- 1Department of Biotechnology, Modern College of Arts, Science and Commerce (Savitribai Phule Pune University), Pune, India
- 2Department of Environmental Science, Savitribai Phule Pune University, Pune, India
- 3Department of Life Sciences and the National Institute for Biotechnology in the Negev, Ben-Gurion University of the Negev, Beer-Sheva, Israel
- 4Ethnopharmacology and Natural Product Research Laboratory, Department of Life Sciences, Presidency University, Kolkata, India
- 5The Fred Wyszkowski Cancer Research Laboratory, Department of Biology, Technion-Israel Institute of Technology, Haifa, Israel
- 6Department of Pharmaceutical Sciences, College of Pharmacy and Health Sciences, St. John’s University, Queens, NY, United States
- 7Department of Microbiology, Weifang Medical University, Weifang, China
Antibiotic resistance or microbial drug resistance is emerging as a serious threat to human healthcare globally, and the multidrug-resistant (MDR) strains are imposing major hurdles to the progression of drug discovery programs. Newer antibiotic-resistance mechanisms in microbes contribute to the inefficacy of the existing drugs along with the prolonged illness and escalating expenditures. The injudicious usage of the conventional and commonly available antibiotics in human health, hygiene, veterinary and agricultural practices is proving to be a major driver for evolution, persistence and spread of antibiotic-resistance at a frightening rate. The drying pipeline of new and potent antibiotics is adding to the severity. Therefore, novel and effective new drugs and innovative therapies to treat MDR infections are urgently needed. Apart from the different natural and synthetic drugs being tested, plant secondary metabolites or phytochemicals are proving efficient in combating the drug-resistant strains. Various phytochemicals from classes including alkaloids, phenols, coumarins, terpenes have been successfully demonstrated their inhibitory potential against the drug-resistant pathogens. Several phytochemicals have proved effective against the molecular determinants responsible for attaining the drug resistance in pathogens like membrane proteins, biofilms, efflux pumps and bacterial cell communications. However, translational success rate needs to be improved, but the trends are encouraging. This review highlights current knowledge and developments associated challenges and future prospects for the successful application of phytochemicals in combating antibiotic resistance and the resistant microbial pathogens.
Introduction
The injudicious use of antibiotics for human healthcare has led to the fast emergence, persistence and spread of the antibiotic or antimicrobial resistance, a condition in which microbes develop resistance against the spectrum of conventional antibiotics, also known as multidrug resistance and these microbes are typically known as antibiotic- or drug-resistant pathogens (Yu et al., 2020). It has left the medical community with a shrunken pool of therapeutic options against these strains. The infections associated with antibiotic resistant pathogens are usually accompanied by substantial morbidity and mortality along with the massive economic burden on global healthcare (Centers for Disease Control and Prevenation, 2019). Unfortunately, MDR pathogens are receiving progressive identification in community-acquired infections (Koulenti et al., 2020). Along with the excessive antibiotics usage in human healthcare, antibiotics used in veterinary also owe to the emergence of antibiotic resistance (Smet et al., 2009). Further, subsequent contamination of soil, sediments and water bodies accelerate the process of development and spread of antibiotic resistant strains in the environment (Gothwal and Shashidhar, 2015; Ben Said et al., 2016). Besides, the waste generated by pharma-industries, hospitals as well as livestock producers carries the unmonitored quantities of antimicrobials, which stimulate the multi-drug resistance in the environment, with prospective resistome-spill over to humans and animals (Oyekale and Oyekale, 2017; World Bank, 2017). Looking at the speed of drug resistance emergence and its serious threat to the global health, development of new drugs to treat such infections is the top priority for the World Health Organization (WHO) (The Lancet Infectious Diseases, 2017).
The WHO has published the first-ever list of antibiotic-resistant priority pathogens, describing a catalogue of twelve bacterial families posing the greatest threat to human health (World Health Organization, 2017). The list covers the resistant pathogens in three tiers as per the urgency of new antibiotics. The first group represents critical priority pathogens, which includes Acinetobacter baumannii, Pseudomonas aeruginosa and members of Enterobacteriaceae (Klebsiella, Escherichia coli, Serratia and Proteus). The second group is a high priority group with the pathogens including Enterococcus faecium, Staphylococcus aureus, Helicobacter pylori, Campylobacter spp., Salmonellae, and Neisseria gonorrhoeae. The last group contains medium priority pathogens such as Streptococcus pneumoniae, Haemophilus influenzae, and Shigella spp. (World Health Organization, 2017). Based on their drug-resistance profile, pathogens can be categorized as multidrug resistant (MDR), extensively drug-resistant (XDR) or pandrug-resistant (PDR) as defined by European Centre for Disease Prevention and Control (ECDC) and the Centers for Disease Control and Prevention (CDC) (Magiorakos et al., 2012). These MDR pathogens are typically associated with nosocomial infections and their trends have been observed concerning certain medical conditions. For instance, retrospective analysis based on five-year single cohort epidemiological observations of MDR infections in oncological patients showed that the carbapenem-resistant Klebsiella pneumoniae, methicillin-resistant Staphylococcus aureus (MRSA) and carbapenem-resistant Acinetobacter baumannii were most frequently isolated strains, with 32% infection-associated mortality (Perdikouri et al., 2019). Diabetic foot infections have exhibited an association with biofilm-forming resistant strains (Vatan et al., 2018). Therefore, conducting a simultaneous process of curing the disease as well as combating the related MDR pathogens is very complex and challenging.
There is an urgent need for new antibacterial agents to fight against the threat of microbial antibiotic resistance. Unfortunately, the progress in this direction is notably slow (Fischbach and Walsh, 2009). Out of the alternative and effective options against MDR pathogens, plants might hold the key in providing the enormous range of the chemotherapeutics in form of their secondary metabolites with an ability to tackle bacterial infections (Anand et al., 2019; Anand et al., 2020). These secondary metabolites or phytochemicals include the members of alkaloids, flavonoids, quinones, coumerins and many more (Mbaveng et al., 2015; Anand et al., 2019; Anand et al., 2020; Mohammed et al., 2021). The current review depicts the potential role of the plant secondary metabolites or phyto-antimicrobials against the antibiotic resistant pathogens. The review incorporates the major molecular determinants of the antibiotic resistance and the bactericidal as well as antibiotic-resistance reversal potentials of phytochemicals. The mini review describes the successful attempts of the applications of phytochemicals in the fight against the antibiotic resistant bacterial pathogens, especially of clinical origin.
Major Determinants of Antibiotic Resistance
Several mechanisms have been identified in the microbial pathogens involved in their self-defense machinery active against the antimicrobial drugs, antibiotics and pesticides. These mechanisms are often active simultaneously in pathogenic microbes, especially with antibiotic resistant phenotypes, ensuring their protection against a wide range of antibiotics. With each application of treatment options, the likelihood of being less effective against both intended and other targets increases when used in the future. The applications of antimicrobial drugs trigger a complex interaction involving these drug-resistance determinants. Interestingly, these mechanisms have also been observed in antibiotic-producing organisms and the genetic determinants coding for the defense machinery are found in clusters with antibiotic biosynthesis-related genes. This forms a complex co-regulated network in pathogens (Mak et al., 2014). These regulated machineries are which mediates the pathogen defense mechanistically diverse and possibly one of the main causes of the origin of antibiotic resistance (Munita and Arias, 2016). Figure 1 depicts the major tactics of antibiotic-resistant strains against wide-spectrum and class of antibiotics, as discussed in the following sections. The evolution of antibiotic resistance can be considered into two categories, the first one being the molecular redundancy, where organisms try to adapt their physiology to newer (and challenging) environments on receiving the signals, and second being the molecular infidelity, where stressed microbial cells become more receptive to foreign DNA besides certain stresses can also induce rapid mutations. Microbes are highly adaptable living organisms with tremendous abilities to mutate in response to their environments. These mutated bacterial strains produce populations with drug-resistance characteristics and can transfer drug-resistance carrying genes and genetic elements to other non-resistant bacteria in the surroundings, through horizontal gene transfer mechanisms. Following sections discuss the main antibiotic resistance determinants in bacteria with antibiotic resistance phenotypes.
Modification or Destruction of Antibiotics
One of the effective strategies in bacteria for coping up with the antibiotics is production of enzymes which modifies the active moieties of the antibiotics via addition of chemical groups or which destroys the antibiotics. This prevents the interaction between the antibiotics and their respective targets in bacteria (Fischbach and Walsh, 2009). The enzymes with capability to induce chemical alterations in antibiotics are well-known in both Gram-negative and Gram-positive pathogens. Most of these antibiotics impacted by the chemical alterations exert their mode of action via inhibiting the protein synthesis at ribosomal level (Wilson, 2014). Most frequently observed chemical modifications of the antibiotics include phosphorylation (chloramphenicol, aminoglycosides), adenylation (lincosamides, aminoglycosides) and acetylation (streptogramins, chloramphenicol, aminoglycosides) of the antibiotics. Some of the most studied examples of antibiotic modifying enzyme include aminoglycoside-modifying enzymes (AMEs) and chloramphenicol acetyltransferases, which are usually harbored in the mobile genetic elements. Irrespective of the biochemical reactions, this alteration results in steric hindrance, which in turn reduces the antibiotic avidity for its target, and thus increasing the minimal inhibitory concentrations (MIC) during the treatment (Munita and Arias, 2016). On the other hand, there is a production of potent bacterial enzymes, good enough to destruct the applied antibiotics. These enzymes typically belong to the class of β-lactamases and destroy the amide bond of the β-lactam ring, making the antimicrobial ineffective. Multiple form of these enzymes usually denoted as ‘bla’, are disseminated on the mobile genetic elements (Tooke et al., 2019). The β-lactamases can be classified in two different ways; either based on their amino acid sequences or based on their biochemical function and substrate specificities (Bush and Jacoby, 2010; Bush, 2013). The β-lactamases are progressively becoming the prime choice for the empirical therapy of nosocomial infections and their interactions with lactams like carbapenems is of a particular interest while studying the resistance (Tooke et al., 2019). Besides this, there are some other types of enzymes, categorized as Extended Spectrum β-lactamases (ESBLs) and having an ability to hydrolyze and deactivate the antibiotics such as penicillins, third-generation cephalosporins and monobactams. The spread of Extended Spectrum β-lactamases is strongly associated with the conjugative plasmids (ur Rahman, 2018).
Cell Permeability and Drug-Efflux Pumps
In order to exert the effective antibiotic activity, it is necessary for the antibiotics to enter the bacterial cell via penetrating the cell membrane and further interact with the respective cellular targets (Mapara et al., 2015). To restrict the entry of antibiotics inside the cells, bacteria have evolved the mechanisms, which changes the cellular permeability. This usually includes reduced or differentially regulated expression of porins; the outer membrane channels used by the antibiotics to cross the outer membrane (Pagès et al., 2008). Several kinds of porin were identified and classified based on their structure, selectivity as well as regulation of their expression levels. Some of the well-characterized bacterial porins include OmpC, OmpF and PhoE from E. coli and OprD from P. aeruginosa (Nikaido, 2003). On the other hand, bacterial efflux pumps have emerged as vital transporters in resistant strains, which transport the antibiotic molecules out of the cell usually in non-specific manner and reduces the antibiotic concentration side the cell (Shriram et al., 2018). On the basis of the energy source, the bacterial efflux pumps are categorized in two super-families; 1) ATP-binding cassette (ABC) multidrug transporters, and 2) secondary transporters using proton motive force (PMF) as an energy source. The secondary transporters are further sub-classed into four families, namely 1) major facilitator superfamily (MFS), 2) resistance-nodulation-cell division (RND), 3) multidrug and toxic compound extrusion (MATE), and 4) small-MDR (SMR) family (Putman et al., 2000; Fernández and Hancock, 2012; Sun et al., 2014). Many of these classes are well characterized in various pathogens with respect to their substrate specificities (reviewed by Shriram et al. (2018)). Some of the most widely analyzed bacterial efflux pump system includes MexAB-OprM from P. aeruginosa, AcrAB-TolC from E. coli, NorA from S. aureus and KnpEF from K. pneumoniae. The pathogens over-representing the efflux pumps on their membranes facilitates the effective elimination of the antibiotics from the inner cellular compartment and subsequently retracts the effective interaction between antibiotics and their targets to attain the resistance. The reduced rate of the antibiotic influx via altering the cell permeability through regulated porin presentations on cell membrane and increasing the efficacy of antibiotic efflux via active efflux pump is effectively causing the development of antibiotic resistance.
Modification of the Antibiotic Targets
Another strategy employed by the MDR pathogens to exterminate the effects of antibiotics includes the modification of the antibiotic target sites, which prevents the optimal interaction between antibiotics and targets. Such types of target modifications may include 1) enzymatic modification of the binding sites on the targets (addition of methyl group), 2) replacement of the original targets and 3) mutation (point) in the target-coding genes. Irrespective of the type of target modification, the ultimate result is decreased affinity of the antibiotics towards the targets and subsequent development of the resistance (Munita and Arias, 2016). For instance, the target modification resistance has been studied during the development of rifampin resistance due to the point mutation in rpoB subunit of the RNA polymerase (Floss and Yu, 2005). The development of macrolide resistance was observed due to the methylation of macrolide-target in the ribosome. The process is catalyzed by an enzyme encoded by the erm genes (erythromycin ribosomal methylation) (Leclercq, 2002), and methicillin resistance in S. aureus due to the acquisition of an exogenous penicillin-binding proteins (PBP2a) encoded by mecA gene (Moellering, 2012).
Additionally, the acquisition of foreign genetic material by bacteria via horizontal gene transfer facilitates the antibiotic resistance development event. The antibiotic resistance related genes have been observed to be transferred from resistant to non-resistant strains via horizontal gene transfer events such as transformation, transduction or conjugation. In such kind of resistance development, mobile genetic elements as well as integrons plays pivotal role in effective transfer of resistance related genes (Thomas and Nielsen, 2005; Manson et al., 2010).
Horizontal Gene-Transfer (HGT) and Mobile Genetic Elements (MGEs)
The horizontal gene transfer is one of the effective processes of microbial evolution and adaptation which allows rapid procurement of new metabolic abilities and microbial phenotypes. During the process of horizontal gene transfer, there is an inter-generational transfer of the genetic material (Wiedenbeck and Cohan, 2011; Hall et al., 2017). Interestingly, the horizontal gene transfer can lead to prompt adaptation of pathogens to the environment harboring high antibiotic levels (Saak et al., 2020). These adaptations can be in the form of acquisition of active of virulence or new metabolic pathways. The genes responsible for such events are usually present of the mobile genetic elements. These mobile genetic elements are the DNA pieces with an ability to move within the host genome or between the genomes of two different (donor and recipient) cells. The bacterial mobile genetic elements are represented by plasmids, phages as well as transposable elements which can be differed based on their mode of mobilization as well as structure (Saak et al., 2020).
