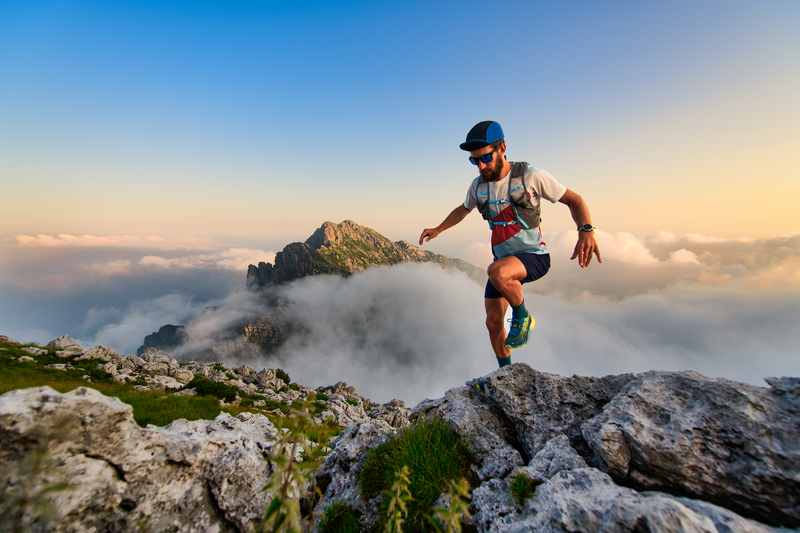
94% of researchers rate our articles as excellent or good
Learn more about the work of our research integrity team to safeguard the quality of each article we publish.
Find out more
ORIGINAL RESEARCH article
Front. Pharmacol. , 22 September 2021
Sec. Cardiovascular and Smooth Muscle Pharmacology
Volume 12 - 2021 | https://doi.org/10.3389/fphar.2021.715466
Infarcted myocardium is predisposed to cause lethal ventricular arrhythmias that remain the main cause of death in patients suffering myocardial ischemia. Liver-derived fibroblast growth factor 21 (FGF21) is an endocrine regulator, which exerts metabolic actions by favoring glucose and lipids metabolism. Emerging evidence has shown a beneficial effect of FGF21 on cardiovascular diseases, but the role of FGF21 on ventricular arrhythmias following myocardial infarction (MI) in humans has never been addressed. This study was conducted to investigate the pharmacological effects of FGF21 on cardiomyocytes after MI in humans. Patients with arrhythmia in acute MI and healthy volunteers were enrolled in this study. Serum samples were collected from these subjects on day 1 and days 7–10 after the onset of MI for measuring FGF21 levels using ELISA. Here, we found that the serum level of FGF21 was significantly increased on day 1 after the onset of MI and it returned to normal on days 7–10, relative to the Control samples. In order to clarify the regulation of FGF21 on arrhythmia, two kinds of arrhythmia animal models were established in this study, including ischemic arrhythmia model (MI rat model) and nonischemic arrhythmia model (ouabain-induced guinea pig arrhythmia model). The results showed that the incidence and duration time of ischemic arrhythmias in rhbFGF21-treated MI rats were significantly reduced at different time point after MI compared with normal saline-treated MI rats. Moreover, the onset of the first ventricular arrhythmias was delayed and the numbers of VF and maintenance were attenuated by FGF21 compared to the rhbFGF21-untreated group in the ouabain model. Consistently, in vitro study also demonstrated that FGF21 administration was able to shorten action potential duration (APD) in hydrogen peroxide-treated AC16 cells. Mechanically, FGF21 can ameliorate the electrophysiological function of AC16 cells, which is characterized by rescuing the expression and dysfunction of cardiac sodium current (INa) and inward rectifier potassium (Ik1) in AC16 cells induced by hydrogen peroxide. Moreover, the restorative effect of FGF21 on NaV1.5 and Kir2.1 was eliminated when FGF receptors were inhibited. Collectively, FGF21 has the potential role of ameliorating transmembrane ion channels remodeling through the NaV1.5/Kir2.1 pathway by FGF receptors and thus reducing life-threatening postinfarcted arrhythmias, which provides new strategies for antiarrhythmic therapy in clinics.
Acute myocardial infarction (AMI) patients are prone to a variety of arrhythmias, among which ventricular arrhythmia has a higher incidence, and it is also one of the important factors leading to sudden death and endangering the lives of patients. In the United States, 80% of sudden deaths are caused by arrhythmia, and 20% of them have a history of myocardial infarction (MI) (Kallergis and Vardas, 2007). Mechanistically, abnormal electrophysiological changes including action potential (AP) prolongation and ion channel imbalance play critical roles in arrhythmia generation in the post-MI heart (Qin et al., 1996; Yang et al., 2015). Increasing evidence suggested that the imbalance of ion channel currents including Na+ current (Kelly et al., 2018), L-type Ca2+ current (Pessina et al., 2018), and various K+ currents (Decher et al., 2017; Ponce-Balbuena et al., 2018) leads to a prolongation of QT interval and contributes to serious ventricular arrhythmias in heart diseases (Al-Akchar and Siddique, 2017; Schwartz et al., 2020). The prolongation of AP repolarization is a hallmark of cardiomyocytes isolated from MI hearts and might lead to the dispersion of repolarization and development of after-depolarization (Tan et al., 1995). It has been reported that the main voltage-gated sodium channel expressed in cardiomyocytes is NaV1.5, and dysfunction of NaV1.5 will cause changes in the flux and duration of sodium current INa, leading to various types of arrhythmias (Makara et al., 2014; Wei et al., 2016). In addition, IK1 mainly forms the repolarizing current during the terminal phase of the AP, thus serving as the primary conductance controlling the resting membrane potential (RMP) in ventricular myocytes, and ventricular myocytes from the border zone of MI hearts undergo a significant reduction of potassium channels in both its activity and expression in mouse, rat, and human beings (Liao et al., 2013; Ponce-Balbuena et al., 2018). However, how to rescue the dysfunction of ion channel and prevent sudden cardiac death in heart diseases remains to be resolved.
