- 1Department of Chemistry, School of Forensic Medicine, China Medical University, Shenyang, China
- 2Key Laboratory of Forensic Bio-evidence Sciences, Shenyang, China
- 3China Medical University Center of Forensic Investigation, Shenyang, China
- 4China Medical University-The Queen’s University of Belfast Joint College, China Medical University, Shenyang, China
- 5First Department of Clinical Medicine, China Medical University, Shenyang, China
- 6Physical College, Liaoning University, Shenyang, China
- 7Second Department of Clinical Medicine, China Medical University, Shenyang, China
- 8Department of Biochemistry and Molecular Biology, China Medical University, Shenyang, China
Ferroptosis, a new iron- and reactive oxygen species–dependent form of regulated cell death, has attracted much attention in the therapy of various types of tumors. With the development of nanomaterials, more and more evidence shows the potential of ferroptosis combined with nanomaterials for cancer therapy. Recently, there has been much effort to develop ferroptosis-inducing nanomedicine, specially combined with the conventional or emerging therapy. Therefore, it is necessary to outline the previous work on ferroptosis-inducing nanomedicine and clarify directions for improvement and application to cancer therapy in the future. In this review, we will comprehensively focus on the strategies of cancer therapy based on ferroptosis-inducing nanomedicine currently, elaborate on the design ideas of synthesis, analyze the advantages and limitations, and finally look forward to the future perspective on the emerging field.
1 Introduction
Cancer is a public health problem worldwide, which cannot be ignored currently, with the incidence gradually increasing year by year. As one of the deadliest diseases in the world, its prevalence has been more than 10 million mortalities annually (Siegel et al., 2021). Current cancer conventional treatments include surgery, radiotherapy, and chemotherapy which have various shortcomings affecting the effective treatment (Johnstone et al., 2002; Esposito et al., 2019; Poon et al., 2021). With the deep understanding of precision medicine recently, targeted therapy and immunotherapy have great progress in cancer treatment (Pham et al., 2018; Adams et al., 2019; Chen et al., 2020a; Rana and Bhatnagar, 2021). However, due to off-target effect, potential toxicity, and drug delivery barriers, these emerging treatment methods fell short of expectation and do not have wide clinical applications.
Ferroptosis, as a form of iron-dependent regulatory cell death, has played an important role in tumor suppression and treatment (Xu et al., 2021a). Targeting ferroptosis has become a promising tumor treatment strategy. In 2001, a unique regulated cell death form by the oxidative stress of nerve cells had been found (Tan et al., 2001). It was first put forward to the conception of ferroptosis by Stockwell in 2012 (Dixon et al., 2012). Different from existing forms of cell death such as apoptosis, autophagy, necrosis, and pyrolysis, the process of ferroptosis mainly includes the iron-dependent Fenton reaction and lipid peroxidation–producing ROS (reactive oxygen species) (Lu et al., 2018; Hassannia et al., 2019; Mou et al., 2019). On the one hand, the iron (III) in the ferritin complex can enter the cell through the glycoprotein transferrin and its carrier protein transferrin receptor (Xu et al., 2021b). Imported iron is reduced and dissociated from the complex to form iron (II). Increased iron promotes the Fenton reaction between iron and hydrogen peroxide (H2O2), which in turn generates ROS (Liu et al., 2021a; Vitalakumar et al., 2021; Xie and Guo, 2021). On the other hand, the polyunsaturated fatty acid (PUFA-LPs-OH) in the cell membrane can be oxidized by ROS and lipoxygenase to form lipid peroxidation (PUFA-LPs-OOH), which generates toxic lipid-free radicals resulting in cell death (Xie et al., 2016). The toxic PUFA-LPs-OOH can be reduced to nontoxic PUFA-LPs-OH by glutathione peroxidase 4 (GPX4). On the contrary, glutathione (GSH) can be oxidized to oxidized GSH (GSSG). Therefore, GPX4 is the key regulator for ferroptosis (Seiler et al., 2008; Seibt et al., 2019; Song et al., 2020). The synthesis of GSH in the cell requires the cystine (Cys)/glutamic acid (Glu) transport system with different directions to transfer Cys into the cell. In this process, system Xc-, including SLC7A11 and SLC3A2, plays an important role (Hong et al., 2021; Liang and Sun, 2021; Ma et al., 2021; Ortiz-Rodriguez et al., 2021). Therefore, according to the mechanism of ferroptosis mentioned above, regulation of the Fenton reaction and the inhibiting activity of the GPX4 enzyme are the two most effective strategies for inducing ferroptosis in tumor cells.
With the regulation mechanisms and signaling pathways of ferroptosis clarified, ferroptosis has attracted increasing attention in cancer therapy over the years (Xu et al., 2019; Nguyen et al., 2020; Xu et al., 2021c). Some small molecule ferroptosis inducers have the potential to become drugs on the treatment of cancer (Xu et al., 2021b). These drugs mainly include system Xc-inhibitors (erastins, sulfasalazine, and sorafenib) and GPX4 inhibitors (RSL3 and altretamine), inducing GPX4 degradation (FIN56) and GSH depletion (buthionine sulfoximine and DPI2) (Lachaier et al., 2014; Yang et al., 2014; Woo et al., 2015; Mai et al., 2017; Gaschler et al., 2018; Wang et al., 2019; Wang et al., 2021a; Cheng et al., 2021; Zhuang et al., 2021). Although some of them have been clinically approved, most of them are in the research stage due to poor solubility, non-specific distribution, and unpredictable side effects. Notably, the rapid development of nanotechnology provides more possibilities for the application of ferroptosis in tumor treatment (Asghari et al., 2019; He et al., 2019; Matos et al., 2019). Because of its unique structure and properties, as carriers, nanomaterials are not only made up for the limitation of traditional drugs but also have introduced new specific features to produce synergy with small molecule drugs, such as generation of ROS or depletion of GSH. Moreover, the nanomaterials even can be the responder as energy synergy therapy.
Due to the potential of ferroptosis in cancer therapy and advantages of nanotechnology in application in the medical field, there are many opportunities and challenges for combination between target ferroptosis and nanotechnology for cancer therapy (Liang et al., 2019). Therefore, it is necessary to summarize the latest work and progress in ferroptosis-inducing nanomedicine for cancer therapy. Meanwhile, the diversity of the biological system and the complexity of clinical application give both challenges and opportunities for further development of ferroptosis-inducing nanomedicine for cancer therapy. Therefore, it is timely to elaborate on the latest advances in this field. According to the mechanism of ferroptosis, from accelerating the Fenton reaction, inhibiting the activity of GPX4, exogenous delivery of lipid peroxides, and combination with conventional therapy, this progress report focused on recent advances of the construction of ferroptosis-inducing nanomedicine and application in cancer therapy.
2 Emerging Nanomedicine-Inducing Ferroptosis for Cancer Therapy
2.1 Inducing Ferroptosis by Accelerating the Fenton Reaction
The Fenton reaction was first described by H. J. H. Fenton in 1894 (Hirschhorn and Stockwell, 2019), whose reaction equation is described as follows (Li et al., 2021a; Wang et al., 2021b) (Yang et al., 2021a):
In tumor cells, H2O2 released by the mitochondria react with endogenous Fe2+/3+ to generate highly toxic hydroxyl radicals and result in tumor cell death via inducing ferroptosis. According to the mechanism of the Fenton reaction, various ferroptosis-inducing nanomedicines for cancer therapy have been developed (Qian et al., 2019). Among them, the design of high-performance nanocatalysts or directly increasing the concentration of reactants to accelerate the Fenton reaction is the most effective strategy.
2.1.1 Accelerating the Fenton Reaction by High-Performance Nanocatalysts
The Fenton reaction is the most direct way to induce ferroptosis. As the important factor to accelerate the reaction, several nanocatalysts based on the Fenton reaction have been developed for cancer treatment (Meng et al., 2020; Wang et al., 2020; Wang et al., 2021c; Kim et al., 2021).
Furthermore, Shi et al. synthesized a new type of single-atom Fe nanocatalyst, in which Fe atoms are isolated in nitrogen-doped carbon, and PEGylation of the outer layer of the catalyst can enhance structural stability and effectively nanocatalyze the Fenton reaction for tumor treatment (Huo et al., 2019). The single-atom Fe catalyst with high catalytic performance would be activated by the weak acidic microenvironment of the tumor and effectively induce the Fenton reaction in the tumor site, which would generate a large amount of toxic hydroxyl radicals (Tian et al., 2021). According to the mechanism of the Fenton reaction, on the one hand, radicals catalyzed by the single-atom Fe nanocatalyst can induce cell apoptosis, and the accumulation of lipid peroxides can lead to ferroptosis of tumor cells as well. The synergistic effect of the catalyst has an impressive tumor suppression outcome. Meanwhile, its good biodegradability and biocompatibility show the potential for application in vivo.
Li et al. (2021b) developed tannic acid (TA) and Fe2+ coated on zeolite imidazole ester skeleton-8 (ZIF-8) self-assembly, which was used as the carrier to encapsulate artemisinin (ART) (Figure 1). In nanomedicine, ART would catalyze the degradation of ferritin via independent autophagy-lysosome pathways, which could increase the amounts of Fe2+ in cells and induce ferroptosis (Chen et al., 2020b). As a carrier, ZIF-8 not only had good biocompatibility but also pH-responsive ability. Drug release experiments showed that ART was only released in pH = 5.0 after 10 h to indicate its tumor-targeted release performance. Meanwhile, increased ROS in the cell, accompanied with decreasing GSH and GPX4, can induce markedly enhanced ferroptosis. The nanomedicine had been demonstrated a better ability of human breast cancer model suppression in vitro and in vivo. The pH-responsive ability of ZIF-8 has already increased the efficacy of artemisinin; if the targets are being introduced into the ZIF-8 shell, it is hopeful to achieve clinical application.