The canonical mechanisms of horizontal gene transfer include transformation, transduction, conjugation and transposition. During the transformation event, the DNA is taken up by the competent cells via proteinaceous DNA uptake machinery (Claverys et al., 2009; Dubnau and Blokesch, 2019). On the other hand, the transposition is achieved by the transposable elements which transpose across the different locations within the genome (Siguier et al., 2017). In bacteria, it is well established that the resistant conferring transposons can integrate themselves in mobile genetic elements such as plasmids and can get shared via horizontal gene transfer (Hedges and Jacob, 1974). Integrons are yet another type of elements that can capture resistant conferring genes. They enable the expression of enable genes via site-specific recombination downstream of a resident promoter (Mazel, 2006). During conjugation, the genetic materials such as extra-chromosomal circular plasmids, linear plasmids or conjugative transposons is transferred through a mating junction formed between two bacterial cells via a physical contact (Wozniak and Waldor, 2010; Dib et al., 2015; Delavat et al., 2017). Lastly, the transduction can occur due to the defects during DNA packaging into viral particles (general transduction), or inaccurate prophages excision from genomes (specialized transduction) (Chiang et al., 2019).
Apart from these canonical mechanisms, new mechanisms of horizontal gene transfer are emerging. For example, gene transfer agents such as the elements common for transduction and transformation were discovered (Westbye et al., 2017). The membrane vesicles formed from the host cell membrane have also displayed the ability to carry the genomic material (chromosomal/plasmid/phage) (Grüll et al., 2018). Such vesicle mediated is considered as vesiduction mode of horizontal gene transfer (Soler and Forterre, 2020). Recently, two new conjugative methods namely, mycoplasma chromosomal transfer (Dordet-Frisoni et al., 2019) and distributive conjugal transfer (Gray and Derbyshire, 2018) were described. Another emerging way is referred as transjugation which is hybrid machinery found in transformation and conjugation (Blesa et al., 2015; Blesa et al., 2017). These transferring events are even more complex as the mobile genetic elements themselves can be incorporated inside one-another. For instance, transposons can be integrated into plasmids or even into other transposons (Sheppard et al., 2016).
All these mechanisms contribute heavily during the horizontal transfer of the genes responsible for the spread of antibiotic resistance. Additionally, the emergence of new machineries involved in the horizontal gene justifying the rapidness in the spread of antibiotic resistance not only in nosocomial infections but also in the environment. The diversity in the mechanisms deciphering the spread of antibiotic resistance related genes and the rapid spread in resistance vindicates the severity of this issue. Hence, there is an urgent requirement of the new/innovative therapeutics to slow or stop the spread of antibiotic resistance.
Phytochemicals for Combating Antibiotic Resistance
Plants are known for their therapeutic potentials, initially in the form of crude extracts or powders and further as purified products (pure active phytomolecules) due to their high potencies (Samy and Gopalakrishnakone, 2010). This can be traced back to 1803 from the report on the isolation of morphine from Papaver somniferum (Schmitz, 1985). Due to their effective applications as food supplements, herbal medicines, cosmetics as well as antibacterial activities, the phytochemicals have gained interest in the scientific community; and numerous phytochemicals have been validated for their antimicrobial potentials including against MDR strains (Shriram et al., 2018; Anand et al., 2019; Yu et al., 2020; Mohammed et al., 2021). However, despite the reports on many phytochemicals, few have been approved by the Food and Drug Administration (FDA) such as codeine, capsaicin, reserpine, paclitaxel, colchicine, to name a few (Kongkham et al., 2020). Some important phytochemicals and their chemical structures are depicted in Figure 2.
The phytochemicals when applied in form of extracts have been demonstrated to impose inhibitory effects against clinical isolates. For example, Dahiya and Purkayastha (2012) tested various solvent-based extracts of medicinal plants including Lemongrass, Neem, Aloe vera, Oregano, Rosemary, Thyme, Tulsi and Bryophyllum against ten MDR clinical isolates (including Gram-positive and Gram-negative bacteria). The study correlated the flavonoids and tannins present in a methanolic extract with the antimicrobial activities against methicillin-resistant S. aureus. Four different extracts of Lawsonia inermis were tested successfully against Gram-negative (E. coli, S. typhi, Klebsiella spp., S. sonnei) and Gram-positive (B. subtilis, S. aureus, S. epidermidis) clinical isolates. Interestingly, not all these extracts induced any sign of toxidrome (Gull et al., 2013). Various solvent extracts of medicinal plants like Curcuma longa, Zingiber officinale and Tinospora cordifolia showed the effective killing of clinical microbes including S. aureus, P. aeruginosa, K. pneumoniae, E. coli, B. subtilis and P. mirabilis (Chakraborty et al., 2014). A summarized list of the antimicrobial potentials of medicinal plant resources is given in Table 1.
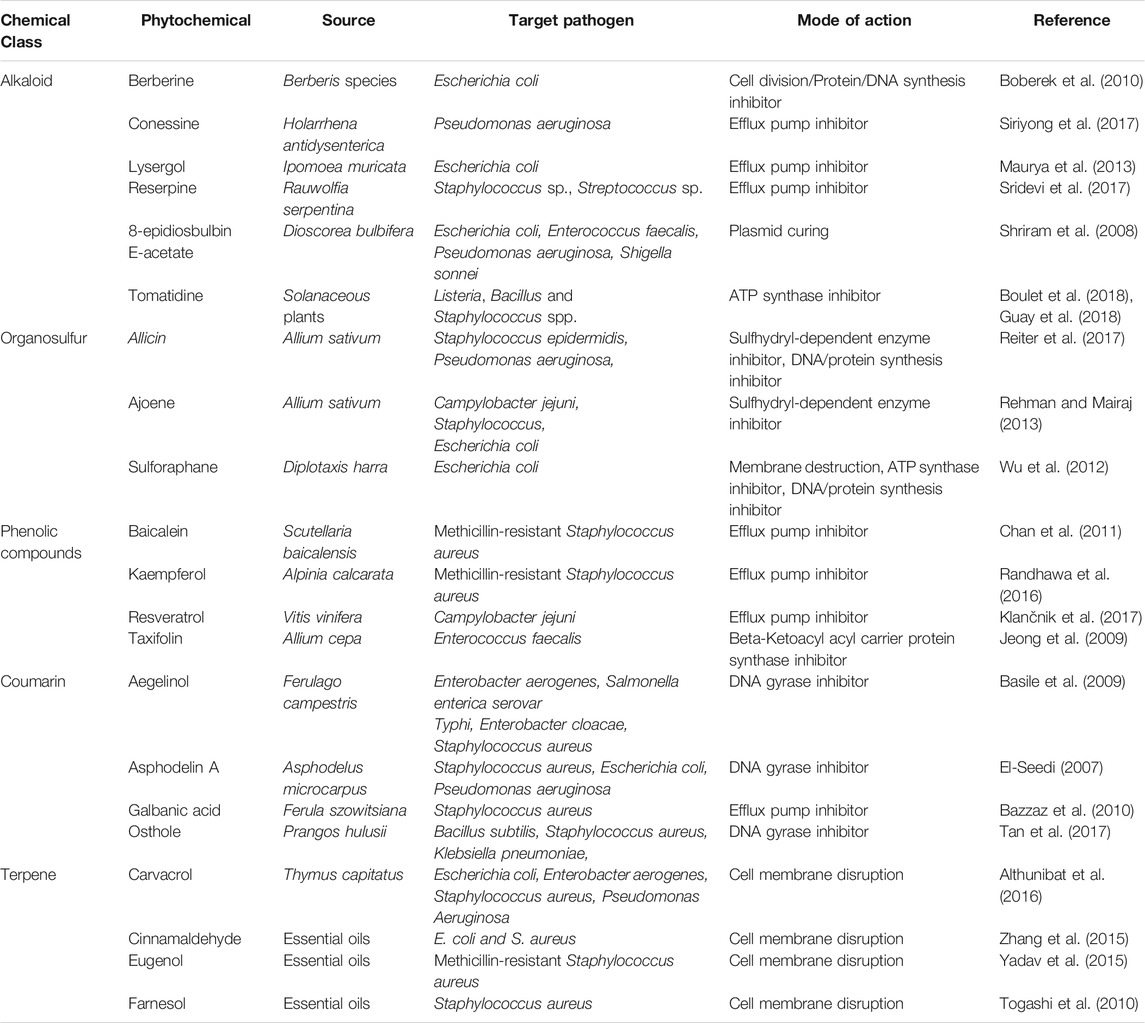
TABLE 1. A summarized list of phytochemicals, their chemical class, source and mode of action against pathogenic strains.
Apart from the extracts representing the complex mixture of many phytochemicals in a single formulation, a number of the single active principles in their purified forms have been tested for their potential against MDR pathogens along with the bacterial mechanisms targeted by them. Such pure compounds have demonstrated their activity either with their sole application or with their synergistic application with the standard antibiotics. For instance, Berberine (isoquinoline alkaloid) found in roots/stem of Berberis species, has exhibited antibacterial activity, which can be correlated with the action of berberine against DNA intercalation, RNA polymerase, gyrase /topoisomerase, as well as the inhibition of cellular division (Iwasa et al., 2001; Yi et al., 2007; Domadia et al., 2008). The aminothiazolyl berberine derivatives have also shown their potential antimicrobial activity against clinically resistant A. baumannii (Gao et al., 2018). This activity was attributed to the inhibition of DNA gyrase via hydrogen bonding. Reserpine (indole alkaloid) from the plant Rauwolfia serpentina has also exhibited potent efflux pump inhibitory activity (Sridevi et al., 2017). Piperine (alkaloid) from Piper nigrum and Piper longum has displayed the inhibitory action against methicillin-resistant S. aureus when co-administered with antibiotics such as ciprofloxacin or gentamicin. This inhibitory effect of piperine was attributed to its efflux pump inhibitory activity against the NorA efflux pumps in the MDR pathogens under consideration (Kumar et al., 2008; Khameneh et al., 2015). Another compound, tomatidine (steroidal alkaloid) from Solanaceous plants (tomato, potato, eggplant etc.), which has demonstrated the antibacterial potential against drug-resistant S. aureus synergistically with aminoglycosides (Mitchell et al., 2012). Tomatidine has also proved its role as antibiotic potentiator for various antibiotics, including ampicillin, cefepime, gentamicin, and ciprofloxacin against pathogens including S. aureus, P. aeruginosa, and Enterococcus faecalis (Soltani et al., 2017). Allicin (diallyl thiosulfinate, organosulfur compound) is found in the plants from the family Alliaceae, especially fin garlic (Allium sativum). The antibacterial potential of this phytochemical has been acknowledged for a long time and its antibacterial activity has been proved against pathogens such as S. epidermidis, methicillin-resistant S. aureus, P. aeruginosa and the periodontitis causing oral pathogens as well as human lung pathogens. Allicin also reported to potentiating the activities of antibiotics ciprofloxacin, tobramycin, and cefoperazone against P. aeruginosa (Cai et al., 2008; Reiter et al., 2017). The possible mechanism of action of allicin is postulated as inhibition of sulfhydryl-dependent enzymes allicin, such as RNA polymerase, thioredoxin reductase and alcohol dehydrogenase (Lanzotti et al., 2014). The isothiocyanates (volatile organosulfur compounds) isolated from the Armoracia rusticana have displayed the strong activity against oral pathogens as well as bactericidal activity against H. pylori via inhibiting the urease activity and decreasing the inflammatory factor of Helicobacter infection (Fahey et al., 2013; Park et al., 2013).
The phenolic compound resveratrol is known for its efflux pump inhibitory activities and is capable of blocking the CmeABC type efflux pumps in Campylobacter jejuni (Klančnik et al., 2017). Kaempferol (flavonoid) and its natural glycoside derivative from Persea lingue proved to increase the antimicrobial potential of ciprofloxacin in a NorA overexpressed S. aureus strain (Holler et al., 2012). Chalcones and catechin gallates are yet another group of phytochemicals with potential efflux pump inhibitory activities (Belofsky et al., 2004; Gibbons et al., 2004). The epigallocatechin gallate from green tea inhibits the DNA gyrase (B subunit) at the ATP binding site (Gradišar et al., 2007).
The frequent location of the genes conferring the antibiotic resistance is a plasmid. These plasmids are usually characterized as self-replicating, circular DNAs coding for various gene groups with different functionality. The phytochemicals targeting such plasmids have also been reported (Buckner et al., 2018). For instance, the 8-epidiosbulbin-E-acetate from Dioscorea bulbifera proved to cure the antibiotic-resistant R-plasmids of clinical isolates of E. faecalis, E. coli, Shigella sonnei and P. aeruginosa with 12–48% curing efficiency (Shriram et al., 2008). There are several more instances of the successful application of the phytochemicals against various MDR pathogens, examples of the phytochemicals along with their potential inhibitory activities are listed in Table 1 and the drug-resistant determinants targeted by the phytochemicals are depicted in Figure 3. The following sections discuss the major types of phytochemicals including alkaloids, phenolics, flavonoids and essential oils, their antimicrobial potentials against drug-resistant microbes and the mode of actions.
Alkaloids
Alkaloids are organic nitrogen heterocyclic compounds with a wide range of chemical structures. Nicotine, morphine, caffeine, and mescaline are examples of alkaloids, which are base-forming water-soluble salts derived from an amino acid (Compean and Ynalvez, 2014). They are found in over 300 plant families, with unique compounds found in just a few of them (e.g., hyoscyamine in Solanaceae) (Evans, 2009). Alkaloids can be found in several parts of the plant, but certain compounds are restricted to a particular type (for example, quinine in cinchona tree bark) (Robbers et al., 1996). Alkaloid-rich biorational plant extracts from various families, including Amaryllidaceae, Burseraceae, Capparaceae, Mimosaceae, Vitaceae, and Tiliaceae, have remarkably demonstrated antibacterial activity against mycobacteria (M. fortuitum, M. tuberculosis, and M. smegmatis), E. coli, S. aureus, S. Typhimurium, K. pneumonia and P. aeruginosa (Thawabteh et al., 2019; Omar et al., 2021).
Alkaloids have shown broad-spectrum antimicrobial activities, and several studies have suggested that these compounds could represent an important role in tackling pathogenesis of a variety of infection agents (Cushnie et al., 2014; Casciaro et al., 2020). Alkaloids are known to target major drug-resistance determinants, especially the powerful EPs. A piperidine-type alkaloid (Piperine) isolated from Piper nigrum and P. longum, prevented the growth of a mutant S. aureus and significantly decreased the MIC values for S. aureus when co-administered with ciprofloxacin (Khan et al., 2006). Co-loaded piperine and gentamicin nanoliposomes were tested for antibacterial activity and EP-inhibitory action in MRSA and the results demonstrated effectiveness in reducing MRSA infection (Khameneh et al., 2015). The use of piperine as an EP inhibitor (EPI) was investigated, and the findings revealed that this compound affected MRSA and S. aureus NorA (388 amino acid protein) EPs (Kumar et al., 2008; Khameneh et al., 2015). Besides, the synthetic piperine analogs including 5-(2,2-dimethyl-chroman-6-yl)-4-methyl-penta-2,4-dienoic acid ethyl ester 5 and 5-(2,2-Dimethyl-chroman-6-yl)-4-methyl-2E,4E-pentadienoic acid pyrrolidide have also been proved their potential as inhibitors of the NorA overexpressing S. aureus (Chopra et al., 2019).
