complications of EVD consist of hypomania, decreased short-term memory, mild cerebellar signs, hyperphagia, insomnia, and mild weakness of the lower limbs (Chertow et al., 2016). Multiple magnetic resonance imaging (MRI) of the brain and multiple punctate microvascular lesions were presented in the white matter (Chertow et al., 2016), experimental examination of CSF, and RNA found in patients affected by EVD (Adekanmbi et al., 2021). In a case study analyzed by Chertow et al., 7 months of monitoring the physical and neurological manifestation in EVD showed that individuals presented decreased executive function and chronic fatigue (Chertow et al., 2016). Consequently, altered mental status, ranging from mild confusion to delirium and hallucinations, might also appear but maybe secondary based on variables, including electrolyte abnormalities and shock (West and Von Saint André -Von Arnim, 2014).
Pathophysiological Mechanisms of Emerging Infectious Diseases
As provided, several dysregulated mechanisms are behind the pathogenesis of emerging infectious diseases.
Pathophysiological Mechanisms of Influenza Virus
After infection, influenza virus replicates mainly in the epithelium of the respiratory tract. The other cell types, comprising immune cells, can be infected by the virus and involve the production of viral protein. Moreover, the efficiency of viral replication varies between cell types. Among humans, the critical site that the hemagglutinin (HA) molecule is efficiently cleaved and producing the viral particles is the epithelium of the respiratory system. Influenza transmission eventuates from respiratory fomite or aerosols of a susceptible individual that comes into contact with other ones. Investigations in ferrets have shown that the soft palate is the primary source of the influenza virus that could be transmitted between people. Significantly, the soft palate is a rich source of α-2,6-linked sialic acids selected by the HA proteins recently detected in human influenza viruses (Lakdawala et al., 2015). The pathophysiology of influenza virus is caused by lung inflammation and involvement of epithelium of the respiratory system, along with immune responses. The inflammation is able to spread systemically and is being manifested as a MOF (Zangrillo et al., 2013; Kalil and Thomas, 2019).
A case report study observed an increase in the levels of CSF neopterin in viral infection-related acute encephalopathy and encephalitis (Nezu et al., 1999), which is generated by IFN-induced inflammatory stimulation (Fredrikson et al., 1987), as IFNs are generated in the CNS. A significant increase in the levels of tumor necrosis factor-α (TNF-α) in CSF of the children with influenza-related encephalitis and encephalopathy was reported by Togashi (1999). The CNS is an immune-privileged organ, and this is associated with mechanisms in avoiding the related function of the immune system (Fabry et al., 1994).
Reactive astrocytes, microglia, and glial cells have been detected as a pathologic sign of immune-mediated illness of the CNS. CD14 molecules are expressed on the surface of microglia (Becher et al., 1996), could be stimulated by lipopolysaccharide, and produce TNF-α by activated T lymphocytes (Becher et al., 1996). Moreover, the glial cells imitate cytokine signaling of macrophages in the CNS (Kong et al., 1997). When the glial cells become overactivated and the alignment of the cytokine network becomes broken down, the reposition of cytokines is increased in the CNS and leads to cytokine storm in the brain. It is reasonable to hypothesize that the pathophysiology of acute influenza-related encephalitis and encephalopathy is the involvement of the glial cells. It overproduces the inflammatory cytokines, the cytokines are gathered in the brain, and afterward, the brain edema is eventuated; then, the degenerative alterations in the neural cells occur. According to the clinical evidence, influenza virus primarily shows modifications in the mental status and then manifests the rapid systemic alterations described by this hypothesis (Yokota et al., 2000).
From another mechanistic point of view, following the entry of influenza virus, related M2 proton channels are activated by low pH of the associated endosome, thereby acidify the viral interior, and weaken the electrostatic interaction, which allows viral uncoating. In this line, inhibiting the function of M2 ion channel prevents the uncoating of the influenza virus. On the other hand, the HA of the influenza virus binds to receptors with neuraminic acid. The enzymatic activity of neuraminidase (NA) releases viruses by removing neuraminic acids from oligosaccharide chains of receptors. So, NA inhibitors (NAIs) are another class of anti-influenza drugs (Mohammadi Pour et al., 2019).
Pathophysiological Mechanisms of DENV
Pathogenesis of DENV disease may be directly related to invasion of the CNS, alterations in the metabolism, and autoimmune reaction (Prabhat et al., 2020). Even though the DENV was conventionally deemed to be nonneurotropic, manifestations of viral particles in the cerebrospinal fluid as well as neurological involvement observed with DENV and also damage to the blood-brain barrier (BBB) owing to DENV disease have been contested by these theories (Li et al., 2017). In recent years, several receptors have been identified to be involved in DENV entry, including claudin-1 cell receptors (Che et al., 2013), lectins (Lo et al., 2016), and carbohydrate molecules (Aoki et al., 2006). Among carbohydrate molecules, sulfated polysaccharides, glycosphingolipids (GSL), and glycosaminoglycans (GAGs) are widely expressed coreceptors for DENV entry and efficiency. The highly sulfated GAGs, heparan sulfates (HS), and heparan sulfates proteoglycans (HSPG) are critical for cellular adhesion to extracellular matrix and binding of polypeptide growth factors (Kato et al., 2010) to facilitate binding of the virus to other receptors and then internalization (Laureti et al., 2018). Besides, in vitro infection of BV2 microglia cell line with DENV resulted in increased expression of proinflammatory cytokines, including IFN-γ, TNF-α, IL-6, IL-1β, IL-10, and monocyte chemoattractant protein-1 (MCP-1), as well as matrix metalloproteinase- (MMP-) 2 and MMP-9 (Bhatt et al., 2015). Current investigations have also confirmed the role of DENV disease on neuroinflammation. The nonstructural 1 antigen (NS1Ag) is a secreted type of glycoprotein (GP) that initiates the cytokine release and acts as a cofactor for the replication of the RNA virus. The natural killer (NK) cells extremely also have a critical role in the pathogenesis of neurological complications of DENV as demonstrated by NK cell’s early activation and eventually activate T helper cells. These T helper cells are divided and transformed into T helper 17 and T helper 9 cells and elevate subsequent release of proinflammatory cytokines such as IL-4, IL-12, IFN-γ, and transforming growth factor-beta (TGF-β). The proinflammatory cytokines in the next step direct to damage the BBB and afterward promote the entrance of other immune mediators into the brain thereby provoke neuroinflammation (Madi et al., 2014; Prabhat et al., 2020).
Pathophysiological Mechanisms of ZIKV
ZIKV attacks and remains in some target host cells such as blood, skin, placental cells, neural stem cells, retina, placental progenitor cells, and neural and gonadal tissues (Lee and Shin, 2019; Serman and Gack, 2019). ZIKV demonstrated tropism for neural stem cells and progenitor cells (Tang et al., 2016). As the most critical entry receptors involved in the entrance of flavivirus, some play key roles, including αvβ3 integrins (Fan et al., 2017), C-type lectin receptors (CLR) (Chen et al., 2012b), phosphatidylserine receptors T-cell immunoglobulin, and mucin domain (TIM), as well as TAM (Meertens et al., 2012). Accordingly, ZIKV employs receptor tyrosine kinases to enter the host cells through endocytosis, and facilitating virus replication. Of those receptors, Axl family receptor tyrosine kinases are intensely expressed in numerous cell types of the cerebral cortex (Richard et al., 2017). ZIKV also enters the placenta through Axl-mediated interaction with endothelial cells of the umbilical vein (Poland et al., 2018) and also replicates in other placenta tissues (Quicke et al., 2016). This infection could lead to impairment of the brain/skull development. Besides, neural progenitor cells seem to be direct targets of ZIKV. For instance, neural stem cells and radial glial cells show immunohistochemical evidence of the Axl entry site (Nowakowski et al., 2016; Kakooza-Mwesige et al., 2019). It is able to access host cells via Axl receptors existing on the membrane of the host cell.
The Zika life cycle in host cells consists of four stages of viral proteins translation, ZIKV RNA replication, viral particle assembly in the endoplasmic reticulum, and virion release (Kohno et al.). ZIKV encodes three structural proteins, that is, called envelope (E), capsid (C), and precursor membrane (prM), and seven nonstructural proteins, including NS1, NS2A, NS2B, NS3, NS4A, NS4B, and NS5. The structural proteins, E, and prM are used by the virus to attach to the neural cell membrane of the host. The nonstructural proteins and C protein localize to different organelles of the neuron such as Golgi apparatus, nucleus and its nucleoli, and cytoplasm lipid droplet, leading to apoptosis, cell cycle arrest, and cell death (Christian et al., 2019; Lee and Shin, 2019).
ZIKV has been exhibited to bring about DNA damage in host cells by breaking double-strand and keeping the host cell in the S phase, preventing replication. These cellular impacts have been presupposed to direct the death of neural cells of the cortex and cause a deficiently developed brain in fetuses who were infected by ZIKV (Hammack et al., 2019). Moreover, ZIKV is considered to use the cellular defense in humans via the nonstructural proteins-mediated interferon antagonism or by reducing the IFN production that is organizing the inhibition of ZIKV replication in human cells (Arora, 2020). Additionally, dysregulation in human embryonic cortical neural progenitor cells (hNPCs) following ZIKV infection revealed a differential expression of genes related to cell cycle dynamics, protein localization, and cell transcription (Tang et al., 2016; Zhang et al., 2016).
Pathophysiological Mechanisms of EBOV
EBOV binds to Tim-1 on T lymphocytes and thereby causes robust inflammatory responses referred to as a cytokine storm (Younan et al., 2017). The aforementioned surge in cytokine/chemokine production, as well as dysregulations in autoimmune responses, plays key roles in the pathogenesis of filoviruses complication possessing a near link with acute neurological symptoms (Wong et al., 2014; Bixler and Goff, 2015). Evidence from the West Africa outbreak and experimental studies also indicated that the EBOV might enter the nervous system (De Greslan et al., 2016). However, revealing the exact mechanisms behind the pathogenesis of EVD remained a significant challenge (Kakooza-Mwesige et al., 2019). EVD cell and tissue tropism are principally identified by the EBOV GP1,2 attachment factors on the surface of the host cell and GP1,2 and intracellular binding to the Niemann–Pick C1 (NPC) intracellular cholesterol transporter 1 receptor (Carette et al., 2011; Côté et al., 2011). Nearly all human cells could get infected, but dendritic cells and mononuclear phagocytes (e.g., microglia, macrophages, and Kupffer cells in the liver) are preliminary EBOV target (Geisbert et al., 1992; Takada et al., 1997; Geisbert et al., 2003a; Ryabchikova and Price, 2004; Bray and Geisbert, 2005; Geisbert et al., 2015). While the preliminary target cells get infected, they likely promote virus dissemination (Schnittler and Feldmann, 1998) and move to the spleen, liver, and regional lymph nodes (Geisbert et al., 2003a). Binding to GP1,2 of EBOV causes activation of infected macrophages evaluated by an in vitro model (Wahl-Jensen et al., 2005). Moreover, in another in vitro model, dendritic cells show a reaction to EVD with partial suppression of histocompatibility complex class II responses, expression of TNF ligand superfamily member 10 (TNFSF10) and tissue factor, reduced secretion of proinflammatory cytokines, and enhanced production of chemokines, for example, IL-8, C-C motif chemokine 2 (CCL2), CCL3, and CCL4 (Hensley et al., 2002; Geisbert et al., 2003b; Bosio et al., 2003; Mahanty et al., 2003; Bosio et al., 2004). Altogether with probable abortive infection (Younan et al., 2019), the TNFSF10 expression and malapropos cytokine responses probably direct to the vast lymphocyte death. This lymphocyte depletion probably attributes to the patients’ susceptibility to EVD towards secondary infections (Geisbert et al., 2000; Geisbert et al., 2003a), hypotension, and ultimately MOF that is general in EVD (Baize et al., 1999; Geisbert et al., 2000; Martines et al., 2015; Jacob et al., 2020). The innate immune systems and systemic adaptive of CNS express pattern recognition receptors (PRR), including TLRs, retinoic acid-inducible gene 1 (RiG-1), and melanoma differentiation-associated protein 5 (MDA-5) that detect viral nucleic acids and promote host antiviral response. Nevertheless, EBOV is recognized and internalized by TLR, MMR, DC-SIGN, CD162, and Scavenger Receptor B as host cell receptors. They also have the potential of escaping immune surveillance by the host systemic and innate immune systems (Denizot et al., 2012). Figure 1 shows emerging infectious diseases and associated dysregulated mediators towards neurological manifestations (Figure 1).
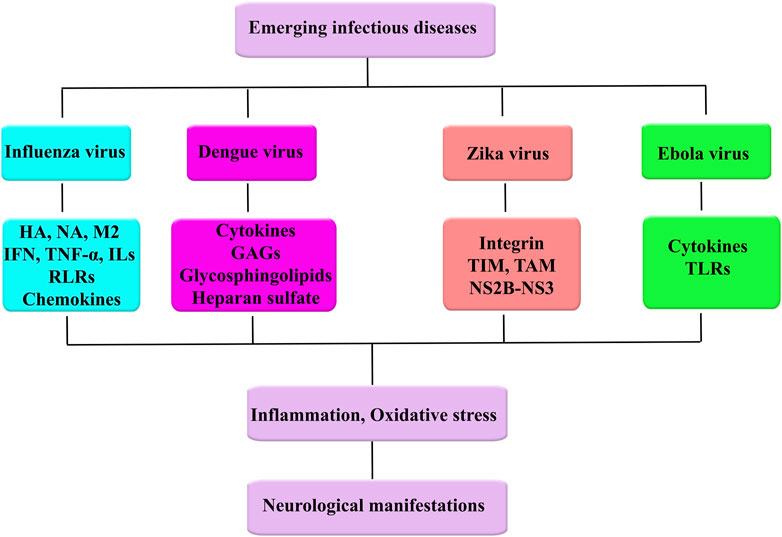
FIGURE 1. Emerging infectious diseases and associated dysregulated mediators towards neurological manifestations.
Conventional Therapeutic Strategies Against Emerging Infectious Diseases: Neuronal Signs and Beyond
To combat the wide complications of emerging viral diseases, conventional strategies are being employed.
Influenza Virus
Lately, all approved anti-influenza drugs intervene in viral protein function and are categorized to the direct-acting antivirals (DAAs) class. The rapid growth of viral resistance has appeared as the prevailing liability of DAAs, in particular when used against RNA viruses with error-prone polymerases such as the influenza viruses (Hussain et al., 2017; Toots and Plemper, 2020) or the respiratory syncytial virus (RSV) (Devincenzo et al., 2014; Chemaly et al., 2020). For instance, amantadine, adamantanes, and rimantadine were the first approved drugs to treat influenza A virus disease. These inhibitors target the viral M2 ion channel, preventing dissociation of the viral ribonucleoprotein (RNP) genome from the matrix protein by blocking M2-mediated diffusion of protons into virions located in maturing endosomes (Jing et al., 2008; Stouffer et al., 2008). Of some strains of influenza A virus, they are also able to impact virion assembly via disturbing M2-mediated pH-equilibration of the organelle Golgi (Jing et al., 2008; Wang et al., 2011).
In spite of the fact that there are no approved biologic drugs for influenza treatment hitherto, neutralizing antibodies (nAbs) have been examined. Therapeutics-based antibodies, in most cases, are well-tolerated and demonstrate desired pharmacokinetic profiles (Ekiert et al., 2012; Tsibane et al., 2012; Laursen and Wilson, 2013). Influenza virus HA was targeted by broadly neutralizing Abs (bnAbs), VIS410, MHAA4548A, and MEDI8852, which are in phase 2 clinical trials. These bnAbs demonstrated some antiviral activities in therapeutic doses and decreased virus replication (Ali et al., 2017; Mcbride et al., 2017; Hershberger et al., 2019). Accordingly, influenza therapeutics currently approved for clinical use are baloxavir, marboxil, oseltamivir, peramivir, and zanamivir (Hayden et al., 1999; Hedrick et al., 2000; Kohno et al., 2010; Heo, 2018).
Immunization based on RNA has appeared as hopeful strategies in comparison with conventional approaches like vaccines (Scorza and Pardi, 2018). Recently influenza vaccines, which have been licensed, exhibited different levels of protection versus seasonal influenza virus strains, though they are insufficient versus pandemic and drifted viruses. Recently, some groups of RNA vaccines showed activity versus influenza virus disease in preclinical models. Moreover, comparative studies displayed the advantages of certain RNA vaccines over the recently utilized inactivated vaccines of influenza virus in animal models. So clinical trials should be initiated and prepare valuable data concerning the translatability of the promising preclinical information to humans (Scorza and Pardi, 2018).
For brain complications of influenza virus, best treatment practice includes initiate antiviral treatment during 48 h of onset (by zanamivir, peramivir, oseltamivir, etc.), administration of a high dose of gamma globulin, and at the same time hormone shock therapy, which decrease the brain metabolism. Plasma exchange therapy is offered when disseminated intravascular coagulation (DIC) and/or MOF exist (Chen et al., 2020). Plasmapheresis and corticosteroids were also administered, but the evidence for their effectiveness is limited (Britton et al., 2017).
DENV
Currently, a chimeric, attenuated vaccine against DENV (from Sanofi-Pasteur, Dengvaxia) (Hadinegoro et al., 2015) was provided by the vaccine strain of YFV as the backbone with structural proteins of precursor membrane E (prE) and prM of DENV from 1 to 4 serotypes (tetravalent) inserted (Vannice et al., 2018). Whereas this demonstrates another essential milestone in the endeavor to control and prevent flavivirus infections, the differences in immune response based on patient age, viral serotype, and preexisting exposure with DENV are ongoing problems in the usage of this therapeutic agent (Katzelnick et al., 2017; Arredondo-García et al., 2018). This vaccination should not be recommended for individuals who have not been previously infected by DENV (Halstead, 2017; Halstead, 2018). DENV vaccines might have implications for the severity of ZIKV, as it is anticipated that there are conserved antigens and hence cross-reactivity (Bernatchez et al., 2019; Elong Ngono and Shresta, 2019).