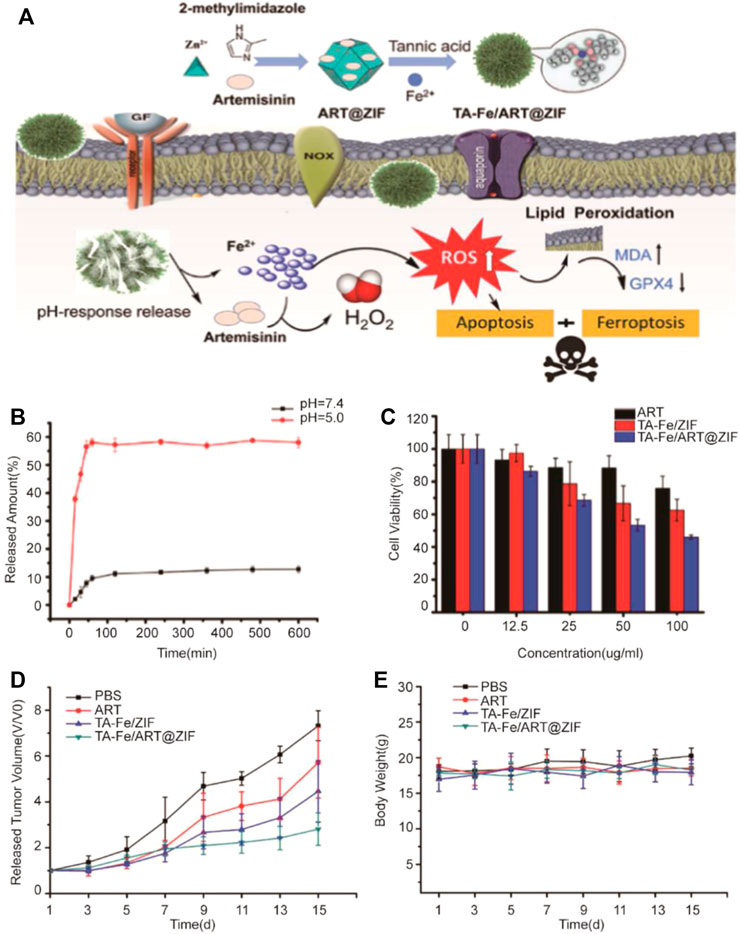
FIGURE 1. (A) Illustration of preparation of nanoparticles and the application in tumor cells; (B) Releasing curve of ART from TA-Fe/ART@ZIF nanoparticles in vitro at pH 7.4 and 5.0; (C) Cytotoxicity of ART, TA-Fe/ZIF, and TA-Fe/ART@ZIF; (D) Relative tumor volume after treated with PBS, ART, TA-Fe/ZIF, and TA-Fe/ART@ZIF nanoparticles; (E) Relative mouse body weight of various groups. (Adapted from Ref. 54 with permission. Copyright © 2021 Nanoscale Research Letters) ART: artemisinin; TA: tannic acid; ZIF: zeolitic imidazolate framework.
2.1.2 Accelerating the Fenton Reaction by Increasing Concentration of Reactants
Shi et al. synthesized amorphous iron nanoparticles (AFeNPs) with suitable particle size and surface properties (Zhang et al., 2016). The AFeNPs can target the mild acidity environment of the tumor and kill the cancer cell by inducing a Fenton reaction overproducing H2O2 in the tumor: the ferrous ion released by the AFeNPs in the tumor, reacting with H2O2 leading to hydroxyl radical generation. The endogenous hydroxyl radicals generated by AFeNPs enabled to kill cancer cells specifically (Tang et al., 2021).
Ji et al. developed the special metal-organic framework (MOF) consisting of FeAc and BDC-NH2. MOF was coated with HA to enhance its biostability. With Fe2+ delivered into breast cancer cells, the Fenton reaction can be triggered and excessive ROS-inducing cell apoptosis is produced (Xu et al., 2020) (Figure 2). The nanomedicine with excellent stability and pH-responsive ability can target tumors due to its acidic microenvironment of cancer and release Fe2+ to tumor cells. The Fenton reaction was induced by excessive Fe2+ to inhibit tumor growth due to ferroptosis. Fe2+-based MOF proved better biocompatibility and inhibitory effects in in vivo experiments.
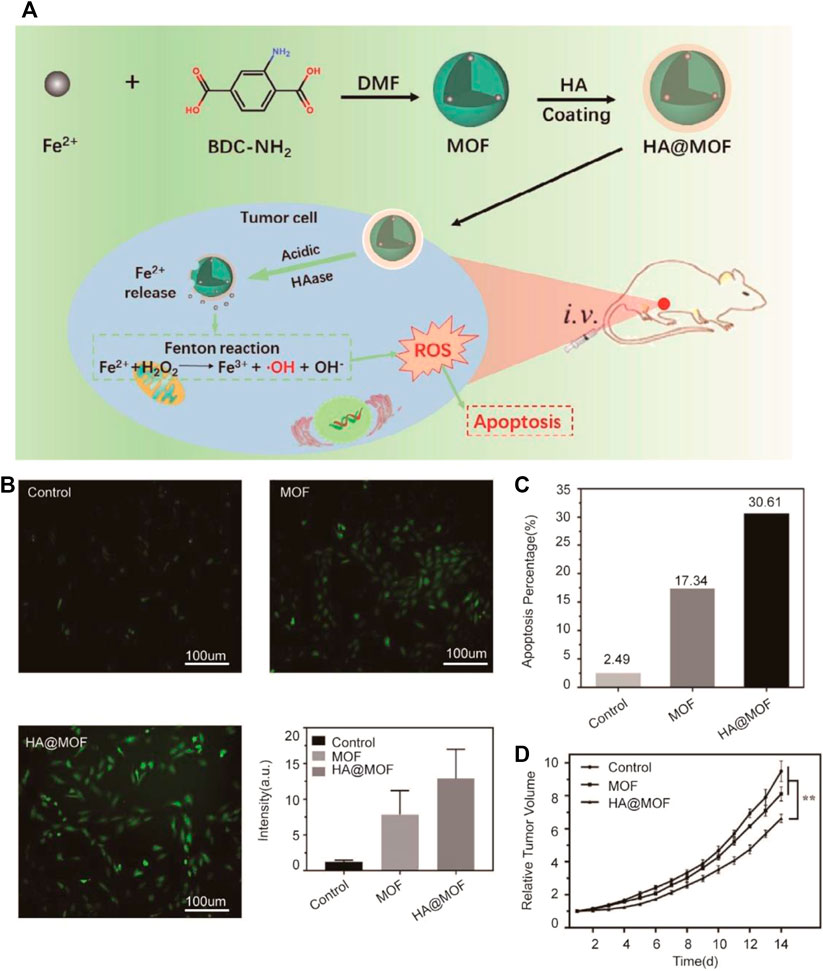
FIGURE 2. (A) Illustration of the synthesis process and mechanism of nanoparticles for tumor therapy. BDC-NH2: 2-aminoterephthalic acid; DMF: N, N-dimethylformamide; MOF: metal-organic framework; HA: hyaluronic acid; (B) Graphs show ROS levels of 4T1 cells after treated with the MOF and HA@MOF for 48 h; untreated 4T1 cells were set as control. A quantitative flow cytometry result was also demonstrated. Scale bar = 100 μm; (C) Quantitative result of 4T1 cell apoptosis after differently treated; (D) Plasma Fe2+ concentrations of SD rats injected with MOF and HA@MOF within 12 h (Adapted from Ref. 58 with permission. Copyright © 2020 Journal of Materials Chemistry B).
Furthermore, increasing the concentration of Fe2+/Fe3+ and H2O2 at the same time is a more effective strategy to accelerate the Fenton reaction. Li and Tang et al. synthesized a Fe3+ metal-organic framework (MOF) based on decorative glucose oxidase (GOx), which was coated with the cancer cell membrane as a cascade nanoreactor for synergistic ferroptosis-starvation anticancer therapy (Wan et al., 2020) (Figure 3). Specifically, NMIL-100, a kind of iron-based MOF, was used as the source of iron to induce ferroptosis and a carrier to load GOx. Furthermore, NMIL-100@GOx was coated by the tumor cell membrane to obtain a nanoreactor. In tumor cells, glucose can be catalyzed by GOx to produce excessive H2O2 for cancer treatment based on ferroptosis, and the glucose reduction caused by GOx, as starvation therapy for tumors, can have a synergistic effect to further inhibit tumors. In mechanism, a large amount of GSH reduced Fe3+ from the nanoreactor to cause the collapse of the MOF structure and release Fe2+ at the tumor site. Then, H2O2 generated by the oxidation of glucose catalyzed by GOx reacted with Fe2+ to produce hydroxyl radicals to promote ferroptosis-inducing cancer treatment. The nanoreactor loading necessary reactants for the Fenton reaction exhibited a better ability of tumor suppression in vivo.
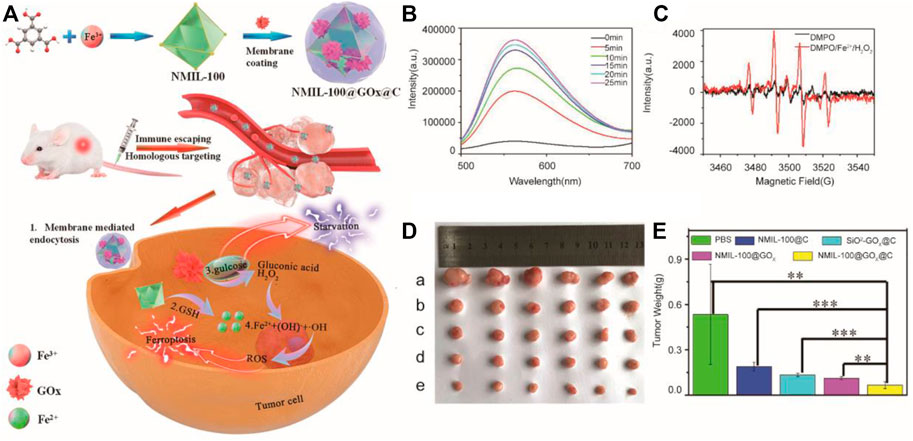
FIGURE 3. (A) Illustration of the synthesis process and mechanism of nanoparticles for tumor therapy; (B) Fluorescence intensity of ER-H2O2 in the NMIL-100@GOx@C solution at different time after addition of glucose; (C) ESR analysis of •OH production using DMPO as the spin trapping agent; (D) Picture of tumors dissected on the 14th day after different treatments (a: PBS, b: NMIL-100@C, c: SiO2−GOx@C, d: NMIL-100@GOx, e: NMIL-100@GOx@C); (E) Average tumor weights in different treatment groups.(Adapted from Ref. 59 with permission. Copyright © 2020 ACS Nano) GOx: glucose oxidase.
