- 1National Institute of Neuroscience, National Center of Neurology and Psychiatry (NCNP), Kodaira, Japan
- 2Biomedical Research Institute, National Institute of Advanced Industrial Science and Technology (AIST), Tsukuba, Japan
AMPA receptors are responsible for fast excitatory synaptic transmission in the mammalian brain. Post-translational protein S-palmitoylation of AMPA receptor subunits GluA1-4 reversibly regulates synaptic AMPA receptor expression, resulting in long-lasting changes in excitatory synaptic strengths. Our previous studies have shown that GluA1 C-terminal palmitoylation-deficient (GluA1C811S) mice exhibited hyperexcitability in the cerebrum and elevated seizure susceptibility without affecting brain structure or basal synaptic transmission. Moreover, some inhibitory GABAergic synapses-targeting anticonvulsants, such as valproic acid, phenobarbital, and diazepam, had less effect on these AMPA receptor palmitoylation-deficient mutant mice. This work explores pharmacological effect of voltage-gated ion channel-targeted anticonvulsants, phenytoin and trimethadione, on GluA1C811S mice. Similar to GABAergic synapses-targeting anticonvulsants, anticonvulsive effects were also reduced for both sodium channel- and calcium channel-blocking anticonvulsants, which suppress excess excitation. These data strongly suggest that the GluA1C811S mice generally underlie the excessive excitability in response to seizure-inducing stimulation. AMPA receptor palmitoylation site could be a novel target to develop unprecedented type of anticonvulsants and GluA1C811S mice are suitable as a model animal for broadly evaluating pharmacological effectiveness of antiepileptic drugs.
Introduction
Various anticonvulsants are clinically used in the treatment of epileptic seizures (Beck and Elger, 2008; Goldenberg, 2010). Antiepileptic drugs (AEDs) affect several pharmacological targets to suppress excessive neuronal firing, resulting in prevention of the seizure spread in the brain. Disrupted excitatory/inhibitory (E/I) balance results in perturbed brain function and repetitive seizures leads to serious epilepsy (Paz and Huguenard, 2015). Despite the appropriate control of neuronal molecules such as ion channels and neurotransmitter receptors is crucial in the maintenance of the E/I balance (Haider and Mccormick, 2009; Isaacson and Scanziani, 2011; Xue et al., 2014), detailed mechanisms underlining epilepsy still remain unclear.
Over the past few decades, many seizure models in rodents have been established for the pharmacological testing of epileptic modifiers (Guillemain et al., 2012; Löscher, 2016; Löscher, 2017). Symptom-mimicking behavioral outcomes in these models provide an indicator of pharmacological effects on epileptic seizure. In addition to acute seizure models using drugs and chemically or electrically kindled rodents, genetically modified mice have been used to discover novel and highly effective anticonvulsants (Marshall et al., 2021). In these researches, many AEDs have been developed so far, which target ion channels, such as voltage-gated sodium channel (VGSC) α-subunits NaV1.1 and NaV1.2 and β-subunit (Kaplan et al., 2016), T-type voltage-gated calcium channel (VGCC) α1H-subunit CaV3.2 (Zamponi et al., 2009), M-type potassium channel subunits (Springer et al., 2021), GABAA receptor α1- and γ2-subunits (Treiman, 2001). These ion channels are implicated in some human epilepsy syndromes (Rogawski and Löscher, 2004). Targets ion channels are diverse and more specific anticonvulsants are in demand for more effective therapies with fewer side effects.
Glutamate is the major excitatory neurotransmitter in the mammalian central nervous system. Majority of the excitatory synapses throughout the mammalian brain contain α-amino-3-hydroxy-5-methyl-4-isoxazole propionate (AMPA)-type glutamate receptors, which are dominantly responsible for fast excitatory synaptic transmission. Therefore, basal excitatory synaptic transmission, synaptic plasticity, and higher brain function are broadly regulated by quantitative controls of postsynaptic AMPA receptor numbers (Collingridge et al., 2004; Shepherd and Huganir, 2007; Kessels and Malinow, 2009; Huganir and Nicoll, 2013). We have already shown that a key modification mechanism of AMPA receptor trafficking into and from excitatory synapses is the posttranslational protein S-palmitoylation of the receptors at their C-termini, the reversible attachment of the most abundant saturated fatty acid, palmitate (Hayashi et al., 2005; Jiang et al., 2006; Lin et al., 2009; Anggono and Huganir, 2012; Lu and Roche, 2012; Thomas and Hayashi, 2013; Lussier et al., 2015). Among the four AMPA receptor subunits GluA1-4 (also known as GluR1-4, GluRA-D or GluRα1-4), GluA1 mainly acts in activity-dependent AMPA receptor trafficking into postsynapses (Shepherd and Huganir, 2007; Heine et al., 2008; Anggono and Huganir, 2012).