ZIKV
Currently, there is no approved antiviral agent or vaccine against ZIKV disease. The major strategy for controlling ZIKV is suppressing mosquito breeding (World Health Organization, 2016). The common treatment is palliative and consists of fluids intake and rest. Accordingly, paracetamol or acetaminophen is used to reduce fever, myalgia, and headache in patients with ZIKV. The usage of salicylates is not offered in childhood to prevent the probability of Reye’s syndrome [Atif et al., 2016; Centers for Disease Control (CDC), 2016]. Based on Bernatchez and coworkers’ study, the antiviral agents based on their targets are categorized into five groups, including blockers of the entrance stage (e.g., nanchangmycin and ZINC33683341). As second pathway, RNA-dependent RNA polymerase (RdRp) nucleoside analogs like adenosine analog 3 (NITD008) and 7-deaza-2′-C-methyladenosine, sofosbuvir, galidesivir, BCX4430, ribavirin, and emetine are hopeful treatments of ZIKV complications. Protease inhibitors include cn-716 (a boronic acid-containing dipeptide inhibitor), NSC157058, and (5-amino-1-((4-methoxyphenyl) sulfonyl)-1H-pyrazol-3-yl benzoate). Consequently, assembly inhibitors include ST-148 as well as endosomal fusion blockers such as quinacrine, chloroquine, mefloquine, and GSK369796 promise antiviral agents against Zika complications. Finally, nucleoside biosynthesis inhibitors like ribavirin, merimepodib, and methotrexate seem to be promising agents to combat associated manifestations of ZIKV (Bernatchez et al., 2019; Elong Ngono and Shresta, 2019).
EBOV
There are still no approved vaccines or medications for the EBOV in the world. Prevalent treatment includes monoclonal antibodies, plasma transfusions, small-molecule antiviral compounds, and vaccines (Bishop, 2015). To find effective therapeutics, which specifically block filovirus family members, many investigations have been accomplished to find antiviral agents to intervene with particular stages of the virus entrance (Picazo and Giordanetto, 2015). Conventional associated drugs are predominantly targeting endosomes and interfere with the events like endosomal trafficking, proteolysis of filovirus GP, interactions with NPC1, and finally fusion. Moreover, the number of cathepsin B/L inhibitors (FY-DMK, CA-074, and CID23631927) or nonspecific cysteine protease (leupeptin, E-64) has also been surveyed for their ability to inhibit EVD by in vitro models (Chandran et al., 2005; Schornberg et al., 2006; Barrientos and Rollin, 2007; Shah et al., 2010; Gnirß et al., 2012). Currently, another kind of cysteine protease inhibitor, K11777, was detected to inhibit EBOV entry in tissue culture (Zhou et al., 2015). Nevertheless, the impact of plenty of these compounds might not translate to in vivo investigations since, as mentioned above, cathepsins B and L are dispensable for in vivo EBOV replication. Finally, specific inhibitors for these enzymes might not prove their efficacy versus the filovirus family in vivo studies (Marzi et al., 2012). Currently, the antibodies, which bind to GP of the EBOV, have been demonstrated to protect nonhuman primates against lethal EBOV challenge (Qiu et al., 2012; Pettitt et al., 2013; Qiu et al., 2014). KZ52, a neutralized monoclonal antibody, was separated from a human survivor of the EBOV (Maruyama et al., 1999; Lee et al., 2008). KZ52 connects to the base part (hot spot) of the prefusion GP1/GP2 and is caused to neutralize the infection in vitro situation (Lee et al., 2008; Dias et al., 2011; Bale et al., 2012). It is worth noting that KZ52 solitary protects guinea pigs and mice against fatal infection but does not protect nonhuman primates (Parren et al., 2002; Rhein and Maury, 2015).
Based on studies, currently, administration of the combination of anti-EBOV GP monoclonal antibodies can protect nonhuman primates against fatal challenges with the EBOV following infection (Qiu et al., 2012; Pettitt et al., 2013; Qiu et al., 2014). One of the effective cocktails is MappBio (MB-003) that consists of the anti-GP antibodies 13F6, 13C6, and 6D8 (Pettitt et al., 2013). The other is Defyrus (ZMAb) that consists of anti-GP antibodies 2G4, 1H3, and 4G7 (Qiu et al., 2012; Audet et al., 2014). Both of these cocktails have been combined to make a drug cocktail of antibodies Z Mapp (2G4, 13C6, and 4G7), which is being accepted as an EBOV therapeutic. The aforementioned antibodies, like 1H3 and 13C6, attach to the glycan cap of GP1, while 2G4 and 4G7 attach to the GP1,2 interface in a similar region as 16 F6 and KZ52, and the first monoclonal antibodies in the cocktail efficiently neutralize virus infection in vitro model (Audet et al., 2014). According to these investigations, the administration of a combination of antibodies that aims at the GP1,2 interface and the glycan cap of GP of EBOV prepares protection in vivo situation (Rhein and Maury, 2015).
Phytochemicals Against Emerging Infectious Diseases: Mechanistic Approaches to Neurological Signs
Emerging infectious diseases are produced by organisms with the capability of transferring from person to person, being a serious threat to human health (Sehgal et al., 2020). Recently scientists investigated the effects of natural products on emerging infectious diseases, including the influenza virus, DENV, Zika, and EBOV (Zheng et al., 2021). Considering the common dysregulated pathophysiological mechanisms of emerging infectious diseases and the capability of some phytochemicals in passing BBB (Fakhri et al., 2020b) urge the need to introduce candidate phytochemicals as potential modulators of peripheral complications, extrapolated to the same dysregulations in CNS.
Influenza Virus
As previously provided, influenza is accompanied by several neurological manifestations such as encephalitis, encephalopathy (Morishima et al., 2002), fever, cough, sore throat, myalgia, headache, diarrhea, numbness, paresthesia, vertigo, drowsiness, weakness, seizures (Leigh, 1946), AIDP, acute disseminated encephalomyelitis, meningitis, transverse myelitis, and changes of consciousness (Asadi-Pooya et al., 2011).
Evidence has shown that green tea catechins and theanine (as flavonoids) can bind to the HA molecule in the influenza virus, thereby inhibit virus adsorption to the host cell, and lead to the inhibition of influenza infection (Matsumoto et al., 2011). It has been also indicated that green tea extracts increase systemic immunity and can cause inhibition of respiratory tract infection and influenza symptoms in healthy humans; tea catechins include epigallocatechin gallate (EGCG), epigallocatechin, epicatechin gallate, epicatechin, (−)-catechin, and (+)-catechin (Yang et al., 2014). During infection with the influenza virus, EGCG and quercetin increased the level of antioxidant enzymes such as catalase (CAT), glutathione (GSH), and superoxide dismutase (SOD) and thereby suppressed oxidative stress (Kumar et al., 2005; Ling et al., 2012). Consequently, EGCG inhibited the ability of HA protein and viral RNA polymerase as well as NA protein to prevent the cleavage of cell surface sialic acid linked to the virus and inhibited internalization (Kim et al., 2013).
As another phenolic compound, betulinic acid has antibacterial, antimalarial, anti-inflammatory, antihelmintic, antinociceptive, and anticancer activities. It also reduced the levels of inflammatory cytokines, such as IFN-γ, to be a therapeutic agent for the treatment of influenza viral infection through anti-inflammatory properties (Hong et al., 2015). Considering the IFN role in the pathogenesis of the influenza virus, isoquercetin (a flavonol) reduced the levels of IFN-γ and iNOS and scavenged free radicals and interfered with NOS activity towards a decline in neurological manifestations (Kim et al., 2010). Isoquercetin inhibited the replication of influenza A and B at the lowest concentration. Besides, synergistic effects of isoquercetin and amantadine were observed by reducing virus titers, viral replication, pathological changes, and emergence of virus resistance (Kim et al., 2010). Other quercetin derivatives also indicated inhibitory properties in the early stage of influenza infection by inhibiting GP of HA in influenza virus and suppressive effect on virus-induced cellular reactive oxygen species (ROS) generation (Nile et al., 2020) as well as immunomodulation (Wu et al., 2016; Li and Wang, 2019). Several studies suggested that TLR4/NF-κB signaling pathway is an essential inflammatory/oxidative stress response caused by different pathogens (Xu et al., 2017). Accordingly, quercetin declined influenza A virus infection via antioxidant potential as well as inhibition of TLR signaling pathway and inhibiting caspase-3 activity (Vaidya et al., 2016). It has been shown that quercetin 3-glucoside is an antiviral agent and indicated more strong anti-influenza A and B activities through blocking the replication and entry of viruses (Nile et al., 2020).
As one class of flavonoids, anthocyanins bind to N1-NA and lead to inhibition of influenza replication (Swaminathan et al., 2014). Baicalin is an anthocyanin with antibacterial, anti-inflammatory, antioxidant, antitumor, antiproliferative, and anticoagulant activity (Xu et al., 2010). It also inhibited the NA activity of influenza A H5N1 and suppressed the level of TLR7, MyD88, NF-κB, and AP-1 (Kannan and Kolandaivel, 2018). Additionally, baicalin suppressed the secretion of TNF-α, IL-1β, IL-6, and IL-8 in H5N1-infected humans and inhibited envelope protein-mediated fusion with chemokine receptors (Hour et al., 2013; Wan et al., 2014). Experimental evidence indicated that baicalin suppressed viral replication in the early phase by triggering macrophage M1 polarization and activating IFN signaling (Geng et al., 2020). Baicalin also inhibited replication of influenza virus A via activation of type I IFN signaling by reducing miR-146a (Li and Wang, 2019). Consequently, baicalin downregulated retinoic acid-inducible gene I- (RIG-I-) like receptors (RLRs) signaling pathway (TNF-α, IFN-γ, IL-1β, IL-2, IL-4, IL-5, IL-6, and IL-10) to combat influenza A virus and improved the prognosis (Pang et al., 2018). As an isoflavone, biochanin A affected cellular signaling pathways resulting in reduced virus-induced activation of extracellular signal-regulated kinases 1/2 (ERK1/2), protein kinase B (Akt), and NF-κB. Furthermore, biochanin A inhibited the virus-induced production of interferon gamma-induced protein 10 (IP-10), IL-6, and IL-8, while baicalein inhibited IL-6 and IL-8 production. In their study, baicalein impaired H5N1 virus replication and interfered with the H5N1-induced production of IL-6, IP-10, and TNF-α (Sithisarn et al., 2013). Baicalin inhibits NA (Ding et al., 2014; Jin et al., 2018) and TLR7/MYD88 signaling pathway activation to suppress inflammation in mice infected with influenza A virus (Wan et al., 2014). Evidence suggested that IFN system acts as an important innate antiviral defense mechanism. Recently, it is observed that Scutellaria baicalensis Georgi (main constituents including baicalin) has inhibitory activity against various viruses and also can inhibit influenza virus replication affecting inducing IFN-γ secretion and also reduced neurological signs (Chu et al., 2015).
Other studies have shown that infection with influenza A virus induces inflammation. Kaempferol has antioxidative and anti-inflammatory effects, thereby declining MAPKs, NF-κB, TNF-α, IL-1β, and blocked ROS generation (Dong et al., 2014; Zhang et al., 2017a). Kaempferol and some other flavonoids have also shown interruption in the influenza life cycle. In vitro posttreatment with kaempferol, quercetin, and catechin hydrate noticeably suppressed viral levels of M2 mRNA/protein. In silico analysis also found that the aforementioned phytochemicals inhibited influenza A virus-induced replication and autophagy (Choi et al., 2019). Additionally, kaempferol has shown inhibitory activity against influenza and other viruses through reducing EV71 activity and thereby suppressed viral protein translation (Tsai et al., 2011).
Experimental evidence indicated that apigenin (a flavone) suppresses the expression of RIG-1 and also leads to the reduction of IFNs and cytokines in influenza virus infection/replication and associated apoptosis (Xu et al., 2020). Resveratrol as another polyphenol inhibited influenza A virus replication and thereby remarkably improved survival and declined damage (Palamara et al., 2005). Resveratrol can cause inhibition of cellular protein kinase (PKC)/MAPK signaling pathway and reduce virus replication, to be a useful candidate for the treatment of neurological signs (Palamara et al., 2005; Kim et al., 2010). Resveratrol showed a direct inhibition of influenza replication and via TLR-9-induced IFN-β production (Lin et al., 2015; Xiao et al., 2015). Based on molecular docking reports, resveratrol derivatives are potential antiviral compounds for developing influenza treatment via NA inhibition. Accordingly, resveratrol and catechin 3-O-gallate showed an inhibitory effect against NAs activity, with IC50 values of 129.8 and 21.3 µM, respectively (Chen et al., 2012a). Considering the results of a docking study, resveratrol and its derivatives (as natural polyphenol) can inhibit the replication of influenza A virus, as well as inhibited intracellular pathways c-Jun N-terminal kinase (JNK) and p38MAPK in the regulation of viral ribonucleoprotein complex (Fioravanti et al., 2012; Li et al., 2015). Evidence has shown that resveratrol and other derivatives have antiviral and antioxidant activities with different mechanisms, such as the inhibition of viral protein synthesis or transcription and modulating viral-related gene expressions or signaling pathways in host cells as it could be useful for the treatment of DENV (Han et al., 2017).
As a triterpene saponin, glycyrrhizin has shown anti-inflammatory and antiviral activities (Finney and Somers, 1958). It declined the level of p38, JNK, and NF-κB (Michaelis et al., 2011), increased NK cell activity, and induced IFN production by T cells (Itoh, 1983). Experimental results indicated that mice receiving lethal doses of the virus survived when treated with glycyrrhizin (Utsunomiya et al., 1997).
As developed, after influenza virus infection, the levels of cytokines were increased, of which geniposide (an iridoid glycoside) significantly inhibited the level of TNF-α, IFN-γ, and IL-6 (Zhang et al., 2017b). Consistently, berberine as a natural alkaloid compound has shown that antioxidant, anti-inflammatory, and anti-influenza effects also inhibited cytopathogenesis and NA activity as well as p38, caspase-3, and NF-κB (Enkhtaivan et al., 2017). It also significantly inhibited the expression of TNF-α and prostaglandin E2 (PGE2) (Cecil et al., 2011). Liu et al. observed that berberine inhibited NLR family pyrin domain containing 3 (NLRP3) inflammasome activation in the influenza virus as well as a decline in ROS generation (Liu et al., 2020). In this line, alkaloids also inhibit the function of HA, NA, and M2 in the structure of the influenza virus (He et al., 2013).
Li et al. indicated that aloe emodin (an anthraquinone) binds to virus envelope and leads to the inhibition of influenza A replication and regulated the level of IFN-γ, IFN-β, and double-stranded-RNA-activated protein kinase in influenza A virus (Li et al., 2014). Dai et al. observed that emodin remarkably inhibits the influenza virus through suppressing the expressions of p38/JNK MAPKs, NF-κB, TLR2, TLR3, TLR4, TLR7, MyD88, and TRAF6, as well as increasing nuclear factor erythroid 2-related factor 2 (Nrf2), heme oxygenase-1 (HO-1), SOD, CAT, and glutathione peroxidase (GPx) (Dai et al., 2017).
As another phytochemical, carvacrol (a phenol) has shown anti-inflammatory, antiviral, and antioxidant effects, with inhibitory effects on influenza virus infection. Carvacrol acts through the regulation of the IFN-γ pathway, modulation of IL-6, IL-17, TGF-β, IL-4, and IL-10, and reduction of TLR7, myeloid differentiation primary response 88 (MyD88), interleukin-1 receptor-associated kinase 4 (IRAK4), TNF receptor-associated factor 6 (TRAF6), and NF-κB (Li et al., 2018; Mahmoodi et al., 2019). In this line, cinnamaldehyde has different biological activities, for instance, antibacterial activity, induction of apoptosis through ROS, and inhibition of NOS (Hayashi et al., 2007).
It has also been shown that quercetin, diosmetin, eriodictyol, kaempferol, and isorhamnetin (Dayem et al., 2015) inhibited influenza infection early phase via interacting with the HA type 2 subunit of the influenza HA protein. In this line, the inhibitory effect of quercetin against both H1N1 and H3N2 virus leads to the inhibition of virus replication towards the reduction of neurological manifestations (Mehrbod et al., 2021). In another study, isorhamnetin 3-glucoside and quercetin 3-rutinoside revealed higher NAI activity in a dose-dependent manner. A molecular docking study showed that flavonol glycosides have higher binding actions towards influenza polymerase membrane GP towards anti-influenza activity (Kim et al., 2019).
The flavonoids extracted from Mosla chinensis Maxim., common name Moslae Herba (MHF), have anti-inflammatory, antioxidant, and antiviral effects of inhibiting TLR7, RIG-1, and AQP5 in the alveolar epithelial cells. It also inhibited the influenza virus and neurological symptoms (Yu et al., 2020). Plants rich in caffeic acids, chlorogenic acids, and related derivatives have shown antiviral effects against NA of influenza virus. However, to escape from gut microbiota metabolization, novel delivery systems are recommended (Karar et al., 2016). The flavonoids quercetin, naringenin, catechin, hispidulin, luteolin, chrysin, vitexin, and kaempferol have the potential for developing novel drugs for controlling influenza, which may help to overcome the clinical challenge of the H1N1 strain (Sadati et al., 2019). Kaempferol derivatives, luteolin, quercetin 3-sophoroside, and chelianthifoline show in vitro antiviral activity with IC50 values ranging from 10.7 to 33.4 µM in comparison to zanamivir 58.3 µM (Lee et al., 2016). These compounds could directly affect the virus itself and inhibit H5N1 viral replication by maintaining cellular redox equilibrium in host cells and blocking the nuclear-cytoplasmic translocation of the viral ribonucleoproteins. They also reduced the expression of late viral proteins related to the inhibition of PKC activity and its dependent pathways. The aforementioned secondary metabolites also showed the potential of downregulating proinflammatory cytokines and protecting organs from the virus- and cytokine-induced oxidative stress by supplying and maintaining sufficient levels of exogenous and endogenous antioxidants (Friel and Lederman, 2006).
Overall, phytochemicals have shown the potential of being used against neurological pathophysiological mechanisms of the influenza virus by targeting the components of the viral life cycle and signaling mediators (Table 1).
