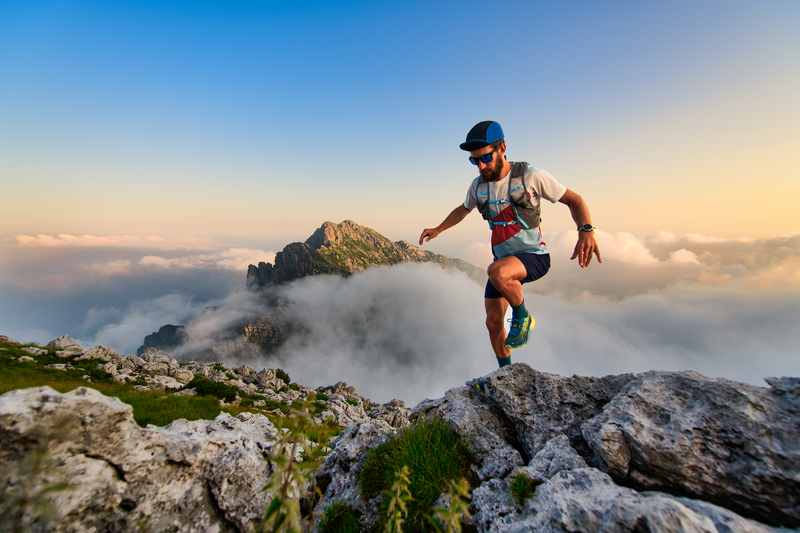
94% of researchers rate our articles as excellent or good
Learn more about the work of our research integrity team to safeguard the quality of each article we publish.
Find out more
REVIEW article
Front. Pharmacol. , 14 July 2021
Sec. Neuropharmacology
Volume 12 - 2021 | https://doi.org/10.3389/fphar.2021.713963
Neurodegenerative disease (NDD), including Alzheimer’s disease, Parkinson’s disease, and amyotrophic lateral sclerosis, are characterized by the progressive loss of neurons which leads to the decline of motor and/or cognitive function. Currently, the prevalence of NDD is rapidly increasing in the aging population. However, valid drugs or treatment for NDD are still lacking. The clinical heterogeneity and complex pathogenesis of NDD pose a great challenge for the development of disease-modifying therapies. Numerous animal models have been generated to mimic the pathological conditions of these diseases for drug discovery. Among them, zebrafish (Danio rerio) models are progressively emerging and becoming a powerful tool for in vivo study of NDD. Extensive use of zebrafish in pharmacology research or drug screening is due to the high conserved evolution and 87% homology to humans. In this review, we summarize the zebrafish models used in NDD studies, and highlight the recent findings on pharmacological targets for NDD treatment. As high-throughput platforms in zebrafish research have rapidly developed in recent years, we also discuss the application prospects of these new technologies in future NDD research.
Neurodegenerative disease (NDD), including Alzheimer’s disease (AD), Parkinson’s disease (PD), and amyotrophic lateral sclerosis (ALS), are progressive disorders with abnormal protein deposition and selective neuronal loss, leading to motor, and/or cognitive impairments and inevitable death (Vaquer-Alicea and Diamond, 2019). NDD has afflicted millions of people worldwide, and is one of the main reasons for the high incidence of mortality in elderly people (Hou et al., 2019). The World Health Organization (WHO) predicts that NDD will become the second leading cause of death after cardiovascular disease by 2040 (Gammon, 2014). Despite huge basic and clinical research efforts, no disease-modifying interventions are available to patients and therapeutic strategies are limited to symptomatic treatments at present. The main challenges in the study of NDD drug development are the diversity of etiology and clinical heterogeneity among individuals. In addition, the lack of biomarkers makes it difficult to identify the disease in the early stages. There is an increasing need for more animal models that can outline the complexity of NND pathogenesis.
Zebrafish (Danio rerio) were initially used to monitor water contamination, teratogenesis and toxic substances. In 1981, Streisinger et al. introduced zebrafish as an effective animal model especially in genetics and developmental biology (Streisinger et al., 1981). Thereafter, zebrafish were widely used by scientists in different fields of research (Lieschke and Currie, 2007). Zebrafish have several experimental advantages. First, their small body size and ease of breeding and maintenance make zebrafish ideal for high-throughput screening for genetic phenotypes and drugs. Second, their rapid development cycle and large spawning numbers greatly reduce time and cost, and provide sufficient samples to minimize differences between individuals. Third, transparent zebrafish embryos and larvae are particularly good for optical manipulation and imaging of neural activity. Finally, high genome homology to humans make zebrafish a valuable model for studying human disease in pharmacology, developmental genetics or other fields. The zebrafish is a perfect model in pharmaceutical science and is sensitive to many pharmacological agents.
Although zebrafish are different to mammals in organization structure of the central nervous system, several cerebral nuclei in the zebrafish brain including the basal ganglia, striatum, hippocampus and amygdala have high homogeneity to mammals (Randlett et al., 2015). The laminated cortex is not developed in zebrafish telencephalon, but the existence of the foxg1 gene which encodes telencephalon transcription factor indicates the high similarity in brain development between zebrafish and human. Neuroendocrine function in zebrafish hypothalamus is very similar to mammals and the zebrafish cerebellum also has a similar layer structure to that in humans. Moreover, the primary neurotransmitter system in zebrafish, including the noradrenergic, serotonergic, dopaminergic and histaminergic systems, show many similarities to the mammalian system (Pitchai et al., 2019). For example, D1 and D3 receptors in the dopamine (DA) system display complete amino acid homology in the binding site between zebrafish and humans, while D2 and D4 receptors show 85–95% homology. Therefore, it is helpful to establish zebrafish models to study the underlying mechanism of neuropsychiatric diseases and develop new medicine for treatment.
In NDD, especially AD, PD, and ALS, zebrafish have been validated as a feasible tool for research use. Advances in novel imaging strategies, tractable behavioral tests and high-throughput drug screening methods in zebrafish, and more new drug targets have recently been discovered for the treatment of NDD. Zebrafish provide a useful platform for drug discovery in NDD that is time- and cost-efficient (Figure 1). In this review, we discuss the application of zebrafish as models in the pharmacology of AD, PD, and ALS.
FIGURE 1. Schematic diagram of the application of zebrafish for high-throughput drug screening in NDD research.
Visible zebrafish behaviors are pivotal indicators of disease model establishment or drug efficacy verification at the organism level. Zebrafish embryos can quickly develop into larvae 72 h post fertilization (hpf). During this process, the complete body plan is established 48 hpf. Zebrafish undergo metamorphosis and are juvenile at 30 days post fertilization (dpf) and the adult phase starts from 90 dpf (Basnet et al., 2019). The brain-blood barrier (BBB) develops from 72 hpf onwards. Furthermore, zebrafish are active in the daytime and have physiological advantages in visual, auditory and olfactory function. Zebrafish and their larvae show different advantages or application in research. For example, large-scale high-throughput behavioral analysis has been carried out in larvae but not adult zebrafish, while adult zebrafish harbor a more sophisticated behavior repertoire than larvae (Basnet et al., 2019). Drug treatment of zebrafish is easy and convenient in the aqueous environment. The efficacy, bioavailability and toxicity of drugs can be evaluated in various steps (Vaz et al., 2018). However, the inadequacy of this model is that this drug administration route is different to humans and mammals where drugs are taken orally. The possibility of drug absorption through the skin or other organs can not be eliminated. This could lead to discrepancies in drug efficacy evaluation. A plethora of zebrafish behaviors in larvae and adult fish highlight zebrafish as a promising model for neurobiological investigations.
To measure the spontaneous swimming behavior of zebrafish, individuals are placed on a light and optical stage with an infrared light source. Digital videos are recorded to track the movement of zebrafish in water horizontally or vertically during a set time session. The swimming pattern of zebrafish can be observed by an automatically drawn trajectory in specialized software systems. Representative swimming behavioral parameters, such as mean velocity, total moving distance, or turning angle are analyzed simultaneously (Wang et al., 2017; Naini et al., 2018). A rough method of measuring zebrafish movement is counting the total number of lines that zebrafish cross. Vertical lines are drawn at equal distances to divide the vessel into several zones. This method is more suitable for adult zebrafish due to their less random behavior compared with larvae (Bretaud et al., 2004).
Zebrafish larvae have a propensity to respond to visual, tactile, or acoustic stimuli by suddenly increasing the velocity and acceleration of movement. The sensorimotor reflexes are induced by touching the head, tail or pectoral fin of the larvae several times. This experiment is usually executed by gently touching the larvae with a fine needle or other blunt tipped equipment (Paquet et al., 2009; Cosacak et al., 2017; Pinho et al., 2019). Immediate swimming behavior after touch is recorded until the tail returns to the resting position. The escape response generally reflects the functional outcome of motor neurons of zebrafish larvae.
Movement pattern affected by alternating light and dark conditions reflects an anxiety-like behavior in zebrafish. Light to dark transition increases zebrafish locomotion while dark to light transition decreases locomotion. Zebrafish larvae can be placed in a 96-well plate with one larva per well for high-throughput drug screening. A chamber with a digital control can change the light and dark periods in cycles and monitor the movement of zebrafish larvae (Pan et al., 2019; Barbereau et al., 2020). Another method in larger adult zebrafish is to assess the time spend in the light compartment or the latency to enter the dark compartment. This test is conducted in a tank separated into equal sized light and dark areas with a plastic barrier. Zebrafish can swim freely between these two areas with barrier lift-up. Each zebrafish is initially placed in the light area, and the number of entries and time spent in the light area are assessed (Rishitha and Muthuraman, 2018; Lv et al., 2019).
Spatial discrimination such as in the T/Y-maze, has been used to characterize learning and memory in zebrafish similar to rodents. The T/Y-maze test utilizes positive stimulus as a reward to initiate motivation of zebrafish if they show the correct response in finding an objective. For example, the T-maze is composed of a starting zone, a long arm, two short arms and two chambers (Pu et al., 2017). Food is employed as a positive stimulus and delivered in one chamber for zebrafish training. The fish are starved for one or two days and then placed in the start zone to conduct the test. The behavior is recorded by camera and the time spent on finding the objective is quantified. A simple apparatus and procedure have been developed based on the T-maze (Williams et al., 2002; Koehler et al., 2018: Koehler et al., 2019). The tank is separated by a central white divider and adequate space at the bottom is reserved for zebrafish to swim freely between the two sections. A red card attached to one end allows fish to distinguish the two halves of the tank. The food is provided 5 s after a light tap at the center of the tank. Zebrafish present on the side with food within 5 s is considered the correct response.
To assess the cognition of zebrafish, locomotor and exploratory activities are observed in the novel tank test. Zebrafish are individually placed in the novel tank and their swimming trajectory is recorded. The tank is divided into three horizontal sections which are defined as the bottom, middle and top areas, respectively. Vertical exploration by zebrafish can be measured by the number of entries and time spent in the bottom or top area. Moreover, anxiety-related behaviors can also be determined by the number and duration of freezing bouts or erratic movement in this test (Nunes et al., 2017; Zanandrea et al., 2018). Cuvettes can be used as novel apparatus which are placed in a parallel arrangement in front of the camera for large sample measurement (Feng et al., 2014).
AD was first reported in 1907 and more than 95% of AD cases occur sporadically in adults who are over 65 years old (Tecalco-Cruz et al., 2020). The AD cases caused by genetic mutations only account for less than 1% while the majority of cases are related to environmental, genetic susceptibility and other factors. The main clinical characters of AD are memory loss, cognitive dysfunction and related comorbidities such as depression or anxiety. Cardinal pathological changes in AD are the death of neurons in brain regions responsible for cognitive functions, the presence of extracellular β-amyloid (Aβ) plaques and intracellular neurofibrillary tangles (NFTs). Based on previous findings, scientists have proposed several hot hypotheses that may induce the development of AD: the amyloid cascade hypothesis, tau hyperphosphorylation, the cholinergic hypothesis, neuroinflammation, and metal ions. Numerous pharmacological and transgenic (Tg) AD models have been established in zebrafish to investigate AD pathogenesis.
Using environmental neurotoxins that trigger AD-like progression is a good approach for investigating AD etiology. Cholinergic dysfunction and cholinergic neuronal loss are observed in the brain of AD patients. Therefore, one hypothesis of AD occurrence is a decline in the synthesis of the neurotransmitter acetylcholine. Scopolamine, an acetylcholine muscarinic receptor antagonist, has been used to establish the zebrafish neurotoxin-induced AD model. Scopolamine can cause learning deficits in zebrafish and the effect can be reversed by acetylcholinesterase inhibitors, such as physostigmine (Kim et al., 2010). Acetylcholinesterase (AChE) and butyrylcholinesterase (BuChE) are enzymes which degrade acetylcholine and are involved in regulation of the cholinergic system. Both enzymes have been proved to contribute to AD progression (Hu et al., 2019).
Although the glutamatergic system and GABAergic system are associated with AD, the underlying mechanisms remain unclear. Glutamatergic neuronal loss in the medial temporal lobe and hippocampal network in AD shows that excitotoxicity of the glutamatergic system is associated with the disease (Greenamyre and Young, 1989). The effects of GABAergic compounds in ameliorating cognitive impairment induced by Aβ demonstrate the contribution of the GABAergic system to AD pathogenesis (Louzada et al., 2004).
Okadaic acid (OKA) is an inhibitor of protein phosphatase 2A (PP2A), which dephosphorylates tau and is associated with cognitive decline. In the AD zebrafish model induced by OKA, Aβ protein aggregation, senile plaque, increased p-tau and kinase glycogen synthase 3β (GSK-3β) are detected in zebrafish brain (Nada et al., 2016). Learning and memory deficits are also observed accompanied by these histological and pathological changes. This model represents most of the neuropathology related to AD and is easy to develop for exploring the mechanisms of AD and drug discovery.