Human fibroblast growth factors (FGFs) are heparin-binding proteins that contain 23 members which can be divided into seven subfamilies based on phylogeny and sequence, which makes up a cytokine superfamily that regulates a plethora of developmental processes, including regulating mitosis, differentiation, development, metabolism, and angiogenic therapeutic applications (Beenken and Mohammadi, 2009; Itoh et al., 2016b). These functions are mediated, in part, by the interaction of FGFs with relatively high-affinity cell-surface receptors, microRNAs (miRNAs), and subsequent alterations in gene expression within responsive cells. FGF21 is a potent insulin-like growth factor that promotes energy expenditure, lipid excretion, and fat utilization (Cheng et al., 2016; Maratos-Flier, 2017). β-Klotho functions as an essential cofactor for FGF21 activity (Yie et al., 2012). Recent studies have shown that FGF21 plays a vital role in the onset and development of cardiovascular diseases. Ferrer-Curriu G et al. (2019) suggested that FGF21 acts as a key factor in the fibrogenesis associated with increased expression levels of β-klotho specifically in the heart (Ferrer-Curriu et al., 2019). In addition, Lin et al. showed that the high level of FGF21 may be associated with poor lipid level in patients with coronary heart disease (Lin et al., 2010). Our previous study uncovered for the first time that FGF21 treatment markedly reduced arrhythmia susceptibility of infarcted hearts in mice by suppressing miR-143 expression and thus mediating the EGR1-SCN5A/KCNJ2 pathway in MI mice (Li et al., 2020). Nevertheless, it remains unclear whether FGF21 has preventive effects on ischemic arrhythmias in humans.
To shed light on this issue, we explored the effect of FGF21 on the ion pathways of myocardial cells after MI and discovered the mechanism of preventing arrhythmia after MI by affecting the ion pathways. Our results unraveled for the first time that FGF21 protects NaV1.5 and Kir2.1 channels by receptors against arrhythmias after MI in humans.
According to the approval of the Harbin Medical University Ethics Committee (HMUIRB20170020), 11 cases were enrolled in the final analysis. Peripheral blood was obtained from eight patients undergoing AMI and three healthy volunteers. AMI group included AMI patients within 24 h after postinfarct onset. Chronic myocardial ischemia (CMI) group included patients with 7th-10th days after postinfarct onset. Healthy volunteers were used as normal control.
Male guinea pigs (220–250 g) were housed under standard animal room conditions (temperature of 22 ± 1°C; humidity of 55–60%). Animals were randomly divided into three groups: Ctl, ouabain, and ouabain + rhbFGF21. The guinea pigs were anesthetized with isoflurane 2–4% (MIDMARK, USA) using a gas chamber. The animals were fixed to the mouse board in a supine position; three different concentrations of rhbFGF21 (1 μg/kg, 5 μg/kg, and 10 μg/kg) were pretreated at 2 h before 0.2 mg/ml ouabain at the same speed (1,000 μl/h) was injected into the external jugular vein of guinea pigs. Standard lead II electrocardiogram (ECG) signals were recorded using bipolar limb leads. Ventricular arrhythmia occurred for the first time, arrhythmia occurs at the time of death, and the arrhythmia numbers were detected by the biological signal analytical system (model BL-420N, TaiMeng Software). The investigation conforms to the Guide for the Care and Use of Laboratory Animals published by the US National Institutes of Health (NIH Publication No. 85–23, revised 1996) and was approved by the Institutional Animal Care and Use Committee of Harbin Medical University.
Male SD rats (180–220 g) were housed under standard animal room conditions (temperature of 22 ± 1°C; humidity of 55–60%). SD rats were randomly divided into two groups: sham and MI groups. The operation of left anterior-descending branch (LAD) coronary artery ligation was performed in MI rats, as previously described (Lindsey et al., 2018). ST-segment elevation in ECGs indicates successful occlusion of the artery. Sham group rats served as surgical controls and were subjected to the same procedures as MI group rats, except the LAD ligation.
Human and rat blood serum were collected for measurement of FGF21 concentrations using an ELISA kit (human, Elabscience Biotechnology Co., Ltd.; Catalog No: E-EL-H0074c, and rat, Elabscience Biotechnology Co., Ltd.; Catalog No: E-EL-R2408c), according to the manufacturer’s instructions with a lower limit of detection of 18.75 pg/ml. Allow samples for 20 min at room temperature before centrifugation at approximately 1,000 g. Add 100 µl of standard, blank, or sample per well and incubate for 90 min at 37°C; add 100 µl Biotinylated Detection Ab working solution and incubate for 1 h at 37°C; and add HRP Conjugate working solution and incubate for 30 min at 37°C. The level of FGF21 was recognized by a biotinylated detection antibody specific for FGF21 and Avidin Horseradish Peroxidase (HRP) conjugate. The optical density (OD) was measured spectrophotometrically at a wavelength of 450 ± 2 nm and finally analyzed according to the instructions of the kits.
Whole-cell patch-clamp recording techniques were used to record Na+ currents, K+ currents, and action potential duration (APD) in AC16 cardiomyocytes as described previously (Ennis et al., 2002; Kao et al., 2016; Li et al., 2020). INa recordings were conducted at room temperature (22–24°C); IK1 and APD recordings were conducted at 37°C using a patch-clamp amplifier (MultiClamp 700B; Axon Instruments Inc. Foster City, CA, United States). APs were recorded in the current-clamp mode by injection of brief current pulses (2 ms; 1 nA) at a stimulation frequency of 1 Hz; the area under the capacitance current curve was divided by the applied voltage step to obtain the total battery capacitance. AP duration at 50% and 90% amplitude repolarization was measured as APD50 and APD90, respectively. AP amplitude (APA) is obtained from the RMP to the peak of AP depolarization.
