- 1Department of Pharmacology and Toxicology, School of Pharmacy, Ardabil University of Medical Sciences, Ardabil, Iran
- 2Traditional Medicine and Hydrotherapy Research Center, Ardabil University of Medical Sciences, Ardabil, Iran
- 3Student Research Committee, School of Medicine, Shahroud University of Medical Sciences, Shahroud, Iran
- 4Students Research Committee, School of Pharmacy, Ardabil University of Medical Sciences, Ardabil, Iran
Oxidative stress and mitochondrial dysfunction are involved in the mechanisms of cardiac toxicity induced by aluminum phosphide (AlP). AlP-induced cardiotoxicity leads to cardiomyocyte death, cardiomyopathy, cardiac dysfunction, and eventually severe heart failure and death. Importantly, protecting cardiomyocytes from death resulting from AlP is vital for improving survival. It has been reported that flavonoids such as myricetin (Myr) act as modifiers of mitochondrial function and prevent mitochondrial damage resulting from many insults and subsequent cell dysfunction. In this study, the ameliorative effect of Myr, as an important antioxidant and mitochondrial protective agent, was investigated in cardiomyocytes and mitochondria isolated from rat heart against AlP-induced toxicity, oxidative stress, and mitochondrial dysfunction. Treatment of AlP (20 μg/ml) significantly increased cytotoxicity; reduced glutathione (GSH) depletion, cellular reactive oxygen species (ROS) formation, malondialdehyde (MDA) level, ATP depletion, caspase-3 activation, mitochondrial membrane potential (ΔΨm) collapse, and lysosomal dysfunction; and decreased the activities of superoxide dismutase (SOD), catalase (CAT), and glutathione peroxidase (GSH-Px) in intact cardiomyocytes. Also, treatment of AlP (20 μg/ml) significantly increased mitochondrial dysfunction and swelling in isolated mitochondria. Myr (80 µM) appeared to ameliorate AlP-induced cytotoxicity in isolated cardiomyocytes; significantly lessened the AlP-stimulated intracellular ROS and MDA production and depletion of GSH; and increased the activities of SOD, CAT, and GSH-Px. Furthermore, Myr (40 and 80 µM) lowered AlP-induced lysosomal/mitochondrial dysfunction, ATP depletion, and caspase-3 activation. In the light of these findings, we concluded that Myr through antioxidant potential and inhibition of mitochondrial permeability transition (MPT) pore exerted an ameliorative role in AlP-induced toxicity in isolated cardiomyocytes and mitochondria, and it would be valuable to examine its in vivo effects.
Introduction
Pesticide poisoning is a global public health problem, and one-third of the suicides in the world is due to self-poisoning (Gunnell et al., 2007). Pesticide poisoning causes more deaths than infections in some parts of developing countries (Eddleston et al., 2002). Due to pesticide poisoning, every year, more than 300,000 deaths occur in the world (Gurjar et al., 2011). The number of annual suicides worldwide due to pesticide self-poisoning is 110,000–168,000 cases (Dandona and Gunnell, 2021). Aluminum phosphide (AlP), organochlorine, and organophosphate compounds are commonly used pesticides around the world. As a common indoor and outdoor pesticide, AlP is used in developing countries, because it is effective, cheap, free from toxic residue, and without effect on seed viability (Gurjar et al., 2011). Due to its low-cost availability, AlP is extensively used as suicidal poison. In developing countries such as Iran and India, AlP is emerging as a common self-poisoning agent (Etemadi-Aleagha et al., 2015; Mehrpour et al., 2018). The toxic effects of AlP on different tissues are associated with phosphine (PH3) gas and oxidative stress (Mehrpour et al., 2012). Phosphine gas induces oxidative stress through mitochondrial dysfunction, inhibition of cytochrome c oxidase in mitochondria and enzymatic antioxidants such as reduced glutathione (GSH), superoxide dismutase (SOD), catalase (CAT), and glutathione peroxidase (GSH-Px) (Bumbrah et al., 2012). Mitochondrial dysfunction, inhibition of cellular respiration, and antioxidant enzymes such as CAT, GR, and SOD can produce free radicals, lipid peroxidation, and oxidative stress. These alterations will lead to cellular injury and cytotoxicity via oxidative stress in different tissues. Among the human tissues, cardiac tissue is more vulnerable to AlP-induced toxicity, oxidative stress, and mitochondrial dysfunction, because the heart is rich in mitochondria and low antioxidant capacity (Sciuto et al., 2016; Tapio, 2016). It has been reported that near 70% of deaths caused by AlP were attributable to cardiovascular disorders (Ames, 2010). The exact mechanism of AlP cardiotoxicity has not yet been determined, but previous studies suggest that mitochondrial dysfunction and oxidative stress play a major role. Therefore, mitochondrial protective agents and antioxidants may play an effective role in reducing cardiac toxicity induced by AlP.
Myricetin (Myr) is a natural flavonoid compound extracted from the leaves and bark of Myrica rubra (Jones et al., 2011). Also, Myr is found in many beverages and foods, including red wine (grapes), teas, vegetables, fruits, berries, and honey (Jones et al., 2011). The health benefits of Myr such as anti-inflammatory, antioxidant, antitumor, antimicrobial, cardioprotective, and other pharmacological effects have been thoroughly investigated over the last decade (Semwal et al., 2016). Recently, due to potential clinical impact of Myr on cardiovascular functions, its cardioprotective effect has attracted attention from the research community (Tran and Wang, 2019). Previous studies have been reported several cardioprotective effects of Myr on isoproterenol (ISO)-induced myocardial infarction, ischemia/reperfusion (I/R)-induced myocardial injury, and endotoxin-induced inflammatory myocardial injury (Wang et al., 2019). These studies suggest that Myr may display beneficial effects again cardiotoxicity induced by drugs and chemicals. Moreover, Myr is well known for its effective reduction of oxidative stress by providing antioxidant benefits (Park et al., 2016). It has been reported that Myr inactivates free radicals such as superoxide anion radical via single electron transfer to form an aryloxy radical (Chobot and Hadacek, 2011). The antioxidant effect of Myr was reported in various animal models and cell-based assays (Barzegar, 2016). In addition, it has been reported that Myr can protect cells from various insults that lead to mitochondria-mediated cytotoxicity, and previous studies demonstrated that this compound attenuates the progression of diseases and toxicity associated with mitochondrial dysfunction and oxidative stress (Lagoa et al., 2011). Due to above beneficial effects of Myr in the reduction of oxidative stress and mitochondrial dysfunction, in this study, we searched the effects of Myr against AlP-induced toxicity, oxidative stress, and mitochondrial dysfunction in isolated cardiomyocytes and mitochondria obtained from rat heart.
Materials and Methods
Animals
Cardiomyocytes were isolated form male Wistar rats (body weight 200–220 g and 8–9 weeks old), which were purchased from the Baqiyatallah University of Medical Sciences (Tehran, Iran) and allowed ad libitum access to tap standard rodent diet and water. The experimental animals received human care in compliance with the Guide for the Care and Use of Laboratory Animals approved by the Ethics Committee of the Ardabil University of Medical Sciences (Ardabil, Iran) with ethics code IR.ARUMS.REC.1397.236. The animals were anesthetized by intraperitoneal injection of combination of ketamine (50 mg/kg) and xylazine (10 mg/kg) and sacrificed by stunning and cervical dislocation. In this study, the male rats were selected due to AlP toxicity ratio in men to women of 2:1 (Moghadamnia, 2012).
Chemicals
Fetal bovine serum (FBS), penicillin and streptomycin solution, Medium 199, Collagenase Type II (product number: C2-BIOC, Sigma), 2′,7′-dichlorofluorescin diacetate (product number: D6883, Sigma), creatine, Hanks’ Balanced Salt Solution (HBSS), potassium chloride, N-(2-hydroxyethyl)piperazine-N′-(2-ethanesulfonic acid) (HEPES), carnitine, rhodamine 123 (product number: R8004, Sigma), taurine (product number: T0625, Sigma), dimethyl sulfoxide (DMSO), Trypan Blue, bovine serum albumin (BSA), sucrose, d-mannitol, 2-amino-2-hydroxymethyl-propane-1,3-diol (TRIS), 2 monopotassium phosphate, ethylenediaminetetraacetic acid (EDTA), 3-(4,5-dimethylthiazol-2-yl)-2,5-diphenyltetrazolium bromide (MTT), sodium succinate, 3-morpholinopropane-1-sulfonic acid (MOPS), magnesium chloride, rotenone, acridine orange (AO), butylated hydroxytoluene (BHT), ethylene glycol-bis(β-aminoethyl ether) (EGTA), Coomassie Brilliant Blue, 5,5′-dithiobis(2-nitrobenzoic acid) (DTNB), Myr (product number: M6760, Sigma), and butylated hydroxytoluene (product number: PHR1117, Sigma) were purchased from Sigma (St. Louis, MO, USA). AlP with a purity of about 99% was gifted from the Samiran Company (Tehran, Iran). AlP was freshly prepared before use and dissolved in DMSO (0.05%).