2.2 Inducing Ferroptosis by Suppressing the Activity of GPX4
GPX4 (Glutathione peroxidase 4) is the key regulator in ferroptosis. Its inhibitor can promote ferroptosis directly. At the same time, the regulation of GSH, as a substrate of GPX4, is another important approach in ferroptosis (Wu et al., 2021).
2.2.1 Suppressing Function of System Xc-
Besides focusing on the Fenton reaction, a research by Xu et al. tried to increase concentration of iron and suppress the function of system Xc-simultaneously (Liu et al., 2021b) (Figure 4). In this study, sorafenib (sor)-loaded Fe-metal organic framework nanoparticles were conjugated with the iRGD peptide to form the multifunctional nanocomposite, MIL-101(Fe)@sor. This nanoparticle can not only effectively induce tumor ferroptosis but also enhance the nanodrug tumor-targeting and penetration abilities. Liver tumors could be eliminated after MIL-101(Fe)@sor nanoparticle treatment, with a significantly prolonged survival period of tumor xenograft mice.
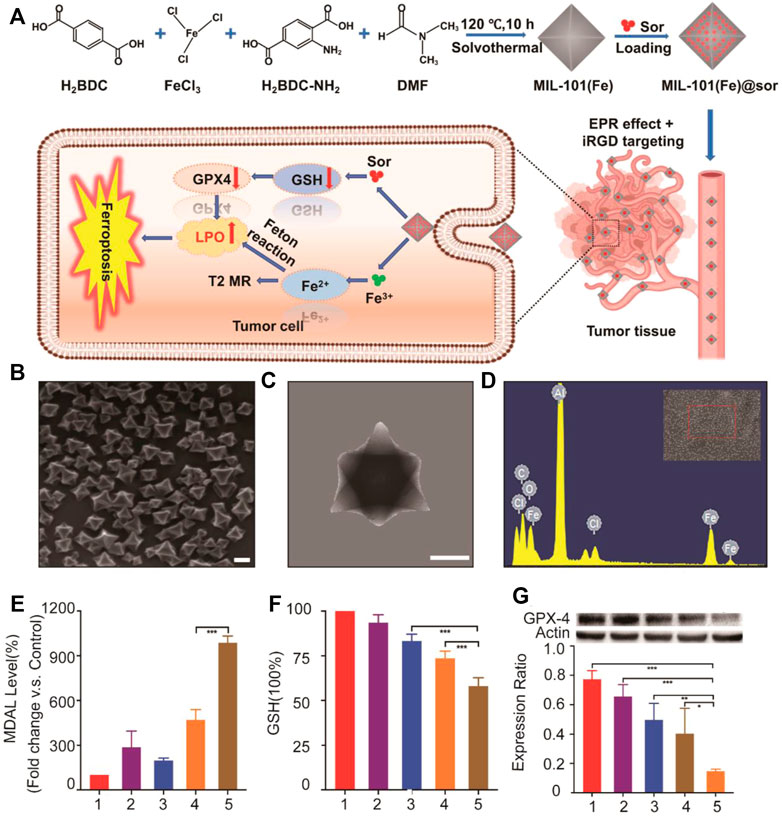
FIGURE 4. (A) Illustration of the synthesis process and mechanism of nanoparticles for liver cancer therapy; (B) SEM and (C) TEM images of MIL-101(Fe) NPs (scale bar: 100 nm); (D) EDS spectrum of MIL-101(Fe) NPs; (E,F) MDA and GSH levels in HepG2 cells after different treatments; (G) WB analysis of the GPX4 expression in HepG2 cells after different treatments. 1–5: control, MIL-101(Fe), sorafenib, MIL-101(Fe)@sor, and MIL-101(Fe)@sor + iRGD groups, respectively. *p < 0.05, **p < 0.01, ***p < 0.001.(Adapted from Ref. 61 with permission. Copyright © 2021 International Journal of Nanomedicine) Sor: sorafenib.
2.2.2 Depletion of GSH
In addition to research studies focusing on the block transport system Xc−, Xu et al. developed nanoparticles (FaPEG-MnMSN@SFB) inducing ferroptosis by both suppressing function of system Xc− and depletion of GSH (Tang et al., 2020) (Figure 5). MnMSN@SFB was synthesized with MnMSN and sorafenib (SFB) by the optimized one-pot Stober’s method. The surface of MnMSN was modified with the FaPEG chain to achieve better stability in circulation and delivery processes. On the one hand, the manganese–oxygen bond (‒Mn‒O‒) in MnMSN leaded to consumption of GSH in the cell. On the other hand, SFB, as an inhibitor of the Xc− transport system could inhibit the synthesis of GSH. Therefore, the nanoparticles exhibited efficient antitumor activity under dual roles. Moreover, apoptosis could be induced by disruption of redox balance producing the synergy effect with ROS-dependent ferroptosis to tumor cells.
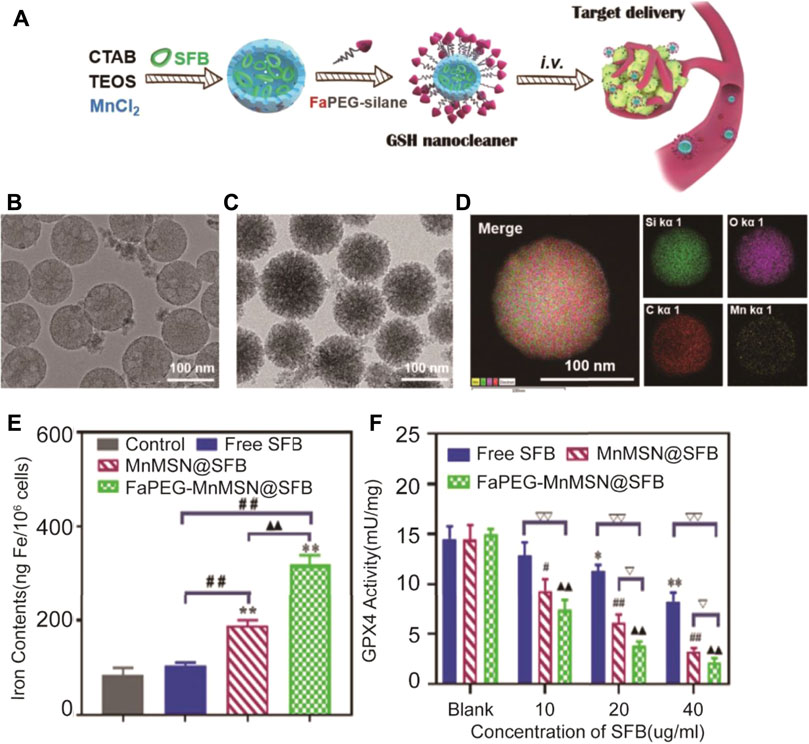
FIGURE 5. Illustration of the synthesis process and mechanism of nanoparticles for tumor therapy; (B) TEM images of MnMSN and (C) FaPEG-MnMSN; (D) Element mappings of FaPEG-MnMSN; (E) Iron contents in HepG2 cells after treated with free SFB, MnMSN@SFB and FaPEG-MnMSN@SFB at the concentration of 20 μg/ml; (F) **p < 0.01 vs. Blank group. ##p < 0.01 vs. cells treated with FaPEG-MnMSN@SFB. ▲▲p < 0.01 vs. cells treated with MnMSN@SFB. GPx4 activity of HepG2 cells after treated with free SFB, MnMSN@SFB and FaPEG-MnMSN@SFB for different concentrations. (Adapted from Ref. 62 with permission. Copyright © 2020 Theranostics) CTAB: cetyltrimethylammonium bromide; TEOS: tetraethyl orthosilicate; SFB: sorafenib tosylate.
3 Emerging Nanomedicine Based on Ferroptosis and Conventional or Emerging Tumor Therapy
Currently, although emerging tumor treatment methods have made some progress, the effect of them is still difficult to be satisfied in the clinical treatment (Lai et al., 2021; Mo et al., 2021; Ou et al., 2021; Wang and Bai, 2021). Ferroptosis has the potential ability to make the synergy effect with conventional or emerging tumor therapy which brings more spark in the treatment of different tumors (Xue et al., 2020).
3.1 Combination of Ferroptosis With Chemotherapy
Chemotherapy is a conventional tumor treatment method. Due to lack of specificity for tumor cells, it brings cytotoxicity to any cells. Besides, drug resistance makes its therapeutic effect limited (Ji et al., 2016; Patil et al., 2016). Li et al. designed a nanolongan delivery system. The oxidized starch-based gel nanoparticle was coated by the upconversion nanoparticle (UCNP) and doxorubicin (Dox) (query). The carboxyl groups on oxidized starch polymers were coordinated with Fe3+ and further decorated with polyethyleneimine (PEI) and 2,3-dimethylmaleic anhydride (DMMA) (Bao et al., 2019) (Figure 6). The negatively charged surface of nanolongan due to DMMA could achieve long circulation after intravenous injection and specially targeted tumor delivery via the EPR effect. After the nanolongan reached the tumor site with a weak acidic microenvironment, the negatively charged surface of nanolongan could be converted with a positive charge due to exfoliation of DMMA, which facilitated its internalization of cancer cells and escape of lysosomes due to the proton-sponge effect. With further near-infrared (NIR) light irradiation, Fe3+ can be converted to Fe2+ by UCNP in the cancer cell, which further promoted the Fenton reaction to induce ferroptosis-generating synergy effect with apoptosis due to Dox.