Our previous studies have revealed that the GluA1 C-terminal palmitoylation regulates seizure susceptibility in vivo (Hayashi, 2021). GluA1 C-terminal palmitoylation-deficient (GluA1 Cys811 to Ser substituted, GluA1C811S) mice showed elevated seizure susceptibility and reduced anticonvulsive effects of AEDs (Itoh et al., 2018). Therefore, our seizure model mouse with single protein modification site-targeted mutation would be useful for effective evaluation of drugs. This may lead to development of unprecedented type of anticonvulsants. To confirm these possibilities, we further investigated pharmacological effects of a diverse group of anticonvulsants on GluA1C811S mice in this report. Our data exhibit that enhanced excitability based on deficiency of AMPA receptor palmitoylation bring less effect of broad AEDs.
Materials and Methods
Experimental Animals
For this study, we used previously generated GluA1C811S mutant mice (Itoh et al., 2018). The intercross of heterozygotes resulted in the production of wild-type, heterozygous, and homozygous offspring at the expected 1:2:1 Mendelian ratio. Wild-type and homozygous adult male mice were used for subsequent analyses. C57BL/6N mice were obtained from Charles River Laboratories Japan. Mice were fed with standard laboratory chow and water in standard animal cages under a 12 h light/dark cycle. Mice were used at the age of 3 months for phenytoin (PHT) and trimethadione (TMZ) experiments and 7 weeks for 2,3-dioxo-6-nitro-7-sulfamoyl-benzo[f]quinoxaline (NBQX) experiment.
All animal care and experiments were performed in accordance with the regulations and institutional guidelines of the National Center of Neurology and Psychiatry (NCNP), Japanese Pharmacological Society, and Japan Neuroscience Society. The technical protocols for animal experiments in this study were approved by the Institutional Review Committees of the National Institute of Neuroscience, NCNP.
Drugs
Pentylenetetrazole (PTZ), PHT, TMZ and NBQX were purchased from Sigma-Aldrich. PHT, 20 mg/kg i.p., was initially dissolved by means of NaOH in distilled water (Klein et al., 2015). Briefly, PHT was dissolved in 75 mM NaOH as a stock solution (50 mg/ml) and then diluted 25 times in phosphate-buffered saline (PBS) for i.p. injection. PTZ, 60 mg/kg i.p., TMZ, 300 mg/kg i.p., and NBQX, 30, 60 or 100 mg/kg i.p., were directly dissolved in PBS. All reagents were prepared at time of use.
Seizure Observation and Scoring
PTZ was administered intraperitoneally at a dose of 60 mg/kg (Itoh et al., 2018). Control mice were injected with PBS. PHT, TMZ or NBQX was administered intraperitoneally for indicated periods before PTZ injection. We examined seizure events during the 20 min observation period after injection. Seizure intensity was scored as follows: stage 0, no response; stage 1, ear, mouth and facial twitching; stage 2, head nodding and convulsive twitching axially through the body; stage 3, limbic myoclonus; stage 4, rearing demonstrated by standing on hind legs and wild jumping; stage 5, generalized tonic-clonic seizures; and stage 6, death (Racine score). The highest seizure scores during the observation period were used for evaluation.
Statistical Analysis
Statistical analyses were performed using Prism 8 (GraphPad Software) and Excel (Microsoft). Two-way ANOVA followed by Fisher’s LSD test was used to compare two groups. All data are expressed as mean ± standard error of the mean (s.e.m.).
Results
Reduced Effects of a Sodium Channel-Targeting Anticonvulsant, Phenytoin, in GluA1C811S Mutant Mice
Our previous studies have revealed that palmitoylation at GluA1 Cys811 suppress hyperexcitation in vivo (Itoh et al., 2018). Compared with wild-type mice, elevated seizure susceptibility was observed in palmitoylation-deficient GluA1C811S mice, when they are administrated with a non-competitive GABAA receptor antagonist, pentylenetetrazole (PTZ). Disturbance of inhibitory GABAergic synapses leads to instability of neuronal network in the brain (Treiman, 2001). Although both wild-type and GluA1C811S mice showed dose dependent effects of PTZ on inhibitory synapse suppression-induced seizure, GluA1C811S mutant mice exhibited severer clonic and/or tonic convulsion, jumping, and wild running (classified in stage 4 or 5, Racine score) during 20 min observation after intraperitoneal PTZ injection at a dose of 60 mg/kg. Our experimental data also showed reduced effects of inhibitory GABAergic synapses-targeting anticonvulsants, valproic acid (VPA), phenobarbital (PB), and diazepam (DZP) on PTZ-induced seizure in GluA1C811S mice.