Solutions and Drugs
Creatine–carnitine–taurine medium (CCT medium) contained the following: 3.6 g of HEPES (25 mM), 655.5 mg of creatine (5 mM), 395.4 mg of carnitine (2 mM), 625.5 mg of taurine (5 mM), and 10 µM of cytosine β-d-arabinofuranoside, and pH was adjusted to 7.4 with NaOH (2 mM) in a sterile medium. Powell medium contained thee following: 6.43 g of NaCl (110 mM), 0.19 g of KCl (2.5 mM), 0.16 g of KH2PO4 (1.2 mM), 0.3 g of MgSO4 7H2O (1.2 mM), 5.96 g of HEPES (25 mM), and 1.98 g of d-(+)-glucose monohydrate (10 mM) in Aqua sterile (double distilled water), and pH was adjusted to 7.4 with NaOH (2 mM) in a sterile medium. Calcium chloride (CaCl2) contained 100 mM of CaCl2. Mitochondrial isolation buffer contained the following: 225 mM of d-mannitol, 75 mM of sucrose, and 0.2 mM of EDTA, and pH was adjusted to 7.4 with NaOH (2 mM). Mitochondrial assay buffer contained the following: 10 mmol/L of NaCl, 140 mmol/L of KCl, 0.5 mmol/L of KH2PO4, 2 mmol/L of MgCl2, 0.5 mmol/L of EGTA, and 20 mmol/L of HEPES and supplemented with 10 mmol/L of succinate and 1 mg/ml of rotenone, and pH adjusted to 7.4. Mitochondrial swelling buffer contained the following: 140 mmol/L of KCl, 2 mmol/L of MgCl2, 10 mmol/L of NaCl, 0.5 mmol/L of KH2PO4, 20 mmol/L of HEPES, and 0.5 mmol/L of EGTA and supplemented with 1 mg/ml of rotenone, and pH was adjusted to 7.4. All AlP and Myr-containing solutions were prepared fresh before the experiments.
Cardiomyocyte Isolation
Cardiomyocytes were isolated from the rat heart as previously described by Nippert et al. (2017)and Ahangari et al. (2020). Briefly, after deep anesthesia was administered to the animals, their hearts were explanted, washed with Powell medium, and cannulated via the aorta in the Langendorff perfusion system. Hearts were perfused with Powell medium at a constant flow rate of 10 ml/min with a peristaltic pump for approximately 5 min (37°C) to wash away the blood and then with 25 ml of warm Powell medium supplemented with collagenase (25 mg in 5 ml), and finally, the cardiac tissues were enzymatically dissociated during 25 min. Ventricles were then separated from the atria, cut in small pieces, and shaken for 10 min in 15 ml of warm Powell medium supplemented with collagenase in the presence of 50 μM of CaCl2. The isolated cardiomyocytes were suspended in CCT medium supplemented with 100 μg/ml of penicillin, 100 μg/ml of streptomycin, and 10% FBS in a humidified air containing 5% CO2 at 37°C.
Experimental Design
The experimental groups were categorized into six groups in the current study. 1) In the control group, cardiomyocytes were treated with 0.05% DMSO for 3 h. 2) In the AlP group, cardiomyocytes were treated with 20 μg/ml of AlP (IC50 3 h) for 3 h according to our previous study (Khezri et al., 2020). 3) In the AlP + Myr group, cardiomyocytes were cotreated with 20 μg/ml of AlP and 20 μM of Myr for 3 h. 4) In the AlP + Myr group, cardiomyocytes were cotreated with 20 μg/ml of AlP and 40 μM of Myr for 3 h. 5) In the AlP + Myr group, cardiomyocytes were cotreated with 20 μg/ml of AlP and 80 μM of Myr for 3 h. 6) In the Myr group, cardiomyocytes were treated with 80 μM of Myr for 3 h.
Measurement of Cytotoxicity
Cytotoxicity was measured by MTT assay in a 96-well plate. After 3 h of exposure to AlP and Myr according to the above groupings, the isolated cardiomyocytes were treated with MTT at 0.5 mg/ml for 2 h. The purple formazan crystals were dissolved in 100 µl of DMSO, and the absorbance was measured at 570 nm. Five independent experiments were performed in triplicate (Khezri et al., 2020).
Caspase-3 Activation
The activation of caspase-3 activity was measured using “Sigma’s caspase 3 assay kit” (CASP-3-C). Briefly, the hydrolysis of substrate peptide, Ac-DEVD-pNA, through caspase-3 in the base was assessed for caspase-3 activation. The released segment of p-nitroaniline has a high absorbance at 405 nm.
ATP/ADP Ratio Assay
ADP/ATP ratio was assessed by ADP/ATP Ratio Assay kit (MAK135, Sigma, USA) in isolated cardiomyocytes using luminometer. ADP/ATP ratio was assessed according to the manufacturer’s instructions (Salimi et al., 2015).
Detection of Reactive Oxygen Species in Cardiomyocytes
Intracellular reactive oxygen species (ROS) generation in cardiomyocytes was detected by staining with fluorescence dye DCFH-DA. Cardiomyocyte ROS level could be monitored by detecting of the fluorescence intensity of DCF, by using flow cytometry. Briefly, after 3 h of exposure to AlP and Myr according to the abovementioned experimental groups, the cardiomyocytes were washed twice with PBS and incubated with 5 µM of DCFH-DA dissolved in CCT medium for 15 min in a dark chamber. Then the fluorescence intensity of DCF was detected by flow cytometry (CyFlow Space-Partec, Sysmex Partec GmbH, Görlitz, Germany) and analyzed by FlowJo software (Eruslanov and Kusmartsev, 2010).
Mitochondrial Membrane Potential Measurement
Mitochondrial membrane potential was measured with a unique cationic dye of rhodamine 123. Briefly, the cardiomyocytes were treated according to the experimental groups described above for 3 h with AlP and Myr. Then the cardiomyocytes were washed twice with PBS and incubated with 5 µM of rhodamine 123 dissolved in CCT medium for 15 min in a dark chamber. Then the fluorescence intensity of rhodamine 123 was detected by flow cytometry (CyFlow Space-Partec, Germany) and analyzed by FlowJo software (Khezri et al., 2020).
Measurement of Lysosomal Membrane Integrity
Lysosomal membrane integrity was measured by staining with fluorescence dye AO. Briefly, the cardiomyocytes were treated according to the experimental groups described above for 3 h with AlP and Myr. Then the cardiomyocytes were washed twice with PBS and incubated with 5 µM of AO dissolved in CCT medium for 15 min in a dark chamber. Then the fluorescence intensity of AO was detected by flow cytometry (CyFlow Space-Partec, Germany) and analyzed by FlowJo software (Khezri et al., 2020).
Determination of Reduced and Oxidized Glutathione Contents
The contents of GSH and oxidized glutathione (GSSG) were measured by the Hissin and Hilf method (Hissin and Hilf, 1976). Briefly, the cardiomyocytes were treated according to the experimental groups described above for 3 h with AlP and Myr. The cardiomyocytes were washed twice with PBS, resuspended in phosphate buffer (0.1 M with pH 7.4), and mechanically lysed using glass homogenizer. The cell lysate was centrifuged for 8,000 × g at 4°C for 10 min, and the supernatants were used for GSH and GSSG determination according to the Hissin and Hilf method 1976) using the enzymatic recycling method with DTNB and glutathione reductase (GR) in a microplate format using a plate reader. For detection of GSSG, 100 μl of supernatant was mixed to 3 ml of reaction solution (150 μM of NADPH, 500 mM of TRIS–HCl buffer, 1 mM of EDTA, glutathione reductase, 10 mM of DTNB, and 3 mM of MgCl2). Also, for detection of GSH, 100 μl of supernatant was mixed with 3 ml of reaction solution (500 mM of TRIS–HCl and 10 mM of DTNB with pH = 8.0). After 15 min of incubation at 25°C, the optical density was measured at 412 nm.
Assay of Lipid Peroxidation in Cardiomyocytes
Lipid peroxidation was measured by production of thiobarbituric acid (TBA) reactive substances (TBARS). Briefly, the cardiomyocytes were treated according to the experimental groups described above for 3 h with AlP and Myr. The cardiomyocytes were washed twice with PBS and mechanically lysed in 1 ml of 0.1% (w/v) trichloroacetic acid (TCA) and centrifuged at 10,000 × g for 10 min. A volume of 200 µl of supernatant was mixed with 400 µl of 20% TCA and 0.5% TBA solution and then boiled at 95°C for 20 min. After cooling on ice and centrifugation at 1,000 × g for 10 min, the absorbance was measured at 532 nm (Beach and Giroux, 1992).