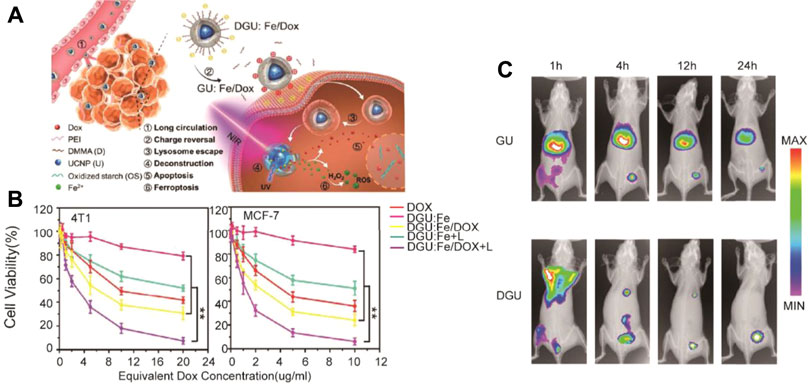
FIGURE 6. Schematic illustration of nanolongan; (B) CCK-8 cytotoxicity analysis of 4T1 and MCF-7 cells treated with different formulations after 24 h of incubation; (C) In vivo imaging of biodistribution of GU and DGU in the tumor-bearing mouse model. (Adapted from Ref. 70 with permission. Copyright © 2019 ACS Nano) UCNP: upconversion nanoparticle; Dox: doxorubicin; PEI: polyethylenimine; DMMA: 2,3-dimethylmaleic anhydride.
3.2 Combination of Ferroptosis With Immunotherapy
Tumor immunotherapy is an emerging tumor therapy method by regulating the tumor microenvironment to enhance the antitumor immunity. It provides a new idea for tumor treatment, but its effectiveness and safety need to be further studied (Fang et al., 2021; George et al., 2021). Studies have shown that immunotherapy-activated T cells can enhance ferroptosis-specific lipid peroxidation in tumor cells, and in turn, increased ferroptosis contributes to the antitumor efficacy of immunotherapy. Therefore, combination of ferroptosis with immunotherapy has been a new strategy for design of nanomedicine (Jiang et al., 2020; Yang et al., 2021b; Liang et al., 2021). In Yao et al.’s research, a nanoactivator (DAR) was constituted by doxorubicin (DOX) (query), tannic-acid (TA), and IR820. During the process of synthesis of DAR, DOX, as a chemotherapeutic drug, interacts with TA, as a disproportionation activator, to form intermediate products (DA) at the first step. Then, DA made the interaction with IR820, as a photothermal therapy agent, to form DAR finally through π–π and electronic interactions (Xiong et al., 2021) (Figure 7). After the nanoactivator is injected into the cell through endocytosis, with the IR820 responding to the laser, DAR can be reassembled quickly to realize the escape of lysosomes and promote the rapid release of endogenous Fe2+ due to the acidic microenvironment of lysosomes. The released Fe2+ accelerated the Fenton reaction to promote ferroptosis and the accumulation of ROS. Excessive ROS further induced the immune response induced by DOX to kill tumor cells. In contrast, immunotherapy would activate the infiltration of CD8+ T cells in tumor cells, and ferroptosis through IFN-γ–related pathways was promoted. The nanoactivator reflected a very significant tumor killing ability through the synergistic effect of immunotherapy and ferroptosis.
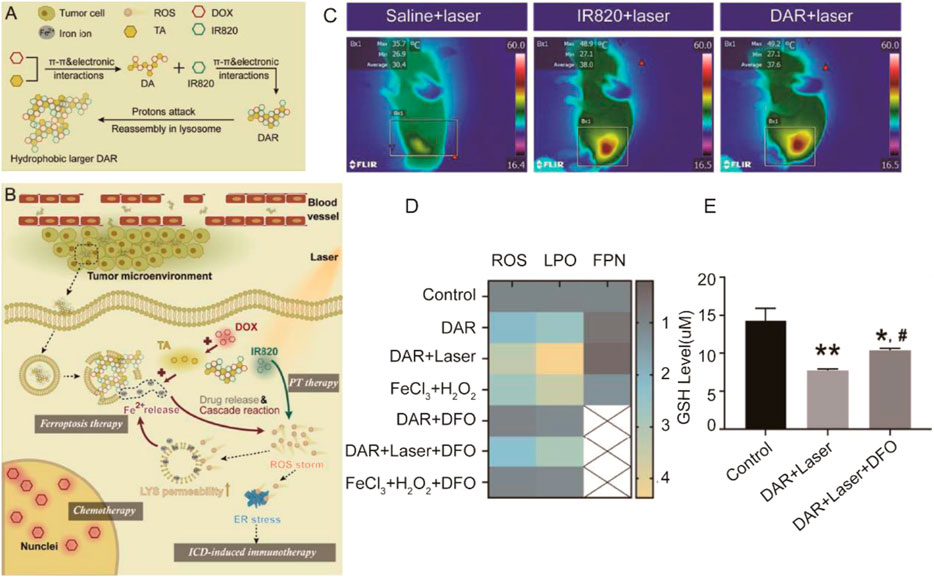
FIGURE 7. (A) Assembly and reassembly processes of DAR; (B) The tumor delivery, tumor cell uptake and intracellular transition, lysosome escape, and laser-promoted drug release processes of DAR. LYS and ER were abbreviations of lysosome and endoplasmic reticulum, respectively; (C) Infrared thermographic images of mice injected with saline; IR820 and DAR were tested at 5 min after laser irradiation; (D) Relative concentration of ROS, LPO, and FPN of MCF7 cultured in different drugs or agents (n = 3); (E) GSH levels of MCF7 cells treated with different formulations (n = 3). *p < 0.05 vs. control; **p < 0.01 vs. control; #p < 0.05 vs. DAR + laser. (Adapted from Ref. 77 with permission. Copyright © 2021 Journal of Controlled release).
A biomimetic magnetosome was prepared by Xie et al., composed of Fe3O4 magnetic nanoclusters (NCs), pre-engineered leukocyte membranes, TGF-β inhibitor (Ti), and PD-1 antibody (Pa) (Zhang et al., 2019). Specially, the NC was coated by leukocyte membranes pre-engineered with azide (N3) with modified Ti and Pa to form Pa-M/Ti-NCs. After intravenous injection, the Fe3O4 magnetic nanoclusters break through the biological barrier and are accumulated to the tumor. The Pa and Ti on the surface of Pa-M/Ti-NCs would increase the amounts of CD4+ T/Treg cells, CD8+ T/Treg cells, and rate of producing M1/M2 to induce immunogenicity of macrophages. In addition, M1 polarization due to immune response increased the amount of H2O2. With the release of ions, it promoted the Fenton reaction with H2O2 and induced ferroptosis of tumor cells.
3.3 Combination of Ferroptosis With Gene Therapy
Tumor gene therapy mainly targets tumor suppressor genes, immunostimulatory genes, and anti-angiogenic factors. Among them, adenovirus carrying p53 has the fastest development in clinical research. However, the specific mechanism, long-term efficacy, and adverse effects of gene therapy are still unclear (Chen et al., 2001; Zhang et al., 2011). In Zhang et al.’s research, breast cancer cells were eradicated by the metal organic network encapsulated with the p53 plasmid (MON-p53) via the ferroptosis/apoptosis hybrid pathway (Zheng et al., 2017) (Figure 8). As we know, p53 is a tumor suppressor, so a “bystander effect” was mediated by MON-p53 to further sensitize cancer cells toward the MON-p53 inducing ferroptosis and gene therapy. MON-p53 treatment has two effects, which both suppressed the tumor growth and prolonged the lifespan of tumor-bearing mice; it was found in an anticancer experiment.
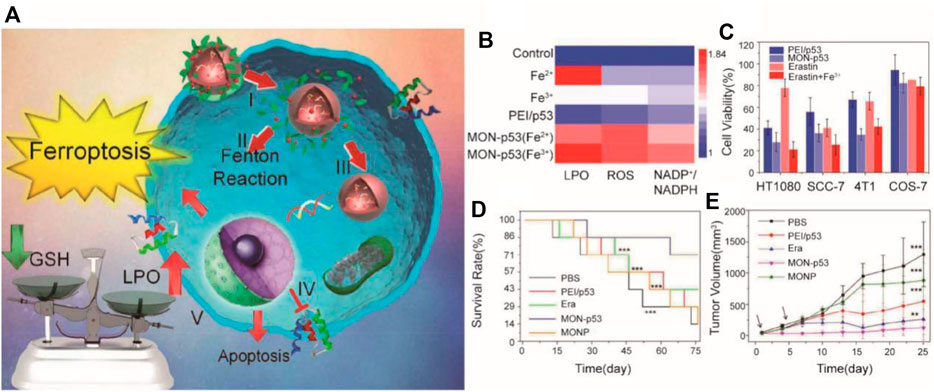
FIGURE 8. (A) Illustration of the synthesis process and mechanism of nanoparticles for tumor therapy; (B) LPO, ROS, and 5 NADP+/NADPH content of HT1080 cells treated with ferric chloride (Fe(III), 10 μM), 6 (Fe(II), 25 μM), PEI/p53, Fe(II)-MON-p53, and Fe(III)-MON-p53; (C) Viability of 7 HT1080 cells, SCC-7 cells, 4T1 cells, and COS7 cells after treatment with PEI/p53, 8 MON-p53, erastin, and erastin + Fe(III); (D) Survival curves of mice receiving injections of Era at a dose of 5 mg/kg, 3 MONP at a dose of 5 mg/kg, and a DNA dose of 0.375 mg/kg (n = 7 for all groups) in 4 HT1080 tumor-bearing mice; (E) HT1080 tumor volume curves of mice at the first 25 days (Adapted from Ref. 81 with permission. Copyright © 2017 Nano Letters) MON: metal organic network.