Berberine, an isoquinoline alkaloid from Berberis species roots and stem bark, besides being the main active ingredient in Cortex phellodendri and Rhizoma coptidis, has been used in herbal medicine for centuries. This phytochemical has antibacterial, antifungal, antiprotozoal, and antiviral properties. The antibacterial action mechanism of Berberine includes DNA intercalation, targeting RNA polymerase, gyrase, and topoisomerase IV, besides cell division inhibition (Yi et al., 2007; Kang et al., 2015; Peng et al., 2015). In addition, its antibacterial properties are also associated with the inhibition of the FtsZ (Filamenting temperature-sensitive mutant Z) protein, cell division protein (Boberek et al., 2010). FtsZ inhibitors could be considered a novel class of antibacterial agents with the ability to demonstrate broad-spectrum activity. This compound has also been shown to inhibit bacterial cell function through a variety of mechanisms, including cell structure damage, protein and DNA synthesis inhibitors, which ultimately leading to bacterial death (Feng et al., 2016). In addition, synthetic derivatives, such as 9, 13-disubstituted berberines, have also displayed potent antistaphylococcal activities (Wang F. et al., 2017). Ungeremine, an isoquinoline alkaloid that was discovered in a methanol extract of Pancratium illyricum L. bulbs, and has been shown to possess antibacterial properties. By targeting and inhibiting the bacterial topoisomerase IA, this compound can cause a significant increase in DNA cleavage (Casu et al., 2011; Schrader et al., 2013).
Quinoline alkaloids (such as masculine, kokusagine and dictamnine) isolated from the stem bark of Teclea afzelii has shown strong antibacterial activities (Kuete et al., 2008). Quinolone alkaloids, natural and synthetic, have been reported to inhibit type II topoisomerase enzymes, and as a result, inhibited DNA replication (Heeb et al., 2011). Reserpine, an indole alkaloid obtained from the Rauwolfia serpentina plant and is a well-known natural compound with strong EPI activity (Abdelfatah and Efferth, 2015). It has been demonstrated that the overexpression of EP was the key mechanism of resistance to fluoroquinolones in resistant Stenotrophomonas maltophilia and the addition of reserpine reduced the antibiotic resistance capabilities (Jia et al., 2015). Tomatidine, a steroidal alkaloid found in solanaceous plants such as eggplant (Solanum melongena), potato (Solanum tuberosum), and tomato (Solanum lycopersicum), and has been shown to have significant antibacterial activity against S. aureus whether used alone or in conjunction with aminoglycosides (Jiang et al., 2016).
Further, several studies have demonstrated the synergistic effects of alkaloids when used with antibiotics, for instance, tomatidine and aminoglycosides against drug-resistant S. aureus strains (Mitchell et al., 2012). Tomatidine could be accounted as an antibiotic potentiator for many antibiotics like ampicillin, cefepime, gentamicin, and ciprofloxacin, and against both Gram-negative and Gram-positive bacteria like P. aeruginosa, S. aureus, and Enterococcus faecalis infections (Soltani et al., 2017). Chanoclavine, a tricyclic ergot alkaloid isolated from the seeds of Ipomoea muricata shown synergistic effects when combined with tetracycline in the treatment of MDR E. coli. EP, which appears to be similar to the ATPase-dependent ones, has been discovered to be inhibited by this compound (Dwivedi et al., 2019). Holarrhena antidysenterica is a member of the Apocynaceae family that has historically been used to treat a variety of ailments, including fever, diarrhea, bacterial infections, and dysentery (Siriyong et al., 2017). The barks of H. antidysenterica are full of alkaloids especially the steroidal alkaloid conessine which is responsible for its therapeutic potential (Zahara et al., 2020). A previous in vitro study demonstrated that conessine restores the inhibitory activity of rifampicin and novobiocin against drug-resistant Acinetobacter baumannii (Phatthalung et al., 2012; Chusri et al., 2014; Siriyong et al., 2016). In comparison to the constituent monotherapies, combination therapies of steroidal alkaloids or conessine with levofloxacin improved bacterial inhibition in vitro and restored antibiotic efficacy in vivo (Siriyong et al., 2018). Furthermore, this compound showed EPI activity against AdeIJK EP, which is involved in the efflux of several antibiotics in A. baumannii (Damier-Piolle et al., 2008; Siriyong et al., 2016). Sanguinarine is a benzophenanthridine alkaloid obtained from the rhizomes of Sanguinaria canadensis, has long been known to have broad antimicrobial and anti-inflammatory properties. The antibacterial activity of this alkaloid is mediated by the disruption of bacterial FtsZ Z-ring formation and inhibition of bacterial cytokinesis (Kelley et al., 2012). A combination of sanguinarine, vancomycin, and EDTA as well as a combination of sanguinarine, streptomycin and EDTA were tested against both Gram-negative and Gram-positive bacteria, including MDR phenotypes, in two recent studies (Hamoud et al., 2014, 2015). These experiments revealed that sanguinarine has potent antibacterial activity against all strains tested; additive and synergistic effects were observed for all sanguinarine + sanguinarine and EDTA + vancomycin + EDTA combinations against gram-negative bacteria. Except for MRSA, the combination of sanguinarine + EDTA + streptomycin demonstrated synergistic activity against almost all strains. Further, the activity of sanguinarine against MRSA strains was investigated besides its mechanism of action. Treatment of bacteria with sanguinarine has been shown to cause the release of membrane-bound cell wall autolytic enzymes, resulting in cell lysis; however, transmission electron microscopy (TEM) of MRSA has revealed alterations in the formation of septa. Overall, the results revealed a possible sanguinarine mechanism of action against MRSA by compromising the cytoplasmic membrane (Obiang-Obounou et al., 2011). Moreover, this compound was proposed to display antimycobacterial properties against Mycobacterium aurum and Mycobacterium smegmatis, two model mycobacteria species (Newton et al., 2002).
Phenolic Compounds
Plant phenolic compounds are regarded as crucial natural bioactive molecules due to their wide-spectrum, striking pharmacological activities. Plant phenolics have an aromatic ring with one or more hydroxyl groups in its molecular structure, and it can be a simple or polymerized molecule. Plant phenolics are classified into many groups based on their structural characteristics, with flavonoids, phenolic acids, and non-flavonoids being the most common (de Souza et al., 2019). They have proven potent in the treatment of many chronic diseases, including bacterial infection, cancer, diabetes, and cardiovascular disease, in terms of human health (Del Rio et al., 2013; Zacchino et al., 2017; Lima et al., 2019). Plant-phenolics have been shown to exhibit antimicrobial properties against a wide range of microorganisms, sensitize MDR strains to bacteriostatic or bactericidal antibiotics, and are promising natural antimicrobial weapons (Miklasińska-Majdanik et al., 2018). Due to their direct antimicrobial action and antibiotic modulation activities, dietary polyphenols have recently been demonstrated as chemopreventive and therapeutic agents (Makarewicz et al., 2021). Phenols, including pyrogallol and catechol, demonstrated antimicrobial activity against several microorganisms, including both Gram-positive and Gram-negative bacteria. Pyrogallol, for example, had a MIC range of 2.4–2500 μg/ml and pyrocatechol had a MIC range of 4.9–312.5 μg/ml against many periodontitis-causing microorganisms (Shahzad et al., 2015). Moreover, the halogenated catechols have also demonstrated the intrinsic inhibitory potential against five MDR strains via inhibiting the bacterial fatty acid synthesis (Liu et al., 2021). Taguri et al. (2006) evaluated 22 polyphenolic compounds for antibacterial activity against Gram-negative and Gram-positive bacteria and the results showed that pyrogallol-based compounds were more effective than resorcinol or catechol (Taguri et al., 2006).
Certain phenolic acids, such as Caffeic acid, exhibit antibacterial properties. In comparison to ampicillin, which demonstrated MICs of 0.1 and 3.2 μg/ml, Caffeic acid demonstrated an MIC of 1600 μg/ml against E. coli and S. aureus (Lim et al., 2016). Moreover, gallic acid has been shown to exhibit antibacterial properties against Enterococcus faecalis (Aires et al., 2013). It has also showed strong antibacterial potentials against Streptococcus pneumonia, P. aeruginosa, Moraxella catarrhalis, S. aureus, Enterococcus faecalis, E. coli, and Streptococcus agalactiae strains (Cueva et al., 2012). Gallic acid was found to have strong antibacterial activity against Campylobacter and bactericidal activity against two Campylobacter coli strains with MIC of 61.5–125 μg/ml (Sarjit et al., 2015).
Ferulic acid and gallic acid exhibited antibacterial properties against many bacterial isolates, where Ferulic acid showed MICs of 100 μg/ml against P. aeruginosa and E. coli, and 1250 μg/ml and 1100 μg/ml against L. monocytogenes and S. aureus while gallic acid demonstrated MIC equal to 2000 μg/ml against L. monocytogenes, 500 μg/ml against P. aeruginosa, 1500 μg/ml against E. coli, and 1750 μg/ml against S. aureus, respectively (Borges et al., 2013). Both ferulic acid and gallic acid damage the cell walls of E. coli, P. aeruginosa, S. aureus, causing local damage and cellular material leakage (Borges et al., 2013). On the other hand, the ferulic acid derivatives, namely 4-((E)-2-(diethylcarbamoyl)vinyl)-2-methoxyphenyl acetate and (E)-methyl 3-(4-((p-tolylcarbamoyl)methoxy)-3-methoxyphenyl)acrylate have also displayed their putative activity as EPI to inhibit the growth of NorA Eps in MRSA (Sundaramoorthy et al., 2018). Caffeic acid, protocatechuic acid, vanillic acid (4-hydroxy-3-methoxybenzoic acid), p-hydroxybenzoic acid or 4-Hydroxybenzoic acid, p-coumaric acid, syringic acid, and ferulic acid, which are found in wild polish mushrooms, displayed intermediate antibacterial activity against a variety of Gram-negative and Gram-positive bacteria, with MIC ranging from 156 to 5000 μg/ml (Nowacka et al., 2015). Galla rhois synthesizes major metabolites called methyl gallate, which demonstrated anti-Salmonella activity against multiple Salmonella strains with MIC ranging from 3.9 to 125 μg/ml (Choi et al., 2014). With MIC of 30 μg/ml, methyl gallate showed strong antibacterial activity against Shigella flexneri and E. coli (Madikizela et al., 2013). Ellagic acid, caffeic acid, hyperoside, ferulic acid, (+)-catechin, and sophorin (rutin) were found to be abundant in three Potentilla species, which were screened for antibacterial activities. With MIC values of 0.78–6.25 mg/ml, P. fruticosa demonstrated strong inhibitory effects against the fungus Candida albicans along with Gram-negative and Gram-positive bacteria (Wang et al., 2013).
The combination of gallic acid (10 μg/ml) and isoquercitrin (10 μg/ml) was effective in inhibiting S. aureus growth, despite their individual MIC being 10 times higher (Soberón et al., 2014). The ethyl acetate (EtOAc) fraction from the ethanol extract of Searsia chirindensis (Rhus chirindensis) which included myricetin-3-O-arabinopyranoside, methyl gallate, quercetin-3-O-arabinofuranoside, myricetin-3-O-rhamnoside, and kaempferol-3-O-rhamnoside showed the most active antibacterial fraction. The MICs of these compounds against E. coli, S. aureus, Campylobacter jejuni, and S. flexneri ranged from 30 to 250 μg/ml (Madikizela et al., 2013).
Flavonoids
Plant flavonoids are the 2-phenyl-benzo-γ-pyrane nucleus with two benzene ring-containing plant phenolic compounds with promising antimicrobial/antimicrobial-potentiator activities. Many classes of flavonoids, such as flavonols, flavanols, flavanones, isoflavonoids, chalcones and dihydrochalcones have been identified as allelochemicals, which inhibits the microbial growth (reviewed by Górniak et al., 2019). Along with these chemicals, the chalcone derivatives such as fluorinated-/chlorinated-/steroidal-/ferrocene-chalcones have also displayed potent antimicrobial activities against various MDR strains (Xu et al., 2019). Cushnie et al. (2008) demonstrated the catechin mediated membrane disruption in methicillin-resistant Staphylococcus aureus (MRSA) which causes potassium leakage and successive loss of the cell membrane integrity. Similarly, the investigation by Budzyńska et al. (2011) suggested the lipophilic nature of the 3-arylideneflavanones, which causes the aggregation of the bacterial cells and alters the membrane integrity and making the membranes more permeable in S. aureus and E. faecalis clinical isolates. Flavonoids are also proved as inhibitors of the quorum sensing and biofilm formation. The quercetin-mediated inhibition of biofilm formation was observed in P. aeruginosa PAO1 with reduced expression levels of the quorum sensing related genes (lasI, lasR, rhlI and rhlR) along with reduced production of virulence factors such as elastase, protease, and pyocyanin (Ouyang et al., 2016). Additionally, the antimicrobial activities of flavonoids have also displayed the association with nucleic acid synthesis. For examples, the flavonoids such as chrysin and kaempferol restrict the DNA gyrase (essential enzyme in DNA replication) activity in E. coli (Wu et al., 2013). Similarly, another important element during the DNA replication, the helicases (DnaB and RecBCD) are also proved to be inhibited by the flavonoids such as morin and myricetin (Xu et al., 2001). Apart from the direct antibacterial activities of flavonoids, there are also promising results indicating the role of flavonoids as resistant-reversal agents. For instance, Christena et al. (2015) demonstrated the synergistic role of pinostrobin-a with antibiotic ciprofloxacin during the growth inhibition in resistant strains of P. aeruginosa and E. coli, where pinostrobin displayed the efflux pump inhibition activity against the NorA efflux pumps. Likewise, the aglycone flavonoids such as myricetin, hesperetin and phloretin displayed the biofilm formation inhibition, which was postulated to the disturbed functioning of the msrA and norA efflux systems in Staphylococcus strains (Lopes et al., 2017). Lin et al. (2005) have shown the activity of twelve different flavonoids against the ESBL producing resistant strains of K. pneumoniae. Collectively this specifies the antimicrobial and resistant reversal potential of the flavonoids.
Essential Oils
Essential oils (EOs) represent a mixture of several low mass plant natural products or phytochemicals. EOs are well-documented for their strong antimicrobial potentials and are used often in traditional medicinal practices (Yu et al., 2020). These are lipophilic, volatile plant products that can be extracted from various plant parts, mainly flowers and fruits. However, their spread is limited to only a number of plant families. They are derived mainly from terpenes and terpenoids, and aromatic, aliphatic aldehydes and phenols. Besides, short-chain aliphatic hydrocarbon derivatives also constitute EOs (Turek and Stintzing, 2013). EOs are looked upon as a pool of antimicrobial agents (Solórzano-Santos and Miranda-Novales, 2012; Lahmar et al., 2017).
EOs have been well-documented for their striking antibacterial activities against both Gram-positive and negative pathogens (Yap et al., 2014). Interestingly, EOs have shown both direct-killing (bactericidal) and re-potentiating or re-sensitizing of antibiotics potentials against pathogenic microbes (Brehm-Stecher and Johnson, 2003; Mayaud et al., 2008). However, considering the vulnerability of EOs for conversion or degradation events, their stability is decisive for their quality and pharmacological potencies (Turek and Stintzing, 2013). But EOs are often reported to be safe for consumption as well as for vital host tissues (Helander et al., 1998).