In this study, we proceeded to test other anti-epileptic agents, which have different pharmacological points of action. First, we examined phenytoin (PHT), a hydantoin derivative, which acts as an anticonvulsant by blocking VGSCs. PHT administered intraperitoneally at an effective dose of 20 mg/kg significantly suppress PTZ-induced seizures in wild-type mice (Figure 1: 4.86 ± 0.14, n = 14 for PBS-injected wild-type mice; 2.70 ± 0.33, n = 12 for PHT-injected wild-type mice) as previously reported (Klein et al., 2015; Song et al., 2018). On the other hand, PHT showed reduced anticonvulsive effects on PTZ-injected GluA1C811S mice (Figure 1: 4.90 ± 0.07, n = 20 for PBS-injected GluA1C811S mice; 4.58 ± 0.23, n = 12 for PHT-injected GluA1C811S mice, Two-way ANOVA, genotype × treatment interaction F1, 54 = 26.22, p < 0.0001). There was no obvious difference between PBS-injected wild-type and GluA1C811S mice (p = 0.8575). Abnormal freezing behaviors were observed just by injecting PHT at higher dose (50 mg/kg for 30 min) without PTZ administration (data not shown).
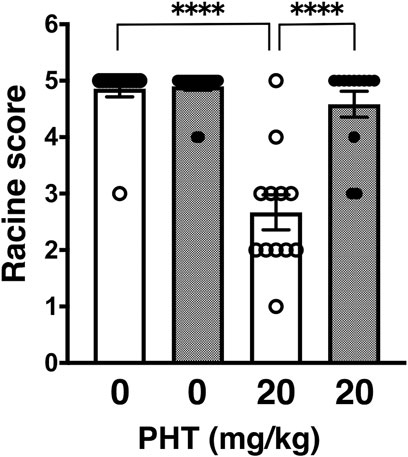
FIGURE 1. Inhibitory effects of phenytoin (PHT) on PTZ-evoked seizure. Effects of PHT on occurrence of generalized tonic-clonic seizures. Seizure scores of adult male wild-type (white dots and white bars) and GluA1C811S (black dots and hashed bars) mice received an administration of PHT at a dose of 0 or 20 mg/kg, 30 min before a single injection of 60 mg/kg PTZ. Four bars: n = 14, 20, 12, 12 mice (left to right). Error bars represent s.e.m. ANOVA with Fisher’s LSD post hoc test. ****p < 0.0001.
Reduced Effects of a Calcium Channel-Targeting Anticonvulsant, Trimethadione, in GluA1C811S Mutant Mice
Next, we tested trimethadione (TMZ), an oxazolidinedione derivative, which possesses anti-seizure activity by inhibiting T-type VGCCs (Zamponi et al., 2009). Similar to other examined anticonvulsants in a series of our studies, TMZ also showed anticonvulsive effect on PTZ-induced seizures in wild-type and GluA1C811S mice at a dose of 300 mg/kg (Barton et al., 2005; Pastore et al., 2014). However, TMZ showed less effect on PTZ-induced seizures in GluA1C811S mice at an effective dose of 300 mg/kg (Figure 2: 4.90 ± 0.07, n = 20 for PBS-injected GluA1C811S mice; 4.00 ± 0.82, n = 6 for TMZ-injected GluA1C811S mice), compared with wild-type mice (Barton et al., 2005; Pastore et al., 2014) (Figure 2: 4.86 ± 0.14, n = 14 for PBS-injected wild-type mice; 1.43 ± 0.37, n = 7 for TMZ-injected wild-type mice, Two-way ANOVA, genotype × treatment interaction F1, 43 = 20.43, p < 0.0001.).
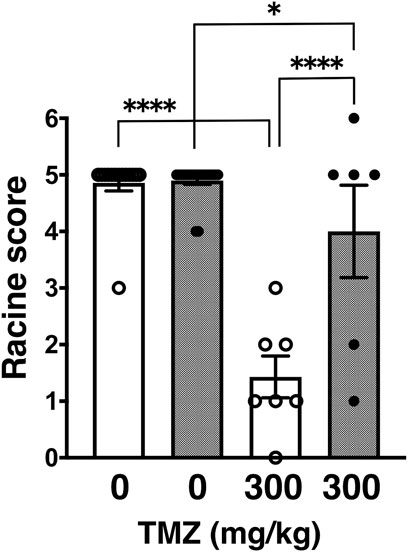
FIGURE 2. Inhibitory effects of trimethadione (TMZ) on PTZ-evoked seizure. Effects of TMZ on occurrence of generalized tonic-clonic seizures. Seizure scores of adult male wild-type (white dots and white bars) and GluA1C811S (black dots and hashed bars) mice received an administration of TMZ at a dose of 0 or 300 mg/kg, 30 min before a single injection of 60 mg/kg PTZ. Four bars: n = 14, 20, 7, 6 mice (left to right). Error bars represent s.e.m. ANOVA with Fisher’s LSD post hoc test. *p < 0.05, ****p < 0.0001.