3.4 Combination of Ferroptosis With Ultrasound Therapy
Ultrasound can pass through the body and focus on deep tumor tissues. High-intensity ultrasound can kill tumors by high temperature. The combination of ultrasound therapy and nanotechnology can be a switch for nanomedicine to achieve precise drug release (Wang et al., 2017; Zhu et al., 2018). A nanomedicine with low-dose ultrasound responding was designed in Hao et al.’s research through combining DOX with the nanoplatform, subsequently incorporating n-heneicosane and polyethylene glycol chains (Fu et al., 2021) (Figure 9). Due to solid–liquid phase change, n-heneicosane could respond to mild high temperatures caused by ultrasound generating ultrasound-responsive cargo. Nanoparticles extravasate into the osteosarcoma tumor through the enhanced permeability and retention (EPR) effect of the tumor. On the one hand, the sensitivity of the apoptosis mediated by DOX of tumors can be caused by ultrasound-responsive DFHHP nanomedicine (DOX-Fe(VI)@HMS-HE-PEG, abbreviated as DFHHP) via alleviating the hypoxic tumor microenvironment by tumor reoxygenation. On the other hand, exogenous iron and systemic GPX4 could cause inactivation-efficient ferroptotic cell death. The US-activatable nanomedicine overcame chemoresistance and suppressed tumor growths by inducing collaborative apoptosis and ferroptosis of hypoxic osteosarcoma in vitro and in vivo.
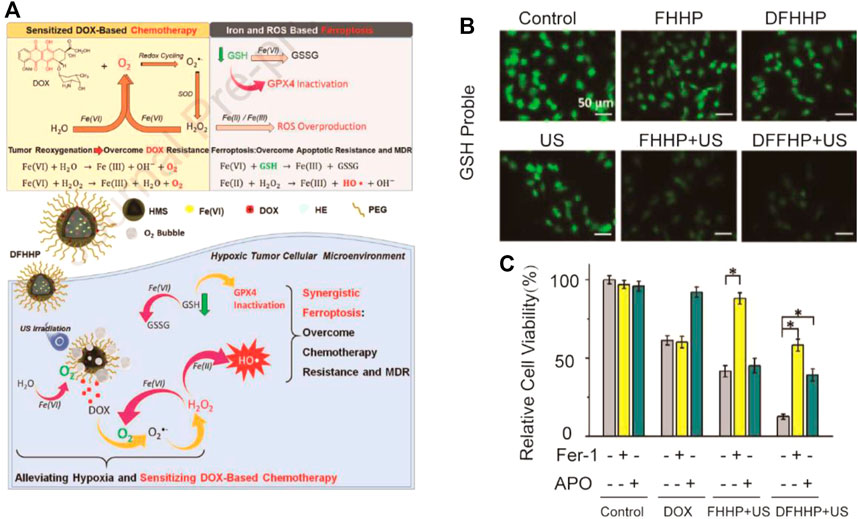
FIGURE 9. (A) Illustration of the synthesis process and mechanism of nanoparticles for tumor therapy; (B) Fluorescence images of Saos-2 cells after different treatments. The cellular GSH level was indicated by Thiol-Tracker Violet. Cells in fresh culture media were used as the control group; (C) In vitro cytotoxicity of DOX, FHHP + US, and DFHHP + US against Saos-2 cells after the pretreatment with the ferroptosis inhibitor ferrostatin-1 (Fer-1) or apoptosis inhibitor Ac-DEVD-CHO (Apo). (Adapted from Ref. 84 with permission. Copyright © 2020 Biomaterials).
3.5 Combination of Ferroptosis With Photodynamic Therapy
Photodynamic therapy uses lasers of specific wavelengths to activate photosensitizers selectively retained in tumor tissues to selectively kill tumor cells (Patrice, 1991; Blasi et al., 2018). Zhao et al. provided a new treatment strategy for triple-negative breast cancer (TNBC) with azobenzene combretastatin A4 (Azo-CA4) (Zhu et al., 2020). Near-infrared light was converted to UV light by upconverting nanocarriers (UCNPs) to activate Azo-CA4, which being physically encapsulated in the lipid (LP) bilayer and was loaded in UCNPs. After irradiation, the viability of TNBC cells was significantly reduced by UCNP@LP (Azo-CA4) nanocarriers through both apoptosis and ferroptosis. On the one hand, microtubule breakdown and cell cycle arrest at the G2/M phase was induced by photoisomerization of Azo-CA4. On the other hand, the UV light–induced reduction of Fe3+ to Fe2+ caused ferroptosis, which facilitated the peroxidation of lipids. The tumor growth of xenograft mice was significantly suppressed by UCNP@LP (Azo-CA4).
A novel theranostic nanoplatform was developed in Zheng et al.’s research for tumor chemodynamic–photothermal therapy and 3D imaging diagnosis (Hu et al., 2020). By the synthesis of Prussian blue cubes (PB) and in situ reduction of iron platinum nanoparticles (FePt-NPs) in a facile way and coating with targeting ligands (hyaluronic acid) and NH2-PEG, the nanoplatform was successfully fabricated. The nanoplatform has two functions: it decomposes endogenous H2O2 into ROS, which is highly cytotoxic to tumor cells and produces a multifunctional theranostic agent for multimodal imaging and therapies. Moreover, the specific tumor-targeting ability to the nanoplatform was given by HA, with excellent biocompatibility and biological degradability, due to binding with CD44 and CD168. The tumor growth of xenograft mice was effectively suppressed by intravenous injection of the nanoplatform, indicating that tumor growth efficaciously inhibited the nanoplatform, with relatively high biosafety.
4 Conclusion and Prospective
Cancer is a public health problem worldwide, whose incidence of tumor increases year by year. At present, traditional treatment of tumor is usually combined with chemotherapy, radiotherapy, and other methods. However, there are large side effects, easy to produce drug resistance, and other problems during the process of treatment. Therefore, in recent years, gene therapy, immunotherapy, and other emerging treatment methods have been widely studied. However, the biosecurity, specific mechanisms, and long-term therapeutic effects of these emerging treatments need to be further studied. Ferroptosis is a new form of regulated cell death that is defined in 2012. In recent years, a large amount of research has focused on the mechanisms of ferroptosis. Based on these mechanisms, especially for the Fenton reaction and suppressing activity of GPX4, ferroptosis has provided a new idea for cancer therapy. However, tumor cells can adapt to the microenvironment through metabolic change; some small molecules, such as the ferroptosis inducers, that merely increase the concentration of ROS in tumor cells or inhibit the activity of GPX4 cannot achieve strong and lasting antitumor effects. In a way, the small molecules lack tumor specificity, and they are easier to be eliminated during blood circulation.
With the development of nanomaterial technology, ferroptosis-inducing nanomedicines have attracted more attention. Therefore, it is timely to outline the latest advances in ferroptosis-inducing nanomedicines. The combination inducing ferroptosis with nanotechnology enhances the stability, biosecurity, targeting, and controlled release of drugs in the body. Specifically, the advantages of ferroptosis-inducing nanomedicines include three aspects. First, the nanomaterials with a regulated size can facilitate passive targeting to the tumor microenvironment (EPR effect). By modifying the nanoparticles with specific antibodies against the tumor surface, the active targeting of nanomedicine to tumors can also be achieved. Second, the large hydrodynamic size of nanomaterials can reduce the renal clearance and prolong the half-life in the blood. Moreover, ferroptosis-inducing nanomedicines combined with other therapies, such as chemotherapy, gene therapy, ultrasound therapy, and photodynamic therapy, can achieve more effective treatments with the synergy effect. To a certain extent, ROS produced by ferroptosis can regulate the tumor microenvironment and further induce cell apoptosis, enhance ability to kill tumor cells of nanomedicine, or even reverse tumor resistance. In addition, a large number of studies have also shown that autophagy is indeed induced by the ferroptosis inducer, and autophagy can enhance ferroptosis in cancer cells by degradation of ferritin (Hou et al., 2016; Torii et al., 2016; Park and Chung, 2019). Chen et al. constructed trehalose-loaded mSiO2@MnOx-mPEG (TreMMM) nanoparticles, its combination of GSH consumption-induced ferroptosis and trehalose-induced autophagy by nanomedicine design, providing a new strategy for the design of ferroptosis-inducing nanomedicines (Yang et al., 2021c). Besides, several other key molecules in the NRF2 pathway or RAS/MAPK pathway are related to ferroptosis, which provides an opportunity to find out various targets to design nanomedicines (Yagoda et al., 2007; Roh et al., 2017; Wang et al., 2021d).
Although ferroptosis-inducing nanomedicines have been developed rapidly, the potential clinical application remains to be further investigated. On the one hand, most of the current research focuses on cells or animal models; there might be a difference between the human body and the models used in most research, such as cells and animals. It is questionable that whether ferroptosis-inducing nanomedicines have effective treatments in the human body. On the other hand, whether ferroptosis-inducing nanomedicines have potential toxic and side effects that should be further investigated. Moreover, existing research of ferroptosis-inducing nanomedicines is mainly a benefit to breast cancer and liver cancer. This may be due to the high incidence of these tumors, which is suitable as the model for tumor therapy. Meanwhile, different tumors have different sensitivities to the ferroptosis-inducing nanomedicines because of their adaptability to ROS. Therefore, it is necessary to further investigate the mechanisms of tumor resistance. Importantly, the research on the mechanism of gene therapy and immunotherapy, as emerging treatment methods, is not thorough enough. Whether the combination with inducing ferroptosis will cause other side effects remains to be further studied. In summary, although ferroptosis-inducing nanomedicines have achieved the positive antitumor effect on the research, the treatment of tumors based on ferroptosis is in the initial stage. In the future, many issues need to be clarified in combination of biochemistry, oncology, and material technology to design the effective and safe cancer therapy strategies based on ferroptosis.
Author Contributions
LH designed the study and revised the manuscript. YW provided information and prepared the manuscript. TL was responsible for information collection.
Funding
This work was partly supported by the 2021 Basic Scientific Research Project of Colleges and Universities of Liaoning Provincial Department of Education (LJKZ0787), China Postdoctoral Science Foundation (2019M661160), and Innovation and Entrepreneurship Training Program for College Students of China Medical University (S202110159038).
Conflict of Interest
The authors declare that the research was conducted in the absence of any commercial or financial relationships that could be construed as a potential conflict of interest.
Publisher’s Note
All claims expressed in this article are solely those of the authors and do not necessarily represent those of their affiliated organizations, or those of the publisher, the editors, and the reviewers. Any product that may be evaluated in this article, or claim that may be made by its manufacturer, is not guaranteed or endorsed by the publisher.