Analysis of Antioxidant Enzymes in Cardiomyocytes
The cardiomyocytes were seeded in a 24-well plate at a density of 1 × 105 cells/ml and treated according to the experimental groups described above for 3 h with AlP and Myr. Then, the cardiomyocytes were washed with PBS and centrifuged at 300 × g for 10 min. The collected cells were crushed by ultrasonic wave, and the cell lysates were resuspended. SOD, CAT, and GSH-Px activities were determined with a microplate reader according to the protocol of the detection kit. The activity of SOD was measured by the xanthine oxidase method. The activity of GSH-Px was detected by the colorimetric method. The activity of CAT activity was detected by the visible spectrophotometer method.
Isolation of Mitochondria
Cardiac mitochondria were isolated from rat heart by differential centrifugation of homogenates with minor modification as described previously (Schulz et al., 2015). Briefly, after deep anesthesia was administered to the experimental animals, their hearts were explanted, washed with normal saline, and cut into mall slices by surgical scissors and cleared from extra tissues. The sliced tissues were homogenized in the isolation buffer (components are mentioned in Solutions and Drugs section) using a glass homogenizer, and then the cell lysates were centrifuged at 1,000 × g for 10 min. The pellet containing nuclei and undisrupted cells was removed, and the supernatant containing mitochondrial fraction were centrifuged again at 10,000 × g for 10 min at 4°C. All solutions and equipment were kept on ice bath during the isolation process. Mitochondria were stored in ice, and mitochondrial protein concentration was measured by the Bradford assay using serum albumin as standard (Mersa et al., 2020). The integrity and purity of isolated mitochondria were measured by succinate dehydrogenase (SDH) and lactate dehydrogenase (LDH) assays.
Measurement of Mitochondrial Succinate Dehydrogenase Activity
The mitochondrial SDH activity was measured through MTT reduction at 570 nm. Briefly, isolated mitochondria (1,000 μg/ml) were incubated in a 96-well plate with total volume of 100 µl/well, in assay buffer, and treated according to the experimental groups described above for 1 h with AlP and Myr. After incubation, 0.4% MTT was added and incubated at 37°C for 30 min. Finally, the formazan crystals were dissolved in 100 µl of DMSO, and the optical density was measured at 570 nm (Mersa et al., 2020).
Measurement of Mitochondrial Swelling
Mitochondrial swelling was measured with a plate reader (BioTek, Winooski, VT, USA) that measured absorbance at 540 nm in a swelling buffer. Briefly, isolated mitochondria (1,000 μg/ml) were incubated in a 96-well plate with total volume of 100 µl/well, in swelling buffer (140 mmol/L of KCl, 2 mmol/L of MgCl2, 10 mmol/L of NaCl, 0.5 mmol/L of KH2PO4, 20 mmol/L of HEPES, and 0.5 mmol/L of EGTA and supplemented with 1 mg/ml of rotenone, and pH was adjusted to 7.4) and treated according to the experimental groups described above for 1 h with AlP and Myr. The absorbance of the samples was monitored for 1 h in 15-min intervals at 540 nm. Reduction of absorbance is related to increase in mitochondrial swelling (Zhao et al., 2010).
Data Analysis
Differences between groups were assessed using the one-way ANOVAs and two-way ANOVAs followed by post-hoc Tukey’s and Bonferroni’s tests, respectively (GraphPad Prism 5; GraphPad Software, San Diego, CA, USA). Values are shown as means ± SD. p ≤ 0.05 was considered significant.
Results
Ameliorative Efficacy of Myricetin in Aluminum Phosphide-Induced Cytotoxicity in Cardiomyocytes
To determine the cytotoxicity of AlP and the ameliorative effect of Myr on isolated cardiomyocytes, the cell viability was evaluated after 3-h exposure by MTT assay. Cell viability of isolated cardiomyocytes markedly decreased following incubation with 20 μg/ml of AlP. To evaluate the ameliorative effect of Myr in AlP-induced cytotoxicity, isolated cardiomyocytes were cotreated for 3 h with the indicated concentrations of Myr. The results of the MTT assay following 80 µM of Myr cotreatment indicated a significantly increased cell viability as compared with cells treated with AlP alone. Cotreatment with 80 μM of Myr increased the cell viability to 81% ± 4.2% (Figure 1A). As positive control for cell death, 5 μM of staurosporine (STS) was used.
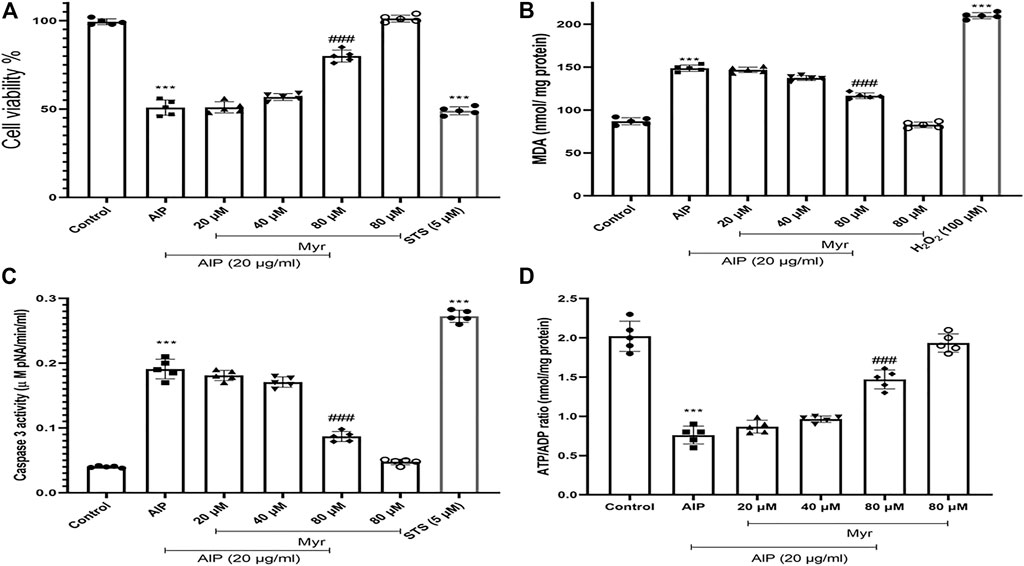
FIGURE 1. Ameliorative effect of Myr on AlP-induced cytotoxicity in isolated cardiomyocytes. (A) Isolated cardiomyocytes were treated or cotreated with the indicated concentrations (20 μg/ml) of AlP and Myr (20, 40, and 80 µM) for 3 h. Cell viability was examined after incubation using MTT assay. Myr inhibits AlP-induced cytotoxicity in isolated cardiomyocytes. (B) Isolated cardiomyocytes were treated or cotreated with Myr (20, 40, and 80 μM) and AlP (20 μg/ml) for 3 h. MDA production was measured by production of thiobarbituric acid (TBA) reactive substances (TBARS). (C) Isolated cardiomyocytes (106 cells/ml) were incubated in CCT medium in conventional condition (37°C and 5% CO2-air) for 3 h. Caspase-3 activity was determined by Sigma-Aldrich kit. Columns represent caspase-3 activity (μM pNA/min/ml) in isolated cardiomyocytes. (D) ATP/ADP ratios were determined by Luciferin/Luciferase assay as described in the Materials and Methods. Values represent mean ± SD (n = 3) of three independent experiments. ***p < 0.001 versus control; ###p < 0.001 versus AlP-treated cardiomyocytes, one-way ANOVA, Tukey’s test. Myr, myricetin; AlP, aluminum phosphide; MDA, malondialdehyde; STS, staurosporine; CCT, creatine–carnitine–taurine.
Myricetin Inhibits Aluminum Phosphide-Induced Malondialdehyde Production
Malondialdehyde (MDA) as an end product of lipid oxidation is considered to be a reliable indicator of ROS formation and oxidative stress. The MDA levels were measured as previously described in the Materials and Methods section to investigate the effect of Myr on AlP-induced lipid peroxidation. A significant elevation of the MDA contents was observed in isolated cardiomyocytes with 20 μg/ml of AlP compared with the control group, whereas cotreatment with 80 µM of Myr exhibited a significant decrease in lipid peroxidation (Figure 1B). The results showed that cotreatment of isolated cardiomyocytes with Myr inhibited AlP-induced MDA production, alleviated lipid peroxidation of the cell membrane, and reduced cell damage. Hydrogen peroxide (H2O2) was used as a positive control (100 µM).
Myricetin Inhibits Aluminum Phosphide-Induced Caspase-3 Activation
As shown in Figure 1C, activation of caspase-3 was observed following treatment of isolated cardiomyocytes with AlP, while Myr (80 µM) significantly reduced AlP-induced caspase-3 activation in the isolated cardiomyocytes. As positive control for caspase-3 activation, 5 μM of STS was used.
Myricetin Inhibits Aluminum Phosphide-Induced ATP Depletion
ATP/ADP ratio was assessed by ADP/ATP Ratio Assay kit (MAK135, Sigma, USA) in isolated cardiomyocytes using luminometer. As shown in Figure 1D, ATP/ADP ratio significantly (p < 0.001) decreased by AlP, while Myr significantly inhibited AlP-ATP depletion in the isolated cardiomyocytes (Figure 1D).