Anticonvulsive Effects of a AMPA Receptor Blocker, NBQX, in GluA1C811S Mutant Mice
NBQX is a highly selective competitive antagonist of AMPA- and kainite-type glutamate receptors, which is widely used because of its well-established anticonvulsant activity in rodent seizure models. The vast majority of fast excitatory synaptic transmission is mediated by AMPA receptors (Collingridge et al., 2004; Shepherd and Huganir, 2007; Kessels and Malinow, 2009; Huganir and Nicoll, 2013). Basically, NBQX is expected to inhibit synaptic AMPA receptor activities, whether AMPA receptors are palmitoylated or not. Namely, NBQX suppress excitatory currents through surface-expressing AMPA receptors on postsynapses, which should consist of both palmitoylated and depalmitoylated receptors (Nakagawa, 2010). Intraperitoneal application of NBQX as a representative of glutamatergic excitatory synapse-targeting anticonvulsant for indicated periods brought significant anticonvulsive effects on both wild-type and GluA1C811S mice (Figure 3A: 30 mg/kg NBQX; Figure 3B: 60 mg/kg NBQX; Figure 3C: 100 mg/kg NBQX). While anticonvulsive effects were hardly observed at a low dose (Figure 3A: 4.58 ± 0.21, n = 19 for 0 min, 5.00 ± 0.00, n = 6 for 5 min, 4.60 ± 0.40, n = 5 for 15 min, 5.00 ± 0.00, n = 4 for 30 min, PBS-injected wild-type mice; 5.00 ± 0.00, n = 19 for 0 min, 4.38 ± 0.42, n = 8 for 5 min, 5.00 ± 0.00, n = 4 for 15 min, 5.00 ± 0.00, n = 4 for 30 min, NBQX-injected GluA1C811S mice, Two-way ANOVA, effect of treatment F3, 61 = 0.364, p = 0.7792), higher concentration of NBQX suppresses PTZ-induced seizure (Figure 3B: 4.58 ± 0.21, n = 19 for 0 min, 4.57 ± 0.30, n = 7 for 5 min, 3.45 ± 0.47, n = 11 for 15 min, 4.13 ± 0.58, n = 8 for 30 min, PBS-injected wild-type mice; 5.00 ± 0.00, n = 19 for 0 min, 3.83 ± 0.75, n = 6 for 5 min, 3.70 ± 0.54, n = 10 for 15 min, 3.67 ± 0.55, n = 9 for 30 min, NBQX-injected GluA1C811S mice, Two-way ANOVA, effect of treatment F3, 81 = 4.829, p = 0.0038; Figure 3C: 4.58 ± 0.21, n = 19 for 0 min, 3.50 ± 0.50, n = 6 for 5 min, 3.71 ± 0.47, n = 7 for 15 min, 5.00 ± 0.00, n = 3 for 30 min, PBS-injected wild-type mice; 5.00 ± 0.00, n = 19 for 0 min, 4.17 ± 0.54, n = 6 for 5 min, 3.50 ± 0.67, n = 6 for 15 min, 4.67 ± 0.33, n = 3 for 30 min, NBQX-injected GluA1C811S mice, Two-way ANOVA, effect of treatment F3, 63 = 6.941, p = 0.0004). Our data showed that there was no significant difference between palmitoylatable wild-type and non-palmitoylated GluA1C811S mice at each time point (Figure 3A: Two-way ANOVA, genotype × treatment interaction F3, 61 = 2.157, p = 0.1023; Figure 3B: Two-way ANOVA, genotype × treatment interaction F3, 81 = 0.9806, p = 0.4061; Figure 3C: Two-way ANOVA, genotype × treatment interaction F3, 63 = 0.5016, p = 0.6825). PTZ-induced seizures cannot be fully suppressed by NBQX and mice treated with 100 mg/kg NBQX for 30 min seem to exhibit less anticonvulsive effect at a glance. These are because some NBQX-treated mice fell down or stayed immobile in a low position after standing up on hind legs in our observation. According to the Racine score, stage 4 or 5 was recorded in these cases. These abnormal physical reactions induced by application of NBQX at high concentration for long periods may cause the poor numerically outcome for NBQX anticonvulsive score at the condition.