Metal ions such as aluminum are thought to be neurotoxin and induce AD morbidity (Wang et al., 2016; Wang et al., 2020a). Zebrafish exposed to aluminum trichloride (AlCl3) show increased AChE activity at concentrations ranging from 50 to 250 μM and locomotor activity deficiency in acid conditions of pH 5.8 (Senger et al., 2011). This indicates that aluminum-induced AD is due to increased AChE activity. A recent study assessed the neurotoxic role of lead (Pb), a heavy metal, in zebrafish fibroblast cells (Lee and Freeman, 2020). The findings suggested that Pb exposure results in de novo DNA copy number alterations in a dose-dependent manner. Although most of the genes in the copy number alterations regions were related to the amyloid precursor protein (APP) molecular pathway, whether Pb is involved in neurological disease needs further elucidation, particularly in AD.
Aβ is generated from APP which is a type I transmembrane glycoprotein. β- and γ-secretases participate in the cascade cleavage of APPβ to form Aβ peptides, whereas α- and γ-secretases are involved in P3 peptide production from APPα. Various isoforms of Aβ with different lengths are produced, especially the toxic peptides Aβ42 and Aβ40. Oligomers, protofibrils, and fibrils generated by the accumulation of Aβ finally lead to Aβ plaque formation. Cerebroventricular microinjection of Aβ42 into zebrafish brain induces Aβ protein accumulation, apoptotic cell death, microglia activation and synaptic degeneration (Bhattarai et al., 2017). Islet amyloid polypeptide (IAPP) or Aβ microinjection into zebrafish embryos can produce an AD-like animal model to study amyloidosis (Nery et al., 2014). This method provides an acute AD model for cost-effective and efficient neuropathology study and drug screening in the future. The Swedish mutant APP is known to cause familial AD and zebrafish harbor two genes (appa and appb) that are similar to human APP (Musa et al., 2001). Although APP gene is evolutionary conserved in vertebrates, the function of appb is more paramount than appa since loss of appb not only impair locomotor behavior, motor neuron patterning and formation, Mauthner cell development but also disrupt cell adhesion during early development in zebrafish (Abramsson et al., 2013; Banote et al., 2016; Banote et a2020). Tg Swedish mutant APP zebrafish with appb promoter successfully express the disease-causing protein in brain, heart, eyes and vasculature. This model exhibits AD-like behavioral symptoms and cerebral β-amyloidosis, as well as neuronal loss and an enlarged perivascular space (Pu et al., 2017). Clearly elucidating the feature of zebrafish gene duplication would pave the road for mechanism exploration of key disease related genes which are potentially lethal in mammals.
NTFs are another typical hallmark of AD and are composed of highly phosphorylated tau. Tau is a microtubule-associated protein which is involved in modulating microtubule assembly and stabilization (Uddin et al., 2020). Hyperphosphorylated tau protein tends to form NTFs and affects microtubule normal function, microtubule dynamics and axonal transport (Sonawane and Chinnathambi, 2018). A stable Tg zebrafish model with mutations on tau encoding gene MAPT (microtubule-associated protein tau), such as Tau-P301L and Tau-A152T, has been established to mimic AD key pathological features of tauopathies. The mutations are found in AD patients with frontotemporal dementia. Tg zebrafish models under the control of a neuronal-specific HuC promoter is used to study AD pathogenesis and drug discovery. Gal4/UAS-based human Tau-P301L-Tg zebrafish show tau hyperphosphorylation, aggregation, degeneration, and behavioral deficiency (Paquet et al., 2009). In Gal4/UAS-based human Tau-A152T-Tg zebrafish, higher tau phosphorylation, neurodegeneration and impaired proteasome activity have been detected. Autophagy plays a role in tau clearance in this Tg model (Lopez et al., 2017). However, in Cre/LoxP-based Tau-P301L-Tg adult zebrafish, no oligomers, NTFs or symptoms of tauopathies were observed only tau hyperphosphorylation (Cosacak et al., 2017). It is possible that different Tg systems or development stages of zebrafish influence the model traits. In adult human brain, six isoforms of tau due to alternative splicing have been identified. Lack of microtubule-binding repeat, encoded by exon 10, leads to the generation of three isoforms with three repeats (3R), while its inclusion produces another three isoforms with four repeats (4R). An additional 29 or 58 amino acids encoded by exons 2, or exons 2 and 3 distinguish three isoforms with the same repeats (Goedert and Spillantini, 2019). In zebrafish, two paralogues by duplication of an ancestral teleost MAPT ortholog, mapta encodes tau isoforms with four, five or six repeats and maptb mainly encodes isoforms with 3R. Mapta and maptb display different expression patterns in certain brain regions during develoment, suggesting a subfunctionalization role of MAPT orthologue after duplication. For instance, mapta mRNA is present in ganglion neurons of the retina while maptb is not (Chen et al., 2009). Wu et al. attempted to overexpress zebrafish 3R or human 4R in zebrafish to observe tauopathy pathogenicity. They found both wild-type and truncated forms are prone to aggregate and increase neuronal death (Wu et al., 2016). More efforts are needed to develop AD-like models by modifying the expression of different tau isoforms. Protein presenilins (PSEN) 1 and 2 are highly homologous proteins, that act as potential catalytic subunits of γ-secretase (Walter et al., 1998). Mutations in their coding genes PSEN1 and PSEN2, have been identified in early-onset familial AD patients. Mutant PSEN1 or PSEN2 have been identified to increase longer and highly fibrillogenic Aβ formation in the brain to induce the onset of AD (Kabir et al., 2020). The zebrafish orthologs of PSEN1 and PSEN2 are psen1 and psen2. Deletion of psen1 in zebrafish causes histamine neuronal loss, and the histaminergic system is suggested to mediate cognitive functions in AD (Sundvik et al., 2013). psen1 splicing interference in zebrafish can induce early-onset AD phenotypes, such as cognitive deficit, Aβ42 aggregation and synaptic reduction (Nery et al., 2017). Blocking psen2 translation, results in defects of zebrafish embryo development in the central nervous system with Notch signaling interference (Nornes et al., 2009). Moreover, the zebrafish genome edited to model heterozygous K115fs mutation of human PSEN2 exhibits accelerated brain aging and microglia-associated immune responses without obvious histopathology (Hin et al., 2020). Further solid evidence of PSEN1 and PSEN2 function in zebrafish is mandatory to allow the application of this Tg model in drug discovery.
GSK3 is known to phosphorylate tau on multiple sites and its isoform GSK-3β is crucial for abnormal tau hyperphosphorylation. GSK3β in zebrafish is functionally similar to human GSK3β. The GSK-3β inhibitor, lithium chloride can reverse cognitive deficits and tau hyperphosphorylation in an in vivo AD model, which was established by injecting Aβ42 into the hindbrain ventricle of zebrafish embryos (Nery et al., 2014). Lithium chloride is also used as a positive control to protect zebrafish locomotors or dephosphorylation for tauopathy in the Tau-P301L-Tg zebrafish model (Naini et al., 2018; Barbereau et al., 2020). Another form of lithium, lithium carbonate (Li2CO3), has been demonstrated to prevent memory impairment and AChE activity in zebrafish induced by scopolamine (Zanandrea et al., 2018). The commercially available compound, TDZD-8 can reduce zebrafish mortality rate and improve cognitive impairment induced by OKA. Protein levels of p-tau, PP2A and the ratio of active: inactive GSK3β are also reduced following treatment with TDZD-8 (Koehler et al., 2019). The synthesized GSK3 inhibitor AR-534 can reduce tau hyperphosphorylation in the Tau-P301L mutation zebrafish model (Paquet et al., 2009). In discovering new GSK inhibitors, AR-A014418, compounds 9e and 26 days, Monte et al. used zebrafish embryos to evaluate the safety and permeability of these inhibitors (Monte et al., 2011; Lo Monte et al., 2013). The results corroborate the use of GSKβ inhibitors in the treatment of AD. However, there is a lack of evidence to decide which GSKβ inhibitor is most efficacious. Notably, GSK3 inhibitor treatment results in zebrafish embryos lacking eyes through activation of the Wnt signaling pathway (Atilla-Gokcumen et al., 2006; Zhong et al., 2009).
Regulation of the cholinergic system can suppress cholinesterase activity and provide guidance in AD treatment. A number of drugs have been identified and investigated in terms of this mechanism. Cotinine and 6-hydroxy-l-nicotine were found to attenuate zebrafish anxiety-like behavior and memory impairment in a scopolamine-induced model. Oxidative stress and AChE activity were also reduced in the brain of zebrafish (Boiangiu et al., 2021). In the same zebrafish model with memory impairment, Thymus vulgaris L. essential oil (TEO) ameliorated zebrafish amnesia and anxiety, reduced AChE activity and brain antioxidant capacity. The compounds contained in TEO are mainly thymol and p-cymene as shown by gas chromatography analysis (Capatina et al., 2020). Linarin, an active ingredient of the flavonoid glycoside, can protect against AlCl3-induced zebrafish dyskinesia by inhibiting AChE activity (Pan et al., 2019). Molecular docking simulation showed that linarin can bind with AChE active sites. Compounds designed from 3-(4-aminophenyl)-coumarin, especially compound 4m, alleviated behavioral symptoms and blocked AChE and BuChE activity (Hu et al., 2019). Compound 4m is suggested to be an interesting lead for drug design in AD treatment.
Tau hyperphosphorylation prevention allows the discovery of novel anti-tauopathy drugs against AD. A cyclin-dependent kinase 5 (CDK5) inhibitor, LDC8, can protect against neurodegeneration, neuroinflammation and synapse density in the Aβ42-induced AD zebrafish model by modulating the phosphorylation of microtubule-associated proteins (Reinhardt et al., 2019). Heparan sulfate is a highly sulfated polysaccharide which is associated with tau aggregates in AD (Naini and Soussi-Yanicostas, 2018). Treatment with surfen or its derivative oxalyl surfen, both possess heparan sulfate antagonist properties, can decrease tau hyperphosphorylation and rescue neuronal and behavioral deficits in Tg [HuC:hTauP301L; DsRed] Tg zebrafish (Naini et al., 2018). Decreased tau hyperphosphorylation observed with certain GSK-3β inhibitors suggests p-tau inhibition which is probably a common way of alleviating AD (Paquet et al., 2009; Nery et al., 2014; Zanandrea et al., 2018; Koehler et al., 2019).
A number of drugs are promising as they show multi-effects in AD-associated symptoms and neuropathology attenuation. 3-arylcoumarin is a compound which has a coumarin skeleton and an aryl structure at the 3-position. 3-arylcoumarin derivatives show dual cholinesterase and monoamine oxidase (MAO) inhibition and improved juvenile zebrafish movement in the AlCl3-induced zebrafish model (Yang et al., 2019). Ferulic acid is an antioxidant which possesses a variety of functions, including amyloid structure elimination. Dyskinesia and the reaction capacity of AlCl3-induced zebrafish recovered following administration of the synthesized ferulic acid derivative, TM-10. TM-10 shows influence on BuChE, MAO and self-induced Aβ aggregation. Moreover, memory loss is improved by TM-10 in scopolamine-induced zebrafish and vascular injury is protected in Aβ40-induced zebrafish (Sang et al., 2019). The newly designed and synthesized cinnamic acid hybrid, compound 4e, can inhibit Aβ42 accumulation, and shows reversible BuChE activity and MAO-B inhibitory activity. Compound 4e improves dyskinesia recovery rate and reaction capacity in AlCl3-induced AD zebrafish models (Wang et al., 2021). A natural sulfur amino acid metabolite, LKE, rescued the cognitive defects and cell apoptosis in the dorsal lateral pallium in the OKA-induced AD zebrafish model (Koehler et al., 2018). In addition, LKE increased BDNF expression, phosphorylation of PKB/Akt and cAMP response element binding protein (CREB).
Studies have also been conducted to develop nanomedicine against AD or human amyloid diseases and the zebrafish model has been used for economic and high-throughput drug screening. In the Aβ toxicity induced zebrafish larvae model, casein coated-gold nanoparticles (βCas AuNPs) were designed and tested (Javed et al., 2019). βCas AuNPs can sequester intracerebral Aβ42 to mitigate Aβ toxicity in a nonspecific and chaperone-like manner. Disappearance of amyloid plaque formation, reduced reactive oxygen species (ROS) and the rescue of synaptophysin loss were observed. Moreover, βCas AuNPs rescued the mobility and cognitive function in the adult zebrafish model of Aβ toxicity. Carbon quantum dots (CQDs) are zero-dimensional, carbon-based nanostructures for carrying drugs (Koppel et al., 2020). CQDs can rescue impaired zebrafish embryonic hatching and suppress the production of ROS in the organism. Carbon dots in this study function as an Aβ inhibitor by deactivating β-secretase 1 (BACE1) (Han et al., 2017).
Although the γ-secretase inhibitor (GSI), DAPT, was developed to prevent Aβ production via interference with PSEN function, potential toxic side effects were observed (Nornes et al., 2009). GSIs not only inhibit Aβ generation in zebrafish embryos, but also impact the Notch signaling pathway which plays a paramount role during embryonic development and throughout adulthood. However, there are also GSIs, such as the JLK isocoumarin inhibitors, which aim to reduce Aβ production without interfering with the Notch signaling pathway (Petit et al., 2003; Arslanova et al., 2010; Yang et al., 2010). Therefore, AD-related drugs should be designed to minimize side effects without triggering an unwanted pathway.
All the compounds described above are summarized in Table 1.
PD is a common neurodegenerative disease, affecting 2–3% of the population aged over 65 years (Hou et al., 2019). It is mainly characterized by motor deficits including bradykinesia, rigidity, resting tremor and abnormal gait. PD patients are also affected by non-motor symptoms such as hyposmia, depression, constipation and sleep disorder. The major pathological features of PD are neuronal loss in the substantia nigra pars compacta (SNpc) and the presence of Lewy bodies (LBs) consisting of misfolded α-synuclein (α-syn). The etiology of PD is still not known, but both genetic and environmental factors are thought to affect the progression of PD. At present, there is still no ideal treatment to delay the development of the disease. Dopamine precursor (l-DOPA) replacement is the most effective treatment for improving motor function; however, long-term application of the drug will worsen dyskinesia (Armstrong and Okun, 2020). PD is a heterogeneous disease with various pathophysiological changes in individuals. No model can accurately mimic all aspects of PD and different models possess their own unique advantages. Zebrafish have been utilized to investigate both neurotoxic and genetic effects in PD for more than 20 years and show great value in screening potential therapeutic drugs.