Myricetin Inhibits Aluminum Phosphide-Induced Reactive Oxygen Species Formation
Intracellular ROS by AlP-induced was monitored by DCFH-DA in isolated cardiomyocytes using flow cytometry. As shown in Figure 2, incubation with AlP for 3 h led to an increase in DCF fluorescence intensity and a shift of DCF peak rightward, which is proportionate to the amount of ROS generated. The result showed that exposure to 20 μg/ml of AlP increased intracellular ROS generation in isolated cardiomyocytes and shifted the peak rightward as compared with the untreated cardiomyocytes. However, cotreatment with Myr (40 and 80 µM) effectively reduced AlP-induced ROS production, as evidenced by the lower DCF fluorescence intensity in Myr-cotreated cardiomyocytes, and shifted the peak leftward as compared with AlP group alone. BHT (50 µM), a known antioxidant, was added to verify that the antioxidant effect of Myr inhibits ROS formation. H2O2 was used as a positive control (100 µM).
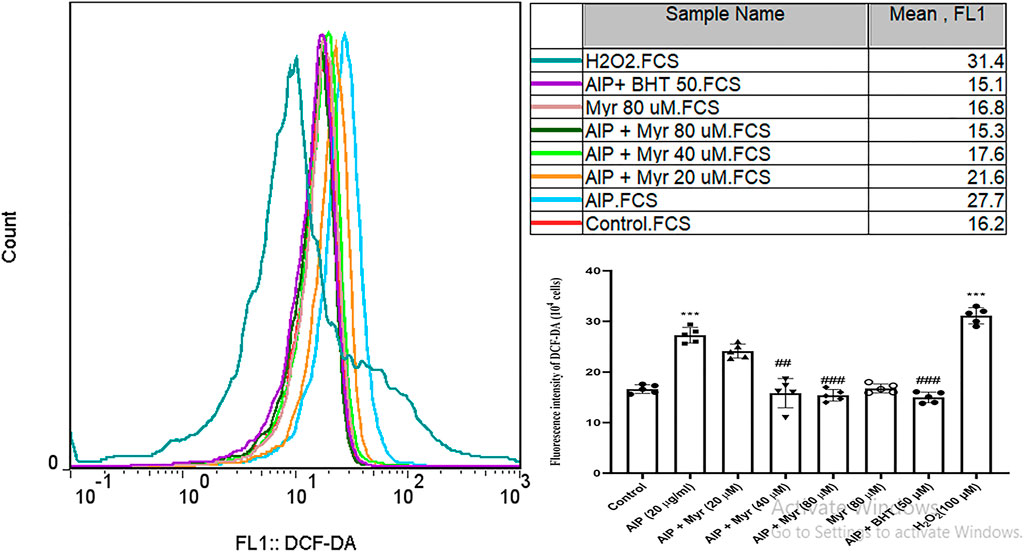
FIGURE 2. Myr inhibits AlP-induced ROS production in isolated cardiomyocytes. As shown, the fluorescence intensity of DCF is increased after exposure to AlP. The peak is moved to the right as compared with control, while cotreatment of Myr (40 and 80 µM) with AlP showed that the fluorescence intensity of DCF is decreased, and the peaks are moved to the left. Values represent mean ± SD (n = 3) of three independent experiments, ***p < 0.001 compared with control; ##p < 0.01; ###p < 0.001 compared with AlP-treated cardiomyocytes, one-way ANOVA, Tukey’s test. Myr, myricetin; AlP, aluminum phosphide; ROS, reactive oxygen species; DCF-DA, 2ʹ,7ʹ-dichlorofluorescin diacetate; BHT, butylated hydroxytoluene; H2O2, hydrogen peroxide.
Myricetin Inhibits Aluminum Phosphide-Induced Mitochondrial Membrane Potential Collapse
Mitochondrial membrane potential collapse by AlP-induced was monitored by rhodamine 123 in isolated cardiomyocytes using flow cytometry. As shown in Figure 3, incubation with AlP for 3 h led to an increase in rhodamine 123 fluorescence intensity, which is proportionate to collapse of mitochondrial membrane potential. The result demonstrated that exposure to 20 μg/ml of AlP increased the mean of rhodamine 123 fluorescence intensity in isolated cardiomyocytes compared with the untreated cardiomyocytes. However, cotreatment with Myr (40 and 80 µM) effectively reduced AlP-induced mitochondrial membrane potential collapse, as evidenced by the lower rhodamine 123 fluorescence intensity in Myr-cotreated cardiomyocytes compared with AlP group alone. Cyclosporine A (5 µM), a PTP inhibitor, was added to verify PTP dependence of mitochondrial swelling. CaCl2 (100 µM), a known inducer of mitochondrial permeability transition (MPT), was used as a positive control.
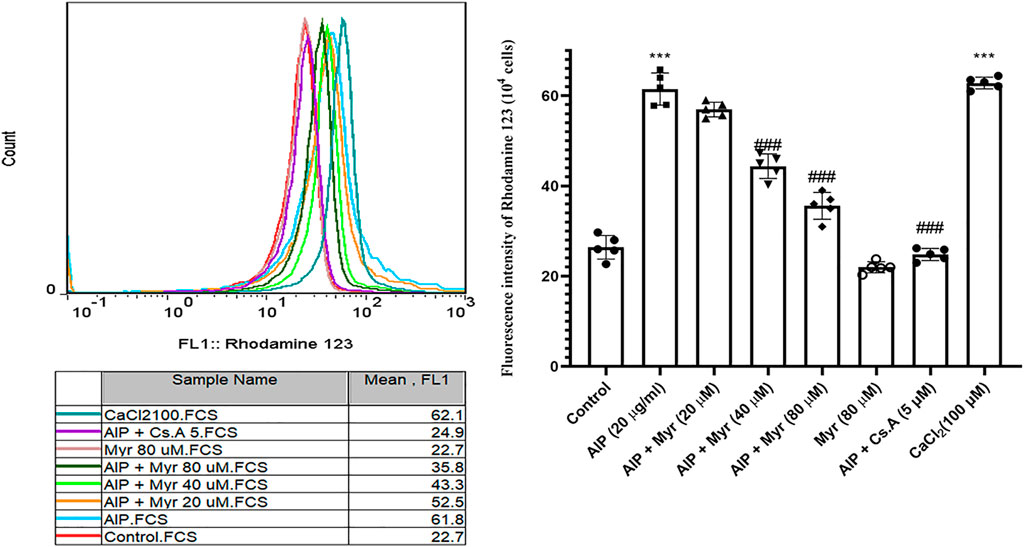
FIGURE 3. Myr inhibits AlP-induced mitochondrial membrane potential (ΔΨm) collapse in isolated cardiomyocytes. Isolated cardiomyocytes were treated or cotreated with the indicated concentrations (20 μg/ml) of AlP and Myr (20, 40, and 80 µM) for 3 h. Mitochondrial membrane potential (ΔΨm) collapse was examined after incubation using rhodamine 123 staining. Representative fluorescence intensity of rhodamine 123 staining. Data presented are the mean ± SD (n = 3 per group). ***p < 0.001 compared with control; ##p < 0.01; ###p < 0.001 compared with AlP-treated cardiomyocytes, one-way ANOVA, Tukey’s test. Myr, myricetin; AlP, aluminum phosphide; Cs.A, cyclosporine; CaCl₂, calcium chloride.
Myricetin Inhibits Aluminum Phosphide-Induced Lysosomal Damages
AlP-induced lysosomal membrane destabilization was monitored by AO in isolated cardiomyocytes using flow cytometry. As showed in Figure 4, incubation with AlP for 3 h led to an increase in AO fluorescence intensity, which is proportionate to lysosomal damages. The result indicated that exposure to 20 μg/ml of AlP increased the mean of AO fluorescence intensity in isolated cardiomyocytes compared with the untreated cardiomyocytes. However, cotreatment with Myr (40 and 80 µM) effectively reduced AlP-induced lysosomal membrane destabilization, as evidenced by the lower AO fluorescence intensity in Myr-cotreated cardiomyocytes compared with AlP group alone. tert-Butyl hydroperoxide (t-BuOOH) at concentration of 0.5 mM, a classical lysosomal membrane permeabilization inducer that causes lysosomal damage via oxidative stress, was used as positive control.