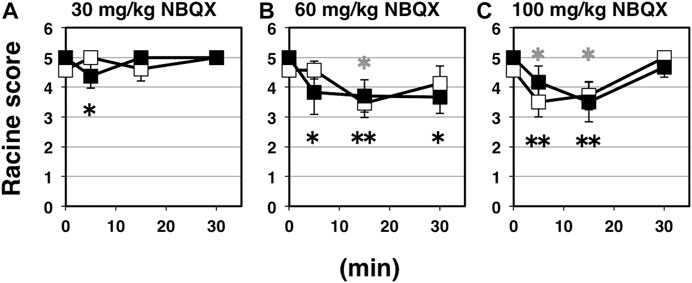
FIGURE 3. Inhibitory effects of NBQX on PTZ-evoked seizure. (A) Time course of NBQX effects at doses of 30 mg/kg. Seizure scores of 7 weeks old male wild-type (white squares) and GluA1C811S (black squares) mice received an administration of NBQX at a dose of 0 or 30 mg/kg for indicated periods before a single injection of 60 mg/kg PTZ. Four white squares (wt): n = 19, 6, 5, 4 mice, four black squares [GluA1C811S]: n = 19, 8, 4, 4 mice (left to right). (B) Time course of NBQX effects at doses of 60 mg/kg. Seizure scores of 7 weeks old male wild-type (white squares) and GluA1C811S (black squares) mice received an administration of NBQX at a dose of 0 or 60 mg/kg for indicated periods before a single injection of 60 mg/kg PTZ. Four white squares (wt): n = 19, 7, 11, 8 mice, four black squares [GluA1C811S]: n = 19, 6, 10, 9 mice (left to right). (C) Time course of NBQX effects at doses of 100 mg/kg. Seizure scores of 7 weeks old male wild-type (white squares) and GluA1C811S (black squares) mice received an administration of NBQX at a dose of 0 or 100 mg/kg for indicated periods before a single injection of 60 mg/kg PTZ. Four white squares (wt): n = 19, 6, 7, 3 mice, four black squares [GluA1C811S]: n = 19, 6, 6, 3 mice (left to right). Error bars represent s.e.m. ANOVA with Fisher’s LSD post hoc test. *p < 0.05, **p < 0.01, compared to PBS controls. Colorless asterisks indicate wild-type and black asterisks indicate GluA1C811S mice.
Discussion
Epilepsy is characterized by unpredictable, unprovoked recurrent seizures, resulting from the abnormally synchronous activity of excitatory neurons in a focal area of the cerebrum. The sudden rush of electrical activity may convey throughout the entire brain in some cases. Epilepsy can last from undetectable short periods to long periods that appears sporadically and continuously evoke vigorous shaking (Kwan and Brodie, 2000; Grone and Baraban, 2015; Staley, 2015). Epileptic seizures are associated with excessive cortical excitability resulting from imbalances between excitation and inhibition in some focal regions of the cerebrum. Disturbance of neuronal network stability induce many types of epileptic seizure and various medicines are selected, depending on the symptom.
Besides brain tumors and traumatic brain injuries, genetic dysfunctions are the predominant determinants for epilepsy (Guillemain et al., 2012; Löscher, 2016; Löscher, 2017; Marshall et al., 2021). More than a hundred genes have been identified as causative genes for epilepsy to date and a lot of epilepsy-related mutations locate on loci coding ion channels (Helbig and Ellis, 2020). Accumulated efforts aimed at improved therapies have revealed many monogenic and combination of factors. As it has been studied for a long time, major excitatory neurotransmission is mediated by glutamate in the mammalian brain. Especially, AMPA receptor palmitoylation-dependent regulation of glutamatergic synapses is a prevailing mechanism to control the excitatory synaptic strength in the maintenance of network stability (Hayashi, 2021). In addition to our previous verification using AMPA receptor palmitoylation-deficient (GluA1C811S) mice with GABAergic synapses-targeting anticonvulsants, we further showed in this report that reduced anticonvulsive effects are observed when GluA1C811S mice are treated by voltage-gated ion channel-blocking anticonvulsants, PHT (Figure 1) and TMZ (Figure 2). Difference of anticonvulsive effect between PHT and TMZ may depend on each target. VGSCs and T-type VGCCs show non-overlapping distribution in the complex cerebral network, in which glutamatergic synaptic transmissions occupy most there (Zamponi et al., 2009; Kaplan et al., 2016; Wang et al., 2017). VGSCs are the basic ion channels for neuronal excitability, which are crucial for the resting potential and the generation and propagation of action potentials in neurons (Wang et al., 2017). As clinical treatment, PHT is useful for the prevention of tonic-clonic seizures and focal seizures, but not absence seizures in human (Kaplan et al., 2016). On the other hand, TMZ is most commonly used to treat epileptic conditions that are resistant to other treatments. When excitatory AMPA receptors themselves are inhibited by pre-injected NBQX, which ubiquitously suppress excitation of glutamatergic synapses in the whole brain, similar level of anticonvulsive effects was observed in both wild-type and palmitoylation-deficient GluA1C811S mice (Figure 3). This means that both palmitoylated and depalmitoylated AMPA receptors were inhibited by NBQX on excitatory synapses in the brain.