Acknowledgments
My supervisors Wenhui Su (Shcool of Life Science, Department of Biochemistry and Molecular Biology, China Medical University, Shenyang 110122, China) and Yiling Li (First Department of Clinical Medicine, China Medical University, Shenyang, China) did offer me so much help that I feel so grateful to them.
References
Adams, S., Gatti-Mays, M. E., Kalinsky, K., Korde, L. A., Sharon, E., Amiri-Kordestani, L., et al. (2019). Current Landscape of Immunotherapy in Breast Cancer: A Review. JAMA Oncol. 5 (8), 1205–1214. doi:10.1001/jamaoncol.2018.7147
Asghari, F., Khademi, R., Esmaeili Ranjbar, F., Faridi Majidi, Z. R., and Majidi, R. F. (2019). Application of Nanotechnology in Targeting of Cancer Stem Cells: A Review. Int. J. Stem Cell 12 (2), 227–239. doi:10.15283/ijsc19006
Bao, W., Liu, X., Lv, Y., Lu, G. H., Li, F., Zhang, F., et al. (2019). Nanolongan with Multiple On-Demand Conversions for Ferroptosis-Apoptosis Combined Anticancer Therapy. Acs Nano 13 (1), 260–273. doi:10.1021/acsnano.8b05602
Blasi, M. A., Pagliara, M. M., Lanza, A., Sammarco, M. G., Caputo, C. G., Grimaldi, G., et al. (2018). Photodynamic Therapy in Ocular Oncology. Biomedicines 6 (1), 17. doi:10.3390/biomedicines6010017
Chen, G. Q., Benthani, F. A., Wu, J., Liang, D., Bian, Z. X., and Jiang, X. (2020). Artemisinin Compounds Sensitize Cancer Cells to Ferroptosis by Regulating Iron Homeostasis. Cell Death Differ 27 (1), 242–254. doi:10.1038/s41418-019-0352-3
Chen, M., Hu, S., Li, Y., Jiang, T. T., Jin, H., and Feng, L. (2020). Targeting Nuclear Acid-Mediated Immunity in Cancer Immune Checkpoint Inhibitor Therapies. Signal. Transduct Target. Ther. 5 (1), 270. doi:10.1038/s41392-020-00347-9
Chen, Q. R., Zhang, L., Gasper, W., and Mixson, A. J. (2001). Targeting Tumor Angiogenesis with Gene Therapy. Mol. Genet. Metab. 74 (1-2), 120–127. doi:10.1006/mgme.2001.3223
Cheng, Q., Bao, L., Li, M., Chang, K., and Yi, X. (2021). Erastin Synergizes with Cisplatin via Ferroptosis to Inhibit Ovarian Cancer Growth In Vitro and In Vivo. J. Obstet. Gynaecol. Res. 47, 2481–2491. doi:10.1111/jog.14779
Dixon, S. J., Lemberg, K. M., Lamprecht, M. R., Skouta, R., Zaitsev, E. M., Gleason, C. E., et al. (2012). Ferroptosis: An Iron-dependent Form of Nonapoptotic Cell Death. Cell 149 (5), 1060–1072. doi:10.1016/j.cell.2012.03.042
Esposito, A., Viale, G., and Curigliano, G. (2019). Safety, Tolerability, and Management of Toxic Effects of Phosphatidylinositol 3-Kinase Inhibitor Treatment in Patients with Cancer: A Review. JAMA Oncol. 5 (9), 1347–1354. doi:10.1001/jamaoncol.2019.0034
Fang, Y., Yu, A., Ye, L., and Zhai, G. (2021). Research Progress in Tumor Targeted Immunotherapy. Expert Opin. Drug Deliv. 18 (8), 1067–1090. doi:10.1080/17425247.2021.1882992
Fu, J., Li, T., Yang, Y., Jiang, L., Wang, W., Fu, L., et al. (2021). Activatable Nanomedicine for Overcoming Hypoxia-Induced Resistance to Chemotherapy and Inhibiting Tumor Growth by Inducing Collaborative Apoptosis and Ferroptosis in Solid Tumors. Biomaterials 268, 120537. doi:10.1016/j.biomaterials.2020.120537
Gaschler, M. M., Andia, A. A., Liu, H., Csuka, J. M., Hurlocker, B., Vaiana, C. A., et al. (2018). FINO2 Initiates Ferroptosis through GPX4 Inactivation and Iron Oxidation. Nat. Chem. Biol. 14 (5), 507–515. doi:10.1038/s41589-018-0031-6
George, A., Sahin, I., Carneiro, B. A., Dizon, D. S., Safran, H. P., and El-Deiry, W. S. (2021). Strategies to Sensitize Cancer Cells to Immunotherapy. Hum. Vaccin. Immunother. 17 (8), 2595–2601. doi:10.1080/21645515.2021.1891817
Hassannia, B., Vandenabeele, P., and Vanden Berghe, T. (2019). Targeting Ferroptosis to Iron Out Cancer. Cancer Cell 35 (6), 830–849. doi:10.1016/j.ccell.2019.04.002
He, Z., Liu, K., Byrne, H. J., Cullen, P. J., Tian, F., and Curtin, J. F. (2019). Combination Strategies for Targeted Delivery of Nanoparticles for Cancer Therapy. Elsevier, 191–219. doi:10.1016/b978-0-12-814029-1.00008-9
Hirschhorn, T., and Stockwell, B. R. (2019). The Development of the Concept of Ferroptosis. Free Radic. Biol. Med. 133, 130–143. doi:10.1016/j.freeradbiomed.2018.09.043
Hong, T., Lei, G., Chen, X., Li, H., Zhang, X., Wu, N., et al. (2021). PARP Inhibition Promotes Ferroptosis via Repressing SLC7A11 and Synergizes with Ferroptosis Inducers in BRCA-Proficient Ovarian Cancer. Redox Biol. 42, 101928. doi:10.1016/j.redox.2021.101928
Hou, W., Xie, Y., Song, X., Sun, X., Lotze, M. T., Zeh, H. J., et al. (2016). Autophagy Promotes Ferroptosis by Degradation of Ferritin. Autophagy 12 (8), 1425–1428. doi:10.1080/15548627.2016.1187366
Hu, Z., Wang, S., Dai, Z., Zhang, H., and Zheng, X. (2020). A Novel Theranostic Nano-Platform (PB@FePt-HA-g-PEG) for Tumor Chemodynamic-Photothermal Co-therapy and Triple-Modal Imaging (MR/CT/PI) Diagnosis. J. Mater. Chem. B 8 (24), 5351–5360. doi:10.1039/d0tb00708k
Huo, M., Wang, L., Wang, Y., Chen, Y., and Shi, J. (2019). Nanocatalytic Tumor Therapy by Single-Atom Catalysts. Acs Nano 13 (2), 2643–2653. doi:10.1021/acsnano.9b00457
Ji, Z. H., Peng, K. W., and Li, Y. (2016). Intraperitoneal Free Cancer Cells in Gastric Cancer: Pathology of Peritoneal Carcinomatosis and Rationale for Intraperitoneal Chemotherapy/hyperthermic Intraperitoneal Chemotherapy in Gastric Cancer. Transl Gastroenterol. Hepatol. 1, 69. doi:10.21037/tgh.2016.08.03
Jiang, Q., Wang, K., Zhang, X., Ouyang, B., Liu, H., Pang, Z., et al. (2020). Platelet Membrane-Camouflaged Magnetic Nanoparticles for Ferroptosis-Enhanced Cancer Immunotherapy. Small 16 (22), e2001704. doi:10.1002/smll.202001704
Johnstone, R. W., Ruefli, A. A., and Lowe, S. W. (2002). Apoptosis: A Link between Cancer Genetics and Chemotherapy. Cell 108 (2), 153–164. doi:10.1016/s0092-8674(02)00625-6
Kim, S., Seo, J. H., Jeong, D. I., Yang, M., Lee, S. Y., Lee, J., et al. (2021). Fenton-like Reaction, Glutathione Reduction, and Photothermal Ablation-Built-In Hydrogels Crosslinked by Cupric Sulfate for Loco-Regional Cancer Therapy. Biomater. Sci. 9 (3), 847–860. doi:10.1039/d0bm01470b
Lachaier, E., Louandre, C., Godin, C., Saidak, Z., Baert, M., Diouf, M., et al. (2014). Sorafenib Induces Ferroptosis in Human Cancer Cell Lines Originating from Different Solid Tumors. Anticancer Res. 34 (11), 6417–6422.