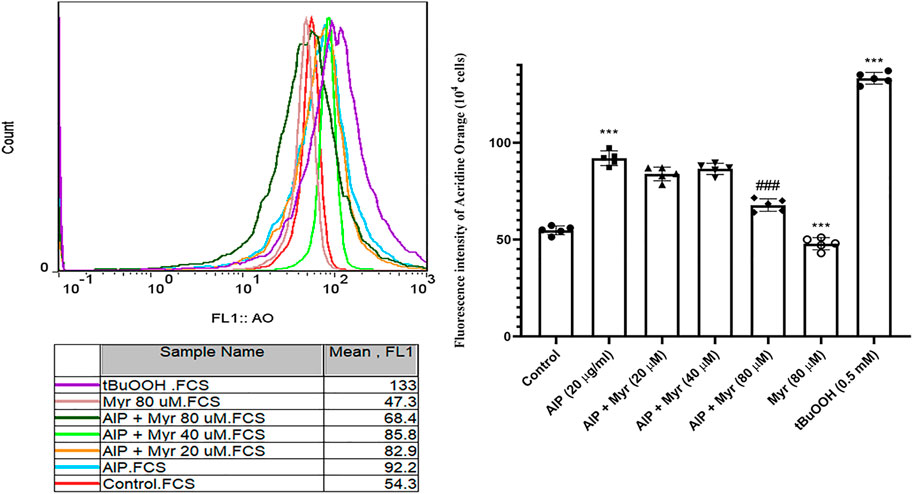
FIGURE 4. Myr inhibits AlP-induced lysosomal dysfunction in isolated cardiomyocytes. Isolated cardiomyocytes were treated or cotreated with the indicated concentrations (20 μg/ml) of AlP and Myr (20, 40, and 80 µM) for 3 h. Lysosomal membrane stability was examined after incubation using acridine orange fluorescence dye. Data are mean ± SD (n = 3) of three independent experiments. ***p < 0.001 significantly different from control, ##p < 0.01 significantly different from AlP-treated cardiomyocytes, one-way ANOVA, Tukey’s test. Myr, myricetin; AlP, aluminum phosphide; AO, acridine orange; t-BuOOH, tert-butyl hydroperoxide.
Ameliorative Effects of Myricetin on Aluminum Phosphide-Induced Glutathione Depletion
Since AlP is a glutathione-depleting agent, we investigated the effects of AlP and Myr on GSH level by measuring the GSH and GSSG. A significant reduction in GSH was observed after AlP treatment in isolated cardiomyocytes. Myr cotreatment (40 and 80 µM) caused a significant recovery in the GSH level (Figure 5A). The GSSG levels was significantly increased in AlP-treated cardiomyocytes. Myr cotreatment (40 and 80 µM), however, caused a significant reduction in the GSSG level (Figure 5B). The results showed that Myr at 40 and 80 μM significantly prevented (p < 0.001) the depletion of GSH level caused AlP by in isolated cardiomyocytes.
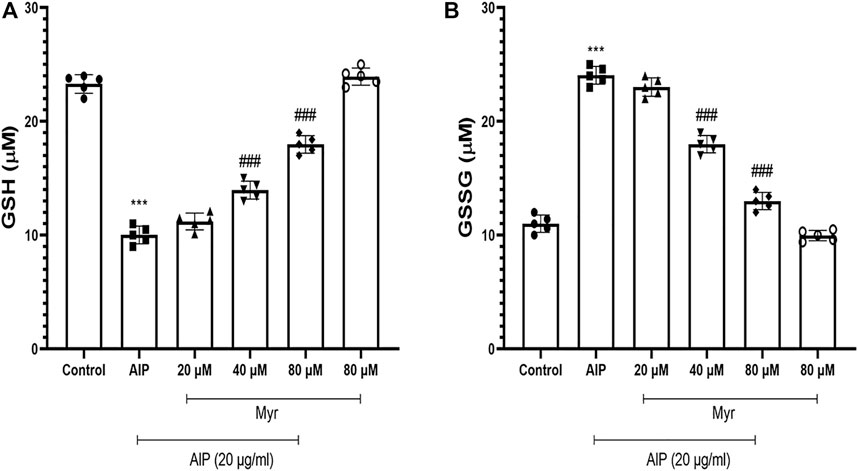
FIGURE 5. Myr inhibits AlP-induced GSH depletion in isolated cardiomyocytes. Isolated cardiomyocytes were treated or cotreated with the indicated concentrations (20 μg/ml) of AlP and Myr (20, 40, and 80 µM) for 3 h. GSH and GSSG levels were measured as previously described in the Materials and Methods section. (A) GSH content significantly decreased AlP-treated isolated cardiomyocytes, while cotreatment of Myr (40 and 80 µM) with AlP obviously increased the GSH content in isolated cardiomyocytes. (B) AlP significantly increased the GSSG content in isolated cardiomyocytes after 3 h of exposure, while Myr (40 and 80 µM) significantly decreased the GSSG content in the presence of AlP. Data are mean ± SD (n = 3) of three independent experiments. ***p < 0.001 significantly different from control, ###p < 0.001 significantly different from AlP-treated cardiomyocytes, one-way ANOVA, Tukey’s test. Myr, myricetin; AlP, aluminum phosphide; GSH, reduced glutathione; GSSG; oxidized glutathione.
Effect of Aluminum Phosphide and Myricetin on Antioxidant Enzyme in Cardiomyocytes
To determine whether AlP and Myr have effects on the levels of antioxidant enzymes, GSH-Px, CAT, and SOD were measured in the cell lysates. The activities of GSH-Px, CAT, and SOD were remarkably decreased in the AlP group compared with the control group (p < 0.01). Myr cotreatment (80 µM) with AlP (20 μg/ml) could increase the activities of SOD, CAT, and GSH-Px compared with that in the AlP group (p < 0.01) (Table 1).
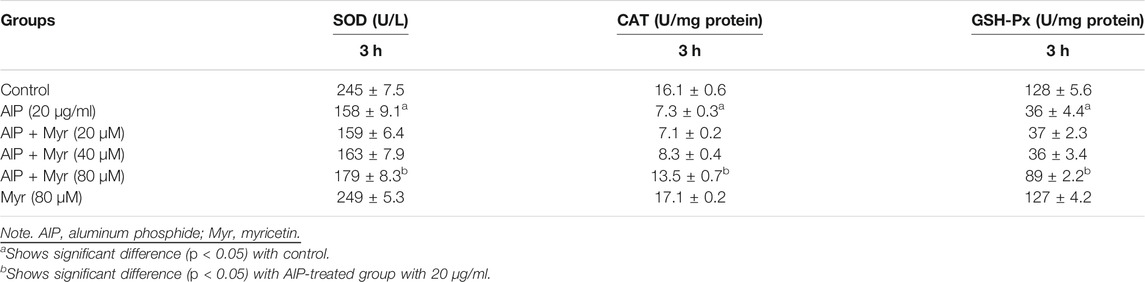
TABLE 1. Effects of AlP at concentration 20 μg/ml and Myr at concentrations of 20, 40, and 80 µM + AlP on superoxide dismutase (SOD), catalase (CAT), and glutathione peroxidase (GSH-Px) levels of rat heart isolated cardiomyocytes at 3 h.
Ameliorative Efficacy of Myricetin in Aluminum Phosphide-Induced Mitochondrial Dysfunction
To determine the mitochondrial dysfunction of AlP and the ameliorative effect of Myr on isolated mitochondria, the SDH activity was evaluated after 1-h exposure by MTT assay. The SDH activity in isolated mitochondria markedly decreased following incubation with 20 μg/ml of AlP. To evaluate the ameliorative effect of Myr in AlP-induced mitochondrial dysfunction, isolated mitochondria were cotreated for 1 h with the indicated concentrations of Myr (20, 40, and 80 μM). The results of the SDH activity following 40 and 80 µM of Myr cotreatment indicated a significant increase of the mitochondrial activity compared with mitochondria treated with AlP alone. Cotreatment with 80 and 40 μM of Myr increased the mitochondrial activity to 67% ± 5.1% and 74% ± 4.9%, respectively, as compared with mitochondria treated with AlP alone (Figure 6A).
