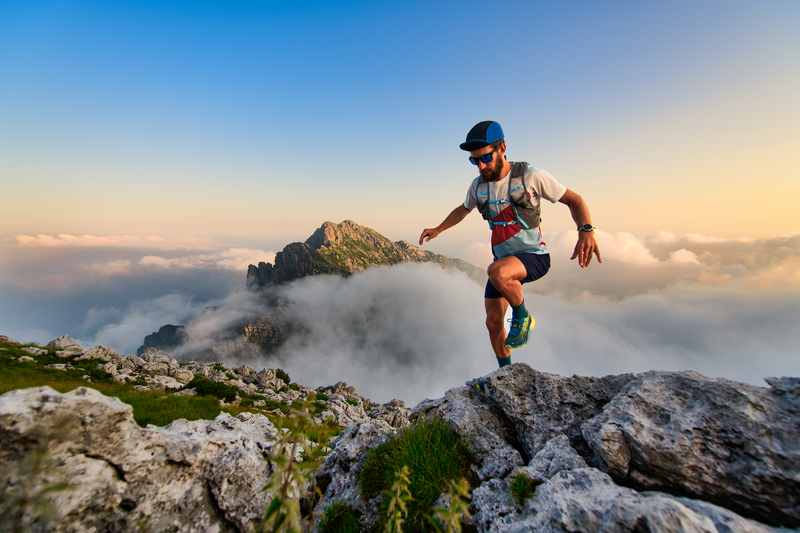
95% of researchers rate our articles as excellent or good
Learn more about the work of our research integrity team to safeguard the quality of each article we publish.
Find out more
ORIGINAL RESEARCH article
Front. Pharmacol. , 27 October 2021
Sec. Pharmacology of Anti-Cancer Drugs
Volume 12 - 2021 | https://doi.org/10.3389/fphar.2021.729367
This article is part of the Research Topic Apoptosis Induction/Suppression: A Feasible Approach for Natural Products to Treatment of Diseases, Volume II View all 28 articles
There is an urgent need for novel agents for colorectal cancer (CRC) due to the increasing number of cases and drug-resistance related to current treatments. In this study, we aim to uncover the potential of chaetocin, a natural product, as a chemotherapeutic for CRC treatment. We showed that, regardless of 5-FU-resistance, chaetocin induced proliferation inhibition by causing G2/M phase arrest and caspase-dependent apoptosis in CRC cells. Mechanically, our results indicated that chaetocin could induce reactive oxygen species (ROS) accumulation and activate c-Jun N-terminal kinase (JNK)/c-Jun pathway in CRC cells. This was confirmed by which the JNK inhibitor SP600125 partially rescued CRC cells from chaetocin induced apoptosis and the ROS scavenger N-acetyl-L-cysteine (NAC) reversed both the chaetocin induced apoptosis and the JNK/c-Jun pathway activation. Additionally, this study indicated that chaetocin could down-regulate the expression of CD47 at both mRNA and protein levels, and enhance macrophages phagocytosis of CRC cells. Chaetocin also inhibited tumor growth in CRC xenograft models. In all, our study reveals that chaetocin induces CRC cell apoptosis, irrelevant to 5-FU sensitivity, by causing ROS accumulation and activating JNK/c-Jun, and enhances macrophages phagocytosis, which suggests chaetocin as a candidate for CRC chemotherapy.
Colorectal cancer (CRC) is one of the most frequently diagnosed cancer in the world (Sung et al., 2021). The clinical options available for CRC include surgery, radiotherapy, chemotherapy, targeted therapy and immunotherapy (Dekker et al., 2019). 5-FU remains the first-line chemo drug for CRC, but resistance and adverse side effects significantly limit its efficacy (Biagi et al., 2011). Although molecular targeted agents like cetuximab, a epidermal growth factor receptor inhibitor, have been approved for CRC patients, high mutation rates of KRAS and BRAF result in unsatisfactory effects of these drugs (Gunjur, 2019). Due to the particular feature of CRC, the effectiveness of immunotherapy includes PD-1/PD-L1 inhibitors is really limited on CRC patients (Ganesh et al., 2019). Therefore, novel agents with better efficacy are urgently needed for CRC treatment.
Reactive oxygen species (ROS) has been proven to participate in many biological events, including cell growth, differentiation and death through interacting with multiple signaling pathways (Holmström and Finkel, 2014). Considerable evidence has showed that various diseases like diabetes mellitus, atherosclerosis and cancer are closely related to the accumulation of ROS (Reuter et al., 2010). Cancer initiation, metastasis and drug resistance may benefit from the moderate increase in ROS levels, but excessive ROS accumulation causes cancer cell death, and cancer cells preserves a higher concentration of ROS comparing to that in their normal counterparts (Tafani et al., 2016; Galadari et al., 2017). Therefore, cancer cells are more vulnerable to ROS accumulation, and redox-targeted agents may be highly efficacious in inducing cancer cell death and can be developed for cancer treatment.
With the deeper understanding of tumor microenvironment, immune checkpoint inhibitors (ICIs) have achieved rapid development (Liu, 2019). Although ICIs including ipilimumab (targets CTLA-4), nivolumab (targets PD-1), and atezolizumab (targets PD-L1) are also approved for CRC by the Food and Drug Administration, due to their limitations such as poor efficiency and drug resistance in CRC treatment, it is also of great importance to study on new immune checkpoint and inhibitors (Franke et al., 2019; Sclafani, 2019). Cluster of differentiation 47 (CD47), also called integrin related proteins (IAP), is a member of the immunoglobulin superfamily. CD47, by binding to signal-regulatory protein α (SIRPα), transmits the signal “Don’t Eat Me” to avoid phagocytosis by immune system (Matlung et al., 2017; Russ et al., 2018). In addition, it is verified that tumor cells overexpress CD47 to escape from immune surveillance in various malignant tumors such as leukemia, lymphoma, breast and colon cancer, and antagonism CD47 has significant anti-tumor effects (Horrigan, 2017; Lian et al., 2019; Hu et al., 2020). As a result, CD47 is considered as an emerging immune checkpoint molecule to be studied. Although some CD47 antibodies are in clinical development currently (Jalil et al., 2020), due to the liabilities exhibited in clinical trials and the limitation of antibodies in modification, searching for small organic molecules that inhibit CD47 should be another effective strategy for the development of immunotherapy (Miller et al., 2019; Burgess et al., 2020).
Natural products are valuable sources for new cancer drug discovery and development. More than 50% of the approved drugs for cancer treatment are of natural origin (Newman and Cragg, 2020). Therefore, exploring efficient anticancer drugs from natural products seems to be an attractive strategy. The natural product chaetocin, which is produced by a species of fungi called Chaetomium (Sekita et al., 1981) has a potent inhibitory effect on various type of cancers, from the result of number of studies (Isham et al., 2007; Lee et al., 2011; Chaib et al., 2012; Dixit et al., 2014; Liu et al., 2015; Wen et al., 2019). Several studies showed that the anticancer effects of chaetocin depended on the accumulation of ROS (Isham et al., 2007; Tibodeau et al., 2009; Dixit et al., 2014; Li et al., 2019; Ozyerli-Goknar et al., 2019; Wen et al., 2019). The growth inhibition of chaetocin in several CRC cell lines has been reported (Isham et al., 2012), indicating the potential of chaetocin as a chemotherapeutic agent. However, the mechanism behind such effects is unclear, and it has not been investigated whether chaetocin could overcome the resistance to 5-FU and whether chaetocin has immunomodulatory effects.