Lai, W. H., Fang, C. Y., Chou, M. C., Lin, M. C., Shen, C. H., Chao, C. N., et al. (2021). Peptide-guided JC Polyomavirus-like Particles Specifically Target Bladder Cancer Cells for Gene Therapy. Sci. Rep. 11 (1), 11889. doi:10.1038/s41598-021-91328-7
Li, F., Zhao, H., Shao, R., Zhang, X., and Yu, H. (2021). Enhanced Fenton Reaction for Xenobiotic Compounds and Lignin Degradation Fueled by Quinone Redox Cycling by Lytic Polysaccharide Monooxygenases. J. Agric. Food Chem. 69, 7104–7114. doi:10.1021/acs.jafc.1c01684
Li, Z., Wu, X., Wang, W., Gai, C., Zhang, W., Li, W., et al. (2021). Fe(II) and Tannic Acid-Cloaked MOF as Carrier of Artemisinin for Supply of Ferrous Ions to Enhance Treatment of Triple-Negative Breast Cancer. Nanoscale Res. Lett. 16 (1), 37. doi:10.1186/s11671-021-03497-z
Liang, C., Zhang, X., Yang, M., and Dong, X. (2019). Recent Progress in Ferroptosis Inducers for Cancer Therapy. Adv. Mater. 31 (51), e1904197. doi:10.1002/adma.201904197
Liang, H., Wu, X., Zhao, G., Feng, K., Ni, K., and Sun, X. (2021). Renal Clearable Ultrasmall Single-Crystal Fe Nanoparticles for Highly Selective and Effective Ferroptosis Therapy and Immunotherapy. J. Am. Chem. Soc. 143 (38), 15812–15823. doi:10.1021/jacs.1c07471
Liang, J., and Sun, Z. (2021). Overexpression of Membranal SLC3A2 Regulates the Proliferation of Oral Squamous Cancer Cells and Affects the Prognosis of Oral Cancer Patients. J. Oral Pathol. Med. 50 (4), 371–377. doi:10.1111/jop.13132
Liu, J., Kang, R., and Tang, D. (2021a). Signaling Pathways and Defense Mechanisms of Ferroptosis. Febs J. doi:10.1111/febs.16059
Liu, X., Zhu, X., Qi, X., Meng, X., and Xu, K. (2021b). Co-Administration of iRGD with Sorafenib-Loaded Iron-Based Metal-Organic Framework as a Targeted Ferroptosis Agent for Liver Cancer Therapy. Int. J. Nanomedicine 16, 1037–1050. doi:10.2147/ijn.s292528
Lu, B., Chen, X. B., Ying, M. D., He, Q. J., Cao, J., and Yang, B. (2018). The Role of Ferroptosis in Cancer Development and Treatment Response. Front. Pharmacol. 8, 992. doi:10.3389/fphar.2017.00992
Ma, L., Zhang, X., Yu, K., Xu, X., Chen, T., Shi, Y., et al. (2021). Targeting SLC3A2 Subunit of System XC− Is Essential for m6A Reader YTHDC2 to Be an Endogenous Ferroptosis Inducer in Lung Adenocarcinoma. Free Radic. Biol. Med. 168, 25–43. doi:10.1016/j.freeradbiomed.2021.03.023
Mai, T. T., Hamaï, A., Hienzsch, A., Cañeque, T., Müller, S., Wicinski, J., et al. (2017). Salinomycin Kills Cancer Stem Cells by Sequestering Iron in Lysosomes. Nat. Chem. 9 (10), 1025–1033. doi:10.1038/nchem.2778
Matos, A. I., Carreira, B., Peres, C., Moura, L. I. F., Conniot, J., Fourniols, T., et al. (2019). Nanotechnology Is an Important Strategy for Combinational Innovative Chemo-Immunotherapies against Colorectal Cancer. J. Control. Release 307, 108–138. doi:10.1016/j.jconrel.2019.06.017
Meng, X., Zhang, X., Liu, M., Cai, B., He, N., and Wang, Z. (2020). Fenton Reaction-Based Nanomedicine in Cancer Chemodynamic and Synergistic Therapy. Appl. Mater. Today 21, 100864. doi:10.1016/j.apmt.2020.100864
Mo, D. C., Huang, J. F., Luo, P. H., Huang, S. X., and Wang, H. L. (2021). The Efficacy and Safety of Combination Therapy with Immune Checkpoint Inhibitors in Non-small Cell Lung Cancer: A Meta-Analysis. Int. Immunopharmacol 96, 107594. doi:10.1016/j.intimp.2021.107594
Mou, Y., Wang, J., Wu, J., He, D., Zhang, C., Duan, C., et al. (2019). Ferroptosis, a New Form of Cell Death: Opportunities and Challenges in Cancer. J. Hematol. Oncol. 12 (1), 34. doi:10.1186/s13045-019-0720-y
Nguyen, T. H. P., Mahalakshmi, B., and Velmurugan, B. K. (2020). Functional Role of Ferroptosis on Cancers, Activation and Deactivation by Various Therapeutic Candidates-An Update. Chem. Biol. Interact 317, 108930. doi:10.1016/j.cbi.2019.108930
Ortiz-Rodriguez, J. M., Nerozzi, C., Bucci, D., Mislei, B., Mari, G., Tamanini, C., et al. (2021). The Inhibition of Spermatic Cystine/glutamate Antiporter xCT (SLC7A11) Influences the Ability of Cryopreserved Stallion Sperm to Bind to Heterologous Zonae Pellucidae. Theriogenology 167, 24–31. doi:10.1016/j.theriogenology.2021.03.002
Ou, X., Ma, Q., Yin, W., Ma, X., and He, Z. (2021). CRISPR/Cas9 Gene-Editing in Cancer Immunotherapy: Promoting the Present Revolution in Cancer Therapy and Exploring More. Front Cel Dev Biol 9, 674467. doi:10.3389/fcell.2021.674467
Park, E., and Chung, S. W. (2019). ROS-mediated Autophagy Increases Intracellular Iron Levels and Ferroptosis by Ferritin and Transferrin Receptor Regulation. Cell Death Dis 10, 822. doi:10.1038/s41419-019-2064-5
Patil, V. M., Noronha, V., Joshi, A., Banavali, S. D., Muddu, V., and Prabhash, K. (2016). Preoperative Chemotherapy and Metronomic Scheduling of Chemotherapy in Locally Advanced Oral Cancers. Oncology 91 (Suppl. 1), 35–40. doi:10.1159/000447579
Patrice, T. (1991). Challenges for Photodynamic Therapy in the Treatment of Gastrointestinal Tumours. J. Photochem. Photobiol. B 9 (3-4), 372–374. doi:10.1016/1011-1344(91)80173-f
Pham, T., Roth, S., Kong, J., Guerra, G., Narasimhan, V., Pereira, L., et al. (2018). An Update on Immunotherapy for Solid Tumors: A Review. Ann. Surg. Oncol. 25 (11), 3404–3412. doi:10.1245/s10434-018-6658-4
Poon, D. J. J., Tay, L. M., Ho, D., Chua, M. L. K., Chow, E. K., and Yeo, E. L. L. (2021). Improving the Therapeutic Ratio of Radiotherapy against Radioresistant Cancers: Leveraging on Novel Artificial Intelligence-Based Approaches for Drug Combination Discovery. Cancer Lett. 511, 56–67. doi:10.1016/j.canlet.2021.04.019
Qian, X., Zhang, J., Gu, Z., and Chen, Y. (2019). Nanocatalysts-augmented Fenton Chemical Reaction for Nanocatalytic Tumor Therapy. Biomaterials 211, 1–13. doi:10.1016/j.biomaterials.2019.04.023
Rana, A., and Bhatnagar, S. (2021). Advancements in Folate Receptor Targeting for Anti-cancer Therapy: A Small Molecule-Drug Conjugate Approach. Bioorg. Chem. 112, 104946. doi:10.1016/j.bioorg.2021.104946
Roh, J. L., Kim, E. H., Jang, H., and Shin, D. (2017). Nrf2 Inhibition Reverses the Resistance of Cisplatin-Resistant Head and Neck Cancer Cells to Artesunate-Induced Ferroptosis. Redox Biol. 11, 254–262. doi:10.1016/j.redox.2016.12.010
Seibt, T. M., Proneth, B., and Conrad, M. (2019). Role of GPX4 in Ferroptosis and its Pharmacological Implication. Free Radic. Biol. Med. 133, 144–152. doi:10.1016/j.freeradbiomed.2018.09.014
Seiler, A., Schneider, M., Förster, H., Roth, S., Wirth, E. K., Culmsee, C., et al. (2008). Glutathione Peroxidase 4 Senses and Translates Oxidative Stress into 12/15-lipoxygenase Dependent- and AIF-Mediated Cell Death. Cell Metab 8 (3), 237–248. doi:10.1016/j.cmet.2008.07.005
Siegel, R. L., Miller, K. D., Fuchs, H. E., and Jemal, A. (2021). Cancer Statistics. Ca-a Cancer J. Clinicians 71, 7–33. doi:10.3322/caac.21669
Song, X., Wang, X., Liu, Z., and Yu, Z. (2020). Role of GPX4-Mediated Ferroptosis in the Sensitivity of Triple Negative Breast Cancer Cells to Gefitinib. Front. Oncol. 10, 597434. doi:10.3389/fonc.2020.597434
Tan, S., Schubert, D., and Maher, P. (2001). Oxytosis: A Novel Form of Programmed Cell Death. Curr. Top. Med. Chem. 1 (6), 497–506. doi:10.2174/1568026013394741
Tang, H., Li, C., Zhang, Y., Zheng, H., Cheng, Y., Zhu, J., et al. (2020). Targeted Manganese Doped Silica Nano GSH-Cleaner for Treatment of Liver Cancer by Destroying the Intracellular Redox Homeostasis. Theranostics 10 (21), 9865–9887. doi:10.7150/thno.46771
Tang, Z., Zhao, P., Wang, H., Liu, Y., and Bu, W. (2021). Biomedicine Meets Fenton Chemistry. Chem. Rev. 121 (4), 1981–2019. doi:10.1021/acs.chemrev.0c00977
Tian, Q., Xue, F., Wang, Y., Cheng, Y., An, L., Yang, S., et al. (2021). Recent Advances in Enhanced Chemodynamic Therapy Strategies. Nano Today 39, 101162. doi:10.1016/j.nantod.2021.101162
Torii, S., Shintoku, R., Kubota, C., Yaegashi, M., Torii, R., Sasaki, M., et al. (2016). An Essential Role for Functional Lysosomes in Ferroptosis of Cancer Cells. Biochem. J. 473, 769–777. doi:10.1042/bj20150658
Vitalakumar, D., Sharma, A., and Flora, S. J. S. (2021). Ferroptosis: A Potential Therapeutic Target for Neurodegenerative Diseases. J. Biochem. Mol. Toxicol. 35, e22830. doi:10.1002/jbt.22830
Wan, X., Song, L., Pan, W., Zhong, H., Li, N., and Tang, B. (2020). Tumor-Targeted Cascade Nanoreactor Based on Metal-Organic Frameworks for Synergistic Ferroptosis-Starvation Anticancer Therapy. Acs Nano 14 (9), 11017–11028. doi:10.1021/acsnano.9b07789
Wang, B., Zhang, X., Wang, Z., and Shi, D. (2020). Ferroptotic Nanomaterials Enhance Cancer Therapy via Boosting Fenton-reaction. J. Drug Deliv. Sci. Techn. 59, 101883. doi:10.1016/j.jddst.2020.101883
Wang, L., and Bai, L. (2021). Progress on Tumor Immune Checkpoints and Their Inhibitors in Tumor Therapy. Xi Bao Yu Fen Zi Mian Yi Xue Za Zhi 37 (7), 663–670.