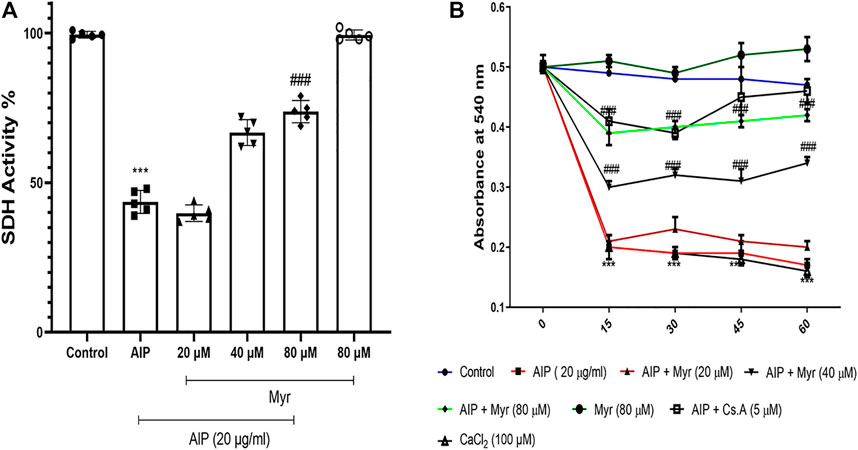
FIGURE 6. Myr inhibits AlP-induced mitochondrial dysfunction (A) and swelling (B) in isolated mitochondria. (A) Isolated mitochondria were treated or cotreated with the indicated concentrations (20 μg/ml) of AlP and Myr (20, 40 and 80 µM) for 1 h. Succinate dehydrogenase activity was evaluated by MTT assay. Data showed AlP (20 μg/ml) to significantly decrease SDH activity as compared with control, while Myr (40 and 80 µM) significantly increased SDH activity as compared with AlP-treated cardiomyocytes. (B) Isolated mitochondria were treated or cotreated with the indicated concentrations (20 μg/ml) of AlP and Myr (20, 40, and 80 µM) for 1 h. Mitochondrial swelling was evaluated by monitoring absorbance at 540 nm. Data showed that AlP (20 μg/ml) significantly induced mitochondrial swelling as compared with control, while Myr (40 and 80 µM) significantly inhibits mitochondrial swelling as compared with AlP-treated cardiomyocytes. Data are mean ± SD (n = 3) of three independent experiments. ***p < 0.001 significantly different from control, ##p < 0.01, ###p < 0.001 significantly different from AlP-treated cardiomyocytes, one-way ANOVA and two-way ANOVA followed by post-hoc Tukey and Bonferroni test. Myr, myricetin; AlP, aluminum phosphide; SDH, succinate dehydrogenase activity.
Myricetin Inhibits Aluminum Phosphide-Induced Mitochondrial Swelling
We monitored the ameliorative effects of Myr against AlP-induced mitochondrial swelling as an indicator of MPT pore opening. To determine the mitochondrial swelling of AlP and the ameliorative effect of Myr on isolated mitochondria, the absorbance at 540 nm was evaluated during 1-h exposure. The mitochondrial swelling markedly increased following incubation with 20 μg/ml of AlP. To evaluate the ameliorative effect of Myr in AlP-induced mitochondrial swelling, isolated mitochondria were cotreated for 1 h with the indicated concentrations of Myr (20, 40, and 80 μM). The results of the mitochondrial swelling following 40 and 80 µM of Myr cotreatment indicated a significant decrease of the mitochondrial swelling compared with mitochondria treated with AlP alone (Figure 6B). Cyclosporine A (5 µM), a MPT pore (PTP) inhibitor, was added to verify PTP dependence of mitochondrial swelling. CaCl2 (100 µM), a known inducer of MPT, was used as a positive control.
Discussion
There are many investigations that showed the underlying mechanisms of AlP-induced toxicity. Mitochondrial dysfunction and oxidative stress are the major mechanisms in performed experimental studies (Valmas et al., 2008; Kariman et al., 2012; Anand et al., 2013; Sciuto et al., 2016). Oxidative stress and mitochondrial damages induced by AlP have been demonstrated in nematodes, insects, mammalian cell lines, and animals (Valmas et al., 2008; Sciuto et al., 2016). Although the underlying mechanisms of AlP is not well understood, it has been found that phosphine inhibits aerobic respiration in a number of tissues and species (Anand et al., 2013). Previous studies on submitochondrial particles and isolated mitochondria have disclosed that mitochondrial complex IV (cytochrome c oxidase) of the electron transport chain is inhibited by AlP (Anand et al., 2013; Sciuto et al., 2016). Our results on isolated cardiomyocytes and mitochondria showed that AlP induces mitochondrial toxicity and oxidative stress. Our findings are in accordance with previous reports that showed AlP that causes oxidative damages and mitochondrial dysfunction (Jahedsani et al., 2020; Khezri et al., 2020).
Antioxidants and mitochondrial protective agents are the simple and most significant defense system of the human body to counteract oxidative stress and mitochondrial dysfunction (Kurutas, 2015). The nonenzymatic agents including glutathione and enzymatic antioxidants such thiol-containing enzymes, SOD, and CAT are of great importance in the human body (Kurutas, 2015). Our results in the current study are in accordance with previous reports that have shown that AlP reduces the antioxidant molecules such as glutathione (Hsu et al., 2000). The action of AlP in the different tissues and species reported in previous studies and isolated cardiomyocytes in our study correlates well with depletion of glutathione, caspase-3 activation, ROS formation, and lipid peroxidation (Kariman et al., 2012). Decreased glutathione in isolated cardiomyocytes strongly suggested the involvement of ROS formation and lipid peroxidation in AlP cytotoxicity. Depletion of glutathione in the cardiomyocytes predisposes cells to oxidant damage, lipid peroxidation, and cytotoxicity (Mohamed et al., 2000). It has been proved that antioxidants such as melatonin can stop most of the oxidative damage induced by AlP in rat cardiac tissues and proprietorially preserve the levels of glutathione and mitochondrial function (Asghari et al., 2017). Other antioxidants such as N-acetylcysteine (NAC), which have antioxidant properties and reload cellular glutathione, have been proposed to reduce the cardiac toxicity induced by AlP (Tehrani et al., 2013). Sine myocardial suppression is a distinguishing feature of AlP poisoning via mitochondrial damages and oxidative stress, the simple and most significant strategy to reduce AlP-induced cardiotoxicity is to use antioxidant and mitochondrial protective agents (Akkaoui et al., 2007). These antioxidant compounds can reduce the toxicity of AlP, either directly or by reloading antioxidant defenses such as glutathione.
In various cell-based assays and animal models, the antioxidant effect of Myr was demonstrated. It has been reported that Myr has a protective effect through inactivation of H2O2-induced radicals as well as regulation of programmed cell death or apoptosis (Mansuri et al., 2014). Likewise, Myr reduced oxidative stress induced by hydrogen peroxide yeast cells and led to a reduction in protein carbonylation and intracellular oxidation (Mansuri et al., 2014). The beneficial effects of Myr on vascular endothelial dysfunction have been reported in human umbilical vein endothelial cells (Yi et al., 2011). Also, in several animal models, the antioxidant effect of Myr was also observed. It has been reported that Myr decreases the generation of myeloperoxidase, MDA, and nitric oxide while increasing the activity of glutathione peroxide and SOD in animal models (Zhao et al., 2013). Moreover, the antioxidant effects of Myr on cardiovascular function have been reported in animal models. Tiwari et al. have reported that Myr significantly inhibits the effects of histopathological alterations of ISO on heart rate, the levels of different cardiac marker enzymes, including aspartate aminotransferase (AST), creatine kinase (CK), LDH, CAT, and SOD as well alterations in electrocardiographic patterns and vascular reactivity in Wistar rats (Tiwari et al., 2009). Our results on isolated cardiomyocytes showed that Myr can inhibit oxidative stress, ROS formation, and depletion of glutathione and ATP and can increase the activities of SOD, CAT, and GSH-Px, which have the main role in AlP-induced cardiotoxicity. These results are in accordance with previous reports in cellar and animal studies (Tiwari et al., 2009; Yi et al., 2011; Taheri et al., 2020).
In various cell-based assays and animal models, the mitochondrial protective effects of Myr were demonstrated. The protective effects of Myr have been reported in preventing methylmercury-induced mitochondrial toxicity by blocking ROS formation and lipid peroxidation (Franco et al., 2010). Also in animal models, the mitochondrial protective effects of Myr on hypoxia-induced mitochondrial impairments were reported, and Myr attenuated acute hypoxia-induced mitochondrial impairment (Zou et al., 2015). At the mitochondrial level, AlP can rapidly inhibit oxidative respiration by up to 70% and perturb mitochondrial conformation, severely decreasing mitochondrial membrane potential (Valmas et al., 2008). It has been reported that AlP mainly inhibits complex IV and decreases complex I and complex II activity, resulting in decreased ATP production and increased ROS generation (Dua and Gill, 2004). These studies are in accordance with our results on isolated mitochondria in the current study (Dua and Gill, 2004). On the other hand, it has been proved that flavonoids such as Myr can suppress mitochondrial ROS production by directly chelating the trace elements and inhibiting enzymes (mitochondria complexes) involved in ROS formation (Kicinska and Jarmuszkiewicz, 2020). The Myr analogs are acacetin, chrysin, apigenin, luteolin, kaempferol, naringenin, and quercetin, which have shown similar effects (Taheri et al., 2020). Recently, we showed that apigenin and chrysin have a similar effect on isolated cardiomyocytes and mitochondria against AlP (Jahedsani et al., 2020; Khezri et al., 2020). It has been reported that quercetin, a very similar analog to Myr, can act as an inhibitor of the MPT pore, and the same effect of Myr was proved in this study (De Marchi et al., 2009). On the other hand, it has been reported that these inhibitors of the MPT pore can play an effective role in reducing myocardial damage (Hausenloy et al., 2002).
In the current study, we proved that Myr can inhibit mitochondrial dysfunction induced by AlP in isolated mitochondria and cardiomyocytes, resulting in decreased ROS generation, MDA level, and lysosomal damages and increased cell viability. For the futures studies, it is suggested that more researches be done on the effect of Myr on AlP-induced cardiotoxicity, and the findings of this study should be confirmed by animal and human studies.