In last few years, several authors have reported antimicrobial activities of EOs from different plants against several antibiotic-resistant strains. Naveed et al. (2013) reported the EOs of Cinnamomum verum barks exhibit strong antibacterial activities against MDR phenotypes of S. typhi, E. coli and S. aureus. Similarly, Sharopov et al. (2015) observed strong activities of Origanum tyttanthum EOs against MRSA. Similarly, Mentha piperita, Coriandrum sativum, and Pimpinella anisum EOs also exhibited antimicrobial potentials against S. aureus and E. coli (Bazargani and Rohloff, 2016). The EOs of Petroselinum crispum and Ocimum basilicum were found to be potent antimicrobials against Vibrio strains (Snoussi et al., 2016). Pogostemon heyneanus and Cinnamomum tamala EOs were found to have anti-biofilm and anti-virulent properties against MRSA strains (Rubini et al., 2018). Carum carvi, C. sativum, Cuminum cyminum EOs were reported to have notable antibacterial activities against E. coli and S. aureus (Khalil et al., 2018). Bučková et al. (2018) reported striking antimicrobial activities of EOs from several plants such as oregano, thyme, and tea tree beside others against drug-resistant strains of P. aeruginosa, E. coli, Enterobacter cloacae, Morganella morganii and Proteus mirabilis with considerably low MIC (0.005–0.5%).
In addition to direct bacterial killing (bactericidal) potentials, plant EOs have also been explored recently for their actions as adjuvants with antibiotic for drug-resistance-reversal or re-sensitizing/re-potentiating potentials. For instance, Benameur et al. (2019) assessed the susceptibility of blaESBL producing Enterobacteriaceae to Slovakian Thymus vulgaris EOs with/out antibiotic (cefotaxime), and observed a synergistic interaction between EOs and the antibiotic against blaSHV-12 producing MDR E. coli. Further, the peppermint oil exhibited synergistic impacts when used with gentamicin against ESBL- and NDM-1-producing Klebsiella pneumoniae strains (Kwiatkowski et al., 2018).
EOs have demonstrated their potentials in targeting and/or disturbing the most prevalent drug-resistance determining mechanisms of microbes including cell wall, cell membrane and permeability, drug efflux pumps, mobile genetic elements, quorum sensing and biofilm (Yu et al., 2020). Bacterial cell membranes work as the first barrier against antimicrobial agents, which any antibiotic should overcome for its interaction with the target (Martinez de Tejada et al., 2012). Bacterial cell membrane permeability play key regulatory roles in the entry and intracellular concentrations of antibiotics (Martinez de Tejada et al., 2012; Bueno, 2016). Bacterial strains have evolved effective ways to reform their membrane permeability mainly through altering the fatty acid- and membrane proteins, in order to have a check on the cellular influx of the applied antibiotics (Burt and Reinders, 2003; Mrozik et al., 2004). However, interestingly, EOs, being hydrophobic in nature, interact with membrane lipids and mitochondria and disturb the cellular structure ultimately resulting in higher membrane permeability. Besides, owing to their lipophilic nature, EOs can impact the amount and structure of unsaturated fatty acids in bacterial cell membranes (Nazzaro et al., 2013). Because of this, bacterial cells get unable to have a check on the seepage of key cellular molecules/ions from the bacterial cells when treated with EOs (Yu et al., 2020). Several recent reports have confirmed the strong potentials of plant EOs in targeting these critical drug-resistance determinants. M. alternifolia EOs increased the bacterial membrane permeability (Cox et al., 2000). Wang J. et al. (2017) reported Dodartia orientalis EOs effective against S. aureus, E. coli, and Salmonella enteritidis, as the EOs successfully disrupted the bacterial cell structure and resisted the biofilm formation. Further, Cinnamon EOs altered the cell microstructure, membrane permeability and integrity, attributed for striking bactericidal potencies against E. coli and Staphylococcus strains (Zhang et al., 2015). Ocimum gratissimum EOs acted as permeabilizer and showed significant antimicrobial potencies against P. aeruginosa and S. aureus (Hyldgaard et al., 2012). Coriandrum sativum EOs disrupted the cell membrane permeability and inhibited the MDR uropathogenic E. coli (Scazzocchio et al., 2019). This altered membrane permeability was attributed to apparent damages to the cellular integrity and functions (Scazzocchio et al., 2019).
Similarly, EOs have been characterized by several research groups as potent bacterial drug efflux inhibitors (EPIs) (reviewed by Yu et al. (2020)). Limaverde et al. (2017) reported Chenopodium ambrosioides EOs as EPIs of S. aureus. EOs from Salvia fruticosa, S. officinalis and S. sclarea significantly reduced the expression levels of tet(K) gene and the reduced drug (tetracycline) efflux from tetracycline resistant Staphylococcus epidermidis (Chovanová et al., 2015). EOs from C. ambrosioides and Thymus vulgaris also showed notable inhibitory activities against the NorA EPs in S. aureus (de Morais Oliveira-Tintino et al., 2018) and ciprofloxacin resistant S. aureus (Salehzadeh et al., 2018). At sub-MIC levels, the norA pump showed lower EtBr efflux, along with down-regulation of norA gene (Salehzadeh et al., 2018). Saviuc et al. (2016) showed EPI and membrane permeabilization-mediated synergistic effects of Rosmarinus officinalis EOs and eucalyptol against drug-resistant A. baumannii and P. aeruginosa phenotypes. T. broussonetii, T. maroccanus, T. pallidus and R. officinalis EOs exhibited strong EPI-mediated chemosensitizers-restoring activities against MDR phenotypes of E. coli, Enterobacter aerogenes, K. pneumoniae, S. enterica serotype Typhimurium and P. aeruginosa (Fadli et al., 2011). Eugenol is an important antimicrobial terpene which can be found in the EOs of plants such as cinnamon or cloves. The study by Muniz et al. (2021) reported the antibacterial activity of eugenol and its derivatives including isoeugenol, allylbenzene, 4-allylanisole, and 4-allyl-2,6-dimethoxyphenol against the S. aureus NorA EPs.
Mobile genetic elements, in particular plasmids, are well known for their role in horizontal gene transfer of resistance genes and thus the spread of antibiotic resistance. EOs have been assessed for their bactericidal as well as resistance reversal potentials attributed mainly to their abilities to eliminate R-plasmids. Schelz et al. (2006) reported potent antibacterial and plasmid-curing activities of EOs of several plants besides purified turpentine oil against S. epidermidis and E. coli strains. Si et al. (2008) reported strong antibacterial activities of oregano EOs against MDR ESBL E. coli strain, and the EOs exhibited additive effects when combined with antibiotics like amoxicillin, polymycin, and lincomycin, and the activities were attributed to the plasmid affecting abilities of EOs. A combination of antibiotics and EOs of cinnamon bark, tea tree, peppermint and lavender significantly decreased the drug-resistance level in E. coli (Yap et al., 2013). In an interesting study, Thymus vulgaris EOs lowered the virulence and abilities to spread the drug-resistance in E. coli (Skalicka-Woźniak et al., 2018).
Biofilm formation and quorum sensing are reported to be highly effective approaches evolved by the bacteria for conferring drug-resistance, its persistence and spread. Therefore, targeting bacterial biofilms and quorum sensing are emerging as effective approaches for combating drug resistance. However, eradicating or inhibiting biofilm is challenging. However, there are recent reports on anti-biofilm and anti-quorum sensing activities of EOs. For instance, Merghni et al. (2018) reported biofilm and quorum-sensing inhibitory activities of EO from Eucalyptus globulus against MRSA strains. Strong biofilm inhibitory activities were reported from some important individual constituents of EOs against community-associated MRSA strains (Espina et al., 2015). The Plectranthus amboinicus EOs also displayed striking biofilm inhibitory activities against antibiotic resistant S. aureus (Vasconcelos et al., 2017). Similarly, strong biofilm and quorum sensing inhibitory activities were reported from EOs of T. daenensis and Satureja hortensis against S. aureus (Sharifi et al., 2018). A study by Alibi et al. (2020) have demonstrated the successful application of the EOs from Cinnamomum verum, Thymus vulgaris and Eugenia caryophyllata against to inhibit growth of 105 MDR clinical isolates via significant anti-biofilm and anti-quorum sensing activities.
Conclusion and Future Perspectives
Antibiotic-resistant pathogens are causing major concern to the current global healthcare system. Most of these pathogens are notably developed via the nosocomial sources and the speed of progression and spread of antibiotic-resistant strains is unprecedented and extraordinarily pacey. The development of new/alternative and effective course of treatments against drug resistant pathogens is therefore emerging as a top priority globally. The active principles of plant origin, referred to as phytochemicals have emerged as an alternative to the conventional antibiotics to treat such antibiotic resistant pathogen originated infections. Many phytochemicals have demonstrated their potential as bactericidal agents or antimicrobial agents potentiating the activity of existing antibiotics (antibiotic reversal agents). These phytochemicals are proving to the promising alternatives in response to the shrinking pool of conventional antibiotics. These phytochemicals have proved to inhibit the major resistance-gaining determinant such as efflux pumps, replication machineries, cell permeability and other events vital for sustenance and resistance of the pathogen. The combinatorial application of these phytochemicals has proved well effective against antibiotic-resistant strains. These phytochemicals have displayed themselves as future drugs and knowledge that is more scientific in this regard is essential. Even though there are numerous successful examples of phytochemicals combating antibiotic resistant infections, the translational success or commercial application of such phytochemicals is very low and needs a drastic improvement. Hence, there is the utmost need for fast-track research, clinical approval and application of these phytochemicals to battle against the MDR associated clinical complications.
Author Contributions
VK, ZL, and Z-SC conceived the idea. TK, UA, AD, YA drafted and revised the manuscript. TK prepared the tables and figures. All the authors made substantial contribution to the manuscript revision and approved it for publication.
Funding
This work was funded by the Natural Science Foundation of Shandong Province, China (ZR2019MC059).
Conflict of Interest
The authors declare that the research was conducted in the absence of any commercial or financial relationships that could be construed as a potential conflict of interest.
Acknowledgments
VK acknowledges the use of facilities created under DST-FIST and DBT Star College implemented at Modern College of Arts, Science and Commerce, Ganeshkhind, Pune, India.
References
Abdelfatah, S. A. A., and Efferth, T. (2015). Cytotoxicity of the Indole Alkaloid Reserpine from Rauwolfia Serpentina against Drug-Resistant Tumor Cells. Phytomedicine 22, 308–318. doi:10.1016/j.phymed.2015.01.002
Aires, A., Marques, E., Carvalho, R., Rosa, E., and Saavedra, M. (2013). Evaluation of Biological Value and Appraisal of Polyphenols and Glucosinolates from Organic Baby-Leaf Salads as Antioxidants and Antimicrobials against Important Human Pathogenic Bacteria. Molecules 18, 4651–4668. doi:10.3390/molecules18044651
Alibi, S., Ben Selma, W., Ramos-Vivas, J., Smach, M. A., Touati, R., Boukadida, J., et al. (2020). Anti-oxidant, Antibacterial, Anti-biofilm, and Anti-Quorum Sensing Activities of Four Essential Oils against Multidrug-Resistant Bacterial Clinical Isolates. Curr. Res. Transl. Med. 68 (2), 59–66. doi:10.1016/j.retram.2020.01.001
Althunibat, O. Y., Qaralleh, H., Al-Dalin, S. Y. A., Abboud, M., Khleifat, K., Majali, I. S., et al. (2016). Effect of Thymol and Carvacrol, the Major Components of Thymus Capitatus on the Growth of Pseudomonas aeruginosa. J. Pure Appl. Microbiol. 10, 367–374.