The ionic currents were measured in the voltage-clamp mode. Electrode resistance ranged from 2.0 to 3.0 MΩ. The isolated cells were placed in an inverted microscope (IX-70; Olympus) and infused with Tyrode solution. The bath solution for Na + currents contained (in mM) 145 NaCl, 4.5 CsCl, 1.5 MgCl2, one CaCl2, 5 HEPES, 5 glucose, and 0.1 CdCl2 (pH 7.35 with CsOH); the pipette solution contained (in mM) 10 NaF, 110 CsF, 20 CsCl, 10 EGTA, and 10 HEPES (pH 7.35 with CsOH). The external solution for recording K+ currents contained (in mM) 136 NaCl, 5.4 KCl, 0.33 NAH2PO4, one MgCl2.6H2O, 1.8 CaCl2, 5 HEPES, and 10 glucose (pH 7.37 with NaOH). The pipette solution for recording K+ currents contained (in mM) 20 KCl, 110 L-Aspartate, 5 HEPES, one MgCl2, 5 Na2ATP, and 10 EGTA (pH 7.20 with KOH). The experiments were controlled by using pClampex 10.6 software (Axon Instruments Inc., Foster City, CA, United States). Currents were acquired by Digidata 1440A converter (Axon Instruments Inc., Foster City, CA, United States), low-pass filtered at 5 kHz, and stored on a computer. A single current was standardized to membrane capacity to control cell size differences and expressed as current density (pA/pF). Data analysis was accomplished using pClampfit 10.4 software (Axon Instruments Inc., Foster City, CA, United States).
Western blot analysis was performed on total proteins extracted from AC16 cells. Sixty milligrams of protein was equally loaded on 10% SDS-polyacrylamide gel and then transferred onto a nitrocellulose filter membrane. After getting the target bands, the membranes were blocked by 5% filtered nonfat milk for 2 h and then incubated with the indicated primary antibodies overnight at 4°C, including rabbit anti-NaV1.5 antibody (ASC-005, Alomone Labs, Israel, 1: 200), rabbit anti-Kir2.1 antibody (APC-026, Alomone Labs, Israel, 1: 200) followed by incubation with the corresponding secondary antibody for 1 h at room temperature. Protein levels were quantified using the Odyssey Infrared Imaging System (LI-COR, 4607 Superior Street, P.O. Box 4,000, Lincoln, Nebraska, United States) by measuring proteins’ gray values and the data was normalized to β-actin (TA-09, Zhongshanjinqiao, Beijing, China, 1:1,000) as an internal control.
AC16 cells were fixed in 4% paraformaldehyde (PFA) and permeabilized with 0.4% Triton-X at room temperature for 20 min. The samples were coincubated with NaV1.5 (ASC-005, Alomone Labs, Israel, 1:200), Kir2.1 (APC-026, Alomone Labs, Israel, 1: 200), and α-actinin (ab50599, Abcam, Cambridge, United Kingdom, 1:100) antibody at 4°C overnight. Then the AC16 cells were incubated with the appropriate secondary antibody at room temperature for 2 h and the nuclei were stained with DAPI (Beyotime, Shanghai, China, 1:1,000) for 10 min. Immunofluorescence was observed under a confocal laser scanning microscope (Nikon 80i, Otawara, Tochigi, Japan).
Data are presented as mean ± SEM. Two-tailed Student’s t-test and ANOVA were used to compare the difference among each group. p-value less than 0.05 was defined as statistical significance. All statistical analyses were carried out using GraphPad Prism 8.0 (GraphPad Software Inc., San Diego, CA, United States).
Patients with arrhythmia in acute MI and healthy volunteers were enrolled in this study. Serum samples were collected from these subjects on day 1 and days 7–10 after the onset of MI for measuring FGF21 levels using ELISA. The results showed that the serum level of FGF21 was significantly increased on day 1 after the onset of MI and it returned to normal on days 7–10, relative to the Control samples (Figure 1A). In our rat model of MI, the serum FGF21 level reached the maximum value within approximately 24 h (acute MI phase) after the onset of MI. Thereafter, from 1 week to 4 weeks after MI, it began to decrease compared with the acute MI phase, but it remained high for 1 week (Figure 1B). The elevation in MI serum does not necessarily mean that the FGF21 concentration is increased in the interstitial space within the heart; the results in Figure 1C showed that the level of FGF21 in heart tissue homogenate was significantly increased in the acute MI phase, which began to decrease in chronic MI period, relative to acute MI phase. It raises the possibility that FGF21 might be involved in regulating the pathological process of MI.
FIGURE 1. Serum FGF21 levels in patients and rats with myocardial infarction. (A) The FGF21 levels in peripheral blood serum from healthy volunteers (Control, n = 3) and myocardial infarction (MI) subjects (n = 8) on the first day (AMI) and 7th-10th day (CMI, n = 3). Two-tailed Student’s t-test was performed, *p < 0.05. (B) Comparison of serum FGF21 levels in rats between MI and sham operation group (sham). n = 3–7, *p < 0.05 and **p < 0.01. (C) Comparison of FGF21 levels in rat heart tissues between MI and sham group. n = 6, *p < 0.05 and **p < 0.01.
Therefore, in order to ascertain whether FGF21 exerts a protective effect on ischemic arrhythmia, we administered FGF21 to MI model rats and recorded the electrocardiogram of each group in the previous study. The results showed that the duration time of ventricular arrhythmias in rhbFGF21-treated MI rats was significantly reduced at 15 min, 1 week, and 4 weeks after MI compared with normal saline-treated MI rats. Moreover, the incidence of ventricular arrhythmias was also obviously decreased in the rhbFGF21-treated MI rats as compared to the MI group (Li et al., 2020). These data suggested that FGF21 has a protective effect on ischemic arrhythmias after MI.
Secondly, we used ouabain-induced arrhythmia of the guinea pig model, a classic nonischemic arrhythmia model, to further explore whether FGF21 has a regulatory effect on malignant ventricular arrhythmia and sudden cardiac death. We investigated that the onset of the first ventricular arrhythmias (arrhythmia in incubation period) was delayed by FGF21 compared to the rhbFGF21-untreated group in the ouabain model (Supplementary Figures S1A,B). At the same time, it was found that the survival time of guinea pigs induced by ouabain can be obviously ameliorated with the increase of FGF21 concentration (Supplementary Figure S1C). In addition, the numbers of VF and maintenance were attenuated by rhbFGF21 pretreatment compared to the rhbFGF21-untreated group (Supplementary Figure S1D,E), but these were not statistically significant.