In the present study, we investigated whether the chaetocin’s proliferation inhibition and apoptosis inducing effects extended to CRC cells. In addition, whether this effect would be influenced by 5-FU sensitivity and translated well to animal studies. Mechanism wise, we showed that chaetocin induced apoptosis is channeled by JNK/c-Jun in CRC cells and also chaetocin’s effect in immune surveillance modulation through CD47. Together, the results from this study highlight the potential of chaetocin as a novel chemo agent for CRC patients.
Chaetocin (#C9492, Sigma-Aldrich, St Louis, MO, United States), z-VAD-fmk (#ALX-260–020, Enzo, New York, NY, United States), N-acetyl-L-cysteine (NAC) (#A7250, Sigma-Aldrich) and SP600125 (#S5567, Sigma-Aldrich) were dissolved in DMSO to form the 10mM, 50mM, 0.5M and 20 mM solution respectively. These reagents were stored at −20°C. Primary antibodies against PARP (#9542), caspase-3 (#9662), cleaved-caspase-3 (#9661), caspase-8 (#9746), caspase-9 (#9508), BCL-2 (#15071), BCL-XL (#2764), MCL-1 (#4572), XIAP (#14334), JNK (#9252) and phospho-JNK (Thr183/Tyr185) (#4668) were purchased from Cell Signaling Technology (Beverly, MA, United States). Anti-c-Jun (#ab131497), anti-Ser63 phosphor-c-Jun (#ab28807), anti-CD47 (#ab9089) anti-α-tubulin (#ab233661) and β-actin (#ab8226) were purchased from Abcam (Cambridge, MA, United States), FITC anti-human CD47 (#323106) was purchased from Biolegend (San Diego, CA, United States). Anti-mouse immunoglobulin G (#B900620) and anti-rabbit immunoglobulin G (#B900610) horseradish peroxidase-conjugated secondary antibodies were purchased from Proteintech Group (Chicago, IL, United States).
LIM1215 cell line was obtained from the European Collection of Authenticated Cell Cultures (Salisbury, Wiltshire, United Kingdom). The other CRC cell lines were purchased from Culture Collection of Chinese Academy of Science (Shanghai, China). The 5-FU-resistant HCT-15/5FU-R cell line was established from HCT-15 as previously described (Wen et al., 2015; Wen et al., 2016; Wang et al., 2020). DLD-1, LS174T, Caco-2, LIM1215, HCT-8, SW620, HCT116 and SW480 cells were cultured in RPMI 1640 (Gibco Life Technologies, Carlsbad, CA, United States). HT-29, LoVo, RKO, HCT-15 and HCT-15/5FU-R cells were cultured in DMEM (Gibco Life Technologies) supplemented with 10% fetal bovine serum (Gibco Life Technologies), 100 units/mL penicillin and 10 μg/ml streptomycin (Gibco Life Technologies). Incubations were controlled in a humidified atmospheric environment of 5% CO2 at 37°C.
To test CRC cell viability after chaetocin treatment, CCK-8 assay (Nanjing KeyGen Biotech Co., Ltd., Nanjing, Jiangsu, China) was performed. Cells seeded in 96-well plates were left to grow overnight. The next day, chaetocin at various concentrations were added, and incubated for 24 h. Cells were assessed after 4 h of incubation with 10 μL of CCK-8 solution. The results were carried out using Varioskan Flash multimode reader (Thermo Fisher Scientific, Waltham, MA, United States) at 450 nm.
500 cells/well of HCT116 and LS174T cells and 1,000 cells/well of LIM1215 and HCT–15/5-FU-R cells were seeded in 6-well plates. Chaetocin at various concentration were then added. Plates were left for incubation for different duration. Before imaging, we fixed cells using ice-cold methanol for 5 min and then cells were stained using 0.1% crystal violet. Colonies were counted from images obtained by Epson scanner (Suwa, Nagano, Japan).
To dynamically monitor cell proliferation, xCELLigence system from Roche Applied Science (Mannheim, Baden-Wuerttemberg, Germany) was utilized. Briefly, cells in 100 μL of media were seeded into E-plate and incubated overnight. Chaetocin treatment took place the next day. The impedance measurement was performed according to the manufacturer’s manual. The measurable impedance should increase when cells proliferate.
FACSCanto II flow cytometry (BD Biosciences) with Propidium Iodide (PI, BD Biosciences, Franklin Lakes, NJ, United States) staining was performed. After chaetocin treatment, cells were collected, washed before fixed overnight with 66% cold ethanol at 4°C. After discarding the ethanol, cells were washed with PBS, followed by staining with PI.
Flow cytometry with Annexin V-FITC/PI staining (Nanjing KeyGen Biotech Co., Ltd.) was used to identify the apoptotic population of CRC cells. After chaetocin treated, both suspended and attached cells were collected, washed with PBS and stained in a working solution (500 μL of binding buffer with 5 μL of Annexin V-FITC and 5 μL of PI) for 15 min at room temperature in the dark. FACSCanto II flow cytometry (BD Biosciences) were then carried out, while cells with positive Annexin V-FITC staining were considered as apoptotic cells.
HCT116 cells and LS174T were treated with 0.5 and 1 μM chaetocin for 24 and 12 h respectively. After PBS washed, cells were then incubated with anti-CD47-FITC antibody for 15 min on ice in the dark. The expression of cell surface CD47 was analyzed using FACSCanto II flow cytometry (BD Biosciences).
Total RNA was extracted from chaetocin-treated CRC cells using Trizol reagent (Ambion, Carlsbad, CA, United States). cDNA was then synthesized from 500 ng RNA of each sample using the PrimeScript RT reagent Kit (TaKaRa, Dalian, Liaoning, China). Real-time PCR using SYBR Premix Ex Taq II Kit (TaKaRa) was performed according to manufacturer’s instruction. The specific primers for real-time PCR are as follows: CD47 forward, 5′-TGG TGG GAA ACT ACA CTT GCG-3′; CD47 reverse, 5′- CGT GCG GTT TTT CAG CTC TAT-3′; actin forward, 5′-GTG ACG TTG ACA TCC GTA AAG A-3′; actin reverse, 5′- GCC GGA CTC ATC GTA CTC C-3′; actin was chosen as endogenous control and the CD47 gene expression was analyzed using 2−ΔΔCt method. The CD47 gene expression is shown as fold change of control.
The mitochondrial membrane potential of CRC cells was measured using the JC-1 mitochondrial membrane potential assay kit (Nanjing KeyGen Biotech Co., Ltd.), following the manufacturer’s protocol. In brief, chaetocin treated cells were stained at 37°C for 20 min, then rinsed, using solutions provided in the kit. The fluorescence intensity results were obtained using FACSCanto II flow cytometry (BD Biosciences), whilst mitochondrial JC-1 aggregates which stained red were considered as mitochondria from health cells.
The cells were incubated with 10 μM of 2′,7′-dichlorofluorescin diacetate (DCFH-DA) (Beyotime Institute of Biotechnology, Shanghai, China), a fluorescent probe, at 37°C for 30 min. Following chaetocin treatments, cells were washed with serum-free media and resuspended in 500 μL of PBS before fluorescence measurements using flow cytometry (BD Biosciences).