Anand, U., Jacobo-Herrera, N., Altemimi, A., and Lakhssassi, N. (2019). A Comprehensive Review on Medicinal Plants as Antimicrobial Therapeutics: Potential Avenues of Biocompatible Drug Discovery. Metabolites 9, 258. doi:10.3390/metabo9110258
Anand, U., Nandy, S., Mundhra, A., Das, N., Pandey, D. K., and Dey, A. (2020). A Review on Antimicrobial Botanicals, Phytochemicals and Natural Resistance Modifying Agents from Apocynaceae Family: Possible Therapeutic Approaches against Multidrug Resistance in Pathogenic Microorganisms. Drug Resist. Updates 51, 100695. doi:10.1016/j.drup.2020.100695
Basile, A., Sorbo, S., Spadaro, V., Bruno, M., Maggio, A., Faraone, N., et al. (2009). Antimicrobial and Antioxidant Activities of Coumarins from the Roots of Ferulago Campestris (Apiaceae). Molecules 14, 939–952. doi:10.3390/molecules14030939
Bazargani, M. M., and Rohloff, J. (2016). Antibiofilm Activity of Essential Oils and Plant Extracts against Staphylococcus aureus and Escherichia coli Biofilms. Food Control 61, 156–164. doi:10.1016/j.foodcont.2015.09.036
Bazzaz, B. S. F., Memariani, Z., Khashiarmanesh, Z., Iranshahi, M., and Naderinasab, M. (2010). Effect of Galbanic Acid, a Sesquiterpene Coumarin from Ferula Szowitsiana, as an Inhibitor of Efflux Mechanism in Resistant Clinical Isolates of Staphylococcus aureus. Braz. J. Microbiol. 41, 574–580. doi:10.1590/S1517-83822010000300006
Belofsky, G., Percivill, D., Lewis, K., Tegos, G. P., and Ekart, J. (2004). Phenolic Metabolites ofDaleaversicolorthat Enhance Antibiotic Activity against Model Pathogenic Bacteria. J. Nat. Prod. 67, 481–484. doi:10.1021/np030409c
Ben Said, L., Jouini, A., Alonso, C. A., Klibi, N., Dziri, R., Boudabous, A., et al. (2016). Characteristics of Extended-Spectrum β-lactamase (ESBL)- and pAmpC Beta-Lactamase-Producing Enterobacteriaceae of Water Samples in Tunisia. Sci. Total Environ. 550, 1103–1109. doi:10.1016/j.scitotenv.2016.01.042
Benameur, Q., Gervasi, T., Pellizzeri, V., Pľuchtová, M., Tali-Maama, H., Assaous, F., et al. (2019). Antibacterial Activity of Thymus Vulgaris Essential Oil Alone and in Combination with Cefotaxime against blaESBL Producing Multidrug Resistant Enterobacteriaceae Isolates. Nat. Product. Res. 33, 2647–2654. doi:10.1080/14786419.2018.1466124
Blesa, A., César, C. E., Averhoff, B., and Berenguer, J. (2015). Noncanonical Cell-To-Cell DNA Transfer in Thermus Spp. Is Insensitive to Argonaute-Mediated Interference. J. Bacteriol. 197, 138–146. doi:10.1128/JB.02113-14
Blesa, A., Baquedano, I., Quintáns, N. G., Mata, C. P., Castón, J. R., and Berenguer, J. (2017). The Transjugation Machinery of Thermus Thermophilus: Identification of TdtA, an ATPase Involved in DNA Donation. PLOS Genet. 13, e1006669. doi:10.1371/journal.pgen.1006669
Boberek, J. M., Stach, J., and Good, L. (2010). Genetic Evidence for Inhibition of Bacterial Division Protein FtsZ by Berberine. PLoS One 5, e13745. doi:10.1371/journal.pone.0013745
Borges, A., Ferreira, C., Saavedra, M. J., and Simões, M. (2013). Antibacterial Activity and Mode of Action of Ferulic and Gallic Acids against Pathogenic Bacteria. Microb. Drug Resist. 19, 256–265. doi:10.1089/mdr.2012.0244
Boulet, M. L., Isabelle, C., Guay, I., Brouillette, E., Langlois, J. P., JacquesÉ, P., et al. (2018). Tomatidine Is a lead Antibiotic Molecule that Targets Staphylococcus aureus ATP Synthase Subunit C. Antimicrob. Agents Chemother. 62, e02197-17. doi:10.1128/AAC.02197-17
Brehm-Stecher, B. F., and Johnson, E. A. (2003). Sensitization of Staphylococcus aureus and Escherichia coli to Antibiotics by the Sesquiterpenoids Nerolidol, Farnesol, Bisabolol, and Apritone. Antimicrob. Agents Chemother. 47, 3357–3360. doi:10.1128/AAC.47.10.3357-3360.2003
Bučková, M., Puškárová, A., Kalászová, V., Kisová, Z., and Pangallo, D. (2018). Essential Oils against Multidrug Resistant Gram-Negative Bacteria. Biologia 73, 803–808. doi:10.2478/s11756-018-0090-x
Buckner, M. M. C., Ciusa, M. L., and Piddock, L. J. V. (2018). Strategies to Combat Antimicrobial Resistance: Anti-plasmid and Plasmid Curing. FEMS Microbiol. Rev. 42, 781–804. doi:10.1093/femsre/fuy031
Budzynska, A., Rozalski, M., Karolczak, W., Wieckowska-Szakiel, M., Sadowska, B., and Rozalska, B. (2011). Synthetic 3-Arylidenefl Avanones as Inhibitors of the Initial Stages of Biofilm Formation by Staphylococcus aureus and Enterococcus faecalis. Z. Naturforsch. C 66, 104–114. doi:10.1515/znc-2011-3-40310.5560/znc.2011.66c0104
Bueno, J. (2016). Antimicrobial Adjuvants Drug Discovery, the Challenge of Avoid the Resistance and Recover the Susceptibility of Multidrug-Resistant Strains. J. Microb. Biochem. Technol. 8, 169–176. doi:10.4172/1948-5948.1000281
Burt, S. A., and Reinders, R. D. (2003). Antibacterial Activity of Selected Plant Essential Oils against Escherichia coli O157:H7. Lett. Appl. Microbiol. 36, 162–167. doi:10.1046/j.1472-765X.2003.01285.x
Bush, K., and Jacoby, G. A. (2010). Updated Functional Classification of β-Lactamases. Antimicrob. Agents Chemother. 54, 969–976. doi:10.1128/AAC.01009-09
Bush, K. (2013). The ABCD's of β-lactamase Nomenclature. J. Infect. Chemother. 19, 549–559. doi:10.1007/s10156-013-0640-7
Cai, Y., Wang, R., An, M.-M., Liang, B.-B., and Fang, Y. (2008). In Vitro bactericidal Activity of Allicin Combined with Cefoperazone, Tobramycin and Ciprofloxacin. Int. J. Antimicrob. Agents 31, 179–180. doi:10.1016/j.ijantimicag.2007.10.009
Casciaro, B., Mangiardi, L., Cappiello, F., Romeo, I., Loffredo, M. R., Iazzetti, A., et al. (2020). Naturally-Occurring Alkaloids of Plant Origin as Potential Antimicrobials against Antibiotic-Resistant Infections. Molecules 25, 3619. doi:10.3390/molecules25163619
Casu, L., Cottiglia, F., Leonti, M., De Logu, A., Agus, E., Tse-Dinh, Y.-C., et al. (2011). Ungeremine Effectively Targets Mammalian as Well as Bacterial Type I and Type II Topoisomerases. Bioorg. Med. Chem. Lett. 21, 7041–7044. doi:10.1016/j.bmcl.2011.09.097
Centers for Disease Control and Prevenation (2019). Biggest Threats and Data | Antibiotic/Antimicrobial Resistance| CDC. Available at: https://www.cdc.gov/drugresistance/biggest-threats.html (Accessed February 12, 2021).
Chakraborty, B., Nath, A., Saikia, H., and Sengupta, M. (2014). Bactericidal Activity of Selected Medicinal Plants against Multidrug Resistant Bacterial Strains from Clinical Isolates. Asian Pac. J. Trop. Med. 7, S435–S441. doi:10.1016/S1995-7645(14)60271-6
Chan, B. C. L., Ip, M., Lau, C. B. S., Lui, S. L., Jolivalt, C., Ganem-Elbaz, C., et al. (2011). Synergistic Effects of Baicalein with Ciprofloxacin against NorA Over-expressed Methicillin-Resistant Staphylococcus aureus (MRSA) and Inhibition of MRSA Pyruvate Kinase. J. Ethnopharmacol. 137, 767–773. doi:10.1016/j.jep.2011.06.039
Chiang, Y. N., Penadés, J. R., and Chen, J. (2019). Genetic Transduction by Phages and Chromosomal Islands: The New and Noncanonical. PLOS Pathog. 15, e1007878. doi:10.1371/journal.ppat.1007878
Choi, J.-G., Mun, S.-H., Chahar, H. S., Bharaj, P., Kang, O.-H., Kim, S.-G., et al. (2014). Methyl Gallate from Galla Rhois Successfully Controls Clinical Isolates of Salmonella Infection in Both In Vitro and In Vivo Systems. PLoS One 9, e102697. doi:10.1371/journal.pone.0102697
Chopra, B., Dhingra, K. A., and Dhar, K. L. (2019). Synthesis and Characterization of Piperine Analogs as Potent Staphylococcus aureus NorA Efflux Pump Inhibitors. Chem. Methodol. 3 (1), 104–114. doi:10.22034/chemm.2018.143459.1068
Chovanová, R., Mezovská, J., Vaverková, Š., and Mikulášová, M. (2015). The Inhibition the Tet(K) Efflux Pump of Tetracycline Resistant Staphylococcus Epidermidis by Essential Oils from Three Salvia Species. Lett. Appl. Microbiol. 61, 58–62. doi:10.1111/lam.12424
Christena, L. R., Subramaniam, S., Vidhyalakshmi, M., Mahadevan, V., Sivasubramanian, A., and Nagarajan, S. (2015). Dual Role of Pinostrobin-A Flavonoid Nutraceutical as an Efflux Pump Inhibitor and Antibiofilm Agent to Mitigate Food Borne Pathogens. RSC Adv. 5, 61881–61887. doi:10.1039/C5RA07165H
Chusri, S., Siriyong, T., Na-Phatthalung, P., and Voravuthikunchai, S. P. (2014). Synergistic Effects of Ethnomedicinal Plants of Apocynaceae Family and Antibiotics against Clinical Isolates of Acinetobacter Baumannii. Asian Pac. J. Trop. Med. 7, 456–461. doi:10.1016/S1995-7645(14)60074-2
Claverys, J.-P., Martin, B., and Polard, P. (2009). The Genetic Transformation Machinery: Composition, Localization, and Mechanism. FEMS Microbiol. Rev. 33, 643–656. doi:10.1111/j.1574-6976.2009.00164.x
Compean, K. L., and Ynalvez, R. A. (2014). Antimicrobial Activity of Plant Secondary Metabolites: A Review. Res. J. Med. Plant 8, 204–213. doi:10.3923/rjmp.2014.204.213
Cox, S. D., Mann, C. M., Markham, J. L., Bell, H. C., Gustafson, J. E., Warmington, J. R., et al. (2000). The Mode of Antimicrobial Action of the Essential Oil of Melaleuca Alternifolia (tea Tree Oil). J. Appl. Microbiol. 88, 170–175. doi:10.1046/j.1365-2672.2000.00943.x
Cueva, C., Mingo, S., Muñoz-González, I., Bustos, I., Requena, T., del Campo, R., et al. (2012). Antibacterial Activity of Wine Phenolic Compounds and Oenological Extracts against Potential Respiratory Pathogens. Lett. Appl. Microbiol. 54, 557–563. doi:10.1111/j.1472-765X.2012.03248.x
Cushnie, T. P. T., Taylor, P. W., Nagaoka, Y., Uesato, S., Hara, Y., and Lamb, A. J. (2008). Investigation of the Antibacterial Activity of 3-O-Octanoyl-(-)-Epicatechin. J. Appl. Microbiol. 105, 1461–1469. doi:10.1111/j.1365-2672.2008.03881.x
Cushnie, T. P. T., Cushnie, B., and Lamb, A. J. (2014). Alkaloids: An Overview of Their Antibacterial, Antibiotic-Enhancing and Antivirulence Activities. Int. J. Antimicrob. Agents 44, 377–386. doi:10.1016/j.ijantimicag.2014.06.001
Dahiya, P., Dahiya, P., and Purkayastha, S. (2012). Phytochemical Screening and Antimicrobial Activity of Some Medicinal Plants against Multi-Drug Resistant Bacteria from Clinical Isolates. Indian J. Pharm. Sci. 74, 443–450. doi:10.4103/0250-474X.108420
Damier-Piolle, L., Magnet, S., Brémont, S., Lambert, T., and Courvalin, P. (2008). AdeIJK, a Resistance-Nodulation-Cell Division Pump Effluxing Multiple Antibiotics in Acinetobacter Baumannii. Antimicrob. Agents Chemother. 52, 557–562. doi:10.1128/AAC.00732-07
de Morais Oliveira-Tintino, C. D., Tintino, S. R., Limaverde, P. W., Figueredo, F. G., Campina, F. F., da Cunha, F. A. B., et al. (2018). Inhibition of the Essential Oil from Chenopodium Ambrosioides L. And α-terpinene on the NorA Efflux-Pump of Staphylococcus aureus. Food Chem. 262, 72–77. doi:10.1016/j.foodchem.2018.04.040
de Souza, E. L., dos Santos, A. S., de Albuquerque, T. M. R., Lacerda Massa, N. M., and de Brito Alves, J. L. (2019). Potential Interactions Among Phenolic Compounds and Probiotics for Mutual Boosting of Their Health-Promoting Properties and Food Functionalities - A Review. Crit. Rev. Food Sci. Nutr. 59, 1645–1659. doi:10.1080/10408398.2018.1425285
Del Rio, D., Rodriguez-Mateos, A., Spencer, J. P. E., Tognolini, M., Borges, G., and Crozier, A. (2013). Dietary (Poly)phenolics in Human Health: Structures, Bioavailability, and Evidence of Protective Effects against Chronic Diseases. Antioxid. Redox Signaling 18, 1818–1892. doi:10.1089/ars.2012.4581
Delavat, F., Miyazaki, R., Carraro, N., Pradervand, N., and van der Meer, J. R. (2017). The Hidden Life of Integrative and Conjugative Elements. FEMS Microbiol. Rev. 41, 512–537. doi:10.1093/femsre/fux008
Dib, J. n. R., Wagenknecht, M., FarÃ, M. a. E., and Meinhardt, F. (2015). Strategies and Approaches in Plasmidome Studiesâ€"uncovering Plasmid Diversity Disregarding of Linear Elements?. Front. Microbiol. 6, 463. doi:10.3389/fmicb.2015.00463
Domadia, P. N., Bhunia, A., Sivaraman, J., Swarup, S., and Dasgupta, D. (2008). Berberine Targets Assembly of Escherichia coli Cell Division Protein FtsZ. Biochemistry 47, 3225–3234. doi:10.1021/bi7018546
Dordet-Frisoni, E., Faucher, M., Sagné, E., Baranowski, E., Tardy, F., Nouvel, L. X., et al. (2019). Mycoplasma Chromosomal Transfer: A Distributive, Conjugative Process Creating an Infinite Variety of Mosaic Genomes. Front. Microbiol. 10, 2441. doi:10.3389/fmicb.2019.02441
Dubnau, D., and Blokesch, M. (2019). Mechanisms of DNA Uptake by Naturally Competent Bacteria. Annu. Rev. Genet. 53, 217–237. doi:10.1146/annurev-genet-112618-043641
Dwivedi, G. R., Maurya, A., Yadav, D. K., Singh, V., Khan, F., Gupta, M. K., et al. (2019). Synergy of Clavine Alkaloid 'chanoclavine' with Tetracycline against Multi-Drug-Resistant E. coli. J. Biomol. Struct. Dyn. 37, 1307–1325. doi:10.1080/07391102.2018.1458654
El-Seedi, H. R. (2007). Antimicrobial Arylcoumarins from Asphodelus Microcarpus. J. Nat. Prod. 70, 118–120. doi:10.1021/np060444u
Espina, L., Pagán, R., López, D., and García-Gonzalo, D. (2015). Individual Constituents from Essential Oils Inhibit Biofilm Mass Production by Multi-Drug Resistant Staphylococcus aureus. Molecules 20, 11357–11372. doi:10.3390/molecules200611357
Evans, W. C., and Evans, D. (2009). “Alkaloids,” in Trease And Evans’s Pharmacognosy,”. Editor W. Evans (London, UK: Elsevier), 353–415. doi:10.1016/b978-0-7020-2933-2.00026-5
Fadli, M., Chevalier, J., Saad, A., Mezrioui, N.-E., Hassani, L., and Pages, J.-M. (2011). Essential Oils from Moroccan Plants as Potential Chemosensitisers Restoring Antibiotic Activity in Resistant Gram-Negative Bacteria. Int. J. Antimicrob. Agents 38, 325–330. doi:10.1016/j.ijantimicag.2011.05.005
Fahey, J. W., Stephenson, K. K., Wade, K. L., and Talalay, P. (2013). Urease from Helicobacter pylori Is Inactivated by Sulforaphane and Other Isothiocyanates. Biochem. Biophys. Res. Commun. 435, 1–7. doi:10.1016/j.bbrc.2013.03.126
Feng, R., Qu, J., Zhou, W., Wei, Q., Yin, Z., Du, Y., et al. (2016). Antibacterial Activity and Mechanism of Berberine on Avian Pasteurella Multocida. Int. J. Clin. Exp. Med. 9, 22886–22892.