Neurotoxins induce rapid loss of nigrostriatal dopaminergic neurons in animal models. The most commonly used neurotoxins include 6-hydroxydopamine (6-OHDA), 1-methyl-4-phenyl-1,2,3,6-tetrahydropyridine (MPTP), rotenone, and paraquat. 6-OHDA is a hydroxylated compound of DA which is highly oxidizable and it can produce harmful ROS in the brain. Intracranial administration of 6-OHDA can successfully model motor disturbance and lead to a decrease in DA and noradrenaline levels in adult zebrafish (Anichtchik et al., 2004). Further studies have elucidated the neuronal oxidation and dopaminergic neuron loss in this model (Vijayanathan et al., 2017). Exposure to 6-OHDA also decreases tyrosine hydroxylase (TH) level and locomotor activity in zebrafish larvae or embryos (Parng et al., 2007; Feng et al., 2014). A recent study also revealed that 6-OHDA can induce ferroptosis in zebrafish brain (Sun et al., 2020).
MPTP was first discovered when addicted individuals with MPTP administration suddenly showed parkinsonian symptoms (Langston et al., 1983). It is oxidized into 1-methyl-4-phenylpyridinium ion (MPP+) by the MAO-B in astrocytes, and MPP+ is then quickly absorbed by dopaminergic neurons through the dopamine transporter and inhibits mitochondrial respiration complex I, leading to apoptosis and necrosis of DA neurons. Several studies have reported that MPTP administration kills DA neurons in embryonic, larval, and adult zebrafish (Anichtchik et al., 2004; Bretaud et al., 2004; Lam et al., 2005). Using MPTP-induced zebrafish models combined with Tg fluorescent labeling, studies have explored the topographic pattern of monoaminergic neuronal loss and the dynamics of mitochondrial transport after MPTP or MPP+ exposure (Wen et al., 2008; Xi et al., 2011; Noble et al., 2015; Dukes et al., 2016; Kalyn et al., 2019). These studies have deepened our understanding of the progression of PD and provided a good platform for the establishment of high-throughput drug screening in zebrafish.
Rotenone is a pesticide commonly used in farming and fishing. Researchers have found that chronic exposure to rotenone is associated with a higher risk of PD (Betarbet et al., 2000). Administration of rotenone in zebrafish can reduce DA neurons (Bretaud et al., 2004). Encouragingly, studies in recent years have found that the rotenone-based zebrafish model can mimic both motor and non-motor PD-like symptoms (Wang et al., 2017; Lv et al., 2019). This may provide a new approach to studying the pathology of early stage PD.
Paraquat, also a pesticide, has high capability to generate superoxide in mitochondria. Paraquat induces neurodegenerative phenotypes and the reduction of DA levels in zebrafish (Nellore and Nandita, 2015; Nunes et al., 2017), while the loss of DA neurons has not been reported in this model. Researchers have also developed mitochondria-targeted redox cycler paraquat (named MitoPQ) to selectively induce mitochondrial superoxide production, and model metabolic and neurodegenerative disease phenotypes (Robb et al., 2015). This toxin could be a useful tool for investigating novel drug targets for improving mitochondrial function (Pinho et al., 2019).
Zebrafish are also becoming a popular tool for evaluating the influence of environmental factors on PD pathogenesis. Exposure of zebrafish embryos to graphene-family nanomaterials has been reported to induce neurotoxicity and alter the expression of PD-related genes (Cao et al., 2021). Coincidentally, zinc oxide nanomaterials disrupt locomotor activity and induce apoptosis and neuronal loss in zebrafish (Jin et al., 2021). More recently, other researchers have also suggested that long-term exposure to a heterocyclic amine from a wide range of pollutants, contributes to the potential risk of PD (Li et al., 2021).
Although neurotoxic models are feasible for defining potential symptomatic benefits for drug development, it is difficult to model the molecular pathology of PD. Family studies have discovered more than 20 risk genes associated with PD (Blauwendraat et al., 2020). In recent years, genome-wide association studies (GWAS) have also identified 90 independent risk variants which contribute to sporadic PD (Blauwendraat et al., 2020). The easiest approach used to study loss-of-function mutations in zebrafish is the injection of antisense morpholino oligonucleotides. Later, Tg and viral zebrafish models based on the genetic causes were widely used to study PD pathobiology and evaluate potential therapeutics.
SNCA (encoding α-syn, which is the major component of LBs) was the first gene found to be unequivocally linked to familial PD. Although zebrafish do not express α-syn ortholog, they express β-, γ1-, and γ2-synucleins and γ1-synuclein has a similar function to α-syn. Knockdown of β- and γ1-synucleins results in abnormal development of dopaminergic neurons and reduces DA levels, and expression of human α-syn in zebrafish can revert the phenotypes (Milanese et al., 2012). Combined with neurotoxic models, a recent study found that insufficient expression of human α-syn can amplify cytoplasmic peroxide flux and oxidative stress, causing motor abnormalities prior to neuronal loss (Kang et al., 2018; Van Laar et al., 2020). α-syn is still an appealing target for disease modification in PD. However, all existing zebrafish PD models can only produce a dopaminergic cell-loss phenotype and neither exhibits the formation of LBs.
Mutations in the leucine-rich repeat kinase 2 (LRRK2) gene are implicated in both familial and sporadic cases of PD and the LRRK2-G2019S mutation is the most common (Blauwendraat et al., 2020). Zebrafish express a homolog of the human LRRK2 gene, which contains all the functional domains. Disruption of LRRK2 in zebrafish leads to severe developmental defects and neuron loss (Prabhudesai et al., 2016). However, studies do not show consistent results on the effects of depleting the WD40 domain of LRRK2 (Sheng et al., 2010; Ren et al., 2011). To date, no zebrafish model has been generated to elucidate the effects of increased kinase activity of LRRK2 which is highly relevant to PD etiology.
Parkin is an E3 ubiquitination ligase encoded by PARK2 gene. It mediates the ubiquitination process of many proteins including α-syn, and promotes the proteasome-dependent degradation and mitochondrial autophagy. Therefore, parkin plays an important role in the elimination of damaged proteins and misfolded proteins. Reduction of parkin activity in zebrafish leads to a significant decrease in the number of dopaminergic neurons, and parkin knockdown zebrafish embryos show specific impairment of the mitochondrial respiratory chain complex I (Flinn et al., 2009; Fett et al., 2010). Moreover, increased expression of parkin protected zebrafish from cell death (Fett et al., 2010).
DJ-1 (encoded by PARK7) appears to have multiple roles in protecting neurons from oxidative stress and loss-of-function of the protein can cause a rare form of early-onset PD. Knockdown of DJ-1 using antisense morpholino oligonucleotides in zebrafish embryos does not reduce the number of dopaminergic neurons, but leads to increased susceptibility of dopaminergic neurons to H2O2 (Bretaud et al., 2007). The loss of DJ-1 in the mouse does not reduce the number of dopaminergic neurons and locomotor activity; however, PARK7 knockout zebrafish produce a reliable phenotype of PD (Edson et al., 2019; Hughes et al., 2020).
PINK1 is another mitochondrial-related gene linked to early-onset PD. Like PARK7, knockdown of PINK1 alone results in reduced dopaminergic neurons in zebrafish, and the models can successfully mimic PD pathology when incorporated with other PD-risk factors (Zhang et al., 2017; Brown et al., 2021). Flinn et al. also identified a fish line with a premature stop mutation (Y431*) in the Pink1 kinase domain, which induces mitochondrial dysfunction and loss of dopaminergic neurons (Flinn et al., 2013; Soman et al., 2017).
In addition, a variety of zebrafish Tg models have been generated based on the PD-associated genes recently. The loss-of-function of the PARLA gene decreases dopaminergic neurons mainly in the olfactory bulb (Merhi et al., 2021). VPS41 knockout causes lysosomal abnormalities as well as microglial and cerebellar dysfunction (Sanderson et al., 2021). NUS1 knockdown zebrafish exhibit movement deficits caused by defective efflux of cholesterol from lysosomes (Yu et al., 2021). Zebrafish Tg models tend to be an effective tool to classify PD into different subtypes according to different pathogenic factors.
By employing the above models, a multitude of drugs have been screened out and proved to be potentially effective in treating PD (Table 2). MAO-B is one of the enzymes that deaminate monoamine neurotransmitters like dopamine, noradrenaline, and serotonin in the brain. The age-related high expression of MAO-B is associated with mitochondrial dysfunction and reduction in the viability of DA neurons. Moreover, recent studies have also shown that α-syn can bind and activate MAO-B, accelerating PD progression. MAO-B inhibitors, such as l-deprenyl and rasagiline, show potential anti-dyskinetic and neuroprotective effects in PD zebrafish models (McKinley et al., 2005; Cronin and Grealy, 2017; Vaz et al., 2020). MAO-B inhibitors have been used clinically as an alternative to l-DOPA. Recently, more MAO-B inhibitors with high potency and selectivity have been designed to improve their therapeutic benefits and decrease the secondary effects. Studies in zebrafish models should be carried out to provide further evidence and accelerate the drug development process.
Mitochondria have an important role in energy metabolism and preserve redox homeostasis in response to stress. Excessive mitochondrial dysfunction is implicated in the pathology of PD. Combining Pink1 deficiency with rotenone, Zhang et al. developed a multifactorial zebrafish drug-screening method to compromise mitochondrial function and identified trifluoperazine and other piperazine phenothiazines as protective compounds (Zhang et al., 2017). Another study found that administration of melatonin can rescue zebrafish embryos from the MPTP-induced PD phenotype by restoring the parkin/Pink1/DJ-1/MUL1 function (Díaz-Casado et al., 2016). These studies will pave the way for future PD therapies that specifically target mitochondria.
Autophagy is a catabolic process that removes damaged organelles and aggregated proteins via the autophagy-lysosome pathway. Many studies have demonstrated that α-syn aggregates are mainly degraded via autophagy. In zebrafish, down-regulation of autophagy-related gene 5 (ATG5) results in the upgrade of PD-associated proteins, abnormal locomotor behavior and neuronal loss, while ATG5 overexpression alleviated these PD pathological features (Hu et al., 2017). This study revealed that up-regulation of autophagy-related genes may be an effective way of preventing PD progression. For instance, trifluoperazine can slow neurodegeneration via enhancing autophagy in response to mitochondrial stress (Zhang et al., 2017).
An imbalance of calcium homeostasis plays a vital role in PD pathogenesis. The vulnerability of dopaminergic cells in PD is related to overloaded cytosolic and mitochondrial calcium. A study reported that pharmacological inactivation of the mitochondrial calcium uniporter (MCU) by Ruthenium red, prevents neuronal loss in PINK1-Y431* mutant zebrafish (Soman et al., 2017). However, isradipine, a calcium channel blocker, did not reverse 6-OHDA-induced phenotypes in a zebrafish model (Cronin and Grealy, 2017). These studies assume that the effects of calcium channels may vary in PD with different etiologies. Owing to the importance of calcium in PD pathology, we suggest that future studies should be performed to verify the effects of more types of calcium channels, including synaptic receptors, in zebrafish models.
Although the relationship between neuroinflammation and PD is unclear, a growing body of evidence supports the immune-targeted therapies as promising PD treatments. An epidemiological study has confirmed the beneficial role of non-steroidal anti-inflammatory drugs in PD. Many researchers use zebrafish models to verify whether anti-inflammatory drugs are effective in improving the motor symptoms of PD. Minocycline, a tetracycline analog prevents the locomotor deficits and neuronal loss in the 6-OHDA-induced zebrafish model (Cronin and Grealy, 2017). Recent studies have reported that rifampicin, a macrocyclic antibiotic, improves neuroinflammation and mitochondrial function (Yurtsever et al., 2020) and LP17, a synthetic peptide blocker of triggering receptors expressed on myeloid cells 1 (TREM-1), shows neuroprotective effects via activation of autophagy and anti-inflammatory activity (Feng et al., 2019).
In addition, numerous compounds extracted from natural products have been screened out and verified to be effective in ameliorating PD pathology using zebrafish models (Abidar et al., 2020; Chen et al., 2020; Duan et al., 2020; Xiong et al., 2020; Zhang et al., 2020; Zhao et al., 2020; Kesh et al., 2021a; Kesh et al., 2021b; Kozioł et al., 2021; Santo et al., 2021; Wu et al., 2021). Although the effective composition of some extracts is still to be determined, fewer side effects of natural derivates compared with synthesized molecules due to their compatibility with humans should be noted. Certain natural products are promising in disease treatment as they also have an effect on autophagy, apoptosis, inflammation, or oxidative stress pathways (Zhang et al., 2020; Kesh et al., 2021b; Santo et al., 2021; Wu et al., 2021). Recently, Vaz et al. tested 1600 FDA-approved bioactive drugs in the 6-OHDA-induced zebrafish model and highlighted novel molecules with antiparkinsonian potential (Vaz et al., 2020). These drugs could possibly become adjuvant medicine in the future treatment of PD. New approaches such as nanoparticles for drug discovery are also being developed in zebrafish models. For example, a nanocrystal formulation of resveratrol protects DA neurons against cytotoxicity induced by MPP+ with no significant toxic effects on zebrafish embryos and larvae (Xiong et al., 2020).
ALS is a neurodegenerative disease pathologically characterized by progressive loss of upper motor neurons from the cortex or lower motor neurons from the spinal cord and brainstem. The clinical features of ALS patients are first manifested by muscle weakness, hyperreflexia, fasiculation, atrophy, spasticity, and eventually resulting in paralysis. The pathophysiology mechanism of ALS may be due to excitotoxicity, the occurrence of astrogliosis and microgliosis, failure of proteostasis, mitochondrial dysfunction, neuroinflammation and so on (Van Damme et al., 2017). Familial ALS only accounts for 10% of all cases, while most ALS cases are sporadic. Genetic mutations and environmental factors are suggested to be the causes of ALS (Morrice et al., 2018a). Based on these findings, Tg and toxin-induced animal models of ALS have been established to investigate the disease pathogenesis or discover potential drugs.