To define the functional impact of FGF21 on the currents of NaV1.5 and Kir2.1, INa and IK1 were recorded using a whole-cell patch clamp. Figure 2A and Figure 2B display the representative current traces generated by INa and IK1 channels in AC16 cells treated with rhbFGF21 75 nM for 24 h with or without H2O2 treatment. We found that FGF21 restored the reduction of INa current density at the tested voltages from −25 to 10 mV compared with the H2O2 group, whereas there is no difference of INa density between Control and rhbFGF21-infected cells alone (Figure 2A). Moreover, at the test potentials of −120 mV to −40 mV, the densities of IK1 recorded in AC16 cells with FGF21 plus H2O2 treatment were significantly increased compared to that with H2O2 administration alone (Figure 2B). We also assessed the effect of FGF21 on the APD of AC16 cells treated with rhbFGF21 75 nM for 24 h with or without H2O2 treatment (Figure 2C). Figures 2D,E show that the action potential amplitude (APA) and the absolute value of RMP of H2O2 treatment cells decreased significantly, which were attenuated by rhbFGF21 administration. Moreover, the H2O2 treatment cells also displayed a significant prolongation of APD (∼182.81%, p < 0.01), APD50 (∼156.25%, p < 0.01), and APD90 (∼256.72%, p < 0.01). Nevertheless, rhbFGF21-treated cells showed shorter APD (∼61.47%, p < 0.01), APD50 (∼58.44%, p < 0.01), and APD90 (∼56.7%, p < 0.01) than those in H2O2 group, and no significant difference was found between Control and rhbFGF21 treatment alone (Figures 2F–H). These results indicate that FGF21 can restore the function of INa and IK1 currents and ameliorate the electrophysiological remodeling of cardiomyocytes induced by hydrogen peroxide.
FIGURE 2. Effects of FGF21 on Na+ channel (INa) and K+ channel (IK1) currents of AC16 human cardiomyocytes. (A, B) The current density-voltage relationships curves of whole-cell sodium current (INa) and potassium current (IK1) were recorded in AC16 cells treatment with H2O2 and H2O2+rhbFGF21. *p < 0.05 vs. Control. (C) Exemplar superimposed traces of APD recorded from myocytes, and comparison of average data of APD (F), APD50(G), and APD90(H) measured AC16 human cardiomyocytes from Control, rhbFGF21, H2O2, and H2O2+rhbFGF21. One-way ANOVA with Tukey’s multiple comparisons test was used to compare the difference between multiple groups, *p < 0.05; **p < 0.01. (D, E) The APA and RMP of AC16 cells from Control, rhbFGF21, H2O2, and H2O2+rhbFGF21 group recorded by patch-clamp technique. n = 3–7, **p < 0.01.
To further clarify if the restoration of depressed INa and IK1 by rhbFGF21 is due to the upregulation of NaV1.5 and Kir2.1, we investigated the effects of FGF21 on the expression of NaV1.5 and Kir2.1 in the hydrogen peroxide-treated human AC16 cells using Western blot assay. As shown in Figure 3A, the treatment of rhbFGF21 elevated the NaV1.5 and Kir2.1 protein levels in H2O2-treated AC16 cells. Moreover, immunocytochemical staining proved the presence of NaV1.5 and Kir2.1 channels on the membrane of AC16 cells, and rhbFGF21 reversed the decrease of NaV1.5 and Kir2.1 protein expression by H2O2 treatment (Figure 3B).
FIGURE 3. Effects of FGF21 on NaV1.5 and Kir2.1 expression in human AC16 cells. (A) Protein level of NaV1.5 and Kir2.1 in AC16 human cardiomyocytes treatment with H2O2 (100 μmol) for 6 h, n = 3–4 batches of cells, *p < 0.05; **p < 0.01. Analysis by one-way ANOVA, mean ± SEM. (B) Immunofluorescence staining of NaV1.5 and Kir2.1 expression in AC16 human cardiomyocytes treatment with H2O2. *p < 0.05; **p < 0.01. Analysis by one-way ANOVA, mean ± SEM. Scale bar indicates 100 μm.
Previous studies suggest that FGF21 binds FGF21 receptors (FGFR) for activating intracellular signaling pathways that ultimately generate biological effects. Consistently, our study showed that the expression of NaV1.5 and Kir2.1 in AC16 cardiomyocytes was obviously reduced after hydrogen peroxide treatment. Notably, in the presence of TKI258, FGF21 failed to increase the expression of NaV1.5 and Kir2.1 protein in AC16 cells significantly (Figure 4A). Similar effect of FGF21 was also observed in immunofluorescence staining (Figure 4B). It implies that FGF21 regulates electrophysiological properties of cardiomyocytes via FGFR-mediated NaV1.5 and Kir2.1 pathways.
FIGURE 4. Effect of FGFR on NaV1.5 and Kir2.1 expression. (A) Protein level of NaV1.5 and Kir2.1 in AC16 human cardiomyocytes in Control, H2O2, H2O2+rhbFGF21, and H2O2+rhbFGF21 + TKI258 group, n = 4 batches of cells, *p < 0.05; **p < 0.01. Analysis by one-way ANOVA, mean ± SEM. (B) Immunofluorescence staining of NaV1.5 and Kir2.1 expression in AC16 human cardiomyocytes in each group. *p < 0.05; **p < 0.01. Analysis by one-way ANOVA, mean ± SEM. Scale bar indicates 100 μm.
In this study, we demonstrated for the first time that circulating levels of FGF21 rise substantially with MI. It is interesting that rhbFGF21 administration ameliorated the electrophysiological remodeling in H2O2-treated AC16 cells by increasing voltage-gated Na+ channel and K+ channel activity which is associated with FGFR-mediated upregulation of NaV1.5 and Kir2.1 expression and shortening of APD. The present study provides new insights into the role of FGF21 in postinfarct arrhythmias and suggests the potential application of FGF21 in the prevention of human cardiac sudden death.