Fernández, L., and Hancock, R. E. W. (2012). Adaptive and Mutational Resistance: Role of Porins and Efflux Pumps in Drug Resistance. Clin. Microbiol. Rev. 25, 661–681. doi:10.1128/CMR.00043-12
Fischbach, M. A., and Walsh, C. T. (2009). Antibiotics for Emerging Pathogens. Science 325, 1089–1093. doi:10.1126/science.1176667
Floss, H. G., and Yu, T.-W. (2005). RifamycinMode of Action, Resistance, and Biosynthesis. Chem. Rev. 105, 621–632. doi:10.1021/cr030112j
Gao, W.-W., Gopala, L., Bheemanaboina, R. R. Y., Zhang, G.-B., Li, S., and Zhou, C.-H. (2018). Discovery of 2-aminothiazolyl Berberine Derivatives as Effectively Antibacterial Agents toward Clinically Drug-Resistant Gram-Negative Acinetobacter Baumanii. Eur. J. Med. Chem. 146, 15–37. doi:10.1016/j.ejmech.2018.01.038
Gibbons, S., Moser, E., and Kaatz, G. W. (2004). Catechin Gallates Inhibit Multidrug Resistance (MDR) inStaphylococcus Aureus. Planta Med. 70, 1240–1242. doi:10.1055/s-2004-835860
Górniak, I., Bartoszewski, R., and Króliczewski, J. (2019). Comprehensive Review of Antimicrobial Activities of Plant Flavonoids. Phytochem. Rev. 18, 241–272. doi:10.1007/s11101-018-9591-z
Gothwal, R., and Shashidhar, T. (2015). Antibiotic Pollution in the Environment: A Review. Clean. Soil Air Water 43, 479–489. doi:10.1002/clen.201300989
Gradišar, H., Pristovšek, P., Plaper, A., and Jerala, R. (2007). Green Tea Catechins Inhibit Bacterial DNA Gyrase by Interaction with its ATP Binding Site. J. Med. Chem. 50, 264–271. doi:10.1021/jm060817o
Gray, T. A., and Derbyshire, K. M. (2018). Blending Genomes: Distributive Conjugal Transfer in Mycobacteria, a Sexier Form of HGT. Mol. Microbiol. 108, 601–613. doi:10.1111/mmi.13971
Grüll, M. P., Mulligan, M. E., and Lang, A. S.. (2018). Small Extracellular Particles with Big Potential for Horizontal Gene Transfer: Membrane Vesicles and Gene Transfer Agents. FEMS Microbiol Lett 365, fny192. doi:10.1093/femsle/fny192
Guay, I., Boulanger, S., Isabelle, C., Brouillette, E., Chagnon, F., Bouarab, K., et al. (2018). Tomatidine and Analog FC04-100 Possess Bactericidal Activities against Listeria, Bacillus and Staphylococcus Spp. BMC Pharmacol. Toxicol. 19, 7. doi:10.1186/s40360-018-0197-2
Gull, I., Sohail, M., Aslam, M., and Athar, M. (2013). Phytochemical, Toxicological and Antimicrobial Evaluation of Lawsonia Inermis Extracts against Clinical Isolates of Pathogenic Bacteria. Ann. Clin. Microbiol. Antimicrobials 12, 36. doi:10.1186/1476-0711-12-36
Hall, J. P. J., Brockhurst, M. A., and Harrison, E. (2017). Sampling the mobile Gene Pool: Innovation via Horizontal Gene Transfer in Bacteria. Phil. Trans. R. Soc. B 372, 20160424. doi:10.1098/rstb.2016.0424
Hamoud, R., Reichling, J., and Wink, M. (2014). Synergistic Antimicrobial Activity of Combinations of Sanguinarine and EDTA with Vancomycin against Multidrug Resistant Bacteria. Drug Metab. Lett. 8, 119–128. doi:10.2174/187231280802150212100742
Hamoud, R., Reichling, J., and Wink, M. (2015). Synergistic Antibacterial Activity of the Combination of the Alkaloid Sanguinarine with EDTA and the Antibiotic Streptomycin against Multidrug Resistant Bacteria. J. Pharm. Pharmacol. 67, 264–273. doi:10.1111/jphp.12326
Hedges, R. W., and Jacob, A. E. (1974). Transposition of Ampicillin Resistance from RP4 to Other Replicons. Mol. Gen. Genet. 132, 31–40. doi:10.1007/BF00268228
Heeb, S., Fletcher, M. P., Chhabra, S. R., Diggle, S. P., Williams, P., and Cámara, M. (2011). Quinolones: From Antibiotics to Autoinducers. FEMS Microbiol. Rev. 35, 247–274. doi:10.1111/j.1574-6976.2010.00247.x
Helander, I. M., Alakomi, H.-L., Latva-Kala, K., Mattila-Sandholm, T., Pol, I., Smid, E. J., et al. (1998). Characterization of the Action of Selected Essential Oil Components on Gram-Negative Bacteria. J. Agric. Food Chem. 46, 3590–3595. doi:10.1021/jf980154m
Holler, J. G., Christensen, S. B., Slotved, H.-C., Rasmussen, H. B., Guzman, A., Olsen, C.-E., et al. (2012). Novel Inhibitory Activity of the Staphylococcus aureus NorA Efflux Pump by a Kaempferol Rhamnoside Isolated from Persea Lingue Nees. J. Antimicrob. Chemother. 67, 1138–1144. doi:10.1093/jac/dks005
Hyldgaard, M., Mygind, T., and Meyer, R. L. (2012). Essential Oils in Food Preservation: Mode of Action, Synergies, and Interactions with Food Matrix Components. Front. Microbio. 3, 12. doi:10.3389/fmicb.2012.00012
Iwasa, K., Moriyasu, M., Yamori, T., Turuo, T., Lee, D.-U., and Wiegrebe, W. (2001). In Vitro Cytotoxicity of the Protoberberine-type Alkaloids. J. Nat. Prod. 64, 896–898. doi:10.1021/np000554f
Jeong, K.-W., Lee, J.-Y., Kang, D.-I., Lee, J.-U., Shin, S. Y., and Kim, Y. (2009). Screening of Flavonoids as Candidate Antibiotics against Enterococcus faecalis. J. Nat. Prod. 72, 719–724. doi:10.1021/np800698d
Jia, W., Wang, J., Xu, H., and Li, G. (2015).Resistance of Stenotrophomonas Maltophilia to Fluoroquinolones: Prevalence in a University Hospital and Possible Mechanisms. Int. J. Environ. Res. Public Health, 12, 5177–5195. doi:10.3390/ijerph120505177
Jiang, Q.-W., Chen, M.-W., Cheng, K.-J., Yu, P.-Z., Wei, X., and Shi, Z. (2016). Therapeutic Potential of Steroidal Alkaloids in Cancer and Other Diseases. Med. Res. Rev. 36, 119–143. doi:10.1002/med.21346
Kang, S., Li, Z., Yin, Z., Jia, R., Song, X., Li, L., et al. (2015). The Antibacterial Mechanism of Berberine againstActinobacillus Pleuropneumoniae. Nat. Product. Res. 29, 2203–2206. doi:10.1080/14786419.2014.1001388
Kelley, C., Zhang, Y., Parhi, A., Kaul, M., Pilch, D. S., and LaVoie, E. J. (2012). 3-Phenyl Substituted 6,7-dimethoxyisoquinoline Derivatives as FtsZ-Targeting Antibacterial Agents. Bioorg. Med. Chem. 20, 7012–7029. doi:10.1016/j.bmc.2012.10.009
Khalil, N., Ashour, M., Fikry, S., Singab, A. N., and Salama, O. (2018). Chemical Composition and Antimicrobial Activity of the Essential Oils of Selected Apiaceous Fruits. Future J. Pharm. Sci. 4, 88–92. doi:10.1016/j.fjps.2017.10.004
Khameneh, B., Iranshahy, M., Ghandadi, M., Ghoochi Atashbeyk, D., Fazly Bazzaz, B. S., et al. (2015). Investigation of the Antibacterial Activity and Efflux Pump Inhibitory Effect of Co-loaded Piperine and Gentamicin Nanoliposomes in Methicillin-resistantStaphylococcus Aureus. Drug Dev. Ind. Pharm. 41, 989–994. doi:10.3109/03639045.2014.920025
Khan, I. A., Mirza, Z. M., Kumar, A., Verma, V., and Qazi, G. N. (2006). Piperine, a Phytochemical Potentiator of Ciprofloxacin against Staphylococcus aureus. Antimicrob. Agents Chemother. 50, 810–812. doi:10.1128/AAC.50.2.810-812.2006
Klančnik, A., Šikić Pogačar, M., Trošt, K., Tušek Žnidarič, M., Mozetič Vodopivec, B., and Smole Možina, S. (2017). Anti-Campylobacter Activity of Resveratrol and an Extract from Waste Pinot Noir Grape Skins and Seeds, and Resistance of Camp. Jejuni Planktonic and Biofilm Cells, Mediated via the CmeABC Efflux Pump. J. Appl. Microbiol. 122, 65–77. doi:10.1111/jam.13315
Kongkham, B., Prabakaran, D., and Puttaswamy, H. (2020). Opportunities and Challenges in Managing Antibiotic Resistance in Bacteria Using Plant Secondary Metabolites. Fitoterapia 147, 104762. doi:10.1016/j.fitote.2020.104762
Koulenti, D., Fragkou, P. C., and Tsiodras, S. (2020). Editorial for Special Issue "Multidrug-Resistant Pathogens". Microorganisms 8, 1383. doi:10.3390/microorganisms8091383
Kuete, V., Wansi, J. D., Mbaveng, A. T., Kana Sop, M. M., Beng, V. P., Etoa, F. X., et al. (2008). Antimicrobial Activity of the Methanolic Extract and Compounds from Teclea Afzelii (Rutaceae). South Afr. J. Bot. 74, 572–576. doi:10.1016/j.sajb.2008.02.004
Kumar, A., Khan, I. A., Koul, S., Koul, J. L., Taneja, S. C., Ali, I., et al. (2008). Novel Structural Analogues of Piperine as Inhibitors of the NorA Efflux Pump of Staphylococcus aureus. J. Antimicrob. Chemother. 61, 1270–1276. doi:10.1093/jac/dkn088
Kwiatkowski, P., Pruss, A., Grygorcewicz, B., Wojciuk, B., Dołęgowska, B., Giedrys-Kalemba, S., et al. (2018). Preliminary Study on the Antibacterial Activity of Essential Oils Alone and in Combination with Gentamicin against Extended-Spectrum β-Lactamase-Producing and New Delhi Metallo-β-Lactamase-1-Producing Klebsiella pneumoniae Isolates. Microb. Drug Resist. 24, 1368–1375. doi:10.1089/mdr.2018.0051
Lahmar, A., Bedoui, A., Mokdad-Bzeouich, I., Dhaouifi, Z., Kalboussi, Z., Cheraif, I., et al. (2017). Reversal of Resistance in Bacteria Underlies Synergistic Effect of Essential Oils with Conventional Antibiotics. Microb. Pathog. 106, 50–59. doi:10.1016/j.micpath.2016.10.018
Lanzotti, V., Scala, F., and Bonanomi, G. (2014). Compounds from Allium Species with Cytotoxic and Antimicrobial Activity. Phytochem. Rev. 13, 769–791. doi:10.1007/s11101-014-9366-0
Leclercq, R. (2002). Mechanisms of Resistance to Macrolides and Lincosamides: Nature of the Resistance Elements and Their Clinical Implications. Clin. Infect. Dis. 34, 482–492. doi:10.1086/324626
Lim, A., Subhan, N., Jazayeri, J. A., John, G., Vanniasinkam, T., and Obied, H. K. (2016). Plant Phenols as Antibiotic Boosters: In Vitro Interaction of Olive Leaf Phenols with Ampicillin. Phytother. Res. 30, 503–509. doi:10.1002/ptr.5562
Lima, M. C., Paiva de Sousa, C., Fernandez-Prada, C., Harel, J., Dubreuil, J. D., de Souza, E. L., et al. (2019). A Review of the Current Evidence of Fruit Phenolic Compounds as Potential Antimicrobials against Pathogenic Bacteria. Microb. Pathog. 130, 259–270. doi:10.1016/j.micpath.2019.03.025
Limaverde, P. W., Campina, F. F., da Cunha, F. A. B., Crispim, F. D., Figueredo, F. G., Lima, L. F., et al. (2017). Inhibition of the TetK Efflux-Pump by the Essential Oil of Chenopodium Ambrosioides L. And α-terpinene against Staphylococcus aureus IS-58. Food Chem. Toxicol. 109, 957–961. doi:10.1016/j.fct.2017.02.031
Lin, R.-D., Chin, Y.-P., and Lee, M.-H. (2005). Antimicrobial Activity of Antibiotics in Combination with Natural Flavonoids against Clinical Extended-Spectrumβ-Lactamase (ESBL)-producingKlebsiella Pneumoniae. Phytother. Res. 19, 612–617. doi:10.1002/ptr.1695
Liu, B., Zhou, C., Zhang, Z., Roland, J. D., and Lee, B. P. (2021). Antimicrobial Property of Halogenated Catechols. Chem. Eng. J. 403, 126340. doi:10.1016/j.cej.2020.126340
Lopes, L. A. A., dos Santos Rodrigues, J. B., Magnani, M., de Souza, E. L., and de Siqueira-Júnior, J. P. (2017). Inhibitory Effects of Flavonoids on Biofilm Formation by Staphylococcus aureus that Overexpresses Efflux Protein Genes. Microb. Pathogenesis 107, 193–197. doi:10.1016/j.micpath.2017.03.033
Madikizela, B., Aderogba, M. A., and Van Staden, J. (2013). Isolation and Characterization of Antimicrobial Constituents of Searsia Chirindensis L. (Anacardiaceae) Leaf Extracts. J. Ethnopharmacol. 150, 609–613. doi:10.1016/j.jep.2013.09.016