Environmental factors such as β-N-methylamino-l-alanine (BMAA) and bisphenol A (BPA) have been demonstrated to be involved in ALS etiology (Gois et al., 2020). BPA is an industrial plasticizer which is thought to be a potential trigger in ALS pathogenesis. It can cause motor neuron degeneration, affect locomotor activity, reduce neuromuscular junction (NMJ) integrity and motor neuron-specific cell death in zebrafish embryos (Morrice et al., 2018b). BMAA is a non-proteinogenic neurotoxic amino acid produced by cyanobacteria. Zebrafish show neurodegeneration symptoms when exposed to BMAA, including neural development disruption and learning and memory ability deficiency (Chiu et al., 2011; Wang et al., 2020b). The mixture of cyanotoxins, BMAA and microcystin leucine and arginine (MCLR), cause severe neurotoxicity and upregulation of TDP-43 in zebrafish than individual toxins (Martin et al., 2021). Together, this suggesting a link between cyanotoxins and ALS. Furthermore, the combined effects of ALS causative genes and environmental factors have also been investigated in ALS zebrafish models. Exposure to BPMM changes the motor neuron growth characteristics in zebrafish with G93R-SOD1 mutation (Sher, 2017). However, neurotoxin-induced ALS-like models are used less than Tg models in drug discovery.
Mutations on the genes, SOD1 (Cu/Zn superoxide dismutase 1), TARDBP (TAR DNA-binding protein 43), FUS/TLS (fused in sarcoma/translocated in liposarcoma), and C9ORF72 have been identified in ALS patients. SOD1 is an enzyme which catalyzes the detoxification of superoxide. Mutation SOD1-G93A impacts zebrafish motor neuron outgrowth, axonal branching, NMJ integrity, motor neuron survival and motor activity. SOD1-G93R mutation shows slowly progressive motor degeneration without muscle denervation in zebrafish (Sakowski et al., 2012; Morrice et al., 2018a). Good representation of ALS phenotype in zebrafish carrying these two mutations suggests a latent model for further drug screening. TDP43 is a nuclear protein which is associated with protein aggregation of motor neurons in ALS patients and is involved in axonal transport. TARDBP-A315T mutation in zebrafish shows motor dysfunction and motor axon abnormality (Morrice et al., 2018a). Knockdown of TDP43 ortholog tdp-43 in zebrafish not only causes early defects of motor phenotype with NMJ disassembly, but also decreases AChE expression in total fish (Campanari et al., 2021). Overexpression of tdp-43 in spinal motor neurons halts axonal outgrowth coupled with protein TDP-43 cytoplasmic mis-localization instead of aggregation (Asakawa et al., 2020). FUS/TLS gene encodes sarcoma fusion protein, which is responsible for RNA-binding. A truncated mutation of FUS-R495X in zebrafish leads to cytoplasmic protein accumulation in motor neurons as well as oxidative stress (Bosco et al., 2010). Depletion of FUS ortholog in zebrafish models results in the main characteristics of ALS physiopathology, including impaired motor abilities, shortened motor neuron length and NMJ fragmentation (Bourefis et al., 2020). The C9ORF72 gene possesses intronic hexanucleotide-repeat (GGGGCC) expansions, which can be translated into aggregation-prone DPR (dipeptide-repeat) proteins, thus leading to neuronal toxicity (Kramer et al., 2018). Shaw et al. generated two zebrafish lines expressing C9orf72 hexanucleotide-repeat expansions and validated their disease-like symptoms of ALS (Shaw et al., 2018). Zebrafish from another group stably expressing C9orf72-associated 100 Gly-Arg repeats in motor neurons also displayed a reduction in motor neuron length and swimming capability (Swaminathan et al., 2018).
Zebrafish models also accelerate the development of new drug candidates for the treatment of ALS (Table 3).
Modulation of ion channels or currents sheds light on ALS treatment and drug discovery. L-type calcium channel agonists, FPL64176 or Bay K8644 to treat ALS have been evaluated in TARDBP Tg zebrafish with G348C mutation. Mutant TARDBP zebrafish showed impaired locomotor function, increased motoneuron excitability, decreased fidelity of synaptic transmission, and abnormal structures at NMJ. Calcium channel agonists treatment of zebrafish can rescue all these behavioral and cellular deficiencies (Armstrong and Drapeau, 2013). The neuroleptic compound, pimozide functions to antagonize T-type Ca2+ channels. Pimozide not only reduces motor defects, rescues orphaned presynaptic endings and stabilizes neuromuscular transmission in zebrafish expressing mutant TDP-43, but also reduces motor deficits in human SOD1-G93A and FUS-R521H Tg zebrafish (Patten et al., 2017). A correlative study screened out one potent compound TRVA242 from a pool of pimozide derivatives. TRVA242 prevented locomotor defects in four Tg zebrafish models, a human TARDBP-G348C mutant model, human SOD1-G93A mutant model, zebrafish tardbp loss-of-function model and a C9ORF72 model with 100 times Gly-Arg repeat. Moreover, TRVA242 restores aberrant spinal motor neuron outgrowth, recovers NMJ structural abnormalities, and rescues NMJ synaptic deficits (Bose et al., 2019). Notably, riluzole, which can inhibit persistent sodium current INaP, reverses the abnormal motor phenotype, normalizes motor axon length, reduces the frequency of spontaneous tail coiling and depolarization frequency of spinal neurons in zebrafish embryos with SOD1-G93R mutation (Benedetti et al., 2016). Another study corroborated the mutant SOD1-G93R zebrafish as a valid ALS animal model for high-throughput drug screening and provided evidence for riluzole in reducing neuronal stress (McGown et al., 2016).
Mechanisms of ALS prevention are complicated and not as distinct as those for AD or PD. Oxidative stress, inflammatory, autophagy or other pathway interference seems effective in alleviating ALS symptoms. In identifying other potential neuroprotective compounds, methylene blue, which has been previously demonstrated to inhibit TDP-43 aggregates formation, was found to protect against oxidative stress and impaired motor phenotypes in mutant human TARDBP-G348C or FUS-R521H zebrafish models (Vaccaro et al., 2012). 1-Fe, acting as an corrole iron complex and catalytic antioxidant, also shows improved locomotor activity in SOD1-G93R mutant zebrafish (Soll et al., 2021). Interestingly, in a recent study, two ALS Tg zebrafish models, SOD1-G93R and TARDBP-G348C, were used to evaluate the combined efficacy of ciprofloxacin and celecoxib. Ciprofloxacin is an approved synthetic antibiotic and celecoxib is an approved nonsteroidal anti-inflammatory drug. Combination treatment improved zebrafish larvae performance and motor neuron axonopathy, and recovered the ramified morphology of microglia and NMJ structures (Goldshtein et al., 2020). These results provide a novel view of drug combination as an effective and promising treatment for ALS. Paik et al. predicted terbutaline sulfate (TS), widely used for asthma, to be a promising candidate for ALS by analyzing electronic medical records and genomics data. TS can prevent axonal defects and NMJ degeneration in a dose-dependent manner in TARDBP-Q331K-Tg zebrafish. The therapeutic effects of TS are mediated by activating β2-adrenergic receptors (Paik et al., 2015). Mutation of SQSTM1 has been recently reported in ALS patients. SQSTM1 knockdown in zebrafish causes locomotor deficits and axonopathy of motor neurons. However, treatment with rapamycin, an mTOR-dependent autophagy activator, rescues motor deficiency (Lattante et al., 2015). A method based on reducing C9ORF72 DPR toxicity is used for drug screening. The ALS-related polypeptide, PR20 is a synthetic polymer of DPR and has been demonstrated to be toxic in K562 cells and mouse primary neurons (Kramer et al., 2018). PFI-1 BET (bromo and extra-terminal) is a bromodomain inhibitor and Na-Phen is a histone deacetylase inhibitor. Both of these inhibitors can increase the viability of zebrafish embryos in vivo when exposed to PR20 (Corman et al., 2019). A tryptophan residue at position 32 (W32) in SOD1 is suggested to contribute to SOD1 induced toxicity in ALS. Zebrafish embryo with an uncommon SOD1-W32S variant shows axonopathy and motor neuron deficits. A uracil-like nucleoside compound, telbivudine, was predicted to interact with W32 residue. Telbivudine can rescue the phenotypes induced by SOD1-WT or disease-associated mutant in a dose-dependent manner (DuVal et al., 2019).
Huntington’s disease (HD) is a monogenic neurodegenerative disease caused by the mutation encoding for an abnormal trinucleotide that leads to glutamine (CAG) expansion at the Huntingtin (HTT) protein (Walker, 2007). Deficient HTT expression in zebrafish results in a variety of developmental defects and disruption in iron homeostasis (Lumsden et al., 2007). Zebrafish embryos expressing the expanded polyglutamine repeat versions cause various pathologies including early cell death and neurodegeneration. Overexpression of the C-terminal Hsp70-interacting protein (CHIP) can suppress aggregation and toxicity of pathogenic HTT fragment (Miller et al., 2005). The zebrafish HD model has also been used to screen for the molecular chaperones with anti-prion aggregation and toxicity effects (Schiffer et al., 2007). Furthermore, recent study revealed that the inhibitors of phosphodiesterase 5 (PDE5), which upregulated intracellular levels of cGMP, reduced aggregates in zebrafish larvae expressing human huntingtin with 71 glutamine repeats (VerPlank et al., 2020). We expect that the zebrafish model of HD will substantially improve our understanding of the disease and promote the development of novel drug discovery in HD.
Prion diseases are characterized by the aggregation of a pathogenic misfolded form of prion protein (PrP) which has a gain of toxic function. Moreover, accumulating evidence show that PrP may act as a receptor for protein aggregates and play a key role in the pathogenesis of other common neurodegenerative disorders (Watts et al., 2018). Using genetic zebrafish models, researchers have found that APP may control neuronal excitability via their interaction with the prion protein (Kanyo et al., 2020). Longer Aβ oligomers or protofibrils can also directly bind to PrP and thereby act to modulate synapses (Um et al., 2012). Blockade of the binding can prevent sleep induction in zebrafish, resulting in a signaling cascade to exacerbate AD progression (Özcan et al., 2020). Taking together, PrP protein could be a potential pharmaceutical target for the treatment of prion diseases and other neurodegenerative disorders.
As the number of people affected by NND is increasing, finding an effective way of treating the disease is urgently needed. However, only symptomatic treatment is available to date. Current AD therapies can only temporarily improve learning and memory by maintaining the levels of ACh and cholinergic transmission by AChE inhibitors or regulating glutamatergic transmission by blockers of N-methyl-d-aspartate (NMDA) receptors. Administration of l-DOPA, dopamine agonists, MAO inhibitors, catechol-O-methyltransferase (COMT) inhibitors and anticholinergics has been shown to temporarily relieve motor and nonmotor PD symptoms but without halting PD progression. In ALS, FDA-approved drugs (riluzole and edaravone) only provide a modest survival benefit. A number of pharmacological drugs designed for NDD treatment have failed in clinical trials over the past several decades due to the clinical heterogeneity of the disease. In addition, disease-modifying treatments can only be effective in the early stage of the disease because most NDD pathologies are irreversible. Therefore, we need to generate more pre-clinical NDD animal models to mimic the alteration in different clinical subtypes and in different stages of the disease. To accomplish this, the zebrafish model is essential. Zebrafish have a number of advantages and provide an important foundation for the determination of NDD pathogenesis and drug discovery. In vitro chemical screening provides much higher throughput than in vivo, while in vivo models can simulate complex systems in the human body such as the BBB. Zebrafish have been used in both in vitro and in vivo drug screening, and numerous new compounds have been verified to be effective against NDD.
However, there is still the challenge of translating these drugs into clinical use. First, there is a big gap between zebrafish and humans in terms of NDD morbidity and drug treatment. Even in Tg zebrafish models, the pathological or behavioral phenotypes are varied due to different genes or mutations. Second, the pathogenesis of NDD involves a host of environmental and genetic factors; thus, classifying the disease into different subtypes. It may be necessary to personalize the treatment approach. Third, the lack of sensitive biomarkers limits the identification of NDD at pre-symptomatic stages, in which drug treatment is likely to show disease-modifying efficacy. In addition, many drugs screened out in zebrafish models have a short therapeutic window. It is difficult for these drugs to access the BBB before degradation without suitable drug delivery approaches.
Small molecules from natural extracts or designed targeting a critical pathway are both feasible for NDD treatment. Although many compounds show efficiency at different levels in alleviating zebrafish pathological or aberrant behavioral features in NDD, comprehensive testing should be carried out to fully understand the mechanisms of a new drug. It is unknown how many NDD abnormal symptoms these drugs have an effect on. This would help us to predict the viability of drugs for further trials. In addition, more molecular mechanisms should be identified in order to develop or discover new drugs besides existing inhibitors or targeting proteins in NDD. Drugs with little or no specificity should also be investigated and improved. Lastly, pharmacological drug safety of compounds should be determined. Zebrafish will be helpful in the transfer of drugs from the laboratory to the clinic.
XW and J-BZ: conceptualization, original draft preparation and editing; K-JH: Visualization; FW: editing; C-FL: editing and supervision.
This work was supported by Jiangsu Provincial Key R&D Program (BE2018658), Jiangsu Provincial Medical Key Discipline Project (ZDXKB2016022), Discipline Construction Program of the Second Affiliated Hospital Soochow University (XKTJ-XK202001), Postgraduate Research & Practice Innovation Program of Jiangsu Province (KYCX21_2977) and the Priority Academic Program Development of Jiangsu Higher Education Institutions (PAPD).
The authors declare that the research was conducted in the absence of any commercial or financial relationships that could be construed as a potential conflict of interest.