RIPA lysis buffer (Cell Signaling Technology) containing protease and phosphatase inhibitors (Nanjing KeyGen Biotech Co., Ltd.) was used to extract proteins from chaetocin treated cells. BCA assay (Pierce, Thermo Fisher Scientific) was implemented to ensure a normalised protein concentration. Equal amounts of protein were resolved by SDS-PAGE and were then transferred to nitrocellulose membranes (Merck Millipore, Burlington, MA, United States). Next, the membranes were first blocked by 5% nonfat dry milk, followed by primary antibody incubation overnight at 4°C. After washing the membranes with TBST the next day, HRP-conjugated secondary antibody was applied and left for incubation for 1 h at room temperature before ECL detection. α-tubulin was used as loading control.
Preparation of human macrophages: monocyte-differentiated macrophages were prepared as described previously (Lou et al., 2019). Monocytes extraction from blood of healthy adult donor was done using MACS using anti-CD14 microbeads (Miltenyi Biotec, Auburn, CA, United States). Monocytes were then cultured in RPMI 1640 (Gibco Life Technologies) supplemented with 10% fetal bovine serum (Gibco Life Technologies), 100 units/mL penicillin and 10 μg/ml streptomycin (Gibco Life Technologies), 200 mM glutamine (Gibco Life Technologies) and 25 mM HEPES (Gibco Life Technologies) and treated with 25 ng/ml human recombinant macrophage colony-stimulating factor (M-CSF) for 7 days to differentiate to macrophages.
Preparation of tumor cells: Live HCT116 cells were stained with 5 μM carboxyfluorescein diacetate succinimidyl ester (CFSE) at 37°C for 15 min after 0.5 μM chaetocin treatment for 24 h. After washed with ice-cold D-PBS, stained cells were resuspended in serum-free RPMI 1640.
For in vitro phagocytosis assay, 1 × 105 macrophages were added to 2 × 105 CFSE-stained tumor cells per well in the 24-well plate for 24 h incubation. Cells were then stained with CD14-APC (Ebiosciences, San Diego, CA, United States) and phagocytosis was analyzed by flow cytometry. CD14-APC plus CFSE positive cells were determined as macrophages which had successfully phagocytized tumor cells.
5 × 106 HCT116 cells were injected subcutaneously into the flank of five-week-old female BALB/c nude mice from Guangdong Laboratory Animals Monitoring Institute. After waited 1 week for measurable tumors to form (approximately 50 mm3), the animals were divided randomly into two groups (n = 6 each group) which was either treated with vehicle (10% DMSO, 20% cremophor EL and 70% NaCl, i. p.) or chaetocin (0.5 mg/kg, i. p.) every day for 18 days. Every other day after treatment began, the body weight of the animals and tumor size were taken. The tumor volumes were estimated as the following formula: a2 × b × 0.5, where a is the smallest diameter and b is the diameter perpendicular to a. At the end of the study, the tumors from sacrificed mice were removed, weighed and stored for further experiments. All animal studies conducted were approved by the Institutional Animal Care and Use Committee of Guangdong Laboratory Animals Monitoring Institute.
All experiments were performed at least in triplicate, and the results were shown as mean ± SD where applicable. One-way analysis of variance followed by Tukey’s test by GraphPad Prism 7.00 (GraphPad Software Inc., La Jolla, CA, United States) was used to analyze significant differences among the results. *p < 0.05 was considered statistically significant.
Chaetocin has a thiodioxopiperazine structure as shown in Supplementary Figure 1 (Wen et al., 2019). Firstly, the cytotoxic effects of chaetocin were tested on various CRC cells. After treated with chaetocin for 24 h at increasing concentrations, from 0 to 2 μM, CCK8 results showed a progressive inhibition in cell viability across a dozen of various CRC cell lines, with IC50 values between 0.052 and 0.64 μM (Figure 1A and Table 1). Real-time cell analysis showed that the normalized cell index was inhibited by chaetocin in a dose-dependent manner in HCT116, LS174T and LIM1215 cells (Figure 1B), indicating that the cell proliferation of CRC cells was markedly restrained by chaetocin. Moreover, colony formation assay also revealed a decrease in both size and numbers in CRC colonies (Figure 1C). Collectively, our results demonstrated that chaetocin suppressed CRC cell growth in vitro. To determine whether chaetocin achieved this by inducing cell cycle arrest, cell cycle distribution analysis was carried out using flow cytometry. As shown in Figure 1D, after HCT116, LS174T and LIM1215 cells were treated with chaetocin for 12 h, the population of HCT116, LS174T and LIM1215 cells at the G2/M phase increased substantially, indicating that chaetocin Inhibits CRC cell proliferation by prompting G2/M phase arrest.
FIGURE 1. Chaetocin Inhibits cell proliferation and causes G2/M phase arrest. (A) Followed by treated with chaetocin at increasing concentrations for 24 h, CCK8 assay was used to measure the viability of CRC cells.(B) Real-time cell analysis results on the proliferation of HCT116, LS174T and LIM1215 cells. (C) After treated with chaetocin for indicated days, number of new colonies formed were counted. (D) Cell cycle distribution analysis on CRC cell lines after 12 h chaetocin treatment at various concentrations detected by PI-stained flow cytometry. For (C) and (D), results were shown as mean ± SD of three independent experiments. *p < 0.05, **p < 0.01, ***p < 0.001, versus control group.
Our previous studies have shown that chaetocin induces apoptosis in gastric cancer cell lines HGC-27 and AGS (Wen et al., 2019), whether the same would occur in CRC cells was investigated. After chaetocin treated, as shown in Figure 2A, flow cytometry exhibited that chaetocin increased the apoptotic cell populations in HCT116, LS174T and LIM1215 cells, and the apoptotic population increases as the duration of treatment lengthened. Because caspase pathway activation is crucial to apoptosis (Mandal et al., 2020), caspase family proteins were then assessed by western blot analysis. Chaetocin decreased the levels of the precursor forms of caspase-3, -8, and -9 but increased cleavage of those proteins in HCT116, LS174T and LIM1215 cells (Figure 2B). Consistently, chaetocin induced PARP cleavage which is the known downstream protein of caspase pathway (Figure 2B), illustrating that chaetocin induces CRC cell apoptosis which may depend on caspase activation. When apoptosis occurs, the integrity of the mitochondrial membrane will decrease, a phenomenon recognized as an indicator of apoptosis (Lindsay et al., 2011). As shown in Figure 2C, the integrity of the mitochondrial membrane was disrupted in HCT116, LS174T and LIM1215 cells after chaetocin treatment. To further confirm this, western blot was performed to evaluate the expression of several antiapoptotic proteins (BCL-2, BCL-XL, MCL-1 and XIAP) in CRC cells lines (Figure 2D). The decrease in antiapoptotic proteins expression further established that chaetocin induces apoptosis in CRC cells. Lastly, z-VAD-fmk, a pan-caspase inhibitor, was implemented as a pretreatment. After the caspase pathway was restrained by z-VAD-fmk (Supplementary Figure 2), the apoptotic cell population following treatment with chaetocin was significantly restored in CRC cells (Figure 2E), indicating that chaetocin-elicited apoptosis of CRC cells depends on caspase activation.
FIGURE 2. Chaetocin induces caspase-dependent apoptosis in CRC cells. (A) Flow cytometry results with Annexin V-FITC/PI staining on apoptotic population of HCT116, LS174T and LIM1215 after chaetocin treatment. (B) Western blot analysis of PARP, caspase-3, capase-9 and caspase-8 expression levels in HCT116, LS174T and LIM1215 cells. (C) Flow cytometry results with JC-1 staining on three CRC cell lines. (D) Western blot results to evaluate the protein level of BCL-2, BCL-XL, MCL-1 and XIAP in CRC cell lines. (E) A 2 h z-VAD-fmk pretreatment could rescue some HCT116 and LS174T cells from apoptosis induced by chaetocin. For (A), (C) and (E), results were shown as mean ± SD of three independent experiments. *p < 0.05, **p < 0.01, ***p < 0.001. For (A) and (C), comparison was against 0 h group.