Magiorakos, A.-P., Srinivasan, A., Carey, R. B., Carmeli, Y., Falagas, M. E., Giske, C. G., et al. (2012). Multidrug-resistant, Extensively Drug-Resistant and Pandrug-Resistant Bacteria: an International Expert Proposal for Interim Standard Definitions for Acquired Resistance. Clin. Microbiol. Infect. 18, 268–281. doi:10.1111/j.1469-0691.2011.03570.x
Mak, S., Xu, Y., and Nodwell, J. R. (2014). The Expression of Antibiotic Resistance Genes in Antibiotic-Producing Bacteria. Mol. Microbiol. 93, 391–402. doi:10.1111/mmi.12689
Makarewicz, M., Drożdż, I., Tarko, T., and Duda-Chodak, A. (2021). The Interactions between Polyphenols and Microorganisms, Especially Gut Microbiota. Antioxidants 10, 188. doi:10.3390/antiox10020188
Manson, J. M., Hancock, L. E., and Gilmore, M. S. (2010). Mechanism of Chromosomal Transfer of Enterococcus faecalis Pathogenicity Island, Capsule, Antimicrobial Resistance, and Other Traits. Proc. Natl. Acad. Sci. 107, 12269–12274. doi:10.1073/pnas.1000139107
Mapara, N., Sharma, M., Shriram, V., Bharadwaj, R., Mohite, K. C., and Kumar, V. (2015). Antimicrobial Potentials of Helicteres Isora Silver Nanoparticles against Extensively Drug-Resistant (XDR) Clinical Isolates of Pseudomonas aeruginosa. Appl. Microbiol. Biotechnol. 99, 10655–10667. doi:10.1007/s00253-015-6938-x
Martinez de Tejada, G., Sanchez-Gomez, S., Razquin-Olazaran, I., Kowalski, I., Kaconis, Y., Heinbockel, L., et al. (2012). Bacterial Cell Wall Compounds as Promising Targets of Antimicrobial Agents I. Antimicrobial Peptides and Lipopolyamines. Cdt 13, 1121–1130. doi:10.2174/138945012802002410
Maurya, A., Dwivedi, G. R., Darokar, M. P., and Srivastava, S. K. (2013). Antibacterial and Synergy of Clavine Alkaloid Lysergol and its Derivatives against Nalidixic Acid-ResistantEscherichia Coli. Chem. Biol. Drug Des. 81, 484–490. doi:10.1111/cbdd.12103
Mayaud, L., Carricajo, A., Zhiri, A., and Aubert, G. (2008). Comparison of Bacteriostatic and Bactericidal Activity of 13 Essential Oils against Strains with Varying Sensitivity to Antibiotics. Lett. Appl. Microbiol. 47, 167–173. doi:10.1111/j.1472-765X.2008.02406.x
Mazel, D. (2006). Integrons: Agents of Bacterial Evolution. Nat. Rev. Microbiol. 4, 608–620. doi:10.1038/nrmicro1462
Mbaveng, A. T., Sandjo, L. P., Tankeo, S. B., Ndifor, A. R., Pantaleon, A., Nagdjui, B. T., et al. (2015). Antibacterial Activity of Nineteen Selected Natural Products against Multi-Drug Resistant Gram-Negative Phenotypes. Springerplus 4, 823. doi:10.1186/s40064-015-1645-8
Merghni, A., Noumi, E., Hadded, O., Dridi, N., Panwar, H., Ceylan, O., et al. (2018). Assessment of the Antibiofilm and Antiquorum Sensing Activities of Eucalyptus Globulus Essential Oil and its Main Component 1,8-cineole against Methicillin-Resistant Staphylococcus aureus Strains. Microb. Pathog. 118, 74–80. doi:10.1016/j.micpath.2018.03.006
Miklasińska-Majdanik, M., Kępa, M., Wojtyczka, R., Idzik, D., and Wąsik, T. (2018). Phenolic Compounds Diminish Antibiotic Resistance of Staphylococcus Aureus Clinical Strains. Int. J. Environ. Res. Public Health 15, 2321. doi:10.3390/ijerph15102321
Mitchell, G., Lafrance, M., Boulanger, S., Seguin, D. L., Guay, I., Gattuso, M., et al. (2012). Tomatidine Acts in Synergy with Aminoglycoside Antibiotics against Multiresistant Staphylococcus aureus and Prevents Virulence Gene Expression. J. Antimicrob. Chemother. 67, 559–568. doi:10.1093/jac/dkr510
Moellering, R. C. (2012). MRSA: the First Half century. J. Antimicrob. Chemother. 67, 4–11. doi:10.1093/jac/dkr437
Mohammed, M. J., Anand, U., Altemimi, A. B., Tripathi, V., Guo, Y., and Pratap-Singh, A. (2021). Phenolic Composition, Antioxidant Capacity and Antibacterial Activity of White Wormwood (Artemisia Herba-alba). Plants 10, 164. doi:10.3390/plants10010164
Mrozik, A., Piotrowska-Seget, Z., and Łabużek, S. (2004). Changes in Whole Cell-Derived Fatty Acids Induced by Naphthalene in Bacteria from Genus Pseudomonas. Microbiol. Res. 159, 87–95. doi:10.1016/j.micres.2004.02.00110.1016/j.micres.2004.02.001
Munita, J. M., and Arias, C. A.. (2016). Mechanisms of Antibiotic Resistance. Microbiol. Spectr. 4, 481–511. doi:10.1128/microbiolspec.VMBF-0016-2015
Muniz, D. F., dos Santos Barbosa, C. R., de Menezes, I. R. A., de Sousa, E. O., Pereira, R. L. S., Júnior, J. T. C., et al. (2021). In Vitro and In Silico Inhibitory Effects of Synthetic and Natural Eugenol Derivatives against the NorA Efflux Pump in Staphylococcus aureus. Food Chem. 337, 127776. doi:10.1016/j.foodchem.2020.127776
Naveed, R., Hussain, I., Tawab, A., Tariq, M., Rahman, M., Hameed, S., et al. (2013). Antimicrobial Activity of the Bioactive Components of Essential Oils from Pakistani Spices against Salmonella and Other Multi-Drug Resistant Bacteria. BMC Complement. Altern. Med. 13, 265. doi:10.1186/1472-6882-13-265
Nazzaro, F., Fratianni, F., De Martino, L., Coppola, R., and De Feo, V. (2013). Effect of Essential Oils on Pathogenic Bacteria. Pharmaceuticals 6, 1451–1474. doi:10.3390/ph6121451
Newton, S. M., Lau, C., Gurcha, S. S., Besra, G. S., and Wright, C. W. (2002). The Evaluation of Forty-Three Plant Species for In Vitro Antimycobacterial Activities; Isolation of Active Constituents from Psoralea Corylifolia and Sanguinaria canadensis. J. Ethnopharmacol. 79, 57–67. doi:10.1016/S0378-8741(01)00350-6
Nikaido, H. (2003). Molecular Basis of Bacterial Outer Membrane Permeability Revisited. Microbiol. Mol. Biol. Rev. 67, 593–656. doi:10.1128/MMBR.67.4.593-656.2003
Nowacka, N., Nowak, R., Drozd, M., Olech, M., Los, R., and Malm, A. (2015). Antibacterial, Antiradical Potential and Phenolic Compounds of Thirty-One Polish Mushrooms. PLoS ONE 10, e0140355. doi:10.1371/journal.pone.0140355
Obiang-Obounou, B. W. B., Kang, O. H., Choi, J. G., Keum, J. H., Kim, S. B., Mun, S. H., et al. (2011). The Mechanism of Action of Sanguinarine against Methicillin-Resistant Staphylococcus aureus. J. Toxicol. Sci. 36, 277–283. doi:10.2131/jts.36.277
Omar, F., Tareq, A. M., Alqahtani, A. M., Dhama, K., Sayeed, M. A., EmranBin, T. B., et al. (2021). Plant-Based Indole Alkaloids: A Comprehensive Overview from a Pharmacological Perspective. Molecules 26, 2297. doi:10.3390/molecules26082297
Ouyang, J., Sun, F., Feng, W., Sun, Y., Qiu, X., Xiong, L., et al. (2016). Quercetin Is an Effective Inhibitor of Quorum Sensing, Biofilm Formation and Virulence Factors in Pseudomonas aeruginosa. J. Appl. Microbiol. 120, 966–974. doi:10.1111/jam.13073
Oyekale, A. S., and Oyekale, T. O. (2017). Healthcare Waste Management Practices and Safety Indicators in Nigeria. BMC Public Health 17, 1–13. doi:10.1186/s12889-017-4794-6
Pagès, J.-M., James, C. E., and Winterhalter, M. (2008). The Porin and the Permeating Antibiotic: a Selective Diffusion Barrier in Gram-Negative Bacteria. Nat. Rev. Microbiol. 6, 893–903. doi:10.1038/nrmicro1994
Park, H.-W., Choi, K.-D., and Shin, I.-S. (2013). Antimicrobial Activity of Isothiocyanates (ITCs) Extracted from Horseradish (Armoracia Rusticana) Root against Oral Microorganisms. Biocontrol Sci. 18, 163–168. doi:10.4265/bio.18.163
Peng, L., Kang, S., Yin, Z., Jia, R., Song, X., Li, L., et al. (2015). Antibacterial Activity and Mechanism of Berberine against Streptococcus Agalactiae. Int. J. Clin. Exp. Pathol. 8, 5217–5223.
Perdikouri, E. I. A., Arvaniti, K., Lathyris, D., Apostolidou Kiouti, F., Siskou, E., Haidich, A. B., et al. (2019). Infections Due to Multidrug-Resistant Bacteria in Oncological Patients: Insights from a Five-Year Epidemiological and Clinical Analysis. Microorganisms 7, 277. doi:10.3390/microorganisms7090277
Phatthalung, P. N., Chusri, S., and Voravuthikunchai, S. P. (2012). Thai Ethnomedicinal Plants as Resistant Modifying Agents for Combating Acinetobacter Baumannii Infections. BMC Complement. Altern. Med. 12, 1052. doi:10.1186/1472-6882-12-56
Putman, M., van Veen, H. W., and Konings, W. N. (2000). Molecular Properties of Bacterial Multidrug Transporters. Microbiol. Mol. Biol. Rev. 64, 672–693. doi:10.1128/mmbr.64.4.672-693.2000
Randhawa, H. K., Hundal, K. K., Ahirrao, P. N., Jachak, S. M., and Nandanwar, H. S. (2016). Efflux Pump Inhibitory Activity of Flavonoids Isolated from Alpinia Calcarata against Methicillin-Resistant Staphylococcus aureus. Biologia 71, 484–493. doi:10.1515/biolog-2016-0073
Rehman, F., and Mairaj, S. (2013). Antimicrobial Studies of Allicin and Ajoene. Int. J. Pharm. Bio Sci. 4, 1095–1105.
Reiter, J., Levina, N., van der Linden, M., Gruhlke, M., Martin, C., and Slusarenko, A. (2017). Diallylthiosulfinate (Allicin), a Volatile Antimicrobial from Garlic (Allium Sativum), Kills Human Lung Pathogenic Bacteria, Including MDR Strains, as a Vapor. Molecules 22, 1711. doi:10.3390/molecules22101711
Robbers, J., Speedie, M., and Tyler, V. (1996). “Chapter 9: Alkaloids,” in Pharmacognosy And Pharmacobiotechnology”. Editors J. Robbers, M. Speedie, and V. Tyler (London, UK: (Williams & Wilkins), 144–185.
Rubini, D., Banu, S. F., Nisha, P., Murugan, R., Thamotharan, S., Percino, M. J., et al. (2018). Essential Oils from Unexplored Aromatic Plants Quench Biofilm Formation and Virulence of Methicillin Resistant Staphylococcus aureus. Microb. Pathog. 122, 162–173. doi:10.1016/j.micpath.2018.06.028
Saak, C. C., Dinh, C. B., and Dutton, R. J. (2020). Experimental Approaches to Tracking mobile Genetic Elements in Microbial Communities. FEMS Microbiol. Rev. 44, 606–630. doi:10.1093/femsre/fuaa025
Salehzadeh, A., Sadat, M., Doulabi, H., Sohrabnia, B., and Jalali, A. (2018). Journal of Genetic Resources the Effect of Thyme (Thymus Vulgaris) Extract on the Expression of norA Efflux Pump Gene in Clinical Strains of Staphylococcus aureus. J. Genet. 4, 26–36. doi:10.22080/jgr.2018.13900.1099
Samy, R. P., and Gopalakrishnakone, P. (2010). Therapeutic Potential of Plants as Anti-Microbials for Drug Discovery. Evid. based Complement. Altern. Med. 7, 283–294. doi:10.1093/ecam/nen036
Sarjit, A., Wang, Y., and Dykes, G. A. (2015). Antimicrobial Activity of Gallic Acid against Thermophilic Campylobacter Is Strain Specific and Associated with a Loss of Calcium Ions. Food Microbiol. 46, 227–233. doi:10.1016/j.fm.2014.08.002
Saviuc, C., Gheorghe, I., Coban, S., Drumea, V., Chifiriuc, M. C., Banu, O., et al. (2016). Rosmarinus Officinalis Essential Oil and Eucalyptol Act as Efflux Pumps Inhibitors and Increase Ciprofloxacin Efficiency against Pseudomonas aeruginosa and Acinetobacter Baumannii MDR Strains. Rom. Biotechnol. Lett. 21, 11783.
Scazzocchio, F., Mondì, L., Ammendolia, M. G., Goldoni, P., Comanducci, A., Marazzato, M., et al. (2017). Coriander (Coriandrum Sativum) Essential Oil: Effect on Multidrug Resistant Uropathogenic Escherichia coli. Nat. Product. Commun. 12, 623–626. doi:10.1177/1934578x1701200438
Schelz, Z., Molnar, J., and Hohmann, J. (2006). Antimicrobial and Antiplasmid Activities of Essential Oils. Fitoterapia 77, 279–285. doi:10.1016/j.fitote.2006.03.013
Schmitz, R. (1985). Friedrich Wilhelm Sertürner and the Discovery of Morphine. Pharm. Hist. 27, 61–74.