Abidar, S., Boiangiu, R. S., Dumitru, G., Todirascu-Ciornea, E., Amakran, A., Cioanca, O., et al. (2020). The Aqueous Extract from Ceratonia Siliqua Leaves Protects against 6-hydroxydopamine in Zebrafish: Understanding the Underlying Mechanism. Antioxidants (Basel) 9, 1–21. doi:10.3390/antiox9040304
Abramsson, A., Kettunen, P., Banote, R. K., Lott, E., Li, M., Arner, A., et al. (2013). The Zebrafish Amyloid Precursor Protein-B Is Required for Motor Neuron Guidance and Synapse Formation. Dev. Biol. 381, 377–388. doi:10.1016/j.ydbio.2013.06.026
Alavi Naini, S. M., and Soussi-Yanicostas, N. (2018). Heparan Sulfate as a Therapeutic Target in Tauopathies: Insights from Zebrafish. Front. Cell Dev. Biol. 6, 1–17. doi:10.3389/fcell.2018.00163
Alavi Naini, S. M., Yanicostas, C., Hassan-Abdi, R., Blondeel, S., Bennis, M., Weiss, R. J., et al. (2018). Surfen and Oxalyl Surfen Decrease Tau Hyperphosphorylation and Mitigate Neuron Deficits In Vivo in a Zebrafish Model of Tauopathy. Transl. Neurodegener. 7, 1–9. doi:10.1186/s40035-018-0111-2
Anichtchik, O. V., Kaslin, J., Peitsaro, N., Scheinin, M., and Panula, P. (2004). Neurochemical and Behavioural Changes in Zebrafish Danio rerio after Systemic Administration of 6-hydroxydopamine and 1-Methyl-4-Phenyl-1,2,3,6-Tetrahydropyridine. J. Neurochem. 88, 443–453. doi:10.1111/j.1471-4159.2004.02190.x
Armstrong, G. A., and Drapeau, P. (2013). Calcium Channel Agonists Protect against Neuromuscular Dysfunction in a Genetic Model of TDP-43 Mutation in ALS. J. Neurosci. 33, 1741–1752. doi:10.1523/JNEUROSCI.4003-12.2013
Armstrong, M. J., and Okun, M. S. (2020). Diagnosis and Treatment of Parkinson Disease: A Review. JAMA 323, 548–560. doi:10.1001/jama.2019.22360
Arslanova, D., Yang, T., Xu, X., Wong, S. T., Augelli-Szafran, C. E., and Xia, W. (2010). Phenotypic Analysis of Images of Zebrafish Treated with Alzheimer's Gamma-Secretase Inhibitors. BMC Biotechnol. 10, 24. doi:10.1186/1472-6750-10-24
Asakawa, K., Handa, H., and Kawakami, K. (2020). Optogenetic Modulation of TDP-43 Oligomerization Accelerates ALS-Related Pathologies in the Spinal Motor Neurons. Nat. Commun. 11, 1004. doi:10.1038/s41467-020-14815-x
Atilla-Gokcumen, G. E., Williams, D. S., Bregman, H., Pagano, N., and Meggers, E. (2006). Organometallic Compounds with Biological Activity: a Very Selective and Highly Potent Cellular Inhibitor for Glycogen Synthase Kinase 3. Chembiochem 7, 1443–1450. doi:10.1002/cbic.200600117
Banote, R. K., Chebli, J., Şatır, T. M., Varshney, G. K., Camacho, R., Ledin, J., et al. (2020). Amyloid Precursor Protein-B Facilitates Cell Adhesion during Early Development in Zebrafish. Sci. Rep. 10, 10127. doi:10.1038/s41598-020-66584-8
Banote, R. K., Edling, M., Eliassen, F., Kettunen, P., Zetterberg, H., and Abramsson, A. (2016). β-Amyloid Precursor Protein-B Is Essential for Mauthner Cell Development in the Zebrafish in a Notch-dependent Manner. Dev. Biol. 413, 26–38. doi:10.1016/j.ydbio.2016.03.012
Barbereau, C., Yehya, A., Silhol, M., Cubedo, N., Verdier, J. M., Maurice, T., et al. (2020). Neuroprotective Brain-Derived Neurotrophic Factor Signaling in the TAU-P301l Tauopathy Zebrafish Model. Pharmacol. Res. 158, 104865. doi:10.1016/j.phrs.2020.104865
Basnet, R. M., Zizioli, D., Taweedet, S., Finazzi, D., and Memo, M. (2019). Zebrafish Larvae as a Behavioral Model in Neuropharmacology. Biomedicines 7, 23. doi:10.3390/biomedicines7010023
Benedetti, L., Ghilardi, A., Rottoli, E., De Maglie, M., Prosperi, L., Perego, C., et al. (2016). INaP Selective Inhibition Reverts Precocious Inter- and Motorneurons Hyperexcitability in the Sod1-G93r Zebrafish ALS Model. Sci. Rep. 6, 1–20. doi:10.1038/srep24515
Betarbet, R., Sherer, T. B., MacKenzie, G., Garcia-Osuna, M., Panov, A. V., and Greenamyre, J. T. (2000). Chronic Systemic Pesticide Exposure Reproduces Features of Parkinson's Disease. Nat. Neurosci. 3, 1301–1306. doi:10.1038/81834
Bhattarai, P., Thomas, A. K., Zhang, Y., and Kizil, C. (2017). The Effects of Aging on Amyloid-Β42-Induced Neurodegeneration and Regeneration in Adult Zebrafish Brain. Neurogenesis 4, e1322666. doi:10.1080/23262133.2017.1322666
Blauwendraat, C., Nalls, M. A., and Singleton, A. B. (2020). The Genetic Architecture of Parkinson's Disease. Lancet Neurol. 19, 170–178. doi:10.1016/S1474-4422(19)30287-X
Boiangiu, R. S., Mihasan, M., Gorgan, D. L., Stache, B. A., and Hritcu, L. (2021). Anxiolytic, Promnesic, Anti-acetylcholinesterase and Antioxidant Effects of Cotinine and 6-Hydroxy-L-Nicotine in Scopolamine-Induced Zebrafish (Danio rerio) Model of Alzheimer’s Disease. Antioxidants (Basel, Switzerland) 10, 212. doi:10.3390/antiox10020212
Bosco, D. A., Lemay, N., Ko, H. K., Zhou, H., Burke, C., Kwiatkowski, T. J., et al. (2010). Mutant FUS Proteins that Cause Amyotrophic Lateral Sclerosis Incorporate into Stress Granules. Hum. Mol. Genet. 19, 4160–4175. doi:10.1093/hmg/ddq335
Bose, P., Tremblay, E., Maios, C., Narasimhan, V., Armstrong, G. A. B., Liao, M., et al. (2019). The Novel Small Molecule TRVA242 Stabilizes Neuromuscular Junction Defects in Multiple Animal Models of Amyotrophic Lateral Sclerosis. Neurotherapeutics 16, 1149–1166. doi:10.1007/s13311-019-00765-w
Bourefis, A. R., Campanari, M. L., Buee-Scherrer, V., and Kabashi, E. (2020). Functional Characterization of a FUS Mutant Zebrafish Line as a Novel Genetic Model for ALS. Neurobiol. Dis. 142, 104935. doi:10.1016/j.nbd.2020.104935
Bretaud, S., Allen, C., Ingham, P. W., and Bandmann, O. (2007). p53-dependent Neuronal Cell Death in a DJ-1-Deficient Zebrafish Model of Parkinson's Disease. J. Neurochem. 100, 1626–1635. doi:10.1111/j.1471-4159.2006.04291.x
Bretaud, S., Lee, S., and Guo, S. (2004). Sensitivity of Zebrafish to Environmental Toxins Implicated in Parkinson's Disease. Neurotoxicol. Teratol. 26, 857–864. doi:10.1016/j.ntt.2004.06.014
Brown, S. J., Boussaad, I., Jarazo, J., Fitzgerald, J. C., Antony, P., Keatinge, M., et al. (2021). PINK1 Deficiency Impairs Adult Neurogenesis of Dopaminergic Neurons. Sci. Rep. 11, 6617. doi:10.1038/s41598-021-84278-7
Campanari, M.-L., Marian, A., Ciura, S., and Kabashi, E. (2021). TDP-43 Regulation of AChE Expression Can Mediate ALS-like Phenotype in Zebrafish. Cells 10, 221. doi:10.3390/cells10020221
Cao, Z., Su, M., Wang, H., Zhou, L., Meng, Z., Xiong, G., et al. (2021). Carboxyl Graphene Oxide Nanoparticles Induce Neurodevelopmental Defects and Locomotor Disorders in Zebrafish Larvae. Chemosphere 270, 128611. doi:10.1016/j.chemosphere.2020.128611
Capatina, L., Todirascu-Ciornea, E., Napoli, E. M., Ruberto, G., Hritcu, L., and Dumitru, G. (2020). Thymus Vulgaris Essential Oil Protects Zebrafish against Cognitive Dysfunction by Regulating Cholinergic and Antioxidants Systems. Antioxidants (Basel, Switzerland) 9, 1083. doi:10.3390/antiox9111083
Chen, M., Martins, R. N., and Lardelli, M. (2009). Complex Splicing and Neural Expression of Duplicated Tau Genes in Zebrafish Embryos. J. Alzheimers. Dis. 18, 305–317. doi:10.3233/JAD-2009-1145
Chen, Y., Li, G., Law, H. C. H., Chen, H., and Lee, S. M. (2020). Determination of Oxyphylla A Enantiomers in the Fruits of Alpinia Oxyphylla by a Chiral High-Performance Liquid Chromatography-Multiple Reaction Monitoring-Mass Spectrometry Method and Comparison of Their In Vivo Biological Activities. J. Agric. Food Chem. 68, 11170–11181. doi:10.1021/acs.jafc.0c04031
Chiu, A. S., Gehringer, M. M., Welch, J. H., and Neilan, B. A. (2011). Does α-amino-β-methylaminopropionic Acid (BMAA) Play a Role in Neurodegeneration? Int. J. Environ. Res. Public Health 8, 3728–3746. doi:10.3390/ijerph8093728
Corman, A., Jung, B., Häggblad, M., Bräutigam, L., Lafarga, V., Lidemalm, L., et al. (2019). A Chemical Screen Identifies Compounds Limiting the Toxicity of C9ORF72 Dipeptide Repeats. Cell Chem. Biol. 26, 235–e5. doi:10.1016/j.chembiol.2018.10.020
Cosacak, M. I., Bhattarai, P., Bocova, L., Dzewas, T., Mashkaryan, V., Papadimitriou, C., et al. (2017). Human TAUP301L Overexpression Results in TAU Hyperphosphorylation without Neurofibrillary Tangles in Adult Zebrafish Brain. Sci. Rep. 7, 12959. doi:10.1038/s41598-017-13311-5
Cronin, A., and Grealy, M. (2017). Neuroprotective and Neuro-Restorative Effects of Minocycline and Rasagiline in a Zebrafish 6-Hydroxydopamine Model of Parkinson's Disease. Neuroscience 367, 34–46. doi:10.1016/j.neuroscience.2017.10.018
Díaz-Casado, M. E., Lima, E., García, J. A., Doerrier, C., Aranda, P., Sayed, R. K., et al. (2016). Melatonin Rescues Zebrafish Embryos from the Parkinsonian Phenotype Restoring the parkin/PINK1/DJ-1/MUL1 Network. J. Pineal Res. 61, 96–107. doi:10.1111/jpi.12332
Duan, W. J., Liang, L., Pan, M. H., Lu, D. H., Wang, T. M., Li, S. B., et al. (2020). Theacrine, a Purine Alkaloid from Kucha, Protects against Parkinson's Disease through SIRT3 Activation. Phytomedicine 77, 153281. doi:10.1016/j.phymed.2020.153281
Dukes, A. A., Bai, Q., Van Laar, V. S., Zhou, Y., Ilin, V., David, C. N., et al. (2016). Live Imaging of Mitochondrial Dynamics in CNS Dopaminergic Neurons In Vivo Demonstrates Early Reversal of Mitochondrial Transport Following MPP(+) Exposure. Neurobiol. Dis. 95, 238–249. doi:10.1016/j.nbd.2016.07.020
DuVal, M. G., Hinge, V. K., Snyder, N., Kanyo, R., Bratvold, J., Pokrishevsky, E., et al. (2019). Tryptophan 32 Mediates SOD1 Toxicity in a In Vivo Motor Neuron Model of ALS and Is a Promising Target for Small Molecule Therapeutics. Neurobiol. Dis. 124, 297–310. doi:10.1016/j.nbd.2018.11.025
Edson, A. J., Hushagen, H. A., Frøyset, A. K., Elda, I., Khan, E. A., Di Stefano, A., et al. (2019). Dysregulation in the Brain Protein Profile of Zebrafish Lacking the Parkinson's Disease-Related Protein DJ-1. Mol. Neurobiol. 56, 8306–8322. doi:10.1007/s12035-019-01667-w
Feng, C.-W., Chen, N.-F., Sung, C.-S., Kuo, H.-M., Yang, S.-N., Chen, C.-L., et al. (2019). Therapeutic Effect of Modulating TREM-1 via Anti-inflammation and Autophagy in Parkinson's Disease. Front. Neurosci. 13, 1–18. doi:10.3389/fnins.2019.00769
Feng, C. W., Wen, Z. H., Huang, S. Y., Hung, H. C., Chen, C. H., Yang, S. N., et al. (2014). Effects of 6-hydroxydopamine Exposure on Motor Activity and Biochemical Expression in Zebrafish (Danio rerio) Larvae. Zebrafish 11, 227–239. doi:10.1089/zeb.2013.0950
Fett, M. E., Pilsl, A., Paquet, D., van Bebber, F., Haass, C., Tatzelt, J., et al. (2010). Parkin Is Protective against Proteotoxic Stress in a Transgenic Zebrafish Model. PLoS One 5, e11783. doi:10.1371/journal.pone.0011783
Flinn, L., Mortiboys, H., Volkmann, K., Köster, R. W., Ingham, P. W., and Bandmann, O. (2009). Complex I Deficiency and Dopaminergic Neuronal Cell Loss in Parkin-Deficient Zebrafish (Danio rerio). Brain 132, 1613–1623. doi:10.1093/brain/awp108