It was previously reported that chaetocin induces apoptosis through the accumulation of ROS in gastric cancer, glioma, and myeloma cells (Isham et al., 2007; Dixit et al., 2014; Wen et al., 2019). The fluorescent probe DCFH/DA was first used to validate that the ROS level rises after chaetocin treatment in HCT116 and LS174T cells (Figure 3A). Next, a ROS scavenger, NAC was used. Flow cytometry analysis showed that NAC almost abolished chaetocin-induced apoptosis in HCT116 and LS174T cells (Figure 3B). Moreover, PARP and caspase-3 cleavage induced by chaetocin were reversed when cells were cotreated with NAC (Figure 3C). These results suggest that Chaetocin-induced apoptosis in CRC cells relies on ROS accumulation.
FIGURE 3. Chaetocin-induced ROS accumulation is required for cell apoptosis. (A) ROS levels of HCT116 and LS174T cells were verified using flow cytometry, after DCFH-DA labeling and chaetocin treatments. HCT116 and LS174T cells were pretreated with NAC for 2 h and then cotreated with chaetocin for another indicated times. (B) Flow cytometry analysis on apoptotic CRC cells. (C) Western blot of PARP and caspase-3 protein level. For (A) and (B), results were shown as mean ± SD of three independent experiments. *p < 0.05, **p < 0.01, ***p < 0.001, versus control group.
It is well known that ROS induce cancer cell apoptosis by activating the JNK/c-Jun pathway (Aggarwal et al., 2019). To explore whether chaetocin-induced apoptosis take on the same ROS/JNK/c-Jun route, several experiments were carried out. First, western blot results showed a markedly increase in JNK and c-Jun phosphorylation after chaetocin treatment (Figure 4A), suggesting JNK/c-Jun pathway activation. SP600125, owing to its ability to suppress JNK/c-Jun pathway, was then used to prove that chaetocin-induced cell death depends on JNK/c-Jun activation. As shown by the results of flow cytometry and western blot, SP600125 partially restored chaetocin-induced apoptosis in HCT116 and LS174T cells (Figures 4B,C). We finally investigated if ROS accumulation induced by chaetocin relates to JNK/c-Jun activation. As shown in Figure 4D, NAC restored the abnormal increase in phosphorylation of JNK and c-Jun after chaetocin treatment. The above results suggest that Chaetocin induces cell death through the ROS/JNK/c-Jun axis.
FIGURE 4. The involvement of ROS/JNK/c-Jun in ROS-mediated cell apoptosis. (A) The protein level of JNK and c-Jun in HCT116 and LS174T cells after chaetocin treatment by western blot. CRC cells were pretreated with SP600125 for 2 h before chaetocin treatment for indicated hours. (B) Cell apoptosis was measured by flow cytometry. Results were shown as mean ± SD of three independent experiments. **p < 0.01, ***p < 0.001. (C) Expression of PARP and caspase-3 was analyzed by western blot. (D) The expression levels of JNK and c-Jun after NAC pretreatment for 2 h and then cotreatment of chaetocin for 12 h in HCT116 and LS174T cells were analyzed by western blot.
We then explored whether chaetocin’s effect could stretch into 5-FU-resistant CRC cells, using cell lines that has already been established by our group (Wen et al., 2015; Wen et al., 2016). A similar and decent IC50 value of 0.054 μM was exhibited in the CCK8 cell viability assay (Figure 5A). Moreover, the same dose-dependent colony formation inhibition pattern could be observed in Figure 5B as well as the Increase in G2/M population as shown in Figure 5C. Flow cytometry and western blot results also certified that chaetocin induced apoptosis in HCT-15/5FU-R cells by caspase activation like that in normal CRC cells Figures 5D,E. These results illustrated that chaetocin could by pass 5-FU resistance in CRC cells.
FIGURE 5. Chaetocin overcomes 5-FU resistance in CRC cells. (A) The CCK8 cell viability assay on 5-FU resistant and sensitive HCT-15 cell lines. (B) After 7 days chaetocin treatment, colony formation result of HCT-15/5FU-R cells. (C) Cell cycle distribution results of HCT-15/5FU-R cells after 12 h chaetocin treatment at indicated concentration were analyzed by flow cytometry with PI staining. (D) Apoptotic population analysis by flow cytometry with Annexin V-FITC/PI staining of HCT-15 and HCT-15/5FU-R cells, after 0.5 μM chaetocin. (E) Western blot results on PARP and caspase-3 expression level in HCT-15/5FU-R cells. For a, (B), (C) and (D), results were shown as mean ± SD of three independent experiments. *p < 0.05, **p < 0.01, ***p < 0.001, versus control group.
To check that chaetocin induces cell death through the same pathway in 5-FU resistant strains of CRC, similar experiments were performed in HCT-15/5FU-R cells. Similar increase in ROS was detected in HCT-15/5FU-R cells (Figure 6A). NAC overturned the apoptotic effect induced by chaetocin (Figure 6B). The above results established that chaetocin induces accumulation of ROS and ultimately leads to apoptosis. Moreover, the increase in phosphorylation of JNK and c-Jun confirms that chaetocin leads to JNK/c-Jun pathway activation in HCT-15/5FU-R cells (Figure 6C). Also SP600125 was able to partially reverse the effect of chaetocin-induced apoptosis (Figures 6D,E). In addition, chaetocin was no longer able to induce JNK/c-Jun activation after the ROS in cells were scavenged by NAC (Figure 6F). The above results illustrated that chaetocin Induces apoptosis in HCT-15/5FU-R cells through the same ROS/JNK/c-Jun axis as in 5-FU-sensitive CRC cell lines.
FIGURE 6. Chaetocin-induced apoptosis in HCT-15/5FU-R cells is associated with the ROS/JNK/c-Jun pathway. (A) The ROS levels of HCT-15/5FU-R cells. Results were shown as mean ± SD of three independent experiments. ***p < 0.001, versus control group. (B) HCT-15/5FU-R cells apoptosis induced by chaetocin were rescued by 2 h of NAC pretreatment (***p < 0.001). (C) The expression level JNK and c-Jun protein in 5-FU-resistant CRC cells by western blot after chaetocin treatment. (D) HCT-15/5FU-R cells were cotreated with chaetocin for 24 h after 2 h SP600125 preincubation. Flow cytometry were used analyze the rescued population (***p < 0.001). (E) After pretreated with SP600125 for 2 h and then cotreated with chaetocin for another 6 h, the expression of JNK and c-Jun in HCT-15/5FU-R cells were analyzed by western blot. (F) The expression levels of JNK and c-Jun in HCT-15/5FU-R cells were evaluated by western blot after 2 h of NAC pretreatment followed by chaetocin cotreatment for 12 h.