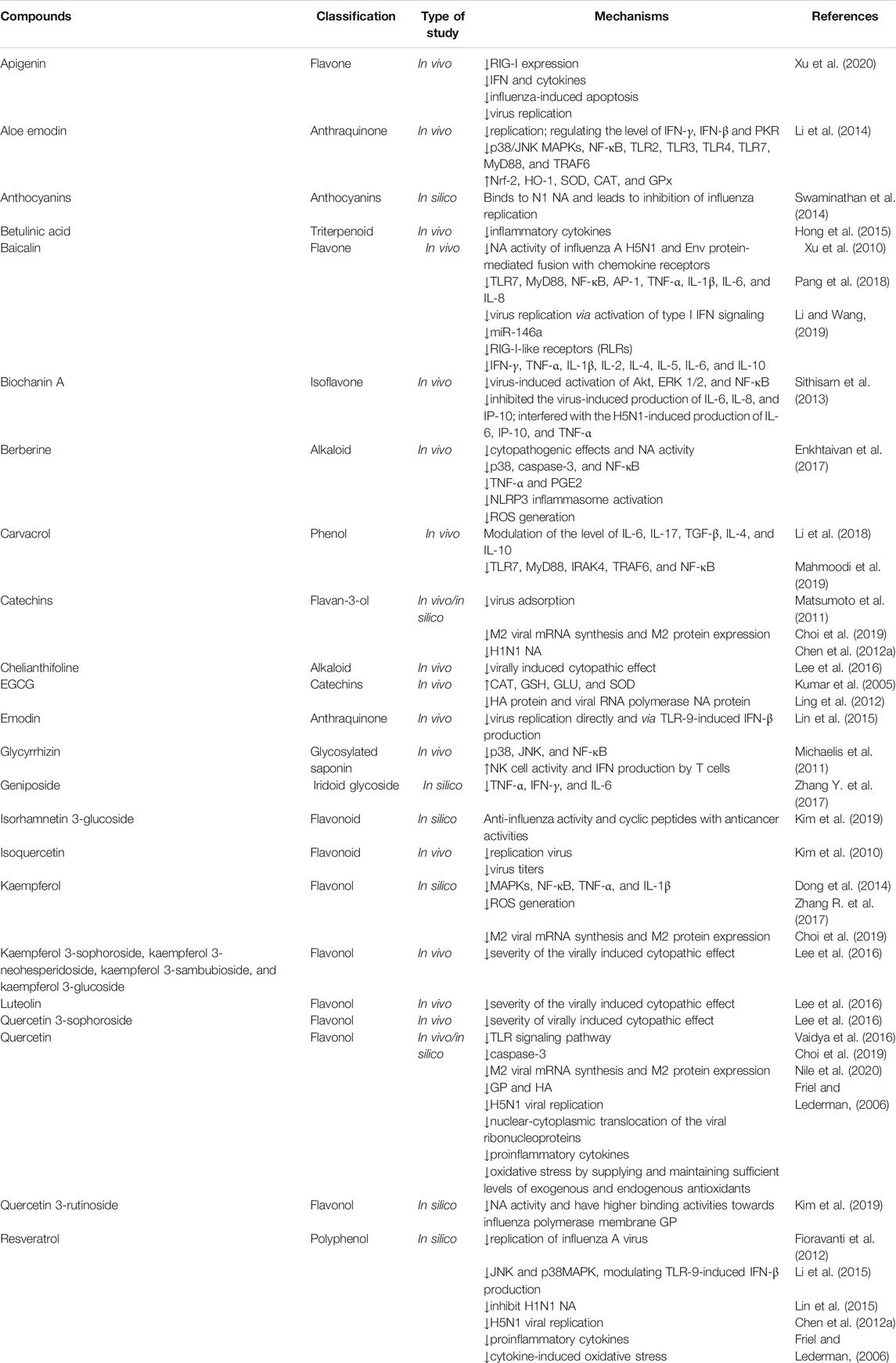
TABLE 1. Preclinical evidence on the use of candidate phytochemicals against influenza virus and related neuropharmacological effects.
DENV
DENV is accompanied by encephalopathy, encephalitis, meningitis, myositis, myelitis, acute disseminated encephalomyelitis, neuromyelitis optica, optic neuritis (Prabhat et al., 2020), GBS, neuroophthalmic complications, intracranial hemorrhage, cerebral edema, hyponatremia, hypokalemia, and cerebral anoxia (Schlindwein et al., 2020). Several reports indicated various phytochemicals with antiviral activity against DENV such as quercetin, daidzein, naringin, hesperetin, glabranine, and 7-O-methyle glabranin (Zandi et al., 2011). In this regard, quercetin has been shown to inhibit dengue polymerase enzyme with an IC50 value of 3.6 μM and lead to the inhibition of DENV replication (Coulerie et al., 2014). Anusuya et al. showed that quercetin and similar structural phytochemicals have antiviral effects against DENV RdRp, based on an in silico study (Anusuya and Gromiha, 2017). In this line, Manjula and coworkers investigated that quercetagetin, quercetin, myricetin, and kaempferol have an anti-DENV effect against DENV NS5 methyltransferase RNA capping site by using an RNA intervention mechanism (Manjula and Kumaradhas, 2020). It has been shown that quercetin and fisetin have antiviral activities, confirmed by in silico evidence. As reported by Jasso-Miranda et al., quercetin and fisetin interact with E, NS1, NS3, and NS5 proteins, thereby inhibiting a different process in the viral replicative cycle. Consequently, quercetin inhibited IL-6 and TNF-α and changed the level of IL-10 and IFN-γ in DENV-2 (Jasso-Miranda et al., 2019).
Luteolin as another natural compound inhibited DENV replication by blocking the later phase of DENV viral lifecycle in infected cells (Peng et al., 2017) and inhibiting the cellular proprotein convertase furin viral NS3 protease (Peng et al., 2018). Frabasile et al. have shown that naringenin can affect replication and/or maturation of the DENV life cycle, which leads to inhibition of viruses (Frabasile et al., 2017). As developed by Calvo et al., EGCG has antiviral effects against DENV, evaluated by docking studies in interacting with the function of proteins at multiple binding sites (Vázquez-Calvo et al., 2017). In silico evidence has shown that hirsutine (an alkaloid) has anti-DENV activity, as it interferes with the late phase of the DENV lifecycle (Hishiki et al., 2017).
Based on molecular docking evidence quercetagetin, kaempferol 3-O-rutinoside, rutin, hyperoside, and epicatechin can inhibit DENV RNA and lead to the reduction of replication and neurological sign (Dwivedi et al., 2020). It has been shown that DENV-2 induced IL-6, IFN-γ, and IL-10, and quercetin, naringin, catechin, and fisetin can change signaling pathways in the innate response to reduce neurological disorders being hopeful candidates for therapy of neurological disorders (Igbe et al., 2017).
It has been shown that ubiquitin-proteasome system reduced the structural E-protein that could affect DENV infection. In this line, curcumin can cause the accumulation of viral proteins and promote the accumulation of ubiquitin conjugated proteins, which reduces DENV infection (Padilla-S et al., 2014). Salidroside increases the level of double-stranded RNA-dependent protein kinase and phosphorylated eukaryotic initiation factor 2 (p-eIF2a), which leads to the suppressed synthesis of viral proteins and declined level of NF-κB, towards suppressing viral replication during the early phase of DENV infection (Loaiza-Cano et al., 2021).
Nordihydroguaiaretic acid has shown inhibitory effects on the DENV. Besides, this phenolic lignan and its methylated derivative inhibited flaviviruses by impairing viral replication regarding suppressing encephalopathy. Since the multiplication of flavivirus is highly dependent on the metabolism of host cell lipid, the antiviral effect of nordihydroguaiaretic acid is associated with its ability to disturb the lipid metabolism through interfering with the sterol regulatory element-binding proteins pathway (Merino-Ramos et al., 2017).
In general, various phytochemicals have the potential of being used against neuronal signs of DENV by suppressing associated dysregulated pathways (Table 2).
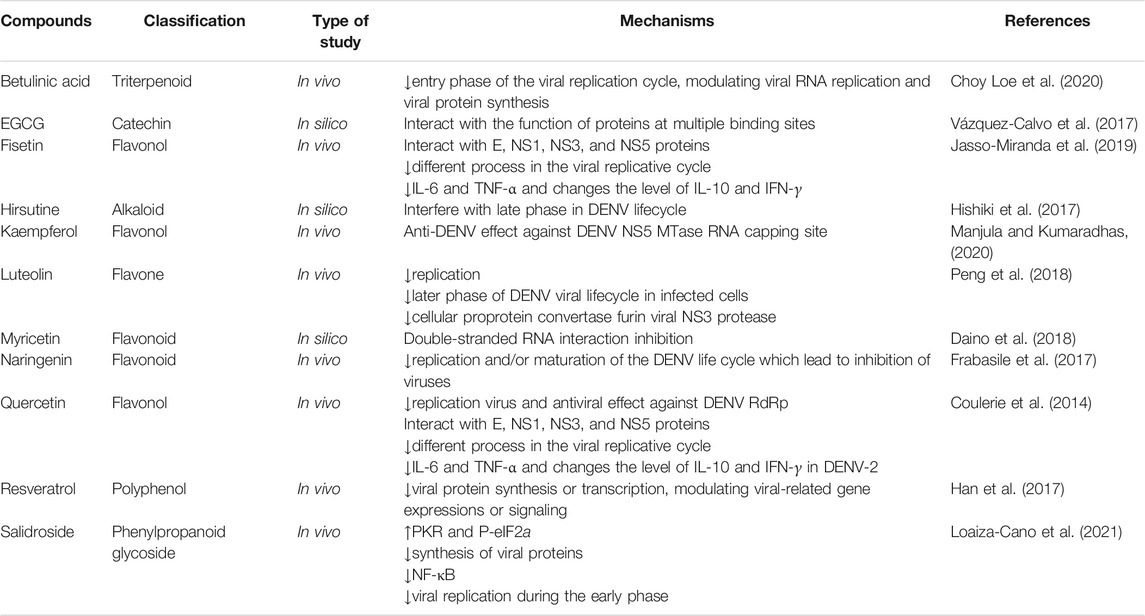
TABLE 2. Preclinical evidence on the use of candidate phytochemicals against DENV and related neuropharmacological responses.
ZIKV
ZIKV is related to fetal malformations such as craniosynostosis, intrauterine growth restriction, craniofacial malformations, pulmonary hypoplasia, arthrogryposis, and severe ventriculomegaly secondary to midbrain damage with aqueduct atresia or stenosis; the occipital lobe sometimes acquires a cystic appearance and moderate ventriculomegaly with the presence of shallow grooves or agyria (De Melo Marques et al., 2019). Similar to other emerging infectious disease, ZIKV is affected by phytochemicals through different mechanisms to prevent associated neuronal signs. Loe et al. indicated that betulinic acid (a triterpenoid) suppressed the entry phase of the viral replication cycle and also can affect viral RNA replication and viral protein synthesis, as it has antiviral effects against other RNA viruses. One of them is ZIKV, a flavivirus related to DENV (Choy Loe et al., 2020). Mohd and coworkers observed that resveratrol inhibited the ZIKV and suppressed the early stage of virus entry into the host cells via inactivating the phosphorylation of the epidermal growth factor receptor (EGFR) (Mohd et al., 2019). Similarly, Suroengrit and colleges observed that chrysin has potent inhibitory effects on ZIKV (Suroengrit et al., 2017).
Recently, it has been suggested that quercetin and other derivatives can affect viral entry process viruses (Qiu et al., 2016) and suppress DENV-2 replication by inhibiting viral RNA polymerase (Fanunza et al., 2020). Consequently, another flavonoid EGCG has an antiviral effect against ZIKV as it inhibited the entry into the host cell and also can inhibit essential phases in the replication cycle viruses to bind the nucleoside-triphosphatase (NTPase) site in ZIKV (Kumar et al., 2020). Studies have also shown that EGCG and baicalin bind to the virus E-protein and can inhibit the entry of ZIKV into host cells (Wang et al., 2019). Also, baicalein and baicalin have antiviral activity against ZIKV infections (Oo et al., 2019). Another in silico evidence has shown curcumin, rutin, sanggenon, delphinidin, isoquercetin, naringenin, and EGCG as antiviral activity against the ZIKV NS2B-NS3 protease activity and can inhibit replication of the virus to reduce neurological sign (Mounce et al., 2017; Wong et al., 2017; Ahmed et al., 2020; Albuquerque de Oliveira Mendes et al., 2020; Yadav et al., 2021).
Evidence indicates that delphinidin and curcumin inhibited ZIKV infection via blocking the virus entry phase (Clain et al., 2019). As evaluated by a docking study, naringenin also inhibited ZIKV infection in human cells. It prevented NS2B-NS3 protease activity of ZIKV via the formation of hydrogen bonds between the phenol hydrogens of naringenin and amino acid of the virus protease (De Oliveira Mendes et al., 2020). Lim et al. investigated several other polyphenol compounds which belong to flavonols, flavanols, flavones, and flavanones such as luteolin, chrysin, myricetin, ampelopsin, astragalin, rutin, icaritin, hesperidin, pyrogallol, pyrocatechol, caffeine, gallic acid against ZIKV NS2B-NS3 proteases, and observed the potential of these compounds in the inhibition of ZIKV replication (Lim et al., 2017). In a molecular docking study, some flavonoids amentoflavone, fisetin, isorhamnetin, and theaflavin 3-gallate have shown potential inhibitory activity against ZIKV NS3-NS2B protease (Bhargava et al., 2019; Zou et al., 2020; Eberle et al., 2021; Lima et al., 2021; Yadav et al., 2021). Roy et al. based on in silico evidence indicated that several natural products such as myricetin, gossypol, naringenin, apigenin, luteolin, isorhamnetin, daidzein, resveratrol, and catechin inhibited Zika NS2B-NS3 via binding to a pocket on the active site and suppressed replication of ZIKV to be useful for the treatment of neurological diseases (Roy et al., 2017; Cataneo et al., 2019; Gao et al., 2019).
Silymarin and pinocembrin, as other natural compounds, also inhibit the production of ZIKV in the early phase (Le Lee et al., 2019; Da Silva et al., 2020). Evidence indicated that cephalotaxine (an alkaloid) has anti-ZIKV activity and disrupts the life cycle of ZIKV, and cephalotaxine has the potential to be developed as a therapeutic agent against ZIKV (Lai et al., 2020). Recently, it has been shown a polyphenol-rich extract from Aphloia theiformis (Vahl) Benn. can suppress ZIKV and DENV infection via inhibiting the virus entry phase (Clain et al., 2019).
Overall, by targeting ZIKV proteases, phytochemicals seem to be promising candidates in combating emerging infectious diseases. Additionally, modulation of TAM may be a critical way for phytochemicals to combat ZIKV.
EBOV
Potential plant-derived secondary metabolites are shown to combat EVD. In this regard, curcumin blocks cytokine storm by suppressing IL-1, IL-6, and TNF-α, which correlates with clinical manifestations of Ebola (Sordillo and Helson, 2015). Additionally, bisdemethoxycurcumin, demethoxycurcumin, and tetrahydrocurcumin are other major metabolites of curcumin with proven antiviral activity. In this line, bisdemethoxycurcumin indicated maximum inhibition of Ebola viral proteins among the curcuminoids (Baikerikar, 2017).
Recently, quercetin and other derivatives have been identified as affecting the entry process of EBOV (Qiu et al., 2016). Another flavonoid derivative called quercetin 3-β-O-D-glucoside protected against Ebola in vivo. Moreover, it was shown that this quercetin derivative inhibited the early steps of viral entry (Qiu et al., 2016). The mechanism of action of quercetin was to restore the IFN-I signaling cascade by a direct interfere with EBOV VP24 binding to karyopherin-α and thereby restoring IFN gene transcription and phosphorylated signal transducer and activator of transcription 1 (STAT1) nuclear transport (Fanunza et al., 2020).
Other studies indicated that genistein has antiviral effects and inhibited EBOV replication (Kolokoltsov et al., 2012). Ellagic acid, myricetin, and some other phytochemicals (Setlur et al., 2017) as anti-Ebola compounds act through inhibiting virus entry (Cui et al., 2018) and RNA interaction, respectively (Daino et al., 2018). An in vitro antiviral effect of oleandrin acts as a novel cardiac glycoside against the EBOV (Newman et al., 2020). Some preclinical evidence suggests the effectiveness of cannabinoids in viral diseases (Mabou Tagne et al., 2020). Altogether, phytochemicals have shown a promising future against EVD.
Table 3 indicates the preclinical evidence on the use of candidate phytochemicals against ZIKV and EBOV and related neuronal manifestations. Figure 2 and Figure 3 indicate selected chemical structures of phenolic compounds, alkaloids, and miscellaneous compounds in combating neuronal signs of emerging infectious diseases.
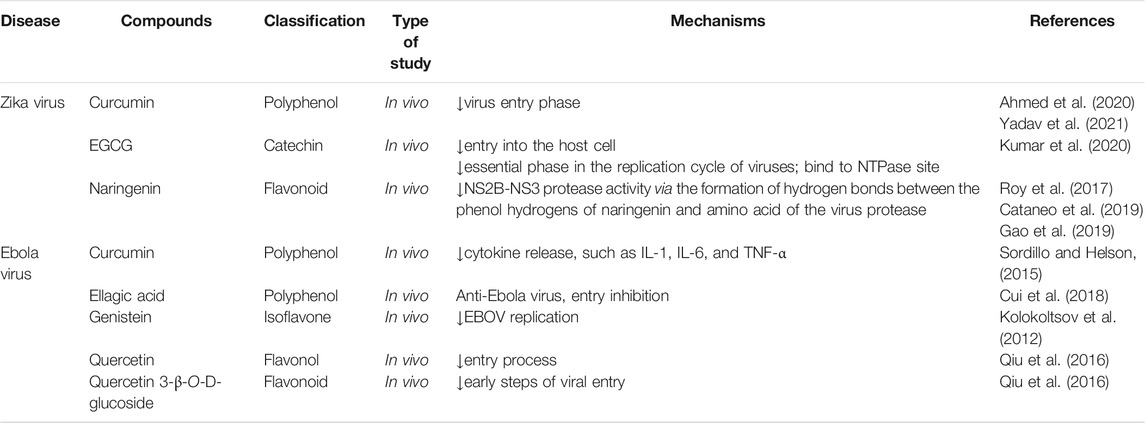
TABLE 3. Some preclinical evidence on the use of candidate phytochemicals against ZIKV and EBOV and neuropharmacological responses.