Myocardium infarction was accompanied by the increased heterogeneity of conduction (Ng et al., 2016; Aronis et al., 2020; He et al., 2020), structural remodeling (Deniset et al., 2019), neurohumoral changes (Heeneman et al., 1997), alterations in transcription factor (Mccarroll et al., 2018), and ion channel protein expression and distribution (Speich et al., 1980), which alters cardiac electrical activity and often triggers malignant ventricular arrhythmias. Lots of experimental and clinical trials have reported that the incidence of ventricular arrhythmias and cardiac sudden death remains at high risk after MI (Pouleur et al., 2010). This highlights the need for further understanding of key mechanisms and development of innovative effective therapies for ventricular arrhythmias.
FGF21 is a typical endocrine signal, while function is a paracrine signal in hearts. Cardiac FGF21 secretion is obviously lower than hepatic FGF21 secretion (Itoh et al., 2016a). FGF21 is abundantly secreted by cardiac cells in response to cardiac stress, including cardiac hypertrophy, myocardial ischemia, heart failure, and diabetic cardiomyopathy (Planavila et al., 2015). Recently, increasing evidence has suggested that FGF21 plays a critical role in cardiovascular protection (Pan et al., 2018). However, the contribution of FGF21 to arrhythmias and electrical remodeling after MI in humans still remains unclear.
In order to clarify the regulation of FGF21 on arrhythmia, two kinds of arrhythmia animal models were established in our study, including ischemic arrhythmia model (MI rat model) and nonischemic arrhythmia model (ouabain-induced guinea pig arrhythmia model). Firstly, we administered rhbFGF21 to MI rats and recorded the electrocardiogram of each group in our previous study (Li et al., 2020). The results showed that the incidence and duration time of ischemic arrhythmias in rhbFGF21-treated MI rats were significantly reduced at different time point after MI. These data suggested that FGF21 has a protective effect on ischemic arrhythmias after MI. Based on the observation of ischemic arrhythmia in the MI rat model, we further clarify that the protective effect of FGF21 on arrhythmia depends on the pathological process of alleviating myocardial ischemia. We used ouabain-induced arrhythmia of the guinea pig model, a classic nonischemic arrhythmia model, to further explore the regulatory effect of FGF21 on arrhythmia. Ouabain is known as G-strophanthin, which belongs to cardiac glycoside drugs. Cardiac glycoside inhibits Na+-K+-ATP enzyme, thus increasing [Na]i and decreasing [K]i in cardiomyocytes and causing more Ca2+ in cardiomyocytes (Nosztray et al., 1989; Diederich et al., 2017). Ouabain-induced toxicity shows a variety of arrhythmias, including tachyarrhythmia, bradycardia, ventricular fibrillation, and even cardiac arrest, by causing dysfunction of ion channels in cardiomyocytes. We found that rhbFGF21 significantly prolonged the latency of ouabain-induced ventricular fibrillation and extend the survival time of guinea pigs with malignant arrhythmia. Therefore, we hypothesized that the protective effect of FGF21 on arrhythmia may be related to maintaining the functional balance of ion channels or stabilizing the myocardial cell membrane.
It has been reported that the inwardly rectifying potassium current (IK1) is considerable for maintenance of the cell RMP and modulating cardiomyocytes repolarization by altering transmembrane currents (Amoros et al., 2013; Klein et al., 2017; Ponce-Balbuena et al., 2018), and the voltage-gated Na + current (INa) produces a rapid depolarizing current during the upstroke of the AP (Shy et al., 2013). Both a reducing potassium currents and an inappropriate delay in the entry of sodium currents into cardiomyocytes may contribute to the QT interval prolongation during the post-infarct ventricular arrhythmias (Goldenberg and Moss, 2017). Consistently with previous reports, our study showed that FGF21 restored the decreased IK1 currents and the decrease of INa currents in H2O2-treated AC16 cells compared to the normal group. Moreover, FGF21 treatment also caused larger APA of the AP and acceleration of cardiac depolarization in ventricular myocytes. In addition, the increasing P wave amplitude and the shortening of the QRS complex in surface ECGs were found in conscious rats pretreated with rhbFGF21. Moreover, we also investigated that the manipulation of IK1 density had a comparatively greater impact on APD90 because outward IK1 is larger at voltages in the vicinity of APD90. These results suggested that FGF21 plays a protective role in electrical disturbance H2O2-treated AC16 cells at least through restoring IK1 and INa.
However, our study has limitations. IK1-mediated potassium ion balance is the main component of the RMP and we found that FGF21 can significantly restore the 4-phase RMP of AC16 cardiomyocytes, so in this study, we mainly tested the effect of FGF21 on the IK1 current. In addition, our results in human AC16 cardiomyocytes are consistent with previously published results which found that FGF21 can also significantly restore the IK1 current of isolated ventricular myocytes in mice (Li et al., 2020). IKr (rapidly activated delayed rectifier potassium current) and IKs (slowly activated delayed rectifier potassium current) cooperate with IK1 to mediate the process of cardiomyocyte repolarization. Because we did not functionally analyze IKr and IKs with patch clamp, we cannot exclude potential contribution of FGF21 function on other ion channels in our cell model which should be defined in future studies. In addition, through the patch-clamp technique, we found that FGF21 could restore the sodium current level of cardiomyocytes. However, the electrophysiological activities of cells are not only dependent on the expression level of ion channels but also closely related to their functions. In the process of detecting the APD, we found that FGF21 upregulated the resting potential of AC16 cardiomyocytes treated with hydrogen peroxide, as well as the levels of APA and Vmax. Sodium channels are voltage-gated ion channels which open when the membrane potential reaches a certain threshold. When the RMP decreases, it is difficult to stimulate the membrane potential to reach the threshold value of the sodium channel and the activity of the sodium channel will decrease accordingly. These results suggest that FGF21 may indirectly affect the inactivation status of sodium channels by ameliorating the changes of resting potential. Although our detection also found that FGF21 could restore the expression of Nav1.5 protein, the physiological correlation between FGF21 and the change of INa channel expression needs to be further explored.