Flinn, L. J., Keatinge, M., Bretaud, S., Mortiboys, H., Matsui, H., De Felice, E., et al. (2013). TigarB Causes Mitochondrial Dysfunction and Neuronal Loss in PINK1 Deficiency. Ann. Neurol. 74, 837–847. doi:10.1002/ana.23999
Gammon, K. (2014). Neurodegenerative Disease: Brain Windfall. Nature 515, 299–300. doi:10.1038/nj7526-299a
Goedert, M., and Spillantini, M. G. (2019). Ordered Assembly of Tau Protein and Neurodegeneration. Adv. Exp. Med. Biol. 1184, 3–21. doi:10.1007/978-981-32-9358-8_1
Gois, A. M., Mendonça, D. M. F., Freire, M. A. M., and Santos, J. R. (2020). In Vitro AND In Vivo MODELS OF AMYOTROPHIC LATERAL SCLEROSIS: AN UPDATED OVERVIEW. Brain Res. Bull. 159, 32–43. doi:10.1016/j.brainresbull.2020.03.012
Goldshtein, H., Muhire, A., Petel Légaré, V., Pushett, A., Rotkopf, R., Shefner, J. M., et al. (2020). Efficacy of Ciprofloxacin/Celecoxib Combination in Zebrafish Models of Amyotrophic Lateral Sclerosis. Ann. Clin. Transl. Neurol. 7, 1883–1897. doi:10.1002/acn3.51174
Greenamyre, J. T., and Young, A. B. (1989). Excitatory Amino Acids and Alzheimer's Disease. Neurobiol. Aging 10, 593–602. doi:10.1016/0197-4580(89)90143-7
Han, X., Jing, Z., Wu, W., Zou, B., Peng, Z., Ren, P., et al. (2017). Biocompatible and Blood-Brain Barrier Permeable Carbon Dots for Inhibition of Aβ Fibrillation and Toxicity, and BACE1 Activity. Nanoscale 9, 12862–12866. doi:10.1039/c7nr04352j
Hin, N., Newman, M., Kaslin, J., Douek, A. M., Lumsden, A., Nik, S. H. M., et al. (2020). Accelerated Brain Aging towards Transcriptional Inversion in a Zebrafish Model of the K115fs Mutation of Human PSEN2. PLoS One 15, e0227258. doi:10.1371/journal.pone.0227258
Hou, Y., Dan, X., Babbar, M., Wei, Y., Hasselbalch, S. G., Croteau, D. L., et al. (2019). Ageing as a Risk Factor for Neurodegenerative Disease. Nat. Rev. Neurol. 15, 565–581. doi:10.1038/s41582-019-0244-7
Hu, Y. H., Yang, J., Zhang, Y., Liu, K. C., Liu, T., Sun, J., et al. (2019). Synthesis and Biological Evaluation of 3-(4-Aminophenyl)-Coumarin Derivatives as Potential Anti-alzheimer's Disease Agents. J. Enzyme Inhib. Med. Chem. 34, 1083–1092. doi:10.1080/14756366.2019.1615484
Hu, Z. Y., Chen, B., Zhang, J. P., and Ma, Y. Y. (2017). Up-regulation of Autophagy-Related Gene 5 (ATG5) Protects Dopaminergic Neurons in a Zebrafish Model of Parkinson's Disease. J. Biol. Chem. 292, 18062–18074. doi:10.1074/jbc.M116.764795
Hughes, G. L., Lones, M. A., Bedder, M., Currie, P. D., Smith, S. L., and Pownall, M. E. (2020). Machine Learning Discriminates a Movement Disorder in a Zebrafish Model of Parkinson's Disease. Dis. Model. Mech. 13, dmm045815. doi:10.1242/dmm.045815
Javed, I., Peng, G., Xing, Y., Yu, T., Zhao, M., Kakinen, A., et al. (2019). Inhibition of Amyloid Beta Toxicity in Zebrafish with a Chaperone-Gold Nanoparticle Dual Strategy. Nat. Commun. 10, 3780. doi:10.1038/s41467-019-11762-0
Jin, M., Li, N., Sheng, W., Ji, X., Liang, X., Kong, B., et al. (2021). Toxicity of Different Zinc Oxide Nanomaterials and Dose-dependent Onset and Development of Parkinson's Disease-like Symptoms Induced by Zinc Oxide Nanorods. Environ. Int. 146, 106179. doi:10.1016/j.envint.2020.106179
Kabir, M. T., Uddin, M. S., Setu, J. R., Ashraf, G. M., Bin-Jumah, M. N., and Abdel-Daim, M. M. (2020). Exploring the Role of PSEN Mutations in the Pathogenesis of Alzheimer's Disease. Neurotox. Res. 38, 833–849. doi:10.1007/s12640-020-00232-x
Kalyn, M., Hua, K., Mohd Noor, S., Wong, C. E. D., and Ekker, M. (2019). Comprehensive Analysis of Neurotoxin-Induced Ablation of Dopaminergic Neurons in Zebrafish Larvae. Biomedicines 8, 1. doi:10.3390/biomedicines8010001
Kang, S. S., Ahn, E. H., Zhang, Z., Liu, X., Manfredsson, F. P., Sandoval, I. M., et al. (2018). α-Synuclein Stimulation of Monoamine Oxidase-B and Legumain Protease Mediates the Pathology of Parkinson's Disease. EMBO J. 37, e98878. doi:10.15252/embj.201798878
Kanyo, R., Leighton, P. L. A., Neil, G. J., Locskai, L. F., and Allison, W. T. (2020). Amyloid-β Precursor Protein Mutant Zebrafish Exhibit Seizure Susceptibility that Depends on Prion Protein. Exp. Neurol. 328, 113283. doi:10.1016/j.expneurol.2020.113283
Kesh, S., Kannan, R. R., Sivaji, K., and Balakrishnan, A. (2021b). Hesperidin Downregulates Kinases Lrrk2 and Gsk3β in a 6-OHDA Induced Parkinson's Disease Model. Neurosci. Lett. 740, 135426. doi:10.1016/j.neulet.2020.135426
Kesh, S., Kannan, R. R., and Balakrishnan, A. (2021a). Naringenin Alleviates 6-hydroxydopamine Induced Parkinsonism in SHSY5Y Cells and Zebrafish Model. Comp. Biochem. Physiol. C: Toxicol. Pharmacol. 239, 108893. doi:10.1016/j.cbpc.2020.108893
Kim, Y. H., Lee, Y., Kim, D., Jung, M. W., and Lee, C. J. (2010). Scopolamine-induced Learning Impairment Reversed by Physostigmine in Zebrafish. Neurosci. Res. 67, 156–161. doi:10.1016/j.neures.2010.03.003
Koehler, D., Shah, Z. A., Hensley, K., and Williams, F. E. (2018). Lanthionine Ketimine-5-Ethyl Ester Provides Neuroprotection in a Zebrafish Model of Okadaic Acid-Induced Alzheimer's Disease. Neurochem. Int. 115, 61–68. doi:10.1016/j.neuint.2018.02.002
Koehler, D., Shah, Z. A., and Williams, F. E. (2019). The GSK3β Inhibitor, TDZD-8, Rescues Cognition in a Zebrafish Model of Okadaic Acid-Induced Alzheimer's Disease. Neurochem. Int. 122, 31–37. doi:10.1016/j.neuint.2018.10.022
Koppel, K., Tang, H., Javed, I., Parsa, M., Mortimer, M., Davis, T. P., et al. (2020). Elevated Amyloidoses of Human IAPP and Amyloid Beta by Lipopolysaccharide and Their Mitigation by Carbon Quantum Dots. Nanoscale 12, 12317–12328. doi:10.1039/d0nr02710c
Kozioł, E., Skalicka-Woźniak, K., Michalak, A., Kaszubska, K., and Budzyńska, B. (2021). Xanthotoxin Reverses Parkinson's Disease-like Symptoms in Zebrafish Larvae and Mice Models: a Comparative Study. Pharmacol. Rep. 73, 122–129. doi:10.1007/s43440-020-00136-9
Kramer, N. J., Haney, M. S., Morgens, D. W., Jovičić, A., Couthouis, J., Li, A., et al. (2018). CRISPR-Cas9 Screens in Human Cells and Primary Neurons Identify Modifiers of C9ORF72 Dipeptide-Repeat-Protein Toxicity. Nat. Genet. 50, 603–612. doi:10.1038/s41588-018-0070-7
Lam, C. S., Korzh, V., and Strahle, U. (2005). Zebrafish Embryos Are Susceptible to the Dopaminergic Neurotoxin MPTP. Eur. J. Neurosci. 21, 1758–1762. doi:10.1111/j.1460-9568.2005.03988.x
Langston, J. W., Ballard, P., Tetrud, J. W., and Irwin, I. (1983). Chronic Parkinsonism in Humans Due to a Product of Meperidine-Analog Synthesis. Science 219, 979–980. doi:10.1126/science.6823561
Lattante, S., De Calbiac, H., Le Ber, I., Brice, A., Ciura, S., and Kabashi, E. (2015). Sqstm1 Knock-Down Causes a Locomotor Phenotype Ameliorated by Rapamycin in a Zebrafish Model of ALS/FTLD. Hum. Mol. Genet. 24, 1682–1690. doi:10.1093/hmg/ddu580
Lee, J., and Freeman, J. L. (2020). Exposure to the Heavy-Metal Lead Induces DNA Copy Number Alterations in Zebrafish Cells. Chem. Res. Toxicol. 33, 2047–2053. doi:10.1021/acs.chemrestox.0c00156
Li, Z., Cao, P., Meng, H., Li, D., Zhang, Y., Li, Y., et al. (2021). Long-term Exposure to 2-Amino-3-Methylimidazo[4,5-F]quinoline Can Trigger a Potential Risk of Parkinson's Disease. J. Hazard. Mater. 412, 125230. doi:10.1016/j.jhazmat.2021.125230
Lieschke, G. J., and Currie, P. D. (2007). Animal Models of Human Disease: Zebrafish Swim into View. Nat. Rev. Genet. 8, 353–367. doi:10.1038/nrg2091
Lo Monte, F., Kramer, T., Gu, J., Brodrecht, M., Pilakowski, J., Fuertes, A., et al. (2013). Structure-based Optimization of Oxadiazole-Based GSK-3 Inhibitors. Eur. J. Med. Chem. 61, 26–40. doi:10.1016/j.ejmech.2012.06.006
Lopez, A., Lee, S. E., Wojta, K., Ramos, E. M., Klein, E., Chen, J., et al. (2017). A152T Tau Allele Causes Neurodegeneration that Can Be Ameliorated in a Zebrafish Model by Autophagy Induction. Brain 140, 1128–1146. doi:10.1093/brain/awx005
Louzada, P. R., Lima, A. C. P., Mendonca‐Silva, D. L., Noël, F., De Mello, F. G., and Ferreira, S. T. (2004). Taurine Prevents the Neurotoxicity of β‐amyloid and Glutamate Receptor Agonists: Activation of GABA Receptors and Possible Implications for Alzheimer's Disease and Other Neurological Disorders. FASEB j. 18, 511–518. doi:10.1096/fj.03-0739com
Lumsden, A. L., Henshall, T. L., Dayan, S., Lardelli, M. T., and Richards, R. I. (2007). Huntingtin-deficient Zebrafish Exhibit Defects in Iron Utilization and Development. Hum. Mol. Genet. 16, 1905–1920. doi:10.1093/hmg/ddm138
Lv, D. J., Li, L. X., Chen, J., Wei, S. Z., Wang, F., Hu, H., et al. (2019). Sleep Deprivation Caused a Memory Defects and Emotional Changes in a Rotenone-Based Zebrafish Model of Parkinson's Disease. Behav. Brain Res. 372, 112031. doi:10.1016/j.bbr.2019.112031
Martin, R. M., Bereman, M. S., and Marsden, K. C. (2021). BMAA and MCLR Interact to Modulate Behavior and Exacerbate Molecular Changes Related to Neurodegeneration in Larval Zebrafish. Toxicol. Sci. 179, 251–261. doi:10.1093/toxsci/kfaa178
McGown, A., Shaw, D. P., and Ramesh, T. (2016). ZNStress: A High-Throughput Drug Screening Protocol for Identification of Compounds Modulating Neuronal Stress in the Transgenic Mutant sod1G93R Zebrafish Model of Amyotrophic Lateral Sclerosis. Mol. Neurodegener. 11, 56–11. doi:10.1186/s13024-016-0122-3
McKinley, E. T., Baranowski, T. C., Blavo, D. O., Cato, C., Doan, T. N., and Rubinstein, A. L. (2005). Neuroprotection of MPTP-Induced Toxicity in Zebrafish Dopaminergic Neurons. Brain Res. Mol. Brain Res. 141, 128–137. doi:10.1016/j.molbrainres.2005.08.014
Merhi, R., Kalyn, M., Zhu-Pawlowsky, A., and Ekker, M. (2021). Loss of Parla Function Results in Inactivity, Olfactory Impairment, and Dopamine Neuron Loss in Zebrafish. Biomedicines 9, 205. doi:10.3390/biomedicines9020205
Milanese, C., Sager, J. J., Bai, Q., Farrell, T. C., Cannon, J. R., Greenamyre, J. T., et al. (2012). Hypokinesia and Reduced Dopamine Levels in Zebrafish Lacking β- and γ1-synucleins. J. Biol. Chem. 287, 2971–2983. doi:10.1074/jbc.M111.308312
Miller, V. M., Nelson, R. F., Gouvion, C. M., Williams, A., Rodriguez-Lebron, E., Harper, S. Q., et al. (2005). CHIP Suppresses Polyglutamine Aggregation and Toxicity In Vitro and In Vivo. J. Neurosci. 25, 9152–9161. doi:10.1523/JNEUROSCI.3001-05.2005
Monte, F. L., Kramer, T., Boländer, A., Plotkin, B., Eldar-Finkelman, H., Fuertes, A., et al. (2011). Synthesis and Biological Evaluation of Glycogen Synthase Kinase 3 (GSK-3) Inhibitors: An Fast and Atom Efficient Access to 1-Aryl-3-Benzylureas. Bioorg. Med. Chem. Lett. 21, 5610–5615. doi:10.1016/j.bmcl.2011.06.131