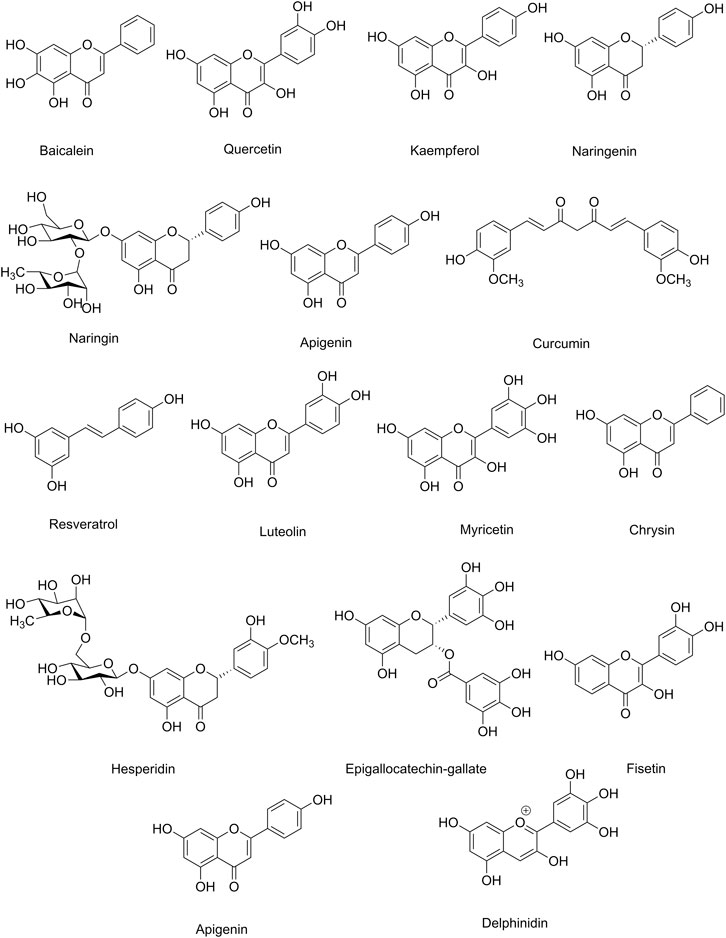
FIGURE 2. Selected chemical structures of phenolic compounds in combating neuronal signs of emerging infectious diseases.
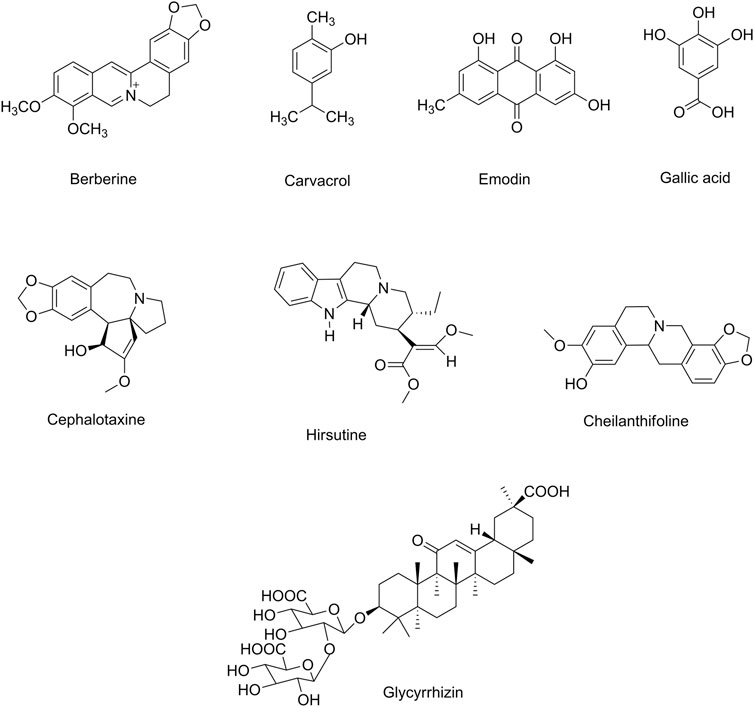
FIGURE 3. Selected chemical structures of alkaloids and miscellaneous compounds in combating neuronal signs of emerging infectious diseases.
Conclusion
Phytochemicals have been excellent sources of alternative therapeutic agents and lead compounds in combating viral diseases. As a top global priority, novel plant-derived antiviral agents are promising candidates in combating neuronal manifestations of emerging infectious diseases. Consequently, inflammatory/oxidative pathways are critical targets, including ILs, TLR, NF-κB, MAPK, iNOS, and AQP, as well as several enzymes involved in the virus life cycle (Figure 4). The potential of phytochemicals in demonstrating antiviral effects through inhibiting the viral life cycle indicates a promising future to find novel antiviral lead compounds against neuronal signs of emerging viral diseases. Previously, we have analyzed the capability of these phytochemicals in passing BBB towards neuroprotective responses (Fakhri et al., 2020b), which showed hopeful results for most plant-derived secondary metabolites in modulating the aforementioned dysregulated pathways in CNS. Additionally, to drawback, the pharmacokinetic limitation of phytochemicals, novel drug delivery systems, could pave the road towards neuroprotection against emerging viral diseases. In our previous study, we also provided that the potential of flavonoids, terpenes/terpenoids, chalcones, and alkaloids has been shown in targeting angiotensin-converting enzyme 2 (ACE2) and spike proteins against neurological signs of coronavirus disease 2019 (COVID-19) and found promising results in combating pathophysiological mechanisms of the virus.
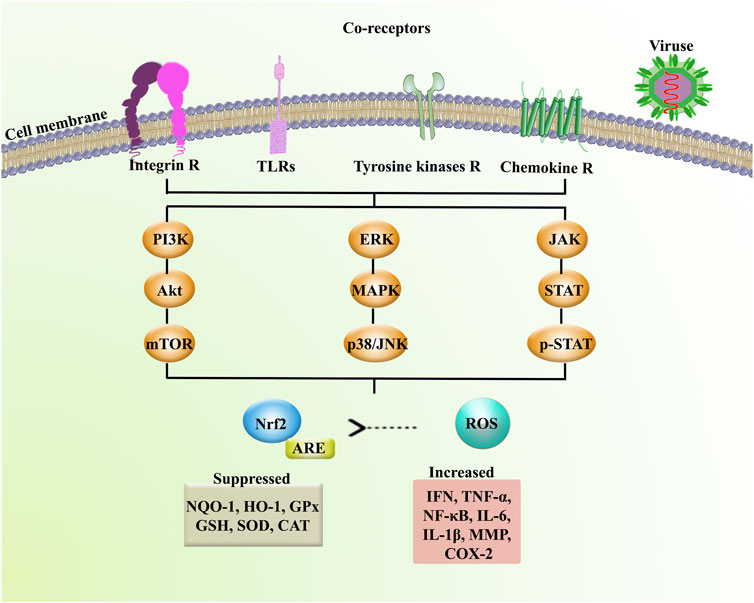
FIGURE 4. Receptors and signaling mediators involved in neuronal signs of emerging infectious diseases. Akt: protein kinase B, ARE: antioxidant response element, CAT: catalase, Chemokine R: chemokine receptor, COX-2: cyclooxygenase-2, ERK: extracellular signal-regulated kinase, GPx: glutathione peroxidase, GSH: glutathione, HO-1: heme oxygenase-1, IFN: interferon, IL: interleukin, Integrin R: integrin receptor, JAK: Janus kinase, mTOR: mammalian target of rapamycin, MMP: matrix metalloproteinase, NF-κB: nuclear factor-kappa B, NQO-1: NAD(P)H Quinone Dehydrogenase 1, Nrf2: nuclear factor erythroid 2-related factor 2, p38: p38 mitogen-activated protein kinase, p-STAT: phospho-signal transducer and activator of transcription, SOD: superoxide dismutase, STAT: signal transducer and activator of transcription, TLRs: toll-like receptors, TNF-α: tumor necrosis factor-α, and Tyrosine kinase R: tyrosine kinase receptor.
In the present review, a mechanistic approach has been employed on plant-derived antiviral compounds with related pharmacological mechanisms, as alternative therapies against neuronal signs of emerging infections (Figure 5). Future research areas should include additional in vitro and in vivo experimentation to highlight the significant pathophysiological mechanisms of viral diseases. Introducing other effective and novel plant-derived antiviral lead compounds through modulating such dysregulated pathways could pave the road in combating viral infections. It should be followed by well-controlled clinical trials to assess phytochemicals as multi-target alternative agents. Such reports will help reveal more applications of phytochemicals in the prevention, management, and treatment of emerging viral diseases.
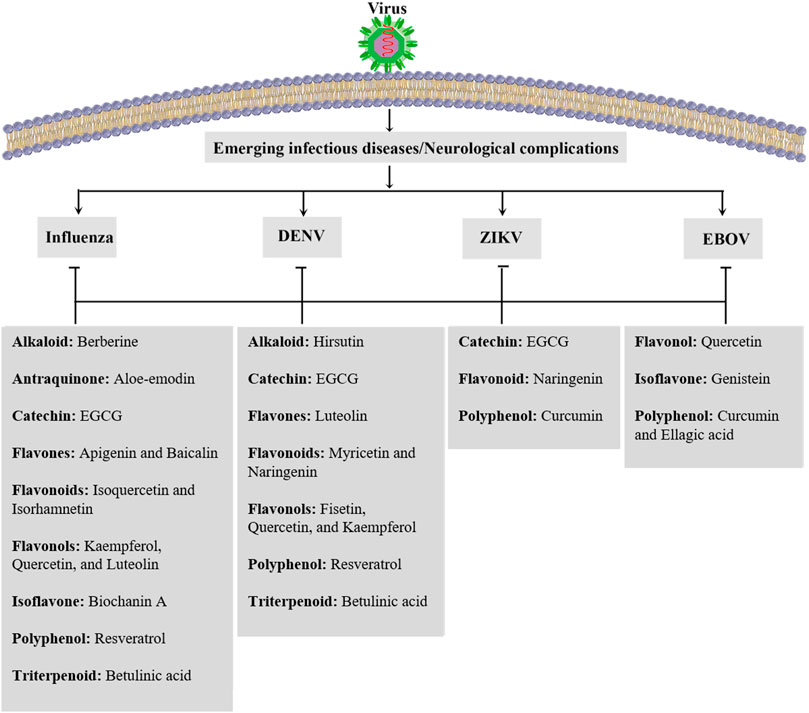
FIGURE 5. Candidate phytochemicals in combating neurological signs of emerging infectious diseases. DENV: dengue virus, EBOV: Ebola virus, EGCG: epigallocatechin gallate, and ZIKV: Zika virus.
Author Contributions
Conceptualization, software, writing—original draft, and writing—review and editing, SF. Writing—original draft, PP and SP. Conceptualization and writing—review and editing, MF and JE.
Funding
JE gratefully acknowledges funding from CONICYT (PAI/ACADEMIA N° 79160109).
Conflict of Interest
The authors declare that the research was conducted in the absence of any commercial or financial relationships that could be construed as a potential conflict of interest.
Publisher’s Note
All claims expressed in this article are solely those of the authors and do not necessarily represent those of their affiliated organizations or those of the publisher, the editors, and the reviewers. Any product that may be evaluated in this article or claim that may be made by its manufacturer is not guaranteed or endorsed by the publisher.
References
Adekanmbi, O., Ilesanmi, O., and Lakoh, S. (2021). Ebola: A Review and Focus on Neurologic Manifestations. J. Neurol. Sci. 421, 117311. doi:10.1016/j.jns.2021.117311
Ahmed, S. R., Banik, A., Anni, S. M., and Chowdhury, M. M. H. (2020). Plant Derived Bioactive Compounds as Potential Inhibitors of ZIKA Virus: an In Silico Investigation. bioRxiv [Epub ahead of print]. doi:10.1101/2020.11.11.378083
Albuquerque De Oliveira Mendes, L., Ponciano, C. S., Depieri Cataneo, A. H., Wowk, P. F., Bordignon, J., Silva, H., et al. (2020). The Anti-zika Virus and Anti-tumoral Activity of the Citrus Flavanone Lipophilic Naringenin-Based Compounds. Chem. Biol. Interact 331, 109218. doi:10.1016/j.cbi.2020.109218
Ali, O., Takas, T., Nyborg, A., Jensen, K., Dubovsky, F., and Mallory, R. (2017). A Phase 2a Study to Evaluate the Safety of MEDI8852 in Outpatient Adults with Acute, Uncomplicated Influenza A. Open Forum Infect. Dis. 4, S519. doi:10.1093/ofid/ofx163.1352
Anusuya, S., and Gromiha, M. M. (2017). Quercetin Derivatives as Non-nucleoside Inhibitors for Dengue Polymerase: Molecular Docking, Molecular Dynamics Simulation, and Binding Free Energy Calculation. J. Biomol. Struct. Dyn. 35, 2895–2909. doi:10.1080/07391102.2016.1234416
Aoki, C., Hidari, K. I., Itonori, S., Yamada, A., Takahashi, N., Kasama, T., et al. (2006). Identification and Characterization of Carbohydrate Molecules in Mammalian Cells Recognized by Dengue Virus Type 2. J. Biochem. 139, 607–614. doi:10.1093/jb/mvj067
Arora, H. S. (2020). A to Z of Zika Virus: a Comprehensive Review for Clinicians. Glob. Pediatr. Health 7, 2333794X20919595. doi:10.1177/2333794X20919595
Arredondo-García, J., Hadinegoro, S., Reynales, H., Chua, M., Medina, D. R., Chotpitayasunondh, T., et al. (2018). Four-year Safety Follow-Up of the Tetravalent Dengue Vaccine Efficacy Randomized Controlled Trials in Asia and Latin America. Clin. Microbiol. Infect. 24, 755–763. doi:10.1016/j.cmi.2018.01.018
Asadi-Pooya, A. A., Yaghoubi, E., Nikseresht, A., Moghadami, M., and Honarvar, B. (2011). The Neurological Manifestations of H1N1 Influenza Infection; Diagnostic Challenges and Recommendations. Iran J. Med. Sci. 36, 36–39.
Atif, M., Azeem, M., Sarwar, M. R., and Bashir, A. (2016). Zika Virus Disease: a Current Review of the Literature. Infection 44, 695–705.
Audet, J., Wong, G., Wang, H., Lu, G., Gao, G. F., Kobinger, G., et al. (2014). Molecular Characterization of the Monoclonal Antibodies Composing ZMAb: a Protective Cocktail against Ebola Virus. Sci. Rep. 4, 6881. doi:10.1038/srep06881
Baikerikar, S. (2017). Curcumin and Natural Derivatives Inhibit Ebola Viral Proteins: An In Silico Approach. Pharmacognosy Res. 9, S15–S22. doi:10.4103/pr.pr_30_17
Baize, S., Leroy, E. M., Georges-Courbot, M.-C., Capron, M., Lansoud-Soukate, J., Debré, P., et al. (1999). Defective Humoral Responses and Extensive Intravascular Apoptosis Are Associated with Fatal Outcome in Ebola Virus-Infected Patients. Nat. Med. 5, 423–426. doi:10.1038/7422
Bale, S., Dias, J. M., Fusco, M. L., Hashiguchi, T., Wong, A. C., Liu, T., et al. (2012). Structural Basis for Differential Neutralization of Ebolaviruses. Viruses 4, 447–470. doi:10.3390/v4040447
Bandeira, A. C., Gois, L. L., Campos, G. S., Sardi, S., Yssel, H., Vieillard, V., et al. (2020). Clinical and Laboratory Findings of Acute Zika Virus Infection in Patients from Salvador during the First Brazilian Epidemic. Braz. J. Infect. Dis. 24, 405–411. doi:10.1016/j.bjid.2020.08.005
Barrientos, L. G., and Rollin, P. E. (2007). Release of Cellular Proteases into the Acidic Extracellular Milieu Exacerbates Ebola Virus-Induced Cell Damage. Virology 358, 1–9. doi:10.1016/j.virol.2006.08.018
Becher, B., Fedorowicz, V., and Antel, J. (1996). Regulation of CD14 Expression on Human Adult central Nervous System-Derived Microglia. J. Neurosci. Res. 45, 375–381. doi:10.1002/(SICI)1097-4547(19960815)45:4<375:AID-JNR6>3.0.CO;2-6
Beckman, D., Seelke, A., Morrison, J. H., and Bliss-Moreau, E. (2020). Novel Approaches to Study the Zika Virus in the Brain. J. Neurosci. Res. 98, 227–228. doi:10.1002/jnr.24499
Bernatchez, J. A., Tran, L. T., Li, J., Luan, Y., Siqueira-Neto, J. L., and Li, R. (2019). Drugs for the Treatment of Zika Virus Infection. J. Med. Chem. 63, 470–489. doi:10.1021/acs.jmedchem.9b00775
Bhargava, S., Patel, T., Gaikwad, R., Patil, U. K., and Gayen, S. (2019). Identification of Structural Requirements and Prediction of Inhibitory Activity of Natural Flavonoids against Zika Virus through Molecular Docking and Monte Carlo Based QSAR Simulation. Nat. Prod. Res. 33, 851–857. doi:10.1080/14786419.2017.1413574
Bhatt, R. S., Kothari, S. T., Gohil, D. J., D’souza, M., and Chowdhary, A. S. (2015). Novel Evidence of Microglial Immune Response in Impairment of Dengue Infection of CNS. Immunobiology 220, 1170–1176. doi:10.1016/j.imbio.2015.06.002
Billioux, B. J., Smith, B., and Nath, A. (2016). Neurological Complications of Ebola Virus Infection. Neurotherapeutics 13, 461–470. doi:10.1007/s13311-016-0457-z
Bishop, B. M. (2015). Potential and Emerging Treatment Options for Ebola Virus Disease. Ann. Pharmacother. 49, 196–206. doi:10.1177/1060028014561227
Bixler, S. L., and Goff, A. J. (2015). The Role of Cytokines and Chemokines in Filovirus Infection. Viruses 7, 5489–5507. doi:10.3390/v7102892
Bosio, C. M., Aman, M. J., Grogan, C., Hogan, R., Ruthel, G., Negley, D., et al. (2003). Ebola and Marburg Viruses Replicate in Monocyte-Derived Dendritic Cells without Inducing the Production of Cytokines and Full Maturation. J. Infect. Dis. 188, 1630–1638. doi:10.1086/379199
Bosio, C. M., Moore, B. D., Warfield, K. L., Ruthel, G., Mohamadzadeh, M., Aman, M. J., et al. (2004). Ebola and Marburg Virus-like Particles Activate Human Myeloid Dendritic Cells. Virology 326, 280–287. doi:10.1016/j.virol.2004.05.025
Bowen, L., Smith, B., Steinbach, S., Billioux, B., Summers, A., Azodi, S., et al. (2016). Survivors of Ebola Virus Disease Have Persistent Neurological Deficits (S53. 003). Neurology 86, 16. supplement.