In recent years, more and more studies have shown that CD47 could be one of the key immune checkpoint molecules in tumor therapy (Lian et al., 2019; Hayat et al., 2019). The present study showed that chaetocin not only reduced the expression of CD47 at mRNA levels (Figure 7A), but also inhibited the expression of total CD47 protein and membrane CD47 protein in CRC cells (Figures 7B,C). The highly expressed CD47 on the surface enables tumor cells to evade from immune surveillance of macrophages (Russ et al., 2018). Our results showed that macrophages had an enhanced phagocytosis of HCT116 cells which were pretreated with chaetocin (Figure 7D), indicating that chaetocin could reduce the expression of CD47 in CRC cells and enhance macrophages phagocytosis of CRC cells. As ROS/JNK/c-Jun involvement in chaetocin treatment was established in our study, whether the expression of CD47 was down-regulated through ROS/JNK/c-Jun signaling pathway was then investigated. When NAC was used to scavenge ROS in chaetocin-treated HCT116 cells, the inhibitory effects of CD47 at mRNA and protein levels were both partially reversed (Figures 7E,F), indicating that ROS accumulation down-regulated the expression of CD47 in CRC cells at transcriptional levels. Although SP600125 did not reverse the reduction of CD47 at mRNA level, it reversed the reduction of CD47 at protein level in HCT116 cells (Figures 7G,H), indicating a post-transcriptional regulation of JNK/c-Jun on CD47. All these results illustrated that chaetocin decreased the expression of CD47 at both mRNA and protein levels in CRC cells and enhanced macrophages phagocytosis.
FIGURE 7. Chaetocin decreases the expression of CD47 in CRC cells and enhances the phagocytosis of macrophages. (A) The expression of CD47 at mRNA levels was detected by qPCR after HCT116 and LS174T cells treated with chaetocin (0.5 μM chaetocin for HCT116 cells and 1 μM chaetocin for LS174T cells) for the indicated times. *p < 0.05, ***p < 0.001. (B) The expression of total CD47 protein was analyzed by western blot after chaetocin treatment. (C) The expression of membrane CD47 protein was detected by flow cytometry after HCT116 and LS174T were treated as described (*p < 0.05, **p < 0.01). (D) HCT116 cells were pretreated with 0.5 μM chaetocin for 24 h and then co-cultured with macrophages for another 24 h, the phagocytosis of HCT116 cells by macrophages was detected by flow cytometry. *p < 0.05. HCT116 cells were pretreated with 1 mM NAC for 2 h and then co-treated with 0.5 μM chaetocin for another 12 h (E) The mRNA level of CD47 was detected by qPCR. *p < 0.05, ***p < 0.001. (F) The expression of total CD47 protein was detected by western blot. SP600125 rescue analysis was done as previously mentioned. (G) mRNA expression of CD47 detection by qPCR (***p < 0.001). (H) The amount of CD47 protein expressed was evaluated by western blot.
The role of chaetocin in tumor growth was assessed in vivo using a nude mouse xenograft model by subcutaneously inoculating them with HCT116 cells. Mice were treated with vehicle and chaetocin (0.5 mg/kg) by intraperitoneal injection every day for 18 days. As shown in Figures 8A–C, the volume and weight of tumor in HCT116 xenografts were effectively suppressed in the chaetocin-treated group compared with control group, suggesting that chaetocin effects tumor proliferation both in vitro and in vivo. The protein levels of pro-caspase-3 were decreased in chaetocin-treated tumors, while p-JNK levels were increased (Figure 8D), indicating that chaetocin induces cell apoptosis like in vitro, undertaking the JNK/c-Jun pathway in CRC cell xenografts.
FIGURE 8. Chaetocin inhibits the growth of CRC cell xenografts. Nude mice subcutaneously inoculated with HCT116 cells were subjected to chaetocin (0.5 mg/kg/day) treatment for 18 days, control group was treated with vehicle. (A) Tumor growth curves were recorded every other day.(B) and (C) The image of tumor tissue at the end of the experiment. (D) The protein levels of pro-caspase-3 and JNK in tumor tissues were detected by western blot. For (A) and (C), results were shown as mean ± SD of six mice in each group. *p < 0.05, versus vehicle group.
Novel agents for CRC treatment are urgently needed to be developed, and natural products are important sources for new cancer drug discovery and development. In the present study, by investigating the natural product chaetocin, we found that chaetocin not only caused cell cycle arrest and induced cell death both in vitro and in vivo with decent efficacy, it also downregulated CD47 and the consequent phagocytosis by macrophages in CRC cells. This study highlights the promising role of chaetocin for CRC treatment.
It has been reported that basal ROS levels are higher in CRC than that in the normal counterparts (Haklar et al., 2001; Rainis et al., 2007), and CRC cells are more vulnerable to the accumulation of ROS, inducing intracellular ROS accumulation should be an efficient strategy for CRC treatment. Chaetocin has been found to cause ROS accumulation and hence leads to significant growth inhibition in various cancer cells such as gastric cancer cells, glioma and leukemia cells (Isham et al., 2007; Truitt et al., 2014; Wen et al., 2019). Consistent with these reports, we found that chaetocin also significantly increased ROS levels in CRC cells (Figure 3A) and chaetocin-induced CRC cells apoptosis was almost abrogated by the ROS scavenger NAC (Figures 3B,C).
Tibodeau et al. has reported that chaetocin is a competitive inhibitor of thioredoxin reductase (TRXR), an enzyme that decrease intracellular ROS levels in an extracellular model (Tibodeau et al., 2009), which is consistent with the results of our previous study that chaetocin lead to ROS accumulation by inactivating TRXR in GC cells (Wen et al., 2019). But in our CRC cells, chaetocin was unable to inactivate with TRXR (data not shown), even though the ROS levels in CRC cells with chaetocin treatment rose up distinctly. These results indicated that chaetocin elevated ROS in different ways owning to the model, and the direct target of chaetocin to elevate ROS in CRC cells remains to be verified. Hypoxia-inducible factor-1α (HIF-1α) have also been reported to be the target of chaetocin in hepatoma (Lee et al., 2011), however, this HIF-1α-targeting mechanism does not hold in other cancer cells (Isham et al., 2012). Similarly, Chaetocin has also been reported to directly inhibit the methyl-transferase for lysine nine tri-methylation on histone H3 (SUV39H1) in acute myeloid leukemia (Lai et al., 2015), but some other studies reported that the inhibition of chaetocin in SU(VAR)three to nine was regulated by the accumulation of ROS induced by chaetocin (Chaib et al., 2012; Dixit et al., 2014; Zhang et al., 2017). Thus, we speculated that chaetocin exerts its multiple functions by inducing ROS accumulation.
It is widely known that JNK is an important downstream effector of ROS and induces apoptosis mainly by affecting cell mitochondria (Castro-Caldas et al., 2012; Sinha et al., 2013). Consistent with the report that chaetocin induces apoptosis via JNK activation in glioma cells (Dixit et al., 2014), chaetocin-activated JNK and c-Jun in CRC cells were also detected in our study (Figure 4A). Moreover, ROS inhibitor NAC reversed both the JNK/c-Jun activation and apoptosis (Figure 4D) further strengthened our speculation that chaetocin-induced CRC cell apoptosis through the ROS/JNK/c-Jun pathway. However, since SP600125, a JNK inhibitor, could not fully rescue CRC cells from apoptosis, this calls for future studies to fully reveal the whole picture of chaetocin-induced apoptosis in CRC cells.
Although 5-FU is able to induce cancer cell death by upregulating ROS levels, by genetic and epigenetic alterations, 5-FU-resistant CRC cells avoid the death threshold induced by the accumulation of ROS (Hwang et al., 2001; Kang et al., 2014). In this case, 5-FU-resistant CRC cells were able to withstand higher level of ROS, and hence may render them more sensitive to redox-targeted agents (Kang et al., 2014; Yao et al., 2017), which is consistent with our results (Supplementary Figure 4 and Figures 5A,D). Therefore, chaetocin may be a promising agent in overcoming 5-FU resistance.