Morrice, J. R., Gregory-Evans, C. Y., and Shaw, C. A. (2018a). Animal Models of Amyotrophic Lateral Sclerosis: A Comparison of Model Validity. Neural Regen. Res. 13, 2050–2054. doi:10.4103/1673-5374.241445
Morrice, J. R., Gregory-Evans, C. Y., and Shaw, C. A. (2018b). Modeling Environmentally-Induced Motor Neuron Degeneration in Zebrafish. Sci. Rep. 8, 4890. doi:10.1038/s41598-018-23018-w
Musa, A., Lehrach, H., and Russo, V. A. (2001). Distinct Expression Patterns of Two Zebrafish Homologues of the Human APP Gene during Embryonic Development. Dev. Genes Evol. 211, 563–567. doi:10.1007/s00427-001-0189-9
Nada, S. E., Williams, F. E., and Shah, Z. A. (2016). Development of a Novel and Robust Pharmacological Model of Okadaic Acid-Induced Alzheimer's Disease in Zebrafish. CNS Neurol. Disord. Drug Targets 15, 86–94. doi:10.2174/1871527314666150821105602
Nellore, J., and P., N. (2015). Paraquat Exposure Induces Behavioral Deficits in Larval Zebrafish during the Window of Dopamine Neurogenesis. Toxicol. Rep. 2, 950–956. doi:10.1016/j.toxrep.2015.06.007
Nery, L. R., Eltz, N. S., Hackman, C., Fonseca, R., Altenhofen, S., Guerra, H. N., et al. (2014). Brain Intraventricular Injection of Amyloid-β in Zebrafish Embryo Impairs Cognition and Increases Tau Phosphorylation, Effects Reversed by Lithium. PLoS One 9, e105862. doi:10.1371/journal.pone.0105862
Nery, L. R., Silva, N. E., Fonseca, R., and Vianna, M. R. M. (2017). Presenilin-1 Targeted Morpholino Induces Cognitive Deficits, Increased Brain Aβ1-42 and Decreased Synaptic Marker PSD-95 in Zebrafish Larvae. Neurochem. Res. 42, 2959–2967. doi:10.1007/s11064-017-2327-4
Noble, S., Godoy, R., Affaticati, P., and Ekker, M. (2015). Transgenic Zebrafish Expressing mCherry in the Mitochondria of Dopaminergic Neurons. Zebrafish 12, 349–356. doi:10.1089/zeb.2015.1085
Nornes, S., Newman, M., Wells, S., Verdile, G., Martins, R. N., and Lardelli, M. (2009). Independent and Cooperative Action of Psen2 with Psen1 in Zebrafish Embryos. Exp. Cell Res. 315, 2791–2801. doi:10.1016/j.yexcr.2009.06.023
Nunes, M. E., Müller, T. E., Braga, M. M., Fontana, B. D., Quadros, V. A., Marins, A., et al. (2017). Chronic Treatment with Paraquat Induces Brain Injury, Changes in Antioxidant Defenses System, and Modulates Behavioral Functions in Zebrafish. Mol. Neurobiol. 54, 3925–3934. doi:10.1007/s12035-016-9919-x
Özcan, G. G., Lim, S., Leighton, P., Allison, W. T., and Rihel, J. (2020). Sleep Is Bi-directionally Modified by Amyloid Beta Oligomers. Elife 9, e53995. doi:10.7554/eLife.53995
Paik, H., Chung, A.-Y., Park, H.-C., Park, R. W., Suk, K., Kim, J., et al. (2015). Repurpose Terbutaline Sulfate for Amyotrophic Lateral Sclerosis Using Electronic Medical Records. Sci. Rep. 5, 1–8. doi:10.1038/srep08580
Pan, H., Zhang, J., Wang, Y., Cui, K., Cao, Y., Wang, L., et al. (2019). Linarin Improves the Dyskinesia Recovery in Alzheimer's Disease Zebrafish by Inhibiting the Acetylcholinesterase Activity. Life Sci. 222, 112–116. doi:10.1016/j.lfs.2019.02.046
Paquet, D., Bhat, R., Sydow, A., Mandelkow, E. M., Berg, S., Hellberg, S., et al. (2009). A Zebrafish Model of Tauopathy Allows In Vivo Imaging of Neuronal Cell Death and Drug Evaluation. J. Clin. Invest. 119, 1382–1395. doi:10.1172/JCI37537
Parng, C., Roy, N. M., Ton, C., Lin, Y., and McGrath, P. (2007). Neurotoxicity Assessment Using Zebrafish. J. Pharmacol. Toxicol. Methods 55, 103–112. doi:10.1016/j.vascn.2006.04.004
Patten, S. A., Aggad, D., Martinez, J., Tremblay, E., Petrillo, J., Armstrong, G. A. B., et al. (2017). Neuroleptics as Therapeutic Compounds Stabilizing Neuromuscular Transmission in Amyotrophic Lateral Sclerosis. JCI insight 2, 1–20. doi:10.1172/jci.insight.97152
Petit, A., Pasini, A., Alves da Costa, C., Ayral, E., Hernandez, J. F., Dumanchin-Njock, C., et al. (2003). JLK Isocoumarin Inhibitors: Selective Gamma-Secretase Inhibitors that Do Not Interfere with Notch Pathway In Vitro or In Vivo. J. Neurosci. Res. 74, 370–377. doi:10.1002/jnr.10747
Pinho, B. R., Reis, S. D., Hartley, R. C., Murphy, M. P., and Oliveira, J. M. A. (2019). Mitochondrial Superoxide Generation Induces a Parkinsonian Phenotype in Zebrafish and Huntingtin Aggregation in Human Cells. Free Radic. Biol. Med. 130, 318–327. doi:10.1016/j.freeradbiomed.2018.10.446
Pitchai, A., Rajaretinam, R. K., and Freeman, J. L. (2019). Zebrafish as an Emerging Model for Bioassay-Guided Natural Product Drug Discovery for Neurological Disorders. Medicines 6, 61. doi:10.3390/medicines6020061
Prabhudesai, S., Bensabeur, F. Z., Abdullah, R., Basak, I., Baez, S., Alves, G., et al. (2016). LRRK2 Knockdown in Zebrafish Causes Developmental Defects, Neuronal Loss, and Synuclein Aggregation. J. Neurosci. Res. 94, 717–735. doi:10.1002/jnr.23754
Pu, Y. Z., Liang, L., Fu, A. L., Liu, Y., Sun, L., Li, Q., et al. (2017). Generation of Alzheimer's Disease Transgenic Zebrafish Expressing Human APP Mutation under Control of Zebrafish Appb Promotor. Curr. Alzheimer Res. 14, 668–679. doi:10.2174/1567205013666161201202000
Randlett, O., Wee, C. L., Naumann, E. A., Nnaemeka, O., Schoppik, D., Fitzgerald, J. E., et al. (2015). Whole-brain Activity Mapping onto a Zebrafish Brain Atlas. Nat. Methods 12, 1039–1046. doi:10.1038/nmeth.3581
Reinhardt, L., Kordes, S., Reinhardt, P., Glatza, M., Baumann, M., Drexler, H. C. A., et al. (2019). Dual Inhibition of GSK3β and CDK5 Protects the Cytoskeleton of Neurons from Neuroinflammatory-Mediated Degeneration In Vitro and In Vivo. Stem Cell Rep. 12, 502–517. doi:10.1016/j.stemcr.2019.01.015
Ren, G., Xin, S., Li, S., Zhong, H., and Lin, S. (2011). Disruption of LRRK2 Does Not Cause Specific Loss of Dopaminergic Neurons in Zebrafish. PLoS One 6, e20630. doi:10.1371/journal.pone.0020630
Rishitha, N., and Muthuraman, A. (2018). Therapeutic Evaluation of Solid Lipid Nanoparticle of Quercetin in Pentylenetetrazole Induced Cognitive Impairment of Zebrafish. Life Sci. 199, 80–87. doi:10.1016/j.lfs.2018.03.010
Robb, E. L., Gawel, J. M., Aksentijević, D., Cochemé, H. M., Stewart, T. S., Shchepinova, M. M., et al. (2015). Selective Superoxide Generation within Mitochondria by the Targeted Redox Cycler MitoParaquat. Free Radic. Biol. Med. 89, 883–894. doi:10.1016/j.freeradbiomed.2015.08.021
Sakowski, S. A., Lunn, J. S., Busta, A. S., Oh, S. S., Zamora-Berridi, G., Palmer, M., et al. (2012). Neuromuscular Effects of G93A-SOD1 Expression in Zebrafish. Mol. Neurodegener. 7, 44. doi:10.1186/1750-1326-7-44
Sanderson, L. E., Lanko, K., Alsagob, M., Almass, R., Al-Ahmadi, N., Najafi, M., et al. (2021). Bi-allelic Variants in HOPS Complex Subunit VPS41 Cause Cerebellar Ataxia and Abnormal Membrane Trafficking. Brain 144, 769–780. doi:10.1093/brain/awaa459
Sang, Z., Wang, K., Han, X., Cao, M., Tan, Z., and Liu, W. (2019). Design, Synthesis, and Evaluation of Novel Ferulic Acid Derivatives as Multi-Target-Directed Ligands for the Treatment of Alzheimer’s Disease. ACS Chem. Neurosci. 10, 1008–1024. doi:10.1021/acschemneuro.8b00530
Santo, G. D., de Veras, B. O., Rico, E., Magro, J. D., Agostini, J. F., Vieira, L. D., et al. (2021). Hexane Extract from SpoSndias Mombin L. (Anacardiaceae) Prevents Behavioral and Oxidative Status Changes on Model of Parkinson's Disease in Zebrafish. Comp. Biochem. Physiol. C. Toxicol. Pharmacol. 241, 108953. doi:10.1016/j.cbpc.2020.108953
Schiffer, N. W., Broadley, S. A., Hirschberger, T., Tavan, P., Kretzschmar, H. A., Giese, A., et al. (2007). Identification of Anti-prion Compounds as Efficient Inhibitors of Polyglutamine Protein Aggregation in a Zebrafish Model. J. Biol. Chem. 282, 9195–9203. doi:10.1074/jbc.M607865200
Senger, M. R., Seibt, K. J., Ghisleni, G. C., Dias, R. D., Bogo, M. R., and Bonan, C. D. (2011). Aluminum Exposure Alters Behavioral Parameters and Increases Acetylcholinesterase Activity in Zebrafish (Danio rerio) Brain. Cell Biol. Toxicol. 27, 199–205. doi:10.1007/s10565-011-9181-y
Shaw, M. P., Higginbottom, A., McGown, A., Castelli, L. M., James, E., Hautbergue, G. M., et al. (2018). Stable Transgenic C9orf72 Zebrafish Model Key Aspects of the ALS/FTD Phenotype and Reveal Novel Pathological Features. Acta Neuropathol. Commun. 6, 125. doi:10.1186/s40478-018-0629-7
Sheng, D., Qu, D., Kwok, K. H., Ng, S. S., Lim, A. Y., Aw, S. S., et al. (2010). Deletion of the WD40 Domain of LRRK2 in Zebrafish Causes Parkinsonism-like Loss of Neurons and Locomotive Defect. Plos Genet. 6, e1000914. doi:10.1371/journal.pgen.1000914
Sher, R. B. (2017). The Interaction of Genetics and Environmental Toxicants in Amyotrophic Lateral Sclerosis: Results from Animal Models. Neural Regen. Res. 12, 902–905. doi:10.4103/1673-5374.208564
Soll, M., Goldshtein, H., Rotkopf, R., Russek-blum, N., and Gross, Z. (2021). A Synthetic SOD/Catalase Mimic Compound for the Treatment of ALS. Antioxidants (Basel) 10, 827. doi:10.3390/antiox10060827
Soman, S., Keatinge, M., Moein, M., Da Costa, M., Mortiboys, H., Skupin, A., et al. (2017). Inhibition of the Mitochondrial Calcium Uniporter Rescues Dopaminergic Neurons in Pink1-/- Zebrafish. Eur. J. Neurosci. 45, 528–535. doi:10.1111/ejn.13473
Sonawane, S. K., and Chinnathambi, S. (2018). Prion-Like Propagation of Post-Translationally Modified Tau in Alzheimer's Disease: A Hypothesis. J. Mol. Neurosci. 65, 480–490. doi:10.1007/s12031-018-1111-5
Streisinger, G., Walker, C., Dower, N., Knauber, D., and Singer, F. (1981). Production of Clones of Homozygous Diploid Zebra Fish (Brachydanio Rerio). Nature 291, 293–296. doi:10.1038/291293a0
Sun, Y., He, L., Wang, T., Hua, W., Qin, H., Wang, J., et al. (2020). Activation of P62-Keap1-Nrf2 Pathway Protects 6-Hydroxydopamine-Induced Ferroptosis in Dopaminergic Cells. Mol. Neurobiol. 57, 4628–4641. doi:10.1007/s12035-020-02049-3
Sundvik, M., Chen, Y. C., and Panula, P. (2013). Presenilin1 Regulates Histamine Neuron Development and Behavior in Zebrafish, danio Rerio. J. Neurosci. 33, 1589–1597. doi:10.1523/JNEUROSCI.1802-12.2013
Swaminathan, A., Bouffard, M., Liao, M., Ryan, S., Callister, J. B., Pickering-Brown, S. M., et al. (2018). Expression of C9orf72-Related Dipeptides Impairs Motor Function in a Vertebrate Model. Hum. Mol. Genet. 27, 1754–1762. doi:10.1093/hmg/ddy083
Tecalco-Cruz, A. C., Ramírez-Jarquín, J. O., Alvarez-Sánchez, M. E., and Zepeda-Cervantes, J. (2020). Epigenetic Basis of Alzheimer Disease. World J. Biol. Chem. 11, 62–75. doi:10.4331/wjbc.v11.i2.62
Uddin, M. S., Kabir, M. T., Rahman, M. S., Behl, T., Jeandet, P., Ashraf, G. M., et al. (2020). Revisiting the Amyloid Cascade Hypothesis: From Anti-aβ Therapeutics to Auspicious New Ways for Alzheimer's Disease. Int. J. Mol. Sci. 21, 5858. doi:10.3390/ijms21165858