Bray, M., and Geisbert, T. W. (2005). Ebola Virus: the Role of Macrophages and Dendritic Cells in the Pathogenesis of Ebola Hemorrhagic Fever. Int. J. Biochem. Cel Biol 37, 1560–1566. doi:10.1016/j.biocel.2005.02.018
Britton, P. N., Dale, R. C., Blyth, C. C., Macartney, K., Crawford, N. W., Marshall, H., et al. (2017). Influenza-associated Encephalitis/encephalopathy Identified by the Australian Childhood Encephalitis Study 2013–2015. Pediatr. Infect. Dis. J. 36, 1021–1026. doi:10.1097/INF.0000000000001650
Carette, J. E., Raaben, M., Wong, A. C., Herbert, A. S., Obernosterer, G., Mulherkar, N., et al. (2011). Ebola Virus Entry Requires the Cholesterol Transporter Niemann–Pick C1. Nature 477, 340–343. doi:10.1038/nature10348
Cataneo, A. H. D., Kuczera, D., Koishi, A. C., Zanluca, C., Silveira, G. F., De Arruda, T. B., et al. (2019). The Citrus Flavonoid Naringenin Impairs the In Vitro Infection of Human Cells by Zika Virus. Sci. Rep. 9, 1–15. doi:10.1038/s41598-019-52626-3
Cecil, C. E., Davis, J. M., Cech, N. B., and Laster, S. M. (2011). Inhibition of H1N1 Influenza A Virus Growth and Induction of Inflammatory Mediators by the Isoquinoline Alkaloid Berberine and Extracts of Goldenseal (Hydrastis canadensis). Int. Immunopharmacol 11, 1706–1714. doi:10.1016/j.intimp.2011.06.002
Centers for Disease Control (CDC) (2016). Zika: The Basics of the Virus and How to Protect against it. Atlanta, GA, USA: Centers for Disease Control.
Chandran, K., Sullivan, N. J., Felbor, U., Whelan, S. P., and Cunningham, J. M. (2005). Endosomal Proteolysis of the Ebola Virus Glycoprotein Is Necessary for Infection. Science 308, 1643–1645. doi:10.1126/science.1110656
Che, P., Tang, H., and Li, Q. (2013). The Interaction between Claudin-1 and Dengue Viral prM/M Protein for its Entry. Virology 446, 303–313. doi:10.1016/j.virol.2013.08.009
Chemaly, R. F., Dadwal, S. S., Bergeron, A., Ljungman, P., Kim, Y.-J., Cheng, G.-S., et al. (2020). A Phase 2, Randomized, Double-Blind, Placebo-Controlled Trial of Presatovir for the Treatment of Respiratory Syncytial Virus Upper Respiratory Tract Infection in Hematopoietic-Cell Transplant Recipients. Clin. Infect. Dis. 71, 2777–2786. doi:10.1093/cid/ciz1166
Chen, K.-T., Zhou, W.-L., Liu, J.-W., Zu, M., He, Z.-N., Du, G.-H., et al. (2012a). Active Neuraminidase Constituents of Polygonum Cuspidatum against Influenza A (H1N1) Influenza Virus. Zhongguo Zhong Yao Za Zhi 37, 3068–3073.
Chen, Q., Li, P., Li, S., Xiao, W., Yang, S., and Lu, H. (2020). Brain Complications with Influenza Infection in Children. J. Behav. Brain Sci. 10, 129–152. doi:10.4236/jbbs.2020.103008
Chen, S.-T., Liu, R.-S., Wu, M.-F., Lin, Y.-L., Chen, S.-Y., Tan, D. T.-W., et al. (2012b). CLEC5A Regulates Japanese Encephalitis Virus-Induced Neuroinflammation and Lethality. Plos Pathog. 8, e1002655. doi:10.1371/journal.ppat.1002655
Chertow, D. S., Nath, A., Suffredini, A. F., Danner, R. L., Reich, D. S., Bishop, R. J., et al. (2016). Severe Meningoencephalitis in a Case of Ebola Virus Disease: a Case Report. Ann. Intern. Med. 165, 301–304. doi:10.7326/M15-3066
Choi, J.-G., Lee, H., Kim, Y. S., Hwang, Y.-H., Oh, Y.-C., Lee, B., et al. (2019). Aloe Vera and its Components Inhibit Influenza A Virus-Induced Autophagy and Replication. Am. J. Chin. Med. 47, 1307–1324. doi:10.1142/S0192415X19500678
Choy Loe, M. W., Hao, E., Chen, M., Li, C., Hua Lee, R. C., Yu Zhu, I. X., et al. (2020). Betulinic Acid Exhibits Antiviral Effects against Dengue Virus Infection. Antivir. Res. 184, 104954. doi:10.1016/j.antiviral.2020.104954
Christian, K. M., Song, H., and Ming, G.-L. (2019). Pathophysiology and Mechanisms of Zika Virus Infection in the Nervous System. Annu. Rev. Neurosci. 42, 249–269. doi:10.1146/annurev-neuro-080317-062231
Chu, M., Xu, L., Zhang, M.-B., Chu, Z.-Y., and Wang, Y.-D. (2015). Role of Baicalin in Anti-influenza Virus A as a Potent Inducer of IFN-Gamma. Biomed. Res. Int. 2015, 263630. doi:10.1155/2015/263630
Clain, E., Haddad, J. G., Koishi, A. C., Sinigaglia, L., Rachidi, W., Desprès, P., et al. (2019). The Polyphenol-Rich Extract from Psiloxylon Mauritianum, an Endemic Medicinal Plant from Reunion Island, Inhibits the Early Stages of Dengue and Zika Virus Infection. Int. J. Mol. Sci. 20, 1860. doi:10.3390/ijms20081860
Côté, M., Misasi, J., Ren, T., Bruchez, A., Lee, K., Filone, C. M., et al. (2011). Small Molecule Inhibitors Reveal Niemann–Pick C1 Is Essential for Ebola Virus Infection. Nature 477, 344–348. doi:10.1038/nature10380
Coulerie, P., Maciuk, A., Eydoux, C., Hnawia, E., Lebouvier, N., Figadère, B., et al. (2014). New Inhibitors of the DENV-NS5 RdRp from Carpolepis Laurifolia as Potential Antiviral Drugs for Dengue Treatment. Rec. Nat. Prod. 8, 286–289.
Cui, Q., Du, R., Anantpadma, M., Schafer, A., Hou, L., Tian, J., et al. (2018). Identification of Ellagic Acid from Plant Rhodiola Rosea L. As an Anti-ebola Virus Entry Inhibitor. Viruses 10, 152. doi:10.3390/v10040152
Da Silva, I., Frontera, J., Bispo De Filippis, A., and Moreira do Nascimento, O. J.RIO-GBS-ZIKV Research Group (2017). Neurologic Complications Associated with the Zika Virus in Brazilian Adults. JAMA Neurol. 74, 1190–1198. doi:10.1001/jamaneurol.2017.1703
Da Silva, T. F., Ferraz, A. C., Almeida, L. T., Da Silva Caetano, C. C., Camini, F. C., Lima, R. L. S., et al. (2020). Antiviral Effect of Silymarin against Zika Virus In Vitro. Acta Trop. 211, 105613. doi:10.1016/j.actatropica.2020.105613
Dai, J.-P., Wang, Q.-W., Su, Y., Gu, L.-M., Zhao, Y., Chen, X.-X., et al. (2017). Emodin Inhibition of Influenza A Virus Replication and Influenza Viral Pneumonia via the Nrf2, TLR4, p38/JNK and NF-kappaB Pathways. Molecules 22, 1754. doi:10.3390/molecules22101754
Daino, G. L., Frau, A., Sanna, C., Rigano, D., Distinto, S., Madau, V., et al. (2018). Identification of Myricetin as an Ebola Virus VP35-Double-Stranded RNA Interaction Inhibitor through a Novel Fluorescence-Based Assay. Biochemistry 57, 6367–6378. doi:10.1021/acs.biochem.8b00892
Dayem, A. A., Choi, H. Y., Kim, Y. B., and Cho, S.-G. (2015). Antiviral Effect of Methylated Flavonol Isorhamnetin against Influenza. PLoS One 10, e0121610. doi:10.1371/journal.pone.0121610
De Greslan, T., Billhot, M., Rousseau, C., Mac Nab, C., Karkowski, L., Cournac, J.-M., et al. (2016). Ebola Virus–Related Encephalitis. Clin. Infect. Dis. 63, 1076–1078. doi:10.1093/cid/ciw469
De Melo Marques, V., Santos, C. S., Santiago, I. G., Marques, S. M., Brasil, M. D. G. N., Lima, T. T., et al. (2019). Neurological Complications of Congenital Zika Virus Infection. Pediatr. Neurol. 91, 3–10. doi:10.1016/j.pediatrneurol.2018.11.003
De Oliveira Mendes, L. A., Ponciano, C. S., Cataneo, A. H. D., Wowk, P. F., Bordignon, J., Silva, H., et al. (2020). The Anti-zika Virus and Anti-tumoral Activity of the Citrus Flavanone Lipophilic Naringenin-Based Compounds. Chem. Biol. Interact 331, 109218. doi:10.1016/j.cbi.2020.109218
Denizot, M., Neal, J. W., and Gasque, P. (2012). Encephalitis Due to Emerging Viruses: CNS Innate Immunity and Potential Therapeutic Targets. J. Infect. 65, 1–16. doi:10.1016/j.jinf.2012.03.019
Devincenzo, J. P., Whitley, R. J., Mackman, R. L., Scaglioni-Weinlich, C., Harrison, L., Farrell, E., et al. (2014). Oral GS-5806 Activity in a Respiratory Syncytial Virus challenge Study. N. Engl. J. Med. 371, 711–722. doi:10.1056/NEJMoa1401184
Dias, J. M., Kuehne, A. I., Abelson, D. M., Bale, S., Wong, A. C., Halfmann, P., et al. (2011). A Shared Structural Solution for Neutralizing Ebolaviruses. Nat. Struct. Mol. Biol. 18, 1424–1427. doi:10.1038/nsmb.2150
Ding, Y., Dou, J., Teng, Z., Yu, J., Wang, T., Lu, N., et al. (2014). Antiviral Activity of Baicalin against Influenza A (H1N1/H3N2) Virus in Cell Culture and in Mice and its Inhibition of Neuraminidase. Arch. Virol. 159, 3269–3278. doi:10.1007/s00705-014-2192-2
Dong, W., Wei, X., Zhang, F., Hao, J., Huang, F., Zhang, C., et al. (2014). A Dual Character of Flavonoids in Influenza A Virus Replication and Spread through Modulating Cell-Autonomous Immunity by MAPK Signaling Pathways. Sci. Rep. 4, 7237. doi:10.1038/srep07237
Dwivedi, V. D., Bharadwaj, S., Afroz, S., Khan, N., Ansari, M. A., Yadava, U., et al. (2020). Anti-dengue Infectivity Evaluation of Bioflavonoid from Azadirachta indica by Dengue Virus Serine Protease Inhibition. J. Biomol. Struct. Dyn. 39, 1417–1430. doi:10.1080/07391102.2020.1734485
Eberle, R. J., Olivier, D. S., Pacca, C. C., Avilla, C. M. S., Nogueira, M. L., Amaral, M. S., et al. (2021). In Vitro study of Hesperetin and Hesperidin as Inhibitors of Zika and Chikungunya Virus Proteases. PLoS One 16, e0246319. doi:10.1371/journal.pone.0246319
Ekiert, D. C., Kashyap, A. K., Steel, J., Rubrum, A., Bhabha, G., Khayat, R., et al. (2012). Cross-neutralization of Influenza A Viruses Mediated by a Single Antibody Loop. Nature 489, 526–532. doi:10.1038/nature11414
Elong Ngono, A., and Shresta, S. (2019). Cross-reactive T Cell Immunity to Dengue and Zika Viruses: New Insights into Vaccine Development. Front. Immunol. 10, 1316. doi:10.3389/fimmu.2019.01316
Enkhtaivan, G., Muthuraman, P., Kim, D. H., and Mistry, B. (2017). Discovery of Berberine Based Derivatives as Anti-influenza Agent through Blocking of Neuraminidase. Bioorg. Med. Chem. 25, 5185–5193. doi:10.1016/j.bmc.2017.07.006
Fabry, Z., Raine, C. S., and Hart, M. N. (1994). Nervous Tissue as an Immune Compartment: the Dialect of the Immune Response in the CNS. Immunol. Today 15, 218–224. doi:10.1016/0167-5699(94)90247-X
Fakhri, S., Moradi, S. Z., Farzaei, M. H., and Bishayee, A. (2020a). Modulation of Dysregulated Cancer Metabolism by Plant Secondary Metabolites: A Mechanistic Review. Semin. Cancer Biol. S1044-579X (20), 30040–30047. doi:10.1016/j.semcancer.2020.02.007
Fakhri, S., Piri, S., Majnooni, M. B., Farzaei, M. H., and Echeverría, J. (2020b). Targeting Neurological Manifestations of Coronaviruses by Candidate Phytochemicals: A Mechanistic Approach. Front. Pharmacol. 11, 621099. doi:10.3389/fphar.2020.621099
Fan, W., Qian, P., Wang, D., Zhi, X., Wei, Y., Chen, H., et al. (2017). Integrin αvβ3 Promotes Infection by Japanese Encephalitis Virus. Res. Vet. Sci. 111, 67–74. doi:10.1016/j.rvsc.2016.12.007
Fanunza, E., Iampietro, M., Distinto, S., Corona, A., Quartu, M., Maccioni, E., et al. (2020). Quercetin Blocks Ebola Virus Infection by Counteracting the VP24 Interferon-Inhibitory Function. Antimicrob. Agents Chemother. 64, e00530–20. doi:10.1128/AAC.00530-20
Ferreira, M. L. B., De Brito, C. a. A., De Oliveira França, R. F., Moreira, Á. J. P., De Morais Machado, M. Í., Da Paz Melo, R., et al. (2020). Neurological Disease in Adults with Zika and Chikungunya Virus Infection in Northeast Brazil: a Prospective Observational Study. Lancet Neurol. 19, 826–839. doi:10.1016/S1474-4422(20)30232-5
Finney, R., and Somers, G. (1958). The Anti-inflammatory Activity of Glycyrrhetinic Acid and Derivatives. J. Pharm. Pharmacol. 10, 613–620. doi:10.1111/j.2042-7158.1958.tb10349.x
Fioravanti, R., Celestino, I., Costi, R., Crucitti, G. C., Pescatori, L., Mattiello, L., et al. (2012). Effects of Polyphenol Compounds on Influenza A Virus Replication and Definition of Their Mechanism of Action. Bioorg. Med. Chem. 20, 5046–5052. doi:10.1016/j.bmc.2012.05.062
Fischer, W. A., Uyeki, T. M., and Tauxe, R. V. (2015). Ebola Virus Disease: What Clinicians in the United States Need to Know. Am. J. Infect. Control. 43, 788–793. doi:10.1016/j.ajic.2015.05.005
Frabasile, S., Koishi, A. C., Kuczera, D., Silveira, G. F., Verri, W. A., Dos Santos, C. N. D., et al. (2017). The Citrus Flavanone Naringenin Impairs Dengue Virus Replication in Human Cells. Sci. Rep. 7, 41864. doi:10.1038/srep41864
Francis, M. E., King, M. L., and Kelvin, A. A. (2019). Back to the Future for Influenza Preimmunity—Looking Back at Influenza Virus History to Infer the Outcome of Future Infections. Viruses 11, 122. doi:10.3390/v11020122
Fredrikson, S., Eneroth, P., and Link, H. (1987). Intrathecal Production of Neopterin in Aseptic Meningo-Encephalitis and Multiple Sclerosis. Clin. Exp. Immunol. 67, 76–81.