Schrader, K. K., Avolio, F., Andolfi, A., Cimmino, A., and Evidente, A. (2013). Ungeremine and its Hemisynthesized Analogues as Bactericides against Flavobacterium Columnare. J. Agric. Food Chem. 61, 1179–1183. doi:10.1021/jf304586j
Shahzad, M., Millhouse, E., Culshaw, S., Edwards, C. A., Ramage, G., and Combet, E. (2015). Selected Dietary (Poly)phenols Inhibit Periodontal Pathogen Growth and Biofilm Formation. Food Funct. 6, 719–729. doi:10.1039/C4FO01087F
Sharifi, A., Mohammadzadeh, A., Zahraei Salehi, T., and Mahmoodi, P. (2018). Antibacterial, Antibiofilm and Antiquorum Sensing Effects of Thymus Daenensis and Satureja Hortensis Essential Oils against Staphylococcus aureus Isolates. J. Appl. Microbiol. 124, 379–388. doi:10.1111/jam.13639
Sharopov, F., Braun, M., Gulmurodov, I., Khalifaev, D., Isupov, S., and Wink, M. (2015). Antimicrobial, Antioxidant, and Anti-inflammatory Activities of Essential Oils of Selected Aromatic Plants from Tajikistan. Foods 4, 645–653. doi:10.3390/foods4040645
Sheppard, A. E., Stoesser, N., Wilson, D. J., Sebra, R., Kasarskis, A., Anson, L. W., et al. (2016). Nested Russian Doll-Like Genetic Mobility Drives Rapid Dissemination of the Carbapenem Resistance Gene Bla KPC. Antimicrob. Agents Chemother. 60, 3767–3778. doi:10.1128/AAC.00464-16
Shriram, V., Jahagirdar, S., Latha, C., Kumar, V., Puranik, V., Rojatkar, S., et al. (2008). A Potential Plasmid-Curing Agent, 8-epidiosbulbin E Acetate, from Dioscorea Bulbifera L. Against Multidrug-Resistant Bacteria. Int. J. Antimicrob. Agents 32, 405–410. doi:10.1016/j.ijantimicag.2008.05.013
Shriram, V., Khare, T., Bhagwat, R., Shukla, R., and Kumar, V. (2018). Inhibiting Bacterial Drug Efflux Pumps via Phyto-Therapeutics to Combat Threatening Antimicrobial Resistance. Front. Microbiol. 9, 2990. doi:10.3389/fmicb.2018.02990
Si, H., Hu, J., Liu, Z., and Zeng, Z.-l. (2008). Antibacterial Effect of Oregano Essential Oil Alone and in Combination with Antibiotics against Extended-Spectrum β-lactamase-producing Escherichia coli. FEMS Immunol. Med. Microbiol. 53, 190–194. doi:10.1111/j.1574-695X.2008.00414.x
Siguier, P., Gourbeyre, E., and Chandler, M. (2017). Known Knowns, Known Unknowns and Unknown Unknowns in Prokaryotic Transposition. Curr. Opin. Microbiol. 38, 171–180. doi:10.1016/j.mib.2017.06.005
Siriyong, T., Chusri, S., Srimanote, P., Tipmanee, V., and Voravuthikunchai, S. P. (2016). Holarrhena Antidysenterica Extract and its Steroidal Alkaloid, Conessine, as Resistance-Modifying Agents against Extensively Drug-Resistant Acinetobacter Baumannii. Microb. Drug Resist. 22, 273–282. doi:10.1089/mdr.2015.0194
Siriyong, T., Srimanote, P., Chusri, S., Yingyongnarongkul, B.-e., Suaisom, C., Tipmanee, V., et al. (2017). Conessine as a Novel Inhibitor of Multidrug Efflux Pump Systems in Pseudomonas aeruginosa. BMC Complement. Altern. Med. 17, 405. doi:10.1186/s12906-017-1913-y
Siriyong, T., Voravuthikunchai, S. P., and Coote, P. J. (2018). Steroidal Alkaloids and Conessine from the Medicinal Plant Holarrhena Antidysenterica Restore Antibiotic Efficacy in a Galleria Mellonella Model of Multidrug-Resistant Pseudomonas aeruginosa Infection. BMC Complement. Altern. Med. 18, 285. doi:10.1186/s12906-018-2348-9
Skalicka-Woźniak, K., Walasek, M., Aljarba, T. M., Stapleton, P., Gibbons, S., Xiao, J., et al. (2018). The Anticonvulsant and Anti-plasmid Conjugation Potential of Thymus Vulgaris Chemistry: An In Vivo Murine and In Vitro Study. Food Chem. Toxicol. 120, 472–478. doi:10.1016/j.fct.2018.07.045
Smet, A., Martel, A., Persoons, D., Dewulf, J., Heyndrickx, M., Cloeckaert, A., et al. (2009). Comparative Analysis of Extended-Spectrum- -Lactamase-Carrying Plasmids from Different Members of Enterobacteriaceae Isolated from Poultry, Pigs and Humans: Evidence for a Shared -lactam Resistance Gene Pool?. J. Antimicrob. Chemother. 63, 1286–1288. doi:10.1093/jac/dkp101
Snoussi, M., Dehmani, A., Noumi, E., Flamini, G., and Papetti, A. (2016). Chemical Composition and Antibiofilm Activity of Petroselinum crispum and Ocimum Basilicum Essential Oils against Vibrio Spp. Strains. Microb. Pathogenesis 90, 13–21. doi:10.1016/j.micpath.2015.11.004
Soberón, J. R., Sgariglia, M. A., Dip Maderuelo, M. R., Andina, M. L., Sampietro, D. A., Vattuone, M. A., et al. (2014). Antibacterial Activities of Ligaria Cuneifolia and Jodina Rhombifolia Leaf Extracts against Phytopathogenic and Clinical Bacteria. J. Biosci. Bioeng. 118, 599–605. doi:10.1016/j.jbiosc.2014.04.018
Soler, N., and Forterre, P. (2020). Vesiduction: the Fourth Way of HGT. Environ. Microbiol. 22, 2457–2460. doi:10.1111/1462-2920.15056
Solórzano-Santos, F., and Miranda-Novales, M. G. (2012). Essential Oils from Aromatic Herbs as Antimicrobial Agents. Curr. Opin. Biotechnol. 23, 136–141. doi:10.1016/j.copbio.2011.08.005
Soltani, R., Fazeli, H., Bahri Najafi, R., and Jelokhanian, A. (2017). Evaluation of the Synergistic Effect of Tomatidine with Several Antibiotics against Standard and Clinical Isolates of Staphylococcus aureus, Enterococcus faecalis, Pseudomonas aeruginosa and Escherichia coli. Iran. J. Pharm. Res. IJPR 16, 290–296.
Sridevi, D., Shankar, C., Prakash, P., Park, J., and Thamaraiselvi, K. (2017). Inhibitory Effects of Reserpine against Efflux Pump Activity of Antibiotic Resistance Bacteria. Chem. Biol. Lett. 4, 69–72.
Sun, J., Deng, Z., and Yan, A. (2014). Bacterial Multidrug Efflux Pumps: Mechanisms, Physiology and Pharmacological Exploitations. Biochem. Biophys. Res. Commun. 453, 254–267. doi:10.1016/j.bbrc.2014.05.090
Sundaramoorthy, N. S., Mitra, K., Ganesh, J. S., Makala, H., Lotha, R., Bhanuvalli, S. R., et al. (2018). Ferulic Acid Derivative Inhibits NorA Efflux and in Combination with Ciprofloxacin Curtails Growth of MRSA In Vitro and In Vivo. Microb. Pathog. 124, 54–62. doi:10.1016/j.micpath.2018.08.022
Taguri, T., Tanaka, T., and Kouno, I. (2006). Antibacterial Spectrum of Plant Polyphenols and Extracts Depending upon Hydroxyphenyl Structure. Biol. Pharm. Bull. 29, 2226–2235. doi:10.1248/bpb.29.2226
Tan, N., Yazıcı-Tütüniş, S., Bilgin, M., Tan, E., and Miski, M. (2017). Antibacterial Activities of Pyrenylated Coumarins from the Roots of Prangos Hulusii. Molecules 22, 1098. doi:10.3390/molecules22071098
Thawabteh, A., Juma, S., Bader, M., Karaman, D., Scrano, L., Bufo, S., et al. (2019). The Biological Activity of Natural Alkaloids against Herbivores, Cancerous Cells and Pathogens. Toxins 11, 656. doi:10.3390/toxins11110656
The Lancet Infectious Diseases (2017). Antibiotic Research Priorities: Ready, Set, Now Go. Lancet Infect. Dis. 17, 349. doi:10.1016/S1473-3099(17)30140-8
Thomas, C. M., and Nielsen, K. M. (2005). Mechanisms of, and Barriers to, Horizontal Gene Transfer between Bacteria. Nat. Rev. Microbiol. 3, 711–721. doi:10.1038/nrmicro1234
Togashi, N., Hamashima, H., Shiraishi, A., Inoue, Y., and Takano, A. (2010). Antibacterial Activities AgainstStaphylococcus Aureusof Terpene Alcohols with Aliphatic Carbon Chains. J. Essent. Oil Res. 22, 263–269. doi:10.1080/10412905.2010.9700321
Tooke, C. L., Hinchliffe, P., Bragginton, E. C., Colenso, C. K., Hirvonen, V. H. A., Takebayashi, Y., et al. (2019). β-Lactamases and β-Lactamase Inhibitors in the 21st Century. J. Mol. Biol. 431, 3472–3500. doi:10.1016/j.jmb.2019.04.002
Turek, C., and Stintzing, F. C. (2013). Stability of Essential Oils: A Review. Compr. Rev. Food Sci. Food Saf. 12, 40–53. doi:10.1111/1541-4337.12006
ur Rahman, S., Ali, T., Ali, I., Khan, N. A., Han, B., and Gao, J. (2018). The Growing Genetic and Functional Diversity of Extended Spectrum Beta-Lactamases. Biomed. Res. Int. 2018, 1–14. doi:10.1155/2018/9519718
Vasconcelos, S. E. C. B., Melo, H. M., Cavalcante, T. T. A., Júnior, F. E. A. C., de Carvalho, M. G., Rodrigues Menezes, F. G., et al. (2017). Plectranthus Amboinicus Essential Oil and Carvacrol Bioactive against Planktonic and Biofilm of Oxacillin- and Vancomycin-Resistant Staphylococcus aureus. BMC Complement. Altern. Med. 17, 462. doi:10.1186/s12906-017-1968-9
Vatan, A., Saltoglu, N., Yemisen, M., Balkan, I. I., Surme, S., Demiray, T., et al. (2018). Association between Biofilm and Multi/extensive Drug Resistance in Diabetic Foot Infection. Int. J. Clin. Pract. 72, e13060. doi:10.1111/ijcp.13060
Wang, S.-S., Wang, D.-M., Pu, W.-J., and Li, D.-W. (2013). Phytochemical Profiles, Antioxidant and Antimicrobial Activities of Three Potentilla Species. BMC Complement. Altern. Med. 13, 321. doi:10.1186/1472-6882-13-321
Wang, F., Wei, F., Song, C., Jiang, B., Tian, S., Yi, J., et al. (2017). Dodartia Orientalis L. Essential Oil Exerts Antibacterial Activity by Mechanisms of Disrupting Cell Structure and Resisting Biofilm. Ind. Crops Prod. 109, 358–366. doi:10.1016/j.indcrop.2017.08.058
Wang, J., Yang, T., Chen, H., Xu, Y.-N., Yu, L.-F., Liu, T., et al. (2017). The Synthesis and Antistaphylococcal Activity of 9, 13-disubstituted Berberine Derivatives. Eur. J. Med. Chem. 127, 424–433. doi:10.1016/j.ejmech.2017.01.012
Westbye, A. B., Beatty, J. T., and Lang, A. S. (2017). Guaranteeing a Captive Audience: Coordinated Regulation of Gene Transfer Agent (GTA) Production and Recipient Capability by Cellular Regulators. Curr. Opin. Microbiol. 38, 122–129. doi:10.1016/j.mib.2017.05.003
Wiedenbeck, J., and Cohan, F. M. (2011). Origins of Bacterial Diversity through Horizontal Genetic Transfer and Adaptation to New Ecological Niches. FEMS Microbiol. Rev. 35, 957–976. doi:10.1111/j.1574-6976.2011.00292.x
Wilson, D. N. (2014). Ribosome-targeting Antibiotics and Mechanisms of Bacterial Resistance. Nat. Rev. Microbiol. 12, 35–48. doi:10.1038/nrmicro3155
World Bank (2017). Drug-Resistant Infections: A Threat to Our Economic Future. Available at: https://www.worldbank.org/en/topic/health/publication/drug-resistant-infections-a-threat-to-our-economic-future (Accessed February 12, 2021).
World Health Organization (2017). WHO Publishes List of Bacteria for Which New Antibiotics Are Urgently Needed. Available at: https://www.who.int/news/item/27-02-2017-who-publishes-list-of-bacteria-for-which-new-antibiotics-are-urgently-needed (Accessed February 12, 2021).
Wozniak, R. A. F., and Waldor, M. K. (2010). Integrative and Conjugative Elements: Mosaic mobile Genetic Elements Enabling Dynamic Lateral Gene Flow. Nat. Rev. Microbiol. 8, 552–563. doi:10.1038/nrmicro2382
Wu, H. Z., Fei, H. J., Zhao, Y. L., Liu, X. J., Huang, Y. J., and Wu, S. W. (2012). [Antibacterial Mechanism of Sulforaphane on Escherichia coli]. Sichuan Da Xue Xue Bao Yi Xue Ban 43, 386–390. Available at: http://www.ncbi.nlm.nih.gov/pubmed/22812243
Wu, T., Zang, X., He, M., Pan, S., and Xu, X. (2013). Structure-Activity Relationship of Flavonoids on Their Anti-Escherichia coli Activity and Inhibition of DNA Gyrase. J. Agric. Food Chem. 61, 8185–8190. doi:10.1021/jf402222v
Xu, H., Ziegelin, G., Schroder, W., Frank, J., Ayora, S., Alonso, J., et al. (2001). Flavones Inhibit the Hexameric Replicative Helicase RepA. Nucleic Acids Res. 29, 5058–5066. doi:10.1093/nar/29.24.5058
Xu, M., Wu, P., Shen, F., Ji, J., and Rakesh, K. P. (2019). Chalcone Derivatives and Their Antibacterial Activities: Current Development. Bioorg. Chem. 91, 103133. doi:10.1016/j.bioorg.2019.103133
Yadav, M. K., Chae, S.-W., Im, G. J., Chung, J.-W., and Song, J.-J. (2015). Eugenol: A Phyto-Compound Effective against Methicillin-Resistant and Methicillin-Sensitive Staphylococcus aureus Clinical Strain Biofilms. PLoS One 10, e0119564. doi:10.1371/journal.pone.0119564
Yap, P. S. X., Lim, S. H. E., Hu, C. P., and Yiap, B. C. (2013). Combination of Essential Oils and Antibiotics Reduce Antibiotic Resistance in Plasmid-Conferred Multidrug Resistant Bacteria. Phytomedicine 20, 710–713. doi:10.1016/j.phymed.2013.02.013
Yap, P. S. X., Yiap, B. C., Ping, H. C., and Lim, S. H. E. (2014). Essential Oils, A New Horizon in Combating Bacterial Antibiotic Resistance. Tomicroj 8, 6–14. doi:10.2174/1874285801408010006
Yi, Z.-B., Yan Yu, Y., Liang, Y.-Z., and Bao Zeng, B. (2007). Evaluation of the Antimicrobial Mode of Berberine by LC/ESI-MS Combined with Principal Component Analysis. J. Pharm. Biomed. Anal. 44, 301–304. doi:10.1016/j.jpba.2007.02.018
Yu, Z., Tang, J., Khare, T., and Kumar, V. (2020). The Alarming Antimicrobial Resistance in ESKAPEE Pathogens: Can Essential Oils Come to the rescue?. Fitoterapia 140, 104433. doi:10.1016/j.fitote.2019.104433
Zacchino, S. A., Butassi, E., LibertoDi, M. D., Raimondi, M., Postigo, A., and Sortino, M. (2017). Plant Phenolics and Terpenoids as Adjuvants of Antibacterial and Antifungal Drugs. Phytomedicine 37, 27–48. doi:10.1016/j.phymed.2017.10.018
Zahara, K., Panda, S. K., Swain, S. S., and Luyten, W. (2020). Metabolic Diversity and Therapeutic Potential of Holarrhena Pubescens: An Important Ethnomedicinal Plant. Biomolecules 10, 1341. doi:10.3390/biom10091341
Keywords: antibiotics, antimicrobial, efflux pumps, medicinal plants, multidrug resistance, phytomolecules, drug resistance reversal agents
Citation: Khare T, Anand U, Dey A, Assaraf YG, Chen Z-S, Liu Z and Kumar V (2021) Exploring Phytochemicals for Combating Antibiotic Resistance in Microbial Pathogens. Front. Pharmacol. 12:720726. doi: 10.3389/fphar.2021.720726
Received: 04 June 2021; Accepted: 28 June 2021;
Published: 21 July 2021.
Edited by:
Ji-Ye Yin, Central South University, ChinaReviewed by:
Haichan Niu, Roivant Sciences GmbH, SwitzerlandTianshun Zhang, University of Minnesota Twin Cities, United States
Copyright © 2021 Khare, Anand, Dey, Assaraf, Chen, Liu and Kumar. This is an open-access article distributed under the terms of the Creative Commons Attribution License (CC BY). The use, distribution or reproduction in other forums is permitted, provided the original author(s) and the copyright owner(s) are credited and that the original publication in this journal is cited, in accordance with accepted academic practice. No use, distribution or reproduction is permitted which does not comply with these terms.
*Correspondence: Zhe-Sheng Chen, Y2hlbnpAc3Rqb2hucy5lZHU=; Zhijun Liu, emhpanVuLmxpdUB3Zm1jLmVkdQ==; Vinay Kumar, dmluYXkua3VtYXJAbW9kZXJuY29sbGVnZWdrLm9yZw==, b3JjaWQub3JnLzAwMDAtMDAwMi05NTY5LTI0MTE=