Um, J. W., Nygaard, H. B., Heiss, J. K., Kostylev, M. A., Stagi, M., Vortmeyer, A., et al. (2012). Alzheimer Amyloid-β Oligomer Bound to Postsynaptic Prion Protein Activates Fyn to Impair Neurons. Nat. Neurosci. 15, 1227–1235. doi:10.1038/nn.3178
Vaccaro, A., Patten, S. A., Ciura, S., Maios, C., Therrien, M., Drapeau, P., et al. (2012). Methylene Blue Protects against TDP-43 and FUS Neuronal Toxicity in C. elegans and D. rerio. PLoS One 7, e42117. doi:10.1371/journal.pone.0042117
Van Damme, P., Robberecht, W., and Van Den Bosch, L. (2017). Modelling Amyotrophic Lateral Sclerosis: Progress and Possibilities. Dis. Model. Mech. 10, 537–549. doi:10.1242/dmm.029058
Van Laar, V. S., Chen, J., Zharikov, A. D., Bai, Q., Di Maio, R., Dukes, A. A., et al. (2020). α-Synuclein Amplifies Cytoplasmic Peroxide Flux and Oxidative Stress Provoked by Mitochondrial Inhibitors in CNS Dopaminergic Neurons In Vivo. Redox Biol. 37, 101695. doi:10.1016/j.redox.2020.101695
Vaquer-Alicea, J., and Diamond, M. I. (2019). Propagation of Protein Aggregation in Neurodegenerative Diseases. Annu. Rev. Biochem. 88, 785–810. doi:10.1146/annurev-biochem-061516-045049
Vaz, R. L., Outeiro, T. F., and Ferreira, J. J. (2018). Zebrafish as an Animal Model for Drug Discovery in Parkinson's Disease and Other Movement Disorders: A Systematic Review. Front. Neurol. 9, 347. doi:10.3389/fneur.2018.00347
Vaz, R. L., Sousa, S., Chapela, D., van der Linde, H. C., Willemsen, R., Correia, A. D., et al. (2020). Identification of Antiparkinsonian Drugs in the 6-hydroxydopamine Zebrafish Model. Pharmacol. Biochem. Behav. 189, 172828. doi:10.1016/j.pbb.2019.172828
VerPlank, J. J. S., Tyrkalska, S. D., Fleming, A., Rubinsztein, D. C., and Goldberg, A. L. (2020). cGMP via PKG Activates 26S Proteasomes and Enhances Degradation of Proteins, Including Ones that Cause Neurodegenerative Diseases. Proc. Natl. Acad. Sci. U. S. A. 117, 14220–14230. doi:10.1073/pnas.2003277117
Vijayanathan, Y., Lim, F. T., Lim, S. M., Long, C. M., Tan, M. P., Majeed, A. B. A., et al. (2017). 6-OHDA-Lesioned Adult Zebrafish as a Useful Parkinson's Disease Model for Dopaminergic Neuroregeneration. Neurotox. Res. 32, 496–508. doi:10.1007/s12640-017-9778-x
Walker, F. O. (2007). Huntington's Disease. The Lancet 369, 218–228. doi:10.1016/S0140-6736(07)60111-1
Walter, J., Grünberg, J., Schindzielorz, A., and Haass, C. (1998). Proteolytic Fragments of the Alzheimer's Disease Associated Presenilins-1 and -2 Are Phosphorylated In Vivo by Distinct Cellular Mechanisms. Biochemistry 37, 5961–5967. doi:10.1021/bi971763a
Wang, K., Shi, J., Zhou, Y., He, Y., Mi, J., Yang, J., et al. (2021). Design, Synthesis and Evaluation of Cinnamic Acid Hybrids as Multi-Target-Directed Agents for the Treatment of Alzheimer's Disease. Bioorg. Chem. 112, 104879. doi:10.1016/j.bioorg.2021.104879
Wang, L., Yin, Y. L., Liu, X. Z., Shen, P., Zheng, Y. G., Lan, X. R., et al. (2020a). Current Understanding of Metal Ions in the Pathogenesis of Alzheimer's Disease. Transl. Neurodegener. 9, 10. doi:10.1186/s40035-020-00189-z
Wang, S., Qiu, J., Zhao, M., Li, F., Yu, R., and Li, A. (2020b). Accumulation and Distribution of Neurotoxin BMAA in Aquatic Animals and Effect on the Behavior of Zebrafish in a T-Maze Test. Toxicon 173, 39–47. doi:10.1016/j.toxicon.2019.11.005
Wang, Y., Liu, W., Yang, J., Wang, F., Sima, Y., Zhong, Z. M., et al. (2017). Parkinson's Disease-like Motor and Non-motor Symptoms in Rotenone-Treated Zebrafish. Neurotoxicology 58, 103–109. doi:10.1016/j.neuro.2016.11.006
Wang, Z., Wei, X., Yang, J., Suo, J., Chen, J., Liu, X., et al. (2016). Chronic Exposure to Aluminum and Risk of Alzheimer's Disease: A Meta-Analysis. Neurosci. Lett. 610, 200–206. doi:10.1016/j.neulet.2015.11.014
Watts, J. C., Bourkas, M. E. C., and Arshad, H. (2018). The Function of the Cellular Prion Protein in Health and Disease. Acta Neuropathol. 135, 159–178. doi:10.1007/s00401-017-1790-y
Wen, L., Wei, W., Gu, W., Huang, P., Ren, X., Zhang, Z., et al. (2008). Visualization of Monoaminergic Neurons and Neurotoxicity of MPTP in Live Transgenic Zebrafish. Dev. Biol. 314, 84–92. doi:10.1016/j.ydbio.2007.11.012
Williams, F. E., White, D., and Messer, W. S. (2002). A Simple Spatial Alternation Task for Assessing Memory Function in Zebrafish. Behav. Process. 58, 125–132. doi:10.1016/s0376-6357(02)00025-6
Wu, B.-K., Yuan, R.-Y., Lien, H.-W., Hung, C.-C., Hwang, P.-P., Chen, R. P.-Y., et al. (2016). Multiple Signaling Factors and Drugs Alleviate Neuronal Death Induced by Expression of Human and Zebrafish Tau Proteins In Vivo. J. Biomed. Sci. 23, 1–14. doi:10.1186/s12929-016-0237-4
Wu, W., Han, H., Liu, J., Tang, M., Wu, X., Cao, X., et al. (2021). Fucoxanthin Prevents 6-OHDA-Induced Neurotoxicity by Targeting Keap1. Oxid. Med. Cel. Longev. 2021, 6688708. doi:10.1155/2021/6688708
Xi, Y., Yu, M., Godoy, R., Hatch, G., Poitras, L., and Ekker, M. (2011). Transgenic Zebrafish Expressing green Fluorescent Protein in Dopaminergic Neurons of the Ventral Diencephalon. Dev. Dyn. 240, 2539–2547. doi:10.1002/dvdy.22742
Xiong, S., Liu, W., Zhou, Y., Mo, Y., Liu, Y., Chen, X., et al. (2020). Enhancement of Oral Bioavailability and Anti-parkinsonian Efficacy of Resveratrol through a Nanocrystal Formulation. Asian J. Pharm. Sci. 15, 518–528. doi:10.1016/j.ajps.2019.04.003
Yang, J., Zhang, P., Hu, Y., Liu, T., Sun, J., and Wang, X. (2019). Synthesis and Biological Evaluation of 3-arylcoumarins as Potential Anti-alzheimer's Disease Agents. J. Enzyme Inhib. Med. Chem. 34, 651–656. doi:10.1080/14756366.2019.1574297
Yang, T., Arslanova, D., Xu, X., Li, Y. M., and Xia, W. (2010). In Vivo manifestation of Notch Related Phenotypes in Zebrafish Treated with Alzheimer's Amyloid Reducing Gamma-Secretase Inhibitors. J. Neurochem. 113, 1200–1209. doi:10.1111/j.1471-4159.2010.06681.x
Yu, S.-H., Wang, T., Wiggins, K., Louie, R. J., Merino, E. F., Skinner, C., et al. (2021). Lysosomal Cholesterol Accumulation Contributes to the Movement Phenotypes Associated with NUS1 Haploinsufficiency. Genet. Med. doi:10.1038/s41436-021-01137-6
Yurtsever, İ., Üstündağ, Ü. V., Ünal, İ., Ateş, P. S., and Emekli-Alturfan, E. (2020). Rifampicin Decreases Neuroinflammation to Maintain Mitochondrial Function and Calcium Homeostasis in Rotenone-Treated Zebrafish. Drug Chem. Toxicol. doi:10.1080/01480545.2020.1846549
Zanandrea, R., Abreu, M. S., Piato, A., Barcellos, L. J. G., and Giacomini, A. C. V. V. (2018). Lithium Prevents Scopolamine-Induced Memory Impairment in Zebrafish. Neurosci. Lett. 664, 34–37. doi:10.1016/j.neulet.2017.11.010
Zhang, S., Yu, Z., Xia, J., Zhang, X., Liu, K., Sik, A., et al. (2020). Anti-Parkinson's Disease Activity of Phenolic Acids from Eucommia Ulmoides Oliver Leaf Extracts and Their Autophagy Activation Mechanism. Food Funct. 11, 1425–1440. doi:10.1039/c9fo02288k
Zhang, Y., Nguyen, D. T., Olzomer, E. M., Poon, G. P., Cole, N. J., Puvanendran, A., et al. (2017). Rescue of Pink1 Deficiency by Stress-dependent Activation of Autophagy. Cell Chem. Biol. 24, 471–e4. doi:10.1016/j.chembiol.2017.03.005
Zhao, Y., Han, Y., Wang, Z., Chen, T., Qian, H., He, J., et al. (2020). Rosmarinic Acid Protects against 1-Methyl-4-Phenyl-1,2,3,6-Tetrahydropyridine-Induced Dopaminergic Neurotoxicity in Zebrafish Embryos. Toxicol. Vitro 65, 104823. doi:10.1016/j.tiv.2020.104823
Zhong, H., Zou, H., Semenov, M. V., Moshinsky, D., He, X., Huang, H., et al. (2009). Characterization and Development of Novel Small-Molecules Inhibiting GSK3 and Activating Wnt Signaling. Mol. Biosyst. 5, 1356–1360. doi:10.1039/b905752h
6-OHDA 6-hydroxydopamine
α-syn α-synuclein
AChE acetylcholinesterase
AD alzheimer’s disease
ALS amyotrophic lateral sclerosis
AlCl3 aluminum trichloride
Aβ β-amyloid
APP amyloid precursor protein
ASOs antisense oligonucleotides
ATG5 autophagy-related gene 5
BACE1 β-secretase 1
BBB blood-brain barrier
BET bromo and extra-terminal
BSP bromosporine
BuChE butyrylcholinesterase
CaP-lipid NPs calcium phosphate lipid coated nanoparticles
CDK5 cyclin-dependent kinase 5
CQDs carbon quantum dots
DA dopamine
FA 4-hydroxy-3-methoxycinnamic acid
FUS fused in sarcoma
Hpf hours post fertilization
IAPP islet amyloid polypeptide
GSI γ-secretase inhibitor
GSK 3β kinase glycogen synthase 3β
LBs Lewy bodies
L-BMAA beta-N-methylamino-l-alanine
LKE lanthionine ketimine-5-ethyl ester
LRRK2 leucine-rich repeat kinase 2
MAO monoamine oxidase
MAPT microtubule-associated protein tau
MCU mitochondrial calcium uniporter
MPP+ 1-methyl-4-phenylpyridinium ion
MPTP 1-methyl-4-phenyl-1,2,3,6-tetrahydropyridine
Na-Phen sodium phenylbutyrate
NDD neurodegenerative disease
NFTs neurofibrillary tangles
NMJ neuromuscular junction
OKA okadaic acid
PD Parkinson’s disease
SNpc substantia nigra pars compacta
SOD1 superoxide dismutase 1
ROS reactive oxygen species
TARDBP TAR DNA-binding protein 43
TDZD-8 4-benzyl-2-methyl-1, 2, 4-thiadiazolidine-3, 5-dione
Tg transgenic model
TH tyrosine hydroxylase
TLS translocated in liposarcoma
Keywords: zebrafish, neurodegenerative disease, animal model, drug discovery, alzheimer’s disease, parkinson’s disease, amyotrophic lateral sclerosis
Citation: Wang X, Zhang J-B, He K-J, Wang F and Liu C-F (2021) Advances of Zebrafish in Neurodegenerative Disease: From Models to Drug Discovery. Front. Pharmacol. 12:713963. doi: 10.3389/fphar.2021.713963
Received: 24 May 2021; Accepted: 30 June 2021;
Published: 14 July 2021.
Edited by:
Francisco Lopez-Munoz, Camilo José Cela University, SpainReviewed by:
Vijay Kumar, Amity University, IndiaCopyright © 2021 Wang, Zhang, He, Wang and Liu. This is an open-access article distributed under the terms of the Creative Commons Attribution License (CC BY). The use, distribution or reproduction in other forums is permitted, provided the original author(s) and the copyright owner(s) are credited and that the original publication in this journal is cited, in accordance with accepted academic practice. No use, distribution or reproduction is permitted which does not comply with these terms.
*Correspondence: Chun-Feng Liu, bGl1Y2h1bmZlbmdAc3VkYS5lZHUuY24=
†These authors have contributed equally to this work
Disclaimer: All claims expressed in this article are solely those of the authors and do not necessarily represent those of their affiliated organizations, or those of the publisher, the editors and the reviewers. Any product that may be evaluated in this article or claim that may be made by its manufacturer is not guaranteed or endorsed by the publisher.
Research integrity at Frontiers
Learn more about the work of our research integrity team to safeguard the quality of each article we publish.