Friel, H., and Lederman, H. (2006). A Nutritional Supplement Formula for Influenza A (H5N1) Infection in Humans. Med. Hypotheses 67, 578–587. doi:10.1016/j.mehy.2006.02.040
Gao, Y., Tai, W., Wang, N., Li, X., Jiang, S., Debnath, A. K., et al. (2019). Identification of Novel Natural Products as Effective and Broad-Spectrum Anti-zika Virus Inhibitors. Viruses 11, 1019. doi:10.3390/v11111019
Geisbert, T., Jahrling, P., Hanes, M., and Zack, P. (1992). Association of Ebola-Related Reston Virus Particles and Antigen with Tissue Lesions of Monkeys Imported to the United States. J. Comp. Pathol. 106, 137–152. doi:10.1016/0021-9975(92)90043-t
Geisbert, T. W., Hensley, L. E., Gibb, T. R., Steele, K. E., Jaax, N. K., and Jahrling, P. B. (2000). Apoptosis Induced In Vitro and In Vivo during Infection by Ebola and Marburg Viruses. Lab. Invest. 80, 171–186. doi:10.1038/labinvest.3780021
Geisbert, T. W., Hensley, L. E., Larsen, T., Young, H. A., Reed, D. S., Geisbert, J. B., et al. (2003a). Pathogenesis of Ebola Hemorrhagic Fever in Cynomolgus Macaques: Evidence that Dendritic Cells Are Early and Sustained Targets of Infection. Am. J. Pathol. 163, 2347–2370. doi:10.1016/S0002-9440(10)63591-2
Geisbert, T. W., Strong, J. E., and Feldmann, H. (2015). Considerations in the Use of Nonhuman Primate Models of Ebola Virus and Marburg Virus Infection. J. Infect. Dis. 212, S91–S97. doi:10.1093/infdis/jiv284
Geisbert, T. W., Young, H. A., Jahrling, P. B., Davis, K. J., Kagan, E., and Hensley, L. E. (2003b). Mechanisms Underlying Coagulation Abnormalities in Ebola Hemorrhagic Fever: Overexpression of Tissue Factor in Primate Monocytes/macrophages Is a Key Event. J. Infect. Dis. 188, 1618–1629. doi:10.1086/379724
Geng, P., Zhu, H., Zhou, W., Su, C., Chen, M., Huang, C., et al. (2020). Baicalin Inhibits Influenza A Virus Infection via Promotion of M1 Macrophage Polarization. Front. Pharmacol. 11, 01298. doi:10.3389/fphar.2020.01298
Gnirß, K., Kühl, A., Karsten, C., Glowacka, I., Bertram, S., Kaup, F., et al. (2012). Cathepsins B and L Activate Ebola but Not Marburg Virus Glycoproteins for Efficient Entry into Cell Lines and Macrophages Independent of TMPRSS2 Expression. Virology 424, 3–10. doi:10.1016/j.virol.2011.11.031
Hadinegoro, S. R., Arredondo-García, J. L., Capeding, M. R., Deseda, C., Chotpitayasunondh, T., Dietze, R., et al. (2015). Efficacy and Long-Term Safety of a Dengue Vaccine in Regions of Endemic Disease. N. Engl. J. Med. 373, 1195–1206. doi:10.1056/NEJMoa1506223
Halstead, S. B. (2017). Dengvaxia Sensitizes Seronegatives to Vaccine Enhanced Disease Regardless of Age. Vaccine 35, 6355–6358. doi:10.1016/j.vaccine.2017.09.089
Halstead, S. B. (2018). Safety Issues from a Phase 3 Clinical Trial of a Live-Attenuated Chimeric Yellow Fever Tetravalent Dengue Vaccine. Hum. Vaccin. Immunother. 14, 2158–2162. doi:10.1080/21645515.2018.1445448
Hammack, C., Ogden, S. C., Madden, J. C., Medina, A., Xu, C., Phillips, E., et al. (2019). Zika Virus Infection Induces DNA Damage Response in Human Neural Progenitors that Enhances Viral Replication. J. Virol. 93, e00638–19. doi:10.1128/JVI.00638-19
Han, Y. S., Penthala, N. R., Oliveira, M., Mesplède, T., Xu, H., Quan, Y., et al. (2017). Identification of Resveratrol Analogs as Potent Anti-dengue Agents Using a Cell-Based Assay. J. Med. Virol. 89, 397–407. doi:10.1002/jmv.24660
Hayashi, K., Imanishi, N., Kashiwayama, Y., Kawano, A., Terasawa, K., Shimada, Y., et al. (2007). Inhibitory Effect of Cinnamaldehyde, Derived from Cinnamomi Cortex, on the Growth of Influenza A/PR/8 Virus In Vitro and In Vivo. Antivir. Res 74, 1–8. doi:10.1016/j.antiviral.2007.01.003
Hayden, F. G., Atmar, R. L., Schilling, M., Johnson, C., Poretz, D., Paar, D., et al. (1999). Use of the Selective Oral Neuraminidase Inhibitor Oseltamivir to Prevent Influenza. N. Engl. J. Med. 341, 1336–1343. doi:10.1056/NEJM199910283411802
He, J., Qi, W. B., Wang, L., Tian, J., Jiao, P. R., Liu, G. Q., et al. (2013). Amaryllidaceae Alkaloids Inhibit Nuclear-To-Cytoplasmic export of Ribonucleoprotein (RNP) Complex of Highly Pathogenic Avian Influenza Virus H5N1. Influenza Other Respir. Viruses 7, 922–931. doi:10.1111/irv.12035
Hedrick, J. A., Barzilai, A., Behre, U., Henderson, F. W., Hammond, J., Reilly, L., et al. (2000). Zanamivir for Treatment of Symptomatic Influenza A and B Infection in Children Five to Twelve Years of Age: a Randomized Controlled Trial. Pediatr. Infect. Dis. J. 19, 410–417. doi:10.1097/00006454-200005000-00005
Hensley, L. E., Young, H. A., Jahrling, P. B., and Geisbert, T. W. (2002). Proinflammatory Response during Ebola Virus Infection of Primate Models: Possible Involvement of the Tumor Necrosis Factor Receptor Superfamily. Immunol. Lett. 80, 169–179. doi:10.1016/s0165-2478(01)00327-3
Heo, Y.-A. (2018). Baloxavir: First Global Approval. Drugs 78, 693–697. doi:10.1007/s40265-018-0899-1
Hershberger, E., Sloan, S., Narayan, K., Hay, C. A., Smith, P., Engler, F., et al. (2019). Safety and Efficacy of Monoclonal Antibody VIS410 in Adults with Uncomplicated Influenza A Infection: Results from a Randomized, Double-Blind, Phase-2, Placebo-Controlled Study. EBioMedicine 40, 574–582. doi:10.1016/j.ebiom.2018.12.051
Hishiki, T., Kato, F., Tajima, S., Toume, K., Umezaki, M., Takasaki, T., et al. (2017). Hirsutine, an Indole Alkaloid of Uncaria Rhynchophylla, Inhibits Late Step in Dengue Virus Lifecycle. Front. Microbiol. 8, 1674. doi:10.3389/fmicb.2017.01674
Ho, J. Y., Liew, Y. K., Loh, J., and Sohil, P. (2020). Case Report: Mononeuritis Multiplex in the Course of Dengue Fever. BMC Infect. Dis. 20, 696. doi:10.1186/s12879-020-05430-8
Hong, E.-H., Song, J. H., Kang, K. B., Sung, S. H., Ko, H.-J., and Yang, H. (2015). Anti-influenza Activity of Betulinic Acid from Zizyphus Jujuba on Influenza A/PR/8 Virus. Biomol. Ther. (Seoul). 23, 345–349. doi:10.4062/biomolther.2015.019
Hour, M.-J., Huang, S.-H., Chang, C.-Y., Lin, Y.-K., Wang, C.-Y., Chang, Y.-S., et al. (2013). Baicalein, Ethyl Acetate, and Chloroform Extracts of Scutellaria Baicalensis Inhibit the Neuraminidase Activity of Pandemic 2009 H1N1 and Seasonal Influenza A Viruses. Evid. Based Complement. Alternat Med. 2013, 750803. doi:10.1155/2013/750803
Hussain, M., Galvin, H. D., Haw, T. Y., Nutsford, A. N., and Husain, M. (2017). Drug Resistance in Influenza A Virus: the Epidemiology and Management. Infect. Drug Resist. 10, 121–134. doi:10.2147/IDR.S105473
Igbe, I., Shen, X.-F., Jiao, W., Qiang, Z., Deng, T., Li, S., et al. (2017). Dietary Quercetin Potentiates the Antiproliferative Effect of Interferon-α in Hepatocellular Carcinoma Cells through Activation of JAK/STAT Pathway Signaling by Inhibition of SHP2 Phosphatase. Oncotarget 8, 113734. doi:10.18632/oncotarget.22556
Inan, S. (2019). “The Potential Role of Nutraceuticals in Inflammation and Oxidative Stress,” in Nutraceuticals-Past, Present and Future (IntechOpen). doi:10.5772/intechopen.83797
Itoh, K. (1983). “Augmentation of NK Activity by Several Anti-inflammatory Agents,” in Int Cong Series: Excerpta Medica (Amsterdam), 460–464.
Jacob, S. T., Crozier, I., Fischer, W. A., Hewlett, A., Kraft, C. S., De La Vega, M.-A., et al. (2020). Ebola Virus Disease. Nat. Rev. Dis. Primers 6, 13. doi:10.1038/s41572-020-0147-3
Jain, A., and Lodha, R. (2020). Influenza Associated Neurological Diseases in Children. Indian J. Pediatr. 87, 889–890. doi:10.1007/s12098-020-03511-9
Jasso-Miranda, C., Herrera-Camacho, I., Flores-Mendoza, L. K., Dominguez, F., Vallejo-Ruiz, V., Sanchez-Burgos, G. G., et al. (2019). Antiviral and Immunomodulatory Effects of Polyphenols on Macrophages Infected with Dengue Virus Serotypes 2 and 3 Enhanced or Not with Antibodies. Infect. Drug Resist. 12, 1833. doi:10.2147/IDR.S210890
Jin, J., Chen, Y., Wang, D., Ma, L., Guo, M., Zhou, C., et al. (2018). The Inhibitory Effect of Sodium Baicalin on Oseltamivir-Resistant Influenza A Virus via Reduction of Neuraminidase Activity. Arch. Pharm. Res. 41, 664–676. doi:10.1007/s12272-018-1022-6
Jing, X., Ma, C., Ohigashi, Y., Oliveira, F. A., Jardetzky, T. S., Pinto, L. H., et al. (2008). Functional Studies Indicate Amantadine Binds to the Pore of the Influenza A Virus M2 Proton-Selective Ion Channel. Proc. Natl. Acad. Sci. U S A. 105, 10967–10972. doi:10.1073/pnas.0804958105
Kakooza-Mwesige, A., Tshala-Katumbay, D., and Juliano, S. L. (2019). Viral Infections of the central Nervous System in Africa. Brain Res. Bull. 145, 2–17. doi:10.1016/j.brainresbull.2018.12.019
Kalil, A. C., and Thomas, P. G. (2019). Influenza Virus-Related Critical Illness: Pathophysiology and Epidemiology. Crit. Care 23, 258. doi:10.1186/s13054-019-2539-x
Kannan, S., and Kolandaivel, P. (2018). The Inhibitory Performance of Flavonoid Cyanidin-3-Sambubiocide against H274Y Mutation in H1N1 Influenza Virus. J. Biomol. Struct. Dyn. 36, 4255–4269. doi:10.1080/07391102.2017.1413422
Karar, M. G. E., Matei, M.-F., Jaiswal, R., Illenberger, S., and Kuhnert, N. (2016). Neuraminidase Inhibition of Dietary Chlorogenic Acids and Derivatives–Potential Antivirals from Dietary Sources. Food Funct. 7, 2052–2059. doi:10.1039/c5fo01412c
Kato, D., Era, S., Watanabe, I., Arihara, M., Sugiura, N., Kimata, K., et al. (2010). Antiviral Activity of Chondroitin Sulphate E Targeting Dengue Virus Envelope Protein. Antivir. Res 88, 236–243. doi:10.1016/j.antiviral.2010.09.002
Katzelnick, L. C., Gresh, L., Halloran, M. E., Mercado, J. C., Kuan, G., Gordon, A., et al. (2017). Antibody-dependent Enhancement of Severe Dengue Disease in Humans. Science 358, 929–932. doi:10.1126/science.aan6836
Kazmi, S. S., Ali, W., Bibi, N., and Nouroz, F. (2020). A Review on Zika Virus Outbreak, Epidemiology, Transmission and Infection Dynamics. J. Biol. Res. (Thessalon). 27, 5. doi:10.1186/s40709-020-00115-4
Kim, D. H., Park, G. S., Nile, A. S., Kwon, Y. D., Enkhtaivan, G., and Nile, S. H. (2019). Utilization of Dianthus Superbus L and its Bioactive Compounds for Antioxidant, Anti-influenza and Toxicological Effects. Food Chem. Toxicol. 125, 313–321. doi:10.1016/j.fct.2019.01.013
Kim, M., Kim, S.-Y., Lee, H. W., Shin, J. S., Kim, P., Jung, Y.-S., et al. (2013). Inhibition of Influenza Virus Internalization by (−)-Epigallocatechin-3-Gallate. Antivir. Res. 100, 460–472. doi:10.1016/j.antiviral.2013.08.002
Kim, Y., Narayanan, S., and Chang, K.-O. (2010). Inhibition of Influenza Virus Replication by Plant-Derived Isoquercetin. Antivir. Res 88, 227–235. doi:10.1016/j.antiviral.2010.08.016
Kohno, S., Kida, H., Mizuguchi, M., and Shimada, J.S-021812 Clinical Study Group (2010). Efficacy and Safety of Intravenous Peramivir for Treatment of Seasonal Influenza Virus Infection. Antimicrob. Agents Chemother. 54, 4568–4574. doi:10.1128/AAC.00474-10
Kolokoltsov, A. A., Adhikary, S., Garver, J., Johnson, L., Davey, R. A., and Vela, E. M. (2012). Inhibition of Lassa Virus and Ebola Virus Infection in Host Cells Treated with the Kinase Inhibitors Genistein and Tyrphostin. Arch. Virol. 157, 121–127. doi:10.1007/s00705-011-1115-8
Kong, L.-Y., Lai, C., Wilson, B. C., Simpson, J. N., and Hong, J.-S. (1997). Protein Tyrosine Kinase Inhibitors Decrease Lipopolysaccharide-Induced Proinflammatory Cytokine Production in Mixed Glia, Microglia-Enriched or Astrocyte-Enriched Cultures. Neurochem. Int. 30, 491–497. doi:10.1016/s0197-0186(96)00086-1
Kumar, D., Sharma, N., Aarthy, M., Singh, S. K., and Giri, R. (2020). Mechanistic Insights into Zika Virus NS3 Helicase Inhibition by Epigallocatechin-3-Gallate. ACS Omega 5, 11217–11226. doi:10.1021/acsomega.0c01353
Kumar, P., Khanna, M., Srivastava, V., Tyagi, Y. K., Raj, H. G., and Ravi, K. (2005). Effect of Quercetin Supplementation on Lung Antioxidants after Experimental Influenza Virus Infection. Exp. Lung Res. 31, 449–459. doi:10.1080/019021490927088
Lai, Z.-Z., Ho, Y.-J., and Lu, J.-W. (2020). Cephalotaxine Inhibits Zika Infection by Impeding Viral Replication and Stability. Biochem. Biophys. Res. Commun. 522, 1052–1058. doi:10.1016/j.bbrc.2019.12.012
Lakdawala, S. S., Jayaraman, A., Halpin, R. A., Lamirande, E. W., Shih, A. R., Stockwell, T. B., et al. (2015). The Soft Palate Is an Important Site of Adaptation for Transmissible Influenza Viruses. Nature 526, 122–125. doi:10.1038/nature15379
Laureti, M., Narayanan, D., Rodriguez-Andres, J., Fazakerley, J. K., and Kedzierski, L. (2018). Flavivirus Receptors: Diversity, Identity, and Cell Entry. Front. Immunol. 9, 2180. doi:10.3389/fimmu.2018.02180
Laursen, N. S., and Wilson, I. A. (2013). Broadly Neutralizing Antibodies against Influenza Viruses. Antivir. Res 98, 476–483. doi:10.1016/j.antiviral.2013.03.021
Le Lee, J., Loe, M. W. C., Lee, R. C. H., and Chu, J. J. H. (2019). Antiviral Activity of Pinocembrin against Zika Virus Replication. Antivir. Res 167, 13–24. doi:10.1016/j.antiviral.2019.04.003
Lee, I.-K., Hwang, B. S., Kim, D.-W., Kim, J.-Y., Woo, E.-E., Lee, Y.-J., et al. (2016). Characterization of Neuraminidase Inhibitors in Korean Papaver Rhoeas Bee Pollen Contributing to Anti-influenza Activities In Vitro. Planta Med. 82, 524–529. doi:10.1055/s-0041-111631
Lee, J. E., Fusco, M. L., Hessell, A. J., Oswald, W. B., Burton, D. R., and Saphire, E. O. (2008). Structure of the Ebola Virus Glycoprotein Bound to an Antibody from a Human Survivor. Nature 454, 177–182. doi:10.1038/nature07082
Lee, J. K., and Shin, O. S. (2019). Advances in Zika Virus–Host Cell Interaction: Current Knowledge and Future Perspectives. Int. J. Mol. Sci. 20, 1101. doi:10.3390/ijms20051101
Leigh, A. D. (1946). Infections of the Nervous System Occurring during an Epidemic of Influenza B. Br. Med. J. 2, 936–938. doi:10.1136/bmj.2.4485.936
Li, C., Fang, J. S., Lian, W. W., Pang, X. C., Liu, A. L., and Du, G. H. (2015). In Vitro Antiviral Effects and 3DQSAR Study of Resveratrol Derivatives as Potent Inhibitors of Influenza H1N1 Neuraminidase. Chem. Biol. Drug Des. 85, 427–438. doi:10.1111/cbdd.12425
Li, G.-H., Ning, Z.-J., Liu, Y.-M., and Li, X.-H. (2017). Neurological Manifestations of Dengue Infection. Front Cel Infect Microbiol 7, 449. doi:10.3389/fcimb.2017.00449
Li, Q., Pang, P., Zheng, K., Sun, L., Wang, J., and Chen, X. (2018). Xin-Jia-Xiang-Ru-Yin Alleviated H1N1-Induced Acute Lung Injury and Inhibited the IFN-γ-Related Regulatory Pathway in Summer Flu. Biomed. Pharmacother. 108, 201–207. doi:10.1016/j.biopha.2018.09.022
Li, R., and Wang, L. (2019). Baicalin Inhibits Influenza Virus A Replication via Activation of Type I IFN Signaling by Reducing miR-146a. Mol. Med. Rep. 20, 5041–5049. doi:10.3892/mmr.2019.10743
Li, S.-W., Yang, T.-C., Lai, C.-C., Huang, S.-H., Liao, J.-M., Wan, L., et al. (2014). Antiviral Activity of Aloe-Emodin against Influenza A Virus via Galectin-3 Up-Regulation. Eur. J. Pharmacol. 738, 125–132. doi:10.1016/j.ejphar.2014.05.028
Lim, H.-J., Nguyen, T. T. H., Kim, N. M., Park, J.-S., Jang, T.-S., and Kim, D. (2017). Inhibitory Effect of Flavonoids against NS2B-NS3 Protease of ZIKA Virus and Their Structure Activity Relationship. Biotechnol. Lett. 39, 415–421. doi:10.1007/s10529-016-2261-6
Lima, C. S., Mottin, M., De Assis, L. R., Mesquita, N., Sousa, B. K. P., Coimbra, L. D., et al. (2021). Flavonoids from Pterogyne Nitens as Zika Virus NS2B-NS3 Protease Inhibitors. Bioorg. Chem. 109, 104719. doi:10.1016/j.bioorg.2021.104719