Different from what Lu et al. reported that chaetocin downregulated PD-L1 by inhibiting a histone-lysine N-methyltransferase 2A (Lu et al., 2017), our study indicated that chaetocin downregulated the expression of CD47 at both mRNA and protein levels via the accumulation of ROS. However, SP600125 was only able to reverse the inhibitory effect of chaetocin on CD47 at protein level, not mRNA level, in CRC cells (Figures 7G,H), which indicated that JNK/c-Jun could not regulate the expression of CD47 in CRC cells transcriptionally. It has been reported that the mRNA expression of CD47 could be regulated by NF-κB pathway in liver cancer cells (Lo et al., 2015) and Wnt/β-catenin in glioma cell (Gowda et al., 2018), which could both be regulated by ROS (Vallée and Lecarpentier, 2018; Capece et al., 2019). Combining the results of this study with those reported by others, here is a speculation that chaetocin regulates the expression of CD47 by regulating NF-κB and Wnt/β-catenin pathways via the accumulation of ROS. However, the regulation of CD47 by chaetocin in CRC cells remains further exploration. Although the enhancement of macrophage phagocytosis of CRC cells induced by chaetocin is shown in our study, this effect of chaetocin still needs further verification in animals.
This study not only showed the cytotoxic effects of chaetocin on killing CRC cells ignoring 5-FU resistance, but also showed the reduction of CD47 and enhancement of macrophages phagocytosis of CRC cells induced by chaetocin (As shown in the graph abstract). Our research confirmed the excellent anti-tumor effect of chaetocin in CRC with “double dimensions”, highlighting the potential of chaetocin as a promising agent for CRC treatment.
The original contributions presented in the study are included in the article/Supplementary Material, further inquiries can be directed to the corresponding authors.
The studies involving human participants were reviewed and approved by The Medical Ethics Committee of the Sixth Affiliated Hospital of Sun Yat-sen University, Guangzhou, China. Written informed consent for participation was not required for this study in accordance with the national legislation and the institutional requirements. The animal study was reviewed and approved by The Public Health Service Policy on Humane Care of Laboratory Animals and approved by the Institution Animal Care and Use Committee (IACUC) at Guangdong Laboratory Animals Monitoring Institute.
HW: Conceptualization, Investigation, Writing—original draft. CW: Conceptualization, Validation, Writing—review and editing. SC: Investigation, Methodology. WL: Investigation, Writing - review and editing. QQ: Methodology, Writing - review and editing. LH: Investigation. FW: Investigation, Validation. JC: Validation. WY: Writing - review and editing. WL: Project administration. JP: Resources. XY: Conceptualization, Supervision. HL: Conceptualization, Supervision.
This work was supported by China Postdoctoral Science Foundation (grant number 2020M683114); National Natural Science Foundation of China (grant number 81672413); Project to Attract High Level Foreign Experts (grant number G20190019023) and National Key Clinical Discipline.
The authors declare that the research was conducted in the absence of any commercial or financial relationships that could be construed as a potential conflict of interest.
All claims expressed in this article are solely those of the authors and do not necessarily represent those of their affiliated organizations, or those of the publisher, the editors and the reviewers. Any product that may be evaluated in this article, or claim that may be made by its manufacturer, is not guaranteed or endorsed by the publisher.
The Supplementary Material for this article can be found online at: https://www.frontiersin.org/articles/10.3389/fphar.2021.729367/full#supplementary-material
Aggarwal, V., Tuli, H. S., Varol, A., Thakral, F., Yerer, M. B., Sak, K., et al. (2019). Role of Reactive Oxygen Species in Cancer Progression: Molecular Mechanisms and Recent Advancements. Biomolecules 9. doi:10.3390/biom9110735
Biagi, J. J., Raphael, M. J., Mackillop, W. J., Kong, W., King, W. D., and Booth, C. M. (2011). Association between Time to Initiation of Adjuvant Chemotherapy and Survival in Colorectal Cancer: a Systematic Review and Meta-Analysis. JAMA 305, 2335–2342. doi:10.1001/jama.2011.749
Burgess, T. L., Amason, J. D., Rubin, J. S., Duveau, D. Y., Lamy, L., Roberts, D. D., et al. (2020). A Homogeneous SIRPα-CD47 Cell-Based, Ligand-Binding Assay: Utility for Small Molecule Drug Development in Immuno-Oncology. PLoS One 15, e0226661. doi:10.1371/journal.pone.0226661
Capece, D., Verzella, D., Di Francesco, B., Alesse, E., Franzoso, G., and Zazzeroni, F. (2019). NF-kappaB and Mitochondria Cross Paths in Cancer: Mitochondrial Metabolism and beyond. Semin. Cel Dev Biol 98, 118–128. doi:10.1016/j.semcdb.2019.05.021
Castro-Caldas, M., Carvalho, A. N., Rodrigues, E., Henderson, C., Wolf, C. R., and Gama, M. J. (2012). Glutathione S-Transferase Pi Mediates MPTP-Induced C-Jun N-Terminal Kinase Activation in the Nigrostriatal Pathway. Mol. Neurobiol. 45, 466–477. doi:10.1007/s12035-012-8266-9
Chaib, H., Nebbioso, A., Prebet, T., Castellano, R., Garbit, S., Restouin, A., et al. (2012). Anti-leukemia Activity of Chaetocin via Death Receptor-dependent Apoptosis and Dual Modulation of the Histone Methyl-Transferase SUV39H1. Leukemia 26, 662–674. doi:10.1038/leu.2011.271
Dekker, E., Tanis, P. J., Vleugels, J. L. A., Kasi, P. M., and Wallace, M. B. (2019). Colorectal Cancer. Lancet 394, 1467–1480. doi:10.1016/S0140-6736(19)32319-0
Dixit, D., Ghildiyal, R., Anto, N. P., and Sen, E. (2014). Chaetocin-induced ROS-Mediated Apoptosis Involves ATM-YAP1 axis and JNK-dependent Inhibition of Glucose Metabolism. Cell Death Dis 5, e1212. doi:10.1038/cddis.2014.179
Franke, A. J., Skelton, W. P., Starr, J. S., Parekh, H., Lee, J. J., Overman, M. J., et al. (2019). Immunotherapy for Colorectal Cancer: A Review of Current and Novel Therapeutic Approaches. J. Natl. Cancer Inst. 111, 1131–1141. doi:10.1093/jnci/djz093