In conclusion, the results of current study demonstrated that AlP can directly cause toxicity in cardiac mitochondria and cardiomyocytes, which are associated with cytotoxicity, mitochondrial toxicity, reduction of antioxidant molecules, ROS formation, oxidative stress, and lysosomal dysfunction, which significantly attenuated by Myr as an antioxidant and mitochondrial protective agent in isolated cardiomyocytes and mitochondria. In the light of these findings, we concluded that Myr through antioxidant potential and inhibition of MPT pore exerted an ameliorative role in AlP-induced toxicity in isolated cardiomyocytes and mitochondria, and it would be valuable to examine in vivo effects.
Data Availability Statement
The original contributions presented in the study are included in the article/Supplementary Material, Further inquiries can be directed to the corresponding author.
Ethics Statement
The animal study was reviewed and approved by Ardabil University of Medical Sciences, Deputy of Research with ethics code IR.ARUMS.REC.1397.236.
Author Contributions
AS designed the study, performed all the cell experiments, processed the data, performed the statistical analysis, wrote the original draft preparation, and revised the manuscript. ZJ and MS participated in collected data.
Funding
This study was supported by the Ardabil University of Medical Sciences, Deputy of Research.
Conflict of Interest
The authors declare that the research was conducted in the absence of any commercial or financial relationships that could be construed as a potential conflict of interest.
Publisher’s Note
All claims expressed in this article are solely those of the authors and do not necessarily represent those of their affiliated organizations, or those of the publisher, the editors, and the reviewers. Any product that may be evaluated in this article, or claim that may be made by its manufacturer, is not guaranteed or endorsed by the publisher.
Supplementary Material
The Supplementary Material for this article can be found online at: https://www.frontiersin.org/articles/10.3389/fphar.2021.719081/full#supplementary-material
References
Ahangari, R., Khezri, S., Jahedsani, A., Bakhshii, S., and Salimi, A. (2020). Ellagic Acid Alleviates Clozapine‑induced Oxidative Stress and Mitochondrial Dysfunction in Cardiomyocytes. Drug Chem. Toxicol. 11 , 1–9. doi:10.1080/01480545.2020.1850758
Akkaoui, M., Achour, S., Abidi, K., Himdi, B., Madani, A., Zeggwagh, A. A., et al. (2007). Reversible Myocardial Injury Associated with Aluminum Phosphide Poisoning. Clin. Toxicol. 45, 728–731. doi:10.1080/15563650701517350
Ames, B. N. (2010). Optimal Micronutrients Delay Mitochondrial Decay and Age-Associated Diseases. Mech. Ageing Dev. 131, 473–479. doi:10.1016/j.mad.2010.04.005
Anand, R., Sharma, D. R., Verma, D., Bhalla, A., Gill, K. D., and Singh, S. (2013). Mitochondrial Electron Transport Chain Complexes, Catalase and Markers of Oxidative Stress in Platelets of Patients with Severe Aluminum Phosphide Poisoning. Hum. Exp. Toxicol. 32, 807–816. doi:10.1177/0960327112468909
Asghari, M. H., Moloudizargari, M., Baeeri, M., Baghaei, A., Rahimifard, M., Solgi, R., et al. (2017). On the Mechanisms of Melatonin in Protection of Aluminum Phosphide Cardiotoxicity. Arch. Toxicol. 91, 3109–3120. doi:10.1007/s00204-017-1998-6
Barzegar, A. (2016). Antioxidant Activity of Polyphenolic Myricetin In Vitro Cell- Free and Cell-Based Systems. Mol. Biol. Res. Commun. 5, 87–95.
Beach, D. C., and Giroux, E. (1992). Inhibition of Lipid Peroxidation Promoted by Iron(III) and Ascorbate. Arch. Biochem. Biophys. 297, 258–264. doi:10.1016/0003-9861(92)90670-r
Bumbrah, G. S., Krishan, K., Kanchan, T., Sharma, M., and Sodhi, G. S. (2012). Phosphide Poisoning: a Review of Literature. Forensic Sci. Int. 214, 1–6. doi:10.1016/j.forsciint.2011.06.018
Chobot, V., and Hadacek, F. (2011). Exploration of Pro-oxidant and Antioxidant Activities of the Flavonoid Myricetin. Redox Rep. 16, 242–247. doi:10.1179/1351000211Y.0000000015
Dandona, R., and Gunnell, D. (2021). Pesticide Surveillance and Deaths by Suicide. Lancet Glob. Health 9, e738–e739. doi:10.1016/S2214-109X(21)00174-1
De Marchi, U., Biasutto, L., Garbisa, S., Toninello, A., and Zoratti, M. (2009). Quercetin Can Act Either as an Inhibitor or an Inducer of the Mitochondrial Permeability Transition Pore: A Demonstration of the Ambivalent Redox Character of Polyphenols. Biochim. Biophys. Acta 1787, 1425–1432. doi:10.1016/j.bbabio.2009.06.002
Dua, R., and Gill, K. D. (2004). Effect of Aluminium Phosphide Exposure on Kinetic Properties of Cytochrome Oxidase and Mitochondrial Energy Metabolism in Rat Brain. Biochim. Biophys. Acta 1674, 4–11. doi:10.1016/j.bbagen.2004.05.003
Eddleston, M., Karalliedde, L., Buckley, N., Fernando, R., Hutchinson, G., Isbister, G., et al. (2002). Pesticide Poisoning in the Developing World-Aa Minimum Pesticides List. Lancet 360, 1163–1167. doi:10.1016/s0140-6736(02)11204-9
Eruslanov, E., and Kusmartsev, S. (2010). “Identification of ROS Using Oxidized DCFDA and Flow-Cytometry,” in Advanced Protocols in Oxidative Stress II. (Springer), 57–72. doi:10.1007/978-1-60761-411-1_4
Etemadi-Aleagha, A., Akhgari, M., and Iravani, F. S. (2015). Aluminum Phosphide Poisoning-Related Deaths in Tehran, Iran, 2006 to 2013. Medicine 94, e1637. doi:10.1097/md.0000000000001637
Franco, J. L., Posser, T., Missau, F., Pizzolatti, M. G., Dos Santos, A. R., Souza, D. O., et al. (2010). Structure-activity Relationship of Flavonoids Derived from Medicinal Plants in Preventing Methylmercury-Induced Mitochondrial Dysfunction. Environ. Toxicol. Pharmacol. 30, 272–278. doi:10.1016/j.etap.2010.07.003
Gunnell, D., Eddleston, M., Phillips, M. R., and Konradsen, F. (2007). The Global Distribution of Fatal Pesticide Self-Poisoning: Systematic Review. BMC Public Health 7, 357–415. doi:10.1186/1471-2458-7-357
Gurjar, M., Baronia, A. K., Azim, A., and Sharma, K. (2011). Managing Aluminum Phosphide Poisonings. J. Emerg. Trauma Shock 4, 378–384. doi:10.4103/0974-2700.83868
Hausenloy, D. J., Maddock, H. L., Baxter, G. F., and Yellon, D. M. (2002). Inhibiting Mitochondrial Permeability Transition Pore Opening: A New Paradigm for Myocardial Preconditioning? Cardiovasc. Res. 55, 534–543. doi:10.1016/s0008-6363(02)00455-8
Hissin, P. J., and Hilf, R. (1976). A Fluorometric Method for Determination of Oxidized and Reduced Glutathione in Tissues. Anal. Biochem. 74, 214–226. doi:10.1016/0003-2697(76)90326-2
Hsu, C., Han, B., Liu, M., Yeh, C., and Casida, J. E. (2000). Phosphine-Induced Oxidative Damage in Rats: Attenuation by Melatonin. Free Radic. Biol. Med. 28, 636–642. doi:10.1016/s0891-5849(99)00277-4
Jahedsani, A., Khezri, S., Ahangari, M., Bakhshii, S., and Salimi, A. (2020). Apigenin Attenuates Aluminum Phosphide-Induced Cytotoxicity via Reducing Mitochondrial/Lysosomal Damages and Oxidative Stress in Rat Cardiomyocytes. Pestic. Biochem. Physiol. 167, 104585. doi:10.1016/j.pestbp.2020.104585
Jones, J. R., Lebar, M. D., Jinwal, U. K., Abisambra, J. F., Koren, J., Blair, L., et al. (2011). The Diarylheptanoid (+)-aR,11S-Myricanol and Two Flavones from Bayberry (Myrica cerifera) Destabilize the Microtubule-Associated Protein Tau. J. Nat. Prod. 74, 38–44. doi:10.1021/np100572z