Lin, C.-J., Lin, H.-J., Chen, T.-H., Hsu, Y.-A., Liu, C.-S., Hwang, G.-Y., et al. (2015). Polygonum Cuspidatum and its Active Components Inhibit Replication of the Influenza Virus through Toll-like Receptor 9-induced Interferon Beta Expression. PLoS One 10, e0117602. doi:10.1371/journal.pone.0117602
Ling, J.-X., Wei, F., Li, N., Li, J.-L., Chen, L.-J., Liu, Y.-Y., et al. (2012). Amelioration of Influenza Virus-Induced Reactive Oxygen Species Formation by Epigallocatechin Gallate Derived from green tea. Acta Pharmacol. Sin 33, 1533–1541. doi:10.1038/aps.2012.80
Liu, H., You, L., Wu, J., Zhao, M., Guo, R., Zhang, H., et al. (2020). Berberine Suppresses Influenza Virus-Triggered NLRP3 Inflammasome Activation in Macrophages by Inducing Mitophagy and Decreasing Mitochondrial ROS. J. Leukoc. Biol. 108, 253–266. doi:10.1002/JLB.3MA0320-358RR
Lo, Y.-L., Liou, G.-G., Lyu, J.-H., Hsiao, M., Hsu, T.-L., and Wong, C.-H. (2016). Dengue Virus Infection Is through a Cooperative Interaction between a Mannose Receptor and CLEC5A on Macrophage as a Multivalent Hetero-Complex. PLoS One 11, e0166474. doi:10.1371/journal.pone.0166474
Loaiza-Cano, V., Monsalve-Escudero, L. M., Martinez-Gutierrez, M., and Sousa, D. P. D. (2021). Antiviral Role of Phenolic Compounds against Dengue Virus: A Review. Biomolecules 11, 11. doi:10.3390/biom11010011
Mabou Tagne, A., Pacchetti, B., Sodergren, M., Cosentino, M., and Marino, F. (2020). Cannabidiol for Viral Diseases: Hype or Hope? Cannabis Cannabinoid Res. 5, 121–131. doi:10.1089/can.2019.0060
Madi, D., Achappa, B., Ramapuram, J. T., Chowta, N., Laxman, M., and Mahalingam, S. (2014). Dengue Encephalitis–A Rare Manifestation of Dengue Fever. Asian Pac. J. Trop. Biomed. 4, S70–S72. doi:10.12980/APJTB.4.2014C1006
Mahanty, S., Hutchinson, K., Agarwal, S., Mcrae, M., Rollin, P. E., and Pulendran, B. (2003). Cutting Edge: Impairment of Dendritic Cells and Adaptive Immunity by Ebola and Lassa Viruses. J. Immunol. 170, 2797–2801. doi:10.4049/jimmunol.170.6.2797
Mahmoodi, M., Amiri, H., Ayoobi, F., Rahmani, M., Taghipour, Z., Ghavamabadi, R. T., et al. (2019). Carvacrol Ameliorates Experimental Autoimmune Encephalomyelitis through Modulating Pro-and Anti-inflammatory Cytokines. Life Sci. 219, 257–263. doi:10.1016/j.lfs.2018.11.051
Manjula, S., and Kumaradhas, P. (2020). Evaluating the Suitability of RNA Intervention Mechanism Exerted by Some Flavonoid Molecules against Dengue Virus MTase RNA Capping Site: a Molecular Docking, Molecular Dynamics Simulation, and Binding Free Energy Study. J. Biomol. Struct. Dyn. 38, 3533–3543. doi:10.1080/07391102.2019.1666744
Martines, R. B., Ng, D. L., Greer, P. W., Rollin, P. E., and Zaki, S. R. (2015). Tissue and Cellular Tropism, Pathology and Pathogenesis of Ebola and Marburg Viruses. J. Pathol. 235, 153–174. doi:10.1002/path.4456
Maruyama, T., Rodriguez, L. L., Jahrling, P. B., Sanchez, A., Khan, A. S., Nichol, S. T., et al. (1999). Ebola Virus Can Be Effectively Neutralized by Antibody Produced in Natural Human Infection. J. Virol. 73, 6024–6030. doi:10.1128/JVI.73.7.6024-6030.1999
Marzi, A., Reinheckel, T., and Feldmann, H. (2012). Cathepsin B & L Are Not Required for Ebola Virus Replication. Plos Negl. Trop. Dis. 6, e1923. doi:10.1371/journal.pntd.0001923
Matsumoto, K., Yamada, H., Takuma, N., Niino, H., and Sagesaka, Y. M. (2011). Effects of green tea Catechins and Theanine on Preventing Influenza Infection Among Healthcare Workers: a Randomized Controlled Trial. BMC Complement. Altern. Med. 11, 15. doi:10.1186/1472-6882-11-15
Mattia, J. G., Vandy, M. J., Chang, J. C., Platt, D. E., Dierberg, K., Bausch, D. G., et al. (2016). Early Clinical Sequelae of Ebola Virus Disease in Sierra Leone: a Cross-Sectional Study. Lancet Infect. Dis. 16, 331–338. doi:10.1016/S1473-3099(15)00489-2
Mcbride, J. M., Lim, J. J., Burgess, T., Deng, R., Derby, M. A., Maia, M., et al. (2017). Phase 2 Randomized Trial of the Safety and Efficacy of MHAA4549A, a Broadly Neutralizing Monoclonal Antibody, in a Human Influenza A Virus challenge Model. Antimicrob. Agents Chemother. 61, e01154–17. doi:10.1128/AAC.01154-17
Meertens, L., Carnec, X., Lecoin, M. P., Ramdasi, R., Guivel-Benhassine, F., Lew, E., et al. (2012). The TIM and TAM Families of Phosphatidylserine Receptors Mediate Dengue Virus Entry. Cell Host Microbe 12, 544–557. doi:10.1016/j.chom.2012.08.009
Mehrbod, P., Hudy, D., Shyntum, D., Markowski, J., Łos, M. J., and Ghavami, S. (2021). Quercetin as a Natural Therapeutic Candidate for the Treatment of Influenza Virus. Biomolecules 11, 10. doi:10.3390/biom11010010
Merino-Ramos, T., De Oya, N. J., Saiz, J.-C., and Martín-Acebes, M. A. (2017). Antiviral Activity of Nordihydroguaiaretic Acid and its Derivative Tetra-O-Methyl Nordihydroguaiaretic Acid against West Nile Virus and Zika Virus. Antimicrob. Agents Chemother. 61, e00376–17. doi:10.1128/AAC.00376-17
Michaelis, M., Geiler, J., Naczk, P., Sithisarn, P., Leutz, A., Doerr, H. W., et al. (2011). Glycyrrhizin Exerts Antioxidative Effects in H5N1 Influenza A Virus-Infected Cells and Inhibits Virus Replication and Pro-inflammatory Gene Expression. PloS one 6, e19705. doi:10.1371/journal.pone.0019705
Mlakar, J., Korva, M., Tul, N., Popović, M., Poljšak-Prijatelj, M., and Mraz, J. (2016). Zika Virus Associated with Microcephaly. N. Engl. J. Med. 374, 951–958. doi:10.1056/NEJMoa1600651
Mobula, L. M., Macdermott, N., Hoggart, C., Brantly, K., Plyler, L., Brown, J., et al. (2018). Clinical Manifestations and Modes of Death Among Ebola Virus Disease Patients, Monrovia, Liberia, 2014. Am. J. Trop. Med. Hyg. 98, 1186–1193. doi:10.4269/ajtmh.17-0090
Mohammadi Pour, P., Fakhri, S., Asgary, S., Farzaei, M. H., and Echeverria, J. (2019). The Signaling Pathways, and Therapeutic Targets of Antiviral Agents: Focusing on the Antiviral Approaches and Clinical Perspectives of Anthocyanins in the Management of Viral Diseases. Front. Pharmacol. 10, 1207. doi:10.3389/fphar.2019.01207
Mohd, A., Zainal, N., Tan, K.-K., and Abubakar, S. (2019). Resveratrol Affects Zika Virus Replication In Vitro. Sci. Rep. 9, 14336. doi:10.1038/s41598-019-50674-3
Morishima, T., Togashi, T., Yokota, S., Okuno, Y., Miyazaki, C., Tashiro, M., et al. (2002). Encephalitis and Encephalopathy Associated with an Influenza Epidemic in Japan. Clin. Infect. Dis. 35, 512–517. doi:10.1086/341407
Mounce, B. C., Cesaro, T., Carrau, L., Vallet, T., and Vignuzzi, M. (2017). Curcumin Inhibits Zika and Chikungunya Virus Infection by Inhibiting Cell Binding. Antivir. Res 142, 148–157. doi:10.1016/j.antiviral.2017.03.014
Munoz, L. S., Garcia, M. A., Gordon-Lipkin, E., Parra, B., and Pardo, C. A. (2018). Emerging Viral Infections and Their Impact on the Global burden of Neurological Disease. Semin. Neurol. 38, 163–175. doi:10.1055/s-0038-1647247
Murthy, J. (2010). Neurological Complications of Dengue Infection. Neurol. India 58, 581–584. doi:10.4103/0028-3886.68654
Mylonaki, E., Harrer, A., Pilz, G., Stalzer, P., Otto, F., Trinka, E., et al. (2020). Neurological Complications Associated with Influenza in Season 2017/18 in Austria- a Retrospective Single center Study. J. Clin. Virol. 127, 104340. doi:10.1016/j.jcv.2020.104340
Naseri, R., Farzaei, F., Fakhri, S., El-Senduny, F. F., Altouhamy, M., Bahramsoltani, R., et al. (2019). Polyphenols for Diabetes Associated Neuropathy: Pharmacological Targets and Clinical Perspective. Daru 27, 781–798. doi:10.1007/s40199-019-00289-w
Newman, R. A., Sastry, K. J., Arav-Boger, R., Cai, H., Matos, R., and Harrod, R. (2020). Antiviral Effects of Oleandrin. J. Exp. Pharmacol. 12, 503–515. doi:10.2147/JEP.S273120
Nezu, J.-I., Tamai, I., Oku, A., Ohashi, R., Yabuuchi, H., Hashimoto, N., et al. (1999). Primary Systemic Carnitine Deficiency Is Caused by Mutations in a Gene Encoding Sodium Ion-dependent Carnitine Transporter. Nat. Genet. 21, 91–94. doi:10.1038/5030
Nile, S. H., Kim, D. H., Nile, A., Park, G. S., Gansukh, E., and Kai, G. (2020). Probing the Effect of Quercetin 3-glucoside from Dianthus Superbus L against Influenza Virus Infection-In Vitro and In Silico Biochemical and Toxicological Screening. Food Chem. Toxicol. 135, 110985. doi:10.1016/j.fct.2019.110985
Nowakowski, T. J., Pollen, A. A., Di Lullo, E., Sandoval-Espinosa, C., Bershteyn, M., and Kriegstein, A. R. (2016). Expression Analysis Highlights AXL as a Candidate Zika Virus Entry Receptor in Neural Stem Cells. Cell Stem Cell 18, 591–596. doi:10.1016/j.stem.2016.03.012
Oo, A., Teoh, B. T., Sam, S. S., Bakar, S. A., and Zandi, K. (2019). Baicalein and Baicalin as Zika Virus Inhibitors. Arch. Virol. 164, 585–593. doi:10.1007/s00705-018-4083-4
Padilla-S, L., Rodríguez, A., Gonzales, M. M., Gallego-G, , J. C., and Castaño-O, J. C. (2014). Inhibitory Effects of Curcumin on Dengue Virus Type 2-infected Cells In Vitro. Arch. Virol. 159, 573–579. doi:10.1007/s00705-013-1849-6
Paksu, M. S., Aslan, K., Kendirli, T., Akyildiz, B. N., Yener, N., Yildizdas, R. D., et al. (2018). Neuroinfluenza: Evaluation of Seasonal Influenza Associated Severe Neurological Complications in Children (A Multicenter Study). Childs Nerv Syst. 34, 335–347. doi:10.1007/s00381-017-3554-3
Palamara, A. T., Nencioni, L., Aquilano, K., De Chiara, G., Hernandez, L., Cozzolino, F., et al. (2005). Inhibition of Influenza A Virus Replication by Resveratrol. J. Infect. Dis. 191, 1719–1729. doi:10.1086/429694
Pan American Health Organization/World Health Organization (PAHO/WHO) (2016). Epidemiological Update: Neurological Syndrome, Congenital Anomalies and Zika Virus Infection. Available at: https://www.paho.org/hq/dmdocuments/2016/2016-jan-17-cha-epi-update-zika-virus.pdf (accesed July 14, 2021).
Panda, P. K., Sharawat, I. K., Bolia, R., and Shrivastava, Y. (2020). Case Report: Dengue Virus–Triggered Parkinsonism in an Adolescent. Am. J. Trop. Med. Hyg. 103, 851–854. doi:10.4269/ajtmh.20-0039
Pang, P., Zheng, K., Wu, S., Xu, H., Deng, L., Shi, Y., et al. (2018). Baicalin Downregulates RLRs Signaling Pathway to Control Influenza a Virus Infection and Improve the Prognosis. Evid. Based Complement. Alternat Med. 2018, 4923062. doi:10.1155/2018/4923062
Parren, P. W., Geisbert, T. W., Maruyama, T., Jahrling, P. B., and Burton, D. R. (2002). Pre-and Postexposure Prophylaxis of Ebola Virus Infection in an Animal Model by Passive Transfer of a Neutralizing Human Antibody. J. Virol. 76, 6408–6412. doi:10.1128/jvi.76.12.6408-6412.2002
Pathak, V. K., and Mohan, M. (2019). A Notorious Vector-Borne Disease: Dengue Fever, its Evolution as Public Health Threat. J. Fam. Med Prim Care 8, 3125–3129. doi:10.4103/jfmpc.jfmpc_716_19
Peng, M., Swarbrick, C. M. D., Chan, K. W.-K., Luo, D., Zhang, W., Lai, X., et al. (2018). Luteolin Escape Mutants of Dengue Virus Map to prM and NS2B and Reveal Viral Plasticity during Maturation. Antivir. Res 154, 87–96. doi:10.1016/j.antiviral.2018.04.013
Peng, M., Watanabe, S., Chan, K. W. K., He, Q., Zhao, Y., Zhang, Z., et al. (2017). Luteolin Restricts Dengue Virus Replication through Inhibition of the Proprotein Convertase Furin. Antivir. Res 143, 176–185. doi:10.1016/j.antiviral.2017.03.026
Pereira, H., Dos Santos, S. P., Amâncio, A., De Oliveira-Szejnfeld, P. S., Flor, E. O., De Sales Tavares, J., et al. (2020). Neurological Outcomes of Congenital Zika Syndrome in Toddlers and Preschoolers: a Case Series. Lancet Child. Adolesc. Health 4, 378–387. doi:10.1016/S2352-4642(20)30041-9
Pettitt, J., Zeitlin, L., Kim, D. H., Working, C., Johnson, J. C., Bohorov, O., et al. (2013). Therapeutic Intervention of Ebola Virus Infection in Rhesus Macaques with the MB-003 Monoclonal Antibody Cocktail. Sci. Transl Med. 5, 199ra113. doi:10.1126/scitranslmed.3006608
Picazo, E., and Giordanetto, F. (2015). Small Molecule Inhibitors of Ebola Virus Infection. Drug Discov. Today 20, 277–286. doi:10.1016/j.drudis.2014.12.010
Poland, G. A., Kennedy, R. B., Ovsyannikova, I. G., Palacios, R., Ho, P. L., and Kalil, J. (2018). Development of Vaccines against Zika Virus. Lancet Infect. Dis. 18, e211–e219. doi:10.1016/S1473-3099(18)30063-X
Prabhat, N., Ray, S., Chakravarty, K., Kathuria, H., Saravana, S., Singh, D., et al. (2020). Atypical Neurological Manifestations of Dengue Fever: a Case Series and Mini Review. Postgrad. Med. J. 96, 759–765. doi:10.1136/postgradmedj-2020-137533
Qiu, X., Audet, J., Wong, G., Pillet, S., Bello, A., Cabral, T., et al. (2012). Successful Treatment of Ebola Virus–Infected Cynomolgus Macaques with Monoclonal Antibodies. Sci. Transl Med. 4, 138ra181. doi:10.1126/scitranslmed.3003876
Qiu, X., Kroeker, A., He, S., Kozak, R., Audet, J., Mbikay, M., et al. (2016). Prophylactic Efficacy of Quercetin 3-β-O-D-Glucoside against Ebola Virus Infection. Antimicrob. Agents Chemother. 60, 5182–5188. doi:10.1128/AAC.00307-16
Qiu, X., Wong, G., Audet, J., Bello, A., Fernando, L., Alimonti, J. B., et al. (2014). Reversion of Advanced Ebola Virus Disease in Nonhuman Primates with ZMapp. Nature 514, 47–53. doi:10.1038/nature13777
Quicke, K. M., Bowen, J. R., Johnson, E. L., Mcdonald, C. E., Ma, H., O’neal, J. T., et al. (2016). Zika Virus Infects Human Placental Macrophages. Cell Host Microbe 20, 83–90. doi:10.1016/j.chom.2016.05.015
Qureshi, A. I., Chughtai, M., Loua, T. O., Pe Kolie, J., Camara, H. F. S., Ishfaq, M. F., et al. (2015). Study of Ebola Virus Disease Survivors in Guinea. Clin. Infect. Dis. 61, 1035–1042. doi:10.1093/cid/civ453
Radzišauskienė, D., Vitkauskaitė, M., Žvinytė, K., and Mameniškienė, R. (2021). Neurological Complications of Pandemic A (H1N1) 2009pdm, Postpandemic A (H1N1) V, and Seasonal Influenza A. Brain Behav. 11, e01916. doi:10.1002/brb3.1916
Rhein, B. A., and Maury, W. J. (2015). Ebola Virus Entry into Host Cells: Identifying Therapeutic Strategies. Curr. Clin. Microbiol. Rep. 2, 115–124. doi:10.1007/s40588-015-0021-3
Richard, A. S., Shim, B.-S., Kwon, Y.-C., Zhang, R., Otsuka, Y., Schmitt, K., et al. (2017). AXL-dependent Infection of Human Fetal Endothelial Cells Distinguishes Zika Virus from Other Pathogenic Flaviviruses. Proc. Natl. Acad. Sci. U S A. 114, 2024–2029. doi:10.1073/pnas.1620558114
Rojas, M., Monsalve, D. M., Pacheco, Y., Acosta-Ampudia, Y., Ramírez-Santana, C., Ansari, A. A., et al. (2020). Ebola Virus Disease: An Emerging and Re-emerging Viral Threat. J. Autoimmun. 106, 102375. doi:10.1016/j.jaut.2019.102375
Rowe, A. K., Bertolli, J., Khan, A. S., Mukunu, R., Muyembe-Tamfum, J., Bressler, D., et al. (1999). Clinical, Virologic, and Immunologic Follow-Up of Convalescent Ebola Hemorrhagic Fever Patients and Their Household Contacts, Kikwit, Democratic Republic of the Congo. J. Infect. Dis. 179, S28–S35. doi:10.1086/514318
Roy, A., Lim, L., Srivastava, S., Lu, Y., and Song, J. (2017). Solution Conformations of Zika NS2B-NS3pro and its Inhibition by Natural Products from Edible Plants. PLoS One 12, e0180632. doi:10.1371/journal.pone.0180632
Russo, F. B., Jungmann, P., and Beltrão-Braga, P. C. B. (2017). Zika Infection and the Development of Neurological Defects. Cell Microbiol 19, e12744. doi:10.1111/cmi.12744
Ryabchikova, E. I., and Price, B. B. (2004). Ebola and Marburg Viruses: A View of Infection Using Electron Microscopy. Columbus, Ohio, USA: Battelle Press.