Wang, R., Su, Q., Yin, H., Wu, D., Lv, C., and Yan, Z. (2021a). Inhibition of SRSF9 Enhances the Sensitivity of Colorectal Cancer to Erastin-Induced Ferroptosis by Reducing Glutathione Peroxidase 4 Expression. Int. J. Biochem. Cel Biol. 134, 105948. doi:10.1016/j.biocel.2021.105948
Wang, S., Li, Q., Stubbings, W. A., Li, L., Qin, J., and Li, H. (2021b). The Effect of Fenton Reaction Using H2O2 and Water Control on the Distribution and Accumulation of as Speciation within the Soil-rice System. Chemosphere 274, 129633. doi:10.1016/j.chemosphere.2021.129633
Wang, S., Wu, H., Sun, K., Hu, J., Chen, F., Liu, W., et al. (2021c). A Novel pH-Responsive Fe-MOF System for Enhanced Cancer Treatment Mediated by the Fenton Reaction. New J. Chem. 45 (6), 3271–3279. doi:10.1039/d0nj05105e
Wang, S., Yi, X., Wu, Z., Guo, S., Dai, W., Wang, H., et al. (2021d). CAMKK2 Defines Ferroptosis Sensitivity of Melanoma Cells by Regulating AMPK‒NRF2 Pathway. J. Invest. Dermatol. doi:10.1016/j.jid.2021.05.025
Wang, W., Green, M., Choi, J. E., Gijón, M., Kennedy, P. D., Johnson, J. K., et al. (2019). CD8+ T Cells Regulate Tumour Ferroptosis during Cancer Immunotherapy. Nature 569 (7755), 270–274. doi:10.1038/s41586-019-1170-y
Wang, Z., He, Q., Zhao, W., Luo, J., and Gao, W. (2017). Tumor-homing, pH- and Ultrasound-Responsive Polypeptide-Doxorubicin Nanoconjugates Overcome Doxorubicin Resistance in Cancer Therapy. J. Control. Release 264, 66–75. doi:10.1016/j.jconrel.2017.08.017
Woo, J. H., Shimoni, Y., Yang, W. S., Subramaniam, P., Iyer, A., Nicoletti, P., et al. (2015). Elucidating Compound Mechanism of Action by Network Perturbation Analysis. Cell 162 (2), 441–451. doi:10.1016/j.cell.2015.05.056
Wu, C., Liu, Z., Chen, Z., Xu, D., Chen, L., Lin, H., et al. (2021). A Nonferrous Ferroptosis-like Strategy for Antioxidant Inhibition-Synergized Nanocatalytic Tumor Therapeutics. Sci. Adv. 7 (39), eabj8833. doi:10.1126/sciadv.abj8833
Xie, B., and Guo, Y. (2021). Molecular Mechanism of Cell Ferroptosis and Research Progress in Regulation of Ferroptosis by Noncoding RNAs in Tumor Cells. Cell Death Discov 7 (1), 101. doi:10.1038/s41420-021-00483-3
Xie, Y., Hou, W., Song, X., Yu, Y., Huang, J., Sun, X., et al. (2016). Ferroptosis: Process and Function. Cel Death Differ 23 (3), 369–379. doi:10.1038/cdd.2015.158
Xiong, H., Wang, C., Wang, Z., Lu, H., and Yao, J. (2021). Self-assembled Nano-Activator Constructed Ferroptosis-Immunotherapy through Hijacking Endogenous Iron to Intracellular Positive Feedback Loop. J. Control. Release 332, 539–552. doi:10.1016/j.jconrel.2021.03.007
Xu, G., Wang, H., Li, X., Huang, R., and Luo, L. (2021b). Recent Progress on Targeting Ferroptosis for Cancer Therapy. Biochem. Pharmacol. 190, 114584. doi:10.1016/j.bcp.2021.114584
Xu, T., Ding, W., Ji, X., Ao, X., Liu, Y., Yu, W., et al. (2019). Molecular Mechanisms of Ferroptosis and its Role in Cancer Therapy. J. Cel Mol Med 23 (8), 4900–4912. doi:10.1111/jcmm.14511
Xu, X., Chen, Y., Zhang, Y., Yao, Y., and Ji, P. (2020). Highly Stable and Biocompatible Hyaluronic Acid-Rehabilitated Nanoscale MOF-Fe2+ Induced Ferroptosis in Breast Cancer Cells. J. Mater. Chem. B 8 (39), 9129–9138. doi:10.1039/d0tb01616k
Xu, Z., Peng, B., Liang, Q., Chen, X., Cai, Y., Zeng, S., et al. (2021c). Construction of a Ferroptosis-Related Nine-lncRNA Signature for Predicting Prognosis and Immune Response in Hepatocellular Carcinoma. Front. Immunol. 12, 719175. doi:10.3389/fimmu.2021.719175
Xu, Z., Peng, B., Liang, Q., Chen, X., Cai, Y., Zeng, S., et al. (2021a). Construction of a Ferroptosis-Related Nine-lncRNA Signature for Predicting Prognosis and Immune Response in Hepatocellular Carcinoma. Front. Immunol. 12, 12. doi:10.3389/fimmu.2021.719175
Xue, C. C., Li, M. H., Zhao, Y., Zhou, J., Hu, Y., Cai, K. Y., et al. (2020). Tumor Microenvironment-Activatable Fe-Doxorubicin Preloaded Amorphous CaCO3 Nanoformulation Triggers Ferroptosis in Target Tumor Cells. Sci. Adv. 6 (18), eaax1346. doi:10.1126/sciadv.aax1346
Yagoda, N., von Rechenberg, M., Zaganjor, E., Bauer, A. J., Yang, W. S., Fridman, D. J., et al. (2007). RAS-RAF-MEK-dependent Oxidative Cell Death Involving Voltage-dependent Anion Channels. Nature 447 (7146), 864–868. doi:10.1038/nature05859
Yang, J., Ma, S., Xu, R., Wei, Y., Zhang, J., Zuo, T., et al. (2021). Smart Biomimetic Metal Organic Frameworks Based on ROS-Ferroptosis-Glycolysis Regulation for Enhanced Tumor Chemo-Immunotherapy. J. Control. Release 334, 21–33. doi:10.1016/j.jconrel.2021.04.013
Yang, J., Ding, L., Yu, L., Wang, Y., Ge, M., Jiang, Q., et al. (2021). Nanomedicine Enables Autophagy-Enhanced Cancer-Cell Ferroptosis. Sci. Bull. 66 (5), 464–477. doi:10.1016/j.scib.2020.10.021
Yang, W. S., SriRamaratnam, R., Welsch, M. E., Shimada, K., Skouta, R., Viswanathan, V. S., et al. (2014). Regulation of Ferroptotic Cancer Cell Death by GPX4. Cell 156 (1-2), 317–331. doi:10.1016/j.cell.2013.12.010
Yang, Y., Tian, Q., Wu, S., Li, Y., Yang, K., Yan, Y., et al. (2021). Blue Light-Triggered Fe2+-Release from Monodispersed Ferrihydrite Nanoparticles for Cancer Iron Therapy. Biomaterials 271, 120739. doi:10.1016/j.biomaterials.2021.120739
Zhang, C., Bu, W., Ni, D., Zhang, S., Li, Q., Yao, Z., et al. (2016). Synthesis of Iron Nanometallic Glasses and Their Application in Cancer Therapy by a Localized Fenton Reaction. Angew. Chem. Int. Ed. Engl. 55 (6), 2101–2106. doi:10.1002/anie.201510031
Zhang, C., Wang, Q. T., Liu, H., Zhang, Z. Z., and Huang, W. L. (2011). Advancement and Prospects of Tumor Gene Therapy. Chin. J. Cancer 30 (3), 182–188. doi:10.5732/cjc.010.10074
Zhang, F., Li, F., Lu, G. H., Nie, W., Zhang, L., Lv, Y., et al. (2019). Engineering Magnetosomes for Ferroptosis/Immunomodulation Synergism in Cancer. Acs Nano 13 (5), 5662–5673. doi:10.1021/acsnano.9b00892
Zheng, D. W., Lei, Q., Zhu, J. Y., Fan, J. X., Li, C. X., Li, C., et al. (2017). Switching Apoptosis to Ferroptosis: Metal-Organic Network for High-Efficiency Anticancer Therapy. Nano Lett. 17 (1), 284–291. doi:10.1021/acs.nanolett.6b04060
Zhu, J., Dai, P., Liu, F., Li, Y., Qin, Y., Yang, Q., et al. (2020). Upconverting Nanocarriers Enable Triggered Microtubule Inhibition and Concurrent Ferroptosis Induction for Selective Treatment of Triple-Negative Breast Cancer. Nano Lett. 20 (9), 6235–6245. doi:10.1021/acs.nanolett.0c00502
Zhu, L., Zhao, H., Zhou, Z., Xia, Y., Wang, Z., Ran, H., et al. (2018). Peptide-Functionalized Phase-Transformation Nanoparticles for Low Intensity Focused Ultrasound-Assisted Tumor Imaging and Therapy. Nano Lett. 18 (3), 1831–1841. doi:10.1021/acs.nanolett.7b05087
Keywords: ferroptosis, nanomedicine, cancer therapy, combination strategies, ROS
Citation: Wang Y, Liu T, Li X, Sheng H, Ma X and Hao L (2021) Ferroptosis-Inducing Nanomedicine for Cancer Therapy. Front. Pharmacol. 12:735965. doi: 10.3389/fphar.2021.735965
Received: 14 July 2021; Accepted: 23 November 2021;
Published: 20 December 2021.
Edited by:
Sandeep Mittal, The University of Texas MD Anderson Cancer Center, United StatesReviewed by:
Xiao-Yuan Mao, Central South University, ChinaZhijie Xu, Central South University, China
Copyright © 2021 Wang, Liu, Li, Sheng, Ma and Hao. This is an open-access article distributed under the terms of the Creative Commons Attribution License (CC BY). The use, distribution or reproduction in other forums is permitted, provided the original author(s) and the copyright owner(s) are credited and that the original publication in this journal is cited, in accordance with accepted academic practice. No use, distribution or reproduction is permitted which does not comply with these terms.
*Correspondence: Liang Hao, aGFvbGlhbmdkdXRAMTYzLmNvbQ==
†These authors have contributed equally to this work