Galadari, S., Rahman, A., Pallichankandy, S., and Thayyullathil, F. (2017). Reactive Oxygen Species and Cancer Paradox: To Promote or to Suppress. Free Radic. Biol. Med. 104, 144–164. doi:10.1016/j.freeradbiomed.2017.01.004
Ganesh, K., Stadler, Z. K., Cercek, A., Mendelsohn, R. B., Shia, J., Segal, N. H., et al. (2019). Immunotherapy in Colorectal Cancer: Rationale, Challenges and Potential. Nat. Rev. Gastroenterol. Hepatol. 16, 361–375. doi:10.1038/s41575-019-0126-x
Gowda, P., Patrick, S., Singh, A., Sheikh, T., and Sen, E. (2018). Mutant Isocitrate Dehydrogenase 1 Disrupts PKM2-β-Catenin-BRG1 Transcriptional Network-Driven CD47 Expression. Mol. Cel Biol 38, e00001. doi:10.1128/MCB.00001-18
Gunjur, A. (2019). Targeted Therapy for BRAF-Mutant Colorectal Cancer. Lancet Oncol. 20, e618. doi:10.1016/S1470-2045(19)30644-8
Haklar, G., Sayin-Ozveri, E., Yüksel, M., Aktan, A. O., and Yalçin, A. S. (2001). Different Kinds of Reactive Oxygen and Nitrogen Species Were Detected in colon and Breast Tumors. Cancer Lett. 165, 219–224. doi:10.1016/s0304-3835(01)00421-9
Hayat, S. M. G., Bianconi, V., Pirro, M., Jaafari, M. R., Hatamipour, M., and Sahebkar, A. (2019). CD47: Role in the Immune System and Application to Cancer Therapy. Cel Oncol (Dordr) 43 (1), 19–30. doi:10.1007/s13402-019-00469-5
Holmström, K. M., and Finkel, T. (2014). Cellular Mechanisms and Physiological Consequences of Redox-dependent Signalling. Nat. Rev. Mol. Cel Biol 15, 411–421. doi:10.1038/nrm3801
Horrigan, S. K. (2017). Replication Study: The CD47-Signal Regulatory Protein Alpha (SIRPa) Interaction Is a Therapeutic Target for Human Solid Tumors. Elife 6, e18173. doi:10.7554/eLife.18173
Hu, T., Liu, H., Liang, Z., Wang, F., Zhou, C., Zheng, X., et al. (2020). Tumor-intrinsic CD47 Signal Regulates Glycolysis and Promotes Colorectal Cancer Cell Growth and Metastasis. Theranostics 10, 4056–4072. doi:10.7150/thno.40860
Hwang, P. M., Bunz, F., Yu, J., Rago, C., Chan, T. A., Murphy, M. P., et al. (2001). Ferredoxin Reductase Affects P53-dependent, 5-Fluorouracil-Induced Apoptosis in Colorectal Cancer Cells. Nat. Med. 7, 1111–1117. doi:10.1038/nm1001-1111
Isham, C. R., Tibodeau, J. D., Bossou, A. R., Merchan, J. R., and Bible, K. C. (2012). The Anticancer Effects of Chaetocin Are Independent of Programmed Cell Death and Hypoxia, and Are Associated with Inhibition of Endothelial Cell Proliferation. Br. J. Cancer 106, 314–323. doi:10.1038/bjc.2011.522
Isham, C. R., Tibodeau, J. D., Jin, W., Xu, R., Timm, M. M., and Bible, K. C. (2007). Chaetocin: a Promising New Antimyeloma Agent with In Vitro and In Vivo Activity Mediated via Imposition of Oxidative Stress. Blood 109, 2579–2588. doi:10.1182/blood-2006-07-027326
Jalil, A. R., Andrechak, J. C., and Discher, D. E. (2020). Macrophage Checkpoint Blockade: Results from Initial Clinical Trials, Binding Analyses, and CD47-Sirpα Structure-Function. Antib Ther. 3, 80–94. doi:10.1093/abt/tbaa006
Kang, K. A., Piao, M. J., Kim, K. C., Kang, H. K., Chang, W. Y., Park, I. C., et al. (2014). Epigenetic Modification of Nrf2 in 5-Fluorouracil-Resistant colon Cancer Cells: Involvement of TET-dependent DNA Demethylation. Cel Death Dis 5, e1183. doi:10.1038/cddis.2014.149
Lai, Y. S., Chen, J. Y., Tsai, H. J., Chen, T. Y., and Hung, W. C. (2015). The SUV39H1 Inhibitor Chaetocin Induces Differentiation and Shows Synergistic Cytotoxicity with Other Epigenetic Drugs in Acute Myeloid Leukemia Cells. Blood Cancer J. 5, e313. doi:10.1038/bcj.2015.37
Lee, Y. M., Lim, J. H., Yoon, H., Chun, Y. S., and Park, J. W. (2011). Antihepatoma Activity of Chaetocin Due to Deregulated Splicing of Hypoxia-Inducible Factor 1α Pre-mRNA in Mice and In Vitro. Hepatology 53, 171–180. doi:10.1002/hep.24010
Li, Z., Huang, L., Wei, L., Hou, Z., Ye, W., and Huang, S. (2019). Chaetocin Induces Caspase-dependent Apoptosis in Ovarian Cancer Cells via the Generation of Reactive Oxygen Species. Oncol. Lett. 18, 1915–1921. doi:10.3892/ol.2019.10507
Lian, S., Xie, X., Lu, Y., and Jia, L. (2019). Checkpoint CD47 Function on Tumor Metastasis and Immune Therapy. Onco Targets Ther. 12, 9105–9114. doi:10.2147/OTT.S220196
Lindsay, J., Esposti, M. D., and Gilmore, A. P. (2011). Bcl-2 Proteins and Mitochondria-Sspecificity in Membrane Targeting for Death. Biochim. Biophys. Acta 1813, 532–539. doi:10.1016/j.bbamcr.2010.10.017
Liu, D. (2019). Cancer Biomarkers for Targeted Therapy. Biomark Res. 7, 25. doi:10.1186/s40364-019-0178-7
Liu, X., Guo, S., Liu, X., and Su, L. (2015). Chaetocin Induces Endoplasmic Reticulum Stress Response and Leads to Death Receptor 5-dependent Apoptosis in Human Non-small Cell Lung Cancer Cells. Apoptosis 20, 1499–1507. doi:10.1007/s10495-015-1167-4
Lo, J., Lau, E. Y., Ching, R. H., Cheng, B. Y., Ma, M. K., Ng, I. O., et al. (2015). Nuclear Factor Kappa B-Mediated CD47 Up-Regulation Promotes Sorafenib Resistance and its Blockade Synergizes the Effect of Sorafenib in Hepatocellular Carcinoma in Mice. Hepatology 62, 534–545. doi:10.1002/hep.27859
Lou, Q., Liu, R., Yang, X., Li, W., Huang, L., Wei, L., et al. (2019). miR-448 Targets Ido1 and Regulates CD8+ T Cell Response in Human colon Cancer. J. Immunother. Cancer 7, 210. doi:10.1186/s40425-019-0691-0
Lu, C., Paschall, A. V., Shi, H., Savage, N., Waller, J. L., Sabbatini, M. E., et al. (2017). The MLL1-H3K4me3 Axis-Mediated PD-L1 Expression and Pancreatic Cancer Immune Evasion. J. Natl. Cancer Inst. 109, djw283. doi:10.1093/jnci/djw283
Mandal, R., Barrón, J. C., Kostova, I., Becker, S., and Strebhardt, K. (2020). Caspase-8: The Double-Edged Sword. Biochim. Biophys. Acta Rev. Cancer 1873, 188357. doi:10.1016/j.bbcan.2020.188357
Matlung, H. L., Szilagyi, K., Barclay, N. A., and van den Berg, T. K. (2017). The CD47-Sirpα Signaling axis as an Innate Immune Checkpoint in Cancer. Immunol. Rev. 276, 145–164. doi:10.1111/imr.12527
Miller, T. W., Amason, J. D., Garcin, E. D., Lamy, L., Dranchak, P. K., Macarthur, R., et al. (2019). Quantitative High-Throughput Screening Assays for the Discovery and Development of SIRPα-CD47 Interaction Inhibitors. PLoS One 14, e0218897. doi:10.1371/journal.pone.0218897