These experimental data presented in this report indicate that single protein modification site such as AMPA receptor palmitoylated cysteine become a novel target to develop unprecedented type of anticonvulsants. Moreover, our GluA1C811S mouse could be useful as a general animal model to evaluate newly developed AED candidates, by comparing with current drugs. Anticonvulsants are also increasingly being used in the treatment of mental disorders and neuropathic pain (Keck et al., 1998; Rogawski and Löscher, 2004; Joshi et al., 2019). Abnormalities are associated with accumulating brain damage and neurological dificits. Palmitoylation-deficient GluA1C811S mice may be widely applicable to test drugs to treat brain disorders in the future.
Data Availability Statement
The original contributions presented in the study are included in the article/supplementary material, further inquiries can be directed to the corresponding author.
Ethics Statement
The animal study was reviewed and approved by the Institutional Review Committees of the National Institute of Neuroscience, NCNP.
Author Contributions
TH designed study; MI, AI, MY, and TH performed experiments and analyzed data; TH drafted the manuscript.
Funding
This work was supported in part by Grants-in-Aid from the Ministry of Education, Culture, Sports, Science and Technology of Japan (MEXT)/Japan Society for the Promotion of Science (JSPS) (Grant numbers 22680029, 23650187, 24111512, 16K07078), RRIME and FORCE from Japan Agency for Medical Research and Development (AMED) (Grant number JP18gm5910009, JP20gm4010004), the Takeda Science Foundation, the Mitsubishi Foundation, the Brain Science Foundation, the Suzuken Memorial Foundation, and the Astellas Foundation for Research on Metabolic Disorders.
Conflict of Interest
The authors declare that the research was conducted in the absence of any commercial or financial relationships that could be construed as a potential conflict of interest.
Publisher’s Note
All claims expressed in this article are solely those of the authors and do not necessarily represent those of their affiliated organizations, or those of the publisher, the editors and the reviewers. Any product that may be evaluated in this article, or claim that may be made by its manufacturer, is not guaranteed or endorsed by the publisher.
Acknowledgments
We are grateful to colleagues for valuable discussion, encouragement, and excellent administrative assistance. P. Kumar kindly proofread the manuscript.
References
Anggono, V., and Huganir, R. L. (2012). Regulation of AMPA Receptor Trafficking and Synaptic Plasticity. Curr. Opin. Neurobiol. 22, 461–469. doi:10.1016/j.conb.2011.12.006
Barton, M. E., Eberle, E. L., and Shannon, H. E. (2005). The Antihyperalgesic Effects of the T-type Calcium Channel Blockers Ethosuximide, Trimethadione, and Mibefradil. Eur. J. Pharmacol. 521, 79–85. doi:10.1016/j.ejphar.2005.08.017
Beck, H., and Elger, C. E. (2008). Epilepsy Research: A Window onto Function to and Dysfunction of the Human Brain. Dialogues Clin. Neurosci. 10, 7–15. doi:10.31887/dcns.2008.10.1/hbeck
Collingridge, G. L., Isaac, J. T., and Wang, Y. T. (2004). Receptor Trafficking and Synaptic Plasticity. Nat. Rev. Neurosci. 5, 952–962. doi:10.1038/nrn1556
Goldenberg, M. M. (2010). Overview of Drugs Used for Epilepsy and Seizures: Etiology, Diagnosis, and Treatment. P T 35, 392–415.