Sadati, S. M., Gheibi, N., Ranjbar, S., and Hashemzadeh, M. S. (2019). Docking Study of Flavonoid Derivatives as Potent Inhibitors of Influenza H1N1 Virus Neuraminidase. Biomed. Rep. 10, 33–38. doi:10.3892/br.2018.117
Schlindwein, M. a. M., Bandeira, I. P., Breis, L. C., Rickli, J. M., Demore, C. C., Chara, B. S., et al. (2020). Dengue Fever and Neurology: Well beyond Hemorrhage and Strokes. Preprints 2020, 2020050421. doi:10.20944/preprints202005.0421.v1
Schnittler, H.-J., and Feldmann, H. (1998). Marburg and Ebola Hemorrhagic Fevers: Does the Primary Course of Infection Depend on the Accessibility of Organ-specific Macrophages? Clin. Infect. Dis. 27, 404–406. doi:10.1086/517704
Schornberg, K., Matsuyama, S., Kabsch, K., Delos, S., Bouton, A., and White, J. (2006). Role of Endosomal Cathepsins in Entry Mediated by the Ebola Virus Glycoprotein. J. Virol. 80, 4174–4178. doi:10.1128/JVI.80.8.4174-4178.2006
Scorza, F. B., and Pardi, N. (2018). New Kids on the Block: RNA-Based Influenza Virus Vaccines. Vaccines 6, 20. doi:10.3390/vaccines6020020
Sehgal, M., Ladd, H. J., and Totapally, B. (2020). Trends in Epidemiology and Microbiology of Severe Sepsis and Septic Shock in Children. Hosp. Pediatr. 10, 1021–1030. doi:10.1542/hpeds.2020-0174
Serman, T. M., and Gack, M. U. (2019). Evasion of Innate and Intrinsic Antiviral Pathways by the Zika Virus. Viruses 11, 970. doi:10.3390/v11100970
Setlur, A. S., Naik, S. Y., and Skariyachan, S. (2017). Herbal Lead as Ideal Bioactive Compounds against Probable Drug Targets of Ebola Virus in Comparison with Known Chemical Analogue: A Computational Drug Discovery Perspective. Interdiscip. Sci. 9, 254–277. doi:10.1007/s12539-016-0149-8
Shah, P. P., Wang, T., Kaletsky, R. L., Myers, M. C., Purvis, J. E., Jing, H., et al. (2010). A Small-Molecule Oxocarbazate Inhibitor of Human Cathepsin L Blocks Severe Acute Respiratory Syndrome and Ebola Pseudotype Virus Infection into Human Embryonic Kidney 293T Cells. Mol. Pharmacol. 78, 319–324. doi:10.1124/mol.110.064261
Sharma, V., Sharma, M., Dhull, D., Sharma, Y., Kaushik, S., and Kaushik, S. (2020). Zika Virus: an Emerging challenge to Public Health Worldwide. Can. J. Microbiol. 66, 87–98. doi:10.1139/cjm-2019-0331
Sithisarn, P., Michaelis, M., Schubert-Zsilavecz, M., and Cinatl, J. (2013). Differential Antiviral and Anti-inflammatory Mechanisms of the Flavonoids Biochanin A and Baicalein in H5N1 Influenza A Virus-Infected Cells. Antivir. Res 97, 41–48. doi:10.1016/j.antiviral.2012.10.004
Solbrig, M. V., and Perng, G.-C. (2015). Current Neurological Observations and Complications of Dengue Virus Infection. Curr. Neurol. Neurosci. Rep. 15, 29. doi:10.1007/s11910-015-0550-4
Sordillo, P. P., and Helson, L. (2015). Curcumin Suppression of Cytokine Release and Cytokine Storm. A Potential Therapy for Patients with Ebola and Other Severe Viral Infections. In Vivo. 29, 1–4.
Stouffer, A. L., Acharya, R., Salom, D., Levine, A. S., Di Costanzo, L., Soto, C. S., et al. (2008). Structural Basis for the Function and Inhibition of an Influenza Virus Proton Channel. Nature 451, 596–599. doi:10.1038/nature06528
Suroengrit, A., Yuttithamnon, W., Srivarangkul, P., Pankaew, S., Kingkaew, K., Chavasiri, W., et al. (2017). Halogenated Chrysins Inhibit Dengue and Zika Virus Infectivity. Sci. Rep. 7, 13696. doi:10.1038/s41598-017-14121-5
Swaminathan, K., Müller, P., and Downard, K. M. (2014). Substituent Effects on the Binding of Natural Product Anthocyanidin Inhibitors to Influenza Neuraminidase with Mass Spectrometry. Anal. Chim. Acta 828, 61–69. doi:10.1016/j.aca.2014.04.021
Takada, A., Robison, C., Goto, H., Sanchez, A., Murti, K. G., Whitt, M. A., et al. (1997). A System for Functional Analysis of Ebola Virus Glycoprotein. Proc. Natl. Acad. Sci. U S A. 94, 14764–14769. doi:10.1073/pnas.94.26.14764
Takia, L., Saini, L., Keshavan, S., Angurana, S. K., Nallasamy, K., Suthar, R., et al. (2020). Neurological Manifestations of Influenza A (H1N1): Clinical Features, Intensive Care Needs, and Outcome. Indian J. Pediatr. 87, 803–809. doi:10.1007/s12098-020-03297-w
Tang, H., Hammack, C., Ogden, S. C., Wen, Z., Qian, X., Li, Y., et al. (2016). Zika Virus Infects Human Cortical Neural Progenitors and Attenuates Their Growth. Cell Stem Cell 18, 587–590. doi:10.1016/j.stem.2016.02.016
Togashi, T. (1999). IL-6 and TNF-α in Cerebrospinal Fluid from Infantile Encephalitis-Encephalopathy Patients during Influenza Seasons. J. Jpn. Pediatr. Soe 103, 16–19.
Toots, M., and Plemper, R. K. (2020). Next-generation Direct-Acting Influenza Therapeutics. Transl Res. 220, 33–42. doi:10.1016/j.trsl.2020.01.005
Tsai, F.-J., Lin, C.-W., Lai, C.-C., Lan, Y.-C., Lai, C.-H., Hung, C.-H., et al. (2011). Kaempferol Inhibits Enterovirus 71 Replication and Internal Ribosome Entry Site (IRES) Activity through FUBP and HNRP Proteins. Food Chem. 128, 312–322. doi:10.1016/j.foodchem.2011.03.022
Tsibane, T., Ekiert, D. C., Krause, J. C., Martinez, O., Crowe, J. E., Wilson, I. A., et al. (2012). Influenza Human Monoclonal Antibody 1F1 Interacts with Three Major Antigenic Sites and Residues Mediating Human Receptor Specificity in H1N1 Viruses. Plos Pathog. 8, e1003067. doi:10.1371/journal.ppat.1003067
Tun, M. M. N., Muthugala, R., Nabeshima, T., Rajamanthri, L., Jayawardana, D., Attanayake, S., et al. (2020). Unusual, Neurological and Severe Dengue Manifestations during the Outbreak in Sri Lanka, 2017. J. Clin. Virol. 125, 104304. doi:10.1016/j.jcv.2020.104304
Utsunomiya, T., Kobayashi, M., Pollard, R. B., and Suzuki, F. (1997). Glycyrrhizin, an Active Component of Licorice Roots, Reduces Morbidity and Mortality of Mice Infected with Lethal Doses of Influenza Virus. Antimicrob. Agents Chemother. 41, 551–556. doi:10.1128/AAC.41.3.551
Vaidya, B., Cho, S.-Y., Oh, K.-S., Kim, S. H., Kim, Y. O., Jeong, E.-H., et al. (2016). Effectiveness of Periodic Treatment of Quercetin against Influenza A Virus H1N1 through Modulation of Protein Expression. J. Agric. Food Chem. 64, 4416–4425. doi:10.1021/acs.jafc.6b00148
Van Den Pol, A. N., Mao, G., Yang, Y., Ornaghi, S., and Davis, J. N. (2017). Zika Virus Targeting in the Developing Brain. J. Neurosci. 37, 2161–2175. doi:10.1523/JNEUROSCI.3124-16.2017
Vannice, K. S., Wilder-Smith, A., Barrett, A. D., Carrijo, K., Cavaleri, M., De Silva, A., et al. (2018). Clinical Development and Regulatory Points for Consideration for Second-Generation Live Attenuated Dengue Vaccines. Vaccine 36, 3411–3417. doi:10.1016/j.vaccine.2018.02.062
Vázquez-Calvo, Á., Jiménez De Oya, N., Martín-Acebes, M. A., Garcia-Moruno, E., and Saiz, J.-C. (2017). Antiviral Properties of the Natural Polyphenols Delphinidin and Epigallocatechin Gallate against the Flaviviruses West Nile Virus, Zika Virus, and Dengue Virus. Front. Microbiol. 8, 1314. doi:10.3389/fmicb.2017.01314
Ventura, C. V., and Ventura, L. O. (2018). Ophthalmologic Manifestations Associated with Zika Virus Infection. Pediatrics 141, S161–S166. doi:10.1542/peds.2017-2038E
Wahl-Jensen, V., Kurz, S. K., Hazelton, P. R., Schnittler, H.-J., Ströher, U., Burton, D. R., et al. (2005). Role of Ebola Virus Secreted Glycoproteins and Virus-like Particles in Activation of Human Macrophages. J. Virol. 79, 2413–2419. doi:10.1128/JVI.79.4.2413-2419.2005
Wan, Q., Wang, H., Han, X., Lin, Y., Yang, Y., Gu, L., et al. (2014). Baicalin Inhibits TLR7/MYD88 Signaling Pathway Activation to Suppress Lung Inflammation in Mice Infected with Influenza A Virus. Biomed. Rep. 2, 437–441. doi:10.3892/br.2014.253
Wang, J., Qiu, J. X., Soto, C., and Degrado, W. F. (2011). Structural and Dynamic Mechanisms for the Function and Inhibition of the M2 Proton Channel from Influenza A Virus. Curr. Opin. Struct. Biol. 21, 68–80. doi:10.1016/j.sbi.2010.12.002
Wang, L., Liang, R., Gao, Y., Li, Y., Deng, X., Xiang, R., et al. (2019). Development of Small-Molecule Inhibitors against Zika Virus Infection. Front. Microbiol. 10, 2725. doi:10.3389/fmicb.2019.02725
West, T. E., and Von Saint André-Von Arnim, A. (2014). Clinical Presentation and Management of Severe Ebola Virus Disease. Ann. Am. Thorac. Soc. 11, 1341–1350. doi:10.1513/AnnalsATS.201410-481PS
Wong, G., He, S., Siragam, V., Bi, Y., Mbikay, M., Chretien, M., et al. (2017). Antiviral Activity of Quercetin-3-β-O-D-Glucoside against Zika Virus Infection. Virol. Sin 32, 545–547. doi:10.1007/s12250-017-4057-9
Wong, G., Kobinger, G. P., and Qiu, X. (2014). Characterization of Host Immune Responses in Ebola Virus Infections. Expert Rev. Clin. Immunol. 10, 781–790. doi:10.1586/1744666X.2014.908705
World Health Organization (2016). Current Zika Product Pipeline. Geneva (SW): World Health Organization. Available at: http://www.who.int/blueprint/priority-diseases/key-action/zika-rd-pipeline.pdf (accessed July 14, 2021).
Wu, W., Li, R., Li, X., He, J., Jiang, S., Liu, S., et al. (2016). Quercetin as an Antiviral Agent Inhibits Influenza A Virus (IAV) Entry. Viruses 8, 6. doi:10.3390/v8010006
Xiao, Y., Chen, H., Song, C., Zeng, X., Zheng, Q., Zhang, Y., et al. (2015). Pharmacological Activities and Structure-Modification of Resveratrol Analogues. Pharmazie. 70, 765–771.
Xu, G., Dou, J., Zhang, L., Guo, Q., and Zhou, C. (2010). Inhibitory Effects of Baicalein on the Influenza Virus In Vivo Is Determined by Baicalin in the Serum. Biol. Pharm. Bull. 33, 238–243. doi:10.1248/bpb.33.238
Xu, M.-J., Liu, B.-J., Wang, C.-L., Wang, G.-H., Tian, Y., Wang, S.-H., et al. (2017). Epigallocatechin-3-gallate Inhibits TLR4 Signaling through the 67-kDa Laminin Receptor and Effectively Alleviates Acute Lung Injury Induced by H9N2 Swine Influenza Virus. Int. Immunopharmacol 52, 24–33. doi:10.1016/j.intimp.2017.08.023
Xu, X., Miao, J., Shao, Q., Gao, Y., and Hong, L. (2020). Apigenin Suppresses Influenza A Virus-Induced RIG-I Activation and Viral Replication. J. Med. Virol. 92, 3057–3066. doi:10.1002/jmv.26403
Yadav, R., Selvaraj, C., Aarthy, M., Kumar, P., Kumar, A., Singh, S. K., et al. (2021). Investigating into the Molecular Interactions of Flavonoids Targeting NS2B-NS3 Protease from ZIKA Virus through In-Silico Approaches. J. Biomol. Struct. Dyn. 39, 272–284. doi:10.1080/07391102.2019.1709546
Yang, Z.-F., Bai, L.-P., Huang, W.-B., Li, X.-Z., Zhao, S.-S., Zhong, N.-S., et al. (2014). Comparison of In Vitro Antiviral Activity of tea Polyphenols against Influenza A and B Viruses and Structure–Activity Relationship Analysis. Fitoterapia 93, 47–53. doi:10.1016/j.fitote.2013.12.011
Yokota, S., Imagawa, T., Miyamae, T., Ito, S. I., Nakajima, S., Nezu, A., et al. (2000). Hypothetical Pathophysiology of Acute Encephalopathy and Encephalitis Related to Influenza Virus Infection and Hypothermia Therapy. Pediatr. Int. L 42, 197–203. doi:10.1046/j.1442-200x.2000.01204.x
Younan, P., Iampietro, M., Nishida, A., Ramanathan, P., Santos, R. I., Dutta, M., et al. (2017). Ebola Virus Binding to Tim-1 on T Lymphocytes Induces a Cytokine Storm. mBio 8, e00845–17. doi:10.1128/mBio.00845-17
Younan, P., Santos, R. I., Ramanathan, P., Iampietro, M., Nishida, A., Dutta, M., et al. (2019). Ebola Virus-Mediated T-Lymphocyte Depletion Is the Result of an Abortive Infection. Plos Pathog. 15, e1008068. doi:10.1371/journal.ppat.1008068
Yu, W.-Y., Li, L., Wu, F., Zhang, H.-H., Fang, J., Zhong, Y.-S., et al. (2020). Moslea Herba Flavonoids Alleviated Influenza A Virus-Induced Pulmonary Endothelial Barrier Disruption via Suppressing NOX4/NF-Κb/mlck Pathway. J. Ethnopharmacol 253, 112641. doi:10.1016/j.jep.2020.112641
Zandi, K., Teoh, B.-T., Sam, S.-S., Wong, P.-F., Mustafa, M. R., and Abubakar, S. (2011). Antiviral Activity of Four Types of Bioflavonoid against Dengue Virus Type-2. Virol. J. 8, 560. doi:10.1186/1743-422X-8-560
Zangrillo, A., Biondi-Zoccai, G., Landoni, G., Frati, G., Patroniti, N., Pesenti, A., et al. (2013). Extracorporeal Membrane Oxygenation (ECMO) in Patients with H1N1 Influenza Infection: a Systematic Review and Meta-Analysis Including 8 Studies and 266 Patients Receiving ECMO. Crit. Care 17, R30. doi:10.1186/cc12512
Zhang, F., Hammack, C., Ogden, S. C., Cheng, Y., Lee, E. M., Wen, Z., et al. (2016). Molecular Signatures Associated with ZIKV Exposure in Human Cortical Neural Progenitors. Nucleic Acids Res. 44, 8610–8620. doi:10.1093/nar/gkw765
Zhang, R., Ai, X., Duan, Y., Xue, M., He, W., Wang, C., et al. (2017). Kaempferol Ameliorates H9N2 Swine Influenza Virus-Induced Acute Lung Injury by Inactivation of TLR4/MyD88-Mediated NF-Κb and MAPK Signaling Pathways. Biomed. Pharmacother. 89, 660–672. doi:10.1016/j.biopha.2017.02.081
Zhang, Y., Yao, J., Qi, X., Liu, X., Lu, X., and Feng, G. (2017). Geniposide Demonstrates Anti-inflammatory and Antiviral Activity against Pandemic A/Jiangsu/1/2009 (H1N1) Influenza Virus Infection In Vitro and In Vivo. Antivir. Ther. 22, 599–611. doi:10.3851/IMP3152
Zheng, K., Wu, S.-Z., Lv, Y.-W., Pang, P., Deng, L., Xu, H.-C., et al. (2021). Carvacrol Inhibits the Excessive Immune Response Induced by Influenza Virus A via Suppressing Viral Replication and TLR/RLR Pattern Recognition. J. Ethnopharmacol 268, 113555. doi:10.1016/j.jep.2020.113555
Zhou, Y., Vedantham, P., Lu, K., Agudelo, J., Carrion, R., Nunneley, J. W., et al. (2015). Protease Inhibitors Targeting Coronavirus and Filovirus Entry. Antivir. Res 116, 76–84. doi:10.1016/j.antiviral.2015.01.011
Keywords: influenza, dengue, Zika virus, ebola, neurological manifestation, natural products, signaling pathway, pharmacology
Citation: Fakhri S, Mohammadi Pour P, Piri S, Farzaei MH and Echeverría J (2021) Modulating Neurological Complications of Emerging Infectious Diseases: Mechanistic Approaches to Candidate Phytochemicals. Front. Pharmacol. 12:742146. doi: 10.3389/fphar.2021.742146
Received: 15 July 2021; Accepted: 23 September 2021;
Published: 26 October 2021.
Edited by:
Celso Alves, Polytechnic of Leiria, PortugalReviewed by:
Juan Carlos Sepúlveda-Arias, Technological University of Pereira, ColombiaFernanda Majolo, Universidade do Vale do Taquari—Univates, Brazil
Copyright © 2021 Fakhri, Mohammadi Pour, Piri, Farzaei and Echeverría. This is an open-access article distributed under the terms of the Creative Commons Attribution License (CC BY). The use, distribution or reproduction in other forums is permitted, provided the original author(s) and the copyright owner(s) are credited and that the original publication in this journal is cited, in accordance with accepted academic practice. No use, distribution or reproduction is permitted which does not comply with these terms.
*Correspondence: Mohammad Hosein Farzaei, bWguZmFyemFlaUBnbWFpbC5jb20=; Javier Echeverría, amF2aWVyLmVjaGV2ZXJyaWFtQHVzYWNoLmNs