Newman, D. J., and Cragg, G. M. (2020). Natural Products as Sources of New Drugs over the Nearly Four Decades from 01/1981 to 09/2019. J. Nat. Prod. 83, 770–803. doi:10.1021/acs.jnatprod.9b01285
Ozyerli-Goknar, E., Sur-Erdem, I., Seker, F., Cingöz, A., Kayabolen, A., Kahya-Yesil, Z., et al. (2019). The Fungal Metabolite Chaetocin Is a Sensitizer for Pro-apoptotic Therapies in Glioblastoma. Cel Death Dis 10, 894. doi:10.1038/s41419-019-2107-y
Rainis, T., Maor, I., Lanir, A., Shnizer, S., and Lavy, A. (2007). Enhanced Oxidative Stress and Leucocyte Activation in Neoplastic Tissues of the colon. Dig. Dis. Sci. 52, 526–530. doi:10.1007/s10620-006-9177-2
Reuter, S., Gupta, S. C., Chaturvedi, M. M., and Aggarwal, B. B. (2010). Oxidative Stress, Inflammation, and Cancer: How Are They Linked. Free Radic. Biol. Med. 49, 1603–1616. doi:10.1016/j.freeradbiomed.2010.09.006
Russ, A., Hua, A. B., Montfort, W. R., Rahman, B., Riaz, I. B., Khalid, M. U., et al. (2018). Blocking "don't Eat Me" Signal of CD47-Sirpα in Hematological Malignancies, an In-Depth Review. Blood Rev. 32, 480–489. doi:10.1016/j.blre.2018.04.005
Sclafani, F. (2019). MEK and PD-L1 Inhibition in Colorectal Cancer: a Burning Blaze Turning into a Flash in the pan. Lancet Oncol. 20, 752–753. doi:10.1016/S1470-2045(19)30076-2
Sekita, S., Yoshihira, K., Natori, S., Udagawa, S., Muroi, T., Sugiyama, Y., et al. (1981). Mycotoxin Production by Chaetomium Spp. And Related Fungi. Can. J. Microbiol. 27, 766–772. doi:10.1139/m81-119
Sinha, K., Das, J., Pal, P. B., and Sil, P. C. (2013). Oxidative Stress: the Mitochondria-dependent and Mitochondria-independent Pathways of Apoptosis. Arch. Toxicol. 87, 1157–1180. doi:10.1007/s00204-013-1034-4
Sung, H., Ferlay, J., Siegel, R. L., Laversanne, M., Soerjomataram, I., Jemal, A., et al. (2021). Global Cancer Statistics 2020: GLOBOCAN Estimates of Incidence and Mortality Worldwide for 36 Cancers in 185 Countries. CA: A Cancer Journal for Clinicians.
Tafani, M., Sansone, L., Limana, F., Arcangeli, T., De Santis, E., Polese, M., et al. (2016). The Interplay of Reactive Oxygen Species, Hypoxia, Inflammation, and Sirtuins in Cancer Initiation and Progression. Oxid Med. Cel Longev 2016, 1–18. doi:10.1155/2016/3907147
Tibodeau, J. D., Benson, L. M., Isham, C. R., Owen, W. G., and Bible, K. C. (2009). The Anticancer Agent Chaetocin Is a Competitive Substrate and Inhibitor of Thioredoxin Reductase. Antioxid. Redox Signal. 11, 1097–1106. doi:10.1089/ars.2008.2318
Truitt, L., Hutchinson, C., DeCoteau, J. F., and Geyer, C. R. (2014). Chaetocin Antileukemia Activity against Chronic Myelogenous Leukemia Cells Is Potentiated by Bone Marrow Stromal Factors and Overcomes Innate Imatinib Resistance. Oncogenesis 3, e122. doi:10.1038/oncsis.2014.37
Vallée, A., and Lecarpentier, Y. (2018). Crosstalk between Peroxisome Proliferator-Activated Receptor Gamma and the Canonical WNT/β-Catenin Pathway in Chronic Inflammation and Oxidative Stress during Carcinogenesis. Front. Immunol. 9, 745. doi:10.3389/fimmu.2018.00745
Wang, H., Wen, C., Chen, S., Wang, F., He, L., Li, W., et al. (2020). Toosendanin-induced Apoptosis in Colorectal Cancer Cells Is Associated with the κ-opioid Receptor/β-Catenin Signaling axis. Biochem. Pharmacol. 177, 114014. doi:10.1016/j.bcp.2020.114014
Wen, C., Chen, J., Zhang, D., Wang, H., Che, J., Qin, Q., et al. (2016). Pseudolaric Acid B Induces Mitotic Arrest and Apoptosis in Both 5-Fluorouracil-Sensitive and -resistant Colorectal Cancer Cells. Cancer Lett. 383, 295–308. doi:10.1016/j.canlet.2016.09.007
Wen, C., Huang, L., Chen, J., Lin, M., Li, W., Lu, B., et al. (2015). Gambogic Acid Inhibits Growth, Induces Apoptosis, and Overcomes Drug Resistance in Human Colorectal Cancer Cells. Int. J. Oncol. 47, 1663–1671. doi:10.3892/ijo.2015.3166
Wen, C., Wang, H., Wu, X., He, L., Zhou, Q., Wang, F., et al. (2019). ROS-mediated Inactivation of the PI3K/AKT Pathway Is Involved in the Antigastric Cancer Effects of Thioredoxin Reductase-1 Inhibitor Chaetocin. Cel Death Dis 10, 809. doi:10.1038/s41419-019-2035-x
Yao, C. W., Kang, K. A., Piao, M. J., Ryu, Y. S., Fernando, P. M. D. J., Oh, M. C., et al. (2017). Reduced Autophagy in 5-Fluorouracil Resistant Colon Cancer Cells. Biomol. Ther. (Seoul) 25, 315–320. doi:10.4062/biomolther.2016.069
Keywords: chaetocin, colorectal cancer, ROS, JNK/c-Jun pathway, apoptosis, CD47
Citation: Wang H, Wen C, Chen S, Li W, Qin Q, He L, Wang F, Chen J, Ye W, Li W, Peng J, Yang X and Liu H (2021) ROS/JNK/C-Jun Pathway is Involved in Chaetocin Induced Colorectal Cancer Cells Apoptosis and Macrophage Phagocytosis Enhancement. Front. Pharmacol. 12:729367. doi: 10.3389/fphar.2021.729367
Received: 23 June 2021; Accepted: 11 October 2021;
Published: 27 October 2021.
Edited by:
Wei Peng, Chengdu University of Traditional Chinese Medicine, ChinaReviewed by:
Feng Wang, Affiliated Hospital of Nantong University, ChinaCopyright © 2021 Wang, Wen, Chen, Li, Qin, He, Wang, Chen, Ye, Li, Peng, Yang and Liu. This is an open-access article distributed under the terms of the Creative Commons Attribution License (CC BY). The use, distribution or reproduction in other forums is permitted, provided the original author(s) and the copyright owner(s) are credited and that the original publication in this journal is cited, in accordance with accepted academic practice. No use, distribution or reproduction is permitted which does not comply with these terms.
*Correspondence: Huanliang Liu, bGl1aHVhbmxAbWFpbC5zeXN1LmVkdS5jbg==; Xiangling Yang, eWFuZ3hsMjhAbWFpbC5zeXN1LmVkdS5jbg==
†These authors have contributed equally to this work.
Disclaimer: All claims expressed in this article are solely those of the authors and do not necessarily represent those of their affiliated organizations, or those of the publisher, the editors and the reviewers. Any product that may be evaluated in this article or claim that may be made by its manufacturer is not guaranteed or endorsed by the publisher.
Research integrity at Frontiers
Learn more about the work of our research integrity team to safeguard the quality of each article we publish.