Grone, B. P., and Baraban, S. C. (2015). Animal Models in Epilepsy Research: Legacies and New Directions. Nat. Neurosci. 18, 339–343. doi:10.1038/nn.3934
Guillemain, I., Kahane, P., and Depaulis, A. (2012). Animal Models to Study Aetiopathology of Epilepsy: What are the Features to Model? Epileptic Disord. 14, 217–225. doi:10.1684/epd.2012.0528
Haider, B., and Mccormick, D. A. (2009). Rapid Neocortical Dynamics: Cellular and Network Mechanisms. Neuron 62, 171–189. doi:10.1016/j.neuron.2009.04.008
Hayashi, T. (2021). Post-Translational Palmitoylation of Ionotropic Glutamate Receptors in Excitatory Synaptic Functions. Br. J. Pharmacol. 178, 784–797. doi:10.1111/bph.15050
Hayashi, T., Rumbaugh, G., and Huganir, R. L. (2005). Differential Regulation of AMPA Receptor Subunit Trafficking by Palmitoylation of Two Distinct Sites. Neuron 47, 709–723. doi:10.1016/j.neuron.2005.06.035
Heine, M., Thoumine, O., Mondin, M., Tessier, B., Giannone, G., and Choquet, D. (2008). Activity-Independent and Subunit-Specific Recruitment of Functional AMPA Receptors at Neurexin/neuroligin Contacts. Proc. Natl. Acad. Sci. U S A. 105, 20947–20952. doi:10.1073/pnas.0804007106
Helbig, I., and Ellis, C. A. (2020). Personalized Medicine in Genetic Epilepsies - Possibilities, Challenges, and New Frontiers. Neuropharmacology 172, 107970. doi:10.1016/j.neuropharm.2020.107970
Huganir, R. L., and Nicoll, R. A. (2013). AMPARs and Synaptic Plasticity: The Last 25 Years. Neuron 80, 704–717. doi:10.1016/j.neuron.2013.10.025
Isaacson, J. S., and Scanziani, M. (2011). How Inhibition Shapes Cortical Activity. Neuron 72, 231–243. doi:10.1016/j.neuron.2011.09.027
Itoh, M., Yamashita, M., Kaneko, M., Okuno, H., Abe, M., Yamazaki, M., et al. (2018). Deficiency of AMPA Receptor-Palmitoylation Aggravates Seizure Susceptibility. J. Neurosci. 38 (47), 10220–10235. doi:10.1523/JNEUROSCI.1590-18.2018
Jiang, J., Suppiramaniam, V., and Wooten, M. W. (2006). Posttranslational Modifications and Receptor-Associated Proteins in AMPA Receptor Trafficking and Synaptic Plasticity. Neurosignals 15, 266–282. doi:10.1159/000105517
Joshi, A., Bow, A., and Agius, M. (2019). Pharmacological Therapies in Bipolar Disorder: A Review of Current Treatment Options. Psychiatr. Danub 31, 595–603.
Kaplan, D. I., Isom, L. L., and Petrou, S. (2016). Role of Sodium Channels in Epilepsy. Cold Spring Harb Perspect. Med. 6, a022814. doi:10.1101/cshperspect.a022814
Keck, P. E., McElroy, S. L., and Strakowski, S. M. (1998). Anticonvulsants and Antipsychotics in the Treatment of Bipolar Disorder. J. Clin. Psychiatry 59 (Suppl. 6), 74–82.
Kessels, H. W., and Malinow, R. (2009). Synaptic AMPA Receptor Plasticity and Behavior. Neuron 61, 340–350. doi:10.1016/j.neuron.2009.01.015
Klein, S., Bankstahl, M., and Löscher, W. (2015). Inter-Individual Variation in the Effect of Antiepileptic Drugs in the Intrahippocampal Kainate Model of Mesial Temporal Lobe Epilepsy in Mice. Neuropharmacology 90, 53–62. doi:10.1016/j.neuropharm.2014.11.008
Kwan, P., and Brodie, M. J. (2000). Early Identification of Refractory Epilepsy. N. Engl. J. Med. 342, 314–319. doi:10.1056/NEJM200002033420503
Lin, D. T., Makino, Y., Sharma, K., Hayashi, T., Neve, R., Takamiya, K., et al. (2009). Regulation of AMPA Receptor Extrasynaptic Insertion by 4.1N, Phosphorylation and Palmitoylation. Nat. Neurosci. 12, 879–887. doi:10.1038/nn.2351
Löscher, W. (2017). Animal Models of Seizures and Epilepsy: Past, Present, and Future Role for the Discovery of Antiseizure Drugs. Neurochem. Res. 42, 1873–1888. doi:10.1007/s11064-017-2222-z
Löscher, W. (2016). The Search for New Screening Models of Pharmacoresistant Epilepsy: Is Induction of Acute Seizures in Epileptic Rodents a Suitable Approach? Neurochem. Res. 42, 1926–1938. doi:10.1007/s11064-016-2025-7
Lu, W., and Roche, K. W. (2012). Posttranslational Regulation of AMPA Receptor Trafficking and Function. Curr. Opin. Neurobiol. 22, 470–479. doi:10.1016/j.conb.2011.09.008