Previous studies have shown that FGF21 binds to the FGF21 receptor (FGFR) to activate intracellular signaling pathways that ultimately produce biological effects (Zhao et al., 2019). Consistently, our study showed that TKI258, an FGFR antagonist, may decrease the expression of NaV1.5/Kir2.1 attenuated by FGF21 in AC16 cells. This means that FGF21 may regulate the electrophysiological properties of cardiomyocytes through FGFR. However, the deep mechanism by which FGF21 regulates the function of sodium and potassium ion channels through receptors and whether FGF21 regulates ion remodeling in AC16 cardiomyocytes through other pathways still need to be further explored.
In summary, we discovered for the first time that FGF21 protects the NaV1.5 and Kir2.1 channels through FGFR and this may contribute to preventing arrhythmia after MI in humans. These findings provide evidence for the antiarrhythmic effect of FGF21 and reveal the potential application prospects of FGF21 in the treatment of arrhythmia after MI in humans.
The datasets presented in this study can be found in online repositories. The names of the repository/repositories and accession number(s) can be found in the article/Supplementary Material.
The animal study conforms to the Guide for the Care and Use of Laboratory Animals published by the US National Institutes of Health (NIH Publication No. 85–23, revised 1996) and was approved by the Institutional Animal Care and Use Committee of Harbin Medical University.
The studies involving human participants were reviewed and approved by the Harbin Medical University Ethics Committee. The patients/participants provided their written informed consent to participate in this study. The animal study was reviewed and approved by the Institutional Animal Care and Use Committee of Harbin Medical University.
NW, BY, JL, and YL designed and conducted the experiments and wrote the manuscript. YL provided the clinical samples. RZ, HX, DH, HC, and YX completed the design and operation of animal experiments. HY, YS, JL, and SL were mostly involved in the patch-clamp experiment. NX, YX, YZ, and ML completed the molecular biology experiment. PL, ML, QL, and ZZ performed the cell culture. XL and WD participated in the revision of the article.
The presented work was supported by the Heilongjiang Touyan Innovation Team Program and the National Nature Science Foundation of China 81573425 and 81773733 to NW; the National Nature Science Youth Foundation of China Grant No. 82003751 to JL; the Natural Science Foundation of Heilongjiang Province H2016010 to NW; the Foundation of Heilongjiang Postdoctoral LBH-Z18166 to JL; the Basic Scientific Research Foundation of Universities in Heilongjiang Province 31041180028 to JL; and the Outstanding Youth Fostering Foundation of Vihaan Academician to NW.
The authors declare that the research was conducted in the absence of any commercial or financial relationships that could be construed as a potential conflict of interest.
All claims expressed in this article are solely those of the authors and do not necessarily represent those of their affiliated organizations, or those of the publisher, the editors and the reviewers. Any product that may be evaluated in this article, or claim that may be made by its manufacturer, is not guaranteed or endorsed by the publisher.
The Supplementary Material for this article can be found online at: https://www.frontiersin.org/articles/10.3389/fphar.2021.715466/full#supplementary-material
Al-Akchar, M., and Siddique, M. S. (2017). Rhythm, QT Prolongation, StatPearls. (Treasure Island (FL)).
Amorós, I., Dolz-Gaitón, P., Gómez, R., Matamoros, M., Barana, A., and De La Fuente, M. G. (2013). Propafenone Blocks Human Cardiac Kir2.X Channels by Decreasing the Negative Electrostatic Charge in the Cytoplasmic Pore. Biochem. Pharmacol. 86 (2), 267–278. doi:10.1016/j.bcp.2013.04.023
Aronis, K. N., Ali, R. L., Prakosa, A., Ashikaga, H., Berger, R. D., and Hakim, J. B. (2020). Accurate Conduction Velocity Maps and Their Association with Scar Distribution on Magnetic Resonance Imaging in Patients with Postinfarction Ventricular Tachycardias. Circ. Arrhythm Electrophysiol. 13 (4), e007792. doi:10.1161/CIRCEP.119.007792
Beenken, A., and Mohammadi, M. (2009). The FGF Family: Biology, Pathophysiology and Therapy. Nat. Rev. Drug Discov. 8 (3), 235–253. doi:10.1038/nrd2792
Cheng, P., Zhang, F., Yu, L., Lin, X., He, L., and Li, X. (2016). Physiological and Pharmacological Roles of FGF21 in Cardiovascular Diseases. J. Diabetes Res. 2016, 1540267. doi:10.1155/2016/1540267
Decher, N., Kiper, A. K., and Rinne, S. (2017). Stretch-activated Potassium Currents in the Heart: Focus on TREK-1 and Arrhythmias. Prog. Biophys. Mol. Biol. 130:223-232. doi:10.1016/j.pbiomolbio.2017.05.005
Deniset, J. F., Belke, D., Lee, W. Y., Jorch, S. K., Deppermann, C., and Hassanabad, A. F. (2019). Gata6(+) Pericardial Cavity Macrophages Relocate to the Injured Heart and Prevent Cardiac Fibrosis. Immunity 51 (1), 131–140.e135. doi:10.1016/j.immuni.2019.06.010
Diederich, M., Muller, F., and Cerella, C. (2017). Cardiac Glycosides: From Molecular Targets to Immunogenic Cell Death. Biochem. Pharmacol. 125, 1–11. doi:10.1016/j.bcp.2016.08.017
Ennis, I. L., Li, R. A., Murphy, A. M., Marban, E., and Nuss, H. B. (2002). Dual Gene Therapy with SERCA1 and Kir2.1 Abbreviates Excitation without Suppressing Contractility. J. Clin. Invest. 109 (3), 393–400. doi:10.1172/JCI13359
Ferrer-Curriu, G., Redondo-Angulo, I., Guitart-Mampel, M., Ruperez, C., Mas-Stachurska, A., and Sitges, M. (2019). Fibroblast Growth Factor-21 Protects against Fibrosis in Hypertensive Heart Disease. J. Pathol. 248 (1), 30–40. doi:10.1002/path.5226
Goldenberg, I., and Moss, A. J. (2017). Risk Stratification for Sudden Cardiac Death in Individuals without Structural Disease: Implications from Studies of Rare Genetic Arrhythmic Disorders. Circ. Arrhythm Electrophysiol. 10 (10), e005774. doi:10.1161/CIRCEP.117.005774
He, S., Wu, J., Li, S. H., Wang, L., Sun, Y., and Xie, J. (2020). The Conductive Function of Biopolymer Corrects Myocardial Scar Conduction Blockage and Resynchronizes Contraction to Prevent Heart Failure. Biomaterials 258, 120285. doi:10.1016/j.biomaterials.2020.120285
Heeneman, S., Smits, J. F., Leenders, P. J., Schiffers, P. M., and Daemen, M. J. (1997). Effects of Angiotensin II on Cardiac Function and Peripheral Vascular Structure during Compensated Heart Failure in the Rat. Arterioscler Thromb. Vasc. Biol. 17 (10), 1985–1994. doi:10.1161/01.atv.17.10.1985
Itoh, N., Nakayama, Y., and Konishi, M. (2016a). Roles of FGFs as Paracrine or Endocrine Signals in Liver Development, Health, and Disease. Front Cel Dev Biol 4, 30. doi:10.3389/fcell.2016.00030
Itoh, N., Ohta, H., Nakayama, Y., and Konishi, M. (2016b). Roles of FGF Signals in Heart Development, Health, and Disease. Front. Cel Dev Biol. 4, 110. doi:10.3389/fcell.2016.00110
Kallergis, E. M., and Vardas, P. E. (2007). Primary Prevention of Sudden Cardiac Death: Apart from the Defibrillator, what Is Important in Patients with Myocardial Infarction or Heart Failure? Hellenic J. Cardiol. 48 (2), 89–93.