Kariman, H., Heydari, K., Fakhri, M., Shahrami, A., Dolatabadi, A. A., Mohammadi, H. A., et al. (2012). Aluminium Phosphide Poisoning and Oxidative Stress: Serum Biomarker Assessment. J. Med. Toxicol. 8, 281–284. doi:10.1007/s13181-012-0219-1
Khezri, S., Sabzalipour, T., Jahedsani, A., Azizian, S., Atashbar, S., and Salimi, A. (2020). Chrysin Ameliorates Aluminum Phosphide-Induced Oxidative Stress and Mitochondrial Damages in Rat Cardiomyocytes and Isolated Mitochondria. Environ. Toxicol. 35, 1114–1124. doi:10.1002/tox.22947
Kicinska, A., and Jarmuszkiewicz, W. (2020). Flavonoids and Mitochondria: Activation of Cytoprotective Pathways? Molecules 25, 3060. doi:10.3390/molecules25133060
Kurutas, E. B. (2015). The Importance of Antioxidants Which Play the Role in Cellular Response Against Oxidative/Nitrosative Stress: Current State. Nutr. J. 15, 71–22. doi:10.1186/s12937-016-0186-5
Lagoa, R., Graziani, I., Lopez-Sanchez, C., Garcia-Martinez, V., and Gutierrez-Merino, C. (2011). Complex I and Cytochrome C Are Molecular Targets of Flavonoids that Inhibit Hydrogen Peroxide Production by Mitochondria. Biochim. Biophys. Acta 1807, 1562–1572. doi:10.1016/j.bbabio.2011.09.022
Mansuri, M. L., Parihar, P., Solanki, I., and Parihar, M. S. (2014). Flavonoids in Modulation of Cell Survival Signalling Pathways. Genes Nutr. 9, 400. doi:10.1007/s12263-014-0400-z
Mehrpour, O., Akbari, A., Jahani, F., Amirabadizadeh, A., Allahyari, E., Mansouri, B., et al. (2018). Epidemiological and Clinical Profiles of Acute Poisoning in Patients Admitted to the Intensive Care Unit in Eastern Iran (2010 to 2017). BMC Emerg. Med. 18, 30–39. doi:10.1186/s12873-018-0181-6
Mehrpour, O., Jafarzadeh, M., and Abdollahi, M. (2012). A Systematic Review of Aluminium Phosphide Poisoning. Arh Hig Rada Toksikol 63, 61–73. doi:10.2478/10004-1254-63-2012-2182
Mersa, A., Atashbar, S., Ahvar, N., and Salimi, A. (2020). 1, 25‐dihydroxyvitamin D3 Prevents Deleterious Effects of Erythromycin on Mitochondrial Function in Rat Heart Isolated Mitochondria. Clin. Exp. Pharmacol. Physiol. 47, 1554–1563. doi:10.1111/1440-1681.13328
Moghadamnia, A. A. (2012). An Update on Toxicology of Aluminum Phosphide. Daru 20, 25–28. doi:10.1186/2008-2231-20-25
Mohamed, H. E., El-Swefy, S. E., and Hagar, H. H. (2000). The Protective Effect of Glutathione Administration on Adriamycin-Induced Acute Cardiac Toxicity in Rats. Pharmacol. Res. 42, 115–121. doi:10.1006/phrs.1999.0630
Nippert, F., Schreckenberg, R., and Schlüter, K.-D. (2017). Isolation and Cultivation of Adult Rat Cardiomyocytes. J. Vis. Exp. (128), e56634. doi:10.3791/56634
Park, K.-S., Chong, Y., and Kim, M. K. (2016). Myricetin: Biological Activity Related to Human Health. Appl. Biol. Chem. 59, 259–269. doi:10.1007/s13765-016-0150-2
Salimi, A., Ayatollahi, A., Seydi, E., Khomeisi, N., and Pourahmad, J. (2015). Direct Toxicity of Amyloid Beta Peptide on Rat Brain Mitochondria: Preventive Role of Mangifera indica and Juglans regia. Toxicol. Environ. Chem. 97, 1057–1070. doi:10.1080/02772248.2015.1079016
Schulz, S., Lichtmannegger, J., Schmitt, S., Leitzinger, C., Eberhagen, C., Einer, C., et al. (2015). “A Protocol for the Parallel Isolation of Intact Mitochondria from Rat Liver, Kidney, Heart, and Brain,” in Proteomic Profiling (Springer), 75–86. doi:10.1007/978-1-4939-2550-6_7
Sciuto, A. M., Wong, B. J., Martens, M. E., Hoard-Fruchey, H., and Perkins, M. W. (2016). Phosphine Toxicity: a story of Disrupted Mitochondrial Metabolism. Ann. N. Y Acad. Sci. 1374, 41–51. doi:10.1111/nyas.13081
Semwal, D. K., Semwal, R. B., Combrinck, S., and Viljoen, A. (2016). Myricetin: A Dietary Molecule with Diverse Biological Activities. Nutrients 8, 90. doi:10.3390/nu8020090
Taheri, Y., Suleria, H. A. R., Martins, N., Sytar, O., Beyatli, A., Yeskaliyeva, B., et al. (2020). Myricetin Bioactive Effects: Moving from Preclinical Evidence to Potential Clinical Applications. BMC Complement. Med. Ther. 20, 241–314. doi:10.1186/s12906-020-03033-z
Tapio, S. (2016). Pathology and Biology of Radiation-Induced Cardiac Disease. J. Radiat. Res. 57, 439–448. doi:10.1093/jrr/rrw064
Tehrani, H., Halvaie, Z., Shadnia, S., Soltaninejad, K., and Abdollahi, M. (2013). Protective Effects of N-Acetylcysteine on Aluminum Phosphide-Induced Oxidative Stress in Acute Human Poisoning. Clin. Toxicol. (Phila) 51, 23–28. doi:10.3109/15563650.2012.743029
Tiwari, R., Mohan, M., Kasture, S., Maxia, A., and Ballero, M. (2009). Cardioprotective Potential of Myricetin in Isoproterenol-Induced Myocardial Infarction in Wistar Rats. Phytother Res. 23, 1361–1366. doi:10.1002/ptr.2688
Tran, D. H., and Wang, Z. V. (2019). Glucose Metabolism in Cardiac Hypertrophy and Heart Failure. J. Am. Heart Assoc. 8, e012673. doi:10.1161/JAHA.119.012673
Valmas, N., Zuryn, S., and Ebert, P. R. (2008). Mitochondrial Uncouplers Act Synergistically with the Fumigant Phosphine to Disrupt Mitochondrial Membrane Potential and Cause Cell Death. Toxicology 252, 33–39. doi:10.1016/j.tox.2008.07.060
Wang, L., Wu, H., Yang, F., and Dong, W. (2019). The Protective Effects of Myricetin against Cardiovascular Disease. J. Nutr. Sci. Vitaminol. 65, 470–476. doi:10.3177/jnsv.65.470
Yi, L., Jin, X., Chen, C. Y., Fu, Y. J., Zhang, T., Chang, H., et al. (2011). Chemical Structures of 4-Oxo-Flavonoids in Relation to Inhibition of Oxidized Low-Density Lipoprotein (LDL)-induced Vascular Endothelial Dysfunction. Int. J. Mol. Sci. 12, 5471–5489. doi:10.3390/ijms12095471
Zhao, J., Hong, T., Dong, M., Meng, Y., and Mu, J. (2013). Protective Effect of Myricetin in Dextran Sulphate Sodium-Induced Murine Ulcerative Colitis. Mol. Med. Rep. 7, 565–570. doi:10.3892/mmr.2012.1225
Zhao, Y., Ye, L., Liu, H., Xia, Q., Zhang, Y., Yang, X., et al. (2010). Vanadium Compounds Induced Mitochondria Permeability Transition Pore (PTP) Opening Related to Oxidative Stress. J. Inorg. Biochem. 104, 371–378. doi:10.1016/j.jinorgbio.2009.11.007
Keywords: cardiomyopathy, poisoning, flavonoids, antioxidant, mitochondrial dysfunction
Citation: Salimi A, Jamali Z and Shabani M (2021) Antioxidant Potential and Inhibition of Mitochondrial Permeability Transition Pore by Myricetin Reduces Aluminium Phosphide-Induced Cytotoxicity and Mitochondrial Impairments. Front. Pharmacol. 12:719081. doi: 10.3389/fphar.2021.719081
Received: 01 June 2021; Accepted: 13 October 2021;
Published: 09 November 2021.
Edited by:
Manuela Oliverio, University of Catanzaro, ItalyReviewed by:
Vinod Kumar Bhaskara Pillai, University of Chicago, United StatesHelen E. Collins, University of Louisville, United States
Copyright © 2021 Salimi, Jamali and Shabani. This is an open-access article distributed under the terms of the Creative Commons Attribution License (CC BY). The use, distribution or reproduction in other forums is permitted, provided the original author(s) and the copyright owner(s) are credited and that the original publication in this journal is cited, in accordance with accepted academic practice. No use, distribution or reproduction is permitted which does not comply with these terms.
*Correspondence: Ahmad Salimi, c2FsaW1pa2RAeWFob28uY29t