Lussier, M. P., Sanz-Clemente, A., and Roche, K. W. (2015). Dynamic Regulation of N-Methyl-D-Aspartate (NMDA) and α-Amino-3-hydroxy-5-methyl-4-isoxazolepropionic Acid (AMPA) Receptors by Posttranslational Modifications. J. Biol. Chem. 290, 28596–28603. doi:10.1074/jbc.R115.652750
Marshall, G. F., Gonzalez-Sulser, A., and Abbott, C. M. (2021). Modelling Epilepsy in the Mouse: Challenges and Solutions. Dis. Models Mech. 14, dmm047449. doi:10.1242/dmm.047449
Nakagawa, T. (2010). The Biochemistry, Ultrastructure, and Subunit Assembly Mechanism of AMPA Receptors. Mol. Neurobiol. 42, 161–184. doi:10.1007/s12035-010-8149-x
Pastore, V., Wasowski, C., Higgs, J., Mangialavori, I. C., Bruno-Blanch, L. E., and Marder, M. (2014). A Synthetic Bioisoster of Trimethadione and Phenytoin Elicits Anticonvulsant Effect, Protects the Brain Oxidative Damage Produced by Seizures and Exerts Antidepressant Action in Mice. Eur. Neuropsychopharmacol. 24, 1405–1414. doi:10.1016/j.euroneuro.2014.04.005
Paz, J. T., and Huguenard, J. R. (2015). Microcircuits and Their Interactions in Epilepsy: Is the Focus Out of Focus? Nat. Neurosci. 18, 351–359. doi:10.1038/nn.3950
Rogawski, M. A., and Löscher, W. (2004). The Neurobiology of Antiepileptic Drugs for the Treatment of Nonepileptic Conditions. Nat. Med. 10, 685–692. doi:10.1038/nm1074
Shepherd, J. D., and Huganir, R. L. (2007). The Cell Biology of Synaptic Plasticity: AMPA Receptor Trafficking. Annu. Rev. Cel Dev Biol 23, 613–643. doi:10.1146/annurev.cellbio.23.090506.123516
Song, H., Tufa, U., Chow, J., Sivanenthiran, N., Cheng, C., Lim, S., et al. (2018). Effects of Antiepileptic Drugs on Spontaneous Recurrent Seizures in a Novel Model of Extended Hippocampal Kindling in Mice. Front. Pharmacol. 9, 451. doi:10.3389/fphar.2018.00451
Springer, K., Varghese, N., and Tzingounis, A. V. (2021). Flexible Stoichiometry: Implications for KCNQ2- and KCNQ3-Associated Neurodevelopmental Disorders. Dev. Neurosci., 1–10. doi:10.1159/000515495
Staley, K. (2015). Molecular Mechanisms of Epilepsy. Nat. Neurosci. 18, 367–372. doi:10.1038/nn.3947
Thomas, G. M., and Hayashi, T. (2013). Smarter Neuronal Signaling Complexes from Existing Components: How Regulatory Modifications Were Acquired During Animal Evolution: Evolution of Palmitoylation-dDpendent Regulation of AMPA-Type Ionotropic Glutamate Receptors. Bioessays 35, 929–939. doi:10.1002/bies.201300076
Treiman, D. M. (2001). GABAergic Mechanisms in Epilepsy. Epilepsia 42 (Suppl. 3), 8–12. doi:10.1046/j.1528-1157.2001.042suppl.3008.x
Wang, J., Ou, S. W., and Wang, Y. J. (2017). Distribution and Function of Voltage-Gated Sodium Channels in the Nervous System. Channels (Austin) 11, 534–554. doi:10.1080/19336950.2017.1380758
Xue, M., Atallah, B. V., and Scanziani, M. (2014). Equalizing Excitation-Inhibition Ratios across Visual Cortical Neurons. Nature 511, 596–600. doi:10.1038/nature13321
Keywords: phenytoin, trimethadione, anticonvulsant, palmitoylation, AMPA receptor, synapse, excitability
Citation: Iizumi M, Oota-Ishigaki A, Yamashita M and Hayashi T (2021) Reduced Effect of Anticonvulsants on AMPA Receptor Palmitoylation-Deficient Mice. Front. Pharmacol. 12:711737. doi: 10.3389/fphar.2021.711737
Received: 19 May 2021; Accepted: 05 August 2021;
Published: 18 August 2021.
Edited by:
Habibeh Khoshbouei, University of Florida, United StatesReviewed by:
Jocelyn Widagdo, The University of Queensland, AustraliaChengwen Zhou, Vanderbilt University, United States
Copyright © 2021 Iizumi, Oota-Ishigaki, Yamashita and Hayashi. This is an open-access article distributed under the terms of the Creative Commons Attribution License (CC BY). The use, distribution or reproduction in other forums is permitted, provided the original author(s) and the copyright owner(s) are credited and that the original publication in this journal is cited, in accordance with accepted academic practice. No use, distribution or reproduction is permitted which does not comply with these terms.
*Correspondence: Takashi Hayashi, dGFrYXNoaS5oYXlhc2hpQGFpc3QuZ28uanA=