Kao, Y. H., Hsu, J. C., Chen, Y. C., Lin, Y. K., Lkhagva, B., and Chen, S. A. (2016). ZFHX3 Knockdown Increases Arrhythmogenesis and Dysregulates Calcium Homeostasis in HL-1 Atrial Myocytes. Int. J. Cardiol. 210, 85–92. doi:10.1016/j.ijcard.2016.02.091
Kelly, A., Salerno, S., Connolly, A., Bishop, M., Charpentier, F., and Stolen, T. (2018). Normal Interventricular Differences in Tissue Architecture Underlie Right Ventricular Susceptibility to Conduction Abnormalities in a Mouse Model of Brugada Syndrome. Cardiovasc. Res. 114 (5), 724–736. doi:10.1093/cvr/cvx244
Klein, M. G., Shou, M., Stohlman, J., Solhjoo, S., Haigney, M., and Tidwell, R. R. (2017). Role of Suppression of the Inward Rectifier Current in Terminal Action Potential Repolarization in the Failing Heart. Heart Rhythm 14 (8), 1217–1223. doi:10.1016/j.hrthm.2017.04.001
Li, J., Xu, C., Liu, Y., Li, Y., Du, S., and Zhang, R. (2020). Fibroblast Growth Factor 21 Inhibited Ischemic Arrhythmias via Targeting miR-143/EGR1 axis. Basic Res. Cardiol. 115 (2), 9. doi:10.1007/s00395-019-0768-4
Liao, S. Y., Tse, H. F., Chan, Y. C., Mei-Chu Yip, P., Zhang, Y., and Liu, Y. (2013). Overexpression of Kir2.1 Channel in Embryonic Stem Cell-Derived Cardiomyocytes Attenuates Posttransplantation Proarrhythmic Risk in Myocardial Infarction. Heart Rhythm 10 (2), 273–282. doi:10.1016/j.hrthm.2012.10.008
Lin, Z., Wu, Z., Yin, X., Liu, Y., Yan, X., and Lin, S. (2010). Serum Levels of FGF-21 Are Increased in Coronary Heart Disease Patients and Are Independently Associated with Adverse Lipid Profile. PLoS One 5 (12), e15534. doi:10.1371/journal.pone.0015534
Lindsey, M. L., Bolli, R., Canty, J. M., Du, X. J., Frangogiannis, N. G., and Frantz, S. (2018). Guidelines for Experimental Models of Myocardial Ischemia and Infarction. Am. J. Physiol. Heart Circ. Physiol. 314 (4), H812–H838. doi:10.1152/ajpheart.00335.2017
Makara, M. A., Curran, J., Little, S. C., Musa, H., Polina, I., and Smith, S. A. (2014). Ankyrin-G Coordinates Intercalated Disc Signaling Platform to Regulate Cardiac Excitability In Vivo. Circ. Res. 115 (11), 929–938. doi:10.1161/CIRCRESAHA.115.305154
Maratos-Flier, E. (2017). Fatty Liver and FGF21 Physiology. Exp. Cel Res 360 (1), 2–5. doi:10.1016/j.yexcr.2017.05.006
Mccarroll, C. S., He, W., Foote, K., Bradley, A., Mcglynn, K., and Vidler, F. (2018). Runx1 Deficiency Protects against Adverse Cardiac Remodeling after Myocardial Infarction. Circulation 137 (1), 57–70. doi:10.1161/CIRCULATIONAHA.117.028911
Ng, F. S., Kalindjian, J. M., Cooper, S. A., Chowdhury, R. A., Patel, P. M., and Dupont, E. (2016). Enhancement of Gap Junction Function during Acute Myocardial Infarction Modifies Healing and Reduces Late Ventricular Arrhythmia Susceptibility. JACC Clin. Electrophysiol. 2 (5), 574–582. doi:10.1016/j.jacep.2016.03.007
Nosztray, K., Szabó, J., and Szegi, J. (1989). [Effect of Propranolol on Ouabain-Sensitive Na+,K+-ATPase Activity of the Rat Heat]. Acta Pharm. Hung 59 (5), 193–200.
Pan, X., Shao, Y., Wu, F., Wang, Y., Xiong, R., and Zheng, J. (2018). FGF21 Prevents Angiotensin II-Induced Hypertension and Vascular Dysfunction by Activation of ACE2/Angiotensin-(1-7) Axis in Mice. Cell Metab 27 (6), 1323–1337.e1325. doi:10.1016/j.cmet.2018.04.002
Pessina, F., Gamberucci, A., Chen, J., Liu, B., Vangheluwe, P., and Gorelli, B. (2018). Negative Chronotropism, Positive Inotropism and Lusitropism of 3,5-Di-T-Butyl-4-Hydroxyanisole (DTBHA) on Rat Heart Preparations Occur through Reduction of RyR2 Ca(2+) Leak. Biochem. Pharmacol. 155, 434–443. doi:10.1016/j.bcp.2018.07.026
Planavila, A., Redondo-Angulo, I., and Villarroya, F. (2015). FGF21 and Cardiac Physiopathology. Front. Endocrinol. (Lausanne) 6, 133. doi:10.3389/fendo.2015.00133
Ponce-Balbuena, D., Guerrero-Serna, G., Valdivia, C. R., Caballero, R., Diez-Guerra, F. J., and Jimenez-Vazquez, E. N. (2018). Cardiac Kir2.1 and NaV1.5 Channels Traffic Together to the Sarcolemma to Control Excitability. Circ. Res. 122 (11), 1501–1516. doi:10.1161/CIRCRESAHA.117.311872
Pouleur, A. C., Barkoudah, E., Uno, H., Skali, H., Finn, P. V., and Zelenkofske, S. L. (2010). Pathogenesis of Sudden Unexpected Death in a Clinical Trial of Patients with Myocardial Infarction and Left Ventricular Dysfunction, Heart Failure, or Both. Circulation 122 (6), 597–602. doi:10.1161/CIRCULATIONAHA.110.940619
Qin, D., Zhang, Z. H., Caref, E. B., Boutjdir, M., Jain, P., and El-Sherif, N. (1996). Cellular and Ionic Basis of Arrhythmias in Postinfarction Remodeled Ventricular Myocardium. Circ. Res. 79 (3), 461–473. doi:10.1161/01.res.79.3.461
Schwartz, P. J., Ackerman, M. J., Antzelevitch, C., Bezzina, C. R., Borggrefe, M., and Cuneo, B. F. (2020). Inherited Cardiac Arrhythmias. Nat. Rev. Dis. Primers 6 (1), 58. doi:10.1038/s41572-020-0188-7
Shy, D., Gillet, L., and Abriel, H. (2013). Cardiac Sodium Channel NaV1.5 Distribution in Myocytes via Interacting Proteins: the Multiple Pool Model. Biochim. Biophys. Acta 1833 (4), 886–894. doi:10.1016/j.bbamcr.2012.10.026
Speich, M., Bousquet, B., and Nicolas, G. (1980). Concentrations of Magnesium, Calcium, Potassium, and Sodium in Human Heart Muscle after Acute Myocardial Infarction. Clin. Chem. 26 (12), 1662–1665. doi:10.1093/clinchem/26.12.1662
Tan, H. L., Hou, C. J., Lauer, M. R., and Sung, R. J. (1995). Electrophysiologic mechanisms of the long QT interval syndromes and torsade de pointes. Ann. Intern. Med. 122 (9), 701–714. doi:10.7326/0003-4819-122-9-199505010-00009
Wei, X., Zhu, A., Zhang, Y., Yao, S., and Mao, W. (2016). Pre- and Delayed Treatments with Ranolazine Ameliorate Ventricular Arrhythmias and Nav1.5 Downregulation in Ischemic/Reperfused Rat Hearts. J. Cardiovasc. Pharmacol. 68 (4), 269–279. doi:10.1097/FJC.0000000000000412
Yang, K. C., Kyle, J. W., Makielski, J. C., and Dudley, S. C. (2015). Mechanisms of Sudden Cardiac Death: Oxidants and Metabolism. Circ. Res. 116 (12), 1937–1955. doi:10.1161/CIRCRESAHA.116.304691
Yie, J., Wang, W., Deng, L., Tam, L. T., Stevens, J., and Chen, M. M. (2012). Understanding the Physical Interactions in the FGF21/FGFR/beta-Klotho Complex: Structural Requirements and Implications in FGF21 Signaling. Chem. Biol. Drug Des. 79 (4), 398–410. doi:10.1111/j.1747-0285.2012.01325.x
Keywords: myocardial infarction, ventricular arrhythmias, ion channel, AC16, action potential duration, FGF21 (fibroblast growth factor 21)
Citation: Li J, Li Y, Liu Y, Yu H, Xu N, Huang D, Xue Y, Li S, Chen H, Liu J, Li Q, Zhao Y, Zhang R, Xue H, Sun Y, Li M, Li P, Liu M, Zhang Z, Li X, Du W, Wang N and Yang B (2021) Fibroblast Growth Factor 21 Ameliorates NaV1.5 and Kir2.1 Channel Dysregulation in Human AC16 Cardiomyocytes. Front. Pharmacol. 12:715466. doi: 10.3389/fphar.2021.715466
Received: 27 May 2021; Accepted: 18 August 2021;
Published: 22 September 2021.
Edited by:
Xiaoqiang Yao, The Chinese University of Hong Kong, ChinaReviewed by:
Guillermo Avila, Centro de Investigaciones y Estudios Avanzados, Instituto Politécnico Nacional de México (CINVESTAV), MexicoCopyright © 2021 Li, Li, Liu, Yu, Xu, Huang, Xue, Li, Chen, Liu, Li, Zhao, Zhang, Xue, Sun, Li, Li, Liu, Zhang, Li, Du, Wang and Yang. This is an open-access article distributed under the terms of the Creative Commons Attribution License (CC BY). The use, distribution or reproduction in other forums is permitted, provided the original author(s) and the copyright owner(s) are credited and that the original publication in this journal is cited, in accordance with accepted academic practice. No use, distribution or reproduction is permitted which does not comply with these terms.
*Correspondence: Baofeng Yang, eWFuZ2JmQGVtcy5ocmJtdS5lZHUuY24=; Ning Wang, d2FuZ25pbmdAZW1zLmhyYm11LmVkdS5jbg==
†These authors have contributed equally to this work and share first authorship
Disclaimer: All claims expressed in this article are solely those of the authors and do not necessarily represent those of their affiliated organizations, or those of the publisher, the editors and the reviewers. Any product that may be evaluated in this article or claim that may be made by its manufacturer is not guaranteed or endorsed by the publisher.
Research integrity at Frontiers
Learn more about the work of our research integrity team to safeguard the quality of each article we publish.